- Sorbonne Université, CNRS UMR8246, INSERM U1130, Neuroscience Paris Seine – Institut de Biologie Paris Seine, Paris, France
Infertility is becoming a major public health problem, with increasing frequency due to medical, environmental and societal causes. The increasingly late age of childbearing, growing exposure to endocrine disruptors and other reprotoxic products, and increasing number of medical reproductive dysfunctions (endometriosis, polycystic ovary syndrome, etc.) are among the most common causes. Fertility relies on fine-tuned control of both neuroendocrine function and reproductive behaviors, those are critically regulated by sex steroid hormones. Testosterone and estradiol exert organizational and activational effects throughout life to establish and activate the neural circuits underlying reproductive function. This regulation is mediated through estrogen receptors (ERs) and androgen receptor (AR). Estradiol acts mainly via nuclear estrogen receptors ERα and ERβ. The aim of this review is to summarize the genetic studies that have been undertaken to comprehend the specific contribution of ERα and ERβ in the neural circuits underlying the regulation of the hypothalamic-pituitary-gonadal axis and the expression of reproductive behaviors, including sexual and parental behavior. Particular emphasis will be placed on the neural role of these receptors and the underlying sex differences.
1 Introduction
In mammals, fertility allows the perpetuation of the species. Fertility relies on a fine regulation of reproductive function involving both adequate neuroendocrine regulation of the hypothalamic-pituitary-gonadal (HPG) axis, and synchronized expression of male and female sexually dimorphic reproductive behaviors.
Within the HPG axis, the pulsatile release of gonadotropin releasing hormone (GnRH) from hypothalamic GnRH neurons in the hypothalamic-pituitary portal system activates the neuroendocrine secretion of the gonadotropins luteinizing hormone (LH) and follicle-stimulating hormone (FSH) (Figure 1). LH and FSH stimulate the gonads and trigger gametogenesis and secretion of gonadal steroid hormones. Sex steroid hormones in turn exert a feedback control on the HPG axis (1). In males, testicular testosterone exerts a negative feedback control on hypothalamic GnRH and pituitary LH release (2). In females, estradiol negatively regulates hypothalamic GnRH secretion for most of the estrous cycle, except during the proestrus phase. During this phase, the growth of ovarian follicles is associated with a rise in circulating estradiol concentration that exerts hypothalamic and pituitary positive feedbacks, leading to the LH discharge necessary for ovulation and induction of female receptivity (3, 4). GnRH neurons activity is finely regulated by a complex neural network including kisspeptin-, neurokinin B-, glutamate- and GABA-expressing neurons. Glial cells, especially astrocytes and tanycytes, also participate in this regulation (5). Kisspeptin neurons are located in two distinct hypothalamic regions, the arcuate nucleus (ARC) and the rostral periventricular area of the third ventricle (RP3V). ARC kisspeptin neurons, referred to as KNDy neurons because of their coexpression of kisspeptin, neurokinin B and dynorphin, participate in estradiol negative feedback and coordination of GnRH pulses (6). RP3V kisspeptin neurons are essential to the regulation of estradiol positive feedback driving the generation of the female LH surge (7). This positive feedback is lost in males. It is a primary example of sexual dimorphism.
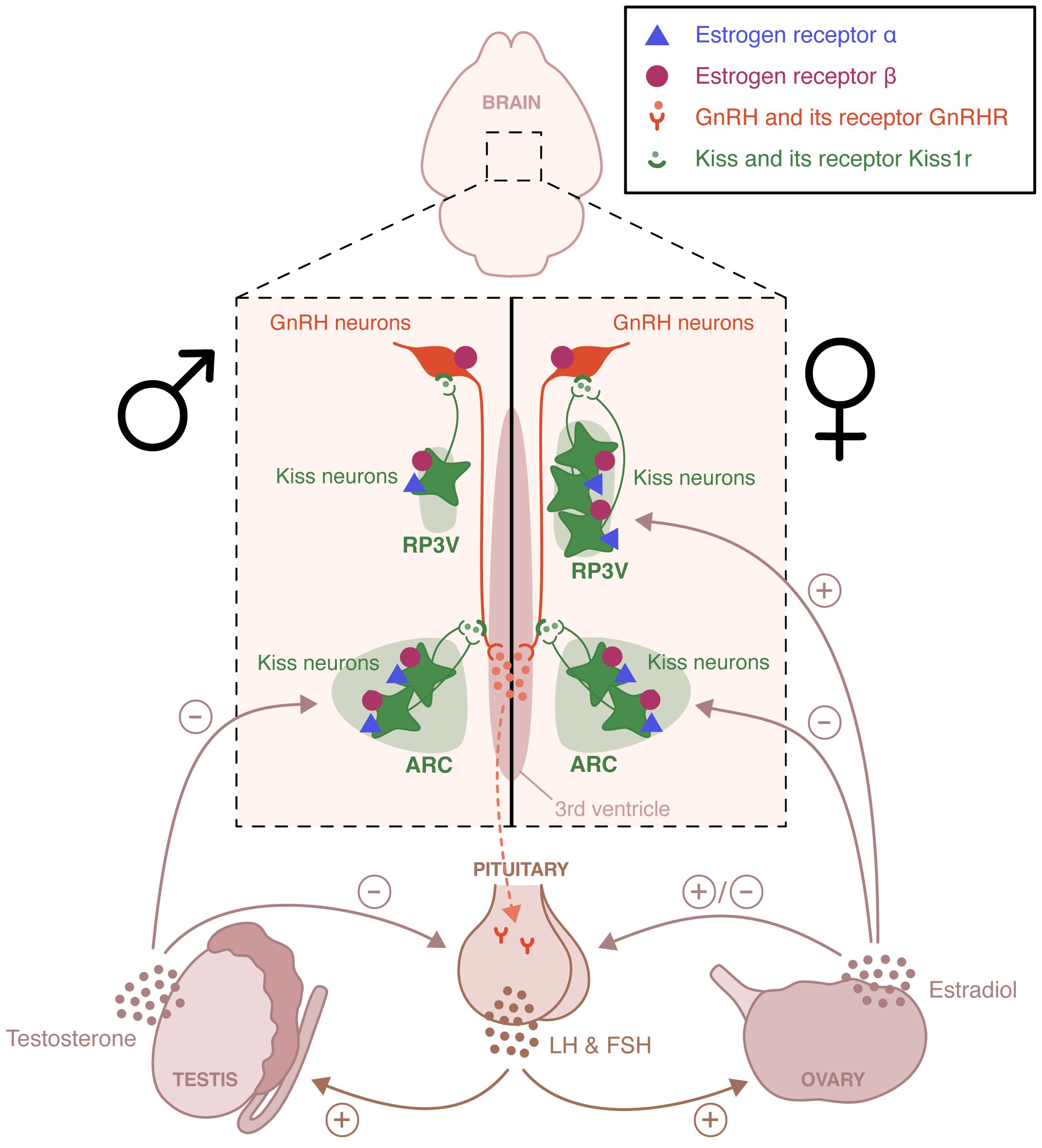
Figure 1 Schematic representation of the neural circuits involved in the estradiol regulation of the hypothalamic-pituitary-gonadal axis. Estrogen receptor (ER) α and ERβ are expressed in the rostral periventricular area of the third ventricle (RP3V) and the arcuate nucleus (ARC) of both males and females. These two hypothalamic nuclei are essential to the control of estradiol positive and negative feedback, respectively. The loss of the positive feedback in males is an example of sexual dimorphism. ERβ, unlike ERα is also expressed in gonadotropin-releasing hormone (GnRH) neurons and appears to participate to the pubertal activation of the hypothalamic-pituitary-axis. LH, Luteinizing hormone; FSH, follicle-stimulating hormone.
In addition to a functional HPG axis, fertility requires appropriate and optimal display of reproductive behaviors, including sexual and parental behavior. Sexual behavior is sexually dimorphic. In rodents, it includes an appetitive phase, during which both sexes actively stimulate their partners by releasing pheromones and displaying appetitive behaviors. These behaviors include ano-genital investigations, series of approaches and solicitations, as well as male emission of ultrasonic vocalizations. These are followed by a consummatory phase, where male mounts are associated with female display of the lordosis posture, favoring intromission (8). Males express a continuous sexual activity, while females are only receptive during the proestrus phase of the estrous cycle, after the sequential rise in estrogen and progesterone (9). Sexual behavior relies on the activation of complex, sexually dimorphic neural circuits (10)(Figure 2). It is triggered by the detection of sensory stimuli, especially pheromones, that are detected by chemosensory neurons located in the vomeronasal organ and the main olfactory epithelium (11, 12). These neurons project to the main olfactory bulb (MOB) and the accessory olfactory bulb (AOB), which innervates among other structures, the medial amygdala (MeA) (13, 14). Neurons from the MeA then project to the bed nucleus of the stria terminalis (BNST), the medial preoptic area (mPOA), and the ventromedial nucleus of the hypothalamus (VMH). In males, the mPOA plays a critical role in the expression of sexual behavior. Projections from the hypothalamic paraventricular nucleus (PVN) are sent to the spinal centers that promote erection and ejaculation (15, 16). In females, the VMH is essential for the activation of sexual behavior. It sends projections to the periaqueductal gray matter (PAG) that project to the brainstem and spinal cord, which innervate the axial muscles involved in the lordosis posture (17). There is also an inhibitory circuit for lordosis behavior involving the ARC and the POA (18).
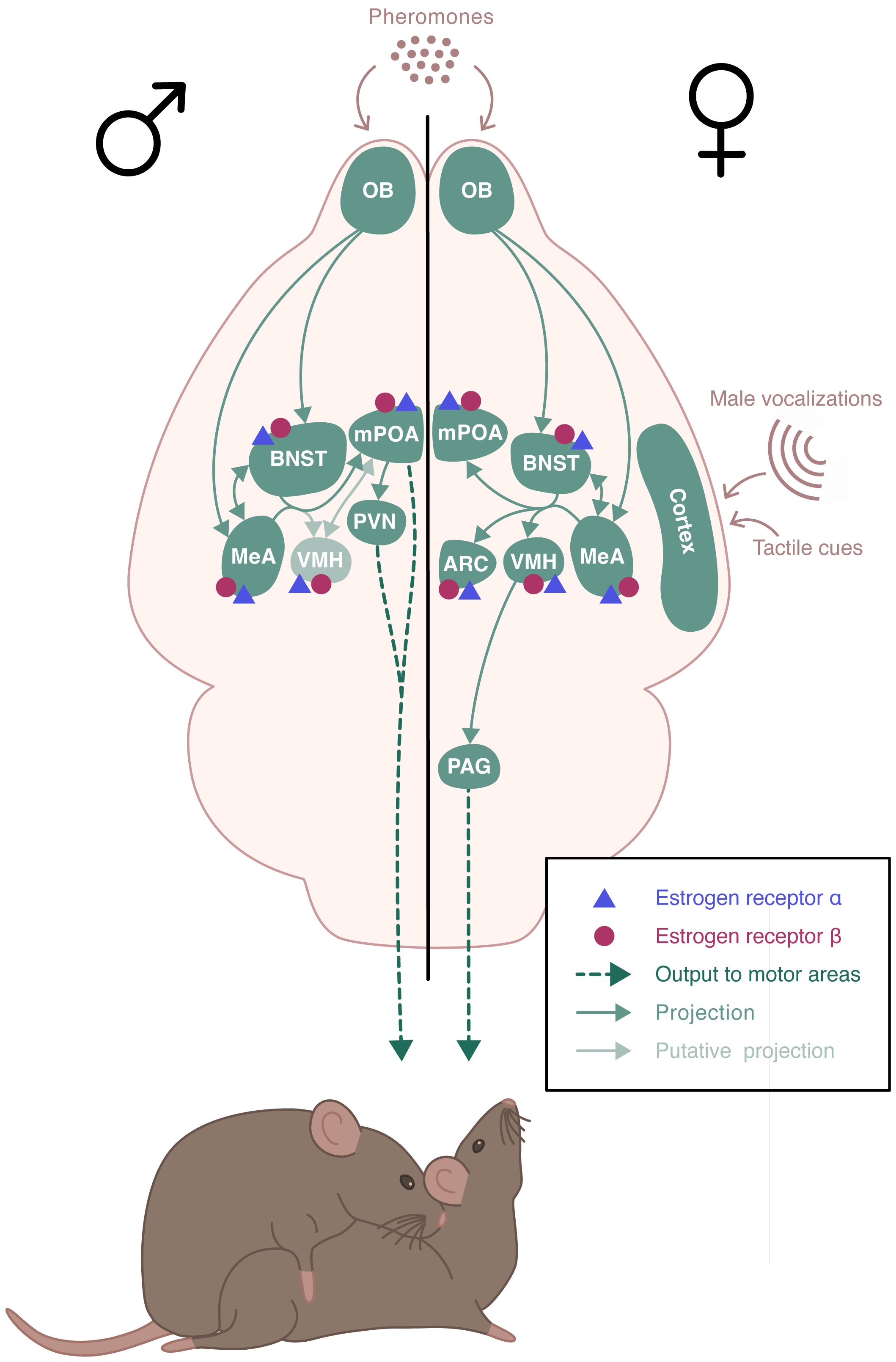
Figure 2 Schematic representation of the neural circuits involved in the estradiol regulation of sexual behaviors. Estrogen receptor (ER) α and ERβ receptors are expressed throughout the neural circuit involved in the expression of sexual behaviors in a sexually dimorphic manner. Sexual behavior is triggered by pheromones that are detected by chemosensory neurons. These neurons project to the olfactory bulb (OB) which innervates the medial amygdala (MeA) and the bed nucleus of the stria terminalis (BNST), which send projections to the medial preoptic area (mPOA) and the ventromedial nucleus of the hypothalamus (VMH). In males, mPOA plays a critical role in the expression of sexual behavior. Projections from the hypothalamic paraventricular nucleus (PVN) are sent to the spinal centers that promote erection and ejaculation. In females, VMH is essential for the activation of sexual behavior. It sends projections to the periaqueductal gray matter (PAG) that project to the brainstem and spinal cord, which innervate the axial muscles involved in the lordosis posture. There is also an inhibitory circuit for lordosis behavior involving the ARC and the POA.
Parental behavior occurs primarily in females and is minimal or absent in males of many mammalian species. In rodents, parental behavior is exhibited by the mother, with the exception of few species that display biparental care, such as the prairie vole or the California mouse (19). Parental behavior defines all behaviors exhibited to increase pups’ chances of survival and development (20). Typically, rodents tend to avoid pups, or even exhibit aggressive behavior and infanticide towards them. A behavioral “switch” occurs in the early days of gestation, with females displaying an intensive nest building and increased aggressivity towards intruders. At birth, dams show a strong interest in newborns and become highly responsive to gustatory, olfactory, and tactile cues. Dams also rapidly respond to ultrasonic vocalizations emitted by pups, by retrieving them safely to the nest (21, 22). Pup’s sensory information is integrated into a neural circuitry that includes the MeA and the BNST (Figure 3). These regions project to the mPOA that promotes pup attractivity. In turn, the mPOA projects to various downstream regions that facilitate the establishment and maintenance of maternal behavior, including the PVN rich in oxytocinergic neurons, and the ventral tegmental area (VTA) of the dopaminergic system (23). Males and virgin or non-lactating females of uniparental species can also exhibit parental behavior. This occurs spontaneously in most strains of laboratory mice, although the level of pup care is much lower than that given by dams (24). The neural pathway underlying paternal behavior seems to be similar to the one for maternal behavior, with mPOA as a key region (19).
