- 1Guang’anmen Hospital, China Academy of Chinese Medical Sciences, Beijing, China
- 2China-Japan Friendship Hospital, Institute of Medical Science, Beijing, China
Diabetic kidney disease (DKD) is a major microvascular complication of diabetes and a leading cause of end-stage renal disease worldwide. Autophagy plays an important role in maintaining cellular homeostasis in renal physiology. In DKD, the accumulation of advanced glycation end products induces decreased renal autophagy-related protein expression and transcription factor EB (TFEB) nuclear transfer, leading to impaired autophagy and lysosomal function and blockage of autophagic flux. This accelerates renal resident cell injury and apoptosis, mediates macrophage infiltration and phenotypic changes, ultimately leading to aggravated proteinuria and fibrosis in DKD. Natural polyphenols show promise in treating DKD by regulating autophagy and promoting nuclear transfer of TFEB and lysosomal repair. This review summarizes the characteristics of autophagy in DKD, and the potential application and mechanisms of some known natural polyphenols as autophagy regulators in DKD, with the goal of contributing to a deeper understanding of natural polyphenol mechanisms in the treatment of DKD and promoting the development of their applications. Finally, we point out the limitations of polyphenols in current DKD research and provide an outlook for their future research.
1 Introduction
Diabetic kidney disease (DKD) is a major microvascular complication of diabetes mellitus. As the incidence of diabetes continues to rise, DKD has become one of the fastest-growing causes of chronic kidney disease and its associated morbidity and mortality (1, 2). Simultaneously, the occurrence and severity of kidney disease increase the risk of adverse health outcomes, including cardiovascular disease and cancer, and premature mortality in patients with diabetes (3, 4). There is, therefore, an urgent need to improve the diagnosis and management of DKD. DKD pathogenesis is complex, with various metabolic and hemodynamic alterations involved in the development and progression of DKD (5).
Autophagy protects against DKD development by regulating cellular metabolism and organelle homeostasis, as well as degrading and recycling damaged proteins, macromolecules, and organelles (6). Furthermore, under diabetic conditions, autophagy interacts with multiple intracellular stress signals to maintain cellular integrity and contributes to the clearance of damaged proteins and organelles (7). Conditional knockout mice of autophagy-related genes (Atg5- or Atg7-KO mice) in renal resident cells further demonstrate the essential role of basal autophagy in cellular homeostasis (8–10). However, autophagy is suppressed in DKD, and dysregulation of autophagy and lysosomal homeostasis, therefore, aggravates podocyte injury, glomerulosclerosis, and fibrosis in DKD (11, 12). Autophagy defects in DKD are thought to be caused primarily by advanced glycation end products (AGEs). AGEs are a complex and heterogeneous group of compounds that originate as heterogeneous molecules from the nonenzymatic products of glucose reactions or other saccharide derivatives with proteins or lipids (13). Their presence in cells and tissues can be detected by several methods including competitive immunoassay, skin autofluorescence, and stable isotopic dilution analysis liquid chromatography and tandem mass spectrometry (14). AGEs are endocytosed by renal proximal tubules and degraded by lysosomes, but they can also form inside different renal cell types. High glucose (HG), AGE-rich diet, and decreased renal clearance all have the potential to accelerate AGE formation and accumulation in DKD (15). This may lead to apoptosis and inflammation and thereby lead to DKD progression (16). Accumulating evidence supports the causative role of AGEs in autophagy defects in DKD (17). Conversely, autophagy is thought to play a protective role against AGEs-induced apoptosis. The p62-dependent autophagy, for example, was shown to facilitate the removal of AGEs, and the absence of p62 accelerated the accumulation of AGEs in the soluble and insoluble fractions (18).
Although great efforts have been made to develop effective therapies for DKD, delaying its progression to end-stage renal disease (ESRD) remains a great challenge. Natural polyphenols, which are abundant in fruits, vegetables, spices, and herbs, are known for their health benefits on DKD by improving detachment and apoptosis of podocytes, tubulointerstitial fibrosis, proliferation, excessive matrix production of mesangial cells, and infiltration and phenotypic changes of macrophages, potentially by improving autophagy and lysosomal function (19).
In this review, we critically evaluate the strengths and limitations of natural polyphenols, focusing on their regulation of autophagy to provide a clinical reference for the treatment of DKD.
2 Dysregulation of autophagy in DKD
Autophagy and lysosomal dysfunction of renal resident cells are important pathological factors affecting DKD progression. In this section, we discuss the characteristics and regulatory role of autophagy in the onset and progression of DKD and highlight the importance of autophagy regulation in renal resident cells and renal macrophages (Figure 1).
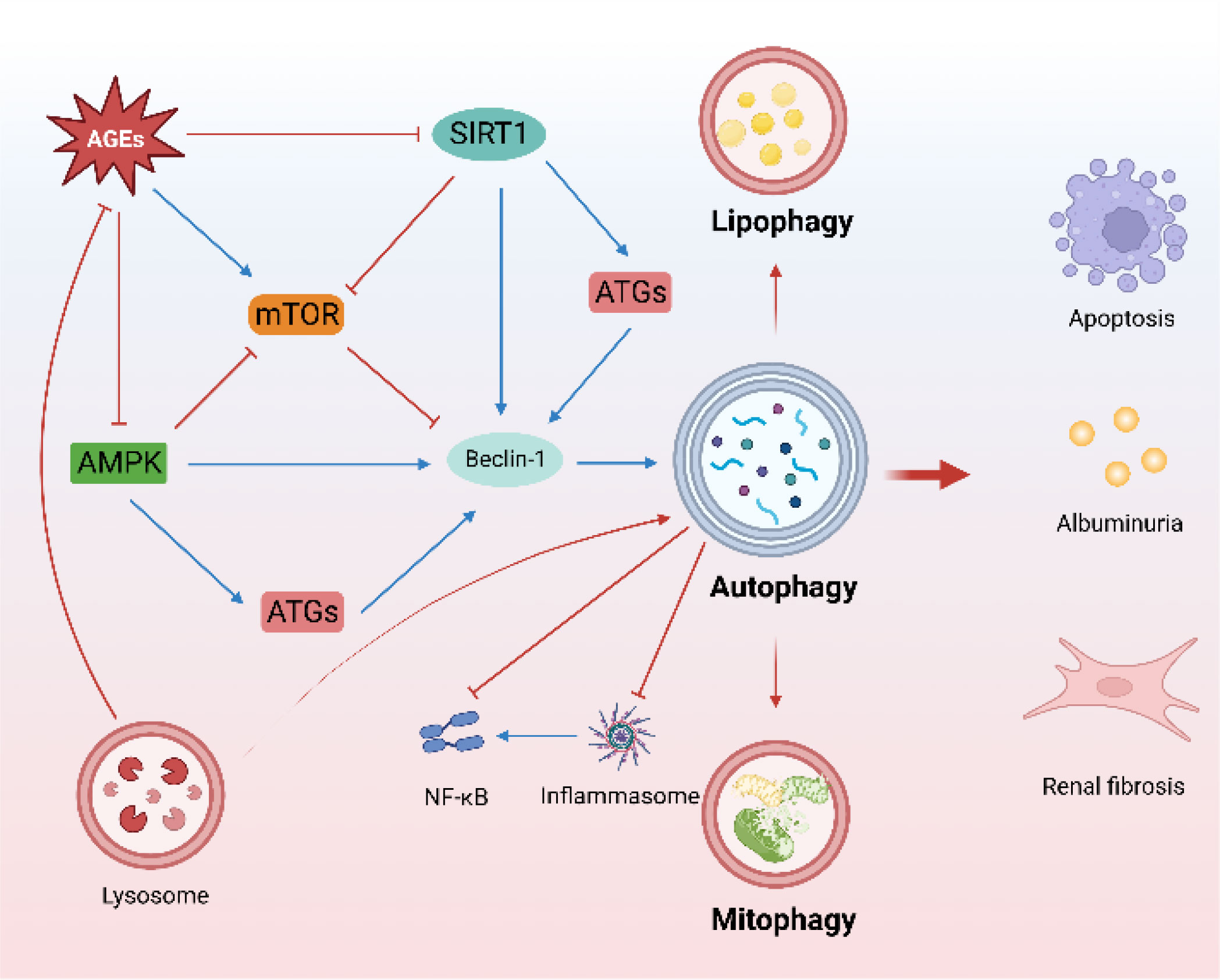
Figure 1 Characteristics of autophagy in DKD. (The mTOR, AMPK, and SIRT1-regulated autophagy pathway is an important protective mechanism for DKD. Mitophagy and lipophagy are critical to DKD. There is a close crosstalk between autophagy and inflammation. Lysosomes promote the degradation of AGEs).
2.1 Autophagy regulatory pathways
Mammalian target of rapamycin (mTOR), AMP-activated protein kinase (AMPK), and Sirtuin 1 (SIRT1) are the main autophagy-regulatory pathways in DKD (20) and maintain cellular homeostasis in DKD. mTOR complex 1 (mTORC1) activation is involved in the early stages of DKD (21, 22). Furthermore, specific activation of mTORC1 in podocytes induces DKD-like renal damage, including podocyte effacement, glomerular basement membrane thickening, mesangial expansion, epithelial-mesenchymal transdifferentiation, and proteinuria (23). Increased mTORC1 activity in the proximal tubule in diabetes induces renal fibrosis and renal function decline (24), and targeted mTORC1 inhibition by rapamycin and sodium-glucose cotransporter 2 inhibitors (SGLT2i) is renoprotective in DKD (24–26). In contrast to mTORC1, AMPK is a positive regulator of autophagy. AMPK can regulate autophagy by direct phosphorylation modification, as well as induce autophagy by inhibiting mTORC1. Loss of AMPK aggravates proteinuria in DKD (27). AMPK activation ameliorates apoptosis and fibrosis in DKD (28, 29), and the effect of metformin mitigates renal oxidative stress and fibrosis in DKD is also associated with activating AMPK (30). Notably, AMPK also plays an important role in maintaining mitochondrial homeostasis and optimizing oxidative phosphorylation to maintain energy homeostasis in DKD (31). A recent study found that AMPK plays a central role in the amelioration of kidney injury in diabetes nephropathy (DN) by the vitamin D (VD)-vitamin D receptor (VDR). VD-VDR activates AMPK to regulate autophagy in DN in a calcium-dependent manner (32). SIRT1, an NAD+-dependent deacetylase, plays a protective role in kidney disease (31). SIRT1 can directly deacetylate Beclin1 to activate autophagy (33) and regulate the expression of autophagy-related proteins (34), as well as regulate autophagy by serving as a substrate (35, 36). Induced SIRT1-overexpression in podocytes attenuates proteinuria and glomerular injury in DKD (37, 38). Furthermore, SIRT1 mediates communication between proximal tubules and podocytes, contributing to maintaining the nicotinamide mononucleotide concentration around glomeruli, which is essential for preventing podocyte injury and proteinuria in DKD (39, 40).
2.2 Mitophagy and lipophagy
Mitophagy, which contributes to mitochondrial quality, is an important selective autophagy mechanism in DKD, as impaired mitochondrial function and abnormal mitochondrial accumulation are involved in DKD onset and progression (41, 42). Mitophagy inhibition aggravates tubulointerstitial inflammation and premature tubular cell aging (43, 44). Similarly, mitophagy has also been implicated in podocyte energy metabolism, inflammation, and apoptosis (45), and promoting mitophagy homeostasis contributes to the amelioration of podocyte injury in DKD (46).
Lipid metabolism disorders and autophagy imbalance are the main characteristics of DKD, both of which are rapidly developing areas of research (47, 48). Ectopic lipid deposition (ELD) accelerates renal resident cell injury and senescence (49). Lipophagy improves ELD and attenuates lipotoxicity-induced kidney injury (50). Furthermore, lipophagy reduces cholesterol influx and ameliorates lipotoxicity-induced podocyte injury and tubular injury in DKD (51, 52).
