- Department of Physiology and Biophysics, University of Mississippi Medical Center, Jackson, MS, United States
Recent evidence from our lab and others suggests that metabolic reprogramming of immune cells drives changes in immune cell phenotypes along the inflammatory-to-reparative spectrum and plays a critical role in mediating the inflammatory responses to cardiac injury (e.g. hypertension, myocardial infarction). However, the factors that drive metabolic reprogramming in immune cells are not fully understood. Extracellular vesicles (EVs) are recognized for their ability to transfer cargo such as microRNAs from remote sites to influence cardiac remodeling. Furthermore, conditions such as obesity and metabolic syndrome, which are implicated in the majority of cardiovascular disease (CVD) cases, can skew production of EVs toward pro-inflammatory phenotypes. In this mini-review, we discuss the mechanisms by which EVs may influence immune cell metabolism during cardiac injury and factors associated with obesity and the metabolic syndrome that can disrupt normal EV function. We also discuss potential sources of cardio-protective and anti-inflammatory EVs, such as brown adipose tissue. Finally, we discuss implications for future therapeutics.
1 Role of immune cells and immunometabolism in cardiac injury and remodeling
Immune cells, including neutrophils, macrophages, and B and T lymphocytes, play a critical role in inflammation and repair after cardiac injury (1). While small resident populations of immune cells reside in the healthy heart, the majority of immune cells in the injured heart derive from extra-cardiac sources, primarily from the spleen (2). An acute inflammatory response is necessary to initiate the proper healing response to cardiac injury. Immune cells play a wide range of roles in the injured heart, including phagocytosis of necrotic cells and coordinating remodeling of the vasculature and extracellular matrix.
Recent studies have highlighted the importance of cardiac immunometabolism, the study of metabolic pathways that contribute to immune cell phenotypes (1, 3). The current paradigm, broadly speaking, is that inflammatory subsets of monocytes/macrophages or T cells (i.e. M1-like macrophages, Ly6C-high monocytes, Th1/Th17 cells) rely mainly on glycolysis, while anti-inflammatory/reparative subsets (M2-like macrophages, Ly6C-low/resident cardiac macrophages, Tregs) rely more on mitochondrial oxidative phosphorylation (OXPHOS). Glycolysis can allow cells to survive in a hypoxic environment, such as the ischemic heart, and permits activation of the pentose phosphate pathway (4). Macrophages and T cells can also program their metabolism to glycolysis even when oxygen is present, a phenomenon known as the Warburg effect. This glycolytic switch is mediated by activation of hypoxia-inducible factor-1 alpha (HIF-1α), which is activated by hypoxia or non-hypoxic signals such as toll-like receptor-4 (TLR4). Conversely, the mitochondrial tricarboxylic acid (TCA) cycle and OXPHOS promote pathways in immune cells that favor anti-inflammatory polarization (1).
2 Role of extracellular vesicles in cardiovascular health and disease
While immunometabolism appears to be important for immune cell responses to cardiac injury, little is known of the upstream signaling mechanisms that drive metabolic reprogramming. One potential unexplored mechanism is through extracellular vesicles (EVs), which are released as membrane bound vesicles to mediate inter-organ communication in many physiological and pathological processes (Figure 1) (5). Due to their small size (30 nm – 4 μm) and the fact that EVs contain cargo that can behave as endocrine signaling molecules, there is much interest in EVs as therapeutic agents (5). EVs can also mediate communication between different cell populations of the cardiovascular system controlling normal tissue function or propagating injury signals during cardiovascular diseases. In contrast, certain EVs have also been identified to mediate cardioprotective mechanisms and have been proposed as promising drug delivery systems due to their low immunogenicity, toxicity and strong ability to cross cell membranes (6).
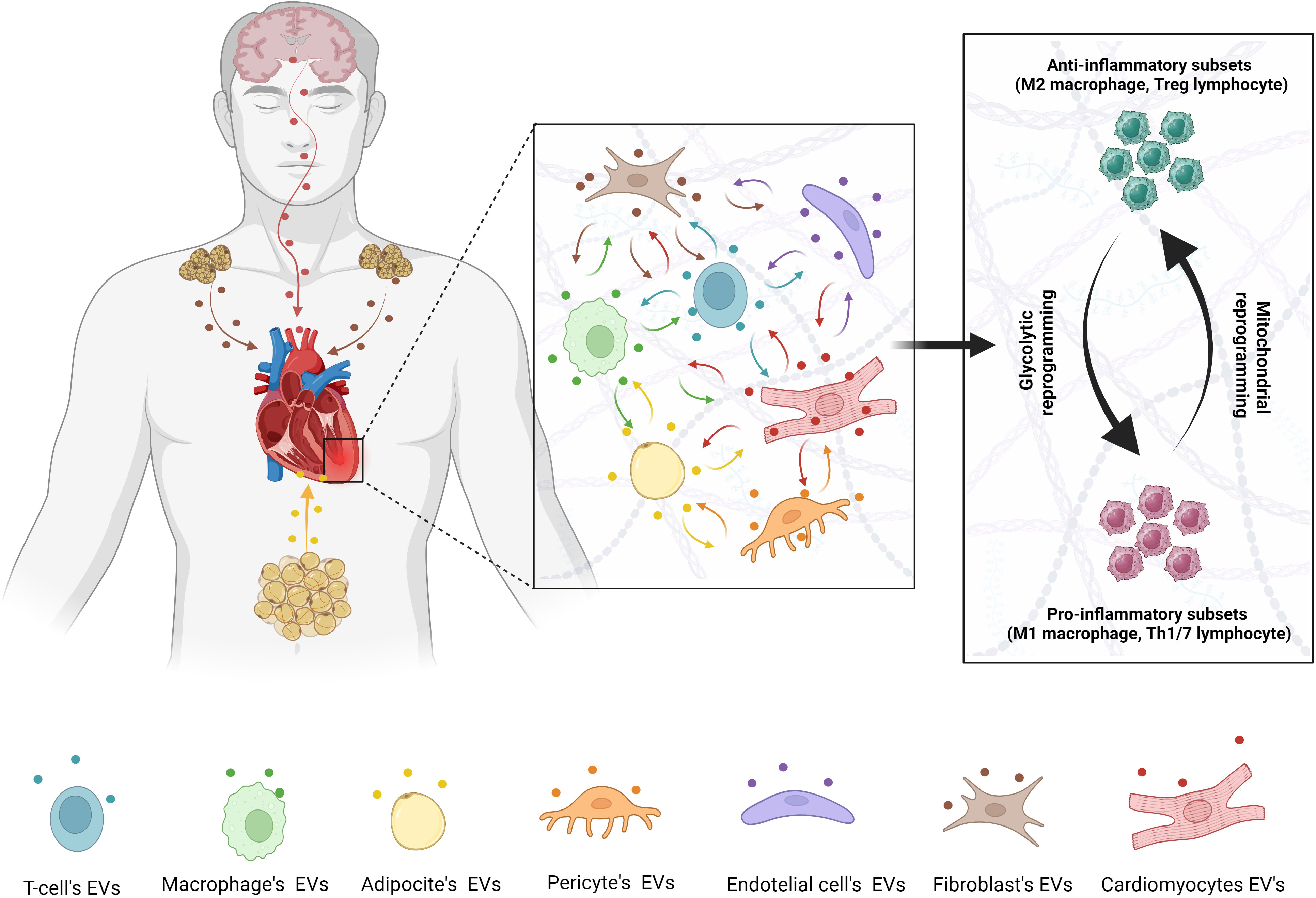
Figure 1 Metabolic regulation of inflammatory pathways during cardiac injury. Metabolic switching between glycolysis and mitochondrial oxidative phosphorylation is regulated by EVs from different cells population and distant organs.
