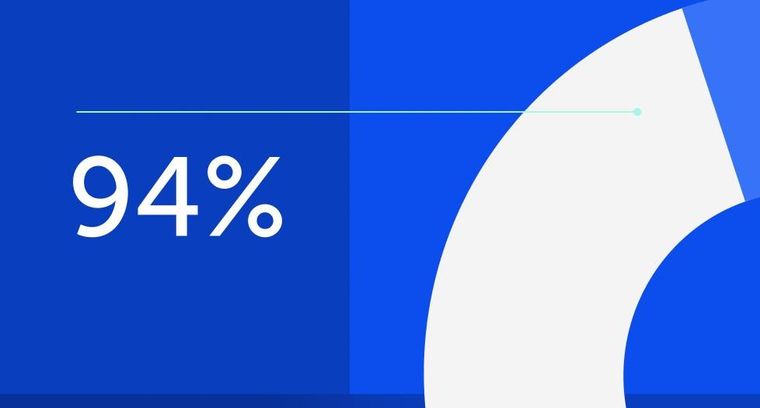
94% of researchers rate our articles as excellent or good
Learn more about the work of our research integrity team to safeguard the quality of each article we publish.
Find out more
REVIEW article
Front. Endocrinol., 09 May 2023
Sec. Diabetes: Molecular Mechanisms
Volume 14 - 2023 | https://doi.org/10.3389/fendo.2023.1114424
This article is part of the Research TopicPharmacogenomics and Pharmacomicrobiomics in Type 2 Diabetes Mellitus (T2DM)View all 10 articles
Type 2 diabetes mellitus (T2DM) is a metabolic disorder characterized by hyperglycemia and insulin resistance. The incidence of T2DM is increasing globally, and a growing body of evidence suggests that gut microbiota dysbiosis may contribute to the development of this disease. Gut microbiota-derived metabolites, including bile acids, lipopolysaccharide, trimethylamine-N-oxide, tryptophan and indole derivatives, and short-chain fatty acids, have been shown to be involved in the pathogenesis of T2DM, playing a key role in the host-microbe crosstalk. This review aims to summarize the molecular links between gut microbiota-derived metabolites and the pathogenesis of T2DM. Additionally, we review the potential therapy and treatments for T2DM using probiotics, prebiotics, fecal microbiota transplantation and other methods to modulate gut microbiota and its metabolites. Clinical trials investigating the role of gut microbiota and its metabolites have been critically discussed. This review highlights that targeting the gut microbiota and its metabolites could be a potential therapeutic strategy for the prevention and treatment of T2DM.
Diabetes mellitus (DM) is considered one of the most serious public healthcare challenges in the world, with more than 536.6 million people aged 20-79 years (prevalence estimated at 10.5%) reported to have diabetes in 2021. This number is projected to rise to 783.2 million (prevalence estimated at 12.2%) by 2045 (1). Type 2 diabetes mellitus (T2DM), accounting for 90% of cases, is the most prevalent type and is characterized by hyperglycemia and insulin resistance (2, 3). The risk factors that contribute to the onset of T2DM are complex and have not been fully elucidated. Obesity, sedentary lifestyle, and genetic susceptibility are recognized as significant risk factors for T2DM progression (4). An increasing number of studies have shown a clear link between the dysregulated gut microbiota and the development of T2DM (5, 6). Understanding these interactions may lead to novel therapeutic implications for T2DM.
The gut microbiota is a complex and dynamic entity composed of trillions of microorganisms that live in close symbiosis with their host, consisting of hundreds of different species of bacteria, primarily distributed among nine phyla (7–9). It is dominated by the phylum Firmicutes, Bacteroidetes, Proteobacteria, Actinobacteria, and Fusobacteria, which account for 90% of the total human microbiota (10, 11). Gut microbiota is strongly influenced by geographic location, age, lifestyle, diet, and even the mode of birth (12–14). Furthermore, variations in gut microbiota can lead to changes in metabolites, such as bile acids (BAs), branched-chain amino acids (BCAA), short-chain fatty acids (SCFAs), lipopolysaccharides (LPS), trimethylamine (TMA), and propionic acid imidazole (PAI) (15). A study has demonstrated that an increase in trimethylamine-N-oxide (TMAO), a conversion product of TMA in the liver, predicts a high mortality risk in patients with T2DM (16). Although peripheral blood BAs levels do not predict the transition from impaired fasting glucose (IFG) to new-onset diabetes (NOD) (17), Tamara et al. reported a non-absorbable polymeric bile acids chelator (SAR442357) that ameliorated hyperglycemia in preclinical animal models of diabetes by reducing intestinal luminal bile acids levels and delaying the development of DM (18). These clinical reports suggest that gut microbiota and its metabolites may be significantly associated with T2DM progression (19, 20).
Current research generally concludes that gut microbial metabolites can influence the development of T2DM by modulating physiological processes such as β-cell dysfunction, chronic low-grade inflammation, oxidative stress, and dysmetabolism of lipids and glucose (21). For example, SCFAs can decrease the expression of pro-inflammatory cytokines by inhibiting NF-κB activation and IκBα degradation, improving glucose control, and mitigating the development of T2DM (22–24). Conversely, elevated TMAO levels impair glucose tolerance by blocking the hepatic insulin signaling pathway, causing systemic inflammation in adipose tissue, and accelerating the development of diabetes. Although these studies indicate that gut microbial metabolites play a role in the development of T2DM, a systematic summary of the molecular mechanisms involved is still lacking. This review aims to summarize the molecular links between microbiota-derived metabolites and the pathogenesis of T2DM, and discuss recent clinical trials and treatments for T2DM. A better understanding of the interactions between gut microbiota and T2DM could provide insights into T2DM prevention and therapy.
Gut microbial metabolites are compounds produced by gut microbiota during the digestion of food. These metabolites, including SCFAs, tryptophan metabolites, TMAO, LPS and BAs, have been shown to play a crucial role in the development of T2DM (25). Most of the metabolites can enter the systemic circulation and act as signaling molecules via various receptors, which further regulate multiple metabolic pathways.
SCFAs are major products of the anaerobic fermentation of resistant starch and fiber by the gut microbiota (Figure 1). Only a small fraction of SCFAs in the gastrointestinal tract is taken up from the diet. Butyric, acetic, and propionic acids constitute the most prevalent SCFAs in the body (26). SCFAs can be originally produced by thick-walled flora, including Clostridium perfringens IV and XIV a. These substances then enter the colonic epithelium via H-dependent or sodium-dependent monocarboxylate transport proteins to provide energy for their production (27). The remaining SCFAs that are then released from the intestine into circulation via the liver and portal system and contribute to the development of several diseases such as obesity, insulin resistance, T2DM, etc. (28).
Figure 1 The main mechanisms of SCFAs regulating metabolism and inflammation in T2DM. SCFAs are produced by the conversion of dietary fiber by gut microbiota and subsequently enter cells directly or act on transmembrane receptors such as FFAR2, FFAR3 and GPR109A, which are involved in improving T2DM related pathways, such as fatty acid oxidation, glucose metabolism and inflammation response. Meanwhile, SCFAs can inhibit the release of inflammatory factors such as TNF-α and IL-1β triggered by LPS through the NK-κB pathway, thus alleviating the inflammatory response. SCFAs, short-chain fatty acids; FFAR2, Free Fatty Acid Receptor 2; FFAR3, Free Fatty Acid Receptor 3; GPR109A, G-protein-coupled receptor 109A; TLR4, Toll-like receptor 4; LPS, Lipopolysaccharide; AMPK, Adenosine 5’-monophosphate (AMP)-activated protein kinase; cAMP, Cyclic adenosine monophosphate; PKA, protein kinase A system; HSL, hormone-sensitive lipase; FFA, free fatty acid; PGC-1α, Peroxisome proliferator-activated receptor-γ coactivator-1α; PPAR, peroxisome proliferator activated receptor; ATGL, Adipose triglyceride lipase; UCP1/2/3, uncoupling protein1/2/3; ATP, Adenosine triphosphate; IGN, intestinal gluconeogenesis; PYY, peotide YY; GLP-1, glucagon-like peptide-1; GLUT4, glucose transporter 4; HDACs, Histone Deacetylases; IL-10, Interleukin-10; IL-18, Interleukin-18; NF-κB, nuclear factor kappa-B; MAPK, mitogen-activated protein kinase; ERK1/2, extracellular regulated protein kinases; TNFα, Tumor necrosis factor α; IL-1β, Interleukin-1β; iNOS, Inducible nitric oxide synthase.
As members of the fatty acid family, SCFAs can serve as substrates for lipid synthesis. It has been shown that SCFAs can activate AMPK, promote the induction of PGC-1α expression and activate peroxisome proliferator-activated receptor (PPAR), thereby regulating the fatty acid oxidation process (29, 30). Additionally, many studies have also pointed out that important lipid metabolic signals such as cAMP (31), adipose triglyceride lipase (ATGL, the main enzyme of lipolysis) (32), and uncoupling protein (UCP) (33) are also regulated by SCFAs. SCFAs have been found to play a role in hyperglycemic syndrome through G protein-coupled receptors (GPRCs) (34). The most crucial SCFAs receptors are the G protein-coupled receptors free fatty acid receptor 2 (FFAR2), free fatty acid receptor 2 (FFAR3), and G-protein-coupled receptor 109A (GPR109A). Extracellular signal-regulated kinase 1 or 2 (ERK1/2), intracellular calcium activation, cyclic adenosine monophosphate (cAMP), and G protein (Gq or Gi/o) are downstream signaling molecules that FFAR influences the absorption of nutrients (35, 36). FFAR2 (GPR43) is mainly expressed in white adipocytes, islet α and β cells, intestinal enteroendocrine cells, and immune cells (37, 38). Butyrate can inhibit histone deacetylase (HDAC) expression by activating FFAR2, thereby having an inhibitory effect on the inflammatory response (39). FFAR3 (GPR41) is expressed in white adipocytes, immune cells, pancreatic islet α and β cells, and intestinal enteroendocrine cells (40, 41). GPR109A is a G protein-coupled receptor for nicotinate and has poor sensitivity for butyrate (42, 43).
