- Department of Animal Sciences, The Robert H. Smith Faculty of Agriculture, Food and Environment, The Hebrew University of Jerusalem, Rehovot, Israel
The manipulation of the somatotropic axis, governing growth, has been a focus of numerous transgenic approaches aimed at developing fast-growing fish for research, medicine and aquaculture purposes. However, the excessively high growth hormone (GH) levels in these transgenic fish often result in deformities that impact both fish health and consumer acceptance. In an effort to mitigate these issues and synchronize exogenous GH expression with reproductive processes, we employed a novel transgenic construct driven by a tilapia luteinizing hormone (LH) promoter. This approach was anticipated to induce more localized and lower exogenous GH secretion. In this study, we characterized the growth and reproduction of these transgenic LHp-GH zebrafish using hormonal and physiological parameters. Our findings reveal that LHp-GH fish exhibited accelerated growth in both length and weight, along with a lower feed conversion ratio, indicating more efficient feed utilization, all while maintaining unchanged body proportions. These fish demonstrated higher expression levels of LH and GH in the pituitary and elevated IGF-1 levels in the liver compared to wild-type fish. An examination of reproductive function in LHp-GH fish unveiled lower pituitary LH and FSH contents, smaller follicle diameter in female gonads, and reduced relative fecundity. However, in transgenic males, neither the distribution of spermatogenesis stages nor sperm concentrations differed significantly between the fish lines. These results suggest that coupling exogenous GH expression with endogenous LH expression in females directs resource investment toward somatic growth at the expense of reproductive processes. Consequently, we conclude that incorporating GH under the LH promoter represents a suitable construct for the genetic engineering of commercial fish species, providing accelerated growth while preserving body proportions.
Introduction
The fundamental processes of energy homeostasis, growth, and reproduction, which are essential for fitness and survival, are intimately related. Growth is a polygenetic trait influenced by several physiological pathways connected to energy usage, metabolism, and reproduction (1). The somatotropic axis, which regulates growth in all vertebrates, has, among others, two main components: growth hormone (GH) and insulin-like growth factor-1 (IGF-1). However, the control of the release of GH from the pituitary is complicated and combines growth hormone-releasing hormone (GHRH), gonadotropin-releasing hormone (GnRH), and dopamine, which upregulate GH expression, and somatostatin, which inhibits GH expression (2–6). GH induces elevated free fatty acid levels, hyperplastic muscle growth, and stimulates appetite (7). In addition, GH is responsible for localized secretion of IGF-1 in fat, muscle, and bone tissues, as well as for IGF-1 secretion from the liver into the bloodstream. In fish, IGF-1 affects muscle growth, protein synthesis, and myoblast proliferation (1). In mammals, it has been shown to directly regulate bone growth and density (8). IGF-1 also mediates a negative feedback loop within the somatotropic axis (7).
As mentioned, somatic growth is tightly coupled with reproductive cycles. The hypothalamic-pituitary-gonadal axis produces the hormonal network that regulates reproductive function. GnRH, which is released from the hypothalamus, stimulates the synthesis and release of the gonadotropins, luteinizing hormone (LH) and follicle-stimulating hormone (FSH) from the anterior pituitary. These act individually or cooperatively on the gonads to regulate steroidogenesis and gametogenesis. Whereas FSH stimulates the growth of ovarian follicles in females and spermatogenesis in males, LH induces ovulation in females and androgen secretion in males (9, 10).
Unlike mammals, whose growth stops when they reach sexual maturity, fish grow throughout their life span (11), switching from somatic growth to reproduction and vice versa. The coordination of these two processes requires communication and reciprocal regulation between the somatotropic and hypothalamic-pituitary-gonadal axes (12). For example, GH is regarded as a secondary reproduction hormone (10). In female catfish, GH injections stimulate ovarian growth and development (13), and in rainbow trout, plasma GH levels are associated with gonadal maturation (14). Furthermore, in killifish, GH stimulates E2 synthesis by ovarian tissue (15). IGF-1 has been shown to induce LH synthesis and release in vitro by pituitary cells of juvenile female eels (16) and to increase FSH content and GnRH-stimulated FSH release from juvenile coho salmon pituitary in vitro (17). Moreover, IGF-I increased FSH and LH sensitivity to GnRH in rainbow trout in vitro (18). In goldfish, E2 stimulates GH secretion throughout the reproductive cycle of female goldfish. Furthermore, GnRH3 stimulates GH release in a teleost (19, 20). These interactions constitute the crosstalk between the two axes and could control the energy shifts between somatic growth and reproduction.
The somatotropic axis is a perfect candidate for manipulation through transgenesis to produce fast-growing organisms for research and agriculture, particularly aquaculture. Fish provide a large portion of the global protein intake by humans (21). Given the constant growth in human population and the depletion of natural fish stocks worldwide, supply increasingly relies on cultured fish. Transgenic lines of fast-growing fish have been created from various teleosts, such as coho salmon (22) and domestic and wild rainbow trout (23), expressing the GH-I gene of sockeye salmon driven by the metallothionein-B promoter from the same species. Other attempts included driving GH expression in Mutiara catfish by cytomegalovirus (24), an “all body” GH expression driven by a β-actin gene promoter in common carp (25), GH expressed under the regulation of medaka β-actin in tilapia (26), and zebrafish containing a carp β-actin promoter driving the expression of the marine silverside fish GH (27).
The strategy of driving GH using an “all body”, highly active promoter has produced very fast-growing fish that suffer from health-related problems and abnormal morphological changes (23, 28–30). To solve these issues, we employed a different approach to GH transgenesis in this study that reduces exogenous GH expression by using a less active and, more specifically, localized promoter. Moreover, exogenous GH expression was induced by LH promoter to couple between somatic growth and reproductive processes. In addition to possibly redirecting metabolic resources from sexual maturation and reproduction to somatic growth and thereby ensuring uninterrupted growth, we postulated that this approach would also provide a more conventional transport system for the hormone to reach its target tissues. We used zebrafish, an established model organism with a fully sequenced genome (31, 32), which is widely used to study aging (33), immune system (34), endocrine models (35), heart arrhythmia (36), and cancer research (37), as well as to search for neuropeptides regulating reproduction (38). To characterize this novel line of transgenic zebrafish, we compared hormonal and physiological parameters of growth and reproduction to those of wild-type fish. Our results indicate that the new construct drives increased somatic growth while suppressing reproductive functions without altering body proportions.
Methods
Transgenic construct
For this study, we generated a new transgenic line of zebrafish, Tg(LHp : GH, cmlc2:EGFP), by using Tol2kit (39) as described in Invitrogen Multisite Gateway Manual. Tilapia LH promoter (NC_031978.2) (40) and tilapia GH (NC_031969.2) DNA fragments were amplified by PCR. The LH promoter was inserted into pDONRP4-P1R, and the tilapia GH was cloned into pDONR221, through BP recombination. The 5′-entry clone (p5E), middle-entry clone (pME), and a 3′-entry clone (p3E), which contained a polyA sequence, were then recombined through an LR reaction into the expression vector pDestTol2CG, which finally carries the tiLH promoter, tilapia GH, GFP heart marker and tol2-recognition sequences.
In order to perform the transgenesis, eggs were promptly collected post-spawning and were injected with a mixture of expression plasmid, transposase mRNA, phenol red, and DEPC-treated molecular biology water. After hatching, larvas were screened for EGFP fluorescent marker in the heart using a fluorescence stereo microscope. EGFP-positive embryos were grown and mated as possible founders. Heterozygous mutants were generated by crossing the F0 pLH-GH with WT fish. Then, verified F1 male and female fish were crossed to generate homozygous transgenic fish.
Fish maintenance, measurements and sample collection
Transgenic zebrafish were maintained in a stand-alone unit with central filtration and heating (28 ± 1°C). The fish were fed in excess (food weight for day >3% averaged body weight) twice daily with commercial feed (Aquazone Ltd., Tzofit, Israel). Fish from the F1 generation of both sexes were placed in the same aquarium for breeding. Fertilized eggs were collected the next morning and incubated for 4 days. Larvae were screened and separated into transgenic (LHp-GH) and wild-type (WT), then transferred into brackish (5 ppt) water in stand-alone tanks and fed with rotifers (Brachionus plicatilis) until 14 days post-fertilization (dpf). Then, larvae were transferred to fresh water and fed with 1 ml of artemia until 28 dpf, after which the fish were fed once a day with 300 mg of commercial feed per tank. Overall, fish from the F2 generation were grown for 3 months to reach sexual maturation in two experimental cycles.
Fish length and weight were measured weekly. Weekly weight values were used to calculate the feed conversion ratio [FCR; feed intake (mg)/weight gain (mg)] and specific growth rate (SGR); % day-1 ln (W)- ln (W0)/ΔT X100, where W is the weight at the end of the experimental period (in mg), W0 is the weight at the beginning of the experimental period (in mg), and ΔT is the experimental period (in days). At the age of 3 months, the fish were anesthetized and dissected. The gonads, liver, and visceral fat tissue were weighed in order to calculate the gonadosomatic index (GSI), hepatosomatic index (HSI), and visceral fat somatic index (VFSI), respectively. Somatic indices were calculated as the percentage of the tissue weight from the body weight (BW).
Brains and livers were collected in Eppendorf Safe-Lock tubes and placed in liquid nitrogen for subsequent total RNA extraction. From each fish, one gonad was collected for total RNA extraction, and the other was fixed in Bouin’s fluid for histology, as described previously in Biran et al. (2008) (38). Pituitaries were collected from WT and LHp-GH fish, and half were prepared for total RNA extraction, and the other half were placed in DDW and kept at -20°C for subsequent enzyme-linked immunosorbent assays (ELISA).
