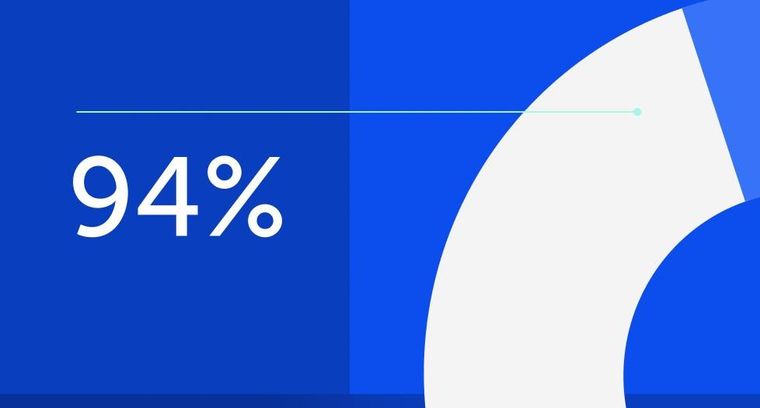
94% of researchers rate our articles as excellent or good
Learn more about the work of our research integrity team to safeguard the quality of each article we publish.
Find out more
REVIEW article
Front. Endocrinol., 29 April 2024
Sec. Renal Endocrinology
Volume 15 - 2024 | https://doi.org/10.3389/fendo.2024.1336402
Diabetic kidney disease (DKD), a significant complication associated with diabetes mellitus, presents limited treatment options. The progression of DKD is marked by substantial lipid disturbances, including alterations in triglycerides, cholesterol, sphingolipids, phospholipids, lipid droplets, and bile acids (BAs). Altered lipid metabolism serves as a crucial pathogenic mechanism in DKD, potentially intertwined with cellular ferroptosis, lipophagy, lipid metabolism reprogramming, and immune modulation of gut microbiota (thus impacting the liver-kidney axis). The elucidation of these mechanisms opens new potential therapeutic pathways for DKD management. This research explores the link between lipid metabolism disruptions and DKD onset.
Diabetic kidney disease (DKD) is a common microvascular complication of diabetes mellitus (1). DKD is characterized by glomerulopathy with diffuse and nodular tethered dilatation and thickening of the glomerular basement membranes, accompanied by tubular atrophy, interstitial inflammation, fibrosis, glomerular endothelial injury, podocyte loss, and glomerular vascular hyalinopathy (2). DKD pathogenesis is complex and is associated with glucose and lipid metabolism disorders and stress (3, 4). Treatments, such as glycemic control and urinary albumin reduction, do not fundamentally alter the course of DKD (5). The latest evidence-based guidelines recommend angiotensin-converting enzyme inhibitors (ACEIs)/angiotensin receptor blockers (ARBs) and novel hypoglycemic agents, such as dipeptidyl peptidase-4 inhibitors, sodium-glucose transporter 2 inhibitors, and sodium-glucose transporter 2 inhibitors. Sodium-glucose transporter 2 inhibitors and glucagon-like peptide 1 agonists (6–8) have not been found to slow down the progression of DKD to end-stage renal disease (1). Therefore, exploring the pathogenesis of DKD and identifying targets for intervention are important clinical goals. Lipid metabolism disorders, one of the pathogenic mechanisms of DKD, mainly involve abnormalities in lipid metabolism, such as triglycerides (TGs), cholesterol (CHOL), and lipid droplets (LDs) (9–11). Recently, there have been numerous studies on the mechanisms of lipid metabolism disorder lipophagy in DKD. Ferroptosis, the programmed death in DKD, is a direct result of lipid peroxidation, which is closely related to lipid metabolism disorders, especially disorders in the regulation of fatty acids (FAs) (12, 13). Another mode of programmed death is associated with defects in the autophagy-lysosomal system and abnormal lipid accumulation in podocytes in DKD (14, 15). The reprogramming of lipid metabolism also results in dysfunctional lipid uptake and oxidation, especially of FAs, which are exacerbated in DKD (16, 17). In addition, an imbalance of gut microbiota and increased permeability of the intestinal barrier, which is one of the pathological manifestations of DKD, and its involvement in immune imbalance, especially involving the liver-kidney axis, affect lipid metabolism, such as bile acid (BA) metabolism, and the immune imbalance aggravates renal injury (18–20). Recent studies related to lipid metabolism disorders in human and animal models of DKD have revealed that the deposition of toxic FAs metabolites leads to ectopic lipid deposition in podocytes and tubular epithelial cells, interstitial fibrosis, and DKD (17, 21–23). The causes and pathogenesis of lipid metabolism disorders in DKD have not yet been fully elucidated. Therefore, studying the mechanism of lipid metabolism changes in DKD and how to slow down the development of DKD through the regulation of different targets has become a hot research topic.
Abnormalities in the metabolism of TG, CHOL, sphingolipids, phospholipids (PLs), LDs, and BAs are key factors in DKD progression. Both the quality and quantity of lipids are associated with this process and produce reactive oxygen species (ROS), which exacerbate oxidative stress, inflammation, and cell death (24).
Abnormal TG metabolism in DKD is mainly characterized by abnormal uptake and oxidation of FAs. Fatty acid transport proteins(FATPs),cluster of differentiation 36 (CD36), and fatty acid-binding protein (FABP) are correlated with FA uptake in DKD. FATPs control FAs uptake, and fatty acid transport protein 2 (FATP2) deficiency improves renal outcomes (25, 26). fatty acid transport protein 4(FATP4) levels in diabetic mice are correlated with lipid accumulation in DKD (27). CD36 is a transmembrane glycoprotein that mediates oxidized low-density lipoprotein (LDL) uptake. An increase in CD36 levels is strongly associated with kidney injury in DKD (28–32). Increased CD36 expression in mouse kidneys promotes TG accumulation in the kidney (33). fatty acid-binding protein1(FABP1), another protein associated with abnormal lipid uptake in DKD, is a reliable marker of the onset and progression of DKD (34–37).
Fatty acid oxidation (FAO) is the primary pathway that reduces the renal lipid content. The expression of FAO genes, including peroxisome proliferators-activated receptors α (PPARα), acyl coenzyme A dehydrogenase, and acyl-CoA oxidase 1/2(ACOX1/2), was significantly reduced (33, 38).
Non esterified fatty acids (NEFA) and essential fatty acid (EFA) changes are also important in DKD. In the early stages of DKD, NEFAs increase and EFAs decrease (39). Other NEFAs (Monounsaturated 16:1/18:1 FAs, omega-6/7/9 in the serum, and 10-nitrooleic acid in the urine) are also consistently elevated in DKD (40). In addition, long-chain free fatty acid levels were reduced in rats with DKD (41).
CHOL synthesis, endocytosis, and exocytosis are all closely associated with DKD. Studies have demonstrated that increased expression of sterol regulatory element-binding proteins (SREBP) and isoforms associated with CHOL synthesis that mediate intracellular CHOL sensing leads to renal damage in DKD (42–46) and plays a role in the accumulation of LDs (47). Increased expression of SREBP and its isoforms in glomeruli of patients with DKD leads to renal injury (48–50). Inhibition of CHOL efflux and increased CHOL influx in DKD cells increases free CHOL levels, which activate sterol O-acyltransferase 1 to form cholesteryl esters (ChEs) that are stored in LDs, causing excessive accumulation of CHOL in podocytes (22, 44, 51). In contrast, induction of CHOL efflux ameliorates DKD progression and DKD-like glomerulosclerosis (38, 52). ATP-binding cassette transporter A1 (ABCA1), which promotes CHOL efflux, and another CHOL efflux scavenger receptor, BI (SR-BI), were found to be significantly inhibited in DKD (53).
Expression of the sphingolipids metabolites ceramide (Cer), sphingosine-1-phosphate (S1P), Ceramide-1-phosphate (C1P) is specific to DKD. Long-chain Cer and ultra-long-chain Cer levels are elevated in DKD (54–56). Renal S1P levels are elevated in diabetic mice (57, 58), and sphingosine kinase, which produces S1P, exhibits increased expression and activity (59). Receptor signaling for S1P is specifically expressed during glomerular injury (60, 61). Sphingomyelin phosphodiesterase acid-like 3b (SMPDL3b) is increased in DKD mice in association with a C1P-deficient state in podocytes (62–64). In addition, increased ganglioside GM3(GM3) in the renal cortex during the early stages of diabetes alters pro-survival receptor-related Automatic Kernel Tunables (Akt) and Protein kinase B signaling to exacerbate DKD (65–68). This suggests a role for sphingolipids in the development of DKD.
PLs are key structural components of all cellular lipid bilayers that contain multiple fatty acyl groups and are potential biomarkers of DKD (69–72). Phosphatidylethanolamine (PE), phosphatidylinositol (PI), phosphocholine (PC) and sphingomyelin (SM) were significantly altered (73, 74). Levels of two lysolecithins (PC and lysophosphatidic acid (LPA)) and Sphingomyelin (SM) (d18:1/16:0) were found to be significantly elevated in the glomeruli of diabetic mice (75–77), and the levels of glucose-modified aminoketoses (Amadori-PEs) were even higher in the renal cortex. PI (40:6) levels tended to decrease in the serum of patients with type 2 DKD (78). In addition, it has been shown that diabetic mice also show reduced relative abundance of Cardiolipin (CL) and its subpopulations in the proximal tubules of the renal cortex (79).
LDs are cellular reservoirs of CHOL and acylglycerols (80). LDs alleviate DKD by preventing lipotoxicity and lipid apoptosis (81–83) or enhancing autophagic pathways (84). Increased accumulation of LDs in DKD was found (22, 52, 85),and an increase in LDs in glomerular and/or tubular cells of the kidneys of hyperglycemic mice was accompanied by an increase in markers of oxidative stress (xanthine oxidoreductase (XOR) and nitrotyrosine with tail-interacting protein of 47 kDa (TIP47)) (86). The expression of perilipin 2 (PLIN2), a family of lipoproteins present in the coating of LDs, is significantly upregulated in DKD pedunculated cells (27, 87).
BAs are oxidized hepatic enzymes derived from CHOL and are found mainly in the enterohepatic circulatory system; they may be directly involved in the regulation of blood glucose (88) or indirectly involved through the gut-kidney axis, improving lipid metabolism to protect the kidney (89). BAs and total CHOL were negatively correlated with the severity of DKD, and BAs may ameliorate DKD through the activation of receptors and downstream signaling pathways in the glomerular cells. The farnesoid X Receptor (FXR) pathway and takeda G protein-coupled receptor 5 (TGR5), which are directly activated by BAs, are highly expressed in the kidney after activation and can play a role in slowing down renal injury (90–94). However, the association between BAs and DKD remains unclear. (Figure 1)
Figure 1 Characteristics of lipid metabolism changes in DKD. The metabolic abnormalities of TG, CHOL, sphingolipids, PLs, LDs, and BAs were mainly reflected in the metabolic abnormalities of TG, which were reflected in the uptake and oxidation process of FAs. The abnormalities of CHOL were related to its own synthesis, endocytosis, and exocytosis. The expression of the metabolites of sphingolipids, Cer, S1P, and C1P was specific to DKD. The metabolic abnormalities of PLs (PC, LPA, SM, CL, PE, and PI) were significantly altered in kidney-associated cell membranes. Changes in LDs were mainly associated with the accumulation of Lipid in DKD cells. BAs may delay renal injury through direct activation of the FXR pathway and TGR5 membrane receptors. The upregulation of CD36 expression facilitated triglyceride accumulation in the kidney, while the increase in SMPDL3b was linked to ceramide-1-phosphate deficiency in podocytes. The coordinated actions of SOAT1/ACAT1, ABCG1/SR-B1, and ABCG1/SR-B were involved in lipid droplet accumulation. However, dysregulation of Akt and protein kinase B signaling by Cer/GM3 exacerbated lipid metabolism abnormalities in renal podocytes, tubule cells, and mesangial cells. These processes are closely associated with the intestine, liver, and blood vessels.
MR refers to the ability of cells to adapt their metabolic processes in response to changing environmental conditions (95) and MR in DKD mainly manifests as renal lipid accumulation (96). Among these, abnormal metabolic pathways of TG, CHOL, sphingolipids, LDs, and BAs are key aspects of MR in DKD.
Renal TG accumulation in patients with DKD is associated with the dysregulated expression of genes involved in lipid metabolism (97). Renal biopsies from patients with DKD showed decreased expression of genes encoding PPAR-α and PPARδ and their downstream acyl-coenzyme A oxidase and carnitine palmitoyl transferase (CPT1) involved in the fatty acid β-oxidation pathway, and SREBP, a transcription factor regulating FA synthesis, induced fluorescent antibody serum neutralization (FASN) and acetyl Coenzyme A(CoA) carboxylation. The expression of genes involved in the fatty acid β-oxidation pathway, such as (22),and SREBP, a transcription factor regulating the synthesis of FAs, induced FASN and acetyl CoA carboxylase to increase the cytosolic TG content (98, 99),and it was found that Streptozotocin(STZ)-induced diabetic rat renal cortex and DKD patients’ renal tubules increased their TG content and increased sterol regulatory element-binding proteins 1(SREBP-1) expression in the renal tubular epithelium of STZ-induced diabetic rats (100–103). In addition, elevated renal TC was also associated with decreased PPAR-α and PPAR-δ expression, which also led directly to decreased FAO (44), showing a direct pathway between decreased FAO and net accumulation of lipids in the renal cortex of patients with DKD.
