- 1Croucher Institute for Environmental Sciences, Department of Biology, Hong Kong Baptist University, Hong Kong, Hong Kong SAR, China
- 2State Key Laboratory in Environmental and Biological Analysis, Hong Kong Baptist University, Hong Kong, Hong Kong SAR, China
Introduction: Multiple factors can contribute to sub-fecundity, including genetics, lifestyle, and environmental contaminants. PFASs are characterized as “forever chemicals” due to their ubiquitous contamination and their persistence in the environment, wildlife, and humans. Numerous studies have demonstrated that PFAS exposure adversely affects multiple bodily functions, including liver metabolism and gonadal function. It is unclear, however, how the disruption of hepatic fatty acid metabolism affects testicular function.
Methods: In this study, male mice were administered 0.3 and 3 μg/g body weight of PFOS for 21 days.
Results: Our data showed that PFOS exposure caused hepatic steatosis, as evidenced by significant increases in triglyceride levels, expression of ATP-citrate lyase, and fatty acid synthase, as well as fasting insulin levels. PFOS perturbed the expression levels of hepatokines, of which fibroblast growth factor-21 (Fgf-21), leukocyte cell-derived chemotaxin-2 (Lect-2), and retinol-binding protein-4 (Rbp-4) were significantly reduced, whereas angiopoietin-like 4 (Angptl4) was noticeably increased. While Rbp-4 and Fgf-21 are known to contribute to spermatogenesis and testosterone synthesis. In PFOS-exposed groups, testicular ATP, and testosterone decreased significantly with a significant increase in the expression of peroxisome proliferator-activated receptor-coactivator 1α. Mass spectrophotometry imaging revealed the localization of PFOS in testes, along with significant increases in fatty acid metabolites. These included arachidonic acid, dihomo-α-linolenic acid, dihomo-γ-linolenic acid, oxidized ceramide, diacylglycerol, phosphatidylcholine, and phosphatidylethanolamine, which are associated with inflammation and post-testicular causes of infertility.
Discussion: This study revealed potential links between PFOS-elicited changes in hepatic metabolism and their impacts on testicular biology. This study provides insights into alternative targets elicited by PFOS that can be used to develop diagnostic and therapeutic strategies for improving testicular dysfunction.
Introduction
Lifestyle, environment, and genetic factors all contribute to sub-fecundity (1). Increasing evidence suggests that environmental chemical contaminants (e.g., heavy metals and anthropogenic chemicals) disrupt testicular physiology. Among different environmental chemical pollutants, per- and poly-fluoroalkyl substances (PFASs) are one of the prioritized family, known to perturb metabolic and reproductive health (2, 3). PFASs are coined as “forever chemicals” that contaminate public water systems, and linger in the environment, wildlife, and humans (4). The legacy PFAS, including PFOA, PFOS, PFNA, PFHxS share in common the lipophobic C-F chain and hydrophilic functional groups (5). PFASs are used in industrial and consumer products and have unique physicochemical properties, including heat and oil resistance, water repellence, and surfactant properties. They exhibited proteinophilic attraction toward albumin and various fatty acid binding proteins, resulting in their long biological half-lives and bioaccumulation in humans (6, 7). In recent decades, the direct effects of PFOS on testicular physiology have been studied in animal and cell culture models, revealing that it disrupted hormonal signaling in the testicles and perturbed the dynamics of tight junctional protein during spermatogenesis, which significantly affected males’ fertility and health (8–10). Even so, little is known about how the systemic impact of PFASs affects testicular function.
Over the past few years, patients seeking reproductive health care increasingly suffer from metabolic disorders, including obesity and insulin resistance (11–13). Since nutritional and hormonal factors influence energy metabolism and reproductive activity, to improve fertility rates, it is crucial to understand the underlying correlation of metabolic syndrome (14). The liver is a primary metabolic tissue that maintains energy and nutrient balance. Further, the liver metabolizes hormones and chemical contaminants, which may be degraded for excretion or bio-activated for more significant toxicity (15). Changes in metabolism affect hormone signals and nutrient flow, which can directly or indirectly affect gonadal function (16). One of the most notable effects of PFOS is the disruption of hepatic liver energy homeostasis, particularly fatty acid metabolism and the signaling of nuclear receptors (17–19). It is unclear how the disruption is related to the perturbing effect of testicular function. It was hypothesized that the disruption in fatty acid metabolism caused by PFASs could affect systemic energy metabolism and fatty acid metabolites in the testes. An integrated approach involving mass-spectrometry imaging, gene expression analysis, and biochemical testing were used in this study to investigate how PFAS affects the mammalian liver and testes. An analysis of the association between PFAS-induced metabolic perturbations and testicular dysfunction was conducted.
Materials and methods
Animals
Male CD-1 mice (8-10 weeks old) were kept in polypropylene cages at 23-24°C and 12 hours of light/dark cycle. The procedure for animal handling was followed according to guidelines and regulations approved by the animal ethics committee (REC/20-21/0234) of Hong Kong Baptist University. Perfluorooctane sulfonate (PFOS, CAS 1763-23-1, Sigma-Aldrich, 98% purity) was dissolved in dimethyl sulfoxide (Sigma-Aldrich) before mixing with corn oil. In the treatment regime, mice with body weights were randomly divided into three groups (control, low, or high-dose PFOS treatment groups) using a random number generator. The animals were provided access to food (LabDiet, 5001, Laboratory Rodent Diet) and water (in glass bottles). The exposed groups received either 0.3 or 3 μg/g of body weight (bw)/day of PFOS for 21 days by oral gavage (Cadence Science). Corn oil was administered to the control group. The low exposure dose is equivalent to human occupational exposure (20). Overnight fasting was performed on day 20. In the next morning, cervical dislocations were performed, and blood samples were drawn. Livers and testes were collected, snap-frozen in liquid nitrogen, and stored at -80°C.
RNA extraction and real-time quantitative PCR (qPCR)
Total RNA was extracted from tissue samples using TRIzol reagent (Invitrogen) according to the manufacturer’s instruction. RNA concentration and quality were measured by BioDrop (Biochrom) and then reverse transcribed to cDNA using SuperScript VILO cDNA Synthesis Kit (Applied Biosystems). Gene expression was analyzed by real-time PCR using Fast SYBR™ Green Master Mix (Applied Biosystems) with StepOnePlus PCR system (Life Technologies) using gene-specific primers (Supplementary Table 1A). Relative expression was calculated by normalizing to actin using the 2-ΔΔCt method. The specificity of the amplicon was verified using melting curve analysis and agarose gel electrophoresis.
Western blot
Tissues were homogenized in RIPA buffer (50 mM Tris-HCl, pH 7.4, 150 mM NaCl, 2 mM EDTA, 0.1% SDS, and 1% NP-40) containing Halt™ Protease and Phosphatase Inhibitor Cocktail (Thermo Fisher Scientific). Tissue homogenates were then sonicated for 8 sec, 5 cycles on ice, followed by centrifugation at 13000 xg, at 4°C for 15min. Protein concentration was determined using the DC Protein Assay Kit II (BioRad). Protein samples were separated by SDS-PAGE and transferred to a PVDF membrane (BioRad). Membranes were blocked with 5% non-fat milk in PBST for 1 hr at room temperature, incubated with primary antibody (Supplementary Table 1B) overnight at 4°C and then incubated with the HRP-conjugated secondary antibody (BioRad) for 1 hr at room temperature. SuperSignal™ West Pico PLUS chemiluminescent substrate (Thermo Scientific) was used to develop the signals.