All experimental procedures followed the Animal Care and Use Guidelines of the Hebrew University and were approved by the local Administrative Panel on Laboratory Animal Care.
Immunohistochemistry of zebrafish pituitaries
Immunofluorescent staining for tilapia GH and carp LHβ was generally performed as outlined previously (41). Briefly, tissue samples were fixed in 4% paraformaldehyde, immersed in a cryoprotecting agent (30% sucrose), and frozen in an OCT embedding compound. Samples were sectioned at a thickness of 15 μm on a cryostat and collected on Superfrost slides. The sections were blocked with 5% normal goat serum (NGS) for 1 hour to reduce non-specific reactions and then incubated with rabbit anti-tiGH (diluted 1:500) (42) or rabbit anti-carp LHβ (diluted 1:200) (43) for 16 hours at 4°C. Antibodies were diluted in PBS with 1% BSA and 0.3% Triton X-100. The slides were rinsed three times with PBS for 5 minutes and were incubated for 2 hours at room temperature with goat anti-rabbit antibodies conjugated to Alexa488 or Alexa495 fluorophore.
For double-labeled staining, sections were blocked again with NGS (1X 5% PBS, 0.3% NGS in Triton X-100) for 1 hour at room temperature to saturate the open binding sites on the first secondary antibody with IgG. The slides were rinsed three times with PBS for 5 minutes and then incubated for 1 hour with an excess of unconjugated Fab Goat Anti-Rabbit IgG (Enco) diluted at 1:65 in an antibody dilution buffer. The slides were rinsed again three times, and the second primary antibody was applied (anti-rtiGH or anti-cLHβ) for 16 hours at 4°C. After an additional wash, the slides were incubated with the second secondary antibody for 2 hours at 25°C. After washing, slides were mounted with anti-fade solution (2% propyl gallate, 75% glycerol, in PBS) and imaged with confocal microscopy. All staining processes were performed in the dark.
ELISA for gonadotropins and GH
A specific and homologous ELISA for carp LH (cLH), carp FSH (cFSH) and tilapia GH (tiGH) measurements had previously been established in our lab (42, 44–46) The binding of antibodies for cLH and cFSH to zebrafish LH and FSH were validated via immunohistochemical staining (46).
The competitive ELISA for LH and FSH was performed by using primary antibodies against recombinant cFSHβ at a 1:50,000 dilution, recombinant LHβ at a 1:14,000 dilution and recombinant tiGH at a 1:15,000 dilution (45, 46) and (43, 45, 46), respectively. Recombinant cFSHβα, cLHβα and tiGH were adopted as a standard, while the wells were coated with cFSHβ (50 ng/ml), cLHβ (100 ng/ml) and tiGH (25 ng/ml). A second antibody, GAR-HRP (Zotal Israel), was added at a 1:10,000 dilution, and the presence of enzyme complexes was visualized by the addition of 100 μl/well of a 3, 3’, 5, 5’-tetramethylbenzidine peroxidase substrate (KPL, Zotal, Israel). The reaction was carried out in complete darkness at room temperature, and absorbance was recorded at 450 nm after 15 minutes using a Sunrise ELISA reader (Tecan, Switzerland). The sensitivity for the measurements was 15.84 pg/ml for LH, 0.24 pg/ml for FSH and 35.0 pg/ml for GH. The inter-assay coefficient of variation (CV) was 14.8, 12.5, and 13%, while intra-assay CV was 7.2, 8, and 8% for LH, FSH and GH, respectively. The parallelism between the standard curve and serial dilutions of zebrafish pituitary extract was detected for both FSH and LH ELISAs (Supplementary Figure 1).
LHβ, FSHβ and tiGH content in the pituitary were measured by ELISA as described above. Pituitary extracts were prepared in 0.1% PBS. The pituitary extracts were diluted for LH, FSH and GH measurements at 1:25. Each sample was run in duplicate.
RNA extraction and real-time PCR
RNA extraction and real-time PCR were performed generally according to (38, 45). Briefly, total RNA was extracted from the pituitaries using Trizol reagent (GIBCO, USA) according to the manufacturer’s protocol. cDNA (8 ng/µl RNA for pituitary; 40 ng/µl RNA for brain and liver). was prepared with the Verso cDNA Synthesis Kit (Thermo Fisher Scientific, Waltham, MA, USA) according to the manufacturer’s instructions. mRNA levels were normalized by the comparative threshold cycle method against the housekeeping gene elongation factor 1α (ef1a; NM_131263). The real-time PCR procedure was conducted as previously described (38, 47). Serial dilutions were prepared from a pituitary cDNA sample, and the efficiencies of specific gene amplifications were compared by plotting Ct versus log (template). The PCR mixture contained 3 μl of a diluted cDNA sample, 400 nM of each primer, and 10 μl of Platinum SYBR Green qPCR SuperMix-UDG (Invitrogen) in a final volume of 20 μl. Amplification was carried out in a LightCycler96™ Real-Time PCR System (Roche) according to the manufacturer’s protocol. The cDNAs of the genes were amplified simultaneously in separate wells in duplicates, and the results were analyzed with the LightCycler96 software. A dissociation-curve analysis was conducted after each real-time experiment to confirm the presence of only one product. To eliminate false positives, a reverse-transcriptase negative control (RT-PCR) was run for each template and primer pair. Primer pair sequences and their slope and R2 values are listed in Table 1.
Gonad histology
Gonadal histology was performed generally according to Mizrahi et al., 2019 (45). Images of histological slides of female gonads were analyzed using ImageJ FIJI software to determine follicle diameter. Imaged slides of male gonads were analyzed using CPCe 4.1 software to randomly assign 70 points, classify cell stage in spermatogenesis, and quantify stage frequencies according to Mizrahi et al., 2019 (45).
Reproductive performances and fertility
Female reproductive performance was evaluated generally according to the formula RF=eggs (n)/body weight (mg). The relative fecundity, i.e., the ratio between the number of eggs spawned and female body weight, was calculated. Four-month-old sibling females of both lines were housed together. Mating of one female with one WT male was allowed for 1 hour. The female’s genotype was verified using the EGFP heart marker. Upon successful mating, we collected and counted the eggs, weighed the females, and housed them in a different tank. Following unsuccessful mattings, females were returned to the communal tank and were mated again on a different day.
Male fertility was assessed by measuring sperm concentration (48). Males were separated from females for 3 days before sperm collection. The night before sperm collection, males were housed with females in a mating tank, separated by a net. In the morning, male fish were anesthetized in tricaine and gently dried to remove excess water on the papilla. Sperm was collected immediately by placing a microcapillary tube on the papilla and carefully pressing the fish’s abdomen. The volume of extracted semen was calculated using a reference capillary, which contained a known volume of water. Sperm samples that contained urine were discarded. The sperm count was determined using a hemocytometer.
Statistical analyses
Data are presented as mean ± SEM. Gaussian distribution tests (Kolmogorov–Smirnov and Shapiro–Wilk test) and unequal variance test (F-test) were performed with GraphPad Prism 8.0 software. Non-normally distributed data were analyzed using the non-parametric Mann-Whitney U test. Welch’s t-test was used to evaluate the significance of differences between group means when sample variances were not equal, but data distribution was normal. When sample variances were equal, a t-test was used. Statistical significance was defined as P < 0.05.
Results
Verification of transgene expression
To overcome the limitations of current fast-growing transgenic fish, particularly those related to abnormal morphology, we have developed a transgenic zebrafish expressing tilapia GH under the tilapia LH promoter (LHp-GH), that was previously tested (40). We chose the LH promoter rather than the FSH one since, for both tilapia and zebrafish, we found before a higher abundance of LH cells in the pituitary compared to FSH (40, 49). Additionally, our observations suggest that LH pituitary content exceeds that of FSH by 1-2 orders of magnitude (46, 50). This trend was reaffirmed in the current study, where the average LH content in wild-type zebrafish pituitaries was 10 times higher than FSH within the same group.
To verify the presence of GH in LH cells in the pituitary of the transgenic fish, we performed immunohistochemistry analysis on pituitaries from LHp-GH and WT fish using specific and validated antibodies against GH and LH (Figure 1). Results showed an overlap between cells that synthesized GH (stained in green) and those that synthesized LH (stained in magenta) in LHp-GH fish pituitaries. By contrast, the two hormones were detected in different pituitary areas in WT fish.
