- 1Department of Radiobiology and Molecular Genetics, VINČA Institute of Nuclear Sciences - National Institute of the Republic of Serbia, University of Belgrade, Belgrade, Serbia
- 2Clinic for Internal Medicine, Department of Endocrinology and Diabetes, Zemun Clinical Hospital, School of Medicine, University of Belgrade, Belgrade, Serbia
- 3Computational Bioscience Research Center (CBRC), King Abdullah University of Science and Technology (KAUST), Thuwal, Saudi Arabia
- 4Computer Science Program, Computer, Electrical and Mathematical Sciences and Engineering Division (CEMSE), King Abdullah University of Science and Technology (KAUST), Thuwal, Saudi Arabia
Introduction: Cardiovascular (CV) disorders are steadily increasing, making them the world’s most prevalent health issue. New research highlights the importance of insulin-like growth factor 1 (IGF-1) for maintaining CV health
Methods: We searched PubMed and MEDLINE for English and non-English articles with English abstracts published between 1957 (when the first report on IGF-1 identification was published) and 2022. The top search terms were: IGF-1, cardiovascular disease, IGF-1 receptors, IGF-1 and microRNAs, therapeutic interventions with IGF-1, IGF-1 and diabetes, IGF-1 and cardiovascular disease. The search retrieved original peer-reviewed articles, which were further analyzed, focusing on the role of IGF-1 in pathophysiological conditions. We specifically focused on including the most recent findings published in the past five years.
Results: IGF-1, an anabolic growth factor, regulates cell division, proliferation, and survival. In addition to its well-known growth-promoting and metabolic effects, there is mounting evidence that IGF-1 plays a specialized role in the complex activities that underpin CV function. IGF-1 promotes cardiac development and improves cardiac output, stroke volume, contractility, and ejection fraction. Furthermore, IGF-1 mediates many growth hormones (GH) actions. IGF-1 stimulates contractility and tissue remodeling in humans to improve heart function after myocardial infarction. IGF-1 also improves the lipid profile, lowers insulin levels, increases insulin sensitivity, and promotes glucose metabolism. These findings point to the intriguing medicinal potential of IGF-1. Human studies associate low serum levels of free or total IGF-1 with an increased risk of CV and cerebrovascular illness. Extensive human trials are being conducted to investigate the therapeutic efficacy and outcomes of IGF-1-related therapy.
Discussion: We anticipate the development of novel IGF-1-related therapy with minimal side effects. This review discusses recent findings on the role of IGF-1 in the cardiovascular (CVD) system, including both normal and pathological conditions. We also discuss progress in therapeutic interventions aimed at targeting the IGF axis and provide insights into the epigenetic regulation of IGF-1 mediated by microRNAs.
1 Introduction
Cardiovascular diseases (CVDs) are the leading cause of morbidity and mortality globally, accounting for 32% of all deaths (1). Coronary artery disease (CAD) is the most common comorbidity, and severe complications of this vascular pathology are heart attack, arrhythmias, chronic nephropathy, and ischemic stroke (2, 3). Peripheral artery disease (PAD), also characterized by atherosclerotic plaque formation, manifests as lesions and pain in the lower extremities (4, 5). Other heart and blood vessel disorders include deep vein thrombosis, pulmonary embolism, and rheumatic and congenital heart disease (1, 6, 7). The principal risk factors for CVDs are genetic predispositions, metabolic syndrome (MetS), inadequate dietary and lifestyle habits, and physical inactivity (8). Insulin resistance, diabetes mellitus (DM), dyslipidemia, elevated blood pressure, and visceral and abdominal obesity represent predisposing factors for the MetS occurrence, which strongly correlates with cerebral and abdominal aneurysms, CAD, PAD, and stroke (9, 10).
Insulin-like growth factor-1 (IGF-1) is a polypeptide growth factor with a structure comparable to insulin. IGF-1 is responsible for cell differentiation, maturation, growth, and proliferation in almost all body organs (11–13). Numerous reports advocate the hypothesis that IGF-1 participates in the homeostasis of cardiovascular (CV) physiology. In particular, IGF-1’s roles in the CV system include maintaining cellular homeostasis by regulating vascular vasoconstriction/vasodilatation, cardiac apoptosis and autophagy, and inflammatory responses (14–21). Furthermore, IGF-1 exerts significant anti-inflammatory and anti-oxidant effects on the vasculature, decreasing atherosclerotic plaque burden (22). In addition, IGF-1 possesses anti-atherogenic properties reflected in its role in modulating endothelial junction protein levels and promoting angiogenesis in endothelial cells (ECs) (23, 24). With the rising prevalence of CVDs, IGF-1’s involvement in CV system functioning is attracting substantial research interest. This review discusses recent research on the roles of IGF-1 in physiological and pathological conditions such as CV and metabolic diseases. We review IGF-1’s roles in atherosclerosis, CAD, PAD, hypertension, and diabetes. Furthermore, we discuss progress in therapeutic interventions that target the IGF axis and provide novel insights into the epigenetic regulation of IGF-1 mediated by microRNAs (miRNAs).
2 IGF-1 system
Salmon and Daughaday (25) discovered IGF-1 in 1957 and initially described it as a “sulfation factor” that stimulated the incorporation of 35Sulfate by costal cartilage. In 1976 it was renamed “insulin growth factor-1” by Rinderknecht and Humbel to reflect its structural resemblance to proinsulin (26). IGF-1 contains 70 amino acids (AAs), has a molecular weight of 7.649 kDa and belongs to a family of insulin-related single-chain peptides (27, 28). The IGF-1 coding gene is on chromosome 12 in humans (29). IGF-1 possesses three disulfide bridges (between AAs 6 and 48; 18 and 61; 47 and 52) and binds to both IGF-1 receptor (IGF-1R) (with a high affinity) and insulin receptors (IR) (with a low-affinity) (30). IGF-1 is mainly synthesized in the liver and exhibits endocrine, paracrine, and autocrine activity (31). As the primary mediator of the anabolic and mitogenic activity of growth hormone (GH), IGF-1 plays a significant role in cellular physiology in childhood and adolescence (32, 33). The primary IGF-1 role is its endocrine activity, but it can also be secreted by tissues other than the liver, in which it acts locally and expresses a paracrine function (12, 34–36). IGF-1 is a negative regulator of pituitary GH production (Figure 1) (35). IGF-1R is present in almost every cell in the organism, and it contains two alpha and two beta subunits associated with disulfide bonds. Beta subunits have tyrosine kinase domains, whose activation is mediated by the binding of IGF-1 to alpha subunits, and it is associated with various signaling pathways, including PI3K/Akt and Raf/MEK/ERK cascade (37, 38) (Figure 2). The IGF-1R gene is positioned on chromosome 15q26 and has approximately 70% similarity with the IR gene (39, 40). In addition to IGF-1R, insulin-like growth factor binding proteins (IGFBPs) are required for IGF-1 bioavailability because they interact with IGF-1 with the same affinity as IGF-1R (41, 42). There are six IGFBPs types (containing approximately 200–300 AAs) that regulate IGF signaling and prolong its half-life, and they bind more than 90% of IGF-1 present in circulation (about 1% of IGF-1 in circulation are in a free-active form), simultaneously blocking its binding to IR (42, 43). Among all known IGFBPs, the most abundant is IGFBP-3, with a concentration of 100 nM/L in adult serum, and represents an essential protein in the IGF pathway. The IGF-1/IGFBP-3 ratio is used as a clinical predictor of metabolic syndrome (MetS) (11, 44). It is assumed that IGFBPs could act in either IGF-dependent or independent manner, and due to different IGFBP expression profiles in various tissues, they are investigated as diagnostic biomarkers and potential therapeutic agents for different pathological disorders (45–47).
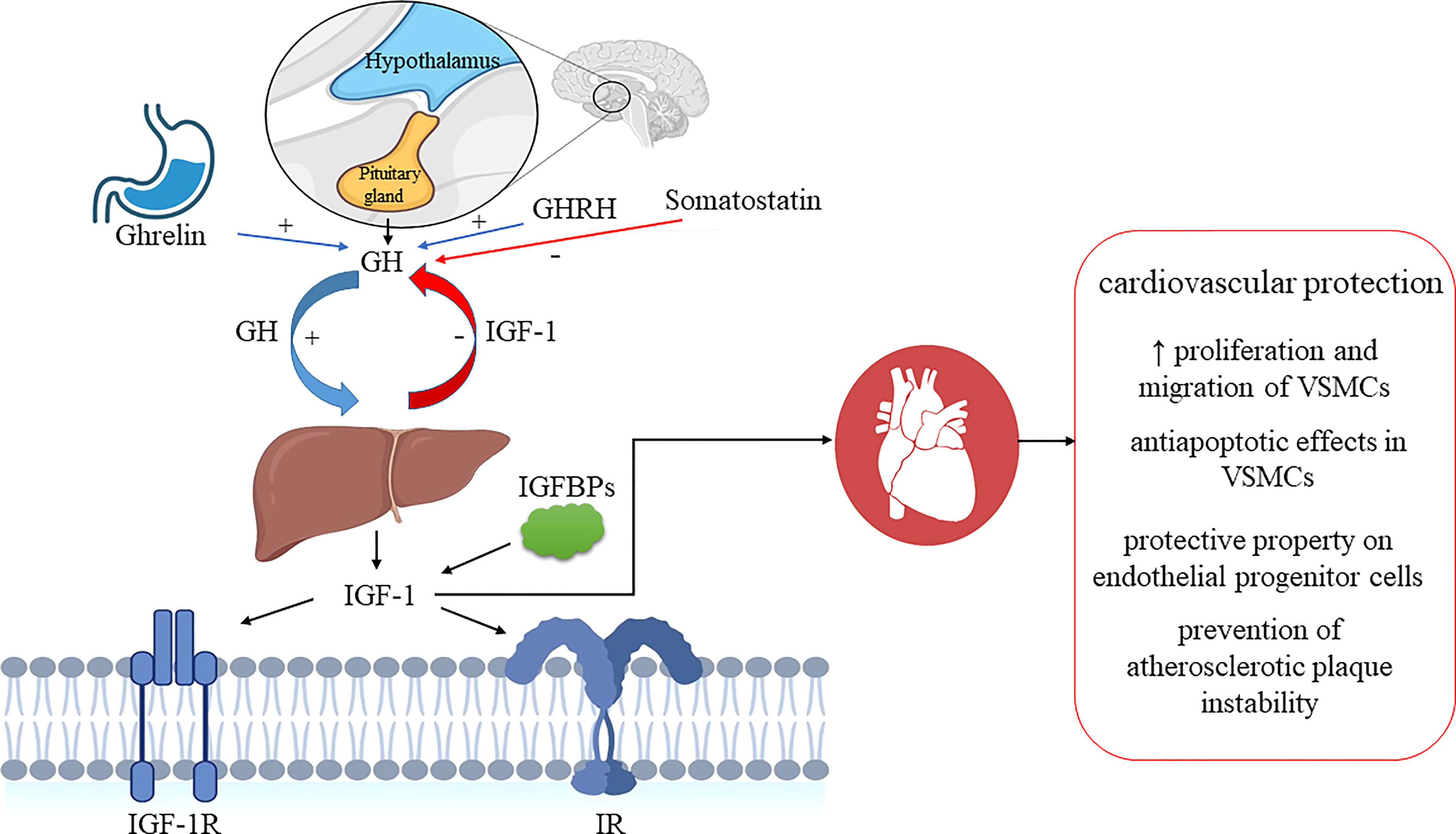
Figure 1 IGF-1 system and GH/IGF-1 axis regulation. Ghrelin and GHRH stimulate pituitary secretion of GH, while somatostatin inhibits it. GH stimulates IGF1 secretion in the liver, and IGF-1 exerts negative feedback and inhibits GH secretion. GH, growth hormone; GHRH, growth hormone-releasing hormone; IGF-1, insulin-like growth factor-1; IGF-1R, insulin-like growth factor receptor-1; IGFBPs, insulin-like growth factor binding proteins; IR, insulin receptor; VSMCs, vascular smooth muscle cells. Biorender.com was used to generate part of the Figure.
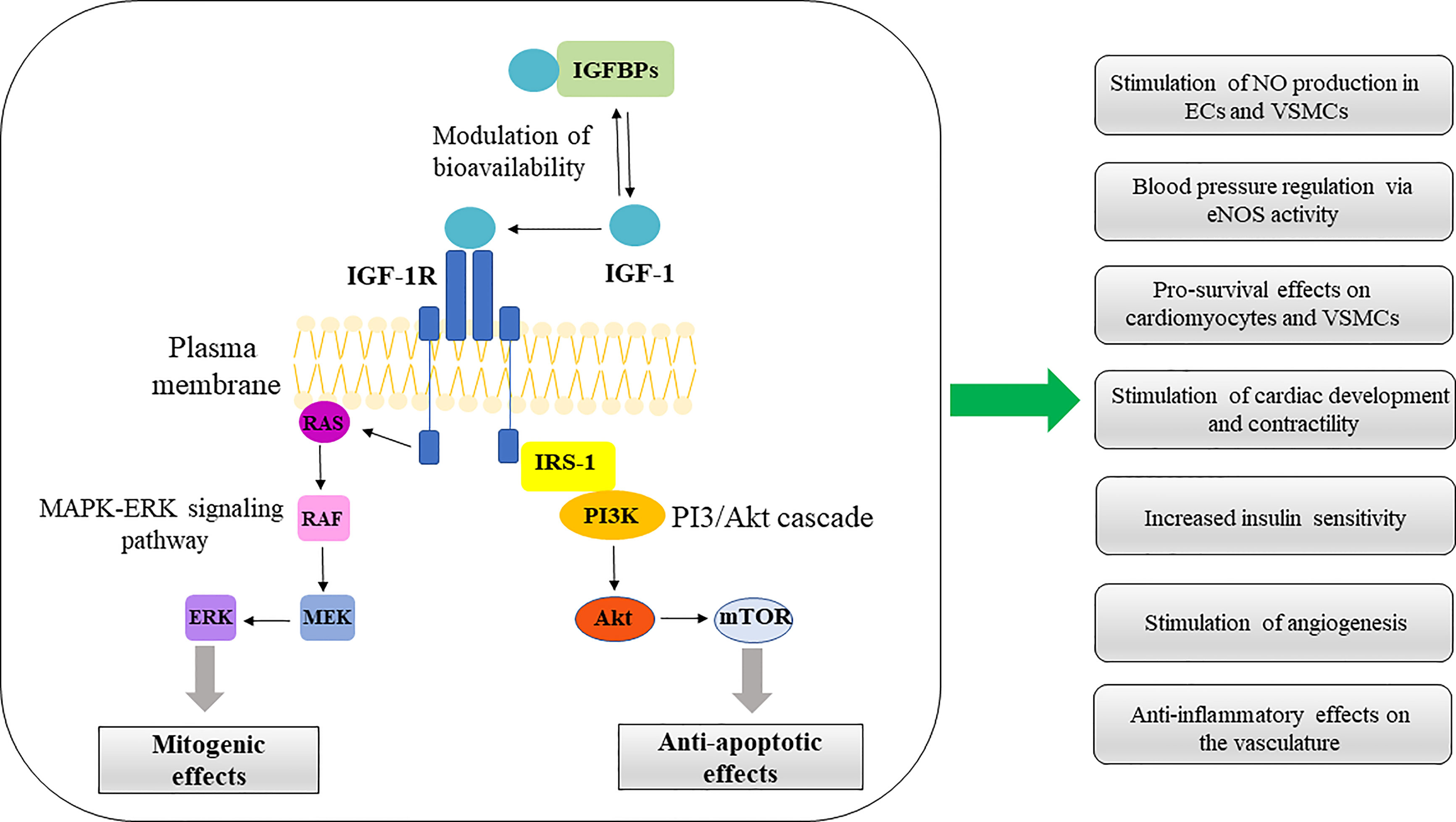
Figure 2 IGF-1 signaling pathways in CV system. ERK/MAPK and PI3K/Akt represent the main pathways involved in IGF-1 signal transduction. Activation of these pathways confer multiple protective effects on the CV system, summarized in the diagram. IGFBPs, insulin-like growth factor binding proteins; IGF-1, insulin-like growth factor-1; IGF-1R, insulin-like growth factor receptor-1; RAS, rat sarcoma protein; RAF, rapidly accelerated fibrosarcoma kinase; MEK, mitogen-activated protein kinase kinase; ERK, extracellular signal-regulated kinase; IRS1, Insulin receptor substrate-1; PI3K, phosphatidylinositol-3 kinase; Akt, serine/threonine kinase (protein kinase B); mTOR, mammalian target of rapamycin; NO, nitric oxide; VSMCs, vascular smooth muscle cells; eNOS, endothelial nitric oxide synthase.