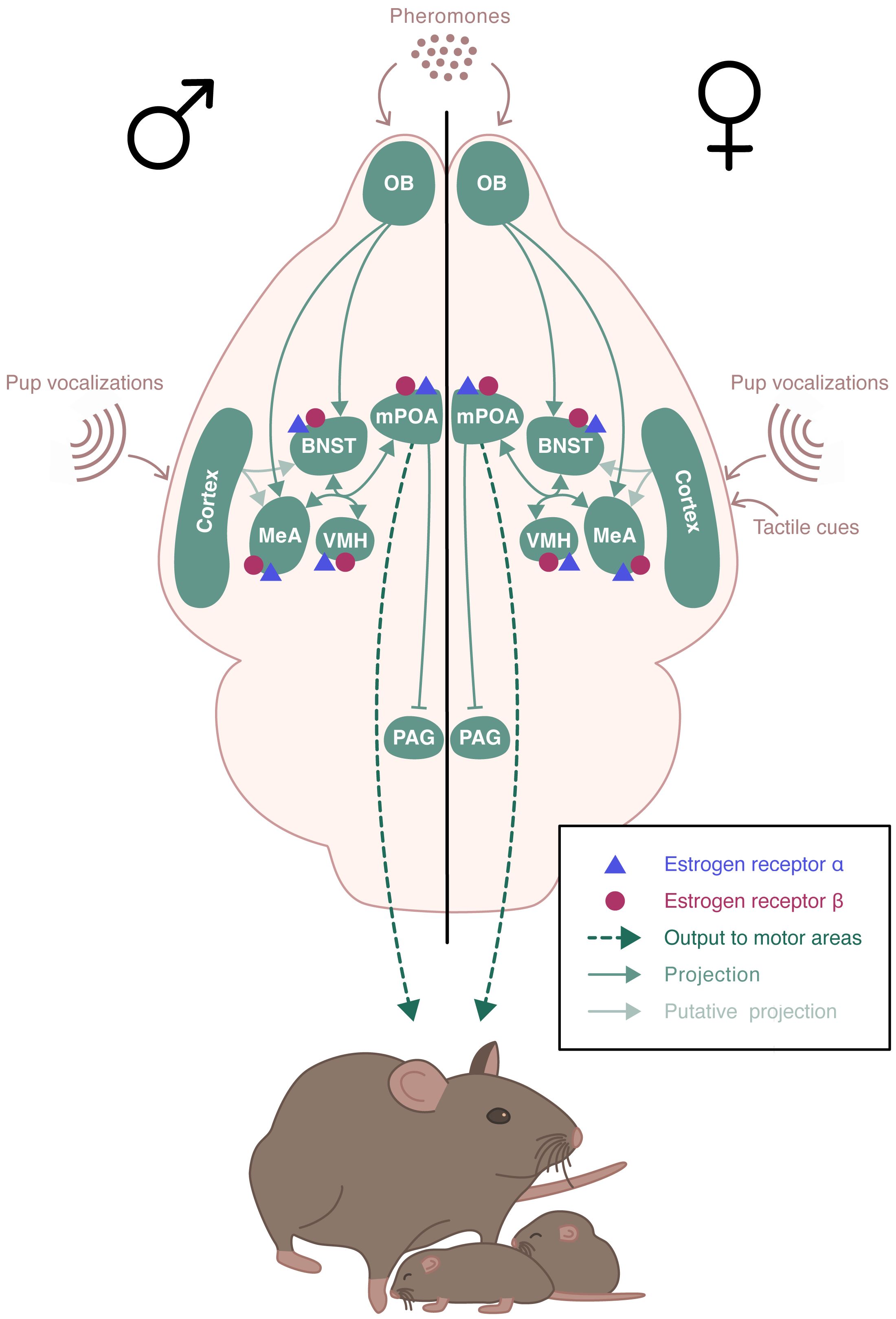
Figure 3 Schematic representation of the neural circuits involved in the estradiol regulation of parental behavior. ERα and ERβ receptors are expressed in the brain regions involved in the expression of parental behavior. Pup’s sensory information is integrated into a neural circuitry that includes the medial amygdala (MeA) and the bed nucleus of the stria terminalis (BNST). These regions project to the medial preoptic area (mPOA) which send projections to various downstream regions including the hypothalamic paraventricular nucleus (PVN) and regions of the dopaminergic system. The neural pathway underlying paternal behavior remains unclear, but it seems to be similar to the one for maternal behavior, with mPOA as a key region.
The reproductive function and behavior are tightly regulated by sex steroid hormones. The sexually dimorphic hormonal control of mating was first demonstrated by the pioneer work of Phoenix and collaborators in 1959 (25). This study, and those that followed, demonstrated the existence of both organizational and activational effects of sex steroids (26). Androgens and estrogens act at specific periods of development to organize, in a sex-dependent manner, the neural circuits controlling reproductive function and behavior, which are then activated by sex steroids in adulthood.
Organizational changes occur during sensitive periods of development. Although initially assumed to affect only males during perinatal life, increasing evidence supports that organization takes place in both sexes at multiple developmental windows, including the perinatal, postnatal, and pubertal periods. It is well known that perinatal masculinization of the male brain results from two testosterone bursts occurring before and after birth. In addition, brain feminization in females appears to occur later as circulating estradiol increases during postnatal and prepubertal development (27–31). These organizational and activational effects of testosterone and estradiol are mediated through their receptors: estrogen receptors (ERs) and androgen receptor (AR). In males, testosterone can also be aromatized due to neural expression of cytochrome p450 aromatase into neural 17β-estradiol, which then acts on ERs (32, 33).
The present review focuses on the neural role of the ERs in male and female reproduction (for a review of the role of AR in the central nervous system see (34)). In particular, we will review genetic evidence using transgenic mouse models and recent technological tools that have provided a better understanding of the role of ERs in estradiol-induced regulation of the HPG axis and reproductive behaviors.
2 Estrogen receptors: types and neural expression
In the mouse, ERα (66 kDa) and ERβ (54 kDa) are encoded by estrogen receptor 1 (Esr1) and estrogen receptor 2 (Esr2) genes located on chromosomes 10 and 12, respectively (35, 36). These receptors belong to the nuclear receptor superfamily and act as transcription factors. The primary regulation of gene expression involves direct binding of the receptor to EREs sequences as it is the case for example for progesterone receptor (Pgr) (37), Kiss-1 metastasis suppressor (Kiss1) (38), dynorphin (Dyn) (39) and brain derived neurotrophic factor (Bdnf) (40). In addition to its slow genomic action, rapid effects of estradiol have been observed. These nongenomic effects are generally initiated by the binding of estradiol to membrane receptors including the classical ERs and other receptors such as estrogen receptor alpha delta 4 (ERαΔ4), membrane estrogen receptor G alpha q (mER-Gαq), G protein-coupled receptor 30 (GPR30), estrogen receptor X (ER-X) and saxitoxin binding protein (STXBP) (41). Binding of estradiol to these receptors activates intracellular signaling pathways such as the mitogen-activated-protein kinase (MAPK), protein kinase C (PKC), protein kinase A (PKA), or phosphotidyl inositol 3 kinase (PI-3K) pathways (42). Mechanisms by which these membrane ERs (mERs) activate these pathways remain unclear. For example, in female rats, mER interaction with membrane metabotropic glutamate receptors (mGluR) was shown to participate in lordosis behavior, through μ-opioid receptor internalization and activation of the fast calcium response, and could also affect long term genetic expression via CREB phosphorylation (43–46). The present review focuses on nuclear ERα and ERβ given their critical and well-identified roles in male and female reproduction.
ERα and ERβ are expressed throughout the neural circuits that control reproductive function and behavior. In adult mice, Esr1 mRNA and protein have been detected in the BNST, VMH, mPOA and ARC (47–49). Esr2 mRNA is also present, less abundantly than Esr1, in the VMH, mPOA and ARC (50–52). Given the lack of selectivity of anti-ERβ antibodies (49, 53), several transgenic ERβ-enhanced green fluorescent protein (EGFP)/-red fluorescent protein (RFP) mouse lines were used and confirmed that the localization of the ERβ protein was similar to that of the transcript (48, 54, 55). During the development, ERα and ERβ are present in the mouse nervous system as early as embryonic day (E) 13 (56). ERα and ERβ mRNA and protein levels change considerably during development in a regional and sex-specific manner. For example, in the anteroventral periventricular nucleus (AVPV), the number of ERα-expressing cells decreases from postnatal day (PND) 0 to PND14 in females, then increases with age, in contrast to males, which show no significant variations over time. In this region, the number of ERβ-expressing cells is highest at PND0 in both sexes and decreases with age in males but not in females (54, 55). In the VMH, ERα-expressing cells are present in both sexes with maximal expression at PND0, followed by a reduction from PND0 to PND14. Then, females show an increase in expression at the end of the pubertal period (PND42-PND56). In males, ERα-expressing cells remain low, and are therefore lower than in females. The number of ERβ-expressing cells in the VMH is also highest at PND0 for both sexes, but more important in females than in males at PND0 and PND7. A sharp decrease is then observed in both sexes that abolishes sex differences after PND7 (54, 55). In the BNST, a greater number of ERα-expressing cells has been described in adult female compared to male mice (57). Indeed, ERα-expression increases in female during development, while remaining constant in males (except for a transient increase at PND28). ERβ-expressing cells remains constant in females, whereas it gradually increases in males to reach its highest level at PND42 and PND56 (55).
3 ERα versus ERβ in estradiol-induced regulation of the hypothalamic-pituitary-gonadal axis
Many studies have focused on the sites of action of estradiol in the regulation of the HPG axis. Does it act directly on GnRH-expressing neurons or indirectly via other cells within the GnRH network? This question was raised by data showing that GnRH neurons do not express Esr1. Of note, they also do not express Ar. Nevertheless, these neurons do express Esr2 and present detectable levels of ERβ protein in mice, rats, and humans (58–62) (Figure 1).
3.1 Role of ERα in the regulation of the HPG axis
3.1.1 Models of ubiquitous Esr1 deletion
Mouse models with ubiquitous deletion for Esr1 (ERαKO) were obtained by homologous recombination targeting exon 2 (63) or exon 3 (64–69). ERαKO males showed a structurally normal urogenital tract, but with a strong decrease of testis weight and sperm count (63, 70). Females ERαKO uterus and vagina were hypoplastic with no cyclic morphological changes. They exhibited a polycystic ovary phenotype with an absence of corpora lutea, and anovulation (66). Both ERαKO males and females were infertile (63–66, 70). Circulating levels of testosterone were slightly increased in ERαKO males but circulating LH levels were unchanged showing no alteration of the negative feedback (70–72). In contrary, ERαKO females showed no negative or positive feedback of estradiol. Indeed, basal LH level was greatly increased in intact ERαKO female, and estradiol treatment in ovariectomized ERαKO females did not trigger the LH surge, unlike wildtypes (68, 69, 72–75).
The role of non-classical ERα signaling pathways was also investigated using a mouse model carrying a mutation in the ERα DNA recognition sequence that abolishes ERα signaling through ERE binding mechanisms (ERα+/AA animals (76);). ERα+/AA males were fertile and showed normal testosterone levels suggesting that ERE-independent ERα signaling is sufficient for some male reproductive function (77). Mutant females, however, were sterile due to uterine defects and anovulation (76). A knocking mutant allele model that selectively restores ERE-independent signaling in ERαKO mice (ERα-/AA animals) revealed that these signaling were able to partially restore estrogen negative feedback on LH secretion, but were not sufficient to mediate estrogen positive feedback, increase GnRH neuron firing to generate the LH surge or mediate spontaneous ovulation (75, 78).
3.1.2 Models of brain specific Esr1 deletion
ERα is thus essential for fertility in both sexes and required for both estradiol positive and negative feedback in females. Nevertheless, ERα is present in many peripheral tissues including male and female reproductive tracts, thus the effects described in ERαKO animals, especially the infertility, do not allow to distinguish between neural and peripheral effects. Studies using more restricted deletion models are reported below.
A mouse line selectively deleted for Esr1 in forebrain neurons (including in the cortex, hippocampus, BNST, amygdala, olfactory bulb, striatum, thalamus and hypothalamus) and pituitary was generated by crossing mice carrying loxP sites on either side of exon 3 of Esr1 with mice expressing Cre recombinase under the control of the calcium/calmodulin-dependent protein kinase IIα promoter (ERαfl/fl; CamKIIα-Cre) (69). Both male and female mutants were infertile. ERαfl/fl; CamKIIα-Cre females showed strong abnormalities of their reproductive organs, an absence of estrous cycle and defect in ovulation (69, 79). Similar to ubiquitous ERαKO mice, estradiol injection in mutant females did not trigger the LH surge (69). Basal LH level of ERαfl/fl; CamKIIα-Cre female was no different from that of control females, but mutant mice did not show an increase in circulating LH levels after ovariectomy (79). The importance of ERα in adulthood in this negative feedback was revealed using an inducible tamoxifen-based Cre-LoxP model, which triggered more than 50% reduction in the number of ERα-expressing cells (79). This treatment resulted in lack of estrous cyclicity and disruption of negative feedback. Indeed, the increase in LH observed after ovariectomy was decreased in mutant mice, and estradiol treatment failed to restore baseline LH levels. These results revealed the importance of ERα in forebrain/pituitary in regulating both positive and acute negative feedback of estradiol.
Deletion of Esr1 selectively in neural cells was generated by crossing ERαfl/fl females with males expressing the Cre recombinase under the control of the promoter and nervous system-specific enhancer of nestin (ERαNescre (80)). This model allowed the deletion of Esr1 in both neuronal and glial cells but not in the pituitary. Females ERαNescre presented early puberty initiation with advanced vaginal opening and first estrus. In adulthood, mutant females showed a diminution in ovary weight with absence of corpora lutea and estrous cycle arrest. This was accompanied with a decrease in the number of kisspeptin cells in the RP3V in females. An increase in uterine weight was also reported, related to elevated levels of circulating estradiol in mutant females, similar to what has been observed in other ERαKO mouse model. In adult males, seminal vesicles weight and testosterone level were increased in mutant mice compared to controls, but no modification in testis weight was observed. In contrary to females, mutant males were fertile. Thus, while neural ERα is critical for normal cyclicity, fertility and regulation of both estradiol positive and negative feedback on LH secretion, sex differences are observed, with a more critical role in females than in males. Several studies, described below, aimed to decipher the specific cell population and neural circuits involved in ERα-mediated regulation of estradiol. Because ERα is not present in GnRH neurons, estradiol appears to act indirectly via afferent circuits.
Kisspeptin neurons have been widely studied for their potent activation of GnRH neurons and are known to be regulated by estradiol through ERα. ERα is expressed in around two-third of RP3V kisspeptin-expressing cells and nearly all ARC kisspeptin cells (81). Estradiol inhibits kisspeptin expression in the ARC while activating its expression in the RP3V (38, 82). This opposing regulation of estradiol on kisspeptin expression was abolished in ERαKO mice (38). To investigate further, a mouse model to delete Esr1 specifically in kisspeptin expressing cells (KERKO) was generated by crossing mice floxed for exon 3 of Esr1 with mice expressing Cre recombinase under the control of kisspeptin promoter (81, 83, 84). Mice carrying this mutation showed advanced vaginal opening, disruption of ovarian cyclicity, complete absence of corporal lutea leading to infertility. Adult females KERKO exhibited a reduced LH secretory response to ovariectomy compared to control suggesting an alteration of the estradiol negative feedback. Nevertheless, estradiol treatment following ovariectomy was still able to reduce LH levels (82). Estradiol injection failed to induce the LH surge in KERKO animals indicating a loss of estradiol positive feedback (82). In addition, KERKO mice lost the LH response to kisspeptin and showed a decreased LH response to GnRH injections (85). Along with these findings, electrophysiological studies demonstrated that RP3V kisspeptin cell activity was increased during estradiol positive feedback in control animals, but to a lesser extent in KERKO females (85). In contrast, ARC kisspeptin neurons activity was reduced during estradiol positive feedback in controls, but instead increased in KERKO females. These results were further confirmed using calcium imaging with protein-based indicators (GCaMP) fiber photometry approach, where KERKO mice showed an increased ARC Kiss1-expressing cell activity compared with intact controls, that was similar to ovariectomized controls (84). Thus, in females, ERα is required in kisspeptin neurons for complete maturation of the HPG axis and control of estradiol negative and positive feedback on GnRH/LH secretion. Although not studied in detail, the authors mentioned that KERKO males did not show modification in testicular weight or alterations in the LH response to castration (83), suggesting that ERα in kisspeptin neurons is not indispensable for estradiol regulation of male HPG axis.
KERKO animals have Esr1 deleted in both RP3V and ARC kisspeptin neuronal populations. The role of ERα in KNDy neurons of the ARC was studied using a mouse model deleted for Esr1 in Tac2-expressing cells, by crossing Tac2Cre mice with Esr1flox animals (ERαTac2KO (81)). Nearly all Tac2-expressing cells co-express ERα. ERαTac2KO females presented morphological abnormalities in ovaries and uteri, precocious puberty and impaired cyclicity. Basal LH levels were elevated in mutant animals compared to control showing an impairment of estradiol negative feedback (81). The role of this cell population in the estradiol positive feedback needs to be further detailed. It is important to note that Kiss1 has been detected in ovaries and Tac3 in both pituitary and ovaries (86–88). Thus, it is likely that in these KERKO and ERαTac2KO models, Esr1 was deleted in some cells outside the brain.
