- 1Center for Reproductive Health, School of Pharmaceutical Sciences, Hangzhou Medical College (Zhejiang Academy of Medical Sciences), Hangzhou, China
- 2Engineering Research Center of Novel Vaccine of Zhejiang Province, Hangzhou Medical College, Hangzhou, China
- 3School of Basic Medical Sciences and Forensic Medicine, Hangzhou Medical College, Hangzhou, China
- 4School of Pharmaceutical Sciences, Hangzhou Medical College, Hangzhou, China
- 5Department of Urology and Andrology, Sir Run-Run Shaw Hospital, Zhejiang University School of Medicine, Hangzhou, China
Intercellular adhesion molecule-1 (ICAM-1) is a transmembrane glycoprotein expressed on immune, endothelial, and epithelial cells. Its ectodomain can be proteolytically cleaved to release a circulating soluble form called sICAM-1. Clinical studies demonstrate sICAM-1 is upregulated in various diseases and associated with disease severity. Research has identified sICAM-1 as a regulator of the blood-testis barrier (BTB) and spermatogenesis. Overexpression of sICAM-1 weakened the BTB in vitro and in vivo, downregulated junction proteins including N-cadherin, γ-catenin, and connexin 43, and caused germ cell loss. This contrasts with barrier-strengthening effects of membrane-bound ICAM-1. sICAM-1 may act as a molecular switch enabling germ cells to open BTB and Sertoli-germ cell adhesion for transport across the seminiferous epithelium. While the mechanism remains unclear, reduced SRC family kinase (SFK) signaling was observed following sICAM-1 overexpression. SRC promotes BTB protein endocytosis and degradation, influences cytoskeletal dynamics, and affects cell polarity. As sICAM-1 overexpression phenocopies SRC inhibition, SRC may operate downstream of sICAM-1 in regulating BTB dynamics and spermatogenesis. Investigating sICAM-1’s structure-function regions and downstream targets will elucidate the molecular mechanisms of junction disruption. This knowledge could enable strategies targeting sICAM-1/SRC to modulate BTB permeability and treat male infertility or diseases involving endothelial/epithelial barrier dysfunction.
1 Introduction
Male infertility is a major global health challenge according to a recent World Health Organization (WHO) report analyzing infertility prevalence worldwide from 1990 to 2021. The report found that around 17.5% of adults, approximately 1 in 6 people, experience infertility, with comparable rates across high-, middle-, and low-income countries. Male infertility negatively impacts the physical, psychological, and social well-being of men of reproductive age worldwide. A separate study estimated that over 56 million men suffered from infertility up to 2019, representing a 76.9% increase from 1990 (1). In addition to impacts on reproductive capacity, male infertility also causes substantial psychosocial distress and introduces treatment costs (2, 3).
Multiple factors can contribute to male infertility, including abnormalities in sperm function and quality, as well as failure to produce sperm. However, the precise molecular mechanisms remain poorly understood, making diagnosis and treatment challenging. Improved semen parameters after treatment do not guarantee identifying the underlying causes (4–8). Studies show close links between male fertility and overall health. Infertility increases risks of illnesses unrelated to reproduction, such as cancer, diabetes, and cardiovascular disease. Infertile men also face higher hospitalization and mortality rates when seriously ill (9–11). This correlation suggests a coordinated regulatory system involving male reproduction and other body systems. Shared effector molecules and regulatory proteins may underlie different disease processes.
One such molecule is intercellular adhesion molecule-1 (ICAM-1), which exists in both membrane-bound and circulating soluble forms (sICAM-1). In rodent testes, germ cells appear to secrete sICAM-1 to alter Sertoli cell adhesion as they move across the seminiferous epithelium during spermatogenesis. Overexpression of sICAM-1 in rat testes severely impairs the blood-testis barrier (BTB) function and germ cell adhesion (12–14). Clinical evidence has associated elevated sICAM-1 levels with diverse pathological states, including male infertility (Table 1). This positions sICAM-1 as a putative shared effector that links reproductive and systemic damage. However, the mechanism behind sICAM-1’s action remains unclear. A comprehensive investigation into sICAM-1’s impacts on testis function and on Sertoli and germ cell adhesion is needed. Deeper insights into sICAM-1’s role and mechanisms could provide therapeutic targets for infertility while elucidating systemic disease mechanisms. This could significantly advance infertility prevention and treatment, as well as therapies targeting common regulatory pathways for systemic diseases.
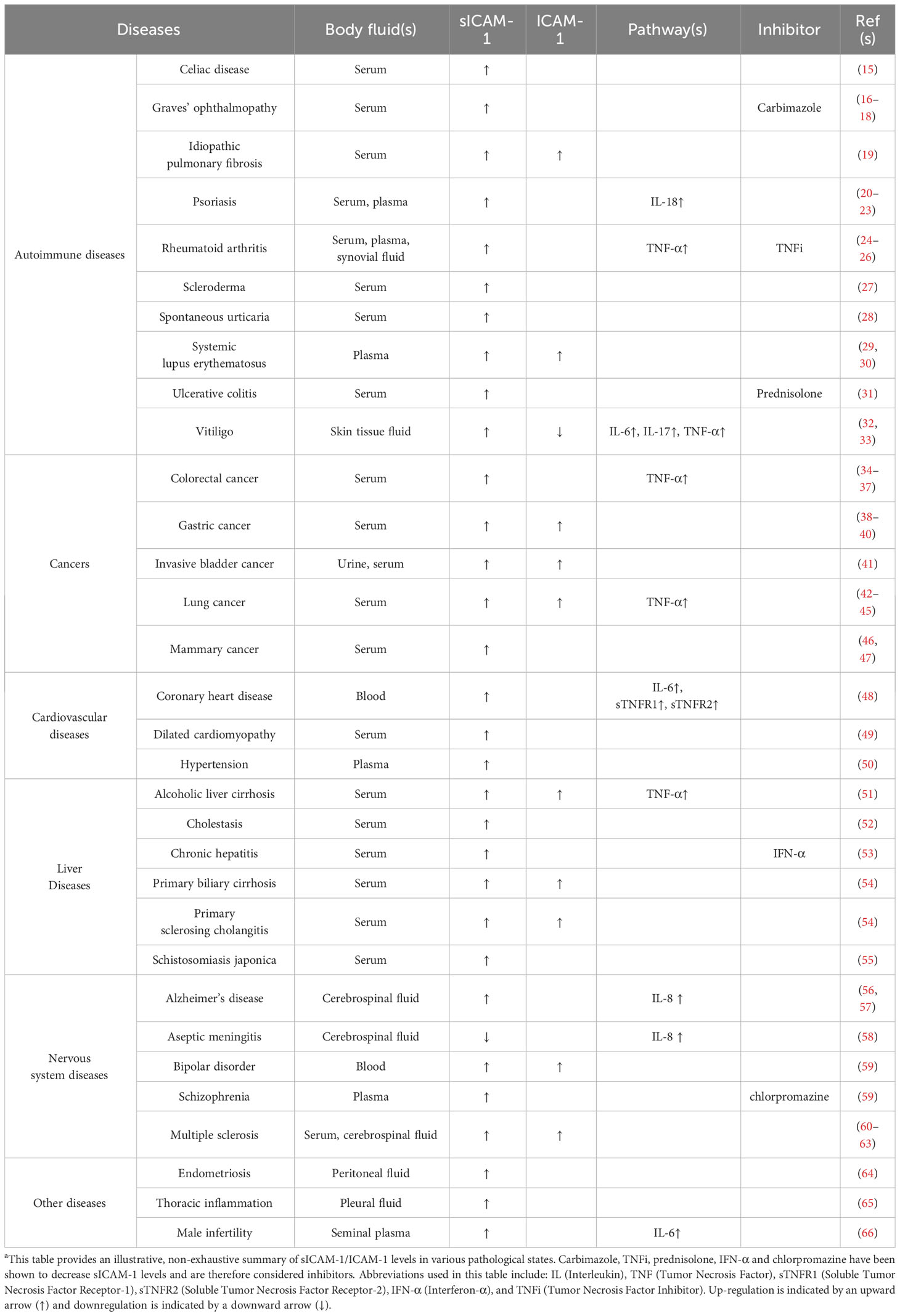
Table 1 sICAM-1/ICAM-1 expression in body fluids across different pathological conditionsa.
2 Background of sICAM-1
2.1 Origins and variants
ICAM-1, also known as Cluster of Differentiation 54 (CD54), is a single-chain transmembrane glycoprotein that consists of an extracellular region with five immunoglobulin (Ig)-like domains, a transmembrane segment, and a short cytoplasmic tail. ICAM-1 is expressed at relatively low levels by immune cells, endothelial cells, epithelial cells, and other normal tissues. However, multiple inflammatory stimuli, including cytokines such as tumor necrosis factor (TNF)-α, interleukin (IL)-1, and interferon (IFN)-γ, as well as lipopolysaccharide (LPS), can increase ICAM-1 expression through transcription (67–70).
Under inflammatory or cellular stress conditions, the ectodomain of ICAM-1 can be proteolytically cleaved and shed from the cell surface. This releases the soluble form, sICAM-1, into extracellular fluids. Pro-inflammatory cytokines enhance this shedding, leading to increased sICAM-1 levels. Circulating in body fluids like blood, sICAM-1 is a truncated form of ICAM-1 that consists solely of the five Ig-like extracellular domains D1-D5, without the transmembrane and cytoplasmic regions (Figure 1A). Being heavily glycosylated, the molecular weights of ICAM-1 and sICAM-1 can vary between 75-115 kDa and 50-90 kDa respectively (13, 70–72).
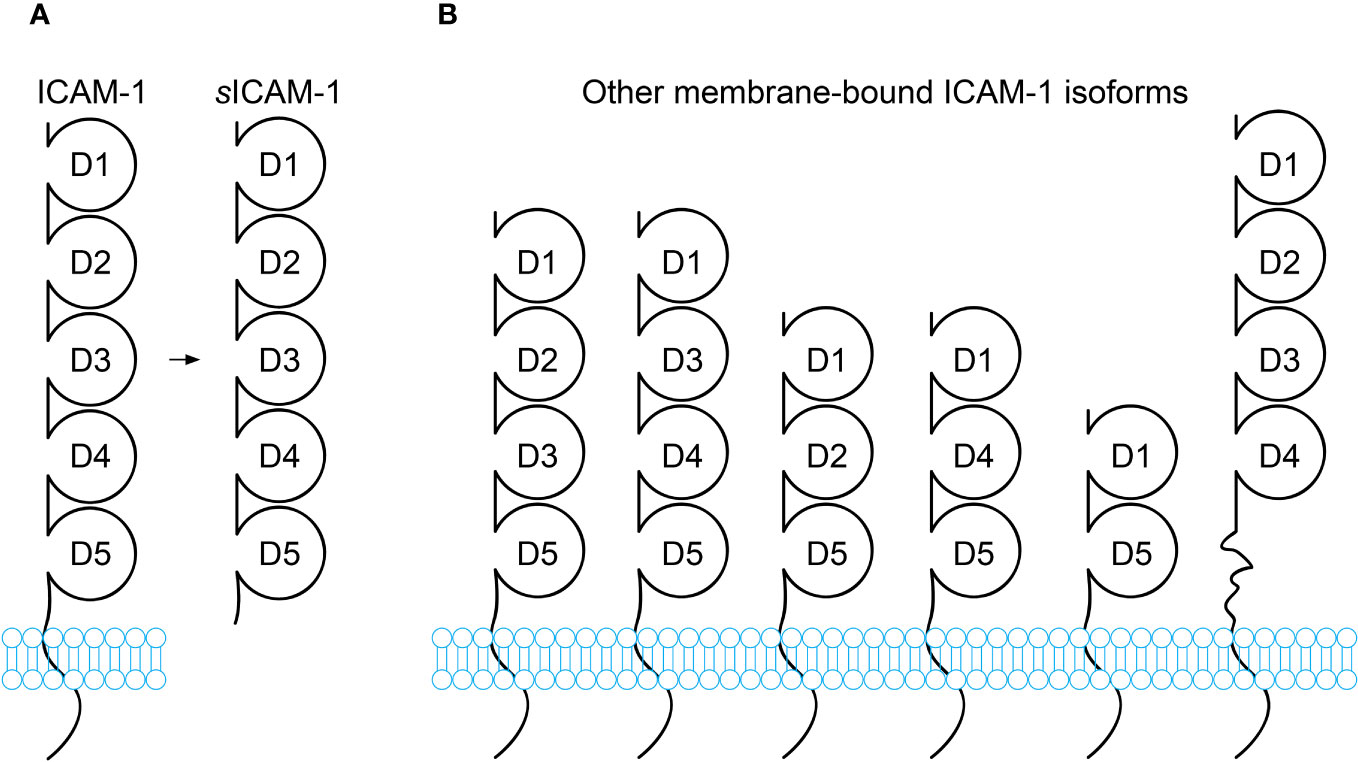
Figure 1 Schematic diagram of ICAM-1 isoforms. (A) Full-length ICAM-1 is a transmembrane protein containing 5 immunoglobulin(Ig)-like extracellular domains (D1-D5), a transmembrane domain, and a short cytoplasmic domain. Soluble ICAM-1 (sICAM-1) lacks the transmembrane and cytoplasmic domains. (B) In addition to the full-length isoform, alternative splicing generates transmembrane ICAM-1 isoforms with truncated extracellular regions containing 2, 3, or 4 Ig-like domains. While these alternatively spliced isoforms have been reported, most functional research pertains to full-length ICAM-1. The isoforms likely differ in tissue distribution, expression levels, and functions. Some may only appear under certain conditions, such as pathological conditions.
