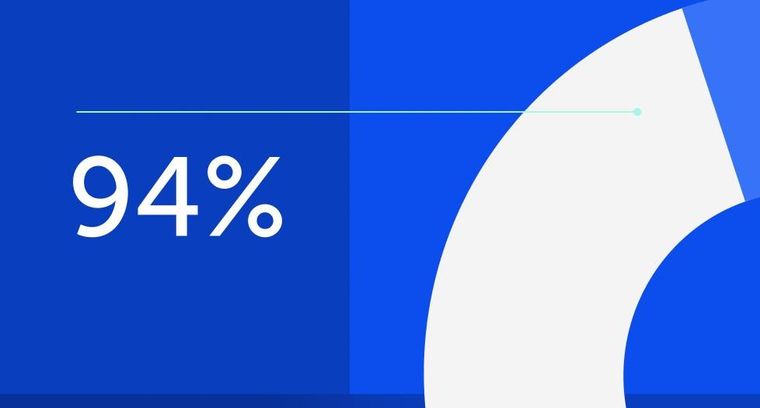
94% of researchers rate our articles as excellent or good
Learn more about the work of our research integrity team to safeguard the quality of each article we publish.
Find out more
REVIEW article
Front. Endocrinol., 16 February 2023
Sec. Obesity
Volume 14 - 2023 | https://doi.org/10.3389/fendo.2023.1114767
This article is part of the Research TopicSystemic Regulation of Organ Homeostasis and Implications of Hormones and Immunity, Volume IIView all 17 articles
Brown adipose tissue (BAT), a thermoregulatory organ known to promote energy expenditure, has been extensively studied as a potential avenue to combat obesity. Although BAT is the opposite of white adipose tissue (WAT) which is responsible for energy storage, BAT shares thermogenic capacity with beige adipose tissue that emerges from WAT depots. This is unsurprising as both BAT and beige adipose tissue display a huge difference from WAT in terms of their secretory profile and physiological role. In obesity, the content of BAT and beige adipose tissue declines as these tissues acquire the WAT characteristics via the process called “whitening”. This process has been rarely explored for its implication in obesity, whether it contributes to or exacerbates obesity. Emerging research has demonstrated that BAT/beige adipose tissue whitening is a sophisticated metabolic complication of obesity that is linked to multiple factors. The current review provides clarification on the influence of various factors such as diet, age, genetics, thermoneutrality, and chemical exposure on BAT/beige adipose tissue whitening. Moreover, the defects and mechanisms that underpin the whitening are described. Notably, the BAT/beige adipose tissue whitening can be marked by the accumulation of large unilocular lipid droplets, mitochondrial degeneration, and collapsed thermogenic capacity, by the virtue of mitochondrial dysfunction, devascularization, autophagy, and inflammation.
Adipose tissue is an endocrine organ that has become the central focus in research toward a better understanding of the pathological mechanisms associated with obesity (1, 2). The latter is characterized by adipose tissue hypertrophy “adipocytes expansion” and hyperplasia “increase adipocytes number” caused by a chronic imbalance between energy intake and expenditure (1, 3). There are three distinct types of adipocytes that have been well studied in mammals (4): (i.) white adipocytes comprised of large single lipid droplet and few mitochondria, and this form of adipose is predominately useful to facilitate the storage of excess energy in a form of fats (5), and (ii.) brown adipocytes, and (iii.) beige or brite adipocytes rich in mitochondria, which are important organelles for regulating thermogenesis, mainly via the action of uncoupling protein 1 (UCP1) (5). Although brown and beige adipocytes share thermogenic capacity, several characteristics established that both are distinct cell types, which are different in terms of origin, anatomical location, and plasticity (6, 7). Of note, beige adipocytes emerge from white adipose tissue (WAT) depots via the process called “browning” (8, 9).
The (re)discovery of brown (BAT) and beige adipose tissue has garnered interest as therapeutic targets to promote energy expenditure and counteract complications linked with obesity (7). Notably, previous conversations have emphasized the importance of sufficient vasculature to improve mitochondrial function in BAT and alleviate obesity-associated complications (10). The vascular rarefaction in BAT was associated with the dysfunction and loss of BAT commonly referred to as “whitening” (11). It is well accepted that both BAT and beige adipose tissue are subjected to a whitening effect which is common in obesity, whereby they acquire unilocular cells that gradually lose all the brown characteristics and assume WAT characteristics (12). Moreover, the whitening of adipose tissue is accompanied by lipid accumulation due to reduced substrate oxidation and the loss of mitochondria through the impairment of molecular mechanisms regulating thermogenesis, as well as those involving autophagy and mitophagy (12). Others have demonstrated that brown-to-white adipose tissue conversion may activate undesirable metabolic complications such as inflammatory response and, the much-explored pyrin domain-containing protein 3 (NLRP3) inflammasome (13). Recently, BAT whitening was defined as a long-term obesity complication that displayed a progressive severity upon chronic intake of a high-fat diet (HFD) during the pathogenesis of obesity (14). Thus, with the rapidly rising prevalence of obesity (15), there is an urgent need to understand the causative and underlying factors implicated in BAT/beige adipose tissue whitening, including elucidating the molecular drivers of their plasticity. This encompasses deciphering pathophysiological mechanisms that implicate the development and aggravation of obesity, as shown by the fact that dysfunctional adipose tissue in obesity leads to a variety of secondary metabolic complications.
The current review elaborates on the prominent mechanisms involved in BAT/Beige adipose tissue whitening, while a sharp focus is placed on the impact of critical factors that contribute to obesity, such as diet, age, temperature, and various chemical substances. Importantly, an overview of BAT and beige adipose tissue, and their physiological relevance is given to highlight vital mechanisms implicated in the process of thermogenesis.
As aforementioned, adipocytes are broadly classified into three distinct types: (i) brown adipocytes, (ii) white adipocytes, and (iii) beige or brite adipocytes (Figure 1) (4). These cells differ in terms of function and morphology, and their localization on various fat depots in mice and humans (16, 17). Importantly, brown adipocytes are enriched in the BAT depot, whereas both white and beige adipocytes are found within WAT depots (18). Predominantly, beige adipocytes emerge in subcutaneous WAT depots, including the anterior and inguinal subcutaneous WAT in mice (18, 19). In contrast to WAT, BAT was mainly viewed as the key site for upholding thermal homeostasis during cold adaptation in human infants (20). Despite a century of studies on neonatal BAT, the knowledge about BAT physiological features and the mechanisms by which this tissue regulates body temperature homeostasis in human neonates is scanty, mainly due to the lack of appropriate methods for such investigations. Recently, studies have utilized infrared thermography as a suitable non-invasive technique to investigate neonatal BAT activity (21, 22). It was reported that a single short-term cold exposure during the first day of life improves body temperature adaptation (21). The later rediscovered BAT and recruitable beige adipose tissue in adult humans which was initially thought to exist in newborn babies and hibernating animals only have highlighted the potential influence of this tissue in improving human health (23). This is attributable to the thermogenic and endocrine functions of these tissues to promote energy expenditure and secrete metabolic regulating molecules, called batokines, such as vascular endothelial growth factors (VEGF)-A, bone morphogenic protein 8b (BMP8b), neuregulin 4 (NRG4) and fibroblast growth factor 21 (FGF21) (24). Recent research has indicated that disease progression is consistent with impairment of gene expression levels of batokines regulating sympathetic neurite outgrowth, vascularization and glucose metabolism in animal model of T2D (25). Thus, the physiological functions of BAT and beige adipose tissue have spurred a new wave of interest in their effects on modulating metabolism. This is supported by mounting research on the health benefits of BAT, especially in combating obesity (26–28). Owing to this, several selective markers of adipose tissues have been identified and characterized as potential biomarkers in obesity, as reviewed by Pilkington et al. (29).
Figure 1 Schematic overview showing the characteristics of brown, white, and beige/brite adipocytes, including their localization, morphology, physiological function, and marker genes. In brief, the anatomical location of these adipocytes differs, except for white and beige adipocytes which are found within WAT depots. In terms of morphology and function, brown adipocytes are comprised of small multilocular lipid droplets, high mitochondrial content, and increased UCP1 expression, which can be also induced in beige adipocytes to promote thermogenesis and enhance energy expenditure, features that are important for the treatment of obesity. In sharp contrast, white adipocytes have fewer mitochondria and large unilocular lipid droplets to facilitate the storage of energy in a form of fats. Of note, these adipocytes have similar and distinct marker genes which could play an important role in tracking metabolic complications linked with obesity. Cidea, cell death-inducing DNA fragmentation factor-like effector A; CD137, tumour necrosis factor receptor superfamily, member 9; Dio2, Iodothyronine deiodinase 2; Lhx8, LIM homeobox protein 8; Pgc1A, peroxisome proliferator-activated receptor coactivator 1 alpha; Prdm16, PR domain-containing 16; Tcf21, Transcription factor 21; TMEM26, transmembrane protein 26; UCP1, uncoupling protein 1; WAT, white adipose tissue; Rb1, RB transcriptional corepressor 1; Zic1, zinc finger protein of the cerebellum.
Although brown, white, and beige adipocytes are derived from similar mesenchymal stem cells, they originate from different precursor cells with unique marker genes, as shown in Figure 2 (30–33). Genetic-lineage tracing indicates that white adipocytes descend from Myf5- (PDGFRα+, CD29, CD44+) progenitors of mesenchymal stem cells (34, 35), while brown adipocytes originate from Myf5+ (CD34+/CD29, MYF5-, PAX3+) progenitors which differentiate into mature brown adipocytes (36–38). Interestingly, the existence of beige adipocytes which resemble white adipocytes in having a low basal expression of UCP1, but like classical brown adipocytes respond to thermogenic stimuli with high UCP1 expression (39). These cells are distinct types of thermogenic fat cells that originate from two distinct processes, de novo differentiation from Myf5- progenitor cells and transdifferentiation from white adipocytes via a process called “browning” (4). A study by Oguri et al. (40) showed that CD81+, Sca1+, PDGFRα+ adipocyte progenitors give rise to beige adipocytes and CD81 loss causes obesity, insulin resistance, and inflammation in mice. In addition to canonical beige adipocytes, a study of adipocyte heterogeneity has demonstrated that there is a subpopulation of thermal stress-induced glycolytic beige adipocytes that emerge from inguinal WAT (41, 42). Although cells in the MyoD1+ lineage do not typically give rise to any adipocytes, it was reported that glycolytic beige adipocytes descend from MyoD1+ progenitors that emerge within a stromal vascular fraction of inguinal WAT when beta-3 adrenergic receptor (β-AR) signaling is inhibited (41, 42). Thus, this has reignited the interest in studying and understanding the role of WAT browning in obesity (26, 28, 43). While white adipocytes transdifferentiate into beige adipocytes, both brown and beige adipocytes can transdifferentiate into white adipocytes through a process called “whitening”, which is quite common in obesity (44, 45). By now, it is evident that the differentiation process constitutes an essential component for understanding the developmental fate and function of BAT, which explains the increased exploration of these transcriptional factors in preclinical models of obesity (46–48).
Figure 2 Differentiation and transdifferentiation trajectories of major adipocyte populations, including brown and white adipocytes, as well as canonical and glycolytic beige/brite adipocytes. Generally, brown adipocytes are derived from Myf5+ progenitors, whereas white and beige adipocytes originate from Myf5- progenitors that descend from mesenchymal stem cells. New advances on adipocyte origin show that there is another subpopulation of beige adipocytes known as glycolytic beige adipocytes, which are derived from MyoD+ progenitors within the stromal vascular fraction of inguinal WAT in response to thermal stress. During differentiation secretory factors BMP-7 and transcriptional factor PRDM16 induce Myf5+ cell commitment to brown preadipocytes whereas BMP-4, BMP-10, and FGF10 induce Myf5- cell commitment to white preadipocytes. On the other hand, BMP-7 induces a commitment of beige adipocyte precursors to canonical beige preadipocytes. Differentiation of all types of preadipocytes into mature adipocytes is driven by PPARγ, and C/EBPs, whereas brown and beige adipocytes require the expression of additional factors, including PRDM16 and PGC1α. Moreover, GABPα regulates the differentiation of glycolytic beige adipocytes. During transdifferentiation, white adipocytes convert to beige adipocytes following cold exposure or β3-AR stimulation. Likewise, brown and beige adipocytes can transdifferentiate into white-like adipocytes, a process termed whitening. β3-AR, beta-3 adrenergic receptor; BMP, bone morphogenetic protein; C/EBPs, CCAAT/enhancer-binding proteins; GABPα, GA-binding protein alpha; PGC1α, peroxisome proliferator-activated receptor coactivator 1 alpha; PPARγ, peroxisome proliferator-activated receptor-gamma; PRDM16, PR domain-containing 16; UCP1, Uncoupling protein 1.
Of note, bone morphogenetic protein (BMP)-7, a secretory protein that acts as an autocrine/paracrine mediator, promotes the differentiation of both brown and beige adipocytes in mice (49, 50). Recently, Townsend et al. (51) demonstrated that BMP7-loaded silk hydrogels into the subcutaneous WAT of mice induced brown adipogenesis in committed and uncommitted progenitor cells, which in turn increased energy expenditure and reduced weight gain in mice. In human-neck adipose-derived stromal cells, BMP7 enhanced mitochondrial DNA content, concomitant with increased gene expression of proliferator-activated receptor coactivator 1 alpha (PGC1α), a transcriptional co-regulator, responsible for mitochondrial biogenesis (52). Amongst other transcriptional factors, PR domain containing 16 (PRDM16) plays a key role in determining the fate of brown adipocyte differentiation (53). An elegant study by Seale et al. (54) has demonstrated that the loss of PRDM16 in brown adipose precursors results in the loss of brown adipocyte characteristics, which in turn promotes skeletal muscle differentiation instead of brown adipocyte differentiation. Importantly, PRDM16 is not only required for determining brown adipocyte fate but also for regulating thermogenic programming and maintenance of brown adipocyte identity (55). Adipogenic transcriptional factors, such as CCAAT enhancer-binding proteins (C/EBPs), and peroxisome proliferator-activated receptor (PPAR)-γ have long been known to play a central role in regulating adipocyte differentiation in almost all types of adipocytes (56–58). To further highlight the significance of studying adipocyte differentiation in obesity, a recently published protocol (59) compares white, beige, and brown adipocyte differentiation, further characterizing the expression of distinct transcriptional factors that are involved in thermogenesis. In fact, findings from this study indicate that differentiated pre-adipocytes from interscapular BAT has a higher thermogenic potential and expression levels of UCP1 with compared to WAT-derived cells. A comprehensive characterization of mature brown adipocyte subpopulations using single-nucleus ribonucleic acid (RNA) sequencing identified a rare subpopulation of adipocytes that increases in abundance at higher temperatures, suggesting a lower thermogenic activity (60). This subpopulation regulates the activity of neighboring adipocytes via acetate-mediated modulation of their thermogenic capacity (60).