Esr1 is also expressed in GABAergic and glutamatergic neurons in key hypothalamic regions including the RP3V and the ARC. These neurons are known to project directly to GnRH neurons. In females, mouse models deleted for Esr1 in GABAergic neurons (Vgat-ires-Cre;Esr1lox/lox) showed no modification of the age of puberty onset or estradiol regulation of the negative feedback. Indeed, basal LH levels were unchanged and mutant mice responded similarly to control to ovariectomy and estradiol replacement. However, Vgat-ires-Cre; Esr1lox/lox females presented abnormal cyclicity, infertility and did not show the normal estradiol positive feedback rise in LH (89). In males, deletion of Esr1 in GABAergic neurons did not modify testicular weight, serum testosterone levels or fertility compared to controls, suggesting that this population is not necessary for proper functioning of the male HPG axis (90).
In parallel, the deletion of Esr1 in glutamatergic neurons in females (Vglut2-ires-Cre;Esr1lox/lox) induced advanced puberty onset, disturbed cyclicity and infertility. Mutant females showed an impairment of estradiol negative feedback with elevated basal LH levels compared to control and abnormal response to ovariectomy and estradiol replacement. In addition, Vglut2-ires-Cre;Esr1lox/lox females were unable to present an LH surge from estradiol injection, illustrating a lack of estradiol positive feedback in these animals (89). Although studied with less detail, Vglut2-ires-Cre;Esr1lox/lox males were fertile, showed normal testicular weight but with a significant increase in serum testosterone levels compared with control animals, suggesting a role for this neuronal population in the control of negative feedback in males (90). These results highlight the existence of strong sex differences in the role of Esr1 in GABAergic and glutamatergic neuron for the control of the HPG axis.
3.1.3 Models of region-specific and time-dependent Esr1 deletion
The genetic models discussed above all have the caveat that ERα was deleted early during development, and thus did not allow for a distinction between developmental (organizational) and adult (activational) roles of this receptor. In addition, these models did not target a specific region, resulting in a lack of precision in understanding the neural network. Targeted viral vector injection in adulthood has permitted the reduction of Esr1 expression in a hypothalamic nucleus-specific and time-dependent manner.
Ablation of Esr1 specifically in the ARC has been achieved by stereotactic injection of an adeno-associated virus containing Cre recombinase (AAV-Cre) specifically into ERαflox/flox mice (91). The deletion of 60–90% of ERα-expressing cells in the ARC induced a disturbance in ovarian cyclicity. Basal LH level in mutant mice was similar to those in control females, but the increase in LH after ovariectomy was reduced, suggesting an activational role of ERα-expressing cells in the ARC for estradiol negative feedback (91).
More recently, the combination of CRISPR-Cas9 technique with targeted injection of viral vectors has enabled the deletion of Esr1 in RP3V or ARC kisspeptin-expressing cells in adult females (AAV-Esr1) (84, 92). Esr1 deletion in RP3V kisspeptin neurons in adulthood did not modify estrus cyclicity but blunted the estradiol-induced LH surge confirming the important role of this neural population in the control of estradiol positive feedback. This effect was linked to a decrease in electrophysiological excitability of RP3V kisspeptin neurons in RP3V-AAV-Esr1 females compared to controls (92). Using the same technique, Esr1 was deleted, in adulthood, in ARC kisspeptin neurons (ARC-AAV-Esr1). Depending on the study, these females showed either disrupted (92) or normal estrous cyclicity (84). A reduced LH response to kisspeptin or GnRH treatment compared to control was observed in Wang et al. (2019). Variability of effects was observed with regards to negative feedback regulation. No modification of LH pulse frequency or basal LH levels was observed between ARC-AAV-Esr1 and control females (92). In this study, CRISPR-mediated Esr1 knockdown was achieved in 60% of cell population. Mc Quillan et al. (2022) observed that the phenotype of the animals depended on the efficacy of the knockdown generated by CRISPR technology. Indeed, an Esr1 knockdown greater than 70–80% in ARC kisspeptin neurons was necessary to generate an increased pattern of synchronization similar to that observed in control animals after ovariectomy (84). Thus, the high percentage of Esr1 deletion required to alter LH secretion could explain the difference between the effects observed in these two studies. These results show that ERα in RP3V and ARC kisspeptin cells has activational roles in regulating estradiol positive and negative feedback, respectively.
3.2 Role of ERβ in the regulation of the HPG axis
ERβ, unlike ERα, is expressed in GnRH neurons. In addition to the indirect regulation of the HPG axis via ERα-expressing neurons, in particular kisspeptin neurons, estradiol could act directly on GnRH neurons via ERβ.
3.2.1 Models of ubiquitous Esr2 deletion
The two first models of ubiquitous deletion of Esr2 were obtained by homologous recombination by targeting exon 3 of Esr2 (66, 93). ERβKO males had normal urogenital tract, testicular function and spermatogenesis and were fertile (66, 93). Nevertheless, castrated ERβKO males showed higher LH levels than wildtypes indicating a role for ERβ in estradiol negative feedback (94). In females, ERβKO induced variable reproductive phenotypes ranging from mild hypofertility to complete infertility (66, 93). Genital tracts of ERβKO females were similar to control animals (66). Adult ERβKO ovaries were macroscopically normal and had normal antral follicles, but with fewer corpora lutea than controls, suggesting less efficient ovulation due to impaired ovarian function (66, 93). Ubiquitous deletion of Esr2 had no major impact on female positive and negative feedbacks exerted by estradiol. Basal LH levels of ERβKO females were either normal (73) or slightly increased (74). Treatment of ovariectomized females with estradiol resulted in a similar induction of the LH surge in both control and mutant mice (69). Furthermore, the distribution and number of GnRH neurons were unchanged and the opposite estradiol regulation of kisspeptin expression in the RP3V and ARC was maintained in ERβKO mice (38, 69). ERβ appeared, however, to be involved in the rapid action of estradiol on GnRH neurons. Rapid modulation of the phosphorylation state of the cAMP response element-binding protein (CREB) in GnRH neurons was abolished in mutant females (95). Importantly, these models were later shown to still express transcripts from alternative splicing of Esr2 (53), which could be the cause of these variable fertility phenotypes. Therefore, a line devoid of any Esr2 transcripts was generated using the Cre/loxP technique (named ERβSTL−/L− (96)). Mutant males and females from this model showed complete infertility. In females, it was due to drastic impairment of cyclicity and ovarian function. In males, the reason was unclear, as testis and epididymis histology and apparent motility of spermatozoa appeared normal (96).
3.2.2 Models of brain specific Esr2 deletion
ERβ is highly expressed in both female and male urogenital tracts, including the ovary, prostate and epididymis (97, 98). Thus, similarly to ERα, the infertility induced by the complete and ubiquitous deletion of Esr2 did not allow the study of its neural effects. Although fewer in number than for ERα, more restrictive approaches have been used.
A mouse model selectively deleted for Esr2 in the forebrain and pituitary has been generated by crossing Esr2flox mice (96) with CamKIIα-Cre mice (79). Mutant females showed normal cyclicity, basal LH levels and a normal increase in LH level after ovariectomy. However, their inability to reduce LH secretion after acute estradiol injection suggested a contribution of forebrain/pituitary ERβ in acute negative feedback of estradiol (79).
The role of neural ERβ was studied using an ERβNesCre mouse line (99). Mutant ERβNesCre females presented delayed puberty initiation with delayed vaginal opening and first estrus and delay of uterine growth (99). This pubertal delay was linked to a delay in postnatal expression of kisspeptin neurons in the RP3V. In adulthood, the number of GnRH neurons in the POA was unchanged, as was kisspeptin-immunoreactivity in the RP3V and ARC. Adult ERβNesCre females showed no change in estradiol levels, nor in LH response to ovariectomy, and had regular estrous cyclicity and fertility (99). The initiation of male puberty has not yet been evaluated in this model. In adulthood, ERβNesCre males were fertile, with seminal vesicles weights and testosterone levels unchanged from control littermates (100).
Selective Esr2 deletion in GnRH neurons (GnRH-Cre;ERβloxP/loxP) did not disrupt cyclicity, basal LH level, or LH response to estradiol. However, there was a reduced increase in LH secretion after ovariectomy in mutant mice compared to controls, suggesting a minor role for ERβ-expressing GnRH neurons in controlling estradiol negative feedback (79). Another study using a different mouse model observed a more drastic phenotype in GnRH-ERβ-KO (GERβKO) females, which exhibited ovarian morphology abnormalities, delayed puberty initiation, impaired fertility and reduced basal and surge LH levels without altering estradiol-negative feedback (101). While studied in less details, GERβKO males showed normal basal LH levels and no change in the timing of preputial separation (101). Although not totally clear, the use of different genetic background strains and knockout approaches could explain some of these discrepancies. Lastly, another study demonstrated that the rapid action of estradiol to phosphorylate CREB in GnRH neurons involved ERβ expressed by GnRH neurons themselves (102).
Overall, these genetic studies showed that neural ERα is essential for fertility and both positive and negative feedback controls exerted by estradiol on the HPG axis in females. These studies also suggest that neural ERα participates in the prepubertal regulation of the female HPG axis, necessary for its activation at puberty. The lack of studies in males makes it difficult to draw definite conclusions, although neural ERα does not appear to be essential for maintaining fertility in males. Its role in estradiol negative feedback remains to be clarified. Regarding neural ERβ, further work will be needed to understand the discrepancies observed in the different mouse models generated. Nevertheless, neural ERβ appears to be less critical for fertility than ERα, since it seems to play a more subtle role in regulating estradiol positive and negative feedback through both classical and non-classical signaling pathways. Interestingly, ERβ appears to play an activating role in puberty initiation, and additional studies will be crucial to understanding the underlying mechanisms of action.
4 ERα versus ERβ in estradiol-induced regulation of sexual behavior
Alongside their role in regulating the HPG axis, ERα and ERβ receptors are present throughout the neural circuits involved in the expression of sexual behavior (Figure 2). The specific involvement of either receptor in the regulation of sexual behavior by estradiol has been addressed in genetic studies presented below.
4.1 Role of ERα in the regulation of sexual behavior
The predominant expression of ERα in brain regions involved in the expression of sexual behavior, including the MeA, BNST, VMH, MPOA and ARC, has made it the primary target of research on ERs.
4.1.1 Models of ubiquitous Esr1 deletion
All studies carried out on ubiquitous ERαKO mouse lines showed a very strong disturbance of sexual behavior in both intact and hormonal-replaced males and females. ERαKO males show altered sexual behavior manifested by a sharp decrease in frequency of intromissions and an increase in latencies (103–106). In addition, no (106, 107) or little ejaculation (70, 103) was observed in ERαKO males. Different results have been reported regarding the number and latency times of mounts, which were found to be equivalent (103) or reduced (106) compared to wildtype mice. Various observations were also made regarding the role of ERα in regulating olfactory preference in males. ERαKO male mice were first shown to have similar interest in the odors of receptive female mice as controls (108). Other studies have observed altered olfactory preference for receptive females in ERαKO males compared to controls (71, 109, 110).
In ERαKO females, lordosis behavior was severely impaired compared to wildtype mice (63, 111–114). This effect was linked to a marked reduction in induction of progesterone receptor (PR) expression by estradiol in the VMH of mutant females (113). In addition, ERαKO females exhibited a strong deficit in proceptive behavioral interactions. While the number of mount attempts by males was similar between mutant and wildtype females, ERαKO mice vigorously rejected mounts from males that were unable to intromit (112, 114). However, stud males were equally attracted to ERαKO as to wildtype females during preference tests, suggesting that ERα was not critical to female attractivity (114). It is important to note that ubiquitous Esr1 deletion induced elevated levels of estrogen and testosterone in mutant animals compared to wildtypes, which may affected brain functions through developmental processes (70, 71, 115).
The role of ERα in ERE-independent mechanisms of estradiol was investigated using the ERα-/AA mice model. In this model, ERα signaling through ERE binding mechanisms was abolished (76, 77). ERα-/AA males showed a strong deficit in sexual behaviors suggesting that ERE-independent ERα signaling was not sufficient to maintain sexual behavior in males and that genomic action of estradiol is critical (77). Another model harboring a mutation of the ERα palmitoylation site, which prevents membrane ERα signaling, showed no change in female sexual behavior but a strong reduction in male sexual behavior, suggesting an involvement of ERα membrane-initiated estrogen signaling in the organization of male sexual behavior (116).
4.1.2 Models of brain specific Esr1 deletion
To understand the specific role of neural ERα in the regulation of sexual behavior, several studies have used more restricted deletion models.
Sexual behavior was assessed in both ERαNesCre males and females (80). Female sexual behavior, under normalized hormonal levels, was completely abolished with mutant females never exhibiting lordosis posture in response to male mounts. This impairment was linked with a drastic decrease in the number of progesterone receptor (PR)-expressing cells in the VMH of mutant animals compared to control littermates. In males, neural Esr1 deletion induced a less severe behavioral phenotype (80). Mutant males were able to emit courtship vocalizations although with reduced number and duration of syllables. They also initiated mounts, intromissions and reached ejaculation, but with disturbance in the numbers and latencies of the events, provoking a less effective sexual behavior compared to control littermates and to mutants lacking both neural Esr1 and Ar (80). Olfactory preference tests showed that both ERαNesCre females and males presented similar preference toward males or receptive females as control littermates, suggesting an effect of Esr1 deletion downstream the olfactory system (80). In particular, immunohistochemical analyses showed feminized calbindin and tyrosine hydroxylase neuronal populations of the mPOA, suggesting an implication of ERα in the perinatal organization of the neural circuitry underlying male sexual behavior (80).
To determine the role of ERα in either excitatory (glutamatergic) versus inhibitory (GABAergic) neurons, Vglut2-Cre;Esr1lox/lox and Vgat-Cre;Esr1lox/lox males were subjected to sexual behavior phenotyping (90). To date, female sexual behavior has not been tested in these models. In adult males, ERα-expressing neurons are mostly GABAergic in the mPOA, BNST and posterior dorsal MeA while they are predominantly glutamatergic in the VMHvl and posterior ventral MeA. Analysis demonstrated that the percentage of animals displaying mounting, intromission and ejaculation behaviors during the 30 min test was unchanged between Vglut2-Cre;Esr1lox/lox and wildtype males. In contrast, Vgat-Cre;Esr1lox/lox males showed similar mounting and intromission behaviors, but with a deficit in the ability to ejaculate, with only 25% of mutant males reaching it compared to controls, suggesting a role for GABAergic signaling in controlling male sexual behavior (90).
4.1.3 Models of region-specific and time dependent Esr1 deletion
Further studies addressed the developmental versus activational roles of ERα and the hypothalamic nuclei involved.
In males, using viral mediated RNA interference technique, deletion of Esr1 specifically in adulthood or during prepuberty, in the mPOA or VMH, altered components of male sexual behavior illustrated by a reduced number of mounts and intromissions during the 30-minute test (29, 117). In contrast, adult deletion in the MeA did not affect these behaviors, while prepubertal deletion resulted in a reduced number of mounts and intromissions in adulthood. Ejaculation was not analyzed in this paradigm (29, 117). These results suggested that ERα within the mPOA and VMH is essential for the activational role of estradiol on male sexual behavior, whereas ERα in the MeA may not be crucial in adulthood but is necessary for the organization of the neural circuits underlying sexual behavior during prepuberty. Going further, calcium recordings of Esr1+ neurons activity in the mPOA, using fiber photometry, confirmed that mPOA-Esr1+ neurons were activated during male mounts. Optogenetic stimulation of these neurons elicited mounting behavior, while the inhibition reduced it (118, 119). mPOA-Esr1+ neurons are mostly GABAergic (80%), and specific optogenetic activation of mPOA-Esr1+-Vgat+ neurons increased the mounting response compared to activation of all mPOA-Esr1+ neurons (119). Importantly, in both studies, activation of mPOA-Esr1+ or mPOA-Esr1+-Vgat+ neurons promoted male-typical mounting behavior also in females suggesting that female adult mPOA maintains the functional neural circuits to execute this behavior (118, 119). Thus, it is suggested that sexually dimorphic activation of mPOA-Esr1+ cells would underlie sex differences in the expression of reproductive behaviors. Indeed, as detailed in section 5, the activation of mPOA-Esr1+ cells also regulate female-typical pup retrieval behavior.