The origin and generation of sICAM-1 is not fully understood. It is thought to primarily occur through the proteolytic cleavage of ICAM-1’s ectodomain by proteases. Proteases like serine proteases, matrix metalloproteinases (MMPs), and members of the “a disintegrin and metalloproteinase” (ADAM) family may cleave at different sites on ICAM-1. Both the ICAM-1 cleavage by different proteases and the differences in glycosylation could produce variants of sICAM-1 with subtle structural differences. Alternative mRNA splicing can also generate other variants of sICAM-1. Six other splice variants of ICAM-1 have been reported, varying in their combination and number of Ig domains (Figure 1B) (73). These variants are more susceptible to proteolytic cleavage and may contribute additional sICAM-1. Some studies suggest mRNAs that encode sICAM-1 directly may exist (13, 74). Each of these mechanisms could give rise to sICAM-1 variants that differ in structure and function. Studies using isoform-deficient mice found they have sharply contrasting disease phenotypes. The ability of ICAM-1 variants to bind ligands like lymphocyte function-associated antigen-1 (LFA-1) in vitro also varies depending on present Ig domains. Expression of isoforms differs between cell types and may change with inflammation (73). However, it remains unclear if these isoforms could differentially regulate ligand interactions, dimerization, intracellular signaling and disease outcomes through their structural variations expressed on different cell types. It is still unknown if any hypothetical fragments have unique roles. Nevertheless, the only soluble form found in body fluids contains domains D1-D5, appearing most physiologically relevant (13, 70, 74). Further work is needed to characterize and compare sICAM-1 variants from splicing versus protease cleavage to understand their properties and potential functions. This may provide insight into the complex origins of sICAM-1.
2.2 General functions and disease associations
sICAM-1 plays complex roles in regulating inflammation and immunity. It is proposed that sICAM-1 retains the characteristics of membrane-bound ICAM-1 and can compete with ICAM-1 for binding to the integrin receptor LFA-1. As a competitive inhibitor, sICAM-1 can influence leukocyte adhesion and migration by inhibiting ICAM-1-mediated interactions between leukocytes and endothelial cells. This potentially weakens the body’s immunity (70, 74, 75). The concentration of sICAM-1 affects cytokine release, immune cell adhesion, angiogenesis and tissue repair in different ways. At low concentrations, sICAM-1 can promote cytokine release and immune cell activation. In contrast, high levels of sICAM-1 may limit leukocyte adhesion while promoting angiogenesis and tissue repair (70). Specific genetic variations in the ICAM-1 gene can also affect sICAM-1 levels (69). Additionally, sICAM-1 acts as a signaling molecule. The intensity of signaling induced by sICAM-1 is regulated by the completeness of N-glycosylation and sialylation. Sialylation does not affect ICAM-1 binding to LFA-1 in astrocytes. However, it is critical for the signaling function of sICAM-1 in inducing production of the inflammatory chemokine MIP-2/CXCL2 (macrophage inflammatory protein 2/C-X-C motif chemokine ligand 2). Fully sialylated sICAM-1 induces a more rapid, robust, and prolonged MIP-2 response compared to nonsialylated or high mannose glycoforms. Sialylation may regulate receptor interactions and signaling kinetics of sICAM-1 (76).
sICAM-1 has been detected in various human body fluids including serum, cerebrospinal fluid, bile, amniotic fluid, and urine (71, 72, 74, 77–80). Elevated sICAM-1 levels correlate with disease severity and prognosis in numerous diseases like cancer, cardiovascular disease, autoimmune disorders, nervous system disease, inflammation, and viral infections (70, 72, 80–86). For instance, recent studies have found that higher levels of sICAM-1 in the serum of COVID-19 patients are positively associated with disease severity and can even predict the risk of mortality (81, 82). In chronic pain patients, serum sICAM-1 levels have been found to significantly correlate with pain intensity. However, in acute experimental pain models with healthy volunteers, sICAM-1 levels did not directly correlate with perceived pain levels. Instead, sICAM-1 underwent short-term changes after acute nociceptive stimuli (87). Additionally, an increase in sICAM-1 levels in seminal plasma may be associated with immune infertility in men (66).
Unlike ICAM-1 whose cellular expression is difficult to clinically assess, sICAM-1 in body fluid is easily measurable and is often used as a common marker for inflammatory diseases (71, 74, 77–79). However, the mechanism behind its action is still unclear. Efforts to therapeutically modulate sICAM-1 levels have shown limited efficacy in treating diseases so far. Currently, most research focuses on exploring relationships between sICAM-1 levels in fluids and disease onset, progression, and prognosis (75, 82, 84, 88) (Table 1). Alternatively, studies have generally viewed ICAM-1 and sICAM-1 as ubiquitous adhesion molecules expressed on epithelial or endothelial cell surfaces in response to environmental stimuli. It is believed that high levels of sICAM-1 may stem from corresponding vascular endothelial dysfunction (70, 72). For example, research has demonstrated that high sICAM-1 levels in cerebrospinal fluid are associated with increased phosphorylation of the microtubule-associated protein tau, which is implicated in blood-brain barrier (BBB) dysfunction, and with elevated levels of total tau protein. However, few studies have examined the links between sICAM-1 and endothelial barrier permeability. Limited existing findings suggest that sICAM-1 can influence the transport of immune cells across the BBB and their communication with surrounding cells (72, 89–92).
The precise impact of sICAM-1 on germ cell transport across the BTB and throughout the seminiferous epithelium remains unclear. It also remains unknown whether sICAM-1 acts as a key signaling molecule for communication between germ cells and surrounding Sertoli cells. At present, these mechanisms remain mysterious, as only one study to date has linked sICAM-1 to spermatogenesis and BTB permeability (12). In the upcoming section of this review, cell adhesion and junction dynamics in the testis will first be introduced. Hypothetical molecular mechanisms for the role of sICAM-1 during spermatogenesis will then be proposed. Exploring potential explanations could help further the understanding of how sICAM-1 may regulate both the BTB and germ cell adhesion. Ultimately, this may provide useful insights to advance future research on sICAM-1’s function. While questions remain, continued investigation of sICAM-1’s involvement holds promise to elucidate the intricate process of spermatogenesis.
3 Cell adhesion and junction dynamics in the testis
3.1 Cell junctions facilitate cell adhesion
Cell migration and morphogenesis, which are central to developmental processes, require dynamic changes in cell adhesion properties. Fundamentally, cell adhesion refers to interactions between cells (cell-cell adhesion) and between cells and the extracellular matrix (ECM, cell-ECM adhesion). These interactions play a pivotal role in maintaining tissue integrity, homeostasis, and function. Cell adhesion is primarily facilitated through specialized structures known as cell junctions, which include tight junctions (TJ), adherens junctions (AJ), desmosomes, and gap junctions (GJ). These junctions work in concert to provide structural stability, coordinate cellular behavior, and maintain tissue architecture. Nonetheless, each junction type serves unique and indispensable roles in connecting cells, facilitating intercellular signaling, and providing cell adhesion. TJ, composed of integral membrane proteins such as occludin and claudins, interact with the actin cytoskeleton through adapter proteins like ZO-1, thereby forming a permeability barrier through tight sealing between cells. This restricts the passage of molecules and plays an integral part during the development and remodeling of epithelial tissue. AJ, with core components like cadherins/catenins and nectins/afadin complexes, typically form first between cells during epithelial development, providing mechanical attachment. This is followed by formation of TJ at the apical region to AJ, sealing the paracellular space. At desmosomes, the adhesion proteins desmoglein and desmocollin provide linkage to intermediate filaments within cells. GJ, formed by clustering of connexins, create intercellular channels that directly transfer molecules between cells (93–97).
3.2 Specialized junctions in the testis
In the adult mammalian testis, spermatogenesis exemplifies the vital role of cell adhesion and junction dynamics. This highly coordinated and cyclical process involves the continual division and differentiation of germ cells, which are tightly bound to the surrounding Sertoli cells within seminiferous tubules. Cell-cell adhesion is mediated by TJ, ectoplasmic specializations (ES), GJ, and desmosomes, all relying on Sertoli cells (98). ES are unique testis-specific actin-based AJ structure located at basal sites between adjacent Sertoli cells. Characterized by their hexagonal actin arrays sandwiched between the Sertoli cell plasma membrane and endoplasmic reticulum, basal ES comprise part of the BTB. They coexist and intermix with the other three junction types (TJ, GJ, and desmosomes) to form the intricate, multifaceted BTB structure (99–102), as depicted in Figure 2 showing the different junction types labeled with colors.
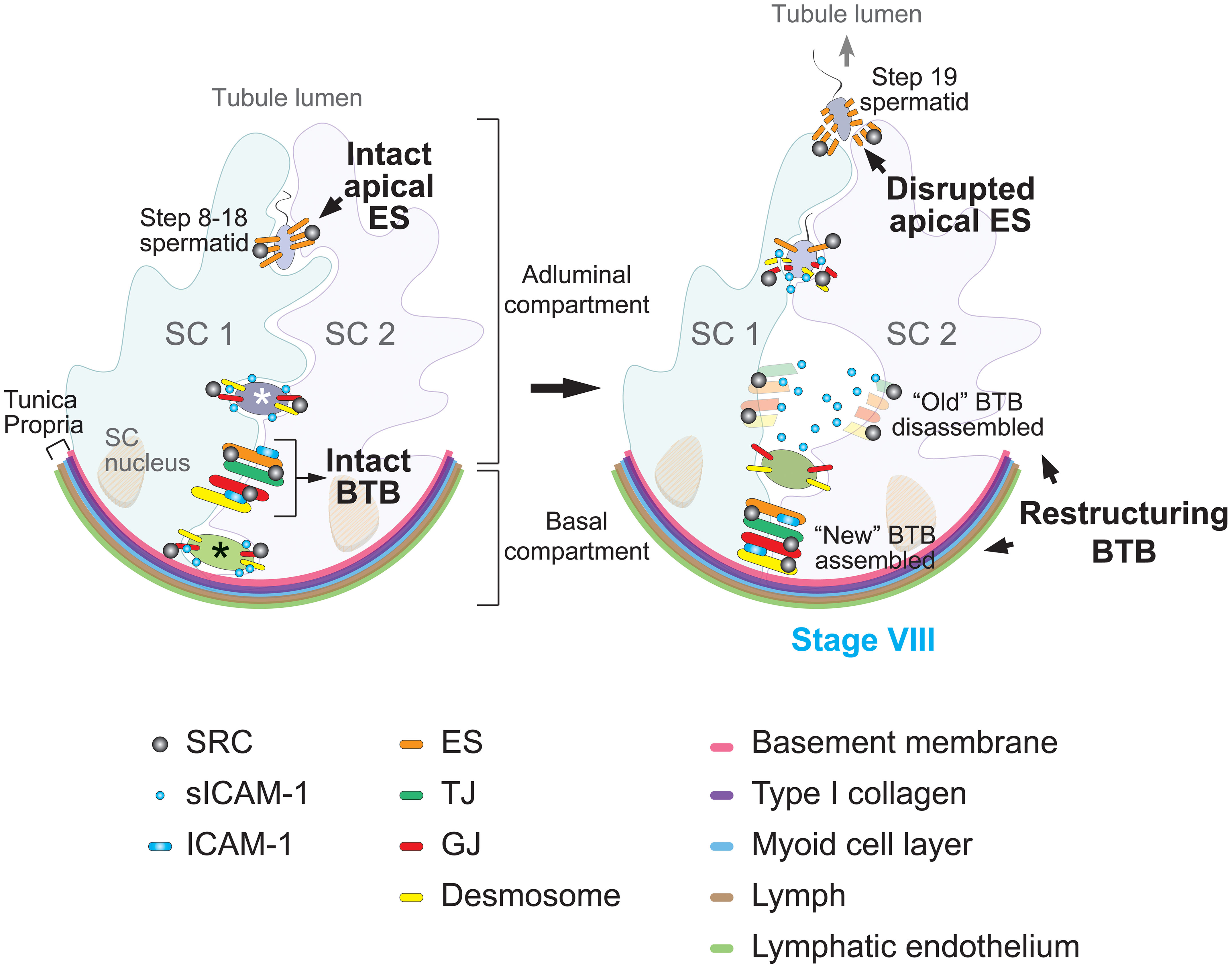
Figure 2 Proposed model of sICAM-1 and SRC signaling in regulating blood-testis barrier (BTB) dynamics and spermatogenesis. In the rat seminiferous tubule (left), the seminiferous epithelium is composed of Sertoli cells (SC) and germ cells at different developmental stages. The BTB, formed between adjacent Sertoli cells (SC 1 and SC 2), divides the epithelium into basal and adluminal compartments. Less mature germ cells (such as spermatogonia and preleptotene spermatocytes, marked with a black asterisk) reside in the basal compartment, while more advanced germ cells (such as primary and secondary spermatocytes, as well as round spermatids, marked with a white asterisk) reside in the adluminal compartment. The BTB comprises four distinct cell junction types, color-coded in the diagram: basal ectoplasmic specializations (ES), tight junctions (TJ), gap junctions (GJ), and desmosomes. Sertoli cells also form apical ES with step 8 and beyond spermatids, acting as the primary anchoring junction until spermiation. Pre-step 8 germ cells are linked to Sertoli cells through GJ and desmosomes. Src functions as a pivotal regulator of both the BTB and Sertoli-germ cell adhesion. At stage VIII of the seminiferous epithelial cycle (right), the BTB restructures to enable transit of preleptotene spermatocytes. This includes the formation of a “new” BTB beneath spermatocytes and the disintegration of the “old” BTB above the spermatocytes, enabling spermatocyte passage into the adluminal compartment without compromising the BTB. Concurrently, the apical ES is broken down to release mature sperm, specifically the step 19 spermatid. During these events, SRC facilitates BTB remodeling and regulates the dynamics of Sertoli-germ cell junctions, particularly apical ES disintegration. Through protein endocytosis and degradation pathways, SRC promotes transport of spermatocytes across the BTB and release of mature spermatozoa from the seminiferous epithelium. Previous studies show membrane-bound ICAM-1 promotes BTB assembly, whereas its soluble form sICAM-1 expressed by germ cells impairs the BTB to facilitate spermatocyte transit. Overexpression of sICAM-1 also disrupts GJ and desmosomes between Sertoli and germ cells, causing loss of immature germ cells. SRC may act downstream of sICAM-1 to regulate BTB restructuring and Sertoli-germ cell adhesion during spermatogenesis.