Testosterone ELISA Kit, blood insulin, glucose and ATP determination
Adult male mouse were killed by cervical dislocation. Blood samples were collected and centrifuged at 1000 xg at 4°C for 10 min to collect sera, which were then stored at -80°C. Testosterone level in serum was measured using testosterone ELISA kit (Cayman Chemical). Briefly, 25 μl of serum sample or standard was mixed with 25 μl of testosterone AChE Tracer and 25 μl of testosterone ELISA antiserum in the mouse anti-rabbit IgG coated-well. The reaction mixture was discarded, and the wells were washed at room temperature on an orbital shaker (Thermo Fisher Scientific). Ellman’s Reagent (200μl) was added in each well and incubated for 90 minutes at room temperature in dark on the orbital shaker. Absorbance at 412 nm of each wells were measured using EnSight Multimode Plate Reader (PerkinElmer). The fasting blood glucose levels were measured using an Accu-check Glucometer (Roche, US). Serum was prepared by centrifugation of clotted blood at 1000 xg at 4°C for 10 min. Insulin level was measured with Ultrasensitive Insulin ELISA kit (10-1132-01, Mercodia, Sweden) according to the manufacturer’s instruction.
Testis samples were homogenized in luciferase cell culture lysis 5X reagent (Promega) and centrifuged at 13,000 ×g at 4°C for 15 min. The supernatants were collected for ATP measurement, using ATP Determination Kit (Invitrogen) according to the manufacturer’s instruction. Luminescence was measured by EnSight Multimode Plate Reader (PerkinElmer). ATP level was then normalized with the protein concentration of each sample using DC Protein Assay Kit II (BioRad).
AFADESI-mass spectrophotometry imaging
Testes were isolated from adult male mice and snap-frozen in liquid nitrogen. The testis was mounted on a cryostat specimen chunk (Thermo Fisher Scientific, U.S.) and sliced at 14 μm in thickness using the Cryostar NX70 (Thermo Fisher Scientific). AFADESI-MSI (air-flow assisted desorption electrospray ionization) was applied to a frozen section mounted on a microscopic slide (Citotest, Jiangsu, China). The images were analyzed using an Orbitrap Exploris™ 120 mass spectrometer (Thermo Fisher Scientific, Bremen, Germany) and the AFAI-MSI image platform (Viktor, Beijing, China). A negative ion mode analysis was performed, with signals ranging from 100-1000m/z at a resolution of 60000. A spray solvent mixture of acetonitrile and dimethylformamide (3:1, v/v), was applied at 2 ml/min, with sprayer voltages at -2500V. The X- and Y-direction scanning speeds were 430 mm/s and 150 mm/s. A 140-psi gas flow and 350°C ion transfer tube temperature were used. MSConvert (Nature Biotechnology Commentary) and imzMLConverter (Thermo Fisher Scientific, U.S.) were used to convert MS data to mzML and imzML formats. SCiLSTM Lab (Bremen, Germany) was used to visualize data. The sections fixed in 4% PFA were then stained with hematoxylin.
Statistical analysis
A statistical mean and standard deviation were used to present the data. The GraphPad Prism version 8.0 was used for statistical analyses. Students’-tests were used to evaluate the physiological and gene expression data. A p-value < 0.05 was considered statistically significant.
Results
The exposure regime revealed a significant increase of liver weights and relative liver weights at the high-dose (3μg/g) of PFOS exposure while there was no noticeable effect on the changes of body weights among the control and PFOS treatment groups (Figure 1A). Additionally, hepatic triglyceride levels were found to be significantly increased at the high-dose exposure (Figure 1B). To underpin the underlying process of PFOS-elicited perturbation to hepatic lipid metabolism, western blot analysis of key metabolic enzymes for lipogenesis were conducted. The data revealed significant upregulation in the expression levels of ATP citrate lyase (ACLY), acetyl CoA carboxylase (ACC), phosphorylated ACC, and fatty acid synthase (FASN) at the high-dose PFOS-exposed groups (Figure 1C). The expression of ACLY and the ratio of pACC to ACC (Supplementary Figure 1A) did not change significantly.
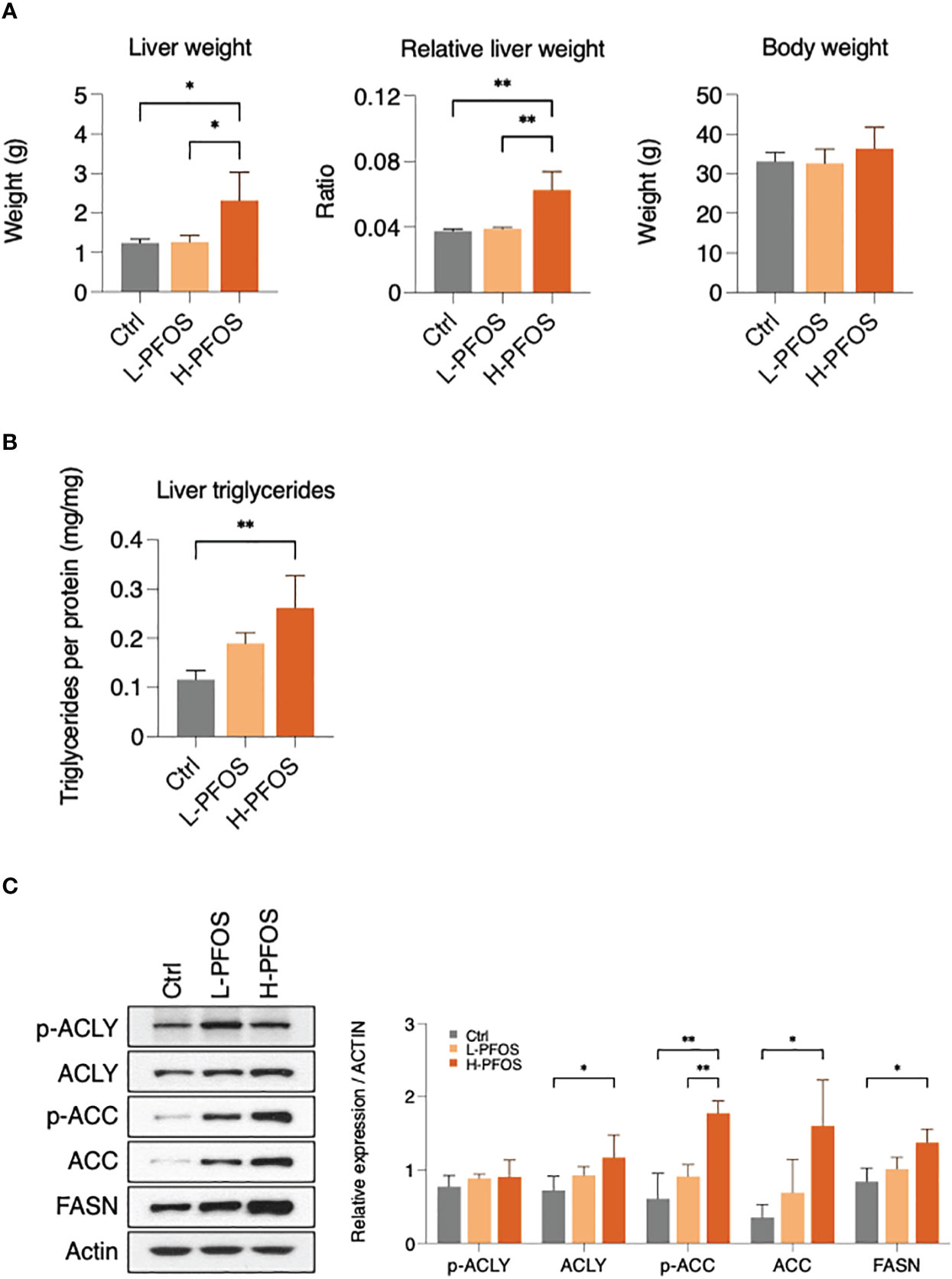
Figure 1 Effect of PFOS exposure on liver fat metabolism at day 21. Mice were administered 0.3 μg/g/day (L-PFOS) and 3 μg/g/day (H-PFOS) of PFOS by gavage for 21 days, and the liver was dissected and assessed. (A) The absolute and relative liver weights were significantly increased in H-PFOS mice compared to control and L-PFOS mice. (B) The triglyceride content was increased in H-PFOS compared to control, and (C) the protein expression of lipogenesis enzymes, including ATP citrate synthase (ACLY), phosphorylated ACLY (6X active), acetyl-CoA carboxylase (ACC), phosphorylated ACC (inactive form), and fatty acid synthase (FASN), were significantly upregulated in the PFOS-exposed groups. Actin served as the endogenous control. Graphs show the mean ± S.D. (*p<0.05, **p<0.01).