A consistently growing body of literature indicates that elevating the thermogenic capacity of BAT through activation, recruitment, or BAT transplant could be an ideal approach to mitigate obesity (Figure 3) (61–63). The thermogenesis in BAT and beige adipose tissue is facilitated by UCP1 or thermogenin, a mitochondrial inner membrane protein that uncouples substrate oxidation from ATP synthesis, thereby dissipating excess energy as heat (64). In addition to a well-recognized UCP1-dependent thermogenic mechanism, there is a newly identified UCP1-independent thermogenic mechanism that could potentially offer a new target for the treatment of obesity and type 2 diabetes, especially targeting UCP1-negative adipocytes in the elderly and people with obesity (65–67). The UCP1-independent thermogenic mechanism involves ATP-dependent Calcium (Ca2+) cycling by Sarco/endoplasmic reticulum Ca2+-ATPase 2b, which enhances energy expenditure and glucose oxidation in beige adipocytes (68). A study by Okamatsu-Ogura et al. (68) has discovered that cold exposure induces UCP1-independent active lipid metabolism in BAT of UCP1-knockout mice. Recently, Oeckl et al. (69) identified the futile ATP-consuming triglyceride/fatty acid cycle as a central process that contributes to thermogenesis in BAT of UCP1-knockout mice. To date, UCP1 is the most investigated mitochondrial carrier protein, involved in the modulation of oxidative phosphorylation, with its isoforms like UCP2 also found in other tissues like the heart where they protect against oxidative stress (70). By now, it is well-accepted that cold exposure is a classical stimulus that utilizes glucose and fatty acids as substrates for adaptive thermogenesis (71). In humans, cold exposure increases glucose uptake and non-esterified fatty acid turnover, suggesting that activation of thermogenesis can help to improve plasma glucose clearance (71). Apart from the cold exposure, thermogenic stimuli, such as dietary compounds, physical exercise, and other various agents, including glucagon-like peptide-1 (GLP-1), thyroid hormones (THs), liraglutide and thiazolidinediones, are known to upregulate the genes involved in thermogenesis and induce WAT browning (26).
Figure 3 Thermogenic activation of brown and beige adipocytes by various exogeneous and endogenous stimuli. Traditionally, cold exposure and other dietary compounds activate thermogenesis via NE, excreted by the SNS, or through direct activation of AMPK. Inactivation of hypothalamic AMPK by thermogenic stimuli like estradiol, GLP-1, liraglutide or thyroid hormones, stimulate SNS resulting in the release of NE and stimulation of β3-AR which subsequently increase cAMP and activate PKA. Moreover, elevated cAMP activates CREB causing the transcription of thermogenic genes such as PPARγ, DIO2, PRDM16, PGC1α, and CIDEA. On the other hand, the cAMP-PKA signaling pathway promotes lipolysis just like AMPK through the phosphorylation of ATGL and HSL. Liberated FFA contributes to the upregulation of UCP1 and increases thermogenesis. In addition, enhanced TAG-derived fatty acids uptake upregulates CPT1, resulting in increased FFA transport into mitochondria. Glucose act as a substrate to generate FFA via FSAN, which ultimately contributes to heat production. Upon β3-AR stimulation, adenylate cyclase cAMP/PKA also activates mTORC1 and inhibits Parkin to repress autophagy/mitophagy, as a means to maintain beige adipocytes. Inversely, AMPK promotes promote autophagy/mitophagy to maintain mitochondrial health via the phosphorylating of ULK1 in brown adipocytes. AMPK, AMP-activated protein kinase; β3-AR, Beta-3 adrenergic receptor; PPARγ, peroxisome proliferator-activated receptor-gamma; CIDEA, cell death-inducing DNA fragmentation factor-like effector A; C/EBPs, CCAAT/enhancer-binding proteins; cAMP, cyclic adenosine monophosphate-protein kinase A; DIO2, Iodothyronine deiodinase 2; FASN, fatty acid synthase; FFA, free fatty acids; GLUTs, glucose transporters; HSL, hormone-sensitive lipase; NE, norepinephrine; PKA, protein kinase A; PRDM16, PR domain-containing 16; PGC1α, peroxisome proliferator-activated receptor coactivator 1 alpha; UCP1, Uncoupling protein 1; SNS, sympathetic nervous system; T4, thyroxine; T3, triiodothyronine; TAG, triacylglyceride.
In general, thermogenesis is modulated by stimulating the sympathetic nervous system (SNS), which is in part regulated through the release of norepinephrine (NE) to activate β3-AR signaling in response to various stimuli (72). Amongst all other well-known mechanisms that regulate thermogenesis, the cyclic adenosine monophosphate-protein kinase A (cAMP-PKA) signaling pathway, and AMP-activated protein kinase (AMPK) are classical mechanisms that have been studied in depth (72). Upon activation, PKA phosphorylates adipose triglyceride lipase (ATGL) and hormone-sensitive lipase (HSL), enzymes that promote lipolysis, leading to the breakdown of triacylglyceride (TAG) to free fatty acids (FFA), again via AMPK (72–74). Resultant FFA can be further broken down and oxidized in the mitochondria by carnitine palmitoyltransferase 1 (CPT1) to produce heat via UCP1 (75). Of note, glucose transporter (GLUT)-1 and GLUT4 are expressed in human BAT to facilitate glucose uptake by brown adipocytes (76, 77). In response to chronic cold-exposure, glucose uptake by BAT is utilized for the fatty acid synthesis and β-oxidation process (78). Alternatively, cAMP-PKA signaling modulates the phosphorylation of cAMP-response element binding protein (CREB), which in turn promotes the transcription of mitochondrial biogenic and thermogenic genes, such as PGC1α, PRDM16, PPARγ, and UCP1 (79). Lipid-droplet-associated protein also known as cell death-inducing DNA fragmentation factor-like effector A (CIDEA) regulates UCP1 transcription for browning and thermogenesis in human adipocytes (80). In specific, CIDEA inhibits liver X receptor (LXR), a repression of UCP1 enhancer activity, and strengthens the binding of PPARγ to UCP1 enhancer, promoting UCP1 transcription in CIDEA knockout primary human adipocytes (80). Another prominent mechanism that is modulated by the kinase enzymes, PKA and AMPK is the regulation of autophagy “degradation of cellular components” or mitophagy “selective degradation of mitochondria” which plays an important role in BAT/beige adipose tissue plasticity (12, 81, 82). In BAT, activation of thermogenesis is paralleled by a reduction in the autophagic degradative activity, which converges at PKA that activates the rapamycin complex 1 (mTORC1) and inhibits Parkin transcription (83, 84). Moreover, melanocyte-inducing transcription factor (MITF) and forkhead box O3 (FOXO3) were identified as downstream autophagy-related transcription factors that are downregulated by cAMP-PKA during autophagy regression in beige adipocytes (83).
Inversely, AMPK is required for the efficient removal of damaged mitochondria or mitophagy through phosphorylation of unc-51-like kinase 1 (ULK1) as a means to maintain BAT and induce browning (85). Thus, targeting AMPK might be a plausible approach for the treatment of various metabolic diseases as recently reviewed by López (86). Dietary components such as polyphenols have been reported to activate the SNS and stimulate the release of THs, which in turn increase thermogenesis and induce WAT browning via the AMPK-PGC1α/Sirt1 and PPAR signaling pathways (87, 88). Apart from that, dietary intake alone is known to induce thermogenesis by increasing postprandial energy expenditure, contributing 5–15% of total daily energy expenditure (89). Other data has uncovered that WAT browning is a highly dynamic physiological process, in which O-linked b-N-acetylglucosamine (O-GlcNAc) signaling in agouti-related protein (AgRP) neurons is essential for suppressing thermogenesis to conserve energy upon fasting (90). In terms of hormonal control, both insulin and leptin act on hypothalamic or proopiomelanocortin neurons to promote WAT browning (91). Interestingly, overexpression of glucose-regulated protein 78 kDa/binding immunoglobulin protein (GRP78) in the ventromedial nucleus of the hypothalamus (VMH) alleviated ER stress and obesity; however, this was not dependent on leptin but it was related to the increased thermogenic activation of BAT and WAT browning (92). GLP-1, a hormone that controls satiation and glucose metabolism via vagal afferent neurons was reported to stimulate BAT thermogenesis by regulating sirtuin 1 (SIRT1) (93), and hypothalamic AMPK (94). Metabolism-activating hormones thyroxine (T4) and triiodothyronine (T3) which are mainly secreted by the thyroid gland convert from T4 to T3 by type II iodothyronine deiodinase (DIO2) promoting UCP1 expression in BAT (95). Importantly, THs target the hypothalamus in the brain to modulate energy balance via AMPK (96, 97). Specifically, THs act on the VMH to regulate the thermogenic program in BAT (98, 99). For instance, it was reported that the central T3 promotes hepatic lipogenesis and thermogenic program in BAT through the parasympathetic nervous system and SNS, respectively (98). These effects were coordinated by distinct signaling pathways in the VMH, JNK1, and ceramides/endoplasmic reticulum stress under the control of AMPK (98). Other fundamental hormones include estradiol, a female reproductive hormone, which inhibits AMPK leading to the activation of BAT thermogenesis via estrogen receptor alpha selectively in the VMH (100).
Autophagy is a fundamental lysosomal catabolic process that degrades and recycles damaged cellular components such as lipids, proteins, and organelles for cellular survival in stress conditions (101–103). Although selective autophagic degradation of mitochondria termed “mitophagy” seems to be beneficial to eliminate damaged mitochondria from accumulated reactive oxygen species (ROS) in active BAT, there are some conflicting results in the literature regarding the importance of this process during the activation of BAT by cold exposure (84, 104–107). For example, Cairó et al. (107) reported that cold-induced thermogenic activation of BAT in mice was linked with autophagy repression, in part by upregulating cAMP-PKA pathway in NE-exposed brown adipocytes. Surprisingly, chronic cold exposure promoted autophagy/mitophagy in primary brown adipocytes and BAT from mice (105, 106). Yau et al. (106) showed similar effect on the activation of autophagy in BAT from mice in response to cold exposure, and this was marked by increased mRNA expression of the autophagic genes, including, sequestosome 1 (Sqstm1), autophagy-related (Atg)-5, Atg7, and Ulk1. This effect promoted β-oxidation, mitochondrial turnover, and thermogenesis in BAT (106). These results further indicate that autophagic processes in BAT are tightly regulated in response to cold exposure, as increasingly discussed (12, 83, 84).
Another hypothesis implementing the role of mitophagy on BAT/beige adipose tissue plasticity also prevails, and it is linked to the process of adipose tissue whitening (13, 83, 108, 109). For example, thermoneutrality reacclimatization after chronic cold exposure or withdrawal of thermogenic stimuli activate autophagic response and promotes the transition of BAT/beige adipose tissue to white-like adipose tissue in mice (83). Obviously, there are some distinct differences between BAT and beige adipose tissue whitening, such as that the brown adipocytes “disappear” from WAT depots and acquire unilocular phenotype with loss of UCP1 expression while brown adipocytes display an increased lipid accumulation, followed by protein degradation, and loss of protein synthesis without disappearance (12). Alternatively, reversal of beige-to-white adipocytes is tightly coupled with autophagy-mediated mitochondrial clearance after withdrawal of the external cues in mice (83). Notably, beige adipocytes progressively lose their morphological structure and molecular characteristics to acquire white-like characteristics bypassing an intermediate precursor stage (83). Inversely, inhibition of autophagy by UCP1+ adipocyte-specific deletion of Atg5 or Atg12 maintained functional beige adipocytes even after stimuli withdrawal (83). More evidence showed that inhibition of Parkin-mediated mitophagy underlies the maintenance of beige adipocyte in mice (108). Interestingly, physiological conditions such as aging can promote autophagy, which result to the loss of BAT activity. Indeed, upregulation of autophagy in BAT of mice is consistent with age-dependent decline of BAT activity and reduced metabolic rate (109). However, inhibition of mitophagy by BAT-specific deletion of the Atg7 gene could improve insulin sensitivity and energy metabolism, as well as maintained higher mitochondrial content by suppressing mitochondrial clearance (109).
Beyond dysregulation of autophagy, inflammation is another instrumental process that may negatively influence BAT activity and compromise the metabolic rate of this tissue. Although the relationship between autophagy and inflammation is relatively complex, they both constitute a natural response to stress conditions. Apparently, elevated inflammatory status impairs brown adipocyte metabolic activity and promotes whitening (110). Kotzbeck et al. (13) showed that BAT whitening is coupled with a decreased mitochondrial content as whitened adipocytes become dysfunctional as they render low-grade inflammatory state that eventually leads to cell death. To further highlight the link between dysregulated autophagy and inflammation, it was demonstrated that activation of the NLRP3 inflammasome and increased expression of inflammatory markers, including F4/80, tumor necrosis factor-alpha (TNF-α), interleukin (IL)-1β, IL-10, IL-18, monocyte chemoattractant protein 1 (Mcp-1), and caspase-1 was linked with Atgl-deficiency in mice (13). Other studies showed that deletion of transcription factor nuclear factor erythroid-2, like-1 (Nfe2l1) induced ER stress, inflammation, mitochondrial dysfunction, insulin resistance, and whitening in BAT (111, 112). Altogether, mitophagic maintenance of the healthy network of mitochondria in BAT and beige adipose tissue is crucial for cell survival but requires a balanced remodeling system of mitochondrial biogenesis and degradation.