EVs can carry many different types of bioactive molecules within their membrane, including proteins, lipids, DNA, microRNAs (miRNAs), long non-coding RNAs (lncRNAs), circulating RNAs (cirRNAs) and mitochondria (7). The phenotype of the parent cell and its environment dictates EV’s cargo and how it will influence the target cell and inter-tissue crosstalk. EVs are generated by 3 different process: 1) fusion of multivesicular bodies with the cell membrane of the parent cell (exosomes, 30 – 100 nm), 2) outward budding of the parent cell membrane (microvesicles, 200 nm – 1 μm) and 3) membrane decomposition of apoptotic cells (apoptotic bodies, 1 – 4 μm) (8). Regardless of the biogenesis pathway, EVs contain in their membrane ligands inherited from their donor cells that could, theoretically, be used to identify the source of circulating EVs. However, studies are needed to elucidate specific molecular makers/ligands that each cell type shares with their EVs.
The content and mode of EVs interaction and/or entry into the target cell determine their functional effects. For example, EVs can trigger signaling pathways through interaction with receptors in recipient cells without being internalized (9). Also, EVs can directly enter into the cytoplasm of the recipient cell through endocytosis, phagocytosis or membrane fusion, subsequently releasing their contents into the cytoplasm (9). However, the most efficient way to transfer EVs content to the target cell in the context of immunometabolism modulation remains elusive.
Studies investigating EVs as potential biomarkers for cardiac diseases found that their cargo can be used as a signature for specific diseases. For example, circulating levels of EV-enclosed miR-126 and miR-199a can predict the occurrence of cardiovascular events in patients with stable coronary artery disease (10). Thus, EVs hold great potential to monitor and treat cardiovascular diseases but there are still many unanswered questions related to the regulatory mechanisms of EV biogenesis, selective sorting of cargo, and cell-specific EV uptake.
2.1 Inter-cellular communication during cardiac injury
The cardiovascular system is formed by a complex network of different cell types including cardiomyocytes, fibroblasts, endothelial cells, pericytes, adipocytes and immune cells. EVs play an important role in their interactions to maintain cardiac structure and function in healthy conditions. However, these cells also release EVs to propagate injury signals during disease. Evidence for a potential role of EVs during cardiac injury is suggested by increased circulating levels of EVs in patients with myocardial infarction (MI) as well as murine and porcine models of MI (11–13). EVs also accumulate in the heart early after MI where they may play a crucial role in regulating inflammation during the first 24 hr after MI (14).
EVs derived from necrotic cardiomyocytes are engulfed by phagocytic monocytes to promote release of cytokines such as IL-6, CCL2, and CCL7 (14). Endothelial cells are also potent sources of EVs after MI, as evidenced by cell surface expression of endothelial markers such as CD31, ICAM-1, PCAM-1, and P and E selectins (13). Endothelial-derived EVs are also engulfed by infiltrating monocytes, in which they promote inflammatory and migratory immune cell responses. Injecting naïve mice with these MI-derived EVs promoted monocyte mobilization from the spleen. For example, endothelial-EVs from diabetic mice impair angiogenesis and re-vascularization after MI and skeletal muscle ischemia (15, 16).
EV-mediated communication between immune cells and fibroblasts is proposed to be involved in cardiac fibrosis. Macrophages exposed to high glucose, mimicking a diabetic environment, produce EVs containing Human antigen R that stimulates fibroblasts to increase collagen production (17), thus enhancing cardiac fibrosis. Also, injection of CD4+ T cell-derived EVs carrying miR-142-3p exacerbates the effects of MI on cardiac function and infarct size expansion in mice (18). On the other hand, cardiac fibroblasts also produce EVs that propagate hypertrophic signals to cardiomyocytes during cardiac pressure overload (19). In addition, EVs produced by activated fibroblasts (myofibroblasts) may mediate endothelial dysfunction during cardiac fibrosis via miR-200a-3q (20).
