- 1GMU-GIBH Joint School of Life Sciences, The Guangdong-Hong Kong-Macau Joint Laboratory for Cell Fate Regulation and Diseases, Guangzhou Laboratory, Guangzhou Medical University, Guangzhou, China
- 2Zhongshan School of Medicine, Sun Yat-Sen University, Guangdong, China
Thermogenic fat, consisting of brown and beige adipocytes, dissipates energy in the form of heat, in contrast to the characteristics of white adipocytes that store energy. Increasing energy expenditure by activating brown adipocytes or inducing beige adipocytes is a potential therapeutic strategy for treating obesity and type 2 diabetes. Thus, a better understanding of the underlying mechanisms of thermogenesis provides novel therapeutic interventions for metabolic diseases. In this review, we summarize the recent advances in the molecular regulation of thermogenesis, focusing on transcription factors, epigenetic regulators, metabolites, and non-coding RNAs. We further discuss the intercellular and inter-organ crosstalk that regulate thermogenesis, considering the heterogeneity and complex tissue microenvironment of thermogenic fat.
Introduction
Obesity is a chronic and complex condition resulting from an imbalance of excessive energy intake and insufficient energy expenditure, and it is tightly associated with type 2 diabetes, cardiovascular disease, nonalcoholic fatty liver disease (NAFLD), and other metabolic diseases (1). Adipose tissue is a metabolically active organ with significant roles in regulating whole-body energy homeostasis, whose dysfunction causes obesity and related metabolic disorders. Mammals have been shown to possess two classes of fat cells—white and thermogenic adipocytes. White adipocyte contains a large lipid droplet and a few mitochondria and plays an essential role in energy storage in triglycerides. In contrast, thermogenic adipocytes possess multilocular lipid droplets and higher amounts of mitochondria and dissipate energy in the form of heat.
Thermogenic adipocytes consist of brown adipocytes and beige adipocytes. Brown adipocytes are characterized by marker gene uncoupling protein 1 (Ucp1), which uncouples oxidative respiration from ATP synthesis, resulting in energy dissipation as heat (2). The brown adipose tissue (BAT) is predominantly located in the interscapular region of infants and rodents. UCP1-positive multilocular adipocytes were also found in cervical and supraclavicular regions in human adults using positron-emission tomography and computed tomography (PET/CT) imaging (3, 4). Importantly, BAT activity is inversely correlated with body mass index (BMI) and age in humans (5, 6). Moreover, Ucp1-deficient mice gain more weight than wild-type mice under thermoneutral conditions (7, 8), while transplantation of mouse BAT or CRISPR-enhanced human or mouse brown-like adipocytes improves glucose tolerance and insulin sensitivity in recipient mice (9, 10). These data suggest the importance of BAT in regulating energy metabolism and homeostasis both in mice and humans. In regard to beige adipocytes, they are predominantly spread in inguinal white adipose tissue (iWAT), and induced in response to cold environment, exercise training or activation of β-adrenergic receptors (β-AR) in mice (11). Intriguingly, the gene profile of mouse beige adipocyte is very similar to that of human BAT in the supraclavicular region during cold exposure (12). Induction of browning in iWAT by transgenic expression of PR domain-containing 16 (Prdm16) increases Ucp1 mRNA level and protects the mice from diet-induced obesity (13). Therefore, inducing the formation of beige adipocytes may serve as an alternative therapeutic strategy for combating obesity and metabolic diseases.
In this review, we summarize the cell autonomous and non-cell autonomous regulation of the biogenesis and function of thermogenic fat, which will facilitate the development of new therapies for metabolic diseases.
Molecular regulations of thermogenesis of brown and beige adipocytes
Brown adipocyte and beige adipocyte share similar functions in energy expenditure and thermogenesis, and various molecular events involve in the cell fate determination of thermogenic fat and thermogenesis, including transcriptional regulation, epigenetic modulation, non-coding RNA regulation and metabolic reprogramming (Figure 1).
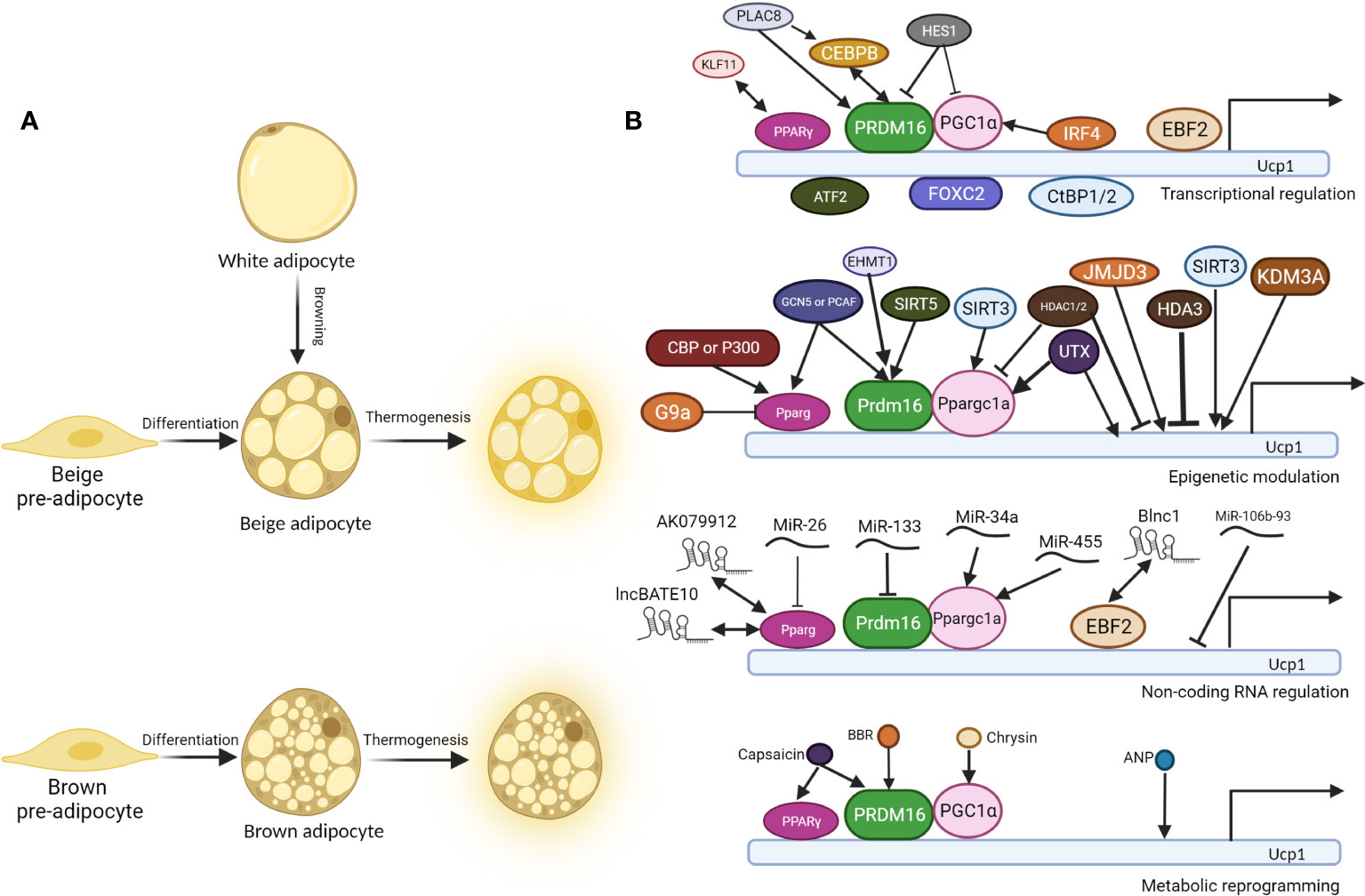
Figure 1 Molecular regulation of thermogenesis of brown and beige adipocytes. (A). Beige pre-adipocyte and brown pre-adipocyte differentiate into beige adipocyte and brown adipocyte respectively. In specific conditions, white adipocytes convert into beige adipocytes, a process called “browning”. Under cold exposure or other signal induction, differentiated brown and beige adipocytes undergo thermogenesis, accompanied by higher glucose and fatty acid uptake, UCP1 expression, and uncoupled respiration. (B). Regulatory mechanisms behind thermogenesis of brown and beige adipocytes including the following 4 parts: 1. Transcriptional regulation; 2. Epigenetic modulation; 3. Non-coding RNA regulation; 4. Metabolic reprogramming. UCP1 is one of the most critical thermogenic genes, and its expression is critical for uncoupled cellular respiration. There are three core regulators in the thermogenesis program regulation: PPARγ, PRDM16, and PGC1α, and most other regulators regulate thermogenesis through them. Double-headed arrows indicate protein interaction and complex formation, while arrow-headed and bar-headed lines show inducing and inhibiting effects.
Transcriptional regulation of thermogenesis in brown and beige adipocytes
The cell fate determination of thermogenic fat is regulated by various adipocyte-specific lineage-determining transcription factors and co-factors as shown in Table 1. There are three core regulators in the regulation of thermogenesis of beige and brown adipocyte, proliferator-activated receptor γ(PPARγ), PRDM16 and peroxisome proliferator-activated receptor γ coactivator 1 α (PGC1α). PPARγ was indispensable for the function of both white and brown adipocytes. PPARγ ligands induce the browning of white adipocytes with the cooperation of PRDM16 (30). PRDM16 is highly expressed in brown adipocyte cells, and overexpression of PRDM16 leads to the browning of white adipocytes. Consistently, knock down of PRDM16 causes to the loss of brown fat cell identity (32). PGC1α also plays essential roles in energy metabolism and homeostasis. Although mice without PGC1α underwent normal brown fat differentiation, it accompanied with decreased thermogenic genes induction (25).
As will discussed in more details below, there are more than 30 transcriptional regulators identified to positively or negatively regulate the formation and function of beige and brown adipocytes, and most of them function through the above three core regulators. CCAAT enhancer-binding protein beta (C/EBPβ) forms a transcriptional complex with PRDM16 to induce brown fat cell determination and differentiation (16). In contrast, CCAAT enhancer-binding protein alpha (C/EBPα) acts collaboratively with other corepressors C-terminal-binding protein 1/2 (CtBP1/2) to repress the expression of white fat genes (15). Early B-cell factor 2 (EBF2), a selective marker of brown and beige precursors (50), regulates the cell fate determination of brown fat precursor cells and the expression of thermogenic genes (17). Brown adipocytes isolated from mice with Ebf2 deficiency exhibit diminished mitochondrial density and larger lipid droplets (51). Interferon regulatory factor 4 (IRF4), which is induced by cold and cAMP, interacts with PGC1α to promote the expression of PRDM16 and then drive the expression of thermogenic genes (20). Claussnitzer et al. found that rs1421085 T-to-C single-nucleotide variant disrupts the function of AT-rich interative domain-containing protein 5B (ARID5B) that repress the expression of Iroquois homeobox protein 3 (IRX3) and Iroquois homeobox protein 5 (IRX5), which further result in a shift from beige adipocytes to white adipocytes (21). Loft et al. reported that kruepple-like factor 11 (KLF11), which is induced by PPARγ agonists, acts in cooperation with PPARγ to activate beige-selective gene program (23). Zinc finger transcription factors also play important roles in thermogenesis. Gupta et al. reported that zinc finger protein 423 (Zfp423) expression is enriched in white adipocytes compared to brown adipocytes and is repressed upon cold exposure (46). Zfp423 inhibits the activity of EBF2 and suppress PRDM16 activation to maintain white adipocyte identity, and loss of adipocyte Zfp423 induces an EBF2 NuRD-to-BAF coregulator switch and promotes thermogenic genes (47). Dempersmier et al. stated that zinc finger protein 516 (Zfp516) directly binds to the proximal region of the Ucp1 promoter and activates its expression to induce white fat cell browning and the development of brown fat cells (49). Taken together, the formation and function of thermogenic fat greatly rely on a complex transcriptional network coordinated by a set of core transcriptional factors.
Epigenetic modulation behind thermogenesis of brown and beige adipocytes
Adipogenesis is involved with complicated epigenetic remodeling that mainly include histone modification and DNA methylation, the two fundamental processes that play crucial roles in the regulation of gene expression and genome stability. In general, Histone modifications modulate chromatin structure, influencing gene accessibility and transcriptional activity, while DNA methylation directly modifies the DNA sequence, leading to gene silencing. A lot of studies have demonstrated the roles of epigenetic modulators in regulating the formation and function of thermogenic adipocytes (Table 2). In this review, we specifically focused on the role of histone modification, including histone acetylation, histone deacetylation, histone methylation, and histone demethylation.
Epigenetic modulators catalyze the formation of active epigenetic markers in the regulatory regions of corresponding genes to positively regulate their expression. CREB binding protein (CBP) and histone acetyltransferase p300 (P300), which catalyze histone acetylation of H3K27, improve the expression of PPARγ and then promote adipocyte differentiation and white adipocyte browning (52). General control of amino acid synthesis 5-like 2 (GCN5) and P300/CBP-associated factor (PCAF), which acetylate histone H3K9, also facilitate brown adipogenesis through positively regulating the expression of Pparγand Prdm16 (53).
In regard to histone deacetylation, epigenetic modulators erase pre-settled active epigenetic marker at the regulatory regions of thermogenic genes to negatively regulate their expression. Histone deacetylases (HDAC1, HDAC2, HDAC3, HDAC9 and HDAC11) exert their influences on thermogenesis through deacetylation of H3K27ac (79). HDAC1 and HDAC2 negatively regulate brown adipocyte thermogenic program through decreasing acetylation of histone H3 lysine 27, an active epigenetic marker, on the promoter regions of Ucp1 and Pgc1α to inhibit their expression (54). Ferrari et al. showed HDAC3 deletion induce WAT browning through increased H3K27ac modification at the enhancer region of Pparγ and Ucp1 (55). However, other study revealed that HDAC3 primes Ucp1 and the thermogenic transcriptional program to maintain the brown adipose tissue identity through deacetylation of PGC1α by HDAC3 (80). Bagchi et al. reported that HDAC11 suppresses WAT browning through physical association with bromodomain-containing protein 2 (BRD2) (57). Other histone deacetylases, including NAD-dependent protein deacetylases-SIRT1, SIRT2, SIRT3, SIRT5, SIRT6, and SIRT7, catalyze the deacetylation of H3K9ac, and/or H4K16ac (58, 81, 82). Shi et al. found that SIRT3 positively correlated with the expression of Pgc1α and Ucp1, and SIRT3 activates mitochondria functions and adaptive thermogenesis in brown adipose (61). Shuai et al. found SIRT5 promoted the browning of subcutaneous white adipose tissue through regulating H3K9me2 and H3K9me3 modification at the promoter regions of Pparγ and Prdm16 (62). Moreover, ten-eleven translocation (TET) proteins, oxidize 5-methylcytosines and promote specific DNA demethylation (83), were found to inhibit β3-AR dependent thermogenic genes’ expression and white fat browning through indirectly recruiting histone deacetylases to the promoter regions of concerning genes (63).
Histone methylation exerts essential roles in regulating chromatin functional states and usually includes two types of amino acids modification, lysine methyl-transferation and arginine methyl-transferation. Several studies have linked histone methylation with thermogenesis (79). Euchromatic histone methyltransferase 1 (EHMT1), which could catalyze methylation of histone 3 lysine 9 (H3K9me2 and me3), promotes adaptive thermogenesis through stabilizing PRDM16 protein (67). Lysine methyltransferase 5C (KMT5C), a H4K20 methyltransferase, positively regulates thermogenesis through regulating the expression of transformation related protein 53 (Trp53), a repressor of thermogenic program (69). DOT1-like (DOT1L), a lysine 79 of histone H3 (H3K79) methyltransferase, inhibits thermogenic adipocyte differentiation and function through repressing the expression of brown adipocyte tissue-selective genes (70).
