- 1Department of Foundations of Medicine, NYU Grossman Long Island School of Medicine, Mineola, NY, United States
- 2Department of Zoology, School of Biological Sciences, Central University of Kerala, Kasargod, Kerala, India
- 3Division of Biomedical Sciences, School of Medicine, University of California, Riverside, Riverside, CA, United States
Spermatogenesis is a multi-step biological process where mitotically active diploid (2n) spermatogonia differentiate into haploid (n) spermatozoa via regulated meiotic programming. The alarming rise in male infertility has become a global concern during the past decade thereby demanding an extensive profiling of testicular gene expression. Advancements in Next-Generation Sequencing (NGS) technologies have revolutionized our empathy towards complex biological events including spermatogenesis. However, despite multiple attempts made in the past to reveal the testicular transcriptional signature(s) either with bulk tissues or at the single-cell, level, comprehensive reviews on testicular transcriptomics and associated disorders are limited. Notably, technologies explicating the genome-wide gene expression patterns during various stages of spermatogenic progression provide the dynamic molecular landscape of testicular transcription. Our review discusses the advantages of single-cell RNA-sequencing (Sc-RNA-seq) over bulk RNA-seq concerning testicular tissues. Additionally, we highlight the cellular heterogeneity, spatial transcriptomics, dynamic gene expression and cell-to-cell interactions with distinct cell populations within the testes including germ cells (Gc), Sertoli cells (Sc), Peritubular cells (PTc), Leydig cells (Lc), etc. Furthermore, we provide a summary of key finding of single-cell transcriptomic studies that have shed light on developmental mechanisms implicated in testicular disorders and male infertility. These insights emphasize the pivotal roles of Sc-RNA-seq in advancing our knowledge regarding testicular transcriptional landscape and may serve as a potential resource to formulate future clinical interventions for male reproductive health.
1 Introduction
During the past two decades, an alarming rise in infertility particularly in developed/developing countries has become a matter of great concern (1). Around 15% of couples suffering from infertility globally, out of which 50%, is exclusively caused by the male partner (2). Male infertility is a multifaceted pathological condition with diverse manifestations, ranging from total absence of testicular sperm to specific changes in sperm quality (3). Chromosomal aberrations (like Klinefelter syndrome 47XXY) or variance (like 9qh+ heteromorphism) and other genetic mutations contribute upto 15-20% of total male infertility; however around 30-50% of cases remain untreatable due to idiopathic in origin/nature (4–6). Therefore, the mechanistic details of testicular spermatogenesis need to be reexamined at the cellular and molecular level (7, 8).
Spermatogenesis is gonadotropin regulated, highly synchronized, developmental programme involving multi-step events like stem cell renewal/differentiation, epigenomic remodeling, and meiotic divisions for generating millions of spermatozoa inside testes (9–11). The continuity of this process throughout adult life is supported by an active spermatogonial stem cell (SSC) population that critically maintains the key balance between the capacity of self-renewal (to sustain the stem cell population) and differentiation [to produce spermatogonial progenitor cells (SPCs), which subsequently develop into mature sperm via meiotic divisions] (12–14). Therefore, the cellular and molecular landscape associated with the SSC micro-environment turns out to be critical for regulating male fertility. The rapid intrinsic speed (around 1000-1500 sperm per heartbeat in adult men) of spermatogenic progression significantly highlights the degree of orchestration governing the transcriptional dynamics of testicular cells (15–17). Figure 1 represents the histological architecture of the testis showing arrays of multiple developing germ cells (Gc) (from SSC to mature motile sperms) alongside with visual representation of the different stages and cellular interactions essential for spermatogenesis.
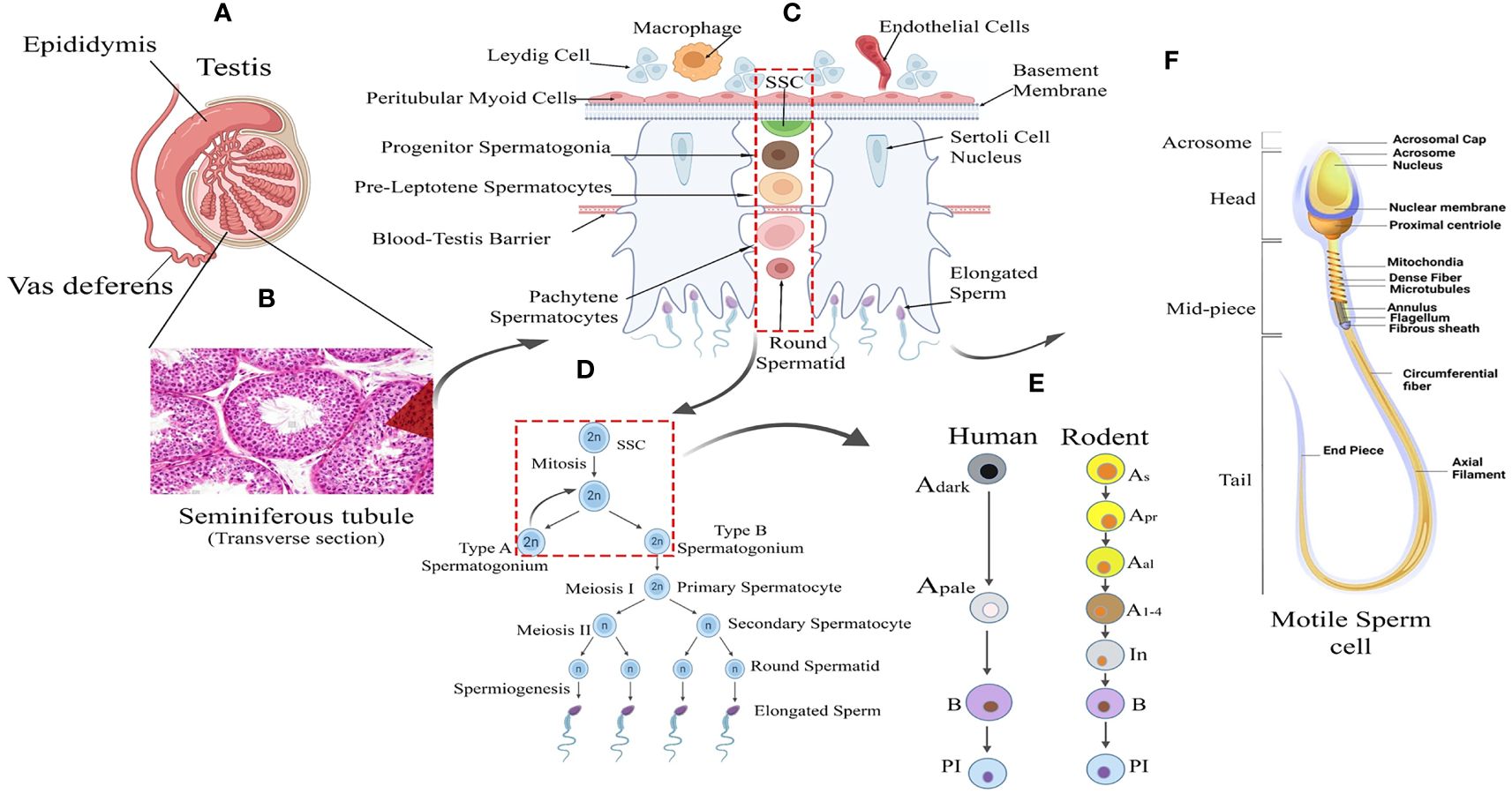
Figure 1 Testicular tissue architecture and Spermatogenic Development. (A) Testis Anatomy: Cross-section illustrating the testis with internal cellular composition and associated structures: the epididymis and vas deferens. (B) Seminiferous Tubule: Histological image showing the transverse section of a seminiferous tubule where spermatogenesis occurs. (C) Spermatogenesis Overview: Schematic representation of spermatogenesis within the seminiferous tubule, from spermatogonial stem cells (SSC) to mature spermatozoa. (D) Sperm Maturation: Detailed progression of sperm development from type A spermatogonium through meiosis to form mature sperm. (E) Human vs. Rodent Spermatogenesis: Comparative stages of spermatogenesis between humans and rodents, highlighting the differentiation of spermatogonial cells. (F) Sperm Cell Morphology: Detailed diagram of a motile sperm cell, showcasing its various structural components from head to tail.
Functional and/or anatomical defects in testicular somatic and/or Gc lead to spermatogenic impairment (with a qualitative and quantitative decline in sperm count clinically defined as azoospermia, oligozoospermia, teratozoospermia, and asthenospermia, etc.) causing male sub-infertility/infertility (18). Azoospermia, (the most severe form where semen without sperm) are of two types: obstructive azoospermia (OA) and non-obstructive azoospermia (NOA). OA stems from normal sperm production but obstructed sperm delivery, while NOA, accounting for around 10% of male infertility, results from lack of spermatogenesis in the testis (19). Three specific clinical categories are described in NOA as primary testicular failure like Sertoli cell only syndrome (SCOS)/Gc aplasia, Gc maturational arrest and hypo-spermatogenesis (20, 21). Supplementary Table 1 highlights (A) clinical categories of semen quality, (B) etiologies and frequencies of male infertility and (C) the hallmarks of primary testicular failure.
Notably, multiple genetic complexities involving over 2,000 spermatogenic genes observed in different forms of male infertility having substantial variations in semen quality and testicular histology (5, 22). Over recent decades assisted reproductive techniques (ART) e.g.- in vitro fertilization (IVF) and intra-cytoplasmic sperm injection (ICSI) have successfully facilitated biological fatherhood for men with extremely low sperm counts (3). However, addressing the fundamental basis of poor sperm quality and quantity remains challenging due to a limited understanding of the intricate molecular and cellular interactions driving human sperm production (18, 23).
An impressive progress in deploying high-throughput sequencing technologies in biomedical research has been observed during the past decade (24). Within this scenario, both bulk and single-cell RNA-sequencing (Sc-RNA-seq) collectively have emerged as revolutionary tools, revealing the molecular complexities governing testicular physiology and elucidating mechanisms underlying testicular dysregulation/disorders (25). However, analyzing bulk RNAs from diverse cell types faces adverse challenges in detecting expression data in rare cell populations, possibly diluting the presence of uncommon transcripts. In contrast, Sc-RNA-seq generates distinct RNA fingerprints for each cell (at single-cell resolution) type, transforming our understanding of differential transcriptomic profiles from complex tissues like the testis and therefore found to be critically informative in the field of clinical endocrinology, especially in deciphering infertility (26, 27). Table 1 summarizes the advantages of the Sc-RNA-seq strategy over the conventional bulk transcriptomic approach.
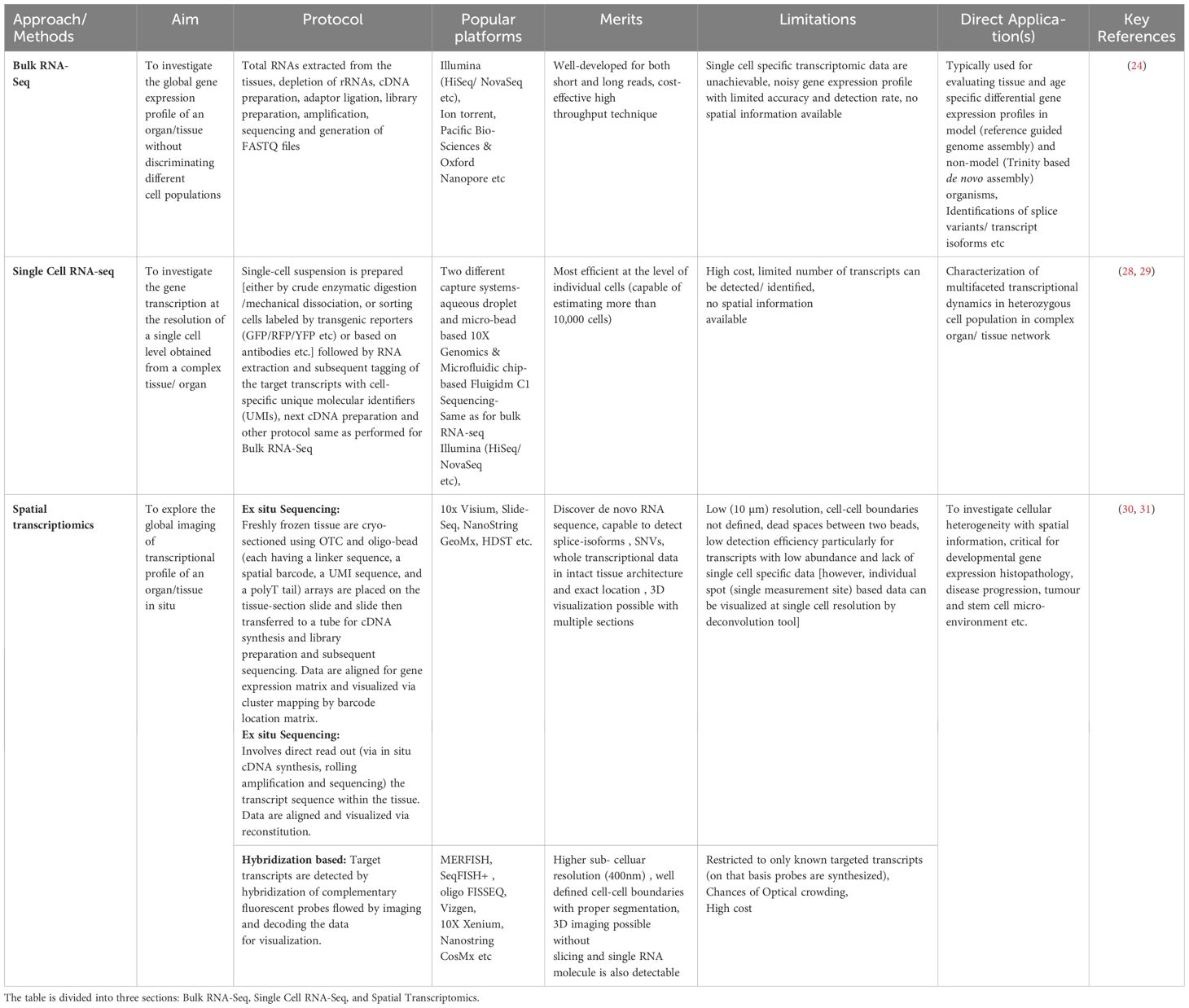
Table 1 Summarizes the essential aspects of different RNA-Seq strategies, including their aims, key features, popular platforms, merits, limitations, and direct applications.
Despite being critically relevant, comprehensive reviews of recent literature revealing the dynamic landscape of single-cell testicular transcriptomics are currently limited (25, 32). The current study dissects the spermatogenic landscapes viz., SSC/SPC niche, and cellular transcriptional dynamics during the meiotic onset and progression. Finally, this review attempts to reveal the intricate tapestry of testicular development and disorders through next-generation sequencing technologies.
2 Methodologies adopted for Sc-RNA-seq
The Sc-RNA-seq generates high-resolution transcriptomic data that precisely identifies minor transcriptomic variations at a single-cell level from a complex organ (33, 34). This tool has significant implications for gonadal development and disorders [viz., infertility, Disorders/Differences of Sex Development (DSDs), and cancer] (26, 27). Sc-RNA-seq produces transcriptomic data employing methods like conventional cellular purification via enzyme-based crude mechanical isolation or transgenic reporter gene/antibody-based sorting of target cells by sophisticated magnetic-activated cell sorter (MACS) or fluorescence-activated cell sorter (FACS) columns (35). Figure 2 represents the fundamental working principles of bulk and Sc-RNA-seq.
