- 1Grupo Reproducción, Departamento de Microbiología y Parasitología, Facultad de Medicina, Universidad de Antioquia - UdeA, Medellín, Colombia
- 2Semillero de Investigación en Alteraciones de la Gestación y Autoinmunidad (SIAGA), Universidad de Antioquia - UdeA, Medellín, Colombia
- 3Grupo de Investigación en Trombosis, Facultad de Medicina, Universidad de Antioquia - UdeA, Medellín, Colombia
- 4Departamento de Obstetricia y Ginecología, Facultad de Medicina, Universidad de Antioquia - UdeA, Medellín, Colombia
The microbiome -defined as the microbiota (bacteria, archaea, lower and higher eukaryotes), their genomes, and the surrounding environmental conditions- has a well-described range of physiological functions. Thus, an imbalance of the microbiota composition -dysbiosis- has been associated with pregnancy complications or adverse fetal outcomes. Although there is controversy about the existence or absence of a microbiome in the placenta and fetus during healthy pregnancy, it is known that gut microbiota can produce bioactive metabolites that can enter the maternal circulation and may be actively or passively transferred through the placenta. Furthermore, the evidence suggests that such metabolites have some effect on the fetus. Since the microbiome can influence the epigenome, and modifications of the epigenome could be responsible for fetal programming, it can be experimentally supported that the maternal microbiome and its metabolites could be involved in fetal programming. The developmental origin of health and disease (DOHaD) approach looks to understand how exposure to environmental factors during periods of high plasticity in the early stages of life (e.g., gestational period) influences the program for disease risk in the progeny. Therefore, according to the DOHaD approach, the influence of maternal microbiota in disease development must be explored. Here, we described some of the diseases of adulthood that could be related to alterations in the maternal microbiota. In summary, this review aims to highlight the influence of maternal microbiota on both fetal development and postnatal life, suggesting that dysbiosis on this microbiota could be related to adulthood morbidity.
1 Introduction
The microbiome comprises a community of microorganisms that inhabit a body space or environment, as well as their genomes and surrounding environmental conditions (1). Some of the functions attributed to microbiota are promoting the development of the immune and central nervous systems, protecting against the invasion of pathogens in the body’s mucous membranes, modulating metabolic processes, and synthesizing active compounds, among others (2, 3).
There is substantial controversy regarding the presence of microorganisms in prenatal intrauterine locations and the consequent environment of sterility in which the fetus develops: the traditional dogma that the human fetal environment is sterile and that the neonate microbiome is acquired during and after birth (4–11) stands against the evidence supporting that the healthy human placenta harbors a unique low-biomass microbiome composed of non-pathogenic commensal microbiota (12–14); although recent studies indicate that the detected microbial signals are likely the result of contamination during sample collection or during DNA extraction and sequencing (15–17). That controversy about the existence or absence of a microbiome in the placenta and fetus of a healthy pregnancy is not the focus of this review. We invite you to check out the recent papers on the subject (18–20).
In contrast, relevant to the scope of this article, current evidence supports that microbiota-derived metabolites or pathogenic microorganisms may be transferred through the placenta during fetal development, which has adverse outcomes for the fetus. Dysbiosis in the gut microbiota (GM) and dysfunction in the integrity of the gut barrier have been associated with multiple diseases. In such cases, the microbial communities and derived metabolites can enter the extraintestinal tissues or the host circulation, exert physiological effects, and activate several signaling pathways, contributing to disease development (21–23).
The microbiome is a new factor in the developmental origin of health and disease (DOHaD). Since microbiota, pathogenic microorganisms, and their derived metabolites influence the epigenome, exploring the evidence about epigenetic mechanisms activated in response to maternal microbiota alterations is gaining relevance. Once it has been understood that the microbiome (and its effects on the metabolome) can influence fetal programming, scenarios can be addressed in which it is possible to associate changes in the maternal microbiome and the development of disease in offspring. In summary, this review aims to highlight the influence of maternal microbiota on fetal development and postnatal life, which can lead to an understanding of how dysbiosis on maternal microbiota can be related to adulthood morbidity.
2 Microbiome alterations in pregnancy disorders
The exact contribution of the maternal microbiome to pregnancy complications or adverse fetal outcomes is not entirely understood. It is widely accepted that some gestational disorders are involved with fetal complications (24, 25), fetal programming, and the origin of adult chronic noncommunicable diseases, in as much as changes in the intrauterine environment can affect the metabolic regulation and development of specific tissues, such as adipose tissue and the cardiovascular system (26, 27). Setting aside the discussion about the presence or absence of the placental microbiome in the context of healthy pregnancy, studies have reported changes in the GM profile in pregnant women with gestational disorders such as preeclampsia (PE), gestational diabetes mellitus (GDM), fetal growth restriction (FGR), maternal obesity, among others. Supplementary Table 1 describes the profile of the microbiota in such gestational alterations (28–43).
Besides, increased levels of plasma lipopolysaccharide (LPS) in PE patients were found, and recently the gut microbiota associated with the LPS synthesis in combination with increased placental LPS levels were reported (44). Another study in antibiotic-treated mice colonized with fecal microbiota from FGR patients confirmed the previous findings of altered gut microbiome in FGR patients and showed that maternal gut dysbiosis can induce placental impairment (40). The maternal gut microbiota influence on fetal-placental growth through the effects of elevated maternal B.breve-derived acetate was shown in mice: its interaction with maternal gut mucosa, and the effects on both placental and fetal metabolism (45). Subsequently, this translocation of bacteria or their derived metabolites from the gut to the placenta may induce changes in the placental structure and functionality under pathological conditions, and consequently, the gut-placenta axis has been proposed to play a crucial role in the etiology of PE (46, 47).
3 Maternal microbial metabolites translocation to the fetus
Gut microbiota can produce bioactive metabolites, including trimethylamine (TMA), trimethylamine N-oxide (TMAO), and short-chain fatty acids (SCFAs) (48). Those small molecules can enter the maternal circulation and may be actively or passively transferred throughout the placenta during fetal development (49–51). The SCFAs regulate intestinal barrier integrity and contribute to placental integrity and vascularization (52). The plasma levels of TMAO in pregnant women were found to be variable depending on the pregnancy trimester, and the levels during late pregnancy were significantly associated with a risk for developing PE (53). In addition, increased levels of LPS and TMAO, and decreased levels of SCFAs were found in plasma and feces respectively in women with PE (30, 54, 55). Since GM and its metabolites play critical roles in inflammation and hypertension, a biomarker panel consisting of intestinal bacteria and SCFAs was described as a potential tool to estimate the risk of PE (31).