In CHOL metabolism, low-density lipoprotein receptor (LDLr) and 3-hydroxy-3-methylglutaryl-CoA (HMG-CoA) were involved in CHOL uptake and synthesis, respectively. The expression of LDLr and HMG-CoA reductase was significantly elevated in DKD, whereas the expression of genes involved in CHOL efflux, including ABCA1, ATP-binding cassette transporter G1(ABCG1), and apoipoprotein E (apoE), was significantly reduced (38, 104, 105). Moreover, sterol regulatory element-binding proteins 2(SREBP-2) activates LDLr and HMG-CoA reductase, enhancing CHOL uptake and synthesis (46, 106). ABCA1 mediates CHOL transport to apolipoprotein A-I (Apo A-I) for further efflux, and strong downregulation of ABCA1 mRNA was observed in DKD, leading to the inhibition of CHOL efflux in pedicle cells (52, 107).
In terms of sphingolipids metabolism, the rs267734 gene variant of Ceramide synthases 2 (CerS2) in patients with DKD resulted in increased proteinuria (108), and polymorphisms in the Sphingosine-1-phosphate lyase 1(SGPL1) gene encoding S1P lyase 1 were associated with reduced enzymatic activity of S1P lyase 1 and the development of nephropathy. In mice, knockdown of the Sgpl1 gene encoding S1P lyase 1 resulted in loss of peduncles and severe proteinuria (109, 110). Studies on C1P have shown that increased SMPDL3b expression in DKD mice is associated with podocyte C1P deficiency (62). SMPDL3b expression is elevated in the glomeruli of patients with DKD (63), whereas SMPDL3b overexpression in podocytes leads to S1P accumulation (64).
Accumulation of LDs in DKD may be related to abnormal protein expression in the coating of LDs. It was found that variations in Perilipin 1(PLIN1) can lead to DKD-like renal injury (111). Clinical studies have shown that the polymorphism rs4578621 in the Perilipin(PLIN) gene is associated with type 2 diabetes mellitus, and the expression of Perilipin 2(PLIN2) is upregulated in the kidneys of diabetic db/db mice (103, 112),which may be the reason.
In BA metabolism, the ATP-binding cassette transporter C 3 (Abcc3) encodes multidrug resistance-associated protein 3 (MRP3), and Abcc4 encodes MRP4. Both of transport taurine and glycine conjugates of bile acids and unconjugated bile acid cholate into the bloodstream. Solute carrier organic anion transporter family member 1A1 (Slco1a1) encodes organic anion transport peptide 1A1 (OATP1A1), which transports unconjugated and conjugated bile acids into the cell (113),and type 2 diabetic db/db mice exhibit decreased Slco1a1 and increased Abcc3 and Abcc4 expression in the kidney, resulting in the loss of bound and unconjugated bile acids and bile salts from the cells (114, 115). (Figure 2)
Figure 2 MR in DKD. The main manifestations were abnormal reprogramming of TG, CHOL, sphingolipids, LDs, and BAs metabolic pathways in renal mesangial cells, renal tubular cells, and podocytes. TG abnormalities were associated with increased expression of FA synthesis transcription factors and decreased expression of proteins of the fatty acid β-oxidation pathway (PPAR-α, PPAR-δ, and SREBP). The abnormalities in CHOL metabolism were related to the abnormal expression of genes encoding CHOL uptake and synthesis proteins (ABCA1, ABCG1, and apoE).Abnormalities in sphingolipids metabolism were associated with deletion of the SGPL1 gene and increased expression of the SMPDL3b protein. Changes in LDs were associated with increased expression of PLIN2. Changes in BAs metabolism were associated with increased expression of the genes encoding BAs transporter proteins (Abcc3, Abcc4, and Slco1a1).
Lipid metabolism disorders in DKD are primarily characterized by disturbances in FA, BA, and CHOL metabolism (116, 117). DKD serum is markedly decreased in l-methionine, which can be methylated in vivo to produce L-(+)-cysteine. The latter is one of the three amino acids used for the synthesis of glutathione (GSH) (118). Elevated Fatty Acid Binding Protein 4(FABP4) expression may lead to altered lipid deposition in DKD and is associated with ferroptosis (119). Elevated expression of FABP4 was found in HG-HK2 cells from patients with DKD who showed iron deposition in renal tubules and loss of mitochondrial cristae, whereas Carnitine Palmitoyl transferase 1A(CPT1A), glutathione peroxidase 4, ferritin heavy chain (FTH), and ferritin light chain (FTL) were found to be elevated in HG-HK2 cells from patients with DKD who showed iron deposition in renal tubules and loss of mitochondrial cristae. FTH and FTL decrease and promotes ferroptosis, leading to renal tubular injury. Simultaneous inhibition of FABP4 restores FAO, thereby reducing lipid accumulation and peroxidation while increasing CPT1A expression, which, in turn, inhibits ferroptosis and reduces renal injury and fibrosis (120). Acyl-CoA synthetase long-chain family4 (ACSL4) is overexpressed in DKD (121), and FA regulation modulates lipid metabolism to affect ferroptosis. The upregulation of ACSL4 increased Arachidonoyl-Phosphatidylethanolamine (AA-PE) and Adrenoyl-Phosphatidylethanolamine (AdA-PE) levels, promoted ferroptosis, and exacerbated tubular fibrosis in DKD (122). In addition, ACSL4 inhibition may ameliorate renal injury by decreasing the levels of lipid peroxidation products and inhibiting ferroptosis (123). CD36 expression is increased in patients with DKD (124). CD36 transports polyunsaturated fatty acids (PUFAs), which are essential for lipid peroxidation, intracellularly, and its increased expression has been shown to correlate with ROS production (1). CD36 has been shown to promote proximal tubular fibrosis under hyperglycemic conditions, which may be mediated by its regulation of ferroptosis suppressor function in the proximal tubular cells. CD36 has been shown to promote proximal tubular fibrosis under hyperglycemic conditions. This may promote ferroptosis by regulating the ubiquitination of ferroptosis suppressor protein 1 in proximal tubular cells (125, 126). A correlation has been found between BA metabolism and glomerulosclerosis and tubulointerstitial fibrosis in DKD (127, 128), possibly through the inhibition of ligand-activated nuclear receptor Farnesoid X Receptor(FXR)/retinoid X receptor activation, which is strongly associated with ferroptosis (129). In addition, compared to soybean oil (SO) and linoleic acid (LN), which are rich in PUFAs, peanut oil (PO), lined oil (LO), and rapeseed oil (RO), which are rich in saturated fatty acids and monosaturated fatty acids, are highly likely to reduce the reabsorption of BAs in the colon, which has a different impact on BA metabolism. This may be related to changes in the gut microbiota structure of DKD (130). Impairment of CHOL efflux and the accumulation of CHOL lead to glomerulosclerosis and podocyte ferroptosis in early DKD, and is related to the reduction of ABCA1, the main protein of CHOL efflux (131, 132). The interaction between CHOL metabolism and ferroptosis (133, 134) may be a potential cause of DKD progression, suggesting that ferroptosis is correlated with FAs, BAs, CHOL metabolism disorders, and DKD development.
Autophagy is an intracellular pathway that maintains cellular homeostasis by degrading cytoplasmic components via autolysosome formation of autolysosomes (135). Studies have shown that the development of DKD is associated with defective renal autophagy (119, 136–140). In DKD, the microtubule-associated protein 1A/1b-light chain 3 (LC3-II) is dependent on phagocytosis of endoplasmic reticulum membranes to form lipid autophagosomes, which with their cargoes, mainly composed of ChE and TG, fuse with lysosomes to form autophagic lysosomes, in which the cargo is degraded to produce FAs, a process known as lipophagy (141–147). The accumulation of ChE and FAs metabolites in DKD podocytes has been implicated in the pathogenesis of glomerular dysfunction and lipotoxicity in DKD (148). LDs, as reservoirs of excess lipids, inhibit lipotoxicity, and over-activation of lipophagy can promote renal fibrosis (81, 149). Adipose triglyceride lipase (ATGL) is a critically important signaling node for lipophagy, and sirtuin 1 (SIRT1) acts as a key mediator downstream of ATGL whose role is to promote lipophagy (150) and decreased expression of SIRT1 in the kidney promotes DKD (151–153). In addition, LDs are subject to a variety of cellular factors that can influence the development of lipophagy. Lipophagy is regulated by the nutritional state of the cell and proteins that detect changes in the nutritional stores (154). Mechanistic target of rapamycin complex 1(mTORC1) inhibits lipophagy, and activation of AMP-activated protein kinase (AMPK) promotes lipophagy (155, 156). Specific activation of mTORC1 in the podocytes in DKD leads to many changes in DKD, including increased albuminuria, podocyte loss, and thylakoid membrane expansion, while AMPK in DKD decreased autophagic activity in podocytes and increased cytotoxicity and apoptosis (157, 158).
Sphingolipids may be a regulator of lipophagy (159). The sphingolipids metabolite C1P also regulates renal autophagy (160, 161). Cer itself induces autophagy, and treatment with exogenous C1P can upregulate the expression of beclin1, leading to autophagy through c-Jun N-terminal kinase (JNK) activation (162), whereas AMPK can initiate autophagy either by phosphorylating beclin1 or by blocking mTORC1 (163, 164). This indicates an important role for the AMPK/mTOR pathway in autophagy. CHOL removal also plays a crucial role in autophagosome initiation (165, 166). It was found that STZ induced a decrease in autophagic activity in podocyte cells after diabetes, which ultimately led to the development of DKD (167), and the abnormalities of sphingolipids and CHOL exhibited by lipid metabolism disorder in patients with DKD may be responsible for the inhibition of autophagy in DKD podocyte cells. The above suggests that in DKD, lipophagy abnormalities and autophagy inhibition caused by sphingolipids and CHOL metabolism disorders are closely related to the development of DKD.
Gut microorganisms produce metabolites, such as short-chain FAs (SCFAs), which are involved in the synthesis and metabolism of the human body and in the immunomodulatory processes of the body (168, 169). The gut microbiota influences both innate and adaptive immune systems. During innate immunity, the gut microbiota of Bacteroides, Bifidobacterium, Lactobacillus, and Aspergillus are involved in the maturation of the immune system (170). SCFAs, metabolites of gut microbiota, are involved in immunomodulation by regulating nuclear factor kappa-B (NF-кB) signaling in neutrophils, eosinophils, and macrophages in the gut and by strengthening the physical barrier of the gut (171–174). During adaptive immunity, Lactobacillus, Clostridium, Bifidobacterium, and Enterococcus in the gut can reduce inflammatory responses by producing lipid metabolites and reducing tumor necrosis factor α (TNF-α), and inflammatory mediators interleukin-1(IL-1), interleukin-6 (IL-6), and interleukin-18(IL-18) (175–178).
In DKD, the gut microbiota are associated with immune dysregulation, lipid metabolism disorders, and DKD development (172–174, 179, 180). The gut microbiota of Lactobacillus, Clostridium, Bifidobacterium, and Enterococcus (175) can control BAs as lipid metabolism modulators to modulate the adaptive immunosuppression of inflammatory responses by altering CHOL secretion (177, 178). The gut-liver-kidney axis is the pathway by which lipid metabolites are metabolized in the liver through intestinal absorption and excreted from the kidney (181). Organic anion transporter 3(OAT3) is mainly expressed in the kidney, and the absence of OAT3 alters the normal metabolite transport function in the gut-liver-kidney axis, resulting in the accumulation of endogenous lipid metabolites, such as bile acids and lipids, and G-protein-coupled receptor 35(GPR35), which is a key receptor for lipid metabolism. The coupled GPR35 is associated with inflammation (182–184). This suggests that the disturbance of the gut microbiota and imbalance of immune homeostasis in patients with DKD may affect lipid metabolism through the gut-liver-kidney axis and ultimately contribute to the development of DKD (Figure 3).
Figure 3 Mechanism of lipid metabolism changes in DKD. The main manifestations are abnormalities of ferroptosis, lipophagy, and immunoregulation of gut microbiota in renal mesangial cells, renal tubular cells, and podocytes. Ferroptosis abnormalities are reflected in the transport of PUFAs and changes in the enzymatic response to the LPO process. Abnormalities in lipophagy are associated with abnormalities in cytosolic C1P and CHOL metabolism, leading to the regulation of autophagy by the JNK/AMPK/mTOR channel activation to regulate autophagy in LDs. Abnormalities in gut microbiota immunoregulation are reflected in disorders of gut-derived SCFAs, BAs, and immune factors (TNF-α,IL-1, IL-6, and IL-18) via the NF-кB, OAT3 pathway directly contributing to renal inflammation and lipid accumulation in DKD.
Atorvastatin effectively reduced the levels of low-density lipoprotein CHOL (LDL-C), creatinine (CREA), and urinary albumin and creatinine (UACR) and downregulated the expression of the inflammatory factors TNF-α, monocyte chemoattractant protein-1 (MCP-1), and IL-6 expression in renal tissues, which ameliorates renal injury and delays the progression of DKD by reducing morphological lesions and renal fibrosis and increases transforming growth factor beta (TGF-β) and collagen I staining (185).
Fenofibrate decreased TG content and lipid accumulation in DKD and increased activation of the AMPK/FOXA2/medium-chain acyl-CoA dehydrogenase pathway, significantly reducing renal function and tubular cell apoptosis and slowing DKD progression (186).
