- 1Department of Ultrasound, The Second Affiliated Hospital of Fujian Medical University, Quanzhou, China
- 2Departments of Medical Imaging, Quanzhou Medical College, Quanzhou, China
- 3Centre of Neurological and Metabolic Research, The Second Affiliated Hospital of Fujian Medical University, Quanzhou, China
- 4Diabetes and Metabolism Division, Garvan Institute of Medical Research, Darlinghurst, Sydney, NSW, Australia
Stem cells have self-renewal, replication, and multidirectional differentiation potential, while progenitor cells are undifferentiated, pluripotent or specialized stem cells. Stem/progenitor cells secrete various factors, such as cytokines, exosomes, non-coding RNAs, and proteins, and have a wide range of applications in regenerative medicine. However, therapies based on stem cells and their secreted exosomes present limitations, such as insufficient source materials, mature differentiation, and low transplantation success rates, and methods addressing these problems are urgently required. Ultrasound is gaining increasing attention as an emerging technology. Low-intensity pulsed ultrasound (LIPUS) has mechanical, thermal, and cavitation effects and produces vibrational stimuli that can lead to a series of biochemical changes in organs, tissues, and cells, such as the release of extracellular bodies, cytokines, and other signals. These changes can alter the cellular microenvironment and affect biological behaviors, such as cell differentiation and proliferation. Here, we discuss the effects of LIPUS on the biological functions of stem/progenitor cells, exosomes, and non-coding RNAs, alterations involved in related pathways, various emerging applications, and future perspectives. We review the roles and mechanisms of LIPUS in stem/progenitor cells and exosomes with the aim of providing a deeper understanding of LIPUS and promoting research and development in this field.
1 Introduction
Stem cells, found in various tissues and organs, are undifferentiated cell populations originating from the early stages of embryonic development. They possess a limited capacity for self-renewal and demonstrate the ability to undergo multidirectional differentiation, giving rise to diverse specialized cell types. They can be classified into embryonic stem cells, induced pluripotent stem cells, and adult stem cells, with adult stem cells exhibiting a more limited differentiation potential. Stem cells undergo differentiation into progenitor cells, which possess a greater potential for differentiation but have limited self-renewal ability. Progenitor cells are characterized as undifferentiated cells with a higher proliferative capacity and the capability to differentiate into specific cell lineages (1). Mesenchymal stem cells (MSCs) are pluripotent tissue stem cells that can differentiate into a variety of mesodermal tissue types and are present as a type of adult stem cell in various mature tissues in vivo, such as the bone marrow, adipose, umbilical cord, and teeth, and they represent the most widely studied class of stem cells (2). Stem cells can exert paracrine effects by secreting factors, such as cytokines and inflammatory factors, and immunomodulatory effects by interacting with immune cells. Their low immunogenicity makes them immune to rejection at the time of transplantation (3). In some cases, inflammatory stimuli cause MSCs to suppress or enhance the ability of the immune response to localize to the site of inflammation, thus exerting an anti-inflammatory effect (4).
Exosomes are extracellular vesicles with a bilayer membrane structure, diameter of 40–100 nm, and density of 1.13–1.19 g/mL. They contain proteins, mRNAs, miRNAs, and DNA. Exosomes are present in nearly all cells and body fluids and are generally obtained from various types of cells, such as stem cells. Exosomes are important for intercellular communication, and they are involved in the integration of cells in physiological and pathological states by delivering biomolecules, thereby causing a series of biochemical changes in receptor molecules. They function in immune regulation (5), reproduction (6), tumor angiogenesis (7), cell differentiation and regeneration (8), apoptosis (9), and inflammatory responses (10). MiRNAs are endogenous non-coding single-stranded RNAs of 21–23 nucleotides that bind directly to the 3′-untranslated regions (3′ UTRs) of target mRNAs and regulate post-transcriptional gene expression negatively or positively (11). Most cells can secrete miRNAs, as can MSC-derived exosomes (MSC-EXOs). MSC-EXOs help restore dynamic cellular homeostasis by delivering proteins, lipids, and other information and affect the proliferation, differentiation, migration, and other behaviors of stem cells through various mechanisms that lead to altered signaling pathways (12, 13).
All of these different types of stem/progenitor cells have promising clinical applications in regenerative medicine (14). MSC-EXOs have the same properties as stem cells but lower immunogenicity, tumorigenicity, and infectivity, and they express MSC surface molecules CD90, CD44, and CD73; moreover, MSCs and extracellular vesicles have similar miRNA expression profiles (15, 16). Therefore, MSC-EXOs are widely studied and applied in regenerative medicine and various diseases (17). Studies have elucidated the biological roles of miRNA-mediated MSC-EXO effects in various tissues. A recent review concluded that MSC-EXO miRNAs have a dual role in the cancer microenvironment, where they significantly inhibit tumorigenesis and transfer between donor and recipient cells, leading to cancer chemoresistance, thus providing new ideas for identifying strategies for overcoming cancer drug resistance (18).
Endometrium-derived MSC-EXOs with high levels of miRNAs are involved in the regulation of macrophage polarization, T-cell activation, and inflammatory cytokine transcription by the immune system (19). MSC-EXO miR-140-5p regulates the mTOR pathway by targeting IGF1R (20), thereby inhibiting the osteogenic differentiation of MSCs. In addition, exosomes and their secreted miRNAs can serve as carriers for drug delivery and have been applied in various disease treatments (21, 22). However, stem cell and exosome therapies still have limitations, such as insufficient sources, mature differentiation, low transplantation success, and insufficient target organ homing, which limit their clinical application (23, 24). In addition, the treatment mechanism is not fully understood in many cases and the amount of treatment required for different diseases has not been standardized (25). To address these issues, methods to improve homing rates are increasingly being explored.
Low-intensity pulsed ultrasound (LIPUS) is a special type of ultrasound output in the form of pulsed waves with frequencies of 1 to 3 MHz and intensities less than 1 w/cm2, and it can be used as a non-invasive physical stimulus for therapeutic applications (26). LIPUS has a low thermal effect because of its low intensity and pulsed output mode; thus, it presents limited thermal effects while delivering acoustic energy to the target tissue. Its mechanical and cavitation effects provide low-intensity mechanical vibrational stimuli that interact with cells and trigger numerous intracellular changes, such as the release of cytokines and signaling molecules. These changes can alter the cellular microenvironment and affect biological behaviors, such as cell proliferation, differentiation, and migration, leading to tissue repair and regeneration (27). LIPUS is a promising treatment modality that was initially used for skeletal muscle disorders and has been shown to promote fracture healing, cartilage repair in osteoarthritis (OA), and tendon ligament injury recovery (28, 29). A recent review summarized the role and mechanism of LIPUS in the repair of peripheral nerve injury (PNI), and as a non-invasive stimulation method, LIPUS is expected to be a successful alternative treatment for PNI with great advantages and application prospects (30). In recent years, studies have found that LIPUS has important effects on stem/progenitor cells and exosomesis emerging as an important tool for enhancing stem cell therapy (Figure 1).
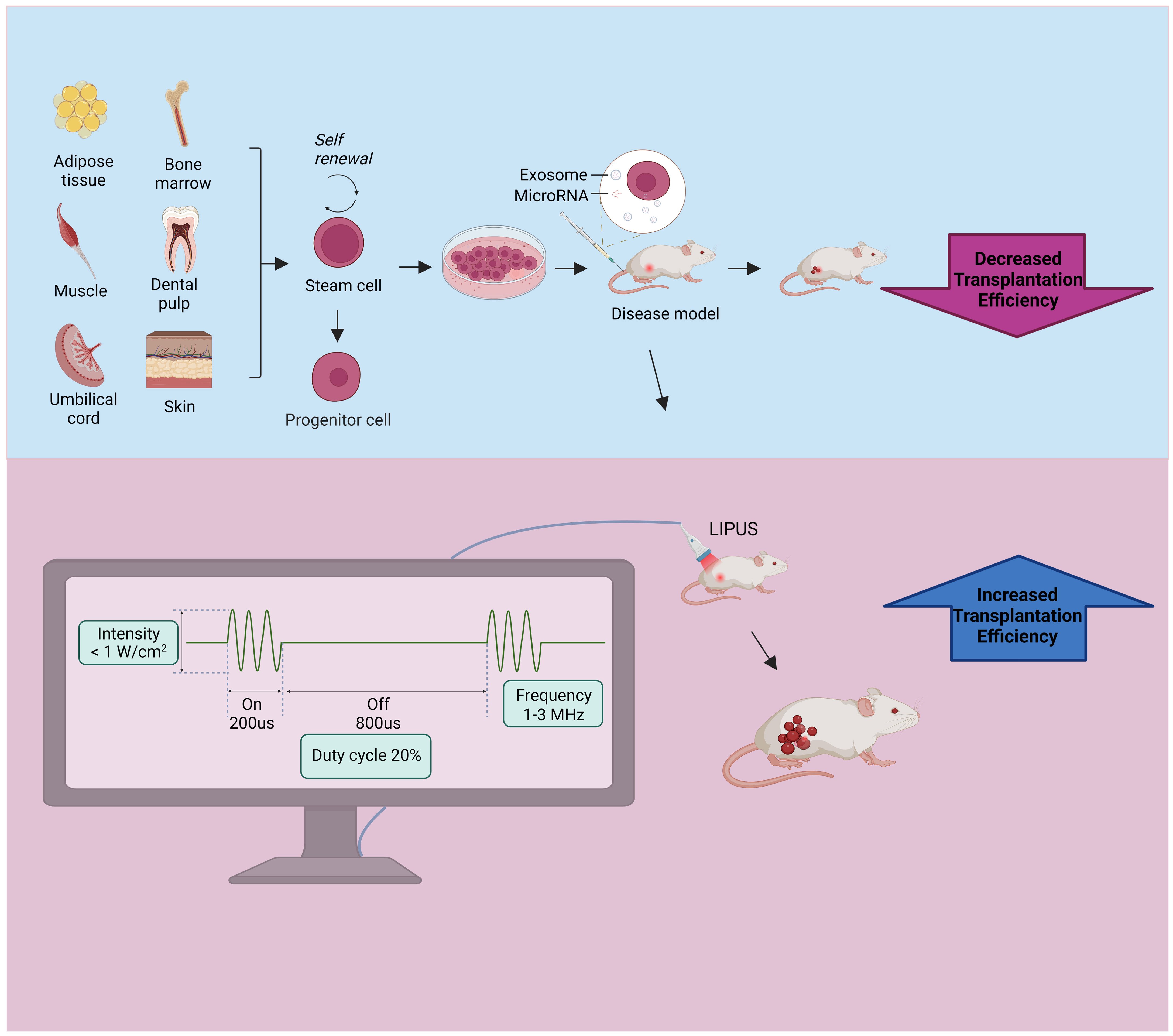
Figure 1 Stem cell and exosome injections are used to treat disease models, and further exposure to LIPUS can increase transplantation efficiency. The stem cell source is shown in the upper left, the lower left shows the common parameters of LIPUS, which features an intensity of less than 1 W/cm2, a frequency of 1-3 MHz, and a duty cycle of 20%. LIPUS, low-intensity pulsed ultrasound.
Herein, we review the research progress in the use of LIPUS for stem/progenitor cell exosomes from three aspects: (1) the effects of LIPUS on the biological functions of stem/progenitor cells and exosomes; (2) the signal transduction pathways in stem/progenitor cells and exosomes affected by LIPUS; and (3) the prospects of clinical applications of LIPUS combined with stem/progenitor cells and exosomes. This review aims to provide a deeper understanding of LIPUS and promote research and development in this field.
2 Effects of LIPUS on the biological functions of stem/progenitor cells
Studies have revealed that LIPUS can affect stem/progenitor cell differentiation, migration, and proliferation and exosome functions through mechanical stimulation (31–33). These biological functions are described in detail below and summarized in Figure 2 and Tables 1–4.