Pericytes are another cardiac cell population that release EVs during cardiac injury, especially in ischemic diseases. These EVs are involved in cardiac fibroblast and macrophage proliferation (21). Also, crosstalk between pericytes and endothelial cells during inflammation in the heart is mediated by EVs in a bi-directional manner (22).
Epicardial adipose is another source of EVs that acts locally on the myocardium, atria and coronary arteries. Recently, these EVs were identified with proinflammatory, profibrotic and proarrhythmic properties that contribute to the development of atrial myopathy and atrial fibrillation (23). Altogether, these studies support an important role of EVs in the pathophysiology of cardiac diseases and demonstrate a strong interaction among different cell populations that is mediated by EVs and their cargos. How EVs sort their cargo in distinct disease models remains to be elucidated, although some studies suggest the participation of distinct RNA-biding sites and protein post-translational modifications including ubiquitination (6).
2.2 Inter-organ communication during cardiac injury and cardioprotection
Studies using transgenic animals expressing membrane-target fluorescent markers (mT/mG mice) (24) allowed in vivo tracing of EVs and showed that EVs produced in one organ can be detected in another organ during pathophysiologic processes. These findings suggest that EVs mediate not only inter-cellular but also inter-organ communications. For example, EVs produced by the heart of animals with congestive heart failure were detected in the brain, contributing to sympathetic excitation mediated by oxidative stress (25).
White adipose tissue (WAT) is proposed to be an important source of EVs with cardiac effects as demonstrated by studies showing that WAT from obese mice induces macrophage activation in a TLR4-dependent manner favoring cardiometabolic complications (26). Also, injection of lean mice with WAT-derived EVs, produced in response to obesity-associated stress, shifted tissue-resident macrophage toward a proinflammatory phenotype (26). In a diet-induced obese mouse model, WAT-derived EVs showed increased levels of miRNA-130b-3p, which exacerbated cardiac ischemia-reperfusion injury due to downregulation of adenosine monophosphate kinase (AMPK) (27).
Contrary to the detrimental effects of WAT-derived EVs in the heart, the brown adipose tissue (BAT) has been shown to be a potent source of EVs with potential cardioprotective and anti-inflammatory properties (28). Exercise activates release of small EVs from BAT enriched with cardioprotective miRNAs such as miR-125b-5p, miR-128-3p, and miR-30d-5p, which inhibit cardiac myocyte apoptosis following I/R injury via inhibition of the TNF receptor associated 6/TNF receptor superfamily member 1B signaling pathway (28). BAT-EVs are also enriched with mitochondrial components that improve OXPHOS and restore cardiac function in obese mice (29). Similarly, Lin et al. demonstrated a cardioprotective effect mediated by EVs communication from BAT to myocytes and cardiac fibroblasts (30). However other studies proposed that during obesity BAT-EVs become enriched with inflammatory proteins as well as the glucose transporter GLUT1, which mediates glucose uptake and glycolytic reprogramming in macrophages (1, 31). Furthermore, during hypertension, BAT-EVs may transport inducible nitric oxide synthase (iNOS) to the heart, which could aggravate cardiac remodeling and hypertrophy (30). Thus, while BAT-EVs appear to be cardioprotective in the healthy state, they may become maladaptive in obese or hypertensive subjects.
Another extracardiac source of EVs with cardioprotective effects are mesenchymal stem cells (MSCs) which reside in the bone marrow (7, 32). MSC-derived EVs have attracted attention as a potential therapeutic for MI due to their anti-apoptotic effect on cardiomyocytes, and their anti-inflammatory/pro-reparative effect on immune cells such as macrophages (33). Thus, harnessing the beneficial MSC-EVs while inhibiting cardiac myocyte and endothelial EVs from the injured heart may be a promising therapeutic strategy.
Together these studies emphasize the importance of the cell environment in determining EV’s cargo and their function on the target cell. Further investigation is needed to understand how EVs are attracted by the target cell in the distant organ.
3 Potential interactions of extracellular vesicles and immune cell metabolism in the heart
EVs clearly play an important role in regulating inflammation during cardiac injury. However how they impact immunometabolic reprogramming is still not well understood. Recent studies suggest that EVs are involved in macrophage polarization in response to different metabolic environments, thus contributing to the pathological process of cardiovascular diseases. In this section we summarize how different EV’s cargo can contribute to this process (Table 1).
3.1 MiRNAs
Metabolic reprogramming requires finely tuned activation and simultaneous repression of multiple cell signaling pathways, metabolites, and genes that can be regulated by multiple miRNAs (34). Activation of HIF-1α is recognized as a critical mediator of glycolytic reprogramming, while AMPK is a major regulator of reprogramming to OXPHOS metabolism (34, 35). Several miRNAs that regulate inflammation also regulate HIF-1α and several glycolytic enzymes. For example, miR-223 targets HIF1a and PFK1 to inhibit lipopolysaccharide (LPS)-induced glycolysis and M1-like polarization in vitro and in mice with sepsis (36). Alternatively, miR-33 targets AMPK and carnitine palmitoyltransferase 1a (CPT1a) to decrease fatty acid oxidation and enhance glycolysis, promoting macrophage M1 polarization (37).
Some miRNAs more directly target immunometabolic pathways. For example, miR-22 is induced by TLR signaling, and reduces glycolysis/M1 polarization by targeting GLUT1 (38). Other miRNAs can target other glycolytic genes, such as hexokinase-2, phosphoglucose isomerase, and enolase (39). In CD4+ T cells, miR-142 regulates the MBD2-MYC axis to promote glycolysis and exacerbate inflammation during myocarditis (40). Multiple miRNAs also regulate lactate dehydrogenase (LDHA) in CD4+ T cells, thus favoring their reliance on OXPHOS and improving their ability to kill viruses such as HIV (35). Several miRNAs have also been discovered to regulate mitochondrial function and metabolic pathways, termed the mitomiRs (41). Collectively, miRNAs that inhibit glycolysis appear to decrease inflammation, while those that inhibit fatty acid oxidation/OXPHOS promote inflammation.