SCFAs have been extensively studied in the field of metabolic diseases. In a previous study, it was demonstrated that propionate upregulated peptide YY (PYY) and glucagon-like peptide-1 (GLP-1) expression in the colonic tissue, leading to weight loss and significantly reduced blood glucose levels (44). SCFAs activate FFAR2 on enteroendocrine L cells, thereby enhancing the release of GLP-1 and PYY (45). FFAR3 is expressed in vagal sensory neurons and cross-talks with cholecystokinin (CCK) to alter food intake (46). By regulating AMPK, GPR109A promotes Nrf2 nuclear import and induces autophagy, resulting in anti-inflammatory effect (47). It also regulates lipid metabolism and inhibits lipolysis in adipose tissue (48). A recent study also shows that SCFAs may contribute to the development of diabetes through DNA methylation (49).
Lipopolysaccharide (LPS) is an important feature on the cell wall of gram-negative bacteria and plays an important role in the pathogenesis of T2DM. LPS exhibits an interactive relationship with SCFAs (Figure 1) (50, 51). The amount of LPS can be used to predict the development of many inflammatory diseases associated with participation in natural immunity (52). It has been shown that ecological dysregulation due to high fat intake also upregulates LPS concentrations, resulting in the release of TNF, IL-1, and IL-6 and systemic inflammation (53). The development of endotoxemia will trigger the host’s immune response, entering a pro-inflammatory state, which may contribute to metabolic diseases, such as T2DM.
Toll-like receptor 4 (TLR-4) has been identified as an important receptor of LPS, which belongs to a family of transmembrane receptors. Upon TLR-4 activation, transcription of inflammatory cytokines such as TNF-α, IL-1, and IL-6 are enhanced via NF-κB and MAPK pathways. These inflammatory cytokines are significantly elevated in patients with T2DM, subsequently resulting in insulin resistance and pancreatic β-cell dysfunction (54).
Bile acids (BAs), including chenodeoxycholic acids (CDCA) and cholic acids (CA), are synthesized in liver from cholesterol (55). There are two pathways of BAs synthesis: the classical pathway and the alternative pathway. CDCA is effectively catalyzed by mitochondrial sterol 27-hydroxylase (CYP27A1) for oxygenation of the carbon chain of corticosteroids, while the production of CA is determined by sterol 12-hydroxylase (CYP8B1) (56). The bile salts export pump (BSEP) then secretes bile salts that have been coupled with the amino-acid taurine or glycine into the digestive system, where they are converted into secondary BAs by gut microbiota. In the intestine, CA and CDCA can be converted into deoxycholic acids (DCA) and lithocholic acid (LCA) respectively by the action of bacterial bile salt hydrolase (BSH) and 7α-dehydroxylase enzyme (57). Clostridium perfringens is a bacterium that is capable of synthesizing the 7α-dehydroxylase enzyme (58). BSH is an enzyme produced by various strains of gut microbiota, including Staphylococcus, Neococcus, Enterococcus, Bifidobacterium, Clostridium perfringens, and Parasiticum (59). BAs are signaling molecules, which regulate insulin sensitivity and inflammation in T2DM via farnesoid X receptor (FXR) and Takeda G protein-coupled receptor 5 (TGR5) (Figure 2) (60–62). Meanwhile, BAs are also the ligands of vitamin D receptor (VDR) (63), progesterone X receptor (PXR) (64), membrane receptor sphingosine-1 phosphate receptor 2 (S1PR2) (65, 66) and play significant role in regulating inflammation and immune functions.
Figure 2 The main mechanisms of BAs regulating glucose homeostasis in T2DM. This figure illustrates the metabolism and transformation of bile acids in the liver, intestine, pancreas, and brown adipose tissue, and the mechanisms by which they regulate glucose homeostasis through the two major bile acid receptors, FXR and TGR5.CYP7A1, Cholesterol 7-alpha hydroxylase; CYP8B1, sterol 12α-hydroxylase; CA, cholic acid; CDCA, chenodeoxycholic acid; T/G, taurine/glycine; BSEP, bile salt export pump; SHP, small heterodimer; JNK/ERK, c-Jun N-terminal kinase/extracellular regulated protein kinases; FFGR4, FGF receptor 4; FGF19/15, fibroblast growth factor 19/15; ASBT, apical sodium-dependent bile acid transporter; PI3K, phosphatidylinositol-3-kinases; Akt, protein kinase B; mTOR, mammalian target of rapamycin; Cers,cermides;SREBP1, sterol-regulatory element binding proteins 1; FXR, farnesoid X receptor; NTCP, sodium taurocholate cotransporting polypeptide; OATP, Organic Anion Transporting Polypeptide; OSTα/β, organosolute transport proteins α and β; DCA, deoxycholic acid; LCA, lithic bile acids; TGR5, Takeda G protein-coupled receptor 5; cAMP, Cyclic adenosine monophosphate; DIO2, deiodinase type 2; T4, thyroxine; T3, triiodothyronine; GLP-1, glucagon-like peptide-1.
FXR is mainly expressed in the liver and intestine, and CDCA is the most potentially endogenous agonist of FXR (67). Activation of intestinal FXR induces the expression and secretion of fibroblast growth factor (FGF)15/19, which subsequently enters into liver via enterohepatic circulation (68). Serum FGF15/19 activates hepatic FGF receptor 4 (FGFR4)/-klotho complex, which in turn inhibits cholesterol 7-alpha hydroxylase (CYP7A1) transcription and reduces bile acid synthesis (69, 70). Additionally, it has been reported that clostridia-rich microbiota can promote BAs synthesis by suppressing intestinal FGF19 production (71). Activation of hepatic FXR promotes transcriptional activity of the small heterodimer (SHP), which in turn represses the expression of CYP7A1 expression and reduces bile acid synthesis. One of the downstream targets of FXR is insulin receptor substrate 1 (IRS1)-AKT-phosphatidylinositol 3 kinase (PI3K) pathway, which plays a crucial role in insulin signaling. While both intestinal and hepatic FXR signaling are involved in regulating bile acid homeostasis, they have distinct functions in lipids synthesis and absorption (72). Semi-synthetic bile acid, such as obeticholic acid (OCA), has been shown to be 30 times more effective in activating FXR than CDCA (73). OCA has been found to inhibit bile acid production, improve oxidative stress and liver fibrosis, and decrease hepatic cholesterol and triglyceride content (74, 75). In a study by Sunder et al. (NCT00501592), patients treated with 25 mg OCA showed that insulin sensitivity increased by 28.0% from baseline (76). These studies suggest that OCA may serve as a novel target in alleviating liver inflammation and insulin resistance.
TGR5 (also known as Gpbar-1) is a G protein-coupled receptor. TGR5 is widely expressed in various tissues, including the liver, adipose tissue and intestine, and plays important roles in regulating energy metabolism. TGR5 activation in enteroendocrine L cells can increase glucagon-like peptide-1 (GLP-1) secretion, leading to improved glucose homeostasis and insulin sensitivity (77). TGR5 activation in adipose tissue induces the expression of thyroid hormone deiodinase type 2 (DIO2), which converts inactive thyroxine (T4) into active thyroid hormone (T3) and enhances energy expenditure (78, 79).
Bile acid binding resins and bile acid chelator are used to regulate the BAs pathway (80). Bile acid binding resins work by binding to bile acids in the intestine, preventing their reabsorption and promoting their excretion in the feces. This leads to a reduction in the amount of bile acids in circulation, which in turn stimulates the liver to synthesize more bile acids from cholesterol. A clinical study of 40 Japanese patients with T2DM (NCT038934220) found that colestimide altered bile acid composition and increased the CA ratio, which enhanced energy metabolism, improved blood glucose levels, and alleviated diabetes via TGR5-cAMP-Dio2 pathway (81, 82). Berberine ursodeoxycholate (BUDCA) (83) has been shown to improve glycemic control and lower serum LDL-cholesterol level (NCT03656744) (84, 85). However, it should be noted that bile acid chelator may decrease the hydrophobicity of BAs and increase the risk of gallstone formation (86).
Tryptophan is an essential amino acid and can be transformed by gut microbiota into molecules, such as indole and its derivatives, including indole-3- lactate (ILA), indole-3-propionic acid (IPA) and indole-3-acetaldehyde (IAld) (Figure 3). The tryptophan metabolites have been implicated in the pathogenesis of T2DM (87, 88). Indole stimulates GLP-1 secretion from intestinal L cells, resulting in insulin release and reduced blood glucose levels. IPA has been shown to have anti-inflammatory and antiseptic properties by acting on the aryl hydrocarbon receptor (AhR) (89–91). In vivo, administration of indole decreased hepatic steatosis and inflammation in rats fed high fat diet. And indole decreased lipid accumulation and stimulates inflammatory responses in vitro (92). Activation of AhR by tryptophan metabolites has been shown to have a variety of physiological effects, including regulation of immune responses, inflammation, and cell differentiation (93).
Figure 3 Role and mechanism of tryptophan metabolic pathway associated with T2DM. This figure depicts the conversion of tryptophan through the action of the gut microbiota, the products of which are involved in T2DM. ILA, indole-3-lactate; IPA, Indole 3-propionic acid;ILDH, indole-3-lactate dehydrogenase;ArAT Aromatic amino acid aminotransferase, IAld, indole-3-acetaldehyde; AhR, aryl hydrocarbon receptor; GLP-1, glucagon-like peptide-1.
Trimethylamine N-Oxide (TMAO) has been implicated in the pathogenesis of T2DM and related complications (94). Studies have shown that TMAO may contribute to the development of T2DM by promoting insulin resistance, impairing glucose tolerance, and inducing inflammation (95, 96). High level of TMAO may be associated with mild cognitive impairment, cardiovascular events in patients with T2DM (97–99).
TMAO is produced by gut bacteria from dietary nutrients such as egg and meat products (100). In intestine, gut microbiota breakdown choline, carnitine, or betaine into trimethylamine (TMA) and dimethylamine (DMA), which are absorbed into the bloodstream and transported to the liver (95).. In the liver, TMA and DMA are oxidized by the enzyme flavin-containing monooxygenase 3 (FMO3) to produce TMAO (101). Notably, a diet high in animal-based foods is associated with higher TMAO levels. The increase in circulating TMAO is thought to be possibly related to several factors, including: 1) dietary choline or carnitine content, 2) kidney function, 3) liver function, and 4) gut microbiota composition.