2.1 Roles of IGF-1, IGF-1R, and IGFBPs in vascular smooth and endothelial cells
IGF-1 and IGF-1R are expressed by vascular smooth muscle cells (VSMCs), endothelial cells (ECs), and macrophages (34), and together with different IGFBPs, they are considered significant regulators of atherosclerosis pathophysiology whose expression is downregulated in atherosclerotic lesions (48–50). IGF-1 participates in the proliferation and migration of VSMCs, and according to numerous data, decreased IGF-1 and IGFBPs serum levels have been associated with carotid atherosclerosis (51–53). In addition, decreased circulating levels of IGF-1 and IGFBP3 have been correlated with a greater incidence of ischemic heart and cerebrovascular stroke (53, 54). In a recent case-control study with more than 4000 participants, it was proposed that the IGF-1 signaling pathway contributes to the risk of stroke occurrence (55). Atherosclerotic plaque stability and dimensions are partly regulated by VSMCs, whose apoptosis and structural alterations influence plaque rupture (56–58). Interestingly, one of the proposed IGF-1 mechanisms in the context of CVDs is the prevention of atherosclerotic plaque instability through VSMCs phenotype modulation and anti-apoptotic effects (59, 60). IGF-1 gene expression in VSMCs is dependent on numerous factors, including thrombin, tumour necrosis factor-alpha (TNFα), angiotensin II, oxidized low-density lipoprotein (ox-LDL), and reactive oxidative species (ROS) (61–66). As previously mentioned, IGF-1 bioavailability is controlled through six IGFBPs. For instance, IGFBP4 is the principal IGFBP produced by VSMCs, and its decreased expression in VSMCs may increase IGF-1 bioavailability (67). IGFBP-1 affected SMC proliferation and was implicated in regulating plaque stability (68). Furthermore, IGFBP2 enhances endothelial cell chemotaxis (69), while IGFBP3 is related to regenerative potential in cardiac atrial appendage stem cells (70). Endothelial dysfunction is an important part of CVDs pathologies and related comorbidities, such as atherosclerosis, hypertension, diabetes, and obesity development and prognosis, as endothelium manages vascular homeostasis (71). IGF-1R moderates EC function through its impact on nitric oxide (NO) bioavailability since it can form hybrid receptors with the insulin receptor (IR) and consequently influence endothelial cell sensitivity to insulin (72). In the rat model of acute renal failure, IGF-1 demonstrated NO-mediated ameliorative action on renal function, possibly due to NO’s vasodilatation effects (73). Also, IGF-1 manifests a protective property on endothelial progenitor cells by preventing its dysfunction caused by oxLDLs via eNOS/NO signaling pathway (74). In addition, one of the proposed novel IGF-1 protective features regarding endothelial function is the upregulation of endothelial junction protein levels (24).
According to the literature data, IGF-1 participates in the regulation of peripheral resistance (75). This important growth factor contributes to pressure regulation since it increases blood flow in rats through vasodilatation, and hypertension has been observed in IGF-1 genetically deficient mice (76, 77). New findings suggest that matrix metalloproteinases receptor cleavage has a notable role in the ineffective IGF-1 signaling in hypertension and might be significant for peripheral vascular resistance (19). Interestingly, manipulation of IGF-1 levels in the peripheral circulation and its increased levels in the brain could be used to recover early manifestations of cerebral artery occlusion (78). Regarding human studies, IGF-1 infusion reduced peripheral resistance in patients with chronic heart failure, suggesting its acute impact on the CV system (79). Besides, IGF-1 serum and plasma levels in humans are considered valuable biomarkers for CVD development. For instance, low serum IGF-1 is linked to hypertension and early CV complications in female patients with rheumatoid arthritis (80), whereas elevated IGF-1 plasma levels are correlated with a declined risk for hypertension incidence in non-diabetic women patients (81).
IGF-1 has been linked to changes in cardiac structure. IGF-1, in particular, stimulates collagen type I synthesis in VSMCs and its extracellular accumulation (82). Moreover, IGF-1 could moderate the myocardium structure since it inhibits apoptosis in hypoxic cardiomyocytes (47). Despite this, there is no conclusive evidence that IGF-1 can modify cardiomyocytes in healthy heart tissue in addition to its observed overexpression (75, 83).
2.2 MiRNA-mediated regulation of IGF-1 and IGF-1R expression
Several studies have reported miRNAs-mediated epigenetic regulation of the expression and secretion of IGF-1 and its receptor, IGF-1R. MiRNAs are small, single-stranded RNAs that post-transcriptionally alter the expression of target genes (84, 85) and typically exert their regulatory effects in a context-dependent manner by binding to cis-elements located in the 3’ untranslated region (3’UTR) of target protein-coding mRNAs. In some cases, miRNAs may bind to 5’UTR or coding regions, affecting mRNA stability and degradation (85, 86). The expression of as many as 1/3 of the genes in the human genome is regulated by miRNAs. Several thousands of miRNAs have been identified so far, representing one of the most abundant gene expression regulators with a role in almost every biological process in multicellular organisms (87, 88). A single miRNA hypothetically may bind to more than a hundred target mRNAs, and several miRNAs can cooperate to finely tune the expression of the same transcript (89, 90).
So far, several miRNAs have been implicated in regulating IGF-1 expression and signaling (91). miR-320 was demonstrated to affect IGF-1 expression and insulin sensitivity in adipocytes by modulating the insulin signaling pathways (92). miR-126, whose levels are significantly decreased in multiple tissues in type 2 diabetes mellitus (T2DM), has also been reported to affect the expression of IGF-1 and IGF-2 (93). MiR-133a stimulated IGF-1R expression by prolonging the IGF-1R mRNA half-life. In atherosclerosis induced by apolipoprotein-E deficiency, decreased miR-133a expression is associated with lower IGF-1R levels and suppressive VSMC growth. Administration of miR-133a precursor increased IGF-1R levels and promoted IGF-1-induced VSMC survival and growth (94). In addition, a specific miRNA may exert multiple regulatory effects by binding to several targets of metabolic signaling pathways, illustrated by the example of let-7 that binds to 3’UTR regions of INSR, IRS-2, and IGF-1R and regulates their expression (95). Also, in cardiac and skeletal muscles, miR-1 controls the expression of both IGF-1 and IGF-1R (96). miR-143-3p is significantly upregulated in the serum of T2DM patients and various tissues of obese mice, such as skeletal muscle, pancreas, and heart. miR-143-3p was reported to regulate the IGF-2R receptor and may contribute to insulin resistance observed during MetS (97).
3 Effects of IGF-1 in physiological conditions
The classical view of IGF-1 as an essential growth factor is expanded by findings of its significant metabolic effects whereby IGF-1 stimulation of its receptor serves as a signal that notifies cells about the availability of adequate nutrients, thus coordinating protein, fat, and carbohydrate metabolism in various cell types. IGF-1 stimulates protein synthesis via the PI-3 kinase pathway in tissue cell culture. IGF-1 receptor activation is followed by a cascade of reactions starting with tyrosine kinase-mediated phosphorylation of insulin receptor substrate-1 (IRS-1) (98). IRS-1 is an adaptor protein that provides a binding site for PI-3 kinase, whose activation stimulates Akt (Figure 2). Consequent TSC-2 suppression and mTORC1 complex activation stimulates phosphorylation of p70S6 kinase and 4E-BP1 translational repressor. AMP kinase activated by nutrient restriction modulates this process by phosphorylating serine residue 794 on IRS-1, which prevents its activation and leads to the inhibition of PI-3 kinase and IGF-1-stimulated protein synthesis (99). IGF-1 also stimulates amino acid transport and protein synthesis in skeletal muscle while inhibiting protein breakdown (100, 101). IGF-1 induces skeletal muscle hypertrophy by blocking the transcriptional upregulation of the ubiquitin-ligases MuRF1 and MAFbx (also called atrogin-1), which serve as key mediators of skeletal muscle atrophy (101). This finding is supported by research demonstrating that genetically altered mice expressing atrogin complex constitutively show resistance to the anticatabolic effects of IGF-1 (101). Another study reported that IGF-1 partially antagonizes the cytokines activated in catabolic states that initiate muscle breakdown through MURF1 and MAF box induction (102). IGF-1-mediated inhibition of MAFbx upregulation by treatment reduces proteasome formation and targeting of proteins for degradation, thus lowering the rate of catabolism (103).
It has been proposed that IGF-1 may be a primary factor that maintains protein synthesis during intervals between meals, whereas insulin is a major factor that stimulates skeletal muscle anabolism following meal ingestion (104). This is supported by findings that IGF-1 treatment stimulates protein synthesis while having little effect on proteolysis and catabolism in healthy volunteers (105). However, IGF-1 can suppress proteolysis at high concentrations, even in normally fed subjects. In contrast, insulin inhibits proteolysis in muscle tissue at very low concentrations, and high concentrations are required to stimulate protein synthesis.
IGF-1’s ability to modulate insulin and GH actions allows it to affect carbohydrate metabolism. Specifically, IGF-1 reduces serum GH levels and affects insulin suppression of gluconeogenesis in the liver. Furthermore, increasing free fatty acid uptake in muscle IGF-1 indirectly enhances hepatic insulin action (106). GH was found to stimulate the PI-3 kinase p85 subunit synthesis (107), which leads to the suppression of p110 subunit activity and results in decreased insulin action (108). IGF-1 has been shown to stimulate glucose transport into muscle via IGF-1 receptors or insulin/IGF-1 hybrid receptors, and a high concentration of free IGF-1 has been shown to suppress gluconeogenesis in mice directly (109–111). Deletion of the insulin receptor in a mouse model showed decreased blood glucose levels in response to IGF-1 (112), whereas in vivo reduction of serum IGF-1 by 80% impaired glucose tolerance (113). Thus, IGF-1 enhances insulin sensitivity and decreases blood glucose, but its primary effects are increasing fatty acid oxidation in muscle, leading to the decreased hepatic free fatty acid influx and increased insulin suppression of liver glucose output (104).
IGF-1’s role in stimulating the uptake and oxidation of free fatty acid in skeletal muscle was confirmed by in vivo experiments with transgenic mice overexpressing a dominant-negative IGF-1R in skeletal muscle (MKR mice). These mice showed a significant decrease in glucose uptake when stimulated with either insulin or IGF-1, indicating a loss of function of both the IR and IGF-1R. Furthermore, MKR mice were insulin-resistant, had high serum and tissue lipid levels, and developed diabetes at a young age (114). In another study, MKR mice were crossed with mice overexpressing a fatty acid translocase, CD36, in skeletal muscle (115). Normalization of the rate of fatty acid oxidation in skeletal muscle and normalization of hyperinsulinemia, hyperglycemia, and significant improvement in hepatic insulin sensitivity was observed in the double-transgenic MKR/CD36 mice (115). These findings suggest the central role of IGF-1 in skeletal muscle fatty acid transport. They are also supported by the finding that long-term administration of recombinant IGF-1 in humans with GH receptor deficiency is associated with decreased fat mass, increased lipolysis, and a faster rate of lipid oxidation (116).
4 Effects of IGF-1 in pathophysiological conditions
It is extensively documented that IGF-1 exerts profound effects on CV and metabolic disorders. IGF-1 modulates CV function by stimulating angiogenesis (117, 118) and promoting anti-apoptotic and anti-inflammatory actions (119, 120). Also, IGF-1 activates nitric oxide synthase (NOS) via Akt-catalyzed phosphorylation, which stimulates NO production in ECs and VSMCs, leading to improved cardiac contractility (121). Furthermore, several studies suggest that IGF-1 may indirectly affect the CV system by increasing insulin sensitivity (113, 122–124). In the context of metabolic disorders, it has been shown that IGF-1 ameliorates insulin sensitivity (123, 124).
Moreover, perturbed IGF-1 levels are associated with insulin resistance, glucose intolerance, increased T2DM risk and cardiovascular morbidity and mortality (125, 126). IGF-1 independently correlates with coronary microvascular impairment, suggesting the crucial role of this growth factor in the increased CV risk associated with acromegaly (127). Also, a recent study reports profoundly reduced serum/plasma IGF-1 levels in patients with obstructive sleep apnea-hypopnea syndrome (OSAHS), a disease with severe CV and metabolic consequences that result in increased mortality of comorbid conditions such as coronary heart disease and cardiac failure (128). The following sections summarize the major findings concerning IGF-1 levels and pathophysiological conditions such as atherosclerosis, CAD, PAD, stroke, hypertension, and diabetes.
