- 1Department of Veterinary Clinical Sciences, College of Veterinary Medicine, Iowa State University, Ames, IA, United States
- 2Department for Small Animals, Veterinary Teaching Hospital, College of Veterinary Medicine, University of Leipzig, Leipzig, SN, Germany
- 3Redox Regulation Laboratory, Department of Zoology, College of Basic Science and Humanities, Odisha University of Agriculture and Technology, Bhubaneswar, India
- 4Department of Life Sciences, Hemchandracharya North Gujarat University, Patan, Gujarat, India
Inflammatory bowel disease (IBD) is a chronic, relapsing gastrointestinal (GI) disorder characterized by intestinal inflammation. The etiology of IBD is multifactorial and results from a complex interplay between mucosal immunity, environmental factors, and host genetics. Future therapeutics for GI disorders, including IBD, that are driven by oxidative stress require a greater understanding of the cellular and molecular mechanisms mediated by reactive oxygen species (ROS). In the GI tract, oxidative stressors include infections and pro-inflammatory responses, which boost ROS generation by promoting the production of pro-inflammatory cytokines. Nuclear factor kappa B (NF-κB) and nuclear factor erythroid 2–related factor 2 (Nrf2) represent two important signaling pathways in intestinal immune cells that regulate numerous physiological processes, including anti-inflammatory and antioxidant activities. Natural antioxidant compounds exhibit ROS scavenging and increase antioxidant defense capacity to inhibit pro-oxidative enzymes, which may be useful in IBD treatment. In this review, we discuss various polyphenolic substances (such as resveratrol, curcumin, quercetin, green tea flavonoids, caffeic acid phenethyl ester, luteolin, xanthohumol, genistein, alpinetin, proanthocyanidins, anthocyanins, silymarin), phenolic compounds including thymol, alkaloids such as berberine, storage polysaccharides such as tamarind xyloglucan, and other phytochemicals represented by isothiocyanate sulforaphane and food/spices (such as ginger, flaxseed oil), as well as antioxidant hormones like melatonin that target cellular signaling pathways to reduce intestinal inflammation occurring with IBD.
1 Introduction
Inflammatory bowel disease (IBD) in humans comprises at least two chronic inflammatory intestinal disorders, defined as Crohn’s disease (CD) and ulcerative colitis (UC). Lesions of CD may occur in the small or large intestine but most commonly involve the colon and rectum as discontinuous areas of transmural inflammation. In contrast, UC affects only the colon and rectum continuously, with inflammation restricted to the mucosa (1). The clinical course of CD is associated with intestinal granulomas, strictures, and fistulae, while these lesions are absent in UC. The underlying mechanism for IBD is believed to result from dysregulated immune responses to environmental factors and the intestinal microbiota in genetically susceptible people (2). These disorders impact millions of people worldwide, with the prevalence of disease in Americans expected to rise by 229% by 2030, relative to the number of diagnoses in 2010 (3).
Scientific evidence that increased levels of reactive oxygen species (ROS) but decreased levels of antioxidants contribute to disease pathogenesis establishes a link between ROS and IBD (4, 5). The intestinal mucosa is lined with an epithelial cell monolayer which separates the anaerobic lumen from the highly metabolic lamina propria. Therefore, the intestinal epithelial cells function under a physiological oxygen gradient that is relatively steep (reaching from physiologic hypoxia to physioxia) compared to other cell types. Moreover, during active IBD, there is a significant metabolic shift towards hypoxia seen with mucosal inflammation (pathologic hypoxia). In vitro and in vivo studies have demonstrated that the activation of the transcription factor hypoxia-inducible factor (HIF) functions as a warning signal in several murine disease models. For example, HIF-1, which is increasingly stabilized in inflammatory lesions, protects against inflammation and IBD by triggering the transcription of several genes that allow the intestinal epithelial cells to operate as an efficient barrier (4). While HIF-1 facilitates adaptive responses to oxidative stress (OS) via nuclear translocation and gene expression regulation, it is well known that mitochondrial HIF-1α protects against OS-induced apoptosis. Several studies have shown that nuclear factor erythroid 2–related factor 2 (Nrf2) helps the anti-inflammatory process by coordinating the recruitment of inflammatory cells and regulating gene expression via the antioxidant response element (ARE) (Figure 1) (6). A decrease in the expression of antioxidant/phase II detoxifying enzymes, such as UDP-glucurosyltransferase 1A1, NAD(P)H-quinone reductase-1, heme-oxygenase-1, and glutathione S-transferase Mu-1 was linked to the increased severity of colitis in Nrf2-deficient mice (7), while Nrf2 overexpression was reported to improve UC (8).
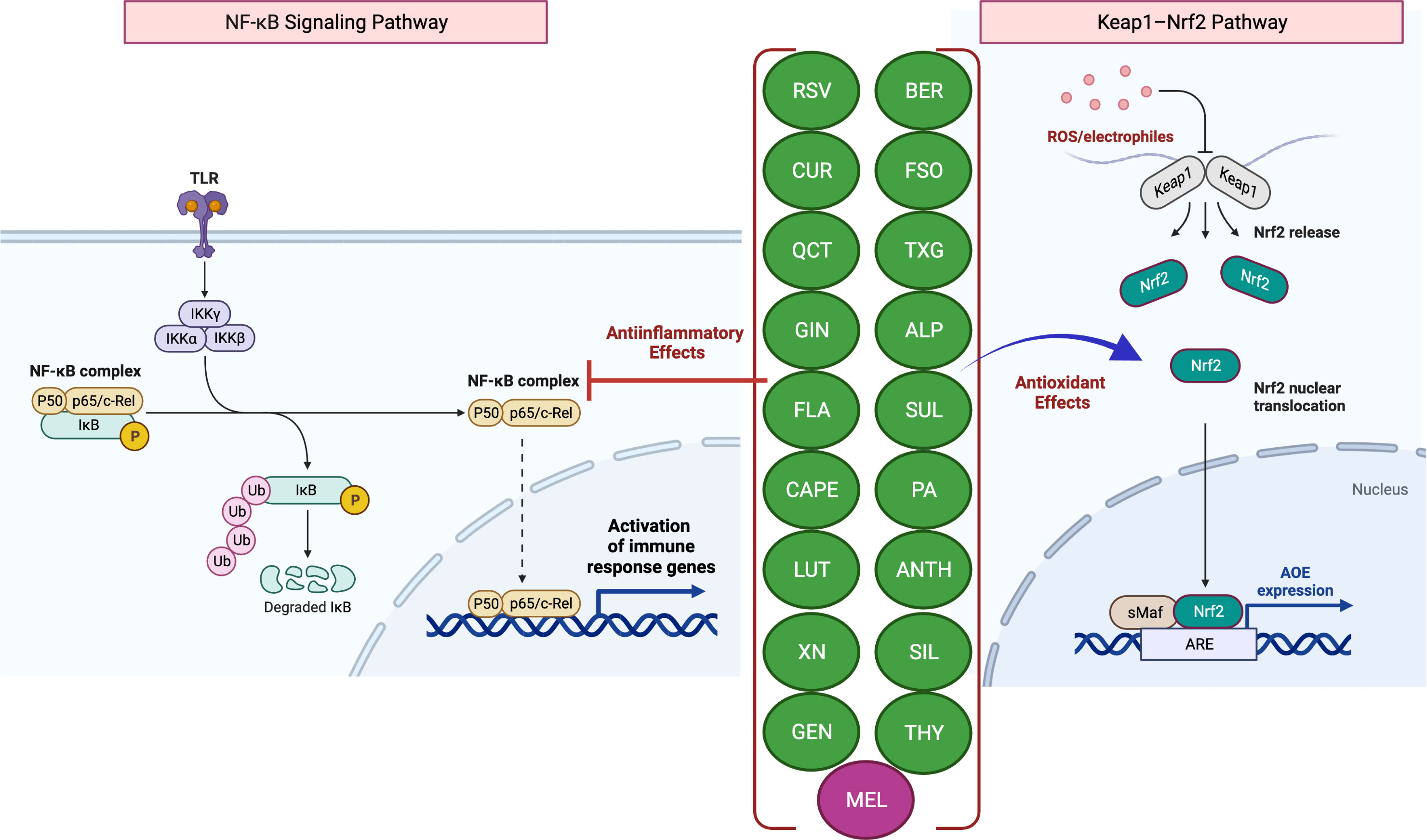
Figure 1 Antioxidant and anti-inflammatory effects of phytochemicals and hormones. Anti-inflammatory mechanisms involve the modulation of nuclear factor-kappa B (NF-κB) pathways, such as the downstream pro-inflammatory effects mediated by Toll-like receptor (TLR) activation. Activation (release of IκB inhibition) and nuclear translocation of NF-κB inhibition, which result in the transcription of several pro-inflammatory genes, can be inhibited by several phytochemicals and hormones (left panel). Kelch-like ECH-associated protein-1 (Keap1)-induced activation and nuclear translocation of nuclear factor erythroid 2-related factor 2 (Nrf2), resulting in an increased expression of antioxidant enzymes (AOEs), can also be inhibited by phytochemicals and hormones (right panel). In the presence of OS, Keap1 relinquishes its binding to Nrf2, thereby enabling the translocation of Nrf2 into the nucleus. Subsequently, Nrf2 forms a complex with small Maf (sMaf) proteins, resulting in the formation of Nrf2/sMaf heterodimer, which then binds to the Antioxidant Response Element (ARE) located on different stress-related gene targets. The figure was produced with BioRender (www.biorender.com; accessed on 17th July 2023). Resveratrol (RSV); Curcumin (CUR); Quercetin (QCT); Ginger (GIN); Flavonoids (FLA); Caffeic acid phenethyl ester (CAPE); Luteolin; (LUT); Xanthohumol (XN); Genistein (GEN); Berberine (BER); Flaxseed oil (α-linolenic acid) (FSO); Sulforaphane (SUL); Tamarind xyloglucan (TXG); Alpinetin (ALP); Proanthocyanidins (PA); Anthocyanins (ANTH); Silymarin (SIL); Thymol (THY); Melatonin (MEL); ubiquitin (Ub); phosphorylation (P); IκB kinase (Iκκ); Mammalian NF-κB family members: NF-κB1 (p50), c-Rel and RelA (p65).
2 Concepts of cellular and ROS damage
The intestinal mucosa in people with IBD (e.g., CD) is typically infiltrated with numerous inflammatory cells, including neutrophils, macrophages, and lymphocytes (9, 10). Uncontrolled immune responses are driven by the excessive activity of effector T-lymphocytes and their increased production of pro-inflammatory cytokines (e.g., tumor necrosis factor-alpha (TNF-α), interleukin (IL)-1β, and IL-6) and chemokines, which, along with other inflammatory mediators, cause tissue damage and perpetuate the inflammatory response (11). Typically, the equilibrium between proinflammatory cytokines (such as TNF-α, IL-1, IL-6, IL-8, IL-17, and IL-23) and anti-inflammatory cytokines, such as IL-5, IL-10, IL-11, and transforming growth factor β (TGF-β) is closely regulated within the GI mucosa (12). The pathogenesis of IBD is characterized by an imbalance between T helper (Th) cells and regulatory T cells, specifically the impaired tolerance of regulatory T cells. While CD is distinguished by inflammation mediated by Th1 cells, which results in the overproduction of IL-12, IL-17, and IL-23, UC is marked by cytokines such as IL-4, IL-5, IL-10, and IL-13, which are produced by Th2-type T cells (13).
The active inflammatory process is coupled with the generation and release of ROS from infiltrating immune cells. Principal ROS produced by inflammatory cells include superoxide (O2 •–), hydroxyl radical (·OH), hydroperoxyl radical (HO2·), nitric oxide (NO), and singlet oxygen (1O2) (14). Furthermore, ROS upregulates genes involved in innate and adaptive immune responses to amplify mucosal inflammation (12). ROS and other inflammatory markers released in the inflamed mucosal environment cause progressive cellular and molecular damage, resulting in increased tissue destruction. The most common cellular targets for ROS include cell membrane lipids, proteins, and DNA which causes lipid peroxidation (LPx), enzymatic dysfunction, and DNA damage, respectively (15–17). OS in IBD occurs due to an imbalance between oxidant and antioxidant substances in affected tissues (18). This review also evaluates the role of antioxidants and hormones in the crosstalk between OS and inflammation in IBD.
3 Clinical studies in companion animals
Canine chronic inflammatory enteropathies (CIE) refer to a group of intestinal disorders characterized by persistent or recurrent gastrointestinal (GI) signs and variable intestinal inflammation (19, 20). The prevalence of CIE in referral veterinary practice is estimated at 2%, and it is generally subclassified by the response to different therapeutic trials (20). The different disease phenotypes include food-responsive enteropathy (FRE), antibiotic-responsive enteropathy (AE), steroid-responsive enteropathy (SRE), often termed idiopathic IBD, and nonresponsive enteropathy (NRE) (20–22). While the cause of canine CIE is unknown, it is also recognized as a multifactorial disorder resulting from a complex interplay among the environment (e.g., diet, microbiome), mucosal immunity, and host genetics that initiates and drives chronic intestinal inflammation, like human IBD (19).
There are few clinical studies evaluating the role of OS in dogs with CIE. In one case-control study, colonic lavage analytes as markers of mucosal inflammation were compared between healthy dogs and dogs with biopsy-confirmed idiopathic IBD (23). Polyethylene glycol solution was administered into the colon via rectal balloon catheter prior to colonoscopy and was then analyzed for total protein, IgG, and nitrite concentrations and myeloperoxidase (MPO) activity. Results showed that mean nitrite and IgG concentrations were higher in lavage samples from idiopathic IBD dogs compared with samples from healthy dogs. Serum metabolite profiles have also demonstrated a potential relevance of OS in the pathogenesis of dogs affected by idiopathic IBD (24). Using an untargeted metabolomic approach, gluconic acid lactone and hexuronic acid increased in the serum of idiopathic IBD dogs when compared to samples from healthy dogs. Gluconic acid is an oxidized derivative of glucose that can scavenge free radicals, and hexuronic acid is a biologically active form of vitamin C that functions as an antioxidant by donating electrons. Interestingly, there were no significant changes in serum metabolite profiles in dogs with idiopathic IBD following medical therapy, despite clinical improvement.
Several other studies have investigated serum biomarkers of OS in dogs with CIE at diagnosis and in response to medical treatment. In one study, trolox equivalent antioxidant capacity (TEAC), cupric reducing antioxidant capacity (CUPRAC), ferric reducing ability of the plasma (FRAP), total thiol concentrations, and paraoxonase-1 (PON1) activity were evaluated in serum to determine the antioxidant response in dogs with idiopathic IBD. Additionally, ferrous oxidation-xylenol orange (FOX), thiobarbituric acid reactive substances (TBARS), and ROS concentrations in serum were determined (25). The mean concentrations of all antioxidant biomarkers except FRAP were lower, and the oxidant markers were higher in the sera of dogs with idiopathic IBD than in healthy controls. Another study showed lower serum fatty acid concentrations in dogs with CIE than in healthy dogs, indicating dysregulation of both pro-inflammatory (arachidonic acid and cyclooxygenase pathways) and anti-inflammatory (omega-3 essential fatty acids) mediators (26). Perturbations in these mediators in the face of chronic intestinal inflammation are a recognized feature of IBD in people (27).
Differences in systemic phospholipids were reported in another study involving dogs with idiopathic IBD and FRE (28). Overall, disease severity and treatment (e.g., elimination diet alone for FRE versus elimination diet and immunosuppressive dose of prednisolone for idiopathic IBD) were the most significant variables affecting phospholipid profiles at diagnosis. After treatment, a shift of phospholipid species from phosphatidylcholine to lysophosphatidylcholine was observed for both disease groups, presumably caused by an increase in anti-inflammatory lipid mediators (lipoxins and resolvins). The effects of dietary supplements and diet therapy on metabolomic changes in dogs with CIE have been investigated in other treatment trials. In one controlled trial, dogs with idiopathic IBD were randomized to treatment with either a hydrolyzed diet alone or a hydrolyzed diet supplemented with prebiotics (PRE) and glycosaminoglycans (GAG) (29). Results indicated that the majority of metabolomic changes involved several different lipid classes (glycerophospholipids, sphingolipids, and di- and triglycerides) and that both treatments increased beneficial metabolites in serum lipid profiles. In addition, co-treatment with PRE + GAG was associated with the greatest increase in lipid metabolites suggesting a possible additional beneficial effect in dogs with idiopathic IBD. Another randomized controlled trial in dogs with idiopathic IBD involved combination therapy with hydrolyzed diet and oral chondroitin sulfate + PRE versus hydrolyzed diet alone (30). The supplement group showed decreased serum levels of paraoxonase-1 after 60 days of treatment, whereas the placebo group showed reduced serum total antioxidant capacity after 120 days. A decrease in the intestinal histologic score was observed only in the supplement group post-treatment. Additionally, breed-specific changes in the fecal metabolomic profile have been reported in Yorkshire Terriers, which show an increased susceptibility to CIE and protein-losing enteropathy. Here, changes in bile acid, fatty acid, and sterol metabolism that only partially recovered with successful treatment were observed (31).
While most studies have investigated the pathomechanisms of OS in chronic gastroenteritis, other studies have evaluated the role of antioxidants in acute enteropathy and in mitigating OS induced by surgery. In one study, a comparison of the OS status of dogs with uncomplicated acute diarrhea (AD) was compared to healthy controls (32). Both cohorts were screened for clinical and laboratory abnormalities as well as routine redox indices (reactive oxygen metabolites [dROMs], serum antioxidant capacity [SAC], and the oxidative stress index [OSi]). Dogs with AD showed increased levels of dROMs and OSi values (calculated as the ratio between dROMs and SAC) as compared to control indices.
Summarizing, different metabolomic studies in dogs with CIE also show disturbances in serum and fecal metabolites reflective of OS at diagnosis. Notably, disturbances in lipid metabolism appear to be a common denominator across multiple studies. Moreover, treatment using a hydrolyzed diet with or without different dietary supplements improves several different measures of OS in most animals showing clinical remission. Given the importance of these metabolites in mediating chronic intestinal inflammation, additional well-designed and sufficiently powered clinical trials in dogs with CIE are warranted.
4 Oxidative stress and antioxidant defenses
The most prevalent antioxidant signaling pathway is the Kelch-like epichlorohydrin-related protein-1 (Keap1)/Nrf2-ARE signaling pathway (33, 34). As an inactive compound with its cytosolic repressor, Keap1, Nrf2 is sequestered in the cytoplasm. In response to OS that oxidizes two SH groups, Nrf2 is dissociated from the inhibitory protein Keap1 and translocated to the nucleus, where it binds ARE to activate the transcription of antioxidant genes (33, 34) (Figure 1).
The production of ROS is a natural consequence of biological metabolism (Figure 2). The beneficial effects of ROS are seen in a variety of physiological processes at low and moderate concentrations, including the killing of invading pathogens, the healing of wounds, and the repair of damaged tissues. Aerobic organisms possess a wide range of antioxidants that are critical to their survival. Antioxidants can be classified as either enzymatic or non-enzymatic, depending on their functions. While the antioxidant defense enzyme superoxide dismutase (SOD) converts the superoxide anion to hydrogen peroxide, catalase (CAT), peroxiredoxins (Prxs), and glutathione peroxidases (GPx) are examples of antioxidant enzymes (AOEs) that catalyze the breakdown of hydrogen peroxide (35, 36). Recent studies investigating refined kinetic measurements show that Prxs remove more than 90% of cellular peroxides in comparison to other antioxidant defense enzymes like CAT and GPx (35). Copper and iron ion-sequestering molecules, heme oxygenase, lipoic acid, uric acid, coenzyme Q, and bilirubin are all examples of non-enzymatic antioxidants present in vivo (37). In the non-enzymatic antioxidant defense system, glutathione (GSH) plays a crucial function as the most abundant cytosolic thiol. Glutathione can protect cells from free radicals and pro-oxidant damage because it also serves as a cofactor for other antioxidant and detoxifying enzymes, including GPx, glutathione S-transferases (GST), and glyoxalases. Thioredoxin (Trx) serves as a co-substrate molecule for Prxs, and its reducing capabilities are essential to the antioxidative activities of Prxs. Trx and GSH need glutathione reductase (GR) and thioredoxin reductase (TR), in addition to NADPH, to retain their reducing capabilities (38). During the process of GST-mediated detoxification of electrophilic compounds and xenobiotics, GSH functions as a cofactor (39). Following detoxifying interactions of vitamin E with lipid peroxyl radicals (LOO·), GSH can replenish the vitamin E pool (40). Antioxidants that can regenerate their original qualities through interactions with other antioxidants are referred to as an “antioxidant network” (41). Growing research suggests that pathological states characterized by elevated ROS levels are associated with diminished enzymatic and non-enzymatic antioxidant activity (34, 39, 42–59). The signaling pathways of nuclear factor kappa B (NF-κB), mitogen-activated protein kinase (MAPK), and signal transducer and activator of transcription 3 (STAT3) are among the major targets that can be influenced by ROS. Therefore, these pathways play a crucial role in defending against the effects of OS and can be used to identify antioxidant food ingredients or to develop therapeutics for diseases such as IBD. Myeloperoxidase is frequently overexpressed in a variety of inflammatory disorders (60, 61), including chronic gastroenteritis. As a lysosomal enzyme, MPO is secreted into the phagosome of neutrophils after degranulation, where it catalyzes the formation of strong oxidants, such as hypohalous acid (HOX; X = Cl or Br) with potent antibacterial properties. When generated at an improper location, time, or concentration, HOX can potentially damage host tissue. MPO-mediated damage is associated with a number of disorders in people, including IBD (60, 62). High leukocyte infiltration in the inflamed mucosa generates high levels of ROS, which triggers OS and causes cellular and tissue damage seen with inflammation (63).
