- 1National Health Commission of the People's Republic of China (NHC) Key Lab of Reproduction Regulation (Shanghai Institute for Biomedical and Pharmaceutical Technologies), School of Pharmacy, Fudan University, Shanghai, China
- 2Reproductive Medicine Center, Zhongshan Hospital, Fudan University, Shanghai, China
Disruption of iron homeostasis plays a negative role in follicle development. The dynamic changes in follicle growth are dependent on Hippo/YAP signaling and mechanical forces. However, little is known about the liaison between iron overload and the Hippo/YAP signalling pathway in term of folliculogenesis. Here, based on the available evidence, we established a hypothesized model linking excessive iron, extracellular matrix (ECM), transforming growth factor-β (TGF-β) and Hippo/Yes-associated protein (YAP) signal regarding follicle development. Hypothetically, the TGF-β signal and iron overload may play a synergistic role in ECM production via YAP. We speculate that the dynamic homeostasis of follicular iron interacts with YAP, increasing the risk of ovarian reserve loss and may enhance the sensitivity of follicles to accumulated iron. Hence, therapeutic interventions targeting iron metabolism disorders, and Hippo/YAP signal may alter the consequences of the impaired developmental process based on our hypothesis, which provides potential targets and inspiration for further drug discovery and development applied to clinical treatment.
Introduction
The regulation of iron homeostasis is particularly indispensable for maintaining dynamic reciprocity of cellular biological redox (1). Disrupted iron metabolism triggers iron overload, which acts as a catalyst for redox reactions, leading to the accumulation of lipid peroxides that induce cytotoxicity (2, 3). Follicle development expresses rapid cell proliferation and increased steroid generation, which is essential for iron demands (4). Conversely, iron overload results in a loss of antioxidant defenses, which affects follicle development (5).
Primordial follicle pools are limited and mostly in a dormant state (6, 7). The development process cannot be reversed once primordial follicles are properly activated; this plays a decisive role in maintaining female reproductive life span (8–11). Follicle development goes through different stages, which involves a series of complex processes. Extracellular matrix (ECM), an essential component of the ovarian microenvironment (12) governed by the TGF-β pathway (13) and iron metabolism (14), can dynamically regulate follicle assembly, activation, and dormancy (15, 16). Iron overload in the follicular fluid of patients with endometriosis leads to impaired oocyte maturation, possibly because granulosa cells with ferroptosis releasing exosomes that inhibit oocyte development (17). Mice with ovarian endometriosis showed significant fibrosis in the ovary, accompanied by increased iron accumulation, resulting in follicular oxidative stress and a dramatically decrease in litter size (18). As an upstream effector, ECM controls the dynamics of the Hippo pathway (19), this is derived from the appreciation of mechanical operations that interfere with ovarian Hippo signal transduction and promote follicle growth (20). In reproductive diseases affecting follicle development such as premature ovarian insufficiency (POI), Yes-associated protein (YAP, also known as YAP1), a key effector of the Hippo pathway, has been reported as a susceptibility gene of it (21, 22). The interactive mode between the Hippo pathway and the TGF-β signal is crucial for follicle development events (20, 23, 24). Therefore, it is highly likely that crosstalk connecting iron metabolism and the two pathways regulates follicle fate. However, a body of evidence merely concentrates on how iron overload induces intracellular oxidative stress. This review explores novel potential therapeutic targets with clinical significance and application value in improving female fertility by deducing a hypothetical mechanism linking excessive iron with the ECM-mediated Hippo pathway in terms of follicle maturation and development.
Method
We used multiple strategies to identify major research publications written in English before July 2022 regarding iron metabolism, extracellular matrix, Hippo/YAP pathway, and follicle development. Under the premise of ensuring the preciseness of this study, we have conducted extensive screening on PubMed and/or Google Scholar using the following keywords alone or in combination: iron, iron overload, ferroptosis, follicles, extracellular matrix (ECM), actin, collagen, fibronectin, mechanical transduction, mechanical stress, Hippo pathway, Transforming growth factor-β (TGF-β), SMAD, BMP, Hepcidin, Fibrosis, premature ovarian insufficiency (POI), drug therapy, coenzyme Q10, resveratrol, melatonin, etc. We also searched the bibliography for other related articles. Overall, we reviewed most of the relevant articles and included them appropriately. Figures were generated via www.biorender.com
Mechanism of iron overload and ferroptosis
Ferroptosis is mediated by intracellular iron overload (25). As a new type of iron-dependent cell death, it is characterized by mitochondrial collapse, the compression of membrane contents, and the accumulation of lipid peroxidation (26, 27). Exposure to excess iron, or polyunsaturated fatty acids (PUFAs) such as arachidonic acid and adrenal acid that activate membrane remodeling enzymes such as acyl-CoA synthase long chain family 4 (ACSL4), which is involved in catalyzing the acetylation of long-chain PUFAs to produce lipid peroxides esterified by interaction with membrane phospholipids (27–29). Ferroptosis, therefore, is strongly driven by ACSL4 (30). Transferrin receptor (TFRC) is a type II transmembrane receptor responsible for the uptake of cellular iron through receptor-mediated endocytosis (31). Dysfunction of iron uptake, transport, and storage, a major contributor to ferroptosis sensitivity, results from over-active TFRC mediating the internalization of iron load (32–34). TFRC knockdown by the lentivirus system inhibits erastin-induced ferroptosis (26). Similarly, suppressed TFRC reduces the risk of ferroptosis induced by amino acid/cysteine (35). In addition, nicotinamide adenine dinucleotide phosphate (NADPH) oxidase (NOXs), including NOX1, NOX2, NOX3, and NOX4, produces oxidative radicals and is the main source of the intracellular lipid reactive oxygen species (ROS) (36). Increased ROS levels lead to the accumulation of lipid peroxidation and ferroptosis (37). The antioxidant glutathione (GSH) is a powerful scavenger of lipid peroxidation products and acts as a cofactor of glutathione peroxidase 4 (GPX4) to restrain the progress of ferroptosis (37). One of the main mechanisms of ferroptosis may be triggered by impaired GSH metabolism (38). High concentrations of homocysteine were reported to induce GPX4 methylation leading to oxidative stress and ferroptosis by promoting methylase expression (39).
Negative role of iron overload in follicle development
Numerous research focus on the correlation between iron overload and follicle development confirmed in a variety of experimental models. Iron accumulation and ferroptosis may occur in the early stage of follicle growth (40). Direct evidence comes from Hu et al. Via establishing an iron overload model induced by ferric ammonium citrate (FAC), the impaired quality of porcine oocytes was observed (5). Li et al. (41) found that transferrin was significantly reduced with increased iron concentration (P <0.05) in follicle fluid samples of advanced endometriosis. Direct negative effects on mouse oocytes with in vitro maturation were determined (41). Decreased oocyte quality, impaired ovarian reserve, and reduced ability of embryonic development can be induced by excessive intracellular ROS accumulation (42–46). In women with thalassemia, iron overload has a toxic effect on the anterior pituitary, leading to hypogonadotropic hypogonadism, low gonadotropin secretion, reduced antral follicle count, and fertility ability (47, 48).
Mechanism of iron overload occurrence in follicle development-related diseases
Abnormal follicle development is the main phenotype of the onset of POI and PCOS. Women with POI lose ovarian activity and develop endocrine disturbances before the age of 40 (49). Iatrogenic factors such as chemotherapy have toxic effects on the reproductive system of young women (50). The main pathogenic mechanism may be through inducing oocyte apoptosis or destroying granulosa cell function, leading to primordial follicle loss, increase in atretic follicle and ovarian tissue fibrosis (51, 52). Accumulation of iron and lipid peroxide are involved in the pathological progress of fibrosis diseases (53, 54). Du et al. reported the association between ferroptosis and POI; this is mediated by cisplatin (55). The process involves lipid peroxidation promoting ferroptosis in granulosa cells, thus causing follicle development disorders and ovarian tissue fibrosis, ultimately perturbing ovarian function and fertility (55). Wang et al. (22)reported that BNC1 gene deficiency triggers oocyte ferroptosis via the NF2-YAP pathway and the pharmacologic inhibition of YAP signaling or ferroptosis significantly rescues POI. BNC1 is essential for maintaining mitochondrial function and oocyte lipid metabolism, while deficiency of BNC1 triggers iron-dependent POI. Perturbed expression of NF2 in BNC1 mutant mice results in dysregulation of oocyte redox homeostasis. Transcriptome data of Wang et al. showed that ferroptosis was involved in BNC1-induced POI. This uncover a pathologic mechanism of POI based on ferroptosis via YAP pathway.
Moreover, the clinical symptoms of polycystic ovary syndrome (PCOS) include oligomenorrhea, amenorrhea, hirsutism, increased risk of type 2 diabetes mellitus and insulin resistance (56, 57). Increased ROS production and decreased antioxidant capacity were observed in ovarian granulosa cells of PCOS patients; this is the cause of miscarriage and infertility in PCOS women (58, 59). PCOS-induced rats result in the activation of ferroptosis cascade and mitochondrial dysfunction in both uterus and placenta, which is associated with the triggering of GPX4/glutathione regulated lipid peroxidation and mitochondria-mediated ferroptosis (60). Both cross-sectional case-control studies (61), and meta-analysis (62) revealed high serum levels of ferritin, iron concentration, and hepcidin (an important regulator of iron homeostasis) in PCOS patients. As PCOS is associated with insulin resistance (63), disrupted iron metabolism may involve endocrine and metabolic disturbances rather than defects in hepcidin production. Therefore, it is not surprising that iron overload is associated with follicle dysplasia in PCOS patients. In sum, iron overload is tightly related to follicle development disorder characterized by ferroptosis, lipid peroxidation and oxidative stress.
Hippo/YAP pathway regulates follicle development
Hippo pathway controls cell fate and organ growth through kinase complexes with evolutionarily conserved profiles (19). Kinase cascades are formed by mammalian sterile-20 like serine/threonine kinase 1/2 (MST 1/2), Salvador (SAV), and large tumor suppressor 1/2 (LATS 1/2). Downstream core effector YAP and transcriptional co-activator PDZ-binding motif (TAZ) are suppressed by the phosphorylation of these kinase complexes. Phosphorylated YAP/TAZ complex resulting in its cytoplasmic ubiquitin and degradation leads to nuclear transcription failure (64). In contrast, disrupted Hippo signaling urge unphosphorylated YAP/TAZ locates in the nucleus and induces expression of CCN family growth factors and baculoviral inhibitors of apoptosis repeat-containing (BIRC) apoptosis inhibitors by primarily binding to transcriptional enhanced associate domain (TEAD) transcription factors, to achieve the purpose of follicle development (20).
Evidence supports the key value of Hippo signaling pathway in follicle activation and growth. MST1/2, LATS1/2, and YAP/TAZ are expressed at different stages of follicle development in mouse and human ovaries (65–68). In vitro research reported that LATS1, during follicle development, can directly phosphorylate forkhead L2 (FOXL2), a vital regulator expressed in granulosa cells participating in follicle maturation, thereby controlling its transcriptional activity (69). Reduced primordial and activated follicles were observed in Lats1 mutant (deletion) mouse ovaries, indicating its significance in maintaining ovarian reserve (70). YAP/TAZ signaling pathway is active in vivo (71), and supports proper follicle growth (72). Impaired follicle development and oocyte maturation were observed in Foxl2-driven Yap-deficient mice (73). Nagashima et al. observed abnormal follicle development (reduced preantral follicle number) in connective tissue growth factor (CTGF, a member of the CCN family) ovarian and uterine conditional knockout (cKO) mice (74). Additionally, the YAP gene is highly expressed in ovaries of PCOS women (75). This may be due to the increased YAP mRNA and protein levels caused by hypomethylation of the YAP promoter in PCOS patients (76). This is consistent with the hypothesis proposed by Dupont et al., that cell fate is induced by the rigid ECM where YAP/TAZ is active, and therefore YAP/TAZ function is required (77). In sum, the tangled Hippo web is an indispensable factor in follicle development obstruction.
