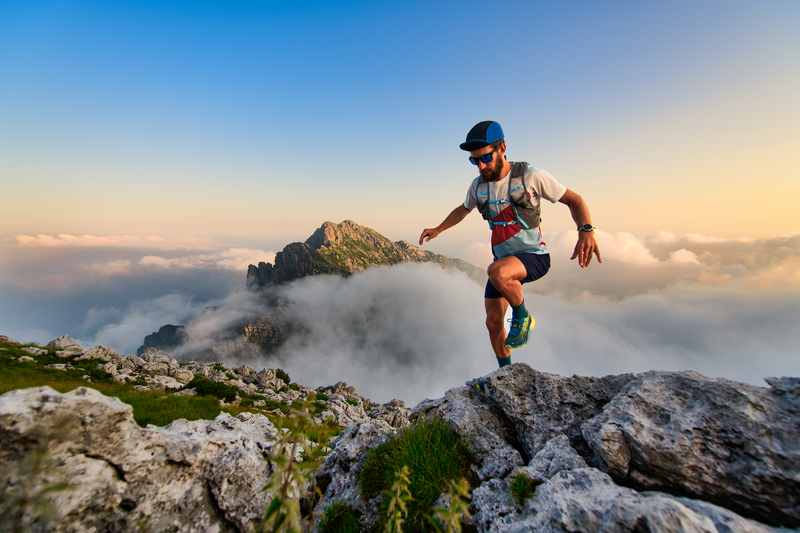
94% of researchers rate our articles as excellent or good
Learn more about the work of our research integrity team to safeguard the quality of each article we publish.
Find out more
REVIEW article
Front. Endocrinol. , 17 June 2024
Sec. Endocrinology of Aging
Volume 15 - 2024 | https://doi.org/10.3389/fendo.2024.1417007
This article is part of the Research Topic Genetics and Epigenetics in Ovarian Aging View all 6 articles
Ovarian aging is a complex process characterized by a decline in oocyte quantity and quality, directly impacting fertility and overall well-being. Recent researches have identified mitochondria as pivotal players in the aging of ovaries, influencing various hallmarks and pathways governing this intricate process. In this review, we discuss the multifaceted role of mitochondria in determining ovarian fate, and outline the pivotal mechanisms through which mitochondria contribute to ovarian aging. Specifically, we emphasize the potential of targeting mitochondrial dysfunction through innovative therapeutic approaches, including antioxidants, metabolic improvement, biogenesis promotion, mitophagy enhancement, mitochondrial transfer, and traditional Chinese medicine. These strategies hold promise as effective means to mitigate age-related fertility decline and preserve ovarian health. Drawing insights from advanced researches in the field, this review provides a deeper understanding of the intricate interplay between mitochondrial function and ovarian aging, offering valuable perspectives for the development of novel therapeutic interventions aimed at preserving fertility and enhancing overall reproductive health.
Ovarian aging is a complex process characterized by a gradual decline in both the quantity and quality of oocytes, a phenomenon that has significant implications for female fertility (1). As women age, the impact of aging on the ovaries becomes increasingly pronounced, particularly after the mid-30s (2). This age-related decline in ovarian function not only results in reduced fertility and an increased risk of pregnancy complications but also significantly impacts critical elements like hormonal balance, bone health, cardiovascular well-being, cognitive function (3). Understanding the mechanisms driving ovarian aging is crucial for the development of interventions aimed at preserving fertility and potentially mitigating the impact of age-related fertility decline on women’s overall health.
Mitochondria, widely known as the cellular “powerhouses”, assume a fundamental role in energy generation through oxidative phosphorylation (4). Considering that the oocyte is the body’s most mitochondria-rich cell, these organelles bear significant responsibility in fostering its development, promoting follicular growth, and orchestrating hormone regulation—all crucial factors in ensuring successful reproduction (5). While several other factors, such as vascular network defects, hormonal dysregulation, genetic and epigenetic alterations, and environmental/lifestyle influences, have been identified as contributing to the aging of the ovary, mitochondrial dysfunction appears to be a more central and significant driver of this process (2, 6). Moreover, preserving mitochondrial function not only holds promise for enhancing reproductive outcomes but also for delaying aging in women. Exploring therapeutic strategies targeted at maintaining mitochondrial health could offer significant opportunities for addressing age-related fertility decline and promoting reproductive and overall well-being in women.
In this review, we focus on the intricate interplay between mitochondrial function and ovarian aging, and the mechanisms through which mitochondrial health affects oocyte and ovarian follicle quality, and how it contributes to age-related fertility decline. Furthermore, we explore potential therapeutic strategies aimed at preserving mitochondrial function to enhance reproductive outcomes for women as they age.
Mitochondria are double-membraned organelles found within the cytoplasm of eukaryotic cells. The outer membrane is porous, allowing the passage of ions and small molecules. In contrast, the inner membrane is impermeable and houses the electron transport chain (ETC), which is a combination of 5 protein-lipid enzyme complexes, electron carriers coenzyme Q10 (CoQ10) and cytochrome c. The ETC generates ATP by transferring electrons to and from these complexes and creating a proton gradient across the inner membrane. Moreover, the inner membrane forms invaginations called cristae, increasing the surface area for energy production (7). The space between the two membranes, known as the intermembrane space, and the innermost compartment of mitochondrion, known as the matrix, are crucial for the transport of protons during oxidative phosphorylation (4). In addition, mitochondria are semiautonomous organelles that have their own DNA. Mitochondrial DNA (mtDNA) contains 37 genes coding for 13 mitochondrial proteins, which are part of the central subunits of the ETC (8).
Cellular senescence, a hallmark of aging, is primarily characterized by an irreversible cell cycle arrest, where upregulation of cyclin-dependent kinase inhibitors such as p16, Rb, p21, and p53 plays a pivotal role (9). This regulatory cessation of cell division is associated with increased expression of genes that enforce this arrest. Concurrently, mitochondrial dysfunction emerges as a critical aspect of aging. Mitochondria are acknowledged not only as the powerhouse of the cell, providing essential energy but also for their involvement in a spectrum of cellular processes including cell cycle regulation, apoptosis, and metabolic control (10, 11). Given the integral nature of these functions, mitochondrial dysfunction undeniably contributes to the aging phenotype. This is particularly relevant in oocytes, which are among the most mitochondria-enriched cells in the organism (5), underscoring the significance of mitochondrial health in ovarian aging and the broader implications for reproductive capacity and overall vitality. Ovarian cells, particularly oocytes and granulosa cells, have high metabolic demands to support their specialized functions, such as oogenesis and steroidogenesis. These cellular processes require mitochondria to provide sufficient energy through oxidative phosphorylation (12, 13). However, ovarian cells possess lower antioxidant defense capabilities compared to other cell types, rendering them more susceptible to mtDNA damage induced by oxidative stress (14). The accumulation of mtDNA mutations and depletion, in turn, exacerbates mitochondrial dysfunction in ovarian cells during the aging process (15).
Nearly two decades ago, Hayflick et al. elucidated the connection between mitochondria and the cellular senescent phenotype (16). A growing body of evidence supports the notion that senescent ovarian cells undergo structural, functional and dynamic changes in their mitochondria (17) (Figure 1). For example, senescent cumulus cells demonstrate increased abnormal mitochondrial numbers and decreased mitochondrial activity, characterized by reduced mitochondrial membrane potential, elevated proton leakage, and heightened generation of reactive oxygen species (ROS) (18, 19). Furthermore, senescent ovarian cells also show dramatic changes in mitochondrial morphology. The mitochondria in these cells are in a state of hyper-fusion, while a healthy mitochondrial network constantly undergoes fission and fusion events to meet metabolic needs and allow for the removal of dysfunctional entities (20).
Figure 1 An overview of mitochondrial dysfunction that occurs during ovarian aging. Senescent ovarian cells can be characterized by a number of distinct changes to their homeostatic mechanisms; specifically mitochondrial biogenesis is exacerbated, mitophagy is decreased and mitochondrial dynamics favor fusion, making the mitochondrial networks highly perfused. Metabolically, the ETC is disrupted, this leads to alterations in the AMP/ATP ratio and the NAD+/NADH ratio, as well as enhanced production of ROS.ROS can damage mitochondria as well as induce nuclear DNA damage. Similarly, ROS can cause damage to proteins, lipids and other components, affecting the normal function of ovarian cells.MtDNA mutation increased and mtDNA copy number decreased in senescent ovarian cells.