BA inhibits phospho-inhibitor of kappa Balpha (IκBα) degradation and NF-κB activity and reduces Fibronectin (FN) expression. It inhibited the DNA-binding activity and transcriptional activity of NF-κB in high glucose-induced glomerular mesangial cells, enhanced the interaction between IκBα and β-arrestin 2 in mesangial cells, and prevented diabetic renal fibrosis by stabilizing the NF-κB inhibitory protein, IκBα, to inhibit NF-κB activation (187).
Liraglutide is a novel hypoglycemic drug. Inhibition of SREBP-1 and Fatty Acid Synthase (FAS) increases ATGL and hormone-sensitive lipase protein expression levels, promoting AMPK phosphorylation to attenuate ectopic lipid deposition in renal tubules, improving PA-induced lipid accumulation in renal tubular epithelial cells, inhibiting lipid synthesis, and promoting lipolysis (188). Liraglutide increased the expression of phosphorylated (p)-eNOS and p-AMPK in the glomeruli, downregulated the expression of p-mTOR, increased the renal expression of LC3B-II, activated autophagy, ameliorated DKD kidney injury, and decreased urinary albumin and Liver-type Fatty Acid Binding Protein (L-FABP) levels (21).
ALA plays a role as an antioxidant in the mitochondrial dehydrogenase reaction, which improves the antioxidant status and lipid distribution, and reduces inflammation by regulating lipid levels, enhancing the body’s antioxidant capacity, protecting vascular endothelial function, and activating the renal cystathionine gamma-lyase/hydrogen sulfide pathway to delay DKD (189).
AdipoRon is an active synthetic lipocalin receptor agonist. AdipoRon ameliorates DKD by activating the intracellular Ca2+/Liver Kinase B1(LKB1)-AMPK/PPARα pathway to ameliorate glomerular endothelial cells(GECs) and podocyte injury (190). AdipoRon reduces palmitate-induced lipotoxicity in the kidney by improving lipid metabolism, especially in GECs and podocytes, and reduces oxidative stress and apoptosis, and preventing renal injury, thereby improving endothelial dysfunction and delaying DKD progression in type 2 diabetic nephropathy (191).
APOD is an essential component of plasma lipoproteins and plays an important role in plasma lipoprotein metabolism. Increased apoD and apoA-IV help counteract the chemical modification of high-density lipoprotein (HDL) by advanced glycation end products (AGEs) and carbamylation, which contributes to the loss of function of HDL in maturing DKD, thereby delaying DKD (192).
Metrnl is a recently discovered hormone produced by skeletal muscles and adipose tissue in response to exercise and cold exposure. Metrnl-specific overexpression or recombinant Metrnl administration in the kidney regulates renal tubular lipid metabolism through mitochondrial homeostasis mediated by the Sirtuin 3(Sirt3)-AMPK/uncoupling protein 1(UCP1) signaling axis, alleviates renal injury, and delays DKD in diabetic mice (193).
Lipin-1 inhibits adipose synthesis, upregulates FAO, attenuates proximal tubular epithelial cell injury in tubulointerstitial fibrosis, and delays DKD by promoting proliferator-activated receptor-gamma co-activator-1alpha(PGC-1α)/PPARα-mediated Carnitine Palmitoyltransferase 1 Alpha(Cpt1α)/hepatocyte nuclear factor 4alpha signaling and upregulating SREBPs (194).
Leptin is a 167-amino acid lipoprotein that plays a role in the regulation of energy metabolism. It attenuates lipid deposition present in the kidney by activating AMPK phosphorylation, which upregulates insulin-induced gene 1 (Insig-1) expression in PA-induced renal tubular epithelial cell lines(NRK-52E) and delays DKD (195).
ABCA1 is one of the most important proteins involved in the maintenance of CHOL homeostasis. In the human renal glomerular endothelial cell line cultured under high-glucose and high-CHOL conditions, ABCA1 deficiency increases cellular CHOL deposition, leads to inflammation and apoptosis, disrupts the endothelial glycoconjugate barrier, and induces endoplasmic reticulum stress (ERS). In contrast, ABCA1 overexpression enhances CHOL efflux or inhibits ERS in vitro, significantly prevents high glucose- and high-CHOL-stimulated glomerular endothelial injury, and delays DKD (196).
MaR1 is a widespread anti-inflammatory lipid mediator, and serum MaR1 concentrations are negatively correlated with hemoglobin A1c, diabetes duration, UACR, neutrophils, and the neutrophil-lymphocyte ratio and positively correlated with HDL CHOL (HDL-C) and estimated glomerular filtration rate. MaR1 alleviated the pathological progression of hyperglycemia, UACR, and DKD through the leucine-rich repeat domain-containing G protein-coupled receptor 6(LGR6)-mediated cyclic adenosine monophosphate-superoxide dismutase-2 antioxidant pathway (197).
Ad reverses the effects of nanocapsules on ameliorating mitochondrial damage by knocking down the overexpression of Neuronatin (Nnat) or translocator protein(TSPO) to improve lipid metabolism and inhibit TSPO activity, thereby enhancing mitochondrial function. It protects hexokinase 2(HK2) from high glucose (HG) stimulation. It also effectively controls blood glucose and lipid levels, improves renal function, inhibits ROS overproduction, protects mitochondria from damage, improves lipid deposition in renal tissues, and downregulates the expression of lipogenic proteins SEBP-1 and Adipose Differentiation-Related Protein (ADRP) in DKD mice (198).
SAR442357 is a newly developed non-absorbable polymeric sequestering agent with optimal phosphate and bile salt sequestration properties. Long-term treatment of diabetes mellitus type 2(T2DM) obese Zucker fatty/spontaneously hypertensive heart failure F1 hybrid (ZSF1) leads to enhanced segregation of BAs and phosphates in the gut, improved glycemic control, reduced serum CHOL, and delayed DKD progression (199).
Effects of Complement Factor B(CFB) on lipid metabolism in developing DKD, Cfb-knockout diabetic mice had significantly less vas deferens interstitial injury and less Cer biosynthesis. Cfb knockout further blocked the transcription of Ceramides (CERs) by inhibiting the NF-κB signaling pathway, which inhibited the activation of the complement alternative pathway and attenuated renal injury in DKD, especially vas deferens mesenchymal injury. CERs regulated the biosynthesis of Cer (200). (Table 1)
Table 1 Therapeutic advances related to conventional drug-targeted lipid metabolism in diabetic kidney disease.
BBR is a potent compound from TCM (201) that can reverse lipid metabolism disorders and ameliorate kidney injury in patients with DKD. BBR stabilizes mitochondrial morphology in podocytes by eliminating PA-induced activation with dynamin related protein 1 (202).BBR peroxisome Peroxisome Proliferator-Activated Receptor Gamma Coactivator 1-Alpha(PGC-1α) signaling pathway activation promotes mitochondrial energetic homeostasis and FAO in podocytes, and PGC-1α-mediated mitochondrial bioenergetics can play a key role in lipid disorder-induced podocyte injury and development of DKD in mice (203).
Breviscapine is a purified flavonoid extract of Erigeron breviscapus (204), which attenuates dyslipidemia by decreasing 24-h urine protein, serum creatinine (Scr),and blood urea nitrogen levels; modulates lipid profiles by increasing levels of TC, TG, and HDL; and protects against kidney injury (205).
MDG-1 is a polysaccharide derived from TCM japonicas (206). MDG-1 reduced blood glucose, TG, Blood Urea Nitrogen (BUN), and albumin levels by activating the phosphatidylinositol-3 kinase/Akt signaling pathway and significantly suppressed the expression of TGF-β1 and connective tissue growth factor. MDG-1 attenuated glomerular mesangial dilatation and tubulointerstitial fibrosis in diabetic mice. MDG-1 ameliorated DKD by reducing hyperglycemia, hyperinsulinemia, and hyperlipidemia and by inhibiting intracellular signaling pathways (207).
FYGL is a water-soluble substance extracted from Ganoderma lucidum, highly branched proteoglycan that protects tissues from oxidative stress damage (208). FYGL significantly inhibited HG/PA-induced proliferation of HBZY-1 cells, ROS generation, and malondialdehyde (MDA) production; promoted Superoxide Dismutase(SOD) activity; and suppressed the expression of NADPH oxidase 1(NOX1), NADPH oxidase 4(NOX4), mitogen-activated protein kinase, NF-κB, and pro-fibronectin expression. It significantly alleviates lipid metabolism disorders and protects the kidneys from oxidative stress-induced dysfunction, delaying DKD (209).
NGR1 is a novel saponin from Panax notoginseng, a TCM for the adjuvant treatment of DKD (210),which demonstrated that NGR1 treatment increased serum lipids in db/db mice, reduced AGE-induced mitochondrial damage, limited the increase in ROS, reduced apoptosis in HK-2 cells, promoted the expression of nuclear factor erythroid 2-related factor 2(Nrf2) and heme oxygenase-1(HO-1) to abrogate apoptosis-inducing and TGF-β signaling by ROS, attenuated histological abnormalities in the kidney, reduced glomerular volume in DKD, inhibited oxidative stress-induced apoptosis and renal fibrosis, and delayed DKD (211).
The TCM Tripterygium wilfordii (TWH) has been used clinically to treat renal diseases (212). Triptolide, the main active ingredient of TWH, can reduce the 24-h urine total protein quantity (24-h UTP), resulting in decreased renal MDA and nitrotyrosine expression, downregulation of renal oxidative carbonyl protein (OCP) expression, and elevated renal SOD to delay DKD (213). In addition, triptolide is an active diterpene purified from the TCM TWH, which can ameliorate hyperlipidemia and albuminuria in db/db diabetic mice, alleviate glomerular hypertrophy and pedunculated cell injury, and attenuate inflammation and oxidative stress in the kidneys (214).
Mulberry extract is considered a potential therapeutic drug for diabetes (215). Mulberry extract can lead to a significant reduction in serum TG and very low-density lipoprotein CHOL and HDL-C concentrations, improve plasma GSH and Malondialdehyde (MDA), and delay the development of DKD (216).
PJ has been shown to exert a therapeutic effect on DKD (217). PJ can reduce hyperlipidemia, serum BUN, and 24-h UTP in diabetic mice by modulating unsaturated FAs, glycerophospholipid metabolism, and purine metabolism; protect against pathological changes in renal tissues; and prevent apoptosis of renal cells by modulating the beclin-2/caspase 3 apoptosis signaling pathway to delay DKD (218).
RSV, an extract of the Chinese herb Tiger Balm, is a naturally occurring polyphenolic compound that reduces blood glucose and lipid concentrations and is a Sirt1 agonist (219). Resveratrol improves circulating lipids and renal dysfunction, reduces lipid deposition in the kidney by modulating the junctional adhesion molecule-like protein/Sirt1 lipid synthesis pathway, and ameliorates DKD (220).
CCP is a fungus that parasitizes ghost moth larvae, modulates lipids, and improves DKD (221). CCP increases Lactobacillus and Anaplasma community abundance while decreasing the abundance of LPS-producing bacteria and reducing the levels of serum TNF-α, IL-1β, and IL-6 in mice. Significant improvements in 24-h urine output levels and urinary protein, albumin to creatinine ratio, and Scr levels and a decrease in glomerular mesangial zone collagen fibers and lipid accumulation were observed in renal tissue samples (222).
MLB, an aqueous extract of Salvia miltiorrhiza, an erect perennial herb of the genus Salvia and family Labiatae, is a potential therapeutic agent for kidney disease (223). The abundance of Shigella and Aspergillus species and fecal BAs levels in the rat intestine were significantly reduced by MLB intervention, suggesting that MLB may restore the integrity of the intestinal barrier and inhibit the release of BA-induced inflammatory cells through localized modulation of the gut microbiota and BA metabolism, slowing renal injury (224). (Table 2)
Table 2 Therapeutic advances related to herbal targeting of lipid metabolism in diabetic kidney disease.
The altered manifestations of lipid metabolism disorder present in DKD have been gradually clarified, and the mechanisms affecting this process mainly include cellular ferroptosis, lipophagy, reprogramming of lipid metabolism, and immune modulation of the gut microbiota (involving the liver-kidney axis) in the body, which, in turn, accelerates the progression of DKD, a process that suggests one of the relationships between lipid metabolism disorder and DKD. Recent research has suggested that interventions targeting altered lipid metabolism disorder may help improve DKD prognosis. However, this requires further clarification of the specific targets of the interventions; otherwise, the interventions may not be effective. In addition, compared with known lipid-lowering drugs, natural drugs have the advantage of multiple targets and multiple pathways in the treatment of DKD, but their mechanism of action and scope of application have not yet been clarified. Most of the existing studies have focused on the monomers of TCM, whereas the complexity of the components of TCM prescriptions commonly used in the clinic makes it difficult to elucidate the mechanism of action. The role of lipid-lowering as a target of natural drug action warrants further study of the relevant mechanisms between DKD and these two diseases and provides a potential research direction for the effective treatment of DKD.
Computer modeling and simulation technologies are now pivotal in identifying therapeutic targets for diseases like cancer, liver fibrosis, and Takotsubo syndrome (TTS) (225–230). Future studies can leverage cost-effective methods like quantitative lipid analysis with ES-MSI and incorporate molecular dynamics (MD) simulations alongside computational bioinformatics to investigate lipid metabolism’s role in diabetic kidney disease (DKD) and natural drug effects on lipid disorders. Starting with MD simulations for atomic-level molecular interaction insights, crucial for DKD’s molecular understanding, followed by computational tools to analyze complex data for genetic and protein stability patterns, this approach aims to elucidate lipid metabolism’s link to DKD and refine therapeutic targets by analyzing lipid profile shifts and SNPs.