4.1 IGF-1 and CVD
The imbalance between pro- and anti-atherogenic factors results in atherosclerosis development. Numerous cell types, including inflammatory cells (macrophages), VSMs, and ECs, are directly or indirectly involved in the pathogenesis of atherosclerosis. Their byproducts promote endothelial dysfunction and accelerate the atherosclerotic process (129–131). IGF-1, one of the byproducts, exhibits pro- and anti-atherogenic properties (14). The risk of atherosclerotic CV disease was lower in healthy persons with high free IGF-1 levels in the blood (22, 132). Accordingly, the risk of developing acute coronary syndrome has also been linked to low IGF-1 levels (53, 133, 134). Lower IGF-1 concentrations in individuals with acute myocardial infarction were linked to a higher incidence of post-myocardial infarction consequences and a worse prognosis (133, 135). A study by Stavropoulou et al. showed that the serum IGF-1 levels in infarcted rats were initially decreased (24 h up to 1 week) and remained unaltered during the late postinfarction period (4 to 8 weeks). Interestingly, this study reported significant upregulation of IGF-1 expression in rat myocardium after coronary artery infarction. The fact that an increase in serum IGF-1 levels did not accompany it suggested that increased IGF-1 expression serves the needs of rat myocardium locally, participating in the postinfarction repair processes (136).
The preferred laboratory models for atherosclerotic diseases are murine and pig models. Murine atherosclerotic models are primarily used at the initial phase of pre-clinical research, as opposed to pig models, which are reserved for later experimental stages (i.e. testing the pharmacokinetics, pharmacodynamics and side-effects of experimental anti-atherosclerotic drugs). Pigs were found to have lipid profiles and atherosclerotic coronary artery involvement that were very similar to humans (137).
The pre-clinical studies are mostly based on animal mechanical injury models demonstrating a link between increased IGF-1 and neointimal proliferation (138–141). IGF-1 inhibition inhibits neointimal proliferation and could be clinically used to treat restenosis after coronary artery angioplasty (142).
There has been little research into the role of IGF-1 in hypercholesterolemic animal models of atherosclerosis with no mechanical injury (143). In general, such animal models revealed that low circulating IGF-1 levels are associated with more extensive atherosclerosis (144), whereas high circulating IGF-1 levels are not (9). Sukhanov et al. demonstrated that IGF-1 infusion reduced atherosclerotic lesion extension (aortic root plaque area by 30%), vascular oxidative stress, inflammation, and atherosclerotic plaque macrophage infiltration in a murine Apoe-/- model (145). Shai et al. demonstrated that chronic IGF-1 overexpression in smooth muscle cells (SMCs) did not increase overall plaque extensiveness and exhibited features of stable plaques in Apoe-/- mice fed a Western diet (146). Interestingly, IGF-1’s atheroprotective effects are largely independent of SMCs (146). IGF-1R deficiency in Apoe-/- mice macrophages increases atherosclerotic extensiveness and destabilizes plaque stability by lowering SMCs and collagen content, according to the pleiotropic effects of IGF-1 on various vascular wall cells (119). IGF-1 signaling disruption determines macrophage activation into the pro-inflammatory M1 phenotype and reduces lipid efflux, promoting lipid accumulation in macrophages (119, 147). In contrast, Snarski et al. demonstrated in a macrophage-specific IGF-1 Apoe-/- mouse model that macrophage-derived IGF-1 inhibited the expression of chemokine (C-X-C motif) ligand 12 (CXCL12), reduced monocyte recruitment to plaques, and favoured macrophage cholesterol efflux (60). Recombinant human IGF-1, given over 6 months at a dose FDA-approved for long-term treatment of growth failure in children with severe primary IGF-1 deficiency, reduced coronary artery atherosclerosis and promoted a stable plaque phenotype in a pig model of familial hypercholesterolemia (FH) (148). IGF-1 suppression of systemic inflammation and oxidative stress provides such effects. In plaques, IGF-1 suppressed gene expression of FOS/FOSB transcription factors, CXCL14, and matrix metalloproteinase 9 (MMP9), according to a spatial transcriptomics study (148).
The clinical indicator of atherosclerosis across the body and in the brain is the common carotid artery intima-media thickness (CC-IMT). Extensive research has shown a strong association between CC-IMT and IGF-1 levels (52, 149–152). Intriguingly, Sirbu et al. (153) proposed that elevated IGF-1 levels might promote plaque stability in advanced atherosclerosis while stimulating smooth muscle hyperplasia in early atherosclerosis. The same study reported that the measure of insulin resistance, HOMA-IR, and total IGF-1 z-score, is associated with increased CC-IMT (153). Also, a positive correlation between CC-IMT and serum IGF-1/IGFBP-3 ratio was reported (52, 150). Furthermore, a negative association of IGF-1 with CC-IMT was observed only in patients with low vitamin D levels (152) and was supported by findings of a comparative study of obese individuals and healthy controls showing that IGF-1 protects against CC-IMT when serum vitamin D levels are low (154). Recent findings show that vitamin D3 treatment activates the IGF-1 promoter and enhances IGF-1R signaling, accompanied by enhanced mesenchymal stem cells-induced angiogenesis and increased vascularization in vitro and in vivo (155).
The PRIME prospective cohort study investigating the relationship between IGF-1 and CAD in 10,600 patients reported that IGF-1 levels negatively correlated with age, markers of inflammation, waist circumference, and tobacco consumption (156). The study revealed that participants with the acute coronary syndrome had significantly lower baseline IGF-1 levels, and those in the highest quartile for IGF-1 levels had a 55% lower relative risk of myocardial infarction (156). Several other studies confirmed a relationship between elevated IGF-1 levels and a decreased prevalence or incidence of CAD (156–158). Additionally, it was found that individuals with early-onset CAD had considerably lower IGF-1 levels (157).
Increased IGF-1 levels protect against ischemic strokes, as evidenced by the lower prevalence or incidence of ischemic stroke (54, 159, 160). For instance, the lowest ischemic stroke incidence was observed for the patients in the highest quartile of circulating IGF-1 (232 ± 41.04 ng/ml) according to a prospective study based on observational data from 757 participants of the Framingham Study (54). This correlation was especially pronounced in patients with insulin resistance and subjects with the highest waist/hip ratio, leading to the conclusion that low circulating IGF-1 levels may be associated with an increased risk of ischemic stroke in obese and diabetic patients due to higher insulin resistance (54).
Vasodilation and reduced vasoconstriction, anti-apoptotic and pro-survival effects on cardiomyocytes and vascular bed cells, tissue remodeling effects, and stimulation of cardiac development and contractility represent positive effects of IGF-1 on the CV system (Figure 2) (14). Even though the majority of research demonstrates a clear correlation between low serum IGF-1 levels and an increased risk of CV diseases (15, 123, 161), specific conflicting findings can be ascribed to methodological problems in assessing total and free IGF-1 levels (161, 162). For instance, it was reported that the risk of CV events such as acute coronary syndrome was reduced in the presence of higher IGF-1 levels (156, 163). Furthermore, the fact that therapy with IGF-1 or its analogues had a favorable effect on patients with chronic CV diseases recognized the role of IGF-1 in the CV system (164, 165). In line with that, Rotterdam and Framingham’s studies revealed that IGF-1 levels are negatively linked with the incidence of CV events (166, 167). Based on a dose-response analysis, every 45 μg/mL increase in IGF-1 correlates with a 9% reduction in overall CV events risk for both genders (163). In addition, a longitudinal study in a large number of subjects prone to hypertension and CVD demonstrated a protective association between IGF-1 and hypertension, CV and all-cause mortality (168).
4.1.2 IGF-1 and PAD
At present, there is limited availability of data regarding the potential association of IGF-1 with PAD (169). In a study by Urbonaviciene et al., serum IGF-1 and IGFBP-2 levels were measured in 440 patients with lower-extremity PAD, revealing an increased risk of CVD mortality was associated with an increase of 100 μg/l of baseline IGFBP-2 value. Furthermore, the study concluded that elevated IGFBP-2 levels in patients with PAD were independently associated with long-term CVD mortality. However, the addition of IGFBP-2 to a model containing conventional CVD risk factors did not improve the risk prediction of CVD mortality (169).
In another cross-sectional study, circulating IGF-1, IGFBP-3, and labile acid subunit (ALS) essential for maintaining normal serum IGF-1 and IGFBP-3 concentrations (170), were measured in PAD patients and healthy controls. In addition, IGF-1, C-reactive protein (CRP), IGFBP-3, and ALS were measured in blood from the aorta and femoral vein of the affected limb in a subset of patients subjected to peripheral angiography. PAD patients had decreased levels of IGFBP-3, and measurements in the affected limb revealed a negative correlation between CRP and IGF-1 venous-arterial difference, whereas a positive correlation between CRP and IGFBP-3 venous-arterial difference was observed. This study established a connection between systemic levels of IGF axis components and the presence of PAD, suggesting that inflammation of the affected limb in PAD patients affects the transfemoral IGF-1 and IGFBP-3 concentrations (171).
4.1.3 IGF-1 and hypertension
IGF-1 mediates the regulation of blood pressure by stimulating endothelial nitric oxide synthase (eNOS) activity and consequent nitric oxide (NO) production in ECs that promotes vasodilatation and increases blood flow (172–174). In rats and mice with genetic IGF-1 deficiency, an elevated mean blood pressure is observed, affirming the IGF-1’s role in blood flow and pressure regulation (76, 174, 175). Increased circulating IGF-1 levels in hypertensive subjects were shown to promote adaptive structural alterations such as ventricular hypertrophy and vascular remodeling (176). In addition, a recent study reports an association between plasma IGF-1 levels and intraventricular septal thickening (177). The reduced vasodilation response to IGF-1 1 was reported in hypertension (178, 179) which may exert a considerable effect in the development of arterial hypertension. It has been suggested that increased matrix metalloproteinase (MMPs) activity in hypertension results in the proteolytic cleavage of the extracellular IGF-1R α subunit and the observed lack of receptor sensitivity (19). Several studies support this notion by showing that increased proteinase activity plays a vital role in organ damage and the loss of cellular function in hypertension (180–183).
However, literature analysis of human studies shows conflicting reports on the association between blood pressure regulation and IGF-1 levels. For instance, several studies on a smaller number of patients showed higher IGF-1 levels in hypertensive patients compared to normotensive subjects (184–187), whereas several cross-sectional studies indicated a neutral relationship between blood pressure and IGF-1 (52, 134, 188–190). Nevertheless, several more recent large cross-sectional studies reported a significant inverse correlation between blood pressure and IGF-1 (123, 191–196). Also, prospective studies confirm the inverse association of IGF-1 with systolic blood pressure and significantly reduced risk for incident hypertension in non-diabetic female subjects (81).
4.1.4 IGF-1, diabetes, and hyperinsulinemia
A link between decreased circulating IGF-1 and MetS components, such as dyslipidemia and DM, has been observed (15, 161) but remains questionable due to conflicting findings in the literature. It has been established that IGF-1 improves insulin sensitivity (123, 124), and higher IGF-1 levels are associated with fasting insulin levels and insulin resistance (197). The risk of developing T2DM and CAD was discovered to be genetically predisposed in people with elevated serum IGF-1 levels (197). More specifically, persons with insulin levels above the median and high levels of free IGF-1 have a higher chance of developing T2DM, whereas those below the median have a lower risk (198).
On the other hand, one study found no link between IGF-1 levels and T2DM (199). Additional longitudinal studies provided evidence of a strong association between increased incidence of insulin resistance and T2DM in subjects with either low or high IGF-1 serum levels (200, 201). The findings suggested that the association of IR and T2DM with low IGF-1 levels is due to the insulin-like actions of IGF-1 that promote hypoglycemia and IGF-1 suppression of growth hormone secretion, which causes insulin resistance (202, 203).
5 Therapeutic interventions that target the IGF axis
Due to its central role in IGF signaling, the IGF-1R has been studied as a target for therapeutic interventions using several strategies that were developed for clinical studies, such as anti-IGF-1R antibodies, IGF-1/2 neutralizing antibodies, nucleic acid-based approaches, small molecule tyrosine kinase inhibitors (TKIs), and IGF ligand TRAPs. Notably, most studies were performed in the context of potential novel treatments for various cancers, and the effects of such therapeutic approaches on cardiovascular and metabolic diseases are still not sufficiently reported in the literature. IGF-1R was the first tyrosine kinase targeted by a monoclonal neutralizing antibody (alpha-IR-3) that blocked the receptor binding domain (204). However, concerns about toxicity resulting from potential INSR co-inhibition halted further clinical evaluation of this approach (205). Nucleic acid-based approaches such as antisense oligonucleotides (ASOs), small interfering RNAs (siRNAs), and dominant-negative receptors successfully blocked IGF-1 signaling in vitro and in vivo. ASO-mediated downregulation of IGF-1 or IGF-1R showed promising inhibitory effects on the IGF axis in vivo (206, 207). For instance, chronic intravenous administration of IGF-1R ASO in spontaneously hypertensive rats (SHR) was employed to study the effects of a functional deficit in IGF-1 signaling (208). IGF-1 ASO administration decreased IGF-IR expression in conductance and resistance blood vessels, reduced aortic IGF-1R density, and angiotensin II type 1 receptor expression (208).
RNA interference (RNAi) technology based on the use of short (20–25 bp) double-stranded siRNAs that recruit the RNA-induced silencing complex (RISC) can be programmed to target virtually any nucleic acid sequence. Typically, siRNAs post-transcriptionally silence target genes via mRNA degradation, but they can also interact with the transcriptional machinery to induce transcriptional repression and may participate in epigenetic modifications (209, 210). Bohula et al. designed an IGF-1R siRNAs that efficiently silenced the IGF1R gene (211). However, the potential clinical use of such siRNA molecules requires their stabilization and improved delivery to improve their effectiveness upon in vivo administration (212).
