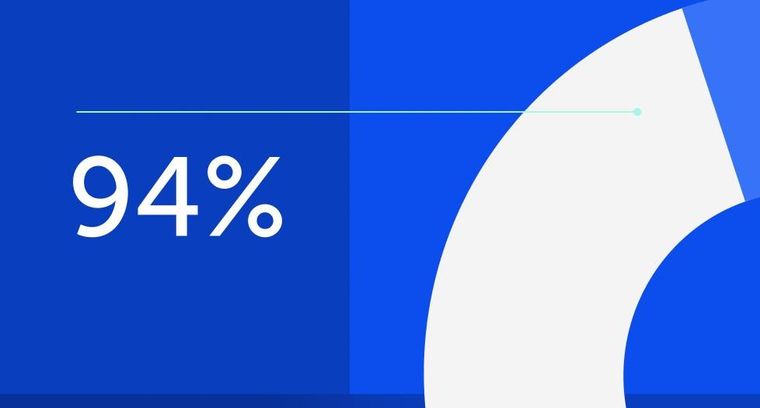
94% of researchers rate our articles as excellent or good
Learn more about the work of our research integrity team to safeguard the quality of each article we publish.
Find out more
REVIEW article
Front. Endocrinol., 25 January 2024
Sec. Reproduction
Volume 14 - 2023 | https://doi.org/10.3389/fendo.2023.1268990
This article is part of the Research TopicImpaired Receptivity of Thin Endometrium: the Mechanism, Hormone Intervention and StrategiesView all 8 articles
The endometrium is a resilient and highly dynamic tissue, undergoing cyclic renewal in preparation for embryo implantation. Cyclic endometrial regeneration depends on the intact function of several cell types, including parenchymal, endothelial, and immune cells, as well as adult stem cells that can arise from endometrial or extrauterine sources. The ability of the endometrium to undergo rapid, repeated regeneration without scarring is unique to this tissue. However, if this tissue renewal process is disrupted or dysfunctional, women may present clinically with infertility due to endometrial scarring or persistent atrophic/thin endometrium. Such disorders are rate-limiting in the treatment of female infertility and in the success of in vitro fertilization because of a dearth of treatment options specifically targeting the endometrium. A growing number of studies have explored the potential of adult stem cells, including mesenchymal stem cells (MSCs), to treat women with disorders of endometrial regeneration. MSCs are multipotent adult stem cells with capacity to differentiate into cells such as adipocytes, chondrocytes, and osteoblasts. In addition to their differentiation capacity, MSCs migrate toward injured sites where they secrete bioactive factors (e.g. cytokines, chemokines, growth factors, proteins and extracellular vesicles) to aid in tissue repair. These factors modulate biological processes critical for tissue regeneration, such as angiogenesis, cell migration and immunomodulation. The MSC secretome has therefore attracted significant attention for its therapeutic potential. In the uterus, studies utilizing rodent models and limited human trials have shown a potential benefit of MSCs and the MSC secretome in treatment of endometrial infertility. This review will explore the potential of MSCs to treat women with impaired endometrial receptivity due to a thin endometrium or endometrial scarring. We will provide context supporting leveraging MSCs for this purpose by including a review of mechanisms by which the MSC secretome promotes regeneration and repair of nonreproductive tissues.
The endometrium is a highly dynamic tissue, undergoing ~400 repeated cycles of proliferation, differentiation, shedding, and regeneration over a woman’s reproductive lifespan. Cyclic regeneration of the endometrium is a prerequisite for embryo implantation and requires hormonal stimulation, inflammation, angiogenesis, and reepithelization of the uterine lining following menses (1). The ability of the endometrium to undergo rapid, repeated regeneration during the proliferative phase, without scarring, is unique to this tissue and is an absolute requirement for the establishment of a human pregnancy. The secretory phase of the endometrial cycle commences after ovulation, during which time local inflammatory events involving immune cells, cytokines, and chemokines play a crucial role in decidualization of the endometrium in preparation for implantation (2).
Embryo implantation is defined as the process by which a blastocyst adheres to and invades the endometrium, prompting development of the feto-maternal interface (3). This process requires a complex crosstalk between a competent embryo and a receptive endometrium. Endometrial receptivity is acquired during a specific time frame post-ovulation in the menstrual cycle, when genotypic and phenotypic changes in endometrial cells result in a conducive environment for blastocyst implantation. This timeframe, known as the “window of implantation”, is also marked by cellular expression of various cytokines, growth factors, and prostaglandins necessary for synchronized crosstalk between the blastocyst and endometrium (3, 4). Beyond this timeframe, the endometrium is considered refractory to implantation or “nonreceptive”, during which time the embryo cannot establish contact, and successful implantation will not occur (4).
Despite significant advancements in assisted reproductive technologies (ART) over the past few decades, embryo implantation remains a rate limiting step in achieving success after in vitro fertilization (IVF). In modern practice, where high quality (and often euploid) embryos are identified and selectively transferred, impaired endometrial receptivity may contribute to as many as two thirds of embryo implantation failures (5). Impaired regeneration of the endometrium predisposes to a thin endometrium, which is an important contributor to impaired endometrial receptivity during IVF cycles (6). Based on a meta-analysis of 22 studies, 2.4% of women undergoing IVF are affected by thin endometrium, typically defined as an endometrial thickness less than 7 mm on transvaginal ultrasound (7). Asherman’s syndrome is one common cause of impaired endometrial regeneration and thin endometrium, and is characterized by intrauterine adhesions causing menstrual abnormalities, infertility, and/or recurrent pregnancy loss (8). The prevalence of Asherman’s syndrome varies drastically by subpopulation, ranging from 2.8% to 45.5% among infertile women (9). Most cases result from trauma to the gravid uterus, with significantly fewer cases resulting from trauma to the nongravid uterus or genital infections such as tuberculosis (10). The inflammation and fibrosis caused by these insults impairs regeneration of the endometrial lining. Thin endometrium due to any cause also increases with age, with an incidence as high as 25% of women undergoing IVF over age 40 (11).
Regardless of the etiology of thin endometrium, it is well established that embryo transfers performed in the setting of thin endometrium have decreased implantation and clinical pregnancy rates (12). A 2014 meta-analysis found that women with an endometrial thickness < 7.0mm had lower clinical pregnancy rates than women with an endometrial thickness of 7.0mm and above (7). More specifically, a retrospective cohort analysis of more than 40,000 embryo transfers found that clinical pregnancy and live birth rates decreased with each millimeter decline in endometrial thickness below 8 mm in fresh IVF-embryo transfer cycles, and below 7 mm in frozen-thawed embryo transfer cycles (13). In women who conceive, these pregnancies are subject to impaired placentation (14) and associated pregnancy complications, including early spontaneous miscarriage (15), preterm birth (16, 17), low birth weight (16, 17), hypertensive disorders of pregnancy (16, 18), placental abruption (16) and ectopic pregnancy (15).
Despite the scope of the clinical problem, therapeutic options for thin endometrium remain extremely limited. Numerous therapeutic options have been evaluated for thin endometrium; multiple medication regimens including high dose estradiol, vitamin E and pentoxifylline, mid-luteal GnRH agonist, tamoxifen, and sildenafil have been investigated (19), with modest and varying degrees of success. Immunomodulatory or pro-inflammatory methods, such as endometrial scratching (20), stem cell transplant, and intrauterine granulocyte-colony stimulating factor (G-CSF) instillation have also been employed (19). However, despite the profound limitations that thin endometrium imposes on ART and pregnancy outcomes, the optimal therapy for this condition has not yet been established.
A growing number of studies have explored the potential of mesenchymal stem cells (MSCs), a specific adult stem cell type, to treat women with disorders of endometrial regeneration. MSCs are multipotent adult stem cells with capacity to differentiate into cells of multiple lineages (21, 22). In addition to their differentiation capacity, MSCs migrate toward injured sites where they secrete bioactive factors that modulate biological processes critical for tissue regeneration (23–25). The MSC secretome has therefore attracted significant attention for its therapeutic potential in regenerative medicine, including in the uterus. Our objective herein is to provide a brief review of MSC biology and explore the potential of MSCs to treat women with impaired endometrial regeneration, based on mechanisms elucidated via a growing number of studies in rodent and human models.
Mesenchymal stem cells (MSCs), alternatively known as mesenchymal stromal cells, are multi-lineage, non-hematopoietic stem cells with self-renewing properties. First discovered by Alexander Friedenstein in the 1960s, MSCs are found throughout multiple adult and fetal tissues including bone marrow, adipose, dental pulp, umbilical cord, placenta, endometrial tissue, and menstrual blood (21, 26, 27). MSCs from all tissue sources are characterized by the expression of CD29, CD44, CD73, CD105, and CD166; while lacking hematopoietic stem cell markers such as CD34 and CD45 (23). MSCs generate various connective tissues including bone, fat, muscle, and cartilage; and have been found to generate various other functional cell types including myocytes and varying subsets of neurons (21, 22). Beyond their capacity to differentiate into various cell types, MSCs are associated with multiple endocrine and paracrine functions, including angiogenesis, homing to areas of inflammation, and systemic modulation of immune responses – both pro- and anti-inflammatory in nature, depending on the microenvironment – via their secretome, including soluble factors, neuropeptides, and microvesicles (Figure 1) (23–25). Critically, MSCs can cross allogeneic barriers, permitting MSCs derived from any patient to be used as an off-the-shelf technology for third party recipients (25).
Figure 1 MSCs for treatment of endometrial disorders. (I) Mesenchymal stem cells (MSCs) can be isolated from various adult tissues, including bone marrow, adipose, placenta, umbilical cord, dental pulp, endometrial tissue, and menstrual blood for autologous use. These cells can be directly administered to a patient with or without minimal modification or can be expanded in vitro prior to administration. (II) Once ready for injection, the MSCs can be introduced systemically (i.e. intravenously) or locally to exert their responses. (III) MSC effects can be due to a combination of their (A) differentiation potential, (B) secretome, and (C) licensing to an anti-inflammatory phenotype. These functions in conjunction make MSCs an attractive candidate for treating endometrial disorders. Figure created with Biorender.com.
Isolation of MSCs for expansion or direct use can be accomplished through minimally invasive procedures including bone marrow aspiration, abdominoplasty, lipoaspiration, and collections of other discard tissues such as the placenta and menstrual blood (28, 29). From here, the cells can be used as a heterogeneous population or can be enriched for specific population(s) of MSCs. Together with their ease of isolation and capacity to cross allogeneic barriers, this further reduces safety and ethical concerns toward MSC use, adding to the MSCs’ clinical appeal.
To date MSCs are listed in use in over two thousand clinical trials (clinicaltrials.gov), capitalizing on the aforementioned properties of MSCs – primarily their ability to home to areas of inflammation, reduce inflammation locally and/or systemically, and promote tissue regeneration (23, 25, 30–33). Beyond these currently listed applications, MSCs are being studied as potential therapy in conditions such as cancer, diabetes mellitus, and end-stage liver disease. In the subsequent sections we will discuss how MSCs modulate the immune system’s inflammatory response and promote tissue regeneration, and we will also discuss emerging uses of MSCs in endometrial dysfunction.
Migration of MSCs from either their microenvironmental niche or site of administration to the target tissue or organ is a fundamental characteristic of MSCs that facilitates their response to inflammation and tissue regeneration. MSC homing, which refers to MSC migration toward a target tissue, is facilitated by an array of cytokines and chemokines released at the site of inflammation (23, 28). MSCs express a wide array of cytokine and chemokine receptors permitting their migration and response to rapidly changing microenvironments (34, 35). Of note, MSCs express CXCL12, the receptor for SDF1α, permitting their chemo-attraction to sites of inflammation (36). Beyond SDF1α, other yet undefined cytokines are believed to be part of this chemoattraction; these cytokines could therefore be targeted for direct therapy, such as in the case of immune regulation, which will be discussed below, or as a mechanism to deliver another therapy, e.g., drug delivery (37). Once at their site, MSCs can be directly involved in tissue regeneration, but MSCs predominantly exert their function via their secretome (24, 25, 38). The efficiency of exogenous MSC mobilization to the target tissue significantly impacts the therapeutic efficacy of these cells.
Despite its importance, MSC mobilization is very inefficient (<10%), leading to debate over optimal routes of administration to improve mobilization for different applications (38). Local administration of MSCs to the target organ or tissue is one route that may enhance MSC effect. However, this mode of delivery of MSCs can be highly invasive, and the MSCs often escape from the site of administration (39). Intravenous administration has also been suggested; however, most of the cells administered intravenously are entrapped in the lung due to the MSCs’ large size and their expression of adhesion molecules such as VCAM1 and VLA-4 which can bind lung epithelial cells, with estimates of only 1% of the cells reaching their target organ (40–42). The half-life of these entrapped MSCs is short – under four days – supporting the role of the MSC secretome in the observed clinical effects of MSCs (43–45).
