- 1Department of Plastic and Burn Surgery, West China Hospital, Sichuan University, Chengdu, China
- 2Department of Plastic & Reconstructive Surgery, Shanghai Ninth People’s Hospital, Shanghai Jiao Tong University School of Medicine, Shanghai, China
Adipose tissue (AT) serves as an energy-capacitive organ and performs functions involving paracrine- and endocrine-mediated regulation via extracellular vesicles (EVs) secretion. Exosomes, a subtype of EVs, contain various bioactive molecules with regulatory effects, such as nucleic acids, proteins, and lipids. AT-derived exosomes (AT-exos) include exosomes derived from various cells in AT, including adipocytes, adipose-derived stem cells (ADSCs), macrophages, and endothelial cells. This review aimed to comprehensively evaluate the impacts of different AT-exos on the regulation of physiological and pathological processes. The contents and functions of adipocyte-derived exosomes and ADSC-derived exosomes are compared simultaneously, highlighting their similarities and differences. The contents of AT-exos have been shown to exert complex regulatory effects on local inflammation, tumor dynamics, and insulin resistance. Significantly, differences in the cargoes of AT-exos have been observed among diabetes patients, obese individuals, and healthy individuals. These differences could be used to predict the development of diabetes mellitus and as therapeutic targets for improving insulin sensitivity and glucose tolerance. However, further research is needed to elucidate the underlying mechanisms and potential applications of AT-exos.
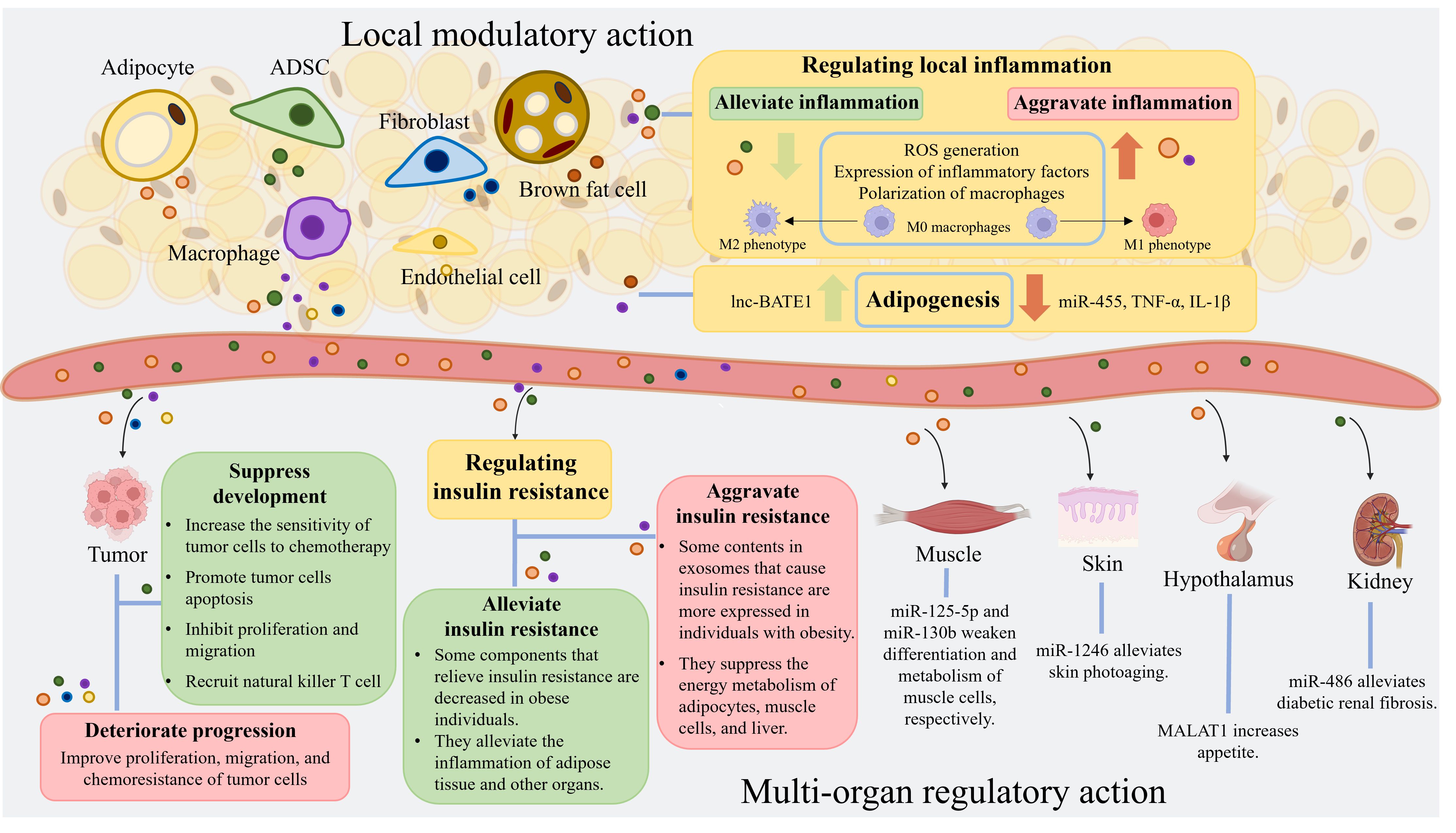
Graphical Abstract. Adipose tissue comprises a variety of cells, including adipocytes, adipose-derived stem cells, macrophages, fibroblasts, and endothelial cells. These cells produce exosomes that collectively constitute adipose tissue-derived exosomes. These exosomes from adipose tissue are rich in RNA, proteins, lipids, and other components. They have diverse regulatory effects on local inflammation, adipogenesis, tumor progression, and insulin resistance levels. Furthermore, adipose tissue-derived exosomes can impact the physiological and pathological processes of muscles, skin, hypothalamus, and kidneys. (Created with BioRender.com). ADSC, adipose-derived stem cell; miRNA, microRNA; ROS, reactive oxygen species; lnc-BATE1, brown adipose tissue enriched long non-coding RNA 1; TNF-α, tumor necrosis factor-α; IL-1β, interleukin-1β; MALAT1, metastasis-associated lung adenocarcinoma transcript 1.ADSC, adipose-derived stem cell; miRNA, microRNA; ROS, reactive oxygen species; lnc-BATE1, brown adipose tissue enriched long non-coding RNA 1; TNF-α, tumor necrosis factor-α; IL-1β, interleukin-1β; MALAT1, metastasis-associated lung adenocarcinoma transcript 1.
1 Introduction
Adipose tissue (AT) is an important energy storage organ and a vital endocrine organ that regulates the functions of other tissues and organs by secreting various signaling molecules via extracellular vesicles (EVs). These EVs affect neighboring tissues [skin (1) and muscles (2)] and distant organs (heart, lung, liver, and pancreas (3–6)). A study involving 101 patients revealed that 0.2% of serum EVs, which are derived from tissue (7), contain high levels of adipocyte proteins and adipokines (8). AT plays a specific role in the secretion of EVs harboring adipocyte proteins and adipokines, which could have systemic effects on various diseases and physiological processes.
Secretion of EVs is an important mechanism through which cells exert regulatory effects and interact with other cells and organs. EVs are categorized as exosomes, ectosomes, and apoptotic EVs based on their mode of secretion (9); this classification system differs from the previous method of categorizing EVs by size (larger EVs > 200 nm and smaller EVs < 200 nm) (10). Exosomes are formed through the inward budding of the cytomembrane (similar to endocytosis) and organelle membrane, involving the endoplasmic reticulum, Golgi apparatus, and other organelles (11). As a subtype of EVs, exosomes perform primary regulatory and therapeutic functions through their contents, including RNAs (microRNA (miRNA), long noncoding RNA (lncRNA), circular RNA, and mRNA), DNA, proteins, and lipids (12). In addition, mitochondrial components and ceramides have been identified as exosomal cargoes that participate in the regulation of the Wnt and MAPK signaling pathways (13).
AT is composed of various cells, including adipocytes, adipose-derived stem cells (ADSCs), AT macrophages (ATMs), endothelial cells, progenitors, and preadipocytes. Many studies have focused on adipocyte-derived exosomes (adipocyte-exos), ADSC-derived exosomes (ADSC-exos), and ATM-derived exosomes (ATM-exos) and studied the roles of these exosomes separately. Adipocyte-exos account for a major proportion of AT-derived exosomes (AT-exos). Additionally, ADSC-exos have been shown to have meaningful therapeutic effects on multiple diseases (14–17). Extensive evidence has demonstrated that exosomes derived from ADSCs and adipocytes can regulate localized inflammation and metabolic diseases (3, 17), promote wound healing (18, 19), and affect tumor growth and migration (20, 21). However, ADSC-exos are significantly more effective at promoting angiogenesis and protecting the heart and blood vessels than adipocyte-exos. The diameters, different surface features, and major RNAs and lipid components of adipocyte-exos and ADSC-exos are summarized in Figure 1. The majority of proteins in adipocyte-exos are mitochondrial fatty acid oxidase enzymes, such as trifunctional enzyme subunit alpha and hydroxyacyl-coenzyme A dehydrogenase (22). ADSC-exos contain various growth factors and enzymes associated with glycolysis, such as phosphoglucomutase, phosphoglycerate kinase, glyceraldehyde 3-phosphate dehydrogenase, enolase, and pyruvate kinase m2 isoform (23), as well as enzymes with dephosphorylation functions (24). Moreover, ATM-exos can play a regulatory role in insulin resistance (25). Furthermore, all of the cells mentioned above produce exosomes, which constitute AT-exos. In summary, AT-exos is complex.
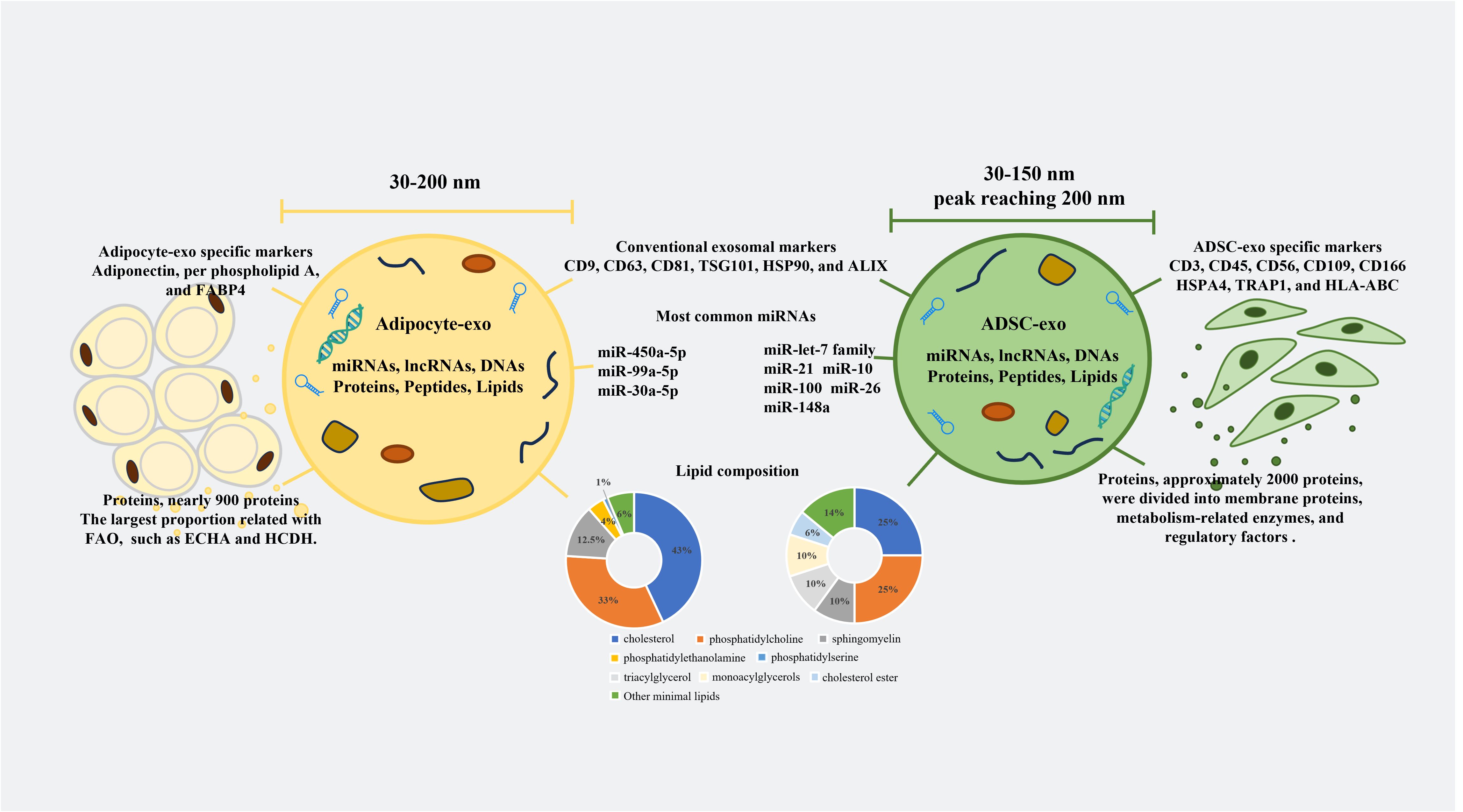
Figure 1. Differences between adipocyte-exos and ADSC-exos. The diameters of the adipocyte-exos were from 30 nm to 200 nm. However, the diameters of the ADSC-exos ranged from 30 nm to 150 nm, with the peak diameters reaching 200 nm. In addition to conventional exosomal markers, both adipocyte-exos and ADSC-exos have unique exosomal markers. The compositions of nucleic acids, proteins, and lipids in the adipocyte-exos and ADSC-exos were also different. FABP4, fatty acid binding protein 4; FAO, fatty acid oxidation; ECHA, trifunctional enzyme subunit alpha; HCDH, hydroxy carboxylic acid dehydrogenase; TSG101, tumor susceptibility gene 101; HSP90, heat shock protein 90; ALIX, ALG-2 interacting protein X; HSPA4, heat shock protein A4; TRAP1, tumor necrosis factor receptor-associated protein 1; HLA-ABC, human leukocyte antigen-ABC.
