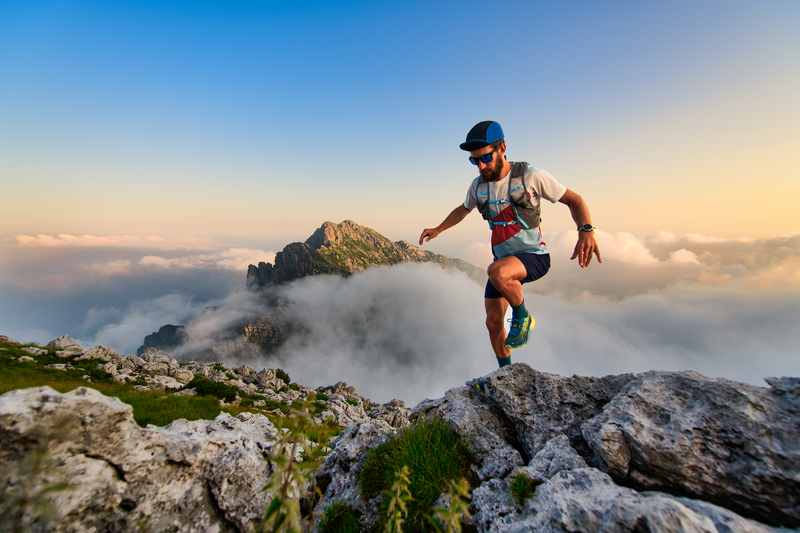
95% of researchers rate our articles as excellent or good
Learn more about the work of our research integrity team to safeguard the quality of each article we publish.
Find out more
REVIEW article
Front. Endocrinol. , 17 July 2023
Sec. Cancer Endocrinology
Volume 14 - 2023 | https://doi.org/10.3389/fendo.2023.1202493
This article is part of the Research Topic Extracellular Vesicles in Cancer Treatment and Diagnosis View all 8 articles
Breast cancer is one of the leading causes of cancer-related death in women. Currently, the treatment of breast cancer is limited by the lack of effectively targeted therapy and patients often suffer from higher severity, metastasis, and resistance. Extracellular vesicles (EVs) consist of lipid bilayers that encapsulate a complex cargo, including proteins, nucleic acids, and metabolites. These bioactive cargoes have been found to play crucial roles in breast cancer initiation and progression. Moreover, EV cargoes play pivotal roles in converting mammary cells to carcinogenic cells and metastatic foci by extensively inducing proliferation, angiogenesis, pre-metastatic niche formation, migration, and chemoresistance. The present update review mainly discusses EVs cargoes released from breast cancer cells and tumor-derived EVs in the breast cancer microenvironment, focusing on proliferation, metastasis, chemoresistance, and their clinical potential as effective biomarkers.
Breast cancer is the most frequently diagnosed cancer in women and severely affects their physical and mental health. It is additionally the leading cause of cancer-related deaths in women (1). Therefore, clarifying mechanisms underlying the development of breast cancer is currently the focus of basic research (2, 3). Extracellular vesicles (EVs) are involved in intracellular communication by carrying important bioactive molecules and play a crucial role in breast cancer development (4–6). Although their nomenclature remains controversial, EVs are classified based on their size, biogenesis, or release pathway into the following: exosomes of endosomal origin, microvesicles of plasma membrane origin (referred to as small EVs [SEVs]), and apoptotic EVs (7). EVs are thought to be carriers to transfer the contents and mediate a wide range of biological responses in intercellular communication, cancer development, and cancer metastasis in the immune system. In addition, they are extensively involved in information exchange within the organism by transferring functional bio-signaling molecules, such as nucleic acids, lipids, proteins, and even pharmacological compounds (8).
Over the past decades, researchers have been investigating the role of EVs in breast cancer. Studies have demonstrated that EVs are extensively involved in the major pathways implicated in breast cancer development, including proliferation, migration, tumor microenvironment (TME), and drug resistance (9, 10). Moreover, multiple clinical studies have demonstrated the potential applications of EVs in the treatment and diagnosis of breast cancer (11–13). This review attempts to provide a theoretical basis for the treatment and diagnosis of breast cancer by focusing on the promotive role of EVs in breast cancer development, the potential of EVs as breast cancer biomarkers, the mechanistic action of EVs in regulating drug resistance, and the role of EVs in anti-cancer treatment.
Accumulating evidence has demonstrated a strong link between glucose homeostasis and the growth of breast cancer cells. Cao et al. have demonstrated that miR-122, an EVs cargo derived from breast cancer cells, inhibits glycolysis and ATP-dependent insulin exocytosis and disrupts the critical function of islets to maintain normal blood glucose levels. This effect further suppresses insulin secretion, augments endogenous glucose production, impairs glucose tolerance, and disrupts fasting hyperglycemia. As a result, all these effects on glucose homeostasis could promote the rapid growth of breast cancer (5, 14, 15). In addition, breast cancer cells have been found to deliberately deviate trajectories during migration with the help of EVs and hyaluronic acid. This explains the role of EVs and hyaluronic acid in guiding the migration and metastasis of tumor cells (16). A study by Eckhard et al. confirmed the role of exosomal Rab27a in breast cancer development and metastasis, which indicated that targeting Rab27a reduced the release of exosomes, thus retarding breast cancer growth (17). Wanessa et al. have demonstrated that adhesion receptors, previously thought to be expressed only at the cellular level, are additionally present on SEVs membranes and thus participate in processes such as adhesion and uptake. Specifically, αvβ3 integrins, adhesion receptors present on SEVs, secreted by triple-negative breast cancer cells (MDA -MB-231) have been reported to accelerate invasion and organ-specific metastasis of breast cancer cells. Moreover, the downregulation of αvβ3 integrin expression further reduces the expression of the pro-oncoprotein CD63 present on the SEVs membrane, thereby inhibiting breast cancer progression (18). A in vitro study by Bertolini et al. highlighted that SEVs released from breast cancer cells activated NF-κB pathway in breast epithelium cells under hypoxic conditions, then promoted the release of inflammatory cytokines and mitochondria-mediated cell migration, which enabled the disruption of the mammary glandular vesicle structure. Simultaneously, this hypoxic microenvironment in patients with breast cancer commonly contributed to local carcinogenic and oncogenic inflammatory changes (19). Another study has found that Caveolin-1 is upregulated in metastatic breast cancer cell-secreted EVs and fosters breast cancer invasion and metastasis through the adhesion proteins (20). Besides, Wu et al. found that the bone marrow mesenchymal stem cell (BMSC)-derived EVs could be internalized by breast cancer cells and significantly improve the proliferation ability of these breast cancer cells (21). Adipocytes are the most abundant stromal cell component in breast cancer tissues and support the survival of metastatic breast cancer cells at distant sites (22). Reportedly, exosomes from adipose tissue-derived MSCs are internalized by breast cancer cells (MCF7), which activate the Hippo pathway to facilitate the proliferation and migration of breast cancer cells (23). Genomic instability (GI) is a major driver of tumorigenesis (24, 25). Specifically, genomic changes tend to affect cellular phenotypes and are evolutionary hallmarks of most cancers (26). As illustrated in the work of Siqi et al., three miRNAs (miR-421, miR-128-1, and miR128-2) were identified from 18 GI-associated miRNAs contained in EVs from serum of breast cancer patients, which were defined as GI-derived miRNA signature (miGISig). By analyzing its correlation with patient clinicopathological data from publicly available breast cancer -associated datasets (such as TCGA) and in vitro functional experiments, miGISig was found to be closely associated with poor prognosis of patients, indicating its high diagnostic potential for breast cancer (27). These results suggest that EVs derived from breast cancer cells can stimulate the growth and migration of tumor cells, which in turn facilitates the malignant progression of tumors.
Angiogenesis, the formation of new blood vessels from pre-existing blood vessels, is an important cause of tumor growth and blood-borne metastasis. This is a dynamically complex process involving multiple mechanisms, regulated by a variety of cancer related molecules (28). In healthy tissues, angiogenesis is tightly regulated by a precise balance between stimulating and inhibiting signals. When this balance is disturbed, abnormal vascular growth occurs and is a major cause of breast cancer invasion and metastasis (29). EVs are important mediators of angiogenesis through constant communication with the environment through a variety of para-secretory factors and cell-to-cell and cell-to-matrix interactions (30). Moreover, EVs released by primary tumors also promote angiogenesis and cancer progression. Aslan et al. have found that docosahexaenoic acid downregulates the expression of pro-angiogenic genes, such as HIF1-α, TGF-β, SOX2, Snail1, Snail2, and VEGFR, in breast cancer cell-derived exosomes. Furthermore, the docosahexaenoic acid inhibits tumor angiogenesis by upregulating tumor-suppressing miRNAs (miR-101, miR-199, and miR-342) and downregulating oncomiRs (miR-382 and miR-21) in exosomes (31). In addition, MSC-derived EVs repress the expression of vascular endothelial growth factor (VEGF) by miR-100/mTOR/HIF1α signal axis, ultimately retarding the formation of capillary-like tubules in endothelial cells (32). Meanwhile, MSC-derived exosomes deliver miR-16 to repress the expression of its target VEGF, thus curtailing angiogenesis in breast cancer (33). Sayantan et al. have demonstrated that, in primary breast cancer cell-derived SEVs, annexin II induces angiogenesis both in vitro and in vivo by upregulating the expression of the tissue fibrinogen activator tPA, which in turn promotes lung and brain metastases of breast cancer (34). Moreover, breast cancer cell-derived exosomes stimulate endothelial cell angiogenesis in the TME via the circHIPK3/miR-124-3p/MTDH axis (35). These data suggest that breast cancer cell-derived EVs can stimulate angiogenesis in the TME, which in turn promote breast cancer development and progression. Although many studies have demonstrated the effect of EVs on angiogenesis, based on the presented findings and clinical experiences, we can easily imply that another severe adverse effect associated with EVs administration may be an increased risk of thrombosis. Therefore, further preclinical models are needed to better evaluate the risk and benefit and more attention should also be paid to this point.
