- 1China Japan Friendship Hospital (Institute of Clinical Medical Sciences), Chinese Academy of Medical Sciences & Peking Union Medical College, Beijing, China
- 2Department of Orthopaedic Surgery, China Japan Friendship Hospital, Beijing, China
Bone health encompasses not only bone mineral density but also bone architecture and mechanical properties that can impact bone strength. While specific dietary interventions have been proposed to treat various diseases such as obesity and diabetes, their effects on bone health remain unclear. The aim of this review is to examine literature published in the past decade, summarize the effects of currently popular diets on bone health, elucidate underlying mechanisms, and provide solutions to neutralize the side effects. The diets discussed in this review include a ketogenic diet (KD), a Mediterranean diet (MD), caloric restriction (CR), a high-protein diet (HP), and intermittent fasting (IF). Although detrimental effects on bone health have been noticed in the KD and CR diets, it is still controversial, while the MD and HP diets have shown protective effects, and the effects of IF diets are still uncertain. The mechanism of these effects and the attenuation methods have gained attention and have been discussed in recent years: the KD diet interrupts energy balance and calcium metabolism, which reduces bone quality. Ginsenoside-Rb2, metformin, and simvastatin have been shown to attenuate bone loss during KD. The CR diet influences energy imbalance, glucocorticoid levels, and adipose tissue, causing bone loss. Adequate vitamin D and calcium supplementation and exercise training can attenuate these effects. The olive oil in the MD may be an effective component that protects bone health. HP diets also have components that protect bone health, but their mechanism requires further investigation. In IF, animal studies have shown detrimental effects on bone health, while human studies have not. Therefore, the effects of diets on bone health vary accordingly.
1 Introduction
Diet is an indispensable component of our daily life, and its impact on the human body has been the subject of extensive research. Over the years, different dietary interventions have been considered as lifestyle interventions that can prevent or treat various diseases such as obesity, cardiovascular disease, epilepsy, and metabolic diseases (1–4). Nutrients participate in every physiological process, regulate metabolism, and play critical roles in each system of the human body, including the skeletal system (5). Various types of diets have different effects on bone health. In this review, we aim to summarize the influences and potential mechanisms of several currently popular diets on bone health, based on both animal and human studies. These diets include the ketogenic diet (KD), the Mediterranean diet (MD), caloric restriction (CR), a high-protein diet (HP), and intermittent fasting (IF). Information about these diets can be found in Table 1.
2 Method
In this review, we conducted a search of Web of Science’s core database from January 2012 to November 2022, to identify published articles about the effect of different kinds of diets on bone health. The topics were utilized when searching included “ketogenic diet”, “Mediterranean diet”, “caloric restriction”, “high-protein diet”, and “intermittent fasting”, combined with “bone” or “calcium”. All relevant randomized controlled trials (RCTs), observational studies, and reviews were screened and integrated. Case studies, letters, and conference papers or reports were excluded. Table 2 summarizes most of the cited human studies and Table 3 summarizes most of the cited animal studies.
3 Ketogenic diets and bone health
3.1 The definition of ketogenic diets
KDs are characterized by a low intake of carbohydrates and a normal to high intake of fat, leading to increased utilization of ketones or fats in the body, similar to changes that occur during periods of starvation. Typically, these diets recommend that only 5% of calories come from carbohydrates, while 75% come from fats and 20% from protein, though the total calorie intake and ratio of energy sources can be adjusted based on individual needs.
3.2 Effects of ketogenic diets on bone health
3.2.1 Evidence from animal studies
Most studies have predominantly shown that the KD has an unfavorable effect on bone health. In mice, Wu et al. used the Micro-CT technique and a three-point bending test to assess the bone quality of 8-week-old mice fed a 4:1 KD for 12 weeks. The results indicated that both the cancellous and cortical bone architecture of long bones were compromised (6). A further study on the vertebrae also found a decrease in bone quality (54). Aikawa et al. researched the skeletal systems of aged mice that underwent exercise training and were fed a KD during the experiment, reporting that KD impaired bone mass, trabecular microstructure, and compromised the benefits regarding bone health after exercise (7). Zengin et al. found that a 4-week consumption of an “Atkin-style” KD diet or low protein KD could impair the bone quality of adult male rats. Their femur trabecular bone volume was relatively low, while this effect was not seen in female rats (8). Ding et al. reported that the bone loss was more significant in the appendicular rather than axial bone of rats fed a 3:1 KD (9). Meanwhile, Liu et al. found that a KD can delay the spinal fusion of rats after surgery (10) and confirmed that the microstructures and properties of cancellous bone deteriorated as a result of the interrupted balance of bone resorption and formation (55). Rats fed with KD had significantly lower alkaline phosphatase (ALP) activity and higher tartrate-resistant acid phosphatase (TRAP) activity, and the osteogenic ability of their bone marrow stromal cells was also found to be impaired (56). By measuring TRAP, collagen type I (CoLI), and osteocalcin (OCN) staining, mice fed a KD were found to have upregulated osteoclast activities. When combined with ovariectomy, the osteoblast activities were found to be downregulated (6).
Attention has been drawn to how to relieve the side effect of KD on bone health. Liu et al. found that the bone quality loss induced by KD can be relieved by ginsenoside-Rb2, which inhibits bone resorption and osteogenic differentiation. Metformin can also reduce bone loss by enhancing osteoblast proliferation and inhibiting osteoclast differentiation (11, 57). Zhou et al. demonstrated the protective effect of simvastatin on the bones of mice that were compromised by KD, and the mechanism may be the facilitation of osteoblast differentiation and inhibition of osteoclast differentiation (58). Previous studies have reported that simvastatin can induce the expression of bone morphogenetic protein (BMP)-2, which improves bone formation (59).
3.2.2 Evidence from human studies
3.2.2.1 KD in epileptic children
The alteration of bone health in children treated with KD has been studied since the 1970s. Five epileptic children treated with KD therapy were reported to have disordered mineral metabolism, and their bone mass and serum 25-OHD levels were found to be decreased compared to the normal control (25). In another observational study, researchers investigated the bone health of 29 epileptic children aged 0.5–6.5 years who persisted with a KD for at least 6 months. After measuring with dual-energy x-ray absorptiometry (DXA), they reported a decrease of 0.16 units of bone mineral density Z score per year relative to age-matched children (26). In terms of the Modified Atkins Diet (MAD), the intake of protein is not restricted and the KD ratio is 1:1–2:1. A recent study reported that a 24-month MAD did not significantly affect the bone mass and height of children who were diagnosed with intractable epilepsy, glucose transporter type 1 deficiency syndrome, or pyruvate dehydrogenase complex deficiency (27). Most notably, the causes of damaged bone mineral status in epileptic children can also include medication side effects, seizures, and mobile ability (60); thus, more high-level evidence is required to determine the extent of how much KD is to blame for impaired bone growth in epileptic children.
3.2.2.2 KD in adults
The recent applications of the KD in adults were mainly focused on the treatment of metabolic diseases including obesity, diabetes, and glucose transporter 1 deficiency syndrome (GLUT-1 DS). Regarding obesity, a study observed 20 adult obese patients who were treated with the KD for 4 months, and with the measurement of DXA, both their bone mineral content (BMC) and bone mineral density (BMD) were statistically unchanged (28). In terms of diabetes, a study enrolled 262 type2 diabetes patients who were treated with a low-carbonate KD to achieve and sustain nutrition ketosis (blood BHB level of 0.5–3.0 mmol/L). It was found that their spine BMD remained stable from baseline to a 2-year follow-up (29). In regard to GTUT-1 DS, the alteration of bone mass of three adult patients who were treated with normocaloric 3:1 KD for 5 years was observed, and the BMD of all three patients decreased in the first 3 years and remained stable thereafter. At the 5-year follow-up, all patients’ BMDs were in the normal range (30). In a study with healthy participants, 21 adult resistance-trained women were randomly assigned to a non-KD or low-carbohydrate KD group for 8 weeks. The results revealed that the KD group displayed a significant increase in BMD after 8 weeks, while the NKD group showed no significant change. However, no statistical significance was found between the 2 groups (31). In recent years, most studies on adults proved that a KD could improve disease conditions and reduce harm to bone health. However, detrimental effects on bone quality especially in children should be given great consideration. Additionally, because the proportion and type of fat in KD were not always recorded and controlled in current studies, and the duration of KD intervention varied among studies, more high-level studies with standardized study methods and large sample sizes are necessary to reach a final judgement.