2.3 Autophagy and kidney inflammation
Crosstalk between autophagy and inflammation is widespread and important in many diseases (53), including DKD. Inflammation plays a key role in DKD onset and progression, and autophagy plays multiple roles in the inflammatory response (54). The accumulation of AGEs triggered the inflammatory response in DKD (55), and autophagy as an important regulator ameliorated renal inflammation by promoting the degradation of AGEs (56). Furthermore, autophagy-related gene 5 (ATG5) ablation was found to impair autophagy and enhance NF-κβ activation (10). Notably, lysosomal rupture also leads to inflammasome activation, further aggravating inflammation (57). TFEB, an important transcription factor for autophagy and lysosomal regulation, plays a multifaceted role in regulating macrophage activation and control cytokine/chemokine transcription (58). NLRP3 inflammasome is an important player in the regulation of inflammation. NLRP3 inflammasome activation impairs glomerular autophagy in DKD, and NLRP3 inhibition or deletion is sufficient to restore autophagy in podocytes (59), highlighting the close relationship between inflammation and autophagy in DKD. Thus, targeted elimination of the crosstalk between autophagy and inflammation has a promising therapeutic effect on DKD (35, 60, 61).
2.4 Lysosomal dyshomeostasis
Lysosomes play invaluable roles in various types of autophagy and cell death (62), are dynamic regulators of cellular and organismal homeostasis, and are responsible for the degradation of cellular content (63). Lysosomes contribute to cellular metabolism, membrane repair, and immune signal transduction (64) and also communicate extensively with other organelles, including mitochondria (65) and the nucleus (66), by establishing membrane contact sites and functional interactions. Lysosome-related research on DKD is advancing rapidly. The accumulation of AGEs triggers lysosomal membrane permeability and lysosomal dysfunction (67). Conversely, lysosomal biogenesis promotes degradation of AGEs in DKD (55). Restoring lysosomal function to activate autophagy improves podocyte damage in DKD (12), and a similar effect has been observed in renal tubules (55). Interestingly, recent studies have found that tunneling nanotubes (TNT) mediated the exchange of autophagosomes and lysosomes between podocytes to allow healthy podocyte components to replace damaged organelles, and the inhibition of TNT accelerated lysosomal dysfunction and apoptosis in podocytes (68). The inactivation of TFEB, a master transcription factor that drives lysosomal functions, is closely related to lysosomal insufficiency and dysfunction in DKD (69). AGEs inhibit the nuclear translocation and activity of TFEB (70). Pharmacologically targeting TFEB activation ameliorates tubular and podocyte injury, apoptosis, and inflammation in DKD (69–71). Maintaining lysosomal homeostasis may therefore be a potential therapeutic approach for DKD (7, 72).
3 Autophagy and renal homeostasis
Autophagy functions differently in various cell types. In this section, we review studies on autophagy in four important cell types implicated in DKD: podocytes, tubular epithelial cells, mesangial cells, endothelial cells, and macrophages (Table 1, Figure 2).
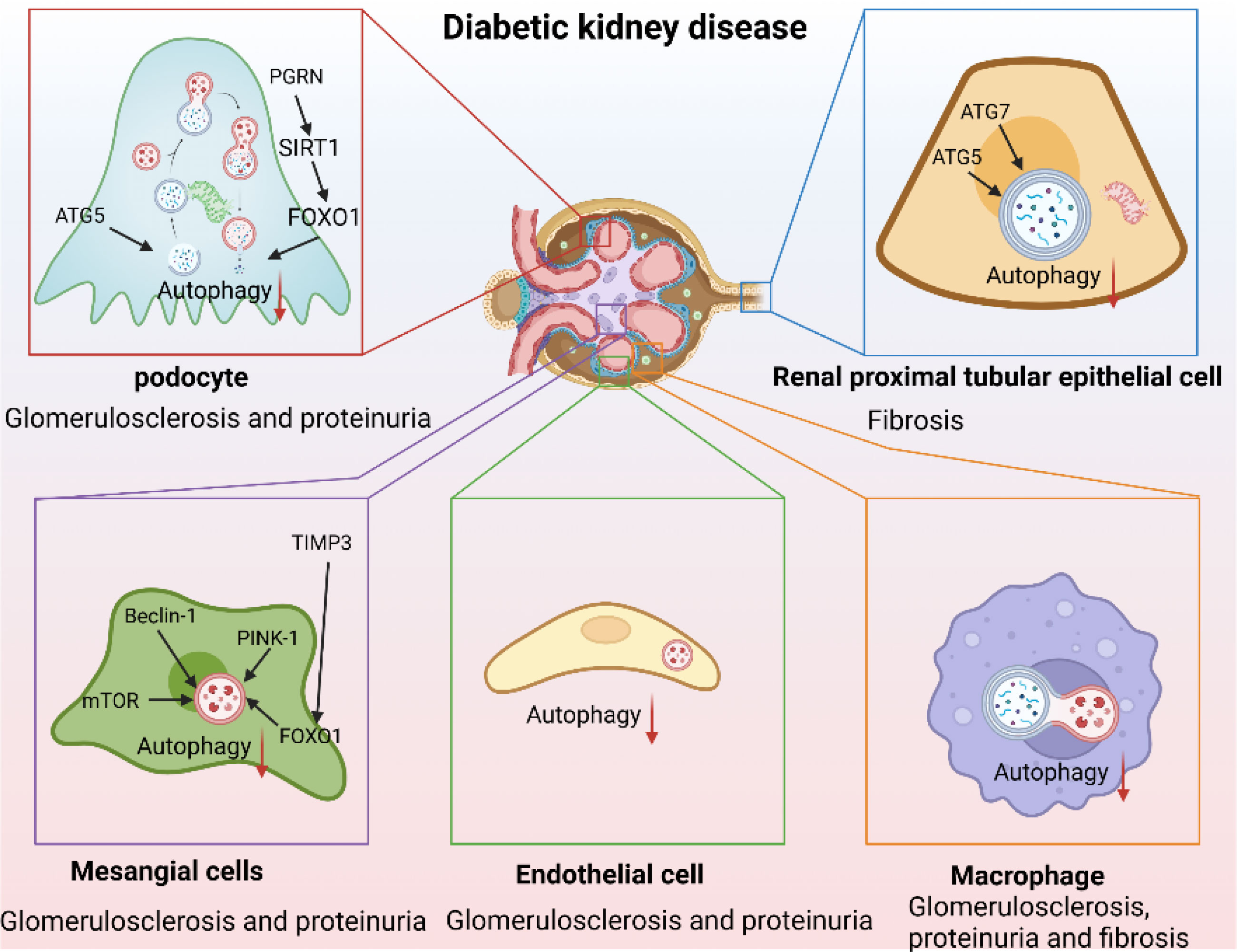
Figure 2 Autophagy as a therapeutic target in DKD. (Autophagy is inhibited in DKD. Autophagy-lysosome dysfunction mediates podocyte injury leading to glomerulosclerosis and massive proteinuria and mediates renal proximal tubular epithelial cells injury aggravating renal fibrosis, and mediates mesangial cell injury and endothelial-to-mesenchymal transition promoting the production of mesangial matrix and aggravating glomerulosclerosis and proteinuria. In addition, inhibition of macrophage autophagy aggravated renal inflammation leading to glomerulosclerosis, proteinuria, and renal fibrosis).
3.1 Autophagy and podocyte injury
Podocytes are terminally differentiated glomerular epithelial cells that play key roles in maintaining the integrity of the glomerular filtration barrier. Podocyte injury and effacement are the main causes of glomerulosclerosis and massive proteinuria in patients with DKD. The physiological function of podocytes requires high levels of autophagy (73). Podocyte-autophagy inhibition occurs in the early stages of DKD and leads to the progression of DKD and massive proteinuria (11). Loss of autophagy was found to simultaneously accelerate podocyte and endothelial injury, leading to disruption of the glomerular filtration barrier and glomerulosclerosis (74). Mitophagy is also involved in podocyte injury. For example, progranulin (PGRN) deficiency aggravates podocyte injury and proteinuria in DN mice, while elevated PGRN levels maintain podocyte mitochondrial homeostasis by mediating mitochondrial biogenesis and mitophagy via the SIRT1-PGC-1α/FoxO1 pathway (46). Similarly, forkhead-box class O1, a transcription factor, reduces podocyte injury in DKD by regulating mitophagy (88). Recent evidence suggests that autophagy aids in the reduction of ELD and the amelioration of lipotoxicity-mediated podocyte injury in DKD (51). Notably, lysosomes are involved in the processing of endocytosed albumin in podocytes, lysosomal dysfunction may contribute to podocyte injury, albuminuria, and glomerulosclerosis (89). AGE-stimulation leads to decreased lysosomal enzyme activity, TFEB inactivation, and lysosomal membrane permeabilization in podocytes (12). This results in an inhibition of the autophagic flux, resulting in podocyte actin cytoskeletal disorganization and loss of slit membrane integrity. Pharmacological attenuation of autophagy and lysosomal dysfunction with drugs such as rapamycin, reduces proteinuria and ameliorates podocyte injury in DKD (90). These studies highlight the importance of autophagic flux in maintaining podocyte homeostasis.
3.2 Autophagy and renal proximal tubular epithelial cells
Although DKD is traditionally characterized by glomerulopathy, many patients with DKD who develop ESRD do not show increased proteinuria (91), illustrating the importance of renal proximal tubular epithelial cell (PTEC) damage for the onset and progression of DKD. Phenotypic changes in PTECs are early manifestations of DKD (92, 93), and the severity of tubulointerstitial lesions strongly correlates with renal outcomes (94). The autophagy-lysosome pathway is important in PTEC, although, unlike in podocytes, the basal level of autophagy in renal PTECs is very low (8). Mice with proximal tubule-specific ATG5 or ATG7 deletion exhibit exacerbated renal function impairment and premature renal senescence (8, 9). Furthermore, deleting sodium-glucose cotransporter 2 (SGLT2), a master regulator of renal tubular glucose reabsorption, reduces renal p62/SQSTM1 accumulation, suggesting that glucose uptake may contribute to autophagy inhibition in PTECs (95). AGEs, which are elevated by long-term HG levels, are degraded by endocytosis in PTEC lysosomes and gradually accumulates with the PTEC autophagy impairment (14). The AGE-overload, in turn, disrupts lysosomal function and autophagic flux, aggravating PTEC injury (55, 67). Impaired mitophagy plays a fundamental role in DKD pathogenesis, and mitophagy deficiency in PTECs leads to tubular cell injury and accelerated senescence (43). MitoQ, a mitochondria-targeted anti-oxidant, attenuates tubular injury and improves renal function by enhancing mitophagy (96). It is important to note that tubular injury often coincides with glomerular injury in DKD, forming tubuloglomerular feedback (TGF) (97), and autophagy plays an indispensable role in TGF through Megalin and SIRT1 (75, 98). Collectively, these studies indicate that PTEC autophagy plays an important renoprotective role in DKD.