Cancer metastasis can be promoted by forming a supportive TME at secondary organ sites, called PMN, wherein the EVs secreted by tumor cells can modulate TME changes (36). Busatto et al. have suggested that EVs secreted from brain metastatic cells of breast cancer induce low-density lipoprotein aggregation, which accelerates uptake of these metastatic breast cancer cells by monocytes, and promotes the formation of a PMN in the brain (37). Reportedly, EVs secreted by the triple-negative breast cancer cell lines MDA-MB-231 and SUM159 have been found to induce lung fibroblasts to express the extracellular matrix (ECM) proteins fibronectin, tenascin-c, and periostin, thereby contributing to the formation of PMN in the brain (38). Moreover, LC3-loaded EVs released by breast cancer cells could induce lung fibroblasts to produce CCL2 by the HSP60-TLR2-MyD88-NF-κB pathway. Then, CCL2 recruits monocytes to the lung and subsequently form a pulmonary PMN characterized by monocytes, high infiltration of macrophages, immunosuppression, and enhanced vascular permeability, ultimately accelerating the formation of breast cancer lung metastasis (39). Lin28B has been considered a potent inducer of breast cancer metastasis, and neutrophil N2 conversion is a key step in the suppression of T-cell function. Specifically, Qi et al. have found that Lin28B is involved in neutrophil recruitment and N2 conversion through let-7s-carrying exosomes to alter the immune status in the TME, thereby establishing an immunosuppressive PMN and ultimately inducing lung metastasis of breast cancer (40). According to the findings of Wu et al., miR-19a and integrin-binding sialoprotein (IBSP) expression in breast cancer cells fail to alter the in vitro growth or migration ability of breast cancer cells. However, following encapsulation and release by exosomes, IBSP can attract osteoclasts and form an osteoclast-rich microenvironment to assist the delivery of exosomal miR-19a to osteoclasts, thus further inducing osteoclast formation. This subsequently augment PMN formation and bone metastasis in estrogen receptor (ER)-positive breast cancer (41). As previously reported, exosomes secreted by the mildly metastatic MDA-MB-231 cell line and the highly metastatic SCP28 breast cancer cell line could release miR-21 and subsequently stimulate PMN formation by upregulating programmed cell death (PD) 4 protein levels, whereby strengthening osteoclast differentiation and activation, ultimately leading to bone metastasis of breast cancer (42). From above findings, we could imply that the formation of PMN caused by tumor-derived EVs could significantly assist in organ colonization and metastasis of breast cancer, such as brain, lung and bone. As a result, patients died from tumor metastasis. In the future, we should focus on developing the novel methods to block the formation of PMN caused by tumor-derived EVs, and effectively prevent the happening of organ-specific tumor metastasis.
Despite significant advances in novel anti-cancer therapies for advanced breast cancer in recent years, many patients with breast cancer remain incurable and metastasis remains the leading cause of death in patients with advanced breast cancer (43, 44). Tumor metastasis is a multistep process involving the spread of cancer cells from the primary tumor to the blood or lymphatic system, then survival in circulation, subsequent migration to target organs, and eventually distant implantation and proliferation (45, 46). These effects require tight regulation of the cellular mechanisms by which tumor cells detach from the primary tumor and transfer to the metastatic site, in which EVs also play a crucial role (47, 48). The work of Shechter et al. has exhibited that highly metastatic or chemo-resistant breast cancer cells release more EVs than breast cancer cells with low metastatic potential. Additionally, these EVs reduce cell adhesion by substantially expressing CD44, which subsequently disrupt actin filament structure and ultimately induces tumor spread, thus contributing to breast cancer metastasis (49).
Bone is the most likely site of metastasis for all molecular subtypes of breast cancer. Patients with bone metastases often have other serious complications, such as severe bone pain, fractures, severe hypercalcemia, and nerve compression syndrome, This seriously affects the life expectancy and the quality of life (50). Under normal conditions, bones undergo a dynamic balance of bone resorption and bone formation mediated by osteoclasts and osteoblasts, respectively. However, bone metastases usually show an imbalance in this process (51), and osteolytic bone metastases is the most common type (52). EVs also promote this process. EVs can introduce miR-940 into the bone to promote osteogenic differentiation of mesenchymal stem cells by targeting Rho GTase-activating protein 1 (ARHGAP1) and FAM134A. As a result, promoting osteolytic bone metastasis (53). MDA-MB-231 triple-negative breast cancer cells also secrete EVs loaded with miR-218 to block osteoblast differentiation, tip the balance toward osteolysis, and form metastatic bone niches to facilitate osteolytic bone metastasis (54). Meanwhile, ER+ breast cancer cells can also produce EVs loaded with miR-19a, and promote osteolytic bone metastasis by inhibiting PTEN expression and inducing NF-κB and AKT pathways (41). So, in the future, targeting EVs to inhibit the formation of osteolytic bone metastasis may be a promising research direction in the field of cancer metastasis.
Lung is another important target organ of frequent breast cancer metastasis. Pulmonary capillaries consist of endothelial cells surrounded by basal membranes and adjacent alveolar cells. Tumor cells need to adhere to this endothelial membrane and penetrate into the lung parenchyma to establish a metastatic tumor (55, 56). Tumor-derived EVs secreted by highly metastatic breast cancer cells could load miR-122 to increase nutrient availability in lung metastatic cancer cells by down-regulating the glycolytic enzyme pyruvate kinase in lung fibroblasts, as a result, facilitating the formation and development of breast cancer lung metastasis (57). EVs also mediate miRNA transfer from cancer cells to other cells for tumor microenvironmental remodeling and promote the formation of pre-metastatic niches in lung. miR-138-5p and miR-183-5p loaded by EVs and secreted by cancer cells can regulate the activity of tumor-associated macrophages (TAM) by targeting KDM6B and PPP2CA respectively, and to promote the formation of breast cancer lung metastasis (58, 59). Besides miRNA, other non-coding RNA loaded in EVs also contribute to the formation of breast cancer lung metastasis. Abnormal expression of lncRNA in EVs promoted the formation of lung metastasis microenvironment (60, 61). Moreover, Exosome circPSMA1 could up-regulate Akt1 expression and affect the expression of downstream genes such as β-catenin and cyclin D1. circPSMA1/miR637/Akt1/β-catenin (cyclin D1) signal axis promotes the lung metastasis of triple-negative breast cancer (62). We believe that blocking the formation of lung-specific breast cancer metastasis could also affectively improve the survival outcomes of patients with breast cancer.
Brain metastasis occur in about 10%–30% of patients with breast cancer (63). Since most chemotherapy drugs can’t cross the blood-brain barrier (BBB), brain metastasis is refractory to conventional chemotherapy and exhibit extremely poor survival outcomes (64, 65). Therefore, preventing breast cancer cells from infiltrating the BBB is an important step in preventing and treating the brain metastases of breast cancer. Specifically, cytoskeleton-associated proteins, which are involved in endothelial cell adhesion and migration at the BBB, are important targets in preventing breast cancer metastases into the brain (66). The tight association between the increased expression of the cytoskeleton-associated protein tubulin tyrosine ligase like 4 (TTLL4) and brain metastasis of breast cancer was proposed by Arnold et al. They reported that TTLL4-dependent β-tubulin glutamylation increased the secretion of EVs synthesized by multivesicular bodies. These EVs increased the ability of breast cancer cells to adhere to BBB endothelial cells as well as the permeability of these endothelial cells, ultimately promoting breast cancer brain metastasis (67). Furthermore, EVs originated from vascular endothelial cells were found to induce the adhesion of triple-negative breast cancer cells to endothelial cells, and this process could be enhanced by cirGal-3. Further mechanistic exploration revealed that the enhanced glycolysis of endothelial cells induced by cirGal-3 could increase ICAM-1 expression; and EVs-dependent delivery of cirGal-3 to triple-negative breast cancer cells (MDA-MB-231) increased adhesion between the two cell types, ultimately leading to brain metastases of breast cancer (68). Moreover, Sirkisoon et al. have revealed that miR-1290-containing EVs derived from breast cancer activate astrocytes in brain TME via the FOXA2-CNTF axis. This subsequently augmented intracranial colonization and growth of breast cancer cells, leading to the progression of brain metastasis (69).
However, how do breast cancer cells acquire the ability to release specific EVs cargo to maintain the plasticity of breast cancer and the rapid spread of tumor metastasis? Further research should be performed to answer this complex question and more strategies should be proposed to block the process.
Tumor development is not only influenced by intrinsic characteristics but also regulated by signals from the TME. In addition, a constant exchange of information between cancer cells and the TME is crucial to sustain tumor growth, angiogenesis, and spread. Tumor stromal cells, including fibroblasts, immune inflammatory cells, vascular endothelial cells, and others play a crucial role in tumor response to therapy and influence tumor cell proliferation, invasion, and metastasis. Interactions between cellular and non-cellular components of the TME underly pro-tumorigenic signaling, which augments tumor growth and metastasis (70–72). Specifically, EVs play an important role in these regulatory processes of tumor progression by mediating signaling from malignant tumor cells to non-tumor cells and the ECM (6, 73–75).
Cancer-associated fibroblasts (CAFs) are a group of activated fibroblasts in the TME with significant heterogeneity and plasticity. Particularly, CAFs are involved in tumor progression-related regulatory processes, such as the regulation of tumorigenesis, metastasis, and therapeutic resistance (76). Fibroblasts can engulf EVs secreted by breast cancer cells, in turn, fibroblasts are activated to become CAFs and stimulate the carcinogenic phenotype of breast cancer (77). Moreover, EVs released from CAFs can be engulfed by breast cancer cells to subsequently drive their migration, invasion, proliferation, epithelial-mesenchymal transition (EMT), chemoresistance, and ECM remodeling (78). Prior evidence by Baroni et al. suggests that triple-negative breast cancer cells stimulate the conversion of fibroblasts to CAFs through EVs cargo miR-9. Moreover, these newly transformed CAFs can secrete miR-9 to augment breast cancer invasion and metastasis by increasing E-cadherin expression (79). Specifically, Vu et al. found that the transfer of miR-125b-containing EVs from breast cancer cells to normal fibroblasts significantly increased the expression of the CAFs activation markers Acta2, MMP2, and MMP3, thereby promoting the conversion of CAFs phenotype (80). Concurring results by Yang et al. demonstrated that EVs released from breast cancer cells (MDA-MB-231 and MCF-7), which transferred miR-146a to normal fibroblasts, then activated the Wnt/β-catenin pathway and subsequently induced the malignant phenotypes of CAFs (81). Nevertheless, Tao et al. highlighted that EVs released from CAFs can impede the growth and metastasis of breast cancer cells by inhibiting EMT and proliferation via upregulating miR-1-3p expression (82). We can easily imply from these reported results that both breast cancer cells and CAFs can secrete EVs to regulate breast cancer progression, but the specific oncogenic or tumor-suppressive effect depends on the different effector proteins. However, more depth mechanisms underlying the effect of EVs on the behaviors of CAFs require further investigation.
Chronic inflammation is an important driver of tumorigenesis and progression (83, 84). Neutrophils are the most abundant leukocytes in the immune system and the first to migrate to sites of inflammation (85). These cancer-associated neutrophils exhibit both anti-tumor (N1) and pro-tumor (N2) phenotypes, with N1 neutrophils having a stronger cytotoxic effect on tumor cells and N2 neutrophils supporting tumor cell growth, invasion, and metastasis (86). As identified by Amorim et al., EVs released from breast cancer cells (MDA-MB-231) induced the N2-phenotype transformation of neutrophils. These MDA-MB-231-secreted EVs enhanced the production of neutrophil extracellular traps, interleukin (IL)-8, and VEGF from N2 neutrophils, as well as increased the expression of Arg-1, MMP9, and the N2 neutrophil marker CD184, which subsequently promoted breast cancer cell invasion (87). Reportedly, PD-L1 is overexpressed in breast cancer cells and is considered a key tumor immunosuppressor (88, 89). Specifically, PD-L1 maintains the immunosuppressive microenvironment by interacting with PD-1 on lymphocytes (90, 91). CAF-derived exosomes overexpress miR-92 via YAP1, and this increases PD-L1 expression to impair the function of tumor-infiltrated immune cells to drive breast cancer progression (88, 92). As previously documented by Xie et al, breast cancer cell-derived EVs transfer active TGF-β type II receptors (TβRII) to activate TGF-β signaling in target cells. The uptake of TβRII-containing EVs by breast cancer cells with low metastatic potential triggers EMT, which enhances the stemness and metastatic ability of breast cancer cells. Meanwhile, EVs-dependent delivery of TβRII to CD8+ T cells also induces the activation of SMAD3 and TCF1, further causes CD8+ T cell depletion and subsequent failure of tumor immunotherapy (93). Besides, Njock et al. identified that three miRNAs (miR-142-5p, miR-183-5p, and miR-222-3p) were released from endothelial cells in the TME and were transferred via EVs to macrophages. In a mouse model of breast cancer, EVs enriched with these miRNAs contributed to the differentiation of macrophages toward the immunosuppressive M2 phenotype, which augmented breast cancer growth (94).