Histone demethylases catalyze histone demethylation that usually correlates with enhanced adipogenesis and white adipocyte browning. LSD1, lysine-specific demethylase 1, increases the content of beige adipocytes in aging inguinal white adipose tissue through activating the expression of proliferator-activated receptor alpha (Pparα) (71). Similarly, lysine-specific demethylase 2 (LSD2) plays its vital roles primarily at the early stage of brown adipocyte differentiation, and its deletion in vivo was accompanied with compromised expression of thermogenic genes (72). Tateishi et al. demonstrated lysine-specific demethylase 3A (KDM3A) positively regulates Pparα and Ucp1 expression, and KDM3A-deficient mice developed obesity and hyperlipidemia (74). Pan et al. revealed that JmjC domain-containing protein 3 (JMJD3) demethylases repressive mark H3K27me3 at the promoter regions of Ucp1 and Cell death-inducing DFFA-like effector a (Cidea) in order to activate thermogenic program and induce white adipocyte browning (77). Moreover, UTX, ubiquitously transcribed tetratricopeptide repeat on chromosome X, catalyzes demethylation of H3K27me2/3 at the promoter region of Ucp1 and Pgc1α to positively regulate their expression and promote brown adipocyte thermogenic genes expression (78). Altogether, various epigenetic remodelers act through altering histone acetylation and methylation dynamics to regulate the thermogenic program in response to the external stimuli.
Non-coding RNAs regulation of thermogenesis of brown and beige adipocytes
Non-coding RNAs, including microRNAs (miRNAs) and long non-coding RNAs (lncRNAs), play important roles in the development and physiology of white, brown and beige adipocytes, and non-coding RNAs themselves can serve as markers of different adipocyte tissue depots (Table 3).
miRNAs usually exert their functions on regulating thermogenesis through complementary reaction with the UTR regions of mRNA transcripts of effector genes. MiR-26 is upregulated during human adipogenesis and induces brown adipocyte differentiation through directly targeting ADAM metallopeptidase domain 17 (ADAM17) (84). MiR-30b/c target 3’UTR of receptor-interacting protein 140 (RIP140), a negative regulator of thermogenic genes, to promote brown adipose tissue function and the development of beige fat (91). MiR-32 is highly expressed during cold exposure, and increases fibroblast growth factor 21 (Fgf21) expression through repressing the expression of transducer of ErbB-2.1 (Tob1), which further promotes white fat cell browning and BAT thermogenesis (92). Ge et al. showed miR-34a inhibits white adipocytes browning through targeting fibronectin type III domain-containing protein 5 (Fndc5) expression (93), while Fu et al. demonstrated miR-34a promotes the deacetylation of PGC1α and its activation by targeting fibroblast growth factor receptor 1 (FGFR1), klotho beta-like protein (βKL) and NAD-dependent protein deacetylase sirtuin-1 (SIRT1) (94). MiR-106b-93 cluster negatively regulate the expression of Ucp1 and promote the lipid content in differentiated brown adipocytes (95). Giroud et al. reported miR-125b prevents beige adipocyte formation through decreasing mitochondrial biogenesis (96). miR-133 targets 3’ UTR of Prdm16 to repress its expression that lead to impaired brown fat differentiation and WAT browning (97, 98). MicroRNA 155 is down-regulated during brown preadipocyte differentiation and inhibition of miR-155 enhances brown adipocyte differentiation and white adipocytes browning. Mechanistically, miR-155 forms a bistable feedback loop with CEBP-β (99). MiR-193b–365, referred to as miR-193b and miR-365, showed two contradictory results, that Sun et al. found that blocking of miR-193b–365 impair brown adipocyte adipogenesis by upregulating the expression of runt-related transcriptional factor 1 translocation partner 1 (Runx1t1) (102), while Feuermann et al. reported that miR-193b–365 are not required for the differentiation and development of BAT (103). The detailed roles of miR-193b–365 in vivo and in vitro need to be further clarified.
The regulation of lncRNAs in the thermogenesis of brown and beige adipocytes are mainly through interacting with other important transcription factors such as PGC1α, EBF2, and PPARγ (113). Recent study identified Blnc1 as a vital lncRNA in promoting the function of brown and beige adipocytes, and then further experiments demonstrated Blnc1 acts synergistically with EBF2 to drive thermogenic gene program (108). Similarly, lncRNA-AK079912 was also reported to play a positive role in brown preadipocyte differentiation and white adipocytes browning, which is mediated by PPARγ (109). A brown adipose tissue-enriched lncRNA, lncBATE10, was found to be differently regulated in cold or exercise conditions, and it regulates brown adipose tissue gene program through decoying the repressor factor-CUGBP Elav-like family member 1 (CELF1) from Pgc1α’s mRNA elements (110). In together, the influences of lncRNAs on the regulatory network of brown and beige adipocytes differentiation remain elusive, and especially their direct roles in affecting core transcriptional factors of thermogenic program need to be further elucidated. In summary, miRNAs and lncRNAs, the tight regulators of gene expression, play an indispensable role in regulating brown and beige adipogenesis, which further complicates the regulatory network of thermogenesis.
Metabolic reprogramming behind thermogenesis of brown and beige adipocytes
The development and function of thermogenic fat involves intensive metabolic reprogramming (114). Table 4 summarized the nutrients and metabolites that regulates thermogenesis. Notably, most of the studies were conducted in rodent models and their implications in human need to be further explored.
Wu et al. reported that NAFLD patients treated with Berberine (BBR) for 1 month exhibited increased brown adipocyte mass and activity in mice, since BBR promotes the DNA demethylation of Prdm16 promoter to activate its expression (117). Dietary capsaicin induces white adipocyte browning through facilitating the interaction and activation of PPARγ and PRDM16, depending on transient receptor potential vanilloid 1 (TRPV1) channels (119). Chlorogenic acid (CGA), a Chinese traditional medicine, induces brown adipocyte thermogenesis through promoting mitochondria function and glucose uptake (121). Lone et al. and Wang et al. demonstrated that curcumin promotes browning of white adipocytes through upregulating Ucp1 expression (125, 126). Ellagic Acid (EA), located mainly in fruits and plant extracts, also increases iWAT browning through decreasing the expression of Zfp423 and aldehyde dehydrogenase family 1 member a1 (Aldh1a1) and increasing thermogenic genes expression (128). Epicatechin (Epi), a cacao flavanol, can induce white adipose tissue browning through improving mitochondrial function and upregulating the expression of key thermogenic genes (131).
Apart from the aforementioned nutrients and small molecules that regulate thermogenesis of brown and beige adipocytes, there are other metabolites performing the similar functions, including flavan-3-Alcohol, fucoxanthin, irisin, leptin, luteolin, Menthol Neuregulin 4 (Nrg4), Prostaglandin (PG), Purple Sweet Potato (PSP), Quercetin, Resveratrol, Rice Bran, Sesamol, Taurine, Telmisartan, and 3-Hydroxydaidzein (134, 135, 137–140, 142–153, 155), which will be discussed in details in the below sections.
Intercellular communications within thermogenic fat
As extensively discussed in a recent review (156), thermogenic fat consists of various cell types or cell states in stromal vascular fractions (SVFs) and mature adipocytes, identified by state-of-art single-cell RNA-sequencing (scRNA-seq) or single nuclei RNA-sequencing (snRNA-seq) in mice (157–165) and humans (157, 162, 165–168). These subpopulations of thermogenic fat, including immune cells, endothelial cells, neurons, smooth muscle cells, Schwann cells, and a few other cell types, create a unique adipose niche and regulate adipose tissue function, such as thermogenic fat turnover, expansion, and remodeling (156). Here we focus on the intercellular crosstalk between thermogenic fat cells and endothelial cells, immune cells, and neurons (Figure 2).
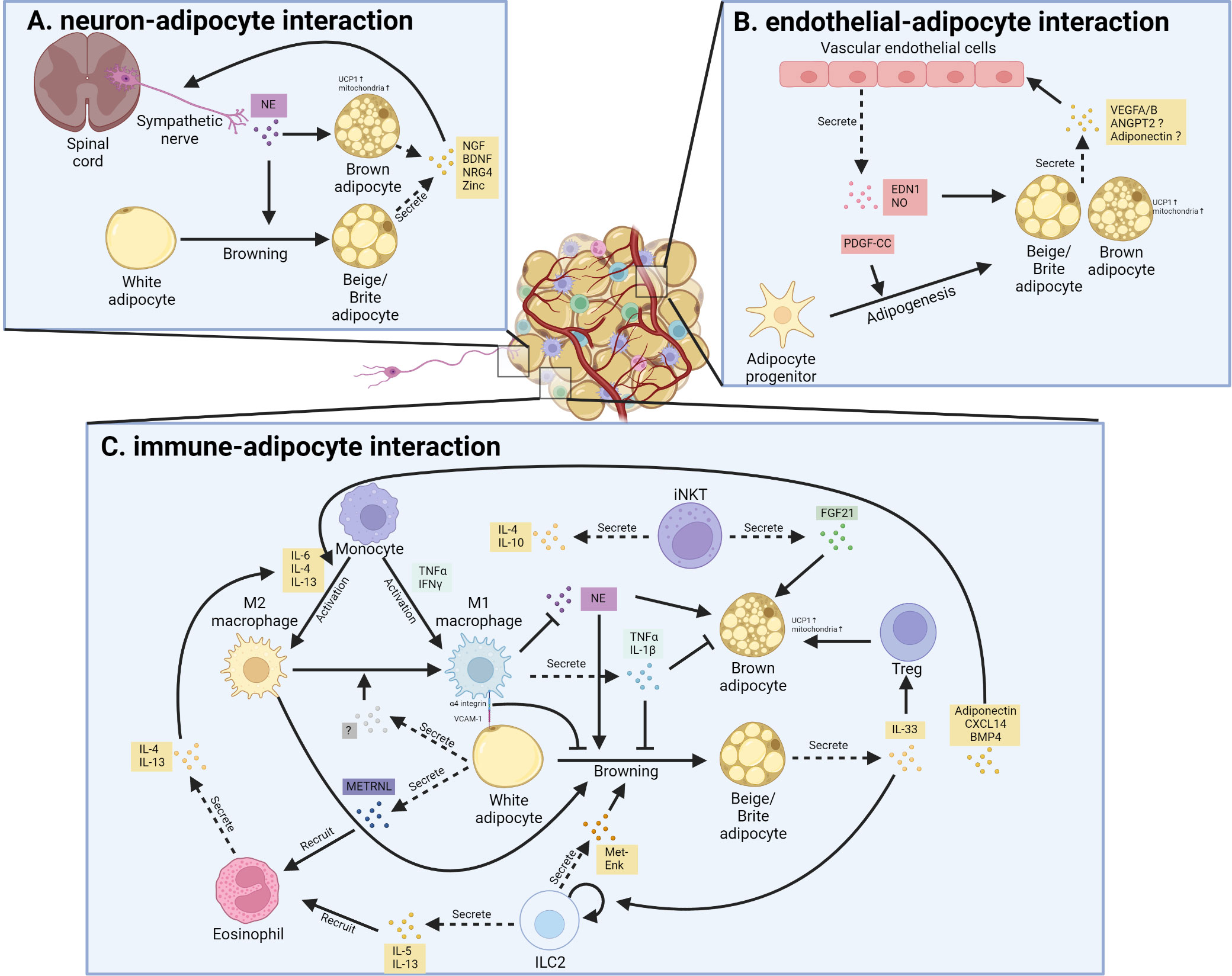
Figure 2 Cellular interaction between thermogenic adipocytes and resident cells. (A). Interaction between sympathetic nerve and thermogenic adipocyte. Sympathetic nerve secretes norepinephrine (NE) that promotes white adipocyte browning and brown adipocyte activation; in turn, beige adipocytes and brown adipocytes promote nerve remodeling through secreting neurotrophic factor, including nerve growth factor (NGF), brain-derived neurotrophic factor (BDNF), neuregulin-4 (NRG4) as well as Zinc. (B). Interaction between vascular endothelial cells and thermogenic adipocyte. Vascular endothelial cells secrete endothelin 1 (EDN1) and nitric oxide (NO) to promote the thermogenic function of brown and beige adipocytes. Besides, the secreted EDN1 and platelet-derived growth factor C (PDGF-C) also regulate the adipogenesis of preadipocytes. Reciprocally, thermogenic adipocytes and their progenitors secrete several factors that promote angiogenesis in adipose tissue. ANGPT2, angiopoietin 2; VEGF, vascular endothelial growth factor. (C). Interaction between resident immune cells and thermogenic adipocytes. Various cytokines and signals mediate the bi-directional communication between thermogenic fat and different kinds of immune cells.
Endothelial cells in the thermogenic adipose tissue
Adipose tissue, especially BAT, is one of the most vascularized tissues in the body (169). A lot of stimuli, including cold, diet, exercise, and nutrition state, modulate angiogenesis and vascular remodeling in adipose tissue. Vascular Endothelial Growth Factor A (VEGFA) and Vascular Endothelial Growth Factor B (VEGFB) are two important angiogenic factors in adipose tissue in response to cold or β3-AR activation. BAT-specific overexpression of VEGFA increases vascularization and improves thermogenesis in mice after cold exposure, and protects mice against diet-induced obesity (170). Similarly, VEGFB promotes the proliferation of endothelial cells and fatty lipid oxidation in thermogenic fat in mice, providing a novel cure strategy for obesity and diabetes diseases (171). Besides, Seki et al. revealed that endothelial-specific Vegfr2-/- mice showed impaired angiogenesis as well as reduced browning of iWAT, which is modulated through the endothelial cells-derived platelet-derived growth factor-CC (PDGF-CC)-induced signaling pathway, since administration of PDGF-CC upregulated the expression level of Ucp1 and promoted browning of iWAT both in mice and humans (172). Endothelial cells-secreted endothelin 1 (EDN1) and nitric oxide inhibit biogenesis and the function of brown and beige adipocytes in vitro (173, 174). In contrast, endothelial deficiency of lysosomal acid lipase (LAL) impairs vascularization and thermogenesis in BAT and WAT (175). The decreased production of vasodilatory factors and increased vasoconstricting factors production, due to dysfunction of endothelial cells, lead to insulin resistance and diabetes (176). The diverse functions of endothelial cells suggest the existence of different subpopulations. Indeed, Sun et al. observed two distinct types of endothelial cells in human deep-neck BAT using scRNA-seq (162). Vijay et al. also identified three types of endothelial cells in human WAT, with the largest population of endothelial cells defined as fatty-acid-handling microvascular endothelial cells and another subpopulation was lymphatic-derived (167). However, delineating the exact role of each subpopulation of endothelial cells in thermogenic fat needs further investigation. Taken together, these bidirectional communications between thermogenic fat and endothelial cells maintain the adipose homeostasis, and dysfunction of them cause metabolic disorders.
Immune cells in the thermogenic adipose tissue
Several types of immune cells reside in adipose tissue, including macrophages, natural killer (NK) cells, lymphocytes, dendritic cells, neutrophils, eosinophils, T cells, and mast cells, which play an important role in regulating metabolic homeostasis (177, 178). The adipose immune cells composition is highly variable in response to the nutritional status, as well as environmental stimuli (179).
Among the immune cells that infiltrate into obese adipose tissue, macrophages are functionally and numerically dominant. Activated macrophages are divided into two main categories, M1 macrophages and M2 macrophages. M1 macrophages produce pro-inflammatory cytokines and chemokines, while M2 macrophages secrete anti-inflammatory cytokines that alleviate inflammation. Several studies show that activated M1-like macrophages facilitate the infiltration of other immune cells into obese adipose tissues and impairs insulin sensitivity (180). In detail, studies identified TNFα as a pro-inflammatory cytokine produced from M1 macrophages that suppresses the emergence of thermogenic adipocytes in mice (181). It was also reported that the direct contact between M1 macrophage and white adipocyte could inhibit the browning process as well as Ucp1 expression in iWAT of mice, mainly though the direct adhesion between α4-integrin in activated M1 macrophage and vascular cell adhesion molecule 1 (Vcam-1) in adipocytes (182). In contrast to M1 macrophages, M2 macrophages exert positive effects on brown adipocyte activity and WAT browning (183). Signal transducer and activator of transcription 6 (Stat6)-deficient or macrophage-specific interleukin-4 receptor α (Ilr4α) knockout mice exhibited impaired BAT thermogenic response, suggesting the positive role of M2 macrophages in BAT thermogenesis, which is further supported by the specific depletion of Ilr4α in myeloid cells of mice (184, 185). M2 macrophages could produce catecholamine to sustain adaptive thermogenesis, which may also reflect the situations in WAT browning, as similar recruitment of M2 macrophages were also found in iWAT of cold-induced mice (185, 186). Another study demonstrated that a fraction of M1 macrophages were concentrated around the sympathetic nerve endings in the adipose tissue of obese people (187). Such macrophages are called sympathetic neuron-associated macrophages (SAM), which can transport catecholamine released from sympathetic nerve endings into the cell body and degrade it through monoamine oxidase A, thereby inhibiting the browning of iWAT induced by sympathetic nerve in obese mice (187, 188). Mutually, thermogenic fat could also secrete batokines to regulate the activation and function of macrophages. CXC Motif Chemokine Ligand 14 (CXCL14), one of the batokines secreted by brown adipocytes, promotes the M2 macrophage phenotype in adipose tissue and leads to WAT browning, and Cxcl14-deficient mice show impaired BAT activity and altered glucose homeostasis in response to cold exposure (189). Adiponectin is another adipokine that promotes the activation of M2 macrophages and then results in cold-induced browning of WAT in mice (190). Adipose-secreted bone morphogenetic protein 4 (BMP4) also increase the accumulation of M2 macrophages and induce beige fat biogenesis in iWAT of mice (191). Moreover, adipocytes deficient in fatty acid synthase (iAdFASNKO) show increased macrophage polarization, and ablation of macrophage from iWAT in iAdFASNKO mice inhibit beige adipogenesis (161).
