- 1Department of Endocrinology, Hospital of Chengdu University of Traditional Chinese Medicine, Chengdu, China
- 2School of Clinical Medicine, Chengdu University of Traditional Chinese Medicine, Chengdu, China
- 3School of Sports Medicine and Health, Chengdu Sports University, Chengdu, China
- 4Jiangsu Key Laboratory for Functional Substance of Chinese Medicine, School of Pharmacy, Nanjing University of Chinese Medicine, Nanjing, China
- 5College of lntegrated Traditional Chinese and Western Medicine, Hunan University of Chinese Medicine, Hunan, China
Diabetic peripheral neuropathy (DPN) is a complication of diabetes mellitus that lacks specific treatment, its high prevalence and disabling neuropathic pain greatly affects patients’ physical and mental health. Schwann cells (SCs) are the major glial cells of the peripheral nervous system, which play an important role in various inflammatory and metabolic neuropathies by providing nutritional support, wrapping axons and promoting repair and regeneration. Increasingly, high glucose (HG) has been found to promote the progression of DPN pathogenesis by targeting SCs death regulation, thus revealing the specific molecular process of programmed cell death (PCD) in which SCs are disrupted is an important link to gain insight into the pathogenesis of DPN. This paper is the first to review the recent progress of HG studies on apoptosis, autophagy, pyroptosis, ferroptosis and necroptosis pathways in SCs, and points out the crosstalk between various PCDs and the related therapeutic perspectives, with the aim of providing new perspectives for a deeper understanding of the mechanisms of DPN and the exploration of effective therapeutic targets.
Introduction
Diabetes, a serious chronic disease, is growing worldwide and is now one of the top ten leading causes of death worldwide (1). Worldwide, an estimated 537 million adults between the ages of 20 and 79 have diabetes, and the number is expected to increase to 643 million by 2030 (2). Persistent hyperglycemia, dyslipidemia, and insulin deficiency or dysfunction contribute to the multiple chronic complications of diabetes mellitus, and the vascular and neuronal systems, as providers of nutrients to all organs as well as connectors, play an important role in the development of diabetes mellitus complications (3). Diabetic peripheral neuropathy (DPN) as the most common complication of diabetes mellitus (4), is a debilitating and complex neurologic condition that has gained widespread attention due to its prevalence of up to 50% in patients with type 1 and type 2 diabetes mellitus (5). It affects patients’ quality of life by causing numbness, neuropathic pain, and severe lower extremity amputations (6), more studies have found that diabetic autonomic neuropathy increases the risk of death by more than twofold (7). Despite the numerous studies on the treatment of DPN, current treatments are limited to improved glycemic control, lifestyle and dietary interventions, and pain management, it remains the only diabetic microangiopathy that lacks specific therapy (8). Therefore, an in-depth exploration of the specific pathogenesis of DPN is essential to facilitate the prevention and treatment of the disease. Further studies have revealed that cellular therapies may be an effective way to address the underlying causes of neuropathy, and cellular therapies for both MSC and pulp stem cell transplantation point to the realization of a neurorestorative effect through the protection of Schwann cells (SCs) (9, 10), At this juncture, studies have substantiated that human tonsil-derived mesenchymal stem cells (MSCs) that have undergone differentiation into Schwann cells (TMSC-SCs) have the capacity to facilitate nerve regeneration in animal models of peripheral nerve injury. Additionally, Schwann cell-derived exosomes (SCEVs) have been demonstrated to possess therapeutic potential for the management of DPN (11, 12), the results of these studies indicate that SCs may play a significant role in the pathogenesis of peripheral neuropathy.
SCs, as the main glial cells in the peripheral nervous system, possess regenerative properties of interest and have important roles in the nervous system in coordinating the gradual formation of peripheral nerves, ensuring neuronal survival, axonal support and myelination, and promoting neurodegeneration and regeneration (13, 14). In addition, SCs can influence neuronal responses to stimuli, respond rapidly in the face of injury and coordinate intraneural changes, playing a key role in the development and maintenance of neuropathic pain (15, 16). The current study found that in DPN patients, SCs are subjected to high glucose (HG), which results in pathologically edematous cytoplasm, proliferation of cell bases, and reduction of neurotrophic factors, leading to mitochondrial dysfunction, localized oxidative damage, and the formation of immature cellular phenotypes, which in turn disrupts their support of neurons triggering neuropathy (4, 17). With more in-depth studies, HG was found to have a significant impact on the death regulation process of SCs.
Programmed cell death (PCD) is an active death process (e.g., apoptosis, autophagy, pyroptosis, ferroptosis, and necroptosis) that occurs after a cell receives a certain stimulus or signal in order to maintain the homeostasis of the internal environment (18), this process is important for the elimination of damaged cells, and the disruption of the process will result in the impairment of the function of normal cells and organs or even the inability to sustain the basic life (19). The effect of HG on this process in SCs may be an important part of the pathogenesis of DPN, which also suggests that intervening in the death of SCs by targeting and alleviating the disrupted PCDs pathway may serve as an effective way to treat DPN. Therefore, our narrative review highlights the current molecular mechanisms and understanding of HG damage to common PCDs in SCs, including apoptosis, autophagy, pyroptosis, ferroptosis, and necroptosis, to characterize the underlying mechanisms of diabetes-induced damage to SCs, highlight new insights, and provide perspectives on preventing as well as treating DPN by targeting the mode of death of SCs.
SCs are an important link in the pathogenesis of DPN: the role of axons
SCs encapsulate all axons in peripheral nerves and maintain the long-term functional integrity of axons (20), they have also been shown to provide trophic support to axons (21). Given their close association with axons, axonal damage due to abnormal function and metabolism of SCs is an important component of neuropathy. Increased polyol pathway flux is a recognized pathway for HG-induced DPN pathogenesis (22, 23). Aldose reductase (AR), the rate-limiting enzyme of the polyol pathway, is highly expressed by SCs in the peripheral nervous system, and overactivity of the polyol pathway in diabetic conditions, especially in SCs, leads to multiple metabolic abnormalities related to DPN (24). Overactivity of this pathway and altered biology of SCs may also be important for impaired axonal regeneration, one of the characteristics of the DPN (25). The lipid metabolism of SCs can transfer long-chain fatty acids (LCFA) to the mitochondria for β-oxidation to produce acetyl-coenzyme A, which enters the TCA cycle and eventually contributes to the production of OxPhos and ATP. In patients with type 2 diabetes mellitus, when there is an overload of the substrate, the remaining acetyl-coenzyme A is unable to enter the new round of β-oxidation and the TCA cycle, and is converted to acyl-carnitine. Carnitine accumulates in SCs and is eventually released into axons, causing axonal toxicity and nerve damage (26, 27). The maintenance of Ca2+ homeostasis plays an important role in axon function and integrity (28), whereas acyl-carnitine allow extracellular Ca2+ to enter axons, leading to abnormal axonal mitochondrial function and the onset of apoptosis (29, 30). Functionally, HG leads to the overproduction of advanced glycosylation end products (AGEs), and the structure and function of SCs can be altered by AGE-induced changes in key proteins, lipids, and nucleic acids, which in turn affect axons, leading to the development of neuropathy (31, 32). SCs mitochondrial function has been shown to be critical for maintaining axon survival, and studies have shown that HG is able to alter mitochondrial respiration, which may lead to SCs mitochondrial dysfunction (33, 34). In conclusion, the above process suggests that SCs may play a non-negligible role in the progression of DPN pathogenesis by acting axonal (Figure 1).
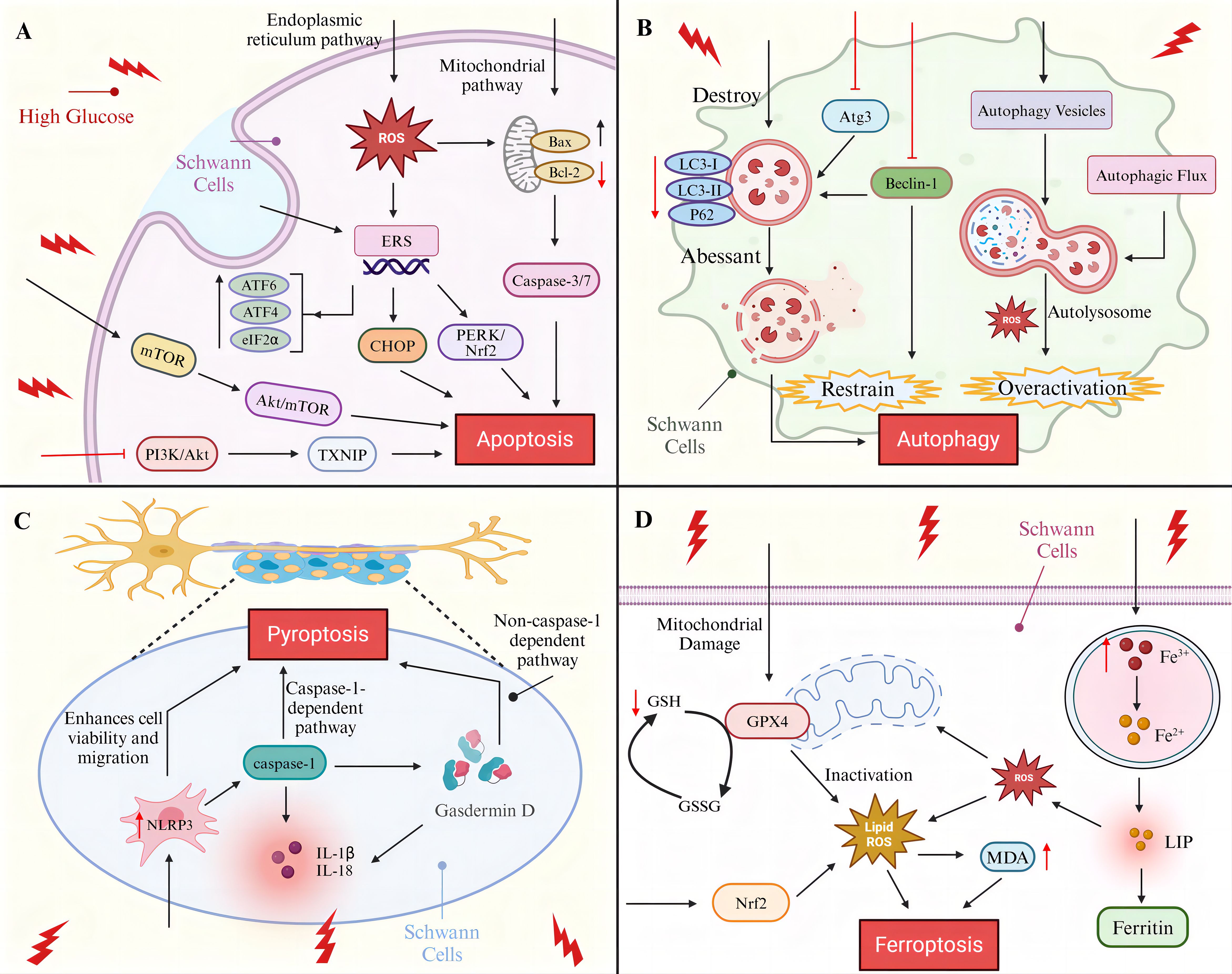
Figure 1. Programmed cell death of SCs in diabetic peripheral neuropathy. (A) SCs apoptosis pathway (B) SCs autophagy molecular pathway (C) SCs pyroptosis-associated signaling pathway (D) SCs ferroptosis mechanisms.