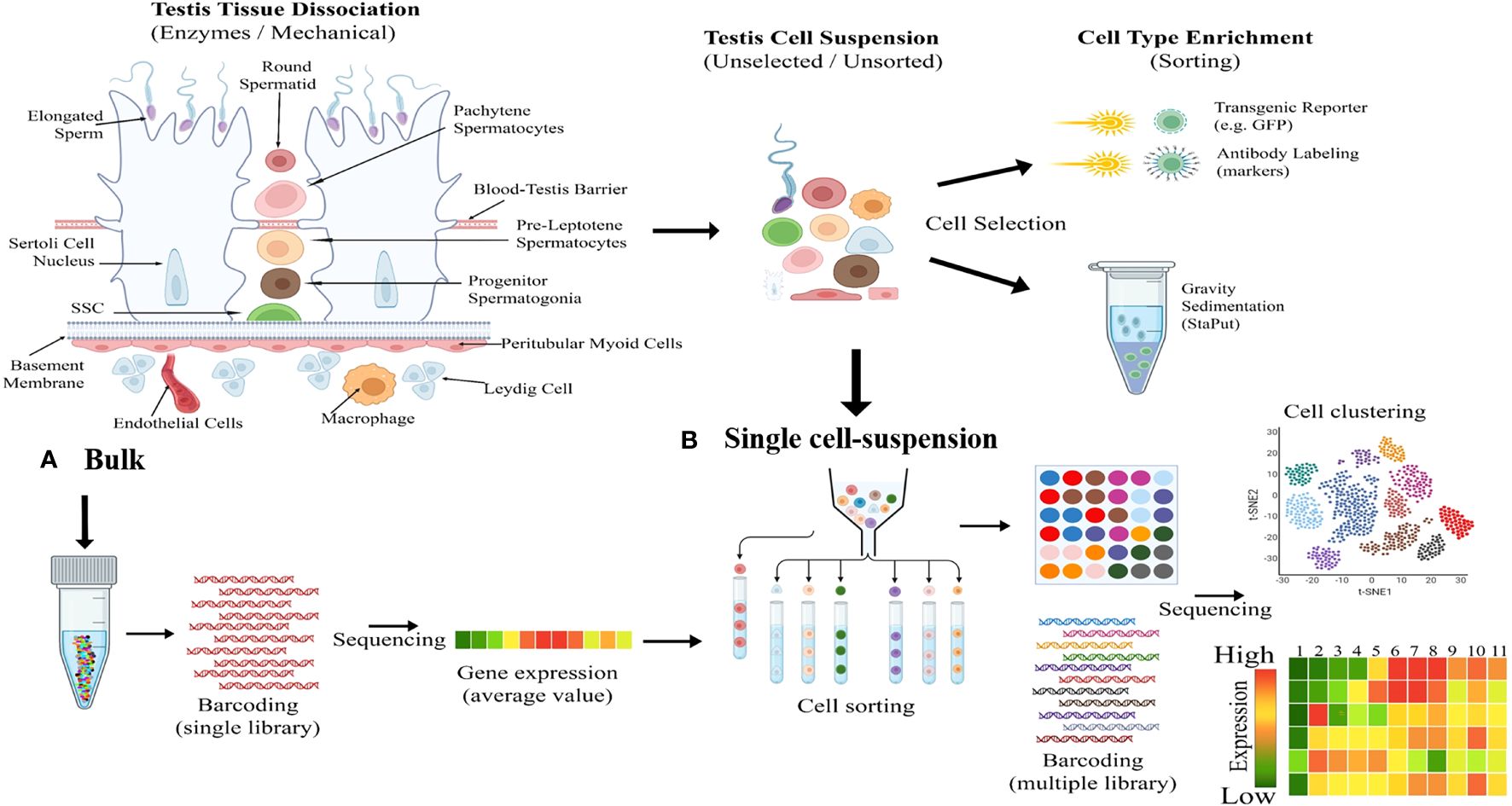
Figure 2 Illustrating fundamental difference between bulk and single-cell RNA sequencing (Sc-RNA-seq). (A) Bulk Processing: The left diagram illustrates the enzymatic and mechanical dissociation of testicular tissue into a heterogeneous mixture of cells including Sertoli cells, Leydig cells, spermatogonia, spermatocytes, spermatids etc. Following dissociation, cells are processed in bulk, as shown by the central test tube graphic, including DNA barcoding and sequencing to yield average gene expression profiles. (B) Single Cell-Suspension and Enrichment: The top middle flow diagram transitions from an unselected testicular cell suspension to cell type enrichment via methods like transgenic reporters (e.g., GFP) and antibody labeling etc. The bottom middle panel depicts the conversion of the unselected cellular mixture into a single-cell suspension, followed by cell sorting based on type-specific markers, with each cell type represented by a different color. The right side shows the cell clustering outcome, visualized by a t-SNE plot, and the subsequent sequencing of individual cells resulting in a detailed gene expression heatmap, with colors indicating expression levels from high (red) to low (green).
Single-cell isolation and sequencing can be achieved at two different platforms, first droplet-based 10× Genomics Chromium where individual cells get encapsulated in aqueous droplets along with micro-beads loaded with barcoded primers (36) and second Fluigidm C1, where individual cells are physically captured on microfluidic chips (37). After generating Sc-RNA-seq libraries, 10x Genomics utilizes 3′ end-counting unique molecular identifiers (UMIs), a genetic barcode that ensures a distinct cell population is traced and categorized as a specific lineage within a particular tissue/organ (32). In contrast, Fluidigm C1 libraries are automatically generated having full-length transcriptomes with all possible mRNA isoforms/variants (due to alternative splicing/transcription start site, etc.) (37). Notably, a new method Smart-seq2 has been developed recently having enhanced yield and length of cDNA libraries with better reverse transcription, template switching, and pre-amplification, improving the accuracy of transcript detection at a cheaper cost (38). Figure 3 schematically illustrates the available Sc-RNA-seq platforms and downstream workflow for data analyses.
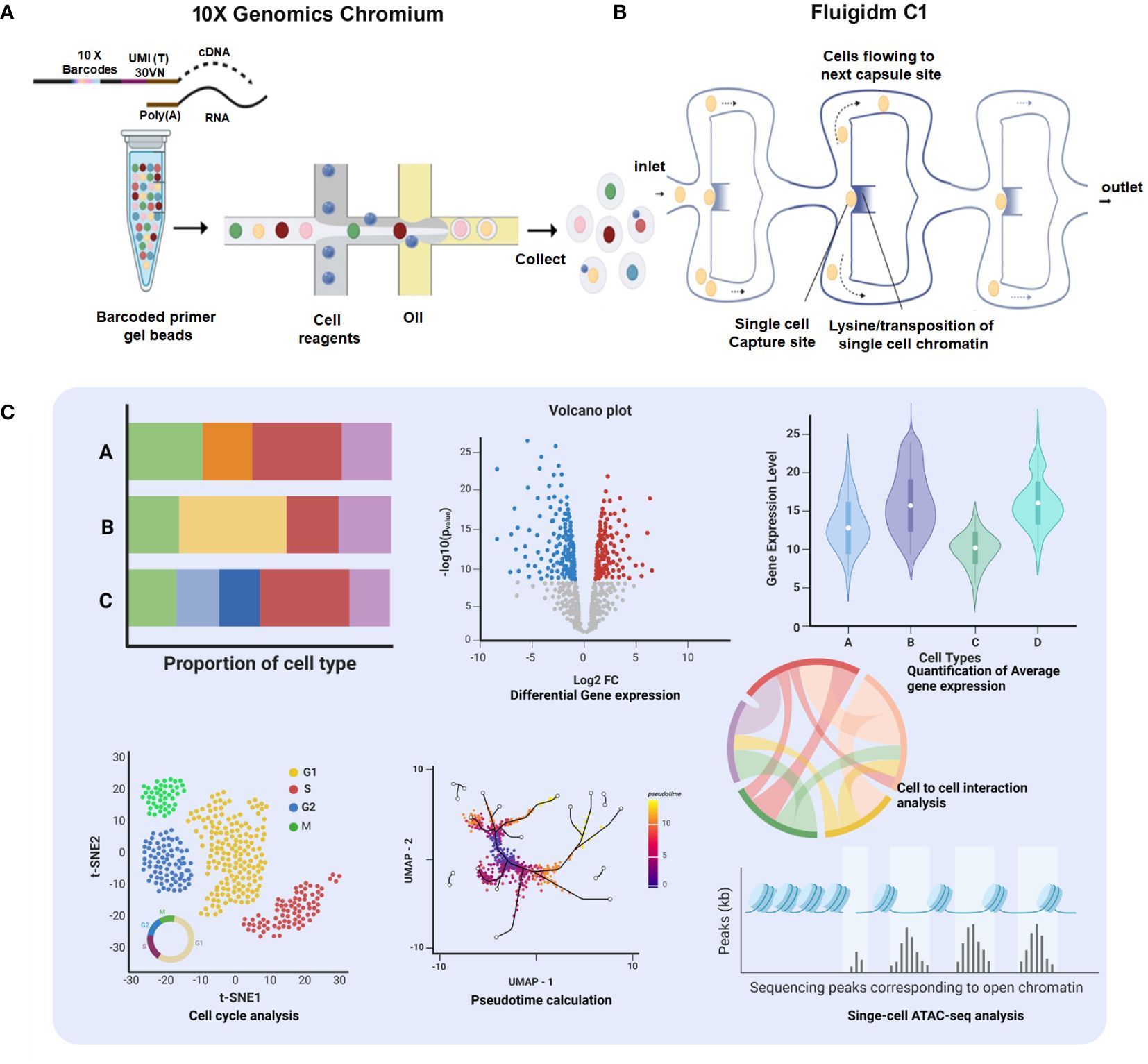
Figure 3 Comprehensive platforms of Single-Cell Transcriptomics. (A) 10× Genomics Chromium: The workflow initiates with encapsulation of individual cells with barcoded primer gel beads into oil droplets to generate Gel bead-in-emulsions (GEMs), ready for collecting and processing. Subsequent steps include sequencing, which generates data for proportion of cell type bar charts, a volcano plot showing differential gene expression, and a violin plot depicting quantification of average gene expression across cell types. (B) Fluigidm C1: Individual cells are physically captured on microfluidic chips. (C) Data Integration and Analysis: The t-SNE plot illustrates cell cycle analysis of individual cells. UMAP with pseudotime calculation provides insights into the developmental trajectory of the cells. The chord diagram highlights cell-to-cell interaction analysis, elucidating the complex interplay between different cell types.
Sc-RNA-seq data are plotted in bi-dimensional space and interpreted by methods like t-Distributed Stochastic Neighbor Embedding (t-SNE) and/or Uniform Manifold Approximation and Projection (UMAP) (33, 34). Although, these methods clearly depict cellular heterogeneity, Sc-RNA-seq data demonstrate cellular condition in a non-functional state as the tissue architecture gets dissociated/disaggregated (39). Therefore, new technology has been developed that captures the spatial transcriptomic data from the tissues via individual-cell laser-capture micro-dissection or multiplexed in situ hybridization (39). However, this method also shows limited sensitivity and depends on prior information on types of cells and/or genes to be targeted/analyzed. Recent Slide-seq technology overcomes these limitations and is considered to be the most suitable for generating high-throughput spatial transcriptomic information at 10-μm resolution (40). Figure 4 depicts the workflow of four popular methodologies being deployed for spatial transcriptomics.
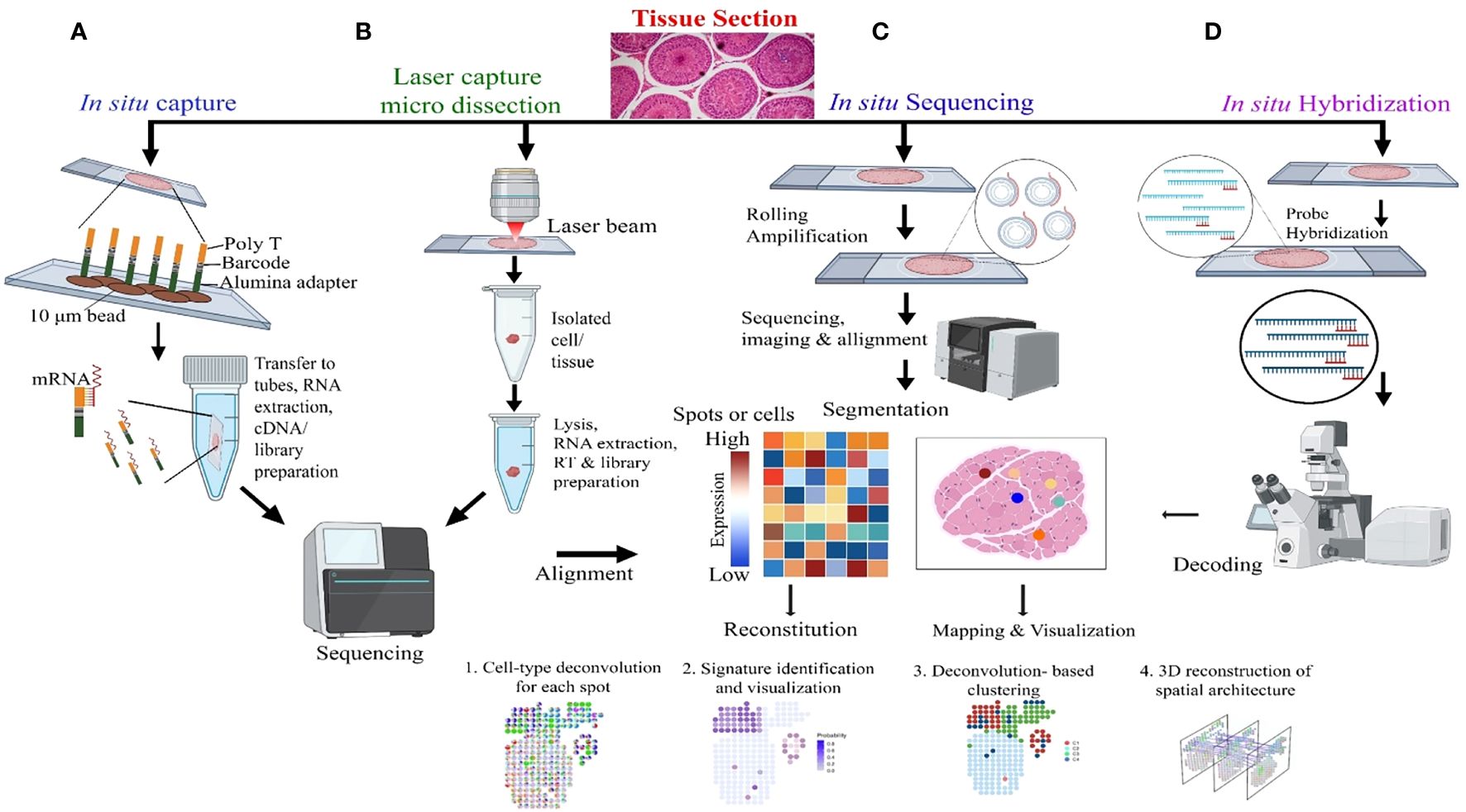
Figure 4 Molecular Profiling of Spatial transcriptomics. (A) In situ Capture: Cells are captured on a chip with poly-T barcoded beads, allowing for mRNA binding and subsequent steps of RNA extraction, cDNA synthesis, and library preparation, followed by sequencing. (B) Laser Capture Micro-dissection: This panel demonstrates the isolation of specific cells or tissue regions using a laser beam, with subsequent lysis, RNA extraction, reverse transcription (RT), and library preparation for sequencing and alignment. (C) In situ Sequencing: Rolling amplification is performed on tissue sections, followed by sequencing, imaging, and alignment. The heatmap represents gene expression levels in specific spots or cells, which is used for reconstitution, mapping, and visualization of cell types and states. (D) In situ Hybridization: Hybridization probes target specific RNA in tissue sections, followed by decoding through microscopy, which leads to the identification of mRNA transcripts and allows for 3D reconstruction of spatial architecture within the tissue.