The following sections will elucidate the detailed mechanisms by which mitochondrial dysfunction influences the senescence phenotype in ovarian cells.
Free Radical Theory of Aging, introduced in 1956, proposes that the accumulated damage caused by free radicals, which are produced during normal metabolic processes, contributes to the aging process (21). ROS, including free radicals, are believed to induce oxidative damage, affecting cellular structures and molecules over time (22). These ROS also act as signaling molecules, influencing various cellular processes such as cell differentiation, proliferation, and apoptosis. However, an elevation in ROS production, often linked to mitochondrial dysfunction, can result in oxidative stress, leading to harm to cellular components and contributing to the aging processes (23).
Mitochondria primarily produce superoxide, hydrogen peroxide, and hydroxyl radicals through the electron transport chain during oxidative phosphorylation, serving as major ROS sources (24). The mechanism underlying ROS-induced aging involves the development of a growth arrest phenotype due to mitochondrial damage, excessive ROS generation, and activation of the DNA damage response through the p53/p21 pathway (25). This imbalance between ROS production and antioxidant systems can lead to oxidative stress, causing damage to lipids, proteins, and DNA, particularly in telomeric regions. Moreover, this imbalance hampers ATP production, triggering AMP-activated protein kinase (AMPK) activation, which in turn leads to cell cycle arrest and the induction of senescence (26). Elevated ROS levels, particularly in the ovaries, can impact cellular components, leading to diminished ovarian reserves and affecting oocyte developmental competence (27). Understanding these intricate connections between mitochondria and ROS is crucial for unraveling the complexities of ovarian aging and reproductive longevity (Figure 2).
Figure 2 Mitochondrial OXPHOS and ROS production in ovarian aging. In the process of ovarian aging, the balance between oxidation and antioxidant systems is disrupted. Mitochondria display aberrant OXPHOS that produces excessive amounts of ROS that damage lipids, proteins and DNA, specially telomeric regions. And reduced ATP level activates AMPK leading to cell cycle arrest and senescence induction. Oxidative phosphorylation (OXPHOS), AMP-activated protein kinase (AMPK), Reactive Oxygen Species (ROS), superoxide (·O2-), hydrogen peroxide (H2O2), and hydroxyl radicals (·OH), Retinoblastoma protein (Rb).
The study by Lim highlights the correlation between aging and increased oxidative damage in the ovarian tissue, coupled with a decline in antioxidant gene expression (28). Additionally, research by Smits et al. confirms oxidative damage and mitochondrial dysfunction in oocytes of advanced maternal age, even at the primordial follicle stage (29).
Sirtuins, being a family of nicotinamide adenine dinucleotide (NAD+)-dependent histone deacetylases, consist of seven SIR2 homologs (SIRT1-7) in mammals (30). Sirt3 is specifically located in the mitochondrial matrix and plays a crucial role in enhancing mitochondrial resilience to ROS (31). Deficiency of Sirt3 in female mice accelerates ovarian aging, affecting mitochondrial function and oocyte quality (32). Within mitochondria, peroxiredoxin 3 (Prdx3) regulates mitochondrial ROS (33), and reduced Prdx3 expression in aged mouse oocytes increases susceptibility to oxidative stress (27).
Granulosa cells (GCs), constituting the predominant cell population in the ovary, require stable mitochondria for their growth, proliferation, and division (34). Tamura et al. reported a notable decrease in the proportion of active mitochondria in an oxidative stress-induced model of GCs (35). Mitochondrial dysfunction disrupts the bi-directional interaction between oocytes and GCs, hindering cell growth and development (5). Additionally, mutations in Immp2l, which encodes a protein integral to the inner mitochondrial membrane, contribute to ovarian aging via the ROS-Wnt/β-catenin pathway, exacerbating oxidative stress in GCs (36).
Mitochondrial quality control is a fundamental cellular process essential for preserving the integrity and functionality of mitochondria. This regulatory network encompasses three key mechanisms: mitochondrial biogenesis, fusion and fission dynamics, and mitophagy.
Mitochondrial biogenesis involves the growth of functional mitochondria by producing new components like proteins, lipids, and mtDNA. These processes are crucial for cellular energy production, adapting to changes in energy demands, and the overall health of cells and tissues. Mitochondria have their own DNA (mtDNA) and hence biogenesis of mitochondria requires a coordination of nuclear DNA and mtDNA, both of which encode for mitochondria proteins (37). Notably, mitochondrial biogenesis plays a critical role in supporting the energy requirements of growing oocytes and determining the size of the follicular pool in the ovaries, which is a key factor in ovarian aging and reproductive longevity (38).
The dynamic balance of mitochondrial dynamics involving fission and fusion is essential for maintaining mitochondrial function (39). The fusion of damaged and undamaged mitochondria can act as a defensive mechanism, diluting the extent of damage. Conversely, mitochondrial fission facilitates the isolation and subsequent elimination of impaired mitochondria through mitophagy (40). Therefore, the meticulous orchestration of fission and fusion is of paramount importance for the health of oocytes and follicles (41). Research findings have demonstrated that the loss of the mitochondrial fission protein Dynamin-related protein 1 (Drp1), which is encoded by the gene Fission 1 (Fis1), results in a decline in oocyte quality (42). Mitofusins, particularly Mitofusin 1 (Mfn1) and Mitofusin 2 (Mfn2), are integral to the process of mitochondrial fusion and play essential roles in ovarian function and female fertility. Oocyte-specific deletion of Mfn1 has been associated with diminished ovarian follicular reserve, emphasizing its crucial role in maintaining ovarian function and fertility (43). Additionally, Mfn2 has been linked to the regulation of oocyte development and quality through its influence on meiosis and mitochondrial function (44).
Mitophagy, the process of degrading damaged or dysfunctional mitochondria, plays a crucial role in maintaining cellular health by regulating mitochondrial numbers and ensuring a population of healthy mitochondria (41). Successful mitophagy necessitates mitochondrial fission, which segregates the compromised mitochondria. These are then encased in autophagosomes and subsequently merged with lysosomes for degradation (45). Defective mitophagy can lead to the accumulation of damaged mitochondria, contributing to increased oxidative stress and impaired energy production in the ovaries, consequently affecting oocyte quality and fertility.
During aging, there is a discernible deterioration in mitochondrial quality control, characterized by enhanced mitochondrial biogenesis, a shift in dynamics favoring fusion over fission, and a decrease in mitophagy (46). This progressive decline leads to an accumulation of mitochondria within senescent ovarian cells, reflected by an increased mitochondrial mass, a heightened state of fused mitochondria, and an elongated mitochondrial morphology (47) (Figure 3).
Figure 3 Mitochondrial quality control in ovarian aging. During ovarian aging, there is a discernible deterioration in mitochondrial quality control, characterized by enhanced mitochondrial biogenesis, a shift in dynamics favoring fusion over fission, and a decrease in mitophagy. Asa a consequence, senescent ovarian cells display an increased mitochondrial mass and mitochondria with a hyperfused and elongated morphology. Mitofusin 1 (Mfn1), Mitofusin 2 (Mfn2), Dynamin-related protein 1 (Drp1).
Overall, mitochondrial quality control holds significant importance in preserving ovarian health and managing fertility issues associated with aging. Understanding and maintaining the delicate balance of mitochondrial biogenesis, dynamics, and mitophagy is critical for addressing ovarian aging and preserving fertility (37, 48, 49).
Mitochondrial DNA (mtDNA) is a unique genetic material inherited exclusively from the mother, encoding 13 core proteins critical for the efficiency of the mitochondrial energy-generating system (50). The dysfunction of mtDNA, whether quantitative or qualitative, may arise from various factors such as oxidative stress, environmental factors, and the natural aging process (51). Additionally, due to its proximity to the ETC and the consequent generation of ROS, mtDNA may be more susceptible to mutational burden, with a mutation rate approximately 25 times higher than nuclear DNA (52).
Ross et al. explore the association of acquired and inherited mtDNA mutations with premature aging, particularly focusing on ovarian aging, using a series of mouse mutant models. Their findings reveal that maternally transmitted mtDNA mutations induce mild aging phenotypes in mice with a normal nuclear genome, anticipating reduced fertility and exacerbating premature aging phenotypes (53). Additionally, acquired (somatic) mtDNA mutations in humans may result from oxidative damage or the inherent error rate of mtDNA polymerase gamma (54, 55). Human oocytes have been found to harbor mitochondrial point mutations, with a higher accumulation in aged oocytes, as indicated by the analysis of human oocytes for specific mutations (50).
