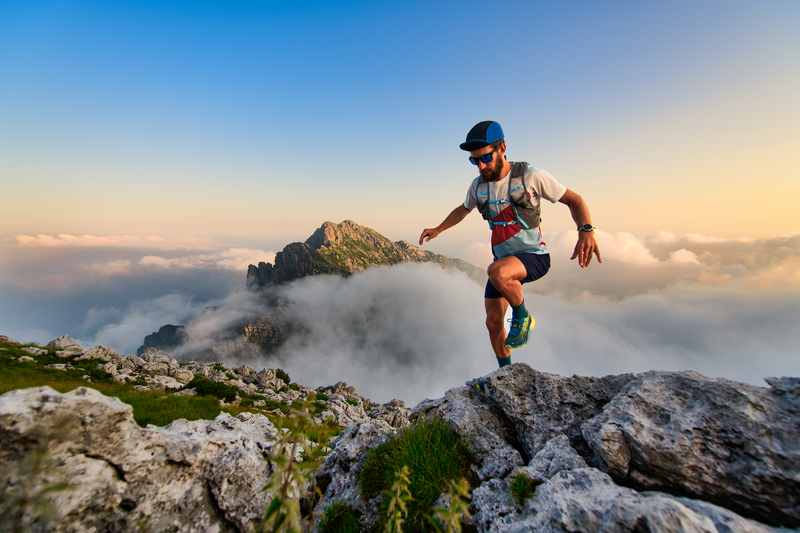
95% of researchers rate our articles as excellent or good
Learn more about the work of our research integrity team to safeguard the quality of each article we publish.
Find out more
SYSTEMATIC REVIEW article
Front. Endocrinol. , 30 January 2024
Sec. Thyroid Endocrinology
Volume 14 - 2023 | https://doi.org/10.3389/fendo.2023.1323284
This article is part of the Research Topic Effects of Prenatal Exposure to Endocrine-Disrupting Chemicals View all 6 articles
We present a comprehensive overview of changes in thyroxine (T4) and thyroid stimulating hormone (TSH) serum concentrations after pre-gestational, gestational and/or lactation exposures of rodents to various chemicals that affect the thyroid hormone system. We show that T4 and TSH changes consistent with the idealized view of the hypothalamic-pituitary-thyroid (HPT) feedback loop (T4 decrements accompanied by TSH increases) are observed with only a relatively small set of chemicals. Most substances affect concentrations of various thyroid hormones without increasing TSH. Studies of altered T4 concentrations after gestational exposures are limited to a relatively small set of chemicals in which pesticides, pharmaceuticals and industrial chemicals are under-represented. Our risk-of-bias analysis exposed deficits in T4/TSH analytics as a problem area. By relating patterns of T4 – TSH changes to mode-of-action (MOA) information, we found that chemicals capable of disrupting the HPT feedback frequently affected thyroid hormone synthesis, while substances that produced T4 serum decrements without accompanying TSH increases lacked this ability, but often induced liver enzyme systems responsible for the elimination of TH by glucuronidation. Importantly, a multitude of MOA leads to decrements of serum T4. The current EU approaches for identifying thyroid hormone system-disrupting chemicals, with their reliance on altered TH serum levels as indicators of a hormonal mode of action and thyroid histopathological changes as indicators of adversity, will miss chemicals that produce T4/T3 serum decreases without accompanying TSH increases. This is of concern as it may lead to a disregard for chemicals that produce developmental neurotoxicity by disrupting adequate T4/T3 supply to the brain, but without increasing TSH.
The thyroid hormone system is involved in the regulation of many vital processes, including lipid metabolism and brain development. These processes are critically dependent on the availability of thyroid hormones (TH) in target tissues, at the correct amount and at the correct time. Mis-timed delivery and over- or under-supply of TH can have adverse and irreversible consequences (1). The complexity of the thyroid hormone system is considerable, involving multiple steps including receptor binding, transport, cellular uptake and hormone conversion-steps controlling the formation of the active hormone, tri-iodothyronine (T3) in target cells. This complexity poses enormous challenges for the identification of chemicals capable of disrupting the proper functioning of the system, here termed thyroid hormone system-disrupting chemicals (THSDC).
Current approaches for the identification of THSDC in the European Union (EU) (2) rely on determinations of altered TH serum levels (T4 and T3), which are taken as indicators of a hormonal mode of action. In addition, adverse effects need to be demonstrated. In the absence of other signs of adversity (e.g. neurodevelopmental toxicity), histopathological changes in the thyroid gland are seen as satisfying the adversity criterion. Such changes are mainly the result of elevated TSH levels.
The rationale of this approach can be traced to concepts of target organ toxicity for the thyroid, and to an idealized view of the canonical hypothalamic-pituitary-thyroid (HPT) axis feedback loop. In this view, insufficient TH output by the thyroid stimulates the release of thyrotropin-releasing hormone (TRH) in hypothalamic neurons and of TSH in pituitary thyrotropes. In turn, TSH induces the thyroid to produce TH, ultimately restoring T4 and TSH to normal serum concentrations. Persistently elevated TSH concentrations can lead to histopathological changes in the thyroid, shifting the gland to a trajectory from follicular cell proliferation to certain cancers (3). Other factors, including iodine deficiency or mutations can also contribute to these changes.
T4 and TSH serum levels consistent with this idealized view of the HPT axis are, however, not always observed. The most striking examples include chemicals such as PCBs, PBDEs and perfluorinated compounds which cause large T4 serum decrements without the expected increases in TSH levels (4–8). Although the reasons for these enigmatic patterns remain to be fully elucidated, they suggest that the underlying mechanisms of action involve parts of the thyroid hormone system that are extraneous to the HPT axis. In any case, the concern is that current EU approaches for the testing and evaluation of THSDCs, with their reliance on adverse changes in thyroid gland histopathology, will miss chemicals which exhibit patterns of hormonal changes incompatible with the idealized view of the HPT axis, yet can disrupt the thyroid hormone system, with negative consequences for healthy brain development.
The aim of our study is to produce a comprehensive overview of the T4 and TSH changes in rodents seen after gestational/lactational exposures to a wide variety of chemicals. Relying on the peer-reviewed scientific literature only, we wanted to compare the effects of different chemicals to discover the most frequently observed patterns, to establish whether different patterns were observed with the same chemical, to compare the effects between dams and pups and to understand how the ways in which the thyroid hormone system was perturbed (here referred to as mode of action, MoA) relate to these patterns. To achieve this, we conducted a systematic evidence mapping with confidence rating of experimental studies. One outcome of this work will be to provide an improved basis for regulatory bodies to consider a wider range of MoA in the assessment of chemicals capable of disrupting the thyroid hormone system.
The systematic review methodology was developed following the COSTER recommendations and reported in accordance with the Preferred Reporting Item for Systematic Reviews and Meta-Analysis (PRISMA) checklist (9). The detailed protocol for the present systematic review can be found in the open-access repository Zenodo, uploaded in 2021 (https://zenodo.org/record/5528557#.Y_dAOybP02w). The initial protocol uploaded to the repository was written to compile data for humans and laboratory animals (adults and offspring). However, after careful review of all the studies obtained, we decided to first focus on pre-gestational, gestational and lactational studies in rodent models. Due to the large numbers of articles, we left epidemiological data and rodent adult studies for subsequent systematic reviews.
The literature search for peer-reviewed articles was conducted in 3 scientific databases: PubMed, Web of Science and Scopus, using a search strategy with terms describing the thyroid hormone system and endocrine-disrupting chemicals to capture all pertinent information on the effects of chemicals on T4/TSH. Seven references were added through recommendation by experts, and these were designated “ad hoc” (AD) studies.
