- 1Institut Universitaire de Cardiologie et de Pneumologie de Québec, Quebec, QC, Canada
- 2Faculté de Pharmacie, Université Laval, Québec, QC, Canada
- 3Faculté de Medicine, Université Laval, Québec, QC, Canada
- 4Montreal Diabetes Research Center, Montreal, QC, Canada
Histamine is a biogenic amine that acts as a neuromodulator within the brain. In the hypothalamus, histaminergic signaling contributes to the regulation of numerous physiological and homeostatic processes, including the regulation of energy balance. Histaminergic neurons project extensively throughout the hypothalamus and two histamine receptors (H1R, H3R) are strongly expressed in key hypothalamic nuclei known to regulate energy homeostasis, including the paraventricular (PVH), ventromedial (VMH), dorsomedial (DMH), and arcuate (ARC) nuclei. The activation of different histamine receptors is associated with differential effects on neuronal activity, mediated by their different G protein-coupling. Consequently, activation of H1R has opposing effects on food intake to that of H3R: H1R activation suppresses food intake, while H3R activation mediates an orexigenic response. The central histaminergic system has been implicated in atypical antipsychotic-induced weight gain and has been proposed as a potential therapeutic target for the treatment of obesity. It has also been demonstrated to interact with other major regulators of energy homeostasis, including the central melanocortin system and the adipose-derived hormone leptin. However, the exact mechanisms by which the histaminergic system contributes to the modification of these satiety signals remain underexplored. The present review focuses on recent advances in our understanding of the central histaminergic system’s role in regulating feeding and highlights unanswered questions remaining in our knowledge of the functionality of this system.
Introduction
Histamine is a small biological molecule (biogenic amine) that is widely distributed throughout the body. Although probably best recognized for its importance in arousal regulation and allergic inflammatory reactions, histamine plays a role in a diverse range of biological functions. This includes the regulation of energy balance, sleep and wakefulness, thermoregulation, gastrointestinal function, immune responses, and learning and memory (1–3). Within the central nervous system (CNS), a population of neurons located in the posterior hypothalamus provide the sole source of neuronal histamine to the brain (4–6) and can be identified based on the expression of histidine decarboxylase (HDC), the enzyme required for histamine synthesis (7, 8). These histaminergic neurons project extensively throughout the CNS, and strongly innervate multiple hypothalamic nuclei known to influence energy homeostasis and feeding behaviors (9–11). While histamine is known to impact food intake via its actions in the hypothalamus (12, 13), the precise mechanisms by which it does so are still being uncovered. The present review focuses on recent advances in understanding of the central histaminergic system’s role in regulating food intake, including potential interactions with satiety signals and neuropeptide/neurotransmitter systems implicated in energy balance regulation.
Histaminergic neurons
Histaminergic neuron somas are confined to the tuberomammillary nucleus (TMN) in the posterior hypothalamus (Figure 1) but have widespread projections that extensively innervate the CNS. This includes major brain regions including the cortex, brainstem, hippocampus, striatum, nucleus accumbens, amygdala, and substantia nigra, as well as multiple intrahypothalamic projections (9–11, 14). The diffuse projection patterns correlate with the multiple functions associated with histaminergic neurons, which have been comprehensively reviewed elsewhere (1, 3, 15). In contrast to the diffuse and well characterized projections of histaminergic neurons, difficulties occurred with initial attempts to identify afferent inputs to the histaminergic neurons, likely due to the inherent limitations of retrograde tracing studies, including potential spread to surrounding tissue and labeling of fibers of passage (16, 17). However, significant afferent input to the histaminergic neurons has since been identified, with inputs originating from the ventrolateral preoptic area (VLPO) (17–19) and the lateral hypothalamus (20–23). Importantly, the TMN can be subdivided into 3-5 different subregions depending on the classification method used (9, 24, 25). While histaminergic neurons are usually acknowledged to reside in the ‘TMN core’, their distribution within the hypothalamus (including the dorsal and bridge regions of the TMN) is much more widespread than typically appreciated (Figure 1), with some degree of variability observed between species (26, 27). However, the anatomical location of the histaminergic neurons and distribution of fibers throughout the brain appears comparable in humans to that described in rodents (28).
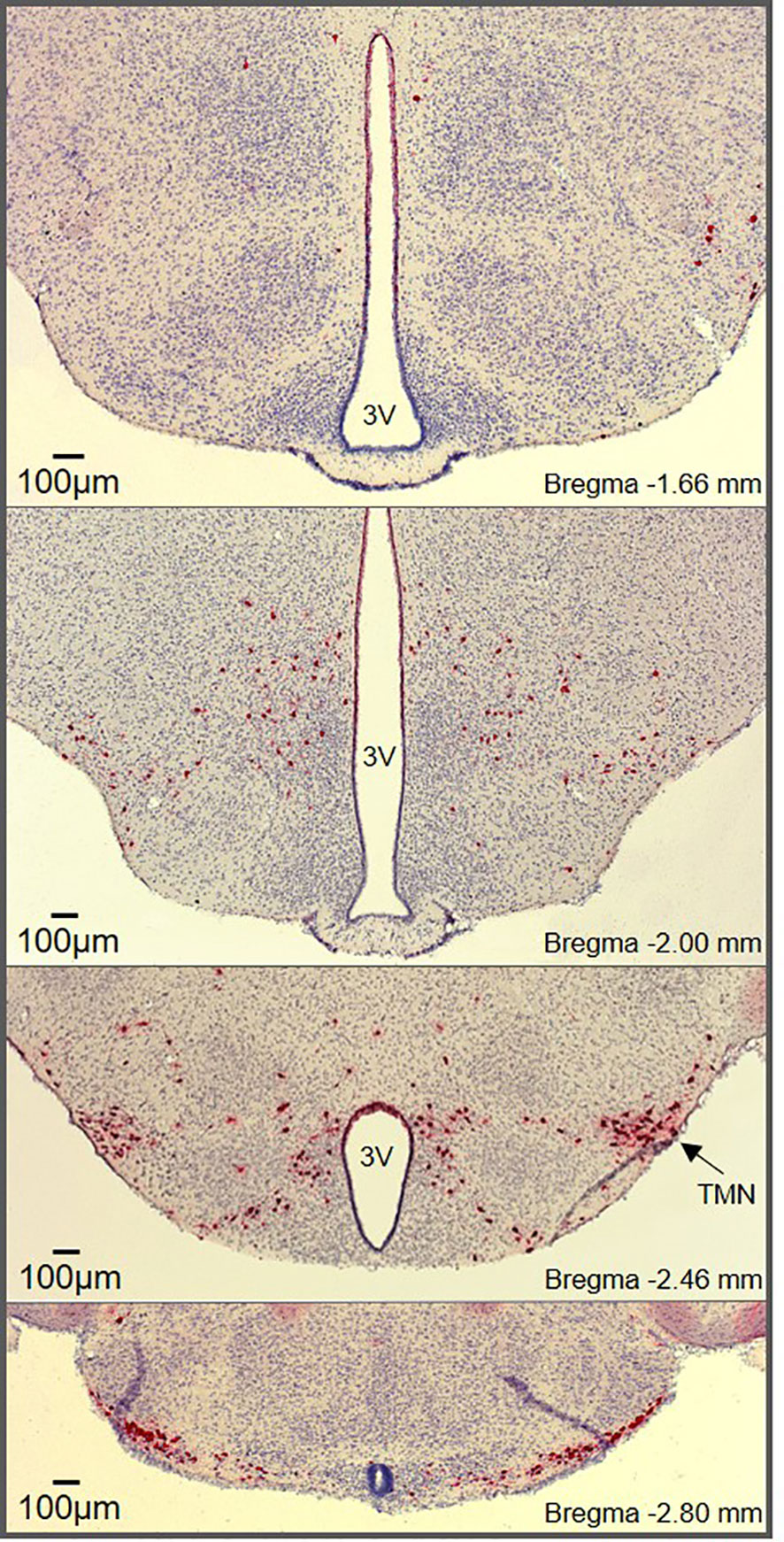
Figure 1 Histaminergic neuron distribution throughout the hypothalamus. RNAscope® in situ hybridization (ISH) targeting Hdc shows histaminergic neurons (red) densely packed in the core region of the tuberomammillary nucleus (marked TMN) along with diffusely scattered histaminergic neurons throughout the hypothalamus. The RNAscope® ISH was performed on hypothalamic brain slices (25µm) from male mice according to the manufacturer’s instructions (Advanced Cell Diagnostics, Inc., USA).
The location of the histaminergic neurons within the hypothalamus raises the potential for their involvement in the regulation of feeding. Many of the histaminergic neurons lay in close proximity to the third ventricle or are located on the ventral surface of the brain, suggesting, like other hypothalamic neurons, a potential for the detection of circulating hormones and neuropeptides (29). Moreover, histaminergic neuron fibers densely innervate the hypothalamus, including key hypothalamic nuclei known to regulate energy balance. While early studies required colchicine treatment to visualize histamine containing neurons (4), targeting of HDC (the enzyme required for histamine synthesis) allowed for the identification of dense fiber networks throughout the hypothalamus (6). Studies examining HDC immunoreactivity alone or in combination with paired retrograde tracer studies reveal high to very high density of histaminergic fibers in hypothalamic regions that regulate energy homeostasis. This includes the paraventricular nucleus of the hypothalamus (PVH), ventromedial hypothalamus (VMH), lateral hypothalamus (LH), dorsomedial hypothalamus (DMH) and the arcuate nucleus (ARC) (6, 9, 10). Use of newer immunohistochemical methods, with increased sensitivity for the visualization of histamine immunoreactive fibers and terminals, provided additional support for a moderate density of histaminergic fibers in the PVH, VMH, DMH, LH, and ARC (11). While identification of fiber tracts does not necessarily indicate functional connections, the presence of histamine receptors in these regions supports the role of histamine in regulating the activity of key metabolic neurons located in these areas of the hypothalamus.
Histamine receptor signaling
Histamine exerts its pleiotropic effects by binding to four subtypes of histamine receptors (HR), three of which are located within the brain (H1R, H2R, and H3R) (30–33). HRs belong to the family of G protein–coupled receptors (GPCRs), which interact with G proteins located in the plasma membrane. When a ligand binds to a GPCR, it causes a conformational change that triggers the interaction between the GPCR and nearby heterotrimeric G proteins. This promotes the exchange of a GDP for a GTP on the Gα subunit, resulting in its dissociation from Gβγ (34). There are four main families of Gα subunits: Gαi, Gαq, Gαs, and Gα12 (35). Gα subunits and Gβγ can activate different signaling pathways.
Identified in 1966, the H1R subclass of histamine receptors (gene symbol: HRH1) primarily couples to Gαq, resulting in the activation of the phospholipase C (PLC) signaling pathway (36–40) (Figure 2). This leads to the subsequent cleavage of phosphatidylinositol 4,5-bisphosphate (PIP2) into diacyl glycerol (DAG) and inositol 1,4,5-trisphosphate (IP3). These second messengers respectively activate protein kinase C (PKC) and promote the mobilization of Ca2+ (41). Accumulation of IP3, DAG and Ca2+ following histamine was shown to be prevented with the H1R inverse antagonist pyrilamine (42–45), while the H1R inverse agonist chlorpheniramine was reported to block the stimulatory effect of histamine on PLC and Ca2+ (46), confirming the involvement of H1R in the Gαq-dependent actions of histamine. One report also suggests that activation of H1R by histamine increases cAMP levels through Gβγ, an effect that is prevented by the H1R inverse agonist pyrilamine (47). As such, H1R activation and stimulation of it signaling cascade has excitatory effects and is associated with membrane depolarization in neurons (48–52).