Regarding the role of the VMH in regulating male sexual behavior, additional studies showed that unlike the mPOA, adult inhibition of the VMHvl or VMHvl-Esr1+ neurons did not disrupt mounting behavior (120). This suggests that while the mPOA is critical for the expression of male-typical mounting behavior, the VMH may contribute but is not necessary. Although defensive behaviors are not the focus of this review, in males the VMH and especially ERα expressing neurons in this region have been shown to control aggressive behavior (119–121).
Another region known to control sexual behavior is the BNST, a major upstream region of the mPOA and the VMH. Esr1+ neurons of the principal subdivision of the BNST (BNSTpr) were chemogenetically silenced during ongoing social behaviors. The results showed reduced male mounting behavior towards a female when silencing took place during the approach phase, but this reduction was not observed during female mounting (122). However, silencing during the attack interrupted this behavior. Going further with optogenetic silencing of BNST projections, the authors showed that the appetitive phase of male sexual behavior (sniffing to mount) depended primarily on the activity of BNSTpr-mPOA projections, while aggression was triggered by BNST-VMHvl projections. BNST is also a node for olfactory sex recognition, with optogenetic inhibition of BNST-Esr1+ neurons reducing olfactory preference for females over males (122, 123). Interestingly, single-cell calcium measurements showed that different BNST-Esr1+ cell populations responded to male versus female stimuli. The same was observed in the mPOA and VMH-Esr1+ populations. BNSTpr-Esr1+ neurons were therefore found involved in controlling the transition between the appetitive and consummatory phases of male sexual behavior (122).
In females, the ablation of Esr1 specifically in the VMH, in adulthood, was performed by RNA interference, using AAV vectors encoding for small hairpin RNA (shRNA) targeting the Esr1 gene. An 80% reduction in Esr1 expression abolished proceptive as well as lordosis behaviors in female mice (124) and rats (125), in association with a reduced estradiol-induced PR expression in this region (124). In the VMH, the neurons expressing Pgr (coding for PR) co-express Esr1. This neuronal population has been shown to project to the RP3V, the POA and the PAG (126). Interestingly, projections to RP3V showed strong structural plasticity, with more projections in females in estrus stage than in diestrus, suggesting a role for this neuronal population in linking sexual behavior and ovulation. This hormone-driven change was not observed for projections to the POA or PAG. Going further, using electrophysiological and optogenetics studies, the authors showed that these VMH-RP3V projections were mostly glutamatergic, and that ovarian sex hormones enhanced these excitatory projections by increasing the number of glutamatergic synapses formed into RP3V neurons (126).
Regarding the role of MeA or POA in female sexual behavior, the suppression of ERα in the MeA of female rats did not show any effects (125), but the reduction of Esr1 expression specifically in the mPOA increased lordosis behavior in mutant females compared to controls, without modification of proceptive behaviors (127). These results suggested that ERα is involved in the inhibitory action of the mPOA on lordosis behavior. Surprisingly, a study in female mice showed that reduction of Esr1 expression in the mPOA induced a small decrease in receptive behaviors compared to control (128). However, these experiments were performed on gonadally intact female mice that expressed low levels of lordosis behaviors, making it difficult to compare the estradiol-induced effects between control and mutants.
4.2 Role of ERβ in the regulation of sexual behavior
Although ERβ is less expressed than ERα, it is found in most regions involved in the expression of sexual behavior. Fewer studies have investigated its function in the regulation of sexual behavior.
4.2.1 Models of ubiquitous Esr2 deletion
Behavioral characterization of the first lines of ERβKO mice (93) showed no disturbance in any aspects of male (latency and number of mounts and intromissions) or female sexual behaviors (lordosis quotient) evaluated at adulthood (129, 130). Olfactory preference was also unaffected in ERβKO males (130). Nevertheless, a role for ERβ in the defeminization of the brain has been suggested by the increase in female-like lordosis behavior displayed by adult ERβKO males when primed with estradiol and progesterone (130). In addition, a delay in behavioral pubertal maturation was observed, with ERβKO males showing a delay in the age of first ejaculatory behavior (94). Interestingly, ERβKO displayed elevated testosterone levels in pubertal and young adults (5–12 weeks), not found in older mice, supporting a potential role for ERβ during pubertal development (131). It is important to note that in this ERβKO mouse line, some Esr2 transcripts were still present. Different results were observed using the genetic model devoid of all Esr2 transcripts (ERβSTL−/L− (96);). Indeed, in this model, mutant males exhibited mild alteration of sexual behavior, with an increase in the number of mounts and intromissions and in the latency to ejaculate compared to controls. Differences observed between controls and mutants declined with sexual experience (132). In females, ERβSTL−/L− mice showed reduced attractiveness and lordosis behavior (132).
4.2.2 Models of brain specific Esr2 deletion
The first studies were carried out in female rats, in which adult intracerebroventricular administration of an Esr2 antisense oligonucleotide showed no effect on lordosis behavior (133). Then, the role of neural ERβ in regulating sexual behavior was evaluated using ERβNesCre males and females (99, 100). In males, neural Esr2 deletion had no impact on the expression of sexual behavior, with mutant animals displaying the full range of sexual behaviors and achieving ejaculation similarly to wildtypes (100). No change in olfactory preference was observed either. In contrast to what was observed in ubiquitous ERβKO males, the analysis of female-like lordosis behavior in castrated ERβNesCre males primed with estradiol and progesterone did not reveal any significant change between mutants and controls (100). Regarding the females, ERβNesCre animals displayed unchanged olfactory preference and lordosis behavior compared with controls (99). These results showed that neural ERβ is not necessary for the proper expression of male and female sexual behaviors at adulthood.
4.2.3 Model of region-specific and time dependent Esr2 deletion
Few studies have gone further in discriminating between potential developmental versus activational roles of ERβ, and hypothalamic neural pathways involved.
In males, site-specific knockdown of ERβ in MeA and mPOA was obtained by injecting small-hairpin RNA (shRNA)-associated adenoviruses (AAV) into these regions during prepuberty (PND21) or at adulthood (134). Prepubertal or adult silencing of Esr2 in mPOA or MeA did not alter the latencies and numbers of mounts and intromissions in adult males. An alteration in olfactory preference towards receptive versus non-receptive females was observed with adult Esr2 silencing. No alteration was observed when tested for preference between a receptive female and a male (134). Going further, fiber photometry recording did show higher activity of MeA-ERβ+ cells when sniffing a receptive female than when sniffing a non-receptive one or a gonadally intact male. Interestingly, chemogenetic inhibition of these neurons abolished the preference for a receptive female over a non-receptive female, but did not change the preference for a receptive female over a male. The authors also identified that MeA-ERβ+ neuronal projections to the BNST participate in this olfactory preference based on female receptivity but not on sex (135). In the MeA, ERβ appeared to be involved in distinguishing receptive states of female mice.
Very few studies have been done in females. In rats, the silencing of ERβ by injection of an shRNA-associated AAV in the VMH, did not modify the lordosis response (136). In addition, in female mice, injection of shRNA-associated AAV into the dorsal raphe nucleus to specifically knockdown ERβ in adulthood, did not modify the lordosis response on the day of estrus, but led to a sustained lordosis response on the day following behavioral estrus (137). The same observations have been made previously in ubiquitous ERβKO females, suggesting a role for ERβ in the inhibitory regulation of female sexual behavior outside the estrus phase (129).
Collectively, these genetic studies have demonstrated the crucial role of neural ERα in regulating both male and female sexual behavior. Its roles and mechanisms of action are extremely complex and depend on sex, age and brain region. Recent elegant studies, using a combination of innovative techniques, have paved the way for establishing the precise neural pathways at play in the control of these behaviors. Fewer studies have focused on the role of ERβ, as the data indicate a less important role for this receptor in the control of sexual behavior. Indeed, ERβ appears to be more involved in the control of other social and mood related behaviors (99, 138). Nevertheless, studies suggest that ERβ participates in the pubertal organization of neural circuits involved in the control of sexual behavior, at least in males (94, 131). Further studies will be instrumental to go deeper into the mechanism of action and establish whether the same is true in females.
5 Estradiol regulation of parental behavior
In addition to their role in regulating sexual behaviors, several neural regions that express ERα and ERβ are also known to control parental behavior, especially the POA, VMH, BNST, and MeA. Parental behavior is crucial for species survival and offspring development (Figure 3). These brain regions exhibited an increase in Fos expression, a marker of neural activation, in response to the display of maternal behavior. Between 25% and 45% of these Fos+ immunoreactive cells co-expressed ERα, suggesting that maternal behavior possibly involved neural ERα activity (139). Furthermore, natural variations in the level of maternal care were associated with changes in Esr1 expression, but not that of Esr2 (140). This suggests a potential role of ERα in regulating maternal behavior, which was further investigated in the genetic studies described below. To date, no genetic studies have addressed the role of ERβ in the expression of parental behavior.
5.1 Role of ERα in the regulation of parental behavior
5.1.1 Models of ubiquitous Esr1 deletion
Studies using ubiquitous ERαKO animals were performed in mice (104, 111, 112) and more recently in rats (141, 142). Due to the infertility of ERαKO animals, analysis of parental behavior was carried out in nulliparous animals following a pup sensitization process, which involves repeated exposure of the animals to pups produced by donor lactating mothers. Maternal behavior of ERαKO mice was strongly disturbed in both intact and gonadectomized females. Mutant females displayed impaired pup-retrieving behavior and a high level of infanticide (111, 112). In male mice, ubiquitous Esr1 deletion had no effects on pup retrieval, but ERαKO males showed a high percentage of infanticide, that was abolished after gonadectomy (104). The authors suggested that the increase in testosterone levels observed in ERαKO males could be sufficient to promote infanticide, by acting on AR. Surprisingly, in rats, juvenile and adult ERαKO females showed no modification in maternal behavior. The latency score for retrieving and regrouping the pups and adopting a crouched position over them was identical in mutant and control animals (141, 142). Only a slight impairment was observed when the experimental tests were performed in a novel cage (142). The discrepancy with the data obtained in mice is not yet understood, but could result from different underlying mechanisms controlling maternal behavior in the two species. Moreover, deletion strategies diverged between the two ERαKO animal lines, with homologous recombination targeting exon 2 in mice (63, 143), and zinc finger nucleases (ZFNs)-mediated genome editing targeting exon 3 in rats (144). In juvenile male rats, ubiquitous Esr1 deletion did not alter the pup retrieval latency score compared with controls. The level of infanticide was not analyzed in these animals (141).
The discrepancies observed in these models underline the need to conduct genetic research under physiological conditions and to use more targeted approaches of Esr1 deletion in the neural regions of interest. Maternal behavior was only studied in nulliparous females, which did not experience the hormonal changes that occur during gestation and parturition in preparation for behavioral processing. Moreover, males and females lacked stimulation of the ERα signaling pathway during embryonic, postnatal and pubertal development periods, which could interfere with the organization of the neural pathways underlying parental behavior.
5.1.2 Models of region-specific and time dependent Esr1 deletion
Over the last decades, a growing number of studies have focused on region-specific deletions of Esr1, with particular emphasis on the mPOA, the main region controlling parental behavior.
In female mice, reduction of Esr1 expression in the mPOA, via targeted viral-vector mediated siRNA silencing, significantly diminished pup retrieving behavior in sexually naive females (128). A similar behavioral impairment was observed when mPOA-Esr1+ cells were ablated (118) or chemogenetically inhibited (145) in naive and lactating females. In addition, experiments performed optogenetic inhibition of mPOA-Esr1+ neurons at specific time points in the behavioral sequence. This study demonstrated the critical role of these neurons in promoting pup contacts once females have initiated pup approach, and in facilitating the completion of pup retrieval once females have initiated this behavior (118). However, inhibition of these mPOA-Esr1+ neurons after the females had initiated crouching over the pups had no effect on this behavior. Conversely, optogenetic activation of mPOA-Esr1+ cells increased pup retrieval in both naive and lactating females (118, 145). With regards to the expression of other maternal behaviors, the reduction in Esr1+ expression in the mPOA decreased the time the female spent licking and nursing the pups without affecting maternal aggression (128). Fang et al., 2018 also reported that chemogenetic inhibition of mPOA-Esr1+ cells had no effect on sniffing, grooming, and crouching over the pups (145).
In the mPOA, galanin-expressing neurons (mPOA-gal) are crucial for the expression of parental behavior in mice (146, 147). They represent about 20% of mPOA neurons, and the majority of them express ERα. A recent study showed that AAV-mediated ablation of Esr1 in mPOA-gal neurons drastically reduced the expression of pregnancy-induced maternal behaviors, including intensive nest building and pup retrieval behaviors, that occur from the first days of gestation. The parental behavior of these animals remained impaired after birth, indicating that these effects could not be compensated for by the effect of the hormonal milieu during parturition (148). Interestingly, the authors also demonstrated that estradiol play a role in the neural modeling of the mPOA-gal neurons during pregnancy, by transiently silencing mPOA-gal neurons and contradictively increasing their excitability. The deletion of Esr1 in mPOA-gal neurons prevented this remodeling (148). These results indicated that within the mPOA, ERα signaling appears to facilitate pup care behaviors.
Other studies have focused on other parameters of parental behavior, such as increased interest in the pups leading to a reduction in infanticide, while there is an increased aggressivity towards intruders. In this context, Mei et al. (2023) uncovered a neural circuit between the BNSTp and the mPOA that modulates the display of female infanticide towards pups (149). Indeed, although the mechanism underlying this state-dependent switch remains unclear, sexually naive females often kill their pups, while lactating females show maternal care. Interestingly, chemogenetic inhibition of mPOA-Esr1+ cells projecting to the BNSTp increased pup attacks by females, while chemogenetic activation of these cells reduced infanticide. On the contrary, blocking the input from BNSTp-Esr1+ to the mPOA suppressed infanticide, while activating this projection suppressed maternal behavior. Furthermore, in vitro current-clamp recording showed that BNSTp-Esr1+ neuronal population switched from excitable in naive females to less excitable in lactating dams, in contrast to mPOA-Esr1+ cells which became more excitable in lactating dams than in naive females (149). These results support the existence of a neural circuitry remodeling between mPOA and BNSTp during motherhood that is dependent on estrogen signaling. In particular, there appears to be reciprocal inhibition between these two regions to control the levels of infanticide versus maternal behavior.
On the other hand, females typically display low levels of aggression, except during gestation and lactation, when dams show high levels of maternal aggression towards perceived threats to their offspring (150). This behavior is displayed from the first days of gestation, in response to the hormonal changes occurring with pregnancy. The ventrolateral part of the VMH (VMHvl) has been identified as a key hypothalamic region controlling maternal aggression in females. The same region has been extensively studied for its role in territorial aggression in males (151). Importantly, the role of VMHvl neural population in controlling female aggression is dependent on the genetic background and the reproductive state of the animals. Focusing on experiments using lactating females only, it has been showed that VMHvl-Esr1+ cells are critical for maternal aggression. Indeed, using the GCaMP fiber photometry approach, it was shown that VMHvl-Esr1+ cells were activated during attacks by a lactating female towards a juvenile or adult male intruder, but not when the lactating female was investigating or retrieving pups. Moreover, inhibition of VMHvl-Esr1+ cells in these mice drastically decreased aggression against intruders (152). Further studies, described below in 6., have used omics analyses to identify specific neural populations located in the POA and VMH which mediate maternal behaviors, and have differentiated them from cell clusters specifically activated during sexual behavior.
Collectively, these studies provide evidence that ERα regulates maternal behavior in mice. Within the mPOA, ERα appears to facilitate pup care behaviors, while it seems implicated in regulating infanticide in the BNSTp, and maternal aggression in the VMHvl. Because mouse strains used in laboratory experiments are a monoparental species, the investigation of paternal behavior remains very limited. One study in male mice showed that ablation of mPOA-Esr1+ neurons had no effect on pup retrieval behavior, whereas optogenetic activation of these cells induced this behavior (118). These results suggested that unlike in females, mPOA-Esr1+ neurons may not be necessary for the display of pup retrieval in males. Nevertheless, additional studies are needed to establish the role of estradiol in the regulation of paternal behavior, especially using biparental species like prairie voles or Californian mice.
