- 1College of Traditional Chinese Medicine, Changchun University of Traditional Chinese Medicine, Changchun, China
- 2Guang’an Men Hospital of China Academy of Chinese Medical Sciences, Beijing, China
- 3Fangshan Hospital, Beijing University of Chinese Medicine, Beijing, China
Diabetic nephropathy (DN) and diabetic retinopathy (DR), as microvascular complications of diabetes mellitus, are currently the leading causes of end-stage renal disease (ESRD) and blindness, respectively, in the adult working population, and they are major public health problems with social and economic burdens. The parallelism between the two in the process of occurrence and development manifests in the high overlap of disease-causing risk factors and pathogenesis, high rates of comorbidity, mutually predictive effects, and partial concordance in the clinical use of medications. However, since the two organs, the eye and the kidney, have their unique internal environment and physiological processes, each with specific influencing molecules, and the target organs have non-parallelism due to different pathological changes and responses to various influencing factors, this article provides an overview of the parallelism and non-parallelism between DN and DR to further recognize the commonalities and differences between the two diseases and provide references for early diagnosis, clinical guidance on the use of medication, and the development of new drugs.
Introduction
The International Diabetes Federation (IDF) Diabetes Map 2021 shows that the world’s adult diabetic population has reached 537 million in 2021, while the adult population with the disease grows by 1.7% to a projected 783 million in 2045 (1). Complications of diabetes mellitus can be categorized into acute and chronic complications, and chronic complications caused by long-term exposure to hyperglycemia can be categorized into microangiopathy, macrovascular disease, and neuropathy (2, 3). Among them, diabetic nephropathy (DN) and diabetic retinopathy (DR), as highly prevalent microvascular complications of diabetes, have become the leading causes of end-stage renal disease (ESRD) and blindness in adult patients, and the disease puts enormous economic pressure on the healthcare system as well as on the patients themselves (4–7). The study provides a comprehensive and up-to-date assessment of the current and 2045 global prevalence of DR through the largest meta-analysis to date, showing that the global prevalence of DR in the diabetic population is estimated to be 22.27%, in 2020, the number of adults with DR will be approximately 103.12 million globally. It will increase by 55.6% to 160.5 million in 2045 (6). DN is now the leading cause of ESRD in the adult working population, accounting for approximately 50% of developed countries (7). A meta-analysis of the prevalence of comorbid DN in the Chinese type 2 diabetes mellitus type 2 (T2DM) population (79,364 study subjects) showed that nearly 1/5 of diabetic patients have comorbid DN (8). It has been reported that type 1 diabetes mellitus (T1DM) patients with DR and T2DM patients without DR are 13.39 and 3.51 times more likely to develop DN, respectively. The prevalence of DN increases with the increasing severity of DR, and the two diseases are closely related (9). A cross-sectional study based on 26,809 patients in five primary hospitals in China showed prevalence rates of DN and DR of 32.3% and 34.6%, respectively, with a positive predictive value of 47.4% for DN (10). Increases in DR severity were strongly associated with increases in DN severity (11).
DN and DR have parallelism and non-parallelism in disease onset and progression. The parallelism of the two diseases includes the mutual predictive effect of predicting the development of the other diseases through one of them, the four aspects of common causative factors, similar pathogenesis, and common use of medication. Non-parallelism includes asynchrony of onset, variability of pathogenic factors, different pathogenic mechanisms, and medications. Exploring the parallelism of two diseases can, on the one hand, predict the development of the other diseases through one disease and take timely and effective preventive measures to prevent the development and deterioration of the disease; on the other hand, the use of generic drugs to treat the two diseases through the parallelism of the diseases can reduce the use of drugs, optimize the management of the disease and the use of medication, and alleviate the financial burden of the patients and the country. This paper reviews the parallelism and non-parallelism of the two diseases, recognizes the commonalities and differences between the two diseases, and guides the selection of clinical drugs, with a view to early diagnosis, delaying the onset and progression of the two diseases, improving patients’ quality of life, and reducing the burden on society.
Parallelism between DN and DR development
Microvascular pathological changes in diabetes mellitus include the thickening of capillary basement membranes due to hyperglycemia (12), increased permeability and dysfunction of endothelial cells (13–15), and vascular smooth muscle cell dysfunction (16, 17). Despite their functional differences, the kidney and the eye share similarities in developmental pathways and molecular structure (18, 19); the glomerulus and choroid have similar structures and vascular networks (19); the internal blood–retina barrier (BRB) and the glomerular filtration barrier have similar developmental pathways (20); the renin–angiotensin–aldosterone system (RAAS) found in both organs, the eye and the kidney (21, 22), leads to parallelism in their pathogenesis. In the following, the parallelism of the two diseases’ development will be described in terms of their mutual predictive effects, disease-influencing factors, identical pathogenesis, and common medication.
Reciprocal predictive effects of DN and DR
DN and DR are closely related as both microvascular complications of diabetes mellitus (23). The severity of renal impairment in DN correlates with the severity of ocular damage in DR (24). The presence of DR can be diagnosed by fundus examination. In contrast, the kidneys are hidden in the abdomen of the human body, so doctors cannot accurately see the specific situation, and the gold standard for diagnosing renal disease is renal puncture biopsy (25). However, renal puncture biopsy is an invasive test, which is often difficult for the majority of patients to accept, so the use of fundus examination to predict renal disease is increasingly becoming the primary means of clinical doctors to screen for DN. At the same time, proteinuria in patients with DN is also often used to predict the severity of DN and predict the development of DR.
DN predicts DR
In a cross-sectional study, 250 T2DM patients with DN diagnosed by renal biopsy were divided into two groups: 130 patients in the DN without DR group and 120 patients in the DN and concomitant DR group. Logistic regression analysis was performed on the above 250 patients to clarify the risk factors for DR, and the results showed that the risk of DR was significantly associated with the risk of developing DR by proteinuria, hematuria, estimated glomerular filtration rate (eGFR) baseline correction, glomerulopathy severity, and DM history >10 years were significantly associated with the risk of developing DR (26). Through a cross-sectional study of 1,102 T2DM patients aged not less than 30 years recruited in Korea in 2010–2011, the results showed that grouped according to DR severity, non-proliferative diabetic retinopathy (NPDR), severe NPDR, and proliferative diabetic retinopathy (PDR), with their early morning field urine samples retained for urinary albumin–creatinine ratio (ACR) measurement, the results showed that the optimal cutoff value of ACR for predicting DR was 2.26 mg/mmol (20 μg/mg), and as the severity of DR increased, ACR ≥ 2.26 mg/mmol tended to increase, and the risk of severe NPDR and PDR also increased, demonstrating that ACR is an independent risk factor for DR (27). Also, in DR patients, even in the absence of proteinuria, we can predict subclinical DN based on eGFR (11).
DR predicts DN
DR was found to be associated with an increased risk of DN prevalence in patients with T2DM through a prospective study. However, the predictive value of DR for the risk of DN in patients with T2DM was relatively low, possibly since the predictive value of DR for DN may be affected by aspects such as mean age, proportion of men, and study quality (28). In a 25-year follow-up based on a cohort study of 184 patients from Denmark, it was suggested that PDR in patients with T1DM may be an independent marker of long-term nephropathy development (29). A deep learning model validated with 115,344 retinal fundus photographs from 57,672 patients indicates that chronic kidney disease staging and eGFR prediction can be performed by retinal fundus image testing and that the model evaluates the level of agreement between the algorithm’s predicted GFR and the measured eGFR by means of a Bland–Altman plot. The results showed that the artificial intelligence (AI) model was able to extract the information for predicting GFR and embed it skillfully in the fundus images, and the deployment of AI fundus diagnostic system can be used as a non-invasive, high-throughput, and low-cost screening tool in the early stage of renal disease regardless of geographic display to effectively predict the progression of renal disease (30). A cross-sectional study shows that DR, especially PDR, is an independent predictor of kidney disease (9).
Risk factors associated with DN and DR
Age, male gender, hypertension, diabetes duration, diabetic neuropathy, DN, diabetic foot ulcers, and foot amputation were used as independent risk factors for DR based on two cross-sectional studies in urban hospitals in China (31, 32). A cross-sectional study based on 13,473 diabetic patients complemented postprandial glucose, glycosylated hemoglobin (HbAlc), triglycerides, and low-density lipoprotein as independent risk factors for DR, with preprandial glucose, postprandial glucose, and HbAlc all being significant predictors of the development or progression of DR (33), which showed that each 1% increase in HbAlc was associated with a 40% increase in the risk of microvascular events (34). In addition, anemia (35, 36), pregnancy (37), smoking (38), genetic factors (39), and microalbuminuria (40, 41) have been shown in multiple trials to increase the risk of DR. Meta-analysis of multiple prospective cohort studies suggests that obesity is a risk factor for DR (42, 43).
Studies have shown that elevated blood glucose levels, long duration of diabetes, hypertension, obesity, and dyslipidemia can contribute to the development of DN (44). Elevated microalbuminuria levels increase the risk of DN (45, 46). Numerous epidemiologic investigations have shown that genetic susceptibility contributes to the development of DN (47–49). A systematic evaluation and meta-analysis based on 20 cohorts identified age, body mass index, smoking, DR, glycosylated hemoglobin, systolic blood pressure, high-density lipoprotein, triglycerides, and urinary albumin/creatinine ratio as risk factors for the development of DN (50). The study results show a substantial overlap in the causative risk factors of the two diseases.
Similar pathogenesis of DN and DR
DN and DR are microvascular complications of diabetes mellitus, and the pathological features of retinal tissue in patients with DR include loss of pericytes and endothelial cells, destruction of BRB, and retinal neovascularization (51, 52). Pathological changes in DN may include glomerular tunica dilatation, basement membrane thickening, glomerular endothelial injury, podocyte injury, and tubular junction abnormalities (53–56). Diabetes-induced endothelial dysfunction in microangiopathy is characterized by decreased nitric oxide (NO) bioavailability, increased oxidative stress, imbalance between vascular endothelial growth factor (VEGF) and NO, and impaired endothelial function repair (57). In the diabetic microvascular system, intracellular hyperglycemia induces vascular endothelial injury through various pathophysiological processes, including inflammation, endothelial cell–reticulocyte/pericyte conversation, and exocytosis (58). In addition, several key regulators such as cell adhesion molecule (CAM), VEGF family, and Notch signaling are involved in developing DN and DR diseases (58). Since the eye and the kidney share similarities in organizational structure and physiological function, exposure to the same risk factors often leads to the occurrence of the two diseases, with simultaneous similarities in pathogenesis (Figure 1).
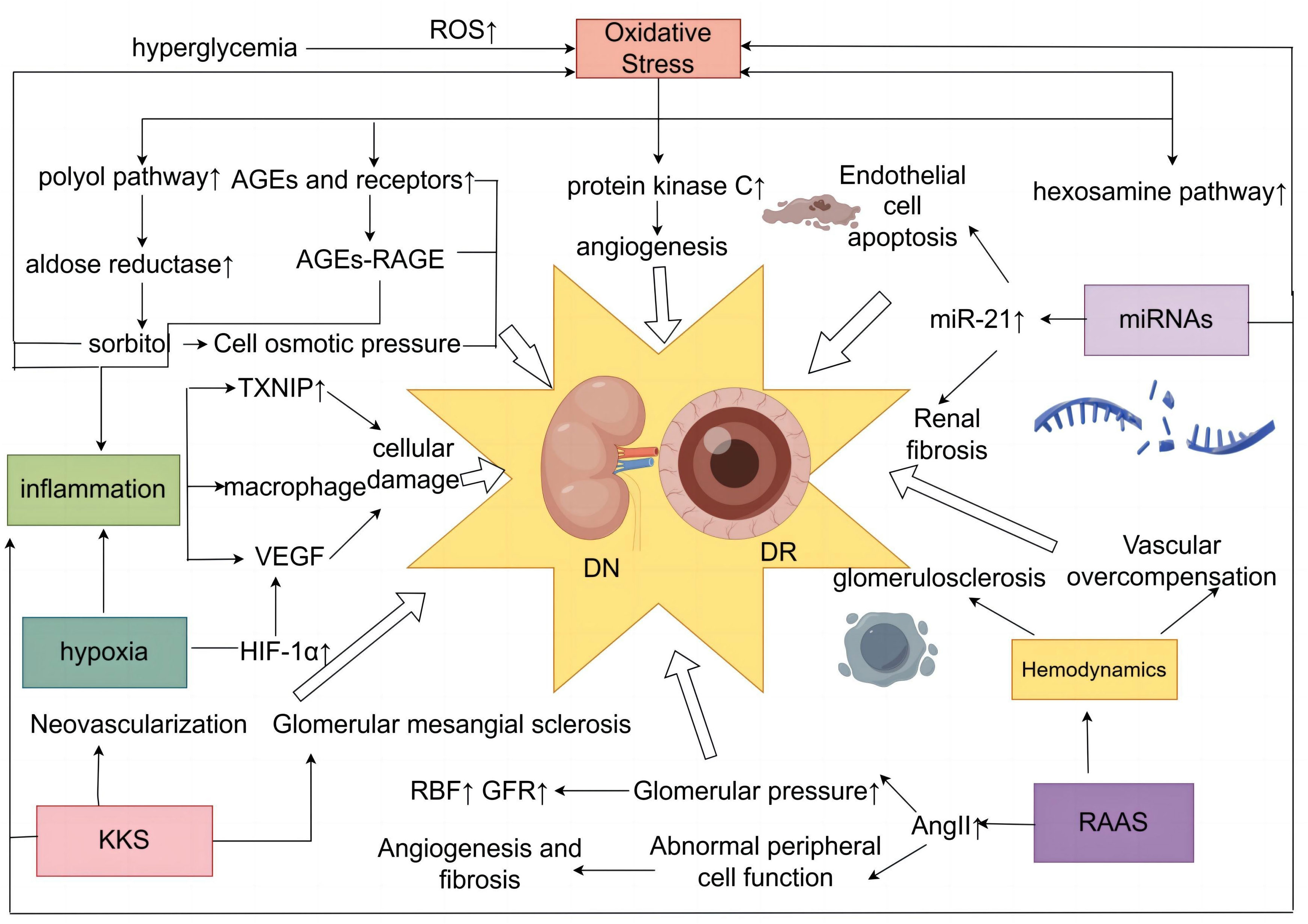
Figure 1 Diagram of the common mechanism of DN and DR. DN, diabetic nephropathy; DR, diabetic retinopathy.