3.2 LncRNAs and circRNAs
The role of EVs enriched with long noncoding (lnc) RNA and circular (circ) RNA on macrophages phenotypic changes is beginning to be elucidated. However, the interaction of these subtypes of non-coding RNAs (ncRNAs) with metabolic pathways that control immune functions is not well established.
LncRNAs are the longest type of ncRNA that participate in gene transcription, translation and post-translational modification (42). Recent studies have shown that lncRNA can act as a sponge for miRNA and alter specific signaling pathways involved in macrophage polarization (43). For example, EV’s resealed by endothelial progenitor cells promote M2 macrophage polarization by suppressing miR-9-5p on SIRT1 through the transfer on lncRNA taurine upregulated gene 1 (TUG1), an important lncRNA that is downregulated during sepsis (44). In patients with coronary atherosclerosis, EVs carrying lncRNA-MRGPRF-6:1 promote M1 macrophage polarization by activating TLR4, myeloid differentiation factor-8, and mitogen-activated protein kinase (TLR4-MyD88-MAPK) (45). Also, blockade of lnc-RNA-ASLNCS5088-enriched EVs dampens the effect of M2 macrophages in fibroblast activation (46).
CircRNAs are evolutionary conserved, stable and endogenous ncRNAs with important biological effects on protein activity regulation, epigenetic modulation, and transcription and post-transcriptional events (47). Yang et al. demonstrated that EVs derived from hypoxic-pretreated adipose stem cell enclose circ-RNA-Rps5 that promotes M2 macrophage polarization by targeting SIRT7 and miR-124-3p during acute ischemic stroke (48). Conversely, Wang et al. showed that M2 macrophage-derived EVs carrying circUbe3a promote cardiac fibroblasts proliferation, migration and phenotypic transformation enhancing fibrosis after acute myocardial infarction (49). Hence, these studies emphasize the importance of additional research on new lncRNAs and circRNAs enclosed into EVs and their role on cardiovascular diseases.
3.3 Proteins
Recent proteomics analyses of various EVs with resolution down to the single EV level have transformed our knowledge of EV-protein transport (50). Multiple studies suggest that EV transport of metabolic enzymes may influence immunometabolism. EVs from cancer cells can transport signaling proteins such as latent membrane protein-1 to cancer-associated fibroblasts, which activate the NF-κB-glycolysis pathway (51). Glycolytic enzymes are often enriched in EVs, regardless of origin (51, 52). EVs use glycolysis as an ATP source while also delivering glycolytic enzymes to recipient cells. Cardiomyocytes secrete EVs that can deliver the glucose transporter GLUT1, the major glucose transporter in macrophages (1), to neighboring cells to enhance glycolysis (53). Monocyte-derived EVs can also transport GLUT1 in addition to pro-inflammatory cargo, thus supporting inflammation through activation of glycolytic pathways (54). Conversely, EVs can also transport enzymes involved in fatty acid oxidation (55), which promotes anti-inflammatory pathways in macrophages and T cells (1).
3.4 Metabolites and lipids
EVs also carry other cargo that could influence immunometabolic phenotypes, including metabolites such as sugars, lipids, and amino acids (56). While most studies have focused on the protein/nucleic acid composition of EVs until recently, several studies have begun to focus on the EV lipid and small metabolite composition (56–58).
Obesity and metabolic disorders often lead to excess fat deposition in several organs including the heart. Within the adipose tissue, lipids regulate immune cells such as adipose tissue macrophages (ATM). Accumulation of lipids inside ATM activates a program of lysosomal catabolism, a process associated with systemic metabolic complications such as insulin resistance (59). Aiming to study how lipids are transferred from adipocytes to ATM, Flaherty III et al. conducted a series of experiments showing that adipocytes release EVs enriched with lipids, specifically triacylglycerol and monoacylglycerides, that were taken up by ATM (59), which can lead to metabolic disorders and development of cardiac diseases.
EVs enriched with ribose-5-phosphate and pentose phosphate pathways enzymes, may support immune cell proliferation during MI (4, 56). Indeed, many other small sugar, amino acid, and nucleotide metabolites have been discovered in EVs, depending on the source, size, and cardiovascular disease context (60).
3.5 Mitochondria
EVs can also transport mitochondrial enzymes, mitochondrial DNA, parts of the electron transport chain, mitochondrial fragments, and whole mitochondria (61). The role of these “mitoEVs” carry many implications for inflammatory pathways, which are tightly regulated by mitochondrial function (62). For example, mitochondrial damage-associated molecular patterns (mtDAMPs), which are released from damaged heart muscle, can trigger inflammation via the cGAS/STING/NF-κB pathway (62). Delivery of whole mitochondria to macrophages via EVs also plays a critical role in macrophage polarization (63). Damaged mitochondria can be packaged into EVs and sent to neighboring cells for repair (61). For example, MSCs undergoing mitophagy package damaged mitochondria into EVs, which are delivered to neighboring macrophages that complete the process of mitophagy, resulting in enhanced OXPHOS and M2-like polarization (64). Furthermore, healthy mitochondria from apoptotic cells can be “recycled” after being taken up by macrophages, in which they promote an M2-like phenotype (61). Conversely, macrophages can “donate” their mitochondria to injured or dysfunctional tissue, such as neurons (65). Thus, the role of mitoEVs in inflammation depends on the size, source, and contents of the EVs.