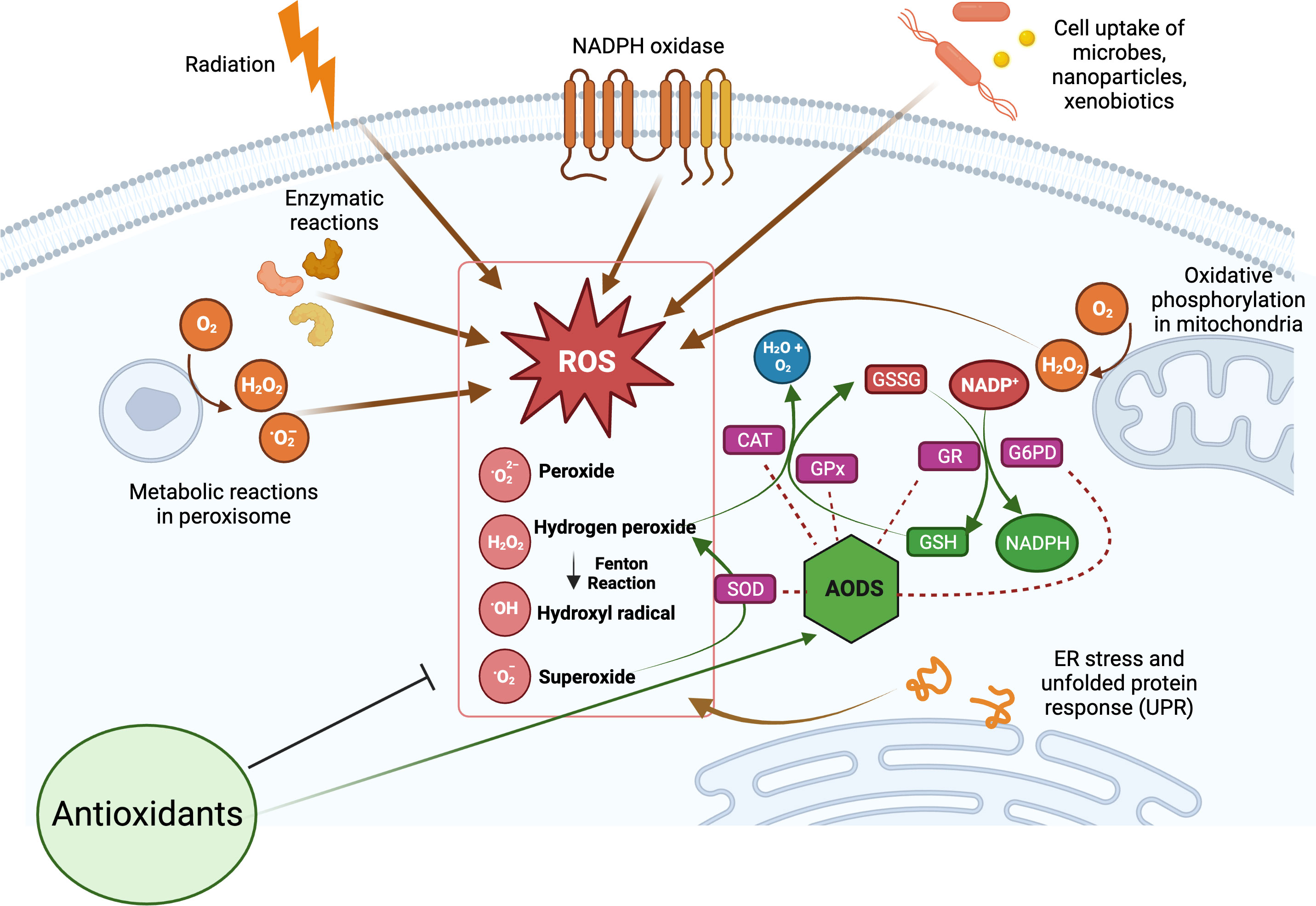
Figure 2 Overview of cellular reactive oxygen species (ROS) generation and their scavenging by antioxidant defense system (AODS). Supplemental antioxidants can help lower oxidative stress by scavenging free radicals, blocking enzymes that produce ROS, or stimulating AODS enzymes and molecules. The figure was produced with BioRender (www.biorender.com; accessed on 17th July 2023). Superoxide dismutase (SOD); catalase (CAT); glutathione peroxidases (GPx); glutathione reductase (GR); reduced glutathione (GSH); oxidized glutathione (GSSG); glucose-6-phosphate dehydrogenase (G6PD).
5 ROS generation in the gastrointestinal tract
The GI tract is one of the primary sources of ROS. Although epithelium acts as a physical and antimicrobial barrier, ingested materials and enteric pathogens can promote inflammation by stimulating the production of proinflammatory cytokines, which further contribute to OS. Oxidative stress is a contributing factor in the development of several GI pathological disorders, such as gastroduodenal ulcers, GI cancer, and IBD. Acute and chronic GI disorders in humans and animal models are characterized by increased ROS production or decreased counteracting antioxidant mechanisms, both of which disrupt redox homeostasis (12, 32, 64, 65).
Oxidative stress-induced damage in chronic intestinal disorders is associated with mucosal infiltration by activated leukocytes, which produce excessive ROS that overwhelm the tissue’s antioxidant defenses and perpetuate or exacerbate mucosal inflammation. Several ROS generated by unstable types of oxygen, including the superoxide ion, hydrogen peroxide, and hydroxyl radicals, are the principal pro-oxidant molecules (12).
The intestinal epithelium has been acknowledged as a crucial factor in the development of IBD due to its dual nature of exhibiting both immune and organ-specific functions. In the context of mucosal inflammation, the activation of NADPH oxidase (NOX) and inducible nitric oxide synthase (iNOS) by inflammatory cytokines leads to the production of superoxide and nitric oxide by intestinal epithelial cells (IECs), neutrophils, and macrophages (12). IECs generate an increased amount of ROS/RNS through the activation of NOX and iNOS. The presence of excessive ROS has the potential to cause harm to cytoskeleton proteins, which may modify tight junctions to increase intestinal permeability. Ultimately, this disrupted intestinal epithelial barrier leads to further mucosal inflammation (66). Thus, the initiation of IBD can be attributed to inflammation of the GI tract caused by OS. The microvascular network encircling the epithelial cells can attract inflammatory mediators causing more tissue damage and an escalation of intestinal inflammation. Morphologic lesions associated with intestinal inflammation include goblet cell depletion, decreased production of mucous, development of ulcers and/or hyperplasia of colonic crypt cells (12, 67).
Both ROS and RNS have been implicated in IBD pathogenesis, with a particular role in CD initiation and progression (68). With inflammation, the production of ROS by leukocytes and monocytes increases along with prostaglandins and leukotrienes (e.g., eicosanoids derived from arachidonic acid metabolism) (69, 70). ROS in the GI tract is produced by infiltrating neutrophils and macrophages, as well as IECs. Elevated blood levels of 8-hydroxy-2′-deoxyguanosine (8-OHdG) may serve as a biomarker for OS in people with IBD (13). In murine colitis models, systemic depletion of macrophages or neutrophils results in decreased ROS/RNS production, reduced concentrations of proinflammatory cytokines, and mitigation of intestinal inflammation (71).
These ROS promote cell damage and harm tissue integrity by preventing the accumulation of antioxidant defenses in host cells. For example, oxidative damage is observed in the intestinal tissues and peripheral blood leukocytes of patients with CD (72). Moreover, CD patients have lower levels of antioxidant vitamins A, C, E, and beta-carotene in their blood and intestinal mucosa, as well as reduced activity of key cellular AOEs such as glutathione peroxidase and SOD (73). Oxidative stress and redox signaling pathways, especially that involving NF-ĸB, are also involved in active IBD (Figure 1). Since the redox status of mucosal glutathione is associated with inflammation and disease progression, impaired mucosal antioxidant defenses likely contribute to the development of UC (74).
Chronic NF-ĸB stimulation promotes cellular infiltration and mucosal inflammation by increasing the transcription of proinflammatory cytokines (e.g., IL-6, IL-8, IL-16, and TNF-α) and by degrading the intestinal barrier through increased apoptosis of intestinal epithelial cells (Figure 3) (75), up-regulation of metalloproteinases which digest mucosal cells, and the release of ROS metabolites that activate NF-ĸB to further impair barrier stability (76). Matrix metalloproteinases (MMPs), a disintegrin and metalloproteinase (ADAMs), and tissue inhibitors of metalloproteinases (TIMPs) are involved in the regulation of the inflammatory response (77). The intestinal mucosa of IBD patients demonstrates an up-regulation of MMPs and ADAM17 (TNF-α converting enzyme; TACE), which is commonly correlated with disease severity but is not accompanied by an up-regulation of TIMP (78). It seems possible that the expression of different MMPs in IBD is affected by the imbalance between oxidants and antioxidants, given the importance of OS in the etiology of the disease (79). In addition to normalizing the intracellular redox state, antioxidants directly influence the regulation of MAPK and transcription factors and can reduce the production of MMPs, restoring their levels to normal (79).
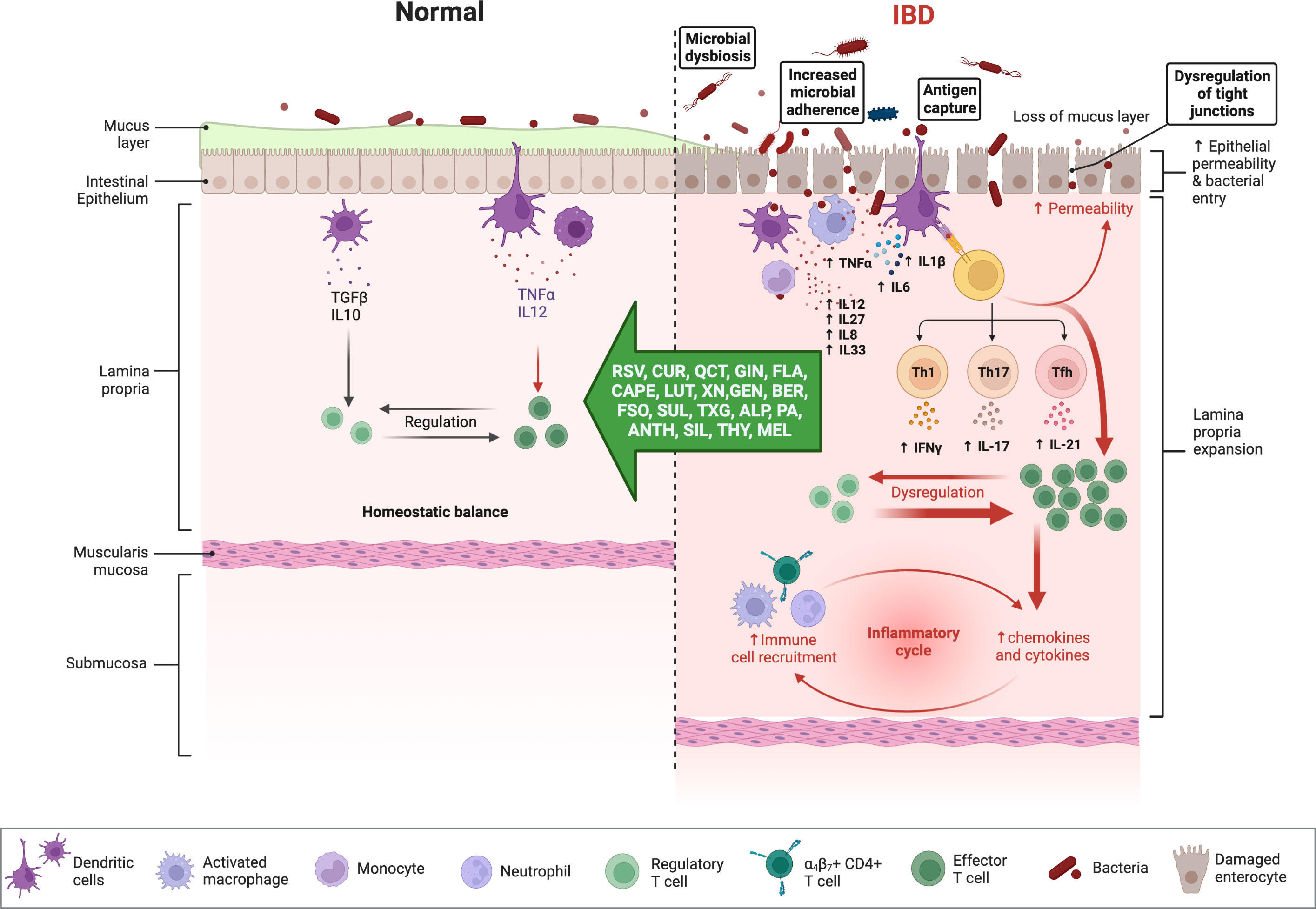
Figure 3 Immune responses in IBD and anti-inflammatory effects of phytochemicals and hormones. Whereas the physiologic state of the gastrointestinal (GI) immune system is dominated by immune tolerance, which maintains homeostatic balance, disturbances with IBD are associated with an exaggerated (i.e., pro-inflammatory) immune response, intestinal dysbiosis, and compromised intestinal barrier function. Pro-inflammatory mediators can perpetuate and exacerbate these dysregulated immune responses, while several phytochemicals and hormones can shift this imbalance toward homeostasis. The figure was produced with BioRender (www.biorender.com; accessed on 17th July 2023). Resveratrol (RSV); Curcumin (CUR); Quercetin (QCT); Ginger (GIN); Flavonoids (FLA); Caffeic acid phenethyl ester (CAPE); Luteolin; (LUT); Xanthohumol (XN); Genistein (GEN); Berberine (BER); Flaxseed oil (α-linolenic acid) (FSO); Sulforaphane (SUL); Tamarind xyloglucan (TXG); Alpinetin (ALP); Proanthocyanidins (PA); Anthocyanins (ANTH); Silymarin (SIL); Thymol (THY); Melatonin (MEL); interferon-gamma (IFNγ); tumor necrosis factor-alpha (TNF-α); transforming growth factor β (TGF-β); interleukin (IL). Naive CD4 T cells differentiated Th1, Th17, Tfh (follicular T helper), and Treg (T regulatory) subsets.
One of the well-established signaling pathways that play a vital role in the modulation of mucosal immunological tolerance relevant to the pathogenesis of IBD is the Janus kinase/signal transducer and activator of transcription (JAK/STAT) system (80). To ensure effective intestinal immunity, the JAK/STAT pathway modulates the proportions of effector to regulatory T cell numbers, intestinal epithelial cells and myeloid cells in the mucosa. In IBD, pro-inflammatory cytokines deliver their signal through cytoplasmic JAKs, which, once phosphorylated, associate with another class of cytoplasmic proteins called STATs. Subsequently, STATs are phosphorylated and translocated into the nucleus, where they enhance the transcription of target genes (including TGF-β, TNF-α, IL-2, IL-6, IL-8, MMP9, Intercellular Adhesion Molecule 1 (ICAM-1/CD54), STAT1, and STAT3) (81).
6 Role of antioxidants for IBD-related therapeutic applications and hormonal intervention
Oxidative/nitrosative stress is a significant pathophysiologic factor that plays a role in the initiation and progression of IBD. Overproduction of ROS is stimulated, and consequently, OS is triggered during inflammation because of the large number of cytokines and chemokines secreted by inflammatory cells. Considering this, therapeutic interventions incorporating substances possessing antioxidant and anti-inflammatory properties may be considered. Multiple antioxidant therapeutic strategies are being investigated because of the importance of OS in the pathophysiology of IBD to eliminate ROS, enhance AOE activities, and inhibit abnormal redox signaling (Figure 2). Colonic malondialdehyde (MDA) level rises because of OS, LPx, and free radical chain reactions that damage the intestinal mucosal barrier and activate inflammatory mediators. The use of antioxidants in people with uncomplicated GI disorders has been proposed as a potential alternative therapy to the use of anti-inflammatory/immunomodulatory drugs (82). The aim of antioxidant therapies is to mitigate the adverse effects of traditional treatments and to enhance the patient’s quality of life. However, the safety of synthetic antioxidants has been a subject of debate over time, despite their extensive utilization as a viable alternative to natural antioxidants (83–85). Numerous studies have documented a correlation between the prolonged consumption of synthetic antioxidants and certain health problems, such as GI disorders and increased cancer susceptibility (83–85). The utilization of natural antioxidants as a substitute for synthetic products is a noteworthy strategy, given that they are employed within the confines of regulatory thresholds (85).
6.1 Phenolic and polyphenolic compounds
Flavonoids, phenolic acids, lignans, and stilbenes are examples of polyphenols, a family of phytochemicals found in many plant diets. A growing body of research has demonstrated that natural polyphenols effectively mitigate the severity of intestinal inflammation and OS in the early stages of IBD (82, 85–89). Polyphenol-rich diets may ameliorate the pathophysiology of disorders where excessive production of ROS plays a role in disease progression (82). The phytochemicals that inhibit Toll-like receptor 4 (TLR4) activation were shown to cause reduced lipopolysaccharide (LPS)-mediated expression of cyclooxygenase-2 (COX-2), NF-κB, and pro-inflammatory cytokines (Figure 1; Supplementary Table 1). Numerous studies have shown the efficacy of phytochemicals against TLR4-mediated inflammation (Figure 1) (137, 138). Flavonoids such as quercetin, catechin, and silymarin have proven therapeutic efficacy in the treatment of IBD (Figure 3) (139, 140). Here, they operate as effective antioxidants and cellular modulators of the protein kinase and lipid kinase signaling pathways that drive chronic intestinal inflammation (139, 140). Dogs with idiopathic IBD also have up-regulated activity of the JAK/STAT pathway marked by phosphorylated STAT3 (pSTAT3) overexpression (141). Numerous studies have demonstrated that consuming antioxidants found naturally in plants can neutralize harmful free radicals and protect against certain diseases.
6.1.1 Resveratrol
The polyphenol resveratrol (RSV, trans-3,5,4’-trihydroxystilbene) is found in grapes, soybeans, berries, nuts, and pomegranates, among other natural sources (142). In rodent models of IBD, the antioxidant potential of RSV has been investigated. In one trinitrobenzene sulphonic acid (TNBS)-induced colitis study, pretreatment of rats with RSV reduced histologic inflammation and MDA levels but increased GPx activity compared to markers in the TNBS and vehicle groups (91) (Supplementary Table 1).
MPO is responsible for tissue damage in IBD and is inhibited effectively by resveratrol and its derivatives (143). Inhibition of IL-1, IL-6, and TNF-α release from macrophages, iNOS expression and subsequent NO production, prostaglandin production, cyclooxygenase (COX) enzyme activity, apoptosis, and MPO activity are the potential mechanisms by which RSV exerts its anti-inflammatory effect (90, 91) (Supplementary Table 1). The action of resveratrol on ROS generation may involve a direct radical scavenger effect or an effect on the activation of NADPH oxidase (90).
It has been shown that the NF-κB pathway is linked to both colitis and colon cancer development as a consequence of chronic intestinal inflammation (144). Additionally, inflammation in the colon inhibits the activity of the silent information regulator 1 (SIRT-1) gene and increases the activity of NF-κB. By activating SIRT-1 and down-regulating NF-κB activation, RSV plays a vital role in the regulation of inflammation that mediates colitis and colon cancer (145) (Supplementary Table 1).
6.1.2 Curcumin
Curcumin, a polyphenol extracted from Curcuma longa rhizomes, has a wide variety of beneficial antioxidant, anti-inflammatory, immunomodulatory, neuroprotective, hepatoprotective, anti-cancer, antiproliferative, and chemopreventive properties (34, 49, 50, 146–148). Curcumin reduces OS and improves intestinal barrier integrity and mitochondrial functions by inducing Parkin-dependent mitophagy via AMPK (adenosine 5’-monophosphate activated protein kinase) activation and TFEB (transcription factor EB) nuclear translocation (149). As a result of its ability to inhibit the expression of transcription factors and pro-inflammatory cytokines such as TNF-α, IL-1, IL-6, IL-8, IL-12, IL-1β, and monocyte chemoattractant protein-1 (MCP-1), curcumin has demonstrated anti-inflammatory properties (Supplementary Table 1). Curcumin can also compete with LPS for TLR4 receptor activation, thereby inhibiting the TLR4/myeloid differentiation 88 (MyD88)/NF-κB signaling pathways (150, 151). Curcumin can reduce LPS-induced inflammation in vascular smooth muscle cells via TLR4-MAPK/NF-κB pathways by inhibiting ROS generation (93). This effect is mediated through curcumin’s effects on TLR4 as it specifically prevents the LPS-induced generation of MCP-1, TNF-α, and NO (93). Curcumin reduces TNBS-induced colitis in rats by inhibiting the TLR4/NF-κB signaling pathway and pro-inflammatory IL-27 expression (94).
Oral supplementation with curcumin reduces OS generated by hyperthyroidism in rats, as shown by decreased LPx and protein carbonyl (PC) levels and increased SOD and CAT activities in tissues (34, 39, 49). In vitro studies show that curcumin can boost cellular glutathione levels by stimulating the transcription of two Gcl genes (Gclc and Gclm) encoding glutamate cysteine ligase, which is the rate-limiting enzyme in glutathione synthesis (49, 152). It has been reported that curcumin may reduce OS by modulating Nrf2 and KEAP1 function in the rat heart during altered thyroid states (153). Oral delivery of nanoparticles containing curcumin in dextran sulfate sodium (DSS)-induced colitis in Guinea pigs was associated with a substantial drop in tissue LPx and PC levels, leukocyte infiltration, and TNF-α production (95). Antioxidant balance is also regulated by curcumin in rats with UC by lowering both colonic MPO activity and total NO content as well as increasing colonic GST activity and GSH contents when administered prior to the DSS challenge (154). Curcumin has been shown to decrease OS markers MPO and MDA levels and cell apoptosis in different animal models of colitis (155–158) (Supplementary Table 1).
6.1.3 Quercetin
Quercetin (QCT), a member of the flavonols (a subclass of flavonoids), is a polyphenolic molecule found in plants and has demonstrated anti-inflammatory, antioxidant, and antitumoral effects. Several studies have shown that quercetin can suppress the expression of inflammatory mediators and cytokines such as COX-2, NO, NF-kB, prostaglandin E2 (PGE2), iNOS, TNF-α, IL-1β, and IL-6 that are generated through the LPS-TLR4 pathway (159) (Supplementary Table 1). LPS-induced inflammation is suppressed by quercetin-rich Myrsine seguinii ethanolic extract, which inhibits Src- and Syk-mediated phosphoinositide 3-kinase (PI3K) tyrosine phosphorylation and the TLR4/MyD88/PI3K signaling pathways (160). This extract also suppressed iNOS, a high-output Ca++-independent NOS stimulated by cytokines (161), and COX-2 gene expression through reduced NF-κB and activator protein (AP-1) stimulation (160).
Modulation of the stress response genes, including the antioxidant enzyme GPX1, was detected after LPS stimulation of the IBD enteroids and colonoids (57). Organoids have more advantages than conventional models and have been employed in fundamental and clinical research, such as for genetic and infectious diseases, regenerative medicine, and accurate and reliable drug screening (162–168). Murine colitis-derived intestinal organoids stimulated by LPS show reduced mRNA expression of inflammatory mediators such as TNF-α and lipocalin-2 (LCN2) when treated with quercetin (96). The anti-inflammatory action of quercetin was also accompanied by a decrease in the expression of C/EBP-β, a transcription factor that induces the expression of several inflammatory mediators, including TNF-α (169). Human apoB promoter analysis shows that a CCAAT enhancer-binding protein (C/EBP)-response element is critical for the action of quercetin. Through its interaction with C/EBPβ, quercetin has the potential to inhibit the recruitment of co-activators. Quercetin also suppresses apolipoprotein B (apoB) expression by inhibiting the transcription of C/EBPβ (169). Several polyphenols, including quercetin, affect the expression of tight junction (TJ) proteins of the intestinal epithelium (88). Using a DSS-colitis murine model, quercetin restored the expression of zonula occludens-1, occludin, junctional adhesion molecule-A, and claudin-3 (88).
There have been several studies on animal models of UC that provide evidence for the use of quercetin to treat IBD (92, 170). Oral quercetin (doses ranging from 25 to 100 mg/kg for 11 days) was associated with decreases in loss of body weight loss, rectal bleeding, and macroscopic and biochemical intestinal damage. It is possible that the antioxidant activity of QCT reduces LPx and OS by regulating nitrites and nitrates, increasing glutathione (GSH), and decreasing MPO activity in the colonic mucosa (92, 170) (Supplementary Table 1). Additional research using animal models demonstrates that QCT activity is mediated through the inhibition of TNF-α expression. By regulating the anti-inflammatory effects and bactericidal activity of macrophages through heme oxygenase-1 (HO-1)-dependent pathway, QCT may reduce the severity of experimental colitis (170, 171). Dietary delivery of QCT to restore intestinal homeostasis and intestinal normobiosis is a potentially promising treatment for IBD (171). Quercetin inhibits the apical efflux of N-acetyl 5-aminosalicylic acid (Ac-5-ASA) from Caco-2 cells, which was mediated by multidrug resistance-associated protein 2. This suggests that using QCT as an additional therapy may contribute to reduced dosages of sulfasalazine required for therapeutic action while reducing adverse drug effects (172). In another murine colitis model study (173), quercetin-loaded microcapsule-treated mice showed significantly more 2,2’-Azino-bis (3-ethylbenzothiazole-6-sulfonic acid) (ABTS) radical cation scavenging and ferric reducing activity as compared to colitis control mice. Results indicated that the QCT-treated mice had significant reduction in neutrophil influx (MPO activity), edema, and colonic macroscopic and histologic inflammation. Finally, this treatment reduced the levels of the pro-inflammatory cytokines IL-1β and IL-33 while maintaining anti-inflammatory cytokine IL-10 levels, and maintained the levels of endogenous antioxidants in the colons of colitic mice (173)(Supplementary Table 1).