ES are also found at apical sites between Sertoli cells and spermatids, termed apical ES. Once formed, apical ES serve as the sole anchoring junction at these sites until spermiation. Different Sertoli-germ cell junction types predominate during specific maturation stages, enabling diverse germ cell activities (98–105). In rodent models, early germ cells including spermatogonial stem cells, spermatogonia, spermatocytes, and pre-step 8 spermatids, primarily connect to Sertoli cells through GJ and desmosomes. However, as germ cells mature into step 8 and beyond spermatids, apical ES takes precedence (99–101, 103, 104). This junctional shift underscores the complexity and specificity of cell-cell interactions within the dynamic environment of the testis. At apical ES sites, hexagonal actin filament bundles are restricted to the Sertoli cell side. Apical ES anchor spermatids and guide their orientation and bidirectional movement in the epithelium, potentially via linkage to microtubule motors like kinesins and dyneins (106–110).
3.3 BTB dynamics
The BTB is a defining feature of the testis, fundamentally consisting of specialized cell junction structures that create a selectively permeable barrier within the seminiferous epithelium. It separates the epithelium into adluminal (apical) and basal compartments (Figure 2) and constitutes one of the tightest tissue barriers in the body. The BTB prevents substances in the blood like drugs and antibodies from accessing the adluminal compartment, providing an immunological and physical shield to developing germ cells. However, the BTB must be dynamic—continuous reorganization of intricate junctional complexes enables spermatocyte transit from the basal to adluminal compartment, a critical step in sperm development. This meticulous BTB remodeling facilitates extensive germ cell transport while maintaining barrier integrity (102).
The BTB’s constant restructuring without compromising its immunological barrier function is made possible by coordinated interplay between its cell junction components. As shown in Figure 2, movement of preleptotene spermatocytes across the BTB involves localized assembly of “new” junctions below transiting spermatocytes, followed by disassembly of “old” junctions above them. Accordingly, junctional proteins undergo endocytosis, then intracellular transport including protein degradation, recycling back to the cell surface, and transcytosis across cells (102, 105, 111–113). This intricately orchestrated BTB dynamics enables the extensive germ cell development needed for the remarkable sperm production capacity of the mammalian testes.
4 Detection and roles of sICAM-1 in the rat testis
4.1 Expression of sICAM-1
A previous study has shown that both Sertoli cells and germ cells express membrane-bound ICAM-1 in the rat testis, as examined using commercial antibodies against the cytoplasmic region of ICAM-1 (12). During stage VIII of the seminiferous epithelial cycle, ICAM-1 expression at the BTB significantly increases when examined by immunofluorescence microscopy, coinciding with BTB restructuring and the transit of preleptotene spermatocytes across it as they differentiate into leptotene and zygotene spermatocytes. This suggests ICAM-1 involvement in spermatocyte transport across the BTB. However, these antibodies cannot recognize sICAM-1. Using a custom polyclonal antibody targeting the extracellular D2-D3 domain of ICAM-1, the authors detected sICAM-1 in rat testes. The identified sICAM-1 comprised all five Ig-like domains and had a molecular weight of around 70 kDa. This is lower than the full-length ICAM-1 in rat testes with a molecular weight of approximately 97 kDa. The antibody also detected additional protein fragments with lower molecular weights, indicating potential alternative forms of sICAM-1 may be present (12). By immunoblotting analysis, sICAM-1 was found to be highly expressed in germ cells (12). This suggests germ cells may secrete sICAM-1 to regulate Sertoli cell adhesion and facilitate their own crossing of the BTB and transport in the epithelium (12–14).
4.2 Contrasting roles of sICAM-1 and ICAM-1
The authors further discovered that overexpression of sICAM-1, via a plasmid containing only the extracellular domains D1-D5, exerted an opposing effect on BTB permeability compared to overexpression of the full-length membrane-bound ICAM-1 (12). While overexpression of full-length ICAM-1 strengthens the BTB, overexpression of sICAM-1 severely impairs BTB function and causes loss of adhesion between spermatocytes and round spermatids with supportive Sertoli cells within the seminiferous epithelium (12–14).
Specifically, overexpression studies in a Sertoli cell culture model, which mimics the BTB in vitro, found ICAM-1 and sICAM-1 have antagonistic effects on Sertoli cell barrier function (12). ICAM-1 strengthened the barrier, mimicking “new” BTB assembly, whereas sICAM-1 weakened it, corresponding to “old” BTB disassembly during restructuring (Figure 2). Moreover, overexpressed sICAM-1 downregulated expression of BTB proteins including N-cadherin (an ES protein), γ-catenin (the only known protein present at both ES and desmosomes, also called plakoglobin (114–116)), and connexin 43 (a GJ protein). These differential effects were verified in vivo, where sICAM-1 overexpression in rat testes disrupted the BTB, downregulated N-cadherin and connexin 43, and caused loss of spermatocytes and round spermatids (step 1-7 spermatids). Compared to membrane-bound ICAM-1, sICAM-1 may act as a molecular switch and promoter of germ cell transit across the BTB. By interfering with Sertoli-germ cell junctions and BTB protein expression, sICAM-1 may facilitate transport of germ cells during differentiation.
In summary, sICAM-1 dually assists germ cell movement by disrupting adhesion at ES, desmosomes and GJ, and downregulating proteins like N-cadherin, γ-catenin and connexin 43 (Figure 2). This contrasts with membrane ICAM-1 which reinforces adhesion. sICAM-1 thus fine-tunes BTB dynamics to support spermatogenesis.
5 The mechanisms of sICAM-1 regulation of cell junctions
5.1 sICAM-1 downregulates SRC signaling pathways
The mechanisms by which sICAM-1 downregulates the expression of BTB and cell adhesion proteins, or “opens” cell junctions in the testis remain unclear. Overexpression studies in cultured primary Sertoli cells provide insights. sICAM-1 overexpression also reduced levels of signaling molecules important in SRC signaling, including SRC, PYK2, p-SRC-Y530 and p-PYK2-Y402 (12). This implies SRC pathways likely mediate intracellular sICAM-1 effects. Phosphorylation at tyrosine 530 (Y530) of SRC renders the kinase in an inactive confirmation (117). Thus, reduced p-SRC-Y530, in the context of decreased total SRC, indicates lower inactive and overall SRC levels, suggesting either a general decline in SRC activity, or a shift in the normal balance between active and inactive SRC conformations. As a known SRC substrate, PYK2 autophosphorylation at tyrosine 402 (Y402) recruits SRC to further regulate PYK2 activity (118). Diminished SRC and p-PYK2-Y402 levels indicate potential downregulation of PYK2 signaling involved in cell adhesion, migration, and possibly calcium-induced signaling events (119, 120). However, more research on PYK2 in the testis is needed. Collectively, this data shows sICAM-1 overexpression decreases SRC-related signaling pathways. This supports SRC pathways as probable intracellular mediators of sICAM-1.
5.2 Role of SRC in regulating BTB dynamics
SRC family kinases (SFKs), a family of non-receptor tyrosine kinases, play crucial roles in various cellular processes through signal transduction. Key members involved in the testis include SRC and YES. SFKs are known to regulate cell adhesion by modulating adhesion complexes and cytoskeletal rearrangement. They also participate in junction remodeling by phosphorylating junction proteins. Additionally, SFKs regulate endocytosis through phosphorylating endocytic vesicle proteins (121–124).
Previous studies have shown SFKs, particularly SRC, are critical modulators of BTB dynamics and spermatogenesis. SRC alters the phosphorylation state of BTB and apical ES proteins. This triggers endocytosis and intracellular transport of the junctional components, controlling opening/closing of the BTB and dissociation of spermatozoa from Sertoli cells via disruption of the apical ES (98, 102, 105). Specifically, during stage VIII of the seminiferous epithelial cycle in rodent testes, BTB restructuring coincides with sperm release through apical ES disruption (Figure 2). SRC promotes BTB disintegration and apical ES disruption by inducing protein endocytosis and degradation, mediating both “old” BTB disassembly during spermatocyte transit and loss of mature sperm association with Sertoli cells during spermiation. SRC also facilitates adhesion between immature germ cells and Sertoli cells. In summary, SRC signaling plays multifaceted regulatory roles on BTB and Sertoli-germ cell interactions throughout spermatogenesis (124–129). While its precise interaction with sICAM-1 requires further clarification, they may coordinately influence critical junction events.
5.3 Similar phenotypes suggest SRC mediates sICAM-1 effects
Previous research shows that SRC contributes to the organization and remodeling of F-actin structures in Sertoli cells (124, 128). Excess sICAM-1 undermines SRC signaling in rat testes, severely impacting the structure and alignment of actin filaments (F-actin) (12), corresponding to SRC knockdown phenotypes (128). In other words, overexpressing sICAM-1 or inhibiting SRC produce similar results on Sertoli cell F-actin cytoskeleton.
Additionally, SRC is known to regulate intracellular protein transport, facilitating junction dynamics and aiding “old” BTB disassembly during spermatocyte transit (124–128). It binds and interacts with specific BTB protein complexes, such as N-cadherin/β-/γ-catenin, desmoglein-2/γ-catenin, and connexin 43/plakophilin-2 (102, 124, 130). SRC phosphorylation of N-cadherin, β-catenin, or γ-catenin leads to catenin dissociation from N-cadherin at the BTB sites, resulting in cadherin/catenin complex degradation, breakdown of basal ES at the BTB, and consequently increasing BTB permeability (13, 102, 130–132). These SRC-induced effects closely resemble effects of overexpressing sICAM-1, which downregulates BTB proteins like the N-cadherin/γ-catenin complex, impairing overall BTB integrity.
