- 1College Of Pharmacy, Shandong University of Traditional Chinese Medicine, Jinan, Shandong, China
- 2College Of Traditional Chinese Medicine, Shandong University of Traditional Chinese Medicine School, Jinan, Shandong, China
- 3Medical College, Shandong University of Traditional Chinese Medicine, Jinan, Shandong, China
- 4The First Clinical College, Shandong University of Traditional Chinese Medicine, Jinan, Shandong, China
This paper explores the significant role of epigenetics in women’s reproductive health, focusing on the impact of environmental factors. It highlights the crucial link between epigenetic modifications—such as DNA methylation and histones post-translational modifications—and reproductive health issues, including infertility and pregnancy complications. The paper reviews the influence of pollutants like PM2.5, heavy metals, and endocrine disruptors on gene expression through epigenetic mechanisms, emphasizing the need for understanding how dietary, lifestyle choices, and exposure to chemicals affect gene expression and reproductive health. Future research directions include deeper investigation into epigenetics in female reproductive health and leveraging gene editing to mitigate epigenetic changes for improving IVF success rates and managing reproductive disorders.
1 Introduction
The World Health Organization (WHO) identifies Sexual and Reproductive Health (SRH) as integral to achieving the highest standard of health. Women’s reproductive health remains a significant global concern, impacting not only physical well-being but also societal development, economic growth, and public health. Women’s unique physiological structures and responses, including the glandular folds of their internal and external genitalia in a moist environment, create conditions conducive to pathogen survival. The presence of androgens in the body may promote the growth and proliferation of pathogens, significantly increasing the risk of tumor development (1). During menstruation, the disruption of the endometrial lining and the increased susceptibility to various infections during sexual intercourse further exacerbate women’s reproductive health challenges (2). According to WHO data, 40% of Chinese women suffer from various degrees of reproductive tract infections, with the prevalence among married women reaching up to 70%. This indicates that approximately 300 million women in China face reproductive health issues, a figure significantly higher than the incidence rate of common colds. The repercussions of reproductive health issues on women’s lives and careers are profound, causing immense distress and suffering. Common gynecological conditions include endometriosis, uterine fibroids, ovarian cysts, vaginitis, cervicitis, cervical erosion, pelvic inflammatory disease, adnexitis, functional uterine bleeding, breast diseases, infertility, and menstrual disorders. Notably, endometriosis affects 10% (190 million) of women of reproductive age worldwide (3). The majority of gynecological diseases, along with psychological factors such as work stress and environmental conditions, can adversely affect ovarian function. This leads to metabolic disorders, endocrine disruptions, and imbalances in estrogen and progesterone levels, triggering conditions such as melasma, wrinkles, constipation, acne, obesity, hyperlipidemia, and even carcinogenesis, accompanied by mental lethargy (4, 5). Hence, reproductive system issues directly impact human health.
Over the past decade, notable progress has been made in the prevention and treatment strategies for common diseases of the female reproductive system. However, current research on the impact of environmental factors through epigenetic mediation on women’s reproductive health remains fragmented. In this review, we summarize the latest findings on the influence of environmental factors on epigenetics and, consequently, on women’s reproductive health. This includes an overview of several common types of epigenetics and the potential cellular and molecular mechanisms involved. Furthermore, we discuss the impact of both internal and external environmental factors on the female reproductive system. Therefore, our aim is to gain a more comprehensive understanding of the pathophysiological processes and potential mechanisms related to female infertility diseases, with the goal of enhancing fertility and pregnancy outcomes in women of childbearing age.
2 An introduction to epigenetics
Epigenetics refers to the transmission of genetic information that does not involve changes to the DNA sequence. It operates through chemical modifications on the genome, such as histones post-translational modifications, DNA methylation, and hydroxymethylation, thereby altering the way genes are expressed. The mechanism by which DNA methylation leads to gene silencing is not yet fully understood; however, three main forms are commonly considered. First, DNA methylation affects the transcriptional activity of genes. It can directly hinder the recognition and binding of transcription factors to specific DNA sequences, thus inhibiting gene transcription (6). Second, the mechanism by which methylation leads to gene silencing involves the methylation of CpG islands located in promoters or other regulatory regions playing a role in gene repression. Methylation of CpG islands in non-coding promoter regions recruits sequence-specific methylated DNA binding proteins and histone deacetylases (HDACs), forming complexes that suppress transcription by obstructing the binding of transcription factors to their target sequences, thereby affecting transcription (7, 8). Finally, DNA methylation can alter chromatin structure to suppress gene expression, where highly methylated promoters cause chromatin to condense, further affecting transcription (9).
Epigenetic modifications can regulate the splicing and expression patterns of genes (10). For instance, numerous studies have shown that histones post-translational modifications can regulate the binding and activity of splicing factors, thus influencing gene splicing. Results obtained from real-time quantitative Polymerase Chain Reaction(PCR) analysis of 16 epigenetic remodeling markers in the epidermal cells of 14 patients after in vitro amplification compared to freshly isolated epidermal cells (ISO), indicated a significant reduction in the transcription levels of genes involved in DNA methylation and histones post-translational modifications in cells cultured to the second generation of keratinocyte formation.
Beyond the aforementioned effects, epigenetic modifications can also regulate genomic stability and genetic memory, enabling cells to stably express specific phenotypes (11). Further research and understanding of the mechanisms of epigenetics are crucial for unraveling important processes in organism development, environmental adaptation, and disease onset.
3 The potential impact of environmental factors on women’s reproductive health
Infertility is commonly defined as “a disease of the male or female reproductive system characterized by the failure to achieve a successful pregnancy after more than one year of regular, unprotected sexual intercourse.” External harmful environmental factors can impair women’s fertility. Non-gaseous pollutants, such as PM2.5, have a certain impact on female reproduction(12, 13). Gaseous pollutants (such as ozone (O3), sulfur dioxide (SO2), and nitrogen oxides (NOx)) can affect the endocrine system of women of childbearing age, leading to infertility and pregnancy complications, including reduced ovarian reserve (14, 15), uterine fibroids (16), and preeclampsia (17, 18).
Harmful chemicals also impact women’s reproductive health (19). Polycyclic aromatic hydrocarbons have been shown to interact with estrogen receptors, activating the aryl hydrocarbon receptor leading to changes in steroid functionality and anti-estrogenic activity, resulting in adverse pregnancy outcomes such as preterm birth, miscarriage, and embryonic developmental arrest (20).Studies indicate that the widespread use of the heavy metal cadmium can cause endocrine disruption in women, potentially directly affecting the development of oocytes, the development of the uterus, and ovarian function, leading to decreased fertility, spontaneous miscarriage, and other reproductive issues (21).
4 Defining the purpose and scope of a literature review
In recent years, a growing body of evidence suggests that environmental exposures can leave epigenetic marks on genes, with various environmental factors proven to induce global or specific epigenetic changes (266). In this review, we discuss the interactions between environmental factors related to women’s reproductive health risks and epigenetics. This includes more common epigenetic processes such as DNA methylation, histones post-translational modifications, and non-coding RNA. Key roles in genetic regulation are played by external environmental factors like heavy metals (cadmium), polycyclic aromatic hydrocarbons, air pollutants, and internal factors such as dietary and nutritional elements, as well as the influence of parental care and climate factors on epigenetics. Moreover, we comment on the regulation of female reproductive functions through epigenetics, such as the pathogenesis and regulatory processes related to Polycystic Ovary Syndrome (PCOS), Premature Ovarian Insufficiency (POI), and endometriosis mediated by epigenetics, elucidating the function of epigenetics as a mediator, bridging environmental factors and female reproduction. Besides the adverse effects on female reproductive functions, epigenetic processes and abnormal epigenetic marks can also impact offspring health. Therefore, understanding how maternal environmental factors can affect offspring health through epigenetic mechanisms is crucial for preventing and managing environmentally related women’s reproductive health issues and accelerating the application of epigenetics in reproductive medicine.
5 The fundamentals of epigenetics
Epigenetics, also referred to as character genetics, exogenetics, paragenetics, postgenetics, or topogenetics, such as DNA methylation and histone modifications. DNA methylation is termed a carrier of epigenetic information, whereas variations and modifications of histones can directly or indirectly impact the structure of local chromatin. These chemical modifications to chromatin are both inheritable and reversible.
5.1 Epigenetic mechanisms
5.1.1 DNA methylation
DNA methylation is the most prevalent epigenetic regulatory mechanism, where methyl groups are covalently bonded to cytosine residues in DNA sequences through enzyme-mediated reactions catalyzed by specific methyltransferases. In vertebrates, three methylation states of DNA are recognized: a persistent hypomethylation state (22), an induced demethylation state, and a hypermethylation state, which is notably observed in the methylation modifications of the inactivated X chromosome (23). Recent studies have demonstrated that genome-wide hypermethylation can impede the epithelial-to-mesenchymal transition, thereby further inhibiting the healing of chronic wounds (24).
In mammals, DNA methylation involves the covalent transfer of a methyl group to the C-5 position of the cytosine ring in CpG dinucleotides. As a form of chemical modification, DNA methylation alters the structure of the cytosine residues, creating the “fifth base” - 5-methylcytosine (5mC), which is the most significant form of DNA methylation in mammals. DNA methylation is essential for the organism’s normal growth and development, including the formation of genetic imprints(11
) and the promotion of dispersed chromatin to become condensed. DNA methylation also impacts the normal expression of genes (25), leading to a decrease in gene transcriptional activity. The mechanism by which DNA methylation impedes gene transcription is complex: it interferes with the binding of transcription factors to promoters, thus blocking transcription (1). Moreover, transcription factors can recognize methylated DNA and reactivate gene transcription (26). DNA methylation is catalyzed by DNA methyltransferases (DNMTs), with DNMT1 and DNMT3 being the active enzymes responsible for establishing and maintaining DNA methylation (27). DNA methyltransferases add methyl groups to CpG islands using S-adenosyl methionine (SAM) as a substrate. Zhu Bing was the first to use the methylation profile of mouse oocytes to confirm that DNMT1 indeed functions as an initiating DNA methyltransferase (28).
De novo methylation refers to the addition of methyl groups to previously unmethylated cytosines under the action of DNMT3 methyltransferases. DNMT3A and DNMT3B are the primary enzymes for mammalian DNA methylation, known as de novo methyltransferases (29). DNMT3A-mediated DNA methylation plays an indispensable role in the spermatogenesis of male germ cells (30),Mutations in DNMT3A render it insensitive to the inhibition of H3K4me3, resulting in the aberrant methylation of promoter subgroups marked by H3K4me3 in mouse embryonic stem cells (ESCs). This aberrant methylation leads to the downregulation of associated genes (31).While the ectopic expression of DNMT3B can enhance the genome-wide methylation level of haploid embryonic stem cells, shorten the transition from the G2 phase to the M phase of cell mitosis, alleviate spontaneous diploidization of haploid cells, and extend the survival time of semiclone mice (32).Weinberg et al. (33) demonstrated that H3K36me2 is essential for the recruitment and maintenance of DNA methylation in intergenic regions by DNMT3A. In contrast, Shirane et al. (34) showed that H3K36me2, deposited by NSD1, plays a crucial role in de novo methylation in germ cells. Typically, de novo methyltransferases preferentially bind to CpG-rich regions that are not protected by H3K4 methylation. These enzymes with methyltransferase activity prioritize the methylation of unmethylated CpG dinucleotides. For instance, the flexible N-terminal guides DNMT3AA1 to its bivalent target catalytic methyltransferase domain, thereby regulating DNA methylation and gene expression (35). DNMT3L, while having no catalytic activity, can interact with DNMT3A and DNMT3B to regulate their activity (36) and is expressed only in specific rodents like mice, rats, gerbils, and hamsters (37). In human oocytes, it is transcriptionally silent and involved in the regulation of repetitive elements and imprinting in germ cells. Although DNMT3L cannot bind the methyl donor S-adenosyl-L-methionine (SAM), it facilitates the interaction of SAM with DNMT3A2, aiding the de novo methyltransferases (38).
DNMT1 is the key enzyme for maintaining DNA methylation (39), preserving the methylation pattern from the parental DNA strand to the daughter strand through cell division and DNA replication (40). The C-terminal domain of DNMT1 consists of two subdomains: the Target Recognition Domain (TRD), which identifies hemimethylated cytosines, and the methyltransferase domain.
DNMT1 preferentially methylates hemimethylated DNA, a process primarily facilitated by the TRD domain recognizing cytosines within hemimethylated DNA. Once the DNA methylation pattern is established, the DNA methyltransferase DNMT1 maintains this pattern during DNA replication. The faithful replication of the DNA methylation pattern during cell division makes it an ideal mechanism for preserving epigenetic memory.
A recent review on DNA methylation proposed a regulatory model for DNMTs, where de novo methyltransferases adopt an autoinhibitory conformation until they are locally activated upon binding to the N-terminal tail of histone H3. Additional research indicates that this regulatory principle is applicable not only to classical de novo methyltransferases but also to DNMT1 (41). In this context, the replication foci targeting sequence (RFT) domain interacts with conformational activators, such as UHRF1, thereby exposing the catalytic site (42, 43).
DNA methylation can suppress the activity of certain genes, while DNA demethylation induces the reactivation and expression of genes (267). TET enzymes play a key role in the DNA demethylation process (44), including TET1, TET2, and TET3. These enzymes convert 5-methylcytosine (5mC) into 5-hydroxymethylcytosine (5hmC) (45), which can be further oxidized to 5-formylcytosine (5fC) and 5-carboxylcytosine (5caC). Subsequently, the TET and base excision repair (BER) pathways intervene in the repair process, converting these modified bases back to unmethylated cytosine, thereby achieving DNA demethylation. Although the BER pathway is commonly considered the final step in DNA demethylation, there remains controversy regarding the specific enzymes and chemical intermediates formed during this process (46). The implications of DNA demethylation are increasingly recognized; for instance, aberrant DNA hypermethylation has been detected in the leptotene spermatocytes of some patients with non-obstructive azoospermia, suggesting that DNA demethylation can influence male meiotic recombination and fertility (47).
5.1.2 Histone post-translational modification
Histones are primarily composed of a globular domain (48) and tails that protrude outside the nucleosome, made up of basic amino acids forming a fundamental structural protein. Two H2A-H2B dimers and one H3-H4 tetramer assemble into a histone octamer. Histones and DNA are the basic components of the nucleosome, providing appropriate sites for DNA winding when nuclear DNA is in a highly condensed state. Timothy J. Richmond and others determined the crystal structure of the chromatin nucleosome core particle, with a 2.8A high-resolution X-ray structure detailing the internal binding mode of the histone octamer and the superhelical organization of its surrounding 146 base pairs of DNA (49). Unmethylated nucleosome DNA spontaneously extends, forming four superhelical turns (50), a phenomenon that strongly evidences the crucial role of histone tails in maintaining the overall nucleosome structure (51).As illustrated in Figure 1, the diagram represents the spectrum of histones post-translational modifications. histones post-translational modifications mainly include methylation, acetylation, ubiquitination, sumoylation, and citrullination.
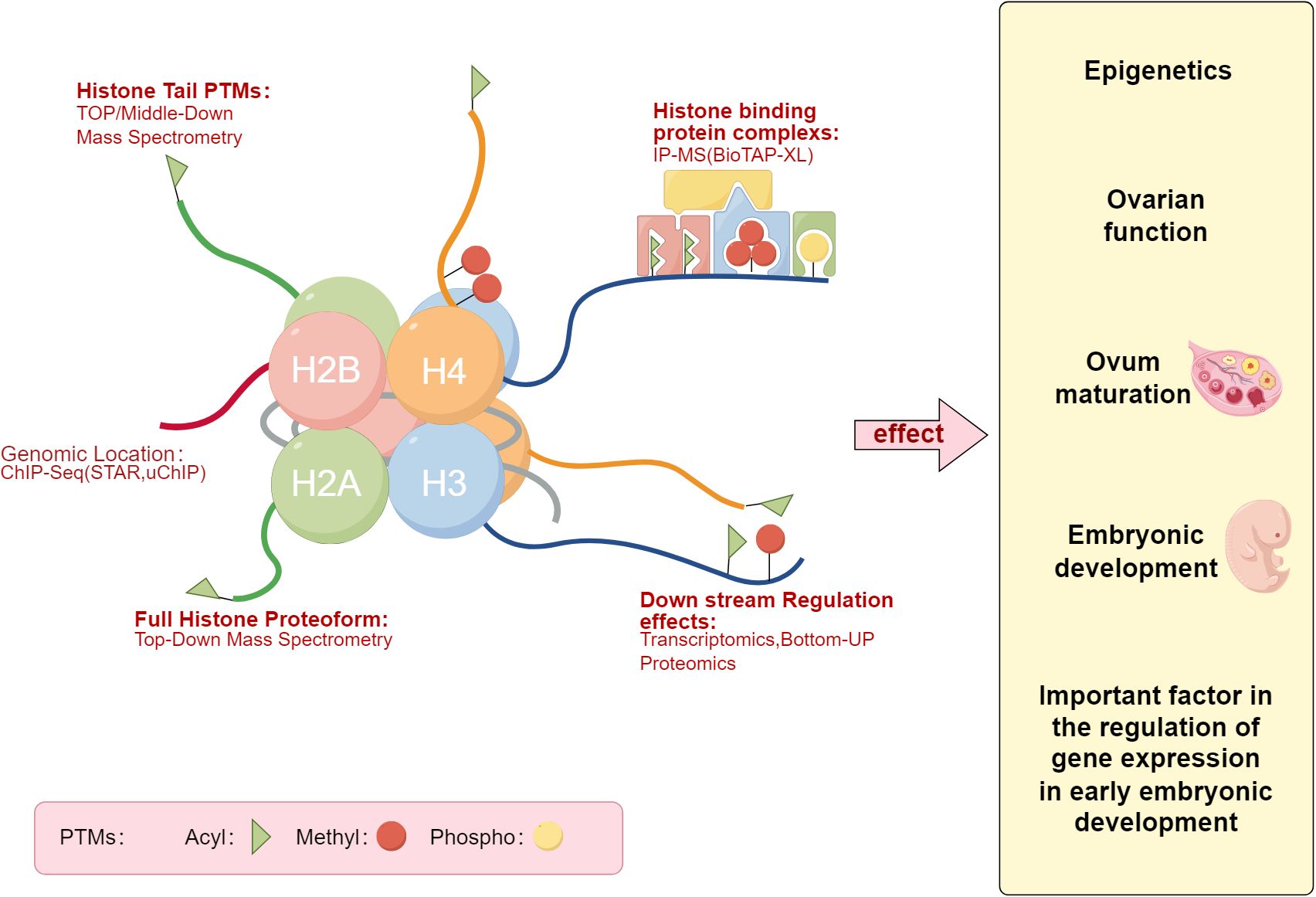
Figure 1. Histones post-translational modifications Map. The left panel describes the patterns of histones post-translational modifications, while the right panel lists the potential impacts of histones post-translational modifications on the pregnancy process, such as embryonic development (Draw by figdraw.).
Methylation of histones that wrap DNA in eukaryotic chromosomes can have significant implications for human health. It occurs on all basic residues, including arginine (52), lysine (53), and histidine (54). Protein methylation can occur at the N-, O-, and S-centers of amino acid residues, with arginine and lysine residues being the most common sites of histone methylation. The effects of methylation on gene activity vary (55), depending on the modified amino residue (56), the genetic context of methylation, its level and pattern. Amino acid methylation primarily changes chromatin structure by increasing or decreasing DNA-histone interactions, leading to the activation or suppression of gene transcription. The core histone H3 plays a key role in nucleosome structure and gene expression regulation, being a primary site of histone methylation, along with other core histones like H2A, H2B, and H4, which are central components of the nucleosome and participate in histone methylation (57). During histone methylation, S-adenosylmethionine (SAM) (58) serves as the substrate, and under the catalysis of histone methyltransferases (HMTs, also known as “writers”), it transfers its methyl group to the lysine residues of histones (59). On the ϵ-amino group of lysine, it can be monomethylated, dimethylated, or trimethylated (60–62), referred to as me1, me2, and me3 (63). Currently, extensive research has been conducted on methylated histones, including H3K4, H3K9, H3K27, H3K36, H3K79, and H4K20 (64). Studies show that H3K4, H3K36, and H3K79 are mainly found in transcriptionally active regions of chromatin, playing a role in activating gene transcription. Conversely, H3K9, H3K27, and H4K20 often act as markers for gene transcription repression, closely associated with gene silencing (65–68).