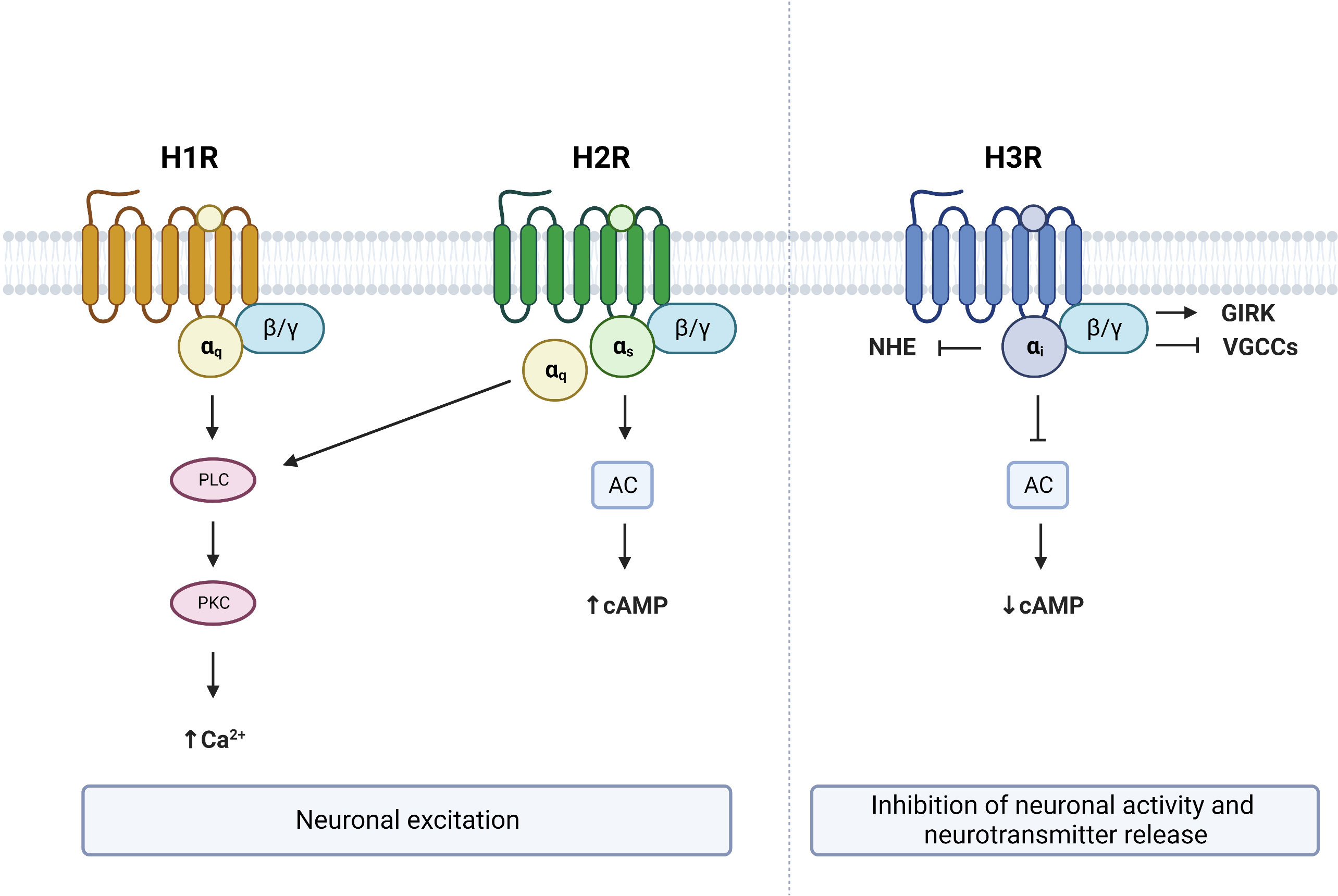
Figure 2 G protein–coupled receptor (GPCR) signaling from brain expressed histamine receptors. Histamine activation of H1R and H2R lead to neuronal excitation via Gαq and/or Gαs dependent mechanisms respectively. Activation of H3R leads to neuronal inhibition and suppression of neurotransmitter release. PLC, phospholipase C; PKC, protein kinase C; AC, adenylate cyclase; NHE, sodium–proton exchanger; GIRK, G protein-gated inwardly rectifying potassium channels; VGCCs, voltage-gated calcium channels; cAMP, cyclic adenosine monophosphate. Figure created with BioRender.com.
The H2R subclass of histamine receptors (gene symbol: HRH2), often referred to as the histamine gastric receptor, couples to both Gαq and Gαs proteins (39, 53). As a consequence, histamine binding to H2R stimulates both PLC and adenylate cyclase (AC) through Gαq and Gαs proteins respectively (53) (Figure 2). Activation of Gαs proteins in turn increases cAMP, an effect that is prevented by the HRH2 antagonist lafutidine (54). Increased cytosolic cAMP then leads to the activation of protein kinase A (PKA), which has been shown to stimulate neurons (55). Therefore, histamine binding to H2Rs also has excitatory actions within the brain, and results in depolarization of neurons through increased Ca2+, cAMP and PKA (56, 57).
The H3R subclass of histamine receptors (gene symbol: HRH3, previously known as GPCR97) primarily couples to Gαi proteins (39, 58) and functions as an inhibitory auto- or hetero-receptor in the brain (59–62). Activation of H3R results in AC inhibition and a subsequent reduction of cAMP levels (63, 64) (Figure 2). In contrast to the excitatory effects of H1R and H2R, binding of the H3R by histamine results in a suppression of neuronal activity and inhibition of neurotransmitter release (15, 60, 65, 66). Several mechanisms can contribute to the inhibitory effects of H3R. First, the Gβγ subunit of Gαi-coupled receptors has been shown to activate G protein-gated inwardly rectifying potassium (GIRK) channels (66, 67). Second, H3R activation can reduce neurotransmitter release by inhibiting N- and P/Q-type voltage-gated calcium channels again through the Gβγ subunit (68, 69). Third, H3R activation has been shown to reduce the activity of the sodium–proton exchanger (NHE), which is under the control of Gαi (70, 71). Therefore, activation of H3R has opposite effects on neuronal activity to that of H1R or H2R activation.
Central histaminergic system and the regulation of feeding
Histamine synthesis
The central histaminergic system has been implicated in the regulation of food intake through multiple different strategies used to manipulate the system. This includes altering the body’s ability to produce histamine. Genetic knock-out of histidine decarboxylase (HDC-KO) has been used to prevent the synthesis of histamine, resulting in histamine deficient mice. Studies using these mice suggest that HDC-KO animals are more susceptible to develop obesity as they age, or after consumption of a high fat diet (72–74). Detailed analyses of food intake in these mice are lacking, however, one study suggests that HDC-KO mice are not hyperphagic, but have an increased feed efficiency (72). However, the increased body weight in HDC-KO mice could be confounded by the decreased locomotor activity observed in these animals (75–77). An alternate method to deplete histamine is the use of α-Fluromethyl-[S]-histidine (α-FMH) which is a suicide inhibitor of histamine synthesis. Chemical inhibition of histamine synthesis with α-FMH has consistently been associated with an increase in food intake (78–82), suggesting that overall, histamine may be anorexigenic. However, such genetic or chemical methods preventing histamine synthesis provide limited and unspecific information regarding histamine’s ability to influence feeding, due to a loss of histaminergic tone at all histamine receptors simultaneously.
H1R activation suppresses food intake
The H1R is generally accepted to mediate the suppression of food intake induced by histamine (Figure 3). Early studies demonstrated that intracerebroventricular (ICV) injection of H1R antagonists stimulated feeding (12, 83). Moreover, the effects of pharmacological strategies increasing brain histamine levels, which are associated with a suppression of food intake, are attenuated, or abolished in the presence of H1R antagonists (78, 84). These actions are consistent with the increased food intake and weight gain seen with first-generation antihistamines used to treat allergies (85–87) which are all inverse agonists of the H1R (88).
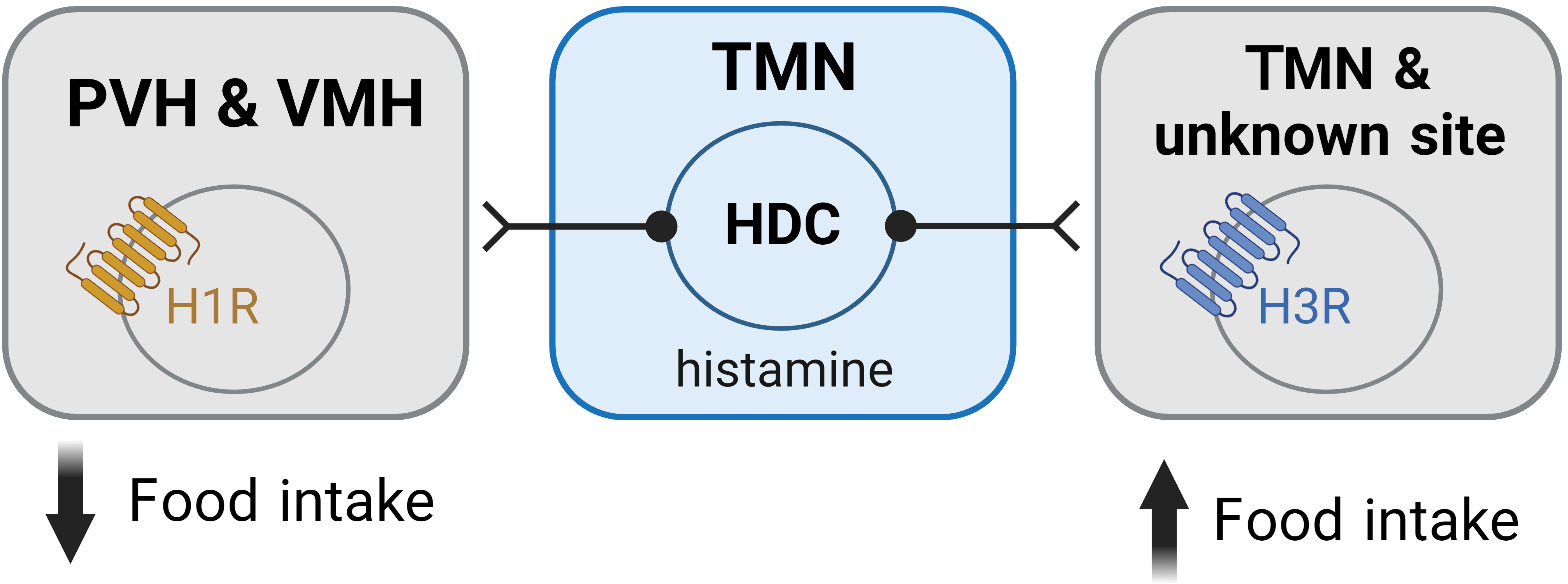
Figure 3 Histamine mediates its effects on feeding via activation of histamine receptors within the hypothalamus. Activation of the H1R is associated with an anorexigenic effect and is believed to be mediated via H1Rs expressed in the PVH and VMH. In contrast, activation of the H3R is orexigenic and occurs via autoinhibition of the histaminergic neurons. H3Rs in unidentified sites may also contribute to the orexigenic effects of H3R activation. Figure created with BioRender.com.
More recently, global H1R knockout (H1R-KO) mice have been developed (89) and food intake and body weight studies in these mice overwhelmingly backed up that obtained with pharmacological ligands targeting the H1R. The ability of α-FMH to stimulate food intake is lost in H1R-KO mice (79) and histamine’s ability to suppress food intake and body weight is reduced in H1R-KO mice compared to control mice (90). Moreover, the ability of betahistine, which enhances histamine levels and acts as an agonist of H1R, to reduce food intake and body weight is absent in H1R-KO mice (91). Together, these data strongly support the idea that histamine’s actions at the H1R are anorexigenic. Interestingly, H1R-KO mice do not show any changes in food intake or body weight when fed a standard chow diet (90, 92). However, with age or high fat diet feeding, H1R-KO mice accumulate fat mass and develop obesity (90, 92, 93), which is consistent with what was seen in mice completely deficient of histamine (HDC-KO) as discussed above. Additionally, H1R-KO mice display a decreased anorexigenic response to thyrotropin releasing hormone (TRH), neurotensin, nesfatin-1, and estradiol (94–97), suggesting that the H1R may contribute to the suppression of feeding normally induced by these anorexigenic peptides.
While the ability of histamine to suppress feeding is well demonstrated to occur via H1R, the exact neuronal populations and mechanisms responsible for these effects are not well understood. Studies where H1R antagonists were directly infused into different hypothalamic nuclei have demonstrated that blockade of H1R only in the paraventricular (PVH) and ventromedial (VMH) hypothalamus stimulate feeding (12, 13) (Figure 3). Similarly, micro infusion of α-FMH to decrease local histamine concentrations, only has effects on food intake when infused in the PVH and VMH (78, 82, 98). While H1R agonists have been shown to induce markers of cell activity (c-Fos) only in the PVH (91), extracellular recording techniques demonstrate that the H1R antagonist chlorpheniramine inhibits neurons in the VMH (83). Despite these studies suggesting that the PVH and VMH are the sites where H1R activation has its anorexigenic effects, future studies are required to further elucidate the mechanisms involved, including the chemical phenotype of the cells in these nuclei mediating the anorexigenic effects of H1R activation.