Excess sICAM-1 in rat testes also led to a disordered arrangement of post-step 8 spermatids within the seminiferous tubules. The sperm heads, rather than maintaining a neat alignment towards the basement membrane, exhibited a loss in polarity. The SRC-PYK2 pathway is associated with cell polarity and is likely involved in germ cell transport in the seminiferous epithelium (126, 133, 134). As sICAM-1 overexpression reduced the protein levels of SRC and PYK2, it may impede the SRC-PYK2 signaling in Sertoli cells, potentially impairing germ cell polarity and their movement within the seminiferous epithelium. Evidence also shows that intraperitoneal injection of the SRC inhibitor PP1 into rats induces sloughing of spermatocytes and round spermatids from the seminiferous epithelium (130). This phenotype of germ cell loss mimics the effects of excessive sICAM-1 in the testis. Together, sICAM-1 overexpression reduces SRC-related signaling while phenocopying global SRC inhibition outcomes, such as disoriented spermatids and their premature leaving. Thus, when secreted by germ cells, sICAM-1 appears to target SRC in Sertoli cells, affecting Sertoli-germ cell adhesion.
5.4 Summary and future directions
In conclusion, the evidence presented here demonstrates that sICAM-1 and SRC both contribute to junction disassembly and F-actin cytoskeleton remodeling. sICAM-1 overexpression diminishes activity of the tyrosine phosphorylation pathways driven by SRC and its related kinases. This impairs GJ and/or desmosomes, leading to BTB dysfunction and premature germ cell release. This pattern mirrors the effects seen when overall SRC activity is suppressed. Regarding F-actin cytoskeleton, the consequences of sICAM-1 overexpression parallel those of an SRC deficiency. Evidence also indicates sICAM-1 overexpression reduces levels of SRC and PYK2, possibly inhibiting downstream signaling in Sertoli cells, which could impair germ cell polarity and transport by disrupting SRC-PYK2. SRC thus mediates sICAM-1 impacts on BTB integrity, Sertoli-germ cell adhesion, and underlying cytoskeleton. When germ cells secrete sICAM-1 at the Sertoli-Sertoli or Sertoli-germ cell interface, SRC in Sertoli cells responds, so as to facilitate germ cell transport via cytoskeletal modulation and/or junction restructuring (Figure 3).
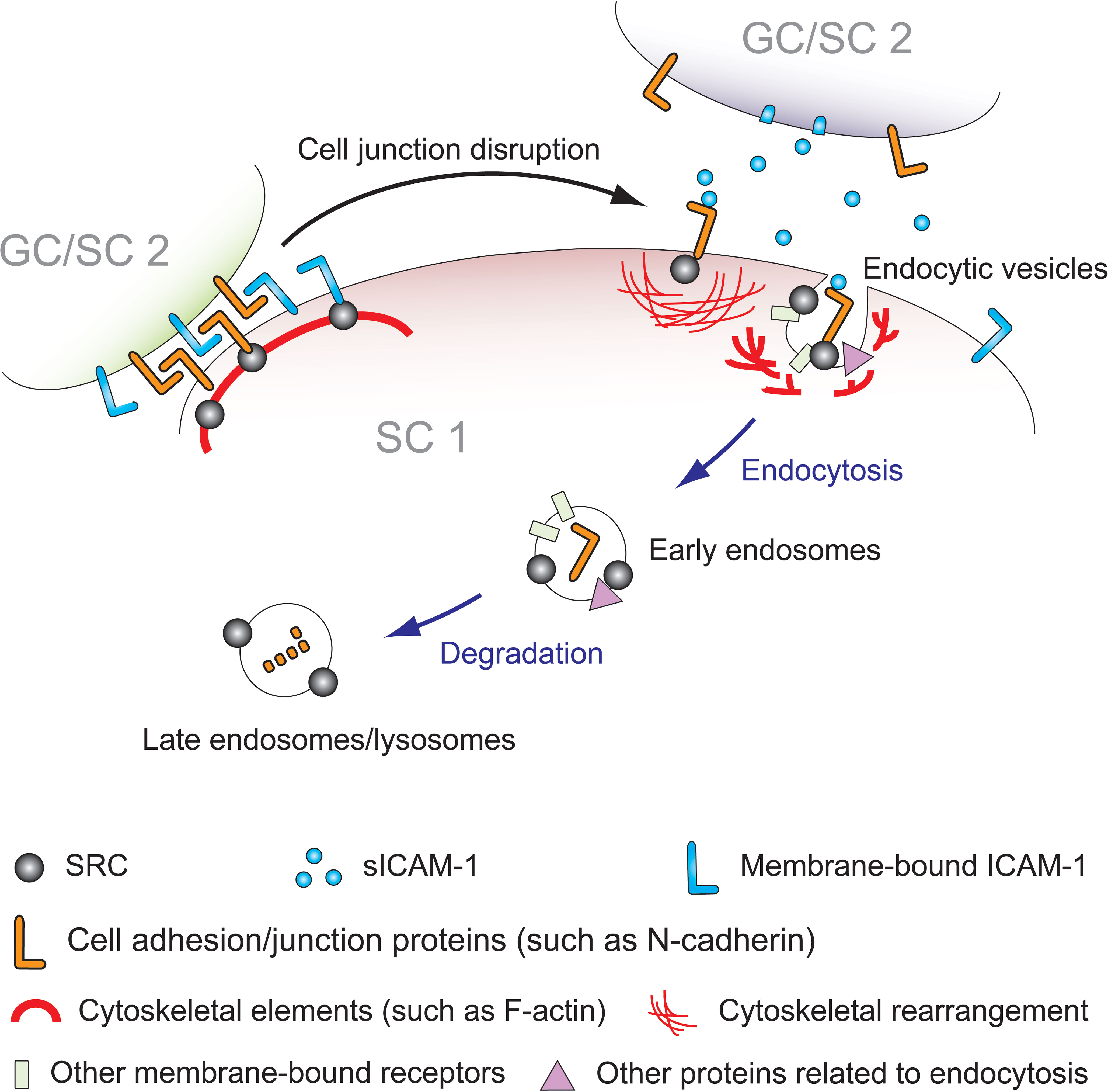
Figure 3 Schematic model depicting the mechanism of sICAM-1 and SRC interplay during Sertoli-Sertoli (SC 1 and SC 2) and Sertoli-germ (SC 1 and GC) cell adhesion. In mammalian testes, germ cell adhesion and the dynamic reorganization of the blood-testis barrier (BTB) primarily rely on protein endocytosis and intracellular transport of internalized proteins, including protein degradation, recycling, and transcytosis. SRC is known to regulate adhesion between immature germ cells and Sertoli cells, as well as the release of mature sperm from the seminiferous epithelium, playing a role in cell junction dissociation and restructuring. Previous studies have shown that SRC promotes endocytosis and degradation of BTB proteins in Sertoli cells, disassembling the “old” BTB. Overexpression of sICAM-1 in primary Sertoli cells and rat testes damages the BTB, GJ, and desmosomes, leading to increased BTB permeability, loss of immature germ cells, and decreased expression levels of SRC, p-SRC-Y530, and SRC substrate PYK2/p-PYK2-Y402. This affects the F-actin cytoskeleton, consistent with the phenotype of SRC deficiency or inhibition. As sICAM-1 is a germ cell-secreted extracellular signal, SRC likely acts downstream as the intracellular mediator of sICAM-1 effects on Sertoli cells, propagating sICAM-1 disruption of the BTB and Sertoli-germ cell adhesion. This model illustrates that at Sertoli-Sertoli (BTB) and Sertoli-germ cell junctions, sICAM-1 signals through cell adhesion proteins to recruit SRC, triggering cytoskeletal reorganization and adhesion protein endocytosis and degradation to regulate the BTB and spermatogenesis.
Future directions could explore these proposed mechanisms further. Research has indicated that the four predominant cytoskeletal structures in the testis—namely, microfilaments (F-actin), microtubules (MT, composed of tubulin polymers), intermediate filaments (e.g., vimentin filaments), and septins—interact with and influence each other (135–137). Additional investigations may delve into the impacts of sICAM-1 overexpression on these various cytoskeletal structures, as well as the subsequent changes in the Sertoli cell cytoskeleton after modulating SRC expression or activity. Approaches that alter SRC might offer a way to counteract the adverse outcomes instigated by sICAM-1 overproduction, such as BTB compromise, premature germ cell loss, and defects in spermatid polarity.
Further studies could also investigate transcription factors and broader gene expression changes resulting from sICAM-1-mediated effects on SRC signaling. The testis has specialized transcription complexes that coordinate the spermatogenic differentiation program (138). SRC can phosphorylate and/or activate various transcription factors like forkhead box class O proteins (FOXO), signal transducer and activator of transcription (STAT) proteins, and nuclear factor-κB (NF-κB) (122, 139–141). Low sICAM-1 levels may also trigger NF-κB and ERK activation, releasing inflammatory cytokines (70). However, limited information exists on specific testicular transcription factors regulated by SRC or the sICAM-1/SRC pathway. It would be interesting to decipher how main transcriptional factors are regulated in response to changes in phosphorylation status of the SRC kinase signaling pathway, and also understand the potential effect of signaling mediated by sICAM-1 on the transcriptional networks that are activated or downregulated in response to changes in sICAM-1/ICAM-1 ratios during spermatogenesis. Profiling genome-wide expression changes at defined spermatogenic stages after sICAM-1 exposure could reveal roles of particular transcription factors and gene networks altered by SRC-mediated signals. Integrating transcriptomic and proteomic data will enable constructing detailed signaling cascades from surface sICAM-1/ICAM-1 ratios and adhesive interactions to nuclear transcriptional responses governing spermatogenesis and cytoskeletal remodeling.
In summary, sICAM-1 and SRC are pivotal regulators of BTB function and spermatogenesis. Overproduction of sICAM-1 mirrors the effects of suppressing SRC, implying that SRC may be positioned downstream of sICAM-1, governing the Sertoli cell cytoskeleton, BTB stability, and the adhesion of germ cells. A more in-depth understanding of the sICAM-1/SRC signaling mechanisms promises to yield valuable insights for manipulating BTB permeability and addressing male infertility.
6 Understanding sICAM-1 interactions and knowledge gaps
6.1 sICAM-1 interactions and binding partners
ICAM-1 and its soluble form sICAM-1 must interact with various partners to mediate their functions. One of the best characterized binding partners is LFA-1, a member of the β2-integrin family. As the classical interacting protein of ICAM-1, studies show that sICAM-1 can competitively bind to LFA-1 similarly to ICAM-1 (142, 143). However, the precise role of this interaction in the testis remains unclear. While LFA-1 has been detected in mouse testicular germ cells, it does not appear to directly bind ICAM-1 in this tissue (144). This suggests there may be alternative, testis-specific binding partners for both ICAM-1 and sICAM-1 that influence their function locally. Co-immunoprecipitation experiments shows ICAM-1 physically associates with actin cytoskeletal filaments as well as several important tight junction proteins in the rat testis, including occludin and N-cadherin (12). These proteins represent plausible interacting partners for ICAM-1 and sICAM-1 at testicular junctional sites. However, it remains unknown whether the associations are direct or indirect. Further studies are still needed to conclusively identify the ligand(s) that ICAM-1 and sICAM-1 bind to on adjacent Sertoli and germ cells.
Questions also remain regarding the precise mechanisms and functional outcomes of these putative interactions in the testicular environment. Do ICAM-1 and sICAM-1 directly engage occludin, N-cadherin or other proteins at junctions? Elucidating their binding modes of action could provide crucial insights into how these molecules regulate testicular permeability and germ cell transport.
6.2 Functional regions of sICAM-1
Beyond its binding partners, understanding the specific domains that facilitate interactions is important for elucidating ICAM-1 and sICAM-1 function. Examining their signaling mechanisms further illuminates the roles of these molecules in the testis.
Structural analyses indicate the D1 domain of ICAM-1 predominantly mediates its binding to transmembrane partners like LFA-1. Additionally, the D3 or D3-D4 regions interact with other β2-integrin family members such as Mac-1 and p150,95 (74). As sICAM-1 likely binds extracellular targets on adjacent Sertoli cells, one hypothesis is that sICAM-1 engages N-cadherin extracellularly and recruits SRC signaling through N-cadherin’s cytoplasmic tail, thereby initiating SRC signaling intracellularly. Analyzing individual or combined sICAM-1 fragments could help identify key motifs enabling these interactions. Understanding the specific domains involved in partner binding and signaling initiation provides insights into the mechanisms by which ICAM-1 and sICAM-1 exert their functions in the testis.