Oxidative stress
Oxidative stress plays a vital role in the development of microvascular and cardiovascular complications in diabetes mellitus (59). Excessive production of reactive oxygen species (ROS) is a major cause of oxidative stress (60–62). Hyperglycemia plays a central role in the development of microvascular complications in diabetes mellitus (63), with a 37% reduction in the incidence of microvascular end-point events for every 1% reduction in HbAlc (64), and a sustained state of hyperglycemia in the cell induces an overproduction of mitochondrial ROS (65). This increased production of ROS is a central and primary mediator of diabetic tissue damage, with this single upstream mediator leading to the activation of five major pathways involved in the pathogenesis of complications, including activation of the polyol pathway (66), increased formation of advanced glycosylation end products (AGEs) (67), increased expression of receptors for AGEs and their activating ligands (68), activation of protein kinase C (PKC) isoforms (69), and enhancement of the hexosamine pathway (70), which are present in the mechanisms of DN and DR production (65, 71) Hyperglycemia-induced ROS triggers renal fibrosis and inflammation and causes significant tissue damage by promoting lipid peroxidation, DNA damage and protein modification, and mitochondrial dysfunction, inducing DN (72). Overproduction of ROS in the diabetic retina leads to retinal cell damage by altering cell signaling, ultimately leading to DR (73).
AGEs aggregated in the hyperglycemic state play an important role in DR (74). AGEs can induce the cross-linking of extracellular matrix proteins through direct action, leading to endothelial dysfunction and neural and vascular components (75, 76). AGEs also interact with specific receptors and binding proteins to induce oxidative stress and cellular dysfunction involved in the progression of DN (77). The polyol pathway contains aldose reductase (AR), mainly found in tissues such as the retina, lens, and kidney (78). Abnormally elevated blood glucose can lead to activation of the AR, which oxidizes glucose to sorbitol and can induce or exacerbate intracellular oxidative stress (79–81), promoting diabetic microangiopathy. Hyperglycemia triggers the glycolytic pathway, which further enhances the synthesis of di-glycerol (DAG), and PKC is activated as a target protein kinase for di-glycerol (82). Total DAG levels in vascular tissues are increased in the retina (83, 84) and glomeruli (85, 86) of diabetic patients. PKC activation impairs glomerular blood flow and filtration, causing renal hypertrophy and glomerular hyperplasia and contributing to the onset and progression of DN, which is mainly characterized by proteinuria (87, 88). PKC-mediated upregulation of extracellularly regulated protein kinases ERK1/2 and matrix metalloproteinases (MMPs) accelerates cell proliferation, migration, maturation, and formation of new blood vessels (89). PKC activation affects blood flow, causes retinal microvascular constriction, induces neovascularization, and promotes the development of DR (90).
Inflammatory response
Chronic subclinical inflammation underlies the vascular pathology of DR, and the stimulated inflammation of leukocyte adhesion to the retinal vascular system and the role of leukocytes in leukocyte stasis with risk of capillary closure suggest that this condition may represent a form of low-grade inflammation (91, 92). A study has shown that DR vascular leakage and no perfusion are temporally and spatially associated with retinal leukocyte stasis in a streptozotocin-induced diabetic rat model (93). NLRP1, NLRP3, NLRC4, and AIM2 inflammatory vesicles can lead to DR pathogenesis (94). DN is a chronic low-grade inflammatory disease (95, 96). Proinflammatory cytokines, chemokines and their receptors, adhesion molecules, and transcription factors are involved in the development and progression of DN (97). Low-grade inflammation accumulates extracellular matrix in the glomerular basement membrane by stimulating endothelial cells and podocytes, while excessive extracellular matrix deposition can cause an acute phase reaction (98). Nuclear factor κB (NF-κB), one of the major factors involved in the inflammatory response, is a crucial link in regulating chemokines, cell adhesion proteins, inflammatory cytokines, and other molecules associated with DN mechanisms (99). Meanwhile, thioredoxin-interacting protein (TXNIP), macrophages, and various cytokines are involved in the pathogenesis of DN and DR.
TXNIP is highly expressed in diabetic retina (100–103). TXNIP, as an early response gene in the high-glucose environment, can induce AGE receptor expression; at the same time, high glucose-induced TXNIP expression enhances AGE receptor expression, leading to feedback in the TXNIP expression loop, which produces persistent chromatin remodeling and sustained inflammatory gene expression in diabetic retinal capillary endothelial cells (101), which can induce NLRP3 expression, increase IL-1β production in Müller cells (103, 104), and promote DR development. At the same time, hyperglycemia upregulates TXNIP expression and induces ROS production and inflammatory and fibrotic responses in diabetic kidneys, leading to dysregulation of autophagy and contributing to the development of DN (101, 105). Macrophages were recruited in rat glomeruli early after the onset of hyperglycemia (106). Macrophages cause tissue damage and sclerosis by producing ROS, cytokines, and proteases (107, 108). Macrophage-restricted protein tyrosine phosphatase 1B (PTP1B) is a crucial regulator of inflammation in the metabolic syndrome resulting from insulin resistance, and aberrant regulation of PTP1B may underlie retinal microvascular disease (109). Several cytokines are involved in the pathogenesis of DN and DR, among which VEGF is one of the essential cytokines (110, 111). Activation of VEGF leads to neovascularization and glomerular injury, and loss of late-stage podocytes induces a decrease in VEGF signaling, which can trigger vascular thinning and renal fibrosis and promote the DN process (58). The generation of neovascularization and enhanced vascular permeability in the retina are associated with the upregulation of VEGF, which is involved in pathological retinal neovascularization and increased vascular permeability, and its mechanism of action is to induce vascular endothelial cells to divide and proliferate through the action of secreting high levels of affinity receptors on retinal vascular endothelial cells (112, 113).
Hemodynamic changes
Early diabetes is characterized by increased blood flow, which is reflected in the kidneys as glomerular hyperfiltration (114). Abnormally elevated blood glucose leads to increased permeability of the glomerular filtration membrane, which results in an increased glomerular filtration rate (115). Hyperfiltration and hyperperfusion can stimulate the proliferation of glomerular mesangial cells, increase the mesangial matrix, damage the endothelial cells, and increase platelet aggregation, leading to the formation of micro-arteriolar flow. It can also cause inflammatory cell infiltration, increase the apoptosis of the mesangial cells, and so on. Thus, glomerulosclerosis is constantly progressing, and the loss of renal units is progressive, inducing the DN (22, 115). High blood glucose levels in the RAAS and its elevated angiotensin levels led to a slowing of renal blood flow, impaired glomerular filtration barrier, and proteinuria, leading to DN. At the same time, the pathological changes of DN can lead to renal small, micro-arteriolar sclerosis and micro-arteriolar constriction enhancement, so the renal blood flow is reduced, and glomerular sclerosis is exacerbated, leading to renal insufficiency (22, 116). Studies have shown that diabetic patients have elevated blood and serum viscosity and elevated red blood cell aggregation and adhesion, which increase the resistance to blood flow and reduce its stability in the flow process, leading to slow blood flow and decreased blood flow rate and triggering circulatory disorders (117). From a hemodynamic perspective, ocular blood flow is determined by the balance between ocular perfusion pressure and vascular resistance (118). Prolonged hyperglycemia leads to endothelial dysfunction, increased vascular resistance, abnormal retinal perfusion, and exacerbation of retinal ischemia and hypoxia (119). As feedback from local ischemia, excessive ischemic dilatation as well as uneven capillary resistance cause other vessels to undergo overcompensation, resulting in a vicious cycle that promotes the development of DR (120).
Hyperactivation of RAAS
Increased RAAS activity is an essential factor in the development of DN, and the end product of this system is angiotensin II (AngII), whose damaging effects include vasoconstriction, increased aldosterone secretion, growth, fibrosis, thrombosis, inflammation, and oxidative (121). Local expression of RAAS is upregulated by glomerular capillary hypertension associated with hyperglycemia-induced hyperfiltration state (122). Hyperactivation of RAAS leads to renal injury by pro-fibrotic and pro-inflammatory factors (123). High glucose leads to overactivation of the RAAS, causing AngII to activate AT1 receptors, and AngII is involved in glucose- and lipid-induced oxidative stress, inflammation, and apoptosis via AT1 receptors. It produces elevated glomerular hydrostatic pressure, proteinuria, and structural damage with sclerosis and fibrosis and promotes the development of DN (124, 125). Studies have shown that patients with DR have higher than average concentrations of reninogen, renin, and AngII in the vitreous humor (126). The presence of its own independent RAAS in the retina regulates blood flow and intraocular pressure (IOP) (127); its key effector molecule, AngII, modulates pericyte function (128); the retinal RAS is activated to stimulate growth factors, such as VEGF, which leads to vascular leakage, pericyte migration, angiogenesis, fibrosis, and exacerbation of DR (129).
Kallikrein–kinin system
The kallikrein–kinin system (KKS) exerts multiple effects on vascular and neuronal tissues through activation of B1 and B2 receptors, which are constitutively expressed in most tissues, and B1 receptors, which are induced to be expressed under stress conditions such as inflammation and diabetes mellitus (130, 131). In the eye, the KKS is one of the identified pathways in the vitreous of patients with PDR and diabetic macular edema (DME) (132). The KKS plays a key role in the pathophysiology of DR, including vascular inflammation and hyperpermeability, oxidative stress, vasodilation, retinal thickening, and neovascularization (133). Activated plasma kinin-releasing enzymes in the retinal and vitreous fluids of patients with PDR may directly contribute to the development of interstitial swelling and macular edema in these patients (134). In the kidney, B2 receptor deficiency has been shown to increase albumin excretion and glomerular thylakoid sclerosis in diabetic mice starting with the Akita mutation (130). Diabetic test mice lacking bradykinin B2 receptors show a significant increase in dominant albuminuria with increasing glomerular mesangial sclerosis (135). Bradykinin has been shown to have NO-releasing properties, induce endothelium-dependent vasodilation, exert antifibrotic and antihypertrophic effects, and stimulate glucose uptake (136). The study suggests a novel role for the KKS in preventing salt-induced and hypertension-induced kidney injury by inhibiting oxidative stress and inflammatory responses (137).
MiRNAs
MiRNAs play an essential role in the pathogenesis of microvascular diabetic complications DR and DN by regulating various pathways including inflammation, apoptosis, and oxidative stress (138). Growing evidence suggests that altered genomic DNA methylation, chromatin histone modifications, and non-coding RNA dysregulation are involved in the pathogenesis of DN (139). Dysregulation of miRNAs promotes DR progression by affecting pathways such as inflammation, oxidative stress, endothelial apoptosis, and vascular cell function (138).
Studies have shown that plasma miR-21 expression is elevated during the development of T2DM combined with DR and can be used to indicate the severity of T2DM combined with DR (140). The high glucose-induced upregulation of miR-21 expression in retinal endothelial cells indirectly regulates NF-κB expression and promotes apoptosis in retinal endothelial cells (141), as well as having a pro-angiogenic effect (142, 143). Serum and renal tissue miR-21 was found to be significantly elevated with the progression of DN (144). MiR-21 promotes epithelial–mesenchymal transition (EMT) and extracellular matrix (ECM) deposition through the downregulation of bone morphogenetic protein 7 (BMP-7), and EMT and ECM deposition in renal tubular epithelial cells is critical for DN pathogenesis (145). Significant histopathological changes in miR-21 expression in DN kidney biopsies can fully reflect the key role of miR-21 in the development of DN (146). Meanwhile, the pathogenic role of miR-21 in DN can also manifest as renal fibrosis (147) and inflammation (148).
Hypoxia
Hypoxia plays a vital role in diabetic complications and occurs in tissues central to the development of diabetes (pancreatic β-cells and adipose tissue) and in tissues susceptible to diabetic complications (nerves, retina, heart, blood vessels, kidneys, and wounds) (149). Hypoxia-inducible factor 1α (HIF-1α) gene polymorphisms may be associated with diabetes and diabetic complications (150). HIF-1α is a transcription factor that is expressed in response to decreased cellular oxygen partial pressure and activated to participate in angiogenesis, glycolysis, and regulation of vascular tone (151) The cellular adaptive response to hypoxia is mediated by hypoxia-inducible factor-1α, and hyperglycemia leads to decreased stability and low transcriptional potency of the HIF-1α protein in response to hypoxia (152), which also leads to a pseudo-hypoxic state that activates HIF-1α activity to adapt to hypoxia (153). Hypoxia is present in the diabetic kidney (154, 155), and renal tubular hypoxia is due to a combination of increased energy demand and decreased perfusion with non-hypoxia-related forces that drive the development of tubular atrophy and interstitial fibrosis, promoting the progression of DN in a vicious cycle (156). The maintenance of normal retinal function depends on a continuous supply of oxygen and the ability to detect and respond to localized hypoxia rapidly (157). Hypoxia occurs frequently during the development of DR (158, 159), and hyperglycemia-induced endothelial dysfunction, as well as inhibition of endothelial NO synthase and disturbances in vascular self-regulation, can lead to the development of hypoxia in retinal tissue (160). Oxygen (O2) is essential for the retina (161, 162), and reduced retinal blood flow in diabetic patients can lead to retinal hypoxia (163). Retinal hypoxia can cause neuroretinal dysfunction and degeneration, which directly lead to vision loss (164) and promote the development of DR.
Co-medication of DN and DR
Because of the similarity between DN and DR in terms of pathogenic factors and pathogenesis, there is partial concordance in terms of the clinical use of drugs. Active control of blood glucose, blood pressure, blood lipids, and other related risk factors can slow down the development of the disease process, so the treatment of the two diseases used in the choice of glucose control drugs, antihypertensive drugs, lipid regulating drugs, and other related drugs have essential consistency (165). In addition, some of the drugs also have the effect of DN and DR at the same time, and the therapeutic effects of some of the drugs are listed below.
Inhibition of aberrant activation of the polyol pathway: the aldose reductase inhibitor epalrestat
AR plays a crucial role in the etiology of long-term diabetic microvascular complications such as retinopathy, nephropathy, and neuropathy (166). Epalrestat is a carboxylic acid derivative that inhibits aldose reductase in the polyol pathway, and the ability of epalrestat to reduce intracellular sorbitol accumulation has been linked to the pathogenesis of microvascular complications in diabetes mellitus (167). A 3-year multicenter aldose reductase inhibitor-diabetic complications trial in Japan, which included 594 patients, showed that epalrestat was particularly effective in patients with reasonable glycemic control and mild microvascular disease (168). Patients treated with epalrestat have also been shown to slow the progression of diabetic retinopathy–nephropathy, possibly due to the inhibition of oxidative and inflammatory stress through the inhibition of the polyol pathway by epalrestat (169).
