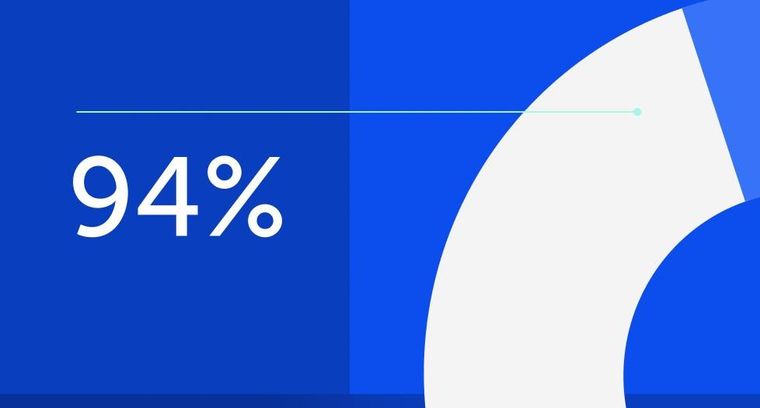
94% of researchers rate our articles as excellent or good
Learn more about the work of our research integrity team to safeguard the quality of each article we publish.
Find out more
MINI REVIEW article
Front. Endocrinol., 29 April 2024
Sec. Diabetes: Molecular Mechanisms
Volume 15 - 2024 | https://doi.org/10.3389/fendo.2024.1351497
This article is part of the Research TopicAdvances in the Research of Diabetic Nephropathy Volume IIIView all 5 articles
Diabetic nephropathy (DKD) is a common chronic complication of diabetes mellitus and an important cause of cardiovascular-related death. Oxidative stress is a key mechanism leading to diabetic nephropathy. However, the current main therapeutic approach remains combination therapy and lacks specific therapies targeting oxidative stress. With the development of nanotechnology targeting ROS, therapeutic fluids regarding their treatment of diabetic nephropathy have attracted attention. In this review, we provide a brief overview of various ROS-based nanomaterials for DKD, including ROS-scavenging nanomaterials, ROS-associated nanodelivery materials, and ROS-responsive nanomaterials. In addition, we summarize and discuss key factors that should be considered when designing ROS-based nanomaterials, such as biosafety, efficacy, targeting, and detection and monitoring of ROS.
Diabetic kidney disease (DKD) is the most severe microvascular complication of diabetes, constituting a predominant cause of end-stage renal disease (ESRD) in many developed countries (1). However, the current prevention and treatment strategy of DKD mainly aims to control blood glucose and blood pressure comprehensively. While employing renin–angiotensin–aldosterone system antagonists and glucose cotransporter-2 inhibitors has demonstrated efficacy in retarding the initiation of DKD, a significant subset of patients still exhibits a continuous trajectory of renal deterioration (2, 3). Moreover, many small molecule drugs for DKD usually require high concentrations, which may lead to non-specific uptake and adverse side effects. Therefore, specific treatments for DKD should attract our attention (4).
Many factors are believed to contribute to the development of DKD, with oxidative stress being an essential pathological mechanism. Moreover, hyperglycemia is a primary reason for promoting excessive reactive oxygen species (ROS) production in oxidative stress (5). Not only do ROS orchestrate cellular equilibrium, but they also serve as primary instigators of cellular aberrations, thus accentuating disease manifestations (6). For individuals diagnosed with DKD, ROS production surges, overpowering the neutralizing capabilities of antioxidants. This ensues a precarious imbalance between pro-oxidative and antioxidative mechanisms, culminating in oxidative stress, amplified apoptosis, and consequential renal fibrosis (7). Hence, strategies focusing on mitigating oxidative stress and neutralizing excessive ROS present promising avenues for DKD management. Given the nanotechnological advancements, many nanomaterials endowed with unique ROS modulatory properties have been synthesized (8). These ROS-centric nanomaterials can either stimulate or mitigate ROS production, aiming for therapeutic modulation.
In recent years, nanotechnology has experienced significant advancements in the field of targeted tumor therapy. Nanoparticles (NPs) have emerged as versatile tools that can effectively modulate the levels of reactive oxygen species (ROS) and encapsulate a diverse array of therapeutic agents, including small molecule drugs, peptides, and nucleic acids. These nanoformulations enable the sustained and controlled release of therapeutic payloads in vivo, offering enhanced precision and efficacy in cancer treatment (9). NPs as drug carriers have many advantages, such as high stability (i.e., long shelf life), high carrier capacity (many drug molecules can be added to the particle–matrix), and capability to deliver both hydrophilic and hydrophobic substances through different routes such as oral administration, inhalation, and so on. These carriers can also be designed to enable controlled (sustained) release of drugs from the matrix (10). Furthermore, tailoring parameters like size, morphology, charge, and surface properties of these nanoscale delivery systems, keeping in view the physiological or pathological nuances of the kidney, can enhance kidney-centric therapeutic interventions (11).
In this review, we discussed and summarized the applications of different types of ROS-based nanomaterials in DKD, including ROS-scavenging nanomaterials, ROS-related nanodrug delivery materials, and ROS-responsive nanomaterials. Common applications in current research are highlighted. In addition, to give full play to the role of ROS nanomaterials, we also summarized the issues that need to be considered in the preparation of ROS nanomaterials. We hope this short review can provide some valuable hints and references for the development of more effective and safer ROS-based nanomaterials in the future.
We searched PubMed using the keywords “diabetic kidney disease,” “ROS,” “kidney disease,” and “nanomaterials” to obtain current relevant literature. Literature related to nanomaterials targeting ROS-related mechanisms for the treatment of diabetic kidney disease and other kidney diseases is discussed, while nanomaterials targeting other pathogenic mechanisms are not discussed. In addition, since there is a large body of literature on the use of ROS for the treatment of other renal diseases, which is not the endpoint of this review, only a few references are cited to elaborate on them.
ROS-scavenging nanoparticles can be broadly categorized based on their operative mechanisms into three distinct classes (12): enzyme-mimicking nanoparticles (nano-enzymes), free radical trapper nanoparticles, and redox ROS-scavenging nanoparticles. Common enzyme-like NPs include noble metal nanoparticles [Au, Ag, Cu (13–15), etc.], metal oxide nanoparticles [CeO2, Fe3O4, MnO2 (16–18), etc.], Prussian blue graphene oxide (19), etc. These nanoparticles typically exhibit multi-enzyme activities akin to peroxidase (POD), catalase (CAT), and superoxide dismutase (SOD), facilitating ROS neutralization. While SOD typically catalyzes the decomposition of O2− to H2O2, POD and CAT catalyze the decomposition of H2O2 into water. Free radical trapper NPs capture ROS via the single electron on nitroxide or conjugated double bonds (20, 21). The primary mechanism of redox ROS-scavenging NPs is related to the stimulation of redox activity by targeting various substances, thus achieving the purpose of scavenging ROS (22, 23) (Figure 1).
