- 1Department of Ophthalmology, The First Affiliated Hospital of Zhengzhou University, Zhengzhou, Henan, China
- 2Department of Ophthalmology, The Second Xiangya Hospital, Central South University, Changsha, Hunan, China
- 3Hunan Clinical Research Centre of Ophthalmic Disease, Changsha, Hunan, China
- 4Department of Ophthalmology, The First Affiliated Hospital of Henan University of Chinese Medicine, Zhengzhou, Henan, China
- 5Manchester Royal Eye Hospital, Manchester University NHS Foundation Trust, Manchester, United Kingdom
- 6Division of Pharmacy and Optometry, School of Health Sciences, Faculty of Biology, Medicine and Health, The University of Manchester, Manchester, United Kingdom
Thyroid-associated ophthalmopathy (TAO) is an autoimmune condition affecting the eyes, characterized by proptosis, extraocular muscle involvement, and in severe cases, vision impairment including diplopia, optic neuropathy, and potential blindness. The exact etiology of TAO remains elusive; however, increased oxidative stress and decreased antioxidant capacity are pivotal in its pathogenesis. Elevated oxidative stress not only directly damages orbital tissues but also influences thyroid function and autoimmune responses, exacerbating tissue destruction. This review explores the role of oxidative stress in TAO, elucidates its mechanisms, and evaluates the efficacy and limitations of antioxidant therapies in managing TAO. The findings aim to enhance understanding of oxidative stress mechanisms in TAO and propose potential antioxidant strategies for future therapeutic development.
1 Introduction
Thyroid-associated ophthalmopathy (TAO), also known as Graves’ ophthalmopathy, is an autoimmune condition affecting the eyes, orbit, and surrounding structures (1). TAO presents with a spectrum of ocular manifestations in clinical practice. Symptoms include eyelid retraction, proptosis, forward displacement of the eyeball, periorbital edema, conjunctival injection, and dysfunction of the extraocular muscles (2). Additionally, TAO can impair vision, causing diplopia, reduced visual acuity, and in severe cases, blindness (3). The severity of TAO varies among individuals, significantly impacting quality of life (4). Patients often experience visual disturbances, facial deformities, psychological distress, and increased risk of suicide (5). It is considered the most prevalent orbital disease in adults. While typically associated with Graves’ disease—an autoimmune disorder causing hyperthyroidism—TAO can also occur in patients with normal thyroid function or hypothyroidism, affecting 25% to 58% of those with Graves’ disease (6). Therefore, extensive research is essential to elucidate TAO’s pathogenesis, identify new therapeutic targets, and establish precise treatment strategies.
Despite the intricate interplay of genetic, environmental, and immune factors, the exact mechanisms underlying TAO remain incompletely understood (7). Autoantibodies, such as thyroid stimulating immunoglobulin, likely initiate autoimmune reactions by binding to thyroid stimulating hormone receptors on orbital fibroblasts (8). This triggers production of proinflammatory cytokines, fibroblasts activation, and recruitment of immune cells, resulting in tissue inflammation, swelling, and fibrosis (9). Recent studies have implicated oxidative stress in the pathogenesis of TAO (10). Oxidative stress arises when the production of reactive oxygen species (ROS) exceeds the body’s antioxidant defense mechanisms (11). Patients with TAO exhibit elevated oxidative stress markers, including increased ROS levels, diminished antioxidant capacity, and accumulation of oxidative byproducts in ocular tissues (12, 13). These findings suggest that immune dysfunction and inflammation induced by oxidative stress play significant roles in ocular manifestations associated with TAO.
Oxidative stress contributes to TAO through multiple mechanisms. Firstly, it directly damages ocular tissues, provoking inflammation and immune cell infiltration (14). Secondly, oxidative stress impacts thyroid function and autoimmune responses, indirectly influencing ocular pathology (15). Furthermore, interactions between oxidative stress, inflammation, and autophagy may exacerbate TAO (16). Researchers are actively investigating the role of oxidative stress pathways in TAO to develop novel therapeutic approaches. Currently, antioxidant therapies aimed at neutralizing free radicals have shown promising results in clinical settings (17). Future efforts should focus on elucidating oxidative stress mechanisms in TAO and advancing treatment strategies to enhance patient outcomes and quality of life.
2 Oxidative stress in TAO
2.1 Basic concept of oxidative stress
Oxidative stress occurs when there is an imbalance between ROS production and the antioxidant defense systems capacity to neutralize them (18). ROS are natural byproducts of cellular metabolism and play essential roles in physiological processes (19). Maintaining ROS within a balanced range is crucial for cellular homeostasis; excess ROS or insufficient antioxidants can lead to oxidative damage and cellular dysfunction (20).
Oxidative stress plays an important role in the pathomechanism of TAO. Oxidative stress is the disruption of the balance between oxygen free radicals and antioxidant systems in the body, leading to cellular damage and tissue injury. Studies have shown that oxidative stress not only directly damages orbital tissues, but also promotes inflammatory responses and fibrosis by activating various signaling pathways (21). Inflammatory response is another important pathological mechanism of TAO. There is a large infiltration of inflammatory cells, such as T cells, B cells, and macrophages, in the orbital tissues of TAO patients. These inflammatory cells further exacerbate orbital tissue damage by secreting various inflammatory factors, such as interleukin-6 (IL-6) and tumor necrosis factor-α (TNF-α) (22). In addition, fibrosis is the final pathological change in the pathologic process of TAO. In TAO, activation and differentiation of fibroblasts are critical steps in the development of fibrosis. Studies have shown that transforming growth factor-β1 (TGF-β1) is an important regulator of TAO fibrosis, which promotes the proliferation of fibroblasts and collagen synthesis by activating the Smad signaling pathway (23). Moreover, oxidative stress and inflammatory responses can also promote fibrosis by activating the TGF-β1 signaling pathway (24). Thus, oxidative stress, inflammatory response, and fibrosis are key mechanisms that are interrelated and mutually reinforcing in the pathologic process of thyroid-related eye disease.
Various lifestyle factors contribute to oxidative stress, potentially triggering the development of TAO, including smoking, environmental exposures, and sleep disturbances (25). Under normal conditions, the body’s antioxidant defenses regulate ROS levels effectively (26). Enzymatic antioxidants such as superoxide dismutase (SOD), catalase, and glutathione peroxidase play pivotal roles in converting ROS like superoxide anions (O2-) into less harmful substances such as hydrogen peroxide (H2O2), which are subsequently detoxified by catalase and glutathione peroxidase (27, 28). Non-enzymatic antioxidants like vitamins E and C, along with glutathione, function as electron donors or scavengers to directly neutralize ROS, thus maintaining cellular redox balance and preventing oxidative damage (29).
Oxidative stress disrupts cellular redox balance, leading to excessive production of oxidants that initiate harmful oxidative reactions. Further investigations reveals that oxidative stress can be categorized as follows (Figure 1):
1. Mitochondrial oxidative stress: Mitochondria, crucial for cellular energy production, are primary targets of oxidative stress. Dysfunction in mitochondria can lead to electron leakage in the electron transport chain, resulting in overproduction of ROS such as superoxide anions and hydrogen peroxide (30). These oxidative species can damage mitochondrial DNA, peroxidize membrane lipids, oxidize proteins, and exacerbate mitochondrial dysfunction, creating a detrimental cycle (31).
2. Endoplasmic reticulum oxidative stress: The endoplasmic reticulum (ER), responsible for protein synthesis and folding, is vulnerable to oxidative stress. Disruption in ER function or sudden accumulation of misfolded proteins triggers ER stress responses (32). Oxidative stress in the ER can induce protein oxidation, thiol oxidation, alter calcium ion homeostasis, and perturb cellular signal transduction (33).
3. Lysosomal oxidative stress: Lysosomes, involved in cellular waste degradation, contain enzymes and metal ions that can generate oxidative compounds such as hydrogen peroxide and hydroxyl radicals under oxidative stress conditions (34, 35). Elevated oxidative stress can damage lysosomal membranes and disrupt the acidic internal environment essential for lysosomal function.
4. Nuclear oxidative stress: The nucleus, housing genetic material, is susceptible to oxidative damage. Oxidative stress can induce nuclear DNA damage, alter chromatin structure, interfere with gene transcription, and increase cellular susceptibility to DNA damage (36, 37).
5. Environmental exposure, smoking and late nights: Environmental pollutants, including air pollutants, heavy metals, and chemicals, contribute to oxidative stress by inducing ROS production through oxidation reactions upon exposure via respiratory, digestive, or dermal routes (38). Tobacco smoke, containing harmful chemicals like carbon monoxide and polycyclic aromatic hydrocarbons, directly increases ROS formation upon inhalation, significantly impacting antioxidant defenses and causing cellular damage (39). Sleep disturbances, such as chronic sleep deprivation or circadian rhythm disruption from staying up late, elevate oxidative stress by altering antioxidant enzyme function, impairing immune responses, and promoting inflammation (40).
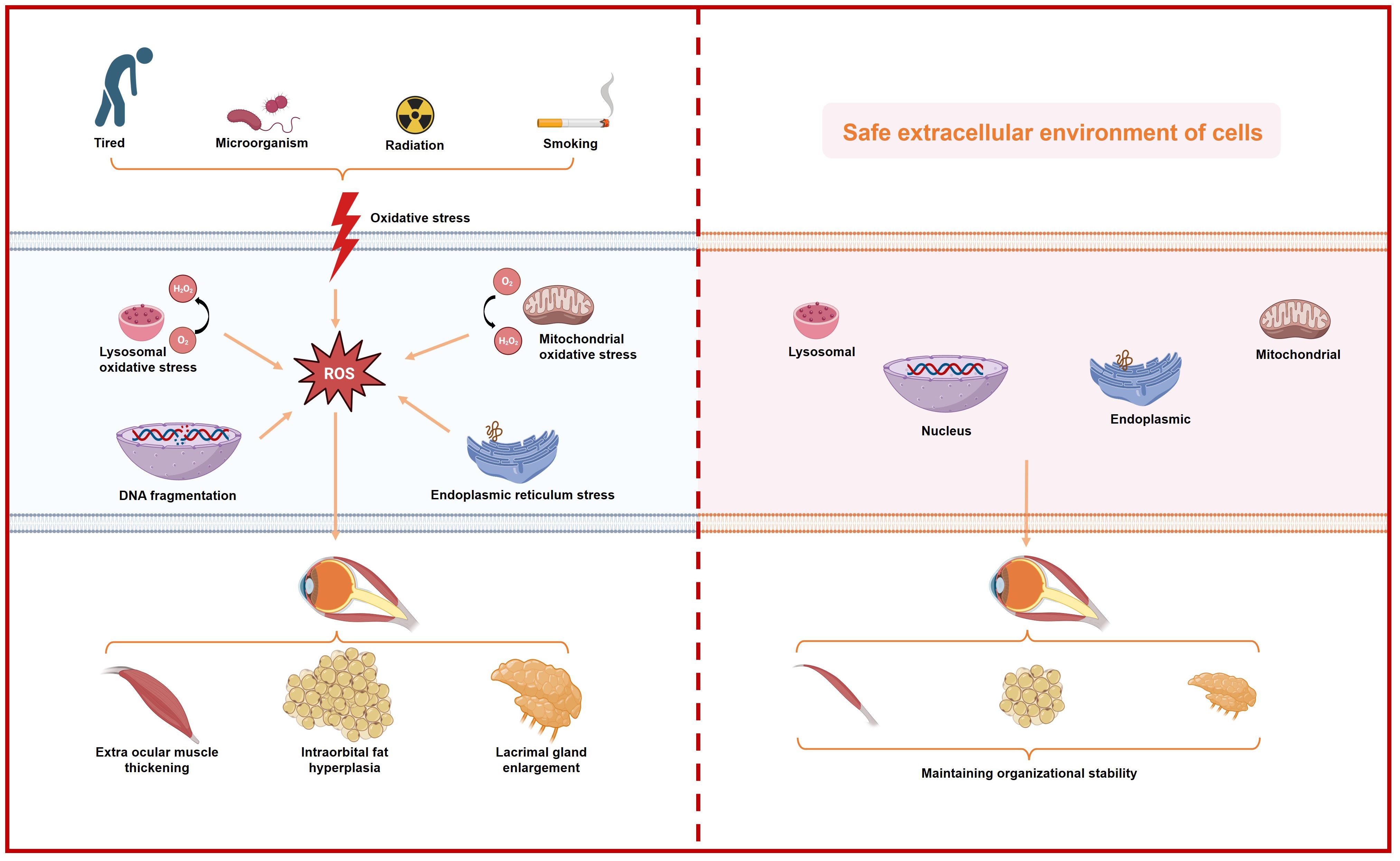
Figure 1 Schematic representation of the increase in intracellular oxidative stress caused by various external factors and its impact on individual organelles, ultimately leading to ocular histopathology in patients with thyroid-associated ophthalmopathy.