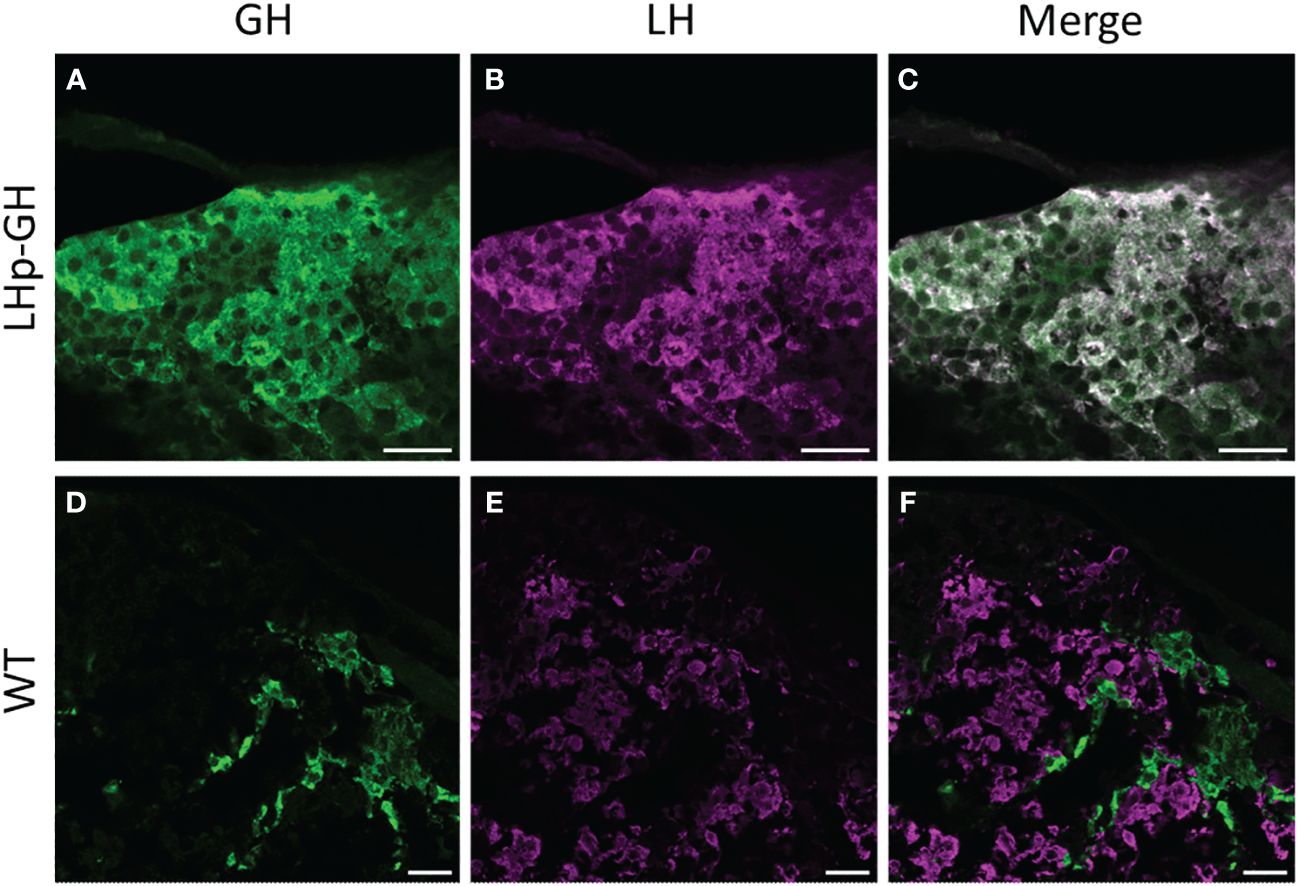
Figure 1 Distribution of LH and GH in the pituitary of zebrafish Tg(tiLHp:tiGH, cmlc2:EGFP) and WT. Confocal microscopy images of immunohistochemical staining of (A, D) GH (green); (B,E) LH (magenta); (C) merge of (A, B); (F) merge of (D, E). (A–C) Tg(LHp-tiGH); (D–F) WT. Scale bar = 20 µm.
Growth performances
To determine the effect of the transgene on growth, we compared length and weight between LHp-GH and WT fish. From 28 dpf onwards, the mean length of LHp-GH fish was significantly greater than that of WT fish, with the exception of a single measurement at 49 dpf, when the mean length of the LHp-GH fish was higher but not statistically significant (Figure 2A; t-test). Likewise, LHp-GH fish were significantly heavier than the WT fish at 21 dpf and from 63 dpf until the end of the experiment (Figure 2B).
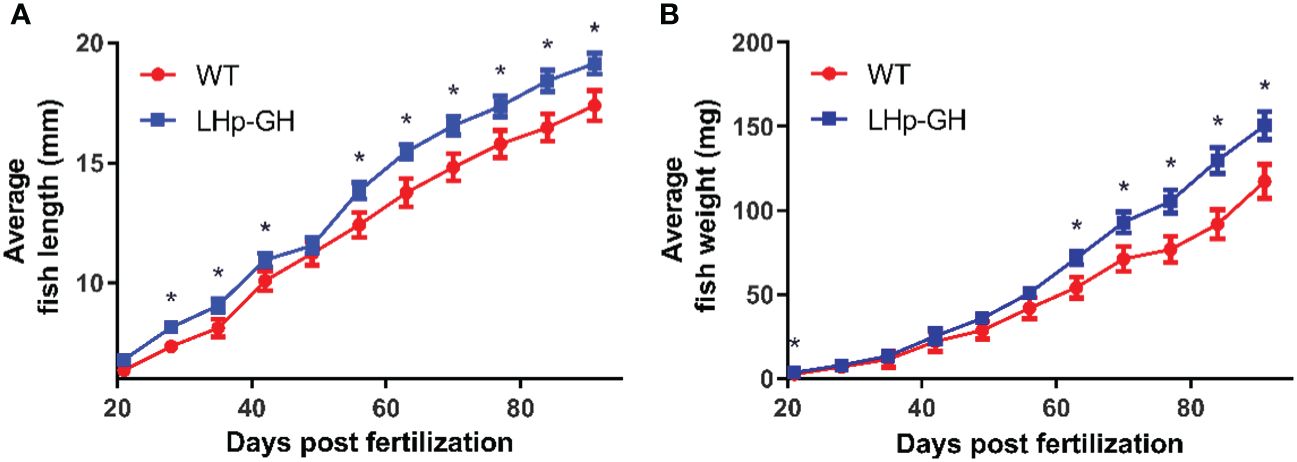
Figure 2 Accelerated weight and length gain in LHp-GH compared to WT fish. Weekly length (A) and weight (B) gain of LHp-GH fish (blue) and wild type (WT; red) fish. Fish were grown for 3 months and fed in excess (food weight for day >3% averaged body weight). n ranged between 85 to 55, depending on the week. The results are combined data from two repeats of the experiment. Asterisks indicate significant differences (P-value < 0.05).
To evaluate the effects of the transgene on fish physiology, we calculated several somatic indices. The GSI is a well-established indicator of reproductive activity and sexual maturation, the HSI informs on the level of vitellogenin synthesis in the liver, and the visceral FSI reveals the accumulation and degradation of fat tissue, reflecting metabolic function. The results showed no significant difference between LHp-GH and WT fish in any index (Figure 3). These findings show that the increase in body mass did not derive from changes in gonadal, hepatic, or fat tissue mass and is more likely to result from an increase in muscle mass, bone mass or both. Examination of gross morphology showed no apparent external changes in body proportion or deformities in LHp-GH fish compared to WT fish.
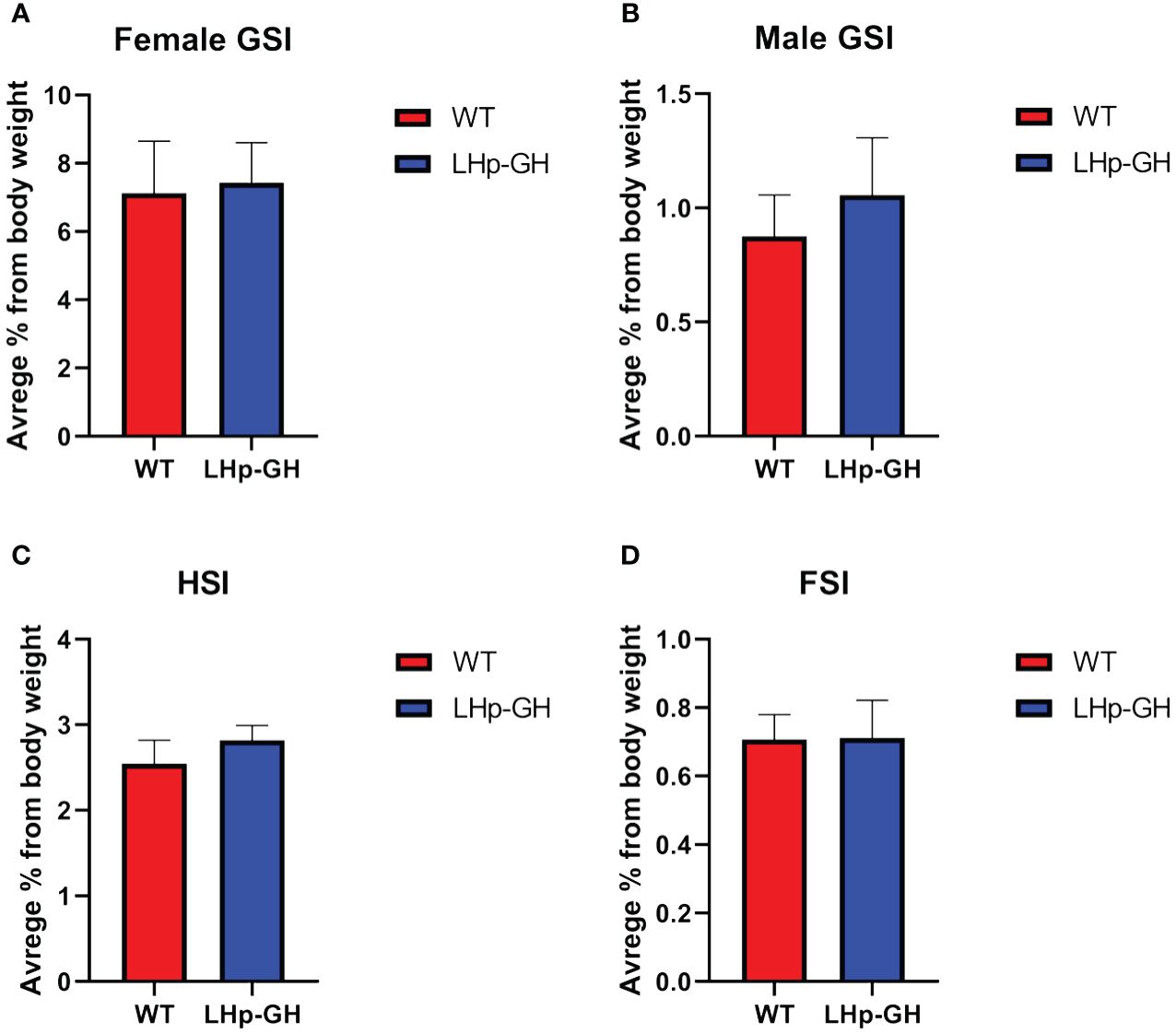
Figure 3 Comparisons of somatic indices between genotypes. Graph showing somatic indices in LHp-GH and WT fish. (A) GSI comparison in females (LHp-GH, n=14; WT, n=8). (B) GSI comparison in males (LHp-GH, n=15; WT, n=7). (C) HSI comparison between lines (LHp-GH, n=30; WT, n=19). (D) FSI comparison between lines (LHp-GH, n=23; WT, n=12). Values represent the mean ± SEM.