In designing the removal of ROS nanomaterials, we need to consider preventing leakage of the remover. If antioxidants are prematurely released from nanocarriers before arriving at the intended site, they may culminate in diminished therapeutic efficacy or even engender adverse reactions (24). Some studies have shown that the drug delivery platform based on nanomaterials can be stabilized by enhancing the molecular interactions, such as hydrophobic interaction, electrostatic interaction, van der Waals force, hydrogen bond, covalent bond, and the encapsulation between drugs and nanomaterials (25, 26). Moreover, enhancing the active-targeting proficiency of nanomaterials is imperative. Active targeting based on ligand–receptor recognition may show better efficacy than passive targeting in human cancer therapy, and several active-targeting nanomedicines have progressed into clinical trials (27). Active targeting is based on the recognition and binding of ligands to cell surface receptors, and they can accomplish site-specific delivery by modifying their surface ligands, which can recognize overexpressed receptors in pathological tissues, thereby promoting the selective uptake of nanomedicines (28). Introducing specific stimuli within the antioxidant delivery system can curtail the premature release of encapsulated antioxidants. Notably, the prime merit of stimulus-responsive release systems lies in their spatial–temporal control of localized drug release, offering a promising mechanism for drug release activation.
Several ROS-scavenging nanomaterials have been investigated for their potential therapeutic effects on DKD. Alomari and his team (29) explored the therapeutic efficacy of 50-nm gold nanoparticles (AuNPs) in treating DKD. Diabetic rats were treated intraperitoneally with 50 nm of AuNPs (2.5 mg/kg) daily for 7 weeks. Their findings substantiated the robust therapeutic potential of AuNPs in DKD management. The AuNPs exhibited noteworthy preventive capabilities against hyperglycemia-induced detrimental effects in renal tissue, mainly by mitigating the accumulation of extracellular matrix proteins and ameliorating podocyte injuries. This was achieved by suppressing growth factors, inflammation, and angiogenesis markers. Remarkably, AuNP treatments led to a significant reduction in renal oxidative stress, as evidenced by enhanced activities of renal SOD and CAT and a decreased malondialdehyde (MDA) tissue level. These outcomes align with preceding in-vivo investigations that attributed antioxidative and antihyperglycemic properties to AuNPs in diabetic animal models.
In another study, Tong and associates (30) formulated a hollow mesoporous silica nanocomposite (HMSN) particle infused with trace cerium oxide by coprecipitation. This nanocomposite demonstrated capabilities in averting ROS-induced DKD pathology and showcased an impressive drug-loading capacity. The nanoparticles were infused with metformin (MET), culminating in the creation of multifunctional nanoparticles (MET-HMSN-CeO2). DKD rats were treated with intraperitoneal administration of MET-HMSN-CeO2 daily for 8 weeks. The cyclical transition between Ce3+ and Ce4+ within this system’s mixed-valence ceria augments its antioxidative potential by extending ROS-scavenging activity. The therapeutic potential of these nanoparticles was validated on a streptozotocin-induced renal injury rat model and a high-glucose-induced NRK-52E cell line. The findings underscored their potential to alleviate DKD symptoms through oxidative stress reduction, inhibition of cellular apoptosis, and renal injury protection in both in-vitro and in-vivo settings. Building on this line of inquiry, Iftekhar Hassan et al. (31) delved into the therapeutic merits of selenium nanoparticles (Se-NPs) with inherent antioxidant properties in addressing nephropathy in the progeny of diabetic rats. The study found that Se-NPs could modulate oxidative stress and inflammation-triggered diabetic conditions in maternal rats, shielding their offspring from diabetes and its associated nephropathy. These research findings underscore the immense potential of targeted removal of excessive ROS in the treatment of DKD.
Apart from nanomaterials exhibiting ROS-scavenging capacities, there are drugs endowed with antioxidant potential that, when combined with nanoparticles, can amplify both the drug’s target specificity and its stability. Manna and coworkers (32) pioneered the synthesis of AuNPs using pomegranate peel ellagitannins (PPE-AuNPs). The pomegranate peel extract (PPE) is rich in polyphenolic compounds like flavonoids and condensed and hydrolyzable tannin variants, exhibiting pronounced antioxidant activity through their free radical-scavenging mechanisms. Yet, these bioactive molecules inherently face stability issues and are susceptible to spontaneous enzymatic hydrolysis. By constructing PPE-AuNPs, these bioactive entities are rendered more stable. Diabetic mice were treated with different concentrations of PPE-AuNPs via intraperitoneal injection. Studies revealed that PPE-AuNP could holistically overturn the oxidative milieu, curbing protein glycation, scavenging ROS, and deactivating the MAPK/NF-κB/STAT3-induced inflammatory stress. Importantly, PPE-AuNPs were observed to invoke an endogenous antioxidant response, stabilizing renal homeostasis in DKD. Moreover, Tong et al. (33) designed a nanoparticle complex by crafting the poly(ethylene glycol)-block-(poly(ethylenediamine 1-glutamate)-graft-poly(ϵ-benzyloxycarbonyl-1-lysine)) (PEG-b-(PELG-g-PZLL)) carrier loaded with quercetin, abbreviated as QUE/PEG-b-(PELG-g-PZLL) or QUE/P by a previous method (34). Quercetin, a plant flavonoid, is recognized for its formidable in-vitro antioxidant characteristics. Emerging research posits quercetin as a potent countermeasure against DKD. Nonetheless, its hydrophobicity, suboptimal bioavailability, and transient half-life impede its in-vivo application. The constructed PEG-b-(PELG-g-PZLL) showed potential in elevating the serum quercetin concentration in Sprague–Dawley rats, extending its circulatory lifespan. SD rats were administrated a dose of 10 mg/kg of QUE/P via abdominal subcutaneous injection. In the DKD rat model, both QUE and QUE/P demonstrated remedial effects, evident from renal recovery, metabolic modulation, and curtailed oxidative stress, denoted by reduced MDA levels and augmented SOD activity. Lastly, Akram Ahangarpour and associates (35) inspected the remedial efficacy of myricitrin and its solid lipid nanoparticles (SLNs) vis-a-vis DKD instigated by streptozotocin-nicotinamide (STZ-NA) in mice. Myricitrin, a pivotal flavonol glycoside, showcases potent counteractions against elevated glucose, oxidants, inflammation, and apoptosis. Yet, its oral uptake efficacy remains subpar. In this context, SLNs emerge as an avant-garde nanodelivery mechanism, bolstering the oral bioavailability of flavonoids. In tandem with its SLN version, indications suggest that myricitrin offers tangible improvements in DKD by mitigating oxidative strain and augmenting antioxidant enzyme levels in diabetic mice treated with SLN. These nanomaterials enhance drug stability and bioavailability by loading drugs with antioxidant effects and treat DKD by exerting their antioxidant activity.