While the browning of WAT has long been a growing area of interest in obesity research, whitening of BAT as an obesity-related complication with metabolic and health implications has been receiving less attention. Latterly, there is cumulative emerging evidence on BAT whitening, and it demonstrates that this phenomenon is multifactorial in origin. Notably, it has long been established that risk factors such as diet, age, genetics, as well as some chemicals can negatively influence the health of the general population (113). Risk factors such as excessive intake of an unhealthy diet can directly contribute to adiposity or weight gain (114), whereas aging is an undeniable major consequence that has long been linked with deteriorated health (115). Although acknowledged to significantly affect metabolic health, precise pathological mechanisms involved in this process are not completely understood. Interestingly, experimental evidence indicates that factors such as diet, thermoneutrality, age, and genetics can induce the whitening of BAT, and exacerbate obesity (Table 1).
Table 1 Summary of evidence reporting on BAT/beige adipose tissue whitening-induced by diet, thermoneutrality, age, and genetics.
It is acknowledged that the consumption of a diet rich in fat and cholesterol, and sugar, is the most common cause of obesity and metabolic syndrome (130, 131). In fact, animal models are a good example of the impact of such a diet, with evidence indicating that feeding rats two individual components of a western-style diet 60% HFD and 55% high fructose diet can cause fat accumulation, drive a state of insulin resistance and other metabolic complications (132–134). Based on growing experimental evidence, exposing rodents to HFD-feeding can cause several pathological abnormalities that include adipocyte hyperplasia and hypertrophy (132). The latter is linked with the state of inflammation, whereby adipose tissue expansion results in the release of proangiogenic cytokines, such as leptin, adiponectin, VEGF, TNF-α, and transforming growth factor beta (TGF-β) angiopoietin; such evidence has been extensively reviewed elsewhere (135, 136). Amongst other cytokines, VEGF-A is a major proangiogenic factor that is commonly downregulated in obesity (137–140). In fact, adipose tissue vascularization regulates chronic inflammation and systemic insulin sensitivity (141). In obese subjects, adipose tissue displays capillary rarefaction and hypoxia, which are paralleled with the infiltration of macrophages and the release of inflammatory cytokines (137, 142, 143). Several lines of evidence showed that HFD and high-fat high-sugar diet induce BAT/beige adipocytes dysfunction and whitening through vascular rarefaction linked with the state of inflammation (116–121).
Although the whitening is less studied in beige adipose tissue compared to BAT, the whitening of beige adipocytes was observed in HFD-fed C57BL/6 mice, and it was marked by enlarged adipocyte size and reduced expression of UCP1 (117). In classical BAT, HFD-feeding progressively induced fat deposition in BAT, which resulted in the expansion and whitening of BAT in female CD-1 mice (64). Similarly, chronic HFD feeding in mice resulted in progressive BAT whitening, which was associated with low energy expenditure, and down-regulation gene expression of Bmp8b, Nrg4, Vegfa involved in regulating vascularization, as well as upregulation of inflammasome and endoplasmic reticulum stress (14). Furthermore, BAT from HFD-fed C57BL/6J mice displayed lipid accumulation and insulin resistance, which were accompanied by reduced glucose and triglyceride-derived fatty acids uptake (118). Of note, Ucp1 gene expression was unaltered, whereas the expression of PGC1α and protein kinase B (PKB) were suppressed, suggesting the impairment of mitochondrial biogenesis and insulin sensitivity (118). More insights on the mechanism showed that HFD-induced iBAT whitening in C57 BL/6 mice, which was accompanied by decreased expression of the genes promoting vascularization, thermogenesis, fatty acids oxidation including VEGF-A, Ucp1, β3-AR, Cidea, and carnitine palmitoyltransferase (CPT) (119). In the same study, they demonstrated that counteracting BAT whitening using PPARα agonists can help to ameliorate the complications associated with obesity (119). The combination of HFD and high-sugar diet impaired BAT function in Wistar rats (120). The evidence of whitening in these animals was marked by the increased adipocytes area and decreased expression of BAT markers, such as FGF21, PPARγ, SIRT1, and CPT1, as well as the genes involved in the insulin signaling including insulin receptor substrate 2 (IRS-2), and glucose transporter (GLUT)-4 (120).
Amid finding the connection between the pathogenesis of obesity and its risk factors, maternal nutrition has become a target to understand the development of obesity and beyond (144). Such nutrition has a significant contribution to the developmental origins of metabolic complications upon growth to adulthood (145). Although adipose tissue plays an utmost important role in newborns as a regulator of energy homeostasis and thermogenesis (146, 147), it is not clear how maternal nutrition affects offspring adipose tissue function. Recently, Savva et al. (121) investigated the impact of maternal HFD on adipose tissue programming in male and female C57Bl6/J mice offspring. Interestingly, only male offspring exhibited a whitening of BAT and impaired metabolic profile whereas female counterparts presented with enhanced thermogenesis and cell differentiation in BAT (121), which can be attributed to the presence of estrogen in females (148). On the other hand, the whitening of BAT in male offspring was accompanied by the upregulation of the genes involved in lipid metabolisms such as Cd36, Cpt1, Cidea, and Pparγ, as well as the downregulation of BAT markers including DIO2, UCP1, PGC1α and PRDM16 (121).
Ambient temperature has a strong impact on body metabolism and energy expenditure, which in turn affects the morphology and thermogenesis function of BAT. Although the thermoneutral condition of approximately 22°C is the standard living environmental condition for humans, it is known to cause BAT involution and adiposity in rodents (149). However, the effect of seasonal changes on BAT thermogenesis and plasticity is still not well understood in humans. Apparently during winter season, human subcutaneous WAT display increased mRNA expression of UCP1, PGC1α, and transmembrane protein 26 (TMEM26), along with other genes involved in energy utilization and lipolysis, such as adiponectin, acetyl CoA carboxylase (ACC), and HSL (150). However, this effect was suppressed by excessive lipid accumulation and inflammation in obesity (150). In healthy men, whole-body energy expenditure and cold-induced thermogenesis were assessed in both summer and winter, using fluorodeoxyglucose (FDG)-positron emission tomography (PET) combined with computed tomography (CT) (151). Cold-induced thermogenesis was increased in winter compared to summer in a BAT-dependent manner, suggesting that the metabolic activity of human BAT is maximal in winter (151). In terms of glucose metabolism, it was reported that winter swimmers displayed no BAT glucose uptake at a thermal comfort zone while winter swimmers have higher cold-induced thermogenesis than control subjects in young healthy men (152).
In mice, a thermoneutral zone of 30°C is used for thermally humanizing mice BAT, which shows a remarkable resemblance to human BAT (153, 154). It has been reported that thermoneutral housing of mice in conjunction with or without a high-calorie diet, strongly reduces metabolic capacity and increases lipid accumulation in BAT, leading to a “white-like” appearance (13, 122, 123). Indeed, A/J mice housed at 30°C with HFD displayed paler and larger brown or beige adipocytes (122). This was accompanied by reduced SNS and thermogenic program which were evident by decreased tyrosine hydroxylase and norepinephrine turnover, as well as the decreased mRNA expression of UCP1, PGC1α, DIO2, elongation of very long chain fatty acids protein 3 (Elovl3) and cyclooxygenase (Cox)-1 (122). Other evidence showed that BAT whitening can be linked to the recruitment of immune cells involved in pro-inflammation and mitophagy.
An ambient temperature of 28 showed that interscapular and mediastinal BAT from C57Bl/6j mice acquired a white-like unilocular adipocyte phenotype, which involved increased macrophage infiltration, formation of crown-like structures, and degenerating mitochondria, marked by adipose triglyceride lipase (Atgl)-deficiency (13). A study by Sass et al. (123), showed that thermoneutral adaptation at 30 induced BAT whitening which was characterized by increased unilocular adipocytes and mitochondrial degradation in Wild-type mice. This was also accompanied by the decreased gene expression of thermogenic markers, including UCP1, PGC1α, cytochrome c oxidase subunit 4 isoform 1 (Cox4i1), cytochrome c oxidase subunit 7A (Cox7a), and cytochrome c oxidase subunit 8B (Cox8b), while the expression levels of the autophagy-regulating transcription factor EB (TFEB) was continuously increased (123). Moreover, the inhibition of autophagy reversed the whitening in BAT (123). This agrees with the previous evidence indicating that suppression of brown adipocyte autophagy improves energy metabolism in part by regulating mitochondrial turnover in mice (109).
Aging has long been implicated in adipose tissue dysfunction and increased risk of obesity (155). A considerable decline in BAT and beige adipose tissue with advancing years and increasing body fat percentage has been determined (156). Generally, aging is closely associated with low-grade systemic inflammation, and alterations in endocrine signals (157); mechanisms that are linked with BAT dysfunction upon aging (156, 158). Several mechanisms that might contribute to the age-related decline in BAT activity have been studied in animals and humans. For instance, Berry et al. (159) have demonstrated that mouse and human beige progenitor cells undergo an age-related senescence-like phenotype that accounts for age-dependent beiging failure; however, genetically or pharmacologically activation of p38/Ink4a-Arf pathway rejuvenated beige progenitors and restored beiging potential. Tajima et al. (160) identified mitochondria lipoylation as a molecular process underlying the age-related decline in BAT thermogenesis of mice, implying that a defect in mitochondrial lipoylation and fuel oxidation in BAT, leads to glucose intolerance and obesity upon aging. Conversely, α-lipoic acid supplementation enhanced mitochondrial lipoylation which in turn restored BAT function in aged mice (160).
A notable observation was made by the decline in BAT content and activity linked with the whitening during adiposity in rodents and rabbits (161). In female C57BL/6 mice aged (6-12 months old), BAT displayed a morphological change toward the fat storage phenotype with increased lipid droplet size and area (124). This was accompanied by the loss of clusters of beige adipocytes from subcutaneous and visceral WAT (124). To elucidate the underlying mechanism, a recent study by Pan et al. (125) reported an increase of unilocular lipid droplets and senescent T cells infiltration which induces BAT whitening via interferon (IFN)-γ secretion in 18-month-old and 3-month-old mice. Moreover, IFN-γ lead to the inhibition of brown pre-adipocyte differentiation which contributed to adipose tissue remodeling in aged mice (125). This was further verified using stromal vascular fraction cells isolated from BAT and T cells co-culture, which showed a reduction in UCP1, PPARγ, PGC1α, Plin1, and adiponectin gene expression (125). Although subcutaneous and visceral WAT supposedly comprised of beige adipocytes were enlarged, UCP1 was poorly detected in young mice and it was not detected in old mice, suggesting that there was a complete loss of thermogenic capacity upon aging. A switch from brown/beige- to white-like adipocytes was observed in FVB mice after 12- months of age (128). Moreover, the transcriptional profile of adipose-derived stromal cells mirrors these changes both at mRNA and microRNA transcriptional levels through E2F transcription factor 1 (E2F1) and nuclear factor kappa B (NFkβ) (128).
In 2 years old (aged) rabbits, BAT presented large unilocular lipid droplets with a dramatic decrease in transcriptional copy numbers of the mitochondrial genes, including cytochrome B, COX2, and NADH dehydrogenase 1 (126). Importantly, long non-coding RNAs (lncRNAs) were highly expressed in the BAT of aged rabbits. When assessed using in vitro model, these lncRNAs appeared to cause impairment in brown adipocyte differentiation. Presumably, lncRNAs suppressed the expression of brown adipocyte transcriptional factors, however, this requires further investigation (126). Recently, Huang et al. (127) have demonstrated that rabbit BAT involutes in a manner similar to humans with the analogous progenitor hierarchy. A progressively whitening with adipocyte hypertrophy and loss of UCP1 expression in the interscapular, subscapular, and suprascapular BAT depots of the rabbits was readily seen from 3 weeks of age and full conversion to WAT-like tissues at 12 weeks (127). This BAT whitening was associated with the restricted adipogenic capacity of follistatin-like 1 (Fstl1+) progenitors (127). Moreover, deletion of the Fstl1 gene or ablation of Fstl1+ progenitors in mice induced BAT paucity (127). This suggested that lncRNAs can be one of the molecular drivers of BAT whitening upon aging. However, this requires confirmation in human studies.
The concept of the genetic alteration as the cause of obesity has been progressively investigated over the past two decades (162). Based upon the years of discoveries, the genetic causes of obesity can be broadly classified into polygenic and monogenic (162). Specifically, polygenic obesity (or common obesity) is caused by multiple gene mutations or polymorphisms that promote weight gain (163). On the other hand, monogenic obesity which is inherited in a Mendelian pattern is typically rare and is characterized by early-onset, high severity, and a single gene mutation in the leptin-melanocortin pathway (162, 163). For these reasons, most people with obesity have certain mutated genes that predispose them to gain excess weight.
To gain a profound understanding of genetic obesity, animal models of obesity and type 2 diabetes such as leptin receptor-deficient db/db mice, and Zucker fatty fa/fa rats have been widely utilized (161). Over the past few years, these models have been also used to study the impact of genetic obesity on the morphology and function of BAT. However, the special focus herein is on BAT whitening. A study by Lapa et al. (129) showed that BAT from Zucker diabetic fatty fa/fa rats, displayed a ubiquitous white adipose-like tissue phenotype, with large unilocular lipid droplets and impaired glucose utilization at 14 weeks of age. This BAT involution was accompanied by the increased synthesis and accumulation of intracellular fatty acids, as well as the decreased expression of UCP1 (129). Although the underlying molecular mechanisms have not been elucidated so far, iBAT from db/db mice displays whitening and crown-like structure formation at 13 weeks of age (13).
