- 1Department of Pharmacy, The Affiliated Hospital of Southwest Medical University, Luzhou, China
- 2School of Pharmacy, Southwest Medical University, Luzhou, China
- 3Department of Pharmacy, Chengdu Seventh People’s Hospital, Chengdu, Sichuan, China
Over the past few decades, increasing prevalence of obesity caused an enormous medical, social, and economic burden. As the sixth most important risk factor contributing to the overall burden of disease worldwide, obesity not only directly harms the human body, but also leads to many chronic diseases such as diabetes, cardiovascular diseases (CVD), nonalcoholic fatty liver disease (NAFLD), and mental illness. Weight loss is still one of the most effective strategies against obesity and related disorders. Recently, the link between intestinal microflora and metabolic health has been constantly established. Butyrate, a four-carbon short-chain fatty acid, is a major metabolite of the gut microbiota that has many beneficial effects on metabolic health. The anti-obesity activity of butyrate has been demonstrated, but its mechanisms of action have not been fully described. This review summarizes current knowledge of butyrate, including its production, absorption, distribution, metabolism, and the effect and mechanisms involved in weight loss and obesity-related diseases. The aim was to contribute to and advance our understanding of butyrate and its role in obesity. Further exploration of butyrate and its pathway may help to identify new anti-obesity.
1 Introduction
Over the past few decades, obesity and related health problems have increased with the accessibility to high calorie foods with low nutritional value and changes in lifestyle (1, 2). In the early 2000s, 1.1 billion adults and 10% of children were classified as overweight or obese (3). According to the last data from the World Health Organization, the number of overweight or obese people has risen to more than 1.9 billion adults aged 18 years and older and over 18% of children (4). Obesity is measured by the body mass index (BMI), which is body weight/height squared (kg/m2), and people with a BMI ≥30 kg/m2 are classified as obese (5). Obesity has been officially recognized as a disease since 1985, and ongoing research has shown that obesity is a serious threat to human health (6). Current evidence confirms that obesity is associated not only with chronic illnesses such as diabetes, atherosclerosis, and cardiovascular disease (CVD) (7–9), but also with cancer (10) and cognitive impairment (11). Beyond this, obesity also severely decreases both physical and mental aspects of the quality of life for people (12, 13). Thus, we need to focus more on the health risks and therapeutic approaches to obesity.
Abnormal or excess accumulation of fat that leads to obesity and impairs health (4), results from an energy imbalance, with more calories consumed than expended (14), and most interventions to control or treat obesity involve calorie restriction (15). Evidence provided by an increasing number of confirms that the composition of the gut microbiota influences the energy metabolism and metabolic health of the human host (16). Changes in human gut microbiota have been linked to obesity, and weight loss influences the composition of the gut microbiota (17). More importantly, supplementation with Akkermansia muciniphila (a kind of intestinal microflora) in overweight and obese human volunteers was able to improve obesity or obesity-related phenotypes (18). Short-chain fatty acids (SCFAs), which constitute the most abundant metabolites of microbial fermentation from undigested dietary carbohydrates, are crucial mediators between microbiota and host metabolism (19). The most abundant SCFAs in the human body include acetate (C2), propionate (C3) and butyrate (C4) and they are also the most abundant anions in the colon (20). Among SCFAs, acetate shows more obesogenic effect. Research has shown that chronic increase in acetate would promote chronic hyperinsulinemia, hyperphagia, and weight gain and the associated sequelae of obesity by the activation of the parasympathetic nervous system (21). Acetate also contributes to the synthesis of cholesterol and the synthesis of lipids in liver (22, 23). Recently, evidence indicate that local acetate inhibits brown fat function (24). Propionate has been relatively poorly studied in relation to obesity, although metabolic benefits have also been reported. In SCFA, butyrate has the most important systemic effects. There are a large number of reports that butyrate is inextricably related to obesity and weight loss. Butyrate is known to have beneficial effects on cellular energy metabolism and intestinal homeostasis (25), and to mediate regulation of whole-body energy homeostasis by the gut microbiota (26). Evidence points to the involvement of decreased butyrate production in metabolic diseases such as diabetes (27). Studies of microbiota composition have revealed that diabetic and obese patients have lower levels of butyrate-producing bacteria, while dietary supplementation with butyrate ameliorates inflammation, insulin resistance and weight gain (28, 29). However, the amount of butyrate in stools has been found to be higher in overweight and obese volunteers than in lean volunteers (30, 31), which led some researchers to believe that butyrate may contribute to the obesogenic phenotype (32). However, fecal concentrations may not accurately reflect physiological concentrations since <10% of butyrate production is excreted in feces and mice studies suggested that the obese microbiota actually has a reduced capacity to produce butyrate (33). The specific effect of butyrate on obesity remains unclear. This review aims to contribute to further related research in the future by summarizing current knowledge about the butyrate, especially its effects and mechanisms of action in obesity.
2 In vivo process of butyrate
2.1 Production source of butyrate
Some studies showed that dietary fiber can exert similar effects with butyrate via enhanced gut butyrate production (34–36). Thus, an understanding of how butyrate is produced in the gut can help to better understand its effects. It is well known that butyrate is produced from carbohydrate polymers via glycolysis by gut microbiota, such as Eubacterium rectale, Faecalibacterium prausnitzii (37, 38). The fermentation substrates are dietary fibers that are transported to the colon after escaping digestion in the upper gastrointestinal tract (38), and are also commonly known as a kind of dietary fibers including undigested plant polysaccharides, resistant starch (RS), and nondigestible oligosaccharides (NDOs) (39, 40). The undigested plant polysaccharides are usually classified into two types: water-soluble polysaccharides and water-insoluble polysaccharides (38). Water-insoluble fibers such as lignin, cellulose, and some hemicelluloses are resistant to fermentation (41). Water-insoluble polysaccharides are usually hydrolyzed to smaller soluble fragments, and are then fermented to produce butyrate (42). Water-soluble polysaccharides, such as pectin,β-glucans, FOS, inulin and gums, tend to be more completely fermented by colonic microflora (41). Most fiber-containing foods contain about one-third soluble and two-thirds insoluble fiber (43). Resistant starch that was able to resist the digestion in the small intestine will arrive at the colon where they will be fermented by the gut microbiota, producing a variety of products including butyrate (44). RS is usually present in cereal grains, seeds, cooked starch, and starch-containing foods like potato, barley, wheat and corn (44, 45). The nondigestible oligosaccharides (NDOs) are typically saccharides containing between 3 and 10 sugar moieties, including isomalt-oligosaccharides (IMOs), galacto-oligosaccharides (GOSs), XOSs, and some pseudo-oligosaccharides, such as acarbose (38, 46).
Butyrate is also formed as products from peptide and amino acid fermentation, although amino acid-fermenting bacteria have been estimated to constitute less than 1% of the large intestinal microbiota (47). The amino acid which can produce butyrate from microbial fermentation include glutamate, lysine, histidine, cysteine, serine and methionine (48). Apart from that, butyrate also occurs naturally in dairy products, like whole cow’s milk (~0.1 g/100g), butter (~3 g/100 g), cheese (especially goat’s cheese (~1-1.8 g/100 g), parmesan (~1.5 g/100 g) (49), and human breast milk(uptake of an estimated amount of approximately 30mg/kg in a breast-fed baby) (50).
2.2 Gut microbiota and pathway of butyrate production
Previously described butyrate-produced microbiota in the human gastrointestinal intestinal tract were commonly distributed in the phylum Firmicutes and the order Clostridiales (38). Most of these producing bacteria are in four main families: Clostridiaceae, Eubacteriaceae, Lachnospiraceae, and Ruminococcaceae (37, 51, 52). Most butyrate-produced microbiota in the order Clostridiales are widely distributed in several clusters, including clusters IV, XIVa, XVI, and I (53). Moreover, other typical butyrogenic species like Roseburia spp, Anaerostipes spp, Clostridium spp, Ruminococcus spp, Coprococcus spp and Butyrivibrio spp are widely distributed across cluster XIVa, and Butyricicoccus pullicaecorum, Subdoligranulum variabile, Anaerotruncus colihominis, and Papillibacter cinnamivorans are cluster IV (51, 52, 54).
Butyrate is produced from carbohydrates by glycolysis that involves the combination of two molecules of acetyl-CoA to form acetoacetyl-CoA followed by stepwise reduction to butyryl-CoA (37). In addition to acetyl-CoA, there are three other pathways known to produce butyrate, glutarate, 4-aminobutyrate, and lysine and all pathways coalesce at a central energy-generating step where crotonyl-CoA is transformed into butyryl-CoA, catalyzed by the electron-transferring flavoprotein butyryl-CoA dehydrogenase (52). Two different pathways are known for the final step in butyrate formation from butyryl-CoA, which proceeds either via butyryl-CoA: acetate CoA-transferase or via phosphotransbutyrylase and butyrate kinase (51). A screen of 38 butyrate-producing human gut isolates found that the butyryl-CoA: acetate CoA-transferase route was far more prevalent in this ecosystem than the butyrate kinase route (55).
2.3 Factors affecting butyrate production
At first, the solubility of fermentation substrate significantly affects the fermentation of NDCs (38). Soluble NDCs are generally more susceptible to fermentation by gut microbiota than insoluble NDCs (56), and highly fermentable to be rapidly consumed by microbes (57). In addition to solubility, carbohydrate chain length also affects butyrate production (38). Generally, NDCs with longer chains have relatively lower utilization rate and are more resistant to intestinal fermentation, which results in a more distal type of metabolism (58). In contrast, NDCs with a shorter chain are more accessible to the microflora, which produces butyrate more rapidly (58, 59). Differences in the orientation and the position of the glycosidic bond and monomeric composition of NDCs may affect the production of butyrate (38).
The gut environment also impacts butyrate production. Particularly, the gut pH tremendously affects the concentration of butyrate because of differing tolerance to low pH of the major bacterial functional groups that comprise the human colonic microbiota (60). PH modulates microbial colonization in the upper gastrointestinal tract (61), and affects the metabolic activity and composition of microbial community (62). Some studies have shown that butyrate formation is affected by lowering of the gut pH (5.5) (60, 63, 64). Butyrate producers are also sensitive to iron availability, while butyrate production is enhanced at high iron concentrations (65, 66). Furthermore, the concentration of intestinal gases, like the oxygen and hydrogen also influences butyrate formation (67, 68).
2.4 Butyrate absorption
Butyrate is mainly considered be absorbed via active transport mediated by monocarboxylate transporters (MCTs) (69). MCT is coupled to a transmembrane H+-gradient that aids the transport and absorption of butyrate (70, 71). Specific isoforms of MCTs that are expressed in the colonic cell membrane that faces the lumen recognize butyrate as a substrate (72). However, the driving force for the uphill entry of butyrate from the lumen into colonocytes via MCTs is very little, because the magnitude of the transmembrane H+ gradient across the colonocyte apical membrane is puny (73). Out of the four functional MCTs, butyrate is mainly the substrate of transporters MCT1 (SLC16A1) and MCT4 (SLC16A3) (74). MCT1 is expressed both in the apical membrane and basolateral membrane of colonic epithelium whereas MCT4 specifically in the basolateral membrane (75). In terms of structure, human MCT1 consists of 500 amino acids with 12 putative transmembrane domains, with both amino- and carboxy-termini positioned on the membrane’s cytoplasmic side (76). MCT1 is highly expressed in Caco-2 cell and play a major role in the apical uptake of butyrate (77, 78). MCT4 consist of 465 amino acids and 12 transmembrane domains (79). MCT4 was shown to be a high-affinity butyrate transporter in gut epithelial cells (80). Beyond that, solute carrier (SLC) family 5 member 8 (SLC5A8), which is a Na+-coupled co-transporter and also known as sodium-coupled monocarboxylate transporter 1 (SMCT1), facilitates the transport and absorption of butyrate (81, 82). SMCT1 consists of 610 amino acids (83), primarily distributing in kidney and intestine (84). Butyrate is more readily transported by SLC5A8,which is relatively a high-affinity transporter with affinities for the butyrate in the sub-millimolar range (73). Some studies have also reported simple passive diffusion as a convincing model of butyrate transport (85).