Innate lymphoid type 2 cells (ILC2s), another group of adipose resident immune cells, also activate M2 macrophage and regulate thermogenesis in brown and beige adipocytes (192). Activation of ILC2s in the iWAT of mice strongly stimulates the biogenesis of beige fat (193). Mechanistically, ILC2 activation leads to the proliferation of adipocyte precursors and their commitment to the beige fat lineage in mice (193). ILC2 cells also secrete peptide methionine-enkephalin (Met-Enk), which directly targets subcutaneous white adipocytes to induce their browning (194). Moreover, ILC2s respond to the stimulation of interleukin (IL)-33 and produce IL-13 and IL-4 to promote the browning of iWAT in mice, although the cellular origin and signal pathways involved in the endogenous IL-33 production in adipose tissue remain unidentified (193). Consistent with this, Il-33 deficient mice in iWAT have fewer beige adipocyte formations and larger white adipocyte compared to control mice (194). In a recent study, the unique ILC populations were profiled in human WAT (168), which suggests ILC3s may play a similar role as ILC2 in adipose homeostasis, but function as a more important mediator of adipose tissue inflammation and obesity (168, 194).
Eosinophils are the main IL-4-producing cells in iWAT of mice, and play a key role in the thermogenesis and metabolic homeostasis (195). METRNL, a circulating factor meteorin-like hormone, is induced after exercise and cold exposure in the skeletal muscle and adipose tissue of mice, respectively (196). METRNL promotes alternative activation of adipose tissue macrophages and thermogenic and anti-inflammatory gene programs in iWAT through an eosinophil-dependent increased Il-4 expression, and blocking IL4/IL13 signaling abrogates METRNL-induced browning of iWAT in mice (196). Moreover, eosinophils-derived IL-4 directly work on PDGFRα+ adipocyte precursors to induce beige adipogenesis both in vitro and in vivo (193). In response to chemokine ligand 11 (CCL11) stimulation, eosinophils are recruited to iWAT and promote type 2 immune responses and beige adipogenesis in mice (197).
Neurons in the thermogenic adipose tissue
BAT is highly innervated by the complex sympathetic nervous system, which can transmit signals from the central nervous system to BAT (198). BAT thermogenesis is triggered by the release of norepinephrine from its sympathetic nerve terminals, which binds to β3-AR that result in the activation of UCP1 (198). Sympathetic innervation increases after cold exposure in BAT and subcutaneous WAT both in mice and human adults (199). More detailed analysis revealed that sympathetic arborizations in iWAT cover 90% of individual adipocytes, and the sympathetic arborizations are important for the cold-induced browning of iWAT in mice (200). Mutually, the thermogenic fat also regulates the sympathetic innervation and neuron activity. Overexpression of PRDM16 in mice significantly increase the number of sympathetic parenchymal nerve fibers infiltrating the iWAT compared with that in wild-type mice, although the exact mechanism of the recruitment of sympathetic nerves in iWAT remain elusive (200). A recent study revealed that mice lack of fatty acid synthase in fat (iAdFASNKO) activated the sympathetic nerve fiber to result in browning in iWAT of mice (161). Zeng et al. reported that thermogenic adipocytes express mammal-specific endoplasmic reticulum membrane protein (Calsyntenin-3β), which promotes the secretion of S100b from brown adipocytes and stimulates neurite outgrowth in mice (201). Luan group further demonstrated that thermogenic adipocytes secrete zinc that promotes sympathetic innervation, and administration of zinc ameliorates obesity by promoting sympathetic neuron-induced thermogenesis in mice (202). These studies revealed the beneficial and critical role of sympathetic innervation in maintenance of thermogenic fat in response to cold exposure and other environmental challenge.
Inter-organ communications around thermogenic fat
The coordination of multiple tissues and organs is very important for maintaining systemic homeostasis and responding to nutritional and environmental challenges, and its dysregulation leads to various metabolic disorders (203–205). The thermogenic fat function as an endocrine organ by secreting specific factors (brown adipokines or batokines) and interact with distant organs that express the corresponding receptors, and vice versa (Figure 3).
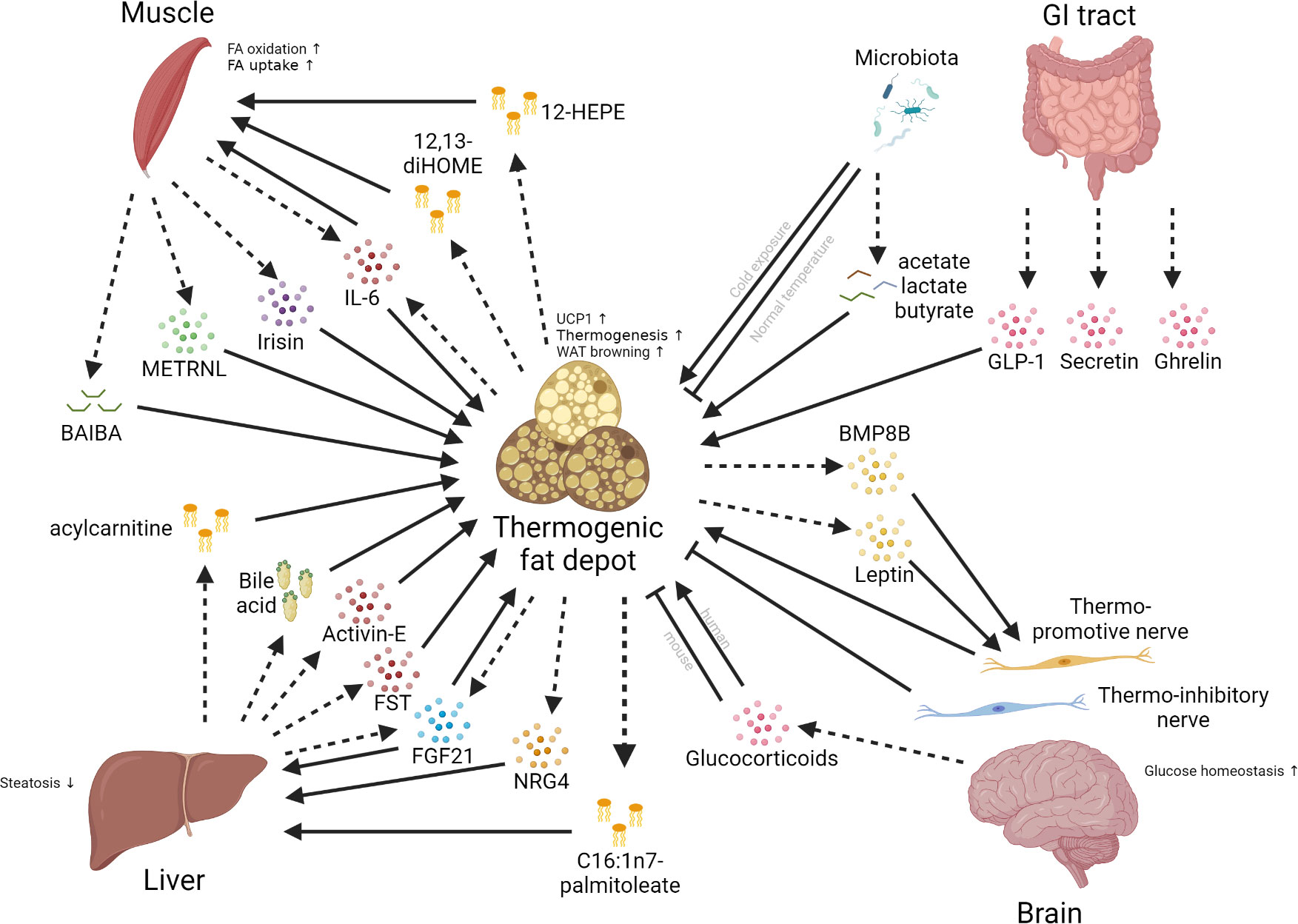
Figure 3 Inter-organ communications between thermogenic fat depots and different organs. Multiple organs, such as the brain, liver, muscle, and gut, can have crosstalk with thermogenic fat depots. The communications between these organs and the thermogenic fat depot mainly involve the secretion of different kinds of molecules, including peptide hormones, lipokines, glucocorticoids, and bile acids. Dashed arrows mean the secretion of factors; solid arrows mean positive effects; blunt-end lines mean inhibitory effects.
Brain-thermogenic fat communication
Besides the local effects of nerve on the thermogenic fat, the brain-thermogenic fat communication axis plays an important role in regulating systemic energy balance. Adipose tissue transmits the message to the brain via secreted factors and sensory innervation (206, 207). Leptin, an adipokine, is mainly produced by the obese (ob) gene in adipocytes, and regulates the balance of energy via decreasing food intake and inducing energy expenditure (208, 209). Although the role of leptin in regulating energy balance is well known, the underlying mechanism is still elusive. Recent work has shown that leptin target the melanocortin receptor 4 (MC4R) and melanocortin receptor 3 (MC3R) in the brain of mice (210, 211). Mc4r-deficient mice exhibit reduced upregulation of Ucp1 in BAT exposed to cold condition or high-fat food (212). In contrast, central administration of MC3/4-R agonists MTII promote Ucp1 mRNA expression in mice (213), suggesting the role of MC4R-expressing neuronal populations in regulating BAT thermogenesis. It was also shown that leptin and insulin act synergically on hypothalamic neurons to promote iWAT browning in mice (214). Bone morphogenetic protein 8b (BMP8b), a factor induced by nutritional and thermogenic stimuli in mature BAT and hypothalamus, is also involved in central control of BAT thermogenesis, and central BMP8B treatment increases sympathetic activation of BAT in mice, depending on the hypothalamic AMP-activated protein kinase (AMPK) activation (215).
Central control could also inhibit the browning process, as fasting and chemical-genetic activation of orexigenic agouti-related protein (AgRP) neurons in the hypothalamus suppress iWAT browning in mice (216). Mechanistically, the levels of O-linked β-N-acetylglucosamine (O-GlcNAc) transferase and O-GlcNAc modification in AgRP neurons are increased after fasting in mice, thus promoting neuronal excitability and inhibiting iWAT browning (216). It was also reported that glucocorticoids, a class of steroid hormones synthesized in the adrenal cortex, also suppress Ucp1 expression and BAT thermogenesis in mice (217). In contrast, the glucocorticoids promote UCP1 expression in human brown adipocytes and increase glucose uptake and energy expenditure in response to mild cold condition (218). Understanding the species-specific action of glucocorticoid on BAT thermogenesis will provide not only the understanding for BAT-brain axis, but also new therapeutic strategy for maintaining energy homeostasis. Overall, these studies show the differential effects of central control of function of thermogenic fat, mainly depending on the different types of neurons.
Liver-thermogenic fat communication
The liver is a metabolic organ important for glucose and lipid metabolism, whose dysfunction leads to many kinds of metabolic diseases. The interaction between the liver and thermogenic fat are mainly mediated by peptide hormones, lipokines as well as bile acids. Fibroblast growth factor 21 (FGF21) is a circulating peptide hormone, which is mainly expressed in the liver in response to starvation or exercise and induced in BAT and WAT when fasted or exposed to cold environment both in mice and humans (219). FGF21 not only acts locally in an endocrine and autocrine manner, but also travels to distant organs to exert its role by secreting into the bloodstream (220). Studies showed that administration of FGF21 increases energy expenditure and improves insulin sensitivity in mice (221). Owen et al. further revealed that FGF21 improves energy expenditure through enhanced sympathetic nerve activity in BAT of mice (222). Moreover, the administration of recombinant FGF21 for 6 weeks in diabetic rhesus monkeys lead to a significant decline in glucose level, body weight, and circulating lipids levels (223). Similarly, Activin-E, a member of transforming growth factor beta (TGFβ) superfamily, is primarily produced by the liver and functions as a hepatokine to activate thermogenesis both in iWAT and BAT of mice (224, 225). Follistatin (Fst), which binds and neutralizes the activity of TGFβ superfamily, is secreted by the liver and promotes brown preadipocyte differentiation and cold-induced brown thermogenesis in mice, although the autocrine effect could not be excluded, since Fst is also induced in brown adipocytes in response to cold (226–228).
On the other hand, brown adipocytes secrete batokines to regulate the functions of the liver. As discussed above, FGF21 mediate the bi-directional crosstalk between BAT and the liver in mice (204, 221, 222). Besides, brown adipocyte-derived Neuregulin 4 (Nrg4), a member of the epidermal growth factor (EGF) family of ligands, attenuates hepatic lipogenic signaling and protects mice against diet-induced insulin resistance and hepatic steatosis (142). In mice, acute psychological stress induces IL6 secretion from brown adipocytes and then promotes hyperglycemia through hepatic enhanced gluconeogenesis (229). Other reports revealed that some adipokines, such as adiponectin, suppress hepatic injury induced by alcohol intake in mice model (230).
Another class of molecules that mediate the communication between the liver and thermogenic fat are lipokines, which can be secreted both by the adipose tissue and the liver (231, 232). Through quantitative and systemic lipidomic analyses, Cao et al. identified C16:1n7-palmitoleate as an adipose tissue-derived lipid hormone that functions as an important regulator of metabolic homeostasis, such as suppression of hepatosteatosis in mice (231). Similarly, using non-targeted liquid chromatography-mass spectrometry-based lipidomics, Simcox et al. identified that acylcarnitine,produced by the mouse liver in response to cold exposure, transports to BAT to induce UCP1-dependent uncoupling respiration and heat production (232). Bile acids also participate in the communication between the liver and thermogenic fat. TGR5, a G-protein-coupled receptor, could bind to the bile acids transported to brown or beige adipocytes from the liver and induce cold-induced thermogenesis in mice (233–235). BAT also regulate liver inflammation, although the exact pathway governing this crosstalk remains unclear. Previous studies showed that Ucp1-/- mice exhibits decreased capacity to clear succinate from both the liver and the circulation, thus driving liver inflammation through the interaction with stellate cells and macrophages (236, 237). Collectively, these studies show that the intensive crosstalk between the liver and thermogenic fat mediated by various circulating factors, including peptide hormones, lipokines as well as bile acids.
Skeletal muscle-thermogenic fat communication
Upon muscle contraction, skeletal muscles produce and release circulating cytokines and other peptides, known as myokines, which exert endocrine effects and mediate the communication between muscle and other organs (238–240). In reciprocal, cold- or exercise-induced batokines from thermogenic fat also regulate the function of skeletal muscle.
The earliest identified and most studied myokine is IL-6, which can increase up to 100 folds in circulation during physical exercise (241). Daily injection of IL-6 for 1 week significantly increases Ucp1 mRNA levels in iWAT of mice (242). Moreover, administration of recombinant human IL-6 enhances lipolysis as well as fatty acid oxidation both in healthy young and elderly humans (243, 244). Consistent with this, elevated IL-6 secretion is also observed in differentiating human beige adipocytes, and blockage of IL-6 receptor by specific antibody inhibits human brown adipocyte differentiation (245). Irisin is another myokine that mediates the communication between skeletal muscle and thermogenic fat, which is secreted from skeletal muscle in a PGC1α-dependent manner and stimulates Ucp1 expression and thermogenesis both in vitro and in vivo (246). Irisin is also induced by cold exposure in human and promotes brown fat thermogenesis in collaboration with FGF21, representing a cold-activated endocrine axis regulating both shivering and non-shivering thermogenesis (247). METRNL is released by skeletal muscle and adipose tissue after exercise or upon cold exposure respectively, and significantly promotes browning of WAT depots (183), stimulates energy expenditure and improves glucose tolerance, which is mediated by the recruitment of resident eosinophil in WAT depots of mice (196). Roberts et al. identified β-aminoisobutyric acid (BAIBA), a myokine secreted after exercise, increases the expression of brown adipocyte marker genes and induces a brown adipocyte-like phenotype both in human iPSC-derived white adipocytes and in white adipose depot of mice (248).