Apoptosis
Apoptosis is the most prominent and widely studied mode of PCD during development (35), the process relies on the activation of a family of cysteine proteases to form an irreversible apoptotic pathway that is morphologically characterized by cell shrinkage or fragmentation, chromosome condensation, plasma membrane vesiculation, and DNA fragmentation (36, 37). The process of apoptosis is regulated by three major pathways: the mitochondrial apoptotic pathway, which is primarily controlled by the Bcl-2 family of proteins; the death receptor pathway, which is characterized by the binding of death receptors to ligands; and the endoplasmic reticulum (ER) apoptotic pathway, which consists of three apoptotic pathways activated by the UPR (IRE1α, PERK, and ATF6 pathways) (19). HG was identified as a crucial initiator of neuronal apoptosis, and HG was found to induce late neural tube apoptosis during gestation in mice (38). The administration of HG to adult gerbils subjected to forebrain ischemia results in the exacerbation of neuronal apoptosis (39). HG causes apoptosis in rat superior cervical ganglion neurons in vitro (40).
The apoptosis of SCs was successfully induced by HG. It was demonstrated that HG could cause the early activation of caspase-3/7 in SCs in vitro and in vivo, inducing caspase-dependent apoptosis in SCs (41). The results of an in vivo study demonstrated that the apoptosis rate of RSC96 cells treated with HG for 48 h was increased by 9.72-fold, confirming that high glucose also elevated the mitochondrial pathway apoptosis-related Bax/Bcl-2 and cleaved caspase-9/caspase-9 ratios, and suggested that knockdown of histone deacetylase (HDAC1) could effectively inhibit HG-promoted mitochondrial apoptosis (42). Endoplasmic reticulum stress (ERS) is an important pathway for apoptosis, and C/EBP homologous protein (CHOP) is the most important apoptotic factor induced by ERS (43), Wu et al. found that the ratio of ERS-activated pro-apoptotic protein CHOP to anti-apoptotic protein ORP150 increased under long-term HG conditions (after 16 weeks), leading to apoptosis (44). The RNA-dependent protein kinase-like ER kinase (PERK) pathway, one of the three apoptotic pathways of the endoplasmic reticulum, PERK/nuclear factor-E2-related factor 2 (Nrf2) play important roles in oxidative stress and ERS (45). It has been demonstrated that the PERK/Nrf2 pathway is activated in the DPN model, thereby promoting apoptosis through the up-regulation of CHOP expression. However, the intervention of traditional Chinese medicine TangLuoning has been shown to reduce the level of CHOP, thereby inhibiting ERS (46). In addition, Salem et al. found an increase in the relative expression of ERS pathway markers ATF6, PERK, ATF4, eIF2α, and CHOP in SCs in the HG environment as determined by protein blotting, suggesting that ERS active (47). Padilla et al. experimental study found that HG-conditioned culture increased lipotoxic damage in SCs and confirmed that this process first occurs by triggering ERS leading to apoptosis (48). In a study conducted by Li et al., it was discovered that excessive ERS in SCs under a HG environment was associated with the inhibition of PI3K/Akt/glycogen synthase kinase 3 beta (GSK3β) and ERK1/2 signaling pathways. Additionally, the administration of nerve growth factor (NGF) was observed to regulate neuronal growth and promote the restoration of DPN. Furthermore, the aforementioned study demonstrated that NGF administration inhibited ERS through the activation of the aforementioned pathways, thereby achieving a protective effect on SCs (49). Oxidative stress is another key pathway for HG-mediated apoptosis in SCs. As the main source and target of reactive oxygen species (ROS) (50), the function of mitochondria is affected by the content of ROS. Elevated ROS cause apoptosis by increasing the permeability of the outer mitochondrial membrane leading to the release of pro-apoptotic factors, such as cytochrome c, caspase, etc. (51–53). The study found that the level of ROS in HG-treated SCs was significantly higher than that of the control by 1.54 times, suggesting that HG may lead to SCs apoptosis by inducing mitochondrial oxidative stress damage (54), whereas, salvianolic acid B (SalB) and geranylgeranyl exhibited inhibitory effects on HG-induced oxidative stress and mitochondrial depolarization in a dose-dependent manner, thereby protecting SCs from apoptosis (55, 56). More studies have shown that long-term HG similarly leads to ROS accumulation in the endoplasmic reticulum and induces ERS (57), interestingly ERS has the potential to contribute to elevated mitochondrial ROS levels, an undesirable interaction that accelerates cell death (46). CXC motif chemokines are involved in neuronal injury, CXC motif chemokine ligand 2 (CXCL2) expression is significantly increased in HG-induced SCs, CXCL2 knockdown increases SCs viability and reduces cleaved caspase 3/9 expression and associated apoptosis (58).
In addition to the above regulatory pathways, the role of HG on signaling pathways is critical in inducing apoptosis in SCs. One of the downstream targets of AMPK, rapamycin (mTOR), plays a role in the apoptosis and autophagy of SCs in the development of DPN disease (59). A study conducted on RSC96 cells revealed that the Akt/mTOR signaling pathway was inhibited and apoptosis and autophagy were increased under HG conditions. Furthermore, the inhibition of the mTOR kinase protein complex mTORC1 in RSC96 cells promoted apoptosis by silencing PARTOR or RICTOR. Additionally, animal experiments yielded similar results, demonstrating that in the sciatic nerves of diabetic mice, the phosphorylation of mTOR decreased, and the apoptosis of Schwann cells increased in diabetic mice (60–62). PI3K/Akt signaling is strongly associated with the prevention of apoptosis (63), thioredoxin interacting protein (TXNIP) plays an important role in inflammatory response, apoptosis (64), Zhang et al. found that HG promotion of SCs apoptosis was associated with TXNIP upregulation and was shown to be achieved by inhibiting the PI3K/Akt pathway (65), whereas artesunate (ART) was shown to inhibit SCs apoptosis and promote SCs viability by acting on the PI3K/AKT/mTOR signaling pathway in ex vivo experiments (66). Extracellular signal-regulated kinase (EPK) as a multifunctional protein kinase, activation of the EPK pathway has been shown to be involved in apoptosis in glial cells (67, 68). Liu et al. found that the decrease in SCs viability and the increase in SCs apoptosis under HG conditions paralleled the increase in ERK phosphorylation levels, suggesting that EPK activation may play a role in HG-induced SCs apoptosis (69), but the exact mechanism remains to be further clarified.
Additionally, dyslipidemia and insulin deficiency are significant predisposing factors for the development of DPN. Diabetes mellitus complicated by dyslipidemia with elevated oxidized low-density lipoprotein (oxLDL) levels affects the progression of DPN. In vitro experiments have demonstrated that the intervention of HG with oxLDL leads to the activation of the Caspase-3 pathway by inducing the overactivation of Toll-like receptor 4 (TLR4) signaling in SCs, which promotes the development of apoptosis (70). Insulin-like growth factor-1 (IGF-1) is a polypeptide protein that exhibits molecular similarity to insulin and is capable of exerting a range of metabolic effects analogous to those of insulin (71). Previous studies have demonstrated that IGF-1 plays a regulatory role in the viability of developing SCs and has been shown to protect SCs from apoptosis through caspase activation of PI3-K signaling, which is located upstream. Subsequent studies have found that high levels of IGF-1 (10 nm) are protective, whereas cells treated with 0.3 nm IGF-I succumbed to cellular apoptosis (41, 72, 73). A recent clinical study has confirmed that there is an association between IGF-1 deficiency and DPN. Furthermore, the study has indicated that there is a potential risk factor for DPN in patients with lower levels of IGF-1. The findings demonstrate that patients with DPN have significantly lower levels of IGF-1 compared to patients without DPN (74). Moreover, an in vivo experiment demonstrated that islet transplantation effectively reversed sciatic nerve injury in diabetic mice. Additionally, islet cells exhibited a protective effect on RSC96 cells under HG conditions through the activation of the mTOR/S6 kinase 1 (S6K1) pathway (75). It can be reasonably deduced that the investigation of pertinent therapeutic modalities that target lipids and insulin is crucial to enhance the progression of DPN lesions.
Autophagy
Autophagy is an important intracellular degradation and recycling process that renews and generates new building blocks and energy for the cell by recycling and degrading intracellular components and damaged organelles, thus acting as a dynamic recycling system (76, 77). Normal autophagy sessions in the nervous system facilitate the maintenance of neuronal integrity as well as synaptic plasticity (78), autophagy also promotes recovery and regeneration of peripheral nerve function by improving myelin and axonal regeneration (79). Not only that, but studies in recent years have shown that autophagy is involved in the formation of neuropathic pain, and that insufficient autophagic activity may lead to neuropathic pain development (80). The autophagy mechanism of SCs has even been shown to favor scar reduction and myelin formation, which is important in preventing or delaying the onset and chronicity of neuropathic pain and neuropathy (81, 82). It has even been suggested that autophagy dysfunction in SCs may be the root cause of DPN (83). Therefore, an in-depth understanding of the disruption of the autophagic mechanism of SCs by HG is extremely important for DPN as well as neuropathic pain.