AT-exos potentially exert regulatory and therapeutic effects on numerous diseases, such as diabetic wounds (19), insulin resistance (26), angiogenesis (27), and nonalcoholic steatohepatitis (28). Different components in AT-exos may regulate the same disease or physiological process in various directions. For different populations, the cargoes delivered by AT-exos and their regulatory effects can vary greatly. For instance, the quantity of AT-exos, especially adipocyte-exos, in obese individuals is significantly increased (29). Moreover, substances within adipocyte-exos, ADSC-exos, and ATM-exos that can alleviate tissue inflammation and mitigate insulin resistance are markedly reduced (30), while those that exacerbate tissue inflammation and worsen insulin resistance are noticeably increased in adipocyte-exos and ATM-exos (31). Consequently, the AT-exos in obese individuals significantly intensify inflammation in local AT and lead to systemic insulin resistance. Because AT-exos is complex, focusing on the effects of AT-exos will provide a more comprehensive and objective understanding than studying one type of cell-derived exosomes in isolation when studying the impact of AT on other tissues and organs.
2 The regulation of inflammation by exosomes derived from AT in the local microenvironment
In the local microenvironment, adipocyte-exos, ADSC-exos, and ATM-exos carry multiple miRNAs and proteins that participate in regulating inflammation. Among these cargoes, some RNAs and proteins can alleviate inflammation, while others can exacerbate inflammation. Notably, the ability of AT-exos to regulate inflammation varies among different populations. AT-exos from obese and aged individuals are more likely to exacerbate inflammation (17, 32).
2.1 The influence of RNAs derived from AT-exos on regulating inflammation
Some RNAs in adipocyte-exos and ADSC-exos can alleviate inflammation in the local microenvironment. Nine adipocyte-exo-derived miRNAs (miR-26a, miR-92a (33), miR-126, miR-143, miR-193a, miR-193b, miR-652, miR-let-7a, and miR-let-7d) can repress the production of C-C motif ligand 2 (CCL2), which induces the polarization of macrophages to the proinflammatory phenotype and is present in higher levels in the serum of obese individuals than in lean individuals (34). miRNAs in ADSC-exos exert a significant effect on inducing M2 macrophage polarization. Activation of NOD-like receptor protein 3 was also proven to be suppressed by ADSC-derived EVs in previous studies (35, 36). In addition, compared to those in old mice, the ADSC-exos in young mice contained higher levels of miR-125b-5p, miR-214-3p, and miR-let7c-5p, which may explain the decreased expression of inflammatory markers and the regeneration of muscle and kidney in old mice injected with ADSC-exos from young mice (17).
AT-exos, including both adipocyte-exos and ADSC-exos, also contain miRNAs that exacerbate inflammation in the AT microenvironment. Moreover, a study confirmed that ADSC-exos also promote macrophage infiltration by upregulating the levels of monocyte chemoattractant protein-1 and macrophage inflammatory protein-1α in a fat transfer experiment (37).
2.2 The effects of exosomal proteins and lipids on the regulation of inflammation
The proteins in adipocyte-exos that are implicated in the regulation of inflammation include adipokines (adiponectin, leptin, resistin, and autotaxin), cytokines (interleukin (IL)-6, IL-1β, IL-8, CCL2, CCL5, and TNF-α), and adipsin (38, 39). Among the proteins in adipocyte-exos, adiponectin significantly affects anti-inflammation and is present in higher levels in the AT space and plasma of lean individuals (40). These cytokines in adipocyte-exos have apparent proinflammatory effects (40). For ADSCs, ADSC-exos carry proinflammatory cytokines (IL-1β, IL-7, IL-8, IL-9, IL-11, IL-12, IL-15, IL-17, IFN-γ, and TNF-α) and anti-inflammatory factors (IL-1Ra, IL-4, IL-10, and IL-13) (41), while IL-2, IL-6, and adipsin have both proinflammatory and anti-inflammatory characteristics (42, 43).
Upon the stimulation of M1 polarization, compared with adiponectin-negative EVs, adiponectin-positive EVs derived from adipocytes promote monocyte differentiation into ATM through the secretion of TNF-α, macrophage colony-stimulating factor, and retinol-binding protein 4 (44). A separate study indicated that retinol-binding protein 4 in adipocyte-exos facilitates the M1 polarization of monocytes and the production of proinflammatory cytokines. Furthermore, levels of exosomal retinol-binding protein 4 are elevated in obese mice (30).
The functional RNAs, proteins, and lipids in exosomes derived from AT that regulate the inflammation of the microenvironment are listed in Table 1.
3 The regulatory effect of exosomes derived from AT on tumors
AT-exos, including adipocyte-exos, ADSC-exos, ATM-exos, endothelial cell-derived exosomes, and exosomes from other AT-derived cells, can affect both adjacent and distant tumor cells. To promote tumor growth, adipocyte-exos can be transported into adjacent tumor cells, such as breast cancer (BC) cells, and improve the proliferation, migration, and chemoresistance of BC cells; this effect is related to stimulating the expression of YAP and TAZ, which are two key downstream proteins of the Hippo signaling pathway (70). In a study on triple-negative BC, cancer-associated adipocytes deliver more C-X-C motif ligand 8 to adjacent BC cells compared to normal adipocytes. C-X-C motif ligand 8 upregulates the expression of the PI3K/AKT/mTOR pathway in tumor cells, promotes the proliferation and epithelial-mesenchymal transition of tumor cells, and enhances the expression of PD-L1 on the BC cell membrane, which is detrimental to the long-term prognosis of patients (71). Fatty acid binding protein 4 derived from adipocyte-exos, expressed at higher levels in metastatic ovarian cancer patients than in primary ovarian cancer patients, is considered a marker of metastatic tumor disease and a therapeutic target (72). Some miRNAs, such as let-7-a-1, miR-21, and miR-1260b, are significantly enriched in ADSC-exos from patients with cancer (73). Moreover, ADSC-exos promote tumor progression by facilitating the proliferation and migration of cancer cells. In comparison to those in the control group, ADSC-exos activates the Wnt signaling pathway in the MCF7 BC cell line and promotes the migration of cancer cells (74). Fewer microvesicles originating from the endothelial cells of BC patients are implicated in better clinical outcomes after chemotherapy (75). Endothelial cell-derived exosomes may also contribute to cancer progression. On the unfavorable aspects of tumors, ADSC-exo-derived miRNAs can suppress tumor growth by increasing the sensitivity of cancer cells to chemotherapy, reducing the expression of drug-resistance genes, promoting cancer cell apoptosis, inhibiting the tumor proliferation and migration, and recruiting natural killer T cells. ADSC-exos can recruit natural killer T cells and promote their antitumor responses in the tumor microenvironment. In an N1S1-induced hepatocellular carcinoma model, mice treated with ADSC-exos had markedly smaller tumor volumes and more circulating and intratumoral natural killer T cells than the control mice (76). In brief, AT-exos can suppress tumor development and promote the proliferation, migration, and drug resistance of tumor cells through the delivery of miRNAs, proteins, and lipids. The contents in various AT-exos affecting tumor progression are summarized in Figure 2. The mechanisms underlying their effects are presented in Table 2.
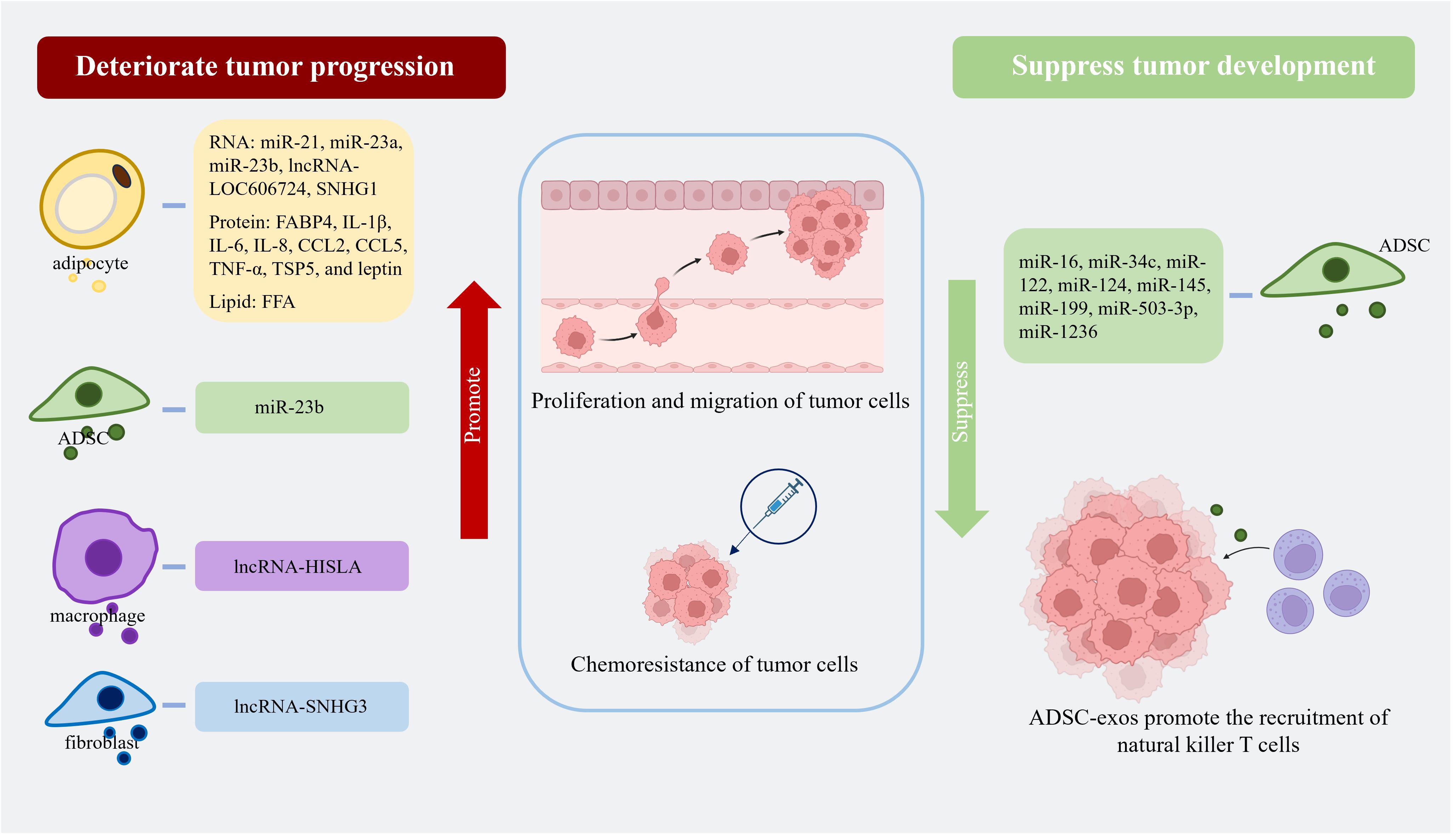
Figure 2. Adipocyte-exos, ADSC-exos, ATM-exos, and fibroblast-derived exosomes all contain substances that can promote tumor progression by enhancing the proliferation, migration, and chemoresistance of tumor cells. ADSC-exos are rich in various miRNAs that can suppress tumor growth by increasing the sensitivity of tumor cells to chemotherapy drugs, promoting apoptosis, inhibiting proliferation and migration, and recruiting natural killer T cells. (Created with BioRender.com). lncRNA-SNHG1, lncRNA-small nucleolar RNA host gene 1; FABP4, fatty acid binding protein 4; IL-1β, interleukin-1β; IL-6, interleukin-6; IL-8, interleukin-8; CCL2, C-C motif ligand 2; CCL5, C-C motif ligand 5; TNF-α, tumor necrosis factor-α; TSP5, thrombospondin family protein 5; FFA, free fatty acid; lncRNA-HISLA, lncRNA-HIF-1α-stabilizing long noncoding RNA; lncRNA-SNHG3, lncRNA-small nucleolar RNA host gene 3.
4 The effects of exosomes derived from AT on obesity and insulin resistance
As the most important energy storage organ in the human body, AT also performs strong endocrine regulatory functions. AT-exos have been proven to play a regulatory role in the sensitivity of the body to insulin through multiple signaling pathways and are strongly correlated with individual obesity.