Animal studies have shown that lower mtDNA copy number is associated with aging, as evidenced by old mice (56) and cows (57) with decreased fecundity having significantly less mtDNA in their oocytes compared to younger counterparts. Furthermore, the association between mtDNA copy number in cumulus cells and reproductive potential has been observed in human studies, indicating its relevance to the reproductive potential of women of advanced reproductive age (58). XY Zhou et al. reported that elevated levels of cell free mtDNA may be associated with premature ovarian insufficiency, shedding light on the potential role of mitochondrial dysfunction in the pathophysiology of this condition (59).
The mitochondrial unfolded protein response (UPRmt) is a cellular mechanism designed to maintain mitochondrial homeostasis by addressing the accumulation of unfolded or misfolded proteins within the mitochondrial matrix (60). Activated in response to mitochondrial stress, UPRmt orchestrates processes such as protein refolding, degradation of damaged proteins, and the promotion of mitochondrial biogenesis to ensure a stable mitochondrial environment (61). ClpP (caseinolytic peptidase P), a protease enzyme located in the mitochondrial matrix, plays a key role in UPRmt (62).
Studies have shown that ClpP deficiency can lead to impaired mitochondrial stress response, increasing apoptosis in GCs, and negatively impacting ovarian function and reproductive health (63, 64). Moreover, the absence of ClpP also accelerates the depletion of ovarian follicle reserve by over-activating the mTOR pathway (65). Under electron microscopy, mitochondria in Clpp-deficient cumulus cells have a smaller aspect ratio (length/width) and have a larger coverage area (mitochondrial area/cytoplasmic area). These ultrastructural changes were accompanied with diminished expression of mitochondrial dynamics genes (66). This underlines the intricate relationship among ClpP, mitochondrial dynamics, and reproductive health.
Targeting mitochondrial dysfunction represents a promising tool to prevent ovarian aging. Here, we explore the potential of targeting mitochondrial dysfunction through innovative therapeutic approaches, including antioxidants, metabolic improvement, biogenesis promotion, enhanced mitophagy, mitochondrial replacement therapy (MRT) and traditional Chinese medicine (TCM) treatments. Also, growth hormone appears to enhance mitochondrial function and reduce aneuploidy in aged mice, highlighting its potential as a therapeutic avenue for mitigating ovarian aging and enhancing reproductive outcomes (67–69). We summarize the current major mitochondrial therapies in Table 1, and while they show great potential in alleviating ovarian aging, there are still some challenges and limitations in practical application.
Mitochondrial-targeted antioxidant therapies have garnered significant attention as a key strategy to alleviate the adverse impact of mitochondrial ROS accumulation, given their central role in driving ovarian aging (85, 86). The antioxidant system comprises a complex interplay of various components, including both enzymes and non-enzymatic substances. Key enzymes within this system encompass superoxide dismutase (SOD), catalase (CAT), glutathione (GSH) and glutathione peroxidase (GSH-Px). Non-enzymatic elements predominantly consist of melatonin, as well as vitamins C, D, and E, and essential trace elements such as copper, zinc, and selenium (27). Consequently, interventions involving the supplementation of antioxidants like vitamins C, D, and E, upregulation of SOD and CAT through sirtuins (87), and the administration of chemical and natural compounds with documented antioxidant properties (e.g., phycocyanin (88), fisetin, and curcumin) have been employed to mitigate oxidative stress and inhibit cellular senescence across various cell types.
Focusing on ovarian cells, interventions involving resveratrol, melatonin, and Secoisolariciresinol diglucoside (SDG) have shown efficacy in reducing intracellular ROS levels. Resveratrol, a compound found in plants like grapes and berries, has gained attention for its potential health benefits, particularly its anti-aging properties (89). Studies suggest that resveratrol can modulate oxidative stress mechanisms, potentially slowing follicular atresia and mitigating the effects of aging on ovarian tissues (90, 91). Its purported effects involve the activation of pathways such as Nrf2-ARE, thereby enhancing antioxidant defense and reducing oxidative damage (70). Similarly, melatonin has emerged as a pivotal player in addressing ovarian aging (92). Studies indicate its potential in ameliorating age-related ovarian decline by enhancing antioxidant defense, protecting oocytes from damage caused by oxidative stress, and preserving mitochondrial function, ultimately impacting ovarian tissue health, follicular development, and oocyte quality (71). Additionally, SDG has exhibited notable scavenging ability against ROS, which gradually accumulates in ovarian tissues. Research by He et al. in 2021 suggests that SDG possesses beneficial effects on aging ovaries, as it has been shown to improve ovarian reserve by inhibiting oxidative stress (72).
Furthermore, mitochondrial-targeted coenzyme Q10 (MitoQ), a unique antioxidant specifically directed to mitochondria, has demonstrated protective effects against oxidative damage in ovarian tissue. Such protection suggests a potential to retard the process of ovarian aging, highlighting MitoQ’s significance in reproductive health interventions (73). A recent study conducted by Wang et al., showed that the supplementation of alpha-ketoglutarate (α-KG) to aging mice over a 4-month period demonstrated marked improvements in ovarian reserve, manifested by increased follicle numbers and enhanced oocyte quality. These benefits were evidenced by reduced fragmentation rates, decreased ROS levels, and a reduced frequency of abnormal spindle assembly (74). Furthermore, α-KG administration exhibited positive effects on post-ovulated aging oocyte quality and early embryonic development by enhancing mitochondrial functions, and reducing ROS accumulation and abnormal spindle assembly.
Recent researches have elucidated that during cell aging, metabolism shifts towards increased mitochondrial oxidative metabolism, accompanied by enhanced mitochondrial biogenesis (93, 94). This metabolic shift results in a decline in NAD+ levels and an elevation in AMP and ADP. Although the increase in mitochondrial biogenesis can offset the loss of healthy mitochondria due to mitochondrial stress, sustained stress experienced by mitochondria during cellular senescence may expose newly synthesized mitochondria to oxidative stress, creating a positive feedback loop that enhances ROS production and exacerbates mitochondrial damage. Therefore, adopting strategies to improve mitochondrial metabolism, enhance mitochondrial biogenesis, and limit ROS production is critical in preventing ovarian cell senescence and prolonging reproductive life (95).
Low levels of NAD+ have been demonstrated to be associated with mitochondrial metabolism disorders and the senescence of stem cells, whereas elevated NAD+ levels have been linked to enhanced mitochondrial function (96, 97). The study by Yang et al. found that the deletion of enzymes responsible for de novo NAD (+) biosynthesis led to a significant acceleration of ovarian aging, highlighting the pivotal role of these enzymes in maintaining ovarian health and function (98). Correspondingly, compounds that raise NAD+ levels, such as nicotinamide mononucleotide (NMN), nicotinamide (NAM), and nicotinamide riboside (NR), acting as precursors of NAD+, are acknowledged for their potential in retarding the aging process (75).
Several interventions have also shown promise in stimulating mitochondrial biogenesis and combatting ovarian aging, including exercise (99), controlled exposure to ROS, and nutritional therapy (100), involving specific nutrients such as CoQ10 (101) and resveratrol. In addition to its well-known antioxidant properties, resveratrol also plays a crucial role in enhancing mitochondrial biogenesis by activating the AMPK/SIRT1/PGC-1α axis, with PGC-1α being the key regulatory factor of mitochondrial biogenesis (76, 102). These approaches may offer potential avenues for maintaining ovarian health and counteracting age-related ovarian decline.
In addition, researches have shown that the tumor suppressor High Mobility Group (HMG)-box transcription factor 1 (HBP1) enhances mitochondrial function, reduces apoptosis of GCs, and significantly preserves ovarian reserve by regulating gene expression, protein interactions, and cellular physiological processes involved in mitochondrial biogenesis. These findings underscore the consequential role of HBP1 in extending the reproductive lifespan (77).
While increased mitochondrial biogenesis can be a beneficial adaptive response, uncontrolled or excessive biogenesis can also lead to deleterious consequences, such as increased NADH, decreased NAD+, and elevated AMP and ADP levels (103). Therefore, a careful balance must be struck when targeting mitochondrial biogenesis as a strategy to improve mitochondrial metabolism and function in the context of ovarian aging.
Enhancing mitophagy is an area of active research with potential therapeutic implications for addressing age-related fertility issues and improving reproductive health (104–106). Therapeutic interventions aimed at enhancing mitophagy encompass lifestyle modifications, dietary therapies, and pharmacological approaches.