6.1.4 Green tea flavonoids
Currently, there is no established tolerable upper limit for flavonoids in the Dietary Reference Intake framework (174). However, the consumption of flavonoids in quantities naturally present in foods does not pose any toxicity concerns (175). A high consumption of flavonoids may increase the likelihood of iron deficiency in populations with suboptimal iron levels (e.g., the elderly). Nonetheless, in Western societies where sufficient intake of heme iron and ascorbic acid occurs, the likelihood of developing anemia is minimal (175). The bioavailability of flavonoids is limited, as only a small fraction (i.e., less than 10% of the ingested quantity) can attain peak concentrations in the bloodstream within a few hours The bioavailability of flavonoids may be subject to various factors, such as ingestion of dietary fiber, macro and micronutrients, the duration of GI transit times, and composition of the gut microbiota (176, 177).
Flavonoids in green tea have been shown to regulate the expression of pro-inflammatory genes that target TLRs and inhibit downstream MyD88- and Toll/IL-1R domain-containing adaptor-inducing IFN-β (TRIF)-dependent signaling pathways (89). Epigallocatechin-3-gallate (EGCG), a flavonoid present in green tea, reduces TNF-α and also gene expression and the effects of nitric oxide synthase (NOS) and COX in murine RAW 264.7 macrophages (97). Treatment with EGCG-docosapentaenoic acid (DPA) esters has been shown to reduce the production of the pro-inflammatory mediators like nitric oxide (NO) and PGE2 via down-regulation of iNOS and COX-2 gene expression. Other EGCG ester derivatives (stearic acid, eicosapentaenoic acid, and docosahexaenoic acids) also have anti-inflammatory activity in murine RAW 264.7 macrophages (97). The mechanism of action is believed to be the inhibition of downstream TLR signaling affecting the MyD88- and/or TRIF-dependent pathways with activation of NF-κB. A study also demonstrated that EGCG blocks both the MyD88-dependent and TRIF-dependent signaling pathways of TLRs in RAW264.7 cells (89). In TRIF-dependent signaling pathways of TLR3 and TLR4, the molecular target of EGCG is TANK-binding kinase1 (TBK1), resulting in the decrease of interferon regulatory factor 3 (IRF3) activation, as TBK1 is the downstream kinase of TRIF and phosphorylates IRF3 resulting in its activation (89).
The combination of EGCG and piperine (piperine for enhancing the bioavailability of EGCG) significantly decreased weight loss, improved the clinical course, and increased overall survival in the DSS-murine model of colitis (87). Reduced severity of the colitis was linked to improved histology scores and reduced colonic MDA and MPO activity (Supplementary Table 1). The combination of EGCG and piperine improved SOD and GPx expression and suppressed the generation of proinflammatory cytokines in vitro. It appears that the powerful antioxidative potential of EGCG is responsible for its anti-inflammatory effects in the DSS-murine model of colitis. Lipid peroxidation occurs during IBD inflammation when ROS are not neutralized, altering the permeability and selectivity of the cell membrane and the activity of transmembrane transporters, receptors, and enzymes (87). DSS-colitis mice that had been administered EGCG and piperine had increased levels of AOEs (SOD and GPx), indicating that the antioxidant capacity had improved (87) (Supplementary Table 1).
6.1.5 Caffeic acid phenethyl ester
The anti-inflammatory, anti-cancer, and antioxidant effects of caffeic acid phenethyl ester (CAPE) have been studied extensively (98, 99, 178–181). Caffeic acid phenethyl ester inhibits LPS-induced IL-12 production and NF-κB activation in monocyte-derived dendritic cells (178). Caffeic acid phenethyl ester prevents the activation of TLR4 by interfering with the interaction between the TLR4/MD2 complex (180). In LPS-induced breast cancer cells, CAPE can down-regulate the expression of TLR4, NF-kB p65, TRIF, MyD88, and IRAK4 while stimulating cell apoptosis and autophagy (181). In gingival fibroblasts, CAPE suppresses LPS-induced production of IL-6, IL-8, iNOS, COX-2, TLR4/MyD88 mediated NF-κB, and phosphorylation of PI3K and protein kinase B (PKB, or Akt) (179).
FA-97 (caffeic acid phenethyl ester 4-O-glucoside) is a new synthetic CAPE derivative shown to attenuate body weight loss, colon length shortening, increased colonic inflammatory cell infiltration, and pro-inflammatory cytokine production (99). While FA-97 increased overall antioxidant capacity in DSS-treated mice and LPS-treated BMDMs and RAW 264.7 cells, it also decreased ROS and MDA production. The mechanism of action of FA-97 is believed to be the activation of the Nrf2/HO-1 pathway in both in vivo and in vitro models. FA-97 activates Nrf2 and promotes its nuclear translocation to increase expression of its downstream target proteins HO-1 and NAD(P)H:quinone oxidoreductase (NQO-1), which reduces ROS. It also inhibits the NF-κB and AP-1 signaling to suppress the expression of pro-inflammatory cytokines IL-1, IL-6, TNF-α, and IL-12 (Supplementary Table 1). In addition to ameliorating DSS-induced colitis, FA-97 promotes normal epithelial barrier function (98, 99).
6.1.6 Luteolin
The flavonoid compound luteolin, found in various plant extracts, has been shown to have anti-inflammatory, antioxidant, anti-metastasis, and apoptosis-inducing properties. Luteolin has been reported to reduce LPS-stimulated expression of NF-κB, TNF-α, and ICAM-1 and TBK1-kinase activity via the MyD88-independent signaling pathway (182). Luteolin inhibited the expression of target genes IL-6, IL-12, IL-27, TNF-α, IP-10, IFNβ, CXCL9 (C-X-C motif chemokine ligand 9) in macrophages by inhibiting IRF3 and NF-κB activation (Supplementary Table 1). Luteolin was able to suppress the ligand-independent activation of IRF3 or NF-κB triggered by TLR4, TRIF, or TBK1. Luteolin also reduces the amount of TBK1-dependent gene expression by inhibiting TBK1-kinase activity, and IRF3 dimerization and phosphorylation. Moreover, luteolin structural analogs, such as quercetin, chrysin, and eriodictyol, also inhibit TBK1-kinase activity and TBK1-target gene expression. These findings indicate that TBK1 is a unique target of anti-inflammatory flavonoids resulting in the inhibition of the TRIF-dependent signaling pathway (182).
LPS-induced inflammatory responses are controlled at the transcriptional level through the MAPK and NF-κB pathways (100, 183, 184). Toll-like receptors trigger NF-κB and MAPK cascades responses to LPS stimulation, causing the production of ROS, increased MPO expression, and expression of pro-inflammatory molecules and chemokines. In mice with LPS-induced acute lung injury (ALI), luteolin reduced the activation of ERK, p38MAPK, and JNK in lung tissue. The protective action of luteolin is due to its ability to block MAPK pathways, which prevents the activation of NF-κB and the degradation of IκB. Upon administration of LPS, luteolin pretreatment prevents these inflammatory processes from developing (100). In addition, the results in mice indicate that the activities of SOD and CAT increased following pretreatment with luteolin versus LPS treatment alone. MPO levels are reduced in LPS-induced acute lung injury (ALI) when pretreated with luteolin. This mechanism of action is believed to be due to luteolin suppression of LPS-induced ALI-related MDA generation in the lungs (100) (Supplementary Table 1).
6.1.7 Xanthohumol
Xanthohumol (XN), a compound derived from hop plants, is a prenylated chalcone with, antioxidant, anti-cancer, and anti-inflammatory activities via inhibition of TLR4/MD-2 complex (185). Downstream, XN suppresses macrophage iNOS expression and NO, and interferon-gamma (IFN-γ) production (186, 187). Pretreatment with XN in DSS-treated mice decreased the severity of diarrhea, hematochezia, rectal bleeding, and reduced colon length. Moreover, XN protected against epithelial cell injury, cellular infiltration, pro-inflammatory cytokines, and crypt changes following the DSS challenge (101). Pre-treatment with XN prior to DSS exposure resulted in decreased MDA levels and COX-2 expression suggesting a protective effect against experimental colitis (101) (Supplementary Table 1). Moreover, nuclear factor of kappa light polypeptide gene enhancer in B-cells inhibitor, alpha (IκBα) phosphorylation, nuclear translocation of p65, p50, and p105 NF-κB subunits, and NF-κB DNA-binding transcriptional activity were suppressed by XN treatment of DSS-treated mice and H2O2- or LPS-treated IEC-6 cells (101).
XN protects mice from DSS-induced colitis and H2O2- or LPS-treated IEC-6 injury, possibly by the interaction between the α, β-unsaturated carbonyl moiety of XN and Cys99 in IKKβ, and thus by its ability to inhibit the IKKβ/NF-κB signaling pathway. Additionally, XN inhibited the activation of the canonical NF-κB pathway, as evidenced by the downregulation of NF-κB target genes, like A1a, A20, Bcl-xL, and c-myc (101) (Supplementary Table 1). These collective results indicate that XN may be a promising therapeutic agent for the prevention or treatment of colitis (188).
6.1.8 Genistein
A soy isoflavone, genistein, is a powerful antioxidant and anti-inflammatory agent (102–105, 189). Following treatment with genistein, reduced levels of IL-6, TNF-α, and NF-κB activation have been observed in in vitro and in vivo studies (104, 189). Genistein also inhibited BV2 microglia LPS-induced NO production, prostaglandin E2 release and expression of inflammatory cytokines (IL-1β, TNF-α), TLR4, and MyD88 expression (102). Expression of interferon beta (IFN-β), IL-1α, IL-1β, IL-6, IL-10, TNF-α, colony-stimulating factor 2 (CSF-2), colony-stimulating factor 3 (CSF-3), chemokines CCL2 (chemokine ligand 2), and CXCL10 (C-X-C motif chemokine ligand 10), transcription factor NF-κB, IκBα, and COX-2 are all decreased in genistein-pretreated LPS-induced RAW264.7 macrophages (103) (Supplementary Table 1). In humans, six months of daily oral administration of genistein has been shown to decrease TNF-α levels decreased in obese postmenopausal women (106). Dietary supplementation with genistein lowered the expression of vascular adhesion molecule-1 (VCAM-1), a major cell adhesion molecule involved in inflammation (190), and F4/80 positive macrophages in the aorta of TNF-α-treated C57BL/6 mice (104). Human umbilical vein endothelial cells (HUVECs) in culture showed significant increases in the activities of GR, GPx, GST, NQO-1, SOD, and CAT when treated with 50 µM genistein (104) (Supplementary Table 1). Due to its ability to stimulate IL-1β secretion, the NLRP3 inflammasome has been linked to IBD in experimental models as well as human studies (191). In the DSS murine colitis model, treatment with genistein significantly slowed weight loss and reduced colon shortening, infiltration of inflammatory cells as well as the production of pro-inflammatory mediators in both serum and colon (105). Genistein’s protective properties may stem from the ubiquitination of NLRP3 caused by the interaction of cAMP with the protein. It has been shown that genistein can inhibit the NLRP3 inflammasome via TGR5-cAMP signaling in human macrophages (105).
6.1.9 Alpinetin
Alpinetin, a naturally occurring dihydroflavone (192), ameliorates clinical severity (disease activity index; DAI), colonic shortening, histological scores, and MPO activity in DSS-treated mice (108). The expression of occludin and zonula occludens-1 and SOD activity were increased, while the MDA amount was decreased by alpinetin in DSS-treated mice. Also, Nrf2/HO-1 signaling pathways were activated by alpinetin in DSS-treated mice (108). Alpinetin exhibits its anti-inflammatory activities by suppressing TLR4/IκBα/NF-κB signaling (107). In that study, alpinetin decreased the expression levels of IL-1β, IL-6 and TNF-α (Supplementary Table 1) in LPS-treated RAW 264.7 macrophages in vitro and in the in vivo LPS-induced acute lung injury murine model (107) by disrupting ERK/p38MAPK signaling (193).
6.1.10 Proanthocyanidins
Grape seed proanthocyanidin extract (GSPE) is composed of approximately 90% proanthocyanidins, including oligomers (74.8%), dimers (6.6%), trimers (5.0%) and tetramers (2.9%) (194). Inhibiting the expression of NF-κB-targeted genes is a primary mechanism by which GSPs exert their antioxidant and anticarcinogenic actions (109, 195, 196). It is proposed that GSPs inhibit NF-κB activation by inhibiting the activation of IκK (inhibitor of κB kinase) to prevent phosphorylation-induced degradation of IκBα (196). The effect of GSPs on enhancing antioxidant enzymatic defense was confirmed by their ability to boost colonic SOD activity (109, 195).
Nutritional and pharmacological dosages of proanthocyanidins protect against endotoxin (LPS)-induced intestinal inflammation, OS, and intestinal permeability in colitic rats (197). Notably, area-specific LPS-induced inflammation in the intestine has been identified, and a unique gene signature may be useful to determine the affected intestinal region (57). Treatment with GSPE reduces MPO and COX-2 activity, modifies the gene expression of ileal inflammatory and permeability proteins, and reduces ROS, MDA, and NO levels, and iNOS activities (Supplementary Table 1) in the large intestine (109, 197). Also, GSPE treatment enhances SOD, GPx activity, and glutathione levels in colon tissues and serum of TNBS-induced colitis in rats (109) (Supplementary Table 1).
6.1.11 Anthocyanins
The antioxidant properties of anthocyanins, as well as their ability to influence gut microbiota and to down-regulate the immune response, have significant implications for reducing intestinal inflammation (198, 199). In human monocytic THP-1 cells, treatment with bilberry extract (BE), rich in anthocyanins, inhibited IFN-γ activation of STAT1 and STAT3 and lowered mRNA expression and/or secretion of MCP-1, TNF-α, IL-6, and ICAM-1 (110). The effects of genetically engineered tomato extracts (enriched in anthocyanins) (200) showed that the extract reduced the activation of epithelial cells SAPK/JNK (stress-activated protein kinase/c-Jun NH (2)-terminal kinase) and p38MAPK signaling pathways. Furthermore, pro-inflammatory cytokines and chemokines, including TNF-α and IL-10, were inhibited by anthocyanin-rich tomato extracts (200). It has been shown that anthocyanin-rich fractions from red raspberries (112) and purple carrots (111) decrease the production of iNOS, COX-2, IL-1β, and IL-6 in LPS/IFN-γ activated macrophages in vitro. In murine colitis, anthocyanins extracted from the Chinese plant Dioscorea alata L (201). decreased DAI (body weight loss, fecal occult blood, and fecal consistency), increased the gene expression of tight junction proteins and reduced OS markers (MPO and iNOS) along with IFN-γ and TNF-α levels. Red raspberry berry (RB) powder (202) can attenuate the effects of DSS treatment by preventing weight loss, neutrophil infiltration, colon shortening by inhibiting IL-1, IL-6, IL-17, and TNF- α and COX-2 levels in inflamed tissues (Supplementary Table 1). Supplementation with RB restored CAT levels to normal, decreased xanthine oxidase (XO) levels and expression of MCP-1, and thus reduced neutrophil infiltration and ROS formation (202). Similarly, when an extract of blueberry anthocyanins (BBA) (203) was used, disease activity, MPO and MDA levels were reduced in murine colitis. In addition to decreasing serum prostaglandin E2 levels, BBA reduces OS marked by increased SOD and CAT levels. Moreover, mRNA expression of NF-κB, IFN-γ, COX-2, IL-1β, and iNOS was reduced, indicating that blueberry’s protective effect is at least partially mediated by the inhibition of inflammatory mediators (203). Similarly, it has been shown that colitis symptoms (colon shortening, DAI, loss of appetite, and weight gain), decreased SOD, and increased MPO can be mitigated and that GPx and GR activities (Supplementary Table 1) can be increased by administering grape pomace extract (GPE) (86). Anti-inflammatory effects of GPE were observed in DSS-treated mice as evidenced by reduced IL-1β, IL-1α, IL-6, and IFN-γ and also TNF-α activities. Gene expression for the p65 subunit of NF-κB, TNF-α, and COX-2 was reduced, which down-regulates the production of pro-inflammatory cytokines by GPE (86). Similarly, cocoa (anthocyanidins/anthocyanins) supplementation (204) decreased MDA, increased SOD, CAT, GPx, and GRx, and reduced iNOS and COX-2 gene expression in murine colitis. Moreover, cocoa is able to stimulate Nrf-2 transcription factor-activated expression of NQO1 and UDP-GT to reduce OS. Cocoa supplementation can also lower CD68+ neutrophils, MPO, TNF-α, IL-1β and IL-17 and suppress STAT-3 activation in colitic tissues (204) (Supplementary Table 1).
One randomized, placebo-controlled trial involving healthy humans found that eating anthocyanins-rich, purple-fleshed potatoes for six weeks reduced the levels of inflammatory markers like IL-6 and C-reactive protein (CRP) and the oxidative DNA-damage marker 8-hydroxydeoxyguanosine (8-HdG) (205). In another study, patients with mild to moderate UC were given an anthocyanin-rich bilberry preparation for six weeks and showed improvements in endoscopic Mayo score, histologic Riley index, and fecal calprotectin levels for intestinal inflammation (206).
6.1.12 Silymarin
Silymarin (SM), an extract from milk thistle (Silybum marianum), has been shown to have anti-inflammatory and antioxidant properties. Silymarin decreases inflammation by inhibiting NF-κB pathways and by optimizing the redox balance in the cell through activating AOEs and non-enzymatic antioxidants via Nrf2 activation (207). Studies show that SM increases the total antioxidant capacity of colonic tissue to reduce LPx levels, neutrophilic infiltration and pro-inflammatory cytokine production in TNBS-induced colitic rats. Also, treatment with SM in TNBS-colitic rodents dramatically decreased colonic NF-κB activity, levels of IL-1β, TNF-α, TBARS and MPO activity (113) (Supplementary Table 1). In one randomized, double-blind, placebo-controlled clinical trial, 35/38 UC patients administered SM for 6 months achieved remission. Moreover, remission was accompanied by improved patient biochemical parameters such as decreased erythrocyte sedimentation rates, increased hemoglobin levels, and decreased DAI scores (208). However, the bioavailability of oral SM appears to vary widely between species and is impacted by preparation methods (209–211). Thus, care should be taken when designing experimental trials and extrapolating results between species and preparations.
6.1.13 Thymol
Essential oils from Thymus, Origanum, and Lippia species are rich in thymol (2-isopropyl-5-methylphenol), a monoterpene phenol derivative of cymene (212). Thymol has demonstrated a wide range of biological and pharmacological activities, such as anti-inflammatory, antioxidant, anti-tumor, and antimicrobial effects (213). Thymol exhibits gastroprotective effects on both acute and chronic ulcer models by modulating the enhancement of mucus production, prostaglandin synthesis, and activation of ATP-sensitive K(+) channels (212). Thymol mitigates the reduction of trans-epithelial electrical resistance (TEER) in a porcine IPEC-J2 monolayer cell model stimulated with LPS by increasing ZO-1 and actin expression (118). In the DSS-induced murine colitis model, thymol mitigates intestinal damage induced by DSS by up-regulating the expression of tight junction protein claudin-3 (119). Similarly, thymol enhances the tight junction integrity and induces up-regulation of cyclooxygenase-1 (COX1) activity in Caco-2 cells (115).
Along with improving barrier function, thymol can decrease the production of ROS and the expression of pro-inflammatory cytokines following stimulation with LPS (118). Thymol exhibits the ability to attenuate increased MPO and MDA levels induced by LPS, as well as the expression of NF-κB, in a murine model of acute lung injury (214) and in colonic homogenates in murine colitis (215). Thymol exhibits inhibitory effects on the expression of TLR4 and the activation of NF-κB signaling in mice treated with acetic acid to induce colitis (117, 120). Thymol has been found to exhibit inhibitory effects on p38 phosphorylation, and it disrupts the activation of the MAPK signaling pathway, thereby contributing to the maintenance of immune homeostasis (216). Moreover, thymol exhibits inhibitory effects on the activation of p-p38, p-JNK, and p-ERK induced by LPS in RAW264.7 cells (116). Consequently, thymol effectively suppresses the production of various inflammatory cytokines such as NO, IL-6, TNF-α and COX-2 (116), thus exhibiting the ability to attenuate the inflammatory response through the inhibition of MAPK signaling pathway. Similarly, thymol had the potential to mitigate inflammatory responses by regulating the expression of JNK, AP-1, STAT-3, and nuclear factors of activated T-cells (NFATs) in LPS-stimulated J774.1 mouse macrophages (117).
6.2 Alkaloids
6.2.1 Berberine
Berberine, an isoquinoline alkaloid from Berberis aristata, has been used for decades to treat intestinal parasites and enteropathogenic diarrhea due to its bactericidal activity, its ability to inhibit protozoan growth, and also to inhibit enterotoxin-induced intestinal electrolyte secretion (217–219). Several in vivo studies confirm its anti-inflammatory role in decreasing the expression of IL-1β, TNF-α, iNOS, ICAM-1, IL-6, and NF-κB activation (220) (Supplementary Table 1). Berberine reduces damage, inflammation scores, MPO activity, and colon shortening caused by oral DSS ingestion. Moreover, berberine has been shown to decrease levels of the pro-inflammatory cytokines IFN-γ, TNF-α, KC (keratinocyte chemoattractant or CXCL1), and IL-17 and to maintain colon epithelial barrier function in DSS-treated mice. Additionally, berberine enhanced apoptosis of colonic macrophages and decreased proinflammatory cytokine production in colonic macrophages and epithelial cells of DSS-treated mice. Berberine suppresses Src activation and TLR4-mediated cell motility in LPS-stimulated macrophages (121). Berberine also inhibits the activation of MAPK and NF-κB signaling pathways that stimulate proinflammatory cytokine production in both colonic macrophages and epithelial cells from DSS-treated mice (122). Multiple cellular kinases and signaling pathways, including AMPK, MAPKs, and Nrf2 and NF-κB pathways, are involved in berberine’s antioxidant and anti-inflammatory activities (221). By suppressing the expression of NADPH oxidase, a key enzyme in the generation of ROS in cells, berberine can mitigate OS (222). Superoxide anion production in LPS-stimulated macrophages was suppressed, while SOD activity normally was restored following berberine treatment. Since it can suppress gp91phox (a plasma membrane subunit of NADPH oxidase) expression and boost SOD activity, berberine is able to restore cellular redox activity (222) (Supplementary Table 1). In this instance, it is possible that berberine enhances SOD expression through the silent mating type information regulation 2 homolog 1 (SIRT1)/Forkhead Box Class O (FOXO) pathway (221). In other experiments, berberine has been shown to directly bind to PLA2G4A and inhibit the MAPK/JNK signaling pathway to suppress PLA2G4A activity in murine colitis (223). A double-blind placebo-controlled phase I trial (123) demonstrated that berberine reduced colonic tissue inflammation (Geboes score) (224) in mesalamine-treated UC patients. However, it had no effect on inflammatory biomarkers in other tissues or blood (123).
6.3 Storage polysaccharides
6.3.1 Tamarind xyloglucan
Tamarind xyloglucan (TXG), a nanofiber extracted from tamarind, is a novel antioxidant that prevents DSS-induced colitis in mice (124, 125). TXG protects the colon by reducing the total inflammatory index, infiltration of inflammatory cells, submucosal edema, goblet cell loss, epithelial erosion, granulation tissue, epithelial hyperplasia, and crypt irregularity, abscesses and loss (124, 125). While TXG reduces inflammation by reducing TNF-α and increasing anti-inflammatory IL-10, it reduces OS by lowering levels of MDA, superoxide anion production, and the expression of iNOS, NOX, COX-2, and p47 (125) (Supplementary Table 1).