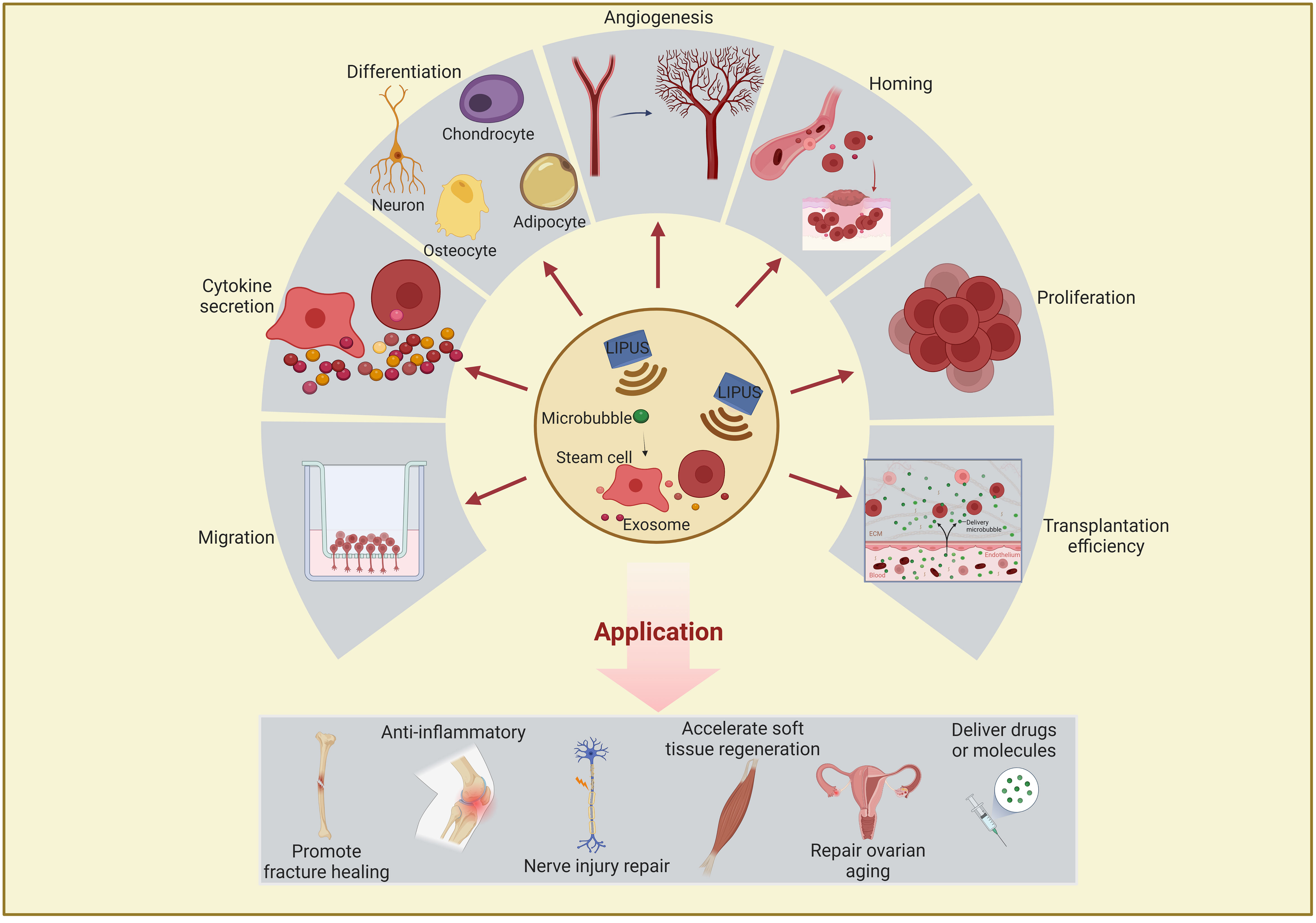
Figure 2 Schematic representation of the effects and applications of LIPUS or combined with microbubble on stem/progenitor cells and exosomes. LIPUS affects stem/progenitor cells and exosomes in the following areas: differentiation, proliferation, migration, homing, transplantation efficiency, cytokine secretion, and angiogenesis regulation. These functions are closely related to therapeutic applications in regenerative medicine and often used to promote fracture healing, anti-inflammation, nerve injury repair, soft tissue regeneration, and drug delivery. LIPUS, low-intensity pulsed ultrasound.
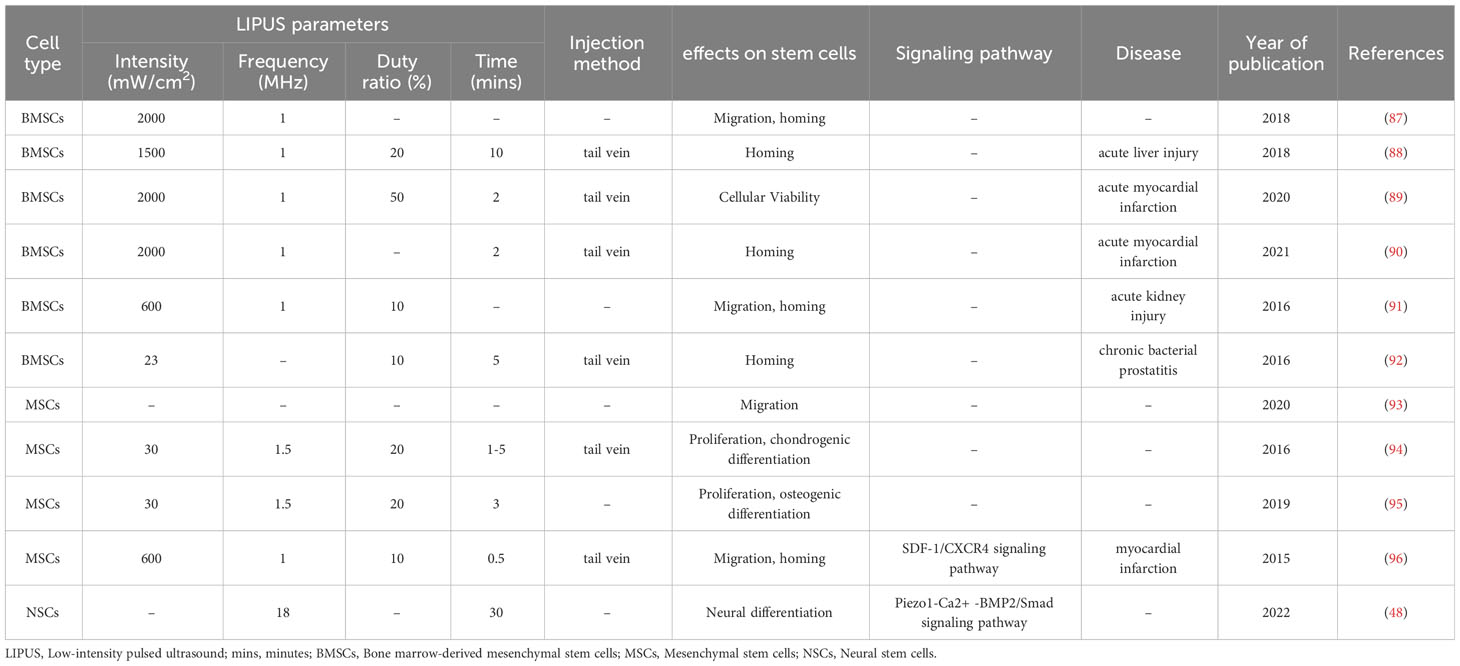
Table 3 The effect of LIPUS combined with microbubbles on the biological function of stem/progenitor cells.
2.1 Effects of LIPUS on the differentiation of stem/progenitor cells
2.1.1 Bone marrow-derived MSCs (BMSCs)
BMSCs are multipotent stem cells. An et al. (36) stimulated rat BMSCs with 100 mW/cm2 LIPUS and found increases in mineralized nodules in the extracellular matrix and the expression levels of osteogenic-related genes encoding the bone-bridging protein osteopontin (OPN), osteocalcin (OCN), bone morphogenetic protein-2 (BMP-2), alkaline phosphatase (ALP), Runt-related transcription factor 2 (Runx2), and type 1 collagen, suggesting that LIPUS promotes the osteogenic differentiation of BMSCs on titanium surfaces. Zhang et al. (39) found that LIPUS reverses the effects of radiation on the osteogenic differentiation of rat mandible-derived BMSCs. Yao et al. (104) reported a cyclic arginine-glycine-aspartate-modified nanobubble that could actively target BMSCs via integrin receptors and was used in combination with LIPUS to further enhance the osteogenic differentiation and bone formation of BMSCs induced by LIPUS. He et al. (38) used LIPUS to irradiate BMSCs with a frequency of 0.6 MHz, a duty cycle of 20%, and an intensity of 30 mW/cm2 and showed that LIPUS treatment promoted the differentiation efficiency and rate of BMSCs. Song et al. (40) and Li et al. (37) treated BMSCs with hepatocyte growth factor and found that LIPUS significantly upregulated the levels of the liver markers alpha-fetoprotein, cytokeratin 18, albumin, and glycogen in the BMSCs, indicating that LIPUS-induced BMSC differentiation towards hepatocytes. Autophagy is involved in regulating BMSC differentiation into chondrocytes. Xia et al. (34) and Wang et al. (35) found that LIPUS inhibited autophagy in BMSCs by regulating the integrin pathway and autophagy to promote the chondrogenic differentiation of BMSCs.
In summary, these studies suggest that LIPUS can regulate the differentiation of BMSCs towards osteogenic, hepatocytic, and chondrogenic lineages. The effects of LIPUS appear to be mediated through various cellular signaling pathways, gene expression, and autophagy. However, further research is needed to elucidate the mechanisms and potential applications of LIPUS-induced BMSC differentiation.
2.1.2 Adipose-derived stem cells (ADSCs)
ADSCs from subcutaneous fat are more readily available than BMSCs and have a greater capacity for proliferation and differentiation (105). Yue et al. (42) and Zhang et al. (44) isolated mouse and human ADSCs in vitro and stimulated the cells in vitro with a certain intensity of LIPUS. These authors performed protein blotting analysis of osteogenic-related genes and found that LIPUS promoted mineralized nodule formation and upregulated the osteogenic-related genes Runx2, OCN, ALP, and OPN, bone sialo protein, and heat shock protein (HSP) 70, HSP90, BMP-2, and BMP proteins. These results suggest that LIPUS stimulation can enhance the osteogenic differentiation of ADSCs by upregulating the expression of HSP70 and HSP90 and activating the BMP signaling pathway. Fu et al. (43) cultured mouse ADSCs in medium containing adipogenic reagents and stimulated them with 30 mW/cm2 LIPUS, and an analysis of adipogenic genes and proteins revealed that LIPUS upregulated the adipocyte lipogenic factors peroxisome proliferator-activated receptor γ and adiponectin, suggesting that LIPUS promotes the lipogenic differentiation of ADSCs.