Meanwhile, batokines from thermogenic fat also regulate the function of skeletal muscle. 12,13-dihydroxy-9Z-octadecenoic acid (12,13-diHOME), a lipokine secreted from BAT when exposed to cold or exercise in mice and human, increases skeletal muscle fatty acid oxidation and uptake (249, 250). 12-hydroxyeicosapentaenoic acid (12-HEPE), a 12-lipoxygenase-derived lipokine that is secreted in response to cold exposure and β-AR signaling, also promotes glucose uptake in muscle as well as BAT in mice (251). These studies clearly show the mutually regulatory network between skeletal muscle and thermogenic fat to maintain thermogenic fat homeostasis.
GI tract-thermogenic fat communication
The gastrointestinal tract (GI tract) plays a very important role in thermogenesis through gut microbiota or directly secreting factors from intestinal cells (252). In a study that compared the metabolic profiling between germ-free mice and conventional mice, Mestdagh et al. revealed increased lipolysis while reduced lipogenesis in BAT of germ-free mice (253). Suarez et al. also showed that depletion of microbiota, either by antibiotic treatment or in germ-free mice, promote the browning of iWAT and perigonadal visceral adipose tissue in lean mice, obese mice and high-fat diet-fed mice (254). However, Zietak et al. found cold exposure markedly alter the microbiome composition, and cold-adapted microbiota improved energy metabolism (255). Transplantation of the gut microbiota from cold-induced mice to germ-free mice increase insulin sensitivity, cold tolerance, and browning of WAT (256). Other study revealed that acetate and lactate from the gut microbiota promote the browning of iWAT of mice after intermittent fasting, although the underlying mechanism remains unclear (257). Of note, administration of the bacterial metabolite butyrate also increases the thermogenic capacity of the germ-free mice (258).
Besides gut microbiota, the GI tract also secrete various factors to regulate thermogenesis. Secretin, secreted by the gut and upregulated during fasting, increases lipolysis and inhibits glucose uptake in mice (259). Li et al. revealed that secretin mediates a gut-BAT-brain axis, which stimulates brown fat thermogenesis and satiation in mice (260). The similar role of secretin is also observed in human (261). Glucagon-like peptide 1 (GLP-1), a peptide released from enteroendocrine cells in the gut, increases insulin secretion in beta cells and activates BAT thermogenesis in mice (262). GLP-1 has also been proved to increase satiety and reduce energy intake in human (263). GLP-1 agonists significantly induce BAT thermogenesis and promote browning of iWAT in mice (264). Numerous evidences support that GLP-1 agonists decrease the risk of developing cardiovascular disease in diabetes and obesity both in mice and humans (265). Ghrelin, another growth-hormone-releasing acylated peptide from stomach, also modulates thermogenesis in BAT as well as lipid utilization in WAT, possibly through the gut-brain-BAT axis, as this occurs when ghrelin was centrally administered in mice (266–269). Further studies need to investigate whether and how thermogenic fat could influence the gut homeostasis, as this has not been explored in depth so far.
Conclusion
Understanding of the development route of thermogenic fat will provides novel therapeutic interventions for metabolic diseases. In this review, we discussed the regulatory network of thermogenic fat at the molecular and cellular levels, respectively. The molecular regulation of thermogenic fat mainly involves transcriptional regulation, epigenetic regulation, non-coding RNA regulation and metabolic reprogramming. Among these regulators, PPARγ, PRDM16 and PGC1α represent the core regulators, as most of the other regulators regulate the thermogenesis depending on them. Besides, thermogenic fat is also educated by other cell types within adipose depots or other organs. These complex and comprehensive regulatory networks help to maintain the functionality of thermogenic fat in response to kinds of changes of the environment. This holds a promising strategy for inducing artificial thermogenesis to counteract obesity in vivo. For example, recent study has shown that thermogenesis could be induced through local hyperthermia therapy, mainly through the HSF1-A2B1 transcriptional axis (270). However, whether this kind of induced thermogenesis represents a new specific regulatory network or converges on the core regulators still needs to be identified. In future, more advanced technology, such as spatial transcriptomics and epigenomics methodologies, should be applied to this field to better delineate the development route of thermogenic fat.
Author contributions
All authors listed have made a substantial, direct, and intellectual contribution to the work and approved it for publication.
Funding
WH was supported by the Natural Science Foundation of China (82270868).
Acknowledgments
The authors thank the members of the laboratory of WH for valuable discussions and proofreading. The authors also acknowledge the many investigators who have contributed to this area of research and whose work, in many cases, could not be cited owing to the limitation of references allowed in this Review. The figures were created by Biorender.
Conflict of interest
The authors declare that the research was conducted in the absence of any commercial or financial relationships that could be construed as a potential conflict of interest.
Publisher’s note
All claims expressed in this article are solely those of the authors and do not necessarily represent those of their affiliated organizations, or those of the publisher, the editors and the reviewers. Any product that may be evaluated in this article, or claim that may be made by its manufacturer, is not guaranteed or endorsed by the publisher.
References
1. Tomiyama AJ. Stress and obesity. Annu Rev Psychol (2019) 70:703–18. doi: 10.1146/annurev-psych-010418-102936
2. Cannon B, Nedergaard J. Brown adipose tissue: function and physiological significance. Physiol Rev (2004) 84(1):277–359. doi: 10.1152/physrev.00015.2003
3. Cypess AM, Lehman S, Williams G, Tal I, Rodman D, Goldfine AB, et al. Identification and importance of brown adipose tissue in adult humans. N Engl J Med (2009) 360(15):1509–17. doi: 10.1056/NEJMoa0810780
4. Virtanen KA, Lidell ME, Orava J, Heglind M, Westergren R, Niemi T, et al. Functional brown adipose tissue in healthy adults. N Engl J Med (2009) 360(15):1518–25. doi: 10.1056/NEJMoa0808949
5. Yoneshiro T, Aita S, Matsushita M, Kayahara T, Kameya T, Kawai Y, et al. Recruited brown adipose tissue as an antiobesity agent in humans. J Clin Invest (2013) 123(8):3404–8. doi: 10.1172/JCI67803
6. Saito M, Okamatsu-Ogura Y, Matsushita M, Watanabe K, Yoneshiro T, Nio-Kobayashi J, et al. High incidence of metabolically active brown adipose tissue in healthy adult humans: effects of cold exposure and adiposity. Diabetes (2009) 58(7):1526–31. doi: 10.2337/db09-0530
7. Zhang Z, Funcke JB, Zi Z, Zhao S, Straub LG, Zhu Y, et al. Adipocyte iron levels impinge on a fat-gut crosstalk to regulate intestinal lipid absorption and mediate protection from obesity. Cell Metab (2021) 33(8):1624–39 e9. doi: 10.1016/j.cmet.2021.06.001
8. Feldmann HM, Golozoubova V, Cannon B, Nedergaard J. UCP1 ablation induces obesity and abolishes diet-induced thermogenesis in mice exempt from thermal stress by living at thermoneutrality. Cell Metab (2009) 9(2):203–9. doi: 10.1016/j.cmet.2008.12.014
9. Tsagkaraki E, Nicoloro SM, DeSouza T, Solivan-Rivera J, Desai A, Lifshitz LM, et al. CRISPR-enhanced human adipocyte browning as cell therapy for metabolic disease. Nat Commun (2021) 12(1):6931. doi: 10.1038/s41467-021-27190-y
10. Stanford KI, Middelbeek RJ, Townsend KL, An D, Nygaard EB, Hitchcox KM, et al. Brown adipose tissue regulates glucose homeostasis and insulin sensitivity. J Clin Invest (2013) 123(1):215–23. doi: 10.1172/JCI62308
11. Peres Valgas da Silva C, Hernandez-Saavedra D, White JD, Stanford KI. Cold and exercise: therapeutic tools to activate brown adipose tissue and combat obesity. Biol (Basel) (2019) 8(1):9-37. doi: 10.3390/biology8010009
12. Sharp LZ, Shinoda K, Ohno H, Scheel DW, Tomoda E, Ruiz L, et al. Human BAT possesses molecular signatures that resemble beige/brite cells. PloS One (2012) 7(11):e49452. doi: 10.1371/journal.pone.0049452
13. Seale P, Conroe HM, Estall J, Kajimura S, Frontini A, Ishibashi J, et al. Prdm16 determines the thermogenic program of subcutaneous white adipose tissue in mice. J Clin Invest (2011) 121(1):96–105. doi: 10.1172/JCI44271
14. Cao W, Daniel KW, Robidoux J, Puigserver P, Medvedev AV, Bai X, et al. p38 mitogen-activated protein kinase is the central regulator of cyclic AMP-dependent transcription of the brown fat uncoupling protein 1 gene. Mol Cell Biol (2004) 24(7):3057–67. doi: 10.1128/MCB.24.7.3057-3067.2004
15. Vernochet C, Peres SB, Davis KE, McDonald ME, Qiang L, Wang H, et al. C/EBPalpha and the corepressors CtBP1 and CtBP2 regulate repression of select visceral white adipose genes during induction of the brown phenotype in white adipocytes by peroxisome proliferator-activated receptor gamma agonists. Mol Cell Biol (2009) 29(17):4714–28. doi: 10.1128/MCB.01899-08
16. Kajimura S, Seale P, Kubota K, Lunsford E, Frangioni JV, Gygi SP, et al. Initiation of myoblast to brown fat switch by a PRDM16-C/EBP-beta transcriptional complex. Nature (2009) 460(7259):1154–8. doi: 10.1038/nature08262
17. Rajakumari S, Wu J, Ishibashi J, Lim HW, Giang AH, Won KJ, et al. EBF2 determines and maintains brown adipocyte identity. Cell Metab (2013) 17(4):562–74. doi: 10.1016/j.cmet.2013.01.015
18. Cederberg A, Grønning LM, Ahrén B, Taskén K, Carlsson P, Enerbäck S. FOXC2 is a winged helix gene that counteracts obesity, hypertriglyceridemia, and diet-induced insulin resistance. Cell (2001) 106(5):563–73. doi: 10.1016/S0092-8674(01)00474-3
19. Bi P, Shan T, Liu W, Yue F, Yang X, Liang XR, et al. Inhibition of notch signaling promotes browning of white adipose tissue and ameliorates obesity. Nat Med (2014) 20(8):911–8. doi: 10.1038/nm.3615
20. Kong X, Banks A, Liu T, Kazak L, Rao Rajesh R, Cohen P, et al. IRF4 is a key thermogenic transcriptional partner of PGC-1α. Cell (2014) 158(1):69–83. doi: 10.1016/j.cell.2014.04.049
21. Claussnitzer M, Dankel SN, Kim KH, Quon G, Meuleman W, Haugen C, et al. FTO obesity variant circuitry and adipocyte browning in humans. N Engl J Med (2015) 373(10):895–907. doi: 10.1056/NEJMoa1502214
22. Laber S, Cox RD. Commentary: FTO obesity variant circuitry and adipocyte browning in humans. Front Genet (2015) 6:318. doi: 10.3389/fgene.2015.00318
23. Loft A, Forss I, Siersbaek MS, Schmidt SF, Larsen AS, Madsen JG, et al. Browning of human adipocytes requires KLF11 and reprogramming of PPARgamma superenhancers. Genes Dev (2015) 29(1):7–22. doi: 10.1101/gad.250829.114
24. McDonald ME, Li C, Bian H, Smith BD, Layne MD, Farmer SR. Myocardin-related transcription factor a regulates conversion of progenitors to beige adipocytes. Cell (2015) 160(1-2):105–18. doi: 10.1016/j.cell.2014.12.005
25. Uldry M, Yang W, St-Pierre J, Lin J, Seale P, Spiegelman BM. Complementary action of the PGC-1 coactivators in mitochondrial biogenesis and brown fat differentiation. Cell Metab (2006) 3(5):333–41. doi: 10.1016/j.cmet.2006.04.002
26. Lin J, Wu PH, Tarr PT, Lindenberg KS, St-Pierre J, Zhang CY, et al. Defects in adaptive energy metabolism with CNS-linked hyperactivity in PGC-1alpha null mice. Cell (2004) 119(1):121–35. doi: 10.1016/j.cell.2004.09.013
27. Puigserver P, Wu Z, Park CW, Graves R, Wright M, Spiegelman BM. A cold-inducible coactivator of nuclear receptors linked to adaptive thermogenesis. Cell (1998) 92(6):829–39. doi: 10.1016/S0092-8674(00)81410-5
28. Tiraby C, Tavernier G, Lefort C, Larrouy D, Bouillaud F, Ricquier D, et al. Acquirement of brown fat cell features by human white adipocytes. J Biol Chem (2003) 278(35):33370–6. doi: 10.1074/jbc.M305235200
29. Jimenez-Preitner M, Berney X, Uldry M, Vitali A, Cinti S, Ledford JG, et al. Plac8 is an inducer of C/EBPβ required for brown fat differentiation, thermoregulation, and control of body weight. Cell Metab (2011) 14(5):658–70. doi: 10.1016/j.cmet.2011.08.008
30. Ohno H, Shinoda K, Spiegelman BM, Kajimura S. PPARγ agonists induce a white-to-brown fat conversion through stabilization of PRDM16 protein. Cell Metab (2012) 15(3):395–404. doi: 10.1016/j.cmet.2012.01.019
31. Qiang L, Wang L, Kon N, Zhao W, Lee S, Zhang Y, et al. Brown remodeling of white adipose tissue by SirT1-dependent deacetylation of ppargamma. Cell (2012) 150(3):620–32. doi: 10.1016/j.cell.2012.06.027
32. Seale P, Kajimura S, Yang W, Chin S, Rohas LM, Uldry M, et al. Transcriptional control of brown fat determination by PRDM16. Cell Metab (2007) 6(1):38–54. doi: 10.1016/j.cmet.2007.06.001
33. Seale P, Bjork B, Yang W, Kajimura S, Chin S, Kuang S, et al. PRDM16 controls a brown fat/skeletal muscle switch. Nature (2008) 454(7207):961–7. doi: 10.1038/nature07182
34. Harms MJ, Ishibashi J, Wang W, Lim HW, Goyama S, Sato T, et al. Prdm16 is required for the maintenance of brown adipocyte identity and function in adult mice. Cell Metab (2014) 19(4):593–604. doi: 10.1016/j.cmet.2014.03.007
35. Scimè A, Grenier G, Huh MS, Gillespie MA, Bevilacqua L, Harper ME, et al. Rb And p107 regulate preadipocyte differentiation into white versus brown fat through repression of PGC-1alpha. Cell Metab (2005) 2(5):283–95. doi: 10.1016/j.cmet.2005.10.002
36. Powelka AM, Seth A, Virbasius JV, Kiskinis E, Nicoloro SM, Guilherme A, et al. Suppression of oxidative metabolism and mitochondrial biogenesis by the transcriptional corepressor RIP140 in mouse adipocytes. J Clin Invest (2006) 116(1):125–36.