Many studies are focusing on regulating the TMA lytic enzymes to reduce TMAO levels. For example, 3,3-dimethyl-1-butanol (DMB), which is a structural analog of choline, can inhibit microbial TMA formation. It has been shown that DMB significantly reduces TMAO levels in mice fed a high choline or carnitine diet, thereby inhibiting diet-enhanced atherosclerosis (102). Metformin has also been found to decrease TMAO concentration in db/db mice (103). In addition, it has been demonstrated that berberine reduces TMAO levels by regulating TMA lytic enzymes via remodeling gut microbiota (104).
Antagonist against TMAO, another metabolite of the gut microbiota, has also been shown to improve glucose homeostasis and metabolism disorders (105). Taurisolo, a novel grape pomace polyphenolic extract, significantly decreased serum TMAO levels in healthy subjects (106). It implies that polyphenols can lower TMAO levels, thereby alleviating impaired glucose tolerance and improving adipose tissue inflammation in patients with T2DM (107).
TMAO has been implicated as a novel risk factor for cardiovascular events related to obesity and T2DM. Targeting gut microbiota and TMAO production may serve as potential therapeutic approaches for the treatment of T2DM.
As we gain a deeper understanding of the relationship between gut microbiota and T2DM, more and more therapies are emerging that aim to regulate the gut microbiota and its metabolites (Supplementary Table 1). Recent approach to regulate gut microbiota for T2DM therapy focuses on probiotics, prebiotics, synbiotics, fecal microbial transplantation, diet intervention, bacteriophages, microbiota-targeted drugs and postbiotics (Figure 4).
Figure 4 Potential therapy and treatments for T2DM by regulating gut microbiota and its metabolites. Recent approaches to regulate gut microbiota for T2DM therapy focuses on probiotics, prebiotics, synbiotics, fecal microbial transplantation, diet intervention, bacteriophages, microbiota-targeted drugs and postbiotics. SCFAs, short-chain fatty acids; FMT, Fecal Microbiota Transplantation; BAs,bile acids; LPS, Lipopolysaccharide; IL-10, Interleukin-10; GLP-1, glucagon-like peptide-1; ZO-1, zonula occludens-1.
T2DM has been linked to dysbiosis of gut microbiota (108). Probiotics such as Bifidobacterium, Lactobacillus, prebiotics such as oligofructose and inulin, as well as synbiotics (a combination of the two) all play a significant role in the development of T2DM.
Probiotics are live microorganisms that provide beneficial effects to the host when adequately administered. Probiotics have been shown to improve glucose metabolism and insulin sensitivity in patients with T2DM. A combination of Bifidobacterium lactis LMG P-28149 and Lactobacillus rhamnosus LMG S-28148 increased PPARγ expression and enhanced insulin sensitivity in high-fat diet (HFD) induced obese mice (109). It has been shown that Bifidobacterium longum and Lactobacillus upregulated GLP-1 and IL-10 expression in patients with obesity or T2DM, and suppressed lipid accumulation in adipocytes (3, 110). In addition, Lactobacillus fermentum MCC2760 increased the expression of glucose transporter 4 (GLUT4), GLP-1 and ZO-1, improving glucose tolerance in HFD mice (111).
Inulin is a type of prebiotic fiber that cannot be digested by the human body. It has been demonstrated that inulin is fermented by microbiota to produce SCFAs in the colon (112, 113). In a clinical trial (NCT02009670), consumption of inulin promotes SCFAs production and improves lipid oxidation, resulting in a significant improvement in glycemic control (114). Another study (NCT00750438) shows that inulin-propionate ester supplementation significantly increases colonic propionate levels, and prevents weight gain by promoting GLP-1 secretion (115).
Synbiotics, which combine probiotics and prebiotics, have the potential to provide more significant benefits than when used separately. For instance, when Lactobacillus paracasei N1115 was combined with oligofructose, it was observed to down-regulate the expression of TLR4 and NF-κB, while up-regulating the p38 MAPK pathway (116). It is important to note that synbiotics currently lack FDA statements, and further clinical validation is required to determine the optimal ratio of probiotics and their safety and efficacy.
Despite probiotics have been shown to have a potential role for T2DM, fecal microbiota transplantation (FMT) has advantage of entire gut microbiota transplantation. FMT has been recommended for the prevention of chronic Clostridium difficile infections since 2013, and it has also shown beneficial effects in ulcerative colitis and even metabolic diseases such as T2DM (117).
Studies have demonstrated that FMT treatment in mice reduces glucose levels, improves insulin sensitivity, and reduces islet cell apoptosis (117). Transplantation of normal human fecal flora into diabetic mice was reported to ameliorate glucose disorders by altering bacterial composition to produce more SCFAs and stimulating GLP-1 releasing via GPR43 receptor (118, 119). In contrast, mice transplanted with gut microbiota from patients with T2DM were found to disrupt blood glucose by regulating BAs metabolism (120). Study by Anne et al. reported that transplanting gut microbiota from lean donors to patients with T2DM could improve insulin sensitivity (121). Similarly, Su et al. showed that the predominant gut microbiota of T2DM patients shifted from bacteroides to Prevotella after FMT (122), with a significant increase in beneficial organisms (e.g., bifidobacteria) and a significant decrease in harmful organisms (e.g., Bilobacteria) in a 90-day open-label controlled trial (122). However, it should be noted that FMT may be ineffective or even cause side effects due to the complex composition of the gut microbiota. Elaine et al. reported that FMT had no clinically significant metabolic effects in a clinical study (NCT02530385) (123), possibly due to the small sample size of the trial. Adverse events such as diarrhea, constipation, abdominal pain, and infections have also been reported with FMT (124, 125).
Although FMT is a promising treatment for T2DM, more convincing evidence is needed to confirm the source of donors and frequency of FMT. The adverse effects of dangerous bacteria in the flora, the resilience of the gut microbiota, and the uncertain clinical result of microbiota modifications need more investigation (126, 127).
A healthy diet helps patients with T2DM improve glycemic control. Research indicates that a weight loss about 15 kg induced by calorie restriction (CR) lead to remissions of T2DM in about 80% patients with obesity and T2DM (128, 129).
In the high-fat diet (HFD) group, an increase in LPS and TMAO and a decrease in SCFAs have been observed, which can affect the host metabolism and immunity. The significant elevation of Escherichia coli, Klebsiella, and Shigella in the HFD group and the decrease of Lactobacillus and Lactobacillus may provide an early warning for the development of T2DM. However, HFD can lead to an increase in the number of β-cells and induce a decrease of islet infiltration, protecting from the development of diabetes (130). This phenomenon may be related to impairment of immune checkpoints (ICPs) and reduced T-cell attack on pancreatic β-cells, which requires further investigation (131).
Interventions such as calorie restriction (CR), very low-calorie-ketogenic (VLCK), and fasting-mimicking diets (FMDs) have been utilized in metabolic diseases such as obesity and T2DM. CR was found to alter the microbiota and reprogram the metabolism, resulting in a different serum bile acid profile characterized by elevated ratio of non-12α-hydroxylated bile acids (132). The mechanism of CR induced glucose homeostasis may be related to GLP-1 secretion via TGR5/cAMP signaling pathway (133). Additionally, CR can reshape the gut microbiota composition and promote SCFAs production to exert anti-inflammatory effect. VLCK may induce elevating plasma concentrations of acetoacetic acid (ACA) and β-hydroxybutyric acid (β-OHB), and activation of white adipose tissue (WAT) lipolysis (134, 135). Ketogenic diets also alter the gut microbiota and reduce inflammatory Th17 cells (136). These studies indicate that more personalized diet interventions may be utilized for prevention and treatment of T2DM.
Gut microbiota contains not only bacteria but also a large number of viruses (dominated by bacteriophages) (137). Bacteriophages specifically infect bacteria in a host-specific manner and are associated with metabolic diseases. For example, altered viral taxonomic composition and reduced viral-bacterial correlation were observed in patients with obesity and T2DM (137, 138). In a previous study, the fecal virome from mice on a low-fat diet was transplanted into the intestine of mice on a high-fat diet. It was observed that the obese mice gained weight more slowly, and their glucose tolerance remained similar to that of mice on a low-fat diet (139). Bacteriophages therapy have been demonstrated to improve clinical healing of diabetic wounds and have less severe impact on the ecosystem than antibiotics (140).
A growing number of studies highlight the possibility that bacteriophages might modify their host genetics through the lysogenic pathway, leading to either an increase or decrease of metabolites levels. For instance, the abundance of Klebsiella phage (vB KpnP SU552A) was found to be negatively correlated with tryptophan levels, indicating that targeting the tryptophan metabolic pathway by phages could regulate indole derivatives and potentially inhibit AhR to prevent insulin resistance (89–91, 141). However, further research is required to fully understand the role of bacteriophages in the treatment of T2DM, including larger clinical studies to confirm their efficacy.
Microbiota-targeted drugs are a newly proposed class of drugs that aim to modulate the metabolites of gut microbiota. However, direct targeting of metabolites can have a significant effect on gastrointestinal function in clinical practice. Therefore, researchers are investigating how to target specific gut microbiota without affecting the gastrointestinal function.
Gut microbiota-derived metabolites play a central role in the host-microbe crosstalk (142, 143). Using a mini-gut model to screen drugs, THIP hydrochloride, methenamine, and mesna have been identified as promising new gut microbiota therapeutics (144). Amuc _1100, a specific outer membrane from Akkermansia muciniphila, has been shown to improve metabolism, insulin resistance and dyslipidemia (145, 146). THIP hydrochloride also has the effect of reducing the inflammatory response by decreasing the overgrowth of Akkermansia muciniphila, which can cause damage to the intestinal barrier. Urotropine significantly enhances the abundance of Veillonellaceae, which converts lactate into SCFAs (147). In addition, Mesna has been shown to decrease the number of Verrucomicrobiaceae and Akkermansia muciniphila while enhancing SCFA synthesis and decreasing endotoxin production. These changes may contribute to alleviating oxidative stress levels and chronic inflammation (148–150).
Postbiotics are the byproducts of the metabolic processes of probiotic bacteria, including exopolysaccharides, γ-aminobutyric acid (GABA), and extracellular vesicles (EV) (151). For example, exopolysaccharide has been found to inhibit adipogenesis and pancreatic α-amylase by activating the AMPK signaling pathway (152, 153). It has been reported that GABA improves glucose intolerance, β-cell mass, and inflammatory response (154–156). EV from Aeromonas aeruginosa was found to improve intestinal barrier function and glucose tolerance in HFD-induced T2DM mice (157). Meanwhile, in this paper, we use a table (Supplementary Table 2) to summarize in as much detail as possible some information about completed or ongoing gut microbial metabolites clinical trials to show the latest progress of current gut microbial metabolites clinical studies.
