- 1School of Engineering Medicine, Beihang University, Beijing, China
- 2Key Laboratory of Big Data-based Precision Medicine (Beihang University), Ministry of Industry and Information Technology, Beijing, China
- 3School of Medicine, The Chinese University of Hong Kong, Shenzhen, Guangdong, China
- 4School of Life Sciences, Westlake University, Hangzhou, China
Extracellular vesicles (EVs) are nanovesicles containing bioactive molecules including proteins, nucleic acids and lipids that mediate intercellular and inter-organ communications, holding promise as potential therapeutics for multiple diseases. Adipose tissue (AT) serves as a dynamically distributed energy storage organ throughout the body, whose accumulation leads to obesity, a condition characterized by infiltration with abundant immune cells. Emerging evidence has illustrated that EVs secreted by AT are the novel class of adipokines that regulate the homeostasis between AT and peripheral organs. However, most of the studies focused on the investigations of EVs derived from adipocytes or adipose-derived stem cells (ADSCs), the summarization of functions in cellular and inter-organ crosstalk of EVs directly derived from adipose tissue (AT-EVs) are still limited. Here, we provide a systemic summary on the key components and functions of EVs derived from healthy adipose tissue, showing their significance on the tissue recovery and metabolic homeostasis regulation. Also, we discuss the harmful influences of EVs derived from obese adipose tissue on the distal organs. Furthermore, we elucidate the potential applications and constraints of EVs from healthy patients lipoaspirates as therapeutic agents, highlighting the potential of AT-EVs as a valuable biological material with broad prospects for future clinical use.
1 Introduction
EVs are small membrane-bound vesicles that released by almost all cell types. These vesicles carry the functional cargoes to their target cells, thus regulating intercellular and inter-organ crosstalk. EVs are crucial in the regulation of biological processes during physiological or pathological states, making them potentially clinically relevant. Especially, owing to their high biocompatibility, low immunogenicity, and extensive delivery range, EVs are being recognized as promising therapeutic agents for treating various diseases such as cardiovascular disorders and tumors.
Adipose tissue, a complex organ for energy storage, plays a central role in mediating systemic metabolism and maintaining energy homeostasis. The excessive accumulation of adipose tissue leads to obesity, which is a metabolic disorder characterized by chronic inflammation that triggers multiple diseases, including type 2 diabetes, heart disease and stroke (1). In addition to its primary function, adipose tissue has been well recognized to be an endocrine organ that produce adipokines, which regulate energy homeostasis and immune response (2, 3). In recent years, EVs from adipose tissue have been clarified as a novel category of adipokines. These EVs regulate systemic metabolism and distal organ function by delivering bioactive cargoes through autocrine, paracrine, and endocrine manners. Moreover, in obesity, the composition of EVs derived from adipose tissue are significantly altered, thus influencing the metabolic state and cell viability of recipient cells. To date, most studies on adipose tissue-derived EVs have focused on investigating the functions and applications of EVs derived from adipocytes or ADSCs. However, there is still a lack of systematic characterization and comprehensive summary regarding the composition and biological functions of EVs obtained directly from healthy or obese adipose tissue. Especially, given the accessibility of adipose tissue, evaluating the feasibility of utilizing AT-EVs as therapeutic agents could provide valuable insights for advancing their clinical applications.
In this review, we summarize the roles of EVs derived from healthy adipose tissue as mediators in intercellular and inter-organ crosstalk. We also discuss the roles of AT-EVs in facilitating the progression of multiple diseases under obesity. Moreover, we outline the procedures and principles of AT-EVs isolation for further investigation. Finally, we emphasize the composition and functions of lipoaspirate-EVs in biological processes, highlighting their potential for clinical translation.
2 Biogenesis and clinical application of EVs
Based on the biogenesis, EVs are primarily divided into three classes: exosomes, microvesicles, and apoptotic bodies (4) (Figure 1). Exosomes are small EVs (sEV) with diameter ranging from 50 to 150 nm, generated through the endocytic pathway, beginning as early endosomes formed by inward invagination of the plasma membrane. These early endosomes mature into late endosomes, which then form multivesicular bodies (MVBs). MVBs fuse with lysosomes or autophagosomes for degradation or with cell membrane to release the intraluminal vesicles (ILVs) as exosomes (5). Microvesicles, also referred to as large EVs (lEV) (6), ranging from 150 nm to over 1,000 nm, with an average size of 250-400 nm (7). They differ from exosomes as they are formed directly from the plasma membrane surface instead of originating from the endocytic pathway. Apoptotic bodies are the largest type of EVs, ranging in size from 1 to 5 µm. They form when programmed cell death is triggered by normal physiological signals or pathological processes, leading to cellular budding and the creation of apoptotic protrusions (5), and ultimately forming apoptotic bodies (5). Typically, apoptotic bodies are recognized by macrophages and cleared through phagocytosis (8).
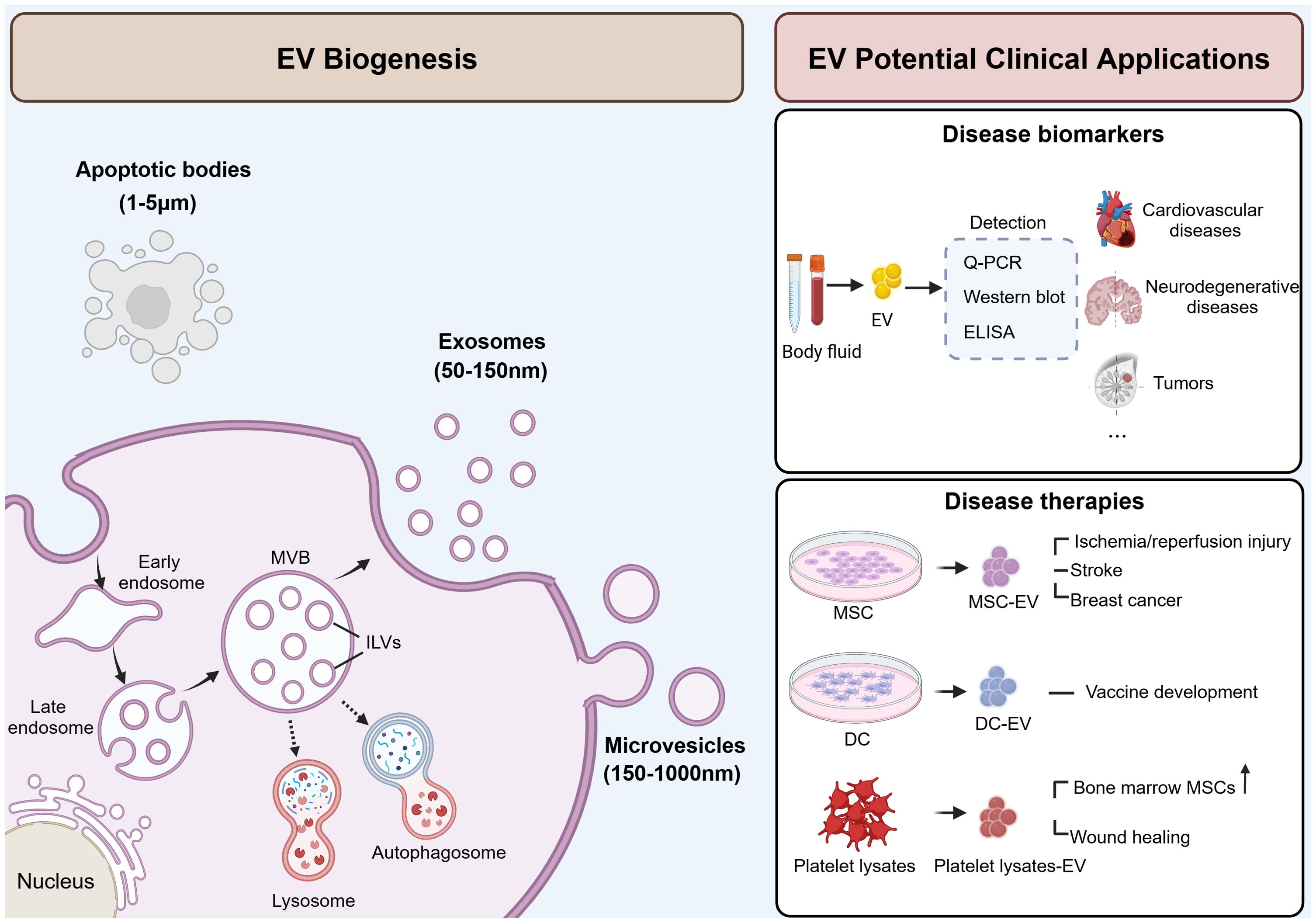
Figure 1. Schematic illustration of EV biogenesis and potential clinical applications. EVs are secreted into extracellular space and can be classified into three subgroups: exosomes (50-150 nm), microvesicles (150-1000 nm), and apoptotic bodies (1-5 µm). Exosomes are formed through the endocytic pathway and secreted by the fusion of MVBs with the plasma membrane. Microvesicles are formed directly from the plasma membrane. And apoptotic bodies are formed by cellular budding and the creation of apoptotic protrusions. EVs can be used as disease biomarkers and therapies. EVs derived from blood or body fluid carry specific cargoes that reflecting the state of parent cells, making them potential biomarkers for multiple diseases. Also, EVs derived from MSC, DC or platelet lysate also serve as promising therapeutic agents in various diseases. MVB, multivesicular body; ILV, intraluminal vesicles; ELISA, enzyme-linked immunosorbent assay; MSC, mesenchymal stem cells; DC, dendritic cells. This figure was created by using BioRender (https://biorender.com/).
In the physiological state, EVs serve as mediators that play a role in cell communication (9), tissue development and maintenance (10, 11), as well as immune response (12, 13). Meanwhile, EVs are also involved in the progression of diseases such as tumors, neurodegenerative diseases, and cardiovascular diseases. Due to their ability to transport disease-related biological information and their detectability in blood, EVs hold promise as potential diagnostic biomarkers. Crucially, active research is underway to harness the therapeutic potential of EVs for treating various diseases based on their biological functions. For example, EVs derived from mesenchymal stem cells (MSCs) have demonstrated therapeutic effects in models of myocardial ischemia/reperfusion injury (14), stroke (15), and breast cancer (16). Additionally, EVs from dendritic cells (DC) showed promise for vaccine development against infectious diseases such as tuberculosis (17). Moreover, EVs isolated from platelet lysates have been found to enhance the proliferation and migration of bone marrow-derived MSCs (18), and accelerate wound healing (19). EVs offer several advantages when used as therapeutic agents. Firstly, they exhibit low immunogenicity when employed in autologous applications, resulting in minimal potential side effects. Secondly, their lipid membrane composition facilitates fusion with target cells while preventing degradation. Finally, due to their modifiability, they can serve as efficient nanocarriers for loading functional miRNAs, thus enhancing the therapeutic efficacy. In conclusion, EVs hold promise in clinical applications, and the development of EV-related agents may provide therapeutic strategies for more diseases.
3 Healthy and obese adipose tissue
Adipose tissues are mainly categorized into two types, white adipose tissue (WAT) and brown adipose tissue (BAT). WAT is mainly composed of white adipocytes, with large unilocular/large lipid droplets and relatively few mitochondria (20). WAT maintains whole-body energy homeostasis by tightly regulating the balance of lipid storage and mobilization (21, 22). Additionally, WAT secretes a variety of adipokines, including adiponectin, leptin, omentin, resistin, as well as a variety of cytokines, such as tumor necrosis factor-α (TNF-α), IL-6, and monocyte chemoattractant protein-1 (MCP-1), all of which are essential for maintaining metabolic homeostasis in the body. WAT are classified into two types according to the location: subcutaneous adipose tissue (SAT) depot and visceral adipose tissue (VAT) depot (23). SAT is located in the abdomen, hip and thigh, and leg circumference. VAT is located surround the visceral organs, including pericardium, omentum, mesentery, retroperitoneum, and gonads (24). SAT is protective, whereas VAT located in intra-abdominal organs is detrimental due to its strong association with metabolic disorders, including obesity and diabetes (25).