Compared to those in healthy and lean individuals, AT-exos in the obese population exhibit significant differences. First, in terms of quantity, the AT of obese individuals and patients with insulin resistance can produce more exosomes (103). Ceramide can promote the membrane curvature of EVs and exert a significant effect on the vesicle budding process. Inhibiting ceramide production can reduce the biogenesis of EVs (104). Moreover, the budding process is influenced by palmitic acid and phospholipase D (105). Obese individuals have a greater variety of ceramides in AT (106) and excessive palmitic acid in enlarged adipocytes (107), which are conducive to the budding of multivesicular bodies in adipocytes, especially in obese individuals. Furthermore, the increased production of exosomes in AT of obese individuals mainly occurs due to increased production by adipocytes, with no significant increase by the other types of cells (108). The number of adipocyte-exos in the circulation of obese mice was approximately twofold higher than that in lean mice (29). Chronic mild inflammation, which is a biological stimulus of AT associated with obesity, might facilitate the secretion of adipocyte-exos (109). However, increased adipocyte-exos could accelerate the excretion of harmful cytoplasmic substances and prevent cellular senescence. The contents and cargoes of adipocyte-exos can also impact other cells and organs throughout the body through paracrine or endocrine effects. The implications of this phenomenon on the human body are intricate and cannot be conclusively defined as beneficial or detrimental. Moreover, the mechanism underlying the increased production of adipocyte-exos in obesity remains incompletely understood (110). The expression profiles of AT-exos, including the levels of miRNAs, proteins, and lipids, also vary between obese individuals and healthy individuals.
4.1 Differentially expressed miRNAs in obese and healthy individuals
As previously mentioned, the following miRNAs are upregulated in the adipocyte-exos of obese individuals: miR-23b, miR-27a, miR-99b, miR-122, miR-140-5p, miR-142-3p, miR-192, miR-222, miR-378a, and miR-4429 (3, 111–114). Moreover, the expression of miR-15a, miR-26a, miR-30c, miR-92a, miR-126, miR-130b, miR-138, miR-143, miR-145, miR-148b, miR-193a, miR-193b, miR-221, miR-223, miR-423-5p, miR-520c-3p, miR-652, miR-4269, miR-let-7af, and miR-let-7d is significantly decreased in the adipocyte-exos of obese individuals (31, 34, 115–119). Among the decreased miRNAs, nine could inhibit the expression of CCL2 to suppress the M1 polarization of macrophages and alleviate inflammation when expressed at higher levels in healthy individuals (34). The influence and working mechanisms of these differential miRNAs are summarized in Table 3.
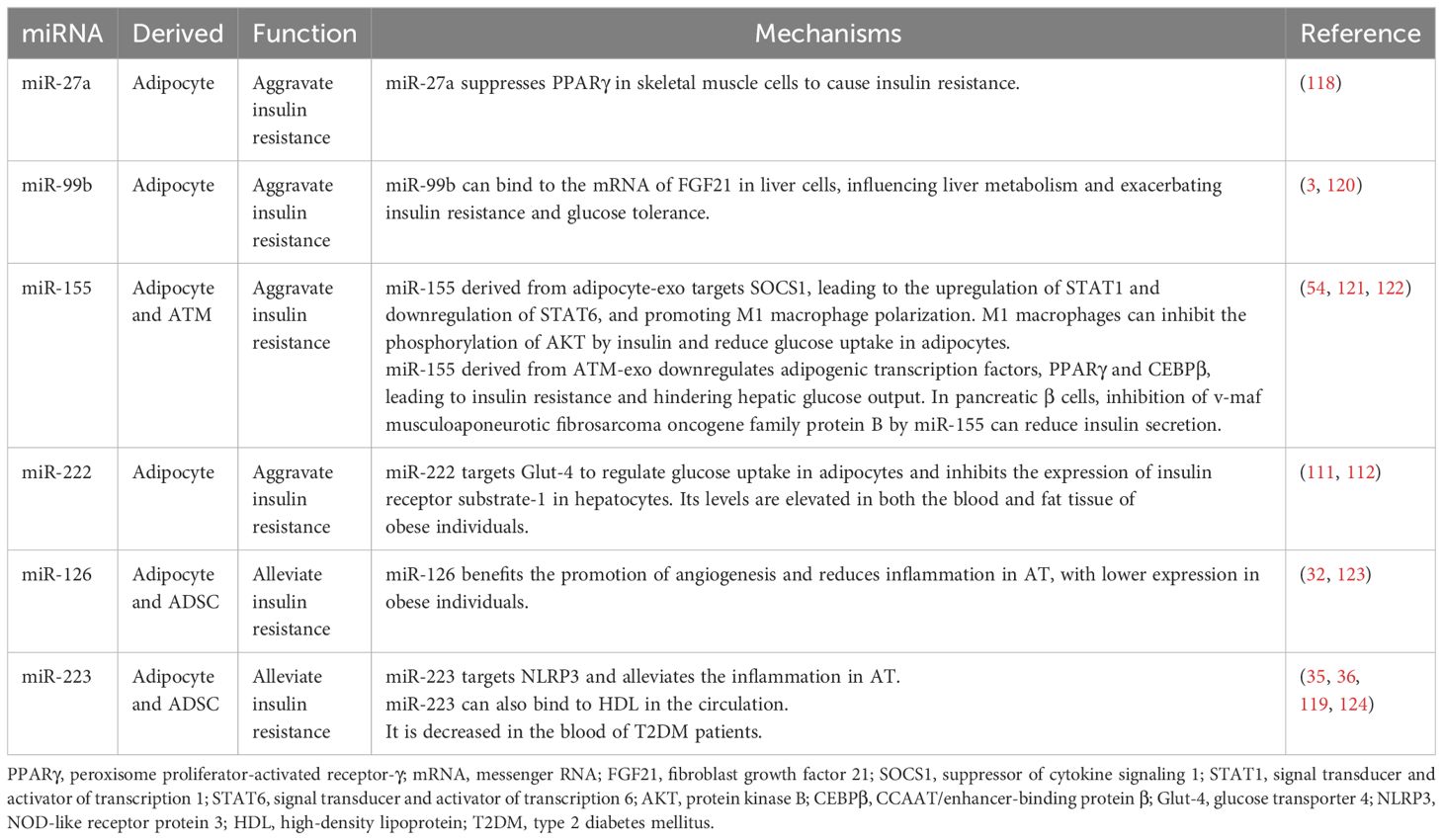
Table 3. The miRNAs in exosomes derived from AT with different expressions are related to insulin resistance and obesity.
Taken together, these data show that the aberrant expression of these miRNAs in obese individuals could worsen inflammation in AT, leading to obesity. Several plasma miRNAs derived from adipocytes could be considered predictive factors of type 2 diabetes mellitus. For example, increased miR-15b levels and decreased miR-138 levels could be considered characteristic of obese individuals compared with individuals in the normal control group (125, 126). Furthermore, a combined analysis of circulating miR-15a, miR-423-5p, and miR-520c-3p, which are downregulated in obese individuals, could predict whether a man has morbid obesity with an accuracy of up to 93.5% (115).
4.2 Differential levels of proteins and lipids between obese individuals and healthy individuals
Some proteins and lipids derived from AT-exos are also different and associated with inflammation in these two groups. As previously stated, adipocyte-exo derived IL-1β, IL-6, IL-8, CCL2, CCL5, TNF-α (3), resistin, and retinol-binding protein 4 (30) are found to be expressed at higher levels in obese individuals. These proteins are considered to be proinflammatory factors and are associated with insulin resistance, and these proteins can also facilitate the M1 polarization of monocytes (30, 40). Furthermore, macrophage migration inhibitory factor, which is expressed at higher levels in the adipocyte-exos from obese individuals (127), is an upstream regulator of the inflammatory cascade and triggers inflammatory responses via migration inhibitory factor signaling pathways (128). Moreover, the plasma levels of adipsin and neuregulin 4 (Nrg4) are decreased in overweight individuals (129, 130). Nrg4 is shown to protect against type 2 diabetes mellitus and non-alcoholic fatty liver disease because Nrg4 can positively regulate ErbB3 and ErbB4 signaling in hepatocytes and inhibit LXR and SREBP1c, promoting lipogenesis (130). In Nrg4-deficient mice with nonalcoholic steatohepatitis, cell death, inflammation, fibrosis, and liver injury are more serious because Nrg4 can positively regulate ErbB3 and ErbB4 to repress the ubiquitination and proteasomal degradation of c-FLIPL to reduce cell death (131). However, the level of palmitoleate, which has certain anti-inflammatory and insulin-sensitizing effects, is downregulated in obese and insulin-resistant mice (132). The proteins and lipids in adipocyte-exos associated with energy metabolism, lipogenesis, and insulin sensitivity are listed in Table 4. Moreover, the signal transducer and activator of transcription 3 in ADSC-exos can alleviate AT inflammation and promote the beiging of white AT to improve insulin sensitivity and glucose intolerance during high-fat diet consumption (61).
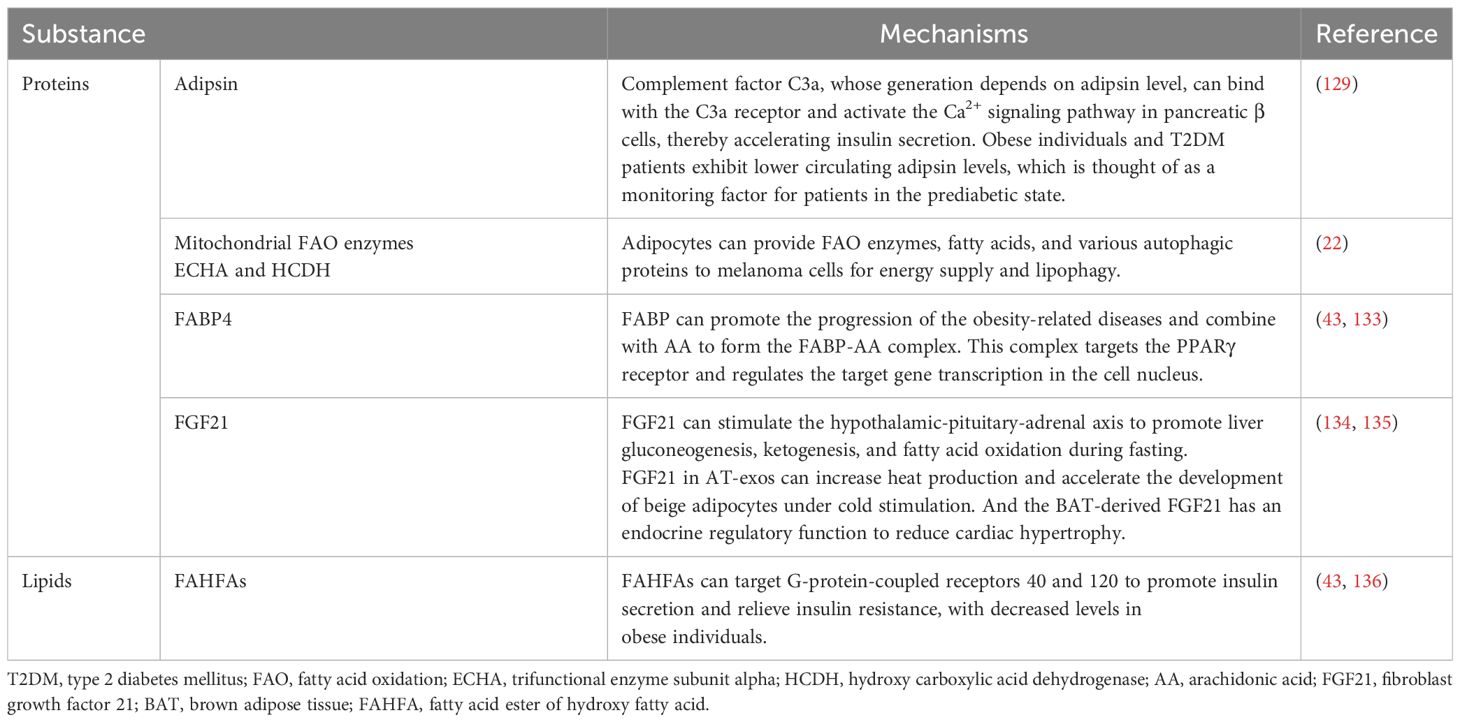
Table 4. Proteins and lipids derived from adipocyte-exos are associated with metabolism and insulin resistance.
In addition to adipocytes and ADSCs, AT is instrumental in regulating the insulin resistance and glucose sensitivity of individuals. In mouse experiments, injecting ATM-exos from obese mice into lean mice decreased insulin sensitivity and induced glucose tolerance. In contrast, ATM-exos obtained from lean mice improved glucose tolerance and insulin sensitivity when administered to obese mice (25, 121). AT contains multiple types of macrophages that secrete different exosomes according to their unique phenotype. Exosomes from M1 macrophages induce insulin resistance in adipocytes, whereas M2 macrophage-derived exosomes improve insulin sensitivity and glucose tolerance (137). IL-4 derived from THP-1 macrophages can decrease miR-33 expression and upregulate miR-21, miR-99a, miR-146b, and miR-378a in both adipocytes and macrophages to promote lipophagy and oxidative phosphorylation, and these effects are accompanied by enhanced insulin sensitivity (138). High expression of glypican-4 in preadipocytes can result in insulin resistance in the initial stage of obesity (139). Additionally, the increased expression of short stature homeobox 2, which is linked to imbalanced fat storage in subcutaneous adipocytes compared to visceral adipocytes (140), can decrease the expression of the β3 adrenergic receptor, leading to reduce lipolysis (141). According to the findings of these studies, subcutaneous preadipocytes in the obese population can promote more proliferation and accumulation of AT. The cargoes in AT-exos associated with insulin resistance are recapitulated in Figure 3.