In a non-obese model of T2D, which shares a susceptibility locus on chromosome 10 like in humans, lower cell density and higher adipocyte area were recorded in Goto-Kakizaki rats (120). Interestingly, glucose uptake in BAT was impaired in both baseline and even after 30 min of stimulation with 1 mg/kg CL316,243, a β3-adrenergic agonist (120). Subsequently, there was an increased expression of genes involved in fatty acid oxidation (CPT1 and CPT2), BAT metabolism (Sirt1 and PGC1α), but decreased gene expression of GLUT-1 compared to other experimental groups (120). Possibly, impairment of the β3-adrenergic response could suggest an increased expression of the above-mentioned genes, acting as a compensatory mechanism.
In parallel with other risk factors, chemicals such as endocrine-disrupting chemicals (EDCs) are known to significantly contribute to the high prevalence of obesity (164). These chemicals are found in a wide spectrum of consumer products, like tobacco, flame retardants, and pesticides which people are most likely to be exposed to in their daily life through ingestion, inhalation, or direct dermal contact (164). The potential targets for EDCs are the glucocorticoid and mineralocortcoid receptors, which are members of the steroid receptor subfamily that mediate the actions of glucocorticoids and mineralocorticoids, the main classes of corticosteroids (165). These chemicals can act directly on adipose tissue to induce hypertrophy and dysfunction of this tissue (166). Several lines of evidence have demonstrated that exposure to certain chemicals can negatively impact the phenotype and physiological functions of BAT and beige adipose tissue by inducing whitening (Table 2).
Table 2 Summary of evidence reporting on BAT/beige adipose tissue whitening induced by chemicals like bevacizumab, nicotine, dechlorane plus, serotonin and glucocorticoid.
Bevacizumab, a recombinant humanized anti-vascular endothelial growth factor (VEGF) antibody, is trailed in retinopathy of premature infants (175). Although anti-VEGF agents are the first-line treatment for various angiogenesis-related retinal diseases, it is not clear how the anti-VEGF antibody can accelerate the risks of systemic complications after intravitreal injection in premature infants (176). A study by Jo et al. (167) showed that intravitreally injection with anti-VEGF antibody (1 μg/eye) increases lipid droplet accumulation and induces the loss of vascular network in neonatal C57BL/6 mice. In addition to reduced VEGF levels, this was accompanied by the downregulation of mitochondria-related genes PGC1α and UCP1 (167). Since BAT is a highly vascularized tissue, it is evident that anti-VEGF agents interfere with BAT vascularization, which in turn induces BAT whitening and dysfunction.
Nicotine is a chemical that is widely found in tobacco, and it has been associated with many health problems (177). Accordingly, epidemiological studies have reported that maternal smoking during pregnancy might be a serious risk factor for childhood obesity (178). Of major concern, maternal nicotine exposure has become a growing risk factor for the health of the offspring and the origin of chronic diseases beyond infancy. For example, prenatal or lactation exposure to nicotine is associated with increased gonadal and inguinal subcutaneous WAT depots and dysfunction in offspring (179). However, the impact of nicotine on BAT structure and function is not well understood. Previously, it was reported that nicotine exposure in adult mice increases BAT thermogenesis and promotes weight loss via the inactivation of hypothalamic AMPK (180), and induces WAT browning through the hypothalamic κ opioid receptor (181). Paradoxically, maternal nicotine exposure induces BAT dysfunction and weight gain in both male offspring (168, 169).
In male rat offspring exposed to 1 mg/kg nicotine during pregnancy and lactation, BAT displayed a whitening phenotype characterized by lipid droplet accumulation and impaired mitochondrial structure (168). Moreover, the expression of BAT structure and function-related genes such as PRDM16, PGC1α, UCP1, and CPT2 was decreased. Similarly (169), female rat offspring exposed to 1 mg/kg nicotine during pregnancy and lactation presented white-like adipocytes, impaired angiogenesis, and abnormal mitochondrial structure in iBAT. This was accompanied by a down-regulation of brown-like genes PGC1α, UCP1, Prdm16, and Cidea, as well as a decrease in BAT secretion of pro-angiogenic factors including VEGF, VEGF receptor 2, hepatocyte growth factor (Hgf), neuropeptide (Npy), and resistin (169). This was further confirmed in vitro using C3H10T1/2 cells, showing a reduction of beige “brown-like” phenotype and angiogenesis, as well as brown-like gene expression of PGC1α and UCP1. Altogether, this evidence suggests that nicotine disrupts angiogenesis in the early development stage and impairs blood vessel formation to induce BAT whitening through downregulation of the PGC1α–UCP1 signals.
Dechlorane plus, an endocrine-disrupting chemical found in flame retardants, is a potential obesogen (182). Apparently, dechlorane plus can induce adiposity by promoting adipogenesis in cultured adipocytes via PPARγ independent mechanism (183). Consistently, Peshdary et al. (170) reported that 1000 μg/kg dechlorane plus induces a WAT-like phenotype and disrupts the function of BAT in part by downregulation of UCP1 mRNA expression in C57BL/6 mice. These outcomes are in line with UCP1 gene knockout in mice suggesting that loss of UCP1 could result in the whitening of BAT (184, 185). However, more research on the impact of dechlorane plus on BAT and beige adipose tissue function is warranted to better understand its influence on obesity and other related diseases.
Endogenous chemicals such as neurotransmitters like serotonin are known to regulate adipogenesis (186). A study by Rozenblit-Susan et al. (171) demonstrated that serotonin (10 μM) induces whitening in palmitic acid-exposed HIB1B adipocytes by shifting their metabolism to lipogenesis rather than lipid oxidation in part by, suppressing brown adipocytes differentiation and inducing beige adipocytes transdifferentiation into white adipocytes. Moreover, this was confirmed by the downregulation of brown adipocyte differentiation markers, such as Prdm16, Bmp7, and Pparγ (171). Consistently, expression of BAT markers such as UCP1 and FGF21, as well as pAMPK/AMPK ratio were downregulated while genes regulating lipogenesis fatty acid synthase (FASN), leptin, and adiponectin were upregulated (171). The observed effects of serotonin require further investigations in human adipose tissue, supposedly similar effects attained in humans could have major implications on obesity reducing WAT and increasing BAT activity.
The class of steroid hormones glucocorticoids and corticosterone are known to modulate glucose homeostasis in humans and rodents, respectively (187). Hypercortisolism caused by either endogenous overproduction of glucocorticoids or exogenous administration of glucocorticoids as anti-inflammatory medication can induce the development of obesity. A study by Gasparini et al. (172) reported that corticosterone (250 μg/day) induces intracellular lipid accumulation and reduces UCP1 expression in BAT of CD1 mice. Interestingly, no significant changes were observed in PGC1α, FASN, or acetyl-coa carboxylase alpha (Acaca) expression, implying that changes in lipid accumulation did not directly involve mitochondrial biogenesis, adipogenesis, or lipogenesis in BAT (172).
Several potent synthetic glucocorticoids including dexamethasone have been developed for pharmacological use (187). For example, dexamethasone has been implicated in the study of adipogenesis (188). Here, Deng et al. (173) showed that exposing C57BL/6 mice to 5 mg/kg dexamethasone for one week induced autophagy and lipid droplet expansion in iBAT. Similar outcomes were observed in vitro using brown adipocytes precursor cells. Notably, dexamethasone increased ATG7 expression, in part by increasing the expression of B cell translocation gene 1 (BTG1) that stimulates the activity of CREB1 (173). Consistently, UCP1 expression was downregulated, while the expression of WAT marker genes RB transcriptional corepressor 1 (Rb1), nuclear-receptor-interacting protein 1 (Nrip1), and Rbl1/p107 (RB transcriptional corepressor like 1) were upregulated (173). Other evidence showed that chronic exposure to corticosterone can induce the whitening of BAT in C57BL/6 mice, this was evident by increased adipocyte area and elevated expressions of UCP1 in BAT (174). Of note, the whitened phenotype has not been previously associated with increased uncoupling proteins under chronic stress, however, Bel et al. (174) suggested that the increased UCP1 expression could be a compensatory mechanism under certain stress.
Although the remodeling of BAT and beige adipose tissue through whitening appears to be more common in obesity, it remains unclear how this maladaptive process occurs. Several lines of evidence have demonstrated that the BAT/beige adipose tissue whitening is multifactorial in origin. Indeed, various factors such as diet, age, genetics, thermoneutrality, and chemical exposure have been shown to greatly influence the whitening of adipose tissue by targeting different mechanisms (Figure 4). Apart from these factors, chronic exposure to high levels of particulate matter, a complex mixture of solid and liquid particles derived from human activities and natural sources, also promotes the whitening of BAT, as reviewed by Guardia and Shin (110). Although the activity of BAT has been strongly linked with the protection against obesity, fatty liver, and T2D (189), the dysfunction or whitening of BAT in obesity, may contribute to or exacerbate other metabolic complications (190). For instance, recent evidence has demonstrated that severe hyperprolactinemia can also promote BAT whitening and aggravates HFD-induced metabolic dysregulations (191). This suggests that the whitening could represent one of the complications implicated in the pathogenesis of obesity, and it can lead to other secondary complications.
Figure 4 Summary of the proposed mechanisms by which multiple factors induce the whitening of brown (BAT) and beige adipose tissue. Factors such as high fat diet (HFD), bevacizumab, and nicotine induce the whitening by suppressing pro-angiogenic cytokines such as VEGF and VEGF-A, which inhibit angiogenesis and impair vascularization in BAT and beige adipose tissue, whereas thermoneutral zone and dexamethasone (Dex) exposure increase ATG7 and TFEB, which stimulate autophagy or mitophagy. Other factors such as aging, and elevated serotonin induce BAT/beige adipose tissue whitening by increasing INF-γ, and LncRNAs, and decreasing BMP-7, which in turn inhibits brown adipocyte differentiation. Moreover, dechlorane plus (DP) contributes to whitening in part by inhibiting UCP1 expression. ATG7, autophagy-related 7; LncRNAs, long non-coding RNAs; BMP-7, bone morphogenetic protein 7; BTG1, B cell translocation gene 1; IFN-γ, interferon-gamma; UCP1, uncoupling protein 1; TFEB, transcription factor EB; VEGF, vascular endothelial growth factor.
Of note, experimental evidence discussed in this review indicates that autophagy, inflammation, and impairment of angiogenesis “vascularization” are central processes implicated in BAT/beige adipose tissue whitening (83, 108, 167, 169). For example, the most prominent mechanisms implicated in adipose tissue whitening include the inhibition of VEGFs, PGC1α, and BMP-7 which impair vascularization, mitochondrial biogenesis, and brown adipocyte differentiation, respectively. This may lead to more sophisticated processes like infiltration of INF-γ secreting T cells, increased autophagy, and impaired substrate metabolism. Such evidence is in line with the finding from gene knockout models, which suggested that the whitening of BAT is under the control of β-AR (13), BMP (192), and mitochondrial transcription factor A (193), and other genes regulating BAT function (194–198), as well as miRNAs that regulate multiple processes including the differentiation and function of brown adipocytes (199, 200). Based on the current evidence, there are several potential marker genes that are involved in regulating BAT/beige adipose tissue whitening (Table 3). However, this requires further investigations in both non-clinical and clinical settings. Particular attention should be placed on identifying plausible therapeutic avenues to prevent or reverse adipose tissue whitening in obesity. This includes assessing and understanding the therapeutic effects of prominent agents like metformin in targeting the adipose tissue to manage obesity-associated complications (201).
KZ, PVD, and SM-M- concept and original draft. KZ and SXM performed the literature search, study selection, and data extraction. KZ, PD, SXHM, BBN, SEM, BUJ, TMN and SEM-M - manuscript writing and approval of the final draft. All authors contributed to the article and approved the submitted version.
This work was funded by the National Research Foundation (NRF) Support for NRF rated scientist 113674 to Sithandiwe E. Mazibuko-Mbeje. Funding from North-West University is also acknowledged. The work reported herein was made possible through funding by the South African Medical Research Council (SAMRC) through its Division of Research of Capacity Development under the Early Investigators Programme from the South African National Treasury (funding number: HDID8682/MB2022/EIP052). Baseline funding from the Northwest University is also acknowledged. The content hereof is the sole responsibility of the authors and do not necessarily represent the official views of the SAMRC. Also, all the content expressed in this review is the official views of the authors and do not represent that of the North-West University.
KZ and SXHM are funded by the SAMRC through its Division of Research Capacity Development under the internship scholarship programme from funding received from the South African National Treasury. Grant holders acknowledge that opinions, findings, and conclusions, or recommendations expressed in any publication generated by the SAMRC.
The authors declare that the research was conducted in the absence of any commercial or financial relationships that could be construed as a potential conflict of interest.
All claims expressed in this article are solely those of the authors and do not necessarily represent those of their affiliated organizations, or those of the publisher, the editors and the reviewers. Any product that may be evaluated in this article, or claim that may be made by its manufacturer, is not guaranteed or endorsed by the publisher.