Additionally, Akkermansia muciniphila and its metabolites (propionate and butyrate) were found to significantly ameliorate PE symptoms in a rat model by promoting autophagy and M2 polarization of macrophages in the placental bed, and propionate also promoted trophoblast invasion (31). The efficacy and safety of SCFAs in preventing insulin resistance and inflammation associated with GDM have been investigated; in this condition, metabolomic studies have been focused on metabolites derived from amino acids, carbohydrates, lipids, purines, uric acid, bile acid, and their related metabolic pathways (55–57).
The effect of a complete microbiota on the metabolite composition in placental and fetal tissues was evaluated recently using germ-free mice models, in which several compounds were found to be depleted in the fetal intestine and/or placenta (See Supplementary Table 1). Furthermore, the maternal microbiota strongly affects the host metabolism in the placenta and in the fetus, not only by direct production of metabolites but also by a persistent impact on host physiology (58). Regarding metabolite transport, the ABCG5 and ABCG8 transporters expressed in the placenta, liver, and intestine are known to limit the uptake of plant-derived sterols, which are themselves subject to microbiota metabolism. However, it remains unclear how far selective placental expression of any of these transporters leads to regulating fetal exposure to maternal microbial metabolites (59).
As demonstrated by gene expression and functional approaches, the solute transport proteins from the OATP and OCT families (formerly SLC21 and SLC22, respectively), and also the ABCG2 family, expressed in the placenta, are involved in the transfer of biliary products (from maternal hepatic synthesis, microbial metabolism in the maternal gut, and also from fetal synthesis) across the basal membrane in the placenta to be transformed and eliminated by the maternal liver; this transfer confers protection against high levels of bile acids in the fetoplacental unit (59, 60). Microbial constituents, such as aryl hydrocarbon ligands, induce transcriptional changes in the fetal gut, enhancing the cellularity of the innate immune system (61). Butyrate regulates the differentiation of Th17 and Th1 cells (62); maternal retinoic acid (RA) induces the generation of fetal type 3 innate lymphoid cells and, therefore, secondary lymphoid organs development (63); and Clostridia spp. in the maternal gut can modulate the RA levels by suppressing the expression of Rdh7 in the mouse intestinal epithelial cells (64).
The absence of a healthy microbiota is associated with deficits in immune and neuronal development, impaired stress adaptation, and metabolic dysfunction later in life, as demonstrated by several studies in germ-free animals [reviewed by (65)]. Therefore, although the existence of a placental or fetal microbiome is still controversial, and the passage of microorganisms from the mother to the fetus during in-utero development is uncertain, the evidence seems to suggest that at least the metabolites derived from the mother´s microbiome or pathogenic microorganisms have some effect on the fetus. Indeed, this evidence is supported by data showing how changes in the maternal fecal metabolome are reflected in the neonatal metabolome (66).
Some of the above-mentioned metabolites, their main known effects, and their recognized transport mechanisms are illustrated in Figure 1.
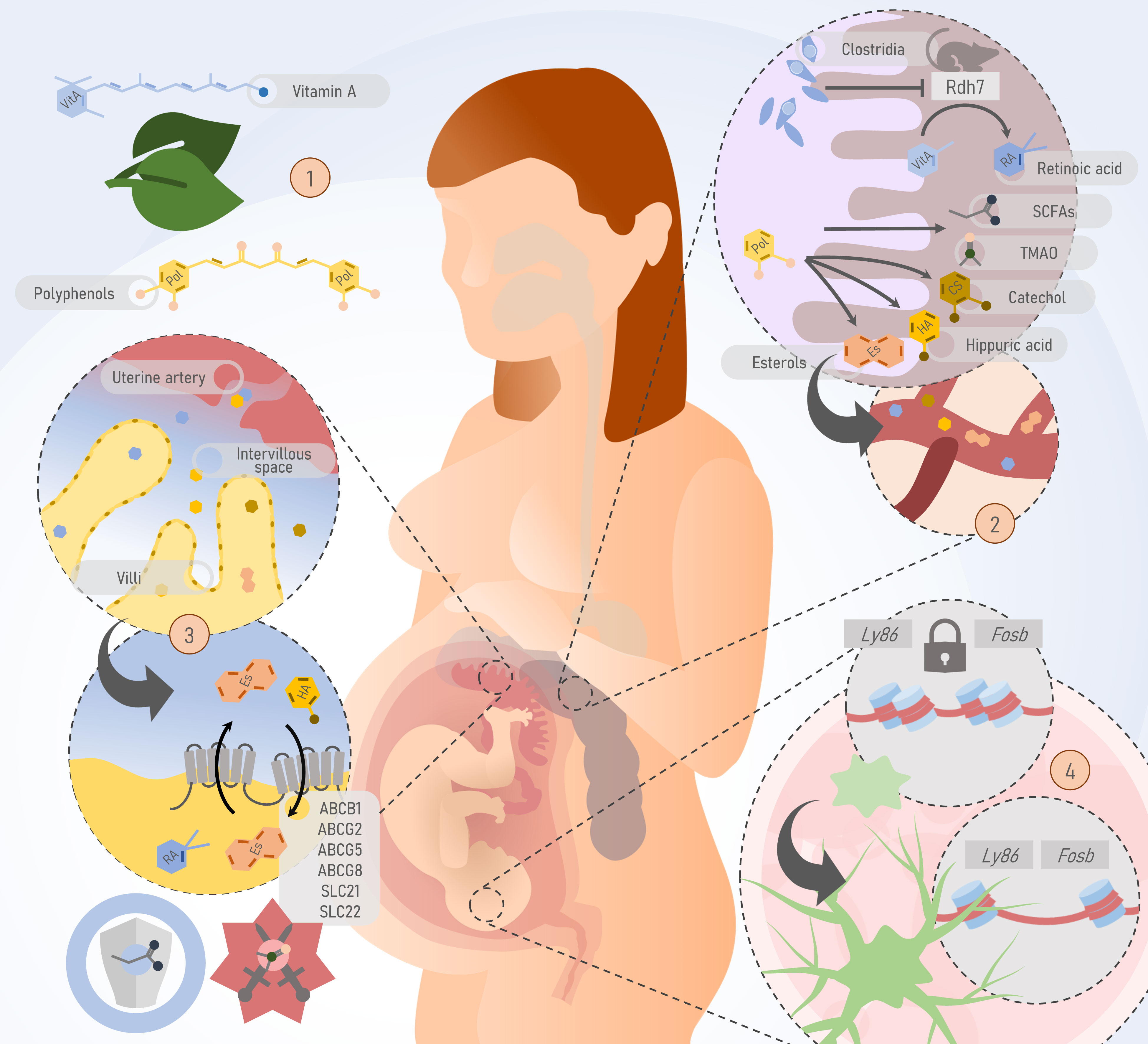
Figure 1 Potential pathways by which the maternal microbiota or its by-products could pass the feto-placental barrier and potentially impact fetal development and postnatal life. 1) Substrates of gut microbiome metabolism such as polyphenols and vitamin A are obtained through the diet. 2) In the gut, the maternal microbiome produces short-chain fatty acids (SCFAs), trimethylamine N-oxide (TMAO), and participates in the metabolism of polyphenols into other metabolites such as sterols, catechol, and hippuric acid. These molecules, derived from the metabolism of the gut microbiome, are passively and actively transferred to the bloodstream. In murine models, bacteria of the Clostridia class inhibit the Rdh7 enzyme, responsible for the metabolism of vitamin A in its active form, retinoic acid. 3) At the maternal-fetal interface, ATP-binding cassettes on the apical surface of the syncytiotrophoblast are responsible for the bidirectional transport of metabolites produced by the gut microbiome. SCFAs contribute to placental integrity and development, while TMAO is associated with preeclampsia. 4) Evidence from murine models shows that the absence of the maternal microbiome has a deleterious impact on processes such as microglial development through epigenetic mechanisms. Thus, microbiome-specific differentially accessible regions have been identified. For example, mouse embryos with a normal microbiome experience increased accessibility of key loci for microglial function such as Ly86 and Fosb, during development. This process does not occur in embryos from germ-free (GF) mice. Rdh7, Retinol dehydrogenase 7; Fap2, Fibroblast Activation Protein-2; SCFAs, short chain fatty acids; TMAO, Trimethylamine N-oxide; ABC, ATP binding cassette; SLC, Solute Carrier family.