6.4 Other phytochemicals
6.4.1 Sulforaphane
Sulforaphane, found in cruciferous vegetables, contains a wide range of antibacterial, antioxidant, anti-inflammatory, and immunomodulatory properties (225, 226). Treatment of rats with colitis using sulforaphane reduces NO and MDA levels, accompanied by increased GPx and reduced glutathione levels, demonstrating its therapeutic antioxidant properties (8). Treatment with sulforaphane increases the levels of Nrf2 and HO-1 (8) (Supplementary Table 1). It has been observed that HO-1, an antioxidant defense protein downstream of Nrf-2, prevents oxidative damage to colonic tissue (99). Sulforaphane possesses anti-inflammatory characteristics by decreasing TNF-α and IL-6 levels, inhibiting COX-2 expression, TLR4 oligomerization, TLR4/MyD88 pathway and blocking the degradation of IL-1R-associated kinase-1, NF-κB, and IFN regulatory factor 3 activation (227, 228).
6.5 Food/spices
6.5.1 Flaxseed oil (α-linolenic acid)
Flaxseed oil is an herbal product with a high α-linolenic acid content, and it has been shown to reduce colonic damage in DSS-induced colitis by modulating inflammatory factors, oxidative state, and the cecal microbiota imbalances. DSS-colitic rat colon showed low SOD activity and GSH levels, increased MPO activity and MDA levels, and flaxseed oil dose-dependently alleviated this condition. Compared to the DSS-treated group, flaxseed oil treatment for six weeks raised SOD activity and GSH levels while decreasing MDA levels and MPO activity (127) (Supplementary Table 1). A metabolite of linoleic acid, 10-hydroxy-cis-12-octadecenoic acid (HYA), inhibits TNF-α expression and DSS-induced alterations in the expression of TJs such as occludin, zonula occludens-1, and myosin light chain kinase. The metabolite HYA partially restores the integrity of the intestinal epithelial barrier via the GPR40-MEK-ERK pathway, as reported in human Caco-2 cells and the murine DSS-colitis model (126).
6.5.2 Ginger
Ginger rhizomes, a rich source of many active compounds, including gingerols, shogaols, gingediols, zingerone, dehydrozingerone, gingerinone, and diarylheptanoids, have a wide range of anti-inflammatory, analgesic, antioxidant, and anti-cancer effects (129, 151, 229). S-[6]-gingerol inhibits the expression of the inflammatory mediators IL-6, IL-8, and serum amyloid A1 (SAA1) in cytokine-stimulated human HuH7 hepatocyte cells by inhibiting the NF-κB/COX-2 pathway to reduce OS (129). Concentration-dependent inhibition of LPS-induced IL-1β, IL-6, TNF-α and PGE2 levels was demonstrated with 6-shogaol, the most bioactive component of ginger (230). 6-Shogaol also reduces the phosphorylation and nuclear translocation of NF-κB p65, thus preventing LPS-induced NF-κB activation. In LPS-induced murine RAW 264.7 macrophages, 6-shogaol was reported to inhibit protein and mRNA expression of iNOS and COX-2 (128). By inhibiting the phosphorylation of inhibitor κB (IκB)α and p65 and the subsequent degradation of IκBα, 6-shogaol decreased the LPS-induced activity of NF-κB. PI3K/Akt and extracellular signal-regulated kinase 1/2 activation by LPS is also blocked by 6-shogaol, although the p38MAPK activation is not (128). Since TLR4 dimerization mediated by LPS is necessary for the activation of downstream signaling pathways, including NF-κB, 6-shogaol blocks this process and prevents NF-κB activation (231). Also, 6-shogaol can block TLR-mediated signaling pathways directly at the receptor (231). In LPS-induced macrophages, 6-shogaol suppresses the MyD88-dependent signaling pathway by inhibiting IκB kinase activity and TRIF-dependent signaling pathways that target TBK1 (232). Compounds extracted from ginger were tested for their ability to stimulate phagocytosis and suppress nitric oxide generation in the RAW 264.7 cell line induced by LPS (229). Significant reductions in both LPS-induced nitric oxide production and inducible nitric oxide synthase expression were observed when 6-shogaol, 1-dehydro-10-gingerdione, and 10-gingerdione were applied (229). Macrophages are essential components of the immune system as they are responsible for the elimination of necrotic cells. This function is critical as it prevents the release of harmful contents from these cells, thereby minimizing a proinflammatory response (233). RAW 264.7 cells treated with 1-dehydro-10-gingerdione (1D10G) showed increased phagocytic activity similar to stimulation by LPS. Also, RAW 264.7 cells treated with 6-gingerol, 8-gingerol, 10-gingerol, 6-paradol, 10-gingerdione, 1,7-bis-(4’ hydroxyl-3’ methoxyphenyl)-5-methoxyhepthan-3-one, and methoxy-10-gingerol exhibited increases in phagocytic activity (229). 1D10G directly decreased the catalytic activity of cell-free IκB kinase β (IKKβ) in RAW 264.7 macrophages activated with the TLR4 agonist LPS. In LPS-activated macrophages, 1D10G inhibited TLR4-mediated expression of TNF-α, NF-κB, IL-1β, IRF3, IFN-β and IP-10 (234). Furthermore, in macrophages triggered by the TLR agonists LPS or TNF-α, D10G irreversibly blocks cytoplasmic IKKβ-catalysed IκBα phosphorylation and IKKβ vector elicited NF-κB transcriptional activity to minimize inflammatory signals (235). Substitution of Cys (179) with Ala in the activation loop of IKKβ abrogates these effects suggesting a direct interaction site of D10G. Lastly, D10G reduced NF-κB activation in LPS-stimulated macrophages and decreased the expression of iNOS, COX-2, and IL-6 (235).
In mice with DSS-induced colitis, ginger alleviates the pathological lesions and reduces the expression of IL-6 and iNOS (131). This study also showed that ginger has an anti-inflammatory effect like that of the anti-inflammatory medication sulfasalazine (SASP), reducing DAI and preventing further weight loss (131). Ginger reduces IBD activity by targeting IL-17, IFN-γ, and TNF-α, while increasing the anti-inflammatory cytokines IL-10, IL-22, and TGF-β (236) (Supplementary Table 1).
Ginger extract exhibited the antioxidant effects in IL-1β-mediated OS in human C28I2 chondrocyte cells by stimulating the expression of several AOEs, decreasing IL-1β-induced ROS production, LPx, and by reducing apoptosis (Bax/Bcl-2 ratio, and caspase-3 activity) (130) (Supplementary Table 1). Patients with active mild to moderate UC who ingested 2000 mg/day of dried ginger powder in a randomized, placebo-controlled clinical trial had lowered MDA without affecting serum total antioxidant capacity (132).
6.6 Hormones and their anti-inflammatory and antioxidant roles in IBD
There is growing recognition that the endocrine system plays an important role in the pathogenesis of IBD through various mechanisms (237). Hormone actions influence seemingly every phase of inflammatory and immunological responses, and the intestinal tract is the largest endocrine gland of the body, secreting a vast amount of peptides with paracrine or endocrine function. In sites of inflammation, several hormone receptors have been found to be present in the reactive structures, which are known to have both pro- and anti-inflammatory effects. Signals are generated upon the binding of hormone molecules to specific hormone receptors; these receptors are found on the surface of endothelial and inflammatory cells and play a role in both pro- (such as insulin receptors) (238, 239) and anti-inflammatory (glucocorticoid receptors) (240–242) responses, respectively. The activity of the adrenal cortex is responsible for mediating the indirect anti-inflammatory effects that are caused by glucagon and thyroid hormones (243). Therefore, inflammation is not only a local response but also a hormone-controlled process that occurs locally (paracrine regulation) and throughout the body (endocrine modulation).
One study involving 1,203 females (64% diagnosed with CD,34% diagnosed with UC) reported increased symptoms during their menstrual cycle. Symptoms were comparable among CD and UC cohorts except for pregnant women, where symptoms worsened (244). Women with UC have increased symptoms as compared to women with CD. Understanding the significance of hormones in the context of IBD is crucial for identifying potential approaches to managing hormonal-associated symptoms in women with IBD. The primary endocrine manifestations of IBD include growth failure, metabolic bone disease, alterations in lipid and carbohydrate metabolism, pubertal delay, and hypogonadism (237). These manifestations are interrelated, and their complex development is influenced by intestinal inflammation and the individual’s nutritional status.
6.6.1 Sex hormones
Estrogens have been shown to increase AOEs by upregulating their expression (34, 147, 245). The antioxidant impact of estrogen is thought to be the principal method by which this hormone protects various tissues from oxidative damage (246–248). It has been postulated that sex hormones such as estrogen, progesterone, and androgen contribute to the pathophysiology of sexual dimorphism in human IBD (249). These control the behavior of many different types of immune cells, including lymphocytes, macrophages, granulocytes, and mast cells. Multiple clinical features of the disease, including intestinal barrier disintegration and mucosal immune activation, may be modulated by sex hormones, as shown in both clinical and experimental models (249). There is growing interest in the potential regulation of the intestinal microbiota by sex hormones. Estrogens have an effect on the microbicidal activity that is carried out by MPO in polymorphonuclear leukocytes (250). In CD, the G protein-coupled estrogen receptor (GPER) appears to be a powerful therapeutic target in maintaining remission because it promotes anti-inflammatory effects. Reducing mortality, improving macroscopic and microscopic scores, and lowering CRP levels were all achieved with GPER activation in a TNBS-induced CD murine model (251). Immunohistochemistry verified that estrogen signaling inhibits intestinal inflammation. Genes involved in signal transduction and immunological response, as well as the expression of certain miRNAs (miR-145, miR-148-5p, and miR-592), were shown to be altered in tandem with GPER activation, as was the extracellular-signal-regulated kinase (ERK) signaling pathway (251).
Increased expression of tight junction proteins was a mechanism by which progesterone and estrogen facilitated wound healing and epithelial barrier function in intestinal epithelial cells, as reported using 2D cell lines and IBD patient-derived inflammatory organoid models (252). These sex hormones also inhibited the generation of pro-inflammatory cytokines in intestinal epithelial models and greatly decreased endoplasmic reticulum (ER)-stress. Pregnancy hormones like estrogen and progesterone have been shown to have beneficial effects on disease activity by positively modulating the intestinal epithelial lining (252).
6.6.2 Glucagon
Glucagon decreased iNOS expression and plasma levels of nitrite/nitrate in LPS-treated rats (253). Glucagon is responsible for inducing the antioxidant response by increasing GSH levels and reducing both protein carbonyl and 3-nitrotyrosine (254) or by upregulating AOEs as indicated by high levels of CAT expression in the α cells of diabetic vs. non-diabetic murine models (255).
6.6.3 Enterohormones
Glucagon-like peptide-1 (GLP-1) and GLP-2 are important enterohormones that mediate local and systemic effects (gut-brain-periphery axis), contribute to glucose homeostasis, and modulate GI functions such as the intestinal absorption of lipids and antioxidant defense (256). The effects of these molecules critically depend upon nitric oxide synthase (NOS) in the enteric nervous system (ENS) and the intestinal microbiome (257).
Activation of the GLP-1 receptor has anti-inflammatory, antioxidant, and anti-apoptotic effects, which include a reduction of the pro-inflammatory actions of advanced glycation end products (258) and of the accumulation of intracellular ROS, the release of NO, and GPx and SOD production (259). Moreover, it has been proposed that GLP-1 plays a critical role in the production of NO (260), and intestinal GLP-1 production can be increased by the ingestion of grape-seed procyanidin extract (GSPE), resveratrol, and curcumin (261–264). GSPE also increases intestinal peptide YY (PYY) and varied cholecystokinin (CCK) secretion, which modulates food intake (261, 265, 266).
The enterotrophic peptide hormone glucagon-like peptide 2 (GLP-2) was shown to abrogate OS and improve intestinal antioxidant capacity by reducing LPS-induced increases in intestinal IL-1β and oxidized glutathione levels (267) and increasing intestinal SOD activity and reduced-glutathione levels (268, 269) (Supplementary Table 1). Additionally, GLP-2 has a protective effect on the function of the intestinal barrier (270), intestinal IgA production (270), NO-regulated intestinal perfusion (271), and stabilizes the expression and function of intestinal xenobiotic transporters (267). Because intestinal barrier function is critically impaired in IBD and CIE, complex neutraceuticals that provide antioxidants and can enhance intestinal GLP-2 secretion, and thus support enterocyte tight junctions and intestinal barrier integrity (e.g., berberine, soy flavonoids, pre-/probiotics, soluble fiber, glutamine), may be useful to restore and maintain intestinal health (272).
Given the effects of GLP-1 and GLP-2 in maintaining intestinal homeostasis, including cross-talk with the immune and central nervous systems (273, 274), they appear to present potentially beneficial treatment targets in IBD and CIE.
Reduced levels of serum motilin and gastrin, together with lower pro-inflammatory cytokines and reduced tissue NF-κB and COX-2 levels, and increased serum somatostatin, vasoactive intestinal peptide, and tissue SOD, NO, and MDA levels were associated with genistein- and daidzein-rich fermented soy (shuidouchi) in an experimental animal model (275). Similar antioxidative, anti-inflammatory, and enterohormone signature-modulating effects have been demonstrated in experimental mice receiving unsaturated fatty acid-rich silkworm pupa oil (276).
6.6.4 Glucocorticoids
The presence of glucocorticoid receptors in endothelial and inflammatory cells (240–243), as well as increased concentrations of circulating glucocorticoids at the onset of inflammation downregulating inflammatory responses, is proof that glucocorticoids are modulators of inflammatory responses (243, 277). Adrenalectomized animals also showed greater microvascular responses to inflammatory mediators and cell migration to inflamed regions (243, 278). Glucocorticoids have powerful anti-inflammatory and immunosuppressive effects, such as lowering cytokine production or activity, reducing microvascular responses to inflammatory mediators, preventing leukocyte accumulation at inflamed sites, impeding phagocytic functions and microbicidal capacity of polymorphonuclear leukocytes, preventing the recruitment of mononuclear phagocytes to injured areas, and interfering with immune function (243, 278–280).
6.6.5 Thyroid hormones
Thyroid hormones are associated with the oxidative and antioxidative status of an organism because of their role in regulating oxidative metabolism and in the formation of ROS (34, 39, 50–55, 281). Rats with thyroid dysfunction, produced by hormone injection or thyroidectomy, were evaluated for their ability to respond to noxious stimuli in an effort to determine the processes through which thyroid gland activity can influence the development of inflammatory reactions (243, 282, 283). Rats with hypothyroidism exhibit typical inflammatory responses, whereas animals with a sustained excess of circulating thyroid hormones exhibit consistently suppressed inflammatory responses (282, 283).
6.6.6 Corticotropin releasing hormone
A study was conducted to investigate the impact of administering Corticotropin releasing hormone (CRH) to induce psychosocial stress in DSS-treated colitic mice, specifically by examining the potential enhancement of autophagy in intestinal macrophages. The inflammatory challenges associated with IBD led to increased autophagy in both intestinal macrophages and murine bone marrow-derived macrophages, and these effects were further increased by CRH (284).
6.6.7 Adipokines
IBD is distinguished by symptoms such as reduced appetite, inadequate nutrition, changes in body composition, and the enlargement of mesenteric white adipose tissue (mWAT) (285, 286). Adipokines, namely leptin, adiponectin, and resistin, play a significant role in anorexia, malnutrition, changes in body composition, and hypertrophy of mWAT (285, 286). Studies have demonstrated that there is an increased expression of leptin, adiponectin, and resistin in mWAT in individuals diagnosed with CD.
6.6.7.1 Leptin
Leptin serves as a regulator of diverse immune and inflammatory reactions in addition to its metabolic and endocrine roles. Leptin has the ability to initiate activation and alter the pattern of cytokine production, favoring a Th1 response by promoting the release of IL-2 and IFN-γ while inhibiting the secretion of IL-4 (287). Additionally, it directly stimulates the expression and release of IL-1a, IL-1b, IL-6, and TNFα by T-cells (288). Leptin was found to be expressed and released into the intestinal lumen by inflamed colonic epithelial cells in patients diagnosed with UC. Leptin, in turn, elicited damage to the epithelial wall and infiltration of neutrophils (289). There was a higher level of leptin mRNA expression in the mesenteric white adipose tissue (mWAT) of patients with IBD compared to the control group suggesting that leptin may play a role in the inflammatory process by increasing the expression of mesenteric TNFα (290). The study found that individuals with IBD had lower levels of serum leptin compared to a control group of healthy individuals. This difference was observed regardless of factors such as sex, age, CRP levels, years since diagnosis, and disease activity and localization. The study found that individuals with a BMI greater than or equal to 25 exhibited significantly lower levels of serum leptin compared to individuals with a BMI below 25 (286). The administration of infliximab, an anti-TNFα biologic agent, to individuals diagnosed with CD resulted in an elevation in leptinaemia levels. This suggests that TNFα plays a significant role in suppressing the production of leptin in patients with CD (291).
6.6.7.2 Adiponectin
Adiponectin production and TNFα are mutually suppressed, and their actions are antagonistic in the target tissues (292). Additionally, in vitro studies have shown that IL-6 reduces adiponectin levels (292). The induction of adiponectin resulted in the production of IL-10 and interleukin-1 receptor antagonist (IL-1Ra) in human peripheral blood mononuclear cells (PBMC), macrophages, and dendritic cells (DC). Additionally, it hindered the production of IFN-γ in macrophages. The macrophages treated with adiponectin demonstrated a decreased ability to engulf particles and a diminished immune response to cells from a different individual (293). Previous studies have suggested a potential protective role of adiponectin against OS (294). Conversely, it has been observed that OS leads to a reduction in adiponectin secretion in 3T3-L1 adipocytes (295). Adiponectin can inhibit the enhanced cytotoxic activity of natural killer (NK) cells that are induced by IL-2, as well as the production of IFN-γ (296). Studies conducted using the adiponectin knock-out (KO) murine model revealed that adiponectin KO mice exhibited a notably more severe form of colitis in comparison to their wild-type counterparts. However, the severity of colitis was significantly reduced when adiponectin was supplemented. Additionally, it was observed that adiponectin exhibited inhibitory effects on the production of IL-8 in HT-29 cells stimulated with LPS, suggesting that adiponectin may possess a direct anti-inflammatory impact on intestinal epithelial cells (297). However, other studies showed contradictory results on the effect of adiponectin on the development of colitis and restoration of inflammation (298). There is an elevated secretion of adiponectin from mWAT in patients with CD who have undergone surgery, in comparison to patients with diverticulitis and colon carcinoma. Also, serum adiponectin levels are increased in patients with IBD versus healthy controls (286).
6.6.7.3 Resistin
The secretion of resistin from mWAT in patients who underwent surgery for colon cancer or diverticulitis was observed to be significantly lower when compared to the secretion from the adipose tissue adjacent to the affected intestine in patients with CD (285). It was noted that the administration of steroids led to a reduction in resistin production in CD patients (299). The levels of serum resistin in patients with IBD (with active disease and also with quiescent disease) are elevated in comparison to healthy controls.
6.6.7.4 Ghrelin
In chronic DSS-induced colitis in ghrelin KO mice, due to the absence of endogenous ghrelin, the DAI was reduced, and the infiltration of neutrophils was delayed compared to wild-type (300). The introduction of ghrelin either at the onset of the disease or a few days after colitis had developed resulted in ameliorating both the clinical and histopathologic severity of the disease. This therapeutic effect was observed alongside the suppression of inflammatory and Th1-driven autoimmune response, as well as elevated levels of IL-10 (301). Ghrelin mRNA expression and its receptor were found to be increased in TNBS-induced colitic murine models. Serum ghrelin levels were increased in patients with IBD, regardless of whether the disease was active or in remission and were higher in male versus female patients. Ghrelin levels were higher in patients with ileal CD compared to those with colonic CD. In contrast, Peracchi et al. (302) demonstrated that individuals with active IBD exhibited elevated levels of circulating ghrelin in comparison to both healthy controls and patients in a state of remission.
6.6.8 Type 2 diabetes and IBD
Previous research conducted on animals has indicated that the presence of peroxisome proliferator-activated receptor γ (PPAR γ) in the intestinal epithelial cells, macrophages, and T cells of mice with experimental IBD demonstrates immunoregulatory effects and potentially plays a role in the prevention of intestinal inflammation (303, 304). The administration of the gamma subtype of peroxisome proliferator-activated receptors (PPARγ) ligands has been demonstrated to reduce the production of inflammatory cytokines, such as IL-1β and TNF-α, as well as inhibit the proliferation of inflammatory cells and the expression of specific adhesion molecules (305). The thiazolidinedione (TZD) antidiabetic medications for the treatment of type 2 diabetes function as ligands for the gamma subtype of PPARs. Several trials with TZD in the context of IBD have yielded intriguing outcomes (237, 306, 307). In a multicenter randomized, double-blind, placebo-controlled clinical trial, the effectiveness of rosiglitazone in managing UC with mild to moderate activity has been demonstrated (306). Here, 17% of patients who received treatment with rosiglitazone were able to achieve remission. Significant clinical improvement was observed in as short as 4 weeks, with enhanced quality of life by the 8th week (306). In another study, it was determined that TZD does not confer any discernible benefits in comparison to alternative oral antidiabetic drugs with regard to the prevention of UC-related flares. Nevertheless, the utilization of TZD may diminish the probability of experiencing more severe disease flares that necessitate oral steroid treatment (307).
6.6.9 Melatonin
Melatonin (N-acetyl-5-methoxytryptamine) has several unique scavenging effects making it an exceptionally potent direct free radical scavenger and multifunctional antioxidant (34, 308, 309). Melatonin is an electron-rich molecule that can react with free radicals to produce stable compounds that are excreted in the urine (310). Melatonin is frequently referred to as a suicidal or terminal antioxidant because it eliminates free electrons from the system throughout its chemical rearrangement, and each of the rearrangement products is again a potent antioxidant. Melatonin acts as an indirect antioxidant, increasing AOEs, including SOD, CAT, GPx, GR (311, 312), and glucose-6-phosphate dehydrogenase (G6PD) (313). Increased intracellular levels of the antioxidant GSH are generated as a consequence of melatonin stimulating γ-glutamyl cysteine synthetase (the rate-limiting enzyme in GSH formation) and GR (the enzyme converting GSSG to GSH) (314, 315). Melatonin also potentiates the function of the mitochondrial electron transport chain, which reduces free radical generation and electron leakage (316). Melatonin inhibits the production of free radicals by acting as a negative modulator of several pro-oxidant enzymes including 5-lipoxygenase, 12-lipoxygenase, and NO synthase (309). It also prevents mitochondrial damage and reduces NF-κB signaling, suggesting a repair mechanism for intestinal injury caused by OS (317). Decreased levels of hydroxyl radical (HO•), peroxynitrite (ONOO-), RO2•, and singlet oxygen, and decreased expression of COX-2 and iNOS and NF-kB activation are all antioxidant effects of melatonin (318, 319), pointing to the possibility that melatonin is beneficial in UC by lowering inflammation and regulating OS. Pineal-derived melatonin and de novo synthesis in the GI tract both produce melatonin which helps regulate gut immunity and intestinal barrier integrity. Due to its antiapoptotic action and ability to decrease bacterial translocation across epithelia, melatonin can limit mucosal damage (73). Animal studies have demonstrated that melatonin treatment reduces inflammation by blocking the production of IL-10, IFN-γ, TNF-α, IL-6, and NO• (320) (Supplementary Table 1). In murine colitis, melatonin reduces the generation of ROS and reactive nitrogen species (RNS), characterized by lowering colonic MDA levels and MPO activity and improved antioxidant defenses with increased GSH and SOD levels in the colon (134).