The upstream signal ECM governs follicle development via Hippo/YAP pathway
ECM is an extracellular network composed of macromolecules, which is related to the biomechanical support—the exchange of extracellular signals governing folliculogenesis and appropriate oocyte maturation (78). Disturbance of ECM homeostasis may lead to interference of biochemical pathways responsible for connecting cumulus-oocyte complex (COC), leading to impaired follicle development (79). Dynamic interactions exist between the ovarian biomechanics and microenvironment. Deposition and remodeling of matrix components are associated with early follicle activation (80). Primordial follicles are compressed and are in a state of intensive mechanical stress resulting from surrounding rigid ECM secreted by granulosa cells (15). The stiff physical environment—collagen-rich ovarian cortex is believed to be essential for maintaining follicle dormancy (64). Conversely, primordial follicles are activated on a soft matrix caused by ECM-degrading enzymes (15), which is consistent with the principle of ovarian fragmentation to improve rigid ovarian environments (20). A randomized controlled trial (RCT) assigned 34 women with poor ovarian response to receiving ovarian fragmentation or with no intervention. Although no significant difference was found in IVF outcomes, follicle activation was observed in patients undergoing ovarian fragmentation surgery (81). The Hippo pathway is extracellularly regulated by mechanical forces generated by ECM upstream signal (19), which is transduced by cytoplasmic actin (82). As a multifunctional protein, actin forms microfilaments to regulate follicle activation and development (65, 83–85). Once the globular actin (G-actin) polymerizes in stress fibers to form the filamentous actin (F-actin), the Hippo signaling pathway was disrupted (86). Cheng et al. successfully stimulated follicle growth in mice using jasplakinolide (JASP) or sphingosine-1-phosphate (S1P), which promoted actin polymerization and increased the F-actin/G-actin ratio, as well as subsequently activated YAP nuclear accumulation following the increase of CCN2 transcriptional level (83). Similarly, S1P addition to the culture medium reduced follicle atresia and improved the quality of primordial follicle (87–89). Hence, as an extracellular mechanical signal, ECM stiffness effectively regulates YAP/TAZ nuclear shuttling via dynamically intracellular actin dynamics, which controls the expression of CCN growth factors and BIRC apoptosis inhibitors in the nucleus, thus determining follicle fate. These findings pave the way for ECM protein levels to be a possible therapeutic target for follicle dysplasia.
Iron-mediated ECM remodeling and follicle development
In the presence of excessive ECM, reduced matrix elasticity and rigid stiffness-induced mechanical stress are generated, thus interfering with the normal cellular biological behavior (90, 91). Positive evidence exhibits that within acceptable thresholds, iron regulates ECM production, including the synthesis of collagen (14, 92) and elastin (93). Higher intracellular iron levels may perturb the expression of genes encoding ECM components (94), suggesting that iron levels mediate matrix remodeling and degradation. Bunda et al. (93) demonstrated that elastin production was positively promoted in the range of 2-20μM iron concentration, yet reduced elastin output was observed when concentration goes out of scope. This is confirmed in the pathological mechanism of fibrosis. Several studies have reported the effect of iron metabolism on fibrosis progress. As a pathological phenomenon of chronic liver disease, liver fibrosis is characterized by excessive deposition of ECM with a typical observation of iron overload (95). Liver iron concentration over 60µmol/g can lead to abnormal function of hepatic stellate cell (HSC), and the threshold for transformation from fibrosis to cirrhosis is over 250µmol/g (96). Zhu et al. established a mouse model of liver fibrosis using carbon tetrachloride, suggesting a link between ferroptosis and liver fibrosis in HSC (97). Yuan et al. showed that triggering HSC ferroptosis alleviates liver damage and fibrosis since HSC is a major contributor to generating fibrosis (98). As a key signal mediating fibrosis, studies showed that iron increases the expression of TGF-β and collagen genes in HSC, thereby inducing fibrosis development (99, 100). Furthermore, during folliculogenesis, integrin mechanoreceptors on the plasma membrane of granulosa cells sense collagen concentration in cortical ECM and regulate the mechanoconduction cascade of Hippo signaling pathway according to the degree of rigidity (20, 85). Concentrations of other ECM components in follicular fluid, such as laminin and fibronectin, were positively correlated with oocyte competence in preovulation events (101). Oocytes synthesize bone morphogenetic protein 15 (BMP-15) and growth differentiation factor 9 (GDF9) belonging to the transforming growth factor β (TGFβ) superfamily, which seek for their respective receptors on granulosa cells to produce a soft visco-elastic ECM via SMAD2/3 or SMAD1/5/8 signalling, thus biomechanically paving the way for COC expansion (102, 103). Fibronectin levels in human follicle fluid are closely related to follicle size and oocyte maturation, indicating its significance in the process of folliculogenesis (104). Alahari et al. reported that under the condition of preeclampsia, iron regulates fibronectin assembly in primary mesenchymal stem cells, and abnormal ECM deposition blocks the migration of HTR-8/SVneo cells (105). In the course of mouse ovulation, the induction of granulosa cell luteinization and cumulus expansion depends on the fibronectin-integrin pathway (106). As a core component mediating the interaction between cells and ECM, the integrin family is crucial in delivering extracellular signal—ECM into cells (107). Cell detaching from ECM regards as a trigger of ferroptosis (108–110). α6β4 integrin has been reported to protect cancer cells from ferroptosis induced by erastin (a ferroptosis inducer) and ECM detachment, possibly due to inhibition of ACSL4 expression by α6β4 (108). Hence, iron overload produces abnormal ECM structures that lead to decreased cell proliferation, adhesion, and motility. The consequence of ECM overproduction is most likely a synergistic action launched by iron overload and TGF-β signal activation. However, whether iron overload is an independent factor contributing to increased ECM output, especially during follicle development, remains to be further investigated.
The association of Hippo/YAP with TGF-β signaling mediates ECM remodeling and has potential implications for follicle development referring iron metabolism
Although lacking studies on follicle development models, correlations between iron metabolism and YAP have been reported in other tissues. YAP is a major downstream effector that mediates oxidative stress or ROS initiation, and phosphorylation of YAP induced by 10μM emodin suppressed ferroptosis and oxidative liver injury (111). YAP is a transcriptional stimulator of ferroptosis-activating genes ACSL4 and TFRC (112); it can directly bind to the TFRC promoter region and target TFRC expression to regulate iron levels (113). TFRC has been reported to be expressed in oocytes (114, 115) and is regulated by estrogen (116). Elevated TFRC mRNA expression was found in patients with endometriosis with reduced oocyte retrieval (117), suggesting adverse outcomes in follicles exposed to a potentially toxic iron-related environment. Therefore, we speculated that the activation of TFRC induced by active YAP might be one of the causes of follicles with iron overload.
Further, the regulatory effect of TAZ on NOXs has been demonstrated, yet the consequences are still controversial. In renal cell carcinoma, activated TAZ up-regulates NOX4 levels and subsequently increases intracellular lipid ROS levels inducing ferroptosis (118). Similarly, TAZ directly regulates the target gene angiopoietin-like 4 (ANGPTL4) and causes ferroptosis in ovarian cancer by activating NOX2 (119). However, a study on oxidative damage of uterine decidua illustrates that TAZ acts as an antioxidant role in restoring mitochondrial function by inhibiting NOX-stimulated ROS production to improve oxidative damage in stromal cells (120). NOXs appear to exert an effect on oocyte developmental stimulation. In a study on oocyte senescence, the fact that reduced NOX4 expression was observed in women more than 40 years old (121). Another study reported that FSH-induced oocyte maturation in vitro is dependent on NOX-mediated ROS activation (122). Therefore, we speculate that appropriate ROS level-mediated oocyte maturation is of great significance, yet excessive ROS undoubtedly accelerates adverse events induced by iron overload. However, evidence is still needed on how TAZ regulates NOXs in follicle development.
Moreover, TGF-β superfamily is involved in a series of biological events during folliculogenesis (123). Activated TGF-β receptors directly phosphorylate SMAD proteins launching nuclear gene transcription (124). SMAD2/3 is normally activated by TGF-β receptors (125). SMAD1/5/8, on the other hand, is induced by BMPs ligand, which mainly mediates hepcidin expression (126). SMAD4 forms a complex with phosphorylated SMAD2/3 or SMAD1/5/8, which translocates to the nucleus and binds to promoters triggering the transcription of numerous genes (124, 126). TGF-β is an effective component that enhances the production of ECM proteins and plays a key role in matrix remodeling (127). TGF-β/SMAD2/3 activation is a typical fibrosis pathway that mediates ECM deposition (13, 127). Further, crosstalk between TGF-β and Hippo signaling plays a synergistic role in transcriptional regulation (24). YAP and TAZ bind to SMAD proteins and are involved in the regulation of BMP or TGF-β signaling through different mechanisms (24). The two WW domains of YAP effectively bind to the PPxY motif in SMAD1 (128). In response to TGF-β signaling, TAZ and YAP are regulated by heteromeric SMAD2/3/4 complexes and determine their intracellular sublocalization (125). TGF-β and Hippo signals converge on transcriptional regulation of common target genes, for instance, CTGF, a component of surrounding ECM production that facilitates follicle assembly and maturation (129, 130).
TGF-β and Hippo pathway, therefore, are jointly aligned in the light of determining follicle fate through ECM remodelling, and may regard as key factors contributing to iron homeostasis.
The hypothetical model
We emphasize that dynamic changes in terms of iron metabolism, ECM production, Hippo, and TGF-β/SMAD signaling pathways are involved in follicle development. The indisputable fact is that negative effort is made by excessive iron on follicle development. The activation of the TGF-β signal was shown to be caused by excess iron (100), and activated TGF-β up-regulates TFRC through the Hippo signaling pathway, enhancing intracellular accumulation of unstable iron and promoting fibrosis transition (131). Therefore, we proposed that overloaded iron and TGF-β signal have a synergistic effect through positive feedback to jointly stimulate the production of follicle-surrounding ECM, which is secreted by granulosa cells (23, 132). Increase in YAP/TAZ expression and its nuclear localization has been demonstrated and is regulated by TGF-β in patients with fibrosis (131, 133, 134). Through the binding of YAP/TAZ and SMADs, crosstalk occurs, promoting YAP/TAZ nuclear translocation under the activation of SMADs (125). ECM production is likely to be induced to increase, suggesting a possible scenario where matrix remodeling and mechanical stress occurr. The rigid ovarian cortex is wide open to compresse follicles; this is involved in the formation of actin stress fiber and integrin-mediated ECM-cell interactions. These changes increase mechanical forces and matrix stiffness on the periphery of follicles. Mechanical transduction also participates in nuclear dynamics in the regulation of YAP (135). The stress fibers generated on the stiff matrix deliver mechanical forces to the nucleus, followed by the flattened nuclear shape (15). Reduced mechanical limitations in nuclear pores appears to increase the YAP nuclear accumulation. By comparison, mechanical forces hardly transduced to the nucleus on the soft matrix, where nuclear YAP shuttling from cytoplasmic import via nucleus pore is equalized. The temporarily activated YAP/TAZ ultimately may not combat the rigid ovarian environment on account of positive cooperativity of TGF-β signal and iron overload, resulting in decreased follicle developmental potential (Figure 1).