Current research on the KD indicates that its effects on bone metabolism vary among different age groups and genders. In terms of obese patients, Carter et al. compared 15 obese patients who underwent KD treatment with another 15 matched obese patients without diet intervention for 3 months. No significant difference was found in the comparison of their bone-specific alkaline phosphatase (BSAP) and urinary cross-linked N-telopeptides of type I collagen (UNTx), indicating a negative effect on bone turnover rate in obese patients (32). As to the world-class athletes who underwent a short-term KD for 3.5 weeks, bone resorption markers (cross-linked C-terminal telopeptide of type I collagen, CTX) increased, while the bone formation marker (procollagen 1 N-terminal propeptide, P1NP) decreased (33). Since bone is the major reservoir of calcium, calcium metabolism can provide another perspective on how a KD affects bone metabolism. Current studies have revealed that a KD could decrease calcium digestibility, release calcium from bone to blood, and promote abnormal excretion of calcium. Hawkes et al. observed cases of epileptic children who were treated with KD therapy and then expanded the research into a multi-center study. In general, it was found that children developed hypercalcemia after an average of 2.1 years. Furthermore, moderately elevated urinary calcium excretion, and low levels of serum alkaline phosphatase, PTH, and 1,25-dihydroxyvitamin D were also noticed (Figure 1) (61, 62).
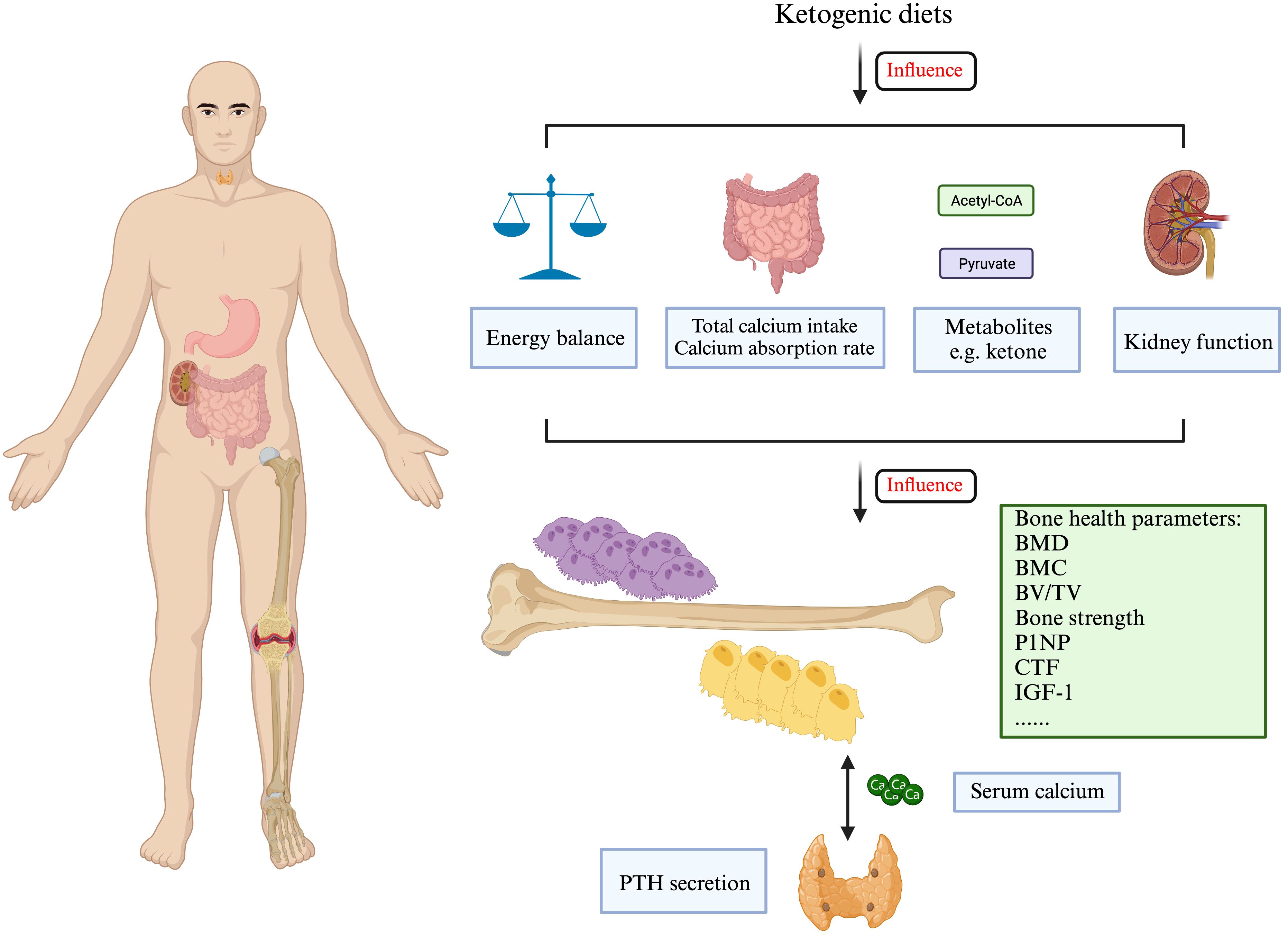
Figure 1 The ketogenic diets may affect bone formation and resorption in multiple ways. Created with BioRender.com.
Since previous studies have noticed the compromised bone quality of patients treated with KD, research on how to reduce or reverse the side effect of a KD on bone were then conducted, and several antiosteoporosis drugs were reported to be effective. Draaisma et al. conducted a retrospective observational cohort study on epileptic children treated with KD and bisphosphonate for over 6 months; DXA scans were taken to assess the bone mass, and the result showed that bisphosphonate may have a positive effect on the bone mass (34).
4 Mediterranean diet and bone health
4.1 The definition of a Mediterranean diet
The MD was first defined as being low in saturated fat and high in vegetable oils in the 1960s and has been continuously revised since then. The modern concept of the MD describes it as a diet that includes a high intake of extra virgin olive oil, vegetable, and fruit; a moderate intake of fish and other meat, dairy products, and red wine; and a low intake of eggs and sweets (63). The most recent definition of the MD was released in 2010 by the Mediterranean Diet Foundation (64) (Table 4). The MD has been shown to be effective in a variety of diseases, like cardiovascular disease and cancer, as well as in bone health. Its protective effect was due to its antioxidant and anti-inflammatory active molecules such as polyphenols (65, 66).
4.2 The Mediterranean diet and bone metabolism
4.2.1 Evidence from animal studies
Until now, only a handful of animal studies have been conducted to reveal the effect of the MD on bone health. Olive oil and vitamin D, which are abundant in the MD, were proven to resist the bone loss induced by estrogen deprivation by regulating the inflammation and oxidative stress status in mice (12). A variety of olive compounds have been studied and proven to have a protective effect on bone. Puel et al. injected ovariectomized rats with osteoporosis (15 mg/kg) with oleuropein, a common component of the MD. After 100 days, the injected rats had a doubled BMD compared with the untreated ovariectomized group. Another study published in 2008 focused on tyrosol and hydroxytyrosol, the main olive oil phenolic compounds. The results showed that ovariectomized rats after 84 days of tyrosol and hydroxytyrosol treatment had higher blood concentrations of osteocalcin and BMD than untreated ovariectomized rats (13, 67).
4.2.2 Evidence from human studies
A study about the MD in children with celiac disease found that it could improve bone health. It was found that both bone mineral content and bone mineral density in these children were significantly increased with high MD adherence than those with low MD adherence. The adherence to the MD was evaluated using the Mediterranean Diet Quality Index in Children and Adolescents (KIDMED) survey. Participants are classified into three categories: (1) high MD adherence (≥8 points), (2) medium MD adherence (4–7 points), and (3) low MD adherence (≤3 points) (35). Another study revealed that compared to a basal diet, male adolescents who adopted an MD as the main meal had a significant improvement in calcium absorption and retention (36). In the meantime, Julian et al. showed that the MD was not associated with BMD (37). Several studies have demonstrated that perimenopausal women with MD had more BMD and trabecular density (38) and less probability of osteoporosis (68–72) than women without. Meanwhile, several studies have indicated that the MD was associated with a reduced risk of fracture, especially in hip fracture (73–75). A case–control study in 2014 that included nearly 700 elderly Chinese persons conducted from 2009 to 2013 with hip fracture showed that a high score in diet-quality scales such as aMed was significantly associated with a decreased risk of hip fractures (76), and a high score in diet-quality scales was often associated with the MD. Other studies revealed that high compliance with the MD was associated with higher BMD and less risk of incident falls (39, 77–79). The protective effect of this diet may be related to the intake of vitamin D3, calcium ions, and the elevated levels of parathyroid hormone in the body (80). However, not all studies found benefits in the MD for bone health. A study conducted from 2000 to 2010 on elderly French persons found no link between the diet and the risk of bone fractures, possibly due to race or environment (40). In the study, individuals with an incident fracture at any of the three sites had a higher mean MeDi score, which assesses MD adherence, at baseline than those who remained free of fracture. Specifically, greater fruit consumption (i.e., >14 servings/week) was significantly associated with a doubled 8-year risk of hip fracture, and a lower intake of dairy products (i.e., <17.0 servings/week in men and <17.9 servings/week in women) was associated with a doubled risk of wrist fracture. It has also been suggested that the olive oil in the MD may reduce the risk of osteoporosis by reducing chronic inflammation (81).