4 Gaps in knowledge and future implications
While several studies have implicated roles for EVs and their contents in regulating immunometabolism, the extent to which this occurs during cardiac injury remains unclear. Inflammation is a promising target for preventing adverse HF outcomes (66), although optimizing the therapeutic window remains a challenge. Targeting metabolism in immune cells is a promising therapeutic option due to availability of bioactive molecules that are well tolerated, such as NAD+ (67). Combining the potential delivery of “immunometabolic agents” with the specificity of EVs could provide a safe, effective method of delivering miRNAs, enzymes, functional mitochondria, or drugs to target immunometabolic pathways. However, several challenges still remain. There are multiple different types of EVs that may act on immune cells in the injured heart, based on size, cell surface expression, cargo, and source (33). Additionally, EVs carry a plethora of molecules with unknown effects, and changes in the donor cell environment can alter EVs cargo, thus complicating the predictability of EVs-based therapies (68). Furthermore, EVs can have a short plasma half-life imposing another challenge with regard the establishment of dose efficiency and off-target effects (68). Technical challenges also need to be overcome, including better loading of the therapeutic agents into EVs, targeting to specific cells within the same organ, and efficient delivery and uptake by target cells. Further understanding of these parameters during different types of cardiac injury, at different stages of disease, in different pre-clinical models (e.g. large animal models), and the impact of certain risk factors (e.g. obesity/diabetes) are still needed. As these fields continue to co-evolve and mature, it is likely that new therapeutic approaches for treating heart disease will emerge.
Author contributions
AO: Writing – original draft, Writing – review & editing. JdC: Writing – original draft, Writing – review & editing. AdS: Writing – original draft, Writing – review & editing. JH: Writing – original draft, Writing – review & editing. AM: Writing – original draft, Writing – review & editing.
Funding
The author(s) declare financial support was received for the research, authorship, and/or publication of this article. The authors were funded by the following National Institutes of Health grants: NHLBI-1R01HL166737 and 1R01HL163076; NIGMS-P30GM149404, P20GM104357, and U54GM115428.
Conflict of interest
The authors declare that the research was conducted in the absence of any commercial or financial relationships that could be construed as a potential conflict of interest.
Publisher’s note
All claims expressed in this article are solely those of the authors and do not necessarily represent those of their affiliated organizations, or those of the publisher, the editors and the reviewers. Any product that may be evaluated in this article, or claim that may be made by its manufacturer, is not guaranteed or endorsed by the publisher.
References
1. Mouton AJ, Li X, Hall ME, Hall JE. Obesity, hypertension, and cardiac dysfunction: novel roles of immunometabolism in macrophage activation and inflammation. Circ Res (2020) 126(6):789–806. doi: 10.1161/CIRCRESAHA.119.312321
2. Ma Y, Mouton AJ, Lindsey ML. Cardiac macrophage biology in the steady-state heart, the aging heart, and following myocardial infarction. Transl Res (2018) 191:15–28. doi: 10.1016/j.trsl.2017.10.001
3. DeBerge M, Chaudhary R, Schroth S, Thorp EB. Immunometabolism at the heart of cardiovascular disease. JACC Basic Transl Sci (2023) 8(7):884–904. doi: 10.1016/j.jacbts.2022.12.010
4. Mouton AJ, Aitken NM, Moak SP, do Carmo JM, da Silva AA, Omoto ACM, et al. Temporal changes in glucose metabolism reflect polarization in resident and monocyte-derived macrophages after myocardial infarction. Front Cardiovasc Med (2023) 10:1136252. doi: 10.3389/fcvm.2023.1136252
5. Liu C, Bayado N, He D, Li J, Chen H, Li L, et al. Therapeutic applications of extracellular vesicles for myocardial repair. Front Cardiovasc Med (2021) 8:758050. doi: 10.3389/fcvm.2021.758050
6. Martins-Marques T, Girão H. The good, the bad and the ugly: the impact of extracellular vesicles on the cardiovascular system. J Physiol (2023) 601(22):4837–52. doi: 10.1113/JP282048
7. Fu S, Zhang Y, Li Y, Luo L, Zhao Y, Yao Y. Extracellular vesicles in cardiovascular diseases. Cell Death Discovery (2020) 6:68. doi: 10.1038/s41420-020-00305-y
8. Neves KB, Rios FJ, Sevilla-Montero J, Montezano AC, Touyz RM. Exosomes and the cardiovascular system: role in cardiovascular health and disease. J Physiol (2023) 601(22):4923–36. doi: 10.1113/JP282054
9. Abels ER, Breakefield XO. Introduction to extracellular vesicles: biogenesis, RNA cargo selection, content, release, and uptake. Cell Mol Neurobiol (2016) 36(3):301–12. doi: 10.1007/s10571-016-0366-z
