- Department of Pharmacology, Faculty of Medicine, Universiti Kebangsaan Malaysia, Cheras, Malaysia
Osteoporosis and osteoarthritis continue to pose significant challenges to the aging population, with limited preventive options and pharmacological treatments often accompanied by side effects. Amidst ongoing efforts to discover new therapeutic agents, tocotrienols (TTs) have emerged as potential candidates. Derived from annatto bean and palm oil, TTs have demonstrated efficacy in improving skeletal and joint health in numerous animal models of bone loss and osteoarthritis. Mechanistic studies suggest that TTs exert their effects through antioxidant, anti-inflammatory, Wnt-suppressive, and mevalonate-modulating mechanisms in bone, as well as through self-repair mechanisms in chondrocytes. However, human clinical trials in this field remain scarce. In conclusion, TTs hold promise as agents for preventing osteoporosis and osteoarthritis, pending further evidence from human clinical trials.
1 Introduction
Vitamin E encompasses a range of compounds falling into two primary families: tocopherols (TPs) and tocotrienols (TTs). Both families share a common molecular structure consisting of a chromanol ring and a long carbon tail. However, a key distinction lies in the carbon tail composition, with TTs featuring three unsaturated bonds while TPs have a saturated carbon tail. This structural variation confers unique biological properties to TTs not shared by TPs. Furthermore, each family can be subdivided into four homologues (α-, β-, γ-, and δ-) based on the position of the methyl group on the chromanol ring (1, 2). Natural sources, such as oil palm (~30% αTP, ~70% mixed TTs) (3), and annatto bean (negligible TP, ~10% γTT, ~90% δTT) (4), contain varying compositions of TTs and TPs (Figure 1). These differences in composition may influence interactions between vitamin E homologues and their biological activities within natural mixtures.
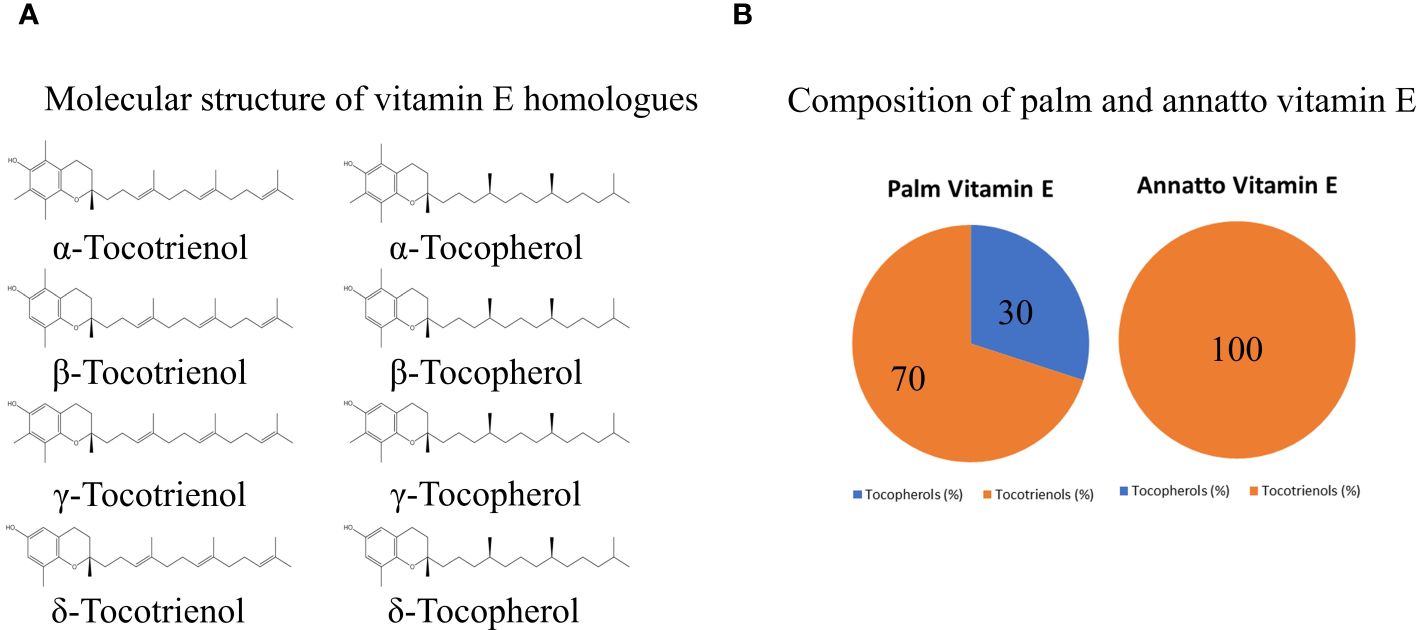
Figure 1 The molecular structure of vitamin E homologues (A) and exemplary composition of vitamin E from palm and annatto bean (B).
TTs or TT-enriched vitamin E fractions have demonstrated protective effects against bone and joint degeneration (5), which are particularly relevant in societies grappling with age-related health challenges. Osteoporosis and osteoarthritis are significant musculoskeletal conditions associated with aging, contributing substantially to healthcare burdens, with 16.6 million and 189.49 million disability-adjusted life-years, respectively, according to the latest Global Burden of Disease Survey in 2019 (6, 7). Despite lifestyle interventions, there remains a limited array of pharmacological preventive agents for both conditions (8, 9). TTs represent a promising avenue to address this gap by potentially serving as an effective preventive agent.
