- 1College of Basic Medicine, Jining Medical University, Jining, Shandong, China
- 2State Key Laboratory of Stem Cell and Reproductive Biology, Institute of Zoology, Chinese Academy of Sciences, Beijing, China
- 3The Collaborative Innovation Center, Jining Medical University, Jining, Shandong, China
- 4Lin He’s Academician Workstation of New Medicine and Clinical Translation, Jining Medical University, Jining, Shandong, China
In mammals, gonadal somatic cell lineage differentiation determines the development of the bipotential gonad into either the ovary or testis. Sertoli cells, the only somatic cells in the spermatogenic tubules, support spermatogenesis during gonadal development. During embryonic Sertoli cell lineage differentiation, relevant genes, including WT1, GATA4, SRY, SOX9, AMH, PTGDS, SF1, and DMRT1, are expressed at specific times and in specific locations to ensure the correct differentiation of the embryo toward the male phenotype. The dysregulated development of Sertoli cells leads to gonadal malformations and male fertility disorders. Nevertheless, the molecular pathways underlying the embryonic origin of Sertoli cells remain elusive. By reviewing recent advances in research on embryonic Sertoli cell genesis and its key regulators, this review provides novel insights into sex determination in male mammals as well as the molecular mechanisms underlying the genealogical differentiation of Sertoli cells in the male reproductive ridge.
1 Introduction
In mammals, sex determination is the process by which the reproductive ridges develop into ovaries or testes. The genital ridge, a bipotential structure functioning as a precursor to the gonads, comprises germ cells surrounded by the coelomic epithelium (1). The directed migration of primordial germ cells (PGCs) to the genital ridge is critical for reproductive system development (2). However, the fate of PGCs that reach the genital ridge and differentiate into oogonia or spermatogonia is determined by the surrounding embryonic somatic cells (3). As early as 1967, Ohno proposed that in higher vertebrates, gonadal sexual differentiation is independent of the germ cells themselves and that the fate of the gonads is dominated by steroid hormone-producing molecules (4). Based on this perspective, in 1972, Krzysztof Boczkowski proposed that the main difference between male and female gonadal differentiation in mammals lies in the different mechanisms of gonadal differentiation and development in males and females. According to Boczkowski, the most important step in the process of male differentiation is the development of mesenchymal cells and hormone secretion (5). Palmer and Burgoyne’s 1991 study confirmed that Sertoli cells play a central role in testicular determination (6). Since then, research has been primarily focused on the regulatory mechanisms of Sertoli cell lineage differentiation in the process of sex determination. Somatic and germ cells are both present in the fetal gonads, and the process of sex determination is dominated by somatic cells, which contribute to the formation of the testes or ovaries through spatiotemporally defined expression of relevant genes. Sertoli cells play a key role in testicular determination and gonadal development (7, 8). Sexual differentiation refers to the development of any physical or behavioral characteristics that are unique to either males or females (9). Two duct systems exist in mammals, Wolffian ducts and Müllerian ducts, which eventually develop into male and female ducts, respectively (9, 10). During sex differentiation, one of the duct systems degenerates while the other continues to develop into the corresponding duct system (9, 10). In males, the Müllerian duct degenerates under the influence of AMH secreted by testicular Sertoli cells, and the Wolffian duct develops into the adult male reproductive tract under the action of androgens produced by Leydig cells (9, 10). Disorders of sex development (DSD) are linked to alterations in the development and functionality of Sertoli cells (11). In people with 46, XY, DSD, the development and function of Sertoli cells is dysfunctional, resulting in reduced levels of AMH, which prevents the Müllerian duct from degenerating into the uterus and fallopian tubes (11). This gender development disorder is known as persistent müllerian duct syndrome (PMDS). Two morphologically distinct Leydig subpopulations, fetal (FLC) and adult Leydig cells (ALC), are present during testicular development (12, 13). In the testis, the first wave of cells entering the gonad produces Sertoli cells that later promote the differentiation of steroidogenic cells entering the gonad into Leydig cells (14). Leydig cells are present in the testicular interstitial compartment and regulate male fertility. FLC population formation has multiple cell origins, including the somatic epithelium (15). Among them, FLC progenitor cells originating from the somatic epithelium gradually lose Notch activation upon entry into the fetal testis and thus differentiate directly into FLC (16). The number of FLCs increases until the fetus is born, peaks at birth, and then decreases gradually (13, 17). During pubertal testicular maturation, ALC gradually replace FLC (13, 18). After male puberty, Sertoli cells are the major somatic cell component in the testis and directly regulate the proliferation and differentiation of germ cells, support the typical spermathecal structure, and induce germ cells to ensure male spermatogenic output (19–22). The timing of Sertoli cell differentiation ultimately determines the number of Sertoli cells and the upper limit of male fecundity (23, 24). USF proteins (including USF1 and USF2) and cell-division control protein 42 (CDC42) regulate Sertoli cell proliferation and differentiation, thereby affecting the final number of Sertoli cells in the testis and imposing limits on fertility (25, 26). In addition, downregulation of Tetraspanin 8, Sostdc1, and miR-92a-3p in pubertal Sertoli cells results in is the maintenance of normal sperm quantity and quality (27, 28). In this process, downregulation of miR-92a-3p is induced by gonadotropins, and gonadotropin promotes the proliferation of immature Sertoli cells and targets Sertoli cells to support the expansion and differentiation of pre-meiotic germ cells (28–30). Several theories have been proposed on the mechanism underlying the origin of Sertoli cells; however, further studies are warranted to explore their embryonic origin. An increasing number of studies have reported on the molecular mechanisms of gonadal differentiation and the associated regulatory factors. Nonetheless, most studies are based on relatively independent studies of one or a few nodal factors and lack a systematic analysis linking the many regulatory factors. For this reason, in this review, we provide a systematic account of the molecular mechanisms of the numerous regulatory genes that contribute to the lineage differentiation process of Sertoli cells and the correlations among them. In this review, we discuss the mechanisms involved in embryonic Sertoli cell development, explore the gene defects associated with Sertoli cell development in DSD models, and highlight the critical factors in Sertoli cell lineage differentiation, providing an updated research perspective on the diagnosis and treatment of DSD and related diseases in the early embryonic stage.
2 Genital ridge formation in mammals
In mouse embryos, genital ridge formation begins around 10.5 days post coitum (dpc), continuing until 11.5–12.0 dpc. This developmental period aligns with the beginning of gonadal sex differentiation (31, 32). Concurrently, at approximately 9.5 dpc, the ventral epithelial layer of the mesonephros thickens in pairs, causing the basement membrane to protrude and disintegrate. Within the coelomic epithelium, certain cells proliferate and differentiate into the mesenchyme, migrating toward the dorsomedial mesenchymal region. These morphological alterations initially manifest at the cephalic end before gradually extending caudally to form the genital ridge (33).
PGCs originate from the embryonic ectoderm and migrate to reach the genital ridge (34) at approximately 10.5 dpc (35). Upon arrival, PGCs are enveloped by somatic cells derived from the coelomic epithelium. Steroidogenic factor-1 (SF1) is an essential transcription factor for genital ridge formation and development. In mice, SF1 expression is first detected in the urogenital ridge at 9 dpc (36), localizes to the adrenal primordium at 11 dpc, and concentrates in the adrenal cortex at 13 dpc (37). Its pattern of expression in humans is similar (38, 39). SF1 deficiency disrupts the testicular pathway, leading to ectopic ovarian development and potentially causing partial or complete inversion of the male-female gonadal system post-birth (40). In XY sex-determining region Y-box 9 (SOX9)-CRE-SF1 cKO mice at 12.5–13.5 dpc, testicular marker levels are significantly decreased, whereas ovarian marker levels are increased. At 10.0 dpc, GATA binding protein 4 (GATA4) is a genital ridge (genetic ridge) formation marker that is expressed only in somatic cells and is required for the initiation of genital ridge formation (32). Previously, it was widely believed that germ cell licensing occurred independently of gonadal somatic cells (41–43). However, Hu et al. showed that the PGC transition to meiotic germ cells is likely to depend on gonadal somatic cells and that systemic knockout of GATA4 prevents somatic epithelial proliferation in the somatic lumen, leading to rupture of the basement membrane and the absence of germinal ridges, thus causing germline cells to remain at the PGC stage, ultimately losing the ability to differentiate between sexes (32, 44). In addition, GATA4 also promotes SF1 expression in progenitor cells (44).
The expression of several genes is necessary for the growth and maintenance of the genital ridge, including LIM Homeobox 9 (LHX9), Wilms’ Tumor 1 (WT1), and Empty Spiracles Homeobox 2 (EMX2) (32). LHX9 is involved in a variety of developmental processes, including gonadogenesis. During sex differentiation, LHX9 upregulates SF1 and promotes the proliferation of somatic epithelial cells and disintegration of the basement membrane, thereby contributing to the formation of the germinal ridge and proliferation of somatic cells in the germinal crest (45, 46). Mice with a conditional knockout of LHX9 in the gonads display normal germ cell migration with reduced SF1 expression, while somatic cells in the germinal ridge are unable to proliferate and form discrete gonads (46). WT1 plays a critical role in lineage specification and the maintenance of gonadal somatic cells and acts as a transcription factor required for somatic cell survival in the genital ridge. It is initially expressed in the mesoderm of the 9 dpc genital ridge region and subsequently in the somatic epithelium. In addition, it could potentially be expressed in the mesenchymal cells of the urogenital tract ridges at 9.5 dpc (34). Deletion of WT1 results in embryonic mortality, apoptosis of genital ridge somatic cells, and metanephros defects. In contrast, a lack of EMX2 impedes the migration of epithelial cells through the basement membrane (47). After these processes, sex-determining gene expression is initiated, which determines the sex and fate of its germ cells as the bipotential genital ridge differentiates into either a testis or an ovary (33, 45).
3 Role of Sertoli cell lineage differentiation in male sex determination
The sex-determining region Y (SRY) on the Y chromosome, which controls the development of a male phenotype, is key to determining mammal sex. If SRY is expressed, testes form and most male secondary sexual characteristics develop under the influence of hormones secreted by the testes. GATA4 is a critical transcription factor required for SRY expression in Sertoli progenitor cells (48). GATA4 binds to the SRY promoter in a mitogen-activated protein kinase (MAPK)-dependent manner in vivo, thereby ensuring the timely expression of SRY (49, 50). SRY acts as a switch that prompts the development of the germinal ridge into the testis. During embryogenesis, SRY expression triggers the differentiation of Sertoli cells into Sertoli progenitor cells that would otherwise form granulosa cells in the ovary. Following SRY expression, several testis-specific events are elicited, including the development of a testis-specific vascular system.
During mouse embryonic development between 10.5 and 12.5 dpc, when the genital ridge remains undifferentiated, SRY is temporarily expressed in XY embryos, which peaks at approximately 11.5 dpc (51). Consequently, SOX9 is rapidly translocated from the cytoplasm to the nucleus. SRY, along with SF1, activates SOX9 expression (52) by binding to the testicular enhancer core (TESCO) sequence. SOX9 expression triggers a cascade of transcriptional events and promotes testicular formation (53, 54). SRY uses both cell-autonomous mechanisms and prostaglandin D2 (PGD2)-mediated signaling mechanisms to stimulate SOX9 expression and induce the differentiation of Sertoli cells in vivo (55).
Activated SOX9 activates SRY expression (54). Once a certain threshold is reached, SOX9 inhibits SRY expression and promotes its expression by interacting with SF1 (56). If either SRY or SOX9 activation fails to occur, the development of granulosa cells and ovarian pathways is initiated instead (57, 58). The expression of SOX9 also enhances the expression of testicular development-related factors, such as prostaglandin D2 synthase (PTGDS), anti-Müllerian hormone (AMH), WT1, GATA4, and ex-determining region Y-box 8 (SOX8), while concurrently inhibiting the expression of ovarian determinants, including WNT4, R-spondin 1 (RSPO1), and β-catenin (51, 54, 59). Moreover, SOX9 can further enhance its own expression by activating the PGD2 and fibroblast growth factor 9 (FGF9) signaling pathways (60), thereby forming a positive feedback loop (51).