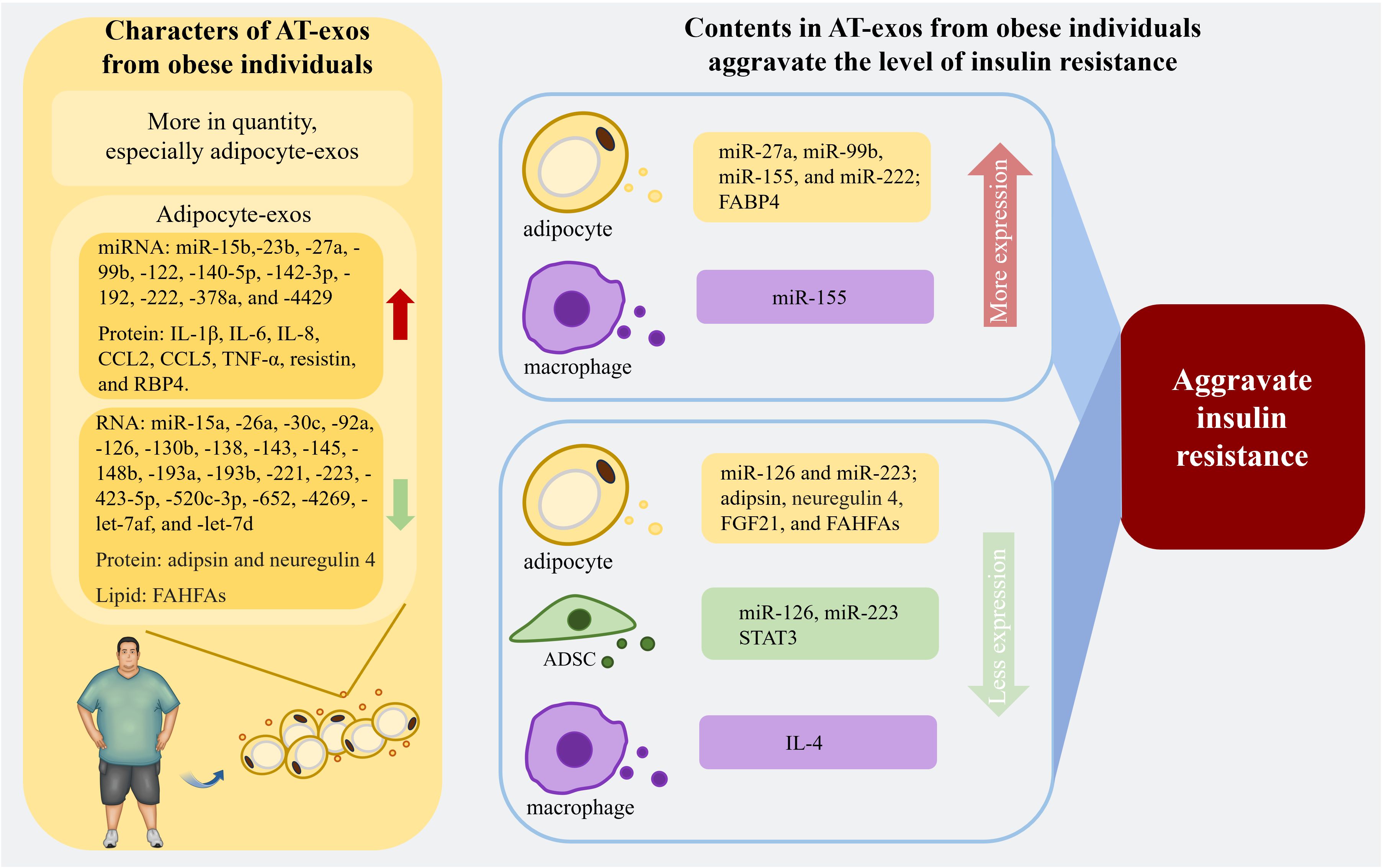
Figure 3. The properties of AT-exos from the obese population and the contents in AT-exos derived from obese individuals are related to the exacerbation of insulin resistance. With an increased quantity, the adipocyte-exos of obese individuals exhibit significant alterations compared to those of normal individuals. The upregulation of certain microRNAs and proteins within these exosomes can exacerbate insulin resistance. Conversely, the downregulation of various RNAs, proteins, and lipid can alleviate insulin resistance, thereby significantly worsening the insulin resistance in obese individuals. Exosomes from ADSCs and macrophages also contain substances that can regulate insulin resistance levels. miR-155 from ATM-exos can inhibit adipogenesis and reduce insulin secretion from pancreatic β cells, aggravating insulin resistance. (Created with BioRender.com). miR-126, miR-223, and STAT3 in ADSC-exos, as well as IL-4 from ATM-exos, are associated with the reduction of inflammation, thereby alleviating insulin resistance. IL-1β, interleukin-1β; IL-6, interleukin-6; IL-8, interleukin-8; CCL2, C-C motif ligand 2; CCL5, C-C motif ligand 5; TNF-α, tumor necrosis factor-α; RBP4, retinol binding protein 4; FAHFAs, fatty acid ester of hydroxy fatty acids; FABP4, fatty acid binding protein 4; FGF21, fibroblast growth factor 21; STAT3, signal transducer and activator of transcription 3; IL-1β, interleukin-1β.
The modulatory influences of adipocyte-exos, ADSC-exos, and ATM-exos on inflammation, tumor progression, and insulin resistance are concisely detailed in Table 5.
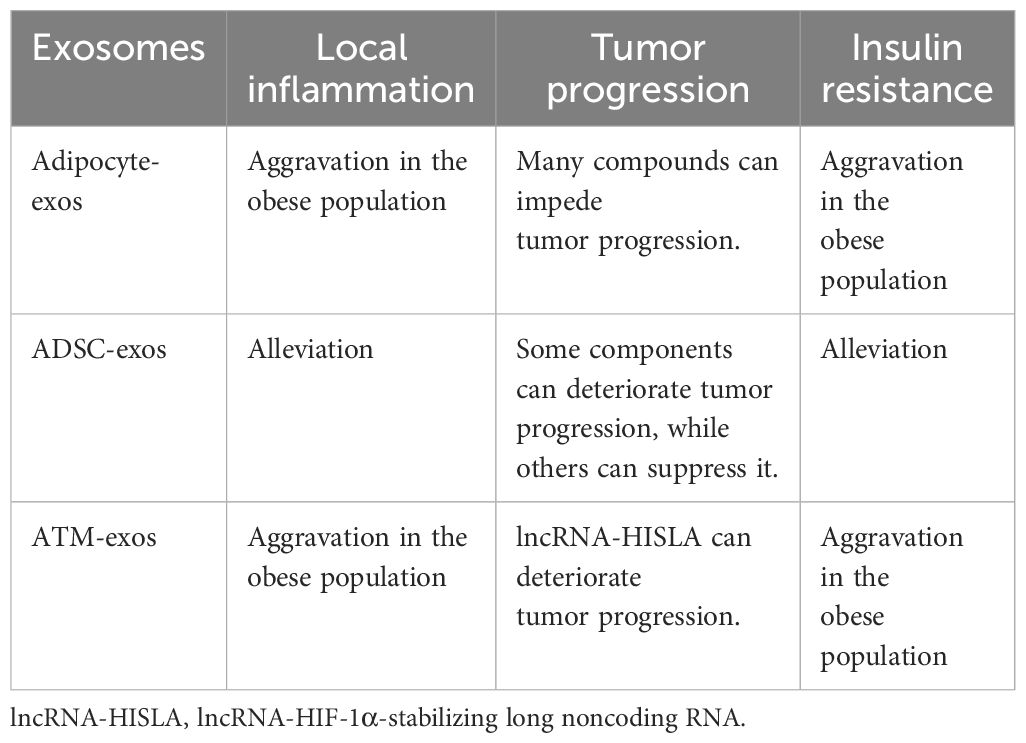
Table 5. Brief overview of adipocyte-exos, ADSC-exos, and ATM-exos regulating inflammation, tumor progression, and insulin resistance.
5 The regulating action of exosomes derived from AT on other tissues and organs
In addition to affecting inflammation, tumors, and insulin resistance, AT can also have regulatory functions on adipocytes themselves, muscle, skin, hypothalamus, and kidney through the delivery of exosomes in the progression of adipogenesis, myodystrophy, skin photoaging, appetite, and renal fibrosis. AT exosomal RNAs play a crucial role in the regulation of adipogenesis (142, 143) and the transformation between white adipocytes and brown adipocytes, such as miR-92a, miR-155, miR-193b, miR-196a, miR-337, and miR-455 (144). Knockout of Dicer, the miRNA-processing enzyme, causes lipodystrophy of visceral and subcutaneous adipocytes, serious insulin resistance, and ‘whitening’ of brown adipocytes in the mouse scapular region (145, 146). Adipocyte-exos could deliver miRNAs to skeletal muscle cells, regulating the metabolism and differentiation of muscle cells. The contents of AT-exos in myodystrophy patients are also different from those in normal individuals (2, 147, 148). Numerous studies have shown that ADSC-exos are beneficial to inflammation-related diseases, which has been confirmed in skin photoaging and renal fibrosis (149, 150). The effective RNAs in AT-exos are listed in Table 6.
Meanwhile, the proteins and lipids in AT-exos also act as regulators in the physiological and pathological processes. Proteins in adipocyte-exos, known as exoadipokines, are associated with inflammation and fibrosis, affect on signaling pathways, and membrane proteins (157). The proteins in both adipocyte-exos and ADSC-exos, as cargoes, can be categorized into regulatory factors and metabolism-related enzymes (110, 158). The enzymes in ADSC-exos can convert adenosine monophosphate to adenosine to activate the PI3K/Akt pathway, exerting a protective effect on myocarditis and arrhythmias (159). Additionally, in tissue infections, ADSCs release the antibacterial peptides and proteins, such as antibacterial peptide LL-37, hepcidin, β-defensin-2, and lipocalin-2, which inhibit the synthesis of DNA, RNA, and valid proteins in infected cells to facilitate the cell-killing process and restore the balance of infection and inflammation (160–162). Lipids in exosomes also play a role in inducing the differentiation of immune cells and regulating gene expression. Lipids in adipocyte-exos promote the differentiation of monocytes from bone marrow into ATM (29). ADSC-exos contain various bioactive lipids, including monounsaturated fatty acids, polyunsaturated fatty acids, and multiple saturated fatty acids. These fatty acids, such as arachidonic acid, prostaglandins, lysophosphatidylcholine, leukotrienes, phosphatidic acid, and docosahexaenoic acid (133), facilitate the transmission of information between cells through different signaling pathways. Arachidonic acid, a common ω-6 polyunsaturated fatty acid, participates in the biosynthesis of prostaglandin. The prostaglandin E2-EP3, found in bone marrow mesenchymal stem cells with selective secretion (163), can induce acute inflammation performance (164). Furthermore, prostaglandin E2 can also accelerate tumor cell proliferation (165). In clinical trials, high expression of docosahexaenoic acid is related to better chemotherapy effect in BC and non-small-cell lung cancer patients (166).
6 Clinical implications of AT-exos
Exosomes, which are characterized by low immunogenicity, regulate recipient cells by delivering cargoes to achieve cell-free therapy. Both utilizing the substances contained in exosomes and delivering drugs through exosomes are beneficial methods for disease treatment. AT-exos were proven to be therapeutic for wound healing by promoting the proliferation and migration of fibroblasts and HaCaT cells as well as angiogenesis (19). AT-exo-derived lnc-H19 (167) and miR-221-3p (27) can promote cell proliferation and vascularization, respectively. Furthermore, AT-exos have potential therapeutic benefits for liver injury, cardiac fibrosis, metabolic syndrome, and tumors (168, 169). As mentioned above, AT-exos are present at different levels in obese and diabetic people than in healthy people and could serve as a basis for identifying potentially diabetic patients from healthy or obese people.
However, there are several limitations in the clinical application of AT-exos. First, the stability of AT-exos during storage is challenging because exosomes are susceptible to environmental conditions during storage and degrade over time, which limits their long-term storage and wide application. Moreover, there is no consensus about preparation methods and quality control standards of AT-exos, which leads to uncertainty about the quality and efficacy of exosomes, limiting their reliability and reproducibility in clinical application. Finally, although AT-exos are considered relatively safe, they have potential safety concerns and side effects, such as immune responses or other adverse reactions. The safety, dosage, and treatment options of AT-exos need to be further studied and evaluated in additional animal and clinical experiments.
Compared with AT-exos, ADSC-exos have been extensively studied in clinical applications, and they have strong and wide-ranging impacts. In addition to promoting cell proliferation and migration as well as angiogenesis in wound healing (170), ADSC-exos have great curative effects on inflammation-related diseases (Crohn’s disease, idiopathic pulmonary fibrosis, COVID-19, arthritis, autoimmune diabetes, etc.), myocardial ischemia, and delayed photoaging (3, 150, 160, 171). Unlike ADSC-exos, some studies have shown that AT-exos in obese people could increase inflammation and exacerbate disease (172, 173). So, the efficacy of AT-exos from obese people in the treatment of inflammatory diseases is still controversial. Ultimately, compared with ADSC-exos, AT-exos have great advantages in terms of preparation. AT-exos are directly extracted from AT without the need for cell expansion, meaning that the time required for AT-exo preparation is significantly shorter than that required for ADSC-exo preparation. AT-exos, which are exosomes in mature tissue, are significantly more abundant than ADSC-exos produced by primary ADSCs that are extracted from the same volume of AT.
7 Conclusions and prospects
AT-exos, which are produced by various AT cells, mediate paracrine and endocrine regulation of the local microenvironment and distant organs by delivering nucleic acids, proteins, and lipids. Among AT-exos, adipocyte-exos and ADSC-exos have positive impacts on wound healing and cardiovascular protection, while their regulatory effects on inflammation and tumors are complicated. Furthermore, the contents of AT-exos significantly differ among healthy individuals, obese individuals, and diabetic patients. These differences could serve as targets for identifying patients with early-stage diabetes. Modulating the expression of insulin-related genes may improve the insulin sensitivity of patients and contribute to the treatment of diabetes and obesity.