Autophagy in SCs was shown to be inhibited in HG environments, microtubule-associated protein light chain 3 (LC3) was a key readout for cellular autophagy levels (84), Du et al. found that autophagy markers LC3-II/LC3-I and P62 were significantly reduced in rat SCs in HG environment compared to normal glucose-treated cells (85). Zhang et al. improved autophagy inhibition in SCs by knocking down TXNIP in HG-cultured SCs, effectively preventing the HG-induced downregulation of LC3-II/LC3-I ratio (65). Qu et al. not only found that LC3 protein expression was down-regulated in SCs in the HG environment, but also observed the appearance of morphologically and structurally abnormal autophagosomes in the cells, suggesting that autophagy is disrupted in SCs (86). Beclin-1 as an important gene in the autophagic process, Beclin-1/VPS34 controls phagocytosis in response to stress signaling pathways in the ER and other membranes (87), Yuan et al. experimentally found that HG reduced the expression of beclin-1 and autophagy-related gene Atg3 in SCs, nevertheless, strychnine treatment via the AMPK pathway negated the correlation and augmented the expression of the SCs autophagosome marker LC3-II, effectively inhibiting autophagy occurrence (88). Similarly, Gao et al. demonstrated that rats with DPN induced by HG exhibited reduced Beclin-1 and LC3-II/LC3-I ratios in SCs. They proposed that translocator protein (TSPO) agonists exert a therapeutic effect on DPN through the regulation of autophagy in SCs (89). In addition, oxidative stress is also involved in the autophagic process, and the HG-induced increase in ROS levels may contribute to autophagic dysfunction in SCs (83). More interestingly, it was found that there may be over-activation of autophagy in SCs by HG, Wei et al. observed abundant autophagic vesicles and enhanced autophagic flux in SCs from the HG group (90), autophagy hyperactivity was also observed in the streptozotocin (STZ)-induced rat model of DPN in Wang et al., these effects were also found to potentially increase apoptosis in SCs and demyelination of sciatic nerves in rats with DPN (91). A recent in vivo and ex vivo study revealed that phosphorylated mTOR and p62 expression was markedly elevated in SCs treated with HG, indicating that HG may facilitate autophagy by influencing mitochondrial function, whereas the combination of cannabidiol (CBD) and Beta-Caryophyllene (BC) pretreatment demonstrated a substantial protective effect against mitochondrial autophagy in SCs (92).
Pyroptosis
Neuroinflammation is emerging as a pivotal factor in diabetes-induced peripheral neuropathy. Chronic HG has been linked to advanced glycation end products AGEs formation, which in turn activates intracellular signaling and promotes the expression of pro-inflammatory transcription factors and the release of various inflammatory cytokines (93). The results of animal experiments indicated that elevated serum TNFα expression in DPN rats and elevated sciatic nerve IL-6 and IL-1β levels in diabetic rats were associated with neuropathy, this suggests that inflammatory factors may play a role in the pathogenesis of neuropathy (94, 95). Moreover, several clinical studies have identified elevated levels of numerous inflammatory markers, including CRP, TNF-α, IL-1RA, sTNFR1, and sTNFR2, along with elevated serum NF-κB levels, in patients with DPN compared to patients with T2DM without neuropathy, suggesting that patients with DPN exhibit a more pronounced inflammatory response (96–98). More significantly, elevated plasma levels of pro-inflammatory factors have been demonstrated to play a pivotal role in predicting the incidence of DPN. Of these factors, the IL-6 inflammatory factor has been shown to be particularly relevant, exhibiting a distinct difference between painful and painless DPN (99, 100). The presented evidence indicates a strong correlation between inflammatory processes and DPN.
Pyroptosis is a cleavage PCD triggered by inflammatory vesicles (101), it is dependent on members of the Gasdermin protein family to form plasma membrane pores and shares DNA breakage, nuclear condensation and caspase-dependent morphological alterations with apoptosis (102). The process of pyroptosis mainly involves the caspase-1-dependent pathway (classical pathway) and the caspase-1-independent pathway (non-classical pathway), and is closely related to the secretion and activation of the pro-inflammatory cytokines interleukin-1β (IL-1β) and IL-18, which play an important role in inflammatory response and immune defense through the release of inflammatory mediators and the recruitment of immune cells (103–105). Pyroptosis has been shown to play a crucial role in the development of diabetes and its complications (106, 107). NLRP3 inflammasome is a cytoplasmic multiprotein complex that is commonly aberrantly activated in inflammatory diseases such as diabetes to induce pyroptosis (108). Cheng et al. observed a significant increase in the protein expression of NLRP3 and Gasdermin D in SCs under HG (25 mM) environment, which promoted the development of SCs pyroptosis (109);Wang et al. found that taurine deoxycholic acid (TUDCA) effectively inhibited pyroptosis in HG-stimulated SCs by targeting NLRP3 expression to enhance cell viability and migration (110). Immunofluorescence staining and quantitative RT-PCR confirmed that pyroptosis-related proteins cleaved-GSDMD, NLRP3, caspase-1, IL-1β, and IL-18 were significantly enhanced in HG-stimulated SCs (106). These findings strongly suggest that HG induces the onset of SCs pyroptosis and that pyroptotic SCs inhibit neuronal function and promote an inflammatory response (111), thus inhibiting pyroptosis in SCs has implications for neurons. Furthermore, NF-κB plays a pivotal role in the pathogenesis of inflammatory neuropathies. It is a crucial mediator of immune, inflammatory, and apoptotic responses, which are initiated by the activation of the RAGE pathway. A study of 45 diabetic patients with peroneal nerve biopsies revealed that RAGE was upregulated in SCs, this suggests that RAGE may be a key regulator of apoptosis or pyroptosis in SCs. However, the precise mechanism by which RAGE exerts its effects remains to be elucidated (112, 113). A recent study demonstrated that the Ras-related protein Rab32 induces significant oxidative stress by disrupting the mitochondria of SCs, thereby exacerbating SCs pyroptosis in peripheral nerve injury, it would be beneficial to investigate whether Rab32 plays a role in the death process of SCs in DPN (114).
Ferroptosis
Diabetes mellitus as a lifelong metabolic disease due to the presence of disturbances in cellular metabolism leading to ferroptosis and ferritin autophagy (115). Ferroptosis is an iron-dependent regulated necrosis that is considered a byproduct of cellular metabolism because of its close association with oxygen, iron, and lipid metabolism (116), it is dependent on the metabolites reactive oxygen species (ROS), phospholipids containing polyunsaturated fatty acid chains (PUFA-PL), and the transition metal iron, and exhibits biochemical features of iron accumulation and unrestricted lipid peroxidation, as well as morphologic alterations such as loss of plasma membrane integrity, mitochondrial abnormalities, cytoplasmic swelling, and moderate chromatin condensation (117, 118). Briefly, Fe3+ is deoxygenated to catalytically active Fe2 + and released into the cytoplasmic labile iron pool (LIP) to be utilized or stored as ferritin in the cell. when the intracellular Fe2+ overload exceeds the caching capacity of ferritin, and intracellular free iron generates ROS through the fenton reaction, and glutathione (GSH), one of the critically important antioxidants in cells, is catalyzed by peroxidase to oxidized glutathione (GSSG) during lipid peroxidation, and inhibit lipid peroxidation-induced ferroptosis (19). The typical ferroptosis mechanism is mediated by glutathione peroxidase 4 (GPX4), and inactivation or knockdown of GPX4 leads to the onset of ferroptosis (119). It was confirmed that serum GPX4 and GSH were significantly reduced and lipid peroxides MDA were significantly elevated in DPN patients, suggesting that ferroptosis levels were elevated in DPN patients, and it was found that HG induced ferroptosis in SCs through the inhibition of a pathway directly downstream of ROS (NRF2 signaling pathway) (120). Hu et al. found that and honokiol reduced HG-induced ferroptosis and SCs mitochondrial dysfunction by acting on the AMPK/SIRT1/PGC-1α axis and downstream gene expression profiles (121). Even though some studies have shown a crossover between the underlying pathologic mechanisms of DPN and ferroptosis (115), and the above experiments have confirmed that HG exerts an effect on ferroptosis in SCs, However, overall more research is needed on the mechanisms of ferroptosis in DPN. For example, a recent report indicates that arachidonic acid 15-lipoxygenase (ALOX15), which is highly correlated with ferroptosis, is highly expressed in human Schwann cells, and also has an important role in functional changes in the DPN, as well as in demyelination, in light of these findings, it would be beneficial to investigate whether HG influences ferroptosis in SCs by modulating ALOX15 activity (122).
Necroptosis
Necroptosis is a regulated mode of cell death induced by environmental stimuli, which is mainly regulated by the TNF-α receptor system and ultimately directly activates and regulates the inflammatory response through the loss of membrane integrity and the efflux of intracellular content (123, 124). MLKL, a key mediator of necroptosis, is phosphorylated by RIP3 kinase and disrupts plasma membrane integrity leading to necrosis (125). Guo et al. demonstrated that MLKL mediates DPN demyelination by in vivo and in vitro observations and found that MLKL levels were increased in the gastrocnemius muscle of STZ-induced diabetic mice and that MLKL signaling was prominent in the myelin sheaths of patients with DPN (126), Demyelination of SCs is associated with a “sock and glove” pattern in patients with DPN, and the process described above involves MLKL-mediated damage to SCs, which may be the result of necroptosis in SCs (127), but Guo et al. did not detect necroptotic apoptotic MLKL phosphorylation in diabetic patients (126). The component nuclear pore protein Seh1 plays a role in protecting the homeostasis of SCs by maintaining genomic integrity, and it has been shown that interactions following Seh1 ablation trigger ZBP1-dependent necroptosis in SCs leading to peripheral neuropathy (128), however, whether HG has an effect on this link is not clear.
Cross-targeting cell death patterns and therapeutic perspectives
Although each of these pathways has specific mechanisms and outcomes, different cell death regulatory pathways crosstalk with each other. Crosstalk between apoptosis and autophagy is regulated by multiple factors such as Ca2+ signaling, Bcl-2 and caspase-3/8 factors, and oxidative stress (129–131), Beclin-1 is a direct bridge between the two signaling pathways and plays an important role in the communication between autophagy and apoptosis (132). The calcium mimetic cinacalcet has been shown to regulate Ca2+ levels and increase Beclin-1 and Bcl-2/Bax expression in SCs in the diabetic sciatic nerve, and to play an important role in the restoration and amelioration of DPN through the regulation of apoptosis and autophagy (133). In addition to its important role in apoptosis, ER is likewise at the crossroads of two pathways, apoptosis and autophagy, in the diabetes process (134), Melatonin (MEL) as an antioxidant and anti-inflammatory agent was found to play a role in the ER major stress response (135). Negi et al. found that MEL can regulate neuroinflammation through activation of the Nrf2 pathway (136), another experimental and clinical study confirmed the protective effect of MEL on glial cells and its beneficial effect on DPN, but the specific therapeutic regimen of administration needs to be further clarified (137). Pro-inflammatory cytokines similarly link autophagy to apoptosis and have been shown to be associated with neuropathic pain (138), This phenomenon suggests that we may be able to further explore whether there are pro-inflammatory cytokine-related drugs to treat neuropathic pain in DPN patients by improving the apoptosis and autophagy link.