4 Microbiome, metabolome, and epigenome
Epigenetics is the set of factors and molecules that modify gene expression while not implying changes in the genome sequence itself. Generally, epigenetic changes occur through modifications in DNA accessibility (i.e., DNA methylation or post-translational changes of the histones). The main interest behind epigenetics lies in the fact that, since these modifications are not directly scripted in the genetic code of the subject, even when they can be heritable material across generations, they are commonly associated with environmental cues that can make different the phenotype of two genotypically identical individuals (67). Notably, the epigenome has been proposed by some authors as one of the main factors responsible for fetal programming [reviewed by (67)], and one of the factors directly impacting the epigenome is the microbiome and its alterations.
The most apparent scenario in which microorganisms and their products can directly interact with the epigenome is in the context of an adaptive immune response. It is known that, in chronic infections, exhausted lymphocytes exhibit a unique epigenetic signature. This pattern includes decreased accessibility to memory-dependent loci such as IL-15 and IL-7R, and increased accessibility to exhaustion-related loci such as EOMES and NFATC2. More importantly, even after infection clearance, some epigenetic features persist as “scars” (68).
The capability of microorganisms to modify the host epigenome has also been confirmed beyond a pathological context and in other cell types. For example, the culture of enteroids with altered Schaedler flora, a selection of 8 dominant microorganisms in the mouse intestinal microbiome, showed that some of its components influence circadian oscillations of the epithelium through the secretion of SCFAs. These, in turn, inhibit histone deacetylases of epithelial cells, a major player in epigenetic control, ultimately leading to alterations in the expression of Bmal1 and PER2 (69).
It is known that microbiome and pathogenic microorganisms have an impact on the epigenome, and it has been proposed that modifications of the epigenome could be responsible for fetal programming; however, could it be possible that the maternal (or fetal) microbiome or infections have an impact on fetal programming through epigenetic mechanisms? In a study that followed 700 Danish children during the first six years of their life, it was described that those children who exhibited allergic rhinitis at the age of six had previously presented a microbiome of reduced diversity in the upper respiratory tract after one week of life. This finding was related to a DNA methylation pattern in mucosal cells that demonstrates a particular epigenetic signature (70). Notably, these features are accompanied by a dysfunctional response of neonatal cells to microbial products at birth, suggesting that the epigenetic characteristics of these cells are the product of in-utero training of the innate immune system (71). The influence of the maternal microbiome on fetal development and postnatal life has been already confirmed in experiments. In contrast to the offspring of C57BL/6J mice with a non-altered microbiome, the offspring of germ-free mice exhibit alterations in microglia development (72).
Thus, the discussion of whether the maternal microbiome and its metabolites could be involved in fetal programming by means of epigenetic changes, in the context of DOHaD, seems to favor an affirmative answer based on what has been reported in animal models.
5 Diseases of adulthood and their relationship to maternal microbiota
The DOHaD concept proposes that exposure to environmental factors during periods of high plasticity in the early stages of life, including the gestational period, can program the risk of the offspring developing diseases (73). The maternal microbiota is one of these factors due to its influence on the microbiome profile of the neonate (74). Just as the hygiene hypothesis raises the relevance of early exposure to microbial environments for the proper development of the immune system, the microbial hypothesis postulates the central role that this exposure plays in the composition of the microbiome, resulting in differential regulation of the immune system (75). Since the primary source of microorganisms that will colonize and shape the microbiota of the newborn comes from the maternal origin, it is worth asking whether there is a connection between dysbiosis of the maternal microbiota and the development of diseases in adulthood, such as metabolic and cardiovascular diseases, neurodevelopment alterations or changes in the immune system. Supplementary Table 1 shows different studies describing this relationship (10).