Different molecular engineering approaches have been employed to increase MSC homing to specific sites, including overexpression of cytokine receptors and downregulation of adhesion molecules (46–51). Another approach shown to enhance MSC homing is magnetic guidance to the target tissue, whereby the MSCs are labeled with magnetic carbon nanotubules prior to administration (52). Notably these studies have largely found decreased lung entrapment and increased homing of MSCs to the target tissues without significant effect on the viability of the cells or their capacity to differentiate (48, 52). It is crucial to keep in mind that when it comes to enhancing the homing of MSCs and their response, the approach will differ depending on the target organ or tissue, the specific disease being treated, and the source and pre-treatment of the MSCs: understanding these nuances is key to developing effective treatments and improving patient outcomes.
As described above, there is little evidence to attribute the basis of MSC clinical effect to their tissue engraftment: only a small percentage of MSCs reach their target organ, and even this small population does not survive long-term (24). Thus, the primary mechanism of the MSCs is likely their release of soluble factors, including cytokines and microvesicles (MVs).
Among classes of MVs, exosomes are the most investigated. Exosomes are MVs of 30-150 nm in diameter, enriched in proteins, miRNA, lipids, and other regulatory molecules; they have been identified in a wide array of body fluids such as blood (including plasma and serum), urine, saliva, cerebrospinal fluid, pleural effusion, and ascites; and are considered representative of the cells from which they were derived [discussed in (53)]. Due to their source-cell representation, circulating MVs are under investigation for diagnostic purposes, evaluating for biomarkers for the presence and type of aberrant cells – including malignancy, injury, and other disorders (53–56). It thus stands to reason that the MVs derived from MSCs of different source tissues, and different treatments and exposures in vitro, would differ from one another (57, 58). Due to their similarity in effect with MSCs themselves, MSC-derived exosomes (MSC-Exos) are under clinical investigation for treatment of similar pathologies (59).
MSC-Exos have been associated with anti-inflammatory and reparative functions (58, 60–63). Over 2000 proteins have been identified within MSC-Exos (57, 58). These include cytokines (e.g. IL-10, IL-6, TGF-β, TNF-α), chemokines (e.g. MCP1, CXCL14, MCP3, SDF-1α), and trophic factors (e.g. FGF, HGF, IGF1, VEGF) (58). Like their parent cells, MSC-Exos can suppress effector T cells, dendritic cell (DC) maturation, M1 macrophages, and natural killer cells (NKs); while enhancing regulatory T cells and M2 macrophages (59, 64–66). Similarly, these cargo contribute to MSC-Exos regenerative capacity, enhancing osteogenesis, chondrogenesis, and angiogenesis (63, 67–69). It should be noted, however, that both direct and indirect forms of intercellular communication between MSCs and target cells have been reported: for example gap junctional intercellular communication (GJIC) for direct intercellular communication, and release of MVs for indirect intercellular communication (70–73). Both of these means of communication, however, have the potential to transfer similar cargo, including proteins and miRNAs to target cells (74).
Within their microenvironment, MSCs can be licensed, or educated, into anti-inflammatory cells (32, 75). While the process of MSC licensing is poorly defined, specific factors are known to be necessary – namely IFNγ, in the presence of other pro-inflammatory cytokines (e.g. TNF-α, IL-1α, IL-1β) (76–78).
The immunomodulatory properties of MSCs were not well understood until recent studies showed that MSCs could impair the function of both innate and adaptive immune system cell proliferation (79, 80). These studies paved the way for scientists to shift their focus from the MSCs’ multiple-lineage and regenerative properties toward understanding the immune regulator capacity of these cells. MSCs regulate the immune system response through the release of MVs and soluble factors that impact the ability of the innate and adaptive immune cells such as myeloid DC and T-cells to respond to an infection (76, 81, 82). MSCs impair DC function by preventing their transition from immature to mature DCs, thus preventing presentation of antigen to naïve T-lymphocytes and decreased release of pro-inflammatory cytokines (e.g. TNF-α) (82, 83). MSCs also decrease NK cytotoxicity through decreased IFNγ release and decreased NK proliferation, in part through downregulating expression of NKp30 and NKG2D in NK cells (76, 84). MSCs further regulate the immune system by impairing the inflammatory response induced by the cells of the adaptive immune system. For example, MSCs inhibit T-lymphocyte differentiation by increasing IL-4 and decreasing IFNγ secretion (76). The decrease of pro-inflammatory cytokines allows T-lymphocytes to differentiate toward anti-inflammatory Th2 rather than pro-inflammatory Th1 phenotype (43, 76, 85). MSCs also act on macrophages by polarization from pro-inflammatory M1 to anti-inflammatory M2 phenotype (64, 86, 87). These findings have relevance to MSC function in the uterus, as MSCs isolated from human endometrium and menstrual fluid have immunomodulatory properties (88). Thus, it has been postulated, although unproven, that similar interactions between MSCs and uterine immune cells (e.g. uterine NK (uNK) cells and macrophages) play a role in promoting the immune microenvironment required for endometrial regeneration (89).
MSCs, in addition to their crucial role in immune modulation, also play an essential role in maintaining host tissues’ homeostasis by replacing dead and dysfunctional tissue. The capacity of MSCs to sustain host homeostasis through the repair and replacement of dead and dysfunctional cells is predominantly attributed to their secretome, as discussed above. The effectiveness of MSC response to inflammation or injury is conditioned by their ability to home to the site of insult or injury. However, once MSCs are recruited to the site of inflammation, they can directly or indirectly interact with the affected tissue by GJIC or paracrine factors, as described earlier. Local inflammatory cytokines (e.g. TNF-α, IFNγ) signal MSCs to release immunomodulatory, pro-angiogenic, regenerative, and neuroprotective factors including TGFβ1, VEGF, HGF, SDF-1, IGF-1, and angi-1 (43, 62, 90, 91). These effects can be accomplished via the MSCs’ endogenous cargo or can be engineered to enhance these effects (21, 25, 92, 93). It is worth noting that MSCs are also being investigated as a drug delivery tool for gene therapy associated with these and other functions (73, 94–96). The self-renewing capacity of MSCs permits the MSCs to act as self-maintaining drug delivery vehicles at a site of inflammation so long as the microenvironment remains permissive to the MSCs (43, 97, 98).
Although research to date predominantly attributes MSCs’ effects to their secretome rather than cellular replacement, that is not to say that such function is not possible. MSCs have been found to generate osteocytes, adipocytes, chondrocytes, myocytes, and functional neurons in vitro (21, 22, 99–101). Numerous groups are investigating ways to accomplish similar direct reprogramming of MSCs and other cells in vivo, for example differentiating fibroblasts into neurons (102). With respect to endometrial regeneration, though paracrine mechanisms predominate, human in vitro studies indicate that MSCs may contribute as cellular progenitors of human endometrial stromal fibroblasts and decidual cells (103, 104).
Menstruation, defined as shedding of the superficial (i.e., functionalis) layer of the endometrium in the absence of embryo implantation, occurs in only a restricted number of mammalian species: humans and certain non-human primates, the spiny mouse, several bat species, and elephant shrews (105). The human endometrium rapidly regenerates an entire tissue layer post menstruation, exhibiting multiple parallels with processes required for cutaneous wound healing (e.g. inflammation, tissue remodeling, fibroblast migration, and re-epithelialization) (106). Indeed, menstruation may be considered a model of tissue “self-damage” (107) followed by rapid, repeated regeneration without fibrosis in healthy women. The remarkable ability of the healthy endometrium to regenerate without fibrosis and scarring therefore lends itself as a unique model of scar-free wound healing (108).
In general, scar formation in repair of damaged tissues (e.g. skin) involves transformation of stromal cells into myofibroblasts, a key mechanism underlying fibrosis and scar formation. In contrast, post-menstrual repair of the endometrial “wound” occurs via tissue-specific mechanisms that prevent fibrosis and result in an entirely renewed and scarless functionalis layer. These mechanisms include: 1) unique ECM interactions mediating cell migration and re-epithelialization of the endometrium (109), and 2) soluble factors secreted in utero during menstruation which prevent transformation of endometrial stromal cells into myofibroblasts (107). Re: the latter mechanism, whether MSCs are one source of these soluble factors responsible for scar-free, post-menstrual cyclic endometrial repair in healthy women is not definitively known. Indeed, the endometrium itself, as well as menstrual fluid, are established sources of MSCs, among other stem/progenitor cell types, which secrete bioactive soluble and insoluble factors for potential clinical application in cutaneous wound healing [(reviewed in (88)]. MSCs, including those isolated from human endometrium, are thus an emerging therapeutic agent for addressing the inflammation, proliferation, and remodeling aspects of cutaneous wound healing – including reduced scar formation (88, 110–113). An expanding body of literature now demonstrates similar MSC and MSC-Exos-enhanced mechanisms in the endometrium, modulating inflammation, angiogenesis, and tissue remodeling. For these reasons, MSCs are under investigation in the treatment of disorders of endometrial regeneration, as seen in women with Asherman’s syndrome and thin endometrium refractory to exogenous estrogen. The following sections will expand on current research evaluating therapeutic value of MSCs on endometrial repair.
The particular ability of MSCs to cross allogeneic barriers has permitted delivery of human MSCs to rodents to study effects on endometrial regeneration and receptivity. Thus, an ever-growing number of studies have been conducted in rodents to explore the efficacy of MSCs, isolated from various rodent and human tissue sources, in treating thin endometrium. In the section below, we summarize a number of recent and pertinent studies which demonstrate various endometrial injury models, MSC sources, modes of delivery, and morphologic and functional outcomes of interest. As noted earlier, limited engraftment in recipient tissues and short retention time are major obstacles in MSC-based therapies. Several methods of MSC delivery have thus been tested in rodent models, including systemic delivery by tail vein injection, local injection directly into the uterus, or administration via a matrix system. These studies, summarized in Table 1, largely utilize three models to simulate endometrial injury (although other models have been described); injection of ethanol to achieve a thin endometrium, and mechanical or electrocoagulation injury of the endometrial surface to achieve the fibrosis typically seen in women with Asherman’s syndrome.
The earliest rodent studies to determine the effect of MSCs on endometrial regeneration were performed with systemic delivery of MSCs after inducing endometrial injury. These studies, summarized below, utilized MSCs from various sources and largely demonstrated improvement in endometrial thickness, gland number, and angiogenesis, as well as decreased fibrosis. Studies differ in terms of time point(s) studied post injury tracking of MSCs to the uterus, and assessment of fertility; those that perform mating studies largely demonstrated improvement in fertility in animals receiving systemically delivered MSCs.
In one of the earliest studies using a rat model of ethanol-induced endometrial injury (114), recipients of bone marrow-derived mesenchymal stem cells (BM-MSCs) post injury had a significantly thicker endometrium after 3 estrous cycles than saline controls. BM-MSC recipients also demonstrated increased expression of endometrial receptivity markers integrin αvβ3 and leukemia inhibiting factor (LIF), increased expression of endometrial bFGF and IL-6 mRNA (anti-inflammatory cytokines), and decreased expression of TNF-α, IL-1β mRNA (pro-inflammatory cytokines), indicating immunomodulation as one likely mechanism underlying reparative responses post endometrial injury. Subsequent studies found promising results after systemic BM-MSC delivery in terms of homing of cells to the uterus and improvements in fibrosis and endometrial morphology (115, 116). Mating studies demonstrated improved fertility in BM-MSC recipients, though not restored to that of uninjured controls (116). Interestingly, although BM-MSCs were localized in the endometrium of recipients up to 3 estrous cycles (115) or 3 weeks (116) post BM-MSC injection, none were noted in pregnant uteri of BM-MSC recipients (116), highlighting functional improvements despite short-lived retention of cells in the target tissue.
Given the well-established role of MSC extracellular vesicles (EVs) in mediating tissue repair, recent studies have characterized the role of BM-MSC-EVs in endometrial repair (117, 118). Xiao et al. (117) demonstrated an exosome-mediated repair process in a rat model of Asherman syndrome; improvements in morphology (decreased fibrosis, increased endometrial thickness and gland number) in BM-MSC recipients were mediated by exosomal miR-340. In a subsequent study, direct comparison between recipients of BM-MSCs vs. BM-MSC-EVs revealed similar improvements in endometrial thickness, gland numbers, and fibrosis, however improved fertility (number of deliveries and cumulative number of pups) was only noted in BM-MSC recipients (118). Thus, though EVs have emerged as a promising “off-the-shelf” cell-free therapy, further investigation is warranted to produce a dose and subpopulation of EVs that will elicit functional improvement comparable to the MSCs from which they were derived.
Studies using alternative sources of MSCs also yield promising results in rodent endometrial injury models. In a study exploring the therapeutic potential of human menstrual blood-derived MSCs (MenSCs), Zhang et al. demonstrated short term retention (up to 7d) of human MSCs in mouse recipient tissues, as well as increased endometrial thickness and microvessel density relative to saline controls (119). Improved fertility was noted with increased conception rates as well as a larger number of embryos. More recently, Zhang et al. employed human umbilical cord-derived MSC (UC-MSCs) as an alternative MSC source (120) and similarly found significant increases in endometrial thickness, gland number, and implantation sites, although not restored to that of uninjured controls. Complementary in vitro studies demonstrated the capacity of UC-MSCs to migrate toward injured rat endometrial stromal cells (ESCs) and enhance ESC proliferation (120), while bioinformatics analyses noted reconstruction of ECM, regulation of inflammatory molecules, cell proliferation and apoptosis as pathways associated with UC-MSC-mediated repair processes. All well-established properties of MSCs, these studies elucidated candidate mechanisms underlying UC-MSC-induced endometrial repair.