The establishment of the concept of PANoptosis (which refers to the inflammatory PCD pathway regulated by the PANoptosome complex, encompassing pyroptosis, apoptosis, and necroptosis, and which cannot be explained by one or the other alone) strongly suggests that there is a broad crosstalk between apoptosis, pyroptosis, and necroptosis (139, 140). NLRP3 inflammasome is important for the crosstalk between the three, CASP8-NLRP3 can connect pyroptosis with apoptosis; MLKL and macrophage potassium efflux-mediated activation of NLRP3 inflammasome crosstalk pyroptosis with necroptosis (summarized in (141)). ZBP1-NLRP3 inflammatory vesicles were even shown to be key components of PANoptosis (142). In addition, NLRP3 inflammatory vesicles play a role in peripheral neuropathic pain, which has been highlighted as having the potential to be a therapeutic target for neuropathic pain (143). Taurine deoxycholic acid (TUDCA), loganin, and CXCL2 blocking therapy all have the effect of modulating NLRP3, and all three have also been shown to improve SCs mortality in the HG environment (58, 109, 110). Caspases are dominant in apoptosis and pyroptosis, apoptotic Caspase-3 and 8 mediate pyroptosis by cleaving Gasdermin and thus Caspase-8 is even more important to maintain the balance between apoptosis and necroptosis (141, 144), thus, strict adjustment of the level of caspase in SCs is important to improve PCD sessions.
The crosstalk between autophagy and ferroptosis at the molecular level was revealed by Hou et al. They demonstrated that autophagy triggers ferroptosis through degradation of ferritin, and found that autophagy mediated by autophagy-associated genes Atg5 and Atg7 likewise led to ferroptosis (145). The GPX4 essential cofactor GSH not only plays an important role in ferroptosis, but GSH depletion also induces a significant increase in autophagy (146), whereas erythropoietin (EPO) was able to increase the level of total GSH, reduce ROS levels, and improve cell viability in SCs in the HG environment (147). The human tumor suppressor protein p53 not only regulates autophagy in a dual manner, nuclear p53 is revealed to stimulate ferroptosis in a transcription-dependent manner (148, 149), Li et al. also showed that p53 may be a key molecule in the ferroptosis - apoptosis crosstalk (150), and as previous studies have long found that p53 is critical for neuronal death (151, 152), we believe that p53 may be a potential therapeutic target. Erastin acts as a ferroptosis activator, which not only induces ferroptosis, but also causes apoptosis to occur (153). The combined action of erastin and the apoptotic agent TRAIL was shown to mediate apoptosis via activation of caspases (154), suggesting a possible mechanism of interaction between ferroptosis and apoptosis. Furthermore, ER and mitochondria are key organelles in PCD, Lee et al. found that ferroptosis-induced ERS crosstalks ferroptosis with apoptosis by increasing the expression of the pro-apoptotic molecule PUMA (155). The Bax-mediated mitochondrial pathway not only affects apoptosis but also mediates ferroptosis by acting upstream of SLC7A11 and GPX4 (156). In addition, Nrf2, as an important participant in redox and inflammatory responses, not only mediates the process of apoptosis, but also participates in the regulation of autophagy and ferroptosis during disease therapy (157). The expression of Nrf2 has been shown to promote SCs-mediated neurological recovery in DPN (158), whereas salvianolic acid A, paeoniflorin, and the traditional Chinese medicine Tangluoning were all found to have a modulatory effect on Nrf2 expression in SCs in the HG environment (46, 159, 160).
Concluding remarks
SCs have an extremely important role in the peripheral nervous system, and the disruption of the death process of SCs by HG is an important part of DPN occurrence and development. In this review, we discuss the specific molecular mechanisms by which several forms of PCD in SCs are affected (Figure 2), and exemplifies some of the drugs that ameliorate the death of SCs through the corresponding linkages (Table 1). For example, HG induces apoptosis in SCs through multiple pathways involving disruption of mitochondrial function, endoplasmic reticulum stress, as well as oxidative stress and signaling pathways; inhibition or over-activation of autophagy is achieved by decreasing the expression of LC3-II/LC3-I, beclin-1, and autophagy genes in SCs, or by increasing autophagy fluxes. Among them, HG-induced inflammatory response is the culprit for the pyroptosis of SCs, and the aberrant activation of NLRP3 inflammatory vesicles is the key link for the induction of pyroptosis and PANoptosis, which suggests that targeting the NLRP3 inflammatory vesicles is a reliable therapeutic pathway. Unfortunately, although a few studies have observed the effects of HG on ferroptosis and necroptosis in SCs, the molecular mechanisms involved are relatively homogeneous and more and deeper mechanistic explorations are lacking, thus these two pathways need to be further explored, especially necroptosis. We conclude by describing the relevant targets of crosstalk of these PCDs, pointing out that some of the drugs targeting the targets are effective in mitigating DPN progression and neuroinflammation, but there is still a therapeutic void for important targets like p53.
It is worth noting that despite significant progress in potential research on the cellular and molecular levels of SCs death, nevertheless, apart from a few encouraging preclinical studies, there is a dearth of clinical studies targeting the Schwann cell pathway for the treatment of DPN. The majority of the promising therapeutic agents currently available remain in ex vivo studies lacking robust clinical validation. Consequently, there is an imperative need for broader and more comprehensive clinical exploration as a means of combating DPN. In addition, although SCs autophagy and NLRP3 were found to be possible therapeutic directions for neuropathic pain, the specific effective mechanisms in DPN need to be further clarified. In conclusion, we believe that there is an urgent need to explore DPN therapies targeting different forms of PCDs in HG-induced SCs and the molecular mechanisms that crosstalk with each other, and, despite the many challenges, we strongly believe that thorough experimental and clinical studies can make the protection of SCs PCDs an important target for the intervention and treatment of DPN.
Author contributions
LW: Writing – original draft, Methodology, Conceptualization. XW: Writing – review & editing, Methodology. XL: Writing – review & editing, Methodology. JZ: Writing – review & editing, Methodology. XZ: Writing – review & editing, Methodology. QC: Writing – review & editing, Supervision, Resources.
Funding
The author(s) declare financial support was received for the research, authorship, and/or publication of this article. This work was supported by the Administration of Traditional Chinese Medicine of Sichuan Province (No. 2023zd020) and the Chengdu Science and Technology Project (No. 2023-YF09-00052-SN) and The College Students’ Innovative Entrepreneurial Training Plan Program (No.202310633008).
Conflict of interest
The authors declare that the study was conducted in the absence of any business or financial relationship that could be interpreted as a potential conflict of interest.
Publisher’s note
All claims expressed in this article are solely those of the authors and do not necessarily represent those of their affiliated organizations, or those of the publisher, the editors and the reviewers. Any product that may be evaluated in this article, or claim that may be made by its manufacturer, is not guaranteed or endorsed by the publisher.