5 Caloric restriction diet
5.1 The definition of a caloric restriction diet
Caloric restriction (CR) diet is classically defined as a diet with reduced caloric intake, which is approximately 20%–30% below average and does not cause malnutrition during the diet intervention. CR was reported to have the ability not only to reduce weight (82) but also to improve aging-related outcomes (83). However, CR was previously considered as a risk factor for compromising bone quality, and the mechanism behind this phenomenon might be the alteration in bone metabolism, hormones, and weight bearing. Consequently, researchers have tried to introduce a number of remedies to reduce dietary bone damage, including vitamin D intake, high-protein intake, and exercise training (84). Studies in the last decade have gained more results on this topic, which will be reviewed below.
5.2 Effects of a caloric restriction diet on bone mass
5.2.1 Evidence from animal studies
Most of the recent animal studies on CR and bone health have once again confirmed the degraded bone mineral condition in rats, mice, and rhesus monkeys. A study on obese female rats implemented a −35% CR diet for 4 months; the BMD, trabecular, and cortical bone volume and bone strength were found to be decreased (14). Another study researched the effect of CR on bone and discovered that the starting age of CR application was found to be crucial to determine its effects. Younger mice showed a more significant loss under CR in terms of cortical bone, cortical BMD, and thickness, compared to senile mice. Long-term CR showed beneficial effects on vertebrae trabecular BMD and BV/TV, which were considered as a reorganization and compensation for the bone loss in cortical bone (15). Issues have also been proposed on whether the decrease of BMD was a pathological process or an adaptation to weight loss. The study on rhesus monkeys indicated that the alteration might be an adaptation process. It was reported that despite the fact that BMD was lower after CR, the alteration of bone turnover markers was not significant; thus, the decreased BMD may be associated with the lower mechanical load generated by a smaller body size, rather than pathological osteopenia (16).
Regarding the mechanism of how CR affects bone health, recent studies focused most on CR-induced bone marrow adipose tissue (BMAT) alterations. BMAT could respond to CR-induced energy imbalance, cause volume expansion and metabolic or endocrinal change (17), and then cause bone loss. The trigger factors of the alterations in BMAT have been widely studied, and the roles of corticoid have been clarified the most. The uprising of serum glucocorticoid as a result of CR was considered to be relevant (85). The effect of leptin was still unclear, and low serum leptin level was found to be insufficient for BMAT expansion (85). Additionally, despite daily leptin supplementation suppression of BMAT formation in CR mice, it does not attenuate BMD loss or the impairment of bone microstructure; thus, the roles of leptin in bone–fat interaction remain unclear (86). Development of insulin resistance during CR was also found to coincide with BMAT expansion (87). Another study revealed that the preservation of BMAT during CR might be related to its characteristics of beta-adrenergic stimuli resistance compared to white adipose tissue (WAT) (88). Furthermore“, it was proposed that bone–hypothalamus–pituitary–adrenal crosstalk might occur, which may regulate BMAT during CR (89). The alteration of BMAT affected bone health in multiple ways. The expansion of BMAT stored fat, which might take up space in bone marrow, and BMAT released biotic factors that modulated bone turnover. However, whether or not BMAT expansion itself was necessitated in bone loss remains controversial since the amount of expansion might not always be related to the extent of bone loss (87, 90, 91). Adiponectin, secreted by WAT and BMAT, was found to be increased in mice and non-obese adults in CR situations, and overexpression of adiponectin might interfere with glucose metabolism and sympathetic tone, which further affects bone cells and induces bone loss (41, 92). Regarding bone metabolic status, a human study revealed the bone metabolic responses to CR, and based on the evaluation of blood samples, P1NP concentration decreased while CTX concentration remained unchanged, and IGF-1 and leptin levels were decreased, which suggested that CR might induce bone loss and decrease bone formation rather than increase bone resorption (53) (Figure 2).
5.2.2 Evidence from human studies
Regarding the determination of whether CR impairs bone quality, while several 2-year studies in non-obese patients, including an RCT study, kept the affirmative opinion (41, 93), other studies found that whether the loss of bone quality existed or not was related to gender differences and the extent of weight loss. An RCT study observed 424 obese and overweight participants, and found decreased BMD in the femoral neck in all patients, while postmenopausal women also showed decreased BMD in the spine. Male patients showed increased BMD in the spine. All participants also underwent regular exercise, which may influence the analysis of the results (42). Another study that investigated 38 men showed that moderate weight loss (−7.9 ± 4.4%) using CR would not decrease BMD at any site or decrease cortical and trabecular bone and geometry (43). Meanwhile, studies have also focused on determining the timing of bone loss during CR. A study on postmenopausal women has shown that the BMD loss did not recover in 2 years after a 6-month CR intervention (44). Moreover, evidence has also shown that bone mineral loss and bone turnover would not recover even after weight regain (45). Furthermore, regarding the assessment tools for the evaluation of BMD in humans who underwent CR diet intervention, aside from the gold standard of DXA, other new predictive tools were investigated. A multiple regression analysis study collected data from 107 obese adults with CR. The researchers selected the changes in thigh muscle volume, lean body mass, osteocalcin, P1NP, CTX, and one-repetition maximum strength as variables. After stepwise multiple linear regression analysis, they demonstrated that changes in thigh muscle volume were positively correlated with changes in hip BMD and were its independent predictor (46).
5.3 Methods for attenuating CR-induced bone loss
Considering the potential side effects of CR on bone, researchers have recently focused on interventions that might attenuate such effects, including exercise, vitamin D and calcium supplementation, and other nutrient supplementation.
In terms of exercise, more recent literature confirmed the positive effect of exercise during CR, while a few animal studies disagreed with this opinion. In studies with positive opinions on the subject, RCTs involving overweight/obese adults have found that aerobic training (AT) for 3 months (94) and resistance training (RT) for 5 months (95) were beneficial to weight-bearing bones’ BMD during CR. However, RT might be more effective in bone quality reservation than AT according to the comparison between the BMDs of RT+CR and AT+CR. The potential mechanism needs to be discussed in the future (95). The level of serum sclerostin was found to be higher in participants with exercise training during CR, which might positively influence bone quality (96, 97). In contrast with human studies, animal studies have revealed varying results. Five-month-old female rats that were fed with CR with exercise for 12 weeks have better BMD, BMC, and lean mass compared to rats only fed with CR (98, 99). Another study found that obese rats fed with CR and subjected to exercise for 3 months can attenuate bone volume decrease at the distal femur (100). Nebot et al. proposed a mixed exercise-training protocol with CR and reported that it induced weight loss while preserving bone quality (19). A few studies also pointed out the negative effects of exercise on bone during CR. Hitori et al. conducted a study that randomly divided 14-week-old mature male rats into a control group, a CR group, an exercise group, and a CR+exercise group, and found no significant difference in femur and tibia BMD and trabecular bone volume between groups after 13 weeks (101). They then conducted a similar study on 4-week-old rats; the results indicated that 13-week exercise with CR was detrimental to bone microstructure and strength (102). A more recent study fed 10-week-old rats with CR and subjected them to exercise for 6 weeks. Bone quality was found to be compromised with increased cortical porosity. Exercise also suppressed MAT formation, interrupting its function as an energy supply source to bone formation during CR (103).