Oxidative stress in TAO is a well-studied component, implicated in disease initiation and progression (41). Evidence suggests that oxidative stress from immune cells, orbital fibroblasts, and adipocytes infiltrating the orbit contributes to TAO pathogenesis (42). Understanding these mechanisms is critical for developing targeted therapeutic strategies to mitigate oxidative stress and improve outcomes in TAO patients (43).
2.2 Relationships between oxidative stress and TAO
The relationship between oxidative stress and TAO involves intricate mechanisms influenced by multiple factors. Imbalance between ROS production and the antioxidant defense system is implicated in TAO pathogenesis (44). Increased ROS production and compromised antioxidant capacity can lead to cellular damage and dysfunction in TAO. Understanding these mechanisms requires exploring oxidative stress at the organelle level and its impact on inflammation and fibrosis, critical components in TAO pathophysiology (45, 46). Oxidative stress perpetuates inflammation, exacerbating tissue damage in a vicious cycle. Additionally, genetic factors and smoking contribute to heightened oxidative stress in orbital tissues of TAO patients (47).
2.2.1 Mitochondrial oxidative stress and TAO
1. Mitochondrial dysfunction: Mitochondria, pivotal for cellular energy production, are implicated in TAO due to their role in thyroid hormone synthesis and metabolism, which consume substantial energy. Impaired mitochondrial function exacerbates hyperthyroidism associated with ocular manifestations in Graves’ disease patients (48, 49).
2. Oxidative stress damage: During oxidative stress, mitochondria generate excessive free radicals and oxidants, disrupting cellular redox balance of cells. This can compromise mitochondrial membrane integrity and DNA, affecting normal ocular tissue function in TAO (50).
3. Inflammatory response: Oxidative stress promotes persistent inflammatory reactions that contribute to ocular tissue damage in TAO (51).
Mitochondrial oxidative stress likely plays a significant role in TAO pathogenesis by influencing thyroid function, damaging ocular tissues, and perpetuating inflammation. Further research on mitochondrial oxidative stress is crucial for understanding the underlying mechanisms of TAO and developing targeted treatments.
2.2.2 Endoplasmic reticulum oxidative stress and TAO
ER oxidative stress occurs when the ER is challenged by various insults, leading to impaired ER function and intracellular oxidative stress (52). Several aspects highlight the connection between TAO and ER oxidative stress:
1. Autoimmune Response Induction: In TAO, thyrotropin receptor antibodies activate thyroid cell receptors, leading to hyperthyroidism and ocular complications. ER oxidative stress increases intracellular free radical generation, triggering inflammation and autoimmune responses (32, 53).
2. Inflammatory response: ER oxidative stress induces the release of inflammatory factors like tumor necrosis factor-α (TNF-α), interleukin-6 (IL-6), which contribute to inflammation, edema, and fibrosis in ocular tissues associated with TAO (54, 55). Furthermore, ER oxidative stress disrupts calcium ion homeostasis, crucial for cell signaling and regulation (56). Calcium imbalance further exacerbates inflammation and fibrosis in TAO (57, 58).
3. Oxidative stress damage: ER oxidative stress increases intracellular oxidative stress, disrupting cellular redox balance. Abnormal protein folding due to ER dysfunction can trigger cell apoptosis and fibrosis, exacerbating disease progression in TAO (59, 60).
Understanding the interplay between ER oxidative stress and TAO requires further basic and clinical research to validate these mechanisms and develop targeted therapeutic strategies.
2.2.3 Lysosomal oxidative stress and TAO
Lysosomes play critical roles in cellular degradation, regulation, and immunity (61). Lysosomal oxidative stress, arising from redox imbalance, contributes to ROS overproduction and inflammation (62). Several factors link lysosomal oxidative stress to TAO:
1. Inflammatory response: Lysosomal oxidative stress triggers inflammatory reactions, involving infiltration and activation of immune cells, exacerbating tissue inflammation and damage in TAO (63). Inflammatory molecules like TNF-α and interleukin-1β are released, contributing to ocular tissue inflammation and fibrosis (64).
2. Apoptosis: Oxidative stress from lysosomal enzymes can induce cell apoptosis, further complicating TAO pathology by promoting tissue damage (65).
3. Immune regulation imbalance: Lysosomal oxidative stress disrupts antigen degradation and presentation, potentially exacerbating autoimmune responses in TAO (66, 67). Lysosomal dysfunction may contribute to adipose tissue hyperplasia and proliferation in orbital tissues associated with TAO (68).
4. Impact on other organelles: Lysosomal oxidative stress can interact with other organelles, such as the ER and mitochondria, affecting cellular signaling and function (34).
Further research is necessary to elucidate the role of lysosomal oxidative stress in thyroid-related eye conditions, confirming and expanding upon existing theoretical frameworks and research findings.
2.2.4 Nuclear oxidative stress and TAO
The nucleus, housing genetic material and regulatory molecules, is vulnerable to oxidative stress-induced damage (69). Nuclear oxidative stress affects TAO through several mechanisms:
1. DNA damage: ROS directly damage DNA in orbital fibroblasts of TAO patients, causing strand breaks, base modifications, and oxidative lesions that disrupt gene expression and DNA repair mechanisms (70).
2. Regulation of transcription factor activity: Oxidative stress influences transcription factor activity, regulating genes involved in inflammation, cell proliferation, apoptosis, and other processes relevant to TAO pathology (71–73).
3. Protein oxidation and modification: Oxidative conditions in the nucleus lead to protein oxidation and modification, altering protein structure and function critical for nuclear processes in TAO (74).
4. Cell cycle and apoptosis regulation: Oxidative stress impacts cell survival and apoptosis pathways, influencing cell cycle progression and apoptotic signaling relevant to TAO progression (75, 76).
Nuclear oxidative stress disrupts cellular functions essential for maintaining ocular tissue integrity in TAO. Further research is essential to comprehensively understand these processes and their implications for therapeutic interventions.
2.3 The role of oxidative stress due to environmental exposure, smoking and late nights in the development of TAO
Several factors contribute to the onset of thyroid-associated eye conditions, including environmental exposure, tobacco use, and sleep patterns.
1. Environmental exposure: Air pollutants, heavy metals, chemicals, and radiation constitute environmental exposure. These pollutants enter the body through various pathways, such as the respiratory tract, digestive system, and skin (77). Research links environmental exposure to TAO, where pollutants induce ROS through oxidation reactions, increasing intracellular oxidative stress (78, 79). This oxidative stress activates the immune system, triggering autoimmune reactions that contribute to TAO development (80).
2. Smoking: Smoking is identified as a significant risk factor for TAO development (81). Chemicals in tobacco smoke initiate oxidative reactions, releasing reactive oxygen radicals that elevate oxidative stress levels in cells. Additionally, smoking induces vasoconstriction and inflammatory responses that exacerbate symptoms of thyroid-related eye disease (82).
3. Late nights: Sleep deprivation disrupts the body’s circadian rhythm and adversely affects immune function and metabolism (83). Insufficient sleep leads to increased free radical production, reduced antioxidant enzyme activity, and oxidative stress reactions. Elevated oxidative pressure due to late nights can influence thyroid function and contribute to orbital abnormalities observed in TAO.
Overall, factors like environmental exposure, smoking, and disrupted sleep patterns significantly contribute to the development of thyroid-related eye conditions by increasing free radicals, reducing antioxidants, and disrupting circadian rhythms. Therefore, reducing environmental exposure, quitting smoking and maintaining healthy sleep habits are crucial in preventing and managing thyroid-related eye diseases. Additionally, patients are advised to pursue other effective treatments including medication, surgery, and lifestyle improvements to promote remission and recovery.
2.4 Oxidative stress in the process of inflammation and fibrosis in TAO
2.4.1 Oxidative stress and inflammatory pathways in TAO
Oxidative stress plays a pivotal role in the inflammatory pathways associated with TAO. Overproduction of ROS triggers inflammatory responses, prominently involving the nuclear factor kappa-B (NF-κB) pathway, a key regulator of inflammation (84, 85). Activation of NF-κB leads to increased levels of inflammatory cytokines such as IL-1β, IL-6, and TNF-α, contributing to persistent inflammation in TAO (86, 87). Moreover, oxidative stress upregulates adhesion molecules like intercellular adhesion molecule-1 (ICAM-1) and vascular cell adhesion molecule-1 (VCAM-1) on orbital fibroblasts and endothelial cells, facilitating immune cell recruitment and adhesion to inflamed orbital tissue (88, 89). Additionally, oxidative stress activates MAPKs (mitogen-activated protein kinases) including extracellular signal-regulated kinases, c-Jun N-terminal kinases (JNK), and p38 MAPK, which regulate cell growth, differentiation, and inflammation (90, 91). Activation of MAPKs in TAO results in the production of pro-inflammatory mediators and activation of fibroblasts, perpetuating inflammation and fibrosis within the orbit (92).
2.4.2 Oxidative stress and fibrosis of TAO
Oxidative stress also influences the fibrotic processes observed in TAO. ROS stimulate the production of transforming growth factor-β (TGF-β), a major mediator of fibrosis (93, 94). TGF-β promotes transformation of orbital fibroblasts into myofibroblasts, leading to excessive production and accumulation of extracellular matrix components such as collagen and fibronectin (95).
Moreover, oxidative stress disrupts the balance between matrix metalloproteinases (MMPs) and tissue inhibitors of metalloproteinases (TIMPs), critical for collagen turnover and tissue remodeling (96). Excess ROS inhibit MMP activity and expression, impairing collagen degradation and contributing to fibrotic changes observed in TAO, such as increased orbital fat volume and fibrotic tissue expansion (97).
3 Therapeutic agents for oxidative stress in TAO and their potential therapeutic targets
3.1 Application of antioxidants
TAO exhibits dysregulation of oxidative stress pathways, which can be targeted to mitigate oxidative damage and restore redox balance (Figure 2). One strategy involves the application of antioxidants, which scavenge ROS and reduce oxidative damage in orbital tissues (98).