2.1.3 Other stem/progenitor cells
Kusuyama et al. (46) extracted periodontal ligament stem cells (PDLSCs) from three healthy third molars and used LIPUS to intervene with BMP9 to induce PDLSC differentiation, which was found to be effective in promoting osteogenic differentiation under inflammatory conditions. LIPUS with an intensity of 90 mW/cm2 and a frequency of 1.5 MHz promoted the osteogenic differentiation of PDLSCSs both in vitro and in vivo (47, 50). Hu et al. (49) demonstrated that 50 mW/cm2 LIPUS promoted the endothelial differentiation and angiogenesis of PDLSCs under inflammatory or non-inflammatory conditions. Lee et al. (56) demonstrated for the first time that intense dual-frequency LIPUS exposure promotes neural stem/progenitor cell differentiation and growth factor utilization more than single-frequency LIPUS owing to the cavitation effect. Recently, they found that dual-frequency ultrasound regulates calcium channels via the downstream extracellular signal-regulated kinase 1/2 (ERK1/2) pathway, thereby promoting the differentiation of functional neural stem/progenitor cell neurons and the secretion of brain-derived neurotrophic factor (BDNF) (58). Furthermore, they found that dual-frequency ultrasound affects cancer stem cells (CSCs) by inducing CSC differentiation and reducing drug resistance and invasiveness; thus, it represents an alternative therapeutic option for treating human tumors (57). Wu et al. (53) found that LIPUS at an intensity of 69.3 mW/cm2 and a frequency of 1 MHz not only reduced astrocyte differentiation but also stimulated neuronal differentiation in vitro by modulating the Notch signaling pathway. Xia et al. (55) applied a rat sciatic nerve injury model and demonstrated that LIPUS may regulate the proliferation of induced pluripotent stem cell-derived neural crest stem cells (iPSC-NCSCs) via the focal adhesion kinase (FAK)-ERK1/2 signaling pathway and neural differentiation, suggesting that LIPUS may be a useful alternative approach in the field of neural regeneration. In 2022, Hua et al. (54) demonstrated for the first time that LIPUS promoted osteogenic differentiation in iPSC-derived MSCs, and the optimal parameters were an intensity of 40 mW/cm2, a frequency of 1.5 MHz, and a duty cycle of 50%.
2.2 Effects of LIPUS on the proliferation of stem/progenitor cells
2.2.1 MSCs
Huang et al. (68) found that LIPUS at 30 mW/cm2 upregulated the gene expression of proliferation-related proteins cyclin D1, c-Myc, and stromal cell-derived factor (SDF)-1α in ADSCs, thereby promoting their proliferation and extending their duration in an undifferentiated state. The proliferative effect of LIPUS on ADSCs has also been demonstrated by Min et al. (69). The effect of LIPUS on the proliferation of PDLSCs has been demonstrated in several studies. For example, Gao et al. (79, 80) found that LIPUS promoted the proliferation of MSCs of different origins by activating different MAPK pathways in an intensity- and cell-specific-dependent manner and revealed that these odontogenic MSCs all had Piezo membrane ion channels sensitive to mechanical stimulation, suggesting that the proliferation of LIPUS-stimulated odontogenic MSCs may involve piezoelectric regulation of ERK1/2 signaling. Han et al. (67) treated rat MSCs with LIPUS at a power of 60 mW/cm2 and a frequency of 1.5 MHz in combination with BMP-2 and assessed relevant proliferation and osteogenic indicators. They found that the combination more strongly promoted cell proliferation, and they validated the effect of the combination treatment in a rabbit distraction osteogenesis model. Ling et al. (75) isolated MSCs from human placental amnion and stimulated them with LIPUS at 30 mw/cm2 and found that LIPUS promoted the transition of MSCs from the G0/G1 phase to the S and G2/M phases and the proliferation of cells. Recently, Ren et al. (81) isolated MSCs from human umbilical cord and found that LIPUS was effective in stimulating cell proliferation and secretory activity in vitro; moreover, LIPUS-treated MSCs effectively reduced thyroid cell apoptosis and excessive autoimmune antibody accumulation, and improved thyroid function in a rat experimental autoimmune thyroiditis model.
2.2.2 Other stem/progenitor cells
Salgarella et al. (52) exposed C2C12 myogenic cells to different regimens of LIPUS and found that stimulation at 1 W/cm2 and 3 MHz maximized cell proliferation. Puts et al. (83) found that stimulation of mouse C2C12 mesenchymal precursors with LIPUS at 44.5 mW/cm2 and 3.6 MHz promoted cell proliferation. Detection of Yes-associated protein (YAP), which acts as a mechanosensor in C2C12 cell fate, revealed increased levels of YAP in the nucleus, whereas silencing of YAP expression eliminated the beneficial effects of LIPUS, suggesting that LIPUS enhances cell proliferation potential by regulating YAP function, which is essential for tissue regeneration processes. The installation of LIPUS modules in a bioreactor for large-scale production of 3D tissue structures based on embryonic stem cells promoted stem cell proliferation, osteogenic mineralized tissue formation, and cavity filling in a rabbit cranial defect model (84). Moghadam et al. (82) found that LIPUS with a mechanical index of 0.4 was effective in increasing the proliferation rate of spermatogonial stem cells within 7 days of culture while a higher mechanical index was detrimental to the cells, suggesting that LIPUS may be an option for the treatment of male oligospermia.
2.3 Effects of LIPUS on the migration of stem/progenitor cells
The effect of LIPUS on stem/progenitor cell migration has been confirmed by many studies. Wang et al. (77) isolated PDLSCs from premolar teeth and found that LIPUS at an intensity of 90 mW/cm2 promoted PDLSC migration based on wound healing and Transwell assays. Subsequently, they injected BMSCs via the tail vein, irradiated the defective areas in rats directly with 30 mW/cm2 LIPUS, and assessed alveolar bone regeneration using micro-computed tomography and found that LIPUS promoted periodontal alveolar bone regeneration based on the BMSCs, suggesting that LIPUS provided treatment by improving the homing and migration of BMSCs (60). Chen et al. (59) applied LIPUS at 30 mW/cm2 to stimulate BMSCs in vitro and then injected the BMSCs into rats with femoral defects followed by LIPUS intervention. Their study revealed that LIPUS promoted BMSC migration in vitro and in vivo, which was possibly associated with FAK-ERK1/2 pathway activation. In 2021, Xia et al. (65) also demonstrated that LIPUS promoted MSC migration using in vivo and in vitro experiments and found that LIPUS at 50 mW/cm2 activated autophagy, which could be inhibited by the use of autophagy inhibitors. In a rat knee OA model, the combined application of LIPUS and BMSCs significantly promoted OA cartilage repair, and this effect was attenuated by autophagy inhibitors, suggesting that LIPUS promotes BMSC migration and OA cartilage repair through autophagy regulation.
2.4 Effects of LIPUS on other biological functions of stem/progenitor cells
In addition to its effects on stem/progenitor cell differentiation, proliferation, and migration, LIPUS affects other biological functions, such as cell viability, cytokine secretion, and tissue homing. In recent years, the optimization of MSC homing and their secretion of therapeutic molecules have been explored, with LIPUS representing an emerging technology (106). One study revealed that LIPUS increased the survival rate of rat BMSCs by 19.57%, with a further 5.36% increase after the optimization of LIPUS parameters to 6.92 V, 1.02 MHz, and 7.3 min (61). LIPUS-stimulated BMSCs showed increased BDNF expression and cell viability in vitro, and better functional recovery was found in a rat spinal cord injury model treated with LIPUS combined with BMSCs, suggesting a possible application of LIPUS in the treatment of spinal cord injury (62). Song et al. (86) stimulated glioma stem cells (GSCs) with LIPUS at 300 mW/cm2 and 1.5 MHz, and showed that LIPUS resulted in diminished expression of GSC biomarkers and promoted GSC escape from G0 quiescence. They further performed experiments on nude mice and confirmed that LIPUS enhanced the sensitivity of GSCs to temozolomide both in vivo and in vitro. To promote angiogenic tissue regeneration, Kang et al. (71) prepared a scaffold composed of collagen and acetyl hyaluronate for coculture of human ADSCs and umbilical vein endothelial cells (HUVECs). Under LIPUS stimulation at 15.5 mW/cm2 in vitro, 1.85-fold and 1.5-fold increases in collagen and cell growth were observed, respectively. These effects were validated in a rat angiogenesis model, indicating that LIPUS can promote the angiogenic capacity and therapeutic potential of scaffold-based cocultured ADSCs/HUVECs.
2.5 Effects of LIPUS combined with microbubbles on stem/progenitor cells
Ultrasound targeted microbubble destruction (UTMD) is a promising technique for non-invasive targeted therapy. Microbubbles are an ultrasound imaging contrast agent that are injected to trigger microbubble cavitation combined with low frequency ultrasound to increase biofilm permeability in the vicinity of the microbubble, thereby facilitating the delivery of biomolecules to cells through blood vessels and achieving targeted therapeutic effects. In recent years, numerous studies have confirmed the effects of UTMD on stem/progenitor cells, particularly in improving their homing ability and transplantation efficiency, effectively addressing the limitations of inadequate homing of seed cell target organs and low transplantation success. Osborn et al. (95) used 30 mW/cm2 LIPUS in combination with microbubbles to intervene in hMSCs and found that it significantly enhanced the proliferation of cells in 3D-printed scaffolds. The effects of LIPUS combined with microbubbles on the homing, migration, and survival of stem/progenitor cells have also been demonstrated in many studies. In animal models of chronic bacterial prostatitis, acute liver injury, and acute myocardial infarction, LIPUS combined with microbubbles promoted the homing of BMSCs to damaged tissues, thus enhancing treatment efficacy (88–90, 92). Cui et al. (93) injected microbubbles and MSCs into rats with brain defects and subjected them to LIPUS transcranial irradiation of the cerebral ischemic zone. They found that the MSCs attached to and crossed the blood-brain barrier of the cerebral vasculature to reach the brain parenchyma to improve neurobehavioral functions. As an excellent delivery vehicle, microbubbles not only protect biomolecules from endogenous clearance during transport but also allow for controlled release in target organs through inertial cavitation and have promising applications.
3 Effects of LIPUS on exosomes
The effects of LIPUS on MSC-EXOs have also been demonstrated. MiRNAs are closely related to various physiological and pathological processes in humans, and the effect of LIPUS on miRNAs has been confirmed in numerous studies.
3.1 Effects of LIPUS on stem/progenitor cell-derived exosomes
Xia et al. (98) recently isolated MSCs from rat bone, cocultured them with OA chondrocytes, and intervened with an inhibitor of exosome release. They then stimulated the cells with LIPUS and detected exosome release and other autophagy markers by transmission electron microscopy. The results suggested that LIPUS promotes exosome release from MSCs via autophagy activation. In a rat knee OA model, they observed that LIPUS significantly enhanced the positive effect of MSCs on OA cartilage, which was significantly blocked by the exosome release inhibitor GW4869. In vitro and in vivo experiments showed that LIPUS enhances the therapeutic effect of MSCs in OA cartilage repair by a mechanism related to the promotion of autophagy-mediated exosome release. The study revealed that LIPUS did not impact the shape and size of exosomes, but it increased the release of exosomes from MSCs for the appropriate duration of stimulation. However, the authors did not investigate how LIPUS enhances autophagy in MSCs to increase the number of exosomes within them, and how LIPUS affects interactions and communication between MSCs and OA chondrocytes through the exosome release pathway to further promote cartilage repair. Future research should delve into these areas to provide valuable insights and guidance for utilizing LIPUS in cartilage repair applications.
Li et al. (103) found that LIPUS stimulation resulted in a 3.66-fold increase in exosome release from BMSCs, and they demonstrated the enhanced anti-inflammatory effects of LIPUS-stimulated BMSC-EXOs both in vivo and in vitro. BMSC RNA-Seq analysis revealed that LIPUS enhanced the cytoskeletal activity of BMSC, leading to increased secretion of bioactive factors, including exosomes. Small RNA-Seq analysis of LIPUS-stimulated BMSC-EXOs identified differentially expressed miRNAs mainly involved in cellular activities. LIPUS-stimulated BMSC up-/down-regulated genes may inhibit inflammation through different mechanisms, whereas miR-328-5p and miR-4876-3p up-regulated in LIPUS-stimulated exosomes target the MAPK signaling pathway to regulate inflammatory responses. However, these mechanisms require further experimental validation. Investigating the interactions between LIPUS-stimulated BMSC-derived exosomes and target cells such as immune cells or damaged tissues is essential to understand their anti-inflammatory effects. This study may involve exploring the uptake mechanisms and downstream signaling pathways activated by LIPUS-stimulated exosomes in recipient cells.