In terms of nutrient supplementation, vitamin D and calcium supplementation provided the most significant findings. Over the last 10 years, four RCT studies have revealed different results. A 6-month supplementation of 400 IU vitamin D and 800 mg calcium per day can improve tibial bone properties, which was measured by quantitative CT in young male jockeys who usually undertake CR and high volumes of physical activities (104). Another RCT study observed that a 6-week intake of 1,200 mg calcium and 400 IU vitamin D supplementation in healthy or obese participants during CR could elevate their osteocalcin level and improve insulin sensitivity, which might benefit bone formation (105). Regarding the dose of vitamin D supplementation, an RCT double-blind study found that when calcium intake is 1,200 mg per day, either 10 or 63 μg of vitamin D per day is sufficient to maintain the calcium balance during CR. Calcium balance was evaluated with the parameter of true fractional calcium absorption (TFCA) (106). However, another RCT study revealed that a 12-month vitamin D supplementation (2000 IU/day) did not result in different changes in BMD from placebo in women participating in a weight loss program with CR. It is worth noting that all participants also took part in 225 min/week of aerobic exercise (107). Other nutrient supplementation researched by recent studies included special protein regimens (soy or casein) and Omega-3 polyunsaturated fatty acid (n-3 PUFA) supplementation. However, both of them could not improve bone quality during CR according to the study results (108, 109).
6 The effect of high-protein diets on bone health
According to the Recommended Dietary Allowances (RDA) published by the National Research Council (US) in 1989, 0.8 g/kg body weight/day of protein is sufficient for adults, while high-protein (HP) diets refer to diets that contain more than 0.8 g/kg body weight/day of protein. HP diets have gained attention since they have been widely used in the treatment of obesity and diabetes. Furthermore, it is believed that HP diets may improve athletes’ performance and body posture by increasing muscle mass (110–112). However, controversial topics of whether and how HP diets influence bone health still remain. During HP diets, serum IGF-1 and bone matrix collagen synthesis were upregulated, while PTH secretion was downregulated. These factors were beneficial to bone formation. On the other hand, HP diets could also produce more acid during protein metabolism, which could impair bone formation. Overall, the protective effect appears to outweigh the detrimental effect (113).
Animal studies on a moderate-high protein diet showed its positive effect on bone, while even higher protein diets did not seem to further improve bone quality. In a study involving 5-week-old rats fed with different levels of dietary protein and that underwent different levels of exercise, it was found that the BMD of tibia and femoral breaking force were lower in the low-protein-diet group (18). Another study examined 6-week-old obese Zuker rats for 2 months, showing that the combination of an HP diet (25% protein) and exercise enhances the trabecular bone microarchitecture and BMD, while leaving the bone turnover markers unchanged (114). However, a study that tracked 3-week-old rats for 3 weeks did not find any improvement in bone length and bone formation biomarkers in the high-protein group (26% protein) compared with the low-protein group (12% protein) (20). In another study, the HP diet (40% protein) did not improve bone quality more than the moderate-protein diet (20% protein) in rats with high-level exercise (18).
In human studies, no detrimental effects on bone health were found during an HP diet. Researchers compared the HP diet with habitual diets in 24 exercise-trained women for half a year. The results proved that neither the whole-body BMD nor lumbar BMD were significantly different after intervention (47). In another large-sample study, the protein intake of 12,812 subjects with femoral BMD and T scores from the National Health and Nutrition Examination Survey (NHANES) were analyzed. It was demonstrated that BMD and T-scores were positively correlated with the amount of protein intake (48). Another analysis also studied the NHANES database and extracted data from 4,447 subjects. The result showed that diets with a higher percentage of energy from protein were associated with higher T-scores (49). However, the two studies did not record the duration of HP diets. In another aspect, there are studies that have shown that HP diets do not attenuate bone loss in patients with chronic kidney disease and low energy availability (48, 50).
7 Intermittent fasting and bone health
Intermittent fasting (IF) is defined as dieting with periodic fasting and non-fasting (115), which includes complete alternate-day fasting, modified fasting regimens, time-restricted feeding (TRF), religious fasting, and Ramadan fasting, thus improving metabolic profiles and reducing the risk of obesity and related diseases (116). Although the intake of calcium was reported to be relatively lower in IF (117), the actual effects of IF on bone health were unclear.
In animal studies, evidence showed the detrimental effects of IF on bone mass and bone remodeling. A study that researched 16 pregnant female rats reported that rats fed with an IF regimen showed a decrease in cortical thickness of the vertebra and the ability of bone remodeling according to the osteoclast count (21). They further observed the offspring of eight pregnant rats fed with IF and found thyroid abnormalities that may be associated with the decrease in bone remodeling ability (22). Another study observed Alzheimer’s disease-induced estrogen-deficient rats, showing that IF could aggravate BMD loss (23). It was also determined that IF may attenuate the detrimental effects of KD on bone. The combination of IF and KD was named Every-other-day ketogenic diet. It was reported that this diet would not impair bone microstructure and strength compared to a normal KD in a rat study (56).
Current human studies showed no detrimental effects in either BMD measurement or bone turnover markers. In terms of IF without energy restriction, a randomized study enrolled 24 healthy lean midlife/older adults, while 10 participants were randomized to stick to their normal feeding pattern for 6 weeks and then transition to a 6-week TRF. The other 14 participants were randomized to stick to the 6-week TRF and then transition to their normal diet pattern; the TRF required participants to consume all meals within a 8-h time window, and the caloric intake was within a regular range to avoid weight loss. In the study results, researchers did not find significant differences between the normal feeding group and the TRF group (51). In terms of IF with energy restriction, another study investigated 16 lean participants for 3 days; on day 1, they consumed a 24-h diet with or without energy restriction (25% of the estimated energy requirement), followed by a standardized breakfast and ad libitum lunch and dinner on day 2, and fasting overnight and return on day 3. Their CTX, PINP, and PTH levels were measured on all 3 days, with no differences found between the groups, which indicated that a 24-h severe energy restriction did not affect bone metabolism (52).
8 Conclusion
This review presents an overview of the current knowledge on the effects of a KD, an MD, an HP diet, IF, and CR on bone health. We suggest that several problems should be solved first before further addressing the following: (i) Related studies lack standardization of the dietary intervention, which includes the proportion and type of fat in a KD, the energy restriction rate and the nutrition structure of CR, the proportion and resources of protein in HP, the types of IF, the duration of the intervention, and whether calcium supplementation can meet the minimum daily requirement. (ii) The method used for measuring bone quality also lacks standardization, which includes the bone site of measurement and the selection of the assessment tool such as x-ray, computed tomography (CT), or DXA. (iii) Sometimes, studies displayed conflicting results in human and animals; further explanation is needed to address this. (iv) More high-level evidence studies, such as an RCT and meta-analysis of different forms of dietary interventions, should be carried out with a standardized protocol and long-term follow-up. In summary, in a KD and CR, detrimental effects on bone quality were more significant, and attenuation methods were proposed. In contrast, most of the relevant studies on MDs and HP diets showed a positive or non-effective impact on bone health. In IF, recent human studies and animal studies showed different results. Although numerous researchers have been working on this topic for a long period of time, current lines of evidence on human and animal studies were still not sufficient to reach a final solid conclusion.
Author contributions
YP: Writing – original draft. ZZ: Writing – original draft. CH: Writing – review & editing. WW: Writing – review & editing.
Funding
The author(s) declare financial support was received for the research, authorship, and/or publication of this article. This study was supported by the National Natural Science Foundation of China (grant number 81972107).
Conflict of interest
The authors declare that the research was conducted in the absence of any commercial or financial relationships that could be construed as a potential conflict of interest.
Publisher’s note
All claims expressed in this article are solely those of the authors and do not necessarily represent those of their affiliated organizations, or those of the publisher, the editors and the reviewers. Any product that may be evaluated in this article, or claim that may be made by its manufacturer, is not guaranteed or endorsed by the publisher.