By modulating autophagy and Nrf2 signaling pathways, melatonin slows the progression of colitis-associated colon cancer in mice. In this colon cancer model, melatonin decreased the levels of inflammatory markers IL-6, IL-17, TNF-α, NF-κB, COX-2 and STAT3, as well as reduced DNA damage and OS indicated by reduced TBARS and increased GSH levels (Supplementary Table 1). The decrease in the expression of Beclin-1 and the LC3-II/LC3-I ratio, along with an increased expression of p62, indicate melatonin-inhibited cancer-associated autophagy. These results are like those showing upregulation of Nrf2 and AOEs NQO-1 and HO-1 in melatonin-treated mice with colon cancer (321). Another study investigated the role of oral melatonin therapy on OS in dogs before and after ovariohysterectomy (OHE) (135). The levels of SOD, GPx, and CAT were increased, and MDA decreased in ovariohysterectomized dogs (Supplementary Table 1) that received melatonin compared to those of the control group. These preliminary findings suggest that melatonin administration may reduce OS induced by OHE in dogs. Adjuvant melatonin treatment may aid in maintaining remission in patients with UC (136). In this clinical trial, patients receiving melatonin adjuvant treatment-maintained remission (e.g., lower disease activity scores and inflammatory biomarkers such as CRP levels with increased hemoglobin concentrations) over 12 months of observation (136).
7 Conclusion
Oxidative stress plays a crucial role in the pathogenesis of IBD and is closely associated with the development of intestinal inflammation in humans and animals. Therapeutic strategies involving natural antioxidant products have shown benefit in numerous human studies and animal models. An improved comprehension of the free radical biology mediating GI disease is essential for developing effective future treatments to reduce intestinal inflammation and improve the quality of life in affected individuals. Also, hormonal intervention holds potential importance in the context of IBD and cannot be disregarded. In this review, we have discussed the major antioxidant and anti-inflammatory properties of various phytochemicals and hormones in IBD. We have also discussed significant evidence-based observations, including the results from clinical trials using natural antioxidants or their modified formulations. Based on the existing scientific evidence, it appears likely that future therapies will include antioxidants with standard treatments or even as an alternative medical option in humans and animals with IBD.
Author contributions
Writing—original draft preparation, revisions, figures, review, and editing DKS, Writing—review, and editing RMH, BP, AP, VKY, DW, AEJ. All authors contributed to the article and approved the submitted version.
Acknowledgments
The authors acknowledge the support of the Department of Veterinary Clinical Sciences (VCS) Core Lab, College of Veterinary Medicine, Iowa State University, Ames, Iowa, USA.
Conflict of interest
AEJ is one of the founders of a biopharmaceutical start-up company, 3D Health Solutions, Inc.
The remaining authors declare that the research was conducted in the absence of any commercial or financial relationships that could be constructed as a potential conflict of interest.
Publisher’s note
All claims expressed in this article are solely those of the authors and do not necessarily represent those of their affiliated organizations, or those of the publisher, the editors and the reviewers. Any product that may be evaluated in this article, or claim that may be made by its manufacturer, is not guaranteed or endorsed by the publisher.
Supplementary material
The Supplementary Material for this article can be found online at: https://www.frontiersin.org/articles/10.3389/fendo.2023.1217165/full#supplementary-material
References
1. Seyedian SS, Nokhostin F, Malamir MD. A review of the diagnosis, prevention, and treatment methods of inflammatory bowel disease. J Med Life (2019) 12:113. doi: 10.25122/JML-2018-0075
2. Sartor RB. Mechanisms of Disease: pathogenesis of Crohn’s disease and ulcerative colitis. Nat Clin Pract Gastroenterol Hepatol (2006) 3(7):390–407. doi: 10.1038/ncpgasthep0528
3. Kaplan GG, Windsor JW. The four epidemiological stages in the global evolution of inflammatory bowel disease. Nat Rev Gastroenterol Hepatol (2021) 18:56. doi: 10.1038/S41575-020-00360-X
4. Colgan SP, Taylor CT. Hypoxia: an alarm signal during intestinal inflammation. Nat Rev Gastroenterol Hepatol (2010) 7:281. doi: 10.1038/NRGASTRO.2010.39
5. Iborra M, Moret I, Rausell F, Bastida G, Aguas M, Cerrillo E, et al. Role of oxidative stress and antioxidant enzymes in Crohn’s disease. Biochem Soc Trans (2011) 39:1102–6. doi: 10.1042/BST0391102
6. Ahmed SMU, Luo L, Namani A, Wang XJ, Tang X. Nrf2 signaling pathway: Pivotal roles in inflammation. Biochim Biophys Acta (BBA) - Mol Basis Dis (2017) 1863:585–97. doi: 10.1016/J.BBADIS.2016.11.005
7. Khor TO, Huang MT, Kwon KH, Chan JY, Reddy BS, Kong AN. Nrf2-deficient mice have an increased susceptibility to dextran sulfate sodium–induced colitis. Cancer Res (2006) 66:11580–4. doi: 10.1158/0008-5472.CAN-06-3562
8. Alattar A, Alshaman R, Al-Gayyar MMH. Therapeutic effects of sulforaphane in ulcerative colitis: effect on antioxidant activity, mitochondrial biogenesis and DNA polymerization. Redox Rep (2022) 27:128. doi: 10.1080/13510002.2022.2092378
9. Naito Y, Takagi T, Yoshikawa T. Molecular fingerprints of neutrophil-dependent oxidative stress in inflammatory bowel disease. J Gastroenterol (2007) 42:787–98. doi: 10.1007/S00535-007-2096-Y
10. Ina K, Kusugami K, Yamaguchi T, Imada A, Hosokawa T, Ohsuga M, et al. Mucosal interleukin-8 is involved in neutrophil migration and binding to extracellular matrix in inflammatory bowel disease. Am J Gastroenterol (1997) 92(8):1342–46.
11. Strober W, Fuss IJ. Proinflammatory cytokines in the pathogenesis of inflammatory bowel diseases. Gastroenterology (2011) 140:1756–1767.e1. doi: 10.1053/J.GASTRO.2011.02.016
12. Tian T, Wang Z, Zhang J. Pathomechanisms of oxidative stress in inflammatory bowel disease and potential antioxidant therapies. Oxid Med Cell Longev (2017) 2017:4535194. doi: 10.1155/2017/4535194
13. Uniken Venema WTC, Voskuil MD, Dijkstra G, Weersma RK, Festen EAM. The genetic background of inflammatory bowel disease: from correlation to causality. J Pathol (2017) 241:146–58. doi: 10.1002/PATH.4817
14. Rice-Evans C, Burdon R. Free radical-lipid interactions and their pathological consequences. Prog Lipid Res (1993) 32:71–110. doi: 10.1016/0163-7827(93)90006-I
15. Fang YZ, Yang S, Wu G. Free radicals, antioxidants, and nutrition. Nutrition (2002) 18:872–9. doi: 10.1016/S0899-9007(02)00916-4
16. Darley-Usmar V, Halliwell B. Blood radicals: reactive nitrogen species, reactive oxygen species, transition metal ions, and the vascular system. Pharm Res (1996) 13:649–62. doi: 10.1023/A:1016079012214
17. Floyd RA. Role of oxygen free radicals in carcinogenesis and brain ischemia. FASEB J (1990) 4:2587–97. doi: 10.1096/FASEBJ.4.9.2189775
18. Bourgonje AR, Feelisch M, Faber KN, Pasch A, Dijkstra G, van Goor H. Oxidative stress and redox-modulating therapeutics in inflammatory bowel disease. Trends Mol Med (2020) 26:1034–46. doi: 10.1016/J.MOLMED.2020.06.006
19. Jergens AE, Heilmann RM. Canine chronic enteropathy—Current state-of-the-art and emerging concepts. Front Vet Sci (2022) 9:923013/BIBTEX. doi: 10.3389/FVETS.2022.923013/BIBTEX
20. Dandrieux JRS. Inflammatory bowel disease versus chronic enteropathy in dogs: are they one and the same? J Small Anim Pract (2016) 57:589–99. doi: 10.1111/JSAP.12588
21. Allenspach K, Culverwell C, Chan D. Long-term outcome in dogs with chronic enteropathies: 203 cases. Veterinary Rec (2016) 178:368–8. doi: 10.1136/VR.103557
22. Rodolphe J, Dandrieux S, Mansfield CS. Chronic enteropathy in canines: prevalence, impact and management strategies. Veterinary Medicine: Res Rep (2019) 10:203–14. doi: 10.2147/VMRR.S162774
23. Gunawardana SC, Jergens AE, Ahrens FA, Niyo Y. Colonic nitrite and immunoglobulin G concentrations in dogs with inflammatory bowel disease. J Am Vet Med Assoc (1997) 211:318–21.
24. Minamoto Y, Otoni CC, Steelman SM, Büyükleblebici O, Steiner JM, Jergens AE, et al. Alteration of the fecal microbiota and serum metabolite profiles in dogs with idiopathic inflammatory bowel disease. Gut Microbes (2015) 6:33–47. doi: 10.1080/19490976.2014.997612/SUPPL_FILE/KGMI_A_997612_SM2973.XLSX
25. Rubio CP, Martínez-Subiela S, Hernández-Ruiz J, Tvarijonaviciute A, Cerón JJ, Allenspach K. Serum biomarkers of oxidative stress in dogs with idiopathic inflammatory bowel disease. Veterinary J (2017) 221:56–61. doi: 10.1016/J.TVJL.2017.02.003
26. Walker HK, Boag AM, Ottka C, Lohi H, Handel I, Gow AG, et al. Serum metabolomic profiles in dogs with chronic enteropathy. J Vet Intern Med (2022) 36:1752–9. doi: 10.1111/JVIM.16419
27. Charpentier C, Chan R, Salameh E, Mbodji K, Ueno A, Coëffier M, et al. Dietary n-3 PUFA may attenuate experimental colitis. Mediators Inflammation (2018) 2018:8430614. doi: 10.1155/2018/8430614
28. Kalenyak K, Heilmann RM, van de Lest CHA, Brouwers JF, Burgener IA. Comparison of the systemic phospholipid profile in dogs diagnosed with idiopathic inflammatory bowel disease or food-responsive diarrhea before and after treatment. PloS One (2019) 14:e0215435. doi: 10.1371/JOURNAL.PONE.0215435
29. Ambrosini YM, Neuber S, Borcherding D, Seo YJ, Segarra S, Glanemann B, et al. Treatment with hydrolyzed diet supplemented with prebiotics and glycosaminoglycans alters lipid metabolism in canine inflammatory bowel disease. Front Vet Sci (2020) 7:451/BIBTEX. doi: 10.3389/FVETS.2020.00451/BIBTEX
30. Segarra S, Martínez-Subiela S, Cerdà-Cuéllar M, Martínez-Puig D, Muñoz-Prieto A, Rodríguez-Franco F, et al. Oral chondroitin sulfate and prebiotics for the treatment of canine Inflammatory Bowel Disease: a randomized, controlled clinical trial. BMC Vet Res (2016) 12:49. doi: 10.1186/S12917-016-0676-X
31. Galler AI, Suchodolski JS, Steiner JM, Sung CH, Hittmair KM, Richter B, et al. Microbial dysbiosis and fecal metabolomic perturbations in Yorkshire Terriers with chronic enteropathy. Sci Rep (2022) 12(1):1–17. doi: 10.1038/s41598-022-17244-6
32. Candellone A, Girolami F, Badino P, Jarriyawattanachaikul W, Odore R. Changes in the oxidative stress status of dogs affected by acute enteropathies. Vet Sci (2022) 9(6):276. doi: 10.3390/VETSCI9060276
33. Erlank H, Elmann A, Kohen R, Kanner J. Polyphenols activate Nrf2 in astrocytes via H2O2, semiquinones, and quinones. Free Radic Biol Med (2011) 51:2319–27. doi: 10.1016/J.FREERADBIOMED.2011.09.033
34. Sahoo DK, Chainy GBN. Hormone-linked redox status and its modulation by antioxidants. Vitam Horm (2023) 121:197–246. doi: 10.1016/BS.VH.2022.10.007
35. Perkins A, Nelson KJ, Parsonage D, Poole LB, Karplus PA. Peroxiredoxins: guardians against oxidative stress and modulators of peroxide signaling. Trends Biochem Sci (2015) 40:435. doi: 10.1016/J.TIBS.2015.05.001
36. Adimora NJ, Jones DP, Kemp ML. A model of redox kinetics implicates the thiol proteome in cellular hydrogen peroxide responses. Antioxid Redox Signal (2010) 13:731. doi: 10.1089/ARS.2009.2968
37. Halliwell B, Gutteridge JMC. Antioxidant defences synthesized in vivo. Free Radicals Biol Med (2015) (5th edn) 77–152. doi: 10.1093/ACPROF:OSO/9780198717478.003.0003
38. Espinosa-Diez C, Miguel V, Mennerich D, Kietzmann T, Sánchez-Pérez P, Cadenas S, et al. Antioxidant responses and cellular adjustments to oxidative stress. Redox Biol (2015) 6:183. doi: 10.1016/J.REDOX.2015.07.008
39. Chainy GBN, Sahoo DK. Hormones and oxidative stress: an overview. Free Radic Res (2020) 54(1):1–26. doi: 10.1080/10715762.2019.1702656
40. Conrad M, Kagan VE, Bayir H, Pagnussat GC, Head B, Traber MG, et al. Regulation of lipid peroxidation and ferroptosis in diverse species. Genes Dev (2018) 32:602–19. doi: 10.1101/GAD.314674.118
41. Kurutas EB. The importance of antioxidants which play the role in cellular response against oxidative/nitrosative stress: current state. Nutr J (2016) 15(1):71. doi: 10.1186/S12937-016-0186-5
42. Valko M, Leibfritz D, Moncol J, Cronin MTD, Mazur M, Telser J. Free radicals and antioxidants in normal physiological functions and human disease. Int J Biochem Cell Biol (2007) 39:44–84. doi: 10.1016/J.BIOCEL.2006.07.001
43. Sahoo DK. Alterations of testicular selenium-dependent and independent glutathione peroxidase activities during experimentally l-thyroxine induced hyperthyroidism and n-propyl thiouracil induced hypothyroidism in adult rats. Res Rev Biosci (2012) 6(3):85–91.
44. Sahoo DK. Increased germ cell apoptosis during testicular development and maturation by experimentally induced transient and persistent hypothyroidism. Webmedcentral (2013) 4:209. doi: 10.9754/JOURNAL.WPLUS.2013.00209
45. Sahoo DK, Chainy GBN. Tissue specific response of antioxidant defence systems of rat to experimentally-induced hyperthyroidism. Natl Acad Sci Lett (2007) 30(7-8):247–50.
46. Sahoo DK, Roy A. Compromised rat testicular antioxidant defence system by hypothyroidism before puberty. Int J Endocrinol (2012) 2012:637825. doi: 10.1155/2012/637825
47. Sahoo DK, Roy A, Chainy GBN. PTU-induced neonatal hypothyroidism modulates antioxidative status and population of rat testicular germ cells. Natl Acad Sci Lett (2006) 29(3-4):133–5.
48. Sahoo DK, Roy A, Chainy GBN. Rat testicular mitochondrial antioxidant defence system and its modulation by aging. Acta Biol Hung (2008) 59(4):413–24. doi: 10.1556/ABiol.59.2008.4.3
49. Sahoo DK, Roy A, Chainy GBN. Protective effects of vitamin E and curcumin on L-thyroxine-induced rat testicular oxidative stress. Chem Biol Interact (2008) 176:121–8. doi: 10.1016/J.CBI.2008.07.009
50. Sahoo DK, Jena S, Chainy GBN. Thyroid dysfunction and testicular redox status: an intriguing association. Oxidants Antioxidants Impact Oxid Status Male Reprod (2019) (1st edn) 149–70. doi: 10.1016/B978-0-12-812501-4.00015-8
51. Sahoo DK, Roy A, Bhanja S, Chainy GBN. Experimental hyperthyroidism-induced oxidative stress and impairment of antioxidant defence system in rat testis. Indian J Exp Biol (2005) 43(11):1058–67.
52. Sahoo DK, Roy A, Chattopadhyay S, Chainy GBN. Effect of T3 treatment on glutathione redox pool and its metabolizing enzymes in mitochondrial and post-mitochondrial fractions of adult rat testes. Indian J Exp Biol (2007) 45(4):338–46.
53. Chattopadhyay S, Sahoo DK, Subudhi U, Chainy GBN. Differential expression profiles of antioxidant enzymes and glutathione redox status in hyperthyroid rats: A temporal analysis. Comp Biochem Physiol - C Toxicol Pharmacol (2007) 146(3):383–91. doi: 10.1016/j.cbpc.2007.04.010
54. Sahoo DK, Roy A, Bhanja S, Chainy GBN. Hypothyroidism impairs antioxidant defence system and testicular physiology during development and maturation. Gen Comp Endocrinol (2008) 156(1):63–70. doi: 10.1016/j.ygcen.2007.11.007
55. Chattopadhyay S, Sahoo DK, Roy A, Samanta L, Chainy GBN. Thiol redox status critically influences mitochondrial response to thyroid hormone-induced hepatic oxidative injury: A temporal analysis. Cell Biochem Funct (2010) 28(2):126–34. doi: 10.1002/cbf.1631
56. Ilango S, Sahoo DK, Paital B, Kathirvel K, Gabriel JI, Subramaniam K, et al. A review on annona muricata and its anticancer activity. Cancers (2022) 14:4539. doi: 10.3390/CANCERS14184539
57. Sahoo DK, Borcherding DC, Chandra L, Jergens AE, Atherly T, Bourgois-Mochel A, et al. Differential transcriptomic profiles following stimulation with lipopolysaccharide in intestinal organoids from dogs with inflammatory bowel disease and intestinal mast cell tumor. Cancers (Basel) (2022) 14:3525. doi: 10.3390/CANCERS14143525/S1
58. Chhabria S, Mathur S, Vadakan S, Sahoo DK, Mishra P, Paital B. A review on phytochemical and pharmacological facets of tropical ethnomedicinal plants as reformed DPP-IV inhibitors to regulate incretin activity. Front Endocrinol (Lausanne) (2022) 13:1027237. doi: 10.3389/FENDO.2022.1027237
59. Halliwell B. Reactive species and antioxidants. Redox biology is a fundamental theme of aerobic life. Plant Physiol (2006) 141:312. doi: 10.1104/PP.106.077073
60. Pattison D, Davies M. Reactions of myeloperoxidase-derived oxidants with biological substrates : gaining chemical insight into human inflammatory diseases. Curr Med Chem (2006) 13:3271–90. doi: 10.2174/092986706778773095
61. Hansberry DR, Shah K, Agarwal P, Agarwal N. Fecal myeloperoxidase as a biomarker for inflammatory bowel disease. Cureus (2017) 9(1):e1004. doi: 10.7759/CUREUS.1004
62. Klebanoff SJ. Myeloperoxidase: friend and foe. J Leukoc Biol (2005) 77:598–625. doi: 10.1189/JLB.1204697
63. Wang Z, Li S, Cao Y, Tian X, Zeng R, Liao DF, et al. Oxidative stress and carbonyl lesions in ulcerative colitis and associated colorectal cancer. Oxid Med Cell Longev (2016) 2016:9875298. doi: 10.1155/2016/9875298
64. Jeon YD, Lee JH, Lee YM, Kim DK. Puerarin inhibits inflammation and oxidative stress in dextran sulfate sodium-induced colitis mice model. BioMed Pharmacother (2020) 124:109847. doi: 10.1016/J.BIOPHA.2020.109847
65. Marseglia L, D’Angelo G, Manti S, Aversa S, Reiter RJ, Antonuccio P, et al. Oxidative stress-mediated damage in newborns with necrotizing enterocolitis: A possible role of melatonin. Am J Perinatol (2015) 32:905–9. doi: 10.1055/S-0035-1547328
66. Rao R, Baker RD, Baker SS. Inhibition of oxidant-induced barrier disruption and protein tyrosine phosphorylation in Caco-2 cell monolayers by epidermal growth factor. Biochem Pharmacol (1999) 57:685–95. doi: 10.1016/S0006-2952(98)00333-5
67. Carr AP, Díaz-Regañón D, Gabriel V, Livania V, Liu D, Ahmed BH, et al. Changes of enterocyte morphology and enterocyte: goblet cell ratios in dogs with protein-losing and non-protein-losing chronic enteropathies. Veterinary Sci (2023) 10:417. doi: 10.3390/VETSCI10070417
68. Mangerich A, Dedon PC, Fox JG, Tannenbaum SR, Wogan GN. Chemistry meets biology in colitis-associated carcinogenesis. Free Radic Res (2013) 47:958. doi: 10.3109/10715762.2013.832239
69. Cornejo-García JA, Perkins JR, Jurado-Escobar R, García-Martín E, Agúndez JA, Viguera E, et al. Pharmacogenomics of prostaglandin and leukotriene receptors. Front Pharmacol (2016) 7:316. doi: 10.3389/FPHAR.2016.00316
70. Babbs CF. Oxygen radicals in ulcerative colitis. Free Radic Biol Med (1992) 13:169–81. doi: 10.1016/0891-5849(92)90079-V
71. Muthupalani S, Ge Z, Feng Y, Rickman B, Mobley M, Mccabe A, et al. Systemic macrophage depletion inhibits helicobacter bilis-induced proinflammatory cytokine-mediated typhlocolitis and impairs bacterial colonization dynamics in a BALB/c Rag2–/– mouse model of inflammatory bowel disease. Infect Immun (2012) 80:4388. doi: 10.1128/IAI.00530-12
72. Moret-Tatay I, Iborra M, Cerrillo E, Tortosa L, Nos P, Beltrán B. Possible biomarkers in blood for Crohn’s disease: Oxidative stress and microRNAs - Current evidences and further aspects to unravel. Oxid Med Cell Longev (2016) 2016:2325162. doi: 10.1155/2016/2325162
73. Balmus I, Ciobica A, Trifan A, Stanciu C. The implications of oxidative stress and antioxidant therapies in Inflammatory Bowel Disease: Clinical aspects and animal models. Saudi J Gastroenterol (2016) 22:3. doi: 10.4103/1319-3767.173753
74. Biasi F, Leonarduzzi G, Oteiza PI, Poli G. Inflammatory bowel disease: mechanisms, redox considerations, and therapeutic targets. Antioxid Redox Signal (2013) 19:1711. doi: 10.1089/ARS.2012.4530
75. Qiu W, Wu B, Wang X, Buchanan ME, Regueiro MD, Hartman DJ, et al. PUMA-mediated intestinal epithelial apoptosis contributes to ulcerative colitis in humans and mice. J Clin Invest (2011) 121:1722–32. doi: 10.1172/JCI42917
76. Andresen L, Jørgensen VL, Perner A, Hansen A, Eugen-Olsen J, Rask-Madsen J. Activation of nuclear factor κB in colonic mucosa from patients with collagenous and ulcerative colitis. Gut (2005) 54:503–9. doi: 10.1136/GUT.2003.034165
77. Fontani F, Domazetovic V, Marcucci T, Vincenzini MT, Iantomasi T. MMPs, ADAMs and their natural inhibitors in inflammatory bowel disease: involvement of oxidative stress. J Clin Gastroenterol Treat (2017) 3(1):039. doi: 10.23937/2469-584X/1510039
78. Brynskov J, Foegh P, Pedersen G, Ellervik C, Kirkegaard T, Bingham A, et al. Tumour necrosis factor α converting enzyme (TACE) activity in the colonic mucosa of patients with inflammatory bowel disease. Gut (2002) 51:37–43. doi: 10.1136/GUT.51.1.37
79. Fontani F, Domazetovic V, Marcucci T, Vincenzini MT, Iantomasi T. Clinical gastroenterology and treatment MMPs, ADAMs and their natural inhibitors in inflammatory bowel disease: involvement of oxidative stress. J Clin Gastroenterol Treat (2017) 2017:39.