3.3 Autophagy and mesangial cells
Mesangial cells (MCs) play an important role in maintaining the structural integrity of the glomerular microvascular bed and mesangial matrix homeostasis (99) by eliciting multiple biological responses to injury, including matrix remodeling and crosstalk with neighboring cells (100). MC hypertrophy, basement membrane thickening, and mesangial matrix expansion induced by HG levels are the earliest pathological features of DKD (101). However, the role of autophagy in MCs in DKD remains unclear. AGE-stimulation of MCs leads to time-dependent changes in LC3II and p62 expression (76). Similarly, HG-stimulation of MCs suppresses autophagy-related protein levels, including mTOR, Beclin1, P62, PINK1, and Parkin. Moreover, inhibition of autophagy in MCs is also found to accelerate AGE-induced senescence in MCs (80, 81) and aggravate renal inflammation and fibrosis in DKD (78). A loss of the tissue inhibitor of metalloproteinase 3 (TIMP3) aggravates basement membrane thickening, mesangial expansion, proteinuria, and interstitial fibrosis in DKD, resulting in decreased expression of FoxO1 and autophagy-related genes, while re-expression of TIMP3 in MCs attenuates these effects (102). Activation of MC autophagy can repair AGEs-induced MC damage by clearing reactive oxygen species (ROS), which are important mediators of AGE-induced MC-apoptosis (79), and improve glomerular mesangial expansion and extracellular matrix deposition, thereby improving DKD (77). Although these studies provide important evidence for the protective role of autophagy in MCs, the potential therapeutic value of autophagy in MCs in DKD requires further research.
3.4 Autophagy and glomerular endothelial cells
Glomerular endothelial cells (GECs) injury plays a key role in the early occurrence and development of DKD (103). High glucose, ROS accumulation, and autophagy inhibition mediate the loss of glycocalyx and GECs dysfunction, leading to endothelial permeability and apoptosis, thereby driving albuminuria and early renal injury (104, 105). Importantly, injured GECs accelerates renal progression by forming crosstalk with adjacent glomerular cells (82, 106). Conditional knockout of Atg5 of GECs showed capillary loops thickening and accumulation of ROS, which eventually developed significant glomerulosclerosis (107). In addition, knockout of Atg5 GECs leads to capillary rarefaction and endothelial-to-mesenchymal transition (EndMT) and accelerating the fibrosis and progression of DN (74, 83, 108). Interestingly, the injured of GECs promoted podocyte dysfunction (109). In turn, autophagy of podocytes plays a renoprotective role against DKD related structural endothelial injury (110). Activating the AMPK pathway was proved to improve the renal injury of DKD by improving autophagy of GECs (29, 111), suggesting the important role of autophagy of GECs in improving DKD.
3.5 Macrophage autophagy in DKD
DKD is a chronic inflammatory disease characterized by massive inflammatory cell infiltration and overexpression of proinflammatory factors. Increased macrophage infiltration is observed in the kidneys of DN mice and patients with DKD. Macrophage infiltration and phenotypic changes are significantly associated with proteinuria and fibrosis in DKD (112). Furthermore, communication between macrophages and renal resident cells, such as podocytes and PTECs, may influence DKD progression (113, 114). Emerging evidence suggests that macrophage autophagy plays a crucial role in macrophage polarization, chronic inflammation, and organ fibrosis (115). In DKD, HG-stimulation results in macrophage-derived exosomes targeting and inhibiting PTEC autophagy (86), and M2 macrophage-derived exosomes activate autophagy to ameliorate podocyte injury in DKD (87). Conversely, autophagy can regulate changes in the macrophage phenotype (85), and autophagy inhibition enhances macrophage adhesion and migration (84). Furthermore, studies have reported that TFEB activation promotes macrophage polarization toward the M2 type, suppresses inflammation, and improves kidney injury in DKD (71). Targeting autophagy regulation in macrophages has been well-studied in many diseases (116, 117). Macrophage autophagy may therefore be a promising therapeutic target for DKD.
4 Polyphenols used to regulate autophagy in DKD
Dietary polyphenols are a widespread class of secondary plant metabolites. The potential of polyphenols to restore SIRT1 and NAD+ metabolism in kidney diseases has received significant attention (118). In addition, epigenetic regulation of autophagy is an important mechanism for maintaining homeostasis. Natural polyphenols can reverse epigenetic alterations of autophagy and delay the progression of DKD (119). Resveratrol was found to regulate SIRT1 and DNA-methyltransferase (DNMT) and exhibited potential regulatory capacity on DKD (120, 121). Similarly, quercetin has also been found to regulate the expression of multiple chromatin modifiers (including DNMTs, histone deacetylases, histone acetyltransferases, and histone methyltransferases) (122). In this section, we review natural polyphenols as autophagy regulators, including the regulation of mitophagy and promoting TFEB-nuclear transfer. The reviewed compounds and their specific effects on autophagy regulation are summarized in Table 2 (Figure 3).
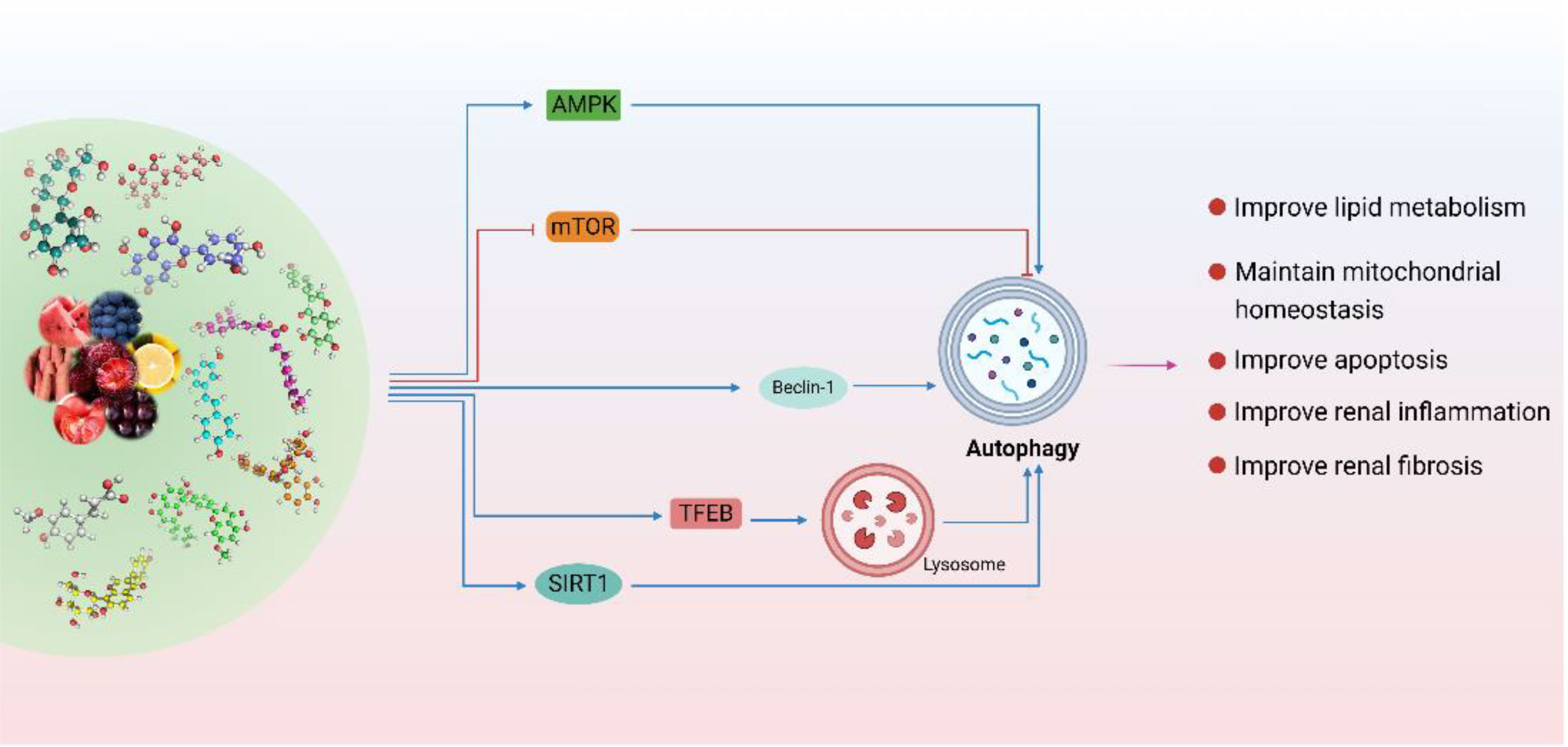
Figure 3 Polyphenols are used to regulate autophagy in DKD. (Natural polyphenols are potential autophagy regulators. Polyphenols are mainly through the SIRT1 pathway, mTOR pathway, and AMPK pathway is involved in the regulation of various links of autophagy. In addition, polyphenols can also improve the autophagy-lysosome pathway by activating TFEB and promoting its nuclear translocation. In conclusion, polyphenols play an important role in improving DKD by regulating autophagy-lysosome pathway to improve renal inflammation, lipid metabolism, mitochondrial homeostasis, apoptosis, and fibrosis.
4.1 Resveratrol
Resveratrol (RSV), a potent, natural SIRT1-agonist, reduces oxidative stress and AGE production, inhibits endoplasmic reticulum (ER) stress, and ameliorates lipotoxicity and inflammation, thereby effectively protecting renal function from DKD without significant side effects, and is widely recommended as a dietary supplement for DKD treatment (148). RSV is also an effective autophagy regulator in DKD, promoting autophagy by activating SIRT1, thereby ameliorating DKD (123). RSV enhance autophagy to improve insulin resistance, lipid metabolism, and renal function in DKD (124), and protects against HG-induced podocyte apoptosis, whereas inhibition of autophagy reverses this therapeutic effect (125). Further research found that RSV can further improve autophagy and apoptosis in podocytes by up-regulating miR-18a-5p (126) and down-regulating miR-383-5p (125). Furthermore, RSV, in combination with vitamin E, improves the lysosome-dependent autophagy pathway, thereby ameliorating AGE-mediated podocyte actin cytoskeleton damage (12).
4.2 Curcumin
Curcumin, a bioactive polyphenolic compound found in turmeric, exhibits anti-inflammatory, anti-oxidant, autophagy-enhancing, anti-apoptotic, and anti-fibrotic properties in DKD (149). Moderate doses of curcumin induce autophagy, whereas high doses induce lysosomal membrane permeabilization, leading to cell death (150). Curcumin has been described as a pharmacological inhibitor of the mTOR-signaling pathway in many diseases (151, 152). Curcumin also activates TFEB, thereby enhancing autophagy and lysosomal activity (153, 154). In DKD, curcumin suppresses p-mTOR levels, thereby promoting autophagy and alleviating podocyte epithelial-to-mesenchymal transition (127), and ameliorates podocyte apoptosis via Beclin1/UVRAG/Bcl2 pathway (128). In addition, autophagy has also been shown to reduce AGEs induced apoptosis in tubule cells (129).
4.3 Puerarin
Puerarin, an isoflavone extracted from Pueraria lobata, is widely used in traditional Chinese medicine. Clinical and basic studies have shown that puerarin exerts renoprotective effects (155, 156) and that autophagy is the primary mechanism by which puerarin alleviates DKD. Puerarin activates autophagy to promote podocyte functional protein expression under ER stress in DKD (130); acts as a SIRT1-agonist, ameliorating podocyte injury, and proteinuria by activating SIRT1 (40); and promotes heme oxygenase 1 and SIRT1 expression and decreases liver kinase B1 acetylation, thereby activating autophagy to protect podocytes (131).
4.4 Kaempferol
Kaempferol, a natural flavanol common in traditional medicines, fruits, and vegetables, is a histone deacetylase (HDAC) inhibitor (157) and a promising autophagy modulator exhibiting therapeutic effects in many diseases (158–160). Kaempferol can not only promote the expression of autophagy-related genes (161) but also promote autophagy in macrophages and inhibit NLRP3 inflammasome activation (162). Recent machine-learning screening has also identified kaempferol as a potent mitophagy inducer for treating Alzheimer’s disease (163). Kaempferol can regulate the AMPK/mTOR pathway to promote autophagy, thereby alleviating mesangial matrix expansion, glomerular basement membrane thickening, and podocyte loss or fusion in DKD (132). Further studies have found that kaempferol also ameliorates ROS generation and mitochondrial damage by AGE accumulation in mesangial cells through autophagy (133).