References
1. Kopp W. How western diet and lifestyle drive the pandemic of obesity and civilization diseases. Diabetes Metab Syndr Obes (2019) 12:2221–36. doi: 10.2147/DMSO.S216791
2. Meira ID, Romao TT, do Prado HJP, Kruger LT, Pires MEP, da Conceicao PO. Ketogenic diet and epilepsy: what we know so far. Front Neurosci (2019) 13. doi: 10.3389/fnins.2019.00005
3. Satija A, Hu FB. Plant-based diets and cardiovascular health. Trends Cardiovasc Med (2018) 28(7):437–41. doi: 10.1016/j.tcm.2018.02.004
4. Giugliano D, Esposito K. Mediterranean diet and metabolic diseases. Curr Opin Lipidol (2008) 19(1):63–8. doi: 10.1097/MOL.0b013e3282f2fa4d
5. Merlotti D, Cosso R, Eller-Vainicher C, Vescini F, Chiodini I, Gennari L, et al. Energy metabolism and ketogenic diets: what about the skeletal health? A narrative review and a prospective vision for planning clinical trials on this issue. Int J Mol Sci (2021) 22(1):435. doi: 10.3390/ijms22010435
6. Wu X, Huang Z, Wang X, Fu Z, Liu J, Huang Z, et al. Ketogenic diet compromises both cancellous and cortical bone mass in mice. Calcified Tissue Int (2017) 101(4):412–21. doi: 10.1007/s00223-017-0292-1
7. Aikawa Y, Yamashita T, Nakai N, Higashida K. Low-carbohydrate, high-fat diet, and running exercise influence bone parameters in old mice. J Appl Physiol (2022) 132(5):1204–12. doi: 10.1152/japplphysiol.00789.2021
8. Zengin A, Kropp B, Chevalier Y, Junnila R, Sustarsic E, Herbach N, et al. Low-carbohydrate, high-fat diets have sex-specific effects on bone health in rats. Eur J Nutr (2016) 55(7):2307–20. doi: 10.1007/s00394-015-1040-9
9. Ding J, Xu X, Wu X, Huang Z, Kong G, Liu J, et al. Bone loss and biomechanical reduction of appendicular and axial bones under ketogenic diet in rats. Exp Ther Med (2019) 17(4):2503–10. doi: 10.3892/etm.2019.7241
10. Liu Q, Wang X, Huang Z, Liu J, Ding J, Xu X, et al. Ketogenic diet delays spinal fusion and decreases bone mass in posterolateral lumbar spinal fusion: an in vivo rat model. Acta Neurochirurgica (2018) 160(10):1909–16. doi: 10.1007/s00701-018-3616-7
11. Liu Q, Zhou J, Yang Z, Xie C, Huang Y, Ling L, et al. The ginsenoside exhibits antiosteoporosis effects in ketogenic-diet-induced osteoporosis via rebalancing bone turnover. Front Pharmacol (2020) 11:593820. doi: 10.3389/fphar.2020.593820
12. Tagliaferri C, Davicco MJ, Lebecque P, Georgé S, Amiot MJ, Mercier S, et al. Olive oil and vitamin D synergistically prevent bone loss in mice. PloS One (2014) 9(12):e115817. doi: 10.1371/journal.pone.0115817
13. Puel C, Mathey J, Agalias A, Kati-Coulibaly S, Mardon J, Obled C, et al. Dose-response study of effect of oleuropein, an olive oil polyphenol, in an ovariectomy/inflammation experimental model of bone loss in the rat. Clin Nutr (2006) 25(5):859–68. doi: 10.1016/j.clnu.2006.03.009
14. Shen CL, Zhu WB, Gao WM, Wang S, Chen LX, Chyu MC. Energy-restricted diet benefits body composition but degrades bone integrity in middle-aged obese female rats. Nutr Res (2013) 33(8):668–76. doi: 10.1016/j.nutres.2013.05.008
15. Behrendt AK, Kuhla A, Osterberg A, Polley C, Herlyn P, Fischer DC, et al. Dietary restriction-induced alterations in bone phenotype: effects of lifelong versus short-term caloric restriction on femoral and vertebral bone in C57BL/6 mice. J Bone Mineral Res (2016) 31(4):852–63. doi: 10.1002/jbmr.2745
16. Colman RJ, Beasley TM, Allison DB, Weindruch R. Skeletal effects of long-term caloric restriction in rhesus monkeys. Age (2012) 34(5):1133–43. doi: 10.1007/s11357-011-9354-x
17. Li ZR, Bowers E, Zhu JX, Yu H, Hardij J, Bagchi DP, et al. Lipolysis of bone marrow adipocytes is required to fuel bone and the marrow niche during energy deficits. eLife (2022) 11:32. doi: 10.7554/eLife.78496.sa2
18. Takeda S, Kobayashi Y, Park JH, Ezawa I, Omi N. Effect of different intake levels of dietary protein and physical exercise on bone mineral density and bone strength in growing male rats. J Nutr Sci Vitaminol (2012) 58(4):240–6. doi: 10.3177/jnsv.58.240
19. Nebot E, Martinez R, Kapravelou G, Sanchez C, Llopis J, Aranda P, et al. Combination of caloric restriction and a mixed training protocol as an effective strategy to counteract the deleterious effects in trabecular bone microarchitecture caused by a diet-induced obesity in sprague dawley rats. Nutrients (2022) 14(18):18. doi: 10.3390/nu14183672
20. Tirapegui J, Ribeiro SML, Pires ISD, Rogero MM. Effects of two different levels of dietary protein on body composition and protein nutritional status of growing rats. Nutrients (2012) 4(9):1328–37. doi: 10.3390/nu4091328
21. Kamel M, Badraoui R, Sahnoun Z, Rebai T, Aouidet A. Intermittent fasting effect on bone maturation and remodeling in young rats. Nutr Clin Metab (2008) 22(2):44–51. doi: 10.1016/j.nupar.2008.04.001
22. Kamel M, Barkia A, Nasri M, Rebai T, Sahnound Z, Kassis M, et al. Effects of food restriction imposed to adult rats on the bone growth and the histological structure of the thyroid in their youth. Pathol Biol (2012) 60(5):296–300. doi: 10.1016/j.patbio.2011.08.001
23. Shin BK, Kang S, Kim DS, Park S. Intermittent fasting protects against the deterioration of cognitive function, energy metabolism and dyslipidemia in Alzheimer’s disease-induced estrogen deficient rats. Exp Biol Med (2018) 243(4):334–43. doi: 10.1177/1535370217751610
24. Xu XL, Ding JY, Wu XH, Huang ZC, Kong GG, Liu Q, et al. Bone microstructure and metabolism changes under the combined intervention of ketogenic diet with intermittent fasting: an in vivo study of rats. Exp Animals (2019) 68(3):371–80. doi: 10.1538/expanim.18-0084
25. Hahn TJ, Halstead LR, Devivo DC. Disordered mineral metabolism produced by ketogenic diet therapy. Calcified Tissue Int (1979) 28(1):17–22. doi: 10.1007/BF02441213
26. Simm P, Bicknell-Royle J, Nation J, Draffin K, Stewart KG, Cameron F, et al. The effect of the ketogenic diet on the developing skeleton. Epilepsia (2013) 54:5–. doi: 10.1016/j.eplepsyres.2017.07.014
27. Svedlund A, Hallbook T, Magnusson P, Dahlgren J, Swolin-Eide D. Longitudinal study of bone mass in swedish children treated with modified ketogenic diet. Hormone Res Paediatrics (2018) 90:169–70. doi: 10.1159/000492307
28. Gomez-Arbelaez D, Bellido D, Castro AI, Ordonez-Mayan L, Carreira J, Galban C, et al. Body composition changes after very-low-calorie ketogenic diet in obesity evaluated by 3 standardized methods. J Clin Endocrinol Metab (2017) 102(2):488–98. doi: 10.1210/jc.2016-2385
29. Athinarayanan SJ, Adams RN, Hallberg SJ, McKenzie AL, Bhanpuri NH, Campbell WW, et al. Long-term effects of a novel continuous remote care intervention including nutritional ketosis for the management of type 2 diabetes: A 2-year non-randomized clinical trial. Front Endocrinol (2019) 10. doi: 10.3389/fendo.2019.00348
30. Bertoli S, Trentani C, Ferraris C, De Giorgis V, Veggiotti P, Tagliabue A. Long-term effects of a ketogenic diet on body composition and bone mineralization in GLUT-1 deficiency syndrome: A case series. Nutrition (2014) 30(6):726–8. doi: 10.1016/j.nut.2014.01.005
31. Vargas-Molina S, Carbone L, Romance R, Petro JL, Schoenfeld BJ, Kreider RB, et al. Effects of a low-carbohydrate ketogenic diet on health parameters in resistance-trained women. Eur J Appl Physiol (2021) 121(8):2349–59. doi: 10.1007/s00421-021-04707-3
32. Carter JD, Vasey FB, Valeriano J. The effect of a low-carbohydrate diet on bone turnover. Osteoporosis Int (2006) 17(9):1398–403. doi: 10.1007/s00198-006-0134-x