Histone acetylation is a reversible process where acetyl groups are transferred by histone acetyltransferases (HATs), with lysine acetylation being a common form of histone acetylation. Simply put, this process involves adding an acetyl group to the positively charged lysine residues. Histone acetylation decreases the electrostatic affinity between histones and negatively charged DNA, loosening chromatin structure and activating open chromatin regions (69), making it easier for transcription factors and RNA polymerase to access DNA, thereby promoting transcription (70). Histone 1 is the most commonly acetylated protein, and H2A, H2B, H3, and H4 form the core of the nucleosome, referred to as core histones. Acetylation modifications within these core histones affect the dynamics of the nucleosome core particle (71), with the acetylation of H2B, H3, and H4 having minimal impact on the dynamics of the nucleosome core particle. In contrast, H2A shows significantly increased dynamics after acetylation modification (72). Histone acetylation primarily occurs at the amino-terminal lysine sites of H3 and H4, including H3K9ac, H3K14ac, H3K18ac, H3K23ac, H3K27ac, H3K56ac, H4K5ac, H4K8ac, H4K16ac, and H4K20ac (73), catalyzed by HATs such as CBP/p300, MOF, HBO1, or KAT6A (74). Previous extensive research has revealed a link between histone acetylation levels and the expression of pro-inflammatory cytokines and other antimicrobial products (75), with acetylases playing an indispensable role.
Acetyl-CoA is the donor of the acetyl group, produced by metabolic enzymes in the nucleus and having a direct effect on histone acetylation (76, 77). To date, studies have shown that three enzymes are crucial for maintaining acetyl-CoA levels: the Acyl-CoA Synthetase Short-Chain Family Member 2 (ACSS2) (78–80). Extensive research indicates that ACSS2 catalyzes the synthesis of acetyl-CoA from acetate, recruited to neuronal sites associated with organismal memory, crucial for memory consolidation (81). Furthermore, ACSS2 is an enzyme required for alcohol-induced neuron-specific gene expression and alcohol-associated associative learning (82). In summary, extensive research highlights the key role of ACSS2 in enhancing spatial memory and regulating histone acetylation.
Zhang et al. (83) were the first to discover histone lactylation, where lactate-derived histone lysine lactylation emerged as a novel epigenetic modification and demonstrated that histone lactylation directly stimulates chromatin gene transcription. Wan et al. reported the formation of cyclic immonium ions of lactylated lysine during tandem mass spectrometry analysis, enabling the identification of protein lactylation. Their findings highlighted that lactylation is common on glycolytic enzymes and is conserved on Aldolase A. Additionally, widespread lactylation was identified on Reductase SDR Family Member 7(DHRS7) in a draft of the human tissue proteome (84).
5.1.3 Non-coding RNA
In eukaryotes, approximately 90% of genes are transcribed, of which only 1%-2% are responsible for encoding proteins, with the majority being transcribed into non-coding RNAs (ncRNAs). ncRNAs refer to RNA molecules transcribed from genes that do not have the capacity to encode proteins.
ncRNAs can be primarily classified into two forms: small ncRNAs (SncRNAs, 18-200 bp) and long ncRNAs (lncRNAs, >200 bp), neither of which can be translated into proteins. SncRNAs include small nucleolar RNAs (snoRNAs), PIWI-interacting RNAs (piRNAs), small interfering RNAs (siRNAs), microRNAs (miRNAs), circular RNAs (circRNAs), and extracellular RNAs (exRNAs) (85, 86). Extensive research on ncRNA biology has demonstrated that ncRNAs play a critical regulatory role in shaping cellular activity. ncRNAs include molecules that act as oncogenes or tumor suppressors, and their aberrant expression has been linked to carcinogenesis and metastasis regulated by epigenetic mechanisms (87–89). ncRNAs can regulate the transcription of individual genes or entire transcriptional programs, affecting the expression of hundreds to thousands of genes (90–92).
MicroRNA (miRNA) is one of the most extensively studied ncRNAs, consisting of single-stranded molecules approximately 20-24 bp in length. Thousands of miRNAs can function as tumor suppressors or oncogenes by base-pairing with complementary sequences in the 3’UTR of target mRNAs, inhibiting gene translation and leading to gene silencing (93). Competitive endogenous RNAs (ceRNAs) are common post-transcriptional regulators. Notably, long non-coding RNAs (lncRNAs) and circular RNAs (circRNAs) within the ceRNA category can induce gene silencing, affecting gene expression and thereby influencing tumor progression (94). lncRNAs regulate various physiological processes such as tumor cell invasion, migration, proliferation, and tumor microenvironment (TME) remodeling by modulating mRNA processes and gene transcription (95, 96). The unique circular structure of circRNAs, resulting from the back-splicing of pre-mRNA, confers them with exceptional stability. CircRNAs, as endogenous ncRNAs with their linear transcriptional 3’ and 5’ ends removed, are implicated in biological processes related to tumor suppression and carcinogenesis (97, 98).
5.2 Advances in research methods and techniques in epigenetics
Current research in epigenetics primarily focuses on three aspects: DNA methylation, histones post-translational modifications, and non-coding RNA. The methodologies for studying DNA methylation (99) are categorized into two main types. The first type examines the overall level of DNA methylation, including techniques such as whole-genome bisulfite sequencing (WGBs), methylation 450K array, and immunoprecipitation techniques (MeDIP). The second type investigates DNA methylation at specific sites; after bisulfite treatment, unmethylated cytosines are converted into uracil, followed by detection methods such as methylation-specific PCR (MSP), reduced representation bisulfite sequencing (RRBS), and methylation-sensitive high-resolution melting (MS-HRM).
Classic methods for detecting histones post-translational modifications (100) include chromatin immunoprecipitation followed by sequencing (ChIP-seq), Cut&Tag, and Edman degradation. Edman degradation, a low-throughput sequencing method, is generally limited to analyzing the N-terminal 50 amino acids of proteins and cannot detect multiple proteins simultaneously; it is used for analyzing chemically unmodified N-terminal α-amino acids. Immunosequencing methods cannot detect unknown modification sites. More widely used methods for histones post-translational modifications detection now include mass spectrometry (MS) and ELISA techniques.
Research methods for non-coding RNA mainly include transcriptome sequencing (RNA-seq), Northern blotting, fluorescence in situ hybridization (FISH), and RNA-binding protein immunoprecipitation (RIP-seq). Currently, Solexa high-throughput sequencing (101) is extensively used. This method can simultaneously analyze hundreds of millions of nucleotide fragments, offering low cost, high precision, and requires a small sample volume. Additionally, ACAT-seq, as a crucial tool for understanding chromatin states and epigenetic regulation, aids researchers in analyzing chromatin accessibility, chromatin structure and three-dimensional organization, and the association between epigenetic changes and diseases. An overview of these common epigenetic research methods, including their advantages, disadvantages, and applicability, is summarized in Table 1.
5.2.1 Environmental chemical substances
Heavy metal pollution has become a significant and widespread environmental issue that poses multiple hazards to human health. Cadmium, a typical heavy metal, exerts its reproductive toxicity, carcinogenicity, and other toxic effects by mediating changes in epigenetic modifications to regulate gene expression (268). Cadmium exposure can affect the overall level of genomic methylation. It leads to abnormal expression of DNMTs enzymes, with significant reductions observed in the mRNA and protein levels of DNMT1, DNMT3A, and DNMT3B, thereby inducing global DNA hypomethylation in vitro (124). Similarly, cadmium exposure can also cause DNA hypermethylation to regulate gene expression. Aigner GP et al. (125), using earthworms as a model organism, revealed cadmium-induced hypermethylation of adenine and cytosine in spot imprinting, thus elucidating the time- and dose-dependent effects of Cd on global and gene-specific DNA methylation and its potential mechanisms.
Research has shown that continuous exposure of Drosophila melanogaster to cadmium during growth results in a significant increase in H3K4me3 levels and a significant decrease in H3K9me3 and H3K27me3 levels in third-instar larvae of the offspring. The expression of histone methylation-related genes dSet-1, ash1, and Lsd1 is significantly increased. Cadmium-induced wing phenotypic defects can be inherited by offspring, suggesting a potential transgenerational effect related to histone methylation’s epigenetic regulation (126).
Chen et al. (127)studied 31-week-old Hy-Line Brown hens fed with dietary cadmium chloride (150 mg/kg) and found a close association between miR-33 and cadmium toxicity. Cadmium toxicity significantly inhibited the expression of miR-33 and significantly increased the mRNA and protein levels of AMP-activated protein kinase(AMPK), altering the expression pattern of the miR-33-AMPK axis in the spleen and causing dysregulation of the miRNA-33-AMPK axis.
Mercury (Hg) is also a heavy metal that, due to its toxicity and widespread human exposure, has become a significant public health concern. Mercury is found in a variety of sources including seafood, household products, medical devices, and cosmetics, making it a common occupational hazard. Mercury pollution and volcanic eruptions are significant sources of its presence in soil, water, and the atmosphere. High doses of mercury are widely recognized as neurotoxic substances that negatively affect the female reproductive system (128).
Studies have shown that whole-body exposure to 2.5 mg/m³ of mercury for 6 hours per day over 6-8 weeks leads to prolonged estrous cycles in treated female rats and increased mortality rates in offspring (129). Davis et al. (130) found immature corpora lutea in rats exposed to mercury vapor. Lamperti et al. (131) observed inhibition of follicle maturation after injecting HgCl2 into hamsters. Dansereau et al. directly demonstrated that female minks exposed to a dietary concentration of 1.0 microgram/gram of mercury had fewer births compared to those exposed to 0.5 micrograms/gram and 0.1 micrograms/gram (132).
The theory of endocrine disruptors was initially proposed in the 1990s, identifying certain exogenous chemicals that interfere with the endogenous hormonal axis (133). These chemicals can interact in various ways within the body and affect different physiological areas. They include a variety of substances found in the environment, such as various pesticides, industrial chemicals (like Bisphenol A and phthalates), and dioxins. The presence and persistence of endocrine-disrupting chemicals (EDCs) in the environment impact organisms, and increasing evidence suggests that EDCs may be etiologically linked to the development and severity of diseases. The reproductive system is a primary target for most endocrine disruptors (134). Universal exposure during early development has been linked to the incidence of female cancers, especially reproductive organ cancers such as breast and ovarian cancer (135). In particular, in utero exposure might affect processes that initiate tumor growth years later. Additionally, some gynecological diseases are associated with exposure to various environmental toxins, particularly during critical developmental stages. Bisphenol A (BPA) was the first synthetic chemical found to cause selective estrogen receptor modulation, particularly as a hormone-like pollutant. Recent studies have shown that prenatal exposure to BPA might cause a phenotype similar to endometriosis in mice (136). Phthalates and BPA are chemicals widely present in many products, such as food packaging and household items. They are typical endocrine disruptors present in various substances and are frequently exposed to the public. Inhalation, ingestion, and skin contact are all possible exposure pathways (137). Urine analysis is a feasible method to confirm human exposure to these chemicals. Studies have shown that endometriosis is associated with elevated levels of phthalate metabolites and BPA metabolites in bodily fluids (138).
In the environment, pesticides are also endocrine disruptors. Current data indicate that approximately 2.5 million tons of pesticides enter the environment annually (139). Pesticides tend to accumulate in the environment due to their lipophilicity, long half-lives, and long mobility, causing significant environmental pollution (140). Pesticides can enter the human body through inhalation or skin penetration, but the highest toxicity is from ingesting contaminated food or water, including fish, meat and dairy products (especially the high-fat parts), drinking water, indoor and environmental air, and dust and soil (141, 142). Dichloro-Diphenyl-Trichloroethane(DDT), one of the most widely used pesticides, can bind with lipids and accumulate in adipose tissue (143). Long-term presence of pesticides in the human body can affect fertility and alter the levels of male and female reproductive hormones. These chemicals have anti-androgenic and estrogen-like properties (144), which may lead to stillbirth, birth defects, spontaneous abortions, and infertility. Animal studies suggest that daily exposure to DDT during prenatal and postnatal development could cause gender differences in steroid levels, possibly through direct interference by DDT and its impact on the hypothalamic-pituitary system (144). Research has also found that the disruptive effects of DDT involve competition with testosterone and damage to androgen receptor binding and signal transduction, as well as an association with increased estrogen synthesis (145). These changes in hormone secretion may be related to reproductive problems and physical diseases in later life, as excessive estrogen secretion and an imbalanced testosterone/estradiol ratio are associated with increased risks of feminization, metabolic disorders, estrogen-related cancers, and cardiovascular diseases (146).
Among the environmental pollutants that have been confirmed to promote transgenerational inheritance of epigenetic phenotypes, endocrine disruptors constitute a heterogeneous group of substances capable of interfering with hormone signaling pathways, directly altering germ cell epigenetic modifications, and changing metabolism and reproductive function (147). For instance, exposure to BPA increases DNA methylation and histone acetylation in zebrafish testicular cells (148). Male zebrafish exposed to BPA early in spermatogenesis and analyzed for F1 embryos showed increased histone acetylation induced by BPA, resulting in cardiac toxicity (149). Interestingly, research has identified three pairs of miRNA-mRNA involved in hypoxia-induced reproductive disorders, including novel miRNA-525-DIAPH2, novel miRNA-525-myocardium, and novel miRNA-525-RAI14, indicating for the first time that miRNAs may participate in hypoxia-induced reproductive disorders through transgenerational inheritance (147).
Furthermore, exposure to other endocrine disruptors such as organic compounds like benzo[a]pyrene (150) and air pollution particulate matter like CO2 (151) is associated with epigenetic changes, mediating DNA methylation, histones post-translational modifications, RNA expression alterations, and inducing human cancers and other diseases. These changes can be transgenerationally inherited and manifest as alterations in fertility, metabolic function, or behavioral traits.
Environmental pollution’s impact on human health is becoming increasingly apparent, with particulate matter (PM) pollution now recognized as one of the most critical public health risks. Particulate matter pollution encompasses various airborne particles, ranging in size from a few micrometers to visible particles up to 100 micrometers. Long-term exposure to environmental particulate matter can lead to cardiac and pulmonary diseases. Most studies focus on particulate matter with an aerodynamic diameter less than 10 micrometers (PM10) or less than 2.5 micrometers (PM2.5), which may adversely affect fetal development, the normal course of pregnancy, and lead to premature birth (152).
Research indicates that high concentrations of PM10 are closely associated with an increased risk of pregnancy complications throughout the gestation period and its various stages. Particularly during the late stages of pregnancy, exposure to high levels of PM2.5 significantly increases the risk of pregnancy complications. In the middle stage of pregnancy and throughout the entire gestational period, every 10 μg/m³ increase in PM10 concentration increases the risk of preterm birth (PTB) by 24% and 27%, respectively. Additionally, exposure to high concentrations of PM10 during the mid-pregnancy stage increases the risk of gestational diabetes mellitus (GDM) by 30%. For PM2.5, every 5 μg/m³ increase in concentration raises the risk of GDM by 15% in the mid-pregnancy stage and 25% throughout the entire pregnancy. In the first three months of pregnancy, exposure to high concentrations of PM10 and PM2.5 increases the risk of having a small for gestational age (SGA) infant by 96% and 26%, respectively (153).
5.2.2 Other factors
There are reports indicating a positive correlation between the highly prevalent sexually transmitted protozoan parasite richomonas vaginalis and vaginal and cervical neoplasms in women, as well as prostate cancer in men (154). Infection with T. vaginalis significantly alters the structure of the vaginal microbiome, shifting from a lactobacilli-dominated community to one that favors the widespread transmission of bacterial vaginosis (155). This parasite releases metabolites, such as indoles, which aid in the survival of intracellular spreading bacteria like Chlamydia trachomatis, which has been independently associated with cancer (156). Given the positive correlation between bacterial vaginosis and precancerous lesions of the cervix (157), it is necessary to conduct research to clarify the role of the microbiome in T. vaginalis -associated vaginal carcinogenesis, whether as a cofactor or a necessary factor.
Climate factors such as global warming (158) can also lead to transgenerational transmission of specific histones post-translational modifications, DNA methylation modifications, and other epigenetic marks. This article provides a concise summary of common environmental factors along with their epigenetic impacts and mechanisms of action, as presented in Table 2 and Figure 2.
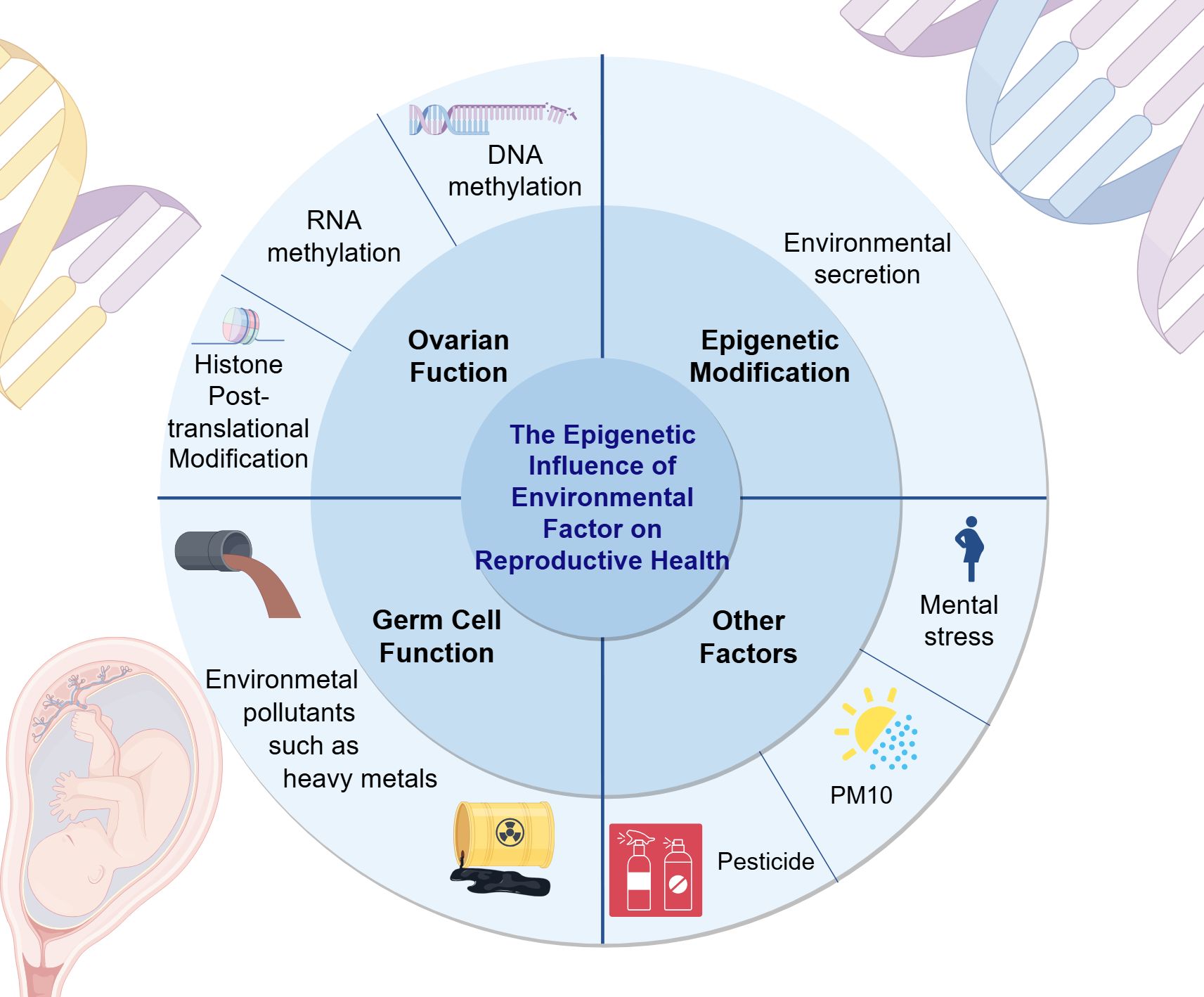
Figure 2. The epigenetic impact of environmental factors on reproductive health. This figure elucidates the influence of environmental factors on reproductive health from three aspects: ovarian function, epigenetic modifications, germ cell function, and other examples are given to illustrate the impact of environmental factors on reproductive health. For example, the negative effects of PM10 and pesticides on women’s reproductive health. (Draw by figdraw.).