Another molecular approach is based on the expression of the dominant-negative IGF-1R receptor (dnIGF-1R), which makes a complex with a wild-type half receptor and can bind a ligand but lacks kinase activity, thus blocking the function of endogenous IGF-1R. Since IGF-1R is a heterotetramer in which one ligand molecule binds into a pocket formed by two IGF-1R α subunits (213, 214), dnIGF-1R were constructed by expression of IGF-1R residues 1–950 that are present at the cell surface or residues 1–486 that encode soluble receptor (215–217). Similarly, dominant negative IGF-1R inhibition was achieved using an integrin binding-defective mutant of IGF-1 (218). Further advancement in IGF-1 signaling inhibition was achieved by the development of small molecule tyrosine kinase inhibitors (TKIs) that block IGF-1R kinase activity (219). However, these TKIs also inhibited INSR due to a high level of sequence homology between IGF-1R and INSR-A/B kinase domains (220–222) which may lead to adverse effects such as hyperinsulinemia (223). Also, the short half-life of TKIs may result in transient inhibition of target receptors, and maintenance of an efficient dosing regimen is associated with a risk of toxicity (224–226).
In the specific context of IGF-1-based therapeutic approaches for the treatment of metabolic diseases, it should be mentioned that recombinant human IGF-1 (rhIGF-1) has been studied as a potential treatment for diabetes. There is evidence that rhIGF-1 administration improved glycemic control but was associated with severe adverse effects such as diabetic retinopathy aggravation (227). Although this approach to diabetes treatment has been abandoned, IGFBP-1 and -2 have recently emerged as potential targets for insulin sensitivity modulation and treating diabetes and obesity (202). Another therapeutic approach with promising potential has been recently proposed by Wang and colleagues, who reported that significant elevation of IGF-1 levels could be achieved by combination treatment with tadalafil (TAD) and hydroxychloroquine HCQ. TAD is a phosphodiesterase 5 inhibitor that exerts cardioprotective effects against ischemia/reperfusion (I/R) injury in diabetic mice, whereas HCQ is an antimalarial and anti-inflammatory drug that reduces hyperglycemia in diabetic patients. The observed increase in insulin and IGF-1 levels upon TAD+HCQ treatment resulted in the activation of the Akt/mTOR signaling pathway. As a result, it was proposed that concurrent TAD and HCQ treatment could be a readily available novel pharmacotherapeutic approach for protection against myocardial I/R injury in T2DM (228).
Sodium-glucose transporter-2 inhibitors (SGLT-2is) and glucagon-like peptide-1 receptor agonists (GLP-1RAs) exhibit multiple metabolic and CV effects that have a positive impact on diabetic state and metabolism in general, such as reduction of hyperglycemia, fat mass and bone remodeling, natriuresis weight loss, and anti-atherosclerosis (229). Increasing evidence indicates the involvement of the IGF axis in pleiotropic responses elicited by SGLT-2is and GLP-1RAs. For instance, GLP-1RAs stimulate pro-survival responses in tissues where the IGF-1/IGF-1R plays an important role, such as pancreatic β-cells and the heart. SGLT-2is effect on the IGF axis is poorly documented, but it has been speculated that they promote increased ketogenesis mediated by elevated circulating GH levels (229). The association of SGLT-2is and GLP-1RAs with the IGF axis represents an exciting novel pharmacological strategy for the modulation of IGF-1 levels, which requires further validation for potential application in the treatment of CVD and metabolic diseases (229).
6 Conclusions
IGF-1 has numerous beneficial and protective effects on the CV system, including anti-apoptotic and pro-survival effects on cardiomyocytes and vascular bed cells, tissue remodeling effects, cardiac development and contractility stimulation, vasodilation and decreased vasoconstriction. Although the majority of available research data demonstrate a correlation between low serum levels of IGF-1 and an increased risk of CV diseases, conflicting findings can be ascribed to methodological problems in assessing total and free IGF-1 levels and the lack of standardization of the IGF-1 assays used in different studies. Therefore, future efforts should focus on introducing measures to standardize methods for measurements of total and free serum IGF-1 to facilitate the interpretation of correlations between IGF-1 and different parameters of cardiovascular and metabolic diseases. The intriguing possibility of targeting the IGF axis for treating cardiovascular and metabolic disorders remains uncertain due to adverse effects observed in pre-clinical and clinical studies. Recent findings regarding miRNAs-mediated regulation of IGF-1 expression open new avenues for designing and developing nucleic acid-based therapeutic agents that can finely tune IGF-1 levels and confer protective effects in cardiovascular and metabolic disease-associated conditions.
Data availability statement
The original contributions presented in the study are included in the article/supplementary material. Further inquiries can be directed to the corresponding author.
Author contributions
MM- wrote the article; ZG -wrote the article; JR-wrote the article, ME - wrote the article, XG - wrote and critically reviewed the article and EI - wrote and critically reviewed the article. All authors contributed to the article and approved the submitted version.
Funding
This work is part of the collaboration between the Department of Radiobiology and Molecular Genetics, “VINČA” Institute of Nuclear Sciences - National Institute of the Republic of Serbia, University of Belgrade, Belgrade, Serbia, Clinic for Internal Medicine, Department of Endocrinology and Diabetes, Zemun Clinical Hospital, School of Medicine, University of Belgrade, Belgrade, Serbia, and KAUST. The research was funded by the Ministry of Education, Science and Technological Development of the Republic of Serbia (Contract No#451-03-9/2021-14/200017) and King Abdullah University of Science and Technology (KAUST) through grant awards Nos. BAS/1/1624-01-01, FCC/1/1976-20-01, FCC/1/1976-26-01, and Contract No#OSR 4129.
Conflict of interest
The authors declare that the research was conducted in the absence of any commercial or financial relationships that could be construed as a potential conflict of interest.
Publisher’s note
All claims expressed in this article are solely those of the authors and do not necessarily represent those of their affiliated organizations, or those of the publisher, the editors and the reviewers. Any product that may be evaluated in this article, or claim that may be made by its manufacturer, is not guaranteed or endorsed by the publisher.
References
1. Organization WH. Cardiovascular diseases (CVDs). Available at: https://www.who.int/en/news-room/fact-sheets/detail/cardiovascular-diseases-(cvds).
2. Roffi M, Patrono C, Collet JP, Mueller C, Valgimigli M, Andreotti F, et al. 2015 ESC Guidelines for the management of acute coronary syndromes in patients presenting without persistent ST-segment elevation: Task force for the management of acute coronary syndromes in patients presenting without persistent ST-segment elevation of the European society of cardiology (ESC). Eur Heart J (2016) 37(3):267–315. doi: 10.1093/eurheartj/ehv320
3. Sdogkos E, Xanthopoulos A, Giamouzis G, Skoularigis J, Triposkiadis F, Vogiatzis I. Diagnosis of coronary artery disease: potential complications of imaging techniques. Acta Cardiol. (2022) 77(4):279–82. doi: 10.1080/00015385.2021.1911467
4. Lanéelle D, Sauvet G, Guillaumat J, Trihan JE, Mahé G. Gender differences in the medical treatment of peripheral artery disease. J Clin Med (2021) 10(13):2855. doi: 10.3390/jcm10132855
5. Mietus CJ, Lackner TJ, Karvelis PS, Willcockson GT, Shields CM, Lambert NG, et al. Abnormal microvascular architecture, fibrosis, and pericyte characteristics in the calf muscle of peripheral artery disease patients with claudication and critical limb ischemia. J Clin Med (2020) 9(8):2575. doi: 10.3390/jcm9082575
6. Liu M, Lu L, Sun R, Zheng Y, Zhang P. Rheumatic heart disease: Causes, symptoms, and treatments. Cell Biochem Biophys. (2015) 72(3):861–3. doi: 10.1007/s12013-015-0552-5
7. Scarvelis D, Wells PS. Diagnosis and treatment of deep-vein thrombosis. CMAJ (2006) 175(9):1087–92. doi: 10.1503/cmaj.060366
8. Flora GD, Nayak MK. A brief review of cardiovascular diseases, associated risk factors and current treatment regimes. Curr Pharm Des (2019) 25(38):4063–84. doi: 10.2174/1381612825666190925163827
9. Onesi SO, Ignatius UE. Metabolic syndrome: Performance of five different diagnostic criterias. Indian J Endocrinol Metab (2014) 18(4):496–501. doi: 10.4103/2230-8210.137494
10. Montazerifar F, Bolouri A, Mahmoudi Mozaffar M, Karajibani M. The prevalence of metabolic syndrome in coronary artery disease patients. Cardiol Res (2016) 7(6):202–8. doi: 10.14740/cr507w
11. Jones JI, Clemmons DR. Insulin-like growth factors and their binding proteins: biological actions. Endoc Rev (1995) 16(1):3–34. doi: 10.1210/edrv-16-1-3
12. Wrigley S, Arafa D, Tropea D. Insulin-like growth factor 1: At the crossroads of brain development and aging. Front Cell Neurosci (2017) 11:14. doi: 10.3389/fncel.2017.00014
13. Isenović E, Muniyappa R, Milivojević N, Rao Y, Sowers JR. Role of PI3-kinase in isoproterenol and IGF-1 induced ecNOS activity. Biochem Biophys Res Commun (2001) 285(4):954–8. doi: 10.1006/bbrc.2001.5246
14. Obradovic M, Zafirovic S, Soskic S, Stanimirovic J, Trpkovic A, Jevremovic D, et al. Effects of IGF-1 on the cardiovascular system. Curr Pharm Des (2019) 25(35):3715–25. doi: 10.2174/1381612825666191106091507
15. Puche JE, Castilla-Cortázar I. Human conditions of insulin-like growth factor-I (IGF-I) deficiency. J Trans Med (2012) 10(1):224. doi: 10.1186/1479-5876-10-224
16. Ren J, Samson WK, Sowers JR. Insulin-like growth factor I as a cardiac hormone: physiological and pathophysiological implications in heart disease. J Mol Cell Cardiol (1999) 31(11):2049–61. doi: 10.1006/jmcc.1999.1036
17. Troncoso R, Vicencio JM, Parra V, Nemchenko A, Kawashima Y, Del Campo A, et al. Energy-preserving effects of IGF-1 antagonize starvation-induced cardiac autophagy. Cardiovasc Res (2012) 93(2):320–9. doi: 10.1093/cvr/cvr321
18. Higashi Y, Gautam S, Delafontaine P, Sukhanov S. IGF-1 and cardiovascular disease. Growth hormone IGF Res (2019) 45:6–16. doi: 10.1016/j.ghir.2019.01.002
19. Cirrik S, Schmid-Schönbein GW. IGF-1 receptor cleavage in hypertension. Hyperten Res (2018) 41(6):406–13. doi: 10.1038/s41440-018-0023-7
20. Lin M, Liu X, Zheng H, Huang X, Wu Y, Huang A, et al. IGF-1 enhances BMSC viability, migration, and anti-apoptosis in myocardial infarction via secreted frizzled-related protein 2 pathway. Stem Cell Res Ther (2020) 11(1):22. doi: 10.1186/s13287-019-1544-y
21. Nederlof R, Reidel S, Spychala A, Gödecke S, Heinen A, Lautwein T, et al. Insulin-like growth factor 1 attenuates the pro-inflammatory phenotype of neutrophils in myocardial infarction. Front Immunol (2022) 13:908023. doi: 10.3389/fimmu.2022.908023
22. Higashi Y, Quevedo HC, Tiwari S, Sukhanov S, Shai SY, Anwar A, et al. Interaction between insulin-like growth factor-1 and atherosclerosis and vascular aging. Front hormone Res (2014) 43:107–24. doi: 10.1159/000360571
23. Lin S, Zhang Q, Shao X, Zhang T, Xue C, Shi S, et al. IGF-1 promotes angiogenesis in endothelial cells/adipose-derived stem cells co-culture system with activation of PI3K/Akt signal pathway. Cell Proliferation (2017) 50(6). doi: 10.1111/cpr.12390
24. Higashi Y, Sukhanov S, Shai S-Y, Danchuk S, Snarski P, Li Z, et al. Endothelial deficiency of insulin-like growth factor-1 receptor reduces endothelial barrier function and promotes atherosclerosis in apoe-deficient mice. Am J Physiology-Heart Circulatory Physiol (2020) 319(4):H730–H43. doi: 10.1152/ajpheart.00064.2020
25. Salmon WD Jr., Daughaday WH. A hormonally controlled serum factor which stimulates sulfate incorporation by cartilage in vitro. J Lab Clin Med (1957) 49(6):825–36.