Various cells within AT generate exosomes that perform diverse regulatory functions. Considering AT-exos as a whole is valuable for studying the regulatory effect of AT on other organs in the body. In order to better understand the regulatory role of AT-exos and take advantage of AT-exos, further studies could focus on the following directions: (1) Identifying appropriate patients with inflammation-related diseases for treatment with autologous AT-exos due to their complex regulatory effects of AT-exos on inflammation. (2) Investigating whether AT-exos exacerbate tumor progression and increase the risk of recurrence in BC patients with fat breast augmentation after resection. (3) contents, such as miRNAs, proteins, and lipids, in AT-exos can be used to predict whether obese patients will suffer from diabetes, although the expression of miRNAs is easily affected by stimulation. (4) Enhancing the separation and purification techniques of exosomes from various cellular sources to facilitate the development of targeted therapies and achieve desired treatment outcomes. (5) Improving methods for the preparation and storage of AT-exos to ensure uniformity, which will greatly enhance the clinical application of AT-exos. Addressing these key questions will pave the way for the development and more efficient use of autologous AT-exos for treating diseases in clinical practice.
Author contributions
YW: Conceptualization, Writing – original draft. QL: Writing – review & editing, Funding acquisition, Supervision. SZ: Funding acquisition, Supervision, Writing – review & editing. PT: Supervision, Writing – review & editing.
Funding
The authors declare financial support was received for the research, authorship, and/or publication of this article. This study was supported by grants from the National Natural Science Foundation of China (82272287); Cross-disciplinary Research Fund of Shanghai Ninth People’s Hospital, Shanghai Jiao Tong University School of Medicine (JYJC202215); Shanghai Clinical Research Center of Plastic and Reconstructive Surgery supported by Science and Technology Commission of Shanghai Municipality (Grant No. 22MC1940300); project list of “National Double First-Class” and “Shanghai-Top-Level” high education initiative at Shanghai Jiao Tong University School of Medicine.
Conflict of interest
The authors declare that the research was conducted in the absence of any commercial or financial relationships that could be construed as a potential conflict of interest.
Publisher’s note
All claims expressed in this article are solely those of the authors and do not necessarily represent those of their affiliated organizations, or those of the publisher, the editors and the reviewers. Any product that may be evaluated in this article, or claim that may be made by its manufacturer, is not guaranteed or endorsed by the publisher.
References
1. Kruglikov IL, Zhang Z, Scherer PE. The role of immature and mature adipocytes in hair cycling. Trends Endocrinol Metabol: TEM. (2019) 30:93–105. doi: 10.1016/j.tem.2018.11.004
2. Ojima K, Muroya S, Wada H, Ogawa K, Oe M, Takimoto K, et al. Immature adipocyte-derived exosomes inhibit expression of muscle differentiation markers. FEBS Open Bio. (2021) 11:768–81. doi: 10.1002/2211-5463.13100
3. Huang Z, Xu A. Adipose extracellular vesicles in intercellular and inter-organ crosstalk in metabolic health and diseases. Front Immunol. (2021) 12:608680. doi: 10.3389/fimmu.2021.608680
4. Pan Y, Hui X, Hoo RLC, Ye D, Chan CYC, Feng T, et al. Adipocyte-secreted exosomal microRNA-34a inhibits M2 macrophage polarization to promote obesity-induced adipose inflammation. J Clin Invest. (2019) 129:834–49. doi: 10.1172/jci123069
5. Gao J, Li X, Wang Y, Cao Y, Yao D, Sun L, et al. Adipocyte-derived extracellular vesicles modulate appetite and weight through mTOR signalling in the hypothalamus. Acta physiologica (Oxford England). (2020) 228:e13339. doi: 10.1111/apha.13339
6. Gesmundo I, Pardini B, Gargantini E, Gamba G, Birolo G, Fanciulli A, et al. Adipocyte-derived extracellular vesicles regulate survival and function of pancreatic β cells. JCI Insight. (2021) 6:e141962. doi: 10.1172/jci.insight.141962
7. Li Y, He X, Li Q, Lai H, Zhang H, Hu Z, et al. EV-origin: Enumerating the tissue-cellular origin of circulating extracellular vesicles using exLR profile. Comput Struct Biotechnol J. (2020) 18:2851–9. doi: 10.1016/j.csbj.2020.10.002
8. Connolly KD, Wadey RM, Mathew D, Johnson E, Rees DA, James PE. Evidence for adipocyte-derived extracellular vesicles in the human circulation. Endocrinology. (2018) 159:3259–67. doi: 10.1210/en.2018-00266
9. Jeppesen DK, Zhang Q, Franklin JL, Coffey RJ. Extracellular vesicles and nanoparticles: emerging complexities. Trends Cell Biol. (2023) 33:667–81. doi: 10.1016/j.tcb.2023.01.002
10. Théry C, Witwer KW, Aikawa E, Alcaraz MJ, Anderson JD, Andriantsitohaina R, et al. Minimal information for studies of extracellular vesicles 2018 (MISEV2018): a position statement of the International Society for Extracellular Vesicles and update of the MISEV2014 guidelines. J Extracell Vesic. (2018) 7:1535750. doi: 10.1080/20013078.2018.1535750
11. Verweij FJ, Bebelman MP, George AE, Couty M, Bécot A, Palmulli R, et al. ER membrane contact sites support endosomal small GTPase conversion for exosome secretion. J Cell Biol. (2022) 221:e202112032. doi: 10.1083/jcb.202112032
12. Yu W, Hurley J, Roberts D, Chakrabortty SK, Enderle D, Noerholm M, et al. Exosome-based liquid biopsies in cancer: opportunities and challenges. Ann Oncol: Off J Eur Soc Med Oncol. (2021) 32:466–77. doi: 10.1016/j.annonc.2021.01.074
13. Crewe C, Joffin N, Rutkowski JM, Kim M, Zhang F, Towler DA, et al. An endothelial-to-adipocyte extracellular vesicle axis governed by metabolic state. Cell. (2018) 175:695–708.e13. doi: 10.1016/j.cell.2018.09.005
14. Al-Ghadban S, Bunnell BA. Adipose tissue-derived stem cells: immunomodulatory effects and therapeutic potential. Physiol (Bethesda Md). (2020) 35:125–33. doi: 10.1152/physiol.00021.2019
15. Kawada-Horitani E, Kita S, Okita T, Nakamura Y, Nishida H, Honma Y, et al. Human adipose-derived mesenchymal stem cells prevent type 1 diabetes induced by immune checkpoint blockade. Diabetologia. (2022) 65:1185–97. doi: 10.1007/s00125-022-05708-3
16. Alió Del Barrio JL, de la Mata A, De Miguel MP, Arnalich-Montiel F, Nieto-Miguel T, El Zarif M, et al. Corneal regeneration using adipose-derived mesenchymal stem cells. Cells. (2022) 11:2549. doi: 10.3390/cells11162549
17. Sanz-Ros J, Romero-García N, Mas-Bargues C, Monleón D, Gordevicius J, Brooke RT, et al. Small extracellular vesicles from young adipose-derived stem cells prevent frailty, improve health span, and decrease epigenetic age in old mice. Sci Adv. (2022) 8:eabq2226. doi: 10.1126/sciadv.abq2226
18. Wang J, Wu H, Peng Y, Zhao Y, Qin Y, Zhang Y, et al. Hypoxia adipose stem cell-derived exosomes promote high-quality healing of diabetic wound involves activation of PI3K/Akt pathways. J Nanobiotechnol. (2021) 19:202. doi: 10.1186/s12951-021-00942-0
19. Pan C, Xu P, Zheng Y, Wang Y, Chen C, Fu S, et al. Preparation of therapy-grade extracellular vesicles from adipose tissue to promote diabetic wound healing. Front Bioeng Biotechnol. (2023) 11:1129187. doi: 10.3389/fbioe.2023.1129187
20. Liu Y, Tan J, Ou S, Chen J, Chen L. Adipose-der ived exosomes deliver miR-23a/b to regulate tumor growth in hepatocellular cancer by targeting the VHL/HIF axis. J Physiol Biochem. (2019) 75:391–401. doi: 10.1007/s13105-019-00692-6
21. Lin Z, Wu Y, Xu Y, Li G, Li Z, Liu T. Mesenchymal stem cell-derived exosomes in cancer therapy resistance: recent advances and therapeutic potential. Mol Cancer. (2022) 21:179. doi: 10.1186/s12943-022-01650-5
22. Clement E, Lazar I, Attané C, Carrié L, Dauvillier S, Ducoux-Petit M, et al. Adipocyte extracellular vesicles carry enzymes and fatty acids that stimulate mitochondrial metabolism and remodeling in tumor cells. EMBO J. (2020) 39:e102525. doi: 10.15252/embj.2019102525
23. Lai RC, Chen TS, Lim SK. Mesenchymal stem cell exosome: a novel stem cell-based therapy for cardiovascular disease. Regenerative Med. (2011) 6:481–92. doi: 10.2217/rme.11.35
24. Alcedo KP, Rouse MA, Jung GS, Fu D, Minor M, Willcockson HH, et al. CD73 maintains hepatocyte metabolic integrity and mouse liver homeostasis in a sex-dependent manner. Cell Mol Gastroenterol Hepatol. (2021) 12:141–57. doi: 10.1016/j.jcmgh.2021.01.016
25. Liu T, Sun YC, Cheng P, Shao HG. Adipose tissue macrophage-derived exosomal miR-29a regulates obesity-associated insulin resistance. Biochem Biophys Res Commun. (2019) 515:352–8. doi: 10.1016/j.bbrc.2019.05.113
26. Xourafa G, Korbmacher M, Roden M. Inter-organ crosstalk during development and progression of type 2 diabetes mellitus. Nat Rev Endocrinol. (2024) 20:27–49. doi: 10.1038/s41574-023-00898-1
27. Li X, Ballantyne LL, Yu Y, Funk CD. Perivascular adipose tissue-derived extracellular vesicle miR-221-3p mediates vascular remodeling. FASEB journal: Off Publ Fed Am Societies Exp Biol. (2019) 33:12704–22. doi: 10.1096/fj.201901548R
28. Fuchs A, Samovski D, Smith GI, Cifarelli V, Farabi SS, Yoshino J, et al. Associations among adipose tissue immunology, inflammation, exosomes and insulin sensitivity in people with obesity and nonalcoholic fatty liver disease. Gastroenterology. (2021) 161:968–81.e12. doi: 10.1053/j.gastro.2021.05.008
29. Flaherty SE 3rd, Grijalva A, Xu X, Ables E, Nomani A, Ferrante AW Jr. A lipase-independent pathway of lipid release and immune modulation by adipocytes. Sci (New York NY). (2019) 363:989–93. doi: 10.1126/science.aaw2586
30. Deng ZB, Poliakov A, Hardy RW, Clements R, Liu C, Liu Y, et al. Adipose tissue exosome-like vesicles mediate activation of macrophage-induced insulin resistance. Diabetes. (2009) 58:2498–505. doi: 10.2337/db09-0216
31. Castaño C, Kalko S, Novials A, Párrizas M. Obesity-associated exosomal miRNAs modulate glucose and lipid metabolism in mice. Proc Natl Acad Sci USA. (2018) 115:12158–63. doi: 10.1073/pnas.1808855115
32. Togliatto G, Dentelli P, Gili M, Gallo S, Deregibus C, Biglieri E, et al. Obesity reduces the pro-angiogenic potential of adipose tissue stem cell-derived extracellular vesicles (EVs) by impairing miR-126 content: impact on clinical applications. Int J Obes. (2016) 40:102–11. doi: 10.1038/ijo.2015.123
33. Kulyté A, Belarbi Y, Lorente-Cebrián S, Bambace C, Arner E, Daub CO, et al. Additive effects of microRNAs and transcription factors on CCL2 production in human white adipose tissue. Diabetes. (2014) 63:1248–58. doi: 10.2337/db13-0702
34. Arner E, Mejhert N, Kulyté A, Balwierz PJ, Pachkov M, Cormont M, et al. Adipose tissue microRNAs as regulators of CCL2 production in human obesity. Diabetes. (2012) 61:1986–93. doi: 10.2337/db11-1508
35. Huang JH, Fu CH, Xu Y, Yin XM, Cao Y, Lin FY. Extracellular vesicles derived from epidural fat-mesenchymal stem cells attenuate NLRP3 inflammasome activation and improve functional recovery after spinal cord injury. Neurochem Res. (2020) 45:760–71. doi: 10.1007/s11064-019-02950-x
