- 1Centre for Emerging Diseases, Department of Biotechnology, Jaypee Institute of Information Technology, Noida, India
- 2Departments of Genetics, Dermatology and Pathology, Heersink School of Medicine, University of Alabama at Birmingham, Birmingham, AL, United States
- 3Center for Women’s Reproductive Health, Heersink School of Medicine, University of Alabama at Birmingham, Birmingham, AL, United States
Ovarian aging is a major health concern for women. Ovarian aging is associated with reduced health span and longevity. Mitochondrial dysfunction is one of the hallmarks of ovarian aging. In addition to providing oocytes with optimal energy, the mitochondria provide a co-substrate that drives epigenetic processes. Studies show epigenetic alterations, both nuclear and mitochondrial contribute to ovarian aging. Both, nuclear and mitochondrial genomes cross-talk with each other, resulting in two ways orchestrated anterograde and retrograde response that involves epigenetic changes in nuclear and mitochondrial compartments. Epigenetic alterations causing changes in metabolism impact ovarian function. Key mitochondrial co-substrate includes acetyl CoA, NAD+, ATP, and α-KG. Thus, enhancing mitochondrial function in aging ovaries may preserve ovarian function and can lead to ovarian longevity and reproductive and better health outcomes in women. This article describes the role of mitochondria-led epigenetics involved in ovarian aging and discusses strategies to restore epigenetic reprogramming in oocytes by preserving, protecting, or promoting mitochondrial function.
1 Introduction
The decline in reproductive aging is one of the main major risk factors associated with women’s health and higher morbidity and treatment-related adverse events for numerous aging-related anomalies (1, 2). Ovarian aging affects women’s reproductive function. Indeed, ovarian aging is linked to 22 age-related illnesses, which can be roughly classified into metabolic, cardiovascular, orthopedic, cancer, and neurological/cognitive disorders (3). Expanding recent research has shown that epigenetic modifications, in addition to genetic variables, are crucial for the onset and advancement of ovarian aging (1).
Epigenetic changes are inherited changes and without affecting the original DNA make-up, epigenetic modifications could lead to changed gene expression. These epigenetic changes are reported in both nuclear as well as mitochondrial DNA (mtDNA) though the understanding of mtDNA epigenetics has only recently gained recognition (4). Both these genomes communicate with each other and thus generate a coordinated bi-directional anterograde and retrograde gene response involving epigenetic processes (5).
Altered mitochondrial function influence ovarian function (6) via epigenetic modifications (7). The nuclear genome contains dynamic epigenetic marks, and a healthy mitochondrial metabolism is required to supply the intermediary metabolites required for the production and alteration of these epigenetic markers and thus regulate gene expression. Key metabolites that serve as co-substrates for epigenetic activities, include ATP, α-ketoglutarate (α-KG; also known as 2-oxoglutarate, 2-OG), β-nicotinamide adenine dinucleotide (NAD+), and acetyl coenzyme A (acetyl CoA). These metabolites are provided by mitochondria (8). Mitochondrial dysfunction (such as due to the reduction in mtDNA content and ATP levels) has been suggested as one of the characteristics of aging, and there is mounting evidence linking it to ovarian aging (6, 9). Further research into how to preserve, protect, and promote mitochondrial function in aging ovaries is needed to develop mitochondrial therapeutics for ovarian longevity to improve women’s reproductive health and reduce the risk of menopause-associated diseases.
This article primarily highlights the role of mitochondria in the epigenetic regulation of ovarian function and aging and provides strategies to prevent or delay the risk of major health issues associated with reproductive aging in females. We propose that a deeper comprehension of the crucial responsibilities, mitochondria play in regulating epigenetics in the ovaries, may lead to clinical advances in preventing or delaying ovarian aging. We describe approaches to target the epigenetic reprogramming in oocytes, to reduce the associated health risks.
2 Ovarian function and factors responsible for its aging
Ovarian aging is described as a biological loss of ovarian function, which results in menopause and infertility (10). Ovarian aging is characterized by a progressive decline in the number and quality of the follicles in the uterus. Unlike men, who reproduce indefinitely, women have few primary follicles that begin to decline as soon as they are born (11). Menopause occurs in most women about age 51 years of age; early menopause occurs before the age of 45 (12). Fertility is significantly impacted by the depletion of oocytes available for ovulation and fertilization. Consequently, menopause is a critical stage of reproductive aging that lasts for several decades and impacts women’s health (2).
As women navigate the diverse stages of life, their reproductive journey unfolds against the backdrop of intricate biological processes, genetic influences, and environmental factors. The gradual decline in ovarian function and fertility is underpinned by mitochondrial dysfunction, genomic instability, oxidative stress, genetic alterations, aneuploidy, epigenetic changes, gradual reduction in telomere length, senescence, stem cell depletion, and disrupted intercellular communication (1, 6). Together, these factors collectively contribute to the diminishing ovarian aging and reproductive health with advancing age, as summarized below (Figure 1).
2.1 Oxidative stress
Even though reactive oxygen species (ROS) are necessary for the physiological function of the ovary such as follicular growth and ovulation, higher levels of ROS are associated with cellular dysfunction and biomolecule damage, a major factor in ovarian aging. Studies indicate the age-related accumulation of ROS-induced biomolecular damage to DNA, lipids, and proteins in ovarian cells (13). Furthermore, the interaction of the biomolecules with ROS generates harmful products which further induce oxidative damage to the ovarian micro-environment. Advanced glycation end-products (AGEs) are one such product, generated through irreversible non-enzymatic interactions between amino groups in proteins, lipids, and nucleic acids with reducing carbohydrates like glucose. Oxidative damage is believed to be caused by the interaction between AGE and the AGE receptor (RAGE) (13). Furthermore, AGEs aggravate oxidative stress in the ovary by interacting with ROS and causing oxidative damage to the ovarian microenvironment. ROS and AGE interactions are linked to ovarian aging and reproductive dysfunction because AGEs activate proinflammatory pathways, degrade oocyte quality, and cause oxidative stress in the ovary. Interactions between AGE and RAGE receptors may also affect oocyte quality and vascular endothelial growth factor (VEGF) production, which may affect the outcomes of in vitro fertilization (IVF) and other reproductive treatments. Together, oxidative stress and AGE decrease oocyte function as quality, which contributes to ovarian aging. Thus it is critical to maintain a redox balance for reproductive health (10). Overproduction of ROS reduces antioxidant reserves, leading to oxidative stress, damage to DNA, and early aging (14). Another study found that long-term ROS exposure in mice reduces oocyte quality and fertility (15). Antioxidant therapy such as N-acetyl-L-cysteine (NAC) can reduce ROS damage, improve oocyte quality, and extend reproductive longevity (15).
2.2 Impaired DNA repair responses linked to genetic alterations and nutritional imbalance
Ovarian aging is regulated by the intricate relationship between genetic variables and DNA repair mechanisms. Genetic factors influence the age at which natural menopause (NM) begins. Over 100 of the genes like FMR1, MYADML, BRCA1/2, MCM8, POLG, NOBOX, PTEN, FIGLA, FOXO3, GPR3, POF1B, etc. have been associated with the start of premature ovarian insufficiency (POI) (16) (14). Recent studies including genome-wide association studies (17–19) also suggest the role of metabolic signaling networks and DNA damage response (DDR) pathways, in controlling ovarian aging. Studies indicate that early menopause and reduced oocyte reserves are also observed to be associated with dysfunctional DNA repair of mutated BRCA1 and BRCA2 genes, otherwise commonly linked to breast cancer (20–22). Ovarian aging is also accelerated by abnormalities in checkpoint signaling pathways. For instance, cell cycle checkpoint kinase 2 (CHK2) is vital for responding to DNA double-strand breaks (DSBs) and variations in CHK2 have been linked to NM (23). This highlights the significance of preserving genomic integrity to sustain ovarian function and fertility throughout the course of a female’s lifetime (14).
One of the top ranking reproductive disorder is POI and nutrients imbalance can cause such disorder. The components of nutrients found in daily meals include vitamins, carbohydrates, fat and lipoprotein, protein and polypeptide, and fruits and vegetables that contain phytoestrogens. Because of their proliferative, anti-inflammatory, antioxidant, and mitochondria protective properties during the menopausal transition, these nutrients are considered to be important factor (24).
2.3 Telomere shortening and aneuploidy
One of the effects of cell division is the shortening of telomeres, which are protective caps on chromosomes. Telomeres that are too short can abuse cell dysfunction and encourage oocyte death (11). The telomere length affects the onset of menopause as well as influences reproductive lifespan, making it an important factor in the advancement of ovarian age. Studies have indicated that women with shorter telomeres are linked to premature menopause and infertility, whereas those with longer telomeres usually have longer fertility and delayed menopause (25–27). Ovarian telomere attrition is caused by many factors such as oxidative stress and decreased telomere activity, which result in chromosomal abnormalities, aneuploidy in embryos, and decreased ovarian reserve (14, 28–30).
Aneuploidy, or an inappropriate number of chromosomes in oocytes, impacts the development of the embryo and results in miscarriage or implantation failure, especially in older women (31, 32). An increased risk of aneuploidy in aged oocytes is caused by discrepancies in chromatin segregation during meiosis I and II, spindle impairments, and improper kinetochore attachments (33, 34). A further risk factor is the age-related decrease in cohesiveness between sister chromatids and homologous chromosomes (34, 35). Improper chromosome segregation is made more difficult by the presence of univalent, which is the result of premature bivalent segregation (34, 36). These results highlight the intricate processes that underlie aneuploidy in mature oocytes (14).
2.4 Stem cell exhaustion
The supply of proliferating follicles is replenished by ovarian stem cells. Age-related decreases in the biomass of these stem cells can further reduce oocyte production (37). Our understanding of adult ovarian biology has been completely transformed by the identification of oogonial or germline stem cells (OSCs) in female mammals, which suggests a possible treatment for ovarian aging. These adult stem cells remain tissue-specific and have been the subject of several studies on a variety of mammalian species, including humans. These studies have revealed their characteristics and functions, including the ability to produce viable offspring both in vitro and in vivo through the fertilization of functioning oocytes. Recent research suggests OSC population heterogeneity may vary as they become more mature, maybe as a result of oxidative damage or genetic abnormalities (38). Despite significant debate regarding the OSC extraction process, studies have confirmed the existence of OSC smaller populations expressing certain surface markers, such as CD61 and ALDH activity (39, 40). Transcriptional profiling also identifies processes that are evolutionarily conserved and underlie the inactivity of OSC with aging, providing insight into possible targets for ovarian aging mitigation (37).
2.5 Altered intercellular communication
For the oocyte to function properly, communication between different cell types within the ovaries is important. Age-related alterations in this communication have the potential to impair oocyte health and follicular development (16). Alterations in cell connections during follicular development play a significant role in the etiology of both primary ovarian insufficiency (POI) and polycystic ovary syndrome (PCOS). Patients with PCOS have decreased expression of growth factors like GDF-9 along with altered gap junction (GJ) activity in cumulus-oocyte complexes (COCs), which may be a factor in abnormal follicular development. Moreover, in PCOS and POI, communication may be impaired by the refutation of transzonal projections (TZPs) during ovulation, which is controlled by the receptor for the epidermal growth factor signaling pathway (41). Oocyte competency and quality are also impacted by aging-related alterations in cellular communications in follicles. Findings on long non-coding RNAs (lncRNAs) in follicular fluid have shown that exosomes mediate intercellular communication, which provides insights into the pathophysiology of PCOS. The results of assisted reproduction may be improved by increasing cell interaction in developed follicles through approaches like collagenase therapy (42). Additionally, single-cell sequencing studies open up new research directions by illuminating the molecular dynamics driving follicular development and related disorders (43–45).
2.6 Epigenetic changes
Changes that affect the expression of genes without changing the DNA pattern itself are known as epigenetic changes that affect gene expression patterns essential to oocyte growth and function (11). Complex epigenetic modifications that go beyond sequence changes in DNA involve gene silencing, DNA modifications, and the impact of non-coding RNAs (ncRNA). Enzymes such as DNMT1, DNMT3A, and DNMT3B regulate DNA methylation, which is necessary for gene expression and cellular processes and may be involved in the aging process of the ovaries (10, 23, 46). Age-related alterations in histone modifications, such as acetylation and methylation, affect gene regulation and chromosomal integrity. Furthermore, ovarian diseases like PCOS and POF, follicle growth, and oocyte maturation are related to ncRNAs (47, 48). Though research on a variety of mammalian species has shown their varied roles and potential as therapeutic targets, their specific significance in natural ovarian aging is unclear (10). Such changes and their effects will be discussed in later sections.
2.7 Mitochondrial dysfunction, autophagy and loss of proteostasis
Mitochondria that produce energy are multifunctional organelles (6). As a hallmark of aging, mitochondrial dysfunction impairs oocyte development (49). Mitochondrial dysfunction can lead to aneuploidy because of abnormal chromosomal segregation during cell division and irregular spindle formation during fertilization. Impaired embryonic mitochondrial inheritance can affect the quality of oocytes and compromise female fertility (49).
Mitochondrial dysfunction also includes impaired autophagy, which is associated with ovarian aging and affect the health of oocytes. It is essential for eliminating damaged mitochondria and preserving oocyte quality for mitochondria to undergo autophagy, particularly via the PINK1-Parkin pathway and receptors such as BNIP3, NIX, and FUNDC1. Reduced fertility is partly caused by age-related abnormalities in this biological process. Improving mitochondrial autophagy can lead to better reproductive outcomes and higher-quality oocytes. Accurately quantifying mitochondrial autophagy is difficult, however. Overall, ovarian aging is associated with a decrease in mitochondrial quality control, including autophagy. This reduction in mitochondrial function and energy supply has an effect on the health of oocytes. Furthermore, in naturally aged mouse oocytes, the loss of proteostasis can impair microtubule stabilization and meiotic integrity, exacerbating the oocyte quality reduction (50)
3 Role of mitochondria in ovarian function
Besides many functions mitochondria support the energetic demands of ovarian cells, impacting oocyte maturation and overall fertility. Dysregulation of mitochondrial function influences ovarian health and reproductive outcomes (6, 51) The reproductive senescence, marked by the depletion of ovarian follicles includes the oocytes’ apoptosis as well as the follicular cells around them (52). Mitochondria, known to regulate apoptosis (53), are centrally involved in follicular atresia (54). In addition, the generation of steroid hormones like progesterone and estrogen, which are essential for luteal support, ovulation, and follicle formation, is also tightly regulated by mitochondria. Thus, healthy mitochondrial activity is important for overall ovarian function and longevity (Figure 2).
Mitochondrial dysfunction in oocytes disrupts ATP supply, impairing meiotic progression and increasing the risk of chromosome segregation errors, leading to aneuploidy. It also alters metabolic pathways, affecting nutrient uptake and biosynthesis critical for oocyte growth (55). Consequently, oocytes with impaired ATP production exhibit reduced developmental competence, resulting in decreased rates of fertilization, poor quality of the embryo, and compromised implantation potential (49) The majority of ROS produced internally, a hazardous end outcome of OXPHOS, are produced by dysfunctional mitochondria. This occurs when electrons are leaking from the respiratory chain pathway, causing accumulation of free radicals and disrupting the healthy functioning of mitochondria (56) Oocyte quality and ovarian function are impacted by damaged mitochondria resulting in a decline of mtDNA content and reductions in ATP synthesis (57, 58). Moreover, deterioration of mitochondrial function and a reduction in mitochondria-produced ATP transferred through mitochondrial permeability transition pores, which is triggered by oxidative stress, can promote spindle disassembly in mouse oocytes, a prominent factor leading to aneuploidy (59).