During testicular development, FGF9 plays a critical role in two distinct steps (61). Initially, it stimulates the proliferation of cell populations that generate Sertoli progenitor cells (61). Within these progenitor cells, FGF9 facilitates the nuclear localization of fibroblast growth factor receptor 2 (FGFR2) (62). In mice, FGF9/FGFR2 signaling inhibits WNT4, antagonizes female development by repressing female-promoting genes, and drives testicular differentiation (63, 64). FGFR2 expression in Sertoli cells coincides with SRY expression and aligns with the male developmental markers (FGF9-activated receptors) of SOX9 downstream of SRY (61), suggesting that FGFR2 has a mediating role in Sertoli progenitor cells across various stages of testicular development (62). In mice, the deletion of FGFR2 in the embryonic gonad is identical to that of FGF9 and indicates sex reversal from male to female (62). Furthermore, knocking out either FGF9 or its receptor FGFR2 leads to significant changes in the somatic niche, including reduced AMH expression, fewer SOX9-positive Sertoli cells, and alterations in the spermatic cord structure, including decreased AMH expression, decreased number of SOX9-positive Sertoli cells, and altered spermatic cord structure (65). Activation of the PI3K/AKT signaling pathway promotes proliferation and prevents apoptosis in immature Sertoli cells (66, 67). In immature Sertoli cells, FGF binds to its receptor FGFR and activates PI3K expression (68). PI3K phosphorylates PIP2 to produce PIP3 (66), which then activates AKT1 by directly inducing AKT1 phosphorylation or activating PDK1 and mTORC2 to phosphorylate different sites on AKT1 (66). Consequently, AKT activates the expression of its downstream gene mTORC1 (66), which in turn activates the expression of p70S6K and EMI1 in the nucleus (69). rpS6, a substrate of p70S6K, facilitates MYC protein expression, thereby upregulating Cyclin A1/2 expression (66, 70). EMI1 maintains SKP2 levels by inhibiting SKP2 degradation, which upregulates Cyclin D1/3-CDK4, Cyclin E1/3, and PCNA, ultimately promoting the proliferation and survival of immature Sertoli cells (66).
Doublesex and mab-3-related transcription factor 1 (DMRT1) expression is triggered at 12.5 dpc, promoting the proliferation of Sertoli cells and maintaining male characteristics (71). DMRT1 inhibits the expression of WNT4 and Forkhead box L2 (FOXL2), and its overexpression transcriptionally activates the expression of PTGDS, FGFR2, and glial cell-derived neurotrophic factor (GDNF). In mice with conditionally deleted DMRT1, Sertoli cells transdifferentiate into granulosa cells, and the ovaries undergo recombination (72) (Figure 1; Table 1).
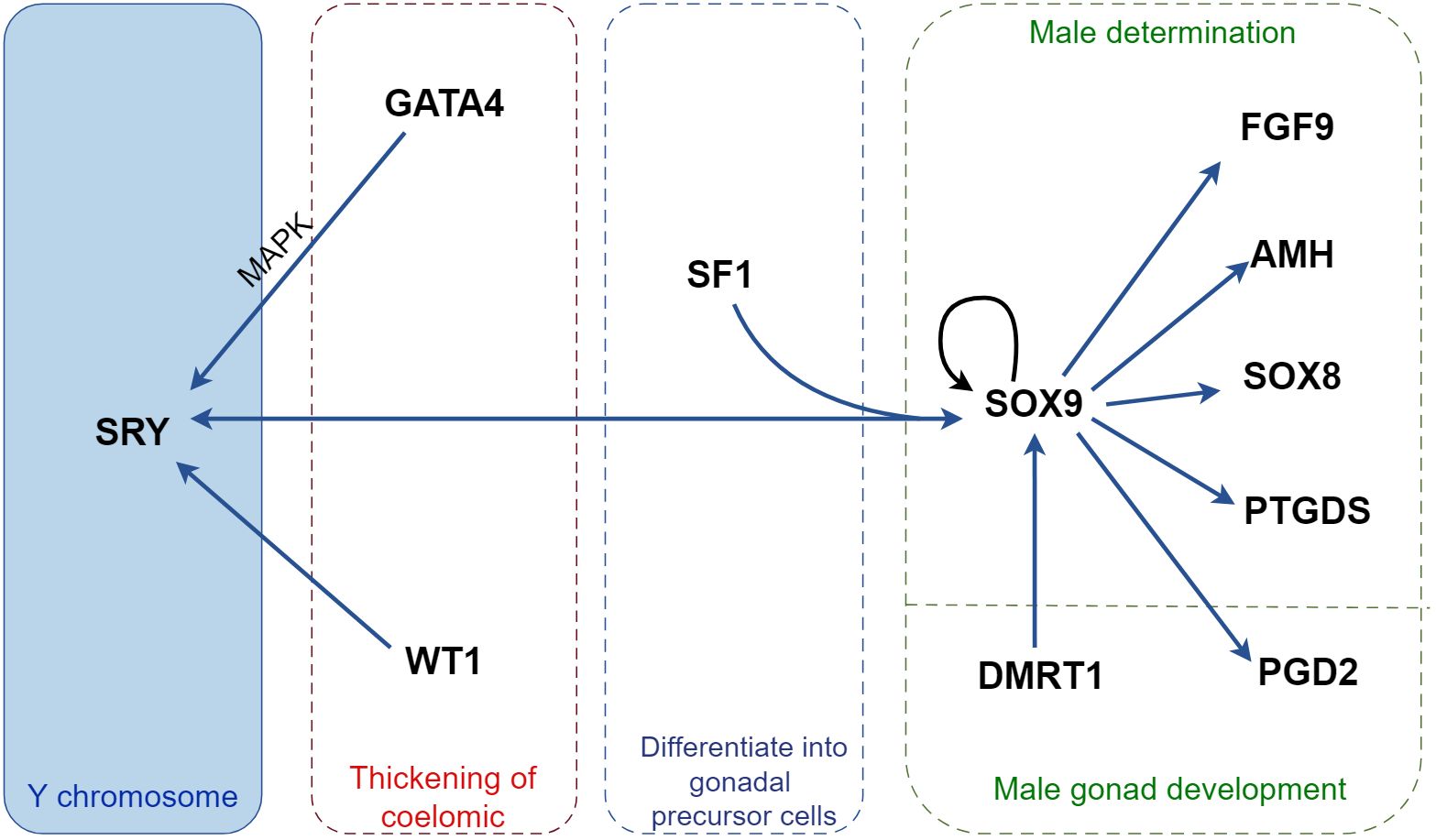
Figure 1 Regulatory mechanisms of cytokines in male sex determination in mice. SRY, the only sex-determining gene located on the Y chromosome, binds to GATA4 in a MAPK-dependent manner to facilitate its own on-time expression and cooperates with SF1 to promote SOX9 expression. Activated SOX9 induces SRY expression and forms a positive feedback pathway with WT1 and DMRT1 to promote further SOX9 expression. SOX9 promotes the expression of testis development-associated factors, including FGF9, AMH, SOX8, PTGDS, and PGD2, facilitating embryonic development into a male phenotype. MAPK, mitogen-activated protein kinase; SOX9, sex-determining region Y-box 9; AMH, anti-Müllerian hormone; DMRT1, doublesex and mab-3 related transcription factor 1; FGF9, fibroblast growth factor 9; GATA4, GATA binding protein 4; PGD2, prostaglandin D2; PTGDS, prostaglandin D synthase; SF1, steroidogenic factor-1; SRY, sex-determining region Y; WT1, Wilms’ tumor 1.
Between 11.5 and 12.5 dpc, cells of the male gonads form testicular cords after undergoing extensive cellular rearrangements (73). The reorganization of Sertoli cells, the vascular system, and Leydig cells simultaneously orchestrate testicular cord morphogenesis. Sertoli cells drive testicular morphogenesis under the guidance of the vascular system (74, 75). In mice, somatic vasculature and interstitial microvasculature in the male gonad are formed by vascular endothelial cells migrating from the mesonephros during testis formation (74, 76, 77). Vascular endothelial cell-mesenchymal interactions determine the fate of testicular cord formation (74, 78, 79). Furthermore, macrophages are present in the fetal testis and promote neoplastic morphogenesis of the testicular cord by controlling vascularization and tissue pruning, as well as removing somatic cells and errant germ cells after testicular structures are established (80).
4 Abnormalities in the molecular regulation of Sertoli cell lineage differentiation are involved in DSD: insights into genetic contributions
During mammalian embryogenesis, Sertoli cells play a vital role in the determination of the testes and the comprehensive development of the gonads, acting as the central components of testicular functionality. However, in patients with DSD, the typical development and function of Sertoli cells are often disrupted. To date, over 60 genes have been associated with 46,XY DSD (81).
A study identified 57 mutations across nine genes in 56 patients with 46,XY DSD (82). In approximately 60% of the patients, a variant of AR, SRD5A2, or SF1 was found to cause 46,XY DSD (82). Recently, seven patients carrying mutations in genes known to be pathogenic for 46,XY DSD were identified; four of these mutations were in DHX37, two in MYRF, and one in PPP2R3C (82). Furthermore, HSD17B3, SRD5A2, and AR are associated with androgen synthesis and action, and PPP2R3C and MYRF are linked to syndromic 46,XY DSD, whereas SRY, DHX37, GATA4, and SF1 are related to testicular determination and development (82). Despite these insights, the genetic etiology of >50% of DSD patients remain unclear, implying the possible existence of numerous unidentified sex-determining genes. As a result, we will dissect the key regulators controlling Sertoli cell lineage differentiation during embryonic development and their mechanisms of action one by one.
WT1, SF1, SOX9, and DMRT1 play major roles in embryonic development. WT1 promotes the lineage differentiation of Sertoli cells through the non-canonical Wnt/planar cell polarity (PCP) pathway and the expression of downstream SF1 by acting as an upstream factor (83). SF1 participates in the differentiation of the Sertoli cells along with WT1, initiating gonadal and testicular differentiation and promoting the expression of its downstream gene SOX9 (84). SOX9 plays a role in the early specification of Sertoli cells and maturation of testicular cords and promotes the expression of the downstream gene DMRT1. DMRT1 influences Sertoli cell maturation and polarity and maintains the Sertoli cell phenotype in postnatal testes. In addition, WT1, SF1, SOX9, and DMRT1 interact during embryonic development, influence the genealogy and differentiation of Sertoli cells, and promote embryonic sex differentiation.
4.1 WT1
During embryonic development, WT1 is expressed in the somatic epithelial and inferior Leydig cells of the urogenital ridge (85). It has been postulated to influence the destiny of WT1+ somatic cells in the testes, directing their differentiation into either Sertoli cells or FLC (71) (Figure 2A). Moreover, the presence of WT1 in pro-Sertoli cells fosters a bias toward Sertoli cell differentiation (71). Its overexpression in the adrenal gland inhibits the development of steroidogenic cells (86), thereby counteracting the expression of steroidogenesis-related enzymes and modulating the balance between the mesenchyme and the epithelium, as well as the Sertoli cell lineage. Our findings suggested that WT1 deficiency can trigger the transformation of Sertoli cells into Leydig cells (87). In contrast, WT1 overexpression in Leydig cells induces the expression of Sertoli cell-specific genes while inhibiting steroidogenic genes (87).