Next, to assess growth in the LHp-GH fish compared to the WT, we calculated FCR and SGR values based on the mean weight of the fish as measured weekly. Examination showed a significant difference in weekly FCR values between the fish lines (Figure 4A, p-value ≤ 0.0005, Wilcoxon matched-pairs test). LHp-GH fish weekly FCR values averaged 1.65, while WT fish averaged 2.32. we were not able to detect significant changes between the weekly SGR values of the two lines (Figure 4B).
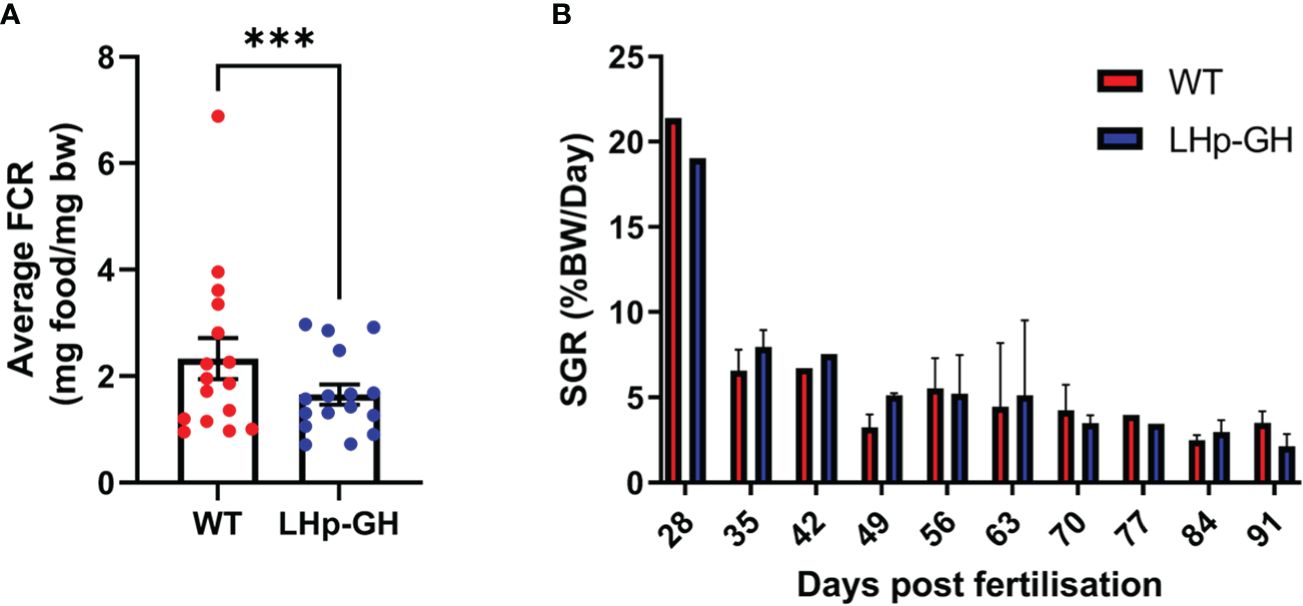
Figure 4 Comparison of FCR and SGR between LHp-GH and WT fish. (A) Teekly FCR values of the fish lines. The weekly FCR values from both experiments are matched and compared (Wilcoxon matched-pairs test; whiskers represent SEM; n=16 (Three asterisks indicate p-value < 0.001). (B) Dfferences in the mean weekly SGR for each week of the experiment (n=4) (Wilcoxon matched-pairs test; whiskers represent SD).
Gonadotropins and growth hormone content in pituitaries of LHp-GH and WT fish
To determine the effect of the transgene on the main hormones related to reproduction and growth, we measured zebrafish LH and FSH and tilapia GH levels in the pituitaries of LHp-GH and WT fish. The results showed significantly higher LH and FSH pituitary content in WT fish, while significantly higher levels of GH were measured in LHp-GH fish (Figure 5; Mann-Whitney U-test). Comparison between sexes showed that LH content was higher in females than in males, whereas FSH and GH levels were similar in males and females (Supplementary Figure 2).
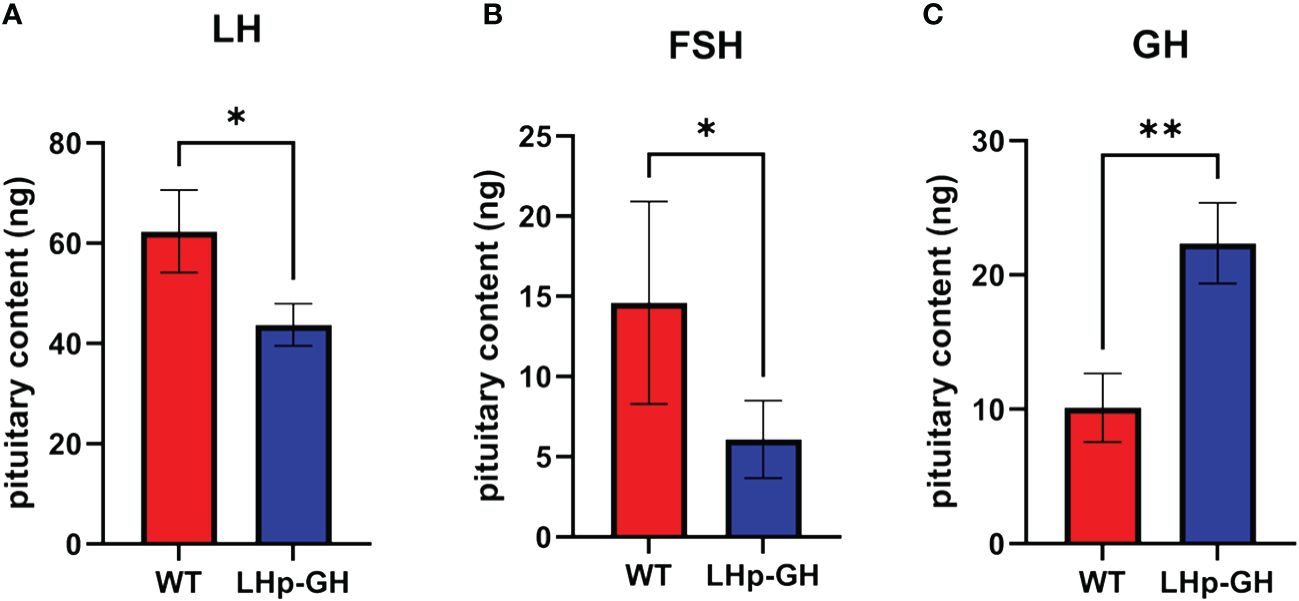
Figure 5 GtH and GH pituitary content in LHp-GH and WT zebrafish. (A) LH content in pituitaries of LHp-GH and WT fish; (B) FSH content in pituitaries of LHp-GH and WT fish; (C) GH content in pituitaries of LHp-GH and WT fish; for LHp-GH fish n=13, for WT fish n=6. Data are presented as mean ± SEM; Asterisks indicate p-value<0.05, double asterisks indicate p-value<0.01.
Expression analysis of growth and reproduction genes
Next, we tested the mRNA levels of essential genes along the growth and reproduction axes. In the brain we tested for: G protein-coupled estrogen receptor 1 (gper1, NM_001128723.1), neuropeptide Y (npy, NM_131074.2), androgen receptor (ar, NM_001083123.1), gonadotropin-releasing hormone 2 (gnrh2, NM_181439.4), gonadotropin-releasing hormone 3 (gnrh3, NM_182887.2), and somatostatin 1, tandem duplicate 2 (NM_001386222.1). in the pituitary we tested for: tilapia growth hormone 1 (tigh, XM_003442542.5), zebrafish growth hormone 1 (zfgh, NM_001020492.2), zebrafish luteinizing hormone (zflh, NM_205622.2), zebrafish follicle stimulating hormone (zffsh NM_205624.1), zebrafish prolactin (zfprl, NM_181437.3), and zebrafish thyroid stimulation hormone (zftsh, NM_181494.2). in the liver we tested for insulin-like growth factor 1 (ifg-1, NM_131825.2). RT-PCR analysis of fish brains showed no significant difference in mRNA levels between LHp-GH and WT fish for any of the examined genes (Figure 6A; Mann-Whitney test). As expected, LHp-GH fish displayed significantly higher tiGH in the pituitary, further validating the construct activity (Figure 6C; Mann-Whitney test). No other examined gene differed significantly in pituitary expression between the fish lines. However, IGF-1 levels in the liver were significantly higher in LHp-GH than WT fish (Figure 6B).