Liao et al. (97) stimulated BMSC-EXOs with LIPUS at 30 mW/cm2 and 1.5 MHz and found that it promotes the anti-inflammatory properties of BMSC-EXOs in OA. This enhancement effect further improved the synthesis and proliferation of extracellular matrix in chondrocytes, thereby promoting the regeneration of articular cartilage. This study suggests that LIPUS may exert its effects by inhibiting the nuclear factor-κB (NF-κB) pathway activated by IL-1β. However, further studies are needed to explore the specific targets, doses, and intrinsic mechanisms of LIPUS-mediated BMSC-EXOs. In addition, this study was conducted in 6-week-old pups. Young rats have a greater capacity for regeneration and their skeletal system is not yet fully developed. Therefore, it may be worth considering whether it is necessary to conduct experiments on adult animals. Li et al. (99) treated bone marrow dendritic cells (BMDCs) with LIPUS using the same parameters and incubated HUVECs with their secreted exosomes and found that the exosomes from the LIPUS-treated BMDCs were enriched in miR-16 and miR-21 and phagocytosed by the HUVECs, prevented tumor necrosis factor (TNF)-α-induced HUVEC activation, and downregulated the expression of cell adhesion molecules. These changes prevented TNFα-induced activation of the NF-κB signaling pathway and ultimately suppressed endothelial inflammation. However, this study was limited to cellular level investigations and further studies are needed to explore the conditions required for in vivo experiments. Moreover, investigating the effects of LIPUS stimulation on exosome uptake by HUVECs and the subsequent modulation of cell adhesion molecule expression to inhibit endothelial cell activation would deepen our understanding of the potential of LIPUS-stimulated exosomes for the treatment of relevant diseases.
3.2 Effect of LIPUS on miRNAs
MiRNAs are involved in various physiological and pathological processes in humans, and the effect of LIPUS on miRNAs has been confirmed in numerous studies. Costa et al. (100) first investigated the role of LIPUS as a promoter of miRNA expression, identified miR-31-5p as a LIPUS-inducible miRNA through bioinformatics analysis, and confirmed these findings by gain- and loss-of-function experiments. In vitro studies showed that miR-31-5p is capable of inducing hypoxia and cytoskeletal responses. Conversely, combined treatment with LIPUS and miR-31-5p inhibitors eliminated the hypoxic response, suggesting that miR-31-5p may be a LIPUS-mechanosensitive miRNA. Thus, LIPUS may be a novel therapeutic option to promote or eliminate the hypoxic response and cytoskeletal organization of hMSCs during bone regeneration. Chen et al. (102) stimulated periodontal ligament cells with LIPUS at 90 mW/cm2 and 1.5 MHz and found that it promoted the accumulation of cellular forkhead box O1 (FOXO1) protein and upregulated the expression of the osteogenic-related genes ALP and Runx2. MiR-182 downregulated FOXO1 through post-transcriptional regulation. Overexpression of miR-182 reversed LIPUS-induced FOXO1 expression and osteogenic differentiation, whereas LIPUS repressed miR-182 expression, which played a crucial role in promoting osteogenic differentiation, thus providing insights for applying LIPUS to periodontal bone defects.
Yang et al. (101) used the UTMD method to transfect miR-let7b into ovarian CSCs. Flow cytometry revealed that UTMD increased the transfection rate of miR-let-7b and the late apoptosis rate of CD133+ ovarian CSCs and decreased surface marker expression of CD133-expressing stem cells. These findings suggest that UTMD-mediated miRNA delivery may be a promising platform for the treatment of CSCs.
MiRNAs in exosomes are often mediators of the ultimate biological effects during LIPUS-promoted secretion. One study revealed that LIPUS remarkably increased the secretory and anti-inflammatory effects of BMSC-EXOs. RNA-sequencing analysis revealed significant upregulation of miR-328-5p and miR-487b-3p, thereby inhibiting the MAPK signaling pathway (103). This result suggests an important role for these two miRNAs in the mechanism underlying the enhancement of the anti-inflammatory effect, which provides insights into inflammation-related disease treatment.
4 Mechanisms involved in the biological effects of LIPUS on stem/progenitor cells and exosomes
The mechanisms by which LIPUS affects the biological functions of stem/progenitor cells and exosomes are complex and not fully understood, although they are likely related to its mechanical and cavitation effects. The mechanical vibrations produced by LIPUS allow for micromechanical interactions with cells, triggering a series of intracellular biochemical events, such as the release of cytokines and signaling molecules, which in turn alter the cellular microenvironment and lead to changes in signaling pathways that affect a range of cellular biological behaviors (27). Currently known signaling pathways regulated by LIPUS include the MAPK, ERK, phosphoinositide 3-kinases (PI3K)/protein kinase B, stromal cell-derived factor 1 (SDF-1)/C-X-C chemokine receptor type 4 (CXCR4), and NF-κB signaling pathways, as shown in Figure 3.
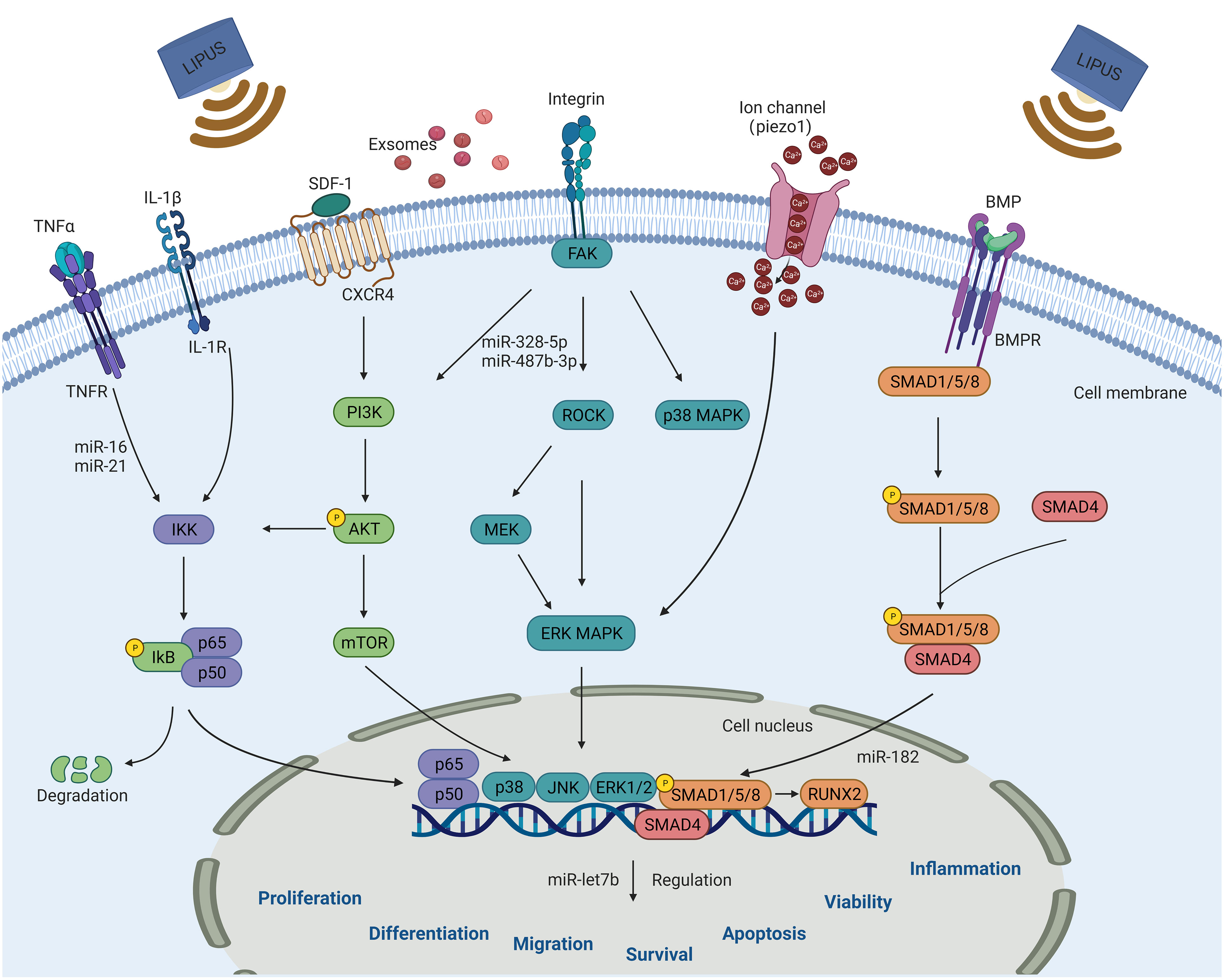
Figure 3 Schematic diagram of signaling pathways that can be activated by LIPUS in steam/progenitor cells for regulating cell biological functions, including proliferation, differentiation, migration, survival, apoptosis, vitality and inflammation. These pathways include the NF-κB, PI3K/AKT, MAPK, BMP/Smad, SDF-1/CXCR4, and Piezo-mediated signaling pathways. LIPUS, low-intensity pulsed ultrasound.
4.1 MAPK signaling pathway
The MAPK signaling pathway plays an important role in regulating physiopathological processes, such as cell growth, differentiation, stress, and inflammatory responses, and its three important downstream components are ERK, Jun amino-terminal kinase (JNK), and p38. Tabuchi et al. (63) exposed mouse BMSCs to 25 mW/cm2 LIPUS and found a significant increase in immediate early gene expression, whereas the use of MAPK/ERK inhibitors prevented LIPUS-induced expression of FOS and EGR1. Wang et al. (70) investigated the changes in cell viability and apoptosis of ADSCs after different doses of LIPUS irradiation and found that a high dose of LIPUS (210 mW/cm2) promoted ADSC apoptosis while a low dose (70 mW/cm2) increased ADSC viability. The use of p38 MAPK activity inhibitors rescued the apoptotic effect of high doses of LIPUS, suggesting that p38 MAPK plays a key role in the effect of LIPUS on ADSCs. The effect of LIPUS on stem/progenitor cell proliferation and its mechanisms have been demonstrated in several studies. Using different pathway blockers, Gao et al. (79, 80) found that LIPUS promoted the proliferation of different types of MSCs via different MAPK signaling pathways and activated JNK MAPK signaling in BMSCs, ERK1/2 signaling in dental pulp stem cells (DPSCs), and JNK and p38 signaling in PDLSCs. They later demonstrated that LIPUS-stimulated proliferation of DPSCs involved Piezo-mediated regulation of ERK1/2 MAPK signaling. Xia et al. (55) found that LIPUS promoted the proliferation and neural differentiation of iPSC-NCSCs in rats with sciatic nerve injury, probably via the FAK-ERK1/2 signaling pathway.
Lee et al. (58) recently found that dual-frequency ultrasound could precisely regulate calcium channels via the downstream ERK1/2 signaling pathway, thereby promoting neuronal differentiation and BDNF secretion in NSCs. Another study revealed that the combination of LIPUS and ADSCs applied to rats with diabetes mellitus and erectile dysfunction significantly improved the treatment efficacy and promoted vascular endothelial growth factor secretion by ADSCs via the Piezo-ERK pathway (74).
4.2 PI3K/AKT signaling pathway
LIPUS has been shown to promote the proliferation of amniotic MSCs. Ling et al. (75) demonstrated that this proliferative effect induced by LIPUS was significantly reduced when ERK1/2 and PI3K inhibitors were used, suggesting the involvement of the ERK1/2 and PI3K/AKT signaling pathways. Specifically, PI3K, which is generally activated by upstream signaling, triggers a signal upon activation that not only alters the AKT protein structure but also activates downstream substrate alterations. These changes ultimately regulate crucial cellular processes such as proliferation, differentiation, migration, and other functions. These findings were further supported by a study conducted by Xie et al. (33), confirming the important role of the ERK1/2 and PI3K/AKT signaling pathways in LIPUS-mediated cell proliferation. In addition, the PI3K/AKT signaling pathway is involved in the effect of LIPUS on the survival of CSCs. The PI3K/AKT/mTOR pathway is often active in glioblastoma, and suppressing the cell survival of glioblastoma CSCs plays a key role in tumor therapy. Tutak et al. (85) found that high doses of anticancer drugs combined with LIPUS inhibited mTOR expression and reduced cell viability, and they concluded that the combined action of the drugs and LIPUS was achieved via the PI3K/AKT/mTOR signaling pathway.