References
1. Antini C, Caixeta R, Luciani S, Hennis AJM. Diabetes mortality: trends and multi-country analysis of the Americas from 2000 to 2019. Int J Epidemiol. (2024) 53:dyad182. doi: 10.1093/ije/dyad182
2. Khatun S, Prasad Bhagat R, Dutta R, Datta A, Jaiswal A, Halder S, et al. Unraveling HDAC11: Epigenetic orchestra in different diseases and structural insights for inhibitor design. Biochem Pharmacol. (2024) 225:116312. doi: 10.1016/j.bcp.2024.116312
3. Yu MG, Gordin D, Fu J, Park K, Li Q, King GL. Protective factors and the pathogenesis of complications in diabetes. Endocr Rev. (2024) 45:227–52. doi: 10.1210/endrev/bnad030
4. Gonçalves NP, Vægter CB, Andersen H, Østergaard L, Calcutt NA, Jensen TS. Schwann cell interactions with axons and microvessels in diabetic neuropathy. Nat Rev Neurol. (2017) 13:135–47. doi: 10.1038/nrneurol.2016.201
5. Selvarajah D, Kar D, Khunti K, Davies MJ, Scott AR, Walker J, et al. Diabetic peripheral neuropathy: advances in diagnosis and strategies for screening and early intervention. Lancet Diabetes Endocrinol. (2019) 7:938–48. doi: 10.1016/s2213-8587(19)30081-6
6. Pop-Busui R, Boulton AJ, Feldman EL, Bril V, Freeman R, Malik RA, et al. Diabetic neuropathy: A position statement by the American diabetes association. Diabetes Care. (2017) 40:136–54. doi: 10.2337/dc16-2042
7. Soedamah-Muthu SS, Chaturvedi N, Witte DR, Stevens LK, Porta M, Fuller JH. Relationship between risk factors and mortality in type 1 diabetic patients in Europe: the EURODIAB Prospective Complications Study (PCS). Diabetes Care. (2008) 31:1360–6. doi: 10.2337/dc08-0107
8. Feldman EL, Callaghan BC, Pop-Busui R, Zochodne DW, Wright DE, Bennett DL, et al. Diabetic neuropathy. Nat Rev Dis Primers. (2019) 5:42. doi: 10.1038/s41572-019-0097-9
9. Han JW, Choi D, Lee MY, Huh YH, Yoon YS. Bone marrow-derived mesenchymal stem cells improve diabetic neuropathy by direct modulation of both angiogenesis and myelination in peripheral nerves. Cell Transplant. (2016) 25:313–26. doi: 10.3727/096368915x688209
10. Omi M, Hata M, Nakamura N, Miyabe M, Ozawa S, Nukada H, et al. Transplantation of dental pulp stem cells improves long-term diabetic polyneuropathy together with improvement of nerve morphometrical evaluation. Stem Cell Res Ther. (2017) 8:279. doi: 10.1186/s13287-017-0729-5
11. Ghosh M, Pearse DD. Schwann cell-derived exosomal vesicles: A promising therapy for the injured spinal cord. Int J Mol Sci. (2023) 24:17317. doi: 10.3390/ijms242417317
12. Yum Y, Park S, Nam YH, Yoon J, Song H, Kim HJ, et al. Therapeutic effect of schwann cell-like cells differentiated from human tonsil-derived mesenchymal stem cells on diabetic neuropathy in db/db mice. Tissue Eng Regener Med. (2024) 21:761–76. doi: 10.1007/s13770-024-00638-0
13. Taveggia C, Feltri ML. Beyond wrapping: canonical and noncanonical functions of schwann cells. Annu Rev Neurosci. (2022) 45:561–80. doi: 10.1146/annurev-neuro-110920-030610
14. Bosch-Queralt M, Fledrich R, Stassart RM. Schwann cell functions in peripheral nerve development and repair. Neurobiol Dis. (2023) 176:105952. doi: 10.1016/j.nbd.2022.105952
15. Campana WM. Schwann cells: activated peripheral glia and their role in neuropathic pain. Brain Behav Immun. (2007) 21:522–7. doi: 10.1016/j.bbi.2006.12.008
16. Wei Z, Fei Y, Su W, Chen G. Emerging role of schwann cells in neuropathic pain: receptors, glial mediators and myelination. Front Cell Neurosci. (2019) 13:116. doi: 10.3389/fncel.2019.00116
17. Naruse K. Schwann cells as crucial players in diabetic neuropathy. Adv Exp Med Biol. (2019) 1190:345–56. doi: 10.1007/978-981-32-9636-7_22
18. Liu C, Mao X, Meng L, Li J. Stresses make microbe undergo programmed cell death: Mechanisms and opportunities. Food Res Int. (2022) 157:111273. doi: 10.1016/j.foodres.2022.111273
19. Li Z, Li D, Chen R, Gao S, Xu Z, Li N. Cell death regulation: A new way for natural products to treat osteoporosis. Pharmacol Res. (2023) 187:106635. doi: 10.1016/j.phrs.2022.106635
20. Nave KA, Trapp BD. Axon-glial signaling and the glial support of axon function. Annu Rev Neurosci. (2008) 31:535–61. doi: 10.1146/annurev.neuro.30.051606.094309
21. Nave KA. Myelination and support of axonal integrity by glia. Nature. (2010) 468:244–52. doi: 10.1038/nature09614
22. Gabbay KH, Merola LO, Field RA. Sorbitol pathway: presence in nerve and cord with substrate accumulation in diabetes. Science. (1966) 151:209–10. doi: 10.1126/science.151.3707.209
23. Tomlinson DR, Holmes PR, Mayer JH. Reversal, by treatment with an aldose reductase inhibitor, of impaired axonal transport and motor nerve conduction velocity in experimental diabetes mellitus. Neurosci Lett. (1982) 31:189–93. doi: 10.1016/0304-3940(82)90115-X
24. Niimi N, Yako H, Takaku S, Chung SK, Sango K. Aldose reductase and the polyol pathway in schwann cells: old and new problems. Int J Mol Sci. (2021) 22:1031. doi: 10.3390/ijms22031031
25. Sango K, Mizukami H, Horie H, Yagihashi S. Impaired axonal regeneration in diabetes. Perspective on the underlying mechanism from in vivo and in vitro experimental studies. Front Endocrinol (Lausanne). (2017) 8:12. doi: 10.3389/fendo.2017.00012
26. McCoin CS, Knotts TA, Adams SH. Acylcarnitines–old actors auditioning for new roles in metabolic physiology. Nat Rev Endocrinol. (2015) 11:617–25. doi: 10.1038/nrendo.2015.129
27. Feldman EL, Nave KA, Jensen TS, Bennett DLH. New horizons in diabetic neuropathy: mechanisms, bioenergetics, and pain. Neuron. (2017) 93:1296–313. doi: 10.1016/j.neuron.2017.02.005
28. George EB, Glass JD, Griffin JW. Axotomy-induced axonal degeneration is mediated by calcium influx through ion-specific channels. J Neurosci. (1995) 15:6445–52. doi: 10.1523/jneurosci.15-10-06445.1995
29. Viader A, Sasaki Y, Kim S, Strickland A, Workman CS, Yang K, et al. Aberrant Schwann cell lipid metabolism linked to mitochondrial deficits leads to axon degeneration and neuropathy. Neuron. (2013) 77:886–98. doi: 10.1016/j.neuron.2013.01.012
30. Fernyhough P. Mitochondrial dysfunction in diabetic neuropathy: a series of unfortunate metabolic events. Curr Diabetes Rep. (2015) 15:89. doi: 10.1007/s11892-015-0671-9
31. Yagihashi S, Kamijo M, Baba M, Yagihashi N, Nagai K. Effect of aminoguanidine on functional and structural abnormalities in peripheral nerve of STZ-induced diabetic rats. Diabetes. (1992) 41:47–52. doi: 10.2337/diab.41.1.47
32. Vincent AM, Perrone L, Sullivan KA, Backus C, Sastry AM, Lastoskie C, et al. Receptor for advanced glycation end products activation injures primary sensory neurons via oxidative stress. Endocrinology. (2007) 148:548–58. doi: 10.1210/en.2006-0073
33. Zhang L, Yu C, Vasquez FE, Galeva N, Onyango I, Swerdlow RH, et al. Hyperglycemia alters the schwann cell mitochondrial proteome and decreases coupled respiration in the absence of superoxide production. J Proteome Res. (2010) 9:458–71. doi: 10.1021/pr900818g
34. Viader A, Golden JP, Baloh RH, Schmidt RE, Hunter DA, Milbrandt J. Schwann cell mitochondrial metabolism supports long-term axonal survival and peripheral nerve function. J Neurosci. (2011) 31:10128–40. doi: 10.1523/jneurosci.0884-11.2011
35. Yi CH, Yuan J. The Jekyll and Hyde functions of caspases. Dev Cell. (2009) 16:21–34. doi: 10.1016/j.devcel.2008.12.012
36. Kerr JFR, Wyllie AH, Currie AR. Apoptosis: A basic biological phenomenon with wideranging implications in tissue kinetics. Br J Cancer. (1972) 26:239–57. doi: 10.1038/bjc.1972.33
37. Degterev A, Yuan J. Expansion and evolution of cell death programmes. Nat Rev Mol Cell Biol. (2008) 9:378–90. doi: 10.1038/nrm2393
38. Fine EL, Horal M, Chang TI, Fortin G, Loeken MR. Evidence that elevated glucose causes altered gene expression, apoptosis, and neural tube defects in a mouse model of diabetic pregnancy. Diabetes. (1999) 48:2454–62. doi: 10.2337/diabetes.48.12.2454
39. Kondo F, Kondo Y, Makino H, Ogawa N. Delayed neuronal death in hippocampal CA1 pyramidal neurons after forebrain ischemia in hyperglycemic gerbils: amelioration by indomethacin. Brain Res. (2000) 853:93–8. doi: 10.1016/s0006-8993(99)02256-8
40. Russell JW, Feldman EL. Insulin-like growth factor-I prevents apoptosis in sympathetic neurons exposed to high glucose. Horm Metab Res. (1999) 31:90–6. doi: 10.1055/s-2007-978704
41. Delaney CL, Russell JW, Cheng H-L, Feldman EL. Insulin-like growth factor-I and over-expression of bcl-xL prevent glucose-mediated apoptosis in schwann cells. J Neuropathol Exp Neurol. (2001) 60:147–60. doi: 10.1093/jnen/60.2.147
42. Jin T, Wang Z, Fan F, Wei W, Zhou C, Zhang Z, et al. HDAC1 promotes mitochondrial pathway apoptosis and inhibits the endoplasmic reticulum stress response in high glucose-treated schwann cells via decreased U4 spliceosomal RNA. Neurochem Res. (2024). doi: 10.1007/s11064-024-04200-1
43. O’Brien PD, Hinder LM, Sakowski SA, Feldman EL. ER stress in diabetic peripheral neuropathy: A new therapeutic target. Antioxid Redox Signal. (2014) 21:621–33. doi: 10.1089/ars.2013.5807
44. Wu Y-B, Li H-Q, Ren M-S, Li W-T, Lv X-Y, Wang L. CHOP/ORP150 ratio in endoplasmic reticulum stress: A new mechanism for diabetic peripheral neuropathy. Cell Physiol Biochem. (2013) 32:367–79. doi: 10.1159/000354444
45. Zhu YF, Li XH, Yuan ZP, Li CY, Tian RB, Jia W, et al. Allicin improves endoplasmic reticulum stress-related cognitive deficits via PERK/Nrf2 antioxidative signaling pathway. Eur J Pharmacol. (2015) 762:239–46. doi: 10.1016/j.ejphar.2015.06.002
46. Yang X, Yao W, Liu H, Gao Y, Liu R, Xu L. Tangluoning, a traditional Chinese medicine, attenuates in vivo and in vitro diabetic peripheral neuropathy through modulation of PERK/Nrf2 pathway. Sci Rep. (2017) 7:1014. doi: 10.1038/s41598-017-00936-9
47. Salem HMA, Chok KC, Koh RY, Ng PY, Tiong YL, Chye SM. Melatonin ameliorates high glucose-induced autophagy in Schwann cells. Int J Biochem Mol Biol. (2023) 14:25–31.