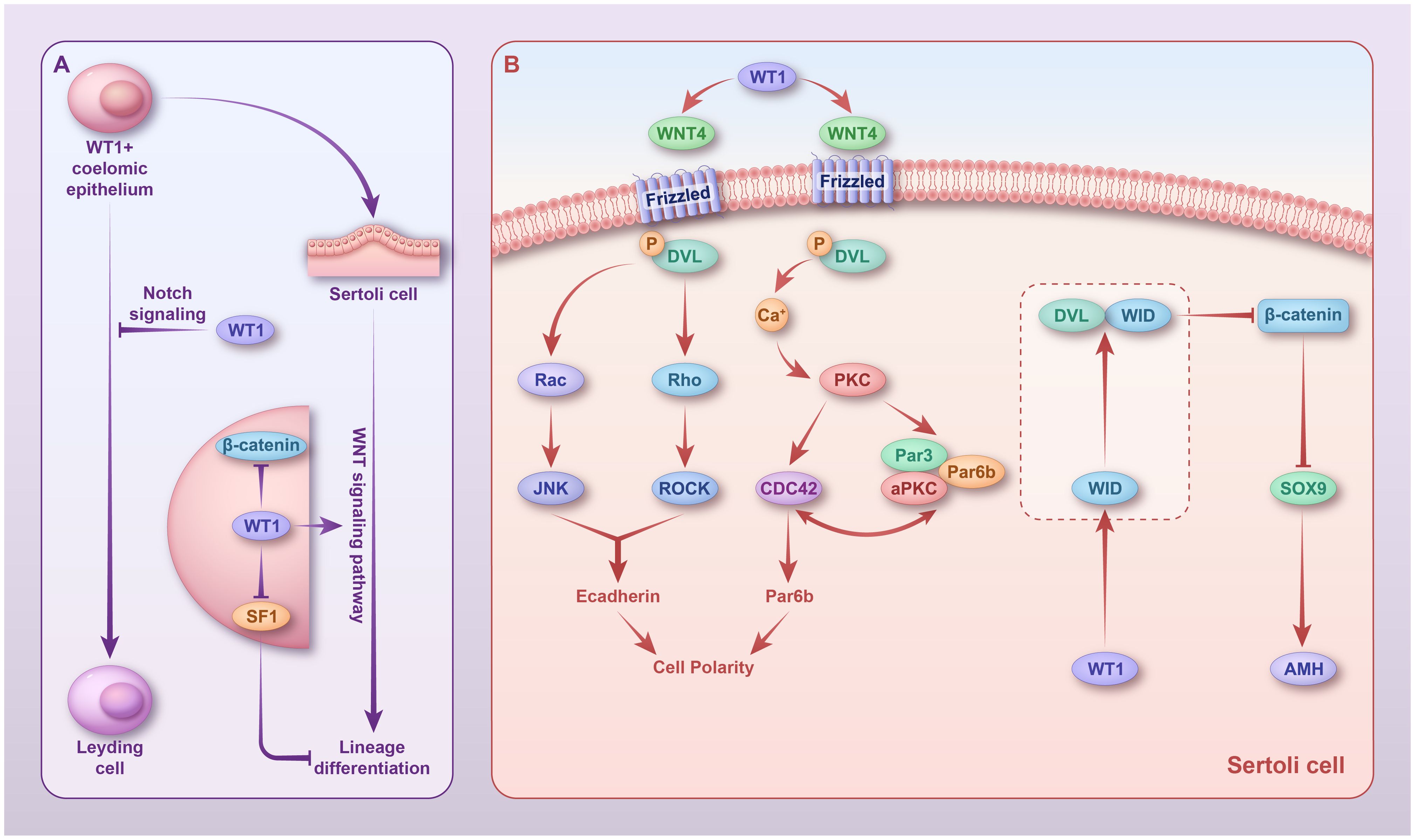
Figure 2 Regulatory mechanisms of WT1 in sex differentiation. (A) In fetal WT1+ somatic cells, WT1 inhibits their differentiation into Leydig cells by activating the Notch pathway. In Sertoli cells, WT1 persists and promotes the lineage differentiation of Sertoli cells by inhibiting SF1 and activating the non-canonical Wnt pathway. (B) In Sertoli cells, WT1 activates WNT4, which activates two non-canonical Wnt pathways. In the Wnt/PCP signaling pathway, phosphorylated DVL activates Rac and Rho; Rac1 activates JNK; and RHO activates the related helix protein ROCK. JNK and ROCK then work together to regulate cell polarity. In the Wnt/Ca+ signaling pathway, Ca2+ activates PKC, which further stimulates CDC42 and promotes actin polymerization, thereby affecting cell polarity. WT1 promotes the expression of its target gene, WID, which forms a complex with DVL to inhibit WNT3a expression and, thus, β-catenin signaling. However, whether this mechanism exists in Sertoli cells remains to be confirmed. WT1, Wilms’ tumor 1; DVL, phosphorylated Disheveled.
WT1 regulates Sertoli cell polarity via the activation of two non-canonical Wnt signaling pathways, the Wnt-PCP signaling pathway and the Wnt/Ca+ signaling pathway, thereby promoting lineage differentiation and spermatogenesis of Sertoli cells (83) (Figure 2B). In the Wnt/PCP signaling pathway, phosphorylated Disheveled (DVL) activates Rac and Rho, Rac1 activates Jun N-terminal kinase (JNK), and Ras homolog gene-family (RHO) activates the related helical protein ROCK. Together, JNK and ROCK regulate cell polarity. In the Wnt/Ca+ signaling pathway, Ca2+ activates protein kinase C (PKC), which in turn stimulates CDC42, thereby promoting actin polymerization and affecting cell polarity (88). Using a conditional knockout WT1 mouse model, we found that WT1 inactivation resulted in massive spermatogenic cell death in adult mice, with only Sertoli cells present in most seminiferous tubules (89).
Histological studies have revealed that WT1-deficient testes have compromised blood-testis barrier integrity (89). Knockdown of endogenous WT1 in neonatal calf Sertoli cells using RNAi technology does not impact the proliferative capacity of Sertoli cells but rather downregulates genes associated with cell polarity (par-6 family cell polarity regulator beta and E-cadherin) and Wnt signaling (WNT4 and WNT11) (90).
β-Catenin is an important effector of the canonical Wnt signaling pathway and a regulator of cell adhesion; hence, its inhibition during embryonic development is critical for testicular development (91). During testis development, WT1 inhibits β-catenin signaling in Sertoli cells; thus, its deletion increases β-catenin expression in Sertoli cells, both in vitro and in vivo (91). In addition, WT1 promotes the expression of its target gene, WID, which forms a complex with DVL to inhibit WNT3a expression (92) and, thus, β-catenin signaling. Whether this mechanism exists in Sertoli cells remains unclear. Although the classical Wnt/β-catenin signaling pathway is not involved in the lineage differentiation of Sertoli cells, it has been shown to affect spermatogenesis in adult mice (93). The presence of β-catenin in Sertoli cells maintains testicular development; however, activation of the Wnt/β-catenin signaling pathway leads to defective spermatogenesis. WT1 inhibits the activation of the Wnt/β-catenin signaling pathway in the testis, ensuring normal spermatogenesis. In mice, the expression of the stabilized form of β-catenin in Sertoli cells disrupts the germ cell microenvironment, leading to failure of spermatogenesis (94). In humans, abnormal accumulation of β-catenin in Leydig cells can lead to spermatogenesis failure, leading to non-obstructive azoospermia and infertility (95).
WT1 regulates the development of FLC and Leydig progenitor cell lineage via Notch signaling and inhibits WT1+ Leydig cell differentiation into FLC (96, 97). A subpopulation of WT1+ progenitor cells differentiated into Sertoli cells upon SOX9 expression, whereas WT1+ cells lacking SOX9 differentiated into Leydig cells of the testis (71). In mesenchymal progenitor cells, WT1 promotes the expression of the Hes family bHLH transcription factor 1 (HES1) and jagged canonical Notch ligand 1 (JAG1) (96) by promoting the expression of NOTCH2 and NOTCH3 (97). HES1 prevents mesenchymal progenitor cells from differentiating into FLC by inhibiting the transcription of the cell cycle inhibitor gene cyclin-dependent kinase inhibitor 1B (CDKN1B) (98, 99). Hedgehog signaling that occurs after Sertoli cell differentiation, promotes the expression of GLI1 in HES1+ Leydig cell progenitors (71). Eventually, Leydig cells develop into steroid-producing embryonic mesenchymal or non-steroidogenic cells. Notably, the population of steroid-producing embryonic Leydig cells is limited by Notch2 signaling from neighboring cells, and non-steroidogenic progenitor cells give rise to adult mesenchymal cells after puberty (71). In addition, Notch signaling can maintain the expression of its progenitor cell markers, such as LHX9 and NES (96). Specifically, deleting WT1 in Sertoli cells at 14.5 dpc during fetal testis development shifts the FLC differentiation state from Leydig progenitors (97). This deletion results in structural disruption of the testis, persistence of FLC-like clusters, failure of adult Leydig cell development, loss of gene expression associated with Leydig cell development (100), and, ultimately, male sterility (101). In addition, WT1 influences Sertoli cell lineage differentiation by suppressing SF1 expression. We have previously reported that WT1 directly binds to the promoter region and suppresses SF1 expression (102). Remarkably, WT1 deletion before sex determination upregulates SF1 expression and impedes the differentiation of supporting cells (102). In pre-pubertal testicular development, WT1 interacts with heterogeneous nuclear ribonucleoprotein U (HNRNPU) in Sertoli cells, binds to endogenous WT1, and activates the transcription function of WT1 on its target genes (103), thereby maintaining the testicular microenvironment. HNRNPU knockout leads to testicular atrophy in mice (101).
4.2 SF1
In the developing gonad, SF1 initiates gonadal and testicular differentiation in conjunction with several other transcription factors, including WT1, GATA, EMX2, and SOX9 (84) (Figure 3). Furthermore, researchers have proposed that WT1 might be essential for controlling basal level SF1 expression in the undifferentiated genital ridge and antagonizing SF1 expression in post-sex determination Sertoli cells (102).
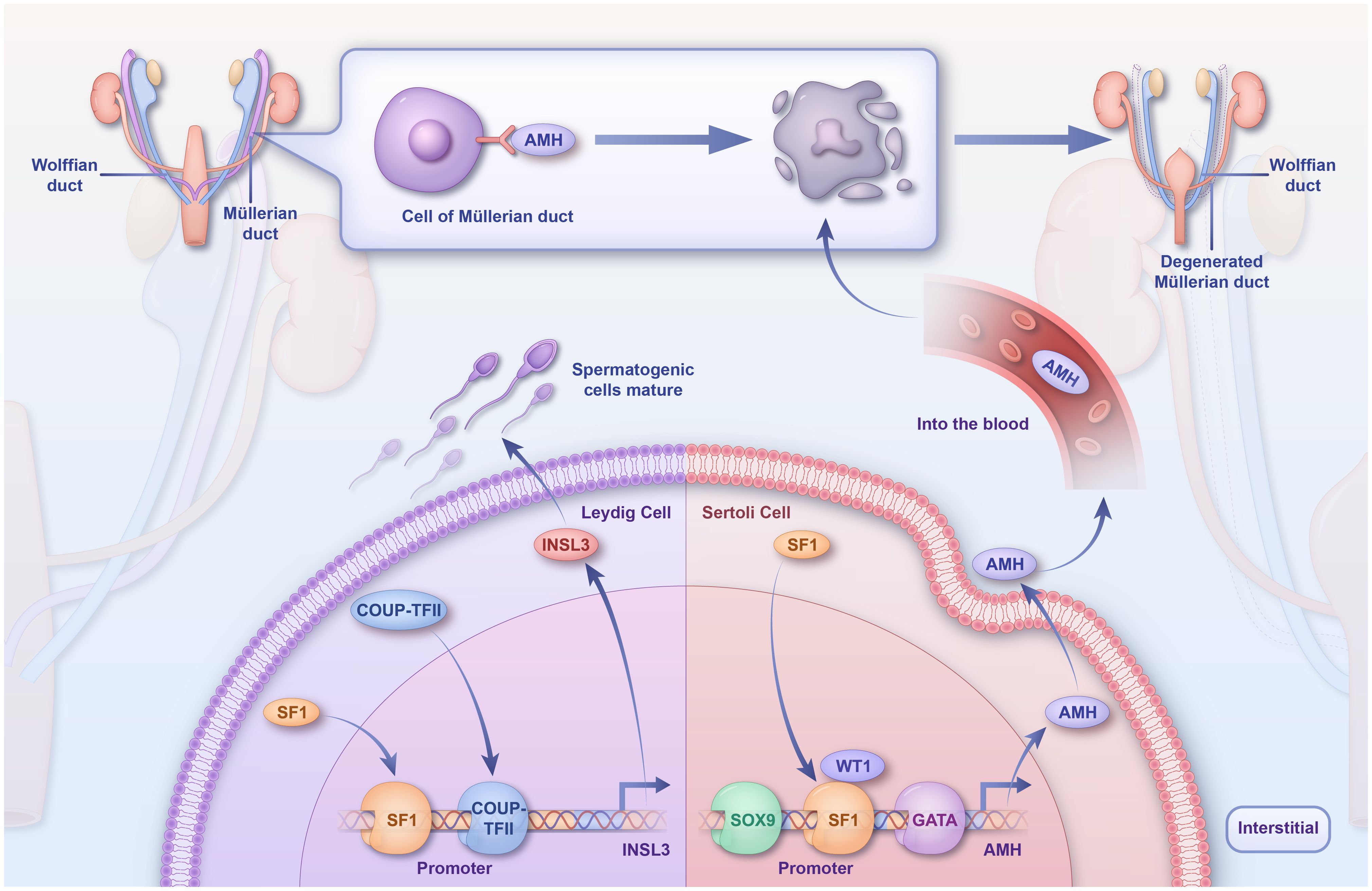
Figure 3 Regulatory mechanisms of SF1 in sex differentiation. SF1 directly binds TES, which synergistically activates TESCO with SRY, leading to a rapid upregulation of SOX9. SF1 then binds to TES along with SOX9 to maintain SOX9 expression in Sertoli cells. SF1 activates the AMH promoter in vivo and inhibits the production of Müllerian tubules, and COUP-TFII and SF1 synergistically activate the INSL3 promoter, which promotes spermatogonial cell development and maturation. TES, testis-specific enhancer of SOX9; TESCO, testicular enhancer core; SF1, steroidogenic factor-1; SOX9, sex-determining region Y-box 9.
