- Section on Molecular Morphogenesis, Eunice Kennedy Shriver National Institute of Child Health and Human Development (NICHD), National Institutes of Health (NIH), Bethesda, MD, United States
Amphibian metamorphosis resembles mammalian postembryonic development, a period around birth when many organs mature into their adult forms and when plasma thyroid hormone (T3) concentration peaks. T3 plays a causative role for amphibian metamorphosis. This and its independence from maternal influence make metamorphosis of amphibians, particularly anurans such as pseudo-tetraploid Xenopus laevis and its highly related diploid species Xenopus tropicalis, an excellent model to investigate how T3 regulates adult organ development. Studies on intestinal remodeling, a process that involves degeneration of larval epithelium via apoptosis and de novo formation of adult stem cells followed by their proliferation and differentiation to form the adult epithelium, have revealed important molecular insights on T3 regulation of cell fate during development. Here, we review some evidence suggesting that T3-induced activation of cell cycle program is important for T3-induced larval epithelial cell death and de novo formation of adult intestinal stem cells.
Introduction
The development of vertebrate intestine, like many other organs, takes place in two phases, the initial formation of a neonatal/juvenile form and subsequent maturation into the adult form. This second phase often occurs during postembryonic development, a perinatal period when plasma thyroid hormone (T3) level peaks (1–3). This period corresponds the first 2-3 weeks after birth in mouse and metamorphosis in amphibians such as the highly related anurans pseudo-tetraploid Xenopus laevis and diploid Xenopus tropicalis (Note that due to the conservations between the two species, we will simply refer to both as Xenopus unless specified, although earlier studies on anuran metamorphosis were mainly on Xenopus laevis while more recent ones, particularly gene knockout studies, have been on Xenopus tropicalis). Importantly, maturation of the intestine during this second phase appears to be highly conserved (4–10). For example, the mouse intestine has villi but no crypts, where adult stem cells reside, at birth and develop crypts during the first 3 weeks after birth when T3 levels are high. Similarly, the intestine in a premetamorphic Xenopus tadpole, when there is little or no T3, is also simple in structure, consisting of mostly a single layer of epithelial cells, surrounded by thin layers of connective tissue and muscles (Figure 1A) (12–17). As T3 levels rises after stage 54 (about 4 weeks of age) (18, 19), metamorphosis begins and larval epithelial cells undergo programmed cell death (12, 13, 20). Some larval epithelial cells undergo dedifferentiation during metamorphosis to form clusters of cells that proliferate rapidly and express well-known adult intestinal stem cell markers such as Lgr5 by climax of metamorphosis, e.g., stage 61 (about 6-7 weeks of age) (Figure 1A) (11, 21, 22). By the end of metamorphosis or stage 66 (about 2 months after fertilization), these proliferating stem cells differentiate to form a multi-folded epithelium surrounded by elaborate connective tissue and muscles (4, 16, 17, 23, 24). In the adult frog, the intestinal stem cells are localized at the bottom of the epithelial fold while cell death occurs mainly at the crest of the fold, similar to those taking place in the crypt-villus unit in adult mammalian intestine (16, 25).
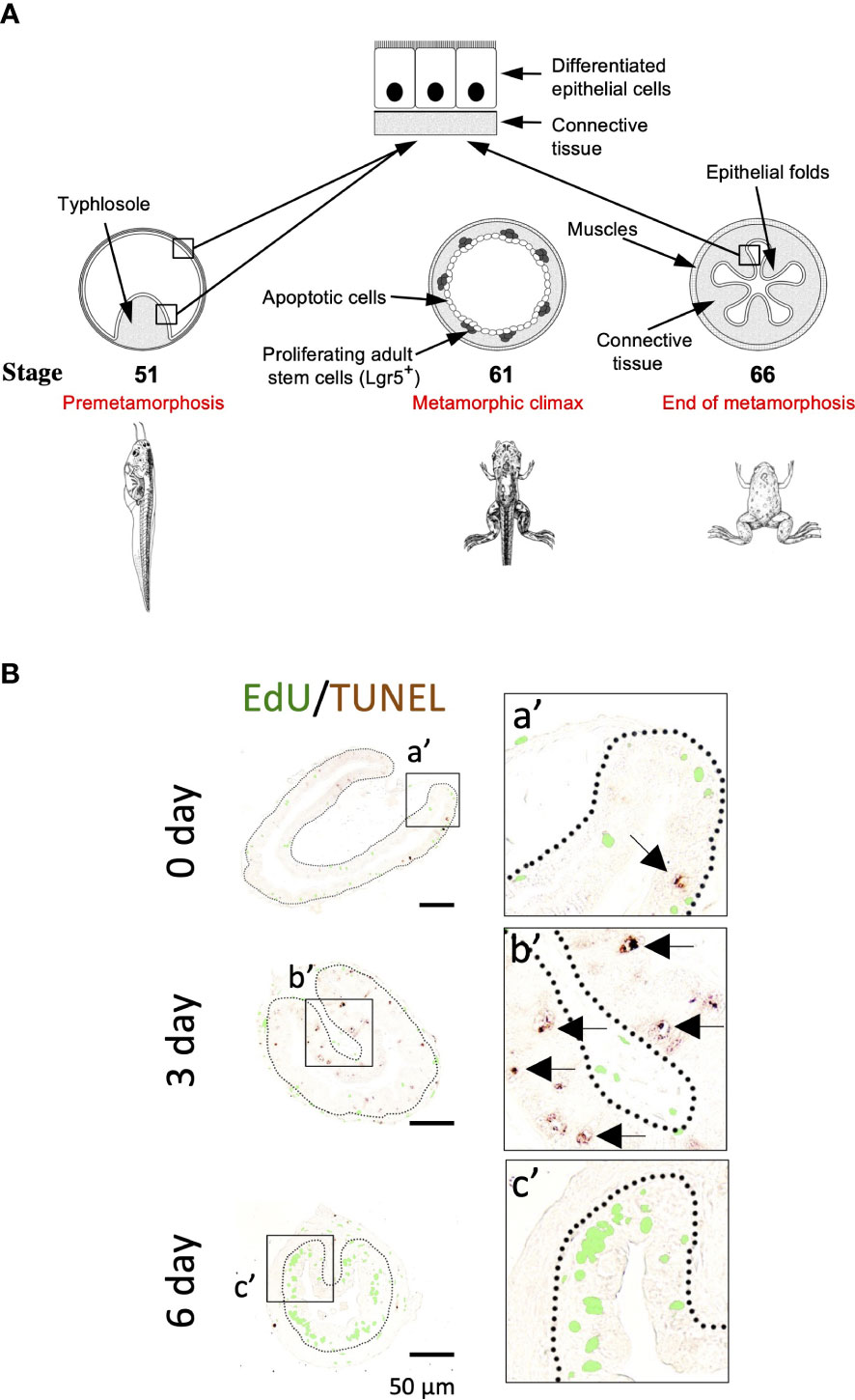
Figure 1 (A). Schematic diagram of Xenopus intestinal metamorphosis. Both tadpole and frog intestine are structurally simple, consisting of mainly three tissue layers: inner epithelium, connective tissue, and outer muscle layers. The tadpole intestine is much simpler, with only a single epithelial fold, the typhlosole. In contrast, the frog intestine has multiple epithelial folds with elaborate connective tissue and muscle layers. The major events underlying the change from tadpole to frog intestine during metamorphosis include the apoptosis of essentially all larval epithelial cells, as indicated by circles. Concurrently, the adult epithelial stem cells, with high level expression of known stem cell markers such as Lgr5, are formed de novo through dedifferentiation of some larval epithelial cells and rapidly proliferate at the climax metamorphosis, as indicated by the dots. The connective tissue and muscle cells also develop extensively during metamorphosis. (B). Apoptotic and proliferating cells are non-overlapping epithelial cells during T3-induced intestinal metamorphosis. Premetamorphic Xenopus laevis tadpoles at stage 54 were treated with 10 nM T3 for 0, 3, or 6 days and sacrificed one hour after injection with EdU to label proliferating cells. Intestinal cross-sections were double stained for EdU and by TUNEL for apoptotic cells. A higher magnification of the boxed areas labeled with a’-c’ is shown on the right. The dotted lines depict the epithelium-mesenchyme boundary. Note that apoptosis as shown by the TUNEL signal in the epithelium occurred prior to the appearance of the clusters (islets) of EdU-labeled cells and in distinct epithelial cells during T3 treatment (c’). Arrows point to some apoptotic cells. See (11) for more details.