Not long after the earliest studies demonstrating reparative effects of BM-MSCs delivered via tail vein (114), subsequent studies investigated the effect of local intrauterine MSC delivery on endometrial repair in endometrial injury models. Using various sources of MSCs, these studies demonstrated many of the same improvements after local transplantation that were seen with systemic administration, including increased endometrial thickness, gland number, vessel density, decreased fibrosis, and improved fertility.
In rat models of endometrial injury, two studies investigating effects of local intrauterine BM-MSC delivery demonstrated significantly increased endometrial thickness and higher expression of receptivity markers integrin αvβ3 and LIF in BM-MSC recipients (121, 122). Zhao et al. (121) also found downregulated expression of proinflammatory cytokines TNF-α and IL-1β, and upregulated anti-inflammatory cytokines bFGF and IL-6 in BM-MSC recipients, suggesting immunomodulatory mechanisms similar to those in rats treated systemically with MSCs. Mating studies by Wang et al. (122) demonstrated a significantly higher number of fetuses in the injured horn of BM-MSC recipients than in control animals receiving PBS. These studies provided support for local intrauterine BM-MSC administration to promote endometrial regeneration, potentially via immunomodulatory effects, and restore fertility after endometrial injury.
Looking at alternative MSC sources, Gan et al. performed one of the few studies to date exploring the use of human amniotic mesenchymal stromal cell (AMSC) transplantation in a rodent model of Asherman syndrome (123). In addition to improved thickness and gland numbers, the endometrium of AMSC recipients had significantly decreased expression of pro-inflammatory (e.g. TNF-α) and pro-fibrotic (TGF-β and COL1A1) cytokines, and increased expression of anti-inflammatory and angiogenic cytokines. This was the first study to demonstrate a role for AMSCs in endometrial regeneration; however, only short-term effects at one week post-transplant were studied. Fertility studies were not performed, thus their overall efficacy compared to more frequently tested sources of MSCs remains to be established.
More recently, Shao et al. explored the therapeutic potential of rat adipose-derived stem cells (ASCs) in a rat model of ethanol-induced endometrial injury (124). At 30 days, recipients of intrauterine ASCs had improved endometrial morphology including increased microvessel density, as well as increased expression of estrogen receptor (ER)α, ERβ, progesterone receptor (PR), LIF and integrin αvβ3 protein levels. Pregnancy rates were also improved in ASC-transplanted rats, indicating that ASC transplant could restore a functional endometrium to support embryo implantation.
To investigate the utility of a cell-free approach, several recent studies explored the effect of intrauterine MSC-exos delivery on endometrial repair (125, 126). In a mouse model of intrauterine adhesions, exosomes isolated from BM-MSC (BM-MSC-exos) promoted endometrial repair by increasing proliferation of endometrial epithelial cells and migration of stromal cells. Antifibrotic effects appeared to be mediated by exosomal miR-29a, via reduction in αSMA, Collagen I, Smad2, and Smad3 expression in murine endometrial stromal fibroblasts. To determine efficacy of a uterine source of MSCs, Saribas et al. explored the effects of locally delivered uterine MSC (UMSC) and their exosomes (UMSC-exos) on rat endometrial regeneration (126). Isolated from whole newborn rat uteri, these MSCs cannot be assumed to be comparable to MSCs isolated from human endometrium or menstrual fluid. Interestingly, although vessel density and cellular proliferation were similarly improved in UMSC and UMSC-exos recipients, fibrotic area was decreased only in uteri of the UMSC-exos group. A more recent study explored the utility of human umbilical cord MSCs and their exosomes (UC-MSC-exos) delivered via intrauterine infusion in a rat model of endometrial injury (127). In addition to morphologic improvements, pregnancy rates were significantly improved in the UC-MSC (60%) and UC-MSC-exos groups (80%), compared with zero pregnancies in untreated controls. UC-MSC and UC-MSC-exos inhibited TGF-β1/SMAD 2/3 (profibrotic) signaling in human endometrial stromal cells (HESCs) and upregulated HESC migration and proliferation in vitro, providing mechanistic insights into the functional improvements seen in the rat in vivo studies. Taken together, these studies elucidated multiple mechanisms by which local administration of MSC-exos could mediate endometrial repair after injury.
Though MSCs administered either systemically or locally have demonstrated favorable effects on endometrial repair in rodent models, few have directly compared the efficacy of systemic vs. local intrauterine administration. One comparison of rat BM-MSCs administered either systemically or locally in an intrauterine adhesion model (128) demonstrated significant and similar improvement in fibrosis and gland numbers in both the systemic and local treatment groups when compared to PBS-treated controls. A more recent study directly compared engraftment, cell retention, and therapeutic efficacy of rat BM-MSCs in a model of ethanol-induced injury, delivered either systemically or intrauterine (129). With either delivery method, BM-MSCs were localized to the endometrial stroma rather than epithelium and were equally effective in improving endometrial thickness, vessel, and gland density, and reducing fibrosis. However, the authors concluded intra-arterial delivery to be superior, given longer retention time and higher expression of VEGF and integrin αvβ3 protein in the endometrium. Fertility studies were not performed.
Zhang et al. utilized a combined systemic and local approach in a rat model, delivering human UC-MSCs locally at the time of endometrial injury, followed by 1-3 systemic injections of UC-MSCs (130). Endometrial morphology and fibrosis significantly improved in all UC-MSC-treated groups. Mating studies demonstrated the highest number of implantation sites in those receiving three systemic MSC injections in addition to intrauterine MSCs, though none recovered fertility to that of uninjured controls. An alternate model, taking a cell-free approach, tested the efficacy of a combined delivery system using locally delivered UC-MSC-extracellular vesicles (EVs) administered in the uterus on the day of endometrial injury and intraperitoneally 3 times at 5 day intervals with or without oral estrogen (131). Uteri of animals receiving UC-MSC-EVs with or without estrogen therapy exhibited many of the improvements noted in other studies (improvements in fibrosis, gland number and VEGF expression, significant decrease in expression of proinflammatory cytokines) relative to untreated animals or those receiving estrogen alone. Unfortunately, this study did not compare UC-MSCs alone to UC-MSC-EVs, and fertility after treatment was not tested.
A majority of the studies performed explore only 1-2 methods of delivery or one source of MSCs; however, Monsef et al. performed a unique study comparing administration of MSCs locally and systemically, as well as the performance of MSCs from either rat bone marrow or rat adipose tissue (132). Although MSC delivery by either method and from either source resulted in significant improvement in endometrial thickness after injury, local transplantation of both types of MSCs appeared to be more effective than systemic administration of ASCs, and systemic BM-MSCs were more effective than systemic ASCs in improving endometrial thickness. Systemic BM-MSC delivery, however, resulted in the fewest MSC visualized in the endometrium relative to other MSC groups. Both routes of delivery significantly increased endometrial VEGF expression and decreased collagen deposition, with the greatest improvement in fibrosis seen in animals receiving local ASC delivery, leading the investigators to conclude that ASCs may be a superior option for endometrial repair than BM-MSCs. Additional comparative studies, including assessment of fertility in MSC recipients, are needed to validate these findings.
Given limited retention of MSCs in the endometrium after systemic or local delivery, a number of studies have investigated novel delivery systems to optimize delivery of MSCs (or their secretome), promote cell retention in the endometrium, and improve the effects on endometrial repair. A variety of matrices have been tested in combination with intrauterine delivery of MSCs derived from various tissues. Some studies utilizing these models demonstrate improved retention of cells in the uterus as well as improved fertility; all demonstrate positive effects on endometrial morphology after injury. However, in part due to heterogeneity in study design, only certain studies demonstrate superiority of the matrix over delivery of MSCs alone in promoting endometrial repair after injury.
In one of the initial studies evaluating matrix-based MSC delivery in a rat model of endometrial injury, Ding et al. explored the regenerative effects of rat BM-MSCs loaded on a scaffold of collagen, a basic structure of extracellular matrix and a widely used biomaterial in tissue regeneration (133). After 90d, animals receiving collagen/BM-MSCs had significantly thicker endometrium and greater degree of neovascularization relative to animals receiving either PBS or collagen alone. Mating studies demonstrated a higher pregnancy rate in collagen/BM-MSC recipients relative to collagen and untreated controls. However, lack of a BM-MSC treatment group precluded evaluation of potential benefits of collagen/BM-MSCs above BM-MSCs alone.
Multiple studies have tested novel matrix delivery systems for MSCs isolated from human pregnancy tissues (e.g. umbilical cord, placenta, amnion) (134–138), given their low immunogenicity and ease in collection at delivery. Lin et al. evaluated MSCs isolated from human placenta (HP-MSC) encapsulated in hyaluronic acid (HA) hydrogel for intrauterine delivery in a mouse model of endometrial injury (134). HP-MSC-HA complex significantly increased endometrial thickness and gland numbers, decreased fibrosis, and promoted cellular proliferation, though not significantly above that of HP-MSC alone. Further, despite prolonged MSC retention time in the HP-MSC-HA group, mating studies demonstrated a significant increase in implantation sites in recipients of HP-MSC with or without HA encapsulation; thus, from a functional standpoint, the superiority of administering MSCs within this matrix delivery system remained unproven.
In another study of novel delivery systems, Wang et al. investigated the efficacy of seeding human UC-MSCs on human acellular amniotic matrix (AAM) in a rat model (135). After 3 estrous cycles, endometrial thickness and gland numbers were significantly improved in the UC-MSC-AAM group relative to the AAM-treated group. Similar to findings from previous studies, UC-MSC-AAM delivery was also associated with upregulation of anti-inflammatory cytokines (IL-4, IL-10) and downregulation of pro-inflammatory cytokines (IL-2, TNFα, IFNγ) in the endometrium. Despite these effects, mating studies indicated zero pregnancies in rats transplanted with UC-MSC-AAM or AAM alone, ultimately raising doubt regarding any functional improvements afforded by this transplant method.
Xu et al. (136) noted limitations of thicker scaffolds, including restriction of nutrient diffusion, which can cause low viability and decreased colonization of MSCs in vivo. They thus tested the use of MSC-laden Matrigel microspheres in a rat uterine injury model, to improve nutrient diffusion and to facilitate MSC encapsulation and ease in transplant via injection (136). Rats receiving UC-MSC-laden Matrigel microspheres exhibited a significant increase in endometrial thickness, gland number, blood vessel density, and pregnancy rates relative to a control group receiving Matrigel alone. Though promising for a novel and less invasive MSC delivery system, lack of a UC-MSC treatment group again precluded a direct comparison of UC-MSCs delivered via this matrix delivery system above that of MSCs alone.
Thermosensitive biomaterials have gained attention for MSC delivery, transitioning from an easily injected liquid to a biodegradable hydrogel when exposed to body temperature. Zhou et al. tested a thermosensitive biodegradable hydrogel FDA-approved for clinical use, Pluronic F127 (PF-127), to deliver UC-MSCs into the uterus after ethanol-induced injury (137). Recipients of PF-127-encapsulated UC-MSCs had significantly increased endometrial gland numbers and angiogenesis relative to saline controls; these effects were not seen after delivery of UC-MSCs nor PF-127 alone. Huang et al. also explored use of a thermoresponsive biomaterial, polyethylene glycol citrate-co-N-isopropylacrylamide (PPCN) mixed with gelatin (PPCNg) to improve cell adhesion and survival of amniotic MSCs (AMSCs) (138). Endometrial thickness and gland numbers were found to be highest, and fibrosis decreased, in the AMSC-PPCNg group relative to AMSC or PPCNg only. Most notably, rats receiving AMSC-PPCNg treatment had the highest pregnancy rate (100%), as compared with 75% and 25% in the AMSC and PPCNg only groups, respectively. In both of these studies, retention of MSCs in the uterus was prolonged when delivered in a thermosensitive matrix; these studies provided compelling evidence supporting a novel delivery matrix for MSCs, warranting additional studies to determine safety and efficacy.
Given the limitations of cell-based MSC therapies (e.g. potential risk of tumor formation, low engraftment rates, storage and transportation logistics), and relative benefits of matrix-based delivery systems, a few studies have evaluated therapeutic effects of the MSC secretome delivered via matrix. Liu et al. (139), for example, evaluated the therapeutic effect of BM-MSC-secretome delivered via hyaluronic acid (HA) matrix in a rat model of endometrial injury. The HA+MSC-secretome group showed a significant improvement in endometrial thickness and gland number, and significant increase in number of implantation sites, in comparison to control and MSC-secretome only groups. In vitro studies demonstrated ability of the BM-MSC-secretome to promote angiogenesis, as well as proliferation and migration of human endometrial epithelial cells, providing mechanisms underlying the improvements seen in their in vivo studies. In another matrix-based model, Xin et al. employed the use of a collagen scaffold for intrauterine UC-MSC-exos delivery after uterine injury in rats (140). UC-MSC-exos (delivered with or without the scaffold) significantly increased gland number and endometrial thickness, reduced fibrosis, and improved fertility, with a significantly higher number of implantation sites seen in UC-MSC-exos recipients. Consistent with known functions of MSC-exos, recipients of UC-MSC-exos exhibited polarization of endometrial macrophages to the anti-inflammatory M2 phenotype, likely mediated in part by exosomal miR-223-3p, supporting the role of MSC-exos cargoes in promoting endometrial repair. Collectively, these studies incorporated favorable aspects of two MSC-based delivery methods (cell-free/secretome-based combined with matrix for sustained release) to ameliorate endometrial morphology and function after injury.