5.2 Lack of models of Esr2 deletions
No genetic study has addressed the role of ERβ in the regulation of parental behavior. Yet this receptor appears to be tightly associated with oxytocin, a key hormone that facilitates maternal behavior. It has been shown that estrogen treatment increased oxytocin gene expression in the rat paraventricular nucleus (153). Oxytocin neurons in this region were found essential for the regulation of emotional and social behaviors, including parental care (for reviews: Acevedo-Rodriguez, Mani & Handa, (154); Neumann, (155)). In the paraventricular nucleus, approximately 84% of oxytocin neurons co-express ERβ, a colocalization not observed in other oxytocinergic regions such as the supraoptic nucleus (156). Estradiol treatment also increased oxytocin expression in the paraventricular nucleus of both male and female mice. This effect was not observed in ERβKO animals (157, 158). These data highlight the need for genetic studies to unravel the implication of ERβ in the regulation of parental behavior, notably in relation to oxytocin.
6 Molecular profiling of brain circuits controlling sex- and reproductive state- dependent reproductive behaviors
In recent years, several innovative techniques have allowed the identification of specific neural populations that control especially male versus female behaviors in the main regions controlling reproductive behaviors (POA, VMH, BNST and MeA).
Combination of single-cell RNA-seq (scRNA-seq) and multiplexed error-robust fluorescence in situ hybridization (MERFISH) analysis of the POA allowed the identification of 70 transcriptomic cell types (T-types), including T-types preferentially activated in females or males during specific social behaviors, particularly parenting, aggression, and mating (159). In this study, by including fos probes in MERFISH measurements, it was shown that cell clusters activated by parenting and mating (i.e. enriched in fos-positive cells) appeared to belong to two transcriptionally distinct cell populations localized into distinct preoptic nuclei (159). Interestingly, Esr1 expression has been found in nearly all behaviorally activated clusters. With regard to mating, it was shown that some clusters enriched in fos-positive cells were activated in both sexes after mating, while a few clusters exhibited sexually dimorphic activation (159).
Several clusters expressing Esr1 in the VMH have also been found to be enriched in a sexually dimorphic manner. For example, Esr1+ cells located in the VMHvl have been shown to co-express PR and control especially female sexual behavior and male aggression (120, 160–162). In males, calcium imaging analysis of VMHvl-Esr1+ neurons showed distinct cell populations activated by male versus female stimuli during resident-intruder assays. Intriguingly, these differences appeared with social and sexual experience, since in naive animals, male and female intruders activated overlapping neuronal populations (163). Going further into synaptic connectivity, viral-genetic tracing in Esr1-Cre male and female mice revealed that most inputs and outputs of VMHvl-Esr1+ neurons were located in the hypothalamus and extended amygdala with a high degree of bidirectional connectivity (164). scRNA-seq analysis identified 17 different sub-types of cells in the VMHvl, including seven Esr1+ T-types (162). Among them, the authors identified two anatomically distinct subsets of VMHvl-Esr1+ neurons, which preferably project to dPAG or mPOA, and would therefore play a role in the control of behavior, or provide feedback to hypothalamic and amygdala circuits, respectively (162, 164). A female-specific cluster has also been identified and subsequently confirmed to be specifically activated during mating (162, 165). Indeed, in females, a reproductive state-dependent switch in female behavior has been revealed, with molecularly distinct subpopulations of VMHvl-Esr1+ neurons excited during maternal aggression or sexual behavior (152, 165). The use of activity-dependent single-cell RNA sequencing allowed the identification of VMHvl T-types specifically activated in either lactating females exhibiting attack or virgins exhibiting lordosis (165). Among these clusters, using several optogenetic manipulations, the authors identified two transcriptomically distinct VMHvl-Esr1+ subtypes and were able to assign causal roles in mating versus aggression in virgins and lactating mothers, respectively. Interestingly, aggression-specific cells displayed changes in bulk-calcium activity depending on the reproductive status, showing an increase in response to social cues (male or female intruders) across the transition from virginity to lactation (165).
A broader approach used translating ribosome affinity purification and sequencing (TRAPseq), and scRNA-seq to identify differentially expressed genes (DEGs) between males and females (comprising females at two estrous stages, estrus and diestrus), specifically in Esr1+ neuronal population. These analyses were carried out in the BNSTpr, MeA, POA and VMHvl. The authors identified 1,415 DEGs between sexes and estrous stages, divided in 137 Esr1+ cell types (166). Almost all Esr1+ cell types co-expressed PR and AR, showing a strong hormonal influence on these populations. This study also confirmed that BNSTpr and POA are mostly GABAergic, the MeA composed of both excitatory and inhibitory neurons, whereas the VMHvl is largely glutamatergic. Nevertheless, a small population of Esr1+ inhibitory neurons was identified in the VMHvl (166). The authors focused on the behavioral role of two specific cell types.
First, BNSTpr-Esr1+-Tac1+ GABAergic neurons were found to be the most enriched in males compared with females, independently of their estrous stage. Chemogenetic inhibition of this population in adult males reduced sex recognition, mounting and intromission during mating, but also attacks during aggression. Interestingly, these effects were not observed when inhibiting BNSTpr-Esr1+-Tac1- neurons, showing the specific involvement of cells expressing both Esr1 and Tac1 in BNSTpr for the regulation of male reproductive behaviors (166). Further studies showed that BNSTpr-Esr1+-Tac1+ neurons project in the POA to POA-Tacr1+ neurons (167) and that these projections regulated male mating but not aggression. POA-Tacr1+ neurons co-express Esr1, and fiber photometry imaging showed that these neurons were activated during mounting and intromission toward females, but not activated during male-male attacks. The neuropeptide substance P encoded by the tachykinin 1 gene (Tac1), is the ligand for the tachykinin receptor 1 (Tac1r). Its release by BNSTpr-Tac1+ cells was shown to potentiate the activation of POA-Tacr1+ neurons and initiate male mating. The authors also revealed that POA-Tacr1+ projections to VTA and PAG control male sexual behavior (167).
Secondly, in females, the authors identified the VMHvl-Esr1+-Cckar+ glutamatergic cell type enriched in females in estrus compared to diestrus. Chemogenetic inhibition of this population highly reduced lordosis behavior without altering maternal behaviors, including pup retrieval and maternal aggression. An additional viral strategy showed that these VMHvl-Esr1+-Cckar+ neurons project preferentially to the AVPV and that these projections peak at estrus. These effects were specific to Esr1+-Cckar+ cells and were not observed in VMHvl-Esr1+-Cckar- neurons. Interestingly, there was no change in the number of projections to the PAG or POA, indicating a specific projection pattern for this neuronal population. In addition, inhibiting VMHvl-Esr1+-Cckar- neurons did not affect lordosis behavior in female but inhibited male mounting behavior and abolished maternal aggression (166).
Altogether these innovative techniques open the way to deciphering the specific neural pathways that control sexually dimorphic reproductive behaviors. Indeed, discrete cell populations appear to be involved in the activation/repression of specific behaviors, according to sex and hormonal state.
7 Discussion and perspectives
Survival of the species relies on the proper regulation of the HPG axis and optimal expression of sexual and parental behavior. These reproductive function and behaviors are strongly influenced by hormonal regulation, especially by estradiol. Extensive research has been carried out since the 1950s, leading to a better comprehension of the specific role of ERα and ERβ and their underlying mechanisms of action. Importantly, all this research points to the existence of an extremely complex neural circuit, which is regulated in different ways according to sex, hormonal status, and age. Recent technological progress has rendered increasingly possible the differentiation of these different parameters in order to obtain a precise view of the neural populations and neural circuits involved in these neuroendocrine and behavioral functions.
The data presented here show that neural ERα is mandatory for the activation and functioning of the gonadotropic axis, expression of sexual and parental behavior in females. In males, it plays a critical role, but it is not indispensable. Indeed, testosterone and its neural metabolite estradiol act in a complementary manner via both AR and ERs in males, with a crucial role established for AR (34, 80). The role of neural ERβ is not entirely clear, but it appears that it is not required for fertility and instead plays a more subtle role in modulating the HPG axis in adults of both sexes. Nevertheless, it is important to bear in mind that the majority of studies analyzing the role of estradiol in regulating the HPG axis have been carried out in females, and that further studies in males are needed to achieve a more thorough understanding. Evidence reported so far has revealed the important role of ERα signaling in the control of maternal behaviors. Further studies, especially in males, will be instrumental in gaining a better understanding of the estradiol regulation of parental behavior.
The recent studies monitoring the activity of Esr1+ cell populations during very specific reproductive behaviors, in different brain regions, as a function of sex and/or hormonal state highlight the existence of a strong neuroplasticity of the brain circuits controlling distinct reproductive behaviors. Interestingly, within the same hypothalamic nucleus, sexually dimorphic activation of different cell clusters corresponds to sexually dimorphic expression of behaviors. Further studies are needed to understand the role of estradiol and other sex steroids in the organization of these neural circuits. Indeed, most of the region-specific deletions have been carried-out in adulthood, but as techniques improve, it is becoming possible to make these genetic modifications at younger ages. It would be particularly interesting with regard to ERβ, whose role in adulthood seems more subtle, but for which several indications point to an implication during pubertal development. It is important to note that, while outside the scope of this review, ERβ is known to play important roles in estradiol regulation of emotional state and social behaviors (99, 138).
Finally, a better understanding of ERs signaling pathways in the regulation of reproductive function and behaviors has an important translational perspective. Indeed, human fertility is under the control of estrogens and ESR1 and/or ESR2 gene mutations and polymorphisms have been associated with reproductive defects in both men and women, including abnormal timing of puberty and infertility (168–175). Loss-of-function mutations in ESR1 induce estrogen resistance in both men and women. The first men case reported normal pubertal development with normal male genitalia and sperm density (168). In addition, the patient had tall stature and delayed skeletal maturation and osteoporosis, with at adulthood a bone age of 15 years old. Indeed, while not in the scope of this review, it is well known that estrogen plays critical role in bone development and mineralization during puberty in both sexes. Testosterone concentrations were normal while estrogen and FSH and LH serum levels were increased. He indicated having sexual interests and had normal functioning, including morning erections and nocturnal emissions. The treatment with estrogen had no detectable effect (168). A loss-of-function ESR1 mutation was identified in a woman without breast development, primary amenorrhea, a small uterus and multicystic ovaries (170). She also had elevated estrogen serum levels, mildly elevated gonadotropins and delayed bone age. Estrogen treatment did not change breast development but diminished ovarian and cyst size (170). Subsequently, few other ESR1 mutations (2 females and 1 male) were identified describing similar clinical phenotypes (174). All suggested estrogen resistance syndrome and were in accordance with the reproductive phenotype of Esr1 ubiquitous knockout mouse models described in section 4.1.1. ESR2 mutations are even rarer. Monoallelic and biallelic ESR2 variants have been identified in one syndromic and two nonsyndromic 46, XY patients with differences of sex development, presenting absent gonadal development or partial and complete gonadal dysgenesis. This suggested a role for ERβ in early gonadal development (175). In addition, a point mutation in ESR2 was identified in a young woman with absent puberty without breast development, a small infantile uterus, no detectable ovaries and severe osteoporosis (173). In contrast to ESR1-deficient patients, estrogen levels were low. Estrogen and progestin replacement therapy enabled breast development, menarche and uterine maturation (173). These results suggest that ESR2 is also necessary for human ovarian development and that ESR1 is not sufficient to support ovarian function in human. ESR1 and ESR2 are expressed in human gonads, making it difficult to distinguish peripheral from central effects in these patients. Some studies have also associated ESR1 and ESR2 gene polymorphisms with infertility and assisted reproduction outcomes (171, 172). Further studies will be needed to understand the neural role of ERα and ERβ in regulating reproductive function in humans. The acquisition of greater knowledge could lead to the development of sex- and age-specific therapeutic strategies for fertility disorders, which represent a major public health issue as their frequency are increasing due to medical, environmental and societal causes.
Author contributions
TT: Writing – original draft, Writing – review & editing. NA: Writing – original draft, Writing – review & editing. SMK: Writing – review & editing. LN: Writing – original draft, Writing – review & editing.
Funding
The author(s) declare financial support was received for the research, authorship, and/or publication of this article. This work was supported by a French Society of Endocrinology Research award 2023 (to LN) and the Agence Nationale de la Recherche (Phtailure, 2018) in France (to SM-K).
Conflict of interest
The authors declare that the research was conducted in the absence of any commercial or financial relationships that could be construed as a potential conflict of interest.
Publisher’s note
All claims expressed in this article are solely those of the authors and do not necessarily represent those of their affiliated organizations, or those of the publisher, the editors and the reviewers. Any product that may be evaluated in this article, or claim that may be made by its manufacturer, is not guaranteed or endorsed by the publisher.
Abbreviations
AAV, Associated adenovirus; AOB, Accessory olfactory bulb; AR, Androgen receptor; ARC, Arcuate nucleus; AVPV, Anteroventral periventricular nucleus; BNST, Bed nucleus of the stria terminalis; BNSTpr, Principal subdivision of the BNST; DEG, Differentially expressed genes; EGFP, Enhanced green fluorescent protein; ER, Estrogen receptor; ERαΔ4, Estrogen receptor alpha delta 4; ER-X, Estrogen receptor X; FSH, Follicle-stimulating hormone; GnRH, Gonadotropin-releasing hormone; GPR30, G protein-coupled receptor 30; HPG, Hypothalamic-pituitary-gonadal; KNDy, Kisspeptin neurokininB dynorphin; LH, Luteinizing hormone; MAPK, Mitogen-activated-protein kinase; MeA, Medial amygdala; mER, Mebrane estrogen receptor; MERFISH, Multiplexed error-robust fluorescence in situ hybridization; mER-Gαq, Membrane estrogen receptor G alpha q; mGluR, Membrane metabotropic glutamate receptor; MOB, Main olfactory bulb; mPOA, Medial preoptic area; PAG, Periaqueductal gray matter; PI-3K, Phosphotidyl inositol 3 kinase; PKA, Protein kinase A; PKC, Protein kinase C; PND, Postnatal day; POA, Preoptic area; PVN, Paraventricular nucleus; RFP, Red fluorescent protein; RP3V, Rostral periventricular area of the third ventricle; scRNA-seq, Single-cell RNA-seq; shRNA, Small-hairpin RNA; STXBP, Saxitoxin binding protein; TRAPseq, Translating ribosome affinity purification and sequencing; T-types, Transcriptomic cell types; VMH, Ventromedial nucleus of the hypothalamus; VTA, Ventral tegmental area; ZFN, Zinc finger nuclease.