3.1.1 Clinical application of therapeutic drugs
3.1.1.1 Selenium
Selenium a trace element found in foods like Brazil nuts, tuna, shrimp, meat, eggs, cereals and grains (99), plays a crucial role in thyroid function (100). Selenoproteins, including glutathione peroxidase, thioredoxin reductase, and iodothyronine deiodinase, function as antioxidants regulating redox reactions (101, 102). Selenium also reduces inflammatory cytokine production by preventing NF-B from binding to its gene promoter (103). Consequently, selenium is considered an important supplement in TAO clinical treatment.
3.1.1.2 Pentoxifylline
A xanthine derivative primarily used to treat peripheral vascular diseases (104), pentoxifylline acts as an antioxidant by eliminating excess ROS, thereby reducing inflammation-related damage (105). In TAO patients, pentoxifylline inhibits the proliferation and synthesis of glycosaminoglycans (GAGs) by extraocular myofibroblasts (106). It exerts immunomodulatory effects on cytokine production (107). Suitable for patients with moderate to severe TAO who have normal thyroid function, pentoxifylline can be used without corticosteroids (108). However, further clinical and experimental validation is required to confirm its specific dosage and effectiveness.
3.1.1.3 Allopurinol and Nicotinamide
In vitro studies show that allopurinol and nicotinamide prevent superoxide-induced growth of orbital fibroblasts in TAO patients (109). Nicotinamide reduces the expression of human leukocyte antigen-DR and ICAM-1 stimulated by cytokines in orbital fibroblasts during the active phase of TAO (110). Additionally, it suppresses the growth of orbital fibroblasts and reduces HSP72 expression induced by superoxide (111). A clinical trial found that 82% of patients with mild to moderately severe, recently diagnosed active TAO experienced a decrease in the severity of orbital lesions after three months of oral allopurinol and nicotinamide treatment, compared to 27% in the control group (112). Further clinical and experimental evidence is necessary to confirm the efficacy and safety of nicotinamide and allopurinol in TAO treatment.
3.1.1.4 Enalapril
This ACE inhibitor, commonly used for hypertension, protects tissues from oxidative damage by enhancing antioxidant defenses and decreasing ROS levels (113). Enalapril supports glutathione-dependent antioxidant protection (114). In vitro studies demonstrated its antiproliferative and anti-hyaluronic acid (HA) secretion effects in both TAO patient groups and non-TAO control fibroblasts (115). A clinical trial with 12 individuals with mild TAO showed improvements in eye bulging, CAS scores, eyelid retraction, and overall well-being compared to the control group (116). Enalapril’s positive impact on TAO is likely due to its antioxidant properties, inhibition of orbital fibroblast growth, and reduction of HA accumulation. However, the limited sample size necessitates additional research to confirm its efficacy in moderate and severe TAO cases.
3.1.1.5 Statins
Statins, inhibitors of 3-hydroxy-3-methylglutaryl coenzyme A reductase, are widely used to treat hypercholesterolemia (117). They reduce oxidative stress by lowering blood lipids, eliminating free radicals, and inhibiting vascularity (118). Evidence suggests that statins may reduce the risk of thyroid-related adverse events in patients with thyroid dysfunction (119, 120). Clinical trials have demonstrated that oral atorvastatin can improve the quality of life and reduce eye lesions in active TAO patients with hypercholesterolemia compared to intravenous corticosteroids (121). Statins may prevent the onset and progression of TAO in newly diagnosed thyroid dysfunction patients, warranting further clinical trials to investigate their prophylactic potential (122). The therapeutic feasibility of statins may be explained by the potential link between TAO and increased free fatty acid load, which leads to ROS and proinflammatory cytokine release implicated in TAO pathogenesis (123).
3.1.2 Vitamins, enzymes and amino acids
3.1.2.1 Vitamin C
Ascorbic acid, essential for wound healing and collagen synthesis, act as a reducing agent in redox reactions (124). Vitamin E: This fat-soluble antioxidant scavenges ROS in the body (125). Ubiquinone (Coenzyme Q10): Essential for aerobic respiration, it prevents free radicals interaction with antioxidant molecules in the body (126). N-acetylcysteine: A precursor to glutathione, it helps decrease free radical generation during oxidative stress (127). Melatonin: Released by the pineal gland to regulate circadian rhythm melatonin scavenges free radicals and enhances antioxidant activity (128).
N-acetylcysteine and vitamin C inhibit H2O2-induced fibroblast proliferation and reduce TGF-β1, IL-1β, and superoxide anion expression in TAO fibroblasts (129). Studies on primary cultures of orbital fibroblasts from TAO patients have shown that vitamin C, N-acetylcysteine and melatonin reduce H2O2-stimulated glutathione disulphide (GSSG) release, potentially benefiting orbital lesion treatment (130). Additionally, vitamin C and E supplementation improved clinical symptoms and clinical scores in TAO patients by reducing oxidative stress markers (131). These antioxidants inhibit TAO fibroblast proliferation, with N-acetylcysteine and melatonin selectively blocking IFN-γ release (132). They also reduce HA and IL-1β levels in orbital fibroblasts of TAO patients and normal controls (133). Despite promising results, the complexity of the body’s circulatory metabolism necessitates in-depth clinical studies to evaluate each antioxidant’s therapeutic efficacy.
3.1.3 Plant extracts
Natural plant extracts with antioxidant properties, such as polyphenols, curcumin, resveratrol and quercetin, show potential benefits in reducing oxidative stress and inflammation in TAO (134). The compounds act as ROS scavengers, inhibit ROS-generating enzymes, and activate the endogenous antioxidant system (135). The effectiveness of these compounds in reducing oxidative stress and improving tissue damage in TAO models has been demonstrated in preclinical studies (136).
3.1.3.1 Quercetin
A polyphenolic flavonoid found in fruits, vegetables, red wine and tea, quercetin has antioxidant, anti-inflammatory, and antiproliferative properties (137, 138). In TAO, quercetin regulates inflammatory pathways, extracellular matrix molecule accumulation, and adipose tissue growth in primary cultured orbital fibroblasts. It suppresses the production of ICAM-1, IL-6, IL-8, and cyclooxygenase 2 mRNA, and prevents IL-1β-induced elevations of these molecules (139). Therefore, quercetin’s effects may alleviate clinical symptoms of orbital lesions in TAO patients.
3.1.3.2 β-carotene
A carotenoid widely found in plants; β-carotene is an antioxidant with neuroprotective effects (140, 141). It reduces H2O2-induced GSSG production, IL-1β levels, and cell proliferation in TAO orbital fibroblasts (142). However, overconsumption can cause carotenosis, an orange pigmentation of the skin, and may increase lung cancer risk in smokers (143, 144). Further investigation is needed to evaluate β-carotene’s long-term therapeutic effects and safety in TAO.
3.1.3.3 Tea polyphenols (TP)
Derived from tea leaves, TP compounds, primarily catechins, provide antioxidant and anti-inflammatory benefits (145, 146). Epigallocatechin-3-gallate, the main component of green tea extract, reduces IL-1β and exerts anti-inflammatory effects in TAO orbital fibroblasts (147). TP also modulates NF-κB/NLRP3 pathway, reducing IL-6, IL-1β, and MCP-1 expression in LPS-induced TAO orbital fibroblasts (148). However, TP treatment for TAO remains experimental, requiring further research to confirm its effectiveness and safety.
3.1.3.4 Resveratrol
A non-flavonoida phenolic compound from red grapes, berries, and peanuts, resveratrol has antioxidant, anticancer, anti-inflammatory, anti-apoptosis, and free radical scavenging properties (149). In TAO, it reduces ROS generated by cigarette smoke extract or H2O2 and during adipogenesis in primary cultured orbital fibroblasts (150). Although promising in animal studies, there is no reliable evidence of resveratrol’s benefits for human health. Further research is necessary to understand its mechanism and therapeutic role in TAO.
3.1.3.5 Curcumin
A plant polyphenol from turmeric with demonstrated antioxidant and anti-inflammatory activity in vitro, in vivo, and in humans (151, 152). In vitro studies show curcumin inhibits cell proliferation, adipogenesis, ROS production, and IL-1β-induced inflammatory responses in TAO patients’ orbital fibroblasts (153). While its anticancer effects are well-documented, curcumin’s poor human absorption and bioavailability limit its clinical use (154, 155). Additional studies on efficacy and safety are required.
3.1.3.6 Caffeine (1,3,7-trimethylxanthine)
A plant alkaloid, which is commonly found in beverages such as coffee, tea, chocolate, and cola (156). Numerous studies have identified it as an oxygen radical scavenger, demonstrating both in vitro and in vivo antioxidant activity in animals and humans, and suggesting it may reduce inflammatory responses (157, 158). In the context of treating TAO, caffeine appears to inhibit orbital changes by reducing ROS production in response to cellular oxidative stress and exhibiting anti-adipogenic effects (159). However, excessive caffeine consumption can lead to adverse effects, including addiction, nervousness, irritability, anxiety, tremors, muscle twitching, insomnia, and heart palpitations (160). Therefore, determining the appropriate dosage to alleviate TAO-related orbital lesions without inducing adverse effects is an important area for future research.
3.1.3.7 Gynostemma pentaphyllum
Gynostemma pentaphyllum is a herbaceous climbing plant, and its derivatives, particularly gypenosides (Gyps), have long been used as safe and effective natural remedies for various diseases. Gyps exhibit different oral bioavailability values and a limited ability to cross the blood-brain barrier (161). Gyps have been shown to reduce oxidative stress induced by H2O2 in orbital fibroblasts from TAO patients, thereby decreasing cellular autophagy and apoptosis, and consequently minimizing orbital tissue damage (162). Additionally, Gyps inhibit the activation of the Toll-like receptor 4/NF-κB signaling pathway and the TGF-β1/SMAD2/SMAD4 signaling pathway in orbital fibroblasts, exerting anti-inflammatory and antioxidant effects (163). While Gyps have demonstrated protective effects on orbital fibroblasts in experimental settings, improving their bioavailability remains a key focus for their clinical application.
3.2 Regulation of organismal oxidative stress
Dysregulation of oxidative stress pathways plays a critical role in the pathogenesis of TAO (Figure 3). Targeting these pathways has emerged as a potential therapeutic strategy to mitigate oxidative damage, reduce inflammation, and improve clinical outcomes in TAO. One approach involves regulating enzyme systems responsible for ROS production, such as NADPH oxidase and xanthine oxidase. These enzymes produce ROS as by-products of their normal physiological function, and their upregulation or overactivation can lead to oxidative stress in TAO (164). Inhibiting or modulating these enzymes has been shown to reduces ROS production and subsequent oxidative damage (165).