The skeletal effects of TTs have been extensively reviewed in previous studies (10–12). This review, however, aims to discuss recent advancements in establishing TTs as a therapeutic agent against osteoporosis and osteoarthritis. It will synthesize and evaluate recent evidence concerning the use of individual TTs or mixtures in preclinical models and human studies. Additionally, the review will outline future directions for research in this field.
2 Osteoporosis
Osteoporosis arises from an imbalance in bone remodeling, characterized by excessive bone resorption by osteoclasts and inadequate bone formation by osteoblasts. This imbalance leads to a net loss of bone mass, compromised microarchitecture, and reduced strength, consequently heightening the risk of fractures (13). The pathogenesis of osteoporosis involves various endogenous and exogenous factors, including sex hormone deficiency, excess glucocorticoids, and oxidative stress (14). Recent research indicates that increased fat mass may also negatively impact bone health by inducing inflammation and disrupting the hypothalamic-pituitary-adrenal axis (15).
2.1 Effects of TTs on osteoporosis
Studies on animals generally suggest that TT mixtures (60 mg/kg body weight) can improve bone structural histomorphometry in normal rats and those with induced bone loss from factors such as estrogen deficiency, testosterone deficiency, nicotine, and glucocorticoids (Table 1). These improvements are attributed to increased osteoblast numbers and bone formation activities, as well as decreased osteoclast numbers and bone resorption activities (16, 19, 22, 31, 34). Recent investigations employing micro-computed tomography have explored the effects of TT mixtures on bone microstructure. Annatto TT significantly improved some trabecular microstructural indices in rats subjected to orchidectomy and androgen deprivation therapy (16, 20). Additionally, studies showed that annatto and palm TTs preserved trabecular microstructure in rats fed a high-fat, high-carbohydrate diet (25, 35).
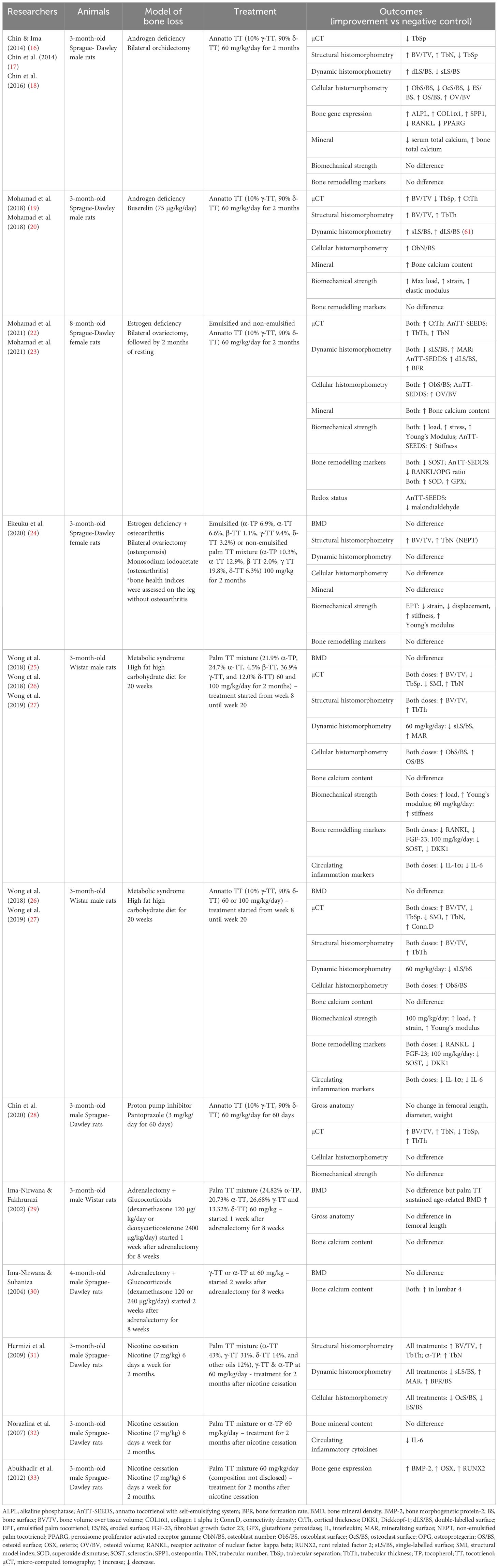
Table 1 Effects of tocotrienol on bone health indices in several representative animal model of osteoporosis.
However, the effects of TTs on bone mineral density, calcium content, and biomechanical strength assessed through various methods such as dual-energy X-ray absorptiometry and atomic absorption spectrophotometry, are less consistent. These inconsistencies may be attributed to variations in the models of bone loss utilized and the duration of treatment (10).
There is a caveat in the previous studies, whereby most have utilized sexually mature rats aged three months old as a model. While commonly employed in osteoporosis research due to their cost-effectiveness and accessibility, these rats may not fully replicate osteoporosis in humans. Unlike humans, rats experience continuous longitudinal skeletal growth because they do not undergo growth plate closure (36). Thus, the use of growing rat might represent a stunted bone growth model rather than an osteoporosis model due to ongoing bone modeling processes.
Furthermore, the bone protective effects of annatto TT have been investigated in postmenopausal women with osteopenia. A 12-week study involving supplementation with 430 mg or 860 mg of annatto TT showed significant decreases in urine N-terminal telopeptide (NTX) levels (a bone resorption marker), circulating receptor activator of nuclear factor kappa-B ligand (RANKL) levels, and RANKL/osteoprotegerin ratios, as well as an increase in bone alkaline phosphatase/NTX ratio (a bone formation marker) compared to a placebo control group (37). However, long-term studies examining the effects of annatto TT on bone mineral density have yet to be conducted.