Additionally, the disruption of the membrane potential of mitochondria and dissemination of apoptotic factors contribute to cell death (60). This compromises cellular function, leading to impaired follicle development and decreased oocyte quality, contributing to declining fertility. Understanding mtPTPs could help develop interventions to preserve ovarian function and extend reproductive lifespan (61). A study demonstrated that ROS production and mPTP opening were increased in the premature ovarian failure group. As a result of this dysfunction in mitochondria, granulosa cell apoptosis occurred which increased the rate of aging in the ovaries (62)
Mitochondria are dynamic organelles that undergo constant fission and fusion. Large dynamin GTPases (DRP1, OPA1, MFN1, and MFN2) anchored in mitochondrial membranes mediate mitochondrial dynamics. Smaller mitochondria are produced by fission, which may promote cell division but also raise ROS levels. Fusion, on the other hand, lessens the aggregation of mtDNA mutations and damaged proteins by fostering communication between mitochondria and other cellular constituents (63). For organelles to closely complement one another and meet cellular energy needs, mitochondrial fusion is necessary (64). Ovarian function can be significantly impacted by mitochondrial proteins, like Dynamin-related protein 1 (Drp1) and Mitofusin 1 (Mfn1). While Drp1 deletion causes oocyte maturation problems that affect oocyte quality (65), Mfn1 deletion speeds up the loss of ovarian follicles (66). Premature ovarian insufficiency (POI), and normal ovarian aging demonstrate decreased mitochondrial biogenesis (6, 41)
Mitochondrial biogenesis ensures a sufficient amount of energy for implantation and the growth of the embryo (65, 67). The transcription factor A of mitochondria is primarily responsible for regulating the expression of mtDNA. TFAM, a ubiquitous transcription factor that targets the mitochondria and promotes mtDNA transcription and replication, and by respiratory factors 1 and 2 of the nucleus (NRF1 and NRF2), which regulate mtDNA expression and the expression of the respiratory chain components encoded by the nucleus. This ensures the essential association between the nuclear and mitochondrial genomes (68).
Dependent on NAD+, silent information regulator 1 (SIRT1) becomes an important regulator of mitochondrial dynamics. Throughout follicle development it regulates mitochondrial biogenesis, protects against oxidative stress, and upholds energy balance (69) Peroxisome proliferator-activated receptor-gamma co-activator 1 alpha (PGC1α) is deacetylated by SIRT1, highlighting its significance in regulating mitochondrial activity. TFAM is a downstream target molecule of the SIRT1/PGC1α pathway. Through the AMP-activated protein kinase (AMPK) pathway (70, 71), SIRT1 further modifies the mitochondrial landscape within ovarian granulosa cells by deacetylating PGC1α. This modulates the quality and function of mitochondria (72, 73).
Cumulus granulosa cells (cGCs) depend on mitochondria for vital energy for both growth and metabolic processes (74). In addition to supplying energy, mitochondria have a strong influence over important biological processes like autophagy, apoptosis, and antioxidant defense, all of which are vital for reproductive processes (75). Because they are the main originator of reactive oxygen species (ROS), they have a significant effect on important reproductive parameters like granulosa cell proliferation, follicular development, and oocyte quality (76). Research shows that females with decreased ovarian reserve (DOR) have lower expression of PGC-1α in oocyte and cumulus cells and lower activity of Sirtuin 3 (SIRT3) compared to those with normal ovarian reserve. This emphasizes a potential connection between subpar oocyte quality in IVF treatments and impaired mitochondrial biogenesis during oocyte maturation (41)
During egg development, mitochondrial biogenesis in the ovaries rises, guaranteeing enough ATP for fertilization and embryonic development. Poor oocyte quality may be a result of reduced mitochondrial biogenesis (77). In mouse models, for example, the lack of Caseinolytic Peptidase P (Clpp), a mitochondrial matrix peptidase that is responsible for quality control and proteostasis of mitochondria, causes an early reduction in ovarian follicles; this may be because the mTOR pathway is activated more than usual (78). This mTOR pathway causes aberrant mitochondrial biogenesis and replicates damaged mitochondria altering their function and in turn altering the reproductive functions. Using processes like Clpp-mediated proteostasis, mitochondria also govern the regulation of protein quality. Ovarian age is influenced by genetic differences in mtDNA. Addressing infertility and age-related ovarian decline requires an understanding of these mitochondrial processes (72).
Maternal transmission of mitochondria is a common process across various organisms, predominantly facilitated by the exclusive transmission of mtDNA through oocytes (79, 80). Over time, as women age, mitochondrial function declines. This deterioration in mitochondrial function impairs oocyte competence and follicular function, contributing significantly to the reduction in ovarian reserve and fertility observed with advancing maternal age (81). Mutations have been discovered through studies in mitochondrial DNA that could be connected to the emergence of PCOS (82)
In addition to determining the age of oocyte development and ovarian aging, mitochondria also affect the quantity and quality of ovocytes (83). Comprehending these pathways is crucial to formulate tactics to prevent untimely ovarian aging; yet, creating pharmacological remedies to revitalize mitochondria in this situation is still difficult. There are several implications associated with the abnormal functioning of mitochondria. Any anomaly can cause defects in the genetic, metabolic, and epigenetic factors associated with ovarian function.
4 Epigenetics of ovarian aging
Ovarian aging is significantly influenced by epigenetic modifications, which influence on expression of gene and cellular processes essential for ovarian function and reproductive health (84, 85). Age-related reduction in ovarian function can be attributed to dysregulated expression of genes inculpated in follicular development, steroid formation, DNA repair, and apoptosis caused by changes in epigenetic markers (1, 86) The quality of oocytes generated by the ovaries is influenced by epigenetic variations (87) Aging oocytes with aberrant epigenetic patterns increase the risk of infertility (88, 89), miscarriage, and birth abnormalities by causing genomic instability, mitochondrial dysfunction, and changed gene expression depiction (90, 91). These conditions also compromise the quality of the oocytes (92).
Reduced reproductive capacity and infertility arise from dysregulation of epigenetic pathways by altering the biological age, which can also speed up the onset of menopause, and hinder follicle recruitment and maturation (93, 94). By modifying the expression of genes that promote apoptosis or minimizing the expression of proteins that inhibit apoptosis in granulosa and oocyte cells, epigenetic modifications can accelerate follicular atresia (95). The ovarian reaction to environmental stimuli, such as exposure to endocrine-disrupting substances, stress, and nutritional variables, is also mediated by epigenetic changes (96). Damage from the environment can cause epigenetic changes in the ovaries, which can interfere with normal gene expression patterns and cellular processes linked to ovarian aging and declining fertility (97). The epigenetic changes in the nuclear and mitochondrial genome are responsible for altering ovarian function and reproductive longevity (Figure 3).
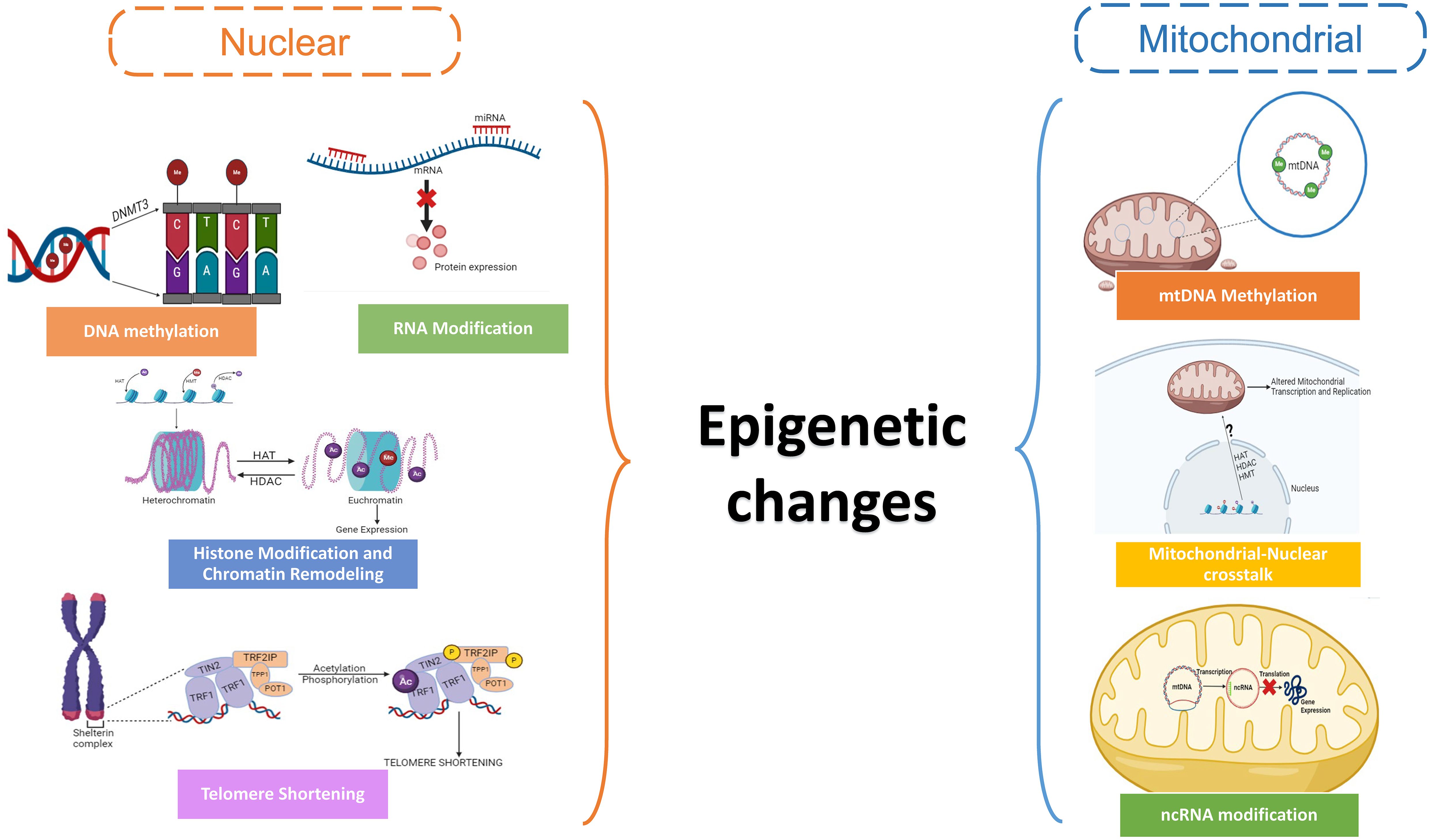
Figure 3. Schematic showing the epigenetic changes in the nuclear and mitochondrial genome in oocytes.
4.1 Epigenetic clocks
The simple unit of measurement for time, chronological age, is the number of years that an individual has been alive from birth (98). But throughout our lives, our body undergoes certain biological changes determined by various biological markers and functional abilities of the body that comprise the biological age of an individual (99). We can determine the biological age of an individual by analyzing the pattern of DNA methylation (as discussed later in the section) via the concept of epigenetic clocks. These clocks are predicated on the hypothesis that specific DNA methylation patterns undergo predictable changes with aging, mirroring cellular aging (100). Researchers can create models or algorithms that estimate a person’s biological age by examining these patterns. Numerous epigenetic clocks have been created to estimate the age of the ovaries and forecast the length of the reproductive life (101). By including nuclear epigenetic modifications, these clocks offer insights into the rate of ovarian aging and possible declines in fertility. The Horvath clock, created by Steve Horvath in 2013, is one of the most widely used epigenetic clocks. It is based on DNA methylation levels found by genome-wide DNA methylation profiling at specific CpG sites throughout the gene pool. The Horvath clock is renowned for its accuracy in estimating biological age across a range of populations and is relevant to a range of tissues and cell types. It has also demonstrated the ability to forecast several age-related events, such as the probability of death and susceptibility to disease (102). In 2013, another epigenetic clock was created by Gregory Hannum called the Hannum clock. It uses DNA methylation patterns to measure biological age, just like the Horvath clock. However, because the Hannum clock is specifically trained on blood sample methylation data, it’s especially applicable to research involving blood-based biomarkers. The Hannum clock is associated with the risk of mortality and age-related disorders, and it has been demonstrated to accurately predict chronological age (103). Newer addition to this, second-generation clocks have come into consideration. Developed by Morgan Levine in 2018, the GrimAge clock improves biological age prediction by incorporating other aging-related biomarkers beyond DNA methylation. The GrimAge clock takes into account plasma protein levels linked to aging, including metabolic health indicators and inflammatory markers, in addition to DNA methylation patterns. This multifaceted method improves age prediction accuracy and sheds light on the molecular mechanisms underlying aging and diseases associated with aging (104). Another epigenetic clock called the PhenoAge clock was created to calculate biological age and forecast health outcomes connected to aging. Unlike conventional clocks, it includes DNA methylation data together with clinical indicators including blood cell counts, renal function, and inflammatory markers (105). These epigenetic clocks have been an asset in obtaining key findings on how epigenetic changes affect aging (106).
4.2 Epigenetic changes in the nuclear genome
When women age, their ovaries’ nuclei change their epigenetic architecture. These changes are known as nuclear epigenetic modifications in ovarian aging. DNA methylation, histone modifications, chromatin remodeling, non-coding RNA regulation along with telomere dynamics are among the nuclear epigenetic changes associated with ovarian aging (107).
4.2.1 DNA Methylation
The process of adding methyl groups to the residues of cytosine in CpG dinucleotides is known as DNA methylation. Gene expression profiles change when ovarian aging occurs due to changes in DNA methylation patterns (108). Genes essential for ovarian function may be silenced by hypermethylation of promoter regions, whereas genes linked to aging may be abnormally activated by hypomethylation (5). During oocyte maturation, DNA methylation in the female germline of mice is reprogrammed, mainly by enzymes called DNA methyltransferases 3 (DNMT3s) (109). But, during oogenesis- the development of egg cells, both internal and external variables (9) have the potential to stop this process of epigenetic reprogramming. For instance, it has been demonstrated that maternal obesity and diabetes in mice change the DNA methylation patterns in oocytes, which may have an impact on the well-being of the offspring. Likewise, variations in DNA methylation across different organs and disease susceptibility have been connected to anomalies in one-carbon metabolism that are implicated in DNA methylation (110).
4.2.2 Histone modification
Multiple post-translational changes of histone proteins, such as the processes of acetylation, methylation, phosphorylation, and ubiquitination, affect the chromatin structure and the expression of genes. Histone changes in ovarian aging can impact chromatin accessibility to transcriptional machinery, which in turn can control the gene expression profiling related to hormone synthesis, cellular senescence, and follicular development (111). Aging is also linked to changes in histone modification levels, including H3K9me3 and H3K9ac, in both living things and lab conditions. These changes are controlled by enzymes like histone methyltransferases (HMTs), histone demethylases (HDMs), histone acetyltransferases (HATs), and histone deacetylases (HDACs) (112). HDACs are categorized into classes I, II, III (sirtuins or SIRTs) (113) (114), and IV, whereas HATs are grouped into type A and B superfamilies. Important epigenetic enzymes involved in oocyte aging that shield germ cells from oxidative damage include SIRTs, particularly SIRT1. By controlling the chromatin state, lowering oxidative stress, and preventing age-related illnesses, SIRT1 maintains genomic integrity. In aged oocytes, SIRT1, 2, and 3 restore aberrant mitochondrial distribution, mostly by controlling ROS. In addition, SIRT1 activity rises with aging in reproductive cells, delaying DNA damage and senescence (115). Reducing ovarian reserve or prolonged maternal age in women can have an impact on the ovarian microenvironment due to the regulation of metabolic processes by SIRT3 and SIRT5, which are localized in mitochondria (116)
Studies indicate that the regulation of longevity and aging is influenced by H3K4me3, which is linked to active gene expression, and H3K27me3, which is linked to gene silencing. While H3K4me3 levels can change with age based on the species and environment, H3K27me3 levels show distinct patterns in many organisms (117).
