- Department of Head and Neck Surgery section II, The Third Affiliated Hospital of Kunming Medical University, Kunming, Yunnan, China
Like the ovaries and prostate, the thyroid exhibits characteristic hormone secretion and regulation. Thyroid cancer (TC), especially differentiated thyroid carcinoma, has typical sex-specific and age-specific hormone-driven clinical features. Previous research has primarily focused on the effects of thyroid stimulating hormone, thyroid hormones, and estrogens on the onset and progression of TC, while the roles of growth hormone (GH), androgens, and glucocorticoids have largely been overlooked. Similarly, few studies have investigated the interactions between hormones and hormone systems. In fact, numerous studies of patients with acromegaly have shown that serum levels of GH and insulin-like growth factor-1 (IGF-1) may be associated with the onset and progression of TC, although the influences of age, sex, and other risk factors, such as obesity and stress, remain unclear. Sex hormones, the GH/IGF axis, and glucocorticoids are likely involved in the onset and progression of TC by regulating the tumor microenvironment and metabolism. The aim of this review was to clarify the roles of hormones and hormone systems in TC, especially papillary thyroid carcinoma, as references for further investigations.
Introduction
Precise production of hormones and regulation of hormone systems are essential for homeostasis and physical functions. Hormone dysregulation contributes to the onset and progression of many pathologies, including cancers. The hypothalamus plays a central role in regulation of both pituitary hormones and important hormone systems, especially the hypothalamic-pituitary-adrenal (HPA), hypothalamic-pituitary-gonadal (HPG), and hypothalamic-pituitary-thyroidal (HPT) axes, in various physiological and pathological processes.
Sex steroids control gonadal development, metabolism, and immunity. The HPG axis regulates secretion of sex hormones via activation of gonadotropin-releasing hormone, which is followed by release of follicle-stimulating hormone and luteinizing hormone from the anterior pituitary. Secretion of sex hormones is dynamic and influenced by various factors, such as emotional changes, stress, diet, and obesity.
Growth hormone (GH) secreted by the pituitary regulates development and metabolism of carbohydrates, proteins, and fats. Serum GH levels peak during early childhood and puberty, then gradually decrease with age. Stressors, such as low blood sugar and intense exercise, stimulate the release of GH (1). Moreover, GH indirectly stimulates production of insulin-like growth factor-1 (IGF-1) in the liver and kidneys, which regulates DNA production and cell division. The interaction between GH and IGF-1 may underlie physiological and pathological cell growth and proliferation.
The HPA axis regulates stress responses, energy balance, and immune function. In response to physical or psychological stress, corticotropin-releasing hormone (CRH) produced by the hypothalamus binds to receptors on the adrenal cortex to control the release of glucocorticoids. The HPA axis is regulated by negative feedback inhibition from glucocorticoids as well as genomic and non-genomic factors. Glucocorticoids are necessary for maintenance of normal physiological functions and processes, including metabolism, immune responses, mood, cognitive functions, reproduction, and development (2).
Thyroid cancer (TC) is the most common cancer of the endocrine system (3). The prevalence of TC is influenced by age, sex, inheritance, and radiation exposure. While most TC patients have normal levels of thyroid hormones (THs) and thyroid stimulating hormone (TSH), many studies have confirmed an association between changes in thyroid function and the onset and development of TC. Notably, TSH promotes growth of thyroid follicular cells, which potentially impact the onset and progression of TC. Similarly, both TSH and THs are associated with certain invasive clinicopathological characteristics and postoperative recurrence of TC (4). TH replacement therapy is the primary approach for long-term management of TC. After total thyroidectomy or lobectomy, TH replacement therapy is initiated to restore euthyroidism and serum TSH levels. However, aggressive TSH-suppressive therapy has limited or no benefits for many patients with differentiated thyroid carcinoma (DTC) (5). Therefore, hormonal regulation of TC is not limited to TSH and THs.
Many malignant tumors exhibit sex-specific differences in occurrence, malignancy, aggressiveness, and prognosis. Unlike endocrine organs, such as the mammary glands, prostate, ovaries, and testes, the thyroid plays a crucial physiological role in both males and females. Although changes to THs and TSH levels in patients with TC are very subtle, variations in levels of TSH, triiodothyronine (T3), and thyroxine (T4) have different effects on the occurrence and development of TC between males and females (6). Although the incidence is higher among females, TC tends to be more aggressive in males (7). TC in males typically presents with a higher propensity for extrathyroidal invasion, lymph node metastasis, and even distant metastasis. A retrospective clinical study reported that TC was more invasiveness in children and adolescents (8). Notably, more than half of adolescent males with TC have lymph node metastasis, while the number of adults and the elderly with TC and lymph node metastasis has decreased. In addition, acromegaly, which is characterized by high GH and IGF-1 levels, and has been associated with greater risks for thyroid diseases, including TC (9). Moreover, the association between stress, obesity, abnormal blood pressure and TC has been increasingly recognized (10). Therefore, the aim of this review is to clarify the functions of hormone and hormone systems in TC and the influences of age, sex, and other risk factors.
Regulation of thyroid development and hormone secretion
The thyroid is the largest endocrine gland in the human endocrine system and indispensable for cellular differentiation and growth. The HPT axis serves as the main regulator of thyroid growth and the production and release of THs. The physiological functions of TSH, especially iodide uptake and production of THs, are activated by binding to the thyrotropin receptor (TSHR) (11). Additionally, various other factors, such as IGF-1, transforming Growth Factor β,GH, and prolactin, are involved in development and growth of the thyroid (12–14).
Exposure to chlordecone, a endocrine-disrupting chemical that mimics female hormones, has been associated with increased levels of TSH and sex steroid hormones in utero (15). With sufficient iodine levels, the thyroid regulates the “growth spurt” that occurs at the onset of puberty of euthyroid children and adolescents, but seems to be regulated by significant increases in circulating sex steroids rather than TSH (16). Meanwhile, variations in sex steroid levels were reported to impact thyroid growth and TSH regulation in rats (17).
GH replacement therapy has been shown to increase serum T3 levels and the total volume of the thyroid gland in GH-deficient adults (18). IGF-1 is a significant modulator of the TSH response in adult thyroid cell differentiation and has an additive effect with TSH (19). Cheung et al. (20) found that IGF-1 promoted thyroid cell proliferation by potentiating the mitogenic activities of TSH. Goretzki et al. (21) demonstrated that TSH enhanced production of thyroid-specific autocrine IGF-1, which is crucial to growth of thyrocytes. Another study by Goretzki et al. (22) supported these findings and showed that the effects of TSH are concentration dependent in tumor cells. For example, TSH at 100 mIU/ml promoted growth of tumor cells, but had an inhibitory effect at higher concentrations. This study also found that TSH regulation of thyrocyte growth is controlled by locally produced IGF-1 (22). These findings emphasize the regulatory role of IGF-1 in the growth of human thyrocytes. Moreover, T3 is also necessary for secretion of GH secretion. However, IGF-1 is reported to suppress GH gene expression through a short negative feedback loop involving T3. Moreover, circulating levels of IGF-1 and TSH receptors have been associated with Graves’ disease (23).
In addition, cortisol levels influence secretion of THs. Cai et al. (24) demonstrated that serum levels of TSH and free T4 were significantly decreased in cortisol-producing adenomas (CPAs) as compared to healthy controls and asymptomatic adrenal incidentalomas. This study also revealed a negative association of cortisol, TSH, and T4 serum levels with CPAs. Furthermore, adrenalectomy was shown to reverse decreased serum levels of both TSH and free T4. However, GH and prolactin levels remained unchanged from baseline levels. These findings suggest that GH, IGF-1, sex hormones, and cortisol control thyroid development and secretion of THs (Figure 1).
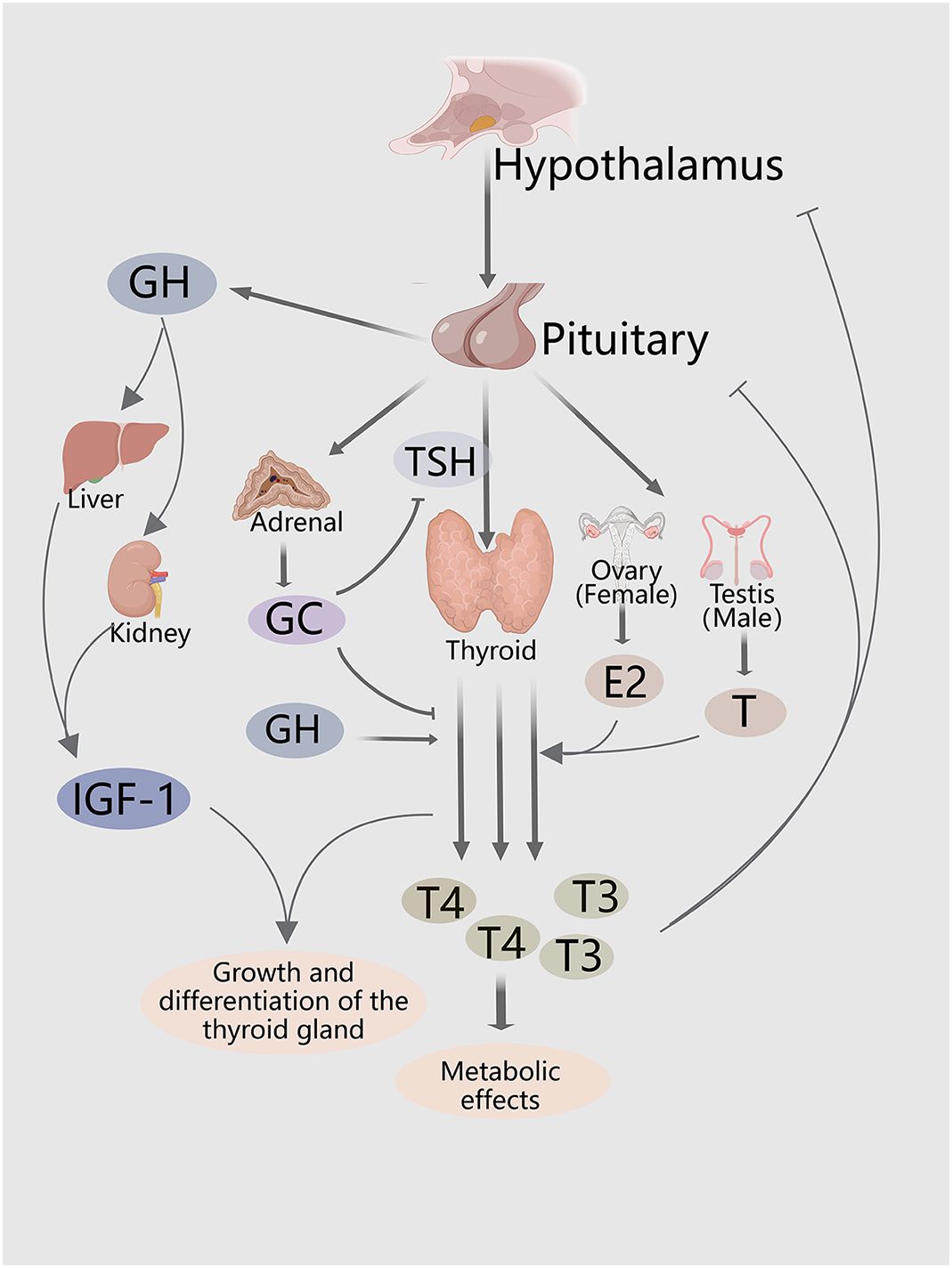
Figure 1 Hormones and hormone systems both directly and indirectly regulate thyroid growth, and thyroid hormone secretion. The HPT axis regulates thyroid proliferation and production of THs. The GH/IGF-1 axis and sex steroids indirectly promote secretion of THs, and thyroid growth. HPA axis inhibits secretion of THs and TSH.
HPG axis and sex hormones
The incidence of TC is 3–5-fold greater in females than males (7, 25, 26). Endogenous estrogens play complex and ambiguous roles in the development of DTC, especially among females during perimenopause (27). Furthermore, higher levels of testosterone and androstenedione increase the risk of DTC in pre/perimenopausal women, but not men or postmenopausal women (28), suggesting that regulation of sex hormones in TC is complex and dynamic.
Estrogen, progesterone, and receptors
Estrogen is an important steroid hormone that regulates development and immune function. Polymorphisms of the estrogen receptor (ER) influence the responses of tissue to estrogens and contribute to oncogenesis (29). The involvement of the ER and progesterone receptor (PR) in TC has been established. For instance, a study by Vannucchi et al. (30) found that more than two-thirds of 182 patients with papillary thyroid cancer (PTC) were positive for estrogen receptor alpha (ERα) and PR. Meanwhile, Eldien et al. (31) reported that upregulated expression of ER and PR, in addition to advanced age, were significantly associated with primary TC. Additionally, pregnancy and delivery can influence the persistence and recurrence of TC (32).