Myeloid-derived suppressor cells (MDSCs) are closely associated with breast cancer progression (95, 96). Jiang et al. highlights that miR-9 and miR-181a shuttled by EVs from breast cancer cells could activate the JAK/STAT signaling pathway by targeting SOCS3 and PIAS3. This fosters the expansion of early MDSCs and compromises T-cell proliferation and promotes T-cell apoptosis, which subsequently accelerates the growth of breast cancer cells both in vivo and in vitro (97). Biswas et al. further indicated that exosomes secreted by MSCs can harbor high levels of TGF-β, which subsequently induced the malignant progression of breast cancer by facilitating the differentiation of immature MDSCs into M2 macrophages with stronger immunosuppressive activity. Exosomes derived from MSCs, but not from breast cancer cells, contain significant amounts of TGF-β, C1q, and semaphorins. Moreover, the induced PD-L1 overexpression in immature myelomonocyte precursors and CD206+ macrophages, and the fostered differentiation of MHC class II macrophages enhance Arg-1 activity and IL-10 secretion to promote myeloid tolerance activity. This ultimately leads to the formation of immunosuppressive M2 macrophages and results in the rapid growth of breast cancer cells (98). The interactions between EVs cargoes and immune cells significantly contribute to the rapid progression of breast cancer, and also facilitate to the formation of immunosuppressive tumor microenvironment.
Tumor cells undergo EMT before the occurrence of tumor metastasis. During EMT, tumor cells lose epithelial features such as cell adhesion and acquire mesenchymal features, which allow them to migrate and metastasis to occur (99–101). When tumor cells reach the metastatic site, they then undergo the reverse process called mesenchymal-epithelial transformation, which allows these cells to colonize their metastatic site (102–104). However, in the early stages of metastatic spread, disseminated tumor cells may remain dormant in the G0/G1 phase to accumulate the capacity for bone marrow metastasis; this process is also observed in metastatic breast cancer cells (105). Casson et al. revealed that bone marrow MSC (BMSC)-derived EVs increase the adhesion capacity of ER+ breast cancer cells (MCF7) to a dormant epithelial cell phenotype. This facilitates their colonization in the bone marrow in a circulating quiescent state, ultimately leading to bone metastases of breast cancer and resistance to chemotherapeutic agents (106). Further evidence has suggested that breast cancer cells augment BMSCs to release EVs containing miR-222 and miR-223. These EVs, in turn, stimulate the dormancy of disseminated breast cancer cells, causing resistance to chemotherapeutic agents. Based on this, Bliss et al. found that, in a mouse model, targeting dormant breast cancer cells with miR-222 and miR-223 antagonists (delivered by EVs secreted by BMSC) significantly improved the susceptibility of breast cancer cells to carboplatin and also extended the survival time of mice (107). Therefore, we believe that targeting dormant breast cancer cells by EVs may be an effective strategy to improve the response to routine therapy and even prevent breast cancer recurrence and metastasis.
Connexin-46 (Cx46) is highly expressed in EVs released from breast cancer cells and can enforce the interactions between EVs and receptor cells, thereby enhancing the migratory and invasive ability of breast cancer cells. This highlights the potential of EVs-Cx46 as a malignancy marker of breast cancer and as a potential target for breast cancer therapy (108). Inflammatory breast cancer is a rare and highly aggressive malignant tumor of the breast that is easily misdiagnosed as mastitis, which results in delayed treatment (109). The EVs extracted from the plasma of patients with inflammatory breast cancer were found to have three miRNAs (miR-181b-5p, miR-222-3p, and let-7a-5p). The receiver operating characteristic curve analysis revealed an area under the curve (AUC) of > 0.9, which indicated that the three miRNAs could be extremely promising diagnostic biomarkers for patients with inflammatory breast cancer (110).
Axillary lymph node (ALN) metastasis is one of the most important prognostic factors in early-stage breast cancer, and sentinel lymph node biopsy (SLNB) is the main method to assess ALN status (111). Nevertheless, the false-negative rate of SLNB and the presence of associated complications such as postoperative lymphedema limit the use of SLNB (112, 113). Therefore, breast cancer patients without ALN metastasis would greatly benefit from avoiding SLNB and instead accurately assessing ALN status preoperatively. A study by Wang et al. has revealed that miR-363-5p was expressed at lower levels in plasma exosomes of patients with ALN-positive breast cancer than in patients with ALN-negative breast cancer. Moreover, miR-363-5p in plasma exosomes has a high diagnostic value in distinguishing ALN-positive patients from ALN-negative patients. In addition, high expression of miR-363-5p in plasma exosomes appreciably prolonged the survival of patients with breast cancer. These results confirmed that miR-363-5p-containing plasma exosomes exert oncogenic effects in breast cancer and have predictive value for non-invasive LN staging and breast cancer prognosis, indicating its potential as a diagnostic marker for breast cancer (114).
Liquid biopsy is an ideal method for studying the risk of recurrence and early detection of systemic dissemination in patients with tumors (115, 116). Multiple miRNAs contained in breast cancer cell-secreted exosomes can be used as novel breast cancer biomarkers (117, 118). Based on this, Baldasici et al. postulated a new method for the early diagnosis of breast cancer using non-invasive detection and isolation of miRNAs in tumor-derived exosomes. They identified exosomal miRNAs for use as biomarkers of breast cancer metastasis, including miRNAs associated with lymph node metastasis (miR-363-5p, miR-370-3p, miR-222, miR-148a, miR-3662, miR-146a, miR-129, and miR-188-5p), with bone metastasis (miR-21 and miR-218-5p), with brain metastases (miR-576-3p, miR-130a-3p, and miR-181c), and with distant metastases without organ specificity (miR-105, miR-200c, miR-141, and miR-7641) (119).
Of note, Buentzel et al. identified eight metabolites with a significant discriminatory power using mass spectrometric analysis of microvesicles in the blood of patients with breast cancer and healthy controls. Among these, high concentrations of lysoPCaC26:0 and PCaaC38:5 were strongly associated with poor prognosis in patients with breast cancer. In addition, an analysis of metabolites specific to breast cancer subtypes revealed 24 metabolites with significantly different expression in plasma micro-vesicles of patients with luminal A and luminal B type breast cancer, indicating the role of these metabolites in differentiating the molecular subtypes of breast cancer. Thus, metabolites in the plasma microvesicles of these patients might be promising biomarkers for breast cancer and are closely associated with breast cancer progression (120). In another study, Cai et al. analyzed mRNAs carried by EVs in patients with breast cancer and those with benign breast lesions, using a second-generation sequencing method. They identified eight mRNAs with high diagnostic values for breast cancer, namely HLA-DRB1, HAVCR1, ENPEP, TIMP1, CD36, MARCKS, DAB2, and CXCL14. The combined AUC of these mRNAs for breast cancer diagnosis was 0.718. Validation in other cohorts confirmed the high diagnostic potential of these mRNAs for breast cancer (combined AUC of validation cohort: 0.737). The study by Chen et al. noted significantly higher phosphorylation of proteins such as RALGAPA2, PKG1, and TJP2 in plasma EVs of patients vs. healthy controls. In addition, these phosphorylated proteins were considered to be viable markers for breast cancer and could assist in tumor screening and surveillance (9, 121). These results implied that certain cargoes of EVs can be used as biomarkers for breast cancer, with high clinical value in the diagnosis, treatment, and even survival outcomes. The main EVs cargoes which can be applied as biomarkers in breast cancer are also summarized and presented in Table 1.
Table 1 The EVs cargoes which can be applied as biomarkers in breast cancer mentioned in this review.
Resistance to treatment is a significant clinical challenge in the treatment of patients with breast cancer as it is often associated with treatment failure (122, 123). Moreover, bidirectional regulation between breast cancer cells and the TME has been established to be an important factor in breast cancer treatment resistance, with crosstalk between EVs and the immune microenvironment playing an important role (124–126). Abad et al. have delineated that EVs secreted by chemo-resistant triple-negative breast cancer cells could transfer mitochondria to chemo-sensitive cancer cells, thereby increasing their chemoresistance. In addition, EVs carrying mitochondria from drug-resistant triple-negative breast cancer cells promoted chemoresistance by increasing the level of mtDNA with mutations in the mtND4 gene, which was responsible for tumorigenesis. Furthermore, the exosome releasing inhibitor GW4869 was found to inhibit mitochondrial transport and subsequently reduce chemotherapy resistance in triple-negative breast cancer cells (127).
As over 70% of breast cancer are hormone receptor-positive (128), and endocrine therapy remains the most effective treatments for ER+ breast cancer (129, 130). Tamoxifen is the gold standard of endocrine therapy in breast cancer; however, tamoxifen resistance has been a major concern for clinicians (131, 132). Recent studies have demonstrated that EVs play a crucial role in conferring resistance to endocrine therapy. Moreover, miRNAs can be expressed as a “rich cocktail” and delivered to cells with exosomes. Among them, miRNAs involved in the negative regulation of ERs, such as miR-181a-2, when delivered to target cells, could further induce the rearrangement or interdiction of hormonal signaling or the activation of the PI3K/AKT signaling pathway, ultimately reducing the sensitivity of ER+ breast cancer cells to endocrine therapy (133). In a study, Wang et al. reported the upregulation of transient receptor potential canonical 5 (TRPC5) expression as an important factor for chemoresistance in breast cancer cells. Specifically, chemo-resistant breast cancer cells can release EVs containing TRPC5, which is then delivered to chemo-sensitive cells, subsequently leading to acquired chemoresistance. Further studies have found that TRPC5 levels in plasma EVs are significantly correlated with TRPC5 levels in breast cancer tissues and chemotherapy response. Moreover, elevated TRPC5 levels in plasma EVs after chemotherapy have been reported to strongly predict acquired chemoresistance in patients with breast cancer. Based on these findings, TRPC5 levels in plasma EVs after chemotherapy may serve as a valid predictive marker for acquired chemoresistance (134). A study by Wang et al. has noted an increased level of long non-coding RNA H19 in EVs derived from adriamycin-resistant breast cancer cells compared to control cells. Moreover, repression of H19 levels in EVs potentiates the sensitivity of breast cancer cells to adriamycin. This implied that H19 carried by EVs can influence adriamycin resistance in breast cancer cells (135). The use of aromatase inhibitors (AIs) is the standard endocrine therapy for postmenopausal patients with ER+ breast cancer (136, 137); however, its efficacy is limited owing to the development of drug resistance. A study by Augimeri et al. demonstrated that AI-resistant MCF7 cells released more EVs to participate in AI resistance than control ER+ MCF7 cells. The proteomic analysis further confirmed that Rab GTPases, an important vesicular transport regulatory protein in tumors, was expressed at significantly high levels in EVs from AI-resistant MCF7 cells, which may be a major factor causing the increased secretion of EVs as well as AI resistance (138).