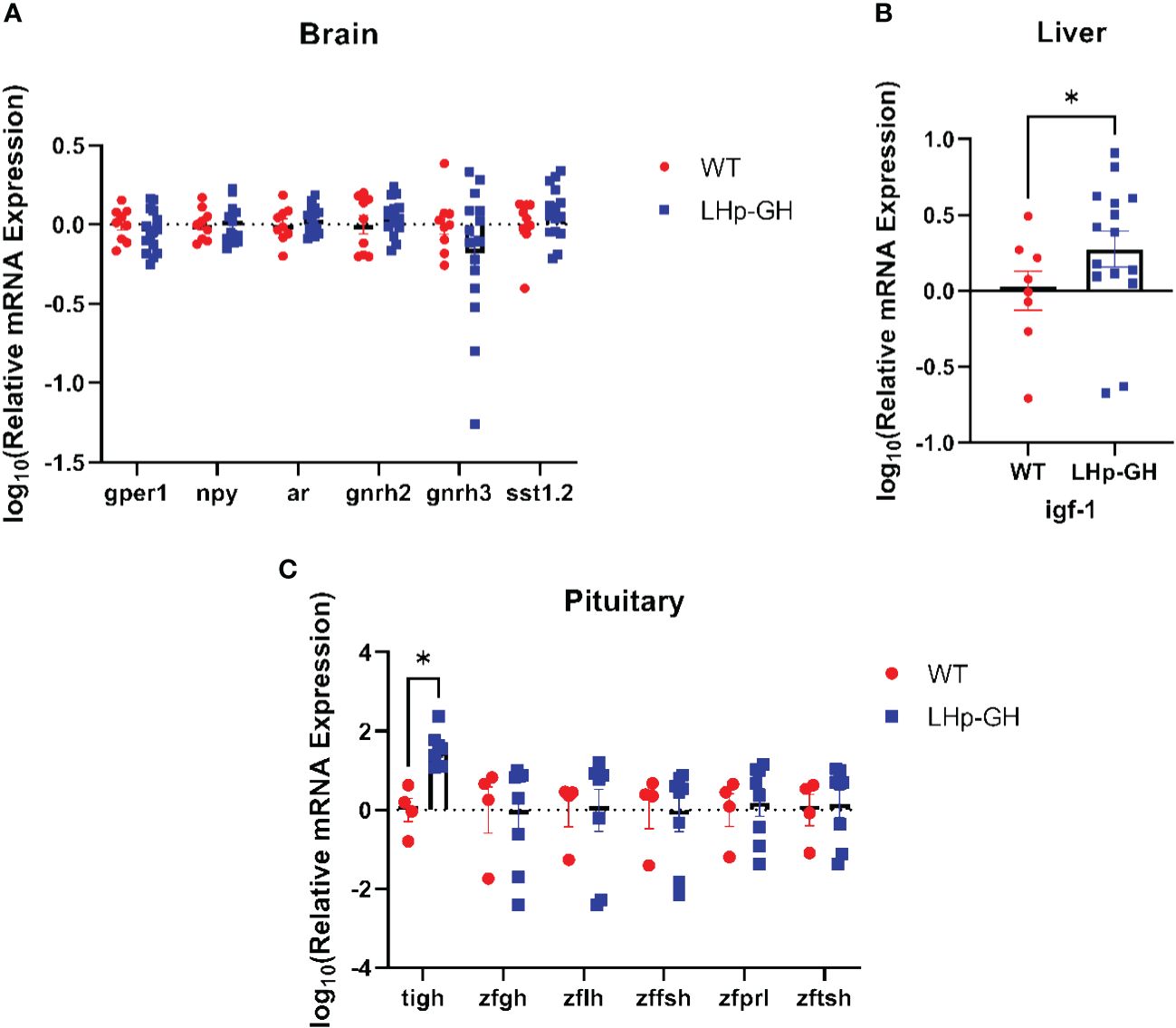
Figure 6 Comparison of mRNA expression of growth and reproduction genes. Expression levels of various genes as measured by real-time RT-PCR analysis in the brain (A), liver (B) and pituitary (C) of LHp-GH and WT fish. Levels of expression were normalized to that of the housekeeping gene ef1a. Statistical significance was measured using two-way ANOVA (brain: LHp-GH fish n=16, WT fish n=9; pituitary: LHp-GH fish n=8, WT fish n=4; liver: LHp-GH fish n=15, WT fish n=8); asterisks indicate significant differences.
Follicle maturation and frequency of spermatogenesis stages
To determine the effect of elevated GH levels on the development of gonads, we performed image analysis on histological sections of ovaries and testes (Figures 7A, B). Because female zebrafish are batch spawners and spawn throughout the year, they constantly carry follicles at various stages of maturation. Accordingly, the mean follicle diameter is highly varied and could not be used to measure follicle maturation. Instead, we calculated the mean of the 10 largest follicle diameters closest to ovulation, thus reducing the variation considerably. The results showed that the mean diameter of these follicles in the gonad was significantly lower in LHp-GH fish than in WT fish (Figure 7C; Mann-Whitney test). We saw a similar trend in relative fecundity, as LHp-GH females spawned fewer eggs relative to their body weight (Figure 7D; t-test). In males, neither the distribution of spermatogenesis stages nor sperm concentrations differed significantly between the fish lines (Supplementary Figure 3).
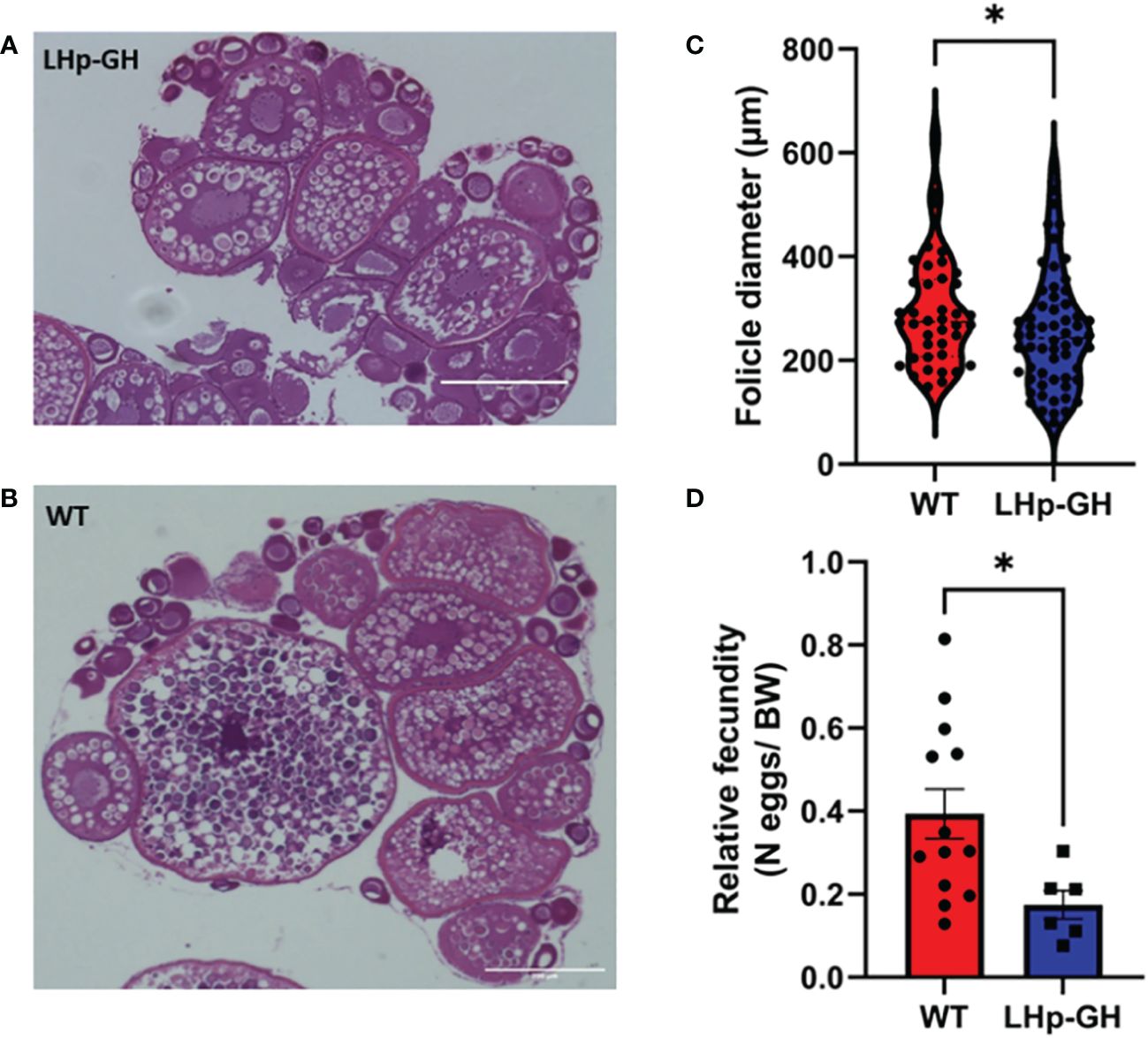
Figure 7 Gonad follicle size and relative fecundity in female LHp-GH vs. WT fish. Examples of stained and imaged gonadal sections from female LHp-GH (A) or WT fish (B); scale bars, 400 µm. Mean follicle diameter of the 10 largest follicles in each slide in the gonads of female LHp-GH and WT fish (C). Mean relative fecundity of female LHp-GH and WT fish (D). (LHp-GH; n=60, WT; n=40; t-test). Data are presented as mean ± SEM. Asterisks in (C, D) indicate significant differences.