4.2.3 Chromatin remodeling
One of the main reasons for ovarian aging is alterations in chromatin structure. Changes in the compactness and arrangement of chromatin as women age might affect DNA accessibility to factors associated with transcription and regulatory proteins, which can affect the expression of genes (118). The aging-related reduction in ovarian function and fertility may be attributed in part to dysregulated chromatin remodeling. The control of gene expression during critical procedures such as corpus luteum development, ovulation, oocyte maturation, and the process of follicle formation and development depends on chromatin remodeling (119). The expression of particular genes at particular periods closely controls these activities, and chromatin remodeling helps to modulate gene expression by facilitating or obstructing other protein regulators and transcription factors access to the DNA (120).
The structure of chromatin in the ovaries is altered by several chromatin remodeling complexes and enzymes, including chromatin remodeling complexes dependent on ATP (like SWI/SNF, ISWI, and CHD complexes) (121, 122) and histone modifiers (like histone acetyltransferases, histone deacetylases, histone methyltransferases, and histone demethylases. Histone methyltransferases (HMTs) (123) and UHRF1 (124) are examples of epigenetic factors that interact with DNA replication machinery components, like the Proliferating cell nuclear antigen (PCNA) clamp (125), to repair chromatin structure after replication. Histone proteins can have chemical changes added or removed by these enzymes and complexes, changing the approachability of DNA to transcriptional machinery. By these pathways, chromatin remodeling regulates gene expression, which is important for ovarian development, hormone signaling, follicle growth, ovulation, and other activities that are necessary for healthy ovarian function. Infertility, PCOS (polycystic ovary syndrome), and ovarian cancer are among the reproductive illnesses that can result from the dysregulation of chromatin remodeling processes in ovaries (126).
4.2.4 RNA Modifications
Without changing the DNA sequence, epigenetic modifications in RNA are chemical changes that take place post-transcriptionally and control gene expression and other biological functions. The most common change is mRNA alteration, N6-methyladenosine (m6A), which affects translation, splicing, and RNA stability.5-methylcytosine (m5C) is a component of several RNA species, it regulates translation, affects RNA stability, and determines the fate of cells. RNA stability, translation efficiency, and structure are all impacted by N1-methyladenosine (m1A). Pseudouridine (Ψ) is abundant in non-coding RNAs and affects the structure and function of RNA. On ribose sugars, 2’-O-methylation (2’-O-Me) takes place, which influences the stability of RNA and its interactions with proteins. Adenosine-to-inosine (A-to-I) editing, a process that is mediated by ADAR enzymes, modifies the sequence and structure of RNA and has an impact on the production and function of proteins. These alterations play key roles in development, cellular homeostasis, and the pathophysiology of illness by dynamically regulating RNA processing, localization, and function (127). Non-coding RNAs control the transcriptional and post-transcriptional stages of gene expression but do not code for proteins. Examples of these RNA molecules are microRNAs (miRNAs) and long non-coding RNAs (lncRNAs) (128). Non-coding RNAs can interact with chromatin-modifying complexes within the nuclei of ovarian cells, thereby regulating gene expression patterns that are necessary for follicular development, oocyte maturation, and overall ovarian function. The age-related loss in ovarian function and fertility can be attributed to the dysregulation of non-coding RNAs in ovarian aging, which can affect gene expression networks (129)
4.2.5 Telomere dynamics
At the tip of chromosomes, repeated DNA sequences called telomeres shield the ends against fusion and breakdown. Because of the end replication issue, telomeres shrink within the nucleus of ovarian cells with every cell division (130, 131). A protein complex called shelterin, attaches to telomeric DNA to shield telomeres against deterioration and improper DNA repair procedures. Telomere function and stability are impacted by epigenetic alterations, such as protein phosphorylation and acetylation within the shelterin complex, which control the complex’s interactions with telomeric DNA and related components (132). Cellular senescence and aging are linked to telomere shortening. Telomere dynamics in ovarian aging can affect follicular cells’ ability to replicate, which can decrease follicle formation and reduce ovarian reserve.
4.3 Epigenetic changes in mitochondrial genome
The epigenetic management of mitochondrial DNA (mtDNA) and mitochondrial function within ovarian cells varies as women age, a process known as mitochondrial epigenetic modifications in ovarian aging. Although mitochondria have DNA that is distinct from nuclear DNA, they can experience epigenetic changes that can affect how they function and speed up the aging process in ovaries (4) Recent research has revealed low amounts of methylation in mtDNA by using techniques like bisulfite sequencing (133) and methylated DNA immunoprecipitation (MeDIP) (134), especially in the regulatory D-loop region, despite the genetic material being long thought to be unmethylated. The control of mitochondrial transcription and replication has been linked to methylation of mtDNA. Mutations in mtDNA methylation patterns may arise with ovarian age, impacting the expression and function of mitochondrial genes. Age-related ovarian function reduction and mitochondrial malfunction have been linked to altered mtDNA methylation (135). The expression and function of mitochondrial genes can be impacted by modifications to the packing and structure of mitochondrial DNA. Nucleoids, which are arranged into nucleoprotein complexes from mitochondrial DNA, are crucial for transcription and mtDNA preservation (136). Ovarian cells may have mitochondrial malfunction and reduced energy production as a result of changes in nucleoid structure and organization in aging mitochondria, which may affect the efficiency of replication and transcription of the mtDNA (137).
Despite the absence of histones in mitochondria, there is evidence that histone-modifying enzymes encoded in nuclear DNA localize to mitochondria and may alter the mtDNA’s epigenetic state (138). Mitochondrial transcription and replication can be influenced by histone changes which can impact mitochondrial function. A variety of non-coding RNAs, such as long non-coding RNAs (lncRNAs) and microRNAs (miRNAs), are said to be linked to the regulation of mitochondrial function (139). Aging tissues have been shown to exhibit dysregulation of ncRNAs, and it is conceivable that changes in ncRNAs that target mitochondria may be a factor in the malfunctioning of mitochondria during ovarian aging (140). There are various metabolic components of the mitochondria that contribute to the regulation of the above processes, potentially reducing the effect of these epigenetic changes.
5 Mitochondrial metabolites regulate epigenetic changes
Mitochondrial metabolites play an important role in cellular function and influence epigenetic alterations. These metabolites, such as acetyl-CoA, alpha-ketoglutarate, and S-adenosylmethionine, serve as cofactors and catalyst substrates for enzymes that modify DNA and histones, thereby affecting gene expression (Figure 4). Alterations in mitochondrial metabolite levels can impact follicular development, oocyte maturation, and overall ovarian function. Dysregulation of these metabolites may contribute to conditions such as polycystic PCOS and POI. Therefore, studies aiming to understand the interplay between mitochondrial metabolites and epigenetic modifications can offer insightful information on ovarian function, aging, and longevity (Table 1).
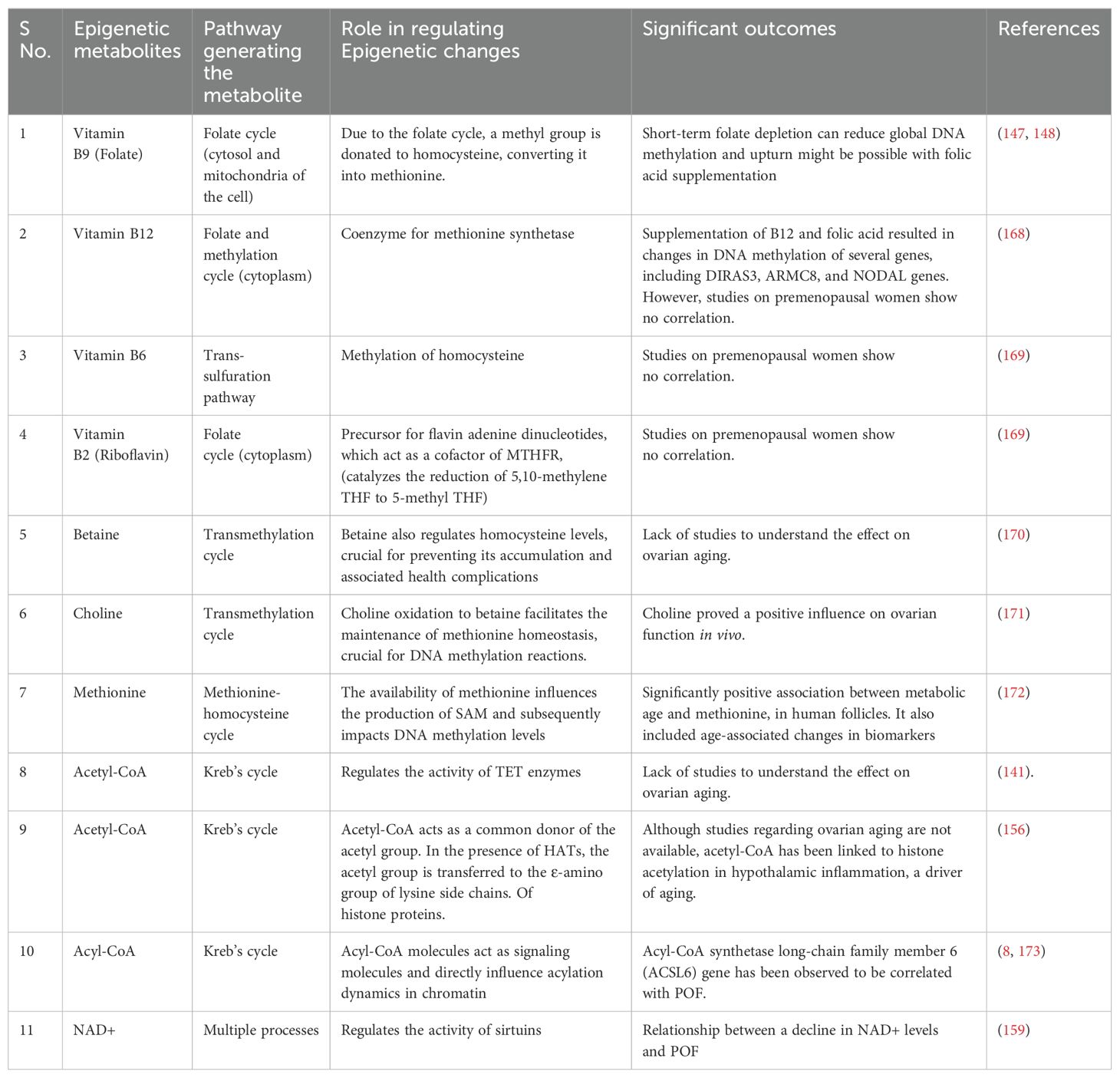
Table 1. Studies summarizing the role of mitochondrial metabolites in regulating the epigenetic changes.
5.1 Mitochondrial metabolite regulating DNA methylation
Ovarian aging is a multidimensional process marked by declining fertility and alterations in oocyte quality (106). Central to this phenomenon are epigenetic modifications, particularly DNA methylation, which wield significant influence over gene expression during pivotal stages of ovarian development. Metabolites, small molecules deeply entrenched in cellular processes, serve as crucial substrates or cofactors in the intricate machinery governing DNA methylation. Grasping the profound impact of these metabolites on ovarian aging offers valuable insights into potential avenues for therapeutic intervention.
DNA methylation, a fundamental epigenetic mechanism, entails the addition of methyl groups to cytosine residues within CpG dinucleotides (141). This biochemical modification primarily occurs at gene promoters and regulatory regions, exerting profound effects on gene expression dynamics. Within aging ovaries, perturbations in DNA methylation patterns emerge, affecting genes crucial for oocyte development, follicular growth, and hormonal regulation.
At the forefront of DNA methylation regulation stands DNA methyltransferase 1 (DNMT1), an enzyme pivotal for preserving methylation patterns. DNMT1 utilizes S-adenosylmethionine (SAM) as a substrate to transfer methyl groups onto the DNA molecule, a process that generates S-adenosylhomocysteine (SAH), subsequently converted to homocysteine (Hcy) (142, 143). This enzymatic cascade ensures the faithful replication of methylation patterns during DNA replication, safeguarding epigenetic inheritance. Beyond DNMT1, a cadre of other enzymes orchestrates the dynamic regulation of DNA methylation patterns. DNMTs, including DNMT3a and DNMT3b, serve as de novo methyltransferases, laying down methyl marks during embryogenesis and cellular differentiation (144, 145). Their concerted actions establish tissue-specific methylation patterns and fine-tune gene regulatory networks critical for ovarian function. In a 2020 study, Uysal and Öztürk analyzed the ovaries of mice at various stages of aging, including prepubertal, pubertal, post-pubertal, and aged (146). Their findings indicated a consistent and significant decline in the expression of DNMT1, DNMT3a, and DMNT3l global DNA methylation patterns, as well as genes at the mRNA and protein levels, as the mice transitioned from youth to old age. These observations led them to infer that the notable changes in the expression of DNMT and levels of global DNA methylation during the process of ovarian aging could potentially be a contributing factor to the onset of infertility in females in their later years.
The ten-eleven translocation (TET) dioxygenases emerge as key players in DNA demethylation, oxidizing methyl groups of cytosine residues. This passive demethylation process, occurring during DNA replication, facilitates the reintroduction of unmethylated cytosines into the DNA molecule, contributing to epigenetic plasticity. Regulation of TET enzyme activity hinges upon AMP-activated protein kinase (AMPK)-mediated phosphorylation, sensitive to cellular energy status and glucose levels. Perturbations in AMPK signaling, particularly under hyperglycemic conditions, disrupt TET enzyme function, altering DNA methylation dynamics.
Metabolites derived from metabolic pathways intricately modulate DNA methylation processes. Acetyl-CoA, a central metabolite in cellular energy metabolism, emerges as a critical regulator of TET enzyme activity. Generated from pyruvate within the Krebs cycle, acetyl-CoA influences the function of TET enzymes, thereby shaping DNA methylation patterns (141).
Folate (Vitamin B9), an indispensable member of the B-vitamin complex, assumes a central role in DNA methylation by serving as a primary methyl donor. Its involvement in one-carbon metabolism, pivotal for methyl group supply, underscores its significance in epigenetic regulation. The folate cycle, primarily occurring in mitochondria, facilitates the synthesis of DNA and RNA building blocks while intricately linked to the methionine cycle, critical for S-adenosylmethionine (SAM) production. Genetic polymorphisms, such as the MTHFR C677T variant, disrupt folate metabolism, impacting DNA methylation patterns. Studies reveal dynamic aberrations in DNA methylation levels globally in response to folate intake, emphasizing the direct influence of dietary factors on epigenetic modifications. Research indicates that folate intake impacts DNA methylation levels in postmenopausal female lymphocytes. A folate-deficient diet led to decreased methylation, while a folate-rich diet increased it (147). In older women (60-85 years), a moderately folate-depleted diet resulted in reduced leukocyte DNA methylation, which didn’t significantly improve even with a folate-replete diet (148). A study also found that a 10-week folic acid supplement intervention increased DNA methylation in leukocytes by 31% (7). These findings imply that short-term folate depletion can reduce methylation of DNA globally, and recovery might be possible with folic acid supplementation, although its effectiveness may decrease with age.
Vitamin B12, another essential member of the B-vitamin complex, interfaces with DNA methylation through its involvement in one-carbon metabolism. Acting as a coenzyme for methionine synthase, vitamin B12 facilitates the conversion of homocysteine to methionine, a pivotal step in DNA methylation reactions. Kok (149) found that Numerous genes’ DNA methylation changed as a result of long-term folic acid and vitamin B12 supplements. After the intervention, there was a difference in the methylation of 163 sites from the baseline. Between the intervention and placebo groups, there were notable differences in six locations. Notably, alterations were discovered in the genes NODAL, ARMC8, and DIRAS3, which are connected to early embryonic development and carcinogenesis.