26. Rinderknecht E, Humbel RE. Polypeptides with nonsuppressible insulin-like and cell-growth promoting activities in human serum: isolation, chemical characterization, and some biological properties of forms I and II. Proc Natl Acad Sci United States Ame (1976) 73(7):2365–9. doi: 10.1073/pnas.73.7.2365
27. Laron Z. Insulin-like growth factor 1 (IGF-1): a growth hormone. Mol Pathol (2001) 54(5):311–6. doi: 10.1136/mp.54.5.311
28. Rinderknecht E, Humbel RE. The amino acid sequence of human insulin-like growth factor I and its structural homology with proinsulin. J Biol Chem (1978) 253(8):2769–76. doi: 10.1016/S0021-9258(17)40889-1
29. Delafontaine P. Insulin-like growth factor I and its binding proteins in the cardiovascular system. Cardiovasc Res (1995) 30(6):825–34. doi: 10.1016/S0008-6363(95)00163-8
30. Bailes J, Soloviev M. Insulin-like growth factor-1 (IGF-1) and its monitoring in medical diagnostic and in sports. Biomolecules (2021) 11(2):217. doi: 10.3390/biom11020217
31. Yakar S, Liu J-L, Stannard B, Butler A, Accili D, Sauer B, et al. Normal growth and development in the absence of hepatic insulin-like growth factor I. Proc Natl Acad Sci (1999) 96(13):7324–9. doi: 10.1073/pnas.96.13.7324
32. Baserga R. The IGF-I receptor in cancer research. Exp Cell Res (1999) 253(1):1–6. doi: 10.1006/excr.1999.4667
33. Badr M, Hassan T, Tarhony SE, Metwally W. Insulin-like growth factor-1 and childhood cancer risk. Oncol Let (2010) 1(6):1055–9. doi: 10.3892/ol.2010.169
34. Delafontaine P, Song YH, Li Y. Expression, regulation, and function of IGF-1, IGF-1R, and IGF-1 binding proteins in blood vessels. Arteriosclerosis thrombosis Vasc Biol (2004) 24(3):435–44. doi: 10.1161/01.ATV.0000105902.89459.09
35. Al-Samerria S, Radovick S. The role of insulin-like growth factor-1 (IGF-1) in the control of neuroendocrine regulation of growth. Cells (2021) 10(10). doi: 10.3390/cells10102664
36. Gahete MD, Córdoba-Chacón J, Lin Q, Brüning JC, Kahn CR, Castaño JP, et al. Insulin and IGF-I inhibit GH synthesis and release in vitro and in vivo by separate mechanisms. Endocrinology (2013) 154(7):2410–20. doi: 10.1210/en.2013-1261
37. Gusscott S, Jenkins CE, Lam SH, Giambra V, Pollak M, Weng AP. IGF1R derived PI3K/AKT signaling maintains growth in a subset of human T-cell acute lymphoblastic leukemias. PloS One (2016) 11(8):e0161158. doi: 10.1371/journal.pone.0161158
38. Obradovic M, Stanimirovic J, Panic A, Bogdanovic N, Sudar-Milovanovic E, Cenic-Milosevic D, et al. Regulation of Na+/K+-ATPase by estradiol and IGF-1 in cardio-metabolic diseases. Curr Pharm Des (2017) 23(10):1551–61. doi: 10.2174/1381612823666170203113455
39. Ullrich A, Gray A, Tam AW, Yang-Feng T, Tsubokawa M, Collins C, et al. Insulin-like growth factor I receptor primary structure: comparison with insulin receptor suggests structural determinants that define functional specificity. EMBO J (1986) 5(10):2503–12. doi: 10.1002/j.1460-2075.1986.tb04528.x
40. Adams TE, Epa VC, Garrett TP, Ward CW. Structure and function of the type 1 insulin-like growth factor receptor. Cell Mol Life Sci (2000) 57(7):1050–93. doi: 10.1007/PL00000744
41. Jehle PM, Schulten K, Schulz W, Jehle DR, Stracke S, Manfras B, et al. Serum levels of insulin-like growth factor (IGF)-I and IGF binding protein (IGFBP)-1 to-6 and their relationship to bone metabolism in osteoporosis patients. Eur J Internal Med (2003) 14(1):32–8. doi: 10.1016/S0953-6205(02)00183-8
42. Allard JB, Duan C. IGF-binding proteins: Why do they exist and why are there so many? Front Endocrinol (2018) 9. doi: 10.3389/fendo.2018.00117
43. Dai W, Kamei H, Zhao Y, Ding J, Du Z, Duan C. Duplicated zebrafish insulin-like growth factor binding protein-5 genes with split functional domains: evidence for evolutionarily conserved IGF binding, nuclear localization, and transactivation activity. FASEB J (2010) 24(6):2020–9. doi: 10.1096/fj.09-149435
44. Varma Shrivastav S, Bhardwaj A, Pathak KA, Shrivastav A. Insulin-like growth factor binding protein-3 (IGFBP-3): Unraveling the role in mediating IGF-independent effects within the cell. Front Cell Dev Biol (2020) 8. doi: 10.3389/fcell.2020.00286
45. Durai R, Yang SY, Seifalian AM, Goldspink G, Winslet MC. Role of insulin-like growth factor binding protein-4 in prevention of colon cancer. World J Surg Oncol (2007) 5(1):1–8. doi: 10.1186/1477-7819-5-128
46. Hoeflich A, David R, Hjortebjerg R. Current IGFBP-related biomarker research in cardiovascular disease-we need more structural and functional information in clinical studies. Front Endocrinol (2018) 9:388. doi: 10.3389/fendo.2018.00388
47. Tang X, Jiang H, Lin P, Zhang Z, Chen M, Zhang Y, et al. Insulin-like growth factor binding protein-1 regulates HIF-1α degradation to inhibit apoptosis in hypoxic cardiomyocytes. Cell Death Discovery. (2021) 7(1):242. doi: 10.1038/s41420-021-00629-3
48. Bayes-Genis A, Conover CA, Schwartz RS. The insulin-like growth factor axis: A review of atherosclerosis and restenosis. Circ Res (2000) 86(2):125–30. doi: 10.1161/01.RES.86.2.125
49. Grant MB, Wargovich TJ, Ellis EA, Tarnuzzer R, Caballero S, Estes K, et al. Expression of IGF-I, IGF-I receptor and IGF binding proteins-1, -2, -3, -4 and -5 in human atherectomy specimens. Regul peptides. (1996) 67(3):137–44. doi: 10.1016/S0167-0115(96)00124-3
50. Steffensen LB, Conover CA, Oxvig C. PAPP-a and the IGF system in atherosclerosis: ' 'what's up, ' 'what's down? Am J Physiology-Heart Circulatory Physiol (2019) 317(5):H1039–H49. doi: 10.1152/ajpheart.00395.2019
51. Arnqvist HJ, Bornfeldt KE, Chen Y, Lindström T. The insulin-like growth factor system in vascular smooth muscle: interaction with insulin and growth factors. Metabo: Clin Experiment (1995) 44(10 Suppl 4):58–66. doi: 10.1016/0026-0495(95)90222-8
52. Kawachi S, Takeda N, Sasaki A, Kokubo Y, Takami K, Sarui H, et al. Circulating insulin-like growth factor-1 and insulin-like growth factor binding protein-3 are associated with early carotid atherosclerosis. Arteriosclerosis thrombosis Vasc Biol (2005) 25(3):617–21. doi: 10.1161/01.ATV.0000154486.03017.35
53. Juul A, Scheike T, Davidsen M, Gyllenborg J, Jørgensen T. Low serum insulin-like growth factor I is associated with increased risk of ischemic heart disease: a population-based case-control study. Circulation (2002) 106(8):939–44. doi: 10.1161/01.CIR.0000027563.44593.CC
54. Saber H, Himali JJ, Beiser AS, Shoamanesh A, Pikula A, Roubenoff R, et al. Serum insulin-like growth factor 1 and the risk of ischemic stroke: The framingham study. Stroke (2017) 48(7):1760–5. doi: 10.1161/STROKEAHA.116.016563
55. Yao Y, Zhu H, Zhu L, Fang Z, Fan Y, Liu C, et al. A comprehensive contribution of genetic variations of the insulin-like growth factor 1 signalling pathway to stroke susceptibility. Atherosclerosis (2020) 296:59–65. doi: 10.1016/j.atherosclerosis.2020.01.009
56. Clarke MC, Figg N, Maguire JJ, Davenport AP, Goddard M, Littlewood TD, et al. Apoptosis of vascular smooth muscle cells induces features of plaque vulnerability in atherosclerosis. Nat Med (2006) 12(9):1075–80. doi: 10.1038/nm1459
57. Harman JL, Jørgensen HF. The role of smooth muscle cells in plaque stability: Therapeutic targeting potential. Br J Pharmacol (2019) 176(19):3741–53. doi: 10.1111/bph.14779
58. Yurdagul A Jr. Crosstalk between macrophages and vascular smooth muscle cells in atherosclerotic plaque stability. Arteriosclerosis thrombosis Vasc Biol (2022) 42(4):372–80. doi: 10.1161/ATVBAHA.121.316233
59. von der Thüsen JH, Borensztajn KS, Moimas S, van Heiningen S, Teeling P, van Berkel TJ, et al. IGF-1 has plaque-stabilizing effects in atherosclerosis by altering vascular smooth muscle cell phenotype. Am J Pathol (2011) 178(2):924–34. doi: 10.1016/j.ajpath.2010.10.007
60. Snarski P, Sukhanov S, Yoshida T, Higashi Y, Danchuk S, Chandrasekar B, et al. Macrophage-specific IGF-1 overexpression reduces CXCL12 chemokine levels and suppresses atherosclerotic burden in apoe-deficient mice. Arteriosclerosis thrombosis Vasc Biol (2022) 42(2):113–26. doi: 10.1161/ATVBAHA.121.316090
61. Du J, Brink M, Peng T, Mottironi B, Delafontaine P. Thrombin regulates insulin-like growth factor-1 receptor transcription in vascular smooth muscle. Circ Res (2001) 88(10):1044–52. doi: 10.1161/hh1001.090840
62. Zhang L, Yue Y, Ouyang M, Liu H, Li Z. The effects of IGF-1 on TNF-α-Treated DRG neurons by modulating ATF3 and GAP-43 expression via PI3K/Akt/S6K signaling pathway. Neurochem Res (2017) 42(5):1403–21. doi: 10.1007/s11064-017-2192-1
63. Yu X, Xing C, Pan Y, Ma H, Zhang J, Li W. IGF-1 alleviates ox-LDL-induced inflammation via reducing HMGB1 release in HAECs. Acta Biochim Biophys Sinica. (2012) 44(9):746–51. doi: 10.1093/abbs/gms059
64. Ock S, Ham W, Kang CW, Kang H, Lee WS, Kim J. IGF-1 protects against angiotensin II-induced cardiac fibrosis by targeting αSMA. Cell Death Dis (2021) 12(7):688. doi: 10.1038/s41419-021-03965-5
65. Handayaningsih AE, Iguchi G, Fukuoka H, Nishizawa H, Takahashi M, Yamamoto M, et al. Reactive oxygen species play an essential role in IGF-I signaling and IGF-i-induced myocyte hypertrophy in C2C12 myocytes. Endocrinology (2011) 152(3):912–21. doi: 10.1210/en.2010-0981
66. Isenovic ER, Meng Y, Jamali N, Milivojevic N, Sowers JR. Ang II attenuates IGF-1-stimulated na+, k(+)-ATPase activity via PI3K/Akt pathway in vascular smooth muscle cells. Int J Mol Med (2004) 13(6):915–22. doi: 10.3892/ijmm.13.6.915
67. Anwar A, Zahid AA, Phillips L, Delafontaine P. Insulin-like growth factor binding protein-4 expression is decreased by angiotensin II and thrombin in rat aortic vascular smooth muscle cells. Arteriosclerosis thrombosis Vasc Biol (2000) 20(2):370–6. doi: 10.1161/01.ATV.20.2.370
68. Wang J, Razuvaev A, Folkersen L, Hedin E, Roy J, Brismar K, et al. The expression of IGFs and IGF binding proteins in human carotid atherosclerosis, and the possible role of IGF binding protein-1 in the regulation of smooth muscle cell proliferation. Atherosclerosis (2012) 220(1):102–9. doi: 10.1016/j.atherosclerosis.2011.10.032
69. Png K, Halberg N, Yoshida M, Tavazoie S. A microRNA regulon that mediates endothelial recruitment and metastasis by cancer cells. Nature (2012) 481:190–4. doi: 10.1038/nature10661
70. Fanton Y, Houbrechts C, Willems L, Daniëls A, Linsen L, Ratajczak J, et al. Cardiac atrial appendage stem cells promote angiogenesis in vitro and in vivo. J Mol Cell Cardiol (2016) 97:235–44. doi: 10.1016/j.yjmcc.2016.06.005
71. Sun HJ, Wu ZY, Nie XW, Bian JS. Role of endothelial dysfunction in cardiovascular diseases: The link between inflammation and hydrogen sulfide. Front Pharmacol (2019) 10:1568. doi: 10.3389/fphar.2019.01568
72. Gatenby VK, Imrie H, Kearney M. The IGF-1 receptor and regulation of nitric oxide bioavailability and insulin signalling in the endothelium. Pflugers Archiv Eur J Physiol (2013) 465(8):1065–74. doi: 10.1007/s00424-013-1218-z
73. Noguchi S, Kashihara Y, Ikegami Y, Morimoto K, Miyamoto M, Nakao K. Insulin-like growth factor-I ameliorates transient ischemia-induced acute renal failure in rats. J Pharmacol Exp Ther (1993) 267(2):919–26.