5.1 Cardio-metabolic diseases
Cardio-metabolic diseases are a group of chronic noncommunicable conditions of great relevance due to their recent increase in prevalence and high mortality rate. This mainly includes cardiovascular disease, atherosclerotic disease, and diabetes, as well as associated conditions such as obesity and dyslipidemia, which may constitute risk factors for the onset of the disease (73).
The cardiovascular risk is configured by several modifiable factors, such as diet, smoking, sedentary lifestyle, and hypertension, among others (76). One of the mechanisms proposed in the study of the association between diet and cardiovascular risk is the potential of eating habits to modify the GM and consequently contribute to increased risk through changes in the metabolic pattern of these bacteria. It has been proposed that the decrease in the production of SCFAs and the increase in the secretion of TMAO are the main mechanisms involved in developing cardio-metabolic diseases (77).
Regarding the risk of developing diabetes mellitus in postnatal life, along with the role of maternal feeding during pregnancy, the antecedent of GDM is related to the presence of metabolic disorders in adulthood. One study comparing the microbiota of children from healthy mothers with children from mothers with GDM, found marked changes in microbial diversity in the healthy group (33); on the other hand, a study reported a similarity between the microbiota profiles of mothers with GDM and their children, postulating a vertical transmission of the microbiota (34).
The intestinal microbiota has also been linked to lipid metabolism. In mouse models, it has been recognized that factors such as a diet with a high intake of fatty acids and cholesterol, and the presence of dyslipidemia during pregnancy, are correlated with an increased risk of dyslipidemia and obesity in the offspring.
Multiple studies have shown a positive association between GM dysbiosis profiles and the development of obesity in adults (42, 78). It has also been reported that the microbial profiles of children are susceptible to changes due to the mode of delivery, constituting a risk factor for childhood obesity (43, 79, 80).
5.2 Neurodevelopment and neuropsychiatric disorders
Axonal development disruptions have been associated with maternal dysbiosis. In a murine model with dysbiosis induced by antibiotics, it has been described a decrease in levels of Netrin-G1A, and a reduction of axons at thalamocortical level, with consequent impairment in tactile and thermal sensitivity. Also, neuropsychiatric disorders such as autism have been associated with maternal dysbiosis. Multiple studies reported differences in microbial profiles among children with and without autism, and prenatal exposure to antibiotics has been postulated as a main risk factor. It has been proposed that maternal dysbiosis impacts sensitive periods of a child’s metabolic programming, which induce changes in the production of essential metabolites for neurodevelopment, as TMAO. This mechanism has been also proposed in the association between maternal obesity and anxiety disorder in the offspring (See Supplementary Table 1).
5.3 Allergy and Atopic diseases
Studies carried out with germ-free mouse models have allowed us to recognize the importance of microbial exposure offered by the GM on the process of development of the immune system in postnatal life, as well as the implication that the lack of exposure has on the increased risk for the development of allergic and autoimmune diseases (81, 82). In this model, and those with a low-diversity microbiota, increased plasma IgE levels and surface-bound IgE on mast cells were found, making these individuals more susceptible to anaphylaxis when challenged with ovalbumin injections (83).
A central change in the pathogenesis of asthma corresponds to the modification of the Th1/Th2 ratio, which a low diversity of the GM may induce (84). One study compared three germ-free mice and a control group with varying exposure to germs at different stages such as fetal, lactation, and childhood. It was evidenced that the control group, which presented a greater diversity of the intestinal microbiota, did not present clinical responses during ovalbumin inhalation, and the serum levels of IFN-γ, as well as the IFN-γ/IL-4 ratio, were significantly higher compared to the other groups. In contrast, the groups of germ-free mice showed clinical responses like asthma attacks and histological changes of alveolar inflammation.
Another study investigated the effect of administering bacterial lysates to pregnant mice on the susceptibility of offspring to develop allergic diseases, finding significant changes related to the Th1/Th2 ratio regulation, such as a higher TLR2 and TLR4 expression in the lung tissue, higher percentage of CD4+/CD35+/Foxp3+ cells in the spleen, as well as higher IL-10 concentration in plasma of the progeny of the intervention group. In contrast, the control progeny showed elevated IL-4 and IL-5 concentrations and GATA3 expression. An essential element recognized in the mothers of the intervention group was the decrease in histone deacetylase 9 expression, suggesting possible epigenetic mechanisms by which the microbiota reduces the risk of developing allergic diseases (10).
6 Concluding remarks
Although the passage of microorganisms from the mother to the fetus is uncertain, the evidence suggests that their derived metabolites have some effect on the fetus. Then, the influence of maternal microbiota on the offspring can be explored through the effects of their bioactive metabolites. The most studied metabolites are those byproducts of the gut microbial metabolism, such as SCFAs, TMA, and TMAO, whose variations along pregnancy are associated with complications such as PE. The microbial capability to modify the host functions is also evidenced by its interaction with the epigenome, suggesting that the maternal microbiome and its metabolites could be involved in fetal programming through epigenetic mechanisms. Then, this introduces the discussion about how dysbiosis in the maternal microbiota can be related to adulthood morbidity. The current knowledge points to the existence of a connection between dysbiosis of the maternal microbiota, and the development of diseases in adulthood, such as metabolic and cardiovascular diseases, neurodevelopment alterations, or changes in the immune system.
Author contributions
JR-T: Conceptualization, Data curation, Investigation, Writing – original draft, Writing – review & editing. DÁ: Conceptualization, Data curation, Investigation, Writing – original draft, Writing – review & editing. AJ: Conceptualization, Data curation, Investigation, Writing – original draft, Writing – review & editing. AA: Conceptualization, Data curation, Investigation, Writing – original draft, Writing – review & editing.
Funding
The author(s) declare financial support was received for the research, authorship, and/or publication of this article. Estrategia de Sostenibilidad Grupo Reproducción, Universidad de Antioquia UdeA, 2021.
Acknowledgments
The authors are grateful for the valuable proofreading service kindly performed by MSc. Juanita Álvarez Jaramillo and James Samuelson.
Conflict of interest
The authors declare that the research was conducted in the absence of any commercial or financial relationships that could be construed as a potential conflict of interest.
Publisher’s note
All claims expressed in this article are solely those of the authors and do not necessarily represent those of their affiliated organizations, or those of the publisher, the editors and the reviewers. Any product that may be evaluated in this article, or claim that may be made by its manufacturer, is not guaranteed or endorsed by the publisher.