Moreover, vitamin B6 and riboflavin contribute to DNA methylation by supporting the folate cycle. These B vitamins aid in methyl group transfer, modulating DNA methylation dynamics and the profiles of gene expression. Choline and betaine, integral components of one-carbon metabolism, indirectly influence DNA methylation dynamics (150). Choline oxidation to betaine facilitates the maintenance of methionine homeostasis, crucial for DNA methylation reactions. Inadequate choline intake during pregnancy disrupts offspring DNA methylation patterns, highlighting the importance of maternal nutrition in epigenetic programming (151). Betaine also regulates homocysteine levels, crucial for preventing its accumulation and associated health complications. The intricate interplay between these metabolites underscores their collective impact on DNA methylation dynamics and ovarian aging processes (152).
Lastly, methionine, an indispensable amino acid, serves as a critical precursor of SAM, which is the main methyl donor in processes involving DNA methylation. Fluctuations in methionine levels exert profound effects on DNA methylation patterns, underscoring its pivotal function in the control of epigenetics. The production of SAM from methionine is essential for DNA methylation, as SAM is required by DNA methyltransferases (DNMTs) to catalyze the addition of methyl groups to DNA (150). Therefore, the availability of methionine influences the production of SAM and subsequently impacts DNA methylation levels. Increasing dietary intake of methionine has been speculated to potentially enhance DNA methylation by providing more substrate for SAM production.
The serine cycle, which is involved in de novo ATP synthesis, plays a crucial role in DNA methylation. Regardless of the methionine status, the serine cycle contributes to DNA methylation by providing the necessary ATP for the generation of SAM from methionine. This highlights the interconnectedness of various metabolic pathways in regulating DNA methylation processes.
5.2 Mitochondrial metabolite regulating histone modification
Histone modification is a key epigenetic mechanism that regulates gene expression. It involves the addition or removal of chemical groups to histone proteins, which are responsible for packaging DNA into a compact, organized structure called chromatin (9). The most common types of histone modifications include methylation, acetylation, phosphorylation, and ubiquitination (107). These modifications can alter the structure of the chromatin, thereby influencing gene accessibility and transcriptional activity. In the context of aging, changes in histone modifications have been observed in various organisms and tissues. For instance, alterations in histone acetylation and methylation patterns have been associated with ovarian aging (153). These findings suggest those histone modifications, and the enzymes that regulate them, could be potential targets for interventions aimed at modulating the aging process.
Amongst the metabolites that regulate histone acetylation, acetyl-CoA serves as the primary acetyl donor. Histone acetylation is catalyzed by histone acetyltransferases (HATs), which transfer an acetyl group from acetyl-CoA to specific lysine residues on histone proteins. The availability of acetyl-CoA is sensitive to nutrient availability and metabolic reprogramming, which can impact histone acetylation patterns and gene expression (154, 155). Acetyl-CoA is at the intersection of diverse metabolic pathways, and its availability is influenced by various metabolic processes. The crosstalk between metabolism and histone acetylation mediated by acetyl-CoA highlights the intricate relationship between cellular metabolism and epigenetic regulation. In 2021, Bradshaw examined that nuclear acetyl-CoA also plays a role in promoting longevity by increasing histone acetylation (156). Inhibitors of class I histone deacetylases (HDACs) increase longevity through increased histone acetylation.
Likewise, Acyl-CoA is a group of metabolic intermediates that play a crucial role in cellular metabolism and energy production. Acyl-CoA molecules serve as substrates for various enzymatic reactions, including fatty acid oxidation, fatty acid synthesis, and the tricarboxylic acid (TCA) cycle. Concentrations of different acyl-CoA metabolites in cells are tightly correlated with the relative abundances of acyl marks on histones. These metabolites act as signaling molecules and directly influence acylation dynamics in chromatin, linking cellular metabolism with epigenetic regulation (157). The Acyl-CoA synthetase long-chain family member 6 (ACSL6) gene has been connected to early ovarian failure (POF), according to a study (8). The study was conducted using genome-wide association research using dense single nucleotide polymorphisms as genetic markers and linkage disequilibrium-based methodology. The ACSL6 gene, located on chromosome 5q31, was found to be associated with POF, and disease-sensitive haplotypes were identified.
NAD+ is a critical coenzyme engaged in several cellular functions, including metabolism, DNA repair, and gene expression regulation. In the context of histone modifications, NAD+ has a major part in controlling the activity of sirtuins, a class of histone deacetylases that remove acetyl groups from histone proteins (158). NAD+ availability influences the deacetylation of histones by sirtuins, thereby affecting chromatin structure and gene expression. Changes in NAD+ levels can impact the ratio of acetylation to deacetylation in histones, leading to alterations in gene transcription and cellular responses to environmental cues. A recent study establishes causality between several age-related illnesses and a reduction in NAD+ levels. It also highlights promising results from strategies using NAD+ precursors to promote NAD+ biosynthesis, which could substantially improve oocyte quality and alleviate ovarian aging (159). In summary, NAD+ influences oocyte quality by enhancing energy production, DNA repair, and cellular resilience. Its anti-inflammatory effects and impact on SIRT1 activation contribute to alleviating ovarian aging. Researchers continue to explore NAD+ as a potential therapeutic avenue for reproductive health (160).
5.3 Mitochondrial metabolite regulating chromatin remodeling
The term “chromatin remodeling” describes the dynamic alterations in the composition and arrangement of chromatin, the complex of proteins and DNA that make up chromosomes in the nucleus of a cell. It involves the alteration of the accessibility of DNA to the cellular machinery, allowing for the regulation of the expression of genes and other genome-associated processes. This remodeling might not always be enzymatic and may use metabolites as regulators.
A by-product of amino acid metabolism, lipid metabolism, and glycolysis, methylglyoxal (MGO) can form covalent adducts with nucleophilic functional groups on histones and DNA. Chronic illnesses and aging are intimately associated with the production of MGO adducts known as advanced glycation end products (AGEs). MGO glycation of histones can destabilize the nucleosome structure and disrupt the landscape of chromatin modifications (161). A study focused on the accumulation of AGEs in the follicular fluid (FF), a liquid that surrounds the ovum in the ovaries. This fluid plays an important role in the maturation of the egg and its readiness for fertilization. The concentration of AGEs in the FF could potentially affect various aspects of assisted reproduction, such as ovarian responsiveness, embryo quality, follicular development, fertilization, embryonic development, and achievement of pregnancy (162).
In addition, 2-oxoglutarate has been found to encourage naïve embryonic stem cells (ESC cells to renew them) by increasing histone H3K27me3 demethylation. It has been observed that the addition of 2-oxoglutarate potentially increases the expression of pluripotency genes in induced pluripotent stem cell (iPSC) reprogramming by removing H3K9me3 and H3K36me3 marks (163). A study suggested that α-KG treatment can ameliorate detrimental effects on female rats’ fertility, potentially as a result of ovarian granulosa cell apoptosis being reduced and glycolysis being restored (164).
5.4 Mitochondrial metabolite regulating RNA modification
RNA modification refers to the process of chemically modifying RNA molecules, which can impact their structure, stability, and function. Methylation, which involves adding a methyl group to the RNA molecule, is one of the most prevalent RNA modifications. This modification is facilitated by a class of enzymes referred to as writers, which include proteins such as Mettl3, Mettl14, and Mettl16 (165).
m6A is a common RNA modification known as N6-methyladenosine. It is done when a methyl group is added to the adenosine base of RNA molecules. m6A modification is essential for controlling a variety of aspects of RNA metabolism, including stability of the RNA, splicing, translation, and degradation. It has a role in controlling the expression of genes. and cellular processes (165). m6A modification is reversible, and metabolites operate as substrates and/or allosteric regulators to directly affect the reaction’s flow. Metabolic processes, such as one-carbon metabolism and the tricarboxylic acid cycle, produce metabolites that affect the activities of m6A writers and erasers. For example, S-adenosyl methionine (SAM) is a methyl donor for m6A modification, while S-adenosyl homocysteine (SAH) inhibits writer activity (166).
A recent study suggests that m6A modification poses a significant role in female reproductive health and disease, and further research in this area could lead to improved understanding and potential therapeutic strategies (167).
6 Ovarian aging confers high risk of diseases
Hormonal, tissue, and cellular changes are all part of ovarian aging, which also includes a notable reduction in the number and quality of oocytes (174). These alterations have systemic impacts on many organs throughout the body in addition to impairing reproductive function. Decrease in important hormones like progesterone and estrogen impact many physiological functions and increase age-related diseases like osteoporosis, cardiovascular disease, and cognitive decline (175). Numerous age-related conditions, such as metabolic syndrome, osteoporosis, cardiovascular disease, cognitive decline, and several malignancies, are brought on faster by menopause (176). These diseases are more likely to occur during the transition to menopause, which is characterized by a considerable decrease in estrogen levels. After menopause, the risk of cardiovascular disease increases two to three times, annual bone loss is one to two percent, and 30 to 60 percent of postmenopausal women have metabolic syndrome (177). Postmenopausal women also have a significantly increased risk of mood disorders, ovarian and breast malignancies, and cognitive deterioration. These figures highlight how critical it is to manage the health issues that come with aging and menopause in women (178). Thus, beyond fertility, ovarian aging has a wider impact on women’s aging process by gradually lowering their quality of life and health (175).
POF is a significant health concern that brings about the cessation of ovarian function before the age of natural menopause. It affects up to one in 100 women, including one in 1000 under thirty years old (179). This condition is associated with a multitude of health problems and complications. One of the most pressing issues associated with POF is infertility, as the premature dissipation of ovarian activity leads to a decrease in the number of viable eggs, making natural conception a challenge. In addition to fertility issues, women with POF experience a hormonal imbalance characterized by lower levels of estrogen (180). This imbalance can manifest in several signs and symptoms, including vaginal dryness, nocturnal sweats, hot flashes, and decreased sexual desire. Moreover, this hormonal imbalance can lead to mood changes, including depression and anxiety (181).
Furthermore, low estrogen levels associated with POF can increase the risk of several health aberrations, such as osteoporosis, urogenital atrophy, cardiovascular disease, and hypothyroidism (180, 182). The diagnosis of POF can also have a significant psychological impact, leading to feelings of anger, depression, and anxiety, and can affect a woman’s self-esteem and body image.
Lastly, the symptoms associated with POF and the resulting infertility can significantly impact a woman’s quality of life. In conclusion, POF is a complex condition with far-reaching implications for a woman’s health, fertility, and overall quality of life. To comprehend this illness better and create efficient remedies, further research is required (183).
Gilmer (184), described how a drop in estrogen levels increases the risk of cardiovascular diseases as the said hormone is necessary to maintain healthy blood vessels and cholesterol levels. Furthermore, estrogen also possesses neuroprotective effects, and its decline during menopause is linked to a higher risk of neurodegenerative illnesses and cognitive impairment as seen in Alzheimer’s disease. It is also associated with an increase in the risk of musculoskeletal disorders such as osteoporosis and osteoarthritis. An essential component to retaining bone density is estrogens., and its decline during menopause can contribute to bone loss and increased fracture risk. Menopausal women are also at an increased chance of developing metabolic diseases like diabetes and obesity as hormonal changes can affect insulin sensitivity and metabolism, leading to disruptions in blood sugar regulation.
Further, delaying the process of ovarian aging can hold potential benefits (140). It can extend the reproductive lifespan of women, providing more time for family planning (123, 185). Women with delayed ovarian aging may experience a slower decline in reproductive function and may have a higher chance of maintaining regular menstrual cycles, endocrine homeostasis (186), and hormonal balance (187). They are also at a lower risk for a range of conditions, including cardiovascular disease, dementia, retinal disease, depression, and osteoporosis. It may also be associated with a lower risk of certain age-related diseases, such as breast and ovarian cancer. Studies have shown that genetic factors linked to delayed ovarian aging, such as BRCA mutations, may confer benefits in terms of enhanced fertility and improved fitness (3). Additionally, delayed ovarian aging may have evolutionary advantages. It may allow for the selection of higher-quality oocytes and embryos, leading to improved reproductive success and offspring quality (185).
Several pharmacological strategies have been explored to help delay ovarian aging which include antioxidants such as vitamins C, E, N-acetyl-L-cysteine, and proanthocyanidin; coenzymes Q10 to help with mitochondrial dysfunction and calorie restriction mimetics like metformin to prolong the delaying process (188). These work by following different mechanisms such as reducing oxidative stress using anti-oxidants, targeting mitochondrial dysfunction, mimicking calorie restriction strategies, using telomerase activators, and inhibiting the mTORC1 pathway to prevent over-activation of the primordial follicle pool, which is associated with ovarian aging (189).
7 Strategies to preserve ovarian function for ovarian longevity
As per the previous discussion, multiple factors such as Genetic alterations, epigenetic factors, ROS and oxidative stress, aneuploidy, mitochondrial dysfunction, and telomere shortening are responsible for ovarian aging. However, mitochondrial dysfunction and epigenetic alterations seem to be some of the potential factors responsible for ovarian aging. Hence in the recent past, a significant number of studies have been conducted to alter the cellular energy and/or epigenetic profile of ovarian cells (Figure 5).
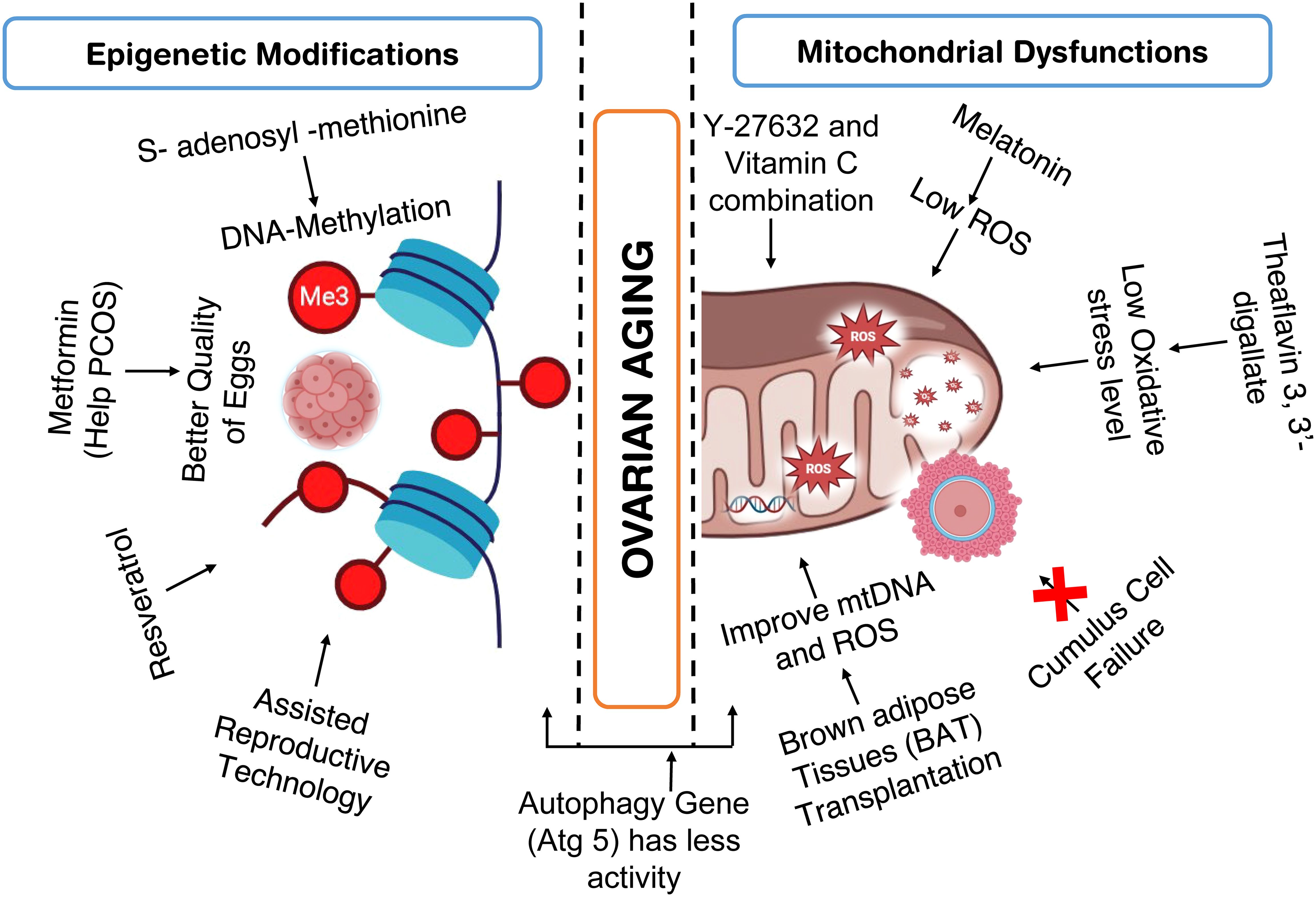
Figure 5. Studies conducted to improve ovarian health by addressing epigenetic modification and mitochondrial dysfunction.