T3 not only has peak levels during postembryonic development but also plays critical roles during this period, with T3 deficiency causing severely developmental problems in all vertebrates including human (1, 2, 26, 27). T3 is both necessary and sufficient for anuran metamorphosis. Thus, preventing the synthesis of endogenous T3 allows Xenopus tadpoles to remain in tadpole form for years while wild type animals typically finish metamorphosis by around 2 months of age (1, 2, 15). Conversely, treating premetamorphic Xenopus tadpoles with physiological levels of T3 in the rearing water causes precociously metamorphosis. Making use of the ability to easily manipulate anuran metamorphosis by controlling the availability of T3 to tadpoles or even organ or primary cell cultures and the advancement in genetic technologies, especially gene-editing for knockout studies in the diploid Xenopus tropicalis (28–35), we and others have been studying the molecular mechanism by which T3 regulates cell fate and tissue transformation during metamorphosis. Here, we review some recent studies on intestinal remodeling, with an emphasis on the potential role of cell cycle activation in larval epithelial cell death and adult stem cell development.
T3 induces larval epithelial cell death and adult stem cell development in an organ autonomous manner via T3 receptor
Intestinal remodeling, just like any other events during metamorphosis, requires T3. Treatment of premetamorphic Xenopus tadpoles with T3 leads to premature intestinal metamorphosis (16). The most noticeable changes during intestinal metamorphosis are the nearly 90% reduction in the length of the small intestine, while the most dramatic tissue transformation occurs in the epithelium with the larva epithelial cells induced to undergo apoptosis by T3, followed by rapid proliferation of newly formed cell clusters in the epithelium (Figure 1B) (11). Importantly, these proliferating cells express high levels of known markers of adult mammalian intestinal stem cells such as Lgr5 (Figure 1B) (11), suggesting that T3 induces the formation of adult stem cells during metamorphosis.
Importantly, T3-treatment of intestinal organ cultures from premetamorphic tadpoles also leads to larval epithelial cell death and de novo formation of adult stem cells, indicating that the adult stem cells develop organ-autonomously in an T3-dependent manner. More importantly, by using recombinant organ cultures generated from isolated intestinal epithelium and non-epithelial tissues (the rest of the intestine after separating the epithelium) from premetamorphic wild type tadpoles and transgenic tadpoles expressing GFP ubiquitously, we have shown that T3-induced stem cells originate from larval epithelium, likely due to dedifferentiation of some larval epithelial cells (23) since there has been no evidence of pre-existing epithelial stem cells in the tadpole intestine and that the differentiated larval epithelial cells are capable of proliferation (16, 36). These findings also indicate that T3 is both necessary and sufficient for larval intestinal cell death and adult stem cell development during metamorphosis.
T3 functions mainly by binding to T3 receptors (TRs) to regulate target gene transcription (37–40). There are two types of TR genes, TRα and TRβ, in all vertebrates. TRs can activate or repress target gene transcription in the presence or absence of T3, respectively, by binding, as heterodimers with 9-cis retinoic acid receptors (RXRs), to specific DNA sequences called T3-response elements (TREs) within target genes (41–45). Earlier studies on the expression patterns and molecular properties of TRs in Xenopus laevis have led to a dual function model for TRs during Xenopus metamorphosis (46). According to the model, TRs are mainly unliganded in premetamorphic tadpoles when there is little or no T3 and thus repress target genes to prevent precocious metamorphosis. During metamorphosis when T3 level is high, T3 binds to TR to activate target genes, thus leading to tadpole metamorphosis. Extensive molecular and transgenic studies in Xenopus laevis and gene knockout studies in Xenopus tropicalis have provided strong support for this model (47–58).
A critical role of TR in intestinal metamorphosis was demonstrated by studies with recombinant organ cultures made of intestinal epithelium and non-epithelial tissues from premetamorphic wild type tadpoles or transgenic ones containing a heat shock-inducible dominant positive TR (dpTR) that cannot bind to T3 but functions like a constitutively liganded TR (59, 60). In these recombinant organ cultures, T3 signaling can be activated in either the epithelium or non-epithelial tissues or both by heat shock treatment of the organ cultures without the presence of T3. Such studies have revealed that dpTR expression in both epithelium and non-epithelium can induce intestinal metamorphosis, including larval epithelial apoptosis and adult intestinal stem cell formation and their subsequent proliferation and differentiation, in the absence of T3 (60). These findings indicate that TR is sufficient for mediating all effects of T3 for intestinal metamorphosis, including larval epithelial cell death and adult stem cell development. Interestingly, activating T3-signaling by expressing dpTR in either the epithelium or non-epithelial tissues alone can induce larval epithelial degeneration, indicating that larval epithelial apoptosis can be induced by T3-signaling both cell autonomously and via cell-cell interaction. However, dpTR expression in either the epithelium or the non-epithelial tissues alone fails to induce the formation of adult stem cells, although dpTR expression in the epithelium alone results in dedifferentiation of some larval epithelial cells (60). These findings suggest that epithelial T3-signaling induces larval epithelial cell dedifferentiation while T3-signaling in the non-epithelial tissues is required to help such dedifferentiated cells to develop into stem cells, likely via the formation of a proper stem cell niche through cell-cell and/or cell-ECM (extracellular matrix) interactions (60–63).
TR is not needed for adult intestinal morphogenesis but is essential for larval epithelial cell death and adult intestinal stem cell development during metamorphosis
The role of endogenous TR in regulating intestinal metamorphosis was first suggested by transgenic studies with dominant negative mutant TRs that cannot bind to T3. The expression of such dominant negative TRs was found to inhibits Xenopus laevis metamorphosis, including intestinal remodeling (50, 61, 64, 65). Since the dominant negative TRs compete functionally against endogenous wild type TR that can bind T3, the findings are not surprising given the causative role of T3 in all aspects of metamorphosis but demonstrate an essential role for TR to mediate the metamorphic effects of T3, including larval intestinal cell death and adult stem cell development.