As the liver is the primary metabolic tissue, PFOS-induced disruptions in energy metabolism are likely to have a systemic effect. Fasting insulin and glucose levels were measured on day 21 of post-PFOS exposure. A significant increase in serum insulin levels was observed in the high-dose PFOS group, but no significant changes were observed in fasting blood glucose levels (Figure 2A). In western blot analysis, high-dose PFOS treatment significantly reduced hepatic expression levels of insulin receptor (IR) but not the insulin-like growth factor-1 receptor (IGF-1R) (Figure 2B). Considering the liver’s role in regulating systemic energy homeostasis, the expression levels of hepatokines, the major liver-to-tissue messengers that responds to perturbed energy metabolism, were investigated. Upon PFOS exposure, there was a dose-dependent reduction in the expression levels of the hepatokines, fibroblast growth factor-21 (Fgf-21), leukocyte cell derived chemotaxin-2 (Lect-2), retinol binding protein-4 (Rbp-4), but a significant induction of angiopoietin-like 4 (Angptl4) (Figure 2C). The expression levels of the other measured hepatokines, Angptl-3, Angptl-6, Selenop, and Smoc-1 showed no noticeable differences (Supplementary Figure 1B).
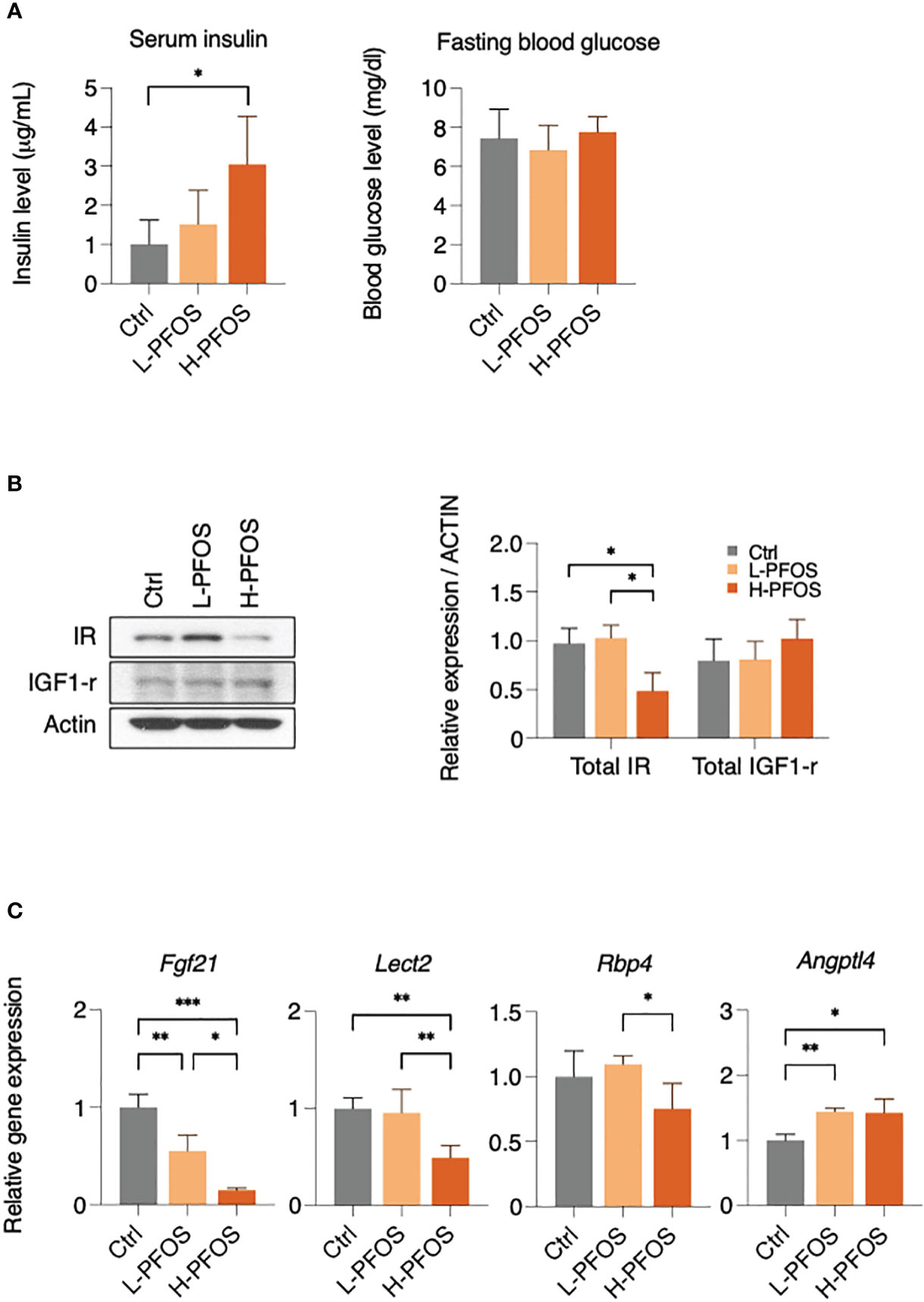
Figure 2 PFOS exposure perturb insulin signaling and expression of hepatokines in liver. (A) Serum insulin levels were significantly increased in H-PFOS compared to control, with no noticeable changes observed in fasting blood glucose levels. (B) The expression of insulin receptor (IR) was significantly downregulated. (C) A significant decrease in gene expression of the hepatokines, fibroblast growth factor 21 (Fgf-21) and leukocyte cell-derived chemotaxin 2 (Lect-2) were noted in PFOS-exposed groups, and a decrease of retinol binding protein 4 (RBP-4) was noted among L-PFOS and H-PFOS groups in livers. Conversely, the gene expression of angiopoietin-like 4 (Angptl-4) was increased in the H-PFOS compared to control. Actin served as the endogenous control. Graphs show the mean ± S.D. (*p<0.05, **p<0.01, ***p<0.001).