Supplementary material
The Supplementary Material for this article can be found online at: https://www.frontiersin.org/articles/10.3389/fendo.2023.1304727/full#supplementary-material.
References
1. Berg G, Rybakova D, Fischer D, Cernava T, Vergès MCC, Charles T, et al. Microbiome definition re-visited: old concepts and new challenges. Microbiome. (2020) 8(1):103. doi: 10.1186/s40168-020-00875-0
2. Gomaa EZ. Human gut microbiota/microbiome in health and diseases: a review. Antonie Van Leeuwenhoek. (2020) 113(12):2019–40. doi: 10.1007/s10482-020-01474-7
3. Hou K, Wu ZX, Chen XY, Wang JQ, Zhang D, Xiao C, et al. Microbiota in health and diseases. Signal Transduct Target Ther (2022) 7(1):135. doi: 10.1038/s41392-022-00974-4
4. Perez-Muñoz ME, Arrieta MC, Ramer-Tait AE, Walter J. A critical assessment of the “sterile womb” and “in utero colonization” hypotheses: implications for research on the pioneer infant microbiome. Microbiome. (2017) 5(1):1–19. doi: 10.1186/s40168-017-0268-4
5. Rehbinder EM, Lødrup Carlsen KC, Staff AC, Angell IL, Landrø L, Hilde K, et al. Is amniotic fluid of women with uncomplicated term pregnancies free of bacteria. Am J Obstet Gynecol. (2018) 219(3):289.e1–289.e12. doi: 10.1016/j.ajog.2018.05.028
6. Leiby JS, McCormick K, Sherrill-Mix S, Clarke EL, Kessler LR, Taylor LJ, et al. Lack of detection of a human placenta microbiome in samples from preterm and term deliveries. Microbiome. (2018) 6(1):1–11. doi: 10.1186/s40168-018-0575-4
7. de Goffau MC, Lager S, Sovio U, Gaccioli F, Cook E, Peacock SJ, et al. Human placenta has no microbiome but can contain potential pathogens. Nature. (2019) 572(7769):329–34. doi: 10.1038/s41586-019-1451-5
8. Theis KR, Romero R, Winters AD, Greenberg JM, Gomez-Lopez N, Alhousseini A, et al. Does the human placenta delivered at term have a microbiota? Results of cultivation, quantitative real-time PCR, 16S rRNA gene sequencing, and metagenomics. Am J Obstet Gynecol. (2019) 220(3):267.e1–267.e39. doi: 10.1016/j.ajog.2018.10.018
9. Kuperman AA, Zimmerman A, Hamadia S, Ziv O, Gurevich V, Fichtman B, et al. Deep microbial analysis of multiple placentas shows no evidence for a placental microbiome. BJOG Int J Obstet Gynaecol. (2020) 127(2):159–69. doi: 10.1111/1471-0528.15896
10. Liu J, Tu C, Yu J, Chen M, Tan C, Zheng X, et al. Maternal microbiome regulation prevents early allergic airway diseases in mouse offspring. Pediatr Allergy Immunol Off Publ Eur Soc Pediatr Allergy Immunol (2020) 31(8):962–73. doi: 10.1111/pai.13315
11. Kennedy KM, Gerlach MJ, Adam T, Heimesaat MM, Rossi L, Surette MG, et al. Fetal meconium does not have a detectable microbiota before birth. Nat Microbiol (2021) 6(7):865–73. doi: 10.1038/s41564-021-00904-0
12. Aagaard K, Ma J, Antony KM, Ganu R, Petrosino J, Versalovic J. The placenta harbors a unique microbiome. Sci Transl Med (2014) 6(237):237ra65–237ra65. doi: 10.1126/scitranslmed.3008599
13. Stinson L, Hallingström M, Barman M, Viklund F, Keelan J, Kacerovsky M, et al. Comparison of bacterial DNA profiles in mid-trimester amniotic fluid samples from preterm and term deliveries. Front Microbiol (2020) 11:415. doi: 10.3389/fmicb.2020.00415
14. Stupak A, Gęca T, Kwaśniewska A, Mlak R, Piwowarczyk P, Nawrot R, et al. Comparative analysis of the placental microbiome in pregnancies with late fetal growth restriction versus physiological pregnancies. Int J Mol Sci (2023) 24(8):6922. doi: 10.3390/ijms24086922
15. Lauder AP, Roche AM, Sherrill-Mix S, Bailey A, Laughlin AL, Bittinger K, et al. Comparison of placenta samples with contamination controls does not provide evidence for a distinct placenta microbiota. Microbiome. (2016) 4(1):29. doi: 10.1186/s40168-016-0172-3
16. Bushman FD. De-discovery of the placenta microbiome. Am J Obstet Gynecol. (2019) 220(3):213–4. doi: 10.1016/j.ajog.2018.11.1093
17. Olomu IN, Pena-Cortes LC, Long RA, Vyas A, Krichevskiy O, Luellwitz R, et al. Elimination of ‘kitome’ and ‘splashome’ contamination results in lack of detection of a unique placental microbiome. BMC Microbiol (2020) 20(1):157. doi: 10.1186/s12866-020-01839-y
18. Briana DD, Papaevangelou V, Malamitsi-Puchner A. The jury is still out on the existence of a placental microbiome. Acta Paediatr (2021) 110(11):2958–63. doi: 10.1111/apa.16048
19. Zakis DR, Paulissen E, Kornete L, Kaan AM, EA N, Zaura E. The evidence for placental microbiome and its composition in healthy pregnancies: A systematic review. J Reprod Immunol (2022) 149:103455. doi: 10.1016/j.jri.2021.103455
20. Kennedy KM, de Goffau MC, Perez-Muñoz ME, Arrieta MC, Bäckhed F, Bork P, et al. Questioning the fetal microbiome illustrates pitfalls of low-biomass microbial studies. Nature. (2023) 613(7945):639–49. doi: 10.1038/s41586-022-05546-8
21. Tang WHW, Kitai T, Hazen SL. Gut microbiota in cardiovascular health and disease. Circ Res (2017) 120(7):1183–96. doi: 10.1161/CIRCRESAHA.117.309715
22. Al Khodor S, Shatat IF. Gut microbiome and kidney disease: a bidirectional relationship. Pediatr Nephrol Berl Ger. (2017) 32(6):921–31. doi: 10.1007/s00467-016-3392-7