Brown adipose tissue is more vascularized and sympathetic innervated, accounting for only a small portion (only ≈4.3%) of AT, and is mainly composed of brown adipocytes (26). Brown adipocytes contain many multilocular/small lipid droplets and significantly more cristae-dense mitochondria (27). Human BAT is located in the supraclavicular, axilla, neck, around the aorta, and in the subscapular region (28, 29). The primary function of BAT is mediating the non-shivering thermogenesis through uncoupling protein 1 (UCP1, also known as thermogenin), which is specifically expressed in brown adipocytes and located in the inner mitochondrial membrane (30). Recent studies have shown that beige adipose tissue, characterized by high mitochondrial content and the expression of UCP1, also participate in thermogenesis (31). In addition to regulating thermogenesis, BAT also secretes adipokines, known as brown adipokines or batokines, including metabolites, lipids, peptides, and miRNAs (32). These adipokines regulate energy balance and glucose homeostasis through crosstalk with various peripheral tissues, such as the liver, skeletal muscle, gut, central nervous system, and immune cells (33). Collectively, although the comprehensive understanding of BAT is still unclear, current evidence has demonstrated the great potential of BAT in the treatment of metabolic disorders in vivo.
Excessive accumulation of adipose tissue leads to obesity, which triggers numerous metabolic disorders such as type 2 diabetes, metabolic syndrome, and cardiovascular diseases (34, 35). In obese adipose tissue, mitochondrial function is significantly impaired, as evidenced by reduced oxidative metabolism (36, 37), compromised mitochondrial fatty acid oxidation (38), and decreased levels of glucose oxidation-related metabolites (39), leading to a decline in oxygen consumption and increased production of reactive oxygen species (ROS) (40). Adipokine levels are disrupted in obese adipose tissues, with decreased adiponectin and omentin, and increased cytokines including leptin, resistin, and FGF21 (41). These changes lead to chronic inflammation, characterized by elevated circulating IL-6 and TNFα (42, 43), indicating a pro-inflammatory phenotype in the immune microenvironment (44).
4 Adipose tissue-derived EVs
4.1 Cellular origin of adipose tissue-derived EVs
Although adipocytes are primarily responsible for fat storage, which is the main function of adipose tissue, they constitute only 15% to 30% of the total cell population. The majority of adipose tissue is composed of the stromal vascular fraction (SVF), which contains diverse cell types, including immune cells, fibroblasts, vascular cells, preadipocytes, and stem cells (45). The cellular origin of AT-EVs includes most of these cell types. Adipocyte-derived EVs are marked with adiponectin, resistin, perilipin A, and fatty acid binding protein 4 (FABP4) (46, 47). They induce the differentiation of monocytes into adipose tissue macrophages (ATMs) by secretion of mixed pro-inflammatory and anti-inflammatory cytokines (48). ADSC-EVs induce macrophages to adopt an anti-inflammatory phenotype, offering potential treatment for obesity-related metabolic dysregulation (49–51). EVs derived from ATMs also involve in regulating inflammatory responses and metabolic homeostasis. A study revealed that ATM-EVs carrying miRNAs was transferred to insulin target cells via paracrine or endocrine manners, significantly influencing insulin sensitivity and glucose homeostasis (52). Additionally, under the stimulation of glucagon, endothelial cells secrete EVs containing blood-derived signals that target adipocytes, participating in the response to systemic nutritional status changes (53).
In summary, AT-EVs are a heterogeneous mixture from various cell types, each carrying distinct cargoes and performing diverse functions. Many studies currently used adipocyte-derived EVs or ADSC-EVs to represent the components or functions of the AT-EVs. However, the crucial information relevant to the actual in vivo conditions are potentially overlooked. Therefore, to accurately understand EV functions and roles in physiology and pathology, it is essential to study EVs directly derived from adipose tissue.
4.2 Isolation of adipose tissue-derived EVs
Here, we collect the procedures of commonly used AT-EVs isolation in present studies (as shown in the Table 1), and further summarize the proper protocol for AT-EVs isolation (Figure 2).
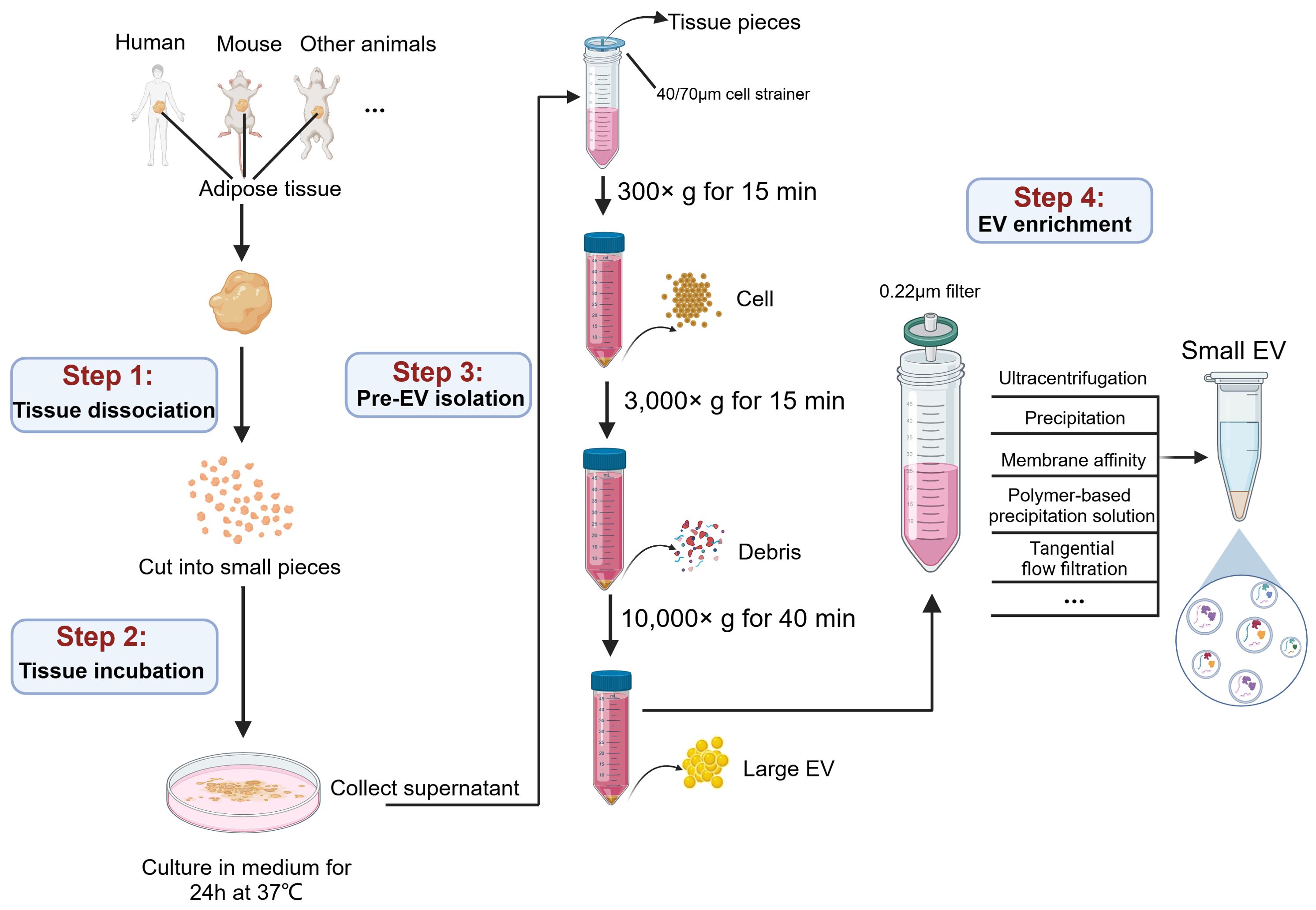
Figure 2. A graphic workflow for the isolation procedures of AT-EVs. The adipose tissues separated from human or other experimental animals were cut into small pieces on ice and incubated in medium for 24 h at 37 °C. Filtration and low force centrifugation (300 ×g for 15 min and 3,000 ×g for 15 min) were used to remove tissue pieces, cells and debris. After centrifugation at 10,000 ×g for 40 min, the large EVs were collected. The subsequent supernatant, after filtration through a 0.22 µm filter, the small EVs were isolated by multiple methods, including ultracentrifugation, precipitation, membrane affinity, polymer-based precipitation solution, and tangential flow filtration. This figure was created by using BioRender (https://biorender.com/).
Briefly, to obtain EVs from tissues as naturally as possible, a common approach involves isolating tissue explants from living tissues and culturing them in vitro for a short time (54). This approach results in the culture medium containing tissue-derived EVs, which is then isolated using techniques similar to those used for EVs from cell culture supernatants. Currently, this approach for isolating tissue-derived EVs has been applied to various tissues, including lung (55), brain (56, 57), heart (58), liver (59), muscle (60), tumor (61–63), as well as adipose tissue (64). However, the processing details vary depending on the specific characteristics of each tissue. Here we summarize the EV isolation procedure for adipose tissue, which involves four steps: tissue dissociation, tissue incubation, pre-EV isolation and EV enrichment.
4.2.1 Tissue dissociation
To minimize the tissue degradation, fresh tissue samples should be immediately immersed in cold PBS after extraction from patients or animals, and then promptly transferred to the laboratory for subsequent experimental procedures (65). Since disrupting cellular integrity result in the release of intracellular vesicles that contaminates tissue-derived EVs, methods causing significant tissue damage, such as filtering (66, 67), vortexing (68), blending, or homogenization (66, 67), should be avoided. For adipose tissue, many studies performed tissue dissociation by directly cutting fresh adipose tissue into small slices (0.1 mm3) and minimizing the mechanical pressure applied to the tissue. This method is recommended for isolating EVs from various tissue types (69).
4.2.2 Tissue incubation
Various enzymes, including collagenases (collagenase I, II, III, and IV) (70, 71), papain (72), and neutral protease (Dispase II) (55)are used to incubate with tissue for more thorough dispersion. In addition, DNase I is commonly added to prevent DNA aggregation (73). However, many studies on adipose tissue incubation use non-enzymatic treatments, which allows for the spontaneous release of EVs from the tissue interstitium. This gentler approach significantly minimizes damage to tissues and cells. Typically, this approach involves incubating sliced adipose tissue in a suitable culture medium for 24-48 hours under standard cell culture conditions, with a maximum incubation period of up to 72 hours.
4.2.3 Pre-EV isolation
Following incubation, the majority of EVs in adipose tissue have been released into the culture medium, requiring subsequent enrichment of EVs from the supernatant. After removing the residual solid tissue fragments through 40 or 70 μm filters, the supernatants are subjected to differential centrifugation to remove cells and cell debris.