Beyond their capacity to combat oxidative stress, ROS-responsive nanomaterials also present promising therapeutic prospects for treating DKD. These nanomaterials are primed to react to elevated ROS concentrations at the site of the lesion, initiating targeted drug release (36). Such materials have found substantial utility in oncology treatments. Contemporary nanomedicine has embraced ROS-responsive functional groups like sulfur ether, peroxalate ester, and thioketal groups, all of which can undergo cleavage at high ROS levels (37). Yet, as an emergent therapeutic strategy targeting the kidneys, these materials’ in-vivo degradability and potential nephrotoxicity need to be more adequately explored. There needs to be more research on applying these nanomaterials specifically to DKD, underlining the need for more comprehensive studies in this area.
In addition, we explored other kidney-targeted nanoparticle systems that have been applied to non-DKD. Based on the pathogenesis of chronic kidney disease (CKD), mitigating excessive ROS and restoring the redox equilibrium emerge as pivotal therapeutic strategies. Adhikari and coworkers (38) delved into the therapeutic potential of spinel-structured, citrate-functionalized Mn3O4 nanoparticles (C-Mn3O4 NPs) as a redox remedy against CKD. There is existing evidence suggesting that C-Mn3O4 efficiently catalyzes free radical reactions in vitro, particularly those involving hydrogen peroxide. Furthermore, within the hausmannite structure of C-Mn3O4 NPs, the dynamic interplay among Mn3+, Mn4+, and Mn2+ is crucial for sustaining a redox balance. This research underscored the salience of a nanoparticle’s redox regulatory activity in CKD treatment both at the cellular and organismal scales. Their findings showed that C-Mn3O4 NPs can redress the redox imbalance in HEK293 cells under H2O2-evoked oxidative stress, bolster the cellular antioxidant defense arsenal, and mitigate damage to the glomeruli and tubulointerstitial structures in CKD-afflicted mice. The ability of C-Mn3O4 NPs to balance the redox state has certain potential applications in the treatment of DKD.
Ni and coworkers (39) introduced molybdenum-based polyoxometalate (POM) nanoclusters, innovative nano-antioxidants geared toward kidney protection. Notably, POM tends to accumulate in the kidney over extended durations (40). Under specific redox conditions, the Mo ions in POM fluctuate between Mo5+ and Mo6+ valences, positioning them as effective ROS scavengers (41). Furthermore, in certain reducing and oxidizing conditions, Mo ions in POM exhibit variable valence between Mo5+ and Mo6+ and can scavenge harmful ROS (42). This study further demonstrated the efficient ability of POM nanoclusters to scavenge ROS in vitro. In other acute kidney injury (AKI) mouse models, renal function in AKI mice was improved when treated with POM nanoclusters, and the dynamic curves of 68Ga-EDTA in the blood pool, kidneys, and bladder were almost identical to that of normal mice, indicating that POM nanoclusters can scavenge ROS and produce therapeutic effects. The protective effect of POM nanoclusters against AKI in animal models suggests their therapeutic potential in treating AKI and other ROS-related diseases. Furthermore, Nagasaki and his colleagues designed pH-responsive NPs containing nitrogen oxide radicals to act as ROS scavengers, effectively alleviating AKI (43). This shows that direct removal of excess ROS is one of the effective treatments for AKI.
These nanomaterials have shown remarkable effects in dealing with ROS production and clearance, and although current research models do not focus on DKD, their ability to target kidneys and balance REDOX and antioxidant stress gives them some potential in treating DKD. In future studies, we believe that more in-depth research and systematic comparative analysis are needed to select the most suitable nanoparticle system for DKD therapy. Developments in this area will provide strong support for more effective and safer treatments for DKD.
The serendipitous discovery of nanomaterials’ renal targeting and selective accumulation has opened new therapeutic avenues for renal pathologies. Owing to their inherent kidney-targeting abilities, these nanomaterials can preferentially amass in diseased tissues, minimizing potential side effects on the rest of the body and highlighting an innovative perspective for the management of DKD. However, to ensure that the role of ROS-based nanomaterials is achieved, we need to pay attention to the biosafety, therapeutic efficacy, and targeting of the materials in the design process, and at the same time, we also need to dynamically monitor the changes of ROS in vivo to facilitate adaptation to disease states.
The potential toxicity of various biocatalytic and antioxidant nanostructures is a critical reason to impede their clinical applications. Research has indicated that polymer nanoparticles, lipid-based nanoparticles (LNPs), certain metal oxide nanoparticles, and carbon-based nanomaterials exhibit favorable biocompatibility. Polymer nanoparticles, comprising various synthetic and natural polymers such as polylactic acid (PLA) and polycaprolactone (PCL), can be utilized to formulate biocompatible nanoparticles. These polymers possess tunable degradation properties, contributing to the reduction of potential toxicity. Many polymer particles are sufficiently small (<20 nm) that they ought to cross the glomerular filtration barrier. In mice, a PEG-PLL carrier significantly enhanced the delivery of fluorescently tagged siRNA to the kidneys, compared with a naked siRNA control (44). LNPs are typically <100 nm in diameter, can be engineered to traverse the phospholipid cell membrane, and have very little toxicity and immunogenicity (45). Some metal oxide nanoparticles, such as silicon dioxide (SiO2) and iron oxide (Fe2O3), and carbon-based nanomaterials, including carbon nanotubes and graphene, can exhibit good biocompatibility under specific conditions. Of course, nanomaterials’ biocompatibilities are always associated with particle sizes, compositions, surface groups, degradability, and metabolic products, and there is an urgent need to fully disclose the toxicity of different types of biocatalytic and antioxidant nanostructures in the long term in animal-level observations to provide a comprehensive evaluation on their biocompatibilities.