7.1 Targeting mitochondria energetics of ovarian cells
Mitochondria are critical targets for preserving ovarian function (6, 190). Potential the mitochondrial membrane potential and mitochondrial oxidative activity complex decline with age, as do endogenous antioxidant system activities, indicating that redox imbalance plays a more prominent character in mature cells and tissues (191).
Melatonin treatment reduces the production of mitochondrial reactive oxygen species (mROS), prevents cell death, stops the collapse of the mitochondrial membrane potential, and maintains the functions of the respiratory chain complex in ovarian mitochondria (192). As melatonin, a circadian hormone reduces oxidative stress and inflammatory reactions to enhance the ability of endometrium to respond and preserve uterine homeostasis (2). Because of its anti-inflammatory and antioxidant qualities, melatonin is recognized to offer anti-aging benefits (193). In addition to controlling the body’s seasonal and circadian rhythms, melatonin (MT) can also delay the aging of ovaries, control their biological rhythm, encourage the development of follicles, and enhance the quality and rate of fertilization of oocytes. Additionally, the effects of MT on a number of ovarian-related disorders were examined, with ovarian aging and polycystic ovary syndrome (PCOS) receiving special attention (194). Supplemental melatonin use may help prevent infertility later in life, ease the frustration of women who are delaying having children, lessen the need for IVF-ET procedures, and assist in resolving the issue of non-aging-related infertility in women for the duration of their reproductive years (193). Female mice given melatonin showed increased mitochondrial antioxidant activity, lowering the risk of oxidative damage of the mitochondria caused by free radicals (192), Notably, melatonin administration increases SIRT3 activity and enhances the binding capability of FoxO3a to the regulators of both enzymes, namely superoxide dismutase 2 (SOD2) and catalase (CAT) (192).
Comparably, Theaflavin 3, 3’-gallate (TF3), extracted from black tea and serves as a bioactive polyphenol, possesses antioxidant qualities that may aid in maintaining the primordial follicle pool, restoring the estrous cycle, and boosting the number of progenies in old mice (190). TF3 exhibits a positive correlation with oocyte retrieval, oxidative stress levels, and spindle abnormalities. These findings suggest that TF3 might positively affect female reproduction and ovarian function by delaying ovarian aging, as evidenced by its ability to maintain ovarian reserve, shorten the estrous cycle, and increase litter size (190).
A study examined the relationship between aging-related declines in ovarian activity and fertility and the mitochondrial function of cumulus cells (CCs). Researchers discovered that older age groups of women with reduced ovarian reserve contained less and lower-quality eggs recovered. Their cumulus cells also had reduced energy output, altered structural characteristics, and decreased activity all indicators of mitochondrial malfunction. This study suggests that a major contributing element to the age-related loss in female fertility may be a mitochondrial failure in cumulus cells (195, 196).
Recent studies indicate that Vit C and Y27632 directly affect mitochondrial biogenesis and enhance their ability to maintain the youth of cellular aging. These agents were employed to dual-control both the metabolism of energy and mitochondrial mass. Y-27632 and vitamin C combination were found to effectively improve the quality of old cells (197). Increasing mitochondrial fusion appears to mitigate excessive mitochondrial fragmentation in old cells, reduces oxidative stress, and maintains mitochondrial membrane potential (197).
7.2 Approaches to alter the epigenetic profile of ovarian cells
Bacon reported that age and reproductive senescence are associated with a decrease in global DNA methylation. While supplementing with the S-adenosyl-methionine precursor methionine delayed the onset of perimenopause, treatment with the DNA-methyltransferase-1 inhibitor expedited the shift to reproductive senescence or menopause. DNA methylation of genes necessary for melatonin and circadian pathways, hormone and glutamate signaling, and other signaling pathways was found through genome-wide epigenetic study. Certain epigenetic modifications in these signaling pathways shed light on the cause of neurological disorders linked to perimenopause, like insomnia (198).
Further studies looked into the possibility of a connection between aging rats’ declining ovarian function and the cellular housekeeping mechanism of autophagy (199). They measured hormone levels, the activity of autophagy genes, and DNA methylation, a sign of gene silencing, to compare young, middle-aged, and elderly rats. The study discovered that all of the autophagy genes they looked at had less activity and that older rats had a lower level of estrogen. Notably, the age-related rise in methylation of the DNA was observed in the autophagy gene Atg5. This implies that autophagy in aging ovaries may be suppressed by DNA methylation, which could lead to a lack of ovaries’ ability to function (199).
Olsen determined the epigenetic profiles of mural granulosa cells (MGCs), which are involved in follicle development, from women with varying levels of ovarian reserve (normal, diminished, and high). They compared DNA methylation patterns between groups and found that MGCs from women with diminished ovarian reserve showed significantly higher variability in DNA methylation compared to the control group; these variable sites were enriched in genes essential for the development of follicles. However, leukocytes, or white blood cells, did not show any differences between groups concerning ovarian reserve. This suggests that decreased ovarian reserve is linked to particular changes in the epigenetic profile of MGCs, which may be linked to premature aging in these cells (200).
Another study analyzed the potential benefits of metformin on egg quality in mice using a DHEA-induced PCOS model. Treatment with metformin seemed to offset the effects, of DHEA possibly through lowering epigenetic changes and oxidative stress. This shows that metformin may be a useful medication to help PCOS-affected women produce better-quality eggs (201).
Resveratrol can mitigate altered DNA methylation and decreased gene activity in the ovaries of aging mice. However, the research also showed that Tet2, a gene, is necessary for these improvements. Tet2-deficient mice did not benefit from resveratrol treatment in the same ways. This implies that Tet2 is necessary for the action of resveratrol (202).
An additional study by (191) examining the relationship between epigenetic senescence and infertility found that mothers who conceived using Assisted Reproductive Technology (ART) aged more quickly than mothers who conceived naturally.
8 Conclusions
The ovary is the first organ to age in the women’s body, affecting fertility and driving overall health and disease in women. In addition to natural ovarian aging in all women, premature ovarian insufficiency (POI) is reported in up to 2% of women, amounting to about 80 million worldwide. Indeed, germ-line mutations in at least eight nuclear genes, including POLG1, known to perform mitochondrial function, are reported in women suffering from POI (203) (204).
The defective mitochondria are the most significant marker for aging (1). Additionally, the mtDNA content in oocytes is also reported to decline with age (6). The content of mtDNA in the oocytes is an important criterion behind the fertilization of oocytes as well as embryonic development. mtDNA is mostly lower in the oocytes of aged women or those with diminished ovarian reserve, in comparison to those of less age and have normal ovarian reserve (205). Diminished ovarian reserve is also associated with decreased mtDNA content in the cumulus cells compared to that in women with normal ovarian reserve (71). The mtDNA content is also reported to be lesser in the unfertilized oocytes of women having different infertility problems (206). Similarly, oocytes of cows older than 15 years are also reported to have a significantly lower copy number of mtDNA than oocytes of younger animals (207). The low copy number of mtDNA is also observed in poor responders to IVF. In physiologically menopausal women, mtDNA levels are lower than normal (77). Based on the positive association between the mt DNA content of oocytes and developmental proficiency and the evidence discussed in this article, we envision that the increase in mitochondrial biogenesis through various pharmacologic interventions may have a significant effect on ovarian function and longevity. A comprehensive and strategic effort is required to screen and identify agents that protect, preserve, or promote mitochondrial function in oocytes to preserve ovarian function, delay menopause, and provide better health outcomes.
Author contributions
SM: Writing – review & editing, Supervision, Resources, Writing – original draft, Conceptualization. VS: Resources, Writing – original draft, Data curation. CS: Writing – original draft, Resources. AK: Writing – original draft, Resources. KS: Writing – review & editing, Supervision, Resources, Project administration, Funding acquisition, Conceptualization.
Funding
The author(s) declare financial support was received for the research, authorship, and/or publication of this article. SM, VS, CS, and AK are supported by institutional funds. KS research is supported by NIH grant 5R21OD031970, funding from Nathan Shock Center for Biology of Aging, University of Alabama at Birmingham and Breast Cancer Foundation of Alabama.
Acknowledgments
SM, VS, CS, and AK acknowledge JIIT, Noida, for providing the entire infrastructure to complete this project.
Conflict of interest
KS is the scientific founder of Yuva Biosciences and serves as Chief Scientific Advisor.
The remaining authors declare that the research was conducted in the absence of any commercial or financial relationships that could be construed as a potential conflict of interest.
Publisher’s note
All claims expressed in this article are solely those of the authors and do not necessarily represent those of their affiliated organizations, or those of the publisher, the editors and the reviewers. Any product that may be evaluated in this article, or claim that may be made by its manufacturer, is not guaranteed or endorsed by the publisher.
References
1. López-Otín C, Blasco MA, Partridge L, Serrano M, Kroemer G. The hallmarks of aging. Cell. (2013) 153:1194–217. doi: 10.1016/j.cell.2013.05.039
2. Wu Y, Li M, Zhang J, Wang S. Unveiling uterine aging: Much more to learn. Ageing Res Rev. (2023) 86:101879. doi: 10.1016/j.arr.2023.101879
3. French JD, Dunn J, Smart CE, Manning N, Brown MA. Disruption of BRCA1 function results in telomere lengthening and increased anaphase bridge formation in immortalized cell lines. Genes Chromosomes Cancer. (2006) 45:277–89. doi: 10.1002/gcc.20290
4. van der Wijst MGP, Rots MG. Mitochondrial epigenetics: an overlooked layer of regulation? Trends Genetics: TIG. (2015) 31:353–6. doi: 10.1016/j.tig.2015.03.009
5. Tomizawa S-I, Nowacka-Woszuk J, Kelsey G. DNA methylation establishment during oocyte growth: mechanisms and significance. Int J Dev Biol. (2012) 56:867–75. doi: 10.1387/ijdb.120152gk
6. Chiang JL, Shukla P, Pagidas K, Ahmed NS, Karri S, Gunn DD, et al. Mitochondria in ovarian aging and reproductive longevity. Ageing Res Rev. (2020) 63:101168. doi: 10.1016/j.arr.2020.101168
7. Pufulete M. Effect of folic acid supplementation on genomic DNA methylation in patients with colorectal adenoma. Gut. (2005) 54:648–53. doi: 10.1136/gut.2004.054718
8. Kang H, Lee SK, Kim MH, Choi H, Lee SH, Kwack K. Acyl-CoA synthetase long-chain family member 6 is associated with premature ovarian failure. Fertility Sterility. (2009) 91:1339–43. doi: 10.1016/j.fertnstert.2008.03.035
9. Ge ZJ, Schatten H, Zhang CL, Sun QY. Oocyte ageing and epigenetics. Reproduction. (2015) 149:R103–14. doi: 10.1530/rep-14-0242
10. Wang J, Sun X, Yang Z, Li S, Wang Y, Ren R, et al. Epigenetic regulation in premature ovarian failure: A literature review. Front Physiol. (2023) 13:998424. doi: 10.3389/fphys.2022.998424
11. Park SU, Walsh L, Berkowitz KM. Mechanisms of ovarian aging. Reproduction. (2021) 162:R19–33. doi: 10.1530/rep-21-0022
12. Stuenkel CA. Ovarian insufficiency: Clinical spectrum and management challenges. J Women’s Health (2002). (2024) 33:397–406. doi: 10.1089/jwh.2023.0942
13. Twarda-Clapa A, Olczak A, Białkowska AM, Koziołkiewicz M. Advanced glycation end-products (AGEs): Formation, chemistry, classification, receptors, and diseases related to AGEs. Cells (Basel Switzerland). (2022) 11:1312. doi: 10.3390/cells11081312
14. Zhu Z, Xu W, Liu L. Ovarian aging: mechanisms and intervention strategies. Med Rev (Berlin Germany). (2023) 2:590–610. doi: 10.1515/mr-2022-0031
15. Liu J, Liu M, Ye X, Liu K, Huang J, Wang L, et al. Delay in oocyte aging in mice by the antioxidant N-acetyl-l-cysteine (NAC). Hum Reprod (Oxford England). (2012) 27:1411–20. doi: 10.1093/humrep/des019
16. Tesarik J, Galán-Lázaro M, Mendoza-Tesarik R. Ovarian aging: Molecular mechanisms and medical management. Int J Mol Sci. (2021) 22:1371. doi: 10.3390/ijms22031371