H2R activation does not influence food intake
When it comes to central H2Rs, there is limited evidence indicating that they have any role in regulating feeding. Importantly, H2R antagonists administered ICV or directly to hypothalamic regions have no effect on food intake (12, 83, 99, 100). Furthermore, the H2R antagonist ranitidine has been shown to have no effect on histamine-induced suppression of food intake, whereas both H1R and H3R antagonists influenced this effect (84). It should be noted that while H2R antagonists can influence feeding when taken orally or infused directly into the gut, peripheral mechanisms including H2R effects on gastric acid secretion and gut hormones likely contribute to these effects (101–103). Furthermore, it is unsurprising that centrally expressed H2Rs do not influence food intake given that these receptors are most strongly expressed in extrahypothalamic regions such as the cortex, hippocampus, striatum, basal ganglia, and amygdala (30, 33, 104). Together, these data strongly suggest that central H2Rs do not contribute to the homeostatic regulation of food intake.
H3R activation stimulates food intake
Pharmacological studies indicate that activation of the H3R is orexigenic, with H3R agonist delivery directly to the brain stimulating food intake (105–107) (Figure 3). In line with these observations, blockade or inverse agonism of the H3R suppresses food intake (78, 82, 84, 100, 107, 108). The capacity of H3R inverse agonists/antagonists to reduce food intake has also been shown to minimize weight gain occurring in models of diet-induced obesity and to reduce body weight in obese rodents (108–112). Moreover, H3R inverse agonists/antagonists suppress food intake in conditions associated with an increased orexigenic drive, i.e., in the fasted state or following neuropeptide Y (NPY) administration (105, 113). In one study, food intake in rats that received a single dose of thioperamide, a H3R antagonist, was significantly less for two days compared to controls (82). The suppression of food intake induced by H3R antagonists has also been demonstrated to occur in non-rodent species including pigs and non-human primates (114). Together, these studies demonstrate that histamine’s actions at the H3R stimulate feeding, and blockade of this receptor is associated with anorexigenic effects.
The ability of H3R inverse agonists/antagonists to suppress food intake is largely assumed to occur by removing the normal auto-inhibition of histaminergic neurons (Figure 3), thereby increasing histamine levels and enhancing action at the anorexigenic H1R (78, 105, 115). However, the H3R also functions as a heteroreceptor and has been shown to be expressed in several brain regions other than the TMN (32, 58, 60, 116). Importantly, the H3R can suppress the release of multiple neurotransmitters including serotonin (117, 118), dopamine (119, 120), noradrenaline (118, 121), acetylcholine (122, 123) and GABA (124–126), neurotransmitters that are implicated in the regulation of feeding. This raises the potential for H3R inverse agonism/antagonism to influence food intake via transmitters other than histamine (Figure 3). However, such a possibility has not been comprehensively assessed.
In comparison with pharmacological studies targeting the H3R, experiments using global H3R-KO mice have generated diverging and less consistent findings. H3R-KO mice were shown to consume more food and have an increase in body weight from approximately 10 weeks of age (127). Such findings seem counterintuitive considering that the KO of H3Rs should remove the auto-inhibition of the histaminergic neurons and enhance anorexigenic actions at the H1R. However, it has been demonstrated that H3R-KO mice actually have decreased histamine levels in the hypothalamus and cortex (127, 128) potentially contributing to this effect. In contrast to the food intake effects reported by Takahashi et al. (127), others have suggested that a decrease in food intake occurs in H3R-KO mice, however, food intake was normalized to body weight, making any absolute changes difficult to assess (129). While genetic mouse models can reveal important insights into the mechanistic underpinnings of physiology and behavior, developmental and compensatory actions can occur, especially in relation to fundamental processes such as eating. Moreover, the function of the H3R as a heteroreceptor adds another level of complexity, whereby knockout of H3R could simultaneously influence multiple neurotransmitter systems. Despite some conflicting results obtained in knockout animals, it is clear that the H3R plays an important role in regulating food intake, and its activation is generally orexigenic.
Histaminergic system and interaction with key metabolic signals
Leptin
In addition to histamine’s ability to influence feeding, the central histaminergic system has been suggested to interact with other signals reflective of the metabolic state. This includes leptin, a hormone produced by adipose tissue that acts in the CNS to regulate energy metabolism (130). Circulating leptin levels occur in proportion to fat mass and decrease with periods of fasting (131, 132), therefore, acting as a signal of energy reserves to the brain. Exogenous leptin administration is associated with a suppression of food intake, a reduction in body weight, and an upregulation of uncoupling protein 1 (UCP1) expression in adipose tissue depots, all of which have been suggested to require a fully functioning histaminergic system (79, 80, 92, 133). Studies in which histamine synthesis was chemically inactivated failed to observe the normal leptin-induced suppression of food intake and decrease in body weight (79, 80, 133). These effects have been linked to the H1R, as studies performed in mice globally lacking H1R show similar effects. In H1R-KO mice, leptin’s effect on food intake and body weight is suppressed or absent compared to that seen in control animals (79, 92). Additionally, leptin’s ability to decrease body fat percentage and upregulate UCP1 in brown adipose tissue was suppressed in H1R-KO mice (92). Moreover, genetic disruption of histamine synthesis (HDC-KO mice) leads to impairments in leptin sensing and regulation (72, 74, 134). While these studies suggest that the histaminergic system may mediate some of the anorexigenic effects of leptin, the mechanisms by which the histaminergic system regulates the actions of leptin in the CNS remains to be determined. Interestingly, the core region of the TMN, where the histaminergic neurons reside, does not express the long form of the leptin receptor (LepR) (135), which likely precludes direct effects of leptin on the histaminergic neurons themselves. In contrast, LepR is expressed in sub-populations of neurons located in the lateral hypothalamus (136–138) a region known to directly and indirectly influence the histaminergic neurons (139, 140). However, the potential for leptin to influence the activity of histaminergic neurons via presynaptic inputs has not previously been investigated. Future studies are required to determine the sites and mechanisms by which histamine and leptin signaling may converge within the brain.
Melanocortin system
The central melanocortin system is one of the best-characterized brain circuits regulating food intake and energy expenditure (141–144). Melanocortin peptides, derived from the proopiomelanocortin (POMC) pre-prohormone, form a crucial component of this system and act at cognate melanocortin receptors to influence energy balance (145, 146). Importantly, recent work has identified that histaminergic neurons are sensitive to activation of the melanocortin 4 receptor (MC4R) (147). Using single neuron ex vivo electrophysiological recordings from genetically identified histaminergic (HDC) neurons, we demonstrated that approximately 40% of histaminergic neurons are excited by the non-selective MC3R/MC4R agonist melanotan II (MTII) or a selective MC4R agonist (THIQ) (147). These MC4R-mediated effects were shown to modify glutamatergic tone to the histaminergic neurons (147). Moreover, the interaction between the melanocortin and histaminergic systems was shown to be important for feeding regulation. Chemogenetic inhibition of the histaminergic neurons using an inhibitory Designer Receptor Exclusively Activated by Designer Drugs (DREADD) approach (148–150), enhanced the anorexigenic response to central infusion of MTII (147). This study found that melanocortin system activation results in unabated anorexia once the histaminergic neurons are silenced and suggests that, under normal conditions, the melanocortin-dependent activation of histaminergic neurons acts naturally as a negative feedback loop of the anorexigenic effects of the melanocortin system (147). Despite this important observation demonstrating histaminergic neurons are sensitive to key metabolic signals conveyed by the melanocortin system, the downstream mechanisms by which histaminergic neurons restrain the anorexigenic effects of melanocortin system activation remain to be identified.
Other appetite-related hormones
The ability of other appetite-related hormones to influence the activity and function of the histaminergic neurons has not been intensively investigated. One previous study suggested that ghrelin may activate the histaminergic neurons, as increased c-Fos expression, an indirect marker of cellular activity, was observed in the TMN following central administration of ghrelin (151). However, the receptor for ghrelin, the growth hormone secretagogue receptor (GHSR), is not expressed in the TMN (152) and Ghsr mRNA is not detected in transcriptomic profiling of histaminergic neurons (153). This likely prevents any direct post-synaptic modulation of histaminergic neurons by ghrelin. Similarly, in vivo work has suggested that the histaminergic system is influenced by glucagon-like peptide-1 (GLP-1), as central GLP-1 infusion has been shown to increase histamine and histamine metabolite levels in the hypothalamus (154). The same study also indicated that the histaminergic system was required for the full anorexigenic effect of GLP-1, as inhibition of histamine synthesis (with α-FMH) attenuated the GLP-1 induced suppression of food intake (154). While there are descriptions of GLP-1 receptor (GLP-1R) expression in the TMN (155) and tuberal region (156), and GLP-1R agonists have been reported to activate (c-Fos) in the ventral region of the TMN (157) and the tuberal region (158), single cell sequencing fails to detect Glp1r mRNA in histaminergic neurons (153). Interestingly, the LH, a region strongly innervating the TMN, has been shown to express Glp1r mRNA (155, 156) and is involved in mediating some of the anorexigenic effects of GLP-1 (159). Thus, any influence of GLP-1 on the histaminergic neurons may be indirect via neurons of the LH.
The pancreatic hormone insulin may also have a role in regulating histaminergic neuron function. One study demonstrated that a very small percentage of histaminergic neurons displayed c-Fos expression following insulin-induced hypoglycemia (160). Further work would be required to delineate whether histaminergic neuron activation in these conditions was mediated by the hypoglycemia or insulin itself. However, histaminergic neurons have been shown to express the insulin receptor (153). Another metabolically relevant neuropeptide known to target the histaminergic neurons is orexin (also known as hypocretin). Orexin is a potent stimulator of feeding and the neurons synthesizing this orexigenic neuropeptide are located in the LH (161, 162). Importantly, histaminergic neurons express the orexin receptor type 2 (OxR2/Hcrt2) (153, 163) and are excited by orexin-A (164, 165). Orexin actions on histaminergic neurons have largely been demonstrated to influence arousal control (165, 166). However, it is important to note that the orexin neurons also co-express glutamate and can signal to the downstream histaminergic neurons via glutamatergic currents (22, 167). Moreover, the glutamatergic tone at histaminergic neurons, arising from the LH, has been linked to the modulation of food intake (147). While it is interesting to speculate about the different functional consequences of orexin neuronal transmission to histaminergic neurons, delineating such multifunctionality remains understudied. Overall, it appears that the ability of histaminergic neurons to detect, and interact with, metabolic signals occur via indirect (presynaptic) mechanisms, or via actions downstream of the histaminergic neurons themselves, i.e. on neurons expressing the histamine receptors.
Medications regulating body weight via the histaminergic system
Psychiatric medications for the treatment of schizophrenia
Supporting the importance of histamine receptors in the regulation of energy balance, antipsychotic medications that interact with the histaminergic system are associated with clinically significant weight gain (168, 169). Notably, the atypical antipsychotics with the largest weight gain profiles, olanzapine and clozapine, also display high affinities for the H1R (170–173). Atypical antipsychotics act to antagonize histamine’s endogenous actions at the H1R, which may partially explain the increased food intake seen with these medications (174–177). While the exact mechanisms underlying atypical antipsychotic-induced weight gain remain somewhat elusive, these medications have been shown to downregulate hypothalamic expression of the H1R (178). In addition, atypical antipsychotics have been shown to increase orexigenic neuropeptide Y (NPY) expression and activate the cellular energy sensor AMP-activated protein kinase (AMPK) in the hypothalamus, effects that are dependent on functional H1Rs (171, 179). Moreover, combination therapies including betahistine, a H1R agonist/H3R antagonist, have been shown to reduce weight gain in people treated with olanzapine (180). Although the histaminergic system is not the only transmitter system implicated in atypical antipsychotic-induced weight gain, strong evidence suggests its ability to influence food intake, and sensitivity to these medications, plays a contributing role.
Therapeutic potential for the treatment of obesity
Following the cloning of the H3R in 1999 (58), numerous ligands were developed to manipulate the function of the receptor, and the H3R was subsequently proposed as a potential therapeutic target for the treatment of obesity (81, 116, 181, 182). In addition to food intake effects of H3R antagonists/inverse agonists, as discussed in this review, pre-clinical work demonstrated that these compounds also improve metabolic health and are associated with decreased body weight and fat mass, improved glucose homeostasis, and increased insulin sensitivity (108–111). These properties saw multiple pharmaceutical companies including Novo Nordisk, Abbott Laboratories, and Gliatech pursue H3R ligands for the treatment of obesity (183). While there was a brief surge in interest in these compounds for their metabolic effects, few ligands transitioned from the pre-clinical stage. Abbott laboratories H3R antagonist (A-331440) was found to have the potential for genotoxic effects which prohibited its further development as an anti-obesity therapeutic (184). Contradictory results were obtained between ligands with some studies failing to demonstrate consistent effects on food intake and anti-obesity properties (81, 185). Additionally, human trials with betahistine, a H1R agonist/H3R antagonist, failed to identify any striking weight loss effects in obese women (186), or on food intake when presented a buffet meal following a single day of betahistine treatment (187). Differences in ligand affinity for the H3R found between species may also contribute to some discrepancies observed among rodent and human studies (63, 188). Even though the pharmaceutical industry appears to have largely withdrawn its interest in pursuing the H3R as an anti-obesity target (189), work endures to optimize H3R ligands and explore their potential to influence food intake and body weight, and H3R antagonists/inverse agonists continue to be proposed for the treatment of obesity (190, 191).