2.5 Butyrate distribution
Up to 95% of butyrate produced by the gut microbiota is absorbed and used in colonocytes and only a very small part is absorbed into the circulation (26) via the hepatic portal vein, which connects the gastrointestinal tract, spleen and liver (86, 87). Butyrate concentrations in the portal vein are ~18µmol/l in a fasting human and 14-64µmol/l in sudden death victims (86, 88), while the concentrations in peripheral blood are low to ~20% of portal vein concentrations (89). Some studies have reported that oral delivery of dietary fiber or butyrate, or colonic infusion of butyrate was able to increase the butyrate concentration in the plasma of circulating blood (90–92). A study that followed the distribution of 11C-labeled butyric acid in baboons found relatively high accumulation of the label in the spleen, and pancreas (93). Another study reported distribution of 13C-labeled butyrate in the intestine, brain, brown adipose tissue (BAT), white adipose tissue (WAT), and especially the brain (94). Liu et al. found slightly elevated butyrate levels in the brains of mice supplemented with live Clostridium butyricum (95).
2.6 Butyrate metabolism and excretion
Butyrate is predominantly metabolized in the colon as an energy substrate, with small concentrations utilized by the liver and kidneys, providing up to 70% of the energy needs of colon cells, and the amount of butyrate metabolized was followed by the excretion of CO2 in breath (96, 97). In colon cells, butyrate is metabolized by mitochondrial β-oxidation to generate NADH, H+ and acetyl-CoA, and in turn can further be used to generate ATP in the citric acid cycle in the mitochondria (97). Butyrate enters into the tricarboxylic acid (TCA) cycle as acetyl-CoA and is converted to citrate, oxaloacetate, triosephosphate, and subsequently in glucose synthesis (98). In addition to the above, butyrate is metabolized to produce fatty acids, cholesterol, and ketone bodies (98). Approximately 10% of butyrate is excreted in the feces (41) and fecal butyrate levels are increased by a diet high in dietary fiber or resistance starches (99).
3 Cellular signaling pathways of butyrate
3.1 G protein-coupled receptors (GPCRs)
Butyrate is the ligand for metabolite-sensing G-protein coupled receptors (GPCRs), mainly GPR43, GPR41, and GPR109a (100), which are also known as free fatty acid receptors 2 (FFAR2), free fatty acid receptors 3 (FFAR3), and Hydroxy-carboxylic acid receptor 2(HCA2), respectively (101, 102). The expression of GRP41/FFAR3 receptors are mainly observed in peripheral nerves, enteroendocrine L and K cells, white adipocytes, pancreatic β- cells, thymus cells, and myeloid dendritic cells, and GRP43/FFAR2 receptors are expressed in white adipocytes, enteroendocrine L cells, intestinal epithelial cells, pancreatic β- cells, and several immune system cells (103). In colonic macrophages and dendritic cells, GPR109A signaling activates the inflammasome pathway, resulting in the differentiation of regulatory T cells and IL-10- producing T cells (104). FFAR2 and FFAR3 are activated by all three major SCFAs (105, 106), while butyrate is the only SCFA that can bind to HCA2 (104). In knockout GPR41 mice, the receptor was found to be involved in the release of peptide YY (PYY), intestinal transit rate, and energy harvesting from food (107). GPR43 knockout mice display weight gain, increased adiposity, and reduced systemic insulin sensitivity, while adipose tissue-specific GPR43 overexpression protects mice against the development of obesity (108). Butyrate directly regulates GPR41-mediated sympathetic nervous system activity and thereby controls body energy expenditure in maintaining metabolic homeostasis (109). These may be associated with the effect of butyrate on obesity.
3.2 Histone deacetylases (HDACs)
Butyrate is also a histone deacetylase inhibitor (HDACi). Anticancer activity of butyrate has been found to be mediated by HDAC inhibition, and includes inhibition of cell proliferation, induction of cell differentiation, apoptosis, and induction or repression of gene expression (110–112). Butyrate also down-regulates proinflammatory effectors by histone deacetylase inhibition to regulate intestinal macrophage function (113). A possible mechanism involves butyrate inhibition of the recruitment of HDACs to the promoter by the transcription factors specificity protein 1/specificity protein 3 (Sp1/Sp3), leading to histone hyperacetylation (112). In addition, studies have shown that, as a histone deacetylase inhibitor, butyrate promoted pancreatic β-cell differentiation, which was seen as having potential for the treatment of diabetes (114, 115).
4 Effect of butyrate on obesity
A growing number of studies have reported effects of butyrate on obesity, involved body weight, fat mass and obesity-related glucose and lipid metabolism (Table 1).
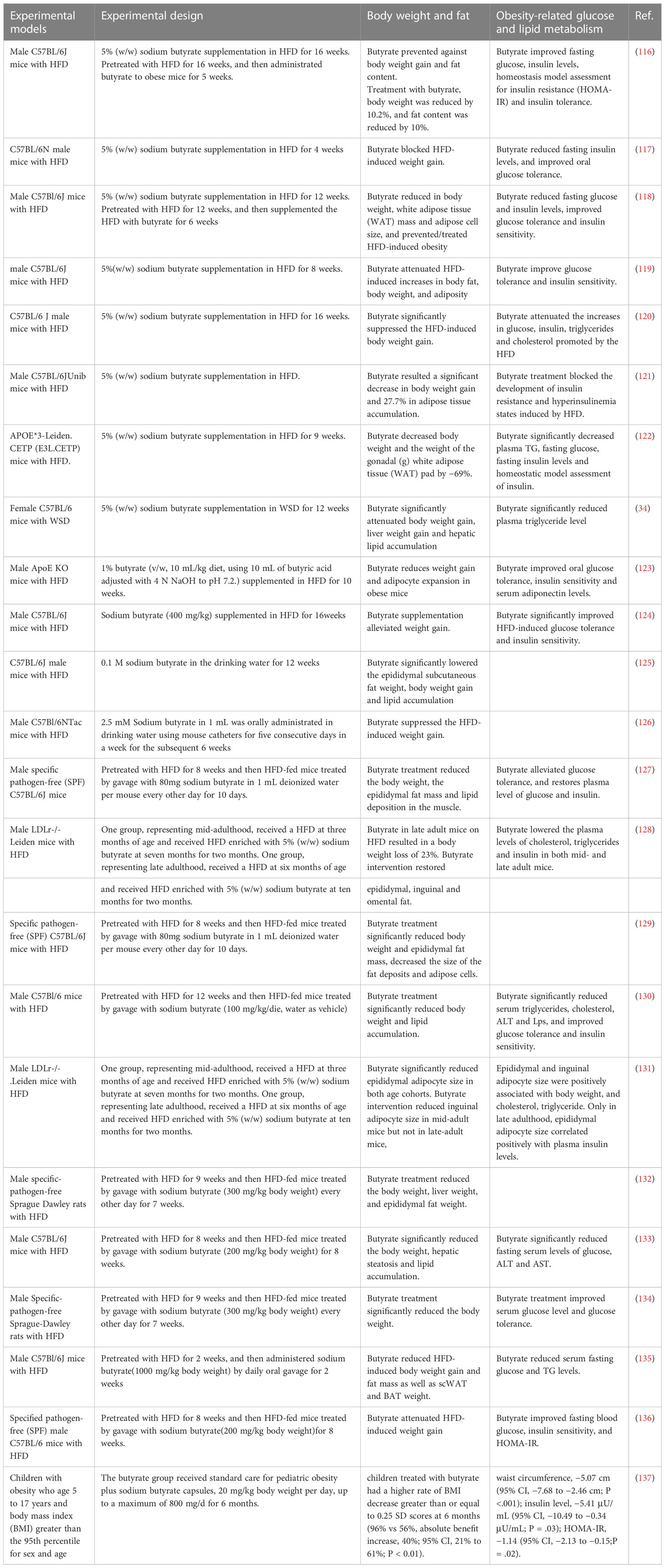
Table 1 Effect of butyrate on obesity, involved body weight, fat mass and obesity-related glucose and lipid metabolism.
4.1 Body weight and fat mass
Most studies showed that dietary supplementation of a high-fat diet (HFD) with 5% (w/w) sodium butyrate significantly reduced body weight gain in mice compared with that induced by the HFD alone (34, 116–122), whatever a long-term supplementation for up to 16weeks (116, 120) or a short-term supplementation for down to 4 weeks (117). Although the dosage of addition of sodium butyrate supplementation varied, it was consistently effective in reducing body weight (123, 124), whether it was supplied in drinking water or administered by gavage (125, 126). Most of these studies also reported that butyrate supplementation can reduce fat mass, suppress adipose tissue accumulation and hepatic lipid accumulation (34, 116–119, 121–123, 125). Such evidence suggests that butyrate prevents diet-induced obesity (DIO) (34, 116–126).
Butyrate was also effective for treating diet-induced obesity (116, 118, 127–136). Studies that pretreated mice or rats with HFD for some time and then treated them by adding sodium butyrate dietary supplementation showed significant decreases in body weight and fat mass (118, 128, 131). In addition to dietary supplementation, more studies that treated HFD-fed mice or rats with oral delivery of sodium butyrate via gavage also significantly reduced body weight and fat mass (116, 127, 129, 130, 132–136). Not only that, an early study revealed that butyrate directly inhibited the proliferation of adipoblasts derived from lean and obese Zucker and WDF rats, and decreased the lipogenic capacity (138). Notably, a study showed that butyrate intervention did not significantly reduce bodyweight in mid-adult mice on HFD but resulted in a body weight loss in late-adult mice on the HFD (128). Another study showed that butyrate intervention reduced inguinal adipocyte size in mid-adult mice but not in late-adult mice (131). The results suggest that the effect of butyrate on obesity is associated with age (128, 131). Recently, a randomized clinical trial about pediatric obesity showed that butyrate decreased the BMI SD scores of obese children (137).
However, butyrate treatment failed to reduce body weight gain and fat mass increased in diabetes (139). It also showed that butyrate did not affect the body weight and fat mass of lean individuals fed a standard diet (121, 123). But in contrast, a study showed that dietary butyrate reduced body weight under standard diet conditions (35). Interestingly, some studies reported that maternal butyrate supplementation increased offspring body weight (140, 141). And the body weight of piglets which were orally gavaged with butyrate from day 4 after birth increased significantly compared with saline-treated control pigs (142). These results suggest that the effect of butyrate on individuals without diet-induced obesity is complex and uncertain.