This streamlined, multidisciplinary approach promises a deeper understanding of DKD’s pathophysiology and treatment, underscoring the importance of merging computational and experimental methods in biomedical research to enhance knowledge and therapeutic developments.
Y-ZH: Writing – review & editing, Writing – original draft, Validation, Supervision, Project administration, Methodology, Investigation, Formal analysis, Data curation, Conceptualization. B-XD: Writing – original draft, Methodology, Data curation. X-YZ: Writing – original draft, Methodology, Data curation. Y-Z-YW: Writing – original draft, Methodology. H-JZ: Writing – review & editing, Validation, Supervision, Resources, Project administration, Methodology, Funding acquisition, Conceptualization. W-JL: Writing – review & editing, Validation, Supervision, Resources, Methodology, Funding acquisition, Conceptualization.
This study was supported by The National Natural Youth Science Foundation of China (No. 82004196), National Natural Science Foundation of China (Grant No. 82374382), and Chinese Medicine Inheritance and Innovation Talent Project-Leading Talent Support Program of National Traditional Chinese Medicine (Grant No. 2018, No. 12), Tongzhou District to promote the medical and health industry development project(Grant No.JX2023YJ025).
We would like to thank Editage (www.editage.cn) for English language editing.
The authors declare that the research was conducted in the absence of any commercial or financial relationships that could be construed as a potential conflict of interest.
All claims expressed in this article are solely those of the authors and do not necessarily represent those of their affiliated organizations, or those of the publisher, the editors and the reviewers. Any product that may be evaluated in this article, or claim that may be made by its manufacturer, is not guaranteed or endorsed by the publisher.
1. Alicic RZ, Rooney MT, Tuttle KR. Diabetic kidney disease: challenges, progress, and possibilities. Clin J Am Soc Nephrol. (2017) 12:2032–45. doi: 10.2215/CJN.11491116
2. Thomas MC, Brownlee M, Susztak K, Sharma K, Jandeleit-Dahm KA, Zoungas S, et al. Diabetic kidney disease. Nat Rev Dis Primers. (2015) 1:15018. doi: 10.1038/nrdp.2015.18
3. Tuttle KR, Agarwal R, Alpers CE, Bakris GL, Brosius FC, Kolkhof P, et al. Molecular mechanisms and therapeutic targets for diabetic kidney disease. Kidney Int. (2022) 102:248–60. doi: 10.1016/j.kint.2022.05.012
4. Zhao C, Li L, Li C, Tang C, Cai J, Liu Y, et al. PACS-2 deficiency in tubular cells aggravates lipid-related kidney injury in diabetic kidney disease. Mol Med. (2022) 28:117. doi: 10.1186/s10020-022-00545-x
5. Pereira PR, Carrageta DF, Oliveira PF, Rodrigues A, Alves MG, Monteiro MP. Metabolomics as a tool for the early diagnosis and prognosis of diabetic kidney disease. Med Res Rev. (2022) 42:1518–44. doi: 10.1002/med.21883
6. Daza-Arnedo R, Rico-Fontalvo JE, Pájaro-Galvis N, Leal-Martínez V, Abuabara-Franco E, Raad-Sarabia M, et al. Dipeptidyl peptidase-4 inhibitors and diabetic kidney disease: A narrative review. Kidney Med. (2021) 3:1065–73. doi: 10.1016/j.xkme.2021.07.007
7. DeFronzo RA, Reeves WB, Awad AS. Pathophysiology of diabetic kidney disease: impact of SGLT2 inhibitors. Nat Rev Nephrol. (2021) 17:319–34. doi: 10.1038/s41581-021-00393-8
8. Kawanami D, Takashi Y. GLP-1 receptor agonists in diabetic kidney disease: from clinical outcomes to mechanisms. Front Pharmacol. (2020) 11:967. doi: 10.3389/fphar.2020.00967
9. Hirano T. Pathophysiology of diabetic dyslipidemia. J Atheroscler Thromb. (2018) 25:771–82. doi: 10.5551/jat.RV17023
10. Chen X, Yin Q, Ma L, Fu P. The role of cholesterol homeostasis in diabetic kidney disease. Curr Med Chem. (2021) 28:7413–26. doi: 10.2174/0929867328666210419132807
11. Mitrofanova A, Fontanella AM, Merscher S, Fornoni A. Lipid deposition and metaflammation in diabetic kidney disease. Curr Opin Pharmacol. (2020) 55:60–72. doi: 10.1016/j.coph.2020.09.004
12. Wu Y, Chen Y. Research progress on ferroptosis in diabetic kidney disease. Front Endocrinol (Lausanne). (2022) 13:945976. doi: 10.3389/fendo.2022.945976
13. Feng X, Wang S, Sun Z, Dong H, Yu H, Huang M, et al. Ferroptosis Enhanced Diabetic Renal Tubular Injury via HIF-1α/HO-1 Pathway in db/db Mice. Front Endocrinol (Lausanne). (2021) 12:626390. doi: 10.3389/fendo.2021.626390
14. Yang D, Livingston MJ, Liu Z, Dong G, Zhang M, Chen JK, et al. Autophagy in diabetic kidney disease: regulation, pathological role and therapeutic potential. Cell Mol Life Sci. (2018) 75:669–88. doi: 10.1007/s00018-017-2639-1
15. Koch EAT, Nakhoul R, Nakhoul F, Nakhoul N. Autophagy in diabetic nephropathy: a review. Int Urol Nephrol. (2020) 52:1705–12. doi: 10.1007/s11255-020-02545-4
16. Xu YX, Pu SD, Li X, Yu ZW, Zhang YT, Tong XW, et al. Exosomal ncRNAs: Novel therapeutic target and biomarker for diabetic complications. Pharmacol Res. (2022) 178:106135. doi: 10.1016/j.phrs.2022.106135
17. Chen X, Han Y, Gao P, Yang M, Xiao L, Xiong X, et al. Disulfide-bond A oxidoreductase-like protein protects against ectopic fat deposition and lipid-related kidney damage in diabetic nephropathy. Kidney Int. (2019) 95:880–95. doi: 10.1016/j.kint.2018.10.038
18. Fang Q, Liu N, Zheng B, Guo F, Zeng X, Huang X, et al. Roles of gut microbial metabolites in diabetic kidney disease. Front Endocrinol (Lausanne). (2021) 12:636175. doi: 10.3389/fendo.2021.636175
19. Linh HT, Iwata Y, Senda Y, Sakai-Takemori Y, Nakade Y, Oshima M, et al. Intestinal bacterial translocation contributes to diabetic kidney disease. J Am Soc Nephrol. (2022) 33:1105–19. doi: 10.1681/ASN.2021060843
20. Chen H, Liu C, Wang Q, Xiong M, Zeng X, Yang D, et al. Renal UTX-PHGDH-serine axis regulates metabolic disorders in the kidney and liver. Nat Commun. (2022) 13:3835. doi: 10.1038/s41467-022-31476-0
21. Su K, Yi B, Yao BQ, Xia T, Yang YF, Zhang ZH, et al. Liraglutide attenuates renal tubular ectopic lipid deposition in rats with diabetic nephropathy by inhibiting lipid synthesis and promoting lipolysis. Pharmacol Res. (2020) 156:104778. doi: 10.1016/j.phrs.2020.104778
22. Herman-Edelstein M, Scherzer P, Tobar A, Levi M, Gafter U. Altered renal lipid metabolism and renal lipid accumulation in human diabetic nephropathy. J Lipid Res. (2014) 55:561–72. doi: 10.1194/jlr.P040501
23. Yang M, Han Y, Luo S, Xiong X, Zhu X, Zhao H, et al. MAMs protect against ectopic fat deposition and lipid-related kidney damage in DN patients. Front Endocrinol (Lausanne). (2021) 12:609580. doi: 10.3389/fendo.2021.609580
24. Mitrofanova A, Burke G, Merscher S, Fornoni A. New insights into renal lipid dysmetabolism in diabetic kidney disease. World J Diabetes. (2021) 12:524–40. doi: 10.4239/wjd.v12.i5.524
25. Tsai IT, Wu CC, Hung WC, Lee TL, Hsuan CF, Wei CT, et al. FABP1 and FABP2 as markers of diabetic nephropathy. Int J Med Sci. (2020) 17:2338–45. doi: 10.7150/ijms.49078
26. Khan S, Gaivin R, Abramovich C, Boylan M, Calles J, Schelling JR. Fatty acid transport protein-2 regulates glycemic control and diabetic kidney disease progression. JCI Insight. (2020) 5. doi: 10.1172/jci.insight.136845
27. Falkevall A, Mehlem A, Palombo I, Heller Sahlgren B, Ebarasi L, He L, et al. Reducing VEGF-B signaling ameliorates renal lipotoxicity and protects against diabetic kidney disease. Cell Metab. (2017) 25:713–26. doi: 10.1016/j.cmet.2017.01.004
28. Susztak K, Ciccone E, McCue P, Sharma K, Böttinger EP. Multiple metabolic hits converge on CD36 as novel mediator of tubular epithelial apoptosis in diabetic nephropathy. PloS Med. (2005) 2:e45. doi: 10.1371/journal.pmed.0020045
29. Feng L, Gu C, Li Y, Huang J. High glucose promotes CD36 expression by upregulating peroxisome proliferator-activated receptor γ Levels to exacerbate lipid deposition in renal tubular cells. BioMed Res Int. (2017) 2017:1414070. doi: 10.1155/2017/1414070
30. Yang X, Wu Y, Li Q, Zhang G, Wang M, Yang H. CD36 promotes podocyte apoptosis by activating the pyrin domain-containing-3 (NLRP3) inflammasome in primary nephrotic syndrome. Med Sci Monit. (2018) 24:6832–9. doi: 10.12659/MSM.909810
31. Kennedy DJ, Chen Y, Huang W, Viterna J, Liu J, Westfall K, et al. CD36 and Na/K-ATPase-α1 form a proinflammatory signaling loop in kidney. Hypertension. (2013) 61:216–24. doi: 10.1161/HYPERTENSIONAHA.112.198770
32. Hua W, Huang HZ, Tan LT, Wan JM, Gui HB, Zhao L, et al. CD36 mediated fatty acid-induced podocyte apoptosis via oxidative stress. PloS One. (2015) 10:e0127507. doi: 10.1371/journal.pone.0127507
33. Kang HM, Ahn SH, Choi P, Ko YA, Han SH, Chinga F, et al. Defective fatty acid oxidation in renal tubular epithelial cells has a key role in kidney fibrosis development. Nat Med. (2015) 21:37–46. doi: 10.1038/nm.3762
34. Ito H, Yamashita H, Nakashima M, Takaki A, Yukawa C, Matsumoto S, et al. Current metabolic status affects urinary liver-type fatty-acid binding protein in normoalbuminuric patients with type 2 diabetes. J Clin Med Res. (2017) 9:366–73. doi: 10.14740/jocmr2934w
35. Thi TND, Gia BN, Thi HLL, Thi TNC, Thanh HP. Evaluation of urinary L-FABP as an early marker for diabetic nephropathy in type 2 diabetic patients. J Med Biochem. (2020) 39(2):224–30. doi: 10.2478/jomb-2019-0037
36. Panduru NM, Forsblom C, Saraheimo M, Thorn L, Bierhaus A, Humpert PM, et al. Urinary liver-type fatty acid-binding protein and progression of diabetic nephropathy in type 1 diabetes. Diabetes Care. (2013) 36:2077–83. doi: 10.2337/dc12-1868
37. Viswanathan V, Sivakumar S, Sekar V, Umapathy D, Kumpatla S. Clinical significance of urinary liver-type fatty acid binding protein at various stages of nephropathy. Indian J Nephrol. (2015) 25:269–73. doi: 10.4103/0971-4065.145097