80. Coskun M, Salem M, Pedersen J, Nielsen OH. Involvement of JAK/STAT signaling in the pathogenesis of inflammatory bowel disease. Pharmacol Res (2013) 76:1–8. doi: 10.1016/J.PHRS.2013.06.007
81. Banerjee S, Biehl A, Gadina M, Hasni S, Schwartz DM. JAK–STAT signaling as a target for inflammatory and autoimmune diseases: current and future prospects. Drugs (2017) 77:521. doi: 10.1007/S40265-017-0701-9
82. Candellone A, Cerquetella M, Girolami F, Badino P, Odore R. Acute diarrhea in dogs: current management and potential role of dietary polyphenols supplementation. Antioxidants (2020) 9:1–17. doi: 10.3390/ANTIOX9080725
83. Botterweck AAM, Verhagen H, Goldbohm RA, Kleinjans J, Van Den Brandt PA. Intake of butylated hydroxyanisole and butylated hydroxytoluene and stomach cancer risk: results from analyses in the Netherlands Cohort Study. Food Chem Toxicol (2000) 38:599–605. doi: 10.1016/S0278-6915(00)00042-9
84. Randhawa S, Bahna SL. Hypersensitivity reactions to food additives. Curr Opin Allergy Clin Immunol (2009) 9:278–83. doi: 10.1097/ACI.0B013E32832B2632
85. Lourenço SC, Moldão-Martins M, Alves VD. Antioxidants of natural plant origins: from sources to food industry applications. Molecules (2019) 24(22):4132. doi: 10.3390/MOLECULES24224132
86. Boussenna A, Cholet J, Goncalves-Mendes N, Joubert-Zakeyh J, Fraisse D, Vasson MP, et al. Polyphenol-rich grape pomace extracts protect against dextran sulfate sodium-induced colitis in rats. J Sci Food Agric (2016) 96:1260–8. doi: 10.1002/JSFA.7214
87. Brückner M, Westphal S, Domschke W, Kucharzik T, Lügering A. Green tea polyphenol epigallocatechin-3-gallate shows therapeutic antioxidative effects in a murine model of colitis. J Crohns Colitis (2012) 6:226–35. doi: 10.1016/J.CROHNS.2011.08.012/2/6-2-FIG043.JPEG
88. Shigeshiro M, Tanabe S, Suzuki T. Dietary polyphenols modulate intestinal barrier defects and inflammation in a murine model of colitis. J Funct Foods (2013) 5:949–55. doi: 10.1016/J.JFF.2013.02.008
89. Youn HS, Lee JY, Saitoh SI, Miyake K, Kang KW, Choi YJ, et al. Suppression of MyD88- and TRIF-dependent signaling pathways of Toll-like receptor by (-)-epigallocatechin-3-gallate, a polyphenol component of green tea. Biochem Pharmacol (2006) 72:850–9. doi: 10.1016/J.BCP.2006.06.021
90. Martinez J, Moreno JJ. Effect of resveratrol, a natural polyphenolic compound, on reactive oxygen species and prostaglandin production. Biochem Pharmacol (2000) 59:865–70. doi: 10.1016/S0006-2952(99)00380-9
91. Yildiz G, Yildiz Y, Ulutas PA, Yaylali A, Ural M. Resveratrol pretreatment ameliorates TNBS colitis in rats. Recent Pat Endocr Metab Immune Drug Discovery (2015) 9:134. doi: 10.2174/1872214809666150806105737
92. Dziąbowska-Grabias K, Sztanke M, Zając P, Celejewski M, Kurek K, Szkutnicki S, et al. Antioxidant therapy in inflammatory bowel diseases. Antioxidants (2021) 10:1–18. doi: 10.3390/ANTIOX10030412
93. Meng Z, Yan C, Deng Q, Gao DF, Niu XL. Curcumin inhibits LPS-induced inflammation in rat vascular smooth muscle cells in vitro via ROS-relative TLR4-MAPK/NF-κB pathways. Acta Pharmacol Sin (2013) 34:901. doi: 10.1038/APS.2013.24
94. Zeng Z, Zhan L, Liao H, Chen L, Lv X. Curcumin improves TNBS-induced colitis in rats by inhibiting IL-27 expression via the TLR4/NF-κB signaling pathway. Planta Med (2013) 79:102–9. doi: 10.1055/S-0032-1328057/BIB
95. Sharma M, Sharma S, Wadhwa J. Improved uptake and therapeutic intervention of curcumin via designing binary lipid nanoparticulate formulation for oral delivery in inflammatory bowel disorder. Artif Cells Nanomed Biotechnol (2019) 47:45–55. doi: 10.1080/21691401.2018.1543191
96. Stallhofer J, Friedrich M, Konrad-Zerna A, Wetzke M, Lohse P, Glas J, et al. Lipocalin-2 is a disease activity marker in inflammatory bowel disease regulated by IL-17A, IL-22, and TNF-α and modulated by IL23R genotype status. Inflammation Bowel Dis (2015) 21:2327–40. doi: 10.1097/MIB.0000000000000515
97. Zhong Y, Chiou YS, Pan MH, Shahidi F. Anti-inflammatory activity of lipophilic epigallocatechin gallate (EGCG) derivatives in LPS-stimulated murine macrophages. Food Chem (2012) 134:742–8. doi: 10.1016/J.FOODCHEM.2012.02.172
98. Khan MN, Lane ME, McCarron PA, Tambuwala MM. Caffeic acid phenethyl ester is protective in experimental ulcerative colitis via reduction in levels of pro-inflammatory mediators and enhancement of epithelial barrier function. Inflammopharmacology (2018) 26:561–9. doi: 10.1007/S10787-017-0364-X
99. Mei Y, Wang Z, Zhang Y, Wan T, Xue J, He W, et al. FA-97, a new synthetic caffeic acid phenethyl ester derivative, ameliorates DSS-induced colitis against oxidative stress by activating nrf2/HO-1 pathway. Front Immunol (2020) 10:2969/FULL. doi: 10.3389/FIMMU.2019.02969/FULL
100. Kuo MY, Liao MF, Chen FL, Li YC, Yang ML, Lin RH, et al. Luteolin attenuates the pulmonary inflammatory response involves abilities of antioxidation and inhibition of MAPK and NFκB pathways in mice with endotoxin-induced acute lung injury. Food Chem Toxicol (2011) 49:2660–6. doi: 10.1016/J.FCT.2011.07.012
101. Cho JM, Yun SM, Choi YH, Heo J, Kim NJ, Kim SH, et al. Xanthohumol prevents dextran sulfate sodium-induced colitis via inhibition of IKKβ/NF-κB signaling in mice. Oncotarget (2018) 9:866. doi: 10.18632/ONCOTARGET.23183
102. Jeong JW, Lee HH, Han MH, Kim GY, Kim WJ, Choi YH. Anti-inflammatory effects of genistein via suppression of the toll-like receptor 4-mediated signaling pathway in lipopolysaccharide-stimulated BV2 microglia. Chem Biol Interact (2014) 212:30–9. doi: 10.1016/J.CBI.2014.01.012
103. Cui S, Bilitewski U. Effect of genistein on the TLR and MAPK transduction cascades in lipopolysaccharide-stimulated macrophages. Chin UB-X bao yu fen zi M yi xue za zhi (2014) 30(3):233–6.
104. Jia Z, Babu PVA, Si H, Nallasamy P, Zhu H, Zhen W, et al. Genistein inhibits TNF-α-induced endothelial inflammation through the protein kinase pathway A and improves vascular inflammation in C57BL/6 mice. Int J Cardiol (2013) 168:2637. doi: 10.1016/J.IJCARD.2013.03.035
105. Chen Y, Le TH, Du Q, Zhao Z, Liu Y, Zou J, et al. Genistein protects against DSS-induced colitis by inhibiting NLRP3 inflammasome via TGR5-cAMP signaling. Int Immunopharmacol (2019) 71:144–54. doi: 10.1016/J.INTIMP.2019.01.021
106. Llaneza P, González C, Fernandez-Iñarrea J, Alonso A, Diaz F, Arnott I, et al. Soy isoflavones, diet and physical exercise modify serum cytokines in healthy obese postmenopausal women. Phytomedicine (2011) 18:245–50. doi: 10.1016/J.PHYMED.2010.07.011
107. Huo M, Chen N, Chi G, Yuan X, Guan S, Li H, et al. Traditional medicine alpinetin inhibits the inflammatory response in Raw 264.7 cells and mouse models. Int Immunopharmacol (2012) 12:241–8. doi: 10.1016/J.INTIMP.2011.11.017
108. Tan Y, Zheng C. Effects of alpinetin on intestinal barrier function, inflammation and oxidative stress in dextran sulfate sodium-induced ulcerative colitis mice. Am J Med Sci (2018) 355:377–86. doi: 10.1016/J.AMJMS.2018.01.002
109. Wang YH, Yang XL, Wang L, Cui MX, Cai YQ, Li XL, et al. Effects of proanthocyanidins from grape seed on treatment of recurrent ulcerative colitis in rats. Can J Physiol Pharmacol (2010) 88:888–98. doi: 10.1139/Y10-071
110. Roth S, Spalinger MR, Müller I, Lang S, Rogler G, Scharl M. Bilberry-derived anthocyanins prevent IFN-γ-induced pro-inflammatory signalling and cytokine secretion in human THP-1 monocytic cells. Digestion (2014) 90:179–89. doi: 10.1159/000366055
111. Olejnik A, Kowalska K, Kidoń M, Czapski J, Rychlik J, Olkowicz M, et al. Purple carrot anthocyanins suppress lipopolysaccharide-induced inflammation in the co-culture of intestinal Caco-2 and macrophage RAW264.7 cells. Food Funct (2016) 7:557–64. doi: 10.1039/C5FO00890E
112. Li L, Wang L, Wu Z, Yao L, Wu Y, Huang L, et al. Anthocyanin-rich fractions from red raspberries attenuate inflammation in both RAW264.7 macrophages and a mouse model of colitis. Sci Rep (2014) 4:6234. doi: 10.1038/SREP06234
113. Esmaily H, Hosseini-Tabatabaei A, Rahimian R, Khorasani R, Baeeri M, Barazesh-Morgani A, et al. On the benefits of silymarin in murine colitis by improving balance of destructive cytokines and reduction of toxic stress in the bowel cells. Cent Eur J Biol (2009) 4:204–13. doi: 10.2478/S11535-008-0053-2/METRICS
114. Koláček M, Muchová J, Dvořáková M, Paduchová Z, Žitňanová I, Čierna I, et al. Effect of natural polyphenols (Pycnogenol) on oxidative stress markers in children suffering from Crohn’s disease–a pilot study. Free Radic Res (2013) 47:624–34. doi: 10.3109/10715762.2013.807508
115. Putaala H, Nurminen P, Tiihonen K. Effects of cinnamaldehyde and thymol on cytotoxicity, tight junction barrier resistance, and cyclooxygenase-1 and -2 expression in Caco-2 cells. J Anim Feed Sci (2017) 26:274–84. doi: 10.22358/JAFS/77058/2017
116. Chen J, Li DL, Xie LN, Ma Y r, Wu PP, Li C, et al. Synergistic anti-inflammatory effects of silibinin and thymol combination on LPS-induced RAW264.7 cells by inhibition of NF-κB and MAPK activation. Phytomedicine (2020) 78:153309. doi: 10.1016/J.PHYMED.2020.153309
117. Gholijani N, Gharagozloo M, Farjadian S, Amirghofran Z. Modulatory effects of thymol and carvacrol on inflammatory transcription factors in lipopolysaccharide-treated macrophages. J Immunotoxicol (2016) 13:157–64. doi: 10.3109/1547691X.2015.1029145
118. Omonijo FA, Liu S, Hui Q, Zhang H, Lahaye L, Bodin JC, et al. Thymol improves barrier function and attenuates inflammatory responses in porcine intestinal epithelial cells during lipopolysaccharide (LPS)-induced inflammation. J Agric Food Chem (2019) 67:615–24. doi: 10.1021/ACS.JAFC.8B05480/ASSET/IMAGES/LARGE/JF-2018-054805_0007.JPEG
119. Mueller K, Blum NM, Mueller AS. Examination of the anti-inflammatory, antioxidant, and xenobiotic-inducing potential of broccoli extract and various essential oils during a mild DSS-induced colitis in rats. ISRN Gastroenterol (2013) 2013:1–14. doi: 10.1155/2013/710856
120. Khazdair MR, Ghorani V, Alavinezhad A, Boskabady MH. Pharmacological effects of Zataria multiflora Boiss L. and its constituents focus on their anti-inflammatory, antioxidant, and immunomodulatory effects. Fundam Clin Pharmacol (2018) 32:26–50. doi: 10.1111/FCP.12331
121. Cheng WE, Ying Chang M, Wei JY, Chen YJ, Maa MC, Leu TH. Berberine reduces Toll-like receptor-mediated macrophage migration by suppression of Src enhancement. Eur J Pharmacol (2015) 757:1–10. doi: 10.1016/J.EJPHAR.2015.03.013
122. Yan F, Wang L, Shi Y, Cao H, Liu L, Kay Washington M, et al. Berberine promotes recovery of colitis and inhibits inflammatory responses in colonic macrophages and epithelial cells in DSS-treated mice. Am J Physiol Gastrointest Liver Physiol (2012) 302:G504. doi: 10.1152/AJPGI.00312.2011
123. Xu L, Zhang Y, Xue X, Liu J, Li ZS, Yang GY, et al. A phase I trial of berberine in Chinese with ulcerative colitis. Cancer Prev Res (2020) 13:117–26. doi: 10.1158/1940-6207.CAPR-19-0258/37877/AM/A-PHASE-I-TRIAL-OF-BERBERINE-IN-CHINESE-WITH
124. Ross EA, Miller MH, Pacheco A, Willenberg AR, Tigno-Aranjuez JT, Crawford KE. Intrarectal xyloglucan administration reduces disease severity in the dextran sodium sulfate model of mouse colitis. Clin Exp Gastroenterol (2021) 14:429–39. doi: 10.2147/CEG.S325945
125. Periasamy S, Lin CH, Nagarajan B, Sankaranarayanan NV, Desai UR, Liu MY. Tamarind xyloglucan attenuates dextran sodium sulfate induced ulcerative colitis: Role of antioxidation. J Funct Foods (2018) 42:327–38. doi: 10.1016/J.JFF.2018.01.014
126. Miyamoto J, Mizukure T, Park SB, Kishino S, Kimura I, HIrano K, et al. A gut microbial metabolite of linoleic acid, 10-hydroxy-cis-12-octadecenoic acid, ameliorates intestinal epithelial barrier impairment partially via GPR40-MEK-ERK pathway. J Biol Chem (2015) 290:2902. doi: 10.1074/JBC.M114.610733
127. Zhou Q, Ma L, Zhao W, Zhao W, Han X, Niu J, et al. Flaxseed oil alleviates dextran sulphate sodium-induced ulcerative colitis in rats. J Funct Foods (2020) 64:103602. doi: 10.1016/J.JFF.2019.103602
128. Pan MH, Hsieh MC, Hsu PC, Ho SY, Lai CS, Wu H, et al. 6-Shogaol suppressed lipopolysaccharide-induced up-expression of iNOS and COX-2 in murine macrophages. Mol Nutr Food Res (2008) 52:1467–77. doi: 10.1002/MNFR.200700515
129. Li XH, McGrath KCY, Tran VH, Li YM, Duke CC, Roufogalis BD, et al. Attenuation of proinflammatory responses by S -[6]-Gingerol via inhibition of ROS/NF-Kappa B/COX2 activation in HuH7 cells. Evidence-Based Complementary Altern Med (2013) 2013:146142. doi: 10.1155/2013/146142
130. Hosseinzadeh A, Bahrampour Juybari K, Fatemi MJ, Kamarul T, Bagheri A, Tekiyehmaroof N, et al. Protective Effect of Ginger (Zingiber officinale Roscoe) Extract against Oxidative Stress and Mitochondrial Apoptosis Induced by Interleukin-1β in Cultured Chondrocytes. Cells Tissues Organs (2017) 204:241–50. doi: 10.1159/000479789
131. Guo S, Geng W, Chen S, Wang L, Rong X, Wang S, et al. Ginger alleviates DSS-induced ulcerative colitis severity by improving the diversity and function of gut microbiota. Front Pharmacol (2021) 12:632569. doi: 10.3389/FPHAR.2021.632569
132. Nikkhah-Bodaghi M, Maleki I, Agah S, Hekmatdoost A. Zingiber officinale and oxidative stress in patients with ulcerative colitis: A randomized, placebo-controlled, clinical trial. Complement Ther Med (2019) 43:1–6. doi: 10.1016/J.CTIM.2018.12.021
133. Skarbaliene J, Mathiesen JM, Larsen BD, Thorkildsen C, Petersen YM. Glepaglutide, a novel glucagon-like peptide-2 agonist, has anti-inflammatory and mucosal regenerative effects in an experimental model of inflammatory bowel disease in rats. BMC Gastroenterol (2023) 23:1–9. doi: 10.1186/S12876-023-02716-4/FIGURES/4
134. Tahan G, Gramignoli R, Marongiu F, Aktolga S, Cetinkaya A, Tahan V, et al. Melatonin expresses powerful anti-inflammatory and antioxidant activities resulting in complete improvement of acetic-acid-induced colitis in rats. Dig Dis Sci (2011) 56:715–20. doi: 10.1007/S10620-010-1364-5/FIGURES/4
135. Salavati S, Mogheiseh A, Nazifi S, Amiri A, Nikahval B. The effects of melatonin treatment on oxidative stress induced by ovariohysterectomy in dogs. BMC Vet Res (2021) 17:1–8. doi: 10.1186/S12917-021-02882-1/FIGURES/3
136. Wisniewska-Jarosinska M, Walecka-Kapica E, Jaworek J. Evaluation of melatonin effectiveness in the adjuvant treatment of ulcerative colitis. Article J Physiol Pharmacol (2011) 62(3):327–4.
137. Hasani-Ranjbar S, Larijani B, Abdollahi M. A systematic review of the potential herbal sources of future drugs effective in oxidant-related diseases. Inflammation Allergy Drug Targets (2009) 8:2–10. doi: 10.2174/187152809787582561
138. Kuzmich NN, Sivak K v., Chubarev VN, Porozov YB, Savateeva-Lyubimova TN, Peri F. TLR4 signaling pathway modulators as potential therapeutics in inflammation and sepsis. Vaccines (Basel) (2017) 5(4):34. doi: 10.3390/VACCINES5040034
139. Liu P, Li Y, Wang R, Ren F, Wang X. Oxidative stress and antioxidant nanotherapeutic approaches for inflammatory bowel disease. Biomedicines (2022) 10(1):85. doi: 10.3390/BIOMEDICINES10010085
140. Williams RJ, Spencer JPE, Rice-Evans C. Flavonoids: Antioxidants or signalling molecules? Free Radic Biol Med (2004) 36:838–49. doi: 10.1016/j.freeradbiomed.2004.01.001
141. Manz A, Allenspach K, Kummer S, Richter B, Walter I, Macho-Maschler S, et al. Upregulation of signal transducer and activator of transcription 3 in dogs with chronic inflammatory enteropathies. J Vet Intern Med (2021) 35:1288–96. doi: 10.1111/JVIM.16141
142. Summerlin N, Soo E, Thakur S, Qu Z, Jambhrunkar S, Popat A. Resveratrol nanoformulations: Challenges and opportunities. Int J Pharm (2015) 479:282–90. doi: 10.1016/J.IJPHARM.2015.01.003
143. Arslan A, Ozcicek F, Cimen FK, Altuner D, Yarali O, Kurt N, et al. Protective effect of resveratrol against methotrexate-induced oxidative stress in the small intestinal tissues of rats. Int J Clin Exp Med (2015) 8(7):10491–500.