Fragility fractures are the consequence of untreated osteoporosis (38). TT’s potential in facilitating fracture healing has been explored in several animal models, where TT administration, alone or in combination with other agents, has been shown to promote callus formation and increase the biochemical strength of the fracture site (39, 40). However, clinical trial evidence regarding TT’s effects in this aspect remains lacking.
2.2 Bone protective mechanisms of TT
The skeletal protective mechanism of TTs has traditionally been attributed to their antioxidant and anti-inflammatory activities. Numerous studies have shown that TTs reduce systemic and skeletal oxidative stress markers in rats with bone loss (23, 41, 42). In vitro exposure of osteoblasts to TTs has also demonstrated a mitigation of negative oxidant effects (43, 44). Moreover, supplementation with TTs has led to a reduction in circulating inflammatory markers, such as interleukin (IL)-1 and IL-6, in animals with induced bone loss (35, 45). In women with osteopenia, metabolic study revealed increased lysophospholipids, but decreased acylcarnitines and catabolites of tryptophan and steroids, indicating suppression of inflammation and oxidative stress (46). However, direct evidence on how TTs influence immune cell populations and activities to achieve bone protective effects is lacking. Recent studies have proposed that TTs may regulate gut microbiota (47), suggesting a potential influence on the gut-bone axis to achieve their anti-osteoporosis potential.
TTs have been shown to promote osteoblast differentiation and bone formation activity in both two- and three-dimensional cultured systems (48–50). Among the homologues, γ and δ-TT were found to be most pro-osteogenic (48). Alpha-TT has been found to suppress osteoclast formation from bone marrow cell and osteoblast cocultures by inhibiting c-FOS expression, attributed to the inhibition of ERK and NF-κB activation (51). Multiple animal studies in ovariectomized mice have demonstrated that TT supplementation reduces RANKL levels and, consequently, the RANKL/OPG ratio, reducing osteoclastogenesis and bone resorption (22, 24). This observation has been supported by randomized controlled trials (37).
Research on the effects of TTs on osteocyte function is actively underway. Osteocytes, acting as bone remodeling mediators and mechanical load sensors, play a pivotal role in regulating bone metabolism. They secrete RANKL and OPG to influence the bone remodeling process, as well as inhibitors of the Wnt signaling pathway critical for osteoblastogenesis, such as Dickkopf-1 (DKK1) and sclerostin (SOST) (52). Studies have shown that TT supplementation increases skeletal mRNA expression of β-catenin, the central transcription factor in the Wnt pathway, in orchidectomized rats (16). Subsequent studies have demonstrated reduced skeletal levels of SOST and DKK1 proteins upon TT supplementation in rats with estrogen deficiency and metabolic syndrome (22, 27). In vitro studies using pre-osteoblasts have shown that δ-TT suppresses critical signaling molecules in the Wnt pathway, stimulating cell proliferation and differentiation (53). Wnt signaling has also been shown to mediate the action of δ-TT in promoting osteoblast migration (54).
The mevalonate pathway, crucial for protein prenylation process of GTPase critical for cellular function, is inhibited by TTs via a mechanism distinct from statins, particularly through post-translational suppression of 3-hydroxy-3-methylglutaryl-coenzyme A reductase (HMGR) (55). Exposure of MC3T3-E1 pre-osteoblast cells to δ-TT resulted in decreased HMGR protein expression and increased differentiation markers. Additionally, annatto TT decreased HMGR mRNA expression while increasing RhoA activity in MC3T3-E1 pre-osteoblast cells, promoting their differentiation (49). Co-supplementation of mevalonate reversed the bone protective effects of δ-TT in ovariectomized mice, indicating the importance of the mevalonate pathway in TT-mediated bone protection (56).
In summary, TTs can promote bone health directly through various signaling pathways (Figure 2), beyond their conventional antioxidant and anti-inflammatory actions. However, more in depth studies are needed to illustrate the signaling cascades involved for better understanding of TTs’ actions.
3 Osteoarthritis
Osteoarthritis is characterized by significant articular cartilage loss, subchondral bone changes, and osteophyte formation, leading to joint pain, swelling, and stiffness (57). Traditionally categorized as non-inflammatory arthritis, recent research has underscored the substantial involvement of inflammation in the local joint environment (58). Wear-and-tear particles generated from cartilage due to accumulated mechanical damage attract macrophages into the joint space. These particles also prompt chondrocytes to release metalloproteinases, exacerbating cartilage breakdown. Moreover, these particles serve as damage-associated molecular patterns, activating toll-like receptor signaling in synoviocytes and chondrocytes, thereby increasing the synthesis of pro-inflammatory cytokines. Initially attempting to compensate for cartilage loss, chondrocytes become hypertrophied before eventually becoming senescent and losing functionality (59).