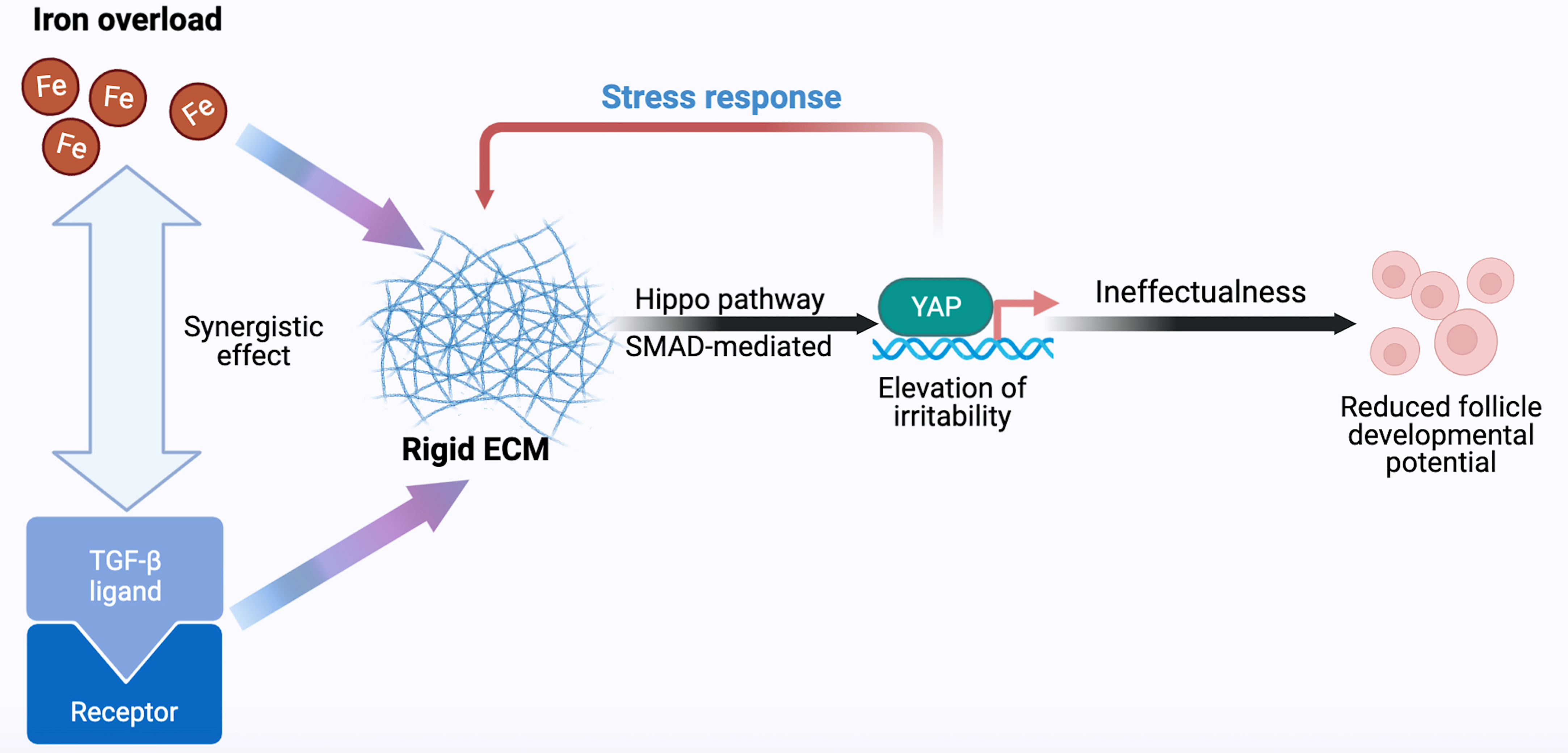
Figure 1 Hypothetical model of follicle developmental regulation. As the main effector, YAP responds to iron, ECM, Hippo, and TGF-β signals, thereby determining the follicle fate. Fe, iron; TGF-β, transforming growth factor-β; ECM, extracellular matrix; YAP, Yes-associated protein.
The Hippo/YAP pathway, TGF-β signal and iron metabolism are illustrated to regulate follicle growth (4, 5, 20, 23, 65), and TFRC is proved to be expressed in follicles and plays a vital role in follicle developmental trajectory (114, 116, 136). Therefore, from the perspective of intracellular environment, we hypothesize that a regulatory mechanism of iron metabolism is expected in follicles. YAP regulates iron metabolism by targeting TFRC (113), we proposed that TFRC mediate extracellular iron transport into the follicle. Increased cytoplasmic free iron concentration causes NOXs-induced Fenton chemistry, which stimulated the overproduction of intracellular ROS (137, 138). YAP/TAZ complex traveling into the nucleus, combine with TEADs transcription factor to promote the expression of ferroptosis-related genes such as TFRC and ACSL4 (131), thus being supposed to accelerate follicle lipid peroxidation and the progression of iron overload. In contrast, the BMP6-mediated SMAD1/5/8-SMAD4 signal enables excessive free iron to be stored through hepcidin (139, 140), thereby being expected to weaken the development of the Fenton reaction in follicles. Restriction of YAP/TAZ nuclear accumulation suppresses follicle development, we assume that follicles may build up resistant to iron overload-induced cytotoxicity. On the other side, Hippo signaling pathway perturbations and YAP/TAZ nuclear translocation/activation triggers follicle development; however, the susceptibility to iron excessive is supposed to be increased. Therefore, we theorize that by virtue of permissive YAP, follicle over-activation and the cytotoxicity of follicle affected by susceptibility to excess iron synergistically lead to the loss of ovarian reserve (Figure 2).
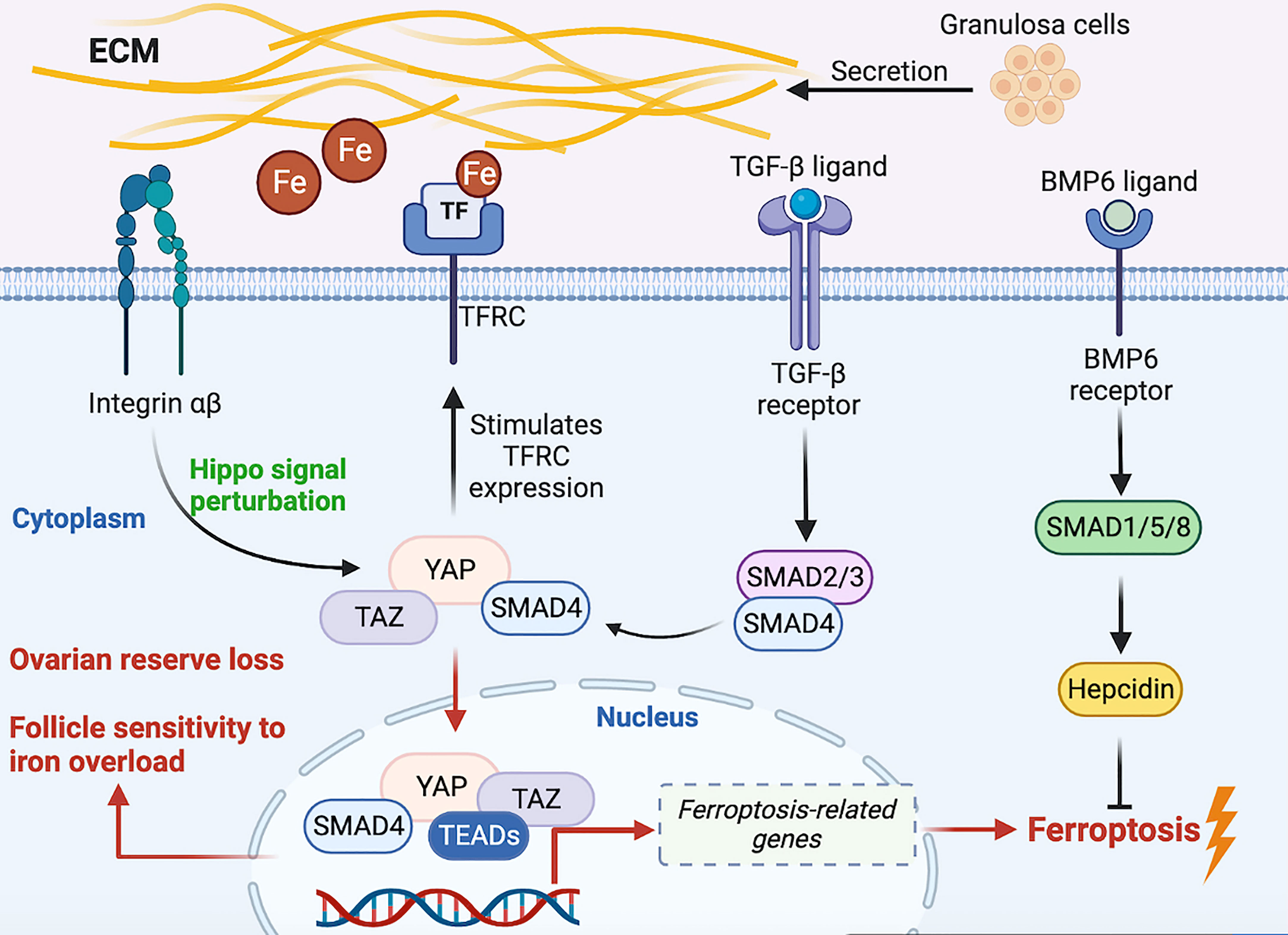
Figure 2 Hypothetical molecular signal model on regulatory iron metabolism of the intra- and extra-oocyte. Fe, iron; TGF-β, transforming growth factor-β; ECM, extracellular matrix; YAP, Yes-associated protein. TAZ, transcriptional co-activator PDZ-binding motif; TEADs, transcriptionally enhanced associate domains; BMP6, bone morphogenetic protein 6.
Therapeutic implications and strategies
The drugs or compounds highlighted here are required for corresponding to the potential targets we have listed above if clinical progress needs to be achieved. In the iron metabolism, ferrostatin-1 acts as an effective component to resist ferroptosis (141, 142), and can lower TFRC levels (143). It has been shown to protect neural tissue (144–146), heart (147–149), liver (53), lung (150), colon (151) and angiotensin II-induced inflammation (145). To study the mechanism of ovarian granulosa cell injury in patients with PCOS, Shi et al. (152) confirmed that ferrostatin-1 has a protective effect on ovarian granulosa cells by regulating methylation mode through the homocysteine-induced KGN cell injury model. Similarly, liproxstatin-1, another ferroptosis inhibitor (141), has been reported to delay the enucleation of rat embryonic erythrocytes and hinder their maturation (143), possibly due to the high iron requirement of erythrocytes to maintain hemoglobin synthesis (153). In addition, iron chelating agents bind free iron to increase iron storage and thus lower intracellular iron levels. Yun et al. reported that the combined addition of GSH and iron-chelating agent can reduce ROS levels and the death of Chinese hamster ovary cells (154). The effect of hepcidin against ferroptosis is similar to that of ferrostatin-1, it can inhibit ferroptosis induced by acute respiratory distress syndrome (ARDS) via reducing iron uptake (155). Elevated serum hepcidin levels were observed in infertile women (156), as well as the trend of increased BNC1 deficiency in POI (22), suggesting a stress response to the regulation of iron metabolism. Therefore, how to fine-regulate endogenous hepcidin levels seem to be a vital target for the treatment of follicle development disorders caused by disrupted iron metabolism in POI patients. As the main ligand controlling the expression of hepcidin, evidence showed that long-term exogenous administration of BMP6 promoted the expression of endogenous hepcidin to improve the serum hepcidin deficiency and biochemical iron overload in Hfe-/- induced hemochromatosis mice (157). TMPRSS6, a type 2 transmembrane serine protease produced by the liver, negatively regulates the expression of hepcidin through BMP/SMAD pathway and participates in the regulation of iron homeostasis (158). Lipid nanoparticles containing TMPRSS6 siRNA increased the expression of hepcidin in the liver and alleviate iron overload in mice with hereditary hemochromatosis and β thalassemia (159).