33. Heikura IA, Burke LM, Hawley JA, Ross ML, Garvican-Lewis L, Sharma AP, et al. A short-term ketogenic diet impairs markers of bone health in response to exercise. Front Endocrinol (2020) 10. doi: 10.3389/fendo.2019.00880
34. Draaisma JMT, Hampsink BM, Janssen M, van Houdt NBM, Linders ETAM, Willemsen MA. The ketogenic diet and its effect on bone mineral density: A retrospective observational cohort study. Neuropediatrics (2019) 50(6):353–8. doi: 10.1055/s-0039-1693059
35. Nestares T, Martín-Masot R, de Teresa C, Bonillo R, Maldonado J, Flor-Alemany M, et al. Influence of mediterranean diet adherence and physical activity on bone health in celiac children on a gluten-free diet. Nutrients (2021) 13(5):1636. doi: 10.3390/nu13051636
36. Seiquer I, Mesías M, Hoyos AM, Galdó G, Navarro MP. A Mediterranean dietary style improves calcium utilization in healthy male adolescents. J Am Coll Nutr (2008) 27(4):454–62. doi: 10.1080/07315724.2008.10719725
37. Julián C, Huybrechts I, Gracia-Marco L, González-Gil EM, Gutiérrez Á, González-Gross M, et al. Mediterranean diet, diet quality, and bone mineral content in adolescents: the HELENA study. Osteoporos Int (2018) 29(6):1329–40. doi: 10.1007/s00198-018-4427-7
38. Pérez-Rey J, Roncero-Martín R, Rico-Martín S, Rey-Sánchez P, Pedrera-Zamorano JD, Pedrera-Canal M, et al. Adherence to a mediterranean diet and bone mineral density in spanish premenopausal women. Nutrients (2019) 11(3):555. doi: 10.3390/nu11030555
39. Cervo MMC, Scott D, Seibel MJ, Cumming RG, Naganathan V, Blyth FM, et al. Adherence to Mediterranean diet and its associations with circulating cytokines, musculoskeletal health and incident falls in community-dwelling older men: The Concord Health and Ageing in Men Project. Clin Nutr (2021) 40(12):5753–63. doi: 10.1016/j.clnu.2021.10.010
40. Feart C, Lorrain S, Ginder Coupez V, Samieri C, Letenneur L, Paineau D, et al. Adherence to a Mediterranean diet and risk of fractures in French older persons. Osteoporos Int (2013) 24(12):3031–41. doi: 10.1007/s00198-013-2421-7
41. Villareal DT, Fontana L, Das SK, Redman L, Smith SR, Saltzman E, et al. Effect of two-year caloric restriction on bone metabolism and bone mineral density in non-obese younger adults: A randomized clinical trial. J Bone Mineral Res (2016) 31(1):40–51. doi: 10.1002/jbmr.2701
42. Tirosh A, de Souza RJ, Sacks F, Bray GA, Smith SR, LeBoff MS. Sex differences in the effects of weight loss diets on bone mineral density and body composition: POUNDS LOST trial. J Clin Endocrinol Metab (2015) 100(6):2463–71. doi: 10.1210/jc.2015-1050
43. Pop LC, Sukumar D, Tomaino K, Schlussel Y, Schneider SH, Gordon CL, et al. Moderate weight loss in obese and overweight men preserves bone quality. Am J Clin Nutr (2015) 101(3):659–67. doi: 10.3945/ajcn.114.088534
44. Von Thun NL, Sukumar D, Heymsfield SB, Shapses SA. Does bone loss begin after weight loss ends? Results 2 years after weight loss or regain in postmenopausal women. Menopause-J N Am Menopause Soc (2014) 21(5):501–8. doi: 10.1097/GME.0b013e3182a76fd5
45. Hinton PS, Rector RS, Linden MA, Warner SO, Dellsperger KC, Chockalingam A, et al. Weight-loss-associated changes in bone mineral density and bone turnover after partial weight regain with or without aerobic exercise in obese women. Eur J Clin Nutr (2012) 66(5):606–12. doi: 10.1038/ejcn.2011.212
46. Armamento-Villareal R, Aguirre L, Napoli N, Shah K, Hilton T, Sinacore DR, et al. Changes in thigh muscle volume predict bone mineral density response to lifestyle therapy in frail, obese older adults. Osteoporosis Int (2014) 25(2):551–8. doi: 10.1007/s00198-013-2450-2
47. Antonio J, Ellerbroek A, Evans C, Silver T, Peacock CA. High protein consumption in trained women: bad to the bone? J Int Soc Sport Nutr (2018) 15:5. doi: 10.1186/s12970-018-0210-6
48. Lee CL, Tsai SF. The impact of protein diet on bone density in people with/without chronic kidney disease: An analysis of the National Health and Nutrition Examination Survey database. Clin Nutr (2020) 39(11):3497–503. doi: 10.1016/j.clnu.2020.03.014
49. Gao SH, Qian X, Huang SC, Deng WX, Li Z, Hu YY. Association between macronutrients intake distribution and bone mineral density. Clin Nutr (2022) 41(8):1689–96. doi: 10.1016/j.clnu.2022.05.019
50. Murphy C, Bilek LD, Koehler K. Low energy availability with and without a high-protein diet suppresses bone formation and increases bone resorption in men: A randomized controlled pilot study. Nutrients (2021) 13(3):12. doi: 10.3390/nu13030802
51. Martens CR, Rossman MJ, Mazzo MR, Jankowski LR, Nagy EE, Denman BA, et al. Short-term time-restricted feeding is safe and feasible in non-obese healthy midlife and older adults. Geroscience (2020) 42(2):667–86. doi: 10.1007/s11357-020-00156-6
52. Clayton DJ, James LJ, Sale C, Templeman I, Betts JA, Varley I. Severely restricting energy intake for 24 h does not affect markers of bone metabolism at rest or in response to re-feeding. Eur J Nutr (2020) 59(8):3527–35. doi: 10.1007/s00394-020-02186-4
53. Papageorgiou M, Martin D, Colgan H, Cooper S, Greeves JP, Tang JCY, et al. Bone metabolic responses to low energy availability achieved by diet or exercise in active eumenorrheic women. Bone (2018) 114:181–8. doi: 10.1016/j.bone.2018.06.016
54. Wu X, Ding J, Xu X, Wang X, Liu J, Jiang J, et al. Ketogenic diet compromises vertebral microstructure and biomechanical characteristics in mice. J Bone Mineral Metab (2019) 37(6):957–66. doi: 10.1007/s00774-019-01002-2
55. Liu Q, Yang Z, Xie C, Ling L, Hu H, Cao Y, et al. The hyperglycemia and hyperketonemia impaired bone microstructures: A pilot study in rats. Front Endocrinol (Lausanne) (2020) 11:590575. doi: 10.3389/fendo.2020.590575
56. Xu X, Ding J, Wu X, Huang Z, Kong G, Liu Q, et al. Bone microstructure and metabolism changes under the combined intervention of ketogenic diet with intermittent fasting: an in vivo study of rats. Exp Animals (2019) 68(3):371–80. doi: 10.1538/expanim.18-0084
57. Liu Q, Xu X, Yang Z, Liu Y, Wu X, Huang Z, et al. Metformin alleviates the bone loss induced by ketogenic diet: an in vivo study in mice. Calcif Tissue Int (2019) 104(1):59–69. doi: 10.1007/s00223-018-0468-3
58. Yang Z, Liu Q, Xu X, Liu Y, Wu X, Huang Z, et al. Simvastatin alleviates the bone loss caused by ketogenic diet: an in vivo study. Chin J Osteoporosis (2019) 25(7):899–904,74. doi: 10.3969/j.issn.1006-7108.2019.07.003
59. Sugiyama M, Kodama T, Konishi K, Abe K, Asami S, Oikawa S. Compactin and simvastatin, but not pravastatin, induce bone morphogenetic protein-2 in human osteosarcoma cells. Biochem Biophys Res Commun (2000) 271(3):688–92. doi: 10.1006/bbrc.2000.2697
60. Shellhaas RA, Joshi SM. Vitamin D and bone health among children with epilepsy. Pediatr Neurol (2010) 42(6):385–93. doi: 10.1016/j.pediatrneurol.2009.12.005
61. Hawkes CP, Levine MA. Ketotic hypercalcemia: A case series and description of a novel entity. J Clin Endocrinol Metab (2014) 99(5):1531–6. doi: 10.1210/jc.2013-4275
62. Hawkes CP, Roy SM, Dekelbab B, Frazier B, Grover M, Haidet J, et al. Hypercalcemia in children using the ketogenic diet: A multicenter study. J Clin Endocrinol Metab (2021) 106(2):E485–E95. doi: 10.1210/clinem/dgaa759
63. Davis C, Bryan J, Hodgson J, Murphy K. Definition of the mediterranean diet; a literature review. Nutrients (2015) 7(11):9139–53. doi: 10.3390/nu7115459
64. Bach-Faig A, Berry EM, Lairon D, Reguant J, Trichopoulou A, Dernini S, et al. Mediterranean diet pyramid today. Science and cultural updates. Public Health Nutr (2011) 14(12A):2274–84. doi: 10.1017/S1368980011002515