37. Christian M, Kiskinis E, Debevec D, Leonardsson G, White R, Parker MG. RIP140-targeted repression of gene expression in adipocytes. Mol Cell Biol (2005) 25(21):9383–91. doi: 10.1128/MCB.25.21.9383-9391.2005
38. Leonardsson G, Steel JH, Christian M, Pocock V, Milligan S, Bell J, et al. Nuclear receptor corepressor RIP140 regulates fat accumulation. Proc Natl Acad Sci USA (2004) 101(22):8437–42. doi: 10.1073/pnas.0401013101
39. Yadav H, Quijano C, Kamaraju AK, Gavrilova O, Malek R, Chen W, et al. Protection from obesity and diabetes by blockade of TGF-beta/Smad3 signaling. Cell Metab (2011) 14(1):67–79. doi: 10.1016/j.cmet.2011.04.013
40. Picard F, Géhin M, Annicotte J-S, Rocchi S, Champy M-F, O'Malley BW, et al. SRC-1 and TIF2 control energy balance between white and brown adipose tissues. Cell (2002) 111(7):931–41. doi: 10.1016/S0092-8674(02)01169-8
41. Gburcik V, Cawthorn WP, Nedergaard J, Timmons JA, Cannon B. An essential role for Tbx15 in the differentiation of brown and "brite" but not white adipocytes. Am J Physiol Endocrinol Metab (2012) 303(8):E1053–60. doi: 10.1152/ajpendo.00104.2012
42. Vernochet C, Mourier A, Bezy O, Macotela Y, Boucher J, Rardin MJ, et al. Adipose-specific deletion of TFAM increases mitochondrial oxidation and protects mice against obesity and insulin resistance. Cell Metab (2012) 16(6):765–76. doi: 10.1016/j.cmet.2012.10.016
43. Villanueva CJ, Vergnes L, Wang J, Drew BG, Hong C, Tu Y, et al. Adipose subtype-selective recruitment of TLE3 or Prdm16 by PPARgamma specifies lipid storage versus thermogenic gene programs. Cell Metab (2013) 17(3):423–35. doi: 10.1016/j.cmet.2013.01.016
44. Pan D, Fujimoto M, Lopes A, Wang Y-X. Twist-1 is a PPARδ-inducible, negative-feedback regulator of PGC-1α in brown fat metabolism. Cell (2009) 137(1):73–86. doi: 10.1016/j.cell.2009.01.051
45. Laurila PP, Soronen J, Kooijman S, Forsstrom S, Boon MR, Surakka I, et al. USF1 deficiency activates brown adipose tissue and improves cardiometabolic health. Sci Transl Med (2016) 8(323):323ra13. doi: 10.1126/scitranslmed.aad0015
46. Gupta RK, Arany Z, Seale P, Mepani RJ, Ye L, Conroe HM, et al. Transcriptional control of preadipocyte determination by Zfp423. Nature (2010) 464(7288):619–23. doi: 10.1038/nature08816
47. Shao M, Zhang Q, Truong A, Shan B, Vishvanath L, Li L, et al. ZFP423 controls EBF2 coactivator recruitment and PPARgamma occupancy to determine the thermogenic plasticity of adipocytes. Genes Dev (2021) 35(21-22):1461–74. doi: 10.1101/gad.348780.121
48. Shao M, Ishibashi J, Kusminski CM, Wang QA, Hepler C, Vishvanath L, et al. Zfp423 maintains white adipocyte identity through suppression of the beige cell thermogenic gene program. Cell Metab (2016) 23(6):1167–84. doi: 10.1016/j.cmet.2016.04.023
49. Dempersmier J, Sambeat A, Gulyaeva O, Paul SM, Hudak CS, Raposo HF, et al. Cold-inducible Zfp516 activates UCP1 transcription to promote browning of white fat and development of brown fat. Mol Cell (2015) 57(2):235–46. doi: 10.1016/j.molcel.2014.12.005
50. Wang W, Kissig M, Rajakumari S, Huang L, Lim HW, Won KJ, et al. Ebf2 is a selective marker of brown and beige adipogenic precursor cells. Proc Natl Acad Sci USA (2014) 111(40):14466–71. doi: 10.1073/pnas.1412685111
51. Angueira AR, Shapira SN, Ishibashi J, Sampat S, Sostre-Colon J, Emmett MJ, et al. Early b cell factor activity controls developmental and adaptive thermogenic gene programming in adipocytes. Cell Rep (2020) 30(9):2869–78 e4. doi: 10.1016/j.celrep.2020.02.023
52. Takahashi N, Kawada T, Yamamoto T, Goto T, Taimatsu A, Aoki N, et al. Overexpression and ribozyme-mediated targeting of transcriptional coactivators CREB-binding protein and p300 revealed their indispensable roles in adipocyte differentiation through the regulation of peroxisome proliferator-activated receptor gamma. J Biol Chem (2002) 277(19):16906–12. doi: 10.1074/jbc.M200585200
53. Jin Q, Wang C, Kuang X, Feng X, Sartorelli V, Ying H, et al. Gcn5 and PCAF regulate PPARgamma and Prdm16 expression to facilitate brown adipogenesis. Mol Cell Biol (2014) 34(19):3746–53. doi: 10.1128/MCB.00622-14
54. Haberland M, Carrer M, Mokalled MH, Montgomery RL, Olson EN. Redundant control of adipogenesis by histone deacetylases 1 and 2. J Biol Chem (2010) 285(19):14663–70. doi: 10.1074/jbc.M109.081679
55. Ferrari A, Longo R, Fiorino E, Silva R, Mitro N, Cermenati G, et al. HDAC3 is a molecular brake of the metabolic switch supporting white adipose tissue browning. Nat Commun (2017) 8(1):93. doi: 10.1038/s41467-017-00182-7
56. Chatterjee TK, Idelman G, Blanco V, Blomkalns AL, Piegore MG Jr., Weintraub DS, et al. Histone deacetylase 9 is a negative regulator of adipogenic differentiation. J Biol Chem (2011) 286(31):27836–47. doi: 10.1074/jbc.M111.262964
57. Bagchi RA, Ferguson BS, Stratton MS, Hu T, Cavasin MA, Sun L, et al. HDAC11 suppresses the thermogenic program of adipose tissue via BRD2. JCI Insight (2018) 3(15):e120159–74. doi: 10.1172/jci.insight.120159
58. Zhou Y, Song T, Peng J, Zhou Z, Wei H, Zhou R, et al. SIRT1 suppresses adipogenesis by activating wnt/beta-catenin signaling in vivo and in vitro. Oncotarget (2016) 7(47):77707–20. doi: 10.18632/oncotarget.12774
59. Mayoral R, Osborn O, McNelis J, Johnson AM, Oh DY, Izquierdo CL, et al. Adipocyte SIRT1 knockout promotes PPARgamma activity, adipogenesis and insulin sensitivity in chronic-HFD and obesity. Mol Metab (2015) 4(5):378–91. doi: 10.1016/j.molmet.2015.02.007
60. Wang F, Tong Q. SIRT2 suppresses adipocyte differentiation by deacetylating FOXO1 and enhancing FOXO1's repressive interaction with PPARgamma. Mol Biol Cell (2009) 20(3):801–8. doi: 10.1091/mbc.e08-06-0647
61. Shi T, Wang F, Stieren E, Tong Q. SIRT3, a mitochondrial sirtuin deacetylase, regulates mitochondrial function and thermogenesis in brown adipocytes. J Biol Chem (2005) 280(14):13560–7. doi: 10.1074/jbc.M414670200
62. Shuai L, Zhang LN, Li BH, Tang CL, Wu LY, Li J, et al. SIRT5 regulates brown adipocyte differentiation and browning of subcutaneous white adipose tissue. Diabetes (2019) 68(7):1449–61. doi: 10.2337/db18-1103
63. Byun S, Lee CH, Jeong H, Kim H, Kwon HM, Park S, et al. Loss of adipose TET proteins enhances beta-adrenergic responses and protects against obesity by epigenetic regulation of beta3-AR expression. Proc Natl Acad Sci USA (2022) 119(26):e2205626119.
64. Lee J, Saha PK, Yang QH, Lee S, Park JY, Suh Y, et al. Targeted inactivation of MLL3 histone H3-Lys-4 methyltransferase activity in the mouse reveals vital roles for MLL3 in adipogenesis. Proc Natl Acad Sci USA (2008) 105(49):19229–34. doi: 10.1073/pnas.0810100105
65. Lee J-E, Wang C, Xu S, Cho Y-W, Wang L, Feng X, et al. H3K4 mono- and di-methyltransferase MLL4 is required for enhancer activation during cell differentiation. eLife (2013) 2:e01503. doi: 10.7554/eLife.01503.027
66. Jang Y, Broun A, Wang C, Park YK, Zhuang L, Lee JE, et al. H3.3K4M destabilizes enhancer H3K4 methyltransferases MLL3/MLL4 and impairs adipose tissue development. Nucleic Acids Res (2019) 47(2):607–20.
67. Ohno H, Shinoda K, Ohyama K, Sharp LZ, Kajimura S. EHMT1 controls brown adipose cell fate and thermogenesis through the PRDM16 complex. Nature (2013) 504(7478):163–7. doi: 10.1038/nature12652
68. Wang L, Xu S, Lee JE, Baldridge A, Grullon S, Peng W, et al. Histone H3K9 methyltransferase G9a represses PPARgamma expression and adipogenesis. EMBO J (2013) 32(1):45–59.
69. Zhao Q, Zhang Z, Rong W, Jin W, Yan L, Jin W, et al. KMT5c modulates adipocyte thermogenesis by regulating Trp53 expression. Proc Natl Acad Sci USA (2020) 117(36):22413–22. doi: 10.1073/pnas.1922548117
70. Shuai L, Li BH, Jiang HW, Yang L, Li J, Li JY. DOT1L regulates thermogenic adipocyte differentiation and function via modulating H3K79 methylation. Diabetes (2021) 70(6):1317–33. doi: 10.2337/db20-1110
71. Duteil D, Tosic M, Willmann D, Georgiadi A, Kanouni T, Schule R. Lsd1 prevents age-programed loss of beige adipocytes. Proc Natl Acad Sci USA (2017) 114(20):5265–70. doi: 10.1073/pnas.1702641114
72. Takase R, Hino S, Nagaoka K, Anan K, Kohrogi K, Araki H, et al. Lysine-specific demethylase-2 is distinctively involved in brown and beige adipogenic differentiation. FASEB J (2019) 33(4):5300–11. doi: 10.1096/fj.201801422RR
73. Guo L, Guo YY, Li BY, Peng WQ, Tang QQ. Histone demethylase KDM5A is transactivated by the transcription factor C/EBPβ and promotes preadipocyte differentiation by inhibiting wnt/β-catenin signaling. J Biol Chem (2019) 294(24):9642–54. doi: 10.1074/jbc.RA119.008419
74. Tateishi K, Okada Y, Kallin EM, Zhang Y. Role of Jhdm2a in regulating metabolic gene expression and obesity resistance. Nature (2009) 458(7239):757–61. doi: 10.1038/nature07777
75. Inagaki T, Tachibana M, Magoori K, Kudo H, Tanaka T, Okamura M, et al. Obesity and metabolic syndrome in histone demethylase JHDM2a-deficient mice. Genes Cells (2009) 14(8):991–1001. doi: 10.1111/j.1365-2443.2009.01326.x
76. Abe Y, Rozqie R, Matsumura Y, Kawamura T, Nakaki R, Tsurutani Y, et al. JMJD1A is a signal-sensing scaffold that regulates acute chromatin dynamics via SWI/SNF association for thermogenesis. Nat Commun (2015) 6:7052. doi: 10.1038/ncomms8052
77. Pan D, Huang L, Zhu LJ, Zou T, Ou J, Zhou W, et al. Jmjd3-mediated H3K27me3 dynamics orchestrate brown fat development and regulate white fat plasticity. Dev Cell (2015) 35(5):568–83. doi: 10.1016/j.devcel.2015.11.002
78. Zha L, Li F, Wu R, Artinian L, Rehder V, Yu L, et al. The histone demethylase UTX promotes brown adipocyte thermogenic program Via coordinated regulation of H3K27 demethylation and acetylation. J Biol Chem (2015) 290(41):25151–63. doi: 10.1074/jbc.M115.662650
79. Nanduri R. Epigenetic regulators of white adipocyte browning. Epigenomes (2021) 5(1):3-21. doi: 10.3390/epigenomes5010003
80. Emmett MJ, Lim HW, Jager J, Richter HJ, Adlanmerini M, Peed LC, et al. Histone deacetylase 3 prepares brown adipose tissue for acute thermogenic challenge. Nature (2017) 546(7659):544–8. doi: 10.1038/nature22819
81. Rifai K, Judes G, Idrissou M, Daures M, Bignon YJ, Penault-Llorca F, et al. SIRT1-dependent epigenetic regulation of H3 and H4 histone acetylation in human breast cancer. Oncotarget (2018) 9(55):30661–78. doi: 10.18632/oncotarget.25771
82. Vaquero A, Scher MB, Lee DH, Sutton A, Cheng HL, Alt FW, et al. SirT2 is a histone deacetylase with preference for histone H4 lys 16 during mitosis. Genes Dev (2006) 20(10):1256–61. doi: 10.1101/gad.1412706
83. Kohli RM, Zhang Y. TET enzymes TDG. And the dynamics of DNA demethylation. Nature (2013) 502(7472):472–9. doi: 10.1038/nature12750
84. Karbiener M, Pisani DF, Frontini A, Oberreiter LM, Lang E, Vegiopoulos A, et al. MicroRNA-26 family is required for human adipogenesis and drives characteristics of brown adipocytes. Stem Cells (2014) 32(6):1578–90. doi: 10.1002/stem.1603
85. Kim SY, Kim AY, Lee HW, Son YH, Lee GY, Lee JW, et al. miR-27a is a negative regulator of adipocyte differentiation via suppressing PPARgamma expression. Biochem Biophys Res Commun (2010) 392(3):323–8. doi: 10.1016/j.bbrc.2010.01.012
86. Kang T, Lu W, Xu W, Anderson L, Bacanamwo M, Thompson W, et al. MicroRNA-27 (miR-27) targets prohibitin and impairs adipocyte differentiation and mitochondrial function in human adipose-derived stem cells. J Biol Chem (2013) 288(48):34394–402. doi: 10.1074/jbc.M113.514372
87. Kang T, Lu W, Xu W, Anderson L, Bacanamwo M, Thompson W, et al. Correction: MicroRNA-27 (miR-27) targets prohibitin and impairs adipocyte differentiation and mitochondrial function in human adipose-derived stem cells. J Biol Chem (2020) 295(48):16468. doi: 10.1074/jbc.AAC120.016601
88. Sun L, Trajkovski M. MiR-27 orchestrates the transcriptional regulation of brown adipogenesis. Metabolism (2014) 63(2):272–82. doi: 10.1016/j.metabol.2013.10.004
89. Lin Q, Gao Z, Alarcon RM, Ye J, Yun Z. A role of miR-27 in the regulation of adipogenesis. FEBS J (2009) 276(8):2348–58. doi: 10.1111/j.1742-4658.2009.06967.x
90. Kong X, Yu J, Bi J, Qi H, Di W, Wu L, et al. Glucocorticoids transcriptionally regulate miR-27b expression promoting body fat accumulation via suppressing the browning of white adipose tissue. Diabetes (2015) 64(2):393–404. doi: 10.2337/db14-0395
91. Hu F, Wang M, Xiao T, Yin B, He L, Meng W, et al. miR-30 promotes thermogenesis and the development of beige fat by targeting RIP140. Diabetes (2015) 64(6):2056–68. doi: 10.2337/db14-1117
92. Ng R, Hussain NA, Zhang Q, Chang C, Li H, Fu Y, et al. miRNA-32 drives brown fat thermogenesis and trans-activates subcutaneous white fat browning in mice. Cell Rep (2017) 19(6):1229–46. doi: 10.1016/j.celrep.2017.04.035
93. Ge X, Sathiakumar D, Lua BJ, Kukreti H, Lee M, McFarlane C. Myostatin signals through miR-34a to regulate Fndc5 expression and browning of white adipocytes. Int J Obes (Lond) (2017) 41(1):137–48. doi: 10.1038/ijo.2016.110
94. Fu T, Seok S, Choi S, Huang Z, Suino-Powell K, Xu HE, et al. MicroRNA 34a inhibits beige and brown fat formation in obesity in part by suppressing adipocyte fibroblast growth factor 21 signaling and SIRT1 function. Mol Cell Biol (2014) 34(22):4130–42. doi: 10.1128/MCB.00596-14