In this review, we discuss the interaction between microbiota-derived metabolites and gut microbiota and their role in T2DM. Currently, there is growing interest in targeting the gut microbiota and its metabolites as a potential therapeutic approach for T2DM. Many approaches have been explored, including the use of probiotics, prebiotics, synbiotics, postbiotics, FMT, dietary interventions, bacteriophages, and microbiota-target drugs.
However, there are still several challenges that need to be addressed. One of the main challenges is the lack of a comprehensive understanding of the complex interactions between the gut microbiota, its metabolites, and the host. The gut microbiota is highly diverse and dynamic, and its composition can be influenced by various factors. Another challenge is the safety and efficacy of targeting the gut microbiota and its metabolites. Although there is growing evidence suggesting that targeting the gut microbiota and its metabolites can have beneficial effects on T2DM, there is also the potential for unintended consequences. In addition, better methods are needed to assess the gut microbiota and its metabolites. Current methods for assessing the gut microbiota and its metabolites, such as 16s rRNA sequencing, metagenomics and chromatography-mass spectrometry, have limitations in terms of resolution and accuracy. Finally, more high-quality clinical trials with larger sample size are needed to verify their safety and efficacy on T2DM. Taken together, a comprehensive understanding the interaction between microbiota-derived metabolites and T2DM will shed light into potential targets for T2DM therapy.
Conceptualization and design: MW and QX. Writing-original draft preparation: JW and KY. Writing-review and editing: JW, KY and HF. Project administration: JW. Funding acquisition: MW and QX. Manuscript revised: MW and QX. All authors have read and agreed to the published version of the manuscript.
This work was supported by Science and Technology Planning Project of Jiangxi Health Commission (202210483) and Science and Technology Planning Project of Jiangxi Health Commission (202210663).
The figures were created using online BioRender software (BioRender.com).
The authors declare that the research was conducted in the absence of any commercial or financial relationships that could be construed as a potential conflict of interest.
All claims expressed in this article are solely those of the authors and do not necessarily represent those of their affiliated organizations, or those of the publisher, the editors and the reviewers. Any product that may be evaluated in this article, or claim that may be made by its manufacturer, is not guaranteed or endorsed by the publisher.
The Supplementary Material for this article can be found online at: https://www.frontiersin.org/articles/10.3389/fendo.2023.1114424/full#supplementary-material
ACA, acetoacetic acids; ATGL, Adipose triglyceride lipase; BA, bile acid; BSEP, bile salts export pump; CA, cholic acids; CDCA, chenodeoxycholic acids; ChREBP, carbohydrate response element binding proteins; CR, Calorie restriction; CYP7A1, Cholesterol 7-alpha hydroxylase; CYP8B1, sterol 12α-hydroxylase; DCA, deoxycholic acid; DIO2, deiodinase type 2; ERK1/2, extracellular regulated protein kinases; FFAR2, Free Fatty Acid Receptor 2; FFAR3, Free Fatty Acid Receptor 3; FXR, farnesoid X receptor; GABAs, γ-aminobutyric acids; GLP-1, glucagon-like peptide-1; GLUT4, glucose transporter 4; GPR109A, G-protein-coupled receptor 109A; HFD, high-fat diet; ICPs, immune checkpoints; IFG, impaired fasting glucose; LCA, lithic bile acid; LPS, lipopolysaccharides; NOD, new-onset diabetes; NTCP, sodium taurocholate cotransporting polypeptide; OATP, Organic Anion Transporting Polypeptide; PAI, propionic acid imidazole; PYY, peotide YY; SCFA, short-chain fatty acid; SHP, small heterodimer; TGR5, Takeda G protein-coupled receptor 5; TLR-4, Toll-like receptor 4; TMA, trimethylamine; TMAO, trimethylamine-N-oxide; UCP1/2/3, uncoupling protein1/2/3; VLCK, very low-calorie-ketogenic.
1. Sun H, Saeedi P, Karuranga S, Pinkepank M, Ogurtsova K, Duncan BB, et al. IDF diabetes atlas: global, regional and country-level diabetes prevalence estimates for 2021 and projections for 2045. Diabetes Res Clin Pract (2022) 183:109119. doi: 10.1016/j.diabres.2021.109119
2. Zheng Y, Ley SH, Hu FB. Global aetiology and epidemiology of type 2 diabetes mellitus and its complications. Nat Rev Endocrinol (2018) 14(2):88–98. doi: 10.1038/nrendo.2017.151
3. Alard J, Cudennec B, Boutillier D, Peucelle V, Descat A, Decoin R, et al. Multiple selection criteria for probiotic strains with high potential for obesity management. Nutrients (2021) 13(3):713. doi: 10.3390/nu13030713
4. Al-Sulaiti H, Diboun I, Agha MV, Mohamed FFS, Atkin S, Dömling AS, et al. Metabolic signature of obesity-associated insulin resistance and type 2 diabetes. J Transl Med (2019) 17(1):348. doi: 10.1186/s12967-019-2096-8
5. Gurung M, Li Z, You H, Rodrigues R, Jump DB, Morgun A, et al. Role of gut microbiota in type 2 diabetes pathophysiology. EBioMedicine (2020) 51:102590. doi: 10.1016/j.ebiom.2019.11.051
6. Zhang Y, Gu Y, Ren H, Wang S, Zhong H, Zhao X, et al. Gut microbiome-related effects of berberine and probiotics on type 2 diabetes (the PREMOTE study). Nat Commun (2020) 11(1):5015. doi: 10.1038/s41467-020-18414-8
7. Eckburg PB, Bik EM, Bernstein CN, Purdom E, Dethlefsen L, Sargent M, et al. Diversity of the human intestinal microbial flora. Science (2005) 308(5728):1635–8. doi: 10.1126/science.1110591
8. Almeida A, Mitchell AL, Boland M, Forster SC, Gloor GB, Tarkowska A, et al. A new genomic blueprint of the human gut microbiota. Nature (2019) 568(7753):499–504. doi: 10.1038/s41586-019-0965-1
9. Li Y, Jin Y, Zhang J, Pan H, Wu L, Liu D, et al. Recovery of human gut microbiota genomes with third-generation sequencing. Cell Death Dis (2021) 12(6):569. doi: 10.1038/s41419-021-03829-y
10. Schloissnig S, Arumugam M, Sunagawa S, Mitreva M, Tap J, Zhu A, et al. Genomic variation landscape of the human gut microbiome. Nature (2013) 493(7430):45–50. doi: 10.1038/nature11711
11. Knudsen JK, Leutscher P, Sørensen S. Gut microbiota in bone health and diabetes. Curr Osteoporos Rep (2021) 19(4):462–79. doi: 10.1007/s11914-020-00629-9
12. Nagpal R, Tsuji H, Takahashi T, Nomoto K, Kawashima K, Nagata S, et al. Ontogenesis of the gut microbiota composition in healthy, full-term, vaginally born and breast-fed infants over the first 3 years of life: a quantitative bird's-eye view. Front Microbiol (2017) 8:1388. doi: 10.3389/fmicb.2017.01388
13. Chi C, Xue Y, Lv N, Hao Y, Liu R, Wang Y, et al. Longitudinal gut bacterial colonization and its influencing factors of low birth weight infants during the first 3 months of life. Front Microbiol (2019) 10:1105. doi: 10.3389/fmicb.2019.01105
14. Vandenplas Y, Carnielli VP, Ksiazyk J, Luna MS, Migacheva N, Mosselmans JM, et al. Factors affecting early-life intestinal microbiota development. Nutrition (2020) 78:110812. doi: 10.1016/j.nut.2020.110812
15. Zhu T, Goodarzi MO. Metabolites linking the gut microbiome with risk for type 2 diabetes. Curr Nutr Rep (2020) 9(2):83–93. doi: 10.1007/s13668-020-00307-3
16. Tang WH, Wang Z, Li XS, Fan Y, Li DS, Wu Y, et al. Increased trimethylamine n-oxide portends high mortality risk independent of glycemic control in patients with type 2 diabetes mellitus. Clin Chem (2017) 63(1):297–306. doi: 10.1373/clinchem.2016.263640
17. Chávez-Talavera O, Wargny M, Pichelin M, Descat A, Vallez E, Kouach M, et al. Bile acids associate with glucose metabolism, but do not predict conversion from impaired fasting glucose to diabetes. Metabolism (2020) 103:154042. doi: 10.1016/j.metabol.2019.154042
18. Castañeda TR, Méndez M, Davison I, Elvert R, Schwahn U, Boldina G, et al. The novel phosphate and bile acid sequestrant polymer SAR442357 delays disease progression in a rat model of diabetic nephropathy. J Pharmacol Exp Ther (2021) 376(2):190–203. doi: 10.1124/jpet.120.000285