Ensuring the biosafety of nanomedicines is fundamental for their biomedical utilization and subsequent clinical translation. While antioxidants at low concentrations provide a protective shield against cellular oxidative damage, elevated concentrations might paradoxically foster ROS production and incite cytotoxic effects (46). A comprehensive assessment of the safety profile of nanomedicines, especially in the context of renal diseases, is imperative to ascertain potential renal impairments or dysfunctions in other organs. The toxicity of NPs is intrinsically linked to their biophysical characteristics, encompassing dimensions, surface area, electrostatic charge, and aggregation state. These attributes influence the dispersal and accumulation of NPs across organ systems and modulate their molecular interplay with an array of proteins and macromolecules (47). Predominant toxic mechanisms stemming from NPs encompass ROS induction, DNA interference, protein structure and functionality perturbations, and membrane integrity disruptions (48). Generally, smaller particles manifest heightened chemical and biological reactivity due to their augmented surface-to-volume ratio. A salient toxicological feature of NPs revolves around amplified ROS production, stemming from the elevated reactivity of nanomaterials—a phenomenon evidenced across diverse nanomaterial categories (49).
Upon systemic introduction, primarily through intravascular routes, NPs exhibit a propensity for deposition within pivotal organs, including the lungs, liver, and kidneys, potentially instigating localized organ-specific toxic effects. The kidneys, serving as cardinal conduits for eliminating metabolic by-products, invariably encounter a multitude of NPs, with diminutive particles particularly prone to renal clearance. This predisposes the renal system to NP accumulation and potential adverse ramifications (6). It is paramount to recognize that ROS-based nanomaterial strategies targeting renal afflictions, most notably DKD, are developing. Biosafety concerns loom large, hindering their prospective advancements. In-vitro evaluations reveal that specific nanoparticles, such as those derived from platinum, silver, titanium dioxide, and silicon, can deleteriously impact the renal system, particularly affecting glomerular and tubular cells. Complementing this, in-vivo investigations elucidate structural distortions within typical renal units and compromised renal functionalities following exposure to these nanoparticles (50). Hence, as we forge ahead in harnessing ROS-based nanomaterials for DKD interventions, meticulous strategies should be delineated for nanomaterial design, ensuring minimized toxicity and optimal biosafety.
The activity and stability of ROS-based nanomaterials, particularly during enzymatic catalysis, can be influenced by factors including particle size, composition, concentration, and environmental parameters such as redox states and pH values. Hence, rigorous examinations of their composition, structure, and other physical and chemical attributes—like size, surface charge, shape, and rigidity—as well as their delivery and administration methods are imperative to achieve the desired pharmacokinetics, target-specific drug delivery, optimal efficacy, and minimized toxicity. Notably, while the oral administration of antioxidant nanomaterials has exhibited subpar effectiveness, intravenous administration encounters a myriad of physiological and biological barriers, including the mononuclear phagocyte system and vascular flow constraints, which can significantly impede target-specific bioavailability (51).
At present, some emerging drugs for the treatment of DKD also have significant therapeutic development prospects, such as antirenal fibrosis drugs (52), activators of the Nrf2 pathway (53), endothelin-1 receptor antagonists (54), and so on. Targeted renal therapy may significantly improve the therapeutic effect by combining appropriate drugs and nanomaterials. Furthermore, it is essential to optimize further the reliability of endogenous stimulus-responsive modalities, including pH (55), temperature (56), and enzymes (57). In recent years, stimuli-responsive nanomaterials have provided an important strategy to maximize the treatment efficacy and reduce the damage to normal tissues, which is another important direction for treating DKD.
It is imperative to achieve efficient delivery to renal tissue to augment the therapeutic potential of nanomedicine for DKD patients. Current renal-targeting nanodrug delivery systems are tailored for various crucial targets, including glomerular endothelial cells (58), glomerular basement membrane (59), podocytes (60), glomerular mesangial cells (61), and renal tubules (62) (Table 1). Despite these advancements, the successful delivery of nanodrugs to renal tissues still needs to be improved. Efforts have been directed toward refining the size, morphology, and surface characteristics of nanodrugs. Nonetheless, enhancing the renal-targeting efficiency of these nanodrugs in clinical settings continues to be challenging, mainly due to the unique structural features of the kidney and the intricacies of the glomerular filtration barrier.
It is widely accepted that NPs with diameters of 3 nm or less permeate tissues non-specifically. Those under 5.5 nm undergo renal clearance, while NPs larger than 15 nm typically resist renal excretion (70). Intriguingly, NPs of varying sizes demonstrate an affinity for different renal compartments: approximately 10 nm-sized NPs localize within the glomerular basement membrane lattice, 30–40 nm NPs are found within the spaces between podocyte foot processes (71), and 60–70 nm NPs distribute across the gaps in the endothelial cells of the peritubular capillaries (72). Mesoscale nanoparticles (MNPs) are nanoparticles with a diameter exceeding 100 nm. Williams et al. synthesized MNPs with a diameter of approximately 400 nm, which avoided uptake by MPS organs and selectively accumulated in the proximal tubules (73). The accumulation efficiency in the kidneys was 26 times higher than in other organs. Histological evidence indicates that the nanoparticles predominantly localize to the basolateral side of the renal tubules rather than the apical side, suggesting that the accumulation of MNPs in the kidneys is facilitated by endocytosis of peritubular capillary endothelial cells. However, the physiological mechanisms still require further investigation. This glomerular filtration-independent delivery strategy may offer a novel approach to the treatment of tubular renal diseases. Beyond merely size and charge, the glomerular filtration propensity of nanoparticles is also influenced by their geometric configuration and flexibility. For instance, while the glomerulus typically resists filtering spheroidal albumin molecules (approximately 50 kDa in size) (74), more elongated molecules, like tannin and hyaluronan—despite possessing comparable molecular weights and charges—can traverse the glomerulus similar to albumin. Notwithstanding these hurdles, there remains a pressing need to innovate biomaterials proficient in targeting renal tissues, primarily through the challenges presented by the glomerular filtration barriers.
In the extensive research field of kidney-targeted nanomaterials, many review articles comprehensively and thoroughly investigated this topic. These reviews covered various aspects, including the types of materials, preparation methods, application areas, and potential biological effects. Chen et al. summarized the physiological and anatomical properties of the kidney and the biological and physicochemical characteristics of drug delivery systems, which affect the ability of drugs to target the kidney, and highlighted the prospects, opportunities, and challenges of nanotechnology in the therapy of kidney diseases (11). Huang et al. presented recent developments of nanoparticles designed for kidney-targeted delivery and briefly introduced targeting strategies for the kidney (75). Alallam et al. highlighted the features of nanomaterials, including NPs and the corresponding hydrogels, in overcoming various barriers to drug delivery to the kidneys. The most common delivery sites and strategies of kidney-targeted drug delivery systems were also discussed (76). Compared with these reviews, the uniqueness of this review lies in its focus on the application of kidney-targeted nanomaterials in DKD. While previous reviews primarily concentrated on the properties of materials and their impact on the kidneys, our review is centered on the potential application of these nanomaterials in patients with DKD, characterized by a highly oxidative stress environment.