An initial pilot search was conducted on September 2020 with an update in July 2021. The PECO statement (Population, Exposure, Comparator, Outcome) was developed to frame the research question and the subsequent screening of the studies (Table 1).
After an initial pilot study, the search string was refined with names of the target chemicals, however, this was modified as we found that such a search strategy could not capture all the literature which may be of relevance. Studies collected in this way were included in the systematic review when they complied with the PECO statement and our inclusion criteria. In the end, we built a final search string which relied on items commonly known to contain potential endocrine-disrupting chemicals (EDCs), avoiding specific names of chemicals. The list of items included was based on the information supplied by the National Institute of Environmental Health Sciences and Endocrine Society websites (10, 11) and (12, 13). We did not apply time limits in our search. The language of the publications was restricted to English. Detailed search terms are presented in Supplementary Material 1.
The systematic review process was managed using the freely available online tool CADIMA (https://www.cadima.info/index.php). Two team members worked independently on the merged list of records from Scopus, Web of Science and Pubmed to conduct title and abstract screening, followed by full-text screening. A consistency check of a subsample of 200 randomly selected studies was performed, in which we achieved a kappa value of 0.55, considered as “fair” based on Cadima criteria. Any potential conflicts among both screeners during the title/abstract or full-text screening were resolved after discussion or by inclusion of a third reviewer.
Articles with no full-text access, book chapters, clinical trials, reviews, systematic reviews, meta-analysis, conference proceedings, opinion articles or letters to editor were excluded. When different studies reported the same research data, we selected the study reporting the most complete dataset.
Only peer-reviewed studies were selected for data extraction and study evaluation. We included all the experimental studies (in vivo) with rodent models (rat and mouse) that measured the levels of T4 (total and/or free) and TSH in serum/plasma of pups/fetus and dams, and pups/fetus or dams alone after chemical administration. Studies with non-mammalian species or marine mammals were excluded, as were studies with chemical administration outside the pre-gestational, gestational or lactational periods. We also excluded studies that used mixtures of different chemicals or when the chemicals were administered by injection, as this route evades liver metabolism. We focused on studies in which individual compounds were tested at 2 or more doses, as studies with only one tested dose may suffer from a higher chance of overlooking TH/TSH dose-response effects. Although studies that used the test chemicals at only one dose did not meet our eligibility criteria, we nevertheless considered their outcomes to investigate whether they revealed response patterns consistent with eligible studies.
Data from the studies were extracted into a template adapted from Martin et al. (14). The corresponding data extraction template can be found in Supplementary Material 2. Briefly, we extracted the following data:
• Meta data (Article title, authors, publication year, journal name, funding source, funding source category).
• Information about the study:
- Animal model (species, strain, Latin name, age at the beginning/end of the treatment, age at the time the TH/TSH were measured).
- Study design (duration, exposure concentration, exposure regimen, dosing route, inclusion of negative and positive control, thyroid histopathology, hepatic T4-UDPGT, measurement method for T4, measurement method for TSH, biological sample measured, statistical methods).
- Chemical characterization (chemical name, acronym, CAS, chemical class, chemical uses, source of the chemical, purity, vehicle, chemical detection: tissue and method).
- Hormone measurements in pups and dams: T4, FT4, TSH (increase “∧”, decrease “∨”, no change “–” and percentage of the change in the treatment groups with respect to the control group).
Percentage changes of T4, fT4 and TSH were calculated with the following formula:
When the TH/TSH data were in the form of plots or images, the freely available online tool WebPlotDigitizer (15) was used to extract the data. Where an article reported TH/TSH levels for more than one chemical, we recorded one entry for every chemical in the data extraction template.
As one of our goals was to elucidate relations between potential MoA and TH/TSH patterns, we retrieved data on other endpoints related to the TH system, including:
- Activity of T4-UDPGT, an enzyme in charge of hepatic catabolism of thyroid hormones.
- Thyroid histopathology.
- Gene and/or protein markers related to the TH axis, for example:
- Liver metabolism: UDP-glucuronosyltransferases (UGTs), sulfotransferases (SULTs), cytochromes P450s (CYPs), constitutive androstane receptor (CAR), pregnane X receptor (PXR),
- Synthesis: sodium-iodide cotransporter (NIS or SLC5a5), thyroid peroxidase (TPO), dual oxidases (DUOXs), thyroglobulin (TG), deiodinases (DIOs).
- Regulation: Thyroid receptors (TRs), TSH receptor (TSHr), thyrotropin-releasing hormone (TRH), thyroid hormone responsive genes (Thrsp or Spot14, ME1, Mdra1), thyroid transcription factors (Nkx2.1, TTF-1, PAX8).
- Distribution, transport and binding: Monocarboxylate transporter 8 (MCT8), organic anion transporting polypeptides (OATPs), pendrin, transthyretin (TTR).
- Enzymatic activity: TPO, DIOs, 7-Ethoxyresorufin-O-deethylase (EROD), 7-pentoxyresorufin O-dealkylase (PROD), 7-benzyloxyresorufin oxidation (BROD), 7-methoxyresorufin O-demethylation (MROD).
- Binding activity/affinity of different TH system components: TTR, TRs.
- Levels of T4 in foetal brain and liver.
- Neurological outcomes in offspring (e.g. motor activity, heterotopia, neurotransmitter alterations).
In addition, we explored the CompTox Chemicals Dashboard database (https://comptox.epa.gov/dashboard/) to retrieve information on EDC bioactivity from the in vitro TH assays (shown in Table 2) and the EFSA report on the establishment of cumulative assessment groups of pesticides (16) to collect information about the effect of pesticides on the thyroid.
The assays were examined for the hit calls in the bioactivity data section (TOXCAST summary). Selection of the “Hit call” showed whether the test chemical was active or inactive. Following (17), a positive hit call is defined as “a biological perturbation having a maximum median response that exceed the cutoff defined for the assay and having data that can be curve-fit”. In addition, when the results were not clear, as was the case when a single endpoint was explored with 2 different assays with conflicting results, we added an “?” in our extraction files. The cytotoxicity limit for each chemical affected the number of active hit calls (18), however, we did not exclude chemicals that may be active only at high concentrations.
The internal validity of the selected studies was appraised with a risk of bias (RoB) assessment adapted from (19, 20; 21) and further developed for this systematic review. When a publication assayed several chemicals, we recorded one RoB entry for each chemical. The RoB tool consisted of a list of 18 questions with 4 response options based on (21) scores: ++ Definitely low risk of bias (dark green); + Probably low risk of bias (light green); ~ Probably high risk of bias (orange); ~~ Definitely high risk of bias (red). The tool was organized within Microsoft Excel and the template can be found in Supplementary Material 3.
Among the 18 questions, we included 8 key questions which had to achieve scores of “definitely” or “probably low risk” to rate a study as “high confidence”. These 8 key elements were:
1 and 2, Reliability and sensitivity of the analytical methods used for T4 and TSH quantification, respectively. As we did not have the resources to conduct a thorough review of this aspect, we scored articles as “~ Probably high risk of bias (orange)” or “~~ Definitely high risk of bias (red)” when i) details about the methodology or the assays were missing, ii) when we were unable to access details of the analytical measurements, for example, at the provider website, iii) when there was no mention of TH analytics, iv) when the assay used was developed for human serum without any further adaptation/validation for the rodent serum matrix. Accordingly, when the names of the assays employed were available, with technical details, we scored studies as “++ Definitely low risk of bias (dark green)” or “+ Probably low risk of bias (light green)”.