17. Milanese C, Bombardieri CR, Sepe S, Barnhoorn S, Payán-Goméz C, Caruso D, et al. DNA damage and transcription stress cause ATP-mediated redesign of metabolism and potentiation of anti-oxidant buffering. Nat Commun. (2019) 10:4887. doi: 10.1038/s41467-019-12640-5
18. Stolk L, Perry JRB, Chasman DI, He C, Mangino M, Sulem P, et al. Meta-analyses identify 13 loci associated with age at menopause and highlight DNA repair and immune pathways. Nat Genet. (2012) 44:260–8. doi: 10.1038/ng.1051
19. Vermeij WP, Dollé MET, Reiling E, Jaarsma D, Payan-Gomez C, Bombardieri CR, et al. Restricted diet delays accelerated ageing and genomic stress in DNA-repair-deficient mice. Nature. (2016) 537:427–31. doi: 10.1038/nature19329
20. Li Q, Engebrecht J. BRCA1 and BRCA2 tumor suppressor function in meiosis. Front Cell Dev Biol. (2021) 9:668309. doi: 10.3389/fcell.2021.668309
21. Lin W, Titus S, Moy F, Ginsburg ES, Oktay K. Ovarian aging in women with BRCA germline mutations. J Clin Endocrinol Metab. (2017) 102:3839–47. doi: 10.1210/jc.2017-00765
22. Titus S, Li F, Stobezki R, Akula K, Unsal E, Jeong K, et al. Impairment of BRCA1-related DNA double-strand break repair leads to ovarian aging in mice and humans. Sci Trans Med. (2013) 5:172ra21. doi: 10.1126/scitranslmed.3004925
23. Bolcun-Filas E, Rinaldi VD, White ME, Schimenti JC. Reversal of female infertility by Chk2 ablation reveals the oocyte DNA damage checkpoint pathway. Sci (New York N.Y.). (2014) 343:533–6. doi: 10.1126/science.1247671
24. Han Q, Chen Z, Du Y. Dietary supplementation for female infertility: Recent advances in the nutritional therapy for premature ovarian insufficiency. Front Microbiol. (2022) 13:1001209. doi: 10.3389/fmicb.2022.1001209
25. Chakravarti D, LaBella KA, DePinho RA. Telomeres: history, health, and hallmarks of aging. Cell. (2021) 184:306–22. doi: 10.1016/j.cell.2020.12.028
26. Kalmbach KH, Fontes Antunes DM, Dracxler RC, Knier TW, Seth-Smith ML, Wang F, et al. Telomeres and human reproduction. Fertility Sterility. (2013) 99:23–9. doi: 10.1016/j.fertnstert.2012.11.039
27. Keefe DL, Liu L, Marquard K. Telomeres and meiosis in health and disease. Cell Mol Life Sciences: CMLS. (2007) 64:139–43. doi: 10.1007/s00018-006-6466-z
28. Hanson BM, Tao X, Zhan Y, Kim JG, Klimczak AM, Herlihy NS, et al. Shorter telomere length of white blood cells is associated with higher rates of aneuploidy among infertile women undergoing in vitro fertilization. Fertility Sterility. (2021) 115:957–65. doi: 10.1016/j.fertnstert.2020.09.164
29. Yamada-Fukunaga T, Yamada M, Hamatani T, Chikazawa N, Ogawa S, Akutsu H, et al. Age-associated telomere shortening in mouse oocytes. Reprod Biol Endocrinology: RB&E. (2013) 11:108. doi: 10.1186/1477-7827-11-108
30. Yu TN, Cheng EH, Tsai HN, Lin PY, Chen CH, Huang CC, et al. Assessment of telomere length and mitochondrial DNA copy number in granulosa cells as predictors of aneuploidy rate in young patients. J Clin Med. (2022) 11:1824. doi: 10.3390/jcm11071824
31. Hassold TJ, Jacobs PA. Trisomy in man. Annu Rev Genet. (1984) 18:69–97. doi: 10.1146/annurev.ge.18.120184.000441
32. Rodriguez-Purata J, Lee J, Whitehouse M, Moschini RM, Knopman J, Duke M, et al. Embryo selection versus natural selection: how do outcomes of comprehensive chromosome screening of blastocysts compare with the analysis of products of conception from early pregnancy loss (dilation and curettage) among an assisted reproductive technology population? Fertility Sterility. (2015) 104:1460–1466.e12. doi: 10.1016/j.fertnstert.2015.08.007
33. Herbert M, Kalleas D, Cooney D, Lamb M, Lister L. Meiosis and maternal aging: Insights from aneuploid oocytes and trisomy births. Cold Spring Harbor Perspect Biol. (2015) 7:a017970. doi: 10.1101/cshperspect.a017970
34. Webster A, Schuh M. Mechanisms of aneuploidy in human eggs. Trends Cell Biol. (2017) 27:55–68. doi: 10.1016/j.tcb.2016.09.002
35. Nagaoka SI, Hassold TJ, Hunt PA. Human aneuploidy: mechanisms and new insights into an age-old problem. Nat Rev Genet. (2012) 13:493–504. doi: 10.1038/nrg3245
36. Sakakibara Y, Hashimoto S, Nakaoka Y, Kouznetsova A, Höög C, Kitajima TS. Bivalent separation into univalents precedes age-related meiosis I errors in oocytes. Nat Commun. (2015) 6:7550. doi: 10.1038/ncomms8550
37. MacDonald JA, Sheehan HC, Piasecki A, Faustino LR, Hauschildt C, Stolzenbach V, et al. Characterization of oogonial stem cells in adult mouse ovaries with age and comparison to in silico data on human ovarian aging. Stem Cells Dev. (2023) 32:99–114. doi: 10.1089/scd.2022.0284
38. Stolzenbach V, Woods DC, Tilly JL. Non-neutral clonal selection and its potential role in mammalian germline stem cell dysfunction with advancing age. Front Cell Dev Biol. (2022) 10:942652. doi: 10.3389/fcell.2022.942652
39. Clarkson YL, McLaughlin M, Waterfall M, Dunlop CE, Skehel PA, Anderson RA, et al. Initial characterisation of adult human ovarian cell populations isolated by DDX4 expression and aldehyde dehydrogenase activity. Sci Rep. (2018) 8:6053. doi: 10.1038/s41598-018-25116-1
40. Moreb J. Aldehyde dehydrogenase as a marker for stem cells. Curr Stem Cell Res Ther. (2008) 3:237–46. doi: 10.2174/157488808786734006
41. Xie J, Xu X, Liu S. Intercellular communication in the cumulus–oocyte complex during folliculogenesis: A review. Front Cell Dev Biol. (2023) 11:1087612. doi: 10.3389/fcell.2023.1087612
42. Kawai T, Shimada M. Pretreatment of ovaries with collagenase before vitrification keeps the ovarian reserve by maintaining cell-cell adhesion integrity in ovarian follicles. Sci Rep. (2020) 10:6841. doi: 10.1038/s41598-020-63948-y
43. Fan X, Moustakas I, Bialecka M, del Valle JS, Overeem AW, Louwe LA, et al. Single-cell transcriptomics analysis of human small antral follicles. Int J Mol Sci. (2021) 22:11955. doi: 10.3390/ijms222111955
44. Long X, Yang Q, Qian J, Yao H, Yan R, Cheng X, et al. Obesity modulates cell-cell interactions during ovarian folliculogenesis. iScience. (2022) 25:103627. doi: 10.1016/j.isci.2021.103627
45. Zhang Y, Yan Z, Qin Q, Nisenblat V, Chang H-M, Yu Y, et al. Transcriptome landscape of human folliculogenesis reveals oocyte and granulosa cell interactions. Mol Cell. (2018) 72:1021–1034.e4. doi: 10.1016/j.molcel.2018.10.029
46. Li E, Zhang Y. DNA methylation in mammals. Cold Spring Harbor Perspect Biol. (2014) 6:a019133–a019133. doi: 10.1101/cshperspect.a019133
47. Butler AE, Ramachandran V, Hayat S, Dargham SR, Cunningham TK, Benurwar M, et al. Expression of microRNA in follicular fluid in women with and without PCOS. Sci Rep. (2019) 9:16306. doi: 10.1038/s41598-019-52856-5
48. Pasquariello R, Manzoni EFM, Fiandanese N, Viglino A, Pocar P, Brevini TAL, et al. Implications of miRNA expression pattern in bovine oocytes and follicular fluids for developmental competence. Theriogenology. (2020) 145:77–85. doi: 10.1016/j.theriogenology.2020.01.027
49. Kasapoğlu I, Seli E. Mitochondrial dysfunction and ovarian aging. Endocrinology. (2020) 161:bqaa001. doi: 10.1210/endocr/bqaa001
50. Ju W, Zhao Y, Yu Y, Zhao S, Xiang S, Lian F. Mechanisms of mitochondrial dysfunction in ovarian aging and potential interventions. Front Endocrinol. (2024) 15:1361289. doi: 10.3389/fendo.2024.1361289
51. Tilly JL, Sinclair DA. Germline energetics, aging, and female infertility. Cell Metab. (2013) 17:838–50. doi: 10.1016/j.cmet.2013.05.007
52. Hussein MR. Apoptosis in the ovary: molecular mechanisms. Hum Reprod Update. (2005) 11:162–78. doi: 10.1093/humupd/dmi001
53. Tait SWG, Green DR. Mitochondria and cell death: outer membrane permeabilization and beyond. Nat Rev Mol Cell Biol. (2010) 11:621–32. doi: 10.1038/nrm2952
54. Ratts VS. Ablation of bcl-2 gene expression decreases the numbers of oocytes and primordial follicles established in the post-natal female mouse gonad. Endocrinology. (1995) 136:3665–8. doi: 10.1210/en.136.8.3665
55. van der Reest J, Nardini Cecchino G, Haigis MC, Kordowitzki P. Mitochondria: Their relevance during oocyte ageing. Ageing Res Rev. (2021) 70:101378. doi: 10.1016/j.arr.2021.101378
56. Turrens JF. Mitochondrial formation of reactive oxygen species. J Physiol. (2003) 552:335–44. doi: 10.1113/jphysiol.2003.049478
57. Wei Y-H, Lee H-C. Oxidative stress, mitochondrial DNA mutation, and impairment of antioxidant enzymes in aging. Exp Biol Med (Maywood N.J.). (2002) 227:671–82. doi: 10.1177/153537020222700901
58. Simsek-Duran F, Li F, Ford W, Swanson RJ, Jones HW, Castora FJ. Age-associated metabolic and morphologic changes in mitochondria of individual mouse and hamster oocytes. PloS One. (2013) 8:e64955. doi: 10.1371/journal.pone.0064955
59. Zhang X, Wu XQ, Lu S, Guo YL, Ma X. Deficit of mitochondria-derived ATP during oxidative stress impairs mouse MII oocyte spindles. Cell Res. (2006) 16:841–50. doi: 10.1038/sj.cr.7310095
60. Halestrap AP. What is the mitochondrial permeability transition pore? J Mol Cell Cardiol. (2009) 46:821–31. doi: 10.1016/j.yjmcc.2009.02.021
61. Aiken CE, Tarry-Adkins JL, Penfold NC, Dearden L, Ozanne SE. Decreased ovarian reserve, dysregulation of mitochondrial biogenesis, and increased lipid peroxidation in female mouse offspring exposed to an obesogenic maternal diet. FASEB Journal: Off Publ Fed Am Societies Exp Biol. (2016) 30:1548–56. doi: 10.1096/fj.15-280800
62. Archer SL. Mitochondrial dynamics — mitochondrial fission and fusion in human diseases. New Engl J Med. (2013) 369:2236–51. doi: 10.1056/nejmra1215233
63. Jiang X, Tai H, Kuang J, Zhang J, Cui S, Lu Y, et al. Jian-Pi-Yi-Shen decoction inhibits mitochondria-dependent granulosa cell apoptosis in a rat model of POF. Aging. (2022), 8321–8345. doi: 10.18632/aging.204320
64. Legros F, Malka F, Frachon P, Lombès A, Rojo M. Organization and dynamics of human mitochondrial DNA. J Cell Sci. (2004) 117:2653–62. doi: 10.1242/jcs.01134
65. Udagawa O, Ishihara T, Maeda M, Matsunaga Y, Tsukamoto S, Kawano N, et al. Mitochondrial fission factor Drp1 maintains oocyte quality via dynamic rearrangement of multiple organelles. Curr Biology: CB. (2014) 24:2451–8. doi: 10.1016/j.cub.2014.08.060
66. Clark H, Knapik LO, Zhang Z, Wu X, Naik MT, Oulhen N, et al. Dysfunctional MDR-1 disrupts mitochondrial homeostasis in the oocyte and ovary. Sci Rep. (2019) 9:9616. doi: 10.1038/s41598-019-46025-x
67. Chiaratti MR, Garcia BM, Carvalho KF, Macabelli CH, Ribeiro FK, da S, et al. Oocyte mitochondria: role on fertility and disease transmission. Anim Reprod. (2018) 15:231–8. doi: 10.21451/1984-3143-ar2018-0069
68. Giles RE, Blanc H, Cann HM, Wallace DC. Maternal inheritance of human mitochondrial DNA. Proc Natl Acad Sci United States America. (1980) 77:6715–9. doi: 10.1073/pnas.77.11.6715
69. Lombard DB, Tishkoff DX, Bao J. Mitochondrial sirtuins in the regulation of mitochondrial activity and metabolic adaptation. In Handbook of Experimental Pharmacology. Histone Deacetylases: Biol Clin Implication. (2011) 163–188. doi: 10.1007/978-3-642-21631-2_8
70. Herzig S, Shaw RJ. AMPK: guardian of metabolism and mitochondrial homeostasis. Nat Rev Mol Cell Biol. (2018) 19:121–35. doi: 10.1038/nrm.2017.95
71. Pacella-Ince L, Zander-Fox DL, Lane M. Mitochondrial SIRT3 and its target glutamate dehydrogenase are altered in follicular cells of women with reduced ovarian reserve or advanced maternal age. Hum Reprod (Oxford England). (2014) 29:1490–9. doi: 10.1093/humrep/deu071
72. Ben-Meir A, Burstein E, Borrego-Alvarez A, Chong J, Wong E, Yavorska T, et al. Coenzyme Q10 restores oocyte mitochondrial function and fertility during reproductive aging. Aging Cell. (2015) 14:887–95. doi: 10.1111/acel.12368
73. Boucret L, Chao de la Barca JM, Moriniere C, Desquiret V, Ferre-L’Hotellier V, Descamps P, et al. Relationship between diminished ovarian reserve and mitochondrial biogenesis in cumulus cells. Hum Reprod (Oxford England). (2015) 30:1653–64. doi: 10.1093/humrep/dev114
74. Ávila J, González-Fernández R, Rotoli D, Hernández J, Palumbo A. Oxidative stress in granulosa-lutein cells from in vitro fertilization patients. Reprod Sci (Thousand Oaks Calif.). (2016) 23:1656–61. doi: 10.1177/1933719116674077
75. Tatone C, Carbone MC, Falone S, Aimola P, Giardinelli A, Caserta D, et al. Age-dependent changes in the expression of superoxide dismutases and catalase are associated with ultrastructural modifications in human granulosa cells. Mol Hum Reprod. (2006) 12:655–60. doi: 10.1093/molehr/gal080
76. Demozay D, Rocchi S, Mas J-C, Grillo S, Pirola L, Chavey C, et al. Fatty aldehyde dehydrogenase. J Biol Chem. (2004) 279:6261–70. doi: 10.1074/jbc.m312062200
77. Bonomi M, Somigliana E, Cacciatore C, Busnelli M, Rossetti R, Bonetti S, et al. Blood cell mitochondrial DNA content and premature ovarian aging. PloS One. (2012) 7:e42423. doi: 10.1371/journal.pone.0042423
78. Richani D, Dunning KR, Thompson JG, Gilchrist RB. Metabolic co-dependence of the oocyte and cumulus cells: essential role in determining oocyte developmental competence. Hum Reprod Update. (2021) 27:27–47. doi: 10.1093/humupd/dmaa043
79. Hutchison CA, Newbold JE, Potter SS, Edgell MH. Maternal inheritance of mammalian mitochondrial DNA. Nature. (1974) 251:536–8. doi: 10.1038/251536a0
80. Carelli V. Keeping in shape the dogma of mitochondrial DNA maternal inheritance. PloS Genet. (2015) 11:e1005179. doi: 10.1371/journal.pgen.1005179
81. Schwartz D, Mayaux MJ. Female fecundity as a function of age. Obstetrical Gynecological Survey. (1982) 37:548–50. doi: 10.1097/00006254-198208000-00019