At 9 dpc, SF1 is first expressed in the gonadal primordium and later persists in the bisexual gonads (54). Moreover, selectively disrupting SF1 in mice impedes gonadal development (84) and induces rupture of the spermatic cord. In conditional knockout SF1 mice, the levels of testicular markers decreased, whereas those of ovarian markers increased between 12.5 and 13.5 dpc (40). By 18.5 dpc, the number of Sertoli and spermatogenic cells decreased considerably, with only a marginal spermatic cord structure remaining (104). In mice testes, Sertoli cells delay maturation (105). These findings highlight the fact that SF1 deletion interrupts the testicular pathway and stimulates ectopic ovarian pathways, leading to partial or complete male-female gonadal inversion post-birth.
During embryonic testis development, SF1 activates the promoter of anti-Müllerian hormone (AMH) in Sertoli cells and is directly involved in sex determination in mammals (106). Deleting SF1 in Sertoli cells results in fewer androgen receptors (58, 105). Persistent Müllerian duct syndrome (PMDS) is a 46,XY DSD usually caused by mutations in AMH or a single-base deletion in the SF1 response element. In patients with PMDS, a regulatory mutation has been identified as a single-base deletion in the SF1 response element of the AMH promoter, significantly diminishing its SF1-binding capacity (107).
In Leydig cells, SF1 regulates the transcription of genes involved in testosterone synthesis and sterol biosynthetic enzymes. Leydig cells secrete 90% of the testosterone in males, which promotes the development and maturation of the sex organs and spermatogenic cells. Dihydrotestosterone, which is catalyzed from testosterone by the enzyme 5α-reductase, leads to prostate and penile growth and fusion of the labial folds (sexual differentiation) (108). Testosterone is synthesized by the steroidogenic enzyme Cyp11a1, which converts cholesterol to pregnenolone. Pregnenolone is then further converted to testosterone by Hsd3b, Cyp17a1, and Hsd17b3 (108). SF1 is involved in testosterone biosynthesis by regulating the transcription of sterol biosynthesis; in Leydig cells, COUP-TFII and SF1 physically interact (109). Insulin-like 3 (INSL3) is a Leydig cell-specific hormone, which, during fetal life, is essential for normal male sex differentiation and testicular descent during the transabdominal period (110). The INSL3 promoter contains species-conserved binding sites for COUP-TFII (-91 bp) and SF1 (-134 bp) (111); hence, COUP-TFII and SF1 can synergistically activate the INSL3 promoter.
SF1 regulates the MDM2/TP53 pathway during development and is pivotal for the survival of fetal Sertoli cells (104). p53, an oncoprotein encoded by TP53, is a transcription factor that regulates the initiation of the cell cycle by controlling the expression of specific genes. SF1 activates p53 by directly regulating MDM2 expression through direct binding to the P1 promoter of MDM2 (112).
4.3 SOX9
In the mammalian testes, SOX9 is critical for the initial differentiation of male Sertoli cells. HNRNPU interacts with SOX9 and enhances the expression of the transcription factors SOX8 and SOX9 in Sertoli cells through direct binding to the promoter regions (101). In both humans and mice, the loss of SOX9 expression leads to the XY embryo developing into a female. Conversely, erroneous activation of SOX9 leads to the embryo developing into a male (60). Furthermore, deletion of SOX9 in XY gonads interferes with the activation of the male-specific markers Mis and P450scc and induces the expression of female-specific markers such as bone morphogenetic protein 2 and follicle-stimulating hormone. SOX9 also influences Sertoli cell differentiation and affects the activation of SOX8 through tissue-specific knockdown methods (113). Animal studies have shown that WT1 can directly or indirectly regulate SOX9 after the cessation of SRY expression. The expression of the testis-determining gene SOX9 is switched off in Sertoli cells after WT1 resection at 14.5 days post-conception (114).
SOX9 is continuously expressed in the testes throughout fetal development. During sex determination, SRY upregulates SOX9 expression in undifferentiated embryonic gonads, thereby promoting the differentiation of coelomic epithelium cells into Sertoli cells (115). Despite the ability of SRY to directly or indirectly activate SOX9, it is not necessarily required for the sustained expression of SOX9. SOX9 was found to be responsible for the rapid disappearance of SRY expression at approximately 12.5 dpc, and SRY expression persisted in XY gonads lacking SOX9 (55). AMH inhibits the expression of Müllerian ductal development. Moreover, SOX9-positive pre-Sertoli cells express AMH and align with spermatogenic cells into primitive cords at 12.5 dpc, which then rapidly transform into more mature testicular cords by 13.5 dpc (116).
The transcription factors SRY and SOX9, along with the RSPO1/WNT4/β-catenin signaling pathway, are involved in an antagonistic pathway for testicular and ovarian development in the mouse embryo, driving the development of common gonadal primordia (117). Rspo1, Wnt4, and β-catenin inhibit testicular cord formation, and targeted deletion of Rspo1 in mice prevents Wnt4 upregulation while triggering the proliferation of androgen-producing cells (118). In the XY gonad, a positive feedback loop between Sox9 and Fgf9 (as well as PGD2) is established to suppress Wnt4/Rspo1 expression in a paracrine manner, thereby promoting Sertoli cell differentiation (118). Using mouse models with double knockouts of SOX9 and WNT4, researchers have found that SOX9, rather than the inhibition of signaling by RSPO1/WNT4/CTNNB1, is vital for the early determination of male Sertoli cells in the testes (117).
Sertoli cells can coordinate the development of all other male-specific cell types. For proper testicular development, it is critical for Sertoli cells to differentiate in adequate numbers, necessitating sustained and sufficient expression of SOX9. At least three mechanisms can effectively maintain the expression of SOX9 in Sertoli cells (Figure 4). In Sertoli cells, SF1 promotes and maintains SOX9 expression. In precursor support cells, SRY and SF1 directly bind to the testis-specific enhancer of SOX9 (TES) and synergistically activate TESCO, leading to rapid upregulation of SOX9 (56, 113, 119, 120). Once SOX9 reaches a critical threshold, SRY is repressed through a SOX9-dependent negative feedback loop. After SRY expression ceases, SF1 binds to enhancers along with SOX9, regulating itself to retain high levels of expression in Sertoli cells (56, 113, 119, 120). The absence of TESCO or TES results in a 60% or 45% decrease in SOX9 expression in XY fetal gonads, respectively, and both have been shown to decrease AMH expression (121). Early testes produce PGD2, which induces the differentiation of pre-Sertoli cells into Sertoli cells. In vivo, SOX9 forms a binding association with the PTGDS promoter and is promptly expressed in Sertoli cells (122). Activation and the continuous presence of SOX9 result in the production of testicular Lipocalin-type PGTDS, leading to the accumulation of PGD2. Subsequently, PGD2 activates the transcription process and aids in the nuclear translocation of SOX9 (123). PGTDS and SOX9 continue to be expressed in FGFR2 mutant follicles, suggesting that the prostaglandin pathway maintains SOX9 expression independently of FGFR2 (124).
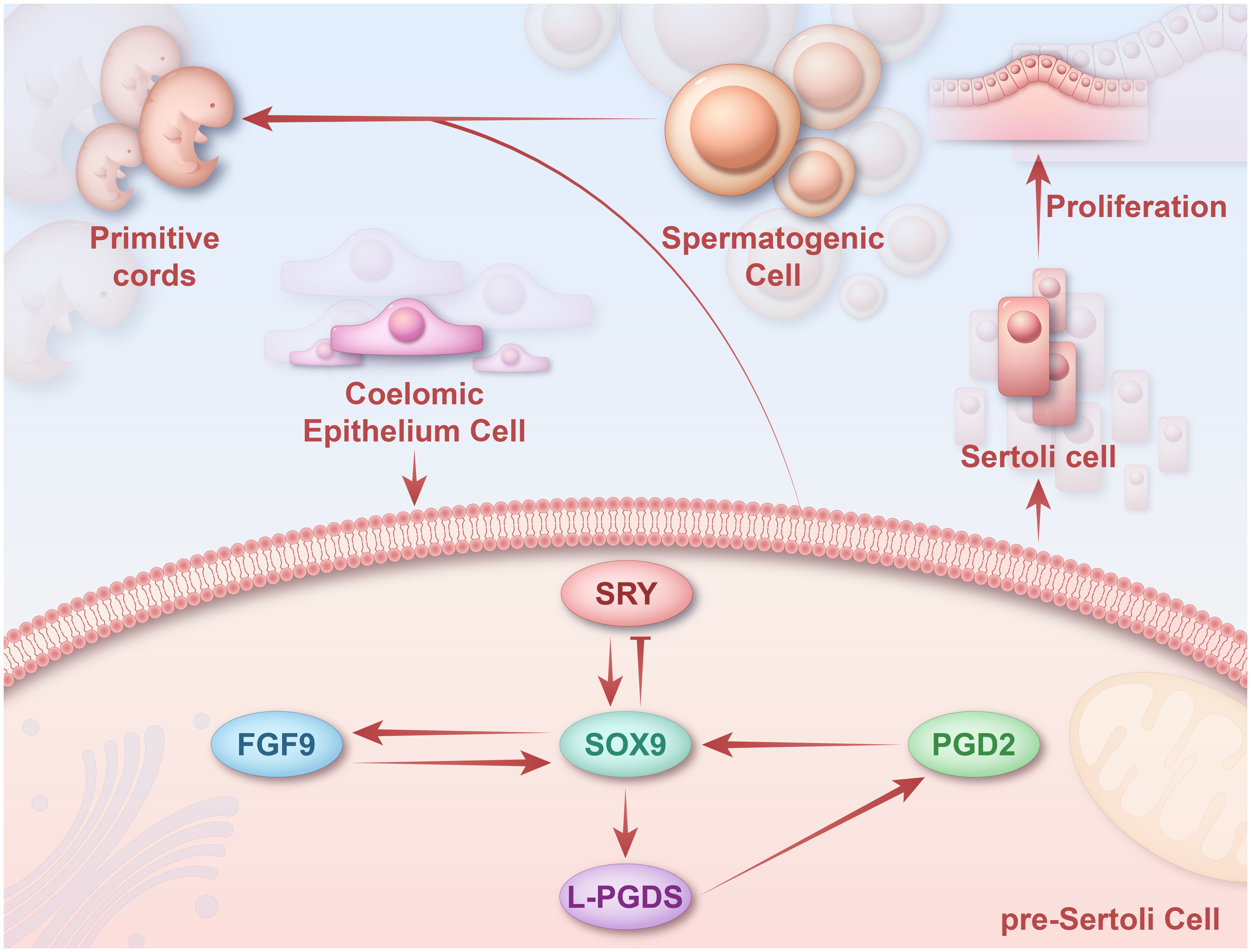
Figure 4 Regulatory mechanisms of SOX9 in sex differentiation. SOX9 can maintain SOX9 expression in Sertoli cells through three pathways mediated by SF1, FGF9/FGFR2, and PGTDS/PGD2. SRY upregulates SOX9 expression in undifferentiated gonads by binding to TES. SOX9 upregulates FGF9 and forms a positive feed-forward loop with FGF9 to maintain SOX9 expression. SOX9 promotes the expression of PTGDS in vivo by binding to the PTGDS promoter. The persistence of SOX9 promotes the production of L-PGDS, which leads to the accumulation of PGD2 and thus activates the transcription of SOX9. Later, these SOX9+ pre-Sertoli cells will form the primitive cords with spermatogenic cells. SOX9, sex-determining region Y-box 9; SRY, sex-determining region Y; FGF9, fibroblast growth factor 9; FGFR2, fibroblast growth factor receptor 2.
In XY gonads, SOX9 and FGF9 form a positive feed-forward loop essential for somatic cell proliferation. SOX9 is responsible for the expression of FGF9, and FGF9 is necessary to sustain the expression of SOX9 (125). In male XY mouse gonads, SOX9 upregulates FGF9, initiating the SOX9/FGF9 feed-forward loop (123). Moreover, SOX9 can enhance SRY expression by activating the FGF9 and PGD2 signaling pathways (60).
DSD is caused by either the deletion or overexpression of SOX9, arising from mutations in the SOX9 coding region or misregulation or disruption of the regulatory region (126). In two patients with 46,XX testicular DSD, an 83.8 kb repeat was detected in the region 600 kb upstream of SOX9 (127). Rearrangements within or around SOX9 can be detected in some patients using chromosome microarray.
In postnatal Sertoli cells, CCAAT enhancer binding protein beta activates SOX9 expression. cAMP-responsive element binding protein 1 is recruited to the proximal promoter region of SOX9 through the cAMP/protein kinase A signaling pathway, leading to CREB1 phosphorylation, which promotes SOX9 expression (128).