10. Jansen F, Yang X, Proebsting S, Hoelscher M, Przybilla D, Baumann K, et al. MicroRNA expression in circulating microvesicles predicts cardiovascular events in patients with coronary artery disease. J Am Heart Assoc (2014) 3(6):e001249. doi: 10.1161/JAHA.114.001249
11. Deddens JC, Vrijsen KR, Colijn JM, Oerlemans MI, Metz CH, van der Vlist EJ, et al. Circulating extracellular vesicles contain miRNAs and are released as early biomarkers for cardiac injury. J Cardiovasc Transl Res (2016) 9(4):291–301. doi: 10.1007/s12265-016-9705-1
12. Khandagale A, Lindahl B, Lind SB, Shevchenko G, Siegbahn A, Christersson C. Plasma-derived extracellular vesicles from myocardial infarction patients inhibits tumor necrosis factor-alpha induced cardiac cell death. Curr Res Transl Med (2022) 70(2):103323. doi: 10.1016/j.retram.2021.103323
13. Akbar N, Digby JE, Cahill TJ, Tavare AN, Corbin AL, Saluja S, et al. Endothelium-derived extracellular vesicles promote splenic monocyte mobilization in myocardial infarction. JCI Insight (2017) 2(17):e2306156. doi: 10.1172/jci.insight.93344
14. Loyer X, Zlatanova I, Devue C, Yin M, Howangyin KY, Klaihmon P, et al. Intra-cardiac release of extracellular vesicles shapes inflammation following myocardial infarction. Circ Res (2018) 123(1):100–6. doi: 10.1161/CIRCRESAHA.117.311326
15. Huang G, Cheng Z, Hildebrand A, Wang C, Cimini M, Roy R, et al. Diabetes impairs cardioprotective function of endothelial progenitor cell-derived extracellular vesicles via H3K9Ac inhibition. Theranostics. (2022) 12(9):4415–30. doi: 10.7150/thno.70821
16. Cheng Z, Naga V Srikanth Garikipati, Truongcao MM, Cimini M, Huang G, Wang C, et al. Serum-derived small extracellular vesicles from diabetic mice impair angiogenic property of microvascular endothelial cells: role of EZH2. J Am Heart Assoc (2021) 10(10):e019755. doi: 10.1161/JAHA.120.019755
17. Govindappa PK, Patil M, Garikipati VNS, Verma SK, Saheera S, Narasimhan G, et al. Targeting exosome-associated human antigen R attenuates fibrosis and inflammation in diabetic heart. FASEB J (2020) 34(2):2238–51. doi: 10.1096/fj.201901995R
18. Bansal SS, Ismahil MA, Goel M, Patel B, Hamid T, Rokosh G, et al. Activated T lymphocytes are essential drivers of pathological remodeling in ischemic heart failure. Circ Heart Fail (2017) 10(3):e003688. doi: 10.1161/CIRCHEARTFAILURE.116.003688
19. Bang C, Batkai S, Dangwal S, Gupta SK, Foinquinos A, Holzmann A, et al. Cardiac fibroblast-derived microRNA passenger strand-enriched exosomes mediate cardiomyocyte hypertrophy. J Clin Invest. (2014) 124(5):2136–46. doi: 10.1172/JCI70577
20. Ranjan P, Kumari R, Goswami SK, Li J, Pal H, Suleiman Z, et al. Myofibroblast-derived exosome induce cardiac endothelial cell dysfunction. Front Cardiovasc Med (2021) 8:676267. doi: 10.3389/fcvm.2021.676267
21. Mayo JN, Bearden SE. Driving the hypoxia-inducible pathway in human pericytes promotes vascular density in an exosome-dependent manner. Microcirculation. (2015) 22(8):711–23. doi: 10.1111/micc.12227
22. Yamamoto S, Niida S, Azuma E, Yanagibashi T, Muramatsu M, Huang TT, et al. Inflammation-induced endothelial cell-derived extracellular vesicles modulate the cellular status of pericytes. Sci Rep (2015) 5:8505. doi: 10.1038/srep08505
23. Shaihov-Teper O, Ram E, Ballan N, Brzezinski RY, Naftali-Shani N, Masoud R, et al. Extracellular vesicles from epicardial fat facilitate atrial fibrillation. Circulation. (2021) 143(25):2475–93. doi: 10.1161/CIRCULATIONAHA.120.052009
24. Muzumdar MD, Tasic B, Miyamichi K, Li L, Luo L. A global double-fluorescent Cre reporter mouse. Genesis. (2007) 45(9):593–605. doi: 10.1002/dvg.20335
25. Tian C, Gao L, Rudebush TL, Yu L, Zucker IH. Extracellular vesicles regulate sympatho-excitation by nrf2 in heart failure. Circ Res (2022) 131(8):687–700. doi: 10.1161/CIRCRESAHA.122.320916
26. Deng ZB, Poliakov A, Hardy RW, Clements R, Liu C, Liu Y, et al. Adipose tissue exosome-like vesicles mediate activation of macrophage-induced insulin resistance. Diabetes. (2009) 58(11):2498–505. doi: 10.2337/db09-0216
27. Gan L, Xie D, Liu J, Bond Lau W, Christopher TA, Lopez B, et al. Small extracellular microvesicles mediated pathological communications between dysfunctional adipocytes and cardiomyocytes as a novel mechanism exacerbating ischemia/reperfusion injury in diabetic mice. Circulation. (2020) 141(12):968–83. doi: 10.1161/CIRCULATIONAHA.119.042640
28. Zhao H, Chen X, Hu G, Li C, Guo L, Zhang L, et al. Small extracellular vesicles from brown adipose tissue mediate exercise cardioprotection. Circ Res (2022) 130(10):1490–506. doi: 10.1161/CIRCRESAHA.121.320458
29. Zhou X, Li Z, Qi M, Zhao P, Duan Y, Yang G, et al. Brown adipose tissue-derived exosomes mitigate the metabolic syndrome in high fat diet mice. Theranostics. (2020) 10(18):8197–210. doi: 10.7150/thno.43968
30. Lin JR, Ding LL, Xu L, Huang J, Zhang ZB, Chen XH, et al. Brown Adipocyte ADRB3 Mediates Cardioprotection via Suppressing Exosomal iNOS. Circ Res (2022) 131(2):133–47. doi: 10.1161/CIRCRESAHA.121.320470