Physical activity has been associated with a greater expression of mitochondrial fission and mitophagy markers, indicating its positive impact on mitochondrial quality control systems (107). Conversely, overfeeding is linked to the accumulation of impaired mitochondria and oxidative stress, underscoring the influence of lifestyle on mitophagy and mitochondrial health (108).
A variety of natural dietary compounds have been identified as potential agents in promoting mitophagy. Polyphenols, flavonoids, spermidine, and trehalose, along with compounds like resveratrol and Urolithin A(UA), are known to facilitate the restoration of normal mitophagy fluxes (109). Resveratrol effectively reinstates mitochondrial autophagy, contributing to the health of mitochondria in ovarian cells (110). UA, in particular, activates mitophagy through the PINK1-Parkin Pathway, which plays a crucial role in enhancing oocyte quality and extending reproductive functionality (78). Recently, Bhansali et al. found that metformin facilitates mitophagy through upregulation of essential mitophagy-related genes, thereby normalizing mitochondrial function (79). This positions metformin as a contender in the effort to decelerate ovarian aging and sustain reproductive health.
There is a complex relationship between mitochondrial quality control and reproductive lifespan. For instance, the daf-2 mutant model has been highlighted for its ability to sustain punctate mitochondrial morphology and execute fission alongside PINK1-mediated mitophagy, processes essential for maintaining oocyte integrity over time (111). Moreover, research has established a connection between elevated PGAM5 expression and age-related changes in mitochondrial dynamics in ovarian cells, suggesting a possible role for PGAM5 in maintaining and regulating mitochondrial function as organisms age (112). However, the development of therapeutic strategies that delay ovarian aging by manipulating mitochondrial dynamics remains an area in need of further exploration.
Mitochondrial replacement therapy (MRT) has emerged as an investigative technique to address mitochondrial dysfunction and its role in ovarian aging and poor oocyte quality. The premise of MRT is to replace defective mitochondria within an oocyte with healthy mitochondria, thereby improving the bioenergetic capacity and overall quality of the oocyte (113).
The primary methods of MRT include autologous mitochondrial transplantation (AMT), cytoplasmic transfer(CT), and germline nuclear transfer(GNT) (114). AMT involves extracting healthy mitochondria from the patient’s own cells and transplanting them into their dysfunctional cells, with the aim of restoring mitochondrial function and improving cellular bioenergetics (80). CT entails the injection of cytoplasm, containing healthy mitochondria, from a donor’s cell into a recipient’s cell (81). GNT offers the possibility to transfer the genetic material of a patient’s oocyte/zygote with compromised cytoplasm to the cytoplasm of an enucleated oocyte/zygote of a healthy donor (82).
In addition to these established techniques, other experimental approaches to MRT are being explored. The CRISPR/Cas9 gene editing technology holds potential for repairing mutated mtDNA in women with aging ovaries (115). Another proposed approach involves the use of stem cells to produce “young” mitochondria. The theory behind this method is that stem cells could be leveraged to generate a fresh supply of healthy mitochondria, which could then be used to supplement or replace dysfunctional mitochondria in aging oocytes (116). However, rigorous testing and evaluation will be essential to ensure the responsible development and implementation of these innovative therapies.
These mitochondrial replacement technologies offer potential avenues for addressing mitochondrial-related disorders and age-related fertility decline, but they also raise important social and ethical considerations (117). Critics argue that MRT, in particular, raises ethical concerns, as it involves the creation of embryos with genetic material from three individuals-the nuclear DNA from the intended parents and the mtDNA from the donor (118). There are also concerns about the long-term safety and efficacy of these interventions, the potential for genetic modifications, and the commercialization and equitable access to these treatments. Careful deliberation and oversight by relevant regulatory bodies and bioethical committees are necessary to address these complex issues (119).
Traditional Chinese medicine (TCM) has long been recognized for its potential in addressing various health concerns, including the issue of ovarian aging. TCM offers a unique perspective and therapeutic approaches to regulate ovarian aging, with a particular emphasis on the role of mitochondria. Some of the key mechanisms through which TCM may contribute to the regulation of ovarian aging include enhancing mitochondrial biogenesis, improving mitochondrial function, modulating mitochondrial dynamics, and regulating mitochondrial quality control (120). For instance, the Qingxin Zishen decoction (QZD) has shown promise in delaying ovarian aging and mitigating age-related ovarian conditions by enhancing ovarian mitochondrial function and regulating ovarian mitochondrial apoptosis in older rats (83), providing potential treatment options for age-related ovarian changes.
Numerous studies have explored the potential of TCM in addressing ovarian aging and related reproductive health concerns. Herbal formulations, such as those containing ingredients like Astragalus, Angelica, and Panax ginseng, have shown promising results in improving ovarian function, increasing the number of follicles, and enhancing oocyte quality by elevating antioxidant capacity, reducing apoptosis and other mechanisms in both animal models and human clinical trials (84, 121). Furthermore, TCM-based interventions have been investigated for their ability to alleviate the symptoms associated with ovarian aging, such as menopausal symptoms, infertility, and the risk of age-related gynecological conditions (122).
While the primary focus of TCM in addressing ovarian aging is on regulating the function and health of the ovaries, the holistic nature of TCM suggests that its interventions may have a broader impact on the body as a whole. TCM practitioners believe in the interconnectedness of all organs and systems within the body, with the goal of restoring balance and harmony. By targeting the underlying imbalances that contribute to ovarian aging, TCM therapies, such as herbal remedies, acupuncture, and lifestyle modifications, may have a positive influence on the overall health and functioning of other organs as well (123).
The mitochondria have emerged as a promising target for regulating ovarian aging, but there are several challenges and limitations that need to be considered. One key challenge is the significant heterogeneity observed in ovarian cells, with respect to their mitochondrial content, morphology, and function (124). This diversity within the ovarian tissue makes it difficult to develop a “one-size-fits-all” approach to mitochondrial-targeted therapies. Another challenge is the effective delivery of mitochondrial-targeted compounds, including some TCM herbs and formulations, to the mitochondria of ovarian cells (125). Developing appropriate delivery systems and compounds is crucial for maximizing the therapeutic potential.
Assessing the impact of mitochondrial-targeted interventions on ovarian function and aging is also difficult, as it requires sophisticated techniques to accurately measure mitochondrial parameters, such as quality control mechanisms including biogenesis and dynamics, within the ovarian tissue. Furthermore, ovarian aging is a multifactorial process, and individual variations in genetic, environmental, and lifestyle factors may influence the mitochondrial function and the responsiveness to certain interventions. Personalized approaches may be necessary to optimize the therapeutic outcomes (126).
Many promising mitochondrial-targeted therapies have also been limited by their potential for off-target toxicity, as mitochondria are essential for the normal functioning of all cells in the body (127). A deeper understanding of the unique metabolic and functional properties of ovarian mitochondria is needed to develop highly specific mitochondrial-targeted therapies that can selectively accumulate in ovarian cells and avoid off-target effects in other tissues.
In summary, considering the heterogeneity of ovarian mitochondria, effective delivery concerns, the need for personalized approaches, and off-target toxicity, a comprehensive and multifaceted approach may be necessary to overcome these obstacles and unlock the full potential of mitochondrial-targeted therapies for addressing ovarian aging.
Mitochondria play a central role in ovarian aging and reproductive health. Elevated reactive oxygen species, impaired mitochondrial quality control network, dysfunctional mtDNA, and decreased efficiency of mitochondrial unfolded protein response collectively contribute to ovarian cell aging. Therapeutic interventions targeting mitochondrial dysfunction are critical to protect ovarian function and support overall reproductive health. In the future, there should be a deeper understanding of the role of mitochondria in ovarian aging and further optimization of treatment methods that target mitochondria to fully utilize the potential of mitochondrial therapies.
Z-HW: Writing – original draft, Writing – review & editing. Z-JW: Writing – review & editing. H-CL: Writing – review & editing. C-YW: Writing – review & editing. Y-QW: Writing – review & editing. YY: Writing – review & editing. CZ: Writing – review & editing. G-YW: Writing – review & editing. J-PW: Writing – original draft, Writing – review & editing.
The author(s) declare that no financial support was received for the research, authorship, and/or publication of this article.
The authors declare that the research was conducted in the absence of any commercial or financial relationships that could be construed as a potential conflict of interest.
All claims expressed in this article are solely those of the authors and do not necessarily represent those of their affiliated organizations, or those of the publisher, the editors and the reviewers. Any product that may be evaluated in this article, or claim that may be made by its manufacturer, is not guaranteed or endorsed by the publisher.