4.4 DMRT1
DMRT1 mRNA initially appears in the germinal ridge of mice at approximately 10.5 dpc, with its abundance significantly increasing by approximately 14.5 dpc. DMRT1 is critical for the maturation and polarity of Sertoli cells. In vivo studies have demonstrated incomplete maturation of Sertoli cells in males lacking DMRT1. Immunohistochemical analyses of the polarity markers ESPIN and NECTIN-2 revealed that DMRT1 is necessary for NECTIN-2 expression in Sertoli cells (129). DMRT1 cooperates with SOX9 to maintain and reprogram sexual cell fates. Remarkably, DMRT1 appears to play the role of a pioneer transcription factor that binds to otherwise “closed” and inaccessible chromatin and facilitates its opening, thereby creating conditions for the binding of other regulators, including SOX9 (130, 131). DMRT1 binds to DNA in a very unusual way and can bind according to different stoichiometries (131).
DMRT1 is essential for sex determination and preservation of the Sertoli cell phenotype in postnatal testes. In mammals, DMRT1 is required to sustain the male gonadal fate (132). Induction of DMRT1 expression in the fetal gonads of XX mice using the WT1-BAC transgenic system is sufficient to stimulate testicular differentiation and foster the development of male secondary sexual characteristics (133). Deletion of DMRT1 results in the sex reversal of mice to female at birth. In male mice, deletion of DMRT1 activates FOXL2 and reprograms Sertoli cells into granular cells, which form follicular cells that produce estrogen, ultimately leading to germ cell feminization (72). The successful nuclear import of DMRT1, along with its interaction with importin-β1, ensures the nuclear retention of DMRT1 and its subsequent influence on the downstream targets of the sexual development cascade (134). DMRT1 inhibition changes the expression of pivotal genes involved in gonadal development, ultimately leading to focal testicular hypoplasia in the human fetus (135).
DMRT1 is crucial in regulating sperm differentiation (136); its deletion in spermatogonia inhibits the cyclic gene expression in Sertoli cells (137). DMRT1 promotes migration and differentiation of mitotic PGCs (early PGCs) to mitotic-blocking PGCs (late PGCs), and prevents premature meiotic initiation by inhibiting retinoic acid signaling and Stra8 expression (138, 139). At the mouse pubertal stage, DMRT1 is able to activate GDNF signaling and interacts with PLZF, which in turn upregulates Sohlh1 expression, thus participating in the process of spermatogonia self-renewal and differentiation (140). In addition, DMRT1 promotes the expression of the receptor tyrosine kinase (RTK) signaling pathway inhibitory protein Spry1. SPRY1 binds to nuclear factor κB1 (NF-κB1), which prevents p65 nuclear translocation, inhibits the activation of the NF-κB signaling pathway, and protects the integrity of the BTB, thereby creating a stable environment for smooth spermatogenesis (141).
Low DMRT1 expression in mouse testes decreases spermatogonia and destabilizes the testicular ecological niche in vivo. The interaction between DMRT1 and promyelocytic leukemia zinc finger (PLZF) proteins is crucial for maintaining male germline stem cell self-renewal (142).
5 Summary conclusion and future direction
Numerous cytokines orchestrate the differentiation of the Sertoli cell lineage during embryonic development. GATA4 ensures the transition of PGCs to meiotic germ cells by promoting the formation of germinal ridges. WT1 affects the polarity of Sertoli cells by regulating the non-canonical Wnt pathway, thereby promoting their lineage differentiation. SF1 is involved in mammalian sex determination by activating the AMH promoter. SOX9 maintains its expression in Sertoli cells through three pathways mediated by SF1, FGF9/FGFR2, and PGTDS/PGD2, which ensures that sufficient Sertoli cells induce testicular development. DMRT1 and SOX9 cooperatively maintain Sertoli cell proliferation; DMRT1 deletion results in male-to-female sex reversal (Figure 5).
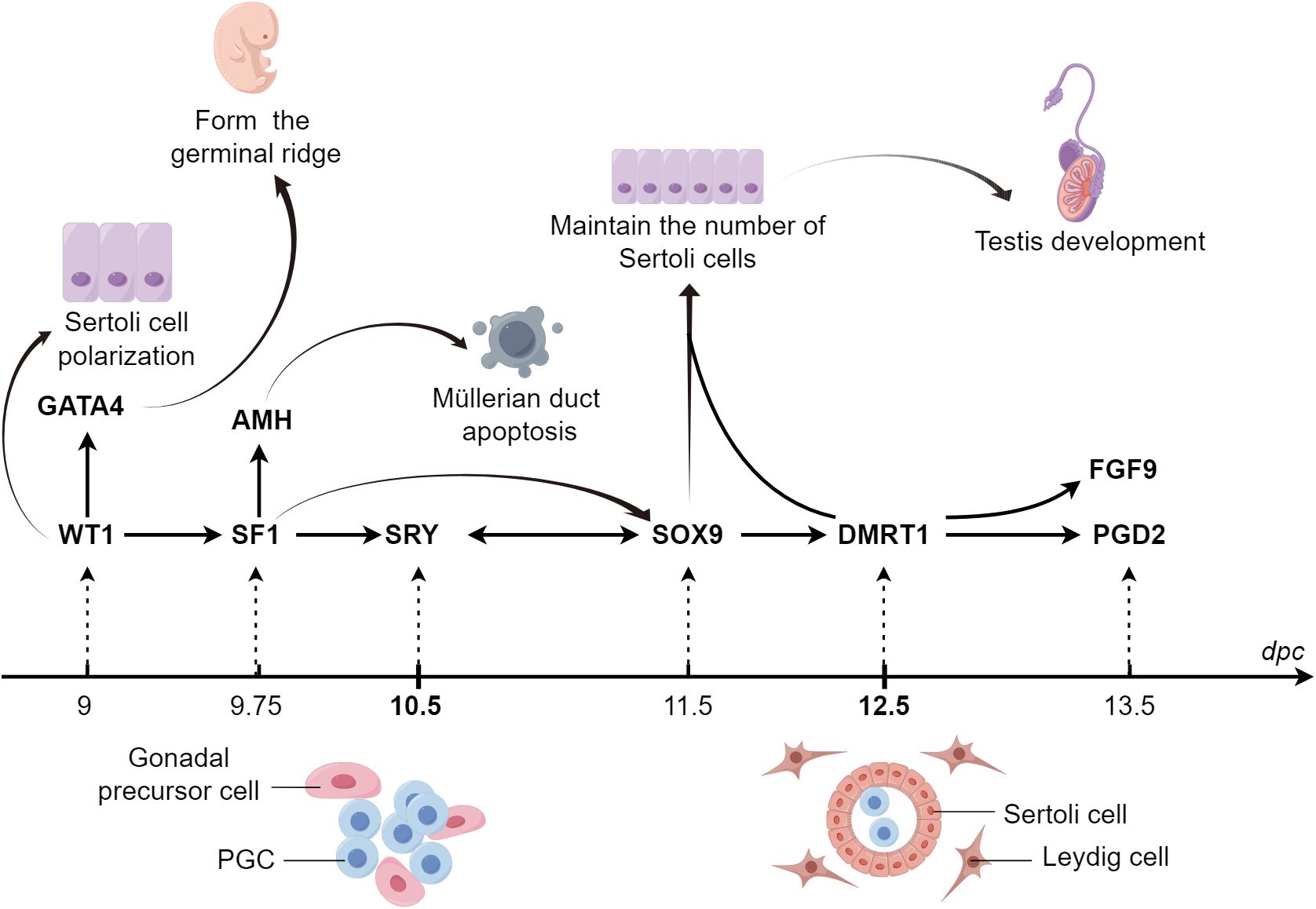
Figure 5 Graphical summary During sex determination in the male embryo, the expression of numerous cytokines at specific times and spaces act together to regulate the lineage differentiation of Sertoli cells. GATA4 ensures the transition of PGCs to meiotic germ cells by promoting the formation of germinal ridges. WT1 influences the polarity of Sertoli cells through the regulation of the non-canonical WNT pathway, which promotes the lineage differentiation of Sertoli cells. SF1 participates in mammalian sex determination through the activation of the promoter of AMH. SOX9 maintains its expression in Sertoli cells through three pathways mediated by SF1, FGF9/FGFR2, and PGDS/PGD2, respectively, thus ensuring that a sufficient number of Sertoli cells are available to induce testicular development. GATA4, GATA binding protein 4; PGCs, primordial germ cells; SF1, steroidogenic factor-1; AMH, anti-Müllerian hormone; SOX9, sex-determining region Y-box 9; FGF9, fibroblast growth factor 9; PGD2, prostaglandin D2; PTGDS, prostaglandin D synthase.
Despite extensive research spanning decades, substantial gaps remain in our understanding of gonadal sex determination, with many vital aspects yet to be fully elucidated. A comprehensive understanding of gonadal development and differentiation depends on the thorough characterization of all cell types involved in this bipotential primordium and the understanding of their interrelations. Moreover, the molecular factors that regulate the various cell lineages emerging from this process remain largely unknown. Although WT1 reportedly negatively regulates the Wnt/β-catenin pathway through its target gene WID in the kidney, whether this mechanism also operates in Sertoli cells to ensure normal spermatogenesis warrants further investigation. In humans, mutations or deletions in the short arm of chromosome 9, where DMRT1 is located, result in varying degrees of sex reversal during the embryonic period. However, mice with inhibited DMRT1 expression are born male and only undergo sex reversal postnatally. Further research is required to pinpoint the causes of these discrepancies.
The development of advanced sequencing technologies, such as single-cell RNA sequencing, not only addresses this need but also provides the field of developmental biology with a unique opportunity to identify previously uncharacterized cell types and rare lineages. Furthermore, a comprehensive understanding of the role of cytokines in Sertoli cell lineage differentiation is vital to elucidate the intricate mechanisms underlying testicular development and sex determination. Further investigation into the precise molecular interactions and signaling pathways involved in cytokine-mediated Sertoli cell differentiation will provide insights into the potential therapeutic targets for sexual development and infertility disorders. In conclusion, in this review, we explored the recent breakthroughs in the development of Sertoli cells and their pivotal regulators to both deepen our understanding of sex determination in male mammals and illuminate the intricate molecular mechanisms governing the differentiation of Sertoli cells within the male reproductive ridge. Nevertheless, it is important to acknowledge the limitations of this review. First, the primary focus of this review was to elucidate the roles of specific factors of particular importance. As a result, we did not provide a comprehensive and in-depth analysis of other related factors. Additionally, due to space constraints, we could only describe the roles of associated factors within specific signaling pathways without delving into the broader implications and relevance of these signaling pathways.
Author contributions
YG: Writing – review & editing, Writing – original draft. ZW: Writing – review & editing. YL: Writing – review & editing. LY: Writing – review & editing. YJ: Writing – review & editing. DD: Writing – review & editing. BT: Writing – review & editing. MC: Writing – review & editing. JY: Writing – review & editing. FG: Writing – review & editing, Writing – original draft.
Funding
The author(s) declare financial support was received for the research, authorship, and/or publication of this article. This work was supported by the Research Start-up Fund of Jining Medical University (600791001, 600980001), the National Key R&D Program of China (2018YFA0107700), and the Research Fund for Lin He’s Academician Workstation of New Medicine and Clinical Translation at Jining Medical University (JYHL2021MS32).
Acknowledgment
We would like to thank Home for Researchers (www.home-forreseachers.com) for their figure coloring service. The authors would also like to express their gratitude to EditSprings (https://www.editsprings.com/) for the expert linguistic services.
Conflict of interest
The authors declare that the research was conducted in the absence of any commercial or financial relationships that could be construed as a potential conflict of interest.
The author(s) declared that they were an editorial board member of Frontiers, at the time of submission. This had no impact on the peer review process and the final decision.
Publisher’s note
All claims expressed in this article are solely those of the authors and do not necessarily represent those of their affiliated organizations, or those of the publisher, the editors and the reviewers. Any product that may be evaluated in this article, or claim that may be made by its manufacturer, is not guaranteed or endorsed by the publisher.