Considerations and future directions
The central histaminergic system has received considerable interest for its ability to regulate energy balance, however, many unanswered questions remain. Generally, histamine is considered an anorexigenic substance, as activation of H1Rs decrease food intake, effects that are believed to be mediated through actions in the PVH and VMH (12, 13). However, these hypothalamic nuclei consist of multiple cell types, and the chemical phenotype or identity of the cells mediating H1R agonism-induced suppression of food intake remain unidentified. Moreover, the view of histamine as an anorexigenic compound seems somewhat contradictory given that feeding occurs during waking hours when histaminergic neurons are active and histamine levels are highest (192–196). It appears that the picture is more complex and likely involves numerous interactions, some of which have yet to be uncovered.
Pharmacological and genetic knockout studies have provided important insights into the functioning of the histaminergic system, but the expression of histamine receptors in both the brain and periphery, and effects of the H3R on multiple neurotransmitter systems, likely complicate the interpretation of some of these findings. The field now requires the ability to manipulate individual histamine receptors in a cell-type specific way (e.g., histamine receptor floxed mice) to further delineate the precise actions of histamine in different nuclei and different cell types, and to overcome some of the inherent limitations of global knockout models.
Evidence also continues to emerge that the histaminergic neurons are heterogeneous. Differences have been demonstrated in their basal electrophysiological properties, transcriptional makeup, and their response to various pharmacological agents (163, 197–199). Such heterogeneity combined with multiple histamine receptors, differentially expressed within the hypothalamus and in multiple cell types, contributes to the complexity of the histaminergic system and highlights multiple ways histamine may serve to influence neuronal activity and food intake. Additionally, histamine itself has been proposed to function more like a neuromodulator or neuropeptide than a classical “neurotransmitter” (1, 200). Histaminergic neurons rarely form close synaptic contacts (201, 202), preventing their potential for traditional fast synaptic signaling to clearly defined post-synaptic targets. Rather, histaminergic neurons are believed to communicate via volume transmission, with histamine being released non-synaptically, allowing it to have longer lasting actions, and modulate neurotransmission at extra synaptic sites similar to other monoamines (203). The ability of histamine to signal in this fashion raises the potential for histaminergic neurons to “prime” other neurons’ responsiveness to additional incoming (metabolic) stimuli during waking hours when histaminergic tone is highest. However, future studies will be required to address such a possibility.
Conclusion
In summary, histamine functions as a neuromodulator in the brain and contributes to the central regulation of energy homeostasis. Its effects on food intake largely depend on the histamine receptor subtype activated, with agonism of H1Rs being anorexigenic and agonism of H3Rs causing an orexigenic response. These important metabolic effects of HR activation contribute towards the weight gain side effects of some common medications and have seen HR ligands proposed as anti-obesity therapeutics. The histaminergic system has also been demonstrated to interact with key metabolic signals in the brain. It is clear that the histaminergic system has a powerful ability to influence food intake. Now we must turn our attention to elucidating the exact mechanisms by which it does so and the circumstances in which histaminergic signaling may contribute to an altered homeostatic drive to eat.
Author contributions
All authors contributed to the article and approved the submitted version.
Funding
This research was supported by the Sentinel North Initiative funded by the Canada First Research Excellence Fund (Partnered Research Chair in Sleep Pharmacometabolism to NJM), the Fonds de recherche du Québec - Santé (FRQS) (J1 Research Scholar award to NJM), the Natural Sciences and Engineering Research Council of Canada (NSERC) (to NJM), the Fondation de l’Insitut Universitaire de Cardiologie et de Pneumologie de Québec (FIUCPQ) (to NJM and MMM), and the Canada Research Chairs Program (to AC).
Conflict of interest
The authors declare that the research was conducted in the absence of any commercial or financial relationships that could be construed as a potential conflict of interest.
Publisher’s note
All claims expressed in this article are solely those of the authors and do not necessarily represent those of their affiliated organizations, or those of the publisher, the editors and the reviewers. Any product that may be evaluated in this article, or claim that may be made by its manufacturer, is not guaranteed or endorsed by the publisher.
References
1. Schwartz JC, Arrang JM, Garbarg M, Pollard H, Ruat M. Histaminergic transmission in the mammalian brain. Physiol Rev (1991) 71(1):1–51. doi: 10.1152/physrev.1991.71.1.1
2. Schneider E, Rolli-Derkinderen M, Arock M, Dy M. Trends in histamine research: new functions during immune responses and hematopoiesis. Trends Immunol (2002) 23(5):255–63. doi: 10.1016/s1471-4906(02)02215-9
3. Haas HL, Sergeeva OA, Selbach O. Histamine in the nervous system. Physiol Rev (2008) 88(3):1183–241. doi: 10.1152/physrev.00043.2007
4. Panula P, Yang HY, Costa E. Histamine-containing neurons in the rat hypothalamus. Proc Natl Acad Sci USA (1984) 81(8):2572–6. doi: 10.1073/pnas.81.8.2572
5. Takeda N, Inagaki S, Shiosaka S, Taguchi Y, Oertel WH, Tohyama M, et al. Immunohistochemical evidence for the coexistence of histidine decarboxylase-like and glutamate decarboxylase-like immunoreactivities in nerve cells of the magnocellular nucleus of the posterior hypothalamus of rats. Proc Natl Acad Sci USA (1984) 81(23):7647–50. doi: 10.1073/pnas.81.23.7647
6. Watanabe T, Taguchi Y, Shiosaka S, Tanaka J, Kubota H, Terano Y, et al. Distribution of the histaminergic neuron system in the central nervous system of rats; a fluorescent immunohistochemical analysis with histidine decarboxylase as a marker. Brain Res (1984) 295(1):13–25. doi: 10.1016/0006-8993(84)90811-4
7. Taylor KM, Snyder SH. Isotopic microassay of histamine, histidine, histidine decarboxylase and histamine methyltransferase in brain tissue. J Neurochem (1972) 19(5):1343–58. doi: 10.1111/j.1471-4159.1972.tb01459.x
8. Green JP, Prell GD, Khandelwal JK, Blandina P. Aspects of histamine metabolism. Agents Actions (1987) 22(1-2):1–15. doi: 10.1007/bf01968810
9. Ericson H, Watanabe T, Kohler C. Morphological analysis of the tuberomammillary nucleus in the rat brain: delineation of subgroups with antibody against l-histidine decarboxylase as a marker. J Comp Neurol (1987) 263(1):1–24. doi: 10.1002/cne.902630102
10. Inagaki N, Yamatodani A, Ando-Yamamoto M, Tohyama M, Watanabe T, Wada H. Organization of histaminergic fibers in the rat brain. J Comp Neurol (1988) 273(3):283–300. doi: 10.1002/cne.902730302
11. Panula P, Pirvola U, Auvinen S, Airaksinen MS. Histamine-immunoreactive nerve fibers in the rat brain. Neuroscience (1989) 28(3):585–610. doi: 10.1016/0306-4522(89)90007-9
12. Sakata T, Ookuma K, Fukagawa K, Fujimoto K, Yoshimatsu H, Shiraishi T, et al. Blockade of the histamine H1-receptor in the rat ventromedial hypothalamus and feeding elicitation. Brain Res (1988) 441(1-2):403–7. doi: 10.1016/0006-8993(88)91423-0
13. Ookuma K, Yoshimatsu H, Sakata T, Fujimoto K, Fukagawa F. Hypothalamic sites of neuronal histamine action on food intake by rats. Brain Res (1989) 490(2):268–75. doi: 10.1016/0006-8993(89)90244-8
14. Lin W, Xu L, Zheng Y, An S, Zhao M, Hu W, et al. Whole-brain mapping of histaminergic projections in mouse brain. Proc Natl Acad Sci USA (2023) 120(14):e2216231120. doi: 10.1073/pnas.2216231120
15. Haas H, Panula P. The role of histamine and the tuberomamillary nucleus in the nervous system. Nat Rev Neurosci (2003) 4(2):121–30. doi: 10.1038/nrn1034
16. Saper CB, Stornetta RL. Central autonomic system. In: Paxinos G, editor. The rat nervous system, 4th Edition. Amsterdam: Academic Press (2015). p. 629–73.
17. Sherin JE, Elmquist JK, Torrealba F, Saper CB. Innervation of histaminergic tuberomammillary neurons by GABAergic and galaninergic neurons in the ventrolateral preoptic nucleus of the rat. J Neurosci Off J Soc Neurosci (1998) 18(12):4705–21. doi: 10.1523/JNEUROSCI.18-12-04705.1998
18. Sherin JE, Shiromani PJ, McCarley RW, Saper CB. Activation of ventrolateral preoptic neurons during sleep. Science (1996) 271(5246):216–9. doi: 10.1126/science.271.5246.216
19. Chung S, Weber F, Zhong P, Tan CL, Nguyen TN, Beier KT, et al. Identification of preoptic sleep neurons using retrograde labelling and gene profiling. Nature (2017) 545(7655):477–81. doi: 10.1038/nature22350
20. Peyron C, Tighe DK, van den Pol AN, de Lecea L, Heller HC, Sutcliffe JG, et al. Neurons containing hypocretin (orexin) project to multiple neuronal systems. J Neurosci Off J Soc Neurosci (1998) 18(23):9996–10015. doi: 10.1523/JNEUROSCI.18-23-09996.1998
21. Chemelli RM, Willie JT, Sinton CM, Elmquist JK, Scammell T, Lee C, et al. Narcolepsy in orexin knockout mice: molecular genetics of sleep regulation. Cell (1999) 98(4):437–51. doi: 10.1016/s0092-8674(00)81973-x
22. Schone C, Cao ZF, Apergis-Schoute J, Adamantidis A, Sakurai T, Burdakov D. Optogenetic probing of fast glutamatergic transmission from hypocretin/orexin to histamine neurons in situ. J Neurosci Off J Soc Neurosci (2012) 32(36):12437–43. doi: 10.1523/JNEUROSCI.0706-12.2012