4.3 SDF-1/CXCR4 signaling pathway
In regenerative medicine, stem cells are recruited to areas of injury to perform their biological functions, and cell migration, homing, and chemotaxis play an important role in this process. The chemokine SDF-1 and its receptor CXCR4 are key factors in regulating stem cell migration. Wei et al. (32) demonstrated that LIPUS upregulated the expression of SDF-1 and CXCR4 in rat MSCs and promoted the migration of MSCs to fracture sites, which could be attenuated by pathway blockers. Li et al. (96) found that the combined application of LIPUS and microbubbles increased the expression of SDF-1 in ischemic myocardium and upregulated CXCR4 expression on the surface of MSCs in vitro and in vivo, suggesting that their combined application may promote MSC homing to repair ischemic myocardium via the SDF-1/CXCR4 signaling pathway. Ling et al. (107) recently found that LIPUS promoted SDF-1-induced migration of human amniotic MSCs via the SDF-1/CXCR4 axis. In the ovaries of rats with chemotherapy-induced premature ovarian insufficiency (POI), SDF-1 levels were significantly elevated and LIPUS-treated MSC homing to the ovaries increased, thereby reducing ovarian damage and improving ovarian function. Conversely, CXCR4 antagonists reduced the number of MSCs homing to POI ovaries, reducing their effectiveness in POI treatment.
4.4 NF-κB signaling pathway
The NF-κB signaling pathway plays an important role in immune regulation, inflammatory and stress responses, and apoptosis. Liao et al. (97) investigated the mechanism by which LIPUS stimulates BMSC-EXOs to promote cartilage regeneration in OA and found that LIPUS-mediated BMSC-EXOs promoted chondrocyte proliferation and extracellular matrix synthesis and inhibited interleukin-1β-induced activation of the NF-κB signaling pathway, thereby suppressing inflammation. However, this study was conducted in 6-week-old mice, which are known to possess enhanced regenerative capacities and have immature skeletal systems. Therefore, this study limits the direct applicability of the results to adult humans or skeletally mature individuals. Another study identified the role for LIPUS in immunomodulation and osteogenesis in hPDLSCs and showed that LIPUS enhanced the immunomodulation and osteogenic differentiation of hPDLSCs by inhibiting the NF-κB signaling pathway in a dose-dependent manner (48).
4.5 Other signaling pathways
In addition to the above pathways, various other pathways have been investigated in relation to the biological functions of LIPUS in stem/progenitor cells and exosomes, such as the Piezo1-Ca2+-BMP2/Smad (108) and Notch (53) signaling pathways. Although these signaling pathways have been elucidated, the mechanisms underlying their interaction and the most important pathways for disease treatment remain unclear. In the future, in vivo implantation models can be used to validate their involvement and investigate their mechanisms of action. For example, in vivo models could be utilized to assess the effects of LIPUS on activation of stem/progenitor cell signaling pathways and subsequent functional outcomes. In addition, investigating the crosstalk between these pathways and other known pathways involved in disease processes would help to elucidate their interconnected roles.
LIPUS stimulation modulates signaling and cellular processes, thereby influencing the synthesis and release of exosomes and the composition, quantity, and cargo of bioactive molecules. This enhances the role of exosomes in intercellular communication and disease therapy. However, further validation of its mechanisms and a comprehensive understanding of its role are needed as research in this area is still in its early stages. Although existing studies suggest that LIPUS has therapeutic potential in exosome production, more research is needed to fully elucidate its detailed mechanisms and clinical applications.
By gaining a comprehensive understanding of these signaling pathways and their interplay, researchers can identify key targets for therapeutic intervention and develop more effective strategies for the treatment of various diseases.
5 Prospects and limitations of LIPUS for clinical applications
LIPUS is an attractive modality for treatment because it is non-invasive and has many advantages, including no risk of infection or tissue damage and no known adverse effects; thus, it is increasingly being explored in basic research and clinical applications. LIPUS has achieved promising results in the treatment of disorders of the skeletal muscular system. Clinical studies have revealed that LIPUS contributes to accelerated bone healing (109), joint function restoration in OA (110), rapid motor recovery in patients with lumbar spondylolisthesis (111), and spinal fusion (112). In addition, studies in preclinical animal models have shown that LIPUS can promote recovery and improve functional outcomes in PNI (30) and holds great potential in the prevention and recovery of traumatic encephalopathy (113–115). Du et al. (116) constructed a first-order characteristic model based on an apparent diffusion coefficient map to evaluate the therapeutic effect of LIPUS on acute craniocerebral injury, and it may be valuable in predicting the therapeutic effect of LIPUS in clinical practice.
The effects of LIPUS on stem cell proliferation, differentiation, migration and the associated signaling pathways have been previously discussed. When combined with stem cells, it can alleviate the problems of insufficient stem cell sources, maturity differentiation, low transplantation success, and insufficient target organ homing. Encouragingly, a large body of evidence suggests that LIPUS combined with stem cell and exosome therapies has positive effects in skeletal muscle system disorders and neurological injury and promising potential in suppressing inflammation and ovarian ageing recovery. In addition, LIPUS combined with microbubbles is often used for drug delivery or gene transfer, where small molecules such as miRNAs are loaded on microbubbles and act on the target organ under the effect of LIPUS cavitation. Compared to conventional methods, LIPUS cavitation can achieve superior therapeutic effects, such as increased sensitivity to chemotherapeutic agents and reduced resistance of CSCs.
Studies have shown satisfactory synergistic effects of LIPUS on stem/progenitor cells, although such methods are still in their infancy. A study registered in the Chinese Clinical Trials Registry in 2019 represented the first clinical study to comprehensively investigate the safety and efficacy of LIPUS for stem cell therapy in OA patients, thus representing the first breakthrough in translating combined LIPUS and stem cell therapy from animal models to clinical practice applications (117). Based on present studies, we recognize that the impact of different LIPUS parameters varies and that the transition from basic to clinical requires additional parameter optimization. Hopefully, a standard report of parameters will be established to help researchers explore this field in depth. Extensive further study is required for basic science research on LIPUS to move towards large-scale clinical dissemination and application.
6 Conclusion and future perspectives
New technologies to enhance the efficacy of stem/progenitor cells are of increasing interest to researchers. The effects of LIPUS on stem/progenitor cells and exosomes and the signaling pathways related to the mechanism have been confirmed by numerous studies. The combination of LIPUS with stem/progenitor cells and exosomes may improve the efficacy of treatment for certain diseases, although it is still in its preliminary stages. This review focused on basic experiments, most of which were cellular experiments. For the translation from basic research to clinical application, the number of animal studies should be increased in the future, with a shift from small animal models to large animal models and then to clinical trials. It is worth noting that in most animal experiments involving the use of stem cells and their extracellular vesicles for therapeutic purposes, tail vein injection or invasive local injections are commonly employed. However, we advocate for non-invasive local injections guided by ultrasound, which offer higher accuracy, safety, and reduced trauma. In addition, different LIPUS parameters have different effects on stem/progenitor cells and exosomes; therefore, identify appropriate therapeutic parameters must be identified. Hopefully, a standardized parameter reporting system will be established to promote more in-depth exploration. Efforts must be made to develop a convenient and inexpensive method to address the bottleneck of low graft success rates in the field of stem cell transplantation. In the future, we hope that more research results will support the positive therapeutic effect of LIPUS combined with stem/progenitor cells and exosomes and the popularization of this method in clinical practice. LIPUS is expected to be an important auxiliary tool to improve the therapeutic effect of stem/progenitor cells and exosomes.
Author contributions
Y-FH: Writing – original draft, Writing – review & editing. X-LW: Writing – original draft. S-PD: Writing – review & editing. Y-LW: Writing – original draft. Q-QH: Writing – original draft. SL: Writing – review & editing. G-RL: Writing – review & editing.
Funding
The author(s) declare financial support was received for the research, authorship, and/or publication of this article. This work was supported by Science and Technology Bureau of Quanzhou (grant number 2020CT003).
Acknowledgments
We would like to thank Editage (www.editage.cn) for English language professional editing of this manuscript.
Conflict of interest
The authors declare that the research was conducted in the absence of any commercial or financial relationships that could be construed as a potential conflict of interest.
Publisher’s note
All claims expressed in this article are solely those of the authors and do not necessarily represent those of their affiliated organizations, or those of the publisher, the editors and the reviewers. Any product that may be evaluated in this article, or claim that may be made by its manufacturer, is not guaranteed or endorsed by the publisher.
Abbreviations
ADSCs, adipose-derived stem cells; AKT, protein kinase B; ALP, alkaline phosphatase; BDNF, brain-derived neurotrophic factor; BMDCs, bone marrow dendritic cells; BMP-2, bone morphogenetic protein-2; BMSCs, bone marrow- derived mesenchymal stem cells; CSCs, cancer stem cells; CXCR4, C-X-C chemokine receptor type 4; ERK1/2, extracellular signal-regulated kinases 1/2; GSCs, glioma stem cells; HSP, heat shock protein; HUVEC, human umbilical vein endothelial cells; iPSC-NCSCs, pluripotent stem cell-derived neural crest stem cells; JNK, Jun N-terminal kinase; LIPUS, low-intensity pulsed ultrasound; MSC-EXO, mesenchymal stem cell derived exosome; MSCs, mesenchymal stem cells; NF-κB, nuclear factor-κB; OA, osteoarthritis; OCN, osteocalcin; OPN, osteopontin; PDLSCs, periodontal ligament stem cells; PI3K, phosphoinositide 3-kinases; PNI, peripheral nerve injury; POI, premature ovarian insufficiency; Runx2, Runt -related transcription factor 2; SDF, stromal cell derived factor; TNF, tumor necrosis factor; UTMD, ultrasonic targeted microbubble destruction; YAP, Yes-associated protein.