48. Padilla A, Descorbeth M, Almeyda AL, Payne K, De Leon M. Hyperglycemia magnifies Schwann cell dysfunction and cell death triggered by PA-induced lipotoxicity. Brain Res. (2011) 1370:64–79. doi: 10.1016/j.brainres.2010.11.013
49. Li R, Wu Y, Zou S, Wang X, Li Y, Xu K, et al. NGF attenuates high glucose-induced ER stress, preventing schwann cell apoptosis by activating the PI3K/akt/GSK3β and ERK1/2 pathways. Neurochem Res. (2017) 42:3005–18. doi: 10.1007/s11064-017-2333-6
50. Marchi S, Giorgi C, Suski JM, Agnoletto C, Bononi A, Bonora M, et al. Mitochondria-ros crosstalk in the control of cell death and aging. J Signal Transduct. (2012) 2012:329635. doi: 10.1155/2012/329635
51. Kuznetsov AV, Margreiter R, Amberger A, Saks V, Grimm M. Changes in mitochondrial redox state, membrane potential and calcium precede mitochondrial dysfunction in doxorubicin-induced cell death. Biochim Biophys Acta. (2011) 1813:1144–52. doi: 10.1016/j.bbamcr.2011.03.002
52. Assaly R, de Tassigny A, Paradis S, Jacquin S, Berdeaux A, Morin D. Oxidative stress, mitochondrial permeability transition pore opening and cell death during hypoxia-reoxygenation in adult cardiomyocytes. Eur J Pharmacol. (2012) 675:6–14. doi: 10.1016/j.ejphar.2011.11.036
53. Hosseini A, Abdollahi M. Diabetic neuropathy and oxidative stress: therapeutic perspectives. Oxid Med Cell Longev. (2013) 2013:168039. doi: 10.1155/2013/168039
54. Tiong YL, Ng KY, Koh RY, Ponnudurai G, Chye SM. Melatonin prevents oxidative stress-induced mitochondrial dysfunction and apoptosis in high glucose-treated Schwann cells via upregulation of Bcl2, NF-κB, mTOR, Wnt signalling pathways. Antioxidants (Basel). (2019) 8:198. doi: 10.3390/antiox8070198
55. Wu Y, Xue B, Li X, Liu H. Puerarin prevents high glucose-induced apoptosis of Schwann cells by inhibiting oxidative stress. Neural Regener Res. (2012) 7:2583–91. doi: 10.3969/j.issn.1673-5374.2012.33.003
56. Sun LQ, Zhao J, Zhang TT, Qu L, Wang X, Xue B, et al. Protective effects of Salvianolic acid B on Schwann cells apoptosis induced by high glucose. Neurochem Res. (2012) 37:996–1010. doi: 10.1007/s11064-011-0695-8
57. Cao SS, Kaufman RJ. Endoplasmic reticulum stress and oxidative stress in cell fate decision and human disease. Antioxid Redox Signal. (2014) 21:396–413. doi: 10.1089/ars.2014.5851
58. Zhang Y, Li C, Wang Z, Wang T, Zhou Y, Zheng L. Blocking CXC motif chemokine ligand 2 ameliorates diabetic peripheral neuropathy via inhibiting apoptosis and NLRP3 inflammasome activation. Biol Pharm Bull. (2023) 46:672–83. doi: 10.1248/bpb.b22-00680
59. Negi G, Kumar A, Sharma SS. Concurrent targeting of nitrosative stress-PARP pathway corrects functional, behavioral and biochemical deficits in experimental diabetic neuropathy. Biochem Biophys Res Commun. (2010) 391:102–6. doi: 10.1016/j.bbrc.2009.11.010
60. Beirowski B, Wong KM, Babetto E, Milbrandt J. mTORC1 promotes proliferation of immature Schwann cells and myelin growth of differentiated Schwann cells. Proc Natl Acad Sci U.S.A. (2017) 114:E4261–e4270. doi: 10.1073/pnas.1620761114
61. Zhu L, Hao J, Cheng M, Zhang C, Huo C, Liu Y, et al. Hyperglycemia-induced Bcl-2/Bax-mediated apoptosis of Schwann cells via mTORC1/S6K1 inhibition in diabetic peripheral neuropathy. Exp Cell Res. (2018) 367:186–95. doi: 10.1016/j.yexcr.2018.03.034
62. Dong J, Li H, Bai Y, Wu C. Muscone ameliorates diabetic peripheral neuropathy through activating AKT/mTOR signalling pathway. J Pharm Pharmacol. (2019) 71:1706–13. doi: 10.1111/jphp.13157
63. Chang F, Lee JT, Navolanic PM, Steelman LS, Shelton JG, Blalock WL, et al. Involvement of PI3K/Akt pathway in cell cycle progression, apoptosis, and neoplastic transformation: a target for cancer chemotherapy. Leukemia. (2003) 17:590–603. doi: 10.1038/sj.leu.2402824
64. Cao X, He W, Pang Y, Cao Y, Qin A. Redox-dependent and independent effects of thioredoxin interacting protein. Biol Chem. (2020) 401:1215–31. doi: 10.1515/hsz-2020-0181
65. Zhang X, Zhao S, Yuan Q, Zhu L, Li F, Wang H, et al. TXNIP, a novel key factor to cause Schwann cell dysfunction in diabetic peripheral neuropathy, under the regulation of PI3K/Akt pathway inhibition-induced DNMT1 and DNMT3a overexpression. Cell Death Dis. (2021) 12:642. doi: 10.1038/s41419-021-03930-2
66. Zhang X, Liang Z, Zhou Y, Wang F, Wei S, Tan B, et al. Artesunate inhibits apoptosis and promotes survival in schwann cells via the PI3K/AKT/mTOR axis in diabetic peripheral neuropathy. Biol Pharm Bull. (2023) 46:764–72. doi: 10.1248/bpb.b22-00619
67. Szydlowska K, Gozdz A, Dabrowski M, Zawadzka M, Kaminska B. Prolonged activation of ERK triggers glutamate-induced apoptosis of astrocytes: neuroprotective effect of FK506. J Neurochem. (2010) 113:904–18. doi: 10.1111/j.1471-4159.2010.06656.x
68. Parthasarathy G, Philipp MT. The MEK/ERK pathway is the primary conduit for Borrelia burgdorferi-induced inflammation and P53-mediated apoptosis in oligodendrocytes. Apoptosis. (2014) 19:76–89. doi: 10.1007/s10495-013-0913-8
69. Liu D, Liang X, Zhang H. Effects of high glucose on cell viability and differentiation in primary cultured schwann cells: potential role of ERK signaling pathway. Neurochemical Res. (2016) 41:1281–90. doi: 10.1007/s11064-015-1824-6
70. Nihei W, Kato A, Himeno T, Kondo M, Nakamura J, Kamiya H, et al. Hyperglycaemia aggravates oxidised low-density lipoprotein-induced schwann cell death via hyperactivation of toll-like receptor 4. Neurol Int. (2024) 16:370–9. doi: 10.3390/neurolint16020027
71. Liu YP, Shao SJ, Guo HD. Schwann cells apoptosis is induced by high glucose in diabetic peripheral neuropathy. Life Sci. (2020) 248:117459. doi: 10.1016/j.lfs.2020.117459
72. Delaney CL, Cheng HL, Feldman EL. Insulin-like growth factor-I prevents caspase-mediated apoptosis in Schwann cells. J Neurobiol. (1999) 41:540–8. doi: 10.1002/(sici)1097-4695(199912)41:4<540::aid-neu9>3.0.co;2-p
73. Syroid DE, Zorick TS, Arbet-Engels C, Kilpatrick TJ, Eckhart W, Lemke G. A role for insulin-like growth factor-I in the regulation of Schwann cell survival. J Neurosci. (1999) 19:2059–68. doi: 10.1523/jneurosci.19-06-02059.1999
74. Zhong J, Lin X, Zheng X, Zhou Y, Huang H, Xu L. Diminished levels of insulin-like growth factor-1 may be a risk factor for peripheral neuropathy in type 2 diabetes patients. J Diabetes Investig. (2024). doi: 10.1111/jdi.14260
75. Zhu X, Xie S, Chen J, Lu Q, Wang X, Duan F, et al. Sildenafil Enhances the Therapeutic Effect of Islet Transplantation for Diabetic Peripheral Neuropathy via mTOR/S6K1 Pathway. Int J Endocrinol. (2023) 2023:8199029. doi: 10.1155/2023/8199029
76. Mizushima N, Komatsu M. Autophagy: renovation of cells and tissues. Cell. (2011) 147:728–41. doi: 10.1016/j.cell.2011.10.026
77. Parzych KR, Klionsky DJ. An overview of autophagy: morphology, mechanism, and regulation. Antioxid Redox Signal. (2014) 20:460–73. doi: 10.1089/ars.2013.5371
78. Nikoletopoulou V, Sidiropoulou K, Kallergi E, Dalezios Y, Tavernarakis N. Modulation of autophagy by BDNF underlies synaptic plasticity. Cell Metab. (2017) 26:230–242.e235. doi: 10.1016/j.cmet.2017.06.005
79. Huang HC, Chen L, Zhang HX, Li SF, Liu P, Zhao TY, et al. Autophagy promotes peripheral nerve regeneration and motor recovery following sciatic nerve crush injury in rats. J Mol Neurosci. (2016) 58:416–23. doi: 10.1007/s12031-015-0672-9
80. Zheng G, Ren J, Shang L, Bao Y. Role of autophagy in the pathogenesis and regulation of pain. Eur J Pharmacol. (2023) 955:175859. doi: 10.1016/j.ejphar.2023.175859
81. Marinelli S, Nazio F, Tinari A, Ciarlo L, D’Amelio M, Pieroni L, et al. Schwann cell autophagy counteracts the onset and chronification of neuropathic pain. Pain. (2014) 155:93–107. doi: 10.1016/j.pain.2013.09.013
82. Ko PY, Yang CC, Kuo YL, Su FC, Hsu TI, Tu YK, et al. Schwann-cell autophagy, functional recovery, and scar reduction after peripheral nerve repair. J Mol Neurosci. (2018) 64:601–10. doi: 10.1007/s12031-018-1056-8
83. Choi SJ, Kim S, Lee WS, Kim DW, Kim CS, Oh SH. Autophagy dysfunction in a diabetic peripheral neuropathy model. Plast Reconstr Surg. (2023) 151:355–64. doi: 10.1097/prs.0000000000009844
84. Barth S, Glick D, Macleod KF. Autophagy: assays and artifacts. J Pathol. (2010) 221:117–24. doi: 10.1002/path.2694
85. Du W, Wang N, Li F, Jia K, An J, Liu Y, et al. STAT3 phosphorylation mediates high glucose—impaired cell autophagy in an HDAC1-dependent and -independent manner in Schwann cells of diabetic peripheral neuropathy. FASEB J. (2019) 33:8008–21. doi: 10.1096/fj.201900127R