Antioxidant: α-lipoic acid
α-Lipoic acid (ALA) is a potent antioxidant (170–172) and is valuable in preventing and treating ocular complications such as diabetic keratopathy and DR (170, 173, 174). ALA has insulin-mimetic and anti-inflammatory activities (175), which increase insulin sensitivity (176), prevent retinal lipid peroxidation in early diabetes (177), inhibit the activation of NF-κB induced by late glycosylation end products in endothelial cells (178), and inhibit apoptosis in retinal capillary cells (179), thereby controlling the development of DR. ALA attenuates oxidative stress by regulating the expression of enzymes involved in the formation of reactive oxygen species (180), prevents glomerular thylakoid matrix expansion (181), inhibits diabetic renal fibrosis by improving mitochondrial function and regulating the expression and activation of isotretinoin receptor α (RXRα) (182), and protects the kidneys by possibly inhibiting neutrophil infiltration, modulating inflammatory mediators (183), and delaying the development of DN.
Inhibitors of the RAS system: angiotensin-converting enzyme inhibitors and angiotensin II receptor blockers
Angiotensin-converting enzyme inhibitor (ACEI) versus angiotensin II receptor blocker (ARB) as first-line therapy for hypertension in DN patients presenting with proteinuria has been shown to have renal and cardiac-related benefits (184). Studies have shown that RAS induces various tissue responses, including vasoconstriction, inflammation, oxidative stress, cellular hypertrophy, proliferation, angiogenesis, and fibrosis, triggering diabetic microangiopathy (185). Mechanisms of ACEI and ARB analogs for DR are mostly related to the RAS system (186). RAS inhibition is the single most effective treatment for slowing the progression of diabetic nephropathy (187). Some experiments indicated that captopril, an ACEI representative drug, and chlorosartan, an ARB representative drug, inhibited hyperglycemia-induced leukocyte retention in the retinal vasculature in rats at 6 weeks and 1 week, respectively; that AngII-induced expression of vascular cell adhesion molecules was inhibited by chlorosartan in experimentally cultured human retinal endothelial cells; that captopril blocks capillary degeneration in the early stages of DR; and that the two drugs inhibit the development of DR by inhibiting the RAS system, which can block the early retinal capillary degeneration and the inflammatory response (188). Meanwhile, an animal study has shown that captopril can reduce oxidative stress in DR patients (189).
Vasoprotective drug: calcium 2,5-dihydroxybenzenesulfonate
Calcium 2,5-dihydroxybenzenesulfonate (CaD) is considered a vasoprotective drug that alleviates microcirculatory and hemorheological abnormalities (190), attenuates diabetes-induced endothelial dysfunction and inflammation (191, 192), and reduces diabetic vascular complications by interfering with acetyl heparin sulfate binding sites to decrease VEGF signaling (193). A systematic review and meta-analysis supports the validity of CaD’s multi-targeted effects on DR through antioxidant, anti-free radical, and vasoprotective effects and inhibition of inflammatory cytokines (194). CaD, as an antioxidant and microvascular protector, has significantly improved the renal impairment of DN. CaD controls the development of DN by alleviating vascular damage, improving microcirculation, delaying renal fibrosis, enhancing the barrier effect on glomerular capillary filtration, preventing or reducing the thickening of the glomerular capillary basement membrane, preventing the disruption of the podocyte process, and maintaining the integrity of the glomerular basement membrane, which then protects the glomerular filtration barrier of DN from being further disrupted and improves the glomerular terminal filtration function (195). CaD prevents DN by downregulating the key apoptotic factor Bim and inhibiting apoptosis in renal proximal tubular epithelial cells (196).
Non-parallelism of DN and DR
As two different organs, the kidney and the eye have their own unique internal environment and physiological processes, each with specific influencing molecules, and the target organs themselves have different pathological changes and responses to various influencing factors, leading to different manifestations of diabetic microangiopathic damage in the two, and at the same time, the diagnostic means of the two also make the two diseases have non-parallelism. The article describes the non-parallelism of the two diseases in terms of the differences in their causative factors, the asynchrony of the disease, the differences in mechanisms, and the different medications used (Figure 2).
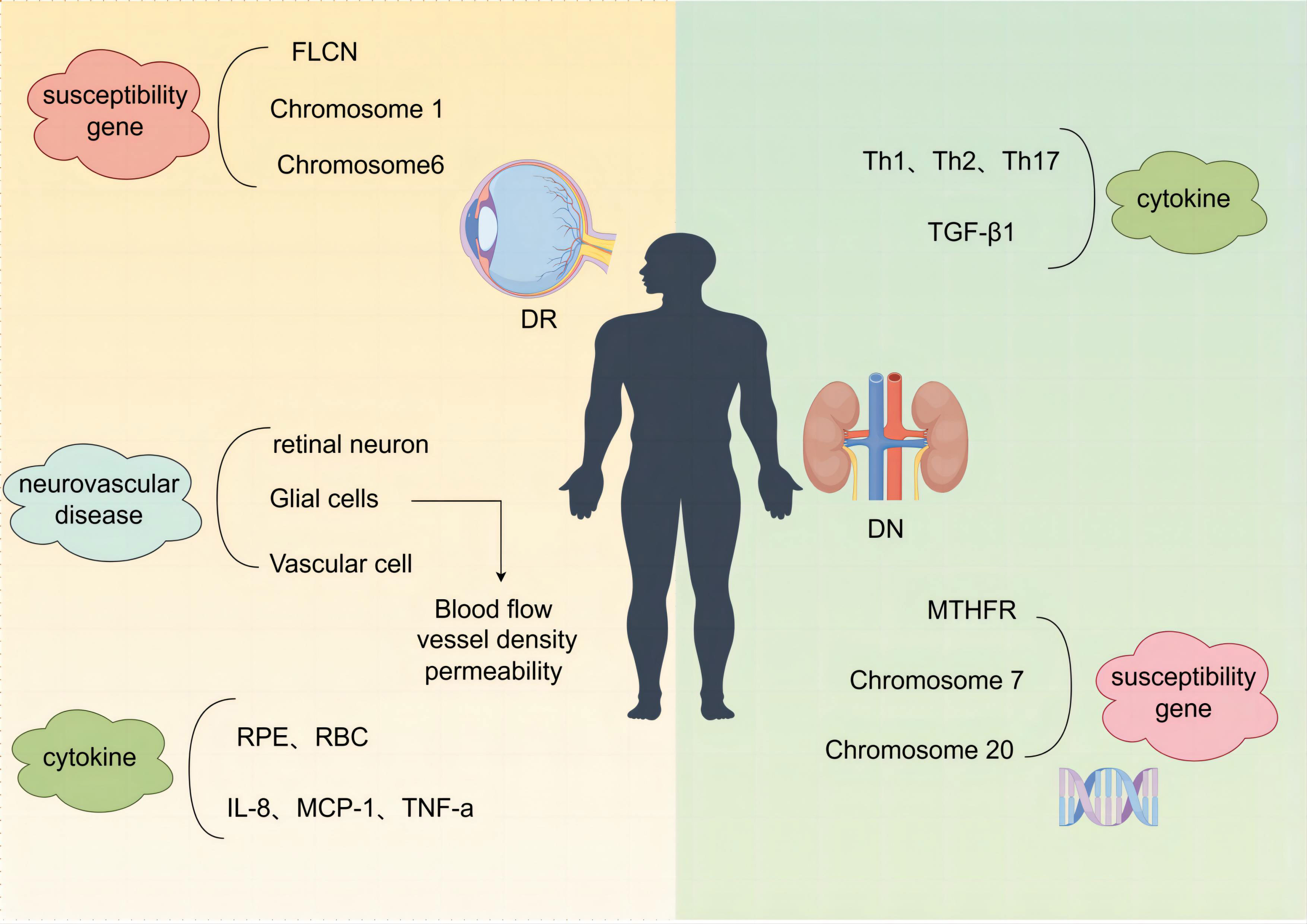
Figure 2 Schematic of the different mechanisms of DN and DR. DN, diabetic nephropathy; DR, diabetic retinopathy.
Asynchrony in the onset of DN and DR
With DN without DR
In a prospective, randomized, blinded research trial of 144 Hispanics and 671 non-Hispanic white patients with non-insulin-dependent diabetes mellitus (NIDDM), it was shown that dominant albuminuria (urinary albumin excretion rate >200 μg/min) (197) was a strong independent risk factor for DR in the Hispanic study population, whereas non-Hispanic white patients did not show such an association, and the study suggests that early care may differ between the two ethnic groups due to differences in economic level as well as healthcare, which may be a possible reason for differences in the prevalence of diabetes complications, particularly DN and DR (198). Renal biopsy is the gold standard for the diagnosis of DN, by which renal disease and its prognostic classification can be accurately diagnosed but may not be performed in diabetic patients due to the limitations of the patient’s age, comorbidities, anticoagulant therapy, and additional costs (199). By screening T2DM patients who underwent renal biopsy, 96 (38.71%) in the DN group and 152 (61.29%) in the non-diabetic renal disease (NDRD) group, it was found that DR was prevalent in 79 (82.3%) and 12 (7.9%), respectively, suggesting that diabetic patients without retinopathy were the most likely to develop NDRD, which suggests that clinically, some DN patients have no DR may be because clinically some patients diagnosed with DN did not undergo renal biopsy, and some patients with NDRD combined with IgE, for example, were included. This suggests that diabetes mellitus without retinopathy has the highest likelihood of developing NDRD, which suggests that some patients with DN are not clinically comorbid with DR possibly because renal biopsy is not practiced in some of the clinically diagnosed patients with DN, and some patients with diabetes mellitus comorbid with NDRD, e.g., IgA nephropathy, are included and therefore do not have DR (200). Of the 98 patients with simple DN, 64 (65.3%) had DR and 34 (34.7%) had no DR, and the analysis concluded that advanced age (p = 0.003) and male gender (p < 0.001) were significantly associated with DN without DR (201).
With DR without DN
In 138 patients with insulin-dependent diabetes mellitus aged between 25 and 34 years with onset before 30 years of age, it was found that nephropathy was rare in patients without retinopathy, but retinopathy was often seen in patients without nephropathy. In both cases, DR was often detected earlier than DN in patients with insulin-dependent diabetes mellitus (202). This may be due to the higher vulnerability of the retina compared to the kidneys and the higher detection rate of DR, as fundus photography or fundography is more accessible to perform and therefore allows for easier detection compared to renal biopsy (203). The trial studied 100 insulin-treated diabetic patients, 35% of whom had PDR without DN changes, suggesting that the two types of microangiopathy, DN and DR, may not evolve similarly (204).
Different Pathogenesis of DN and DR
Different susceptibility genes
The development of DN and DR is hereditary and has familial aggregation, and the extent of disease damage is also determined by the genetic determinants of individual susceptibility as well as the presence of multiple independent risk factors (205–207). In a meta-analysis of genome-wide association studies, the folliculin gene (FLCN) was identified as a susceptibility gene for DR (208). Methylenetetrahydrofolate-reducing gene (MTHFR), a key enzyme regulating nucleotide synthesis and DNA methylation, is a susceptibility gene for DN and may be a risk factor for DN in white people and Africans (209). Chromosome 7 and chromosome 20 are susceptibility genes for DN (210). Chromosome 1 (211) and chromosome 6 (212) are susceptibility genes for DR.
DR: diabetic microvascular complications, neurovascular lesions
The American Diabetes Association defines DR lesion as a highly tissue-specific neurovascular complication (213, 214), a neurovascular disease resulting from the destruction of the retinal neurovascular unit (NVU) (214), which consists of retinal neurons, glial cells, and vascular cells coordinately regulating blood flow, vascular density, and permeability in response to the similarly dynamic demands of the retinal neurons, through the supply of oxygen and nutrients, the recycling of neurotransmitters, and the removal of metabolic wastes, a vascular function whose fine-tuning is essential for maintaining retinal homeostasis (215). Retinal neurodegeneration is involved in the development of microvascular abnormalities as an early event in DR (215, 216). In addition to premature neuronal death, biochemical and structural changes in neurons and glial cells contribute to neurodegeneration, and the functional abnormalities that occur in DR may be due to early changes in neural organization (217).
Different cytokines
The growing agreement that DN is an inflammatory process is because leukocyte infiltration occurs at all stages of kidney damage (96). The involvement of proinflammatory factors, Th1, Th2, and Th17 cytokines and TGF-β1 in the development of DN predicts susceptibility and progression of DN (218, 219). Inflammatory cytokines and oxidative stress-related pathways DR in different systems such as red blood cells (RBC) and retinal pigment epithelium (RPE) cells provide important potential biomarkers (220). Some findings support the association of PDR bleeding with IL-8, MCP-1, TNF-α, and other inflammatory cytokines in atrial fluid (221).
Different medications for DN and DR
DN clinical use
Treatment of DN includes strict control of blood glucose and blood pressure, restriction of protein intake, and maintenance of water–electrolyte and acid–base balance (222). In addition to hypoglycemic effects, dipeptidyl peptidase-IV (DPP-4) inhibitors exert renoprotective effects through antioxidant and anti-inflammatory mechanisms, and anti-fibrotic effects through inhibition of TGF-β-mediated signaling (223). Inhibitors of sodium-dependent glucose transporters 2 (SGLT-2) reduce hyperglycemia while preventing glomerular hyperfiltration and slowing the development of DN (224, 225). Aldosterone is pro-inflammatory and pro-fibrotic and promotes kidney injury (226), and aldosterone can elevate arterial and glomerular pressure (227). Aldosterone receptors are present in glomerular endothelial and epithelial cells, and abnormal aldosterone infusion can lead to proteinuria and glomerulosclerosis by damaging glomerular epithelial cells (228). Clinically available aldosterone receptor antagonists are used to treat DN, and proteinuria continues to be significantly reduced during spironolactone treatment; however, it should be noted that aldosterone receptor antagonists, such as spironolactone, increase the risk of hyperkalemia (229). Currently, a number of new drug targets have recently been identified against the underlying pathophysiological mechanisms of DN that may contribute to the development of new drugs to prevent renal and vascular damage and slow down the progression of DN in patients with DN, including the development of personalized medicines based on genetic and epigenetic variations (230). New therapeutic targets such as the GSK3β signaling pathway (231), NLRP3 inflammatory vesicles (232), PI3K/Akt and JAK/STAT pathways (233), endothelin receptor (234), and DPP-4 (235) are involved in the pathological progression of DN, and there is still an urgent need to identify and validate new drug targets and candidates for better DN therapy.