3.1 Effects of TT on osteoarthritis
The effects of annatto TT and palm TT have been assessed in rats induced with osteoarthritis using intra-articular monosodium iodoacetate (MIA) injection. MIA exposure, acting as a glycolysis inhibitor, induces mitochondrial stress and chondrocyte death (60), producing structural and pain response in rodents akin to osteoarthritis in humans (61, 62). An initial pilot study demonstrated that at 100 mg/kg, annatto TT prevented degenerative joint changes and reduced circulating joint degradation markers, cartilage oligomeric matrix protein, and hyaluronan (63). At a higher dose (150 mg/kg), annatto TT decreased osteocalcin levels and osteoclast surface at the subchondral bone, suggesting suppression of bone remodeling (63). Subsequent analyses evaluated the effects of palm TT mixture alone or in combination with glucosamine sulfate in MIA-induced osteoarthritis in rats (64). Palm TT alone or combined with glucosamine sulfate effectively suppressed circulating cartilage oligomeric matrix protein levels but did not significantly alter Mankin’s scores of the joint. Only combination therapy increased the retention time of rats on inverted mesh wire, a surrogate measure of grip strength, albeit not specific to the affected hindlimb (64). Variations in the anti-osteoarthritis potential between annatto and palm tocotrienol mixtures may stem from differences in tocotrienol homologue composition.
Given the greater prevalence of osteoarthritis among women compared to men, the effects of palm tocotrienol were evaluated in ovariectomized rats (24). After bilateral ovariectomy, rats rested for one month before receiving intra-articular MIA injections. Prophylactic treatment with palm TT mixtures (two formulations: 100 mg/kg unformulated or 50% less TT in emulsified version) commenced immediately post-ovariectomy. Both palm TT formulations prevented joint swelling and cartilage degradation, with only emulsified palm TT preventing grip strength decline. Additionally, both palm TT formulations preserved subchondral bone volume, but only emulsified palm TT significantly increased bone formation rate, while unformulated palm TT decreased osteoclast surface on subchondral bone (24).
Furthermore, the efficacy of palm TT mixture (400 mg/day) on physical function and pain in osteoarthritis patients was compared with glucosamine sulfate (1500 mg/day) over 6 months (65). No significant difference in Western Ontario and McMaster Universities osteoarthritis index (WOMAC) or visual analogue scale scores was observed between groups. However, the palm TT-supplemented group exhibited significantly lower malondialdehyde levels compared to the glucosamine sulfate-supplemented group (65).
3.2 Joint protective mechanisms of TT
The protective mechanisms of annatto TT and palm TT on chondrocytes have been explored in an in vitro study utilizing SW1353 chondrocytes exposed to MIA (66). The findings suggest that TTs mitigate oxidative stress in chondrocytes subjected to MIA-induced damage. Particularly noteworthy is the observation that concurrent exposure to annatto TT and MIA may activate a self-repair mechanism in the SW1353 chondrocyte cell line. This was evidenced by a significant increase in SRY-Related HMG Box Gene 9, collagen 2α1, and aggrecan protein levels, along with a decrease in A disintegrin and metalloproteinase with thrombospondin motifs 4 levels (66) (Figure 2). However, further mechanistic studies are warranted to elucidate the precise mechanisms through which TTs protect cartilage in osteoarthritis.
4 Future directions
Our body preferentially absorbs α-TP over other vitamin E homologues due to the presence of α-TP transport protein (67). Consequently, the presence of α-TP in the diet or vitamin E mixture could potentially interfere with the absorption of TTs (68). Several attempts have been made to enhance the absorption of TTs. One such approach is the utilization of self-emulsifying delivery systems (SEDDS), which aims to increase the bioavailability of TTs by partitioning them into smaller micelles with a larger surface-to-body ratio to facilitate intestinal absorption (21). However, a study by Mohamed et al. (23) showed that while SEDDS increased serum TT levels by fourfold, it did not significantly improve the efficacy of TT in preventing bone loss (23). These findings suggest a potential threshold for the skeletal effects of TT. Similarly, in the study by Shen et al. (37), women with osteopenia who received two doses of annatto tocotrienol (430 mg/day versus 860 mg/day) did not exhibit significant differences in bone remodeling markers (37). Subsequently, Ekeuku et al. (24) compared the bone and joint protective effects of an unformulated palm TT mixture with a commercialized emulsified palm TT mixture containing 50% less TT. They found similar total circulating vitamin E levels and skeletal and joint effects in ovariectomized rats fed with both palm TT formulations (24). These findings suggest that SEDDS could potentially reduce the amount of TTs required to achieve therapeutic effects.
Clinical evidence regarding the musculoskeletal side effects of TTs has been limited thus far. The clinical trial conducted by Shen et al. (37) on women with osteopenia demonstrated the benefits of annatto TT in suppressing bone resorption (37), but whether it can slow down the decline of bone mineral density remains uncertain. Moreover, since all groups in the trials were supplemented with calcium and vitamin D, it is plausible that any differences in skeletal parameters between TT-supplemented and unsupplemented groups may have been minimized. Furthermore, only one trial has been conducted thus far on the effects of palm TT on osteoarthritis. Haflah et al. (65) compared the effects of TT with glucosamine sulfate in improving visual analogue scale scores, which measure pain intensity, as well as WOMAC scores, which assess joint pain, stiffness, and function (65). The lack of difference between the two groups suggests that palm TT may be as effective as glucosamine sulfate in improving joint function and reducing joint pain. However, no clinical studies have been conducted to investigate the structural changes in the joint caused by TT supplementation.
In summary, further research is needed, including exploration of innovative delivery systems and conducting more clinical trials, to fully understand the potential benefits and mechanisms of action of TTs in musculoskeletal health.
5 Conclusion
The accumulated preclinical evidence thus far strongly suggests that TTs could protect bone health in individuals at risk of osteoporosis. However, only one clinical trial has validated the skeletal protective effects of TT. Similarly, a series of studies have been conducted to investigate the promising effects of TT in preventing osteoarthritis, but only one study validated the preclinical findings using questionnaire-based assessment tools. On that note, we need more well-planned randomized controlled trials to translate the putative benefits of TT to patients with osteoporosis and osteoarthritis.