4.2.4 EV enrichment
Large EVs can be precipitated at 10,000 ×g for 40 minutes. The supernatants were collected for the subsequent enrichment of small EVs. Previous studies have considered “ultracentrifugation” as the gold standard method for small EVs isolation (62, 74), and it is also widely recognized for isolating EVs from adipose tissue. However, due to inherent limitations such as incomplete removal of contaminating particles and time-consuming, alternative enrichment methods have been employed in many studies. These methods include precipitation (ExoQuick), membrane affinity (exoEasy Maxi kit) and polymer-based precipitation solution (Total Exosome Isolation TM reagent) (75). For high-throughput isolation, a clinically applicable, cell-free and sterile method of tangential flow filtration (TFF) has been established (76). TFF offers higher efficiency and can process large volumes (>1 L) of liquids, enabling large-scale production (77, 78). In addition, Casadei et al. have developed a novel cross-flow microfiltration method for isolating EVs from liposarcoma, a malignant tumor composed of adipocytes and other cell types (63, 79, 80). Subsequently, small EVs were pelleted and washed with PBS, followed by EV characterization assays, including size distribution determination by nanoparticle tracking analysis (NTA), morphology determination by transmission electron microscopy (TEM), and EV-specific molecules detection using immunoblotting or flow cytometry.
5 Roles of EVs derived from healthy and obese adipose tissue in systemic regulation
5.1 EVs derived from healthy adipose tissue
Given their different origins, WAT-EVs and BAT-EVs exhibit different functions and roles, as shown in Figure 3. WAT is involved in tissue regeneration through secreting considerable amounts of adipokines, including cytokines and growth factors. Recently, evidence illustrated that WAT-EVs may also contribute to skin wound healing through releasing functional miRNAs. Shao et al. discovered that miR-92a-3p, abundant in WAT-EVs, targeted the large tumor suppressor kinase 2 (LATS2) in keratinocytes and fibroblasts (81). This activated the YAP/TAZ signaling pathway, enhancing the proliferation, migration, and extracellular matrix (ECM) deposition of keratinocytes and fibroblasts. And in vivo, WAT-EVs from adult patients undergoing skin flap transplantation treatment promoted wound healing in dorsal skin injured rats. Another example indicated that WAT-EVs promoted soft tissue healing. The WAT-EVs extracted from rat fat pad exhibited preventive effects against bisphosphonate-related osteonecrosis of the jaw (BRONJ) (82). In rats model, the WAT-EV-treated group showed reduced necrotic bones and empty osteocyte lacunae, along with an increase in osteoclasts, collagen fibers, and blood vessels. Moreover, WAT-EVs enhanced the proliferation, migration, and tube formation of human umbilical vein endothelial cells (HUVECs) inhibited by Zoledronic acid (Zol). These findings provide crucial theoretical foundations and insights for crafting innovative treatment strategies in wound healing processes.
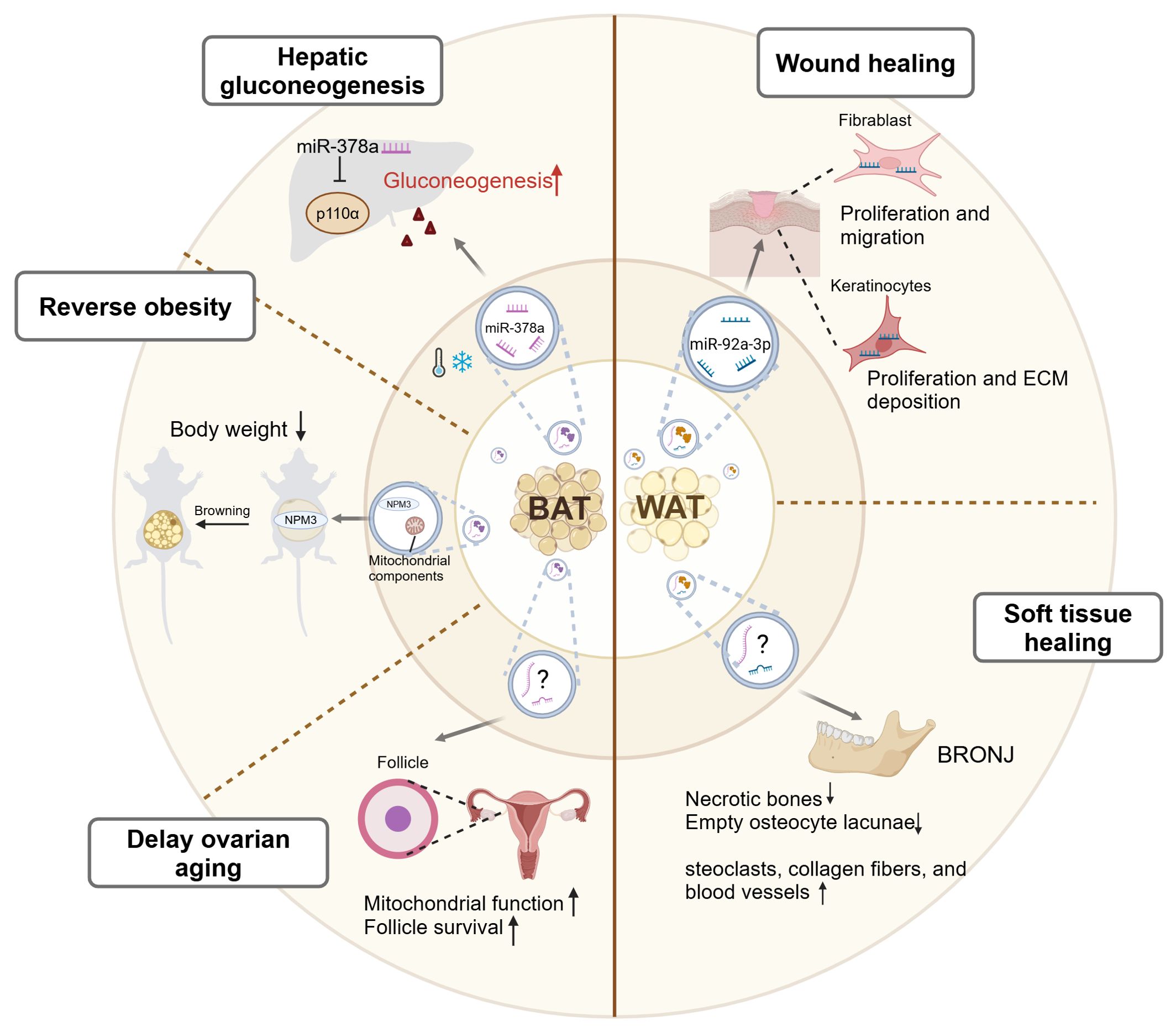
Figure 3. A graphic summary of the mediation of inter-organ crosstalk by BAT-EVs and WAT-EVs. Under cold stress conditions, BAT-EVs regulate systemic glucose metabolism by promoting hepatic gluconeogenesis through the effects of miR-378a. BAT-EVs also reverse obesity through two mechanisms. Firstly, their abundant mitochondrial components promote the oxygen consumption of recipient cells. Secondly, the NPM3 protein in BAT-EVs enhances the expression of browning related genes, thus inducing WAT browning. Additionally, BAT-EVs participate in the maintenance of reproductive system function by enhancing the mitochondrial function, thereby delaying ovarian aging. WAT-EVs improve skin wound healing through the effects of miR-92a-3p, which enhances the proliferation, migration, and ECM deposition of keratinocytes and fibroblasts. WAT-EVs also contribute to soft tissue healing in specific diseases, such as BRONJ. BAT, brown adipose tissue; WAT, white adipose tissue; NPM3, nucleophosmin3; ECM, extracellular matrix; BRONJ, bisphosphonate-related osteonecrosis of the jaw. This figure was created by using BioRender (https://biorender.com/).
BAT is the primary site of non-shivering thermogenesis (NST) in mammals and secretes numerous batokines that control energy metabolism and glucose homeostasis (83). Emerging evidence has shown that BAT-EVs also participate in the regulation of systemic homeostasis. Xu et al. demonstrated that cold-induced activation of BAT-EVs regulated systemic glucose metabolism by promoting hepatic gluconeogenesis (84). They identified that in mice model exposed to cold, miR-378a-3p was selectively packaged into BAT-EVs and delivered to the liver, as detected by in vivo visualization and tracking. Within hepatocytes, miR-378a-3p efficiently suppressed p110α, a key subunit of phosphoinositide 3-kinase (PI3K) that governed glucose metabolism (85), leading to increased glucose production. This study illustrated the intricate role of BAT-EVs in mediating inter-organ communication during cold exposure, thus contributing to the maintenance of systemic glucose metabolism stability (84). Apart from regulating whole-body glucose metabolism, BAT-EVs also participate in fat metabolism. Previous study has confirmed that BAT transplantation alleviated obesity and type I diabetes in various laboratories (86, 87). Zhou et al. discovered that BAT-EVs could also reverse obesity. BAT-EVs were enriched in mitochondrial components, which was consistent with BAT being mitochondria-rich (64). The uptake of BAT-EVs promoted the oxygen consumption of the recipient cells. Intravenous injection of BAT-EVs isolated from young healthy mice reduced body weight, lowered blood glucose levels, and restored cardiac function in high-fat-diet (HFD) mice (64). It is further evidenced by another study that BAT-EVs treatment induced weight reduction through activating the WAT browning. The cargo of BAT-EVs, nucleophosmin3 (NPM3), regulated the stability of PRDM16 mRNA, thereby enhancing the expressions of browning related genes, thus inducing WAT browning (88). This contributed to weight loss, increased energy expenditure, alleviated adipose tissue inflammation, and enhanced insulin sensitivity in HFD mice (88). Additionally, BAT-EVs also participate in the maintenance of reproductive system function. Ovarian aging is centrally drived by mitochondrial dysfunction (89). Zhang et al. demonstrated that injecting BAT-EVs that isolated from young female mice to aging mice enhanced mitochondrial function, promoted follicle survival, improved fertility, and extended ovarian lifespan, highlighting the significant role of BAT-EVs in delaying ovarian aging (90). In summary, BAT-EVs contribute to metabolic homeostasis and maintenance of distal organ functions through their cargoes, including miRNAs, proteins, and mitochondrial components.
Collectively, in healthy individuals, EVs released from both brown and white adipose tissue have a promising application prospect in metabolic regulation, organ function maintenance, and wound healing. Therefore, AT-EVs may be developed as a new cell-free therapy agent in the future. However, it should be noted that EVs derived directly from adipose tissue are a mixture of cellular EVs with complex compositions, which may contain components with opposing functions. Thus, their effects can be difficult to predict, and their roles in various diseases need further exploration.
5.2 EVs derived from obese adipose tissue
Above evidence indicates that EVs derived from healthy adipose tissue serve as pivotal mediators for intercellular communication, facilitating cross-talk between adipose tissue and other organs. However, due to the heightened inflammation in obesity, such communication often yields unfavorable outcomes, including promoting the cancer progression, metabolic disorders, disruption of immune homeostasis, and distal organ dysfunction (Figure 4).