6.3 Differences between membrane-bound ICAM-1 and tailless sICAM-1 signaling
The cytoplasmic tail of ICAM-1 plays a pivotal regulatory role in intracellular signaling, influencing various cellular processes (70). Deletion of this tail impairs ICAM-1 function, reducing adhesion and stress fiber formation. The RKIKK motif within the tail plays a critical part in regulating ICAM-1 dynamics on the cell surface, inducing actin cytoskeleton rearrangement and stress fiber formation through interactions with actin binding proteins (145). Additionally, the tail establishes important interactions with the actin cytoskeleton. It is essential for efficient RhoA activation and also interacts with myosin-II and Rac1, contributing to downstream effects on actin cytoskeleton remodeling and cell adhesion (70, 146, 147). Moreover, it is crucial for the ICAM-1 cleavage process, particularly through tyrosine residues Y474 and Y485 within its cytoplasmic region (148). The tail’s association with proteins like α-actinin, ezrin, and moesin is also pivotal for ICAM-1 localization and functions related to adhesion and migration (149, 150).
In contrast to membrane-bound ICAM-1, as the tailless form, sICAM-1 lacks the ability to directly impact key intracellular signaling entities. For example, sICAM-1 cannot influence SRC or components of the actin cytoskeleton in the same way as ICAM-1. The precise mechanisms of sICAM-1 signaling remain uncertain. It is unclear whether signaling solely involves sICAM-1 functioning as a cleaved and released molecule, or if signaling may still occur through residual interactions between the tail and intracellular proteins after cleavage. Alternatively, sICAM-1 could signal independently upon binding extracellular receptors. Elucidating these uncertainties surrounding sICAM-1 signaling pathways requires further investigation.
6.4 Target molecules of sICAM-1
While sICAM-1 shares similarities with ICAM-1, emerging evidence indicates it functions as a distinct signaling molecule in the testis (12–14). Both Sertoli and germ cells express ICAM-1, but germ cells predominantly express sICAM-1 and not ICAM-1. Since sICAM-1 overexpression alone triggers downstream signaling, it likely transmits signals generated by germ cells to Sertoli cells. One hypothesis is that sICAM-1 functions to open the BTB, enabling spermatocyte transit across. It may also help interrupt and reassemble Sertoli-germ cell adhesion to facilitate transport of developing germ cells through the epithelium. Elevated sICAM-1 associates with decreased SRC signaling and lower levels of specific adhesion proteins such as N-cadherin, connexin 43, and γ-catenin. Thus, sICAM-1 could prompt degradation of BTB/adhesion proteins like N-cadherin via SRC pathways, compromising junction integrity. N-cadherin and other membrane proteins may be direct targets on Sertoli cells that enter SRC-mediated degradation pathways upon receiving extracellular sICAM-1 signals from germ cells (Figure 3). Identifying sICAM-1’s exact molecular targets and deciphering its role in regulating barrier permeability and germ cell movement are fundamental goals for advancing our understanding.
6.5 Linking sICAM-1 to testicular pathology and dysfunction
While studies establish associations between sICAM-1 and barrier integrity proteins, several questions remain regarding its links to pathological conditions in the testis. The inverse relationship between sICAM-1 levels and proteins involved in cell contacts points to its potential involvement in adhesion dysregulation. However, causality has yet to be proven. Exposure to toxicants is known to disrupt the BTB and cause germ cell loss. Given sICAM-1’s role in barrier function, it may mediate some aspects of toxicant susceptibility in the testis. Some studies have shown that air pollutants such as diesel exhaust particles and particulate matter up-regulate sICAM-1 and/or ICAM-1 expression in both humans and animals (151–153). Additionally, drug treatments like statins mostly decreased sICAM-1 and/or ICAM-1 levels in patients (154, 155). However, direct evidence in the testis is still lacking. The precise circumstances influencing sICAM-1 level changes in the testis remain unknown, as does the identification of treatments that could reduce its levels.
To move from correlation to elucidating disease mechanisms, more in-depth investigation of sICAM-1 signaling pathways is needed. Answering questions about how it specifically modifies junction formation and barrier properties, as well as its effects in toxicant exposure models, could help link sICAM-1 functions to pathological barrier breakdown in the testis. Addressing key gaps through techniques like overexpression, knockdown and toxicological models will help determine sICAM-1’s precise role in testis dysfunction. Its intricate regulation of cell adhesions indicates its potential as a target for diagnosing and treating male reproductive conditions.
7 Future avenues and opportunities for sICAM-1 study
The study of sICAM-1, an endogenous regulatory molecule with unique biological activity, presents promising opportunities to advance our understanding of processes beyond its established role in BTB restructuring and spermatogenesis. One exciting area of future research is the BBB. Like the BTB, the BBB’s vital protective function also prevents drug delivery to the brain. sICAM-1’s ability to modulate barrier function makes it a candidate for strategies to enable efficient drug delivery across the BBB, potentially enabling new treatments for neurological disorders. Pairing sICAM-1 fragments with specific drugs could be explored, developing targeted brain delivery approaches. Additionally, sICAM-1’s association with inflammatory diseases like rheumatoid arthritis suggests the possibility of elucidating molecular mechanisms to inform targeted therapies. Its influence on tumor progression and metastasis also indicates significance for cancer research, perhaps leading to innovative therapeutic approaches for patients. Deeper investigation into sICAM-1’s role across tissue systems may provide pivotal insights. For cases of unexplained male infertility or testicular inflammation, exploring BTB damage and the expression of sICAM-1, SRC, and related signals could illuminate underlying causes. In summary, sICAM-1’s diverse implications across biology and medicine offer promising opportunities to advance both scientific understanding and therapeutic innovation.
Author contributions
XX: Conceptualization, Funding acquisition, Resources, Supervision, Writing – original draft, Writing – review & editing. YH: Investigation, Methodology, Writing – review & editing. QL: Investigation, Methodology, Writing – review & editing. DZ: Investigation, Methodology, Writing – review & editing. CC: Methodology, Resources, Validation, Writing – review & editing. YN: Conceptualization, Funding acquisition, Methodology, Resources, Supervision, Writing – review & editing.
Funding
The author(s) declare financial support was received for the research, authorship, and/or publication of this article. This work was supported by grants from the National Natural Science Foundation of China (31371176), Zhejiang Provincial Natural Science Foundation of China (LY21H040005), Zhejiang Provincial Department of Education (Y202045395), Health Commission of Zhejiang Province (2023ZL355), Hangzhou Medical College 2021 Institutional Special Fund (YS2021007).
Conflict of interest
The authors declare that the research was conducted in the absence of any commercial or financial relationships that could be construed as a potential conflict of interest.
Publisher’s note
All claims expressed in this article are solely those of the authors and do not necessarily represent those of their affiliated organizations, or those of the publisher, the editors and the reviewers. Any product that may be evaluated in this article, or claim that may be made by its manufacturer, is not guaranteed or endorsed by the publisher.
References
1. Huang B, Wang Z, Kong Y, Jin M, Ma L. Global, regional and national burden of male infertility in 204 countries and territories between 1990 and 2019: an analysis of global burden of disease study. BMC Public Health (2023) 23(1):2195. doi: 10.1186/s12889-023-16793-3
2. Njagi P, Groot W, Arsenijevic J, Dyer S, Mburu G, Kiarie J. Financial costs of assisted reproductive technology for patients in low- and middle-income countries: a systematic review. Hum Reprod Open (2023) 2023(2):hoad007. doi: 10.1093/hropen/hoad007
3. Lei A, You H, Luo B, Ren J. The associations between infertility-related stress, family adaptability and family cohesion in infertile couples. Sci Rep (2021) 11(1):24220. doi: 10.1038/s41598-021-03715-9
4. Levine H, Jørgensen N, Martino-Andrade A, Mendiola J, Weksler-Derri D, Jolles M, et al. Temporal trends in sperm count: a systematic review and meta-regression analysis of samples collected globally in the 20th and 21st centuries. Hum Reprod Update (2023) 29(2):157–76. doi: 10.1093/humupd/dmac035
5. Liu J, Dai Y, Li Y, Yuan E, Wang Q, Wang X, et al. A longitudinal study of semen quality among Chinese sperm donor candidates during the past 11 years. Sci Rep (2020) 10(1):10771. doi: 10.1038/s41598-020-67707-x
6. Sengupta P, Borges E Jr., Dutta S, Krajewska-Kulak E. Decline in sperm count in European men during the past 50 years. Hum Exp Toxicol (2018) 37(3):247–55. doi: 10.1177/0960327117703690
7. Yuan HF, Shangguan HF, Zheng Y, Meng TQ, Xiong CL, Guan HT. Decline in semen concentration of healthy Chinese adults: evidence from 9357 participants from 2010 to 2015. Asian J Androl (2018) 20(4):379–84. doi: 10.4103/aja.aja_80_17
8. Oud MS, Smits RM, Smith HE, Mastrorosa FK, Holt GS, Houston BJ, et al. A de novo paradigm for male infertility. Nat Commun (2022) 13(1):154. doi: 10.1038/s41467-021-27132-8
9. Burke ND, Nixon B, Roman SD, Schjenken JE, Walters JLH, Aitken RJ, et al. Male infertility and somatic health - insights into lipid damage as a mechanistic link. Nat Rev Urol (2022) 19(12):727–50. doi: 10.1038/s41585-022-00640-y
10. Murshidi MM, Choy JT, Eisenberg ML. Male infertility and somatic health. Urol Clin North Am (2020) 47(2):211–7. doi: 10.1016/j.ucl.2019.12.008
11. Behboudi-Gandevani S, Bidhendi-Yarandi R, Panahi MH, Vaismoradi M, Systematic Review A. and meta-analysis of male infertility and the subsequent risk of cancer. Front Oncol (2021) 11:696702. doi: 10.3389/fonc.2021.696702
12. Xiao X, Cheng CY, Mruk DD. Intercellular adhesion molecule-1 is a regulator of blood-testis barrier function. J Cell Sci (2012) 125(Pt 23):5677–89. doi: 10.1242/jcs.107987
13. Xiao X, Mruk DD, Cheng CY. Intercellular adhesion molecules (ICAMs) and spermatogenesis. Hum Reprod Update (2013) 19(2):167–86. doi: 10.1093/humupd/dms049
14. Mruk DD, Xiao X, Lydka M, Li MW, Bilinska B, Cheng CY. Intercellular adhesion molecule 1: recent findings and new concepts involved in mammalian spermatogenesis. Semin Cell Dev Biol (2014) 29:43–54. doi: 10.1016/j.semcdb.2013.07.003
15. Jelinkova L, Tuckova L, Sanchez D, Krupickova S, Pozler O, Nevoral J, et al. Increased levels of circulating ICAM-1, E-selectin, and IL-2 receptors in celiac disease. Dig Dis Sci (2000) 45(2):398–402. doi: 10.1023/A:1005489316037
16. Massart C, Sonnet E, Gibassier J, Maugendre D, Genetet B. Clinical validity of intercellular adhesion molecule-1 (ICAM-1) and TSH receptor antibodies in sera from patients with Graves' disease. Clinica Chimica Acta (1997) 265(2):157–68. doi: 10.1016/S0009-8981(97)00102-2
17. Sonnet E, Massart C, Gibassier J, Allannic H, Maugendre D. Longitudinal study of soluble intercellular adhesion molecule-1 (ICAM-1) in sera of patients with Graves' disease. J Endocrinol Invest (1999) 22(6):430–5. doi: 10.1007/BF03343586
18. Fukazawa H, Yoshida K, Kaise N, Kiso Y, Sayama N, Mori K, et al. Intercellular adhesion molecule-1 (ICAM-1) in the sera of patients with Graves' disease: correlation with disease activity and treatment status. Thyroid (1995) 5(5):373–7. doi: 10.1089/thy.1995.5.373
19. Shijubo N, Imai K, Aoki S, Hirasawa M, Sugawara H, Koba H, et al. Circulating intercellular adhesion molecule-1 (ICAM-1) antigen in sera of patients with idiopathic pulmonary fibrosis. Clin Exp Immunol (1992) 89(1):58–62. doi: 10.1111/j.1365-2249.1992.tb06877.x
20. Batycka-Baran A, Paprocka M, Krawczenko A, Dus D, Szepietowski JC. Increased number of circulating endothelial cells (CECs) in patients with psoriasis–preliminary report. J Eur Acad Dermatol Venereol (2014) 28(1):116–9. doi: 10.1111/j.1468-3083.2012.04671.x
21. Gangemi S, Merendino RA, Guarneri F, Minciullo PL, DiLorenzo G, Pacor M, et al. Serum levels of interleukin-18 and s-ICAM-1 in patients affected by psoriasis: preliminary considerations. J Eur Acad Dermatol Venereol (2003) 17(1):42–6. doi: 10.1046/j.1468-3083.2003.00647.x
22. De Pita O, Frezzolini A, Cianetti A, De Sanctis G, Fontana L, Bottari V. Squamous cell carcinoma-related antigen (SCCr-Ag), sICAM-1 and beta 2-microglobulin are useful markers of disease activity in psoriasis. Acta Derm Venereol (1999) 79(2):132–5. doi: 10.1080/000155599750011354
23. Borská L, Fiala Z, Krejsek J, Andrýs C, Vokurková D, Hamáková K, et al. Selected immunological changes in patients with Goeckerman's therapy TNF-alpha, sE-selectin, sP-selectin, sICAM-1 and IL-8. Physiol Res (2006) 55(6):699–706. doi: 10.33549/physiolres.930928
24. Zhao J, Ye X, Zhang Z. The predictive value of serum soluble ICAM-1 and CXCL13 in the therapeutic response to TNF inhibitor in rheumatoid arthritis patients who are refractory to csDMARDs. Clin Rheumatol (2020) 39(9):2573–81. doi: 10.1007/s10067-020-05043-1
25. Navarro-Hernandez RE, Oregon-Romero E, Vazquez-Del Mercado M, Rangel-Villalobos H, Palafox-Sanchez CA, Munoz-Valle JF. Expression of ICAM1 and VCAM1 serum levels in rheumatoid arthritis clinical activity. Assoc Genet polymorphisms. Dis Markers (2009) 26(3):119–26. doi: 10.3233/DMA-2009-0621
26. Hussein MR, Fathi NA, El-Din AM, Hassan HI, Abdullah F, Al-Hakeem E, et al. Alterations of the CD4(+), CD8 (+) T cell subsets, interleukins-1beta, IL-10, IL-17, tumor necrosis factor-alpha and soluble intercellular adhesion molecule-1 in rheumatoid arthritis and osteoarthritis: preliminary observations. Pathol Oncol Res (2008) 14(3):321–8. doi: 10.1007/s12253-008-9016-1
27. Delle Sedie A, Riente L, Maggiorini L, Pratesi F, Tavoni A, Migliorini P, et al. Potential biomarkers in patients with systemic sclerosis. Int J Rheum Dis (2018) 21(1):261–5. doi: 10.1111/1756-185X.13196
28. Puxeddu I, Panza F, Pratesi F, Bartaloni D, Casigliani Rabl S, Rocchi V, et al. CCL5/RANTES, sVCAM-1, and sICAM-1 in chronic spontaneous urticaria. Int Arch Allergy Immunol (2013) 162(4):330–4. doi: 10.1159/000354922
29. Tso TK, Huang WN. Elevated soluble intercellular adhesion molecule-1 levels in patients with systemic lupus erythematosus: relation to insulin resistance. J Rheumatol (2007) 34(4):726–30.