3 Fetal testicular morphogenesis
The ontology of testicular germ and somatic cell lineages are developmentally independent (15, 41, 42). The bipotential fetal gonads are formed at the gonadal ridge (GR) during embryonic life [embryonic days (E) 8.0-9.5 in mice and weeks of gestation (W) 3.5-4 in humans] and appear distinctly (by E9.5-10 and W5) with thickening on the ventral side of the mesonephros (43). However, during E6.5- 7.5 and W3 murine and human primordial gem cells (PGCs) emerged from posterior-epiblast and amnion respectively. After getting specified (at E7.5 in mice and W4 in humans), these PGCs start migrating towards the developing GR and finally colonize the nascent gonads by E10.5 in mice and W8 in humans (44). The gonadal PGCs stop proliferation, lose pluripotency to gain competence for meiotic commitment via upregulating RNA binding DAZL protein (45). During E11.5 in mice and W6-7 in humans these nascent gonads undergo sex determination (SD), triggered by Sry expression in pre-Sertoli cells of XY gonads (46). The SD event involves step-wise transcriptional cascades generating male (Sry, Sox9, Fgf9, Amh, Dmrt1 etc.) and female (Wnt4, Rspo1, Foxl2, Runx1, etc.) specific robust genetic programming (47). The hallmark of fetal testicular morphogenesis is completed with the formation of the testicular cord and associated vasculature pattern due to selective (XY specific) migration of the mesonephric endothelial cells by E12.5 in mice and W6.5-7 in humans (48, 49). Notably, any developmental defect in the testicular cord formation or abnormality in Sertoli cells (Sc)/Leydig cells (Lc) differentiation directly leads to spermatogenic impairment and infertility during adulthood. Male Gc fail to enter meiosis due to active degradation of Retinoic acid (RA) by CYP26B1 expressed in fetal murine testes from E12.5-13.5 and transformed into mitotically quiescent (G0 arrested) gonocytes (50, 51). During fetal life, E15.5 in mice and W8.5-9 in humans, tubular supportive Sc, PTc, and interstitial fetal Lc (FLc) all are well demarcated (43).
4 Exploring cellular heterogeneity in the testis
Testis is a highly complex organ showing remarkable cellular heterogeneity constituted by both germline and somatic cells. Multiple somatic cell types [like nourishing Sc within seminiferous tubules (ST), supportive PTc covering the ST, interstitial (in inter-tubular space) steroidogenic Lc, interstitial macrophages, etc.] and diverse arrays of developing Gc [including pre-meiotic SSCs/SPCs (2n), different stages of meiotic Gc like primary spermatocytes (2n), secondary spermatocytes (n), round and elongated spermatids (n) and post-meiotic spermatozoa (n) etc] within ST contribute the tissue–organization of testis (Figure 1). Notably, almost all possible cellular events [like mitosis, meiosis, cellular differentiation, apoptosis, epigenetic remodeling, histone–protamine exchange and meiotic sex chromosome inactivation (MSCI) etc.] are evident in testis (52). Table 2 summarizes distinct/key cell markers of individual testicular cells with respect to the appropriate functional significance regulating male fertility. We here have discussed individual major cell–types contributing to the tissue organization of adult testis.
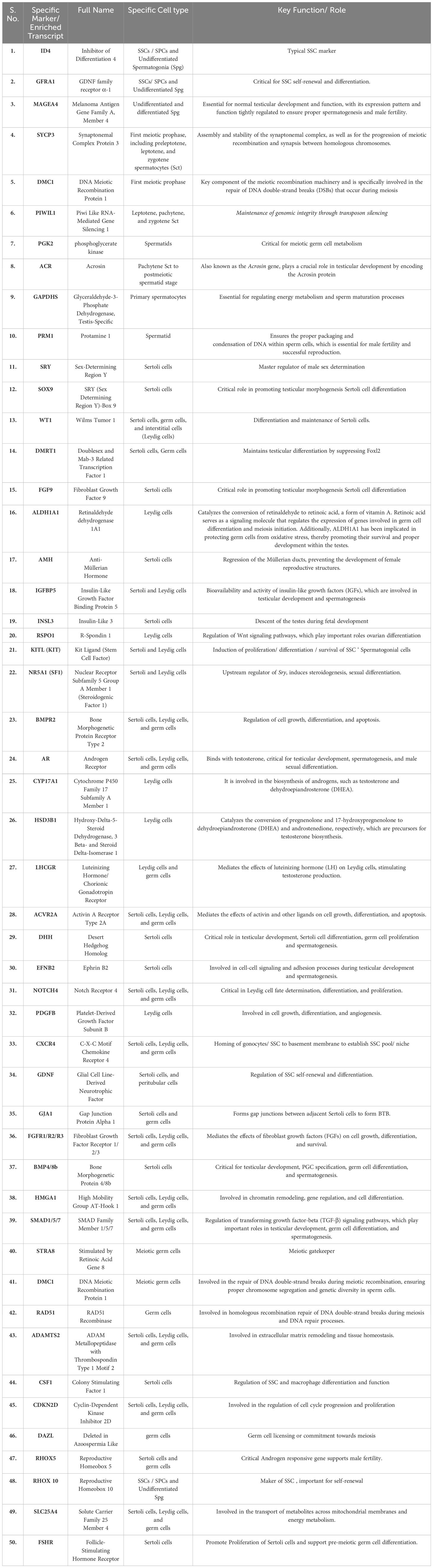
Table 2 Essential Genes Influencing Spermatogenesis Identified Across Multiple Single-Cell RNA Sequencing Research Studies.
4.1 The germ-line cell types
The Gc residing within ST passes through a series of developmental stages [spermatogonia (2n), primary and secondary spermatocytes (n), round and elongated spermatids (n) etc] culminating in the production of mature sperm. Here we discuss different stages of developing Gc.
4.1.1 Primordial germ cells
Primordial germ cells (PGCs) represent the earliest identifiable precursors of Gc. PGCs originate via direct induction of amnion and/or posterior-epiblast (PE) cells from adjacent extra-embryonic ectoderm (EEE) and visceral endoderm (VE) by morphogens like bone morphogenetic proteins (Bmp4 and Bmp8b), Activin A and transcription factors like Blimp1 (Transcriptional repressor B lymphocyte induced maturation protein 1, also known as PR domain-containing protein 1, Prdm1), Prdm14 (PR domain zinc-finger protein 14) and AP2γ (activating enhancer-binding protein 2γ or Tfap2c) and Sox17 (only in humans) etc. (44). The PGCs migrate to colonize the developing GR and subsequently gain the meiotic commitment (lose pluripotency by upregulating DAZL) by E12.5 in mice and W8-9 in humans (50).
4.1.2 SSCs
SSCs are established from gonocytes post-birth (postnatal age 2-3 days in mice) which guarantee the continuity of sperm production throughout adult life (53). In rodents and primates, the duration of spermatogenesis varies among species, (in days; man 64, rhesus monkey 42, mouse 35, and rat 52) typically spanning 35 to 70 days (10, 54). In rodents As (A single spermatogonia) and in primates Apale (proliferative stem/progenitor pool) or Adark (slow diving reserve stem pool) are considered to be the functional SSC populations respectively (13, 14). The key balance between self-renewal and differentiation of these cells remains critical for spermatogenic maintenance throughout adult life (13). The Sc and PTc contribute to establishing the micro-environment of SSC that governs such regulated balance (55).
4.1.3 SPCs
SPCs are constituted with undifferentiated Spermatogonia (Spg), type Apaired or Aaligned connected with common cytoplasmic bridges. In rodents, these pre-meiotic (mitotic) Gc exhibit clonal fragmentation and get differentiated into a transit amplifying (TA) population (A1 to A4) induced by RA and then further transformed to differentiated Spg type B stage having meiotic fate (56). In primates, no such TA population is observed however, in rhesus monkeys (not human) 4 categories of Spg B (B1,B2,B3,B4) are found. In rodents, RA further drives the meiotic entry of Spg B by inducing Stra8, Rec8 and Meiosin expressions in these cells (50, 57).
4.1.4 Meiotic Gc
Meiotic Gc represent the most critical stage of spermatogenesis (58) with two successive divisions. In the first division, diploid (2n) primary spermatocytes transform into haploid (n) secondary spermatocytes followed by in the second division, these spermatocytes further divide to produce round spermatids. Meiotic Gc are pivotal in reducing the chromosome number by half, ensuring that the resulting sperm cells possess the correct genetic material for fertilization upon union with an egg cell. The juxtacrine testosterone (T) signaling through Sc regulates the meiotic progression (59).
4.1.5 Post-meiotic Gc
Post-meiotic Gc maturation mainly involves the transformation of a round spermatid to an elongated structure and such elongated spermatids get condensed and further mature into spermatozoa. In this step, the histone–protamine transition is observed leading to massive hetero-chromatinization leading to transcriptional silencing. However, the functional maturation of male gamete occurs with spermiogenesis, which involves complex cellular changes, viz., development of acrosome and flagellum, etc. Unraveling this metamorphosis is crucial for addressing male fertility concerns and developing strategies for male reproductive health and contraception (60). Figure 5 elucidates the testicular transcriptional dynamics concerning complex cellular heterogeneity. Figure 5A exhibits the differential degrees of heterogeneous/diverse gene expression profiles observed in multiple developmental stages of human (i) spermatogonia, (ii) spermatocytes, (iii) spermatids, and (iv) the entire adult human testis. Figure 5B is the graphical representation of developing Gc-specific marker expression during the entire spermatogenic progression. Figure 6 illustrates the comparative heat maps showing the expression profiles of critical genes involved/associated with different developmental/maturational stages of (A) spermatogonia (B) spermatocytes (C) spermatids and (D) whole adult testis.
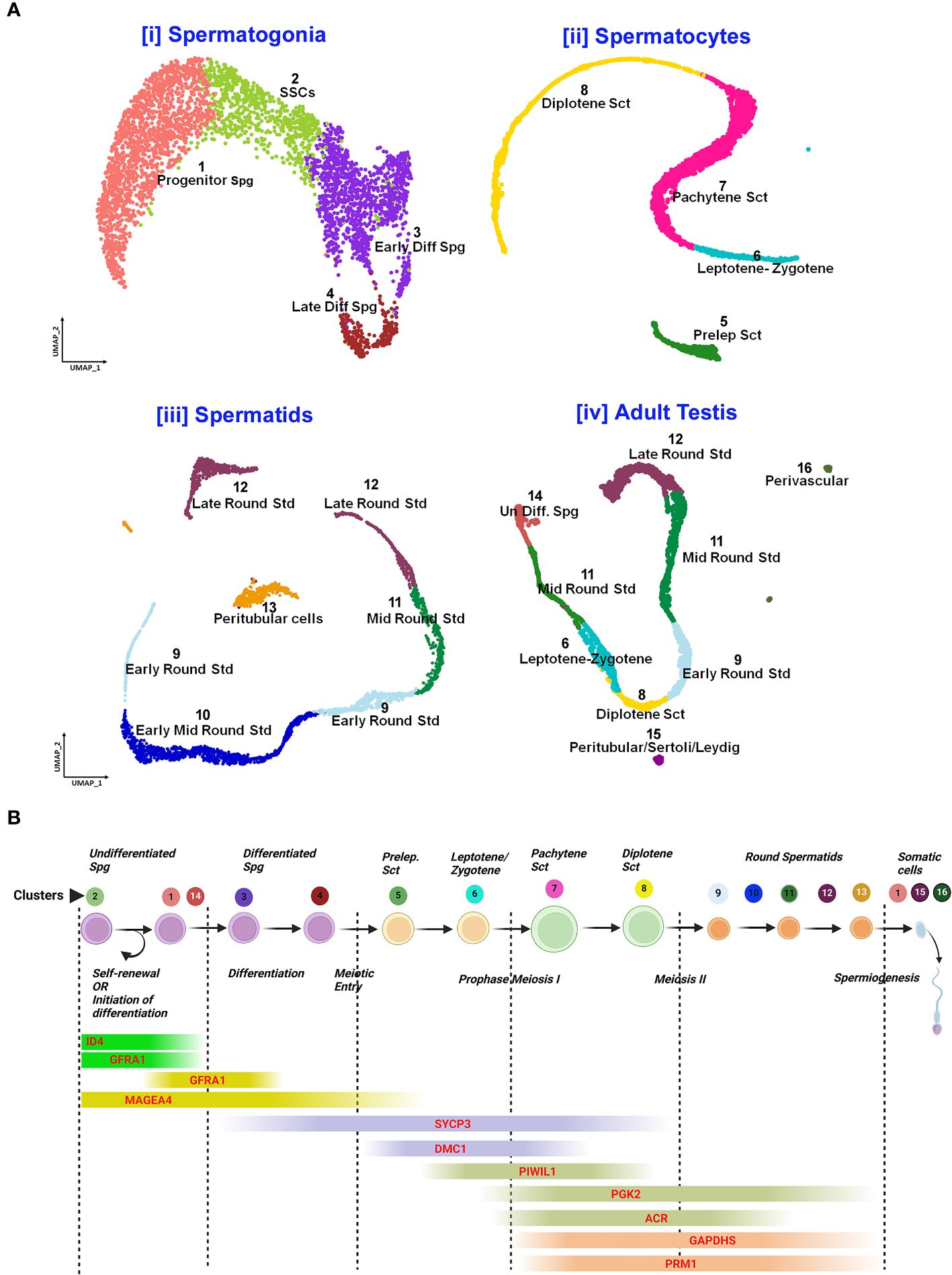
Figure 5 Single-cell analysis of spermatogenesis reveals developmental trajectories and gene expression profiles. (A) UMAP visualization of cell clusters from testicular single-cell RNA sequencing: Cell Differentiation Trajectories: [i] Spermatogonia clusters 1-4 (Progenitor to Late Differentiated), [ii]. Spermatocytes stages 5-8 (Preleptotene to Diplotene), [iii]. Spermatids 9-12 (Early to Late Round), [iv]. Adult Testis Cells 13-16 (Undifferentiated to Perivascular). (B) Gene Expression Markers throughout spermatogenic progression: Sequential expression from self-renewal (GFRAL) to spermiogenesis (PRM1).