The precise functions of female hormones in TC vary across different studies. A nationwide cohort study conducted in Korea (33) found that estrogen and related hormone receptors only slightly promoted progression of TC, while the absence of estrogen did not protect against disease onset. Although the risk of TC is increased after hysterectomy and oophorectomy, there is reportedly no significant correlation between the use of oral contraceptives and disease incidence (34). Moreover, studies on the effects of phytoestrogens on the development of TC have reported mixed results. For example, high intake of coumestrol was correlated to an increased risk of TC, while moderate intake of genistein provided some protection against thyroid macrocarcinomas in females (35).
Estrogen regulates the progression of TC through both classical genomic and non-genomic pathways (26). In the genomic pathway, estrogen enters the cell and forms a complex with ERα and estrogen receptor Beta (ERβ), which binds to estrogen-responsive elements to promote transcription of target genes (36). Additionally, membrane-associated estrogen receptors are involved in non-genomic signaling of estradiol (E2), which stimulates activation of various pathways associated with regulation of the cell cycle, signal transmission from membrane-based receptors, and immune and inflammatory responses (37). In PTC, these pathways are activated either by chromosomal rearrangement of the receptor tyrosine kinase (RTK),or the BRAFV600E mutation (37).
In TC, estrogen and hypoxia promote generation of reactive oxygen species (ROS) through various mechanisms (38). Estrogen/ERα-dependent autophagy has been associated with production of ROS, activation of extracellular signal-regulated kinases 1 and 2 (ERK1/2), and the survival and growth of PTC cells (39). Excessive accumulation of ROS or the lack of proper detoxification mechanisms can be detrimental to cells and contribute to the occurrence of TC. Oxidative damage, particularly to DNA, can promote malignant transformation of thyroid tissues, while 2,4-dienoyl CoA reductase 1 (NADPH) oxidase generates substantial amounts of ROS, thus potentially enhancing the incidence of spontaneous mutations. Faria et al. (40) demonstrated that estrogen via NADPH oxidase 4 stimulates production of ROS, which can penetrate the nucleus, likely contributing to thyroid carcinogenesis, and that the thyroid glands of adult female, as compared to male, rats produce relatively higher amounts of hydrogen peroxide and relatively lower levels of antioxidant enzymes. Furthermore, estrogen and hypoxia have been reported to regulate hypoxia-inducible factor-1 and influence key molecular, cellular, and metabolic processes involved in progression of TC (38).
ER mediates production of ROS and promotes cellular proliferation (41, 42). The expression patterns of ERα and ERβ in TC are complex and multifaceted. PTC tissues have elevated expression of ERα as compared to adjacent non-tumor tissues (39). Kim et al. (43) suggested that ERα may play a role in the association between breast cancer and TC, although ERβ has been shown to act as an oncosuppressor. For instance, Magri et al. (44) reported that ERβ (−), as compared to ERβ (+), tumors were more likely to have vascular invasion. However, Dong et al. (45) found a positive correlation between ERβ2 and Ki-67 in female TC patients during perimenopause. Nevertheless, ERβ2 expression was lower in females of reproductive age with lymph node metastasis of PTC than without and positively associated with vascular endothelial growth factor expression in males aged 18–45 years, but not tumor size, extrathyroidal extension, or metastasis stage (45). Interestingly, undifferentiated thyroid stem and progenitor cells exhibited lower levels of ERβ as compared to differentiated human thyrocytes (46). Thus, low levels of ER expression may indicate dedifferentiation in TC (26).
ERs are expressed by various immune cells in the tumor microenvironment (TME) of TC and play various roles in tumorigenesis and inflammation (41, 47). As important constituents of the TME, immune cells contribute to tumor growth by facilitating metastasis of malignant cells. The BRAFV600E mutation plays an important role in the estrogen responsiveness of TC by regulating ER expression (48). Additionally, a detailed comparison of glycosylation patterns of TC patients and healthy controls provided insights into abnormal changes to glycosylation of the Fc fragment of immunoglobulin (Ig) G1. Interestingly, among females, there were distinct changes in the incidences of most glycosylated forms starting at puberty or menopause that were associated with sex hormones and IgG glycans, with a particularly notable impact of E2 (49).
Androgens and receptors
Xu et al. (50) reported associations between serum levels of sex hormones and the pathological characteristics of PTC in males, as high serum levels of estrogens promoted proliferation of cancer cells, while androgens exhibited protective effects, as least to some extent. This finding is consistent with the clinical phenomenon of the higher incidence of PTC in females. However, the protective roles of androgens against the onset and progression of TC remain controversial (Table 1). Thus, the functions of androgens and androgen receptors (ARs) in the development of TC are complex and multifaceted.
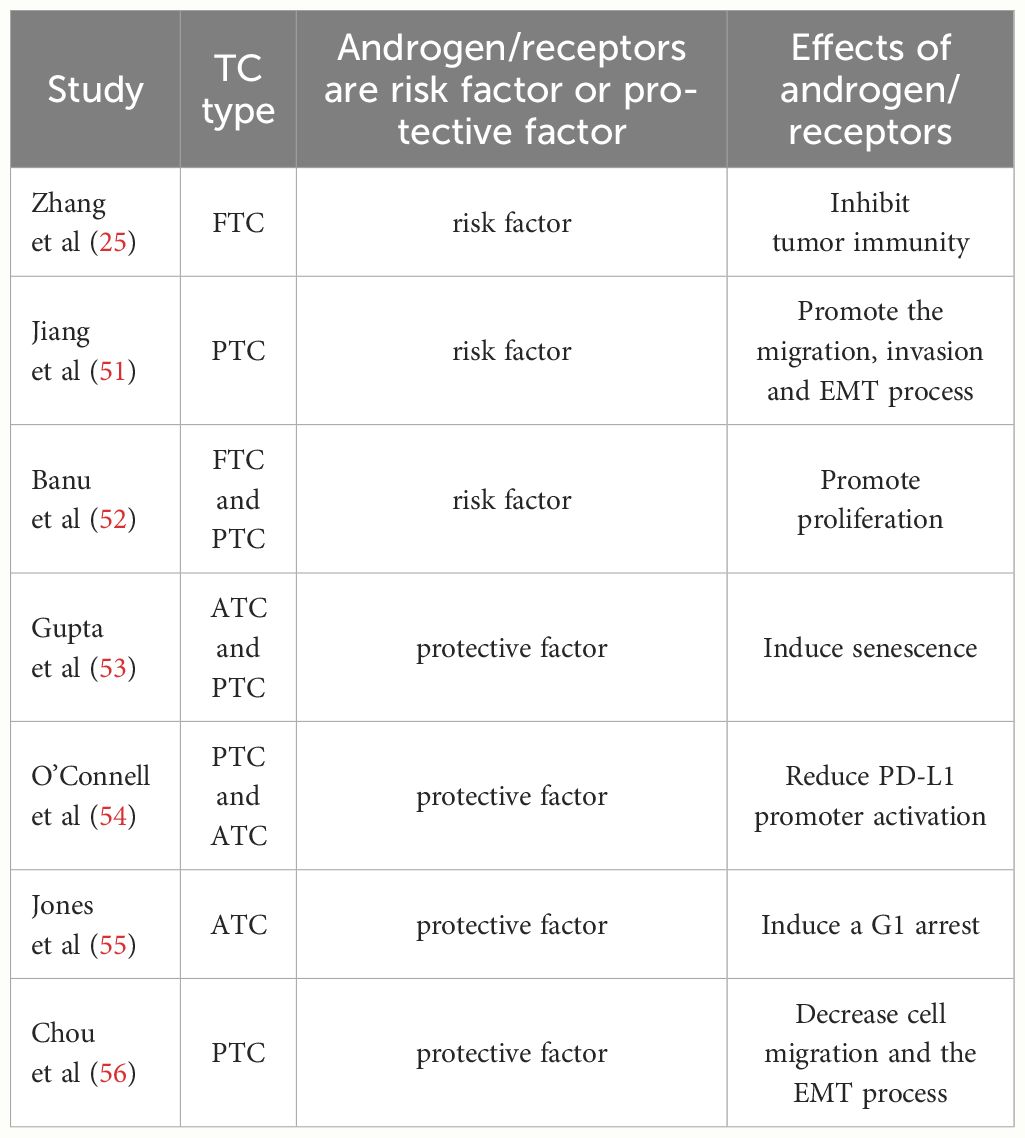
Table 1 Studies that report the role of androgen/receptors in TC in addition to the dual effects of risk and protective factors.
Testosterone is an androgen that plays various physiological roles in the onset and progression of PTC (51). Banu et al. (52) suggested that testosterone, similar to E2, promotes growth and metastasis of PTC cells, although the underlying mechanisms remain unclear. Zhang et al. (25) showed that testosterone regulates progression of TC by reducing expression of tumor suppressor genes and tumor immunity. In addition, Jiang et al. (51) found that testosterone facilitated growth, invasion, and migration of PTC cells and epithelial-mesenchymal transition (EMT). Upregulation of Tnnt1 activates the P38/JNK pathway and promotes malignant behavior in PTC (51). Furthermore, Spirina et al. (57) revealed upregulated expression of AR in PTC, while Magri et al. (44) suggested that AR expression was associated with higher frequencies of capsular invasion. Similarly, Gupta et al. (53) observed increased migration, rather than invasion, by TC cells treated with 5α-dihydrotestosterone, demonstrating that androgens may contribute to the progression of TC.
However, many investigations found that androgens protective against TC (55). High expression of programmed death-ligand 1 (PD-L1) has been associated with aggressive forms of TC (58). O’Connell et al. (54) showed that AR activation decreased PD-L1 expression in TC cells via inhibition of the nuclear factor kB (NF-kB) signaling. Moreover, Gupta et al. (53) provided evidence that activation of AR promotes senescence and apoptosis of malignant TC cells. Similarly, Chou et al. (56) reported that overexpression of AR decreased cell migration and repressed epithelial-mesenchymal transition (EMT) in TC, indicating that the androgen-AR axis may protect males against TC, at least to some extent. The contradictory effects of sex hormones in TC may be explained by the different responsiveness to hormones and the influences of other hormones.
HPA axis and glucocorticoids
For the past six decades, there has been a predominant focus on the immunosuppressive properties of glucocorticoids. However, recent studies have increasingly demonstrated that glucocorticoids also enhance inflammation and immunity (59). Nonetheless, current evidence is insufficient to support the involvement of glucocorticoids in the formation of the TME in TC. Upon binding to an appropriate glucocorticoid receptor (GR), glucocorticoids are involved in various physiological processes, including cell differentiation, metabolism, and proliferation (60). Crucially, glucocorticoids play significant roles in responses to stressors by facilitating energy production, inflammation suppression, and blood pressure regulation. Afrashteh et al. (10) demonstrated that stress and short-temperedness were directly related to the occurrence of TC, while sufficient sleep quantity and good sleep quality appeared to decrease this risk. The HPA axis and glucocorticoids, which serve as the primary regulators of stress, are potential predictors of the risk of TC in individuals experiencing high levels of stress. Lee et al. (61) found higher GR expression in males, as compared to females, with TC. Furthermore, GR expression was significantly higher in TC patients aged >45 years, suggesting that the pathobiological role of GR in TC might be associated with changes to the circadian rhythm of thyroid tumors (61). Additionally, Choi et al. (62) found that urine corticoid levels were slightly higher in male PTC patients.
Invitti et al. (63) reported that the prevalence of nodular thyroid disease was significantly higher in patients with Cushing’s disease. Although the direct cause of thyroid changes in relation to glucocorticoid excess remains unclear, other factors could potentially be involved, such as increased activity of corticotrophic cells or the presence of a growth factor that stimulates both corticotroph and thyrocyte proliferation. In addition, Zhang et al. (64) suggested a possible relationship between GR expression and thyroid adenomas, although this association was not established in TC. Conversely, Melnik et al. (65) reported that the synthetic glucocorticoid dexamethasone suppressed metastasis of follicular thyroid cancer (FTC) cells, but had no effect on benign and recurrent FTC. Relatively few studies have explored potential between the HPA axis and the onset and progression of TC. Although glucocorticoids have different effects on the regulation of stress, immunity, and inflammatory responses in the hormone environment depending on sex and age, these reactions may directly or indirectly participate in the onset and development of TC. Therefore, a thorough evaluation involving a larger patient cohort is needed to clarify the roles of glucocorticoids and GRs in TC.