Due to PFOS’ effects on hepatic metabolism and serum insulin levels, it likely perturbed testicular functions. Western blot analysis showed that there were no significant changes in the expression levels of IR and phosphorylated IR in testes (Supplementary Figure 1C). However, PFOS-exposed groups showed a significant dose-dependent decrease of testicular ATP, associated with a significant increase in the expression of peroxisome proliferator-activated receptor γ coactivator 1α, Pgc1α (Figure 3A), a transcription coactivator in the regulation of cellular energy metabolism. Additionally, there was a significant reduction in testosterone levels in the high-dose PFOS exposed groups (Figure 3B, left panel). Yet, no significant change in testicular weight and epididymal sperm counts were noted (Figure 3B, right panel). Nonetheless, in testing the expression levels of endocrine and paracrine factors, there were no significant changes in the testicular gene expression of follicle-stimulating hormone receptor (Fshr) and luteinizing hormone receptor (Lhr) (Supplementary Figure 2A), growth hormone receptor (Ghr), insulin-like growth factor (Igf) and its receptor, Igfr (Supplementary Figure 2B), hepatocyte growth factor (Hgf) and its receptor, Hgf-r (Supplementary Figure 2C), and the steroidogenic enzymes (Star, Cyp11a1, Cyp17a1, Hsd-3β, & Hsd-17β) (Supplementary Figure 3) as compared with the control. Interesting, a significant reduction in the expression levels of Srd5α2 was noted in the high-dose group vs low-dose group.
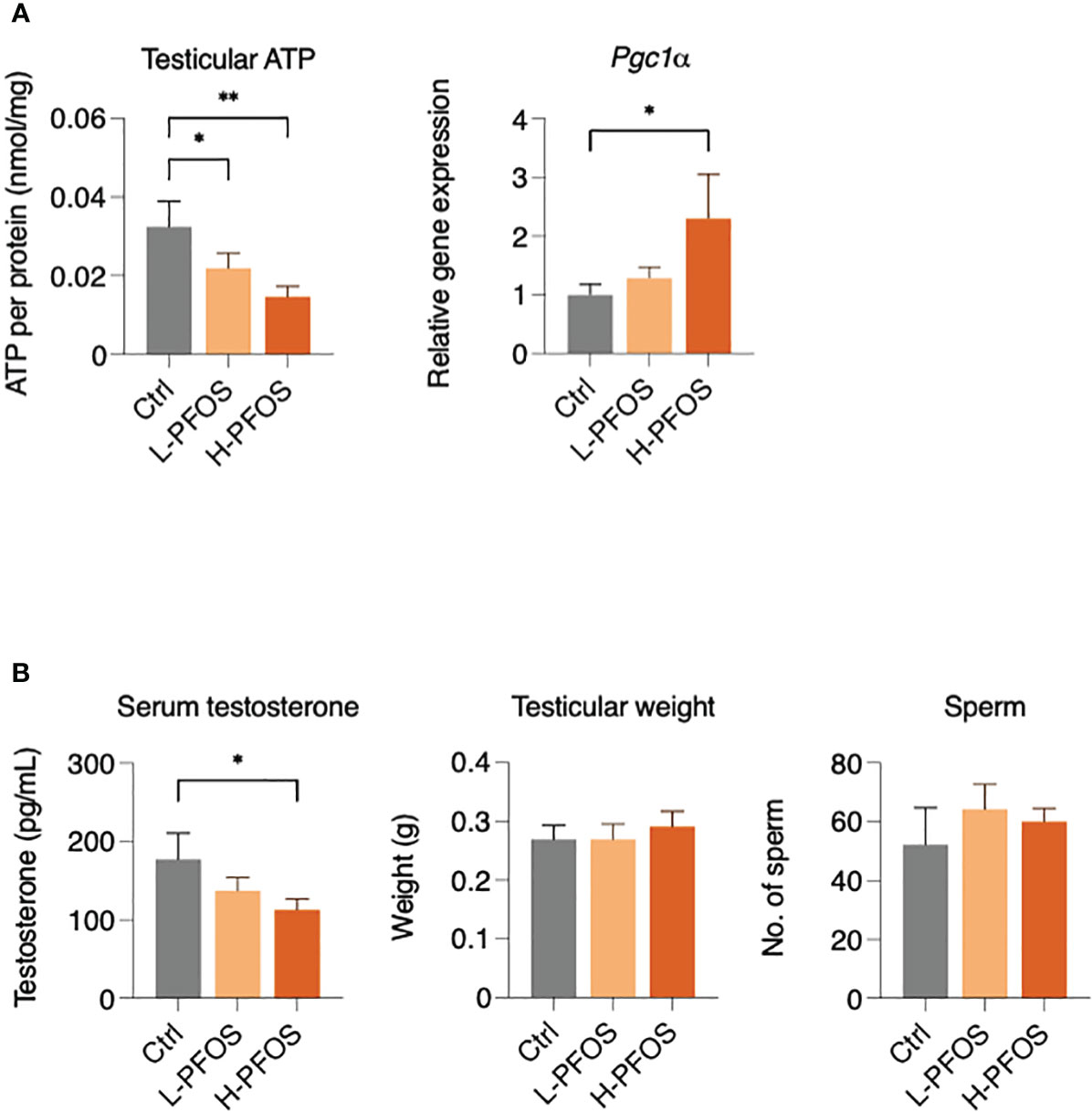
Figure 3 Impact of PFOS on testicular activity, energy metabolism and testosterone expression. (A) Testicular ATP levels were significantly decreased in PFOS-exposed groups. There was an increase in the gene expression of peroxisome proliferator-activated receptor gamma coactivator 1-α (Pgc1α) in H-PFOS groups compared to control. Actin served as the endogenous control. (B) Serum testosterone levels were significantly decreased in H-PFOS compared to control with no noticeable changes observed in testis weight and number of epididymal sperm counts. Graphs show the mean ± S.D. (*p<0.05, **p<0.01).
PFOS is known to perturb fatty acid metabolism via PPARs and fatty acid-mimicry pathways. To unravel the possible impact of PFOS on fatty acid metabolites in testes, we examined PFOS uptake and its perturbation to lipid profiles using MS-imaging. Figure 4A showed a significant increase of PFOS levels in the testes of low- and high-dose exposed mice. MS-imaging data identified significant increases in the levels of the poly-unsaturated fatty acids, eicosa-5, 8, 11-trienoic acid (ETA), eicosa-5, 11, 14-trienoic acid (arachidonic acid, AA), dihomo-α-linolenic acid (DALA), and dihomo-γ-linolenic acid (DGLA) (Figure 4B right panel, Supplementary Table 2), oxidized ceramide (CER) (Figure 4C; Supplementary Table 3), diacylglycerol (DAG) (Figure 4D; Supplementary Table 4), and the phospholipids (phosphatidylcholine & phosphatidylethanolamine) (Figure 4E; Supplementary Table 5). The ion signal of phosphatidylinositol, which is a common and stable component of cell membranes (Figure 4B, left panel), was used for data normalization.