Author contributions
KC: Conceptualization, Funding acquisition, Investigation, Visualization, Writing – original draft.
Funding
The author declares financial support was received for the research, authorship, and/or publication of this article. The author is supported by Fundamental Research Grant Scheme provided by Ministry of Education, Malaysia (Code: FRGS/1/2022/SKK10/UKM/02/3). The author thanks Ministry of Education, Malaysia and Universiti Kebangsaan Malaysia for their supports.
Acknowledgments
The author has used ChatGPT version 3.5 (OpenAI, San Francisco, California, USA) to polish the language of this manuscript, but he is responsible for the content of this manuscript.
Conflict of interest
The author declares that the research was conducted in the absence of any commercial or financial relationships that could be construed as a potential conflict of interest.
The author declared that he was an editorial board member of Frontiers, at the time of submission. This had no impact on the peer review process and the final decision.
Publisher’s note
All claims expressed in this article are solely those of the authors and do not necessarily represent those of their affiliated organizations, or those of the publisher, the editors and the reviewers. Any product that may be evaluated in this article, or claim that may be made by its manufacturer, is not guaranteed or endorsed by the publisher.
References
1. Wong SK, Kamisah Y, Mohamed N, Muhammad N, Masbah N, Fahami NAM, et al. Potential role of tocotrienols on non-communicable diseases: A review of current evidence. Nutrients. (2020) 12:E259. doi: 10.3390/nu12010259
2. Chin KY, Pang KL, Soelaiman IN. Tocotrienol and its role in chronic diseases. Adv Exp Med Biol. (2016) 928:97–130. doi: 10.1007/978-3-319-41334-1_5
3. Sen CK, Rink C, Khanna S. Palm oil-derived natural vitamin E alpha-tocotrienol in brain health and disease. J Am Coll Nutr. (2010) 29:314s–23s. doi: 10.1080/07315724.2010.10719846
4. Frega N, Mozzon M, Bocci F. Identification and estimation of tocotrienols in the annatto lipid fraction by gas chromatography-mass spectrometry. J Am Oil Chemists' Society. (1998) 75:1723–7. doi: 10.1007/s11746-998-0323-1
5. Saud Gany SL, Chin KY, Tan JK, Aminuddin A, Makpol S. Preventative and therapeutic potential of tocotrienols on musculoskeletal diseases in ageing. Front Pharmacol. (2023) 14:1290721. doi: 10.3389/fphar.2023.1290721
6. Cui A, Li H, Wang D, Zhong J, Chen Y, Lu H. Global, regional prevalence, incidence and risk factors of knee osteoarthritis in population-based studies. eClinicalMedicine. (2020) 29:100587. doi: 10.1016/j.eclinm.2020.100587
7. Shen Y, Huang X, Wu J, Lin X, Zhou X, Zhu Z, et al. The global burden of osteoporosis, low bone mass, and its related fracture in 204 countries and territories, 1990–2019. Front Endocrinol (Lausanne). (2022) 13. doi: 10.3389/fendo.2022.882241
8. Deng J, Zong Z, Su Z, Chen H, Huang J, Niu Y, et al. Recent advances in pharmacological intervention of osteoarthritis: A biological aspect. Front Pharmacol. (2021) 12:772678. doi: 10.3389/fphar.2021.772678
9. Pavone V, Testa G, Giardina SMC, Vescio A, Restivo DA, Sessa G. Pharmacological therapy of osteoporosis: A systematic current review of literature. Front Pharmacol. (2017) 8:803. doi: 10.3389/fphar.2017.00803
10. Chin KY, Ima-Nirwana S. The biological effects of tocotrienol on bone: a review on evidence from rodent models. Drug design Dev Ther. (2015) 9:2049–61. doi: 10.2147/DDDT
11. Chin KY, Ima-Nirwana S. The role of tocotrienol in preventing male osteoporosis-A review of current evidence. Int J Mol Sci. (2019) 20:1355. doi: 10.3390/ijms20061355
12. Chin KY, Mo H, Soelaiman IN. A review of the possible mechanisms of action of tocotrienol - a potential antiosteoporotic agent. Curr Drug targets. (2013) 14:1533–41. doi: 10.2174/13894501113149990178
13. Fang H, Deng Z, Liu J, Chen S, Deng Z, Li W. The mechanism of bone remodeling after bone aging. Clin Interv Aging. (2022) 17:405–15. doi: 10.2147/CIA.S349604
14. Chin KY, Ng BN, Rostam MKI, Muhammad Fadzil NFD, Raman V, Mohamed Yunus F, et al. A mini review on osteoporosis: from biology to pharmacological management of bone loss. J Clin Med. (2022) 11:6434. doi: 10.3390/jcm11216434
15. Wong SK, Chin K-Y, Suhaimi F, Ahmad F, Ima-Nirwana S. The relationship between metabolic syndrome and osteoporosis: A review. Nutrients. (2016) 8:347. doi: 10.3390/nu8060347