Discussion
In vertebrates, the somatotropic axis constantly interacts with other physiological pathways that control energy homeostasis, metabolism and reproduction to regulate somatic growth. Thus, transgenic fish that produce high levels of GH have immense potential for science, medicine, and aquaculture. Indeed, transgenic GH lines of various teleosts generated in the last decades display accelerated growth. However, in most cases, a highly active promoter was used to drive the expression of GH, resulting in fish that are more prone to health problems and severe morphological abnormalities (22–27). In this instance, we utilized an alternative GH transgenesis approach, incorporating a less active and, more precisely, localized LH promoter. Upon confirming the construct’s efficacy by demonstrating the expression of exogenous GH in LH cells of the pituitaries in this new LHp-GH zebrafish, we conducted a more in-depth characterization of these novel fish lines. Our results show that they exhibited accelerated growth in length and weight, lower FCR values, and higher expression and production levels of tiGH in the pituitary and of IGF-1 expression in the liver, combined with reduced GtH production levels. Analysis of reproductive parameters unveiled that female LHp-GH fish exhibited smaller gonad follicles and a reduced egg-spawning count compared to weight-matched WT females. This suggests a resource redirection from reproduction to somatic growth in the LHp-GH fish.
Growth performance is of the utmost importance for aquaculture. In the last decades, many newly developed lines of GH transgenic fish from a variety of species, including coho salmon (22), domestic and wild rainbow trout (23), catfish (24), common carp (25), tilapia (26), and zebrafish (27), have displayed intensified growth, as they were heavier and longer than their WT counterparts. The findings of our study are consistent with those previous results. The mean length of the LHp-GH fish was significantly higher throughout most of the experiment. Body weights also differed significantly from the second half of the experiment since LHp-GH fish were heavier than WT fish.
The FCR parameter represents the efficiency with which an organism converts food into body mass, a process that is tightly regulated by the somatotropic axis. The Feed Conversion Ratio (FCR) values in fish bear significant implications for aquaculture, with GH transgenic fish frequently demonstrating lower values. This observation suggests that GH-transgenic fish tend to achieve greater body mass than wild-type (WT) counterparts while consuming an equivalent amount of food (51, 52). Our results show a similar trend, as LHp-GH fish displayed lower FCR values. Combined with the increases in length and weight, these findings strengthen the conclusion that LHp-GH fish devote more energy and resources to somatic growth.
Interestingly, some of our results were inconsistent with those of former studies. For instance, the SGR did not differ between LHp-GH and WT fish. Previous studies measuring SGR in transgenic GH fish reported significantly higher values than WT fish. This was shown in coho salmon in which metallothionein-b promotor drove GH expression (22, 23), in common carp in which grass carp GH driven by β-actin gene promoter of common carp was used (25), and in Nile Tilapia where the medaka β-actin promoter drove tiGH expression (53). One reason for this inconsistency may be the use of different statistical analyses. We calculated SGR values for each week separately and then compared these values between LHp-GH and WT fish by using matched pairs for all weeks, whereas other studies have usually employed a t-test or Mann-Whitney u-test on SGR data from a single period to compare multiple groups or individuals of each treatment (54).
During the experiment, while no significant differences in SGR were observed between the two fish lines, significant differences in length and weight were noted. However, it is possible that there were some specific periods of SGR variation that went undetected. Such transient fluctuations could have contributed to minor disparities in weight, which subsequently amplified throughout the study. It’s important to note that variations in weight can manifest even when SGR values are comparable. This phenomenon arises because expressing growth as a percentage of body weight results in different weight gains among subjects with varying initial body weights.
Somatic indexes, such as the GSI, HSI, and VFSI, provide information about physiological processes such as fat accumulation, gonadal development, environmental adaptation, and general well-being (55). We found no significant change in GSI, HSI, or VFSI between LHp-GH and WT fish. Similar GSI values suggest that reproductive development is strongly coupled with body weight, such that the high GH levels did not direct energy and resources toward somatic growth at the expense of sexual development. Bessey et al. (54) reported similar findings and conclusions after identifying an unchanged GSI in GH transgenic coho salmon (56). However, the decrease in FSH and LH pituitary content and follicle diameter may suggest that such redirection of energy does occur, as has been shown in other studies on fish. For example, it has been shown that common carp that overexpress GH exhibit a reduction in GSI compared to their WT counterparts (57–59). Furthermore, in GH transgenic tilapia, it was observed that the female GSI was lower in transgenic individuals compared to their non-transgenic siblings in both mixed and separate culture conditions. Additionally, transgenic male GSI values were lower in separate cultures than their non-transgenic siblings (60).
In any case, the unchanged somatic indices could imply that the bodies of the LHp-GH fish remained proportional and similar to those of the WT fish and that the weight gain that the LHp-GH fish exhibit is not the result of increment in gonadal, hepatic or fat tissues. If so, it is possible that the conservation of body proportion in our LHp-GH fish may result from lower and localized GH expression, as compared to other GH transgenic fish which might allow the LHp-GH fish to retain their enhanced growth while avoiding body deformation. The unchanged body proportions could be beneficial in aquaculture. In some transgenic fish lines, overexpression of GH under the regulation of a highly active promoter, such as β-actin or metallothionein-B, has caused unwanted deformities, such as acromegaly, abnormal body shape, and changes in organ locations (23, 28–30). These morphological changes might affect fish health and consumer acceptance. Thus, using the LHp : GH construct, which ended with increased fish size while preserving their body proportion, produces more suitable fish for consumption.
Inregard to gonadotropin levels in the pituitary, our findings indicate a notable decrease in LH and FSH pituitary content in LHp-GH fish compared to WT fish. This finding is consistent with the findings of (61) who reported GH inhibition upon LH release from neighboring GH cells in common carp, in addition, elevated igf-1 levels have been shown to increase LH content in the pituitary (16, 62). These two interactions act apparently in opposition to each other. However, in the current study we observed a decrease in gonadotropin levels, indicating that the regulatory effect of GH overexpression on the gonadotropins was more pronounced than the effects of elevated igf-1. Consequently, we can suggest that the observed changes in reproductive parameters were caused by high GH, levels that inhibits the release of LH and FSH.
Concerning gonad morphology, we found that LHp-GH females have smaller follicles than WT females. These results are consistent with previous findings in salmonids (56, 63) Yet, whereas Bessey et al. (54) reported decreased egg diameter but increased egg number in GH transgenic coho salmon, which led to unchanged GSIs, Jonsson et al. (61) showed that Atlantic salmon displayed a phenotypically plastic response to reduction in juvenile resource abundance and growth opportunities by increasing egg size and decreasing fecundity (56, 63). The current study demonstrated a different trend, as both relative fecundity and follicle size decreased. This implies that the alterations in the reproductive parameters of female tilapia may not solely be attributed to elevated GH levels but may also result from the integration of GH with reproductive processes.
Our findings related to endocrine regulation may offer a possible explanation for the observed morphological changes. As expected, gene expression analysis revealed gene changes associated with the somatotropic axis and growth regulation. tiGH expression level was significantly elevated in the pituitary, alongside IGF-1 level in the liver. These two hormones are critical components in the somatotropic axis, and therefore, their upregulation may account for the changes noted in both growth and feed efficiency (1).
Another intriguing revelation from these findings is the interaction between tilapia GH and the zebrafish growth hormone receptor (zfGHr). Considering the considerable phylogenetic distance between these two fish species in the piscine world, it was noteworthy to discover that tiGH could bind to the zfGHr and induce effects such as increased IGF-1 expression levels and growth. These effects likely contributed to the overall enhanced growth performances that were consistently observed throughout the study. Although the exact affinity of zfGHr for tiGH and the efficiency of this binding in mediating effects in target cells are yet to be determined, we can confidently posit that tiGH is indeed capable of binding to zfGHr.
In summary, our primary observations highlight that the LHp-GH zebrafish line demonstrated accelerated growth and more efficient conversion of food into body mass, likely attributed to the heightened activation of the somatic axis. Despite the rapid growth, the preservation of body proportions suggests the viability of employing this transgenic construct in genetic engineering for commercial aquaculture fish species. Furthermore, we suggest that the decline in reproductive parameters results from the co-regulation of GH and LH expression, promoting the redirection of metabolic resources from reproduction to somatic growth. With the exception of fish species that are grown for their gonads or eggs, this shift is a highly desirable trait that further qualifies the LHp : GH construct as a highly potential candidate for effective GH transgenesis.
Data availability statement
The raw data supporting the conclusions of this article will be made available by the authors, without undue reservation.
Ethics statement
The animal study was approved by Animal Care and Use Guidelines of the Hebrew University and were approved by the local Administrative Panel on Laboratory Animal Care. The study was conducted in accordance with the local legislation and institutional requirements.