References
1. Herbison AE. Chapter 11 - physiology of the adult gonadotropin-releasing hormone neuronal network. In: Plant TM, Zeleznik AJ, editors. Knobil and Neill’s Physiology of Reproduction, 4th ed. Academic Press, San Diego (2015). p. 399–467. Available at: https://www.sciencedirect.com/science/article/pii/B9780123971753000119
2. Tilbrook AJ, Clarke IJ. Negative feedback regulation of the secretion and actions of gonadotropin-releasing hormone in males. Biol Reprod. (2001) 64:735–42. doi: 10.1095/biolreprod64.3.735
3. Herbison AE. Estrogen positive feedback to gonadotropin-releasing hormone (GnRH) neurons in the rodent: the case for the rostral periventricular area of the third ventricle (RP3V). Brain Res Rev. (2008) 57:277–87. doi: 10.1016/j.brainresrev.2007.05.006
4. Naftolin F, Garcia-Segura LM, Horvath TL, Zsarnovszky A, Demir N, Fadiel A, et al. Estrogen-induced hypothalamic synaptic plasticity and pituitary sensitization in the control of the estrogen-induced gonadotrophin surge. Reprod Sci Thousand Oaks Calif. (2007) 14:101–16. doi: 10.1177/1933719107301059
5. Naulé L, Maione L, Kaiser UB. Puberty, A sensitive window of hypothalamic development and plasticity. Endocrinology. (2021) 162:bqaa209. doi: 10.1210/endocr/bqaa209
6. Navarro VM, Gottsch ML, Chavkin C, Okamura H, Clifton DK, Steiner RA. Regulation of gonadotropin-releasing hormone secretion by kisspeptin/dynorphin/neurokinin B neurons in the arcuate nucleus of the mouse. J Neurosci Off J Soc Neurosci. (2009) 29:11859–66. doi: 10.1523/JNEUROSCI.1569-09.2009
7. Clarkson J, d’Anglemont de Tassigny X, Moreno AS, Colledge WH, Herbison AE. Kisspeptin-GPR54 signaling is essential for preovulatory gonadotropin-releasing hormone neuron activation and the luteinizing hormone surge. J Neurosci Off J Soc Neurosci. (2008) 28:8691–7. doi: 10.1523/JNEUROSCI.1775-08.2008
8. Beach FA. Sexual attractivity, proceptivity, and receptivity in female mammals. Horm Behav. (1976) 7:105–38. doi: 10.1016/0018-506X(76)90008-8
9. Powers JB. Hormonal control of sexual receptivity during the estrous cycle of the rat. Physiol Behav. (1970) 5:831–5. doi: 10.1016/0031-9384(70)90167-8
10. Mhaouty-Kodja S, Naulé L, Capela D. Sexual behavior: from hormonal regulation to endocrine disruption. Neuroendocrinology. (2018) 107:400–16. doi: 10.1159/000494558
11. Karlson P, Luscher M. Pheromones’: a new term for a class of biologically active substances. Nature. (1959) 183:55–6. doi: 10.1038/183055a0
12. Brennan PA, Zufall F. Pheromonal communication in vertebrates. Nature. (2006) 444:308–15. doi: 10.1038/nature05404
13. Kevetter GA, Winans SS. Connections of the corticomedial amygdala in the golden hamster. I. Efferents of the “vomeronasal amygdala.” J Comp Neurol. (1981) 197:81–98. doi: 10.1002/cne.901970107
14. Kang N, Baum MJ, Cherry JA. A direct main olfactory bulb projection to the ‘vomeronasal’ amygdala in female mice selectively responds to volatile pheromones from males. Eur J Neurosci. (2009) 29:624–34. doi: 10.1111/j.1460-9568.2009.06638.x
15. Hull EM, Dominguez JM. Sexual behavior in male rodents. Horm Behav. (2007) 52:45–55. doi: 10.1016/j.yhbeh.2007.03.030
16. Sakamoto H. Brain–spinal cord neural circuits controlling male sexual function and behavior. Neurosci Res. (2012) 72:103–16. doi: 10.1016/j.neures.2011.11.002
17. Kow LM, Pfaff DW. Physiology of somatosensory and estrogenic control over the lordosis reflex. Exp Brain Res. (1981) Suppl 3:262–73. doi: 10.1007/978-3-642-45525-4_21
18. Sinchak K, Micevych PE. Progesterone blockade of estrogen activation of mu-opioid receptors regulates reproductive behavior. J Neurosci Off J Soc Neurosci. (2001) 21:5723–9. doi: 10.1523/JNEUROSCI.21-15-05723.2001
19. Bales KL, Saltzman W. Fathering in rodents: Neurobiological substrates and consequences for offspring. Horm Behav. (2016) 77:249–59. doi: 10.1016/j.yhbeh.2015.05.021
20. Keller M, Vandenberg LN, Charlier TD. The parental brain and behavior: A target for endocrine disruption. Front Neuroendocrinol. (2019) 54:100765. doi: 10.1016/j.yfrne.2019.100765
21. Numan M, Insel TR. The neurobiology of parental behavior [Internet]. New York: Springer (2003). Available at: http://site.ebrary.com/id/10130203
22. McRae BR, Andreu V, Marlin BJ. Integration of olfactory and auditory cues eliciting parental behavior. J Neuroendocrinol. (2023) 35:e13307. doi: 10.1111/jne.13307
23. Adam N, Mhaouty-Kodja S. Behavioral effects of exposure to phthalates in female rodents: evidence for endocrine disruption? Int J Mol Sci. (2022) 23:2559. doi: 10.3390/ijms23052559
24. Rosenblatt JS. Nonhormonal basis of maternal behavior in the rat. Science. (1967) 156:1512–4. doi: 10.1126/science.156.3781.1512
25. Phoenix CH, Goy RW, Gerall AA, Young WC. Organizing action of prenatally administered testosterone propionate on the tissues mediating mating behavior in the female Guinea pig. Endocrinology. (1959) 65:369–82. doi: 10.1210/endo-65-3-369
26. Wallen K. The Organizational Hypothesis: Reflections on the 50th anniversary of the publication of Phoenix, Goy, Gerall, and Young (1959). Horm Behav. (2009) 55:561–5. doi: 10.1016/j.yhbeh.2009.03.009
27. Lenz KM, McCarthy MM. Organized for sex – steroid hormones and the developing hypothalamus. Eur J Neurosci. (2010) 32:2096–104. doi: 10.1111/j.1460-9568.2010.07511.x
28. Bakker J, Honda SI, Harada N, Balthazart J. The aromatase knock-out mouse provides new evidence that estradiol is required during development in the female for the expression of sociosexual behaviors in adulthood. J Neurosci. (2002) 22:9104–12. doi: 10.1523/JNEUROSCI.22-20-09104.2002
29. Sano K, Nakata M, Musatov S, Morishita M, Sakamoto T, Tsukahara S, et al. Pubertal activation of estrogen receptor α in the medial amygdala is essential for the full expression of male social behavior in mice. Proc Natl Acad Sci USA. (2016) 113:7632–7. doi: 10.1073/pnas.1524907113
30. Juraska JM, Sisk CL, DonCarlos LL. Sexual differentiation of the adolescent rodent brain: Hormonal influences and developmental mechanisms. Horm Behav. (2013) 64:203–10. doi: 10.1016/j.yhbeh.2013.05.010
31. Schulz KM, Zehr JL, Salas-Ramirez KY, Sisk CL. Testosterone Programs Adult Social Behavior before and during, But Not after, Adolescence. Endocrinology. (2009) 150:3690–8. doi: 10.1210/en.2008-1708
32. George FW, Ojeda SR. Changes in aromatase activity in the rat brain during embryonic, neonatal, and infantile development*. Endocrinology. (1982) 111:522–9. doi: 10.1210/endo-111-2-522
33. Roselli CE, Resko JA. Aromatase activity in the rat brain: Hormonal regulation and sex differences. J Steroid Biochem Mol Biol. (1993) 44:499–508. doi: 10.1016/0960-0760(93)90254-T
34. Mhaouty-Kodja S. Role of the androgen receptor in the central nervous system. Mol Cell Endocrinol. (2018) 465:103–12. doi: 10.1016/j.mce.2017.08.001
35. Sluyser M, Rijkers AWM, De Goeij CCJ, Parker M, Hilkens J. Assignment of estradiol receptor gene to mouse chromosome 10. J Steroid Biochem. (1988) 31:757–61. doi: 10.1016/0022-4731(88)90283-X
36. Tremblay GB, Tremblay A, Copeland NG, Gilbert DJ, Jenkins NA, Labrie F, et al. Cloning, chromosomal localization, and functional analysis of the murine estrogen receptor β. Mol Endocrinol. (1997) 11:353–65. doi: 10.1210/mend.11.3.9902
37. Alves SE, Mcewen BS, Hayashi S, Korach KS, Pfaff DW, Ogawa S. Estrogen-regulated progestin receptors are found in the midbrain raphe but not hippocampus of estrogen receptor alpha (ER)? gene-disrupted mice. J Comp Neurol. (2000) 427:185–95. doi: 10.1002/1096-9861(20001113)427:2<185::AID-CNE2>3.0.CO;2-G
38. Smith JT, Cunningham MJ, Rissman EF, Clifton DK, Steiner RA. Regulation of kiss1 gene expression in the brain of the female mouse. Endocrinology. (2005) 146:3686–92. doi: 10.1210/en.2005-0488
39. Gottsch ML, Navarro VM, Zhao Z, Glidewell-Kenney C, Weiss J, Jameson JL, et al. Regulation of Kiss1 and Dynorphin gene expression in the murine brain by classical and nonclassical estrogen receptor pathways. J Neurosci. (2009) 29:9390–5. doi: 10.1523/JNEUROSCI.0763-09.2009
40. Deb P, Chini A, Guha P, Rishi A, Bhan A, Brady B, et al. Dynamic regulation of BDNF gene expression by estradiol and lncRNA HOTAIR. Gene. (2023) 897:148055. doi: 10.1016/j.gene.2023.148055
41. Sinchak K, Wagner EJ. Estradiol signaling in the regulation of reproduction and energy balance. Front Neuroendocrinol. (2012) 33:342–63. doi: 10.1016/j.yfrne.2012.08.004
42. Micevych P, Dominguez R. Membrane estradiol signaling in the brain. Front Neuroendocrinol. (2009) 30:315–27. doi: 10.1016/j.yfrne.2009.04.011
43. Dewing P, Boulware MI, Sinchak K, Christensen A, Mermelstein PG, Micevych P. Membrane estrogen receptor-α Interactions with metabotropic glutamate receptor 1a modulate female sexual receptivity in rats. J Neurosci. (2007) 27:9294–300. doi: 10.1523/JNEUROSCI.0592-07.2007
44. Dewing P, Christensen A, Bondar G, Micevych P. Protein kinase C signaling in the hypothalamic arcuate nucleus regulates sexual receptivity in female rats. Endocrinology. (2008) 149:5934–42. doi: 10.1210/en.2008-0847
45. Grove-Strawser D, Boulware MI, Mermelstein PG. Membrane estrogen receptors activate the metabotropic glutamate receptors mGluR5 and mGluR3 to bidirectionally regulate CREB phosphorylation in female rat striatal neurons. Neuroscience. (2010) 170:1045–55. doi: 10.1016/j.neuroscience.2010.08.012
46. Johnson CS, Mermelstein PG. The interaction of membrane estradiol receptors and metabotropic glutamate receptors in adaptive and maladaptive estradiol-mediated motivated behaviors in females. Int Rev Neurobiol. (2023) 168:33–91. doi: 10.1016/bs.irn.2022.11.001
47. Mozhui K, Lu L, Armstrong WE, Williams RW. Sex-specific modulation of gene expression networks in murine hypothalamus. Front Neurosci. (2012) 6:63/abstract. doi: 10.3389/fnins.2012.00063/abstract
48. Milner TA, Thompson LI, Wang G, Kievits JA, Martin E, Zhou P, et al. Distribution of estrogen receptor β containing cells in the brains of bacterial artificial chromosome transgenic mice. Brain Res. (2010) 1351:74–96. doi: 10.1016/j.brainres.2010.06.038
49. Mitra SW, Hoskin E, Yudkovitz J, Pear L, Wilkinson HA, Hayashi S, et al. Immunolocalization of estrogen receptor β in the mouse brain: comparison with estrogen receptor α. Endocrinology. (2003) 144:2055–67. doi: 10.1210/en.2002-221069
50. Osterlund M, Kuiper GG, Gustafsson JA, Hurd YL. Differential distribution and regulation of estrogen receptor-alpha and -beta mRNA within the female rat brain. Brain Res Mol Brain Res. (1998) 54:175–80. doi: 10.1016/S0169-328X(97)00351-3
51. Shughrue PJ, Lane MV, Merchenthaler I. Comparative distribution of estrogen receptor-alpha and -beta mRNA in the rat central nervous system. J Comp Neurol. (1997) 388:507–25. doi: 10.1002/(SICI)1096-9861(19971201)388:4<507::AID-CNE1>3.0.CO;2-6
52. Orikasa C, Kondo Y, Hayashi S, McEwen BS, Sakuma Y. Sexually dimorphic expression of estrogen receptor beta in the anteroventral periventricular nucleus of the rat preoptic area: implication in luteinizing hormone surge. Proc Natl Acad Sci USA. (2002) 99:3306–11. doi: 10.1073/pnas.052707299
53. Snyder MA, Smejkalova T, Forlano PM, Woolley CS. Multiple ERβ antisera label in ERβ knockout and null mouse tissues. J Neurosci Methods. (2010) 188:226–34. doi: 10.1016/j.jneumeth.2010.02.012
54. Zuloaga DG, Zuloaga KL, Hinds LR, Carbone DL, Handa RJ. Estrogen receptor β expression in the mouse forebrain: age and sex differences. J Comp Neurol. (2014) 522:358–71. doi: 10.1002/cne.23400
55. Lana LC, Hatsukano T, Sano K, Nakata M, Ogawa S. Sex and age differences in the distribution of estrogen receptors in mice. Neurosci Lett. (2023) 793:136973. doi: 10.1016/j.neulet.2022.136973
56. Gerlach JL, McEwen BS, Toran-Allerand CD, Friedman WJ. Perinatal development of estrogen receptors in mouse brain assessed by radioautography, nuclear isolation and receptor assay. Dev Brain Res. (1983) 11:7–18. doi: 10.1016/0165-3806(83)90197-9
57. Kelly DA, Varnum MM, Krentzel AA, Krug S, Forger NG. Differential control of sex differences in estrogen receptor α in the bed nucleus of the Stria terminalis and anteroventral periventricular nucleus. Endocrinology. (2013) 154:3836–46. doi: 10.1210/en.2013-1239
58. Herbison AE, Pape JR. New evidence for estrogen receptors in gonadotropin-releasing hormone neurons. Front Neuroendocrinol. (2001) 22:292–308. doi: 10.1006/frne.2001.0219
59. Hrabovszky E, Shughrue PJ, Merchenthaler I, Hajszán T, Carpenter CD, Liposits Z, et al. Detection of estrogen receptor-beta messenger ribonucleic acid and 125I-estrogen binding sites in luteinizing hormone-releasing hormone neurons of the rat brain. Endocrinology. (2000) 141:3506–9. doi: 10.1210/endo.141.9.7788
60. Hrabovszky E, Kalló I, Szlávik N, Keller E, Merchenthaler I, Liposits Z. Gonadotropin-releasing hormone neurons express estrogen receptor-beta. J Clin Endocrinol Metab. (2007) 92:2827–30. doi: 10.1210/jc.2006-2819
61. Legan SJ, Tsai HW. Oestrogen receptor-alpha and -beta immunoreactivity in gonadotropin-releasing hormone neurones after ovariectomy and chronic exposure to oestradiol. J Neuroendocrinol. (2003) 15:1164–70. doi: 10.1111/j.1365-2826.2003.01115.x
62. Skynner MJ, Sim JA, Herbison AE. Detection of estrogen receptor alpha and beta messenger ribonucleic acids in adult gonadotropin-releasing hormone neurons. Endocrinology. (1999) 140:5195–201. doi: 10.1210/endo.140.11.7146
63. Lubahn DB, Moyer JS, Golding TS, Couse JF, Korach KS, Smithies O. Alteration of reproductive function but not prenatal sexual development after insertional disruption of the mouse estrogen receptor gene. Proc Natl Acad Sci USA. (1993) 90:11162–6. doi: 10.1073/pnas.90.23.11162
64. Antonson P, Omoto Y, Humire P, Gustafsson JÅ. Generation of ERα-floxed and knockout mice using the Cre/LoxP system. Biochem Biophys Res Commun. (2012) 424:710–6. doi: 10.1016/j.bbrc.2012.07.016