16. Chin KY, Ima-Nirwana S. Effects of annatto-derived tocotrienol supplementation on osteoporosis induced by testosterone deficiency in rats. Clin Interv Aging. (2014) 9:1247–59. doi: 10.2147/CIA
17. Chin KY, Abdul-Majeed S, Fozi NF, Ima-Nirwana S. Annatto tocotrienol improves indices of bone static histomorphometry in osteoporosis due to testosterone deficiency in rats. Nutrients. (2014) 6:4974–83. doi: 10.3390/nu6114974
18. Chin KY, Gengatharan D, Mohd Nasru FS, Khairussam RA, Ern SL, Aminuddin SA, et al. The effects of annatto tocotrienol on bone biomechanical strength and bone calcium content in an animal model of osteoporosis due to testosterone deficiency. Nutrients. (2016) 8:E808. doi: 10.3390/nu8120808
19. Mohamad NV, Soelaiman IN, Chin KY. Effects of tocotrienol from Bixa orellana (annatto) on bone histomorphometry in a male osteoporosis model induced by buserelin. Biomedicine pharmacotherapy = Biomedecine pharmacotherapie. (2018) 103:453–62. doi: 10.1016/j.biopha.2018.04.083
20. Mohamad NV, Ima-Nirwana S, Chin KY. Effect of tocotrienol from Bixa orellana (annatto) on bone microstructure, calcium content, and biomechanical strength in a model of male osteoporosis induced by buserelin. Drug design Dev Ther. (2018) 12:555–64. doi: 10.2147/DDDT
21. Alqahtani S, Alayoubi A, Nazzal S, Sylvester PW, Kaddoumi A. Enhanced solubility and oral bioavailability of γ-tocotrienol using a self-emulsifying drug delivery system (SEDDS). Lipids. (2014) 49:819–29. doi: 10.1007/s11745-014-3923-6
22. Mohamad N-V, Ima-Nirwana S, Chin K-Y. Self-emulsified annatto tocotrienol improves bone histomorphometric parameters in a rat model of oestrogen deficiency through suppression of skeletal sclerostin level and RANKL/OPG ratio. Int J Med Sci. (2021) 18:3665–73. doi: 10.7150/ijms.64045
23. Mohamad NV, Ima-Nirwana S, Chin KY. Therapeutic potential of annatto tocotrienol with self-emulsifying drug delivery system in a rat model of postmenopausal bone loss. Biomedicine pharmacotherapy = Biomedecine pharmacotherapie. (2021) 137:111368. doi: 10.1016/j.biopha.2021.111368
24. Ekeuku SO, Nor Muhamad ML, Aminuddin AA, Ahmad F, Wong SK, Mark-Lee WF, et al. Effects of emulsified and non-emulsified palm tocotrienol on bone and joint health in ovariectomised rats with monosodium iodoacetate-induced osteoarthritis. Biomedicine pharmacotherapy = Biomedecine pharmacotherapie. (2024) 170:115998. doi: 10.1016/j.biopha.2023.115998
25. Wong SK, Chin K-Y, Suhaimi FH, Ahmad F, Ima-Nirwana S. The effects of palm tocotrienol on metabolic syndrome and bone loss in male rats induced by high-carbohydrate high-fat diet. J Funct Foods. (2018) 44:246–54. doi: 10.1016/j.jff.2018.03.022
26. Wong S, Chin K-Y, Suhaimi F, Ahmad F, Ima-Nirwana S. The effects of vitamin E from elaeis guineensis (Oil palm) in a rat model of bone loss due to metabolic syndrome. Int J Environ Res Public Health. (2018) 15:1828. doi: 10.3390/ijerph15091828
27. Wong SK, Chin K-Y, Ima-Nirwana S. The effects of tocotrienol on bone peptides in a rat model of osteoporosis induced by metabolic syndrome: the possible communication between bone cells. Int J Environ Res Public Health. (2019) 16:3313. doi: 10.3390/ijerph16183313
28. Chin KY, Thong BKS, Kamalulloh RF, Mohamad NV, Wong SK, Mohd Arlamsyah A, et al. Effects of calcium and annatto tocotrienol supplementation on bone loss induced by pantoprazole in male rats. Drug design Dev Ther. (2020) 14:2561–72. doi: 10.2147/DDDT.S260565
29. Ima-Nirwana S, Fakhrurazi H. Palm vitamin E protects bone against dexamethasone-induced osteoporosis in male rats. Med J Malaysia. (2002) 57:133–41.
30. Ima Nirwana S, Suhaniza S. Effects of tocopherols and tocotrienols on body composition and bone calcium content in adrenalectomized rats replaced with dexamethasone. J Med Food. (2004) 7(1):45–51. doi: 10.1089/109662004322984699
31. Hermizi H, Faizah O, Ima-Nirwana S, Ahmad Nazrun S, Norazlina M. Beneficial effects of tocotrienol and tocopherol on bone histomorphometric parameters in sprague-dawley male rats after nicotine cessation. Calcif Tissue Int. (2009) 84:65–74. doi: 10.1007/s00223-008-9190-x
32. Norazlina M, Lee PL, Lukman HI, Nazrun AS, Ima-Nirwana S. Effects of vitamin E supplementation on bone metabolism in nicotine-treated rats. Singapore Med J. (2007) 48:195–9.