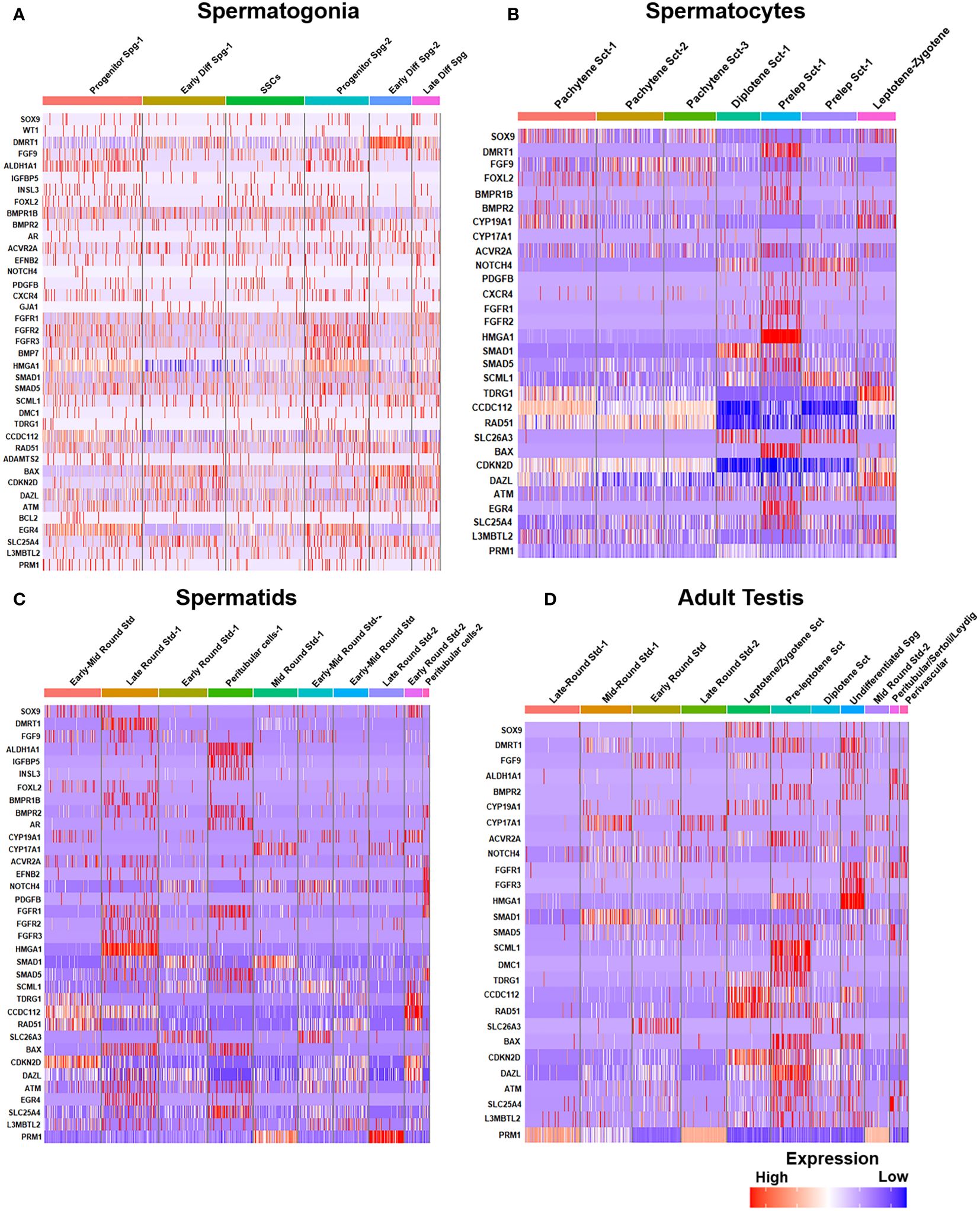
Figure 6 Gene expression profiles during human spermatogenesis. (A) Gene expression heatmap of spermatogonia: The heatmap illustrates the gene expression levels at different steps of spermatogonial development, from pre-meiotic to SSCs. (B) Gene expression heatmap of spermatocytes: Displays the gene expression patterns during the meiotic stages (spermatocytes, from pre-leptotene to post-meiotic). (C) Gene expression heatmap of spermatids: Depicts variations of gene expression in spermatids, highlighting the transition from early to late spermatid stages. (D) Comparative expression heatmap of adult human testis: Compares gene expression across different cell types and stages of sperm development, including leptotene and zygotene spermatocytes and diplotene to secondary spermatocytes.
Notably, Dazl (essential for gametogenic competence to Gc) expression was found to be consistent in all stages of spermatogonial development [like SSCs, progenitor spermatogonia (Spg)-1,2, early differentiating Spg-1,2 and late differentiating Spg] (Figure 6A). However, Dazl expression gets upregulated in high leptotene-zygotene spermatocytes (Sc1-1); declined in subsequent pachytene stage. Essential meiotic genes like Dmc1, Scml1 and Rad51 were found to be elevated in pre-leptotene stage, whereas, RA synthesizing genes like Aldh1a1 was highly enriched in somatic Sc/PTc. Finally, Dmrt1, a critical regulator testicular development was found to get augmented in early differentiating Spg-2, pre-leptotene Sc1-1 and late round spermatid (Std1) (Figure 6).
4.2 The somatic cells
Five distinct somatic cell types (that undergo mitosis but not meiosis) provide the critical structural and nutritional support are discussed in the following section.
4.2.1 Sertoli cells
Sertoli cells (Sc) are the major somatic cells that are the target of both FSH and T, known to dictate spermatogenesis by providing direct structural and nutritional support to all stages of developing Gc within the ST. Sc nurture and regulate the meiotic progression and completion of Gc. Sc-Sc tight junctions establish the blood-testis barrier (BTB) essential for Gc development and their directional movement towards the tubular lumen (61). FSH is critical for Sc proliferation and expansion of the pre-meiotic Gc population, whereas T governs the BTB formation, dynamics and Gc meiosis (4, 62). Sc derived glial cell line-derived neurotrophic factor (GDNF) and stem cell factor (SCF) play crucial role in determining the SSC fate decision from self-renewal to differentiation. Fetal Sc originate from the coelomic epithelium (63) and get specified (at E11.5 in mice and W6-7 in humans) by Sry driven Sox9 expression (43).The development of Sc is distinctly divided into three phases, immature (during fetal/neonatal/perinatal phase), maturing (during juvenile/pre-pubertal life) and mature (at puberty and adulthood) (64–66). Hormonal (FSH & T) stimulations to fetal/neonatal/infant Sc, show limited responses like self-proliferation and local expansion of pre-meiotic Gc but are found to be insufficient to induce robust onset of spermatogonial differentiation. The functional immaturity in terms of hormonal responsiveness of younger Sc is considered the underlying cause. Restricted plasma membrane localization of FSH-Receptor (Fsh-r), inadequate expression of associated Gαs sub-units and poor binding of T with Androgen Receptor (Ar) have been demonstrated in immature Sc during infancy (67–70). Therefore, the functional maturation of pubertal Sc is found to be developmentally critical for inducing Gc differentiation. Such pubertal maturational event in Sc become a prerequisite for spermatogenic onset and male fertility (15, 65). This is further evident by the presence of persistent immature Sc in adult testes that results in severe oligozoospermic conditions with infertility in rodents (71–75) and in humans (64, 76–79).
4.2.2 Peritubular cells
Peritubular cells (PTc) constitute the outermost covering of the ST and further provides structural support by creating peristaltic waves through their contractile elements, potentially prompting fluid movement within the tubule lumen to facilitate the expulsion of spermatozoa (80). PTc are the targets of T signaling and shown to promote Sc maturation. Furthermore, crosstalk between PTc with adjacent Lc play a vital role in spermatogenic development. Along with Sc, these cells govern the maintenance of the SSC micro-environment (81, 82).
4.2.3 Leydig cells
Leydig cells (Lc) are the key steroidogenic cells located within the inter-tubular interstitium and the sole target of LH for T biosynthesis. Lc are critical for male reproductive development viz., differentiation of the genital tract, fetal virilization, pubertal maturation and regulation of fertility during adulthood (82, 83). Two [fetal (FLc) and adult (ALc)] and four (fetal, neonatal, pubertal, and adult) distinct populations of Lc are reported in mice and primates with differential morphology and functions respectively (41). In mice, FLc exhibit dual origins (both coelomic epithelium and notch-active Nestin-positive perivascular cells located at the gonad–mesonephros borders) and first detected as Nr5a1 (also known as Ad4BP/SF-1) positive cells on E12.5 (W9-10 in humans). Murine FLc lack HSD17β3 enzyme and thereby produce only androstenedione (precursor of T) and promote the initial virilization and differentiation of male genitalia. Post-birth by P (post-natal age in days) 7-12, FLc undergo periodic regression and replaced by T-producing ALc during P18-25. Nestin-positive perivascular cells and FLc are the progenitor populations for ALc (84). Recent research indicates that 5-20% of FLc are maintained in adult testis (37). T acts on ALc, PTc and Sc to promote spermatogenesis, however, Gc do not express Ar. Sc shown to be the most critical target of T signaling for regulating male fertility (85). Although the extent of LH responsiveness of ALc remain unaltered with testicular aging, overall T production declines with testicular aging and testicular metabolic complications (86–88).
4.2.4 Macrophages
Macrophages, (being CSF1R+, CD206+ MHCII-) are present in the testicular interstitium and regulate the steroidogenic activity of ALc (84, 89). Another distinct population of adult macrophages (CSF1R-, CD206-, MHCII+) has been observed at various patches of the peritubular region which actively contributes to the SSC niche and promotes spermatogonial differentiation (80, 90). Intriguingly in fetal life, yolk-sac-derived murine macrophages (at E7.5) play a critical role in directing the selective migration of mesonephric endothelial cells towards developing XY gonads (not XX) and directs testicular cord (at E12.5) formation (49). Recent findings indicate that monocytes derived from hematopoietic stem cells (HSCs) of fetal liver get colonized in embryonic mouse testes in Sc dependent manner and further differentiate into testis resident macrophages during postnatal life (84). During adulthood, CD206+ interstitial macrophages promote ALc proliferation and steroidogenesis and thereby indirectly regulate male fertility. Furthermore, these macrophages also maintain the immunosuppressive environment within the testis (91).
4.2.5 Endothelial cells
Endothelial cells (ECs) form the border walls of blood vessels, facilitating nutrient exchange for developing Gc/sperm. Dysfunction in these cells can affect sperm development and overall testicular health, impacting fertility. During murine testicular morphogenesis, a key feature involves sex-specific vascularization where endothelial cells migrate from the neighboring mesonephros into the fetal XY gonads (during E11-12.5), encircling Sc-Gc clusters and prompting the formation of seminiferous cords. These cells are potent sources of vascular endothelial growth factor A (Vegf A) critical for tissue remodeling/homeostasis (92). ECs also contribute to the SSC niche by producing GDNF that sustains SSCs during extended culture periods (93).
Notably, moderate expressions of Dmrt1, Sox9, Wt1, Amh, Dhh and Aldh1a1 were found in murine Sc from E18.5 to P2D upto P7D. However, the transcript levels of the genes essential for induction of Gc differentiation like Scf/Kit-ligand, Gdnf and Csf-1 etc, remained low during this time of testicular development. In consistent with previous reports, Lc specific Insl3, Cyp17a1, Hsd3b1, Lh/cg-receptor and PTc restricted Ar expressions were observed from E18.5 to P7D; whereas meiotic gatekeeper Stra8 expression was enriched at Gc on P7D (Figure 7C). Figures 7A, B show age-dependent dynamic gene expression profiles observed in different cell types during three [(i) E18.5, (ii) P2D (post-natal 2 days of age) & (iii) P7D (post-natal 7 days of age)] distinct developmental phases of murine testicular maturation. Figure 7C depicts the comparative heat maps revealing the expression profiles of key spermatogenic genes in different cell types during (i) fetal (E18.5) testicular developmental and neonatal [(ii) P2D, (iii) P7D] testis maturation.
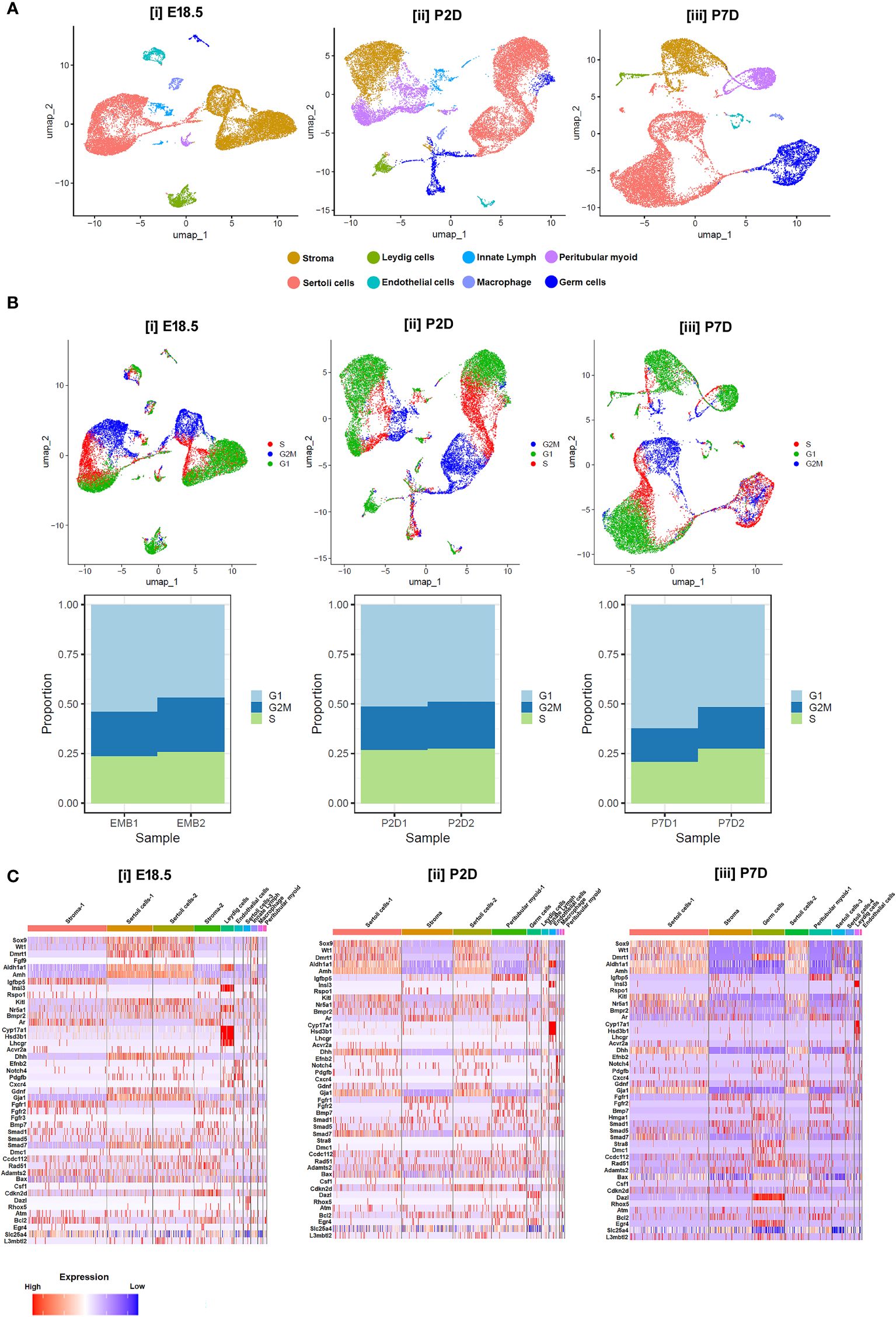
Figure 7 Single-cell transcriptomic analysis of testicular cells at different developmental age groups. (A) UMAP visualizations representing the cellular composition of testicular cells at (i) embryonic days 18.5 (E18.5), (ii) postnatal days 2 (P2D), and (iii) postnatal days 7 (P7D). Each dot represents a single cell, color-coded by identified cell types: stroma (cyan), Leydig cells (yellow), Sertoli cells (dark blue), endothelial cells (green), innate lymphoid cells (purple), peritubular myoid cells (red), macrophages (light blue), and germ cells (orange). (B) UMAP plots displaying cell cycle phases for testicular cells on E18.5, P2D, and P7D. Cells are color-coded according to their phase in the cell cycle: G1 (blue), S (red), G2/M (green), and cells not in cycle (black). Arrows indicate the direction of the developmental trajectory. (C) Heatmaps illustrating the expression profiles of selected marker genes across different cell types on E18.5, P2D, and P7D. Gene expression levels are represented by a gradient from low (blue) to high (red) expression. Beneath each UMAP plot in B, stacked bar charts show the proportion of cells in each cell cycle phase for two replicates at each developmental stage (EMB1, EMB2, P2D1, P2D2, P7D1, P7D2).