23. Chen X, Li P, Liu M, Zheng H, He Y, Chen MX, et al. Gut dysbiosis induces the development of pre-eclampsia through bacterial translocation. Gut. (2020) 69(3):513–22. doi: 10.1136/gutjnl-2019-319101
24. Ribeiro IM, Souto PCS, Borbely AU, Tanabe ELL, Cadavid A, Alvarez AM, et al. The limited knowledge of placental damage due to neglected infections: ongoing problems in Latin America. Syst Biol Reprod Med (2020) 66(3):151–69. doi: 10.1080/19396368.2020.1753850
25. Giachini FR, Galaviz-Hernandez C, Damiano AE, Viana M, Cadavid A, Asturizaga P, et al. Vascular dysfunction in mother and offspring during preeclampsia: contributions from latin-american countries. Curr Hypertens Rep (2017) 19(10):83. doi: 10.1007/s11906-017-0781-7
26. Fall CHD. Fetal programming and the risk of noncommunicable disease. Indian J Pediatr (2013) 80 Suppl 1(0 1):S13–20. doi: 10.1007/s12098-012-0834-5
27. Nobile S, Di Sipio Morgia C, Vento G. Perinatal origins of adult disease and opportunities for health promotion: A narrative review. J Pers Med (2022) 12(2):157. doi: 10.3390/jpm12020157
28. Lv LJ, Li SH, Li SC, Zhong ZC, Duan HL, Tian C, et al. Early-onset preeclampsia is associated with gut microbial alterations in antepartum and postpartum women. Front Cell Infect Microbiol (2019) 9:224. doi: 10.3389/fcimb.2019.00224
29. Qing W, Shi Y, Zhou H, Chen M. Gut microbiota dysbiosis in patients with preeclampsia: A systematic review. Med Microecol. (2021) 10:100047. doi: 10.1016/j.medmic.2021.100047
30. Wang J, Gu X, Yang J, Wei Y, Zhao Y. Gut microbiota dysbiosis and increased plasma LPS and TMAO levels in patients with preeclampsia. Front Cell Infect Microbiol (2019) 9:409. doi: 10.3389/fcimb.2019.00409
31. Jin J, Gao L, Zou X, Zhang Y, Zheng Z, Zhang X, et al. Gut dysbiosis promotes preeclampsia by regulating macrophages and trophoblasts. Circ Res (2022) 131(6):492–506. doi: 10.1161/CIRCRESAHA.122.320771
32. Meijer S, Pasquinelli E, Renzi S, Lavasani S, Nouri M, Erlandsson L, et al. Gut micro- and mycobiota in preeclampsia: bacterial composition differences suggest role in pathophysiology. Biomolecules. (2023) 13(2):346. doi: 10.3390/biom13020346
33. Su M, Nie Y, Shao R, Duan S, Jiang Y, Wang M, et al. Diversified gut microbiota in newborns of mothers with gestational diabetes mellitus. PloS One (2018) 13(10):e0205695. doi: 10.1371/journal.pone.0205695
34. Wang J, Zheng J, Shi W, Du N, Xu X, Zhang Y, et al. Dysbiosis of maternal and neonatal microbiota associated with gestational diabetes mellitus. Gut. (2018) 67(9):1614–25. doi: 10.1136/gutjnl-2018-315988
35. Soderborg TK, Carpenter CM, Janssen RC, Weir TL, Robertson CE, Ir D, et al. Gestational diabetes is uniquely associated with altered early seeding of the infant gut microbiota. Front Endocrinol (2023) 11:603021. doi: 10.3389/fendo.2020.603021
36. Chen T, Qin Y, Chen M, Zhang Y, Wang X, Dong T, et al. Gestational diabetes mellitus is associated with the neonatal gut microbiota and metabolome. BMC Med (2021) 19(1):120. doi: 10.1186/s12916-021-01991-w
37. Dualib PM, Fernandes G, Taddei CR, Carvalho CRS, Sparvoli LG, Bittencourt C, et al. The gut microbiome of obese postpartum women with and without previous gestational diabetes mellitus and the gut microbiota of their babies. Diabetol Metab Syndr (2022) 14(1):194. doi: 10.1186/s13098-022-00954-2
38. Zhu Q, Yang X, Zhang Y, Shan C, Shi Z. Role of the gut microbiota in the increased infant body mass index induced by gestational diabetes mellitus. mSystems. (2022) 7(5):e0046522. doi: 10.1128/msystems.00465-22
39. Tu X, Duan C, Lin B, Li K, Gao J, Yan H, et al. Characteristics of the gut microbiota in pregnant women with fetal growth restriction. BMC Pregnancy Childbirth. (2022) 22(1):297. doi: 10.1186/s12884-022-04635-w
40. Tao Z, Chen Y, He F, Tang J, Zhan L, Hu H, et al. Alterations in the gut microbiome and metabolisms in pregnancies with fetal growth restriction. Microbiol Spectr. (2023) 11(3):e0007623. doi: 10.1128/spectrum.00076-23
41. He X, Li Z, Li X, Zhao H, Hu Y, Han W, et al. The fecal microbiota of gravidas with fetal growth restriction newborns characterized by metagenomic sequencing. Curr Res Transl Med (2023) 71(1):103354. doi: 10.1016/j.retram.2022.103354
42. Collado MC, Isolauri E, Laitinen K, Salminen S. Effect of mother’s weight on infant’s microbiota acquisition, composition, and activity during early infancy: a prospective follow-up study initiated in early pregnancy. Am J Clin Nutr (2010) 92(5):1023–30. doi: 10.3945/ajcn.2010.29877
43. Tun HM, Bridgman SL, Chari R, Field CJ, Guttman DS, Becker AB, et al. Roles of birth mode and infant gut microbiota in intergenerational transmission of overweight and obesity from mother to offspring. JAMA Pediatr (2018) 172(4):368–77. doi: 10.1001/jamapediatrics.2017.5535
44. Tang R, Xiao G, Jian Y, Yuan Q, Jiang C, Wang W. The Gut Microbiota Dysbiosis in Preeclampsia Contributed to Trophoblast Cell Proliferation, Invasion, and Migration via lncRNA BC030099/NF-κB Pathway. Mediators Inflammation (2022) 2022:6367264. doi: 10.1155/2022/6367264