4.2 Obesity-related glucose and lipid metabolism
Butyrate supplementation in HFD can prevent HFD-induced elevation of fasting glucose and insulin levels, and improve hyperglycemia and hyperinsulinism (116–118, 120–122). Butyrate supplementation can also improve glucose tolerance and insulin sensitivity (116–119, 121–124). Moreover, butyrate supplementation can reduce the serum triglycerides (TG) and cholesterol levels promoted by HFD (34, 122, 123). Butyrate treatment also had therapeutic benefits in disorders of glucose and lipid metabolism in obese individuals which were induced by HFD pretreatment (118, 127, 130, 131, 133–136). Butyrate improved the glucose homeostasis and peripheral insulin-resistance induced by diabetes or HFD without changes in body weight and fat mass (139, 143, 144), which indicates that there are other mechanisms underlying butyrate regulation on glucose homeostasis besides variation in body weight and body composition. It is worth mentioning that butyrate always improves the dyslipidemia under most conditions (34, 118, 122, 123, 127, 130, 131, 133–135, 139, 143), even in a randomized crossover trial which treats overweight/obese men with butyrate mixtures (91).
5 Mechanisms of butyrate effect for obesity
The root cause of obesity is that energy intake exceeds energy expenditure (14, 145). Accordingly, the central to obesity treatment are the increase of energy expenditure and the decrease of energy intake (146, 147). Butyrate plays an important role in both energy expenditure and energy intake through a variety of mechanisms (Figure 1).
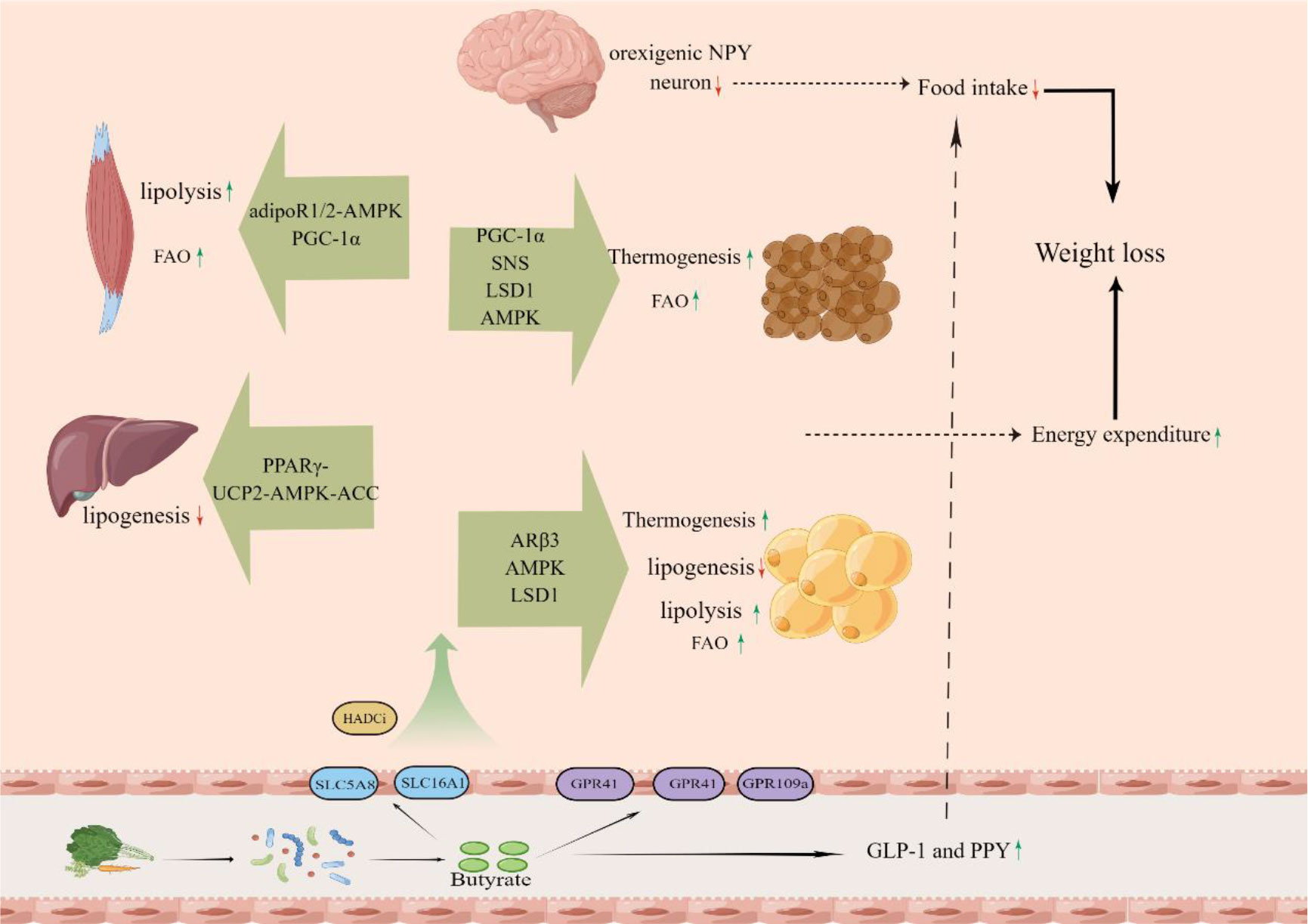
Figure 1 The butyrate, which is produced from dietary fiber by gut microbiota in the colon, is absorbed into the body and increase energy expenditure of muscle, liver, white fat and brown fat and decrease energy intake.
5.1 Thermogenesis of adipose tissue
In some studies, butyrate stimulated thermogenesis of brown adipose tissue (BAT) via the upregulation of uncoupling protein-1 (UCP1) expression, thus increasing energy expenditure and improve HFD-induced obesity (116, 122, 135). BAT, whose brown adipocytes are packed with mitochondria that contain uncoupling protein-1 (UCP1), is a key site of thermogenesis and energy expenditure (148). UCP1, a very important regulatory factor in thermogenesis, which residing in the inner mitochondrial membrane, uncouples mitochondrial respiration from ATP synthesis resulting in thermogenesis (148). It is a significant mechanism for butyrate to promote weight loss by increasing energy expenditure that BAT dissipate chemical energy in the form of heat via UCP1 to regulate body temperature and whole body energy expenditure.
Further studies did find that butyrate can increase the expression of peroxisome proliferator–activated receptor (PPAR)-γ coactivator-1α(PGC-1α) (116), which is a critical regulator of mitochondrial function, and stimulates thermogenesis via the upregulation of UCP1 expression (149). As increased expression of PGC-1α in BAT was reported in a study of the effects of butyrate supplementation, and was positively associated with GPR43 (120). This evidence may suggest that butyrate promote thermogenesis of BAT in partly through activating GPR43 to upregulate PGC-1α. In addition, it has also shown that butyrate stimulated PGC-1α activity by activating AMP-activated protein kinase (AMPK) and inhibiting histone deacetylases (116).
Another convincing mechanism is that butyrate improves BAT thermogenic capacity by increasing sympathetic outflow towards BAT, as butyrate was found to increase the protein expression of tyrosine hydroxylase (TH) (122), a marker of sympathetic nerve activity (150). Furthermore, butyrate treatment did not influence the UCP-1 expression in BAT of vagotomised mice (122), which indicates that the sympathetic nervous system was necessary for butyrate-induced BAT activation.
Recent research identified LSD1, which is an important factor in the regulation of BAT thermogenesis function (151), as a potential mediator of butyrate-induced thermogenesis in BAT, because it can be activated by butyrate to increase UCP1 expression (135). In this study, we can see that LSD1 knockout blocked the butyrate-induced increase in thermogenesis and energy expenditure in BAT (135). The results of experiments with adipocytes also showed that the effects of butyrate on LSD1 and UCP1 were in an AMPK-independent manner (135). These results suggest that butyrate, taken up and metabolized via MCT1 and ACSM3, can directly activate LSD1 to increase the UCP1 expression to mediate thermogenesis in BAT.
Butyrate can change the composition of gut microbiota and improve the disturbed intestinal flora of obese mice (124, 125). Some researches have shown that microbiota depletion impaired thermogenesis of BAT and that butyrate supplementation partially rescued thermogenesis function (94, 135). Microbiota depletion by different cocktails of antibiotics (ABX) or in germfree (GF) mice was reported to impaired the thermogenic capacity of BAT by decreasing the expression of UCP1 (94). It is possibility that butyrate enhance the function of brown fat by regulating the intestinal microflora of obese individuals.
Beige adipocytes that appear in WAT are similar to brown adipocytes in that they release energy as heat and the thermogenesis is mediated by UCP1 (152). Butyrate supplementation can increase the expression of UCP-1 to mediate thermogenesis in WAT and beige adipocyte markers (Tbx1, Tmem26, CD137) (120, 135). GPR43, GPR41 and LSD1 are needed in WAT for butyrate regulation on beiging (120, 135).
5.2 Lipogenesis
It is reported that butyrate can reduce the body weight and fat mass by decreasing lipogenesis in liver and adipose tissue (118, 133). Butyrate supplementation decreased Fatty acid synthase (FAS) expression to reduce lipid synthesis, especially triglycerides (118). This may be attributed to the effect of butyrate in activating AMPK to induce phosphorylation of its downstream target acetyl-CoA carboxylase (ACC) (118, 133). Further, increase of the AMP-to-ATP ratios, which is a direct activator of AMPK, may have been caused by proton leak via UCP2 in butyrate-feed animals, and the disruption of the activity of PPARγ abolished the SCFA-induced increase of the UCP2-pAMPK-pACC pathway activity (118). These results suggested that butyrate activated the UCP2-AMPK-ACC pathway by downregulating the peroxisome proliferator–activated receptor-γ (PPARγ), which can reduce the lipogenesis (118). Other studies suggest that butyrate can inhibit lipid synthesis by inducing the p-AMPK/p-ACC pathway through upregulation of hepatic Glucagon-likepeptide-1 receptor(GLP-1R) (133, 153).
5.3 Adipose lipolysis
Adipose tissue, especially the white adipose tissue, is the largest energy reservoir of body, and has a critical role in the regulation of energy homeostasis (154). Thus, increasing fat mobilization in adipose tissue is an effective strategy to control or treat obesity. A study suggested that butyrate stimulated adipose lipolysis by the phosphorylation of adipose triglyceride lipase (ATGL) and hormone-sensitive lipase (HSL), which are the two major lipases for fat mobilization from triglyceride stores in adipose tissue, and then significantly reduced the epididymal fat mass and body weight (129). Some studies also found that butyrate supplementation reversed the reduction of adipose HSL and lipoprotein lipase (LPL) in obese individuals, causing an increase in the fat hydrolyzed (120). The decrease of lipid content, associated with a significant up-regulation of HSL and LPL was also found in muscle (127).
Butyrate modified histone acetylation on the promoter of beta3-adrenergic receptors (ARβ3) gene, and increased the activation of its downstream signaling molecule cAMP-dependent protein kinase (protein kinase A, PKA) (129). Activating ARβ3, which also belongs to the family of G protein-coupled receptors and is widely expressed in adipose tissues to play important roles in lipolysis (155), stimulates lipolysis by activating phosphorylation of HSL and ATGL (156, 157). HSL and ATGL phosphorylation depend on PKA activation (158, 159). The results suggest that butyrate stimulates adipose lipolysis through histone hyperacetylation-associated AR3β activation in WAT. In muscle, reduced muscle lipid content and up-regulation of Hsl and Lpl mRNA expression are due to butyrate which up-regulates the expression of adiponectin receptors as an HDAC inhibitor (127).