82. Bibi S, Abbas G, Khan MZ, Nawaz T, Ullah Q, Uddin A, et al. The mutational analysis of mitochondrial DNA in maternal inheritance of polycystic ovarian syndrome. Front Endocrinol. (2023) 14:1093353. doi: 10.3389/fendo.2023.1093353
83. May-Panloup P, Boucret L, Chao de la Barca JM, Desquiret-Dumas V, Ferré-L’Hotellier V, Morinière C, et al. Ovarian ageing: the role of mitochondria in oocytes and follicles. Hum Reprod Update. (2016) 22:725–43. doi: 10.1093/humupd/dmw028
84. Li N. MicroRNA-27a activity is not suppressed in porcine oocytes. Front Bioscience (Elite Edition). (2012) E4:2579–85. doi: 10.2741/e574
85. Tatone C, Amicarelli F. The aging ovary—the poor granulosa cells. Fertility Sterility. (2013) 99:12–7. doi: 10.1016/j.fertnstert.2012.11.029
86. López-Otín C, Blasco MA, Partridge L, Serrano M, Kroemer G. Hallmarks of aging: An expanding universe. Cell. (2023) 186:243–78. doi: 10.1016/j.cell.2022.11.001
87. Felsenfeld G. A brief history of epigenetics. Cold Spring Harbor Perspect Biol. (2014) 6:a018200–a018200. doi: 10.1101/cshperspect.a018200
88. Armstrong DT. Effects of maternal age on oocyte developmental competence. Theriogenology. (2001) 55:1303–22. doi: 10.1016/s0093-691x(01)00484-8
89. Klein J, Sauer MV. Assessing fertility in women of advanced reproductive age. Am J Obstetrics Gynecology. (2001) 185:758–70. doi: 10.1067/mob.2001.114689
90. Berkowitz GS, Skovron ML, Lapinski RH, Berkowitz RL. Delayed childbearing and the outcome of pregnancy. New Engl J Med. (1990) 322:659–64. doi: 10.1056/nejm199003083221004
91. Keefe DL, Niven-Fairchild T, Powell S, Buradagunta S. Mitochondrial deoxyribonucleic acid deletions in oocytes and reproductive aging in women. Fertility Sterility. (1995) 64:577–83. doi: 10.1016/s0015-0282(16)57796-6
92. Li Y, Daniel M, Tollefsbol TO. Epigenetic regulation of caloric restriction in aging. BMC Med. (2011) 9:98. doi: 10.1186/1741-7015-9-98
93. Cedars MI. Biomarkers of ovarian reserve–do they predict somatic aging? Semin Reprod Med. (2013) 31:443–51. doi: 10.1055/s-0033-1356480
94. Atwood CS, Bowen RL. The reproductive-cell cycle theory of aging: An update. Exp Gerontology. (2011) 46:100–7. doi: 10.1016/j.exger.2010.09.007
95. Mishra GD, Pandeya N, Dobson AJ, Chung H-F, Anderson D, Kuh D, et al. Early menarche, nulliparity and the risk for premature and early natural menopause. Hum Reprod (Oxford England). (2017) 679–686. doi: 10.1093/humrep/dew350
96. Zhang X, Huangfu Z, Wang S. Review of mendelian randomization studies on age at natural menopause. Front Endocrinol. (2023) 14:1234324. doi: 10.3389/fendo.2023.1234324
97. Tschiderer L, Peters SAE, van der Schouw YT, van Westing AC, Tong TYN, Willeit P, et al. Age at menopause and the risk of stroke: Observational and Mendelian randomization analysis in 204 244 postmenopausal women. J Am Heart Assoc. (2023) 12:18. doi: 10.1161/jaha.123.030280
98. Perheentupa A, Huhtaniemi I. Aging of the human ovary and testis. Mol Cell Endocrinol. (2009) 299:2–13. doi: 10.1016/j.mce.2008.11.004
99. Broekmans FJ, Soules MR, Fauser BC. Ovarian aging: Mechanisms and clinical consequences. Endocrine Rev. (2009) 30:465–93. doi: 10.1210/er.2009-0006
100. Simpson DJ, Chandra T. Epigenetic age prediction. Aging Cell. (2021) 20:e13452. doi: 10.1111/acel.13452
101. Freire-Aradas A, Girón-Santamaría L, Mosquera-Miguel A, Ambroa-Conde A, Phillips C, Casares de Cal M, et al. A common epigenetic clock from childhood to old age. Forensic Sci Int Genet. (2022) 60:102743. doi: 10.1016/j.fsigen.2022.102743
102. Horvath S, Erhart W, Brosch M, Ammerpohl O, von Schönfels W, Ahrens M, et al. Obesity accelerates epigenetic aging of human liver. Proc Natl Acad Sci United States America. (2014) 111:15538–43. doi: 10.1073/pnas.1412759111
103. Hannum G, Guinney J, Zhao L, Zhang L, Hughes G, Sadda S, et al. Genome-wide methylation profiles reveal quantitative views of human aging rates. Mol Cell. (2013) 49:359–67. doi: 10.1016/j.molcel.2012.10.016
104. Lu AT, Quach A, Wilson JG, Reiner AP, Aviv A, Raj K, et al. DNA methylation GrimAge strongly predicts lifespan and healthspan. Aging. (2019) 11:303–27. doi: 10.18632/aging.101684
105. Levine ME, Lu AT, Quach A, Chen BH, Assimes TL, Bandinelli S, et al. An epigenetic biomarker of aging for lifespan and healthspan. Aging. (2018) 10:573–91. doi: 10.18632/aging.101414
106. Knight AK, Spencer JB, Smith AK. DNA methylation as a window into female reproductive aging. Epigenomics. (2024) 16:175–88. doi: 10.2217/epi-2023-0298
107. Wang K, Liu H, Hu Q, Wang L, Liu J, Zheng Z, et al. Epigenetic regulation of aging: implications for interventions of aging and diseases. Signal Transduction Targeted Ther. (2022) 7:374. doi: 10.1038/s41392-022-01211-8
108. Poulos RC, Olivier J, Wong JWH. The interaction between cytosine methylation and processes of DNA replication and repair shape the mutational landscape of cancer genomes. Nucleic Acids Res. (2017) 45:7786–95. doi: 10.1093/nar/gkx463
109. Gu TP, Guo F, Yang H, Wu HP, Xu GF, Liu W, et al. The role of Tet3 DNA dioxygenase in epigenetic reprogramming by oocytes. Nature. (2011) 477:606–10. doi: 10.1038/nature10443
110. Hales BF, Grenier L, Lalancette C, Robaire B. Epigenetic programming: From gametes to blastocyst. Birth Defects Res Part A Clin Mol Teratology. (2011) 91:652–65. doi: 10.1002/bdra.20781
111. Soto-Palma C, Niedernhofer LJ, Faulk CD, Dong X. Epigenetics, DNA damage, and aging. J Clin Invest. (2022) 132:e158446. doi: 10.1172/jci158446
112. Benito E, Urbanke H, Ramachandran B, Barth J, Halder R, Awasthi A, et al. HDAC inhibitor–dependent transcriptome and memory reinstatement in cognitive decline models. J Clin Invest. (2015) 125:3572–84. doi: 10.1172/jci79942
113. Tanner KG, Landry J, Sternglanz R, Denu JM. Silent information regulator 2 family of NAD- dependent histone/protein deacetylases generates a unique product, 1- O- acetyl-ADP-ribose. Proc Natl Acad Sci United States America. (2000) 97:14178–82. doi: 10.1073/pnas.250422697
114. Mostoslavsky R, Chua KF, Lombard DB, Pang WW, Fischer MR, Gellon L, et al. Genomic instability and aging-like phenotype in the absence of mammalian SIRT6. Cell. (2006) 124:315–29. doi: 10.1016/j.cell.2005.11.044
115. Choi YJ, Kang M-H, Hong K, Kim J-H. Tubastatin A inhibits HDAC and Sirtuin activity rather than being a HDAC6-specific inhibitor in mouse oocytes. Aging. (2019) 11:1759–77. doi: 10.18632/aging.101867
116. Caponnetto A, Battaglia R, Ferrara C, Vento ME, Borzì P, Paradiso M. Italian Society of Embryology, Reproduction, Research (SIERR). (2022). Down-regulation of long non-coding RNAs in reproductive aging and analysis of the lncRNA-miRNA-mRNA networks in human cumulus cells. J Assisted Reprod Genet. (2022) 39:919–31. doi: 10.1007/s10815-022-02446-8
117. Price AJ, Manjegowda MC, Kain J, Anandh S, Bochkis IM. Hdac3, Setdb1, and Kap1 mark H3K9me3/H3K14ac bivalent regions in young and aged liver. Aging Cell. (2020) 19:e13092. doi: 10.1111/acel.13092
118. Liu B, Yip KH, Zhou Z. Chromatin remodeling, DNA damage repair and aging. Curr Genomics. (2012) 13:533–47. doi: 10.2174/138920212803251373
119. Pouikli A, Parekh S, Maleszewska M, Nikopoulou C, Baghdadi M, Tripodi I, et al. Chromatin remodeling due to degradation of citrate carrier impairs osteogenesis of aged mesenchymal stem cells. Nat Aging. (2021) 1:810–25. doi: 10.1038/s43587-021-00105-8
120. Stephens AD, Liu PZ, Banigan EJ, Almassalha LM, Backman V, Adam SA, et al. Chromatin histone modifications and rigidity affect nuclear morphology independent of lamins. Mol Biol Cell. (2018) 220–233. doi: 10.1091/mbc.e17-06-0410
121. Zhu D, Wu X, Zhou J, Li X, Huang X, Li J, et al. NuRD mediates mitochondrial stress–induced longevity via chromatin remodeling in response to acetyl-CoA level. Sci Adv. (2020) 6:31. doi: 10.1126/sciadv.abb2529
122. Cavalli G, Heard E. Advances in epigenetics link genetics to the environment and disease. Nature. (2019) 571:489–99. doi: 10.1038/s41586-019-1411-0
123. Zhu D, Li X, Tian Y. Mitochondrial-to-nuclear communication in aging: an epigenetic perspective. Trends Biochem Sci. (2022) 47:645–59. doi: 10.1016/j.tibs.2022.03.008
124. Liu X, Gao Q, Li P, Zhao Q, Zhang J, Li J, et al. UHRF1 targets DNMT1 for DNA methylation through cooperative binding of hemi-methylated DNA and methylated H3K9. Nat Commun. (2013) 4:1563. doi: 10.1038/ncomms2562
125. Peng, Xu X, Wang C, Yang J, Wang S, Dai J, et al. EZH2 promotes DNA replication by stabilizing interaction of POLδ and PCNA via methylation-mediated PCNA trimerization. Epigenet Chromatin. (2018) 11:44. doi: 10.1186/s13072-018-0213-1
126. Diao Z, Ji Q, Wu Z, Zhang W, Cai Y, Wang Z, et al. Correction to ‘SIRT3 consolidates heterochromatin and counteracts senescence’. Nucleic Acids Res. (2021) 49:9004–6. doi: 10.1093/nar/gkab698
127. Chen X, Xu H, Shu X, Song C-X. Mapping epigenetic modifications by sequencing technologies. Cell Death Differentiation. (2023). doi: 10.1038/s41418-023-01213-1
128. Wei J-W, Huang K, Yang C, Kang C-S. Non-coding RNAs as regulators in epigenetics. Oncol Rep. (2017) 37:3–9. doi: 10.3892/or.2016.5236
129. Yang X, Liu M, Li M, Zhang S, Hiju H, Sun J, et al. Epigenetic modulations of noncoding RNA: a novel dimension of Cancer biology. Mol Cancer. (2020) 19:64. doi: 10.1186/s12943-020-01159-9
130. Vaquero-Sedas MI, Vega-Palas MA. On the chromatin structure of eukaryotic telomeres. Epigenetics: Off J DNA Methylation Soc. (2011) 6:1055–8. doi: 10.4161/epi.6.9.16845
131. Ichikawa Y, Nishimura Y, Kurumizaka H, Shimizu M. Nucleosome organization and chromatin dynamics in telomeres. Biomolecular Concepts. (2015) 6:67–75. doi: 10.1515/bmc-2014-0035
132. Mir SM, Samavarchi Tehrani S, Goodarzi G, Jamalpoor Z, Jahanbakhsh A, Khelghati N, et al. Shelterin complex at telomeres: Implications in ageing. Clin Interventions Aging. (2020) 15:827–39. doi: 10.2147/cia.s256425
133. Mechta M, Ingerslev LR, Fabre O, Picard M, Barrès R. Evidence suggesting absence of mitochondrial DNA methylation. Front Genet. (2017) 8:166. doi: 10.3389/fgene.2017.00166
134. Mohn F, Weber M, Schübeler D, Roloff TC. Methylated DNA immunoprecipitation (MeDIP). In methods in molecular biology (Clifton, N.J.). Methods Mol Biol. (2009) 507:55–64. doi: 10.1007/978-1-59745-522-0_5
135. Sharma N, Pasala MS, Prakash A. Mitochondrial DNA: epigenetics and environment. Environ Mol Mutagenesis. (2019) 60:668–82. doi: 10.1002/em.22319
136. Mongelli A, Mengozzi A, Geiger M, Gorica E, Mohammed SA, Paneni F, et al. Mitochondrial epigenetics in aging and cardiovascular diseases. Front Cardiovasc Med. (2023) 10:1204483. doi: 10.3389/fcvm.2023.1204483
137. Song Y, Zhu X-Y, Zhang X-M, Xiong H. Targeted mitochondrial epigenetics: A new direction in Alzheimer’s disease treatment. Int J Mol Sci. (2022) 23:9703. doi: 10.3390/ijms23179703
138. Morin AL, Win PW, Lin AZ, Castellani CA. Mitochondrial genomic integrity and the nuclear epigenome in health and disease. Front Endocrinol. (2022) 13:1059085. doi: 10.3389/fendo.2022.1059085
139. Boughanem H, Böttcher Y, Tomé-Carneiro J, López de las Hazas M-C, Dávalos A, Cayir A, et al. The emergent role of mitochondrial RNA modifications in metabolic alterations. Wiley Interdiscip Rev RNA. (2023) 14:e1753. doi: 10.1002/wrna.1753
140. Cavalcante MB, Sampaio OGM, Câmara FEA, Schneider A, de Ávila BM, Prosczek J, et al. Ovarian aging in humans: potential strategies for extending reproductive lifespan. GeroScience. (2023) 45:2121–33. doi: 10.1007/s11357-023-00768-8
141. Lopes FC. Mitochondrial metabolism and DNA methylation: a review of the interaction between two genomes. Clin Epigenet. (2020) 12:182. doi: 10.1186/s13148-020-00976-5
142. Robertson KD. DNA methylation, methyltransferases, and cancer. Oncogene. (2001) 20(24):3139–55. doi: 10.1038/sj.onc.1204341
143. Arasaradnam RP, Commane DM, Bradburn D, Mathers JC. A review of dietary factors and its influence on DNA methylation in colorectal carcinogenesis. Epigenetics: Off J DNA Methylation Soc. (2008) 3:193–8. doi: 10.4161/epi.3.4.6508
144. Chen T, Ueda Y, Dodge JE, Wang Z, Li E. Establishment and maintenance of genomic methylation patterns in mouse embryonic stem cells by Dnmt3a and Dnmt3b. Mol Cell Biol. (2003) 23:5594–605. doi: 10.1128/mcb.23.16.5594-5605.2003
145. Okano M, Bell DW, Haber DA, Li E. DNA methyltransferases Dnmt3a and Dnmt3b are essential for DE Novo methylation and mammalian development. Cell. (1999) 99:247–57. doi: 10.1016/s0092-8674(00)81656-6
146. Uysal F, Ozturk S. The loss of global DNA methylation due to decreased DNMT expression in the postnatal mouse ovaries may associate with infertility emerging during ovarian aging. Histochem Cell Biol. (2020) 154:301–14. doi: 10.1007/s00418-020-01890-w
147. Jacob RA, Gretz DM, Taylor PC, James SJ, Pogribny IP, Miller BJ, et al. Moderate folate depletion increases plasma homocysteine and decreases lymphocyte DNA methylation in postmenopausal women. J Nutr. (1998) 128:1204–12. doi: 10.1093/jn/128.7.1204
148. Rampersaud GC, Kauwell GPA, Hutson AD, Cerda JJ, Bailey LB. Genomic DNA methylation decreases in response to moderate folate depletion in elderly women. Am J Clin Nutr. (2000) 72:998–1003. doi: 10.1093/ajcn/72.4.998
149. Kok DEG, Dhonukshe-Rutten RAM, Lute C, Heil SG, Uitterlinden AG, van der Velde N, et al. The effects of long-term daily folic acid and vitamin B12 supplementation on genome-wide DNA methylation in elderly subjects. Clin Epigenet. (2015) 7:121. doi: 10.1186/s13148-015-0154-5