95. Wu Y, Zuo J, Zhang Y, Xie Y, Hu F, Chen L, et al. Identification of miR-106b-93 as a negative regulator of brown adipocyte differentiation. Biochem Biophys Res Commun (2013) 438(4):575–80. doi: 10.1016/j.bbrc.2013.08.016
96. Giroud M, Pisani DF, Karbiener M, Barquissau V, Ghandour RA, Tews D, et al. miR-125b affects mitochondrial biogenesis and impairs brite adipocyte formation and function. Mol Metab (2016) 5(8):615–25. doi: 10.1016/j.molmet.2016.06.005
97. Trajkovski M, Ahmed K, Esau CC, Stoffel M. MyomiR-133 regulates brown fat differentiation through Prdm16. Nat Cell Biol (2012) 14(12):1330–5. doi: 10.1038/ncb2612
98. Liu W, Bi P, Shan T, Yang X, Yin H, Wang YX, et al. miR-133a regulates adipocyte browning in vivo. PloS Genet (2013) 9(7):e1003626. doi: 10.1371/journal.pgen.1003626
99. Chen Y, Siegel F, Kipschull S, Haas B, Frohlich H, Meister G, et al. miR-155 regulates differentiation of brown and beige adipocytes via a bistable circuit. Nat Commun (2013) 4:1769. doi: 10.1038/ncomms2742
100. Ignotz RA, Massagué J. Type beta transforming growth factor controls the adipogenic differentiation of 3T3 fibroblasts. Proc Natl Acad Sci USA (1985) 82(24):8530–4. doi: 10.1073/pnas.82.24.8530
101. Kim HJ, Cho H, Alexander R, Patterson HC, Gu M, Lo KA, et al. MicroRNAs are required for the feature maintenance and differentiation of brown adipocytes. Diabetes (2014) 63(12):4045–56. doi: 10.2337/db14-0466
102. Sun L, Xie H, Mori MA, Alexander R, Yuan B, Hattangadi SM, et al. Mir193b-365 is essential for brown fat differentiation. Nat Cell Biol (2011) 13(8):958–65. doi: 10.1038/ncb2286
103. Feuermann Y, Kang K, Gavrilova O, Haetscher N, Jang SJ, Yoo KH, et al. MiR-193b and miR-365-1 are not required for the development and function of brown fat in the mouse. RNA Biol (2013) 10(12):1807–14. doi: 10.4161/rna.27239
104. Mori M, Nakagami H, Rodriguez-Araujo G, Nimura K, Kaneda Y. Essential role for miR-196a in brown adipogenesis of white fat progenitor cells. PloS Biol (2012) 10(4):e1001314. doi: 10.1371/journal.pbio.1001314
105. Oliverio M, Schmidt E, Mauer J, Baitzel C, Hansmeier N, Khani S, et al. Dicer1-miR-328-Bace1 signalling controls brown adipose tissue differentiation and function. Nat Cell Biol (2016) 18(3):328–36. doi: 10.1038/ncb3316
106. Pan D, Mao C, Quattrochi B, Friedline RH, Zhu LJ, Jung DY, et al. MicroRNA-378 controls classical brown fat expansion to counteract obesity. Nat Commun (2014) 5:4725. doi: 10.1038/ncomms5725
107. Zhang H, Guan M, Townsend KL, Huang TL, An D, Yan X, et al. MicroRNA-455 regulates brown adipogenesis via a novel HIF1an-AMPK-PGC1alpha signaling network. EMBO Rep (2015) 16(10):1378–93. doi: 10.15252/embr.201540837
108. Zhao XY, Li S, Wang GX, Yu Q, Lin JD. A long noncoding RNA transcriptional regulatory circuit drives thermogenic adipocyte differentiation. Mol Cell (2014) 55(3):372–82. doi: 10.1016/j.molcel.2014.06.004
109. Xiong Y, Yue F, Jia Z, Gao Y, Jin W, Hu K, et al. A novel brown adipocyte-enriched long non-coding RNA that is required for brown adipocyte differentiation and sufficient to drive thermogenic gene program in white adipocytes. Biochim Biophys Acta Mol Cell Biol Lipids (2018) 1863(4):409–19. doi: 10.1016/j.bbalip.2018.01.008
110. Bai Z, Chai XR, Yoon MJ, Kim HJ, Lo KA, Zhang ZC, et al. Dynamic transcriptome changes during adipose tissue energy expenditure reveal critical roles for long noncoding RNA regulators. PloS Biol (2017) 15(8):e2002176. doi: 10.1371/journal.pbio.2002176
111. Liu J, Zhang C, Zhang B, Sheng Y, Xu W, Luo Y, et al. Comprehensive analysis of the characteristics and differences in adult and newborn brown adipose tissue (BAT): newborn BAT is a more Active/Dynamic BAT. Cells (2020) 9(1):201. doi: 10.3390/cells9010201
112. Schmidt E, Dhaouadi I, Gaziano I, Oliverio M, Klemm P, Awazawa M, et al. LincRNA H19 protects from dietary obesity by constraining expression of monoallelic genes in brown fat. Nat Commun (2018) 9(1):3622. doi: 10.1038/s41467-018-05933-8
113. Zhang B, Xu S, Liu J, Xie Y, Xiaobo S. Long noncoding RNAs: novel important players in adipocyte lipid metabolism and derivative diseases. Front Physiol (2021) 12:691824. doi: 10.3389/fphys.2021.691824
114. Zhang P, He Y, Wu S, Li X, Lin X, Gan M, et al. Factors associated with white fat browning: new regulators of lipid metabolism. Int J Mol Sci (2022) 23(14):7641-70. doi: 10.3390/ijms23147641
115. Kimura H, Nagoshi T, Oi Y, Yoshii A, Tanaka Y, Takahashi H, et al. Treatment with atrial natriuretic peptide induces adipose tissue browning and exerts thermogenic actions in vivo. Sci Rep (2021) 11(1):17466. doi: 10.1038/s41598-021-96970-9
116. Schlueter N, de Sterke A, Willmes DM, Spranger J, Jordan J, Birkenfeld AL. Metabolic actions of natriuretic peptides and therapeutic potential in the metabolic syndrome. Pharmacol Ther (2014) 144(1):12–27. doi: 10.1016/j.pharmthera.2014.04.007
117. Wu L, Xia M, Duan Y, Zhang L, Jiang H, Hu X, et al. Berberine promotes the recruitment and activation of brown adipose tissue in mice and humans. Cell Death Dis (2019) 10(6):468. doi: 10.1038/s41419-019-1706-y
118. Kim S, Choe S, Lee DK. BMP-9 enhances fibroblast growth factor 21 expression and suppresses obesity. Biochim Biophys Acta (2016) 1862(7):1237–46. doi: 10.1016/j.bbadis.2016.04.006
119. Baskaran P, Krishnan V, Ren J, Thyagarajan B. Capsaicin induces browning of white adipose tissue and counters obesity by activating TRPV1 channel-dependent mechanisms. Br J Pharmacol (2016) 173(15):2369–89. doi: 10.1111/bph.13514
120. Fischer K, Ruiz HH, Jhun K, Finan B, Oberlin DJ, van der Heide V, et al. Alternatively activated macrophages do not synthesize catecholamines or contribute to adipose tissue adaptive thermogenesis. Nat Med (2017) 23(5):623–30. doi: 10.1038/nm.4316
121. Han X, Zhang Y, Guo J, You Y, Zhan J, Huang W. Chlorogenic acid stimulates the thermogenesis of brown adipocytes by promoting the uptake of glucose and the function of mitochondria. J Food Sci (2019) 84(12):3815–24. doi: 10.1111/1750-3841.14838
122. Vasileva LV, Savova MS, Amirova KM, Balcheva-Sivenova Z, Ferrante C, Orlando G, et al. Caffeic and chlorogenic acids synergistically activate browning program in human adipocytes: implications of AMPK- and PPAR-mediated pathways. Int J Mol Sci (2020) 21(24):9740-55. doi: 10.3390/ijms21249740
123. Choi JH, Yun JW. Chrysin induces brown fat-like phenotype and enhances lipid metabolism in 3T3-L1 adipocytes. Nutrition (2016) 32(9):1002–10. doi: 10.1016/j.nut.2016.02.007
124. Zuo J, Zhao D, Yu N, Fang X, Mu Q, Ma Y, et al. Cinnamaldehyde ameliorates diet-induced obesity in mice by inducing browning of white adipose tissue. Cell Physiol Biochem (2017) 42(4):1514–25. doi: 10.1159/000479268
125. Lone J, Choi JH, Kim SW, Yun JW. Curcumin induces brown fat-like phenotype in 3T3-L1 and primary white adipocytes. J Nutr Biochem (2016) 27:193–202. doi: 10.1016/j.jnutbio.2015.09.006
126. Wang S, Wang X, Ye Z, Xu C, Zhang M, Ruan B, et al. Curcumin promotes browning of white adipose tissue in a norepinephrine-dependent way. Biochem Biophys Res Commun (2015) 466(2):247–53. doi: 10.1016/j.bbrc.2015.09.018
127. Song Z, Revelo X, Shao W, Tian L, Zeng K, Lei H, et al. Dietary curcumin intervention targets mouse white adipose tissue inflammation and brown adipose tissue UCP1 expression. Obes (Silver Spring) (2018) 26(3):547–58. doi: 10.1002/oby.22110
128. Wang L, Wei Y, Ning C, Zhang M, Fan P, Lei D, et al. Ellagic acid promotes browning of white adipose tissues in high-fat diet-induced obesity in rats through suppressing white adipocyte maintaining genes. Endocr J (2019) 66(10):923–36. doi: 10.1507/endocrj.EJ18-0467
129. Liu R, Li J, Cheng Y, Huo T, Xue J, Liu Y, et al. Effects of ellagic acid-rich extract of pomegranates peel on regulation of cholesterol metabolism and its molecular mechanism in hamsters. Food Funct (2015) 6(3):780–7. doi: 10.1039/C4FO00759J
130. Cheng L, Zhang S, Shang F, Ning Y, Huang Z, He R, et al. Emodin improves glucose and lipid metabolism disorders in obese mice via activating brown adipose tissue and inducing browning of white adipose tissue. Front Endocrinol (Lausanne) (2021) 12:618037. doi: 10.3389/fendo.2021.618037
131. Varela CE, Rodriguez A, Romero-Valdovinos M, Mendoza-Lorenzo P, Mansour C, Ceballos G, et al. Browning effects of (-)-epicatechin on adipocytes and white adipose tissue. Eur J Pharmacol (2017) 811:48–59. doi: 10.1016/j.ejphar.2017.05.051
132. Tanaka N, Takahashi S, Zhang Y, Krausz KW, Smith PB, Patterson AD, et al. Role of fibroblast growth factor 21 in the early stage of NASH induced by methionine- and choline-deficient diet. Biochim Biophys Acta (2015) 1852(7):1242–52. doi: 10.1016/j.bbadis.2015.02.012
133. Fisher FM, Maratos-Flier E. Understanding the physiology of FGF21. Annu Rev Physiol (2016) 78:223–41. doi: 10.1146/annurev-physiol-021115-105339
134. Yamashita Y, Mitani T, Wang L, Ashida H. Methylxanthine derivative-rich cacao extract suppresses differentiation of adipocytes through downregulation of PPARγ and C/EBPs. J Nutr Sci Vitaminol (Tokyo) (2018) 64(2):151–60. doi: 10.3177/jnsv.64.151
135. Maeda H, Hosokawa M, Sashima T, Funayama K, Miyashita K. Fucoxanthin from edible seaweed, undaria pinnatifida, shows antiobesity effect through UCP1 expression in white adipose tissues. Biochem Biophys Res Commun (2005) 332(2):392–7. doi: 10.1016/j.bbrc.2005.05.002
136. Rodríguez AM, Palou A. UCP1 mRNA induction by RU486 in brown adipocytes is followed by marked induction of UCP1 protein levels. Genes Nutr (2007) 2(1):133–4. doi: 10.1007/s12263-007-0035-4
137. Zhang Y, Li R, Meng Y, Li S, Donelan W, Zhao Y, et al. Irisin stimulates browning of white adipocytes through mitogen-activated protein kinase p38 MAP kinase and ERK MAP kinase signaling. Diabetes (2014) 63(2):514–25. doi: 10.2337/db13-1106
138. Commins SP, Watson PM, Frampton IC, Gettys TW. Leptin selectively reduces white adipose tissue in mice via a UCP1-dependent mechanism in brown adipose tissue. Am J Physiol Endocrinol Metab (2001) 280(2):E372–7. doi: 10.1152/ajpendo.2001.280.2.E372
139. Zhang X, Zhang QX, Wang X, Zhang L, Qu W, Bao B, et al. Dietary luteolin activates browning and thermogenesis in mice through an AMPK/PGC1α pathway-mediated mechanism. Int J Obes (Lond) (2016) 40(12):1841–9. doi: 10.1038/ijo.2016.108
140. Liu D, Bordicchia M, Zhang C, Fang H, Wei W, Li JL, et al. Activation of mTORC1 is essential for β-adrenergic stimulation of adipose browning. J Clin Invest (2016) 126(5):1704–16. doi: 10.1172/JCI83532
141. Jiang C, Zhai M, Yan D, Li D, Li C, Zhang Y, et al. Dietary menthol-induced TRPM8 activation enhances WAT "browning" and ameliorates diet-induced obesity. Oncotarget (2017) 8(43):75114–26. doi: 10.18632/oncotarget.20540
142. Wang GX, Zhao XY, Meng ZX, Kern M, Dietrich A, Chen Z, et al. The brown fat-enriched secreted factor Nrg4 preserves metabolic homeostasis through attenuation of hepatic lipogenesis. Nat Med (2014) 20(12):1436–43. doi: 10.1038/nm.3713
143. Pfeifer A. NRG4: an endocrine link between brown adipose tissue and liver. Cell Metab (2015) 21(1):13–4. doi: 10.1016/j.cmet.2014.12.008
144. Ali AT, Hochfeld WE, Myburgh R, Pepper MS. Adipocyte and adipogenesis. Eur J Cell Biol (2013) 92(6-7):229–36. doi: 10.1016/j.ejcb.2013.06.001
145. Paschos GK, Tang SY, Theken KN, Li X, Verginadis I, Lekkas D, et al. Cold-induced browning of inguinal white adipose tissue is independent of adipose tissue cyclooxygenase-2. Cell Rep (2018) 24(4):809–14. doi: 10.1016/j.celrep.2018.06.082
146. Lee SG, Chae J, Kim DS, Lee JB, Kwon GS, Kwon TK, et al. Enhancement of the antiobesity and antioxidant effect of purple sweet potato extracts and enhancement of the effects by fermentation. Antioxidants (Basel) (2021) 10(6):888-900. doi: 10.3390/antiox10060888
147. Pei Y, Otieno D, Gu I, Lee SO, Parks JS, Schimmel K, et al. Effect of quercetin on nonshivering thermogenesis of brown adipose tissue in high-fat diet-induced obese mice. J Nutr Biochem (2021) 88:108532. doi: 10.1016/j.jnutbio.2020.108532
148. Hui S, Liu Y, Huang L, Zheng L, Zhou M, Lang H, et al. Resveratrol enhances brown adipose tissue activity and white adipose tissue browning in part by regulating bile acid metabolism via gut microbiota remodeling. Int J Obes (Lond) (2020) 44(8):1678–90. doi: 10.1038/s41366-020-0566-y
149. Zou Y, Ju X, Chen W, Yuan J, Wang Z, Aluko RE, et al. Rice bran attenuated obesity via alleviating dyslipidemia, browning of white adipocytes and modulating gut microbiota in high-fat diet-induced obese mice. Food Funct (2020) 11(3):2406–17. doi: 10.1039/C9FO01524H
150. Lee DH, Chang SH, Yang DK, Song NJ, Yun UJ, Park KW. Sesamol increases Ucp1 expression in white adipose tissues and stimulates energy expenditure in high-fat diet-fed obese mice. Nutrients (2020) 12(5):1459-73. doi: 10.3390/nu12051459
151. Lin C, Chen J, Hu M, Zheng W, Song Z, Qin H. Sesamol promotes browning of white adipocytes to ameliorate obesity by inducing mitochondrial biogenesis and inhibition mitophagy via beta3-AR/PKA signaling pathway. Food Nutr Res (2021) 65:7577-88.