Taken together, a growing number of rodent studies have demonstrated efficacy of several MSC sources and administration methods for the treatment of thin endometrium and/or intrauterine adhesions. The most widely tested of these include BM-MSCs and UC-MSCs: all show the ability in rodent models to improve endometrial regeneration, and multiple demonstrate improved fertility. Direct comparison between sources are lacking; only one study performed a direct comparison of efficacy between two MSC sources (BM-MSCs and ASCs), and concluded that ASCs may be a superior option for endometrial repair than BM-MSCs (132). Very few studies compare mode of delivery (systemic vs. local administration) and yielded mixed results. Of the various MSC delivery vehicles evaluated, only a few directly compare efficacy of MSCs (or MSC secretome) delivered in a matrix to those delivered alone; in those studies, the data indicate these delivery complexes may be beneficial in prolonging tissue retention of MSCs and improving endometrial regeneration.
These rodent studies have overall improved our understanding of the mechanisms by which MSCs support endometrial regeneration, and many have laid the groundwork for human studies. Both the human and rodent endometrium undergo cyclic remodeling which is highly dependent on ovarian sex steroids and a functional hypothalamic-pituitary-ovarian axis (141). Over the course of either the rodent estrous cycle (4-5 days) or human menstrual cycle (28 days), the endometrium undergoes distinct changes including stromal and epithelial proliferation, apoptosis, extracellular matrix remodeling and leukocyte infiltration, under the influence of estradiol and progesterone (142, 143). Thus, many parallels exist between rodents and women, but the lack of menstruation in rodents renders this species as an imperfect model, albeit convenient and easy to manipulate, for in vivo studies of endometrial regeneration and repair. It is important to keep these similarities and differences in mind when extrapolating the results seen in rodents to findings from human studies. In vitro studies in human tissues, summarized in the section below and Table 2, have provided valuable additional insights into MSC-mediated endometrial repair mechanisms.
Among the first studies to explore paracrine mechanisms by which MSCs mediate human endometrial repair, Xin et al. used a collagen scaffold (CS) loaded with human UC-MSCs and studied the effect on human endometrial stromal cells (HESCs) (140). HESCs exposed to CS-UC-MSCs demonstrated significantly increased proliferative capacity and decreased apoptosis relative to controls, and secreted higher levels of VEGF-α, TGF-β, and insulin-like growth factor 1. These in vitro findings were further validated by the same group in a rat model of uterine injury, in which the application of CS-UC-MSCs into the uterus after injury resulted in improved endometrial morphology and higher pregnancy rates. The endometrium of CS-UC-MSC rat recipients also exhibited higher expression of VEGF-α, TGF-β, and insulin-like growth factor 1, supporting MSC-mediated paracrine upregulation of these growth factors as a mechanism promoting morphologic and functional restoration of injured uteri.
As summarized earlier, MSC-derived exosomes contain cargo, including transcription factors and abundant microRNAs (miRNAs), that regulate expression of related genes in recipient cells and promote repair and regeneration in damaged tissues (117). A number of recent human in vitro studies have thus explored mechanisms by which UC-MSC-exos promote survival and proliferation of HESCs, utilizing various in vitro models of HESC injury (144). Exposure to UC-MSC-exos significantly increased HESC proliferation and decreased apoptosis; mediated via activated PTEN/Akt signaling in mifepristone-injured HESCs, upregulated HESC expression of Bcl-2 (an anti-apoptotic protein) and downregulated cleaved Caspase-3, a marker of apoptotic injury (144). In a similar model, Shi et al. (145) identified miR-7162-3p as an effector of UC-MSC-exosome endometrial repair. Direct binding of miR-7162-3p to apolipoprotein 6 (APOL6), a programmed cell death gene in target HESCs, protected against mifepristone-induced injury. Li et al. also investigated therapeutic potential of UC-MSC-exos in reversing TGFβ1-induced HESC fibrosis (146). Exposure of injured HESCs to UC-MSC-exos resulted in significant down regulation of fibrosis markers α-SMA1 and COL1A1 relative to controls. This finding was mediated at least in part by exosomal miR-145-5p, a negative regulator of the transcription factor ZEB2 which promotes epithelial-mesenchymal transition and fibroblast differentiation. With respect to endometrial epithelial cells (EECs), Wang et al. (147) demonstrated that uptake of UC-MSC-exos inhibited hypoxia-induced apoptosis, migration, reversed epithelial mesenchymal transition (EMT) of EECs, and upregulated EEC proliferation relative to untreated, injured controls. The protective effect of UC-MSC-exos appeared to be mediated by regulation of the miR663-a/CDKN2A axis, the latter an apoptosis regulatory gene. Collectively, these studies provided important insights into mechanisms by which miRNA cargo of UC-MSC-exos could support endometrial repair.
While the studies above largely focused on MSC-mediated mechanisms regulating HESC proliferation, survival, and anti-fibrotic mechanisms, our lab investigated whether the MSC secretome could enhance endometrial stromal cell motility functions, and whether MSCs isolated from either UC or BM would have similar effects (148). Exposure of HESCs to the secretome of either UC- or BM-MSC significantly and similarly increased HESC migration and invasion; although cellular proliferation was also significantly increased, the effect was modest and varied among MSC donors. Expression of CCL2 and HGF mRNA, genes associated with HESC motility, were increased in HESCs exposed to both UC- and BM-MSC, and exposure to recombinant CCL2 significantly increased HESC migration and invasion. These findings indicated both paracrine and autocrine mechanisms involved in MSC secretome-mediated motility, further supporting the potential to leverage the MSC secretome as a cell-free therapy to support endometrial regeneration/repair.
The human endometrium and menstrual fluid are now established sources of MSCs, and multiple in vitro studies have demonstrated the ability of menstrual blood MSCs (MenSCs) to affect phenotype and function of endometrial epithelial and stromal cells. Zhao et al. (149). demonstrated that exposure of EECs to MenSCs increased EEC proliferation and invasive capacity, and increased EEC expression of EGF, FGF, PDGF and MMP3 proteins; these cytokines and growth factors play important roles in cellular motility, proliferation, and tissue remodeling in the endometrium. With respect to effects on HESCs, in a model of mifepristone-induced injury, Zhu et al. demonstrated the ability of MenSCs to upregulate endometrial stromal cell proliferation, migration, and decrease apoptosis via activation of p38 MAPK and AKT signaling pathways (150). MenSCs and their secretory products (e.g., G-CSF) appear to attenuate endometrial fibrosis (151, 152); exposure of HESCs to either MenSCs or MenSC-conditioned medium resulted in downregulation of myofibroblast markers αSMA and collagen I and promoted HESC migration (152). Interestingly, as noted earlier, TGF-β is an inducer of fibrosis in the endometrium, and exposure to MenSCs attenuated TGF-β-mediated increase in profibrotic markers and myofibroblast activation, via activation of the Hippo/TAZ signaling pathway (152). Thus, although untested in vivo, these in vitro studies elucidated candidate MenSC-mediated mechanisms that promote scar-free endometrial repair in healthy tissue and identified potential molecular targets in the therapy of endometrial fibrosis/scarring.
Santamaria et al. were one of the first groups to explore the use of bone marrow-derived stem cells (BMDSCs) in the uterus in a human trial (153). In a prospective, non-randomized study, 16 women with primary infertility and either Asherman’s syndrome (n=11) or endometrial atrophy (EA, n=5) received autologous CD133+ BMDSCs, delivered via intra-arterial catheterization of uterine spiral arterioles, followed by hormone replacement therapy. The endometrium of recipients was assessed prior to and at 3 and 6 months post treatment. Average endometrial thickness increased only modestly, from 4.3 mm to 6.7 mm post-treatment in women with Asherman’s syndrome; and from 4.2 mm to 5.7 mm in women with EA. Of 14 embryo transfers performed, 7 women became pregnant resulting in one live birth and one ongoing pregnancy; 3 patients conceived spontaneously at 2, 4, and 19 months after treatment resulting in one live birth and one ongoing pregnancy. No adverse events were noted with BMDSC treatment. It should be noted that the selection of CD133 as a marker indicates the use of a potentially heterogeneous stem/progenitor population not truly limited to MSCs. Although limited by lack of controls and unproven homing of BMDSCs to the uterus, this was a novel and important proof-of-concept pilot study setting the stage for subsequent trials in humans.
In a subsequent report by Singh et al., 25 women with infertility and Asherman’s syndrome (n=12) or thin endometrium (n=13) underwent transplant of autologous bone marrow-derived mononuclear stem cells (154). Cells were transplanted transmyometrially into the subendometrial zone, followed by 3-6 months of oral estrogen therapy. Of the 7 amenorrheic women, 6 resumed menses after treatment. Average endometrial thickness improved modestly from 3.3 to 5.1 mm at 3 months, without further improvement at 6 and 9 months. Eleven patients underwent IVF, of which only two conceived, resulting in one live birth and one ectopic pregnancy. Two women conceived naturally at 3 years post-transplant, resulting in one live birth each. The infusion of mononuclear cells includes a heterogenous population of hematopoietic and nonhematopoietic bone marrow-derived cells not limited to MSCs; further, lack of controls (and conception at several years post-transplant) precluded the ability to directly attribute pregnancies to the intervention.
In one of the earliest studies using a menstrual source of MSCs in the human uterus, Tan et al. studied the effects of autologous transplantation of MenSCs on endometrial regeneration (155). Unlike prior human trials, in this trial MSC phenotype was confirmed via expression of MSC markers. Seven women with Asherman’s syndrome underwent ultrasound-guided intrauterine transplant of MenSCs on day 16 of the menstrual cycle under ultrasound guidance, followed by oral estrogen for 21 days. Five of 7 patients achieved an endometrial thickness of 7-8 mm and underwent IVF with embryo transfer, of which two conceived. One patient naturally conceived 3.5 months after transplant. In a more recent study, Ma et al. also used MenSCs in 12 patients with refractory Asherman’s syndrome (156). These investigators also characterized MSC phenotype via cell surface marker expression and multilineage differentiation potential. MenSCs were transplanted into the basal layer of the endometrium, followed by 1-2 cycles of hormone therapy. Mean endometrial thickness was significantly improved at 14 days post-transplant, from 3.9 to 7.5 mm. The clinical pregnancy rate was 41.7% (5/12), 4 of which resulted from IVF/embryo transfer and 1 conceived spontaneously. Though promising, live births were not reported in either study, with lack of controls and small sample sizes limiting applicability of the results.
To explore an alternative MSC source, Sudoma et al. performed the first trial with adipose-derived stem cells (ASCs) in 25 women with thin endometrium and at least 3 prior IVF failures (157). MSC phenotype was confirmed via multilineage differentiation assays. Autologous ASCs were injected subendometrially in several locations under ultrasound guidance 3 times at 5–7-day intervals followed by oral estrogen. Endometrial thickness was measured in 3-10 natural cycles post-treatment, or if the patient was menopausal or had ovulatory dysfunction, after 3-10 artificial cycles. 19 women achieving endometrial thickness >7mm underwent embryo transfer. A total of 13 women achieved pregnancy: 11 after embryo transfer and 2 as a result of natural conception, resulting in a total of 9 live births. Compared to prior studies, this cohort of patients experienced the highest pregnancy and live birth rates, but again lacked controls.
Tersoglio et al. performed a study using autologous endometrial MSCs (eMSCs) in 29 patients with thin endometrium and recurrent implantation failure, defined as failed embryo implantation after at least 3 transfers of high-quality blastocysts (158). Autologous endometrial MSCs, characterized by expression of traditional MSC cell surface markers and eMSC marker SUSD2, were delivered transmyometrially after estrogen supplementation for 6-8 weeks. Average endometrial thickness increased from 5.2 mm pre-treatment to 9.9 mm. Of 29 women undergoing embryo transfer, the authors reported 23 clinical and 7 ongoing pregnancies, promising outcomes tempered by lack of controls and the major caveat that in all women, eMSCs were diluted in platelet-rich plasma, bringing into question the therapeutic role of eMSCs themselves.
Zhang et al. explored the use of UC-MSCs on endometrial regeneration in 16 infertile women with thin endometrium (< 5.5 mm) (159). UC-MSCs, confirmed by cell surface marker expression and multilineage differentiation potential, were loaded onto a collagen scaffold and transplanted hysteroscopically into the uterine cavity on the 7-12th day of the menstrual cycle in 2 sequential cycles. Three months after transplantation, average endometrial thickness increased from 4.1 mm to 5.9 mm, with significant increase in CD34+ micro-vessel density, gland number, and expression of ERα, PR, and the proliferative marker Ki67. Of 15 women undergoing 22 frozen embryo transfer (FET) cycles, 3 conceived, 2 of which resulted in live birth; 1 patient had a naturally conceived pregnancy and live birth. In a similar trial, Cao et al. administered intrauterine UC-MSC on a collagen scaffold after hysteroscopic adhesiolysis in 26 women with infertility and intrauterine adhesions (160). Subjects received estradiol for 30 days followed by a single dose of 60 mg progesterone. At 3 months, 10/26 women were noted to have either absent or mild adhesions, with modest increases in average endometrial thickness from 4.5 mm to 5.7 mm and upregulated expression of ERα, proliferative marker Ki67, and angiogenic marker vWF. At 30 months, 10 subjects conceived a pregnancy, resulting in 8 live births, one ongoing pregnancy in third trimester, and one first trimester spontaneous abortion. No adverse events were reported in either trial. Though uncontrolled, these studies provided important safety data and insights into mechanisms by which intrauterine UC-MSC administration, via degraded collagen scaffold, could improve endometrial function.