3. Exposure characterization, in terms of purity of the chemical, method of administration or measurement of real concentrations in the diet or drinking water. Studies with a comprehensive description of the exposure were classified as “++ Definitely low risk of bias (dark green)” or “+ Probably low risk of bias (light green)” when the study included a list of minimal information such as method of administration, concentrations, carrier solvent or duration of the exposure. When those details were missing or where we considered the information regarding the exposure as insufficient, the study was classified as “~ Probably high risk of bias (orange)”. Studies with direct evidence of inconsistent administrations were marked as “~~ Definitely high risk of bias (red)”.
4. Numbers of animals used per dose. Studies using 5 or more animals per experimental group were marked as “++ Definitely low risk of bias (dark green)”, while studies using fewer than 5 animals but with significant effects on TH/TSH levels were classified as “+ Probably low risk of bias (light green)”. Studies with insufficient information or direct evidence of using a lower number of animals per group were marked as “~ Probably high risk of bias (orange)” or “~~ Definitely high risk of bias (red)”, respectively.
5. Inclusion of a positive control for producing hormonal changes. Demonstration of effects with a positive control was taken as evidence of a responsive animal model. Accordingly, when a positive control was ineffective in terms of TH/TSH alterations, the study was ranked as “~~ Definitely high risk of bias (red)”. When a positive control showed an effect or was not included, but the treatment altered TH or TSH, the study was marked as “++ Definitely low risk of bias (dark green)” or “+ Probably low risk of bias (light green)”, respectively. If a positive control was not included and the treatments did not show effects, then, the study was classified as “~ Probably high risk of bias (orange)”.
6. Timing of sampling for TH/TSH measurements. When significant changes of TH/TSH levels were observed and the timing of sampling was in accordance with OECD/EPA test guidelines (2), we considered a study as “++ Definitely low risk of bias (dark green)”. If there were ambiguities in relation to compliance with test guideline recommendations (no specification of guidelines in the methodology), but significant effects were observed, we rated a study as “+ Probably low risk of bias (light green)”. Studies were marked as “~ Probably high risk of bias (orange)” when no significant changes were observed and there were ambiguities regarding adherence with test guidelines. “~~ Definitely high risk of bias (red)” studies with no changes and not adherence to guidelines (22).
7. Use of contemporaneous or historical controls as a comparator of hormonal changes. When a vehicle control was not employed, and data from historical controls were used as a comparator, the study was evaluated as “~~ Definitely high risk of bias (red)”, as identical test conditions cannot be guaranteed (See discussion in 23). When contemporaneous controls were used, we rated a study as “++ Definitely low risk of bias (dark green)”. The categories “+ Probably low risk of bias (light green)” and “~ Probably high risk of bias (orange)” were not used.
8. Use of soy-free animal feed. Soy-containing feeds can produce alterations in the hormonal levels and potentially mask the effects of EDCs (24) and can elicit goitrogenic effects in rats due to isoflavones (25–27). When the diet contained soy-derived components or alfalfa, the study was rated as “~~ Definitely high risk of bias (red)”. In cases where we were unable to trace the components of the diets, we scored the study as “+ Probably low risk of bias (light green)”. When the authors specified the diet as “soy-free”, then the study was ranked “++ Definitely low risk of bias (dark green)”.
To achieve an overall evaluation of each study, we adopted the decision rules detailed in EFSA (28) which encompasses 3 Tiers, as follows:
We placed studies in the highest confidence level (Tier 1, green) when all 8 key elements were scored ++ (Definitely low risk of bias) OR + (probably low risk of bias) AND no more than 1 question not addressing these key elements was scored ~ (probably high risk of bias) or ~~ (definitely high risk of bias).
A medium confidence level (Tier 2 orange) was used for all combinations not covered in Tier 1 or Tier 3.
The lowest confidence level (Tier 3 red) was reserved for studies where any one of the 8 key elements was rated ~ or ~~ OR more than 50% of the questions not addressing these key elements were scored ~ or ~~.
We piloted the RoB scheme by selecting a random sample of 10 studies which was scored independently by 3 different team members. Any conflict regarding RoB outcomes was reviewed and resolved after discussion between the team members.
The outcome of our literature search and screening is shown in Figure 1 (Prisma flow). We retrieved a total of 30,282 records from 3 different databases (Pubmed, Web of Science, Scopus) and 7 additional records through other sources (expert suggestions). After removal of duplicates, 25,113 records were manually screened for relevance (article titles and abstracts). Of these, 23,870 records were identified as not relevant or as not meeting our eligibility criteria, due to: i) exposure factors other than chemicals or their mixtures, ii) incomplete T4 and TSH data; iii) studies in systems other than in vivo experiments, or epidemiological studies. At this stage we also excluded all records that were not primary research papers.
The remaining 1,243 items were subjected to full-text screening based on our PECO criteria (see Table 1). Of those, 532 full-text records were set aside for another evidence mapping focusing on human epidemiology. Of the remaining 711 studies, 628 studies were excluded at this last step as they did not meet our PECO criteria due to i) adult rodent exposure (left aside for a follow-up evidence mapping for adult exposures); ii) non-gavage administration (e.g. injection, inhalation); iii) administration of the chemical outside the gestation and/or lactation period; iv) missing thyroid hormone data; v) use of animals with surgical modifications such as e.g. ovariectomy; vi) use of mixtures or lack of original data; vi) use of non-rodent species (See Supplementary Material 4 for the list of articles for full-text screening with CADIMA reference numbers). Only one study using non-rodent animal models was found (CADIMA reference number in parentheses): ewe (2196). Due to the small number of animals in these non-rodent studies, and the difficulty of comparing the results with those from rodents, we excluded the study. This left a total of 83 records for in-depth analysis (Supplementary Material 5). Of those, a subset of 24 studies were experiments with only one dose group per test compound. These studies were taken out of the main body of the systematic review and dealt with separately. The remaining records were considered the most suitable for our purposes and were prioritized for data extraction and evaluation (Supplementary Material 6 for multiple doses and Supplementary Material 7 for studies with only one dose group).
We evaluated the internal validity of eligible studies by conducting a risk-of-bias analysis (Supplementary Material 8). Because some studies reported the effects of multiple chemicals, we conducted the risk-of-bias analysis with a focus on each tested compound. Accordingly, the unit of evidence was the chemical and not the study.
As shown in Table 3, most “low confidence” units of evidence (Tier 3) did not meet our quality criteria for T4 or TSH analytics and quantification methods (key elements 1 and 2), mainly because of inadequate descriptions of method details, or because of the use of human immunoassays on rat sera without adaptation or validation.
Table 3 Risk of Bias ratings of each unit of evidence for all the questions involved in the risk-of-bias analysis for studies with two or more chemical doses.
Another frequently encountered shortcoming was the use of soy-containing diets (key element 8). Furthermore, a few studies included insufficient numbers of animals per dose group. The quality criteria for all other key elements were generally met. Supplementary Material 9 lists the risk-of-bias outcome for studies with only a single dose exposure group.
Of the 49 studies selected with more than 2 dose groups (representing 59 units of evidence), 12 units of evidence were scored as “definitely low” or “probably low risk” on all the key elements and for most of the remaining elements. We classed these studies as high-confidence studies (Tier 1). While no records were placed in Tier 2, our evaluation returned 47 units of evidence with a low confidence rating, placed in Tier 3 (Table 4). The same analysis was performed for studies with one dose group (Supplementary Materials 9, 10).