GH and GH/IGF axis
Studies investigating the mechanisms of GH in TC have established that growth hormone-releasing hormone (GHRH), a peptide hormone secreted by the hypothalamus that regulates GH synthesis and secretion in the pituitary, plays a role in TC. GHRH expression and GHRH receptor mRNA have been identified in thyroid cells, and inhibition of GHRH has been found to suppress growth and promote apoptosis of TC cells (66). However, the specific functions of GH in these processes remain unclear. Studies exploring the roles of the GH/IGF-1 axis in TC have primarily focused on individuals with acromegaly, who typically exhibit elevated GH and IGF-1 levels. Nonetheless, the results of these studies have yielded conflicting findings. Most research in this area has predominantly concentrated on three main aspects: (i) whether individuals with acromegaly are at a greater risk of TC; (ii) whether the onset and progression of TC in acromegaly patients are associated with GH/IGF-1 levels; and (iii) whether there are any sex differences of TC among patients with acromegaly. However, further investigations are still required to precisely address these questions.
Relationship between GH/IGF excess and TC
Colorectal cancer and thyroid carcinoma, especially PTC, are the most frequently occurring malignant tumors among individuals with acromegaly (67). Most studies (68–71) report an increased risk of TC in acromegaly patients. More aggressive tumor behavior of TC has also been associated with acromegaly (72). However, other studies (73–75) found no significant difference in TC occurrence between acromegaly patients and the control group, despite a higher incidence of thyroid diseases (Table 2).
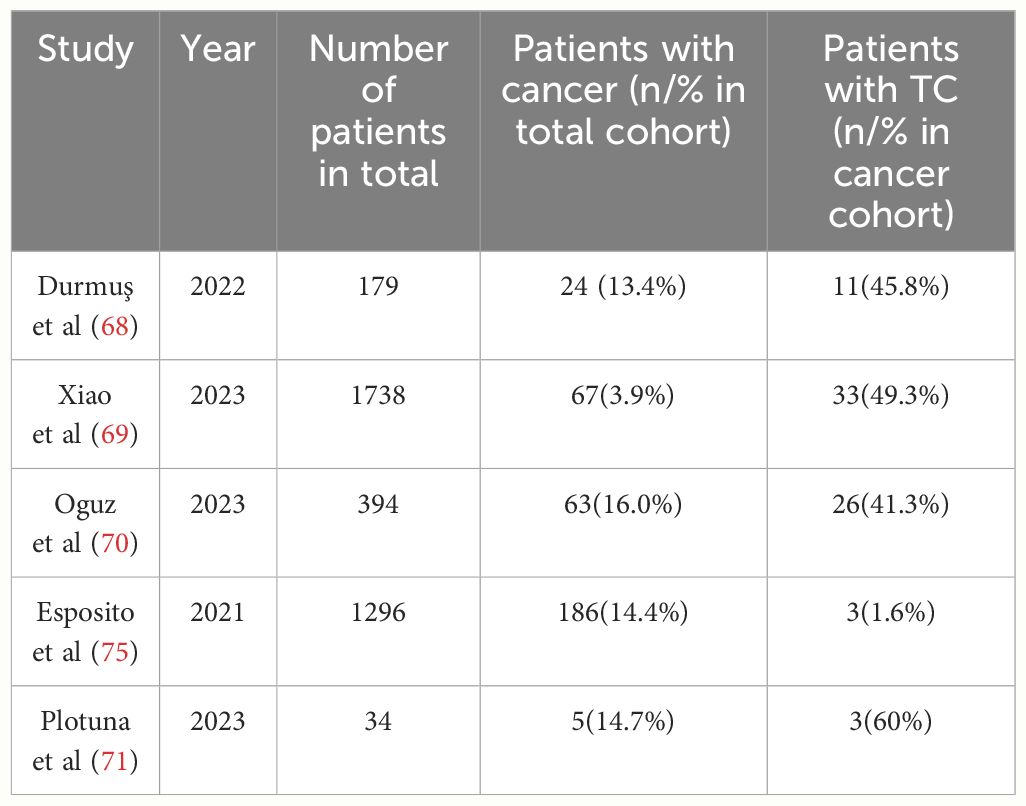
Table 2 Recent single and multicenter retrospective cohort and case-control studies that report the prevalence of TC in acromegaly (The last three years of research in PubMed).
The pathogenesis of TC varies among acromegaly patients. Some studies (9, 76) suggested a potential link between the activation of IGF-1 and the development of TC. Several case series reported that somatostatin receptor ligands reduced IGF-1 levels in acromegaly patients (77). Interestingly, GH and IGF-1 deficiencies have been associated with a lower incidence of malignancy (78). Additionally, mutations to enzymes involved in GH/IGF-1 signaling pathways have been linked to increased carcinogenesis (67), although there are dissenting opinions. For example, Gullu et al. (79) argued that the development of TC in acromegaly patients is more closely related to elevated initial GH levels rather than IGF-1 levels, while Zhao et al. (72) found a high prevalence of a BRAF mutation in PTC patients with acromegaly, suggesting the potential pathogenesis of this subgroup. An cohort study conducted in Italy (80) indicated that the risk of DTC was not correlated to GH/IGF-1 levels, but might be associated with BRAF mutations and overexpression of the aryl hydrocarbon receptor. However, Aydin et al. (81) challenged the notion that the BRAFV600E mutation is a causative factor of DTC among acromegaly patients, citing a relatively lower prevalence of this mutation.
Sex difference and metabolic environment in TC patients with acromegaly
Females generally are at a greater risk of TC than males. However, this association has not been verified in females with acromegaly. A study conducted in Korea (82) reported that TC was the most common malignancy of patients with acromegaly and females were at a greater risk of malignancy, consistent with the prevalence in the overall cohort. However, a similar study (79) reported that the prevalence of TC was higher in males. In most studies, the difference in the incidence of TC between the males and females was not significant. Additionally, in acromegaly patients with controlled disease, GH levels were higher in postmenopausal females than males, while IGF-1 levels were comparable (83).
Xiao et al. (69) found that patients with acromegaly have an increased risk of cancer and acromegaly was associated with diabetes mellitus. GH plays a significant role in glycometabolism and exerts an insulin-sensitizing effect that surpasses the effects of IGF-1 (84). Consequently, long-term exposure to high levels of GH and IGF-1 may lead to insulin resistance and lipodystrophy (85).
In summary, the inconsistent findings of these studies suggest that the predisposition of patients with acromegaly to TC is influenced by factors other than sex hormones. Nonetheless, elevated levels of GH and IGF-1, which are significant hormonal features of acromegaly, may have distinct roles in the development of TC in this population.
IGF signaling in TC
IGF-1/2 and related receptors have been implicated in the pathogenesis of TC. Studies conducted as early as 30 years ago confirmed that IGF-1 promotes growth of human FTC cells (21). More recent data consistently show significant upregulation of IGF-1 and downregulation of insulin-like growth factor-2 (IGF-2) in TC as compared to normal thyroid tissues (86). Similarly, PTC, but not multinodular nontoxic goiter, is associated with elevated concentrations of circulating IGF-1. Furthermore, IGF-1 receptor (IGF-1R) levels are relatively upregulated in PTC and anaplastic thyroid cancer (ATC) (87). In addition, IGF-1 concentrations were positively associated with the risk of DTC (88). Pidchenko et al. (89) demonstrated that elevated levels of IGF-1 and IGF-2 were correlated with increased insulin production in PTC patients. Furthermore, IGF-1, IGF binding protein-3 (IGF-BP3), and adiponectin levels were correlated to various histologic types of TC. For example, IGF-1 and IGF-BP3 levels were upregulated in patients with intrathyroid invasion and associated with the invasive capacity of TC, while IGF-1, IGF-BP3, and adiponectin levels with type 2 diabetes were correlated with tumor size (90). Interestingly, IGF-I and IGF-IR expression was observed in children and adolescents, and malignant features were correlated with IGF-1R (91).
IGF-1 promotes progression of PTC through the signal transducer and activator of transcription 3 (STAT3) signaling pathway (92). A study conducted by Lv et al. (93) to clarify the mechanisms underlying the onset and proliferation of TC demonstrated that release of IGF-1 by M2-like tumor-associated macrophages promoted metastasis and increased the stemness of ATC cells via insulin receptor-A/IGF1R-mediated activation of the phosphoinositide 3-kinase (PI3K)/alpha serine/threonine-protein kinase (AKT)/mammalian target of rapamycin (mTOR) signaling pathway (93). Furthermore, the long non-coding RNA Linc00210 was reported to enhance the malignant potential of TC cells via modulation of the miR-195-5p/IGF1R/Akt axis (94). Additionally, the disrupted in renal carcinoma 3 was shown to alter progression of DTC by regulating IGF signaling (95).
Despite a compelling preclinical rationale for targeting IGFs in TC, the findings of clinical studies thus far have been underwhelming, which could be attributed to the interplay between IGF signaling and other pathways, leading to resistance against targeted agents designed to inhibit specific components of these intricate signaling networks (96).
Overlapping activities and interconnectedness of hormone systems
Hormones not only regulate growth of TC cells through shared pathways, but also interact to modulate regulatory effects. Hormone levels differ between TC patients and healthy controls, as some TC patients exhibit abnormal thyroid function, imbalance of sex hormone levels, and endocrine-related diseases, such as diabetes and acromegaly. As mentioned earlier, the synthesis and secretion of these important hormones are regulated by the hypothalamic-pituitary axis. The unique hormone environment of TC cells directly or indirectly affects hormone secretion by the hypothalamus and pituitary gland. However, single hormone monitoring and management is insufficient to control the occurrence and development of TC.
In TC cells, sex steroids, GH/IGF-1, and TSH/TH activate the PI3K/AKT and mitogen-activated protein kinase (MAPK) pathways (Figure 2). Recent studies have extensively explored the effects of THs, TSH, and sex hormones of TC, but relatively few studies have investigated the impact and interactions of cortisol, GH, and other hormones in TC.
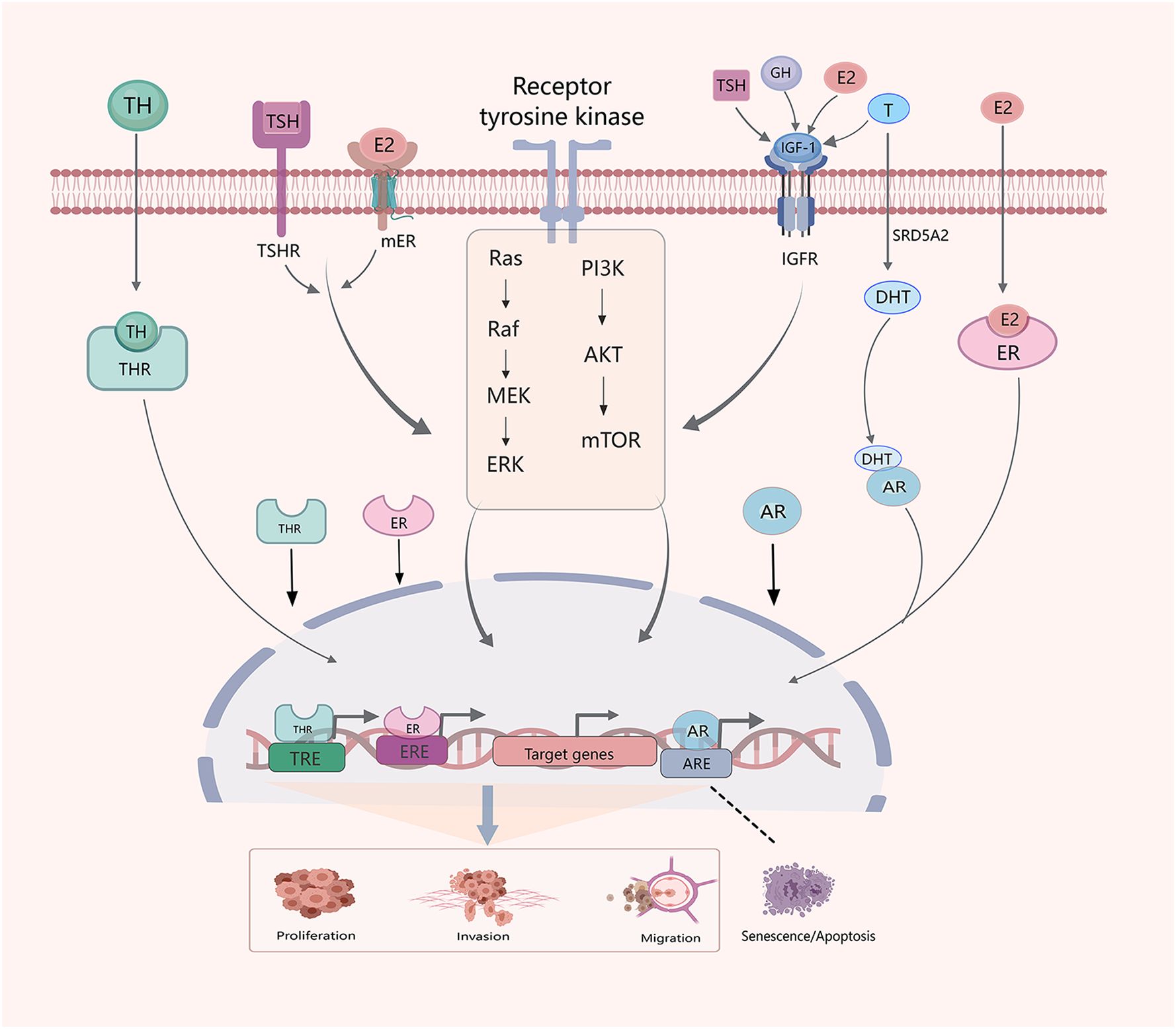
Figure 2 Crosstalk between hormones and hormone systems in the development of TC. Sex hormones, THs, TSH, GH and IGF-1 interact. E2, IGF-1 and TSH influence thyroid development through PI3K/AKT/mTOM and MAPK signaling pathways. Similarly, E2,THs and testosterone (T) regulate progression of TC through classical genomic factors. The SRD5A2 enzyme reduces T to dihydrotestosterone (DHT).