Author contributions
NC-R: Data curation, Formal analysis, Investigation, Methodology, Writing – original draft. NM: Conceptualization, Investigation, Writing – review & editing. BL-S: Conceptualization, Funding acquisition, Resources, Supervision, Writing – review & editing.
Funding
The author(s) declare financial support was received for the research, authorship, and/or publication of this article. The U.S.-Israel Binational Science Foundation (Joint Funding Research Grants # NSF-BSF-1947541).
Conflict of interest
The authors declare that the research was conducted in the absence of any commercial or financial relationships that could be construed as a potential conflict of interest.
Publisher’s note
All claims expressed in this article are solely those of the authors and do not necessarily represent those of their affiliated organizations, or those of the publisher, the editors and the reviewers. Any product that may be evaluated in this article, or claim that may be made by its manufacturer, is not guaranteed or endorsed by the publisher.
Supplementary material
The Supplementary Material for this article can be found online at: https://www.frontiersin.org/articles/10.3389/fendo.2024.1369043/full#supplementary-material
References
1. Dai XY, Zhang W, Zhuo ZJ, He JY, Yin Z. Neuroendocrine regulation of somatic growth in fishes. Sci China Life Sci. (2015) 58:137–47. doi: 10.1007/s11427-015-4805-8
2. Peter RE, Marchant TA. The endocrinology of growth in carp and related species. Aquaculture. (1995) 129:299–321. doi: 10.1016/0044-8486(94)00302-5
3. Lee LTO, Siu FKY, Tam JKV, Lau ITY, Wong AOL, Lin MCM, et al. Discovery of growth hormone-releasing hormones and receptors in nonmammalian vertebrates. Proc Natl Acad Sci U.S.A. (2007) 104:2133–8. doi: 10.1073/pnas.0611008104
4. Melamed P, Eliahu N, Ofir M, Levavi-Sivan B, Smal J, Rentier-Delrue F, et al. The effects of gonadal development and sex steroids on growth hormone secretion in the male tilapia hybrid (Oreochromis niloticus × O. aureus). Fish Physiol Biochem. (1995) 14:267–77. doi: 10.1007/BF00004065
5. Kwong P, Chang JP. Somatostatin inhibition of growth hormone release in goldfish: possible targets of intracellular mechanisms of action. (1997) 446:56–108. doi: 10.1006/gcen.1997.6995
6. Li WS, Lin HR, Wong AOL. Effects of gonadotropin-releasing hormone on growth hormone secretion and gene expression in common carp pituitary. Comp Biochem Physiol Part B. (2002) 132:335–41. doi: 10.1016/S1096-4959(02)00039-8
7. Jönsson E, Björnsson BT. Physiological functions of growth hormone in fish with special reference to its influence on behaviour. Fisheries Science. (2002) 68:742–8. doi: 10.2331/fishsci.68.sup1_742
8. Yakar S, Rosen CJ, Beamer WG, Ackert-Bicknell CL, Wu Y, Liu JL, et al. Circulating levels of IGF-1 directly regulate bone growth and density. J Clin Invest. (2002) 110:771. doi: 10.1172/JCI200215463
9. Molés G, Hausken K, Carrillo M, Zanuy S, Levavi-Sivan B, Gómez A. Generation and use of recombinant gonadotropins in fish. Gen Comp Endocrinol. (2020) 113555. doi: 10.1016/j.ygcen.2020.113555
10. Levavi-Sivan B, Bogerd J, Mananos EL, Gomez A, Lareyre JJ. Perspectives on fish gonadotropins and their receptors. Gen Comp Endocrinol. (2010) 165:412–37. doi: 10.1016/j.ygcen.2009.07.019
11. Finch CE, Pike MC. Maximum life span predictions from the Gompertz mortality model. J Gerontol A Biol Sci Med Sci. (1996) 51A:B183–94. doi: 10.1093/gerona/51A.3.B183
12. Taranger GL, Carrillo M, Schulz RW, Fontaine P, Zanuy S, Felip A, et al. Control of puberty in farmed fish. Gen Comp Endocrinol. (2010) 165:483–515. doi: 10.1016/j.ygcen.2009.05.004
13. Singh AK, Lal B. Seasonal and circadian time-dependent dual action of GH on somatic growth and ovarian development in the Asian catfish, Clarias batrachus (Linn.): Role of temperature. Gen Comp Endocrinol. (2008) 159:98–106. doi: 10.1016/j.ygcen.2008.08.001
14. Holloway AC, Sheridan MA, van der Kraak G, Leatherland JF. Correlations of plasma growth hormone with somatostatin, gonadal steroid hormones and thyroid hormones in rainbow trout during sexual recrudescence. Comp Biochem Physiol - B Biochem Mol Biol. (1999) 123:251–60. doi: 10.1016/S0305-0491(99)00059-0
15. Singh H, Griffith RW, Takahashi A, Kawauchi H, Thomas P, Stegeman JJ. Regulation of gonadal steroidogenesis in Fundulus heteroclitus by recombinant salmon growth hormone and purified salmon prolactin. Gen Comp Endocrinol. (1988) 72:144–53. doi: 10.1016/0016-6480(88)90190-6
16. Huang YS, Rousseau K, Le Belle N, Vidal B, Burzawa-Gerard E, Marchelidon J, et al. Insulin-like growth factor-I stimulates gonadotrophin production from eel pituitary cells: a possible metabolic signal for induction of puberty. J Endocrinol. (1998) 159:43–52. doi: 10.1677/joe.0.1590043
17. Baker DM, Davies B, Dickhoff WW, Swanson P. Insulin-like growth factor I increases follicle-stimulating hormone (FSH) content and gonadotropin-releasing hormone-stimulated FSH release from coho salmon pituitary cells in vitro. Biol Reprod. (2000) 63:865–71. doi: 10.1095/biolreprod63.3.865
18. Weil C, Carré F, Blaise O, Breton B, Le Bail PY. Differential effect of insulin-like growth factor I on in vitro gonadotropin (I and II) and growth hormone secretions in rainbow trout (Oncorhynchus mykiss) at different stages of the reproductive cycle. Endocrinol. (1999) 140:2054–62. doi: 10.1210/endo.140.5.6747
19. Trudeau VL, Somoza GM, Nahorniak CS, Peter RE. Interactions of estradiol with gonadotropin-releasing hormone and thyrotropin-releasing hormone in the control of growth hormone secretion in the goldfish. Neuroendocrinol. (1992) 56:483–90. doi: 10.1159/000126265
20. Zou JJ, Trudeau VL, Cui Z, Brechin J, MacKenzie K, Zhu Z, et al. Estradiol stimulates growth hormone production in female goldfish. Gen Comp Endocrinol. (1997) 106:102–12. doi: 10.1006/gcen.1996.6857
21. FAO. The state of world fisheries and aquaculture 2020(2020). Available at: https://www.fao.org/state-of-fisheries-aquaculture.
22. Devlin RH, D’Andrade M, Uh M, Biagi CA. Population effects of growth hormone transgenic coho salmon depend on food availability and genotype by environment interactions. Proc Natl Acad Sci U.S.A. (2004) 101:9303–8. doi: 10.1073/pnas.0400023101
23. Devlin RH, Biagi CA, Yesaki TY, Smailus DE, Byatt JC. Growth of domesticated transgenic fish. Nature. (2001) 409:781–2. doi: 10.1038/35057314
24. Buwono ID, Iskandar I, Grandiosa R. Growth hormone transgenesis and feed composition influence growth and protein and amino acid content in transgenic G3 mutiara catfish (Clarias gariepinus). Aquaculture Int. (2021) 29:431–51. doi: 10.1007/s10499-020-00628-8
25. Li D, Fu C, Hu W, Zhong S, Wang Y, Zhu Z. Rapid growth cost in “all-fish” growth hormone gene transgenic carp: Reduced critical swimming speed. Chin Sci Bull. (2007) 52:1501–6. doi: 10.1007/s11434-007-0217-x
26. Kobayashi SI, Alimuddin, Morita T, Miwa M, Lu J, Endo M, et al. Transgenic Nile tilapia (Oreochromis niloticus) over-expressing growth hormone show reduced ammonia excretion. Aquaculture. (2007) 270:427–35. doi: 10.1016/j.aquaculture.2007.05.016
27. Silva ACG, Almeida DV, Nornberg BF, Figueiredo MA, Romano LA, Marins LF. Effects of double transgenesis of somatotrophic axis (GH/GHR) on skeletal muscle growth of zebrafish (Danio rerio). Zebrafish. (2015) 12:408. doi: 10.1089/zeb.2015.29001.sil
28. Dunham RA. Utilization of transgenic fish in developing countries: Potential benefits and risks. J World Aquac Soc. (1999) 30:1–11. doi: 10.1111/j.1749-7345.1999.tb00312.x
29. Lu J, Yoshizaki G, Endo M, Takeuchi T. Effect of dietary high amount of calcium and phosphorous on reducing the prevalence of morphological deformities in GH-transgenic Nile tilapia. Fisheries Sci. (2013) 79:647–58. doi: 10.1007/s12562-013-0626-5
30. Venugopal T, Anathy V, Kirankumar S, Pandian TJ. Growth enhancement and food conversion efficiency of transgenic fish Labeo rohita. J Exp Zool A Comp Exp Biol. (2004) 301:477–90. doi: 10.1002/jez.a.78
31. Lieschke GJ, Currie PD. Animal models of human disease: zebrafish swim into view. Nat Rev Genet. (2007) 8:353–67. doi: 10.1038/nrg2091
32. Jekosch K. The zebrafish genome project: sequence analysis and annotation. Methods Cell Biol. (2004) 77:225–39. doi: 10.1016/S0091-679X(04)77012-0
33. Gerhard GS, Cheng KC. A call to fins! Zebrafish as a gerontological model. Aging Cell. (2002) 1:104–11. doi: 10.1046/j.1474-9728.2002.00012.x
34. Traver D, Herbomel P, Patton EE, Murphey RD, Yoder JA, Litman GW, et al. The zebrafish as a model organism to study development of the immune system. In: Advances in immunology, vol. 81. Academic Press Inc (2003). p. 253–330.