Most studies were conducted in the rat; only 3 out of 83 studies used mice (Cadima reference numbers 563 [triclosan], 2343 [PFBS] and 4842 [mancozeb, imidacloprid]). All the test chemicals were administered orally: by diet, via drinking water or intragastrical, during gestation and/or lactation. Most studies covered the period when the thyroid gland develops during gestation (GD9 to GD17 in the rat) and when it becomes functional (GD17 to GD21).
Only a few studies exposed the animals before pregnancy, prior to mating or during mating (Cadima reference numbers 1456, 1247, 2118, 29594, 106, 3257, 4293). We also included 4 articles where free, unbound T4 (fT4) was measured instead of T4 (Cadima reference numbers 299, 1172, 2261 and 11193). Most of the studies extracted the T4 and TSH from serum as recommended in ATA guidelines (29); only 17 studies used plasma to quantify the hormones.
The levels of T4, TSH and, when available, fT4, were reported as an increase or decrease (statistically significant changes) or no change in relation to a control group (no test compound exposure). We calculated the percentage of such changes in relation to control groups for all the test compounds (see Supplementary Material 6 for further details for multiple doses and Supplementary Material 7 for single-dose studies).
We grouped chemicals according to T4 - TSH response patterns observed in dams and pups, as follows: Suppressions of serum T4 levels followed by increases in TSH (Figure 2), decreases of serum T4, with no changes in TSH (Figure 3), and mixed patterns, with either unchanged or increased T4 and varying TSH changes (Figures 4, 5). Supplementary Material 11 lists similar patterns but for studies where only one dose group was used.
Figure 2 Summary of T4 – TSH response patterns with chemicals producing T4 decrements and TSH increases in dams and pups. Shown are records for test compounds with corresponding Cadima reference numbers (see Supplementary Material 5). Light grey cells show responses in dams, white cells in pups. Windows of exposure were labelled BP (pre-mating/mating periods, before pregnancy), GD (gestational days, GD 0 to birth) and PND (postnatal days from 10 to 30 or after 30) and green horizontal bars depict the duration of the exposure to pregnant dams. The period of thyroid development (GD 9 – GD 17, 30) is shown as an orange bar. Black arrowheads “▼” indicate the timing of blood sampling for T4 and TSH measurements. Blue downward arrowheads stand for T4 decrements, light blue “▼” in pups and dark blue “▼” in dams; orange upwards arrowheads show increases of T4 or TSH, light orange “▲”in pups, dark orange “▲” in dams. The absence of change in hormonal levels is represented by “↔”. Where varying responses occurred, we used “?”. Most of the studies reported dosages of test chemicals as mg/kg bw/d, except for PTU administered via the drinking water where doses were expressed as parts per million (ppm) (indicated next to the chemical name). The asterisk (*) indicates studies where FT4 was measured instead of T4. When the exposure (green horizontal bars) reached “≥ PND 30”, this indicates that the dosing period was prolonged until or beyond the PND 30. When the sampling day was placed on the “≥ PND 30”, this indicates that the sampling took place on the 30 PND or after this day (for more details, see the Supplementary Materials 5–7).
Figure 3 Summary of T4 – TSH response patterns with chemicals producing T4 serum decrements with no TSH changes in dams and pups. Symbols and shading as in Figure 2.
Figure 4 Summary of studies where T4 - TSH changes were not observed in dams and pups at various dosages after gestational and perinatal exposures to test chemicals. List of studies showing as main response the unaffected levels of T4 and TSH. Symbols and shading as in Figure 2.
Figure 5 Summary of T4/TSH response patterns in dams and pups for chemicals producing inconsistent effects. Symbols and shading as in Figure 2. (See Supplementary Material 10 for single-dose studies).
Decreased serum T4 with increased TSH is one of the two response patterns which stands out as the most frequently observed (Figure 2). Chemicals such as amitrole (7478 in pups), BPA (2146 in dams and pups), DEHP (527 in pups), PTU (AD6 in dams and pups, AD 7 in pups), TCDD (191 in dams and pups) and triclosan (304 in dams) showed significant changes for T4/TSH at all doses tested. With PTU (AD4 in pups, AD5 in dams and pups, AD6 in dams and pups, AD7 in pups), most of the exposed groups showed significantly reduced serum T4 followed by an increase in TSH (although this was not observed when the PTU doses were low). Some other studies, such as with chlorpyrifos in pups (2118), indomethacin in dams (27596), PFBS (2343 in dams and pups), PTU (930, AD4 and AD5 in dams and pups, AD3 in dams), triclosan in dams (563) and BDE-209 in pups (1172) only reported significant changes for T4 and/or TSH at higher dosages. We noticed that some compounds, such as amitrole (7478), chlorpyrifos (2118) and PTU (930, AD4, AD7) produced different responses in dams and pups (see next section). TCDD (738) administered as single oral dose (200, 800 ng/kg bw) at GD 15 did not show a clear trend for T4 in pups. In this case, T4 was measured at 2 different time points for male and female, PND 21 and PND 49. While TCDD decreased the levels of T4 in both sexes at PND 21, T4 was exclusively augmented in males at PND 49 with the highest concentration. Another example is PFBS (2343) for pups: although there was an increase for TSH with 200 and 500 mg/kg bw/d, significant differences were only reported for PND 30, and not at PND 1 or PND 60.
The other frequently observed response pattern is characterized by T4 serum decrements without corresponding increases in TSH levels (Figure 3). This applies to Aroclor 1254 (1947 in pups), PFHxS (2429 in dams and pups), PFOS (2295 in pups, 1765 and 1247 in dams), 4-OH-CB107 (3341 in pups) and nonylphenol (299 in pups). In some studies T4 decrements were only observed at higher doses (relative to the dose range tested in that particular study), such as with DBDE (14143 in pups), DE-47 (6748 in pups and dams), DE-71 (930, 6748 and 2218 in dams and pups, 5748 in dams), PCB 153 (2215 in pups), PCB 77 (1126 in pups), TCDD (1126 in pups), TBBPA (1456 in pups and dams), triclosan (1657 in pups and dams), and vinclozolin (106 and 3257 in pups).
ETU (166) did not show a clear response pattern in offspring. In female pups, there were decrements of serum T4 at an intermediate dose at three sampling times (PND 23, 42, 75), while in male offspring the levels of T4 remained unchanged (PND 42, and with the lowest and highest dosages). TSH levels did not show a clear response pattern, with an increase at 0.1 mg/kg bw/d in PND 23 males and PND 75 females. A few chemicals produced an increase of TSH solely at higher doses. Examples are DE-71 (5748 for dams, 2218 for pups), perchlorate (18235 and AD2 in dams) and PFOS (1247 for pups).
Differences in the timing of serum T4 and TSH measurements may have produced these varying response patterns. Such cases are highlighted by the “?” symbols in Figure 2B.
In some studies where T4 and TSH were measured the test chemicals produced no alterations, although distinct changes had been reported by others (Figure 4). Examples include cyanamide for pups and dams (7458), BPA (255), genistein (14584), PCB 126 (1126), PCB 153 (1885) and TBBPA (2320) in pups.
Less frequently seen patterns include unchanged T4 levels with increased or decreased TSH (Figure 5), as in the case of HBCD (2320) for the highest concentration, glyphosate (267). Increased serum T4 with unchanged TSH was observed with BPA (1933). In the study of DE-71 (1826), T4 and TSH were measured at PND 21 and PND 60 for 2 different concentrations, 1.7 and 30.6 mg/kg bw/d. However, T4 was significantly decreased only at PND 21 with the highest concentration tested. TSH was increased at the highest dose, at PND 60. Nonylphenol (4293) yielded significant declines in TSH and T4 at the lowest tested dose (2 mg/kg bw/d), observed in both male and female offspring. Additionally, females showed a significant decrease of TSH at the highest dose (50 mg/kg bw/d). There were 2 studies with pregnant rats exposed from GD 6 to GD 19 to 3 different doses (20, 100, 500 mg/kg bw/d) of phthalates, DCHP and DHP (748 and 2863). In study 748, the hormones were measured at PND 20 and PND 32, while study 2863 measured at PND 90 for both male and female offspring. However, the results showed varied responses for TSH and T4 for both chemicals.