36. Yu C, Chen P, Xu J, Liu Y, Li H, Wang L, et al. hADSCs derived extracellular vesicles inhibit NLRP3inflammasome activation and dry eye. Sci Rep. (2020) 10:14521. doi: 10.1038/s41598-020-71337-8
37. Chen B, Cai J, Wei Y, Jiang Z, Desjardins HE, Adams AE, et al. Exosomes are comparable to source adipose stem cells in fat graft retention with up-regulating early inflammation and angiogenesis. Plast Reconstruct Surg. (2019) 144:816e–27e. doi: 10.1097/prs.0000000000006175
38. Zhou X, Zhang J, Lv W, Zhao C, Xia Y, Wu Y, et al. The pleiotropic roles of adipocyte secretome in remodeling breast cancer. J Exp Clin Cancer Res: CR. (2022) 41:203. doi: 10.1186/s13046-022-02408-z
39. Wu Q, Li B, Li Z, Li J, Sun S, Sun S. Cancer-associated adipocytes: key players in breast cancer progression. J Hematol Oncol. (2019) 12:95. doi: 10.1186/s13045-019-0778-6
40. Blaszczak AM, Jalilvand A, Hsueh WA. Adipocytes, innate immunity and obesity: A mini-review. Front Immunol. (2021) 12:650768. doi: 10.3389/fimmu.2021.650768
41. Trzyna A, Banaś-Ząbczyk A. Adipose-derived stem cells secretome and its potential application in “Stem cell-free therapy. Biomolecules. (2021) 11:878. doi: 10.3390/biom11060878
42. Blaber SP, Webster RA, Hill CJ, Breen EJ, Kuah D, Vesey G, et al. Analysis of in vitro secretion profiles from adipose-derived cell populations. J Trans Med. (2012) 10:172. doi: 10.1186/1479-5876-10-172
43. Scheja L, Heeren J. The endocrine function of adipose tissues in health and cardiometabolic disease. Nat Rev Endocrinol. (2019) 15:507–24. doi: 10.1038/s41574-019-0230-6
44. Jussila A, Zhang B, Kirti S, Atit R. Tissue fibrosis associated depletion of lipid-filled cells. Exp Dermatol. (2024) 33:e15054. doi: 10.1111/exd.15054
45. Chang X, Wang L, Wang Z, Wu S, Zhu X, Hu S, et al. TRADD mediates the tumor necrosis factor-induced apoptosis of L929 cells in the absence of RIP3. Sci Rep. (2017) 7:16111. doi: 10.1038/s41598-017-16390-6
46. Song Y, Li H, Ren X, Li H, Feng C. SNHG9, delivered by adipocyte-derived exosomes, alleviates inflammation and apoptosis of endothelial cells through suppressing TRADD expression. Eur J Pharmacol. (2020) 872:172977. doi: 10.1016/j.ejphar.2020.172977
47. Hu J, Jiang Y, Wu X, Wu Z, Qin J, Zhao Z, et al. Exosomal miR-17-5p from adipose-derived mesenchymal stem cells inhibits abdominal aortic aneurysm by suppressing TXNIP-NLRP3 inflammasome. Stem Cell Res Ther. (2022) 13:349. doi: 10.1186/s13287-022-03037-1
48. Liu Y, Zhang Z, Wang B, Dong Y, Zhao C, Zhao Y, et al. Inflammation-stimulated MSC-derived small extracellular vesicle miR-27b-3p regulates macrophages by targeting CSF-1 to promote temporomandibular joint condylar regeneration. Small (Weinheim an der Bergstrasse Germany). (2022) 18:e2107354. doi: 10.1002/smll.202107354
49. Li R, Li D, Wang H, Chen K, Wang S, Xu J, et al. Exosomes from adipose-derived stem cells regulate M1/M2 macrophage phenotypic polarization to promote bone healing via miR-451a/MIF. Stem Cell Res Ther. (2022) 13:149. doi: 10.1186/s13287-022-02823-1
50. Gao Y, Mi N, Zhang Y, Li X, Guan W, Bai C. Uterine macrophages as treatment targets for therapy of premature rupture of membranes by modified ADSC-EVs through a circRNA/miRNA/NF-κB pathway. J Nanobiotechnol. (2022) 20:487. doi: 10.1186/s12951-022-01696-z
51. Zhuang G, Meng C, Guo X, Cheruku PS, Shi L, Xu H, et al. A novel regulator of macrophage activation: miR-223 in obesity-associated adipose tissue inflammation. Circulation. (2012) 125:2892–903. doi: 10.1161/circulationaha.111.087817
52. Niu Q, Wang T, Wang Z, Wang F, Huang D, Sun H, et al. Adipose-derived mesenchymal stem cell-secreted extracellular vesicles alleviate non-alcoholic fatty liver disease via delivering miR-223-3p. Adipocyte. (2022) 11:572–87. doi: 10.1080/21623945.2022.2098583
53. Liu W, Liu A, Li X, Sun Z, Sun Z, Liu Y, et al. Dual-engineered cartilage-targeting extracellular vesicles derived from mesenchymal stem cells enhance osteoarthritis treatment via miR-223/NLRP3/pyroptosis axis: Toward a precision therapy. Bioactive Mater. (2023) 30:169–83. doi: 10.1016/j.bioactmat.2023.06.012
54. Zhang Y, Mei H, Chang X, Chen F, Zhu Y, Han X. Adipocyte-derived microvesicles from obese mice induce M1 macrophage phenotype through secreted miR-155. J Mol Cell Biol. (2016) 8:505–17. doi: 10.1093/jmcb/mjw040
55. Zhao YQ, Ren YF, Li BB, Wei C, Yu B. The mysterious association between adiponectin and endometriosis. Front Pharmacol. (2024) 15:1396616. doi: 10.3389/fphar.2024.1396616
56. Chedid P, Hurtado-Nedelec M, Marion-Gaber B, Bournier O, Hayem G, Gougerot-Pocidalo MA, et al. Adiponectin and its globular fragment differentially modulate the oxidative burst of primary human phagocytes. Am J pathology. (2012) 180:682–92. doi: 10.1016/j.ajpath.2011.10.013
57. Trellakis S, Rydleuskaya A, Fischer C, Canbay A, Tagay S, Scherag A, et al. Low adiponectin, high levels of apoptosis and increased peripheral blood neutrophil activity in healthy obese subjects. Obes facts. (2012) 5:305–18. doi: 10.1159/000339452
58. Duan Y, Zhang S, Xing Y, Wu Y, Zhao W, Xie P, et al. Adiponectin-mediated promotion of CD44 suppresses diabetic vascular inflammatory effects. iScience. (2023) 26:106428. doi: 10.1016/j.isci.2023.106428
59. Qiu W, Wu H, Hu Z, Wu X, Tu M, Fang F, et al. Identification and characterization of a novel adiponectin receptor agonist adipo anti-inflammation agonist and its anti-inflammatory effects in vitro and in vivo. Br J Pharmacol. (2021) 178:280–97. doi: 10.1111/bph.15277
60. Liu Z, Gan L, Zhang T, Ren Q, Sun C. Melatonin alleviates adipose inflammation through elevating α-ketoglutarate and diverting adipose-derived exosomes to macrophages in mice. J pineal Res. (2018) 64:e12455. doi: 10.1111/jpi.12455
61. Zhao H, Shang Q, Pan Z, Bai Y, Li Z, Zhang H, et al. Exosomes from adipose-derived stem cells attenuate adipose inflammation and obesity through polarizing M2 macrophages and beiging in white adipose tissue. Diabetes. (2018) 67:235–47. doi: 10.2337/db17-0356
62. Endo J, Sano M, Isobe Y, Fukuda K, Kang JX, Arai H, et al. 18-HEPE, an n-3 fatty acid metabolite released by macrophages, prevents pressure overload-induced maladaptive cardiac remodeling. J Exp Med. (2014) 211:1673–87. doi: 10.1084/jem.20132011
63. Dayakar A, Chandrasekaran S, Veronica J, Maurya R. Leptin induces the phagocytosis and protective immune response in Leishmania donovani infected THP-1 cell line and human PBMCs. Exp Parasitol. (2016) 160:54–9. doi: 10.1016/j.exppara.2015.12.002
64. Ninou I, Magkrioti C, Aidinis V. Autotaxin in pathophysiology and pulmonary fibrosis. Front Med. (2018) 5:180. doi: 10.3389/fmed.2018.00180
65. Ma H, Li YN, Song L, Liu R, Li X, Shang Q, et al. Macrophages inhibit adipogenic differentiation of adipose tissue derived mesenchymal stem/stromal cells by producing pro-inflammatory cytokines. Cell Biosci. (2020) 10:88. doi: 10.1186/s13578-020-00450-y
66. Su J, Chen X, Huang Y, Li W, Li J, Cao K, et al. Phylogenetic distinction of iNOS and IDO function in mesenchymal stem cell-mediated immunosuppression in mammalian species. Cell Death Differ. (2014) 21:388–96. doi: 10.1038/cdd.2013.149
67. Park HK, Kwak MK, Kim HJ, Ahima RS. Linking resistin, inflammation, and cardiometabolic diseases. Korean J Internal Med. (2017) 32:239–47. doi: 10.3904/kjim.2016.229
68. Schwartz DR, Lazar MA. Human resistin: found in translation from mouse to man. Trends Endocrinol Metabolism: TEM. (2011) 22:259–65. doi: 10.1016/j.tem.2011.03.005
69. Benomar Y, Amine H, Crépin D, Al Rifai S, Riffault L, Gertler A, et al. Central resistin/TLR4 impairs adiponectin signaling, contributing to insulin and FGF21 resistance. Diabetes. (2016) 65:913–26. doi: 10.2337/db15-1029
70. Wang S, Su X, Xu M, Xiao X, Li X, Li H, et al. Exosomes secreted by mesenchymal stromal/stem cell-derived adipocytes promote breast cancer cell growth via activation of Hippo signaling pathway. Stem Cell Res Ther. (2019) 10:117. doi: 10.1186/s13287-019-1220-2
71. Huang R, Wang Z, Hong J, Wu J, Huang O, He J, et al. Targeting cancer-associated adipocyte-derived CXCL8 inhibits triple-negative breast cancer progression and enhances the efficacy of anti-PD-1 immunotherapy. Cell Death Dis. (2023) 14:703. doi: 10.1038/s41419-023-06230-z
72. Tucker SL, Gharpure K, Herbrich SM, Unruh AK, Nick AM, Crane EK, et al. Molecular biomarkers of residual disease after surgical debulking of high-grade serous ovarian cancer. Clin Cancer research: an Off J Am Assoc Cancer Res. (2014) 20:3280–8. doi: 10.1158/1078-0432.ccr-14-0445
73. García-Contreras M, Vera-Donoso CD, Hernández-Andreu JM, García-Verdugo JM, Oltra E. Therapeutic potential of human adipose-derived stem cells (ADSCs) from cancer patients: a pilot study. PloS One. (2014) 9:e113288. doi: 10.1371/journal.pone.0113288
74. Lin R, Wang S, Zhao RC. Exosomes from human adipose-derived mesenchymal stem cells promote migration through Wnt signaling pathway in a breast cancer cell model. Mol Cell Biochem. (2013) 383:13–20. doi: 10.1007/s11010-013-1746-z
75. García Garre E, Luengo Gil G, Montoro García S, Gonzalez Billalabeitia E, Zafra Poves M, García Martinez E, et al. Circulating small-sized endothelial microparticles as predictors of clinical outcome after chemotherapy for breast cancer: an exploratory analysis. Breast Cancer Res Treat. (2018) 169:83–92. doi: 10.1007/s10549-017-4656-z
76. Ko SF, Yip HK, Zhen YY, Lee CC, Lee CC, Huang CC, et al. Adipose-derived mesenchymal stem cell exosomes suppress hepatocellular carcinoma growth in a rat model: apparent diffusion coefficient, natural killer T-cell responses, and histopathological features. Stem Cells Int. (2015) 2015:853506. doi: 10.1155/2015/853506
77. Yoshida K, Yokoi A, Kato T, Ochiya T, Yamamoto Y. The clinical impact of intra- and extracellular miRNAs in ovarian cancer. Cancer Sci. (2020) 111:3435–44. doi: 10.1111/cas.14599
78. Heyn GS, Corrêa LH, Magalhães KG. The impact of adipose tissue-derived miRNAs in metabolic syndrome, obesity, and cancer. Front Endocrinol. (2020) 11:563816. doi: 10.3389/fendo.2020.563816
79. Ono M, Kosaka N, Tominaga N, Yoshioka Y, Takeshita F, Takahashi RU, et al. Exosomes from bone marrow mesenchymal stem cells contain a microRNA that promotes dormancy in metastatic breast cancer cells. Sci Signal. (2014) 7:ra63. doi: 10.1126/scisignal.2005231
80. Phan TG, Croucher PI. The dormant cancer cell life cycle. Nat Rev Cancer. (2020) 20:398–411. doi: 10.1038/s41568-020-0263-0
81. Li Y, Zhao Z, Liu W, Li X. SNHG3 functions as miRNA sponge to promote breast cancer cells growth through the metabolic reprogramming. Appl Biochem Biotechnol. (2020) 191:1084–99. doi: 10.1007/s12010-020-03244-7
82. Yang E, Wang X, Gong Z, Yu M, Wu H, Zhang D. Exosome-mediated metabolic reprogramming: the emerging role in tumor microenvironment remodeling and its influence on cancer progression. Signal Transduct Target Ther. (2020) 5:242. doi: 10.1038/s41392-020-00359-5
83. Chen F, Chen J, Yang L, Liu J, Zhang X, Zhang Y, et al. Extracellular vesicle-packaged HIF-1α-stabilizing lncRNA from tumour-associated macrophages regulates aerobic glycolysis of breast cancer cells. Nat Cell Biol. (2019) 21:498–510. doi: 10.1038/s41556-019-0299-0