4.5 Other polyphenols
Ferulic acid, a phenolic acid present in the seeds and leaves of most plants, has shown satisfactory effects in the treatment of DKD (164). Ferulic acid was shown to improve renal injury in patients with DKD by enhancing autophagy and inhibiting excessive inflammation (135). Similarly, isorhamnetin, found in Acanthus nigricans fruits and Ginkgo biloba leaves, ameliorates renal injury in DN by enhancing autophagy and inhibiting excessive inflammation (136). Cyanidin-3-O-glucoside activates SIRT1 and AMPK to ease HG-induced autophagy inhibition, thereby attenuating podocyte dysfunction and epithelial-mesenchymal transition (134). Phenolics from Physalis peruviana fruits activate the AMPK/mTOR pathway, enhancing autophagy and ameliorating apoptosis and kidney injury in DKD (137). Similarly, ginkgetin reduces HG-induced oxidative stress damage, inflammation, and extracellular matrix deposition in mesangial cells via AMPK/mTOR pathway-mediated autophagy (144). Genistein has also been found to inactivate the mTOR pathway, maintain autophagy-related protein levels, and inhibit HG-induced podocyte injury (141). Bergenin, a plant polyphenol derived from the cortex of Mallotus japonicus (L.f.) Müll.Arg., and defined as a PPARγ-agonist (165), inhibits oxidative stress and reduces extracellular matrix production in DKD mesangial cells by inhibiting mTOR phosphorylation (143). Wogonin targets phosphoinositide 3-kinase (PI3K) to regulate autophagy and inflammation and attenuates tubulointerstitial fibrosis and tubular cell injury in DN (138). In addition, wogonin has also been found to regulate the crosstalk between autophagy and apoptosis to reduce glomerulopathy and podocyte damage (139). Similarly, dihydromyricetin enhances autophagy and attenuates renal interstitial fibrosis in DN via the PI3K/AKT/mTOR pathway (140). Salvianolic acid A restores the actin cytoskeleton rearrangement of glomerular endothelial cells in DN by modulating autophagy and inflammation via the AGE-RAGE-Nox4 axis, thereby ameliorating early renal injury in DN (142). Chrysin inhibits AGEs-induced activation of the mTOR pathway and promotes autophagy to inhibit mesangial cell proliferation, α-smooth muscle actin production, and adhesion in DN (145). Mangiferin, a xanthonoid from Salacia oblonga, can promote the phosphorylation of AMPK and ULK1, inhibit the phosphorylation of mTOR, increase the number of autophagosomes and thereby ameliorate podocyte injury and proteinuria in DN (146). Fisetin has also been found to reduce podocyte injury in DN by restoring autophagy and inhibiting the NLRP3 inflammasome (147). Isorhapontigenin attenuates HG-induced oxidative stress and activates autophagy by stimulating AMPK/Nrf2 pathway, thereby improving the EndMT and podocyte injury of DKD (111).
5 Autophagy as a therapeutic target in DKD
Accumulating evidence indicates that autophagy plays a critical role in both early and late stages of DKD. Pharmacological activation of autophagy has shown invaluable advantages in DKD. Rapamycin can reduce streptozocin (STZ)-induced renal injury by promoting podocyte autophagy and inhibiting apoptosis (166). Sirolimus has also been found to block mTOR to reduce fibrosis and mesangial matrix accumulation in STZ-induced DKD (167). In addition, several effective drugs against DKD, such as SGLT2 and metformin, also enhance autophagy. It has been reported that the amelioration of tubulointerstitial fibrosis by SGLT2i in Akita diabetic mice is entirely dependent on mTORC1, and deletion of mTORC1 reverses the renoprotective effects of SGLT2i (24, 25). Similarly, Metformin has also been found to enhance autophagy of mesangial cell via SIRT1 and AMPK pathways to effectively ameliorate glycolipid metabolic disorders, inflammation, MC proliferation, and extracellular matrix expression in DKD (168, 169). The beneficial effects of some non-pharmacological DKD therapies, such as diet and exercise, are also associated with autophagy regulation. Dietary modification was shown to activate SIRT1 and AMPK and inhibit mTOR to regulate autophagy, thereby playing a crucial role in improving DKD (170, 171). A cross-sectional study involving 229 participants found that exercise can improve proteinuria and plasma lipids in patients with diabetes (172), and this renoprotective effect of exercise was found to be associated with activation of AMPK and inhibition of mTORC1 in Wistar fatty (fa/fa) rats (173).
6 Therapeutic potential of polyphenols targeted autophagy for DKD
Natural polyphenols as autophagy regulators have shown promise in the treatment of DKD. Many clinical studies have demonstrated the protective effects of dietary polyphenols against DKD. For example, resveratrol was shown to assist angiotensin receptor blockers in reducing proteinuria for DKD patients in a randomized double-blind placebo-controlled clinical trial with 60 participants (174). Similarly, curcumin has also been shown to improve urinary microalbumin excretion and inflammation in a randomized, double-blind, and placebo-controlled study that enrolled 40 patients with overt type 2 DKD (175). Other polyphenols have also been shown to similarly ameliorate podocyte injury in clinical studies, such as green tea polyphenols (176). Based on the preclinical evidence that polyphenols improve autophagy presented above, it is plausible to conclude that polyphenols show therapeutic potential to improve DKD by modulating autophagy. However, there are some challenges in replicating the beneficial effects of polyphenols in clinical settings, such as polyphenol absorption and bioavailability, which may be addressed with new methods and technologies, such as nanotechnology (177).
7 Conclusions and future perspectives
Although considerable progress has been made in DKD treatment in recent years, delaying DKD progression remains a global challenge. Autophagy plays a crucial role in DKD onset and progression, with impaired autophagy and lysosomal function aggravating renal resident cell injury and apoptosis, as well as inducing macrophage phenotype changes, resulting in the development of proteinuria and fibrosis in DKD. A growing body of evidence suggests that polyphenol-rich natural products may assist with DKD while causing no serious side effects. The protective effects of polyphenols in DKD involve multiple mechanisms of action, including modulation of inflammation, oxidative stress, autophagy, and mitochondrial quality control. Although polyphenols are generally considered safe, oxidative stress caused by large amounts of polyphenols can have deleterious effects. Additional studies are therefore needed to determine the optimal polyphenol dosage, and extensive human clinical trials are required to evaluate potential side effects.
Author contributions
TL, QJ, PL, and YZ designed the study. FM and YW collected the data. TL, LY, and HM analyzed the data and drafted the manuscript. All authors contributed to the article and approved the final version of the manuscript.
Funding
This study was supported by the National Nature Science Foundation of China (82074393, 82204905, 82205094).
Conflict of interest
The authors declare that the research was conducted in the absence of any commercial or financial relationships that could be construed as a potential conflict of interest.
Publisher’s note
All claims expressed in this article are solely those of the authors and do not necessarily represent those of their affiliated organizations, or those of the publisher, the editors and the reviewers. Any product that may be evaluated in this article, or claim that may be made by its manufacturer, is not guaranteed or endorsed by the publisher.
References
1. Rayego-Mateos S, Rodrigues-Diez R, Fernandez-Fernandez B, Mora-Fernández C, Marchant V, Donate-Correa J, et al. Targeting inflammation to treat diabetic kidney disease: the road to 2030. Kidney Int (2022) 103(2):282–96. doi: 10.1016/j.kint.2022.10.030
2. Forst T, Mathieu C, Giorgino F, Wheeler DC, Papanas N, Schmieder RE, et al. New strategies to improve clinical outcomes for diabetic kidney disease. BMC Med (2022) 20(1):337. doi: 10.1186/s12916-022-02539-2
3. Chan JCN, Lim LL, Wareham NJ, Shaw JE, Orchard TJ, Zhang P, et al. The lancet commission on diabetes: using data to transform diabetes care and patient lives. Lancet (London England) (2021) 396(10267):2019–82. doi: 10.1016/s0140-6736(20)32374-6
4. Brosius FC, Cherney D, Gee PO, Harris RC, Kliger AS, Tuttle KR, et al. Transforming the care of patients with diabetic kidney disease. Clin J Am Soc Nephrology (2021) 16(10):1590–600. doi: 10.2215/cjn.18641120
5. Ricciardi CA, Gnudi L. Kidney disease in diabetes: From mechanisms to clinical presentation and treatment strategies. Metabolism: Clin Experimental (2021) 124:154890. doi: 10.1016/j.metabol.2021.154890
6. Kaushal GP, Chandrashekar K, Juncos LA, Shah SV. Autophagy function and regulation in kidney disease. Biomolecules (2020) 10(1):100. doi: 10.3390/biom10010100
7. Wu M, Zhang M, Zhang Y, Li Z, Li X, Liu Z, et al. Relationship between lysosomal dyshomeostasis and progression of diabetic kidney disease. Cell Death Dis (2021) 12(11):958. doi: 10.1038/s41419-021-04271-w
8. Liu S, Hartleben B, Kretz O, Wiech T, Igarashi P, Mizushima N, et al. Autophagy plays a critical role in kidney tubule maintenance, aging and ischemia-reperfusion injury. Autophagy (2012) 8(5):826–37. doi: 10.4161/auto.19419
9. Kimura T, Takabatake Y, Takahashi A, Kaimori JY, Matsui I, Namba T, et al. Autophagy protects the proximal tubule from degeneration and acute ischemic injury. J Am Soc Nephrology (2011) 22(5):902–13. doi: 10.1681/asn.2010070705