33. Abukhadir SS, Mohamed N, Makpol S, Muhammad N. Effects of palm vitamin e on bone-formation-related gene expression in nicotine-treated rats. Evid Based Complement Alternat Med. (2012) 2012:656025. doi: 10.1155/2012/656025
34. Ekeuku SO, Mohd Ramli ES, Abdullah Sani N, Abd Ghafar N, Soelaiman IN, Chin K-Y. Tocotrienol as a protecting agent against glucocorticoid-induced osteoporosis: A mini review of potential mechanisms. Molecules. (2022) 27:5862. doi: 10.3390/molecules27185862
35. Wong SK, Chin KY, Suhaimi FH, Ahmad F, Ima-Nirwana S. Exploring the potential of tocotrienol from Bixa orellana as a single agent targeting metabolic syndrome and bone loss. Bone. (2018) 116:8–21. doi: 10.1016/j.bone.2018.07.003
36. Allen MR. Preclinical models for skeletal research: how commonly used species mimic (or don't) aspects of human bone. Toxicol Pathol. (2017) 45:851–4. doi: 10.1177/0192623317733925
37. Shen CL, Yang S, Tomison MD, Romero AW, Felton CK, Mo H. Tocotrienol supplementation suppressed bone resorption and oxidative stress in postmenopausal osteopenic women: a 12-week randomized double-blinded placebo-controlled trial. Osteoporos Int. (2018) 29:881–91. doi: 10.1007/s00198-017-4356-x
38. Pisani P, Renna MD, Conversano F, Casciaro E, Di Paola M, Quarta E, et al. Major osteoporotic fragility fractures: Risk factor updates and societal impact. World J Orthop. (2016) 7:171–81. doi: 10.5312/wjo.v7.i3.171
39. Ibrahim N, Khamis MF, Mod Yunoh MF, Abdullah S, Mohamed N, Shuid AN. Targeted delivery of lovastatin and tocotrienol to fracture site promotes fracture healing in osteoporosis model: micro-computed tomography and biomechanical evaluation. PloS One. (2014) 9:e115595. doi: 10.1371/journal.pone.0115595
40. Mohamad S, Shuid AN, Mokhtar SA, Abdullah S, Soelaiman IN. Tocotrienol supplementation improves late-phase fracture healing compared to alpha-tocopherol in a rat model of postmenopausal osteoporosis: a biomechanical evaluation. Evid Based Complement Alternat Med. (2012) 2012:372878. doi: 10.1155/2012/372878
41. Maniam S, Mohamed N, Shuid AN, Soelaiman IN. Palm tocotrienol exerted better antioxidant activities in bone than α-tocopherol. Basic Clin Pharmacol Toxicology. (2008) 103:55–60. doi: 10.1111/j.1742-7843.2008.00241.x
42. Nazrun A, Khairunnur A, Norliza M, Norazlina M, Ima Nirwana S. Effects of palm tocotrienol on oxidative stress and bone strength in ovariectomised rats. Med Health. (2008) 3:83–90.
43. Abd Manan N, Mohamed N, Shuid AN. Effects of low-dose versus high-dose gamma-tocotrienol on the bone cells exposed to the hydrogen peroxide-induced oxidative stress and apoptosis. Evid Based Complement Alternat Med. (2012) 2012:680834. doi: 10.1155/2012/680834
44. Casati L, Pagani F, Limonta P, Vanetti C, Stancari G, Sibilia V. Beneficial effects of δ-tocotrienol against oxidative stress in osteoblastic cells: studies on the mechanisms of action. Eur J Nutr. (2020) 59:1975–87. doi: 10.1007/s00394-019-02047-9
45. Norazlina M, Hermizi H, Faizah O, Nazrun AS, Norliza M, Ima-Nirwana S. Vitamin E reversed nicotine-induced toxic effects on bone biochemical markers in male rats. Arch Med Sci. (2010) 6:505–12. doi: 10.5114/aoms.2010.14460
46. Shen CL, Mo H, Dunn DM, Watkins BA. Tocotrienol supplementation led to higher serum levels of lysophospholipids but lower acylcarnitines in postmenopausal women: A randomized double-blinded placebo-controlled clinical trial. Front Nutr. (2021) 8:766711. doi: 10.3389/fnut.2021.766711
47. Kumareswaran A, Ekeuku SO, Mohamed N, Muhammad N, Hanafiah A, Pang K-L, et al. The effects of tocotrienol on gut microbiota: A scoping review. Life. (2023) 13:1882. doi: 10.3390/life13091882
48. Nur Farhana MF, James Jam J, Chua KH, Ekram A, Chin KY, Ima Nirwana S. Comparing the effects of alpha-tocopherol and tocotrienol isomers on osteoblasts hFOB 1.19 cultured on bovine bone scaffold. Sains Malays. (2021) 50:2319–28. doi: 10.17576/jsm
49. Wan Hasan WN, Chin KY, Abd Ghafar N, Soelaiman IN. Annatto-derived tocotrienol promotes mineralization of MC3T3-E1 cells by enhancing BMP-2 protein expression via inhibiting rhoA activation and HMG-coA reductase gene expression. Drug design Dev Ther. (2020) 14:969–76. doi: 10.2147/DDDT
50. Wan Hasan WN, Abd Ghafar N, Chin KY, Ima-Nirwana S. Annatto-derived tocotrienol stimulates osteogenic activity in preosteoblastic MC3T3-E1 cells: a temporal sequential study. Drug design Dev Ther. (2018) 12:1715–26. doi: 10.2147/DDDT
51. Ha H, Lee J-H, Kim H-N, Lee ZH. α-Tocotrienol inhibits osteoclastic bone resorption by suppressing RANKL expression and signaling and bone resorbing activity. Biochem Biophys Res Commun. (2011) 406:546–51. doi: 10.1016/j.bbrc.2011.02.085