DR clinical use
The main methods of DR treatment include strict control of blood glucose, blood pressure, and blood lipids, medication to improve microcirculation and anti-neovascularization, laser therapy, and vitrectomy (236). Lipid-lowering drug fenofibrate slows DR progression through anti-inflammatory, anti-angiogenic, and retinal neuroprotective effects (237). VEGF inhibitors: VEGF is a hypoxia-induced angiogenic peptide (238) that mediates BRB destruction and angiogenesis in the pathogenesis of DR (239). Clinicians reduce VEGF signaling in the retina by vitreous cavity injections of anti-VEGF biologics. In a series of disease models and clinical studies, it has been shown that multiple pharmaceutical agents that successfully target VEGF significantly improve vascular permeability and retinal edema (240). Anti-VEGF drugs improve DR severity and are the first-line treatment for diabetic macular edema with PDR (241). Current anti-VEGF agents for the treatment of DR include bevacizumab, ranibizumab, and abciximab (240, 242), which have demonstrated their safety in several trials (243). Studies have shown that ranibizumab rapidly and consistently improves vision and reduces the risk of further vision loss in patients with diabetic macular edema (244). The anti-VEGF regimen is usually 1 injection per month for 3 months and every 4–6 weeks if necessary (245). Commonly used drugs for treatment with intravitreal injections of corticosteroids include dexamethasone, triamcinolone acetonide, and flurazepam (246). Studies have shown that dexamethasone is safer, has the lowest risk of causing glaucoma and cataracts, and can be classified as the drug of choice for intraocular corticosteroid injections (247, 248). Corticosteroids target pro-inflammatory mediators in DME, including IL-6, IL-8, MCP-1, ICAM-1, and TNF-α (249), producing anti-inflammatory effects through various mechanisms, including reducing the synthesis of inflammatory mediators and adhesion proteins and lowering VEGF (250). These drugs are commonly used to treat patients with refractory and vision-threatening DR and DME because of the bracketed IOP elevation and cataract risk (251).
Future directions in DR treatment will focus on the following two areas: the development of retinal imaging techniques and the discovery of new molecules for the treatment of refractory patterns of DME (252). The continued refinement of new technologies such as AI (253) and ultra-wide-angle (UWF) retinal imaging (254) provide important support for early screening for DR. New targets such as anti-VEGF (255), anti-inflammatory (249), neuroprotection (190, 194), soluble epoxide hydrolase (sEH) (256), nano molecules (52), and photo-biomodulation (PBM) (257) provide more directions for the development of new therapeutic approaches for DR and suggest that the future trend in the management of DR may be a multi-pathway targeted therapy. Among them, high treatment burden, treatment adherence problems, and under-treatment are the challenges that DR treatment needs to face.
Summary and outlook
DN and DR, as diabetic microvascular comorbidities, are the main factors of end-stage renal disease and blindness, respectively, which bring a heavy economic burden to society. Parallelism and non-parallelism exist in the process of disease development of the two diseases, and the parallelism of the two helps to guide the co-medication of the two diseases. DN and DR homeopathic drugs can be used as a future research direction for the treatment and prevention of the two diseases to reduce the types of medication used by patients, optimize the management, alleviate the burden of hepatic and renal metabolism, and improve the patients’ quality of life, as well as further alleviate the burden of patients and the country’s medical care. Early comprehensive intervention is of great significance. Current research suggests that the two diseases are treated with polyol pathway inhibitors, antioxidants, RAS system inhibitors, and vasoprotective agents, which have a better potential for development, and in the development of new anti-inflammatory drugs, miRNA is a new therapeutic target in seeking breakthroughs. The development of drugs targeting these common molecular pathways may provide new therapeutic approaches for both diseases.
Author contributions
ST: Writing – original draft. XA: Writing – original draft. WS: Writing – original draft. YZ: Writing – original draft. CY: Writing – original draft. XK: Writing – original draft. YS: Writing – original draft. LJ: Writing – original draft. XZ: Writing – original draft. QG: Writing – original draft. HJ: Writing – review & editing. FL: Writing – review & editing.
Funding
The author(s) declare financial support was received for the research, authorship, and/or publication of this article. This study was supported by the National Multidisciplinary Interdisciplinary Innovation Team Project of Traditional Chinese Medicine (ZYYCXTD-D-202001), the Science and Technology Innovation Project of the China Academy of Traditional Chinese Medicine (C12021A02610), the National Natural Science Foundation of China under the Young Science Foundation Project (82305205), and the Clinical Research Center Construction Project of the Guang’anmen Hospital of the China Academy of Traditional Chinese Medicine (Approval No. 2022LYJSZX01~2022LYJSZX29).
Acknowledgments
The images in this article were drawn by Figdraw.
Conflict of interest
The authors declare that the research was conducted in the absence of any commercial or financial relationships that could be construed as a potential conflict of interest.
Publisher’s note
All claims expressed in this article are solely those of the authors and do not necessarily represent those of their affiliated organizations, or those of the publisher, the editors and the reviewers. Any product that may be evaluated in this article, or claim that may be made by its manufacturer, is not guaranteed or endorsed by the publisher.
Glossary
References
1. Sun H, Saeedi P, Karuranga S, Pinkepank M, Ogurtsova K, Duncan BB, et al. IDF Diabetes Atlas: Global, regional and country-level diabetes prevalence estimates for 2021 and projections for 2045. Diabetes Res Clin Pract (2022) 183:109119. doi: 10.1016/j.diabres.2021.109119
2. Skyler SJ. MICROVASCULAR COMPLICATIONS. Endocrinol Metab Clinics ofNorth America (2001) 30(4):833–56. doi: 10.1016/S0889-8529(05)70218-8
3. Forbes JM, Cooper ME. Mechanisms of diabetic complications[. Physiol Rev (2013) 93(1):137–88. doi: 10.1152/physrev.00045.2011
4. Gregg EW, Williams DE, Geiss L. Changes in diabetes-related complications in the United States. New Engl J Med (2014) 371(3):284–7. doi: 10.1056/NEJMc1406009
5. Kobrin Klein BE. Overview of epidemiologic studies of diabetic retinopathy. Ophthalmic Epidemiol (2007) 14(4):179–83. doi: 10.1080/09286580701396720
6. Teo ZL, Tham Y-C, Yu M, Chee ML, Rim TH, Cheung N, et al. Global prevalence of diabetic retinopathy and projection of burden through 2045. Ophthalmology (2021) 128(11):1580–91. doi: 10.1016/j.ophtha.2021.04.027
7. Tuttle KR, Bakris GL, Bilous RW, Chiang JL, de Boer IH, Goldstein-Fuchs J, et al. Diabetic kidney disease: A report from an ADA consensus conference. Diabetes Care (2014) 37(10):2864–83. doi: 10.2337/dc14-1296
8. Zhang X-X, Kong J, Yun K. Prevalence of diabetic nephropathy among patients with type 2 diabetes mellitus in China: A meta-analysis of observational studies. J Diabetes Res (2020) 2020:1–11. doi: 10.1155/2020/2315607
9. El-Asrar AMA, Al-Rubeaan KA, Al-Amro SA, Moharram OA, Kangave D. Retinopathy as a predictor of other diabetic complications. Int Ophthalmol (2001) 24(1):1–11. doi: 10.1023/a:1014409829614
10. Liu Z, Li X, Wang Y, Song Y, Liu Q, Gong J, et al. The concordance and discordance of diabetic kidney disease and retinopathy in patients with type 2 diabetes mellitus: A cross-sectional study of 26,809 patients from 5 primary hospitals in China. Front Endocrinol (2023) 14:1133290. doi: 10.3389/fendo.2023.1133290
11. Saini D, Kochar A, Poonia R. Clinical correlation of diabetic retinopathy with nephropathy and neuropathy. Indian J Ophthalmol (2021) 69(11):3364. doi: 10.4103/ijo.IJO_1237_21
12. Tooke JE. Microcirculation and diabetes. Br Med Bull (1989) 45(1):206–23. doi: 10.1093/oxfordjournals.bmb.a072313
13. Tan KCB, Chow W-S, Ai VHG, Metz C, Bucala R, Lam KS. Advanced glycation end products and endothelial dysfunction in type 2 diabetes. Diabetes Care (2002) 25(6):1055–9. doi: 10.2337/diacare.25.6.1055
14. Hink U, Li H, Mollnau H, Oelze M, Matheis E, Hartmann M, et al. Mechanisms underlying endothelial dysfunction in diabetes mellitus. Circ Res (2001) 88(2):E14–22. doi: 10.1161/01.res.88.2.e14
15. Cheng H, Harris R. Renal endothelial dysfunction in diabetic nephropathy. Cardiovasc Hematological Disorders-Drug Targets (2014) 14(1):22–33. doi: 10.2174/1871529X14666140401110841
16. Madonna R, Balistreri CR, Geng Y-J, De Caterina R. Diabetic microangiopathy: Pathogenetic insights and novel therapeutic approaches. Vasc Pharmacol (2017) 90:1–7. doi: 10.1016/j.vph.2017.01.004
17. Doyon G, Bruemmer D. Vascular smooth muscle cell dysfunction in diabetes: nuclear receptors channel to relaxation. Clin Sci (2016) 130(20):1837–9. doi: 10.1042/CS20160518
18. Izzedine H, Bodaghi B, Launay-Vacher V, Deray G. Eye and kidney: from clinical findings to genetic explanations. J Am Soc Nephrol (2003) 14(2):516–29. doi: 10.1097/01.ASN.0000051705.97966.AD
19. Farrah TE, Dhillon B, Keane PA, Webb DJ, Dhaun N. The eye, the kidney, and cardiovascular disease: old concepts, better tools, and new horizons. Kidney Int (2020) 98(2):323–42. doi: 10.1016/j.kint.2020.01.039
20. Fursova A, Derbeneva AS, Vasilyeva MA, Tarasov MS, Nikulich IF, Galkina EV. Development, clinical manifestations and diagnosis of retinal changes in chronic kidney disease. Vestnik oftal’mologii (2021) 137(1):107. doi: 10.17116/oftalma2021137011107
21. Lovshin JA, Lytvyn Y, Lovblom LE, Katz A, Boulet G, Bjornstad P, et al. Retinopathy and RAAS activation: results from the canadian study of longevity in type 1 diabetes. Diabetes Care (2019) 42(2):273–80. doi: 10.2337/dc18-1809
22. Lin Y-C, Chang Y-H, Yang S-Y, Wu KD, Chu TS. Update of pathophysiology and management of diabetic kidney disease. J Formosan Med Assoc (2018) 117(8):662–75. doi: 10.1016/j.jfma.2018.02.007
23. Li J, Cao Y, Liu W, Wang Q, Qian Y, Lu P. Correlations among diabetic microvascular complications: A systematic review and meta-analysis. Sci Rep (2019) 9(1):3137. doi: 10.1038/s41598-019-40049-z
24. Kotlarsky P, Bolotin A, Dorfman K, Knyazer B, Lifshitz T, Levy J. Link between retinopathy and nephropathy caused by complications of diabetes mellitus type 2. Int Ophthalmol (2015) 35(1):59–66. doi: 10.1007/s10792-014-0018-6
25. Mhamedi SA, Meghraoui H, Benabdelhak M, Bentata Y, Haddiya I. La ponction biopsie rénale: indications, complications et résultats. Pan Afr Med J (2018) 31:44. doi: 10.11604/pamj.2018.31.44.15604
26. Zhang J, Wang Y, Li L, Zhang R, Guo R, Li H, et al. Diabetic retinopathy may predict the renal outcomes of patients with diabetic nephropathy. Renal Failure (2018) 40(1):243–51. doi: 10.1080/0886022X.2018.1456453
27. Lee M-K, Han K-D, Lee J-H, Sohn SY, Hong OK, Jeong JS, et al. Normal-to-mildly increased albuminuria predicts the risk for diabetic retinopathy in patients with type 2 diabetes. Sci Rep (2017) 7(1):11757. doi: 10.1038/s41598-017-11906-6
28. Li Y, Su X, Ye Q, Guo X, Xu B, Guan T, et al. The predictive value of diabetic retinopathy on subsequent diabetic nephropathy in patients with type 2 diabetes: a systematic review and meta-analysis of prospective studies. Renal Failure (2021) 43(1):231–40. doi: 10.1080/0886022X.2020.1866010