With the advancement of gene editing technologies, it became possible to knock out endogenous TR genes in Xenopus. Indeed, individual TRα and TRβ genes or both have been knocked out recently in the diploid Xenopus tropicalis and found to have distinct tissue-dependent effects during metamorphosis (52, 55–58, 66–70). Consistent with the high but relatively constant expression of TRα during intestinal metamorphosis (71), knocking out TRα delayed intestinal remodeling (66, 69, 72). Surprisingly, knocking out TRβ had relatively subtle effect on intestinal remodeling during natural metamorphosis (52, 55), although TRβ expression, which is very low in premetamorphic tadpole intestine, is dramatically upregulated during intestinal metamorphosis (71). On the other hand, when premetamorphic wild type and TRβ knockout tadpoles were induced to metamorphose with exogenous T3 treatment, both larval epithelial cell death and adult intestinal stem cell formation, which occurred within 2-3 days in wild type tadpoles after the treatment, were delayed or inhibited in the TRβ knockout tadpoles (55). These findings suggest that TRα and TRβ have distinct but compensatory roles during intestinal metamorphosis. As T3-induced metamorphosis occurs much faster than the 2-3 weeks required for the premetamorphic tadpoles at stage 54 to develop to the climax (stages 60-62, when larval cell death and adult epithelial stem cell formation occur) during the natural metamorphosis, the compensation by TRα may be too slow to prevent the effects of TRβ knockout on intestinal remodeling during T3-induced metamorphosis but fast enough to prevent any major defect in intestinal remodeling during natural metamorphosis in TRβ knockout animals.
When both TRα and TRβ genes were knocked out in Xenopus tropicalis, the tadpoles could develop to the climax stage 61 and died after about 2 weeks at stage 61, in contrast to wild type tadpoles that develop from stage 61 to the end of metamorphosis in a week (56). While the wild type intestine at stage 61 had extensive larval epithelial cell death and proliferation of new formed adult epithelial stem cells, TR double knockout tadpoles at stage 61 had little larval cell death or adult stem cell proliferation (Figure 2A) (73). In addition, as predicted, T3 treatment of premetamorphic TR double knockout animals had no effect on the intestine (56, 73), in contrast to the wild type animals (Figure 1B). Surprisingly, the intestine of TR double knockout tadpoles developed adult morphology (with numerous epithelial folds and thick layers of connective tissue and muscles) precociously, by as early as stage 58, mimicking the wild type intestine at the end of metamorphosis (stage 66) (Figure 2A) (73). These findings indicate that TR is required for T3-induced larval epithelial cell death and adult epithelial stem cell development but not for adult intestinal morphogenesis during metamorphosis.
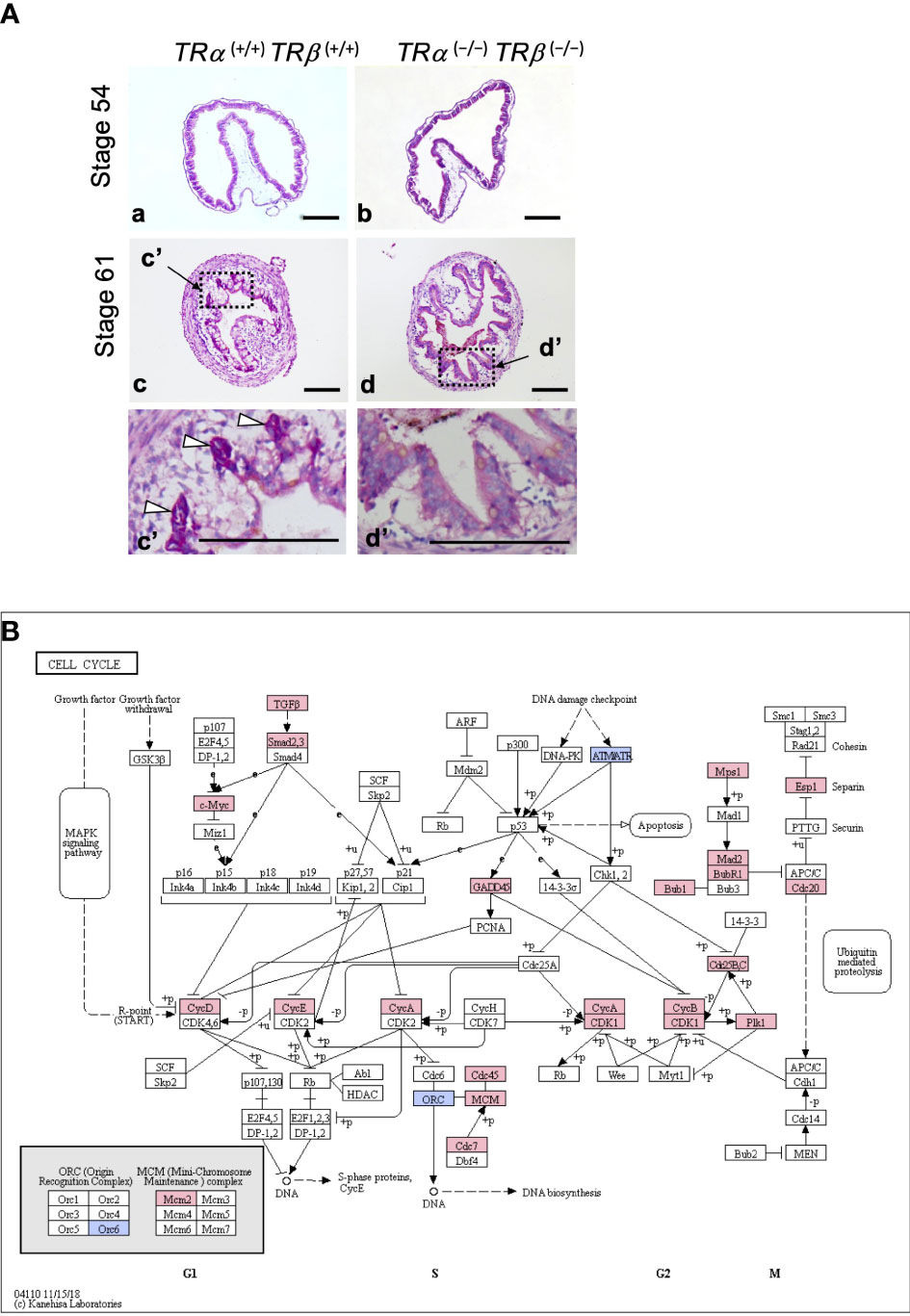
Figure 2 (A) TR double knockout tadpoles have abnormal intestinal morphology with premature adult type epithelial folding. Cross-sections of the intestine of indicated genotypes stages were stained with methyl green-pyronin Y. (a, c): Wild type TRα (+/+)TRβ (+/+); and (b, d): TR double knockout TRα (−/−)TRβ (−/−). Dashed boxes in c and d are shown in higher magnification in c’ and d’, respectively. White arrowheads point to the clusters of proliferating adult epithelial stem cells adjacent to/underneath the degenerating larval epithelium (vacuole-like, poorly stained) at the climax of metamorphosis (stage 61) in wild type tadpoles. Note that the knockout tadpoles lacked such clusters at stage 61 and the epithelium appeared to be uniform without any obvious degeneration, but with numerous folds. Bars: 100 μm. See (73) for details. (B) TRα is required for the activation of many cell cycle genes during early phase of T3-induced intestinal remodeling. Genes regulated by at least 2.0-fold after 18 hours of T3 treatment in stage 54 wild type but not TRα knockout tadpoles were mapped onto the KEGG pathway for cell cycle. Pink boxes indicate upregulation and blue boxes indicate downregulation. Note that most of the regulated genes were upregulated by T3. See (72) for more details.