References
1. Navas A, Magaña-Guerrero FS, Domínguez-López A, Chávez-García C, Partido G, Graue-Hernández EO, et al. Anti-inflammatory and anti-fibrotic effects of human amniotic membrane mesenchymal stem cells and their potential in corneal repair. Stem Cells Transl Med (2018) 7(12):906–17. doi: 10.1002/sctm.18-0042
2. Gao Q, Wang L, Wang S, Huang B, Jing Y, Su J. Bone marrow mesenchymal stromal cells: identification, classification, and differentiation. Front Cell Dev Biol (2021) 9:9787118. doi: 10.3389/fcell.2021.787118
3. Wang YH, Chen EQ. Mesenchymal stem cell therapy in acute liver failure. Gut Liver (2023) 17(5):674–83. doi: 10.5009/gnl220417
4. Wang Y, Chen X, Cao W, Shi Y. Plasticity of mesenchymal stem cells in immunomodulation: pathological and therapeutic implications. Nat Immunol (2014) 15(11):1009–16. doi: 10.1038/ni.3002
5. Xu Z, Cai Y, Liu W, Kang F, He Q, Hong Q, et al. Downregulated exosome-associated gene FGF9 as a novel diagnostic and prognostic target for ovarian cancer and its underlying roles in immune regulation. Aging (Albany NY) (2022) 14(4):1822–35. doi: 10.18632/aging.203905
6. Dimik M, Abeysinghe P, Logan J, Mitchell M. The exosome: a review of current therapeutic roles and capabilities in human reproduction. Drug Delivery Transl Res (2023) 13(2):473–502. doi: 10.1007/s13346-022-01225-3
7. Yang K, Zhou Q, Qiao B, Shao B, Hu S, Wang G, et al. Exosome-derived noncoding RNAs: Function, mechanism, and application in tumor angiogenesis. Mol Ther Nucleic Acids (2022) 27:27983–997. doi: 10.1016/j.omtn.2022.01.009
8. Lee SY, Lee JW. 3D spheroid cultures of stem cells and exosome applications for cartilage repair. Life (Basel) (2022) 12(7):939. doi: 10.3390/life12070939
9. Zhang L, Lin Y, Bai W, Sun L, Tian M. Human umbilical cord mesenchymal stem cell-derived exosome suppresses programmed cell death in traumatic brain injury via PINK1/Parkin-mediated mitophagy. CNS Neurosci Ther (2023) 29(8):2236–58. doi: 10.1111/cns.14159
10. Han J, Zhang Y, Ge P, Dakal TC, Wen H, Tang S, et al. Exosome-derived CIRP: An amplifier of inflammatory diseases. Front Immunol (2023) 14:1066721. doi: 10.3389/fimmu.2023.1066721
11. Pozniak T, Shcharbin D, Bryszewska M. Circulating microRNAs in medicine. Int J Mol Sci (2022) 23(7):3996. doi: 10.3390/ijms23073996
12. Kim HJ, Kim G, Lee J, Lee Y, Kim JH. Secretome of stem cells: roles of extracellular vesicles in diseases, stemness, differentiation, and reprogramming. Tissue Eng Regener Med (2022) 19(1):19–33. doi: 10.1007/s13770-021-00406-4
13. Mani S, Gurusamy N, Ulaganathan T, Paluck AJ, Ramalingam S, Rajasingh J. Therapeutic potentials of stem cell-derived exosomes in cardiovascular diseases. Exp Biol Med (Maywood) (2023) 248(5):15353702231151960. doi: 10.1177/15353702231151960
14. Hoang DM, Pham PT, Bach TQ, Ngo ATL, Nguyen QT, Phan TTK, et al. Stem cell-based therapy for human diseases. Signal Transduct Target Ther (2022) 7(1):272. doi: 10.1038/s41392-022-01134-4
15. LR T, Sánchez-Abarca LI, Muntión S, Preciado S, Puig N, López-Ruano G, et al. MSC surface markers (CD44, CD73, and CD90) can identify human MSC-derived extracellular vesicles by conventional flow cytometry. Cell Commun Signal (2016) 14:142. doi: 10.1186/s12964-015-0124-8
16. Zou XY, Yu Y, Lin S, Zhong L, Sun J, Zhang G, et al. Comprehensive miRNA analysis of human umbilical cord-derived mesenchymal stromal cells and extracellular vesicles. Kidney Blood Press Res (2018) 43(1):152–61. doi: 10.1159/000487369
17. Yaghoubi Y, Movassaghpour A, Zamani M, Talebi M, Mehdizadeh A, Yousefi M. Human umbilical cord mesenchymal stem cells derived-exosomes in diseases treatment. Life Sci (2019) 233:233116733. doi: 10.1016/j.lfs.2019.116733
18. Hussein GM, Mohammed SM, Faris M, Mohammed A, Kadhim MJ, Awadh SA, et al. Find new channel for overcoming chemoresistance in cancers: Role of stem cells-derived exosomal microRNAs. Int J Biol Macromol (2022) 219:219530–537. doi: 10.1016/j.ijbiomac.2022.07.253
19. Leñero C, Kaplan LD, Best TM, Kouroupis D. CD146+ Endometrial-derived mesenchymal stem/stromal cell subpopulation possesses exosomal secretomes with strong immunomodulatory miRNA attributes. Cells (2022) 11(24):4002. doi: 10.3390/cells11244002
20. Tang Y, Sun Y, Zeng J, Yuan B, Zhao Y, Geng X, et al. Exosomal miR-140-5p inhibits osteogenesis by targeting IGF1R and regulating the mTOR pathway in ossification of the posterior longitudinal ligament. J Nanobiotechnol (2022) 20(1):452. doi: 10.1186/s12951-022-01655-8
21. Liu H, Deng S, Han L, Ren Y, Gu J, He L, et al. Mesenchymal stem cells, exosomes and exosome-mimics as smart drug carriers for targeted cancer therapy. Colloids Surf B Biointerfaces (2022) 209(Pt 1):112163. doi: 10.1016/j.colsurfb.2021.112163
22. Guo ZY, Tang Y, Cheng YC. Exosomes as targeted delivery drug system: advances in exosome loading, surface functionalization and potential for clinical application. Curr Drug Delivery (2022). doi: 10.2174/1567201819666220613150814
23. Lou S, Duan Y, Nie H, Cui X, Du J, Yao Y. Mesenchymal stem cells: Biological characteristics and application in disease therapy. Biochimie (2021) 185:1859–21. doi: 10.1016/j.biochi.2021.03.003
24. Berebichez-Fridman R, Montero-Olvera PR. Sources and clinical applications of mesenchymal stem cells: state-of-the-art review. Sultan Qaboos Univ Med J (2018) 18(3):e264–77. doi: 10.18295/squmj.2018.18.03.002
25. Bellin G, Gardin C, Ferroni L, Chachques JC, Rogante M, Mitrečić D, et al. Exosome in cardiovascular diseases: A complex world full of hope. Cells (2019) 8(2):166. doi: 10.3390/cells8020166
26. Searle HK, Lewis SR, Coyle C, Welch M, Griffin XL. Ultrasound and shockwave therapy for acute fractures in adults. Cochrane Database Syst Rev (2023) 3(3):Cd008579. doi: 10.1002/14651858.CD008579.pub4
27. Miller DL, Smith NB, Bailey MR, Czarnota GJ, Hynynen K, Makin IR. Overview of therapeutic ultrasound applications and safety considerations. J Ultrasound Med (2012) 31(4):623–34. doi: 10.7863/jum.2012.31.4.623
28. Jiang X, Savchenko O, Li Y, Qi S, Yang T, Zhang W, et al. A review of low-intensity pulsed ultrasound for therapeutic applications. IEEE Trans BioMed Eng (2019) 66(10):2704–18. doi: 10.1109/TBME.2018.2889669
29. Jia L, Wang Y, Chen J, Chen W. Efficacy of focused low-intensity pulsed ultrasound therapy for the management of knee osteoarthritis: a randomized, double blind, placebo-controlled trial. Sci Rep (2016) 6:635453. doi: 10.1038/srep35453
30. Liu X, Zou D, Hu Y, He Y, Lu J. Research progress of low-intensity pulsed ultrasound in the repair of peripheral nerve injury. Tissue Eng Part B Rev (2023) 29(4):414–28. doi: 10.1089/ten.TEB.2022.0194
31. Amini A, Chien S, Bayat M. Impact of ultrasound therapy on stem cell differentiation - A systematic review. Curr Stem Cell Res Ther (2020) 15(5):462–72. doi: 10.2174/1574888X15666200225124934
32. Wei FY, Leung KS, Li G, Qin J, Chow SK, Huang S, et al. Low intensity pulsed ultrasound enhanced mesenchymal stem cell recruitment through stromal derived factor-1 signaling in fracture healing. PloS One (2014) 9(9):e106722. doi: 10.1371/journal.pone.0106722
33. Xie S, Jiang X, Wang R, Xie S, Hua Y, Zhou S, et al. Low-intensity pulsed ultrasound promotes the proliferation of human bone mesenchymal stem cells by activating PI3K/AKt signaling pathways. J Cell Biochem (2019) 120(9):15823–33. doi: 10.1002/jcb.28853
34. Xia P, Wang X, Qu Y, Lin Q, Cheng K, Gao M, et al. TGF-beta1-induced chondrogenesis of bone marrow mesenchymal stem cells is promoted by low-intensity pulsed ultrasound through the integrin-mTOR signaling pathway. Stem Cell Res Ther (2017) 8(1):281. doi: 10.1186/s13287-017-0733-9
35. Wang X, Lin Q, Zhang T, Wang X, Cheng K, Gao M, et al. Low-intensity pulsed ultrasound promotes chondrogenesis of mesenchymal stem cells via regulation of autophagy. Stem Cell Res Ther (2019) 10(1):41. doi: 10.1186/s13287-019-1142-z
36. An Y, Song Y, Wang Z, Wang J, Wu G, Zhu G, et al. Effect of low-intensity pulsed ultrasound on the biological behaviors of bone marrow mesenchymal stem cells on titanium with different surface topographies. Am J Transl Res (2018) 10(1):67–76.
37. Li F, Liu Y, Cai Y, Li X, Bai M, Sun T, et al. Ultrasound irradiation combined with hepatocyte growth factor accelerate the hepatic differentiation of human bone marrow mesenchymal stem cells. Ultrasound Med Biol (2018) 44(5):1044–52. doi: 10.1016/j.ultrasmedbio.2018.01.005
38. He R, Chen J, Jiang J, Liu B, Liang D, Zhou W, et al. Synergies of accelerating differentiation of bone marrow mesenchymal stem cells induced by low intensity pulsed ultrasound, osteogenic and endothelial inductive agent. Artif Cells Nanomed Biotechnol (2019) 47(1):674–84. doi: 10.1080/21691401.2019.1576704
39. Zhang R, Wang Z, Zhu G, Wu G, Guo Q, Liu H, et al. Low-intensity pulsed ultrasound modulates rhoA/ROCK signaling of rat mandibular bone marrow mesenchymal stem cells to rescue their damaged cytoskeletal organization and cell biological function induced by radiation. Stem Cells Int (2020) 2020:20208863577. doi: 10.1155/2020/8863577
40. Song L, Constanthin PE, Sun T, Li X, Xia Z, An L, et al. Long-term production of glycogen and hepatic-derived, cell-invasion-promoting chemokines by ultrasound-driven hepatic-differentiated human bone marrow mesenchymal stem cells. Radiat Res (2020) 193(4):394–405. doi: 10.1667/RR15421.1
41. Jiang T, Xu T, Gu F, Chen A, Xiao Z, Zhang D. Osteogenic effect of low intensity pulsed ultrasound on rat adipose-derived stem cells in vitro. J Huazhong Univ Sci Technolog Med Sci (2012) 32(1):75–81. doi: 10.1007/s11596-012-0013-y
42. Yue Y, Yang X, Wei X, Chen J, Fu N, Fu Y, et al. Osteogenic differentiation of adipose-derived stem cells prompted by low-intensity pulsed ultrasound. Cell Prolif (2013) 46(3):320–7. doi: 10.1111/cpr.12035
43. Fu N, Yang X, Ba K, Fu Y, Wei X, Yue Y, et al. Low-intensity pulsed ultrasound induced enhanced adipogenesis of adipose-derived stem cells. Cell Prolif (2013) 46(3):312–9. doi: 10.1111/cpr.12031
44. Zhang Z, Ma Y, Guo S, He Y, Bai G, Zhang W. Low-intensity pulsed ultrasound stimulation facilitates in vitro osteogenic differentiation of human adipose-derived stem cells via up-regulation of heat shock protein (HSP)70, HSP90, and bone morphogenetic protein (BMP) signaling pathway. Biosci Rep (2018) 38(3):BSR20180087. doi: 10.1042/BSR20180087