5.3 The regulation of female reproductive function by epigenetics
5.3.1 Ovarian function and epigenetic regulation
PCOS, as a heterogeneous disease leading to reproductive and metabolic disorders in women, is the most common cause of infertility in women of childbearing age (171). A study (172) analyzed the DNA methylation in ovarian tissues of PCOS-like mice, and these findings suggest that PCOS can be transmitted to offspring through changes in DNA methylation in epigenetics, proposing that methylation biomarkers may serve as potential diagnostic indicators for this disease. Another study indicated that the histone deacetylase inhibitor valproic acid can reduce metabolic dysfunction in the skeletal muscles of PCOS rats by inhibiting PDK4/NLRP3 inflammasome (173). Therefore, inhibiting histone acetylation may aid in the diagnosis and treatment of PCOS. Additionally, alterations in non-coding RNAs (ncRNAs) are one of the mechanisms of PCOS (174). In recent years, numerous studies have shown significant abnormalities in the expression of ncRNAs in follicular fluid, serum, ovarian granulosa cells (175), and other tissues of women with PCOS. Therefore, analyzing the abnormal expression of ncRNAs in PCOS patients can serve as diagnostic biomarkers and play crucial roles as therapeutic targets in the treatment of PCOS.
As shown in Figure 3, premature ovarian insufficiency depicts common ovarian dysfunctions and their epigenetic mechanisms. POI refers to the ovarian function decline characterized by elevated gonadotropins and estrogen deficiency in women before the age of 40, mainly manifested as menstrual abnormalities, ultimately progressing to premature ovarian failure (POF) with varying degrees of perimenopausal symptoms (176), affecting the fertility and quality of life of women of reproductive age. N6-methyladenosine (m6A) modification can effectively regulate the epigenetics of mammalian transcriptome. A case-control study (177) measured the m6A content in the RNA of POI patients and controls. Compared with the control group, the m6A content in the granulosa cells of POI patients was significantly increased, accompanied by a significant decrease in FTO mRNA and protein expression levels. The results indicate a strong association between m6A content and the risk of POI, which may impair ovarian function and further lead to complications of POI.
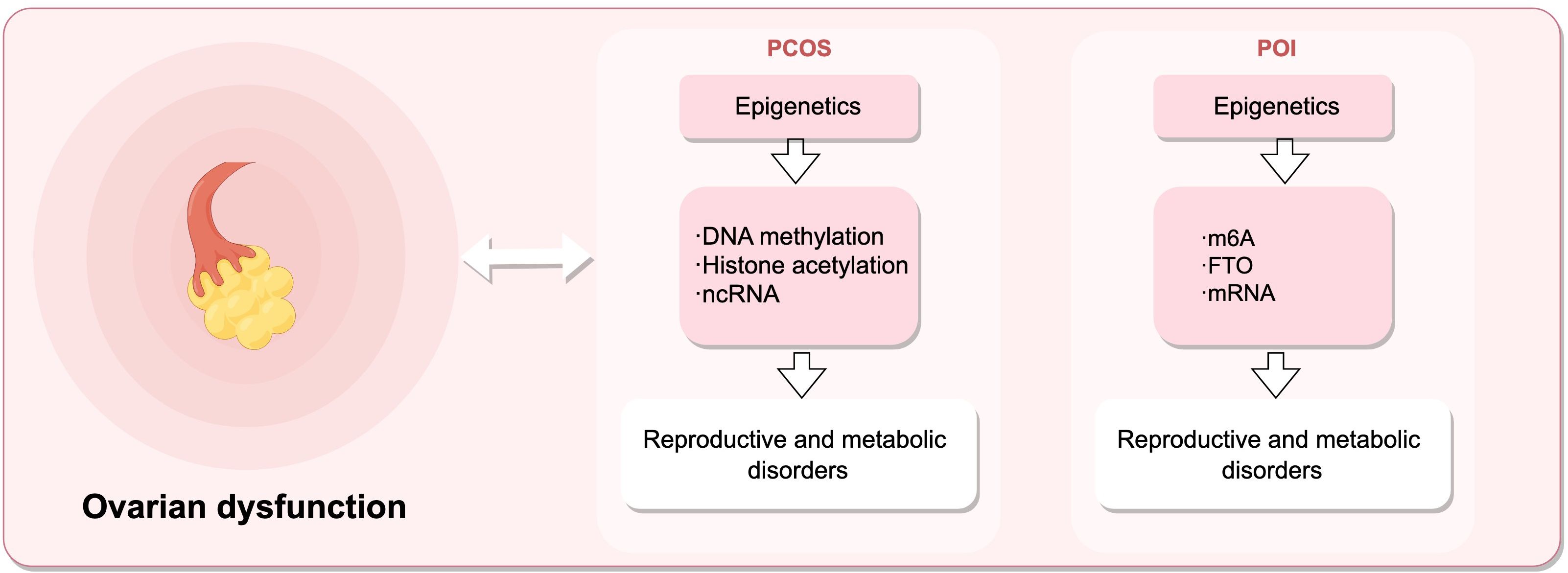
Figure 3. Epigenetic mechanisms regulate female reproductive capacity, leading to ovarian dysfunction. Dysregulation of DNA methylation, histones post-translational modifications, and ncRNAs can result in polycystic ovary syndrome (PCOS), while alterations in m6A, FTO, and mRNA levels contribute to premature ovarian insufficiency (POI). (Draw by figdraw.).
5.3.2 Fertility capability
In non-disease conditions, epigenetics can influence the female reproductive environment, thus affecting fertility. The proper development and maturation of oocytes not only directly impact fertility but also relate to embryo health and the likelihood of successful pregnancy. Appropriate methylation of oocytes is crucial for coordinating gene expression patterns, driving oocyte developmental programs, and ensuring oocyte quality. N6-methyladenosine (m6A) is the most common internal modification of mRNA (178), playing a role in oocyte maturation. Specific knockout of METTL3 in Gdf9-Cre mouse oocytes disrupts normal mRNA methylation, leading to DNA damage, follicular development defects, and ovulation abnormalities. It has been shown that the methyltransferase METTL3 may enhance the stability of m6A modifications on Itsn2, affecting oocyte meiosis (179, 180). Similarly, studies have found that the negative mutant H3.3-K4M specifically expressed in mouse oocytes reduces H3K4 methylation levels, leading to decreased transcriptional activity and increased DNA methylation in oocytes, disrupting oocyte development and female mouse fertility. Early embryos from H3.3-K4M oocytes exhibit developmental arrest and reduced activation of the zygotic genome (181).
Epigenetics also influences the role of hormones in the reproductive system by regulating gene expression, including hormone synthesis, secretion, and receptor sensitivity, thus affecting ovarian function, the uterine environment, and cyclic changes. Research has shown that the estrogen receptor α (ERα) can recruit various coregulators (such as histone modifiers, transcription factors, or other auxiliary proteins), where histone modifiers alter the chromatin structure and organization, regulate gene accessibility and transcriptional activity, and promote chromatin opening, facilitating the binding of ERα and transcription mechanisms to estrogen response elements (ERE) (182). SMYD2 is a proven negative regulator of ERα, primarily inhibiting the activation of estrogen receptor target genes by aiding methylation of ERα protein at the K266 site (183). An epigenetic axis exists between TET2 and ERα, with endogenous TET2 occupying active enhancers and promoting proper recruitment of ERα. DNA demethylation activates enhancers to coordinate transcription programs, enhancing estrogen response (184). Besides influencing reproductive hormone receptors, epigenetics can cause changes in reproductive hormone synthesis. Endometriosis or adenomyosis cells often exhibit aberrant epigenetic programming mechanisms. Binding of NR5A1 to the proximal promoter of the CYP19A1 gene may promote demethylation of the NR5A1 gene promoter region, leading to overexpression of estrogen receptor-b (ESR2), excessive estrogen formation, abnormal interaction of estrogen with ESR2, and progesterone resistance (185). In regulating the internal hormonal environment in women, the hypothalamus serves as a key hub of the endocrine system, regulating the secretion of multiple hormones. By secreting gonadotropin-releasing hormone (GnRH), it controls the anterior pituitary secretion of follicle-stimulating hormone (FSH) and luteinizing hormone (LH) (186). Epigenetic mechanisms regulate the expression of related hypothalamic genes, affecting the synthesis and secretion of GnRH, thereby controlling the release of FSH and LH, stimulating follicular development and ovulation, thus maintaining a normal reproductive cycle (187). A study showed that perinatal exposure to EDCs can reprogram DNA methylation and steroid hormone receptor expression through epigenetic mechanisms to regulate female fertility (188).
When embryos develop to the blastocyst stage, they can enter the uterine cavity and interact with the endometrium, a process regulated by epigenetics. During this process, the miRNA processing enzyme Dicer is upregulated, and microRNA Let-7a is downregulated, enabling the blastocyst to acquire the ability to implant in the uterus (189). During embryo implantation, DNA methylation can regulate the expression of HOXA10. Abnormal DNA methylation can downregulate HOXA10 expression (190), affecting endometrial receptivity. Targeted destruction of HOXA10 in female mice can lead to embryo death and implantation failure (191). Endometrial angiogenesis is a necessary condition for good endometrial receptivity, and a study showed that the KLF4 (Krüppel-like factor 4)-VEGFA (vascular endothelial growth factor A) positive feedback loop is regulated by epigenetics, promoting proliferation and migration of human endometrial microvascular endothelial cells (HEMECs), inhibiting apoptosis, thus enhancing endometrial receptivity (192). Abnormal expression of non-coding RNA miRNAs can lead to defects in human endometrial receptivity. Patients with recurrent implantation failure (RIF) in IVF exhibit lower mRNA levels of cell adhesion molecules, Wnt signaling components, and cell cycle pathways, including N-cadherin, H2AFX, netrin-4, and secreted frizzled-related protein-4 (193).
Endometriosis is a common benign inflammatory gynecological disease characterized by the presence and growth of endometrial-like glands and stroma outside the uterus, leading to pelvic pain and reduced fertility in reproductive-aged women, significantly impacting the quality of life of affected women 194. Epigenetic processes can regulate gene expression during endometrial development throughout the menstrual cycle through various mechanisms, altering the function and morphology of the endometrium. Epigenetic dysregulation plays an important role in the pathogenesis and pathophysiology of endometriosis, with aberrant expression of epigenetic processes found in the endometrium of affected women, holding great potential as therapeutic targets, diagnostic, and prognostic markers (195). DNA methylation is one of the most common epigenetic modifications in endometrial biology, with changes in DNA methylation occurring during different stages of the menstrual cycle (196). As a representative example, high methylation of the HOXA10 gene promoter in the endometrium of women with endometriosis leads to gene silencing, resulting in decreased levels of HOXA10 in the ectopic endometrium, potentially impairing female fertility (197). Similarly, histones post-translational modifications are associated with endometrial function. Reduced protein levels of histone deacetylase 3 (HDAC3) in the ectopic endometrium of infertile women with endometriosis may impair fertility, as HDAC3 is crucial for endometrial receptivity and decidualization. As a typical example, studies by Samartzis, E.P. et al. on the impact of Hdac3 deletion in mouse uteri on fertility demonstrated that Hdac3 deficiency can lead to aberrant transcriptional activation of two direct targets of mouse and human HDAC3, COL1A1 and COL1A2(COL1A1 and COL1A2, genes that encodes the human collagen Iαchain), resulting in implantation and decidualization abnormalities and consequent loss of fertility (198). Thus, aberrant expression of HDACs can better explain the causes and mechanisms of endometriosis. A study evaluating differential expression of microRNAs in serum cultures of severe endometriosis patients and controls found significant dysregulation of six microRNAs. This study confirmed the role of miRNAs in the pathogenesis of endometriosis, demonstrating that serum-derived eMSCs from severe endometriosis patients can induce abnormal expression of miRNAs and their target genes, leading to the development of endometriosis (199). Figure 4 illustrates the common epigenetic mechanisms and associated genes involved in endometriosis.
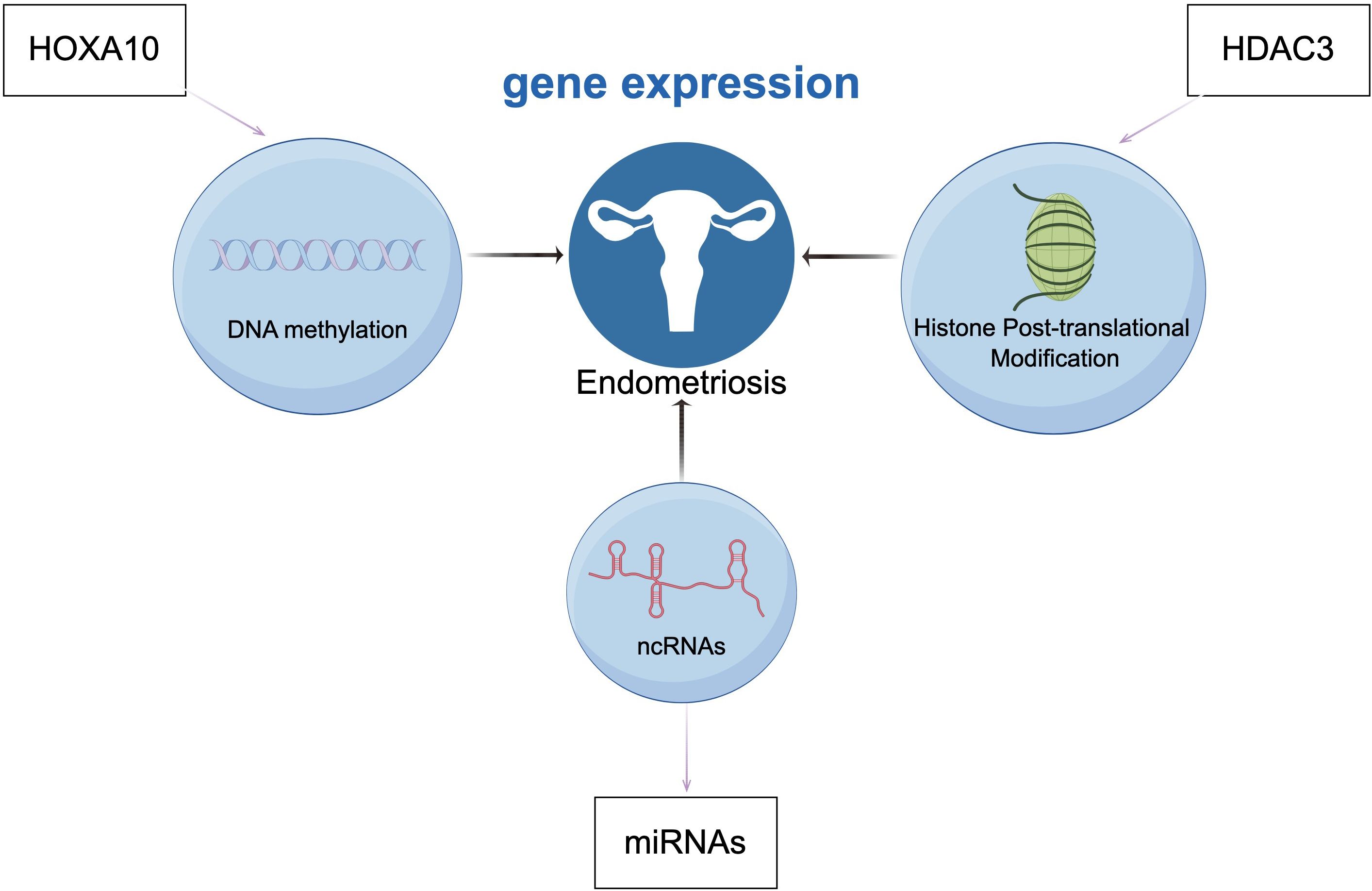
Figure 4. Endometriosis is associated with epigenetic mechanisms related to gene regulation, DNA methylation (HOXA10), histones post-translational modifications (HDAC3), and non-coding RNAs (ncRNAs). (Draw by figdraw.).
Pre-eclampsia is a multisystem disease broadly affecting pregnancy, annually responsible for over 60,000 maternal deaths globally and causing more than 500,000 cases of preterm birth (200). Studies have established an association between the ACVR2A gene and pre-eclampsia. Although single nucleotide polymorphisms (SNPs) related to the ACVR2A gene do not reside within its coding region, and thus do not directly alter the coding sequence of the ACVR2A protein, they may still influence the transcription of the ACVR2A gene by affecting the binding of transcription factors or the regulation by microRNAs. ACVR2A is located on chromosome 2q22, and its encoded type II activin receptor binds the activin A ligand (201). In maternal tissues, activin A promotes decidualization of the endometrial stroma cells and regulates the invasion process of the trophoblast (202); in fetal tissues, it is essential for trophoblast differentiation, placental development, and the functional regulation of the trophoblast (203).
Adenomyosis, a common gynecological disorder in women of reproductive age, is characterized by the aberrant invasion of endometrial tissue into the myometrium and is closely associated with infertility (204). Studies on the expression and localization of DNMT in healthy females and those with adenomyosis revealed heightened immunoreactivity to DNMT1 and DNMT3B in ectopic endometrium (265), whereas DNMT3A staining levels significantly decreased in both eutopic and ectopic endometrium. Beyond DNA methylation, abnormal expression and localization of class I histone deacetylases (HDACs) in the endometrium have also been confirmed (205). Additionally, lower total m6A levels in the myometrium of patients with adenomyosis have been linked to differential expression of METTL3 and ZC3H13 (206), suggesting that m6A RNA methylation regulatory factors may participate in the pathogenesis of adenomyosis through aberrant expression in the endometrium.
Furthermore, improper maintenance of heritable epigenetic markers can result in aberrantly activated or suppressed signaling pathways, leading to malignant tumors in the reproductive system (207). Research has shown that epigenetic modifications like DNA methylation can influence TMB and play an indispensable role in tumor onset, as proposed by Liu B et al., where DNA methylation in the tumor microenvironment (TME) affects the expression levels of certain cervical cancer genes, further influencing the immune response in the TME, thereby inducing cervical cancer (208). Moreover, other reproductive system cancers such as endometrial cancer, ovarian cancer, and uterine fibroids can also arise due to epigenetic induction, leading to infertility and life-threatening conditions for the patient.