5.4 Fatty acid oxidation
Increasing fatty acid oxidation (FAO) is able to reduce fat accumulation and improves obesity (160). The increase in energy expenditure increases FAO because lipids and fatty acid are the main energy substrates for cellular energy expenditure. Some studies showed that butyrate treatment reduced the Respiratory exchange ratio(RER), suggesting an increase in FAO in response to butyrate (116, 118, 135). FAO is associated with expression of carnitine palmitoyltransferase-1 (CPT-1), which includes three isoforms: CPT-1A(liver), CPT-1B (muscle and heart), and CPT-1C (brain) (160, 161). The increases of CPT-1 induced by butyrate are found in muscle, liver and adipose tissue (116, 118, 120, 135). Butyrate treatment significantly reduced muscle content of TG and total cholesterol compared with mice fed a HFD (127). In addition to CPT-1, butyrate also increased the expression of COX-1(cytochrome c oxidase), UCP2 and UCP3, which can facilitate FAO in skeletal muscle (162). Butyrate induced the transformation of skeletal muscle fiber from the glycolytic muscle fiber type to the oxidative type (116), which is rich in mitochondria, red in color, and active in fat oxidation for ATP biosynthesis (163). The increase of FAO induced by butyrate in skeletal muscle may ascribe to the increased expression of PGC-1α (116). Activation of AMPK and inhibition of HDAC may contribute to the PGC-1α regulation (116). As a HDAC inhibitor, butyrate can activate AMPK by enhancing the expression of adiponectin receptors (adipoR1/2) (127). The increase of the FAO induced by butyrate in BAT may attribute to the energy demands of thermogenesis (135). Butyrate can reduce HFD-induced body weight gain and fat mass by enhancing energy expenditure through increased lipid oxidation in WAT (118, 120). The effect in WAT is due to activation of the UCP2-AMPK-ACC pathway by butyrate depends on PPARγ (118). Butyrate has also been shown to increase lipid oxidation by directly activating the AMPK/ACC pathway to reduce fat mass (130).
5.5 Mitochondrial function
A study showed that butyrate reduced lipid accumulation by regulating liver mitochondrial function, reducing liver mitochondrial energy efficiency, and improving the capability of mitochondria to utilize fat as metabolic fuel (130). Butyrate can stimulate mitochondrial oxidative phosphorylation in WAT through histone hyperacetylation-associated ARB3 activation (129). Short-term oral administration of butyrate can alleviate diet-induced obesity in mice by stimulating mitochondrial function in skeletal muscle (127). Butyrate has also been reported to increase the number of mitochondria in skeletal muscle (116).
5.6 Appetite and intake
Studies have shown that butyrate suppressed food intake, which contributed to reduce body weight and fat mass (117, 122). Butyrate administration via intragastric gavage but not intravenous injection significantly reduced acute food intake within 1 hour after refeeding and cumulative food intake over 24 hours (122). Others found that butyrate did not influence fat absorption by the gastrointestinal tract (116). But some studies showed that butyrate did not reduce the food intake (116, 118), and butyrate has unpleasant taste. This is still a controversial issue that butyrate can increase satiety and decrease appetite.
The appetite is mainly affected by the neural circuits and gut hormones (164, 165). A study showed that butyrate reduced the activity of orexigenic neuropeptide Y (NPY) neuron in the hypothalamus and neuron activity within the nucleus tractus solitarius (NTS) and dorsal vagal complex (DVC) in the brainstem (122). Not only that, butyrate-induced satiety and decreasing food intake was completely abolished by subdiaphragmatic vagotomy, which indicated that the gut-brain neural circuit is necessary for butyrate-induced satiety (122). Butyrate can also significantly increase the levels of gut hormones in the colon and plasma such as GLP-1 and PPY, which can reduce the appetite and food intake (91, 117). Evidence indicated that the effect of butyrate in inhibiting weight gain and food intake in Ffar3 knockouts was to the same extent as in wild-type mice, but stimulation of PYY and GLP-1 by butyrate was blunted in the absence of FFAR3 (117). The peptide hormone leptin can also regulate food intake and body mass (166). Butyrate can increase the leptin production in DIO mice (117, 123, 127, 130, 131).
6 Obesity-induced complication
Obesity or overweight is an important determinant of a range of health problems and increases the risk of many related diseases including type 2 diabetes mellitus (T2DM), CVD, nonalcoholic fatty liver disease (NAFLD), impaired cognition, and others (7, 167–169). Butyrate has a role in control of obesity-induced complications not only by its effect on weight loss but also because of many other mechanisms of action.
6.1 Type 2 diabetes mellitus
The epidemic of diabetes mellitus, which is the ninth major cause of death, poses a major global health threat and about 1 in 10 adults worldwide now has type 2 diabetes mellitus (170). Obesity is one of the strongest risk factors and predisposition for type 2 diabetes (169, 171). Gut microbiota play a key role in obesity and diabetes (172). As a main metabolic product of intestinal microbiota, butyrate can improve HFD-induced glucose homeostasis and insulin resistance, which are directly associated with development of diabetes mellitus (116–118, 121, 124, 127, 130). Butyrate activated the hormone signaling such as protein kinase B (PKB/Akt), and increase the expression of glucose transporter (Glut4) (116, 123, 124, 130).
Evidence showed that butyrate can reduce HFD-induced pancreatic beta cell dysfunctions (121, 143), which has the beneficial effect on glucose homeostasis and suppresses the development of diabetes (173). Butyrate can improve pancreatic β cell development, proliferation, and function via the inhibition of HDACs (174, 175), protect pancreatic Beta cells from Cytokine-Induced Dysfunction (176), increase the pancreatic Beta cells viability and prevent pancreatic Beta cell-death during exposure to streptozotocin (177). Increased oxidative stress is one of the important factors which can lead insulin resistance and contribute to the development of T2DM (178). A study showed that butyrate stimulated transcription of downstream antioxidant enzymes via the activation of nuclear factor E2-related factor 2, thus contributing to the amelioration of HFD-induced oxidative stress and insulin resistance (134). Butyrate directly induced intestinal gluconeogenesis via upregulating key enzymes G6PC and PCK1 with a cAMP-dependent mechanism, which can activate hypothalamic nuclei to decrease liver glucose production and regulate insulin sensitivity and glucose homeostasis (35).
6.2 Nonalcoholic fatty liver disease
Over the past four decades, non-alcoholic fatty liver disease has become the most common chronic liver disorder, which has a global prevalence of 25% and is a leading cause of cirrhosis and hepatocellular carcinoma (179). Obesity is closely associated with the rising prevalence and severity of NAFLD (180). Some studies showed that butyrate improved the accumulation of fat in the liver, hepatic steatosis and inflammation, the liver index and serum levels of alanine transaminase (ALT) and aspartate transaminase (AST) induced by diet-induced obesity (34, 118, 121, 125, 132, 133, 144).
Several findings suggested that butyrate alleviates HFD-induced NAFLD by improving mitochondrial function and stimulating fatty acid β oxidation in the liver (118, 132, 181). Moreover, butyrate can inhibit the NF-κB signaling and NLRP3 inflammasome activation by up-regulating hepatic expression of peroxisome proliferator-activated receptor α (PPARα), which contribute to the alleviation of HFD-induced, NAFLD-associated hepatic inflammation (132, 144). Butyrate can protect against high-fat diet-induced oxidative stress in rat liver by promoting expression of nuclear factor E2-related factor 2 (134). Butyrate can decrease the lipopolysaccharide (LPS) and its receptor Toll-like receptor 4 (TLR4) in the liver by repairing HFD-induced damage to the intestinal mucosa and strengthened intestinal tight junctions, which is beneficial for the treatment of NAFLD (34, 136). Evidence showed that improved HFD-induced non-alcoholic steatohepatitis resulted from up-regulation of hepatic GLP-1R expression (133). Meanwhile, a recent study reported that butyrate protected mice against diet-induced NASH and liver fibrosis development by direct inhibition of collagen synthesis in hepatic stellate cells involving suppression of specific non-canonical TGF-β signaling pathways Rho-like GTPases and PI3K/AKT, and other important pro-fibrotic regulators (29). Butyrate also ameliorated NAFLD by upregulating miR-150 to suppress C-X-C Motif Chemokine Receptor 4 expression (182).
6.3 Neuropsychiatric dysfunction
Obesity is associated with an increased risk of neuropsychiatric disorders, including mood disorders, schizophrenia, major neurocognitive disorder or cognitive impairment, and neurodegenerative diseases (NDDs) (183–185). Evidence showed that Butyrate intervention restored HFD-induced spatial memory impairment, brain function, and neuroinflammation within the thalamus, cortex and hippocampus (128). Butyrate can also reverse HFD-induced social deficits and anxiety-like behaviors by regulating microglial homeostasis and reducing dendritic spine density in the bilateral medial prefrontal cortex (mPFC) (186). Analysis of the gut microbiome suggests that these beneficial effects may correlate with gut microbiota composition (128, 186). Butyrate can protect against NDDs by suppressing neurotoxicity and cell death. A study showed that butyrate attenuated the expression of P-53 and the neuroinflammation in the brains of HFD-fed mice (126). Butyrate also upregulated PPARγ/CREB, BDNF, and modulated the Nrf-2/HO-1 pathway in HFD mice brains, which play important roles in neuroprotective effect (126).
7 Conclusion and prospect
There is a lot of evidence supporting that butyrate, as a key mediator of microbiota in host metabolic control, has beneficial effects on obesity, especially weight loss. However, the role of butyrate is still controversial and not clear. First, as we mentioned earlier, the amount of butyrate in stools has been found to be higher in overweight and obese volunteers than in lean volunteers. These contradictory results led some researchers to believe that butyrate may contribute to the obesogenic phenotype. Besides, the range of action of butyrate is a controversial. Because there were many contradictory results about the effect of butyrate on weight loss in diabetic and non-obese individuals. Last but not least, the exact mechanisms of butyrate regulation need to be found out. For example, some studies reported that butyrate didn’t affect food intake, but some reported butyrate reduced appetite. Thus, we’ve done a lot of work on existing mechanisms of butyrate. These issues require largely meticulous and comprehensive studies to fully understand the role of butyrate in host metabolic health. More human clinical studies are needed to prove the effectiveness and specific effect of butyrate on obesity, and more diversified experimental models are needed to determine the effective conditions of butyrate in obesity. Finally, we need more persuasive experimental designs and studies to figure out the exact mechanism by which butyrate acts. Besides, the side effects of butyrate, like nausea and its unpleasant smell, also needed to be considered. It’s important to discover and try to remove the side effect of butyrate. To provide better strategies for obesity, further research based on published studies is needed in the future. However, experimental designs in rodent models may not be transferrable to human situations. The limited available human data are derived from studies with a relatively small sample size and short intervention period and didn’t show encouraging results. Therefore, it is needed to consider whether further research is worthwhile. We just hope that our work can contribute to related research and help someone who are interested in this field.
Author contributions
Select the topic, YH and XY; literature collection, HT and WZ; writing-original draft preparation, KP; investigation and editing, WD and TL. All authors contributed to the article and approved the submitted version.
Funding
This study was supported by research funding from the National Natural Science Foundation of China (No.82104251); Doctoral Research Initiation Fund of Affiliated Hospital of Southwest Medical University (No.21032); Science and Technology Planning Project of Sichuan Province (2022YFS0626-B2); Sichuan Science and Technology Program (2022NSFSC1356).
Conflict of interest
The authors declare that the research was conducted in the absence of any commercial or financial relationships that could be construed as a potential conflict of interest.
Publisher’s note
All claims expressed in this article are solely those of the authors and do not necessarily represent those of their affiliated organizations, or those of the publisher, the editors and the reviewers. Any product that may be evaluated in this article, or claim that may be made by its manufacturer, is not guaranteed or endorsed by the publisher.