1. Marchante M, Ramirez-Martin N, Buigues A, Martinez J, Pellicer N, Pellicer A, et al. Deciphering reproductive aging in women using a NOD/SCID mouse model for distinct physiological ovarian phenotypes. Aging (Albany NY). (2023) 15:10856–74. doi: 10.18632/aging.205086
2. Wu J, Liu Y, Song Y, Wang L, Ai J, Li K. Aging conundrum: A perspective for ovarian aging. Front Endocrinol. (2022) 13. doi: 10.3389/fendo.2022.952471
3. Dong L, Teh DBL, Kennedy BK, Huang Z. Unraveling female reproductive senescence to enhance healthy longevity. Cell Res. (2023) 33:11–29. doi: 10.1038/s41422-022-00718-7
4. San-Millán I. The key role of mitochondrial function in health and disease. Antioxidants. (2023) 12:3. doi: 10.3390/antiox12040782
5. May-Panloup P, Boucret L, Chao de la Barca J-M, Desquiret-Dumas V, Ferré-L'Hotellier V, Morinière C, et al. Ovarian ageing: the role of mitochondria in oocytes and follicles. Hum Reprod Update. (2016) 22:725–43. doi: 10.1093/humupd/dmw028
6. Park SU, Walsh L, Berkowitz KM. Mechanisms of ovarian aging. Reproduction. (2021) 162:R19–r33. doi: 10.1530/REP-21-0022
7. Kasapoğlu I, Seli E. Mitochondrial dysfunction and ovarian aging. Endocrinology. (2020) 161:2. doi: 10.1210/endocr/bqaa001
8. Schirrmacher V. Mitochondria at Work: New Insights into Regulation and Dysregulation of Cellular Energy Supply and Metabolism. Biomedicines. (2020) 8:6. doi: 10.3390/biomedicines8110526
9. Mohamad Kamal NS, Safuan S, Shamsuddin S, Foroozandeh P. Aging of the cells: Insight into cellular senescence and detection Methods. Eur J Cell Biol. (2020) 99:151108. doi: 10.1016/j.ejcb.2020.151108
10. Nunnari J, Suomalainen A. Mitochondria: in sickness and in health. Cell. (2012) 148:1145–59. doi: 10.1016/j.cell.2012.02.035
11. Vakifahmetoglu-Norberg H, Ouchida AT, Norberg E. The role of mitochondria in metabolism and cell death. Biochem Biophys Res Commun. (2017) 482:426–31. doi: 10.1016/j.bbrc.2016.11.088
12. Adhikari D, Lee IW, Yuen WS, Carroll J. Oocyte mitochondria-key regulators of oocyte function and potential therapeutic targets for improving fertility. Biol Reprod. (2022) 106:366–77. doi: 10.1093/biolre/ioac024
13. Sreerangaraja Urs DB, Wu WH, Komrskova K, Postlerova P, Lin YF, Tzeng CR, et al. Mitochondrial function in modulating human granulosa cell steroidogenesis and female fertility. Int J Mol Sci. (2020) 21:12. doi: 10.3390/ijms21103592
14. Shi YQ, Zhu XT, Zhang SN, Ma YF, Han YH, Jiang Y, et al. Premature ovarian insufficiency: a review on the role of oxidative stress and the application of antioxidants. Front Endocrinol (Lausanne). (2023) 14:1172481. doi: 10.3389/fendo.2023.1172481
15. Yang L, Lin X, Tang H, Fan Y, Zeng S, Jia L, et al. Mitochondrial DNA mutation exacerbates female reproductive aging via impairment of the NADH/NAD+ redox. Aging Cell. (2020) 19:1. doi: 10.1111/acel.13206
16. von Zglinicki T, Wan T, Miwa S. Senescence in post-mitotic cells: A driver of aging? Antioxid Redox Signal. (2021) 34:308–23. doi: 10.1089/ars.2020.8048
17. Chapman J, Fielder E, Passos JF. Mitochondrial dysfunction and cell senescence: deciphering a complex relationship. FEBS Lett. (2019) 593:1566–79. doi: 10.1002/1873-3468.13498
18. Lu X, Liu Y, Xu J, Cao X, Zhang D, Liu M, et al. Mitochondrial dysfunction in cumulus cells is related to decreased reproductive capacity in advanced-age women. Fertil Steril. (2022) 118:393–404. doi: 10.1016/j.fertnstert.2022.04.019
19. Mas-Bargues C. Mitochondria pleiotropism in stem cell senescence: Mechanisms and therapeutic approaches. Free Radical Biol Med. (2023) 208:657–71. doi: 10.1016/j.freeradbiomed.2023.09.019
20. Dalle Pezze P, Nelson G, Otten EG, Korolchuk VI, Kirkwood TB, von Zglinicki T, et al. Dynamic modelling of pathways to cellular senescence reveals strategies for targeted interventions. PloS Comput Biol. (2014) 10:e1003728. doi: 10.1371/journal.pcbi.1003728
21. Atayik MC, Çakatay U. Redox signaling and modulation in ageing. Biogerontology. (2023) 24:603–8. doi: 10.1007/s10522-023-10055-w
22. Jomova K, Raptova R, Alomar SY, Alwasel SH, Nepovimova E, Kuca K, et al. Reactive oxygen species, toxicity, oxidative stress, and antioxidants: chronic diseases and aging. Arch Toxicol. (2023) 97:2499–574. doi: 10.1007/s00204-023-03562-9
23. Amorim JA, Coppotelli G, Rolo AP, Palmeira CM, Ross JM, Sinclair DA. Mitochondrial and metabolic dysfunction in ageing and age-related diseases. Nat Rev Endocrinol. (2022) 18:243–58. doi: 10.1038/s41574-021-00626-7
24. Nesci S, Trombetti F, Pagliarani A, Ventrella V, Algieri C, Tioli G, et al. Molecular and supramolecular structure of the mitochondrial oxidative phosphorylation system: implications for pathology. Life (Basel). (2021) 11:24–5. doi: 10.3390/life11030242
25. Huang W, Hickson LJ, Eirin A, Kirkland JL, Lerman LO. Cellular senescence: the good, the bad and the unknown. Nat Rev Nephrol. (2022) 18:611–27. doi: 10.1038/s41581-022-00601-z
26. Martini H, Passos JF. Cellular senescence: all roads lead to mitochondria. FEBS J. (2023) 290:1186–202. doi: 10.1111/febs.16361
27. Yan F, Zhao Q, Li Y, Zheng Z, Kong X, Shu C, et al. The role of oxidative stress in ovarian aging: a review. J Ovarian Res. (2022) 15:2–3. doi: 10.1186/s13048-022-01032-x
28. Lim J, Luderer U. Oxidative damage increases and antioxidant gene expression decreases with aging in the mouse ovary. Biol Reprod. (2010) 84:775–82. doi: 10.1095/biolreprod.110.088583
29. Smits MAJ, Schomakers BV, van Weeghel M, Wever EJM, Wüst RCI, Dijk F, et al. Human ovarian aging is characterized by oxidative damage and mitochondrial dysfunction. Hum Reprod. (2023) 38:2208–20. doi: 10.1093/humrep/dead177
30. Sharma A, Mahur P, Muthukumaran J, Singh AK, Jain M. Shedding light on structure, function and regulation of human sirtuins: a comprehensive review. 3 Biotech. (2023) 13:29. doi: 10.1007/s13205-022-03455-1
31. Afzaal A, Rehman K, Kamal S, Akash MSH. Versatile role of sirtuins in metabolic disorders: From modulation of mitochondrial function to therapeutic interventions. J Biochem Mol Toxicol. (2022) 36:e23047. doi: 10.1002/jbt.23047
32. Zhu J, Yang Q, Li H, Wang Y, Jiang Y, Wang H, et al. Sirt3 deficiency accelerates ovarian senescence without affecting spermatogenesis in aging mice. Free Radic Biol Med. (2022) 193:511–25. doi: 10.1016/j.freeradbiomed.2022.10.324
33. Wang L, Tang J, Wang L, Tan F, Song H, Zhou J, et al. Oxidative stress in oocyte aging and female reproduction. J Cell Physiol. (2021) 236:7966–83. doi: 10.1002/jcp.30468
34. Gao Y, Zou Y, Wu G, Zheng L. Oxidative stress and mitochondrial dysfunction of granulosa cells in polycystic ovarian syndrome. Front Med (Lausanne). (2023) 10:1193749. doi: 10.3389/fmed.2023.1193749
35. Tamura H, Tanabe M, Jozaki M, Taketani T, Sugino N. Antioxidative action of melatonin and reproduction. Glycative Stress Res. (2019) 6:192–7. doi: 10.24659/gsr.6.3_192