84. Wang Z, He J, Bach DH, Huang YH, Li Z, Liu H, et al. Induction of m(6)A methylation in adipocyte exosomal LncRNAs mediates myeloma drug resistance. J Exp Clin Cancer Res: CR. (2022) 41:4. doi: 10.1186/s13046-021-02209-w
85. Guaita-Esteruelas S, Gumà J, Masana L, Borràs J. The peritumoural adipose tissue microenvironment and cancer. The roles of fatty acid binding protein 4 and fatty acid binding protein 5. Mol Cell Endocrinol. (2018) 462:107–18. doi: 10.1016/j.mce.2017.02.002
86. Nieman KM, Kenny HA, Penicka CV, Ladanyi A, Buell-Gutbrod R, Zillhardt MR, et al. Adipocytes promote ovarian cancer metastasis and provide energy for rapid tumor growth. Nat Med. (2011) 17:1498–503. doi: 10.1038/nm.2492
87. Afrin S, Ramaiyer M, Begum UAM, Borahay MA. Adipocyte and adipokines promote a uterine leiomyoma friendly microenvironment. Nutrients. (2023) 15:715. doi: 10.3390/nu15030715
88. Jafari N, Kolla M, Meshulam T, Shafran JS, Qiu Y, Casey AN, et al. Adipocyte-derived exosomes may promote breast cancer progression in type 2 diabetes. Sci Signaling. (2021) 14:eabj2807. doi: 10.1126/scisignal.abj2807
89. Wang L, Tang C, Cao H, Li K, Pang X, Zhong L, et al. Activation of IL-8 via PI3K/Akt-dependent pathway is involved in leptin-mediated epithelial-mesenchymal transition in human breast cancer cells. Cancer Biol Ther. (2015) 16:1220–30. doi: 10.1080/15384047.2015.1056409
90. He JY, Wei XH, Li SJ, Liu Y, Hu HL, Li ZZ, et al. Adipocyte-derived IL-6 and leptin promote breast Cancer metastasis via upregulation of Lysyl Hydroxylase-2 expression. Cell Commun Signal.: CCS. (2018) 16:100. doi: 10.1186/s12964-018-0309-z
91. Chen C, Chang YC, Lan MS, Breslin M. Leptin stimulates ovarian cancer cell growth and inhibits apoptosis by increasing cyclin D1 and Mcl-1 expression via the activation of the MEK/ERK1/2 and PI3K/Akt signaling pathways. Int J Oncol. (2013) 42:1113–9. doi: 10.3892/ijo.2013.1789
92. Montagner A, Polizzi A, Fouché E, Ducheix S, Lippi Y, Lasserre F, et al. Liver PPARα is crucial for whole-body fatty acid homeostasis and is protective against NAFLD. Gut. (2016) 65:1202–14. doi: 10.1136/gutjnl-2015-310798
93. Vallabhaneni KC, Penfornis P, Dhule S, Guillonneau F, Adams KV, Mo YY, et al. Extracellular vesicles from bone marrow mesenchymal stem/stromal cells transport tumor regulatory microRNA, proteins, and metabolites. Oncotarget. (2015) 6:4953–67. doi: 10.18632/oncotarget.3211
94. Wan FZ, Chen KH, Sun YC, Chen XC, Liang RB, Chen L, et al. Exosomes overexpressing miR-34c inhibit Malignant behavior and reverse the radioresistance of nasopharyngeal carcinoma. J Trans Med. (2020) 18:12. doi: 10.1186/s12967-019-02203-z
95. Lou G, Song X, Yang F, Wu S, Wang J, Chen Z, et al. Exosomes derived from miR-122-modified adipose tissue-derived MSCs increase chemosensitivity of hepatocellular carcinoma. J Hematol Oncol. (2015) 8:122. doi: 10.1186/s13045-015-0220-7
96. Lee HK, Finniss S, Cazacu S, Bucris E, Ziv-Av A, Xiang C, et al. Mesenchymal stem cells deliver synthetic microRNA mimics to glioma cells and glioma stem cells and inhibit their cell migration and self-renewal. Oncotarget. (2013) 4:346–61. doi: 10.18632/oncotarget.868
97. Qian C, Wang Y, Ji Y, Chen D, Wang C, Zhang G, et al. Neural stem cell−derived exosomes transfer miR−124−3p into cells to inhibit glioma growth by targeting FLOT2. Int J Oncol. (2022) 61:115. doi: 10.3892/ijo.2022.5405
98. Takahara K, Ii M, Inamoto T, Nakagawa T, Ibuki N, Yoshikawa Y, et al. microRNA-145 mediates the inhibitory effect of adipose tissue-derived stromal cells on prostate cancer. Stem Cells Dev. (2016) 25:1290–8. doi: 10.1089/scd.2016.0093
99. Lou G, Chen L, Xia C, Wang W, Qi J, Li A, et al. MiR-199a-modified exosomes from adipose tissue-derived mesenchymal stem cells improve hepatocellular carcinoma chemosensitivity through mTOR pathway. J Exp Clin Cancer research: CR. (2020) 39:4. doi: 10.1186/s13046-019-1512-5
100. Yu L, Gui S, Liu Y, Qiu X, Zhang G, Zhang X, et al. Exosomes derived from microRNA-199a-overexpressing mesenchymal stem cells inhibit glioma progression by down-regulating AGAP2. Aging. (2019) 11:5300–18. doi: 10.18632/aging.102092
101. Seo M, Kim SM, Woo EY, Han KC, Park EJ, Ko S, et al. Stemness-attenuating miR-503-3p as a paracrine factor to regulate growth of cancer stem cells. Stem Cells Int. (2018) 2018:4851949. doi: 10.1155/2018/4851949
102. Jia Z, Zhu H, Sun H, Hua Y, Zhang G, Jiang J, et al. Adipose mesenchymal stem cell-derived exosomal microRNA-1236 reduces resistance of breast cancer cells to cisplatin by suppressing SLC9A1 and the wnt/β-catenin signaling. Cancer Manage Res. (2020) 12:8733–44. doi: 10.2147/cmar.s270200
103. Kranendonk ME, Visseren FL, van Balkom BW, Nolte-’t Hoen EN, van Herwaarden JA, de Jager W, et al. Human adipocyte extracellular vesicles in reciprocal signaling between adipocytes and macrophages. Obes (Silver Spring Md). (2014) 22:1296–308. doi: 10.1002/oby.20679
104. Trajkovic K, Hsu C, Chiantia S, Rajendran L, Wenzel D, Wieland F, et al. Ceramide triggers budding of exosome vesicles into multivesicular endosomes. Sci (New York NY). (2008) 319:1244–7. doi: 10.1126/science.1153124
105. Egea-Jimenez AL, Zimmermann P. Phospholipase D and phosphatidic acid in the biogenesis and cargo loading of extracellular vesicles. J Lipid Res. (2018) 59:1554–60. doi: 10.1194/jlr.R083964
106. Li Y, Talbot CL, Chaurasia B. Ceramides in adipose tissue. Front Endocrinol. (2020) 11:407. doi: 10.3389/fendo.2020.00407
107. Kim JI, Huh JY, Sohn JH, Choe SS, Lee YS, Lim CY, et al. Lipid-overloaded enlarged adipocytes provoke insulin resistance independent of inflammation. Mol Cell Biol. (2015) 35:1686–99. doi: 10.1128/mcb.01321-14
108. Lazar I, Clement E, Dauvillier S, Milhas D, Ducoux-Petit M, LeGonidec S, et al. Adipocyte exosomes promote melanoma aggressiveness through fatty acid oxidation: A novel mechanism linking obesity and cancer. Cancer Res. (2016) 76:4051–7. doi: 10.1158/0008-5472.can-16-0651
109. Durcin M, Fleury A, Taillebois E, Hilairet G, Krupova Z, Henry C, et al. Characterisation of adipocyte-derived extracellular vesicle subtypes identifies distinct protein and lipid signatures for large and small extracellular vesicles. J Extracell Vesic. (2017) 6:1305677. doi: 10.1080/20013078.2017.1305677
110. Kwan HY, Chen M, Xu K, Chen B. The impact of obesity on adipocyte-derived extracellular vesicles. Cell Mol Life sciences: CMLS. (2021) 78:7275–88. doi: 10.1007/s00018-021-03973-w
111. Ortega FJ, Mercader JM, Moreno-Navarrete JM, Rovira O, Guerra E, Esteve E, et al. Profiling of circulating microRNAs reveals common microRNAs linked to type 2 diabetes that change with insulin sensitization. Diabetes Care. (2014) 37:1375–83. doi: 10.2337/dc13-1847
112. Chartoumpekis DV, Zaravinos A, Ziros PG, Iskrenova RP, Psyrogiannis AI, Kyriazopoulou VE, et al. Differential expression of microRNAs in adipose tissue after long-term high-fat diet-induced obesity in mice. PloS One. (2012) 7:e34872. doi: 10.1371/journal.pone.0034872
113. Santangelo A, Imbrucè P, Gardenghi B, Belli L, Agushi R, Tamanini A, et al. A microRNA signature from serum exosomes of patients with glioma as complementary diagnostic biomarker. J neuro-oncology. (2018) 136:51–62. doi: 10.1007/s11060-017-2639-x
114. Ferrante SC, Nadler EP, Pillai DK, Hubal MJ, Wang Z, Wang JM, et al. Adipocyte-derived exosomal miRNAs: a novel mechanism for obesity-related disease. Pediatr Res. (2015) 77:447–54. doi: 10.1038/pr.2014.202
115. Ortega FJ, Mercader JM, Catalán V, Moreno-Navarrete JM, Pueyo N, Sabater M, et al. Targeting the circulating microRNA signature of obesity. Clin Chem. (2013) 59:781–92. doi: 10.1373/clinchem.2012.195776
116. Can U, Buyukinan M, Yerlikaya FH. The investigation of circulating microRNAs associated with lipid metabolism in childhood obesity. Pediatr Obes. (2016) 11:228–34. doi: 10.1111/ijpo.12050
117. Willeit P, Skroblin P, Moschen AR, Yin X, Kaudewitz D, Zampetaki A, et al. Circulating microRNA-122 is associated with the risk of new-onset metabolic syndrome and type 2 diabetes. Diabetes. (2017) 66:347–57. doi: 10.2337/db16-0731
118. Yu Y, Du H, Wei S, Feng L, Li J, Yao F, et al. Adipocyte-derived exosomal miR-27a induces insulin resistance in skeletal muscle through repression of PPARγ. Theranostics. (2018) 8:2171–88. doi: 10.7150/thno.22565
119. Wen D, Qiao P, Wang L. Circulating microRNA-223 as a potential biomarker for obesity. Obes Res Clin Pract. (2015) 9:398–404. doi: 10.1016/j.orcp.2015.01.006
120. Geng L, Lam KSL, Xu A. The therapeutic potential of FGF21 in metabolic diseases: from bench to clinic. Nat Rev Endocrinology. (2020) 16:654–67. doi: 10.1038/s41574-020-0386-0
121. Ying W, Riopel M, Bandyopadhyay G, Dong Y, Birmingham A, Seo JB, et al. Adipose tissue macrophage-derived exosomal miRNAs can modulate in vivo and in vitro insulin sensitivity. Cell. (2017) 171:372–84.e12. doi: 10.1016/j.cell.2017.08.035
122. Zhu M, Wei Y, Geißler C, Abschlag K, Corbalán Campos J, Hristov M, et al. Hyperlipidemia-induced microRNA-155-5p improves β-cell function by targeting mafb. Diabetes. (2017) 66:3072–84. doi: 10.2337/db17-0313
123. Fish JE, Santoro MM, Morton SU, Yu S, Yeh RF, Wythe JD, et al. miR-126 regulates angiogenic signaling and vascular integrity. Dev Cell. (2008) 15:272–84. doi: 10.1016/j.devcel.2008.07.008
124. Haneklaus M, Gerlic M, Kurowska-Stolarska M, Rainey AA, Pich D, McInnes IB, et al. Cutting edge: miR-223 and EBV miR-BART15 regulate the NLRP3 inflammasome and IL-1β production. J Immunol (Baltimore Md: 1950). (2012) 189:3795–9. doi: 10.4049/jimmunol.1200312
125. Pescador N, Pérez-Barba M, Ibarra JM, Corbatón A, Martínez-Larrad MT, Serrano-Ríos M. Serum circulating microRNA profiling for identification of potential type 2 diabetes and obesity biomarkers. PloS One. (2013) 8:e77251. doi: 10.1371/journal.pone.0077251
126. Hernández-Gómez KG, Avila-Nava A, González-Salazar LE, Noriega LG, Serralde-Zúñiga AE, Guizar-Heredia R, et al. Modulation of microRNAs and exosomal microRNAs after dietary interventions for obesity and insulin resistance: A narrative review. Metabolites. (2023) 13:1190. doi: 10.3390/metabo13121190
127. Kranendonk ME, Visseren FL, van Herwaarden JA, Nolte-’t Hoen EN, de Jager W, Wauben MH, et al. Effect of extracellular vesicles of human adipose tissue on insulin signaling in liver and muscle cells. Obes (Silver Spring Md). (2014) 22:2216–23. doi: 10.1002/oby.20847
128. Sumaiya K, Langford D, Natarajaseenivasan K, Shanmughapriya S. Macrophage migration inhibitory factor (MIF): A multifaceted cytokine regulated by genetic and physiological strategies. Pharmacol Ther. (2022) 233:108024. doi: 10.1016/j.pharmthera.2021.108024
129. Lo JC, Ljubicic S, Leibiger B, Kern M, Leibiger IB, Moede T, et al. Adipsin is an adipokine that improves β cell function in diabetes. Cell. (2014) 158:41–53. doi: 10.1016/j.cell.2014.06.005
130. Wang GX, Zhao XY, Meng ZX, Kern M, Dietrich A, Chen Z, et al. The brown fat-enriched secreted factor Nrg4 preserves metabolic homeostasis through attenuation of hepatic lipogenesis. Nat Med. (2014) 20:1436–43. doi: 10.1038/nm.3713