Trastuzumab emtansine (T-DM1) is an antibody-drug conjugate designed to specifically deliver the cytotoxic drug DM1 (a derivative of maytansine) to HER2+ breast cancer cells (139–141). Despite the significant efficacy of T-DM1 to target HER2+ breast cancer cells, most patients with HER2+ breast cancer treated with T-DM1 experience local recurrence or distant metastases after drug discontinuation. In addition, a proportion of patients with HER2+ breast cancer developed resistance to T-DM1 (141–143). Oya et al. elaborated that this diminished efficacy of antibody-drug conjugate therapy may be ascribed to the binding of T-DM1 to HER2+ breast cancer cell-secreted exosomes, which eventually resulted in treatment resistance (144). In addition, other studies have found that MSC-derived exosomal miR-342-3p inhibited metastasis and chemoresistance of breast cancer cells by targeting ID4 (145). In line with this, Liu et al. observed robust expression of miR-9-5p in EVs derived from ER+ breast cancer cells (MCF7), which could lead to tamoxifen treatment resistance by downregulating the expression of its target ADIPOQ (146). Moreover, prior evidence has clarified that EVs derived from cisplatin-resistant MDA-MB-231 cells have high expression of miR-423-5p. Furthermore, miR-423-5p-enriched EVs could convert cisplatin-sensitive breast cancer cells into cisplatin-resistant breast cancer cells by activating proliferation, metastasis, and anti-apoptosis-related signaling pathways (147). Available evidence suggests that the MDA-MB-231 cell-derived EVs can stimulate chemoresistance by shuttling miR-887-3p to target BTBD7 and thus activate the Notch1/Hes1 signaling pathway (148). As illustrated by Yang et al., adriamycin and paclitaxel can activate breast cancer cells to secrete miR-378a-3p- and miR-378d-enriched EVs. Then, these EVs can be internalized by breast cancer cells and subsequently activate the Wnt and Notch signaling pathways by targeting DKK3 and NUMB via miR-378a-3p and miR-378d. This ultimately led to increased cell stemness and the acquisition of a drug-resistant phenotype (149). These results predicted that EVs cargoes also participated in the formation of drug resistance of breast cancer. Therefore, targeting these EVs cargoes may increase the therapeutic effect of anti-tumor drugs, including chemotherapy, endocrine therapy, targeted therapy and even immune therapy. The roles of EVs in resistance to the treatment of breast cancer were also summarized and presented in Figure 1.
Figure 1 Roles of EVs in resistance to the treatment of breast cancer (A) Drug resistant triple-negative breast cancer cells can secrete EVs including mitochondrion (MC), miRNA (miR-423-5p, miR-887-3p) to drug sensitive triple-negative breast cancer cells. For MC, it can induce gene mutation of mtND4 to cause drug resistance. For miR-423-5p, miR-423-5p-enriched EVs could convert cisplatin-sensitive breast cancer cells into cisplatin-resistant breast cancer cells by activating proliferation, metastasis, and anti-apoptosis-related signaling pathways. For miR-887-3p, it could target BTBP7 and activate Notch/Hes1 pathway to induce drug resistance of triple-negative breast cancer cells. (B) Drug resistant luminal breast cancer cells can also secrete EVs including miR-181a-2, TRPC5, miR-9-5p to drug sensitive luminal breast cancer cells. For miR-181a-2, it can contribute to the interdiction of ER signal and activation of PI3K/AKT to induce drug resistance. TRPC5 could convert cisplatin-sensitive breast cancer cells into cisplatin-resistant breast cancer cells. For miR-9-5p, it can lead to tamoxifen treatment resistance by downregulating the expression of its target gene ADIPOQ. (C) This diminished efficacy of antibody-drug conjugate therapy may be ascribed to the binding of T-DM1 to HER2+ breast cancer cell-secreted exosomes, which eventually resulted in treatment resistance.
Finally, this study examines the important roles that EVs played in breast cancer clinical treatment. Targeting the conversion of the M1 to M2 phenotype of TAMs is an effective breast cancer treatment (150–152). Of note, the M1 phenotype of TAMs exerts pro-cancer effects, whereas the M2 phenotype exerts cancer-suppressive effects. In this regard, Zhao et al. proposed a new system to load docetaxel into M1 TAM-derived exosomes and found that the conversion of the M1 phenotype to the M2 phenotype, thus significantly improving the anti-cancer effect with minimal side effects (153). In addition, another EVs-based drug delivery system demonstrated a good synergistic anti-tumor effect, which increased tumor-killing efficiency by 15% by promoting the drug’s tumor-targeting capabilities (154). Various microbiota has been shown to play a crucial role in cancer progression as well as in treatment (155, 156). A study by An et al. expounded that the combination of Klebsiella pneumoniae-derived EVs and tamoxifen considerably enhanced the therapeutic effect of tamoxifen on ER+ MCF7 cells by downregulating cyclin E2 and p-ERK expression (157). In line with this, evidence has suggested the role of microbiome in mediating breast cancer. Specifically, the microbiome with the ability to regulate estrogen metabolism is known as the estrogenome (158). It modulates estrogen levels in the body and is strongly associated with the development of various cancers, including breast cancer. In their study, An et al. compared the EVs profiles of blood microorganisms sampled from both patients with breast cancer and healthy controls and found that Staphylococcus aureus (S. aureus)-derived EVs could influence the efficacy of tamoxifen by modulating ERK- and AKT-related signaling pathways. Moreover, the combination of S. aureus-derived EVs and tamoxifen synergistically impeded the growth of ER+ breast cancer cells (159).
In addition, Chang et al. have revealed that endocytosis of EVs secreted from Wharton’s Jelly mesenchymal stem cells (WJ-MSCs) into triple-negative breast cancer cells significantly reduced the proliferation potential, stem cell characteristics, tumor formation capacity in nude mice, and metastatic capacity under hypoxic conditions of triple-negative breast cancer cells. This indicated that targeting triple-negative breast cancer with MSC-derived EVs has potential therapeutic effects. Further in-depth mechanistic analysis revealed that WJ-MSC-secreted EVs attenuated the tumorigenic ability of triple-negative breast cancer cells and prevented the formation of immunosuppression in the TME. This was facilitated by transferring miR-125b to triple-negative breast cancer cells and inhibiting HIF1α signaling pathway-related protein expression, which ultimately induced therapeutic effects (160). Using a tissue engineering approach, Gong et al. developed EVs containing therapeutic doses of adriamycin and cholesterol-modified miR-159. Their study revealed that using these EVs to target triple-negative breast cancer cells resulted in good anti-tumor effects (161). Owing to the pharmacologically important characteristics of exosomes, nanoparticles based on cell-derived exosomes, developed using tissue engineering, have considerably contributed to the treatment of tumors (162–164). Shi et al. developed the synthetic multivalent antibodies retargeted exosome platform using tissue engineering. Briefly, they modified exosomes targeting human-derived CD3 and HER2 proteins to attack HER2-expressing breast cancer cells by recruiting CD3+-expressing cytotoxic T cells. This ensured efficient and targeted treatment against HER2+ breast cancer (165). Thus, we believe that, based on the biological properties of EVs, a combination of research methods related to tissue engineering such as the application of nanomaterials and tumor cell biology helps in developing more effective anti-tumor treatments, consequently providing new alternatives for tumor treatment in clinical settings.
EVs play an important role in intercellular signaling, regulating almost all tumor biological properties. In addition, they significantly affect the interaction between tumor cells and TME. Meanwhile, as a novel anti-cancer therapeutic tool, EVs are receiving considerable attention for application in clinical settings. Specifically, using tissue engineering, EVs have been found to have broad clinical application prospects. These advantages of EVs are primarily attributed to their presence at various locations in tumor cells and TME, their unique composition, and their high efficacy in targeting tumor cells. However, the extraction and purification of EVs are still challenging, and their utility as biomarkers and therapeutic targets requires further exploration. In short, this update review mainly focuses on the role of EVs in the malignant progression of breast cancer, the potential of EVs as biomarkers of breast cancer, the mechanism of EVs in regulating drug resistance of breast cancer treatment, and the prospects of EVs in breast cancer treatment. We hope that this update review could provide a theoretical basis for the treatment and diagnosis of breast cancer in the real clinical practice.
XDZ and JL designed this research. XYZ, CZW, JHY, JWB, FLA, YW and XDZ retrieved relevant literature. JL and XDZ wrote this paper. All authors contributed to the article and approved the submitted version.
This study was supported by Doctoral Start-up Foundation of Liaoning Province (2023-BS-048).
The authors declare that the research was conducted in the absence of any commercial or financial relationships that could be construed as a potential conflict of interest.
The handling editor FZ declared a shared parent affiliation with the authors JHY, JWB, FLA, YW, JL, and XDZ at the time of review.
All claims expressed in this article are solely those of the authors and do not necessarily represent those of their affiliated organizations, or those of the publisher, the editors and the reviewers. Any product that may be evaluated in this article, or claim that may be made by its manufacturer, is not guaranteed or endorsed by the publisher.