References
1. Byskov AG. Differentiation of mammalian embryonic gonad. Physiol Rev. (1986) 66:71–117. doi: 10.1152/physrev.1986.66.1.71
2. Nef S, Vassalli JD. Complementary pathways in mammalian female sex determination. J Biol. (2009) 8:74. doi: 10.1186/jbiol173
3. Kamalidehghan B, Habibi M, Afjeh SS, Shoai M, Alidoost S, Almasi Ghale R, et al. The importance of small non-coding RNAs in human reproduction: A review article. Appl Clin Genet. (2020) 13:1–11. doi: 10.2147/TACG
4. Schmid M, Steinlein C. Sex chromosomes, sex-linked genes, and sex determination in the vertebrate class amphibia. EXS. (2001) 91:143–76. doi: 10.1007/978-3-0348-7781-7_8
5. Boczkowski K. Male and female differentiation of the human gonad. Clin Genet. (1973) 4:213–9. doi: 10.1111/j.1399-0004.1973.tb01145.x
6. Palmer SJ, Burgoyne PS. In situ analysis of fetal, prepuberal and adult XX—XY chimaeric mouse testes: Sertoli cells are predominantly, but not exclusively, XY. Development. (1991) 112:265–8. doi: 10.1242/dev.112.1.265
7. Heinrich A, Potter SJ, Guo L, Ratner N, DeFalco T. Distinct roles for rac1 in sertoli cell function during testicular development and spermatogenesis. Cell Rep. (2020) 31:107513. doi: 10.1016/j.celrep.2020.03.077
8. Stukenborg JB, Jahnukainen K, Hutka M, Mitchell RT. Cancer treatment in childhood and testicular function: the importance of the somatic environment. Endocr Connect. (2018) 7:R69–87. doi: 10.1530/EC-17-0382
9. Wilhelm D, Palmer S, Koopman P. Sex determination and gonadal development in mammals. Physiol Rev. (2007) 87:1–28. doi: 10.1152/physrev.00009.2006
10. Zhao F, Yao HH. A tale of two tracts: history, current advances, and future directions of research on sexual differentiation of reproductive tractsdagger. Biol Reprod. (2019) 101:602–16. doi: 10.1093/biolre/ioz079
11. Lucas-Herald AK, Mitchell RT. Testicular sertoli cell hormones in differences in sex development. Front Endocrinol (Lausanne). (2022) 13:919670. doi: 10.3389/fendo.2022.919670
12. Roosen-Runge EC, Anderson D. The development of the interstitial cells in the testis of the albino rat. Acta Anat (Basel). (1959) 37:125–37. doi: 10.1159/000141460
13. Teerds KJ, Huhtaniemi IT. Morphological and functional maturation of Leydig cells: from rodent models to primates. Hum Reprod Update. (2015) 21:310–28. doi: 10.1093/humupd/dmv008
14. Rotgers E, Jorgensen A, Yao HH. At the crossroads of fate-somatic cell lineage specification in the fetal gonad. Endocr Rev. (2018) 39:739–59. doi: 10.1210/er.2018-00010
15. Bhattacharya I, Dey S. Emerging concepts on Leydig cell development in fetal and adult testis. Front Endocrinol (Lausanne). (2022) 13:1086276. doi: 10.3389/fendo.2022.1086276
16. Kumar DL, DeFalco T. A perivascular niche for multipotent progenitors in the fetal testis. Nat Commun. (2018) 9:4519. doi: 10.1038/s41467-018-06996-3
17. Habert R, Lejeune H, Saez JM. Origin, differentiation and regulation of fetal and adult Leydig cells. Mol Cell Endocrinol. (2001) 179:47–74. doi: 10.1016/S0303-7207(01)00461-0
18. Haider SG. Cell biology of Leydig cells in the testis. Int Rev Cytol. (2004) 233:181–241. doi: 10.1016/S0074-7696(04)33005-6
19. Bhattacharya I, Sharma SS, Majumdar SS. Etiology of male infertility: an update. Reprod Sci. (2023) 31(4):942–65. doi: 10.1007/s43032-023-01401-x
20. Griswold MD. 50 years of spermatogenesis: Sertoli cells and their interactions with germ cells. Biol Reprod. (2018) 99:87–100. doi: 10.1093/biolre/ioy027
21. Franca LR, Hess RA, Dufour JM, Hofmann MC, Griswold MD. The Sertoli cell: one hundred fifty years of beauty and plasticity. Andrology. (2016) 4:189–212. doi: 10.1111/andr.12165
22. Bhattacharya I, Sen Sharma S, Majumdar SS. Pubertal orchestration of hormones and testis in primates. Mol Reprod Dev. (2019) 86:1505–30. doi: 10.1002/mrd.23246
23. Wood MA, Mukherjee P, Toocheck CA, Walker WH. Upstream stimulatory factor induces Nr5a1 and Shbg gene expression during the onset of rat Sertoli cell differentiation. Biol Reprod. (2011) 85:965–76. doi: 10.1095/biolreprod.111.093013
24. Sharpe RM, McKinnell C, Kivlin C, Fisher JS. Proliferation and functional maturation of Sertoli cells, and their relevance to disorders of testis function in adulthood. Reproduction. (2003) 125:769–84. doi: 10.1530/rep.0.1250769
25. Wood MA, Walker WH. USF1/2 transcription factor DNA-binding activity is induced during rat Sertoli cell differentiation. Biol Reprod. (2009) 80:24–33. doi: 10.1095/biolreprod.108.070037
26. Heinrich A, Bhandary B, Potter SJ, Ratner N, DeFalco T. Cdc42 activity in Sertoli cells is essential for maintenance of spermatogenesis. Cell Rep. (2021) 37:109885. doi: 10.1016/j.celrep.2021.109885
27. Pradhan BS, Bhattacharya I, Sarkar R, Majumdar SS. Pubertal down-regulation of Tetraspanin 8 in testicular Sertoli cells is crucial for male fertility. Mol Hum Reprod. (2020) 26:760–72. doi: 10.1093/molehr/gaaa055
28. Gupta A, Vats A, Ghosal A, Mandal K, Sarkar R, Bhattacharya I, et al. Follicle-stimulating hormone-mediated decline in miR-92a-3p expression in pubertal mice Sertoli cells is crucial for germ cell differentiation and fertility. Cell Mol Life Sci. (2022) 79:136. doi: 10.1007/s00018-022-04174-9
29. Bhattacharya I, Dey S, Banerjee A. Revisiting the gonadotropic regulation of mammalian spermatogenesis: evolving lessons during the past decade. Front Endocrinol (Lausanne). (2023) 14:1110572. doi: 10.3389/fendo.2023.1110572
30. Edelsztein NY, Valeri C, Lovaisa MM, Schteingart HF, Rey RA. AMH regulation by steroids in the mammalian testis: underlying mechanisms and clinical implications. Front Endocrinol (Lausanne). (2022) 13:906381. doi: 10.3389/fendo.2022.906381
31. Cunha GR, Baskin LS. Human and mouse gonadal development. Differentiation. (2023) 129:1–3. doi: 10.1016/j.diff.2022.09.004
32. Hu YC, Okumura LM, Page DC. Gata4 is required for formation of the genital ridge in mice. PloS Genet. (2013) 9:e1003629. doi: 10.1371/journal.pgen.1003629
33. Tanaka SS, Nishinakamura R. Regulation of male sex determination: genital ridge formation and Sry activation in mice. Cell Mol Life Sci. (2014) 71:4781–802. doi: 10.1007/s00018-014-1703-3
34. Chen M, Cen C, Wang N, Shen Z, Wang M, Liu B, et al. The functions of Wt1 in mouse gonad development and somatic cells differentiationdagger. Biol Reprod. (2022) 107:269–74. doi: 10.1093/biolre/ioac050
35. Karl J, Capel B. Sertoli cells of the mouse testis originate from the coelomic epithelium. Dev Biol. (1998) 203:323–33. doi: 10.1006/dbio.1998.9068
36. Hatano O, Takayama K, Imai T, Waterman MR, Takakusu A, Omura T, et al. Sex-dependent expression of a transcription factor, Ad4BP, regulating steroidogenic P-450 genes in the gonads during prenatal and postnatal rat development. Development. (1994) 120:2787–97. doi: 10.1242/dev.120.10.2787
37. Morohashi K, Iida H, Nomura M, Hatano O, Honda S, Tsukiyama T, et al. Functional difference between Ad4BP and ELP, and their distributions in steroidogenic tissues. Mol Endocrinol. (1994) 8:643–53. doi: 10.1210/mend.8.5.8058072
38. Hanley NA, Ball SG, Clement-Jones M, Hagan DM, Strachan T, Lindsay S, et al. Expression of steroidogenic factor 1 and Wilms’ tumour 1 during early human gonadal development and sex determination. Mech Dev. (1999) 87:175–80. doi: 10.1016/S0925-4773(99)00123-9
39. Ramayya MS, Zhou J, Kino T, Segars JH, Bondy CA, Chrousos GP. Steroidogenic factor 1 messenger ribonucleic acid expression in steroidogenic and nonsteroidogenic human tissues: Northern blot and in situ hybridization studies. J Clin Endocrinol Metab. (1997) 82:1799–806. doi: 10.1210/jcem.82.6.3967
40. Ikeda Y, Tagami A, Maekawa M, Nagai A. The conditional deletion of steroidogenic factor 1 (Nr5a1) in Sox9-Cre mice compromises testis differentiation. Sci Rep. (2021) 11:4486. doi: 10.1038/s41598-021-84095-y
41. Upadhyay S, Zamboni L. Ectopic germ cells: natural model for the study of germ cell sexual differentiation. Proc Natl Acad Sci USA. (1982) 79:6584–8. doi: 10.1073/pnas.79.21.6584
42. Zamboni L, Upadhyay S. Germ cell differentiation in mouse adrenal glands. J Exp Zool. (1983) 228:173–93. doi: 10.1002/jez.1402280204
43. Molyneaux KA, Stallock J, Schaible K, Wylie C. Time-lapse analysis of living mouse germ cell migration. Dev Biol. (2001) 240:488–98. doi: 10.1006/dbio.2001.0436
44. Hu YC, Nicholls PK, Soh YQ, Daniele JR, Junker JP, van Oudenaarden A, et al. Licensing of primordial germ cells for gametogenesis depends on genital ridge signaling. PloS Genet. (2015) 11:e1005019. doi: 10.1371/journal.pgen.1005019
45. Piprek RP, Kloc M, Kubiak JZ. Early development of the gonads: origin and differentiation of the somatic cells of the genital ridges. Results Probl Cell Differ. (2016) 58:1–22. doi: 10.1007/978-3-319-31973-5
46. Birk OS, Casiano DE, Wassif CA, Cogliati T, Zhao L, Zhao Y, et al. The LIM homeobox gene Lhx9 is essential for mouse gonad formation. Nature. (2000) 403:909–13. doi: 10.1038/35002622
47. Kusaka M, Katoh-Fukui Y, Ogawa H, Miyabayashi K, Baba T, Shima Y, et al. Abnormal epithelial cell polarity and ectopic epidermal growth factor receptor (EGFR) expression induced in Emx2 KO embryonic gonads. Endocrinology. (2010) 151:5893–904. doi: 10.1210/en.2010-0915
48. Miyamoto Y, Taniguchi H, Hamel F, Silversides DW, Viger RS. A GATA4/WT1 cooperation regulates transcription of genes required for mammalian sex determination and differentiation. BMC Mol Biol. (2008) 9:44. doi: 10.1186/1471-2199-9-44
49. Larney C, Bailey TL, Koopman P. Switching on sex: transcriptional regulation of the testis-determining gene Sry. Development. (2014) 141:2195–205. doi: 10.1242/dev.107052
50. Gierl MS, Gruhn WH, von Seggern A, Maltry N, Niehrs C. GADD45G functions in male sex determination by promoting p38 signaling and Sry expression. Dev Cell. (2012) 23:1032–42. doi: 10.1016/j.devcel.2012.09.014
51. Cen CH, Chen M, Jiang L, Hou XH, Gao F. The regulation of gonadal somatic cell differentiation during sex determination in mice. Sheng Li Xue Bao. (2020) 72:20–30.