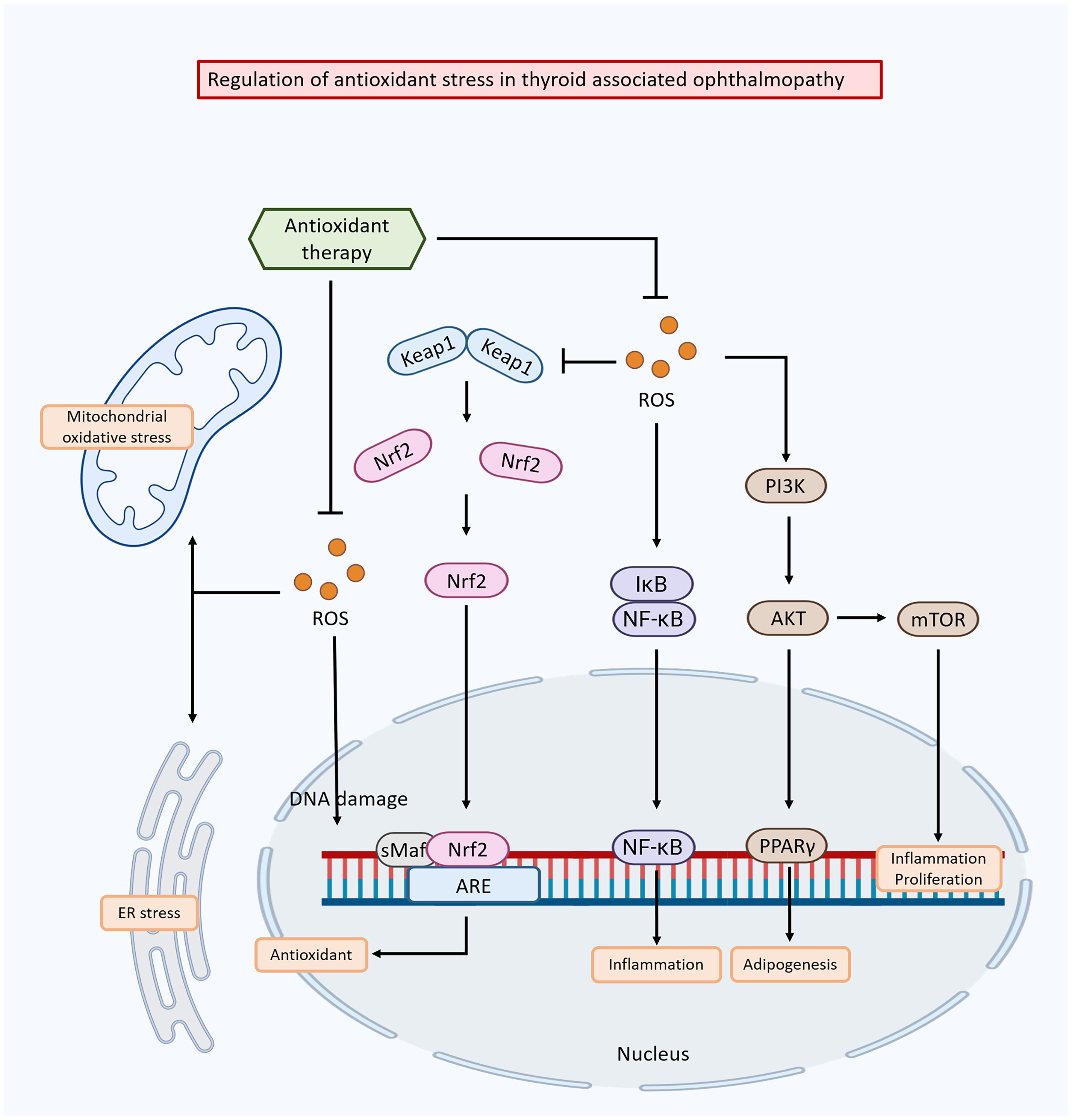
Figure 3 Mechanisms of oxidative stress-induced damage in thyroid-associated ophthalmopathy and the modulation of these effects by anti-oxidative stress drugs.
Another strategy is to enhance the antioxidant defense system to clear ROS and restore redox balance. This can be achieved by activating endogenous antioxidant enzymes such as SOD, catalase, and glutathione peroxidase, which neutralize ROS and prevent oxidative damage (166, 167). The nuclear factor erythroid 2-related factor 2 (Nrf2) pathway is a key transcription factor that regulates the expression of antioxidant-responsive genes, playing a crucial role in this process (168).
The Nrf2 pathway induces broad-spectrum antioxidant responses and mitigates damage from inflammatory factors (169). Under normal conditions, Nrf2 is sequestered in the cytoplasm by its inhibitor protein, Kelch-like ECH-associated protein 1 (Keap1). When oxidative stress occurs, Nrf2 dissociates from Keap1 and translocates to the nucleus, where it binds to antioxidant response elements (AREs) in the promoter regions of specific genes, initiating their transcription process (170). The Keap1/Nrf2 system significant contributes to maintaining redox and metabolic homeostasis, demonstrating positive effects in various disease models, including inflammatory, autoimmune, metabolic, and neurodegenerative conditions (171).
Activation of the Nrf2 pathway shows promising outcomes in TAO. Nrf2 activation attenuates oxidative stress in orbital fibroblasts, reduces inflammation, and ameliorates orbital tissue damage in TAO patients (172). Additionally, Nrf2 activation has been associated with reduced fat aggregation in TAO and improved clinical outcomes (173). Conversely, blocking Nrf2 transcriptional activity in leukemia cells leads to ROS accumulation and cell death via apoptosis (174). Vitamin C, a well-known antioxidant, enhances the sensitivity of cancer cells resistant to imatinib by disrupting the Nrf2/ARE complex, resulting in decreased expression of the catalytic subunit of glutamate-cysteine ligase and lower GSH levels (175).
The regulation of Nrf2 varies across different diseases, and it could be beneficial in identifying biomarkers and potential drug targets for thyroid diseases during TAO treatment. Therefore, key areas for future investigation include exploring the functions and pathways of Nrf2-triggered antioxidants in TAO and examining the potential effectiveness of Nrf2 regulation in modulating autoimmune responses in TAO. Modulation of oxidative stress pathways as therapeutic targets for TAO offers a promising avenue for new interventions. Further studies are needed to elucidate the specific molecular mechanisms of oxidative stress in TAO and to assess the safety and efficacy of therapeutic agents targeting these pathways. Addressing oxidative stress may help attenuate tissue damage, reduce inflammation, and improve clinical outcomes in TAO.
4 Summarization and prospect
Oxidative stress plays a significant role in the pathogenesis of TAO. An imbalance between ROS production and antioxidant defenses lead to oxidative stress, resulting in cellular damage and dysfunction. Multiple factors contribute to the relationship between oxidative stress and TAO, including the activation of inflammatory pathways, genetic and environmental factors, and dysregulation of antioxidant defense mechanisms. The relationship between oxidative stress and TAO is complex and multifaceted. ROS in TAO are produced by infiltrating immune cells, orbital fibroblasts and adipocytes, leading to tissue damage and inflammation. Elevated oxidative stress activates inflammatory pathways, perpetuating the cycle of tissue damage and inflammation in TAO.
Addressing oxidative stress in TAO is critical for developing effective therapeutic strategies. Antioxidants, such as vitamins C and E, and activation of endogenous antioxidant defense mechanisms have shown promise in reducing oxidative stress and inflammation in TAO. Further studies are needed to fully understand the complex mechanisms of oxidative stress and its role in the pathogenesis of TAO. Future research could focus on several areas to enhance our knowledge and improve therapeutic strategies for TAO:
1. Exploring the interactions between oxidative stress and other key pathways: Investigating the interactions between oxidative stress and the inflammation, fibrosis, and immune dysregulation that characterize TAO could lead to a more comprehensive understanding of the disease. Elucidating these interactions could reveal underlying mechanisms and aid in developing targeted therapies that address multiple aspects of the disease.
2. Identification of new therapeutic targets: Identifying new targets within the oxidative stress pathway could provide novel therapeutic opportunities. This could involve targeting specific enzymes or molecules involved in ROS production, such as NADPH oxidase or xanthine oxidase.
3. Modulation of antioxidant defense mechanisms: Exploring ways to enhance endogenous protection against oxidative stress, such as through the Nrf2 pathway, could be beneficial. Understanding the regulation and activation of Nrf2 in TAO could inform new therapeutic strategies.
In conclusion, future research efforts should focus on unraveling the complex interactions between oxidative stress and other key pathways in TAO, identifying new therapeutic targets, and conducting robust clinical studies to validate the effectiveness of antioxidant interventions. By improving our understanding and developing targeted therapeutic approaches, we can endeavor to enhance management strategies and improve the quality of life for TAO patients.
Author contributions
CM: Funding acquisition, Resources, Supervision, Writing – original draft, Writing – review & editing. HL: Data curation, Writing – review & editing. SL: Methodology, Writing – review & editing. XL: Supervision, Writing – original draft.
Funding
The author(s) declare financial support was received for the research, authorship, and/or publication of this article. This study is funded by the Joint construction project of Henan Medical Science and technology (LHGJ20220370) and Natural Science Foundation of Henan (232300420237).
Conflict of interest
The authors declare that the research was conducted in the absence of any commercial or financial relationships that could be construed as a potential conflict of interest.
Publisher’s note
All claims expressed in this article are solely those of the authors and do not necessarily represent those of their affiliated organizations, or those of the publisher, the editors and the reviewers. Any product that may be evaluated in this article, or claim that may be made by its manufacturer, is not guaranteed or endorsed by the publisher.