Percentage changes of T4 and TSH were calculated with respect to the control (see Supplementary Materials 5–7). Significant decrements of T4 (pups) for the first group of chemicals (T4 down, TSH up) varied from -8% (DEPH, 527) to -92% (PTU, AD7) with increments in TSH from 16% (PFBS, 2343) to 750% (PTU, AD4). For the dams, the percentage of change in T4 ranged from -12% (PTU, AD6) to -90% (PTU, AD5), and from 10% (triclosan, 304) to 2843% (PTU, AD5) for TSH.
In the second group of chemicals (T4 down, TSH unmodified), the percentage of T4 in pups varied between -8% (PFOS, 2295) to -100% (PFOS, 1247).
Certain chemicals showed variable T4 – TSH response patterns in different studies (Table 5, Supplementary Material 7 for studies with only one dose group). In many cases, the discrepancies can be explained in terms of differences in the doses administered (e.g. Aroclor 1254, PCB 153, TCDD, DE-71, PTU), treatment duration (TCDD) and hormone measurement time points (Aroclor 1254, DE-71).
However, the divergent patterns seen with BPA and TBBPA are hard to explain. While one study of BPA (255) did not produce any changes in T4 or TSH in pups, T4 decrements with accompanying TSH rises were observed in another BPA experiment (2146), despite a shorter treatment period. A third BPA study (1933), in contrast, found T4 increases without changes in TSH, in line with the observed in vitro TR antagonist properties, although lower doses than in the first BPA study (255) were used.
In the case of TBBPA, both studies used comparable doses, covered the period of thyroid gland development in gestation and conducted hormone measurements at similar time points, yet one (2320) did not find T4 or TSH changes in pups, while the other (1456) observed T4 decrements without changes in TSH. There were differences in the timing of dosing. Study 2320 started dosing at GD10 and study 1456 during the premating period.
By considering studies where TH and TSH were measured in dams and pups, we assessed whether the direction of hormonal changes was similar in dams and pups.
The direction of changes in dams and pups was consistent in studies of BPA (2146), TCDD (191) and PTU (930, AD 4 – 7), with decreased T4 and increased TSH, and BDE-47 (6748), DE-71 (930, 5748, 6748, 2218), perchlorate (18235) or PFHxS (2429) with decrements in T4 and unchanged TSH.
However, other chemicals showed a lack of concordance between dams and pups. Amitrole (7478), Chlorpyrifos (2118), ETU (166), 4-OH-CB107 (3441) were not able to alter the TH in dams but evoked TH changes in the pups reducing T4 with different effects on TSH (see Table 6).
To investigate whether the observed T4 – TSH response patterns can be related to documented modes of action (MOA) of the tested chemicals, we compiled the relevant information for each of the four response patterns for all studies, including single-dose experiments (Table 7). This included histological analyses of the thyroid gland, expression profiles of genes coding for TH axis markers, enzymatic activities, TH-related enzymatic activities and protein levels, T4 levels in the brain and other neurological outcomes (see materials and methods for the name of the assays and Supplementary Materials 6, 7 for the detailed extracted data). Because most studies did not provide MOA data themselves or reported such data incompletely, we extracted additional relevant information from the CompTox Chemical Dashboard database. We excluded 2 studies with the phthalates DHP and DCHP (758, 2863) due to the unclear responses of T4/TH. Both phthalates altered the thyroid histomorphology.
Chemicals which produced T4 decrements with concomitant increases in TSH were frequently capable of inhibiting TH synthesis (Table 7). Examples are BPA, amitrole, chlorpyrifos, fipronil, PTU, triclosan and dimethoate. Based on data extracted from in vitro assays (CompTox), there are indications that BPA, TCDD, chlorpyrifos, fipronil, PTU, triclosan and dimethoate also affect hepatic metabolism by interacting with xenobiotic-sensing receptors (CAR, PXR) and inducing mono-oxygenases (CYP) and conjugating enzymes that glucuronidise TH (UGT). PTU, fipronil, DEHP, triclosan and dimethoate were able to suppress the enzymatic activity or gene expression of DIOs and IYD. PTU and perchlorate also led to decreases in T4 brain concentrations in offspring. Pax8 and Ttf1, transcription factors associated with thyroid development and involved in the regulation of thyroid-related genes, were also altered after DEHP (527) and perchlorate (AD2) exposures.
The chemicals that produced T4 decrements without corresponding TSH increases were frequently able to act as PXR agonist. This is the case for TBBPA, PFOS, Vinclozolin, BDE-47 and Nitrofen. Only PCB 77 was capable of inducing T4 UDPGT (or UGT1A1), which catalyzes T4-glucuronidation. In addition, vinclozolin tended to induce other hepatic enzymes as BROD, EROD and MROD. Interestingly, 4-OH-CB107 showed binding affinity for TTR.
The case of TBBPA is interesting, as its in vitro effect profile would be expected to produce T4 – TSH response patterns different from the observed serum T4 decrements without attendant TSH increases. As an inhibitor of TH synthesis, TBBPA might be expected to also produce TSH increases, while its capability to antagonise the TR would lead to T4 increases with no TSH changes.
A similar pattern would be expected from PFOS, due to its ability to antagonise TRs, while its ability to inhibit NIS would be expected to produce T4 decrements and TSH increases, different from the pattern observed (T4 decrements, no TSH increase).
Following the approaches in (30, 31), we next classified our test compounds according to their capacity to interfere with TH regulation (TH receptors, TRH, TRH receptors, TSH, TSH receptors), TH synthesis (NIS, TPO), TH binding proteins (TTR), TH transporters (MCT8, OATP), control of local TH action (DIO/IYD), and induction of hepatic enzymes (UGTs, SULTs, CYPs, AhR, PXR, CAR, PPARa) (See Table 8 in Supplementary Material 12). This classification shows that several chemicals were able to interact with more than one target of the TH system, e.g. BPA, Aroclor 1254, genistein or chlorpyrifos. Scarce data are available for other markers, such as TH transporters or binding proteins.
We present a comprehensive overview of scientific reports characterizing changes in T4 and TSH serum levels observed after gestational exposures of rodents to various chemicals affecting the thyroid hormone system. By employing generic terms in our search strategy, excluding specific chemical names, we captured over 30,000 records which we subjected to relevance screening. Some relevant records may have been missed because search terms were not present in title or abstract, but we believe this number to be small.
Our evidence mapping shows that studies of T4 and TSH changes after gestational exposures are limited to a relatively small set of chemicals in which pesticides, pharmaceuticals and industrial chemicals are somewhat under-represented. A wider range of substances has been examined in studies of adult exposures (to be reported elsewhere).
The T4 and TSH changes we detected were not significantly affected by issues of between-study reproducibility. Similar changes were observed in multiple independent studies of the same chemical, and any divergent findings could be explained in terms of differences in the administered doses, the duration of dosing or the timing of TH measurements. There was reasonably good concordance between dams and pups, and any differences are likely due to differences in toxicokinetics. Additional factors that can come into play include sensitivity differences between pup and dam, due to their developmental stage which may blur concordances in some cases. The lactational transfer of some chemicals may also confound concordances.