52. Sekido R. SRY: A transcriptional activator of mammalian testis determination. Int J Biochem Cell Biol. (2010) 42:417–20. doi: 10.1016/j.biocel.2009.12.005
53. Stewart MK, Mattiske DM, Pask AJ. Estrogen suppresses SOX9 and activates markers of female development in a human testis-derived cell line. BMC Mol Cell Biol. (2020) 21:66. doi: 10.1186/s12860-020-00307-9
54. Xu CZ, Dai YC, Mohsin A, Hang HF, Zhuang YP, Guo MJ. Mapping molecular pathways for embryonic Sertoli cells derivation based on differentiation model of mouse embryonic stem cells. Stem Cell Res Ther. (2020) 11:85. doi: 10.1186/s13287-020-01600-2
55. Wilhelm D, Martinson F, Bradford S, Wilson MJ, Combes AN, Beverdam A, et al. Sertoli cell differentiation is induced both cell-autonomously and through prostaglandin signaling during mammalian sex determination. Dev Biol. (2005) 287:111–24. doi: 10.1016/j.ydbio.2005.08.039
56. Sekido R, Bar I, Narvaez V, Penny G, Lovell-Badge R. SOX9 is up-regulated by the transient expression of SRY specifically in Sertoli cell precursors. Dev Biol. (2004) 274:271–9. doi: 10.1016/j.ydbio.2004.07.011
57. Dupont S, Capel B. The chromatin state during gonadal sex determination. Sex Dev. (2021) 15:308–16. doi: 10.1159/000520007
58. Xie M, Hu X, Li L, Xiong Z, Zhang H, Zhuang Y, et al. Loss of Raptor induces Sertoli cells into an undifferentiated state in mice. Biol Reprod. (2022) 107:1125–38. doi: 10.1093/biolre/ioac104
59. Haseeb A, Lefebvre V. The SOXE transcription factors-SOX8, SOX9 and SOX10-share a bi-partite transactivation mechanism. Nucleic Acids Res. (2019) 47:6917–31. doi: 10.1093/nar/gkz523
60. Gonen N, Lovell-Badge R. The regulation of Sox9 expression in the gonad. Curr Top Dev Biol. (2019) 134:223–52. doi: 10.1016/bs.ctdb.2019.01.004
61. Schmahl J, Kim Y, Colvin JS, Ornitz DM, Capel B. Fgf9 induces proliferation and nuclear localization of FGFR2 in Sertoli precursors during male sex determination. Development. (2004) 131:3627–36. doi: 10.1242/dev.01239
62. Kim Y, Bingham N, Sekido R, Parker KL, Lovell-Badge R, Capel B. Fibroblast growth factor receptor 2 regulates proliferation and Sertoli differentiation during male sex determination. Proc Natl Acad Sci USA. (2007) 104:16558–63. doi: 10.1073/pnas.0702581104
63. Harpelunde Poulsen K, Nielsen JE, Frederiksen H, Melau C, Juul Hare K, Langhoff Thuesen L, et al. Dysregulation of FGFR signalling by a selective inhibitor reduces germ cell survival in human fetal gonads of both sexes and alters the somatic niche in fetal testes. Hum Reprod. (2019) 34:2228–43. doi: 10.1093/humrep/dez191
64. Jameson SA, Lin YT, Capel B. Testis development requires the repression of Wnt4 by Fgf signaling. Dev Biol. (2012) 370:24–32. doi: 10.1016/j.ydbio.2012.06.009
65. Lundgaard Riis M, Jorgensen A. Deciphering sex-specific differentiation of human fetal gonads: insight from experimental models. Front Cell Dev Biol. (2022) 10:902082. doi: 10.3389/fcell.2022.902082
66. Chen KQ, Wei BH, Hao SL, Yang WX. The PI3K/AKT signaling pathway: How does it regulate development of Sertoli cells and spermatogenic cells? Histol Histopathol. (2022) 37:621–36. doi: 10.14670/HH-18-457
67. Xi H, Ren F, Li Y, Xian M, Wang L, Hu J. FSH inhibits autophagy and lysosomal biogenesis to regulate protein degradation in cultured goat Sertoli cells. Mol Cell Endocrinol. (2022) 540:111505. doi: 10.1016/j.mce.2021.111505
68. Tian RH, Yao CC, Yang C, Zhu ZJ, Li C, Zhi EL, et al. Fibroblast growth factor-5 promotes spermatogonial stem cell proliferation via ERK and AKT activation. Stem Cell Res Ther. (2019) 10(1):40. doi: 10.1186/s13287-019-1139-7
69. Jiao ZJ, Yi W, Rong YW, Kee JD, Zhong WX. MicroRNA-1285 regulates 17beta-estradiol-inhibited immature boar sertoli cell proliferation via adenosine monophosphate-activated protein kinase activation. Endocrinology. (2015) 156:4059–70. doi: 10.1210/en.2014-1982
70. Yang WR, Zhu FW, Zhang JJ, Wang Y, Zhang JH, Lu C, et al. PI3K/akt activated by GPR30 and src regulates 17beta-estradiol-induced cultured immature boar sertoli cells proliferation. Reprod Sci. (2017) 24:57–66. doi: 10.1177/1933719116649696
71. Liu C, Rodriguez K, Yao HH. Mapping lineage progression of somatic progenitor cells in the mouse fetal testis. Development. (2016) 143:3700–10. doi: 10.1242/dev.135756
72. Matson CK, Murphy MW, Sarver AL, Griswold MD, Bardwell VJ, Zarkower D. DMRT1 prevents female reprogramming in the postnatal mammalian testis. Nature. (2011) 476:101–4. doi: 10.1038/nature10239
73. Cool J, DeFalco T, Capel B. Testis formation in the fetal mouse: dynamic and complex de novo tubulogenesis. Wiley Interdiscip Rev Dev Biol. (2012) 1:847–59. doi: 10.1002/wdev.62
74. Combes AN, Wilhelm D, Davidson T, Dejana E, Harley V, Sinclair A, et al. Endothelial cell migration directs testis cord formation. Dev Biol. (2009) 326:112–20. doi: 10.1016/j.ydbio.2008.10.040
75. Coveney D, Cool J, Oliver T, Capel B. Four-dimensional analysis of vascularization during primary development of an organ, the gonad. Proc Natl Acad Sci USA. (2008) 105:7212–7. doi: 10.1073/pnas.0707674105
76. Coveney D, Ross AJ, Slone JD, Capel B. A microarray analysis of the XX Wnt4 mutant gonad targeted at the identification of genes involved in testis vascular differentiation. Gene Expr Patterns. (2007) 7:82–92. doi: 10.1016/j.modgep.2006.05.012
77. Sekido R, Lovell-Badge R. Genetic control of testis development. Sex Dev. (2013) 7:21–32. doi: 10.1159/000342221
78. Cool J, DeFalco TJ, Capel B. Vascular-mesenchymal cross-talk through Vegf and Pdgf drives organ patterning. Proc Natl Acad Sci USA. (2011) 108:167–72. doi: 10.1073/pnas.1010299108
79. Gu X, Li SY, DeFalco T. Immune and vascular contributions to organogenesis of the testis and ovary. FEBS J. (2022) 289:2386–408. doi: 10.1111/febs.15848
80. DeFalco T, Bhattacharya I, Williams AV, Sams DM, Capel B. Yolk-sac-derived macrophages regulate fetal testis vascularization and morphogenesis. Proc Natl Acad Sci USA. (2014) 111:E2384–93. doi: 10.1073/pnas.1400057111
81. Delot EC, Vilain E. Towards improved genetic diagnosis of human differences of sex development. Nat Rev Genet. (2021) 22:588–602. doi: 10.1038/s41576-021-00365-5
82. Zhang W, Mao J, Wang X, Zhao Z, Zhang X, Sun B, et al. The genetic spectrum of a Chinese series of patients with 46, XY disorders of the sex development. Andrology. (2023) 12(1):98–108. doi: 10.1111/andr.13446
83. Chen M, Wang X, Wang Y, Zhang L, Xu B, Lv L, et al. Wt1 is involved in leydig cell steroid hormone biosynthesis by regulating paracrine factor expression in mice. Biol Reprod. (2014) 90:71. doi: 10.1095/biolreprod.113.114702
84. Ozisik G, Achermann JC, Meeks JJ, Jameson JL. SF1 in the development of the adrenal gland and gonads. Horm Res. (2003) 59 Suppl 1:94–8. doi: 10.1159/000067831
85. Pelletier J, Schalling M, Buckler AJ, Rogers A, Haber DA, Housman D. Expression of the Wilms’ tumor gene WT1 in the murine urogenital system. Genes Dev. (1991) 5:1345–56. doi: 10.1101/gad.5.8.1345
86. Bandiera R, Vidal VP, Motamedi FJ, Clarkson M, Sahut-Barnola I, von Gise A, et al. WT1 maintains adrenal-gonadal primordium identity and marks a population of AGP-like progenitors within the adrenal gland. Dev Cell. (2013) 27:5–18. doi: 10.1016/j.devcel.2013.09.003
87. Zhang L, Chen M, Wen Q, Li Y, Wang Y, Wang Y, et al. Reprogramming of Sertoli cells to fetal-like Leydig cells by Wt1 ablation. Proc Natl Acad Sci USA. (2015) 112:4003–8. doi: 10.1073/pnas.1422371112
88. Wang X, Adeniran SO, Wang Z, Li X, Huang F, Ma M, et al. 3, 3’, 5-Triiodo-L-thyronine affects polarity proteins of bovine Sertoli cells via WT1/non-canonical Wnt signaling pathway. Theriogenology. (2020) 148:8–17. doi: 10.1016/j.theriogenology.2020.02.034
89. Wang XN, Li ZS, Ren Y, Jiang T, Wang YQ, Chen M, et al. The wilms tumor gene, wt1, is critical for mouse spermatogenesis via regulation of sertoli cell polarity and is associated with non-obstructive azoospermia in humans. PloS Genet. (2013) 9(8):e1003645. doi: 10.1371/journal.pgen.1003645
90. Wang X, Adegoke EO, Ma M, Huang F, Zhang H, Adeniran SO, et al. Influence of Wilms’ tumor suppressor gene WT1 on bovine Sertoli cells polarity and tight junctions via non-canonical WNT signaling pathway. Theriogenology. (2019) 138:84–93. doi: 10.1016/j.theriogenology.2019.07.007
91. Chang H, Gao F, Guillou F, Taketo MM, Huff V, Behringer RR. Wt1 negatively regulates beta-catenin signaling during testis development. Development. (2008) 135:1875–85. doi: 10.1242/dev.018572
92. Kim MS, Yoon SK, Bollig F, Kitagaki J, Hur W, Whye NJ, et al. A novel Wilms tumor 1 (WT1) target gene negatively regulates the WNT signaling pathway. J Biol Chem. (2010) 285:14585–93. doi: 10.1074/jbc.M109.094334
93. Kerr GE, Young JC, Horvay K, Abud HE, Loveland KL. Regulated Wnt/beta-catenin signaling sustains adult spermatogenesis in mice. Biol Reprod. (2014) 90:3. doi: 10.1095/biolreprod.112.105809
94. Tanwar PS, Kaneko-Tarui T, Zhang L, Rani P, Taketo MM, Teixeira J. Constitutive WNT/beta-catenin signaling in murine Sertoli cells disrupts their differentiation and ability to support spermatogenesis. Biol Reprod. (2010) 82:422–32. doi: 10.1095/biolreprod.109.079335
95. Ghaffari Novin M, Mirfakhraie R, Nazarian H. Aberrant wnt/beta-catenin signaling pathway in testis of azoospermic men. Adv Pharm Bull. (2015) 5:373–7. doi: 10.15171/apb.2015.051
96. Defalco T, Saraswathula A, Briot A, Iruela-Arispe ML, Capel B. Testosterone levels influence mouse fetal Leydig cell progenitors through notch signaling. Biol Reprod. (2013) 88:91. doi: 10.1095/biolreprod.112.106138
97. Wen Q, Wang Y, Tang J, Cheng CY, Liu YX. Sertoli cell wt1 regulates peritubular myoid cell and fetal leydig cell differentiation during fetal testis development. PloS One. (2016) 11:e0167920. doi: 10.1371/journal.pone.0167920
98. Murata K, Hattori M, Hirai N, Shinozuka Y, Hirata H, Kageyama R, et al. Hes1 directly controls cell proliferation through the transcriptional repression of p27Kip1. Mol Cell Biol. (2005) 25:4262–71. doi: 10.1128/MCB.25.10.4262-4271.2005
99. Tang H, Brennan J, Karl J, Hamada Y, Raetzman L, Capel B. Notch signaling maintains Leydig progenitor cells in the mouse testis. Development. (2008) 135:3745–53. doi: 10.1242/dev.024786
100. Wen Q, Zheng Q-S, Li X-X, Hu Z-Y, Gao F, Cheng CY, et al. Wt1 dictates the fate of fetal and adult Leydig cells during development in the mouse testis. Am J Physiology-Endocrinol Metab. (2014) 307:E1131–E43. doi: 10.1152/ajpendo.00425.2014