52. Prideaux M, Findlay DM, Atkins GJ. Osteocytes: The master cells in bone remodelling. Curr Opin Pharmacol. (2016) 28:24–30. doi: 10.1016/j.coph.2016.02.003
53. Xu W, Li Y, Feng R, He P, Zhang Y. γ-Tocotrienol induced the proliferation and differentiation of MC3T3-E1 cells through the stimulation of the Wnt/β-catenin signaling pathway. Food Funct. (2022) 13:398–410. doi: 10.1039/D1FO02583J
54. Casati L, Pagani F, Maggi R, Ferrucci F, Sibilia V. Food for bone: evidence for a role for delta-tocotrienol in the physiological control of osteoblast migration. Int J Mol Sci. (2020) 21:4661. doi: 10.3390/ijms21134661
55. Parker RA, Pearce BC, Clark RW, Gordon DA, Wright JJ. Tocotrienols regulate cholesterol production in mammalian cells by post-transcriptional suppression of 3-hydroxy-3-methylglutaryl-coenzyme A reductase. J Biol Chem. (1993) 268:11230–8. doi: 10.1016/S0021-9258(18)82115-9
56. Deng L, Ding Y, Peng Y, Wu Y, Fan J, Li W, et al. γ-Tocotrienol protects against ovariectomy-induced bone loss via mevalonate pathway as HMG-CoA reductase inhibitor. Bone. (2014) 67:200–7. doi: 10.1016/j.bone.2014.07.006
57. Coaccioli S, Sarzi-Puttini P, Zis P, Rinonapoli G, Varrassi G. Osteoarthritis: new insight on its pathophysiology. J Clin Med. (2022) 11:6013. doi: 10.3390/jcm11206013
58. Chow YY, Chin KY. The role of inflammation in the pathogenesis of osteoarthritis. Mediators Inflamm. (2020) 2020:8293921. doi: 10.1155/2020/8293921
59. Lambert C, Zappia J, Sanchez C, Florin A, Dubuc JE, Henrotin Y. The damage-associated molecular patterns (DAMPs) as potential targets to treat osteoarthritis: perspectives from a review of the literature. Front Med (Lausanne). (2020) 7:607186. doi: 10.3389/fmed.2020.607186
60. Jiang L, Li L, Geng C, Gong D, Jiang L, Ishikawa N, et al. Monosodium iodoacetate induces apoptosis via the mitochondrial pathway involving ROS production and caspase activation in rat chondrocytes in vitro. J Orthop Res. (2013) 31:364–9. doi: 10.1002/jor.22250
61. Pitcher T, Sousa-Valente J, Malcangio M. The monoiodoacetate model of osteoarthritis pain in the mouse. J Vis Exp. (2016) 111:53746. doi: 10.3791/53746-v
62. Ekeuku SO, Ahmad F, Chin K-Y. Changes of grip strength, articular cartilage and subchondral bone in monoiodoacetate-induced osteoarthritis in rats. Sains Malays. (2022) 51:3741–54. doi: 10.17576/jsm
63. Chin KY, Wong SK, Japar Sidik FZ, Abdul Hamid J, Abas NH, Mohd Ramli ES, et al. The effects of annatto tocotrienol supplementation on cartilage and subchondral bone in an animal model of osteoarthritis induced by monosodium iodoacetate. Int J Environ Res Public Health. (2019) 16:2897. doi: 10.3390/ijerph16162897
64. Al-Saadi HM, Chin K-Y, Ahmad F, Mohd Ramli ES, Arlamsyah AM, Japar Sidik FZ, et al. Effects of palm tocotrienol-rich fraction alone or in combination with glucosamine sulphate on grip strength, cartilage structure and joint remodelling markers in a rat model of osteoarthritis. Appl Sci. (2021) 11:8577. doi: 10.3390/app11188577
65. Haflah NH, Jaarin K, Abdullah S, Omar M. Palm vitamin E and glucosamine sulphate in the treatment of osteoarthritis of the knee. Saudi Med J. (2009) 30:1432–8.
66. Pang K-L, Ghafar NA, Soelaiman IN, Chin K-Y. Protective effects of annatto tocotrienol and palm tocotrienol-rich fraction on chondrocytes exposed to monosodium iodoacetate. Appl Sci. (2021) 11:9643. doi: 10.3390/app11209643
67. Hosomi A, Arita M, Sato Y, Kiyose C, Ueda T, Igarashi O, et al. Affinity for alpha-tocopherol transfer protein as a determinant of the biological activities of vitamin E analogs. FEBS letters. (1997) 409:105–8. doi: 10.1016/S0014-5793(97)00499-7
Keywords: bone, cartilage, osteoarthritis, osteoporosis, vitamin E
Citation: Chin K-Y (2024) Updates in the skeletal and joint protective effects of tocotrienol: a mini review. Front. Endocrinol. 15:1417191. doi: 10.3389/fendo.2024.1417191
Received: 16 April 2024; Accepted: 04 June 2024;
Published: 21 June 2024.
Edited by:
Giacomina Brunetti, University of Bari Aldo Moro, ItalyReviewed by:
Bouchra Edderkaoui, Loma Linda Veterans Association for Research and Education, United StatesCopyright © 2024 Chin. This is an open-access article distributed under the terms of the Creative Commons Attribution License (CC BY). The use, distribution or reproduction in other forums is permitted, provided the original author(s) and the copyright owner(s) are credited and that the original publication in this journal is cited, in accordance with accepted academic practice. No use, distribution or reproduction is permitted which does not comply with these terms.
*Correspondence: Kok-Yong Chin, Y2hpbmtva3lvbmdAcHB1a20udWttLmVkdS5teQ==