38. Ducasa GM, Mitrofanova A, Mallela SK, Liu X, Molina J, Sloan A, et al. ATP-binding cassette A1 deficiency causes cardiolipin-driven mitochondrial dysfunction in podocytes. J Clin Invest. (2019) 129:3387–400. doi: 10.1172/JCI125316
39. Han LD, Xia JF, Liang QL, Wang Y, Wang YM, Hu P, et al. Plasma esterified and non-esterified fatty acids metabolic profiling using gas chromatography-mass spectrometry and its application in the study of diabetic mellitus and diabetic nephropathy. Anal Chim Acta. (2011) 689:85–91. doi: 10.1016/j.aca.2011.01.034
40. Zhang Y, Zhang S, Wang G. Metabolomic biomarkers in diabetic kidney diseases–A systematic review. J Diabetes Complications. (2015) 29:1345–51. doi: 10.1016/j.jdiacomp.2015.06.016
41. Zhang B, Wan Y, Zhou X, Zhang H, Zhao H, Ma L, et al. Characteristics of serum metabolites and gut microbiota in diabetic kidney disease. Front Pharmacol. (2022) 13:872988. doi: 10.3389/fphar.2022.872988
42. Jiang T, Liebman SE, Lucia MS, Li J, Levi M. Role of altered renal lipid metabolism and the sterol regulatory element binding proteins in the pathogenesis of age-related renal disease. Kidney Int. (2005) 68:2608–20. doi: 10.1111/j.1523-1755.2005.00733.x
43. Jiang T, Wang Z, Proctor G, Moskowitz S, Liebman SE, Rogers T, et al. Diet-induced obesity in C57BL/6J mice causes increased renal lipid accumulation and glomerulosclerosis via a sterol regulatory element-binding protein-1c-dependent pathway. J Biol Chem. (2005) 280:32317–25. doi: 10.1074/jbc.M500801200
44. Proctor G, Jiang T, Iwahashi M, Wang Z, Li J, Levi M. Regulation of renal fatty acid and cholesterol metabolism, inflammation, and fibrosis in Akita and OVE26 mice with type 1 diabetes. Diabetes. (2006) 55:2502–9. doi: 10.2337/db05-0603
45. Ishigaki N, Yamamoto T, Shimizu Y, Kobayashi K, Yatoh S, Sone H, et al. Involvement of glomerular SREBP-1c in diabetic nephropathy. Biochem Biophys Res Commun. (2007) 364:502–8. doi: 10.1016/j.bbrc.2007.10.038
46. Sun H, Yuan Y, Sun ZL. Cholesterol contributes to diabetic nephropathy through SCAP-SREBP-2 pathway. Int J Endocrinol. (2013) 2013:592576. doi: 10.1155/2013/592576
47. Zhou C, Pridgen B, King N, Xu J, Breslow JL. Hyperglycemic Ins2AkitaLdlr-/- mice show severely elevated lipid levels and increased atherosclerosis: a model of type 1 diabetic macrovascular disease. J Lipid Res. (2011) 52:1483–93. doi: 10.1194/jlr.M014092
48. Woroniecka KI, Park AS, Mohtat D, Thomas DB, Pullman JM, Susztak K. Transcriptome analysis of human diabetic kidney disease. Diabetes. (2011) 60:2354–69. doi: 10.2337/db10-1181
49. Ju W, Greene CS, Eichinger F, Nair V, Hodgin JB, Bitzer M, et al. Defining cell-type specificity at the transcriptional level in human disease. Genome Res. (2013) 23:1862–73. doi: 10.1101/gr.155697.113
50. Liu X, Ducasa GM, Mallela SK, Kim JJ, Molina J, Mitrofanova A, et al. Sterol-O-acyltransferase-1 has a role in kidney disease associated with diabetes and Alport syndrome. ey Int. (2020) 98:1275–85. doi: 10.1016/j.kint.2020.06.040
51. Tang C, Kanter JE, Bornfeldt KE, Leboeuf RC, Oram JF. Diabetes reduces the cholesterol exporter ABCA1 in mouse macrophages and kidneys. J Lipid Res. (2010) 51:1719–28. doi: 10.1194/jlr.M003525
52. Merscher-Gomez S, Guzman J, Pedigo CE, Lehto M, Aguillon-Prada R, Mendez A, et al. Cyclodextrin protects podocytes in diabetic kidney disease. Diabetes. (2013) 62:3817–27. doi: 10.2337/db13-0399
53. Tsun JG, Yung S, Chau MK, Shiu SW, Chan TM, Tan KC. Cellular cholesterol transport proteins in diabetic nephropathy. PloS One. (2014) 9:e105787. doi: 10.1371/journal.pone.0105787
54. Sas KM, Nair V, Byun J, Kayampilly P, Zhang H, Saha J, et al. Targeted lipidomic and transcriptomic analysis identifies dysregulated renal ceramide metabolism in a mouse model of diabetic kidney disease. J Proteomics Bioinform. (2015) Suppl 14. doi: 10.4172/jpb
55. Liu JJ, Ghosh S, Kovalik JP, Ching J, Choi HW, Tavintharan S, et al. Profiling of plasma metabolites suggests altered mitochondrial fuel usage and remodeling of sphingolipid metabolism in individuals with type 2 diabetes and kidney disease. Kidney Int Rep. (2017) 2:470–80. doi: 10.1016/j.ekir.2016.12.003
56. Morita Y, Kurano M, Sakai E, Nishikawa T, Nishikawa M, Sawabe M, et al. Analysis of urinary sphingolipids using liquid chromatography-tandem mass spectrometry in diabetic nephropathy. J Diabetes Investig. (2020) 11:441–9. doi: 10.1111/jdi.13154
57. Nojiri T, Kurano M, Tokuhara Y, Ohkubo S, Hara M, Ikeda H, et al. Modulation of sphingosine-1-phosphate and apolipoprotein M levels in the plasma, liver and kidneys in streptozotocin-induced diabetic mice. J Diabetes Investig. (2014) 5:639–48. doi: 10.1111/jdi.12232
58. Geoffroy K, Troncy L, Wiernsperger N, Lagarde M, El Bawab S. Glomerular proliferation during early stages of diabetic nephropathy is associated with local increase of sphingosine-1-phosphate levels. FEBS Lett. (2005) 579:1249–54. doi: 10.1016/j.febslet.2004.12.094
59. Lan T, Shen X, Liu P, Liu W, Xu S, Xie X, et al. Berberine ameliorates renal injury in diabetic C57BL/6 mice: Involvement of suppression of SphK-S1P signaling pathway. Arch Biochem Biophys. (2010) 502:112–20. doi: 10.1016/j.abb.2010.07.012
60. Awad AS, Rouse MD, Khutsishvili K, Huang L, Bolton WK, Lynch KR, et al. Chronic sphingosine 1-phosphate 1 receptor activation attenuates early-stage diabetic nephropathy independent of lymphocytes. Kidney Int. (2011) 79:1090–8. doi: 10.1038/ki.2010.544
61. Awad AS, Ye H, Huang L, Li L, Foss FW Jr, Macdonald TL, et al. Selective sphingosine 1-phosphate 1 receptor activation reduces ischemia-reperfusion injury in mouse kidney. Am J Physiol Renal Physiol. (2006) 290:F1516–24. doi: 10.1152/ajprenal.00311.2005
62. Mitrofanova A, Mallela SK, Ducasa GM, Yoo TH, Rosenfeld-Gur E, Zelnik ID, et al. SMPDL3b modulates insulin receptor signaling in diabetic kidney disease. Nat Commun. (2019) 10:2692. doi: 10.1038/s41467-019-10584-4
63. Yoo TH, Pedigo CE, Guzman J, Correa-Medina M, Wei C, Villarreal R, et al. Sphingomyelinase-like phosphodiesterase 3b expression levels determine podocyte injury phenotypes in glomerular disease. J Am Soc Nephrol. (2015) 26:133–47. doi: 10.1681/ASN.2013111213
64. Ahmad A, Mitrofanova A, Bielawski J, Yang Y, Marples B, Fornoni A, et al. Sphingomyelinase-like phosphodiesterase 3b mediates radiation-induced damage of renal podocytes. FASEB J. (2017) 31(2):771–80. doi: 10.1096/fj.201600618R
65. Zador IZ, Deshmukh GD, Kunkel R, Johnson K, Radin NS, Shayman JA. A role for glycosphingolipid accumulation in the renal hypertrophy of streptozotocin-induced diabetes mellitus. J Clin Invest. (1993) 91:797–803. doi: 10.1172/JCI116299
66. Vukovic I, Bozic J, Markotic A, Ljubicic S, Ticinovic Kurir T. The missing link - likely pathogenetic role of GM3 and other gangliosides in the development of diabetic nephropathy. Kidney Blood Press Res. (2015) 40:306–14. doi: 10.1159/000368506
67. Hou B, He P, Ma P, Yang X, Xu C, Lam SM, et al. Comprehensive lipidome profiling of the kidney in early-stage diabetic nephropathy. Front Endocrinol (Lausanne). (2020) 11:359. doi: 10.3389/fendo.2020.00359
68. Ene CD, Penescu M, Anghel A, Neagu M, Budu V, Nicolae I. Monitoring diabetic nephropathy by circulating gangliosides. J Immunoassay Immunochem. (2016) 37:68–79. doi: 10.1080/15321819.2015.1050107
69. Du F, Virtue A, Wang H, Yang XF. Metabolomic analyses for atherosclerosis, diabetes, and obesity. biomark Res. (2013) 1:17. doi: 10.1186/2050-7771-1-17
70. Yang Y, Wang J, Qin L, Shou Z, Zhao J, Wang H, et al. Rapamycin prevents early steps of the development of diabetic nephropathy in rats. Am J Nephrol. (2007) 27:495–502. doi: 10.1159/000106782
71. Zhao YY. Metabolomics in chronic kidney disease. Clin Chim Acta. (2013) 422:59–69. doi: 10.1016/j.cca.2013.03.033
72. Zhao YY, Vaziri ND, Lin RC. Lipidomics: new insight into kidney disease. Adv Clin Chem. (2015) 68:153–75. doi: 10.1016/bs.acc.2014.11.002
73. Jiang Z, Liang Q, Luo G, Hu P, Li P, Wang Y. HPLC-electrospray tandem mass spectrometry for simultaneous quantitation of eight plasma aminothiols: application to studies of diabetic nephropathy. Talanta. (2009) 77:1279–84. doi: 10.1016/j.talanta.2008.08.031
74. Karpe F, Dickmann JR, Frayn KN. Fatty acids, obesity, and insulin resistance: time for a reevaluation. Diabetes. (2011) 60:2441–9. doi: 10.2337/db11-0425
75. Smith CA, O’Maille G, Want EJ, Qin C, Trauger SA, Brandon TR, et al. METLIN - a metabolite mass spectral database. Ther Drug Monit. (2005) 27:747–51. doi: 10.1097/01.ftd.0000179845.53213.39
76. Fahy E, Sud M, Cotter D, Subramaniam S. LIPID MAPS online tools for lipid research. Nucleic Acids Res. (2007) 35:W606–12. doi: 10.1093/nar/gkm324
77. Grove KJ, Voziyan PA, Spraggins JM, Wang S, Paueksakon P, Harris RC, et al. Diabetic nephropathy induces alterations in the glomerular and tubule lipid profiles. J Lipid Res. (2014) 55:1375–85. doi: 10.1194/jlr.M049189
78. Zhu C, Liang QL, Hu P, Wang YM, Luo GA. Phospholipidomic identification of potential plasma biomarkers associated with type 2 diabetes mellitus and diabetic nephropathy. Talanta. (2011) 85:1711–20. doi: 10.1016/j.talanta.2011.05.036
79. Zhang G, Zhang J, DeHoog RJ, Pennathur S, Anderton CR, Venkatachalam MA, et al. DESI-MSI and METASPACE indicates lipid abnormalities and altered mitochondrial membrane components in diabetic renal proximal tubules. Metabolomics. (2020) 16:11. doi: 10.1007/s11306-020-1637-8
80. Melo RC, D’Avila H, Wan HC, Bozza PT, Dvorak AM, Weller PF. Lipid bodies in inflammatory cells: structure, function, and current imaging techniques. J Histochem Cytochem. (2011) 59:540–56. doi: 10.1369/0022155411404073