References
1. Wadden TA, Tronieri JS, Butryn ML. Lifestyle modification approaches for the treatment of obesity in adults. Am Psychol (2020) 752:235–51. doi: 10.1037/amp0000517
2. Coronel J, Pinos I, Amengual J. Beta-carotene in obesity research: Technical considerations and current status of the field. Nutrients (2019) 11(4):842. doi: 10.3390/nu11040842
3. Haslam DW, James WPT. Obesity. Lancet (2005) 3669492:1197–209. doi: 10.1016/s0140-6736(05)67483-1
4. Organization WH. Obesity and overweight. Available at: https://www.who.int/en/news-room/fact-sheets/detail/obesity-and-overweight (Accessed October 28).
5. Chou SY, Grossman M, Saffer H. An economic analysis of adult obesity: results from the behavioral risk factor surveillance system. J Health Econ (2004) 233:565–87. doi: 10.1016/j.jhealeco.2003.10.003
6. Conway B, Rene A. Obesity as a disease: no lightweight matter. Obes Rev an Off J Int Assoc Study Obes (2004) 53:145–51. doi: 10.1111/j.1467-789X.2004.00144.x
7. Piche ME, Tchernof A, Despres JP. Obesity phenotypes, diabetes, and cardiovascular diseases. Circ Res (2020) 12611:1477–500. doi: 10.1161/CIRCRESAHA.120.316101
8. Sjöström L, Lindroos AK, Peltonen M, Torgerson J, Bouchard C, Carlsson B, et al. Lifestyle, diabetes, and cardiovascular risk factors 10 years after bariatric surgery. New Engl J Med (2004) 35126:2683–93. doi: 10.1056/NEJMoa035622
9. Schauer PR, Kashyap SR, Wolski K, Brethauer SA, Kirwan JP, Pothier CE, et al. Bariatric surgery versus intensive medical therapy in obese patients with diabetes. New Engl J Med (2012) 36617:1567–76. doi: 10.1056/NEJMoa1200225
10. Kolb R, Sutterwala FS, Zhang W. Obesity and cancer: inflammation bridges the two. Curr Opin Pharmacol (2016) 29:77–89. doi: 10.1016/j.coph.2016.07.005
11. Leigh SJ, Morris MJ. Diet, inflammation and the gut microbiome: Mechanisms for obesity-associated cognitive impairment. Biochim Biophys Acta Mol Basis Dis (2020) 18666:165767. doi: 10.1016/j.bbadis.2020.165767
12. Mannucci E, Petroni ML, Villanova N, Rotella CM, Apolone G, Marchesini G. Clinical and psychological correlates of health-related quality of life in obese patients. Health Qual Life outcomes (2010) 8(1):90. doi: 10.1186/1477-7525-8-90
13. Kolotkin RL, Meter K, Williams GR. Quality of life and obesity. Obes Rev an Off J Int Assoc Study Obes (2001) 24:219–29. doi: 10.1046/j.1467-789x.2001.00040.x
14. Bluher M. Obesity: global epidemiology and pathogenesis. Nat Rev Endocrinol (2019) 155:288–98. doi: 10.1038/s41574-019-0176-8
15. Pant R, Firmal P, Shah VK, Alam A, Chattopadhyay S. Epigenetic regulation of adipogenesis in development of metabolic syndrome. Front Cell Dev Biol (2020) 8619888:619888. doi: 10.3389/fcell.2020.619888
16. Fan Y, Pedersen O. Gut microbiota in human metabolic health and disease. Nat Rev Microbiol (2021) 191:55–71. doi: 10.1038/s41579-020-0433-9
17. Stanislawski MA, Frank DN, Borengasser SJ, Ostendorf DM, Ir D, Jambal P, et al. The gut microbiota during a behavioral weight loss intervention. Nutrients (2021) 13(9):3248. doi: 10.3390/nu13093248
18. Depommier C, Everard A, Druart C, Plovier H, Van Hul M, Vieira-Silva S, et al. Supplementation with akkermansia muciniphila in overweight and obese human volunteers: a proof-of-concept exploratory study. Nat Med (2019) 257:1096–103. doi: 10.1038/s41591-019-0495-2
19. Morrison DJ, Preston T. Formation of short chain fatty acids by the gut microbiota and their impact on human metabolism. Gut Microbes (2016) 73:189–200. doi: 10.1080/19490976.2015.1134082
20. Macfarlane S, Macfarlane GT. Regulation of short-chain fatty acid production. Proc Nutr Soc (2003) 621:67–72. doi: 10.1079/PNS2002207
21. Perry RJ, Peng L, Barry NA, Cline GW, Zhang D, Cardone RL, et al. Acetate mediates a microbiome-brain-beta-cell axis to promote metabolic syndrome. Nature (2016) 5347606:213–7. doi: 10.1038/nature18309
22. Zhao S, Jang C, Liu J, Uehara K, Gilbert M, Izzo L, et al. Dietary fructose feeds hepatic lipogenesis via microbiota-derived acetate. Nature (2020) 5797800:586–91. doi: 10.1038/s41586-020-2101-7
23. Harris K, Kassis A, Major G, Chou CJ. Is the gut microbiota a new factor contributing to obesity and its metabolic disorders? J Obes (2012) 2012(879151):14. doi: 10.1155/2012/879151
24. Sun W, Dong H, Wolfrum C. Local acetate inhibits brown adipose tissue function. Proc Natl Acad Sci U.S.A. (2021) 118(49). doi: 10.1073/pnas.2116125118
25. Guilloteau P, Martin L, Eeckhaut V, Ducatelle R, Zabielski R, Van Immerseel F. From the gut to the peripheral tissues: the multiple effects of butyrate. Nutr Res Rev (2010) 232:366–84. doi: 10.1017/S0954422410000247
26. Zhang L, Liu C, Jiang Q, Yin Y. Butyrate in energy metabolism: There is still more to learn. Trends Endocrinol Metab (2021) 323:159–69. doi: 10.1016/j.tem.2020.12.003
27. Qin J, Li Y, Cai Z, Li S, Zhu J, Zhang F, et al. A metagenome-wide association study of gut microbiota in type 2 diabetes. Nature (2012) 4907418:55–60. doi: 10.1038/nature11450
28. Li Q, Chang Y, Zhang K, Chen H, Tao S, Zhang Z. Implication of the gut microbiome composition of type 2 diabetic patients from northern China. Sci Rep (2020) 101:5450. doi: 10.1038/s41598-020-62224-3
29. Gart E, van Duyvenvoorde W, Toet K, Caspers MPM, Verschuren L, Nielsen MJ, et al. Butyrate protects against diet-induced NASH and liver fibrosis and suppresses specific non-canonical TGF-beta signaling pathways in human hepatic stellate cells. Biomedicines (2021) 9(12):1954. doi: 10.3390/biomedicines9121954
30. Schwiertz A, Taras D, Schafer K, Beijer S, Bos NA, Donus C, et al. Microbiota and SCFA in lean and overweight healthy subjects. Obes (Silver Spring) (2010) 181:190–5. doi: 10.1038/oby.2009.167
31. Nandy D, Craig SJC, Cai J, Tian Y, Paul IM, Savage JS, et al. Metabolomic profiling of stool of two-year old children from the INSIGHT study reveals links between butyrate and child weight outcomes. Pediatr Obes (2022) 171:e12833. doi: 10.1111/ijpo.12833
32. Liu H, Wang J, He T, Becker S, Zhang G, Li D, et al. Butyrate: A double-edged sword for health? Adv Nutr (2018) 91:21–9. doi: 10.1093/advances/nmx009
33. Xiao L, Sonne SB, Feng Q, Chen N, Xia Z, Li X, et al. High-fat feeding rather than obesity drives taxonomical and functional changes in the gut microbiota in mice. Microbiome (2017) 51:43. doi: 10.1186/s40168-017-0258-6
34. Beisner J, Filipe Rosa L, Kaden-Volynets V, Stolzer I, Günther C, Bischoff SC. Prebiotic inulin and sodium butyrate attenuate obesity-induced intestinal barrier dysfunction by induction of antimicrobial peptides. Front Immunol (2021) 12:678360. doi: 10.3389/fimmu.2021.678360
35. De Vadder F, Kovatcheva-Datchary P, Goncalves D, Vinera J, Zitoun C, Duchampt A, et al. Microbiota-generated metabolites promote metabolic benefits. via gut-brain Neural circuits. Cell (2014) 1561-2:84–96. doi: 10.1016/j.cell.2013.12.016
36. Singh DP, Singh S, Bijalwan V, Kumar V, Khare P, Baboota RK, et al. Co-Supplementation of isomalto-oligosaccharides potentiates metabolic health benefits of polyphenol-rich cranberry extract in high fat diet-fed mice via enhanced gut butyrate production. Eur J Nutr (2018) 578:2897–911. doi: 10.1007/s00394-017-1561-5
37. Louis P, Flint HJ. Formation of propionate and butyrate by the human colonic microbiota. Environ Microbiol (2017) 191:29–41. doi: 10.1111/1462-2920.13589
38. Fu X, Liu Z, Zhu C, Mou H, Kong Q. Nondigestible carbohydrates, butyrate, and butyrate-producing bacteria. Crit Rev Food Sci Nutr (2019) 59sup1:S130–S52. doi: 10.1080/10408398.2018.1542587
39. Holscher HD. Dietary fiber and prebiotics and the gastrointestinal microbiota. Gut Microbes (2017) 82:172–84. doi: 10.1080/19490976.2017.1290756
40. Alexander C, Swanson KS, Fahey GC, Garleb KA. Perspective: Physiologic importance of short-chain fatty acids from nondigestible carbohydrate fermentation. Adv Nutr (2019) 104:576–89. doi: 10.1093/advances/nmz004
41. Wong JM, de Souza R, Kendall CW, Emam A, Jenkins DJ. Colonic health: fermentation and short chain fatty acids. J Clin Gastroenterol (2006) 403:235–43. doi: 10.1097/00004836-200603000-00015
42. Robert CL, Bernalier-Donadille A. The cellulolytic microflora of the human colon: evidence of microcrystalline cellulose-degrading bacteria in methane-excreting subjects. FEMS Microbiol Ecol (2003) 461:81–9. doi: 10.1016/s0168-6496(03)00207-1
43. Cummings JH. Short chain fatty acids in the human colon. Gut (1981) 229:763–79. doi: 10.1136/gut.22.9.763
44. Zaman SA, Sarbini SR. The potential of resistant starch as a prebiotic. Crit Rev Biotechnol (2016) 363:578–84. doi: 10.3109/07388551.2014.993590
45. Raigond P, Ezekiel R, Raigond B. Resistant starch in food: a review. J Sci Food Agric (2015) 9510:1968–78. doi: 10.1002/jsfa.6966
46. Xu JL, Liu ZF, Zhang XW, Liu HL, Wang Y. Microbial oligosaccharides with biomedical applications. Mar Drugs (2021) 19(6):350. doi: 10.3390/md19060350
47. Smith EA, Macfarlane GT. Enumeration of human colonic bacteria producing phenolic and indolic compounds: effects of pH, carbohydrate availability and retention time on dissimilatory aromatic amino acid metabolism. J Appl Bacteriol (1996) 813:288–302. doi: 10.1111/j.1365-2672.1996.tb04331.x
48. Smith EA, Macfarlane GT. Dissimilatory amino acid metabolism in human colonic bacteria. Anaerobe (1997) 35:327–37. doi: 10.1006/anae.1997.0121