31. Camino T, Lago-Baameiro N, Sueiro A, Bravo SB, Couto I, Santos FF, et al. Brown adipose tissue sheds extracellular vesicles that carry potential biomarkers of metabolic and thermogenesis activity which are affected by high fat diet intervention. Int J Mol Sci (2022) 23(18):e10826. doi: 10.3390/ijms231810826
32. Guo Y, Yu Y, Hu S, Chen Y, Shen Z. The therapeutic potential of mesenchymal stem cells for cardiovascular diseases. Cell Death Dis (2020) 11(5):349. doi: 10.1038/s41419-020-2542-9
33. You B, Yang Y, Zhou Z, Yan Y, Zhang L, Jin J, et al. Extracellular vesicles: A new frontier for cardiac repair. Pharmaceutics (2022) 14(9):e1848. doi: 10.3390/pharmaceutics14091848
34. Nelson MC, O'Connell RM. MicroRNAs: at the interface of metabolic pathways and inflammatory responses by macrophages. Front Immunol (2020) 11:1797. doi: 10.3389/fimmu.2020.01797
35. Gibson MS, Noronha-Estima C, Gama-Carvalho M. Therapeutic metabolic reprograming using microRNAs: from cancer to HIV infection. Genes (Basel). (2022) 13(2):273. doi: 10.3390/genes13020273
36. Dang CP, Leelahavanichkul A. Over-expression of miR-223 induces M2 macrophage through glycolysis alteration and attenuates LPS-induced sepsis mouse model, the cell-based therapy in sepsis. PloS One (2020) 15(7):e0236038. doi: 10.1371/journal.pone.0236038
37. Gest J, Langston C, Eatroff A. Iron status of cats with chronic kidney disease. J Vet Intern Med (2015) 29(6):1488–93. doi: 10.1111/jvim.13630
38. Kang YJ. MicroRNA-22 regulates the pro-inflammatory responses and M1 polarization of macrophages by targeting GLUT1 and 4-1BBL. J Immunol Res (2023) 2023:2457006. doi: 10.1155/2023/2457006
39. Alshahrani SH, Ibrahim YS, Jalil AT, Altoum AA, Achmad H, Zabibah RS, et al. Metabolic reprogramming by miRNAs in the tumor microenvironment: Focused on immunometabolism. Front Oncol (2022) 12:1042196. doi: 10.3389/fonc.2022.1042196
40. Sun P, Wang N, Zhao P, Wang C, Li H, Chen Q, et al. Circulating Exosomes Control CD4(+) T Cell Immunometabolic Functions via the Transfer of miR-142 as a Novel Mediator in Myocarditis. Mol Ther (2020) 28(12):2605–20. doi: 10.1016/j.ymthe.2020.08.015
41. Duroux-Richard I, Apparailly F, Khoury M. Mitochondrial microRNAs contribute to macrophage immune functions including differentiation, polarization, and activation. Front Physiol (2021) 12:738140. doi: 10.3389/fphys.2021.738140
42. Devaux Y, Zangrando J, Schroen B, Creemers EE, Pedrazzini T, Chang CP, et al. Long noncoding RNAs in cardiac development and ageing. Nat Rev Cardiol (2015) 12(7):415–25. doi: 10.1038/nrcardio.2015.55
43. Ahmad I, Valverde A, Naqvi RA, Naqvi AR. Long non-coding RNAs RN7SK and GAS5 regulate macrophage polarization and innate immune responses. Front Immunol (2020) 11:604981. doi: 10.3389/fimmu.2020.604981
44. Ma W, Zhang W, Cui B, Gao J, Liu Q, Yao M, et al. Functional delivery of lncRNA TUG1 by endothelial progenitor cells derived extracellular vesicles confers anti-inflammatory macrophage polarization in sepsis via impairing miR-9-5p-targeted SIRT1 inhibition. Cell Death Dis (2021) 12(11):1056. doi: 10.1038/s41419-021-04117-5
45. Hu D, Wang Y, You Z, Lu Y, Liang C. lnc-MRGPRF-6:1 promotes M1 polarization of macrophage and inflammatory response through the TLR4-myD88-MAPK pathway. Mediators Inflamm (2022) 2022:6979117. doi: 10.1155/2022/6979117
46. Chen J, Zhou R, Liang Y, Fu X, Wang D, Wang C. Blockade of lncRNA-ASLNCS5088-enriched exosome generation in M2 macrophages by GW4869 dampens the effect of M2 macrophages on orchestrating fibroblast activation. FASEB J (2019) 33(11):12200–12. doi: 10.1096/fj.201901610
47. Collado A, Gan L, Tengbom J, Kontidou E, Pernow J, Zhou Z. Extracellular vesicles and their non-coding RNA cargos: Emerging players in cardiovascular disease. J Physiol (2023) 601(22):4989–5009. doi: 10.1113/JP283200
48. Yang H, Tu Z, Yang D, Hu M, Zhou L, Li Q, et al. Exosomes from hypoxic pre-treated ADSCs attenuate acute ischemic stroke-induced brain injury via delivery of circ-Rps5 and promote M2 microglia/macrophage polarization. Neurosci Lett (2022) 769:136389. doi: 10.1016/j.neulet.2021.136389
49. Wang Y, Li C, Zhao R, Qiu Z, Shen C, Wang Z, et al. CircUbe3a from M2 macrophage-derived small extracellular vesicles mediates myocardial fibrosis after acute myocardial infarction. Theranostics. (2021) 11(13):6315–33. doi: 10.7150/thno.52843
50. Mallia A, Gianazza E, Zoanni B, Brioschi M, Barbieri SS, Banfi C. Proteomics of extracellular vesicles: update on their composition, biological roles and potential use as diagnostic tools in atherosclerotic cardiovascular diseases. Diagnostics (Basel). (2020) 10(10):843. doi: 10.3390/diagnostics10100843
51. Fridman ES, Ginini L, Gil Z. The role of extracellular vesicles in metabolic reprogramming of the tumor microenvironment. Cells. (2022) 11(9):1433. doi: 10.3390/cells11091433