Considerable variations in the timing of hormone measurements became apparent, especially in offspring, and greater consideration should be given to harmonization of timing. Many studies limited hormone measurements in pups to the end of lactation (PND 21) which may have missed some effects, as more pronounced alterations in T4 and TSH are often observed earlier (GD20, PND 4 or 14).
Our risk-of-bias analysis exposes deficiencies in TH and TSH analytics as a potential problem. For most eligible studies, we were unable to exclude bias, mainly because of inadequate descriptions of method details, or because of the use of human immunoassays on rat sera without adaptation or validation.
One aim of this exercise was to support future hazard assessments by producing insights of the spectrum of MOAs involved in TH system disruption. However, most of the studies considered here measured only a few endpoints useful for elucidating potential MOAs. Accordingly, we had to make inferences about MOAs from extraneous sources, which may compromise their validity due to complications with in vitro to in vivo extrapolations and differences in exposure time scales (32–35).
With these provisos in mind, the following patterns became apparent: Gestational exposures to certain chemicals during the time when the thyroid develops can lead to disruptions of the HPT feedback loop, with increased TSH levels in dams and offspring. Our evidence mapping shows that chemicals acting in this way include amitrole, bisphenol A, chlorpyrifos, DEHP, PTU, TCDD and triclosan. A feature common to this group is a capacity to interfere with TH synthesis by inhibiting iodide transport into the thyroid (NIS inhibition) and/or by inhibiting TPO, based on evidence from in vitro assays.
Similar disruptions of the HPT feedback mechanism do not occur with other chemicals also capable of suppressing circulating TH levels after gestational exposures, even though the observed TH decrements often exceed those seen with chemicals that disrupt the HPT axis. Substances that fall into this group include PCBs, PBDEs, PFAS, TBBPA and vinclozolin. With a few exceptions, these chemicals are not able to interfere with TH synthesis. Instead, their MOA appears to be best characterized as induction of microsomal enzyme systems in the liver via PXR and/or CAR which promote the elimination of TH, by glucuronidation.
Other patterns observed after gestational exposures include elevated T4 levels but without TSH changes, consistent with TRb antagonism, and increases in TSH with no changes in T4, suggestive of TH transmembrane transport inhibition (e.g. MCT8). Finally, there were T4 increases accompanied by TSH increases, a phenotype observed in DIO2 knock-out mice (36).
As pointed out in the Results section, the phenotypes expected based on in vitro test outcomes do not always materialize in vivo (an example are the patterns expected from the TR antagonist properties of bisphenol A). In cases where substances interact with multiple molecular targets, the prediction of in vivo phenotypes from in vitro results becomes particularly complex.
The current EU approaches for identifying THSDC rely on determinations of altered TH serum levels as indicators of a hormonal mode of action and on demonstrations of histopathological changes in the thyroid gland required as indicators of adversity. Since thyroid histopathological changes are driven by TSH, chemicals that produce TH alterations without accompanying TSH increases will be missed with the current implementation of the EU criteria. Our evidence mapping shows that this applies to many of the substances for which data after gestational exposures are available. This is of concern as it may lead to overlooking chemicals that produce developmental neurotoxicity by disrupting adequate TH supply to the brain, but without increasing TSH. Examples are Aroclor 1254 (4) and DE-71 (37) which produced changes in motor activity in rat offspring. In both cases, strong T4 decreases in offspring were observed, but TSH levels were left unchanged. Similarly, Aroclor 1254 impaired hearing in offspring (4), again without any TSH changes. These studies suggest that it is the extent of T4 decrements, and not TSH, that is an important predictor of developmental neurotoxicity. Whether this applies to all indicators of developmental neurotoxicity is currently unclear. Periventricular heterotopia, the occurrence of misplaced neurons due to migration deficits, were found in studies with PTU or amitrole which produces T4 decrements (38, 39), but were not seen after gestational PFHxS or triclosan exposures, despite pronounced reductions in T4 serum levels (8).
However, these considerations do not apply to the regulatory regimes in countries outside the EU where endocrine disruptors are not treated differently from other chemicals and where endocrine disruptor criteria do not exist.
In any case, we show that T4 decrements in offspring can arise from a multitude of MOAs. In view of recent evidence of a direct role of T4 in the brain via non-genomic signaling (40), these observations are particularly interesting.
However, TSH increases do not seem to be linked to a similar multitude of MOAs. Disruptions of the HPT axis after gestational exposures appear to be linked to inhibition of TH synthesis and can occur even after relatively modest declines of TH serum levels. In a review of mechanisms of drug metabolizing enzyme inducers and TH alterations, Vansell (41) has drawn attention to another MOA leading to TSH increases. In studies of liver microsomal enzyme system inducers, the most pronounced disruptions of the HPT axis occurred with substances that promoted T3 glucuronidation and transmembrane transporters important in the biliary excretion of TH conjugates, such as Mrp2. Sometimes, strong TSH increases occurred even after only modest T4 serum decreases such as with clobazam which induced UGT1A1/Ugt1a1 (T4 glucuronidation), UGT2B/Ugt2b1, Ugt2b2 (T3 glucuronidation) and MRP2/Mrp2 (biliary excretion of TH conjugates) (42, 43). Our evidence mapping shows that the relevant TH conjugating enzymes and transmembrane transporters are rarely measured in studies of hormonal changes, but more information on these enzyme systems may be helpful in further elucidating the MOAs behind T4 – TSH response patterns.
The original contributions presented in the study are included in the article/Supplementary Material, further inquiries can be directed to the corresponding author.
IF-P: Conceptualization, Data curation, Formal analysis, Investigation, Methodology, Visualization, Writing – original draft, Writing – review & editing. AHB: Conceptualization, Data curation, Formal analysis, Investigation, Methodology, Visualization, Writing – original draft, Writing – review & editing. AK: Conceptualization, Formal analysis, Funding acquisition, Supervision, Writing – original draft, Writing – review & editing.
The author(s) declare financial support was received for the research, authorship, and/or publication of this article. This research was funded by the EU Horizon 2020 program, ATHENA project, grant number 825161, which is gratefully acknowledged.
The authors would like to thank Prof. Dr. Josef Köhrle for his assistance with the TH quantification methods and Dr. Sibylle Ermler for her help with the risk of bias analysis.
The authors declare that the research was conducted in the absence of any commercial or financial relationships that could be construed as a potential conflict of interest.
All claims expressed in this article are solely those of the authors and do not necessarily represent those of their affiliated organizations, or those of the publisher, the editors and the reviewers. Any product that may be evaluated in this article, or claim that may be made by its manufacturer, is not guaranteed or endorsed by the publisher.
This publication reflects only the authors’ view, and the European Commission is not responsible for any use that may be made of the information it contains.