74. Wen HJ, Liu GF, Xiao LZ, Wu YG. Involvement of endothelial nitric oxide synthase pathway in IGF−1 protects endothelial progenitor cells against injury from oxidized LDLs. Mol Med Rep (2019) 19(1):660–6. doi: 10.3892/mmr.2018.9633
75. Isgaard J, Arcopinto M, Karason K, Cittadini A. GH and the cardiovascular system: an update on a topic at heart. Endocrine (2015) 48(1):25–35. doi: 10.1007/s12020-014-0327-6
76. Lembo G, Rockman HA, Hunter JJ, Steinmetz H, Koch WJ, Ma L, et al. Elevated blood pressure and enhanced myocardial contractility in mice with severe IGF-1 deficiency. J Clin Invest (1996) 98(11):2648–55. doi: 10.1172/JCI119086
77. Izhar U, Hasdai D, Richardson DM, Cohen P, Lerman A. Insulin and insulin-like growth factor-I cause vasorelaxation in human vessels in vitro. Coronary artery Dis (2000) 11(1):69–76. doi: 10.1097/00019501-200002000-00012
78. Li X, Yu W, Guan Y, Zou H, Liang Z, Huang M, et al. Peripheral circulation and astrocytes contribute to the MSC-mediated increase in IGF-1 levels in the infarct cortex in a dMCAO rat model. Stem Cells Int (2020) 2020:8853444. doi: 10.1155/2020/8853444
79. Donath MY, Sütsch G, Yan XW, Piva B, Brunner HP, Glatz Y, et al. Acute cardiovascular effects of insulin-like growth factor I in patients with chronic heart failure. J Clin Endocrinol Metab (1998) 83(9):3177–83. doi: 10.1210/jc.83.9.3177
80. Erlandsson MC, Lyngfelt L. Low serum IGF1 is associated with hypertension and predicts early cardiovascular events in women with rheumatoid arthritis. BMC Medicine (2019) 17(1):141. doi: 10.1186/s12916-019-1374-x
81. Zhang L, Curhan GC, Forman JP. Plasma insulin-like growth factor-1 level and risk of incident hypertension in non-diabetic women. J hypertension. (2011) 29(2):229–35. doi: 10.1097/HJH.0b013e32834103bf
82. Blackstock CD, Higashi Y, Sukhanov S, Shai SY, Stefanovic B, Tabony AM, et al. Insulin-like growth factor-1 increases synthesis of collagen type I via induction of the mRNA-binding protein LARP6 expression and binding to the 5' stem-loop of COL1a1 and COL1a2 mRNA. J Biol Chem (2014) 289(11):7264–74. doi: 10.1074/jbc.M113.518951
83. Huang KW, Wang IH, Fu P, Krum H, Bach LA, Wang BH. Insulin-like growth factor-1 directly affects cardiac cellular remodelling via distinct pathways. Int J Cardiol Heart Vascul (2021) 36:100852. doi: 10.1016/j.ijcha.2021.100852
84. Filipowicz W, Bhattacharyya SN, Sonenberg N. Mechanisms of post-transcriptional regulation by microRNAs: are the answers in sight? Nat Rev Genet (2008) 9(2):102–14. doi: 10.1038/nrg2290
85. Bartel DP. MicroRNAs: genomics, biogenesis, mechanism, and function. Cell (2004) 116(2):281–97. doi: 10.1016/S0092-8674(04)00045-5
86. Guo H, Ingolia NT, Weissman JS, Bartel DP. Mammalian microRNAs predominantly act to decrease target mRNA levels. Nature (2010) 466(7308):835–40. doi: 10.1038/nature09267
87. Tétreault N, De Guire V. miRNAs: their discovery, biogenesis and mechanism of action. Clin Biochem (2013) 46(10-11):842–5. doi: 10.1016/j.clinbiochem.2013.02.009
88. Ambros V. The functions of animal microRNAs. Nature (2004) 431(7006):350–5. doi: 10.1038/nature02871
89. Selbach M, Schwanhäusser B, Thierfelder N, Fang Z, Khanin R, Rajewsky N. Widespread changes in protein synthesis induced by microRNAs. Nature (2008) 455(7209):58–63. doi: 10.1038/nature07228
90. Grimson A, Farh KK, Johnston WK, Garrett-Engele P, Lim LP, Bartel DP. MicroRNA targeting specificity in mammals: determinants beyond seed pairing. Mol Cell (2007) 27(1):91–105. doi: 10.1016/j.molcel.2007.06.017
91. Macvanin M, Obradovic M, Zafirovic S, Stanimirovic J, Isenovic ER. The role of miRNAs in metabolic diseases. Curr Med Chem (2022). doi: 10.2174/0929867329666220801161536
92. Ling HY, Ou HS, Feng SD, Zhang XY, Tuo QH, Chen LX, et al. CHANGES IN microRNA (miR) profile and effects of miR-320 in insulin-resistant 3T3-L1 adipocytes. Clin Exp Pharmacol Physiol (2009) 36(9):e32–9. doi: 10.1111/j.1440-1681.2009.05207.x
93. Bai Y, Bai X, Wang Z, Zhang X, Ruan C, Miao J. MicroRNA-126 inhibits ischemia-induced retinal neovascularization via regulating angiogenic growth factors. Exp Mol Pathol (2011) 91(1):471–7. doi: 10.1016/j.yexmp.2011.04.016
94. Gao S, Wassler M, Zhang L, Li Y, Wang J, Zhang Y, et al. MicroRNA-133a regulates insulin-like growth factor-1 receptor expression and vascular smooth muscle cell proliferation in murine atherosclerosis. Atherosclerosis (2014) 232(1):171–9. doi: 10.1016/j.atherosclerosis.2013.11.029
95. Zhu H, Shyh-Chang N, Segrè AV, Shinoda G, Shah SP, Einhorn WS, et al. The Lin28/let-7 axis regulates glucose metabolism. Cell (2011) 147(1):81–94. doi: 10.1016/j.cell.2011.08.033
96. Elia L, Contu R, Quintavalle M, Varrone F, Chimenti C, Russo MA, et al. Reciprocal regulation of microRNA-1 and insulin-like growth factor-1 signal transduction cascade in cardiac and skeletal muscle in physiological and pathological conditions. Circulation (2009) 120(23):2377–85. doi: 10.1161/CIRCULATIONAHA.109.879429
97. Xihua L, Shengjie T, Weiwei G, Matro E, Tingting T, Lin L, et al. Circulating miR-143-3p inhibition protects against insulin resistance in metabolic syndrome via targeting of the insulin-like growth factor 2 receptor. Transl Res (2019) 205:33–43. doi: 10.1016/j.trsl.2018.09.006
98. Myers MG Jr., White MF. Insulin signal transduction and the IRS proteins. Annu Rev Pharmacol Toxicol (1996) 36:615–58. doi: 10.1146/annurev.pa.36.040196.003151
99. Ning J, Clemmons DR. AMP-activated protein kinase inhibits IGF-I signaling and protein synthesis in vascular smooth muscle cells via stimulation of insulin receptor substrate 1 S794 and tuberous sclerosis 2 S1345 phosphorylation. Mol Endocrinol (Baltimore Md). (2010) 24(6):1218–29. doi: 10.1210/me.2009-0474
100. Adamo ML, Farrar RP. Resistance training, and IGF involvement in the maintenance of muscle mass during the aging process. Ageing Res Rev (2006) 5(3):310–31. doi: 10.1016/j.arr.2006.05.001
101. Glass DJ. Skeletal muscle hypertrophy and atrophy signaling pathways. Int J Biochem Cell Biol (2005) 37(10):1974–84. doi: 10.1016/j.biocel.2005.04.018
102. O'Connor JC, McCusker RH, Strle K, Johnson RW, Dantzer R, Kelley KW. Regulation of IGF-I function by pro-inflammatory cytokines: at the interface of immunology and endocrinology. Cell Immunol (2008) 252(1-2):91–110. doi: 10.1016/j.cellimm.2007.09.010
103. Dehoux M, Van Beneden R, Pasko N, Lause P, Verniers J, Underwood L, et al. Role of the insulin-like growth factor I decline in the induction of atrogin-1/MAFbx during fasting and diabetes. Endocrinology (2004) 145(11):4806–12. doi: 10.1210/en.2004-0406
104. Clemmons DR. Metabolic actions of insulin-like growth factor-I in normal physiology and diabetes. Endocrinol Metab Clinics North Ame (2012) 41(2):425–43, vii-viii. doi: 10.1016/j.ecl.2012.04.017
105. Mauras N, Beaufrere B. Recombinant human insulin-like growth factor-I enhances whole body protein anabolism and significantly diminishes the protein catabolic effects of prednisone in humans without a diabetogenic effect. J Clin Endocrinol Metab (1995) 80(3):869–74. doi: 10.1210/jcem.80.3.7533772
106. Yuen KC, Dunger DB. Therapeutic aspects of growth hormone and insulin-like growth factor-I treatment on visceral fat and insulin sensitivity in adults. Diabet Obes Metab (2007) 9(1):11–22. doi: 10.1111/j.1463-1326.2006.00591.x
107. del Rincon JP, Iida K, Gaylinn BD, McCurdy CE, Leitner JW, Barbour LA, et al. Growth hormone regulation of p85alpha expression and phosphoinositide 3-kinase activity in adipose tissue: mechanism for growth hormone-mediated insulin resistance. Diabetes (2007) 56(6):1638–46. doi: 10.2337/db06-0299
108. Barbour LA, Mizanoor Rahman S, Gurevich I, Leitner JW, Fischer SJ, Roper MD, et al. Increased P85alpha is a potent negative regulator of skeletal muscle insulin signaling and induces in vivo insulin resistance associated with growth hormone excess. J Biol Chem (2005) 280(45):37489–94. doi: 10.1074/jbc.M506967200
109. Furling D, Marette A, Puymirat J. Insulin-like growth factor I circumvents defective insulin action in human myotonic dystrophy skeletal muscle cells. Endocrinology (1999) 140(9):4244–50. doi: 10.1210/endo.140.9.7057
110. Henry RR, Abrams L, Nikoulina S, Ciaraldi TP. Insulin action and glucose metabolism in non-diabetic control and NIDDM subjects. comparison using human skeletal muscle cell cultures. Diabetes (1995) 44(8):936–46. doi: 10.2337/diab.44.8.936
111. Pennisi P, Gavrilova O, Setser-Portas J, Jou W, Santopietro S, Clemmons D, et al. Recombinant human insulin-like growth factor-I treatment inhibits gluconeogenesis in a transgenic mouse model of type 2 diabetes mellitus. Endocrinology (2006) 147(6):2619–30. doi: 10.1210/en.2005-1556
112. Le Roith D, Kim H, Fernandez AM, Accili D. Inactivation of muscle insulin and IGF-I receptors and insulin responsiveness. Curr Opin Clin Nutr Metab Care (2002) 5(4):371–5. doi: 10.1097/00075197-200207000-00004
113. Yakar S, Liu JL, Fernandez AM, Wu Y, Schally AV, Frystyk J, et al. Liver-specific igf-1 gene deletion leads to muscle insulin insensitivity. Diabetes (2001) 50(5):1110–8. doi: 10.2337/diabetes.50.5.1110
114. Fernández AM, Kim JK, Yakar S, Dupont J, Hernandez-Sanchez C, Castle AL, et al. Functional inactivation of the IGF-I and insulin receptors in skeletal muscle causes type 2 diabetes. Genes Dev (2001) 15(15):1926–34. doi: 10.1101/gad.908001
115. Héron-Milhavet L, Haluzik M, Yakar S, Gavrilova O, Pack S, Jou WC, et al. Muscle-specific overexpression of CD36 reverses the insulin resistance and diabetes of MKR mice. Endocrinology (2004) 145(10):4667–76. doi: 10.1210/en.2003-1543
116. Mauras N, Martinez V, Rini A, Guevara-Aguirre J. Recombinant human insulin-like growth factor I has significant anabolic effects in adults with growth hormone receptor deficiency: studies on protein, glucose, and lipid metabolism. J Clin Endocrinol Metab (2000) 85(9):3036–42. doi: 10.1210/jcem.85.9.6772
117. Hutter R, Sauter BV, Reis ED, Roque M, Vorchheimer D, Carrick FE, et al. Decreased reendothelialization and increased neointima formation with endostatin overexpression in a mouse model of arterial injury. Circulation (2003) 107(12):1658–63. doi: 10.1161/01.CIR.0000058169.21850.CE
118. Nakao-Hayashi J, Ito H, Kanayasu T, Morita I, Murota S. Stimulatory effects of insulin and insulin-like growth factor I on migration and tube formation by vascular endothelial cells. Atherosclerosis (1992) 92(2-3):141–9. doi: 10.1016/0021-9150(92)90273-J
119. Higashi Y, Sukhanov S, Shai SY, Danchuk S, Tang R, Snarski P, et al. Insulin-like growth factor-1 receptor deficiency in macrophages accelerates atherosclerosis and induces an unstable plaque phenotype in apolipoprotein e-deficient mice. Circulation (2016) 133(23):2263–78. doi: 10.1161/CIRCULATIONAHA.116.021805
120. Li Y, Higashi Y, Itabe H, Song YH, Du J, Delafontaine P. Insulin-like growth factor-1 receptor activation inhibits oxidized LDL-induced cytochrome c release and apoptosis via the phosphatidylinositol 3 kinase/Akt signaling pathway. Arteriosclerosis thrombosis Vasc Biol (2003) 23(12):2178–84. doi: 10.1161/01.ATV.0000099788.31333.DB
121. Burgos JI, Yeves AM, Barrena JP, Portiansky EL, Vila-Petroff MG, Ennis IL. Nitric oxide and CaMKII: Critical steps in the cardiac contractile response to IGF-1 and swim training. J Mol Cell Cardiol (2017) 112:16–26. doi: 10.1016/j.yjmcc.2017.08.014
122. Russell-Jones DL, Bates AT, Umpleby AM, Hennessy TR, Bowes SB, Hopkins KD, et al. A comparison of the effects of IGF-I and insulin on glucose metabolism, fat metabolism and the cardiovascular system in normal human volunteers. Eur J Clin Invest (1995) 25(6):403–11. doi: 10.1111/j.1365-2362.1995.tb01721.x
123. Sesti G, Sciacqua A, Cardellini M, Marini MA, Maio R, Vatrano M, et al. Plasma concentration of IGF-I is independently associated with insulin sensitivity in subjects with different degrees of glucose tolerance. Diabetes Care (2005) 28(1):120–5. doi: 10.2337/diacare.28.1.120
124. Sjögren K, Wallenius K, Liu JL, Bohlooly YM, Pacini G, Svensson L, et al. Liver-derived IGF-I is of importance for normal carbohydrate and lipid metabolism. Diabetes (2001) 50(7):1539–45. doi: 10.2337/diabetes.50.7.1539
125. Hannon AM, Thompson CJ, Sherlock M. Diabetes in patients with acromegaly. Curr Diabetes Rep (2017) 17(2):8. doi: 10.1007/s11892-017-0838-7
126. Lombardi G, Di Somma C, Grasso LF, Savanelli MC, Colao A, Pivonello R. The cardiovascular system in growth hormone excess and growth hormone deficiency. J Endocrinol Invest (2012) 35(11):1021–9. doi: 10.3275/8717
127. Tellatin S, Maffei P, Osto E, Dassie F, Famoso G, Montisci R, et al. Coronary microvascular dysfunction may be related to IGF-1 in acromegalic patients and can be restored by therapy. Atherosclerosis (2018) 269:100–5. doi: 10.1016/j.atherosclerosis.2017.12.019
128. He J, Li X, Yu M. The correlation of serum/plasma IGF-1 concentrations with obstructive sleep apnea hypopnea syndrome: A meta-analysis and meta-regression. Front Endocrinol (2022) 13:922229. doi: 10.3389/fendo.2022.922229
129. Rafieian-Kopaei M, Setorki M, Doudi M, Baradaran A, Nasri H. Atherosclerosis: process, indicators, risk factors and new hopes. Int J Prev Med (2014) 5(8):927–46.