36. He Q, Gu L, Lin Q, Ma Y, Liu C, Pei X, et al. The immp2l mutation causes ovarian aging through ROS-wnt/β-catenin-estrogen pathway: preventive effect of melatonin. Endocrinology. (2020) 161:1. doi: 10.1210/endocr/bqaa119
37. Chiang JL, Shukla P, Pagidas K, Ahmed NS, Karri S, Gunn DD, et al. Mitochondria in ovarian aging and reproductive longevity. Ageing Res Rev. (2020) 63:3. doi: 10.1016/j.arr.2020.101168
38. Aiken CE, Tarry-Adkins JL, Penfold NC, Dearden L, Ozanne SE. Decreased ovarian reserve, dysregulation of mitochondrial biogenesis, and increased lipid peroxidation in female mouse offspring exposed to an obesogenic maternal diet. FASEB J. (2016) 30:1548–56. doi: 10.1096/fj.15-280800
39. Archer SL, Longo DL. Mitochondrial dynamics — Mitochondrial fission and fusion in human diseases. N Engl J Med. (2013) 369:2236–51. doi: 10.1056/NEJMra1215233
40. Sebastián D, Palacín M, Zorzano A. Mitochondrial dynamics: coupling mitochondrial fitness with healthy aging. Trends Mol Med. (2017) 23:201–15. doi: 10.1016/j.molmed.2017.01.003
41. Chan DC. Mitochondrial dynamics and its involvement in disease. Annu Rev Pathol. (2020) 15:235–59. doi: 10.1146/annurev-pathmechdis-012419-032711
42. Adhikari D, Lee IW, Al-Zubaidi U, Liu J, Zhang QH, Yuen WS, et al. Depletion of oocyte dynamin-related protein 1 shows maternal-effect abnormalities in embryonic development. Sci Adv. (2022) 8:eabl8070. doi: 10.1126/sciadv.abl8070
43. Zhang M, Bener MB, Jiang Z, Wang T, Esencan E, Scott Iii R, et al. Mitofusin 1 is required for female fertility and to maintain ovarian follicular reserve. Cell Death Dis. (2019) 10:560. doi: 10.1038/s41419-019-1799-3
44. Zhang M, Bener MB, Jiang Z, Wang T, Esencan E, Scott R, et al. Mitofusin 2 plays a role in oocyte and follicle development, and is required to maintain ovarian follicular reserve during reproductive aging. Aging (Albany NY). (2019) 11:3919–38. doi: 10.18632/aging.v11i12
45. Novak I. Mitophagy: a complex mechanism of mitochondrial removal. Antioxid Redox Signal. (2012) 17:794–802. doi: 10.1089/ars.2011.4407
46. Ng MYW, Wai T, Simonsen A. Quality control of the mitochondrion. Dev Cell. (2021) 56:881–905. doi: 10.1016/j.devcel.2021.02.009
47. Westermann B. Mitochondrial fusion and fission in cell life and death. Nat Rev Mol Cell Biol. (2010) 11:872–84. doi: 10.1038/nrm3013
48. Liu L, Li Y, Chen G, Chen Q. Crosstalk between mitochondrial biogenesis and mitophagy to maintain mitochondrial homeostasis. J BioMed Sci. (2023) 30:86. doi: 10.1186/s12929-023-00975-7
49. Palikaras K, Lionaki E, Tavernarakis N. Coordination of mitophagy and mitochondrial biogenesis during ageing in C. elegans. Nature. (2015) 521:525–8. doi: 10.1038/nature14300
50. Podolak A, Woclawek-Potocka I, Lukaszuk K. The role of mitochondria in human fertility and early embryo development: what can we learn for clinical application of assessing and improving mitochondrial DNA? Cells. (2022) 11:2. doi: 10.3390/cells11050797
51. Leuthner TC, Meyer JN. Mitochondrial DNA mutagenesis: feature of and biomarker for environmental exposures and aging. Curr Environ Health Rep. (2021) 8:294–308. doi: 10.1007/s40572-021-00329-1
52. Hahn A, Zuryn S. Mitochondrial genome (mtDNA) mutations that generate reactive oxygen species. Antioxid (Basel). (2019) 8:3–4. doi: 10.3390/antiox8090392
53. Ross JM, Olson L, Coppotelli G. Mitochondrial dysfunction and protein homeostasis in aging: insights from a premature-aging mouse model. Biomolecules. (2024) 14:1. doi: 10.3390/biom14020162
54. Larsson NG. Somatic mitochondrial DNA mutations in mammalian aging. Annu Rev Biochem. (2010) 79:683–706. doi: 10.1146/annurev-biochem-060408-093701
55. Faraci C, Annis S, Jin J, Li H, Khrapko K, Woods DC. Impact of exercise on oocyte quality in the POLG mitochondrial DNA mutator mouse. Reproduction. (2018) 156:185–94. doi: 10.1530/REP-18-0061
56. Kushnir VA, Ludaway T, Russ RB, Fields EJ, Koczor C, Lewis W. Reproductive aging is associated with decreased mitochondrial abundance and altered structure in murine oocytes. J Assist Reprod Genet. (2012) 29:637–42. doi: 10.1007/s10815-012-9771-5
57. Cree LM, Hammond ER, Shelling AN, Berg MC, Peek JC, Green MP. Maternal age and ovarian stimulation independently affect oocyte mtDNA copy number and cumulus cell gene expression in bovine clones. Hum Reprod. (2015) 30:1410–20. doi: 10.1093/humrep/dev066
58. Korolkova AI, Mishieva NG, Martazanova BA, Burmenskaya OV, Veyukova MA, Ekimov AN, et al. Implications of mitochondrial DNA copy number in cumulus cells in late reproductive-age women. Akusherstvo i ginekologiia. (2019) 2019:108–14. doi: 10.18565/aig
59. Zhou XY, Yang YZ, Zhang J, Zhang XF, Liu YD, Wang Z, et al. Elevated cell-free mitochondria DNA level of patients with premature ovarian insufficiency. BMC Pregnancy Childbirth. (2023) 23:462. doi: 10.1186/s12884-023-05769-1
60. Shen G, Liu W, Xu L, Wang LL. Mitochondrial unfolded protein response and its roles in stem cells. Stem Cells Dev. (2020) 29:627–37. doi: 10.1089/scd.2019.0278
61. De I, Dogra N, Singh S. The mitochondrial unfolded protein response: role in cellular homeostasis and disease. Curr Mol Med. (2017) 17:587–97. doi: 10.2174/1566524018666180308110130
62. Ergun Y, Imamoglu AG, Cozzolino M, Demirkiran C, Basar M, Garg A, et al. Mitochondrial unfolded protein response gene clpp is required for oocyte function and female fertility. Int J Mol Sci. (2024) 25:1. doi: 10.3390/ijms25031866
63. Li G, Gu J, Zhou X, Wu T, Li X, Hua R, et al. Mitochondrial stress response gene Clpp deficiency impairs oocyte competence and deteriorate cyclophosphamide-induced ovarian damage in young mice. Front Endocrinol (Lausanne). (2023) 14:1122012. doi: 10.3389/fendo.2023.1122012
64. Yuan X, Ma W, Chen S, Wang H, Zhong C, Gao L, et al. CLPP inhibition triggers apoptosis in human ovarian granulosa cells via COX5A abnormality-Mediated mitochondrial dysfunction. Front Genet. (2023) 14:1141167. doi: 10.3389/fgene.2023.1141167
65. Wang T, Babayev E, Jiang Z, Li G, Zhang M, Esencan E, et al. Mitochondrial unfolded protein response gene Clpp is required to maintain ovarian follicular reserve during aging, for oocyte competence, and development of pre-implantation embryos. Aging Cell. (2018) 17:e12784. doi: 10.1111/acel.12784
66. Esencan E, Jiang Z, Wang T, Zhang M, Soylemez-Imamoglu G, Seli E. Impaired mitochondrial stress response due to CLPP deletion is associated with altered mitochondrial dynamics and increased apoptosis in cumulus cells. Reprod Sci. (2020) 27:621–30. doi: 10.1007/s43032-019-00063-y
67. Luo Y-Y, Zeng X, Zhu L, Li C, Xie J, Dong Q, et al. Growth hormone reduces aneuploidy and improves oocytes quality by JAK2-MAPK3/1 pathway in aged mice. J Trans Med. (2023) 21:1. doi: 10.1186/s12967-023-04296-z
68. Han L, Tian H, Guo X, Zhang L. Regulation of ovarian function by growth hormone: Potential intervention of ovarian aging. Front Endocrinol (Lausanne). (2022) 13:1072313. doi: 10.3389/fendo.2022.1072313