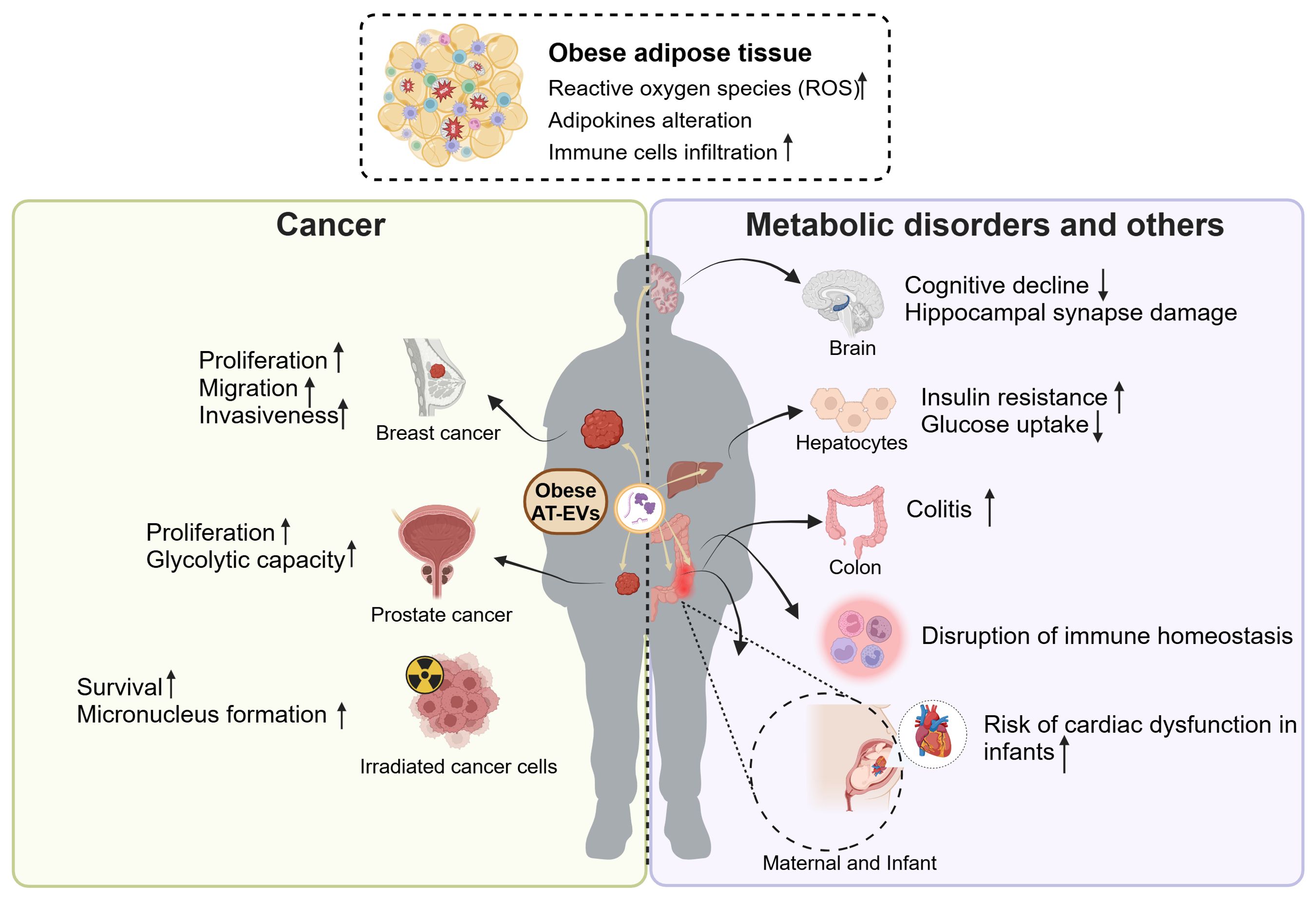
Figure 4. The roles of obese AT-EVs in cancer, metabolic disorders and other diseases. The obese adipose tissue exhibits elevated ROS, with adipokines alteration and abundant immune cells infiltration. The obese AT-EVs promote the progression of several types of cancers, including breast cancer and prostate cancer. Also, obese AT-EVs enhance the formation of micronucleus and promote the irradiated cancer cell survival. Additionally, obese AT-EVs can be transferred to distal organs to induce cognitive decline, insulin resistance, colitis, disruption of immune homeostasis and increasing risk of cardiac dysfunction in infants. ROS, reactive oxygen species; AT-EVs, adipose tissue-derived extracellular vesicles. This figure was created by using BioRender (https://biorender.com/).
5.2.1 Cancer progression
Obesity is increasingly recognized as a risk factor and a poor prognostic indicator for various types of cancer. It exacerbates distant metastasis, thereby amplifying disease severity and mortality rates (91–93). In addition to known mechanisms such as hormones, inflammation, and the immune system (94), emerging evidence suggests that obese AT-EVs are also involved in promoting cancer progression. A study revealed that obese AT-EVs promoted tumor activities and exacerbated the malignancy of breast cancer cells (95). Compared to AT-EVs isolated from lean patients, the AT-EVs from obese patients exhibited higher levels of leptin and MMP9 (closely associated with cell invasion) (96). The treatment of AT-EVs from obese patients enhanced the proliferation, migration, and invasiveness of breast cancer cells. Although this study had limitations, including the absence of in vivo experimental evidence and the unclear specific mechanism, these observations still offered evidence for the crucial role of obese AT-EVs in the tumor microenvironment. Another study demonstrated that obese AT-EVs induced the functional changes of tumors and exacerbated the malignant phenotype of prostate cancer. The EVs from paired omental (OM) and subcutaneous (SC) adipose tissue of patients enhanced the proliferation and glycolytic capacity of immortalized metastatic prostate cancer PC3ML cells by targeting the TWIST1/EMT signaling axis (97). Besides, it was currently found that obese AT-EVs promoted survival of irradiated m5S cells and enhanced micronucleus (MN) formation (98). This illustrated that in radiation therapy, obese AT-EVs promote the survival of irradiated cells with severe DNA damage, potentially leading to poor prognosis. This study partly explained the poor prognosis of obese patients in cancer treatment. Overall, obese AT-EVs contribute to tumor development and progression by increasing the risk of malignancies and leading to poorer prognoses.
5.2.2 Metabolic disorders
Obesity often leads to insulin resistance, characterized by reduced insulin sensitivity in tissues such as the liver and muscles (99). In terms of adipose tissue accumulation site, VAT is more likely to induce insulin resistance compared to SAT (100, 101). Likewise, Deng et al. demonstrated that injecting VAT-EVs from obese mice intravenously induced insulin resistance in recipient mice (102). RBP4 carried by obese VAT-EVs activated macrophage in a paracrine manner and enhanced the production of proinflammatory cytokines IL-6 and TNF-α. The elevated TNF-α in obesity is a vital factor to induce insulin resistance because TNF-α activates IKK, p38 MAPK, JNK, and PKC, which directly target serine residues of insulin receptor substrate (IRS) (103, 104). Another study reported that obese VAT-EVs induced the insulin resistance in hepatocytes. The miR-141-3p in normal AT-EVs contributed to increasing AKT phosphorylation level in recipient cells, thereby promoting insulin signal transduction. While in obese VAT-EVs, the expression of miR-141-3p was decreased, resulting in impaired insulin signaling, and subsequently reducing insulin sensitivity and glucose uptake in vitro (105). In conclusion, obese VAT-EVs serve as a detrimental mediator of intercellular communication, inducing insulin resistance either by recruiting immune cells to release cytokines or by directly secreting cargo molecules that target cells.
5.2.3 Disruption of immune homeostasis
AT-EVs are a crucial source of circulating EVs, involved in immune regulation both inside or outside the adipose tissue microenvironment. Under the stress of obesity, the AT-EVs actively participate in regulating immune metabolic homeostasis or disruption by acting on nearby or distant immune cells. These obese AT-EVs were internalized into immune cells generally by endocytosis, membrane fusion, macropinocytosis, and phagocytosis (106–108). Obese AT-EVs carrying abundant TLR8 were internalized by monocytes and elevated the expression of CD16. This expression alteration subsequently boosted monocyte migration capacity, thereby facilitating leukocyte infiltration in the obese omental tissue (109). These findings may elucidate the roles of obese AT-EVs in immune cells infiltration and activation in obesity. In addition, emerging evidence has illustrated that obese AT-EVs contribute to modulating macrophage polarization. Obese AT-EVs contained higher level of miR-34a, which inhibited adipose-resident macrophages M2 polarization through repressing the expression of Krüppel-like factor 4 (Klf4), thus subsequently suppressing the anti-inflammatory phenotype (110). In addition, miR-155 in obese AT-EVs was upregulated, targeting SOCS1 and modulating the JAK/STAT signaling pathway to mediate M1 macrophage polarization (111). Taken together, the obese AT-EVs contained high heterogeneity of EV contents, which may have complex effects on the differentiation, migration and polarization of immune cells, thus disrupting the immune homeostasis.
5.2.4 Distal organ dysfunction
In addition to affecting nearby organs through paracrine manner, obese AT-EVs can also influence the function of distal organs through endocrine manner. Obese AT-EVs can be remotely transported to the brain and promote insulin resistance related cognitive impairment. Wang et al. illustrated that injection of AT-EVs derived from HFD mice into recipient mice resulted in significant cognitive decline and hippocampal synapse reduction (112). The mechanism is that miR-9-3p in HFD-AT-EVs targeted BDNF (brain-derived neurotrophic factor, which is crucial for typical synaptic functions) in neurons, leading to synaptic damage. These observations may explain the declined brain functions in obesity. Wei et al. found that the VAT-EVs from HFD mice transferred to gastrointestinal system to aggravate the colitis severity in dextran sulfate sodium (DSS) model. The VAT-EVs loaded with miR-155 and other pro-inflammatory miRNAs induced M1 macrophage polarization, subsequently promoting DSS-induced colitis (113). This study showed that obesity changed the miRNAs in the AT-EVs, and transferred the EV phenotype from anti-inflammatory to pro-inflammatory. In addition to influencing different organs within independent individuals, VAT-EVs are also involved in the maternal-fetal communication, regulating placental development and fetal heart function (114). The miRNA profile in VAT-EVs from maternal obesity has been reported to be altered, notably with reduced levels of anti-inflammatory miR-19b. The injection of VAT-EVs from obesity promoted proinflammatory effects, leading to placental inflammation and fetal cardiac development defects (114).
In conclusion, adipose tissue has recently been recognized as an important endocrine organ in the body via active secretion of adipokines. AT-EVs act as a new type of adipokines, influencing the function and homeostasis of other organs. The miRNA or protein profile of AT-EVs were markedly altered in a high-fat diet and subsequent obesity, resulting in a transition from an anti-inflammatory to a pro-inflammatory phenotype in EVs. This phenotypic shift contributes to inflammatory infiltration and a certain level of function impairment. Additionally, targeting miRNAs altered in obese AT-EVs using specific inhibitors can effectively reverse the proinflammatory phenotype, offering a potentially promising therapeutic strategy in the future.
6 Potential clinical implication of adipose tissue-derived EVs
Currently, the most widely studied EVs for clinical applications in adipose tissue are ADSC-derived EVs, which have shown promising therapeutic effects. Recent research has revealed that ADSC-EVs primarily contribute to reducing inflammation, suppressing tumor proliferation and invasion (115, 116), inhibiting fibrosis progression (117), and promoting angiogenesis (118). At present, the isolation strategy for ADSC-EVs has been well-established, and the process can be summarized as follows: the extraction of adipose tissue from the body, separation of ADSCs, proliferation in vitro and purify EVs from the supernatant (119). However, this process is rather cumbersome and time-consuming. Currently, EVs isolated directly from adipose tissue represent a clinically feasible source of adipose tissue-derived EVs, offering a more cost-effective method with higher yields and greater efficiency compared to ADSC-EVs. Especially, Zhang et al. found that the therapeutic effects of AT-EVs surpassed those of ADSC-EVs due to the higher levels of functional miRNAs (120). As we mentioned above, AT-EVs from obese individuals may contain pro-inflammatory factors that promote disease progression. Therefore, only AT-EVs from healthy individuals are suitable as clinically effective therapeutic agents.