10. Peng X, Wang Y, Li H, Fan J, Shen J, Yu X, et al. ATG5-mediated autophagy suppresses NF-κB signaling to limit epithelial inflammatory response to kidney injury. Cell Death Dis (2019) 10(4):253. doi: 10.1038/s41419-019-1483-7
11. Tagawa A, Yasuda M, Kume S, Yamahara K, Nakazawa J, Chin-Kanasaki M, et al. Impaired podocyte autophagy exacerbates proteinuria in diabetic nephropathy. Diabetes (2016) 65(3):755–67. doi: 10.2337/db15-0473
12. Liu WJ, Gan Y, Huang WF, Wu HL, Zhang XQ, Zheng HJ, et al. Lysosome restoration to activate podocyte autophagy: a new therapeutic strategy for diabetic kidney disease. Cell Death Dis (2019) 10(11):806. doi: 10.1038/s41419-019-2002-6
13. Semba RD, Nicklett EJ, Ferrucci L. Does accumulation of advanced glycation end products contribute to the aging phenotype? J Gerontol A Biol Sci Med Sci (2010) 65(9):963–75. doi: 10.1093/gerona/glq074
14. Rabbani N, Thornalley PJ. Advanced glycation end products in the pathogenesis of chronic kidney disease. Kidney Int (2018) 93(4):803–13. doi: 10.1016/j.kint.2017.11.034
15. Zhang XM, Gao Y, Yang MX, Zheng XD, Zhang R, Wu YY, et al. Exploration of noninvasive detection of advanced glycation end products in the lens to screen for diabetic kidney disease. Front Endocrinol (2022) 13:892070. doi: 10.3389/fendo.2022.892070
16. Tan AL, Forbes JM, Cooper ME. AGE. RAGE, and ROS in diabetic nephropathy. Semin Nephrology (2007) 27(2):130–43. doi: 10.1016/j.semnephrol.2007.01.006
17. Peres GB, Schor N, Michelacci YM. Impact of high glucose and AGEs on cultured kidney-derived cells. effects on cell viability, lysosomal enzymes and effectors of cell signaling pathways. Biochimie (2017) 135:137–48. doi: 10.1016/j.biochi.2017.02.004
18. Aragonès G, Dasuri K, Olukorede O, Francisco SG, Renneburg C, Kumsta C, et al. Autophagic receptor p62 protects against glycation-derived toxicity and enhances viability. Aging Cell (2020) 19(11):e13257. doi: 10.1111/acel.13257
19. Liu T, Jin Q, Ren F, Yang L, Mao H, Ma F, et al. Potential therapeutic effects of natural compounds targeting autophagy to alleviate podocyte injury in glomerular diseases. BioMed Pharmacother (2022) 155:113670. doi: 10.1016/j.biopha.2022.113670
20. Yang D, Livingston MJ, Liu Z, Dong G, Zhang M, Chen JK, et al. Autophagy in diabetic kidney disease: regulation, pathological role and therapeutic potential. Cell Mol Life Sci (2018) 75(4):669–88. doi: 10.1007/s00018-017-2639-1
21. Lieberthal W, Levine JS. The role of the mammalian target of rapamycin (mTOR) in renal disease. J Am Soc Nephrology (2009) 20(12):2493–502. doi: 10.1681/asn.2008111186
22. Fantus D, Rogers NM, Grahammer F, Huber TB, Thomson AW. Roles of mTOR complexes in the kidney: implications for renal disease and transplantation. Nat Rev Nephrology (2016) 12(10):587–609. doi: 10.1038/nrneph.2016.108
23. Inoki K, Mori H, Wang J, Suzuki T, Hong S, Yoshida S, et al. mTORC1 activation in podocytes is a critical step in the development of diabetic nephropathy in mice. J Clin Invest (2011) 121(6):2181–96. doi: 10.1172/jci44771
24. Kogot-Levin A, Hinden L, Riahi Y, Israeli T, Tirosh B, Cerasi E, et al. Proximal tubule mTORC1 is a central player in the pathophysiology of diabetic nephropathy and its correction by SGLT2 inhibitors. Cell Rep (2020) 32(4):107954. doi: 10.1016/j.celrep.2020.107954
25. Tomita I, Kume S, Sugahara S, Osawa N, Yamahara K, Yasuda-Yamahara M, et al. SGLT2 inhibition mediates protection from diabetic kidney disease by promoting ketone body-induced mTORC1 inhibition. Cell Metab (2020) 32(3):404–19.e6. doi: 10.1016/j.cmet.2020.06.020
26. Yasuda-Yamahara M, Kume S, Maegawa H. Roles of mTOR in diabetic kidney disease. Antioxidants (Basel Switzerland) (2021) 10(2):321. doi: 10.3390/antiox10020321
27. Dugan LL, You YH, Ali SS, Diamond-Stanic M, Miyamoto S, DeCleves AE, et al. AMPK dysregulation promotes diabetes-related reduction of superoxide and mitochondrial function. J Clin Invest (2013) 123(11):4888–99. doi: 10.1172/jci66218
28. Cheng Y, Zhang X, Ma F, Sun W, Wang W, Yu J, et al. The role of Akt2 in the protective effect of fenofibrate against diabetic nephropathy. Int J Biol Sci (2020) 16(4):553–67. doi: 10.7150/ijbs.40643
29. Lim JH, Kim HW, Kim MY, Kim TW, Kim EN, Kim Y, et al. Cinacalcet-mediated activation of the CaMKKβ-LKB1-AMPK pathway attenuates diabetic nephropathy in db/db mice by modulation of apoptosis and autophagy. Cell Death Dis (2018) 9(3):270. doi: 10.1038/s41419-018-0324-4
30. Han YC, Tang SQ, Liu YT, Li AM, Zhan M, Yang M, et al. AMPK agonist alleviate renal tubulointerstitial fibrosis via activating mitophagy in high fat and streptozotocin induced diabetic mice. Cell Death Dis (2021) 12(10):925. doi: 10.1038/s41419-021-04184-8
31. Clark AJ, Parikh SM. Targeting energy pathways in kidney disease: the roles of sirtuins, AMPK, and PGC1α. Kidney Int (2021) 99(4):828–40. doi: 10.1016/j.kint.2020.09.037
32. Li A, Yi B, Han H, Yang S, Hu Z, Zheng L, et al. (vitamin d receptor) regulates defective autophagy in renal tubular epithelial cell in streptozotocin-induced diabetic mice via the AMPK pathway. Autophagy (2022) 18(4):877–90. doi: 10.1080/15548627.2021.1962681
33. Deng Z, Sun M, Wu J, Fang H, Cai S, An S, et al. SIRT1 attenuates sepsis-induced acute kidney injury via Beclin1 deacetylation-mediated autophagy activation. Cell Death Dis (2021) 12(2):217. doi: 10.1038/s41419-021-03508-y
34. Ji J, Tao P, Wang Q, Li L, Xu Y. SIRT1: Mechanism and protective effect in diabetic nephropathy. Endocrine Metab Immune Disord Drug Targets (2021) 21(5):835–42. doi: 10.2174/1871530320666201029143606
35. Lu Z, Liu H, Song N, Liang Y, Zhu J, Chen J, et al. METTL14 aggravates podocyte injury and glomerulopathy progression through N(6)-methyladenosine-dependent downregulating of Sirt1. Cell Death Dis (2021) 12(10):881. doi: 10.1038/s41419-021-04156-y
36. Fu Y, Sun Y, Wang M, Hou Y, Huang W, Zhou D, et al. Elevation of JAML promotes diabetic kidney disease by modulating podocyte lipid metabolism. Cell Metab (2020) 32(6):1052–62.e8. doi: 10.1016/j.cmet.2020.10.019
37. Hong Q, Zhang L, Das B, Li Z, Liu B, Cai G, et al. Increased podocyte sirtuin-1 function attenuates diabetic kidney injury. Kidney Int (2018) 93(6):1330–43. doi: 10.1016/j.kint.2017.12.008
38. Yasuda I, Hasegawa K, Sakamaki Y, Muraoka H, Kawaguchi T, Kusahana E, et al. Pre-emptive short-term nicotinamide mononucleotide treatment in a mouse model of diabetic nephropathy. J Am Soc Nephrology (2021) 32(6):1355–70. doi: 10.1681/asn.2020081188
39. Hasegawa K, Wakino S, Simic P, Sakamaki Y, Minakuchi H, Fujimura K, et al. Renal tubular Sirt1 attenuates diabetic albuminuria by epigenetically suppressing claudin-1 overexpression in podocytes. Nat Med (2013) 19(11):1496–504. doi: 10.1038/nm.3363
40. Zhong Y, Lee K, He JC. SIRT1 is a potential drug target for treatment of diabetic kidney disease. Front Endocrinol (2018) 9:624. doi: 10.3389/fendo.2018.00624
41. Saxena S, Mathur A, Kakkar P. Critical role of mitochondrial dysfunction and impaired mitophagy in diabetic nephropathy. J Cell Physiol (2019) 234(11):19223–36. doi: 10.1002/jcp.28712
42. Flemming N, Pernoud L, Forbes J, Gallo L. Mitochondrial dysfunction in individuals with diabetic kidney disease: A systematic review. Cells (2022) 11(16):2481. doi: 10.3390/cells11162481
43. Chen K, Dai H, Yuan J, Chen J, Lin L, Zhang W, et al. Optineurin-mediated mitophagy protects renal tubular epithelial cells against accelerated senescence in diabetic nephropathy. Cell Death Dis (2018) 9(2):105. doi: 10.1038/s41419-017-0127-z
44. Chen K, Feng L, Hu W, Chen J, Wang X, Wang L, et al. Optineurin inhibits NLRP3 inflammasome activation by enhancing mitophagy of renal tubular cells in diabetic nephropathy. FASEB J (2019) 33(3):4571–85. doi: 10.1096/fj.201801749RRR
45. Liu S, Yuan Y, Xue Y, Xing C, Zhang B. Podocyte injury in diabetic kidney disease: A focus on mitochondrial dysfunction. Front Cell Dev Biol (2022) 10:832887. doi: 10.3389/fcell.2022.832887
46. Zhou D, Zhou M, Wang Z, Fu Y, Jia M, Wang X, et al. PGRN acts as a novel regulator of mitochondrial homeostasis by facilitating mitophagy and mitochondrial biogenesis to prevent podocyte injury in diabetic nephropathy. Cell Death Dis (2019) 10(7):524. doi: 10.1038/s41419-019-1754-3
47. Nishi H, Nangaku M. Podocyte lipotoxicity in diabetic kidney disease. Kidney Int (2019) 96(4):809–12. doi: 10.1016/j.kint.2019.07.013
48. Schelling JR. The contribution of lipotoxicity to diabetic kidney disease. Cells (2022) 11(20):3236. doi: 10.3390/cells11203236
49. Opazo-Ríos L, Mas S, Marín-Royo G, Mezzano S, Gómez-Guerrero C, Moreno JA, et al. Lipotoxicity and diabetic nephropathy: Novel mechanistic insights and therapeutic opportunities. Int J Mol Sci (2020) 21(7):2632. doi: 10.3390/ijms21072632
50. Yang M, Song P, Zhao L, Wang X. Lipophagy: A potential therapeutic target for diabetic nephropathy. Curr Medicinal Chem (2022). doi: 10.2174/0929867329666220727113129
51. Lu J, Chen PP, Zhang JX, Li XQ, Wang GH, Yuan BY, et al. GPR43 activation-mediated lipotoxicity contributes to podocyte injury in diabetic nephropathy by modulating the ERK/EGR1 pathway. Int J Biol Sci (2022) 18(1):96–111. doi: 10.7150/ijbs.64665
52. Han Y, Xiong S, Zhao H, Yang S, Yang M, Zhu X, et al. Lipophagy deficiency exacerbates ectopic lipid accumulation and tubular cells injury in diabetic nephropathy. Cell Death Dis (2021) 12(11):1031. doi: 10.1038/s41419-021-04326-y
53. Deretic V. Autophagy in inflammation, infection, and immunometabolism. Immunity (2021) 54(3):437–53. doi: 10.1016/j.immuni.2021.01.018
54. Levine B, Mizushima N, Virgin HW. Autophagy in immunity and inflammation. Nature (2011) 469(7330):323–35. doi: 10.1038/nature09782
55. Takahashi A, Takabatake Y, Kimura T, Maejima I, Namba T, Yamamoto T, et al. Autophagy inhibits the accumulation of advanced glycation end products by promoting lysosomal biogenesis and function in the kidney proximal tubules. Diabetes (2017) 66(5):1359–72. doi: 10.2337/db16-0397