69. Hou HY, Wang X, Yu Q, Li HY, Li SJ, Tang RY, et al. Evidence that growth hormone can improve mitochondrial function in oocytes from aged mice. Reproduction. (2018) 157:345–58. doi: 10.1530/REP-18-0529
70. Farkhondeh T, Folgado SL, Pourbagher-Shahri AM, Ashrafizadeh M, Samarghandian S. The therapeutic effect of resveratrol: Focusing on the Nrf2 signaling pathway. BioMed Pharmacother. (2020) 127:110234. doi: 10.1016/j.biopha.2020.110234
71. Yang Y, Cheung HH, Zhang C, Wu J, Chan WY. Melatonin as potential targets for delaying ovarian aging. Curr Drug Targets. (2019) 20:16–28. doi: 10.2174/1389450119666180828144843
72. He X, Wang Y, Wu M, Wei J, Sun X, Wang A, et al. Secoisolariciresinol diglucoside improves ovarian reserve in aging mouse by inhibiting oxidative stress. Front Mol Biosci. (2022) 8. doi: 10.3389/fmolb.2021.806412
73. Gruber J, Fong S, Chen CB, Yoong S, Pastorin G, Schaffer S, et al. Mitochondria-targeted antioxidants and metabolic modulators as pharmacological interventions to slow ageing. Biotechnol Adv. (2013) 31:563–92. doi: 10.1016/j.biotechadv.2012.09.005
74. Wang H, Xu J, Li H, Chen W, Zeng X, Sun Y, et al. Alpha-ketoglutarate supplementation ameliorates ovarian reserve and oocyte quality decline with aging in mice. Mol Cell Endocrinol. (2023) 571:111935. doi: 10.1016/j.mce.2023.111935
75. Katsyuba E, Auwerx J. Modulating NAD(+) metabolism, from bench to bedside. EMBO J. (2017) 36:2670–83. doi: 10.15252/embj.201797135
76. de Oliveira MR, Nabavi SF, Manayi A, Daglia M, Hajheydari Z, Nabavi SM. Resveratrol and the mitochondria: From triggering the intrinsic apoptotic pathway to inducing mitochondrial biogenesis, a mechanistic view. Biochim Biophys Acta. (2016) 1860:727–45. doi: 10.1016/j.bbagen.2016.01.017
77. Dong Z, Huang M, Liu Z, Xie P, Dong Y, Wu X, et al. Focused screening of mitochondrial metabolism reveals a crucial role for a tumor suppressor Hbp1 in ovarian reserve. Cell Death Differ. (2016) 23:1602–14. doi: 10.1038/cdd.2016.47
78. Webb M, Sideris DP. Intimate relations-mitochondria and ageing. Int J Mol Sci. (2020) 21:26–7. doi: 10.3390/ijms21207580
79. Bharath LP, Agrawal M, McCambridge G, Nicholas DA, Hasturk H, Liu J, et al. Metformin enhances autophagy and normalizes mitochondrial function to alleviate aging-associated inflammation. Cell Metab. (2020) 32:44–55.e6. doi: 10.1016/j.cmet.2020.04.015
80. Mobarak H, Heidarpour M, Tsai PJ, Rezabakhsh A, Rahbarghazi R, Nouri M, et al. Autologous mitochondrial microinjection; a strategy to improve the oocyte quality and subsequent reproductive outcome during aging. Cell Biosci. (2019) 9:95. doi: 10.1186/s13578-019-0360-5
81. Sobek A, Tkadlec E, Klaskova E, Prochazka M. Cytoplasmic transfer improves human egg fertilization and embryo quality: an evaluation of sibling oocytes in women with low oocyte quality. Reprod Sci. (2021) 28:1362–9. doi: 10.1007/s43032-020-00371-8
82. Christodoulaki A, Boel A, Tang M, De Roo C, Stoop D, Heindryckx B. Prospects of germline nuclear transfer in women with diminished ovarian reserve. Front Endocrinol (Lausanne). (2021) 12:635370. doi: 10.3389/fendo.2021.635370
83. Chen Y, Wu Y, Zhou Y, Teo YQ, Miao T, Lei W, et al. The delaying effect of Qingxin Zishen decoction on ovarian aging: Examining regulation of ovarian mitochondria apoptosis in aged rats. Exp Gerontol. (2023) 182:1. doi: 10.1016/j.exger.2023.112298
84. Liu Y, Weng W, Gao R, Liu Y. New insights for cellular and molecular mechanisms of aging and aging-related diseases: herbal medicine as potential therapeutic approach. Oxid Med Cell Longev. (2019) 2019:1–25. doi: 10.1155/2019/4598167
85. Rodríguez-Varela C, Labarta E. Clinical application of antioxidants to improve human oocyte mitochondrial function: A review. Antioxidants. (2020) 9:2. doi: 10.3390/antiox9121197
86. Mihalas BP, Redgrove KA, McLaughlin EA, Nixon B. Molecular mechanisms responsible for increased vulnerability of the ageing oocyte to oxidative damage. Oxid Med Cell Longev. (2017) 2017:1–22. doi: 10.1155/2017/4015874
87. van de Ven RAH, Santos D, Haigis MC. Mitochondrial sirtuins and molecular mechanisms of aging. Trends Mol Med. (2017) 23:320–31. doi: 10.1016/j.molmed.2017.02.005
88. Wen X, Han Z, Liu SJ, Hao X, Zhang XJ, Wang XY, et al. Phycocyanin improves reproductive ability in obese female mice by restoring ovary and oocyte quality. Front Cell Dev Biol. (2020) 8:595373. doi: 10.3389/fcell.2020.595373
89. Saad NM, Sekar M, Gan SH, Lum PT, Vaijanathappa J, Ravi S. Resveratrol: latest scientific evidences of its chemical, biological activities and therapeutic potentials. Pharmacogn J. (2020) 12:1779–91. doi: 10.5530/pj
90. Zhou DD, Luo M, Huang SY, Saimaiti A, Shang A, Gan RY, et al. Effects and mechanisms of resveratrol on aging and age-related diseases. Oxid Med Cell Longev. (2021) 2021:9932218. doi: 10.1155/2021/9932218
91. Liu M, Yin Y, Ye X, Zeng M, Zhao Q, Keefe DL, et al. Resveratrol protects against age-associated infertility in mice. Hum Reprod. (2013) 28:707–17. doi: 10.1093/humrep/des437
92. Basini G, Grasselli F. Role of melatonin in ovarian function. Anim (Basel). (2024) 14:5. doi: 10.3390/ani14040644
93. Vasileiou P, Evangelou K, Vlasis K, Fildisis G, Panayiotidis M, Chronopoulos E, et al. Mitochondrial homeostasis and cellular senescence. Cells. (2019) 8:3. doi: 10.3390/cells8070686
94. Long YC, Tan TM, Takao I, Tang BL. The biochemistry and cell biology of aging: metabolic regulation through mitochondrial signaling. Am J Physiol Endocrinol Metab. (2014) 306:E581–91. doi: 10.1152/ajpendo.00665.2013
95. Kwon SM, Hong SM, Lee YK, Min S, Yoon G. Metabolic features and regulation in cell senescence. BMB Rep. (2019) 52:5–12. doi: 10.5483/BMBRep.2019.52.1.291
96. Liang J, Huang F, Song Z, Tang R, Zhang P, Chen R. Impact of NAD+ metabolism on ovarian aging. Immun Age. (2023) 20:70. doi: 10.1186/s12979-023-00398-w
97. Yang Q, Cong L, Wang Y, Luo X, Li H, Wang H, et al. Increasing ovarian NAD(+) levels improve mitochondrial functions and reverse ovarian aging. Free Radic Biol Med. (2020) 156:1–10. doi: 10.1016/j.freeradbiomed.2020.05.003
98. Yang Q, Li H, Wang H, Chen W, Zeng X, Luo X, et al. Deletion of enzymes for de novo NAD(+) biosynthesis accelerated ovarian aging. Aging Cell. (2023) 22:e13904. doi: 10.1111/acel.13904
99. Li J, Wang Z, Li C, Song Y, Wang Y, Bo H, et al. Impact of exercise and aging on mitochondrial homeostasis in skeletal muscle: roles of ROS and epigenetics. Cells. (2022) 11:1. doi: 10.3390/cells11132086
100. Shaum KM, Polotsky AJ. Nutrition and reproduction: is there evidence to support a "Fertility Diet" to improve mitochondrial function? Maturitas. (2013) 74:309–12. doi: 10.1016/j.maturitas.2013.01.011