29. Karlberg C, Falk C, Green A, Sjølie AK, Grauslund J. Proliferative retinopathy predicts nephropathy: a 25-year follow-up study of type 1 diabetic patients. Acta Diabetologica (2012) 49(4):263–8. doi: 10.1007/s00592-011-0304-y
30. Zhang K, Liu X, Xu J, Yuan J, Cai W, Chen T, et al. Deep-learning models for the detection and incidence prediction of chronic kidney disease and type 2 diabetes from retinal fundus images. Nat Biomed Eng (2021) 5(6):533–45. doi: 10.1038/s41551-021-00745-6
31. Yin L, Zhang D, Ren Q, Su X, Sun Z. Prevalence and risk factors of diabetic retinopathy in diabetic patients: A community based cross-sectional study. Medicine (2020) 99(9):e19236. doi: 10.1097/MD.0000000000019236
32. Liu Z, Fu C, Wang W, Xu B. Prevalence of chronic complications of type 2 diabetes mellitus in outpatients - a cross-sectional hospital based survey in urban China. Health Qual Life Outcomes (2010) 8(1):62. doi: 10.1186/1477-7525-8-62
33. Liu Y, Yang J, Tao L, Lv H, Jiang X, Zhang M, et al. Risk factors of diabetic retinopathy and sight-threatening diabetic retinopathy: a cross-sectional study of 13 473 patients with type 2 diabetes mellitus in mainland China. BMJ Open (2017) 7(9):e016280. doi: 10.1136/bmjopen-2017-016280
34. Zoungas S, Chalmers J, Ninomiya T, Li Q, Cooper ME, Colagiuri S, et al. Association of HbA1c levels with vascular complications and death in patients with type 2 diabetes: evidence of glycaemic thresholds. Diabetologia (2012) 55(3):636–43. doi: 10.1007/s00125-011-2404-1
35. Chung JO, Park S-Y, Chung DJ, Chung MY. Relationship between anemia, serum bilirubin concentrations, and diabetic retinopathy in individuals with type 2 diabetes. Medicine (2019) 98(43):e17693. doi: 10.1097/MD.0000000000017693
36. Qiao Q, Keinänen-Kiukaanniemi S, Läärä E. The relationship between hemoglobin levels and diabetic retinopathy. J Clin Epidemiol (1997) 50(2):153–8. doi: 10.1016/S0895-4356(96)00335-6
37. Morrison JL, Hodgson LA, Lim LL, Al-Qureshi S. Diabetic retinopathy in pregnancy: a review: Diabetic retinopathy in pregnancy. Clin Exp Ophthalmol (2016) 44(4):321–34. doi: 10.1111/ceo.12760
38. Cai X, Chen Y, Yang W, Gao X, Han X, Ji L. The association of smoking and risk of diabetic retinopathy in patients with type 1 and type 2 diabetes: a meta-analysis. Endocrine (2018) 62(2):299–306. doi: 10.1007/s12020-018-1697-y
39. Milluzzo A, Maugeri A, Barchitta M, Sciacca L, Agodi A. Epigenetic mechanisms in type 2 diabetes retinopathy: A systematic review. Int J Mol Sci (2021) 22(19):10502. doi: 10.3390/ijms221910502
40. Chawla S, Trehan S, Chawla A, Jaggi S, Chawla R, Kumar V, et al. Relationship between diabetic retinopathy microalbuminuria and other modifiable risk factors. Primary Care Diabetes (2021) 15(3):567–70. doi: 10.1016/j.pcd.2021.01.012
41. Manaviat MR, Afkhami M, Shoja MR. Retinopathy and microalbuminuria in type II diabetic patients. BMC Ophthalmol (2004) 4(1):9. doi: 10.1186/1471-2415-4-9
42. Dhana K, Nano J, Ligthart S, Peeters A, Hofman A, Nusselder W, et al. Obesity and life expectancy with and without diabetes in adults aged 55 years and older in the Netherlands: A prospective cohort study. N.J Wareham PloS Med (2016) 13(7):e1002086. doi: 10.1371/journal.pmed.1002086
43. Zhu W, Wu Y, Meng Y-F, Xing Q, Tao JJ, Lu J. Association of obesity and risk of diabetic retinopathy in diabetes patients: A meta-analysis of prospective cohort studies. Medicine (2018) 97(32):e11807. doi: 10.1097/MD.0000000000011807
44. Tziomalos K, Athyros VG. Diabetic nephropathy: new risk factors and improvements in diagnosis. Rev Diabetic Stud (2015) 12(1–2):110–8. doi: 10.1900/RDS.2015.12.110
45. Viberti GC, Hill RD, Jarrett RJ, Argyropoulos A, Mahmud U, Keen H. Microalbuminuria as A predictor of clinical nephropathy in insulin-dependent diabetes mellitus. Lancet (1982) 319(8287):1430–2. doi: 10.1016/S0140-6736(82)92450-3
46. De Boer IH, Steffes MW. Glomerular filtration rate and albuminuria: twin manifestations of nephropathy in diabetes. J Am Soc Nephrol (2007) 18(4):1036–7. doi: 10.1681/ASN.2007020189
47. Quinn M, Angelico MC, Warram JH, Krolewski AS. Familial factors determine the development of diabetic nephropathy in patients with IDDM. Diabetologia (1996) 39(8):940–5. doi: 10.1007/BF00403913
48. Freedman BI, Tuttle AB, Spray BJ. Familial predisposition to nephropathy in African-Americans with non-insulin-dependent diabetes mellitus. Am J Kidney Dis (1995) 25(5):710–3. doi: 10.1016/0272-6386(95)90546-4
49. Pettitt DJ, Saad MF, Bennett PH, Nelson RG, Knowler WC. Familial predisposition to renal disease in two generations of Pima Indians with Type 2 (non-insulin-dependent) diabetes mellitus. Diabetologia (1990) 33(7):438–43. doi: 10.1007/BF00404096
50. Jiang W, Wang J, Shen X, Lu W, Wang Y, Li W, et al. Establishment and validation of a risk prediction model for early diabetic kidney disease based on a systematic review and meta-analysis of 20 cohorts. Diabetes Care (2020) 43(4):925–33. doi: 10.2337/dc19-1897
51. Ren J, Zhang S, Pan Y, Jin M, Li J, Luo Y, et al. Diabetic retinopathy: Involved cells, biomarkers, and treatments. Front Pharmacol (2022) 13:953691. doi: 10.3389/fphar.2022.953691
52. Liu Y, Wu N. Progress of nanotechnology in diabetic retinopathy treatment. Int J Nanomedicine (2021) 16:1391–403. doi: 10.2147/IJN.S294807
53. Barrera-Chimal J, Jaisser F. Pathophysiologic mechanisms in diabetic kidney disease: A focus on current and future therapeutic targets. Diabetes Obes Metab (2020) 22(S1):16–31. doi: 10.1111/dom.13969
54. Maezawa Y, Takemoto M, Yokote K. Cell biology of diabetic nephropathy: Roles of endothelial cells, tubulointerstitial cells and podocytes. J Diabetes Invest (2015) 6(1):3–15. doi: 10.1111/jdi.12255
55. White KE, Bilous RW, Diabiopsies Study Group. Structural alterations to the podocyte are related to proteinuria in type 2 diabetic patients. Nephrol Dial Transplant (2004) 19(6):1437–40. doi: 10.1093/ndt/gfh129
56. Najafian B, Crosson JT, Kim Y, Mauer M. Glomerulotubular junction abnormalities are associated with proteinuria in type 1 diabetes. J Am Soc Nephrol (2006) 17(4 Suppl 2):S53–60. doi: 10.1681/ASN.2005121342
57. Shi Y, Vanhoutte PM. Macro- and microvascular endothelial dysfunction in diabetes. J Diabetes (2017) 9(5):434–49. doi: 10.1111/1753-0407.12521
58. Yang J, Liu Z. Mechanistic pathogenesis of endothelial dysfunction in diabetic nephropathy and retinopathy. Front Endocrinol (2022) 13:816400. doi: 10.3389/fendo.2022.816400
59. Darenskaya MA, Kolesnikova LI, Kolesnikov SI. Oxidative stress: pathogenetic role in diabetes mellitus and its complications and therapeutic approaches to correction. Bull Exp Biol Med (2021) 171(2):179–89. doi: 10.1007/s10517-021-05191-7
60. Nebbioso M, Pranno F, Pescosolido N. Lipoic acid in animal models and clinical use in diabetic retinopathy. Expert Opin Pharmacotherapy (2013) 14(13):1829–38. doi: 10.1517/14656566.2013.813483
61. Beatty S, Koh H-H, Phil M, Henson D, Boulton M. The role of oxidative stress in the pathogenesis of age-related macular degeneration. Survey Ophthalmol (2000) 45(2):115–34. doi: 10.1016/S0039-6257(00)00140-5
62. Apel K, Hirt H. REACTIVE OXYGEN SPECIES: metabolism, oxidative stress, and signal transduction. Annu Rev Plant Biol (2004) 55(1):373–99. doi: 10.1146/annurev.arplant.55.031903.141701
63. Hanssen KF, Bangstad HJ, Brinchmann-Hansen O, Dahl-Jørgensen K. Blood glucose control and diabetic microvascular complications: long-term effects of near-normoglycaemia. Diabetes Med (1992) 9(8):697–705. doi: 10.1111/j.1464-5491.1992.tb01876.x
64. Stratton IM, Adler AI, Neil HA, Matthews DR, Manley SE, Cull CA, et al. Association of glycaemia with macrovascular and microvascular complications of type 2 diabetes (UKPDS 35): prospective observational study. BMJ. (2000) 321(7258):405–12. doi: 10.1136/bmj.321.7258.405
65. Giacco F, Brownlee M. Oxidative stress and diabetic complications. Circ Res (2010) 107(9):1058–70. doi: 10.1161/CIRCRESAHA.110.223545
66. Garg SS, Gupta J. Polyol pathway and redox balance in diabetes. Pharmacol Res (2022) 182:106326. doi: 10.1016/j.phrs.2022.106326
67. Moldogazieva NT, Mokhosoev IM, Mel’nikova TI, Porozov YB, Terentiev AA. Oxidative stress and advanced lipoxidation and glycation end products (ALEs and AGEs) in aging and age-related diseases. Oxid Med Cell Longevity (2019) 2019:1–14. doi: 10.1155/2019/3085756
68. Wu X-Q, Zhang D-D, Wang Y-N, Tan YQ, Yu XY, Zhao YY. AGE/RAGE in diabetic kidney disease and ageing kidney. Free Radical Biol Med (2021) 171:260–71. doi: 10.1016/j.freeradbiomed.2021.05.025
69. Gopalakrishna R, Jaken S. Protein kinase C signaling and oxidative stress. Free Radical Biol Med (2000) 28(9):1349–61. doi: 10.1016/S0891-5849(00)00221-5
70. Kang Q, Yang C. Oxidative stress and diabetic retinopathy: Molecular mechanisms, pathogenetic role and therapeutic implications. Redox Biol (2020) 37:101799. doi: 10.1016/j.redox.2020.101799
71. Ighodaro OM. Molecular pathways associated with oxidative stress in diabetes mellitus. Biomedicine Pharmacotherapy (2018) 108:656–62. doi: 10.1016/j.biopha.2018.09.058
72. Jha JC, Banal C, Chow BS, Cooper ME, Jandeleit-Dahm K. Diabetes and kidney disease: role of oxidative stress. Antioxid Redox Signal (2016) 25(12):657–84. doi: 10.1089/ars.2016.6664
73. Valle MS, Russo C, Malaguarnera L. Protective role of vitamin D against oxidative stress in diabetic retinopathy. Diabetes/Metabolism Res Rev (2021) 37(8):e3447. doi: 10.1002/dmrr.3447
74. Stitt AW. AGEs and diabetic retinopathy. Invest Opthalmology Visual Sci (2010) 51(10):4867. doi: 10.1167/iovs.10-5881
75. Xu J, Chen LJ, Yu J, Wang HJ, Zhang F, Liu Q, Wu J. Involvement of Advanced Glycation End Products in the Pathogenesis of Diabetic Retinopathy. Cell Physiol Biochem (2018) 48(2):705–717. doi: 10.1159/000491897
76. Zong H, Ward M, Stitt AW. AGEs, RAGE, and diabetic retinopathy. Curr Diabetes Rep (2011) 11(4):244–52. doi: 10.1007/s11892-011-0198-7
77. Thomas MC, Forbes JM, Cooper ME. Advanced glycation end products and diabetic nephropathy. Am J Ther (2005) 12(6):562–72. doi: 10.1097/01.mjt.0000178769.52610.69
78. Cheng H-M, González RG. The effect of high glucose and oxidative stress on lens metabolism, aldose reductase, and senile cataractogenesis. Metabolism (1986) 35(4):10–4. doi: 10.1016/0026-0495(86)90180-0
79. Oates PJ, Mylari BL. Aldose reductase inhibitors: therapeutic implications for diabetic complications. Expert Opin Investigational Drugs (1999) 8(12):2095–119. doi: 10.1517/13543784.8.12.2095
80. Greene DA, Stevens MJ, Obrosova I, Feldman EL. Glucose-induced oxidative stress and programmed cell death in diabetic neuropathy. Eur J Pharmacol (1999) 375(1–3):217–23. doi: 10.1016/S0014-2999(99)00356-8
81. Chung SSM, Ho ECM, Lam KSL, Chung SK. Contribution of polyol pathway to diabetes-induced oxidative stress. J Am Soc Nephrol (2003) 14(suppl_3):S233–6. doi: 10.1097/01.ASN.0000077408.15865.06
82. Wang QJ. PKD at the crossroads of DAG and PKC signaling. Trends Pharmacol Sci (2006) 27(6):317–23. doi: 10.1016/j.tips.2006.04.003
83. Shiba T, Inoguchi T, Sportsman JR, Heath WF, Bursell S, King GL. Correlation of diacylglycerol level and protein kinase C activity in rat retina to retinal circulation. Am J Physiology-Endocrinology Metab (1993) 265(5):E783–93. doi: 10.1152/ajpendo.1993.265.5.E783
84. Xia P, Inoguchi T, Kern TS, Engerman RL, Oates PJ, King GL. Characterization of the mechanism for the chronic activation of diacylglycerol-protein kinase C pathway in diabetes and hypergalactosemia. Diabetes (1994) 43(9):1122–9. doi: 10.2337/diab.43.9.1122
85. Haneda M, Koya D, Kikkawa R. Cellular mechanisms in the development and progression of diabetic nephropathy: Activation of the DAG-PKC-ERK pathway. Am J Kidney Dis (2001) 38(4):S178–81. doi: 10.1053/ajkd.2001.27438