30. Guo Liu RN, Cheng QY, Zhou HY, Li BZ, Ye DQ. Elevated blood and urinary ICAM-1 is a biomarker for systemic lupus erythematosus: A systematic review and meta-analysis. Immunol Invest (2020) 49(1-2):15–31. doi: 10.1080/08820139.2019.1624769
31. Vainer B, Nielsen OH. Correlation between circulating soluble ICAM-1 and prednisolone-induced amelioration of ulcerative colitis. Scand J Gastroenterol (2003) 38(3):283–7. doi: 10.1080/00365520310000609a
32. Yang X, Yan L, Ha D, Qu L, Liu L, Tao Y. Changes in sICAM-1 and GM-CSF levels in skin tissue fluid and expression of IL-6, IL-17 and TNF-α in blood of patients with vitiligo. Exp Ther Med (2019) 17(1):408–12. doi: 10.3892/etm.2018.6937
33. Reichert Faria A, Jung JE, Silva de Castro CC, de Noronha L. Reduced immunohistochemical expression of adhesion molecules in vitiligo skin biopsies. Pathol Res Pract (2017) 213(3):199–204. doi: 10.1016/j.prp.2016.12.019
34. Strzelczyk JK, Cuber P, Bochon B, Gajdzik K, Strzelczyk J, Krakowczyk L, et al. The levels of sICAM-1, sELAM-1, TNFalpha and sTNFR1 proteins in patients with colorectal adenocarcinoma in tumor and corresponding normal mucosa. Acta Biochim Pol (2020) 67(4):579–85. doi: 10.18388/abp.2020_5449
35. Mantur M, Snarska J, Koper O, Dzieciol J, Plonski A, Lemancewicz D. Serum sICAM, sVCAM and sE-selectin levels in colorectal cancer patients. Folia Histochem Cytobiol (2009) 47(4):621–5. doi: 10.2478/v10042-009-0077-0
36. Kang X, Wang F, Xie JD, Cao J, Xian PZ. Clinical evaluation of serum concentrations of intercellular adhesion molecule-1 in patients with colorectal cancer. World J Gastroenterol (2005) 11(27):4250–3. doi: 10.3748/wjg.v11.i27.4250
37. Herrero A, Benedicto A, Romayor I, Olaso E, Arteta B. Inhibition of COX-2 impairs colon cancer liver metastasis through reduced stromal cell reaction. Biomol Ther (Seoul) (2021) 29(3):342–51. doi: 10.4062/biomolther.2020.160
38. Dong Z, Fu S, Xu X, Yang Y, Du L, Li W, et al. Leptin-mediated regulation of ICAM-1 is Rho/ROCK dependent and enhances gastric cancer cell migration. Br J Cancer (2014) 110(7):1801–10. doi: 10.1038/bjc.2014.70
39. Liu YZ, Chen B, She XD. A clinical evaluation of serum concentrations of intercellular adhesion molecule-1 in patients with gastric cancer. World J Gastroenterol (1998) 4(3):225–7. doi: 10.3748/wjg.v4.i3.225
40. Benekli M, Gullu IH, Tekuzman G, Savas MC, Hayran M, Hascelik G, et al. Circulating intercellular adhesion molecule-1 and E-selectin levels in gastric cancer. Br J Cancer (1998) 78(2):267–71. doi: 10.1038/bjc.1998.476
41. Shi BB, Goya N, Okuda H, Ryoji O, Nakazawa H, Toma H. Detection and quantification of soluble intercellular adhesion molecule-1 (sICAM-1) in the serum and urine of patients with bladder cancer. Int J Urol (1998) 5(4):324–8. doi: 10.1111/j.1442-2042.1998.tb00359.x
42. De Vita F, Orditura M, Infusino S, Auriemma A, Catalano G. Increased serum levels of tumor necrosis factor-alpha are correlated to soluble intercellular adhesion molecule-1 concentrations in non-small cell lung cancer patients. Int J Mol Med (1998) 1(3):605–8. doi: 10.3892/ijmm.1.3.605
43. De Vita F, Infusino S, Auriemma A, Orditura M, Catalano G. Circulating levels of soluble intercellular adhesion molecule-1 in non-small cell lung cancer patients. Oncol Rep (1998) 5(2):393–6. doi: 10.3892/or.5.2.393
44. Sprenger A, Schardt C, Rotsch M, Zehrer M, Wolf M, Havemann K, et al. Soluble intercellular adhesion molecule-1 in patients with lung cancer and benign lung diseases. J Cancer Res Clin Oncol (1997) 123(11-12):632–8. doi: 10.1007/s004320050117
45. Grothey A, Heistermann P, Philippou S, Voigtmann R. Serum levels of soluble intercellular adhesion molecule-1 (ICAM-1, CD54) in patients with non-small-cell lung cancer: correlation with histological expression of ICAM-1 and tumour stage. Br J Cancer (1998) 77(5):801–7. doi: 10.1038/bjc.1998.130
46. Eggeman H, Stöblen F, Thill M, Korlach S, Schmid P, Lüftner D, et al. Influence of a dose-dense adjuvant chemotherapy on sVCAM-1/sICAM-1 serum levels in breast cancer patients with 1-3 positive lymph nodes. Anticancer Res (2011) 31(8):2617–22.
47. Blann AD, Byrne GJ, Baildam AD. Increased soluble intercellular adhesion molecule-1, breast cancer and the acute phase response. Blood Coagul Fibrinolysis (2002) 13(2):165–8. doi: 10.1097/00001721-200203000-00012
48. Shai I, Pischon T, Hu FB, Ascherio A, Rifai N, Rimm EB. Soluble intercellular adhesion molecules, soluble vascular cell adhesion molecules, and risk of coronary heart disease. Obes (Silver Spring) (2006) 14(11):2099–106. doi: 10.1038/oby.2006.245
49. Noutsias M, Hohmann C, Pauschinger M, Schwimmbeck P-L, Ostermann K, Rode U, et al. sICAM-1 correlates with myocardial ICAM-1 expression in dilated cardiomyopathy. Int J Cardiol (2003) 91(2-3):153–61. doi: 10.1016/S0167-5273(03)00033-0
50. Niu H, Jiang R, Dong S, Xia L, Fang H. Research on association between levels of serum adiponectin, hs-CRP, and sICAM-1 and hypertensive cerebrovascular complications. BioMed Res Int (2021) 2021:4455038. doi: 10.1155/2021/4455038
51. Nagy I, Mandi Y. Serum and ascitic levels of soluble intercellular adhesion molecule-1 in patients with alcoholic liver cirrhosis: relation to biochemical markers of disease activity and alcohol intake. Alcohol Clin Exp Res (1996) 20(5):929–33. doi: 10.1111/j.1530-0277.1996.tb05273.x
52. Polzien F. G. R., Increased intercellular adhesion molecule-1 serum concentration in cholestasis. J Hepatol (1996) 25(6):877–86. doi: 10.1016/s0168-8278(96)80292-3
53. Lo Iacono O, Garcia-Monzon C, Almasio P, Garcia-Buey L, Craxi A, Moreno-Otero R. Soluble adhesion molecules correlate with liver inflammation and fibrosis in chronic hepatitis C treated with interferon-alpha. Aliment Pharmacol Ther (1998) 12(11):1091–9. doi: 10.1046/j.1365-2036.1998.00412.x
54. Adams DH, Mainolfi E, Burra P, Neuberger JM, Ayres R, Elias E, et al. Detection of circulating intercellular adhesion molecule-1 in chronic liver diseases. Hepatology (1992) 16(3):810–4. doi: 10.1002/hep.1840160330
55. Ellis MK, Li Y, Hou X, Chen H, McManus DP. sTNFR-II and sICAM-1 are associated with acute disease and hepatic inflammation in schistosomiasis japonica. Int J Parasitol (2008) 38(6):717–23. doi: 10.1016/j.ijpara.2007.09.013
56. Wennstrom M, Nielsen HM, Orhan F, Londos E, Minthon L, Erhardt S. Kynurenic Acid levels in cerebrospinal fluid from patients with Alzheimer's disease or dementia with lewy bodies. Int J Tryptophan Res (2014) 7:1–7. doi: 10.4137/IJTR.S13958
57. Bowman GL, Dayon L, Kirkland R, Wojcik J, Peyratout G, Severin IC, et al. Blood-brain barrier breakdown, neuroinflammation, and cognitive decline in older adults. Alzheimers Dement (2018) 14(12):1640–50. doi: 10.1016/j.jalz.2018.06.2857
58. Koper OM, Kaminska J, Sawicki K, Reszec J, Rutkowski R, Jadeszko M, et al. Cerebrospinal fluid and serum IL-8, CCL2, and ICAM-1 concentrations in astrocytic brain tumor patients. Ir J Med Sci (2018) 187(3):767–75. doi: 10.1007/s11845-017-1695-8
59. Muller N. The role of intercellular adhesion molecule-1 in the pathogenesis of psychiatric disorders. Front Pharmacol (2019) 10:1251. doi: 10.3389/fphar.2019.01251
60. Rieckmann P, Altenhofen B, Riegel A, Baudewig J, Felgenhauer K. Soluble adhesion molecules (sVCAM-1 and sICAM-1) in cerebrospinal fluid and serum correlate with MRI activity in multiple sclerosis. Ann Neurol (1997) 41(3):326–33. doi: 10.1002/ana.410410307
61. McDonnell GV, McMillan SA, Douglas JP, Droogan AG, Hawkins SA. Serum soluble adhesion molecules in multiple sclerosis: raised sVCAM-1, sICAM-1 and sE-selectin in primary progressive disease. J Neurol (1999) 246(2):87–92. doi: 10.1007/s004150050313
62. Khoury SJ, Orav EJ, Guttmann CR, Kikinis R, Jolesz FA, Weiner HL. Changes in serum levels of ICAM and TNF-R correlate with disease activity in multiple sclerosis. Neurology (1999) 53(4):758–64. doi: 10.1212/WNL.53.4.758
63. Sharief MK, Noori MA, Ciardi M, Cirelli A, Thompson EJ. Increased levels of circulating ICAM-1 in serum and cerebrospinal fluid of patients with active multiple sclerosis. Correlation with TNF-α and blood-brain barrier damage. J Neuroimmunol (1993) 43(1-2):15–21. doi: 10.1016/0165-5728(93)90070-f
64. Somigliana E, Vigano P, Gaffuri B, Guarneri D, Busacca M, Vignali M. Human endometrial stromal cells as a source of soluble intercellular adhesion molecule (ICAM)-1 molecules. Hum Reprod (1996) 11(6):1190–4. doi: 10.1093/oxfordjournals.humrep.a019353
65. Hoffmann JC, Kruger H, Luhrs J, Hamm H. Detection of soluble adhesion molecules in pleural effusions. Chest (1996) 110(1):107–13. doi: 10.1378/chest.110.1.107
66. Shi B, Wei RX, Cui Y, Zhou J, Zhang XX. [IL-6 and sICAM-1 in seminal plasma relate to male immune infertility]. Zhonghua Nan Ke Xue (2014) 20(12):1098–102.