Overall, pregnancies and live births in women with refractory Asherman’s syndrome and/or thin endometrium, coupled with a lack of treatment-related adverse events, generate enthusiasm for the development of novel MSC-based therapies to improve fertility in women suffering from these challenging conditions. However, major caveats exist, and proper randomized controlled trials are required before MSCs can be considered for clinical use. All of the above are uncontrolled studies with small sample sizes and heterogeneous clinical protocols; thus, none can definitively attribute clinical successes to MSC-based therapies. Heterogeneity among studies in transplanted cell types/cell populations raise uncertainty regarding the specific stem/progenitor cell types involved. Mechanisms responsible for clinical outcomes seen in humans, as well as the optimal mode of delivery, remain unknown. Finally, although MSCs from different tissues of origin are predicted to show slight differences in efficacy, and differing methods of MSC administration are expected to show differences in homing and target tissue residency (43) none of the reviewed trials compared different MSC sources or administration methods within the same cohort of patients. Thus, the optimal MSC source or administration method for ameliorating thin endometrium remains unknown.
The studies described herein demonstrate an ever-expanding amount of work supporting the therapeutic potential of MSCs, derived from reproductive and non-reproductive tissues, in promoting endometrial regeneration and repair after injury. Rodent (in vivo) and human (in vitro) studies have elucidated mechanisms that are consistent with well-established functions of MSCs and their secretome: angiogenesis, amelioration of fibrosis, increased cellular proliferation and motility, and immunomodulation (Figure 2). Although MSCs and their secretory products hold great promise in treating infertility due to a thin or scarred endometrium, caveats remain. Effects seen in rodents may not fully extrapolate to humans. Results generated from human clinical studies to date are limited by small sample size, heterogeneity in protocols, inconsistency in MSC characterization, and critically, lack of controls. The use of cell-based vs. cell-free (secretome-based) therapies each have relative benefits and drawbacks, and the optimal mode of delivery into the human uterus (systemic, local +/-matrix-based) needs to be determined. Before MSCs can be applied for treatment in this context, their isolation and preparation for clinical use will need to adhere to current good manufacturing practice regulations to assure safety, standardization and reproducibility (161). Working to achieve this is a worthwhile endeavor, as endometrial factor infertility remains a rate-limiting step in achieving IVF success. If proven efficacious and safe, MSC-based therapies for thin endometrium will not only improve pregnancy rates; equally and vitally important, they will minimize the risk of placental dysfunction related to thin endometrium, thus setting the stage for healthier pregnancies and live births.
Figure 2 MSC administration methods and mechanisms of action. Mesenchymal stem cells (MSCs) can be delivered via direct administration (+/- matrix) into the uterus, or by systemic delivery, followed by homing of the MSCs to the endometrium. Alternatively, cell-free therapies utilizing the MSC secretome (+/- matrix) may be administered directly into the uterus. At the site of injury within the endometrium, MSC-based therapies promote regeneration via immunomodulation, increased angiogenesis, increased cellular proliferation and motility, decreased apoptosis and downregulation of pro-fibrotic pathways. Figure created with Biorender.com.
MS-N: Writing – original draft, Data curation. YK: Writing – original draft, Data curation. LS: Writing – original draft, Writing – review & editing. OC: Writing – original draft, Data curation. SM: Writing – original draft, Writing – review & editing, Conceptualization, Data curation, Supervision.
The author(s) declare that no financial support was received for the research, authorship, and/or publication of this article.
The authors declare that the research was conducted in the absence of any commercial or financial relationships that could be construed as a potential conflict of interest.
All claims expressed in this article are solely those of the authors and do not necessarily represent those of their affiliated organizations, or those of the publisher, the editors and the reviewers. Any product that may be evaluated in this article, or claim that may be made by its manufacturer, is not guaranteed or endorsed by the publisher.
1. Critchley HOD, Maybin JA, Armstrong GM, Williams ARW. Physiology of the endometrium and regulation of menstruation. Physiol Rev (2020) 100(3):1149–79. doi: 10.1152/physrev.00031.2019
2. Sehring J, Beltsos A, Jeelani R. Human implantation: The complex interplay between endometrial receptivity, inflammation, and the microbiome. Placenta (2022) 117:179–86. doi: 10.1016/j.placenta.2021.12.015
3. Achache H, Revel A. Endometrial receptivity markers, the journey to successful embryo implantation. Hum Reprod Update (2006) 12(6):731–46. doi: 10.1093/humupd/dml004
4. Singh M, Chaudhry P, Asselin E. Bridging endometrial receptivity and implantation: network of hormones, cytokines, and growth factors. J Endocrinol (2011) 210(1):5–14. doi: 10.1530/JOE-10-0461
5. Craciunas L, Gallos I, Chu J, Bourne T, Quenby S, Brosens JJ, et al. Conventional and modern markers of endometrial receptivity: a systematic review and meta-analysis. Hum Reprod Update (2019) 25(2):202–23. doi: 10.1093/humupd/dmy044
6. Zhang X, Li Y, Chen X, Jin B, Shu C, Ni W, et al. Single-cell transcriptome analysis uncovers the molecular and cellular characteristics of thin endometrium. FASEB J (2022) 36(3):e22193. doi: 10.1096/fj.202101579R
7. Kasius A, Smit JG, Torrance HL, Eijkemans MJ, Mol BW, Opmeer BC, et al. Endometrial thickness and pregnancy rates after IVF: a systematic review and meta-analysis. Hum Reprod Update (2014) 20(4):530–41. doi: 10.1093/humupd/dmu011
8. Yu D, Wong YM, Cheong Y, Xia E, Li TC. Asherman syndrome–one century later. Fertil Steril (2008) 89(4):759–79. doi: 10.1016/j.fertnstert.2008.02.096
9. March CM, Israel R, March AD. Hysteroscopic management of intrauterine adhesions. Am J Obstet Gynecol (1978) 130(6):653–7. doi: 10.1016/0002-9378(78)90322-8
10. Dreisler E, Kjer JJ. Asherman’s syndrome: current perspectives on diagnosis and management. Int J Womens Health (2019) 11:191–8. doi: 10.2147/IJWH.S165474
11. Sher G, Herbert C, Maassarani G, Jacobs MH. Assessment of the late proliferative phase endometrium by ultrasonography in patients undergoing in-vitro fertilization and embryo transfer (IVF/ET). Hum Reprod (1991) 6(2):232–7. doi: 10.1093/oxfordjournals.humrep.a137312
12. El-Toukhy T, Coomarasamy A, Khairy M, Sunkara K, Seed P, Khalaf Y, et al. The relationship between endometrial thickness and outcome of medicated frozen embryo replacement cycles. Fertil Steril (2008) 89(4):832–9. doi: 10.1016/j.fertnstert.2007.04.031
13. Liu KE, Hartman M, Hartman A, Luo ZC, Mahutte N. The impact of a thin endometrial lining on fresh and frozen-thaw IVF outcomes: an analysis of over 40 000 embryo transfers. Hum Reprod (2018) 33(10):1883–8. doi: 10.1093/humrep/dey281
14. Ganer Herman H, Volodarsky-Perel A, Ton Nu TN, MaChado-Gedeon A, Cui Y, Shaul J, et al. Pregnancy complications and placental histology following embryo transfer with a thinner endometrium. Hum Reprod (2022) 37(8):1739–45. doi: 10.1093/humrep/deac148
15. Song L, Bu Z, Sun Y. Endometrial thickness and early pregnancy complications after frozen-thawed embryo transfers. Front Endocrinol (Lausanne) (2023) 14:1066922. doi: 10.3389/fendo.2023.1066922
16. Fang Z, Huang J, Mao J, Yu L, Wang X. Effect of endometrial thickness on obstetric and neonatal outcomes in assisted reproduction: a systematic review and meta-analysis. Reprod Biol Endocrinol (2023) 21(1):55. doi: 10.1186/s12958-023-01105-6
17. Zheng Y, Chen B, Dai J, Xu B, Ai J, Jin L, et al. Thin endometrium is associated with higher risks of preterm birth and low birth weight after frozen single blastocyst transfer. Front Endocrinol (Lausanne) (2022) 13:1040140. doi: 10.3389/fendo.2022.1040140
18. Liu X, Wang J, Fu X, Li J, Zhang M, Yan J, et al. Thin endometrium is associated with the risk of hypertensive disorders of pregnancy in fresh IVF/ICSI embryo transfer cycles: a retrospective cohort study of 9,266 singleton births. Reprod Biol Endocrinol (2021) 19(1):55. doi: 10.1186/s12958-021-00738-9
19. Eftekhar M, Tabibnejad N, Tabatabaie AA. The thin endometrium in assisted reproductive technology: An ongoing challenge. Middle East Fertility Soc J (2018) 23(1):1–7. doi: 10.1016/j.mefs.2017.12.006
20. Gnainsky Y, Granot I, Aldo PB, Barash A, Or Y, Schechtman E, et al. Local injury of the endometrium induces an inflammatory response that promotes successful implantation. Fertil Steril (2010) 94(6):2030–6. doi: 10.1016/j.fertnstert.2010.02.022
21. Sherman LS, Shaker M, Mariotti V, Rameshwar P. Mesenchymal stromal/stem cells in drug therapy: New perspective. Cytotherapy (2017) 19(1):19–27. doi: 10.1016/j.jcyt.2016.09.007
22. Rohban R, Pieber TR. Mesenchymal stem and progenitor cells in regeneration: tissue specificity and regenerative potential. Stem Cells Int (2017) 2017:5173732. doi: 10.1155/2017/5173732
23. Le Blanc K, Tammik C, Rosendahl K, Zetterberg E, Ringdén O. HLA expression and immunologic propertiesof differentiated and undifferentiated mesenchymal stem cells. Exp Hematol (2003) 31(10):890–6. doi: 10.1016/S0301-472X(03)00110-3
24. Iso Y, Spees JL, Serrano C, Bakondi B, Pochampally R, Song YH, et al. Multipotent human stromal cells improve cardiac function after myocardial infarction in mice without long-term engraftment. Biochem Biophys Res Commun (2007) 354(3):700–6. doi: 10.1016/j.bbrc.2007.01.045
25. Sherman LS, Condé-Green A, Sandiford OA, Rameshwar P. A discussion on adult mesenchymal stem cells for drug delivery: pros and cons. Ther Deliv (2015) 6(12):1335–46. doi: 10.4155/tde.15.80
26. Friedenstein AJ, Petrakova KV, Kurolesova AI, Frolova GP. Heterotopic of bone marrow. Analysis of precursor cells for osteogenic and hematopoietic tissues. Transplantation (1968) 6(2):230–47.