19. Yang ZD, Guo YS, Huang JS, Gao YF, Peng F, Xu RY, et al. Isomaltulose exhibits prebiotic activity, and modulates gut microbiota, the production of short chain fatty acids, and secondary bile acids in rats. Molecules (2021) 26(9):2464. doi: 10.3390/molecules26092464
20. Jiang W, Lu G, Gao D, Lv Z, Li D. The relationships between the gut microbiota and its metabolites with thyroid diseases. Front Endocrinol (Lausanne) (2022) 13:943408. doi: 10.3389/fendo.2022.943408
21. Scheithauer TPM, Rampanelli E, Nieuwdorp M, Vallance BA, Verchere CB, van Raalte DH, et al. Gut microbiota as a trigger for metabolic inflammation in obesity and type 2 diabetes. Front Immunol (2020) 11:571731. doi: 10.3389/fimmu.2020.571731
22. Chambers ES, Preston T, Frost G, Morrison DJ. Role of gut microbiota-generated short-chain fatty acids in metabolic and cardiovascular health. Curr Nutr Rep (2018) 7(4):198–206. doi: 10.1007/s13668-018-0248-8
23. Xu L, Ota T. Emerging roles of SGLT2 inhibitors in obesity and insulin resistance: focus on fat browning and macrophage polarization. Adipocyte (2018) 7(2):121–8. doi: 10.1080/21623945.2017.1413516
24. Zhao L, Zhang F, Ding X, Wu G, Lam YY, Wang X, et al. Gut bacteria selectively promoted by dietary fibers alleviate type 2 diabetes. Science (2018) 359(6380):1151–6. doi: 10.1126/science.aao5774
25. Wang D, Liu J, Zhou L, Zhang Q, Li M, Xiao X. Effects of oral glucose-lowering agents on gut microbiota and microbial metabolites. Front Endocrinol (Lausanne) (2022) 13:905171. doi: 10.3389/fendo.2022.905171
26. Jørgensen JR, Fitch MD, Mortensen PB, Fleming SE. In vivo absorption of medium-chain fatty acids by the rat colon exceeds that of short-chain fatty acids. Gastroenterology (2001) 120(5):1152–61. doi: 10.1053/gast.2001.23259
27. den Besten G, van Eunen K, Groen AK, Venema K, Reijngoud DJ, Bakker BM. The role of short-chain fatty acids in the interplay between diet, gut microbiota, and host energy metabolism. J Lipid Res (2013) 54(9):2325–40. doi: 10.1194/jlr.R036012
28. Vinolo MA, Rodrigues HG, Nachbar RT, Curi R. Regulation of inflammation by short chain fatty acids. Nutrients (2011) 3(10):858–76. doi: 10.3390/nu3100858
29. Liu T, Song X, An Y, Wu X, Zhang W, Li J, et al. Lactobacillus rhamnosus GG colonization in early life ameliorates inflammaging of offspring by activating SIRT1/AMPK/PGC-1α pathway. Oxid Med Cell Longev (2021) 2021:3328505. doi: 10.1155/2021/3328505
30. Cantó C, Auwerx J. AMP-activated protein kinase and its downstream transcriptional pathways. Cell Mol Life Sci (2010) 67(20):3407–23. doi: 10.1007/s00018-010-0454-z
31. Wu L, Zhou M, Xie Y, Lang H, Li T, Yi L, et al. Dihydromyricetin enhances exercise-induced GLP-1 elevation through stimulating cAMP and inhibiting DPP-4. Nutrients (2022) 14(21):4583. doi: 10.3390/nu14214583
32. Tang T, Song J, Li J, Wang H, Zhang Y, Suo H. A synbiotic consisting of lactobacillus plantarum S58 and hull-less barley β-glucan ameliorates lipid accumulation in mice fed with a high-fat diet by activating AMPK signaling and modulating the gut microbiota. Carbohydr Polym (2020) 243:116398. doi: 10.1016/j.carbpol.2020.116398
33. Wang B, Kong Q, Li X, Zhao J, Zhang H, Chen W, et al. A high-fat diet increases gut microbiota biodiversity and energy expenditure due to nutrient difference. Nutrients (2020) 12(10):3197. doi: 10.3390/nu12103197
34. Kimura I, Ichimura A, Ohue-Kitano R, Igarashi M. Free fatty acid receptors in health and disease. Physiol Rev (2020) 100(1):171–210. doi: 10.1152/physrev.00041.2018
35. Theiler A, Bärnthaler T, Platzer W, Richtig G, Peinhaupt M, Rittchen S, et al. Butyrate ameliorates allergic airway inflammation by limiting eosinophil trafficking and survival. J Allergy Clin Immunol (2019) 144(3):764–76. doi: 10.1016/j.jaci.2019.05.002
36. McNelis JC, Lee YS, Mayoral R, van der Kant R, Johnson AMF, Wollam J, et al. GPR43 potentiates β-cell function in obesity. Diabetes (2015) 64(9):3203–17. doi: 10.2337/db14-1938
37. Smith PM, Howitt MR, Panikov N, Michaud M, Gallini CA, Bohlooly-Y M, et al. The microbial metabolites, short-chain fatty acids, regulate colonic T reg cell homeostasis. Science (2013) 341(6145):569–73. doi: 10.1126/science.1241165
38. Pingitore A, Gonzalez-Abuin N, Ruz-Maldonado I, Huang GC, Frost G, Persaud SJ. Short chain fatty acids stimulate insulin secretion and reduce apoptosis in mouse and human islets in vitro: role of free fatty acid receptor 2. Diabetes Obes Metab (2018) 21(2):330–9. doi: 10.1111/dom.13529
39. Pan P, Oshima K, Huang YW, Agle KA, Drobyski WR, Chen X, et al. Loss of FFAR2 promotes colon cancer by epigenetic dysregulation of inflammation suppressors. Int J Cancer (2018) 143(4):886–96. doi: 10.1002/ijc.31366
40. Grunddal KV, Tonack S, Egerod KL, Thompson JJ, Petersen N, Engelstoft MS, et al. Adhesion receptor ADGRG2/GPR64 is in the GI-tract selectively expressed in mature intestinal tuft cells. Mol Metab (2021) 51:101231. doi: 10.1016/j.molmet.2021.101231
41. Sepahi A, Liu Q, Friesen L, Kim CH. Dietary fiber metabolites regulate innate lymphoid cell responses. Mucosal Immunol (2021) 14(2):317–30. doi: 10.1038/s41385-020-0312-8
42. Moutinho M, Puntambekar SS, Tsai AP, Coronel I, Lin PB, Casali BT, et al. The niacin receptor HCAR2 modulates microglial response and limits disease progression in a mouse model of alzheimer's disease. Sci Transl Med (2022) 14(637):eabl7634. doi: 10.1126/scitranslmed.abl7634
43. Schulz R, Korkut-Demirbaş M, Venturino A, Colombo G, Siegert S. Chimeric GPCRs mimic distinct signaling pathways and modulate microglia responses. Nat Commun (2022) 13(1):4728. doi: 10.1038/s41467-022-32390-1
44. Zhang Y, Li X, Huang G, Wang H, Chen H, Su Y, et al. Propionate stimulates the secretion of satiety hormones and reduces acute appetite in a cecal fistula pig model. Anim Nutr (2022) 10:390–8. doi: 10.1016/j.aninu.2022.06.003
45. Forbes S, Stafford S, Coope G, Heffron H, Real K, Newman R, et al. Selective FFA2 agonism appears to act via intestinal PYY to reduce transit and food intake but does not improve glucose tolerance in mouse models. Diabetes (2015) 64(11):3763–71. doi: 10.2337/db15-0481
46. Cook TM, Gavini CK, Jesse J, Aubert G, Gornick E, Bonomo R, et al. Vagal neuron expression of the microbiota-derived metabolite receptor, free fatty acid receptor (FFAR3), is necessary for normal feeding behavior. Mol Metab (2021) 54:101350. doi: 10.1016/j.molmet.2021.101350
47. Guo W, Li W, Su Y, Liu S, Kan X, Ran X, et al. GPR109A alleviate mastitis and enhances the blood milk barrier by activating AMPK/Nrf2 and autophagy. Int J Biol Sci (2021) 17(15):4271–84. doi: 10.7150/ijbs.62380
48. Geisler CE, Miller KE, Ghimire S, Renquist BJ. The role of GPR109a signaling in niacin induced effects on fed and fasted hepatic metabolism. Int J Mol Sci (2021) 22(8):4001. doi: 10.3390/ijms22084001
49. Guo W, Zhang Z, Li L, Liang X, Wu Y, Wang X, et al. Gut microbiota induces DNA methylation via SCFAs predisposing obesity-prone individuals to diabetes. Pharmacol Res (2022) 182:106355. doi: 10.1016/j.phrs.2022.106355
50. Wang Z, Zhang X, Zhu L, Yang X, He F, Wang T, et al. Inulin alleviates inflammation of alcoholic liver disease via SCFAs-inducing suppression of M1 and facilitation of M2 macrophages in mice. Int Immunopharmacol (2020) 78:106062. doi: 10.1016/j.intimp.2019.106062
51. Saad MJ, Santos A, Prada PO. Linking gut microbiota and inflammation to obesity and insulin resistance. Physiol (Bethesda) (2016) 31(4):283–93. doi: 10.1152/physiol.00041.2015
52. Ojo O, Ojo OO, Zand N, Wang X. The effect of dietary fibre on gut microbiota, lipid profile, and inflammatory markers in patients with type 2 diabetes: a systematic review and meta-analysis of randomised controlled trials. Nutrients (2021) 13(6):1805. doi: 10.3390/nu13061805
53. Zhu Q, An YA, Kim M, Zhang Z, Zhao S, Zhu Y, et al. Suppressing adipocyte inflammation promotes insulin resistance in mice. Mol Metab (2020) 39:101010. doi: 10.1016/j.molmet.2020.101010