81. Urahama Y, Ohsaki Y, Fujita Y, Maruyama S, Yuzawa Y, Matsuo S, et al. Lipid droplet-associated proteins protect renal tubular cells from fatty acid-induced apoptosis. Am J Pathol. (2008) 173:1286–94. doi: 10.2353/ajpath.2008.080137
82. Garbarino J, Padamsee M, Wilcox L, Oelkers PM, D’ AD, Ruggles K, et al. Sterol and diacylglycerol acyltransferase deficiency triggers fatty acid mediated cell death. J Biol Chem. (2009) 284:30994–1005. doi: 10.1074/jbc.M109.050443
83. Greenberg AS, Coleman RA, Kraemer FB, McManaman JL, Obin MS, Puri V, et al. The role of lipid droplets in metabolic disease in rodents and humans. J Clin Invest. (2011) 121:2102–10. doi: 10.1172/JCI46069
84. Olzmann JA, Carvalho P. Dynamics and functions of lipid droplets. Nat Rev Mol Cell Biol. (2019) 20:137–55. doi: 10.1038/s41580-018-0085-z
85. Wang Z, Jiang T, Li J, Proctor G, McManaman JL, Lucia S, et al. Regulation of renal lipid metabolism, lipid accumulation, and glomerulosclerosis in FVBdb/db mice with type 2 diabetes. Diabetes. (2005) 54:2328–35. doi: 10.2337/diabetes.54.8.2328
86. Kiss E, Kränzlin B, Wagenblaβ K, Bonrouhi M, Thiery J, Gröne E, et al. Lipid droplet accumulation is associated with an increase in hyperglycemia-induced renal damage: prevention by liver X receptors. Am J Pathol. (2013) 182:727–41. doi: 10.1016/j.ajpath.2012.11.033
87. Yang W, Luo Y, Yang S, Zeng M, Zhang S, Liu J, et al. Ectopic lipid accumulation: potential role in tubular injury and inflammation in diabetic kidney disease. Clin Sci (Lond). (2018) 132:2407–22. doi: 10.1042/CS20180702
88. Wang X, Chen C, Xie C, Huang W, Young RL, Jones KL, et al. Serum bile acid response to oral glucose is attenuated in patients with early type 2 diabetes and correlates with 2-hour plasma glucose in individuals without diabetes. Diabetes Obes Metab. (2022) 24:1132–42. doi: 10.1111/dom.14683
89. Xiao X, Zhang J, Ji S, Qin C, Wu Y, Zou Y, et al. Lower bile acids as an independent risk factor for renal outcomes in patients with type 2 diabetes mellitus and biopsy-proven diabetic kidney disease. Front Endocrinol (Lausanne). (2022) 13:1026995. doi: 10.3389/fendo.2022.1026995
90. Jiang T, Wang XX, Scherzer P, Wilson P, Tallman J, Takahashi H, et al. Farnesoid X receptor modulates renal lipid metabolism, fibrosis, and diabetic nephropathy. Diabetes. (2007) 56:2485–93. doi: 10.2337/db06-1642
91. Arsenijevic D, Cajot JF, Fellay B, Dulloo AG, Van Vliet BN, Montani JP. Uninephrectomy-induced lipolysis and low-grade inflammation are mimicked by unilateral renal denervation. Front Physiol. (2016) 7:227. doi: 10.3389/fphys.2016.00227
92. Xiong F, Li X, Yang Z, Wang Y, Gong W, Huang J, et al. TGR5 suppresses high glucose-induced upregulation of fibronectin and transforming growth factor-β1 in rat glomerular mesangial cells by inhibiting RhoA/ROCK signaling. Endocrine. (2016) 54:657–70. doi: 10.1007/s12020-016-1032-4
93. Yang Z, Xiong F, Wang Y, Gong W, Huang J, Chen C, et al. TGR5 activation suppressed S1P/S1P2 signaling and resisted high glucose-induced fibrosis in glomerular mesangial cells. Pharmacol Res. (2016) 111:226–36. doi: 10.1016/j.phrs.2016.05.035
94. Wang XX, Edelstein MH, Gafter U, Qiu L, Luo Y, Dobrinskikh E, et al. G Protein-coupled bile acid receptor TGR5 activation inhibits kidney disease in obesity and diabetes. J Am Soc Nephrol. (2016) 27:1362–78. doi: 10.1681/ASN.2014121271
95. Podrini C, Rowe I, Pagliarini R, Costa ASH, Chiaravalli M, Di Meo I, et al. Dissection of metabolic reprogramming in polycystic kidney disease reveals coordinated rewiring of bioenergetic pathways. Commun Biol. (2018) 1:194. doi: 10.1038/s42003-018-0200-x
96. Cargill K, Sims-Lucas S. Metabolic requirements of the nephron. Pediatr Nephrol. (2020) 35:1–8. doi: 10.1007/s00467-018-4157-2
97. Njeim R, Alkhansa S, Fornoni A. Unraveling the crosstalk between lipids and NADPH oxidases in diabetic kidney disease. Pharmaceutics. (2023) 15:1360. doi: 10.3390/pharmaceutics15051360
98. Brown MS, Goldstein JL. The SREBP pathway: regulation of cholesterol metabolism by proteolysis of a membrane-bound transcription factor. Cell. (1997) 89:331–40. doi: 10.1016/s0092-8674(00)80213-5
99. Hao J, Zhu L, Zhao S, Liu S, Liu Q, Duan H. PTEN ameliorates high glucose-induced lipid deposits through regulating SREBP-1/FASN/ACC pathway in renal proximal tubular cells. Exp Cell Res. (2011) 317:1629–39. doi: 10.1016/j.yexcr.2011.02.003
100. Sun L, Halaihel N, Zhang W, Rogers T, Levi M. Role of sterol regulatory element-binding protein 1 in regulation of renal lipid metabolism and glomerulosclerosis in diabetes mellitus. J Biol Chem. (2002) 277:18919–27. doi: 10.1074/jbc.M110650200
101. Wang H, Zhu L, Hao J, Duan H, Liu S, Zhao S, et al. Co-regulation of SREBP-1 and mTOR ameliorates lipid accumulation in kidney of diabetic mice. Exp Cell Res. (2015) 336(1):76–84. doi: 10.1016/j.yexcr.2015.06.006
102. Szolkiewicz M, Chmielewski M, Nogalska A, Stelmanska E, Swierczynski J, Rutkowski B. The potential role of sterol regulatory element binding protein transcription factors in renal injury. J Ren Nutr. (2007) 17:62–5. doi: 10.1053/j.jrn.2006.10.009
103. Jun H, Song Z, Chen W, Zanhua R, Yonghong S, Shuxia L, et al. In vivo and in vitro effects of SREBP-1 on diabetic renal tubular lipid accumulation and RNAi-mediated gene silencing study. Histochem Cell Biol. (2009) 131(3):327–45. doi: 10.1007/s00418-008-0528-2
104. Nosadini R, Tonolo G. Role of oxidized low density lipoproteins and free fatty acids in the pathogenesis of glomerulopathy and tubulointerstitial lesions in type 2 diabetes. Nutr Metab Cardiovasc Dis. (2011) 21:79–85. doi: 10.1016/j.numecd.2010.10.002
105. Lee HS, Lee SK. Intraglomerular lipid deposition in renal disease. Miner Electrolyte Metab. (1993) 19:144–8.
106. Ruan XZ, Varghese Z, Powis SH, Moorhead JF. Dysregulation of LDL receptor under the influence of inflammatory cytokines: a new pathway for foam cell formation. Kidney Int. (2001) 60:1716–25. doi: 10.1046/j.1523-1755.2001.00025.x
107. Attie AD. ABCA1: at the nexus of cholesterol, HDL and atherosclerosis. Trends Biochem Sci. (2007) 32:172–9. doi: 10.1016/j.tibs.2007.02.001
108. Shiffman D, Pare G, Oberbauer R, Louie JZ, Rowland CM, Devlin JJ, et al. A gene variant in CERS2 is associated with rate of increase in albuminuria in patients with diabetes from ONTARGET and TRANSCEND. PloS One. (2014) 9(9):e106631. doi: 10.1371/journal.pone.0106631
109. Lovric S, Goncalves S, Gee HY, Oskouian B, Srinivas H, Choi WI, et al. Mutations in sphingosine-1-phosphate lyase cause nephrosis with ichthyosis and adrenal insufficiency. J Clin Invest. (2017) 127(3):912–28. doi: 10.1172/JCI89626
110. Mitrofanova A, Sosa MA, Fornoni A. Lipid mediators of insulin signaling in diabetic kidney disease. Am J Physiol Renal Physiol. (2019) 317:F1241–52. doi: 10.1152/ajprenal.00379.2019
111. Chen RX, Zhang L, Ye W, Wen YB, Si N, Li H, et al. The renal manifestations of type 4 familial partial lipodystrophy: a case report and review of literature. BMC Nephrol. (2018) 19(1):111. doi: 10.1186/s12882-018-0913-6
112. Saravani R, Noorzehi N, Galavi HR, Ranjbar N, Lotfian Sargazi M. Association of perilipin and insulin receptor substrate-1 genes polymorphism with lipid profiles, central obesity, and type 2 diabetes in a sample of an Iranian population. Iran Red Crescent Med J. (2017) 19:e55100. doi: 10.5812/ircmj
113. Kullak-Ublick GA, Hagenbuch B, Stieger B, Schteingart CD, Hofmann AF, Wolkoff AW, et al. Molecular and functional characterization of an organic anion transporting polypeptide cloned from human liver. Gastroenterology. (1995) 109(4):1274–82. doi: 10.1016/0016-5085(95)90588-X
114. More VR, Wen X, Thomas PE, Aleksunes LM, Slitt AL. Severe diabetes and leptin resistance cause differential hepatic and renal transporter expression in mice. Comp Hepatol. (2012) 11:1. doi: 10.1186/1476-5926-11-1
115. Zhang H, Zhao T, Li Z, Yan M, Zhao H, Zhu B, et al. Transcriptional profile of kidney from type 2 diabetic db/db mice. J Diabetes Res. (2017) 2017:8391253. doi: 10.1155/2017/8391253
116. Atlas D. International diabetes federation[J]. IDF Diabetes Atlas. 7th edn. Brussels, Belgium: International Diabetes Federation (2015) 33(2).
117. Sharma SK, Panneerselvam A, Singh KP, Parmar G, Gadge P, Swami OC. Teneligliptin in management of type 2 diabetes mellitus. Diabetes Metab Syndr Obes. (2016) 9:251–60. doi: 10.2147/DMSO
118. Cansby E, Caputo M, Gao L, Kulkarni NM, Nerstedt A, Ståhlman M, et al. Depletion of protein kinase STK25 ameliorates renal lipotoxicity and protects against diabetic kidney disease. JCI Insight. (2020) 5:e140483. doi: 10.1172/jci.insight.140483
119. Gonzalez CD, Carro Negueruela MP, Nicora Santamarina C, Resnik R, Vaccaro MI. Autophagy dysregulation in diabetic kidney disease: from pathophysiology to pharmacological interventions. Cells. (2021) 10:2497. doi: 10.3390/cells10092497
120. Ao L, Xie Y. Research advance in the mechanism for oxidative stress-induced podocyte injury in diabetic kidney disease. Zhong Nan Da Xue Xue Bao Yi Xue Ban. (2021) 46:1403–8. doi: 10.11817/j.issn.1672-7347.2021.210199
121. Perkovic V, Jardine MJ, Neal B, Bompoint S, Heerspink HJL, Charytan DM, et al. Canagliflozin and renal outcomes in type 2 diabetes and nephropathy. N Engl J Med. (2019) 380(24):2295–306. doi: 10.1056/NEJMoa1811744
122. Abubaker M, Mishra P, Swami OC. Teneligliptin in management of diabetic kidney disease: A review of place in therapy. J Clin Diagn Res. (2017) 11:OE05–9. doi: 10.7860/JCDR/2017/25060.9228
123. Pavkov ME, Collins AJ, Coresh J, Nelson RG. Kidney disease in diabetes. In: Cowie CC, Casagrande SS, Menke A, et al, editors. Diabetes in America. 3rd edn. Bethesda, MD: National Institute of Diabetes and Digestive and Kidney Diseases (US). (2018).