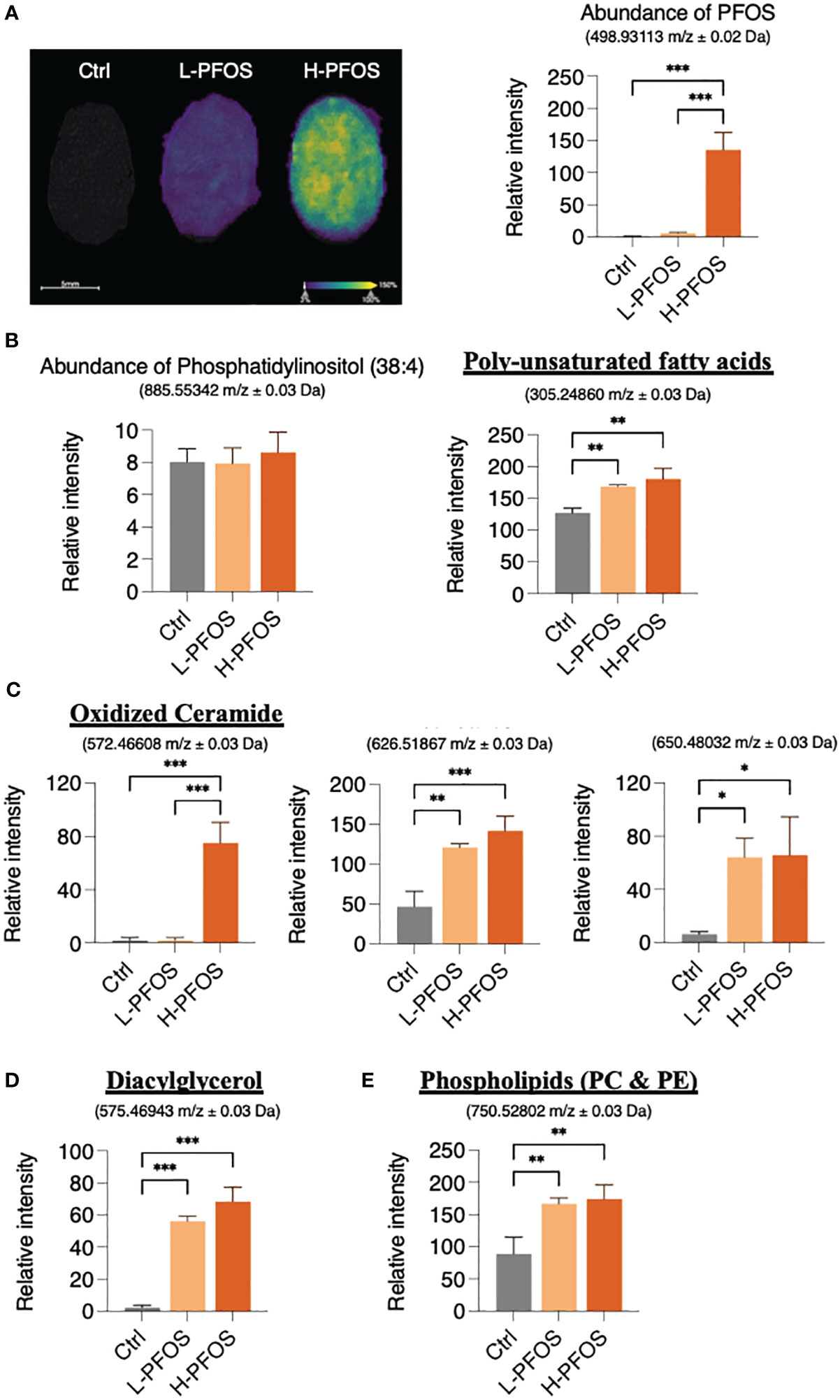
Figure 4 AFADESI-Mass Spectrophotometry Imaging of PFOS and metabolite distribution in testis. (A) Left panel: Ion image of PFOS distribution in testes with overlayed haematoxylin and eosin staining. Right panel: a corresponding graph shows the relative coneceantration of PFOS in the control, L-PFOS and H-PFOS samples. (B) Left panel: The levels of the phosphatidylinositol served for data normalization. Right panel: There was a significant increase in summated levels of the polyunsaturated fatty acids (eicosa-5, 8, 11-trienoic acid, eicosa-5, 11, 14-trienoic acid, dihomo-α-linolenic acid, dihomo-γ-linolenic acid), (C) oxidized ceramides (CER), (D) diacylglycerol (DAG) and (E) phosphatidylcholine (PC) & phosphatidylethanolamine (PE) in PFOS-exposed groups. Graphs show the mean ± S.D. (*p<0.05, **p<0.01, ***p<0.001).
Discussion
With hindsight, the effects of PFOS on both PPAR-dependent and PPAR-independent fatty acid mimicry pathways disrupt multiple metabolic pathways in multiple tissues, resulting in dyslipidemia, insulin resistance, and inflammation (21–24). This study showed that PFOS exposure at the high-dose (3μg/g b.w.) induced hepatic lipid accumulation which exhibited the early sign of non-alcoholic fatty liver disease (NAFLD). The observation is consistent with the previous studies, showing PFOS perturbed lipid metabolism and induced hepatotoxicity (22, 25). The hepatic liver accumulation was associated with the significant increase in the expression levels of ACLY and FASN. A combination of elevated expression of these enzyme activities promoted fatty acid synthesis from cytosolic acetyl-CoA. Despite this, the expression level of phosphorylated ACLY (pACLY) with reported 6-fold higher activity of ACLY (26) remained unchanged. The expression levels of both total ACC (active form) and pACC (inactive form) increased significantly with high-dose PFOS treatment. There was no significant change in the ratio of pACC to ACC, presumably no significant alteration in ACC activity. Nonetheless, it was unanticipated to see a significant increase in pACC with a functional outcome of hepatic lipogenesis in the group receiving high doses of PFOS. In retrospect, AMP-activated protein kinase (AMPK) is the major kinase known to phosphorylate ACC to promote fatty acid oxidation (27). In a recent study, PFOS-induced deranged hepatic metabolism stimulated both lipogenesis and lipid catabolism, as well as an activation of AMPK pathway (28). Therefore, in this study the observation of an increased phosphorylation of ACC might be associated with the dysregulation of hepatic metabolism induced by PFOS. The dysregulation was further demonstrated with a significant reduction in hepatic IR expression and a significant increase in blood insulin levels. This shows that PFOS perturbed insulin signaling in the liver and systemically.
Further illustrating the liver’s effect on bodily function, the secreted hepatic metabolic factors (hepatokines) were measured. In the PFOS-exposed groups, the expression levels of the four hepatokines (Fgf-21, Lect-2, Rbp-4, and Angptl4) were significantly altered. The four hepatokines were found to be associated with the progression of NAFLD (29–33). Apart from being a metabolic regulator, Fgf-21 was also found to play roles in promoting spermatogenesis, protecting germ cells from diabetes-induced apoptosis (34, 35), and increasing sperm motility (36). Lect-2 was linked to tissue inflammatory responses (37), coherent with the early sign of PFOS-elicited liver steatosis (22). Another perturbed hepatokine, Rbp-4 regulated the physiological functions of testosterone receptors in the testicles, including the production of testosterone and spermatogenesis (38). In obese adolescents, Rbp-4 expression was associated with gonadal functions (39). The decreased expression levels of Fgf-21 and Rbp4 imposed negative influence on testicular functions. In fact, fatty liver disease has also been linked to impaired testicular function (40, 41). There were reports indicating an association between NAFLD and low blood levels of testosterone, which caused accumulation of visceral adipose tissues, elevated free fatty acid levels, inflammation, and increased insulin resistance (42, 43). The observations suggest that systemic metabolism and gonadal function are mutually interdependent.