5 A comprehensive review of literature on testicular Sc-cell-RNA-seq revealed the molecular basis of testicular dysfunctions/disorders
The literature search was conducted during December – 2023- February 2024. Initially, systematic inquiries were performed in the PubMed database. Given the review’s primary focus on “single-cell RNA sequencing” and “Testis”, a tailored search strategy was applied to connect these two aspects. In detail, concerning the former aspect, the terms “single-cell” (term A) or “Sc-RNA-seq” (term B) were designated. Each of these terms was paired with one of the subsequent testis-related (the latter aspect-related) or animal model-related search terms, which included “testis” (term 1), “spermatogenesis” (term 2), “testicular development” (term 3), “azoospermia” (term 4), “Klinefelter Syndrome” (term 5), “cryptozoospermia” (term 6), “oligozoospermia” (term 7), “asthenospermia” (term 8), “teratospermia” (term 9), “orchitis” (term 10), “cryptorchidism” (term 11), “male and gonad” (term 12), “Humans” (term 13), “Monkey” (term 14), “Primates” (term 15), “mouse” (term 16), “Rat” (term 17), “Testicular diseases” (term 18), and “Testicular atlas” (term 19).
To reduce the likelihood of overlooking pertinent studies, particularly preprint studies, an additional search is conducted on the GEO dataset (https://www.https://www.ncbi.nlm.nih.gov/geo/) employing combinations of refined above search terms. The searches yielded approximately ~132 records from the last 10 years (2015–2024). Among these, 60 articles contained original Sc-RNA-seq data (from at least one donor) related to human testicular samples, while the remaining 68 articles involved re-analyzed data from prior publications or the datasets from other primates, rodents, livestock animals, or non-mammalian species. Starting in 2015, Guo et al. pioneered Sc-RNA-seq on 233 individual male and female human PGCs from 15 embryos between 4 and 19 weeks of gestation (94), followed by two studies in 2017 that sequenced adult testicular cells (95, 96). Interestingly, despite being from different teams, both adult studies focused on spermatogonia. Since 2018, numerous studies have conducted Sc-RNA-seq on human testicular samples, encompassing both prenatal and postnatal stages, normal and abnormal testes. Figure 8 represents the timeline of literature published/available in PubMed. Table 3 is the chronological summary of original research articles published on Sc-RNA-seq data for normal testicular samples collected from (A) primates (human and monkeys) and (B) rodents. Table 4 discusses country-wise Sc-RNA-seq data on clinical human samples with multiple forms of impaired spermatogenesis/infertility reported so far. Notably, Tables 3, 4 will serve as a critical repositories/valuable resource for Sc-RNA-seq datasets specific to distinct testicular cell types generated to date.
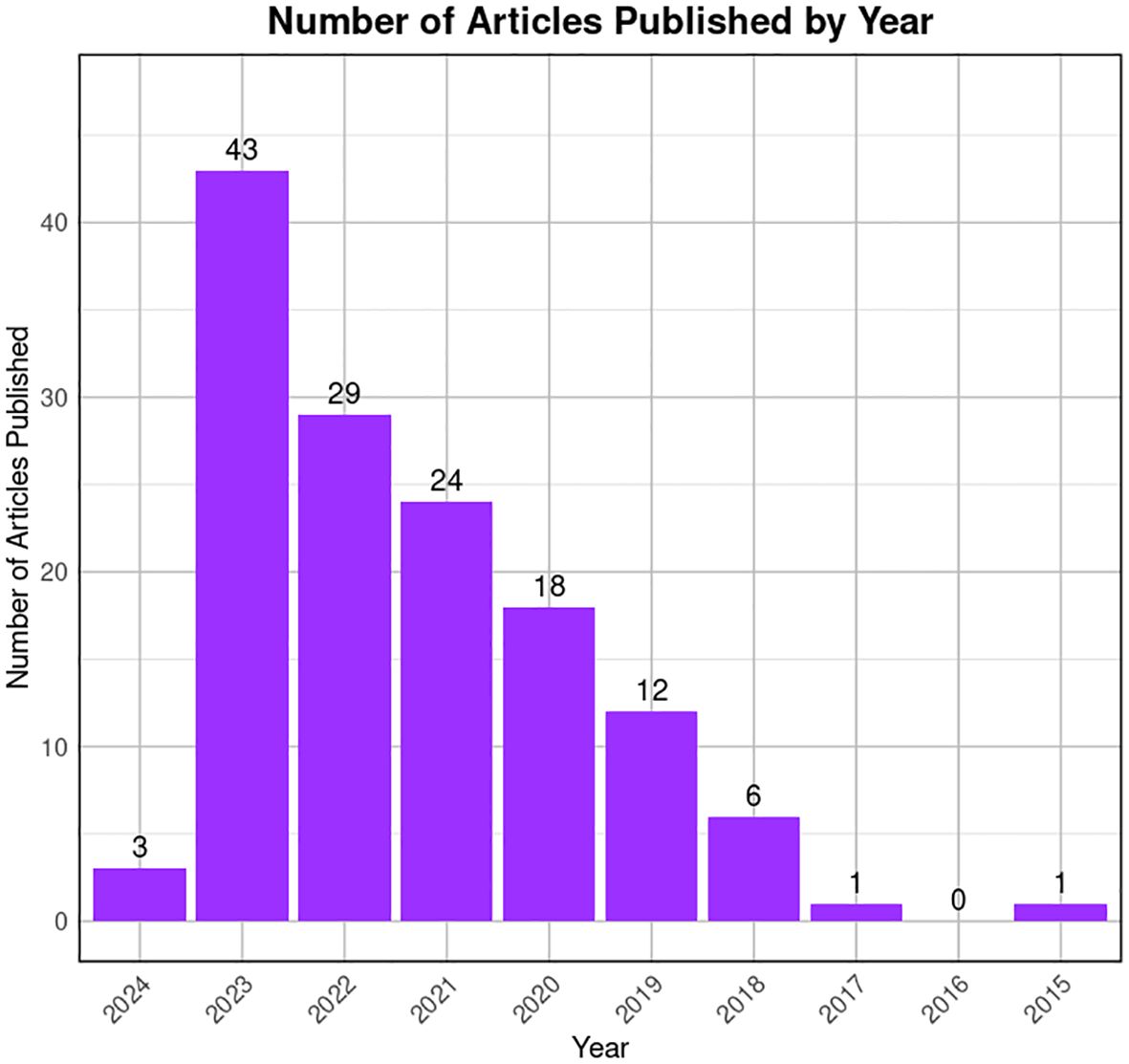
Figure 8 Trends in publication on testicular Sc-RNA-seq studies. The bar graph illustrates the number of articles published by year, from 2015 to 2024 available at PubMed. A noticeable peak is observed in 2022, followed by a decline in subsequent years. The x-axis represents the years, while the y-axis quantifies the number of articles published. The data suggests a period of heightened research activity in 2022, with a gradual decrease in the volume of publications thereafter.
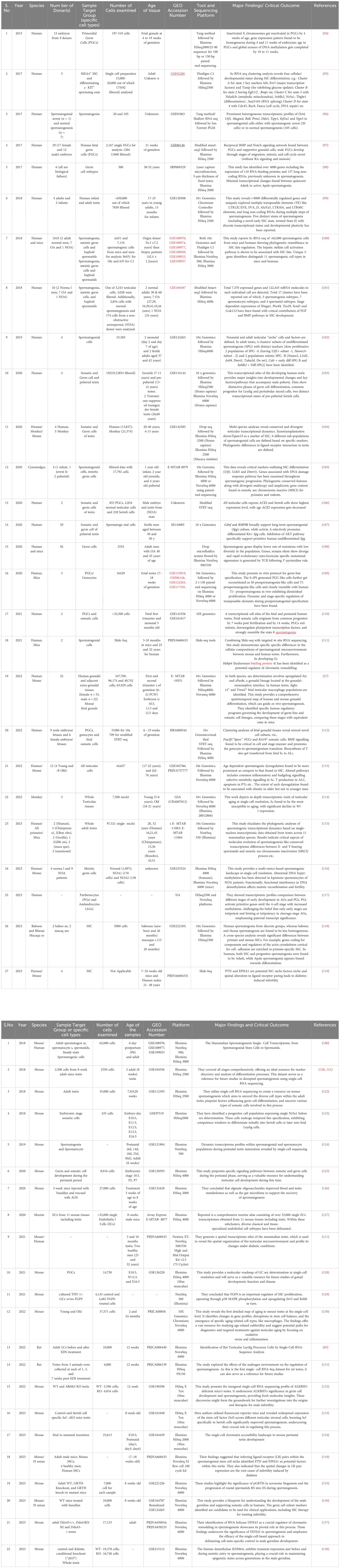
Table 3 Summarizing the key findings of mammalian testicular single-cell RNA-seq datasets. (A). Normal healthy Human/ Monkeys. (B). Exclusively Rodents.
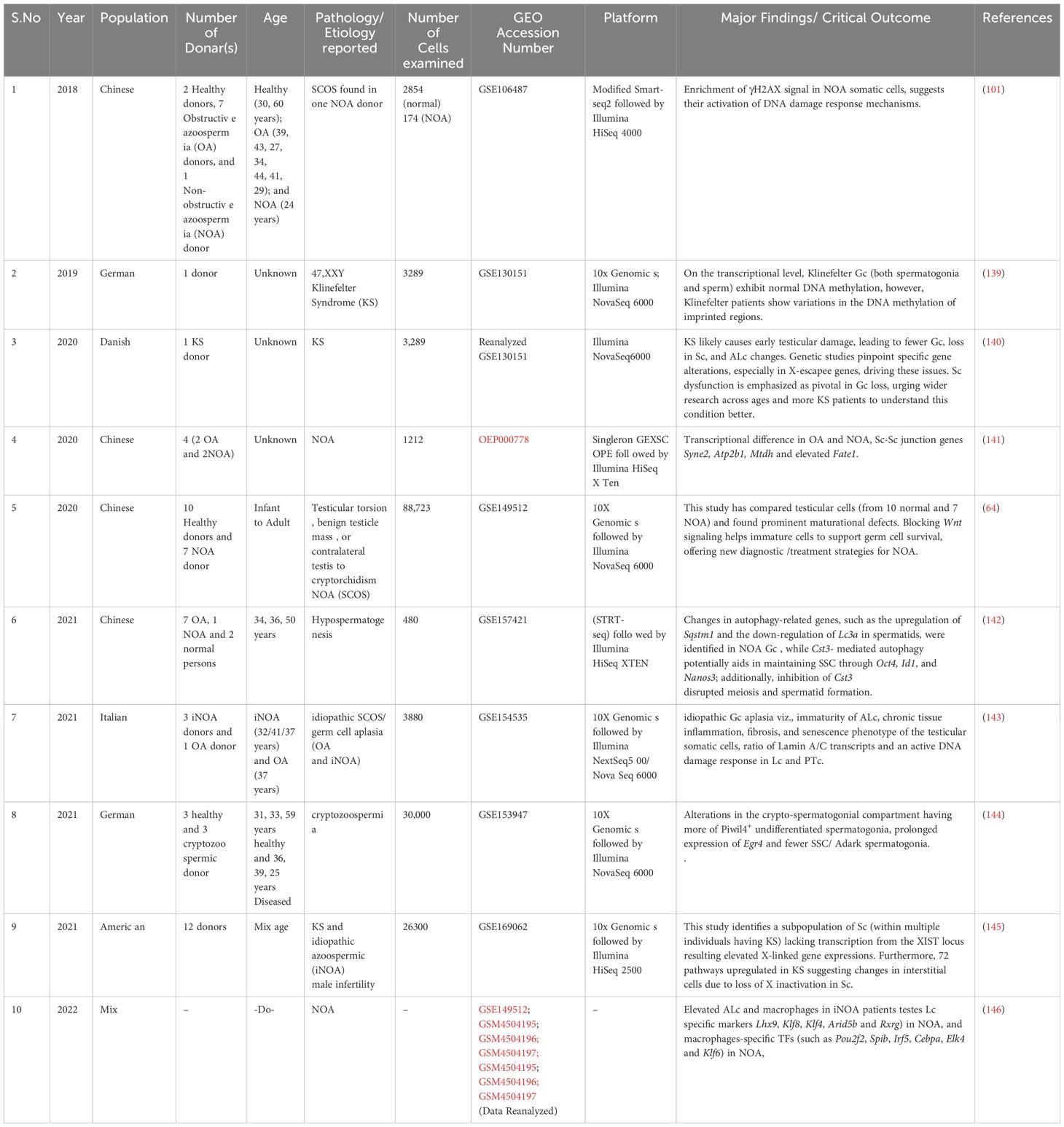
Table 4 List of Human Participants Exhibiting Spermatogenic Dysfunction or Infertility: A Detailed Examination.
6 New progress
This comprehensive review of literature on testicular Sc-RNA-seq highlights the complexity of testicular tissue composition and the uniqueness of cellular transcriptional dynamics. The cutting-edge approach has unveiled a heterogeneous landscape within the testis, providing insights into spermatogenic Gc, somatic PTc and interstitial Lc and supportive Sc associated with SSC microenvironments. Studies employing Sc-RNA-seq have examined the transcriptional profiles of various Gc populations at distinct developmental stages elucidating the molecular regulation of spermatogonial differentiation, meiosis, and sperm maturation. The application of Sc-RNA-seq has not only enriched our understanding of testicular cell heterogeneity but has also identified novel markers and regulatory pathways critical for male infertility and reproductive health (147). Integration of multi-omics data and advanced in-silico analyses offers a comprehensive view of the testicular transcriptomic landscape, fostering discoveries with profound implications for both basic biology and clinical applications in reproductive medicine.
A significant development in the field of testicular transcriptomics requires an inclusive assimilation/integration of data obtained from Sc-RNA-seq analyses with spatial transcriptomic information (32). For example, Chen and colleagues employed the “spatial transcriptomic analysis” technology, creating a spatial atlas that delineates testicular gene expression at near-single-cell resolution in the human testis (111). Similarly, Garcia-Alonso et al. conducted integrated analyses of male gonadal development by fusing Sc-RNA-seq and spatial transcriptomics, offering a more comprehensive and in-depth perspective (27).
7 Current challenges
The integration of these methodologies is anticipated to augment future investigations on the human testis and male infertility, affording a comprehensive understanding of functional/impaired spermatogenesis. Although Sc-RNA-seq analyses have corroborated the notable presence of immune cells (e.g., macrophages - both M1 and M2, T cells, mast cells, and B cells) in the testicular microenvironment, the functional roles (and also transcriptional patterns) of these cells in regulating male infertility development remain elusive (85). However, despite the current high cost of Sc-RNA-seq, several of the studies sincerely lack a direct clinical orientation Therefore, diverse testicular pathologies, viz., cryptorchidism, AZFc deletions, azoospermia post chemo/radiotherapy, and teratospermia, demand focused exploration of Sc-RNA-seq analyses. The ongoing refinement and cost mitigation of this technology holds future promise for clinical applications, such as diagnostics, classification, prediction of sperm retrieval, and evaluation of hormonal treatment efficacy. Figure 9 illustrates the probable applications of Sc-RNA-Seq data towards framing appropriate preclinical studies (both in vitro and in vivo approach) leading to potential future diagnostic (assays/tools/kits) in male reproductive health care.