Uterine fibroids (also known as leiomyomas), the most common benign gynecological tumors among women of reproductive age worldwide, can cause severe anemia, pelvic pain, and infertility by affecting the overall function of the endometrium (209). Compared to normal myometrial layers, uterine fibroids exhibit altered levels of DNA methylation, increased estrogen receptor mRNA, and DNA methyltransferase levels. The activation of the PANKL gene plays a crucial role in the development of uterine fibroids by activating stem cells in the myometrial layer (210), while DNA methylation and MED12 gene mutations form a complex regulatory network affecting the expression of the PANKL gene mediated by progesterone and its receptors. Furthermore, post-translational modifications of histones in uterine fibroid tissues have changed, particularly through genomic activation-related histone acetylation; acetylated histone H3K27 is involved in regulating genes related to cell signaling, transport, angiogenesis, and extracellular matrix formation, promoting the development of uterine fibroids (211). The miR-29 family is associated with the deposition of the extracellular matrix, and the miR-3 family can regulate cyclins, also interacting with long non-coding RNAs (lncRNAs) to promote the production and deposition of the extracellular matrix, providing a physical platform for tumor cell growth, migration, and spread. Overproduction of extracellular matrix components not only increases the hardness and volume of the fibroids but also regulates the TGF-β signaling pathway through miRNA expression, thereby affecting the normal biological functions of the uterine wall, such as menstrual bleeding, fertility, and embryo implantation. In this context, research by Włodarczyk et al. (212) further indicates that abnormal changes in TET protein and 5hmC levels may be key factors leading to the formation of uterine fibroids. Additionally, lncRNAs can directly activate the Wnt/β-catenin pathway through the estrogen receptor, promoting the proliferation of uterine fibroid cells (213).
Ovarian cancer poses a serious threat to female reproductive health, with miRNAs playing a critical role in the pathogenesis and progression of the disease (214). Studies have found that certain miRNAs are aberrantly expressed in ovarian cancer tissues, showing upregulation or downregulation, directly affecting multiple key biological processes including cell proliferation, apoptosis, metastasis, and invasion capabilities (215). For instance, the significant downregulation of miR-126-3p in ovarian cancer cells is closely related to tumor cell proliferation and invasion (216). In the treatment process, aberrant miRNA expression is also associated with resistance of ovarian cancer cells to chemotherapy drugs, potentially leading to disease relapse and progression (217). Additionally, single-gene methylation biomarkers such as RASSF1A and BRCA1 are significant factors in the onset and progression of ovarian cancer. Studies have shown that high methylation levels of BRCA1 and RASSF1A are prevalent in ovarian cancer patients, with 82% of patients exhibiting the same high methylation pattern in serum or plasma BRCA1 and RASSF1A (218). Methylation of these genes is highly correlated with clinical features of ovarian cancer such as FIGO stage, plasma CA-125 levels, and histological type (219).
In the development of cervical cancer (CC), persistent HR-HPV infection, aberrant methylation of the host cell genome and HPV genome DNA in cervical squamous epithelial cells can lead to dysfunction of various tumor suppressor genes, promoting the development of CC (220). The cervix, a critical gateway for nurturing life, is highly sensitive to estrogens, and research in an HPV transgenic mouse model has shown that estrogens and their nuclear receptors, in conjunction with HPV oncogenes, promote the onset of cervical cancer (221). Highly methylated and lowly expressed genes (Hyper-LGs) are significantly enriched in estrogen receptor pathways and the Wnt/β-catenin signaling pathway, affecting estrogen expression (222). Current research on post-translational modifications of CC proteins has focused on histone acetylation. One study showed that histone H3 and H4 acetylation is associated with the activation of HPV16 gene expression levels in CC cells, with histone acetylation levels increasing as HPV16 gene expression increases, thereby advancing the development of CC (223). Moreover, extensive research has demonstrated that lncRNAs are involved in the malignant transformation of cervical epithelial cells. For example, lncRNAMIR210HG is overexpressed in CC tissues and promotes cell proliferation and invasion through hypoxia-inducible factor-1α (HIF-1α) (224).
The endometrium, a hormone-sensitive tissue, undergoes numerous biochemical and morphological changes during the normal menstrual cycle under the control of steroid hormone levels, while abnormal exposure to estrogens can significantly increase the risk of endometrial cancer (225). DNA methylation can affect the functional changes of endometrial tissue, for instance, overall DNA methylation status and progesterone receptor levels are significantly increased during the proliferative phase and decrease at the end of the secretory phase (226). Apoptotic cell death mediated elimination of senescent cells in the functional layer of the endometrium helps maintain cellular homeostasis, thus avoiding apoptosis remains a major issue in the successful treatment of late-stage endometrial cancer. Abnormal changes in DNA methylation can lead to the deregulation of key apoptotic proteins during the development of endometrial cancer, resulting in the development of apoptosis resistance (227).
5.3.3 Specific studies
Women exposed to various chemical, biological, physical, and sociopsychological factors may experience impacts on their reproductive systems. These effects can manifest as changes in sex hormone levels, sexual dysfunction, menstrual disorders, early menopause, delayed menarche, impaired ovarian function, reduced fertility, and adverse pregnancy outcomes. During pregnancy, maternal exposure can disrupt normal fetal development, such as intrauterine growth retardation, preterm birth, birth defects, and impacts on cognitive development and immune function (228). Thakur et al.’s study showed that in areas affected by heavy metals and pesticides, the rate of spontaneous abortions is 20.6 per 1000 live births, and the rate of preterm births is 6.7 per 1000 live births, significantly higher than in non-polluted areas. Petrelli et al. (229) found that the abortion/pregnancy ratio for pesticide applicators was 0.27, compared to 0.07 for food retailers. In a multivariable logistic regression model, after adjusting for the wife’s age and parents’ smoking habits, the odds ratio for spontaneous abortion was 3.8 times higher compared to the control group; considering interaction effects, this ratio increased to 7.6 times. Both men and women exposed to certain pesticides face increased risks of abnormal sperm, reduced fertility, increased spontaneous abortions, male birth defects, birth defects, or fetal growth retardation (230). Logan and Chen (231) noted that exposure to bis(4-chlorophenyl)-1,1,1-trichloroethane (DDT) might reduce the rate of preterm births, thereby lowering infant mortality in malaria control. Additionally, Salazar-García et al. (232) reported that occupational exposure to DDT is associated with an increased risk of birth defects. It has been reported that levels of DDT metabolites (p, p’-DDE) are higher in 100% of infertile women. Furthermore, studies (233) have also found that pesticide exposure can lead to reduced fertility. Moreover, parents working in agriculture may increase the risk of congenital anomalies in their children, such as hemangiomas, orofacial clefts, neurological damage, and musculoskeletal defects.
In conclusion, environmental factors can induce epigenetic changes that affect female reproductive function. Epigenetics serves as a bridge and mediator, establishing a connection between environmental factors and female reproductive function. Below are specific research examples. A cross-sectional study based on 1647 American women aged 20-54 with endometriosis from 1999 to 2006 showed a positive correlation between urinary cadmium levels and the prevalence of endometriosis (234). Additionally, research exposed fruit flies to cadmium during egg development to adulthood, then cultured the offspring in a cadmium-free environment. Under cadmium exposure, the expression of histone methylation-related genes significantly increased in the ovaries of third instar larvae and adult flies, with a marked increase in histone H3K4me3 post-translational modification and a decrease in H3K9me3 and H3K27me3 levels. These changes could be transmitted to the offspring’s ovaries, leading to changes in reproductive ability (126). Female reproductive capacity begins in fetal ovaries, and early steps in folliculogenesis are sensitive to environmental factors. The quality of oocytes is closely linked to the process of folliculogenesis, with a long window of susceptibility to environmental damage. After fertilization, fertilized eggs and pre-implantation embryos undergo extensive epigenetic reprogramming. The FEDEXPO project studied potential transgenerational inheritance based on epigenetic markers in F1 offspring gametes, demonstrating that early and perinatal environments can have adverse effects on female reproductive capacity. Abnormalities in epigenetic processes and imprints may affect the health of future generations (235). Therefore, it is crucial to closely monitor and assess the toxicity and risks of environmental factors, avoid inducing epigenetic changes that may harm female reproductive capacity, and consider the adaptability of future generations.
5.4 The role of epigenetics in female reproductive health
In the field of female reproductive health, epigenetic processes such as DNA methylation and histones post-translational modifications play a pivotal role. These changes are crucial in the transition from the maternal environment of the oocyte to the embryo-driven developmental expression program (236), thereby significantly influencing the regulation of ovarian function, oocyte maturation, and embryo development.
DNA methylation, particularly in the regulation of ovarian function, plays a key role. Alterations in DNA methylation can affect the activity of specific genes, thereby regulating the levels of hormones in the ovaries, which are vital for fertility. For instance, methylation changes in genes that affect oocyte maturation and the ovulatory cycle may lead to fertility-related issues.
Taking m6A as an example, N6-methyladenosine, also known as m6A, is a widely occurring base modification on mRNA and represents the most prevalent form of RNA modification in the human body. Due to the dynamic and reversible regulation of various biological processes by m6A (237), abnormal increases or decreases in its levels can lead to the occurrence of different diseases, including many female reproductive endocrine disorders such as endometriosis, polycystic ovary syndrome, and malignancies of the female reproductive system like endometrial cancer and cervical cancer (172, 198, 238).
In the development of these diseases, DNA methylation and m6A modifications may exert their effects through pathways such as influencing the expression of specific genes or regulating hormone levels in the ovaries. These findings underscore the importance of in-depth research into the role of epigenetics in female reproductive health to better understand, prevent, and treat related diseases.
5.4.1 The epigenetic impact of environmental factors on reproductive health
Environmental factors such as diet, lifestyle, psychological stress, and exposure to chemicals play a significant role in female reproductive health. These factors can alter patterns of DNA methylation and histones post-translational modifications, affecting gene expression and thereby significantly impacting reproductive health.
For instance, long-term exposure to certain environmental pollutants, such as heavy metals, organic pollutants, and endocrine-disrupting chemicals, may lead to changes in the epigenetic markers of eggs and sperm (239, 240). These changes can affect the functionality of reproductive cells, such as the maturation of oocytes and the vitality of sperm, thereby impacting the healthy development of embryos and fertility.
Studies have shown that environmental endocrine disruptors may affect epigenetic modifications, interfering with the development of reproductive cells (241).
Increasing evidence suggests that widely prevalent environmental pollutants known as endocrine-disrupting chemicals (EDCs), such as BPA, polychlorinated biphenyls (PCBs), and phthalates, negatively impact reproductive health in animals and humans and are associated with various diseases, including infertility. EDCs can exhibit estrogen-like activity, mimicking or blocking the actions of endogenous hormones and affecting related genes, thus altering phenotypes. Hormones cause developmental changes in offspring through embryonic methylation and maintain these changes in germ cells. Evidence indicates that exposure to EDCs impacts female reproductive potential, as measured by ovarian reserve and outcomes of assisted reproductive technologies (ART) (242).
In addition to natural pregnancies, women undergoing ART are also susceptible to environmental influences. Studies have examined women who underwent ART and were closely monitored during early pregnancy to explore the association between exposure to types of environmental air pollution and the timing of miscarriages. It was found that higher NO2 exposure was associated with an increased risk of miscarriage within 30 days after a positive Human Chorionic Gonadotropin(HCG) test (243). Beyond miscarriages, immediate maternal complications related to ART are among the more apparent and recognizable issues, such as ovarian hyperstimulation syndrome (OHSS), and risks associated with ART procedures including egg retrieval, embryo transfer, and fetal reduction surgeries (244). Additionally, a range of derived issues such as risks associated with operative anesthesia, endometrial biopsy, and hysteroscopy can impact the individual and potentially affect the offspring.
Despite ART being a core technology for treating infertility in contemporary settings, a woman’s reproductive potential still largely depends on the quality of her oocytes and the maternal environment that supports embryo implantation and development. The influence of the perinatal environment on the epigenetics of developing embryos has become a focal point in research into the effects of the environment, nutrition, and assisted reproductive technologies on human development and health (245).
These findings indicate that environmental factors and lifestyle choices are crucial for maintaining reproductive health, especially in preventing and managing reproductive issues related to the environment. Therefore, understanding how environmental factors influence reproductive health through epigenetic mechanisms is essential for developing effective prevention and intervention strategies.
5.4.2 Epigenetics and female fertility
Moreover, the impact of epigenetic changes on female fertility is a significant area of research. As age increases, the quantity and quality of eggs in the ovaries gradually decline, a phenomenon that may be related to changes in the methylation patterns of specific genes. Age-related changes in the methylation patterns of genes in eggs may affect their maturation process and fertilization capability. The decline in egg quality is not only related to epigenetic changes but also involves abnormalities in mitochondrial function, mutations in nuclear DNA, shortening of telomeres, misalignment of chromosomes, and inactivation of the spindle checkpoint. These changes may lead to early pregnancy loss, neonatal death, or chromosomal aneuploidy genetic diseases such as Down syndrome (246). In terms of epigenetic regulation, METTL3 is a key factor. Knocking out METTL3 severely inhibits the maturation of oocytes, affecting the transition from oocyte to zygote (247). Although the pathogenesis of most patients with ovarian dysfunction is not entirely clear, related experiments indicate (248) that the development of oocytes in Zmettl3m/m zebrafish (a zygotic defect mutant line targeting METTL3 exons) is delayed, with most remaining at an early stage. The m6A modification evidently affects the maturation rate of follicles; the experiment also pointed out that due to the significant reduction in m6A content in the oocytes of Zmettl3m/m zebrafish, the key factors related to in vivo sex hormone synthesis and gonadotropin signaling cannot be normally expressed. Consequently, this leads to a decrease in the secretion of 11-ketotestosterone and 17β-estradiol in the offspring embryos, ultimately causing gamete maturation disorders and reduced fertility. Additionally, the methylation status of some genes may affect the cell cycle regulation and aging of the oocyte (249), thereby impacting its maturation and quality.
5.4.3 The role of epigenetics in pregnancy and embryonic development
During pregnancy and embryonic development, epigenetics plays a crucial role. The nutritional status of pregnant women, environmental exposure, and psychological state can influence the development of the embryo by altering its epigenetic status (250). These factors not only have a significant impact on embryonic development during pregnancy but may also have a profound effect on the long-term health of the offspring, including their metabolic status and disease susceptibility in adulthood.
Epigenetics plays a vital role at every stage of embryonic development. For example, during the early stages of embryonic development (251), the nutrition and environmental factors provided by the mother can regulate the gene expression and development of the embryo by affecting gene methylation and histones post-translational modifications. This epigenetic regulation is not only crucial for the normal development of the embryo but may also affect the long-term health and disease susceptibility of the embryo.
Moreover, the regulation of gene expression during the early stages of embryonic development is highly complex and dynamically ordered, supported by various factors, including transcription factors and epigenetic information such as chromatin accessibility, DNA methylation, and histones post-translational modifications. Transcription factors and epigenetic information (such as chromatin accessibility, DNA methylation, histones post-translational modifications, etc.) are important factors in the regulation of gene expression during early embryonic development (252, 253). These factors work together to ensure the smooth progression and transition of important biological processes at various stages of early embryonic development.
Therefore, in-depth research into the role of epigenetics in pregnancy and embryonic development is of great significance for understanding the mechanisms of normal embryonic development, predicting and preventing developmental abnormalities, and improving the health of offspring.
5.4.4 The epigenetic effects of environmental factors on offspring health
Embryonic development and infancy are two critical periods that are particularly sensitive to environmental factors. During these stages, epigenetic programming is highly susceptible to various environmental factors such as diet, temperature, environmental toxins, maternal behavioral habits, and even childhood experiences of abuse. The epigenetic modifications of imprinted genes induced by these factors may lead to poor development of multiple organs in the fetus and may increase the risk of various diseases in adulthood (254). For instance, Professor Li Jingwen from Fujian Medical University has pointed out that exposure to cadmium during pregnancy in rats has shown effects on the regulation of miRNA and DNA methylation patterns in the offspring’s ovarian granulosa cells, revealing the potential for epigenetic changes to be inherited across generations. This cross-generational impact highlights the significance of environmental factors in affecting the health of offspring, especially how exposure to harmful substances during early pregnancy can have long-lasting effects on the health of the offspring.
The intrauterine environment and the early environment of newborns can provoke permanent responses in fetuses and newborns, thereby increasing their susceptibility to diseases later in life (255). Today, the mode of conception (such as in vitro fertilization), maternal metabolic conditions (such as malnutrition, overnutrition, diabetes), and pregnancy complications (such as preeclampsia and intrauterine growth restriction) are suspected to be negative predictors of long-term health in offspring.
Moreover, as ART become more widespread, while they compensate for familial deficiencies, they also bring subsequent health risks to offspring. During ART, significant epigenetic reprogramming occurs, which is crucial for the normal destiny of the embryo. This epigenetic reprogramming is highly susceptible to changes in environmental conditions, such as those inherent in in vitro fertilization, including in vitro culture, nutrients, lighting, temperature, oxygen tension, embryo-maternal signaling, and the general lack of protection against exogenous elements that could destabilize this process (256). Professor Zhuan Ning Xia of Shanghai Jiao Tong University, using the Shanghai area as an example, found that exposure to pesticides affects not only the reproductive health of women undergoing ART but also adversely affects their offspring. As more infants are born, some involving embryo culture and exposure to potentially inappropriate environmental factors, this could alter the phenotype of the offspring, such as Large Offspring Syndrome in cattle (257).
Like in cattle, ART can facilitate the development of human congenital overgrowth conditions, known as Beckwith-Wiedemann syndrome (BWS), which may later lead to molar pregnancies and embryonal tumor formation. BWS is an overgrowth and embryonal tumor susceptibility disorder linked to genetic or epigenetic abnormalities in the chromosome 11p15.5 region, causing abnormal expression of parental alleles. ART disrupts the DNA methylation of imprinting sites, supporting the notion that ART could lead to imprinting disorders, including BWS. Children conceived through ART are at a 4 to 10 times higher risk of developing BWS compared to those conceived naturally (258). Furthermore, long-term exposure to inappropriate environmental factors aligns with the “DOHaD theory” – that in addition to adult lifestyle and genetic inheritance, early life environmental factors, including nutrition, influence the risk of some non-communicable diseases in adulthood, such as obesity, diabetes, and cardiovascular diseases.
These studies indicate that the environmental and behavioral choices of mothers during pregnancy not only affect their own health but can also have profound effects on the health of their offspring. Therefore, understanding how maternal environmental factors influence the health of offspring through epigenetic mechanisms is of significant importance for the prevention and management of environment-related health issues.
5.4.5 The application of epigenetics in reproductive medicine
The application of epigenetics in the field of reproductive medicine has significantly enhanced our understanding of the fertility process and provided new therapeutic strategies for treating infertility. According to a 2021 study published in “The Lancet,” the current global live birth rate for ART, such as in vitro fertilization (IVF), is below 30%. Epigenetics plays a crucial role in the development of embryos and the trophoblast layer. The use of ART during this critical period introduces a potential window of vulnerability where epigenetic changes can occur. This susceptibility is due to the significant epigenetic reprogramming that embryos undergo during early development, which can be influenced by the ART procedures. These procedures may include culture conditions, media composition, and handling techniques, all of which could potentially impact the epigenetic landscape of developing embryos, leading to lasting effects on gene expression and function. By regulating the methylation status of specific genes in ovarian cells, the success rate of IVF can be effectively improved (259), and the yield of high-quality embryos can be increased. This method increases the chances of fertilization and healthy pregnancy by optimizing the quality of oocytes and the early development of embryos.
Furthermore, research in epigenetics provides a solid scientific foundation for future reproductive health intervention strategies. These interventions could address the issue of declining fertility due to ovarian aging as age increases (260) and may play a role in treating reproductive endocrine diseases. For example, treatments for reproductive endocrine disorders like PCOS might find new directions from an epigenetic perspective.
In the realm of female reproductive health, studies in epigenetics have not only revealed the crucial roles of DNA methylation and histones post-translational modifications in ovarian function, oocyte maturation, and embryonic development but also emphasized the significant impact of environmental factors such as diet, lifestyle, and chemical exposures on gene expression. These impacts may extend to the pregnancy process and the long-term health of offspring.