150. Anderson OS, Sant KE, Dolinoy DC. Nutrition and epigenetics: an interplay of dietary methyl donors, one-carbon metabolism and DNA methylation. J Nutr Biochem. (2012) 23:853–9. doi: 10.1016/j.jnutbio.2012.03.003
151. Guéant JL, Guéant-Rodriguez RM. Maternal folate, methyl donors, one-carbon metabolism, vitamin B12 and choline in foetal programming. In. Diet Nutrition Fetal Programming. (2017), 293–307. doi: 10.1007/978-3-319-60289-9_22
152. Amenyah SD, Hughes CF, Ward M, Rosborough S, Deane J, Thursby S-J, et al. Influence of nutrients involved in one-carbon metabolism on DNA methylation in adults—a systematic review and meta-analysis. Nutr Rev. (2020) 78:647–66. doi: 10.1093/nutrit/nuz094
153. Chamani IJ, Keefe DL. Epigenetics and female reproductive aging. Front Endocrinol. (2019) 10:473. doi: 10.3389/fendo.2019.00473
154. Trefely S, Doan MT, Snyder NW. Crosstalk between cellular metabolism and histone acetylation. Methods Enzymol. (2019). p. 1–21. doi: 10.1016/bs.mie.2019.07.013
155. Wiese M, Bannister AJ. Two genomes, one cell: Mitochondrial-nuclear coordination via epigenetic pathways. Mol Metab. (2020) 38:100942. doi: 10.1016/j.molmet.2020.01.006
156. Bradshaw PC. Acetyl-CoA metabolism and histone acetylation in the regulation of aging and lifespan. Antioxidants (Basel Switzerland). (2021) 10:572. doi: 10.3390/antiox10040572
157. Simithy J, Sidoli S, Yuan Z-F, Coradin M, Bhanu NV, Marchione DM, et al. Characterization of histone acylations links chromatin modifications with metabolism. Nat Commun. (2017) 8:1141. doi: 10.1038/s41467-017-01384-9
158. Fan J, Krautkramer KA, Feldman JL, Denu JM. Metabolic regulation of histone post-translational modifications. ACS Chem Biol. (2015) 10:95–108. doi: 10.1021/cb500846u
159. Liang J, Huang F, Song Z, Tang R, Zhang P, Chen R. Impact of NAD+ metabolism on ovarian aging. Immun Ageing: I A. (2023) 20:70. doi: 10.1186/s12979-023-00398-w
160. Perrone R, Kumaar PVA, Haky L, Hahn C, Riley R, Balough J, et al. CD38 regulates ovarian function and fecundity via NAD+ metabolism. iScience. (2023) 26:107949. doi: 10.1016/j.isci.2023.107949
161. Dai Z, Ramesh V, Locasale JW. The evolving metabolic landscape of chromatin biology and epigenetics. Nat Rev Genet. (2020) 21:737–53. doi: 10.1038/s41576-020-0270-8
162. Roushenas F, Hamdi K, Jafarpour F, Fattahi A, Pashaiasl M, Nasr-Esfahani MH. Follicular fluid advanced glycation end products in assisted reproduction: A systematic review. Clinica Chimica Acta; Int J Clin Chem. (2023) 549:117560. doi: 10.1016/j.cca.2023.117560
163. Yao Z, Chen Y, Cao W, Shyh-Chang N. Chromatin-modifying drugs and metabolites in cell fate control. Cell Proliferation. (2020) 53:e12898. doi: 10.1111/cpr.12898
164. Li T, Liu J, Liu K, Wang Q, Cao J, Xiao P, et al. Alpha-ketoglutarate ameliorates induced premature ovarian insufficiency in rats by inhibiting apoptosis and upregulating glycolysis. Reprod Biomedicine Online. (2023) 46:673–85. doi: 10.1016/j.rbmo.2023.01.005
165. D’Aquila P, De Rango F, Paparazzo E, Mandalà M, Bellizzi D, Passarino G. Impact of nutrition on age-related epigenetic RNA modifications in rats. Nutrients. (2022) 14:1232. doi: 10.3390/nu14061232
166. Kim J, Lee G. Metabolic control of m6A RNA modification. Metabolites. (2021) 11:80. doi: 10.3390/metabo11020080
167. Chen J, Fang Y, Xu Y, Sun H. Role of m6A modification in female infertility and reproductive system diseases. Int J Biol Sci. (2022) 18:3592–604. doi: 10.7150/ijbs.69771
168. Kim K, Mills JL, Michels KA, Chaljub EN, Wactawski-Wende J, Plowden TC, et al. Dietary intakes of vitamin B-2 (Riboflavin), vitamin B-6, and vitamin B-12 and ovarian cycle function among premenopausal women. J Acad Nutr Dietetics. (2019) 120:885–92. doi: 10.1016/j.jand.2019.10.013
169. Kim K, Mills JL, Michels KA, Chaljub EN, Wactawski-Wende J, Plowden TC, et al. Dietary intakes of vitamin B-2 (Riboflavin), vitamin B-6, and vitamin B-12 and ovarian cycle function among premenopausal women. J Acad Nutr Dietetics. (2019) 120:885–92. doi: 10.1016/j.jand.2019.10.013
170. Mohammadi N, Hemmati M, Motlagh B, Biyabani A. Betaine postpones hyperglycemia-related senescence in ovarian and testicular cells: Involvement of RAGE and β-galactosidase. Cell Biochem Funct. (2024) 42:e3973. doi: 10.1002/cbf.3973
171. Zhan X, Fletcher L, Dingle S, Baracuhy E, Wang B, Huber L-A, et al. Choline supplementation influences ovarian follicular development. Front Bioscience-Landmark. (2021) 26:1525–36. doi: 10.52586/5046
172. Huang Y, Tu M, Qian Y, Ma J, Chen L, Liu Y, et al. Age-dependent metabolomic profile of the follicular fluids from women undergoing assisted reproductive technology treatment. Front Endocrinol. (2022) 13:818888. doi: 10.3389/fendo.2022.818888
173. Wang H, Fang J, Kuang X, Miao L, Wang C, Xia G, et al. Activity of long-chain acyl-coA synthetase is required for maintaining meiotic arrest in xenopus laevis1. Biol Reprod. (2012) 87:1–9. doi: 10.1095/biolreprod.112.100511
174. Broekmans FJ, Soules MR, Fauser BC. Ovarian aging: Mechanisms and clinical consequences. Endocrine Rev. (2009) 30:465–93. doi: 10.1210/er.2009-0006
175. Recognizing the importance of ovarian aging research. Nat Aging. (2022) 2:1071–2. doi: 10.1038/s43587-022-00339-0
176. Vos T, Lim SS, Abbafati C, Abbas KM, Abbasi M, Abbasifard M, et al. Global burden of 369 diseases and injuries in 204 countries and territories, 1990–2019: a systematic analysis for the Global Burden of Disease Study 2019. Lancet. (2020) 396:1204–22. doi: 10.1016/s0140-6736(20)30925-9
177. Nechuta S, Paneth N, Velie EM. Pregnancy characteristics and maternal breast cancer risk: a review of the epidemiologic literature. Cancer Causes Control. (2010) 21:967–89. doi: 10.1007/s10552-010-9524-7
178. Faubion SSMMFN, Crandall CJMMMNF, Davis LDFN, Faha EKSRPM, Hodis HN, Lobo RA, et al. The 2022 hormone therapy position statement of The North American Menopause Society. Menopause J North Am Menopause Soc. (2022) 29:767–94. doi: 10.1097/gme.0000000000002028
179. Tucker EJ, Grover SR, Bachelot A, Touraine P, Sinclair AH. Premature ovarian insufficiency: New perspectives on genetic cause and phenotypic spectrum. Endocrine Rev. (2016) 37:609–35. doi: 10.1210/er.2016-1047
180. Rudnicka E, Kruszewska J, Klicka K, Kowalczyk J, Grymowicz M, Skórska J, et al. Premature ovarian insufficiency – aetiopathology, epidemiology, and diagnostic evaluation. Przeglad Menopauzalny. (2018) 17:105–8. doi: 10.5114/pm.2018.78550
181. Rahman R, Panay N. Diagnosis and management of premature ovarian insufficiency. Bailliere’s Best Pract Res Clin Endocrinol Metab. (2021) 35:101600. doi: 10.1016/j.beem.2021.101600
182. Podfigurna-Stopa A, Czyzyk A, Grymowicz M, Smolarczyk R, Katulski K, Czajkowski K, et al. Premature ovarian insufficiency: the context of long-term effects. J Endocrinological Invest. (2016) 39:983–90. doi: 10.1007/s40618-016-0467-z
183. Traub ML, Santoro N. Reproductive aging and its consequences for general health. Ann New York Acad Sci. (2010) 1204:179–87. doi: 10.1111/j.1749-6632.2010.05521.x
184. Gilmer G, Hettinger ZR, Tuakli-Wosornu Y, Skidmore E, Silver JK, Thurston RC, et al. Female aging: when translational models don’t translate. Nat Aging. (2023) 3:1500–8. doi: 10.1038/s43587-023-00509-8
185. Laisk T, Tšuiko O, Jatsenko T, Hõrak P, Otala M, Lahdenperä M, et al. Demographic and evolutionary trends in ovarian function and aging. Hum Reprod Update. (2019) 25:34–50. doi: 10.1093/humupd/dmy031
186. Llarena N, Hine C. Reproductive longevity and aging: Geroscience approaches to maintain long-term ovarian fitness. Journals Gerontology. Ser A Biol Sci Med Sci. (2021) 76:1551–60. doi: 10.1093/gerona/glaa204
187. Ruth KS, Beaumont RN, Tyrrell J, Jones SE, Tuke MA, Yaghootkar H, et al. Genetic evidence that lower circulating FSH levels lengthen menstrual cycle, increase age at menopause and impact female reproductive health. Hum Reprod (Oxford England). (2016) 31:473–81. doi: 10.1093/humrep/dev318
188. Yang L, Chen Y, Liu Y, Xing Y, Miao C, Zhao Y, et al. The role of oxidative stress and natural antioxidants in ovarian aging. Front Pharmacol. (2021) 11:617843. doi: 10.3389/fphar.2020.617843
189. Zhang J, Chen Q, Du D, Wu T, Wen J, Wu M, et al. Can ovarian aging be delayed by pharmacological strategies? Aging. (2019) 11:817–32. doi: 10.18632/aging.101784
190. He J, Yao G, He Q, Zhang T, Fan H, Bai Y, et al. Theaflavin 3, 3 ′ -digallate delays ovarian aging by improving oocyte quality and regulating granulosa cell function. . Oxid Med Cell Longevity. (2021) 2021:1–18. doi: 10.1155/2021/7064179
191. Lee Y, Bohlin J, Page CM, Nustad HE, Harris JR, Magnus P, et al. Associations between epigenetic age acceleration and infertility. Hum Reprod (Oxford England). (2022) 37:2063–74. doi: 10.1093/humrep/deac147
192. Song C, Peng W, Yin S, Zhao J, Fu B, Zhang J, et al. Melatonin improves age-induced fertility decline and attenuates ovarian mitochondrial oxidative stress in mice. Sci Rep. (2016) 6:35165. doi: 10.1038/srep35165
193. Faubion SSMMFN, Crandall CJMMMNF, Davis LDFN, Faha EKSRPM, Hodis HN, MD, Lobo RA, MD, et al. The 2022 hormone therapy position statement of The North American Menopause Society. Menopause J North Am Menopause Soc. (2022) 29:767–94. doi: 10.1097/gme.0000000000002028
194. Guo YM, Sun TC, Wang HP, Chen X. Research progress of melatonin (MT) in improving ovarian function: a review of the current status. Aging. (2021) 13:17930–47. doi: 10.18632/aging.203231
195. Lu X, Liu Y, Xu J, Cao X, Zhang D, Liu M, et al. Mitochondrial dysfunction in cumulus cells is related to decreased reproductive capacity in advanced-age women. Fertility Sterility. (2022) 118:393–404. doi: 10.1016/j.fertnstert.2022.04.019
196. Zhang H, Fang Y, Gao Y, Zeng X, Lu Z, Liu L, et al. Brown adipose tissue-derived exosomes delay fertility decline in aging mice. Front Endocrinol. (2023) 14:1180104. doi: 10.3389/fendo.2023.1180104
197. Su WP, Li CJ, Lin LT, Lin PH, Wen Z-H, Sheu JJC, et al. Boosting mitochondrial function and metabolism in aging female germ cells with dual ROCK/ROS inhibition. Biomedecine Pharmacotherapie Biomedicine Pharmacotherapy. (2023) 163:114888. doi: 10.1016/j.biopha.2023.114888
198. Bacon ER, Mishra A, Wang Y, Desai MK, Yin F, Brinton RD. Neuroendocrine aging precedes perimenopause and is regulated by DNA methylation. Neurobiol Aging. (2019) 74:213–24. doi: 10.1016/j.neurobiolaging.2018.09.029
199. Li Q, Cai M, Wang J, Gao Q, Guo X, Jia X, et al. Decreased ovarian function and autophagy gene methylation in aging rats. J Ovarian Res. (2020) 13:12. doi: 10.1186/s13048-020-0615-0
200. Olsen KW, Castillo-Fernandez J, Chan AC, la Cour Freiesleben N, Zedeler A, Bungum M, et al. Identification of a unique epigenetic profile in women with diminished ovarian reserve. Fertility Sterility. (2021) 115:732–41. doi: 10.1016/j.fertnstert.2020.09.009
201. Amani Abkenari S, Safdarian L, Amidi F, Hosseini A, Aryanpour R, Salahi E, et al. Metformin improves epigenetic modification involved in oocyte growth and embryo development in polycystic ovary syndrome mice model. Mol Reprod Dev. (2021) 88:817–29. doi: 10.1002/mrd.23537
202. Gou M, Li J, Yi L, Li H, Ye X, Wang H, et al. Reprogramming of ovarian aging epigenome by resveratrol. PNAS Nexus. (2023) 2:pgac310. doi: 10.1093/pnasnexus/pgac310
203. Pagnamenta AT, Taanman J-W, Wilson CJ, Anderson NE, Marotta R, Duncan AJ, et al. Dominant inheritance of premature ovarian failure associated with mutant mitochondrial DNA polymerase gamma. Hum Reprod (Oxford England). (2006) 21:2467–73. doi: 10.1093/humrep/del076
204. Tiosano D, Mears JA, Buchner DA. Mitochondrial dysfunction in Primary ovarian insufficiency. Endocrinology. (2019) 160:2353–66. doi: 10.1210/en.2019-00441
205. Konstantinidis M, Alfarawati S, Hurd D, Paolucci M, Shovelton J, Fragouli E, et al. Simultaneous assessment of aneuploidy, polymorphisms, and mitochondrial DNA content in human polar bodies and embryos with the use of a novel microarray platform. Fertility Sterility. (2014) 102:1385–92. doi: 10.1016/j.fertnstert.2014.07.1233
206. Santos TA, El Shourbagy S, St John JC. Mitochondrial content reflects oocyte variability and fertilization outcome. Fertility sterility. (2006) 85:584–91. doi: 10.1016/j.fertnstert.2005.09.017
Keywords: epigenetics, menopause, ovary, aging, mitochondria
Citation: Mani S, Srivastava V, Shandilya C, Kaushik A and Singh KK (2024) Mitochondria: the epigenetic regulators of ovarian aging and longevity. Front. Endocrinol. 15:1424826. doi: 10.3389/fendo.2024.1424826
Received: 28 April 2024; Accepted: 21 October 2024;
Published: 13 November 2024.
Edited by:
Honey V. Reddi, Belay Diagnostics, United StatesReviewed by:
Bei Shi, China Medical University, ChinaShafeeq Ahmed Mohammed, University Hospital Zürich, Switzerland
Yidong Bai, The University of Texas Health Science Center at San Antonio, United States
Copyright © 2024 Mani, Srivastava, Shandilya, Kaushik and Singh. This is an open-access article distributed under the terms of the Creative Commons Attribution License (CC BY). The use, distribution or reproduction in other forums is permitted, provided the original author(s) and the copyright owner(s) are credited and that the original publication in this journal is cited, in accordance with accepted academic practice. No use, distribution or reproduction is permitted which does not comply with these terms.
*Correspondence: Keshav K. Singh, a2tzaW5naEB1YWIuZWR1; Shalini Mani, c2hhbGluaS5tYW5pQGppaXQuYWMuaW4=