152. Guo YY, Li BY, Peng WQ, Guo L, Tang QQ. Taurine-mediated browning of white adipose tissue is involved in its anti-obesity effect in mice. J Biol Chem (2019) 294(41):15014–24. doi: 10.1074/jbc.RA119.009936
153. Jeon EJ, Kim DY, Lee NH, Choi HE, Cheon HG. Telmisartan induces browning of fully differentiated white adipocytes via M2 macrophage polarization. Sci Rep (2019) 9(1):1236. doi: 10.1038/s41598-018-38399-1
154. Jeon EJ, Kim DY, Lee NH, Choi HE, Cheon HG. Author correction: telmisartan induces browning of fully differentiated white adipocytes via M2 macrophage polarization. Sci Rep (2020) 10(1):2165. doi: 10.1038/s41598-020-58948-x
155. Tung Y-C, Chou R-F, Nagabhushanam K, Ho C-T, Pan M-H. 3'-hydroxydaidzein improves obesity through the induced browning of beige adipose and modulation of gut microbiota in mice with obesity induced by a high-fat diet. J Agric Food Chem (2020) 68(49):14513–22. doi: 10.1021/acs.jafc.0c06138
156. Sun W, Modica S, Dong H, Wolfrum C. Plasticity and heterogeneity of thermogenic adipose tissue. Nat Metab (2021) 3(6):751–61. doi: 10.1038/s42255-021-00417-4
157. Emont MP, Jacobs C, Essene AL, Pant D, Tenen D, Colleluori G, et al. A single-cell atlas of human and mouse white adipose tissue. Nature (2022) 603(7903):926–33. doi: 10.1038/s41586-022-04518-2
158. Song A, Dai W, Jang MJ, Medrano L, Li Z, Zhao H, et al. Low- and high-thermogenic brown adipocyte subpopulations coexist in murine adipose tissue. J Clin Invest (2020) 130(1):247–57.
159. Rajbhandari P, Arneson D, Hart SK, Ahn IS, Diamante G, Santos LC, et al. Single cell analysis reveals immune cell-adipocyte crosstalk regulating the transcription of thermogenic adipocytes. Elife (2019) 8:e49501-26. doi: 10.7554/eLife.49501
160. Oguri Y, Shinoda K, Kim H, Alba DL, Bolus WR, Wang Q, et al. CD81 controls beige fat progenitor cell growth and energy balance via FAK signaling. Cell (2020) 182(3):563–77 e20. doi: 10.1016/j.cell.2020.06.021
161. Henriques F, Bedard AH, Guilherme A, Kelly M, Chi J, Zhang P, et al. Single-cell RNA profiling reveals adipocyte to macrophage signaling sufficient to enhance thermogenesis. Cell Rep (2020) 32(5):107998. doi: 10.1016/j.celrep.2020.107998
162. Sun W, Dong H, Balaz M, Slyper M, Drokhlyansky E, Colleluori G, et al. snRNA-seq reveals a subpopulation of adipocytes that regulates thermogenesis. Nature (2020) 587(7832):98–102. doi: 10.1038/s41586-020-2856-x
163. Karlina R, Lutter D, Miok V, Fischer D, Altun I, Schottl T, et al. Identification and characterization of distinct brown adipocyte subtypes in C57BL/6J mice. Life Sci Alliance (2021) 4(1):e202000924-42. doi: 10.26508/lsa.202000924
164. Shamsi F, Piper M, Ho LL, Huang TL, Gupta A, Streets A, et al. Vascular smooth muscle-derived Trpv1(+) progenitors are a source of cold-induced thermogenic adipocytes. Nat Metab (2021) 3(4):485–95. doi: 10.1038/s42255-021-00373-z
165. Angueira AR, Sakers AP, Holman CD, Cheng L, Arbocco MN, Shamsi F, et al. Defining the lineage of thermogenic perivascular adipose tissue. Nat Metab (2021) 3(4):469–84. doi: 10.1038/s42255-021-00380-0
166. Ramirez AK, Dankel SN, Rastegarpanah B, Cai W, Xue R, Crovella M, et al. Single-cell transcriptional networks in differentiating preadipocytes suggest drivers associated with tissue heterogeneity. Nat Commun (2020) 11(1):2117. doi: 10.1038/s41467-020-16019-9
167. Vijay J, Gauthier MF, Biswell RL, Louiselle DA, Johnston JJ, Cheung WA, et al. Single-cell analysis of human adipose tissue identifies depot and disease specific cell types. Nat Metab (2020) 2(1):97–109.
168. Hildreth AD, Ma F, Wong YY, Sun R, Pellegrini M, O'Sullivan TE. Single-cell sequencing of human white adipose tissue identifies new cell states in health and obesity. Nat Immunol (2021) 22(5):639–53. doi: 10.1038/s41590-021-00922-4
169. Cao Y. Angiogenesis and vascular functions in modulation of obesity, adipose metabolism, and insulin sensitivity. Cell Metab (2013) 18(4):478–89. doi: 10.1016/j.cmet.2013.08.008
170. Sun K, Kusminski CM, Luby-Phelps K, Spurgin SB, An YA, Wang QA, et al. Brown adipose tissue derived VEGF-a modulates cold tolerance and energy expenditure. Mol Metab (2014) 3(4):474–83. doi: 10.1016/j.molmet.2014.03.010
171. Hagberg CE, Falkevall A, Wang X, Larsson E, Huusko J, Nilsson I, et al. Vascular endothelial growth factor b controls endothelial fatty acid uptake. Nature (2010) 464(7290):917–21. doi: 10.1038/nature08945
172. Seki T, Hosaka K, Lim S, Fischer C, Honek J, Yang Y, et al. Endothelial PDGF-CC regulates angiogenesis-dependent thermogenesis in beige fat. Nat Commun (2016) 7:12152. doi: 10.1038/ncomms12152
173. Bhattacharya I, Ullrich A. Endothelin-1 inhibits adipogenesis: role of phosphorylation of akt and ERK1/2. FEBS Lett (2006) 580(24):5765–71. doi: 10.1016/j.febslet.2006.09.032
174. Engeli S, Janke J, Gorzelniak K, Böhnke J, Ghose N, Lindschau C, et al. Regulation of the nitric oxide system in human adipose tissue. J Lipid Res (2004) 45(9):1640–8. doi: 10.1194/jlr.M300322-JLR200
175. Fischer AW, Jaeckstein MY, Gottschling K, Heine M, Sass F, Mangels N, et al. Lysosomal lipoprotein processing in endothelial cells stimulates adipose tissue thermogenic adaptation. Cell Metab (2021) 33(3):547–64 e7. doi: 10.1016/j.cmet.2020.12.001
176. Rask-Madsen C, King GL. Mechanisms of disease: endothelial dysfunction in insulin resistance and diabetes. Nat Clin Pract Endocrinol Metab (2007) 3(1):46–56. doi: 10.1038/ncpendmet0366
177. Lumeng CN, Saltiel AR. Inflammatory links between obesity and metabolic disease. J Clin Invest (2011) 121(6):2111–7. doi: 10.1172/JCI57132
178. Olefsky JM, Glass CK. Macrophages, inflammation, and insulin resistance. Annu Rev Physiol (2010) 72:219–46. doi: 10.1146/annurev-physiol-021909-135846
179. Ferrante AW Jr. The immune cells in adipose tissue. Diabetes Obes Metab (2013) 15 Suppl 3(0 3):34–8. doi: 10.1111/dom.12154
180. Liu R, Nikolajczyk BS. Tissue immune cells fuel obesity-associated inflammation in adipose tissue and beyond. Front Immunol (2019) 10:1587. doi: 10.3389/fimmu.2019.01587
181. Sakamoto T, Nitta T, Maruno K, Yeh YS, Kuwata H, Tomita K, et al. Macrophage infiltration into obese adipose tissues suppresses the induction of UCP1 level in mice. Am J Physiol Endocrinol Metab (2016) 310(8):E676–E87. doi: 10.1152/ajpendo.00028.2015
182. Chung KJ, Chatzigeorgiou A, Economopoulou M, Garcia-Martin R, Alexaki VI, Mitroulis I, et al. A self-sustained loop of inflammation-driven inhibition of beige adipogenesis in obesity. Nat Immunol (2017) 18(6):654–64. doi: 10.1038/ni.3728
183. Villarroya F, Cereijo R, Villarroya J, Gavalda-Navarro A, Giralt M. Toward an understanding of how immune cells control brown and beige adipobiology. Cell Metab (2018) 27(5):954–61. doi: 10.1016/j.cmet.2018.04.006
184. Ricardo-Gonzalez RR, Red Eagle A, Odegaard JI, Jouihan H, Morel CR, Heredia JE, et al. IL-4/STAT6 immune axis regulates peripheral nutrient metabolism and insulin sensitivity. Proc Natl Acad Sci USA (2010) 107(52):22617–22. doi: 10.1073/pnas.1009152108
185. Nguyen KD, Qiu Y, Cui X, Goh YP, Mwangi J, David T, et al. Alternatively activated macrophages produce catecholamines to sustain adaptive thermogenesis. Nature (2011) 480(7375):104–8. doi: 10.1038/nature10653
186. Qiu Y, Nguyen KD, Odegaard JI, Cui X, Tian X, Locksley RM, et al. Eosinophils and type 2 cytokine signaling in macrophages orchestrate development of functional beige fat. Cell (2014) 157(6):1292–308. doi: 10.1016/j.cell.2014.03.066
187. Pirzgalska RM, Seixas E, Seidman JS, Link VM, Sánchez NM, Mahú I, et al. Sympathetic neuron-associated macrophages contribute to obesity by importing and metabolizing norepinephrine. Nat Med (2017) 23(11):1309–18. doi: 10.1038/nm.4422
188. Camell CD, Sander J, Spadaro O, Lee A, Nguyen KY, Wing A, et al. Inflammasome-driven catecholamine catabolism in macrophages blunts lipolysis during ageing. Nature (2017) 550(7674):119–23. doi: 10.1038/nature24022
189. Cereijo R, Gavalda-Navarro A, Cairo M, Quesada-Lopez T, Villarroya J, Moron-Ros S, et al. CXCL14, a brown adipokine that mediates brown-Fat-to-Macrophage communication in thermogenic adaptation. Cell Metab (2018) 28(5):750–63 e6. doi: 10.1016/j.cmet.2018.07.015
190. Hui X, Gu P, Zhang J, Nie T, Pan Y, Wu D, et al. Adiponectin enhances cold-induced browning of subcutaneous adipose tissue via promoting M2 macrophage proliferation. Cell Metab (2015) 22(2):279–90. doi: 10.1016/j.cmet.2015.06.004
191. Qian SW, Wu MY, Wang YN, Zhao YX, Zou Y, Pan JB, et al. BMP4 facilitates beige fat biogenesis via regulating adipose tissue macrophages. J Mol Cell Biol (2019) 11(1):14–25. doi: 10.1093/jmcb/mjy011
192. Molofsky AB, Nussbaum JC, Liang HE, Van Dyken SJ, Cheng LE, Mohapatra A, et al. Innate lymphoid type 2 cells sustain visceral adipose tissue eosinophils and alternatively activated macrophages. J Exp Med (2013) 210(3):535–49. doi: 10.1084/jem.20121964
193. Lee MW, Odegaard JI, Mukundan L, Qiu Y, Molofsky AB, Nussbaum JC, et al. Activated type 2 innate lymphoid cells regulate beige fat biogenesis. Cell (2015) 160(1-2):74–87. doi: 10.1016/j.cell.2014.12.011
194. Brestoff JR, Kim BS, Saenz SA, Stine RR, Monticelli LA, Sonnenberg GF, et al. Group 2 innate lymphoid cells promote beiging of white adipose tissue and limit obesity. Nature (2015) 519(7542):242–6. doi: 10.1038/nature14115
195. Wu D, Molofsky AB, Liang HE, Ricardo-Gonzalez RR, Jouihan HA, Bando JK, et al. Eosinophils sustain adipose alternatively activated macrophages associated with glucose homeostasis. Science (2011) 332(6026):243–7. doi: 10.1126/science.1201475
196. Rao RR, Long JZ, White JP, Svensson KJ, Lou J, Lokurkar I, et al. Meteorin-like is a hormone that regulates immune-adipose interactions to increase beige fat thermogenesis. Cell (2014) 157(6):1279–91. doi: 10.1016/j.cell.2014.03.065
197. Huang Z, Zhong L, Lee JTH, Zhang J, Wu D, Geng L, et al. The FGF21-CCL11 axis mediates beiging of white adipose tissues by coupling sympathetic nervous system to type 2 immunity. Cell Metab (2017) 26(3):493–508.e4. doi: 10.1016/j.cmet.2017.08.003
198. Bartness TJ, Vaughan CH, Song CK. Sympathetic and sensory innervation of brown adipose tissue. Int J Obes (Lond) (2010) 34 Suppl 1(0 1):S36–42. doi: 10.1038/ijo.2010.182
199. Muzik O, Mangner TJ, Leonard WR, Kumar A, Granneman JG. Sympathetic innervation of cold-activated brown and white fat in lean young adults. J Nucl Med (2017) 58(5):799–806. doi: 10.2967/jnumed.116.180992
200. Jiang H, Ding X, Cao Y, Wang H, Zeng W. Dense intra-adipose sympathetic arborizations are essential for cold-induced beiging of mouse white adipose tissue. Cell Metab (2017) 26(4):686–92 e3. doi: 10.1016/j.cmet.2017.08.016
201. Zeng X, Ye M, Resch JM, Jedrychowski MP, Hu B, Lowell BB, et al. Innervation of thermogenic adipose tissue via a calsyntenin 3β-S100b axis. Nature (2019) 569(7755):229–35. doi: 10.1038/s41586-019-1156-9
202. Jiang J, Zhou D, Zhang A, Yu W, Du L, Yuan H, et al. Thermogenic adipocyte-derived zinc promotes sympathetic innervation in male mice. Nat Metab (2023) 5(3):481–94. doi: 10.1038/s42255-023-00751-9
203. Priest C, Tontonoz P. Inter-organ cross-talk in metabolic syndrome. Nat Metab (2019) 1(12):1177–88. doi: 10.1038/s42255-019-0145-5
204. Shamsi F, Wang CH, Tseng YH. The evolving view of thermogenic adipocytes - ontogeny, niche and function. Nat Rev Endocrinol (2021) 17(12):726–44. doi: 10.1038/s41574-021-00562-6
205. Yin X, Chen Y, Ruze R, Xu R, Song J, Wang C, et al. The evolving view of thermogenic fat and its implications in cancer and metabolic diseases. Signal Transduct Target Ther (2022) 7(1):324. doi: 10.1038/s41392-022-01178-6
206. Bartness TJ, Liu Y, Shrestha YB, Ryu V. Neural innervation of white adipose tissue and the control of lipolysis. Front Neuroendocrinol (2014) 35(4):473–93. doi: 10.1016/j.yfrne.2014.04.001
207. Morrison SF, Nakamura K. Central mechanisms for thermoregulation. Annu Rev Physiol (2019) 81:285–308. doi: 10.1146/annurev-physiol-020518-114546
208. Picó C, Palou M, Pomar CA, Rodríguez AM, Palou A. Leptin as a key regulator of the adipose organ. Rev Endocr Metab Disord (2022) 23(1):13–30. doi: 10.1007/s11154-021-09687-5
209. Friedman JM, Halaas JL. Leptin and the regulation of body weight in mammals. Nature (1998) 395(6704):763–70. doi: 10.1038/27376
210. Begriche K, Sutton GM, Butler AA. Homeostastic and non-homeostatic functions of melanocortin-3 receptors in the control of energy balance and metabolism. Physiol Behav (2011) 104(4):546–54. doi: 10.1016/j.physbeh.2011.04.007
211. Kishi T, Aschkenasi CJ, Lee CE, Mountjoy KG, Saper CB, Elmquist JK. Expression of melanocortin 4 receptor mRNA in the central nervous system of the rat. J Comp Neurol (2003) 457(3):213–35. doi: 10.1002/cne.10454
212. Voss-Andreae A, Murphy JG, Ellacott KL, Stuart RC, Nillni EA, Cone RD, et al. Role of the central melanocortin circuitry in adaptive thermogenesis of brown adipose tissue. Endocrinology (2007) 148(4):1550–60. doi: 10.1210/en.2006-1389
213. Williams DL, Bowers RR, Bartness TJ, Kaplan JM, Grill HJ. Brainstem melanocortin 3/4 receptor stimulation increases uncoupling protein gene expression in brown fat. Endocrinology (2003) 144(11):4692–7. doi: 10.1210/en.2003-0440
214. Dodd GT, Decherf S, Loh K, Simonds SE, Wiede F, Balland E, et al. Leptin and insulin act on POMC neurons to promote the browning of white fat. Cell (2015) 160(1-2):88–104. doi: 10.1016/j.cell.2014.12.022
215. Whittle AJ, Carobbio S, Martins L, Slawik M, Hondares E, Vazquez MJ, et al. BMP8B increases brown adipose tissue thermogenesis through both central and peripheral actions. Cell (2012) 149(4):871–85. doi: 10.1016/j.cell.2012.02.066
216. Ruan HB, Dietrich MO, Liu ZW, Zimmer MR, Li MD, Singh JP, et al. O-GlcNAc transferase enables AgRP neurons to suppress browning of white fat. Cell (2014) 159(2):306–17. doi: 10.1016/j.cell.2014.09.010
217. Soumano K, Desbiens S, Rabelo R, Bakopanos E, Camirand A, Silva JE. Glucocorticoids inhibit the transcriptional response of the uncoupling protein-1 gene to adrenergic stimulation in a brown adipose cell line. Mol Cell Endocrinol (2000) 165(1-2):7–15. doi: 10.1016/S0303-7207(00)00276-8
218. Ramage LE, Akyol M, Fletcher AM, Forsythe J, Nixon M, Carter RN, et al. Glucocorticoids acutely increase brown adipose tissue activity in humans, revealing species-specific differences in UCP-1 regulation. Cell Metab (2016) 24(1):130–41. doi: 10.1016/j.cmet.2016.06.011
219. Staiger H, Keuper M, Berti L, Hrabe de Angelis M, Haring HU. Fibroblast growth factor 21-metabolic role in mice and men. Endocr Rev (2017) 38(5):468–88. doi: 10.1210/er.2017-00016
220. Cheong LY, Xu A. Intercellular and inter-organ crosstalk in browning of white adipose tissue: molecular mechanism and therapeutic complications. J Mol Cell Biol (2021) 13(7):466–79. doi: 10.1093/jmcb/mjab038
221. Bookout AL, de Groot MH, Owen BM, Lee S, Gautron L, Lawrence HL, et al. FGF21 regulates metabolism and circadian behavior by acting on the nervous system. Nat Med (2013) 19(9):1147–52. doi: 10.1038/nm.3249
222. Owen BM, Ding X, Morgan DA, Coate KC, Bookout AL, Rahmouni K, et al. FGF21 acts centrally to induce sympathetic nerve activity, energy expenditure, and weight loss. Cell Metab (2014) 20(4):670–7. doi: 10.1016/j.cmet.2014.07.012
223. Kharitonenkov A, Wroblewski VJ, Koester A, Chen YF, Clutinger CK, Tigno XT, et al. The metabolic state of diabetic monkeys is regulated by fibroblast growth factor-21. Endocrinology (2007) 148(2):774–81. doi: 10.1210/en.2006-1168
224. Chabicovsky M, Herkner K, Rossmanith W. Overexpression of activin beta(C) or activin beta(E) in the mouse liver inhibits regenerative deoxyribonucleic acid synthesis of hepatic cells. Endocrinology (2003) 144(8):3497–504. doi: 10.1210/en.2003-0388
225. Hashimoto O, Funaba M, Sekiyama K, Doi S, Shindo D, Satoh R, et al. Activin e controls energy homeostasis in both brown and white adipose tissues as a hepatokine. Cell Rep (2018) 25(5):1193–203. doi: 10.1016/j.celrep.2018.10.008
226. Braga M, Reddy ST, Vergnes L, Pervin S, Grijalva V, Stout D, et al. Follistatin promotes adipocyte differentiation, browning, and energy metabolism. J Lipid Res (2014) 55(3):375–84. doi: 10.1194/jlr.M039719
227. Singh R, Braga M, Reddy ST, Lee SJ, Parveen M, Grijalva V, et al. Follistatin targets distinct pathways to promote brown adipocyte characteristics in brown and white adipose tissues. Endocrinology (2017) 158(5):1217–30. doi: 10.1210/en.2016-1607
228. Hansen JS, Rutti S, Arous C, Clemmesen JO, Secher NH, Drescher A, et al. Circulating follistatin is liver-derived and regulated by the glucagon-to-Insulin ratio. J Clin Endocrinol Metab (2016) 101(2):550–60. doi: 10.1210/jc.2015-3668
229. Qing H, Desrouleaux R, Israni-Winger K, Mineur YS, Fogelman N, Zhang C, et al. Origin and function of stress-induced IL-6 in murine models. Cell (2020) 182(2):372–87 e14.