131. Guo L, Zhang P, Chen Z, Xia H, Li S, Zhang Y, et al. Hepatic neuregulin 4 signaling defines an endocrine checkpoint for steatosis-to-NASH progression. J Clin Invest. (2017) 127:4449–61. doi: 10.1172/jci96324
132. Scheja L, Heeren J. Metabolic interplay between white, beige, brown adipocytes and the liver. J hepatology. (2016) 64:1176–86. doi: 10.1016/j.jhep.2016.01.025
133. Deng H, Sun C, Sun Y, Li H, Yang L, Wu D, et al. Lipid, protein, and microRNA composition within mesenchymal stem cell-derived exosomes. Cell Reporgram. (2018) 20:178–86. doi: 10.1089/cell.2017.0047
134. Liang Q, Zhong L, Zhang J, Wang Y, Bornstein SR, Triggle CR, et al. FGF21 maintains glucose homeostasis by mediating the cross talk between liver and brain during prolonged fasting. Diabetes. (2014) 63:4064–75. doi: 10.2337/db14-0541
135. Ruan CC, Kong LR, Chen XH, Ma Y, Pan XX, Zhang ZB, et al. A(2A) receptor activation attenuates hypertensive cardiac remodeling via promoting brown adipose tissue-derived FGF21. Cell Metab. (2018) 28:476–89.e5. doi: 10.1016/j.cmet.2018.06.013
136. Yore MM, Syed I, Moraes-Vieira PM, Zhang T, Herman MA, Homan EA, et al. Discovery of a class of endogenous mammalian lipids with anti-diabetic and anti-inflammatory effects. Cell. (2014) 159:318–32. doi: 10.1016/j.cell.2014.09.035
137. Zhang Y, Shi L, Mei H, Zhang J, Zhu Y, Han X, et al. Inflamed macrophage microvesicles induce insulin resistance in human adipocytes. Nutr Metab. (2015) 12:21. doi: 10.1186/s12986-015-0016-3
138. Phu TA, Ng M, Vu NK, Bouchareychas L, Raffai RL. IL-4 polarized human macrophage exosomes control cardiometabolic inflammation and diabetes in obesity. Mol therapy: J Am Soc Gene Ther. (2022) 30:2274–97. doi: 10.1016/j.ymthe.2022.03.008
139. Heidarianpour A, Keshvari M, Shahidi S, Zarei M. Modulation of GPC-4 and GPLD1 serum levels by improving glycemic indices in type 2 diabetes: Resistance training and hawthorn extract intervention. Heliyon. (2023) 9:e15537. doi: 10.1016/j.heliyon.2023.e15537
140. Reed JN, Huang J, Li Y, Ma L, Banka D, Wabitsch M, et al. Systems genetics analysis of human body fat distribution genes identifies adipocyte processes. Life Sci alliance. (2024) 7:e202402603. doi: 10.26508/lsa.202402603
141. Lee KY, Yamamoto Y, Boucher J, Winnay JN, Gesta S, Cobb J, et al. Shox2 is a molecular determinant of depot-specific adipocyte function. Proc Natl Acad Sci United States America. (2013) 110:11409–14. doi: 10.1073/pnas.1310331110
142. Arner P, Kulyté A. MicroRNA regulatory networks in human adipose tissue and obesity. Nat Rev Endocrinol. (2015) 11:276–88. doi: 10.1038/nrendo.2015.25
143. González-Sánchez GD, Granados-López AJ, López-Hernández Y, Robles MJG, López JA. miRNAs as interconnectors between obesity and cancer. Non-coding RNA. (2024) 10:24. doi: 10.3390/ncrna10020024
144. Liang D, Li G. Pulling the trigger: Noncoding RNAs in white adipose tissue browning. Rev Endocrine Metab Disord. (2024) 25:399–420. doi: 10.1007/s11154-023-09866-6
145. Mori MA, Raghavan P, Thomou T, Boucher J, Robida-Stubbs S, Macotela Y, et al. Role of microRNA processing in adipose tissue in stress defense and longevity. Cell Metab. (2012) 16:336–47. doi: 10.1016/j.cmet.2012.07.017
146. Mori MA, Thomou T, Boucher J, Lee KY, Lallukka S, Kim JK, et al. Altered miRNA processing disrupts brown/white adipocyte determination and associates with lipodystrophy. J Clin Invest. (2014) 124:3339–51. doi: 10.1172/jci73468
147. Li A, Peng R, Sun Y, Liu H, Peng H, Zhang Z. LincRNA 1700020I14Rik alleviates cell proliferation and fibrosis in diabetic nephropathy via miR-34a-5p/Sirt1/HIF-1α signaling. Cell Death disease. (2018) 9:461. doi: 10.1038/s41419-018-0527-8
148. Xia W, Chen H, Chen D, Ye Y, Xie C, Hou M. PD-1 inhibitor inducing exosomal miR-34a-5p expression mediates the cross talk between cardiomyocyte and macrophage in immune checkpoint inhibitor-related cardiac dysfunction. J Immunother cancer. (2020) 8:e001293. doi: 10.1136/jitc-2020-001293
149. Gao W, Yuan LM, Zhang Y, Huang FZ, Gao F, Li J, et al. miR-1246-overexpressing exosomes suppress UVB-induced photoaging via regulation of TGF-β/Smad and attenuation of MAPK/AP-1 pathway. Photochemical photobiological sciences: Off J Eur Photochem Assoc Eur Soc Photobiology. (2023) 22:135–46. doi: 10.1007/s43630-022-00304-1
150. Jin J, Shi Y, Gong J, Zhao L, Li Y, He Q, et al. Exosome secreted from adipose-derived stem cells attenuates diabetic nephropathy by promoting autophagy flux and inhibiting apoptosis in podocyte. Stem Cell Res Ther. (2019) 10:95. doi: 10.1186/s13287-019-1177-1
151. Zhang H, Guan M, Townsend KL, Huang TL, An D, Yan X, et al. MicroRNA-455 regulates brown adipogenesis via a novel HIF1an-AMPK-PGC1α signaling network. EMBO Rep. (2015) 16:1378–93. doi: 10.15252/embr.201540837
152. Alvarez-Dominguez JR, Bai Z, Xu D, Yuan B, Lo KA, Yoon MJ, et al. De novo reconstruction of adipose tissue transcriptomes reveals long non-coding RNA regulators of brown adipocyte development. Cell Metab. (2015) 21:764–76. doi: 10.1016/j.cmet.2015.04.003
153. Zhang Y, Song K, Qi G, Yan R, Yang Y, Li Y, et al. Adipose-derived exosomal miR-210/92a cluster inhibits adipose browning via the FGFR-1 signaling pathway in high-altitude hypoxia. Sci Rep. (2020) 10:14390. doi: 10.1038/s41598-020-71345-8
154. Wang YC, Li Y, Wang XY, Zhang D, Zhang H, Wu Q, et al. Circulating miR-130b mediates metabolic crosstalk between fat and muscle in overweight/obesity. Diabetologia. (2013) 56:2275–85. doi: 10.1007/s00125-013-2996-8
155. Wang YC, Yao X, Ma M, Zhang H, Wang H, Zhao L, et al. miR-130b inhibits proliferation and promotes differentiation in myocytes via targeting Sp1. J Mol Cell Biol. (2021) 13:422–32. doi: 10.1093/jmcb/mjab012
156. Piergentili R, Marinelli E, Cucinella G, Lopez A, Napoletano G, Gullo G, et al. miR-125 in breast cancer etiopathogenesis: an emerging role as a biomarker in differential diagnosis, regenerative medicine, and the challenges of personalized medicine. Non-coding RNA. (2024) 10:16. doi: 10.3390/ncrna10020016
157. Hartwig S, De Filippo E, Göddeke S, Knebel B, Kotzka J, Al-Hasani H, et al. Exosomal proteins constitute an essential part of the human adipose tissue secretome. Biochim Biophys Acta Proteins proteomics. (2019) 1867:140172. doi: 10.1016/j.bbapap.2018.11.009
158. Lv J, Yang S, Lv M, Lv J, Sui Y, Guo S. Protective roles of mesenchymal stem cells on skin photoaging: A narrative review. Tissue Cell. (2022) 76:101746. doi: 10.1016/j.tice.2022.101746
159. Ndzie Noah ML, Adzika GK, Mprah R, Adekunle AO, Koda S, Adu-Amankwaah J, et al. Estrogen downregulates CD73/adenosine axis hyperactivity via adaptive modulation PI3K/Akt signaling to prevent myocarditis and arrhythmias during chronic catecholamines stress. Cell Commun Signal: CCS. (2023) 21:41. doi: 10.1186/s12964-023-01052-0
160. Gentile P, Sterodimas A, Pizzicannella J, Calabrese C, Garcovich S. Research progress on mesenchymal stem cells (MSCs), adipose-derived mesenchymal stem cells (AD-MSCs), drugs, and vaccines in inhibiting COVID-19 disease. Aging disease. (2020) 11:1191–201. doi: 10.14336/ad.2020.0711
161. Krasnodembskaya A, Song Y, Fang X, Gupta N, Serikov V, Lee JW, et al. Antibacterial effect of human mesenchymal stem cells is mediated in part from secretion of the antimicrobial peptide LL-37. Stem Cells (Dayton Ohio). (2010) 28:2229–38. doi: 10.1002/stem.544
162. Sutton MT, Fletcher D, Ghosh SK, Weinberg A, van Heeckeren R, Kaur S, et al. Antimicrobial properties of mesenchymal stem cells: therapeutic potential for cystic fibrosis infection, and treatment. Stem Cells Int. (2016) 2016:5303048. doi: 10.1155/2016/5303048
163. Pizzinat N, Ong-Meang V, Bourgailh-Tortosa F, Blanzat M, Perquis L, Cussac D, et al. Extracellular vesicles of MSCs and cardiomyoblasts are vehicles for lipid mediators. Biochimie. (2020) 178:69–80. doi: 10.1016/j.biochi.2020.07.013
164. Morimoto K, Shirata N, Taketomi Y, Tsuchiya S, Segi-Nishida E, Inazumi T, et al. Prostaglandin E2-EP3 signaling induces inflammatory swelling by mast cell activation. J Immunol (Baltimore Md: 1950). (2014) 192:1130–7. doi: 10.4049/jimmunol.1300290
165. Jiang MJ, Chen YY, Dai JJ, Gu DN, Mei Z, Liu FR, et al. Dying tumor cell-derived exosomal miR-194-5p potentiates survival and repopulation of tumor repopulating cells upon radiotherapy in pancreatic cancer. Mol cancer. (2020) 19:68. doi: 10.1186/s12943-020-01178-6
166. Halma MTJ, Tuszynski JA, Marik PE. Cancer metabolism as a therapeutic target and review of interventions. Nutrients. (2023) 15:4245. doi: 10.3390/nu15194245
167. Yu P, Guo J, Li J, Shi X, Xu N, Jiang Y, et al. lncRNA-H19 in fibroblasts promotes wound healing in diabetes. Diabetes. (2022) 71:1562–78. doi: 10.2337/db21-0724
168. Lin JR, Ding LL, Xu L, Huang J, Zhang ZB, Chen XH, et al. Brown Adipocyte ADRB3 Mediates Cardioprotection via Suppressing Exosomal iNOS. Circ Res. (2022) 131:133–47. doi: 10.1161/circresaha.121.320470
169. Liu Y, Wang C, Wei M, Yang G, Yuan L. Multifaceted roles of adipose tissue-derived exosomes in physiological and pathological conditions. Front Physiol. (2021) 12:669429. doi: 10.3389/fphys.2021.669429
170. Ren S, Chen J, Duscher D, Liu Y, Guo G, Kang Y, et al. Microvesicles from human adipose stem cells promote wound healing by optimizing cellular functions via AKT and ERK signaling pathways. Stem Cell Res Ther. (2019) 10:47. doi: 10.1186/s13287-019-1152-x
171. Woo CH, Kim HK, Jung GY, Jung YJ, Lee KS, Yun YE, et al. Small extracellular vesicles from human adipose-derived stem cells attenuate cartilage degeneration. J Extracell Vesic. (2020) 9:1735249. doi: 10.1080/20013078.2020.1735249
172. Son T, Jeong I, Park J, Jun W, Kim A, Kim OK. Adipose tissue-derived exosomes contribute to obesity-associated liver diseases in long-term high-fat diet-fed mice, but not in short-term. Front Nutr. (2023) 10:1162992. doi: 10.3389/fnut.2023.1162992
Keywords: adipose tissue, adipose-derived stem cell (ADSC), exosome, inflammation, tumor, diabetes
Citation: Wang Y, Li Q, Zhou S and Tan P (2024) Contents of exosomes derived from adipose tissue and their regulation on inflammation, tumors, and diabetes. Front. Endocrinol. 15:1374715. doi: 10.3389/fendo.2024.1374715
Received: 24 January 2024; Accepted: 31 July 2024;
Published: 16 August 2024.
Edited by:
Marcia Hiriart, Universidad Nacional Autonoma de Mexico, MexicoReviewed by:
Zhiqiang Huang, Nanjing University, ChinaXiaosong Chen, Fujian Medical University Union Hospital, China
Copyright © 2024 Wang, Li, Zhou and Tan. This is an open-access article distributed under the terms of the Creative Commons Attribution License (CC BY). The use, distribution or reproduction in other forums is permitted, provided the original author(s) and the copyright owner(s) are credited and that the original publication in this journal is cited, in accordance with accepted academic practice. No use, distribution or reproduction is permitted which does not comply with these terms.
*Correspondence: Qingfeng Li, ZHIubGlxaW5nZmVuZ0B5YWhvby5jb20=; Shuangbai Zhou, c2h1YW5nYmFpemhvdUB5YWhvby5jb20=; Pohching Tan, Ym9qdW5fdGFuQGhvdG1haWwuY29t