1. Wang QA, Tao C, Gupta RK, Scherer PE. Tracking adipogenesis during white adipose tissue development, expansion and regeneration. Nat Med (2013) 19:1338–44. doi: 10.1038/nm.3324
2. Jakab J, Miškić B, Mikšić Š, Juranić B, Ćosić V, Schwarz D, et al. Adipogenesis as a potential anti-obesity target: A review of pharmacological treatment and natural products. Diabetes Metab Syndr Obes (2021) 14:67. doi: 10.2147/DMSO.S281186
3. Choe SS, Huh JY, Hwang IJ, Kim JI, Kim JB. Adipose tissue remodeling: Its role in energy metabolism and metabolic disorders. Front Endocrinol (Lausanne) (2016) 7:30. doi: 10.3389/fendo.2016.00030
4. Giralt M, Villarroya F. White, brown, beige/brite: Different adipose cells for different functions? Endocrinology (2013) 154:2992–3000. doi: 10.1210/en.2013-1403
5. Cedikova M, Kripnerová M, Dvorakova J, Pitule P, Grundmanova M, Babuska V, et al. Mitochondria in white, brown, and beige adipocytes. Stem Cells Int (2016) 2016:6067349. doi: 10.1155/2016/6067349
6. Kajimura S, Spiegelman BM, Seale P. Brown and beige fat: Physiological roles beyond heat-generation. Cell Metab (2015) 22:546. doi: 10.1016/J.CMET.2015.09.007
7. Cheng L, Wang J, Dai H, Duan Y, An Y, Shi L, et al. Brown and beige adipose tissue: a novel therapeutic strategy for obesity and type 2 diabetes mellitus. Adipocyte (2021) 10:48–65. doi: 10.1080/21623945.2020.1870060
8. Lee YH, Kim SN, Kwon HJ, Granneman JG. Metabolic heterogeneity of activated beige/brite adipocytes in inguinal adipose tissue. Sci Rep (2017) 7:39794. doi: 10.1038/SREP39794
9. Keipert S, Jastroch M. Brite/beige fat and UCP1 - is it thermogenesis? Biochim Biophys Acta (2014) 1837:1075–82. doi: 10.1016/J.BBABIO.2014.02.008
10. Bagchi M, Kim LA, Boucher J, Walshe TE, Kahn CR, D’Amore PA. Vascular endothelial growth factor is important for brown adipose tissue development and maintenance. FASEB J (2013) 27:3257–71. doi: 10.1096/FJ.12-221812
11. Shimizu I, Aprahamian T, Kikuchi R, Shimizu A, Papanicolaou KN, Maclauchlan S, et al. Vascular rarefaction mediates whitening of brown fat in obesity. J Clin Invest (2014) 124(5):2099–112. doi: 10.1172/JCI71643DS1
12. Cairó M, Villarroya J. The role of autophagy in brown and beige adipose tissue plasticity. J Physiol Biochem (2020) 76:213–26. doi: 10.1007/S13105-019-00708-1/FIGURES/4
13. Kotzbeck P, Giordano A, Mondini E, Murano I, Severi I, Venema W, et al. Brown adipose tissue whitening leads to brown adipocyte death and adipose tissue inflammation. J Lipid Res (2018) 59:784–94. doi: 10.1194/JLR.M079665
14. Rangel-Azevedo C, Santana-Oliveira DA, Miranda CS, Martins FF, Mandarim-de-Lacerda CA, Souza-Mello V. Progressive brown adipocyte dysfunction: Whitening and impaired nonshivering thermogenesis as long-term obesity complications. J Nutr Biochem (2022) 105:109002. doi: 10.1016/J.JNUTBIO.2022.109002
15. Agha M, Agha R. The rising prevalence of obesity: part a: impact on public health. Int J Surg Oncol (N Y) (2017) 2:17. doi: 10.1097/ij9.0000000000000017
16. Chernukha IM, Fedulova L v., Kotenkova EA. White, beige and brown adipose tissue: structure, function, specific features and possibility formation and divergence in pigs. Foods Raw Materials (2022) 10:10–8. doi: 10.21603/2308-4057-2022-1-10-18
17. Sidossis L, Kajimura S. Brown and beige fat in humans: thermogenic adipocytes that control energy and glucose homeostasis. J Clin Invest (2015) 125:478–86. doi: 10.1172/JCI78362
18. Ikeda K, Maretich P, Kajimura S. The common and distinct features of brown and beige adipocytes. Trends Endocrinol Metab (2018) 29:191. doi: 10.1016/J.TEM.2018.01.001
19. Zhang F, Hao G, Shao M, Nham K, An Y, Wang Q, et al. An adipose tissue atlas: An image-guided identification of human-like BAT and beige depots in rodents. Cell Metab (2018) 27:252–62.e3. doi: 10.1016/J.CMET.2017.12.004
21. González-García I, Urisarri A, Nogueiras R, Diéguez C, Couce ML, López M. An updated view on human neonatal thermogenesis. Nat Rev Endocrinol (2022) 18(5):263–4. doi: 10.1038/s41574-022-00642-1
22. Urisarri A, González-García I, Estévez-Salguero Á, Pata MP, Milbank E, López N, et al. BMP8 and activated brown adipose tissue in human newborns. Nat Commun (2021) 12:1–13. doi: 10.1038/s41467-021-25456-z
23. Virtanen KA. The rediscovery of BAT in adult humans using imaging. Best Pract Res Clin Endocrinol Metab (2016) 30:471–7. doi: 10.1016/j.beem.2016.09.001
24. Ahmad B, Vohra MS, Saleemi MA, Serpell CJ, Fong IL, Wong EH. Brown/Beige adipose tissues and the emerging role of their secretory factors in improving metabolic health: The batokines. Biochimie (2021) 184:26–39. doi: 10.1016/J.BIOCHI.2021.01.015
25. Ziqubu K, Dludla PV, Moetlediwa MT, Nyawo TA, Pheiffer C, Jack BU, et al. Disease progression promotes changes in adipose tissue signatures in type 2 diabetic (db/db) mice: The potential pathophysiological role of batokines. Life Sci (2023) 313:121273. doi: 10.1016/J.LFS.2022.121273
26. Singh R, Barrios A, Dirakvand G, Pervin S. Human brown adipose tissue and metabolic health: Potential for therapeutic avenues. Cells (2021) 10(11):3030. doi: 10.3390/CELLS10113030
27. Carey AL, Kingwell BA. Brown adipose tissue in humans: Therapeutic potential to combat obesity. Pharmacol Ther (2013) 140:26–33. doi: 10.1016/J.PHARMTHERA.2013.05.009
28. Wang CH, Wei YH. Therapeutic perspectives of thermogenic adipocytes in obesity and related complications. Int J Mol Sci (2021) 22(13):7177. doi: 10.3390/ijms22137177
29. Pilkington AC, Paz HA, Wankhade UD. Beige adipose tissue identification and marker specificity–overview. Front Endocrinol (Lausanne) (2021) 12:599134/BIBTEX. doi: 10.3389/FENDO.2021.599134/BIBTEX
30. Carobbio S, Rosen B, Vidal-Puig A. Adipogenesis: New insights into brown adipose tissue differentiation. J Mol Endocrinol (2013) 51(3):T75–85. doi: 10.1530/JME-13-0158
31. Merrick D, Sakers A, Irgebay Z, Okada C, Calvert C, Morley MP, et al. Identification of a mesenchymal progenitor cell hierarchy in adipose tissue. Science (2019) 364(6438):eaav2501. doi: 10.1126/SCIENCE.AAV2501
32. Ladoux A, Peraldi P, Chignon-Sicard B, Dani C. Distinct shades of adipocytes control the metabolic roles of adipose tissues: From their origins to their relevance for medical applications. Biomedicines (2021) 9:1–17. doi: 10.3390/BIOMEDICINES9010040
33. Duerre DJ, Galmozzi A. Deconstructing adipose tissue heterogeneity one cell at a time. Front Endocrinol (Lausanne) (2022) 13:847291. doi: 10.3389/FENDO.2022.847291
34. Russell AP, Crisan M, Léger B, Corselli M, McAinch AJ, O’Brien PE, et al. Brown adipocyte progenitor population is modified in obese and diabetic skeletal muscle. Int J Obes (2012) 36(1):155–8. doi: 10.1038/ijo.2011.85
35. Crisan M, Casteilla L, Lehr L, Carmona M, Paoloni-Giacobino A, Yap S, et al. A reservoir of brown adipocyte progenitors in human skeletal muscle. Stem Cells (2008) 26:2425–33. doi: 10.1634/STEMCELLS.2008-0325
36. Carobbio S, Guénantin AC, Samuelson I, Bahri M, Vidal-Puig A. Brown and beige fat: From molecules to physiology and pathophysiology. Biochim Biophys Acta Mol Cell Biol Lipids (2019) 1864:37–50. doi: 10.1016/J.BBALIP.2018.05.013
37. Van Nguyen TT, Vu VV, Pham PV. Transcriptional factors of thermogenic adipocyte development and generation of brown and beige adipocytes from stem cells. Stem Cell Rev Rep (2020) 16:876–92. doi: 10.1007/S12015-020-10013-W
38. Sanchez-Gurmaches J, Guertin DA. Adipocytes arise from multiple lineages that are heterogeneously and dynamically distributed. Nat Commun (2014) 5:4099. doi: 10.1038/NCOMMS5099
39. Wu J, Boström P, Sparks LM, Ye L, Choi JH, Giang AH, et al. Beige adipocytes are a distinct type of thermogenic fat cell in mouse and human. Cell (2012) 150:366–76. doi: 10.1016/j.cell.2012.05.016
40. Oguri Y, Shinoda K, Kim H, Alba DL, Bolus WR, Wang Q, et al. CD81 controls beige fat progenitor cell growth and energy balance via FAK signaling. Cell (2020) 182:563–77.e20. doi: 10.1016/J.CELL.2020.06.021
41. Joshi J, Beaudoin GAW, Patterson JA, García-García JD, Belisle CE, Chang LY, et al. Thermogenic adipocytes: lineage, function and therapeutic potential. Biochem J (2020) 477:2055–69. doi: 10.1042/BCJ20200298
42. Chen Y, Ikeda K, Yoneshiro T, Scaramozza A, Tajima K, Wang Q, et al. Thermal stress induces glycolytic beige fat formation via a myogenic state. Nature (2019) 565:180–5. doi: 10.1038/S41586-018-0801-Z
43. Whittle A, Relat-Pardo J, Vidal-Puig A. Pharmacological strategies for targeting BAT thermogenesis. Trends Pharmacol Sci (2013) 34:347–55. doi: 10.1016/j.tips.2013.04.004
44. Cinti S. Adipocyte differentiation and transdifferentiation: Plasticity of the adipose organ. J Endocrinol Invest (2002) 25:823–35. doi: 10.1007/BF03344046
45. Shimizu I, Walsh K. The whitening of brown fat and its implications for weight management in obesity. Curr Obes Rep (2015) 4:224–9. doi: 10.1007/s13679-015-0157-8
46. Sim CK, Kim SY, Brunmeir R, Zhang Q, Li H, Dharmasegaran D, et al. Regulation of white and brown adipocyte differentiation by RhoGAP DLC1. PloS One (2017) 12(3):e0174761. doi: 10.1371/journal.pone.0174761
47. Pradhan RN, Bues JJ, Gardeux V, Schwalie PC, Alpern D, Chen W, et al. Dissecting the brown adipogenic regulatory network using integrative genomics. Sci Rep (2017) 7:42130. doi: 10.1038/srep42130
48. Mae J, Nagaya K, Okamatsu-Ogura Y, Tsubota A, Matsuoka S, Nio-Kobayashi J, et al. Adipocytes and stromal cells regulate brown adipogenesis through secretory factors during the postnatal white-to-Brown conversion of adipose tissue in Syrian hamsters. Front Cell Dev Biol (2021) 9:698692. doi: 10.3389/fcell.2021.698692
49. Schulz TJ, Huang P, Huang TL, Xue R, McDougall LE, Townsend KL, et al. Brown-fat paucity due to impaired BMP signalling induces compensatory browning of white fat. Nature (2013) 495(7441):379–83. doi: 10.1038/nature11943
50. Tseng YH, Kokkotou E, Schulz TJ, Huang TL, Winnay JN, Taniguchi CM, et al. New role of bone morphogenetic protein 7 in brown adipogenesis and energy expenditure. Nature (2008) 454(7207):1000–4. doi: 10.1038/nature07221
51. Townsend KL, Pritchard E, Coburn JM, Kwon YM, Blaszkiewicz M, Lynes MD, et al. Silk hydrogel-mediated delivery of bone morphogenetic protein 7 directly to subcutaneous white adipose tissue increases browning and energy expenditure. Front Bioeng Biotechnol (2022) 10:884601. doi: 10.3389/FBIOE.2022.884601
52. Shaw A, Tóth BB, Arianti R, Csomós I, Póliska S, Vámos A, et al. BMP7 increases UCP1-dependent and independent thermogenesis with a unique gene expression program in human neck area derived adipocytes. Pharm (Basel) (2021) 14(11):1078. doi: 10.3390/PH14111078
53. Jiang N, Yang M, Han Y, Zhao H, Sun L. PRDM16 regulating adipocyte transformation and thermogenesis: A promising therapeutic target for obesity and diabetes. Front Pharmacol (2022) 13:870250/BIBTEX. doi: 10.3389/FPHAR.2022.870250/BIBTEX
54. Seale P, Bjork B, Yang W, Kajimura S, Chin S, Kuang S, et al. PRDM16 controls a brown fat/skeletal muscle switch. Nature (2008) 454:961–7. doi: 10.1038/NATURE07182
55. Harms MJ, Ishibashi J, Wang W, Lim HW, Goyama S, Sato T, et al. Prdm16 is required for the maintenance of brown adipocyte identity and function in adult mice. Cell Metab (2014) 19:593–604. doi: 10.1016/j.cmet.2014.03.007
56. Cristancho AG, Lazar MA. Forming functional fat: a growing understanding of adipocyte differentiation. Nat Rev Mol Cell Biol (2011) 12:722–34. doi: 10.1038/NRM3198
57. Lefterova MI, Zhang Y, Steger DJ, Schupp M, Schug J, Cristancho A, et al. PPARgamma and C/EBP factors orchestrate adipocyte biology via adjacent binding on a genome-wide scale. Genes Dev (2008) 22:2941–52. doi: 10.1101/GAD.1709008