56. Kimura T, Isaka Y, Yoshimori T. Autophagy and kidney inflammation. Autophagy (2017) 13(6):997–1003. doi: 10.1080/15548627.2017.1309485
57. Schroder K, Zhou R, Tschopp J. The NLRP3 inflammasome: a sensor for metabolic danger? Sci (New York NY) (2010) 327(5963):296–300. doi: 10.1126/science.1184003
58. Brady OA, Martina JA, Puertollano R. Emerging roles for TFEB in the immune response and inflammation. Autophagy (2018) 14(2):181–9. doi: 10.1080/15548627.2017.1313943
59. Shahzad K, Fatima S, Khawaja H, Elwakiel A, Gadi I, Ambreen S, et al. Podocyte-specific Nlrp3 inflammasome activation promotes diabetic kidney disease. Kidney Int (2022) 102(4):766–79. doi: 10.1016/j.kint.2022.06.010
60. Liu M, Liang K, Zhen J, Zhou M, Wang X, Wang Z, et al. Sirt6 deficiency exacerbates podocyte injury and proteinuria through targeting notch signaling. Nat Commun (2017) 8(1):413. doi: 10.1038/s41467-017-00498-4
61. Dusabimana T, Park EJ, Je J, Jeong K, Yun SP, Kim HJ, et al. Geniposide improves diabetic nephropathy by enhancing ULK1-mediated autophagy and reducing oxidative stress through AMPK activation. Int J Mol Sci (2021) 22(4):1651. doi: 10.3390/ijms22041651
62. Mahapatra KK, Mishra SR, Behera BP, Patil S, Gewirtz DA, Bhutia SK. The lysosome as an imperative regulator of autophagy and cell death. Cell Mol Life Sci (2021) 78(23):7435–49. doi: 10.1007/s00018-021-03988-3
63. Ballabio A, Bonifacino JS. Lysosomes as dynamic regulators of cell and organismal homeostasis. Nat Rev Mol Cell Biol (2020) 21(2):101–18. doi: 10.1038/s41580-019-0185-4
64. Saftig P, Puertollano R. How lysosomes sense, integrate, and cope with stress. Trends Biochem Sci (2021) 46(2):97–112. doi: 10.1016/j.tibs.2020.09.004
65. Deus CM, Yambire KF, Oliveira PJ, Raimundo N. Mitochondria-lysosome crosstalk: From physiology to neurodegeneration. Trends Mol Med (2020) 26(1):71–88. doi: 10.1016/j.molmed.2019.10.009
66. Zhao Q, Gao SM, Wang MC. Molecular mechanisms of lysosome and nucleus communication. Trends Biochem Sci (2020) 45(11):978–91. doi: 10.1016/j.tibs.2020.06.004
67. Liu WJ, Shen TT, Chen RH, Wu HL, Wang YJ, Deng JK, et al. Autophagy-lysosome pathway in renal tubular epithelial cells is disrupted by advanced glycation end products in diabetic nephropathy. J Biol Chem (2015) 290(33):20499–510. doi: 10.1074/jbc.M115.666354
68. Barutta F, Bellini S, Kimura S, Hase K, Corbetta B, Corbelli A, et al. Protective effect of the tunneling nanotube-TNFAIP2/M-sec system on podocyte autophagy in diabetic nephropathy. Autophagy (2023) 19(2):505–24. doi: 10.1080/15548627.2022.2080382
69. Wang S, Jing K, Wu H, Li X, Yang C, Li T, et al. Activation of transcription factor EB alleviates tubular epithelial cell injury via restoring lysosomal homeostasis in diabetic nephropathy. Oxid Med Cell Longev (2022) 2022:2812493. doi: 10.1155/2022/2812493
70. Zhao X, Chen Y, Tan X, Zhang L, Zhang H, Li Z, et al. Advanced glycation end-products suppress autophagic flux in podocytes by activating mammalian target of rapamycin and inhibiting nuclear translocation of transcription factor EB. J Pathol (2018) 245(2):235–48. doi: 10.1002/path.5077
71. Yuan Y, Li L, Zhu L, Liu F, Tang X, Liao G, et al. Mesenchymal stem cells elicit macrophages into M2 phenotype via improving transcription factor EB-mediated autophagy to alleviate diabetic nephropathy. Stem Cells (Dayton Ohio) (2020) 38(5):639–52. doi: 10.1002/stem.3144
72. Meyer-Schwesinger C. Lysosome function in glomerular health and disease. Cell Tissue Res (2021) 385(2):371–92. doi: 10.1007/s00441-020-03375-7
73. Bork T, Liang W, Yamahara K, Lee P, Tian Z, Liu S, et al. Podocytes maintain high basal levels of autophagy independent of mtor signaling. Autophagy (2020) 16(11):1932–48. doi: 10.1080/15548627.2019.1705007
74. Lenoir O, Jasiek M, Hénique C, Guyonnet L, Hartleben B, Bork T, et al. Endothelial cell and podocyte autophagy synergistically protect from diabetes-induced glomerulosclerosis. Autophagy (2015) 11(7):1130–45. doi: 10.1080/15548627.2015.1049799
75. Packer M. Interplay of adenosine monophosphate-activated protein kinase/sirtuin-1 activation and sodium influx inhibition mediates the renal benefits of sodium-glucose co-transporter-2 inhibitors in type 2 diabetes: A novel conceptual framework. Diabetes Obes Metab (2020) 22(5):734–42. doi: 10.1111/dom.13961
76. Chen J, Zhao D, Zhu M, Zhang M, Hou X, Ding W, et al. Paeoniflorin ameliorates AGEs-induced mesangial cell injury through inhibiting RAGE/mTOR/autophagy pathway. BioMed Pharmacother (2017) 89:1362–9. doi: 10.1016/j.biopha.2017.03.016
77. Wen D, Tan RZ, Zhao CY, Li JC, Zhong X, Diao H, et al. Astragalus mongholicus bunge and panax notoginseng (Burkill) F.H. chen formula for renal injury in diabetic nephropathy-In vivo and In vitro evidence for autophagy regulation. Front Pharmacol (2020) 11:732. doi: 10.3389/fphar.2020.00732
78. Gao C, Fan F, Chen J, Long Y, Tang S, Jiang C, et al. FBW7 regulates the autophagy signal in mesangial cells induced by high glucose. BioMed Res Int (2019) 2019:6061594. doi: 10.1155/2019/6061594
79. Xu L, Fan Q, Wang X, Zhao X, Wang L. Inhibition of autophagy increased AGE/ROS-mediated apoptosis in mesangial cells. Cell Death Dis (2016) 7(11):e2445. doi: 10.1038/cddis.2016.322
80. Liu X, Fu B, Chen D, Hong Q, Cui J, Li J, et al. miR-184 and miR-150 promote renal glomerular mesangial cell aging by targeting Rab1a and Rab31. Exp Cell Res (2015) 336(2):192–203. doi: 10.1016/j.yexcr.2015.07.006
81. Shi M, Yang S, Zhu X, Sun D, Sun D, Jiang X, et al. The RAGE/STAT5/autophagy axis regulates senescence in mesangial cells. Cell Signal (2019) 62:109334. doi: 10.1016/j.cellsig.2019.05.019
82. Wang X, Su W, Ma M, Zhu L, Gao R, Qin C, et al. The KLF4-p62 axis prevents vascular endothelial cell injury via the mTOR/S6K pathway and autophagy in diabetic kidney disease. Endokrynologia Polska (2022) 73(5):837–45. doi: 10.5603/EP.a2022.0072
83. Dong R, Zhang X, Liu Y, Zhao T, Sun Z, Liu P, et al. Rutin alleviates EndMT by restoring autophagy through inhibiting HDAC1 via PI3K/AKT/mTOR pathway in diabetic kidney disease. Phytomedicine (2023) 112:154700. doi: 10.1016/j.phymed.2023.154700
84. Jiang Y, Zhao Y, Zhu X, Liu Y, Wu B, Guo Y, et al. Effects of autophagy on macrophage adhesion and migration in diabetic nephropathy. Ren Fail (2019) 41(1):682–90. doi: 10.1080/0886022x.2019.1632209
85. Zhao Y, Guo Y, Jiang Y, Zhu X, Liu Y, Zhang X. Mitophagy regulates macrophage phenotype in diabetic nephropathy rats. Biochem Biophys Res Commun (2017) 494(1-2):42–50. doi: 10.1016/j.bbrc.2017.10.088
86. Zhao J, Chen J, Zhu W, Qi XM, Wu YG. Exosomal miR-7002-5p derived from highglucose-induced macrophages suppresses autophagy in tubular epithelial cells by targeting Atg9b. FASEB J (2022) 36(9):e22501. doi: 10.1096/fj.202200550RR
87. Huang H, Liu H, Tang J, Xu W, Gan H, Fan Q, et al. M2 macrophage-derived exosomal miR-25-3p improves high glucose-induced podocytes injury through activation autophagy via inhibiting DUSP1 expression. IUBMB Life (2020) 72(12):2651–62. doi: 10.1002/iub.2393
88. Li W, Du M, Wang Q, Ma X, Wu L, Guo F, et al. FoxO1 promotes mitophagy in the podocytes of diabetic male mice via the PINK1/Parkin pathway. Endocrinology (2017) 158(7):2155–67. doi: 10.1210/en.2016-1970
89. Carson JM, Okamura K, Wakashin H, McFann K, Dobrinskikh E, Kopp JB, et al. Podocytes degrade endocytosed albumin primarily in lysosomes. PloS One (2014) 9(6):e99771. doi: 10.1371/journal.pone.0099771
90. Hou B, Li Y, Li X, Zhang C, Zhao Z, Chen Q, et al. HGF protected against diabetic nephropathy via autophagy-lysosome pathway in podocyte by modulating PI3K/Akt-GSK3β-TFEB axis. Cell Signal (2020) 75:109744. doi: 10.1016/j.cellsig.2020.109744
91. Piscitelli P, Viazzi F, Fioretto P, Giorda C, Ceriello A, Genovese S, et al. Predictors of chronic kidney disease in type 1 diabetes: a longitudinal study from the AMD annals initiative. Sci Rep (2017) 7(1):3313. doi: 10.1038/s41598-017-03551-w
92. Coughlan MT, Nguyen TV, Penfold SA, Higgins GC, Thallas-Bonke V, Tan SM, et al. Mapping time-course mitochondrial adaptations in the kidney in experimental diabetes. Clin Sci (London Engl 1979) (2016) 130(9):711–20. doi: 10.1042/cs20150838
93. Zeni L, Norden AGW, Cancarini G, Unwin RJ. A more tubulocentric view of diabetic kidney disease. J Nephrology (2017) 30(6):701–17. doi: 10.1007/s40620-017-0423-9
94. Nath KA. Tubulointerstitial changes as a major determinant in the progression of renal damage. Am J Kidney Diseases (1992) 20(1):1–17. doi: 10.1016/s0272-6386(12)80312-x
95. Vallon V, Rose M, Gerasimova M, Satriano J, Platt KA, Koepsell H, et al. Knockout of na-glucose transporter SGLT2 attenuates hyperglycemia and glomerular hyperfiltration but not kidney growth or injury in diabetes mellitus. Am J Physiol Renal Physiol (2013) 304(2):F156–67. doi: 10.1152/ajprenal.00409.2012
96. Xiao L, Xu X, Zhang F, Wang M, Xu Y, Tang D, et al. The mitochondria-targeted antioxidant MitoQ ameliorated tubular injury mediated by mitophagy in diabetic kidney disease via Nrf2/PINK1. Redox Biol (2017) 11:297–311. doi: 10.1016/j.redox.2016.12.022
97. Chen SJ, Lv LL, Liu BC, Tang RN. Crosstalk between tubular epithelial cells and glomerular endothelial cells in diabetic kidney disease. Cell Prolif (2020) 53(3):e12763. doi: 10.1111/cpr.12763
98. Kuwahara S, Hosojima M, Kaneko R, Aoki H, Nakano D, Sasagawa T, et al. Megalin-mediated tubuloglomerular alterations in high-fat diet-induced kidney disease. J Am Soc Nephrol JASN (2016) 27(7):1996–2008. doi: 10.1681/asn.2015020190
99. Abboud HE. Mesangial cell biology. Exp Cell Res (2012) 318(9):979–85. doi: 10.1016/j.yexcr.2012.02.025