References
1. Sohn SY, Ahn JY, Lee MK, Lee JH, Kwon JW, Kweon JM, et al. Risk of non-thyroidal autoimmune diseases in patients with Graves' disease: a nationwide retrospective cohort study. Rheumatol (Oxford). (2024) 708. doi: 10.1093/rheumatology/kead708
2. Bartalena L, Pinchera A, Marcocci C. Management of Graves' ophthalmopathy: reality and perspectives. Endocr Rev. (2000) 21:168–99. doi: 10.1210/edrv.21.2.0393
3. Bartalena L, Fatourechi V. Extrathyroidal manifestations of Graves' disease: a 2014 update. J Endocrinol Invest. (2014) 37:691–700. doi: 10.1007/s40618-014-0097-2
4. Perros P, Žarković M, Azzolini C, Ayvaz G, Baldeschi L, Bartalena L, et al. PREGO (presentation of Graves' orbitopathy) study: changes in referral patterns to European Group On Graves' Orbitopathy (EUGOGO) centres over the period from 2000 to 2012. Br J Ophthalmol. (2015) 99:1531–5. doi: 10.1136/bjophthalmol-2015-306733
5. Estcourt S, Quinn AG, Vaidya B. Quality of life in thyroid eye disease: impact of quality of care. Eur J Endocrinol. (2011) 164:649–55. doi: 10.1530/EJE-11-0055
6. Novaes P, Diniz Grisolia AB, Smith TJ. Update on thyroid-associated Ophthalmopathy with a special emphasis on the ocular surface. Clin Diabetes endocrinology. (2016) 2:19. doi: 10.1186/s40842-016-0037-5
7. Wiersinga WM. Autoimmunity in Graves' ophthalmopathy: the result of an unfortunate marriage between TSH receptors and IGF-1 receptors? J Clin Endocrinol Metab. (2011) 96:2386–94. doi: 10.1210/jc.2011-0307
9. Smith TJ, Kahaly GJ, Ezra DG, Fleming JC, Dailey RA, Tang RA, et al. Teprotumumab for thyroid-associated ophthalmopathy. N Engl J Med. (2017) 376:1748–61. doi: 10.1056/NEJMoa1614949
10. Ma C, Li H, Liu W, Lu S, Li X, Chen J, et al. Therapeutic effect of gypenosides on antioxidant stress injury in orbital fibroblasts of graves' Orbitopathy. J Immunol Res. (2022) 2022:4432584. doi: 10.1155/2022/4432584
11. Pizzino G, Irrera N, Cucinotta M, Pallio G, Mannino F, Arcoraci V, et al. Oxidative stress: harms and benefits for human health. Oxid Med Cell Longev. (2017) 2017:8416763. doi: 10.1155/2017/8416763
12. Lanzolla G, Marcocci C, Marinò M. Oxidative stress in graves disease and graves orbitopathy. Eur Thyroid J. (2020) 9:40–50. doi: 10.1159/000509615
13. Zarković M. The role of oxidative stress on the pathogenesis of graves' disease. J Thyroid Res. (2012) 2012:302537. doi: 10.1155/2012/302537
14. Li SY, Xue RY, Wu H, Pu N, Wei D, Zhao N, et al. Novel role of molecular hydrogen: the end of ophthalmic diseases? Pharm (Basel). (2023) 16:1567. doi: 10.3390/ph16111567
15. Kochman J, Jakubczyk K, Bargiel P, Janda-Milczarek K. The influence of oxidative stress on thyroid diseases. Antioxidants (Basel Switzerland). (2021) 10:1442. doi: 10.3390/antiox10091442
16. Hou TY, Wu SB, Kau HC, Tsai CC. The role of oxidative stress and therapeutic potential of antioxidants in graves' Ophthalmopathy. Biomedicines. (2021) 9:1871. doi: 10.3390/biomedicines9121871
17. Byeon HJ, Kim JY, Ko J, Lee EJ, Don K, Yoon JS. Protein tyrosine phosphatase 1B as a therapeutic target for Graves' orbitopathy in an in vitro model. PloS One. (2020) 15:e0237015. doi: 10.1371/journal.pone.0237015
18. Sharifi-Rad M, Anil Kumar NV, Zucca P, Varoni EM, Dini L, Panzarini E, et al. Lifestyle, oxidative stress, and antioxidants: back and forth in the pathophysiology of chronic diseases. Front Physiol. (2020) 11:694. doi: 10.3389/fphys.2020.00694
19. He L, He T, Ji L, Liu T, Ma X. Antioxidants maintain cellular redox homeostasis by elimination of reactive oxygen species. Cell Physiol Biochem. (2017) 44:532–53. doi: 10.1159/000485089
20. Nita M, Grzybowski A. The role of the reactive oxygen species and oxidative stress in the pathomechanism of the age-related ocular diseases and other pathologies of the anterior and posterior eye segments in adults. Oxid Med Cell Longev. (2016) 2016:3164734. doi: 10.1155/2016/3164734
21. Zhou J, Tripathi M, Ho JP, Widjaja AA, Shekeran SG, Camat MD, et al. Thyroid hormone decreases hepatic steatosis, inflammation, and fibrosis in a dietary mouse model of nonalcoholic steatohepatitis. Thyroid. (2022) 32:725–38. doi: 10.1089/thy.2021.0621
22. Hai YP, Lee ACH, Chen K, Kahaly GJ. Traditional Chinese medicine in thyroid-associated orbitopathy. J Endocrinol Invest. (2023) 46:1103–13. doi: 10.1007/s40618-023-02024-4
23. Yu JH, Wang YH, Liao HF. Research status of potential therapeutic targets for thyroid-associated ophthalmopathy. Zhonghua Yan Ke Za Zhi. (2024) 60:282–8. doi: 10.3760/cma.j.cn112142-20231116-00235
24. Orisaka M, Mizutani T, Miyazaki Y, Shirafuji A, Tamamura C, Fujita M, et al. Chronic low-grade inflammation and ovarian dysfunction in women with polycystic ovarian syndrome, endometriosis, and aging. Front Endocrinol (Lausanne). (2023) 14:1324429. doi: 10.3389/fendo.2023.1324429
25. Aoun T, Danielova Gueorguieva D, Wu KY. Orbital inflammation in thyroid eye disease: stress responses and their implications. Stresses. (2024) 4:54–78. doi: 10.3390/stresses4010004
26. Poljsak B, Šuput D, Milisav I. Achieving the balance between ROS and antioxidants: when to use the synthetic antioxidants. Oxid Med Cell Longev. (2013) 2013:956792. doi: 10.1155/2013/956792
27. Bhattacharyya A, Chattopadhyay R, Mitra S, Crowe SE. Oxidative stress: an essential factor in the pathogenesis of gastrointestinal mucosal diseases. Physiol Rev. (2014) 94:329–54. doi: 10.1152/physrev.00040.2012
28. Nandi A, Yan LJ, Jana CK, Das N. Role of catalase in oxidative stress- and age-associated degenerative diseases. Oxid Med Cell Longev. (2019) 2019:9613090. doi: 10.1155/2019/9613090
29. Pisoschi AM, Pop A. The role of antioxidants in the chemistry of oxidative stress: A review. Eur J Med Chem. (2015) 97:55–74. doi: 10.1016/j.ejmech.2015.04.040
30. Guo C, Sun L, Chen X, Zhang D. Oxidative stress, mitochondrial damage and neurodegenerative diseases. Neural regeneration Res. (2013) 8:2003–14. doi: 10.3969/j.issn.1673-5374.2013.21.009
31. Kowalczyk P, Sulejczak D, Kleczkowska P, Bukowska-Ośko I, Kucia M, Popiel M, et al. Mitochondrial oxidative stress-A causative factor and therapeutic target in many diseases. Int J Mol Sci. (2021) 22:13384. doi: 10.3390/ijms222413384
32. Cao SS, Kaufman RJ. Endoplasmic reticulum stress and oxidative stress in cell fate decision and human disease. Antioxid Redox Signal. (2014) 21:396–413. doi: 10.1089/ars.2014.5851
33. Chong WC, Shastri MD, Eri R. Endoplasmic reticulum stress and oxidative stress: A vicious nexus implicated in bowel disease pathophysiology. Int J Mol Sci. (2017) 18:771. doi: 10.3390/ijms18040771
34. Pivtoraiko VN, Stone SL, Roth KA, Shacka JJJA, Signaling R. Oxidative stress and autophagy in the regulation of lysosome-dependent neuron death. Antioxid Redox Signal. (2009) 11:481–96. doi: 10.1089/ars.2008.2263
35. Zhang Z, Yue P, Lu T, Wang Y, Wei Y, Wei X. Role of lysosomes in physiological activities, diseases, and therapy. J Hematol Oncol. (2021) 14:79. doi: 10.1186/s13045-021-01087-1
36. Casali C, Siciliani S, Galgano L, Biggiogera M. Oxidative stress and nuclear reprogramming: A pilot study of the effects of reactive oxygen species on architectural and epigenetic landscapes. Int J Mol Sci. (2022) 24:153. doi: 10.3390/ijms24010153
37. Snezhkina AV, Kudryavtseva AV, Kardymon OL, Savvateeva MV, Melnikova NV, Krasnov GS, et al. ROS generation and antioxidant defense systems in normal and Malignant cells. Oxid Med Cell Longev. (2019) 2019:6175804. doi: 10.1155/2019/6175804
38. Samet JM, Wages PA. Oxidative stress from environmental exposures. Curr Opin toxicology. (2018) 7:60–6. doi: 10.1016/j.cotox.2017.10.008
39. Caliri AW, Tommasi S, Besaratinia A. Relationships among smoking, oxidative stress, inflammation, macromolecular damage, and cancer. Mutat Res Rev Mutat Res. (2021) 787:108365. doi: 10.1016/j.mrrev.2021.108365
40. Chen S, Xie Y, Li Y, Fan X, Xing F, Mao Y, et al. Sleep deprivation and recovery sleep affect healthy male resident's pain sensitivity and oxidative stress markers: The medial prefrontal cortex may play a role in sleep deprivation model. Front Mol Neurosci. (2022) 15:937468. doi: 10.3389/fnmol.2022.937468
41. Shan Q, Ma F, Wei J, Li H, Ma H, Sun P. Physiological functions of heat shock proteins. Curr Protein Pept Sci. (2020) 21:751–60. doi: 10.2174/1389203720666191111113726
42. Tsai CC, Cheng CY, Liu CY, Kao SC, Kau HC, Hsu WM, et al. Oxidative stress in patients with Graves' ophthalmopathy: relationship between oxidative DNA damage and clinical evolution. Eye (London England). (2009) 23:1725–30. doi: 10.1038/eye.2008.310
43. Li X, Li S, Fan W, Rokohl AC, Ju S, Ju X, et al. Recent advances in graves ophthalmopathy medical therapy: a comprehensive literature review. Int Ophthalmol. (2023) 43:1437–49. doi: 10.1007/s10792-022-02537-6
44. Neag EJ, Smith TJ. 2021 update on thyroid-associated ophthalmopathy. J Endocrinol Invest. (2022) 45:235–59. doi: 10.1007/s40618-021-01663-9
45. Ko J, Kim JY, Lee EJ, Yoon JS. Role of binding immunoglobulin protein (BiP) in Graves' orbitopathy pathogenesis. J Mol Endocrinol. (2021) 66:71–81. doi: 10.1530/JME-20-0155
46. Zhang X, Zhao Q, Li B. Current and promising therapies based on the pathogenesis of Graves' ophthalmopathy. Front Pharmacol. (2023) 14:1217253. doi: 10.3389/fphar.2023.1217253
47. Kau HC, Wu SB, Tsai CC, Liu CJ, Wei YH. Cigarette smoke extract-induced oxidative stress and fibrosis-related genes expression in orbital fibroblasts from patients with graves' Ophthalmopathy. Oxid Med Cell Longev. (2016) 2016:4676289. doi: 10.1155/2016/4676289
48. Yamada M, Li AW, Wall JR. Thyroid-associated ophthalmopathy: clinical features, pathogenesis, and management. Crit Rev Clin Lab Sci. (2000) 37:523–49. doi: 10.1080/10408360091174303
49. Biondi B, Kahaly GJ, Robertson RP. Thyroid dysfunction and diabetes mellitus: two closely associated disorders. Endocr Rev. (2019) 40:789–824. doi: 10.1210/er.2018-00163
50. Wall JR, Stachura I, Kennerdell JH. Mitochondrial abnormalities in eye muscle fiber from three cases of thyroid-associated ophthalmopathy. Thyroid. (2006) 16:1181–3. doi: 10.1089/thy.2006.16.1181
51. El-Kaissi S, Frauman AG, Wall JR. Thyroid-associated ophthalmopathy: a practical guide to classification, natural history and management. Intern Med J. (2004) 34:482–91. doi: 10.1111/j.1445-5994.2004.00662.x
52. Lee SJ, Lee IK, Jeon JH. Vascular calcification-new insights into its mechanism. Int J Mol Sci. (2020) 21:2685. doi: 10.3390/ijms21082685
53. Matsuzawa A, Nishitoh H, Tobiume K, Takeda K, Ichijo H. Physiological roles of ASK1-mediated signal transduction in oxidative stress- and endoplasmic reticulum stress-induced apoptosis: advanced findings from ASK1 knockout mice. Antioxid Redox Signal. (2002) 4:415–25. doi: 10.1089/15230860260196218
54. Kang J, Li Y, Zhao Z, Zhang H. Differentiation between thyroid-associated orbitopathy and Graves' disease by iTRAQ-based quantitative proteomic analysis. FEBS Open bio. (2021) 11:1930–40. doi: 10.1002/2211-5463.13172
55. Huang J, Chen M, Liang Y, Hu Y, Xia W, Zhang Y, et al. Integrative metabolic analysis of orbital adipose/connective tissue in patients with thyroid-associated ophthalmopathy. Front Endocrinol (Lausanne). (2022) 13:1001349. doi: 10.3389/fendo.2022.1001349
56. Song Z, Wang Y, Zhang F, Yao F, Sun C. Calcium signaling pathways: key pathways in the regulation of obesity. Int J Mol Sci. (2019) 20:2768. doi: 10.3390/ijms20112768
57. Bahar E, Kim H, Yoon H. ER stress-mediated signaling: action potential and ca(2+) as key players. Int J Mol Sci. (2016) 17:1558. doi: 10.3390/ijms17091558
58. Chen K, Xie Y, Zhao L, Mo Z. Hyperthyroidism-associated hypercalcemic crisis: A case report and review of the literature. Med (Baltimore). (2017) 96:e6017. doi: 10.1097/MD.0000000000006017
59. Haeri M, Knox BE. Endoplasmic reticulum stress and unfolded protein response pathways: potential for treating age-related retinal degeneration. J ophthalmic Vision Res. (2012) 7:45–59.