A significant challenge in the clinical development of nanomaterials pertains to redox pathology. Diseases associated with oxidative stress often exhibit heterogeneity in various oxidative species’ types, concentrations, and metabolism. Crucially, the redox state evolution, commencing from the onset of the disease, predominantly determines the requisite dosage and periodicity of antioxidants. Consequently, there is an imperative need for specific and sensitive monitoring of ROS dynamics to enrich our comprehension of the pathological significance of ROS in organisms. A widely employed measurement technique is electron paramagnetic resonance (EPR), which is alternately known as electron spin resonance (ESR) or electron magnetic resonance (EMR) spectroscopy. EPR spectrophotometers are adept at determining ROS in vivo, offering high specificity and sensitivity in ROS detection. Its capability for in-vivo ROS detection precludes potential artificial interference. Notably, EPR spectrophotometry can reliably detect free radicals, even at minuscule concentrations (e.g., 1 µM) (77).
However, the specificity of free radical detection through spin trapping may occasionally be indistinct, rendering EPR-based detection of free radical complex, particularly given the transient lifespan and scarce production of free radicals in living tissues. Although nascent technologies, such as fluorescence and photoacoustic imaging, facilitate ROS detection with enhanced sensitivity and specificity, there exists a pressing need to fine-tune imaging probes concerning their biocompatibility, photostability, solubility, organ specificity, imaging depth, and adaptability to biological milieu. At present, the meticulous quantification and analysis of diverse ROS pose a considerable challenge. This underscores the exigency to pioneer advanced techniques capable of discerning and quantifying varied free radicals, thus paving the way for the clinical application of antioxidant therapies. Moreover, specific strategies promise to circumvent the pitfalls associated with conventional antioxidant analyses, furnishing more dependable metrics of nano-antioxidant bioactivity. In summation, a multifaceted detection approach would offer an exhaustive assessment of the antioxidant potential of nanomaterials.
ROS-based nanomaterials have unique properties such as targeting, stability, and adjustable ROS levels, making them competitive candidates for the treatment of DKD. In this review, we discuss the application of different types of ROS-based nanomaterials for the treatment of DKD, namely, ROS-scavenging nanomaterials, ROS-related nanodrug delivery materials, and ROS-responsive nanomaterials, which provide indications for better treatment of DKD.
However, the role of ROS nanomaterials is affected by various factors such as their composition, structure, and physicochemical properties, so we should pay attention to the therapeutic effect, biosafety, targeting, and dynamic monitoring of ROS in the design process. We believe that with the deepening of our understanding of renal pathology and the development of nanotechnology applications, ROS-based nanomaterials have great potential in the treatment of DKD. Despite the promising potential of nanomedicine in the treatment of DKD, the lack of nanoformulations advancing to clinical trials may be attributed to a combination of factors. Technical challenges in nanoparticle design and formulation, concerns regarding safety and efficacy profiles, and barriers to clinical translation could be hindering the progression of nanotherapeutics for DKD. Further research and collaboration are needed to address these challenges and accelerate the development of nanoformulations toward clinical trials for DKD treatment.
QH: Writing – original draft. JT: Writing – original draft. YD: Writing – review & editing. FL: Writing – review & editing.
The author(s) declare that no financial support was received for the research, authorship, and/or publication of this article.
The authors declare that the research was conducted in the absence of any commercial or financial relationships that could be construed as a potential conflict of interest.
All claims expressed in this article are solely those of the authors and do not necessarily represent those of their affiliated organizations, or those of the publisher, the editors and the reviewers. Any product that may be evaluated in this article, or claim that may be made by its manufacturer, is not guaranteed or endorsed by the publisher.
1. Xue R, Gui D, Zheng L, Zhai R, Wang F, Wang N. Mechanistic insight and management of diabetic nephropathy: recent progress and future perspective. J Diabetes Res. (2017) 2017:1839809. doi: 10.1155/2017/1839809
2. Gupta S, Dominguez M, Golestaneh L. Diabetic kidney disease: an update. Med Clinics North America. (2023) 107:689–705. doi: 10.1016/j.mcna.2023.03.004
3. Duan S, Lu F, Song D, Zhang C, Zhang B, Xing C, et al. Current challenges and future perspectives of renal tubular dysfunction in diabetic kidney disease. Front Endocrinol. (2021) 12:661185. doi: 10.3389/fendo.2021.661185
4. Huang Y, Wang J, Jiang K, Chung EJ. Improving kidney targeting: the influence of nanoparticle physicochemical properties on kidney interactions. J Controlled release: Off J Controlled Release Soc. (2021) 334:127–37. doi: 10.1016/j.jconrel.2021.04.016
5. Elmarakby AA, Sullivan JC. Relationship between oxidative stress and inflammatory cytokines in diabetic nephropathy. Cardiovasc Ther. (2012) 30:49–59. doi: 10.1111/j.1755-5922.2010.00218.x