27. Friedenstein AJ, Piatetzky S II, Petrakova KV. Osteogenesis in transplants of bone marrow cells. J Embryol Exp Morphol (1966) 16(3):381–90. doi: 10.1242/dev.16.3.381
28. Conaty P, Sherman LS, Naaldijk Y, Ulrich H, Stolzing A, Rameshwar P. Methods of mesenchymal stem cell homing to the blood-brain barrier. Methods Mol Biol (2018) 1842:81–91. doi: 10.1007/978-1-4939-8697-2_6
29. Sherman LS, Condé-Green A, Naaldijk Y, Lee ES, Rameshwar P. An enzyme-free method for isolation and expansion of human adipose-derived mesenchymal stem cells. J Vis Exp (2019) 154:1–6. doi: 10.3791/59419-v
30. Jung WY, Kang JH, Kim KG, Kim HS, Jang BI, Park YH, et al. Human adipose-derived stem cells attenuate inflammatory bowel disease in IL-10 knockout mice. Tissue Cell (2015) 47(1):86–93. doi: 10.1016/j.tice.2014.12.001
31. Kim YS, Kim JY, Shin DM, Huh JW, Lee SW, Oh YM. Tracking intravenous adipose-derived mesenchymal stem cells in a model of elastase-induced emphysema. Tuberc Respir Dis (Seoul) (2014) 77(3):116–23. doi: 10.4046/trd.2014.77.3.116
32. Potian JA, Aviv H, Ponzio NM, Harrison JS, Rameshwar P. Veto-like activity of mesenchymal stem cells: functional discrimination between cellular responses to alloantigens and recall antigens. J Immunol (2003) 171(7):3426–34. doi: 10.4049/jimmunol.171.7.3426
33. Sherman LS, Munoz J, Patel SA, Dave MA, Paige I, Rameshwar P. Moving from the laboratory bench to patients’ bedside: considerations for effective therapy with stem cells. Clin Transl Sci (2011) 4(5):380–6. doi: 10.1111/j.1752-8062.2011.00283.x
34. Ponte AL, Marais E, Gallay N, Langonné A, Delorme B, Hérault O, et al. The in vitro migration capacity of human bone marrow mesenchymal stem cells: comparison of chemokine and growth factor chemotactic activities. Stem Cells (2007) 25(7):1737–45. doi: 10.1634/stemcells.2007-0054
35. Honczarenko M, Le Y, Swierkowski M, Ghiran I, Glodek AM, Silberstein LE. Human bone marrow stromal cells express a distinct set of biologically functional chemokine receptors. Stem Cells (2006) 24(4):1030–41. doi: 10.1634/stemcells.2005-0319
36. Wynn RF, Hart CA, Corradi-Perini C, O’Neill L, Evans CA, Wraith JE, et al. A small proportion of mesenchymal stem cells strongly expresses functionally active CXCR4 receptor capable of promoting migration to bone marrow. Blood (2004) 104(9):2643–5. doi: 10.1182/blood-2004-02-0526
37. Cornelissen AS, Maijenburg MW, Nolte MA, Voermans C. Organ-specific migration of mesenchymal stromal cells: Who, when, where and why? Immunol Lett (2015) 168(2):159–69. doi: 10.1016/j.imlet.2015.06.019
38. Liesveld JL, Sharma N, Aljitawi OS. Stem cell homing: From physiology to therapeutics. Stem Cells (2020) 38(10):1241–53. doi: 10.1002/stem.3242
39. Braid LR, Wood CA, Wiese DM, Ford BN. Intramuscular administration potentiates extended dwell time of mesenchymal stromal cells compared to other routes. Cytotherapy (2018) 20(2):232–44. doi: 10.1016/j.jcyt.2017.09.013
40. Naderi-Meshkin H, Bahrami AR, Bidkhori HR, Mirahmadi M, Ahmadiankia N. Strategies to improve homing of mesenchymal stem cells for greater efficacy in stem cell therapy. Cell Biol Int (2015) 39(1):23–34. doi: 10.1002/cbin.10378
41. Lee RH, Pulin AA, Seo MJ, Kota DJ, Ylostalo J, Larson BL, et al. Intravenous hMSCs improve myocardial infarction in mice because cells embolized in lung are activated to secrete the anti-inflammatory protein TSG-6. Cell Stem Cell (2009) 5(1):54–63. doi: 10.1016/j.stem.2009.05.003
42. Ren G, Zhao X, Zhang L, Zhang J, L’Huillier A, Ling W, et al. Inflammatory cytokine-induced intercellular adhesion molecule-1 and vascular cell adhesion molecule-1 in mesenchymal stem cells are critical for immunosuppression. J Immunol (2010) 184(5):2321–8. doi: 10.4049/jimmunol.0902023
43. Sherman LS, Romagano MP, Williams SF, Rameshwar P. Mesenchymal stem cell therapies in brain disease. Semin Cell Dev Biol (2019) 95:111–9. doi: 10.1016/j.semcdb.2019.03.003
44. Soukup R, Gerner I, Mohr T, Gueltekin S, Grillari J, Jenner F. Mesenchymal stem cell conditioned medium modulates inflammation in tenocytes: complete conditioned medium has superior therapeutic efficacy than its extracellular vesicle fraction. Int J Mol Sci (2023) 24(13):1–23. doi: 10.3390/ijms241310857
45. Lubis AMT, Aprianto P, Pawitan JA, Priosoeryanto BP, Dewi TIT, Kamal AF. Intra-articular injection of secretome, derived from umbilical cord mesenchymal stem cell, enhances the regeneration process of cartilage in early-stage osteo-arthritis: an animal study. Acta Orthop (2023) 94:300–6. doi: 10.2340/17453674.2023.12359
46. Fischer UM, Harting MT, Jimenez F, Monzon-Posadas WO, Xue H, Savitz SI, et al. Pulmonary passage is a major obstacle for intravenous stem cell delivery: the pulmonary first-pass effect. Stem Cells Dev (2009) 18(5):683–92. doi: 10.1089/scd.2008.0253
47. Kerkelä E, Hakkarainen T, Mäkelä T, Raki M, Kambur O, Kilpinen L, et al. Transient proteolytic modification of mesenchymal stromal cells increases lung clearance rate and targeting to injured tissue. Stem Cells Transl Med (2013) 2(7):510–20. doi: 10.5966/sctm.2012-0187
48. Moeinabadi-Bidgoli K, Mazloomnejad R, Beheshti Maal A, Asadzadeh Aghdaei H, Kazem Arki M, Hossein-Khannazer N, et al. Genetic modification and preconditioning strategies to enhance functionality of mesenchymal stromal cells: a clinical perspective. Expert Opin Biol Ther (2023) 23(6):461–78. doi: 10.1080/14712598.2023.2205017
49. Kim DS, Kim JH, Lee JK, Choi SJ, Kim JS, Jeun SS, et al. Overexpression of CXC chemokine receptors is required for the superior glioma-tracking property of umbilical cord blood-derived mesenchymal stem cells. Stem Cells Dev (2009) 18(3):511–9. doi: 10.1089/scd.2008.0050
50. Haider H, Jiang S, Idris NM, Ashraf M. IGF-1-overexpressing mesenchymal stem cells accelerate bone marrow stem cell mobilization via paracrine activation of SDF-1alpha/CXCR4 signaling to promote myocardial repair. Circ Res (2008) 103(11):1300–8. doi: 10.1161/CIRCRESAHA.108.186742
51. Huang B, Qian J, Ma J, Huang Z, Shen Y, Chen X, et al. Myocardial transfection of hypoxia-inducible factor-1α and co-transplantation of mesenchymal stem cells enhance cardiac repair in rats with experimental myocardial infarction. Stem Cell Res Ther (2014) 5(1):22. doi: 10.1186/scrt410
52. Vittorio O, Quaranta P, Raffa V, Funel N, Campani D, Pelliccioni S, et al. Magnetic carbon nanotubes: a new tool for shepherding mesenchymal stem cells by magnetic fields. Nanomedicine (2011) 6(1):43–54. doi: 10.2217/nnm.10.125
53. Doyle LM, Wang MZ. Overview of extracellular vesicles, their origin, composition, purpose, and methods for exosome isolation and analysis. Cells (2019) 8(7):1–24. doi: 10.3390/cells8070727
54. Zhou H, Pisitkun T, Aponte A, Yuen PS, Hoffert JD, Yasuda H, et al. Exosomal Fetuin-A identified by proteomics: a novel urinary biomarker for detecting acute kidney injury. Kidney Int (2006) 70(10):1847–57. doi: 10.1038/sj.ki.5001874
55. Sandfeld-Paulsen B, Aggerholm-Pedersen N, Bæk R, Jakobsen KR, Meldgaard P, Folkersen BH, et al. Exosomal proteins as prognostic biomarkers in non-small cell lung cancer. Mol Oncol (2016) 10(10):1595–602. doi: 10.1016/j.molonc.2016.10.003
56. Simpson RJ, Lim JW, Moritz RL, Mathivanan S. Exosomes: proteomic insights and diagnostic potential. Expert Rev Proteomics (2009) 6(3):267–83. doi: 10.1586/epr.09.17
57. Deng H, Sun C, Sun Y, Li H, Yang L, Wu D, et al. Lipid, protein, and microRNA composition within mesenchymal stem cell-derived exosomes. Cell Reprogram (2018) 20(3):178–86. doi: 10.1089/cell.2017.0047
58. Harrell CR, Jovicic N, Djonov V, Volarevic V. Therapeutic use of mesenchymal stem cell-derived exosomes: from basic science to clinics. Pharmaceutics (2020) 12(5):1–17. doi: 10.3390/pharmaceutics12050474
59. Lee BC, Kang I, Yu KR. Therapeutic features and updated clinical trials of mesenchymal stem cell (MSC)-derived exosomes. J Clin Med (2021) 10(4):1–19. doi: 10.3390/jcm10040711
60. Reis LA, Borges FT, Simões MJ, Borges AA, Sinigaglia-Coimbra R, Schor N. Bone marrow-derived mesenchymal stem cells repaired but did not prevent gentamicin-induced acute kidney injury through paracrine effects in rats. PloS One (2012) 7(9):e44092. doi: 10.1371/journal.pone.0044092
61. Gatti S, Bruno S, Deregibus MC, Sordi A, Cantaluppi V, Tetta C, et al. Microvesicles derived from human adult mesenchymal stem cells protect against ischaemia-reperfusion-induced acute and chronic kidney injury. Nephrol Dial Transplant (2011) 26(5):1474–83. doi: 10.1093/ndt/gfr015
62. Allahdadi KJ, de Santana TA, Santos GC, Azevedo CM, Mota RA, Nonaka CK, et al. IGF-1 overexpression improves mesenchymal stem cell survival and promotes neurological recovery after spinal cord injury. Stem Cell Res Ther (2019) 10(1):146. doi: 10.1186/s13287-019-1223-z
63. Ren S, Lin Y, Liu W, Yang L, Zhao M. MSC-Exos: Important active factor of bone regeneration. Front Bioeng Biotechnol (2023) 11:1136453. doi: 10.3389/fbioe.2023.1136453
64. Willis GR, Fernandez-Gonzalez A, Anastas J, Vitali SH, Liu X, Ericsson M, et al. Mesenchymal stromal cell exosomes ameliorate experimental bronchopulmonary dysplasia and restore lung function through macrophage immunomodulation. Am J Respir Crit Care Med (2018) 197(1):104–16. doi: 10.1164/rccm.201705-0925OC
65. Sotiropoulou PA, Perez SA, Gritzapis AD, Baxevanis CN, Papamichail M. Interactions between human mesenchymal stem cells and natural killer cells. Stem Cells (2006) 24(1):74–85. doi: 10.1634/stemcells.2004-0359
66. He X, Dong Z, Cao Y, Wang H, Liu S, Liao L, et al. MSC-derived exosome promotes M2 polarization and enhances cutaneous wound healing. Stem Cells Int (2019) 2019:7132708. doi: 10.1155/2019/7132708
67. Liang X, Zhang L, Wang S, Han Q, Zhao RC. Exosomes secreted by mesenchymal stem cells promote endothelial cell angiogenesis by transferring miR-125a. J Cell Sci (2016) 129(11):2182–9. doi: 10.1242/jcs.170373
68. Jia Y, Qiu S, Xu J, Kang Q, Chai Y. Exosomes secreted by young mesenchymal stem cells promote new bone formation during distraction osteogenesis in older rats. Calcif Tissue Int (2020) 106(5):509–17. doi: 10.1007/s00223-019-00656-4
69. Zhang S, Chuah SJ, Lai RC, Hui JHP, Lim SK, Toh WS. MSC exosomes mediate cartilage repair by enhancing proliferation, attenuating apoptosis and modulating immune reactivity. Biomaterials (2018) 156:16–27. doi: 10.1016/j.biomaterials.2017.11.028
70. Arslan F, Lai RC, Smeets MB, Akeroyd L, Choo A, Aguor EN, et al. Mesenchymal stem cell-derived exosomes increase ATP levels, decrease oxidative stress and activate PI3K/Akt pathway to enhance myocardial viability and prevent adverse remodeling after myocardial ischemia/reperfusion injury. Stem Cell Res (2013) 10(3):301–12. doi: 10.1016/j.scr.2013.01.002
71. Lim PK, Bliss SA, Patel SA, Taborga M, Dave MA, Gregory LA, et al. Gap junction-mediated import of microRNA from bone marrow stromal cells can elicit cell cycle quiescence in breast cancer cells. Cancer Res (2011) 71(5):1550–60. doi: 10.1158/0008-5472.CAN-10-2372
72. Chen J, Li C, Chen L. The role of microvesicles derived from mesenchymal stem cells in lung diseases. BioMed Res Int (2015) 2015:985814. doi: 10.1155/2015/985814
73. Bliss SA, Sinha G, Sandiford OA, Williams LM, Engelberth DJ, Guiro K, et al. Mesenchymal stem cell–derived exosomes stimulate cycling quiescence and early breast cancer dormancy in bone marrow. Cancer Res (2016) 76(19):5832–44. doi: 10.1158/0008-5472.CAN-16-1092