67. Gao N, Wang C, Yu Y, Xie L, Xing Y, Zhang Y, et al. LFA-1/ ICAM-1 promotes NK cell cytotoxicity associated with the pathogenesis of ocular toxoplasmosis in murine model. PloS Negl Trop Dis (2022) 16(10):e0010848. doi: 10.1371/journal.pntd.0010848
68. Zhong H, Lin H, Pang Q, Zhuang J, Liu X, Li X, et al. Macrophage ICAM-1 functions as a regulator of phagocytosis in LPS induced endotoxemia. Inflamm Res (2021) 70(2):193–203. doi: 10.1007/s00011-021-01437-2
69. Qiu Z, Wang Y, Zhang Z, Qin R, Peng Y, Tang W, et al. Roles of intercellular cell adhesion molecule-1 (ICAM-1) in colorectal cancer: expression, functions, prognosis, tumorigenesis, polymorphisms and therapeutic implications. Front Oncol (2022) 12:1052672. doi: 10.3389/fonc.2022.1052672
70. Bui TM, Wiesolek HL, Sumagin R. ICAM-1: A master regulator of cellular responses in inflammation, injury resolution, and tumorigenesis. J Leukoc Biol (2020) 108(3):787–99. doi: 10.1002/JLB.2MR0220-549R
71. Witkowska AM, Borawska MH. Soluble intercellular adhesion molecule-1 (sICAM-1): an overview. Eur Cytokine Netw (2004) 15(2):91–8.
72. Müller N. The role of intercellular adhesion molecule-1 in the pathogenesis of psychiatric disorders. Front Pharmacol (2019) 10:1251. doi: 10.3389/fphar.2019.01251
73. Bullard DC, Hu X, Crawford D, McDonald K, Ramos TN, Barnum SR. Expression of a single ICAM-1 isoform on T cells is sufficient for development of experimental autoimmune encephalomyelitis. Eur J Immunol (2014) 44(4):1194–9. doi: 10.1002/eji.201344023
74. Ramos TN, Bullard DC, Barnum SR. ICAM-1: isoforms and phenotypes. J Immunol (2014) 192(10):4469–74. doi: 10.4049/jimmunol.1400135
75. Kuessel L, Wenzl R, Proestling K, Balendran S, Pateisky P, Yotova S, et al. Soluble VCAM-1/soluble ICAM-1 ratio is a promising biomarker for diagnosing endometriosis. Hum Reprod (2017) 32(4):770–9. doi: 10.1093/humrep/dex028
76. D'Addio M, Frey J, Otto VI. The manifold roles of sialic acid for the biological functions of endothelial glycoproteins. Glycobiology (2020) 30(8):490–9. doi: 10.1093/glycob/cwaa008
77. Marlin SD, Staunton DE, Springer TA, Stratowa C, Sommergruber W, Merluzzi VJ. A soluble form of intercellular adhesion molecule-1 inhibits rhinovirus infection. Nature (1990) 344(6261):70–2. doi: 10.1038/344070a0
78. Riccioli A, Filippini A, De Cesaris P, Barbacci E, Stefanini M, Starace G, et al. Inflammatory mediators increase surface expression of integrin ligands, adhesion to lymphocytes, and secretion of interleukin 6 in mouse Sertoli cells. Proc Natl Acad Sci USA (1995) 92(13):5808–12. doi: 10.1073/pnas.92.13.5808
79. Rieckmann P, Michel U, Albrecht M, Brück W, Wöckel L, Felgenhauer K. Soluble forms of intercellular adhesion molecule-1 (ICAM-1) block lymphocyte attachment to cerebral endothelial cells. J Neuroimmunol (1995) 60(1-2):9–15. doi: 10.1016/0165-5728(95)00047-6
80. Yu X, Shang H, Jiang Y. ICAM-1 in HIV infection and underlying mechanisms. Cytokine (2020) 125:154830. doi: 10.1016/j.cyto.2019.154830
81. Tong M, Jiang Y, Xia D, Xiong Y, Zheng Q, Chen F, et al. Elevated expression of serum endothelial cell adhesion molecules in COVID-19 patients. J Infect Dis (2020) 222(6):894–8. doi: 10.1093/infdis/jiaa349
82. Vassiliou AG, Keskinidou C, Jahaj E, Gallos P, Dimopoulou I, Kotanidou A, et al. ICU admission levels of endothelial biomarkers as predictors of mortality in critically ill COVID-19 patients. Cells (2021) 10(1):186. doi: 10.3390/cells10010186
83. İşlek A, Songu M, Balcı MK, Aladağ İ. The Role of Serum Adhesion Molecules s-ICAM-1 and s-VCAM-1 in Chronic Otitis Media Pathogenesis: A Prospective Controlled Study. Indian J Otolaryngol Head Neck Surg (2022) 74(Suppl 3):3610–5. doi: 10.1007/s12070-020-02141-z
84. Hanser S, Mphekgwana PM, Moraba MM, Erasmus L, van Staden M. Increased endothelial biomarkers are associated with HIV antiretroviral therapy and C-reactive protein among a African rural population in Limpopo Province, South Africa. Front Public Health (2022) 10:980754. doi: 10.3389/fpubh.2022.980754
85. Papayianni A, Alexopoulos E, Giamalis P, Gionanlis L, Belechri AM, Koukoudis P, et al. Circulating levels of ICAM-1, VCAM-1, and MCP-1 are increased in haemodialysis patients: association with inflammation, dyslipidaemia, and vascular events. Nephrol Dial Transplant (2002) 17(3):435–41. doi: 10.1093/ndt/17.3.435
86. Sheikh MA, O'Connell KS, Lekva T, Szabo A, Akkouh IA, Osete JR, et al. Systemic cell adhesion molecules in severe mental illness: potential role of intercellular CAM-1 in linking peripheral and neuroinflammation. Biol Psychiatry (2023) 93(2):187–96. doi: 10.1016/j.biopsych.2022.06.029
87. Lüke P, Kraft E, Azad SC. The Short-Term Kinetics of sICAM-1 after Induction of Acute Experimental Pain in Healthy Volunteers. J Clin Med (2021) 10(9):2021. doi: 10.3390/jcm10092021
88. Lino DOC, Freitas IA, Meneses GC, Martins AMC, Daher EF, Rocha JHC, et al. Interleukin-6 and adhesion molecules VCAM-1 and ICAM-1 as biomarkers of post-acute myocardial infarction heart failure. Braz J Med Biol Res (2019) 52(12):e8658. doi: 10.1590/1414-431x20198658
89. Michalicova A, Majerova P, Kovac A. Tau protein and its role in blood-brain barrier dysfunction. Front Mol Neurosci (2020) 13:570045. doi: 10.3389/fnmol.2020.570045
90. Meixensberger S, Kuzior H, Fiebich BL, Süß P, Runge K, Berger B, et al. Upregulation of sICAM-1 and sVCAM-1 Levels in the Cerebrospinal Fluid of Patients with Schizophrenia Spectrum Disorders. Diagnostics (Basel) (2021) 11(7):1134. doi: 10.3390/diagnostics11071134
91. Blair LJ, Frauen HD, Zhang B, Nordhues BA, Bijan S, Lin YC, et al. Tau depletion prevents progressive blood-brain barrier damage in a mouse model of tauopathy. Acta Neuropathol Commun (2015) 3:8. doi: 10.1186/s40478-015-0186-2
92. Majerova P, Michalicova A, Cente M, Hanes J, Vegh J, Kittel A, et al. Trafficking of immune cells across the blood-brain barrier is modulated by neurofibrillary pathology in tauopathies. PloS One (2019) 14(5):e0217216. doi: 10.1371/journal.pone.0217216
93. Garcia MA, Nelson WJ, Chavez N. Cell-cell junctions organize structural and signaling networks. Cold Spring Harb Perspect Biol (2018) 10(4):a029181. doi: 10.1101/cshperspect.a029181
94. Bhat AA, Uppada S, Achkar IW, Hashem S, Yadav SK, Shanmugakonar M, et al. Tight junction proteins and signaling pathways in cancer and inflammation: A functional crosstalk. Front Physiol (2018) 9:1942. doi: 10.3389/fphys.2018.01942
95. Vanslembrouck B, Chen JH, Larabell C, van Hengel J. Microscopic visualization of cell-cell adhesion complexes at micro and nanoscale. Front Cell Dev Biol (2022) 10:819534. doi: 10.3389/fcell.2022.819534
96. Khalili AA, Ahmad MR. A review of cell adhesion studies for biomedical and biological applications. Int J Mol Sci (2015) 16(8):18149–84. doi: 10.3390/ijms160818149
97. Citi S. The mechanobiology of tight junctions. Biophys Rev (2019) 11(5):783–93. doi: 10.1007/s12551-019-00582-7
98. Mruk DD, Cheng CY. Sertoli-Sertoli and Sertoli-germ cell interactions and their significance in germ cell movement in the seminiferous epithelium during spermatogenesis. Endocr Rev (2004) 25(5):747–806. doi: 10.1210/er.2003-0022
99. Grove BD, Vogl AW. Sertoli cell ectoplasmic specializations: a type of actin-associated adhesion junction? J Cell Sci (1989) 93(Pt 2):309–23. doi: 10.1242/jcs.93.2.309
100. Vogl AW, Pfeiffer DC, Mulholland D, Kimel G, Guttman J. Unique and multifunctional adhesion junctions in the testis: ectoplasmic specializations. Arch Histol Cytol (2000) 63(1):1–15. doi: 10.1679/aohc.63.1
101. Lee NP, Cheng CY. Ectoplasmic specialization, a testis-specific cell-cell actin-based adherens junction type: is this a potential target for male contraceptive development? Hum Reprod Update (2004) 10(4):349–69. doi: 10.1093/humupd/dmh026
102. Cheng CY, Mruk DD. The blood-testis barrier and its implications for male contraception. Pharmacol Rev (2012) 64(1):16–64. doi: 10.1124/pr.110.002790
103. Siu MK, Mruk DD, Lee WM, Cheng CY. Adhering junction dynamics in the testis are regulated by an interplay of beta 1-integrin and focal adhesion complex-associated proteins. Endocrinology (2003) 144(5):2141–63. doi: 10.1210/en.2002-221035
104. Russell L. Observations on rat Sertoli ectoplasmic ('junctional') specializations in their association with germ cells of the rat testis. Tissue Cell (1977) 9(3):475–98. doi: 10.1016/0040-8166(77)90007-6
105. Xiao X, Mruk DD, Wong CK, Cheng CY. Germ cell transport across the seminiferous epithelium during spermatogenesis. Physiol (Bethesda) (2014) 29(4):286–98. doi: 10.1152/physiol.00001.2014
106. Yao M, Qu H, Han Y, Cheng CY, Xiao X. Kinesins in mammalian spermatogenesis and germ cell transport. Front Cell Dev Biol (2022) 10:837542. doi: 10.3389/fcell.2022.837542
107. Vaid KS, Guttman JA, Singaraja RR, Vogl AW. A kinesin is present at unique sertoli/spermatid adherens junctions in rat and mouse testes. Biol Reprod (2007) 77(6):1037–48. doi: 10.1095/biolreprod.107.063735
108. Guttman JA, Kimel GH, Vogl AW. Dynein and plus-end microtubule-dependent motors are associated with specialized Sertoli cell junction plaques (ectoplasmic specializations). J Cell Sci (2000) 113(Pt 12):2167–76. doi: 10.1242/jcs.113.12.2167
109. Beach SF, Vogl AW. Spermatid translocation in the rat seminiferous epithelium: coupling membrane trafficking machinery to a junction plaque. Biol Reprod (1999) 60(4):1036–46. doi: 10.1095/biolreprod60.4.1036
110. Dunleavy JEM, O'Bryan MK, Stanton PG, O'Donnell L. The cytoskeleton in spermatogenesis. Reproduction (2019) 157(2):R53–72. doi: 10.1530/REP-18-0457
111. Russell L. Movement of spermatocytes from the basal to the adluminal compartment of the rat testis. Am J Anat (1977) 148(3):313–28. doi: 10.1002/aja.1001480303
112. Smith BE, Braun RE. Germ cell migration across Sertoli cell tight junctions. Science (2012) 338(6108):798–802. doi: 10.1126/science.1219969