100. Avraham S, Korin B, Chung JJ, Oxburgh L, Shaw AS. The mesangial cell - the glomerular stromal cell. Nat Rev Nephrol (2021) 17(12):855–64. doi: 10.1038/s41581-021-00474-8
101. Tung CW, Hsu YC, Shih YH, Chang PJ, Lin CL. Glomerular mesangial cell and podocyte injuries in diabetic nephropathy. Nephrol (Carlton Vic) (2018) 23 Suppl 4:32–7. doi: 10.1111/nep.13451
102. Fiorentino L, Cavalera M, Menini S, Marchetti V, Mavilio M, Fabrizi M, et al. Loss of TIMP3 underlies diabetic nephropathy via FoxO1/STAT1 interplay. EMBO Mol Med (2013) 5(3):441–55. doi: 10.1002/emmm.201201475
103. Lassén E, Daehn IS. Molecular mechanisms in early diabetic kidney disease: Glomerular endothelial cell dysfunction. Int J Mol Sci (2020) 21(24):9456. doi: 10.3390/ijms21249456
104. Mohandes S, Doke T, Hu H, Mukhi D, Dhillon P, Susztak K. Molecular pathways that drive diabetic kidney disease. J Clin Invest (2023) 133(4):e165654. doi: 10.1172/jci165654
105. Jianbing H, Xiaotian L, Jie T, Xueying C, Honge J, Bo Z, et al. The effect of allograft inflammatory factor-1 on inflammation, oxidative stress, and autophagy via miR-34a/ATG4B pathway in diabetic kidney disease. Oxid Med Cell Longev (2022) 2022:1668000. doi: 10.1155/2022/1668000
106. Fu J, Lee K, Chuang PY, Liu Z, He JC. Glomerular endothelial cell injury and cross talk in diabetic kidney disease. Am J Physiol Renal Physiol (2015) 308(4):F287–97. doi: 10.1152/ajprenal.00533.2014
107. Matsuda J, Namba T, Takabatake Y, Kimura T, Takahashi A, Yamamoto T, et al. Antioxidant role of autophagy in maintaining the integrity of glomerular capillaries. Autophagy (2018) 14(1):53–65. doi: 10.1080/15548627.2017.1391428
108. Takagaki Y, Lee SM, Dongqing Z, Kitada M, Kanasaki K, Koya D. Endothelial autophagy deficiency induces IL6 - dependent endothelial mesenchymal transition and organ fibrosis. Autophagy (2020) 16(10):1905–14. doi: 10.1080/15548627.2020.1713641
109. Casalena GA, Yu L, Gil R, Rodriguez S, Sosa S, Janssen W, et al. The diabetic microenvironment causes mitochondrial oxidative stress in glomerular endothelial cells and pathological crosstalk with podocytes. Cell Commun Signal (2020) 18(1):105. doi: 10.1186/s12964-020-00605-x
110. Yoshibayashi M, Kume S, Yasuda-Yamahara M, Yamahara K, Takeda N, Osawa N, et al. Protective role of podocyte autophagy against glomerular endothelial dysfunction in diabetes. Biochem Biophys Res Commun (2020) 525(2):319–25. doi: 10.1016/j.bbrc.2020.02.088
111. Tian H, Zheng X, Wang H. Isorhapontigenin ameliorates high glucose-induced podocyte and vascular endothelial cell injuries via mitigating oxidative stress and autophagy through the AMPK/Nrf2 pathway. Int Urol Nephrology (2023) 55(2):423–36. doi: 10.1007/s11255-022-03325-y
112. Calle P, Hotter G. Macrophage phenotype and fibrosis in diabetic nephropathy. Int J Mol Sci (2020) 21(8):2806. doi: 10.3390/ijms21082806
113. Jiang WJ, Xu CT, Du CL, Dong JH, Xu SB, Hu BF, et al. Tubular epithelial cell-to-macrophage communication forms a negative feedback loop via extracellular vesicle transfer to promote renal inflammation and apoptosis in diabetic nephropathy. Theranostics (2022) 12(1):324–39. doi: 10.7150/thno.63735
114. Ji L, Chen Y, Wang H, Zhang W, He L, Wu J, et al. Overexpression of Sirt6 promotes M2 macrophage transformation, alleviating renal injury in diabetic nephropathy. Int J Oncol (2019) 55(1):103–15. doi: 10.3892/ijo.2019.4800
115. Wen JH, Li DY, Liang S, Yang C, Tang JX, Liu HF. Macrophage autophagy in macrophage polarization, chronic inflammation and organ fibrosis. Front Immunol (2022) 13:946832. doi: 10.3389/fimmu.2022.946832
116. Liu C, Xiao K, Xie L. Progress in preclinical studies of macrophage autophagy in the regulation of ALI/ARDS. Front Immunol (2022) 13:922702. doi: 10.3389/fimmu.2022.922702
117. Chen P, Cescon M, Bonaldo P. Autophagy-mediated regulation of macrophages and its applications for cancer. Autophagy (2014) 10(2):192–200. doi: 10.4161/auto.26927
118. Tovar-Palacio C, Noriega LG, Mercado A. Potential of polyphenols to restore SIRT1 and NAD+ metabolism in renal disease. Nutrients (2022) 14(3):653. doi: 10.3390/nu14030653
119. Yessenkyzy A, Saliev T, Zhanaliyeva M, Masoud AR, Umbayev B, Sergazy S, et al. Polyphenols as caloric-restriction mimetics and autophagy inducers in aging research. Nutrients (2020) 12(5):1344. doi: 10.3390/nu12051344
120. Maugeri A, Barchitta M, Mazzone MG, Giuliano F, Basile G, Agodi A. Resveratrol modulates SIRT1 and DNMT functions and restores LINE-1 methylation levels in ARPE-19 cells under oxidative stress and inflammation. Int J Mol Sci (2018) 19(7):2118. doi: 10.3390/ijms19072118
121. Li F, Guo D, Zhi S, Jia K, Wang Y, Zhang A, et al. Etoposide-induced protein 2.4 ameliorates high glucose-induced epithelial-mesenchymal transition by activating adenosine monophosphate-activated protein kinase pathway in renal tubular cells. Int J Biochem Cell Biol (2022) 142:106117. doi: 10.1016/j.biocel.2021.106117
122. Kedhari Sundaram M, Hussain A, Haque S, Raina R, Afroze N. Quercetin modifies 5'CpG promoter methylation and reactivates various tumor suppressor genes by modulating epigenetic marks in human cervical cancer cells. J Cell Biochem (2019) 120(10):18357–69. doi: 10.1002/jcb.29147
123. Ma L, Fu R, Duan Z, Lu J, Gao J, Tian L, et al. Sirt1 is essential for resveratrol enhancement of hypoxia-induced autophagy in the type 2 diabetic nephropathy rat. Pathol Res Pract (2016) 212(4):310–8. doi: 10.1016/j.prp.2016.02.001
124. Zhao YH, Fan YJ. Resveratrol improves lipid metabolism in diabetic nephropathy rats. Front Bioscience (Landmark edition) (2020) 25(10):1913–24. doi: 10.2741/4885
125. Huang SS, Ding DF, Chen S, Dong CL, Ye XL, Yuan YG, et al. Resveratrol protects podocytes against apoptosis via stimulation of autophagy in a mouse model of diabetic nephropathy. Sci Rep (2017) 7:45692. doi: 10.1038/srep45692
126. Xu XH, Ding DF, Yong HJ, Dong CL, You N, Ye XL, et al. Resveratrol transcriptionally regulates miRNA-18a-5p expression ameliorating diabetic nephropathy via increasing autophagy. Eur Rev Med Pharmacol Sci (2017) 21(21):4952–65.
127. Tu Q, Li Y, Jin J, Jiang X, Ren Y, He Q. Curcumin alleviates diabetic nephropathy via inhibiting podocyte mesenchymal transdifferentiation and inducing autophagy in rats and MPC5 cells. Pharm Biol (2019) 57(1):778–86. doi: 10.1080/13880209.2019.1688843
128. Zhang P, Fang J, Zhang J, Ding S, Gan D. Curcumin inhibited podocyte cell apoptosis and accelerated cell autophagy in diabetic nephropathy via regulating Beclin1/UVRAG/Bcl2. Diabetes Metab Syndrome Obes (2020) 13:641–52. doi: 10.2147/dmso.S237451
129. Wei Y, Gao J, Qin L, Xu Y, Shi H, Qu L, et al. Curcumin suppresses AGEs induced apoptosis in tubular epithelial cells via protective autophagy. Exp Ther Med (2017) 14(6):6052–8. doi: 10.3892/etm.2017.5314
130. Xu X, Chen B, Huang Q, Wu Y, Liang T. The effects of puerarin on autophagy through regulating of the PERK/eIF2α/ATF4 signaling pathway influences renal function in diabetic nephropathy. Diabetes Metab Syndrome Obes (2020) 13:2583–92. doi: 10.2147/dmso.S256457
131. Li X, Zhu Q, Zheng R, Yan J, Wei M, Fan Y, et al. Puerarin attenuates diabetic nephropathy by promoting autophagy in podocytes. Front Physiol (2020) 11:73. doi: 10.3389/fphys.2020.00073
132. Sheng H, Zhang D, Zhang J, Zhang Y, Lu Z, Mao W, et al. Kaempferol attenuated diabetic nephropathy by reducing apoptosis and promoting autophagy through AMPK/mTOR pathways. Front Med (2022) 9:986825. doi: 10.3389/fmed.2022.986825
133. Zhang N, Zhao S, Hong J, Li W, Wang X. Protective effects of kaempferol on d-Ribose-Induced mesangial cell injury. Oxid Med Cell Longev (2019) 2019:7564207. doi: 10.1155/2019/7564207
134. Wang S, Huang Y, Luo G, Yang X, Huang W. Cyanidin-3-O-glucoside attenuates high glucose-induced podocyte dysfunction by inhibiting apoptosis and promoting autophagy via activation of SIRT1/AMPK pathway. Can J Physiol Pharmacol (2021) 99(6):589–98. doi: 10.1139/cjpp-2020-0341
135. Ma R, He Y, Fang Q, Xie G, Qi M. Ferulic acid ameliorates renal injury via improving autophagy to inhibit inflammation in diabetic nephropathy mice. BioMed Pharmacother (2022) 153:113424. doi: 10.1016/j.biopha.2022.113424
136. Matboli M, Ibrahim D, Hasanin AH, Hassan MK, Habib EK, Bekhet MM, et al. Epigenetic modulation of autophagy genes linked to diabetic nephropathy by administration of isorhamnetin in type 2 diabetes mellitus rats. Epigenomics (2021) 13(3):187–202. doi: 10.2217/epi-2020-0353
137. Ezzat SM, Abdallah HMI, Yassen NN, Radwan RA, Mostafa ES, Salama MM, et al. Phenolics from physalis peruviana fruits ameliorate streptozotocin-induced diabetes and diabetic nephropathy in rats via induction of autophagy and apoptosis regression. BioMed Pharmacother (2021) 142:111948. doi: 10.1016/j.biopha.2021.111948
138. Lei L, Zhao J, Liu XQ, Chen J, Qi XM, Xia LL, et al. Wogonin alleviates kidney tubular epithelial injury in diabetic nephropathy by inhibiting PI3K/Akt/NF-κB signaling pathways. Drug design Dev Ther (2021) 15:3131–50. doi: 10.2147/dddt.S310882
139. Liu XQ, Jiang L, Li YY, Huang YB, Hu XR, Zhu W, et al. Wogonin protects glomerular podocytes by targeting bcl-2-mediated autophagy and apoptosis in diabetic kidney disease. Acta pharmacologica Sinica (2022) 43(1):96–110. doi: 10.1038/s41401-021-00721-5
140. Guo L, Tan K, Luo Q, Bai X. Dihydromyricetin promotes autophagy and attenuates renal interstitial fibrosis by regulating miR-155-5p/PTEN signaling in diabetic nephropathy. Bosnian J basic Med Sci (2020) 20(3):372–80. doi: 10.17305/bjbms.2019.4410
141. Wang Y, Li Y, Zhang T, Chi Y, Liu M, Liu Y. Genistein and Myd88 activate autophagy in high glucose-induced renal podocytes in vitro. Med Sci Monitor (2018) 24:4823–31. doi: 10.12659/msm.910868