In testes, metabolic perturbation was evidenced with significant reduction in ATP levels and the increased expression of Pgc-1α, which was reported to protect against oxidative stress and energy metabolism dysfunction in the testes (44). Previous studies had shown that men with fatty liver disease exhibited lower levels of testosterone and sperm count (41, 45, 46). In this study, our data only showed the reduction of blood testosterone levels at the high-dose group. In our previous studies, a higher dose of PFOS exposure (5 μg/g b.w. for 21 days) in mice, caused significant reduction in testosterone levels, sperm count, and sperm swimming activities (8, 9, 47). To decipher the underlying process of the reduced testosterone levels in the high-dose group, the analysis of the steroidogenic enzymes did not reveal noticeable changes, except a significant reduction of Srd5α2 (steroid 5α-reductase 2, a membrane enzyme catalyzes testosterone to dihydrotestosterone). From a pharmacological perspective, an inhibition of Srd5α2 activity might be linked to an increase in serum testosterone levels (48). This may explain an increase in serum testosterone in the high-dose group of our study. In order to further investigate the impact of PFOS on testicular physiology, we focused on the notorious action of PFOS in perturbing fatty acid homeostasis, which may negatively impact testicular functions (49, 50).
The PFOS exposure increased the testicular levels of the poly-unsaturated fatty acids (ETA and AA), the important metabolites in regulating gonadal functions (51, 52). This observation is related to our previous study showing an increase of hydroxyeicosatetraenoic acids in neonatal testes upon PFOS exposure (49). Additionally, PFOS-induced an increase of the poly-unsaturated fatty acids (DGLA and DGLA), which were reported to be directly associated with the sign of tissue inflammation (53, 54). Moreover, our data showed a significant increase in oxidized ceramides and DAG, the important messengers for spermatogenesis, and apoptosis (55, 56). Furthermore, significant increases in total phospholipids, especially phosphatidylcholine, and phosphatidylethanolamine, were associated with the post-testicular causes of infertility (57). Overall, the data suggest that PFOS disrupted fatty acid metabolites’ homeostasis, altering the fatty acid signaling pathway in testes.
This study aimed to better understand the pleiotropic effects of PFOS on tissue functions. As shown by our data, PFOS affects hepatic lipid metabolism, hepatokine expression, blood insulin, testosterone levels, and testicular fatty acid metabolism. This study provided a mechanistic link between disrupted lipid metabolism and perturbed testicular physiology. These findings may help identify PFOS disregard targets and develop targeted interventions to restore and protect testicular function in mammals exposed to these pollutants. As a result of this study, we have gained a better understanding of the pathology, the molecular mechanisms, and the biochemical changes resulting from PFAS-induced testicular toxicity.
Data availability statement
The original contributions presented in the study are included in the article/Supplementary Material. Further inquiries can be directed to the corresponding authors.
Ethics statement
The procedure for animal handling was followed according to guidelines and regulations approved by the animal ethics committee (REC/20-21/0234) of Hong Kong Baptist University. The study was conducted in accordance with the local legislation and institutional requirements.
Author contributions
WL: Data curation, Formal Analysis, Investigation, Writing – review & editing. TL: Formal Analysis, Investigation, Methodology, Software, Writing – review & editing. HT: Investigation, Methodology, Writing – review & editing. TH: Data curation, Investigation, Writing – review & editing. HW: Conceptualization, Investigation, Methodology, Project administration, Supervision, Writing – review & editing. CW: Conceptualization, Funding acquisition, Supervision, Writing – original draft, Writing – review & editing.
Funding
The author(s) declare financial support was received for the research, authorship, and/or publication of this article. This work was supported by the Faculty-niche Research Fund (RC-FNRA-IG-20-21-SCI-01), and the research fund from the State Key Laboratory of Environmental and Biological Analysis, (SKLP_2324_P01) to CW (Hong Kong Baptist University), research fund from Shenzhen Science, Technology and innovation commission (SZSTI, 20180247).
Conflict of interest
The authors declare that the research was conducted in the absence of any commercial or financial relationships that could be construed as a potential conflict of interest.
The author(s) declared that they were an editorial board member of Frontiers, at the time of submission. This had no impact on the peer review process and the final decision.
Publisher’s note
All claims expressed in this article are solely those of the authors and do not necessarily represent those of their affiliated organizations, or those of the publisher, the editors and the reviewers. Any product that may be evaluated in this article, or claim that may be made by its manufacturer, is not guaranteed or endorsed by the publisher.
Supplementary material
The Supplementary Material for this article can be found online at: https://www.frontiersin.org/articles/10.3389/fendo.2023.1302965/full#supplementary-material
References
1. Szabo A, Vancsa S, Hegyi P, Varadi A, Forintos A, Filipov T, et al. Lifestyle-, environmental-, and additional health factors associated with an increased sperm DNA fragmentation: a systematic review and meta-analysis. Reprod Biol Endocrinol (2023) 21(1):5. doi: 10.1186/s12958-023-01054-0
2. Roth K, Petriello MC. Exposure to per- and polyfluoroalkyl substances (PFAS) and type 2 diabetes risk. Front Endocrinol (Lausanne) (2022) 13:965384. doi: 10.3389/fendo.2022.965384
3. Sun Z, Wen Y, Wang B, Deng S, Zhang F, Fu Z, et al. Toxic effects of per- and polyfluoroalkyl substances on sperm: Epidemiological and experimental evidence. Front Endocrinol (Lausanne) (2023) 14:1114463. doi: 10.3389/fendo.2023.1114463
4. Braun JM. Enhancing regulations to reduce exposure to P. N Engl J Med (2023) 388(21):1924–6. doi: 10.1056/NEJMp2303333
5. Shen Y, Wang L, Ding Y, Liu S, Li Y, Zhou Z, et al. Trends in the analysis and exploration of per- and polyfluoroalkyl substances (PFAS) in environmental matrices: A review. Crit Rev Anal Chem (2023), 1–25. doi: 10.1080/10408347.2023.2231535
6. Forsthuber M, Kaiser AM, Granitzer S, Hassl I, Hengstschlager M, Stangl H, et al. Albumin is the major carrier protein for PFOS, PFOA, PFHxS, PFNA and PFDA in human plasma. Environ Int (2020) 137:105324. doi: 10.1016/j.envint.2019.105324