Clinically, lipoaspirates obtained during liposuction represent the most accessible and abundant source of AT-EVs in the body. The isolation of lipoaspirate-EVs has been established. First, the lipoaspirate samples through liposuction from non-obese patients are mechanically dispersed by the non-enzymatic micro-fragmented process. After centrifugation to remove large debris, the EVs in the supernatant is isolated using method TFF. By using this isolation method, a study has compared the donor-matched ADSC-EVs and lipoaspirate-EVs in the EV characterization, yield, components, and function (78) (Figure 5). Both ADSC-EVs and lipoaspirate-EVs had the normal EV structure and size distribution. Notably, lipoaspirate-EVs samples contained lipoprotein-like spherical structures, which was confirmed by size-exclusion chromatography (SCE) that approximately 53% of the nanoparticles was lipoproteins (78). The EV yield from lipoaspirate was approximately 30-fold higher than that of cultured ADSCs (78). In terms of EV composition, the protein level was much higher in ADSC-EVs than lipoaspirate-EVs, however, the latter contained more miRNAs and glycerolipids (triglycerides in particular) (78). The ADSC-EVs and lipoaspirate-EVs exhibited similar protective and anti-inflammatory effects in macrophages. In conclusion, lipoaspirate-EVs are high-yielding, readily available, and possess fundamental EV characteristics, making them promising for large-scale clinical production and application.
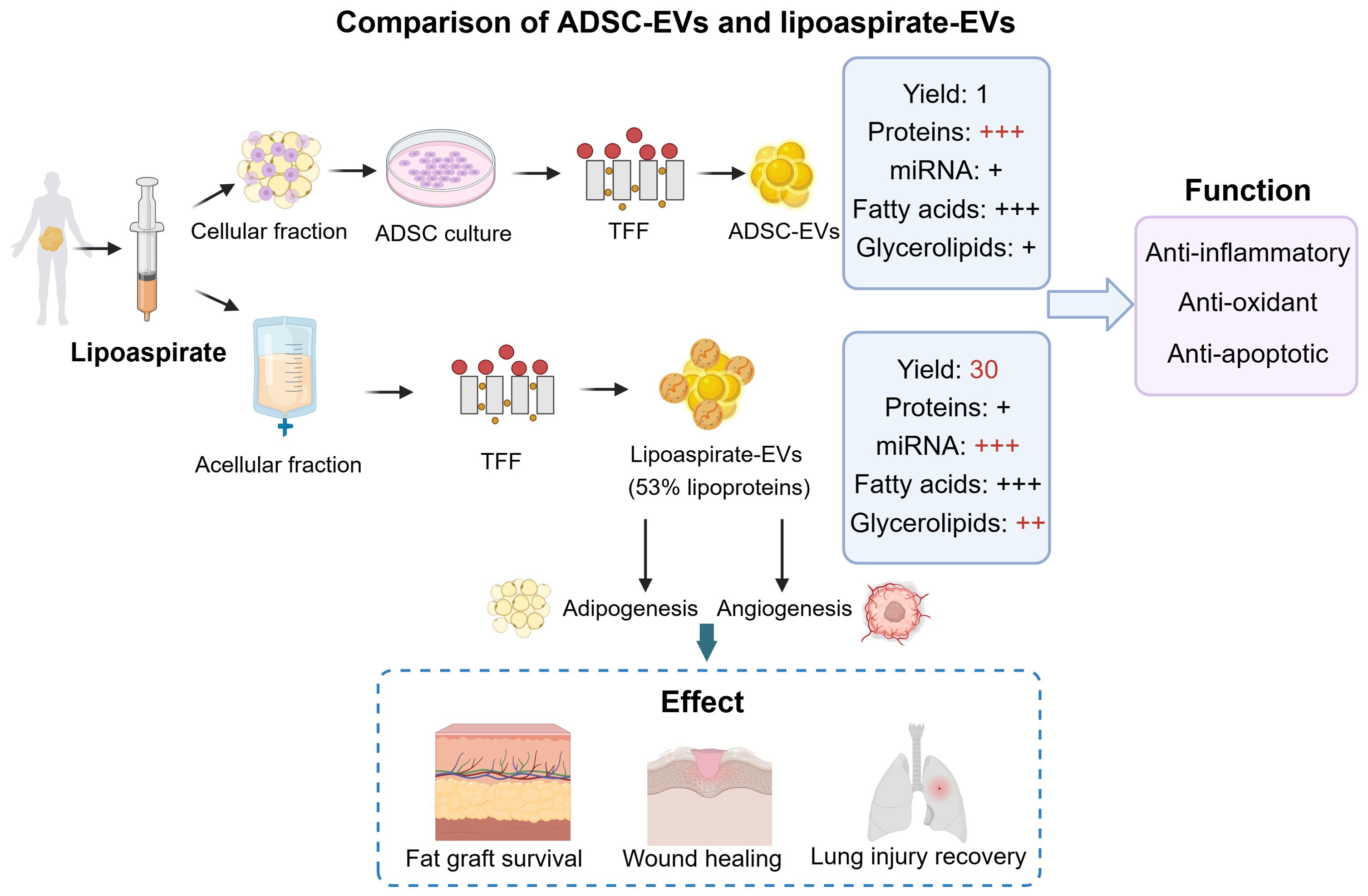
Figure 5. The comparison of ADSC-EVs and lipoaspirate-EVs. The ADSC-EVs were obtained from cell culture and lipoaspirate-EVs were obtained directly from acellular fraction. They vary in the yield, abundance of protein, miRNAs, fatty acids, and glycerolipids. ADSC-EVs and lipoaspirate-EVs both have the function of anti-inflammatory, anti-oxidant, and anti-apoptotic. Additionally, lipoaspirate-EVs have the therapeutic potential in promoting fat graft survival, wound healing, and lung injury recovery. ADSCs, adipose-derived stem cells; TFF, tangential flow filtration. This figure was created by using BioRender (https://biorender.com/).
Growing evidence suggests that lipoaspirate-EVs have significant effects on tissue recovery. For example, it was reported that lipoaspirate-EVs effectively promoted the proliferation and migratory ability of endothelial cells, thus promoting graft survival in a fat grafted mouse model (121). In the same year, another team found that lipoaspirate-EVs promoted angiogenesis of vascular endothelial cells and adipogenic differentiation of ADSCs in vitro and in vivo, resulting in improving the fat retention (122). These studies suggest that lipoaspirate-EVs have great potential as a new autologous component to aid in fat grafting. Furthermore, Wu et al. have developed a novel method of cell-free and EV-riched products (lipoaspirate fluid derived factors and extracellular vesicles, LF-FVs) extracted from human lipoaspirate (123). In vitro, LF-FVs promoted the proliferation and migration of fibroblasts. In vivo, LF-FVs significantly accelerated burn wound healing in rats, improved skin appendage regeneration, and inhibited scar formation. This study indicates that LF-FVs have potential applications in clinical wound healing. Additionally, Yu et al. found that lipoaspirate-EVs was a new type of bioactive substance to protect the microvascular barrier in mice (124). By intravenous injection, lipoaspirate-EVs protected the pulmonary microvascular endothelial cells (PMVEC) barrier integrity, thus reducing lung injury. Taken together, lipoaspirate-EVs, as a novel biomaterial, can be considered as a viable alternative to ADSC-EVs for clinical applications.
7 Conclusions and future perspectives
Emerging evidence from both in vitro and in vivo research has identified AT-EVs as crucial mediators of intercellular communication within adipose tissue through paracrine signaling, and they also participate in inter-organ crosstalk via endocrine signaling. These processes contribute to the maintenance of metabolism, immune response, and tissue repair. However, excessive accumulation of adipose tissue induces chronic inflammation, and the EVs released from this environment lead to adverse effects, including tumor progression, insulin resistance, and dysfunction of other organs. Numerous studies have demonstrated the promising clinical translational potential of AT-EVs. Lipoaspirates extracted from clinic serve as an excellent source of AT-EVs due to its high-yield and efficient isolation. It facilitates both adipogenesis and angiogenesis, making it applicable in the treatment of numerous diseases. To advance the clinical applications of AT-EVs, many challenges remain to be thoroughly explored. First, the sorting mechanism of AT-EV cargoes, particularly under physiological and pathological conditions, remains unclear. A comprehensive understanding of the sorting mechanisms aids in directing the loading of functional molecules into EVs. Second, in the clinical application of lipoaspirate-EVs, it is crucial to evaluate the impact of additional contaminants, such as lipoproteins, on treatment outcomes. Developing a more refined EV isolation method that maximizes purity without affecting yield holds promise for enhancing therapeutic efficacy. Furthermore, investigating appropriate storage methods that preserve the activity of AT-EVs, particularly their mitochondrial components, enhances the potential for future large-scale production applications. Such research has already been initiated in the field. Moreover, exploring the potential of lipoaspirate-EVs as carriers for functional miRNA delivery and to develop strategies for enhancing EV specificity is important. This makes lipoaspirate-EVs a valuable tool in precision medicine and targeted therapy. In conclusion, further advancements in AT-EVs research will provide a more comprehensive understanding of its functions, potentially contributing to the treatment of various diseases in the future.
Author contributions
YH: Writing – original draft, Writing – review & editing. SY:Writing – review & editing, Supervision, Validation. BL: Conceptualization, Writing – original draft, Writing – review & editing, Funding acquisition.
Funding
The author(s) declare financial support was received for the research, authorship, and/or publication of this article. This work was supported by grant from the China Postdoctoral Science Foundation (grant no. 2022M723052 to BL)
Conflict of interest
The authors declare that the research was conducted in the absence of any commercial or financial relationships that could be construed as a potential conflict of interest.
Publisher’s note
All claims expressed in this article are solely those of the authors and do not necessarily represent those of their affiliated organizations, or those of the publisher, the editors and the reviewers. Any product that may be evaluated in this article, or claim that may be made by its manufacturer, is not guaranteed or endorsed by the publisher.