GH/IGF axis and sex hormone/receptors
Centrally, sex hormones regulate pituitary secretion of GH and, peripherally, modulate related signaling pathways (97). Estrogens, for example, can reduce IGF-1 levels by inhibiting GH secretion by hepatocytes, but increase central GH secretion (98). Meanwhile, estrogens suppress GH receptor signaling via inhibition of cytokine receptor signaling (98). However, compounds with estrogen-like properties can inhibit some activities of GH while promoting others. Androgens, on the other hand, affect both GH secretion and activities, and also play a role in stimulating the production of IGF-1 (98). The biological function of estrogens, especially E2, are mainly exerted by interactions with ERs. This process is subject to feedback regulation by GH and IGF-1 (99). In men, testosterone concentrations are positively correlated with the regularity of GH secretion, although both testosterone and GH concentrations tend to decrease with increasing age (100, 101).
The close relationship between nutrient metabolism and IGFs expression was demonstrated as early as 1994 (102). Secretion of GH under fasting conditions has sex-specific ramifications (101, 103). A pediatric study conducted by Cicognani et al. (104) found that sex hormones influence IGF-1 levels, which appears to be mediated through GH secretion. A study by Papatheodorou et al. (105) revealed an association between circulating levels of E2, sex hormone-binding globulin (SHBG), and IGF-BP3 in males in the United States. In addition, both IGF-1and ER mediate various biological processes via the PI3K signaling pathway that are crucial for the onset and progression of TC. Additionally, metformin may be useful for treatment of differentiated or poorly differentiated TC, which may involve regulation through the ER or AR signaling pathway, as demonstrated in prostate cancer (106).
The effects of complicated hormone levels on metabolism, particularly glycoproteins and lipids, have been implicated in progression of TC. Both IGFs and estrogen have been found to influence glycolysis in TC. For example, Huang et al. (107) suggested that the fat mass and obesity-associated protein inhibits expression of apolipoprotein E through insulin-like growth factor binding protein 2-mediated m6A modification, which may inhibit glycolytic metabolism in PTC by modulating the interluekin-6/Janus kinase 2/STAT3 signaling pathway, consequently suppressing tumor growth (107). Similarly, Zhu et al. (108) demonstrated that estrogen increases malignant activities as well as glycolysis in PTC cells by inhibiting expression of FAM111 trypsin-like peptidase B (FAM111B) and proposed that the E2/DNA (cytosine-5)-methyltransferase 3B/FAM111B axis is crucial for regulating the progression of PTC (108). Collectively, these findings suggest crosstalk between sex hormones and the GH/IGF axis in TC, particularly PTC.
TSH/THs and sex hormone/receptors
Extranuclear signaling pathways mediated by THs, estrogens, and androgens regulate various biological processes (109). In certain types of tumors, THs and sex steroids exhibit interacting and overlapping effects. For instance, THs have been shown to influence concentrations of SHBG, as evidenced by elevated testosterone and SHBG levels in males with hyperthyroidism (110). Similarly, the estrogen inhibitor tamoxifen was found to lower serum T3 levels in adult female rats. Moreover, sex-specific relationships between TSH and TH levels were observed in children and adolescents (111). Specifically, this study reported that mean logTSH and freeT3 levels were significantly higher in males than females, while age was negatively correlated with thyroid function in both males and females, and with freeT4 levels in males (111). Currently, there is no evidence linking sex hormones to differences in TSH and TH levels between males and females.
In cancer cells, THs and estrogen signaling display significant crosstalk through promoter cross-reactivity (112). Similarly, THs interact with the AR promoter region and affect the responsiveness of androgen by increasing AR expression. Thyroid response elements have been identified in the promoter regions of AR and several androgen-related genes (113). Estrogens, especially E2, and THs have overlapped biologic effects in TC, although the underlying mechanisms remain unclear. Some reports have suggested that estrogen-related cancers may be permissively modulated by TH and ER, especially ER-positive TC (27). Administration of estrogens may negatively influence treatment of relapsed DTC in both males and females. Estrogens and THs demonstrated an additive effect on tumor growth and development in postmenopausal females with recurrent differentiated tumors after estrogen replacement and TSH suppression (27). Meanwhile, E2 was shown to significantly inhibit TSH-induced differentiation of progenitor cells and expression of the sodium/iodide symporter (46). Furthermore, E2 obviously decreased levels of thyroid differentiation markers, including TSHR, indicating impaired thyroid differentiation (46).
Estrogens and TSH/TH exhibit significant effects on BRAF and p53 activities in TC tumor cells. The BRAFV600E mutation was significantly more common in tumors expressing TSH (114) and was shown to override BRAF-induced senescence, thereby promoting tumor progression via downregulation of p53 expression in PTC (115). Additionally, cell lines carrying the BRAFV600E mutation demonstrated increased metastatic potential in response to E2 (48). In the BRAFV600E group, the ERα/ERβ ratio was elevated in younger participants (≤50 years) (48). Furthermore, E2 was found to synergistically activate the tyrosine kinase pathway in TC cells with the RET fusion and BRAF mutation (37). Moreover, thyroid transcription factor-1, ER, PR, and p53 were co-expressed in most young females (15–34 years) with PTC (116).
Additionally, sex hormones and TSH regulate the development of TC via the MAPK signaling pathway. Estrogen activates MAPK-dependent serine phosphorylation of nuclear ERα. Similarly, a study by Jiang et al. (51) showed that testosterone promotes malignancy via the MAPK (p38/JNK) signaling pathway. TH is a MAPK-dependent growth factor with anti-apoptotic effects. When THs are activated, the MAPK (ERK1/2) signaling pathway induces serine phosphorylation of several nucleoproteins, including nuclear ERβ (117). An in vitro study reported that microRNA 106a, which regulates expression of the TSH receptor, is associated with the proliferation, apoptosis, differentiation, and iodine uptake of TC cells by modulating the MAPK signaling pathway (118). Furthermore, ERs have been shown to modulate the PI3K/AKT/mTOR pathway, thereby influencing proliferation of TC (41). Similarly, activation of TSH-TSHR signaling has been found to play a role in increasing mobility and dedifferentiation of TC cells via crosstalk with the PI3K/AKT/mTOR signaling pathway (119).
IGF1 and TSH/TH
Similar to TSH, IGF-1 is also considered a risk factor in TC (120). An in vitro study (96) demonstrated that IGF-1R enhances TSH-induced activation of thyroid-specific genes, particularly the sodium/iodide symporter. This process is facilitated by the ERK1/2 or AKT pathways. Additionally, TSH interacts with IGF-1 (22). The co-activities of TSH and IGF-1 promote growth of human FTC cells (21). Moreover, TSHR and IGF-1R have been observed to co-immunoprecipitate in both orbital and thyroid tissues, indicating formation of a functional complex (121). Furthermore, TSH and insulin/IGF-I act synergistically to increase proliferation and growth of thyroid cells, which are primarily mediated through the cyclic adenosine monophosphate, PI3K, and MAPK pathways (122, 123).
Crosstalk of hormone systems as a risk factor for TC
Increasing evidence suggests that obesity, stress, and high blood pressure increase the risk of TC (10, 124). Various hormones are also activated in response to these stressors (Figure 3). Obesity is a complex physiological condition that is regulated by various hormones, but can also lead to hormonal imbalances and homeostatic disruptions. In general, obesity, central fat distribution, stress, depression, and unstable blood pressure are closely related and may contribute to several pathological effects, such as hyperinsulinemia, increased aromatase activity, chronic inflammation, altered immune responses, and oxidative stress (125). IGF-1, sex hormones, TSH, and cortisol directly contribute to these processes and play significant roles in thyroid tumorigenesis.
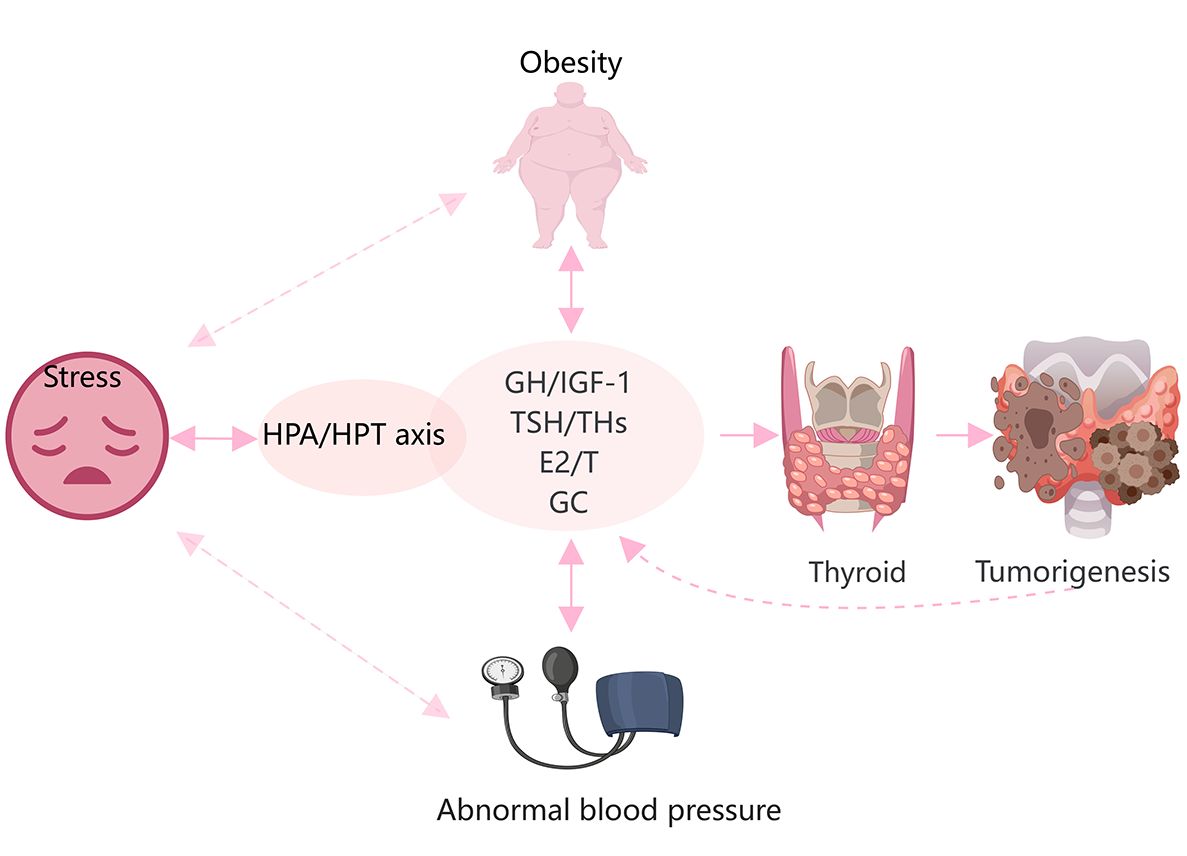
Figure 3 Hypertension, obesity, and stress are closely related to hormonal changes, and promote the onset and progression of TC. Glucocorticoids(GC), THs, sex steroids, and the GH/IGF-1 axis are directly and indirectly involved in the development of obesity and hypertension. Stress is mainly regulated by the HPA and HPT axes. These factors are involved in the occurrence and development of TC.