6. Forrester SJ, Kikuchi DS, Hernandes MS, Xu Q, Griendling KK. Reactive oxygen species in metabolic and inflammatory signaling. Circ Res. (2018) 122:877–902. doi: 10.1161/circresaha.117.311401
7. Jha JC, Banal C, Chow BS, Cooper ME, Jandeleit-Dahm K. Diabetes and kidney disease: role of oxidative stress. Antioxidants Redox Signaling. (2016) 25:657–84. doi: 10.1089/ars.2016.6664
8. Yang B, Chen Y, Shi J. Reactive oxygen species (Ros)-based nanomedicine. Chem Rev. (2019) 119:4881–985. doi: 10.1021/acs.chemrev.8b00626
9. Williams RM, Jaimes EA, Heller DA. Nanomedicines for kidney diseases. Kidney Int. (2016) 90:740–5. doi: 10.1016/j.kint.2016.03.041
10. Gelperina S, Kisich K, Iseman MD, Heifets L. The potential advantages of nanoparticle drug delivery systems in chemotherapy of tuberculosis. Am J Respir Crit Care Med. (2005) 172:1487–90. doi: 10.1164/rccm.200504-613PP
11. Chen Z, Peng H, Zhang C. Advances in kidney-targeted drug delivery systems. Int J pharmaceut. (2020) 587:119679. doi: 10.1016/j.ijpharm.2020.119679
12. Huang X, He D, Pan Z, Luo G, Deng J. Reactive-oxygen-species-scavenging nanomaterials for resolving inflammation. Mat Today Bio. (2021) 11:100124. doi: 10.1016/j.mtbio.2021.100124
13. Liu X, Domingues NP, Oveisi E, Coll-Satue C, Jansman MMT, Smit B, et al. Metal-organic framework-based oxygen carriers with antioxidant activity resulting from the incorporation of gold nanozymes. Biomat Sci. (2023) 11:2551–65. doi: 10.1039/D2BM01405J
14. Gao C, Cheng H, Xu N, Li Y, Chen Y, Wei Y, et al. Poly(Dopamine) and ag nanoparticle-loaded tio(2) nanotubes with optimized antibacterial and ros-scavenging bioactivities. Nanomed (London England). (2019) 14:803–18. doi: 10.2217/nnm-2018-0131
15. Liu T, Xiao B, Xiang F, Tan J, Chen Z, Zhang X, et al. Ultrasmall copper-based nanoparticles for reactive oxygen species scavenging and alleviation of inflammation related diseases. Nat Commun. (2020) 11:2788. doi: 10.1038/s41467-020-16544-7
16. Singh S, Dosani T, Karakoti AS, Kumar A, Seal S, Self WT. A phosphate-dependent shift in redox state of cerium oxide nanoparticles and its effects on catalytic properties. Biomaterials. (2011) 32:6745–53. doi: 10.1016/j.biomaterials.2011.05.073
17. Li P, Zhang W, Zhao J, Meng F, Yue Q, Wang L, et al. Electrochemical antioxidant detection technique based on guanine-bonded graphene and magnetic nanoparticles composite materials. Anal. (2012) 137:4318–26. doi: 10.1039/c2an35270b
18. Pu Y, Wang P, Yang R, Tan X, Shi T, Ma J, et al. Bio-fabricated nanocomposite hydrogel with ros scavenging and local oxygenation accelerates diabetic wound healing. J Mat Chem B. (2022) 10:4083–95. doi: 10.1039/D2TB00343K
19. Cai X, Gao W, Zhang L, Ma M, Liu T, Du W, et al. Enabling pRussian blue with tunable localized surface plasmon resonances: simultaneously enhanced dual-mode imaging and tumor photothermal therapy. ACS nano. (2016) 10:11115–26. doi: 10.1021/acsnano.6b05990
20. Soule BP, Hyodo F, Matsumoto K, Simone NL, Cook JA, Krishna MC, et al. The chemistry and biology of nitroxide compounds. Free Radical Biol Med. (2007) 42:1632–50. doi: 10.1016/j.freeradbiomed.2007.02.030
21. Zhang C, Wang X, Du J, Gu Z, Zhao Y. Reactive oxygen species-regulating strategies based on nanomaterials for disease treatment. Advanced Sci (Weinheim Baden-Wurttemberg Germany). (2021) 8:2002797. doi: 10.1002/advs.202002797
22. Aggarwal BB. Targeting inflammation-induced obesity and metabolic diseases by curcumin and other nutraceuticals. Annu Rev Nutr. (2010) 30:173–99. doi: 10.1146/annurev.nutr.012809.104755
23. Lee Y, Sugihara K, Gillilland MG 3rd, Jon S, Kamada N, Moon JJ. Hyaluronic acid-bilirubin nanomedicine for targeted modulation of dysregulated intestinal barrier, microbiome and immune responses in colitis. Nat Mat. (2020) 19:118–26. doi: 10.1038/s41563-019-0462-9
24. Song G, Liang C, Gong H, Li M, Zheng X, Cheng L, et al. Core-shell mnse@Bi2 se3 fabricated via a cation exchange method as novel nanotheranostics for multimodal imaging and synergistic thermoradiotherapy. Advanced Mat (Deerfield Beach Fla). (2015) 27:6110–7. doi: 10.1002/adma.201503006
25. Wang N, Cheng X, Li N, Wang H, Chen H. Nanocarriers and their loading strategies. Advanced healthcare Mat. (2019) 8:e1801002. doi: 10.1002/adhm.201801002
26. Liu Y, Li K, Liu B, Feng SS. A strategy for precision engineering of nanoparticles of biodegradable copolymers for quantitative control of targeted drug delivery. Biomaterials. (2010) 31:9145–55. doi: 10.1016/j.biomaterials.2010.08.053
27. Zhu Y, Feijen J, Zhong Z. Dual-targeted nanomedicines for enhanced tumor treatment. Nano Today. (2018) 18:65–85. doi: 10.1016/j.nantod.2017.12.007
28. Park DH, Cho J, Kwon OJ, Yun CO, Choy JH. Biodegradable inorganic nanovector: passive versus active tumor targeting in sirna transportation. Angewandte Chemie (International ed English). (2016) 55:4582–6. doi: 10.1002/anie.201510844
29. Alomari G, Al-Trad B, Hamdan S, Aljabali A, Al-Zoubi M, Bataineh N, et al. Gold nanoparticles attenuate albuminuria by inhibiting podocyte injury in a rat model of diabetic nephropathy. Drug delivery Trans Res. (2020) 10:216–26. doi: 10.1007/s13346-019-00675-6
30. Tong Y, Zhang L, Gong R, Shi J, Zhong L, Duan X, et al. A ros-scavenging multifunctional nanoparticle for combinational therapy of diabetic nephropathy. Nanoscale. (2020) 12:23607–19. doi: 10.1039/D0NR06098D
31. Hassan I, Ebaid H, Al-Tamimi J, Habila MA, Alhazza IM, Rady AM. Selenium nanoparticles mitigate diabetic nephropathy and pancreatopathy in rat offspring via inhibition of oxidative stress. J King Saud Univ - Sci. (2021) 33:101265. doi: 10.1016/j.jksus.2020.101265