References
1. Lee MW, Lee M, Oh KJ. Adipose tissue-derived signatures for obesity and type 2 diabetes: adipokines, batokines and microRNAs. J Clin Med. (2019) 8:854. doi: 10.3390/jcm8060854
2. Liang W, Ye DD. The potential of adipokines as biomarkers and therapeutic agents for vascular complications in type 2 diabetes mellitus. Cytokine Growth Factor Rev. (2019) 48:32–9. doi: 10.1016/j.cytogfr.2019.06.002
3. Giralt M, Cereijo R, Villarroya F. Adipokines and the endocrine role of adipose tissues. Handb Exp Pharmacol. (2016) 233:265–82. doi: 10.1007/164_2015_6
4. Jiang X, Lei R. Extracellular lncRNAs secreted and absorbed by cardiomyocytes. J Cell Biochem. (2023) 124:785–96. doi: 10.1002/jcb.30425
5. Cheng L, Hill AF. Therapeutically harnessing extracellular vesicles. Nat Rev Drug Discovery. (2022) 21:379–99. doi: 10.1038/s41573-022-00410-w
6. Théry C, Witwer KW, Aikawa E, Alcaraz MJ, Anderson JD, Andriantsitohaina R, et al. Minimal information for studies of extracellular vesicles 2018 (MISEV2018): a position statement of the International Society for Extracellular Vesicles and update of the MISEV2014 guidelines. J Extracell Vesicles. (2018) 7:1535750. doi: 10.1080/20013078.2018.1535750
7. Koifman N, Biran I, Aharon A, Brenner B, Talmon Y. A direct-imaging cryo-EM study of shedding extracellular vesicles from leukemic monocytes. J Struct Biol. (2017) 198:177–85. doi: 10.1016/j.jsb.2017.02.004
8. Lane JD, Allan VJ, Woodman PG. Active relocation of chromatin and endoplasmic reticulum into blebs in late apoptotic cells. J Cell Sci. (2005) 118:4059–71. doi: 10.1242/jcs.02529
9. Rackov G, Garcia-Romero N, Esteban-Rubio S, Carrión-Navarro J, Belda-Iniesta C, Ayuso-Sacido A. Vesicle-mediated control of cell function: the role of extracellular matrix and microenvironment. Front Physiol. (2018) 9:651. doi: 10.3389/fphys.2018.00651
10. Mathiesen A, Hamilton T, Carter N, Brown M, McPheat W, Dobrian A. Endothelial extracellular vesicles: from keepers of health to messengers of disease. Int J Mol Sci. (2021) 22:4640. doi: 10.3390/ijms22094640
11. Gharbi T, Zhang Z, Yang GY. The function of astrocyte mediated extracellular vesicles in central nervous system diseases. Front Cell Dev Biol. (2020) 8:568889. doi: 10.3389/fcell.2020.568889
12. Ramachandra L, Qu Y, Wang Y, Lewis CJ, Cobb BA, Takatsu K, et al. Mycobacterium tuberculosis synergizes with ATP to induce release of microvesicles and exosomes containing major histocompatibility complex class II molecules capable of antigen presentation. Infect Immun. (2010) 78:5116–25. doi: 10.1128/iai.01089-09
13. Singh PP, Li L, Schorey JS. Exosomal RNA from mycobacterium tuberculosis-infected cells is functional in recipient macrophages. Traffic. (2015) 16:555–71. doi: 10.1111/tra.12278
14. Lai RC, Arslan F, Lee MM, Sze NSK, Choo A, Chen TS, et al. Exosome secreted by MSC reduces myocardial ischemia/reperfusion injury. Stem Cell Res. (2010) 4:214–22. doi: 10.1016/j.scr.2009.12.003
15. Xin H, Li Y, Cui Y, Yang JJ, Zhang ZG, Chopp M. Systemic administration of exosomes released from mesenchymal stromal cells promote functional recovery and neurovascular plasticity after stroke in rats. J Cereb Blood Flow metabolism: Off J Int Soc Cereb Blood Flow Metab. (2013) 33:1711–5. doi: 10.1038/jcbfm.2013.152
16. Lee JK, Park SR, Jung BK, Jeon YK, Lee YS, Kim MK, et al. Exosomes derived from mesenchymal stem cells suppress angiogenesis by down-regulating VEGF expression in breast cancer cells. PloS One. (2013) 8:e84256. doi: 10.1371/journal.pone.0084256
17. Cheng Y, Schorey JS. Exosomes carrying mycobacterial antigens can protect mice against Mycobacterium tuberculosis infection. Eur J Immunol. (2013) 43:3279–90. doi: 10.1002/eji.201343727
18. Torreggiani E, Perut F, Roncuzzi L, Zini N, Baglìo SR, Baldini N. Exosomes: novel effectors of human platelet lysate activity. Eur Cell Mater. (2014) 28:137–51. doi: 10.22203/ecm.v028a11
19. Guo SC, Tao SC, Yin WJ, Qi X, Yuan T, Zhang CQ. Exosomes derived from platelet-rich plasma promote the re-epithelization of chronic cutaneous wounds via activation of YAP in a diabetic rat model. Theranostics. (2017) 7:81–96. doi: 10.7150/thno.16803
20. Sugihara H, Yonemitsu N, Miyabara S, Yun K. Primary cultures of unilocular fat cells: characteristics of growth in vitro and changes in differentiation properties. Differentiation. (1986) 31:42–9. doi: 10.1111/j.1432-0436.1986.tb00381.x
21. Coppack SW, Jensen MD, Miles JM. In vivo regulation of lipolysis in humans. J Lipid Res. (1994) 35:177–93. doi: 10.1016/S0022-2275(20)41207-6
22. Zechner R, Strauss JG, Haemmerle G, Lass A, Zimmermann R. Lipolysis: pathway under construction. Curr Opin Lipidol. (2005) 16:333–40. doi: 10.1097/01.mol.0000169354.20395.1c
23. Spalding KL, Bernard S, Näslund E, Salehpour M, Possnert G, Appelsved L, et al. Impact of fat mass and distribution on lipid turnover in human adipose tissue. Nat Commun. (2017) 8:15253. doi: 10.1038/ncomms15253
24. Bjørndal B, Burri L, Staalesen V, Skorve J, Berge RK. Different adipose depots: their role in the development of metabolic syndrome and mitochondrial response to hypolipidemic agents. J Obes. (2011) 2011:490650. doi: 10.1155/2011/490650
25. Hamdy O, Porramatikul S, Al-Ozairi E. Metabolic obesity: the paradox between visceral and subcutaneous fat. Curr Diabetes Rev. (2006) 2:367–73. doi: 10.2174/1573399810602040367
26. White JD, Dewal RS, Stanford KI. The beneficial effects of brown adipose tissue transplantation. Mol Aspects Med. (2019) 68:74–81. doi: 10.1016/j.mam.2019.06.004
27. Frontini A, Cinti S. Distribution and development of brown adipocytes in the murine and human adipose organ. Cell Metab. (2010) 11:253–6. doi: 10.1016/j.cmet.2010.03.004
28. Soler-Vázquez MC, Mera P, Zagmutt S, Serra D, Herrero L. New approaches targeting brown adipose tissue transplantation as a therapy in obesity. Biochem Pharmacol. (2018) 155:346–55. doi: 10.1016/j.bcp.2018.07.022
29. Cypess AM, White AP, Vernochet C, Schulz TJ, Xue R, Sass CA, et al. Anatomical localization, gene expression profiling and functional characterization of adult human neck brown fat. Nat Med. (2013) 19:635–9. doi: 10.1038/nm.3112
30. McNeill BT, Suchacki KJ, Stimson RH. MECHANISMS IN ENDOCRINOLOGY: Human brown adipose tissue as a therapeutic target: warming up or cooling down? Eur J Endocrinol. (2021) 184:R243–r59. doi: 10.1530/eje-20-1439
31. Ahmad B, Vohra MS, Saleemi MA, Serpell CJ, Fong IL, Wong EH. Brown/Beige adipose tissues and the emerging role of their secretory factors in improving metabolic health: The batokines. Biochimie. (2021) 184:26–39. doi: 10.1016/j.biochi.2021.01.015
32. Villarroya J, Cereijo R, Gavaldà-Navarro A, Peyrou M, Giralt M, Villarroya F. New insights into the secretory functions of brown adipose tissue. J Endocrinol. (2019) 243:R19–r27. doi: 10.1530/joe-19-0295
33. Rodríguez A, Becerril S, Ezquerro S, Méndez-Giménez L, Frühbeck G. Crosstalk between adipokines and myokines in fat browning. Acta Physiol (Oxf). (2017) 219:362–81. doi: 10.1111/apha.12686
34. AlZaim I, Hammoud SH, Al-Koussa H, Ghazi A, Eid AH, El-Yazbi AF. Adipose tissue immunomodulation: A novel therapeutic approach in cardiovascular and metabolic diseases. Front Cardiovasc Med. (2020) 7:602088. doi: 10.3389/fcvm.2020.602088
35. Chait A, den Hartigh LJ. Adipose tissue distribution, inflammation and its metabolic consequences, including diabetes and cardiovascular disease. Front Cardiovasc Med. (2020) 7:22. doi: 10.3389/fcvm.2020.00022
36. Wilson-Fritch L, Nicoloro S, Chouinard M, Lazar MA, Chui PC, Leszyk J, et al. Mitochondrial remodeling in adipose tissue associated with obesity and treatment with rosiglitazone. J Clin Invest. (2004) 114:1281–9. doi: 10.1172/jci21752
37. Schöttl T, Kappler L, Fromme T, Klingenspor M. Limited OXPHOS capacity in white adipocytes is a hallmark of obesity in laboratory mice irrespective of the glucose tolerance status. Mol Metab. (2015) 4:631–42. doi: 10.1016/j.molmet.2015.07.001
38. Kusminski CM, Scherer PE. Mitochondrial dysfunction in white adipose tissue. Trends Endocrinol Metab. (2012) 23:435–43. doi: 10.1016/j.tem.2012.06.004
39. Cummins TD, Holden CR, Sansbury BE, Gibb AA, Shah J, Zafar N, et al. Metabolic remodeling of white adipose tissue in obesity. Am J Physiol Endocrinol Metab. (2014) 307:E262–77. doi: 10.1152/ajpendo.00271.2013
40. Goossens GH, Blaak EE. Adipose tissue dysfunction and impaired metabolic health in human obesity: a matter of oxygen? Front Endocrinol (Lausanne). (2015) 6:55. doi: 10.3389/fendo.2015.00055
41. Newell-Morris LL, Treder RP, Shuman WP, Fujimoto WY. Fatness, fat distribution, and glucose tolerance in second-generation Japanese-American (Nisei) men. Am J Clin Nutr. (1989) 50:9–18. doi: 10.1093/ajcn/50.1.9
42. Bastard JP, Jardel C, Bruckert E, Blondy P, Capeau J, Laville M, et al. Elevated levels of interleukin 6 are reduced in serum and subcutaneous adipose tissue of obese women after weight loss. J Clin Endocrinol Metab. (2000) 85:3338–42. doi: 10.1210/jcem.85.9.6839
43. Pickup JC, Chusney GD, Thomas SM, Burt D. Plasma interleukin-6, tumour necrosis factor alpha and blood cytokine production in type 2 diabetes. Life Sci. (2000) 67:291–300. doi: 10.1016/s0024-3205(00)00622-6
44. Weisberg SP, McCann D, Desai M, Rosenbaum M, Leibel RL, Ferrante AW Jr. Obesity is associated with macrophage accumulation in adipose tissue. J Clin Invest. (2003) 112:1796–808. doi: 10.1172/jci19246
45. Wang T, Sharma AK, Wolfrum C. Novel insights into adipose tissue heterogeneity. Rev Endocr Metab Disord. (2022) 23:5–12. doi: 10.1007/s11154-021-09703-8
46. Eguchi A, Lazic M, Armando AM, Phillips SA, Katebian R, Maraka S, et al. Circulating adipocyte-derived extracellular vesicles are novel markers of metabolic stress. J Mol Med (Berl). (2016) 94:1241–53. doi: 10.1007/s00109-016-1446-8
47. Hubal MJ, Nadler EP, Ferrante SC, Barberio MD, Suh JH, Wang J, et al. Circulating adipocyte-derived exosomal MicroRNAs associated with decreased insulin resistance after gastric bypass. Obes (Silver Spring). (2017) 25:102–10. doi: 10.1002/oby.21709