Obesity is an established risk factor for DTC in females but not males (126). Both obesity and TC are more frequent in females than males worldwide, implying the involvement of estrogens (127, 128). In fact, serum levels of ERα are increased in obese females (128). However, various factors other than sex, such ethnicity and age, especially adolescence, may influence the association between obesity and the risk of TC (125). An imbalance of estrogens and androgens may be responsible for the greater risk of TC in obese individuals (125). As a well-known risk factor for diabetes, obesity is characterized by insulin resistance and hyperinsulinemia (128). IGF-1 and IGF-2 mediate the functions of insulin as well as the obesity process (129). Marcello et al. (126) reported that higher consumption of animal proteins and carbohydrates contribute to obesity and a greater risk of DTC via a mechanism possibly related to upregulation of IGF-1.
Furthermore, the HPA and HPT axes are also involved in the regulation of obesity, stress, and depression (130, 131). Accumulating epidemiological data demonstrate an independent association between TSH levels and obesity (125). Additionally, there is a parallel increase in the incidence of TC among both adults and children in conjunction with the rising levels of stress in contemporary society. Prolonged secretion of glucocorticoids due to stress can potentially induce chronic inflammation and suppress immune responses. Stress can lead to dysregulation of the thyroid gland through crosstalk between the HPA and HPT axes. However, further investigations are needed to determine whether this association is involved in the onset and progression of TC (132).
Hormone regulation in TC at different ages
Hormone levels change with age. Typically, children and adolescents experience hormone surges during periods of rapid growth and development. Adult and middle-aged women usually have relatively stable hormone levels until perimenopause or menopause, when hormone levels once again fluctuate. Hormonal changes may explain why TC cells in adults also exhibit milder characteristics, with tumors less aggressive and less able to migrate than in children and the elderly (Table 3).
Hormone regulation in TC among younger populations
DTC is the most frequent malignant tumor of the endocrine system in children and adolescents. TC, especially DTC, is typically more aggressive in pediatric patients than adults. Nevertheless, the prognosis of pediatric TC patients is often positive, even for those with advanced disease. However, the prognosis of persistent or recurrent disease is often poor (133). Therefore, it is crucial to elucidate the pathogenesis of TC in young individuals and effectively manage associated risk factors to optimize treatment.
At puberty, the HPG axis interacts with the GH/IGF-1 axis to facilitate physical growth spurts (134). In addition, GH and insulinomimetic signals are regulated by sex steroids. Androgens, for example, induce IGF-1 activation in bone, muscle, and skin tissues, while suppressing IGF-1R expression in adipose tissues. Thus, regulation of GH and IGF-1 is determined by the specific type of sex hormone and target tissue. In contrast, GH and insulin upregulate AR and ER in an organ-specific manner. Consequently, interactions of sex steroids with the GH/IGF axis can increase susceptibly to TC in children and teenagers.
Sex steroids, rather than TSH, play more significant roles in thyroid development in children and adolescents (16). Notably, during puberty in females, characteristic changes to glycosylation are associated with sex hormones, particularly E2. These modifications may create a favorable TME for TC (49). A case-control study conducted by Kim et al. (135) revealed a relationship between obesity and TC in a young Korean population (18 years old). Besides obesity, other risk factors for TC include adolescence (125). A case-control study by Suzuki et al. (136) demonstrated a positive association between body weight and an increased risk of TC in children and adolescents, particularity in males. However, post puberty, the risks of TC and persistent disease decrease. The post-puberty period is strongly associated with the clinical behavior of TC and males are at a greater risk for persistent disease (137). High levels of TSH are generally considered a risk factor for TC. However, this regulatory mechanism appears to be more prevalent in adults than teenagers. Suzuki et al. (111) reported that TSH levels were associated with age and thyroid function in children and adolescents with pediatric thyroid carcinoma, and age was negatively correlated with thyroid function in both male and female teenagers. Moreover, lower logTSH levels and higher antithyroglobulin levels were independent risk factors for the development of thyroid nodules and age was positively correlated with free T4 levels in young males (111). These findings suggest that teenage males may be more susceptible to TC than adult males.
ERα expression is lower in adolescents with TC, while hormone receptor levels in females are not related to sex, American Thyroid Association risk score, persistent structural disease, or pubertal status (137). Therefore, female hormones and receptors may not be major factors in the progression of TC at puberty.
Additionally, IGF-1 and IGF-1R are associated with the development of TC in younger populations, as high expression of IGF-1R enhances the aggressiveness of cancer cells (91). Circulating levels of GH, IGF-1, sex hormones, and THs may directly or indirectly affect the expression levels of IGF-1 and IGF-1R in tumor tissues.TC tends to be more aggressive in children and adolescents with higher GH and IGF-1 levels. Moreover, the unique psychological changes and stress experienced during adolescence may amplify the interplay between hormonal systems, particularly the HPA and HPT axes. Hence, further studies are warranted to investigate the characteristics of TC and associated risk factors in children and adolescents.
Hormone regulation in TC among the elderly
According to the American Joint Committee on Cancer, age at diagnosis is associated with staging of DTC and advanced age is associated with compromised prognosis. The pathogenesis of DTC is complex in older patients and not well understood. Thus, further studies are needed to explore the roles of hormone systems in the elderly.
Younger people usually have substantial physiological reserves, which are gradually depleted with aging because of reduced hormone requirements. During senescence, hypopituitarism may be a physiological process. Older patients are more susceptible to growth hormone deficiency, gonadotropin deficiency, and hypothyroidism (138). Pituitary hormone deficiency increases with age. DTC cells are regulated by multiple hormones. Older people experience a relatively nutrient-poor environment due to lower hormone levels and less physiological reserves, which can increase the susceptibility of DTC cells to stress, thereby enhancing invasion and migration.
The prevalence of TC is increased in patients with hypothyroidism (139). Subclinical hypothyroidism was identified as an independent risk factor for extrathyroidal extension in patients with PTC. The risk of extrathyroidal extension associated with subclinical hypothyroidism is reportedly higher in males than females (140). More importantly,hypothyroidism is the most frequent endocrine disease in the elderly, with an increased prevalence in women as compared to men (141, 142). The most frequent cause of hypothyroidism in the elderly is autoimmune thyroiditis (143). Older men have higher baseline TSH levels and lower T3 and free T4 serum levels than younger men. Older people also have lower TSH responses to thyrotropin-releasing hormone. Furthermore, the inhibitory effect of glucocorticoids on TSH secretion is reduced with aging in men (144). This evidence suggests that the elderly, especially older men, may exhibit poorer hormonal responsiveness. The secretion of hypothalamic-pituitary axis and target organ hormones, especially glucocorticoids and TSH, may be chaotic. In this hormone environment, TC cells are more stressful, and the cancer-promoting effects of hormones may be more obvious.
Undifferentiated TC is more common in the elderly. TSH and IGF-1 are major regulators of thyroid cell differentiation in adults (19). IGF-1 is crucial for differentiation of murine embryonic stem cells to thyrocytes (145). The lower level of IGF-1 in the elderly may increase the dedifferentiation of tumor cells in TC, which may explain why 95% of TCs are well differentiated in children and adolescents. For example, high total IGF-2 levels accompanied by low serum levels of IGF-I and GH have been associated with poorly differentiated TC in the elderly (146). In addition, postmenopausal women have increased ERα expression (147), which may be involved in more aggressive behavior. Similarly, decreased estrogen levels and increased levels of follicle-stimulating hormone are associated with epidermal growth factor receptor expression and activation in postmenopausal women with DTC (148). This status is involved in the recurrence of PTC (148). In summary, differences in the hormone environment, including fluctuations in thyroid function, suppression of the GH/IGF-1axis, and changes to estrogen and ER levels, partially explain why TC is more common and aggressive in older people.
Conclusion and future perspectives
The thyroid is a complex endocrine gland that produces a variety of hormones. The onset and progression of TC, particularly DTC, are regulated by various hormone systems. The interactions of various hormones influence thyroid tumorigenesis. In pediatric patients, the development of TC, particularly DTC, is dependent on the interactions of sex hormones and GH/IGF-1, especially during puberty due to specific physiological needs. Thus, further investigations with the use of in vitro and animal models should focus on the mechanisms underlying the crosstalk between various hormones and hormone systems in the onset and progression of TC.
Author contributions
L-HC: Writing – original draft, Writing – review & editing. TX: Writing – original draft. QL: Writing – original draft. Y-RG: Writing – original draft. C-ZS: Writing – review & editing.
Funding
The author(s) declare financial support was received for the research, authorship, and/or publication of this article. This work was supported by grants from the National Natural Science Foundation of China (82360568 and 81960543), and Yunnan Province Basic Research Program (202401AS070011).
Acknowledgments
We would like to acknowledge the reviewers for their helpful comments on this paper. MedPeer (https://www.medpeer.cn) provided the drawing materials and drawing platform.
Conflict of interest
The authors declare that the research was conducted in the absence of any commercial or financial relationships that could be construed as a potential conflict of interest.
Publisher’s note
All claims expressed in this article are solely those of the authors and do not necessarily represent those of their affiliated organizations, or those of the publisher, the editors and the reviewers. Any product that may be evaluated in this article, or claim that may be made by its manufacturer, is not guaranteed or endorsed by the publisher.