101. Wen Y, Ma X, Wang X, Wang F, Dong J, Wu Y, et al. hnRNPU in Sertoli cells cooperates with WT1 and is essential for testicular development by modulating transcriptional factors Sox8/9. Theranostics. (2021) 11:10030–46. doi: 10.7150/thno.66819
102. Chen M, Zhang L, Cui X, Lin X, Li Y, Wang Y, et al. Wt1 directs the lineage specification of sertoli and granulosa cells by repressing Sf1 expression. Development. (2017) 144:44–53. doi: 10.1242/dev.144105
103. Spraggon L, Dudnakova T, Slight J, Lustig-Yariv O, Cotterell J, Hastie N, et al. hnRNP-U directly interacts with WT1 and modulates WT1 transcriptional activation. Oncogene. (2007) 26:1484–91. doi: 10.1038/sj.onc.1209922
104. Anamthathmakula P, Miryala CSJ, Moreci RS, Kyathanahalli C, Hassan SS, Condon JC, et al. Steroidogenic factor 1 (Nr5a1) is required for sertoli cell survival post sex determination. Sci Rep. (2019) 9:4452. doi: 10.1038/s41598-019-41051-1
105. Kato T, Esaki M, Matsuzawa A, Ikeda Y. NR5A1 is required for functional maturation of Sertoli cells during postnatal development. Reproduction. (2012) 143:663–72. doi: 10.1530/REP-11-0365
106. Shen WH, Moore CC, Ikeda Y, Parker KL, Ingraham HA. Nuclear receptor steroidogenic factor 1 regulates the mullerian inhibiting substance gene: a link to the sex determination cascade. Cell. (1994) 77:651–61. doi: 10.1016/0092-8674(94)90050-7
107. Schteingart HF, Picard JY, Valeri C, Marshall I, Treton D, di Clemente N, et al. A mutation inactivating the distal SF1 binding site on the human anti-Mullerian hormone promoter causes persistent Mullerian duct syndrome. Hum Mol Genet. (2019) 28:3211–8. doi: 10.1093/hmg/ddz147
108. Sun J, Lian X, Lv C, Li H, Lin Z, Luo S, et al. Trps1 acts as a regulator of Sf-1 transcription and testosterone synthesis in mouse Leydig cells. Cell Biol Toxicol. (2023) 39(6):3141–57. doi: 10.1007/s10565-023-09823-8
109. Mendoza-Villarroel RE, Di-Luoffo M, Camire E, Giner XC, Brousseau C, Tremblay JJ. The INSL3 gene is a direct target for the orphan nuclear receptor, COUP-TFII, in Leydig cells. J Mol Endocrinol. (2014) 53:43–55. doi: 10.1530/JME-13-0290
110. Bogatcheva NV, Agoulnik AI. INSL3/LGR8 role in testicular descent and cryptorchidism. Reprod BioMed Online. (2005) 10:49–54. doi: 10.1016/S1472-6483(10)60803-6
111. Di-Luoffo M, Pierre KJ, Robert NM, Girard MJ, Tremblay JJ. The nuclear receptors SF1 and COUP-TFII cooperate on the Insl3 promoter in Leydig cells. Reproduction. (2022) 164:31–40. doi: 10.1530/REP-22-0109
112. Hansen S, Hupp TR, Lane DP. Allosteric regulation of the thermostability and DNA binding activity of human p53 by specific interacting proteins. CRC Cell Transform Group J Biol Chem. (1996) 271:3917–24. doi: 10.1074/jbc.271.7.3917
113. Chaboissier MC, Kobayashi A, Vidal VIP, Lutzkendorf S, van de Kant HJG, Wegner M, et al. Functional analysis of Sox8 and Sox9 during sex determination in the mouse. Development. (2004) 131:1891–901. doi: 10.1242/dev.01087
114. Wagner KD, Wagner N, Vidal VP, Schley G, Wilhelm D, Schedl A, et al. The Wilms’ tumor gene Wt1 is required for normal development of the retina. EMBO J. (2002) 21:1398–405. doi: 10.1093/emboj/21.6.1398
115. Koopman P, Gubbay J, Vivian N, Goodfellow P, Lovell-Badge R. Male development of chromosomally female mice transgenic for Sry. Nature. (1991) 351:117–21. doi: 10.1038/351117a0
116. Aksel S, Cao M, Derpinghaus A, Baskin LS, Cunha GR. Ontogeny of mouse Sertoli, Leydig and peritubular myoid cells from embryonic day 10 to adulthood. Differentiation. (2023) 129:96–108. doi: 10.1016/j.diff.2022.02.006
117. Tang FR, Richardson N, Albina A, Chaboissier MC, Perea-Gomez A. Mouse gonad development in the absence of the pro-ovary factor WNT4 and the pro-testis factor SOX9. Cells. (2020) 9(5):1103. doi: 10.3390/cells9051103
118. Chassot AA, Ranc F, Gregoire EP, Roepers-Gajadien HL, Taketo MM, Camerino G, et al. Activation of beta-catenin signaling by Rspo1 controls differentiation of the mammalian ovary. Hum Mol Genet. (2008) 17:1264–77. doi: 10.1093/hmg/ddn016
119. Sekido R, Lovell-Badge R. Sex determination involves synergistic action of SRY and SF1 on a specific Sox9 enhancer. Nature. (2008) 453:930–4. doi: 10.1038/nature06944
120. Ikeda Y, Takeda Y, Shikayama T, Mukai T, Hisano S, Morohashi KI. Comparative localization of Dax-1 and Ad4BP/SF-1 during development of the hypothalamic-pituitary-gonadal axis suggests their closely related and distinct functions. Dev Dyn. (2001) 220:363–76. doi: 10.1002/dvdy.1116
121. Gonen N, Quinn A, O’Neill HC, Koopman P, Lovell-Badge R. Normal levels of sox9 expression in the developing mouse testis depend on the TES/TESCO enhancer, but this does not act alone. PloS Genet. (2017) 13:e1006520. doi: 10.1371/journal.pgen.1006520
122. Wilhelm D, Hiramatsu R, Mizusaki H, Widjaja L, Combes AN, Kanai Y, et al. SOX9 regulates prostaglandin D synthase gene transcription in vivo to ensure testis development. J Biol Chem. (2007) 282:10553–60. doi: 10.1074/jbc.M609578200
123. Moniot B, Declosmenil F, Barrionuevo F, Scherer G, Aritake K, Malki S, et al. The PGD2 pathway, independently of FGF9, amplifies SOX9 activity in Sertoli cells during male sexual differentiation. Development. (2009) 136:1813–21. doi: 10.1242/dev.032631
124. Bagheri-Fam S, Sim H, Bernard P, Jayakody I, Taketo MM, Scherer G, et al. Loss of Fgfr2 leads to partial XY sex reversal. Dev Biol. (2008) 314:71–83. doi: 10.1016/j.ydbio.2007.11.010
125. Siggers P, Carre GA, Bogani D, Warr N, Wells S, Hilton H, et al. A novel mouse Fgfr2 mutant, hobbyhorse (hob), exhibits complete XY gonadal sex reversal. PloS One. (2014) 9:e100447. doi: 10.1371/journal.pone.0100447
126. Sreenivasan R, Gonen N, Sinclair A. SOX genes and their role in disorders of sex development. Sex Dev. (2022) 16:80–91. doi: 10.1159/000524453
127. Hyon C, Chantot-Bastaraud S, Harbuz R, Bhouri R, Perrot N, Peycelon M, et al. Refining the regulatory region upstream of SOX9 associated with 46, XX testicular disorders of sex development (DSD). Am J Med Genet Part A. (2015) 167:1851–8. doi: 10.1002/ajmg.a.37101
128. Diawara M, Arsenault A, Charette SA, Martin LJ. The transcription factors Creb1 and CEBPB regulate Sox9 promoter activity in TM4 Sertoli cells. Gene. (2023) 873:147477. doi: 10.1016/j.gene.2023.147477
129. Agbor VA, Tao S, Lei N, Heckert LL. A Wt1-Dmrt1 transgene restores DMRT1 to sertoli cells of Dmrt1(-/-) testes: a novel model of DMRT1-deficient germ cells. Biol Reprod. (2013) 88:51. doi: 10.1095/biolreprod.112.103135
130. Rahmoun M, Lavery R, Laurent-Chaballier S, Bellora N, Philip GK, Rossitto M, et al. In mammalian foetal testes, SOX9 regulates expression of its target genes by binding to genomic regions with conserved signatures. Nucleic Acids Res. (2017) 45:7191–211. doi: 10.1093/nar/gkx328
131. Lindeman RE, Murphy MW, Agrimson KS, Gewiss RL, Bardwell VJ, Gearhart MD, et al. The conserved sex regulator DMRT1 recruits SOX9 in sexual cell fate reprogramming. Nucleic Acids Res. (2021) 49:6144–64. doi: 10.1093/nar/gkab448
132. Matson CK, Zarkower D. Sex and the singular DM domain: insights into sexual regulation, evolution and plasticity. Nat Rev Genet. (2012) 13:163–74. doi: 10.1038/nrg3161
133. Zhao L, Svingen T, Ng ET, Koopman P. Female-to-male sex reversal in mice caused by transgenic overexpression of Dmrt1. Development. (2015) 142:1083–8. doi: 10.1242/dev.122184
134. Ying M, Chen B, Tian Y, Hou Y, Li Q, Shang X, et al. Nuclear import of human sexual regulator DMRT1 is mediated by importin-beta. Biochim Biophys Acta. (2007) 1773:804–13. doi: 10.1016/j.bbamcr.2007.03.006
135. Bruni V, Roppa K, Scionti F, Apa R, Sestito S, Di Martino MT, et al. A 46,XY female with a 9p24.3p24.1 deletion and a 8q24.11q24.3 duplication: A case report and review of the literature. Cytogenet Genome Res. (2019) 158:74–82. doi: 10.1159/000500619
136. Kobayashi T, Matsuda M, Kajiura-Kobayashi H, Suzuki A, Saito N, Nakamoto M, et al. Two DM domain genes, DMY and DMRT1, involved in testicular differentiation and development in the medaka, Oryzias latipes. Dev Dyn. (2004) 231:518–26. doi: 10.1002/dvdy.20158
137. Matson CK, Murphy MW, Griswold MD, Yoshida S, Bardwell VJ, Zarkower D. The mammalian doublesex homolog DMRT1 is a transcriptional gatekeeper that controls the mitosis versus meiosis decision in male germ cells. Dev Cell. (2010) 19:612–24. doi: 10.1016/j.devcel.2010.09.010
138. Lu Y, Yuan P, Qiao J. DMRT1 drives the human germline forward. Nat Cell Biol. (2023) 25:1408–10. doi: 10.1038/s41556-023-01196-8
139. Irie N, Lee SM, Lorenzi V, Xu H, Chen J, Inoue M, et al. DMRT1 regulates human germline commitment. Nat Cell Biol. (2023) 25:1439–52. doi: 10.1038/s41556-023-01224-7
140. Zhang T, Zarkower D. DMRT proteins and coordination of mammalian spermatogenesis. Stem Cell Res. (2017) 24:195–202. doi: 10.1016/j.scr.2017.07.026
141. Zhang MF, Wan SC, Chen WB, Yang DH, Liu WQ, Li BL, et al. Transcription factor Dmrt1 triggers the SPRY1-NF-kappaB pathway to maintain testicular immune homeostasis and male fertility. Zool Res. (2023) 44:505–21. doi: 10.24272/j.issn.2095-8137.2022.440
Keywords: Sertoli cell, sex determination, mice, spermatogenesis, embryonic cell development, primordial germ cell
Citation: Gao Y, Wang Z, Long Y, Yang L, Jiang Y, Ding D, Teng B, Chen M, Yuan J and Gao F (2024) Unveiling the roles of Sertoli cells lineage differentiation in reproductive development and disorders: a review. Front. Endocrinol. 15:1357594. doi: 10.3389/fendo.2024.1357594
Received: 18 December 2023; Accepted: 07 April 2024;
Published: 18 April 2024.
Edited by:
Bhola Shankar Pradhan, Łukasiewicz Research Network – PORT Polish Center for Technology Development, PolandReviewed by:
Indrashis Bhattacharya, Central University of Kerala, IndiaAjit Singh, Banaras Hindu University, India
Huitao Li, Second Affiliated Hospital and Yuying Children’s Hospital of Wenzhou Medical University, China
Copyright © 2024 Gao, Wang, Long, Yang, Jiang, Ding, Teng, Chen, Yuan and Gao. This is an open-access article distributed under the terms of the Creative Commons Attribution License (CC BY). The use, distribution or reproduction in other forums is permitted, provided the original author(s) and the copyright owner(s) are credited and that the original publication in this journal is cited, in accordance with accepted academic practice. No use, distribution or reproduction is permitted which does not comply with these terms.
*Correspondence: Fei Gao, Z2FvZkBpb3ouYWMuY24=; Jinxiang Yuan, eXVhbmppbnhpYW5nMThAMTYzLmNvbQ==; Min Chen, Y2hlbm1pbkBpb3ouYWMuY24=