1. Bray F, Ferlay J, Soerjomataram I, Siegel RL, Torre LA, Jemal A. Global cancer statistics 2018: GLOBOCAN estimates of incidence and mortality worldwide for 36 cancers in 185 countries. CA Cancer J Clin (2018) 68:394–424. doi: 10.3322/caac.21492
2. Insua-Rodriguez J, Oskarsson T. The extracellular matrix in breast cancer. Adv Drug Delivery Rev (2016) 97:41–55. doi: 10.1016/j.addr.2015.12.017
3. Parhi L, Alon-Maimon T, Sol A, Nejman D, Shhadeh A, Fainsod-Levi T, et al. Breast cancer colonization by fusobacterium nucleatum accelerates tumor growth and metastatic progression. Nat Commun (2020) 11:3259. doi: 10.1038/s41467-020-16967-2
4. Keklikoglou I, Cianciaruso C, Guc E, Squadrito ML, Spring LM, Tazzyman S, et al. Chemotherapy elicits pro-metastatic extracellular vesicles in breast cancer models. Nat Cell Biol (2019) 21:190–202. doi: 10.1038/s41556-018-0256-3
5. Cao M, Isaac R, Yan W, Ruan X, Jiang L, Wan Y, et al. Cancer-cell-secreted extracellular vesicles suppress insulin secretion through miR-122 to impair systemic glucose homeostasis and contribute to tumour growth. Nat Cell Biol (2022) 24:954–67. doi: 10.1038/s41556-022-00919-7
6. Tkach M, Thalmensi J, Timperi E, Gueguen P, Nevo N, Grisard E, et al. Extracellular vesicles from triple negative breast cancer promote pro-inflammatory macrophages associated with better clinical outcome. Proc Natl Acad Sci USA (2022) 119:e2107394119. doi: 10.1073/pnas.2107394119
7. Kim DK, Kang B, Kim OY, Choi DS, Lee J, Kim SR, et al. EVpedia: an integrated database of high-throughput data for systemic analyses of extracellular vesicles. J Extracell Vesicles (2013) 2. doi: 10.3402/jev.v2i0.20384
8. Zhou Y, Xiao Z, Zhu W. The roles of small extracellular vesicles as prognostic biomarkers and treatment approaches in triple-negative breast cancer. Front Oncol (2022) 12:998964. doi: 10.3389/fonc.2022.998964
9. Chen IH, Xue L, Hsu CC, Paez JS, Pan L, Andaluz H, et al. Phosphoproteins in extracellular vesicles as candidate markers for breast cancer. Proc Natl Acad Sci USA (2017) 114:3175–80. doi: 10.1073/pnas.1618088114
10. Li XQ, Zhang R, Lu H, Yue XM, Huang YF. Extracellular vesicle-packaged CDH11 and ITGA5 induce the premetastatic niche for bone colonization of breast cancer cells. Cancer Res (2022) 82:1560–74. doi: 10.1158/0008-5472.CAN-21-1331
11. Namee NM, O'driscoll L. Extracellular vesicles and anti-cancer drug resistance. Biochim Biophys Acta Rev Cancer (2018) 1870:123–36. doi: 10.1016/j.bbcan.2018.07.003
12. Hoshino A, Kim HS, Bojmar L, Gyan KE, Cioffi M, Hernandez J, et al. Extracellular vesicle and particle biomarkers define multiple human cancers. Cell (2020) 182:1044–1061 e18. doi: 10.1016/j.cell.2020.07.009
13. Teles RHG, Yano RS, Villarinho NJ, Yamagata AS, Jaeger RG, Meybohm P, et al. Advances in breast cancer management and extracellular vesicle research, a bibliometric analysis. Curr Oncol (2021) 28:4504–20. doi: 10.3390/curroncol28060382
14. Kang C, Leroith D, Gallagher EJ. Diabetes, obesity, and breast cancer. Endocrinology (2018) 159:3801–12. doi: 10.1210/en.2018-00574
15. Ku HC, Cheng CF. Master regulator activating transcription factor 3 (ATF3) in metabolic homeostasis and cancer. Front Endocrinol (Lausanne) (2020) 11:556. doi: 10.3389/fendo.2020.00556
16. Jorquera-Cordero C, Lara P, Cruz LJ, Schomann T, Van Hofslot A, De Carvalho TG, et al. Extracellular vesicles from M1-polarized macrophages combined with hyaluronic acid and a beta-blocker potentiate doxorubicin's antitumor activity by downregulating tumor-associated macrophages in breast cancer. Pharmaceutics (2022) 14(5):1068. doi: 10.3390/pharmaceutics14051068
17. Alt EU, Worner PM, Pfnur A, Ochoa JE, Schachtele DJ, Barabadi Z, et al. Targeting TRAF3IP2, compared to Rab27, is more effective in suppressing the development and metastasis of breast cancer. Sci Rep (2020) 10:8834. doi: 10.1038/s41598-020-64781-z
18. Altei WF, Pachane BC, Dos Santos PK, Ribeiro LNM, Sung BH, Weaver AM, et al. Inhibition of alphavbeta3 integrin impairs adhesion and uptake of tumor-derived small extracellular vesicles. Cell Commun Signal (2020) 18:158. doi: 10.1186/s12964-020-00630-w
19. Bertolini I, Perego M, Ghosh JC, Kossenkov AV, Altieri DC. NFkappaB activation by hypoxic small extracellular vesicles drives oncogenic reprogramming in a breast cancer microenvironment. Oncogene (2022) 41:2520–5. doi: 10.1038/s41388-022-02280-3
20. Campos A, Salomon C, Bustos R, Diaz J, Martinez S, Silva V, et al. Caveolin-1-containing extracellular vesicles transport adhesion proteins and promote malignancy in breast cancer cell lines. Nanomedicine (Lond) (2018) 13:2597–609. doi: 10.2217/nnm-2018-0094
21. Wu W, Huang R, Ou L, Lei R. Exosomes derived from bone marrow mesenchymal stem cells promote proliferation and migration via upregulation yes-associated protein/transcriptional coactivator with PDZ binding motif expression in breast cancer cells. Chin J Physiol (2022) 65:233–40. doi: 10.4103/0304-4920.359800
22. Annett S, Moore G, Robson T. Obesity and cancer metastasis: molecular and translational perspectives. Cancers (Basel) (2020) 12(12):3798. doi: 10.3390/cancers12123798
23. Wang S, Su X, Xu M, Xiao X, Li X, Li H, et al. Exosomes secreted by mesenchymal stromal/stem cell-derived adipocytes promote breast cancer cell growth via activation of hippo signaling pathway. Stem Cell Res Ther (2019) 10:117. doi: 10.1186/s13287-019-1220-2
24. Shen Z. Genomic instability and cancer: an introduction. J Mol Cell Biol (2011) 3:1–3. doi: 10.1093/jmcb/mjq057
25. Ahmad SS, Ahmed K, Venkitaraman AR. Science in focus: genomic instability and its implications for clinical cancer care. Clin Oncol (R Coll Radiol) (2018) 30:751–5. doi: 10.1016/j.clon.2018.09.001
26. Negrini S, Gorgoulis VG, Halazonetis TD. Genomic instability–an evolving hallmark of cancer. Nat Rev Mol Cell Biol (2010) 11:220–8. doi: 10.1038/nrm2858
27. Bao S, Hu T, Liu J, Su J, Sun J, Ming Y, et al. Genomic instability-derived plasma extracellular vesicle-microRNA signature as a minimally invasive predictor of risk and unfavorable prognosis in breast cancer. J Nanobiotechnology (2021) 19:22. doi: 10.1186/s12951-020-00767-3
28. Li S, Xu HX, Wu CT, Wang WQ, Jin W, Gao HL, et al. Angiogenesis in pancreatic cancer: current research status and clinical implications. Angiogenesis (2019) 22:15–36. doi: 10.1007/s10456-018-9645-2
29. de Heer EC, Jalving M, Harris AL. HIFs, angiogenesis, and metabolism: elusive enemies in breast cancer. J Clin Invest (2020) 130:5074–87. doi: 10.1172/JCI137552
30. Todorova D, Simoncini S, Lacroix R, Sabatier F, Dignat-George F. Extracellular vesicles in angiogenesis. Circ Res (2017) 120:1658–73. doi: 10.1161/CIRCRESAHA.117.309681
31. Aslan C, Maralbashi S, Kahroba H, Asadi M, Soltani-Zangbar MS, Javadian M, et al. Docosahexaenoic acid (DHA) inhibits pro-angiogenic effects of breast cancer cells via down-regulating cellular and exosomal expression of angiogenic genes and microRNAs. Life Sci (2020) 258:118094. doi: 10.1016/j.lfs.2020.118094
32. Pakravan K, Babashah S, Sadeghizadeh M, Mowla SJ, Mossahebi-Mohammadi M, Ataei F, et al. MicroRNA-100 shuttled by mesenchymal stem cell-derived exosomes suppresses in vitro angiogenesis through modulating the mTOR/HIF-1alpha/VEGF signaling axis in breast cancer cells. Cell Oncol (Dordr) (2017) 40:457–70. doi: 10.1007/s13402-017-0335-7
33. Lee JK, Park SR, Jung BK, Jeon YK, Lee YS, Kim MK, et al. Exosomes derived from mesenchymal stem cells suppress angiogenesis by down-regulating VEGF expression in breast cancer cells. PloS One (2013) 8:e84256. doi: 10.1371/journal.pone.0084256
34. Maji S, Chaudhary P, Akopova I, Nguyen PM, Hare RJ, Gryczynski I, et al. Exosomal annexin II promotes angiogenesis and breast cancer metastasis. Mol Cancer Res (2017) 15:93–105. doi: 10.1158/1541-7786.MCR-16-0163
35. Shi P, Liu Y, Yang H, Hu B. Breast cancer derived exosomes promoted angiogenesis of endothelial cells in microenvironment via circHIPK3/miR-124-3p/MTDH axis. Cell Signal (2022) 95:110338. doi: 10.1016/j.cellsig.2022.110338
36. Hoshino A, Costa-Silva B, Shen TL, Rodrigues G, Hashimoto A, Tesic Mark M, et al. Tumour exosome integrins determine organotropic metastasis. Nature (2015) 527:329–35. doi: 10.1038/nature15756
37. Busatto S, Yang Y, Walker SA, Davidovich I, Lin WH, Lewis-Tuffin L, et al. Brain metastases-derived extracellular vesicles induce binding and aggregation of low-density lipoprotein. J Nanobiotechnology (2020) 18:162. doi: 10.1186/s12951-020-00722-2
38. Medeiros B, Goodale D, Postenka C, Lowes LE, Kiser P, Hearn S, et al. Triple-negative primary breast tumors induce supportive premetastatic changes in the extracellular matrix and soluble components of the lung microenvironment. Cancers (Basel) (2020) 12(1):172. doi: 10.3390/cancers12010172
39. Sun X, Wang X, Yan C, Zheng S, Gao R, Huang F, et al. Tumor cell-released LC3-positive EVs promote lung metastasis of breast cancer through enhancing premetastatic niche formation. Cancer Sci (2022) 113:3405–16. doi: 10.1111/cas.15507
40. Qi M, Xia Y, Wu Y, Zhang Z, Wang X, Lu L, et al. Lin28B-high breast cancer cells promote immune suppression in the lung pre-metastatic niche via exosomes and support cancer progression. Nat Commun (2022) 13:897. doi: 10.1038/s41467-022-28438-x
41. Wu K, Feng J, Lyu F, Xing F, Sharma S, Liu Y, et al. Exosomal miR-19a and IBSP cooperate to induce osteolytic bone metastasis of estrogen receptor-positive breast cancer. Nat Commun (2021) 12:5196. doi: 10.1038/s41467-021-25473-y