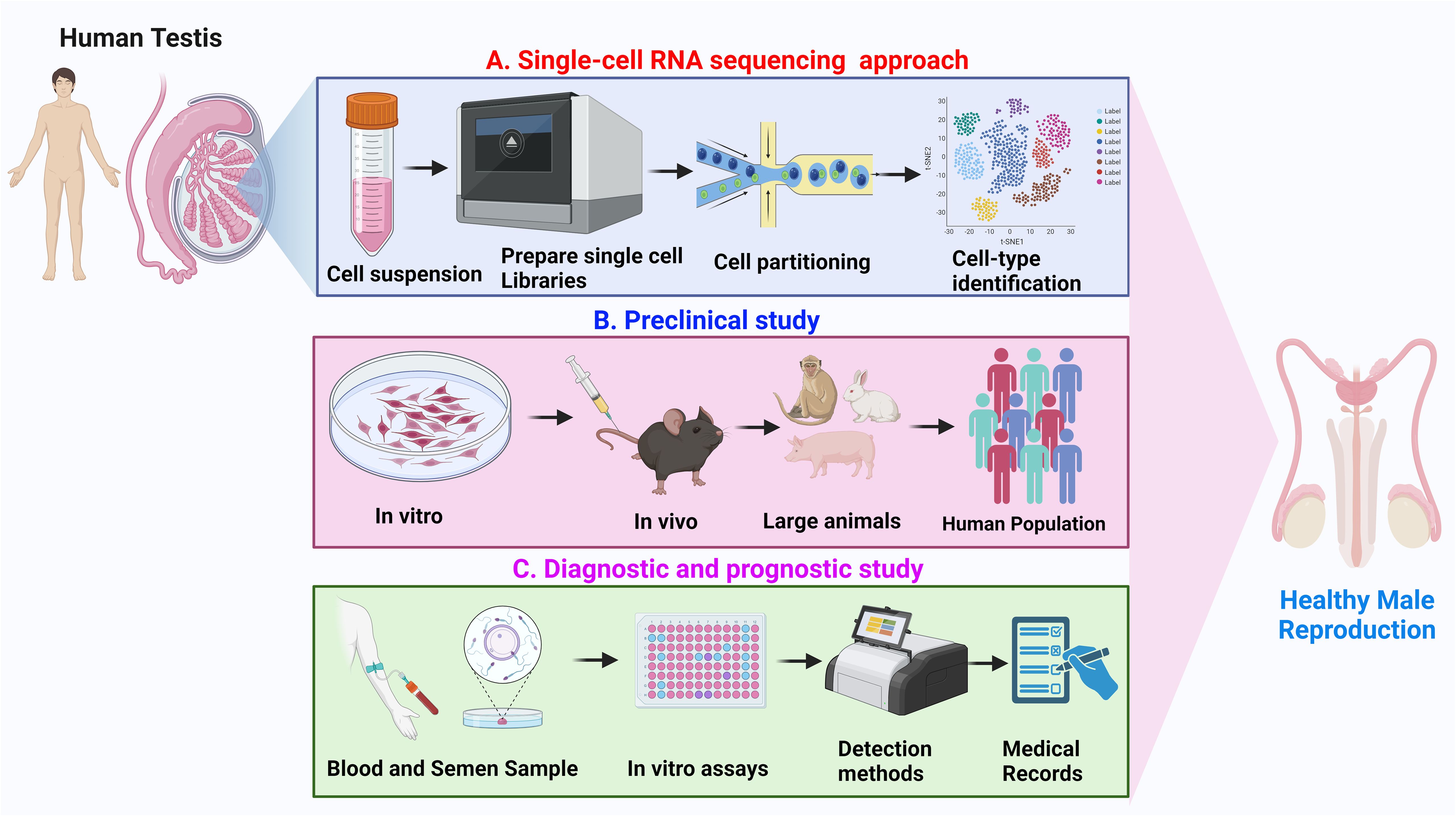
Figure 9 Overview of application of Sc-RNA-seq data. (A) Single-cell RNA sequencing approach: Depicts the workflow from human testicular tissue sample to single-cell suspension, followed by library preparation for RNA sequencing, cell partitioning, and subsequent cell-type identification using clustering analysis. (B) Preclinical study: Represents the preclinical research progression, beginning with in vitro experiments, advancing through in vivo studies in rodents and large animals, and culminating in analysis within a human population. (C) Diagnostic and prognostic study: Illustrates the process of collecting blood and semen samples, performing in vitro assays, utilizing various detection methods, and integrating findings with medical records for the advancement of healthy male reproduction diagnostics and prognostics.
8 Concluding remarks and future directions
Exploring the intricate landscape of testicular function and pathology requires a comprehensive understanding spanning from the fundamental processes of development to the complexities of the disorders. This review has examined the uncharted territory of the testicular single-cell transcriptional atlas, illuminating the diverse cellular populations and their orchestrated molecular dynamics. From the genesis of Gc to the intricate orchestration of somatic cell interactions, this exploration navigates the transcriptional intricacies shaping testicular development. Furthermore, it delves into the disruptive signatures underlying various testicular disorders, shedding light on potential avenues for diagnostic and therapeutic advancements. By unraveling the cellular intricacies from a single-cell perspective, this review aims to provide comprehensive insights bridging the developmental trajectory to the manifestations of disorders within the testicular microenvironment. We also summarize the previous attempts deploying Sc-RNA-Seq on clinical testicular biopsies (Table 4) that have significantly enriched our understanding of testicular physiology e.g.- regulation of SSC micro-environment and testicular somatic cell maturation, etc. Finally, the increasing availability of publicly accessible testicular Sc-RNA-seq datasets provides researchers with greater possibilities for conducting personalized data analyses to address diverse research requirements. We anticipate that this review will not only facilitate the comprehensive understanding of spermatogenic transcription during normal and impaired (sub-fertile/infertile) conditions but also serve as a valuable reference for critical diagnosis and cure for some forms of unexplained/idiopathic male infertility.
Author contributions
MT: Conceptualization, Data curation, Formal analysis, Investigation, Methodology, Software, Writing – review & editing. IB: Visualization, Formal Analysis, Writing – original draft, Writing – review & editing. MM: Data curation, Formal analysis, Writing – review & editing. VB: Data curation, Formal analysis, Writing – review & editing. MC: Conceptualization, Data curation, Investigation, Supervision, Validation, Visualization, Writing – original draft, Writing – review & editing.
Funding
The author(s) declare that no financial support was received for the research, authorship, and/or publication of this article.
Acknowledgments
IB sincerely thanks the past and present Vice-Chancellor, Central University of Kerala, Kasaragod, Kerala, India for necessary administrative support.
Conflict of interest
The authors declare that the research was conducted in the absence of any commercial or financial relationships that could be construed as a potential conflict of interest.
Publisher’s note
All claims expressed in this article are solely those of the authors and do not necessarily represent those of their affiliated organizations, or those of the publisher, the editors and the reviewers. Any product that may be evaluated in this article, or claim that may be made by its manufacturer, is not guaranteed or endorsed by the publisher.
Supplementary material
The Supplementary Material for this article can be found online at: https://www.frontiersin.org/articles/10.3389/fendo.2024.1394812/full#supplementary-material
References
1. Inhorn MC, Patrizio P. Infertility around the globe: new thinking on gender, reproductive technologies and global movements in the 21st century. Hum Reprod Update. (2015) 21:411–26. doi: 10.1093/humupd/dmv016
2. Eisenberg ML, Esteves SC, Lamb DJ, Hotaling JM, Giwercman A, Hwang K, et al. Male infertility. Nat Rev Dis Primers. (2023) 9:49. doi: 10.1038/s41572-023-00459-w
3. Agarwal A, Baskaran S, Parekh N, Cho CL, Henkel R, Vij S, et al. Male infertility. Lancet. (2021) 397:319–33. doi: 10.1016/S0140-6736(20)32667-2
4. Bhattacharya I, Dey S, Banerjee A. Revisiting the gonadotropic regulation of mammalian spermatogenesis: evolving lessons during the past decade. Front Endocrinol (Lausanne). (2023) 14:1110572. doi: 10.3389/fendo.2023.1110572
5. Krausz C, Riera-Escamilla A. Genetics of male infertility. Nat Rev Urol. (2018) 15:369–84. doi: 10.1038/s41585-018-0003-3
6. Tournaye H, Krausz C, Oates RD. Novel concepts in the aetiology of male reproductive impairment. Lancet Diabetes Endocrinol. (2017) 5:544–53. doi: 10.1016/S2213-8587(16)30040-7
7. Cannarella R, Condorelli RA, Mongioì LM, La Vignera S, Calogero AE. Molecular biology of spermatogenesis: novel targets of apparently idiopathic male infertility. Int J Mol Sci. (2020) 21:1728. doi: 10.3390/ijms21051728
8. Majumdar SS, Bhattacharya I. Genomic and post-genomic leads toward regulation of spermatogenesis. Prog Biophys Mol Biol. (2013) 113:409–22. doi: 10.1016/j.pbiomolbio.2013.01.002
9. Russell LD, Ettlin RA, Hikim AP, Clegg ED. Mammalian spermatogenesis. In: Russell LD, Ettlin R, Sinha Hikim AP, Clegg ED, editors. Histological and histopathological evaluation of the testis. Cache River, Florida (1990). p. 1–40.
10. Sharpe RM. Regulation of spermatogenesis. The physiology of reproduction Vol. 1. New York: Raven Press (1994) p. 1363–434.
11. Bhattacharya I, Sharma SS, Majumdar SS. Etiology of male infertility: an update. Reprod Sci. (2023) 1(4):942–65. doi: 10.1007/s43032-023-01401-x
12. Song HW, Wilkinson MF. In vitro spermatogenesis: A long journey to get tails. Spermatogenesis. (2012) 2:238–44. doi: 10.4161/spmg.22069
13. Fayomi AP, Orwig KE. Spermatogonial stem cells and spermatogenesis in mice, monkeys, and men. Stem Cell Res. (2018) 29:207–14. doi: 10.1016/j.scr.2018.04.009
14. Oatley JM, Brinster RL. The germline stem cell niche unit in mammalian testes. Physiol Rev. (2012) 92:577–95. doi: 10.1152/physrev.00025.2011
15. Bhattacharya I, Sen Sharma S, Majumdar SS. Pubertal orchestration of hormones and testis in primates. Mol Reprod Dev. (2019) 86:1505–30. doi: 10.1002/mrd.23246
16. Rabbani M, Zheng X, Manske GL, Vargo A, Shami AN, Li JZ, et al. Decoding the spermatogenesis program: new insights from transcriptomic analyses. Annu Rev Genet. (2022) 56:339–68. doi: 10.1146/annurev-genet-080320-040045
17. Griswold MD. Spermatogenesis: the commitment to meiosis. Physiol Rev. (2016) 96:1–17. doi: 10.1152/physrev.00013.2015
18. Tüttelmann F, Ruckert C, Röpke A. Disorders of spermatogenesis: Perspectives for novel genetic diagnostics after 20 years of unchanged routine. Med Genet. (2018) 30:12–20. doi: 10.1007/s11825-018-0181-7
19. Zhankina R, Baghban N, Askarov M, Saipiyeva D, Ibragimov A, Kadirova B, et al. Mesenchymal stromal/stem cells and their exosomes for restoration of spermatogenesis in non-obstructive azoospermia: a systemic review. Stem Cell Res Ther. (2021) 12:229. doi: 10.1186/s13287-021-02295-9
20. Cioppi F, Rosta V, Krausz C. Genetics of azoospermia. Int J Mol Sci. (2021) 22:3264. doi: 10.3390/ijms22063264
21. Behre HM, Bergmann M, Simoni M, Tüttelmann F. Primary testicular failure. 2015 aug 30. In: Feingold KR, Anawalt B, Blackman MR, Boyce A, Chrousos G, Corpas E, de Herder WW, Dhatariya K, Dungan K, Hofland J, Kalra S, Kaltsas G, Kapoor N, Koch C, Kopp P, Korbonits M, Kovacs CS, Kuohung W, Laferrère B, Levy M, McGee EA, McLachlan R, New M, Purnell J, Sahay R, Shah AS, Singer F, Sperling MA, Stratakis CA, Trence DL, Wilson DP, editors. MDText.com, Inc, South Dartmouth (MA (2000).
22. Matzuk MM, Lamb DJ. The biology of infertility: research advances and clinical challenges. Nat Med. (2008) 14:1197–213. doi: 10.1038/nm.f.1895
23. Neto FTL, Bach PV, Najari BB, Li PS, Goldstein M. Spermatogenesis in humans and its affecting factors. Semin Cell Dev Biol. (2016) 59:10–26. doi: 10.1016/j.semcdb.2016.04.009
24. Stark R, Grzelak M, Hadfield J. RNA sequencing: the teenage years. Nat Rev Genet. (2019) 20:631–56. doi: 10.1038/s41576-019-0150-2
25. Suzuki S, Diaz VD, Hermann BP. What has single-cell RNA-seq taught us about mammalian spermatogenesis? Biol Reprod. (2019) 101:617–34. doi: 10.1093/biolre/ioz088
26. Estermann MA, Smith CA. Applying single-cell analysis to gonadogenesis and DSDs (Disorders/differences of sex development). Int J Mol Sci. (2020) 21:6614. doi: 10.3390/ijms21186614
27. Garcia-Alonso L, Lorenzi V, Mazzeo CI, Alves-Lopes JP, Roberts K, Sancho-Serra C, et al. Single-cell roadmap of human gonadal development. Nature. (2022) 607:540–7. doi: 10.1038/s41586-022-04918-4
28. Jovic D, Liang X, Zeng H, Lin L, Xu F, Luo Y. Single-cell RNA sequencing technologies and applications: A brief overview. Clin Transl Med. (2022) 12:e694. doi: 10.1002/ctm2.694
29. Zhang S, Li X, Lin J, Lin Q, Wong KC. Review of single-cell RNA-seq data clustering for cell-type identification and characterization. RNA. (2023) 29:517–30. doi: 10.1261/rna.078965.121
30. Rao A, Barkley D, França GS, Yanai I. Exploring tissue architecture using spatial transcriptomics. Nature. (2021) 596:211–20. doi: 10.1038/s41586-021-03634-9
31. Zhang Y, Lin X, Yao Z, Sun D, Lin X, Wang X, et al. Deconvolution algorithms for inference of the cell-type composition of the spatial transcriptome. Comput Struct Biotechnol J. (2022) 21:176–84. doi: 10.1016/j.csbj.2022.12.001
32. Dong F, Ping P, Ma Y, Chen XF. Application of single-cell RNA sequencing on human testicular samples: a comprehensive review. Int J Biol Sci. (2023) 19:2167–97. doi: 10.7150/ijbs.82191
33. Cheng C, Chen W, Jin H, Chen X. A review of single-cell RNA-seq annotation, integration, and cell-cell communication. Cells. (2023) 12:1970. doi: 10.3390/cells12151970
34. Slovin S, Carissimo A, Panariello F, Grimaldi A, Bouché V, Gambardella G, et al. Single-cell RNA sequencing analysis: A step-by-step overview. Methods Mol Biol. (2021) 2284:343–65. doi: 10.1007/978-1-0716-1307-8_19
35. Hwang B, Lee JH, Bang D. Single-cell RNA sequencing technologies and bioinformatics pipelines. Exp Mol Med. (2018) 50:1–14. doi: 10.1038/s12276-018-0071-8
36. Zheng GX, Terry JM, Belgrader P, Ryvkin P, Bent ZW, Wilson R, et al. Massively parallel digital transcriptional profiling of single cells. Nat Commun. (2017) 8:14049. doi: 10.1038/ncomms14049
37. Peng X, Wu J, Brunmeir R, Kim SY, Zhang Q, Ding C, et al. TELP, a sensitive and versatile library construction method for next-generation sequencing. Nucleic Acids Res. (2015) 43:e35. doi: 10.1093/nar/gku818
38. Picelli S, Björklund ÅK, Faridani OR, Sagasser S, Winberg G, Sandberg R. Smart-seq2 for sensitive full-length transcriptome profiling in single cells. Nat Methods. (2013) 10:1096–8. doi: 10.1038/nmeth.2639
39. Asp M, Bergenstråhle J, Lundeberg J. Spatially resolved transcriptomes-next generation tools for tissue exploration. Bioessays. (2020) 42:e1900221. doi: 10.1002/bies.201900221
40. Rodriques SG, Stickels RR, Goeva A, Martin CA, Murray E, Vanderburg CR, et al. Slide-seq: A scalable technology for measuring genome-wide expression at high spatial resolution. Science. (2019) 363:1463–7. doi: 10.1126/science.aaw1219
41. Bhattacharya I, Dey S. Emerging concepts on Leydig cell development in fetal and adult testis. Front Endocrinol (Lausanne). (2023) 13:1086276. doi: 10.3389/fendo.2022.1086276
42. Rey R, Josso N, Racine C. Sexual differentiation. In: Feingold KR, Anawalt B, Blackman MR, et al, editors. Endotext. MDText.com, Inc, South Dartmouth (MA (2000). Available at: https://www.ncbi.nlm.nih.gov/books/NBK279001/.