Cell cycle activation by liganded TR is involved in larval epithelial cell death and adult stem cell development
As a transcription factor, TR regulates target gene transcription in a T3-dependent manner. Toward understanding how T3 regulates intestinal remodeling, various approaches have been used to isolate and characterize T3-response genes during Xenopus intestinal metamorphosis (74–78). These studies have revealed, perhaps expectedly, that many genes in signaling pathways known to be important for stem cell proliferation and function are induced by T3 during intestinal remodeling. These pathways include hedgehog pathway (21, 79–82), Wnt signaling (83–85), Notch pathway (86), and BMP signaling (87, 88), etc. In addition, many other genes, such as the methyl-CpG binding domain protein 3 (MBD3) (89) and tRNA methyltransferase-like 1 (Mettl1) (90), that were not previously known to associated with cell death or stem cells, were found to be highly upregulated by T3 during intestinal metamorphosis, suggesting that they may be novel regulators of cell death or stem cells during development.
When global gene expression analyses with RNA-seq were carried out on wild type and TR knockout intestine during metamorphosis, it was revealed that many more genes in gene ontology (GO) terms related to stem cells, cell proliferation, and apoptosis were upregulated in the wild type tadpole intestine at the climax of metamorphosis (stage 61) compared to premetamorphic stage 54 than in the TR double knockout intestine (73), consistent with the massive larval epithelial cell death and formation/proliferation of adult stem cells at the climax of metamorphosis in the wild type but not TR double knockout intestine. In addition, GO and KEGG pathway analyses of the genes that were regulated between stage 54 and stage 61 in the intestine of wild type and TR double knockout animals showed that many GO terms and KEGG pathways, particularly cell cycle/proliferation-related ones, were enriched among the upregulated genes in the wild type but not TR double knockout intestine. In fact, several cell cycle/proliferation-related GO terms were even enriched among genes downregulated at stage 61 compared to stage 54 in the TR double knockout intestine. Furthermore, when GO and KEGG pathway analyses were performed on the genes expressed at higher levels in the intestine of wild type than TR double knockout tadpoles at stage 61, it was again found that many cell cycle-related GO terms/pathways were enriched among these genes (73). These findings suggest that T3-bound TR activates cell cycle programs to facilitate intestinal metamorphosis. Given the rapid proliferation of adult stem cells at the climax of intestinal metamorphosis in the wild type (Figure 1) but not TR double knockout tadpoles, it is not surprising to find the upregulation of genes in the cell cycle program in the wild type intestine compared to the TR double knockout ones at the climax of metamorphosis.
A recent RNA-seq analysis of intestinal gene expression in premetamorphic wild type and TRα knockout tadpoles at stage 54 after 18 hours of T3 treatment suggests that activation of cell cycle program may also be important for T3-induced larval epithelial cell death during metamorphosis (72). During T3-induced metamorphosis, larval epithelial cell death is induced dramatically after 2 days while epithelial cell proliferation is increased significantly only after 3 days, due to the formation of adult epithelial stem cells (Figure 1) (11, 91). Thus, it was expected that T3 would not induce cell proliferation program, e.g., cell cycle activation, in the first 2 days of treatment. Surprisingly, GO and KEGG pathway analyses of the genes regulated by 18 hour T3 treatment of wild type tadpoles showed significant enrichment of GO terms and KEGG pathways related to cell cycle (72). This raises a possibility that the activation of the cell cycle program by T3 is an important early step for T3 to induced larval epithelial cell death (11, 72, 91). Furthermore, when the gene expression in the intestine of wild type and TRα knockout tadpoles with or without 18 hour T3-treatment were compared, it was found that the endogenous TRα was important for the regulation of the cell cycle program (72), including the KEGG cell cycle pathway, where many genes were upregulated by T3 in the wild type but not TRα knockout tadpoles (Figure 2B). This important role of TRα in gene regulation by T3 during the early phase of T3-induced metamorphosis is consistent with the fact that there is little TRβ expression in premetamorphic tadpole intestine (71). Thus, T3 likely activates the cell cycle program via TRα early during metamorphosis to facilitate epithelial cell fate determination in the intestine: apoptosis vs. dedifferentiation into adult stem cells.
Whether cell cycle activation leads to larval epithelial cell death and adult stem cell development remains to be elucidated. As cell cycle activation is typically associated with cell proliferation in development, the discovery of a role of cell cycle activation in developmental cell death was surprising. On the other hand, there has been evidence for involvement of cell cycle regulators in apoptosis. For example, c-Myc, a well-known oncogene that activate target gene transcription and promote cell proliferation, can induce cell death when overexpressed, at least in cell cultures (92–102). It is possible that that over activation of cell cycle pathways may lead to apoptosis in some cells. As the larval intestinal epithelial cells are mitotically active, even though differentiated sufficiently to function in the tadpole (16), T3-induced further activation of the cell cycle/proliferation pathways in such mitotically active yet differentiated cells may force to them to change their fate, either death via apoptosis or dedifferentiation to become adult stem cells to accommodate the faster proliferation needed for intestinal metamorphosis. Such a mechanism may explain why cell cycle/proliferation pathways are activated in the larval epithelium prior to and throughout the two major epithelial transformations, apoptotic larval epithelial degeneration, and de novo formation of the adult epithelium, during T3 intestinal remodeling.
Conclusion
The ability of vertebrate intestinal epithelium for self-renewal throughout adulthood has made the intestine a well-studied model for analyses of the properties and regulation of adult organ-specific stem cells (4–10). The formation of the adult intestinal stem cells during vertebrate development is much less known but appears to be conserved in vertebrates. In both mouse and Xenopus, the formation of the adult intestinal stem cells occurs during postembryonic development (the neonatal period in mouse and metamorphosis in Xenopus) when plasma T3 level peaks, and T3-signaling is important for the development and/or maintenance of the adult intestine (6, 103–111). These suggest a conservation in T3-regulation of adult intestinal development.
Studies on intestinal metamorphosis in Xenopus laevis and Xenopus tropicalis have revealed important and novel insights on how T3 regulates the development of the adult intestine. First, T3 induces de novo formation of adult intestinal epithelial stem cells via larval epithelial cell dedifferentiation. Second, T3-signaling in both the epithelium and non-epithelial tissues are required for adult stem cell development, likely involving the formation of adult stem cell niche. Third, TR is essential to mediate T3 signaling for both larval epithelial cell death and the formation of adult stem cells while adult intestinal morphogenesis does not require TR. Finally, and importantly, global gene expression studies on intestinal development in wild type and TR knockout tadpoles have not only revealed the regulation of diverse GO terms and pathways by T3 during intestinal metamorphosis but also implicate a surprising and novel role of cell cycle activation by T3 in cell fate determination. It would be interesting to test whether the activation of cell cycle by T3 is indeed a prerequisite for larval epithelial cells to choose between apoptosis or dedifferentiation into stem cells during intestinal metamorphosis. Future studies on the potentially conserved functions of the T3-induced GO terms and pathways in the development of the adult intestine in other species should improve our understanding of the development and function of adult stem cells in human intestinal homeostasis and diseases.
Author contributions
All authors contributed to the article and approved the submitted version.