23. Jego S, Glasgow SD, Herrera CG, Ekstrand M, Reed SJ, Boyce R, et al. Optogenetic identification of a rapid eye movement sleep modulatory circuit in the hypothalamus. Nat Neurosci (2013) 16(11):1637–43. doi: 10.1038/nn.3522
24. Kohler C, Swanson LW, Haglund L, Wu JY. The cytoarchitecture, histochemistry and projections of the tuberomammillary nucleus in the rat. Neuroscience (1985) 16(1):85–110. doi: 10.1016/0306-4522(85)90049-1
25. Inagaki N, Toda K, Taniuchi I, Panula P, Yamatodani A, Tohyama M, et al. An analysis of histaminergic efferents of the tuberomammillary nucleus to the medial preoptic area and inferior colliculus of the rat. Exp Brain Res (1990) 80(2):374–80. doi: 10.1007/bf00228164
26. Karlstedt K, Nissinen M, Michelsen KA, Panula P. Multiple sites of l-histidine decarboxylase expression in mouse suggest novel developmental functions for histamine. Dev Dyn (2001) 221(1):81–91. doi: 10.1002/dvdy.1127
27. Airaksinen MS, Panula P. The histaminergic system in the guinea pig central nervous system: an immunocytochemical mapping study using an antiserum against histamine. J Comp Neurol (1988) 273(2):163–86. doi: 10.1002/cne.902730204
28. Panula P, Airaksinen MS, Pirvola U, Kotilainen E. A histamine-containing neuronal system in human brain. Neuroscience (1990) 34(1):127–32. doi: 10.1016/0306-4522(90)90307-p
29. Reiner PB, Semba K, Watanabe T, Wada H. En bloc immunohistochemistry reveals extensive distribution of histidine decarboxylase-immunoreactive neurons on the ventral surface of the rat hypothalamus. Neurosci Lett (1987) 77(2):137–42. doi: 10.1016/0304-3940(87)90575-1
30. Martinez-Mir MI, Pollard H, Moreau J, Arrang JM, Ruat M, Traiffort E, et al. Three histamine receptors (H1, H2 and H3) visualized in the brain of human and non-human primates. Brain Res (1990) 526(2):322–7. doi: 10.1016/0006-8993(90)91240-h
31. Bouthenet ML, Ruat M, Sales N, Garbarg M, Schwartz JC. A detailed mapping of histamine H1-receptors in guinea-pig central nervous system established by autoradiography with [125I]iodobolpyramine. Neuroscience (1988) 26(2):553–600. doi: 10.1016/0306-4522(88)90167-4
32. Pollard H, Moreau J, Arrang JM, Schwartz JC. A detailed autoradiographic mapping of histamine H3 receptors in rat brain areas. Neuroscience (1993) 52(1):169–89. doi: 10.1016/0306-4522(93)90191-h
33. Vizuete ML, Traiffort E, Bouthenet ML, Ruat M, Souil E, Tardivel-Lacombe J, et al. Detailed mapping of the histamine H2 receptor and its gene transcripts in guinea-pig brain. Neuroscience (1997) 80(2):321–43. doi: 10.1016/s0306-4522(97)00010-9
34. Weis WI, Kobilka BK. The molecular basis of G protein-coupled receptor activation. Annu Rev Biochem (2018) 87:897–919. doi: 10.1146/annurev-biochem-060614-033910
35. Pfleger J, Gresham K, Koch WJ. G Protein-coupled receptor kinases as therapeutic targets in the heart. Nat Rev Cardiol (2019) 16(10):612–22. doi: 10.1038/s41569-019-0220-3
36. Ash AS, Schild HO. Receptors mediating some actions of histamine. Br J Pharmacol Chemother (1966) 27(2):427–39. doi: 10.1111/j.1476-5381.1966.tb01674.x
37. Gutowski S, Smrcka A, Nowak L, Wu DG, Simon M, Sternweis PC. Antibodies to the alpha q subfamily of guanine nucleotide-binding regulatory protein alpha subunits attenuate activation of phosphatidylinositol 4,5-bisphosphate hydrolysis by hormones. J Biol Chem (1991) 266(30):20519–24. doi: 10.1016/S0021-9258(18)54955-3
38. Leopoldt D, Harteneck C, Nurnberg B. G Proteins endogenously expressed in sf 9 cells: interactions with mammalian histamine receptors. Naunyn-Schmiedeberg's Arch Pharmacol (1997) 356(2):216–24. doi: 10.1007/pl00005044
39. Harding SD, Armstrong JF, Faccenda E, Southan C, Alexander SPH, Davenport AP, et al. The IUPHAR/BPS guide to PHARMACOLOGY in 2022: curating pharmacology for COVID-19, malaria and antibacterials. Nucleic Acids Res (2022) 50(D1):D1282–D94. doi: 10.1093/nar/gkab1010
40. Leurs R, Traiffort E, Arrang JM, Tardivel-Lacombe J, Ruat M, Schwartz JC. Guinea Pig histamine H1 receptor. II. stable expression in Chinese hamster ovary cells reveals the interaction with three major signal transduction pathways. J Neurochem (1994) 62(2):519–27. doi: 10.1046/j.1471-4159.1994.62020519.x
41. Harding SD, Sharman JL, Faccenda E, Southan C, Pawson AJ, Ireland S, et al. The IUPHAR/BPS guide to PHARMACOLOGY in 2018: updates and expansion to encompass the new guide to IMMUNOPHARMACOLOGY. Nucleic Acids Res (2018) 46(D1):D1091–D106. doi: 10.1093/nar/gkx1121
42. Lo WW, Fan TP. Histamine stimulates inositol phosphate accumulation via the H1-receptor in cultured human endothelial cells. Biochem Biophys Res Commun (1987) 148(1):47–53. doi: 10.1016/0006-291x(87)91074-6
43. Li H, Choe NH, Wright DT, Adler KB. Histamine provokes turnover of inositol phospholipids in guinea pig and human airway epithelial cells via an H1-receptor/G protein-dependent mechanism. Am J Respir Cell Mol Biol (1995) 12(4):416–24. doi: 10.1165/ajrcmb.12.4.7695921
44. De Backer MD, Gommeren W, Moereels H, Nobels G, Van Gompel P, Leysen JE, et al. Genomic cloning, heterologous expression and pharmacological characterization of a human histamine H1 receptor. Biochem Biophys Res Commun (1993) 197(3):1601–8. doi: 10.1006/bbrc.1993.2662
45. Tilly BC, Tertoolen LG, Lambrechts AC, Remorie R, de Laat SW, Moolenaar WH. Histamine-H1-receptor-mediated phosphoinositide hydrolysis, Ca2+ signalling and membrane-potential oscillations in human HeLa carcinoma cells. Biochem J (1990) 266(1):235–43. doi: 10.1042/bj2660235
46. Markwardt KL, Magnino PE, Pang IH. Effect of histamine on phosphoinositide turnover and intracellular calcium in human ciliary muscle cells. Exp Eye Res (1996) 62(5):511–20. doi: 10.1006/exer.1996.0062
47. Maruko T, Nakahara T, Sakamoto K, Saito M, Sugimoto N, Takuwa Y, et al. Involvement of the betagamma subunits of G proteins in the cAMP response induced by stimulation of the histamine H1 receptor. Naunyn-Schmiedeberg's Arch Pharmacol (2005) 372(2):153–9. doi: 10.1007/s00210-005-0001-x
48. Segal M. Histamine modulates reactivity of hippocampal CA3 neurons to afferent stimulation in vitro. Brain Res (1981) 213(2):443–8. doi: 10.1016/0006-8993(81)90251-1
49. McCormick DA, Williamson A. Modulation of neuronal firing mode in cat and guinea pig LGNd by histamine: possible cellular mechanisms of histaminergic control of arousal. J Neurosci Off J Soc Neurosci (1991) 11(10):3188–99. doi: 10.1523/JNEUROSCI.11-10-03188.1991
50. Smith BN, Armstrong WE. The ionic dependence of the histamine-induced depolarization of vasopressin neurones in the rat supraoptic nucleus. J Physiol (1996) 495(Pt 2):465–78. doi: 10.1113/jphysiol.1996.sp021607
51. Reiner PB, Kamondi A. Mechanisms of antihistamine-induced sedation in the human brain: H1 receptor activation reduces a background leakage potassium current. Neuroscience (1994) 59(3):579–88. doi: 10.1016/0306-4522(94)90178-3
52. Billups D, Billups B, Challiss RA, Nahorski SR. Modulation of gq-protein-coupled inositol trisphosphate and Ca2+ signaling by the membrane potential. J Neurosci Off J Soc Neurosci (2006) 26(39):9983–95. doi: 10.1523/JNEUROSCI.2773-06.2006
53. Kuhn B, Schmid A, Harteneck C, Gudermann T, Schultz G. G Proteins of the gq family couple the H2 histamine receptor to phospholipase c. Mol Endocrinol (1996) 10(12):1697–707. doi: 10.1210/mend.10.12.8961278
54. Fukushima Y, Otsuka H, Ishikawa M, Asano T, Anai M, Katsube T, et al. Potent and long-lasting action of lafutidine on the human histamine H(2) receptor. Digestion (2001) 64(3):155–60. doi: 10.1159/000048856
55. Pedarzani P, Storm JF. PKA mediates the effects of monoamine transmitters on the k+ current underlying the slow spike frequency adaptation in hippocampal neurons. Neuron (1993) 11(6):1023–35. doi: 10.1016/0896-6273(93)90216-e
56. Yanovsky Y, Haas HL. Histamine increases the bursting activity of pyramidal cells in the CA3 region of mouse hippocampus. Neurosci Lett (1998) 240(2):110–2. doi: 10.1016/s0304-3940(97)00925-7
57. Selbach O, Brown RE, Haas HL. Long-term increase of hippocampal excitability by histamine and cyclic AMP. Neuropharmacology (1997) 36(11-12):1539–48. doi: 10.1016/s0028-3908(97)00144-5
58. Lovenberg TW, Roland BL, Wilson SJ, Jiang X, Pyati J, Huvar A, et al. Cloning and functional expression of the human histamine H3 receptor. Mol Pharmacol (1999) 55(6):1101–7. doi: 10.1124/mol.55.6.1101
59. Schlicker E, Malinowska B, Kathmann M, Gothert M. Modulation of neurotransmitter release via histamine H3 heteroreceptors. Fundam Clin Pharmacol (1994) 8(2):128–37. doi: 10.1111/j.1472-8206.1994.tb00789.x
60. Nieto-Alamilla G, Marquez-Gomez R, Garcia-Galvez AM, Morales-Figueroa GE, Arias-Montano JA. The histamine H3 receptor: structure, pharmacology, and function. Mol Pharmacol (2016) 90(5):649–73. doi: 10.1124/mol.116.104752
61. Arrang JM, Garbarg M, Schwartz JC. Auto-inhibition of brain histamine release mediated by a novel class (H3) of histamine receptor. Nature (1983) 302(5911):832–7. doi: 10.1038/302832a0
62. Arrang JM, Garbarg M, Schwartz JC. Autoregulation of histamine release in brain by presynaptic H3-receptors. Neuroscience (1985) 15(2):553–62. doi: 10.1016/0306-4522(85)90233-7
63. Lovenberg TW, Pyati J, Chang H, Wilson SJ, Erlander MG. Cloning of rat histamine H(3) receptor reveals distinct species pharmacological profiles. J Pharmacol Exp Ther (2000) 293(3):771–8.
64. Wieland K, Bongers G, Yamamoto Y, Hashimoto T, Yamatodani A, Menge WM, et al. Constitutive activity of histamine h(3) receptors stably expressed in SK-N-MC cells: display of agonism and inverse agonism by H(3) antagonists. J Pharmacol Exp Ther (2001) 299(3):908–14.