74. Valadi H, Ekström K, Bossios A, Sjöstrand M, Lee JJ, Lötvall JO. Exosome-mediated transfer of mRNAs and microRNAs is a novel mechanism of genetic exchange between cells. Nat Cell Biol (2007) 9(6):654–9. doi: 10.1038/ncb1596
75. Kang HS, Habib M, Chan J, Abavana C, Potian JA, Ponzio NM, et al. A paradoxical role for IFN-gamma in the immune properties of mesenchymal stem cells during viral challenge. Exp Hematol (2005) 33(7):796–803. doi: 10.1016/j.exphem.2005.03.012
76. Aggarwal S, Pittenger MF. Human mesenchymal stem cells modulate allogeneic immune cell responses. Blood (2005) 105(4):1815–22. doi: 10.1182/blood-2004-04-1559
77. Ren G, Zhang L, Zhao X, Xu G, Zhang Y, Roberts AI, et al. Mesenchymal stem cell-mediated immunosuppression occurs via concerted action of chemokines and nitric oxide. Cell Stem Cell (2008) 2(2):141–50. doi: 10.1016/j.stem.2007.11.014
78. Valencic E, Loganes C, Cesana S, Piscianz E, Gaipa G, Biagi E, et al. Inhibition of mesenchymal stromal cells by pre-activated lymphocytes and their culture media. Stem Cell Res Ther (2014) 5(1):3. doi: 10.1186/scrt392
79. Di Nicola M, Carlo-Stella C, Magni M, Milanesi M, Longoni PD, Matteucci P, et al. Human bone marrow stromal cells suppress T-lymphocyte proliferation induced by cellular or nonspecific mitogenic stimuli. Blood (2002) 99(10):3838–43. doi: 10.1182/blood.V99.10.3838
80. Li YP, Paczesny S, Lauret E, Poirault S, Bordigoni P, Mekhloufi F, et al. Human mesenchymal stem cells license adult CD34+ hemopoietic progenitor cells to differentiate into regulatory dendritic cells through activation of the Notch pathway. J Immunol (2008) 180(3):1598–608. doi: 10.4049/jimmunol.180.3.1598
81. Uccelli A, Moretta L, Pistoia V. Mesenchymal stem cells in health and disease. Nat Rev Immunol (2008) 8(9):726–36. doi: 10.1038/nri2395
82. Jiang XX, Zhang Y, Liu B, Zhang SX, Wu Y, Yu XD, et al. Human mesenchymal stem cells inhibit differentiation and function of monocyte-derived dendritic cells. Blood (2005) 105(10):4120–6. doi: 10.1182/blood-2004-02-0586
83. Aiello A, Farzaneh F, Candore G, Caruso C, Davinelli S, Gambino CM, et al. Immunosenescence and its hallmarks: how to oppose aging strategically? A review of potential options for therapeutic intervention. Front Immunol (2019) 10:2247. doi: 10.3389/fimmu.2019.02247
84. Spaggiari GM, Capobianco A, Becchetti S, Mingari MC, Moretta L. Mesenchymal stem cell-natural killer cell interactions: evidence that activated NK cells are capable of killing MSCs, whereas MSCs can inhibit IL-2-induced NK-cell proliferation. Blood (2006) 107(4):1484–90. doi: 10.1182/blood-2005-07-2775
85. Bai L, Lennon DP, Eaton V, Maier K, Caplan AI, Miller SD, et al. Human bone marrow-derived mesenchymal stem cells induce Th2-polarized immune response and promote endogenous repair in animal models of multiple sclerosis. Glia (2009) 57(11):1192–203. doi: 10.1002/glia.20841
86. Luz-Crawford P, Djouad F, Toupet K, Bony C, Franquesa M, Hoogduijn MJ, et al. Mesenchymal stem cell-derived interleukin 1 receptor antagonist promotes macrophage polarization and inhibits B cell differentiation. Stem Cells (2016) 34(2):483–92. doi: 10.1002/stem.2254
87. Lu D, Jiao X, Jiang W, Yang L, Gong Q, Wang X, et al. Mesenchymal stem cells influence monocyte/macrophage phenotype: Regulatory mode and potential clinical applications. BioMed Pharmacother (2023) 165:115042. doi: 10.1016/j.biopha.2023.115042
88. Bozorgmehr M, Gurung S, Darzi S, Nikoo S, Kazemnejad S, Zarnani AH, et al. Endometrial and menstrual blood mesenchymal stem/stromal cells: biological properties and clinical application. Front Cell Dev Biol (2020) 8:497. doi: 10.3389/fcell.2020.00497
89. Salamonsen LA, Hutchison JC, Gargett CE. Cyclical endometrial repair and regeneration. Development (2021) 148(17):1–14. doi: 10.1242/dev.199577
90. Gjorgieva D, Zaidman N, Bosnakovski D. Mesenchymal stem cells for anti-cancer drug delivery. Recent Pat Anticancer Drug Discov (2013) 8(3):310–8. doi: 10.2174/15748928113089990040
91. Wang X, Jiang H, Guo L, Wang S, Cheng W, Wan L, et al. SDF-1 secreted by mesenchymal stem cells promotes the migration of endothelial progenitor cells via CXCR4/PI3K/AKT pathway. J Mol Histol (2021) 52(6):1155–64. doi: 10.1007/s10735-021-10008-y
92. Niu J, Yue W, Le-Le Z, Bin L, Hu X. Mesenchymal stem cells inhibit T cell activation by releasing TGF-β1 from TGF-β1/GARP complex. Oncotarget (2017) 8(59):99784–800. doi: 10.18632/oncotarget.21549
93. Shigemoto-Kuroda T, Oh JY, Kim DK, Jeong HJ, Park SY, Lee HJ, et al. MSC-derived extracellular vesicles attenuate immune responses in two autoimmune murine models: type 1 diabetes and uveoretinitis. Stem Cell Rep (2017) 8(5):1214–25. doi: 10.1016/j.stemcr.2017.04.008
94. Greco SJ, Rameshwar P. Mesenchymal stem cells in drug/gene delivery: implications for cell therapy. Ther Deliv (2012) 3(8):997–1004. doi: 10.4155/tde.12.69
95. Munoz JL, Bliss SA, Greco SJ, Ramkissoon SH, Ligon KL, Rameshwar P. Delivery of functional anti-miR-9 by mesenchymal stem cell-derived exosomes to glioblastoma multiforme cells conferred chemosensitivity. Mol Ther Nucleic Acids (2013) 2(10):e126. doi: 10.1038/mtna.2013.60
96. Shah K. Mesenchymal stem cells engineered for cancer therapy. Adv Drug Deliv Rev (2012) 64(8):739–48. doi: 10.1016/j.addr.2011.06.010
97. Lin P, Lin Y, Lennon DP, Correa D, Schluchter M, Caplan AI. Efficient lentiviral transduction of human mesenchymal stem cells that preserves proliferation and differentiation capabilities. Stem Cells Transl Med (2012) 1(12):886–97. doi: 10.5966/sctm.2012-0086
98. Loebinger MR, Eddaoudi A, Davies D, Janes SM. Mesenchymal stem cell delivery of TRAIL can eliminate metastatic cancer. Cancer Res (2009) 69(10):4134–42. doi: 10.1158/0008-5472.CAN-08-4698
99. Cho KJ, Trzaska KA, Greco SJ, McArdle J, Wang FS, Ye JH, et al. Neurons derived from human mesenchymal stem cells show synaptic transmission and can be induced to produce the neurotransmitter substance P by interleukin-1 alpha. Stem Cells (2005) 23(3):383–91. doi: 10.1634/stemcells.2004-0251
100. Greco SJ, Zhou C, Ye JH, Rameshwar P. An interdisciplinary approach and characterization of neuronal cells transdifferentiated from human mesenchymal stem cells. Stem Cells Dev (2007) 16(5):811–26. doi: 10.1089/scd.2007.0011
101. Trzaska KA, Rameshwar P. Dopaminergic neuronal differentiation protocol for human mesenchymal stem cells. Methods Mol Biol (2011) 698:295–303. doi: 10.1007/978-1-60761-999-4_22
102. Torper O, Pfisterer U, Wolf DA, Pereira M, Lau S, Jakobsson J, et al. Generation of induced neurons via direct conversion in vivo. Proc Natl Acad Sci USA (2013) 110(17):7038–43. doi: 10.1073/pnas.1303829110
103. Aghajanova L, Horcajadas JA, Esteban FJ, Giudice LC. The bone marrow-derived human mesenchymal stem cell: potential progenitor of the endometrial stromal fibroblast. Biol Reprod (2010) 82(6):1076–87. doi: 10.1095/biolreprod.109.082867
104. Muñoz-Fernández R, de la Mata C, Requena F, Martín F, Fernandez-Rubio P, Llorca T, et al. Human predecidual stromal cells are mesenchymal stromal/stem cells and have a therapeutic effect in an immune-based mouse model of recurrent spontaneous abortion. Stem Cell Res Ther (2019) 10(1):177. doi: 10.1186/s13287-019-1284-z
105. Catalini L, Fedder J. Characteristics of the endometrium in menstruating species: lessons learned from the animal kingdom†. Biol Reprod (2020) 102(6):1160–9. doi: 10.1093/biolre/ioaa029
106. Cousins FL, Kirkwood PM, Murray AA, Collins F, Gibson DA, Saunders PT. Androgens regulate scarless repair of the endometrial “wound” in a mouse model of menstruation. FASEB J (2016) 30(8):2802–11. doi: 10.1096/fj.201600078R
107. Eremichev R, Kulebyakina M, Alexandrushkina N, Nimiritsky P, Basalova N, Grigorieva O, et al. Scar-free healing of endometrium: tissue-specific program of stromal cells and its induction by soluble factors produced after damage. Front Cell Dev Biol (2021) 9:616893. doi: 10.3389/fcell.2021.616893
108. Kirkwood PM, Shaw IW, Saunders PTK. Mechanisms of scarless repair at time of menstruation: insights from mouse models. Front Reprod Health (2021) 3:801843. doi: 10.3389/frph.2021.801843
109. Evans J, Kaitu’u-Lino T, Salamonsen LA. Extracellular matrix dynamics in scar-free endometrial repair: perspectives from mouse in vivo and human in vitro studies. Biol Reprod (2011) 85(3):511–23. doi: 10.1095/biolreprod.111.090993
110. Elfawy LA, Ng CY, Amirrah IN, Mazlan Z, Wen APY, Fadilah NIM, et al. Sustainable approach of functional biomaterials-tissue engineering for skin burn treatment: A comprehensive review. Pharm (Basel) (2023) 16(5):1–29. doi: 10.3390/ph16050701
111. Gangadaran P, Oh EJ, Rajendran RL, Oh JM, Kim HM, Kwak S, et al. Three-dimensional culture conditioned bone marrow MSC secretome accelerates wound healing in a burn injury mouse model. Biochem Biophys Res Commun (2023) 673:87–95. doi: 10.1016/j.bbrc.2023.05.088
112. Zhao W, Zhang H, Liu R, Cui R. Advances in immunomodulatory mechanisms of mesenchymal stem cells-derived exosome on immune cells in scar formation. Int J Nanomed (2023) 18:3643–62. doi: 10.2147/IJN.S412717
113. Wang M, Xu X, Lei X, Tan J, Xie H. Mesenchymal stem cell-based therapy for burn wound healing. Burns Trauma (2021) 9:tkab002. doi: 10.1093/burnst/tkab002
114. Jing Z, Qiong Z, Yonggang W, Yanping L. Rat bone marrow mesenchymal stem cells improve regeneration of thin endometrium in rat. Fertil Steril (2014) 101(2):587–94. doi: 10.1016/j.fertnstert.2013.10.053
115. Xia L, Meng Q, Xi J, Han Q, Cheng J, Shen J, et al. The synergistic effect of electroacupuncture and bone mesenchymal stem cell transplantation on repairing thin endometrial injury in rats. Stem Cell Res Ther (2019) 10(1):244. doi: 10.1186/s13287-019-1326-6
116. Gao L, Huang Z, Lin H, Tian Y, Li P, Lin S. Bone marrow mesenchymal stem cells (BMSCs) restore functional endometrium in the rat model for severe asherman syndrome. Reprod Sci (2019) 26(3):436–44. doi: 10.1177/1933719118799201
117. Xiao B, Zhu Y, Huang J, Wang T, Wang F, Sun S. Exosomal transfer of bone marrow mesenchymal stem cell-derived miR-340 attenuates endometrial fibrosis. Biol Open (2019) 8(5):1–9. doi: 10.1242/bio.039958
118. Mansouri-Kivaj N, Nazari A, Esfandiari F, Shekari F, Ghaffari M, Pakzad M, et al. Homogenous subpopulation of human mesenchymal stem cells and their extracellular vesicles restore function of endometrium in an experimental rat model of Asherman syndrome. Stem Cell Res Ther (2023) 14(1):61. doi: 10.1186/s13287-023-03279-7
119. Zhang Y, Lin X, Dai Y, Hu X, Zhu H, Jiang Y, et al. Endometrial stem cells repair injured endometrium and induce angiogenesis via AKT and ERK pathways. Reproduction (2016) 152(5):389–402. doi: 10.1530/REP-16-0286
120. Zhang L, Li Y, Dong Y-C, Guan C-Y, Tian S, Lv X-D, et al. Transplantation of umbilical cord-derived mesenchymal stem cells promotes the recovery of thin endometrium in rats. Sci Rep (2022) 12(1):412. doi: 10.1038/s41598-021-04454-7
121. Zhao J, Zhang Q, Wang Y, Li Y. Uterine infusion with bone marrow mesenchymal stem cells improves endometrium thickness in a rat model of thin endometrium. Reprod Sci (2015) 22(2):181–8. doi: 10.1177/1933719114537715