Either controlling ECM overproduction or degrading the rigid ovarian cortex is endowed with clinical significance to solve follicle developmental obstruction. The current mainstream approach is to stimulate follicles through mechanical manipulation combined with in vitro activation therapy. POI ovarian cortex is surgically fragmented followed by autologous transplant, or the follicle development can be partially restored by in vitro culture in the presence of protein kinase B (AKT) stimulator before artificial transplant (65). These disrupted procedures, together with activation in vitro, promote actin polymerization and Hippo signal perturbation, followed by follicle growth (65, 81, 83). this is applied for rendering the successful pregnancy to infertile patients (160, 161). Given the presence of preoperative anxiety and fear of anesthesia in patients (162, 163), seeking for new compounds or drugs as an adjunct or even alternative to surgical therapies needs to be addressed. As mentioned above, treatment of mouse ovarian or human granulosa cells with agents that stimulate actin polymerization, such as JASP and/or S1P, increases nuclear YAP localization and downstream CCN growth factor expression, as well as activates follicle growth. In addition, YAP as a susceptibility gene may serve as a reliable target for screening PCOS (21). Since YAP/TAZ nuclear translocation can promote tumor development, dysregulation of YAP/TAZ signal transduction has been an effective drug target for inducing tumor cell apoptosis (164, 165). However, it remains to be seen whether direct YAP/TAZ regulators can be used in the reproductive field. Widely expressed YAP/TAZ requires specific drug-delivering carriers to gonads tissue following exerting its influence. Although YAP/TAZ nuclear translocation promotes follicle activation, excessive follicle activation due to high consumption of this complex may result in irreversible ovarian reserve failure, including POI disease. Therefore, how to delicately balance nucleocytoplasmic shuttling of YAP/TAZ is still an intractable issue on governing follicle development and dormancy. More evidence is needed on ameliorating the rigid ovarian microenvironment to optimize infertile therapy.
The disorder of iron metabolism in follicles is inseparable from the excessive production of oxidative stress products—cytoplasmic ROS, which can be weakened or reversed via being supplemented with antioxidants. Coenzyme Q10 (CoQ10) is an important lipid-soluble antioxidant in the human body (166). Doll et al. (167),reported that the inhibition of ferroptosis via ferroptosis suppressor protein 1 (FSP1) is mediated by CoQ10, which make up for GPX4 deficiency. FSP1 made NAD(P)H catalyze the regeneration of CoQ10. The FSP1-CoQ10-NAD(P)H pathway synergistically works with GPX4 and glutathione to inhibit ferroptosis. Administration of CoQ10 significantly increases the oocyte maturation rate in women aged 38-46 years, with reduced the oocyte aneuploidy rate and chromosome aneuploidy (168). Similarly, a randomized controlled study involving 186 subjects with reduced ovarian reserve demonstrated that CoQ10 supplementation during IVF cycles improved ovarian response, follicles count, and embryo quality (169). Resveratrol (RSV), another natural non-flavonoid polyphenol compound, can improve oocyte chromosome arrangement and spindle morphology, and have a positive effect on oocyte quality and quantity, as well as increase ovarian reserve to prolong ovarian lifespan (170). The recovery effect of RSV on ferroptosis has been widely established (171–174). RSV significantly restored the consumption of exogenous iron on follicle-stimulating hormone (FSH) and luteinizing hormone (LH), and decreased malondialdehyde (MDA, a product of membrane lipid peroxidation positively associated with ferroptosis) content (175). Additionally, it can effectively reduce oxidative stress and apoptosis of granulosa cells and oocytes in rats (176). In the same study, serum MDA was reduced in the resveratrol treatment group (176), indicating its significance in inhibiting iron overload in oocytes. Moreover, melatonin, as a free radical scavenger and broad-spectrum antioxidant produced by the pineal gland, serves an indispensable role in oocyte maturation, embryo development, and luteinization of granulosa cells (177). Similarly, role of melatonin in resisting iron overload has been extensively studied (178–181). Melatonin treatment significantly improves oocyte quality during IVF cycles, possibly by reducing ROS in oocytes and increasing GSH levels, as well as upregulating the expression of key genes being conducive to follicle developmental potentials, such as BMP-15, GDF-9, and GPX4, with down-regulating the expression of caspase-3 and other apoptotic genes (182).
In short, plenty of research on the mechanism of follicle development disorder remains in animal experiments. Iron overload and related molecular signals can be targeted therapeutically through different mechanisms (22) in POI. Although relevant evidence has yet to be investigated, multiple targets may be regulated via rational drug combination; this provides novel enlightenment for the subsequent drug development process.
Summary and outlook
The present review highlights the significance of iron metabolism in follicle development and theorizes the underlying molecular mechanism that lead to follicle development disorders. Clues to the interaction between the Hippo pathway and iron overload sensitivity are integrated into follicle development events mediated by external environmental stimuli (ECM dynamics). Our hypothesis highlights that the combination of targeting TGF-β and iron overload may play a synergistic role in ECM overloading through the YAP/TAZ. In addition, we theorize a dynamic follicular iron homeostasis interacting with YAP, whose overactivation positively increases the risk of ovarian reserve loss and presumably enhances the follicle sensitivity to excess iron. However, the inferences are based on the existing evidence, and follow-up requires persuasive experimental confirmation and discussion. The mechanism of iron metabolic regulation and related signal transduction pathways applying to follicle development needs to be clarified. The corollary that hepcidin signals in response to follicle growth deserve further investigation. Blocking iron excess by various ferroptosis inhibitors or antioxidants may interfere with the progress of follicle dysplasia and may serve as an effective target for reducing adverse outcomes. We believed that YAP is a highly likely target for regulation of iron metabolism addressing ECM overproduction, which may therapeutically provide new insight into follicle developmental disorder. The role of iron in ECM deposition needs to be further determined; this may contribute to developing an iron-related diagnosis, prognosis, and treatment strategies in terms of aberrant follicle development.
Author contributions
LX and YS contributed to manuscript writing and editing. SL and JD revised the manuscript for important intellectual content. All authors listed have made a substantial, direct, and intellectual contribution to the work and approved it for publication.
Conflict of interest
The authors declare that the research was conducted in the absence of any commercial or financial relationships that could be construed as a potential conflict of interest.
Publisher’s note
All claims expressed in this article are solely those of the authors and do not necessarily represent those of their affiliated organizations, or those of the publisher, the editors and the reviewers. Any product that may be evaluated in this article, or claim that may be made by its manufacturer, is not guaranteed or endorsed by the publisher.
References
1. Wang Y, Zhao Y, Ye T, Yang L, Shen Y, Li H. Ferroptosis signaling and regulators in atherosclerosis. Front Cell Dev Biol (2021) 9:809457. doi: 10.3389/fcell.2021.809457
2. Galaris D, Pantopoulos K. Oxidative stress and iron homeostasis: mechanistic and health aspects. Crit Rev Clin Lab Sci (2008) 45(1):1–23. doi: 10.1080/10408360701713104
3. Klóska D, Kopacz A, Piechota-Polańczyk A, Neumayer C, Huk I, Dulak J, et al. Biliverdin reductase deficiency triggers an endothelial-to-mesenchymal transition in human endothelial cells. Arch Biochem Biophys (2019) 678:108182. doi: 10.1016/j.abb.2019.108182
4. Tonai S, Kawabata A, Nakanishi T, Lee JY, Okamoto A, Shimada M, et al. Iron deficiency induces female infertile in order to failure of follicular development in mice. J Reprod Dev (2020) 66(5):475–83. doi: 10.1262/jrd.2020-074
5. Hu W, Zhang Y, Wang D, Yang T, Qi J, Zhang Y, et al. Iron overload-induced ferroptosis impairs porcine oocyte maturation and subsequent embryonic developmental competence in vitro. Front Cell Dev Biol (2021) 9:673291. doi: 10.3389/fcell.2021.673291
6. Zhang H, Panula S, Petropoulos S, Edsgärd D, Busayavalasa K, Liu L, et al. Adult human and mouse ovaries lack DDX4-expressing functional oogonial stem cells. Nat Med (2015) 21(10):1116–8. doi: 10.1038/nm.3775
7. Monget P, McNatty K, Monniaux D. The crazy ovary. Genes (Basel) (2021) 12(6):928. doi: 10.3390/genes12060928
8. Zhang H, Liu K. Cellular and molecular regulation of the activation of mammalian primordial follicles: somatic cells initiate follicle activation in adulthood. Hum Reprod Update (2015) 21(6):779–86. doi: 10.1093/humupd/dmv037
9. Kallen A, Polotsky AJ, Johnson J. Untapped reserves: controlling primordial follicle growth activation. Trends Mol Med (2018) 24(3):319–31. doi: 10.1016/j.molmed.2018.01.008
10. Zhao Y, Feng H, Zhang Y, Zhang JV, Wang X, Liu D, et al. Current understandings of core pathways for the activation of mammalian primordial follicles. Cells (2021) 10(6):1491. doi: 10.3390/cells10061491
11. Chen YY, Russo DD, Drake RS, Duncan FE, Shalek AK, Goods BA, et al. Single-cell transcriptomics of staged oocytes and somatic cells reveal novel regulators of follicle activation. Reprod (Cambridge England) (2022) 164(2):55–70. doi: 10.1530/REP-22-0053
12. Nagyová E, Němcová L, Camaioni A. Cumulus extracellular matrix is an important part of oocyte microenvironment in ovarian follicles: its remodeling and proteolytic degradation. Int J Mol Sci (2021) 23(1):54. doi: 10.3390/ijms23010054