The Supplementary Material for this article can be found online at: https://www.frontiersin.org/articles/10.3389/fendo.2023.1323284/full#supplementary-material
BPA, Bisphenol A; BROD, 7-benzyloxyresorufin oxidation; CAR, Constitutive androstane receptor; CYP, Cytochrome P-450; DCHP, Dicyclohexyl phthalate; DEHP, Bis(2-ethylhexyl) phthalate; DHP, Diisoheptyl phthalate; DIO, deiodinases; DUOX, Dual oxidases; EDC, endocrine-disrupting chemical; EROD, 7-Ethoxyresorufin-O-deethylase; ETU, Ethylenethiourea; EU, European Union; FT4, Free thyroxine; FT3, Free triiodothyronine; GD, Gestational Day; HBCD, Hexabromocyclododecane; HPT, Hypothalamic–pituitary–thyroid axis; MCT8, Monocarboxylate transporter 8; MOA, Mode of action; MROD, 7-methoxyresorufin O-demethylation; NIS, Sodium/iodide symporter; OATP, Organic anion transporting polypeptide; PBDE, Polybrominated diphenyl ethers; PCB, Polychlorinated biphenyl; PECO, Population; Exposure; Comparator; Outcome; PFBS, Perfluorobutanesulfonic acid; PFHxS, Perfluorohexanesulfonic acid; PFOS, Perfluorooctanesulfonic acid; PND, Postnatal Day; PPAR, Peroxisome proliferator-activated receptors; PROD, 7-pentoxyresorufin O-dealkylase; PTU, Propylthiouracil; PXR, Pregnane X receptor; SULT, sulfotransferases; T4, Thyroxine; T3, Triiodothyronine; TBBPA, Tetrabromobisphenol A; TCDD, 2;3;7;8-Tetrachlorodibenzodioxin; TG, Thyroglobulin; TH, Thyroid Hormone; THRSP, Thyroid hormone responsive protein; THSDC, Thyroid Hormone System Disrupting Chemical; TPO, Thyroid peroxidase; TR, Thyroid receptor; TRH, Thyrotropin-releasing hormone; TSH, Thyroid stimulating hormone; TTR, Transthyretin; UGT, UDP-glucuronosyltransferases; 2-mecarp., 2-Mercaptobenzimidazole.
1. Köhrle J, Frädrich C. Thyroid hormone system disrupting chemicals. Best Pract Res Clin Endocrinol Metab (2021) 35:101562. doi: 10.1016/J.BEEM.2021.101562
2. ECHA (European Chemicals Agency), EFSA (European Food Safety Authority), JRC (Joint Research Centre), Andersson N, Arena M, Auteri D, et al. Guidance for the identification of endocrine disruptors in the context of Regulations (EU) No 528/2012 and (EC) No 1107/2009. EFSA J (2018) 16:e05311. doi: 10.2903/J.EFSA.2018.5311
3. McLeod DSA. Thyrotropin in the development and management of differentiated thyroid cancer. Endocrinol Metab Clin North Am (2014) 43:367–83. doi: 10.1016/j.ecl.2014.02.012
4. Goldey ES, Kehn LS, Lau C, Rehnberg GL, Crofton KM. Developmental exposure to polychlorinated biphenyls (Aroclor 1254) reduces circulating thyroid hormone concentrations and causes hearing deficits in rats. Toxicol Appl Pharmacol (1995) 135:77–88. doi: 10.1006/taap.1995.1210
5. Morse DC, Wehler EK, Wesseling W, Koeman JH, Brouwer A. Alterations in rat brain thyroid hormone status following pre- and postnatal exposure to polychlorinated biphenyls (Aroclor 1254). Toxicol Appl Pharmacol (1996) 136:269–79. doi: 10.1006/taap.1996.0034
6. Luebker DJ, York RG, Hansen KJ, Moore JA, Butenhoff JL. Neonatal mortality from in utero exposure to perfluorooctanesulfonate (PFOS) in Sprague-Dawley rats: dose-response, and biochemical and pharamacokinetic parameters. Toxicology (2005) 215:149–69. doi: 10.1016/J.TOX.2005.07.019
7. Ramhøj L, Hass U, Gilbert ME, Wood C, Svingen T, Usai D, et al. Evaluating thyroid hormone disruption: investigations of long-term neurodevelopmental effects in rats after perinatal exposure to perfluorohexane sulfonate (PFHxS). Sci Rep (2020) 10:2672. doi: 10.1038/s41598-020-59354-z
8. Gilbert ME, o’Shaughnessy KL, Thomas SE, Riutta C, Wood CR, Smith A, et al. Thyroid disruptors: extrathyroidal sites of chemical action and neurodevelopmental outcome-an examination using triclosan and perfluorohexane sulfonate (PFHxS). Toxicol Sci (2021) 183(1):195–213. doi: 10.1093/toxsci/kfab080
9. Page MJ, McKenzie JE, Bossuyt PM, Boutron I, Hoffmann TC, Mulrow CD, et al. The PRISMA 2020 statement: an updated guideline for reporting systematic reviews. Syst Rev (2021) 10:1–11. doi: 10.1186/S13643-021-01626-4/FIGURES/1
10. Endocrine Society. Endocrine-disrupting Chemicals (2023). Available at: https://www.endocrine.org/topics/edc (Accessed 9.27.23).
11. NIEHS. Endocrine Disruptors (2023). Available at: https://www.niehs.nih.gov/health/topics/agents/endocrine/index.cfm (Accessed 9.27.23).
12. Gore AC, Chappell VA, Fenton SE, Flaws JA, Nadal A, Prins GS, et al. EDC-2: the endocrine society’s second scientific statement on endocrine-disrupting chemicals. Endocr Rev (2015) 36:E1–E150. doi: 10.1210/er.2015-1010
13. Bertram MG, Gore AC, Tyler CR, Brodin T. Endocrine-disrupting chemicals. Curr Biol (2022) 32:R727–30. doi: 10.1016/J.CUB.2022.05.063
14. Martin O, Scholze M, Ermler S, McPhie J, Bopp SK, Kienzler A, et al. Ten years of research on synergisms and antagonisms in chemical mixtures: A systematic review and quantitative reappraisal of mixture studies. Environ Int (2021) 146:106206. doi: 10.1016/J.ENVINT.2020.106206
15. Rohatgi A. WebPlotDigitizer (2022). Available at: https://automeris.io/WebPlotDigitizer (Accessed 9.13.23).
16. EFSA, Crivellente F, Hart A, Hernandez-Jerez AF, Hougaard Bennekou S, Pedersen R, et al. Establishment of cumulative assessment groups of pesticides for their effects on the thyroid. EFSA J (2019) 17:e05801. doi: 10.2903/j.efsa.2019.5801
17. Williams AJ, Lambert JC, Thayer K, Dorne J.-L.C.M. Sourcing data on chemical properties and hazard data from the US-EPA CompTox Chemicals Dashboard: A practical guide for human risk assessment. Environ Int (2021) 154:106566. doi: 10.1016/j.envint.2021.106566
18. Silva M, Kwok RKH. Use of computational toxicology tools to predict in vivo endpoints associated with Mode of Action and the endocannabinoid system: A case study with chlorpyrifos, chlorpyrifos-oxon and Δ9Tetrahydrocannabinol. Curr Res Toxicol (2022) 3. doi: 10.1016/J.CRTOX.2022.100064
19. Ermler S, Kortenkamp A. Systematic review of associations of polychlorinated biphenyl (PCB) exposure with declining semen quality in support of the derivation of reference doses for mixture risk assessments. Environ Health (2022) 21:94. doi: 10.1186/s12940-022-00904-5
20. Kortenkamp A, Martin O, Ermler S, Baig A, Scholze M. Bisphenol A and declining semen quality: A systematic review to support the derivation of a reference dose for mixture risk assessments. Int J Hyg Environ Health (2022) 241:113942. doi: 10.1016/J.IJHEH.2022.113942
21. NTP OHAT. Handbook for conducting a literature-based health assessment using OHAT approach for systematic review and evidence integration. (2019).