86. Qu L, Liang X, Gu B, Liu W. Quercetin alleviates high glucose-induced Schwann cell damage by autophagy. Neural Regener Res. (2014) 9:1195–203. doi: 10.4103/1673-5374.135328
87. Glick D, Barth S, Macleod KF. Autophagy: cellular and molecular mechanisms. J Pathol. (2010) 221:3–12. doi: 10.1002/path.2697
88. Yuan Q, Zhang X, Wei W, Zhao J, Wu Y, Zhao S, et al. Lycorine improves peripheral nerve function by promoting Schwann cell autophagy via AMPK pathway activation and MMP9 downregulation in diabetic peripheral neuropathy. Pharmacol Res. (2022) 175:105985. doi: 10.1016/j.phrs.2021.105985
89. Gao N, Ma B, Jia H, Hao C, Jin T, Liu X. Translocator protein alleviates allodynia and improves Schwann cell function against diabetic peripheral neuropathy via activation of the Nrf2-dependent antioxidant system and promoting autophagy. Diabetic Med. (2023) 40:e15090. doi: 10.1111/dme.15090
90. Wei X, Zheng Y, Ai Y, Li B. Regulatory effects of astragaloside IV on hyperglycemia-induced mitophagy in schwann cells. Evid Based Complement Alternat Med. (2022) 2022:7864308. doi: 10.1155/2022/7864308
91. Wang M, Xie M, Yu S, Shang P, Zhang C, Han X, et al. Lipin1 alleviates autophagy disorder in sciatic nerve and improves diabetic peripheral neuropathy. Mol Neurobiol. (2021) 58:6049–61. doi: 10.1007/s12035-021-02540-5
92. Khan I, Kaur S, Rishi AK, Boire B, Aare M, Singh MJB. Cannabidiol and beta-caryophyllene combination attenuates diabetic neuropathy by inhibiting NLRP3 inflammasome/NFκB through the AMPK/sirT3/nrf2 axis. Biomedicines. (2024) 12:1442. doi: 10.3390/biomedicines12071442
93. Chong ZZ, Menkes DL, Souayah N. Targeting neuroinflammation in distal symmetrical polyneuropathy in diabetes. Drug Discov Today. (2024) 29:104087. doi: 10.1016/j.drudis.2024.104087
94. Li W, Prakash R, Chawla D, Du W, Didion SP, Filosa JA, et al. Early effects of high-fat diet on neurovascular function and focal ischemic brain injury. Am J Physiol Regul Integr Comp Physiol. (2013) 304:R1001–1008. doi: 10.1152/ajpregu.00523.2012
95. Shi X, Chen Y, Nadeem L, Xu G. Beneficial effect of TNF-α inhibition on diabetic peripheral neuropathy. J Neuroinflamm. (2013) 10:69. doi: 10.1186/1742-2094-10-69
96. González-Clemente JM, Mauricio D, Richart C, Broch M, Caixàs A, Megia A, et al. Diabetic neuropathy is associated with activation of the TNF-alpha system in subjects with type 1 diabetes mellitus. Clin Endocrinol (Oxf). (2005) 63:525–9. doi: 10.1111/j.1365-2265.2005.02376.x
97. Doupis J, Lyons TE, Wu S, Gnardellis C, Dinh T, Veves A. Microvascular reactivity and inflammatory cytokines in painful and painless peripheral diabetic neuropathy. J Clin Endocrinol Metab. (2009) 94:2157–63. doi: 10.1210/jc.2008-2385
98. Priyadarsini N, Ramachandran M, Behera KK, Kiran S, Devi S. Association of serum NF-κB levels with peripheral neuropathy in type 2 diabetes mellitus patients: a pilot study. Horm Mol Biol Clin Investig. (2024) 45:27–33. doi: 10.1515/hmbci-2022-0105
99. Herder C, Bongaerts BW, Rathmann W, Heier M, Kowall B, Koenig W, et al. Differential association between biomarkers of subclinical inflammation and painful polyneuropathy: results from the KORA F4 study. Diabetes Care. (2015) 38:91–6. doi: 10.2337/dc14-1403
100. Zheng H, Sun W, Zhang Q, Zhang Y, Ji L, Liu X, et al. Proinflammatory cytokines predict the incidence of diabetic peripheral neuropathy over 5 years in Chinese type 2 diabetes patients: A prospective cohort study. EClinicalMedicine. (2021) 31:100649. doi: 10.1016/j.eclinm.2020.100649
101. Kovacs SB, Miao EA. Gasdermins: effectors of pyroptosis. Trends Cell Biol. (2017) 27:673–84. doi: 10.1016/j.tcb.2017.05.005
102. Jorgensen I, Miao EA. Pyroptotic cell death defends against intracellular pathogens. Immunol Rev. (2015) 265:130–42. doi: 10.1111/imr.12287
103. Man SM, Karki R, Kanneganti TD. Molecular mechanisms and functions of pyroptosis, inflammatory caspases and inflammasomes in infectious diseases. Immunol Rev. (2017) 277:61–75. doi: 10.1111/imr.12534
104. Galluzzi L, Vitale I, Aaronson SA, Abrams JM, Adam D, Agostinis P, et al. Molecular mechanisms of cell death: recommendations of the Nomenclature Committee on Cell Death 2018. Cell Death Differentiation. (2018) 25:486–541. doi: 10.1038/s41418-017-0012-4
105. Vasudevan SO, Behl B, Rathinam VA. Pyroptosis-induced inflammation and tissue damage. Semin Immunol. (2023) 69:101781. doi: 10.1016/j.smim.2023.101781
106. Mamun AA, Wu Y, Nasrin F, Akter A, Taniya MA, Munir F, et al. Role of pyroptosis in diabetes and its therapeutic implications. J Inflammation Res. (2021) 14:2187–206. doi: 10.2147/jir.S291453
107. Li X, Xiao GY, Guo T, Song YJ, Li QM. Potential therapeutic role of pyroptosis mediated by the NLRP3 inflammasome in type 2 diabetes and its complications. Front Endocrinol (Lausanne). (2022) 13:986565. doi: 10.3389/fendo.2022.986565
108. Huang Y, Xu W, Zhou R. NLRP3 inflammasome activation and cell death. Cell Mol Immunol. (2021) 18:2114–27. doi: 10.1038/s41423-021-00740-6
109. Cheng YC, Chu LW, Chen JY, Hsieh SL, Chang YC, Dai ZK, et al. Loganin attenuates high glucose-induced schwann cells pyroptosis by inhibiting ROS generation and NLRP3 inflammasome activation. Cells. (2020) 9:1948. doi: 10.3390/cells9091948
110. Wang Q, Li W, Zhang X, Chung SL, Dai J, Jin Z. Tauroursodeoxycholic acid protects Schwann cells from high glucose-induced cytotoxicity by targeting NLRP3 to regulate cell migration and pyroptosis. Biotechnol Appl Biochem. (2024) 71:28–37. doi: 10.1002/bab.2518
111. Wang J, Lu S, Yuan Y, Huang L, Bian M, Yu J, et al. Inhibition of schwann cell pyroptosis promotes nerve regeneration in peripheral nerve injury in rats. Mediators Inflammation. (2023) 2023:9721375. doi: 10.1155/2023/9721375
112. Haslbeck KM, Neundörfer B, Schlötzer-Schrehardtt U, Bierhaus A, Schleicher E, Pauli E, et al. Activation of the RAGE pathway: a general mechanism in the pathogenesis of polyneuropathies? Neurol Res. (2007) 29:103–10. doi: 10.1179/174313206x152564
113. Ratan Y, Rajput A, Pareek A, Pareek A, Kaur R, Sonia S, et al. Recent advances in biomolecular patho-mechanistic pathways behind the development and progression of diabetic neuropathy. Biomedicines. (2024) 12:1390. doi: 10.3390/biomedicines12071390
114. Wang J, Chen P, Han G, Zhou Y, Xiang X, Bian M, et al. Rab32 facilitates Schwann cell pyroptosis in rats following peripheral nerve injury by elevating ROS levels. J Transl Med. (2024) 22:194. doi: 10.1186/s12967-024-04999-x
115. He J, Li Z, Xia P, Shi A, FuChen X, Zhang J, et al. Ferroptosis and ferritinophagy in diabetes complications. Mol Metab. (2022) 60:101470. doi: 10.1016/j.molmet.2022.101470
116. Liang D, Minikes AM, Jiang X. Ferroptosis at the intersection of lipid metabolism and cellular signaling. Mol Cell. (2022) 82:2215–27. doi: 10.1016/j.molcel.2022.03.022
117. Jiang X, Stockwell BR, Conrad M. Ferroptosis: mechanisms, biology and role in disease. Nat Rev Mol Cell Biol. (2021) 22:266–82. doi: 10.1038/s41580-020-00324-8
118. Tang D, Chen X, Kang R, Kroemer G. Ferroptosis: molecular mechanisms and health implications. Cell Res. (2021) 31:107–25. doi: 10.1038/s41422-020-00441-1
119. Friedmann Angeli JP, Schneider M, Proneth B, Tyurina YY, Tyurin VA, Hammond VJ, et al. Inactivation of the ferroptosis regulator Gpx4 triggers acute renal failure in mice. Nat Cell Biol. (2014) 16:1180–91. doi: 10.1038/ncb3064
120. Wu KY, Deng F, Mao XY, Zhou D, Shen WG. Ferroptosis involves in Schwann cell death in diabetic peripheral neuropathy. Open Med (Wars). (2023) 18:20230809. doi: 10.1515/med-2023-0809
121. Hu M, Jiang W, Ye C, Hu T, Yu Q, Meng M, et al. Honokiol attenuates high glucose-induced peripheral neuropathy via inhibiting ferroptosis and activating AMPK/SIRT1/PGC-1α pathway in Schwann cells. Phytother Res. (2023) 37:5787–802. doi: 10.1002/ptr.7984
122. He K, Zhou X, Du H, Zhao J, Deng R, Wang J. A review on the relationship between Arachidonic acid 15-Lipoxygenase (ALOX15) and diabetes mellitus. PeerJ. (2023) 11:e16239. doi: 10.7717/peerj.16239
123. Yuan J, Amin P, Ofengeim D. Necroptosis and RIPK1-mediated neuroinflammation in CNS diseases. Nat Rev Neurosci. (2019) 20:19–33. doi: 10.1038/s41583-018-0093-1
124. Khoury MK, Gupta K, Franco SR, Liu B. Necroptosis in the pathophysiology of disease. Am J Pathol. (2020) 190:272–85. doi: 10.1016/j.ajpath.2019.10.012
125. Sun L, Wang H, Wang Z, He S, Chen S, Liao D, et al. Mixed lineage kinase domain-like protein mediates necrosis signaling downstream of RIP3 kinase. Cell. (2012) 148:213–27. doi: 10.1016/j.cell.2011.11.031