144. Greten FR, Eckmann L, Greten TF, Park JM, Li ZW, Egan LJ, et al. IKKβ links inflammation and tumorigenesis in a mouse model of colitis-associated cancer. Cell (2004) 118:285–96. doi: 10.1016/j.cell.2004.07.013
145. Hofseth LJ, Singh UP, Singh NP, Nagarkatti M, Nagarkatti PS. Taming the beast within: resveratrol suppresses colitis and prevents colon cancer. Aging (Albany NY) (2010) 2:183. doi: 10.18632/AGING.100143
146. Kocaadam B, Şanlier N. Curcumin, an active component of turmeric (Curcuma longa), and its effects on health. Crit Rev Food Sci Nutr (2017) 57:2889–95. doi: 10.1080/10408398.2015.1077195
147. Chainy GBN, Sahoo DK. Hormones and oxidative stress: an overview. Free Radic Res (2019) 54:1–26. doi: 10.1080/10715762.2019.1702656
148. Lestari MLAD, Indrayanto G. Curcumin. Profiles Drug Subst Excip Relat Methodol (2014) 39:113–204. doi: 10.1016/B978-0-12-800173-8.00003-9
149. Cao S, Wang C, Yan J, Li X, Wen J, Hu C. Curcumin ameliorates oxidative stress-induced intestinal barrier injury and mitochondrial damage by promoting Parkin dependent mitophagy through AMPK-TFEB signal pathway. Free Radic Biol Med (2020) 147:8–22. doi: 10.1016/J.FREERADBIOMED.2019.12.004
150. Zhu H t, Bian C, Yuan J c, Chu W h, Xiang X, Chen F, et al. Curcumin attenuates acute inflammatory injury by inhibiting the TLR4/MyD88/NF-κB signaling pathway in experimental traumatic brain injury. J Neuroinflamm (2014) 11:59. doi: 10.1186/1742-2094-11-59
151. Chen CY, Kao CL, Liu CM. The cancer prevention, anti-inflammatory and anti-oxidation of bioactive phytochemicals targeting the TLR4 signaling pathway. Int J Mol Sci (2018) 19:2729. doi: 10.3390/IJMS19092729
152. Dickinson DA, Iles KE, Zhang H, Blank V, Forman HJ. Curcumin alters EpRE and AP-1 binding complexes and elevates glutamate-cysteine ligase gene expression. FASEB J (2003) 17:473–5. doi: 10.1096/FJ.02-0566FJE
153. Mishra P, Paital B, Jena S, Swain SS, Kumar S, Yadav MK, et al. Possible activation of NRF2 by Vitamin E/Curcumin against altered thyroid hormone induced oxidative stress via NFĸB/AKT/mTOR/KEAP1 signalling in rat heart. Sci Rep (2019) 9:1–16. doi: 10.1038/s41598-019-43320-5
154. Arafa HMM, Hemeida RA, El-Bahrawy AIM, Hamada FMA. Prophylactic role of curcumin in dextran sulfate sodium (DSS)-induced ulcerative colitis murine model. Food Chem Toxicol (2009) 47:1311–7. doi: 10.1016/J.FCT.2009.03.003
155. Lin Y, Liu H, Bu L, Chen C, Ye X. Review of the effects and mechanism of curcumin in the treatment of inflammatory bowel disease. Front Pharmacol (2022) 13:908077. doi: 10.3389/FPHAR.2022.908077
156. Topcu-Tarladacalisir Y, Akpolat M, Uz YH, Kizilay G, Sapmaz-Metin M, Cerkezkayabekir A, et al. Effects of curcumin on apoptosis and oxidoinflammatory regulation in a rat model of acetic acid-induced colitis: the roles of c-Jun N-terminal kinase and p38 mitogen-activated protein kinase. J Med Food (2013) 16:296–305. doi: 10.1089/JMF.2012.2550
157. Mouzaoui S, Rahim I, Djerdjouri B. Aminoguanidine and curcumin attenuated tumor necrosis factor (TNF)-α-induced oxidative stress, colitis and hepatotoxicity in mice. Int Immunopharmacol (2012) 12:302–11. doi: 10.1016/J.INTIMP.2011.10.010
158. Lubbad A, Oriowo MA, Khan I. Curcumin attenuates inflammation through inhibition of TLR-4 receptor in experimental colitis. Mol Cell Biochem (2009) 322:127–35. doi: 10.1007/S11010-008-9949-4
159. Spagnuolo C, Moccia S, Russo GL. Anti-inflammatory effects of flavonoids in neurodegenerative disorders. Eur J Med Chem (2018) 153:105–15. doi: 10.1016/J.EJMECH.2017.09.001
160. Yang WS, Jeong D, Yi YS, Lee BH, Kim TW, Htwe KM, et al. Myrsine seguinii ethanolic extract and its active component quercetin inhibit macrophage activation and peritonitis induced by LPS by targeting to Syk/Src/IRAK-1. J Ethnopharmacol (2014) 151:1165–74. doi: 10.1016/J.JEP.2013.12.033
161. Kleinert H, Forstermann U. Inducible nitric oxide synthase. xPharm: Compr Pharmacol Reference (2007), 1–12. doi: 10.1016/B978-008055232-3.60509-4
162. Bedos L, Wickham H, Gabriel V, Zdyrski C, Allbaugh RA, Sahoo DK, et al. Culture and characterization of canine and feline corneal epithelial organoids: A new tool for the study and treatment of corneal diseases. Front Vet Sci (2022) 9:1050467/FULL. doi: 10.3389/FVETS.2022.1050467/FULL
163. Gabriel V, Zdyrski C, Sahoo DK, Dao K, Bourgois-Mochel A, Kopper J, et al. Standardization and maintenance of 3D canine hepatic and intestinal organoid cultures for use in biomedical research. J Visualized Experiments (2022) 179:e63515. doi: 10.3791/63515
164. Minkler S, Lucien F, Kimber MJ, Sahoo DK, Bourgois-Mochel A, Musser M, et al. Emerging roles of urine-derived components for the management of bladder cancer: one man’s trash is another man’s treasure. Cancers (2021) 13:422. doi: 10.3390/CANCERS13030422
165. Kopper JJ, Iennarella-Servantez C, Jergens AE, Sahoo DK, Guillot E, Bourgois-Mochel A, et al. Harnessing the biology of canine intestinal organoids to heighten understanding of inflammatory bowel disease pathogenesis and accelerate drug discovery: A one health approach. Front Toxicol (2021) 0:773953. doi: 10.3389/FTOX.2021.773953
166. Gabriel V, Zdyrski C, Sahoo DK, Dao K, Bourgois-Mochel A, Atherly T, et al. Canine intestinal organoids in a dual-chamber permeable support system. J Vis Exp (2022) 181:e63612. doi: 10.3791/63612
167. Sahoo DK, Martinez MN, Dao K, Gabriel V, Zdyrski C, Jergens AE, et al. Canine intestinal organoids as a novel in vitro model of intestinal drug permeability: A proof-of-concept study. Cells (2023) 12:1269. doi: 10.3390/CELLS12091269
168. Moshksayan K, Harihara A, Mondal S, Hegarty E, Atherly T, Sahoo DK, et al. OrganoidChip facilitates hydrogel-free immobilization for fast and blur-free imaging of organoids. Sci Rep (2023) 13:1–14. doi: 10.1038/s41598-023-38212-8
169. Shimizu M, Li J, Inoue J, Sato R. Quercetin represses apolipoprotein B expression by inhibiting the transcriptional activity of C/EBPβ. PloS One (2015) 10:e0121784. doi: 10.1371/JOURNAL.PONE.0121784
170. Dodda D, Chhajed R, Mishra J, Padhy M. Targeting oxidative stress attenuates trinitrobenzene sulphonic acid induced inflammatory bowel disease like symptoms in rats: Role of quercetin. Indian J Pharmacol (2014) 46:286. doi: 10.4103/0253-7613.132160
171. Ju S, Ge Y, Li P, Tian X, Wang H, Zheng X, et al. Dietary quercetin ameliorates experimental colitis in mouse by remodeling the function of colonic macrophages via a heme oxygenase-1-dependent pathway. Cell Cycle (2018) 17:53. doi: 10.1080/15384101.2017.1387701
172. Kamishikiryo J, Matsumura R, Takamori T, Sugihara N. Effect of quercetin on the transport of N-acetyl 5-aminosalicylic acid. J Pharm Pharmacol (2013) 65:1037–43. doi: 10.1111/JPHP.12062
173. Guazelli CFS, Fattori V, Colombo BB, Georgetti SR, Vicentini FTMC, Casagrande R, et al. Quercetin-loaded microcapsules ameliorate experimental colitis in mice by anti-inflammatory and antioxidant mechanisms. J Nat Prod (2013) 76(2):200–8. doi: 10.1021/np300670w
175. Corcoran MP, McKay DL, Blumberg JB. Flavonoid basics: chemistry, sources, mechanisms of action, and safety. J Nutr Gerontol Geriatr (2012) 31:176–89. doi: 10.1080/21551197.2012.698219
176. Ortega N, Reguant J, Romero MP, Macià A, Motilva MJ. Effect of fat content on the digestibility and bioaccessibility of cocoa polyphenol by an in vitro digestion model. J Agric Food Chem (2009) 57:5743–9. doi: 10.1021/JF900591Q
177. Pérez-Jiménez J, Serrano J, Tabernero M, Arranz S, Díaz-Rubio ME, García-Diz L, et al. Bioavailability of phenolic antioxidants associated with dietary fiber: plasma antioxidant capacity after acute and long-term intake in humans. Plant Foods Hum Nutr (2009) 64:102–7. doi: 10.1007/S11130-009-0110-7
178. Wang LC, Lin YL, Liang YC, Yang YH, Lee JH, Yu HH, et al. The effect of caffeic acid phenethyl ester on the functions of human monocyte-derived dendritic cells. BMC Immunol (2009) 10:39. doi: 10.1186/1471-2172-10-39
179. Li L, Sun W, Wu T, Lu R, Shi B. Caffeic acid phenethyl ester attenuates lipopolysaccharide-stimulated proinflammatory responses in human gingival fibroblasts via NF-κB and PI3K/Akt signaling pathway. Eur J Pharmacol (2017) 794:61–8. doi: 10.1016/J.EJPHAR.2016.11.003
180. Kim SY, Koo JE, Seo YJ, Tyagi N, Jeong E, Choi J, et al. Suppression of Toll-like receptor 4 activation by caffeic acid phenethyl ester is mediated by interference of LPS binding to MD2. Br J Pharmacol (2013) 168:1933–45. doi: 10.1111/BPH.12091
181. Chang H, Wang Y, Yin X, Liu X, Xuan H. Ethanol extract of propolis and its constituent caffeic acid phenethyl ester inhibit breast cancer cells proliferation in inflammatory microenvironment by inhibiting TLR4 signal pathway and inducing apoptosis and autophagy. BMC Complement Altern Med (2017) 17:471. doi: 10.1186/S12906-017-1984-9
182. Lee JK, Kim SY, Kim YS, Lee WH, Hwang DH, Lee JY. Suppression of the TRIF-dependent signaling pathway of Toll-like receptors by luteolin. Biochem Pharmacol (2009) 77:1391–400. doi: 10.1016/J.BCP.2009.01.009
183. Liu S, Feng G, Wang G l, Liu G j. p38MAPK inhibition attenuates LPS-induced acute lung injury involvement of NF-κB pathway. Eur J Pharmacol (2008) 584:159–65. doi: 10.1016/J.EJPHAR.2008.02.009
184. Schuh K, Pahl A. Inhibition of the MAP kinase ERK protects from lipopolysaccharide-induced lung injury. Biochem Pharmacol (2009) 77:1827–34. doi: 10.1016/J.BCP.2009.03.012
185. Chen G, Xiao B, Chen L, Bai B, Zhang Y, Xu Z, et al. Discovery of new MD2-targeted anti-inflammatory compounds for the treatment of sepsis and acute lung injury. Eur J Med Chem (2017) 139:726–40. doi: 10.1016/J.EJMECH.2017.08.036
186. Zhao F, Nozawa H, Daikonnya A, Kondo K, Kitanaka S. Inhibitors of nitric oxide production from hops (Humulus lupulus L.). Biol Pharm Bull (2003) 26:61–5. doi: 10.1248/BPB.26.61
187. Cho YC, Kim HJ, Kim YJ, Lee KY, Choi HJ, Lee IS, et al. Differential anti-inflammatory pathway by xanthohumol in IFN-gamma and LPS-activated macrophages. Int Immunopharmacol (2008) 8:567–73. doi: 10.1016/J.INTIMP.2007.12.017
188. Langley BO, Ryan JJ, Phipps J, Buttolph L, Bray B, Aslan JE, et al. Xanthohumol microbiome and signature in adults with Crohn’s disease (the XMaS trial): a protocol for a phase II triple-masked, placebo-controlled clinical trial. Trials (2022) 23(1):885. doi: 10.1186/S13063-022-06782-Z
189. Gao X, Liu K, Huang F, Zhang D, Guo X, Wang M, et al. Positive and negative regulation of insulin action by genistein in the endothelium. J Nutr Biochem (2013) 24:222–30. doi: 10.1016/J.JNUTBIO.2012.05.008
190. Kong DH, Kim YK, Kim MR, Jang JH, Lee S. Emerging roles of vascular cell adhesion molecule-1 (VCAM-1) in immunological disorders and cancer. Int J Mol Sci (2018) 19(4):1057. doi: 10.3390/IJMS19041057
191. Zhen Y, Zhang H. NLRP3 inflammasome and inflammatory bowel disease. Front Immunol (2019) 10:276. doi: 10.3389/FIMMU.2019.00276
192. Zhao G, Tong Y, Luan F, Zhu W, Zhan C, Qin T, et al. Alpinetin: A review of its pharmacology and pharmacokinetics. Front Pharmacol (2022) 13:814370/BIBTEX. doi: 10.3389/FPHAR.2022.814370/BIBTEX
193. Haijin C, Xiaodong M, Jinlong Y, Zonghai H. Alpinetin attenuates inflammatory responses by interfering toll-like receptor 4/nuclear factor kappa B signaling pathway in lipopolysaccharide-induced mastitis in mice. Int Immunopharmacol (2013) 17:26–32. doi: 10.1016/J.INTIMP.2013.04.030
194. Katiyar SK. Proanthocyanidins from grape seeds inhibit UV–radiation-induced immune suppression in mice: detection and analysis of molecular and cellular targets. Photochem Photobiol (2015) 91:156–62. doi: 10.1111/PHP.12330
195. Wang YH, Ge B, Yang XL, Zhai J, Yang LN, Wang XX, et al. Proanthocyanidins from grape seeds modulates the nuclear factor-kappa B signal transduction pathways in rats with TNBS-induced recurrent ulcerative colitis. Int Immunopharmacol (2011) 11:1620–7. doi: 10.1016/J.INTIMP.2011.05.024
196. Li X, Yang X, Cai Y, Qin H, Wang L, Wang Y, et al. Proanthocyanidins from grape seeds modulate the NF-κB signal transduction pathways in rats with TNBS-induced ulcerative colitis. Molecules (2011) 16:6721–31. doi: 10.3390/MOLECULES16086721
197. Gil-Cardoso K, Comitato R, Ginés I, Ardévol A, Pinent M, Virgili F, et al. Protective effect of proanthocyanidins in a rat model of mild intestinal inflammation and impaired intestinal permeability induced by LPS. Mol Nutr Food Res (2019) 63:1800720. doi: 10.1002/MNFR.201800720
198. Farzaei MH, El-Senduny FF, Momtaz S, Parvizi F, Iranpanah A, Tewari D, et al. An update on dietary consideration in inflammatory bowel disease: anthocyanins and more. Expert Rev Gastroenterol Hepatol (2018) 12:1007–24. doi: 10.1080/17474124.2018.1513322
199. Cassidy A, Rogers G, Peterson JJ, Dwyer JT, Lin H, Jacques PF. Higher dietary anthocyanin and flavonol intakes are associated with anti-inflammatory effects in a population of US adults. Am J Clin Nutr (2015) 102:172–81. doi: 10.3945/AJCN.115.108555
200. Tomlinson ML, Butelli E, Martin C, Carding SR. Flavonoids from Engineered Tomatoes Inhibit Gut Barrier Pro-inflammatory Cytokines and Chemokines, via SAPK/JNK and p38 MAPK Pathways. Front Nutr (2017) 4:61/BIBTEX. doi: 10.3389/FNUT.2017.00061/BIBTEX
201. Chen T, Hu S, Zhang H, Guan Q, Yang Y, Wang X. Anti-inflammatory effects of Dioscorea alata L. anthocyanins in a TNBS-induced colitis model. Food Funct (2017) 8:659–69. doi: 10.1039/C6FO01273F
202. Bibi S, Kang Y, Du M, Zhu MJ. Dietary red raspberries attenuate dextran sulfate sodium-induced acute colitis. J Nutr Biochem (2018) 51:40–6. doi: 10.1016/J.JNUTBIO.2017.08.017
203. Pervin M, Hasnat MA, Lim JH, Lee YM, Kim EO, Um BH, et al. Preventive and therapeutic effects of blueberry (Vaccinium corymbosum) extract against DSS-induced ulcerative colitis by regulation of antioxidant and inflammatory mediators. J Nutr Biochem (2016) 28:103–13. doi: 10.1016/J.JNUTBIO.2015.10.006
204. Pérez-Berezo T, Ramírez-Santana C, Franch A, Ramos-Romero S, Castellote C, Pérez-Cano FJ, et al. Effects of a cocoa diet on an intestinal inflammation model in rats. Exp Biol Med (Maywood) (2012) 237:1181–8. doi: 10.1258/EBM.2012.012083
205. Kaspar KL, Park JS, Brown CR, Mathison BD, Navarre DA, Chew BP. Pigmented potato consumption alters oxidative stress and inflammatory damage in men. J Nutr (2011) 141:108–11. doi: 10.3945/JN.110.128074
206. Biedermann L, Mwinyi J, Scharl M, Frei P, Zeitz J, Kullak-Ublick GA, et al. Bilberry ingestion improves disease activity in mild to moderate ulcerative colitis — An open pilot study. J Crohns Colitis (2013) 7:271–9. doi: 10.1016/J.CROHNS.2012.07.010
207. Surai PF. Silymarin as a natural antioxidant: an overview of the current evidence and perspectives. Antioxidants (Basel) (2015) 4:204–47. doi: 10.3390/ANTIOX4010204
208. Rastegarpanah M, Malekzadeh R, Vahedi H, Mohammadi M, Elahi E, Chaharmahali M, et al. A randomized, double blinded, placebo-controlled clinical trial of silymarin in ulcerative colitis. Chin J Integr Med (2015) 21:902–6. doi: 10.1007/S11655-012-1026-X
209. Zhang Z, Li X, Sang S, McClements DJ, Chen L, Long J, et al. A review of nanostructured delivery systems for the encapsulation, protection, and delivery of silymarin: An emerging nutraceutical. Food Res Int (2022) 156:111314. doi: 10.1016/J.FOODRES.2022.111314
210. Ahmad S, Khan JA, Kausar TN, Mahnashi MH, Alasiri A, Alqahtani AA, et al. Preparation, characterization and evaluation of flavonolignan silymarin effervescent floating matrix tablets for enhanced oral bioavailability. Molecules (2023) 28:2606. doi: 10.3390/MOLECULES28062606
211. Hackett ES, Mama KR, Twedt DC, Gustafson DL. Pharmacokinetics and safety of silibinin in horses. Am J Vet Res (2013) 74:1327–32. doi: 10.2460/AJVR.74.10.1327
212. Ribeiro ARS, Diniz PBF, Pinheiro MS, Albuquerque-Júnior RLC, Thomazzi SM. Gastroprotective effects of thymol on acute and chronic ulcers in rats: The role of prostaglandins, ATP-sensitive K(+) channels, and gastric mucus secretion. Chem Biol Interact (2016) 244:121–8. doi: 10.1016/J.CBI.2015.12.004
213. Salehi B, Mishra AP, Shukla I, Sharifi-Rad M, Contreras M del M, Segura-Carretero A, et al. Thymol, thyme, and other plant sources: Health and potential uses. Phytother Res (2018) 32:1688–706. doi: 10.1002/PTR.6109
214. Wan L, Meng D, Wang H, Wan S, Jiang S, Huang S, et al. Preventive and therapeutic effects of thymol in a lipopolysaccharide-induced acute lung injury mice model. Inflammation (2018) 41:183–92. doi: 10.1007/S10753-017-0676-4
215. Winterbourn CC, Kettle AJ, Hampton MB. Reactive oxygen species and neutrophil function. Annu Rev Biochem (2016) 85:765–92. doi: 10.1146/ANNUREV-BIOCHEM-060815-014442
216. Liang D, Li F, Fu Y, Cao Y, Song X, Wang T, et al. Thymol inhibits LPS-stimulated inflammatory response via down-regulation of NF-κB and MAPK signaling pathways in mouse mammary epithelial cells. Inflammation (2014) 37:214–22. doi: 10.1007/S10753-013-9732-X
217. Sack RB, Froehlich JL. Berberine inhibits intestinal secretory response of Vibrio cholerae and Escherichia coli enterotoxins. Infect Immun (1982) 35:471–5. doi: 10.1128/IAI.35.2.471-475.1982
218. Kaneda Y, Torii M, Tanaka T, Aikawa M. In vitro effects of berberine sulphate on the growth and structure of Entamoeba histolytica, Giardia lamblia and Trichomonas vaginalis. Ann Trop Med Parasitol (1991) 85:417–25. doi: 10.1080/00034983.1991.11812586
219. Amin AH, Subbaiah T v., Abbasi KM. Berberine sulfate: antimicrobial activity, bioassay, and mode of action. Can J Microbiol (1969) 15:1067–76. doi: 10.1139/M69-190
220. Wan X, Chen X, Liu L, Zhao Y, Huang WJ, Zhang Q, et al. Berberine ameliorates chronic kidney injury caused by atherosclerotic renovascular disease through the suppression of NFκB signaling pathway in rats. PloS One (2013) 8(3):e59794. doi: 10.1371/JOURNAL.PONE.0059794
221. Li Z, Geng YN, Jiang JD, Kong WJ. Antioxidant and anti-inflammatory activities of berberine in the treatment of diabetes mellitus. Evid Based Complement Alternat Med (2014) 2014:289264. doi: 10.1155/2014/289264
222. Sarna LK, Wu N, Hwang SY, Siow YL, Karmin O. Berberine inhibits NADPH oxidase mediated superoxide anion production in macrophages. Can J Physiol Pharmacol (2010) 88:369–78. doi: 10.1139/Y09-136
223. Zhai L, Huang T, Xiao HT, Wu PG, Lin CY, Ning ZW, et al. Berberine suppresses colonic inflammation in dextran sulfate sodium–induced murine colitis through inhibition of cytosolic phospholipase A2 activity. Front Pharmacol (2020) 11:576496/FULL. doi: 10.3389/FPHAR.2020.576496/FULL
224. Jauregui-Amezaga A, Geerits A, Das Y, Lemmens B, Sagaert X, Bessissow T, et al. A simplified geboes score for ulcerative colitis. J Crohns Colitis (2017) 11:305–13. doi: 10.1093/ECCO-JCC/JJW154
225. Kaiser AE, Baniasadi M, Giansiracusa D, Giansiracusa M, Garcia M, Fryda Z, et al. Sulforaphane: A broccoli bioactive phytocompound with cancer preventive potential. Cancers (Basel) (2021) 13(19):4796. doi: 10.3390/CANCERS13194796
226. Wei LY, Zhang JK, Zheng L, Chen Y. The functional role of sulforaphane in intestinal inflammation: a review. Food Funct (2022) 13:514–29. doi: 10.1039/D1FO03398K
227. Youn HS, Kim YS, Park ZY, Kim SY, Choi NY, Joung SM, et al. Sulforaphane suppresses oligomerization of TLR4 in a thiol-dependent manner. J Immunol (2010) 184:411–9. doi: 10.4049/JIMMUNOL.0803988
228. Zhang Y, Tan L, Li C, Wu H, Ran D, Zhang Z. Sulforaphane alter the microbiota and mitigate colitis severity on mice ulcerative colitis induced by DSS. AMB Express (2020) 10:119. doi: 10.1186/S13568-020-01053-Z
229. Eun MK, Hye JK, Kim S, Woo HC, Yeon HC, Shi YR, et al. Modulation of macrophage functions by compounds isolated from Zingiber officinale. Planta Med (2009) 75:148–51. doi: 10.1055/S-0028-1088347
230. Han Q, Yuan Q, Meng X, Huo J, Bao Y, Xie G. 6-Shogaol attenuates LPS-induced inflammation in BV2 microglia cells by activating PPAR-γ. Oncotarget (2017) 8:42001–6. doi: 10.18632/ONCOTARGET.16719
231. Ahn S i, Lee JK, Youn HS. Inhibition of homodimerization of toll-like receptor 4 by 6-shogaol. Mol Cells (2009) 27:211–5. doi: 10.1007/S10059-009-0026-Y
232. Park SJ, Lee MY, Son BS, Youn HS. TBK1-targeted suppression of TRIF-dependent signaling pathway of Toll-like receptors by 6-shogaol, an active component of ginger. Biosci Biotechnol Biochem (2009) 73:1474–8. doi: 10.1271/BBB.80738
233. Krysko D v., Denecker G, Festjens N, Gabriels S, Parthoens E, D’Herde K, et al. Macrophages use different internalization mechanisms to clear apoptotic and necrotic cells. Cell Death Differ (2006) 13:2011–22. doi: 10.1038/SJ.CDD.4401900
234. Park SH, Kyeong MS, Hwang Y, Ryu SY, Han SB, Kim Y. Inhibition of LPS binding to MD-2 co-receptor for suppressing TLR4-mediated expression of inflammatory cytokine by 1-dehydro-10-gingerdione from dietary ginger. Biochem Biophys Res Commun (2012) 419:735–40. doi: 10.1016/J.BBRC.2012.02.091
235. Lee HY, Park SH, Lee M, Kim HJ, Ryu SY, Kim ND, et al. 1-Dehydro-[10]-gingerdione from ginger inhibits IKKβ activity for NF-κB activation and suppresses NF-κB-regulated expression of inflammatory genes. Br J Pharmacol (2012) 167:128–40. doi: 10.1111/J.1476-5381.2012.01980.X
236. Sadeghi Poor Ranjbar F, Mohammadyari F, Omidvar A, Nikzad F, Doozandeh Nargesi N, Varmazyar M, et al. Zingiber officinale (Ginger) as a treatment for inflammatory bowel disease: A review of current literature. Front Drug Discovery (2022) 2:1043617. doi: 10.3389/FDDSV.2022.1043617
237. Tigas S, Tsatsoulis A. Endocrine and metabolic manifestations in inflammatory bowel disease. Ann Gastroenterol (2012) 25:37.