42. Yuan X, Qian N, Ling S, Li Y, Sun W, Li J, et al. Breast cancer exosomes contribute to pre-metastatic niche formation and promote bone metastasis of tumor cells. Theranostics (2021) 11:1429–45. doi: 10.7150/thno.45351
43. Kerr AJ, Dodwell D, Mcgale P, Holt F, Duane F, Mannu G, et al. Adjuvant and neoadjuvant breast cancer treatments: a systematic review of their effects on mortality. Cancer Treat Rev (2022) 105:102375. doi: 10.1016/j.ctrv.2022.102375
44. Nolan E, Lindeman GJ, Visvader JE. Deciphering breast cancer: from biology to the clinic. Cell (2023) 186(8):1708–28. doi: 10.1016/j.cell.2023.01.040
45. Welch DR, Hurst DR. Defining the hallmarks of metastasis. Cancer Res (2019) 79:3011–27. doi: 10.1158/0008-5472.CAN-19-0458
46. Liang Y, Zhang H, Song X, Yang Q. Metastatic heterogeneity of breast cancer: molecular mechanism and potential therapeutic targets. Semin Cancer Biol (2020) 60:14–27. doi: 10.1016/j.semcancer.2019.08.012
47. Barenholz-Cohen T, Merkher Y, Haj J, Shechter D, Kirchmeier D, Shaked Y, et al. Lung mechanics modifications facilitating metastasis are mediated in part by breast cancer-derived extracellular vesicles. Int J Cancer (2020) 147:2924–33. doi: 10.1002/ijc.33229
48. Jabbari N, Akbariazar E, Feqhhi M, Rahbarghazi R, Rezaie J. Breast cancer-derived exosomes: tumor progression and therapeutic agents. J Cell Physiol (2020) 235:6345–56. doi: 10.1002/jcp.29668
49. Shechter D, Harel M, Mukherjee A, Sagredo LM, Loven D, Prinz E, et al. Breast cancer-derived microparticles reduce cancer cell adhesion, an effect augmented by chemotherapy. Cells (2020) 9(10):2269. doi: 10.3390/cells9102269
50. Theriault RL, Theriault RL. Biology of bone metastases. Cancer Control (2012) 19:92–101. doi: 10.1177/107327481201900203
51. Wang M, Xia F, Wei Y, Wei X. Molecular mechanisms and clinical management of cancer bone metastasis. Bone Res (2020) 8:30. doi: 10.1038/s41413-020-00105-1
52. Zeng Y, Pan Z, Yuan J, Song Y, Feng Z, Chen Z, et al. Inhibiting osteolytic breast cancer bone metastasis by bone-targeted nanoagent via remodeling the bone tumor microenvironment combined with NIR-II photothermal therapy. Small (2023) 21:e2301003. doi: 10.1002/smll.202301003
53. Hashimoto K, Ochi H, Sunamura S, Kosaka N, Mabuchi Y, Fukuda T, et al. Cancer-secreted hsa-miR-940 induces an osteoblastic phenotype in the bone metastatic microenvironment via targeting ARHGAP1 and FAM134A. Proc Natl Acad Sci USA (2018) 115:2204–9. doi: 10.1073/pnas.1717363115
54. Liu X, Cao M, Palomares M, Wu X, Li A, Yan W, et al. Metastatic breast cancer cells overexpress and secrete miR-218 to regulate type I collagen deposition by osteoblasts. Breast Cancer Res (2018) 20:127. doi: 10.1186/s13058-018-1059-y
55. Medeiros B, Allan AL. Molecular mechanisms of breast cancer metastasis to the lung: clinical and experimental perspectives. Int J Mol Sci (2019) 20(9):2272. doi: 10.3390/ijms20092272
56. Tyagi A, Sharma S, Wu K, Wu SY, Xing F, Liu Y, et al. Nicotine promotes breast cancer metastasis by stimulating N2 neutrophils and generating pre-metastatic niche in lung. Nat Commun (2021) 12:474. doi: 10.1038/s41467-020-20733-9
57. Fong MY, Zhou W, Liu L, Alontaga AY, Chandra M, Ashby J, et al. Breast-cancer-secreted miR-122 reprograms glucose metabolism in premetastatic niche to promote metastasis. Nat Cell Biol (2015) 17:183–94. doi: 10.1038/ncb3094
58. Guo J, Duan Z, Zhang C, Wang W, He H, Liu Y, et al. Mouse 4T1 breast cancer cell-derived exosomes induce proinflammatory cytokine production in macrophages via miR-183. J Immunol (2020) 205:2916–25. doi: 10.4049/jimmunol.1901104
59. Xun J, Du L, Gao R, Shen L, Wang D, Kang L, et al. Cancer-derived exosomal miR-138-5p modulates polarization of tumor-associated macrophages through inhibition of KDM6B. Theranostics (2021) 11:6847–59. doi: 10.7150/thno.51864
60. Feng T, Zhang P, Sun Y, Wang Y, Tong J, Dai H, et al. High throughput sequencing identifies breast cancer-secreted exosomal LncRNAs initiating pulmonary pre-metastatic niche formation. Gene (2019) 710:258–64. doi: 10.1016/j.gene.2019.06.004
61. Yue X, Wu WY, Dong M, Guo M. LncRNA MALAT1 promotes breast cancer progression and doxorubicin resistance via regulating miR-570-3p. BioMed J (2021) 44:S296–304. doi: 10.1016/j.bj.2020.11.002
62. Yang SJ, Wang DD, Zhong SL, Chen WQ, Wang FL, Zhang J, et al. Tumor-derived exosomal circPSMA1 facilitates the tumorigenesis, metastasis, and migration in triple-negative breast cancer (TNBC) through miR-637/Akt1/beta-catenin (cyclin D1) axis. Cell Death Dis (2021) 12:420. doi: 10.1038/s41419-021-03680-1
63. Hosonaga M, Saya H, Arima Y. Molecular and cellular mechanisms underlying brain metastasis of breast cancer. Cancer Metastasis Rev (2020) 39:711–20. doi: 10.1007/s10555-020-09881-y
64. Pedrosa R, Mustafa DA, Soffietti R, Kros JM. Breast cancer brain metastasis: molecular mechanisms and directions for treatment. Neuro Oncol (2018) 20:1439–49. doi: 10.1093/neuonc/noy044
65. Wang Y, Ye F, Liang Y, Yang Q. Breast cancer brain metastasis: insight into molecular mechanisms and therapeutic strategies. Br J Cancer (2021) 125:1056–67. doi: 10.1038/s41416-021-01424-8
66. Schoumacher M, Goldman RD, Louvard D, Vignjevic DM. Actin, microtubules, and vimentin intermediate filaments cooperate for elongation of invadopodia. J Cell Biol (2010) 189:541–56. doi: 10.1083/jcb.200909113
67. Arnold J, Schattschneider J, Blechner C, Krisp C, Schluter H, Schweizer M, et al. Tubulin tyrosine ligase like 4 (TTLL4) overexpression in breast cancer cells is associated with brain metastasis and alters exosome biogenesis. J Exp Clin Cancer Res (2020) 39:205. doi: 10.1186/s13046-020-01712-w
68. Wang L, Du DD, Zheng ZX, Shang PF, Yang XX, Sun C, et al. Circulating galectin-3 promotes tumor-endothelium-adhesion by upregulating ICAM-1 in endothelium-derived extracellular vesicles. Front Pharmacol (2022) 13:979474. doi: 10.3389/fphar.2022.979474
69. Sirkisoon SR, Wong GL, Aguayo NR, Doheny DL, Zhu D, Regua AT, et al. Breast cancer extracellular vesicles-derived miR-1290 activates astrocytes in the brain metastatic microenvironment via the FOXA2–>CNTF axis to promote progression of brain metastases. Cancer Lett (2022) 540:215726. doi: 10.1016/j.canlet.2022.215726
70. Wu T, Dai Y. Tumor microenvironment and therapeutic response. Cancer Lett (2017) 387:61–8. doi: 10.1016/j.canlet.2016.01.043
71. Vitale I, Manic G, Coussens LM, Kroemer G, Galluzzi L. Macrophages and metabolism in the tumor microenvironment. Cell Metab (2019) 30:36–50. doi: 10.1016/j.cmet.2019.06.001
72. Xiao Y, Yu D. Tumor microenvironment as a therapeutic target in cancer. Pharmacol Ther (2021) 221:107753. doi: 10.1016/j.pharmthera.2020.107753
73. Mao Y, Keller ET, Garfield DH, Shen K, Wang J. Stromal cells in tumor microenvironment and breast cancer. Cancer Metastasis Rev (2013) 32:303–15. doi: 10.1007/s10555-012-9415-3
74. Mu W, Rana S, Zoller M. Host matrix modulation by tumor exosomes promotes motility and invasiveness. Neoplasia (2013) 15:875–87. doi: 10.1593/neo.13786
75. Chen F, Chen J, Yang L, Liu J, Zhang X, Zhang Y, et al. Extracellular vesicle-packaged HIF-1alpha-stabilizing lncRNA from tumour-associated macrophages regulates aerobic glycolysis of breast cancer cells. Nat Cell Biol (2019) 21:498–510. doi: 10.1038/s41556-019-0299-0
76. Gieniec KA, Butler LM, Worthley DL, Woods SL. Cancer-associated fibroblasts-heroes or villains? Br J Cancer (2019) 121:293–302. doi: 10.1038/s41416-019-0509-3
77. Augimeri G, Gelsomino L, Plastina P, Giordano C, Barone I, Catalano S, et al. Natural and synthetic PPARgamma ligands in tumor microenvironment: a new potential strategy against breast cancer. Int J Mol Sci (2020) 21(24):9721. doi: 10.3390/ijms21249721
78. Castillo-Sanchez R, Churruca-Schuind A, Martinez-Ival M, Salazar EP. Cancer-associated fibroblasts communicate with breast tumor cells through extracellular vesicles in tumor development. Technol Cancer Res Treat (2022) 21:15330338221131647. doi: 10.1177/15330338221131647
79. Baroni S, Romero-Cordoba S, Plantamura I, Dugo M, D'ippolito E, Cataldo A, et al. Exosome-mediated delivery of miR-9 induces cancer-associated fibroblast-like properties in human breast fibroblasts. Cell Death Dis (2016) 7:e2312. doi: 10.1038/cddis.2016.224
80. Vu LT, Peng B, Zhang DX, Ma V, Mathey-Andrews CA, Lam CK, et al. Tumor-secreted extracellular vesicles promote the activation of cancer-associated fibroblasts via the transfer of microRNA-125b. J Extracell Vesicles (2019) 8:1599680. doi: 10.1080/20013078.2019.1599680
81. Yang SS, Ma S, Dou H, Liu F, Zhang SY, Jiang C, et al. Breast cancer-derived exosomes regulate cell invasion and metastasis in breast cancer via miR-146a to activate cancer associated fibroblasts in tumor microenvironment. Exp Cell Res (2020) 391:111983. doi: 10.1016/j.yexcr.2020.111983
82. Tao S, Li H, Ma X, Ma Y, He J, Gao Y, et al. Elevating microRNA-1-3p shuttled by cancer-associated fibroblasts-derived extracellular vesicles suppresses breast cancer progression and metastasis by inhibiting GLIS1. Cancer Gene Ther (2021) 28:634–48. doi: 10.1038/s41417-020-00244-x
84. Korbecki J, Siminska D, Gassowska-Dobrowolska M, Listos J, Gutowska I, Chlubek D, et al. Chronic and cycling hypoxia: drivers of cancer chronic inflammation through HIF-1 and NF-kappaB activation: a review of the molecular mechanisms. Int J Mol Sci (2021) 22(19):10701. doi: 10.3390/ijms221910701
85. Shaul ME, Fridlender ZG. Cancer-related circulating and tumor-associated neutrophils - subtypes, sources and function. FEBS J (2018) 285:4316–42. doi: 10.1111/febs.14524