32. Manna K, Mishra S, Saha M, Mahapatra S, Saha C, Yenge G, et al. Amelioration of diabetic nephropathy using pomegranate peel extract-stabilized gold nanoparticles: assessment of nf-kappab and nrf2 signaling system. Int J Nanomed. (2019) 14:1753–77. doi: 10.2147/IJN.S176013
33. Tong F, Liu S, Yan B, Li X, Ruan S, Yang S. Quercetin nanoparticle complex attenuated diabetic nephropathy via regulating the expression level of icam-1 on endothelium. Int J nanomedicine. (2017) 12:7799–813. doi: 10.2147/ijn.S146978
34. Tong F, Tang X, Li X, Xia W, Liu D. The effect of insulin-loaded linear poly(Ethylene glycol)-brush-like poly(L-lysine) block copolymer on renal ischemia/reperfusion-induced lung injury through downregulating hypoxia-inducible factor. Int J nanomedicine. (2016) 11:1717–30. doi: 10.2147/ijn.S99890
35. Ahangarpour A, Oroojan AA, Khorsandi L, Kouchak M, Badavi M. Antioxidant, anti-apoptotic, and protective effects of myricitrin and its solid lipid nanoparticle on streptozotocin-nicotinamide-induced diabetic nephropathy in type 2 diabetic male mice. Iranian J basic Med Sci. (2019) 22:1424–31. doi: 10.22038/ijbms.2019.13989
36. Guang-zhao HC LU, Yan-qiang ZHONG, Ying LU, Hao ZOU. Recent progress of reactive oxygen species-responsive drug delivery systems. Acta Pharm Sin. (2017) 52:206–13. doi: 10.16438/j.0513-4870.2016-0923
37. Peng S, Xiao F, Chen M, Gao H. Tumor-microenvironment-responsive nanomedicine for enhanced cancer immunotherapy. Advanced Sci (Weinheim Baden-Wurttemberg Germany). (2022) 9:e2103836. doi: 10.1002/advs.202103836
38. Adhikari A, Mondal S, Chatterjee T, Das M, Biswas P, Ghosh R, et al. Redox nanomedicine ameliorates chronic kidney disease (Ckd) by mitochondrial reconditioning in mice. Commun Biol. (2021) 4:1013. doi: 10.1038/s42003-021-02546-8
39. Ni D, Jiang D, Kutyreff CJ, Lai J, Yan Y, Barnhart TE, et al. Molybdenum-based nanoclusters act as antioxidants and ameliorate acute kidney injury in mice. Nat Commun. (2018) 9:5421. doi: 10.1038/s41467-018-07890-8
40. An J, Niu F, Sim JJ. Cardiovascular and kidney outcomes of spironolactone or eplerenone in combination with acei/arbs in patients with diabetic kidney disease. Pharmacotherapy. (2021) 41:998–1008. doi: 10.1002/phar.2633
41. Zhang C, Bu W, Ni D, Zuo C, Cheng C, Li Q, et al. A polyoxometalate cluster paradigm with self-adaptive electronic structure for acidity/reducibility-specific photothermal conversion. J Am Chem Soc. (2016) 138:8156–64. doi: 10.1021/jacs.6b03375
42. Ni D, Jiang D, Valdovinos HF, Ehlerding EB, Yu B, Barnhart TE, et al. Bioresponsive polyoxometalate cluster for redox-activated photoacoustic imaging-guided photothermal cancer therapy. Nano Lett. (2017) 17:3282–9. doi: 10.1021/acs.nanolett.7b00995
43. Yoshitomi T, Hirayama A, Nagasaki Y. The ros scavenging and renal protective effects of ph-responsive nitroxide radical-containing nanoparticles. Biomaterials. (2011) 32:8021–8. doi: 10.1016/j.biomaterials.2011.07.014
44. Shimizu H, Hori Y, Kaname S, Yamada K, Nishiyama N, Matsumoto S, et al. Sirna-based therapy ameliorates glomerulonephritis. J Am Soc Nephrol: JASN. (2010) 21:622–33. doi: 10.1681/asn.2009030295
45. Hou X, Zaks T, Langer R, Dong Y. Lipid nanoparticles for mrna delivery. Nat Rev Mat. (2021) 6:1078–94. doi: 10.1038/s41578-021-00358-0
46. Poljsak B, Milisav I. The role of antioxidants in cancer, friends or foes? Curr Pharm design. (2018) 24:5234–44. doi: 10.2174/1381612825666190123112647
47. Wu T, Tang M. Review of the effects of manufactured nanoparticles on mammalian target organs. J Appl toxicol: JAT. (2018) 38:25–40. doi: 10.1002/jat.3499
48. Najahi-Missaoui W, Arnold RD, Cummings BS. Safe nanoparticles: are we there yet? Int J Mol Sci. (2020) 22. doi: 10.3390/ijms22010385
49. Sharifi S, Behzadi S, Laurent S, Forrest ML, Stroeve P, Mahmoudi M. Toxicity of nanomaterials. Chem Soc Rev. (2012) 41:2323–43. doi: 10.1039/C1CS15188F
50. Iavicoli I, Fontana L, Nordberg G. The effects of nanoparticles on the renal system. Crit Rev Toxicol. (2016) 46:490–560. doi: 10.1080/10408444.2016.1181047
51. Blanco E, Shen H, Ferrari M. Principles of nanoparticle design for overcoming biological barriers to drug delivery. Nat Biotechnol. (2015) 33:941–51. doi: 10.1038/nbt.3330
52. Seo E, Kang H, Oh YS, Jun HS. Psoralea corylifolia L. Seed extract attenuates diabetic nephropathy by inhibiting renal fibrosis and apoptosis in streptozotocin-induced diabetic mice. Nutrients. (2017) 9. doi: 10.3390/nu9080828
53. Huang Z, Mou Y, Xu X, Zhao D, Lai Y, Xu Y, et al. Novel derivative of bardoxolone methyl improves safety for the treatment of diabetic nephropathy. J med Chem. (2017) 60:8847–57. doi: 10.1021/acs.jmedchem.7b00971
54. Barton M, Yanagisawa M. Endothelin: 30 years from discovery to therapy. Hypertension (Dallas Tex: 1979). (2019) 74:1232–65. doi: 10.1161/hypertensionaha.119.12105
55. Dai L, Li X, Duan X, Li M, Niu P, Xu H, et al. A ph/ros cascade-responsive charge-reversal nanosystem with self-amplified drug release for synergistic oxidation-chemotherapy. Advanced Sci (Weinheim Baden-Wurttemberg Germany). (2019) 6:1801807. doi: 10.1002/advs.201801807
56. Gupta MK, Martin JR, Werfel TA, Shen T, Page JM, Duvall CL. Cell protective, abc triblock polymer-based thermoresponsive hydrogels with ros-triggered degradation and drug release. J Am Chem Soc. (2014) 136:14896–902. doi: 10.1021/ja507626y
57. Daniel KB, Callmann CE, Gianneschi NC, Cohen SM. Dual-responsive nanoparticles release cargo upon exposure to matrix metalloproteinase and reactive oxygen species. Chem Commun (Cambridge England). (2016) 52:2126–8. doi: 10.1039/C5CC09164K