65. Chen M, Wolfe A, Wang X, Chang C, Yeh S, Radovick S. Generation and characterization of a complete null estrogen receptor alpha mouse using Cre/LoxP technology. Mol Cell Biochem. (2009) 321:145–53. doi: 10.1007/s11010-008-9928-9
66. Dupont S, Krust A, Gansmuller A, Dierich A, Chambon P, Mark M. Effect of single and compound knockouts of estrogen receptors alpha (ERalpha) and beta (ERbeta) on mouse reproductive phenotypes. Dev Camb Engl. (2000) 127:4277–91. doi: 10.1242/dev.127.19.4277
67. Feng Y, Manka D, Wagner KU, Khan SA. Estrogen receptor-alpha expression in the mammary epithelium is required for ductal and alveolar morphogenesis in mice. Proc Natl Acad Sci USA. (2007) 104:14718–23. doi: 10.1073/pnas.0706933104
68. Hewitt SC, Kissling GE, Fieselman KE, Jayes FL, Gerrish KE, Korach KS. Biological and biochemical consequences of global deletion of exon 3 from the ER alpha gene. FASEB J Off Publ Fed Am Soc Exp Biol. (2010) 24:4660–7. doi: 10.1096/fj.10-163428
69. Wintermantel TM, Campbell RE, Porteous R, Bock D, Gröne HJ, Todman MG, et al. Definition of estrogen receptor pathway critical for estrogen positive feedback to gonadotropin-releasing hormone neurons and fertility. Neuron. (2006) 52:271–80. doi: 10.1016/j.neuron.2006.07.023
70. Eddy EM, Washburn TF, Bunch DO, Goulding EH, Gladen BC, Lubahn DB, et al. Targeted disruption of the estrogen receptor gene in male mice causes alteration of spermatogenesis and infertility. Endocrinology. (1996) 137:4796–805. doi: 10.1210/endo.137.11.8895349
71. Rissman EF, Wersinger SR, Taylor JA, Lubahn DB. Estrogen receptor function as revealed by knockout studies: neuroendocrine and behavioral aspects. Horm Behav. (1997) 31:232–43. doi: 10.1006/hbeh.1997.1390
72. Wersinger SR, Haisenleder DJ, Lubahn DB, Rissman EF. Steroid feedback on gonadotropin release and pituitary gonadotropin subunit mRNA in mice lacking a functional estrogen receptor alpha. Endocrine. (1999) 11:137–43. doi: 10.1385/ENDO:11:2:137
73. Couse JF, Yates MM, Walker VR, Korach KS. Characterization of the hypothalamic-pituitary-gonadal axis in estrogen receptor (ER) Null mice reveals hypergonadism and endocrine sex reversal in females lacking ERalpha but not ERbeta. Mol Endocrinol Baltim Md. (2003) 17:1039–53. doi: 10.1210/me.2002-0398
74. Dorling AA, Todman MG, Korach KS, Herbison AE. Critical role for estrogen receptor alpha in negative feedback regulation of gonadotropin-releasing hormone mRNA expression in the female mouse. Neuroendocrinology. (2003) 78:204–9. doi: 10.1159/000073703
75. Glidewell-Kenney C, Hurley LA, Pfaff L, Weiss J, Levine JE, Jameson JL. Nonclassical estrogen receptor alpha signaling mediates negative feedback in the female mouse reproductive axis. Proc Natl Acad Sci USA. (2007) 104:8173–7. doi: 10.1073/pnas.0611514104
76. Jakacka M, Ito M, Martinson F, Ishikawa T, Lee EJ, Jameson JL. An estrogen receptor (ER)alpha deoxyribonucleic acid-binding domain knock-in mutation provides evidence for nonclassical ER pathway signaling in vivo. Mol Endocrinol Baltim Md. (2002) 16:2188–201. doi: 10.1210/me.2001-0174
77. McDevitt MA, Glidewell-Kenney C, Weiss J, Chambon P, Jameson JL, Levine JE. Estrogen response element-independent estrogen receptor (ER)-alpha signaling does not rescue sexual behavior but restores normal testosterone secretion in male ERalpha knockout mice. Endocrinology. (2007) 148:5288–94. doi: 10.1210/en.2007-0673
78. Christian CA, Glidewell-Kenney C, Jameson JL, Moenter SM. Classical estrogen receptor alpha signaling mediates negative and positive feedback on gonadotropin-releasing hormone neuron firing. Endocrinology. (2008) 149:5328–34. doi: 10.1210/en.2008-0520
79. Cheong RY, Porteous R, Chambon P, Abrahám I, Herbison AE. Effects of neuron-specific estrogen receptor (ER) α and ERβ deletion on the acute estrogen negative feedback mechanism in adult female mice. Endocrinology. (2014) 155:1418–27. doi: 10.1210/en.2013-1943
80. Trouillet AC, Ducroq S, Naulé L, Capela D, Parmentier C, Radovick S, et al. Deletion of neural estrogen receptor alpha induces sex differential effects on reproductive behavior in mice. Commun Biol. (2022) 5:383. doi: 10.1038/s42003-022-03324-w
81. Greenwald-Yarnell ML, Marsh C, Allison MB, Patterson CM, Kasper C, MacKenzie A, et al. ERα in Tac2 neurons regulates puberty onset in female mice. Endocrinology. (2016) 157:1555–65. doi: 10.1210/en.2015-1928
82. Dubois SL, Acosta-Martínez M, DeJoseph MR, Wolfe A, Radovick S, Boehm U, et al. Positive, but not negative feedback actions of estradiol in adult female mice require estrogen receptor α in kisspeptin neurons. Endocrinology. (2015) 156:1111–20. doi: 10.1210/en.2014-1851
83. Mayer C, Acosta-Martinez M, Dubois SL, Wolfe A, Radovick S, Boehm U, et al. Timing and completion of puberty in female mice depend on estrogen receptor alpha-signaling in kisspeptin neurons. Proc Natl Acad Sci USA. (2010) 107:22693–8. doi: 10.1073/pnas.1012406108
84. McQuillan HJ, Clarkson J, Kauff A, Han SY, Yip SH, Cheong I, et al. Definition of the estrogen negative feedback pathway controlling the GnRH pulse generator in female mice. Nat Commun. (2022) 13:7433. doi: 10.1038/s41467-022-35243-z
85. Wang L, Burger LL, Greenwald-Yarnell ML, Myers MG, Moenter SM. Glutamatergic transmission to hypothalamic kisspeptin neurons is differentially regulated by estradiol through estrogen receptor α in adult female mice. J Neurosci Off J Soc Neurosci. (2018) 38:1061–72. doi: 10.1523/JNEUROSCI.2428-17.2017
86. Ghosh P, Saha SK, Bhattacharya S, Bhattacharya S, Mukherjee S, Roy SS. Tachykinin family genes and their receptors are differentially expressed in the hypothyroid ovary and pituitary. Cell Physiol Biochem Int J Exp Cell Physiol Biochem Pharmacol. (2007) 20:357–68. doi: 10.1159/000107521
87. Pintado CO, Pinto FM, Pennefather JN, Hidalgo A, Baamonde A, Sanchez T, et al. A role for tachykinins in female mouse and rat reproductive function. Biol Reprod. (2003) 69:940–6. doi: 10.1095/biolreprod.103.017111
88. Salehi S, Adeshina I, Chen H, Zirkin BR, Hussain MA, Wondisford F, et al. Developmental and endocrine regulation of kisspeptin expression in mouse Leydig cells. Endocrinology. (2015) 156:1514–22. doi: 10.1210/en.2014-1606
89. Cheong RY, Czieselsky K, Porteous R, Herbison AE. Expression of ESR1 in glutamatergic and GABAergic neurons is essential for normal puberty onset, estrogen feedback, and fertility in female mice. J Neurosci Off J Soc Neurosci. (2015) 35:14533–43. doi: 10.1523/JNEUROSCI.1776-15.2015
90. Wu MV, Tollkuhn J. Estrogen receptor alpha is required in GABAergic, but not glutamatergic, neurons to masculinize behavior. Horm Behav. (2017) 95:3–12. doi: 10.1016/j.yhbeh.2017.07.001
91. Yeo SH, Herbison AE. Estrogen-negative feedback and estrous cyclicity are critically dependent upon estrogen receptor-α expression in the arcuate nucleus of adult female mice. Endocrinology. (2014) 155:2986–95. doi: 10.1210/en.2014-1128
92. Wang L, Vanacker C, Burger LL, Barnes T, Shah YM, Myers MG, et al. Genetic dissection of the different roles of hypothalamic kisspeptin neurons in regulating female reproduction. eLife. (2019) 8:e43999. doi: 10.7554/eLife.43999
93. Krege JH, Hodgin JB, Couse JF, Enmark E, Warner M, Mahler JF, et al. Generation and reproductive phenotypes of mice lacking estrogen receptor beta. Proc Natl Acad Sci USA. (1998) 95:15677–82. doi: 10.1073/pnas.95.26.15677
94. Temple JL, Scordalakes EM, Bodo C, Gustafsson JA, Rissman EF. Lack of functional estrogen receptor beta gene disrupts pubertal male sexual behavior. Horm Behav. (2003) 44:427–34. doi: 10.1016/j.yhbeh.2003.09.002
95. Abrahám IM, Han SK, Todman MG, Korach KS, Herbison AE. Estrogen receptor beta mediates rapid estrogen actions on gonadotropin-releasing hormone neurons in vivo. J Neurosci Off J Soc Neurosci. (2003) 23:5771–7. doi: 10.1523/JNEUROSCI.23-13-05771.2003
96. Antal MC, Krust A, Chambon P, Mark M. Sterility and absence of histopathological defects in nonreproductive organs of a mouse ERbeta-null mutant. Proc Natl Acad Sci USA. (2008) 105:2433–8. doi: 10.1073/pnas.0712029105
97. Kuiper GG, Carlsson B, Grandien K, Enmark E, Häggblad J, Nilsson S, et al. Comparison of the ligand binding specificity and transcript tissue distribution of estrogen receptors alpha and beta. Endocrinology. (1997) 138:863–70. doi: 10.1210/endo.138.3.4979
98. Couse JF, Lindzey J, Grandien K, Gustafsson JA, Korach KS. Tissue distribution and quantitative analysis of estrogen receptor-alpha (ERalpha) and estrogen receptor-beta (ERbeta) messenger ribonucleic acid in the wild-type and ERalpha-knockout mouse. Endocrinology. (1997) 138:4613–21. doi: 10.1210/endo.138.11.5496
99. Naulé L, Robert V, Parmentier C, Martini M, Keller M, Cohen-Solal M, et al. Delayed pubertal onset and prepubertal Kiss1 expression in female mice lacking central oestrogen receptor beta. Hum Mol Genet. (2015) 24:7326–38. doi: 10.1093/hmg/ddv430
100. Naulé L, Marie-Luce C, Parmentier C, Martini M, Albac C, Trouillet AC, et al. Revisiting the neural role of estrogen receptor beta in male sexual behavior by conditional mutagenesis. Horm Behav. (2016) 80:1–9. doi: 10.1016/j.yhbeh.2016.01.014
101. Novaira HJ, Negron AL, Graceli JB, Capellino S, Schoeffield A, Hoffman GE, et al. Impairments in the reproductive axis of female mice lacking estrogen receptor β in GnRH neurons. Am J Physiol Endocrinol Metab. (2018) 315:E1019–33. doi: 10.1152/ajpendo.00173.2018
102. Cheong RY, Kwakowsky A, Barad Z, Porteous R, Herbison AE, Ábrahám IM. Estradiol acts directly and indirectly on multiple signaling pathways to phosphorylate cAMP-response element binding protein in GnRH neurons. Endocrinology. (2012) 153:3792–803. doi: 10.1210/en.2012-1232
103. Ogawa S, Lubahn DB, Korach KS, Pfaff DW. Behavioral effects of estrogen receptor gene disruption in male mice. Proc Natl Acad Sci USA. (1997) 94:1476–81. doi: 10.1073/pnas.94.4.1476
104. Ogawa S, Washburn TF, Taylor J, Lubahn DB, Korach KS, Pfaff DW. Modifications of testosterone-dependent behaviors by estrogen receptor-alpha gene disruption in male mice. Endocrinology. (1998) 139:5058–69. doi: 10.1210/endo.139.12.6358
105. Ogawa S, Chester AE, Hewitt SC, Walker VR, Gustafsson JA, Smithies O, et al. Abolition of male sexual behaviors in mice lacking estrogen receptors alpha and beta (alpha beta ERKO). Proc Natl Acad Sci USA. (2000) 97:14737–41. doi: 10.1073/pnas.250473597
106. Wersinger SR, Sannen K, Villalba C, Lubahn DB, Rissman EF, De Vries GJ. Masculine sexual behavior is disrupted in male and female mice lacking a functional estrogen receptor alpha gene. Horm Behav. (1997) 32:176–83. doi: 10.1006/hbeh.1997.1419
107. Sato T, Matsumoto T, Kawano H, Watanabe T, Uematsu Y, Sekine K, et al. Brain masculinization requires androgen receptor function. Proc Natl Acad Sci USA. (2004) 101:1673–8. doi: 10.1073/pnas.0305303101
108. Ogawa S, Gordan JD, Taylor J, Lubahn D, Korach K, Pfaff DW. Reproductive functions illustrating direct and indirect effects of genes on behavior. Horm Behav. (1996) 30:487–94. doi: 10.1006/hbeh.1996.0052
109. Wersinger SR, Rissman EF. Dopamine activates masculine sexual behavior independent of the estrogen receptor alpha. J Neurosci Off J Soc Neurosci. (2000) 20:4248–54. doi: 10.1523/JNEUROSCI.20-11-04248.2000
110. Gore AC, Wersinger SR, Rissman EF. Effects of female pheromones on gonadotropin-releasing hormone gene expression and luteinizing hormone release in male wild-type and oestrogen receptor-alpha knockout mice. J Neuroendocrinol. (2000) 12:1200–4. doi: 10.1046/j.1365-2826.2000.00578.x
111. Ogawa S, Taylor JA, Lubahn DB, Korach KS, Pfaff DW. Reversal of sex roles in genetic female mice by disruption of estrogen receptor gene. Neuroendocrinology. (1996) 64:467–70. doi: 10.1159/000127154
112. Ogawa S, Eng V, Taylor J, Lubahn DB, Korach KS, Pfaff DW. Roles of estrogen receptor-alpha gene expression in reproduction-related behaviors in female mice. Endocrinology. (1998) 139:5070–81. doi: 10.1210/endo.139.12.6357
113. Kudwa AE, Rissman EF. Double oestrogen receptor alpha and beta knockout mice reveal differences in neural oestrogen-mediated progestin receptor induction and female sexual behaviour. J Neuroendocrinol. (2003) 15:978–83. doi: 10.1046/j.1365-2826.2003.01089.x
114. Rissman EF, Early AH, Taylor JA, Korach KS, Lubahn DB. Estrogen receptors are essential for female sexual receptivity. Endocrinology. (1997) 138:507–10. doi: 10.1210/endo.138.1.4985
115. Couse JF, Curtis SW, Washburn TF, Lindzey J, Golding TS, Lubahn DB, et al. Analysis of transcription and estrogen insensitivity in the female mouse after targeted disruption of the estrogen receptor gene. Mol Endocrinol Baltim Md. (1995) 9:1441–54. doi: 10.1210/mend.9.11.8584021
116. Khbouz B, de Bournonville C, Court L, Taziaux M, Corona R, Arnal JF, et al. Role for the membrane estrogen receptor alpha in the sexual differentiation of the brain. Eur J Neurosci. (2020) 52:2627–45. doi: 10.1111/ejn.14646
117. Sano K, Tsuda MC, Musatov S, Sakamoto T, Ogawa S. Differential effects of site-specific knockdown of estrogen receptor α in the medial amygdala, medial pre-optic area, and ventromedial nucleus of the hypothalamus on sexual and aggressive behavior of male mice. Eur J Neurosci. (2013) 37:1308–19. doi: 10.1111/ejn.12131
118. Wei YC, Wang SR, Jiao ZL, Zhang W, Lin JK, Li XY, et al. Medial preoptic area in mice is capable of mediating sexually dimorphic behaviors regardless of gender. Nat Commun. (2018) 9:279. doi: 10.1038/s41467-017-02648-0
119. Karigo T, Kennedy A, Yang B, Liu M, Tai D, Wahle IA, et al. Distinct hypothalamic control of same- and opposite-sex mounting behaviour in mice. Nature. (2021) 589:258–63. doi: 10.1038/s41586-020-2995-0
120. Lee H, Kim DW, Remedios R, Anthony TE, Chang A, Madisen L, et al. Scalable control of mounting and attack by Esr1+ neurons in the ventromedial hypothalamus. Nature. (2014) 509:627–32. doi: 10.1038/nature13169