238. Bar RS, Hoak JC, Peacock ML. Insulin receptors in human endothelial cells: identification and characterization. J Clin Endocrinol Metab (1978) 47:699–702. doi: 10.1210/JCEM-47-3-699
239. Bar RS, Kahn CR, Koren HS. Insulin inhibition of antibody-dependent cytoxicity and insulin receptors in macrophages. Nature (1977) 265:632–5. doi: 10.1038/265632A0
240. Peers SH, Moon D, Flower RJ. Reversal of the anti-inflammatory effects of dexamethasone by the glucocorticoid antagonist RU 38486. Biochem Pharmacol (1988) 37:556–7. doi: 10.1016/0006-2952(88)90230-4
241. Laue L, Kawai S, Brandon DD, Brightwell D, Barnes K, Knazek RA, et al. Receptor-mediated effects of glucocorticoids on inflammation: enhancement of the inflammatory response with a glucocorticoid antagonist. J Steroid Biochem (1988) 29:591–8. doi: 10.1016/0022-4731(88)90156-2
242. Tsurufuji S, Sugio K, Takemasa F. The role of glucocorticoid receptor and gene expression in the anti-inflammatory action of dexamethasone. Nature (1979) 280:408–10. doi: 10.1038/280408A0
243. Garcia-Leme J, Farsky SP. Hormonal control of inflammatory responses. Mediators Inflammation (1993) 2:181. doi: 10.1155/S0962935193000250
244. Rolston VS, Boroujerdi L, Long MD, McGovern DPB, Chen W, Martin CF, et al. The influence of hormonal fluctuation on inflammatory bowel disease symptom severity—A cross-sectional cohort study. Inflammation Bowel Dis (2018) 24:387. doi: 10.1093/IBD/IZX004
245. Bellanti F, Matteo M, Rollo T, De Rosario F, Greco P, Vendemiale G, et al. Sex hormones modulate circulating antioxidant enzymes: impact of estrogen therapy. Redox Biol (2013) 1:340–6. doi: 10.1016/J.REDOX.2013.05.003
246. Diaz-Flores M, Baiza-Gutman LA, Pedrón NN, Hicks JJ. Uterine glutathione reductase activity: Modulation by estrogens and progesterone. Life Sci (1999) 65:2481–8. doi: 10.1016/S0024-3205(99)00514-7
247. Huh K, Shin US, Choi JW, Lee S. Effect of sex hormones on lipid peroxidation in rat liver. Arch Pharm Res (1994) 17:109–14. doi: 10.1007/BF02974233
248. Persky AM, Green PS, Stubley L, Howell CO, Zaulyanov L, Brazeau GA, et al. Protective effect of estrogens against oxidative damage to heart and skeletal muscle in vivo and in vitro. Proc Soc Exp Biol Med (2000) 223:59–66. doi: 10.1046/J.1525-1373.2000.22308.X
249. Xu L, Huang G, Cong Y, Yu Y, Li Y. Sex-related differences in inflammatory bowel diseases: the potential role of sex hormones. Inflammation Bowel Dis (2022) 28:1766–75. doi: 10.1093/IBD/IZAC094
250. Klebanoff SJ. Effect of estrogens on the myeloperoxidase-mediated antimicrobial system. Infect Immun (1979) 25:153–6. doi: 10.1128/iai.25.1.153-156.1979
251. Jacenik D, Zielińska M, Mokrowiecka A, Michlewska S, Małecka-Panas E, Kordek R, et al. G protein-coupled estrogen receptor mediates anti-inflammatory action in Crohn’s disease. Sci Rep (2019) 9:1–13. doi: 10.1038/s41598-019-43233-3
252. Van Der Giessen J, van der Woude CJ, Peppelenbosch MP, Fuhler GM. A direct effect of sex hormones on epithelial barrier function in inflammatory bowel disease models. Cells (2019) 8:261. doi: 10.3390/CELLS8030261
253. Harbrecht BG, Perpetua M, Fulmer M, Zhang B. Glucagon regulates hepatic inducible nitric oxide synthesis in vivo. Shock (2004) 22:157–62. doi: 10.1097/01.SHK.0000131579.22409.33
254. Alquicer G, Kodrík D, Krishnan N, Večeřa J, Socha R. Activation of insect anti-oxidative mechanisms by mamMalian glucagon. Comp Biochem Physiol B Biochem Mol Biol (2009) 152:226–33. doi: 10.1016/J.CBPB.2008.11.007
255. Bloch K, Shichman E, Vorobeychik M, Bloch D, Vardi P. Catalase expression in pancreatic alpha cells of diabetic and non-diabetic mice. Histochem Cell Biol (2007) 127:227–32. doi: 10.1007/S00418-006-0248-4
256. Grande EM, Raka F, Hoffman S, Adeli K. GLP-2 regulation of dietary fat absorption and intestinal chylomicron production via neuronal nitric oxide synthase (nNOS) signaling. Diabetes (2022) 71:1388–99. doi: 10.2337/DB21-1053
257. Grasset E, Puel A, Charpentier J, Collet X, Christensen JE, Tercé F, et al. A specific gut microbiota dysbiosis of type 2 diabetic mice induces GLP-1 resistance through an enteric NO-dependent and gut-brain axis mechanism. Cell Metab (2017) 25:1075–1090.e5. doi: 10.1016/J.CMET.2017.04.013
258. Chang JT, Liang YJ, Hsu CY, Chen CY, Chen PJ, Yang YF, et al. Glucagon-like peptide receptor agonists attenuate advanced glycation end products-induced inflammation in rat mesangial cells. BMC Pharmacol Toxicol (2017) 18:67. doi: 10.1186/S40360-017-0172-3
259. Spielman LJ, Gibson DL, Klegeris A. Incretin hormones regulate microglia oxidative stress, survival and expression of trophic factors. Eur J Cell Biol (2017) 96:240–53. doi: 10.1016/J.EJCB.2017.03.004
260. Domae C, Nanba F, Maruo T, Suzuki T, Ashida H, Yamashita Y. Black soybean seed coat polyphenols promote nitric oxide production in the aorta through glucagon-like peptide-1 secretion from the intestinal cells. Food Funct (2019) 10:7875–82. doi: 10.1039/C9FO02050K
261. Grau-bové C, González-quilen C, Terra X, Teresa Blay M, Beltrán- Debón R, Jorba-martín R, et al. Effects of flavanols on enteroendocrine secretion. Biomolecules (2020) 10:1–14. doi: 10.3390/BIOM10060844
262. Pegah A, Abbasi-Oshaghi E, Khodadadi I, Mirzaei F, Tayebinia H. Probiotic and resveratrol norMalize GLP-1 levels and oxidative stress in the intestine of diabetic rats. Metabol Open (2021) 10:100093. doi: 10.1016/J.METOP.2021.100093
263. González-Abuín N, Martínez-Micaelo N, Blay M, Ardévol A, Pinent M. Grape-seed procyanidins prevent the cafeteria-diet-induced decrease of glucagon-like peptide-1 production. J Agric Food Chem (2014) 62:1066–72. doi: 10.1021/JF405239P
264. Cao W, Chen X, Chin Y, Zheng J, Lim PE, Xue C, et al. Identification of curcumin as a potential α-glucosidase and dipeptidyl-peptidase 4 inhibitor: Molecular docking study, in vitro and in vivo biological evaluation. J Food Biochem (2022) 46(3):e13686. doi: 10.1111/JFBC.13686
265. Ginés I, Gil-Cardoso K, Terra X, Blay Mt, Pérez-Vendrell AM, Pinent M, et al. Grape seed proanthocyanidins target the enteroendocrine system in cafeteria-diet-fed rats. Mol Nutr Food Res (2019) 63(11):e1800912. doi: 10.1002/MNFR.201800912
266. Casanova-Martí À, González-Abuín N, Serrano J, Blay MT, Terra X, Frost G, et al. Long term exposure to a grape seed proanthocyanidin extract enhances L-cell differentiation in intestinal organoids. Mol Nutr Food Res (2020) 64(16):e2000303. doi: 10.1002/MNFR.202000303
267. Arana MR, Tocchetti GN, ZecChinati F, Londero AS, Dominguez C, Perdomo V, et al. Glucagon-like peptide 2 prevents down-regulation of intestinal multidrug resistance-associated protein 2 and P-glycoprotein in endotoxemic rats. Toxicology (2017) 390:22–31. doi: 10.1016/J.TOX.2017.08.007
268. Arda-Pirincci P, Bolkent S. The role of glucagon-like peptide-2 on apoptosis, cell proliferation, and oxidant-antioxidant system at a mouse model of intestinal injury induced by tumor necrosis factor-alpha/actinomycin D. Mol Cell Biochem (2011) 350:13–27. doi: 10.1007/S11010-010-0678-0
269. Lei Q, Bi J, Wang X, Jiang T, Wu C, Tian F, et al. GLP-2 prevents intestinal mucosal atrophy and improves tissue antioxidant capacity in a mouse model of total parenteral nutrition. Nutrients (2016) 8(1):33. doi: 10.3390/NU8010033
270. Chen J, Dong JT, Li XJ, Gu Y, Cheng ZJ, Cai YK. Glucagon-like peptide-2 protects impaired intestinal mucosal barriers in obstructive jaundice rats. World J Gastroenterol (2015) 21:484–90. doi: 10.3748/WJG.V21.I2.484
271. Guan X, Stoll B, Lu X, Tappenden KA, Holst JJ, Hartmann B, et al. GLP-2-mediated up-regulation of intestinal blood flow and glucose uptake is nitric oxide-dependent in TPN-fed piglets. Gastroenterology (2003) 125:136–47. doi: 10.1016/S0016-5085(03)00667-X
272. McCarty MF, Lerner A. Perspective: prospects for nutraceutical support of intestinal barrier function. Adv Nutr (2021) 12:316–24. doi: 10.1093/ADVANCES/NMAA139
273. Li M, Weigmann B. A novel pathway of flavonoids protecting against inflammatory bowel disease: modulating enteroendocrine system. Metabolites (2022) 12(1):31. doi: 10.3390/METABO12010031
274. Abdalqadir N, Adeli K. GLP-1 and GLP-2 orchestrate intestine integrity, gut microbiota, and immune system crosstalk. Microorganisms (2022) 10(10):2061. doi: 10.3390/MICROORGANISMS10102061
275. Suo H, Feng X, Zhu K, Wang C, Zhao X, Kan J. Shuidouchi (Fermented soybean) fermented in different vessels attenuates HCl/ethanol-induced gastric mucosal injury. Molecules (2015) 20:19748–63. doi: 10.3390/MOLECULES201119654
276. Long X, Zhao X, Wang W, Zhang Y, Wang H, Liu X, et al. Protective effect of silkworm pupa oil on hydrochloric acid/ethanol-induced gastric ulcers. J Sci Food Agric (2019) 99:2974–86. doi: 10.1002/JSFA.9511
277. Leme JG, Schapoval EES. Stimulation of the hypothalamo-pituitary-adrenal axis by compounds formed in inflamed tissue. Br J Pharmacol (1975) 53:75. doi: 10.1111/J.1476-5381.1975.TB07332.X
278. Serda M, Becker FG, Cleary M, Team RM, Holtermann H, The D, et al. Insulin , glucocorticoids and the control of inflammatory responses. Agents Actions (1992) 36:99–118. doi: 10.2/JQUERY.MIN.JS
279. Lew W, Oppenheim JJ, Matsushima K. Analysis of the suppression of IL-1 alpha and IL-1 beta production in human peripheral blood mononuclear adherent cells by a glucocorticoid hormone. J Immunol (1988) 140:1895–902. doi: 10.4049/JIMMUNOL.140.6.1895
280. Snyder DS, Unanue ER. Corticosteroids inhibit murine macrophage Ia expression and interleukin 1 production. J Immunol (1982) 129:1803–5. doi: 10.4049/JIMMUNOL.129.5.1803
281. Venditti P, Di Meo S. Thyroid hormone-induced oxidative stress. Cell Mol Life Sci (2006) 63:414–34. doi: 10.1007/S00018-005-5457-9
282. Cury Y, Garcia-Leme J. The inflammatory response of hyperthyroid and hypothyroid rats. Role of adrenocortical steroids. Agents Actions (1984) 15:377–85. doi: 10.1007/BF01972375
283. Sena L, Torrielli MV, Franzone J, Curzio M, Cirillo R. The influence of experimental hypo- and hyperthyroid states on acute and chronic inflammatory reactions: modified response to non-steroidal anti-inflammatory agents. J Pathol (1981) 135:9–17. doi: 10.1002/PATH.1711350103
284. Zhao SB, Wu JY, He ZX, Song YH, Chang X, Xia T, et al. Corticotropin releasing hormone promotes inflammatory bowel disease via inducing intestinal macrophage autophagy. Cell Death Discovery (2021) 7:1–13. doi: 10.1038/s41420-021-00767-8
285. Karmiris K, Koutroubakis IE, KourouMalis EA. Leptin, adiponectin, resistin, and ghrelin–implications for inflammatory bowel disease. Mol Nutr Food Res (2008) 52:855–66. doi: 10.1002/MNFR.200700050
286. Karmiris K, Koutroubakis IE, Xidakis C, Polychronaki M, Voudouri T, KourouMalis EA. Circulating levels of leptin, adiponectin, resistin, and ghrelin in inflammatory bowel disease. Inflammation Bowel Dis (2006) 12:100–5. doi: 10.1097/01.MIB.0000200345.38837.46
287. Lord GM, Matarese G, Howard JK, Baker RJ, Bloom SR, Lechler RI. Leptin modulates the T-cell immune response and reverses starvation-induced immunosuppression. Nature (1998) 394:897–901. doi: 10.1038/29795
288. Dixit VD, Schaffer EM, Pyle RS, Collins GD, Sakthivel SK, Palaniappan R, et al. Ghrelin inhibits leptin- and activation-induced proinflammatory cytokine expression by human monocytes and T cells. J Clin Invest (2004) 114:57–66. doi: 10.1172/JCI21134
289. Sitaraman S, Liu X, Charrier L, Gu LH, Ziegler TR, Gewirtz A, et al. Colonic leptin: source of a novel proinflammatory cytokine involved in IBD. FASEB J (2004) 18:696–8. doi: 10.1096/FJ.03-0422FJE
290. Barbier M, Vidal H. Overexpression of leptin mRNA in mesenteric adipose tissue in inflammatory bowel diseases. Gastroenterol Clin Biol (2003) 27(11):987–91.
291. Franchimont D, Roland S, Gustot T, Quertinmont E, Toubouti Y, Gervy MC, et al. Impact of infliximab on serum leptin levels in patients with Crohn’s disease. J Clin Endocrinol Metab (2005) 90:3510–6. doi: 10.1210/JC.2004-1222
292. Kern PA, Di Gregorio GB, Lu T, Rassouli N, Ranganathan G. Adiponectin expression from human adipose tissue: relation to obesity, insulin resistance, and tumor necrosis factor-alpha expression. Diabetes (2003) 52:1779–85. doi: 10.2337/DIABETES.52.7.1779
293. Wolf AM, Wolf D, Rumpold H, Enrich B, Tilg H. Adiponectin induces the anti-inflammatory cytokines IL-10 and IL-1RA in human leukocytes. Biochem Biophys Res Commun (2004) 323:630–5. doi: 10.1016/J.BBRC.2004.08.145
294. Nakanishi S, Yamane K, Kamei N, Nojima H, Okubo M, Kohno N. A protective effect of adiponectin against oxidative stress in Japanese Americans: The association between adiponectin or leptin and urinary isoprostane. Metabolism (2005) 54:194–9. doi: 10.1016/j.metabol.2004.08.012
295. Soares AF, Guichardant M, Cozzone D, Bernoud-Hubac N, Bouzaïdi-Tiali N, Lagarde M, et al. Effects of oxidative stress on adiponectin secretion and lactate production in 3T3-L1 adipocytes. Free Radic Biol Med (2005) 38:882–9. doi: 10.1016/J.FREERADBIOMED.2004.12.010
296. Kim K, Kim JK, Han SH, Lim J-S, Kim K, DH C, et al. Adiponectin is a negative regulator of NK cell cytotoxicity. J Immunol (2006) 176:5958–64. doi: 10.4049/JIMMUNOL.176.10.5958
297. Nishihara T, Matsuda M, Araki H, Oshima K, Kihara S, Funahashi T, et al. Effect of adiponectin on murine colitis induced by dextran sulfate sodium. Gastroenterology (2006) 131:853–61. doi: 10.1053/J.GASTRO.2006.06.015
298. Yamamoto K, Kiyohara T, Murayama Y, Kihara S, Okamoto Y, Funahashi T, et al. Production of adiponectin, an anti-inflammatory protein, in mesenteric adipose tissue in Crohn’s disease. Gut (2005) 54:789–96. doi: 10.1136/GUT.2004.046516
299. Paul G, Schäffler A, Neumeier M, Fürst A, Bataillle F, Buechler C, et al. Profiling adipocytokine secretion from creeping fat in Crohn’s disease. Inflammation Bowel Dis (2006) 12:471–7. doi: 10.1097/00054725-200606000-00005
300. De Smet B, Thijs T, Moechars D, Colsoul B, Polders L, Ver Donck L, et al. Endogenous and exogenous ghrelin enhance the colonic and gastric manifestations of dextran sodium sulphate-induced colitis in mice. Neurogastroenterol Motil (2009) 21:59–70. doi: 10.1111/J.1365-2982.2008.01184.X
301. Gonzalez-Rey E, Chorny A, Delgado M. Therapeutic action of ghrelin in a mouse model of colitis. Gastroenterology (2006) 130:1707–20. doi: 10.1053/J.GASTRO.2006.01.041
302. Peracchi M, Bardella MT, Caprioli F, Massironi S, Conte D, Valenti L, et al. Circulating ghrelin levels in patients with inflammatory bowel disease. Gut (2006) 55:432–3. doi: 10.1136/GUT.2005.079483
303. Hontecillas R, Horne WT, Climent M, Guri AJ, Evans C, Zhang Y, et al. Immunoregulatory mechanisms of macrophage PPAR γ in mice with experimental inflammatory bowel disease. Mucosal Immunol (2011) 4:304. doi: 10.1038/MI.2010.75
304. Mohapatra SK, Guri AJ, Climent M, Vives C, Carbo A, Horne WT, et al. Immunoregulatory actions of epithelial cell PPAR γ at the colonic mucosa of mice with experimental inflammatory bowel disease. PloS One (2010) 5(4):e10215. doi: 10.1371/JOURNAL.PONE.0010215
305. Dubuquoy L, Rousseaux C, Thuru X, Peyrin-Biroulet L, ROmano O, Chavatte P, et al. PPARgamma as a new therapeutic target in inflammatory bowel diseases. Gut (2006) 55:1341–9. doi: 10.1136/GUT.2006.093484
306. Lewis JD, Lichtenstein GR, Deren JJ, Sands BE, Hanauer SB, Katz JA, et al. Rosiglitazone for active ulcerative colitis: A randomized placebo-controlled trial. Gastroenterology (2008) 134:688. doi: 10.1053/J.GASTRO.2007.12.012
307. Lund JL, Stürmer T, Porter CQ, Sandler RS, Kappelman MD. Thiazolidinedione Use and Ulcerative Colitis-related Flares: An exploratory analysis of administrative data. Inflammation Bowel Dis (2011) 17:787. doi: 10.1002/IBD.21348
308. Allegra M, Reiter RJ, Tan DX, Gentile C, Tesoriere L, Livrea MA. The chemistry of melatonin’s interaction with reactive species. J Pineal Res (2003) 34:1–10. doi: 10.1034/J.1600-079X.2003.02112.X
309. Hardeland R, Pandi-Perumal SR. Melatonin, a potent agent in antioxidative defense: Actions as a natural food constituent, gastrointestinal factor, drug and prodrug. Nutr Metab (2005) 2:1–15. doi: 10.1186/1743-7075-2-22
310. Ma X, Idle JR, Krausz KW, Gonzalez FJ. Metabolism of melatonin by human cytochromes p450. Drug Metab Dispos (2005) 33:489–94. doi: 10.1124/DMD.104.002410
311. Fischer TW, Kleszczyński K, Hardkop LH, Kruse N, Zillikens D. Melatonin enhances antioxidative enzyme gene expression (CAT, GPx, SOD), prevents their UVR-induced depletion, and protects against the formation of DNA damage (8-hydroxy-2’-deoxyguanosine) in ex vivo human skin. J Pineal Res (2013) 54:303–12. doi: 10.1111/JPI.12018
312. Rodriguez C, Mayo JC, Sainz RM, Antolín I, Herrera F, Martín V, et al. Regulation of antioxidant enzymes: a significant role for melatonin. J Pineal Res (2004) 36:1–9. doi: 10.1046/J.1600-079X.2003.00092.X
313. Hardeland R. Antioxidative protection by melatonin: multiplicity of mechanisms from radical detoxification to radical avoidance. Endocrine (2005) 27:119–30. doi: 10.1385/ENDO:27:2:119
314. Urata Y, Honma S, Goto S, Todoroki S, Iida T, Cho S, et al. Melatonin induces gamma-glutamylcysteine synthetase mediated by activator protein-1 in human vascular endothelial cells. Free Radic Biol Med (1999) 27:838–47. doi: 10.1016/S0891-5849(99)00131-8
315. Winiarska K, Fraczyk T, Malinska D, Drozak J, Bryla J. Melatonin attenuates diabetes-induced oxidative stress in rabbits. J Pineal Res (2006) 40:168–76. doi: 10.1111/J.1600-079X.2005.00295.X
316. Reiter RJ, Tan DX, Rosales-Corral S, Galano A, Zhou XJ, Xu B. Mitochondria: central organelles for melatonin′s antioxidant and anti-aging actions. Molecules (2018) 23:509. doi: 10.3390/MOLECULES23020509
317. Fernandez-Gil B, Abdel Moneim AE, Ortiz F, Shen YQ, Soto-Mercado V, Mendivil-Perez M, et al. Melatonin protects rats from radiotherapy-induced small intestine toxicity. PloS One (2017) 12:e0174474. doi: 10.1371/JOURNAL.PONE.0174474
318. Dong WG, Mei Q, Yu JP, Xu JM, Xiang L, Xu Y. Effects of melatonin on the expression of iNOS and COX-2 in rat models of colitis. World J Gastroenterology: WJG (2003) 9:1307. doi: 10.3748/WJG.V9.I6.1307
319. Trivedi PP, Jena GB. Melatonin reduces ulcerative colitis-associated local and systemic damage in mice: Investigation on possible mechanisms. Dig Dis Sci (2013) 58:3460–74. doi: 10.1007/S10620-013-2831-6/METRICS
320. Moura FA, de Andrade KQ, dos Santos JCF, Araújo ORP, Goulart MOF. Antioxidant therapy for treatment of inflammatory bowel disease: Does it work? Redox Biol (2015) 6:617–39. doi: 10.1016/J.REDOX.2015.10.006
Keywords: antioxidants, ulcerative colitis, Crohn’s disease, IBD, oxidative stress, flavonoids, polyphenols, hormones
Citation: Sahoo DK, Heilmann RM, Paital B, Patel A, Yadav VK, Wong D and Jergens AE (2023) Oxidative stress, hormones, and effects of natural antioxidants on intestinal inflammation in inflammatory bowel disease. Front. Endocrinol. 14:1217165. doi: 10.3389/fendo.2023.1217165
Received: 04 May 2023; Accepted: 07 August 2023;
Published: 28 August 2023.
Edited by:
Sandhya Srikant Visweswariah, Indian Institute of Science (IISc), IndiaReviewed by:
Chittur Srikanth, Regional Centre for Biotechnology (RCB), IndiaAnika E. Wagner, University of Giessen, Germany
Copyright © 2023 Sahoo, Heilmann, Paital, Patel, Yadav, Wong and Jergens. This is an open-access article distributed under the terms of the Creative Commons Attribution License (CC BY). The use, distribution or reproduction in other forums is permitted, provided the original author(s) and the copyright owner(s) are credited and that the original publication in this journal is cited, in accordance with accepted academic practice. No use, distribution or reproduction is permitted which does not comply with these terms.
*Correspondence: Dipak Kumar Sahoo, ZGlwYWtzYWhvbzExQGdtYWlsLmNvbQ==; ZHNhaG9vQGlhc3RhdGUuZWR1; Albert E. Jergens, YWplcmdlbnNAaWFzdGF0ZS5lZHU=