86. Wu L, Saxena S, Awaji M, Singh RK. Tumor-associated neutrophils in cancer: going pro. Cancers (Basel) (2019) 11(4):564. doi: 10.3390/cancers11040564
87. Amorim C, Docasar CL, Guimaraes-Bastos D, Frony AC, Barja-Fidalgo C, Renovato-Martins M, et al. Extracellular vesicles derived from MDA-MB-231 cells trigger neutrophils to a pro-tumor profile. Cells (2022) 11(12):1875. doi: 10.3390/cells11121875
88. Vranic S, Cyprian FS, Gatalica Z, Palazzo J. PD-L1 status in breast cancer: current view and perspectives. Semin Cancer Biol (2021) 72:146–54. doi: 10.1016/j.semcancer.2019.12.003
89. Lee CH, Bae JH, Choe EJ, Park JM, Park SS, Cho HJ, et al. Macitentan improves antitumor immune responses by inhibiting the secretion of tumor-derived extracellular vesicle PD-L1. Theranostics (2022) 12:1971–87. doi: 10.7150/thno.68864
90. Schutz F, Stefanovic S, Mayer L, Von Au A, Domschke C, Sohn C. PD-1/PD-L1 pathway in breast cancer. Oncol Res Treat (2017) 40:294–7. doi: 10.1159/000464353
91. Zhou Y, Yamamoto Y, Takeshita F, Yamamoto T, Xiao Z, Ochiya T. Delivery of miR-424-5p via extracellular vesicles promotes the apoptosis of MDA-MB-231 TNBC cells in the tumor microenvironment. Int J Mol Sci (2021) 22(2):844. doi: 10.3390/ijms22020844
92. Dou D, Ren X, Han M, Xu X, Ge X, Gu Y, et al. Cancer-associated fibroblasts-derived exosomes suppress immune cell function in breast cancer via the miR-92/PD-L1 pathway. Front Immunol (2020) 11:2026. doi: 10.3389/fimmu.2020.02026
93. Xie F, Zhou X, Su P, Li H, Tu Y, Du J, et al. Breast cancer cell-derived extracellular vesicles promote CD8(+) T cell exhaustion via TGF-beta type II receptor signaling. Nat Commun (2022) 13:4461. doi: 10.1038/s41467-022-31250-2
94. Njock MS, O'grady T, Nivelles O, Lion M, Jacques S, Cambier M, et al. Endothelial extracellular vesicles promote tumour growth by tumour-associated macrophage reprogramming. J Extracell Vesicles (2022) 11:e12228. doi: 10.1002/jev2.12228
95. Li W, Tanikawa T, Kryczek I, Xia H, Li G, Wu K, et al. Aerobic glycolysis controls myeloid-derived suppressor cells and tumor immunity via a specific CEBPB isoform in triple-negative breast cancer. Cell Metab (2018) 28:87–103e6. doi: 10.1016/j.cmet.2018.04.022
96. Alshetaiwi H, Pervolarakis N, Mcintyre LL, Ma D, Nguyen Q, Rath JA, et al. Defining the emergence of myeloid-derived suppressor cells in breast cancer using single-cell transcriptomics. Sci Immunol (2020) 5(44):eaay6017. doi: 10.1126/sciimmunol.aay6017
97. Jiang M, Zhang W, Zhang R, Liu P, Ye Y, Yu W, et al. Cancer exosome-derived miR-9 and miR-181a promote the development of early-stage MDSCs via interfering with SOCS3 and PIAS3 respectively in breast cancer. Oncogene (2020) 39:4681–94. doi: 10.1038/s41388-020-1322-4
98. Biswas S, Mandal G, Roy Chowdhury S, Purohit S, Payne KK, Anadon C, et al. Exosomes produced by mesenchymal stem cells drive differentiation of myeloid cells into immunosuppressive M2-polarized macrophages in breast cancer. J Immunol (2019) 203:3447–60. doi: 10.4049/jimmunol.1900692
99. Tsai JH, Yang J. Epithelial-mesenchymal plasticity in carcinoma metastasis. Genes Dev (2013) 27:2192–206. doi: 10.1101/gad.225334.113
100. Yeung KT, Yang J. Epithelial-mesenchymal transition in tumor metastasis. Mol Oncol (2017) 11:28–39. doi: 10.1002/1878-0261.12017
101. Mittal V. Epithelial mesenchymal transition in tumor metastasis. Annu Rev Pathol (2018) 13:395–412. doi: 10.1146/annurev-pathol-020117-043854
102. Zhang Y, Weinberg RA. Epithelial-to-mesenchymal transition in cancer: complexity and opportunities. Front Med (2018) 12:361–73. doi: 10.1007/s11684-018-0656-6
103. Lu W, Kang Y. Epithelial-mesenchymal plasticity in cancer progression and metastasis. Dev Cell (2019) 49:361–74. doi: 10.1016/j.devcel.2019.04.010
104. Pastushenko I, Blanpain C. EMT transition states during tumor progression and metastasis. Trends Cell Biol (2019) 29:212–26. doi: 10.1016/j.tcb.2018.12.001
105. Chong Seow Khoon M. Experimental models of bone metastasis: opportunities for the study of cancer dormancy. Adv Drug Delivery Rev (2015) 94:141–50. doi: 10.1016/j.addr.2014.12.007
106. Casson J, Davies OG, Smith CA, Dalby MJ, Berry CC. Mesenchymal stem cell-derived extracellular vesicles may promote breast cancer cell dormancy. J Tissue Eng (2018) 9:2041731418810093. doi: 10.1177/2041731418810093
107. Bliss SA, Sinha G, Sandiford OA, Williams LM, Engelberth DJ, Guiro K, et al. Mesenchymal stem cell-derived exosomes stimulate cycling quiescence and early breast cancer dormancy in bone marrow. Cancer Res (2016) 76:5832–44. doi: 10.1158/0008-5472.CAN-16-1092
108. Acuna RA, Varas-Godoy M, Berthoud VM, Alfaro IE, Retamal MA. Connexin-46 contained in extracellular vesicles enhance malignancy features in breast cancer cells. Biomolecules (2020) 10(5):676. doi: 10.3390/biom10050676
109. Faldoni FLC, Villacis RAR, Canto LM, Fonseca-Alves CE, Cury SS, Larsen SJ, et al. Inflammatory breast cancer: clinical implications of genomic alterations and mutational profiling. Cancers (Basel) (2020) 12(10):2816. doi: 10.3390/cancers12102816
110. Ahmed SH, Espinoza-Sanchez NA, El-Damen A, Fahim SA, Badawy MA, Greve B, et al. Small extracellular vesicle-encapsulated miR-181b-5p, miR-222-3p and let-7a-5p: next generation plasma biopsy-based diagnostic biomarkers for inflammatory breast cancer. PloS One (2021) 16:e0250642. doi: 10.1371/journal.pone.0250642
111. Lyman GH, Somerfield MR, Bosserman LD, Perkins CL, Weaver DL, Giuliano AE. Sentinel lymph node biopsy for patients with early-stage breast cancer: American society of clinical oncology clinical practice guideline update. J Clin Oncol (2017) 35:561–4. doi: 10.1200/JCO.2016.71.0947
112. Magnoni F, Galimberti V, Corso G, Intra M, Sacchini V, Veronesi P. Axillary surgery in breast cancer: an updated historical perspective. Semin Oncol (2020) 47:341–52. doi: 10.1053/j.seminoncol.2020.09.001
113. Barrio AV, Montagna G, Mamtani A, Sevilimedu V, Edelweiss M, Capko D, et al. Nodal recurrence in patients with node-positive breast cancer treated with sentinel node biopsy alone after neoadjuvant chemotherapy-a rare event. JAMA Oncol (2021) 7:1851–5. doi: 10.1001/jamaoncol.2021.4394
114. Wang X, Qian T, Bao S, Zhao H, Chen H, Xing Z, et al. Circulating exosomal miR-363-5p inhibits lymph node metastasis by downregulating PDGFB and serves as a potential noninvasive biomarker for breast cancer. Mol Oncol (2021) 15:2466–79. doi: 10.1002/1878-0261.13029
115. Tay TKY, Tan PH. Liquid biopsy in breast cancer: a focused review. Arch Pathol Lab Med (2021) 145:678–86. doi: 10.5858/arpa.2019-0559-RA
116. Freitas AJA, Causin RL, Varuzza MB, Calfa S, Hidalgo Filho CMT, Komoto TT, et al. Liquid biopsy as a tool for the diagnosis, treatment, and monitoring of breast cancer. Int J Mol Sci (2022) 23(17):9952. doi: 10.3390/ijms23179952
117. Lakshmi S, Hughes TA, Priya S. Exosomes and exosomal RNAs in breast cancer: a status update. Eur J Cancer (2021) 144:252–68. doi: 10.1016/j.ejca.2020.11.033
118. Nakase I, Takatani-Nakase T. Exosomes: breast cancer-derived extracellular vesicles; recent key findings and technologies in disease progression, diagnostics, and cancer targeting. Drug Metab Pharmacokinet (2022) 42:100435. doi: 10.1016/j.dmpk.2021.100435
119. Baldasici O, Pileczki V, Cruceriu D, Gavrilas LI, Tudoran O, Balacescu L, et al. Breast cancer-delivered exosomal miRNA as liquid biopsy biomarkers for metastasis prediction: a focus on translational research with clinical applicability. Int J Mol Sci (2022) 23(16):9371. doi: 10.3390/ijms23169371
120. Buentzel J, Klemp HG, Kraetzner R, Schulz M, Dihazi GH, Streit F, et al. Metabolomic profiling of blood-derived microvesicles in breast cancer patients. Int J Mol Sci (2021) 22(24):13540 doi: 10.3390/ijms222413540
121. Cai GX, Lin L, Zhai XM, Guo ZW, Wu YS, Ye GL, et al. A plasma-derived extracellular vesicle mRNA classifier for the detection of breast cancer. Gland Surg (2021) 10:2002–9. doi: 10.21037/gs-21-275
122. Hanker AB, Sudhan DR, Arteaga CL. Overcoming endocrine resistance in breast cancer. Cancer Cell (2020) 37:496–513. doi: 10.1016/j.ccell.2020.03.009
123. Garcia-Martinez L, Zhang Y, Nakata Y, Chan HL, Morey L. Epigenetic mechanisms in breast cancer therapy and resistance. Nat Commun (2021) 12:1786. doi: 10.1038/s41467-021-22024-3
124. Shee K, Yang W, Hinds JW, Hampsch RA, Varn FS, Traphagen NA, et al. Therapeutically targeting tumor microenvironment-mediated drug resistance in estrogen receptor-positive breast cancer. J Exp Med (2018) 215:895–910. doi: 10.1084/jem.20171818
125. Cosentino G, Plantamura I, Tagliabue E, Iorio MV, Cataldo A. Breast cancer drug resistance: overcoming the challenge by capitalizing on MicroRNA and tumor microenvironment interplay. Cancers (Basel) (2021) 13(15):3691. doi: 10.3390/cancers13153691
126. Samuels M, Cilibrasi C, Papanastasopoulos P, Giamas G. Extracellular vesicles as mediators of therapy resistance in the breast cancer microenvironment. Biomolecules (2022) 12(1):132. doi: 10.3390/biom12010132
127. Abad E, Lyakhovich A. Movement of mitochondria with mutant DNA through extracellular vesicles helps cancer cells acquire chemoresistance. ChemMedChem (2022) 17:e202100642. doi: 10.1002/cmdc.202100642
128. Vaz-Luis I, Francis PA, Di Meglio A, Stearns V. Challenges in adjuvant therapy for premenopausal women diagnosed with luminal breast cancers. Am Soc Clin Oncol Educ Book (2021) 41:1–15. doi: 10.1200/EDBK_320595