60. Cheng KC, Huang HH, Hung CT, Chen CC, Wu WC, Suen JL, et al. Proteomic analysis of the differences in orbital protein expression in thyroid orbitopathy. Graefes Arch Clin Exp Ophthalmol. (2013) 251:2777–87. doi: 10.1007/s00417-013-2446-9
61. Ballabio A, Bonifacino JS. Lysosomes as dynamic regulators of cell and organismal homeostasis. Nat Rev Mol Cell Biol. (2020) 21:101–18. doi: 10.1038/s41580-019-0185-4
62. Butler D, Bahr BA. Oxidative stress and lysosomes: CNS-related consequences and implications for lysosomal enhancement strategies and induction of autophagy. Antioxidants Redox Signaling. (2006) 8:185–96. doi: 10.1089/ars.2006.8.185
63. Komosińska-Vassev K, Olczyk K, Koźma EM, Winsz-Szczotka K, Olczyk P, Wisowski G. Graves' disease-associated changes in the serum lysosomal glycosidases activity and the glycosaminoglycan content. Clin Chim Acta. (2003) 331:97–102. doi: 10.1016/s0009-8981(03)00090-1
64. Chen YQ, Gao LD, Liu YL, Shen Y, Diao JL, Yang WH, et al. Autophagy in graves' ophthalmopathy. Front Cell Dev Biol. (2023) 11:1158279. doi: 10.3389/fcell.2023.1158279
65. Morshed S, Latif R, Davies TF. Rescue of thyroid cells from antibody induced cell death via induction of autophagy. J Autoimmun. (2022) 126:102746. doi: 10.1016/j.jaut.2021.102746
66. Le S, Fu X, Pang M, Zhou Y, Yin G, Zhang J, et al. The antioxidative role of chaperone-mediated autophagy as a downstream regulator of oxidative stress in human diseases. Technol Cancer Res Treat. (2022) 21:15330338221114178. doi: 10.1177/15330338221114178
67. Smith TJ. Understanding pathogenesis intersects with effective treatment for thyroid eye disease. J Clin Endocrinol Metab. (2022) 107:S13–s26. doi: 10.1210/clinem/dgac328
68. Chen MH, Liao SL, Chen MH, Tsou PL, Shih MJ, Chang TC, et al. Lysosome-related genes are regulated in the orbital fat of patients with graves' ophthalmopathy. Invest Ophthalmol Vis Sci. (2008) 49:4760–4. doi: 10.1167/iovs.08-2020
69. Chittiboyina S, Bai Y, Lelièvre SA. Microenvironment-cell nucleus relationship in the context of oxidative stress. Front Cell Dev Biol. (2018) 6:23. doi: 10.3389/fcell.2018.00023
70. Tsai CC, Wu SB, Cheng CY, Kao SC, Kau HC, Chiou SH, et al. Increased oxidative DNA damage, lipid peroxidation, and reactive oxygen species in cultured orbital fibroblasts from patients with Graves' ophthalmopathy: evidence that oxidative stress has a role in this disorder. Eye (London England). (2010) 24:1520–5. doi: 10.1038/eye.2010.31
71. Ursu HI, Gheorghiu ML. Oxidative stress, nuclear factor-κB pathway and current smoking in graves' Ophthalmopathy. Eur Thyroid J. (2013) 2:69–70. doi: 10.1159/000347235
72. Zheng J, Duan H, You S, Liang B, Chen Y, Huang H. Research progress on the pathogenesis of Graves' ophthalmopathy: Based on immunity, noncoding RNA and exosomes. Front Immunol. (2022) 13:952954. doi: 10.3389/fimmu.2022.952954
73. Mishra S, Maurya VK, Kumar S, Ankita, Kaur A, Saxena SK. Clinical management and therapeutic strategies for the thyroid-associated ophthalmopathy: current and future perspectives. Curr Eye Res. (2020) 45:1325–41. doi: 10.1080/02713683.2020.1776331
74. Mseddi M, Ben Mansour R, Gargouri B, Mnif F, El Ghawi S, Hammami B, et al. Proteins oxidation and autoantibodies' reactivity against hydrogen peroxide and malondialdehyde -oxidized thyroid antigens in patients' plasmas with Graves' disease and Hashimoto Thyroiditis. Chem Biol Interact. (2017) 272:145–52. doi: 10.1016/j.cbi.2017.04.013
75. Koga M, Hiromatsu Y, Jimi A, Inoue Y, Nonaka K. Possible involvement of Fas-mediated apoptosis in eye muscle tissue from patients with thyroid-associated ophthalmopathy. Thyroid. (1998) 8:311–8. doi: 10.1089/thy.1998.8.311
76. Jiang L, Wei R, Diao J, Ding H, Wang W, Ao R. Proteomics of tear in inactive thyroid-associated ophthalmopathy. Acta endocrinologica (Bucharest Romania: 2005). (2021) 17:291–303. doi: 10.4183/aeb.2021.291
77. Ma Y, Liu H, Wu J, Yuan L, Wang Y, Du X, et al. The adverse health effects of bisphenol A and related toxicity mechanisms. Environ Res. (2019) 176:108575. doi: 10.1016/j.envres.2019.108575
78. Romieu I, Castro-Giner F, Kunzli N, Sunyer J. Air pollution, oxidative stress and dietary supplementation: a review. Eur Respir J. (2008) 31:179–97. doi: 10.1183/09031936.00128106
79. Mudway IS, Kelly FJ, Holgate ST. Oxidative stress in air pollution research. Free Radic Biol Med. (2020) 151:2–6. doi: 10.1016/j.freeradbiomed.2020.04.031
80. Ferrari SM, Fallahi P, Antonelli A, Benvenga S. Environmental issues in thyroid diseases. Front Endocrinol (Lausanne). (2017) 8:50. doi: 10.3389/fendo.2017.00050
81. Tavilani H, Nadi E, Karimi J, Goodarzi MT. Oxidative stress in COPD patients, smokers, and non-smokers. Respir Care. (2012) 57:2090–4. doi: 10.4187/respcare.01809
82. Pinilla Lozano I, Maneu V, Campello Blasco L, Kutsyr O, Lax P. Inherited retinal dystrophies: role of oxidative stress and inflammation in their physiopathology and therapeutic implications. Antioxidants (Basel Switzerland). (2022) V11:1086. doi: 10.3390/antiox11061086
83. Hansen DA, Satterfield BC, Layton ME, Van Dongen HPA. Sleep deprivation and sleep-onset insomnia are associated with blunted physiological reactivity to stressors. Mil Med. (2021) 186:246–52. doi: 10.1093/milmed/usaa464
84. Dang X, He B, Ning Q, Liu Y, Guo J, Niu G, et al. Alantolactone suppresses inflammation, apoptosis and oxidative stress in cigarette smoke-induced human bronchial epithelial cells through activation of Nrf2/HO-1 and inhibition of the NF-κB pathways. Respir Res. (2020) 21:95. doi: 10.1186/s12931-020-01358-4
85. Morgan MJ, Liu ZG. Crosstalk of reactive oxygen species and NF-κB signaling. Cell Res. (2011) 21:103–15. doi: 10.1038/cr.2010.178
86. Kim SE, Kim J, Lee JY, Lee SB, Paik JS, Yang SW. Octreotide inhibits secretion of IGF-1 from orbital fibroblasts in patients with thyroid-associated ophthalmopathy via inhibition of the NF-κB pathway. PloS One. (2021) V16:e0249988. doi: 10.1371/journal.pone.0249988
87. Giuliani C, Bucci I, Napolitano G. The role of the transcription factor nuclear factor-kappa B in thyroid autoimmunity and cancer. Front Endocrinol (Lausanne). (2018) 9:471. doi: 10.3389/fendo.2018.00471
88. Habas K, Shang L. Alterations in intercellular adhesion molecule 1 (ICAM-1) and vascular cell adhesion molecule 1 (VCAM-1) in human endothelial cells. Tissue Cell. (2018) 54:139–43. doi: 10.1016/j.tice.2018.09.002
89. Nowak M, Wielkoszyński T, Kos-Kudła B, Marek B, Karpe J, Kajdaniuk D, et al. The blood concentration of intercellular adhesion molecule-1 (sICAM-1) and vascular cell adhesion molecule-1 (sVCAM-1) in patients with active thyroid-associated orbitopathy before and after methylprednisolone treatment. Endokrynol Pol. (2007) 58:487–91.