58. Pu Y, Veiga-Lopez A. PPARγ agonist through the terminal differentiation phase is essential for adipogenic differentiation of fetal ovine preadipocytes. Cell Mol Biol Lett (2017) 22:6. doi: 10.1186/S11658-017-0037-1
59. Rocha AL, Guerra BA, Boucher J, Mori MA. A method to induce Brown/Beige adipocyte differentiation from murine preadipocytes. Bio Protoc (2021) 11(24):e4265. doi: 10.21769/BIOPROTOC.4265
60. Sun W, Dong H, Balaz M, Slyper M, Drokhlyansky E, Colleluori G, et al. snRNA-seq reveals a subpopulation of adipocytes that regulates thermogenesis. Nature (2020) 587:98–102. doi: 10.1038/S41586-020-2856-X
61. Yoneshiro T, Saito M. Activation and recruitment of brown adipose tissue as anti-obesity regimens in humans. Ann Med (2015) 47:133–41. doi: 10.3109/07853890.2014.911595
62. Kuryłowicz A, Puzianowska-Kuźnicka M. Induction of adipose tissue browning as a strategy to combat obesity. Int J Mol Sci (2020) 21:1–28. doi: 10.3390/IJMS21176241
63. Payab M, Abedi M, Foroughi Heravani N, Hadavandkhani M, Arabi M, Tayanloo-Beik A, et al. Brown adipose tissue transplantation as a novel alternative to obesity treatment: a systematic review. Int J Obes (2020) 45(1):109–21. doi: 10.1038/s41366-020-0616-5
64. Song NJ, Chang SH, Li DY, Villanueva CJ, Park KW. Induction of thermogenic adipocytes: Molecular targets and thermogenic small molecules. Exp Mol Med (2017) 49(7):e353. doi: 10.1038/emm.2017.70
65. Chouchani ET, Kazak L, Spiegelman BM. New advances in adaptive thermogenesis: UCP1 and beyond. Cell Metab (2019) 29:27–37. doi: 10.1016/J.CMET.2018.11.002
66. Mottillo EP, Ramseyer VD, Granneman JG. SERCA2b cycles its way to UCP1-independent thermogenesis in beige fat. Cell Metab (2018) 27:7–9. doi: 10.1016/J.CMET.2017.12.015
67. Ikeda K, Yamada T. UCP1 dependent and independent thermogenesis in brown and beige adipocytes. Front Endocrinol (Lausanne) (2020) 11:498. doi: 10.3389/FENDO.2020.00498
68. Okamatsu-Ogura Y, Kuroda M, Tsutsumi R, Tsubota A, Saito M, Kimura K, et al. UCP1-dependent and UCP1-independent metabolic changes induced by acute cold exposure in brown adipose tissue of mice. Metabolism (2020) 113:154396. doi: 10.1016/J.METABOL.2020.154396
69. Oeckl J, Janovska P, Adamcova K, Bardova K, Brunner S, Dieckmann S, et al. Loss of UCP1 function augments recruitment of futile lipid cycling for thermogenesis in murine brown fat. Mol Metab (2022) 61:101499. doi: 10.1016/J.MOLMET.2022.101499
70. Dludla PV, Nkambule BB, Tiano L, Louw J, Jastroch M, Mazibuko-Mbeje SE. Uncoupling proteins as a therapeutic target to protect the diabetic heart. Pharmacol Res (2018) 137:11–24. doi: 10.1016/j.phrs.2018.09.013
71. Ouellet V, Labbé SM, Blondin DP, Phoenix S, Guérin B, Haman F, et al. Brown adipose tissue oxidative metabolism contributes to energy expenditure during acute cold exposure in humans. J Clin Invest (2012) 122:545–52. doi: 10.1172/JCI60433
72. Zhang Z, Yang D, Xiang J, Zhou J, Cao H, Che Q, et al. Non-shivering thermogenesis signalling regulation and potential therapeutic applications of brown adipose tissue. Int J Biol Sci (2021) 17:2853–70. doi: 10.7150/IJBS.60354
73. Kim S-J, Tang T, Abbott M, Viscarra JA, Wang Y, Sul HS. AMPK phosphorylates Desnutrin/ATGL and hormone-sensitive lipase to regulate lipolysis and fatty acid oxidation within adipose tissue. Mol Cell Biol (2016) 36:1961. doi: 10.1128/MCB.00244-16
74. Pagnon J, Matzaris M, Stark R, Meex RCR, Macaulay SL, Brown W, et al. Identification and functional characterization of protein kinase a phosphorylation sites in the major lipolytic protein, adipose triglyceride lipase. Endocrinology (2012) 153:4278–89. doi: 10.1210/EN.2012-1127
75. Calderon-Dominguez M, Sebastián D, Fucho R, Weber M, Mir JF, García-Casarrubios E, et al. Carnitine palmitoyltransferase 1 increases lipolysis, UCP1 protein expression and mitochondrial activity in brown adipocytes. PloS One (2016) 11(7):e0159399. doi: 10.1371/JOURNAL.PONE.0159399
76. Ramage LE, Akyol M, Fletcher AM, Forsythe J, Nixon M, Carter RN, et al. Glucocorticoids acutely increase brown adipose tissue activity in humans, revealing species-specific differences in UCP-1 regulation. Cell Metab (2016) 24:130–41. doi: 10.1016/J.CMET.2016.06.011
77. Orava J, Nuutila P, Lidell ME, Oikonen V, Noponen T, Viljanen T, et al. Different metabolic responses of human brown adipose tissue to activation by cold and insulin. Cell Metab (2011) 14:272–9. doi: 10.1016/J.CMET.2011.06.012
78. Jung SM, Doxsey WG, Le J, Haley JA, Mazuecos L, Luciano AK, et al. In vivo isotope tracing reveals the versatility of glucose as a brown adipose tissue substrate. Cell Rep (2021) 36(4):109459. doi: 10.1016/J.CELREP.2021.109459
79. Tabuchi C, Sul HS. Signaling pathways regulating thermogenesis. Front Endocrinol (Lausanne) (2021) 12:595020/BIBTEX. doi: 10.3389/FENDO.2021.595020/BIBTEX
80. Jash S, Banerjee S, Lee MJ, Farmer SR, Puri V. CIDEA transcriptionally regulates UCP1 for britening and thermogenesis in human fat cells. IScience (2019) 20:73–89. doi: 10.1016/J.ISCI.2019.09.011
81. Ferhat M, Funai K, Boudina S. Autophagy in adipose tissue physiology and pathophysiology. Antioxid Redox Signal (2019) 31:487–501. doi: 10.1089/ARS.2018.7626
82. Desjardins EM, Steinberg GR. Emerging role of AMPK in brown and beige adipose tissue (BAT): Implications for obesity, insulin resistance, and type 2 diabetes. Curr Diabetes Rep (2018) 18(10):80. doi: 10.1007/S11892-018-1049-6
83. Altshuler-Keylin S, Shinoda K, Hasegawa Y, Ikeda K, Hong H, Kang Q, et al. Beige adipocyte maintenance is regulated by autophagy-induced mitochondrial clearance. Cell Metab (2016) 24:402–19. doi: 10.1016/J.CMET.2016.08.002
84. Cairó M, Campderrós L, Gavaldà-Navarro A, Cereijo R, Delgado-Anglés A, Quesada-López T, et al. Parkin controls brown adipose tissue plasticity in response to adaptive thermogenesis. EMBO Rep (2019) 20:e46832. doi: 10.15252/EMBR.201846832
85. Mottillo EP, Desjardins EM, Crane JD, Smith BK, Green AE, Ducommun S, et al. Lack of adipocyte AMPK exacerbates insulin resistance and hepatic steatosis through brown and beige adipose tissue function. Cell Metab (2016) 24:118–29. doi: 10.1016/J.CMET.2016.06.006
86. López M. Hypothalamic AMPK as a possible target for energy balance-related diseases. Trends Pharmacol Sci (2022) 43:546–56. doi: 10.1016/J.TIPS.2022.04.007
87. Saito M, Matsushita M, Yoneshiro T, Okamatsu-Ogura Y. Brown adipose tissue, diet-induced thermogenesis, and thermogenic food ingredients: From mice to men. Front Endocrinol (Lausanne) (2020) 11:222. doi: 10.3389/fendo.2020.00222
88. Zhang X, Li X, Fang H, Guo F, Li F, Chen A, et al. Flavonoids as inducers of white adipose tissue browning and thermogenesis: Signalling pathways and molecular triggers. Nutr Metab (Lond) (2019) 16:47. doi: 10.1186/s12986-019-0370-7
89. Ho KKY. Diet-induced thermogenesis: Fake friend or foe? J Endocrinol (2018) 238:R185–91. doi: 10.1530/JOE-18-0240
90. Ruan HB, Dietrich MO, Liu ZW, Zimmer MR, Li MD, Singh JP, et al. O-GlcNAc transferase enables AgRP neurons to suppress browning of white fat. Cell (2014) 159:306–17. doi: 10.1016/J.CELL.2014.09.010
91. Dodd GT, Decherf S, Loh K, Simonds SE, Wiede F, Balland E, et al. Leptin and insulin act on POMC neurons to promote the browning of white fat. Cell (2015) 160:88–104. doi: 10.1016/J.CELL.2014.12.022
92. Contreras C, González-García I, Seoane-Collazo P, Martínez-Sánchez N, Liñares-Pose L, Rial-Pensado E, et al. Reduction of hypothalamic endoplasmic reticulum stress activates browning of white fat and ameliorates obesity. Diabetes (2017) 66:87–99. doi: 10.2337/DB15-1547
93. Xu F, Lin B, Zheng X, Chen Z, Cao H, Xu H, et al. GLP-1 receptor agonist promotes brown remodelling in mouse white adipose tissue through SIRT1. Diabetologia (2016) 59:1059–69. doi: 10.1007/S00125-016-3896-5
94. Beiroa D, Imbernon M, Gallego R, Senra A, Herranz D, Villarroya F, et al. GLP-1 agonism stimulates brown adipose tissue thermogenesis and browning through hypothalamic AMPK. Diabetes (2014) 63:3346–58. doi: 10.2337/DB14-0302
95. Solmonson A, Mills EM. Uncoupling proteins and the molecular mechanisms of thyroid thermogenesis. Endocrinology (2016) 157:455–62. doi: 10.1210/EN.2015-1803
96. López M, Varela L, Vázquez MJ, Rodríguez-Cuenca S, González CR, Velagapudi VR, et al. Hypothalamic AMPK and fatty acid metabolism mediate thyroid regulation of energy balance. Nat Med (2010) 16:1001–8. doi: 10.1038/NM.2207
97. Contreras C, González-García I, Martínez-Sánchez N, Seoane-Collazo P, Jacas J, Morgan DA, et al. Central ceramide-induced hypothalamic lipotoxicity and ER stress regulate energy balance. Cell Rep (2014) 9:366–77. doi: 10.1016/J.CELREP.2014.08.057
98. Martínez-Sánchez N, Seoane-Collazo P, Contreras C, Varela L, Villarroya J, Rial-Pensado E, et al. Hypothalamic AMPK-ER stress-JNK1 axis mediates the central actions of thyroid hormones on energy balance. Cell Metab (2017) 26:212–29.e12. doi: 10.1016/J.CMET.2017.06.014
99. Martínez-Sánchez N, Moreno-Navarrete JM, Contreras C, Rial-Pensado E, Fernø J, Nogueiras R, et al. Thyroid hormones induce browning of white fat. J Endocrinol (2017) 232:351–62. doi: 10.1530/JOE-16-0425
100. Martínez De Morentin PB, González-García I, Martins L, Lage R, Fernández-Mallo D, Martínez-Sánchez N, et al. Estradiol regulates brown adipose tissue thermogenesis via hypothalamic AMPK. Cell Metab (2014) 20:41–53. doi: 10.1016/J.CMET.2014.03.031
101. Levine B, Klionsky DJ. Development by self-digestion: molecular mechanisms and biological functions of autophagy. Dev Cell (2004) 6:463–77. doi: 10.1016/S1534-5807(04)00099-1
102. Mizushima N, Komatsu M. Autophagy: renovation of cells and tissues. Cell (2011) 147:728–41. doi: 10.1016/J.CELL.2011.10.026
103. Singh R, Kaushik S, Wang Y, Xiang Y, Novak I, Komatsu M, et al. Autophagy regulates lipid metabolism. Nature (2009) 458(7242):1131–5. doi: 10.1038/nature07976
104. Gospodarska E, Nowialis P, Kozak LP. Mitochondrial turnover: a phenotype distinguishing brown adipocytes from interscapular brown adipose tissue and white adipose tissue. J Biol Chem (2015) 290:8243–55. doi: 10.1074/JBC.M115.637785
105. Lu Y, Fujioka H, Joshi D, Li Q, Sangwung P, Hsieh P, et al. Mitophagy is required for brown adipose tissue mitochondrial homeostasis during cold challenge. Sci Rep (2018) 8(1):8251. doi: 10.1038/S41598-018-26394-5
106. Yau WW, Wong KA, Zhou J, Thimmukonda NK, Wu Y, Bay BH, et al. Chronic cold exposure induces autophagy to promote fatty acid oxidation, mitochondrial turnover, and thermogenesis in brown adipose tissue. IScience (2021) 24(5):102434. doi: 10.1016/J.ISCI.2021.102434
107. Cairó M, Villarroya J, Cereijo R, Campderrós L, Giralt M, Villarroya F. Thermogenic activation represses autophagy in brown adipose tissue. Int J Obes (2016) 40(10):1591–9. doi: 10.1038/ijo.2016.115
108. Lu X, Altshuler-Keylin S, Wang Q, Chen Y, Sponton CH, Ikeda K, et al. Mitophagy controls beige adipocyte maintenance through a parkin-dependent and UCP1-independent mechanism. Sci Signal (2018) 11(527):eaap8526. doi: 10.1126/SCISIGNAL.AAP8526
109. Kim D, Kim JH, Kang YH, Kim JS, Yun SC, Kang SW, et al. Suppression of brown adipocyte autophagy improves energy metabolism by regulating mitochondrial turnover. Int J Mol Sci (2019) 20(14):3520. doi: 10.3390/ijms20143520
110. della Guardia L, Shin AC. White and brown adipose tissue functionality is impaired by fine particulate matter (PM 2.5) exposure. J Mol Med (Berl) (2022) 100:665–76. doi: 10.1007/S00109-022-02183-6
111. Bartelt A, Widenmaier SB, Schlein C, Johann K, Goncalves RLS, Eguchi K, et al. Brown adipose tissue thermogenic adaptation requires Nrf1-mediated proteasomal activity. Nat Med (2018) 24(3):292–303. doi: 10.1038/nm.4481