Funding
This work was supported by the intramural Research Program of NICHD, NIH. YS and YT were supported in part by the Japan Society for the Promotion of Science (NIH) Fellowship (No. 29-71715 and 28-71606).
Conflict of interest
The authors declare that the research was conducted in the absence of any commercial or financial relationships that could be construed as a potential conflict of interest.
Publisher’s note
All claims expressed in this article are solely those of the authors and do not necessarily represent those of their affiliated organizations, or those of the publisher, the editors and the reviewers. Any product that may be evaluated in this article, or claim that may be made by its manufacturer, is not guaranteed or endorsed by the publisher.
References
1. Shi Y-B. Amphibian metamorphosis: from morphology to molecular biology. New York: John Wiley & Sons, Inc. (1999).
2. Tata JR. Gene expression during metamorphosis: an ideal model for post-embryonic development. Bioessays (1993) 15:239–48. doi: 10.1002/bies.950150404
3. Atkinson BG. Metamorphosis: model systems for studying gene expression in postembryonic development. Dev Genet (1994) 15:313–9. doi: 10.1002/dvg.1020150402
4. Shi YB, Hasebe T, Fu L, Fujimoto K, Ishizuya-Oka A. The development of the adult intestinal stem cells: insights from studies on thyroid hormone-dependent amphibian metamorphosis. Cell Biosci (2011) 1:30. doi: 10.1186/2045-3701-1-30
5. van der Flier LG, Clevers H, Cells S. Self-renewal, and differentiation in the intestinal epithelium. Annu Rev Physiol (2009) 71:241–60. doi: 10.1146/annurev.physiol.010908.163145
6. Sun G, Shi Y-B. Thyroid hormone regulation of adult intestinal stem cell development: mechanisms and evolutionary conservations. Int J Biol Sci (2012) 8:1217–24. doi: 10.7150/ijbs.5109
7. Sun G, Fu L, Shi Y-B. Epigenetic regulation of thyroid hormone-induced adult intestinal stem cell development during anuran metamorphosis. Cell Biosci (2014) 4:73. doi: 10.1186/2045-3701-4-73
8. Sirakov M, Kress E, Nadjar J, Plateroti M. Thyroid hormones and their nuclear receptors: new players in intestinal epithelium stem cell biology? Cell Mol Life Sci (2014) 71:2897–907. doi: 10.1007/s00018-014-1586-3
9. Clevers H. The intestinal crypt, a prototype stem cell compartment. Cell (2013) 154:274–84. doi: 10.1016/j.cell.2013.07.004
10. Bao L, Shi B, Shi YB. Intestinal homeostasis: a communication between life and death. Cell Biosci (2020) 10:66. doi: 10.1186/s13578-020-00429-9
11. Okada M, Wen L, Miller TC, Su D, Shi YB. Molecular and cytological analyses reveal distinct transformations of intestinal epithelial cells during xenopus metamorphosis. Cell Biosci (2015) 5:74. doi: 10.1186/s13578-015-0065-3
12. McAvoy JW, Dixon KE. Cell proliferation and renewal in the small intestinal epithelium of metamorphosing and adult xenopus laevis. J Exp Zool (1977) 202:129–38. doi: 10.1002/jez.1402020115
13. Marshall JA, Dixon KE. Cell specialization in the epithelium of the small intestine of feeding xenopus laevis tadpoles. J Anat (1978) 126:133–44.
14. Ishizuya-Oka A, Shimozawa A. Development of the connective tissue in the digestive tract of the larval and metamorphosing xenopus laevis. Anat Anz (1987) 164:81–93.
15. Dodd MHI, Dodd JM. The biology of metamorphosis. In: Lofts B, editor. Physiology of the amphibia. New York: Academic Press (1976). p. 467–599.
16. Shi Y-B, Ishizuya-Oka A. Biphasic intestinal development in amphibians: embryogensis and remodeling during metamorphosis. Curr Topics Dev Biol (1996) 32:205–35. doi: 10.1016/S0070-2153(08)60429-9
17. Sterling J, Fu L, Matsuura K, Shi Y-B. Cytological and morphological analyses reveal distinct features of intestinal development during xenopus tropicalis metamorphosis. PloS One (2012) 7:e47407. doi: 10.1371/journal.pone.0047407
18. Leloup J, Buscaglia M. La triiodothyronine: hormone de la métamorphose des amphibiens. C.R. Acad Sci (1977) 284:2261–3.
19. Nieuwkoop PD, Faber J. Normal table of xenopus laevis. North Holland Publishing Amsterdam (1965).
20. Ishizuya-Oka A, Shimozawa A. Programmed cell death and heterolysis of larval epithelial cells by macrophage-like cells in the anuran small intestine in vivo and in vitro. J Morphol (1992) 213:185–95. doi: 10.1002/jmor.1052130205
21. Wen L, Hasebe T, Miller TC, Ishizuya-Oka A, Shi YB. A requirement for hedgehog signaling in thyroid hormone-induced postembryonic intestinal remodeling. Cell Biosci (2015) 5:13. doi: 10.1186/s13578-015-0004-3
22. Ishizuya-Oka A, Shimizu K, Sakakibara S, Okano H, Ueda S. Thyroid hormone-upregulated expression of musashi-1 is specific for progenitor cells of the adult epithelium during amphibian gastrointestinal remodeling. J Cell Sci (2003) 116:3157–64. doi: 10.1242/jcs.00616
23. Ishizuya-Oka A, Hasebe T, Buchholz DR, Kajita M, Fu L, Shi YB. Origin of the adult intestinal stem cells induced by thyroid hormone in xenopus laevis. FASEB J (2009) 23:2568–75. doi: 10.1096/fj.08-128124
24. Schreiber AM, Cai L, Brown DD. Remodeling of the intestine during metamorphosis of xenopus laevis. Proc Natl Acad Sci U.S.A. (2005) 102:3720–5. doi: 10.1073/pnas.0409868102
25. Ishizuya-Oka A, Shi YB. Evolutionary insights into postembryonic development of adult intestinal stem cells. Cell Biosci (2011) 1:37. doi: 10.1186/2045-3701-1-37
26. Hetzel BS. The story of iodine deficiency: an international challenge in nutrition. Oxford: Oxford University Press (1989).