65. Arrang JM, Garbarg M, Lancelot JC, Lecomte JM, Pollard H, Robba M, et al. Highly potent and selective ligands for histamine H3-receptors. Nature (1987) 327(6118):117–23. doi: 10.1038/327117a0
66. Parks GS, Olivas ND, Ikrar T, Sanathara NM, Wang L, Wang Z, et al. Histamine inhibits the melanin-concentrating hormone system: implications for sleep and arousal. J Physiol (2014) 592(10):2183–96. doi: 10.1113/jphysiol.2013.268771
67. Kano H, Toyama Y, Imai S, Iwahashi Y, Mase Y, Yokogawa M, et al. Structural mechanism underlying G protein family-specific regulation of G protein-gated inwardly rectifying potassium channel. Nat Commun (2019) 10(1):2008. doi: 10.1038/s41467-019-10038-x
68. Zamponi GW, Currie KP. Regulation of Ca(V)2 calcium channels by G protein coupled receptors. Biochim Biophys Acta (2013) 1828(7):1629–43. doi: 10.1016/j.bbamem.2012.10.004
69. Takeshita Y, Watanabe T, Sakata T, Munakata M, Ishibashi H, Akaike N. Histamine modulates high-voltage-activated calcium channels in neurons dissociated from the rat tuberomammillary nucleus. Neuroscience (1998) 87(4):797–805. doi: 10.1016/s0306-4522(98)00152-3
70. Silver RB, Mackins CJ, Smith NC, Koritchneva IL, Lefkowitz K, Lovenberg TW, et al. Coupling of histamine H3 receptors to neuronal Na+/H+ exchange: a novel protective mechanism in myocardial ischemia. Proc Natl Acad Sci USA (2001) 98(5):2855–9. doi: 10.1073/pnas.051599198
71. van Willigen G, Nieuwland R, Nurnberg B, Gorter G, Akkerman JW. Negative regulation of the platelet Na+/H+ exchanger by trimeric G-proteins. Eur J Biochem (2000) 267(24):7102–8. doi: 10.1046/j.1432-1327.2000.01813.x
72. Fulop AK, Foldes A, Buzas E, Hegyi K, Miklos IH, Romics L, et al. Hyperleptinemia, visceral adiposity, and decreased glucose tolerance in mice with a targeted disruption of the histidine decarboxylase gene. Endocrinology (2003) 144(10):4306–14. doi: 10.1210/en.2003-0222
73. Jorgensen EA, Vogelsang TW, Knigge U, Watanabe T, Warberg J, Kjaer A. Increased susceptibility to diet-induced obesity in histamine-deficient mice. Neuroendocrinology (2006) 83(5-6):289–94. doi: 10.1159/000095339
74. Kennedy L, Hargrove L, Demieville J, Bailey JM, Dar W, Polireddy K, et al. Knockout of l-histidine decarboxylase prevents cholangiocyte damage and hepatic fibrosis in mice subjected to high-fat diet feeding via disrupted Histamine/Leptin signaling. Am J Pathol (2018) 188(3):600–15. doi: 10.1016/j.ajpath.2017.11.016
75. Dere E, De Souza-Silva MA, Spieler RE, Lin JS, Ohtsu H, Haas HL, et al. Changes in motoric, exploratory and emotional behaviours and neuronal acetylcholine content and 5-HT turnover in histidine decarboxylase-KO mice. Eur J Neurosci (2004) 20(4):1051–8. doi: 10.1111/j.1460-9568.2004.03546.x
76. Kubota Y, Ito C, Sakurai E, Sakurai E, Watanabe T, Ohtsu H. Increased methamphetamine-induced locomotor activity and behavioral sensitization in histamine-deficient mice. J Neurochem (2002) 83(4):837–45. doi: 10.1046/j.1471-4159.2002.01189.x
77. Acevedo SF, Ohtsu H, Benice TS, Rizk-Jackson A, Raber J. Age-dependent measures of anxiety and cognition in male histidine decarboxylase knockout (Hdc-/-) mice. Brain Res (2006) 1071(1):113–23. doi: 10.1016/j.brainres.2005.11.067
78. Ookuma K, Sakata T, Fukagawa K, Yoshimatsu H, Kurokawa M, Machidori H, et al. Neuronal histamine in the hypothalamus suppresses food intake in rats. Brain Res (1993) 628(1-2):235–42. doi: 10.1016/0006-8993(93)90960-u
79. Morimoto T, Yamamoto Y, Mobarakeh JI, Yanai K, Watanabe T, Watanabe T, et al. Involvement of the histaminergic system in leptin-induced suppression of food intake. Physiol Behav (1999) 67(5):679–83. doi: 10.1016/s0031-9384(99)00123-7
80. Toftegaard CL, Knigge U, Kjaer A, Warberg J. The role of hypothalamic histamine in leptin-induced suppression of short-term food intake in fasted rats. Regul Peptides (2003) 111(1-3):83–90. doi: 10.1016/s0167-0115(02)00260-4
81. Yoshimoto R, Miyamoto Y, Shimamura K, Ishihara A, Takahashi K, Kotani H, et al. Therapeutic potential of histamine H3 receptor agonist for the treatment of obesity and diabetes mellitus. Proc Natl Acad Sci USA (2006) 103(37):13866–71. doi: 10.1073/pnas.0506104103
82. Sakata T, Fukagawa K, Ookuma K, Fujimoto K, Yoshimatsu H, Yamatodani A, et al. Hypothalamic neuronal histamine modulates ad libitum feeding by rats. Brain Res (1990) 537(1-2):303–6. doi: 10.1016/0006-8993(90)90373-j
83. Fukagawa K, Sakata T, Shiraishi T, Yoshimatsu H, Fujimoto K, Ookuma K, et al. Neuronal histamine modulates feeding behavior through H1-receptor in rat hypothalamus. Am J Physiol (1989) 256(3 Pt 2):R605–11. doi: 10.1152/ajpregu.1989.256.3.R605
84. Lecklin A, Tuomisto L. The blockade of H1 receptors attenuates the suppression of feeding and diuresis induced by inhibition of histamine catabolism. Pharmacol Biochem Behav (1998) 59(3):753–8. doi: 10.1016/s0091-3057(97)00465-6
85. Orthen-Gambill N. Antihistaminic drugs increase feeding, while histidine suppresses feeding in rats. Pharmacol Biochem Behav (1988) 31(1):81–6. doi: 10.1016/0091-3057(88)90315-2
86. Comer SD, Haney M, Fischman MW, Foltin RW. Cyproheptadine produced modest increases in total caloric intake by humans. Physiol Behav (1997) 62(4):831–9. doi: 10.1016/s0031-9384(97)00246-1
87. Greaves MW. Antihistamines. Dermatol Clin (2001) 19(1):53–62. doi: 10.1016/S0733-8635(05)70229-1
88. Leurs R, Church MK, Taglialatela M. H1-antihistamines: inverse agonism, anti-inflammatory actions and cardiac effects. Clin Exp Allergy (2002) 32(4):489–98. doi: 10.1046/j.0954-7894.2002.01314.x
89. Inoue I, Yanai K, Kitamura D, Taniuchi I, Kobayashi T, Niimura K, et al. Impaired locomotor activity and exploratory behavior in mice lacking histamine H1 receptors. Proc Natl Acad Sci USA (1996) 93(23):13316–20. doi: 10.1073/pnas.93.23.13316
90. Masaki T, Yoshimatsu H, Chiba S, Watanabe T, Sakata T. Central infusion of histamine reduces fat accumulation and upregulates UCP family in leptin-resistant obese mice. Diabetes (2001) 50(2):376–84. doi: 10.2337/diabetes.50.2.376
91. Masaki T, Chiba S, Yasuda T, Noguchi H, Kakuma T, Watanabe T, et al. Involvement of hypothalamic histamine H1 receptor in the regulation of feeding rhythm and obesity. Diabetes (2004) 53(9):2250–60. doi: 10.2337/diabetes.53.9.2250
92. Masaki T, Yoshimatsu H, Chiba S, Watanabe T, Sakata T. Targeted disruption of histamine H1-receptor attenuates regulatory effects of leptin on feeding, adiposity, and UCP family in mice. Diabetes (2001) 50(2):385–91. doi: 10.2337/diabetes.50.2.385
93. Wang KY, Tanimoto A, Yamada S, Guo X, Ding Y, Watanabe T, et al. Histamine regulation in glucose and lipid metabolism via histamine receptors: model for nonalcoholic steatohepatitis in mice. Am J Pathol (2010) 177(2):713–23. doi: 10.2353/ajpath.2010.091198
94. Gotoh K, Masaki T, Chiba S, Higuchi K, Kakuma T, Shimizu H, et al. Hypothalamic neuronal histamine signaling in the estrogen deficiency-induced obesity. J Neurochem (2009) 110(6):1796–805. doi: 10.1111/j.1471-4159.2009.06272.x
95. Gotoh K, Fukagawa K, Fukagawa T, Noguchi H, Kakuma T, Sakata T, et al. Hypothalamic neuronal histamine mediates the thyrotropin-releasing hormone-induced suppression of food intake. J Neurochem (2007) 103(3):1102–10. doi: 10.1111/j.1471-4159.2007.04802.x
96. Gotoh K, Masaki T, Chiba S, Ando H, Shimasaki T, Mitsutomi K, et al. Nesfatin-1, corticotropin-releasing hormone, thyrotropin-releasing hormone, and neuronal histamine interact in the hypothalamus to regulate feeding behavior. J Neurochem (2013) 124(1):90–9. doi: 10.1111/jnc.12066
97. Ohinata K, Shimano T, Yamauchi R, Sakurada S, Yanai K, Yoshikawa M. The anorectic effect of neurotensin is mediated via a histamine H1 receptor in mice. Peptides (2004) 25(12):2135–8. doi: 10.1016/j.peptides.2004.07.009
98. Sakata T, Ookuma K, Fujimoto K, Fukagawa K, Yoshimatsu H. Histaminergic control of energy balance in rats. Brain Res Bull (1991) 27(3-4):371–5. doi: 10.1016/0361-9230(91)90127-6
99. Itowi N, Nagai K, Nakagawa H, Watanabe T, Wada H. Changes in the feeding behavior of rats elicited by histamine infusion. Physiol Behav (1988) 44(2):221–6. doi: 10.1016/0031-9384(88)90142-4
100. Doi T, Sakata T, Yoshimatsu H, Machidori H, Kurokawa M, Jayasekara LA, et al. Hypothalamic neuronal histamine regulates feeding circadian rhythm in rats. Brain Res (1994) 641(2):311–8. doi: 10.1016/0006-8993(94)90160-0
101. Stoa-Birketvedt G, Waldum HL, Vonen B, Florholmen J. Effect of cimetidine on basal and postprandial plasma concentrations of cholecystokinin and gastrin in humans. Acta Physiol Scand (1997) 159(4):321–5. doi: 10.1046/j.1365-201X.1997.00122.x
102. Stoa-Birketvedt G, Lovhaug N, Vonen B, Florholmen J. H2-receptor antagonist reduces food intake and weight gain in rats by non-gastric acid secretory mechanisms. Acta Physiol Scand (1997) 161(4):489–94. doi: 10.1046/j.1365-201X.1997.00249.x
103. Stoa-Birketvedt G. Effect of cimetidine suspension on appetite and weight in overweight subjects. BMJ (1993) 306(6885):1091–3. doi: 10.1136/bmj.306.6885.1091
104. Ruat M, Traiffort E, Bouthenet ML, Schwartz JC, Hirschfeld J, Buschauer A, et al. Reversible and irreversible labeling and autoradiographic localization of the cerebral histamine H2 receptor using [125I]iodinated probes. Proc Natl Acad Sci USA (1990) 87(5):1658–62. doi: 10.1073/pnas.87.5.1658
105. Clapp RH, Luckman SM. Proxyfan acts as a neutral antagonist of histamine H3 receptors in the feeding-related hypothalamic ventromedial nucleus. Br J Pharmacol (2012) 167(5):1099–110. doi: 10.1111/j.1476-5381.2012.02056.x
106. Chiba S, Itateyama E, Sakata T, Yoshimatsu H. Acute central administration of immepip, a histamine H3 receptor agonist, suppresses hypothalamic histamine release and elicits feeding behavior in rats. Brain Res Bull (2009) 79(1):37–40. doi: 10.1016/j.brainresbull.2008.12.012
107. Jethwa PH, Barrett P, Turnbull Y, Enright RA, Warner A, Murphy M, et al. The role of histamine 3 receptors in the control of food intake in a seasonal model of obesity: the Siberian hamster. Behav Pharmacol (2009) 20(2):155–65. doi: 10.1097/FBP.0b013e32832a8099
108. Malmlof K, Zaragoza F, Golozoubova V, Refsgaard HH, Cremers T, Raun K, et al. Influence of a selective histamine H3 receptor antagonist on hypothalamic neural activity, food intake and body weight. Int J Obes (Lond) (2005) 29(12):1402–12. doi: 10.1038/sj.ijo.0803036
109. Kotanska M, Mika K, Regula K, Szczepanska K, Szafarz M, Bednarski M, et al. KSK19 - novel histamine H3 receptor ligand reduces body weight in diet induced obese mice. Biochem Pharmacol (2019) 168:193–203. doi: 10.1016/j.bcp.2019.07.006
110. Kotanska M, Kuder KJ, Szczepanska K, Sapa J, Kiec-Kononowicz K. The histamine H3 receptor inverse agonist pitolisant reduces body weight in obese mice. Naunyn-Schmiedeberg's Arch Pharmacol (2018) 391(8):875–81. doi: 10.1007/s00210-018-1516-2
111. Hancock AA, Bennani YL, Bush EN, Esbenshade TA, Faghih R, Fox GB, et al. Antiobesity effects of a-331440, a novel non-imidazole histamine H3 receptor antagonist. Eur J Pharmacol (2004) 487(1-3):183–97. doi: 10.1016/j.ejphar.2004.01.015