58. Jourde-Chiche N, Fakhouri F, Dou L, Bellien J, Burtey S, Frimat M, et al. Endothelium structure and function in kidney health and disease. Nat Rev Nephrol. (2019) 15:87–108. doi: 10.1038/s41581-018-0098-z
59. Zuckerman JE, Choi CH, Han H, Davis ME. Polycation-sirna nanoparticles can disassemble at the kidney glomerular basement membrane. Proc Natl Acad Sci United States America. (2012) 109:3137–42. doi: 10.1073/pnas.1200718109
60. Wu L, Chen M, Mao H, Wang N, Zhang B, Zhao X, et al. Albumin-based nanoparticles as methylprednisolone carriers for targeted delivery towards the neonatal fc receptor in glomerular podocytes. Int J Mol Med. (2017) 39:851–60. doi: 10.3892/ijmm.2017.2902
61. Guo L, Luo S, Du Z, Zhou M, Li P, Fu Y, et al. Targeted delivery of celastrol to mesangial cells is effective against mesangioproliferative glomerulonephritis. Nat Commun. (2017) 8:878. doi: 10.1038/s41467-017-00834-8
62. Williams RM, Shah J, Tian HS, Chen X, Geissmann F, Jaimes EA, et al. Selective nanoparticle targeting of the renal tubules. Hypertension. (2018) 71:87–94. doi: 10.1161/hypertensionaha.117.09843
63. Leeuwis JW, Nguyen TQ, Dendooven A, Kok RJ, Goldschmeding R. Targeting podocyte-associated diseases. Advanced Drug delivery Rev. (2010) 62:1325–36. doi: 10.1016/j.addr.2010.08.012
64. Hauser PV, Pippin JW, Kaiser C, Krofft RD, Brinkkoetter PT, Hudkins KL, et al. Novel sirna delivery system to target podocytes in vivo. PloS One. (2010) 5:e9463. doi: 10.1371/journal.pone.0009463
65. Hartleben B, Gödel M, Meyer-Schwesinger C, Liu S, Ulrich T, Köbler S, et al. Autophagy influences glomerular disease susceptibility and maintains podocyte homeostasis in aging mice. J Clin Invest. (2010) 120:1084–96. doi: 10.1172/jci39492
66. Choi CH, Zuckerman JE, Webster P, Davis ME. Targeting kidney mesangium by nanoparticles of defined size. Proc Natl Acad Sci United States America. (2011) 108:6656–61. doi: 10.1073/pnas.1103573108
67. Hu JB, Li SJ, Kang XQ, Qi J, Wu JH, Wang XJ, et al. Cd44-targeted hyaluronic acid-curcumin prodrug protects renal tubular epithelial cell survival from oxidative stress damage. Carbohydr polymers. (2018) 193:268–80. doi: 10.1016/j.carbpol.2018.04.011
68. Yu H, Jin F, Liu D, Shu G, Wang X, Qi J, et al. Ros-responsive nano-drug delivery system combining mitochondria-targeting ceria nanoparticles with atorvastatin for acute kidney injury. Theranostics. (2020) 10:2342–57. doi: 10.7150/thno.40395
69. Luo J, Sun J, Luo X, Wei Y, Zheng H, Mu C, et al. Low molecular weight chitosan-based conjugates for efficient rhein oral delivery: synthesis, characterization, and pharmacokinetics. Drug Dev Ind Pharm. (2019) 45:96–104. doi: 10.1080/03639045.2018.1522326
70. Liang X, Wang H, Zhu Y, Zhang R, Cogger VC, Liu X, et al. Short- and long-term tracking of anionic ultrasmall nanoparticles in kidney. ACS nano. (2016) 10:387–95. doi: 10.1021/acsnano.5b05066
71. Hironaka K, Makino H, Yamasaki Y, Ota Z. Pores in the glomerular basement membrane revealed by ultrahigh- resolution scanning electron microscopy. Nephron. (1993) 64(4):647-9. doi: 10.1159/000187418
72. Satchell SC, Braet F. Glomerular endothelial cell fenestrations: an integral component of the glomerular filtration barrier. Am J Physiol Renal Physiol. (2009) 296:F947–56. doi: 10.1152/ajprenal.90601.2008
73. Williams RM, Shah J, Ng BD, Minton DR, Gudas LJ, Park CY, et al. Mesoscale nanoparticles selectively target the renal proximal tubule epithelium. Nano Lett. (2015) 15:2358–64. doi: 10.1021/nl504610d
74. Haraldsson B, Nyström J, Deen WM. Properties of the glomerular barrier and mechanisms of proteinuria. Physiol Rev. (2008) 88:451–87. doi: 10.1152/physrev.00055.2006
75. Huang X, Ma Y, Li Y, Han F, Lin W. Targeted drug delivery systems for kidney diseases. Front bioengineer Biotechnol. (2021) 9:683247. doi: 10.3389/fbioe.2021.683247
76. Alallam B, Choukaife H, Seyam S, Lim V, Alfatama M. Advanced drug delivery systems for renal disorders. Gels (Basel Switzerland). (2023) 9. doi: 10.3390/gels9020115
Keywords: reactive oxygen species (ROS), nanomaterials, diabetic kidney disease, treatment, design considerations
Citation: Huang Q, Tang J, Ding Y and Li F (2024) Application and design considerations of ROS-based nanomaterials in diabetic kidney disease. Front. Endocrinol. 15:1351497. doi: 10.3389/fendo.2024.1351497
Received: 06 December 2023; Accepted: 12 April 2024;
Published: 29 April 2024.
Edited by:
Katsumi Iizuka, Fujita Health University, JapanReviewed by:
Ryan Williams, City College of New York (CUNY), United StatesCopyright © 2024 Huang, Tang, Ding and Li. This is an open-access article distributed under the terms of the Creative Commons Attribution License (CC BY). The use, distribution or reproduction in other forums is permitted, provided the original author(s) and the copyright owner(s) are credited and that the original publication in this journal is cited, in accordance with accepted academic practice. No use, distribution or reproduction is permitted which does not comply with these terms.
*Correspondence: Yunchuan Ding, eXVuY2h1YW5kaW5nQGhvdG1haWwuY29t; Fangping Li, NDcyNjU1MDM3QHFxLmNvbQ==
†These authors have contributed equally to this work
Disclaimer: All claims expressed in this article are solely those of the authors and do not necessarily represent those of their affiliated organizations, or those of the publisher, the editors and the reviewers. Any product that may be evaluated in this article or claim that may be made by its manufacturer is not guaranteed or endorsed by the publisher.
Research integrity at Frontiers
Learn more about the work of our research integrity team to safeguard the quality of each article we publish.