112. Hou Y, Liu Z, Zuo Z, Gao T, Fu J, Wang H, et al. Adipocyte-specific deficiency of Nfe2l1 disrupts plasticity of white adipose tissues and metabolic homeostasis in mice. Biochem Biophys Res Commun (2018) 503:264–70. doi: 10.1016/J.BBRC.2018.06.013
113. Pillon NJ, Loos RJF, Marshall SM, Zierath JR. Metabolic consequences of obesity and type 2 diabetes: Balancing genes and environment for personalized care. Cell (2021) 184:1530–44. doi: 10.1016/j.cell.2021.02.012
114. Nascimento GG, Peres MA, Mittinty MN, Peres KG, Do LG, Horta BL, et al. Diet-induced overweight and obesity and periodontitis risk: An application of the parametric g-formula in the 1982 pelotas birth cohort. Am J Epidemiol (2017) 185:442–51. doi: 10.1093/aje/kww187
115. Fabbri E, Zoli M, Gonzalez-Freire M, Salive ME, Studenski SA, Ferrucci L. Aging and multimorbidity: New tasks, priorities, and frontiers for integrated gerontological and clinical research. J Am Med Dir Assoc (2015) 16:640–7. doi: 10.1016/J.JAMDA.2015.03.013
116. Gao M, Ma Y, Liu D. High-fat diet-induced adiposity, adipose inflammation, hepatic steatosis and hyperinsulinemia in outbred CD-1 mice. PloS One (2015) 10(3):e0119784. doi: 10.1371/journal.pone.0119784
117. Dobner J, Ress C, Rufinatscha K, Salzmann K, Salvenmoser W, Folie S, et al. Fat-enriched rather than high-fructose diets promote whitening of adipose tissue in a sex-dependent manner. J Nutr Biochem (2017) 49:22–9. doi: 10.1016/j.jnutbio.2017.07.009
118. Kuipers EN, Held NM, In het Panhuis W, Modder M, Ruppert PMM, Kersten S, et al. A single day of high-fat diet feeding induces lipid accumulation and insulin resistance in brown adipose tissue in mice. Am J Physiol Endocrinol Metab (2019) 317:E820–30. doi: 10.1152/ajpendo.00123.2019
119. Miranda CS, Silva-Veiga F, Martins FF, Rachid TL, Mandarim-De-Lacerda CA, Souza-Mello V. PPAR-α activation counters brown adipose tissue whitening: a comparative study between high-fat– and high-fructose–fed mice. Nutrition (2020) 78:110791. doi: 10.1016/j.nut.2020.110791
120. Serdan TDA, Masi LN, Pereira JNB, Rodrigues LE, Alecrim AL, Scervino MVM, et al. Impaired brown adipose tissue is differentially modulated in insulin-resistant obese wistar and type 2 diabetic goto-kakizaki rats. Biomedicine Pharmacotherapy (2021) 142:112019. doi: 10.1016/j.biopha.2021.112019
121. Savva C, Helguero LA, González-Granillo M, Melo T, Couto D, Buyandelger B, et al. Maternal high-fat diet programs white and brown adipose tissue lipidome and transcriptome in offspring in a sex- and tissue-dependent manner in mice. Int J Obes (2022) 46:831–42. doi: 10.1038/s41366-021-01060-5
122. Cui X, Nguyen NLT, Zarebidaki E, Cao Q, Li F, Zha L, et al. Thermoneutrality decreases thermogenic program and promotes adiposity in high-fat diet-fed mice. Physiol Rep (2016) 4(10):e12799. doi: 10.14814/phy2.12799
123. Sass F, Schlein C, Jaeckstein MY, Pertzborn P, Schweizer M, Schinke T, et al. TFEB deficiency attenuates mitochondrial degradation upon brown adipose tissue whitening at thermoneutrality. Mol Metab (2021) 47:101173. doi: 10.1016/j.molmet.2021.101173
124. Gonçalves LF, Machado TQ, Castro-Pinheiro C, de Souza NG, Oliveira KJ, Fernandes-Santos C. Ageing is associated with brown adipose tissue remodelling and loss of white fat browning in female C57BL/6 mice. Int J Exp Pathol (2017) 98:100–8. doi: 10.1111/IEP.12228
125. Pan XX, Yao KL, Yang YF, Ge Q, Zhang R, Gao PJ, et al. Senescent T cell induces brown adipose tissue “Whitening” via secreting IFN-γ. Front Cell Dev Biol (2021) 9:637424. doi: 10.3389/fcell.2021.637424
126. Du K, Bai X, Yang L, Shi Y, Chen L, Wang H, et al. De novo reconstruction of transcriptome identified long non-coding RNA regulator of aging-related brown adipose tissue whitening in rabbits. Biol (Basel) (2021) 10(11):1176. doi: 10.3390/biology10111176
127. Huang Z, Zhang Z, Moazzami Z, Heck R, Hu P, Nanda H, et al. Brown adipose tissue involution associated with progressive restriction in progenitor competence. Cell Rep (2022) 39(2):110575. doi: 10.1016/J.CELREP.2022.110575
128. Scambi I, Peroni D, Nodari A, Merigo F, Benati D, Boschi F, et al. The transcriptional profile of adipose-derived stromal cells (ASC) mirrors the whitening of adipose tissue with age. Eur J Cell Biol (2022) 101(2):151206. doi: 10.1016/J.EJCB.2022.151206
129. Lapa C, Arias-Loza P, Hayakawa N, Wakabayashi H, Werner RA, Chen X, et al. Whitening and impaired glucose utilization of brown adipose tissue in a rat model of type 2 diabetes mellitus. Sci Rep (2017) 7(1):16795. doi: 10.1038/s41598-017-17148-w
130. Konda PY, Poondla V, Jaiswal KK, Dasari S, Uyyala R, Surtineni VP, et al. Pathophysiology of high fat diet induced obesity: impact of probiotic banana juice on obesity associated complications and hepatosteatosis. Sci Rep (2020) 10(1):1–17. doi: 10.1038/s41598-020-73670-4
131. Kayode OO. Diet and obesity. In: Psychology and pathophysiological outcomes of eating IntechOpen (2021). doi: 10.5772/INTECHOPEN.98326
132. de Moura e Dias M, dos Reis SA, da Conceição LL, de Oliveira Sediyama CMN, Pereira SS, de Oliveira LL, et al. Diet-induced obesity in animal models: points to consider and influence on metabolic markers. Diabetol Metab Syndr (2021) 13:1–14. doi: 10.1186/S13098-021-00647-2/TABLES/3
133. Lang P, Hasselwander S, Li H, Xia N. Effects of different diets used in diet-induced obesity models on insulin resistance and vascular dysfunction in C57BL/6 mice. Sci Rep (2019) 9(1):19556. doi: 10.1038/s41598-019-55987-x
134. Hariri N, Thibault L. High-fat diet-induced obesity in animal models. Nutr Res Rev (2010) 23:270–99. doi: 10.1017/S0954422410000168
135. Herold J, Kalucka J. Angiogenesis in adipose tissue: The interplay between adipose and endothelial cells. Front Physiol (2021) 11:624903. doi: 10.3389/FPHYS.2020.624903
136. Corvera S, Gealekman O. Adipose tissue angiogenesis: impact on obesity and type-2 diabetes. Biochim Biophys Acta (2014) 1842:463–72. doi: 10.1016/J.BBADIS.2013.06.003
137. Paavonsalo S, Hariharan S, Lackman MH, Karaman S. Capillary rarefaction in obesity and metabolic diseases–Organ-Specificity and possible mechanisms. Cells (2020) 9:2683. doi: 10.3390/CELLS9122683
138. Miranda M, Escoté X, Ceperuelo-Mallafré V, Megía A, Caubet E, Näf S, et al. Relation between human LPIN1, hypoxia and endoplasmic reticulum stress genes in subcutaneous and visceral adipose tissue. Int J Obes (Lond) (2010) 34:679–86. doi: 10.1038/IJO.2009.290
139. Elias I, Franckhauser S, Bosch F. New insights into adipose tissue VEGF-a actions in the control of obesity and insulin resistance. Adipocyte (2013) 2:109. doi: 10.4161/ADIP.22880
140. Pellegata NS, Berriel Diaz M, Rohm M, Herzig S. Obesity and cancer–extracellular matrix, angiogenesis, and adrenergic signaling as unusual suspects linking the two diseases. Cancer Metastasis Rev (2022) 41:517–47. doi: 10.1007/S10555-022-10058-Y
141. Ye J. Adipose tissue vascularization: its role in chronic inflammation. Curr Diabetes Rep (2011) 11:203–10. doi: 10.1007/S11892-011-0183-1
142. O’Rourke RW, White AE, Metcalf MD, Olivas AS, Mitra P, Larison WG, et al. Hypoxia-induced inflammatory cytokine secretion in human adipose tissue stromovascular cells. Diabetologia (2011) 54:1480. doi: 10.1007/S00125-011-2103-Y
143. Fujisaka S, Usui I, Ikutani M, Aminuddin A, Takikawa A, Tsuneyama K, et al. Adipose tissue hypoxia induces inflammatory M1 polarity of macrophages in an HIF-1α-dependent and HIF-1α-independent manner in obese mice. Diabetologia (2013) 56:1403–12. doi: 10.1007/S00125-013-2885-1/FIGURES/4
144. Parlee SD, MacDougald OA. Maternal nutrition and risk of obesity in offspring: The Trojan horse of developmental plasticity. Biochim Biophys Acta Mol Basis Dis (2014) 1842:495–506. doi: 10.1016/j.bbadis.2013.07.007
145. Sarker G, Litwan K, Kastli R, Peleg-Raibstein D. Maternal overnutrition during critical developmental periods leads to different health adversities in the offspring: relevance of obesity, addiction and schizophrenia. Sci Rep (2019) 9(1):17322. doi: 10.1038/s41598-019-53652-x
146. Lidell ME. Brown adipose tissue in human infants. Handb Exp Pharmacol (2019) 251:107–23. doi: 10.1007/164_2018_118
147. Quesada-López T, Gavaldà-Navarro A, Morón-Ros S, Campderrós L, Iglesias R, Giralt M, et al. GPR120 controls neonatal brown adipose tissue thermogenic induction. Am J Physiol Endocrinol Metab (2019) 317:742–50. doi: 10.1152/ajpendo.00081.2019.-Adaptive
148. González-García I, Contreras C, Estévez-Salguero Á, Ruíz-Pino F, Colsh B, Pensado I, et al. Estradiol regulates energy balance by ameliorating hypothalamic ceramide-induced ER stress. Cell Rep (2018) 25:413–23.e5. doi: 10.1016/J.CELREP.2018.09.038
149. Johnson F, Mavrogianni A, Ucci M, Vidal-Puig A, Wardle J. Could increased time spent in a thermal comfort zone contribute to population increases in obesity? Obes Rev (2011) 12:543–51. doi: 10.1111/j.1467-789X.2010.00851.x
150. Kern PA, Finlin BS, Zhu B, Rasouli N, McGehee RE, Westgate PM, et al. The effects of temperature and seasons on subcutaneous white adipose tissue in humans: evidence for thermogenic gene induction. J Clin Endocrinol Metab (2014) 99:E2772–9. doi: 10.1210/JC.2014-2440
151. Yoneshiro T, Matsushita M, Nakae S, Kameya T, Sugie H, Tanaka S, et al. Brown adipose tissue is involved in the seasonal variation of cold-induced thermogenesis in humans. Am J Physiol Regul Integr Comp Physiol (2016) 310:R999–1009. doi: 10.1152/AJPREGU.00057.2015
152. Søberg S, Löfgren J, Philipsen FE, Jensen M, Hansen AE, Ahrens E, et al. Altered brown fat thermoregulation and enhanced cold-induced thermogenesis in young, healthy, winter-swimming men. Cell Rep Med (2021) 2(10):100408. doi: 10.1016/J.XCRM.2021.100408
153. Fischer AW, Cannon B, Nedergaard J. Optimal housing temperatures for mice to mimic the thermal environment of humans: An experimental study. Mol Metab (2018) 7:161–70. doi: 10.1016/j.molmet.2017.10.009
154. Speakman JR, Keijer J. Not so hot: Optimal housing temperatures for mice to mimic the thermal environment of humans. Mol Metab (2013) 2:5–9. doi: 10.1016/j.molmet.2012.10.002
155. Mancuso P, Bouchard B. The impact of aging on adipose function and adipokine synthesis. Front Endocrinol (Lausanne) (2019) 10:137. doi: 10.3389/fendo.2019.00137
156. Zoico E, Rubele S, de Caro A, Nori N, Mazzali G, Fantin F, et al. Brown and beige adipose tissue and aging. Front Endocrinol (Lausanne) (2019) 10:368. doi: 10.3389/fendo.2019.00368
157. Mogilenko DA, Shchukina I, Artyomov MN. Immune ageing at single-cell resolution. Nat Rev Immunol (2021) 22(8):484–98. doi: 10.1038/s41577-021-00646-4
158. Bahler L, Verberne HJ, Admiraal WM, Stok WJ, Soeters MR, Hoekstra JB, et al. Differences in sympathetic nervous stimulation of brown adipose tissue between the young and old, and the lean and obese. J Nucl Med (2016) 57:372–7. doi: 10.2967/jnumed.115.165829
159. Berry DC, Jiang Y, Arpke RW, Close EL, Uchida A, Reading D, et al. Cellular aging contributes to failure of cold-induced beige adipocyte formation in old mice and humans. Cell Metab (2017) 25:166–81. doi: 10.1016/J.CMET.2016.10.023
160. Tajima K, Ikeda K, Chang HY, Chang CH, Yoneshiro T, Oguri Y, et al. Mitochondrial lipoylation integrates age-associated decline in brown fat thermogenesis. Nat Metab (2019) 1:886–98. doi: 10.1038/S42255-019-0106-Z
161. Lutz TA, Woods SC. Overview of animal models of obesity. Curr Protoc Pharmacol (2012) 58:5.61.1–5.61.18. doi: 10.1002/0471141755.ph0561s58
162. Loos RJF, Yeo GSH. The genetics of obesity: from discovery to biology. Nat Rev Genet (2022) 23:120–33. doi: 10.1038/s41576-021-00414-z