Glossary
References
1. Hiller-Sturmhöfel S, Bartke A. The endocrine system: an overview. Alcohol Health Res World. (1998) 22:153–64.
2. Timmermans S, Souffriau J, Libert C. A general introduction to glucocorticoid biology. Front Immunol. (2019) 10:1545. doi: 10.3389/fimmu.2019.01545
3. Davies L, Welch HG. Increasing incidence of thyroid cancer in the United States, 1973-2002. Jama. (2006) 295:2164–7. doi: 10.1001/jama.295.18.2164
4. Liu Y, Huang Y, Mo G, Zhou T, Hou Q, Shi C, et al. Combined prognostic value of preoperative serum thyrotrophin and thyroid hormone concentration in papillary thyroid cancer. J Clin Lab Anal. (2022) 36:e24503. doi: 10.1002/jcla.24503
5. Grani G, Ramundo V, Verrienti A, Sponziello M, Durante C. Thyroid hormone therapy in differentiated thyroid cancer. Endocrine. (2019) 66:43–50. doi: 10.1007/s12020-019-02051-3
6. Huang H, Rusiecki J, Zhao N, Chen Y, Ma S, Yu H, et al. Thyroid-stimulating hormone, thyroid hormones, and risk of papillary thyroid cancer: a nested case–control study. Cancer Epidemiol Biomarkers Prev. (2017) 26:1209–18. doi: 10.1158/1055-9965.EPI-16-0845
7. Nilubol N, Zhang L, Kebebew E. Multivariate analysis of the relationship between male sex, disease-specific survival, and features of tumor aggressiveness in thyroid cancer of follicular cell origin. Thyroid. (2013) 23:695–702. doi: 10.1089/thy.2012.0269
8. Cheng F, Xiao J, Shao C, Huang F, Wang L, Ju Y, et al. Burden of thyroid cancer from 1990 to 2019 and projections of incidence and mortality until 2039 in China: findings from global burden of disease study. Front Endocrinol. (2021) 12:738213. doi: 10.3389/fendo.2021.738213
9. Vargas-Ortega G, Romero-Gameros CA, Rendón-Macias ME, Balcázar-Hernández L, Sosa-Eroza E, Mercado M, et al. Risk factors associated with thyroid nodular disease in acromegalic patients: a case-cohort study in a tertiary center. Growth Hormone IGF Res. (2021) 60:101431. doi: 10.1016/j.ghir.2021.101431
10. Afrashteh S, Fararouei M, Parad M, Mirahmadizadeh A. Sleep quality, stress and thyroid cancer: a case–control study. J Endocrinol Invest. (2022) 45:1219–26. doi: 10.1007/s40618-022-01751-4
11. Brent GA. Mechanisms of thyroid hormone action. J Clin Invest. (2012) 122:3035–43. doi: 10.1172/JCI60047
12. Sornson MW, Wu W, Dasen JS, Flynn SE, Norman DJ, O'Connell SM, et al. Pituitary lineage determination by the Prophet of Pit-1 homeodomain factor defective in Ames dwarfism. Nature. (1996) 384:327–33. doi: 10.1038/384327a0
13. Gesing A, Bartke A, Masternak MM, Lewiński A, Karbownik-Lewińska M. Decreased thyroid follicle size in dwarf mice may suggest the role of growth hormone signaling in thyroid growth regulation. Thyroid Res. (2012) 5:1–6. doi: 10.1186/1756-6614-5-7
14. Brix K, Führer D, Biebermann H. Molecules important for thyroid hormone synthesis and action-known facts and future perspectives. Thyroid Res. (2011) 4:1–6. doi: 10.1186/1756-6614-4-S1-S9
15. Ayhan G, Rouget F, Giton F, Costet N, Michineau L, Monfort C, et al. In utero chlordecone exposure and thyroid, metabolic, and sex-steroid hormones at the age of seven years: A study from the TIMOUN mother-child cohort in Guadeloupe. Front Endocrinol. (2021) 12:771641. doi: 10.3389/fendo.2021.771641
16. Michalaki MA, Mamali I, Tsekouras A, Vlassopoulou B, Anastasiou E, Koukkou EG, et al. Thyroid-stimulating hormone is not the primary regulator of thyroid development in euthyroid children and adolescents living in an iodine-replete area. Hormones. (2018) 17:391–6. doi: 10.1007/s42000-018-0056-y
17. Banu KS, Aruldhas M. Sex steroids regulate TSH-induced thyroid growth during sexual maturation in Wistar rats. Exp Clin Endocrinol Diabetes. (2002) 110:37–42. doi: 10.1055/s-2002-19993
18. Leite N, Salvatori R, Alcântara M, Alcântara P, Oliveira C, Oliveira J, et al. Effects of depot growth hormone replacement on thyroid function and volume in adults with congenital isolated growth hormone deficiency. J Endocrinol Invest. (2012) 35:265–8. doi: 10.3275/7608
19. López-Márquez A, Carrasco-López C, Fernández-Méndez C, Santisteban P. Unraveling the complex interplay between transcription factors and signaling molecules in thyroid differentiation and function, from embryos to adults. Front Endocrinol. (2021) 12:654569. doi: 10.3389/fendo.2021.654569
20. Cheung N, Lou J, Boyages S. Growth hormone does not increase thyroid size in the absence of thyrotropin: a study in adults with hypopituitarism. J Clin Endocrinol Metab. (1996) 81:1179–83. doi: 10.1210/jcem.81.3.8772597
21. Goretzki P, Frilling A, Simon D, Roeher H-D. Growth regulation of normal thyroids and thyroid tumors in man. Hormone-Rel Malignant Tumors. (1990) 118:48–63. doi: 10.1007/978-3-642-83816-3_6
22. Goretzki PE, Simon D, Dotzenrath C, Schulte K-M, Röher H-D. Growth regulation of thyroid and thyroid tumors in humans. World J Surg. (2000) 24:913–22. doi: 10.1007/s002680010174
23. Tsui S, Naik V, Hoa N, Hwang CJ, Afifiyan NF, Sinha Hikim A, et al. Evidence for an association between thyroid-stimulating hormone and insulin-like growth factor 1 receptors: a tale of two antigens implicated in Graves’ disease. J Immunol. (2008) 181:4397–405. doi: 10.4049/jimmunol.181.6.4397
24. Cai R, Zhou W, Jiang L, Jiang Y, Su T, Zhang C, et al. Association between thyroid function and serum cortisol in cortisol-producing adenoma patients. Endocrine. (2020) 69:196–203. doi: 10.1007/s12020-020-02278-5
25. Zhang LJ, Xiong Y, Nilubol N, He M, Bommareddi S, Zhu X, et al. Testosterone regulates thyroid cancer progression by modifying tumor suppressor genes and tumor immunity. Carcinogenesis. (2015) 36:420–8. doi: 10.1093/carcin/bgv001
26. Lu M, Liu H, Zheng B, Sun S, Chen C. Links between breast and thyroid cancer: hormones, genetic susceptibility and medical interventions. Cancers. (2022) 14:5117. doi: 10.3390/cancers14205117
27. Davis PJ, Lin H-Y, Mousa SA, Luidens MK, Hercbergs AA, Wehling M, et al. Overlapping nongenomic and genomic actions of thyroid hormone and steroids. Steroids. (2011) 76:829–33. doi: 10.1016/j.steroids.2011.02.012
28. Rinaldi S, Dossus L, Keski-Rahkonen P, Kiss A, Navionis AS, Biessy C, et al. Circulating endogenous sex steroids and risk of differentiated thyroid carcinoma in men and women. Int J Cancer. (2024) 154:2064–74. doi: 10.1002/ijc.34872
29. Maha R, Kallel I, Charfeddine S, Hamza F, Guermazi F, Rebaï A. Association of polymorphisms in estrogen and thyroid hormone receptors with thyroid cancer risk. J Recept Signal Transduct. (2009) 29:113–8. doi: 10.1080/10799890902845682
30. Vannucchi G, De Leo S, Perrino M, Rossi S, Tosi D, Cirello V, et al. Impact of estrogen and progesterone receptor expression on the clinical and molecular features of papillary thyroid cancer. Eur J Endocrinol. (2015) 173:29–36. doi: 10.1530/EJE-15-0054
31. Eldien MMS, Abdou AG, Rageh T, Abdelrazek E, Elkholy E. Immunohistochemical expression of ER-α and PR in papillary thyroid carcinoma. Ecancermedicalscience. (2017) 11:748. doi: 10.3332/ecancer.2017.748
32. Messuti I, Corvisieri S, Bardesono F, Rapa I, Giorcelli J, Pellerito R, et al. Impact of pregnancy on prognosis of differentiated thyroid cancer: clinical and molecular features. Eur J Endocrinol. (2014) 170:659–66. doi: 10.1530/EJE-13-0903
33. Kim M, Kim BH, Lee H, Nam H, Park S, Jang MH, et al. Thyroid cancer after hysterectomy and oophorectomy: a nationwide cohort study. Eur J Endocrinol. (2021) 184:143–51. doi: 10.1530/EJE-20-0686
34. Rosenblatt KA, Gao DL, Ray RM, Nelson ZC, Wernli KJ, Li W, et al. Oral contraceptives and the risk of all cancers combined and site-specific cancers in Shanghai. Cancer Causes Control. (2009) 20:27–34. doi: 10.1007/s10552-008-9213-y
35. Wang Q, Huang H, Zhao N, Ni X, Udelsman R, Zhang Y. Phytoestrogens and thyroid cancer risk: A population-based case-control study in connecticut. Cancer Epidemiol Biomarkers Prev. (2020) 29:500–8. doi: 10.1158/1055-9965.EPI-19-0456
36. Nilsson S, Makela S, Treuter E, Tujague M, Thomsen J, Andersson Gr, et al. Mechanisms of estrogen action. Physiol Rev. (2001) 81:1535–65. doi: 10.1152/physrev.2001.81.4.1535
37. Derwahl M, Nicula D. Estrogen and its role in thyroid cancer. Endocrine-related Cancer. (2014) 21:T273–83. doi: 10.1530/ERC-14-0053
38. Rajoria S, Hanly E, Nicolini A, George AL, Geliebter J, Shin EJ, et al. Interlinking of hypoxia and estrogen in thyroid cancer progression. Curr Med Chem. (2014) 21:1351–60. doi: 10.2174/0929867321666131201142434
39. Fan D, Liu SY, van Hasselt CA, Vlantis AC, Ng EK, Zhang H, et al. Estrogen receptor α induces prosurvival autophagy in papillary thyroid cancer via stimulating reactive oxygen species and extracellular signal regulated kinases. J Clin Endocrinol Metab. (2015) 100:E561–71. doi: 10.1210/jc.2014-3257
40. Faria CC, Peixoto MS, Carvalho DP, Fortunato RS. The emerging role of estrogens in thyroid redox homeostasis and carcinogenesis. Oxid Med Cell Longev. (2019) 2019:2514312. doi: 10.1155/2019/2514312
41. Denaro N, Romanò R, Alfieri S, Dolci A, Licitra L, Nuzzolese I, et al. The tumor microenvironment and the estrogen loop in thyroid cancer. Cancers. (2023) 15:2458. doi: 10.3390/cancers15092458
42. Fugazzola L, Colombo C, Perrino M, Muzza M. Papillary thyroid carcinoma and inflammation. Front Endocrinol. (2011) 2:88. doi: 10.3389/fendo.2011.00088
43. Kim YA, Kim YA, Cho SW, Song YS, Min HS, Park IA, et al. Increased expression of thyroid hormone receptor alpha and estrogen receptor alpha in breast cancer associated with thyroid cancer. Eur J Surg Oncol. (2021) 47:1316–23. doi: 10.1016/j.ejso.2021.01.015
44. Magri F, Capelli V, Rotondi M, Leporati P, La Manna L, Ruggiero R, et al. Expression of estrogen and androgen receptors in differentiated thyroid cancer: an additional criterion to assess the patient's risk. Endocrine Related Cancer. (2012) 19:463. doi: 10.1530/ERC-11-0389
45. Dong W, Li J, Zhang H, Huang Y, He L, Wang Z, et al. Altered expression of estrogen receptor β2 is associated with different biological markers and clinicopathological factors in papillary thyroid cancer. Int J Clin Exp Pathol. (2015) 8:7149–56.
46. Xu S, Chen G, Peng W, Renko K, Derwahl M. Oestrogen action on thyroid progenitor cells: relevant for the pathogenesis of thyroid nodules. J Endocrinol. (2013) 218:125–33. doi: 10.1530/JOE-13-0029
47. Chakraborty B, Byemerwa J, Krebs T, Lim F, Chang C-Y, McDonnell DP, et al. Estrogen receptor signaling in the immune system. Endocrine Rev. (2023) 44:117–41. doi: 10.1210/endrev/bnac017
48. Kim M, Kim S-j, Ha SY, Xu Z, Han Y, Jee H-G, et al. BRAFV600E mutation enhances estrogen-induced metastatic potential of thyroid cancer by regulating the expression of estrogen receptors. Endocrinol Metab. (2022) 37:879–90. doi: 10.3803/EnM.2022.1563
49. Chen G, Wang Y, Qiu L, Qin X, Liu H, Wang X, et al. Human IgG Fc-glycosylation profiling reveals associations with age, sex, female sex hormones and thyroid cancer. J Proteomics. (2012) 75:2824–34. doi: 10.1016/j.jprot.2012.02.001
50. Xu FZ, Zheng LL, Chen KH, Wang R, Yi DD, Jiang CY, et al. Serum sex hormones correlate with pathological features of papillary thyroid cancer. Endocrine. (2023) 84:148–54. doi: 10.1007/s12020-023-03554-w
51. Jiang C, Xu F, Yi D, Jiang B, Wang R, Wu L, et al. Testosterone promotes the migration, invasion and EMT process of papillary thyroid carcinoma by up-regulating Tnnt1. J Endocrinol Invest. (2024) 47:149–66. doi: 10.1007/s40618-023-02132-1
52. Banu KS, Govindarajulu P, Aruldhas MM. Testosterone and estradiol have specific differential modulatory effect on the proliferation of human thyroid papillary and follicular carcinoma cell lines independent of TSH action. Endocrine Pathol. (2001) 12:315–27. doi: 10.1385/ep:12:3:315
53. Gupta A, Carnazza M, Jones M, Darzynkiewicz Z, Halicka D, O'Connell T, et al. Androgen receptor activation induces senescence in thyroid cancer cells. Cancers (Basel). (2023) 15:2198. doi: 10.3390/cancers15082198
54. O'Connell TJ, Dadafarin S, Jones M, Rodríguez T, Gupta A, Shin E, et al. Androgen activity is associated with PD-L1 downregulation in thyroid cancer. Front Cell Dev Biol. (2021) 9:663130. doi: 10.3389/fcell.2021.663130
55. Jones ME, O'Connell TJ, Zhao H, Darzynkiewicz Z, Gupta A, Buchsbaum J, et al. Androgen receptor activation decreases proliferation in thyroid cancer cells. J Cell Biochem. (2021) 122:1113–25. doi: 10.1002/jcb.29934
56. Chou CK, Chi SY, Chou FF, Huang SC, Wang JH, Chen CC, et al. Aberrant expression of androgen receptor associated with high cancer risk and extrathyroidal extension in papillary thyroid carcinoma. Cancers (Basel). (2020) 12:1109. doi: 10.3390/cancers12051109
57. Spirina L, Chizhevskaya SY, Kondakova I, Choinzonov E. Reception of sex steroid hormones in thyroid papillary cancer tissue and relationship with expression and content of transcription factors Brn-3α and TRIM16. Bull Exp Biol Med. (2018) 166:237–40. doi: 10.1007/s10517-018-4322-4
58. Chintakuntlawar AV, Rumilla KM, Smith CY, Jenkins SM, Foote RL, Kasperbauer JL, et al. Expression of PD-1 and PD-L1 in anaplastic thyroid cancer patients treated with multimodal therapy: results from a retrospective study. J Clin Endocrinol Metab. (2017) 102:1943–50. doi: 10.1210/jc.2016-3756
59. Cain DW, Cidlowski JA. Immune regulation by glucocorticoids. Nat Rev Immunol. (2017) 17:233–47. doi: 10.1038/nri.2017.1
60. Melnik D, Sahana J, Corydon TJ, Kopp S, Nassef MZ, Wehland M, et al. Dexamethasone inhibits spheroid formation of thyroid cancer cells exposed to simulated microgravity. Cells. (2020) 9:367. doi: 10.3390/cells9020367
61. Lee C, Kao H, Lin H, P'eng F, Chi C. Estrogen receptors and glucocorticoid receptors in human well-differentiated thyroid cancer. Int J Mol Med. (1998) 2(2):229–33.