130. Sudar-Milovanovic E, Gluvic Z, Obradovic M, Zaric B, Isenovic ER. Tryptophan metabolism in atherosclerosis and diabetes. Curr Med Chem (2022) 29(1):99–113. doi: 10.2174/0929867328666210714153649
131. Raggi P, Genest J, Giles JT, Rayner KJ, Dwivedi G, Beanlands RS, et al. Role of inflammation in the pathogenesis of atherosclerosis and therapeutic interventions. Atherosclerosis (2018) 276:98–108. doi: 10.1016/j.atherosclerosis.2018.07.014
132. Mosca S, Paolillo S, Colao A, Bossone E, Cittadini A, Iudice FL, et al. Cardiovascular involvement in patients affected by acromegaly: an appraisal. Int J Cardiol (2013) 167(5):1712–8. doi: 10.1016/j.ijcard.2012.11.109
133. Abbas A, Grant PJ, Kearney MT. Role of IGF-1 in glucose regulation and cardiovascular disease. Expert Rev Cardiovasc Ther (2008) 6(8):1135–49. doi: 10.1586/14779072.6.8.1135
134. Laughlin GA, Barrett-Connor E, Criqui MH, Kritz-Silverstein D. The prospective association of serum insulin-like growth factor I (IGF-I) and IGF-binding protein-1 levels with all cause and cardiovascular disease mortality in older adults: the rancho Bernardo study. J Clin Endocrinol Metab (2004) 89(1):114–20. doi: 10.1210/jc.2003-030967
135. Conti E, Carrozza C, Capoluongo E, Volpe M, Crea F, Zuppi C, et al. Insulin-like growth factor-1 as a vascular protective factor. Circulation (2004) 110(15):2260–5. doi: 10.1161/01.CIR.0000144309.87183.FB
136. Stavropoulou A, Halapas A, Sourla A, Philippou A, Papageorgiou E, Papalois A, et al. IGF-1 expression in infarcted myocardium and MGF e peptide actions in rat cardiomyocytes in vitro. Mol Med (Cambridge Mass) (2009) 15(5-6):127–35. doi: 10.2119/molmed.2009.00012
137. Suzuki Y, Yeung AC, Ikeno F. The representative porcine model for human cardiovascular disease. J Biomed Biotechnol (2011) 2011:195483. doi: 10.1155/2011/195483
138. Niu XL, Li J, Hakim ZS, Rojas M, Runge MS, Madamanchi NR. Leukocyte antigen-related deficiency enhances insulin-like growth factor-1 signaling in vascular smooth muscle cells and promotes neointima formation in response to vascular injury. J Biol Chem (2007) 282(27):19808–19. doi: 10.1074/jbc.M610452200
139. Zhu B, Zhao G, Witte DP, Hui DY, Fagin JA. Targeted overexpression of IGF-I in smooth muscle cells of transgenic mice enhances neointimal formation through increased proliferation and cell migration after intraarterial injury. Endocrinology (2001) 142(8):3598–606. doi: 10.1210/endo.142.8.8331
140. Khorsandi MJ, Fagin JA, Giannella-Neto D, Forrester JS, Cercek B. Regulation of insulin-like growth factor-I and its receptor in rat aorta after balloon denudation. evidence for local bioactivity. J Clin Invest (1992) 90(5):1926–31. doi: 10.1172/JCI116070
141. Cercek B, Fishbein MC, Forrester JS, Helfant RH, Fagin JA. Induction of insulin-like growth factor I messenger RNA in rat aorta after balloon denudation. Circ Res (1990) 66(6):1755–60. doi: 10.1161/01.RES.66.6.1755
142. Moses JW, Leon MB, Popma JJ, Fitzgerald PJ, Holmes DR, O'Shaughnessy C, et al. Sirolimus-eluting stents versus standard stents in patients with stenosis in a native coronary artery. New Engl J Med (2003) 349(14):1315–23. doi: 10.1056/NEJMoa035071
143. Harrington SC, Simari RD, Conover CA. Genetic deletion of pregnancy-associated plasma protein-a is associated with resistance to atherosclerotic lesion development in apolipoprotein e-deficient mice challenged with a high-fat diet. Circ Res (2007) 100(12):1696–702. doi: 10.1161/CIRCRESAHA.106.146183
144. Shai SY, Sukhanov S, Higashi Y, Vaughn C, Rosen CJ, Delafontaine P. Low circulating insulin-like growth factor I increases atherosclerosis in ApoE-deficient mice. Am J Physiol Heart Circulatory Physiol (2011) 300(5):H1898–906. doi: 10.1152/ajpheart.01081.2010
145. Sukhanov S, Higashi Y, Shai SY, Vaughn C, Mohler J, Li Y, et al. IGF-1 reduces inflammatory responses, suppresses oxidative stress, and decreases atherosclerosis progression in ApoE-deficient mice. Arteriosclerosis thrombosis Vasc Biol (2007) 27(12):2684–90. doi: 10.1161/ATVBAHA.107.156257
146. Shai SY, Sukhanov S, Higashi Y, Vaughn C, Kelly J, Delafontaine P. Smooth muscle cell-specific insulin-like growth factor-1 overexpression in apoe-/- mice does not alter atherosclerotic plaque burden but increases features of plaque stability. Arteriosclerosis thrombosis Vasc Biol (2010) 30(10):1916–24. doi: 10.1161/ATVBAHA.110.210831
147. Zaric BL, Radovanovic JN, Gluvic Z, Stewart AJ, Essack M, Motwalli O, et al. Atherosclerosis linked to aberrant amino acid metabolism and immunosuppressive amino acid catabolizing enzymes. Front Immunol (2020) 11:551758. doi: 10.3389/fimmu.2020.551758
148. Sukhanov S, Higashi Y, Yoshida T, Danchuk S, Alfortish M, Goodchild T, et al. Insulin-like growth factor I reduces coronary atherosclerosis in pigs with familial hypercholesterolemia. JCI Insight (2023). doi: 10.1172/jci.insight.165713
149. Colao A, Spiezia S, Di Somma C, Pivonello R, Marzullo P, Rota F, et al. Circulating insulin-like growth factor-I levels are correlated with the atherosclerotic profile in healthy subjects independently of age. J Endocrinol Invest (2005) 28(5):440–8. doi: 10.1007/BF03347225
150. Spilcke-Liss E, Friedrich N, Dörr M, Schminke U, Völzke H, Brabant G, et al. Serum insulin-like growth factor I and its binding protein 3 in their relation to intima-media thickness: results of the study of health in pomerania (SHIP). Clin Endocrinol (2011) 75(1):70–5. doi: 10.1111/j.1365-2265.2011.04010.x
151. van den Beld AW, Bots ML, Janssen JA, Pols HA, Lamberts SW, Grobbee DE. Endogenous hormones and carotid atherosclerosis in elderly men. Am J Epidemiol (2003) 157(1):25–31. doi: 10.1093/aje/kwf160
152. Ameri P, Canepa M, Fabbi P, Leoncini G, Milaneschi Y, Mussap M, et al. Vitamin d modulates the association of circulating insulin-like growth factor-1 with carotid artery intima-media thickness. Atherosclerosis (2014) 236(2):418–25. doi: 10.1016/j.atherosclerosis.2014.08.022
153. Sirbu A, Nicolae H, Martin S, Barbu C, Copaescu C, Florea S, et al. IGF-1 and insulin resistance are major determinants of common carotid artery thickness in morbidly obese young patients. Angiology (2016) 67(3):259–65. doi: 10.1177/0003319715586499
154. Abd El-Hafez H, Elrakhawy MM, El-Baiomy AA, El-Eshmawy MM. Carotid intima media thickness is independently associated with Male gender, middle age, and IGF-1 in metabolically healthy obese individuals. ISRN Obes (2014) 2014:545804. doi: 10.1155/2014/545804
155. Chen L, Samanta A, Zhao L, Dudley NR, Buehler T, Vincent RJ, et al. Vitamin D3 induces mesenchymal-to-endothelial transition and promotes a proangiogenic niche through IGF-1 signaling. iScience (2021) 24(4):102272. doi: 10.1016/j.isci.2021.102272
156. Ruidavets JB, Luc G, Machez E, Genoux AL, Kee F, Arveiler D, et al. Effects of insulin-like growth factor 1 in preventing acute coronary syndromes: the PRIME study. Atherosclerosis (2011) 218(2):464–9. doi: 10.1016/j.atherosclerosis.2011.05.034
157. De Lorenzo A, Moreira AS, Souza EG, Oliveira GM. Insulin-like growth factor-1 in early-onset coronary artery disease: Insights into the pathophysiology of atherosclerosis. Int J Cardiol (2016) 202:1–2. doi: 10.1016/j.ijcard.2015.04.032
158. Janssen JA, Stolk RP, Pols HA, Grobbee DE, Lamberts SW. Serum total IGF-I, free IGF-I, and IGFB-1 levels in an elderly population: relation to cardiovascular risk factors and disease. Arteriosclerosis thrombosis Vasc Biol (1998) 18(2):277–82. doi: 10.1161/01.ATV.18.2.277
159. Kaplan RC, McGinn AP, Pollak MN, Kuller LH, Strickler HD, Rohan TE, et al. Association of total insulin-like growth factor-I, insulin-like growth factor binding protein-1 (IGFBP-1), and IGFBP-3 levels with incident coronary events and ischemic stroke. J Clin Endocrinol Metab (2007) 92(4):1319–25. doi: 10.1210/jc.2006-1631
160. Johnsen SP, Hundborg HH, Sørensen HT, Orskov H, Tjønneland A, Overvad K, et al. Insulin-like growth factor (IGF) I, -II, and IGF binding protein-3 and risk of ischemic stroke. J Clin Endocrinol Metab (2005) 90(11):5937–41. doi: 10.1210/jc.2004-2088
161. Akanji AO, Smith RJ. The insulin-like growth factor system, metabolic syndrome, and cardiovascular disease risk. Metab syndrome related Disord (2012) 10(1):3–13. doi: 10.1089/met.2011.0083
162. Aronis KN, Sahin-Efe A, Chamberland JP, Spiro A 3rd, Vokonas P, Mantzoros CS. Chemerin levels as predictor of acute coronary events: a case-control study nested within the veterans affairs normative aging study. Metabo: Clin Experiment (2014) 63(6):760–6. doi: 10.1016/j.metabol.2014.02.013
163. Li T, Zhao Y, Yang X, Feng Y, Li Y, Wu Y, et al. Association between insulin-like growth factor-1 and cardiovascular events: a systematic review and dose-response meta-analysis of cohort studies. J Endocrinol Invest (2022) 45(12):2221–31. doi: 10.1007/s40618-022-01819-1
164. Berryman DE, Glad CA, List EO, Johannsson G. The GH/IGF-1 axis in obesity: pathophysiology and therapeutic considerations. Nat Rev Endocrinol (2013) 9(6):346–56. doi: 10.1038/nrendo.2013.64
165. Song CL, Liu B, Diao HY, Shi YF, Zhang JC, Li YX, et al. Down-regulation of microRNA-320 suppresses cardiomyocyte apoptosis and protects against myocardial ischemia and reperfusion injury by targeting IGF-1. Oncotarget (2016) 7(26):39740–57. doi: 10.18632/oncotarget.9240
166. Vasan RS, Sullivan LM, D'Agostino RB, Roubenoff R, Harris T, Sawyer DB, et al. Serum insulin-like growth factor I and risk for heart failure in elderly individuals without a previous myocardial infarction: the framingham heart study. Ann Internal Med (2003) 139(8):642–8. doi: 10.7326/0003-4819-139-8-200310210-00007
167. Bleumink GS, Rietveld I, Janssen JA, van Rossum EF, Deckers JW, Hofman A, et al. Insulin-like growth factor-I gene polymorphism and risk of heart failure (the Rotterdam study). Am J Cardiol (2004) 94(3):384–6. doi: 10.1016/j.amjcard.2004.04.044
168. Schutte AE, Conti E, Mels CM, Smith W, Kruger R, Botha S, et al. Attenuated IGF-1 predicts all-cause and cardiovascular mortality in a black population: A five-year prospective study. Eur J Prev Cardiol (2016) 23(16):1690–9. doi: 10.1177/2047487316661436
169. Urbonaviciene G, Frystyk J, Urbonavicius S, Lindholt JS. IGF-I and IGFBP2 in peripheral artery disease: results of a prospective study. Scandinavian Cardiovasc J SCJ. (2014) 48(2):99–105. doi: 10.3109/14017431.2014.891760
170. Domené S, Domené HM. The role of acid-labile subunit (ALS) in the modulation of GH-IGF-I action. Mol Cell Endocrinol (2020) 518:111006. doi: 10.1016/j.mce.2020.111006
171. Brevetti G, Colao A, Schiano V, Pivonello R, Laurenzano E, Di Somma C, et al. IGF system and peripheral arterial disease: relationship with disease severity and inflammatory status of the affected limb. Clin Endocrinol (2008) 69(6):894–900. doi: 10.1111/j.1365-2265.2008.03269.x
172. Tsukahara H, Gordienko DV, Tonshoff B, Gelato MC, Goligorsky MS. Direct demonstration of insulin-like growth factor-i-induced nitric oxide production by endothelial cells. Kidney Int (1994) 45(2):598–604. doi: 10.1038/ki.1994.78
173. Wu HY, Jeng YY, Yue CJ, Chyu KY, Hsueh WA, Chan TM. Endothelial-dependent vascular effects of insulin and insulin-like growth factor I in the perfused rat mesenteric artery and aortic ring. Diabetes (1994) 43(8):1027–32. doi: 10.2337/diab.43.8.1027
174. Hasdai D, Rizza RA, Holmes DR Jr., Richardson DM, Cohen P, Lerman A. Insulin and insulin-like growth factor-I cause coronary vasorelaxation in vitro. Hyperten (Dallas Tex 1979) (1998) 32(2):228–34. doi: 10.1161/01.HYP.32.2.228
175. Pendergrass M, Fazioni E, Collins D, DeFronzo RA. IGF-I increases forearm blood flow without increasing forearm glucose uptake. Am J Physiol (1998) 275(2):E345–50. doi: 10.1152/ajpendo.1998.275.2.E345
176. Díez J. Insulin-like growth factor I in essential hypertension. Kidney Int (1999) 55(2):744–59. doi: 10.1046/j.1523-1755.1999.00300.x
177. Chen Y, Cheng X, Li S, Yin Y, Xing S, Guo Y. Insulin-like growth factor-1 levels are associated with interventricular septal thickening. Front Endocrinol (2022) 13:997023. doi: 10.3389/fendo.2022.997023
178. McCallum RW, Hamilton CA, Graham D, Jardine E, Connell JM, Dominiczak AF. Vascular responses to IGF-I and insulin are impaired in aortae of hypertensive rats. J hypertension. (2005) 23(2):351–8. doi: 10.1097/00004872-200502000-00017
179. Vecchione C, Colella S, Fratta L, Gentile MT, Selvetella G, Frati G, et al. Impaired insulin-like growth factor I vasorelaxant effects in hypertension. Hyperten (Dallas Tex 1979). (2001) 37(6):1480–5. doi: 10.1161/01.HYP.37.6.1480
180. DeLano FA, Schmid-Schönbein GW. Proteinase activity and receptor cleavage: mechanism for insulin resistance in the spontaneously hypertensive rat. Hyperten (Dallas Tex 1979). (2008) 52(2):415–23. doi: 10.1161/HYPERTENSIONAHA.107.104356
181. Delano FA, Zhang H, Tran EE, Zhang C, Schmid-Schönbein GW. A new hypothesis for insulin resistance in hypertension due to receptor cleavage. Expert Rev Endocrinol Metab (2010) 5(1):149–58. doi: 10.1586/eem.09.64
182. Delano FA, Chen AY, Wu KI, Tran ED, Rodrigues SF, Schmid-Schönbein GW. the autodigestion hypothesis and receptor cleavage in diabetes and hypertension. Drug Discovery Today Dis models. (2011) 8(1):37–46. doi: 10.1016/j.ddmod.2011.05.002
183. Tran ED, DeLano FA, Schmid-Schönbein GW. Enhanced matrix metalloproteinase activity in the spontaneously hypertensive rat: VEGFR-2 cleavage, endothelial apoptosis, and capillary rarefaction. J Vasc Res (2010) 47(5):423–31. doi: 10.1159/000281582
184. Diéz J, Laviades C. Insulin-like growth factor I and collagen type III synthesis in patients with essential hypertension and left ventricular hypertrophy. J Hum hypertension. (1994) 8 Suppl 1:S21–5.