129. Glassman D, Hignett S, Rehman S, Linforth R, Salhab M. Adjuvant endocrine therapy for hormone-positive breast cancer, focusing on ovarian suppression and extended treatment: an update. Anticancer Res (2017) 37:5329–41. doi: 10.21873/anticanres.11959
130. Zhang A, Wang X, Fan C, Mao X. The role of Ki67 in evaluating neoadjuvant endocrine therapy of hormone receptor-positive breast cancer. Front Endocrinol (Lausanne) (2021) 12:687244. doi: 10.3389/fendo.2021.687244
131. Dittmer J. Nuclear mechanisms involved in endocrine resistance. Front Oncol (2021) 11:736597. doi: 10.3389/fonc.2021.736597
132. Xu Y, Huangyang P, Wang Y, Xue L, Devericks E, Nguyen HG, et al. ERalpha is an RNA-binding protein sustaining tumor cell survival and drug resistance. Cell (2021) 184:5215–5229.e17. doi: 10.1016/j.cell.2021.08.036
133. Andreeva OE, Sorokin DV, Mikhaevich EI, Bure IV, Shchegolev YY, Nemtsova MV, et al. Towards unravelling the role of ERalpha-targeting miRNAs in the exosome-mediated transferring of the hormone resistance. Molecules (2021) 26(21):6661. doi: 10.3390/molecules26216661
134. Wang T, Ning K, Lu TX, Sun X, Jin L, Qi X, et al. Increasing circulating exosomes-carrying TRPC5 predicts chemoresistance in metastatic breast cancer patients. Cancer Sci (2017) 108:448–54. doi: 10.1111/cas.13150
135. Wang X, Pei X, Guo G, Qian X, Dou D, Zhang Z, et al. Exosome-mediated transfer of long noncoding RNA H19 induces doxorubicin resistance in breast cancer. J Cell Physiol (2020) 235:6896–904. doi: 10.1002/jcp.29585
136. Early Breast Cancer Trialists' Collaborative, G. Aromatase inhibitors versus tamoxifen in early breast cancer: patient-level meta-analysis of the randomised trials. Lancet (2015) 386:1341–52. doi: 10.1016/S0140-6736(15)61074-1
137. Kharb R, Haider K, Neha K, Yar MS. Aromatase inhibitors: role in postmenopausal breast cancer. Arch Pharm (Weinheim) (2020) 353:e2000081. doi: 10.1002/ardp.202000081
138. Augimeri G, La Camera G, Gelsomino L, Giordano C, Panza S, Sisci D, et al. Evidence for enhanced exosome production in aromatase inhibitor-resistant breast cancer cells. Int J Mol Sci (2020) 21(16):5841. doi: 10.3390/ijms21165841
139. von Minckwitz G, Huang CS, Mano MS, Loibl S, Mamounas EP, Untch M, et al. Trastuzumab emtansine for residual invasive HER2-positive breast cancer. N Engl J Med (2019) 380:617–28. doi: 10.1056/NEJMoa1814017
140. Cortes J, Kim SB, Chung WP, Im SA, Park YH, Hegg R, et al. Trastuzumab deruxtecan versus trastuzumab emtansine for breast cancer. N Engl J Med (2022) 386:1143–54. doi: 10.1056/NEJMoa2115022
141. Hurvitz SA, Hegg R, Chung WP, Im SA, Jacot W, Ganju V, et al. Trastuzumab deruxtecan versus trastuzumab emtansine in patients with HER2-positive metastatic breast cancer: updated results from DESTINY-Breast03, a randomised, open-label, phase 3 trial. Lancet (2023) 401:105–17. doi: 10.1016/S0140-6736(22)02420-5
142. Verma S, Miles D, Gianni L, Krop IE, Welslau M, Baselga J, et al. Trastuzumab emtansine for HER2-positive advanced breast cancer. N Engl J Med (2012) 367:1783–91. doi: 10.1056/NEJMoa1209124
143. Montemurro F, Delaloge S, Barrios CH, Wuerstlein R, Anton A, Brain E, et al. Trastuzumab emtansine (T-DM1) in patients with HER2-positive metastatic breast cancer and brain metastases: exploratory final analysis of cohort 1 from KAMILLA, a single-arm phase IIIb clinical trial(☆). Ann Oncol (2020) 31:1350–8. doi: 10.1016/j.annonc.2020.06.020
144. Oya K, Shen LT, Maruo K, Matsusaka S. Plasma exchange may enhance antitumor effects by removal of soluble programmed death-ligand 1 and extracellular vesicles: preliminary study. Biomedicines (2022) 10(10):2483. doi: 10.3390/biomedicines10102483
145. Yu S, Zhou Y, Niu L, Qiao Y, Yan Y. Mesenchymal stem cell-derived exosome mir-342-3p inhibits metastasis and chemo-resistance of breast cancer through regulating ID4. Genes Genomics (2022) 44:539–50. doi: 10.1007/s13258-021-01200-1
146. Liu J, Zhu S, Tang W, Huang Q, Mei Y, Yang H. Exosomes from tamoxifen-resistant breast cancer cells transmit drug resistance partly by delivering miR-9-5p. Cancer Cell Int (2021) 21:55. doi: 10.1186/s12935-020-01659-0
147. Dong X, Bai X, Ni J, Zhang H, Duan W, Graham P, et al. Exosomes and breast cancer drug resistance. Cell Death Dis (2020) 11:987. doi: 10.1038/s41419-020-03189-z
148. Wang B, Wang Y, Wang X, Gu J, Wu W, Wu H, et al. Extracellular vesicles carrying miR-887-3p promote breast cancer cell drug resistance by targeting BTBD7 and activating the Notch1/Hes1 signaling pathway. Dis Markers (2022) 2022:5762686. doi: 10.1155/2022/5762686
149. Yang Q, Zhao S, Shi Z, Cao L, Liu J, Pan T, et al. Chemotherapy-elicited exosomal miR-378a-3p and miR-378d promote breast cancer stemness and chemoresistance via the activation of EZH2/STAT3 signaling. J Exp Clin Cancer Res (2021) 40:120. doi: 10.1186/s13046-021-01901-1
150. Ngambenjawong C, Gustafson HH, Pun SH. Progress in tumor-associated macrophage (TAM)-targeted therapeutics. Adv Drug Delivery Rev (2017) 114:206–21. doi: 10.1016/j.addr.2017.04.010
151. Xia Y, Rao L, Yao H, Wang Z, Ning P, Chen X. Engineering macrophages for cancer immunotherapy and drug delivery. Adv Mater (2020) 32:e2002054. doi: 10.1002/adma.202002054
152. Mantovani A, Allavena P, Marchesi F, Garlanda C. Macrophages as tools and targets in cancer therapy. Nat Rev Drug Discovery (2022) 21:799–820. doi: 10.1038/s41573-022-00520-5
153. Zhao Y, Zheng Y, Zhu Y, Li H, Zhu H, Liu T. Docetaxel-loaded M1 macrophage-derived exosomes for a safe and efficient chemoimmunotherapy of breast cancer. J Nanobiotechnology (2022) 20:359. doi: 10.1186/s12951-022-01526-2
154. Zhu L, Dong D, Yu ZL, Zhao YF, Pang DW, Zhang ZL. Folate-engineered microvesicles for enhanced target and synergistic therapy toward breast cancer. ACS Appl Mater Interfaces (2017) 9:5100–8. doi: 10.1021/acsami.6b14633
155. Fernandez MF, Reina-Perez I, Astorga JM, Rodriguez-Carrillo A, Plaza-Diaz J, Fontana L. Breast cancer and its relationship with the microbiota. Int J Environ Res Public Health (2018) 15(8):1747. doi: 10.3390/ijerph15081747
156. Chen J, Douglass J, Prasath V, Neace M, Atrchian S, Manjili MH, et al. The microbiome and breast cancer: a review. Breast Cancer Res Treat (2019) 178:493–6. doi: 10.1007/s10549-019-05407-5
157. An J, Kim JB, Yang EY, Kim HO, Lee WH, Yang J, et al. Bacterial extracellular vesicles affect endocrine therapy in MCF7 cells. Med (Baltimore) (2021) 100:e25835. doi: 10.1097/MD.0000000000025835
158. Kwa M, Plottel CS, Blaser MJ, Adams S. The intestinal microbiome and estrogen receptor-positive female breast cancer. J Natl Cancer Inst (2016) 108(8):djw029. doi: 10.1093/jnci/djw029
159. An J, Kwon H, Lim W, Moon BI. Staphylococcus aureus-derived extracellular vesicles enhance the efficacy of endocrine therapy in breast cancer cells. J Clin Med (2022) 11(7):2030. doi: 10.3390/jcm11072030
160. Chang YH, Vuong CK, Ngo NH, Yamashita T, Ye X, Futamura Y, et al. Extracellular vesicles derived from wharton's jelly mesenchymal stem cells inhibit the tumor environment via the miR-125b/HIF1alpha signaling pathway. Sci Rep (2022) 12:13550. doi: 10.1038/s41598-022-17767-y
161. Armstrong JP, Holme MN, Stevens MM. Re-engineering extracellular vesicles as smart nanoscale therapeutics. ACS Nano (2017) 11:69–83. doi: 10.1021/acsnano.6b07607
162. Duan H, Liu Y, Gao Z, Huang W. Recent advances in drug delivery systems for targeting cancer stem cells. Acta Pharm Sin B (2021) 11:55–70. doi: 10.1016/j.apsb.2020.09.016
163. Chen Q, Li Q, Liang Y, Zu M, Chen N, Canup BSB, et al. Natural exosome-like nanovesicles from edible tea flowers suppress metastatic breast cancer via ROS generation and microbiota modulation. Acta Pharm Sin B (2022) 12:907–23. doi: 10.1016/j.apsb.2021.08.016
164. Shen DD, Pang JR, Bi YP, Zhao LF, Li YR, Zhao LJ, et al. LSD1 deletion decreases exosomal PD-L1 and restores T-cell response in gastric cancer. Mol Cancer (2022) 21:75. doi: 10.1186/s12943-022-01557-1
Keywords: breast cancer, extracellular vesicles, proliferation, metastasis, chemoresistance, biomarkers
Citation: Zhang X, Wang C, Yu J, Bu J, Ai F, Wang Y, Lin J and Zhu X (2023) Extracellular vesicles in the treatment and diagnosis of breast cancer: a status update. Front. Endocrinol. 14:1202493. doi: 10.3389/fendo.2023.1202493
Received: 12 April 2023; Accepted: 30 June 2023;
Published: 17 July 2023.
Edited by:
Feng Zhao, China Medical University, ChinaReviewed by:
Shan Jin, Inner Mongolia Medical University, ChinaCopyright © 2023 Zhang, Wang, Yu, Bu, Ai, Wang, Lin and Zhu. This is an open-access article distributed under the terms of the Creative Commons Attribution License (CC BY). The use, distribution or reproduction in other forums is permitted, provided the original author(s) and the copyright owner(s) are credited and that the original publication in this journal is cited, in accordance with accepted academic practice. No use, distribution or reproduction is permitted which does not comply with these terms.
*Correspondence: Jie Lin, bGlueGlyYW4wMjIxQDE2My5jb20=; Xudong Zhu, eGR6aHVAY211LmVkdS5jbg==
Disclaimer: All claims expressed in this article are solely those of the authors and do not necessarily represent those of their affiliated organizations, or those of the publisher, the editors and the reviewers. Any product that may be evaluated in this article or claim that may be made by its manufacturer is not guaranteed or endorsed by the publisher.
Research integrity at Frontiers
Learn more about the work of our research integrity team to safeguard the quality of each article we publish.