86. Craven PA, Davidson CM. Increase in diacylglycerol mass in isolated glomeruli by glucose from de novo synthesis of glycerolipids. Diabetes (1990) 39(6):667–74. doi: 10.2337/diab.39.6.667
87. Pan D, Xu L, Guo M. The role of protein kinase C in diabetic microvascular complications. Front Endocrinol (2022) 13:973058. doi: 10.3389/fendo.2022.973058
88. Koya D, Haneda M, Kikkawa R, King GL. d-α-tocopherol treatment prevents glomerular dysfunctions in diabetic rats through inhibition of protein kinase C-diacylglycerol pathway. BioFactors (1998) 7(1–2):69–76. doi: 10.1002/biof.5520070110
89. Singh RK, Kumar S, Gautam PK, Tomar MS, Verma PK, Singh SP, et al. Protein kinase C-α and the regulation of diverse cell responses. Biomolecular Concepts (2017) 8(3–4):143–53. doi: 10.1515/bmc-2017-0005
90. Miyata Y, Kase M, Sugita Y, Shimada A, Nagase T, Katsura Y, et al. Protein kinase C-mediated regulation of matrix metalloproteinase and tissue inhibitor of metalloproteinase production in a human retinal müller cells. Curr Eye Res (2012) 37(9):842–9. doi: 10.3109/02713683.2012.682635
91. Serra AM, Waddell J, Manivannan A, Xu H, Cotter M, Forrester JV. CD11b + Bone marrow–derived monocytes are the major leukocyte subset responsible for retinal capillary leukostasis in experimental diabetes in mouse and express high levels of CCR5 in the circulation. Am J Pathol (2012) 181(2):719–27. doi: 10.1016/j.ajpath.2012.04.009
92. Adamis AP. Is diabetic retinopathy an inflammatory disease? Br J Ophthalmol (2002) 86(4):363–5. doi: 10.1136/bjo.86.4.363
93. Miyamoto K, Khosrof S, Bursell S-E, Rohan R, Murata T, Clermont AC, et al. Prevention of leukostasis and vascular leakage in streptozotocin-induced diabetic retinopathy via intercellular adhesion molecule-1 inhibition. Proc Natl Acad Sci (1999) 96(19):10836–41. doi: 10.1073/pnas.96.19.10836
94. Yerramothu P, Vijay AK, Willcox MDP. Inflammasomes, the eye and anti-inflammasome therapy. Eye (2018) 32(3):491–505. doi: 10.1038/eye.2017.241
95. Huang W, Man Y, Gao C, Zhou L, Gu J, Xu H, et al. Short-chain fatty acids ameliorate diabetic nephropathy via GPR43-mediated inhibition of oxidative stress and NF- κ B signaling. Oxid Med Cell Longevity (2020) 2020:1–21. doi: 10.1155/2020/8706898
96. Chen J, Liu Q, He J, Li Y. Immune responses in diabetic nephropathy: Pathogenic mechanisms and therapeutic target. Front Immunol (2022) 13:958790. doi: 10.3389/fimmu.2022.958790
97. Navarro-González JF, Mora-Fernández C, Muros de Fuentes M, García-Pérez J. Inflammatory molecules and pathways in the pathogenesis of diabetic nephropathy. Nat Rev Nephrol (2011) 7(6):327–40. doi: 10.1038/nrneph.2011.51
98. Dalla Vestra M, Mussap M, Gallina P, Bruseghin M, Cernigoi AM, Saller A, et al. Acute-phase markers of inflammation and glomerular structure in patients with type 2 diabetes. J Am Soc Nephrol (2005) 16(3_suppl_1):S78–82. doi: 10.1681/ASN.2004110961
99. Wada J, Makino H. Inflammation and the pathogenesis of diabetic nephropathy. Clin Sci (2013) 124(3):139–52. doi: 10.1042/CS20120198
100. Devi TS, Hosoya K-I, Terasaki T, Singh LP. Critical role of TXNIP in oxidative stress, DNA damage and retinal pericyte apoptosis under high glucose: Implications for diabetic retinopathy. Exp Cell Res (2013) 319(7):1001–12. doi: 10.1016/j.yexcr.2013.01.012
101. Perrone L, Devi TS, Hosoya K, Terasaki T, Singh LP. Thioredoxin interacting protein (TXNIP) induces inflammation through chromatin modification in retinal capillary endothelial cells under diabetic conditions. J Cell Physiol (2009) 221(1):262–72. doi: 10.1002/jcp.21852
102. Singh LP. Thioredoxin interacting protein (TXNIP) and pathogenesis of diabetic retinopathy. J Clin Exp Ophthalmol (2013) 04:10.4172/2155-9570.1000287. doi: 10.4172/2155-9570.1000287
103. Devi TS, Lee I, Hüttemann M, Kumar A, Nantwi KD, Singh LP. TXNIP links innate host defense mechanisms to oxidative stress and inflammation in retinal muller glia under chronic hyperglycemia: implications for diabetic retinopathy. Exp Diabetes Res (2012) 2012:1–19. doi: 10.1155/2012/438238
104. Perrone L, Devi TS, Hosoya K-I, Terasaki T, Singh LP. Inhibition of TXNIP expression in vivo blocks early pathologies of diabetic retinopathy. Cell Death Dis (2010) 1(8):e65–5. doi: 10.1038/cddis.2010.42
105. Dai X, Liao R, Liu C, Liu S, Huang H, Liu J, et al. Epigenetic regulation of TXNIP-mediated oxidative stress and NLRP3 inflammasome activation contributes to SAHH inhibition-aggravated diabetic nephropathy. Redox Biol (2021) 45:102033. doi: 10.1016/j.redox.2021.102033
106. Sassy-Prigent C, Heudes D, Mandet C, Bélair MF, Michel O, Perdereau B. Early glomerular macrophage recruitment in streptozotocin-induced diabetic rats. Diabetes (2000) 49(3):466–75. doi: 10.2337/diabetes.49.3.466
107. Shikata K, Makino H. Role of macrophages in the pathogenesis of diabetic nephropathy. Contrib Nephrol (2001) 134):46–54. doi: 10.1159/000060147
108. Tesch G. Role of macrophages in complications of type 2 diabetes. Clin Exp Pharmacol Physiol (2007) 34(10):1016–9. doi: 10.1111/j.1440-1681.2007.04729.x
109. Forrester JV, Kuffova L, Delibegovic M. The role of inflammation in diabetic retinopathy. Front Immunol (2020) 11:583687. doi: 10.3389/fimmu.2020.583687
110. Capitão M, Soares R. Angiogenesis and inflammation crosstalk in diabetic retinopathy: V ASCULARIZATION IN DR. J Cell Biochem (2016) 117(11):2443–53. doi: 10.1002/jcb.25575
111. Sung SH, Ziyadeh FN, Wang A, Pyagay PE, Kanwar YS, Chen S. Blockade of vascular endothelial growth factor signaling ameliorates diabetic albuminuria in mice. J Am Soc Nephrol (2006) 17(11):3093–104. doi: 10.1681/ASN.2006010064
112. Lechner J, O’Leary OE, Stitt AW. The pathology associated with diabetic retinopathy. Vision Res (2017) 139:7–14. doi: 10.1016/j.visres.2017.04.003
113. Yan H-T, Su G-F. Expression and significance of HIF-1 α and VEGF in rats with diabetic retinopathy. Asian Pacific J Trop Med (2014) 7(3):237–40. doi: 10.1016/S1995-7645(14)60028-6
114. Hasslacher C. Diabetic nephropathy: structural-functional relationships. Contrib Nephrol (1989) 73:24–8; discussion 28-9. doi: 10.1159/000417376
115. Tuttle KR. Back to the future: glomerular hyperfiltration and the diabetic kidney. Diabetes (2017) 66(1):14–6. doi: 10.2337/dbi16-0056
116. Anderson S, Brenner BM. Pathogenesis of diabetic glomerulopathy: Hemodynamic considerations. Diabetes / Metab Rev (1988) 4(2):163–77. doi: 10.1002/dmr.5610040206
117. McMillan DE. Deterioration of the microcirculation in diabetes. Diabetes (1975) 2(10):944–57. doi: 10.2337/diabetes.24.10.944
118. Pappelis K, Jansonius NM. U-shaped effect of blood pressure on structural OCT metrics and retinal blood flow autoregulation in ophthalmologically healthy subjects. Ophthalmology (2021). doi: 10.1101/2021.01.14.21249808
119. Burgansky-Eliash Z, Nelson DA, Bar-Tal OP, Lowenstein A, Grinvald A, Barak A. Reduced retinal blood flow velocity in diabetic retinopathy. Retina (2010) 30(5):765–73. doi: 10.1097/IAE.0b013e3181c596c6
120. Wright WS, Eshaq RS, Lee M, Kaur G, Harris NR. Retinal physiology and circulation: effect of diabetes. Compr Physiol (2020) 10(3):933–74. doi: 10.1002/cphy.c190021
121. Sonkodi S. Treatment of diabetic nephropathy with angiotensin II blockers. Nephrol Dialysis Transplant (2003) 18(90005):21v–23. doi: 10.1093/ndt/gfg1037
122. Brewster UC, Perazella MA. The renin-angiotensin-aldosterone system and the kidney: effects on kidney disease. Am J Med (2004) 116(4):263–72. doi: 10.1016/j.amjmed.2003.09.034
123. Patel S, Rauf A, Khan H, Abu-Izneid T. Renin-angiotensin-aldosterone (RAAS): The ubiquitous system for homeostasis and pathologies. Biomedicine Pharmacotherapy (2017) 94:317–25. doi: 10.1016/j.biopha.2017.07.091
124. Luther JM, Brown NJ. The renin–angiotensin–aldosterone system and glucose homeostasis. Trends Pharmacol Sci (2011) 32(12):734–9. doi: 10.1016/j.tips.2011.07.006
125. Rahimi Z. The role of renin angiotensin aldosterone system genes in diabetic nephropathy. Can J Diabetes (2016) 40(2):178–83. doi: 10.1016/j.jcjd.2015.08.016
126. Funatsu H, Yamashita H, Ikeda T, Mimura T, Shimizu E, Hori S. Relation of diabetic macular edema to cytokines and posterior vitreous detachment. Am J Ophthalmol (2003) 135(3):321–7. doi: 10.1016/S0002-9394(02)01971-2
127. Strain WD, Chaturvedi N. Review: The renin-angiotensin-aldosterone system and the eye in diabetes. J Renin-Angiotensin-Aldosterone System (2002) 3(4):243–6. doi: 10.3317/jraas.2002.045
128. Phipps JA, Dixon MA, Jobling AI, Wang AY, Greferath U, Vessey KA, et al. The renin-angiotensin system and the retinal neurovascular unit: A role in vascular regulation and disease. Exp Eye Res (2019) 187:107753. doi: 10.1016/j.exer.2019.107753
129. Wilkinson-Berka JL. Diabetes and retinal vascular disorders: role of the renin–angiotensin system. Expert Rev Mol Med (2004) 6(15):1–18. doi: 10.1017/S1462399404008129
130. Chiang W-C, Chien C-T, Lin W-W, Lin SL, Chen YM, Lai CF, et al. Early activation of bradykinin B2 receptor aggravates reactive oxygen species generation and renal damage in ischemia/reperfusion injury. Free Radical Biol Med (2006) 41(8):1304–14. doi: 10.1016/j.freeradbiomed.2006.07.011
131. Kayashima Y, Smithies O, Kakoki M. The kallikrein–kinin system and oxidative stress. Curr Opin Nephrol Hypertension (2012) 21(1):92–6. doi: 10.1097/MNH.0b013e32834d54b1
132. Abdulaal M, Haddad NMN, Sun JK, Silva PS. The role of plasma kallikrein–kinin pathway in the development of diabetic retinopathy: pathophysiology and therapeutic approaches. Semin Ophthalmol (2016) 31(1–2):19–24. doi: 10.3109/08820538.2015.1114829
133. Liu J, Feener EP. Plasma kallikrein-kinin system and diabetic retinopathy. bchm (2013) 394(3):319–28. doi: 10.1515/hsz-2012-0316
134. Webb JG. The kallikrein/kinin system in ocular function. J Ocular Pharmacol Ther (2011) 27(6):539–43. doi: 10.1089/jop.2011.0187
135. Kakoki M, Takahashi N, Jennette JC, Smithies O. Diabetic nephropathy is markedly enhanced in mice lacking the bradykinin B2 receptor. Proc Natl Acad Sci (2004) 101(36):13302–5. doi: 10.1073/pnas.0405449101
136. Spillmann F, Van Linthout S, Schultheiss H-P, Tschöpe C. Cardioprotective mechanisms of the kallikrein–kinin system in diabetic cardiopathy. Curr Opin Nephrol Hypertension (2006) 15(1):22–9. doi: 10.1097/01.mnh.0000199009.56799.2b
137. Riad A, Zhuo JL, Schultheiss HP, Tschöpe C. The role of the renal kallikrein–kinin system in diabetic nephropathy. Curr Opin Nephrol Hypertension (2007) 16(1):22–6. doi: 10.1097/MNH.0b013e328011a20c
138. Kaur P, Kotru S, Singh S, Munshi A. miRNA signatures in diabetic retinopathy and nephropathy: delineating underlying mechanisms. J Physiol Biochem (2022) 78(1):19–37. doi: 10.1007/s13105-021-00867-0
139. Kato M, Natarajan R. Epigenetics and epigenomics in diabetic kidney disease and metabolic memory. Nat Rev Nephrol (2019) 15(6):327–45. doi: 10.1038/s41581-019-0135-6
140. Jiang Q, Lyu X-M, Yuan Y, Wang L. Plasma miR-21 expression: an indicator for the severity of Type 2 diabetes with diabetic retinopathy. Bioscience Rep (2017) 37(2):BSR20160589. doi: 10.1042/BSR20160589
141. Chen Q, Qiu F, Zhou K, Matlock HG, Takahashi Y, Rajala RVS, et al. Pathogenic role of microRNA-21 in diabetic retinopathy through downregulation of PPARα. Diabetes (2017) 66(6):1671–82. doi: 10.2337/db16-1246
142. Liu L-Z, Li C, Chen Q, Jing Y, Carpenter R, Jiang Y, et al. MiR-21 induced angiogenesis through AKT and ERK activation and HIF-1α Expression. PloS One (2011) 6(4):e19139. doi: 10.1371/journal.pone.0019139
143. Lu J-M, Zhang Z-Z, Ma X, Fang SF, Qin XH. Repression of microRNA-21 inhibits retinal vascular endothelial cell growth and angiogenesis via PTEN dependent-PI3K/Akt/VEGF signaling pathway in diabetic retinopathy. Exp Eye Res (2020) 190:107886. doi: 10.1016/j.exer.2019.107886