43. Rotgers E, Jørgensen A, Yao HH. At the crossroads of fate-somatic cell lineage specification in the fetal gonad. Endocr Rev. (2018) 39:739–59. doi: 10.1210/er.2018-00010
44. Hancock GV, Wamaitha SE, Peretz L, Clark AT. Mammalian primordial germ cell specification. Development. (2021) 148:dev189217. doi: 10.1242/dev.189217
45. Nicholls PK, Schorle H, Naqvi S, Hu YC, Fan Y, Carmell MA, et al. Mammalian germ cells are determined after PGC colonization of the nascent gonad. Proc Natl Acad Sci U.S.A. (2019) 116:25677–87. doi: 10.1073/pnas.1910733116
46. Spiller C, Koopman P, Bowles J. Sex determination in the mammalian germline. Annu Rev Genet. (2017) 51:265–85. doi: 10.1146/annurev-genet-120215-035449
47. Capel B. Vertebrate sex determination: evolutionary plasticity of a fundamental switch. Nat Rev Genet. (2017) 18:675–89. doi: 10.1038/nrg.2017.60
48. Cool J, DeFalco T, Capel B. Testis formation in the fetal mouse: dynamic and complex de novo tubulogenesis. Wiley Interdiscip Rev Dev Biol. (2012) 1:847–59. doi: 10.1002/wdev.62
49. DeFalco T, Bhattacharya I, Williams AV, Sams DM, Capel B. Yolk-sac-derived macrophages regulate fetal testis vascularization and morphogenesis. Proc Natl Acad Sci U.S.A. (2014) 111:E2384–93. doi: 10.1073/pnas.1400057111
50. Bhattacharya I, Sharma P, Purohit S, Kothiyal S, Das M, Banerjee A. Recent update on retinoic acid-driven initiation of spermatogonial differentiation. Front Cell Dev Biol. (2022) 10:833759. doi: 10.3389/fcell.2022.833759
51. Bowles J, Koopman P. Sex determination in mammalian germ cells: extrinsic versus intrinsic factors. Reproduction. (2010) 139:943–58. doi: 10.1530/REP-10-0075
52. Sekido R, Lovell-Badge R. Genetic control of testis development. Sex Dev. (2013) 7:21–32. doi: 10.1159/000342221
53. Kubota H, Brinster RL. Spermatogonial stem cells. Biol Reprod. (2018) 99:52–74. doi: 10.1093/biolre/ioy077
54. Hermann BP, Sukhwani M, Hansel MC, Orwig KE. Spermatogonial stem cells in higher primates: are there differences from those in rodents? Reproduction. (2010) 139:479–93. doi: 10.1530/REP-09-0255
55. de Rooij DG, Repping S, van Pelt AM. Role for adhesion molecules in the spermatogonial stem cell niche. Cell Stem Cell. (2008) 3:467–8. doi: 10.1016/j.stem.2008.10.010
56. de Rooij DG, Grootegoed JA. Spermatogonial stem cells. Curr Opin Cell Biol. (1998) 10:694–701. doi: 10.1016/s0955-0674(98)80109-9
57. de Rooij DG. The nature and dynamics of spermatogonial stem cells. Development. (2017) 144:3022–30. doi: 10.1242/dev.146571
58. Billmyre KK. Chromosome-specific behaviors during early meiosis. Curr Top Dev Biol. (2023) 151:127–54. doi: 10.1016/bs.ctdb.2022.05.002
59. Larose H, Kent T, Ma Q, Shami AN, Harerimana N, Li JZ, et al. Regulation of meiotic progression by Sertoli-cell androgen signaling. Mol Biol Cell. (2020) 31:2841–62. doi: 10.1091/mbc.E20-05-0334
60. Hao SL, Ni FD, Yang WX. The dynamics and regulation of chromatin remodeling during spermiogenesis. Gene. (2019) 706:201–10. doi: 10.1016/j.gene.2019.05.027
61. Griswold MD. 50 years of spermatogenesis: Sertoli cells and their interactions with germ cells. Biol Reprod. (2018) 99:87–100. doi: 10.1093/biolre/ioy027
62. Walker WH, Cheng J. FSH and testosterone signaling in Sertoli cells. Reproduction. (2005) 130:15–28. doi: 10.1530/rep.1.00358
63. Karl J, Capel B. Sertoli cells of the mouse testis originate from the coelomic epithelium. Dev Biol. (1998) 203:323–33. doi: 10.1006/dbio.1998.9068
64. Zhao L, Yao C, Xing X, Jing T, Li P, Zhu Z, et al. Single-cell analysis of developing and azoospermia human testicles reveals central role of Sertoli cells. Nat Commun. (2020) 11:5683. doi: 10.1038/s41467-020-19414-4
65. Bhattacharya I, Basu S, Pradhan BS, Sarkar H, Nagarajan P, Majumdar SS. Testosterone augments FSH signaling by upregulating the expression and activity of FSH-Receptor in Pubertal Primate Sertoli cells. Mol Cell Endocrinol. (2019) 482:70–80. doi: 10.1016/j.mce.2018.12.012
66. Sharpe RM, McKinnell C, Kivlin C, Fisher JS. Proliferation and functional maturation of Sertoli cells, and their relevance to disorders of testis function in adulthood. Reproduction. (2003) 125:769–84. doi: 10.1530/rep.0.1250769
67. Bhattacharya I, Sharma SS, Sarkar H, Gupta A, Pradhan BS, Majumdar SS. FSH mediated cAMP signalling upregulates the expression of Gα subunits in pubertal rat Sertoli cells. Biochem Biophys Res Commun. (2021) 569:100–5. doi: 10.1016/j.bbrc.2021.06.094
68. Bhattacharya I, Basu S, Sarda K, Gautam M, Nagarajan P, Pradhan BS, et al. Low levels of Gαs and Ric8b in testicular sertoli cells may underlie restricted FSH action during infancy in primates. Endocrinology. (2015) 156:1143–55. doi: 10.1210/en.2014-1746
69. Bhattacharya I, Pradhan BS, Sarda K, Gautam M, Basu S, Majumdar SS. A switch in Sertoli cell responsiveness to FSH may be responsible for robust onset of germ cell differentiation during prepubartal testicular maturation in rats. Am J Physiol Endocrinol Metab. (2012) 303:E886–98. doi: 10.1152/ajpendo.00293.2012
70. Majumdar SS, Sarda K, Bhattacharya I, Plant TM. Insufficient androgen and FSH signaling may be responsible for the azoospermia of the infantile primate testes despite exposure to an adult-like hormonal milieu. Hum Reprod. (2012) 27:2515–25. doi: 10.1093/humrep/des184
71. Pradhan BS, Bhattacharya I, Sarkar R, Majumdar SS. Downregulation of sostdc1 in testicular sertoli cells is prerequisite for onset of robust spermatogenesis at puberty. Sci Rep. (2019) 9:11458. doi: 10.1038/s41598-019-47930-x
72. Pradhan BS, Bhattacharya I, Sarkar R, Majumdar SS. Pubertal down-regulation of Tetraspanin 8 in testicular Sertoli cells is crucial for male fertility. Mol Hum Reprod. (2020) 26:760–72. doi: 10.1093/molehr/gaaa055
73. Gupta A, Vats A, Ghosal A, Mandal K, Sarkar R, Bhattacharya I, et al. Follicle-stimulating hormone-mediated decline in miR-92a-3p expression in pubertal mice Sertoli cells is crucial for germ cell differentiation and fertility. Cell Mol Life Sci. (2022) 79:136. doi: 10.1007/s00018-022-04174-9
74. Heinrich A, Bhandary B, Potter SJ, Ratner N, DeFalco T. Cdc42 activity in Sertoli cells is essential for maintenance of spermatogenesis. Cell Rep. (2021) 37:109885. doi: 10.1016/j.celrep.2021.109885
75. Heinrich A, Potter SJ, Guo L, Ratner N, DeFalco T. Distinct roles for rac1 in sertoli cell function during testicular development and spermatogenesis. Cell Rep. (2020) 31:107513. doi: 10.1016/j.celrep.2020.03.077
76. Ma M, Yang S, Zhang Z, Li P, Gong Y, Liu L, et al. Sertoli cells from non-obstructive azoospermia and obstructive azoospermia patients show distinct morphology, Raman spectrum and biochemical phenotype. Hum Reprod. (2013) 28:1863–73. doi: 10.1093/humrep/det068
77. Plotton I, Sanchez P, Durand P, Lejeune H. Decrease of both stem cell factor and clusterin mRNA levels in testicular biopsies of azoospermic patients with constitutive or idiopathic but not acquired spermatogenic failure. Hum Reprod. (2006) 21:2340–5. doi: 10.1093/humrep/del158
78. Steger K, Rey R, Kliesch S, Louis F, Schleicher G, Bergmann M. Immunohistochemical detection of immature Sertoli cell markers in testicular tissue of infertile adult men: a preliminary study. Int J Androl. (1996) 19:122–8. doi: 10.1111/j.1365-2605.1996.tb00448.x
79. Paduch DA, Hilz S, Grimson A, Schlegel PN, Jedlicka AE, Wright WW. Aberrant gene expression by Sertoli cells in infertile men with Sertoli cell-only syndrome [published correction appears in PLoS One. 2019 Aug 20;14(8):e0221648]. PloS One. (2019) 14:e0216586. doi: 10.1371/journal.pone.0216586
80. Potter SJ, DeFalco T. Role of the testis interstitial compartment in spermatogonial stem cell function. Reproduction. (2017) 153:R151–62. doi: 10.1530/REP-16-0588
81. Mishra J, Gautam M, Dadhich R, Kowtharapu BS, Majumdar SS. Peritubular cells may modulate Leydig cell-mediated testosterone production through a nonclassic pathway. Fertil Steril. (2012) 98:1308–17.e1. doi: 10.1016/j.fertnstert.2012.07.1124
82. Zhang T, Sun P, Geng Q, Fan H, Gong Y, Hu Y, et al. Disrupted spermatogenesis in a metabolic syndrome model: the role of vitamin A metabolism in the gut-testis axis. Gut. (2022) 71:78–87. doi: 10.1136/gutjnl-2020-323347
83. Zirkin BR, Papadopoulos V. Leydig cells: formation, function, and regulation. Biol Reprod. (2018) 99:101–11. doi: 10.1093/biolre/ioy059
84. Gu X, Heinrich A, Li SY, DeFalco T. Testicular macrophages are recruited during a narrow fetal time window and promote organ-specific developmental functions. Nat Commun. (2023) 14:1439. doi: 10.1038/s41467-023-37199-0
85. Guan X, Chen P, Ji M, Wen X, Chen D, Zhao X, et al. Identification of rat testicular leydig precursor cells by single-cell-RNA-sequence analysis. Front Cell Dev Biol. (2022) 10:805249. doi: 10.3389/fcell.2022.805249
86. Choubey M, Ranjan A, Bora PS, Baltazar F, Martin LJ, Krishna A. Role of adiponectin as a modulator of testicular function during aging in mice. Biochim Biophys Acta Mol Basis Dis. (2019) 1865:413–27. doi: 10.1016/j.bbadis.2018.11.019
87. Choubey M, Ranjan A, Bora PS, Krishna A. Protective role of adiponectin against testicular impairment in high-fat diet/streptozotocin-induced type 2 diabetic mice. Biochimie. (2020) 168:41–52. doi: 10.1016/j.biochi.2019.10.014
88. Choubey M, Ranjan A, Krishna A. Adiponectin/AdipoRs signaling as a key player in testicular aging and associated metabolic disorders. Vitam Horm. (2021) 115:611–34. doi: 10.1016/bs.vh.2020.12.024
89. Bhushan S, Meinhardt A. The macrophages in testis function. J Reprod Immunol. (2017) 119:107–12. doi: 10.1016/j.jri.2016.06.008
90. DeFalco T, Potter SJ, Williams AV, Waller B, Kan MJ, Capel B. Macrophages contribute to the spermatogonial niche in the adult testis. Cell Rep. (2015) 12:1107–19. doi: 10.1016/j.celrep.2015.07.015
91. Gu X, Li SY, DeFalco T. Immune and vascular contributions to organogenesis of the testis and ovary. FEBS J. (2022) 289:2386–408. doi: 10.1111/febs.15848
92. Sargent KM, McFee RM, Spuri Gomes R, Cupp AS. Vascular endothelial growth factor A: just one of multiple mechanisms for sex-specific vascular development within the testis? J Endocrinol. (2015) 227:R31–50. doi: 10.1530/JOE-15-0342
93. Bhang DH, Kim BJ, Kim BG, Schadler K, Baek KH, Kim YH, et al. Testicular endothelial cells are a critical population in the germline stem cell niche. Nat Commun. (2018) 9:4379. doi: 10.1038/s41467-018-06881-z
94. Guo F, Yan L, Guo H, Li L, Hu B, Zhao Y, et al. The transcriptome and DNA methylome landscapes of human primordial germ cells. Cell. (2015) 161:1437–52. doi: 10.1016/j.cell.2015.05.015
95. Guo J, Grow EJ, Yi C, Mlcochova H, Maher GJ, Lindskog C, et al. Chromatin and single-cell RNA-seq profiling reveal dynamic signaling and metabolic transitions during human spermatogonial stem cell development. Cell Stem Cell. (2017) 21:533–46. e6. doi: 10.1016/j.stem.2017.09.003
96. Neuhaus N, Yoon J, Terwort N, Kliesch S, Seggewiss J, Huge A, et al. Single-cell gene expression analysis reveals diversity among human spermatogonia. Mol Hum Reprod. (2017) 23:79–90. doi: 10.1093/molehr/gaw079
97. Wang M, Liu X, Chang G, Chen Y, An G, Yan L, et al. Single-cell RNA-seq analysis maps development of human germline cells and gonadal niche interactions. Cell Stem Cell. (2017) 20:858–873.e4. doi: 10.1016/j.stem.2017.03.007
98. Jan SZ, Vormer TL, Jongejan A, Röling MD, Silber SJ, de Rooij DG, et al. Unraveling transcriptome dynamics in human spermatogenesis. Development. (2017) 144:3659–73. doi: 10.1242/dev.152413
99. Guo J, Grow EJ, Mlcochova H, Maher GJ, Lindskog C, Nie X, et al. The adult human testis transcriptional cell atlas. Cell Res. (2018) 28:1141–57. doi: 10.1038/s41422-018-0099-2
100. Hermann BP, Cheng K, Singh A, Roa-De La Cruz L, Mutoji KN, Chen IC, et al. The mammalian spermatogenesis single-cell transcriptome, from spermatogonial stem cells to spermatids. Cell Rep. (2018) 25:1650–1667.e8. doi: 10.1016/j.celrep.2018.10.026
101. Wang M, Liu X, Chang G, Chen Y, An G, Yan L, et al. Single-cell RNA sequencing analysis reveals sequential cell fate transition during human spermatogenesis. Cell Stem Cell. (2018) 23:599–614.e4. doi: 10.1016/j.stem.2018.08.007