35. McGonnell IM, Fowkes RC. Fishing for gene function–endocrine modelling in the zebrafish. J Endocrinol. (2006) 189:425–39. doi: 10.1677/joe.1.06683
36. Milan DJ, MacRae CA. Zebrafish genetic models for arrhythmia. Prog Biophys Mol Biol. (2008) 98:301–8. doi: 10.1016/j.pbiomolbio.2009.01.011
37. Feitsma H, Cuppen E. Zebrafish as a cancer model. Mol Cancer Res. (2008) 6:685–94. doi: 10.1158/1541-7786.MCR-07-2167
38. Biran J, Ben-Dor S, Levavi-Sivan B. Molecular identification and functional characterization of the Kisspeptin/Kisspeptin receptor system in lower vertebrates. Biol Reprod. (2008) 4:776–86. doi: 10.1095/biolreprod.107.066266
39. Kwan KM, Fujimoto E, Grabher C, Mangum BD, Hardy ME, Campbell DS, et al. The Tol2kit: A multisite gateway-based construction kit for Tol2 transposon transgenesis constructs. Dev Dynamics. (2007) 236:3088–99. doi: 10.1002/dvdy.21343
40. Golan M, Biran J, Levavi-Sivan B. A novel model for development, organization, and function of gonadotropes in fish pituitary. Front Endocrinol (Lausanne). (2014) 5. doi: 10.3389/fendo.2014.00182
41. Golan M, Levavi-Sivan B. Social dominance in tilapia is associated with gonadotroph hyperplasia. Gen Comp Endocrinol. (2013) 192:126–35. doi: 10.1016/j.ygcen.2013.04.032
42. Melamed P, Eliahu N, Levavi-Sivan B, Ofir M, Farchi-Pisanty O, Rentier-Delrue F, et al. Hypothalamic and thyroidal regulation of growth hormone in tilapia. Gen Comp Endocrinol. (1995) 97:13–30. doi: 10.1006/gcen.1995.1002
43. Aizen J, Hollander-Cohen L, Shpilman M, Levavi-Sivan B. Biologically active recombinant carp LH as a spawning-inducing agent for carp. J Endocrinol. (2017) 232:391–402. doi: 10.1530/JOE-16-0435
44. Aizen J, Kasuto H, Levavi-Sivan B. Development of specific enzyme-linked immunosorbent assay for determining LH and FSH levels in tilapia, using recombinant gonadotropins. Gen Comp Endocrinol. (2007) 153:323–32. doi: 10.1016/j.ygcen.2007.04.004
45. Mizrahi N, Gilon C, Atre I, Ogawa S, Parhar IS, Levavi-Sivan B. Deciphering direct and indirect effects of neurokinin B and GnRH in the brain-pituitary axis of tilapia. Front Endocrinol. (2019) 1:469. doi: 10.3389/fendo.2019.00469
46. Hollander-Cohen L, Golan M, Aizen J, Shpilman M, Levavi-Sivan B. Characterization of carp gonadotropins: Structure, annual profile, and carp and zebrafish pituitary topographic organization. Gen Comp Endocrinol. (2018) 264:28–38. doi: 10.1016/j.ygcen.2017.11.022
47. Levavi-Sivan B, Biran J, Fireman E. Sex steroids are involved in the regulation of gonadotropin-releasing hormone and dopamine D2 receptors in female tilapia pituitary. Biol Reprod. (2006) 75:642–50. doi: 10.1095/biolreprod.106.051540
48. Jing R, Huang C, Bai C, Tanguay R, Dong Q. Optimization of activation, collection, dilution, and storage methods for zebrafish sperm. Aquaculture. (2009) 290:165–71. doi: 10.1016/j.aquaculture.2009.02.027
49. Golan M, Martin AO, Mollard P, Levavi-Sivan B. Anatomical and functional gonadotrope networks in the teleost pituitary. Sci. Rep. (2016) 6:23777. doi: 10.1038/srep23777
50. Mizrahi N, Levavi-Sivan B. A novel agent for induced spawning using a combination of GnRH analog and an FDA-approved dopamine receptor antagonist. Aquaculture. (2023) 565:739095. doi: 10.1016/j.aquaculture.2022.739095
51. Dwi Buwono I, Junianto J, Iskandar I, Alimuddin A. Growth and expression level of growth hormone in transgenic mutiara catfish second generation. J Biotech Res. (2019) 10:102–9. Available at: http://www.btsjournals.com/assets/2019v10p102-109.pdf.
52. Kurdianto, Alimuddin, Faridah N, Yoshizaki G, Nuryati S, Setiawati M. Growth, Survival, and Body Composition of Transgenic Common Carp Cyprinus carpio 3rd Generation Expressing Tilapia Growth Hormone cDNA. Hayati. (2016) 23:150–4. doi: 10.1016/j.hjb.2016.12.002
53. Lu J, Li J, Furuya Y, Yoshizaki G, Sun H, Endo M, et al. Efficient productivity and lowered nitrogen and phosphorus discharge load from GH-transgenic tilapia (Oreochromis niloticus) under visual satiation feeding. Aquacult. (2009) 293:241–7. doi: 10.1016/j.aquaculture.2009.04.021
54. Yossa R, Sarker PK, Karanth S, Ekker M, Vandenberg GW. Effects of dietary biotin and avidin on growth, survival, feed conversion, biotin status and gene expression of zebrafish Danio rerio. Comp Biochem Physiol B Biochem Mol Biol. (2011) 160:150–8. doi: 10.1016/j.cbpb.2011.07.005
55. Rizzo E, Bazzoli N. Reproduction and embryogenesis. Biol Physiol Freshw Neotropical Fish. (2020) Chapter 13. Editor: Baldisserotto B, Urbinati EC, Cyrino JEP. In iology and Physiology of Freshwater Neotropical Fish. Academic Press. doi: 10.1016/B978-0-12-815872-2.00013-0
56. Bessey C, Devlin RH, Liley NR, Biagi CA. Reproductive performance of growth-enhanced transgenic coho salmon. Trans Am Fish Soc. (2004) 133(5):1205–20. doi: 10.1577/T04-010.1
57. Kapuscinski AR, Hayes KR, Li S, Dana G, Hallerman EM, Schei PJ, et al. Environmental risk assessment of genetically modified organisms. Methodologies Transgenic Fish. (2007) 3.
58. Chen J, Cao M, Zhang A, Shi M, Tao B, Li Y, et al. Growth hormone overexpression disrupts reproductive status through actions on leptin. Front Endocrinol (Lausanne). (2018) 9:131. doi: 10.3389/fendo.2018.00131
59. Cao M, Chen J, Peng W, Wang Y, Liao L, Li Y, et al. Effects of growth hormone over-expression on reproduction in the common carp Cyprinus carpio L. Gen Comp Endocrinol. (2014) 195:47–57. doi: 10.1016/j.ygcen.2013.10.011
60. Rahman MA, Ronyai A, Engidaw BZ, Jauncey K, Hwang GL, Smith A, et al. Growth and nutritional trials on transgenic Nile tilapia containing an exogenous fish growth hormone gene. J Fish Biol. (2001) 59:62–78. doi: 10.1111/j.1095-8649.2001.tb02338.x
61. Zhou H, Wang X, Ko WKW, Wong AOL. Evidence for a novel intrapituitary autocrine/paracrine feedback loop regulating growth hormone synthesis and secretion in grass carp pituitary cells by functional interactions between gonadotrophs and somatotrophs. Endocrinol. (2004) 145:5548–59. doi: 10.1210/en.2004-0362
62. Dees WL, Hiney JK, Srivastava VK. IGF-1 influences gonadotropin-releasing hormone regulation of puberty. Neuroendocrinology. (2021) 111:1151–63. doi: 10.1159/000514217
Keywords: LH, transgenic fish, GH, pituitary, growth
Citation: Cohen-Rothschild N, Mizrahi N and Levavi-Sivan B (2024) Characterization of a novel fast-growing zebrafish: a new approach to growth hormone transgenesis. Front. Endocrinol. 15:1369043. doi: 10.3389/fendo.2024.1369043
Received: 11 January 2024; Accepted: 13 March 2024;
Published: 02 April 2024.
Edited by:
Vera Chesnokova, Cedars Sinai Medical Center, United StatesCopyright © 2024 Cohen-Rothschild, Mizrahi and Levavi-Sivan. This is an open-access article distributed under the terms of the Creative Commons Attribution License (CC BY). The use, distribution or reproduction in other forums is permitted, provided the original author(s) and the copyright owner(s) are credited and that the original publication in this journal is cited, in accordance with accepted academic practice. No use, distribution or reproduction is permitted which does not comply with these terms.
*Correspondence: Berta Levavi-Sivan, QmVydGEuU2l2YW5AbWFpbC5odWppLmFjLmls