142. Hou B, Qiang G, Zhao Y, Yang X, Chen X, Yan Y, et al. Salvianolic acid a protects against diabetic nephropathy through ameliorating glomerular endothelial dysfunction via inhibiting AGE-RAGE signaling. Cell Physiol Biochem (2017) 44(6):2378–94. doi: 10.1159/000486154
143. Qiao S, Liu R, Lv C, Miao Y, Yue M, Tao Y, et al. Bergenin impedes the generation of extracellular matrix in glomerular mesangial cells and ameliorates diabetic nephropathy in mice by inhibiting oxidative stress via the mTOR/β-TrcP/Nrf2 pathway. Free Radical Biol Med (2019) 145:118–35. doi: 10.1016/j.freeradbiomed.2019.09.003
144. Wei L, Jian P, Erjiong H, Qihan Z. Ginkgetin alleviates high glucose-evoked mesangial cell oxidative stress injury, inflammation, and extracellular matrix (ECM) deposition in an AMPK/mTOR-mediated autophagy axis. Chem Biol Drug Design (2021) 98(4):620–30. doi: 10.1111/cbdd.13915
145. Lee EJ, Kang MK, Kim YH, Kim DY, Oh H, Kim SI, et al. Dietary chrysin suppresses formation of actin cytoskeleton and focal adhesion in AGE-exposed mesangial cells and diabetic kidney: Role of autophagy. Nutrients (2019) 11(1):127. doi: 10.3390/nu11010127
146. Wang X, Gao L, Lin H, Song J, Wang J, Yin Y, et al. Mangiferin prevents diabetic nephropathy progression and protects podocyte function via autophagy in diabetic rat glomeruli. Eur J Pharmacol (2018) 824:170–8. doi: 10.1016/j.ejphar.2018.02.009
147. Dong W, Jia C, Li J, Zhou Y, Luo Y, Liu J, et al. Fisetin attenuates diabetic nephropathy-induced podocyte injury by inhibiting NLRP3 inflammasome. Front Pharmacol (2022) 13:783706. doi: 10.3389/fphar.2022.783706
148. Gowd V, Kang Q, Wang Q, Wang Q, Chen F, Cheng KW. Resveratrol: Evidence for its nephroprotective effect in diabetic nephropathy. Adv Nutr (2020) 11(6):1555–68. doi: 10.1093/advances/nmaa075
149. Zhu X, Xu X, Du C, Su Y, Yin L, Tan X, et al. An examination of the protective effects and molecular mechanisms of curcumin, a polyphenol curcuminoid in diabetic nephropathy. BioMed Pharmacother (2022) 153:113438. doi: 10.1016/j.biopha.2022.113438
150. Rainey N, Motte L, Aggarwal BB, Petit PX. Curcumin hormesis mediates a cross-talk between autophagy and cell death. Cell Death Dis (2015) 6(12):e2003. doi: 10.1038/cddis.2015.343
151. Beevers CS, Li F, Liu L, Huang S. Curcumin inhibits the mammalian target of rapamycin-mediated signaling pathways in cancer cells. Int J Cancer (2006) 119(4):757–64. doi: 10.1002/ijc.21932
152. Wu X, Liu Z, Yu XY, Xu S, Luo J. Autophagy and cardiac diseases: Therapeutic potential of natural products. Medicinal Res Rev (2021) 41(1):314–41. doi: 10.1002/med.21733
153. Song JX, Malampati S, Zeng Y, Durairajan SSK, Yang CB, Tong BC, et al. A small molecule transcription factor EB activator ameliorates beta-amyloid precursor protein and tau pathology in alzheimer's disease models. Aging Cell (2020) 19(2):e13069. doi: 10.1111/acel.13069
154. Li X, Zhu R, Jiang H, Yin Q, Gu J, Chen J, et al. Autophagy enhanced by curcumin ameliorates inflammation in atherogenesis via the TFEB-P300-BRD4 axis. Acta Pharm Sin B (2022) 12(5):2280–99. doi: 10.1016/j.apsb.2021.12.014
155. Wang B, Chen S, Yan X, Li M, Li D, Lv P, et al. The therapeutic effect and possible harm of puerarin for treatment of stage III diabetic nephropathy: a meta-analysis. Altern Ther Health Med (2015) 21(1):36–44.
156. Chen X, Yu J, Shi J. Management of diabetes mellitus with puerarin, a natural isoflavone from pueraria lobata. Am J Chin Med (2018) 46(8):1771–89. doi: 10.1142/s0192415x18500891
157. Berger A, Venturelli S, Kallnischkies M, Böcker A, Busch C, Weiland T, et al. Kaempferol, a new nutrition-derived pan-inhibitor of human histone deacetylases. J Nutr Biochem (2013) 24(6):977–85. doi: 10.1016/j.jnutbio.2012.07.001
158. Xiao X, Hu Q, Deng X, Shi K, Zhang W, Jiang Y, et al. Old wine in new bottles: Kaempferol is a promising agent for treating the trilogy of liver diseases. Pharmacol Res (2022) 175:106005. doi: 10.1016/j.phrs.2021.106005
159. Han X, Zhao S, Song H, Xu T, Fang Q, Hu G, et al. Kaempferol alleviates LD-mitochondrial damage by promoting autophagy: Implications in parkinson's disease. Redox Biol (2021) 41:101911. doi: 10.1016/j.redox.2021.101911
160. Kim TW, Lee SY, Kim M, Cheon C, Ko SG. Kaempferol induces autophagic cell death via IRE1-JNK-CHOP pathway and inhibition of G9a in gastric cancer cells. Cell Death Dis (2018) 9(9):875. doi: 10.1038/s41419-018-0930-1
161. Ashrafizadeh M, Tavakol S, Ahmadi Z, Roomiani S, Mohammadinejad R, Samarghandian S. Therapeutic effects of kaempferol affecting autophagy and endoplasmic reticulum stress. Phytotherapy Res (2020) 34(5):911–23. doi: 10.1002/ptr.6577
162. Han X, Sun S, Sun Y, Song Q, Zhu J, Song N, et al. Small molecule-driven NLRP3 inflammation inhibition via interplay between ubiquitination and autophagy: implications for parkinson disease. Autophagy (2019) 15(11):1860–81. doi: 10.1080/15548627.2019.1596481
163. Xie C, Zhuang XX, Niu Z, Ai R, Lautrup S, Zheng S, et al. Amelioration of alzheimer's disease pathology by mitophagy inducers identified via machine learning and a cross-species workflow. Nat Biomed Engineering (2022) 6(1):76–93. doi: 10.1038/s41551-021-00819-5
164. Choi R, Kim BH, Naowaboot J, Lee MY, Hyun MR, Cho EJ, et al. Effects of ferulic acid on diabetic nephropathy in a rat model of type 2 diabetes. Exp Mol Med (2011) 43(12):676–83. doi: 10.3858/emm.2011.43.12.078
165. Yang L, Zheng Y, Miao YM, Yan WX, Geng YZ, Dai Y, et al. Bergenin, a PPARγ agonist, inhibits Th17 differentiation and subsequent neutrophilic asthma by preventing GLS1-dependent glutaminolysis. Acta Pharmacologica Sinica (2022) 43(4):963–76. doi: 10.1038/s41401-021-00717-1
166. Xiao T, Guan X, Nie L, Wang S, Sun L, He T, et al. Rapamycin promotes podocyte autophagy and ameliorates renal injury in diabetic mice. Mol Cell Biochem (2014) 394(1-2):145–54. doi: 10.1007/s11010-014-2090-7
167. Lloberas N, Cruzado JM, Franquesa M, Herrero-Fresneda I, Torras J, Alperovich G, et al. Mammalian target of rapamycin pathway blockade slows progression of diabetic kidney disease in rats. J Am Soc Nephrology (2006) 17(5):1395–404. doi: 10.1681/asn.2005050549
168. Xu J, Chen Y, Xing Y, Ye S. Metformin inhibits high glucose-induced mesangial Cell Prolif, inflammation and ECM expression through the SIRT1-FOXO1-autophagy axis. Clin Exp Pharmacol Physiol (2019) 46(9):813–20. doi: 10.1111/1440-1681.13120
169. Ren H, Shao Y, Wu C, Ma X, Lv C, Wang Q. Metformin alleviates oxidative stress and enhances autophagy in diabetic kidney disease via AMPK/SIRT1-FoxO1 pathway. Mol Cell Endocrinol (2020) 500:110628. doi: 10.1016/j.mce.2019.110628
170. Oza MJ, Laddha AP, Gaikwad AB, Mulay SR, Kulkarni YA. Role of dietary modifications in the management of type 2 diabetic complications. Pharmacol Res (2021) 168:105602. doi: 10.1016/j.phrs.2021.105602
171. Kitada M, Ogura Y, Monno I, Koya D. A low-protein diet for diabetic kidney disease: Its effect and molecular mechanism, an approach from animal studies. Nutrients (2018) 10(5):544. doi: 10.3390/nu10050544
172. Kuo HY, Huang YH, Wu SW, Chang FH, Tsuei YW, Fan HC, et al. The effects of exercise habit on albuminuria and metabolic indices in patients with type 2 diabetes mellitus: A cross-sectional study. Medicina (Kaunas Lithuania) (2022) 58(5):577. doi: 10.3390/medicina58050577
173. Monno I, Ogura Y, Xu J, Koya D, Kitada M. Exercise ameliorates diabetic kidney disease in type 2 diabetic fatty rats. Antioxidants (Basel Switzerland) (2021) 10(11):1754. doi: 10.3390/antiox10111754
174. Sattarinezhad A, Roozbeh J, Shirazi Yeganeh B, Omrani GR, Shams M. Resveratrol reduces albuminuria in diabetic nephropathy: A randomized double-blind placebo-controlled clinical trial. Diabetes Metab (2019) 45(1):53–9. doi: 10.1016/j.diabet.2018.05.010
175. Khajehdehi P, Pakfetrat M, Javidnia K, Azad F, Malekmakan L, Nasab MH, et al. Oral supplementation of turmeric attenuates proteinuria, transforming growth factor-β and interleukin-8 levels in patients with overt type 2 diabetic nephropathy: a randomized, double-blind and placebo-controlled study. Scandinavian J Urol Nephrology (2011) 45(5):365–70. doi: 10.3109/00365599.2011.585622
176. Borges CM, Papadimitriou A, Duarte DA, Lopes de Faria JM, Lopes de Faria JB. The use of green tea polyphenols for treating residual albuminuria in diabetic nephropathy: A double-blind randomised clinical trial. Sci Rep (2016) 6:28282. doi: 10.1038/srep28282
Keywords: natural polyphenols, autophagy, diabetic kidney disease, transcription factor EB, lysosome
Citation: Liu T, Jin Q, Yang L, Mao H, Ma F, Wang Y, Li P and Zhan Y (2023) Regulation of autophagy by natural polyphenols in the treatment of diabetic kidney disease: therapeutic potential and mechanism. Front. Endocrinol. 14:1142276. doi: 10.3389/fendo.2023.1142276
Received: 11 January 2023; Accepted: 24 July 2023;
Published: 10 August 2023.
Edited by:
Huafeng Liu, Affiliated Hospital of Guangdong Medical University, ChinaReviewed by:
Dilip Sharma, The State University of New Jersey, United StatesAkira Sugawara, Tohoku University, Japan
Ranjan Das, Oregon Health and Science University, United States
Copyright © 2023 Liu, Jin, Yang, Mao, Ma, Wang, Li and Zhan. This is an open-access article distributed under the terms of the Creative Commons Attribution License (CC BY). The use, distribution or reproduction in other forums is permitted, provided the original author(s) and the copyright owner(s) are credited and that the original publication in this journal is cited, in accordance with accepted academic practice. No use, distribution or reproduction is permitted which does not comply with these terms.
*Correspondence: Ping Li, bHA4Njc1QDE2My5jb20=; Yongli Zhan, emhhbnlvbmdsaTg4QHNpbmEuY29t