144. Wang J, Duan L, Tian L, Liu J, Wang S, Gao Y, et al. Serum miR-21 may be a Potential Diagnostic Biomarker for Diabetic Nephropathy. Exp Clin Endocrinol Diabetes (2015) 124(07):417–23. doi: 10.1055/s-0035-1565095
145. Liu L, Wang Y, Yan R, Liang L, Zhou X, Liu H, et al. BMP-7 inhibits renal fibrosis in diabetic nephropathy via miR-21 downregulation. Life Sci (2019) 238:116957. doi: 10.1016/j.lfs.2019.116957
146. Liu S, Wu W, Liao J, Tang F, Gao G, Peng J, et al. MicroRNA-21: A critical pathogenic factor of diabetic nephropathy. Front Endocrinol (2022) 13:895010. doi: 10.3389/fendo.2022.895010
147. McClelland AD, Herman-Edelstein M, Komers R, Jha JC, Winbanks CE, Hagiwara S, et al. miR-21 promotes renal fibrosis in diabetic nephropathy by targeting PTEN and SMAD7. Clin Sci (2015) 129(12):1237–49. doi: 10.1042/CS20150427
148. Zhong X, Chung ACK, Chen HY, Dong Y, Meng XM, Li R, et al. miR-21 is a key therapeutic target for renal injury in a mouse model of type 2 diabetes. Diabetologia (2013) 56(3):663–74. doi: 10.1007/s00125-012-2804-x
149. Catrina S-B, Zheng X. Hypoxia and hypoxia-inducible factors in diabetes and its complications. Diabetologia (2021) 64(4):709–16. doi: 10.1007/s00125-021-05380-z
150. Ren H, Luo J-Q, Gao Y-C, Chen MY, Chen XP, Zhou HH, et al. Genetic association of hypoxia inducible factor 1-alpha (HIF1A) Pro582Ser polymorphism with risk of diabetes and diabetic complications. Aging (2020) 12(13):12783–98. doi: 10.18632/aging.103213
151. Semenza GL. Hypoxia-inducible factor 1: master regulator of O2 homeostasis. Curr Opin Genet Dev (1998) 8(5):588–94. doi: 10.1016/S0959-437X(98)80016-6
152. Catrina SB, Okamoto K, Pereira T, Brismar K, Poellinger L. Hyperglycemia regulates hypoxia-inducible factor-1alpha protein stability and function. Diabetes. (2004) 53(12):3226–32. doi: 10.2337/diabetes.53.12.3226
153. M R, D M, Filippo CD, Piegari E, Nappo F, Esposito K, et al. Myocardial infarction in diabetic rats: role of hyperglycaemia on infarct size and early expression of hypoxia-inducible factor 1. Diabetologia (2002) 45(8):1172–81. doi: 10.1007/s00125-002-0882-x
154. Vinovskis C, Li L-P, Prasad P, Tommerdahl K, Pyle L, Nelson RG, et al. Relative hypoxia and early diabetic kidney disease in type 1 diabetes. Diabetes (2020) 69(12):2700–8. doi: 10.2337/db20-0457
155. Packer M. Mechanisms leading to differential hypoxia-inducible factor signaling in the diabetic kidney: modulation by SGLT2 inhibitors and hypoxia mimetics. Am J Kidney Dis (2021) 77(2):280–6. doi: 10.1053/j.ajkd.2020.04.016
156. Gilbert RE. Proximal tubulopathy: prime mover and key therapeutic target in diabetic kidney disease. Diabetes (2017) 66(4):791–800. doi: 10.2337/db16-0796
157. Lange CA, Bainbridge JW. Oxygen sensing in retinal health and disease. Ophthalmologica. (2012) 227(3):115–31. doi: 10.1159/000331418
158. Niu S, Hu J, Lin S, Hong Y. Research progress on exosomes/microRNAs in the treatment of diabetic retinopathy. Front Endocrinol (2022) 13:935244. doi: 10.3389/fendo.2022.935244
159. Ramsey DJ, Arden GB. Hypoxia and dark adaptation in diabetic retinopathy: interactions, consequences, and therapy. Curr Diabetes Rep (2015) 15(12):118. doi: 10.1007/s11892-015-0686-2
160. Hammer M, Vilser W, Riemer T, Mandecka A, Schweitzer D, Kühn U, et al. Diabetic patients with retinopathy show increased retinal venous oxygen saturation. Graefe’s Arch Clin Exp Ophthalmol (2009) 247(8):1025–30. doi: 10.1007/s00417-009-1078-6
161. Linsenmeier RA, Zhang HF. Retinal oxygen: from animals to humans. Prog Retinal Eye Res (2017) 58:115–51. doi: 10.1016/j.preteyeres.2017.01.003
162. Wangsa-Wirawan ND. Retinal oxygen: fundamental and clinical aspects. Arch Ophthalmol (2003) 121(4):547. doi: 10.1001/archopht.121.4.547
163. Wright WS, McElhatten RM, Messina JE, Harris NR. Hypoxia and the expression of HIF-1α and HIF-2α in the retina of streptozotocin-injected mice and rats. Exp Eye Res (2010) 90(3):405–12. doi: 10.1016/j.exer.2009.12.002
164. Werkmeister RM, Schmidl D, Aschinger G, Doblhoff-Dier V, Palkovits S, Wirth M, et al. Retinal oxygen extraction in humans. Sci Rep (2015) 5(1):15763. doi: 10.1038/srep15763
165. Crasto W, Patel V, Davies MJ, Khunti K. Prevention of microvascular complications of diabetes. Endocrinol Metab Clinics North America (2021) 50(3):431–55. doi: 10.1016/j.ecl.2021.05.005
166. Kumar H, Shah A, Sobhia ME. Novel insights into the structural requirements for the design of selective and specific aldose reductase inhibitors. J Mol Modeling (2012) 18(5):1791–9. doi: 10.1007/s00894-011-1195-0
167. He J, Gao H, Yang N, Zhu XD, Sun RB, Xie Y, et al. The aldose reductase inhibitor epalrestat exerts nephritic protection on diabetic nephropathy in db/db mice through metabolic modulation. Acta Pharmacologica Sin (2019) 40(1):86–97. doi: 10.1038/s41401-018-0043-5
168. Hotta N, Akanuma Y, Kawamori R, Matsuoka K, Oka Y, Shichiri M, et al. Long-term clinical effects of epalrestat, an aldose reductase inhibitor, on diabetic peripheral neuropathy. Diabetes Care (2006) 29(7):1538–44. doi: 10.2337/dc05-2370
169. Hotta N, Kawamori R, Fukuda M, Shigeta Y. Long-term clinical effects of epalrestat, an aldose reductase inhibitor, on progression of diabetic neuropathy and other microvascular complications: multivariate epidemiological analysis based on patient background factors and severity of diabetic neuropathy. Diabetic Med (2012) 29(12):1529–33. doi: 10.1111/j.1464-5491.2012.03684.x
170. Gomes MB, Negrato CA. Alpha-lipoic acid as a pleiotropic compound with potential therapeutic use in diabetes and other chronic diseases. Diabetol Metab Syndrome (2014) 6(1):80. doi: 10.1186/1758-5996-6-80
171. Alvarez-Rivera F, Fernández-Villanueva D, Concheiro A, Alvarez-Lorenzo C. α-lipoic acid in soluplus ® Polymeric nanomicelles for ocular treatment of diabetes-associated corneal diseases. J Pharm Sci (2016) 105(9):2855–63. doi: 10.1016/j.xphs.2016.03.006
172. Wu H, Xu G, Liao Y, Ren H, Fan J, Sun Z, et al. Supplementation with antioxidants attenuates transient worsening of retinopathy in diabetes caused by acute intensive insulin therapy. Graefe’s Arch Clin Exp Ophthalmol (2012) 250(10):1453–8. doi: 10.1007/s00417-012-2079-4
173. Chen CL, Cheng WS, Chen JL, Chiang CH. Potential of nonoral α-lipoic acid aqueous formulations to reduce ocular microvascular complications in a streptozotocin-induced diabetic rat model. J Ocular Pharmacol Ther (2013) 29(8):738–45. doi: 10.1089/jop.2012.0147
174. Bierbrauer KL, Comini LR, Leonhard V, Escobar Manzanelli MA, Castelli G, Farfán S, et al. Eudragit films as carriers of lipoic acid for transcorneal permeability. Polymers (2023) 15(7):1793. doi: 10.3390/polym15071793
175. Rochette L, Ghibu S, Muresan A, Vergely C. Alpha-lipoic acid: molecular mechanisms and therapeutic potential in diabetes. Can J Physiol Pharmacol (2015) 93(12):1021–7. doi: 10.1139/cjpp-2014-0353
176. Jacob S, Ruus P, Hermann R, Tritschler HJ, Maerker E, Renn W, et al. Oral administration of RAC-alpha-lipoic acid modulates insulin sensitivity in patients with type-2 diabetes mellitus: a placebo-controlled pilot trial. Free Radic Biol Med (1999) 27(3-4):309–14. doi: 10.1016/S0891-5849(99)00089-1
177. Obrosova IG, Fathallah L, Greene DA. Early changes in lipid peroxidation and antioxidative defense in diabetic rat retina: effect of DL-a-lipoic acid. Eur J Pharmacol (2000) 398(1):139–46. doi: 10.1016/S0014-2999(00)00286-7
178. Bierhaus A, Chevion S, Chevion M, Hofmann M, Quehenberger P, Illmer T, et al. Advanced glycation end product-induced activation of NF-κB is suppressed by α-lipoic acid in cultured endothelial cells. Diabetes (1997) 46(9):1481–90. doi: 10.2337/diab.46.9.1481
179. Kowluru RA, Odenbach S. Effect of long-term administration of α-lipoic acid on retinal capillary cell death and the development of retinopathy in diabetic rats. Diabetes (2004) 53(12):3233–8. doi: 10.2337/diabetes.53.12.3233
180. Bhatti F, Mankhey RW, Asico L, Quinn MT, Welch WJ, Maric C. Mechanisms of antioxidant and pro-oxidant effects of α-lipoic acid in the diabetic and nondiabetic kidney. Kidney Int (2005) 67(4):1371–80. doi: 10.1111/j.1523-1755.2005.00214.x
181. Melhem MF, Craven PA, Liachenko J, DeRubertis FR. Alpha-lipoic acid attenuates hyperglycemia and prevents glomerular mesangial matrix expansion in diabetes. J Am Soc Nephrol (2002) 13(1):108–16. doi: 10.1681/ASN.V131108
182. Zhang HF, Liu HM, Xiang JY, Zhou XC, Wang D, Chen RY, et al. Alpha lipoamide inhibits diabetic kidney fibrosis via improving mitochondrial function and regulating RXRα expression and activation. Acta Pharmacologica Sin (2023) 44(5):1051–65. doi: 10.1038/s41401-022-00997-1
183. Şehirli Ö, Şener E, Çetinel Ş, Yüksel M, Gedik N, Sener G. Alpha-lipoic acid protects against renal ischaemia-reperfusion injury in rats. Clin Exp Pharmacol Physiol (2008) 35(3):249–55. doi: 10.1111/j.1440-1681.2007.04810.x
184. Rossing P, Caramori ML, Chan JCN, Heerspink HJL, Hurst C, Khunti K, et al. Executive summary of the KDIGO 2022 Clinical Practice Guideline for Diabetes Management in Chronic Kidney Disease: an update based on rapidly emerging new evidence. Kidney Int (2022) 102(5):990–9. doi: 10.1016/j.kint.2022.06.013
185. Wilkinson-Berka JL. Angiotensin and diabetic retinopathy. Int J Biochem Cell Biol (2006) 38(5–6):752–65. doi: 10.1016/j.biocel.2005.08.002
186. Senanayake Pd, Bonilha VL, Peterson J W, Yamada Y, Karnik SS, Daneshgari F, et al. Retinal angiotensin II and angiotensin-(1-7) response to hyperglycemia and an intervention with captopril. J Renin-Angiotensin-Aldosterone System (2018) 19(3):147032031878932. doi: 10.1177/1470320318789323
187. Umanath K, Lewis JB. Update on diabetic nephropathy: core curriculum 2018. Am J Kidney Dis (2018) 71(6):884–95. doi: 10.1053/j.ajkd.2017.10.026
188. Zhang J-Z, Xi X, Gao L, Kern TS. Captopril inhibits capillary degeneration in the early stages of diabetic retinopathy. Curr Eye Res (2007) 32(10):883–9. doi: 10.1080/02713680701584123
189. Gao X, Liu K, Hu C, Chen K, Jiang Z. Captopril alleviates oxidative damage in diabetic retinopathy. Life Sci (2022) 290:120246. doi: 10.1016/j.lfs.2021.120246
190. Tejerina T, Ruiz E. Calcium dobesilate: pharmacology and future approaches. Gen Pharmacol (1998) 31(3):357–60. doi: 10.1016/S0306-3623(98)00040-8
191. Zhou Y, Qi C, Li S, Shao X, Mou S, Ni Z. Diabetic nephropathy can be treated with calcium dobesilate by alleviating the chronic inflammatory state and improving endothelial cell function. Cell Physiol Biochem (2018) 51(3):1119–33. doi: 10.1159/000495491
192. Zhou Y, Yuan J, Qi C, Shao X, Mou S, Ni Z. Calcium dobesilate may alleviate diabetes-induced endothelial dysfunction and inflammation. Mol Med Rep (2017) 16(6):8635–42. doi: 10.3892/mmr.2017.7740
193. Njau F, Shushakova N, Schenk H, Wulfmeyer VC, Bollin R, Menne J, et al. Calcium dobesilate reduces VEGF signaling by interfering with heparan sulfate binding site and protects from vascular complications in diabetic mice. PloS One (2020) 15(1):e0218494. doi: 10.1371/journal.pone.0218494
194. Zhang X, Liu W, Wu S, Jin J, Li W, Wang N. Calcium dobesilate for diabetic retinopathy: a systematic review and meta-analysis. Sci China Life Sci (2015) 58(1):101–7. doi: 10.1007/s11427-014-4792-1
195. Liu J, Li S, Sun D. Calcium dobesilate and micro-vascular diseases. Life Sci (2019) 221:348–53. doi: 10.1016/j.lfs.2019.02.023
196. Cai T, Wu XY, Zhang XQ, Shang HX, Zhang ZW, Liao L, et al. Calcium dobesilate prevents diabetic kidney disease by decreasing bim and inhibiting apoptosis of renal proximal tubular epithelial cells. DNA Cell Biol (2017) 36(4):249–55. doi: 10.1089/dna.2016.3276
197. Mogensen CE. Microalbuminuria in diabetic renal disease. In: Mogensen CE, Standl E, editors. Prevention and Treatment of Diabetic Late Complications. New York, NY: Walter de Gruyter (1989).