124. American Diabetes Association. Microvascular complications and foot care: standards of medical care in diabetes. Diabetes Care. (2020) 43:S135–51. doi: 10.2337/dc20-S011
125. Lu Q, Yang L, Xiao JJ, Liu Q, Ni L, Hu JW, et al. Empagliflozin attenuates the renal tubular ferroptosis in diabetic kidney disease through AMPK/NRF2 pathway. Free Radic Biol Med. (2023) 195:89–102. doi: 10.1016/j.freeradbiomed.2022.12.088
126. Wu X, Li H, Wan Z, Wang R, Liu J, Liu Q, et al. The combination of ursolic acid and empagliflozin relieves diabetic nephropathy by reducing inflammation, oxidative stress and renal fibrosis. BioMed Pharmacother. (2021) 144:112267. doi: 10.1016/j.biopha.2021.112267
127. Li D, Li Y. The interaction between ferroptosis and lipid metabolism in cancer. Signal Transduct Target Ther. (2020) 5:108. doi: 10.1038/s41392-020-00216-5
128. Bao WD, Pang P, Zhou XT, Hu F, Xiong W, Chen K, et al. Loss of ferroportin induces memory impairment by promoting ferroptosis in Alzheimer’s disease. Cell Death Differ. (2021) 28:1548–62. doi: 10.1038/s41418-020-00685-9
129. Fang X, Ardehali H, Min J, Wang F. The molecular and metabolic landscape of iron and ferroptosis in cardiovascular disease. Nat Rev Cardiol. (2023) 20:7–23. doi: 10.1038/s41569-022-00735-4
130. Wang J, Liu Y, Wang Y, Sun L. The cross-link between ferroptosis and kidney diseases. Oxid Med Cell Longev. (2021) 2021:6654887. doi: 10.1155/2021/6654887
132. Akatsuka S, Yamashita Y, Ohara H, Liu YT, Izumiya M, Abe K, et al. Fenton reaction induced cancer in wild type rats recapitulates genomic alterations observed in human cancer. PloS One. (2012) 7:e43403. doi: 10.1371/journal.pone.0043403
133. Kim JW, Lee JY, Oh M, Lee EW. An integrated view of lipid metabolism in ferroptosis revisited via lipidomic analysis. Exp Mol Med. (2023) 55:1620–31. doi: 10.1038/s12276-023-01077-y
134. Zielinski ZAM, Pratt DA. Cholesterol autoxidation revisited: debunking the dogma associated with the most vilified of lipids. J Am Chem Soc. (2016) 138:6932–5. doi: 10.1021/jacs.6b03344
135. Kitada M, Koya D. Autophagy in metabolic disease and ageing. Nat Rev Endocrinol. (2021) 17:647–61. doi: 10.1038/s41574-021-00551-9
136. Deretic V. Autophagy in inflammation, infection, and immunometabolism. Immunity. (2021) 54:437–53. doi: 10.1016/j.immuni.2021.01.018
137. Yu J, Liu Y, Li H, Zhang P. Pathophysiology of diabetic kidney disease and autophagy: A review. Med (Baltimore). (2023) 102:e33965. doi: 10.1097/MD.0000000000033965
138. Zhang Z, Sun Y, Xue J, Jin D, Li X, Zhao D, et al. The critical role of dysregulated autophagy in the progression of diabetic kidney disease. Front Pharmacol. (2022) 13:977410. doi: 10.3389/fphar.2022.977410
139. Liu P, Zhu W, Wang Y, Ma G, Zhao H, Li P. Chinese herbal medicine and its active compounds in attenuating renal injury via regulating autophagy in diabetic kidney disease. Front Endocrinol (Lausanne). (2023) 14:1142805. doi: 10.3389/fendo.2023.1142805
140. Li Z, Nakatogawa H. Degradation of nuclear components via different autophagy pathways. Trends Cell Biol. (2022) 32:574–84. doi: 10.1016/j.tcb.2021.12.008
141. Choi AM, Ryter SW, Levine B. Autophagy in human health and disease. N Engl J Med. (2013) 368:1845–6. doi: 10.1056/NEJMc1303158
142. Dikic I, Elazar Z. Mechanism and medical implications of mammalian autophagy. Nat Rev Mol Cell Biol. (2018) 19:349–64. doi: 10.1038/s41580-018-0003-4
143. Singh R, Kaushik S, Wang Y, Xiang Y, Novak I, Komatsu M, et al. Autophagy regulates lipid metabolism. Nature. (2009) 458:1131–5. doi: 10.1038/nature07976
144. Zhao X, Amevor FK, Cui Z, Wan Y, Xue X, Peng C, et al. Corrigendum to “Steatosis in metabolic diseases: A focus on lipolysis and lipophagy”. BioMed Pharmacother. (2023) 163:114842. doi: 10.1016/j.biopha.2023.114842
145. Lan ZQ, Ge ZY, Lv SK, Zhao B, Li CX. The regulatory role of lipophagy in central nervous system diseases. Cell Death Discovery. (2023) 9:229. doi: 10.1038/s41420-023-01504-z
146. Shin DW. Lipophagy: molecular mechanisms and implications in metabolic disorders. Mol Cells. (2020) 43:686–93. doi: 10.14348/molcells.2020.0046
147. Zhang X, Evans TD, Jeong SJ, Razani B. Classical and alternative roles for autophagy in lipid metabolism. Curr Opin Lipidol. (2018) 29:203–11. doi: 10.1097/MOL.0000000000000509
148. Schelling JR. The contribution of lipotoxicity to diabetic kidney disease. Cells. (2022) 11:3236. doi: 10.3390/cells11203236
149. Yan Q, Song Y, Zhang L, Chen Z, Yang C, Liu S, et al. Autophagy activation contributes to lipid accumulation in tubular epithelial cells during kidney fibrosis [published correction appears in Cell Death Discov. 2019 Jul 10;5:116]. Cell Death Discovery. (2018) 4:2. doi: 10.1038/s41420-018-0065-2
150. Sathyanarayan A, Mashek MT, Mashek DG. ATGL promotes autophagy/lipophagy via SIRT1 to control hepatic lipid droplet catabolism. Cell Rep. (2017) 19:1–9. doi: 10.1016/j.celrep.2017.03.026
151. Yacoub R, Lee K, He JC. The role of SIRT1 in diabetic kidney disease. Front Endocrinol (Lausanne). (2014) 5:166. doi: 10.3389/fendo.2014.00166
152. Ji J, Tao P, Wang Q, Li L, Xu Y. SIRT1: mechanism and protective effect in diabetic nephropathy. Endocr Metab Immune Disord Drug Targets. (2021) 21:835–42. doi: 10.2174/1871530320666201029143606
153. Yang L, Li P, Fu S, Calay ES, Hotamisligil GS. Defective hepatic autophagy in obesity promotes ER stress and causes insulin resistance. Cell Metab. (2010) 11:467–78. doi: 10.1016/j.cmet.2010.04.005
154. Pressly JD, Gurumani MZ, Varona Santos JT, Fornoni A, Merscher S, Al-Ali H. Adaptive and maladaptive roles of lipid droplets in health and disease. Am J Physiol Cell Physiol. (2022) 322:C468–81. doi: 10.1152/ajpcell.00239.2021
155. Zhang H, Yan S, Khambu B, Ma F, Li Y, Chen X, et al. Dynamic MTORC1-TFEB feedback signaling regulates hepatic autophagy, steatosis and liver injury in long-term nutrient oversupply. Autophagy. (2018) 14(10):1779–95. doi: 10.1080/15548627.2018.1490850
156. Seo AY, Lau PW, Feliciano D, Sengupta P, Gros MAL, Cinquin B, et al. AMPK and vacuole-associated Atg14p orchestrate μ-lipophagy for energy production and long-term survival under glucose starvation. Elife. (2017) 6:e21690. doi: 10.7554/eLife.21690
157. Brosius FC, Coward RJ. Podocytes, signaling pathways, and vascular factors in diabetic kidney disease. Adv Chronic Kidney Dis. (2014) 21:304–10. doi: 10.1053/j.ackd.2014.03.011
158. Wang Y, Zhao H, Wang Q, Zhou X, Lu X, Liu T, et al. Chinese herbal medicine in ameliorating diabetic kidney disease via activating autophagy. J Diabetes Res. (2019) 2019:9030893. doi: 10.1155/2019/9030893
159. Yang Z, Klionsky DJ. Eaten alive: a history of macroautophagy. Nat Cell Biol. (2010) 12:814–22. doi: 10.1038/ncb0910-814
160. Yamagata M, Obara K, Kihara A. Sphingolipid synthesis is involved in autophagy in Saccharomyces cerevisiae. Biochem Biophys Res Commun. (2011) 410:786–91. doi: 10.1016/j.bbrc.2011.06.061
161. Drexler Y, Molina J, Mitrofanova A, Fornoni A, Merscher S. Sphingosine-1-phosphate metabolism and signaling in kidney diseases. J Am Soc Nephrol. (2021) 32:9–31. doi: 10.1681/ASN.2020050697
162. Scarlatti F, Bauvy C, Ventruti A, Sala G, Cluzeaud F, Vandewalle A, et al. Ceramide-mediated macroautophagy involves inhibition of protein kinase B and up-regulation of beclin 1. J Biol Chem. (2004) 279(18):18384–91. doi: 10.1074/jbc.M313561200
163. Kim SJ, Tang T, Abbott M, Viscarra JA, Wang Y, Sul HS. AMPK phosphorylates desnutrin/ATGL and hormone-sensitive lipase to regulate lipolysis and fatty acid oxidation within adipose tissue. Mol Cell Biol. (2016) 36:1961–76. doi: 10.1128/MCB.00244-16
164. Dodson M, Darley-Usmar V, Zhang J. Cellular metabolic and autophagic pathways: traffic control by redox signaling. Free Radic Biol Med. (2013) 63:207–21. doi: 10.1016/j.freeradbiomed.2013.05.014
165. Ng MYW, Charsou C, Lapao A, Singh S, Trachsel-Moncho L, Schultz SW, et al. The cholesterol transport protein GRAMD1C regulates autophagy initiation and mitochondrial bioenergetics. Nat Commun. (2022) 13(1):6283. doi: 10.1038/s41467-022-33933-2
166. Lin Z, Long F, Kang R, Klionsky DJ, Yang M, Tang D. The lipid basis of cell death and autophagy. Autophagy. (2024) 20(3):469–88. doi: 10.1080/15548627.2023.2259732
167. Yang C, Zhang Z, Liu J, Chen P, Li J, Shu H, et al. Research progress on multiple cell death pathways of podocytes in diabetic kidney disease. Mol Med. (2023) 29(1):135. doi: 10.1186/s10020-023-00732-4
168. Singh A, Mittal M. Neonatal microbiome-a brief review. J Matern Fetal Neona. (2020) 33:3841–8. doi: 10.1080/14767058.2019.1583738
169. McLean MH, Dieguez D Jr, Miller LM, Young HA. Does the microbiota play a role in the pathogenesis of autoimmune diseases? Gut. (2015) 64:332–41. doi: 10.1136/gutjnl-2014-308514
170. Wu HJ, Wu E. The role of gut microbiota in immune homeostasis and autoimmunity. Gut Microbes. (2012) 3:4–14. doi: 10.4161/gmic.19320
171. Sanidad KZ, Amir M, Ananthanarayanan A, Singaraju A, Shiland NB, Hong HS, et al. Maternal gut microbiome-induced IgG regulates neonatal gut microbiome and immunity. Sci Immunol. (2022) 7(72):eabh3816. doi: 10.1126/sciimmunol.abh3816
172. Brown EM, Kenny DJ, Xavier RJ. Gut microbiota regulation of t cells during inflammation and autoimmunity. Annu Rev Immunol. (2019) 37:599–624. doi: 10.1146/annurev-immunol-042718-041841
173. Rooks MG, Garrett WS. Gut microbiota, metabolites and host immunity. Nat Rev Immunol. (2016) 16:341–52. doi: 10.1038/nri.2016.42
174. Chang PV, Hao LM, Offermanns S, Medzhitov R. The microbial metabolite butyrate regulates intestinal macrophage function via histone deacetylase inhibition. Proc Natl Acad Sci USA. (2014) 111(6):2247–52. doi: 10.1073/pnas.1322269111
175. Tian Y, Gui W, Koo I, Smith PB, Allman EL, Nichols RG, et al. The microbiome modulating activity of bile acids. Gut Microbes. (2020) 11(4):979–96. doi: 10.1080/19490976.2020.1732268
176. Lanza M, Filippone A, Ardizzone A, Casili G, Paterniti I, Esposito E, et al. SCFA treatment alleviates pathological signs of migraine and related intestinal alterations in a mouse model of NTG-induced migraine. Cells. (2021) 10:2756. doi: 10.3390/cells10102756
177. McGlone ER, Bloom SR. Bile acids and the metabolic syndrome. Ann Clin Biochem. (2019) 56:326–37. doi: 10.1177/0004563218817798
178. Shao JW, Ge TT, Chen SZ, Wang G, Yang Q, Huang CH, et al. Role of bile acids in liver diseases mediated by the gut microbiome. World J Gastroenterol. (2021) 27(22):3010–21. doi: 10.3748/wjg.v27.i22.3010
179. Chi MX, Ma K, Wang J, Ding Z, Li Y, Zhu S, et al. The immunomodulatory effect of the gut microbiota in kidney disease. J Immunol Res. (2021) 15:5516035. doi: 10.1155/2021/5516035
180. Zaky A, Glastras SJ, Wong MYW, Pollock CA, Saad S. The role of the gut microbiome in diabetes and obesity-related kidney disease. Int J Mol Sci. (2021) 22:9641. doi: 10.3390/ijms22179641
181. Amini Khiabani S, Asgharzadeh M, Samadi Kafil H. Chronic kidney disease and gut microbiota. Heliyon. (2023) 9:e18991. doi: 10.1016/j.heliyon.2023.e18991
182. Bush KT, Wu W, Lun C, Nigam SK. The drug transporter OAT3 (SLC22A8) and endogenous metabolite communication via the gut-liver-kidney axis. J Biol Chem. (2017) 292:15789–803. doi: 10.1074/jbc.M117.796516
183. Tan JK, McKenzie C, Mariño E, Macia L, Mackay CR. Metabolite-sensing G protein-coupled receptors-facilitators of diet-related immune regulation. Annu Rev Immunol. (2017) 35:371–402. doi: 10.1146/annurev-immunol-051116-052235
184. Thorburn AN, Macia L, Mackay CR. Diet, metabolites, and “Western-lifestyle” inflammatory diseases. Immunity. (2014) 40:833–42. doi: 10.1016/j.immuni.2014.05.014
185. Liao D, Liu YQ, Xiong LY, Zhang L. Renoprotective effect of atorvastatin on STZ-diabetic rats through inhibiting inflammatory factors expression in diabetic rat. Eur Rev Med Pharmacol Sci. (2016) 20:1888–93.