102. Sohni A, Tan K, Song HW, Burow D, de Rooij DG, Laurent L, et al. The neonatal and adult human testis defined at the single-cell level. Cell Rep. (2019) 26:1501–1517.e4. doi: 10.1016/j.celrep.2019.01.045
103. Guo J, Nie X, Giebler M, Mlcochova H, Wang Y, Grow EJ, et al. The dynamic transcriptional cell atlas of testis development during human puberty. Cell Stem Cell. (2020) 26:262–276.e4. doi: 10.1016/j.stem.2019.12.005
104. Shami AN, Zheng X, Munyoki SK, Ma Q, Manske GL, Green CD, et al. Single-cell RNA sequencing of human, macaque, and mouse testes uncovers conserved and divergent features of mammalian spermatogenesis. Dev Cell. (2020) 54:529–547.e12. doi: 10.1016/j.devcel.2020.05.010
105. Lau X, Munusamy P, Ng MJ, Sangrithi M. Single-cell RNA sequencing of the cynomolgus macaque testis reveals conserved transcriptional profiles during mammalian spermatogenesis. Dev Cell. (2020) 54:548–566.e7. doi: 10.1016/j.devcel.2020.07.018
106. Liu X, Chen Y, Tang W, Zhang L, Chen W, Yan Z, et al. Single-cell transcriptome analysis of the novel coronavirus (SARS-CoV-2) associated gene ACE2 expression in normal and non-obstructive azoospermia (NOA) human male testes. Sci China Life Sci. (2020) 63:1006–15. doi: 10.1007/s11427-020-1705-0
107. Tan K, Song HW, Thompson M, Munyoki S, Sukhwani M, Hsieh TC, et al. Transcriptome profiling reveals signaling conditions dictating human spermatogonia fate in vitro. Proc Natl Acad Sci U.S.A. (2020) 117:17832–41. doi: 10.1073/pnas.2000362117
108. Xia B, Yan Y, Baron M, Wagner F, Barkley D, Chiodin M, et al. Widespread transcriptional scanning in the testis modulates gene evolution rates. Cell. (2020) 180:248–262.e21. doi: 10.1016/j.cell.2019.12.015
109. Hwang YS, Suzuki S, Seita Y, Ito J, Sakata Y, Aso H, et al. Reconstitution of prospermatogonial specification in vitro from human induced pluripotent stem cells. Nat Commun. (2020) 11:5656. doi: 10.1038/s41467-020-19350-3
110. Guo J, Sosa E, Chitiashvili T, Nie X, Rojas EJ, Oliver E, et al. Single-cell analysis of the developing human testis reveals somatic niche cell specification and fetal germline stem cell establishment. Cell Stem Cell. (2021) 28:764–778.e4. doi: 10.1016/j.stem.2020.12.004
111. Chen H, Murray E, Sinha A, Laumas A, Li J, Lesman D, et al. Dissecting mammalian spermatogenesis using spatial transcriptomics. Cell Rep. (2021) 37:109915. doi: 10.1016/j.celrep.2021.109915
112. Wang R, Liu X, Li L, Yang M, Yong J, Zhai F, et al. Dissecting human gonadal cell lineage specification and sex determination using A single-cell RNA-seq approach. Genomics Proteomics Bioinf. (2022) 20:223–45. doi: 10.1016/j.gpb.2022.04.002
113. Nie X, Munyoki SK, Sukhwani M, Schmid N, Missel A, Emery BR, et al. Single-cell analysis of human testis aging and correlation with elevated body mass index. Dev Cell. (2022) 57:1160–1176.e5. doi: 10.1016/j.devcel.2022.04.004
114. Huang D, Zuo Y, Zhang C, Sun G, Jing Y, Lei J, et al. A single-nucleus transcriptomic atlas of primate testicular aging reveals exhaustion of the spermatogonial stem cell reservoir and loss of Sertoli cell homeostasis. Protein Cell. (2023) 14:888–907. doi: 10.1093/procel/pwac057
115. Murat F, Mbengue N, Winge SB, Trefzer T, Leushkin E, Sepp M, et al. The molecular evolution of spermatogenesis across mammals. Nature. (2023) 613:308–16. doi: 10.1038/s41586-022-05547-7
116. Huang Y, Li L, An G, Yang X, Cui M, Song X, et al. Single-cell multi-omics sequencing of human spermatogenesis reveals a DNA demethylation event associated with male meiotic recombination. Nat Cell Biol. (2023) 25:1520–34. doi: 10.1038/s41556-023-01232-7
117. de Castro P, Vendrell X, Escrich L, Grau N, Gonzalez-Martin R, Quiñonero A, et al. Comparative single-cell transcriptomic profiles of human androgenotes and parthenogenotes during early development. Fertil Steril. (2023) 119:675–87. doi: 10.1016/j.fertnstert.2022.12.027
118. Singh A, Hermann BP. Conserved transcriptome features define prepubertal primate spermatogonial stem cells as adark spermatogonia and identify unique regulators. Int J Mol Sci. (2023) 24:4755. doi: 10.3390/ijms24054755
119. Rajachandran S, Zhang X, Cao Q, Caldeira-Brant AL, Zhang X, Song Y, et al. Dissecting the spermatogonial stem cell niche using spatial transcriptomics. Cell Rep. (2023) 42:112737. doi: 10.1016/j.celrep.2023.112737
120. Lukassen S, Bosch E, Ekici AB, Winterpacht A. Single-cell RNA sequencing of adult mouse testes. Sci Data. (2018) 5:180192. doi: 10.1038/sdata.2018.192
121. Lukassen S, Bosch E, Ekici AB, Winterpacht A. Characterization of germ cell differentiation in the male mouse through single-cell RNA sequencing. Sci Rep. (2018) 8:6521. doi: 10.1038/s41598-018-24725-0
122. Green CD, Ma Q, Manske GL, Shami AN, Zheng X, Marini S, et al. A comprehensive roadmap of murine spermatogenesis defined by single-cell RNA-seq. Dev Cell. (2018) 46:651–667.e10. doi: 10.1016/j.devcel.2018.07.025
123. Stévant I, Neirijnck Y, Borel C, Escoffier J, Smith LB, Antonarakis SE, et al. Deciphering cell lineage specification during male sex determination with single-cell RNA sequencing. Cell Rep. (2018) 22:1589–99. doi: 10.1016/j.celrep.2018.01.043
124. Grive KJ, Hu Y, Shu E, Grimson A, Elemento O, Grenier JK, et al. Dynamic transcriptome profiles within spermatogonial and spermatocyte populations during postnatal testis maturation revealed by single-cell sequencing. PloS Genet. (2019) 15:e1007810. doi: 10.1371/journal.pgen.1007810
125. Tan K, Song HW, Wilkinson MF. Single-cell RNAseq analysis of testicular germ and somatic cell development during the perinatal period. Development. (2020) 147:dev183251. doi: 10.1242/dev.183251
126. Zhao Y, Zhang P, Ge W, Feng Y, Li L, Sun Z, et al. Alginate oligosaccharides improve germ cell development and testicular microenvironment to rescue busulfan disrupted spermatogenesis. Theranostics. (2020) 10:3308–24. doi: 10.7150/thno.43189
127. Kalucka J, de Rooij LPMH, Goveia J, Rohlenova K, Dumas SJ, Meta E, et al. Single-cell transcriptome atlas of murine endothelial cells. Cell. (2020) 180:764–779.e20. doi: 10.1016/j.cell.2020.01.015
128. Mayère C, Neirijnck Y, Sararols P, Rands CM, Stévant I, Kühne F, et al. Single-cell transcriptomics reveals temporal dynamics of critical regulators of germ cell fate during mouse sex determination. FASEB J. (2021) 35:e21452. doi: 10.1096/fj.202002420R
129. Yang F, Whelan EC, Guan X, Deng B, Wang S, Sun J, et al. FGF9 promotes mouse spermatogonial stem cell proliferation mediated by p38 MAPK signalling. Cell Prolif. (2021) 54:e12933. doi: 10.1111/cpr.12933
130. Zhang W, Xia S, Xiao W, Song Y, Tang L, Cao M, et al. A single-cell transcriptomic landscape of mouse testicular aging. J Adv Res. (2023) 53:219–34. doi: 10.1016/j.jare.2022.12.007
131. Guan X, Ji M, Wen X, Huang F, Zhao X, Chen D, et al. Single-cell RNA sequencing of adult rat testes after Leydig cell elimination and restoration. Sci Data. (2022) 9:106. doi: 10.1038/s41597-022-01225-5
132. Hong S, Shen X, Luo C, Sun F. Comparative analysis of the testes from wild-type and Alkbh5-knockout mice using single-cell RNA sequencing. G3 (Bethesda). (2022) 12:jkac130. doi: 10.1093/g3journal/jkac130
133. Peng YJ, Tang XT, Shu HS, Dong W, Shao H, Zhou BO. Sertoli cells are the source of stem cell factor for spermatogenesis. Development. (2023) 150:dev200706. doi: 10.1242/dev.200706
134. Suen HC, Rao S, Luk ACS, Zhang R, Yang L, Qi H, et al. The single-cell chromatin accessibility landscape in mouse perinatal testis development. Elife. (2023) 12:e75624. doi: 10.7554/eLife.75624
135. Kavarthapu R, Anbazhagan R, Pal S, Dufau ML. Single-cell transcriptomic profiling of the mouse testicular germ cells reveals important role of phosphorylated GRTH/DDX25 in round spermatid differentiation and acrosome biogenesis during spermiogenesis. Int J Mol Sci. (2023) 24:3127. doi: 10.3390/ijms24043127
136. Jin C, Wang Z, Li P, Tang J, Jiao T, Li Y, et al. Decoding the spermatogonial stem cell niche under physiological and recovery conditions in adult mice and humans. Sci Adv. (2023) 9:eabq3173. doi: 10.1126/sciadv.abq3173
137. Tan H, Wang W, Zhou C, Wang Y, Zhang S, Yang P, et al. Single-cell RNA-seq uncovers dynamic processes orchestrated by RNA-binding protein DDX43 in chromatin remodeling during spermiogenesis. Nat Commun. (2023) 14:2499. doi: 10.1038/s41467-023-38199-w
138. Walters BW, Rainsford SR, Heuer RA, Dias N, Huang X, de Rooij D, et al. KDM6A/UTX promotes spermatogenic gene expression across generations and is not required for male fertility†. Biol Reprod. (2024) 110:391–407. doi: 10.1093/biolre/ioad141
139. Laurentino S, Heckmann L, Di Persio S, Li X, Meyer Zu Hörste G. High-resolution analysis of germ cells from men with sex chromosomal aneuploidies reveals normal transcriptome but impaired imprinting. Clin Epigenet. (2019) 11:127. doi: 10.1186/s13148-019-0720-3
140. Winge SB, Soraggi S, Schierup MH, Rajpert-De Meyts E, Almstrup K. Integration and reanalysis of transcriptomics and methylomics data derived from blood and testis tissue of men with 47,XXY Klinefelter syndrome indicates the primary involvement of Sertoli cells in the testicular pathogenesis. Am J Med Genet C Semin Med Genet. (2020) 184:239–55. doi: 10.1002/ajmg.c.31793
141. Chen S, An G, Wang H, Wu X, Ping P, Hu L, et al. Human obstructive (postvasectomy) and nonobstructive azoospermia - Insights from scRNA-Seq and transcriptome analysis. Genes Dis. (2020) 9:766–76. doi: 10.1016/j.gendis.2020.09.004
142. Wang M, Xu Y, Zhang Y, Chen Y, Chang G, An G, et al. Deciphering the autophagy regulatory network via single-cell transcriptome analysis reveals a requirement for autophagy homeostasis in spermatogenesis. Theranostics. (2021) 11:5010–27. doi: 10.7150/thno.55645
143. Alfano M, Tascini AS, Pederzoli F, Locatelli I, Nebuloni M, Giannese F, et al. Aging, inflammation and DNA damage in the somatic testicular niche with idiopathic germ cell aplasia. Nat Commun. (2021) 12:5205. doi: 10.1038/s41467-021-25544-0
144. Di Persio S, Tekath T, Siebert-Kuss LM, Cremers JF, Wistuba J, Li X, et al. Single-cell RNA-seq unravels alterations of the human spermatogonial stem cell compartment in patients with impaired spermatogenesis. Cell Rep Med. (2021) 2:100395. doi: 10.1016/j.xcrm.2021.100395
145. Mahyari E, Guo J, Lima AC, Lewinsohn DP, Stendahl AM, Vigh-Conrad KA, et al. Comparative single-cell analysis of biopsies clarifies pathogenic mechanisms in Klinefelter syndrome. Am J Hum Genet. (2021) 108:1924–45. doi: 10.1016/j.ajhg.2021.09.001
146. Tang XJ, Xiao QH, Wang XL, He Y, Tian YN, Xia BT, et al. Single-cell transcriptomics-based study of transcriptional regulatory features in the non-obstructive azoospermia testis. Front Genet. (2022) 13:875762. doi: 10.3389/fgene.2022.875762
Keywords: single-cell RNA-sequencing (Sc-RNA-seq), testis, spatial transcriptomics, spermatogenesis, male infertility
Citation: Tirumalasetty MB, Bhattacharya I, Mohiuddin MS, Baki VB and Choubey M (2024) Understanding testicular single cell transcriptional atlas: from developmental complications to male infertility. Front. Endocrinol. 15:1394812. doi: 10.3389/fendo.2024.1394812
Received: 02 March 2024; Accepted: 14 June 2024;
Published: 11 July 2024.
Edited by:
Maria Eugenia Teves, Virginia Commonwealth University, United StatesReviewed by:
Carlos Córdova-Fletes, Universidad Autonoma de Nuevo León, MexicoShivani Srivastava, Yale University, United States
Copyright © 2024 Tirumalasetty, Bhattacharya, Mohiuddin, Baki and Choubey. This is an open-access article distributed under the terms of the Creative Commons Attribution License (CC BY). The use, distribution or reproduction in other forums is permitted, provided the original author(s) and the copyright owner(s) are credited and that the original publication in this journal is cited, in accordance with accepted academic practice. No use, distribution or reproduction is permitted which does not comply with these terms.
*Correspondence: Mayank Choubey, Y2hvdWJleW1heWFuazQ4QGdtYWlsLmNvbQ==; TWF5YW5rLkNob3ViZXlAbnl1bGFuZ29uZS5vcmc=
†These authors have contributed equally to this work