49. Stilling RM, van de Wouw M, Clarke G, Stanton C, Dinan TG, Cryan JF. The neuropharmacology of butyrate: The bread and butter of the microbiota-gut-brain axis? Neurochemistry Int (2016) 99:110–32. doi: 10.1016/j.neuint.2016.06.011
50. Aitoro R, Paparo L, Amoroso A, Di Costanzo M, Cosenza L, Granata V, et al. Gut microbiota as a target for preventive and therapeutic intervention against food allergy. Nutrients (2017) 9(7):672. doi: 10.3390/nu9070672
51. Louis P, Flint HJ. Diversity, metabolism and microbial ecology of butyrate-producing bacteria from the human large intestine. FEMS Microbiol Lett (2009) 2941:1–8. doi: 10.1111/j.1574-6968.2009.01514.x
52. Vital M, Howe AC, Tiedje JM. Revealing the bacterial butyrate synthesis pathways by analyzing (meta)genomic data. mBio (2014) 52:e00889. doi: 10.1128/mBio.00889-14
53. Walker AW, Duncan SH, Louis P, Flint HJ. Phylogeny, culturing, and metagenomics of the human gut microbiota. Trends Microbiol (2014) 225:267–74. doi: 10.1016/j.tim.2014.03.001
54. Van den Abbeele P, Belzer C, Goossens M, Kleerebezem M, De Vos WM, Thas O, et al. Butyrate-producing clostridium cluster XIVa species specifically colonize mucins in an in vitro gut model. ISME J (2013) 75:949–61. doi: 10.1038/ismej.2012.158
55. Louis P, Duncan SH, McCrae SI, Millar J, Jackson MS, Flint HJ. Restricted distribution of the butyrate kinase pathway among butyrate-producing bacteria from the human colon. J Bacteriol (2004) 1867:2099–106. doi: 10.1128/JB.186.7.2099-2106.2004
56. Jenkins DJ, Vuksan V, Kendall CW, Wursch P, Jeffcoat R, Waring S, et al. Physiological effects of resistant starches on fecal bulk, short chain fatty acids, blood lipids and glycemic index. J Am Coll Nutr (1998) 176:609–16. doi: 10.1080/07315724.1998.10718810
57. Rose DJ, DeMeo MT, Keshavarzian A, Hamaker BR. Influence of dietary fiber on inflammatory bowel disease and colon cancer: importance of fermentation pattern. Nutr Rev (2007) 652:51–62. doi: 10.1111/j.1753-4887.2007.tb00282.x
58. van de Wiele T, Boon N, Possemiers S, Jacobs H, Verstraete W. Inulin-type fructans of longer degree of polymerization exert more pronounced in vitro prebiotic effects. J Appl Microbiol (2007) 1022:452–60. doi: 10.1111/j.1365-2672.2006.03084.x
59. Pylkas AM, Juneja LR, Slavin JL. Comparison of different fibers for in vitro production of short chain fatty acids by intestinal microflora. J Med Food (2005) 81:113–6. doi: 10.1089/jmf.2005.8.113
60. Kettle H, Louis P, Holtrop G, Duncan SH, Flint HJ. Modelling the emergent dynamics and major metabolites of the human colonic microbiota. Environ Microbiol (2015) 175:1615–30. doi: 10.1111/1462-2920.12599
61. Ohland CL, Jobin C. Microbial activities and intestinal homeostasis: A delicate balance between health and disease. Cell Mol Gastroenterol Hepatol (2015) 11:28–40. doi: 10.1016/j.jcmgh.2014.11.004
62. Belenguer A, Duncan SH, Holtrop G, Anderson SE, Lobley GE, Flint HJ. Impact of pH on lactate formation and utilization by human fecal microbial communities. Appl Environ Microbiol (2007) 7320:6526–33. doi: 10.1128/AEM.00508-07
63. Duncan SH, Louis P, Thomson JM, Flint HJ. The role of pH in determining the species composition of the human colonic microbiota. Environ Microbiol (2009) 118:2112–22. doi: 10.1111/j.1462-2920.2009.01931.x
64. Walker AW, Duncan SH, McWilliam Leitch EC, Child MW, Flint HJ. pH and peptide supply can radically alter bacterial populations and short-chain fatty acid ratios within microbial communities from the human colon. Appl Environ Microbiol (2005) 717:3692–700. doi: 10.1128/AEM.71.7.3692-3700.2005
65. Dostal A, Chassard C, Hilty FM, Zimmermann MB, Jaeggi T, Rossi S, et al. Iron depletion and repletion with ferrous sulfate or electrolytic iron modifies the composition and metabolic activity of the gut microbiota in rats. J Nutr (2012) 1422:271–7. doi: 10.3945/jn.111.148643
66. Dostal A, Lacroix C, Bircher L, Pham VT, Follador R, Zimmermann MB, et al. Iron modulates butyrate production by a child gut microbiota. In Vitro. mBio (2015) 66:e01453–15. doi: 10.1128/mBio.01453-15
67. Khan MT, Duncan SH, Stams AJ, van Dijl JM, Flint HJ, Harmsen HJ. The gut anaerobe faecalibacterium prausnitzii uses an extracellular electron shuttle to grow at oxic-anoxic interphases. ISME J (2012) 68:1578–85. doi: 10.1038/ismej.2012.5
68. Wolf PG, Biswas A, Morales SE, Greening C, Gaskins HR. H2 metabolism is widespread and diverse among human colonic microbes. Gut Microbes (2016) 73:235–45. doi: 10.1080/19490976.2016.1182288
69. Dalile B, Van Oudenhove L, Vervliet B, Verbeke K. The role of short-chain fatty acids in microbiota-gut-brain communication. Nat Rev Gastroenterol Hepatol (2019) 168:461–78. doi: 10.1038/s41575-019-0157-3
70. Counillon L, Bouret Y, Marchiq I, Pouyssegur J. Na(+)/H(+) antiporter (NHE1) and lactate/H(+) symporters (MCTs) in pH homeostasis and cancer metabolism. Biochim Biophys Acta (2016) 186310:2465–80. doi: 10.1016/j.bbamcr.2016.02.018
71. Stumpff F. A look at the smelly side of physiology: transport of short chain fatty acids. Pflugers Arch (2018) 4704:571–98. doi: 10.1007/s00424-017-2105-9
72. Cuff MA, Shirazi-Beechey SP. The importance of butyrate transport to the regulation of gene expression in the colonic epithelium. Biochem Soc Trans (2004) 32Pt 6:1100–2. doi: 10.1042/BST0321100
73. Gupta N, Martin PM, Prasad PD, Ganapathy V. SLC5A8 (SMCT1)-mediated transport of butyrate forms the basis for the tumor suppressive function of the transporter. Life Sci (2006) 7821:2419–25. doi: 10.1016/j.lfs.2005.10.028
74. Vijay N, Morris ME. Role of monocarboxylate transporters in drug delivery to the brain. Curr Pharm Des (2014) 2010:1487–98. doi: 10.2174/13816128113199990462
75. Sivaprakasam S, Bhutia YD, Yang S, Ganapathy V. Short-chain fatty acid transporters: Role in colonic homeostasis. Compr Physiol (2017) 81:299–314. doi: 10.1002/cphy.c170014
76. Garcia CK, Li X, Luna J, Francke U. cDNA cloning of the human monocarboxylate transporter 1 and chromosomal localization of the SLC16A1 locus to 1p13.2-p12. Genomics (1994) 232:500–3. doi: 10.1006/geno.1994.1532
77. Hadjiagapiou C, Schmidt L, Dudeja PK, Layden TJ, Ramaswamy K. Mechanism(s) of butyrate transport in caco-2 cells: role of monocarboxylate transporter 1. Am J Physiol Gastrointest Liver Physiol (2000) 2794:G775–80. doi: 10.1152/ajpgi.2000.279.4.G775
78. Goncalves P, Araujo JR, Pinho MJ, Martel F. Modulation of butyrate transport in caco-2 cells. Naunyn Schmiedebergs Arch Pharmacol (2009) 3794:325–36. doi: 10.1007/s00210-008-0372-x
79. Lean CB, Lee EJ. Genetic variations of the MCT4 (SLC16A3) gene in the Chinese and Indian populations of Singapore. Drug Metab Pharmacokinet (2012) 274:456–64. doi: 10.2133/dmpk.dmpk-11-sh-104
80. Kekuda R, Manoharan P, Baseler W, Sundaram U. Monocarboxylate 4 mediated butyrate transport in a rat intestinal epithelial cell line. Dig Dis Sci (2013) 583:660–7. doi: 10.1007/s10620-012-2407-x
81. Cresci GA, Thangaraju M, Mellinger JD, Liu K, Ganapathy V. Colonic gene expression in conventional and germ-free mice with a focus on the butyrate receptor GPR109A and the butyrate transporter SLC5A8. J Gastrointest Surg (2010) 143:449–61. doi: 10.1007/s11605-009-1045-x
82. Singh V, Yang J, Chen TE, Zachos NC, Kovbasnjuk O, Verkman AS, et al. Translating molecular physiology of intestinal transport into pharmacologic treatment of diarrhea: stimulation of na+ absorption. Clin Gastroenterol Hepatol (2014) 121:27–31. doi: 10.1016/j.cgh.2013.10.020
83. Rodriguez AM, Perron B, Lacroix L, Caillou B, Leblanc G, Schlumberger M, et al. Identification and characterization of a putative human iodide transporter located at the apical membrane of thyrocytes. J Clin Endocrinol Metab (2002) 877:3500–3. doi: 10.1210/jcem.87.7.8797
84. Ganapathy V, Thangaraju M, Gopal E, Martin PM, Itagaki S, Miyauchi S, et al. Sodium-coupled monocarboxylate transporters in normal tissues and in cancer. AAPS J (2008) 101:193–9. doi: 10.1208/s12248-008-9022-y
85. Kamp F, Hamilton JA. How fatty acids of different chain length enter and leave cells by free diffusion. Prostaglandins Leukot Essent Fatty Acids (2006) 753:149–59. doi: 10.1016/j.plefa.2006.05.003
86. Peters SG, Pomare EW, Fisher CA. Portal and peripheral blood short chain fatty acid concentrations after caecal lactulose instillation at surgery. Gut (1992) 339:1249–52. doi: 10.1136/gut.33.9.1249
87. Anand S, Mande SS. Host-microbiome interactions: Gut-liver axis and its connection with other organs. NPJ Biofilms Microbiomes (2022) 81:89. doi: 10.1038/s41522-022-00352-6
88. Hamer HM, Jonkers D, Venema K, Vanhoutvin S, Troost FJ, Brummer RJ. Review article: the role of butyrate on colonic function. Aliment Pharmacol Ther (2008) 272:104–19. doi: 10.1111/j.1365-2036.2007.03562.x
89. Jakobsdottir G, Jadert C, Holm L, Nyman ME. Propionic and butyric acids, formed in the caecum of rats fed highly fermentable dietary fibre, are reflected in portal and aortic serum. Br J Nutr (2013) 1109:1565–72. doi: 10.1017/S0007114513000809
90. Boets E, Deroover L, Houben E, Vermeulen K, Gomand SV, Delcour JA, et al. Quantification of in vivo colonic short chain fatty acid production from inulin. Nutrients (2015) 711:8916–29. doi: 10.3390/nu7115440