54. Talepoor AG, Rastegari B, Kalani M, Doroudchi M. Decrease in the inflammatory cytokines of LPS-stimulated PBMCs of patients with atherosclerosis by a TLR-4 antagonist in the co-culture with HUVECs. Int Immunopharmacol (2021) 101(Pt A):108295. doi: 10.1016/j.intimp.2021.108295
55. Hang S, Paik D, Yao L, Kim E, Trinath J, Lu J, et al. Bile acid metabolites control T17 and T cell differentiation. Nature (2019) 576(7785):143–8. doi: 10.1038/s41586-019-1785-z
56. Sayin SI, Wahlström A, Felin J, Jäntti S, Marschall H-U, Bamberg K, et al. Gut microbiota regulates bile acid metabolism by reducing the levels of tauro-beta-muricholic acid, a naturally occurring FXR antagonist. Cell Metab (2013) 17(2):225–35. doi: 10.1016/j.cmet.2013.01.003
57. Huang F, Zheng X, Ma X, Jiang R, Zhou W, Zhou S, et al. Theabrownin from Pu-erh tea attenuates hypercholesterolemia via modulation of gut microbiota and bile acid metabolism. Nat Commun (2019) 10(1):4971. doi: 10.1038/s41467-019-12896-x
58. Tonin F, Otten LG, Arends I. NAD(+) -dependent enzymatic route for the epimerization of hydroxysteroids. Chem Sus Chem (2019) 12(13):3192–203. doi: 10.1002/cssc.201801862
59. Qi X, Yun C, Sun L, Xia J, Wu Q, Wang Y, et al. Gut microbiota-bile acid-interleukin-22 axis orchestrates polycystic ovary syndrome. Nat Med (2019) 25(8):1225–33. doi: 10.1038/s41591-019-0509-0
60. Jia W, Xie G, Jia W. Bile acid-microbiota crosstalk in gastrointestinal inflammation and carcinogenesis. Nat Rev Gastroenterol Hepatol (2018) 15(2):111–28. doi: 10.1038/nrgastro.2017.119
61. Wu Q, Sun L, Hu X, Wang X, Xu F, Chen B, et al. Suppressing the intestinal farnesoid X receptor/sphingomyelin phosphodiesterase 3 axis decreases atherosclerosis. J Clin Invest (2021) 131(9):e142865. doi: 10.1172/JCI142865
62. Shen Y, Lu C, Song Z, Qiao C, Wang J, Chen J, et al. Ursodeoxycholic acid reduces antitumor immunosuppression by inducing CHIP-mediated TGF-β degradation. Nat Commun (2022) 13(1):3419. doi: 10.1038/s41467-022-31141-6
63. Sasaki H, Masuno H, Kawasaki H, Yoshihara A, Numoto N, Ito N, et al. Lithocholic acid derivatives as potent vitamin d receptor agonists. J Med Chem (2021) 64(1):516–26. doi: 10.1021/acs.jmedchem.0c01420
64. Little M, Dutta M, Li H, Matson A, Shi X, Mascarinas G, et al. Understanding the physiological functions of the host xenobiotic-sensing nuclear receptors PXR and CAR on the gut microbiome using genetically modified mice. Acta Pharm Sin B (2022) 12(2):801–20. doi: 10.1016/j.apsb.2021.07.022
65. Yu H. Targeting S1PRs as a therapeutic strategy for inflammatory bone loss diseases-beyond regulating S1P signaling. Int J Mol Sci (2021) 22(9):4411. doi: 10.3390/ijms22094411
66. Wang L, Gong Z, Zhang X, Zhu F, Liu Y, Jin C, et al. Gut microbial bile acid metabolite skews macrophage polarization and contributes to high-fat diet-induced colonic inflammation. Gut Microbes (2020) 12(1):1–20. doi: 10.1080/19490976.2020.1819155
67. Zhang S-Y, Li RJW, Lim Y-M, Batchuluun B, Liu H, Waise TMZ, et al. FXR in the dorsal vagal complex is sufficient and necessary for upper small intestinal microbiome-mediated changes of TCDCA to alter insulin action in rats. Gut (2021) 70(9):1675–83. doi: 10.1136/gutjnl-2020-321757
68. Morstein J, Trads JB, Hinnah K, Willems S, Barber DM, Trauner M, et al. Optical control of the nuclear bile acid receptor FXR with a photohormone. Chem Sci (2020) 11(2):429–34. doi: 10.1039/C9SC02911G
69. Jiao N, Baker SS, Chapa-Rodriguez A, Liu W, Nugent CA, Tsompana M, et al. Suppressed hepatic bile acid signalling despite elevated production of primary and secondary bile acids in NAFLD. Gut (2018) 67(10):1881–91. doi: 10.1136/gutjnl-2017-314307
70. Gulfo J, Rotondo F, Ávalos de León CG, Cornide-Petronio ME, Fuster C, Gracia-Sancho J, et al. FGF15 improves outcomes after brain dead donor liver transplantation with steatotic and non-steatotic grafts in rats. J Hepatol (2020) 73(5):1131–43. doi: 10.1016/j.jhep.2020.05.007
71. Zhao L, Yang W, Chen Y, Huang F, Lu L, Lin C, et al. A clostridia-rich microbiota enhances bile acid excretion in diarrhea-predominant irritable bowel syndrome. J Clin Invest (2020) 130(1):438–50. doi: 10.1172/JCI130976
72. Clifford BL, Sedgeman LR, Williams KJ, Morand P, Cheng A, Jarrett KE, et al. FXR activation protects against NAFLD via bile-acid-dependent reductions in lipid absorption. Cell Metab (2021) 33(8):1671–84.e4. doi: 10.1016/j.cmet.2021.06.012
73. Trivedi PJ, Hirschfield GM, Gershwin ME. Obeticholic acid for the treatment of primary biliary cirrhosis. Expert Rev Clin Pharmacol (2016) 9(1):13–26. doi: 10.1586/17512433.2015.1092381
74. Hirschfield GM, Mason A, Luketic V, Lindor K, Gordon SC, Mayo M, et al. Efficacy of obeticholic acid in patients with primary biliary cirrhosis and inadequate response to ursodeoxycholic acid. Gastroenterology (2015) 148(4):751–61.e8. doi: 10.1053/j.gastro.2014.12.005
75. Zhang DY, Zhu L, Liu HN, Tseng YJ, Weng SQ, Liu TT, et al. The protective effect and mechanism of the FXR agonist obeticholic acid via targeting gut microbiota in non-alcoholic fatty liver disease. Drug Des Devel Ther (2019) 13:2249–70. doi: 10.2147/DDDT.S207277
76. Mudaliar S, Henry RR, Sanyal AJ, Morrow L, Marschall HU, Kipnes M, et al. Efficacy and safety of the farnesoid X receptor agonist obeticholic acid in patients with type 2 diabetes and nonalcoholic fatty liver disease. Gastroenterology (2013) 145(3):574–82.e1. doi: 10.1053/j.gastro.2013.05.042
77. Zheng X, Chen T, Jiang R, Zhao A, Wu Q, Kuang J, et al. Hyocholic acid species improve glucose homeostasis through a distinct TGR5 and FXR signaling mechanism. Cell Metab (2021) 33(4):791–803.e7. doi: 10.1016/j.cmet.2020.11.017
78. Song Y, Xu C, Shao S, Liu J, Xing W, Xu J, et al. Thyroid-stimulating hormone regulates hepatic bile acid homeostasis via SREBP-2/HNF-4α/CYP7A1 axis. J Hepatol (2015) 62(5):1171–9. doi: 10.1016/j.jhep.2014.12.006
79. Jia L, Ma Y, Haywood J, Jiang L, Xue B, Shi H, et al. NPC1L1 deficiency suppresses ileal fibroblast growth factor 15 expression and increases bile acid pool size in high-Fat-Diet-Fed mice. Cells (2021) 10(12):3468. doi: 10.3390/cells10123468
80. Hansen M, Sonne DP, Mikkelsen KH, Gluud LL, Vilsbøll T, Knop FK. Bile acid sequestrants for glycemic control in patients with type 2 diabetes: a systematic review with meta-analysis of randomized controlled trials. J Diabetes Complications (2017) 31(5):918–27. doi: 10.1016/j.jdiacomp.2017.01.011
81. Suzuki T, Oba K, Igari Y, Watanabe K, Matsumura N, Futami-Suda S, et al. Effects of bile-acid-binding resin (colestimide) on blood glucose and visceral fat in Japanese patients with type 2 diabetes mellitus and hypercholesterolemia: an open-label, randomized, case-control, crossover study. J Diabetes Complications (2012) 26(1):34–9. doi: 10.1016/j.jdiacomp.2011.11.008
82. McMurdie PJ, Stoeva MK, Justice N, Nemchek M, Sieber CMK, Tyagi S, et al. Increased circulating butyrate and ursodeoxycholate during probiotic intervention in humans with type 2 diabetes. BMC Microbiol (2022) 22(1):19. doi: 10.1186/s12866-021-02415-8
83. Di Bisceglie AM, Watts GF, Lavin P, Yu M, Bai R, Liu L. Pharmacokinetics and pharmacodynamics of HTD1801 (berberine ursodeoxycholate, BUDCA) in patients with hyperlipidemia. Lipids Health Dis (2020) 19(1):239. doi: 10.1186/s12944-020-01406-4
84. Harrison SA, Gunn N, Neff GW, Kohli A, Liu L, Flyer A, et al. A phase 2, proof of concept, randomised controlled trial of berberine ursodeoxycholate in patients with presumed non-alcoholic steatohepatitis and type 2 diabetes. Nat Commun (2021) 12(1):5503. doi: 10.1038/s41467-021-25701-5
85. Sun R, Kong B, Yang N, Cao B, Feng D, Yu X, et al. The hypoglycemic effect of berberine and berberrubine involves modulation of intestinal farnesoid X receptor signaling pathway and inhibition of hepatic gluconeogenesis. Drug Metab Dispos (2021) 49(3):276–86. doi: 10.1124/dmd.120.000215
86. Feingold KR, Anawalt B, Boyce A, Chrousos G, Dungan K, Grossman A, et al. Cholesterol lowering drugs. Feingold KR, Anawalt B, Boyce A, Chrousos G, de Herder WW, Dhatariya K, editors. Endotext. South Dartmouth (MA: MDText.com, Inc (2000).