13. Meng XM, Nikolic-Paterson DJ, Lan HY. TGF-β: the master regulator of fibrosis. Nat Rev Nephrol (2016) 12(6):325–38. doi: 10.1038/nrneph.2016.48
14. Casco M, Olsen T, Herbst A, Evans G, Rothermel T, Pruett L, et al. Iron oxide nanoparticles stimulates extra-cellular matrix production in cellular spheroids. Bioengineering (Basel Switzerland) (2017) 4(1):4. doi: 10.3390/bioengineering4010004
15. Nagamatsu G, Shimamoto S, Hamazaki N, Nishimura Y, Hayashi K. Mechanical stress accompanied with nuclear rotation is involved in the dormant state of mouse oocytes. Sci Adv (2019) 5(6):eaav9960. doi: 10.1126/sciadv.aav9960
16. Nagamatsu G. Regulation of primordial follicle formation, dormancy, and activation in mice. J Reprod Dev (2021) 67(3):189–95. doi: 10.1262/jrd.2021-040
17. Ni Z, Li Y, Song D, Ding J, Mei S, Sun S, et al. Iron-overloaded follicular fluid increases the risk of endometriosis-related infertility by triggering granulosa cell ferroptosis and oocyte dysmaturity. Cell Death Dis (2022) 13(7):579. doi: 10.1038/s41419-022-05037-8
18. Hayashi S, Nakamura T, Motooka Y, Ito F, Jiang L, Akatsuka S, et al. Novel ovarian endometriosis model causes infertility via iron-mediated oxidative stress in mice. Redox Biol (2020) 37:101726. doi: 10.1016/j.redox.2020.101726
19. Piccolo S, Dupont S, Cordenonsi M. The biology of YAP/TAZ: hippo signaling and beyond. Physiol Rev (2014) 94(4):1287–312. doi: 10.1152/physrev.00005.2014
20. Hsueh AJW, Kawamura K. Hippo signaling disruption and ovarian follicle activation in infertile patients. Fertility Sterility (2020) 114(3):458–64. doi: 10.1016/j.fertnstert.2020.07.031
21. Li T, Zhao H, Zhao X, Zhang B, Cui L, Shi Y, et al. Identification of YAP1 as a novel susceptibility gene for polycystic ovary syndrome. J Med Genet (2012) 49(4):254–7. doi: 10.1136/jmedgenet-2011-100727
22. Wang F, Liu Y, Ni F, Jin J, Wu Y, Huang Y, et al. BNC1 deficiency-triggered ferroptosis through the NF2-YAP pathway induces primary ovarian insufficiency. Nat Commun (2022) 13(1):5871. doi: 10.1038/s41467-022-33323-8
23. Zhou S, Ma Y, Yao J, Zhao A, Xie C, Mi Y, et al. TGF-β1-induced collagen promotes chicken ovarian follicle development via an intercellular cooperative pattern. Cell Biol Int (2021) 45(6):1336–48. doi: 10.1002/cbin.11580
24. Luo K. Signaling cross talk between TGF-β/Smad and other signaling pathways. Cold Spring Harbor Perspect Biol (2017) 9(1):a022137. doi: 10.1101/cshperspect.a022137
25. Zhang P, Chen L, Zhao Q, Du X, Bi M, Li Y, et al. Ferroptosis was more initial in cell death caused by iron overload and its underlying mechanism in parkinson’s disease. Free Radical Biol Med (2020) 152:227–34. doi: 10.1016/j.freeradbiomed.2020.03.015
26. Yang WS, Stockwell BR. Synthetic lethal screening identifies compounds activating iron-dependent, nonapoptotic cell death in oncogenic-RAS-harboring cancer cells. Chem Biol (2008) 15(3):234–45. doi: 10.1016/j.chembiol.2008.02.010
27. Dixon SJ, Lemberg KM, Lamprecht MR, Skouta R, Zaitsev EM, Gleason CE, et al. Ferroptosis: an iron-dependent form of nonapoptotic cell death. Cell (2012) 149(5):1060–72. doi: 10.1016/j.cell.2012.03.042
28. Kagan VE, Mao G, Qu F, Angeli JP, Doll S, Croix CS, et al. Oxidized arachidonic and adrenic PEs navigate cells to ferroptosis. Nat Chem Biol (2017) 13(1):81–90. doi: 10.1038/nchembio.2238
29. Yang WS, Stockwell BR. Ferroptosis: death by lipid peroxidation. Trends Cell Biol (2016) 26(3):165–76. doi: 10.1016/j.tcb.2015.10.014
30. Sha R, Xu Y, Yuan C, Sheng X, Wu Z, Peng J, et al. Predictive and prognostic impact of ferroptosis-related genes ACSL4 and GPX4 on breast cancer treated with neoadjuvant chemotherapy. EBioMedicine (2021) 71:103560. doi: 10.1016/j.ebiom.2021.103560
31. Shen Y, Li X, Dong D, Zhang B, Xue Y, Shang P. Transferrin receptor 1 in cancer: a new sight for cancer therapy. Am J Cancer Res (2018) 8(6):916–31.
32. Tang LJ, Zhou YJ, Xiong XM, Li NS, Zhang JJ, Luo XJ, et al. Ubiquitin-specific protease 7 promotes ferroptosis via activation of the p53/TfR1 pathway in the rat hearts after ischemia/reperfusion. Free Radical Biol Med (2021) 162:339–52. doi: 10.1016/j.freeradbiomed.2020.10.307
33. Hong X, Roh W, Sullivan RJ, Wong KHK, Wittner BS, Guo H, et al. The lipogenic regulator SREBP2 induces transferrin in circulating melanoma cells and suppresses ferroptosis. Cancer Discov (2021) 11(3):678–95. doi: 10.1158/2159-8290.CD-19-1500
34. Wang Y, Tang M. PM2.5 induces ferroptosis in human endothelial cells through iron overload and redox imbalance. Environ pollut (Barking Essex: 1987) (2019) 254(Pt A):112937. doi: 10.1016/j.envpol.2019.07.105
35. Gao M, Monian P, Quadri N, Ramasamy R, Jiang X. Glutaminolysis and transferrin regulate ferroptosis. Mol Cell (2015) 59(2):298–308. doi: 10.1016/j.molcel.2015.06.011
36. Poznyak AV, Grechko AV, Orekhova VA, Khotina V, Ivanova EA, Orekhov AN. NADPH oxidases and their role in atherosclerosis. Biomedicines (2020) 8(7):206. doi: 10.3390/biomedicines8070206
37. Yang WS, SriRamaratnam R, Welsch ME, Shimada K, Skouta R, Viswanathan VS, et al. Regulation of ferroptotic cancer cell death by GPX4. Cell (2014) 156(1-2):317–31. doi: 10.1016/j.cell.2013.12.010
38. Ursini F, Maiorino M. Lipid peroxidation and ferroptosis: the role of GSH and GPx4. Free Radical Biol Med (2020) 152:175–85. doi: 10.1016/j.freeradbiomed.2020.02.027
39. Zhang X, Huang Z, Xie Z, Chen Y, Zheng Z, Wei X, et al. Homocysteine induces oxidative stress and ferroptosis of nucleus pulposus via enhancing methylation of GPX4. Free Radical Biol Med (2020) 160:552–65. doi: 10.1016/j.freeradbiomed.2020.08.029
40. Zhang J, Liu Y, Yao W, Li Q, Liu H, Pan Z. Initiation of follicular atresia: gene networks during early atresia in pig ovaries. Reprod (Cambridge England) (2018) 156(1):23–33. doi: 10.1530/REP-18-0058
41. Li A, Ni Z, Zhang J, Cai Z, Kuang Y, Yu C. Transferrin insufficiency and iron overload in follicular fluid contribute to oocyte dysmaturity in infertile women with advanced endometriosis. Front Endocrinol (2020) 11:391. doi: 10.3389/fendo.2020.00391
42. Tripathi A, Khatun S, Pandey AN, Mishra SK, Chaube R, Shrivastav TG, et al. Intracellular levels of hydrogen peroxide and nitric oxide in oocytes at various stages of meiotic cell cycle and apoptosis. Free Radical Res (2009) 43(3):287–94. doi: 10.1080/10715760802695985
43. Tiwari M, Prasad S, Shrivastav TG, Chaube SK. Calcium signaling during meiotic cell cycle regulation and apoptosis in mammalian oocytes. J Cell Physiol (2017) 232(5):976–81. doi: 10.1002/jcp.25670
44. Yu S, Zhao Y, Feng Y, Zhang H, Li L, Shen W, et al. β-carotene improves oocyte development and maturation under oxidative stress in vitro. In Vitro Cell Dev Biol Anim (2019) 55(7):548–58. doi: 10.1007/s11626-019-00373-0
45. Zhou C, Zhang X, Chen Y, Liu X, Sun Y, Xiong B. Glutathione alleviates the cadmium exposure-caused porcine oocyte meiotic defects via eliminating the excessive ROS. Environ pollut (Barking Essex: 1987) (2019) 255(Pt 1):113194. doi: 10.1016/j.envpol.2019.113194
46. Xiao Y, Yuan B, Hu W, Qi J, Jiang H, Sun B, et al. Tributyltin oxide exposure during in vitro maturation disrupts oocyte maturation and subsequent embryonic developmental competence in pigs. Front Cell Dev Biol (2021) 9:683448. doi: 10.3389/fcell.2021.683448
47. Singer ST, Sweeters N, Vega O, Higa A, Vichinsky E, Cedars M. Fertility potential in thalassemia major women: current findings and future diagnostic tools. Ann New York Acad Sci (2010) 1202:226–30. doi: 10.1111/j.1749-6632.2010.05583.x
48. Uysal A, Alkan G, Kurtoğlu A, Erol O, Kurtoğlu E. Diminished ovarian reserve in women with transfusion-dependent beta-thalassemia major: is iron gonadotoxic? Eur J obstetrics gynecol Reprod Biol (2017) 216:69–73. doi: 10.1016/j.ejogrb.2017.06.038
49. European Society for Human Reproduction and Embryology (ESHRE) Guideline Group on POI; , Webber L, Davies M, Anderson R, Bartlett J, Braat D, et al. ESHRE guideline: management of women with premature ovarian insufficiency. Hum Reprod (2016) 31(5):926–37. doi: 10.1093/humrep/dew027
50. Cattoni A, Parissone F, Porcari I, Molinari S, Masera N, Franchi M, et al. Hormonal replacement therapy in adolescents and young women with chemo- or radio-induced premature ovarian insufficiency: practical recommendations. Blood Rev (2021) 45:100730. doi: 10.1016/j.blre.2020.100730
51. Spears N, Lopes F, Stefansdottir A, Rossi V, De Felici M, Anderson RA, et al. Ovarian damage from chemotherapy and current approaches to its protection. Hum Reprod Update (2019) 25(6):673–93. doi: 10.1093/humupd/dmz027
52. Bedoschi G, Navarro PA, Oktay K. Chemotherapy-induced damage to ovary: mechanisms and clinical impact. Future Oncol (London England) (2016) 12(20):2333–44. doi: 10.2217/fon-2016-0176
53. Yu Y, Jiang L, Wang H, Shen Z, Cheng Q, Zhang P, et al. Hepatic transferrin plays a role in systemic iron homeostasis and liver ferroptosis. Blood (2020) 136(6):726–39. doi: 10.1182/blood.2019002907
54. Zhang Y, Mou Y, Zhang J, Suo C, Zhou H, Gu M, et al. Therapeutic implications of ferroptosis in renal fibrosis. Front Mol Biosci (2022) 9:890766. doi: 10.3389/fmolb.2022.890766
55. Du R, Cheng X, Ji J, Lu Y, Xie Y, Wang W, et al. Mechanism of ferroptosis in a rat model of premature ovarian insufficiency induced by cisplatin. Sci Rep (2023) 13(1):4463. doi: 10.1038/s41598-023-31712-7
56. Palomba S, de Wilde MA, Falbo A, Koster MP, La Sala GB, Fauser BC. Pregnancy complications in women with polycystic ovary syndrome. Hum Reprod Update (2015) 21(5):575–92. doi: 10.1093/humupd/dmv029
57. Azziz R. Polycystic ovary syndrome. Obstetrics gynecol (2018) 132(2):321–36. doi: 10.1097/AOG.0000000000002698
58. Lai Q, Xiang W, Li Q, Zhang H, Li Y, Zhu G, et al. Oxidative stress in granulosa cells contributes to poor oocyte quality and IVF-ET outcomes in women with polycystic ovary syndrome. Front Med (2018) 12(5):518–24. doi: 10.1007/s11684-017-0575-y
59. Bañuls C, Rovira-Llopis S, Martinez de Marañon A, Veses S, Jover A, Gomez M, et al. Metabolic syndrome enhances endoplasmic reticulum, oxidative stress and leukocyte-endothelium interactions in PCOS. Metabol: Clin Exp (2017) 71:153–62. doi: 10.1016/j.metabol.2017.02.012