45. Hu B, Zhang Y, Zhou J, Li J, Deng F, Wang Z, et al. Low-intensity pulsed ultrasound stimulation facilitates osteogenic differentiation of human periodontal ligament cells. PloS One (2014) 9(4):e95168. doi: 10.1371/journal.pone.0095168
46. Kusuyama J, Nakamura T, Ohnishi T, Eiraku N, Noguchi K, Matsuguchi T. Low-intensity pulsed ultrasound (LIPUS) promotes BMP9-induced osteogenesis and suppresses inflammatory responses in human periodontal ligament-derived stem cells. J Orthop Trauma (2017) 31(7):S4. doi: 10.1097/01.bot.0000520897.92470.70
47. Li H, Zhou J, Zhu M, Ying S, Li L, Chen D, et al. Low-intensity pulsed ultrasound promotes the formation of periodontal ligament stem cell sheets and ectopic periodontal tissue regeneration. J BioMed Mater Res A (2021) 109(7):1101–12. doi: 10.1002/jbm.a.37102
48. Lin H, Wang Q, Quan C, Ren Q, He W, Xiao H. Low-intensity pulsed ultrasound enhances immunomodulation and facilitates osteogenesis of human periodontal ligament stem cells by inhibiting the NF-kappaB pathway. Cell Tissue Bank (2022) 24(1):1–14. doi: 10.1007/s10561-022-10010-y
49. Hu R, Yang ZY, Li YH, Zhou Z. LIPUS promotes endothelial differentiation and angiogenesis of periodontal ligament stem cells by activating piezo1. Int J Stem Cells (2022) 15(4):372–83. doi: 10.15283/ijsc22024
50. Li H, Deng Y, Tan M, Feng G, Kuang Y, Li J, et al. Low-intensity pulsed ultrasound upregulates osteogenesis under inflammatory conditions in periodontal ligament stem cells through unfolded protein response. Stem Cell Res Ther (2020) 11(1):215. doi: 10.1186/s13287-020-01732-5
51. El-Bialy T, Alhadlaq A, Wong B, Kucharski C. Ultrasound effect on neural differentiation of gingival stem/progenitor cells. Ann BioMed Eng (2014) 42(7):1406–12. doi: 10.1007/s10439-014-1013-9
52. Salgarella AR, Cafarelli A, Ricotti L, Capineri L, Dario P, Menciassi A. Optimal ultrasound exposure conditions for maximizing C2C12 muscle cell proliferation and differentiation. Ultrasound Med Biol (2017) 43(7):1452–65. doi: 10.1016/j.ultrasmedbio.2017.03.003
53. Wu Y, Gao Q, Zhu S, Wu Q, Zhu R, Zhong H, et al. Low-intensity pulsed ultrasound regulates proliferation and differentiation of neural stem cells through notch signaling pathway. Biochem Biophys Res Commun (2020) 526(3):793–8. doi: 10.1016/j.bbrc.2020.03.142
54. Hua Z, Li S, Liu Q, Yu M, Liao M, Zhang H, et al. Low-Intensity Pulsed Ultrasound Promotes Osteogenic Potential of iPSC-Derived MSCs but Fails to Simplify the iPSC-EB-MSC Differentiation Process. Front Bioeng Biotechnol (2022) 10:10841778. doi: 10.3389/fbioe.2022.841778
55. Xia B, Chen G, Zou Y, Yang L, Pan J, Lv Y. Low-intensity pulsed ultrasound combination with induced pluripotent stem cells-derived neural crest stem cells and growth differentiation factor 5 promotes sciatic nerve regeneration and functional recovery. J Tissue Eng Regener Med (2019) 13(4):625–36. doi: 10.1002/term.2823
56. Lee IC, Wu HJ, Liu HL. Dual-frequency ultrasound induces neural stem/progenitor cell differentiation and growth factor utilization by enhancing stable cavitation. ACS Chem Neurosci (2019) 10(3):1452–61. doi: 10.1021/acschemneuro.8b00483
57. Lee IC, Fadera S, Liu HL. Strategy of differentiation therapy: effect of dual-frequency ultrasound on the induction of liver cancer stem-like cells on a HA-based multilayer film system. J Mater Chem B (2019) 7(35):5401–11. doi: 10.1039/c9tb01120j
58. Lee IC, Lin YC, Liu HL, Liu NC. Dual-frequency ultrasound enhances functional neuron differentiation from neural stem cells by modulating Ca(2+) dynamics and the ERK1/2 signaling pathway. J Cell Physiol (2023) 238(1):137–50. doi: 10.1002/jcp.30911
59. Chen J, Jiang J, Wang W, Qin J, Chen J, Chen W, et al. Low intensity pulsed ultrasound promotes the migration of bone marrow- derived mesenchymal stem cells via activating FAK-ERK1/2 signalling pathway. Artif Cells Nanomed Biotechnol (2019) 47(1):3603–13. doi: 10.1080/21691401.2019.1657878
60. Wang Y, Li J, Zhou J, Qiu Y, Song J. Low-intensity pulsed ultrasound enhances bone marrow-derived stem cells-based periodontal regenerative therapies. Ultrasonics (2022) 121:121106678. doi: 10.1016/j.ultras.2021.106678
61. Yang X, Wu Y, Li J, Yin W, An Y, Wang Y, et al. A pilot study of parameter-optimized low-intensity pulsed ultrasound stimulation for the bone marrow mesenchymal stem cells viability improvement. Comput Math Methods Med (2019) 2019:20198386024. doi: 10.1155/2019/8386024
62. Ning GZ, Song WY, Xu H, Zhu RS, Wu QL, Wu Y, et al. Bone marrow mesenchymal stem cells stimulated with low-intensity pulsed ultrasound: Better choice of transplantation treatment for spinal cord injury: Treatment for SCI by LIPUS-BMSCs transplantation. CNS Neurosci Ther (2019) 25(4):496–508. doi: 10.1111/cns.13071
63. Tabuchi Y, Hasegawa H, Suzuki N, Furusawa Y, Hirano T, Nagaoka R, et al. Low-intensity pulsed ultrasound promotes the expression of immediate-early genes in mouse ST2 bone marrow stromal cells. J Med Ultrasonics (2020) 47(2):193–201. doi: 10.1007/s10396-020-01007-9
64. Aliabouzar M, Lee SJ, Zhou X, Zhang GL, Sarkar K. Effects of scaffold microstructure and low intensity pulsed ultrasound on chondrogenic differentiation of human mesenchymal stem cells. Biotechnol Bioeng (2018) 115(2):495–506. doi: 10.1002/bit.26480
65. Xia P, Wang X, Wang Q, Wang X, Lin Q, Cheng K, et al. Low-intensity pulsed ultrasound promotes autophagy-mediated migration of mesenchymal stem cells and cartilage repair. Cell Transplant (2021) 30:963689720986142. doi: 10.1177/0963689720986142
66. Lorsung RM, Rosenblatt RB, Cohen G, Frank JA, Burks SR. Acoustic radiation or cavitation forces from therapeutic ultrasound generate prostaglandins and increase mesenchymal stromal cell homing to murine muscle. Front Bioeng Biotechnol (2020) 8:870. doi: 10.3389/fbioe.2020.00870
67. Han JJ, Yang HJ, Hwang SJ. Enhanced bone regeneration by bone morphogenetic protein-2 after pretreatment with low-intensity pulsed ultrasound in distraction osteogenesis. Tissue Eng Regener Med (2022) 19(4):871–86. doi: 10.1007/s13770-022-00457-1
68. Huang D, Gao Y, Wang S, Zhang W, Cao H, Zheng L, et al. Impact of low-intensity pulsed ultrasound on transcription and metabolite compositions in proliferation and functionalization of human adipose-derived mesenchymal stromal cells. Sci Rep (2020) 10(1):13690. doi: 10.1038/s41598-020-69430-z
69. Min S, Byeon Y, Kim M, Lee Y, Lee SH, Lee Y, et al. Production enhancement of human adipose-derived mesenchymal stem cells by low-intensity ultrasound stimulation. Sci Rep (2022) 12(1):22041. doi: 10.1038/s41598-022-24742-0
70. Wang Y, Jiang L, Xu T, Su Z, Guo X, Tu J, et al. p38 MAPK signaling is a key mediator for low-intensity pulsed ultrasound (LIPUS) in cultured human omental adipose-derived mesenchymal stem cells. Am J Transl Res (2019) 11(1):418–29.
71. Kang PL, Huang HH, Chen T, Ju KC, Kuo SM. Angiogenesis-promoting effect of LIPUS on hADSCs and HUVECs cultured on collagen/hyaluronan scaffolds. Mater Sci Eng C Mater Biol Appl (2019) 102:10222–33. doi: 10.1016/j.msec.2019.04.045
72. Yue Y, Yang X, Zhang L, Xiao X, Nabar NR, Lin Y, et al. Low-intensity pulsed ultrasound upregulates pro-myelination indicators of Schwann cells enhanced by co-culture with adipose-derived stem cells. Cell Prolif (2016) 49(6):720–8. doi: 10.1111/cpr.12298
73. Chen C, Zhang T, Liu F, Qu J, Chen Y, Fan S, et al. Effect of low-intensity pulsed ultrasound after autologous adipose-derived stromal cell transplantation for bone-tendon healing in a rabbit model. Am J Sports Med (2019) 47(4):942–53. doi: 10.1177/0363546518820324
74. Liu S, Jiang C, Hu J, Chen H, Han B, Xia S. Low-intensity pulsed ultrasound enhanced adipose-derived stem cell-mediated angiogenesis in the treatment of diabetic erectile dysfunction through the piezo-ERK-VEGF axis. Stem Cells Int (2022) 2022:20226202842. doi: 10.1155/2022/6202842
75. Ling L, Wei T, He L, Wang Y, Wang Y, Feng X, et al. Low-intensity pulsed ultrasound activates ERK1/2 and PI3K-Akt signalling pathways and promotes the proliferation of human amnion-derived mesenchymal stem cells. Cell Prolif (2017) 50(6):e12383. doi: 10.1111/cpr.12383
76. Ling L, Feng X, Wei T, Wang Y, Wang Y, Zhang W, et al. Effects of low-intensity pulsed ultrasound (LIPUS)-pretreated human amnion-derived mesenchymal stem cell (hAD-MSC) transplantation on primary ovarian insufficiency in rats. Stem Cell Res Ther (2017) 8(1):283. doi: 10.1186/s13287-017-0739-3
77. Wang Y, Li J, Qiu Y, Hu B, Chen J, Fu T, et al. Low−intensity pulsed ultrasound promotes periodontal ligament stem cell migration through TWIST1−mediated SDF−1 expression. Int J Mol Med (2022) 49(3):38. doi: 10.3892/ijmm.2022.5093
78. El-Bialy T, Alhadlaq A, Lam B. Effect of therapeutic ultrasound on human periodontal ligament cells for dental and periodontal tissue engineering. Open Dent J (2012) 6:6235–239. doi: 10.2174/1874210601206010235
79. Gao Q, Walmsley AD, Cooper PR, Scheven BA. Ultrasound stimulation of different dental stem cell populations: role of mitogen-activated protein kinase signaling. J Endod (2016) 42(3):425–31. doi: 10.1016/j.joen.2015.12.019
80. Gao Q, Cooper PR, Walmsley AD, Scheven BA. Role of piezo channels in ultrasound-stimulated dental stem cells. J Endod (2017) 43(7):1130–6. doi: 10.1016/j.joen.2017.02.022
81. Ren Z, Fang R, Deng W, Long J, Liu D. Effect of umbilical cord mesenchymal stem cell transplantation under LIFPUS pretreatment on thyroid function in EAT rats. Curr Stem Cell Res Ther (2022) 18(2):260–75. doi: 10.2174/1574888X17666220513143100
82. Moghaddam ZH, Mokhtari-Dizaji M, Movahedin M, Ravari ME. Estimation of the distribution of low-intensity ultrasound mechanical index as a parameter affecting the proliferation of spermatogonia stem cells in vitro. Ultrason Sonochem (2017) 2017:37571–581. doi: 10.1016/j.ultsonch.2017.02.013
83. Puts R, Rikeit P, Ruschke K, Knaus P, Schreivogel S, Raum K. Functional regulation of YAP mechanosensitive transcriptional coactivator by Focused Low-Intensity Pulsed Ultrasound (FLIPUS) enhances proliferation of murine mesenchymal precursors. PloS One (2018) 13(10):e0206041. doi: 10.1371/journal.pone.0206041