90. Son Y, Cheong YK, Kim NH, Chung HT, Kang DG, Pae HO. Mitogen-activated protein kinases and reactive oxygen species: how can ROS activate MAPK pathways? J Signal transduction.(2011) 2011:792639. doi: 10.1155/2011/792639
91. Rezatabar S, Karimian A, Rameshknia V, Parsian H, Majidinia M, Kopi TA, et al. RAS/MAPK signaling functions in oxidative stress, DNA damage response and cancer progression. J Cell Physiol. (2019) 234:14951–65. doi: 10.1002/jcp.28334
92. Bartalena L, Tanda ML. Current concepts regarding Graves' orbitopathy. J Intern Med. (2022) 292:692–716. doi: 10.1111/joim.13524
93. Liu RM, Desai LP. Reciprocal regulation of TGF-β and reactive oxygen species: A perverse cycle for fibrosis. Redox Biol. (2015) 6:565–77. doi: 10.1016/j.redox.2015.09.009
94. Krstić J, Trivanović D, Mojsilović S, Santibanez JF. Transforming growth factor-beta and oxidative stress interplay: implications in tumorigenesis and cancer progression. Oxid Med Cell Longev. (2015) 2015:654594. doi: 10.1155/2015/654594
95. Kardalas E, Maraka S, Papagianni M, Paltoglou G, Siristatidis C, Mastorakos G. TGF-β Physiology as a novel therapeutic target regarding autoimmune thyroid diseases: where do we stand and what to expect. Medicina (Kaunas). (2021) 57:621. doi: 10.3390/medicina57060621
96. Kowluru RA, Kanwar M. Oxidative stress and the development of diabetic retinopathy: contributory role of matrix metalloproteinase-2. Free Radic Biol Med. (2009) 46:1677–85. doi: 10.1016/j.freeradbiomed.2009.03.024
97. Kapelko-Słowik K, Słowik M, Szaliński M, Dybko J, Wołowiec D, Prajs I, et al. Elevated serum concentrations of metalloproteinases (MMP-2, MMP-9) and their inhibitors (TIMP-1, TIMP-2) in patients with Graves' orbitopathy. Adv Clin Exp medicine: Off Organ Wroclaw Med University. (2018) 27:99–103. doi: 10.17219/acem/68991
98. Lanzolla G, Marcocci C, Marinò M. Antioxidant therapy in graves' Orbitopathy. Front Endocrinol (Lausanne). (2020) 11:608733. doi: 10.3389/fendo.2020.608733
99. Rayman MP. The importance of selenium to human health. Lancet. (2000) 356:233–41. doi: 10.1016/S0140-6736(00)02490-9
100. Wang F, Li C, Li S, Cui L, Zhao J, Liao L. Selenium and thyroid diseases. Front Endocrinol (Lausanne). (2023) 14:1133000. doi: 10.3389/fendo.2023.1133000
101. Steinbrenner H, Speckmann B, Klotz LO. Selenoproteins: Antioxidant selenoenzymes and beyond. Arch Biochem Biophys. (2016) 595:113–9. doi: 10.1016/j.abb.2015.06.024
102. Marinò M, Dottore GR, Leo M, Marcocci C. Mechanistic pathways of selenium in the treatment of graves' Disease and graves' Orbitopathy. Horm Metab Res. (2018) 50:887–93. doi: 10.1055/a-0658-7889
103. Duntas LH. The evolving role of selenium in the treatment of graves' disease and ophthalmopathy. J Thyroid Res. (2012) 2012:736161. doi: 10.1155/2012/736161
104. Salhiyyah K, Forster R, Senanayake E, Abdel-Hadi M, Booth A, Michaels JA. Pentoxifylline for intermittent claudication. Cochrane Database systematic Rev. (2015) 9:Cd005262. doi: 10.1002/14651858.CD005262.pub3
105. Chavarría AP, Vázquez RRV, Cherit JGD, Bello HH, Suastegui HC, Moreno-Castañeda L, et al. Antioxidants and pentoxifylline as coadjuvant measures to standard therapy to improve prognosis of patients with pneumonia by COVID-19. Comput Struct Biotechnol J. (2021) 19:1379–90. doi: 10.1016/j.csbj.2021.02.009
106. Chang CC, Chang TC, Kao SC, Kuo YF, Chien LF. Pentoxifylline inhibits the proliferation and glycosaminoglycan synthesis of cultured fibroblasts derived from patients with Graves' ophthalmopathy and pretibial myxoedema. Acta Endocrinol (Copenh). (1993) 129:322–7. doi: 10.1530/acta.0.1290322
107. Berman B, Duncan MR. Pentoxifylline inhibits the proliferation of human fibroblasts derived from keloid, scleroderma and morphoea skin and their production of collagen, glycosaminoglycans and fibronectin. Br J Dermatol. (1990) 123:339–46. doi: 10.1111/j.1365-2133.1990.tb06294.x
108. Finamor FE, Martins JR, Nakanami D, Paiva ER, Manso PG, Furlanetto RP. Pentoxifylline (PTX): an alternative treatment in Graves ophthalmopathy (inactive phase): assessment by a disease specific quality of life questionnaire and by exophthalmometry in a prospective randomized trial. Eur J Ophthalmol. (2004) 14:277–83. doi: 10.5301/ejo.2008.5017
109. Bartalena L, Martino E, Marcocci C, Bogazzi F, Panicucci M, Velluzzi F, et al. More on smoking habits and Graves' ophthalmopathy. J Endocrinol Invest. (1989) 12:733–7. doi: 10.1007/BF03350047
110. Marinò M, Menconi F, Rotondo Dottore G, Leo M, Marcocci C. Selenium in graves hyperthyroidism and orbitopathy. Ophthalmic Plast Reconstr Surg. (2018) 34:S105–s110. doi: 10.1097/IOP.0000000000001136
111. Bartalena L, Tanda ML, Piantanida E, Lai A. Oxidative stress and Graves' ophthalmopathy: in vitro studies and therapeutic implications. Biofactors. (2003) 19:155–63. doi: 10.1002/biof.5520190308
112. Bouzas EA, Karadimas P, Mastorakos G, Koutras DA. Antioxidant agents in the treatment of Graves' ophthalmopathy. Am J Ophthalmol. (2000) 129:618–22. doi: 10.1016/S0002-9394(00)00359-7
113. Chandran G, Sirajudeen KN, Yusoff NS, Swamy M, Samarendra MS. Effect of the antihypertensive drug enalapril on oxidative stress markers and antioxidant enzymes in kidney of spontaneously hypertensive rat. Oxid Med Cell Longev. (2014) 2014:608512. doi: 10.1155/2014/608512
114. Kmet O, Filipets N, Kmet T, Hrachova T, Vepriuk Y. Enalapril effect on glutathione chain of the antioxidant system of the brain in rats with scopolamine-induced neurodegeneration. Georgian Med News. (2019) 97–101.
115. Botta R, Lisi S, Marcocci C, Sellari-Franceschini S, Rocchi R, Latrofa F, et al. Enalapril reduces proliferation and hyaluronic acid release in orbital fibroblasts. Thyroid. (2013) 23:92–6. doi: 10.1089/thy.2012.0373
116. Ataabadi G, Dabbaghmanesh MH, Owji N, Bakhshayeshkaram M, Montazeri-Najafabady N. Clinical features of graves' Ophthalmopathy and impact of enalapril on the course of mild graves' Ophthalmopathy: A pilot study. Endocr Metab Immune Disord Drug Targets. (2020) 20:139–48. doi: 10.2174/1389201020666190725113816
117. Istvan ES, Deisenhofer J. Structural mechanism for statin inhibition of HMG-CoA reductase. Science. (2001) 292:1160–4. doi: 10.1126/science.1059344
118. Beltowski J. Statins and modulation of oxidative stress. Toxicol Mech Methods. (2005) 15:61–92. doi: 10.1080/15376520590918766
119. Stein JD, Childers D, Gupta S, Talwar N, Nan B, Lee BJ, et al. Risk factors for developing thyroid-associated ophthalmopathy among individuals with Graves disease. JAMA ophthalmology. (2015) 133:290–6. doi: 10.1001/jamaophthalmol.2014.5103
120. Bartalena L, Piantanida E, Tanda ML. Statins for Graves' orbitopathy: a new tool for prevention and treatment? Lancet Diabetes endocrinology. (2021) 9:726–7. doi: 10.1016/S2213-8587(21)00268-0
121. Lanzolla G, Sabini E, Leo M, Menconi F, Rocchi R, Sframeli A, et al. Statins for Graves' orbitopathy (STAGO): a phase 2, open-label, adaptive, single centre, randomised clinical trial. Lancet Diabetes endocrinology. (2021) 9:733–42. doi: 10.1016/S2213-8587(21)00238-2
122. Malboosbaf R, Maghsoomi Z, Emami Z, Khamseh ME, Azizi F. Statins and thyroid eye disease (TED): a systematic review. Endocrine. (2024) 1–7. doi: 10.1007/s12020-023-03680-5
123. Lanzolla G, Vannucchi G, Ionni I, Campi I, Sileo F, Lazzaroni E, et al. Cholesterol serum levels and use of statins in graves' Orbitopathy: A new starting point for the therapy. Front Endocrinol (Lausanne). (2019) 10:933. doi: 10.3389/fendo.2019.00933
124. Padayatty SJ, Katz A, Wang Y, Eck P, Kwon O, Lee JH, et al. Vitamin C as an antioxidant: evaluation of its role in disease prevention. J Am Coll Nutr. (2003) 22:18–35. doi: 10.1080/07315724.2003.10719272
125. Niki E. Role of vitamin E as a lipid-soluble peroxyl radical scavenger: in vitro and in vivo evidence. Free Radic Biol Med. (2014) 66:3–12. doi: 10.1016/j.freeradbiomed.2013.03.022
126. Saini R. Coenzyme Q10: The essential nutrient. J Pharm Bioallied Sci. (2011) 3:466–7. doi: 10.4103/0975-7406.84471
127. Bavarsad Shahripour R, Harrigan MR, Alexandrov AV. N-acetylcysteine (NAC) in neurological disorders: mechanisms of action and therapeutic opportunities. Brain behavior. (2014) 4:108–22. doi: 10.1002/brb3.208
128. Sharafati-Chaleshtori R, Shirzad H, Rafieian-Kopaei M, Soltani A. Melatonin and human mitochondrial diseases. J Res Med Sci. (2017) 22:2. doi: 10.4103/1735-1995.199092
129. Stan MN, Bahn RS. Risk factors for development or deterioration of Graves' ophthalmopathy. Thyroid. (2010) 20:777–83. doi: 10.1089/thy.2010.1634
130. Rotondo Dottore G, Ionni I, Menconi F, Casini G, Sellari-Franceschini S, Nardi M, et al. Action of three bioavailable antioxidants in orbital fibroblasts from patients with Graves' orbitopathy (GO): a new frontier for GO treatment? J Endocrinol Invest. (2018) 41:193–201. doi: 10.1007/s40618-017-0718-7
131. Farasati Far B, Behnoush AH, Ghondaghsaz E, Habibi MA, Khalaji A. The interplay between vitamin C and thyroid. Endocrinology Diabetes Metab. (2023) 6:e432. doi: 10.1002/edm2.432
132. Moncayo R, Moncayo H. Practical guidelines for diagnosing and treating thyroid disease based on the WOMED metabolic model of disease focusing on glycolysis and coenzyme Q(10) deficiency-A clinical alternative to the 2021 retired clinical practice guidelines of the endocrine society. Diagnostics (Basel Switzerland). (2022) 12:107. doi: 10.3390/diagnostics12010107
133. Marique L, Senou M, Craps J, Delaigle A, Van Regemorter E, Wérion A, et al. Oxidative stress and upregulation of antioxidant proteins, including adiponectin, in extraocular muscular cells, orbital adipocytes, and thyrocytes in graves' Disease associated with orbitopathy. Thyroid. (2015) 25:1033–42. doi: 10.1089/thy.2015.0087
134. Zhao L, Wang H, Du X. The therapeutic use of quercetin in ophthalmology: recent applications. BioMed Pharmacother. (2021) 137:111371. doi: 10.1016/j.biopha.2021.111371
135. Akbarian S, Chaibakhsh S, Kashkouli MB, Karimi N, Abdolalizadeh P, Ghahvehchian H. A systematic review on the role of antioxidants in thyroid eye disease. J Curr ophthalmology. (2022) 34:16–24. doi: 10.4103/joco.joco_266_21