27. Porterfield SP, Hendrich CE. The role of thyroid hormones in prenatal and neonatal neurological development–current perspectives. Endocr Rev (1993) 14:94–106. doi: 10.1210/edrv-14-1-94
28. Young JJ, Cherone JM, Doyon Y, Ankoudinova I, Faraji FM, Lee AH, et al. Efficient targeted gene disruption in the soma and germ line of the frog xenopus tropicalis using engineered zinc-finger nucleases. Proc Natl Acad Sci U.S.A. (2011) 108:7052–7. doi: 10.1073/pnas.1102030108
29. Lei Y, Guo X, Liu Y, Cao Y, Deng Y, Chen X, et al. Efficient targeted gene disruption in xenopus embryos using engineered transcription activator-like effector nucleases (TALENs). Proc Natl Acad Sci U.S.A. (2012) 109:17484–9. doi: 10.1073/pnas.1215421109
30. Lei Y, Guo X, Deng Y, Chen Y, Zhao H. Generation of gene disruptions by transcription activator-like effector nucleases (TALENs) in xenopus tropicalis embryos. Cell Biosci (2013) 3:21. doi: 10.1186/2045-3701-3-21
31. Blitz IL, Biesinger J, Xie X, Cho KW. Biallelic genome modification in F(0) xenopus tropicalis embryos using the CRISPR/Cas system. Genesis (2013) 51:827–34. doi: 10.1002/dvg.22719
32. Nakayama T, Fish MB, Fisher M, Oomen-Hajagos J, Thomsen GH, Grainger RM. Simple and efficient CRISPR/Cas9-mediated targeted mutagenesis in xenopus tropicalis. Genesis (2013) 51:835–43. doi: 10.1002/dvg.22720
33. Nakade S, Tsubota T, Sakane Y, Kume S, Sakamoto N, Obara M, et al. Microhomology-mediated end-joining-dependent integration of donor DNA in cells and animals using TALENs and CRISPR/Cas9. Nat Commun (2014) 5:5560. doi: 10.1038/ncomms6560
34. Shi Z, Wang F, Cui Y, Liu Z, Guo X, Zhang Y, et al. Heritable CRISPR/Cas9-mediated targeted integration in xenopus tropicalis. FASEB J (2015) 29:4914–23. doi: 10.1096/fj.15-273425
35. Wang F, Shi Z, Cui Y, Guo X, Shi YB, Chen Y. Targeted gene disruption in xenopus laevis using CRISPR/Cas9. Cell Biosci (2015) 5:15. doi: 10.1186/s13578-015-0006-1
36. Ishizuya-Oka A, Shi YB. Thyroid hormone regulation of stem cell development during intestinal remodeling. Mol Cell Endocrinol (2008) 288:71–8. doi: 10.1016/j.mce.2008.02.020
37. Lazar MA. Thyroid hormone receptors: multiple forms, multiple possibilities. Endocr Rev (1993) 14:184–93. doi: 10.1210/edrv-14-2-184
38. Yen PM. Physiological and molecular basis of thyroid hormone action. Physiol Rev (2001) 81:1097–142. doi: 10.1152/physrev.2001.81.3.1097
39. Tsai MJ, O'Malley BW. Molecular mechanisms of action of steroid/thyroid receptor superfamily members. Ann Rev Biochem (1994) 63:451–86. doi: 10.1146/annurev.bi.63.070194.002315
41. Jones PL, Shi Y-B. N-CoR-HDAC corepressor complexes: roles in transcriptional regulation by nuclear hormone receptors. In: Workman JL, editor. Current topics in microbiology and immunology: protein complexes that modify chromatin. Berlin: Springer-Verlag (2003). p. 237–68.
42. Perissi V, Jepsen K, Glass CK, Rosenfeld MG. Deconstructing repression: evolving models of co-repressor action. Nat Rev Genet (2010) 11:109–23. doi: 10.1038/nrg2736
43. O'Malley BW, Malovannaya A, Qin J. Minireview: nuclear receptor and coregulator proteomics–2012 and beyond. Mol Endocrinol (2012) 26:1646–50. doi: 10.1210/me.2012-1114
44. Bulynko YA, O'Malley BW. Nuclear receptor coactivators: structural and functional biochemistry. Biochemistry (2011) 50:313–28. doi: 10.1021/bi101762x
45. Shi YB, Matsuura K, Fujimoto K, Wen L, Fu L. Thyroid hormone receptor actions on transcription in amphibia: the roles of histone modification and chromatin disruption. Cell Biosci (2012) 2:42. doi: 10.1186/2045-3701-2-42
46. Sachs LM, Damjanovski S, Jones PL, Li Q, Amano T, Ueda S, et al. Dual functions of thyroid hormone receptors during xenopus development. Comp Biochem Physiol B Biochem Mol Biol (2000) 126:199–211. doi: 10.1016/S0305-0491(00)00198-X
47. Grimaldi A, Buisine N, Miller T, Shi YB, Sachs LM. Mechanisms of thyroid hormone receptor action during development: lessons from amphibian studies. Biochim Biophys Acta (2013) 1830:3882–92. doi: 10.1016/j.bbagen.2012.04.020
48. Buchholz DR, Paul BD, Fu L, Shi YB. Molecular and developmental analyses of thyroid hormone receptor function in xenopus laevis, the African clawed frog. Gen Comp Endocrinol (2006) 145:1–19. doi: 10.1016/j.ygcen.2005.07.009
49. Shi Y-B. Dual functions of thyroid hormone receptors in vertebrate development: the roles of histone-modifying cofactor complexes. Thyroid (2009) 19:987–99. doi: 10.1089/thy.2009.0041
50. Nakajima K, Yaoita Y. Dual mechanisms governing muscle cell death in tadpole tail during amphibian metamorphosis. Dev Dyn (2003) 227:246–55. doi: 10.1002/dvdy.10300
51. Denver RJ, Hu F, Scanlan TS, Furlow JD. Thyroid hormone receptor subtype specificity for hormone-dependent neurogenesis in xenopus laevis. Dev Biol (2009) 326:155–68. doi: 10.1016/j.ydbio.2008.11.005
52. Nakajima K, Tazawa I, Yaoita Y. Thyroid hormone receptor alpha- and beta-knockout xenopus tropicalis tadpoles reveal subtype-specific roles during development. Endocrinology (2018) 159:733–43. doi: 10.1210/en.2017-00601
53. Sachs LM. Unliganded thyroid hormone receptor function: amphibian metamorphosis got TALENs. Endocrinology (2015) 156:409–10. doi: 10.1210/en.2014-2016
54. Nakajima K, Tazawa I, Shi YB. A unique role of thyroid hormone receptor beta in regulating notochord resorption during xenopus metamorphosis. Gen Comp Endocrinol (2019) 277:66–72. doi: 10.1016/j.ygcen.2019.03.006
55. Shibata Y, Tanizaki Y, Shi YB. Thyroid hormone receptor beta is critical for intestinal remodeling during xenopus tropicalis metamorphosis. Cell Biosci (2020) 10:46. doi: 10.1186/s13578-020-00411-5
56. Shibata Y, Wen L, Okada M, Shi YB. Organ-specific requirements for thyroid hormone receptor ensure temporal coordination of tissue-specific transformations and completion of xenopus metamorphosis. Thyroid (2020) 30:300–13. doi: 10.1089/thy.2019.0366
57. Buchholz DR, Shi YB. Dual function model revised by thyroid hormone receptor alpha knockout frogs. Gen Comp Endocrinol (2018) 265:214–8. doi: 10.1016/j.ygcen.2018.04.020
58. Shi YB. Life without thyroid hormone receptor. Endocrinology (2021) 162:bqab028, 1–12. doi: 10.1210/endocr/bqab028
59. Buchholz DR, Tomita A, Fu L, Paul BD, Shi Y-B. Transgenic analysis reveals that thyroid hormone receptor is sufficient to mediate the thyroid hormone signal in frog metamorphosis. Mol Cell Biol (2004) 24:9026–37. doi: 10.1128/MCB.24.20.9026-9037.2004
60. Hasebe T, Buchholz DR, Shi YB, Ishizuya-Oka A. Epithelial-connective tissue interactions induced by thyroid hormone receptor are essential for adult stem cell development in the xenopus laevis intestine. Stem Cells (2011) 29:154–61. doi: 10.1002/stem.560
61. Schreiber AM, Mukhi S, Brown DD. Cell-cell interactions during remodeling of the intestine at metamorphosis in xenopus laevis. Dev Biol (2009) 331:89–98. doi: 10.1016/j.ydbio.2009.04.033
62. Ishizuya-Oka A, Shimozawa A. Connective tissue is involved in adult epithelial development of the small intestine during anuran metamorphosis in vitro. Roux's Arch Dev Biol (1992) 201:322–9. doi: 10.1007/BF00592113
63. Ishizuya-Oka A, Hasebe T. Establishment of intestinal stem cell niche during amphibian metamorphosis. Curr Top Dev Biol (2013) 103:305–27. doi: 10.1016/B978-0-12-385979-2.00011-3