7. Khazaee M, Christie E, Cheng W, Michalsen M, Field J, Ng C. Perfluoroalkyl Acid Binding with Peroxisome Proliferator-Activated Receptors alpha, gamma, and delta, and Fatty Acid Binding Proteins by Equilibrium Dialysis with a Comparison of Methods. Toxics (2021) 9(3):45. doi: 10.3390/toxics9030045
8. Wan HT, Zhao YG, Wong MH, Lee KF, Yeung WS, Giesy JP, et al. Testicular signaling is the potential target of perfluorooctanesulfonate-mediated subfertility in male mice. Biol Reprod (2011) 84(5):1016–23. doi: 10.1095/biolreprod.110.089219
9. Wan HT, Mruk DD, Wong CK, Cheng CY. The apical ES-BTB-BM functional axis is an emerging target for toxicant-induced infertility. Trends Mol Med (2013) 19(7):396–405. doi: 10.1016/j.molmed.2013.03.006
10. Wan HT, Mruk DD, Wong CK, Cheng CY. Perfluorooctanesulfonate (PFOS) perturbs male rat Sertoli cell blood-testis barrier function by affecting F-actin organization via p-FAK-Tyr(407): an in vitro study. Endocrinology (2014) 155(1):249–62. doi: 10.1210/en.2013-1657
11. Westerman R, Kuhnt AK. Metabolic risk factors and fertility disorders: A narrative review of the female perspective. Reprod BioMed Soc Online (2022) 14:66–74. doi: 10.1016/j.rbms.2021.09.002
12. Darand M, Salimi Z, Ghorbani M, Sadeghi N, Babaie S, Hosseinzadeh M. Obesity is associated with quality of sperm parameters in men with infertility: a cross-sectional study. Reprod Health (2023) 20(1):134. doi: 10.1186/s12978-023-01664-2
13. Lakoma K, Kukharuk O, Sliz D. The influence of metabolic factors and diet on fertility. Nutrients (2023) 15(5):1180. doi: 10.3390/nu15051180
14. Arya S, Hansen KR, Peck JD, Wild RA, National Institute of Child, H, Human Development Reproductive Medicine, N. Metabolic syndrome in obesity: treatment success and adverse pregnancy outcomes with ovulation induction in polycystic ovary syndrome. Am J Obstet Gynecol (2021) 225(3):280 e281–280 e211. doi: 10.1016/j.ajog.2021.03.048
15. Gu X, Manautou JE. Molecular mechanisms underlying chemical liver injury. Expert Rev Mol Med (2012) 14:e4. doi: 10.1017/S1462399411002110
16. Rak A, Mellouk N, Froment P, Dupont J. Adiponectin and resistin: potential metabolic signals affecting hypothalamo-pituitary gonadal axis in females and males of different species. Reproduction (2017) 153(6):R215–26. doi: 10.1530/REP-17-0002
17. Bjork JA, Butenhoff JL, Wallace KB. Multiplicity of nuclear receptor activation by PFOA and PFOS in primary human and rodent hepatocytes. Toxicology (2011) 288(1-3):8–17. doi: 10.1016/j.tox.2011.06.012
18. Sen P, Qadri S, Luukkonen PK, Ragnarsdottir O, McGlinchey A, Jantti S, et al. Exposure to environmental contaminants is associated with altered hepatic lipid metabolism in non-alcoholic fatty liver disease. J Hepatol (2022) 76(2):283–93. doi: 10.1016/j.jhep.2021.09.039
19. Taibl KR, Dunlop AL, Barr DB, Li YY, Eick SM, Kannan K, et al. Newborn metabolomic signatures of maternal per- and polyfluoroalkyl substance exposure and reduced length of gestation. Nat Commun (2023) 14(1):3120. doi: 10.1038/s41467-023-38710-3
20. Shi F, Almerick TB, Wan HT, Chan TF, Zhang EL, Lai KP, et al. Hepatic metabolism gene expression and gut microbes in offspring, subjected to in-utero PFOS exposure and postnatal diet challenges. Chemosphere (2022) 308(Pt 1):136196. doi: 10.1016/j.chemosphere.2022.136196
21. Abbott BD, Wolf CJ, Schmid JE, Das KP, Zehr RD, Helfant L, et al. Perfluorooctanoic acid induced developmental toxicity in the mouse is dependent on expression of peroxisome proliferator activated receptor-alpha. Toxicol Sci (2007) 98(2):571–81. doi: 10.1093/toxsci/kfm110
22. Wan HT, Zhao YG, Wei X, Hui KY, Giesy JP, Wong CK. PFOS-induced hepatic steatosis, the mechanistic actions on beta-oxidation and lipid transport. Biochim Biophys Acta (2012) 1820(7):1092–101. doi: 10.1016/j.bbagen.2012.03.010
23. Zhang L, Ren XM, Guo LH. Structure-based investigation on the interaction of perfluorinated compounds with human liver fatty acid binding protein. Environ Sci Technol (2013) 47(19):11293–301. doi: 10.1021/es4026722
24. Behr AC, Plinsch C, Braeuning A, Buhrke T. Activation of human nuclear receptors by perfluoroalkylated substances (PFAS). Toxicol In Vitro (2020) 62:104700. doi: 10.1016/j.tiv.2019.104700
25. Costello E, Rock S, Stratakis N, Eckel SP, Walker DI, Valvi D, et al. Exposure to per- and polyfluoroalkyl substances and markers of liver injury: A systematic review and meta-analysis. Environ Health Perspect (2022) 130(4):46001. doi: 10.1289/EHP10092
26. Potapova IA, El-Maghrabi MR, Doronin SV, Benjamin WB. Phosphorylation of recombinant human ATP:citrate lyase by cAMP-dependent protein kinase abolishes homotropic allosteric regulation of the enzyme by citrate and increases the enzyme activity. Allosteric activation of ATP:citrate lyase by phosphorylated sugars. Biochemistry (2000) 39(5):1169–79. doi: 10.1021/bi992159y
27. Galic S, Loh K, Murray-Segal L, Steinberg GR, Andrews ZB, Kemp BE. AMPK signaling to acetyl-CoA carboxylase is required for fasting- and cold-induced appetite but not thermogenesis. Elife (2018) 7:e32656. doi: 10.7554/eLife.32656
28. Ho TC, Wan HT, Lee WK, Lam TKY, Lin X, Chan TF, et al. Effects of in utero PFOS exposure on epigenetics and metabolism in mouse fetal livers. Environ Sci Technol (2023) 57(40):14892–903. doi: 10.1021/acs.est.3c05207
29. Chen X, Shen T, Li Q, Chen X, Li Y, Li D, et al. Retinol Binding Protein-4 Levels and Non-alcoholic Fatty Liver Disease: A community-based cross-sectional study. Sci Rep (2017) 7:45100. doi: 10.1038/srep45100
30. Yoo HJ, Hwang SY, Choi JH, Lee HJ, Chung HS, Seo JA, et al. Association of leukocyte cell-derived chemotaxin 2 (LECT2) with NAFLD, metabolic syndrome, and atherosclerosis. PloS One (2017) 12(4):e0174717. doi: 10.1371/journal.pone.0174717
31. Polyzos SA, Kountouras J, Mavrouli M, Katsinelos P, Doulberis M, Gavana E, et al. Selenoprotein P in patients with nonalcoholic fatty liver disease. Exp Clin Endocrinol Diabetes (2019) 127(9):598–602. doi: 10.1055/a-0811-9136
32. Praktiknjo M, Djayadi N, Mohr R, Schierwagen R, Bischoff J, Dold L, et al. Fibroblast growth factor 21 is independently associated with severe hepatic steatosis in non-obese HIV-infected patients. Liver Int (2019) 39(8):1514–20. doi: 10.1111/liv.14107
33. Singh AK, Chaube B, Zhang X, Sun J, Citrin KM, Canfran-Duque A, et al. Hepatocyte-specific suppression of ANGPTL4 improves obesity-associated diabetes and mitigates atherosclerosis in mice. J Clin Invest (2021) 131(17):e140989. doi: 10.1172/JCI140989