136. Rotondo Dottore G, Leo M, Casini G, Latrofa F, Cestari L, Sellari-Franceschini S, et al. Antioxidant actions of selenium in orbital fibroblasts: A basis for the effects of selenium in graves' Orbitopathy. Thyroid. (2017) 27:271–8. doi: 10.1089/thy.2016.0397
137. Mckay TB, Karamichos D. Quercetin and the ocular surface: What we know and where we are going. Exp Biol Med (Maywood). (2017) 242:565–72. doi: 10.1177/1535370216685187
138. Williams RJ, Spencer JP, Rice-Evans C. Flavonoids: antioxidants or signalling molecules? Free Radic Biol Med. (2004) 36:838–49. doi: 10.1016/j.freeradbiomed.2004.01.001
139. Yoon JS, Lee HJ, Choi SH, Chang EJ, Lee SY, Lee EJ. Quercetin inhibits IL-1β-induced inflammation, hyaluronan production and adipogenesis in orbital fibroblasts from Graves' orbitopathy. PloS One. (2011) 6:e26261. doi: 10.1371/journal.pone.0026261
140. Hira S, Saleem U, Anwar F, Sohail MF, Raza Z, Ahmad B. β-carotene: A natural compound improves cognitive impairment and oxidative stress in a mouse model of streptozotocin-induced alzheimer's disease. Biomolecules. (2019) 9:441. doi: 10.3390/biom9090441
141. Zhou L, Ouyang L, Lin S, Chen S, Liu Y, Zhou W, et al. Protective role of β-carotene against oxidative stress and neuroinflammation in a rat model of spinal cord injury. Int Immunopharmacol. (2018) 61:92–9. doi: 10.1016/j.intimp.2018.05.022
142. Rotondo Dottore G, Ionni I, Menconi F, Casini G, Sellari-Franceschini S, Nardi M, et al. Antioxidant effects of β-carotene, but not of retinol and vitamin E, in orbital fibroblasts from patients with Graves' orbitopathy (GO). J Endocrinol Invest. (2018) 41:815–20. doi: 10.1007/s40618-017-0809-5
143. Stahl W, Heinrich U, Jungmann H, Von Laar J, Schietzel M, Sies H, et al. Increased dermal carotenoid levels assessed by noninvasive reflection spectrophotometry correlate with serum levels in women ingesting Betatene. J Nutr. (1998) 128:903–7. doi: 10.1093/jn/128.5.903
144. Tanvetyanon T, Bepler G. Beta-carotene in multivitamins and the possible risk of lung cancer among smokers versus former smokers: a meta-analysis and evaluation of national brands. Cancer. (2008) 113:150–7. doi: 10.1002/cncr.23527
145. Oz HS, Chen TS, Mcclain CJ, De Villiers WJ. Antioxidants as novel therapy in a murine model of colitis. J Nutr Biochem. (2005) 16:297–304. doi: 10.1016/j.jnutbio.2004.09.007
146. Cabrera C, Artacho R, Giménez R. Beneficial effects of green tea–a review. J Am Coll Nutr. (2006) 25:79–99. doi: 10.1080/07315724.2006.10719518
147. Lee JY, Paik JS, Yun M, Lee SB, Yang SW. The effect of (-)-epigallocatechin-3-gallate on IL-1β Induced IL-8 expression in orbital fibroblast from patients with thyroid-associated ophthalmopathy. PloS One. (2016) 11:e0148645. doi: 10.1371/journal.pone.0148645
148. Liu W, Ma C, Li HY, Yuan SS, Li KJ. Tea polyphenols reduce inflammation of orbital fibroblasts in graves' Ophthalmopathy via the NF-κB/NLRP3 pathway. Curr Med science. (2023) 43:123–9. doi: 10.1007/s11596-023-2708-7
149. Shakibaei M, Harikumar KB, Aggarwal BB. Resveratrol addiction: to die or not to die. Mol Nutr Food Res. (2009) 53:115–28. doi: 10.1002/mnfr.200800148
150. Kim CY, Lee HJ, Chae MK, Byun JW, Lee EJ, Yoon JS. Therapeutic effect of resveratrol on oxidative stress in graves' Orbitopathy orbital fibroblasts. Invest Ophthalmol Vis Sci. (2015) 56:6352–61. doi: 10.1167/iovs.15-16870
151. Chowdhury I, Banerjee S, Driss A, Xu W, Mehrabi S, Nezhat C, et al. Curcumin attenuates proangiogenic and proinflammatory factors in human eutopic endometrial stromal cells through the NF-κB signaling pathway. J Cell Physiol. (2019) 234:6298–312. doi: 10.1002/jcp.27360
152. Xiao Y, Xia J, Wu S, Lv Z, Huang S, Huang H, et al. Curcumin inhibits acute vascular inflammation through the activation of heme oxygenase-1. Oxid Med Cell Longev. (2018) 2018:3295807. doi: 10.1155/2018/3295807
153. Lee JS, Kim J, Lee EJ, Yoon JS. Therapeutic effect of curcumin, a plant polyphenol extracted from curcuma longae, in fibroblasts from patients with graves' Orbitopathy. Invest Ophthalmol Vis Sci. (2019) 60:4129–40. doi: 10.1167/iovs.19-27376
154. Ojo OA, Adeyemo TR, Rotimi D, Batiha GE, Mostafa-Hedeab G, Iyobhebhe ME, et al. Anticancer properties of curcumin against colorectal cancer: A review. Front Oncol. (2022) 12:881641. doi: 10.3389/fonc.2022.881641
155. Nelson KM, Dahlin JL, Bisson J, Graham J, Pauli GF, Walters MA. The essential medicinal chemistry of curcumin. J Med Chem. (2017) 60:1620–37. doi: 10.1021/acs.jmedchem.6b00975
156. Su SH, Shyu HW, Yeh YT, Chen KM, Yeh H, Su SJ. Caffeine inhibits adipogenic differentiation of primary adipose-derived stem cells and bone marrow stromal cells. Toxicol In Vitro. (2013) 27:1830–7. doi: 10.1016/j.tiv.2013.05.011
157. Lv X, Chen Z, Li J, Zhang L, Liu H, Huang C, et al. Caffeine protects against alcoholic liver injury by attenuating inflammatory response and oxidative stress. Inflammation Res. (2010) 59:635–45. doi: 10.1007/s00011-010-0176-6
158. Jagdeo J, Brody N. Complementary antioxidant function of caffeine and green tea polyphenols in normal human skin fibroblasts. J Drugs dermatology: JDD. (2011) 10:753–61.
159. Ko J, Kim JY, Kim JW, Yoon JS. Anti-oxidative and anti-adipogenic effects of caffeine in an in vitro model of Graves' orbitopathy. Endocr J. (2020) 67:439–47. doi: 10.1507/endocrj.EJ19-0521
160. Grosso G, Micek A, Castellano S, Pajak A, Galvano F. Coffee, tea, caffeine and risk of depression: A systematic review and dose-response meta-analysis of observational studies. Mol Nutr Food Res. (2016) 60:223–34. doi: 10.1002/mnfr.201500620
161. Li K, Ma C, Li H, Dev S, He J, Qu X. Medicinal value and potential therapeutic mechanisms of gynostemma pentaphyllum (Thunb.) makino and its derivatives: an overview. Curr Top Med Chem. (2019) 19:2855–67. doi: 10.2174/1568026619666191114104718
162. Kim BY, Jang SY, Choi DH, Jung CH, Mok JO, Kim CH. Anti-inflammatory and antioxidant effects of selenium on orbital fibroblasts of patients with graves ophthalmopathy. Ophthalmic Plast Reconstr Surg. (2021) 37:476–81. doi: 10.1097/IOP.0000000000001931
163. Li H, Ma C, Liu W, He J, Li K. Gypenosides protect orbital fibroblasts in graves ophthalmopathy via anti-inflammation and anti-fibrosis effects. Invest Ophthalmol Vis Sci. (2020) 61:64. doi: 10.1167/iovs.61.5.64
164. Carvalho DP, Dupuy C. Role of the NADPH oxidases DUOX and NOX4 in thyroid oxidative stress. Eur Thyroid J. (2013) 2:160–7. doi: 10.1159/000354745
165. Burch HB, Lahiri S, Bahn RS, Barnes S. Superoxide radical production stimulates retroocular fibroblast proliferation in Graves' ophthalmopathy. Exp Eye Res. (1997) 65:311–6. doi: 10.1006/exer.1997.0353
166. Komosinska-Vassev K, Olczyk K, Kucharz EJ, Marcisz C, Winsz-Szczotka K, Kotulska A. Free radical activity and antioxidant defense mechanisms in patients with hyperthyroidism due to Graves' disease during therapy. Clin Chim Acta. (2000) 300:107–17. doi: 10.1016/S0009-8981(00)00306-5
167. Bednarek J, Wysocki H, Sowiński J. Oxidative stress peripheral parameters in Graves' disease: the effect of methimazole treatment in patients with and without infiltrative ophthalmopathy. Clin Biochem. (2005) 38:13–8. doi: 10.1016/j.clinbiochem.2004.09.015
168. Renaud CO, Ziros PG, Chartoumpekis DV, Bongiovanni M, Sykiotis GP. Keap1/nrf2 signaling: A new player in thyroid pathophysiology and thyroid cancer. Front Endocrinol (Lausanne). (2019) 10:510. doi: 10.3389/fendo.2019.00510
169. Sabatino L. Nrf2-mediated antioxidant defense and thyroid hormone signaling: A focus on cardioprotective effects. Antioxidants (Basel Switzerland). (2023) 12:1177. doi: 10.3390/antiox12061177
170. Zhang Y, Shi Z, Zhou Y, Xiao Q, Wang H, Peng Y. Emerging substrate proteins of kelch-like ECH associated protein 1 (Keap1) and potential challenges for the development of small-molecule inhibitors of the keap1-nuclear factor erythroid 2-related factor 2 (Nrf2) protein-protein interaction. J Med Chem. (2020) 63:7986–8002. doi: 10.1021/acs.jmedchem.9b01865
171. Cuadrado A, Rojo AI, Wells G, Hayes JD, Cousin SP, Rumsey WL, et al. Therapeutic targeting of the NRF2 and KEAP1 partnership in chronic diseases. Nat Rev Drug Discovery. (2019) 18:295–317. doi: 10.1038/s41573-018-0008-x
172. Thanas C, Ziros PG, Chartoumpekis DV, Renaud CO, Sykiotis GP. The keap1/nrf2 signaling pathway in the thyroid-2020 update. Antioxidants (Basel Switzerland). (2020) 9:1082. doi: 10.3390/antiox9111082
173. Li H, Min J, Chen Y, Li H, Zhang Y. Polydatin attenuates orbital oxidative stress in Graves' orbitopathy through the NRF2 pathway. Chem Biol Interact. (2020) 315:108894. doi: 10.1016/j.cbi.2019.108894
174. Manna A, De Sarkar S, De S, Bauri AK, Chattopadhyay S, Chatterjee M. The variable chemotherapeutic response of Malabaricone-A in leukemic and solid tumor cell lines depends on the degree of redox imbalance. Phytomedicine. (2015) 22:713–23. doi: 10.1016/j.phymed.2015.05.007
Keywords: thyroid-associated ophthalmopathy, oxidative stress, inflammation, therapeutic method, antioxidants
Citation: Ma C, Li H, Lu S and Li X (2024) Thyroid-associated ophthalmopathy: the role of oxidative stress. Front. Endocrinol. 15:1400869. doi: 10.3389/fendo.2024.1400869
Received: 14 March 2024; Accepted: 28 June 2024;
Published: 11 July 2024.
Edited by:
Sijie Fang, Shanghai Jiao Tong University, ChinaReviewed by:
Yue Wang, First Affiliated Hospital of Xi’an Jiaotong University, ChinaLianqun Wu, Fudan University, China
Copyright © 2024 Ma, Li, Lu and Li. This is an open-access article distributed under the terms of the Creative Commons Attribution License (CC BY). The use, distribution or reproduction in other forums is permitted, provided the original author(s) and the copyright owner(s) are credited and that the original publication in this journal is cited, in accordance with accepted academic practice. No use, distribution or reproduction is permitted which does not comply with these terms.
*Correspondence: Chao Ma, Z21jaGFvMjE5QDE2My5jb20=
†These authors have contributed equally to this work and share first authorship