230. Shen H, Jiang L, Lin JD, Omary MB, Rui L. Brown fat activation mitigates alcohol-induced liver steatosis and injury in mice. J Clin Invest (2019) 129(6):2305–17. doi: 10.1172/JCI124376
231. Cao H, Gerhold K, Mayers JR, Wiest MM, Watkins SM, Hotamisligil GS. Identification of a lipokine, a lipid hormone linking adipose tissue to systemic metabolism. Cell (2008) 134(6):933–44. doi: 10.1016/j.cell.2008.07.048
232. Simcox J, Geoghegan G, Maschek JA, Bensard CL, Pasquali M, Miao R, et al. Global analysis of plasma lipids identifies liver-derived acylcarnitines as a fuel source for brown fat thermogenesis. Cell Metab (2017) 26(3):509–22 e6. doi: 10.1016/j.cmet.2017.08.006
233. Velazquez-Villegas LA, Perino A, Lemos V, Zietak M, Nomura M, Pols TWH, et al. TGR5 signalling promotes mitochondrial fission and beige remodelling of white adipose tissue. Nat Commun (2018) 9(1):245. doi: 10.1038/s41467-017-02068-0
234. Broeders EP, Nascimento EB, Havekes B, Brans B, Roumans KH, Tailleux A, et al. The bile acid chenodeoxycholic acid increases human brown adipose tissue activity. Cell Metab (2015) 22(3):418–26. doi: 10.1016/j.cmet.2015.07.002
235. Watanabe M, Houten SM, Mataki C, Christoffolete MA, Kim BW, Sato H, et al. Bile acids induce energy expenditure by promoting intracellular thyroid hormone activation. Nature (2006) 439(7075):484–9. doi: 10.1038/nature04330
236. Mills EL, Pierce KA, Jedrychowski MP, Garrity R, Winther S, Vidoni S, et al. Accumulation of succinate controls activation of adipose tissue thermogenesis. Nature (2018) 560(7716):102–6. doi: 10.1038/s41586-018-0353-2
237. Mills EL, Harmon C, Jedrychowski MP, Xiao H, Garrity R, Tran NV, et al. UCP1 governs liver extracellular succinate and inflammatory pathogenesis. Nat Metab (2021) 3(5):604–17. doi: 10.1038/s42255-021-00389-5
238. Iizuka K, Machida T, Hirafuji M. Skeletal muscle is an endocrine organ. J Pharmacol Sci (2014) 125(2):125–31. doi: 10.1254/jphs.14R02CP
239. Florin A, Lambert C, Sanchez C, Zappia J, Durieux N, Tieppo AM, et al. The secretome of skeletal muscle cells: a systematic review. Osteoarthr Cartil Open (2020) 2(1):100019. doi: 10.1016/j.ocarto.2019.100019
240. Severinsen MCK, Pedersen BK. Muscle-organ crosstalk: the emerging roles of myokines. Endocr Rev (2020) 41(4):594–609. doi: 10.1210/endrev/bnaa016
241. Pedersen BK, Febbraio MA. Muscle as an endocrine organ: focus on muscle-derived interleukin-6. Physiol Rev (2008) 88(4):1379–406. doi: 10.1152/physrev.90100.2007
242. Knudsen JG, Murholm M, Carey AL, Bienso RS, Basse AL, Allen TL, et al. Role of IL-6 in exercise training- and cold-induced UCP1 expression in subcutaneous white adipose tissue. PloS One (2014) 9(1):e84910. doi: 10.1371/journal.pone.0084910
243. van Hall G, Steensberg A, Sacchetti M, Fischer C, Keller C, Schjerling P, et al. Interleukin-6 stimulates lipolysis and fat oxidation in humans. J Clin Endocrinol Metab (2003) 88(7):3005–10. doi: 10.1210/jc.2002-021687
244. Petersen EW, Carey AL, Sacchetti M, Steinberg GR, Macaulay SL, Febbraio MA, et al. Acute IL-6 treatment increases fatty acid turnover in elderly humans in vivo and in tissue culture in vitro. Am J Physiol Endocrinol Metab (2005) 288(1):E155–62. doi: 10.1152/ajpendo.00257.2004
245. Kristof E, Klusoczki A, Veress R, Shaw A, Combi ZS, Varga K, et al. Interleukin-6 released from differentiating human beige adipocytes improves browning. Exp Cell Res (2019) 377(1-2):47–55. doi: 10.1016/j.yexcr.2019.02.015
246. Bostrom P, Wu J, Jedrychowski MP, Korde A, Ye L, Lo JC, et al. A PGC1-alpha-dependent myokine that drives brown-fat-like development of white fat and thermogenesis. Nature (2012) 481(7382):463–8. doi: 10.1038/nature10777
247. Lee P, Linderman JD, Smith S, Brychta RJ, Wang J, Idelson C, et al. Irisin and FGF21 are cold-induced endocrine activators of brown fat function in humans. Cell Metab (2014) 19(2):302–9. doi: 10.1016/j.cmet.2013.12.017
248. Roberts LD, Bostrom P, O'Sullivan JF, Schinzel RT, Lewis GD, Dejam A, et al. Beta-aminoisobutyric acid induces browning of white fat and hepatic beta-oxidation and is inversely correlated with cardiometabolic risk factors. Cell Metab (2014) 19(1):96–108. doi: 10.1016/j.cmet.2013.12.003
249. Lynes MD, Leiria LO, Lundh M, Bartelt A, Shamsi F, Huang TL, et al. The cold-induced lipokine 12,13-diHOME promotes fatty acid transport into brown adipose tissue. Nat Med (2017) 23(5):631–7. doi: 10.1038/nm.4297
250. Stanford KI, Lynes MD, Takahashi H, Baer LA, Arts PJ, May FJ, et al. 12,13-diHOME: an exercise-induced lipokine that increases skeletal muscle fatty acid uptake. Cell Metab (2018) 27(5):1111–20 e3. doi: 10.1016/j.cmet.2018.03.020
251. Leiria LO, Wang CH, Lynes MD, Yang K, Shamsi F, Sato M, et al. 12-lipoxygenase regulates cold adaptation and glucose metabolism by producing the omega-3 lipid 12-HEPE from brown fat. Cell Metab (2019) 30(4):768–83 e7. doi: 10.1016/j.cmet.2019.07.001
252. Clarke G, Stilling RM, Kennedy PJ, Stanton C, Cryan JF, Dinan TG. Minireview: gut microbiota: the neglected endocrine organ. Mol Endocrinol (2014) 28(8):1221–38. doi: 10.1210/me.2014-1108
253. Mestdagh R, Dumas ME, Rezzi S, Kochhar S, Holmes E, Claus SP, et al. Gut microbiota modulate the metabolism of brown adipose tissue in mice. J Proteome Res (2012) 11(2):620–30. doi: 10.1021/pr200938v
254. Suarez-Zamorano N, Fabbiano S, Chevalier C, Stojanovic O, Colin DJ, Stevanovic A, et al. Microbiota depletion promotes browning of white adipose tissue and reduces obesity. Nat Med (2015) 21(12):1497–501. doi: 10.1038/nm.3994
255. Zietak M, Kovatcheva-Datchary P, Markiewicz LH, Stahlman M, Kozak LP, Backhed F. Altered microbiota contributes to reduced diet-induced obesity upon cold exposure. Cell Metab (2016) 23(6):1216–23. doi: 10.1016/j.cmet.2016.05.001
256. Chevalier C, Stojanovic O, Colin DJ, Suarez-Zamorano N, Tarallo V, Veyrat-Durebex C, et al. Gut microbiota orchestrates energy homeostasis during cold. Cell (2015) 163(6):1360–74. doi: 10.1016/j.cell.2015.11.004
257. Li G, Xie C, Lu S, Nichols RG, Tian Y, Li L, et al. Intermittent fasting promotes white adipose browning and decreases obesity by shaping the gut microbiota. Cell Metab (2017) 26(4):672–85.e4. doi: 10.1016/j.cmet.2017.08.019
258. Li B, Li L, Li M, Lam SM, Wang G, Wu Y, et al. Microbiota depletion impairs thermogenesis of brown adipose tissue and browning of white adipose tissue. Cell Rep (2019) 26(10):2720–37 e5. doi: 10.1016/j.celrep.2019.02.015
259. Sumara G, Sumara O, Kim JK, Karsenty G. Gut-derived serotonin is a multifunctional determinant to fasting adaptation. Cell Metab (2012) 16(5):588–600. doi: 10.1016/j.cmet.2012.09.014
260. Li Y, Schnabl K, Gabler SM, Willershauser M, Reber J, Karlas A, et al. Secretin-activated brown fat mediates prandial thermogenesis to induce satiation. Cell (2018) 175(6):1561–74 e12. doi: 10.1016/j.cell.2018.10.016
261. Laurila S, Sun L, Lahesmaa M, Schnabl K, Laitinen K, Klen R, et al. Secretin activates brown fat and induces satiation. Nat Metab (2021) 3(6):798–809. doi: 10.1038/s42255-021-00409-4
262. Krieger JP, Santos da Conceição EP, Sanchez-Watts G, Arnold M, Pettersen KG, Mohammed M, et al. Glucagon-like peptide-1 regulates brown adipose tissue thermogenesis via the gut-brain axis in rats. Am J Physiol Regul Integr Comp Physiol (2018) 315(4):R708–r20. doi: 10.1152/ajpregu.00068.2018
263. Gutzwiller JP, Drewe J, Göke B, Schmidt H, Rohrer B, Lareida J, et al. Glucagon-like peptide-1 promotes satiety and reduces food intake in patients with diabetes mellitus type 2. Am J Physiol (1999) 276(5):R1541–4. doi: 10.1152/ajpregu.1999.276.5.R1541
264. Beiroa D, Imbernon M, Gallego R, Senra A, Herranz D, Villarroya F, et al. GLP-1 agonism stimulates brown adipose tissue thermogenesis and browning through hypothalamic AMPK. Diabetes (2014) 63(10):3346–58. doi: 10.2337/db14-0302
265. Drucker DJ. The cardiovascular biology of glucagon-like peptide-1. Cell Metab (2016) 24(1):15–30. doi: 10.1016/j.cmet.2016.06.009
266. Kojima M, Hosoda H, Date Y, Nakazato M, Matsuo H, Kangawa K. Ghrelin is a growth-hormone-releasing acylated peptide from stomach. Nature (1999) 402(6762):656–60. doi: 10.1038/45230
267. Tschöp M, Smiley DL, Heiman ML. Ghrelin induces adiposity in rodents. Nature (2000) 407(6806):908–13. doi: 10.1038/35038090
268. Yasuda T, Masaki T, Kakuma T, Yoshimatsu H. Centrally administered ghrelin suppresses sympathetic nerve activity in brown adipose tissue of rats. Neurosci Lett (2003) 349(2):75–8. doi: 10.1016/S0304-3940(03)00789-4
269. Theander-Carrillo C, Wiedmer P, Cettour-Rose P, Nogueiras R, Perez-Tilve D, Pfluger P, et al. Ghrelin action in the brain controls adipocyte metabolism. J Clin Invest (2006) 116(7):1983–93. doi: 10.1172/JCI25811
Keywords: thermogenic fat, energy expenditure, transcription factor, epigenetic modification, intercellular regulation, inter-organ crosstalk
Citation: Wang C, Wang X and Hu W (2023) Molecular and cellular regulation of thermogenic fat. Front. Endocrinol. 14:1215772. doi: 10.3389/fendo.2023.1215772
Received: 02 May 2023; Accepted: 14 June 2023;
Published: 03 July 2023.
Edited by:
Endre Károly Kristóf, University of Debrecen, HungaryReviewed by:
Monica Colitti, University of Udine, ItalyRosemari Otton, Universidade Cruzeiro do Sul, Brazil
Copyright © 2023 Wang, Wang and Hu. This is an open-access article distributed under the terms of the Creative Commons Attribution License (CC BY). The use, distribution or reproduction in other forums is permitted, provided the original author(s) and the copyright owner(s) are credited and that the original publication in this journal is cited, in accordance with accepted academic practice. No use, distribution or reproduction is permitted which does not comply with these terms.
*Correspondence: Wenxiang Hu, aHVfd2VueGlhbmdAZ3psYWIuYWMuY24=
†These authors have contributed equally to this work