48. Kranendonk ME, Visseren FL, van Balkom BW, Nolte-’t Hoen EN, van Herwaarden JA, de Jager W, et al. Human adipocyte extracellular vesicles in reciprocal signaling between adipocytes and macrophages. Obes (Silver Spring). (2014) 22:1296–308. doi: 10.1002/oby.20679
49. Bassi ÊJ, Moraes-Vieira PM, Moreira-Sá CS, Almeida DC, Vieira LM, Cunha CS, et al. Immune regulatory properties of allogeneic adipose-derived mesenchymal stem cells in the treatment of experimental autoimmune diabetes. Diabetes. (2012) 61:2534–45. doi: 10.2337/db11-0844
50. González MA, Gonzalez-Rey E, Rico L, Büscher D, Delgado M. Treatment of experimental arthritis by inducing immune tolerance with human adipose-derived mesenchymal stem cells. Arthritis Rheum. (2009) 60:1006–19. doi: 10.1002/art.24405
51. González MA, Gonzalez-Rey E, Rico L, Büscher D, Delgado M. Adipose-derived mesenchymal stem cells alleviate experimental colitis by inhibiting inflammatory and autoimmune responses. Gastroenterology. (2009) 136:978–89. doi: 10.1053/j.gastro.2008.11.041
52. Ying W, Riopel M, Bandyopadhyay G, Dong Y, Birmingham A, Seo JB, et al. Adipose tissue macrophage-derived exosomal miRNAs can modulate in vivo and in vitro insulin sensitivity. Cell. (2017) 171:372–84.e12. doi: 10.1016/j.cell.2017.08.035
53. Crewe C, Joffin N, Rutkowski JM, Kim M, Zhang F, Towler DA, et al. An endothelial-to-adipocyte extracellular vesicle axis governed by metabolic state. Cell. (2018) 175:695–708.e13. doi: 10.1016/j.cell.2018.09.005
54. Mincheva-Nilsson L, Baranov V, Nagaeva O, Dehlin E. Isolation and characterization of exosomes from cultures of tissue explants and cell lines. Curr Protoc Immunol. (2016) 115:14.42.1–21. doi: 10.1002/cpim.17
55. Liu B, Jin Y, Yang J, Han Y, Shan H, Qiu M, et al. Extracellular vesicles from lung tissue drive bone marrow neutrophil recruitment in inflammation. J Extracell Vesicles. (2022) 11:e12223. doi: 10.1002/jev2.12223
56. Zhang Z, Yu K, You Y, Jiang P, Wu Z, DeTure MA, et al. Comprehensive characterization of human brain-derived extracellular vesicles using multiple isolation methods: Implications for diagnostic and therapeutic applications. J Extracell Vesicles. (2023) 12:e12358. doi: 10.1002/jev2.12358
57. Vella LJ, Scicluna BJ, Cheng L, Bawden EG, Masters CL, Ang CS, et al. A rigorous method to enrich for exosomes from brain tissue. J Extracell Vesicles. (2017) 6:1348885. doi: 10.1080/20013078.2017.1348885
58. Wang X, Huang W, Liu G, Cai W, Millard RW, Wang Y, et al. Cardiomyocytes mediate anti-angiogenesis in type 2 diabetic rats through the exosomal transfer of miR-320 into endothelial cells. J Mol Cell Cardiol. (2014) 74:139–50. doi: 10.1016/j.yjmcc.2014.05.001
59. Lee J, Kim SR, Lee C, Jun YI, Bae S, Yoon YJ, et al. Extracellular vesicles from in vivo liver tissue accelerate recovery of liver necrosis induced by carbon tetrachloride. J Extracell Vesicles. (2021) 10:e12133. doi: 10.1002/jev2.12133
60. Matejovič A, Wakao S, Kitada M, Kushida Y, Dezawa M. Comparison of separation methods for tissue-derived extracellular vesicles in the liver, heart, and skeletal muscle. FEBS Open Bio. (2021) 11:482–93. doi: 10.1002/2211-5463.13075
61. Jang SC, Crescitelli R, Cvjetkovic A, Belgrano V, Olofsson Bagge R, Sundfeldt K, et al. Mitochondrial protein enriched extracellular vesicles discovered in human melanoma tissues can be detected in patient plasma. J Extracell Vesicles. (2019) 8:1635420. doi: 10.1080/20013078.2019.1635420
62. Crescitelli R, Lässer C, Lötvall J. Isolation and characterization of extracellular vesicle subpopulations from tissues. Nat Protoc. (2021) 16:1548–80. doi: 10.1038/s41596-020-00466-1
63. Casadei L, Calore F, Braggio DA, Zewdu A, Deshmukh AA, Fadda P, et al. MDM2 derived from dedifferentiated liposarcoma extracellular vesicles induces MMP2 production from preadipocytes. Cancer Res. (2019) 79:4911–22. doi: 10.1158/0008-5472.Can-19-0203
64. Zhou X, Li Z, Qi M, Zhao P, Duan Y, Yang G, et al. Brown adipose tissue-derived exosomes mitigate the metabolic syndrome in high fat diet mice. Theranostics. (2020) 10:8197–210. doi: 10.7150/thno.43968
65. Zhang C, Yang X, Jiang T, Yan C, Xu X, Chen Z. Tissue-derived extracellular vesicles: Isolation, purification, and multiple roles in normal and tumor tissues. Life Sci. (2023) 321:121624. doi: 10.1016/j.lfs.2023.121624
66. Perez-Gonzalez R, Gauthier SA, Kumar A, Levy E. The exosome secretory pathway transports amyloid precursor protein carboxyl-terminal fragments from the cell into the brain extracellular space. J Biol Chem. (2012) 287:43108–15. doi: 10.1074/jbc.M112.404467
67. Yelamanchili SV, Lamberty BG, Rennard DA, Morsey BM, Hochfelder CG, Meays BM, et al. MiR-21 in extracellular vesicles leads to neurotoxicity via TLR7 signaling in SIV neurological disease. PloS Pathog. (2015) 11:e1005032. doi: 10.1371/journal.ppat.1005032
68. Banigan MG, Kao PF, Kozubek JA, Winslow AR, Medina J, Costa J, et al. Differential expression of exosomal microRNAs in prefrontal cortices of schizophrenia and bipolar disorder patients. PloS One. (2013) 8:e48814. doi: 10.1371/journal.pone.0048814
69. Huang Y, Cheng L, Turchinovich A, Mahairaki V, Troncoso JC, Pletniková O, et al. Influence of species and processing parameters on recovery and content of brain tissue-derived extracellular vesicles. J Extracell Vesicles. (2020) 9:1785746. doi: 10.1080/20013078.2020.1785746
70. Jeppesen DK, Fenix AM, Franklin JL, Higginbotham JN, Zhang Q, Zimmerman LJ, et al. Reassessment of exosome composition. Cell. (2019) 177:428–45.e18. doi: 10.1016/j.cell.2019.02.029
71. Polanco JC, Scicluna BJ, Hill AF, Götz J. Extracellular vesicles isolated from the brains of rTg4510 mice seed tau protein aggregation in a threshold-dependent manner. J Biol Chem. (2016) 291:12445–66. doi: 10.1074/jbc.M115.709485
72. Muraoka S, DeLeo AM, Sethi MK, Yukawa-Takamatsu K, Yang Z, Ko J, et al. Proteomic and biological profiling of extracellular vesicles from Alzheimer’s disease human brain tissues. Alzheimers Dement. (2020) 16:896–907. doi: 10.1002/alz.12089
73. Möller K, Stahl T, Boltze J, Wagner DC. Isolation of inflammatory cells from rat brain tissue after stroke. Exp Transl Stroke Med. (2012) 4:20. doi: 10.1186/2040-7378-4-20
74. Gardiner C, Di Vizio D, Sahoo S, Thery C, Witwer KW, Wauben M, et al. Techniques used for the isolation and characterization of extracellular vesicles: results of a worldwide survey. J Extracellular Vesicles. (2016) 5:32945. doi: 10.3402/jev.v5.32945
75. Veerman RE, Teeuwen L, Czarnewski P, Güclüler Akpinar G, Sandberg A, Cao X, et al. Molecular evaluation of five different isolation methods for extracellular vesicles reveals different clinical applicability and subcellular origin. J Extracell Vesicles. (2021) 10:e12128. doi: 10.1002/jev2.12128
76. Busatto S, Vilanilam G, Ticer T, Lin WL, Dickson DW, Shapiro S, et al. Tangential flow filtration for highly efficient concentration of extracellular vesicles from large volumes of fluid. Cells. (2018) 7:273. doi: 10.3390/cells7120273
77. Witwer KW, Buzas EI, Bemis LT, Bora A, Lasser C, Lotvall J, et al. Standardization of sample collection, isolation and analysis methods in extracellular vesicle research. J Extracellular Vesicles. (2013) 2:20360. doi: 10.3402/jev.v2i0.20360
78. Tian M, Ticer T, Wang Q, Walker S, Pham A, Suh A, et al. Adipose-derived biogenic nanoparticles for suppression of inflammation. Small. (2020) 16:e1904064. doi: 10.1002/smll.201904064
79. Casadei L, Sarchet P, de Faria FCC, Calore F, Nigita G, Tahara S, et al. In situ hybridization to detect DNA amplification in extracellular vesicles. J Extracell Vesicles. (2022) 11:e12251. doi: 10.1002/jev2.12251
80. Casadei L, Choudhury A, Sarchet P, Mohana Sundaram P, Lopez G, Braggio D, et al. Cross-flow microfiltration for isolation, selective capture and release of liposarcoma extracellular vesicles. J Extracell Vesicles. (2021) 10:e12062. doi: 10.1002/jev2.12062
81. Shao Z, Xu J, Wang X, Zhou Y, Wang Y, Li Y, et al. Exosomes derived from adipose tissues accelerate fibroblasts and keratinocytes proliferation and cutaneous wound healing via miR-92a/Hippo-YAP axis. J Physiol Biochem. (2024) 80:189–204. doi: 10.1007/s13105-023-00996-8
82. Huang J, Wang L, Tian W. Small extracellular vesicles derived from adipose tissue prevent bisphosphonate-related osteonecrosis of the jaw by promoting angiogenesis. Int J Nanomedicine. (2021) 16:3161–72. doi: 10.2147/ijn.S305361
83. Sidossis L, Kajimura S. Brown and beige fat in humans: thermogenic adipocytes that control energy and glucose homeostasis. J Clin Invest. (2015) 125:478–86. doi: 10.1172/jci78362
84. Xu J, Cui L, Wang J, Zheng S, Zhang H, Ke S, et al. Cold-activated brown fat-derived extracellular vesicle-miR-378a-3p stimulates hepatic gluconeogenesis in male mice. Nat Commun. (2023) 14:5480. doi: 10.1038/s41467-023-41160-6
85. Liu W, Cao H, Ye C, Chang C, Lu M, Jing Y, et al. Hepatic miR-378 targets p110α and controls glucose and lipid homeostasis by modulating hepatic insulin signalling. Nat Commun. (2014) 5:5684. doi: 10.1038/ncomms6684
86. Liu X, Wang S, You Y, Meng M, Zheng Z, Dong M, et al. Brown adipose tissue transplantation reverses obesity in ob/ob mice. Endocrinology. (2015) 156:2461–9. doi: 10.1210/en.2014-1598
87. Gunawardana SC, Piston DW. Reversal of type 1 diabetes in mice by brown adipose tissue transplant. Diabetes. (2012) 61:674–82. doi: 10.2337/db11-0510
88. Zhang Y, Yu M, Dong J, Wu Y, Tian W. Nucleophosmin3 carried by small extracellular vesicles contribute to white adipose tissue browning. J Nanobiotechnology. (2022) 20:165. doi: 10.1186/s12951-022-01381-1
89. Kasapoğlu I, Seli E. Mitochondrial dysfunction and ovarian aging. Endocrinology. (2020) 161:bqaa001. doi: 10.1210/endocr/bqaa001
90. Zhang H, Fang Y, Gao Y, Zeng X, Lu Z, Liu L, et al. Brown adipose tissue-derived exosomes delay fertility decline in aging mice. Front Endocrinol (Lausanne). (2023) 14:1180104. doi: 10.3389/fendo.2023.1180104
91. Vainio H, Kaaks R, Bianchini F. Weight control and physical activity in cancer prevention: international evaluation of the evidence. Eur J Cancer Prev. (2002) 11 Suppl 2:S94–100.