45. Lopez-Tello J, Schofield Z, Kiu R, Dalby MJ, van Sinderen D, Le Gall G, et al. Maternal gut microbiota Bifidobacterium promotes placental morphogenesis, nutrient transport and fetal growth in mice. Cell Mol Life Sci (2022) 79(7):386. doi: 10.1007/s00018-022-04379-y
46. Rubinstein MR, Wang X, Liu W, Hao Y, Cai G, Han YW. Fusobacterium nucleatum promotes colorectal carcinogenesis by modulating E-cadherin/β-catenin signaling via its FadA adhesin. Cell Host Microbe (2013) 14(2):195–206. doi: 10.1016/j.chom.2013.07.012
47. de Goffau MC, Lager S, Salter SJ, Wagner J, Kronbichler A, Charnock-Jones DS, et al. Recognizing the reagent microbiome. Nat Microbiol (2018) 3(8):851–3. doi: 10.1038/s41564-018-0202-y
48. Tang WHW, Li DY, Hazen SL. Dietary metabolism, the gut microbiome, and heart failure. Nat Rev Cardiol (2019) 16(3):137–54. doi: 10.1038/s41569-018-0108-7
49. Braniste V, Al-Asmakh M, Kowal C, Anuar F, Abbaspour A, Tóth M, et al. The gut microbiota influences blood-brain barrier permeability in mice. Sci Transl Med (2014) 6(263):263ra158–263ra158. doi: 10.1126/scitranslmed.3009759
50. Jašarević E, Morrison KE, Bale TL. Sex differences in the gut microbiome-brain axis across the lifespan. Philos Trans R Soc Lond B Biol Sci (2016) 371(1688):20150122. doi: 10.1098/rstb.2015.0122
51. Bolte EE, Moorshead D, Aagaard KM. Maternal and early life exposures and their potential to influence development of the microbiome. Genome Med (2022) 14(1):4. doi: 10.1186/s13073-021-01005-7
52. Cui J, Wang J, Wang Y. The role of short-chain fatty acids produced by gut microbiota in the regulation of pre-eclampsia onset. Front Cell Infect Microbiol (2023) 13:1177768. doi: 10.3389/fcimb.2023.1177768
53. Huang X, Li Z, Gao Z, Wang D, Li X, Li Y, et al. Association between risk of preeclampsia and maternal plasma trimethylamine-N-oxide in second trimester and at the time of delivery. BMC Pregnancy Childbirth. (2020) 20(1):302. doi: 10.1186/s12884-020-02997-7
54. Chang Y, Chen Y, Zhou Q, Wang C, Chen L, Di W, et al. Short-chain fatty acids accompanying changes in the gut microbiome contribute to the development of hypertension in patients with preeclampsia. Clin Sci Lond Engl (1979) 134(2):289–302. doi: 10.1042/CS20191253
55. Cornelius DC, Amaral LM, Wallace K, Campbell N, Thomas AJ, Scott J, et al. Reduced uterine perfusion pressure T-helper 17 cells cause pathophysiology associated with preeclampsia during pregnancy. Am J Physiol Regul Integr Comp Physiol (2016) 311(6):R1192–9. doi: 10.1152/ajpregu.00117.2016
56. Zhao H, Li H, Chung ACK, Xiang L, Li X, Zheng Y, et al. Large-scale longitudinal metabolomics study reveals different trimester-specific alterations of metabolites in relation to gestational diabetes mellitus. J Proteome Res (2019) 18(1):292–300. doi: 10.1021/acs.jproteome.8b00602
57. Roy R, Nguyen-Ngo C, Lappas M. Short-chain fatty acids as novel therapeutics for gestational diabetes. J Mol Endocrinol (2020) 65(2):21–34. doi: 10.1530/JME-20-0094
58. Pessa-Morikawa T, Husso A, Kärkkäinen O, Koistinen V, Hanhineva K, Iivanainen A, et al. Maternal microbiota-derived metabolic profile in fetal murine intestine, brain and placenta. BMC Microbiol (2022) 22(1):46. doi: 10.1186/s12866-022-02457-6
59. Ganal-Vonarburg SC, Hornef MW, Macpherson AJ. Microbial-host molecular exchange and its functional consequences in early mammalian life. Science. (2020) 368(6491):604–7. doi: 10.1126/science.aba0478
60. McIlvride S, Dixon PH, Williamson C. Bile acids and gestation. Mol Aspects Med (2017) 56:90–100. doi: 10.1016/j.mam.2017.05.003
61. Gomez de Agüero M, Ganal-Vonarburg SC, Fuhrer T, Rupp S, Uchimura Y, Li H, et al. The maternal microbiota drives early postnatal innate immune development. Science. (2016) 351(6279):1296–302. doi: 10.1126/science.aad2571
62. Chen L, Sun M, Wu W, Yang W, Huang X, Xiao Y, et al. Microbiota metabolite butyrate differentially regulates th1 and th17 cells’ Differentiation and function in induction of colitis. Inflammation Bowel Dis (2019) 25(9):1450–61. doi: 10.1093/ibd/izz046
63. van de Pavert SA, Ferreira M, Domingues RG, Ribeiro H, Molenaar R, Moreira-Santos L, et al. Maternal retinoids control type 3 innate lymphoid cells and set the offspring immunity. Nature. (2014) 508(7494):123–7. doi: 10.1038/nature13158
64. Grizotte-Lake M, Zhong G, Duncan K, Kirkwood J, Iyer N, Smolenski I, et al. Commensals suppress intestinal epithelial cell retinoic acid synthesis to regulate interleukin-22 activity and prevent microbial dysbiosis. Immunity. (2018) 49(6):1103–1115.e6. doi: 10.1016/j.immuni.2018.11.018
65. Miko E, Csaszar A, Bodis J, Kovacs K. The maternal–fetal gut microbiota axis: physiological changes, dietary influence, and modulation possibilities. Life. (2022) 12(3):424. doi: 10.3390/life12030424
66. Wu LL, Peng WH, Kuo WT, Huang CY, Ni YH, Lu KS, et al. Commensal bacterial endocytosis in epithelial cells is dependent on myosin light chain kinase–activated brush border fanning by interferon-γ. Am J Pathol (2014) 184(8):2260–74. doi: 10.1016/j.ajpath.2014.05.003