62. Choi MH, Moon J-Y, Cho S-H, Chung BC, Lee EJ. Metabolic alteration of urinary steroids in pre-and post-menopausal women, and men with papillary thyroid carcinoma. BMC Cancer. (2011) 11:1–14. doi: 10.1186/1471-2407-11-342
63. Invitti C, Manfrini R, Romanini B, Cavagnini F. High prevalence of nodular thyroid disease in patients with Cushing's disease. Clin Endocrinol. (1995) 43:359–63. doi: 10.1111/j.1365-2265.1995.tb02044.x
64. Zhang X-W, Li Y, Wang Z-L, Li P. Glucocorticoid receptor subunit gene expression in thyroid gland and adenomas. Acta Oncol. (2006) 45:1073–8. doi: 10.1080/02841860600602961
65. Melnik D, Cortés-Sánchez JL, Sandt V, Kahlert S, Kopp S, Grimm D, et al. Dexamethasone selectively inhibits detachment of metastatic thyroid cancer cells during random positioning. Cancers. (2023) 15:1641. doi: 10.3390/cancers15061641
66. Pópulo H, Nunes B, Sampaio C, Batista R, Pinto MT, Gaspar TB, et al. Inhibitory effects of antagonists of growth hormone-releasing hormone (GHRH) in thyroid cancer. Hormones Cancer. (2017) 8:314–24. doi: 10.1007/s12672-017-0307-4
67. Guevara-Aguirre J, Peña G, Acosta W, Pazmiño G, Saavedra J, Soto L, et al. Cancer in growth hormone excess and growth hormone deficit. Endocrine-related Cancer. (2023) 30:e220402. doi: 10.1530/ERC-22-0402
68. Durmuş ET, Atmaca A, Çolak R, Durmuş B. Cancer prevalence and cancer screening in patients with acromegaly: a single center experience. Endocrine. (2022) 77:363–71. doi: 10.1007/s12020-022-03082-z
69. Xiao T, Jiao R, Yang S, Wang Y, Bai X, Zhou J, et al. Incidence and risk factors of cancers in acromegaly: a Chinese single-center retrospective study. Endocrine. (2023) 82:368–78. doi: 10.1007/s12020-023-03447-y
70. Oguz SH, Firlatan B, Sendur SN, Dagdelen S, Erbas T. Follow, consider, and catch: second primary tumors in acromegaly patients. Endocrine. (2023) 80:160–73. doi: 10.1007/s12020-022-03282-7
71. Plotuna I-S, Balas M, Golu I, Amzar D, Vlad A, Moleriu LC, et al. The experience of a single tertiary center regarding benign and Malignant tumors in acromegalic patients. Medicina. (2023) 59:1148. doi: 10.3390/medicina59061148
72. Zhao Y, Wang Y, Zhang X, Jia N, Ma Z, Fu J, et al. Papillary thyroid carcinoma in patients with acromegaly from a single center in China. World Neurosurg. (2021) 149:e22–8. doi: 10.1016/j.wneu.2021.02.101
73. Cankurtaran Y, Örük GG, Tozduman B. Evaluation of thyroid disease and thyroid Malignancy in acromegalic patients. Minerva Endocrinol. (2023) 48:130–9. doi: 10.23736/S2724-6507.21.03363-7
74. Woliński K, Stangierski A, Gurgul E, Bromińska B, Czarnywojtek A, Lodyga M, et al. Thyroid lesions in patients with acromegaly—case-control study and update to the meta-analysis. Endokrynol Polska. (2017) 68:2–6. doi: 10.5603/EP.2017.0001
75. Esposito D, Ragnarsson O, Johannsson G, Olsson DS. Incidence of benign and Malignant tumors in patients with acromegaly is increased: a nationwide population-based study. J Clin Endocrinol Metab. (2021) 106:3487–96. doi: 10.1210/clinem/dgab560
76. Kim HK, Lee JS, Park MH, Cho JS, Yoon JH, Kim SJ, et al. Tumorigenesis of papillary thyroid cancer is not BRAF-dependent in patients with acromegaly. PloS One. (2014) 9:e110241. doi: 10.1371/journal.pone.0110241
77. Dharan SS, Kamaruddin NA. Successful primary medical therapy with somatostatin receptor ligand in acromegaly with thyroid cancer. J ASEAN Fed Endocrine Soc. (2017) 32:169. doi: 10.15605/jafes.032.02.12
78. Danilowicz K, Sosa S, Gonzalez Pernas MS, Bamberger E, Diez SM, Fainstein-Day P, et al. Acromegaly and thyroid cancer: analysis of evolution in a series of patients. Clin Diabetes Endocrinol. (2020) 6:24. doi: 10.1186/s40842-020-00113-4
79. Gullu BE, Celik O, Gazioglu N, Kadioglu P. Thyroid cancer is the most common cancer associated with acromegaly. Pituitary. (2010) 13:242–8. doi: 10.1007/s11102-010-0224-9
80. Mian C, Ceccato F, Barollo S, Watutantrige-Fernando S, Albiger N, Regazzo D, et al. AHR over-expression in papillary thyroid carcinoma: clinical and molecular assessments in a series of Italian acromegalic patients with a long-term follow-up. PloS One. (2014) 9:e101560. doi: 10.1371/journal.pone.0101560
81. Aydin K, Aydin C, Dagdelen S, Tezel GG, Erbas T. Genetic alterations in differentiated thyroid cancer patients with acromegaly. Exp Clin Endocrinol Diabetes. (2015) 124(3):198–202. doi: 10.1055/s-0035-1565061
82. Park KH, Lee EJ, Seo GH, Ku CR. Risk for acromegaly-related comorbidities by sex in Korean acromegaly. J Clin Endocrinol Metab. (2020) 105:e1815–26. doi: 10.1210/clinem/dgz317
83. Dal J, Rosendal C, Karmisholt J, Feldt-Rasmussen U, Andersen MS, Klose M, et al. Sex difference in patients with controlled acromegaly—A multicentre survey. Clin Endocrinol. (2023) 98:74–81. doi: 10.1111/cen.14750
84. Moustaki M, Paschou SA, Xekouki P, Kotsa K, Peppa M, Psaltopoulou T, et al. Secondary diabetes mellitus in acromegaly. Endocrine. (2023) 81(1):1–15. doi: 10.1007/s12020-023-03339-1
85. Freda PU. The acromegaly lipodystrophy. Front Endocrinol. (2022) 13:933039. doi: 10.3389/fendo.2022.933039
86. Karagiannis A, Kassi E, Chatzigeorgiou A, Koutsilieris M. IGF bioregulation system in benign and Malignant thyroid nodular disease: a systematic review. Vivo. (2020) 34:3069–91. doi: 10.21873/invivo.12141
87. Ławnicka H, Motylewska E, Borkowska M, Kuzdak K, Siejka A, Świętosławski J, et al. Elevated serum concentrations of IGF-1 and IGF-1R in patients with thyroid cancers. Biomed Papers-Olomouc. (2020) 164:77–83. doi: 10.5507/bp.2019.018
88. Schmidt JA, Allen NE, Almquist M, Franceschi S, Rinaldi S, Tipper SJ, et al. Insulin-like growth factor-i and risk of differentiated thyroid carcinoma in the European prospective investigation into cancer and nutrition. Cancer Epidemiol Biomarkers Prev. (2014) 23:976–85. doi: 10.1158/1055-9965.EPI-13-1210-T
89. Pidchenko N, Krasnoselskyi M, Mitriaieva N, Grebenik L, Astapieva O, Grushka G, et al. Insulin-like growth factors in the serum of patients with papillary thyroid cancer. Wiad Lek. (2021) 74:1925–30.
90. Pazaitou-Panayiotou K, Panagiotou G, Polyzos SA, Polyzos SA, Mantzoros CS. Serum adiponectin and insulin-like growth factor 1 in predominantly female patients with thyroid cancer: association with the histologic characteristics of the tumor. Endocrine Pract. (2016) 22:68–75. doi: 10.4158/EP15814.OR
91. Gydee H, O'neill JT, Patel A, Bauer AJ, Tuttle RM, Francis GL, et al. Differentiated thyroid carcinomas from children and adolescents express IGF-I and the IGF-I receptor (IGF-IR). Cancers with the most intense IGF-IR expression may be more aggressive. Pediatr Res. (2004) 55:709–15. doi: 10.1203/01.PDR.0000111282.98401.93
92. Yang L, Tan Z, Li Y, Zhang X, Wu Y, Xu B, et al. Insulin-like growth factor 1 promotes proliferation and invasion of papillary thyroid cancer through the STAT3 pathway. J Clin Lab Anal. (2020) 34:e23531. doi: 10.1002/jcla.23531
93. Lv J, Liu C, Chen F-K, Feng Z-P, Jia L, Liu P-J, et al. M2−like tumour−associated macrophage−secreted IGF promotes thyroid cancer stemness and metastasis by activating the PI3K/AKT/mTOR pathway. Mol Med Rep. (2021) 24:604. doi: 10.3892/mmr.2021.12249
94. Du P, Liu F, Liu Y, Shao M, Li X, Qin G, et al. Linc00210 enhances the Malignancy of thyroid cancer cells by modulating miR-195-5p/IGF1R/Akt axis. J Cell Physiol. (2020) 235:1001–12. doi: 10.1002/jcp.29016
95. Wysocki PT, Czubak K, Marusiak AA, Kolanowska M, Nowis D. lncRNA DIRC3 regulates invasiveness and insulin-like growth factor signaling in thyroid cancer cells. Endocrine-Related Cancer. (2023) 30:e230058. doi: 10.1530/ERC-23-0058
96. Manzella L, Massimino M, Stella S, Tirrò E, Pennisi MS, Martorana F, et al. Activation of the IGF axis in thyroid cancer: implications for tumorigenesis and treatment. Int J Mol Sci. (2019) 20:3258. doi: 10.3390/ijms20133258
97. Fernández-Pérez L, de Mirecki-Garrido M, Guerra B, Díaz M, Díaz-Chico JC. Sex steroids and growth hormone interactions. Endocrinología y Nutrición. (2016) 63:171–80. doi: 10.1016/j.endonu.2015.11.004
98. Birzniece V, Ho KK. Sex steroids and the GH axis: implications for the management of hypopituitarism. Best Pract Res Clin Endocrinol Metab. (2017) 31:59–69. doi: 10.1016/j.beem.2017.03.003
99. Veldhuis JD, Roemmich JN, Richmond EJ, Bowers CY. Somatotropic and gonadotropic axes linkages in infancy, childhood, and the puberty-adult transition. Endocrine Rev. (2006) 27:101–40. doi: 10.1210/er.2005-0006
100. Van Den Berg G, Veldhuis JD, Frölich M, Roelfsema F. An amplitude-specific divergence in the pulsatile mode of growth hormone (GH) secretion underlies the gender difference in mean GH concentrations in men and premenopausal women. J Clin Endocrinol Metab. (1996) 81:2460–7. doi: 10.1210/jcem.81.7.8675561
101. Bleach R, Sherlock M, O’Reilly MW, McIlroy M. Growth hormone/insulin growth factor axis in sex steroid associated disorders and related cancers. Front Cell Dev Biol. (2021) 9:630503. doi: 10.3389/fcell.2021.630503
102. Thissen J-P, Ketelslegers J-M, Underwood LE. Nutritional regulation of the insulin-like growth factors. Endocrine Rev. (1994) 15:80–101. doi: 10.1210/edrv-15-1-80
103. Lowe WL Jr., Adamo M, LeRoith D, Roberts CT Jr. Expression and stability of insulin-like growth factor-I (IGF-I) mRNA splicing variants in the GH3 rat pituitary cell line. Biochem Biophys Res Commun. (1989) 162:1174–9. doi: 10.1016/0006-291x(89)90797-3
104. Cicognani A, Cacciari E, Tacconi M, Pascucci MG, Tonioli S, Pirazzoli P, et al. Effect of gonadectomy on growth hormone, IGF-I and sex steroids in children with complete and incomplete androgen insensitivity. Eur J Endocrinol. (1989) 121:777–83. doi: 10.1530/acta.0.1210777
105. Papatheodorou SI, Rohrmann S, Lopez DS, Bradwin G, Joshu CE, Kanarek N, et al. Association between endogenous sex steroid hormones and insulin-like growth factor proteins in US men. Cancer Causes Control. (2014) 25:353–63. doi: 10.1007/s10552-013-0336-4