On the other hand, epigenetic changes are reversible, and understanding the pathogenesis of epigenetic diseases could allow for therapeutic interventions by targeting these modifications. In-depth studies on epigenetics help elucidate the mechanisms of human reproduction and related diseases and offer potential therapeutic approaches. For example, abnormal DNA methylation plays a crucial role in the initiation and progression of endometrial cancer, leading to the silencing of Estrogen Receptor(ER) and Progesterone Receptor(PR) expression, increased genomic DNA instability, activation of oncogenes, and inactivation of tumor suppressor genes. Yanokura et al. (261) found that the abnormal hypermethylation of the CHFR mitotic checkpoint gene in endometrial cancer tumor cells is closely related to the sensitivity to taxane-based drugs, providing new intervention targets and guidance for tumor treatment.
As basic and clinical research advances, epigenetic regulation has been found to be significant in the pathogenesis, diagnosis, treatment, and prognosis assessment of various malignancies. Recent studies have shown that DNMT inhibitors, which competitively inhibit DNMT activity and block methylation reactions, have been effective in treating endometrial cancer in clinical settings (262). Clinical trials indicate that DNA demethylating agents can reverse platinum resistance in ovarian cancer patients, suggesting that epigenetic drug therapy has clinical benefits in treating chemotherapy-resistant or recurrent advanced ovarian cancer (263).
Exploring the epigenetic molecular regulatory mechanisms of human germ cell development not only provides a theoretical basis for inquiries into issues like epigenetic reprogramming of human germ cells, the establishment of pluripotency in early embryos, directed differentiation of stem cells into gametes, and transgenerational inheritance of DNA methylation but also holds significant implications for assessing the safety of assisted reproductive technologies, determining whether reproductive disorders will be inherited by offspring or across generations, researching recurrent miscarriages and embryonic arrest, and studying diseases related to abnormal development of reproductive cells in clinical settings.
In summary, research and applications of epigenetics in the field of reproductive medicine have provided new insights and therapeutic strategies, especially showing great potential in improving IVF and embryo quality.
6 Conclusion and future perspectives
Epigenetics plays a crucial role in women’s reproductive health, and environmental factors also have a potential impact on it by inducing epigenetic changes that affect female reproductive functions. External harmful environmental factors, such as PM2.5 and gaseous pollutants, can impact the female reproductive system, leading to infertility, pregnancy complications, and other issues. Additionally, harmful chemicals such as polycyclic aromatic hydrocarbons and the heavy metal cadmium can affect women’s reproductive health, potentially leading to preterm birth, miscarriage, and halted embryonic development among other adverse pregnancy outcomes. Long-term exposure to these environmental pollutants may lead to changes in the epigenetic markers of eggs and sperm, affecting the function of reproductive cells and thereby impacting the development of the embryo and fertility. Furthermore, environmental endocrine disruptors may affect epigenetic modifications, thereby interfering with the development of reproductive cells. Therefore, environmental factors and lifestyle choices are crucial for maintaining reproductive health.
The revelation of the impact of epigenetics and environmental factors on women’s reproductive health provides new approaches for treating infertility, pregnancy complications, and other diseases. By regulating the methylation status of specific genes in ovarian cells, the success rate of in vitro fertilization can be effectively increased and embryo quality improved. Meanwhile, studying how environmental factors influence reproductive health through epigenetic mechanisms is important for predicting and preventing developmental anomalies and improving offspring health.
Future research directions may include more in-depth studies on the role of epigenetics in women’s reproductive health and intervening in epigenetic changes through gene editing. Technologically, researchers might utilize epigenetics to address issues such as infertility and might also reduce the risk of embryos developing other diseases in adulthood by altering methylation in ovarian cells. For instance, the preconception period, pregnancy, and prebirth are becoming recognized as sensitive periods to the epigenetic impacts of environmental factors, which may increase the risk of chronic diseases in adulthood (including neurodegenerative diseases) (264). Additionally, future research will delve deeper into how environmental factors such as diet, lifestyle, and chemical exposure affect gene expression, and how these impacts, through epigenetic mechanisms, influence the health of offspring, thereby preventing and intervening in women’s reproductive health issues.
Author contributions
XY: Writing – original draft. JX: Writing – original draft. BS: Writing – original draft. RZ: Writing – review & editing. JL: Writing – original draft. YL: Writing – original draft. YM: Methodology, Writing – original draft, Writing – review & editing.
Funding
The author(s) declare that no financial support was received for the research, authorship, and/or publication of this article.
Conflict of interest
The authors declare that the research was conducted in the absence of any commercial or financial relationships that could be construed as a potential conflict of interest.
Publisher’s note
All claims expressed in this article are solely those of the authors and do not necessarily represent those of their affiliated organizations, or those of the publisher, the editors and the reviewers. Any product that may be evaluated in this article, or claim that may be made by its manufacturer, is not guaranteed or endorsed by the publisher.
References
1. Zhang Y, Cheng J, Zhong C, Xia Q, Li Y, Chen P, et al. ESR1 regulates the obesity- and metabolism-differential gene MMAA to inhibit the occurrence and development of hepatocellular carcinoma. Front Oncol. (2022) 12:899969. doi: 10.3389/fonc.2022.899969
2. Vayeda M, Ghanghar V, Desai S, Shah P, Modi D, Dave K, et al. Improving menstrual hygiene management among adolescent girls in tribal areas of Gujarat: an evaluation of an implementation model integrating the government service delivery system. Sex Reprod Health Matters. (2021) 29:1992199. doi: 10.1080/26410397.2021.1992199
3. World Health Organization. Available online at: https://www.who.int/news-room/fact-sheets/detail/endometriosis (Accessed 27 September 2022).
4. Mørch LS, Skovlund CW, Hannaford PC, Iversen L, Fielding S, Lidegaard Ø. Contemporary hormonal contraception and the risk of breast cancer. N Engl J Med. (2017) 377:2228–39. doi: 10.1056/NEJMoa1700732
5. Schairer C, Lubin J, Troisi R, Sturgeon S, Brinton L, Hoover R. Menopausal estrogen and estrogen-progestin replacement therapy and breast cancer risk [published correction appears in JAMA 2000 Nov 22-29;284(20):2597. JAMA. (2000) 283:485–91. doi: 10.1001/jama.283.4.485
6. Kaluscha S, Domcke S, Wirbelauer C, Stadler MB, Durdu S, Burger L, et al. Evidence that direct inhibition of transcription factor binding is the prevailing mode of gene and repeat repression by DNA methylation. Nat Genet. (2022) 54:1895–906. doi: 10.1038/s41588-022-01241-6
7. Okae H, Toh H, Sato T, Hiura H, Takahashi S, Shirane K, et al. Derivation of human trophoblast stem cells. Cell Stem Cell. (2018) 22:50–63. doi: 10.1016/j.stem.2017.11.004
8. Tang L, Zhang YY, Liu WJ, Fu Q, Zhao J, Liu Y. DNA methylation of promoter region inhibits Galectin-1 expression in BMSCs of aged mice. Am J Physiol Cell Physiol. (2024) 326(2):C429–C441. doi: 10.1152/ajpcell.00334.2023
9. Zhong Z, Xue Y, Harris CJ, Wang M, Li Z, Ke Y, et al. MORC proteins regulate transcription factor binding by mediating chromatin compaction in active chromatin regions. Genome Biol. (2023) 24:96. doi: 10.1186/s13059-023-02939-4
10. Kamińska J, Langa P, Deptuła M, Zieliński J, Sachadyn P, Wardowska A, et al. Transcriptional activity of epigenetic remodeling genes declines in keratinocytes after in vitro expansion. Adv Med Sci. (2019) 64:274–9. doi: 10.1016/j.advms.2019.03.001
11. Butz S, Schmolka N, Karemaker ID, Villaseñor R, Schwarz I, Domcke S, et al. DNA sequence and chromatin modifiers cooperate to confer epigenetic bistability at imprinting control regions. Nat Genet. (2022) 54:1702–10. doi: 10.1038/s41588-022-01210-z
12. Luderer U, Lim J, Ortiz L, Nguyen JD, Shin JH, Allen BD, et al. Exposure to environmentally relevant concentrations of ambient fine particulate matter (PM2.5) depletes the ovarian follicle reserve and causes sex-dependent cardiovascular changes in apolipoprotein E null mice. Part Fibre Toxicol. (2022) 19:5. doi: 10.1186/s12989-021-00445-8
13. Zhou S, Xi Y, Chen Y, Zhang Z, Wu C, Yan W, et al. Ovarian dysfunction induced by chronic whole-body PM2.5 exposure. Small. (2020) 16:e2000845. doi: 10.1002/smll.202000845
14. Wu S, Hao G, Zhang Y, Chen X, Ren H, Fan Y, et al. Poor ovarian response is associated with air pollutants: A multicentre study in China. EBioMedicine. (2022) 81:104084. doi: 10.1016/j.ebiom.2022.104084
15. LaPointe S, Lee JC, Nagy ZP, Shapiro DB, Chang HH, Wang Y, et al. Ambient traffic related air pollution in relation to ovarian reserve and oocyte quality in young, healthy oocyte donors. Environ Int. (2024) 183:108382. doi: 10.1016/j.envint.2023.108382
16. Dos Anjos LG, de Almeida BC, Baracat EC, Al-Hendy A, Yang Q, Carvalho KC. Gene expression profile of uterine leiomyoma from women exposed to different air pollution levels in metropolitan cities of sao paulo, Brazil. Int J Mol Sci. (2023) 24:2431. doi: 10.3390/ijms24032431
17. Goin DE, Sudat S, Riddell C, Morello-Frosch R, Apte JS, Glymour MM, et al. Hyperlocalized measures of air pollution and preeclampsia in oakland, california. Environ Sci Technol. (2021) 55:14710–9. doi: 10.1021/acs.est.1c02151
18. Juan-Reyes SS, Gómez-Oliván LM, Juan-Reyes NS, Islas-Flores H, Dublán-García O, Orozco-Hernández JM, et al. Women with preeclampsia exposed to air pollution during pregnancy: Relationship between oxidative stress and neonatal disease - Pilot study. Sci Total Environ. (2023) 871:161858. doi: 10.1016/j.scitotenv.2023.161858
19. Song W, Li A, Sha QQ, Liu SY, Zhou Y, Zhou CY, et al. Maternal exposure to 4-vinylcyclohexene diepoxide during pregnancy induces subfertility and birth defects of offspring in mice. Sci Total Environ. (2023) 859:160431. doi: 10.1016/j.scitotenv.2022.160431
20. Dai M, Huang W, Huang X, Ma C, Wang R, Tian P, et al. BPDE, the migration and invasion of human trophoblast cells, and occurrence of miscarriage in humans: roles of a novel lncRNA-HZ09. Environ Health Perspect. (2023) 131:17009. doi: 10.1289/EHP10477
21. ssah I, Duah MS, Arko-Mensah J, Bawua SA, Agyekum TP, Fobil JN. Exposure to metal mixtures and adverse pregnancy and birth outcomes: A systematic review. Sci Total Environ. (2024) 908:168380. doi: 10.1016/j.scitotenv.2023.168380
22. Yano S, Ishiuchi T, Abe S, Namekawa SH, Huang G, Ogawa Y, et al. Histone H3K36me2 and H3K36me3 form a chromatin platform essential for DNMT3A-dependent DNA methylation in mouse oocytes. Nat Commun. (2022) 13:4440. doi: 10.1038/s41467-022-32141-2
23. Malachowski T, Chandradoss KR, Boya R, Zhou L, Cook AL, Su C, et al. Spatially coordinated heterochromatinization of long synaptic genes in fragile X syndrome. Cell. (2023) 186:5840–58. doi: 10.1016/j.cell.2023.11.019
24. Singh K, Rustagi Y, Abouhashem AS, Tabasum S, Verma P, Hernandez E, et al. Genome-wide DNA hypermethylation opposes healing in patients with chronic wounds by impairing epithelial-mesenchymal transition. J Clin Invest. (2022) 132:e157279. doi: 10.1172/JCI157279
25. He L, Huang H, Bradai M, Zhao C, You Y, Ma J, et al. DNA methylation-free Arabidopsis reveals crucial roles of DNA methylation in regulating gene expression and development. Nat Commun. (2022) 13:1335. doi: 10.1038/s41467-022-28940-2
26. Xuan Lin QX, Sian S, An O, Thieffry D, Jha S, Benoukraf T. MethMotif: an integrative cell specific database of transcription factor binding motifs coupled with DNA methylation profiles. Nucleic Acids Res. (2019) 47:D145–54. doi: 10.1093/nar/gky1005
27. Chen Z, Zhang Y. Role of mammalian DNA methyltransferases in development. Annu Rev Biochem. (2020) 89:135–58. doi: 10.1146/annurev-biochem-103019-102815
28. Li Y, Zhang Z, Chen J, Liu W, Lai W, Liu B, et al. Stella safeguards the oocyte methylome by preventing de novo methylation mediated by DNMT1. Nature. (2018) 564:136–40. doi: 10.1038/s41586-018-0751-5
29. Andrews S, Krueger C, Mellado-Lopez M, Hemberger M, Dean W, Perez-Garcia V, et al. Mechanisms and function of de novo DNA methylation in placental development reveals an essential role for DNMT3B. Nat Commun. (2023) 14:371. doi: 10.1038/s41467-023-36019-9
30. Dura M, Teissandier A, Armand M, Barau J, Lapoujade C, Fouchet P, et al. DNMT3A-dependent DNA methylation is required for spermatogonial stem cells to commit to spermatogenesis. Nat Genet. (2022) 54:469–80. doi: 10.1038/s41588-022-01040-z
31. Noh KM, Wang H, Kim HR, Wenderski W, Fang F, Li CH, et al. Engineering of a histone-recognition domain in dnmt3a alters the epigenetic landscape and phenotypic features of mouse ESCs. ” Mol Cell. (2015) 59,1:89–103. doi: 10.1016/j.molcel.2015.05.017
32. He W, Zhang X, Zhang Y, Zheng W, Xiong Z, Hu X, et al. Reduced self-diploidization and improved survival of semi-cloned mice produced from androgenetic haploid embryonic stem cells through overexpression of dnmt3b. Stem Cell Rep. (2018) 10:477–93. doi: 10.1016/j.stemcr.2017.12.024
33. Weinberg DN, Papillon-Cavanagh S, Chen H, Yue Y, Chen X, Rajagopalan KN, et al. The histone mark H3K36me2 recruits DNMT3A and shapes the intergenic DNA methylation landscape. ” Nat. (2019) 573:281–6. doi: 10.1038/s41586-019-1534-3
34. Kenjiro S, Shirane K, Miura F, Ito T, Lorincz MC. NSD1-deposited H3K36me2 directs de novo methylation in the mouse male germline and counteracts Polycomb-associated silencing. ” Nat Genet. (2020) 52:1088–98. doi: 10.1038/s41588-020-0689-z
35. Gu T, Hao D, Woo J, Huang TW, Guo L, Lin X, et al. The disordered N-terminal domain of DNMT3A recognizes H2AK119ub and is required for postnatal development. Nat Genet. (2022) 54:625–36. doi: 10.1038/s41588-022-01063-6
36. Qin L, Qiao C, Sheen V, Wang Y, Lu J. DNMT3L promotes neural differentiation by enhancing STAT1 and STAT3 phosphorylation independent of DNA methylation. Prog Neurobiol. (2021) 201:102028. doi: 10.1016/j.pneurobio.2021.102028
37. Behluli L, Fontanilla AM, Andessner-Angleitner L, Tolar N, Molina JM, Gahurova L. Expression analysis suggests that DNMT3L is required for oocyte de novo DNA methylation only in Muridae and Cricetidae rodents. Epigenet Chromatin. (2023) 16:43. doi: 10.1186/s13072-023-00518-2
38. Finnegan AI, Kim S, Jin H, Gapinske M, Woods WS, Perez-Pinera P, et al. Epigenetic engineering of yeast reveals dynamic molecular adaptation to methylation stress and genetic modulators of specific DNMT3 family members. Nucleic Acids Res. (2020) 48:4081–99. doi: 10.1093/nar/gkaa161
39. Wang Q, Liang N, Yang T, Li Y, Li J, Huang Q, et al. DNMT1-mediated methylation of BEX1 regulates stemness and tumorigenicity in liver cancer. J Hepatol. (2021) 75:1142–53. doi: 10.1016/j.jhep.2021.06.025
40. Hahm JY, Park JW, Kang JY, Park J, Kim CH, Kim JY, et al. Acetylation of UHRF1 regulates hemi-methylated DNA binding and maintenance of genome-wide DNA methylation. Cell Rep. (2020) 32:107958. doi: 10.1016/j.celrep.2020.107958
41. Mattei AL, Bailly N, Meissner A. DNA methylation: a historical perspective. Trends Genet. (2022) 38:676–707. doi: 10.1016/j.tig.2022.03.010
42. Schneider M, Trummer C, Stengl A, Zhang P, Szwagierczak A, Cardoso MC, et al. Systematic analysis of the binding behaviour of UHRF1 towards different methyl- and carboxylcytosine modification patterns at CpG dyads. PloS One. (2020) 15:e0229144. doi: 10.1371/journal.pone.0229144
43. Fang J, Cheng J, Wang J, Zhang Q, Liu M, Gong R, et al. Hemi-methylated DNA opens a closed conformation of UHRF1 to facilitate its histone recognition. Nat Commun. (2016) 7:11197. doi: 10.1038/ncomms11197
44. Tahiliani M, Koh KP, Shen Y, Pastor WA, Bandukwala H, Brudno Y, et al. Conversion of 5-methylcytosine to 5-hydroxymethylcytosine in mammalian DNA by MLL partner TET1. Sci (New York N.Y.). (2009) 324:930–5:5929. doi: 10.1126/science.1170116
45. Kriaucionis S, Heintz N. The nuclear DNA base 5-hydroxymethylcytosine is present in Purkinje neurons and the brain. Science. (2009) 324:929–30. doi: 10.1126/science.1169786
46. Bhutani N, Burns DM, Blau HM. DNA demethylation dynamics. Cell. (2011) 146:866–72. doi: 10.1016/j.cell.2011.08.042
47. Huang Y, Li L, An G, Yang X, Cui M, Song X, et al. Single-cell multi-omics sequencing of human spermatogenesis reveals a DNA demethylation event associated with male meiotic recombination. Nat Cell Biol. (2023) 25:1520–34. doi: 10.1038/s41556-023-01232-7