185. Laviades C, Gil MJ, Monreal I, González A, Díez J. Is the tissue availability of circulating insulin-like growth factor I involved in organ damage and glucose regulation in hypertension? J hypertension (1997) 15(10):1159–65. doi: 10.1097/00004872-199715100-00014
186. Laviades C, Mayor G, Diez J. Elevated circulating levels of insulin-like growth factor I in essential hypertensive patients with left ventricular hypertrophy. Arch Des maladies du coeur Des vaisseaux. (1991) 84(8):1039–41.
187. Andronico G, Mangano MT, Nardi E, Mulè G, Piazza G, Cerasola G. Insulin-like growth factor 1 and sodium-lithium countertransport in essential hypertension and in hypertensive left ventricular hypertrophy. J hypertension. (1993) 11(10):1097–101. doi: 10.1097/00004872-199310000-00014
188. Colangelo LA, Liu K, Gapstur SM. Insulin-like growth factor-1, insulin-like growth factor binding protein-3, and cardiovascular disease risk factors in young black men and white men: the CARDIA Male hormone study. Am J Epidemiol (2004) 160(8):750–7. doi: 10.1093/aje/kwh289
189. Lawlor DA, Ebrahim S, Smith GD, Cherry L, Watt P, Sattar N. The association of insulin-like-growth factor 1 (IGF-1) with incident coronary heart disease in women: findings from the prospective British women's heart and health study. Atherosclerosis (2008) 201(1):198–204. doi: 10.1016/j.atherosclerosis.2007.12.061
190. Kieć-Wilk B, Stolarz-Skrzypek K, Sliwa A, Zdzienicka A, Kawecka-Jaszcz K. Peripheral blood concentrations of TGFβ1, IGF-1 and bFGF and remodelling of the left ventricle and blood vessels in hypertensive patients. Kardiol Pol (2010) 68(9):996–1002.
191. Schutte AE, Huisman HW, van Rooyen JM, Malan L, Malan NT, Fourie CM, et al. A significant decline in IGF-I may predispose young africans to subsequent cardiometabolic vulnerability. J Clin Endocrinol Metab (2010) 95(5):2503–7. doi: 10.1210/jc.2009-2329
192. Succurro E, Andreozzi F, Sciacqua A, Hribal ML, Perticone F, Sesti G. Reciprocal association of plasma IGF-1 and interleukin-6 levels with cardiometabolic risk factors in non-diabetic subjects. Diabetes Care (2008) 31(9):1886–8. doi: 10.2337/dc08-0553
193. Colao A, Di Somma C, Cascella T, Pivonello R, Vitale G, Grasso LF, et al. Relationships between serum IGF1 levels, blood pressure, and glucose tolerance: an observational, exploratory study in 404 subjects. Eur J Endocrinol (2008) 159(4):389–97. doi: 10.1530/EJE-08-0201
194. Zachariah JP, Xanthakis V, Larson MG, Vita JA, Sullivan LM, Smith HM, et al. Circulating vascular growth factors and central hemodynamic load in the community. Hyperten (Dallas Tex 1979). (2012) 59(4):773–9. doi: 10.1161/HYPERTENSIONAHA.111.179242
195. Capoluongo E, Pitocco D, Lulli P, Minucci A, Santonocito C, Manto A, et al. Inverse correlation between serum free IGF-I and IGFBP-3 levels and blood pressure in patients affected with type 1 diabetes. Cytokine (2006) 34(5-6):303–11. doi: 10.1016/j.cyto.2006.06.007
196. Schutte AE, Volpe M, Tocci G, Conti E. Revisiting the relationship between blood pressure and insulin-like growth factor-1. Hyperten (Dallas Tex 1979). (2014) 63(5):1070–7. doi: 10.1161/HYPERTENSIONAHA.113.03057
197. Larsson SC, Michaëlsson K. IGF-1 and cardiometabolic diseases: a mendelian zrandomization study. Diabetologia (2020) 63(9):1775–82. doi: 10.1007/s00125-020-05190-9
198. Rajpathak SN, He M, Sun Q, Kaplan RC, Muzumdar R, Rohan TE, et al. Insulin-like growth factor axis and risk of type 2 diabetes in women. Diabetes (2012) 61(9):2248–54. doi: 10.2337/db11-1488
199. Drogan D, Schulze MB, Boeing H, Pischon T. Insulin-like growth factor 1 and insulin-like growth factor-binding protein 3 in relation to the risk of type 2 diabetes mellitus: Results from the EPIC-potsdam study. Am J Epidemiol (2016) 183(6):553–60. doi: 10.1093/aje/kwv188
200. Schneider HJ, Friedrich N, Klotsche J, Schipf S, Nauck M, Völzke H, et al. Prediction of incident diabetes mellitus by baseline IGF1 levels. Eur J Endocrinol (2011) 164(2):223–9. doi: 10.1530/EJE-10-0963
201. Friedrich N, Thuesen B, Jørgensen T, Juul A, Spielhagen C, Wallaschofksi H, et al. The association between IGF-I and insulin resistance: a general population study in Danish adults. Diabetes Care (2012) 35(4):768–73. doi: 10.2337/dc11-1833
202. Haywood NJ, Slater TA, Matthews CJ, Wheatcroft SB. The insulin like growth factor and binding protein family: Novel therapeutic targets in obesity & diabetes. Mol Metab (2019) 19:86–96. doi: 10.1016/j.molmet.2018.10.008
203. Osher E, Macaulay VM. Therapeutic targeting of the IGF axis. Cells (2019) 8(8). doi: 10.3390/cells8080895
204. Arteaga CL, Kitten LJ, Coronado EB, Jacobs S, Kull FC Jr., Allred DC, et al. Blockade of the type I somatomedin receptor inhibits growth of human breast cancer cells in athymic mice. J Clin Invest (1989) 84(5):1418–23. doi: 10.1172/JCI114315
205. Li R, Pourpak A, Morris SW. Inhibition of the insulin-like growth factor-1 receptor (IGF1R) tyrosine kinase as a novel cancer therapy approach. J Med Chem (2009) 52(16):4981–5004. doi: 10.1021/jm9002395
206. Trojan J, Johnson TR, Rudin SD, Ilan J, Tykocinski ML, Ilan J. Treatment and prevention of rat glioblastoma by immunogenic C6 cells expressing antisense insulin-like growth factor I RNA. Sci (New York NY). (1993) 259(5091):94–7. doi: 10.1126/science.8418502
207. Resnicoff M, Sell C, Rubini M, Coppola D, Ambrose D, Baserga R, et al. Rat glioblastoma cells expressing an antisense RNA to the insulin-like growth factor-1 (IGF-1) receptor are nontumorigenic and induce regression of wild-type tumors. Cancer Res (1994) 54(8):2218–22.
208. Nguyen TT, Cao N, Short JL, White PJ. Intravenous insulin-like growth factor-I receptor antisense treatment reduces angiotensin receptor expression and function in spontaneously hypertensive rats. J Pharmacol Exp Ther (2006) 318(3):1171–7. doi: 10.1124/jpet.106.103655
209. Elbashir SM, Harborth J, Lendeckel W, Yalcin A, Weber K, Tuschl T. Duplexes of 21-nucleotide RNAs mediate RNA interference in cultured mammalian cells. Nature (2001) 411(6836):494–8. doi: 10.1038/35078107
210. Castel SE, Martienssen RA. RNA Interference in the nucleus: roles for small RNAs in transcription, epigenetics and beyond. Nat Rev Genet (2013) 14(2):100–12. doi: 10.1038/nrg3355
211. Bohula EA, Salisbury AJ, Sohail M, Playford MP, Riedemann J, Southern EM, et al. The efficacy of small interfering RNAs targeted to the type 1 insulin-like growth factor receptor (IGF1R) is influenced by secondary structure in the IGF1R transcript. J Biol Chem (2003) 278(18):15991–7. doi: 10.1074/jbc.M300714200
212. Soutschek J, Akinc A, Bramlage B, Charisse K, Constien R, Donoghue M, et al. Therapeutic silencing of an endogenous gene by systemic administration of modified siRNAs. Nature (2004) 432(7014):173–8. doi: 10.1038/nature03121
213. De Meyts P, Whittaker J. Structural biology of insulin and IGF1 receptors: implications for drug design. Nat Rev Drug discovery. (2002) 1(10):769–83. doi: 10.1038/nrd917
214. Xu Y, Kong GK, Menting JG, Margetts MB, Delaine CA, Jenkin LM, et al. How ligand binds to the type 1 insulin-like growth factor receptor. Nat Commun (2018) 9(1):821. doi: 10.1038/s41467-018-03219-7
215. D'Ambrosio C, Ferber A, Resnicoff M, Baserga R. A soluble insulin-like growth factor I receptor that induces apoptosis of tumor cells in vivo and inhibits tumorigenesis. Cancer Res (1996) 56(17):4013–20.
216. Min Y, Adachi Y, Yamamoto H, Imsumran A, Arimura Y, Endo T, et al. Insulin-like growth factor I receptor blockade enhances chemotherapy and radiation responses and inhibits tumour growth in human gastric cancer xenografts. Gut (2005) 54(5):591–600. doi: 10.1136/gut.2004.048926
217. Samani AA, Chevet E, Fallavollita L, Galipeau J, Brodt P. Loss of tumorigenicity and metastatic potential in carcinoma cells expressing the extracellular domain of the type 1 insulin-like growth factor receptor. Cancer Res (2004) 64(10):3380–5. doi: 10.1158/0008-5472.CAN-03-3780
218. Fujita M, Ieguchi K, Cedano-Prieto DM, Fong A, Wilkerson C, Chen JQ, et al. An integrin binding-defective mutant of insulin-like growth factor-1 (R36E/R37E IGF1) acts as a dominant-negative antagonist of the IGF1 receptor (IGF1R) and suppresses tumorigenesis but still binds to IGF1R. J Biol Chem (2013) 288(27):19593–603. doi: 10.1074/jbc.M113.470872
219. García-Echeverría C, Pearson MA, Marti A, Meyer T, Mestan J, Zimmermann J, et al. In vivo antitumor activity of NVP-AEW541-A novel, potent, and selective inhibitor of the IGF-IR kinase. Cancer Cell (2004) 5(3):231–9. doi: 10.1016/S1535-6108(04)00051-0
220. Favelyukis S, Till JH, Hubbard SR, Miller WT. Structure and autoregulation of the insulin-like growth factor 1 receptor kinase. Nat Struct Biol (2001) 8(12):1058–63. doi: 10.1038/nsb721
221. Mulvihill MJ, Cooke A, Rosenfeld-Franklin M, Buck E, Foreman K, Landfair D, et al. Discovery of OSI-906: a selective and orally efficacious dual inhibitor of the IGF-1 receptor and insulin receptor. Future Med Chem (2009) 1(6):1153–71. doi: 10.4155/fmc.09.89
222. Carboni JM, Wittman M, Yang Z, Lee F, Greer A, Hurlburt W, et al. BMS-754807, a small molecule inhibitor of insulin-like growth factor-1R/IR. Mol Cancer Ther (2009) 8(12):3341–9. doi: 10.1158/1535-7163.MCT-09-0499
223. Weroha SJ, Haluska P. IGF-1 receptor inhibitors in clinical trials–early lessons. J mammary gland Biol neoplasia. (2008) 13(4):471–83. doi: 10.1007/s10911-008-9104-6
224. Jones RL, Kim ES, Nava-Parada P, Alam S, Johnson FM, Stephens AW, et al. Phase I study of intermittent oral dosing of the insulin-like growth factor-1 and insulin receptors inhibitor OSI-906 in patients with advanced solid tumors. Clin Cancer Res (2015) 21(4):693–700. doi: 10.1158/1078-0432.CCR-14-0265
225. Macaulay VM, Middleton MR, Eckhardt SG, Rudin CM, Juergens RA, Gedrich R, et al. Phase I dose-escalation study of linsitinib (OSI-906) and erlotinib in patients with advanced solid tumors. Clin Cancer Res (2016) 22(12):2897–907. doi: 10.1158/1078-0432.CCR-15-2218
226. Oza A, Kaye S, Van Tornout J, Sessa C, Gore M, Naumann RW, et al. Phase 2 study evaluating intermittent and continuous linsitinib and weekly paclitaxel in patients with recurrent platinum resistant ovarian epithelial cancer. Gynecol Oncol (2018) 149(2):275–82. doi: 10.1016/j.ygyno.2018.01.019
227. Moses AC, Young SC, Morrow LA, O'Brien M, Clemmons DR. Recombinant human insulin-like growth factor I increases insulin sensitivity and improves glycemic control in type II diabetes. Diabetes (1996) 45(1):91–100. doi: 10.2337/diab.45.1.91
228. Wang R, Xi L, Kukreja RC. PDE5 inhibitor tadalafil and hydroxychloroquine cotreatment provides synergistic protection against type 2 diabetes and myocardial infarction in mice. J Pharmacol Exp Ther (2017) 361(1):29–38. doi: 10.1124/jpet.116.239087
Keywords: IGF-1, cardiovascular diseases, IGF-1 system, atherosclerosis, diabetes, microRNA
Citation: Macvanin M, Gluvic Z, Radovanovic J, Essack M, Gao X and Isenovic ER (2023) New insights on the cardiovascular effects of IGF-1. Front. Endocrinol. 14:1142644. doi: 10.3389/fendo.2023.1142644
Received: 11 January 2023; Accepted: 26 January 2023;
Published: 09 February 2023.
Edited by:
Gaetano Santulli, Albert Einstein College of Medicine, United StatesReviewed by:
Jörgen Isgaard, University of Gothenburg, SwedenPradeep Kumar Dabla, G B Pant Institute of Postgraduate Medical Education and Research (GIPMER), India
Copyright © 2023 Macvanin, Gluvic, Radovanovic, Essack, Gao and Isenovic. This is an open-access article distributed under the terms of the Creative Commons Attribution License (CC BY). The use, distribution or reproduction in other forums is permitted, provided the original author(s) and the copyright owner(s) are credited and that the original publication in this journal is cited, in accordance with accepted academic practice. No use, distribution or reproduction is permitted which does not comply with these terms.
*Correspondence: Mirjana Macvanin, mirjana.macvanin@vin.bg.ac.rs