106. García-Sáenz M, Lobaton-Ginsberg M, Ferreira-Hermosillo A. Metformin in differentiated thyroid Cancer: molecular pathways and its clinical implications. Biomolecules. (2022) 12:574. doi: 10.3390/biom12040574
107. Huang J, Sun W, Wang Z, Lv C, Zhang T, Zhang D, et al. FTO suppresses glycolysis and growth of papillary thyroid cancer via decreasing stability of APOE mRNA in an N6-methyladenosine-dependent manner. J Exp Clin Cancer Res. (2022) 41:1–18. doi: 10.1186/s13046-022-02254-z
108. Zhu X, Xue C, Kang X, Jia X, Wang L, Younis MH, et al. DNMT3B-mediated FAM111B methylation promotes papillary thyroid tumor glycolysis, growth and metastasis. Int J Biol Sci. (2022) 18:4372. doi: 10.7150/ijbs.72397
109. Hammes SR, Davis PJ. Overlapping nongenomic and genomic actions of thyroid hormone and steroids. Best Pract Res Clin Endocrinol Metab. (2015) 29:581–93. doi: 10.1016/j.beem.2015.04.001
110. Meikle AW. The interrelationships between thyroid dysfunction and hypogonadism in men and boys. Thyroid. (2004) 14:17–25. doi: 10.1089/105072504323024552
111. Suzuki S, Suzuki S, Iwadate M, Matsuzuka T, Shimura H, Ohira T, et al. Possible association between thyroid nodule formation and developmental alterations in the pituitary–thyroid hormone axis in children and adolescents: the fukushima health management survey. Thyroid. (2022) 32:1316–27. doi: 10.1089/thy.2022.0327
112. Halada S, Casado-Medrano V, Baran JA, Baran JA, Lee J, Chinmay P, Bauer AJ, et al. Hormonal crosstalk between thyroid and breast cancer. Endocrinology. (2022) 163:bqac075. doi: 10.1210/endocr/bqac075
113. Torabinejad S, Miro C, Barone B, Imbimbo C, Crocetto F, Dentice M, et al. The androgen-thyroid hormone crosstalk in prostate cancer and the clinical implications. Eur Thyroid J. (2023) 12:e220228. doi: 10.1530/ETJ-22-0228
114. Hernandez BY, Rahman M, Loo LW, Chan OT, Horio D, Morita S, et al. BRAF V600E, hypothyroidism, and human relaxin in thyroid carcinogenesis. J Cancer Res Clin Oncol. (2021) 147:183–94. doi: 10.1007/s00432-020-03401-9
115. Zou M, Baitei E, Al-Rijjal R, Parhar R, Al-Mohanna F, Kimura S, et al. TSH overcomes BrafV600E-induced senescence to promote tumor progression via downregulation of p53 expression in papillary thyroid cancer. Oncogene. (2016) 35:1909–18. doi: 10.1038/onc.2015.253
116. Jung C-K, Choi Y-J, Lee K-Y, Bae J-S, Kim H-J, Yoon S-K, et al. The cytological, clinical, and pathological features of the cribriform-morular variant of papillary thyroid carcinoma and mutation analysis of CTNNB1 and BRAF genes. Thyroid. (2009) 19:905–13. doi: 10.1089/thy.2008.0332
117. Tang H-Y, Lin H-Y, Zhang S, Davis FB, Davis PJ. Thyroid hormone causes mitogen-activated protein kinase-dependent phosphorylation of the nuclear estrogen receptor. Endocrinology. (2004) 145:3265–72. doi: 10.1210/en.2004-0308
118. Shen C-T, Qiu Z-L, Song H-J, Wei W-J, Luo Q-Y. miRNA-106a directly targeting RARB associates with the expression of Na+/I– symporter in thyroid cancer by regulating MAPK signaling pathway. J Exp Clin Cancer Res. (2016) 35:101. doi: 10.1186/s13046-016-0377-0
119. Feng F, Han H, Wu S, Wang H. Crosstalk between abnormal TSHR signaling activation and PTEN/PI3K in the dedifferentiation of thyroid cancer cells. Front Oncol. (2021) 11:718578. doi: 10.3389/fonc.2021.718578
120. Abdellateif MS, Shaarawy S, Elesawy YF, Mansour M, Tharwat E, Ibrahim NH, et al. The role of vitamin D, platelet-derived growth factor and insulin-like growth factor 1 in the progression of thyroid diseases. Asian Pac J Cancer Prevent: APJCP. (2020) 21:2083–9. doi: 10.31557/APJCP.2020.21.7.2083
121. Mo C, Zhong L. The effect of acromegaly on thyroid disease. Endocrine J. (2023) 70:1051–60. doi: 10.1507/endocrj.EJ23-0356
122. Zaballos MA, Santisteban P. FOXO1 controls thyroid cell proliferation in response to TSH and IGF-I and is involved in thyroid tumorigenesis. Mol Endocrinol. (2013) 27:50–62. doi: 10.1210/me.2012-1032
123. Ciampolillo A, Tullio C, Giorgino F. The IGF-I/IGF-I receptor pathway: implications in the pathophysiology of thyroid cancer. Curr Med Chem. (2005) 12:2881–91. doi: 10.2174/092986705774454715
124. Huang L, Feng X, Yang W, Li X, Zhang K, Feng S, et al. Appraising the effect of potential risk factors on thyroid cancer: a mendelian randomization study. J Clin Endocrinol Metab. (2022) 107:e2783–91. doi: 10.1210/clinem/dgac196
125. Pappa T, Alevizaki M. Obesity and thyroid cancer: a clinical update. Thyroid. (2014) 24:190–9. doi: 10.1089/thy.2013.0232
126. Marcello MA, Sampaio AC, Geloneze B, Vasques ACJ, Assumpção LVM, Ward LS. Obesity and excess protein and carbohydrate consumption are risk factors for thyroid cancer. Nutr Cancer. (2012) 64:1190–5. doi: 10.1080/01635581.2012.721154
127. Leeners B, Geary N, Tobler PN, Asarian L. Ovarian hormones and obesity. Hum Reprod Update. (2017) 23:300–21. doi: 10.1093/humupd/dmw045
128. Franchini F, Palatucci G, Colao A, Ungaro P, Macchia PE, Nettore IC. Obesity and thyroid cancer risk: an update. Int J Environ Res Public Health. (2022) 19:1116. doi: 10.3390/ijerph19031116
129. Artim SC, Mendrola JM, Lemmon MA. Assessing the range of kinase autoinhibition mechanisms in the insulin receptor family. Biochem J. (2012) 448:213–20. doi: 10.1042/BJ20121365
130. Du F-M, Kuang H-Y, Duan B-H, Liu D-N, Yu X-Y. Effects of thyroid hormone and depression on common components of central obesity. J Int Med Res. (2019) 47:3040–9. doi: 10.1177/0300060519851624
132. Kyriacou A, Tziaferi V, Toumba M. Stress, thyroid dysregulation, and thyroid cancer in children and adolescents: proposed impending mechanisms. Hormone Res Paediatrics. (2023) 96:44–53. doi: 10.1159/000524477
133. Zanella AB, Scheffel RS, Weinert L, Dora JM, Maia AL. New insights into the management of differentiated thyroid carcinoma in children and adolescents. Int J Oncol. (2021) 58:13. doi: 10.3892/ijo.2021.5193
134. Rogol AD. Sex steroids, growth hormone, leptin and the pubertal growth spurt. Pediatr Neuroendocrinol. (2010) 17:77–85. doi: 10.1159/000262530
135. Kim K-N, Hwang Y, Kim KH, Lee KE, Park YJ, Kim S-j, et al. Adolescent overweight and obesity and the risk of papillary thyroid cancer in adulthood: a large-scale case-control study. Sci Rep. (2020) 10:5000. doi: 10.1038/s41598-020-59245-3
136. Suzuki T, Matsuo K, Hasegawa Y, Hiraki A, Kawase T, Tanaka H, et al. Anthropometric factors at age 20 years and risk of thyroid cancer. Cancer Causes Control. (2008) 19:1233–42. doi: 10.1007/s10552-008-9194-x
137. da Silva Breder JRA, Alves PAG, Araújo ML, Pires B, Valverde P, Bulzico DA, et al. Puberty and sex in pediatric thyroid cancer: could expression of estrogen and progesterone receptors affect prognosis? Eur Thyroid J. (2022) 11:e210090. doi: 10.1530/ETJ-21-0090
138. Curtò L, Trimarchi F. Hypopituitarism in the elderly: a narrative review on clinical management of hypothalamic–pituitary–gonadal, hypothalamic–pituitary–thyroid and hypothalamic–pituitary–adrenal axes dysfunction. J Endocrinol Invest. (2016) 39:1115–24. doi: 10.1007/s40618-016-0487-8
139. Zubčić Ž, Šestak A, Mihalj H, Kotromanović Ž, Včeva A, Prpić T, et al. The association between type 2 diabetes mellitus, hypothyroidism, and thyroid cancer. Acta Cli Croatica. (2020) 59:129–35. doi: 10.20471/acc.2020.59.s1.17
140. Li C, Zhang J, Dionigi G, Sun H. The relationship between subclinical hypothyroidism and invasive papillary thyroid cancer. Front Endocrinol. (2023) 14:1294441. doi: 10.3389/fendo.2023.1294441
141. Mitrou P, Raptis SA, Dimitriadis G. Thyroid disease in older people. Maturitas. (2011) 70:5–9. doi: 10.1016/j.maturitas.2011.05.016
142. Calsolaro V, Niccolai F, Pasqualetti G, Calabrese AM, Polini A, Okoye C, et al. Overt and subclinical hypothyroidism in the elderly: when to treat? Front Endocrinol. (2019) 10:177. doi: 10.3389/fendo.2019.00177
143. Peeters RP. Thyroid hormones and aging. Hormones. (2008) 7:28–35. doi: 10.14310/horm.2002.1111035
144. Habra M, Sarlis NJ. Thyroid and aging. Rev Endocrine Metab Disord. (2005) 6:145–54. doi: 10.1007/s11154-005-1494-9
145. Arufe MC, Lu M, Lin R-Y. Differentiation of murine embryonic stem cells to thyrocytes requires insulin and insulin-like growth factor-1. Biochem Biophys Res Commun. (2009) 381:264–70. doi: 10.1016/j.bbrc.2009.02.035
146. Morioka T, Ohba K, Morita H, Takahashi G, Uchida H, Matsushita A, et al. Non–islet cell tumor-induced hypoglycemia associated with macronodular pulmonary metastases from poorly differentiated thyroid carcinoma. Thyroid. (2014) 24:395–9. doi: 10.1089/thy.2013.0141
147. Rubio GA, Catanuto P, Glassberg MK, Lew JI, Elliot SJ. Estrogen receptor subtype expression and regulation is altered in papillary thyroid cancer after menopause. Surgery. (2018) 163:143–9. doi: 10.1016/j.surg.2017.04.031
Keywords: thyroid cancer, hormone, hormone system, growth hormone, insulin-like growth factor, estrogen, androgen, glucocorticoid
Citation: Chen L-h, Xie T, Lei Q, Gu Y-r and Sun C-z (2024) A review of complex hormone regulation in thyroid cancer: novel insights beyond the hypothalamus–pituitary–thyroid axis. Front. Endocrinol. 15:1419913. doi: 10.3389/fendo.2024.1419913
Received: 19 April 2024; Accepted: 08 July 2024;
Published: 22 July 2024.
Edited by:
Xianquan Zhan, Shandong First Medical University, ChinaReviewed by:
Jeehee Yoon, Chonnam National University Bitgoeul Hospital, Republic of KoreaPatricia De Gortari, National Institute of Psychiatry Ramon de la Fuente Muñiz (INPRFM), Mexico
Copyright © 2024 Chen, Xie, Lei, Gu and Sun. This is an open-access article distributed under the terms of the Creative Commons Attribution License (CC BY). The use, distribution or reproduction in other forums is permitted, provided the original author(s) and the copyright owner(s) are credited and that the original publication in this journal is cited, in accordance with accepted academic practice. No use, distribution or reproduction is permitted which does not comply with these terms.
*Correspondence: Chuan-zheng Sun, scz008@126.com