122. Wang G, Ce R, Jiang J. Effects of bone marrow mesenchymal stem cells on repair and receptivity of damaged endometrium in rats. J Obstet Gynaecol Res (2021) 47(9):3223–31. doi: 10.1111/jog.14888
123. Gan L, Duan H, Xu Q, Tang Y-Q, Li J-J, Sun F-Q, et al. Human amniotic mesenchymal stromal cell transplantation improves endometrial regeneration in rodent models of intrauterine adhesions. Cytotherapy (2017) 19(5):603–16. doi: 10.1016/j.jcyt.2017.02.003
124. Shao X, Ai G, Wang L, Qin J, Li Y, Jiang H, et al. Adipose-derived stem cells transplantation improves endometrial injury repair. Zygote (2019) 27(6):367–74. doi: 10.1017/S096719941900042X
125. Tan Q, Xia D, Ying X. miR-29a in exosomes from bone marrow mesenchymal stem cells inhibit fibrosis during endometrial repair of intrauterine adhesion. ijsc (2020) 13(3):414–23. doi: 10.15283/ijsc20049
126. Saribas GS, Ozogul C, Tiryaki M, Alpaslan Pinarli F, Hamdemir Kilic S. Effects of uterus derived mesenchymal stem cells and their exosomes on asherman’s syndrome. Acta Histochemica (2020) 122(1):151465. doi: 10.1016/j.acthis.2019.151465
127. Zhang S, Wang D, Yang F, Shen Y, Li D, Deng X. Intrauterine injection of umbilical cord mesenchymal stem cell exosome gel significantly improves the pregnancy rate in thin endometrium rats. Cell Transplant (2022) 31:9636897221133345. doi: 10.1177/09636897221133345
128. Wang J, Ju B, Pan C, Gu Y, Zhang Y, Sun L, et al. Application of bone marrow-derived mesenchymal stem cells in the treatment of intrauterine adhesions in rats. Cell Physiol Biochem (2016) 39(4):1553–60. doi: 10.1159/000447857
129. Guo Q, Chang Y, Li J, Zhou C, Huang R, Yang X, et al. Regenerative effects of locally or intra-arterially administered BMSCs on the thin endometrium. Front Bioeng Biotechnol (2022) 10. doi: 10.3389/fbioe.2022.735465
130. Zhang L, Li Y, Guan CY, Tian S, Lv XD, Li JH, et al. Therapeutic effect of human umbilical cord-derived mesenchymal stem cells on injured rat endometrium during its chronic phase. Stem Cell Res Ther (2018) 9(1):36. doi: 10.1186/s13287-018-0777-5
131. Ebrahim N, Mostafa O, El Dosoky RE, Ahmed IA, Saad AS, Mostafa A, et al. Human mesenchymal stem cell-derived extracellular vesicles/estrogen combined therapy safely ameliorates experimentally induced intrauterine adhesions in a female rat model. Stem Cell Res Ther (2018) 9(1):175. doi: 10.1186/s13287-018-0924-z
132. Monsef F, Artimani T, Alizadeh Z, Ramazani M, Solgi G, Yavangi M, et al. Comparison of the regenerative effects of bone marrow/adipose-derived stem cells in the Asherman model following local or systemic administration. J Assist Reprod Genet (2020) 37(8):1861–8. doi: 10.1007/s10815-020-01856-w
133. Ding L, Xa Li, Sun H, Su J, Lin N, Péault B, et al. Transplantation of bone marrow mesenchymal stem cells on collagen scaffolds for the functional regeneration of injured rat uterus. Biomaterials (2014) 35(18):4888–900. doi: 10.1016/j.biomaterials.2014.02.046
134. Lin Y, Dong S, Ye X, Liu J, Li J, Zhang Y, et al. Synergistic regenerative therapy of thin endometrium by human placenta-derived mesenchymal stem cells encapsulated within hyaluronic acid hydrogels. Stem Cell Res Ther (2022) 13(1):66. doi: 10.1186/s13287-022-02717-2
135. Wang S, Shi C, Cai X, Wang Y, Chen X, Han H, et al. Human acellular amniotic matrix with previously seeded umbilical cord mesenchymal stem cells restores endometrial function in a rat model of injury. Mediators Inflamm (2021) 2021:5573594. doi: 10.1155/2021/5573594
136. Xu B, Cao Y, Zheng Z, Galan EA, Hu Z, Ge J, et al. Injectable mesenchymal stem cell-laden matrigel microspheres for endometrium repair and regeneration. Adv Biol (2021) 5(8):2000202. doi: 10.1002/adbi.202000202
137. Zhou S, Lei Y, Wang P, Chen J, Zeng L, Qu T, et al. Human umbilical cord mesenchymal stem cells encapsulated with pluronic F-127 enhance the regeneration and angiogenesis of thin endometrium in rat via local IL-1β Stimulation. Stem Cells Int (2022) 2022:7819234. doi: 10.1155/2022/7819234
138. Huang J, Zhang W, Yu J, Gou Y, Liu N, Wang T, et al. Human amniotic mesenchymal stem cells combined with PPCNg facilitate injured endometrial regeneration. Stem Cell Res Ther (2022) 13(1):17. doi: 10.1186/s13287-021-02682-2
139. Liu F, Hu S, Yang H, Li Z, Huang K, Su T, et al. Hyaluronic acid hydrogel integrated with mesenchymal stem cell-secretome to treat endometrial injury in a rat model of asherman’s syndrome. Adv Healthc Mater (2019) 8(14):1900411. doi: 10.1002/adhm.201900411
140. Xin L, Lin X, Pan Y, Zheng X, Shi L, Zhang Y, et al. A collagen scaffold loaded with human umbilical cord-derived mesenchymal stem cells facilitates endometrial regeneration and restores fertility. Acta Biomaterialia (2019) 92:160–71. doi: 10.1016/j.actbio.2019.05.012
141. Ajayi AF, Akhigbe RE. Staging of the estrous cycle and induction of estrus in experimental rodents: an update. Fertil Res Pract (2020) 6:5. doi: 10.1186/s40738-020-00074-3
142. Evans J, Salamonsen LA. Inflammation, leukocytes and menstruation. Rev Endocrine Metab Disord (2012) 13(4):277–88. doi: 10.1007/s11154-012-9223-7
143. Wood GA, Fata JE, Watson KL, Khokha R. Circulating hormones and estrous stage predict cellular and stromal remodeling in murine uterus. Reproduction (2007) 133(5):1035–44. doi: 10.1530/REP-06-0302
144. Wang J, Hu R, Xing Q, Feng X, Jiang X, Xu Y, et al. Exosomes derived from umbilical cord mesenchymal stem cells alleviate mifepristone-induced human endometrial stromal cell injury. Stem Cells Int (2020) 2020:6091269. doi: 10.1155/2020/6091269
145. Shi Q, Wang D, Ding X, Yang X, Zhang Y. Exosome-shuttled miR-7162-3p from human umbilical cord derived mesenchymal stem cells repair endometrial stromal cell injury by restricting APOL6. Arch Biochem Biophys (2021) 707:108887. doi: 10.1016/j.abb.2021.108887
146. Li X, Duan H, Wang S, Lv C-X. Umbilical cord mesenchymal stem cell-derived exosomes reverse endometrial fibrosis by the miR-145-5p/ZEB2 axis in intrauterine adhesions. Reprod BioMed Online (2023) 46(2):234–43. doi: 10.1016/j.rbmo.2022.05.018
147. Wang H, Liu S, Zhang W, Liu M, Deng C. Human Umbilical Cord Mesenchymal Stem Cell-Derived Exosome Repairs Endometrial Epithelial Cells Injury Induced by Hypoxia via Regulating miR-663a/CDKN2A Axis. Oxid Med Cell Longev (2022) 2022:3082969. doi: 10.1155/2022/3082969
148. Zhao Q, Larios K, Naaldijk Y, Sherman L, Chemerinski A, Okereke K, et al. Mesenchymal stem cell secretome alters gene expression and upregulates motility of human endometrial stromal cells. Reproduction (2023) 166(2):161–74. doi: 10.1101/2022.11.12.516251
149. Zhao M, Chi F, Zhang T, Teng X, Li K. Human menstrual blood-derived mesenchymal stem cells regulation of the EGF/Ras p21 pathway as a potential therapeutic target for thin endometrium. Ann Transl Med (2021) 9(18):1476. doi: 10.21037/atm-21-4652
150. Zhu H, Jiang Y, Pan Y, Shi L, Zhang S. Human menstrual blood-derived stem cells promote the repair of impaired endometrial stromal cells by activating the p38 MAPK and AKT signaling pathways. Reprod Biol (2018) 18(3):274–81. doi: 10.1016/j.repbio.2018.06.003
151. Lin X, Zhang Y, Pan Y, He S, Dai Y, Zhu B, et al. Endometrial stem cell-derived granulocyte-colony stimulating factor attenuates endometrial fibrosis via sonic hedgehog transcriptional activator Gli2. Biol Reprod (2018) 98(4):480–90. doi: 10.1093/biolre/ioy005
152. Zhu H, Pan Y, Jiang Y, Li J, Zhang Y, Zhang S. Activation of the Hippo/TAZ pathway is required for menstrual stem cells to suppress myofibroblast and inhibit transforming growth factor β signaling in human endometrial stromal cells. Hum Reprod (2019) 34(4):635–45. doi: 10.1093/humrep/dez001
153. Santamaria X, Cabanillas S, Cervelló I, Arbona C, Raga F, Ferro J, et al. Autologous cell therapy with CD133+ bone marrow-derived stem cells for refractory Asherman’s syndrome and endometrial atrophy: a pilot cohort study. Hum Reprod (2016) 31(5):1087–96. doi: 10.1093/humrep/dew042
154. Singh N, Shekhar B, Mohanty S, Kumar S, Seth T, Girish B. Autologous bone marrow-derived stem cell therapy for asherman’s syndrome and endometrial atrophy: A 5-year follow-up study. J Hum Reprod Sci (2020) 13(1):31–7. doi: 10.4103/jhrs.JHRS_64_19
155. Tan J, Li P, Wang Q, Li Y, Li X, Zhao D, et al. Autologous menstrual blood-derived stromal cells transplantation for severe Asherman’s syndrome. Hum Reprod (2016) 31(12):2723–9. doi: 10.1093/humrep/dew235
156. Ma H, Liu M, Li Y, Wang W, Yang K, Lu L, et al. Intrauterine transplantation of autologous menstrual blood stem cells increases endometrial thickness and pregnancy potential in patients with refractory intrauterine adhesion. J Obstet Gynaecol Res (2020) 46(11):2347–55. doi: 10.1111/jog.14449
157. Sudoma I, Pylyp L, Kremenska Y, Goncharova Y. Application of autologous adipose-derived stem cells for thin endometrium treatment in patients with failed ART programs. J Stem Cell Ther Transplant (2019) 3:001–8. doi: 10.29328/journal.jsctt.1001013
158. Tersoglio AE, Tersoglio S, Salatino DR, Castro M, Gonzalez A, Hinojosa M, et al. Regenerative therapy by endometrial mesenchymal stem cells in thin endometrium with repeated implantation failure. A novel strategy. JBRA Assist Reprod (2020) 24(2):118–27. doi: 10.5935/1518-0557.20190061
159. Zhang Y, Shi L, Lin X, Zhou F, Xin L, Xu W, et al. Unresponsive thin endometrium caused by Asherman syndrome treated with umbilical cord mesenchymal stem cells on collagen scaffolds: a pilot study. Stem Cell Res Ther (2021) 12(1):420. doi: 10.1186/s13287-021-02499-z
160. Cao Y, Sun H, Zhu H, Zhu X, Tang X, Yan G, et al. Allogeneic cell therapy using umbilical cord MSCs on collagen scaffolds for patients with recurrent uterine adhesion: a phase I clinical trial. Stem Cell Res Ther (2018) 9(1):192. doi: 10.1186/s13287-018-0904-3
Keywords: mesenchymal stem cells, thin endometrium, Asherman’s syndrome, endometrial regeneration, endometrial receptivity
Citation: Saad-Naguib MH, Kenfack Y, Sherman LS, Chafitz OB and Morelli SS (2024) Impaired receptivity of thin endometrium: therapeutic potential of mesenchymal stem cells. Front. Endocrinol. 14:1268990. doi: 10.3389/fendo.2023.1268990
Received: 28 July 2023; Accepted: 26 December 2023;
Published: 25 January 2024.
Edited by:
Xinmei Liu, Fudan University, ChinaReviewed by:
Meixiang Zhang, First Affiliated Hospital of Zhengzhou University, ChinaCopyright © 2024 Saad-Naguib, Kenfack, Sherman, Chafitz and Morelli. This is an open-access article distributed under the terms of the Creative Commons Attribution License (CC BY). The use, distribution or reproduction in other forums is permitted, provided the original author(s) and the copyright owner(s) are credited and that the original publication in this journal is cited, in accordance with accepted academic practice. No use, distribution or reproduction is permitted which does not comply with these terms.
*Correspondence: Sara S. Morelli, bW9yZWxsc2FAbmptcy5ydXRnZXJzLmVkdQ==
Disclaimer: All claims expressed in this article are solely those of the authors and do not necessarily represent those of their affiliated organizations, or those of the publisher, the editors and the reviewers. Any product that may be evaluated in this article or claim that may be made by its manufacturer is not guaranteed or endorsed by the publisher.
Research integrity at Frontiers
Learn more about the work of our research integrity team to safeguard the quality of each article we publish.