60. Zhang Y, Hu M, Jia W, Liu G, Zhang J, Wang B, et al. Hyperandrogenism and insulin resistance modulate gravid uterine and placental ferroptosis in PCOS-like rats. J Endocrinol (2020) 246(3):247–63. doi: 10.1530/JOE-20-0155
61. Kim JW, Kang KM, Yoon TK, Shim SH, Lee WS. Study of circulating hepcidin in association with iron excess, metabolic syndrome, and BMP-6 expression in granulosa cells in women with polycystic ovary syndrome. Fertility sterility (2014) 102(2):548–554.e542. doi: 10.1016/j.fertnstert.2014.04.031
62. Yin J, Hong X, Ma J, Bu Y, Liu R. Serum trace elements in patients with polycystic ovary syndrome: a systematic review and meta-analysis. Front Endocrinol (2020) 11:572384. doi: 10.3389/fendo.2020.572384
63. Diamanti-Kandarakis E, Dunaif A. Insulin resistance and the polycystic ovary syndrome revisited: an update on mechanisms and implications. Endoc Rev (2012) 33(6):981–1030. doi: 10.1210/er.2011-1034
64. Vo KCT, Kawamura K. In vitro activation early follicles: from the basic science to the clinical perspectives. Int J Mol Sci (2021) 22(7):3785. doi: 10.3390/ijms22073785
65. Kawamura K, Cheng Y, Suzuki N, Deguchi M, Sato Y, Takae S, et al. Hippo signaling disruption and akt stimulation of ovarian follicles for infertility treatment. Proc Natl Acad Sci United States America (2013) 110(43):17474–9. doi: 10.1073/pnas.1312830110
66. Xiang C, Li J, Hu L, Huang J, Luo T, Zhong Z, et al. Hippo signaling pathway reveals a spatio-temporal correlation with the size of primordial follicle pool in mice. Cell Physiol biochem: Int J Exp Cell physiol biochem Pharmacol (2015) 35(3):957–68. doi: 10.1159/000369752
67. Abbassi L, Malki S, Cockburn K, Macaulay A, Robert C, Rossant J, et al. Multiple mechanisms cooperate to constitutively exclude the transcriptional Co-activator YAP from the nucleus during murine oogenesis. Biol Reprod (2016) 94(5):102. doi: 10.1095/biolreprod.115.137968
68. Hu LL, Su T, Luo RC, Zheng YH, Huang J, Zhong ZS, et al. Hippo pathway functions as a downstream effector of AKT signaling to regulate the activation of primordial follicles in mice. J Cell Physiol (2019) 234(2):1578–87. doi: 10.1002/jcp.27024
69. Pisarska MD, Kuo FT, Bentsi-Barnes IK, Khan S, Barlow GM. LATS1 phosphorylates forkhead L2 and regulates its transcriptional activity. Am J Physiol Endocrinol Metab (2010) 299(1):E101–109. doi: 10.1152/ajpendo.00534.2009
70. Sun T, Pepling ME, Diaz FJ. Lats1 deletion causes increased germ cell apoptosis and follicular cysts in mouse ovaries. Biol Reprod (2015) 93(1):22. doi: 10.1095/biolreprod.114.118604
71. Bernabé BP, Woodruff T, Broadbelt LJ, Shea LD. Ligands, receptors, and transcription factors that mediate inter-cellular and intra-cellular communication during ovarian follicle development. Reprod Sci (Thousand Oaks Calif) (2020) 27(2):690–703. doi: 10.1007/s43032-019-00075-8
72. Clark KL, George JW, Przygrodzka E, Plewes MR, Hua G, Wang C, et al. Hippo signaling in the ovary: emerging roles in development, fertility, and disease. Endoc Rev (2022) 43(6):1074–96. doi: 10.1210/endrev/bnac013
73. Lv X, He C, Huang C, Wang H, Hua G, Wang Z, et al. Timely expression and activation of YAP1 in granulosa cells is essential for ovarian follicle development. FASEB J (2019) 33(9):10049–64. doi: 10.1096/fj.201900179RR
74. Nagashima T, Kim J, Li Q, Lydon JP, DeMayo FJ, Lyons KM, et al. Connective tissue growth factor is required for normal follicle development and ovulation. Mol Endocrinol (Baltimore Md) (2011) 25(10):1740–59. doi: 10.1210/me.2011-1045
75. Shi Y, Zhao H, Shi Y, Cao Y, Yang D, Li Z, et al. Genome-wide association study identifies eight new risk loci for polycystic ovary syndrome. Nat Genet (2012) 44(9):1020–5. doi: 10.1038/ng.2384
76. Jiang LL, Xie JK, Cui JQ, Wei D, Yin BL, Zhang YN, et al. Promoter methylation of yes-associated protein (YAP1) gene in polycystic ovary syndrome. Medicine (2017) 96(2):e5768. doi: 10.1097/MD.0000000000005768
77. Dupont S, Morsut L, Aragona M, Enzo E, Giulitti S, Cordenonsi M, et al. Role of YAP/TAZ in mechanotransduction. Nature (2011) 474(7350):179–83. doi: 10.1038/nature10137
78. Berkholtz CB, Shea LD, Woodruff TK. Extracellular matrix functions in follicle maturation. Semin Reprod Med (2006) 24(4):262–9. doi: 10.1055/s-2006-948555
79. Fiorentino G, Cimadomo D, Innocenti F, Soscia D, Vaiarelli A, Ubaldi FM, et al. Biomechanical forces and signals operating in the ovary during folliculogenesis and their dysregulation: implications for fertility. Hum Reprod Update (2023) 29(1):1–23. doi: 10.1093/humupd/dmac031
80. Ouni E, Bouzin C, Dolmans MM, Marbaix E, Pyr Dit Ruys S, Vertommen D, et al. Spatiotemporal changes in mechanical matrisome components of the human ovary from prepuberty to menopause. Hum Reprod (Oxford England) (2020) 35(6):1391–410. doi: 10.1093/humrep/deaa100
81. Díaz-García C, Herraiz S, Pamplona L, Subirá J, Soriano MJ, Simon C, et al. Follicular activation in women previously diagnosed with poor ovarian response: a randomized, controlled trial. Fertility Sterility (2022) 117(4):747–55. doi: 10.1016/j.fertnstert.2021.12.034
82. Seo J, Kim J. Regulation of hippo signaling by actin remodeling. BMB Rep (2018) 51(3):151–6. doi: 10.5483/BMBRep.2018.51.3.012
83. Cheng Y, Feng Y, Jansson L, Sato Y, Deguchi M, Kawamura K, et al. Actin polymerization-enhancing drugs promote ovarian follicle growth mediated by the hippo signaling effector YAP. FASEB J (2015) 29(6):2423–30. doi: 10.1096/fj.14-267856
84. Yang Q, Zhu L, Jin L. Human follicle in vitro culture including activation, growth, and maturation: a review of research progress. Front Endocrinol (2020) 11:548. doi: 10.3389/fendo.2020.00548
85. Lunding SA, Andersen AN, Hardardottir L, Olesen H, Kristensen SG, Andersen CY, et al. Hippo signaling, actin polymerization, and follicle activation in fragmented human ovarian cortex. Mol Reprod Dev (2020) 87(6):711–9. doi: 10.1002/mrd.23353
86. Sansores-Garcia L, Bossuyt W, Wada K, Yonemura S, Tao C, Sasaki H, et al. Modulating f-actin organization induces organ growth by affecting the hippo pathway. EMBO J (2011) 30(12):2325–35. doi: 10.1038/emboj.2011.157
87. Li F, Turan V, Lierman S, Cuvelier C, De Sutter P, Oktay K. Sphingosine-1-phosphate prevents chemotherapy-induced human primordial follicle death. Hum Reprod (Oxford England) (2014) 29(1):107–13. doi: 10.1093/humrep/det391
88. Henry L, Fransolet M, Labied S, Blacher S, Masereel MC, Foidart JM, et al. Supplementation of transport and freezing media with anti-apoptotic drugs improves ovarian cortex survival. J Ovarian Res (2016) 9:4. doi: 10.1186/s13048-016-0216-0
89. Guzel Y, Bildik G, Oktem O. Sphingosine-1-phosphate protects human ovarian follicles from apoptosis in vitro. Eur J Obstetrics Gynecol Reprod Biol (2018) 222:19–24. doi: 10.1016/j.ejogrb.2018.01.001
90. Nestor-Bergmann A, Stooke-Vaughan GA, Goddard GK, Starborg T, Jensen OE, Woolner S. Decoupling the roles of cell shape and mechanical stress in orienting and cueing epithelial mitosis. Cell Rep (2019) 26(8):2088–2100.e2084. doi: 10.1016/j.celrep.2019.01.102
91. Molnar K, Labouesse M. The plastic cell: mechanical deformation of cells and tissues. Open Biol (2021) 11(2):210006. doi: 10.1098/rsob.210006
92. Weintraub LR, Goral A, Grasso J, Franzblau C, Sullivan A, Sullivan S. Collagen biosynthesis in iron overload. Ann New York Acad Sci (1988) 526:179–84. doi: 10.1111/j.1749-6632.1988.tb55504.x
93. Bunda S, Kaviani N, Hinek A. Fluctuations of intracellular iron modulate elastin production. J Biol Chem (2005) 280(3):2341–51. doi: 10.1074/jbc.M409897200
94. Templeton DM, Liu Y. Genetic regulation of cell function in response to iron overload or chelation. Biochim Biophys Acta (2003) 1619(2):113–24. doi: 10.1016/S0304-4165(02)00497-X
95. Mehta KJ, Farnaud SJ, Sharp PA. Iron and liver fibrosis: mechanistic and clinical aspects. World J Gastroenterol (2019) 25(5):521–38. doi: 10.3748/wjg.v25.i5.521
96. Philippe MA, Ruddell RG, Ramm GA. Role of iron in hepatic fibrosis: one piece in the puzzle. World J Gastroenterol (2007) 13(35):4746–54. doi: 10.3748/wjg.v13.i35.4746
97. Zhu Y, Zhang C, Huang M, Lin J, Fan X, Ni T. TRIM26 induces ferroptosis to inhibit hepatic stellate cell activation and mitigate liver fibrosis through mediating SLC7A11 ubiquitination. Front Cell Dev Biol (2021) 9:644901. doi: 10.3389/fcell.2021.644901
98. Yuan S, Wei C, Liu G, Zhang L, Li J, Li L, et al. Sorafenib attenuates liver fibrosis by triggering hepatic stellate cell ferroptosis via HIF-1α/SLC7A11 pathway. Cell Prolif (2022) 55(1):e13158. doi: 10.1111/cpr.13158
99. Carthew P, Edwards RE, Smith AG, Dorman B, Francis JE. Rapid induction of hepatic fibrosis in the gerbil after the parenteral administration of iron-dextran complex. Hepatol (Baltimore Md) (1991) 13(3):534–9. doi: 10.1002/hep.1840130322
100. Houglum K, Bedossa P, Chojkier M. TGF-beta and collagen-alpha 1 (I) gene expression are increased in hepatic acinar zone 1 of rats with iron overload. Am J Physiol (1994) 267(5 Pt 1):G908–913. doi: 10.1152/ajpgi.1994.267.5.G908
101. Honda T, Fujiwara H, Yoshioka S, Yamada S, Nakayama T, Egawa M, et al. Laminin and fibronectin concentrations of the follicular fluid correlate with granulosa cell luteinization and oocyte quality. Reprod Med Biol (2004) 3(1):43–9. doi: 10.1111/j.1447-0578.2004.00051.x
102. Chen X, Bonfiglio R, Banerji S, Jackson DG, Salustri A, Richter RP. Micromechanical analysis of the hyaluronan-rich matrix surrounding the oocyte reveals a uniquely soft and elastic composition. Biophys J (2016) 110(12):2779–89. doi: 10.1016/j.bpj.2016.03.023
103. Lo BKM, Archibong-Omon A, Ploutarchou P, Day AJ, Milner CM, Williams SA. Oocyte-specific ablation of n- and O-glycans alters cumulus cell signalling and extracellular matrix composition. Reproduction fertility Dev (2019) 31(3):529–37. doi: 10.1071/RD18209
104. Hung TT, Tsuiki A, Yemini M. Fibronectin in reproduction. Steroids (1989) 54(6):575–82. doi: 10.1016/0039-128X(89)90081-0
105. Alahari S, Farrell A, Ermini L, Park C, Sallais J, Roberts S, et al. JMJD6 dysfunction due to iron deficiency in preeclampsia disrupts fibronectin homeostasis resulting in diminished trophoblast migration. Front Cell Dev Biol (2021) 9:652607. doi: 10.3389/fcell.2021.652607