64. Schreiber AM, Das B, Huang H, Marsh-Armstrong N, Brown DD. Diverse developmental programs of xenopus laevis metamorphosis are inhibited by a dominant negative thyroid hormone receptor. PNAS (2001) 98:10739–44. doi: 10.1073/pnas.191361698
65. Buchholz DR, Hsia VS-C, Fu L, Shi Y-B. A dominant negative thyroid hormone receptor blocks amphibian metamorphosis by retaining corepressors at target genes. Mol Cell Biol (2003) 23:6750–8. doi: 10.1128/MCB.23.19.6750-6758.2003
66. Choi J, Ishizuya-Oka A, Buchholz DR. Growth, development, and intestinal remodeling occurs in the absence of thyroid hormone receptor alpha in tadpoles of xenopus tropicalis. Endocrinology (2017) 158:1623–33. doi: 10.1210/en.2016-1955
67. Choi J, Suzuki KI, Sakuma T, Shewade L, Yamamoto T, Buchholz DR. Unliganded thyroid hormone receptor alpha regulates developmental timing via gene repression as revealed by gene disruption in xenopus tropicalis. Endocrinology (2015) 156:735–44. doi: 10.1210/en.2014-1554
68. Wen L, Shi YB. Unliganded thyroid hormone receptor alpha controls developmental timing in xenopus tropicalis. Endocrinology (2015) 156:721–34. doi: 10.1210/en.2014-1439
69. Wen L, Shibata Y, Su D, Fu L, Luu N, Shi Y-B. Thyroid hormone receptor α controls developmental timing and regulates the rate and coordination of tissue specific metamorphosis in xenopus tropicalis. Endocrinology (2017) 158:1985–98. doi: 10.1210/en.2016-1953
70. Wen L, Shi YB. Regulation of growth rate and developmental timing by xenopus thyroid hormone receptor alpha. Dev Growth Differ (2016) 58:106–15. doi: 10.1111/dgd.12231
71. Wang X, Matsuda H, Shi Y-B. Developmental regulation and function of thyroid hormone receptors and 9-cis retinoic acid receptors during xenopus tropicalis metamorphosis. Endocrinology (2008) 149:5610–8. doi: 10.1210/en.2008-0751
72. Tanizaki Y, Shibata Y, Zhang H, Shi YB. Analysis of thyroid hormone receptor alpha-knockout tadpoles reveals that the activation of cell cycle program is involved in thyroid hormone-induced larval epithelial cell death and adult intestinal stem cell development during xenopus tropicalis metamorphosis. Thyroid (2021) 31:128–42. doi: 10.1089/thy.2020.0022
73. Shibata Y, Tanizaki Y, Zhang H, Lee H, Dasso M, Shi YB. Thyroid hormone receptor is essential for larval epithelial apoptosis and adult epithelial stem cell development but not adult intestinal morphogenesis during xenopus tropicalis metamorphosis. Cells (2021) 10:536. doi: 10.3390/cells10030536
74. Shi Y-B, Brown DD. The earliest changes in gene expression in tadpole intestine induced by thyroid hormone. J Biol Chem (1993) 268:20312–7. doi: 10.1016/S0021-9258(20)80730-3
75. Amano T, Yoshizato K. Isolation of genes involved in intestinal remodeling during anuran metamorphosis. Wound Repair Regener (1998) 6:302–13. doi: 10.1046/j.1524-475X.1998.60406.x
76. Buchholz DR, Heimeier RA, Das B, Washington T, Shi Y-B. Pairing morphology with gene expression in thyroid hormone-induced intestinal remodeling and identification of a core set of TH-induced genes across tadpole tissues. Dev Biol (2007) 303:576–90. doi: 10.1016/j.ydbio.2006.11.037
77. Heimeier RA, Das B, Buchholz DR, Fiorentino M, Shi YB. Studies on xenopus laevis intestine reveal biological pathways underlying vertebrate gut adaptation from embryo to adult. Genome Biol (2010) 11:R55. doi: 10.1186/gb-2010-11-5-r55
78. Fu L, Das B, Matsuura K, Fujimoto K, Heimeier RA, Shi YB. Genome-wide identification of thyroid hormone receptor targets in the remodeling intestine during xenopus tropicalis metamorphosis. Sci Rep (2017) 7:6414. doi: 10.1038/s41598-017-06679-x
79. Hasebe T, Kajita M, Fu L, Shi YB, Ishizuya-Oka A. Thyroid hormone-induced sonic hedgehog signal up-regulates its own pathway in a paracrine manner in the xenopus laevis intestine during metamorphosis. Dev Dyn (2012) 241:403–14. doi: 10.1002/dvdy.23723
80. Hasebe T, Kajita M, Shi YB, Ishizuya-Oka A. Thyroid hormone-up-regulated hedgehog interacting protein is involved in larval-to-adult intestinal remodeling by regulating sonic hedgehog signaling pathway in xenopus laevis. Dev Dyn (2008) 237:3006–15. doi: 10.1002/dvdy.21698
81. Ishizuya-Oka A, Ueda S, Inokuchi T, Amano T, Damjanovski S, Stolow M, et al. Thyroid hormone-induced expression of sonic hedgehog correlates with adult epithelial development during remodeling of the xenopus stomach and intestine. Differentiation (2001) 69:27–37. doi: 10.1046/j.1432-0436.2001.690103.x
82. Stolow MA, Shi YB. Xenopus sonic hedgehog as a potential morphogen during embryogenesis and thyroid hormone-dependent metamorphosis. Nucleic Acids Res (1995) 23:2555–62. doi: 10.1093/nar/23.13.2555
83. Shi YB. Thyroid hormone and intestinal tumor: a wnt connection. Oncotarget (2018) 9:31941. doi: 10.18632/oncotarget.25822
84. Hasebe T, Fujimoto K, Kajita M, Ishizuya-Oka A. Thyroid hormone activates wnt/beta-catenin signaling involved in adult epithelial development during intestinal remodeling in xenopus laevis. Cell Tissue Res (2016) 365:309–18. doi: 10.1007/s00441-016-2396-8
85. Ishizuya-Oka A, Kajita M, Hasebe T. Thyroid hormone-regulated Wnt5a/Ror2 signaling is essential for dedifferentiation of larval epithelial cells into adult stem cells in the xenopus laevis intestine. PloS One (2014) 9:e107611. doi: 10.1371/journal.pone.0107611
86. Hasebe T, Fujimoto K, Kajita M, Fu L, Shi YB, Ishizuya-Oka A. Thyroid hormone-induced activation of notch signaling is required for adult intestinal stem cell development during xenopus laevis metamorphosis. Stem Cells (2017) 35:1028–39. doi: 10.1002/stem.2544
87. Ishizuya-Oka A, Hasebe T, Shimizu K, Suzuki K, Ueda S. Shh/BMP-4 signaling pathway is essential for intestinal epithelial development during xenopus larval-to-adult remodeling. Dev Dyn (2006) 235:3240–9. doi: 10.1002/dvdy.20969
88. Ishizuya-Oka A, Ueda S, Amano T, Shimizu K, Suzuki K, Ueno N, et al. Thyroid-hormone-dependent and fibroblast-specific expression of BMP-4 correlates with adult epithelial development during amphibian intestinal remodeling. Cell Tissue Res (2001) 303:187–95. doi: 10.1007/s004410000291
89. Fu L, Li C, Na W, Shi YB. Thyroid hormone activates xenopus MBD3 gene via an intronic TRE in vivo. Front Biosci (Landmark Ed) (2020) 25:437–51. doi: 10.2741/4812
90. Na W, Fu L, Luu N, Shi YB. Direct activation of tRNA methyltransferase-like 1 (Mettl1) gene by thyroid hormone receptor implicates a role in adult intestinal stem cell development and proliferation during xenopus tropicalis metamorphosis. Cell Biosci (2020) 10:60. doi: 10.1186/s13578-020-00423-1
91. Okada M, Miller TC, Wen L, Shi YB. A balance of mad and myc expression dictates larval cell apoptosis and adult stem cell development during xenopus intestinal metamorphosis. Cell Death Dis (2017) 8:e2787. doi: 10.1038/cddis.2017.198
92. Shi Y, Glynn JM, Guilbert LJ, Cotter TG, Bissonnette RP, Green DR. Role for c-myc in activation-induced apoptotic cell death in T cell hybridomas. Science (1992) 257:212–4. doi: 10.1126/science.1378649
93. Koskinen PJ, Alitalo K. Role of myc amplification and overexpression in cell growth, differentiation and death. Semin Cancer Biol (1993) 4:3–12.