91. Canfora EE, van der Beek CM, Jocken JWE, Goossens GH, Holst JJ, Olde Damink SWM, et al. Colonic infusions of short-chain fatty acid mixtures promote energy metabolism in overweight/obese men: a randomized crossover trial. Sci Rep (2017) 71:2360. doi: 10.1038/s41598-017-02546-x
92. Nakatani M, Inoue R, Tomonaga S, Fukuta K, Tsukahara T. Production, absorption, and blood flow dynamics of short-chain fatty acids produced by fermentation in piglet hindgut during the suckling(-)Weaning period. Nutrients (2018) 10(9):1220. doi: 10.3390/nu10091220
93. Kim SW, Hooker JM, Otto N, Win K, Muench L, Shea C, et al. Whole-body pharmacokinetics of HDAC inhibitor drugs, butyric acid, valproic acid and 4-phenylbutyric acid measured with carbon-11 labeled analogs by PET. Nucl Med Biol (2013) 407:912–8. doi: 10.1016/j.nucmedbio.2013.06.007
94. Li B, Li L, Li M, Lam SM, Wang G, Wu Y, et al. Microbiota depletion impairs thermogenesis of brown adipose tissue and browning of white adipose tissue. Cell Rep (2019) 2610:2720–37 e5. doi: 10.1016/j.celrep.2019.02.015
95. Liu J, Sun J, Wang F, Yu X, Ling Z, Li H, et al. Neuroprotective effects of clostridium butyricum against vascular dementia in mice via metabolic butyrate. BioMed Res Int (2015) 2015:412946. doi: 10.1155/2015/412946
96. Brahe LK, Astrup A, Larsen LH. Is butyrate the link between diet, intestinal microbiota and obesity-related metabolic diseases? Obes Rev an Off J Int Assoc Study Obes (2013) 1412:950–9. doi: 10.1111/obr.12068
97. Astbury SM, Corfe BM. Uptake and metabolism of the short-chain fatty acid butyrate, a critical review of the literature. Curr Drug Metab (2012) 136:815–21. doi: 10.2174/138920012800840428
98. den Besten G, Lange K, Havinga R, van Dijk TH, Gerding A, van Eunen K, et al. Gut-derived short-chain fatty acids are vividly assimilated into host carbohydrates and lipids. Am J Physiol Gastrointest Liver Physiol (2013) 30512:G900–10. doi: 10.1152/ajpgi.00265.2013
99. McOrist AL, Miller RB, Bird AR, Keogh JB, Noakes M, Topping DL, et al. Fecal butyrate levels vary widely among individuals but are usually increased by a diet high in resistant starch. J Nutr (2011) 1415:883–9. doi: 10.3945/jn.110.128504
100. Brown AJ, Goldsworthy SM, Barnes AA, Eilert MM, Tcheang L, Daniels D, et al. The orphan G protein-coupled receptors GPR41 and GPR43 are activated by propionate and other short chain carboxylic acids. J Biol Chem (2003) 27813:11312–9. doi: 10.1074/jbc.M211609200
101. Bindels LB, Dewulf EM, Delzenne NM. GPR43/FFA2: physiopathological relevance and therapeutic prospects. Trends Pharmacol Sci (2013) 344:226–32. doi: 10.1016/j.tips.2013.02.002
102. Macia L, Tan J, Vieira AT, Leach K, Stanley D, Luong S, et al. Metabolite-sensing receptors GPR43 and GPR109A facilitate dietary fibre-induced gut homeostasis through regulation of the inflammasome. Nat Commun (2015) 6:6734. doi: 10.1038/ncomms7734
103. Kimura I, Miyamoto J, Ohue-Kitano R, Watanabe K, Yamada T, Onuki M, et al. Maternal gut microbiota in pregnancy influences offspring metabolic phenotype in mice. Science (2020) 367(6481). doi: 10.1126/science.aaw8429
104. Singh N, Gurav A, Sivaprakasam S, Brady E, Padia R, Shi H, et al. Activation of Gpr109a, receptor for niacin and the commensal metabolite butyrate, suppresses colonic inflammation and carcinogenesis. Immunity (2014) 401:128–39. doi: 10.1016/j.immuni.2013.12.007
105. Ulven T. Short-chain free fatty acid receptors FFA2/GPR43 and FFA3/GPR41 as new potential therapeutic targets. Front Endocrinol (Lausanne) (2012) 3111:111. doi: 10.3389/fendo.2012.00111
106. Yonezawa T, Kurata R, Yoshida K, Murayama MA, Cui X, Hasegawa A. Free fatty acids-sensing G protein-coupled receptors in drug targeting and therapeutics. Curr Med Chem (2013) 2031:3855–71. doi: 10.2174/09298673113209990168
107. Samuel BS, Shaito A, Motoike T, Rey FE, Backhed F, Manchester JK, et al. Effects of the gut microbiota on host adiposity are modulated by the short-chain fatty-acid binding G protein-coupled receptor, Gpr41. Proc Natl Acad Sci USA (2008) 10543:16767–72. doi: 10.1073/pnas.0808567105
108. Kimura I, Ozawa K, Inoue D, Imamura T, Kimura K, Maeda T, et al. The gut microbiota suppresses insulin-mediated fat accumulation via the short-chain fatty acid receptor GPR43. Nat Commun (2013) 4:1829. doi: 10.1038/ncomms2852
109. Kimura I, Inoue D, Maeda T, Hara T, Ichimura A, Miyauchi S, et al. Short-chain fatty acids and ketones directly regulate sympathetic nervous system via G protein-coupled receptor 41 (GPR41). Proc Natl Acad Sci USA (2011) 10819:8030–5. doi: 10.1073/pnas.1016088108
110. Donohoe DR, Collins LB, Wali A, Bigler R, Sun W, Bultman SJ. The warburg effect dictates the mechanism of butyrate-mediated histone acetylation and cell proliferation. Mol Cell (2012) 484:612–26. doi: 10.1016/j.molcel.2012.08.033
111. Steliou K, Boosalis MS, Perrine SP, Sangerman J, Faller DV. Butyrate histone deacetylase inhibitors. Biores Open Access (2012) 14:192–8. doi: 10.1089/biores.2012.0223
112. Davie JR. Inhibition of histone deacetylase activity by butyrate. J Nutr (2003) 1337 Suppl:2485S–93S. doi: 10.1093/jn/133.7.2485S
113. Chang PV, Hao L, Offermanns S, Medzhitov R. The microbial metabolite butyrate regulates intestinal macrophage function via histone deacetylase inhibition. Proc Natl Acad Sci U.S.A. (2014) 1116:2247–52. doi: 10.1073/pnas.1322269111
114. Khan S, Jena G. The role of butyrate, a histone deacetylase inhibitor in diabetes mellitus: experimental evidence for therapeutic intervention. Epigenomics (2015) 74:669–80. doi: 10.2217/epi.15.20
115. Zhang Y, Lei Y, Honarpisheh M, Kemter E, Wolf E, Seissler J. Butyrate and class I histone deacetylase inhibitors promote differentiation of neonatal porcine islet cells into beta cells. Cells (2021) 10(11):3249. doi: 10.3390/cells10113249
116. Gao Z, Yin J, Zhang J, Ward RE, Martin RJ, Lefevre M, et al. Butyrate improves insulin sensitivity and increases energy expenditure in mice. Diabetes (2009) 587:1509–17. doi: 10.2337/db08-1637
117. Lin HV, Frassetto A, Kowalik EJ Jr., Nawrocki AR, Lu MM, Kosinski JR, et al. Butyrate and propionate protect against diet-induced obesity and regulate gut hormones via free fatty acid receptor 3-independent mechanisms. PloS One (2012) 74:e35240. doi: 10.1371/journal.pone.0035240
118. den Besten G, Bleeker A, Gerding A, van Eunen K, Havinga R, van Dijk TH, et al. Short-chain fatty acids protect against high-fat diet-induced obesity via a PPARgamma-dependent switch from lipogenesis to fat oxidation. Diabetes (2015) 647:2398–408. doi: 10.2337/db14-1213
119. Henagan TM, Stefanska B, Fang Z, Navard AM, Ye J, Lenard NR, et al. Sodium butyrate epigenetically modulates high-fat diet-induced skeletal muscle mitochondrial adaptation, obesity and insulin resistance through nucleosome positioning. Br J Pharmacol (2015) 17211:2782–98. doi: 10.1111/bph.13058
120. Lu Y, Fan C, Li P, Lu Y, Chang X, Qi K. Short chain fatty acids prevent high-fat-diet-induced obesity in mice by regulating G protein-coupled receptors and gut microbiota. Sci Rep (2016) 6(37589). doi: 10.1038/srep37589
121. Matheus VA, Monteiro L, Oliveira RB, Maschio DA, Collares-Buzato CB. Butyrate reduces high-fat diet-induced metabolic alterations, hepatic steatosis and pancreatic beta cell and intestinal barrier dysfunctions in prediabetic mice. Exp Biol Med (Maywood) (2017) 24212:1214–26. doi: 10.1177/1535370217708188
122. Li Z, Yi CX, Katiraei S, Kooijman S, Zhou E, Chung CK, et al. Butyrate reduces appetite and activates brown adipose tissue via the gut-brain neural circuit. Gut (2018) 677:1269–79. doi: 10.1136/gutjnl-2017-314050
123. Aguilar EC, da Silva JF, Navia-Pelaez JM, Leonel AJ, Lopes LG, Menezes-Garcia Z, et al. . doi: 10.1016/j.nut.2017.10.007
124. Gao F, Lv YW, Long J, Chen JM, He JM, Ruan XZ, et al. Butyrate improves the metabolic disorder and gut microbiome dysbiosis in mice induced by a high-fat diet. Front Pharmacol (2019) 101040:1040. doi: 10.3389/fphar.2019.01040
125. Fang W, Xue H, Chen X, Chen K, Ling W. Supplementation with sodium butyrate modulates the composition of the gut microbiota and ameliorates high-fat diet-induced obesity in mice. J Nutr (2019) 1495:747–54. doi: 10.1093/jn/nxy324
126. Bayazid AB, Kim JG, Azam S, Jeong SA, Kim DH, Park CW, et al. Sodium butyrate ameliorates neurotoxicity and exerts anti-inflammatory effects in high fat diet-fed mice. Food Chem Toxicol (2022) 159:112743. doi: 10.1016/j.fct.2021.112743
127. Hong J, Jia Y, Pan S, Jia L, Li H, Han Z, et al. Butyrate alleviates high fat diet-induced obesity through activation of adiponectin-mediated pathway and stimulation of mitochondrial function in the skeletal muscle of mice. Oncotarget (2016) 735:56071–82. doi: 10.18632/oncotarget.11267
128. Arnoldussen IAC, Wiesmann M, Pelgrim CE, Wielemaker EM, van Duyvenvoorde W, Amaral-Santos PL, et al. Butyrate restores HFD-induced adaptations in brain function and metabolism in mid-adult obese mice. Int J Obes (Lond) (2017) 416:935–44. doi: 10.1038/ijo.2017.52
129. Jia Y, Hong J, Li H, Hu Y, Jia L, Cai D, et al. Butyrate stimulates adipose lipolysis and mitochondrial oxidative phosphorylation through histone hyperacetylation-associated beta3 -adrenergic receptor activation in high-fat diet-induced obese mice. Exp Physiol (2017) 1022:273–81. doi: 10.1113/EP086114