113. Russell LD. The blood-testis barrier and its formation relative to spermatocyte maturation in the adult rat: a lanthanum tracer study. Anat Rec (1978) 190(1):99–111. doi: 10.1002/ar.1091900109
114. Cowin P, Kapprell HP, Franke WW, Tamkun J, Hynes RO. Plakoglobin: a protein common to different kinds of intercellular adhering junctions. Cell (1986) 46(7):1063–73. doi: 10.1016/0092-8674(86)90706-3
115. Troyanovsky RB, Chitaev NA, Troyanovsky SM. Cadherin binding sites of plakoglobin: localization, specificity and role in targeting to adhering junctions. J Cell Sci (1996) 109(Pt 13):3069–78. doi: 10.1242/jcs.109.13.3069
116. Witcher LL, Collins R, Puttagunta S, Mechanic SE, Munson M, Gumbiner B, et al. Desmosomal cadherin binding domains of plakoglobin. J Biol Chem (1996) 271(18):10904–9. doi: 10.1074/jbc.271.18.10904
117. Martin GS. The hunting of the src. Nat Rev Mol Cell Biol (2001) 2(6):467–75. doi: 10.1038/35073094
118. Dikic I, Tokiwa G, Lev S, Courtneidge SA, Schlessinger J. A role for Pyk2 and Src in linking G-protein-coupled receptors with MAP kinase activation. Nature (1996) 383(6600):547–50. doi: 10.1038/383547a0
119. Lev S, Moreno H, Martinez R, Canoll P, Peles E, Musacchio JM, et al. Protein tyrosine kinase PYK2 involved in Ca(2+)-induced regulation of ion channel and MAP kinase functions. Nature (1995) 376(6543):737–45. doi: 10.1038/376737a0
120. Momin AA, Mendes T, Barthe P, Faure C, Hong S, Yu P, et al. PYK2 senses calcium through a disordered dimerization and calmodulin-binding element. Commun Biol (2022) 5(1):800. doi: 10.1038/s42003-022-03760-8
121. Reinecke J, Caplan S. Endocytosis and the Src family of non-receptor tyrosine kinases. Biomol Concepts (2014) 5(2):143–55. doi: 10.1515/bmc-2014-0003
122. Ortiz MA, Mikhailova T, Li X, Porter BA, Bah A, Kotula L. Src family kinases, adaptor proteins and the actin cytoskeleton in epithelial-to-mesenchymal transition. Cell Commun Signal (2021) 19(1):67. doi: 10.1186/s12964-021-00750-x
123. Parsons SJ, Parsons JT. Src family kinases, key regulators of signal transduction. Oncogene (2004) 23(48):7906–9. doi: 10.1038/sj.onc.1208160
124. Xiao X, Yang Y, Mao B, Cheng CY, Ni Y. Emerging Role for SRC family kinases in junction dynamics during spermatogenesis. Reproduction (2019) 157(3):R85–94. doi: 10.1530/REP-18-0440
125. Xiao X, Mruk DD, Cheng CY. c-Yes regulates cell adhesion at the apical ectoplasmic specialization-blood-testis barrier axis via its effects on protein recruitment and distribution. Am J Physiol Endocrinol Metab (2013) 304(2):E145–59. doi: 10.1152/ajpendo.00422.2012
126. Xiao X, Ni Y, Yu C, Li L, Mao B, Yang Y, et al. Src family kinases (SFKs) and cell polarity in the testis. Semin Cell Dev Biol (2018) 81:46–53. doi: 10.1016/j.semcdb.2017.11.024
127. Xiao X, Mruk DD, Cheng FL, Cheng CY. C-Src and c-Yes are two unlikely partners of spermatogenesis and their roles in blood-testis barrier dynamics. Adv Exp Med Biol (2012) 763:295–317. doi: 10.1007/978-1-4614-4711-5_15
128. Xiao X, Mruk DD, Wong EW, Lee WM, Han D, Wong CK, et al. Differential effects of c-Src and c-Yes on the endocytic vesicle-mediated trafficking events at the Sertoli cell blood-testis barrier: an in vitro study. Am J Physiol Endocrinol Metab (2014) 307(7):E553–62. doi: 10.1152/ajpendo.00176.2014
129. Shupe J, Cheng J, Puri P, Kostereva N, Walker WH. Regulation of Sertoli-germ cell adhesion and sperm release by FSH and nonclassical testosterone signaling. Mol Endocrinol (2011) 25(2):238–52. doi: 10.1210/me.2010-0030
130. Lee NP, Cheng CY. Protein kinases and adherens junction dynamics in the seminiferous epithelium of the rat testis. J Cell Physiol (2005) 202(2):344–60. doi: 10.1002/jcp.20119
131. D'Souza-Schorey C. Disassembling adherens junctions: breaking up is hard to do. Trends Cell Biol (2005) 15(1):19–26. doi: 10.1016/j.tcb.2004.11.002
132. Irby RB, Yeatman TJ. Increased Src activity disrupts cadherin/catenin-mediated homotypic adhesion in human colon cancer and transformed rodent cells. Cancer Res (2002) 62(9):2669–74.
133. Chen H, Xiao X, Lui WY, Lee WM, Cheng CY. Vangl2 regulates spermatid planar cell polarity through microtubule (MT)-based cytoskeleton in the rat testis. Cell Death Dis (2018) 9(3):340. doi: 10.1038/s41419-018-0339-x
134. Li L, Li H, Wang L, Wu S, Lv L, Tahir A, et al. Role of cell polarity and planar cell polarity (PCP) proteins in spermatogenesis. Crit Rev Biochem Mol Biol (2020) 55(1):71–87. doi: 10.1080/10409238.2020.1742091
135. Wen Q, Li N, Xiao X, Lui WY, Chu DS, Wong CKC, et al. Actin nucleator Spire 1 is a regulator of ectoplasmic specialization in the testis. Cell Death Dis (2018) 9(2):208. doi: 10.1038/s41419-017-0201-6
136. Wu S, Lv L, Li L, Wang L, Mao B, Li J, et al. KIF15 supports spermatogenesis via its effects on Sertoli cell microtubule, actin, vimentin, and septin cytoskeletons. Endocrinology (2021) 162(4):bqab010. doi: 10.1210/endocr/bqab010
137. Wang L, Yan M, Wu S, Mao B, Wong CKC, Ge R, et al. Microtubule cytoskeleton and spermatogenesis - Lesson from studies of toxicant models. Toxicol Sci (2020) 177(2):305–15. doi: 10.1016/j.reprotox.2020.05.017
138. Kimmins S, Kotaja N, Davidson I, Sassone-Corsi P. Testis-specific transcription mechanisms promoting male germ-cell differentiation. Reproduction (2004) 128(1):5–12. doi: 10.1530/rep.1.00170
139. Bülow MH, Bülow TR, Hoch M, Pankratz MJ, Jünger MA. Src tyrosine kinase signaling antagonizes nuclear localization of FOXO and inhibits its transcription factor activity. Sci Rep (2014) 4:4048. doi: 10.1038/srep04048
140. Saijo K, Schmedt C, Su IH, Karasuyama H, Lowell CA, Reth M, et al. Essential role of Src-family protein tyrosine kinases in NF-kappaB activation during B cell development. Nat Immunol (2003) 4(3):274–9. doi: 10.1038/ni893
141. Jalal DI, Kone BC. Src activation of NF-kappaB augments IL-1beta-induced nitric oxide production in mesangial cells. J Am Soc Nephrol (2006) 17(1):99–106. doi: 10.1681/ASN.2005070693
142. Harjunpää H, Llort Asens M, Guenther C, Fagerholm SC. Cell adhesion molecules and their roles and regulation in the immune and tumor microenvironment. Front Immunol (2019) 10:1078. doi: 10.3389/fimmu.2019.01078
143. Walling BL, Kim M. LFA-1 in T cell migration and differentiation. Front Immunol (2018) 9:952. doi: 10.3389/fimmu.2018.00952
144. Lam HC. Identification of interacting partners of LFA-1 in the testis. Hong Kong: The University of Hong Kong (2013).
145. Oh HM, Lee S, Na BR, Wee H, Kim SH, Choi SC, et al. RKIKK motif in the intracellular domain is critical for spatial and dynamic organization of ICAM-1: functional implication for the leukocyte adhesion and transmigration. Mol Biol Cell (2007) 18(6):2322–35. doi: 10.1091/mbc.e06-08-0744
146. Thompson PW, Randi AM, Ridley AJ. Intercellular adhesion molecule (ICAM)-1, but not ICAM-2, activates RhoA and stimulates c-fos and rhoA transcription in endothelial cells. J Immunol (2002) 169(2):1007–13. doi: 10.4049/jimmunol.169.2.1007
147. van Buul JD, van Rijssel J, van Alphen FP, Hoogenboezem M, Tol S, Hoeben KA, et al. Inside-out regulation of ICAM-1 dynamics in TNF-alpha-activated endothelium. PloS One (2010) 5(6):e11336. doi: 10.1371/journal.pone.0011336
148. Tsakadze NL, Sen U, Zhao Z, Sithu SD, English WR, D'Souza SE. Signals mediating cleavage of intercellular adhesion molecule-1. Am J Physiol Cell Physiol (2004) 287(1):C55–63. doi: 10.1152/ajpcell.00585.2003
149. Carpén O, Pallai P, Staunton DE, Springer TA. Association of intercellular adhesion molecule-1 (ICAM-1) with actin-containing cytoskeleton and alpha-actinin. J Cell Biol (1992) 118(5):1223–34. doi: 10.1083/jcb.118.5.1223
150. Rahman A, Fazal F. Hug tightly and say goodbye: role of endothelial ICAM-1 in leukocyte transmigration. Antioxid Redox Signal (2009) 11(4):823–39. doi: 10.1089/ars.2008.2204
151. Pope CA 3rd, Bhatnagar A, McCracken JP, Abplanalp W, Conklin DJ, O'Toole T. Exposure to fine particulate air pollution is associated with endothelial injury and systemic inflammation. Circ Res (2016) 119(11):1204–14. doi: 10.1161/CIRCRESAHA.116.309279
152. Takizawa H, Abe S, Ohtoshi T, Kawasaki S, Takami K, Desaki M, et al. Diesel exhaust particles up-regulate expression of intercellular adhesion molecule-1 (ICAM-1) in human bronchial epithelial cells. Clin Exp Immunol (2000) 120(2):356–62. doi: 10.1046/j.1365-2249.2000.01213.x
153. Dondi A, Carbone C, Manieri E, Zama D, Del Bono C, Betti L, et al. Outdoor air pollution and childhood respiratory disease: the role of oxidative stress. Int J Mol Sci (2023) 24(5):4345. doi: 10.3390/ijms24054345
154. Greenwood J, Mason JC. Statins and the vascular endothelial inflammatory response. Trends Immunol (2007) 28(2):88–98. doi: 10.1016/j.it.2006.12.003
Keywords: soluble intercellular adhesion molecule-1 (sICAM-1), Sertoli cells, blood-testis barrier (BTB), testis, spermatogenesis, cytoskeleton, male fertility
Citation: Xiao X, Han Y, Li Q, Zheng D, Cheng CY and Ni Y (2024) Exploring the evolving function of soluble intercellular adhesion molecule-1 in junction dynamics during spermatogenesis. Front. Endocrinol. 14:1281812. doi: 10.3389/fendo.2023.1281812
Received: 23 August 2023; Accepted: 15 December 2023;
Published: 08 January 2024.
Edited by:
Baohua Ma, Northwest A&F University, ChinaReviewed by:
Cristina Corina Clement, Cornell University, United StatesSergio Minucci, University of Campania Luigi Vanvitelli, Italy
James Nyirenda, University of Zambia, Zambia
Copyright © 2024 Xiao, Han, Li, Zheng, Cheng and Ni. This is an open-access article distributed under the terms of the Creative Commons Attribution License (CC BY). The use, distribution or reproduction in other forums is permitted, provided the original author(s) and the copyright owner(s) are credited and that the original publication in this journal is cited, in accordance with accepted academic practice. No use, distribution or reproduction is permitted which does not comply with these terms.
*Correspondence: Xiang Xiao, eHhpYW9AemphbXMuY24=; Ya Ni, bml5YTk5QDEyNi5jb20=