101. Harsini R, Zavareh S, Nasiri M, Seyfi S. The effect of Coenzyme Q10 on mitochondrial biogenesis in mouse ovarian follicles during in vitro culture. Zygote. (2024) 32:14–20. doi: 10.1017/S0967199423000461
102. Yang M, Shen Y, Zhao S, Zhang R, Dong W, Lei X. Protective effect of resveratrol on mitochondrial biogenesis during hyperoxia-induced brain injury in neonatal pups. BMC Neurosci. (2023) 24:27. doi: 10.1186/s12868-023-00797-1
103. Palikaras K, Tavernarakis N. Mitochondrial homeostasis: the interplay between mitophagy and mitochondrial biogenesis. Exp Gerontol. (2014) 56:182–8. doi: 10.1016/j.exger.2014.01.021
104. Guo J, Chiang WC. Mitophagy in aging and longevity. IUBMB Life. (2022) 74:296–316. doi: 10.1002/iub.2585
105. Cox RT, Poulton J, Williams SA. The role of mitophagy during oocyte aging in human, mouse, and Drosophila: implications for oocyte quality and mitochondrial disease. Reprod Fertil. (2021) 2:R113–R29. doi: 10.1530/RAF-21-0060
106. Cota V, Sohrabi S, Kaletsky R, Murphy CT. Oocyte mitophagy is critical for extended reproductive longevity. PloS Genet. (2022) 18:e1010400. doi: 10.1371/journal.pgen.1010400
107. Arribat Y, Broskey NT, Greggio C, Boutant M, Conde Alonso S, Kulkarni SS, et al. Distinct patterns of skeletal muscle mitochondria fusion, fission and mitophagy upon duration of exercise training. Acta Physiol (Oxf). (2019) 225:e13179. doi: 10.1111/apha.13179
108. Salvestrini V, Sell C, Lorenzini A. Obesity may accelerate the aging process. Front Endocrinol (Lausanne). (2019) 10:266. doi: 10.3389/fendo.2019.00266
109. Varghese N, Werner S, Grimm A, Eckert A. Dietary mitophagy enhancer: A strategy for healthy brain aging? Antioxid (Basel). (2020) 9:1. doi: 10.3390/antiox9100932
110. Sebori R, Kuno A, Hosoda R, Hayashi T, Horio Y. Resveratrol decreases oxidative stress by restoring mitophagy and improves the pathophysiology of dystrophin-deficient mdx mice. Oxid Med Cell Longev. (2018) 2018:9179270. doi: 10.1155/2018/9179270
111. Sharma A, Smith HJ, Yao P, Mair WB. Causal roles of mitochondrial dynamics in longevity and healthy aging. EMBO Rep. (2019) 20:e48395. doi: 10.15252/embr.201948395
112. Li CJ, Lin LT, Tsai HW, Wen ZH, Tsui KH. Phosphoglycerate mutase family member 5 maintains oocyte quality via mitochondrial dynamic rearrangement during aging. Aging. Cell. (2022) 21:1. doi: 10.1111/acel.13546
113. Wolf DP, Mitalipov N, Mitalipov S. Mitochondrial replacement therapy in reproductive medicine. Trends Mol Med. (2015) 21:68–76. doi: 10.1016/j.molmed.2014.12.001
114. Rodríguez-Varela C, Herraiz S, Labarta E. Mitochondrial enrichment in infertile patients: a review of different mitochondrial replacement therapies. Ther Adv Reprod Health. (2021) 15:26334941211023544. doi: 10.1177/26334941211023544
115. Fogleman S, Santana C, Bishop C, Miller A, Capco DG. CRISPR/Cas9 and mitochondrial gene replacement therapy: promising techniques and ethical considerations. Am J Stem Cells. (2016) 5:39–52
116. Suhr ST, Chang EA, Tjong J, Alcasid N, Perkins GA, Goissis MD, et al. Mitochondrial rejuvenation after induced pluripotency. PloS One. (2010) 5:e14095. doi: 10.1371/journal.pone.0014095
117. Caicedo A, Aponte PM, Cabrera F, Hidalgo C, Khoury M. Artificial mitochondria transfer: current challenges, advances, and future applications. Stem Cells Int. (2017) 2017:7610414. doi: 10.1155/2017/7610414
118. Appleby JB. The ethical challenges of the clinical introduction of mitochondrial replacement techniques. Med Health Care Philos. (2015) 18:501–14. doi: 10.1007/s11019-015-9656-3
119. D'Amato M, Morra F, Di Meo I, Tiranti V. Mitochondrial transplantation in mitochondrial medicine: current challenges and future perspectives. Int J Mol Sci. (2023) 24:13–5. doi: 10.3390/ijms24031969
120. Yan X, Li N. Research progress on the relationship between mitochondrial dysfunction and premature ovarian failure from the perspective of TCM treatment. J Clin Nurs Res. (2022) 6:69–74. doi: 10.26689/jcnr.v6i4
121. Noh S, Go A, Kim DB, Park M, Jeon HW, Kim B. Role of antioxidant natural products in management of infertility: A review of their medicinal potential. Antioxid (Basel). (2020) 9:33–8. doi: 10.3390/antiox9100957
122. Zhang J, Zhang M, Luo A, Yang S, Shen L, Wang M, et al. Prevention and management of ovarian aging. Ovarian Aging: Springer;. (2023) p:199–238. doi: 10.1007/978-981-19-8848-6
123. Zhao H, Luo Y. Traditional Chinese medicine and aging intervention. Aging dis. (2017) 8:688. doi: 10.14336/AD.2017.1002
124. Kuznetsov AV, Margreiter R. Heterogeneity of mitochondria and mitochondrial function within cells as another level of mitochondrial complexity. Int J Mol Sci. (2009) 10:1911–29. doi: 10.3390/ijms10041911
125. Milane L, Trivedi M, Singh A, Talekar M, Amiji M. Mitochondrial biology, targets, and drug delivery. J Controlled release. (2015) 207:40–58. doi: 10.1016/j.jconrel.2015.03.036
126. Koklesova L, Mazurakova A, Samec M, Kudela E, Biringer K, Kubatka P, et al. Mitochondrial health quality control: measurements and interpretation in the framework of predictive, preventive, and personalized medicine. Epma J. (2022) 13:177–93. doi: 10.1007/s13167-022-00281-6
Keywords: mitochondria, ovarian aging, reproductive health, mitochondrial dysfunction, mitochondrial therapies, infertility
Citation: Wang Z-H, Wang Z-J, Liu H-C, Wang C-Y, Wang Y-Q, Yue Y, Zhao C, Wang G and Wan J-P (2024) Targeting mitochondria for ovarian aging: new insights into mechanisms and therapeutic potential. Front. Endocrinol. 15:1417007. doi: 10.3389/fendo.2024.1417007
Received: 13 April 2024; Accepted: 29 May 2024;
Published: 17 June 2024.
Edited by:
James Harper, Sam Houston State University, United StatesReviewed by:
Frank Castora, Eastern Virginia Medical School, United StatesCopyright © 2024 Wang, Wang, Liu, Wang, Wang, Yue, Zhao, Wang and Wan. This is an open-access article distributed under the terms of the Creative Commons Attribution License (CC BY). The use, distribution or reproduction in other forums is permitted, provided the original author(s) and the copyright owner(s) are credited and that the original publication in this journal is cited, in accordance with accepted academic practice. No use, distribution or reproduction is permitted which does not comply with these terms.
*Correspondence: Ji-Peng Wan, d2FuamlwZW5nc2RAaG90bWFpbC5jb20=
†These authors have contributed equally to this work and share first authorship
Disclaimer: All claims expressed in this article are solely those of the authors and do not necessarily represent those of their affiliated organizations, or those of the publisher, the editors and the reviewers. Any product that may be evaluated in this article or claim that may be made by its manufacturer is not guaranteed or endorsed by the publisher.
Research integrity at Frontiers
Learn more about the work of our research integrity team to safeguard the quality of each article we publish.