48. Ghoneim M, Fuchs HA, Musselman CA. Histone tail conformations: a fuzzy affair with DNA. Trends Biochem Sci. (2021) 46:564–78. doi: 10.1016/j.tibs.2020.12.012
49. Luger K, Mäder AW, Richmond RK, Sargent DF, Richmond TJ. Crystal structure of the nucleosome core particle at 2. 8 A resolution. Nat. (1997) 389:251–60. doi: 10.1038/38444
50. Li S, Peng Y, Landsman D, Panchenko AR. DNA methylation cues in nucleosome geometry, stability and unwrapping. Nucleic Acids Res. (2022) 50:1864–74. doi: 10.1093/nar/gkac097
51. Peng Y, Li S, Landsman D, Panchenko AR. Histone tails as signaling antennas of chromatin. Curr Opin Struct Biol. (2021) 67:153–60. doi: 10.1016/j.sbi.2020.10.018
52. Gao G, Hausmann S, Flores NM, Benitez AM, Shen J, Yang X, et al. The NFIB/CARM1 partnership is a driver in preclinical models of small cell lung cancer. Nat Commun. (2023) 14:363. doi: 10.1038/s41467-023-35864-y
53. Gao Y, Sheng X, Tan D, Kim S, Choi S, Paudel S, et al. Identification of histone lysine acetoacetylation as a dynamic post-translational modification regulated by HBO1. Adv Sci (Weinh). (2023) 10:e2300032. doi: 10.1002/advs.202300032
54. Liao L, He Y, Li SJ, Yu XM, Liu ZC, Liang YY, et al. Lysine 2-hydroxyisobutyrylation of NAT10 promotes cancer metastasis in an ac4C-dependent manner. Cell Res. (2023) 33:355–71. doi: 10.1038/s41422-023-00793-4
55. Hu Z, Wei F, Su Y, Wang Y, Shen Y, Fang Y, et al. Histone deacetylase inhibitors promote breast cancer metastasis by elevating NEDD9 expression. Signal Transduct Target Ther. (2023) 8:11. doi: 10.1038/s41392-022-01221-6
56. Wang ZA, Cole PA. The chemical biology of reversible lysine post-translational modifications. Cell Chem Biol. (2020) 27:953–69. doi: 10.1016/j.chembiol.2020.07.002
57. Jambhekar A, Dhall A, Shi Y. Roles and regulation of histone methylation in animal development. Nat Rev Mol Cell Biol. (2019) 20:625–41. doi: 10.1038/s41580-019-0151-1
58. Haws SA, Yu D, Ye C, Wille CK, Nguyen LC, Krautkramer KA, et al. Methyl-metabolite depletion elicits adaptive responses to support heterochromatin stability and epigenetic persistence. Mol Cell. (2020) 78:210–23. doi: 10.1016/j.molcel.2020.03.004
59. Yi Y, Ge S. Targeting the histone H3 lysine 79 methyltransferase DOT1L in MLL-rearranged leukemias. J Hematol Oncol. (2022) 15:35. doi: 10.1186/s13045-022-01251-1
60. Qiu J, Xu B, Ye D, Ren D, Wang S, Benci JL, et al. Cancer cells resistant to immune checkpoint blockade acquire interferon-associated epigenetic memory to sustain T cell dysfunction. Nat Cancer. (2023) 4:43–61. doi: 10.1038/s43018-022-00490-y
61. Padeken J, Methot SP, Gasser SM. Establishment of H3K9-methylated heterochromatin and its functions in tissue differentiation and maintenance. Nat Rev Mol Cell Biol. (2022) 23:623–40. doi: 10.1038/s41580-022-00483-w
62. Li Z, Duan S, Hua X, Xu X, Li Y, Menolfi D, et al. Asymmetric distribution of parental H3K9me3 in S phase silences L1 elements. Nature. (2023) 623:643–51. doi: 10.1038/s41586-023-06711-3
63. Husmann D, Gozani O. Histone lysine methyltransferases in biology and disease. Nat Struct Mol Biol. (2019) 26:880–9. doi: 10.1038/s41594-019-0298-7
64. Greer EL, Shi Y. Histone methylation: a dynamic mark in health, disease and inheritance. Nat Rev Genet. (2012) 13:343–57. doi: 10.1038/nrg3173
65. Xiao Y, Zhao C, Tai Y, Li B, Lan T, Lai E, et al. STING mediates hepatocyte pyroptosis in liver fibrosis by Epigenetically activating the NLRP3 inflammasome. Redox Biol. (2023) 62:102691. doi: 10.1016/j.redox.2023.102691
66. Gray ZH, Chakraborty D, Duttweiler RR, Alekbaeva GD, Murphy SE, Chetal K, et al. Epigenetic balance ensures mechanistic control of MLL amplification and rearrangement. Cell. (2023) 186:4528–4545.e18. doi: 10.1016/j.cell.2023.09.009
67. Huang X, Zhang X, Zong L, Gao Q, Zhang C, Wei R, et al. Gene body methylation safeguards ribosomal DNA transcription by preventing PHF6-mediated enrichment of repressive histone mark H4K20me3. J Biol Chem. (2021) 297:101195. doi: 10.1016/j.jbc.2021.101195
68. Jiang Q, Stachelscheid J, Bloehdorn J, Pacholewska A, Aszyk C, Grotenhuijs F, et al. Oncogenic role and target properties of the lysine-specific demethylase KDM1A in chronic lymphocytic leukemia. Blood. (2023) 142:44–61. doi: 10.1182/blood.2022017230
69. Zhang J, Wang H, Chen H, Li H, Xu P, Liu B, et al. ATF3 -activated accelerating effect of LINC00941/lncIAPF on fibroblast-to-myofibroblast differentiation by blocking autophagy depending on ELAVL1/HuR in pulmonary fibrosis. Autophagy. (2022) 18:2636–55. doi: 10.1080/15548627.2022.2046448
70. Xie S, Jiang C, Wu M, Ye Y, Wu B, Sun X, et al. Dietary ketone body-escalated histone acetylation in megakaryocytes alleviates chemotherapy-induced thrombocytopenia. Sci Transl Med. (2022) 14:eabn9061. doi: 10.1126/scitranslmed.abn9061
71. Tessarz P, Kouzarides T. Histone core modifications regulating nucleosome structure and dynamics. Nat Rev Mol Cell Biol. (2014) 15:703–8. doi: 10.1038/nrm3890
72. Kim TH, Nosella ML, Bolik-Coulon N, Harkness RW, Huang SK, Kay LE. Correlating histone acetylation with nucleosome core particle dynamics and function. Proc Natl Acad Sci U S A. (2023) 120:e2301063120. doi: 10.1073/pnas.2301063120
73. Voss AK, Thomas T. Histone lysine and genomic targets of histone acetyltransferases in mammals. Bioessays. (2018) 40:e1800078. doi: 10.1002/bies.201800078
74. von Knethen A, Brüne B. Histone deacetylation inhibitors as therapy concept in sepsis. Int J Mol Sci. (2019) 20:346. doi: 10.3390/ijms20020346
75. Li H, Chen X, Xu J, Zhu L, Li C, Sun X, et al. GRP/GRPR enhances alcohol-associated liver injury through the IRF1-mediated Caspase-1 inflammasome and NOX2-dependent ROS pathway. Hepatology. (2024) 79:392–408. doi: 10.1097/HEP.0000000000000531
76. Cai L, Sutter BM, Li B, Tu BP. Acetyl-CoA induces cell growth and proliferation by promoting the acetylation of histones at growth genes. Mol Cell. (2011) 42:426–37. doi: 10.1016/j.molcel.2011.05.004
77. Wellen KE, Hatzivassiliou G, Sachdeva UM, Bui TV, Cross JR, Thompson CB. ATP-citrate lyase links cellular metabolism to histone acetylation. Science. (2009) 324:1076–80. doi: 10.1126/science.1164097
78. Izzo LT, Trefely S, Demetriadou C, Drummond JM, Mizukami T, Kuprasertkul N, et al. Acetylcarnitine shuttling links mitochondrial metabolism to histone acetylation and lipogenesis. Sci Adv. (2023) 9:eadf0115. doi: 10.1126/sciadv.adf0115
79. Morrow MR, Batchuluun B, Wu J, Ahmadi E, Leroux JM, Mohammadi-Shemirani P, et al. Inhibition of ATP-citrate lyase improves NASH, liver fibrosis, and dyslipidemia. Cell Metab. (2022) 34:919–936.e8. doi: 10.1016/j.cmet.2022.05.004
80. Mao Y, Zhang J, Zhou Q, He X, Zheng Z, Wei Y, et al. Hypoxia induces mitochondrial protein lactylation to limit oxidative phosphorylation. Cell Res. (2024) 34:13–30. doi: 10.1038/s41422-023-00864-6
81. Mews P, Donahue G, Drake AM, Luczak V, Abel T, Berger SL. Acetyl-CoA synthetase regulates histone acetylation and hippocampal memory. Nature. (2017) 546:381–6. doi: 10.1038/nature22405
82. Mews P, Egervari G, Nativio R, Sidoli S, Donahue G, Lombroso SI, et al. Alcohol metabolism contributes to brain histone acetylation. Nature. (2019) 574:717–21. doi: 10.1038/s41586-019-1700-7
83. Zhang D, Tang Z, Huang H, Zhou G, Cui C, Weng Y, et al. Metabolic regulation of gene expression by histone lactylation. Nature. (2019) 574:575–80. doi: 10.1038/s41586-019-1678-1
84. Wan N, Wang N, Yu S, Zhang H, Tang S, Wang D, et al. Cyclic immonium ion of lactyllysine reveals widespread lactylation in the human proteome. Nat Methods. (2022) 19:854–64. doi: 10.1038/s41592-022-01523-1
85. Villanueva L, Alvarez-Errico D, Esteller M. The contribution of epigenetics to cancer immunotherapy. Trends Immunol. (2020) 41:676–91. doi: 10.1016/j.it.2020.06.002
86. Slack FJ, Chinnaiyan AM. The role of non-coding RNAs in oncology. Cell. (2019) 179:1033–55. doi: 10.1016/j.cell.2019.10.017
87. An Y, Duan H. The role of m6A RNA methylation in cancer metabolism. Mol Cancer. (2022) 21:14. doi: 10.1186/s12943-022-01500-4
88. Raju GSR, Pavitra E, Bandaru SS, Varaprasad GL, Nagaraju GP, Malla RR, et al. HOTAIR: a potential metastatic, drug-resistant and prognostic regulator of breast cancer. Mol Cancer. (2023) 22:65. doi: 10.1186/s12943-023-01765-3
89. Xue C, Li G, Zheng Q, Gu X, Bao Z, Lu J, et al. The functional roles of the circRNA/Wnt axis in cancer. Mol Cancer. (2022) 21:108. doi: 10.1186/s12943-022-01582-0
90. van Zonneveld AJ, Zhao Q, Rotmans JI, Bijkerk R. Circulating non-coding RNAs in chronic kidney disease and its complications. Nat Rev Nephrol. (2023) 19:573–86. doi: 10.1038/s41581-023-00725-w
91. Welsh SA, Gardini A. Genomic regulation of transcription and RNA processing by the multitasking Integrator complex. Nat Rev Mol Cell Biol. (2023) 24:204–20. doi: 10.1038/s41580-022-00534-2
92. Cornes E, Bourdon L, Singh M, Mueller F, Quarato P, Wernersson E, et al. piRNAs initiate transcriptional silencing of spermatogenic genes during C. elegans germline development. Dev Cell. (2022) 57:180–196.e7. doi: 10.1016/j.devcel.2021.11.025
93. Ruggieri F, Jonas K, Ferracin M, Dengler M, Jäger V, Pichler M. MicroRNAs as regulators of tumor metabolism. Endocr Relat Cancer. (2023) 30:e220267. doi: 10.1530/ERC-22-0267
94. Lin C, Ma M, Zhang Y, Li L, Long F, Xie C, et al. The N6-methyladenosine modification of circALG1 promotes the metastasis of colorectal cancer mediated by the miR-342-5p/PGF signalling pathway [published correction appears in Mol Cancer. Mol Cancer. (2022) 21:80. doi: 10.1186/s12943-022-01560-6
95. Liu Y, Li C, Fang L, Wang L, Liu H, Tian H, et al. Lipid metabolism-related lncRNA SLC25A21-AS1 promotes the progression of oesophageal squamous cell carcinoma by regulating the NPM1/c-Myc axis and SLC25A21 expression. Clin Transl Med. (2022) 12:e944. doi: 10.1002/ctm2.944
96. Luo Y, Huang S, Wei J, Zhou H, Wang W, Yang J, et al. Long noncoding RNA LINC01606 protects colon cancer cells from ferroptotic cell death and promotes stemness by SCD1-Wnt/β-catenin-TFE3 feedback loop signalling. Clin Transl Med. (2022) 12:e752. doi: 10.1002/ctm2.752
97. Zhang N, Zhang X, Xu W, Zhang X, Mu Z. CircRNA_103948 inhibits autophagy in colorectal cancer in a ceRNA manner. Ann N Y. Acad Sci. (2021) 1503:88–101. doi: 10.1111/nyas.14679
98. Xi Y, Shen Y, Wu D, Zhang J, Lin C, Wang L, et al. CircBCAR3 accelerates esophageal cancer tumorigenesis and metastasis via sponging miR-27a-3p. Mol Cancer. (2022) 21:145. doi: 10.1186/s12943-022-01615-8
99. Hattori N, Liu YY, Ushijima T. DNA methylation analysis. Methods Mol Biol. (2023) 2691:165–83. doi: 10.1007/978-1-0716-3331-1_13
100. Ma F, Jiang S, Zhang CY. Recent advances in histone modification and histone modifying enzyme assays. Expert Rev Mol Diagn. (2019) 19:27–36. doi: 10.1080/14737159.2019.1559053
101. Salmen F, De Jonghe J, Kaminski TS, Alemany A, Parada GE, Verity-Legg J, et al. High-throughput total RNA sequencing in single cells using VASA-seq. Nat Biotechnol. (2022) 40:1780–93. doi: 10.1038/s41587-022-01361-8
102. Rauscher GH, Kresovich JK, Poulin M, Yan L, Macias V, Mahmoud AM, et al. Exploring DNA methylation changes in promoter, intragenic, and intergenic regions as early and late events in breast cancer formation. BMC Cancer. (2015) 15:816. doi: 10.1186/s12885-015-1777-9
103. Zou LS, Erdos MR, Taylor DL, Chines PS, Varshney A, McDonnell Genome Institute, et al. BoostMe accurately predicts DNA methylation values in whole-genome bisulfite sequencing of multiple human tissues. BMC Genomics. (2018) 19:390. doi: 10.1186/s12864-018-4766-y
104. Weber M, Davies JJ, Wittig D, Oakeley EJ, Haase M, Lam WL, et al. Chromosome-wide and promoter-specific analyses identify sites of differential DNA methylation in normal and transformed human cells. Nat Genet. (2005) 37:853–62. doi: 10.1038/ng1598
105. Jacinto FV, Ballestar E, Esteller M. Methyl-DNA immunoprecipitation (MeDIP): hunting down the DNA methylome. Biotechniques. (2008) 44:35, 37, 39. doi: 10.2144/000112708
106. Bibikova M, Barnes B, Tsan C, Ho V, Klotzle B, Le JM, et al. High density DNA methylation array with single CpG site resolution. Genomics. (2011) 98:288–95. doi: 10.1016/j.ygeno.2011.07.007
107. Herman JG, Graff JR, Myöhänen S, Nelkin BD, Baylin SB. Methylation-specific PCR: a novel PCR assay for methylation status of CpG islands. Proc Natl Acad Sci U S A. (1996) 93:9821–6. doi: 10.1073/pnas.93.18.9821
108. Masser DR, Berg AS, Freeman WM. Focused, high accuracy 5-methylcytosine quantitation with base resolution by benchtop next-generation sequencing. Epigenet Chromatin. (2013) 6:33. doi: 10.1186/1756-8935-6-33
109. Meissner A, Gnirke A, Bell GW, Ramsahoye B, Lander ES, Jaenisch R. Reduced representation bisulfite sequencing for comparative high-resolution DNA methylation analysis. Nucleic Acids Res. (2005) 33:5868–77. doi: 10.1093/nar/gki901
110. Javadmanesh A, Mojtabanezhad Shariatpanahi A, Shams Davodly E, Azghandi M, Yassi M, Heidari M, et al. MS-HRM protocol: a simple and low-cost approach for technical validation of next-generation methylation sequencing data. Mol Genet Genomics. (2022) 297:1101–9. doi: 10.1007/s00438-022-01906-1
111. Nakato R, Sakata T. Methods for ChIP-seq analysis: A practical workflow and advanced applications. Methods. (2021) 187:44–53. doi: 10.1016/j.ymeth.2020.03.005
112. Teng M. Statistical analysis in chIP-seq-related applications. Methods Mol Biol. (2023) 2629:169–81. doi: 10.1007/978-1-0716-2986-4_9
113. Kaya-Okur HS, Wu SJ, Codomo CA, Pledger ES, Bryson TD, Henikoff JG, et al. CUT&Tag for efficient epigenomic profiling of small samples and single cells. Nat Commun. (2019) 10:1930. doi: 10.1038/s41467-019-09982-5
114. Sidoli S, Kori Y, Lopes M, Yuan Z-F, Kim HJ, Kulej K, et al. One minute analysis of 200 histone posttranslational modifications by direct injection mass spectrometry. Genome Res. (2019) 29:978–87. doi: 10.1101/gr.247353.118
115. Brasil PE, De Castro L, Hasslocher-Moreno AM, Sangenis LH, Braga JU. ELISA versus PCR for diagnosis of chronic Chagas disease: systematic review and meta-analysis. BMC Infect Dis. (2010) 10:337. doi: 10.1186/1471-2334-10-337
116. Kauter J, Damek F, Schares G, Blaga R, Schott F, Deplazes P, et al. Detection of Toxoplasma gondii-specific antibodies in pigs using an oral fluid-based commercial ELISA: Advantages and limitations. Int J Parasitol. (2023) 53:523–30. doi: 10.1016/j.ijpara.2022.11.003
117. Kashina AS, Yates Iii JR. Analysis of arginylated peptides by subtractive edman degradation. Methods Mol Biol. (2023) 2620:153–5. doi: 10.1007/978-1-0716-2942-0_20
118. Ma P, Amemiya HM, He LL, Gandhi SJ, Nicol R, Bhattacharyya RP, et al. Bacterial droplet-based single-cell RNA-seq reveals antibiotic-associated heterogeneous cellular states. Cell. (2023) 186:877–891.e14. doi: 10.1016/j.cell.2023.01.002
119. Cao M, Zhao J, Hu G. Genome-wide methods for investigating long noncoding RNAs. Biomedicine pharmacotherapy = Biomedecine pharmacotherapie. (2019) 111:395–401. doi: 10.1016/j.biopha.2018.12.078
120. Taylor SC, Posch A. The design of a quantitative western blot experiment. BioMed Res Int. (2014) 2014:361590. doi: 10.1155/2014/361590
121. Zhao J, Ohsumi TK, Kung JT, Ogawa Y, Grau DJ, Sarma K, et al. Genome-wide identification of polycomb-associated RNAs by RIP-seq. Mol Cell. (2010) 40:939–53. doi: 10.1016/j.molcel.2010.12.011
122. Jing Y, Lin S, Wang FT, Yu JW, Jiang F. [Detection of Gene Abnormalities in 43 Cases of Chronic Lymphocytic Leukemia by Fluorescence in Situ Hybridization]. Zhongguo Shi Yan Xue Ye Xue Za Zhi. (2018) 26(4):1038–43. doi: 10.7534/j.issn.1009-2137.2018.04.016.
123. Huang J, Ju Z, Li Q, Hou Q, Wang C, Li J, et al. Solexa sequencing of novel and differentially expressed microRNAs in testicular and ovarian tissues in Holstein cattle. Int J Biol Sci. (2011) 7:1016–26. doi: 10.7150/ijbs.7.1016
124. Ghosh K, Chatterjee B, Behera P, Kanade SR. The carcinogen cadmium elevates CpG-demethylation and enrichment of NFYA and E2F1 in the promoter of oncogenic PRMT5 and EZH2 methyltransferases resulting in their elevated expression in vitro. Chemosphere. (2020) 242:125186. doi: 10.1016/j.chemosphere.2019.125186
125. Aigner GP, Pittl V, Fiechtner B, Egger B, Šrut M, Höckner M. Common mechanisms cannot explain time- and dose-dependent DNA methylation changes in earthworms exposed to cadmium. Sci Total Environ. (2022) 812:151468. doi: 10.1016/j.scitotenv.2021.151468
126. Sun L, Mu Y, Xu L, Han X, Gu W, Zhang M. Transgenerational inheritance of wing development defects in Drosophila melanogaster induced by cadmium. Ecotoxicol Environ Saf. (2023) 250:114486. doi: 10.1016/j.ecoenv.2022.114486
127. Chen M, Li X, Fan R, Yang J, Jin X, Hamid S, et al. Cadmium induces BNIP3-dependent autophagy in chicken spleen by modulating miR-33-AMPK axis. Chemosphere. (2018) 194:396–402. doi: 10.1016/j.chemosphere.2017.12.026
128. Sharara FI, Seifer DB, Flaws JA. Environmental toxicants and female reproduction. Fertil Steril. (1998) 70:613–22. doi: 10.1016/S0015-0282(98)00253-2
129. Baranski B, Szymczyk I. Effects of mercury vapors upon reproductive function of the female white rat. Medycyna Pracy. (1973) 24:249–61.