121. Lin D, Boyle MP, Dollar P, Lee H, Lein ES, Perona P, et al. Functional identification of an aggression locus in the mouse hypothalamus. Nature. (2011) 470:221–6. doi: 10.1038/nature09736
122. Yang B, Karigo T, Anderson DJ. Transformations of neural representations in a social behaviour network. Nature. (2022) 608:741–9. doi: 10.1038/s41586-022-05057-6
123. Bayless DW, Yang T, Mason MM, Susanto AAT, Lobdell A, Shah NM. Limbic neurons shape sex recognition and social behavior in sexually naive males. Cell. (2019) 176:1190–205.e20. doi: 10.1016/j.cell.2018.12.041
124. Musatov S, Chen W, Pfaff DW, Kaplitt MG, Ogawa S. RNAi-mediated silencing of estrogen receptor α in the ventromedial nucleus of hypothalamus abolishes female sexual behaviors. Proc Natl Acad Sci USA. (2006) 103:10456–60. doi: 10.1073/pnas.0603045103
125. Spiteri T, Musatov S, Ogawa S, Ribeiro A, Pfaff DW, Agmo A. Estrogen-induced sexual incentive motivation, proceptivity and receptivity depend on a functional estrogen receptor alpha in the ventromedial nucleus of the hypothalamus but not in the amygdala. Neuroendocrinology. (2010) 91:142–54. doi: 10.1159/000255766
126. Inoue S, Yang R, Tantry A, Davis CH, Yang T, Knoedler JR, et al. Periodic remodeling in a neural circuit governs timing of female sexual behavior. Cell. (2019) 179:1393–408. doi: 10.1016/j.cell.2019.10.025
127. Spiteri T, Ogawa S, Musatov S, Pfaff DW, Agmo A. The role of the estrogen receptor α in the medial preoptic area in sexual incentive motivation, proceptivity and receptivity, anxiety, and wheel running in female rats. Behav Brain Res. (2012) 230:11–20. doi: 10.1016/j.bbr.2012.01.048
128. Ribeiro AC, Musatov S, Shteyler A, Simanduyev S, Arrieta-Cruz I, Ogawa S, et al. siRNA silencing of estrogen receptor-α expression specifically in medial preoptic area neurons abolishes maternal care in female mice. Proc Natl Acad Sci. (2012) 109:16324–9. doi: 10.1073/pnas.1214094109
129. Ogawa S, Chan J, Chester AE, Gustafsson JA, Korach KS, Pfaff DW. Survival of reproductive behaviors in estrogen receptor beta gene-deficient (betaERKO) male and female mice. Proc Natl Acad Sci USA. (1999) 96:12887–92. doi: 10.1073/pnas.96.22.12887
130. Kudwa AE, Bodo C, Gustafsson JA, Rissman EF. A previously uncharacterized role for estrogen receptor beta: defeminization of male brain and behavior. Proc Natl Acad Sci USA. (2005) 102:4608–12. doi: 10.1073/pnas.0500752102
131. Nomura M, Durbak L, Chan J, Smithies O, Gustafsson JA, Korach KS, et al. Genotype/age interactions on aggressive behavior in gonadally intact estrogen receptor beta knockout (betaERKO) male mice. Horm Behav. (2002) 41:288–96. doi: 10.1006/hbeh.2002.1773
132. Antal MC, Petit-Demoulière B, Meziane H, Chambon P, Krust A. Estrogen dependent activation function of ERβ is essential for the sexual behavior of mouse females. Proc Natl Acad Sci USA. (2012) 109:19822–7. doi: 10.1073/pnas.1217668109
133. Walf AA, Ciriza I, Garcia-Segura LM, Frye CA. Antisense oligodeoxynucleotides for estrogen receptor-beta and alpha attenuate estradiol’s modulation of affective and sexual behavior, respectively. Neuropsychopharmacol Off Publ Am Coll Neuropsychopharmacol. (2008) 33:431–40. doi: 10.1038/sj.npp.1301416
134. Nakata M, Sano K, Musatov S, Yamaguchi N, Sakamoto T, Ogawa S. Effects of prepubertal or adult site-specific knockdown of estrogen receptor β in the medial preoptic area and medial amygdala on social behaviors in male mice. eNeuro. (2016) 3(2):ENEURO.0155-15.2016. doi: 10.1523/ENEURO.0155-15.2016
135. Takenawa S, Nagasawa Y, Go K, Chérasse Y, Mizuno S, Sano K, et al. Activity of estrogen receptor β expressing neurons in the medial amygdala regulates preference toward receptive females in male mice. Proc Natl Acad Sci USA. (2023) 120:e2305950120. doi: 10.1073/pnas.2305950120
136. Le Moëne O, Stavarache M, Ogawa S, Musatov S, Ågmo A. Estrogen receptors α and β in the central amygdala and the ventromedial nucleus of the hypothalamus: Sociosexual behaviors, fear and arousal in female rats during emotionally challenging events. Behav Brain Res. (2019) 367:128–42. doi: 10.1016/j.bbr.2019.03.045
137. Sano K, Morimoto C, Nataka M, Musatov S, Tsuda MC, Yamaguchi N, et al. The role of estrogen receptor β in the dorsal raphe nucleus on the expression of female sexual behavior in C57BL/6J mice. Front Endocrinol. (2018) 9:243. doi: 10.3389/fendo.2018.00243
138. Dombret C, Naulé L, Trouillet AC, Parmentier C, Hardin-Pouzet H, Mhaouty-Kodja S. Effects of neural estrogen receptor beta deletion on social and mood-related behaviors and underlying mechanisms in male mice. Sci Rep. (2020) 10:6242. doi: 10.1038/s41598-020-63427-4
139. Lonstein JS, Gréco B, De Vries GJ, Stern JM, Blaustein JD. Maternal Behavior Stimulates c-fos Activity within Estrogen Receptor Alpha-Containing Neurons in Lactating Rats. Neuroendocrinology. (2000) 72:91–101. doi: 10.1159/000054576
140. Champagne FA, Weaver ICG, Diorio J, Sharma S, Meaney MJ. Natural variations in maternal care are associated with estrogen receptor α Expression and estrogen sensitivity in the medial preoptic area. Endocrinology. (2003) 144:4720–4. doi: 10.1210/en.2003-0564
141. Moran CR, Gallagher JM, Bridges RS. The role of the estrogen receptor-α gene, Esr1, in maternal-like behavior in juvenile female and male rats. Physiol Behav. (2020) 216:112797. doi: 10.1016/j.physbeh.2020.112797
142. Gallagher JM, Nephew BC, Poirier G, King JA, Bridges RS. Estrogen receptor-alpha knockouts and maternal memory in nulliparous rats. Horm Behav. (2019) 110:40–5. doi: 10.1016/j.yhbeh.2019.02.002
143. Korach KS, Couse JF, Curtis SW, Washburn TF, Lindzey J, Kimbro KS, et al. Estrogen receptor gene disruption: molecular characterization and experimental and clinical phenotypes. Recent Prog Horm Res. (1996) 51:159–86
144. Rumi MAK, Dhakal P, Kubota K, Chakraborty D, Lei T, Larson MA, et al. Generation of Esr1-knockout rats using zinc finger nuclease-mediated genome editing. Endocrinology. (2014) 155:1991–9. doi: 10.1210/en.2013-2150
145. Fang YY, Yamaguchi T, Song SC, Tritsch NX, Lin D. A hypothalamic midbrain pathway essential for driving maternal behaviors. Neuron. (2018) 98:192–207.e10. doi: 10.1016/j.neuron.2018.02.019
146. Wu Z, Autry AE, Bergan JF, Watabe-Uchida M, Dulac CG. Galanin neurons in the medial preoptic area govern parental behaviour. Nature. (2014) 509:325–30. doi: 10.1038/nature13307
147. Kohl J, Babayan BM, Rubinstein ND, Autry AE, Marin-Rodriguez B, Kapoor V, et al. Functional circuit architecture underlying parental behaviour. Nature. (2018) 556:326–31. doi: 10.1038/s41586-018-0027-0
148. Ammari R, Monaca F, Cao M, Nassar E, Wai P, Del Grosso NA, et al. Hormone-mediated neural remodeling orchestrates parenting onset during pregnancy. Science. (2023) 382:76–81. doi: 10.1126/science.adi0576
149. Mei L, Yan R, Yin L, Sullivan RM, Lin D. Antagonistic circuits mediating infanticide and maternal care in female mice. Nature. (2023) 618:1006–16. doi: 10.1038/s41586-023-06147-9
150. Lonstein JS, Gammie SC. Sensory, hormonal, and neural control of maternal aggression in laboratory rodents. Neurosci Biobehav Rev. (2002) 26:869–88. doi: 10.1016/S0149-7634(02)00087-8
151. Mei L, Osakada T, Lin D. Hypothalamic control of innate social behaviors. Science. (2023) 382:399–404. doi: 10.1126/science.adh8489
152. Hashikawa K, Hashikawa Y, Tremblay R, Zhang J, Feng JE, Sabol A, et al. Esr1+ cells in the ventromedial hypothalamus control female aggression. Nat Neurosci. (2017) 20:1580–90. doi: 10.1038/nn.4644
153. Amico JA, Thomas A, Hollingshead DJ. The duration of estradiol and progesterone exposure prior to progesterone withdrawal regulates oxytocin Mrna levels in the paraventricular nucleus of the rat. Endocr Res. (1997) 23:141–56. doi: 10.3109/07435809709031849
154. Acevedo-Rodriguez A, Mani SK, Handa RJ. Oxytocin and estrogen receptor β in the brain: an overview. Front Endocrinol. (2015) 6:160/abstract. doi: 10.3389/fendo.2015.00160/abstract
155. Neumann ID. Brain oxytocin: a key regulator of emotional and social behaviours in both females and males. J Neuroendocrinol. (2008) 20:858–65. doi: 10.1111/j.1365-2826.2008.01726.x
156. Suzuki S, Handa RJ. Estrogen receptor-?, but not estrogen receptor-?, is expressed in prolactin neurons of the female rat paraventricular and supraoptic nuclei: Comparison with other neuropeptides. J Comp Neurol. (2005) 484:28–42. doi: 10.1002/cne.20457
157. Patisaul HB, Scordalakes EM, Young LJ, Rissman EF. Oxytocin, but not oxytocin receptor, is regulated by oestrogen receptor β in the female mouse hypothalamus: regulatory role for ERβ. J Neuroendocrinol. (2003) 15:787–93. doi: 10.1046/j.1365-2826.2003.01061.x
158. Nomura M, McKenna E, Korach KS, Pfaff DW, Ogawa S. Estrogen receptor-β regulates transcript levels for oxytocin and arginine vasopressin in the hypothalamic paraventricular nucleus of male mice. Mol Brain Res. (2002) 109:84–94. doi: 10.1016/S0169-328X(02)00525-9
159. Moffitt JR, Bambah-Mukku D, Eichhorn SW, Vaughn E, Shekhar K, Perez JD, et al. Molecular, spatial, and functional single-cell profiling of the hypothalamic preoptic region. Science. (2018) 362:eaau5324. doi: 10.1126/science.aau5324
160. Yang CF, Chiang MC, Gray DC, Prabhakaran M, Alvarado M, Juntti SA, et al. Sexually dimorphic neurons in the ventromedial hypothalamus govern mating in both sexes and aggression in males. Cell. (2013) 153:896–909. doi: 10.1016/j.cell.2013.04.017
161. Yang T, Yang CF, Chizari MD, Maheswaranathan N, Burke KJ, Borius M, et al. Social control of hypothalamus-mediated male aggression. Neuron. (2017) 95:955–970.e4. doi: 10.1016/j.neuron.2017.06.046
162. Kim DW, Yao Z, Graybuck LT, Kim TK, Nguyen TN, Smith KA, et al. Multimodal analysis of cell types in a hypothalamic node controlling social behavior. Cell. (2019) 179:713–728.e17. doi: 10.1016/j.cell.2019.09.020
163. Remedios R, Kennedy A, Zelikowsky M, Grewe BF, Schnitzer MJ, Anderson DJ. Social behaviour shapes hypothalamic neural ensemble representations of conspecific sex. Nature. (2017) 550:388–92. doi: 10.1038/nature23885
164. Lo L, Yao S, Kim DW, Cetin A, Harris J, Zeng H, et al. Connectional architecture of a mouse hypothalamic circuit node controlling social behavior. Proc Natl Acad Sci USA. (2019) 116:7503–12. doi: 10.1073/pnas.1817503116
165. Liu M, Kim DW, Zeng H, Anderson DJ. Make war not love: The neural substrate underlying a state-dependent switch in female social behavior. Neuron. (2022) 110:841–56. doi: 10.1016/j.neuron.2021.12.002
166. Knoedler JR, Inoue S, Bayless DW, Yang T, Tantry A, Davis C, et al. A functional cellular framework for sex and estrous cycle-dependent gene expression and behavior. Cell. (2022) 185:654–71.e22. doi: 10.1016/j.cell.2021.12.031
167. Bayless DW, Davis CHO, Yang R, Wei Y, de Andrade Carvalho VM, Knoedler JR, et al. A neural circuit for male sexual behavior and reward. Cell. (2023) 186:3862–81.e28. doi: 10.1016/j.cell.2023.07.021
168. Smith EP, Boyd J, Frank GR, Takahashi H, Cohen RM, Specker B, et al. Estrogen resistance caused by a mutation in the estrogen-receptor gene in a man. N Engl J Med. (1994) 331:1056–61. doi: 10.1056/NEJM199410203311604
169. Paskulin DD, Cunha-Filho JS, Paskulin LD, Souza CAB, Ashton-Prolla P. ESR1 rs9340799 is associated with endometriosis-related infertility and in vitro fertilization failure. Dis Markers. (2013) 35:907–13. doi: 10.1155/2013/796290
170. Quaynor SD, Stradtman EW, Kim HG, Shen Y, Chorich LP, Schreihofer DA, et al. Delayed puberty and estrogen resistance in a woman with estrogen receptor α variant. N Engl J Med. (2013) 369:164–71. doi: 10.1056/NEJMoa1303611
171. Ge YZ, Xu LW, Jia RP, Xu Z, Li WC, Wu R, et al. Association of polymorphisms in estrogen receptors (ESR1 and ESR2) with male infertility: a meta-analysis and systematic review. J Assist Reprod Genet. (2014) 31:601–11. doi: 10.1007/s10815-014-0212-5
172. de Mattos CS, Trevisan CM, Peluso C, Adami F, Cordts EB, Christofolini DM, et al. ESR1 and ESR2 gene polymorphisms are associated with human reproduction outcomes in Brazilian women. J Ovarian Res. (2014) 7:114. doi: 10.1186/s13048-014-0114-2
173. Lang-Muritano M, Sproll P, Wyss S, Kolly A, Hürlimann R, Konrad D, et al. Early-onset complete ovarian failure and lack of puberty in a woman with mutated estrogen receptor β (ESR2). J Clin Endocrinol Metab. (2018) 103:3748–56. doi: 10.1210/jc.2018-00769
174. Bernard V, Kherra S, Francou B, Fagart J, Viengchareun S, Guéchot J, et al. Familial multiplicity of estrogen insensitivity associated with a loss-of-function ESR1 mutation. J Clin Endocrinol Metab. (2017) 102:93–9. doi: 10.1210/jc.2016-2749
Keywords: estrogen receptors, hypothalamic-pituitary-gonadal axis, reproductive behaviors, sex steroids, neuroendocrinology
Citation: Torres T, Adam N, Mhaouty-Kodja S and Naulé L (2024) Reproductive function and behaviors: an update on the role of neural estrogen receptors alpha and beta. Front. Endocrinol. 15:1408677. doi: 10.3389/fendo.2024.1408677
Received: 28 March 2024; Accepted: 29 May 2024;
Published: 24 June 2024.
Edited by:
Alexandre Benani, UMR6265 Centre des Sciences du Goût et de l’Alimentation (CSGA) Dijon, FranceReviewed by:
Carolina Dalmasso, University of Kentucky, United StatesRosaria Meccariello, University of Naples Parthenope, Italy
Ebtesam Abdullah Al-Suhaimi, King Abdulaziz and His Companions Foundation for Giftedness and Creativity, Saudi Arabia
Copyright © 2024 Torres, Adam, Mhaouty-Kodja and Naulé. This is an open-access article distributed under the terms of the Creative Commons Attribution License (CC BY). The use, distribution or reproduction in other forums is permitted, provided the original author(s) and the copyright owner(s) are credited and that the original publication in this journal is cited, in accordance with accepted academic practice. No use, distribution or reproduction is permitted which does not comply with these terms.
*Correspondence: Lydie Naulé, bHlkaWUubmF1bGVAc29yYm9ubmUtdW5pdmVyc2l0ZS5mcg==