65. Romero Pérez A, Rivas Velasco A. Adherence to Mediterranean diet and bone health. Nutr Hosp (2014) 29(5):989–96.
66. Tresserra-Rimbau A, Rimm EB, Medina-Remón A, Martínez-González MA, López-Sabater MC, Covas MI, et al. Polyphenol intake and mortality risk: a re-analysis of the PREDIMED trial. BMC Med (2014) 12:77. doi: 10.1186/1741-7015-12-77
67. Puel C, Mardon J, Agalias A, Davicco MJ, Lebecque P, Mazur A, et al. Major phenolic compounds in olive oil modulate bone loss in an ovariectomy/inflammation experimental model. J Agric Food Chem (2008) 56(20):9417–22. doi: 10.1021/jf801794q
68. Correa-Rodríguez M, El Mansouri-Yachou J, Tapia-Haro RM, Molina F, Rus A, Rueda-Medina B, et al. Mediterranean diet, body composition, and activity associated with bone health in women with fibromyalgia syndrome. Nurs Res (2019) 68(5):358–64. doi: 10.1097/NNR.0000000000000361
69. Silva TRD, Martins CC, Ferreira LL, Spritzer PM. Mediterranean diet is associated with bone mineral density and muscle mass in postmenopausal women. Climacteric (2019) 22(2):162–8. doi: 10.1080/13697137.2018.1529747
70. Rivas A, Romero A, Mariscal-Arcas M, Monteagudo C, Feriche B, Lorenzo ML, et al. Mediterranean diet and bone mineral density in two age groups of women. Int J Food Sci Nutr (2013) 64(2):155–61. doi: 10.3109/09637486.2012.718743
71. Kontogianni MD, Melistas L, Yannakoulia M, Malagaris I, Panagiotakos DB, Yiannakouris N. Association between dietary patterns and indices of bone mass in a sample of Mediterranean women. Nutrition (2009) 25(2):165–71. doi: 10.1016/j.nut.2008.07.019
72. Aparicio VA, Ruiz-Cabello P, Borges-Cosic M, Andrade A, Coll-Risco I, Acosta-Manzano P, et al. Association of physical fitness, body composition, cardiometabolic markers and adherence to the Mediterranean diet with bone mineral density in perimenopausal women. The FLAMENCO project. J Sports Sci (2017) 35(9):880–7. doi: 10.1080/02640414.2016.1196825
73. Malmir H, Saneei P, Larijani B, Esmaillzadeh A. Adherence to Mediterranean diet in relation to bone mineral density and risk of fracture: a systematic review and meta-analysis of observational studies. Eur J Nutr (2018) 57(6):2147–60. doi: 10.1007/s00394-017-1490-3
74. Byberg L, Bellavia A, Larsson SC, Orsini N, Wolk A, Michaëlsson K. Mediterranean diet and hip fracture in swedish men and women. J Bone Miner Res (2016) 31(12):2098–105. doi: 10.1002/jbmr.2896
75. Palomeras-Vilches A, Viñals-Mayolas E, Bou-Mias C, Jordà-Castro M, Agüero-Martínez M, Busquets-Barceló M, et al. Adherence to the mediterranean diet and bone fracture risk in middle-aged women: A case control study. Nutrients (2019) 11(10):989–96. doi: 10.3390/nu11102508
76. Zeng FF, Xue WQ, Cao WT, Wu BH, Xie HL, Fan F, et al. Diet-quality scores and risk of hip fractures in elderly urban Chinese in Guangdong, China: a case-control study. Osteoporos Int (2014) 25(8):2131–41. doi: 10.1007/s00198-014-2741-2
77. Chen GD, Dong XW, Zhu YY, Tian HY, He J, Chen YM. Adherence to the Mediterranean diet is associated with a higher BMD in middle-aged and elderly Chinese. Sci Rep (2016) 6:25662. doi: 10.1038/srep25662
78. García-Gavilán JF, Paz-Graniel I, Babio N, Romaguera D, Martínez JA, Martin V, et al. Inflammatory potential of diet and bone mineral density in a senior Mediterranean population: a cross-sectional analysis of PREDIMED-Plus study. Eur J Nutr (2022) 61(3):1445–55. doi: 10.1007/s00394-021-02751-5
79. Erkkilä AT, Sadeghi H, Isanejad M, Mursu J, Tuppurainen M, Kröger H. Associations of Baltic Sea and Mediterranean dietary patterns with bone mineral density in elderly women. Public Health Nutr (2017) 20(15):2735–43. doi: 10.1017/S1368980017001793
80. Jennings A, Cashman KD, Gillings R, Cassidy A, Tang J, Fraser W, et al. A Mediterranean-like dietary pattern with vitamin D3 (10 µg/d) supplements reduced the rate of bone loss in older Europeans with osteoporosis at baseline: results of a 1-y randomized controlled trial. Am J Clin Nutr (2018) 108(3):633–40. doi: 10.1093/ajcn/nqy122
81. Loued S, Berrougui H, Componova P, Ikhlef S, Helal O, Khalil A. Extra-virgin olive oil consumption reduces the age-related decrease in HDL and paraoxonase 1 anti-inflammatory activities. Br J Nutr (2013) 110(7):1272–84. doi: 10.1017/S0007114513000482
82. Liu DY, Huang Y, Huang CSH, Yang SY, Wei XY, Zhang PZ, et al. Calorie restriction with or without time-restricted eating in weight loss. New Engl J Med (2022) 386(16):1495–504. doi: 10.1056/NEJMoa2114833
83. Ravussin E, Redman LM, Rochon J, Das SK, Fontana L, Kraus WE, et al. A 2-year randomized controlled trial of human caloric restriction: feasibility and effects on predictors of health span and longevity. J Gerontol Ser A-Biol Sci Med Sci (2015) 70(9):1097–104. doi: 10.1093/gerona/glv057
84. Shapses SA, Sukumar D. Bone metabolism in obesity and weight loss. In: Cousins RJ, editor. Annual Review of Nutrition, Vol 32. Palo Alto: Annual Reviews (2012). p. 287–309.