106. Kitasaka H, Kawai T, Hoque SAM, Umehara T, Fujita Y, Shimada M. Inductions of granulosa cell luteinization and cumulus expansion are dependent on the fibronectin-integrin pathway during ovulation process in mice. PloS One (2018) 13(2):e0192458. doi: 10.1371/journal.pone.0192458
107. Sun Z, Guo SS, Fässler R. Integrin-mediated mechanotransduction. J Cell Biol (2016) 215(4):445–56. doi: 10.1083/jcb.201609037
108. Brown CW, Amante JJ, Goel HL, Mercurio AM. The α6β4 integrin promotes resistance to ferroptosis. J Cell Biol (2017) 216(12):4287–97. doi: 10.1083/jcb.201701136
109. Brown CW, Amante JJ, Mercurio AM. Cell clustering mediated by the adhesion protein PVRL4 is necessary for α6β4 integrin-promoted ferroptosis resistance in matrix-detached cells. J Biol Chem (2018) 293(33):12741–8. doi: 10.1074/jbc.RA118.003017
110. Brown CW, Amante JJ, Chhoy P, Elaimy AL, Liu H, Zhu LJ, et al. Prominin2 drives ferroptosis resistance by stimulating iron export. Dev Cell (2019) 51(5):575–586.e574. doi: 10.1016/j.devcel.2019.10.007
111. Lee EH, Baek SY, Park JY, Kim YW. Emodin in rheum undulatum inhibits oxidative stress in the liver via AMPK with Hippo/Yap signalling pathway. Pharm Biol (2020) 58(1):333–41. doi: 10.1080/13880209.2020.1750658
112. Wu J, Minikes AM, Gao M, Bian H, Li Y, Stockwell BR, et al. Intercellular interaction dictates cancer cell ferroptosis via NF2-YAP signalling. Nature (2019) 572(7769):402–6. doi: 10.1038/s41586-019-1426-6
113. Zhu G, Murshed A, Li H, Ma J, Zhen N, Ding M, et al. O-GlcNAcylation enhances sensitivity to RSL3-induced ferroptosis via the YAP/TFRC pathway in liver cancer. Cell Death Discov (2021) 7(1):83. doi: 10.1038/s41420-021-00468-2
114. Balboni GC, Vannelli GB, Barni T, Orlando C, Serio M. Transferrin and somatomedin c receptors in the human ovarian follicles. Fertility sterility (1987) 48(5):796–801. doi: 10.1016/S0015-0282(16)59533-8
115. Bretscher MS. Expression and changing distribution of the human transferrin receptor in developing drosophila oocytes and embryos. J Cell Sci (1996) 109(Pt 13):3113–9. doi: 10.1242/jcs.109.13.3113
116. Burch MG, Li C, Albrecht ED, Pepe GJ. Developmental regulation of the expression of the transferrin receptor and Ki67 in oocytes of the baboon fetal ovary by estrogen. Endocrine (2009) 35(2):177–83. doi: 10.1007/s12020-008-9133-3
117. Sanchez AM, Papaleo E, Corti L, Santambrogio P, Levi S, Viganò P, et al. Iron availability is increased in individual human ovarian follicles in close proximity to an endometrioma compared with distal ones. Hum Reprod (Oxford England) (2014) 29(3):577–83. doi: 10.1093/humrep/det466
118. Yang WH, Ding CC, Sun T, Rupprecht G, Lin CC, Hsu D, et al. The hippo pathway effector TAZ regulates ferroptosis in renal cell carcinoma. Cell Rep (2019) 28(10):2501–8.e2504. doi: 10.1016/j.celrep.2019.07.107
119. Yang WH, Huang Z, Wu J, Ding CC, Murphy SK, Chi JT. A TAZ-ANGPTL4-NOX2 axis regulates ferroptotic cell death and chemoresistance in epithelial ovarian cancer. Mol Cancer res: MCR (2020) 18(1):79–90. doi: 10.1158/1541-7786.MCR-19-0691
120. Yu HF, Zheng LW, Yang ZQ, Wang YS, Wang TT, Yue ZP, et al. TAZ as a novel regulator of oxidative damage in decidualization via Nrf2/ARE/Foxo1 pathway. Exp Mol Med (2021) 53(9):1307–18. doi: 10.1038/s12276-021-00655-2
121. Maraldi T, Resca E, Nicoli A, Beretti F, Zavatti M, Capodanno F, et al. NADPH oxidase-4 and MATER expressions in granulosa cells: relationships with ovarian aging. Life Sci (2016) 162:108–14. doi: 10.1016/j.lfs.2016.08.007
122. Chen Q, Zhang W, Ran H, Feng L, Yan H, Mu X, et al. PKCδ and θ possibly mediate FSH-induced mouse oocyte maturation via NOX-ROS-TACE cascade signaling pathway. PloS One (2014) 9(10):e111423. doi: 10.1371/journal.pone.0111423
123. Knight PG, Glister C. TGF-beta superfamily members and ovarian follicle development. Reprod (Cambridge England) (2006) 132(2):191–206. doi: 10.1530/rep.1.01074
124. Massagué J. TGFβ signalling in context. Nat Rev Mol Cell Biol (2012) 13(10):616–30. doi: 10.1038/nrm3434
125. Varelas X, Sakuma R, Samavarchi-Tehrani P, Peerani R, Rao BM, Dembowy J, et al. TAZ controls smad nucleocytoplasmic shuttling and regulates human embryonic stem-cell self-renewal. Nat Cell Biol (2008) 10(7):837–48. doi: 10.1038/ncb1748
126. Parrow NL, Fleming RE. Bone morphogenetic proteins as regulators of iron metabolism. Annu Rev Nutr (2014) 34:77–94. doi: 10.1146/annurev-nutr-071813-105646
127. Saito A, Nagase T. Hippo and TGF-β interplay in the lung field. Am J Physiol Lung Cell Mol Physiol (2015) 309(8):L756–767. doi: 10.1152/ajplung.00238.2015
128. Alarcón C, Zaromytidou AI, Xi Q, Gao S, Yu J, Fujisawa S, et al. Nuclear CDKs drive smad transcriptional activation and turnover in BMP and TGF-beta pathways. Cell (2009) 139(4):757–69. doi: 10.1016/j.cell.2009.09.035
129. Schindler R, Nilsson E, Skinner MK. Induction of ovarian primordial follicle assembly by connective tissue growth factor CTGF. PloS One (2010) 5(9):e12979. doi: 10.1371/journal.pone.0012979
130. Wang DH, Ren J, Zhou CJ, Han Z, Wang L, Liang CG. Supplementation with CTGF, SDF1, NGF, and HGF promotes ovine in vitro oocyte maturation and early embryo development. Domest Anim Endocrinol (2018) 65:38–48. doi: 10.1016/j.domaniend.2018.05.003
131. Pei Z, Qin Y, Fu X, Yang F, Huo F, Liang X, et al. Inhibition of ferroptosis and iron accumulation alleviates pulmonary fibrosis in a bleomycin model. Redox Biol (2022) 57:102509. doi: 10.1016/j.redox.2022.102509
132. Li H, Chang HM, Shi Z, Leung PCK. The p38 signaling pathway mediates the TGF-β1-induced increase in type I collagen deposition in human granulosa cells. FASEB J (2020) 34(11):15591–604. doi: 10.1096/fj.202001377R
133. Noguchi S, Saito A, Nagase T. YAP/TAZ signaling as a molecular link between fibrosis and cancer. Int J Mol Sci (2018) 19(11):3674. doi: 10.3390/ijms19113674
134. Piersma B, Bank RA, Boersema M. Signaling in fibrosis: TGF-β, WNT, and YAP/TAZ converge. Front Med (2015) 2:59. doi: 10.3389/fmed.2015.00059
135. Elosegui-Artola A, Andreu I, Beedle AEM, Lezamiz A, Uroz M, Kosmalska AJ, et al. Force triggers YAP nuclear entry by regulating transport across nuclear pores. Cell (2017) 171(6):1397–1410.e1314. doi: 10.1016/j.cell.2017.10.008
136. Briggs DA, Sharp DJ, Miller D, Gosden RG. Transferrin in the developing ovarian follicle: evidence for de-novo expression by granulosa cells. Mol Hum Reprod (1999) 5(12):1107–14. doi: 10.1093/molehr/5.12.1107
137. Shaeib F, Banerjee J, Maitra D, Diamond MP, Abu-Soud HM. Impact of hydrogen peroxide-driven fenton reaction on mouse oocyte quality. Free Radical Biol Med (2013) 58:154–9. doi: 10.1016/j.freeradbiomed.2012.12.007
138. Máté G, Bernstein LR, Török AL. Endometriosis is a cause of infertility. does reactive oxygen damage to gametes and embryos play a key role in the pathogenesis of infertility caused by endometriosis? Front Endocrinol (2018) 9:725. doi: 10.3389/fendo.2018.00725
139. Silvestri L, Nai A, Dulja A, Pagani A. Hepcidin and the BMP-SMAD pathway: an unexpected liaison. Vitamins hormones (2019) 110:71–99. doi: 10.1016/bs.vh.2019.01.004
140. Hoffmann A, de Souza LV, Seifert M, von Raffay L, Haschka D, Grubwieser P, et al. Pharmacological targeting of BMP6-SMAD mediated hepcidin expression does not improve the outcome of systemic infections with intra-or extracellular gram-negative bacteria in mice. Front Cell infection Microbiol (2021) 11:705087. doi: 10.3389/fcimb.2021.705087
141. Zilka O, Shah R, Li B, Friedmann Angeli JP, Griesser M, Conrad M, et al. On the mechanism of cytoprotection by ferrostatin-1 and liproxstatin-1 and the role of lipid peroxidation in ferroptotic cell death. ACS Cent Sci (2017) 3(3):232–43. doi: 10.1021/acscentsci.7b00028
142. Miotto G, Rossetto M, Di Paolo ML, Orian L, Venerando R, Roveri A, et al. Insight into the mechanism of ferroptosis inhibition by ferrostatin-1. Redox Biol (2020) 28:101328. doi: 10.1016/j.redox.2019.101328
143. Zheng H, Jiang L, Tsuduki T, Conrad M, Toyokuni S. Embryonal erythropoiesis and aging exploit ferroptosis. Redox Biol (2021) 48:102175. doi: 10.1016/j.redox.2021.102175
144. Chu J, Liu CX, Song R, Li QL. Ferrostatin-1 protects HT-22 cells from oxidative toxicity. Neural Regener Res (2020) 15(3):528–36. doi: 10.4103/1673-5374.266060
145. Li S, Zhou C, Zhu Y, Chao Z, Sheng Z, Zhang Y, et al. Ferrostatin-1 alleviates angiotensin II (Ang II)- induced inflammation and ferroptosis in astrocytes. Int Immunopharmacol (2021) 90:107179. doi: 10.1016/j.intimp.2020.107179
146. Liang J, Shen Y, Wang Y, Huang Y, Wang J, Zhu Q, et al. Ferroptosis participates in neuron damage in experimental cerebral malaria and is partially induced by activated CD8(+) T cells. Mol Brain (2022) 15(1):57. doi: 10.1186/s13041-022-00942-7
147. Wu X, Li Y, Zhang S, Zhou X. Ferroptosis as a novel therapeutic target for cardiovascular disease. Theranostics (2021) 11(7):3052–9. doi: 10.7150/thno.54113
148. Li X, Ma N, Xu J, Zhang Y, Yang P, Su X, et al. Targeting ferroptosis: pathological mechanism and treatment of ischemia-reperfusion injury. Oxid Med Cell Longevity (2021) 2021:1587922. doi: 10.1155/2021/1587922
149. Xiao Z, Kong B, Fang J, Qin T, Dai C, Shuai W, et al. Ferrostatin-1 alleviates lipopolysaccharide-induced cardiac dysfunction. Bioengineered (2021) 12(2):9367–76. doi: 10.1080/21655979.2021.2001913
150. Liu P, Feng Y, Li H, Chen X, Wang G, Xu S, et al. Ferrostatin-1 alleviates lipopolysaccharide-induced acute lung injury via inhibiting ferroptosis. Cell Mol Biol Lett (2020) 25:10. doi: 10.1186/s11658-020-00205-0
151. Xu J, Liu S, Cui Z, Wang X, Ning T, Wang T, et al. Ferrostatin-1 alleviated TNBS induced colitis via the inhibition of ferroptosis. Biochem Biophys Res Commun (2021) 573:48–54. doi: 10.1016/j.bbrc.2021.08.018
152. Shi Q, Liu R, Chen L. Ferroptosis inhibitor ferrostatin−1 alleviates homocysteine−induced ovarian granulosa cell injury by regulating TET activity and DNA methylation. Mol Med Rep (2022) 25(4):130. doi: 10.3892/mmr.2022.12645
153. Muckenthaler MU, Rivella S, Hentze MW, Galy B. A red carpet for iron metabolism. Cell (2017) 168(3):344–61. doi: 10.1016/j.cell.2016.12.034
154. Yun Z, Takagi M, Yoshida T. Combined addition of glutathione and iron chelators for decrease of intracellular level of reactive oxygen species and death of Chinese hamster ovary cells. J Biosci Bioeng (2003) 95(2):124–7.