130. Davis BJ, Price HC, O'Connor RW, Fernando R, Rowland AS, Morgan DL. Mercury vapor and female reproductive toxicity. Toxicol Sci. (2001) 59:291–6. doi: 10.1093/toxsci/59.2.291
131. Lamperti AA, Printz RH. Effects of mercuric chloride on the reproductive cycle of the female hamster. Biol Reprod. (1973) 8:378–87. doi: 10.1093/biolreprod/8.3.378
132. Dansereau M, Larivière DN, Du Tremblay D, Belanger D. Reproductive performance of two generations of female semidomesticated mink fed diets containing organic mercury contaminated freshwater fish. Arch Environ Contam Toxicol. (1999) 36:221–6. doi: 10.1007/s002449900464
133. Haugen BR. Drugs that suppress TSH or cause central hypothyroidism. Best Pract Res Clin Endocrinol Metab. (2009) 23(6):793–800. doi: 10.1016/j.beem.2009.08.003
134. Gonsioroski A, Mourikes VE, Flaws JA. Endocrine Disruptors in Water and Their Effects on the Reproductive System. Int J Mol Sci. (2020) 21(6):1929. doi: 10.3390/ijms21061929
135. Rattan S, Flaws JA. The epigenetic impacts of endocrine disruptors on female reproduction across generations. Biol Reprod. (2019) 101:635–44. doi: 10.1093/biolre/ioz081
136. Signorile PG, Spugnini EP, Citro G, Viceconte R, Vincenzi B, Baldi F, et al. Endocrine disruptors in utero cause ovarian damages linked to endometriosis. Front Biosci -Elite. (2012) 4:1724–30. doi: 10.2741/e493
137. WHO. State of the science of endocrine disrupting chemicals 2012. In: Summary for decision-makers. WHO, Geneva, Switzerland (2012).
138. Moreira Fernandez MA, Cardeal ZL, Carneiro MM, André LC. Study of possible association between endometriosis and phthalate and bisphenol A by biomarkers analysis. J Pharm Biomed Anal. (2019) 172:238–42. doi: 10.1016/j.jpba.2019.04.048
139. Ji C, Song Q, Chen Y, Zhou Z, Wang P, Liu J, et al. The potential endocrine disruption of pesticide transformation products (TPs): The blind spot of pesticide risk assessment. Environ Int. (2020) 137:105490. doi: 10.1016/j.envint.2020.105490
140. Kaushal J, Khatri M, Arya SK. A treatise on Organophosphate pesticide pollution: Current strategies and advancements in their environmental degradation and elimination. Ecotoxicol. Environ Saf. (2021) 207:111483. doi: 10.1016/j.ecoenv.2020.111483
141. Mahmood I, Imadi SR, Shazadi K, Gul A, Hakeem KR. Plant, soil and microbes. In: Effects of pesticides on environment. Springer, Berlin/Heidelberg, Germany (2016). p. 253–69.
142. Anand N, Chakraborty P, Ray S. Human exposure to organochlorine, pyrethroid and neonicotinoid pesticides: Comparison between urban and semi-urban regions of India. Environ pollut. (2021) 270:116156. doi: 10.1016/j.envpol.2020.116156
143. Jugan J, Lind PM, Salihovic S, Stubleski J, Karrman A, Lind L, et al. The associations between p,p′-DDE levels and plasma levels of lipoproteins and their subclasses in an elderly population determined by analysis of lipoprotein content. Lipids Health Dis. (2020) 19:249. doi: 10.1186/s12944-020-01417-1
144. Yaglova N, Tsomartova D, Yaglov V. Differences in production of adrenal steroid hormones in pubertal rats exposed to low doses of the endocrine disruptor DDT during prenatal and postnatal development. Biochem (Mosc.) Suppl. Ser B Biomed Chem. (2018) 12:80–6. doi: 10.1134/S1990750818010122
145. Wang L, Qie Y, Yang Y, Zhao Q. Binding and activation of estrogen-related receptor γ: A novel molecular mechanism for the estrogenic disruption effects of DDT and its metabolites. Environ Sci Technol. (2022) 56:12358–67. doi: 10.1021/acs.est.1c08624
146. Yaglova NV, Tsomartova DA, Obernikhin SS, Yaglov VV, Nazimova SV, Tsomartova ES, et al. Differential disrupting effects of prolonged low-dose exposure to dichlorodiphenyltrichloroethane on androgen and estrogen production in males. Int J Mol Sci. (2021) 22:3155. doi: 10.3390/ijms22063155
147. Lai KP, Tim Leung CC, Boncan DAT, Tam N, Lin X, Wang SY, et al. Hypoxia-induced epigenetic transgenerational miRNAs dysregulation involved in reproductive impairment of ovary. Chem Biol Interact. (2022) 367:110176. doi: 10.1016/j.cbi.2022.110176
148. González-Rojo S, Lombó M, Fernández-Díez C, Herráez MP. Male exposure to bisphenol a impairs spermatogenesis and triggers histone hyperacetylation in zebrafish testes. Environ pollut. (2019) 248:368–79. doi: 10.1016/j.envpol.2019.01.127
149. Lombó M, Herráez MP. Paternal inheritance of bisphenol A cardiotoxic effects: the implications of sperm epigenome. Int J Mol Sci. (2021) 22:2125. doi: 10.3390/ijms22042125
150. Mo J, Au DW, Wan MT, Shi J, Zhang G, Winkler C, et al. Multigenerational impacts of benzo[a]pyrene on bone modeling and remodeling in medaka (Oryzias latipes). Environ Sci Technol. (2020) 54:12271–84. doi: 10.1021/acs.est.0c02416
151. Saban JM, Watson-Lazowski A, Chapman MA, Taylor G. The methylome is altered for plants in a high CO2 world: Insights into the response of a wild plant population to multigenerational exposure to elevated atmospheric [CO2. Glob Chang Biol. (2020) 26:6474–92. doi: 10.1111/gcb.15249
152. Wu J, Ren C, Delfino RJ, Chung J, Wilhelm M, Ritz B. Association between local traffic-generated air pollution and preeclampsia and preterm delivery in the south coast air basin of California. Environ Health Perspectives. (2009) 117:1773–9. doi: 10.1289/ehp.0800334
153. Ahn TG, Kim YJ, Lee G, You YA, Kim SM, Chae R, et al. Association between individual air pollution (PM10, PM2.5) exposure and adverse pregnancy outcomes in korea: A multicenter prospective cohort, air pollution on pregnancy outcome (APPO) study. J Korean Med Sci. (2024) 39:e131. doi: 10.3346/jkms.2024.39.e131
154. Twu O, Dessí D, Vu A, Mercer F, Stevens GC, de Miguel N, et al. Trichomonas vaginalis homolog of macrophage migration inhibitory factor induces prostate cell growth, invasiveness, and inflammatory responses. Proc Natl Acad Sci U S A. (2014) 111:8179–84. doi: 10.1073/pnas.1321884111
155. Onderdonk AB, Delaney ML, Fichorova RN. The human microbiome during bacterial vaginosis. Clin Microbiol Rev. (2016) 29:223–38. doi: 10.1128/CMR.00075-15
156. Aiyar A, Quayle AJ, Buckner LR, Sherchand SP, Chang TL, Zea AH, et al. Influence of the tryptophan-indole-IFNγ axis on human genital Chlamydia trachomatis infection: role of vaginal co-infections. Front Cell Infect Microbiol. (2014) 4:72. doi: 10.3389/fcimb.2014.00072
157. Sodhani P, Gupta S, Gupta R, Mehrotra R. Bacterial vaginosis and cervical intraepithelial neoplasia: is there an association or is co-existence incidental? Asian Pac J Cancer Prev. (2017) 18:1289–92. doi: 10.22034/APJCP.2017.18.5.1289
158. Fellous A, Wegner KM, John U, Mark FC, Shama LNS. Windows of opportunity: Ocean warming shapes temperature-sensitive epigenetic reprogramming and gene expression across gametogenesis and embryogenesis in marine stickleback. Glob Chang Biol. (2022) 28:54–71. doi: 10.1111/gcb.15942
159. Bertin G, Averbeck D. Cadmium: cellular effects, modifications of biomolecules, modulation of DNA repair and genotoxic consequences (a review). Biochimie. (2006) 88:1549–59. doi: 10.1016/j.biochi.2006.10.001
160. Carvan MJ 3rd, Kalluvila TA, Klingler RH, Larson JK, Pickens M, Mora-Zamorano FX, et al. Mercury-induced epigenetic transgenerational inheritance of abnormal neurobehavior is correlated with sperm epimutations in zebrafish. PloS One. (2017) 12:e0176155. doi: 10.1371/journal.pone.0176155
161. Bhan A, Sarkar NN. Mercury in the environment: effect on health and reproduction. Rev Environ Health. (2005) 20:39–56. doi: 10.1515/reveh.2005.20.1.39
162. Martos SN, Tang WY, Wang Z. Elusive inheritance: Transgenerational effects and epigenetic inheritance in human environmental disease. Prog Biophys Mol Biol. (2015) 118:44–54. doi: 10.1016/j.pbiomolbio.2015.02.011
163. Hou L, Wang D, Baccarelli A. Environmental chemicals and microRNAs. Mutat Res. (2011) 714:105–12. doi: 10.1016/j.mrfmmm.2011.05.004
164. Cao Y, Calafat AM, Doerge DR, Umbach DM, Bernbaum JC, Twaddle NC, et al. Isoflavones in urine, saliva, and blood of infants: Data from a pilot study on the estrogenic activity of soy formula. J Expo. Sci Environ Epidemiol. (2008) 19:223–34. doi: 10.1038/jes.2008.44
165. Yu L, Rios E, Castro L, Liu J, Yan Y, Dixon D. Genistein: dual role in women's health. Nutrients. (2021) 13:3048. doi: 10.3390/nu13093048
166. Longnecker MP, Klebanoff MA, Zhou H, Brock JW. Association between maternal serum concentration of the DDT metabolite DDE and preterm and small-for-gestational-age babies at birth. Lancet. (2001) 358:110–4. doi: 10.1016/S0140-6736(01)05329-6
167. Gapp K, von Ziegler L, Tweedie-Cullen RY, Mansuy IM. Early life epigenetic programming and transmission of stress-induced traits in mammals: how and when can environmental factors influence traits and their transgenerational inheritance? Bioessays. (2014) 36:491–502. doi: 10.1002/bies.201300116
168. Marín-Palma D, Tabares-Guevara JH, Taborda N, Rugeles MT, Hernandez JC. Coarse particulate matter (PM10) induce an inflammatory response through the NLRP3 activation. J Inflammation (Lond). (2024) 21:15. doi: 10.1186/s12950-024-00388-9
169. Bollati V, Marinelli B, Apostoli P, Bonzini M, Nordio F, Hoxha M, et al. Exposure to metal-rich particulate matter modifies the expression of candidate microRNAs in peripheral blood leukocytes. Environ Health Perspect. (2010) 118:763–8. doi: 10.1289/ehp.0901300
170. Zhang TN, Li D, Wu QJ, Xia J, Wen R, Chen XC, et al. Exposure to nitrogen oxide in the first trimester and risk of cardiovascular-related malformations: A dose-response meta-analysis of observational studies. BioMed Res Int. (2018) 2018:1948407. doi: 10.1155/2018/1948407
171. Jarrett BY, Vanden Brink H, Brooks ED, Hoeger KM, Spandorfer SD, Pierson RA, et al. Impact of right-left differences in ovarian morphology on the ultrasound diagnosis of polycystic ovary syndrome. Fertil Steril. (2019) 112:939–46. doi: 10.1016/j.fertnstert.2019.06.016
172. Mimouni NEH, Paiva I, Barbotin AL, Timzoura FE, Plassard D, Le Gras S, et al. Polycystic ovary syndrome is transmitted via a transgenerational epigenetic process. Cell Metab. (2021) 33:513–530.e8. doi: 10.1016/j.cmet.2021.01.004
173. Olaniyi KS, Areloegbe SE. Acetate circumvents impaired metabolic switch in skeletal muscle of letrozole-induced PCOS rat model by suppression of PDK4/NLRP3. Nutrition. (2023) 107:111914. doi: 10.1016/j.nut.2022.111914
174. Geng X, Zhao J, Huang J, Li S, Chu W, Wang WS, et al. lnc-MAP3K13-7:1 inhibits ovarian GC proliferation in PCOS via DNMT1 downregulation-mediated CDKN1A promoter hypomethylation. Mol Ther. (2021) 29:1279–93. doi: 10.1016/j.ymthe.2020.11.018
175. Liu Y, Zhang S, Chen L, Huang X, Wang M, Ponikwicka-Tyszko D, et al. The molecular mechanism of miR-96-5p in the pathogenesis and treatment of polycystic ovary syndrome. Transl Res. (2023) 256:1–13. doi: 10.1016/j.trsl.2022.12.007
176. Ling L, Feng X, Wei T, Wang Y, Wang Y, Wang Z, et al. Human amnion-derived mesenchymal stem cell (hAD-MSC) transplantation improves ovarian function in rats with premature ovarian insufficiency (POI) at least partly through a paracrine mechanism. Stem Cell Res Ther. (2019) 10:46. doi: 10.1186/s13287-019-1136-x
177. Huang B, Ding C, Zou Q, Wang W, Li H. Cyclophosphamide regulates N6-methyladenosine and m6A RNA enzyme levels in human granulosa cells and in ovaries of a premature ovarian aging mouse model. Front Endocrinol (Lausanne). (2019) 10:415. doi: 10.3389/fendo.2019.00415
178. Zhao BS, Roundtree IA, He C. Post-transcriptional gene regulation by mRNA modifications. Nat Rev Mol Cell Biol. (2017) 18:31–42. doi: 10.1038/nrm.2016.132
179. Mu H, Zhang T, Yang Y, Zhang D, Gao J, Li J, et al. METTL3-mediated mRNA N-methyladenosine is required for oocyte and follicle development in mice. Cell Death Dis. (2021) 12:989. doi: 10.1038/s41419-021-04272-9
180. Liu HB, Muhammad T, Guo Y, Li MJ, Sha QQ, Zhang CX, et al. RNA-binding protein IGF2BP2/IMP2 is a critical maternal activator in early zygotic genome activation. Advanced Sci (Weinheim Baden-Wurttemberg Germany). (2019) 6,15:1900295. doi: 10.1002/advs.201900295
181. Mei NH, Guo SM, Zhou Q, Zhang YR, Liu XZ, Yin Y, et al. H3K4 methylation promotes expression of mitochondrial dynamics regulators to ensure oocyte quality in mice. Adv Sci (Weinh). (2023) 10:e2204794. doi: 10.1002/advs.202204794
182. Wu SC, Zhang Y. Minireview: role of protein methylation and demethylation in nuclear hormone signaling. Mol Endocrinol. (2009) 23:1323–34. doi: 10.1210/me.2009-0131
183. Zhang X, Tanaka K, Yan J, Li J, Peng D, Jiang Y, et al. Regulation of estrogen receptor α by histone methyltransferase SMYD2-mediated protein methylation. Proc Natl Acad Sci U S A. (2013) 110:17284–9. doi: 10.1073/pnas.1307959110
184. Wang L, Ozark PA, Smith ER, Zhao Z, Marshall SA, Rendleman EJ, et al. TET2 coactivates gene expression through demethylation of enhancers. Sci Adv. (2018) 4:eaau6986. doi: 10.1126/sciadv.aau6986
185. Bulun SE, Yildiz S, Adli M, Chakravarti D, Parker JB, Milad M, et al. Endometriosis and adenomyosis: shared pathophysiology. Fertil Steril. (2023) 119:746–50. doi: 10.1016/j.fertnstert.2023.03.006
186. Cui J, Wu F, Yang X, Liu S, Han S, Chen B. Effects of ammonia on hypothalamic-pituitary-ovarian axis in female rabbits. Ecotoxicol Environ Saf. (2021) 227:112922. doi: 10.1016/j.ecoenv.2021.112922
187. Steegers-Theunissen RPM, Wiegel RE, Jansen PW, Laven JSE, Sinclair KD. Polycystic ovary syndrome: A brain disorder characterized by eating problems originating during puberty and adolescence. Int J Mol Sci. (2020) 21:8211. doi: 10.3390/ijms21218211
188. Wang A, Wan Y, Mahai G, Qian X, Li Y, Xu S, et al. Association of prenatal exposure to organophosphate, pyrethroid, and neonicotinoid insecticides with child neurodevelopment at 2 years of age: A prospective cohort study. Environ Health Perspect. (2023) 131:107011. doi: 10.1289/EHP12097
189. Cheong AW, Pang RT, Liu WM, Kottawatta KS, Lee KF, Yeung WS. MicroRNA Let-7a and dicer are important in the activation and implantation of delayed implanting mouse embryos. Hum Reprod. (2014) 29:750–62. doi: 10.1093/humrep/det462
190. Andersson KL, Bussani C, Fambrini M, Polverino V, Taddei GL, Gemzell-Danielsson K, et al. DNA methylation of HOXA10 in eutopic and ectopic endometrium. Hum Reprod. (2014) 29:1906–11. doi: 10.1093/humrep/deu161
191. Taylor HS, Arici A, Olive D, Igarashi P. HOXA10 is expressed in response to sex steroids at the time of implantation in the human endometrium. J Clin Invest. (1998) 101:1379–84. doi: 10.1172/JCI1057
192. Cao C, Zhou Y, Zhang Y, Ma Y, Du S, Fan L, et al. GCN5 participates in KLF4-VEGFA feedback to promote endometrial angiogenesis. iScience. (2022) 25:104509. doi: 10.1016/j.isci.2022.104509
193. Revel A, Achache H, Stevens J, Smith Y, Reich R. MicroRNAs are associated with human embryo implantation defects. Hum Reprod. (2011) 26:2830–40. doi: 10.1093/humrep/der255
194. Li Y, Liu H, Ye S, Zhang B, Li X, Yuan J, et al. The effects of coagulation factors on the risk of endometriosis: a Mendelian randomization study. BMC Med. (2023) 21:195. doi: 10.1186/s12916-023-02881-z
195. Beddows I, Fan H, Heinze K, Johnson BK, Leonova A, Senz J, et al. Cell state of origin impacts development of distinct endometriosis-related ovarian carcinoma histotypes. Cancer Res. (2024) 84:26–38. doi: 10.1158/0008-5472.CAN-23-1362
196. Mortlock S, Houshdaran S, Kosti I, Rahmioglu N, Nezhat C, Vitonis AF, et al. Global endometrial DNA methylation analysis reveals insights into mQTL regulation and associated endometriosis disease risk and endometrial function. Commun Biol. (2023) 6:780. doi: 10.1038/s42003-023-05070-z
197. Peinado FM, Olivas-Martínez A, Iribarne-Durán LM, Ubiña A, León J, Vela-Soria F, et al. Cell cycle, apoptosis, cell differentiation, and lipid metabolism gene expression in endometriotic tissue and exposure to parabens and benzophenones. Sci Total Environ. (2023) 879:163014. doi: 10.1016/j.scitotenv.2023.163014
198. Kim TH, Yoo JY, Choi KC, Shin JH, Leach RE, Fazleabas AT, et al. Loss of HDAC3 results in nonreceptive endometrium and female infertility. Sci Transl Med. (2019) 11:eaaf7533. doi: 10.1126/scitranslmed.aaf7533