186. Tang C, Deng X, Qu J, Miao Y, Tian L, Zhang M, et al. Fenofibrate attenuates renal tubular cell apoptosis by up-regulating MCAD in diabetic kidney disease. Drug Des Devel Ther. (2023) 17:1503–14. doi: 10.2147/DDDT.S405266
187. Wang S, Yang Z, Xiong F, Chen C, Chao X, Huang J, et al. Betulinic acid ameliorates experimental diabetic-induced renal inflammation and fibrosis via inhibiting the activation of NF-κB signaling pathway. Mol Cell Endocrinol. (2016) 434:135–43. doi: 10.1016/j.mce.2016.06.019
188. Yamada S, Tanabe J, Ogura Y, Nagai Y, Sugaya T, Ohata K, et al. Renoprotective effect of GLP-1 receptor agonist, liraglutide, in early-phase diabetic kidney disease in spontaneously diabetic Torii fatty rats. Clin Exp Nephrol. (2021) 25:365–75. doi: 10.1007/s10157-020-02007-2
189. Dugbartey GJ, Alornyo KK, Diaba DE, Adams I. Activation of renal CSE/H2S pathway by alpha-lipoic acid protects against histological and functional changes in the diabetic kidney. BioMed Pharmacother. (2022) 153:113386. doi: 10.1016/j.biopha.2022.113386
190. Choi SR, Lim JH, Kim MY, Kim EN, Kim Y, Choi BS, et al. Adiponectin receptor agonist AdipoRon decreased ceramide, and lipotoxicity, and ameliorated diabetic nephropathy. Metabolism. (2018) 85:348–60. doi: 10.1016/j.metabol.2018.02.004
191. Kim Y, Lim JH, Kim MY, Kim EN, Yoon HE, Shin SJ, et al. The adiponectin receptor agonist adipoRon ameliorates diabetic nephropathy in a model of type 2 diabetes. J Am Soc Nephrol. (2018) 29:1108–27. doi: 10.1681/ASN.2017060627
192. Santana MFM, Lira ALA, Pinto RS, Minanni CA, Silva ARM, Sawada MIBAC, et al. Enrichment of apolipoprotein A-IV and apolipoprotein D in the HDL proteome is associated with HDL functions in diabetic kidney disease without dialysis. Lipids Health Dis. (2020) 19:205. doi: 10.1186/s12944-020-01381-w
193. Zhou Y, Liu L, Jin B, Wu Y, Xu L, Chang X, et al. Metrnl alleviates lipid accumulation by modulating mitochondrial homeostasis in diabetic nephropathy. Diabetes. (2023) 72:611–26. doi: 10.2337/db22-0680
194. Lin S, Wang L, Jia Y, Sun Y, Qiao P, Quan Y, et al. Lipin-1 deficiency deteriorates defect of fatty acid β-oxidation and lipid-related kidney damage in diabetic kidney disease. Transl Res. (2023) 266:1–15. doi: 10.1016/j.trsl.2023.07.004
195. Liu S, Da J, Yu J, Dong R, Yuan J, Yu F, et al. Renal tubule ectopic lipid deposition in diabetic kidney disease rat model and in vitro mechanism of leptin intervention. J Physiol Biochem. (2022) 78:389–99. doi: 10.1007/s13105-022-00874-9
196. Zhang J, Wu Y, Zhang J, Zhang R, Wang Y, Liu F. ABCA1 deficiency-mediated glomerular cholesterol accumulation exacerbates glomerular endothelial injury and dysfunction in diabetic kidney disease. Metabolism. (2023) 139:155377. doi: 10.1016/j.metabol.2022.155377
197. Li X, Xu B, Wu J, Pu Y, Wan S, Zeng Y, et al. Maresin 1 Alleviates Diabetic Kidney Disease via LGR6-Mediated cAMP-SOD2-ROS Pathway. Oxid Med Cell Longev. (2022) 2022:7177889. doi: 10.1155/2022/7177889
198. Yu M, Wang D, Zhong D, Xie W, Luo J. Adropin carried by reactive oxygen species-responsive nanocapsules ameliorates renal lipid toxicity in diabetic mice. ACS Appl Mater Interfaces. (2022) 14:37330–44. doi: 10.1021/acsami.2c06957
199. Castañeda TR, Méndez M, Davison I, Elvert R, Schwahn U, Boldina G, et al. The novel phosphate and bile acid sequestrant polymer SAR442357 delays disease progression in a rat model of diabetic nephropathy. J Pharmacol Exp Ther. (2021) 376:190–203. doi: 10.1124/jpet.120.000285
200. Sun ZJ, Chang DY, Chen M, Zhao MH. Deficiency of CFB attenuates renal tubulointerstitial damage by inhibiting ceramide synthesis in diabetic kidney disease. JCI Insight. (2022) 7:e156748. doi: 10.1172/jci.insight.156748
201. Qin X, Jiang M, Zhao Y, Gong J, Su H, Yuan F, et al. Berberine protects against diabetic kidney disease via promoting PGC-1α-regulated mitochondrial energy homeostasis. Br J Pharmacol. (2020) 177:3646–61. doi: 10.1111/bph.14935
202. Qin X, Zhao Y, Gong J, Huang W, Su H, Yuan F, et al. Berberine protects glomerular podocytes via inhibiting drp1-mediated mitochondrial fission and dysfunction. Theranostics. (2019) 9:1698–713. doi: 10.7150/thno.30640
203. Wang FL, Tang LQ, Yang F, Zhu LN, Cai M, Wei W. Renoprotective effects of berberine and its possible molecular mechanisms in combination of high-fat diet and low-dose streptozotocin-induced diabetic rats. Mol Biol Rep. (2013) 40:2405–18. doi: 10.1007/s11033-012-2321-5
204. Wu R, Liang Y, Xu M, Fu K, Zhang Y, Wu L, et al. Advances in chemical constituents, clinical applications, pharmacology, pharmacokinetics and toxicology of erigeron breviscapus. Front Pharmacol. (2021) 12:656335. doi: 10.3389/fphar.2021.656335
205. Liu X, Yao L, Sun D, Zhu X, Liu Q, Xu T, et al. Effect of breviscapine injection on clinical parameters in diabetic nephropathy: A meta-analysis of randomized controlled trials. Exp Ther Med. (2016) 12:1383–97. doi: 10.3892/etm.2016.3483
206. Udrea AM, Gradisteanu Pircalabioru G, Boboc AA, Mares C, Dinache A, Mernea M, et al. Advanced bioinformatics tools in the pharmacokinetic profiles of natural and synthetic compounds with anti-diabetic activity. Biomolecules. (2021) 11(11):1692. doi: 10.3390/biom11111692
207. Wang Y, Shi LL, Wang LY, Xu JW, Feng Y. Protective effects of MDG-1, a polysaccharide from ophiopogon japonicus on diabetic nephropathy in diabetic KKAy mice. Int J Mol Sci. (2015) 16:22473–84. doi: 10.3390/ijms160922473
208. Swallah MS, Bondzie-Quaye P, Wu Y, Acheampong A, Sossah FL, Elsherbiny SM, et al. Therapeutic potential and nutritional significance of Ganoderma lucidum - a comprehensive review from 2010 to 2022. Food Funct. (2023) 14(4):1812–38. doi: 10.1039/D2FO01683D
209. Pan Y, Zhang Y, Li J, Zhang Z, He Y, Zhao Q, et al. A proteoglycan isolated from Ganoderma lucidum attenuates diabetic kidney disease by inhibiting oxidative stress-induced renal fibrosis both in vitro and in vivo. J Ethnopharmacol. (2023) 310:116405. doi: 10.1016/j.jep.2023.116405
210. Tang X, Huang M, Jiang J, Liang X, Li X, Meng R, et al. Panax notoginseng preparations as adjuvant therapy for diabetic kidney disease: a systematic review and meta-analysis. Pharm Biol. (2020) 58(1):138–45. doi: 10.1080/13880209.2020.1711782
211. Zhang B, Zhang X, Zhang C, Shen Q, Sun G, Sun X. Notoginsenoside R1 Protects db/db Mice against Diabetic Nephropathy via Upregulation of Nrf2-Mediated HO-1 Expression. Molecules. (2019) 24:247. doi: 10.3390/molecules24020247
212. Liang D, Mai H, Ruan F, Fu H. The efficacy of triptolide in preventing diabetic kidney diseases: A systematic review and meta-analysis. Front Pharmacol. (2021) 12:728758. doi: 10.3389/fphar.2021.728758
213. Dong XG, An ZM, Guo Y, Zhou JL, Qin T. Effect of triptolide on expression of oxidative carbonyl protein in renal cortex of rats with diabetic nephropathy. J Huazhong Univ Sci Technolog Med Sci. (2017) 37:25–9. doi: 10.1007/s11596-017-1689-9
214. Gao Q, Shen W, Qin W, Zheng C, Zhang M, Zeng C, et al. Treatment of db/db diabetic mice with triptolide: a novel therapy for diabetic nephropathy. Nephrol Dial Transplant. (2010) 25:3539–47. doi: 10.1093/ndt/gfq245
215. Boers HM, van Dijk TH, Duchateau GS, Mela DJ, Hiemstra H, Hoogenraad AR, et al. Effect of mulberry fruit extract on glucose fluxes after a wheat porridge meal: a dual isotope study in healthy human subjects. Eur J Clin Nutr. (2023) 77(7):741–7. doi: 10.1038/s41430-023-01282-y
216. Taghizadeh M, Soleimani A, Bahmani F, Moravveji A, Asadi A, Amirani E, et al. Metabolic response to mulberry extract supplementation in patients with diabetic nephropathy: a randomized controlled trial. Iran J Kidney Dis. (2017) 11:438–46.
217. Jin D, Zhang Y, Zhang Y, Duan L, Zhou R, Duan Y, et al. Panax ginseng C.A.Mey. as medicine: the potential use of panax ginseng C.A.Mey. as a remedy for kidney protection from a pharmacological perspective. Front Pharmacol. (2021) 12:734151. doi: 10.3389/fphar.2021.734151
218. Wang T, Huang X, Zhai K, Yu J, Li J, Duan H, et al. Integrating metabolomics and network pharmacology to investigate Panax japonicus prevents kidney injury in HFD/STZ-induced diabetic mice. J Ethnopharmacol. (2023) 303:115893. doi: 10.1016/j.jep.2022.115893
219. Salami M, Salami R, Mafi A, Aarabi MH, Vakili O, Asemi Z. Therapeutic potential of resveratrol in diabetic nephropathy according to molecular signaling. Curr Mol Pharmacol. (2022) 15:716–35. doi: 10.2174/1874467215666211217122523
220. Gu W, Wang X, Zhao H, Geng J, Li X, Zheng K, et al. Resveratrol ameliorates diabetic kidney injury by reducing lipotoxicity and modulates expression of components of the junctional adhesion molecule-like/sirtuin 1 lipid metabolism pathway. Eur J Pharmacol. (2022) 918:174776. doi: 10.1016/j.ejphar.2022.174776
221. Liu W, Gao Y, Zhou Y, Yu F, Li X, Zhang N. Mechanism of Cordyceps sinensis and its Extracts in the Treatment of Diabetic Kidney Disease: A Review. Front Pharmacol. (2022) 13:881835. doi: 10.3389/fphar.2022.881835
222. Yang J, Dong H, Wang Y, Jiang Y, Zhang W, Lu Y, et al. Cordyceps cicadae polysaccharides ameliorated renal interstitial fibrosis in diabetic nephropathy rats by repressing inflammation and modulating gut microbiota dysbiosis. Int J Biol Macromol. (2020) 163:442–56. doi: 10.1016/j.ijbiomac.2020.06.153
223. Wojcikowski K, Johnson DW, Gobé G. Medicinal herbal extracts–renal friend or foe? Part two: herbal extracts with potential renal benefits. Nephrol (Carlton). (2004) 9:400–5. doi: 10.1111/j.1440-1797.2004.00355.x
224. Zhao J, Zhang QL, Shen JH, Wang K, Liu J. Magnesium lithospermate B improves the gut microbiome and bile acid metabolic profiles in a mouse model of diabetic nephropathy. Acta Pharmacol Sin. (2019) 40:507–13. doi: 10.1038/s41401-018-0029-3
225. Dutta K, Kravtsov V, Oleynikova K, Ruzov A, Skorb EV, Shityakov S. Analyzing the effects of single nucleotide polymorphisms on hnRNPA2/B1 protein stability and function: insights for anticancer therapeutic design. ACS Omega. (2024) 9:5485–95. doi: 10.1021/acsomega.3c07195
226. Fareed MM, Dutta K, Dandekar T, Tarabonda H, Skorb EV, Shityakov S. In silico investigation of nonsynonymous single nucleotide polymorphisms in BCL2 apoptosis regulator gene to design novel protein-based drugs against cancer. J Cell Biochem. (2022) 123:2044–56. doi: 10.1002/jcb.30330
227. Karnati S, Guntas G, Rajendran R, Shityakov S, Höring M, Liebisch G, et al. Quantitative lipidomic analysis of takotsubo syndrome patients’ Serum. Front Cardiovasc Med. (2022) 9:797154. doi: 10.3389/fcvm.2022.797154
228. Fareed MM, Khalid H, Khalid S, Shityakov S. Deciphering molecular mechanisms of carbon tetrachlorideInduced hepatotoxicity (Fibrosis): A brief systematic review. Curr Mol Med. (2023). doi: 10.2174/0115665240257603230919103539
229. Mugnai ML, Shi Y, Keatinge-Clay AT, Elber R. Molecular dynamics studies of modular polyketide synthase ketoreductase stereospecificity. Biochemistry. (2015) 54:2346–59. doi: 10.1021/bi501401g
Keywords: diabetic kidney disease, ferroptosis, gut microbiota, lipid metabolism, metabolic reprogramming, treatment
Citation: Han Y-Z, Du B-X, Zhu X-Y, Wang Y-Z-Y, Zheng H-J and Liu W-J (2024) Lipid metabolism disorder in diabetic kidney disease. Front. Endocrinol. 15:1336402. doi: 10.3389/fendo.2024.1336402
Received: 10 November 2023; Accepted: 09 April 2024;
Published: 29 April 2024.
Edited by:
Vinod Tiwari, Indian Institute of Technology (BHU), IndiaReviewed by:
Sergey Shityakov, ITMO University, RussiaCopyright © 2024 Han, Du, Zhu, Wang, Zheng and Liu. This is an open-access article distributed under the terms of the Creative Commons Attribution License (CC BY). The use, distribution or reproduction in other forums is permitted, provided the original author(s) and the copyright owner(s) are credited and that the original publication in this journal is cited, in accordance with accepted academic practice. No use, distribution or reproduction is permitted which does not comply with these terms.
*Correspondence: Hui-Juan Zheng, dGNtemhlbmdodWlqdWFuQDE2My5jb20=; Wei-Jing Liu, bGl1d2VpamluZy0xOTc3QGhvdG1haWwuY29t
†These authors have contributed equally to this work
Disclaimer: All claims expressed in this article are solely those of the authors and do not necessarily represent those of their affiliated organizations, or those of the publisher, the editors and the reviewers. Any product that may be evaluated in this article or claim that may be made by its manufacturer is not guaranteed or endorsed by the publisher.
Research integrity at Frontiers
Learn more about the work of our research integrity team to safeguard the quality of each article we publish.