22. Li AA, Makris SL, Marty MS, Strauss V, Gilbert ME, Blacker A, et al. Practical considerations for developmental thyroid toxicity assessments: What’s working, what’s not, and how can we do better? Regul Toxicol Pharmacol RTP (2019) 106:111–36. doi: 10.1016/j.yrtph.2019.04.010
23. Vandenberg LN, Prins GS, Patisaul HB, Zoeller RT. The use and misuse of historical controls in regulatory toxicology: lessons from the CLARITY-BPA study. Endocrinology (2020) 161(5):bqz014. doi: 10.1210/ENDOCR/BQZ014
24. Ruhlen RL, Taylor JA, Mao J, Kirkpatrick J, Welshons WV, vom Saal FS. Choice of animal feed can alter fetal steroid levels and mask developmental effects of endocrine disrupting chemicals. J Dev Orig. Health Dis (2011) 2:36–48. doi: 10.1017/S2040174410000711
25. Chang HC, Doerge DR. Dietary genistein inactivates rat thyroid peroxidase in vivo without an apparent hypothyroid effect. Toxicol Appl Pharmacol (2000) 168:244–52. doi: 10.1006/TAAP.2000.9019
26. Doerge DR, Sheehan DM. Goitrogenic and estrogenic activity of soy isoflavones. Environ Health Perspect (2002) 110:349–53. doi: 10.1289/ehp.02110s3349
27. de Souza dos Santos MC, Gonçalves CFL, Vaisman M, Ferreira ACF, de Carvalho DP. Impact of flavonoids on thyroid function. Food Chem Toxicol (2011) 49:2495–502. doi: 10.1016/J.FCT.2011.06.074
28. EFSA, Croera C, Batke M, Corsini E, FitzGerald RE, Gott D, et al. Testing the study appraisal methodology from the 2017 Bisphenol A (BPA) hazard assessment protocol. EFSA Support Publ (2019) 16:1732E. doi: 10.2903/sp.efsa.2019.EN-1732
29. Bianco AC, Anderson G, Forrest D, Galton VA, Gereben B, Kim BW, et al. American Thyroid Association Guide to investigating thyroid hormone economy and action in rodent and cell models. Thyroid (2014) 24:88–168. doi: 10.1089/thy.2013.0109
30. Howdeshell KL. A model of the development of the brain as a construct of the thyroid system. Environ Health Perspect (2002) 110 Suppl:337–48. doi: 10.1289/ehp.02110s3337
31. OECD. New Scoping Document on in vitro and ex vivo Assays for the Identification of Modulators of Thyroid Hormone Signalling. (2014). doi: 10.1787/9789264274716-en
32. Flippin JL, Hedge JM, DeVito MJ, LeBlanc GA, Crofton KM. Predictive modeling of a mixture of thyroid hormone disrupting chemicals that affect production and clearance of thyroxine. Int J Toxicol (2010) 28:368. doi: 10.1177/1091581809360005
33. Murk AJ, Rijntjes E, Blaauboer BJ, Clewell R, Crofton KM, Dingemans MML, et al. Mechanism-based testing strategy using in vitro approaches for identification of thyroid hormone disrupting chemicals. Toxicol Vitr (2013) 27:1320–46. doi: 10.1016/j.tiv.2013.02.012
34. Hassan I, El-Masri H, Ford J, Brennan A, Handa S, Paul Friedman K, et al. Extrapolating in vitro screening assay data for thyroperoxidase inhibition to predict serum thyroid hormones in the rat. Toxicol Sci (2020) 173:280–92. doi: 10.1093/toxsci/kfz227
35. Carlson JM, Janulewicz PA, Kleinstreuer NC, Heiger-Bernays W. Impact of high-throughput model parameterization and data uncertainty on thyroid-based toxicological estimates for pesticide chemicals. Environ Sci Technol (2022) 56:5620–31. doi: 10.1021/acs.est.1c07143
36. Schneider MJ, Fiering SN, Pallud SE, Parlow AF, St. Germain DL, Galton VA, et al. Targeted disruption of the type 2 selenodeiodinase gene (DIO2) results in a phenotype of pituitary resistance to T4. Mol Endocrinol (Baltimore Md.) (2001) 15:2137–48. doi: 10.1210/mend.15.12.0740
37. Bowers WJ, Wall PM, Nakai JS, Yagminas A, Wade M, Li N. Behavioral and thyroid effects of in utero and lactational exposure of Sprague-Dawley rats to the polybrominated diphenyl ether mixture DE71. Neurotoxicol Teratol (2015) 52:127–42. doi: 10.1016/j.ntt.2015.08.002
38. Gilbert ME, Ramos RL, McCloskey DP, Goodman JH. Subcortical band heterotopia in rat offspring following maternal hypothyroxinaemia: structural and functional characteristics. J Neuroendocrinol (2014) 26:528–41. doi: 10.1111/jne.12169
39. Ramhøj L, Frädrich C, Svingen T, Scholze M, Wirth EK, Rijntjes E, et al. Testing for heterotopia formation in rats after developmental exposure to selected in vitro inhibitors of thyroperoxidase. Environ pollut (2021) 283:117135. doi: 10.1016/j.envpol.2021.117135
40. Schroeder A, Privalsky M. Thyroid hormones, T3 and T4, in the brain. Front Endocrinol (Lausanne) (2014) 5:40. doi: 10.3389/fendo.2014.00040
41. Vansell NR. Mechanisms by which inducers of drug metabolizing enzymes alter thyroid hormones in rats. Drug Metab Dispos (2022) 50:508–17. doi: 10.1124/dmd.121.000498
42. Miyawaki I, Moriyasu M, Funabashi H, Yasuba M, Matsuoka N. Mechanism of clobazam-induced thyroidal oncogenesis in male rats. Toxicol Lett (2003) 145:291–301. doi: 10.1016/j.toxlet.2003.08.002
43. Miyawaki I, Tamura A, Matsumoto I, Inada H, Kunimatsu T, Kimura J, et al. The effects of clobazam treatment in rats on the expression of genes and proteins encoding glucronosyltransferase 1A/2B (UGT1A/2B) and multidrug resistance-associated protein-2 (MRP2), and development of thyroid follicular cell hypertrophy. Toxicol Appl Pharmacol (2012) 265:351–9. doi: 10.1016/j.taap.2012.09.003
Keywords: thyroxine, T4, thyroid stimulating hormone, TSH, endocrine disruptors, systematic review
Citation: Forner-Piquer I, Baig AH and Kortenkamp A (2024) Disruption of the thyroid hormone system and patterns of altered thyroid hormones after gestational chemical exposures in rodents – a systematic review. Front. Endocrinol. 14:1323284. doi: 10.3389/fendo.2023.1323284
Received: 17 October 2023; Accepted: 30 November 2023;
Published: 30 January 2024.
Edited by:
Joanne Rovet, University of Toronto, CanadaReviewed by:
Frédéric Flamant, Université de Lyon, FranceCopyright © 2024 Forner-Piquer, Baig and Kortenkamp. This is an open-access article distributed under the terms of the Creative Commons Attribution License (CC BY). The use, distribution or reproduction in other forums is permitted, provided the original author(s) and the copyright owner(s) are credited and that the original publication in this journal is cited, in accordance with accepted academic practice. No use, distribution or reproduction is permitted which does not comply with these terms.
*Correspondence: Andreas Kortenkamp, YW5kcmVhcy5rb3J0ZW5rYW1wQGJydW5lbC5hYy51aw==
Disclaimer: All claims expressed in this article are solely those of the authors and do not necessarily represent those of their affiliated organizations, or those of the publisher, the editors and the reviewers. Any product that may be evaluated in this article or claim that may be made by its manufacturer is not guaranteed or endorsed by the publisher.
Research integrity at Frontiers
Learn more about the work of our research integrity team to safeguard the quality of each article we publish.