155. Jiao Y, Yong C, Zhang R, Qi D, Wang D. Hepcidin alleviates LPS-induced ARDS by regulating the ferritin-mediated suppression of ferroptosis. Shock (2022) 57(6):274–81. doi: 10.1097/SHK.0000000000001941
156. Moreno-Navarrete JM, López-Navarro E, Candenas L, Pinto F, Ortega FJ, Sabater-Masdeu M, et al. Ferroportin mRNA is down-regulated in granulosa and cervical cells from infertile women. Fertility sterility (2017) 107(1):236–42. doi: 10.1016/j.fertnstert.2016.10.008
157. Corradini E, Schmidt PJ, Meynard D, Garuti C, Montosi G, Chen S, et al. BMP6 treatment compensates for the molecular defect and ameliorates hemochromatosis in hfe knockout mice. Gastroenterology (2010) 139(5):1721–9. doi: 10.1053/j.gastro.2010.07.044
158. Lee P. Role of matriptase-2 (TMPRSS6) in iron metabolism. Acta Haematol (2009) 122(2-3):87–96. doi: 10.1159/000243792
159. Schmidt PJ, Toudjarska I, Sendamarai AK, Racie T, Milstein S, Bettencourt BR, et al. An RNAi therapeutic targeting Tmprss6 decreases iron overload in hfe(-/-) mice and ameliorates anemia and iron overload in murine β-thalassemia intermedia. Blood (2013) 121(7):1200–8. doi: 10.1182/blood-2012-09-453977
160. Suzuki N, Yoshioka N, Takae S, Sugishita Y, Tamura M, Hashimoto S, et al. Successful fertility preservation following ovarian tissue vitrification in patients with primary ovarian insufficiency. Hum Reprod (Oxford England) (2015) 30(3):608–15. doi: 10.1093/humrep/deu353
161. Zhai J, Yao G, Dong F, Bu Z, Cheng Y, Sato Y, et al. In vitro activation of follicles and fresh tissue auto-transplantation in primary ovarian insufficiency patients. J Clin Endocrinol Metab (2016) 101(11):4405–12. doi: 10.1210/jc.2016-1589
162. Aust H, Eberhart L, Sturm T, Schuster M, Nestoriuc Y, Brehm F, et al. A cross-sectional study on preoperative anxiety in adults. J psychosomatic Res (2018) 111:133–9. doi: 10.1016/j.jpsychores.2018.05.012
163. Eberhart L, Aust H, Schuster M, Sturm T, Gehling M, Euteneuer F, et al. Preoperative anxiety in adults - a cross-sectional study on specific fears and risk factors. BMC Psychiatry (2020) 20(1):140. doi: 10.1186/s12888-020-02552-w
164. Andl T, Zhou L, Yang K, Kadekaro AL, Zhang Y. YAP and WWTR1: new targets for skin cancer treatment. Cancer Lett (2017) 396:30–41. doi: 10.1016/j.canlet.2017.03.001
165. Nguyen CDK, Yi C. YAP/TAZ signaling and resistance to cancer therapy. Trends Cancer (2019) 5(5):283–96. doi: 10.1016/j.trecan.2019.02.010
166. Hargreaves I, Heaton RA, Mantle D. Disorders of human coenzyme Q10 metabolism: an overview. Int J Mol Sci (2020) 21(18):6695. doi: 10.3390/ijms21186695
167. Doll S, Freitas FP, Shah R, Aldrovandi M, da Silva MC, Ingold I, et al. FSP1 is a glutathione-independent ferroptosis suppressor. Nature (2019) 575(7784):693–8. doi: 10.1038/s41586-019-1707-0
168. Ma L, Cai L, Hu M, Wang J, Xie J, Xing Y, et al. Coenzyme Q10 supplementation of human oocyte in vitro maturation reduces postmeiotic aneuploidies. Fertility sterility (2020) 114(2):331–7. doi: 10.1016/j.fertnstert.2020.04.002
169. Xu Y, Nisenblat V, Lu C, Li R, Qiao J, Zhen X, et al. Pretreatment with coenzyme Q10 improves ovarian response and embryo quality in low-prognosis young women with decreased ovarian reserve: a randomized controlled trial. Reprod Biol endocrinol: RB&E (2018) 16(1):29. doi: 10.1186/s12958-018-0343-0
170. Liu M, Yin Y, Ye X, Zeng M, Zhao Q, Keefe DL, et al. Resveratrol protects against age-associated infertility in mice. Hum Reprod (Oxford England) (2013) 28(3):707–17. doi: 10.1093/humrep/des437
171. Zhang X, Jiang L, Chen H, Wei S, Yao K, Sun X, et al. Resveratrol protected acrolein-induced ferroptosis and insulin secretion dysfunction via ER-stress- related PERK pathway in MIN6 cells. Toxicology (2022) 465:153048. doi: 10.1016/j.tox.2021.153048
172. Li T, Tan Y, Ouyang S, He J, Liu L. Resveratrol protects against myocardial ischemia-reperfusion injury via attenuating ferroptosis. Gene (2022) 808:145968. doi: 10.1016/j.gene.2021.145968
173. Wang X, Simayi A, Fu J, Zhao X, Xu G. Resveratrol mediates the miR-149/HMGB1 axis and regulates the ferroptosis pathway to protect myocardium in endotoxemia mice. Am J Physiol Endocrinol Metab (2022) 323(1):E21–e32. doi: 10.1152/ajpendo.00227.2021
174. Liu J, Zhang M, Qin C, Wang Z, Chen J, Wang R, et al. Resveratrol attenuate myocardial injury by inhibiting ferroptosis Via inducing KAT5/GPX4 in myocardial infarction. Front Pharmacol (2022) 13:906073. doi: 10.3389/fphar.2022.906073
175. Ahmed MM, Hussein MMA, Saber T, Abd-Elhakim YM. Palliative effect of resveratrol against nanosized iron oxide-induced oxidative stress and steroidogenesis-related genes dysregulation in testicular tissue of adult Male rats. Int J Environ Res Public Health (2022) 19(13):8171. doi: 10.3390/ijerph19138171
176. Li N, Liu L. Mechanism of resveratrol in improving ovarian function in a rat model of premature ovarian insufficiency. J obstetrics gynaecol Res (2018) 44(8):1431–8. doi: 10.1111/jog.13680
177. Tamura H, Takasaki A, Taketani T, Tanabe M, Kizuka F, Lee L, et al. Melatonin as a free radical scavenger in the ovarian follicle. Endoc J (2013) 60(1):1–13. doi: 10.1507/endocrj.EJ12-0263
178. Park WR, Choi B, Kim YJ, Kim YH, Park MJ, Kim DI, et al. Melatonin regulates iron homeostasis by inducing hepcidin expression in hepatocytes. Int J Mol Sci (2022) 23(7):3593. doi: 10.3390/ijms23073593
179. Yang J, Tang Q, Zeng Y. Melatonin: potential avenue for treating iron overload disorders. Ageing Res Rev (2022) 81:101717. doi: 10.1016/j.arr.2022.101717
180. Ma H, Wang X, Zhang W, Li H, Zhao W, Sun J, et al. Melatonin suppresses ferroptosis induced by high glucose via activation of the Nrf2/HO-1 signaling pathway in type 2 diabetic osteoporosis. Oxid Med Cell Longevity (2020) 2020:9067610. doi: 10.1155/2020/9067610
181. Yang F, Yang L, Li Y, Yan G, Feng C, Liu T, et al. Melatonin protects bone marrow mesenchymal stem cells against iron overload-induced aberrant differentiation and senescence. J Pineal Res (2017) 63(3):10.1111/jpi.12422. doi: 10.1111/jpi.12422
Keywords: follicle development, iron overload, ferroptosis, extracellular matrix (ECM), Hippo/YAP pathway
Citation: Xia L, Shen Y, Liu S and Du J (2023) Iron overload triggering ECM-mediated Hippo/YAP pathway in follicle development: a hypothetical model endowed with therapeutic implications. Front. Endocrinol. 14:1174817. doi: 10.3389/fendo.2023.1174817
Received: 27 February 2023; Accepted: 12 April 2023;
Published: 08 May 2023.
Edited by:
Carlos O. Stocco, University of Illinois Chicago, United StatesReviewed by:
Weijie Yang, Zhejiang University, ChinaJitu W. George, University of Nebraska Medical Center, United States
Copyright © 2023 Xia, Shen, Liu and Du. This is an open-access article distributed under the terms of the Creative Commons Attribution License (CC BY). The use, distribution or reproduction in other forums is permitted, provided the original author(s) and the copyright owner(s) are credited and that the original publication in this journal is cited, in accordance with accepted academic practice. No use, distribution or reproduction is permitted which does not comply with these terms.
*Correspondence: Suying Liu, bHN5NjU5MkAxNjMuY29t; Jing Du, ZHVqaW5nNDJAMTI2LmNvbQ==
†These authors have contributed equally to this work