87. Hendrikx T, Schnabl B. Indoles: metabolites produced by intestinal bacteria capable of controlling liver disease manifestation. J Intern Med (2019) 286(1):32–40. doi: 10.1111/joim.12892
88. Qi Q, Li J, Yu B, Moon J-Y, Chai JC, Merino J, et al. Host and gut microbial tryptophan metabolism and type 2 diabetes: an integrative analysis of host genetics, diet, gut microbiome and circulating metabolites in cohort studies. Gut (2022) 71(6):1095–105. doi: 10.1136/gutjnl-2021-324053
89. Dodd D, Spitzer MH, Van Treuren W, Merrill BD, Hryckowian AJ, Higginbottom SK, et al. A gut bacterial pathway metabolizes aromatic amino acids into nine circulating metabolites. Nature (2017) 551(7682):648–52. doi: 10.1038/nature24661
90. Roager HM, Licht TR. Microbial tryptophan catabolites in health and disease. Nat Commun (2018) 9(1):3294. doi: 10.1038/s41467-018-05470-4
91. Venkatesh M, Mukherjee S, Wang H, Li H, Sun K, Benechet Alexandre P, et al. Symbiotic bacterial metabolites regulate gastrointestinal barrier function via the xenobiotic sensor PXR and toll-like receptor 4. Immunity (2014) 41(2):296–310. doi: 10.1016/j.immuni.2014.06.014
92. Delzenne NM, Knudsen C, Beaumont M, Rodriguez J, Neyrinck AM, Bindels LB. Contribution of the gut microbiota to the regulation of host metabolism and energy balance: a focus on the gut-liver axis. Proc Nutr Soc (2019) 78(3):319–28. doi: 10.1017/S0029665118002756
93. Rothhammer V, Quintana FJ. The aryl hydrocarbon receptor: an environmental sensor integrating immune responses in health and disease. Nat Rev Immunol (2019) 19(3):184–97. doi: 10.1038/s41577-019-0125-8
94. de Vos WM, Tilg H, Van Hul M, Cani PD. Gut microbiome and health: mechanistic insights. Gut (2022) 71(5):1020–32. doi: 10.1136/gutjnl-2021-326789
95. Kalagi NA, Thota RN, Stojanovski E, Alburikan KA, Garg ML. Association between plasma trimethylamine n-oxide levels and type 2 diabetes: a case control study. Nutrients (2022) 14(10):2093. doi: 10.3390/nu14102093
96. Li SY, Chen S, Lu XT, Fang AP, Chen YM, Huang RZ, et al. Serum trimethylamine-n-oxide is associated with incident type 2 diabetes in middle-aged and older adults: a prospective cohort study. J Transl Med (2022) 20(1):374. doi: 10.1186/s12967-022-03581-7
97. Croyal M, Saulnier PJ, Aguesse A, Gand E, Ragot S, Roussel R, et al. Plasma trimethylamine n-oxide and risk of cardiovascular events in patients with type 2 diabetes. J Clin Endocrinol Metab (2020) 105(7):2371–80. doi: 10.1210/clinem/dgaa188
98. Xu N, Wan J, Wang C, Liu J, Qian C, Tan H. Increased serum trimethylamine n-oxide level in type 2 diabetic patients with mild cognitive impairment. Diabetes Metab Syndr Obes (2022) 15:2197–205. doi: 10.2147/DMSO.S370206
99. Jia J, Dou P, Gao M, Kong X, Li C, Liu Z, et al. Assessment of causal direction between gut microbiota-dependent metabolites and cardiometabolic health: a bidirectional mendelian randomization analysis. Diabetes (2019) 68(9):1747–55. doi: 10.2337/db19-0153
100. Tang WH, Wang Z, Levison BS, Koeth RA, Britt EB, Fu X, et al. Intestinal microbial metabolism of phosphatidylcholine and cardiovascular risk. N Engl J Med (2013) 368(17):1575–84. doi: 10.1056/NEJMoa1109400
101. Deng Y, Zhou Q, Wu Y, Chen X, Zhong F. Properties and mechanisms of flavin-dependent monooxygenases and their applications in natural product synthesis. Int J Mol Sci (2022) 23(5):2622. doi: 10.3390/ijms23052622
102. Wang Z, Roberts AB, Buffa JA, Levison BS, Zhu W, Org E, et al. Non-lethal inhibition of gut microbial trimethylamine production for the treatment of atherosclerosis. Cell (2015) 163(7):1585–95. doi: 10.1016/j.cell.2015.11.055
103. Kuka J, Videja M, Makrecka-Kuka M, Liepins J, Grinberga S, Sevostjanovs E, et al. Metformin decreases bacterial trimethylamine production and trimethylamine n-oxide levels in db/db mice. Sci Rep (2020) 10(1):14555. doi: 10.1038/s41598-020-71470-4
104. Ma SR, Tong Q, Lin Y, Pan LB, Fu J, Peng R, et al. Berberine treats atherosclerosis via a vitamine-like effect down-regulating choline-TMA-TMAO production pathway in gut microbiota. Signal Transduct Target Ther (2022) 7(1):207. doi: 10.1038/s41392-022-01027-6
105. Steinke I, Ghanei N, Govindarajulu M, Yoo S, Zhong J, Amin RH. Drug discovery and development of novel therapeutics for inhibiting TMAO in models of atherosclerosis and diabetes. Front Physiol (2020) 11:567899. doi: 10.3389/fphys.2020.567899
106. Annunziata G, Maisto M, Schisano C, Ciampaglia R, Narciso V, Tenore GC, et al. Effects of grape pomace polyphenolic extract (Taurisolo(®)) in reducing TMAO serum levels in humans: preliminary results from a randomized, placebo-controlled, cross-over study. Nutrients (2019) 11(1):139. doi: 10.3390/nu11010139
107. Gao X, Liu X, Xu J, Xue C, Xue Y, Wang Y. Dietary trimethylamine n-oxide exacerbates impaired glucose tolerance in mice fed a high fat diet. J Biosci Bioeng (2014) 118(4):476–81. doi: 10.1016/j.jbiosc.2014.03.001
108. Dahiya D, Nigam PS. The gut microbiota influenced by the intake of probiotics and functional foods with prebiotics can sustain wellness and alleviate certain ailments like gut-inflammation and colon-cancer. Microorganisms (2022) 10(3):665. doi: 10.3390/microorganisms10030665
109. Alard J, Lehrter V, Rhimi M, Mangin I, Peucelle V, Abraham AL, et al. Beneficial metabolic effects of selected probiotics on diet-induced obesity and insulin resistance in mice are associated with improvement of dysbiotic gut microbiota. Environ Microbiol (2016) 18(5):1484–97. doi: 10.1111/1462-2920.13181
110. Kim DE, Kim JK, Han SK, Jang SE, Han MJ, Kim DH. Lactobacillus plantarum NK3 and bifidobacterium longum NK49 alleviate bacterial vaginosis and osteoporosis in mice by suppressing NF-κB-Linked TNF-α expression. J Med Food (2019) 22(10):1022–31. doi: 10.1089/jmf.2019.4419
111. Archer AC, Muthukumar SP, Halami PM. Lactobacillus fermentum MCC2759 and MCC2760 alleviate inflammation and intestinal function in high-fat diet-fed and streptozotocin-induced diabetic rats. Probiotics Antimicrob Proteins (2021) 13(4):1068–80. doi: 10.1007/s12602-021-09744-0
112. Birkeland E, Gharagozlian S, Birkeland KI, Valeur J, Måge I, Rud I, et al. Prebiotic effect of inulin-type fructans on faecal microbiota and short-chain fatty acids in type 2 diabetes: a randomised controlled trial. Eur J Nutr (2020) 59(7):3325–38. doi: 10.1007/s00394-020-02282-5
113. Biruete A, Cross TL, Allen JM, Kistler BM, de Loor H, Evenepoel P, et al. Effect of dietary inulin supplementation on the gut microbiota composition and derived metabolites of individuals undergoing hemodialysis: a pilot study. J Ren Nutr (2021) 31(5):512–22. doi: 10.1053/j.jrn.2020.10.003
114. van der Beek CM, Canfora EE, Kip AM, Gorissen SHM, Olde Damink SWM, van Eijk HM, et al. The prebiotic inulin improves substrate metabolism and promotes short-chain fatty acid production in overweight to obese men. Metabolism (2018) 87:25–35. doi: 10.1016/j.metabol.2018.06.009
115. Chambers ES, Viardot A, Psichas A, Morrison DJ, Murphy KG, Zac-Varghese SE, et al. Effects of targeted delivery of propionate to the human colon on appetite regulation, body weight maintenance and adiposity in overweight adults. Gut (2015) 64(11):1744–54. doi: 10.1136/gutjnl-2014-307913
116. Yao F, Jia R, Huang H, Yu Y, Mei L, Bai L, et al. Effect of lactobacillus paracasei N1115 and fructooligosaccharides in nonalcoholic fatty liver disease. Arch Med Sci (2019) 15(5):1336–44. doi: 10.5114/aoms.2019.86611
117. Wang H, Lu Y, Yan Y, Tian S, Zheng D, Leng D, et al. Promising treatment for type 2 diabetes: fecal microbiota transplantation reverses insulin resistance and impaired islets. Front Cell Infect Microbiol (2019) 9:455. doi: 10.3389/fcimb.2019.00455
118. Han X, Wang Y, Zhang P, Zhu M, Li L, Mao X, et al. Kazak faecal microbiota transplantation induces short-chain fatty acids that promote glucagon-like peptide-1 secretion by regulating gut microbiota in mice. Pharm Biol (2021) 59(1):1077–87. doi: 10.1080/13880209.2021.1954667
119. Zhang P-P, Li L-L, Han X, Li Q-W, Zhang X-H, Liu JJ, et al. Fecal microbiota transplantation improves metabolism and gut microbiome composition in db/db mice. Acta Pharmacol Sin (2020) 41(5):678–85. doi: 10.1038/s41401-019-0330-9
120. Wang C, Wang Y, Yang H, Tian Z, Zhu M, Sha X, et al. Uygur type 2 diabetes patient fecal microbiota transplantation disrupts blood glucose and bile acid levels by changing the ability of the intestinal flora to metabolize bile acids in C57BL/6 mice. BMC Endocr Disord (2022) 22(1):236. doi: 10.1186/s12902-022-01155-8
121. Vrieze A, Van Nood E, Holleman F, Salojärvi J, Kootte RS, Bartelsman JF, et al. Transfer of intestinal microbiota from lean donors increases insulin sensitivity in individuals with metabolic syndrome. Gastroenterology (2012) 143(4):913–6.e7. doi: 10.1053/j.gastro.2012.06.031
122. Su L, Hong Z, Zhou T, Jian Y, Xu M, Zhang X, et al. Health improvements of type 2 diabetic patients through diet and diet plus fecal microbiota transplantation. Sci Rep (2022) 12(1):1152. doi: 10.1038/s41598-022-05127-9
123. Yu EW, Gao L, Stastka P, Cheney MC, Mahabamunuge J, Torres Soto M, et al. Fecal microbiota transplantation for the improvement of metabolism in obesity: the FMT-TRIM double-blind placebo-controlled pilot trial. PloS Med (2020) 17(3):e1003051. doi: 10.1371/journal.pmed.1003051