52. Goran Ronquist K. Extracellular vesicles and energy metabolism. Clin Chim Acta (2019) 488:116–21. doi: 10.1016/j.cca.2018.10.044
53. Garcia NA, Moncayo-Arlandi J, Sepulveda P, Diez-Juan A. Cardiomyocyte exosomes regulate glycolytic flux in endothelium by direct transfer of GLUT transporters and glycolytic enzymes. Cardiovasc Res (2016) 109(3):397–408. doi: 10.1093/cvr/cvv260
54. Yang M, Walker SA, Aguilar Diaz de Leon JS, Davidovich I, Broad K, Talmon Y, et al. Extracellular vesicle glucose transporter-1 and glycan features in monocyte-endothelial inflammatory interactions. Nanomedicine. (2022) 42:102515. doi: 10.1016/j.nano.2022.102515
55. Clement E, Lazar I, Attane C, Carrie L, Dauvillier S, Ducoux-Petit M, et al. Adipocyte extracellular vesicles carry enzymes and fatty acids that stimulate mitochondrial metabolism and remodeling in tumor cells. EMBO J (2020) 39(3):e102525. doi: 10.15252/embj.2019102525
56. Harmati M, Bukva M, Boroczky T, Buzas K, Gyukity-Sebestyen E. The role of the metabolite cargo of extracellular vesicles in tumor progression. Cancer Metastasis Rev (2021) 40(4):1203–21. doi: 10.1007/s10555-021-10014-2
57. Lam SM, Zhang C, Wang Z, Ni Z, Zhang S, Yang S, et al. A multi-omics investigation of the composition and function of extracellular vesicles along the temporal trajectory of COVID-19. Nat Metab (2021) 3(7):909–22. doi: 10.1038/s42255-021-00425-4
58. Hayasaka R, Tabata S, Hasebe M, Ikeda S, Hikita T, Oneyama C, et al. Metabolomics of small extracellular vesicles derived from isocitrate dehydrogenase 1-mutant HCT116 cells collected by semi-automated size exclusion chromatography. Front Mol Biosci (2022) 9:1049402. doi: 10.3389/fmolb.2022.1049402
59. Xu X, Grijalva A, Skowronski A, van Eijk M, Serlie MJ, Ferrante AW Jr. Obesity activates a program of lysosomal-dependent lipid metabolism in adipose tissue macrophages independently of classic activation. Cell Metab (2013) 18(6):816–30. doi: 10.1016/j.cmet.2013.11.001
60. Zhang Y, Liang F, Zhang D, Qi S, Liu Y. Metabolites as extracellular vesicle cargo in health, cancer, pleural effusion, and cardiovascular diseases: An emerging field of study to diagnostic and therapeutic purposes. BioMed Pharmacother. (2023) 157:114046. doi: 10.1016/j.biopha.2022.114046
61. Sanz-Ros J, Mas-Bargues C, Romero-Garcia N, Huete-Acevedo J, Dromant M, Borras C. The potential use of mitochondrial extracellular vesicles as biomarkers or therapeutical tools. Int J Mol Sci (2023) 24(8):7005. doi: 10.3390/ijms24087005
62. Di Mambro T, Pellielo G, Agyapong ED, Carinci M, Chianese D, Giorgi C, et al. The tricky connection between extracellular vesicles and mitochondria in inflammatory-related diseases. Int J Mol Sci (2023) 24(9):8181. doi: 10.3390/ijms24098181
63. Amari L, Germain M. Mitochondrial extracellular vesicles - origins and roles. Front Mol Neurosci (2021) 14:767219. doi: 10.3389/fnmol.2021.767219
64. Phinney DG, Di Giuseppe M, Njah J, Sala E, Shiva S, St Croix CM, et al. Mesenchymal stem cells use extracellular vesicles to outsource mitophagy and shuttle microRNAs. Nat Commun (2015) 6:8472. doi: 10.1038/ncomms9472
65. van der Vlist M, Raoof R, Willemen H, Prado J, Versteeg S, Martin Gil C, et al. Macrophages transfer mitochondria to sensory neurons to resolve inflammatory pain. Neuron. (2022) 110(4):613–26 e9. doi: 10.1016/j.neuron.2021.11.020
66. Everett BM, Cornel JH, Lainscak M, Anker SD, Abbate A, Thuren T, et al. Anti-inflammatory therapy with canakinumab for the prevention of hospitalization for heart failure. Circulation. (2019) 139(10):1289–99. doi: 10.1161/CIRCULATIONAHA.118.038010
67. Thorp EB. Cardiac macrophages and emerging roles for their metabolism after myocardial infarction. J Clin Invest (2023) 133(18):e171953. doi: 10.1172/JCI171953
Keywords: myocardial infarction, microRNAs, metabolism, immune system, macrophages
Citation: Omoto ACM, do Carmo JM, da Silva AA, Hall JE and Mouton AJ (2024) Immunometabolism, extracellular vesicles and cardiac injury. Front. Endocrinol. 14:1331284. doi: 10.3389/fendo.2023.1331284
Received: 31 October 2023; Accepted: 19 December 2023;
Published: 08 January 2024.
Edited by:
Gautham Yepuri, New York University, United StatesReviewed by:
Vikas Kumar, New York University, United StatesRamesh Nimma, New York University, United States
Sangeeta Bhallamudi, New York University, United States
Copyright © 2024 Omoto, do Carmo, da Silva, Hall and Mouton. This is an open-access article distributed under the terms of the Creative Commons Attribution License (CC BY). The use, distribution or reproduction in other forums is permitted, provided the original author(s) and the copyright owner(s) are credited and that the original publication in this journal is cited, in accordance with accepted academic practice. No use, distribution or reproduction is permitted which does not comply with these terms.
*Correspondence: Alan J. Mouton, YW1vdXRvbkB1bWMuZWR1