163. Thaker V v. Genetic and Epigenetic Causes of Obesity. n.d. Adolesc Med State Art Rev. (2017) 28(2):379–405.
164. Amato AA, Wheeler HB, Blumberg B. Obesity and endocrine-disrupting chemicals. Endocr Connect (2021) 10:R87–105. doi: 10.1530/EC-20-0578
165. Zhang J, Yang Y, Liu W, Schlenk D, Liu J. Glucocorticoid and mineralocorticoid receptors and corticosteroid homeostasis are potential targets for endocrine-disrupting chemicals. Environ Int (2019) 133(Pt A):105133. doi: 10.1016/j.envint.2019.105133
166. Pestana D, Teixeira D, Meireles M, Marques C, Norberto S, Sá C, et al. Adipose tissue dysfunction as a central mechanism leading to dysmetabolic obesity triggered by chronic exposure to p,p’-DDE. Sci Rep (2017) 7(1):2738. doi: 10.1038/s41598-017-02885-9
167. Jo DH, Park SW, Cho CS, Powner MB, Kim JH, Fruttiger M, et al. Intravitreally injected anti-VEGF antibody reduces brown fat in neonatal mice. PloS One (2015) 10(7):e0134308. doi: 10.1371/journal.pone.0134308
168. Fan J, Ping J, Zhang Wx, Rao Ys, Liu Hx, Zhang J, et al. Prenatal and lactation nicotine exposure affects morphology and function of brown adipose tissue in male rat offspring. Ultrastruct Pathol (2016) 40:288–95. doi: 10.1080/01913123.2016.1223243
169. Chen Hj, Li Gl, Zhang Wx, Fan J, Hu L, Zhang L, et al. Maternal nicotine exposure during pregnancy and lactation induces brown adipose tissue whitening in female offspring. Toxicol Appl Pharmacol (2020) 409:115298. doi: 10.1016/J.TAAP.2020.115298
170. Peshdary V, Styles G, Rigden M, Caldwell D, Kawata A, Sorisky A, et al. Exposure to low doses of dechlorane plus promotes adipose tissue dysfunction and glucose intolerance in male mice. Endocrinol (United States) (2021) 161:1–15. doi: 10.1210/ENDOCR/BQAA096
171. Rozenblit-Susan S, Chapnik N, Froy O. Serotonin prevents differentiation into brown adipocytes and induces transdifferentiation into white adipocytes. Int J Obes (2018) 42:704–10. doi: 10.1038/ijo.2017.261
172. Gasparini SJ, Swarbrick MM, Kim S, Thai LJ, Henneicke H, Cavanagh LL, et al. Androgens sensitise mice to glucocorticoid-induced insulin resistance and fat accumulation. Diabetologia (2019) 62:1463–77. doi: 10.1007/s00125-019-4887-0
173. Deng J, Guo Y, Yuan F, Chen S, Yin H, Jiang X, et al. Autophagy inhibition prevents glucocorticoid-increased adiposity via suppressing BAT whitening. Autophagy (2020) 16:451–65. doi: 10.1080/15548627.2019.1628537
174. Bel JS, Tai TC, Khaper N, Lees SJ. Chronic glucocorticoid exposure causes brown adipose tissue whitening, alters whole-body glucose metabolism and increases tissue uncoupling protein-1. Physiol Rep (2022) 10(9):e15292. doi: 10.14814/PHY2.15292
175. Jin H, Li D, Wang X, Jia J, Chen Y, Yao Y, et al. VEGF and VEGFB play balancing roles in adipose differentiation, gene expression, and function. Endocrinology (2018) 159:2036–49. doi: 10.1210/en.2017-03246
176. Zayek M, Parker K, Rydzewska M, Rifai A, Bhat R, Eyal F. Bevacizumab for retinopathy of prematurity: 2-year neurodevelopmental follow-up. Am J Perinatol (2021) 38:1158–66. doi: 10.1055/s-0040-1710556
177. Mishra A, Chaturvedi P, Datta S, Sinukumar S, Joshi P, Garg A. Harmful effects of nicotine. Indian J Med Paediatric Oncol (2015) 36:24–31. doi: 10.4103/0971-5851.151771
178. Riedel C, Schönberger K, Yang S, Koshy G, Chen YC, Gopinath B, et al. Parental smoking and childhood obesity: Higher effect estimates for maternal smoking in pregnancy compared with paternal smoking-a meta-analysis. Int J Epidemiol (2014) 43:1593–606. doi: 10.1093/ije/dyu150
179. Zhang Wx, Chen Hj, Fan J, Li Gl, Sun A, Lan L, et al. The association between maternal nicotine exposure and adipose angiogenesis in female rat offspring: A mechanism of adipose tissue function changes. Toxicol Lett (2020) 318:12–21. doi: 10.1016/J.TOXLET.2019.10.007
180. Martínez De Morentin PB, Whittle AJ, Fernø J, Nogueiras R, Diéguez C, Vidal-Puig A, et al. Nicotine induces negative energy balance through hypothalamic AMP-activated protein kinase. Diabetes (2012) 61:807–17. doi: 10.2337/DB11-1079
181. Seoane-Collazo P, Liñares-Pose L, Rial-Pensado E, Romero-Picó A, Moreno-Navarrete JM, Martínez-Sánchez N, et al. Central nicotine induces browning through hypothalamic κ opioid receptor. Nat Commun (2019) 10(1):4037. doi: 10.1038/S41467-019-12004-Z
182. Mohajer N, Du CY, Checkcinco C, Blumberg B. Obesogens: How they are identified and molecular mechanisms underlying their action. Front Endocrinol (Lausanne) (2021) 12:780888. doi: 10.3389/fendo.2021.780888
183. Peshdary V, Calzadilla G, Landry A, Sorisky A, Atlas E. Dechlorane plus increases adipogenesis in 3T3-L1 and human primary preadipocytes independent of peroxisome proliferator-activated receptor γ transcriptional activity. Int J Obes (2019) 43:545–55. doi: 10.1038/s41366-018-0072-7
184. Winn NC, Vieira-Potter VJ, Gastecki ML, Welly RJ, Scroggins RJ, Zidon TM, et al. Loss of UCP1 exacerbates Western diet-induced glycemic dysregulation independent of changes in body weight in female mice downloaded from. Am J Physiol Regul Integr Comp Physiol (2017) 312:74–84. doi: 10.1152/ajpregu.00425.2016.-We
185. Winn NC, Grunewald ZI, Gastecki ML, Woodford ML, Welly RJ, Clookey SL, et al. Deletion of UCP1 enhances ex vivo aortic vasomotor function in female but not male mice despite similar susceptibility to metabolic dysfunction. Am J Physiol Endocrinol Metab (2017) 313:E402–12. doi: 10.1152/AJPENDO.00096.2017
186. Oh CM, Namkung J, Go Y, Shong KE, Kim K, Kim H, et al. Regulation of systemic energy homeostasis by serotonin in adipose tissues. Nat Commun (2015) 6:6794. doi: 10.1038/ncomms7794
187. Luijten IHN, Cannon B, Nedergaard J. Glucocorticoids and brown adipose tissue: Do glucocorticoids really inhibit thermogenesis? Mol Aspects Med (2019) 68:42–59. doi: 10.1016/j.mam.2019.07.002
188. Zilberfarb V, Siquier K, Strosberg AD, Issad T. Effect of dexamethasone on adipocyte differentiation markers and tumour necrosis factor-alpha expression in human PAZ6 cells. Diabetologia (2001) 44:377–86. doi: 10.1007/S001250051630
189. Wibmer AG, Becher T, Eljalby M, Crane A, Andrieu PC, Jiang CS, et al. Brown adipose tissue is associated with healthier body fat distribution and metabolic benefits independent of regional adiposity. Cell Rep Med (2021) 2(7):100332. doi: 10.1016/j.xcrm.2021.100332
190. Alcalá M, Calderon-Dominguez M, Serra D, Herrero L, Viana M. Mechanisms of impaired brown adipose tissue recruitment in obesity. Front Physiol (2019) 10:94. doi: 10.3389/fphys.2019.00094
191. Lopez-Vicchi F, de Winne C, Ornstein AM, Sorianello E, Toneatto J, Becu-Villalobos D. Severe hyperprolactinemia promotes brown adipose tissue whitening and aggravates high fat diet induced metabolic imbalance. Front Endocrinol (Lausanne) (2022) 13:883092. doi: 10.3389/FENDO.2022.883092
192. Blázquez-Medela AM, Jumabay M, Rajbhandari P, Sallam T, Guo Y, Yao J, et al. Noggin depletion in adipocytes promotes obesity in mice. Mol Metab (2019) 25:50–63. doi: 10.1016/J.MOLMET.2019.04.004
193. Vernochet C, Damilano F, Mourier A, Bezy O, Mori MA, Smyth G, et al. Adipose tissue mitochondrial dysfunction triggers a lipodystrophic syndrome with insulin resistance, hepatosteatosis, and cardiovascular complications. FASEB J (2014) 28:4408–19. doi: 10.1096/FJ.14-253971
194. Cannavino J, Shao M, An YA, Bezprozvannaya S, Chen S, Kim J, et al. Regulation of cold-induced thermogenesis by the RNA binding protein FAM195A. Proc Natl Acad Sci U.S.A. (2021) 118(23):e2104650118. doi: 10.1073/PNAS.2104650118/SUPPL_FILE/PNAS.2104650118.SD04.XLSX
195. Ahmadian M, Liu S, Reilly SM, Hah N, Fan W, Yoshihara E, et al. ERRγ preserves brown fat innate thermogenic activity. Cell Rep (2018) 22:2849–59. doi: 10.1016/J.CELREP.2018.02.061
196. Cui J, Pang J, Lin YJ, Gong H, Wang ZH, Li YX, et al. Adipose-specific deletion of Kif5b exacerbates obesity and insulin resistance in a mouse model of diet-induced obesity. FASEB J (2017) 31:2533–47. doi: 10.1096/FJ.201601103R
197. Silvester AJ, Aseer KR, Yun JW. Ablation of DJ-1 impairs brown fat function in diet-induced obese mice. Biochimie (2018) 154:107–18. doi: 10.1016/J.BIOCHI.2018.08.005
198. Ahmadian M, Abbott MJ, Tang T, Hudak CSS, Kim Y, Bruss M, et al. Desnutrin/ATGL is regulated by AMPK and is required for a brown adipose phenotype. Cell Metab (2011) 13:739–48. doi: 10.1016/J.CMET.2011.05.002
199. Mori MA, Thomou T, Boucher J, Lee KY, Lallukka S, Kim JK, et al. Altered miRNA processing disrupts brown/white adipocyte determination and associates with lipodystrophy. J Clin Invest (2014) 124:3339–51. doi: 10.1172/JCI73468
200. Lou P, Bi X, Tian Y, Li G, Kang Q, Lv C, et al. MiR-22 modulates brown adipocyte thermogenesis by synergistically activating the glycolytic and mTORC1 signaling pathways. Theranostics (2021) 11:3607–23. doi: 10.7150/THNO.50900
Keywords: brown adipose tissue, beige adipose tissue, whitening, obesity, metabolic complications
Citation: Ziqubu K, Dludla PV, Mthembu SXH, Nkambule BB, Mabhida SE, Jack BU, Nyambuya TM and Mazibuko-Mbeje SE (2023) An insight into brown/beige adipose tissue whitening, a metabolic complication of obesity with the multifactorial origin. Front. Endocrinol. 14:1114767. doi: 10.3389/fendo.2023.1114767
Received: 02 December 2022; Accepted: 06 February 2023;
Published: 16 February 2023.
Edited by:
Magdalene K. Montgomery, The University of Melbourne, AustraliaReviewed by:
Endre Károly Kristóf, University of Debrecen, HungaryCopyright © 2023 Ziqubu, Dludla, Mthembu, Nkambule, Mabhida, Jack, Nyambuya and Mazibuko-Mbeje. This is an open-access article distributed under the terms of the Creative Commons Attribution License (CC BY). The use, distribution or reproduction in other forums is permitted, provided the original author(s) and the copyright owner(s) are credited and that the original publication in this journal is cited, in accordance with accepted academic practice. No use, distribution or reproduction is permitted which does not comply with these terms.
*Correspondence: Sithandiwe E. Mazibuko-Mbeje, MzY1ODgyOTZAbnd1LmFjLnph
Disclaimer: All claims expressed in this article are solely those of the authors and do not necessarily represent those of their affiliated organizations, or those of the publisher, the editors and the reviewers. Any product that may be evaluated in this article or claim that may be made by its manufacturer is not guaranteed or endorsed by the publisher.
Research integrity at Frontiers
Learn more about the work of our research integrity team to safeguard the quality of each article we publish.