34. Jiang X, Zhang C, Xin Y, Huang Z, Tan Y, Huang Y, et al. Protective effect of FGF21 on type 1 diabetes-induced testicular apoptotic cell death probably via both mitochondrial- and endoplasmic reticulum stress-dependent pathways in the mouse model. Toxicol Lett (2013) 219(1):65–76. doi: 10.1016/j.toxlet.2013.02.022
35. Jiang X, Chen J, Zhang C, Zhang Z, Tan Y, Feng W, et al. The protective effect of FGF21 on diabetes-induced male germ cell apoptosis is associated with up-regulated testicular AKT and AMPK/Sirt1/PGC-1alpha signaling. Endocrinology (2015) 156(3):1156–70. doi: 10.1210/en.2014-1619
36. Bourdon G, Estienne A, Chevaleyre C, Rame C, Guerif F, Brun JS, et al. The hepatokine FGF21 increases the human spermatozoa motility. Front Endocrinol (Lausanne) (2022) 13:775650. doi: 10.3389/fendo.2022.775650
37. Takata N, Ishii KA, Takayama H, Nagashimada M, Kamoshita K, Tanaka T, et al. LECT2 as a hepatokine links liver steatosis to inflammation via activating tissue macrophages in NASH. Sci Rep (2021) 11(1):555. doi: 10.1038/s41598-020-80689-0
38. Wang Q, Zhang Q, Li Y, Zhao X, Zhang Y. RBP4 regulates androgen receptor expression and steroid synthesis in Sertoli cells from Bactrian camels. Reprod Domest Anim (2022) 57(4):429–37. doi: 10.1111/rda.14081
39. Condorelli RA, Calogero AE, Vicari E, Mongioi L, Favilla V, Morgia G, et al. The gonadal function in obese adolescents: review. J Endocrinol Invest (2014) 37(12):1133–42. doi: 10.1007/s40618-014-0107-4
40. Lopez-Lemus UA, Garza-Guajardo R, Barboza-Quintana O, Rodriguez-Hernandez A, Garcia-Rivera A, Madrigal-Perez VM, et al. Association between nonalcoholic fatty liver disease and severe male reproductive organ impairment (Germinal epithelial loss): study on a mouse model and on human patients. Am J Mens Health (2018) 12(3):639–48. doi: 10.1177/1557988318763631
41. Hawksworth DJ, Burnett AL. Nonalcoholic fatty liver disease, male sexual dysfunction, and infertility: common links, common problems. Sex Med Rev (2020) 8(2):274–85. doi: 10.1016/j.sxmr.2019.01.002
42. Wang C, Jackson G, Jones TH, Matsumoto AM, Nehra A, Perelman MA, et al. Low testosterone associated with obesity and the metabolic syndrome contributes to sexual dysfunction and cardiovascular disease risk in men with type 2 diabetes. Diabetes Care (2011) 34(7):1669–75. doi: 10.2337/dc10-2339
43. Mody A, White D, Kanwal F, Garcia JM. Relevance of low testosterone to non-alcoholic fatty liver disease. Cardiovasc Endocrinol (2015) 4(3):83–9. doi: 10.1097/XCE.0000000000000057
44. Liu X, Ye J, Wang L, Li Z, Zhang Y, Sun J, et al. Protective effects of PGC-1alpha against lead-induced oxidative stress and energy metabolism dysfunction in testis sertoli cells. Biol Trace Elem Res (2017) 175(2):440–8. doi: 10.1007/s12011-016-0799-8
45. Li Y, Liu L, Wang B, Chen D, Wang J. Nonalcoholic fatty liver disease and alteration in semen quality and reproductive hormones. Eur J Gastroenterol Hepatol (2015) 27(9):1069–73. doi: 10.1097/MEG.0000000000000408
46. Jaruvongvanich V, Sanguankeo A, Riangwiwat T, Upala S. Testosterone, sex hormone-binding globulin and nonalcoholic fatty liver disease: a systematic review and meta-analysis. Ann Hepatol (2017) 16(3):382–94. doi: 10.5604/01.3001.0009.8593
47. Li Z, Lin Z, Ji S, Lai KP, Wan HT, Wong CKC, et al. Perfluorooctanesulfonic acid exposure altered hypothalamic metabolism and disturbed male fecundity. Sci Total Environ (2022) 844:156881. doi: 10.1016/j.scitotenv.2022.156881
48. Traish AM, Krakowsky Y, Doros G, Morgentaler A. Do 5alpha-reductase inhibitors raise circulating serum testosterone levels? A comprehensive review and meta-analysis to explaining paradoxical results. Sex Med Rev (2019) 7(1):95–114. doi: 10.1016/j.sxmr.2018.06.002
49. Lai KP, Lee JC, Wan HT, Li JW, Wong AY, Chan TF, et al. Effects of in utero PFOS exposure on transcriptome, lipidome, and function of mouse testis. Environ Sci Technol (2017) 51(15):8782–94. doi: 10.1021/acs.est.7b02102
50. Varma S, Molangiri A, Kona SR, Ibrahim A, Duttaroy AK, Basak S. Fetal exposure to endocrine disrupting-bisphenol A (BPA) alters testicular fatty acid metabolism in the adult offspring: relevance to sperm maturation and quality. Int J Mol Sci (2023) 24(4):3769. doi: 10.3390/ijms24043769
51. Coniglio JG, Buch D, Grogan WM. Effect of eicosa-5, 8, 11, 14-tetraynoic acid on fatty acid composition of selected organs in the rat. Lipids (1976) 11(2):143–7. doi: 10.1007/BF02532664
52. Suh M, Merrells KJ, Dick A, Taylor CG. Testes of obese rats are highly responsive to n-3 long-chain fatty acids. Br J Nutr (2011) 106(7):1005–12. doi: 10.1017/S0007114511001292
53. Perreault M, Roke K, Badawi A, Nielsen DE, Abdelmagid SA, El-Sohemy A, et al. Plasma levels of 14:0, 16:0, 16:1n-7, and 20:3n-6 are positively associated, but 18:0 and 18:2n-6 are inversely associated with markers of inflammation in young healthy adults. Lipids (2014) 49(3):255–63. doi: 10.1007/s11745-013-3874-3
54. Mustonen AM, Nieminen P. Dihomo-gamma-linolenic acid (20:3n-6)-metabolism, derivatives, and potential significance in chronic inflammation. Int J Mol Sci (2023) 24(3):2116. doi: 10.3390/ijms24032116
55. Futerman AH, Hannun YA. The complex life of simple sphingolipids. EMBO Rep (2004) 5(8):777–82. doi: 10.1038/sj.embor.7400208
56. Furland NE, Zanetti SR, Oresti GM, Maldonado EN, Aveldano MI. Ceramides and sphingomyelins with high proportions of very long-chain polyunsaturated fatty acids in mammalian germ cells. J Biol Chem (2007) 282(25):18141–50. doi: 10.1074/jbc.M700708200
Keywords: mass spectrophotometry imaging, hepatokines, lipogenesis, testosterone, fecundity
Citation: Lee WK, Lam TKY, Tang HC, Ho TC, Wan HT and Wong CKC (2023) PFOS-elicited metabolic perturbation in liver and fatty acid metabolites in testis of adult mice. Front. Endocrinol. 14:1302965. doi: 10.3389/fendo.2023.1302965
Received: 27 September 2023; Accepted: 02 November 2023;
Published: 22 November 2023.
Edited by:
Xiang Xiao, Hangzhou Medical College, ChinaReviewed by:
Huitao Li, Second Affiliated Hospital and Yuying Children’s Hospital of Wenzhou Medical University, ChinaAlessandra Santillo, University of Campania Luigi Vanvitelli, Italy
Copyright © 2023 Lee, Lam, Tang, Ho, Wan and Wong. This is an open-access article distributed under the terms of the Creative Commons Attribution License (CC BY). The use, distribution or reproduction in other forums is permitted, provided the original author(s) and the copyright owner(s) are credited and that the original publication in this journal is cited, in accordance with accepted academic practice. No use, distribution or reproduction is permitted which does not comply with these terms.
*Correspondence: Hin Ting Wan, d2FuaGludGluZ0Boa2J1LmVkdS5oaw==; Chris Kong Chu Wong, Y2tjd29uZ0Boa2J1LmVkdS5oaw==