126. Guo J, Guo Z, Huang Y, Ma S, Yan B, Pan C, et al. Blockage of MLKL prevents myelin damage in experimental diabetic neuropathy. Proc Natl Acad Sci U S A. (2022) 119:e2121552119. doi: 10.1073/pnas.2121552119
127. Belavgeni A, Maremonti F, Stadtmüller M, Bornstein SR, Linkermann A. Schwann cell necroptosis in diabetic neuropathy. Proc Natl Acad Sci U.S.A. (2022) 119:e2204049119. doi: 10.1073/pnas.2204049119
128. Wu M, Li M, Liu W, Yan M, Li L, Ding W, et al. Nucleoporin Seh1 maintains Schwann cell homeostasis by regulating genome stability and necroptosis. Cell Rep. (2023) 42:112802. doi: 10.1016/j.celrep.2023.112802
129. Maiuri MC, Zalckvar E, Kimchi A, Kroemer G. Self-eating and self-killing: crosstalk between autophagy and apoptosis. Nat Rev Mol Cell Biol. (2007) 8:741–52. doi: 10.1038/nrm2239
130. Sorice M. Crosstalk of autophagy and apoptosis. Cells. (2022) 11:1479. doi: 10.3390/cells11091479
131. Sharma P, Kaushal N, Saleth LR, Ghavami S, Dhingra S, Kaur P. Oxidative stress-induced apoptosis and autophagy: Balancing the contrary forces in spermatogenesis. Biochim Biophys Acta Mol Basis Dis. (2023) 1869:166742. doi: 10.1016/j.bbadis.2023.166742
132. Fairlie WD, Tran S, Lee EF. Crosstalk between apoptosis and autophagy signaling pathways. Int Rev Cell Mol Biol. (2020) 352:115–58. doi: 10.1016/bs.ircmb.2020.01.003
133. Chung YC, Lim JH, Oh HM, Kim HW, Kim MY, Kim EN, et al. Calcimimetic restores diabetic peripheral neuropathy by ameliorating apoptosis and improving autophagy. Cell Death Dis. (2018) 9:1163. doi: 10.1038/s41419-018-1192-7
134. Demirtas L, Guclu A, Erdur FM, Akbas EM, Ozcicek A, Onk D, et al. Apoptosis, autophagy & endoplasmic reticulum stress in diabetes mellitus. Indian J Med Res. (2016) 144:515–24. doi: 10.4103/0971-5916.200887
135. Fernández A, Ordóñez R, Reiter RJ, González-Gallego J, Mauriz JL. Melatonin and endoplasmic reticulum stress: relation to autophagy and apoptosis. J Pineal Res. (2015) 59:292–307. doi: 10.1111/jpi.12264
136. Negi G, Kumar A, Sharma SS. Melatonin modulates neuroinflammation and oxidative stress in experimental diabetic neuropathy: effects on NF-κB and Nrf2 cascades. J Pineal Res. (2011) 50:124–31. doi: 10.1111/j.1600-079X.2010.00821.x
137. Oliveira-Abreu K, Cipolla-Neto J, Leal-Cardoso JH. Effects of melatonin on diabetic neuropathy and retinopathy. Int J Mol Sci. (2021) 23:100. doi: 10.3390/ijms23010100
138. Liao MF, Lu KT, Hsu JL, Lee CH, Cheng MY, Ro LS. The role of autophagy and apoptosis in neuropathic pain formation. Int J Mol Sci. (2022) 23:2685. doi: 10.3390/ijms23052685
139. Samir P, Malireddi RKS, Kanneganti TD. The PANoptosome: A deadly protein complex driving pyroptosis, apoptosis, and necroptosis (PANoptosis). Front Cell Infect Microbiol. (2020) 10:238. doi: 10.3389/fcimb.2020.00238
140. Shi C, Cao P, Wang Y, Zhang Q, Zhang D, Wang Y, et al. PANoptosis: A cell death characterized by pyroptosis, apoptosis, and necroptosis. J Inflammation Res. (2023) 16:1523–32. doi: 10.2147/jir.S403819
141. Wang Y, Kanneganti TD. From pyroptosis, apoptosis and necroptosis to PANoptosis: A mechanistic compendium of programmed cell death pathways. Comput Struct Biotechnol J. (2021) 19:4641–57. doi: 10.1016/j.csbj.2021.07.038
142. Zheng M, Kanneganti TD. The regulation of the ZBP1-NLRP3 inflammasome and its implications in pyroptosis, apoptosis, and necroptosis (PANoptosis). Immunol Rev. (2020) 297:26–38. doi: 10.1111/imr.12909
143. Basu P, Maier C, Averitt DL, Basu A. NLR family pyrin domain containing 3 (NLRP3) inflammasomes and peripheral neuropathic pain - Emphasis on microRNAs (miRNAs) as important regulators. Eur J Pharmacol. (2023) 955:175901. doi: 10.1016/j.ejphar.2023.175901
144. Sarhan J, Liu BC, Muendlein HI, Li P, Nilson R, Tang AY, et al. Caspase-8 induces cleavage of gasdermin D to elicit pyroptosis during Yersinia infection. Proc Natl Acad Sci U.S.A. (2018) 115:E10888–e10897. doi: 10.1073/pnas.1809548115
145. Hou W, Xie Y, Song X, Sun X, Lotze MT, Zeh HJ 3rd, et al. Autophagy promotes ferroptosis by degradation of ferritin. Autophagy. (2016) 12:1425–8. doi: 10.1080/15548627.2016.1187366
146. Sun Y, Zheng Y, Wang C, Liu Y. Glutathione depletion induces ferroptosis, autophagy, and premature cell senescence in retinal pigment epithelial cells. Cell Death Dis. (2018) 9:753. doi: 10.1038/s41419-018-0794-4
147. Yu T, Li L, Bi Y, Liu Z, Liu H, Li Z. Erythropoietin attenuates oxidative stress and apoptosis in Schwann cells isolated from streptozotocin-induced diabetic rats. J Pharm Pharmacol. (2014) 66:1150–60. doi: 10.1111/jphp.12244
148. Jiang L, Kon N, Li T, Wang SJ, Su T, Hibshoosh H, et al. Ferroptosis as a p53-mediated activity during tumour suppression. Nature. (2015) 520:57–62. doi: 10.1038/nature14344
149. Zhou Y, Shen Y, Chen C, Sui X, Yang J, Wang L, et al. The crosstalk between autophagy and ferroptosis: what can we learn to target drug resistance in cancer? Cancer Biol Med. (2019) 16:630–46. doi: 10.20892/j.issn.2095-3941.2019.0158
150. Li Y, Cao Y, Xiao J, Shang J, Tan Q, Ping F, et al. Inhibitor of apoptosis-stimulating protein of p53 inhibits ferroptosis and alleviates intestinal ischemia/reperfusion-induced acute lung injury. Cell Death Differ. (2020) 27:2635–50. doi: 10.1038/s41418-020-0528-x
151. Miller FD, Pozniak CD, Walsh GS. Neuronal life and death: an essential role for the p53 family. Cell Death Differ. (2000) 7:880–8. doi: 10.1038/sj.cdd.4400736
152. Jacobs WB, Kaplan DR, Miller FD. The p53 family in nervous system development and disease. J Neurochem. (2006) 97:1571–84. doi: 10.1111/j.1471-4159.2006.03980.x
153. Huo H, Zhou Z, Qin J, Liu W, Wang B, Gu Y. Erastin disrupts mitochondrial permeability transition pore (mPTP) and induces apoptotic death of colorectal cancer cells. PloS One. (2016) 11:e0154605. doi: 10.1371/journal.pone.0154605
154. Lee YS, Kalimuthu K, Park YS, Luo X, Choudry MHA, Bartlett DL, et al. BAX-dependent mitochondrial pathway mediates the crosstalk between ferroptosis and apoptosis. Apoptosis. (2020) 25:625–31. doi: 10.1007/s10495-020-01627-z
155. Lee YS, Lee DH, Choudry HA, Bartlett DL, Lee YJ. Ferroptosis-induced endoplasmic reticulum stress: cross-talk between ferroptosis and apoptosis. Mol Cancer Res. (2018) 16:1073–6. doi: 10.1158/1541-7786.Mcr-18-0055
156. Wu P, Zhang X, Duan D, Zhao L. Organelle-specific mechanisms in crosstalk between apoptosis and ferroptosis. Oxid Med Cell Longev. (2023) 2023:3400147. doi: 10.1155/2023/3400147
157. Hashemi M, Zandieh MA, Ziaolhagh S, Mojtabavi S, Sadi FH, Koohpar ZK, et al. Nrf2 signaling in diabetic nephropathy, cardiomyopathy and neuropathy: Therapeutic targeting, challenges and future prospective. Biochim Biophys Acta Mol Basis Dis. (2023) 1869:166714. doi: 10.1016/j.bbadis.2023.166714
158. Tang W, Chen X, Liu H, Lv Q, Zou J, Shi Y, et al. Expression of nrf2 promotes schwann cell-mediated sciatic nerve recovery in diabetic peripheral neuropathy. Cell Physiol Biochem. (2018) 46:1879–94. doi: 10.1159/000489373
159. Yang X, Yao W, Shi H, Liu H, Li Y, Gao Y, et al. Paeoniflorin protects Schwann cells against high glucose induced oxidative injury by activating Nrf2/ARE pathway and inhibiting apoptosis. J Ethnopharmacol. (2016) 185:361–9. doi: 10.1016/j.jep.2016.03.031
Keywords: diabetic peripheral neuropathy, Schwann cells, hyperglycemia, programmed cell death regulation, neuropathic pain (NP)
Citation: Wu L, Wang XJ, Luo X, Zhang J, Zhao X and Chen Q (2024) Diabetic peripheral neuropathy based on Schwann cell injury: mechanisms of cell death regulation and therapeutic perspectives. Front. Endocrinol. 15:1427679. doi: 10.3389/fendo.2024.1427679
Received: 07 May 2024; Accepted: 24 July 2024;
Published: 12 August 2024.
Edited by:
Xianshuang Liu, Henry Ford Hospital, United StatesReviewed by:
Dilip Sharma, Rutgers, The State University of New Jersey, United StatesEsma Nur Okatan, University of Istinye, Türkiye
Copyright © 2024 Wu, Wang, Luo, Zhang, Zhao and Chen. This is an open-access article distributed under the terms of the Creative Commons Attribution License (CC BY). The use, distribution or reproduction in other forums is permitted, provided the original author(s) and the copyright owner(s) are credited and that the original publication in this journal is cited, in accordance with accepted academic practice. No use, distribution or reproduction is permitted which does not comply with these terms.
*Correspondence: Qiu Chen, Y2hlbnFpdTEwMDVAY2R1dGNtLmVkdS5jbg==