124. Khoruts A, Sadowsky MJ. Understanding the mechanisms of faecal microbiota transplantation. Nat Rev Gastroenterol Hepatol (2016) 13(9):508–16. doi: 10.1038/nrgastro.2016.98
125. Dailey FE, Turse EP, Daglilar E, Tahan V. The dirty aspects of fecal microbiota transplantation: a review of its adverse effects and complications. Curr Opin Pharmacol (2019) 49:29–33. doi: 10.1016/j.coph.2019.04.008
126. Kang M, Choe D, Kim K, Cho B-K, Cho S. Synthetic biology approaches in the development of engineered therapeutic microbes. Int J Mol Sci (2020) 21(22):8744. doi: 10.3390/ijms21228744
127. Bloom PP, Tapper EB, Young VB, Lok AS. Microbiome therapeutics for hepatic encephalopathy. J Hepatol (2021) 75(6):1452–64. doi: 10.1016/j.jhep.2021.08.004
128. Magkos F, Hjorth MF, Astrup A. Diet and exercise in the prevention and treatment of type 2 diabetes mellitus. Nat Rev Endocrinol (2020) 16(10):545–55. doi: 10.1038/s41574-020-0381-5
129. Taheri S, Chagoury O, Zaghloul H, Elhadad S, Ahmed SH, Omar O, et al. Diabetes intervention accentuating diet and enhancing metabolism (DIADEM-i): a randomised controlled trial to examine the impact of an intensive lifestyle intervention consisting of a low-energy diet and physical activity on body weight and metabolism in early type 2 diabetes mellitus: study protocol for a randomized controlled trial. Trials (2018) 19(1):284. doi: 10.1186/s13063-018-2660-1
130. Clark AL, Yan Z, Chen SX, Shi V, Kulkarni DH, Diwan A, et al. High-fat diet prevents the development of autoimmune diabetes in NOD mice. Diabetes Obes Metab (2021) 23(11):2455–65. doi: 10.1111/dom.14486
131. Ding J-T, Yang K-P, Lin K-L, Cao Y-K, Zou F. Mechanisms and therapeutic strategies of immune checkpoint molecules and regulators in type 1 diabetes. Front Endocrinol (Lausanne) (2023) 13. doi: 10.3389/fendo.2022.1090842
132. Li M, Wang S, Li Y, Zhao M, Kuang J, Liang D, et al. Gut microbiota-bile acid crosstalk contributes to the rebound weight gain after calorie restriction in mice. Nat Commun (2022) 13(1):2060. doi: 10.1038/s41467-022-29589-7
133. Brønden A, Knop FK. Gluco-metabolic effects of pharmacotherapy-induced modulation of bile acid physiology. J Clin Endocrinol Metab (2020) 105(1):dgz025. doi: 10.1210/clinem/dgz025
134. Harreiter J, Simmons D, Desoye G, Corcoy R, Adelantado JM, Devlieger R, et al. Nutritional lifestyle intervention in obese pregnant women, including lower carbohydrate intake, is associated with increased maternal free fatty acids, 3-β-Hydroxybutyrate, and fasting glucose concentrations: a secondary factorial analysis of the European multicenter, randomized controlled DALI lifestyle intervention trial. Diabetes Care (2019) 42(8):1380–9. doi: 10.2337/dc19-0418
135. Olson CA, Vuong HE, Yano JM, Liang QY, Nusbaum DJ, Hsiao EY. The gut microbiota mediates the anti-seizure effects of the ketogenic diet. Cell (2018) 173(7):1728–41.e13. doi: 10.1016/j.cell.2018.04.027
136. Ang QY, Alexander M, Newman JC, Tian Y, Cai J, Upadhyay V, et al. Ketogenic diets alter the gut microbiome resulting in decreased intestinal Th17 cells. Cell (2020) 181(6):1263–75.e16. doi: 10.1016/j.cell.2020.04.027
137. Santiago-Rodriguez TM, Hollister EB. Human virome and disease: high-throughput sequencing for virus discovery, identification of phage-bacteria dysbiosis and development of therapeutic approaches with emphasis on the human gut. Viruses (2019) 11(7):656. doi: 10.3390/v11070656
138. Yang K, Niu J, Zuo T, Sun Y, Xu Z, Tang W, et al. Alterations in the gut virome in obesity and type 2 diabetes mellitus. Gastroenterology (2021) 161(4):1257–1269.e13. doi: 10.1053/j.gastro.2021.06.056
139. Rasmussen TS, Mentzel CMJ, Kot W, Castro-Mejía JL, Zuffa S, Swann JR, et al. Faecal virome transplantation decreases symptoms of type 2 diabetes and obesity in a murine model. Gut (2020) 69(12):2122–30. doi: 10.1136/gutjnl-2019-320005
140. Huon J-F, Montassier E, Leroy A-G, Grégoire M, Vibet M-A, Caillon J, et al. Phages versus antibiotics to treat infected diabetic wounds in a mouse model: a microbiological and microbiotic evaluation. mSystems (2020) 5(6):e00542–20141. doi: 10.1128/mSystems.00542-20
141. Yang J, Zheng P, Li Y, Wu J, Tan X, Zhou J, et al. Landscapes of bacterial and metabolic signatures and their interaction in major depressive disorders. Sci Adv (2020) 6(49). doi: 10.1126/sciadv.aba8555
142. Du L, Li Q, Yi H, Kuang T, Tang Y, Fan G. Gut microbiota-derived metabolites as key actors in type 2 diabetes mellitus. BioMed Pharmacother (2022) 149:112839. doi: 10.1016/j.biopha.2022.112839
143. Montassier E, Gastinne T, Vangay P, Al-Ghalith GA, Bruley des Varannes S, Massart S, et al. Chemotherapy-driven dysbiosis in the intestinal microbiome. Aliment Pharmacol Ther (2015) 42(5):515–28. doi: 10.1111/apt.13302
144. Barone M, Rampelli S, Biagi E, Bertozzi SM, Falchi F, Cavalli A, et al. Searching for new microbiome-targeted therapeutics through a drug repurposing approach. J Med Chem (2021) 64(23):17277–86. doi: 10.1021/acs.jmedchem.1c01333
145. Plovier H, Everard A, Druart C, Depommier C, Van Hul M, Geurts L, et al. A purified membrane protein from akkermansia muciniphila or the pasteurized bacterium improves metabolism in obese and diabetic mice. Nat Med (2017) 23(1):107–13. doi: 10.1038/nm.4236
146. Yan J, Sheng L, Li H. : is it the holy grail for ameliorating metabolic diseases? Gut Microbes (2021) 13(1):1984104. doi: 10.1080/19490976.2021.1984104
147. Scheiman J, Luber JM, Chavkin TA, MacDonald T, Tung A, Pham L-D, et al. Meta-omics analysis of elite athletes identifies a performance-enhancing microbe that functions via lactate metabolism. Nat Med (2019) 25(7):1104–9. doi: 10.1038/s41591-019-0485-4
148. Hughes HK, Rose D, Ashwood P. The gut microbiota and dysbiosis in autism spectrum disorders. Curr Neurol Neurosci Rep (2018) 18(11):81. doi: 10.1007/s11910-018-0887-6
149. Hagar HH, Almubrik SA, Attia NM, Aljasser SN. Mesna alleviates cerulein-induced acute pancreatitis by inhibiting the inflammatory response and oxidative stress in experimental rats. Dig Dis Sci (2020) 65(12):3583–91. doi: 10.1007/s10620-020-06072-1
150. Cauli O. Oxidative stress and cognitive alterations induced by cancer chemotherapy drugs: a scoping review. Antioxid (Basel) (2021) 10(7):1116. doi: 10.3390/antiox10071116
151. Jastrząb R, Graczyk D, Siedlecki P. Molecular and cellular mechanisms influenced by postbiotics. Int J Mol Sci (2021) 22(24):13475. doi: 10.3390/ijms222413475
152. Lee J, Park S, Oh N, Park J, Kwon M, Seo J, et al. Oral intake of lactobacillus plantarum l-14 extract alleviates TLR2- and AMPK-mediated obesity-associated disorders in high-fat-diet-induced obese C57BL/6J mice. Cell Prolif (2021) 54(6):e13039. doi: 10.1111/cpr.13039
153. Huang Z, Lin F, Zhu X, Zhang C, Jiang M, Lu Z. An exopolysaccharide from lactobacillus plantarum H31 in pickled cabbage inhibits pancreas α-amylase and regulating metabolic markers in HepG2 cells by AMPK/PI3K/Akt pathway. Int J Biol Macromol (2020) 143:775–84. doi: 10.1016/j.ijbiomac.2019.09.137
154. Li X, Chen L, Zhu X, Lu Z, Lu Y. Effect of γ-aminobutyric acid-rich yogurt on insulin sensitivity in a mouse model of type 2 diabetes mellitus. J Dairy Sci. (2020) 103(9):7719–29. doi: 10.3168/jds.2019-17757
155. Marques TM, Patterson E, Wall R, O’Sullivan O, Fitzgerald GF, Cotter PD, et al. Influence of GABA and GABA-producing lactobacillus brevis DPC 6108 on the development of diabetes in a streptozotocin rat model. Beneficial Microbes (2016) 7(3):409–20. doi: 10.3920/BM2015.0154
156. Patterson E, Ryan PM, Cryan JF, Dinan TG, Ross RP, Fitzgerald GF, et al. Gut microbiota, obesity and diabetes. Postgraduate Med J (2016) 92(1087):286–300. doi: 10.1136/postgradmedj-2015-133285
Keywords: type 2 diabetes mellitus, gut microbiota, gut microbial metabolites, targeted therapy, probiotics
Citation: Wu J, Yang K, Fan H, Wei M and Xiong Q (2023) Targeting the gut microbiota and its metabolites for type 2 diabetes mellitus. Front. Endocrinol. 14:1114424. doi: 10.3389/fendo.2023.1114424
Received: 02 December 2022; Accepted: 28 April 2023;
Published: 09 May 2023.
Edited by:
Yan Shu, University of Maryland, United StatesReviewed by:
Elena Rampanelli, Amsterdam University Medical Center, NetherlandsCopyright © 2023 Wu, Yang, Fan, Wei and Xiong. This is an open-access article distributed under the terms of the Creative Commons Attribution License (CC BY). The use, distribution or reproduction in other forums is permitted, provided the original author(s) and the copyright owner(s) are credited and that the original publication in this journal is cited, in accordance with accepted academic practice. No use, distribution or reproduction is permitted which does not comply with these terms.
*Correspondence: Meilin Wei, d2VpbWVpbGluMTAwN0AxNjMuY29t; Qin Xiong, RWxpbm9yXzIwQGZveG1haWwuY29t
†These authors have contributed equally to this work and share first authorship
Disclaimer: All claims expressed in this article are solely those of the authors and do not necessarily represent those of their affiliated organizations, or those of the publisher, the editors and the reviewers. Any product that may be evaluated in this article or claim that may be made by its manufacturer is not guaranteed or endorsed by the publisher.
Research integrity at Frontiers
Learn more about the work of our research integrity team to safeguard the quality of each article we publish.