198. Estacio R, McFarling E, Biggerstaff S, Jeffers BW, Johnson D, Schrier RW. Overt albuminuria predicts diabetic retinopathy in Hispanics with NIDDM. Am J Kidney Dis (1998) 31(6):947–53. doi: 10.1053/ajkd.1998.v31.pm9631838
199. Bermejo S, García-Carro C, Soler MJ. Diabetes and renal disease-should we biopsy? Nephrol Dial Transplant (2021) 36(8):1384–6. doi: 10.1093/ndt/gfz248
200. Dong Z, Wang Y, Qiu Q, Zhang X, Zhang L, Wu J, et al. Clinical predictors differentiating non-diabetic renal diseases from diabetic nephropathy in a large population of type 2 diabetes patients. Diabetes Res Clin Pract (2016) 121:112–8. doi: 10.1016/j.diabres.2016.09.005
201. Cao X, Gong X, Ma X. Diabetic nephropathy versus diabetic retinopathy in a chinese population: A retrospective study. Med Sci Monit (2019) 25:6446–53. doi: 10.12659/MSM.915917
202. Johansen J, Sjølie AK, Elbøl P, Eshøj O. The relation between retinopathy and albumin excretion rate in insulin-dependent diabetes mellitus: From the Funen County Epidemiology of Type 1 Diabetes Complications Survey. Acta Ophthalmologica (2009) 72(3):347–51. doi: 10.1111/j.1755-3768.1994.tb02771.x
203. Grauslund J, Green A, Sjølie AK. Proliferative retinopathy and proteinuria predict mortality rate in type 1 diabetic patients from Fyn County, Denmark. Diabetologia (2008) 51(4):583–8. doi: 10.1007/s00125-008-0953-8
204. Agardh E, Tallroth G, Bauer B, Cavallin-Sjöberg U, Agardh CD. Retinopathy and nephropathy in insulin-dependent diabetics: an inconsistent relationship? Diabetes Med (1987) 4(3):248–50. doi: 10.1111/j.1464-5491.1987.tb00873.x
205. Cole JB, Florez JC. Genetics of diabetes mellitus and diabetes complications. Nat Rev Nephrol (2020) 16(7):377–90. doi: 10.1038/s41581-020-0278-5
206. Earle K, Walker J, Hill C, Viberti G. Familial clustering of cardiovascular disease in patients with insulin-dependent diabetes and nephropathy. New Engl J Med (1992) 326(10):673–7. doi: 10.1056/NEJM199203053261005
207. Bek T. The risk for developing vision threatening diabetic retinopathy is influenced by heredity to diabetes. Curr Eye Res (2022) 47(9):1322–8. doi: 10.1080/02713683.2022.2067564
208. Skol AD, Jung SC, Sokovic AM, Chen S, Fazal S, Sosina O, et al. Integration of genomics and transcriptomics predicts diabetic retinopathy susceptibility genes. eLife (2020) 9:e59980. doi: 10.7554/eLife.59980
209. Zhou TB, Drummen GP, Jiang ZP, Li HY. Methylenetetrahydrofolate reductase ( MTHFR ) C677T gene polymorphism and diabetic nephropathy susceptibility in patients with type 2 diabetes mellitus. Renal Failure (2015) 37(8):1247–59. doi: 10.3109/0886022X.2015.1064743
210. Imperatore G, Hanson RL, Pettitt DJ, Kobes S, Bennett PH, Knowler WC. Sib-pair linkage analysis for susceptibility genes for microvascular complications among Pima Indians with type 2 diabetes. Pima Diabetes Genes Group Diabetes (1998) 47(5):821–30. doi: 10.2337/diabetes.47.5.821
211. Looker HC, Nelson RG, Chew E, Klein R, Klein BE, Knowler WC, et al. Genome-wide linkage analyses to identify loci for diabetic retinopathy. Diabetes (2007) 56(4):1160–6. doi: 10.2337/db06-1299
212. Lin H-J, Huang Y-C, Lin J-M, Wu JY, Chen LA, Tsai FJ. Association of genes on chromosome 6, GRIK2, TMEM217 and TMEM63B (Linked to MRPL14) with diabetic retinopathy. Ophthalmologica (2013) 229(1):54–60. doi: 10.1159/000342616
213. Solomon SD, Chew E, Duh EJ, Sobrin L, Sun JK, VanderBeek BL, et al. Diabetic retinopathy: A position statement by the american diabetes association. Diabetes Care (2017) 40(3):412–8. doi: 10.2337/dc16-2641
214. Ji L, Tian H, Webster KA, Li W. Neurovascular regulation in diabetic retinopathy and emerging therapies. Cell Mol Life Sci (2021) 78(16):5977–85. doi: 10.1007/s00018-021-03893-9
215. Simó R, Stitt AW, Gardner TW. Neurodegeneration in diabetic retinopathy: does it really matter? Diabetologia (2018) 61(9):1902–12. doi: 10.1007/s00125-018-4692-1
216. Simó R, Hernández C. Neurodegeneration in the diabetic eye: new insights and therapeutic perspectives. Trends Endocrinol Metab (2014) 25(1):23–33. doi: 10.1016/j.tem.2013.09.005
217. Barber AJ. Diabetic retinopathy: recent advances towards understanding neurodegeneration and vision loss. Sci China Life Sci (2015) 58(6):541–9. doi: 10.1007/s11427-015-4856-x
218. Wu CC, Sytwu HK, Lin YF. Cytokines in diabetic nephropathy. Adv Clin Chem (2012) 56:55–74. doi: 10.1016/B978-0-12-394317-0.00014-5
219. Kanwar YS, Sun L, Xie P, Liu FY, Chen S. A glimpse of various pathogenetic mechanisms of diabetic nephropathy. Annu Rev Pathology: Mech Dis (2011) 6(1):395–423. doi: 10.1146/annurev.pathol.4.110807.092150
220. Obadă O, Pantalon AD, Rusu-Zota G, Hăisan A, Lupuşoru SI, Constantinescu D, et al. Aqueous humor cytokines in non-proliferative diabetic retinopathy. Medicina (2022) 58(7):909. doi: 10.3390/medicina58070909
221. Ra H, Lee A, Lee J, Kim I, Baek J. Cytokines associated with hemorrhage in proliferative diabetic retinopathy. Int Ophthalmol (2021) 41(5):1845–53. doi: 10.1007/s10792-021-01746-9
222. Samsu N. Diabetic nephropathy: challenges in pathogenesis, diagnosis, and treatment. M.I Bellini BioMed Res Int (2021) 2021:1–17. doi: 10.1155/2021/1497449
223. Panchapakesan U, Pollock C. The role of dipeptidyl peptidase – 4 inhibitors in diabetic kidney disease. Front Immunol (2015) 6:443. doi: 10.3389/fimmu.2015.00443
224. Vallon V, Rose M, Gerasimova M, Satriano J, Platt KA, Koepsell H, et al. Knockout of Na-glucose transporter SGLT2 attenuates hyperglycemia and glomerular hyperfiltration but not kidney growth or injury in diabetes mellitus. Am J Physiology-Renal Physiol (2013) 304(2):F156–67. doi: 10.1152/ajprenal.00409.2012
225. Vallon V, Gerasimova M, Rose MA, Masuda T, Satriano J, Mayoux E, et al. SGLT2 inhibitor empagliflozin reduces renal growth and albuminuria in proportion to hyperglycemia and prevents glomerular hyperfiltration in diabetic Akita mice. Am J Physiology-Renal Physiol (2014) 306(2):F194–204. doi: 10.1152/ajprenal.00520.2013
226. Brem AS, Morris DJ, Gong R. Aldosterone-induced fibrosis in the kidney: questions and controversies. Am J Kidney Dis (2011) 58(3):471–9. doi: 10.1053/j.ajkd.2011.03.029
227. Ponda MP, Hostetter TH. Aldosterone antagonism in chronic kidney disease. Clin J Am Soc Nephrol (2006) 1(4):668–77. doi: 10.2215/CJN.00120106
228. Viengchareun S, Le Menuet D, Martinerie L, Munier M, Pascual-Le Tallec L, Lombès M. The Mineralocorticoid Receptor: Insights into its Molecular and (Patho)Physiological Biology. Nucl Receptor Signaling (2007) 5(1):nrs.05012. doi: 10.1621/nrs.05012
229. Hou J, Xiong W, Cao L, Wen X, Li A. Spironolactone add-on for preventing or slowing the progression of diabetic nephropathy: A meta-analysis. Clin Ther (2015) 37(9):2086–2103.e10. doi: 10.1016/j.clinthera.2015.05.508
230. Danta CC, Boa AN, Bhandari S, Sathyapalan T, Xu SZ. Recent advances in drug discovery for diabetic kidney disease. Expert Opin Drug Discovery (2021) 16(4):447–61. doi: 10.1080/17460441.2021.1832077
231. Li XZ, Jiang H, Xu L, Liu YQ, Tang JW, Shi JS, et al. Sarsasapogenin restores podocyte autophagy in diabetic nephropathy by targeting GSK3β signaling pathway. Biochem Pharmacol (2021) 192:114675. doi: 10.1016/j.bcp.2021.114675
232. Ren C, Zhou X, Bao X, Zhang J, Tang J, Zhu Z, et al. Dioscorea zingiberensis ameliorates diabetic nephropathy by inhibiting NLRP3 inflammasome and curbing the expression of p66Shc in high-fat diet/streptozotocin-induced diabetic mice. J Pharm Pharmacol (2021) 73(9):1218–29. doi: 10.1093/jpp/rgab053
233. Rayego-Mateos S, Morgado-Pascual JL, Opazo-Ríos L, Guerrero-Hue M, García-Caballero C, Vázquez-Carballo C, et al. Pathogenic pathways and therapeutic approaches targeting inflammation in diabetic nephropathy. Int J Mol Sci (2020) 21(11):3798. doi: 10.3390/ijms21113798
234. Mann JF, Green D, Jamerson K, Ruilope LM, Kuranoff SJ, Littke T, et al. Avosentan for overt diabetic nephropathy. J Am Soc Nephrol (2010) 21(3):527–35. doi: 10.1681/ASN.2009060593
235. Gupta S, Sen U. More than just an enzyme: Dipeptidyl peptidase-4 (DPP-4) and its association with diabetic kidney remodelling. Pharmacol Res (2019) 147:104391. doi: 10.1016/j.phrs.2019.104391
236. Wang W, Lo A. Diabetic retinopathy: pathophysiology and treatments. Int J Mol Sci (2018) 19(6):1816. doi: 10.3390/ijms19061816
237. Simó R, Simó-Servat O, Hernández C. Is fenofibrate a reasonable treatment for diabetic microvascular disease? Curr Diabetes Rep (2015) 15(5):24. doi: 10.1007/s11892-015-0599-0
238. Jin KL, Mao XO, Greenberg DA. Vascular endothelial growth factor: Direct neuroprotective effect in in vitro ischemia. Proc Natl Acad Sci (2000) 97(18):10242–7. doi: 10.1073/pnas.97.18.10242
239. Simó R, Sundstrom JM, Antonetti DA. Ocular anti-VEGF therapy for diabetic retinopathy: the role of VEGF in the pathogenesis of diabetic retinopathy. Diabetes Care (2014) 37(4):893–9. doi: 10.2337/dc13-2002
240. Titchenell PM, Antonetti DA. Using the past to inform the future: anti-VEGF therapy as a road map to develop novel therapies for diabetic retinopathy. Diabetes (2013) 62(6):1808–15. doi: 10.2337/db12-1744
241. Chatziralli I. Ranibizumab for the treatment of diabetic retinopathy. Expert Opin Biol Ther (2021) 21(8):991–7. doi: 10.1080/14712598.2021.1928629
242. Uludag G, Hassan M, Matsumiya W, Pham BH, Chea S, Tuong T, et al. Efficacy and safety of intravitreal anti-VEGF therapy in diabetic retinopathy: what we have learned and what should we learn further? Expert Opin Biol Ther (2022) 22(10):1275–91. doi: 10.1080/14712598.2022.2100694
243. Nicholson BP, Schachat AP. A review of clinical trials of anti-VEGF agents for diabetic retinopathy. Graefe’s Arch Clin Exp Ophthalmol (2010) 248(7):915–30. doi: 10.1007/s00417-010-1315-z
244. Nguyen QD, Brown DM, Marcus DM, Boyer DS, Patel S, Feiner L, et al. Ranibizumab for diabetic macular edema. Ophthalmology (2012) 119(4):789–801. doi: 10.1016/j.ophtha.2011.12.039
245. Chen Y-P, Wu A-L, Chuang C-C, Chen SN. Factors influencing clinical outcomes in patients with diabetic macular edema treated with intravitreal ranibizumab: comparison between responder and non-responder cases. Sci Rep (2019) 9(1):10952. doi: 10.1038/s41598-019-47241-1
246. Guigou S, Pommier S, Meyer F, Hajjar C, Merite PY, Parrat E, et al. Efficacy and safety of intravitreal dexamethasone implant in patients with diabetic macular edema. Ophthalmologica (2015) 233(3–4):169–75. doi: 10.1159/000381356
247. Chatziralli I, Theodossiadis P, Parikakis E, Dimitriou E, Xirou T, Theodossiadis G, et al. Dexamethasone intravitreal implant in diabetic macular edema: real-life data from a prospective study and predictive factors for visual outcome. Diabetes Ther (2017) 8(6):1393–404. doi: 10.1007/s13300-017-0332-x
248. Lattanzio R, Cicinelli MV, Bandello F. Intravitreal steroids in diabetic macular edema. Dev Ophthalmol (2017) 60:78–90. doi: 10.1159/000459691
249. Munk MR, Somfai GM, De Smet MD, Donati G, Menke MN, Garweg JG, et al. The role of intravitreal corticosteroids in the treatment of DME: predictive OCT biomarkers. Int J Mol Sci (2022) 23(14):7585. doi: 10.3390/ijms23147585
250. Sonoda S, Sakamoto T, Shirasawa M, Yamashita T, Otsuka H, Terasaki H. Correlation between reflectivity of subretinal fluid in OCT images and concentration of intravitreal VEGF in eyes with diabetic macular edema. Invest Opthalmology Visual Sci (2013) 54(8):5367. doi: 10.1167/iovs.13-12382
251. Schwartz SG, Scott IU, Stewart MW, Flynn HW Jr. Update on corticosteroids for diabetic macular edema. Clin Ophthalmol (2016) 10:1723–30. doi: 10.2147/OPTH.S115546
252. Striglia E, Caccioppo A, Castellino N, Reibaldi M, Porta M. Emerging drugs for the treatment of diabetic retinopathy. Expert Opin Emerg Drugs (2020) 25(3):261–71. doi: 10.1080/14728214.2020.1801631
253. Huang X, Wang H, She C, Feng J, Liu X, Hu X, et al. Artificial intelligence promotes the diagnosis and screening of diabetic retinopathy. Front Endocrinol (Lausanne) (2022) 13:946915. doi: 10.3389/fendo.2022.946915
254. Choudhry N, Duker JS, Freund KB, Kiss S, Querques G, Rosen R, et al. Classification and guidelines for widefield imaging: recommendations from the international widefield imaging study group. Ophthalmol Retina (2019) 3(10):843–9. doi: 10.1016/j.oret.2019.05.007
255. Arrigo A, Aragona E, Bandello F. VEGF-targeting drugs for the treatment of retinal neovascularization in diabetic retinopathy. Ann Med (2022) 54(1):1089–111. doi: 10.1080/07853890.2022.2064541
256. Fleming I. New lipid mediators in retinal angiogenesis and retinopathy. Front Pharmacol (2019) 10:739. doi: 10.3389/fphar.2019.00739
Keywords: diabetic retinopathy, diabetic nephropathy, parallelism, non-parallelism, mechanism
Citation: Tang S, An X, Sun W, Zhang Y, Yang C, Kang X, Sun Y, Jiang L, Zhao X, Gao Q, Ji H and Lian F (2024) Parallelism and non-parallelism in diabetic nephropathy and diabetic retinopathy. Front. Endocrinol. 15:1336123. doi: 10.3389/fendo.2024.1336123
Received: 10 November 2023; Accepted: 23 January 2024;
Published: 14 February 2024.
Edited by:
Qi Pan, Peking University, ChinaReviewed by:
Anu Grover, Ipca Laboratories, IndiaEberval Gadelha Figueiredo, University of São Paulo, Brazil
Copyright © 2024 Tang, An, Sun, Zhang, Yang, Kang, Sun, Jiang, Zhao, Gao, Ji and Lian. This is an open-access article distributed under the terms of the Creative Commons Attribution License (CC BY). The use, distribution or reproduction in other forums is permitted, provided the original author(s) and the copyright owner(s) are credited and that the original publication in this journal is cited, in accordance with accepted academic practice. No use, distribution or reproduction is permitted which does not comply with these terms.
*Correspondence: Fengmei Lian, bGZtNTY1QHNvaHUuY29t; Hangyu Ji, amloYW5neXVlY2hvQDE2My5jb20=
†These authors have contributed equally to this work