94. Amati B, Land H. Myc-Max-Mad: a transcription factor network controlling cell cycle progression, differentiation and death. Curr Opin Genet Dev (1994) 4:102–8. doi: 10.1016/0959-437X(94)90098-1
95. McMahon SB. MYC and the control of apoptosis. Cold Spring Harb Perspect Med (2014) 4:a014407. doi: 10.1101/cshperspect.a014407
96. Nieminen AI, Partanen JI, Klefstrom J. C-myc blazing a trail of death: coupling of the mitochondrial and death receptor apoptosis pathways by c-myc. Cell Cycle (2007) 6:2464–72. doi: 10.4161/cc.6.20.4917
97. Packham G, Cleveland JL. C-myc and apoptosis. Biochim Biophys Acta (1995) 1242:11–28. doi: 10.1016/0304-419X(94)00015-T
98. Kuchino Y, Asai A, Kitanaka C. Myc-mediated apoptosis. Prog Mol Subcell Biol (1996) 16:104–29. doi: 10.1007/978-3-642-79850-4_7
99. Thompson EB. The many roles of c-myc in apoptosis. Annu Rev Physiol (1998) 60:575–600. doi: 10.1146/annurev.physiol.60.1.575
100. Dang CV. C-myc target genes involved in cell growth, apoptosis, and metabolism. Mol Cell Biol (1999) 19:1–11. doi: 10.1128/MCB.19.1.1
101. Pelengaris S, Rudolph B, Littlewood T. Action of myc in vivo - proliferation and apoptosis. Curr Opin Genet Dev (2000) 10:100–5. doi: 10.1016/S0959-437X(99)00046-5
102. Okada M, Shi YB. The balance of two opposing factors mad and myc regulates cell fate during tissue remodeling. Cell Biosci (2018) 8:51. doi: 10.1186/s13578-018-0249-8
103. Muncan V, Heijmans J, Krasinski SD, Buller NV, Wildenberg ME, Meisner S, et al. Blimp1 regulates the transition of neonatal to adult intestinal epithelium. Nat Commun (2011) 2:452. doi: 10.1038/ncomms1463
104. Harper J, Mould A, Andrews RM, Bikoff EK, Robertson EJ. The transcriptional repressor Blimp1/Prdm1 regulates postnatal reprogramming of intestinal enterocytes. Proc Natl Acad Sci U.S.A. (2011) 108:10585–90. doi: 10.1073/pnas.1105852108
105. Matsuda H, Shi YB. An essential and evolutionarily conserved role of protein arginine methyltransferase 1 for adult intestinal stem cells during postembryonic development. Stem Cells (2010) 28:2073–83. doi: 10.1002/stem.529
106. Plateroti M, Gauthier K, Domon-Dell C, Freund JN, Samarut J, Chassande O. Functional interference between thyroid hormone receptor alpha (TRalpha) and natural truncated TRDeltaalpha isoforms in the control of intestine development. Mol Cell Biol (2001) 21:4761–72. doi: 10.1128/MCB.21.14.4761-4772.2001
107. Flamant F, Poguet AL, Plateroti M, Chassande O, Gauthier K, Streichenberger N, et al. Congenital hypothyroid Pax8(-/-) mutant mice can be rescued by inactivating the TRalpha gene. Mol Endocrinol (2002) 16:24–32. doi: 10.1210/mend.16.1.0766
108. Kress E, Rezza A, Nadjar J, Samarut J, Plateroti M. The frizzled-related sFRP2 gene is a target of thyroid hormone receptor alpha1 and activates beta-catenin signaling in mouse intestine. J Biol Chem (2009) 284:1234–41. doi: 10.1074/jbc.M806548200
109. Plateroti M, Chassande O, Fraichard A, Gauthier K, Freund JN, Samarut J, et al. Involvement of T3Ralpha- and beta-receptor subtypes in mediation of T3 functions during postnatal murine intestinal development. Gastroenterology (1999) 116:1367–78. doi: 10.1016/S0016-5085(99)70501-9
110. Bao L, Roediger J, Park S, Fu L, Shi B, Cheng SY, et al. Thyroid hormone receptor alpha mutations lead to epithelial defects in the adult intestine in a mouse model of resistance to thyroid hormone. Thyroid (2019) 29:439–48. doi: 10.1089/thy.2018.0340
Keywords: programmed cell death, metamorphosis, Xenopus laevis, Xenopus tropicalis, postembryonic development, intestine, thyroid hormone receptor, stem cell
Citation: Tanizaki Y, Shibata Y, Na W and Shi Y-B (2023) Cell cycle activation in thyroid hormone-induced apoptosis and stem cell development during Xenopus intestinal metamorphosis. Front. Endocrinol. 14:1184013. doi: 10.3389/fendo.2023.1184013
Received: 10 March 2023; Accepted: 03 May 2023;
Published: 17 May 2023.
Edited by:
Marco António Campinho, University of Algarve, PortugalReviewed by:
Yoshio Yaoita, Hiroshima University, JapanNicolas Buisine, Muséum National d’Histoire Naturelle, France
Copyright © 2023 Tanizaki, Shibata, Na and Shi. This is an open-access article distributed under the terms of the Creative Commons Attribution License (CC BY). The use, distribution or reproduction in other forums is permitted, provided the original author(s) and the copyright owner(s) are credited and that the original publication in this journal is cited, in accordance with accepted academic practice. No use, distribution or reproduction is permitted which does not comply with these terms.
*Correspondence: Yun-Bo Shi, U2hpQGhlbGl4Lm5paC5nb3Y=