67. Bianco-Miotto T, Craig JM, Gasser YP, van Dijk SJ, Ozanne SE. Epigenetics and DOHaD: from basics to birth and beyond. J Dev Orig Health Dis (2017) 8(5):513–9. doi: 10.1017/S2040174417000733
68. Yates KB, Tonnerre P, Martin GE, Gerdemann U, Al Abosy R, Comstock DE, et al. Epigenetic scars of CD8+ T cell exhaustion persist after cure of chronic infection in humans. Nat Immunol (2021) 22(8):1020–9. doi: 10.1038/s41590-021-00979-1
69. Fawad JA, Luzader DH, Hanson GF, Moutinho TJ, McKinney CA, Mitchell PG, et al. Histone deacetylase inhibition by gut microbe-generated short-chain fatty acids entrains intestinal epithelial circadian rhythms. Gastroenterology. (2022) 163(5):1377–1390.e11. doi: 10.1053/j.gastro.2022.07.051
70. Morin A, McKennan CG, Pedersen CET, Stokholm J, Chawes BL, Malby Schoos AM, et al. Epigenetic landscape links upper airway microbiota in infancy with allergic rhinitis at 6 years of age. J Allergy Clin Immunol (2020) 146(6):1358–66. doi: 10.1016/j.jaci.2020.07.005
71. Vercelli D, Lynch SV. Interactions between host epigenetics and microbiota: Who does what to whom, when, and why? J Allergy Clin Immunol (2023) 151(6):1465–7. doi: 10.1016/j.jaci.2023.01.018
72. Thion MS, Low D, Silvin A, Chen J, Grisel P, Schulte-Schrepping J, et al. Microbiome influences prenatal and adult microglia in a sex-specific manner. Cell. (2018) 172(3):500–516.e16. doi: 10.1016/j.cell.2017.11.042
73. Xu C, Cao Z. Cardiometabolic diseases, total mortality, and benefits of adherence to a healthy lifestyle: a 13-year prospective UK Biobank study. J Transl Med (2022) 20(1):234. doi: 10.1186/s12967-022-03439-y
74. Ferretti P, Pasolli E, Tett A, Asnicar F, Gorfer V, Fedi S, et al. Mother-to-infant microbial transmission from different body sites shapes the developing infant gut microbiome. Cell Host Microbe (2018) 24(1):133–145.e5. doi: 10.1016/j.chom.2018.06.005
75. Ooi DSQ, Tan CPT, Tay MJY, Ong SG, Tham EH, Siah KTH, et al. Developmental Origins of Health and Disease: Impact of environmental dust exposure in modulating microbiome and its association with non-communicable diseases. J Dev Orig Health Dis (2020) 11(6):545–56. doi: 10.1017/S2040174420000549
76. Marzullo P, Di Renzo L, Pugliese G, De Siena M, Barrea L, Muscogiuri G, et al. From obesity through gut microbiota to cardiovascular diseases: a dangerous journey. Int J Obes Suppl. (2020) 10(1):35–49. doi: 10.1038/s41367-020-0017-1
77. Yan Q, Gu Y, Li X, Yang W, Jia L, Chen C, et al. Alterations of the gut microbiome in hypertension. Front Cell Infect Microbiol (2017) 7:381. doi: 10.3389/fcimb.2017.00381
78. Taveras EM, Rifas-Shiman SL, Belfort MB, Kleinman KP, Oken E, Gillman MW. Weight status in the first 6 months of life and obesity at 3 years of age. Pediatrics. (2009) 123(4):1177–83. doi: 10.1542/peds.2008-1149
79. Isolauri E, Salminen S, Rautava S. Early microbe contact and obesity risk: evidence of causality? J Pediatr Gastroenterol Nutr (2016) 63(1S):S3. doi: 10.1097/MPG.0000000000001220
80. Sitarik AR, Havstad SL, Johnson CC, Jones K, Levin AM, Lynch SV, et al. Association between cesarean delivery types and obesity in preadolescence. Int J Obes (2020) 44(10):2023–34. doi: 10.1038/s41366-020-00663-8
81. Olszak T, An D, Zeissig S, Vera MP, Richter J, Franke A, et al. Microbial exposure during early life has persistent effects on natural killer T cell function. Science. (2012) 336(6080):489–93. doi: 10.1126/science.1219328
82. Russell SL, Gold MJ, Hartmann M, Willing BP, Thorson L, Wlodarska M, et al. Early life antibiotic-driven changes in microbiota enhance susceptibility to allergic asthma. EMBO Rep (2012) 13(5):440–7. doi: 10.1038/embor.2012.32
83. Cahenzli J, Köller Y, Wyss M, Geuking MB, McCoy KD. Intestinal microbial diversity during early-life colonization shapes long-term igE levels. Cell Host Microbe (2013) 14(5):559–70. doi: 10.1016/j.chom.2013.10.004
Keywords: microbiota, dysbiosis, pregnancy, microbial metabolites, epigenome, fetal development
Citation: Ruiz-Triviño J, Álvarez D, Cadavid J. ÁP and Alvarez AM (2023) From gut to placenta: understanding how the maternal microbiome models life-long conditions. Front. Endocrinol. 14:1304727. doi: 10.3389/fendo.2023.1304727
Received: 29 September 2023; Accepted: 23 November 2023;
Published: 15 December 2023.
Edited by:
Alicia E. Damiano, University of Buenos Aires, ArgentinaReviewed by:
Ulrike Kemmerling, University of Chile, ChileRicardo E. Fretes, National University of Cordoba, Argentina
Copyright © 2023 Ruiz-Triviño, Álvarez, Cadavid J. and Alvarez. This is an open-access article distributed under the terms of the Creative Commons Attribution License (CC BY). The use, distribution or reproduction in other forums is permitted, provided the original author(s) and the copyright owner(s) are credited and that the original publication in this journal is cited, in accordance with accepted academic practice. No use, distribution or reproduction is permitted which does not comply with these terms.
*Correspondence: Angela M. Alvarez, YW5nZWxhLmFsdmFyZXpnQHVkZWEuZWR1LmNv