92. McDowell SAC, Luo RBE, Arabzadeh A, Doré S, Bennett NC, Breton V, et al. Neutrophil oxidative stress mediates obesity-associated vascular dysfunction and metastatic transmigration. Nat Cancer. (2021) 2:545–62. doi: 10.1038/s43018-021-00194-9
93. Annett S, Moore G, Robson T. Obesity and cancer metastasis: molecular and translational perspectives. Cancers. (2020) 12:3798. doi: 10.3390/cancers12123798
94. Das M, Webster NJG. Obesity, cancer risk, and time-restricted eating. Cancer Metastasis Rev. (2022) 41:697–717. doi: 10.1007/s10555-022-10061-3
95. Ramos-Andrade I, Moraes J, Brandão-Costa RM, Vargas da Silva S, de Souza A, da Silva C, et al. Obese adipose tissue extracellular vesicles raise breast cancer cell Malignancy. Endocr Relat Cancer. (2020) 27:571–82. doi: 10.1530/erc-19-0507
96. Shimoda M. Extracellular vesicle-associated MMPs: A modulator of the tissue microenvironment. Adv Clin Chem. (2019) 88:35–66. doi: 10.1016/bs.acc.2018.10.006
97. Mathiesen A, Haynes B, Huyck R, Brown M, Dobrian A. Adipose tissue-derived extracellular vesicles contribute to phenotypic plasticity of prostate cancer cells. Int J Mol Sci. (2023) 24:1229. doi: 10.3390/ijms24021229
98. Ariyoshi K, Fujishima Y, Goh VST, Nakata A, Kasai K, Yoshida MA, et al. Exosome-like vesicles released from ob/ob mouse adipose tissue enhance cell survival of cells with radiation-induced genomic instability. J Radiat Res. (2023) 64:352–57. doi: 10.1093/jrr/rrac102
99. Polsky S, Ellis SL. Obesity, insulin resistance, and type 1 diabetes mellitus. Curr Opin Endocrinol Diabetes Obes. (2015) 22:277–82. doi: 10.1097/med.0000000000000170
100. Fox CS, Massaro JM, Hoffmann U, Pou KM, Maurovich-Horvat P, Liu CY, et al. Abdominal visceral and subcutaneous adipose tissue compartments: association with metabolic risk factors in the Framingham Heart Study. Circulation. (2007) 116:39–48. doi: 10.1161/circulationaha.106.675355
101. Kanhai DA, Kappelle LJ, van der Graaf Y, Uiterwaal CS, Visseren FL. The risk of general and abdominal adiposity in the occurrence of new vascular events and mortality in patients with various manifestations of vascular disease. Int J Obes (Lond). (2012) 36:695–702. doi: 10.1038/ijo.2011.115
102. Deng ZB, Poliakov A, Hardy RW, Clements R, Liu C, Liu Y, et al. Adipose tissue exosome-like vesicles mediate activation of macrophage-induced insulin resistance. Diabetes. (2009) 58:2498–505. doi: 10.2337/db09-0216
103. Nieto-Vazquez I, Fernández-Veledo S, Krämer DK, Vila-Bedmar R, Garcia-Guerra L, Lorenzo M. Insulin resistance associated to obesity: the link TNF-alpha. Arch Physiol Biochem. (2008) 114:183–94. doi: 10.1080/13813450802181047
104. Qing Z, Xiao-Hui W, Xi-Mei W, Chao-Chun Z. Vitamin C deficiency aggravates tumor necrosis factor α-induced insulin resistance. Eur J Pharmacol. (2018) 829:1–11. doi: 10.1016/j.ejphar.2018.03.044
105. Dang SY, Leng Y, Wang ZX, Xiao X, Zhang X, Wen T, et al. Exosomal transfer of obesity adipose tissue for decreased miR-141-3p mediate insulin resistance of hepatocytes. Int J Biol Sci. (2019) 15:351–68. doi: 10.7150/ijbs.28522
106. Dave KM, Stolz DB, Manickam DS. Delivery of mitochondria-containing extracellular vesicles to the BBB for ischemic stroke therapy. Expert Opin Drug Delivery. (2023) 20:1769–88. doi: 10.1080/17425247.2023.2279115
107. Hayakawa K, Esposito E, Wang X, Terasaki Y, Liu Y, Xing C, et al. Transfer of mitochondria from astrocytes to neurons after stroke. Nature. (2016) 535:551–5. doi: 10.1038/nature18928
108. Peruzzotti-Jametti L, Bernstock JD, Willis CM, Manferrari G, Rogall R, Fernandez-Vizarra E, et al. Neural stem cells traffic functional mitochondria via extracellular vesicles. PloS Biol. (2021) 19:e3001166. doi: 10.1371/journal.pbio.3001166
109. Renovato-Martins M, Matheus ME, de Andrade IR, Moraes JA, da Silva SV, Citelli Dos Reis M, et al. Microparticles derived from obese adipose tissue elicit a pro-inflammatory phenotype of CD16(+), CCR5(+) and TLR8(+) monocytes. Biochim Biophys Acta Mol Basis Dis. (2017) 1863:139–51. doi: 10.1016/j.bbadis.2016.09.016
110. Pan Y, Hui X, Hoo RLC, Ye D, Chan CYC, Feng T, et al. Adipocyte-secreted exosomal microRNA-34a inhibits M2 macrophage polarization to promote obesity-induced adipose inflammation. J Clin Invest. (2019) 129:834–49. doi: 10.1172/jci123069
111. Zhang Y, Mei H, Chang X, Chen F, Zhu Y, Han X. Adipocyte-derived microvesicles from obese mice induce M1 macrophage phenotype through secreted miR-155. J Mol Cell Biol. (2016) 8:505–17. doi: 10.1093/jmcb/mjw040
112. Wang J, Li L, Zhang Z, Zhang X, Zhu Y, Zhang C, et al. Extracellular vesicles mediate the communication of adipose tissue with brain and promote cognitive impairment associated with insulin resistance. Cell Metab. (2022) 34:1264–79.e8. doi: 10.1016/j.cmet.2022.08.004
113. Wei M, Gao X, Liu L, Li Z, Wan Z, Dong Y, et al. Visceral adipose tissue derived exosomes exacerbate colitis severity via pro-inflammatory miRNAs in high fat diet fed mice. ACS Nano. (2020) 14:5099–110. doi: 10.1021/acsnano.0c01860
114. Liu Y, Wang Y, Wang C, Shi R, Zhou X, Li Z, et al. Maternal obesity increases the risk of fetal cardiac dysfunction via visceral adipose tissue derived exosomes. Placenta. (2021) 105:85–93. doi: 10.1016/j.placenta.2021.01.020
115. Gečys D, Skredėnienė R, Gečytė E, Kazlauskas A, Balnytė I, Jekabsone A. Adipose tissue-derived stem cell extracellular vesicles suppress glioblastoma proliferation, invasiveness and angiogenesis. Cells. (2023) 12:1247. doi: 10.3390/cells12091247
116. Felthaus O, Vedlin S, Eigenberger A, Klein SM, Prantl L. Exosomes from adipose-tissue-derived stem cells induce proapoptotic gene expression in breast tumor cell line. Int J Mol Sci. (2024) 25:2190. doi: 10.3390/ijms25042190
117. Song Y, You Y, Xu X, Lu J, Huang X, Zhang J, et al. Adipose-derived mesenchymal stem cell-derived exosomes biopotentiated extracellular matrix hydrogels accelerate diabetic wound healing and skin regeneration. Adv Sci (Weinh). (2023) 10:e2304023. doi: 10.1002/advs.202304023
118. Wang T, Li T, Niu X, Hu L, Cheng J, Guo D, et al. ADSC-derived exosomes attenuate myocardial infarction injury by promoting miR-205-mediated cardiac angiogenesis. Biol Direct. (2023) 18:6. doi: 10.1186/s13062-023-00361-1
119. Hamilton G, Teufelsbauer M. Adipose-derived stromal/stem cells and extracellular vesicles for cancer therapy. Expert Opin Biol Ther. (2022) 22:67–78. doi: 10.1080/14712598.2021.1954156
120. Zhang Y, Yu M, Dai M, Chen C, Tang Q, Jing W, et al. miR-450a-5p within rat adipose tissue exosome-like vesicles promotes adipogenic differentiation by targeting WISP2. J Cell Sci. (2017) 130:1158–68. doi: 10.1242/jcs.197764
121. Nie F, Ding P, Zhang C, Zhao Z, Bi H. Extracellular vesicles derived from lipoaspirate fluid promote fat graft survival. Adipocyte. (2021) 10:293–309. doi: 10.1080/21623945.2021.1932355
122. Nie JY, Zhu YZ, Wang JW, Hu X, Wang ZH, Wu S, et al. Preparing adipogenic hydrogel with neo-mechanical isolated adipose-derived extracellular vesicles for adipose tissue engineering. Plast Reconstr Surg. (2021) 148:212e–22e. doi: 10.1097/prs.0000000000008186
123. Wu Y, Hong P, Liu P, Zhang Q, Zhang Y, Yang B, et al. Lipoaspirate fluid derived factors and extracellular vesicles accelerate wound healing in a rat burn model. Front Bioeng Biotechnol. (2023) 11:1185251. doi: 10.3389/fbioe.2023.1185251
124. Yu Q, Wang D, Fan S, Tang X, He J, Peng J, et al. Protective effects of adipose-derived biogenic nanoparticles on the pulmonary microvascular endothelial barrier in mice with ventilator-induced lung injury via the TRPV4/ROCK1 signalling pathway. Pulm Pharmacol Ther. (2022) 73-74:102123. doi: 10.1016/j.pupt.2022.102123
125. Hong P, Wu Y, Zhang Q, Liu P, Zhang S, Yu M, et al. Identification of thermogenesis-related lncRNAs in small extracellular vesicles derived from adipose tissue. BMC Genomics. (2022) 23:660. doi: 10.1186/s12864-022-08883-0
126. Zhang Y, Dong T, Wang M. Lipidomic landscape of lipokines in adipose tissue derived extracellular vesicles. Front Mol Biosci. (2023) 10:1281244. doi: 10.3389/fmolb.2023.1281244
127. Dong J, Wu B, Tian W. Adipose tissue-derived small extracellular vesicles modulate macrophages to improve the homing of adipocyte precursors and endothelial cells in adipose tissue regeneration. Front Cell Dev Biol. (2022) 10:1075233. doi: 10.3389/fcell.2022.1075233
128. Blandin A, Dugail I, Hilairet G, Ponnaiah M, Ghesquière V, Froger J, et al. Lipidomic analysis of adipose-derived extracellular vesicles reveals specific EV lipid sorting informative of the obesity metabolic state. Cell Rep. (2023) 42:112169. doi: 10.1016/j.celrep.2023.112169
129. Chen M, Zhang F, Chen B, Lau C, Xu K, Tong T, et al. Omics approach to reveal the effects of obesity on the protein profiles of the exosomes derived from different adipose depots. Cell Mol Life Sci. (2022) 79:570. doi: 10.1007/s00018-022-04597-4
Keywords: extracellular vesicles, adipose tissue, obesity, clinical implication, inter-organ communication
Citation: Han Y, Ye S and Liu B (2024) Roles of extracellular vesicles derived from healthy and obese adipose tissue in inter-organ crosstalk and potential clinical implication. Front. Endocrinol. 15:1409000. doi: 10.3389/fendo.2024.1409000
Received: 29 March 2024; Accepted: 12 August 2024;
Published: 29 August 2024.
Edited by:
Alok Raghav, Gachon University, Republic of KoreaReviewed by:
Raphael Pollock, The Ohio State University, United StatesSuchandan Sikder, James Cook University, Australia
Copyright © 2024 Han, Ye and Liu. This is an open-access article distributed under the terms of the Creative Commons Attribution License (CC BY). The use, distribution or reproduction in other forums is permitted, provided the original author(s) and the copyright owner(s) are credited and that the original publication in this journal is cited, in accordance with accepted academic practice. No use, distribution or reproduction is permitted which does not comply with these terms.
*Correspondence: Sheng Ye, eWVzaGVuZ0BidWFhLmVkdS5jbg==; Bowen Liu, bGl1Ym93ZW5AYmptdS5lZHUuY24=