112. Hancock AA, Diehl MS, Fey TA, Bush EN, Faghih R, Miller TR, et al. Antiobesity evaluation of histamine H3 receptor (H3R) antagonist analogs of a-331440 with improved safety and efficacy. Inflamm Res (2005) 54 (Suppl 1):S27–9. doi: 10.1007/s00011-004-0412-z
113. Itoh E, Fujimiya M, Inui A. Thioperamide, a histamine H3 receptor antagonist, suppresses NPY-but not dynorphin a-induced feeding in rats. Regul Peptides (1998) 75-76:373–6. doi: 10.1016/s0167-0115(98)00090-1
114. Malmlof K, Hastrup S, Wulff BS, Hansen BC, Peschke B, Jeppesen CB, et al. Antagonistic targeting of the histamine H3 receptor decreases caloric intake in higher mammalian species. Biochem Pharmacol (2007) 73(8):1237–42. doi: 10.1016/j.bcp.2007.01.034
115. Schneider EH, Neumann D, Seifert R. Modulation of behavior by the histaminergic system: lessons from HDC-, H3R- and H4R-deficient mice. Neurosci Biobehav Rev (2014) 47:101–21. doi: 10.1016/j.neubiorev.2014.07.020
116. Leurs R, Bakker RA, Timmerman H, de Esch IJ. The histamine H3 receptor: from gene cloning to H3 receptor drugs. Nat Rev Drug Discovery (2005) 4(2):107–20. doi: 10.1038/nrd1631
117. Schlicker E, Betz R, Gothert M. Histamine H3 receptor-mediated inhibition of serotonin release in the rat brain cortex. Naunyn-Schmiedeberg's Arch Pharmacol (1988) 337(5):588–90. doi: 10.1007/BF00182737
118. Smits RP, Mulder AH. Inhibitory effects of histamine on the release of serotonin and noradrenaline from rat brain slices. Neurochem Int (1991) 18(2):215–20. doi: 10.1016/0197-0186(91)90188-j
119. Schlicker E, Fink K, Detzner M, Gothert M. Histamine inhibits dopamine release in the mouse striatum via presynaptic H3 receptors. J Neural Transm Gen Sect (1993) 93(1):1–10. doi: 10.1007/BF01244933
120. Medhurst AD, Atkins AR, Beresford IJ, Brackenborough K, Briggs MA, Calver AR, et al. GSK189254, a novel H3 receptor antagonist that binds to histamine H3 receptors in alzheimer's disease brain and improves cognitive performance in preclinical models. J Pharmacol Exp Ther (2007) 321(3):1032–45. doi: 10.1124/jpet.107.120311
121. Schlicker E, Fink K, Hinterthaner M, Gothert M. Inhibition of noradrenaline release in the rat brain cortex via presynaptic H3 receptors. Naunyn-Schmiedeberg's Arch Pharmacol (1989) 340(6):633–8. doi: 10.1007/BF00717738
122. Ichinose M, Barnes PJ. Inhibitory histamine H3-receptors on cholinergic nerves in human airways. Eur J Pharmacol (1989) 163(2-3):383–6. doi: 10.1016/0014-2999(89)90212-4
123. Arrang JM, Drutel G, Schwartz JC. Characterization of histamine H3 receptors regulating acetylcholine release in rat entorhinal cortex. Br J Pharmacol (1995) 114(7):1518–22. doi: 10.1111/j.1476-5381.1995.tb13379.x
124. Garcia M, Floran B, Arias-Montano JA, Young JM, Aceves J. Histamine H3 receptor activation selectively inhibits dopamine D1 receptor-dependent [3H]GABA release from depolarization-stimulated slices of rat substantia nigra pars reticulata. Neuroscience (1997) 80(1):241–9. doi: 10.1016/s0306-4522(97)00100-0
125. Jang IS, Rhee JS, Watanabe T, Akaike N, Akaike N. Histaminergic modulation of GABAergic transmission in rat ventromedial hypothalamic neurones. J Physiol (2001) 534(Pt 3):791–803. doi: 10.1111/j.1469-7793.2001.00791.x
126. Barrett P, van den Top M, Wilson D, Mercer JG, Song CK, Bartness TJ, et al. Short photoperiod-induced decrease of histamine H3 receptors facilitates activation of hypothalamic neurons in the Siberian hamster. Endocrinology (2009) 150(8):3655–63. doi: 10.1210/en.2008-1620
127. Takahashi K, Suwa H, Ishikawa T, Kotani H. Targeted disruption of H3 receptors results in changes in brain histamine tone leading to an obese phenotype. J Clin Invest (2002) 110(12):1791–9. doi: 10.1172/JCI15784
128. Toyota H, Dugovic C, Koehl M, Laposky AD, Weber C, Ngo K, et al. Behavioral characterization of mice lacking histamine H(3) receptors. Mol Pharmacol (2002) 62(2):389–97. doi: 10.1124/mol.62.2.389
129. Nuutinen S, Lintunen M, Vanhanen J, Ojala T, Rozov S, Panula P. Evidence for the role of histamine H3 receptor in alcohol consumption and alcohol reward in mice. Neuropsychopharmacol Off Publ Am Coll Neuropsychopharmacol (2011) 36(10):2030–40. doi: 10.1038/npp.2011.90
130. Campfield LA, Smith FJ, Guisez Y, Devos R, Burn P. Recombinant mouse OB protein: evidence for a peripheral signal linking adiposity and central neural networks. Science (1995) 269(5223):546–9. doi: 10.1126/science.7624778
131. Frederich RC, Hamann A, Anderson S, Lollmann B, Lowell BB, Flier JS. Leptin levels reflect body lipid content in mice: evidence for diet-induced resistance to leptin action. Nat Med (1995) 1(12):1311–4. doi: 10.1038/nm1295-1311
132. Ahima RS, Prabakaran D, Mantzoros C, Qu D, Lowell B, Maratos-Flier E, et al. Role of leptin in the neuroendocrine response to fasting. Nature (1996) 382(6588):250–2. doi: 10.1038/382250a0
133. Yoshimatsu H, Itateyama E, Kondou S, Tajima D, Himeno K, Hidaka S, et al. Hypothalamic neuronal histamine as a target of leptin in feeding behavior. Diabetes (1999) 48(12):2286–91. doi: 10.2337/diabetes.48.12.2286
134. Hegyi K, Fulop KA, Kovacs KJ, Falus A, Toth S. High leptin level is accompanied with decreased long leptin receptor transcript in histamine deficient transgenic mice. Immunol Lett (2004) 92(1-2):193–7. doi: 10.1016/j.imlet.2003.11.029
135. Elmquist JK, Bjorbaek C, Ahima RS, Flier JS, Saper CB. Distributions of leptin receptor mRNA isoforms in the rat brain. J Comp Neurol (1998) 395(4):535–47. doi: 10.1002/(SICI)1096-9861(19980615)395:4<535::AID-CNE9>3.0.CO;2-2
136. Cui H, Sohn JW, Gautron L, Funahashi H, Williams KW, Elmquist JK, et al. Neuroanatomy of melanocortin-4 receptor pathway in the lateral hypothalamic area. J Comp Neurol (2012) 520(18):4168–83. doi: 10.1002/cne.23145
137. Mickelsen LE, Kolling FW, Chimileski BR, Fujita A, Norris C, Chen K, et al. Neurochemical heterogeneity among lateral hypothalamic Hypocretin/Orexin and melanin-concentrating hormone neurons identified through single-cell gene expression analysis. eNeuro (2017) 4(5):1–24. doi: 10.1523/ENEURO.0013-17.2017
138. Leinninger GM, Jo YH, Leshan RL, Louis GW, Yang H, Barrera JG, et al. Leptin acts via leptin receptor-expressing lateral hypothalamic neurons to modulate the mesolimbic dopamine system and suppress feeding. Cell Metab (2009) 10(2):89–98. doi: 10.1016/j.cmet.2009.06.011
139. Venner A, De Luca R, Sohn LT, Bandaru SS, Verstegen AMJ, Arrigoni E, et al. An inhibitory lateral hypothalamic-preoptic circuit mediates rapid arousals from sleep. Curr Biol CB (2019) 29(24):4155–68 e5. doi: 10.1016/j.cub.2019.10.026
140. Bonnavion P, Mickelsen LE, Fujita A, de Lecea L, Jackson AC. Hubs and spokes of the lateral hypothalamus: cell types, circuits and behaviour. J Physiol (2016) 594(22):6443–62. doi: 10.1113/JP271946
141. Cone RD. Anatomy and regulation of the central melanocortin system. Nat Neurosci (2005) 8(5):571–8. doi: 10.1038/nn1455
142. Schwartz MW, Woods SC, Porte D, Seeley RJ, Baskin DG. Central nervous system control of food intake. Nature (2000) 404(6778):661–71. doi: 10.1038/35007534
143. Millington GW. The role of proopiomelanocortin (POMC) neurones in feeding behaviour. Nutr Metab (2007) 4:18. doi: 10.1186/1743-7075-4-18
144. Belgardt BF, Okamura T, Bruning JC. Hormone and glucose signalling in POMC and AgRP neurons. J Physiol (2009) 587(Pt 22):5305–14. doi: 10.1113/jphysiol.2009.179192
145. Ellacott KL, Cone RD. The role of the central melanocortin system in the regulation of food intake and energy homeostasis: lessons from mouse models. Philos Trans R Soc Lond B Biol Sci (2006) 361(1471):1265–74. doi: 10.1098/rstb.2006.1861
146. Mountjoy KG. Pro-opiomelanocortin (POMC) neurones, POMC-derived peptides, melanocortin receptors and obesity: how understanding of this system has changed over the last decade. J Neuroendocrinol (2015) 27(6):406–18. doi: 10.1111/jne.12285
147. Michael NJ, Caron A, Castorena CM, Lee CE, Lee S, Zigman JM, et al. Melanocortin regulation of histaminergic neurons via perifornical lateral hypothalamic melanocortin 4 receptors. Mol Metab (2020) 35:100956. doi: 10.1016/j.molmet.2020.01.020
148. Zhu H, Aryal DK, Olsen RH, Urban DJ, Swearingen A, Forbes S, et al. Cre-dependent DREADD (Designer receptors exclusively activated by designer drugs) mice. Genesis (2016) 54(8):439–46. doi: 10.1002/dvg.22949
149. Roth BL. DREADDs for neuroscientists. Neuron (2016) 89(4):683–94. doi: 10.1016/j.neuron.2016.01.040
150. Armbruster BN, Li X, Pausch MH, Herlitze S, Roth BL. Evolving the lock to fit the key to create a family of G protein-coupled receptors potently activated by an inert ligand. Proc Natl Acad Sci United States America (2007) 104(12):5163–8. doi: 10.1073/pnas.0700293104
151. Nakazato M, Murakami N, Date Y, Kojima M, Matsuo H, Kangawa K, et al. A role for ghrelin in the central regulation of feeding. Nature (2001) 409(6817):194–8. doi: 10.1038/35051587
152. Zigman JM, Jones JE, Lee CE, Saper CB, Elmquist JK. Expression of ghrelin receptor mRNA in the rat and the mouse brain. J Comp Neurol (2006) 494(3):528–48. doi: 10.1002/cne.20823
153. Campbell JN, Macosko EZ, Fenselau H, Pers TH, Lyubetskaya A, Tenen D, et al. A molecular census of arcuate hypothalamus and median eminence cell types. Nat Neurosci (2017) 20(3):484–96. doi: 10.1038/nn.4495
154. Gotoh K, Fukagawa K, Fukagawa T, Noguchi H, Kakuma T, Sakata T, et al. Glucagon-like peptide-1, corticotropin-releasing hormone, and hypothalamic neuronal histamine interact in the leptin-signaling pathway to regulate feeding behavior. FASEB J Off Publ Fed Am Societies Exp Biol (2005) 19(9):1131–3. doi: 10.1096/fj.04-2384fje
155. Ten Kulve JS, van Bloemendaal L, Balesar R, RG IJ, Swaab DF, Diamant M, et al. Decreased hypothalamic glucagon-like peptide-1 receptor expression in type 2 diabetes patients. J Clin Endocrinol Metab (2016) 101(5):2122–9. doi: 10.1210/jc.2015-3291
156. Jensen CB, Pyke C, Rasch MG, Dahl AB, Knudsen LB, Secher A. Characterization of the glucagonlike peptide-1 receptor in Male mouse brain using a novel antibody and In situ hybridization. Endocrinology (2018) 159(2):665–75. doi: 10.1210/en.2017-00812
157. Gu G, Roland B, Tomaselli K, Dolman CS, Lowe C, Heilig JS. Glucagon-like peptide-1 in the rat brain: distribution of expression and functional implication. J Comp Neurol (2013) 521(10):2235–61. doi: 10.1002/cne.23282
158. Salinas CBG, Lu TT, Gabery S, Marstal K, Alanentalo T, Mercer AJ, et al. Integrated brain atlas for unbiased mapping of nervous system effects following liraglutide treatment. Sci Rep (2018) 8(1):10310. doi: 10.1038/s41598-018-28496-6
159. Lopez-Ferreras L, Richard JE, Noble EE, Eerola K, Anderberg RH, Olandersson K, et al. Lateral hypothalamic GLP-1 receptors are critical for the control of food reinforcement, ingestive behavior and body weight. Mol Psychiatry (2018) 23(5):1157–68. doi: 10.1038/mp.2017.187
160. Miklos IH, Kovacs KJ. Functional heterogeneity of the responses of histaminergic neuron subpopulations to various stress challenges. Eur J Neurosci (2003) 18(11):3069–79. doi: 10.1111/j.1460-9568.2003.03033.x