85. Cawthorn WP, Scheller EL, Parlee SD, Pham HA, Learman BS, Redshaw CMH, et al. Expansion of bone marrow adipose tissue during caloric restriction is associated with increased circulating glucocorticoids and not with hypoleptinemia. Endocrinology (2016) 157(2):508–21. doi: 10.1210/en.2015-1477
86. Devlin MJ, Brooks DJ, Conlon C, van Vliet M, Louis L, Rosen CJ, et al. Daily leptin blunts marrow fat but does not impact bone mass in calorie-restricted mice. J Endocrinol (2016) 229(3):295–306. doi: 10.1530/JOE-15-0473
87. Walji TA, Turecamo SE, Sanchez AC, Anthony BA, Abou-Ezzi G, Scheller EL, et al. Marrow adipose tissue expansion coincides with insulin resistance in MAGP1-deficient mice. Front Endocrinol Editorial Mater (2016) 7:9. doi: 10.3389/fendo.2016.00087
88. Scheller EL, Khandaker S, Learman BS, Cawthorn WP, Anderson LM, Pham HA, et al. Bone marrow adipocytes resist lipolysis and remodeling in response to beta-adrenergic stimulation. Bone (2019) 118:32–41. doi: 10.1016/j.bone.2018.01.016
89. Pierce JL, Ding KH, Xu JR, Sharma AK, Yu KL, Arbona ND, et al. The glucocorticoid receptor in osteoprogenitors regulates bone mass and marrow fat. J Endocrinol (2019) 243(1):27–42. doi: 10.1530/JOE-19-0230
90. Hardouin P, Rharass T, Lucas S. Bone marrow adipose tissue: to be or not to be a typical adipose tissue? Front Endocrinol (2016) 7:11. doi: 10.3389/fendo.2016.00085
91. Rendina-Ruedy E, Rosen CJ. Bone-fat interaction. Endocrinol Metabol Clin North Amer (2017) 46(1):41. doi: 10.1016/j.ecl.2016.09.004
92. Zhu JX, Liu C, Jia JL, Zhang CG, Yuan WQ, Leng HJ, et al. Short-term caloric restriction induced bone loss in both axial and appendicular bones by increasing adiponectin. Ann NY Acad Sci (2020) 1474(1):47–60. doi: 10.1111/nyas.14380
93. Romashkan SV, Das SK, Villareal DT, Ravussin E, Redman LM, Rochon J, et al. Safety of two-year caloric restriction in non-obese healthy individuals. Oncotarget (2016) 7(15):19124–33. doi: 10.18632/oncotarget.8093
94. Hosny IA, Elghawabi HS, Younan WBF, Sabbour AA, Gobrial MAM. Beneficial impact of aerobic exercises on bone mineral density in obese premenopausal women under caloric restriction. Skeletal Radiol (2012) 41(4):423–7. doi: 10.1007/s00256-011-1196-1
95. Beavers KM, Beavers DP, Martin SB, Marsh AP, Lyles MF, Lenchik L, et al. Change in bone mineral density during weight loss with resistance versus aerobic exercise training in older adults. J Gerontol Ser A-Biol Sci Med Sci (2017) 72(11):1582–5. doi: 10.1093/gerona/glx048
96. Armamento-Villareal R, Sadler C, Napoli N, Shah K, Chode S, Sinacore DR, et al. Weight loss in obese older adults increases serum sclerostin and impairs hip geometry but both are prevented by exercise training. J Bone Mineral Res (2012) 27(5):1215–21. doi: 10.1002/jbmr.1560
97. Murphy C, Koehler K. Caloric restriction induces anabolic resistance to resistance exercise. Eur J Appl Physiol (2020) 120(5):1155–64. doi: 10.1007/s00421-020-04354-0
98. Metzger CE, Baek K, Swift SN, De Souza MJ, Bloomfield SA. Exercise during energy restriction mitigates bone loss but not alterations in estrogen status or metabolic hormones. Osteoporosis Int (2016) 27(9):2755–64. doi: 10.1007/s00198-016-3590-y
99. Metzger CE, Swift SN, Baek K, De Souza MJ, Bloomfield SA. Fat and lean mass predict bone mass during energy restriction in sedentary and exercising rodents. Front Physiol (2018) 9:11. doi: 10.3389/fphys.2018.01346
100. Cao JJ. Caloric restriction combined with exercise is effective in reducing adiposity and mitigating bone structural deterioration in obese rats. Ann NY Acad Sci (2018) 1433(1):41–52. doi: 10.1111/nyas.13936
101. Hattori S, Park JH, Agata U, Akimoto T, Oda M, Higano M, et al. Influence of food restriction combined with voluntary running on bone morphology and strength in male rats. Calcified Tissue Int (2013) 93(6):540–8. doi: 10.1007/s00223-013-9787-6
102. Hattori S, Park JH, Agata U, Oda M, Higano M, Aikawa Y, et al. Food restriction causes low bone strength and microarchitectural deterioration in exercised growing male rats. J Nutr Sci Vitaminol (2014) 60(1):35–42. doi: 10.3177/jnsv.60.35
103. McGrath C, Sankaran JS, Misaghian-Xanthos N, Sen B, Xie ZH, Styner MA, et al. Exercise degrades bone in caloric restriction, despite suppression of marrow adipose tissue (MAT). J Bone Mineral Res (2020) 35(1):106–15. doi: 10.1002/jbmr.3872
104. Silk LN, Greene DA, Baker MK, Jander CB. Tibial bone responses to 6-month calcium and vitamin D supplementation in young male jockeys: A randomised controlled trial. Bone (2015) 81:554–61. doi: 10.1016/j.bone.2015.09.004
105. Sukumar D, Shapses SA, Schneider SH. Vitamin D supplementation during short-term caloric restriction in healthy overweight/obese older women: Effect on glycemic indices and serum osteocalcin levels. Mol Cell Endocrinol (2015) 410(C):73–7. doi: 10.1016/j.mce.2015.01.002
106. Shapses SA, Sukumar D, Schneider SH, Schlussel Y, Sherrell RM, Field MP, et al. Vitamin D supplementation and calcium absorption during caloric restriction: a randomized double-blind trial. Am J Clin Nutr (2013) 97(3):637–45. doi: 10.3945/ajcn.112.044909
107. Mason C, Tapsoba JD, Duggan C, Imayama I, Wang CY, Korde L, et al. Effects of vitamin D-3 supplementation on lean mass, muscle strength, and bone mineral density during weight loss: A double-blind randomized controlled trial. J Am Geriatr Soc (2016) 64(4):769–78. doi: 10.1111/jgs.14049
108. Duque G, Al Saedi A, Rivas D, Miard S, Ferland G, Picard F, et al. Differential effects of long-term caloric restriction and dietary protein source on bone and marrow fat of the aging rat. J Gerontol Ser A-Biol Sci Med Sci (2020) 75(11):2031–6. doi: 10.1093/gerona/glaa093
109. Razny U, Goralska J, Calder PC, Gruca A, Childs CE, Kapusta M, et al. The Effect of Caloric Restriction with and without n-3 PUFA Supplementation on Bone Turnover Markers in Blood of Subjects with Abdominal Obesity: A Randomized Placebo-Controlled Trial. Nutrients (2021) 13(9):20. doi: 10.3390/nu13093096
110. Westerterp-Plantenga MS, Lemmens SG, Westerterp KR. Dietary protein - its role in satiety, energetics, weight loss and health. Br J Nutr (2012) 108:S105–S12. doi: 10.1017/S0007114512002589
111. Ajala O, English P, Pinkney J. Systematic review and meta-analysis of different dietary approaches to the management of type 2 diabetes. Am J Clin Nutr Rev (2013) 97(3):505–16. doi: 10.3945/ajcn.112.042457
112. Devries MC, Phillips SM. Supplemental protein in support of muscle mass and health: advantage whey. J Food Sci (2015) 80:A8–A15. doi: 10.1111/1750-3841.12802
113. Cao JJ. High dietary protein intake and protein-related acid load on bone health. Curr Osteoporos Rep Rev (2017) 15(6):571–6. doi: 10.1007/s11914-017-0408-6
114. Nebot E, Aparicio VA, Coll-Risco I, Camiletti-Moiron D, Schneider J, Kapravelou G, et al. Effects of a moderately high-protein diet and interval aerobic training combined with strength-endurance exercise on markers of bone metabolism, microarchitecture and turnover in obese Zucker rats. Bone (2016) 92:116–23. doi: 10.1016/j.bone.2016.08.017
115. Veronese N, Reginster JY. The effects of calorie restriction, intermittent fasting and vegetarian diets on bone health. Aging Clin Exp Res Rev (2019) 31(6):753–8. doi: 10.1007/s40520-019-01174-x
116. Patterson RE, Sears DD. Metabolic effects of intermittent fasting. In: Stover PJ, Balling R, editors. Annual Review of Nutrition, Vol 37. Palo Alto: Annual Reviews (2017). p. 371–93.
Keywords: ketogenic diet (KD), Mediterranean diet (MD), caloric restriction (CR), high-protein diet, intermittent fasting (IF), bone health
Citation: Peng Y, Zhong Z, Huang C and Wang W (2024) The effects of popular diets on bone health in the past decade: a narrative review. Front. Endocrinol. 14:1287140. doi: 10.3389/fendo.2023.1287140
Received: 01 September 2023; Accepted: 14 November 2023;
Published: 26 March 2024.
Edited by:
Alberto Falchetti, Italian Auxological Institute (IRCCS), ItalyReviewed by:
Guido Zavatta, University of Bologna, ItalyJuliana Ebling Brondani, Federal University of Minas Gerais, Brazil
Copyright © 2024 Peng, Zhong, Huang and Wang. This is an open-access article distributed under the terms of the Creative Commons Attribution License (CC BY). The use, distribution or reproduction in other forums is permitted, provided the original author(s) and the copyright owner(s) are credited and that the original publication in this journal is cited, in accordance with accepted academic practice. No use, distribution or reproduction is permitted which does not comply with these terms.
*Correspondence: Weiguo Wang, d2FuZ3dlaWd1b0B6cnloeXkuY29tLmNu
†These authors have contributed equally to this work