84. Cha JM, Hwang YS, Kang DK, Lee J, Cooper ES, Mantalaris A. Development of a novel perfusion rotating wall vessel bioreactor with ultrasound stimulation for mass-production of mineralized tissue constructs. Tissue Eng Regener Med (2022) 19(4):739–54. doi: 10.1007/s13770-022-00447-3
85. Tutak I, Ozdil B, Uysal A. Voxtalisib and low intensity pulsed ultrasound combinatorial effect on glioblastoma multiforme cancer stem cells via PI3K/AKT/mTOR. Pathol Res Pract (2022) 239:239154145. doi: 10.1016/j.prp.2022.154145
86. Song S, Ma D, Xu L, Wang Q, Liu L, Tong X, et al. Low-intensity pulsed ultrasound-generated singlet oxygen induces telomere damage leading to glioma stem cell awakening from quiescence. iScience (2022) 25(1):103558. doi: 10.1016/j.isci.2021.103558
87. Qian J, Wang L, Li Q, Sha D, Wang J, Zhang J, et al. Ultrasound-targeted microbubble enhances migration and therapeutic efficacy of marrow mesenchymal stem cell on rat middle cerebral artery occlusion stroke model. J Cell Biochem (2019) 120(3):3315–22. doi: 10.1002/jcb.27600
88. Sun T, Gao F, Li X, Cai Y, Bai M, Li F, et al. A combination of ultrasound-targeted microbubble destruction with transplantation of bone marrow mesenchymal stem cells promotes recovery of acute liver injury. Stem Cell Res Ther (2018) 9(1):356. doi: 10.1186/s13287-018-1098-4
89. Sun Z, Xie Y, Lee RJ, Chen Y, Jin Q, Lv Q, et al. Myocardium-targeted transplantation of PHD2 shRNA-modified bone mesenchymal stem cells through ultrasound-targeted microbubble destruction protects the heart from acute myocardial infarction. Theranostics (2020) 10(11):4967–82. doi: 10.7150/thno.43233
90. Wei X, Zheng Y, Zhang W, Tan J and Zheng H. Ultrasound−targeted microbubble destruction−mediated Galectin−7−siRNA promotes the homing of bone marrow mesenchymal stem cells to alleviate acute myocardial infarction in rats. Int J Mol Med (2021) 47(2):677–87. doi: 10.3892/ijmm.2020.4830
91. Wang G, Zhang Q, Zhuo Z, Wu S, Xu Y, Zou L, et al. Enhanced homing of CXCR-4 modified bone marrow-derived mesenchymal stem cells to acute kidney injury tissues by micro-bubble-mediated ultrasound exposure. Ultrasound Med Biol (2016) 42(2):539–48. doi: 10.1016/j.ultrasmedbio.2015.10.005
92. Yi S, Han G, Shang Y, Liu C, Cui D, Yu S, et al. Microbubble-mediated ultrasound promotes accumulation of bone marrow mesenchymal stem cell to the prostate for treating chronic bacterial prostatitis in rats. Sci Rep (2016) 6:619745. doi: 10.1038/srep19745
93. Cui H, Zhu Q, Xie Q, Liu Z, Gao Y, He Y, et al. Low intensity ultrasound targeted microbubble destruction assists MSCs delivery and improves neural function in brain ischaemic rats. J Drug Target (2020) 28(3):320–9. doi: 10.1080/1061186X.2019.1656724
94. Aliabouzar M, Zhang LG, Sarkar K. Lipid coated microbubbles and low intensity pulsed ultrasound enhance chondrogenesis of human mesenchymal stem cells in 3D printed scaffolds. Sci Rep (2016) 6:637728. doi: 10.1038/srep37728
95. Osborn J, Aliabouzar M, Zhou X, Rao R, Zhang LG, Sarkar K. Enhanced osteogenic differentiation of human mesenchymal stem cells using microbubbles and low intensity pulsed ultrasound on 3D printed scaffolds. Adv Biosyst (2019) 3(2):e1800257. doi: 10.1002/adbi.201800257
96. Li L, Wu S, Liu Z, Zhuo Z, Tan K, Xia H, et al. Ultrasound-targeted microbubble destruction improves the migration and homing of mesenchymal stem cells after myocardial infarction by upregulating SDF-1/CXCR4: A pilot study. Stem Cells Int (2015) 2015:2015691310. doi: 10.1155/2015/691310
97. Liao Q, Li BJ, Li Y, Xiao Y, Zeng H, Liu JM, et al. Low-intensity pulsed ultrasound promotes osteoarthritic cartilage regeneration by BMSC-derived exosomes via modulating the NF-kappaB signaling pathway. Int Immunopharmacol (2021) 97:97107824. doi: 10.1016/j.intimp.2021.107824
98. Xia P, Wang Q, Song J, Wang X, Wang X, Lin Q, et al. Low-intensity pulsed ultrasound enhances the efficacy of bone marrow-derived MSCs in osteoarthritis cartilage repair by regulating autophagy-mediated exosome release. Cartilage (2022) 13(2):19476035221093060. doi: 10.1177/19476035221093060
99. Li X, Li X, Lin J, Sun X, Ding Q. Exosomes derived from low-intensity pulsed ultrasound-treated dendritic cells suppress tumor necrosis factor-induced endothelial inflammation. J Ultrasound Med (2019) 38(8):2081–91. doi: 10.1002/jum.14898
100. Costa V, Carina V, Conigliaro A, Raimondi L, De Luca A, Bellavia D, et al. miR-31-5p is a LIPUS-mechanosensitive microRNA that targets HIF-1alpha signaling and cytoskeletal proteins. Int J Mol Sci (2019) 20(7):1569. doi: 10.3390/ijms20071569
101. Yang C, Li B, Yu J, Yang F, Cai K, Chen Z. Ultrasound microbubbles mediated miR-let-7b delivery into CD133(+) ovarian cancer stem cells. Biosci Rep (2018) 38(5):BSR20180922. doi: 10.1042/BSR20180922
102. Chen D, Xiang M, Gong Y, Xu L, Zhang T, He Y, et al. LIPUS promotes FOXO1 accumulation by downregulating miR-182 to enhance osteogenic differentiation in hPDLCs. Biochimie (2019) 165:165219–228. doi: 10.1016/j.biochi.2019.08.005
103. Li X, Zhong Y, Zhou W, Song Y, Li W, Jin Q, et al. Low-intensity pulsed ultrasound (LIPUS) enhances the anti-inflammatory effects of bone marrow mesenchymal stem cells (BMSCs)-derived extracellular vesicles. Cell Mol Biol Lett (2023) 28(1):9. doi: 10.1186/s11658-023-00422-3
104. Yao H, Zhang L, Yan S, He Y, Zhu H, Li Y, et al. Low-intensity pulsed ultrasound/nanomechanical force generators enhance osteogenesis of BMSCs through microfilaments and TRPM7. J Nanobiotechnol (2022) 20(1):378. doi: 10.1186/s12951-022-01587-3
105. Bacakova L, Zarubova J, Travnickova M, Musilkova J, Pajorova J, Slepicka P, et al. Stem cells: their source, potency and use in regenerative therapies with focus on adipose-derived stem cells - a review. Biotechnol Adv (2018) 36(4):1111–26. doi: 10.1016/j.bioteChadv.2018.03.011
106. Liu DD, Ullah M, Concepcion W, Dahl JJ, Thakor AS. The role of ultrasound in enhancing mesenchymal stromal cell-based therapies. Stem Cells Transl Med (2020) 9(8):850–66. doi: 10.1002/sctm.19-0391
107. Ling L, Hou J, Wang Y, Shu H, Huang Y. Effects of low-intensity pulsed ultrasound on the migration and homing of human amnion-derived mesenchymal stem cells to ovaries in rats with premature ovarian insufficiency. Cell Transplant (2022) 31:19636897221129171. doi: 10.1177/09636897221129171
108. Li J, Zhang Y, Lou Z, Li M, Cui L, Yang Z, et al. Magnetic nanobubble mechanical stress induces the piezo1-ca(2+) -BMP2/smad pathway to modulate neural stem cell fate and MRI/ultrasound dual imaging surveillance for ischemic stroke. Small (2022) 18(23):e2201123. doi: 10.1002/smll.202201123
109. Leighton R, Phillips M, Bhandari M, Zura R. Low intensity pulsed ultrasound (LIPUS) use for the management of instrumented, infected, and fragility non-unions: a systematic review and meta-analysis of healing proportions. BMC Musculoskelet Disord (2021) 22(1):532. doi: 10.1186/s12891-021-04322-5
110. Chen H, Wang Z, Zhang X, Sun M. Effects of low-intensity pulsed ultrasound on knee osteoarthritis: A systematic review and meta-analysis of randomized controlled trials. Clin Rehabil (2022) 36(9):1153–69. doi: 10.1177/02692155221097035
111. Tanveer F, Arslan SA, Darain H, Ahmad A, Gilani SA, Hanif A. Role of low-intensity pulsed ultrasound on lumbar spondylolysis: A systematic review. J Pak Med Assoc (2022) 72(3):522–5. doi: 10.47391/jpma.3320
112. Cottrill E, Downey M, Pennington Z, Ehresman J, Schilling A, Downey M, et al. Low-intensity pulsed ultrasound as a potential adjuvant therapy to promote spinal fusion: systematic review and meta-analysis of the available data. J Ultrasound Med (2021) 40(10):2005–17. doi: 10.1002/jum.15587
113. Tsai SJ. Preventive potential of low intensity pulsed ultrasound for chronic traumatic encephalopathy after repetitive head collisions in contact sports. Med Hypotheses (2020) 134:109422. doi: 10.1016/j.mehy.2019.109422
114. Hung TH, Liu YC, Wu CH, Chen CC, Chao H, Yang FY, et al. Antenatal low-intensity pulsed ultrasound reduces neurobehavioral deficits and brain injury following dexamethasone-induced intrauterine growth restriction. Brain Pathol (2021) 31(6):e12968. doi: 10.1111/bpa.12968
115. Wang T, Ito A, Xu S, Kawai H, Kuroki H, Aoyama T. Low-intensity pulsed ultrasound prompts both functional and histologic improvements while upregulating the brain-derived neurotrophic factor expression after sciatic crush injury in rats. Ultrasound Med Biol (2021) 47(6):1586–95. doi: 10.1016/j.ultrasmedbio.2021.02.009
116. Du D, Gao Y, Zheng T, Yang L, Wang Z, Shi Q, et al. The value of first-order features based on the apparent diffusion coefficient map in evaluating the therapeutic effect of low-intensity pulsed ultrasound for acute traumatic brain injury with a rat model. Front Comput Neurosci (2022) 16:16923247. doi: 10.3389/fncom.2022.923247
117. Nasb M, Liangjiang H, Gong C, Hong C. Human adipose-derived Mesenchymal stem cells, low-intensity pulsed ultrasound, or their combination for the treatment of knee osteoarthritis: study protocol for a first-in-man randomized controlled trial. BMC Musculoskelet Disord (2020) 21(1):33. doi: 10.1186/s12891-020-3056-4
Keywords: stem cells, exosomes, low-intensity pulsed ultrasound, differentiation, proliferation, migration, homing
Citation: He Y-f, Wang X-l, Deng S-p, Wang Y-l, Huang Q-q, Lin S and Lyu G-r (2023) Latest progress in low-intensity pulsed ultrasound for studying exosomes derived from stem/progenitor cells. Front. Endocrinol. 14:1286900. doi: 10.3389/fendo.2023.1286900
Received: 31 August 2023; Accepted: 06 November 2023;
Published: 28 November 2023.
Edited by:
Guifang Zhao, Mayo Clinic Florida, United StatesReviewed by:
Xiaohui Xie, Baylor College of Medicine, United StatesYusheng Li, University of Mississippi, United States
Copyright © 2023 He, Wang, Deng, Wang, Huang, Lin and Lyu. This is an open-access article distributed under the terms of the Creative Commons Attribution License (CC BY). The use, distribution or reproduction in other forums is permitted, provided the original author(s) and the copyright owner(s) are credited and that the original publication in this journal is cited, in accordance with accepted academic practice. No use, distribution or reproduction is permitted which does not comply with these terms.
*Correspondence: Guo-rong Lyu, bGdyX2ZldXNAc2luYS5jb20=; Shu Lin, c2h1bGluMTk1NkAxMjYuY29t