161. Sakurai T, Amemiya A, Ishii M, Matsuzaki I, Chemelli RM, Tanaka H, et al. Orexins and orexin receptors: a family of hypothalamic neuropeptides and G protein-coupled receptors that regulate feeding behavior. Cell (1998) 92(4):573–85. doi: 10.1016/s0092-8674(00)80949-6
162. de Lecea L, Kilduff TS, Peyron C, Gao X, Foye PE, Danielson PE, et al. The hypocretins: hypothalamus-specific peptides with neuroexcitatory activity. PNAS (1998) 95(1):322–7. doi: 10.1073/pnas.95.1.322
163. Mickelsen LE, Flynn WF, Springer K, Wilson L, Beltrami EJ, Bolisetty M, et al. Cellular taxonomy and spatial organization of the murine ventral posterior hypothalamus. eLife (2020) 9:e58901. doi: 10.7554/eLife.58901
164. Eriksson KS, Sergeeva O, Brown RE, Haas HL. Orexin/hypocretin excites the histaminergic neurons of the tuberomammillary nucleus. J Neurosci Off J Soc Neurosci (2001) 21(23):9273–9. doi: 10.1523/JNEUROSCI.21-23-09273.2001
165. Mochizuki T, Arrigoni E, Marcus JN, Clark EL, Yamamoto M, Honer M, et al. Orexin receptor 2 expression in the posterior hypothalamus rescues sleepiness in narcoleptic mice. Proc Natl Acad Sci USA (2000) 108(11):4471–6. doi: 10.1073/pnas.1012456108
166. Huang ZL, Qu WM, Li WD, Mochizuki T, Eguchi N, Watanabe T, et al. Arousal effect of orexin a depends on activation of the histaminergic system. Proc Natl Acad Sci United States America (2001) 98(17):9965–70. doi: 10.1073/pnas.181330998
167. Schone C, Apergis-Schoute J, Sakurai T, Adamantidis A, Burdakov D. Coreleased orexin and glutamate evoke nonredundant spike outputs and computations in histamine neurons. Cell Rep (2014) 7(3):697–704. doi: 10.1016/j.celrep.2014.03.055
168. Wetterling T. Bodyweight gain with atypical antipsychotics. a comparative review. Drug Saf (2001) 24(1):59–73. doi: 10.2165/00002018-200124010-00005
169. Reynolds GP, Kirk SL. Metabolic side effects of antipsychotic drug treatment–pharmacological mechanisms. Pharmacol Ther (2010) 125(1):169–79. doi: 10.1016/j.pharmthera.2009.10.010
170. Kroeze WK, Hufeisen SJ, Popadak BA, Renock SM, Steinberg S, Ernsberger P, et al. H1-histamine receptor affinity predicts short-term weight gain for typical and atypical antipsychotic drugs. Neuropsychopharmacol Off Publ Am Coll Neuropsychopharmacol (2003) 28(3):519–26. doi: 10.1038/sj.npp.1300027
171. Kim SF, Huang AS, Snowman AM, Teuscher C, Snyder SH. Antipsychotic drug-induced weight gain mediated by histamine H1 receptor-linked activation of hypothalamic AMP-kinase. Proc Natl Acad Sci United States America (2007) 104(9):3456–9. doi: 10.1073/pnas.0611417104
172. Wirshing DA, Wirshing WC, Kysar L, Berisford MA, Goldstein D, Pashdag J, et al. Novel antipsychotics: comparison of weight gain liabilities. J Clin Psychiatry (1999) 60(6):358–63. doi: 10.4088/JCP.v60n0602
173. Humbert-Claude M, Davenas E, Gbahou F, Vincent L, Arrang JM. Involvement of histamine receptors in the atypical antipsychotic profile of clozapine: a reassessment in vitro and in vivo. Psychopharmacology (2012) 220(1):225–41. doi: 10.1007/s00213-011-2471-5
174. Gothelf D, Falk B, Singer P, Kairi M, Phillip M, Zigel L, et al. Weight gain associated with increased food intake and low habitual activity levels in male adolescent schizophrenic inpatients treated with olanzapine. Am J Psychiatry (2002) 159(6):1055–7. doi: 10.1176/appi.ajp.159.6.1055
175. Fountaine RJ, Taylor AE, Mancuso JP, Greenway FL, Byerley LO, Smith SR, et al. Increased food intake and energy expenditure following administration of olanzapine to healthy men. Obes (Silver Spring) (2010) 18(8):1646–51. doi: 10.1038/oby.2010.6
176. Davoodi N, Kalinichev M, Korneev SA, Clifton PG. Hyperphagia and increased meal size are responsible for weight gain in rats treated sub-chronically with olanzapine. Psychopharmacol (Berl) (2009) 203(4):693–702. doi: 10.1007/s00213-008-1415-1
177. Coccurello R, Caprioli A, Ghirardi O, Conti R, Ciani B, Daniele S, et al. Chronic administration of olanzapine induces metabolic and food intake alterations: a mouse model of the atypical antipsychotic-associated adverse effects. Psychopharmacol (Berl) (2006) 186(4):561–71. doi: 10.1007/s00213-006-0368-5
178. Han M, Deng C, Burne TH, Newell KA, Huang XF. Short- and long-term effects of antipsychotic drug treatment on weight gain and H1 receptor expression. Psychoneuroendocrinology (2008) 33(5):569–80. doi: 10.1016/j.psyneuen.2008.01.018
179. Chen X, Yu Y, Zheng P, Jin T, He M, Zheng M, et al. Olanzapine increases AMPK-NPY orexigenic signaling by disrupting H1R-GHSR1a interaction in the hypothalamic neurons of mice. Psychoneuroendocrinology (2020) 114:104594. doi: 10.1016/j.psyneuen.2020.104594
180. Poyurovsky M, Fuchs C, Pashinian A, Levi A, Weizman R, Weizman A. Reducing antipsychotic-induced weight gain in schizophrenia: a double-blind placebo-controlled study of reboxetine-betahistine combination. Psychopharmacol (Berl) (2013) 226(3):615–22. doi: 10.1007/s00213-012-2935-2
181. Esbenshade TA, Fox GB, Cowart MD. Histamine H3 receptor antagonists: preclinical promise for treating obesity and cognitive disorders. Mol Interv (2006) 6(2):77–88, 59. doi: 10.1124/mi.6.2.5
182. Hancock AA, Brune ME. Assessment of pharmacology and potential anti-obesity properties of H3 receptor antagonists/inverse agonists. Expert Opin Investig Drugs (2005) 14(3):223–41. doi: 10.1517/13543784.14.3.223
183. Celanire S, Wijtmans M, Talaga P, Leurs R, de Esch IJ. Keynote review: histamine H3 receptor antagonists reach out for the clinic. Drug Discov Today (2005) 10(23-24):1613–27. doi: 10.1016/S1359-6446(05)03625-1
184. Hancock AA, Diehl MS, Faghih R, Bush EN, Krueger KM, Krishna G, et al. In vitro optimization of structure activity relationships of analogues of a-331440 combining radioligand receptor binding assays and micronucleus assays of potential antiobesity histamine H3 receptor antagonists. Basic Clin Pharmacol Toxicol (2004) 95(3):144–52. doi: 10.1111/j.1742-7843.2004.950307.x
185. Barbier AJ, Berridge C, Dugovic C, Laposky AD, Wilson SJ, Boggs J, et al. Acute wake-promoting actions of JNJ-5207852, a novel, diamine-based H3 antagonist. Br J Pharmacol (2004) 143(5):649–61. doi: 10.1038/sj.bjp.0705964
186. Barak N, Greenway FL, Fujioka K, Aronne LJ, Kushner RF. Effect of histaminergic manipulation on weight in obese adults: a randomized placebo controlled trial. Int J Obes (Lond) (2008) 32(10):1559–65. doi: 10.1038/ijo.2008.135
187. Ali AH, Yanoff LB, Stern EA, Akomeah A, Courville A, Kozlosky M, et al. Acute effects of betahistine hydrochloride on food intake and appetite in obese women: a randomized, placebo-controlled trial. Am J Clin Nutr (2010) 92(6):1290–7. doi: 10.3945/ajcn.110.001586
188. Ligneau X, Morisset S, Tardivel-Lacombe J, Gbahou F, Ganellin CR, Stark H, et al. Distinct pharmacology of rat and human histamine H(3) receptors: role of two amino acids in the third transmembrane domain. Br J Pharmacol (2000) 131(7):1247–50. doi: 10.1038/sj.bjp.0703712
189. Provensi G, Blandina P, Passani MB. The histaminergic system as a target for the prevention of obesity and metabolic syndrome. Neuropharmacology (2016) 106:3–12. doi: 10.1016/j.neuropharm.2015.07.002
190. Szczepanska K, Pockes S, Podlewska S, Horing C, Mika K, Latacz G, et al. Structural modifications in the distal, regulatory region of histamine H(3) receptor antagonists leading to the identification of a potent anti-obesity agent. Eur J Med Chem (2021) 213:113041. doi: 10.1016/j.ejmech.2020.113041
191. Kumar A, Pasam VR, Thakur RK, Singh M, Singh K, Shukla M, et al. Novel tetrahydroquinazolinamines as selective histamine 3 receptor antagonists for the treatment of obesity. J Med Chem (2019) 62(9):4638–55. doi: 10.1021/acs.jmedchem.9b00241
192. Vanni-Mercier G, Sakai K, Jouvet M. Neurons specifiques de l'eveil dans l'hypothalamus posterieur du chat. C R Acad Sci III (1984) 298(7):195–200.
193. Mochizuki T, Yamatodani A, Okakura K, Horii A, Inagaki N, Wada H. Circadian rhythm of histamine release from the hypothalamus of freely moving rats. Physiol Behav (1992) 51(2):391–4. doi: 10.1016/0031-9384(92)90157-w
194. Tuomisto L, Tuomisto J. Diurnal variations in brain and pituitary histamine and histamine-n-methyltransferase in the rat and guinea pig. Med Biol (1982) 60(4):204–9.
195. Strecker RE, Nalwalk J, Dauphin LJ, Thakkar MM, Chen Y, Ramesh V, et al. Extracellular histamine levels in the feline preoptic/anterior hypothalamic area during natural sleep-wakefulness and prolonged wakefulness: an in vivo microdialysis study. Neuroscience (2002) 113(3):663–70. doi: 10.1016/s0306-4522(02)00158-6
196. Dong H, Li M, Yan Y, Qian T, Lin Y, Ma X, et al. Genetically encoded sensors for measuring histamine release both in vitro and in vivo. Neuron (2023) 11(10):1564–76.e6. doi: 10.1016/j.neuron.2023.02.024
197. Michael NJ, Zigman JM, Williams KW, Elmquist JK. Electrophysiological properties of genetically identified histaminergic neurons. Neuroscience (2020) 444:183–95. doi: 10.1016/j.neuroscience.2020.06.031
198. Sergeeva OA, Eriksson KS, Sharonova IN, Vorobjev VS, Haas HL. GABA(A) receptor heterogeneity in histaminergic neurons. Eur J Neurosci (2002) 16(8):1472–82. doi: 10.1046/j.1460-9568.2002.02221.x
199. Giannoni P, Passani MB, Nosi D, Chazot PL, Shenton FC, Medhurst AD, et al. Heterogeneity of histaminergic neurons in the tuberomammillary nucleus of the rat. Eur J Neurosci (2009) 29(12):2363–74. doi: 10.1111/j.1460-9568.2009.06765.x
200. Prell GD, Green JP. Histamine as a neuroregulator. Annu Rev Neurosci (1986) 9:209–54. doi: 10.1146/annurev.ne.09.030186.001233
201. Takagi H, Morishima Y, Matsuyama T, Hayashi H, Watanabe T, Wada H. Histaminergic axons in the neostriatum and cerebral cortex of the rat: a correlated light and electron microscopic immunocytochemical study using histidine decarboxylase as a marker. Brain Res (1986) 364(1):114–23. doi: 10.1016/0006-8993(86)90992-3
202. Tohyama M, Tamiya R, Inagaki N, Tagaki H. Morphology of histaminergic neurons with histidine decarboxy-lase as a marker. In: Watanabe T, Wada H, editors. Histaminergic neurons: morphology and function. Boca Raton, FL: CRC Press (1991). p. 107–26.
Keywords: histamine, food intake, hypothalamus, neurometabolism, melanocortin, leptin, histamine receptors, GPCR
Citation: Khouma A, Moeini MM, Plamondon J, Richard D, Caron A and Michael NJ (2023) Histaminergic regulation of food intake. Front. Endocrinol. 14:1202089. doi: 10.3389/fendo.2023.1202089
Received: 07 April 2023; Accepted: 06 June 2023;
Published: 27 June 2023.
Edited by:
Alexandre Benani, Centre National de la Recherche Scientifique (CNRS), FranceCopyright © 2023 Khouma, Moeini, Plamondon, Richard, Caron and Michael. This is an open-access article distributed under the terms of the Creative Commons Attribution License (CC BY). The use, distribution or reproduction in other forums is permitted, provided the original author(s) and the copyright owner(s) are credited and that the original publication in this journal is cited, in accordance with accepted academic practice. No use, distribution or reproduction is permitted which does not comply with these terms.
*Correspondence: Natalie Jane Michael, bmF0YWxpZS5taWNoYWVsQHBoYS51bGF2YWwuY2E=
†These authors contributed equally to this work and share first authorship