199. Moustafa S, Burn M, Mamillapalli R, Nematian S, Flores V, Taylor HS. Accurate diagnosis of endometriosis using serum microRNAs. Am J obstetrics gynecology. (2020) 223:557.e1–557.e11. doi: 10.1016/j.ajog.2020.02.050
200. Ma'ayeh M, Costantine MM. Prevention of preeclampsia. Semin Fetal Neonatal Med. (2020) 25:101123. doi: 10.1016/j.siny.2020.101123
201. Caniggia I, Lye SJ, Cross JC. Activin is a local regulator of human cytotrophoblast cell differentiation. Endocrinology. (1997) 138:3976–86. doi: 10.1210/endo.138.9.5403
202. Moses EK, Lade JA, Guo G, Wilton AN, Grehan M, Freed K, et al. A genome scan in families from Australia and New Zealand confirms the presence of a maternal susceptibility locus for pre-eclampsia, on chromosome 2. Am J Hum Genet. (2000) 67:1581–5. doi: 10.1086/316888
203. Jones RL, Salamonsen LA, Findlay JK. Activin A promotes human endometrial stromal cell decidualization in vitro. J Clin Endocrinol Metab. (2002) 87:4001–4. doi: 10.1210/jcem.87.8.8880
204. Pados G, Gordts S, Sorrentino F, Nisolle M, Nappi L, Daniilidis A. Adenomyosis and infertility: A literature review. Medicina. (2023) 59:1551. doi: 10.3390/medicina59091551
205. Zhai J, Vannuccini S, Petraglia F, Giudice LC. Adenomyosis: mechanisms and pathogenesis. Semin Reprod Med. (2020) 38:129–43. doi: 10.1055/s-0040-1716687
206. Huang E, Chen L. RNA N6-methyladenosine modification in female reproductive biology and pathophysiology. Cell Commun Signal. (2023) 21:53. doi: 10.1186/s12964-023-01078-4
207. Wu Y, Yang R, Lan J, Wu Y, Huang J, Fan Q, et al. Iron overload modulates follicular microenvironment via ROS/HIF-1α/FSHR signaling. Free Radic Biol Med. (2023) 196:37–52. doi: 10.1016/j.freeradbiomed.2022.12.105
208. Liu B, Zhai J, Wang W, Liu T, Liu C, Zhu X, et al. Identification of tumor microenvironment and DNA methylation-related prognostic signature for predicting clinical outcomes and therapeutic responses in cervical cancer. Front Mol Biosci. (2022) 9:872932. doi: 10.3389/fmolb.2022.872932
209. Navarro A, Bariani MV, Yang Q, Al-Hendy A. Understanding the impact of uterine fibroids on human endometrium function. Front Cell Dev Biol. (2021) 9:633180. doi: 10.3389/fcell.2021.633180
210. Yang Q, Mas A, Diamond MP, Al-Hendy A. The mechanism and function of epigenetics in uterine leiomyoma development. Reprod Sci. (2016) 23:163–75. doi: 10.1177/1933719115584449
211. Carbajo-García MC, De Miguel-Gómez L, Juárez-Barber E, Trelis A, Monleón J, Pellicer A, et al. Deciphering the role of histone modifications in uterine leiomyoma:acetylation of h3k27 regulates the expression of genes involved in proliferation,cell signaling,cell transport,angiogenesis and extracellular matrix formation. Biomedicines. (2022) 10(6):1279. doi: 10.3390/biomedicines10061279
212. Włodarczyk M, Nowicka G, Ciebiera M, Ali M, Yang Q, Al-Hendy A. Epigenetic regulation in uterine fibroids—The role of ten-eleven translocation enzymes and their potential therapeutic application. Int J Mol Sci. (2022) 23:2720. doi: 10.3390/ijms23052720
213. Zhou W, Wang G, Li B, Qu J, Zhang Y. LncRNA APTR promotes uterine leiomyoma cell proliferation by targeting erα to activate the Wnt/β-catenin pathway. Front Oncol. (2021) 11:536346. doi: 10.3389/fonc.2021.536346
214. Zhao L, Liang X, Wang L, Zhang X. The role of miRNA in ovarian cancer: an overview. Reprod Sci. (2022) 29:2760–7. doi: 10.1007/s43032-021-00717-w
215. Di Leva G, Croce CM. Roles of small RNAs in tumor formation. Trends Mol Med. (2010) 16:257–67. doi: 10.1016/j.molmed.2010.04.001
216. Xiang G, Cheng Y. MiR-126-3p inhibits ovarian cancer proliferation and invasion via targeting PLXNB2. Reprod Biol. (2018) 18:218–24. doi: 10.1016/j.repbio.2018.07.005
217. Vernon M, Lambert B, Meryet-Figuière M, Brotin E, Weiswald LB, Paysant H, et al. Functional miRNA Screening Identifies Wide-ranging Antitumor Properties of miR-3622b-5p and Reveals a New Therapeutic Combination Strategy in Ovarian Tumor Organoids. Mol Cancer Ther. (2020) 19:1506–19. doi: 10.1158/1535-7163.MCT-19-0510
218. Ibanez de Caceres I, Battagli C, Esteller M, Herman JG, Dulaimi E, Edelson MI, et al. Tumor cell-specific BRCA1 and RASSF1A hypermethylation in serum, plasma, and peritoneal fluid from ovarian cancer patients. Cancer Res. (2004) 64:6476–81. doi: 10.1158/0008-5472.CAN-04-1529
219. SK S, Swamy SN, Premalatha CS, Pallavi VR, Gawari R. Aberrant promoter hypermethylation of RASSF1a and BRCA1 in circulating cell-free tumor DNA serves as a biomarker of ovarian carcinoma. Asian Pac J Cancer Prev. (2019) 20:3001–5. doi: 10.31557/APJCP.2019.20.10.3001
220. Sen P, Ganguly P, Ganguly N. Modulation of DNA methylation by human papillomavirus E6 and E7 oncoproteins in cervical cancer. Oncol Lett. (2018) 15:11–22. doi: 10.3892/ol.2017.7292
221. Chung SH, Franceschi S, Lambert PF. Estrogen and ERalpha: culprits in cervical cancer? Trends Endocrinol Metab. (2010) 21:504–11. doi: 10.1016/j.tem.2010.03.005
222. Ma X, Liu J, Wang H, Jiang Y, Wan Y, Xia Y, et al. Identification of crucial aberrantly methylated and differentially expressed genes related to cervical cancer using an integrated bioinformatics analysis. Biosci Rep. (2020) 40:BSR20194365. doi: 10.1042/BSR20194365
223. Johansson C, Jamal Fattah T, Yu H, Nygren J, Mossberg AK, Schwartz S. Acetylation of intragenic histones on HPV16 correlates with enhanced HPV16 gene expression. Virology. (2015) 482:244–59. doi: 10.1016/j.virol.2015.02.053
224. Du Y, Wei N, Ma R, Jiang SH, Song D. Long noncoding RNA MIR210HG is induced by hypoxia-inducible factor 1α and promotes cervical cancer progression. Am J Cancer Res. (2022) 12:2783–97. doi: 10.21203/rs.3.rs-1207674/v1
225. Guha P, Sen K, Chowdhury P, Mukherjee D. Estrogen receptors as potential therapeutic target in endometrial cancer. J Recept Signal Transduct Res. (2023) 43:19–26. doi: 10.1080/10799893.2023.2187643
226. Ghabreau L, Roux JP, Niveleau A, Fontanière B, Mahe C, Mokni M, et al. Correlation between the DNA global methylation status and progesterone receptor expression in normal endometrium, endometrioid adenocarcinoma and precursors. Virchows Arch. (2004) 445:129–34. doi: 10.1007/s00428-004-1059-4
227. Caplakova V, Babusikova E, Blahovcova E, Balharek T, Zelieskova M, Hatok J. DNA methylation machinery in the endometrium and endometrial cancer. Anticancer Res. (2016) 36:4407–20. doi: 10.21873/anticanres.10984
228. Kumar S, Sharma A, Kshetrimayum C. Environmental & occupational exposure & female reproductive dysfunction. Indian J Med Res. (2019) 150:532–45. doi: 10.4103/ijmr.IJMR_1652_17
229. Petrelli G, Figà-Talamanca I, Tropeano R, Tangucci M, Cini C, Aquilani S, et al. Reproductive male-mediated risk: spontaneous abortion among wives of pesticide applicators. Eur J Epidemiol. (2000) 16:391–3. doi: 10.1023/a:1007630610911
230. Frazier LM. Reproductive disorders associated with pesticide exposure. J Agromedicine. (2007) 12:27–37. doi: 10.1300/J096v12n01_04
231. Rogan WJ, Chen A. Health risks and benefits of bis(4-chlorophenyl)-1,1,1-trichloroethane (DDT). Lancet. (2005) 366:763–73. doi: 10.1016/S0140-6736(05)67182-6
232. Salazar-García F, Gallardo-Díaz E, Cerón-Mireles P, Loomis D, Borja-Aburto VH. Reproductive effects of occupational DDT exposure among male malaria control workers. Environ Health Perspect. (2004) 112:542–7. doi: 10.1289/ehp.112-1241918
233. Reshi MS, Mustafa RA, Javaid D, Haque S. Pesticide Toxicity Associated with Infertility. Adv Exp Med Biol. (2022) 1319:59–69. doi: 10.1007/978-3-031-12966-7_4
234. Hall MS, Talge NM, Upson K. Urinary cadmium and endometriosis prevalence in a US nationally representative sample: results from NHANES 1999-2006. Hum Reprod. (2023) 38:1835–42. doi: 10.1093/humrep/dead117
235. El Fouikar S, Duranthon V, Helies V, Jammes H, Couturier-Tarrade A, Gayrard V, et al. Multigenerational effects of a complex human-relevant exposure during folliculogenesis and preimplantation embryo development: the FEDEXPO study. Toxics. (2023) 11:425. doi: 10.3390/toxics11050425
236. Eckersley-Maslin MA, Alda-Catalinas C, Reik W. Dynamics of the epigenetic landscape during the maternal-to-zygotic transition. Nat Rev Mol Cell Biol. (2018) 19:436–50. doi: 10.1038/s41580-018-0008-z
237. Jin Z, Sheng J, Hu Y, Zhang Y, Wang X, Huang Y. Shining a spotlight on m6A and the vital role of RNA modification in endometrial cancer: a review. Front Genet. (2023) 14:1247309. doi: 10.3389/fgene.2023.1247309
238. Raja MHR, Farooqui N, Zuberi N, Ashraf M, Azhar A, Baig R, et al. Endometriosis, infertility and MicroRNA's: A review. J Gynecol Obstet Hum Reprod. (2021) 50:102157. doi: 10.1016/j.jogoh.2021.102157
239. Nandi S, Tripathi SK, Singh PK, Gupta PSP, Mondal S. Global DNA methylation, DNA methyltransferase and stress-related gene expression in ovine oocytes and embryos after exposure to metabolic stressors. Reprod Domest Anim. (2023) 58:717–25. doi: 10.1111/rda.14341
240. Ibrahim Y, Hotaling J. Sperm epigenetics and its impact on male fertility, pregnancy loss, and somatic health of future offsprings. Semin Reprod Med. (2018) 36:233–9. doi: 10.1055/s-0038-1677047
241. Gaspari L, Paris F, Soyer-Gobillard MO, Kalfa N, Sultan C, Hamamah S. Perturbateurs endocriniens environnementaux et fertilité [Environmental endocrine disruptors and fertility. Gynecol Obstet Fertil Senol. (2022) 50:402–8. doi: 10.1016/j.gofs.2021.09.009
242. Karwacka A, Zamkowska D, Radwan M, Jurewicz J. Exposure to modern, widespread environmental endocrine disrupting chemicals and their effect on the reproductive potential of women: an overview of current epidemiological evidence. Hum Fertil (Camb). (2019) 22:2–25. doi: 10.1080/14647273.2017.1358828
243. Gaskins AJ, Mínguez-Alarcón L, Williams PL, Chavarro JE, Schwartz JD, Kloog I, et al. Ambient air pollution and risk of pregnancy loss among women undergoing assisted reproduction. Environ Res. (2020) 191:110201. doi: 10.1016/j.envres.2020.110201
244. Hilbert SM, Gunderson S. Complications of assisted reproductive technology. Emerg Med Clin North Am. (2019) 37:239–49. doi: 10.1016/j.emc.2019.01.005
245. Lucas E. Epigenetic effects on the embryo as a result of periconceptional environment and assisted reproduction technology. Reprod BioMed Online. (2013) 27:477–85. doi: 10.1016/j.rbmo.2013.06.003
246. Alves da Silva AF, MaChado FB, Pavarino ÉC, Biselli-Périco JM, Zampieri BL, da Silva Francisco JR, et al. Trisomy 21 alters DNA methylation in parent-of-origin-dependent and -independent manners. PloS One. (2016) 11:e0154108. doi: 10.1371/journal.pone.0154108
247. Sui X, Hu Y, Ren C, Cao Q, Zhou S, Cao Y, et al. METTL3-mediated m6A is required for murine oocyte maturation and maternal-to-zygotic transition. Cell Cycle. (2020) 19:391–404. doi: 10.1080/15384101.2019.1711324
248. Xia H, Zhong C, Wu X, Chen J, Tao B, Xia X, et al. Mettl3 mutation disrupts gamete maturation and reduces fertility in zebrafish. Genetics. (2018) 208:729–43. doi: 10.1534/genetics.117.300574
249. Niehrs C, Calkhoven CF. Emerging role of C/EBPβ and epigenetic DNA methylation in ageing. Trends Genet. (2020) 36:71–80. doi: 10.1016/j.tig.2019.11.005
250. Andraos S, de Seymour JV, O'Sullivan JM, Kussmann M. The impact of nutritional interventions in pregnant women on DNA methylation patterns of the offspring: A systematic review. Mol Nutr Food Res. (2018) 62:e1800034. doi: 10.1002/mnfr.201800034
251. Goodrich JM, Reddy P, Naidoo RN, Asharam K, Batterman S, Dolinoy DC. Prenatal exposures and DNA methylation in newborns: a pilot study in Durban, South Africa. Environ Sci Process Impacts. (2016) 18:908–17. doi: 10.1039/c6em00074f
252. Delhaes F, Giza SA, Koreman T, Eastabrook G, McKenzie CA, Bedell S, et al. Altered maternal and placental lipid metabolism and fetal fat development in obesity: Current knowledge and advances in non-invasive assessment. Placenta. (2018) :69:118–124. doi: 10.1016/j.placenta.2018.05.011
253. Bowman CE, Arany Z, Wolfgang MJ. Regulation of maternal-fetal metabolic communication. Cell Mol Life Sci. (2021) 78:1455–86. doi: 10.1007/s00018-020-03674-w
254. Kalish JM, Jiang C, Bartolomei MS. Epigenetics and imprinting in human disease. Int J Dev Biol. (2014) 58:291–8. doi: 10.1387/ijdb.140077mb
255. Joó JG, Karabélyos C, Héjja H, Kornya L, Rigó J Jr. Epigenetikai mechanizmusok élettani és kóros terhességben [Epigenetic mechanisms in physiologic and pathologic pregnancies. Orv Hetil. (2014) 155:566–74. doi: 10.1556/OH.2014.29861
256. Ventura-Juncá P, Irarrázaval I, Rolle AJ, Gutiérrez JI, Moreno RD, Santos MJ. In vitro fertilization (IVF) in mammals: epigenetic and developmental alterations. Sci bioethical implications IVF humans. Biol Res. (2015) 48:68. doi: 10.1186/s40659-015-0059-y
257. Hansen PJ, Dobbs KB, Denicol AC, Siqueira LGB. Sex and the preimplantation embryo: implications of sexual dimorphism in the preimplantation period for maternal programming of embryonic development. Cell Tissue Res. (2016) 363:237–47. doi: 10.1007/s00441-015-2287-4
258. Johnson JP, Beischel L, Schwanke C, Styren K, Crunk A, Schoof J, et al. Overrepresentation of pregnancies conceived by artificial reproductive technology in prenatally identified fetuses with Beckwith-Wiedemann syndrome. J Assist Reprod Genet. (2018) 35:985–92. doi: 10.1007/s10815-018-1228-z
259. Li Piani L, Reschini M, Somigliana E, Ferrari S, Busnelli A, Viganò P, et al. telomere length and DNA methylation as predictors of live birth in in vitro fertilization cycles. PloS One. (2022) 17:e0261591. doi: 10.1371/journal.pone.0261591
260. Ding T, Yan W, Zhou T, Shen W, Wang T, Li M, et al. Endocrine disrupting chemicals impact on ovarian aging: Evidence from epidemiological and experimental evidence. Environ pollut. (2022) 305:119269. doi: 10.1016/j.envpol.2022.119269
261. Muraki Y, Banno K, Yanokura M, Kobayashi Y, Kawaguchi M, Nomura H, et al. Relationship of aberrant DNA hypermethylation of CHFR with sensitivity to taxanes in endometrial cancer. Oncol Rep. (2009) 22(5):967–72. doi: 10.3892/or_00000523
262. Hassanzadeh M, Mahernia S, Caprini G, Fossati G, Adib M, Moakedi F, et al. Epigenetic-based cancer therapeutics: new potential HDAC8 inhibitors. J Biomol Struct Dyn. (2022) 40:297–311. doi: 10.1080/07391102.2020.1813203
263. Shawky H, Tawfik H, Hewidy M. Weekly dose-dense paclitaxel and carboplatin in recurrent ovarian carcinoma: a phase II trial. J Egypt Natl Canc Inst. (2014) 26:139–45. doi: 10.1016/j.jnci.2014.05.001
264. Migliore L, Coppedè F. Gene-environment interactions in Alzheimer disease: the emerging role of epigenetics. Nat Rev Neurol. (2022) 18:643–60. doi: 10.1038/s41582-022-00714-w
265. Liu X, Guo S-W. Aberrant immunoreactivity of deoxyribonucleic acid methyltransferases in adenomyosis. Gynecol Obstet Invest. (2012) 74:100–8. doi: 10.1159/000337718
266. Kim M, Kang D, Kwon MY, Lee HJ, Kim MJ. MicroRNAs as potential indicators of the development and progression of uterine leiomyoma. PloS One. (2022) 17:e0268793.microRNA. doi: 10.1371/journal.pone.0268793
267. Gao Y, Zhou N, Liu J. Ovarian cancer diagnosis and prognosis based on cell-free DNA methylation. Cancer Control. (2024) 31:10732748241255548. doi: 10.1177/10732748241255548
Keywords: epigenetics, women, reproductive, environmental factors, reproductive health
Citation: Yu X, Xu J, Song B, Zhu R, Liu J, Liu YF and Ma YJ (2024) The role of epigenetics in women’s reproductive health: the impact of environmental factors. Front. Endocrinol. 15:1399757. doi: 10.3389/fendo.2024.1399757
Received: 12 March 2024; Accepted: 28 August 2024;
Published: 13 September 2024.
Edited by:
Jeff M. P. Holly, University of Bristol, United KingdomReviewed by:
Xiaowei Nie, Jiangsu Provincial Hospital of Traditional Chinese Medicine, ChinaRita Khoueiry, International Agency For Research On Cancer (IARC), France
Copyright © 2024 Yu, Xu, Song, Zhu, Liu, Liu and Ma. This is an open-access article distributed under the terms of the Creative Commons Attribution License (CC BY). The use, distribution or reproduction in other forums is permitted, provided the original author(s) and the copyright owner(s) are credited and that the original publication in this journal is cited, in accordance with accepted academic practice. No use, distribution or reproduction is permitted which does not comply with these terms.
*Correspondence: Ying Jie Ma, NTQ1OTE0NTUzQHFxLmNvbQ==