130. Mollica MP, Mattace Raso G, Cavaliere G, Trinchese G, De Filippo C, Aceto S, et al. Butyrate regulates liver mitochondrial function, efficiency, and dynamics in insulin-resistant obese mice. Diabetes (2017) 665:1405–18. doi: 10.2337/db16-0924
131. Pelgrim CE, Franx BAA, Snabel J, Kleemann R, Arnoldussen IAC, Kiliaan AJ. Butyrate reduces HFD-induced adipocyte hypertrophy and metabolic risk factors in obese LDLr-/-.Leiden mice. Nutrients (2017) 9(7):714. doi: 10.3390/nu9070714
132. Sun B, Jia Y, Hong J, Sun Q, Gao S, Hu Y, et al. Sodium butyrate ameliorates high-Fat-Diet-Induced non-alcoholic fatty liver disease through peroxisome proliferator-activated receptor alpha-mediated activation of beta oxidation and suppression of inflammation. J Agric Food Chem (2018) 6629:7633–42. doi: 10.1021/acs.jafc.8b01189
133. Zhou D, Chen YW, Zhao ZH, Yang RX, Xin FZ, Liu XL, et al. Sodium butyrate reduces high-fat diet-induced non-alcoholic steatohepatitis through upregulation of hepatic GLP-1R expression. Exp Mol Med (2018) 5012:1–12. doi: 10.1038/s12276-018-0183-1
134. Sun B, Jia Y, Yang S, Zhao N, Hu Y, Hong J, et al. Sodium butyrate protects against high-fat diet-induced oxidative stress in rat liver by promoting expression of nuclear factor E2-related factor 2. Br J Nutr (2019) 1224:400–10. doi: 10.1017/S0007114519001399
135. Wang D, Liu CD, Li HF, Tian ML, Pan JQ, Shu G, et al. LSD1 mediates microbial metabolite butyrate-induced thermogenesis in brown and white adipose tissue. Metabolism (2020) 102:154011. doi: 10.1016/j.metabol.2019.154011
136. Zhou D, Pan Q, Xin FZ, Zhang RN, He CX, Chen GY, et al. Sodium butyrate attenuates high-fat diet-induced steatohepatitis in mice by improving gut microbiota and gastrointestinal barrier. World J Gastroenterol (2017) 231:60–75. doi: 10.3748/wjg.v23.i1.60
137. Coppola S, Nocerino R, Paparo L, Bedogni G, Calignano A, Di Scala C, et al. Therapeutic effects of butyrate on pediatric obesity: A randomized clinical trial. JAMA Netw Open (2022) 512:e2244912. doi: 10.1001/jamanetworkopen.2022.44912
138. Gregoire FM, Magrum L, Johnson PR, Greenwood MR. Positive modulation of the adipoconversion of adipoblasts derived from genetically obese rats by sodium butyrate. Obes Res (1994) 22:110–6. doi: 10.1002/j.1550-8528.1994.tb00636.x
139. Khan S, Jena G. Sodium butyrate reduces insulin-resistance, fat accumulation and dyslipidemia in type-2 diabetic rat: A comparative study with metformin. Chem Biol Interact (2016) 254:124–34. doi: 10.1016/j.cbi.2016.06.007
140. Huang Y, Gao S, Chen J, Albrecht E, Zhao R, Yang X. Maternal butyrate supplementation induces insulin resistance associated with enhanced intramuscular fat deposition in the offspring. Oncotarget (2017) 88:13073–84. doi: 10.18632/oncotarget.14375
141. Zhou J, Gao S, Chen J, Zhao R, Yang X. Maternal sodium butyrate supplement elevates the lipolysis in adipose tissue and leads to lipid accumulation in offspring liver of weaning-age rats. Lipids Health Dis (2016) 151:119. doi: 10.1186/s12944-016-0289-1
142. Lu H, Su S, Ajuwon KM. Butyrate supplementation to gestating sows and piglets induces muscle and adipose tissue oxidative genes and improves growth performance. J Anim Sci (2012) 90 Suppl:4430–2. doi: 10.2527/jas.53817
143. Adeyanju OA, Badejogbin OC, Areola DE, Olaniyi KS, Dibia C, Soetan OA, et al. Sodium butyrate arrests pancreato-hepatic synchronous uric acid and lipid dysmetabolism in high fat diet fed wistar rats. BioMed Pharmacother (2021) 133:110994. doi: 10.1016/j.biopha.2020.110994
144. Mattace Raso G, Simeoli R, Russo R, Iacono A, Santoro A, Paciello O, et al. Effects of sodium butyrate and its synthetic amide derivative on liver inflammation and glucose tolerance in an animal model of steatosis induced by high fat diet. PloS One (2013) 87:e68626. doi: 10.1371/journal.pone.0068626
145. Oussaada SM, van Galen KA, Cooiman MI, Kleinendorst L, Hazebroek EJ, van Haelst MM, et al. The pathogenesis of obesity. Metabolism (2019) 92:26–36. doi: 10.1016/j.metabol.2018.12.012
146. Swift DL, McGee JE, Earnest CP, Carlisle E, Nygard M, Johannsen NM. The effects of exercise and physical activity on weight loss and maintenance. Prog Cardiovasc Dis (2018) 612:206–13. doi: 10.1016/j.pcad.2018.07.014
147. Chao AM, Quigley KM, Wadden TA. Dietary interventions for obesity: clinical and mechanistic findings. J Clin Invest (2021) 131(1):e140065. doi: 10.1172/JCI140065
148. Harms M, Seale P. Brown and beige fat: development, function and therapeutic potential. Nat Med (2013) 1910:1252–63. doi: 10.1038/nm.3361
149. Puigserver P, Wu Z, Park CW, Graves R, Wright M, Spiegelman BM. A cold-inducible coactivator of nuclear receptors linked to adaptive thermogenesis. Cell (1998) 926:829–39. doi: 10.1016/s0092-8674(00)81410-5
150. Nakashima A, Hayashi N, Kaneko YS, Mori K, Sabban EL, Nagatsu T, et al. Role of n-terminus of tyrosine hydroxylase in the biosynthesis of catecholamines. J Neural Transm (Vienna) (2009) 11611:1355–62. doi: 10.1007/s00702-009-0227-8
151. Sambeat A, Gulyaeva O, Dempersmier J, Tharp KM, Stahl A, Paul SM, et al. LSD1 interacts with Zfp516 to promote UCP1 transcription and brown fat program. Cell Rep (2016) 1511:2536–49. doi: 10.1016/j.celrep.2016.05.019
152. Wu J, Bostrom P, Sparks LM, Ye L, Choi JH, Giang AH, et al. Beige adipocytes are a distinct type of thermogenic fat cell in mouse and human. Cell (2012) 1502:366–76. doi: 10.1016/j.cell.2012.05.016
153. Ben-Shlomo S, Zvibel I, Shnell M, Shlomai A, Chepurko E, Halpern Z, et al. Glucagon-like peptide-1 reduces hepatic lipogenesis via activation of AMP-activated protein kinase. J Hepatol (2011) 546:1214–23. doi: 10.1016/j.jhep.2010.09.032
154. Cypess AM. Reassessing human adipose tissue. New Engl J Med (2022) 3868:768–79. doi: 10.1056/NEJMra2032804
155. Granneman JG, Li P, Zhu Z, Lu Y. Metabolic and cellular plasticity in white adipose tissue I: effects of beta3-adrenergic receptor activation. Am J Physiol Endocrinol Metab (2005) 2894:E608–16. doi: 10.1152/ajpendo.00009.2005
156. Ahmadian M, Duncan RE, Sul HS. The skinny on fat: lipolysis and fatty acid utilization in adipocytes. Trends Endocrinol Metab (2009) 209:424–8. doi: 10.1016/j.tem.2009.06.002
157. Granneman JG, Moore HP, Krishnamoorthy R, Rathod M. Perilipin controls lipolysis by regulating the interactions of AB-hydrolase containing 5 (Abhd5) and adipose triglyceride lipase (Atgl). J Biol Chem (2009) 28450:34538–44. doi: 10.1074/jbc.M109.068478
158. Carmen GY, Victor SM. Signalling mechanisms regulating lipolysis. Cell Signal (2006) 184:401–8. doi: 10.1016/j.cellsig.2005.08.009
159. Lampidonis AD, Rogdakis E, Voutsinas GE, Stravopodis DJ. The resurgence of hormone-sensitive lipase (HSL) in mammalian lipolysis. Gene (2011) 4771-2:1–11. doi: 10.1016/j.gene.2011.01.007
160. Serra D, Mera P, Malandrino MI, Mir JF, Herrero L. Mitochondrial fatty acid oxidation in obesity. Antioxid Redox Signal (2013) 193:269–84. doi: 10.1089/ars.2012.4875
161. Schlaepfer IR, Joshi M. CPT1A-mediated fat oxidation, mechanisms, and therapeutic potential. Endocrinology (2020) 161(2):bqz046. doi: 10.1210/endocr/bqz046
162. Betz MJ, Enerback S. Targeting thermogenesis in brown fat and muscle to treat obesity and metabolic disease. Nat Rev Endocrinol (2018) 142:77–87. doi: 10.1038/nrendo.2017.132
163. Egan B, Zierath JR. Exercise metabolism and the molecular regulation of skeletal muscle adaptation. Cell Metab (2013) 172:162–84. doi: 10.1016/j.cmet.2012.12.012
164. Chaudhri OB, Salem V, Murphy KG, Bloom SR. Gastrointestinal satiety signals. Annu Rev Physiol (2008) 70:239–55. doi: 10.1146/annurev.physiol.70.113006.100506
165. Sternson SM, Eiselt AK. Three pillars for the neural control of appetite. Annu Rev Physiol (2017) 79:401–23. doi: 10.1146/annurev-physiol-021115-104948
166. Obradovic M, Sudar-Milovanovic E, Soskic S, Essack M, Arya S, Stewart AJ, et al. Leptin and obesity: Role and clinical implication. Front Endocrinol (Lausanne) (2021) 12585887:585887. doi: 10.3389/fendo.2021.585887
167. Fabbrini E, Sullivan S, Klein S. Obesity and nonalcoholic fatty liver disease: biochemical, metabolic, and clinical implications. Hepatology (2010) 512:679–89. doi: 10.1002/hep.23280
168. Dye L, Boyle NB, Champ C, Lawton C. The relationship between obesity and cognitive health and decline. Proc Nutr Soc (2017) 764:443–54. doi: 10.1017/S0029665117002014
169. The L. Diabetes: a dynamic disease. Lancet (2017) 389(10085):2163. doi: 10.1016/s0140-6736(17)31537-4
170. Zheng Y, Ley SH, Hu FB. Global aetiology and epidemiology of type 2 diabetes mellitus and its complications. Nat Rev Endocrinol (2018) 142:88–98. doi: 10.1038/nrendo.2017.151
171. Al-Sulaiti H, Diboun I, Agha MV, Mohamed FFS, Atkin S, Domling AS, et al. Metabolic signature of obesity-associated insulin resistance and type 2 diabetes. J Transl Med (2019) 171:348. doi: 10.1186/s12967-019-2096-8
172. Meijnikman AS, Gerdes VE, Nieuwdorp M, Herrema H. Evaluating causality of gut microbiota in obesity and diabetes in humans. Endocr Rev (2018) 392:133–53. doi: 10.1210/er.2017-00192
173. Eizirik DL, Pasquali L, Cnop M. Pancreatic beta-cells in type 1 and type 2 diabetes mellitus: different pathways to failure. Nat Rev Endocrinol (2020) 167:349–62. doi: 10.1038/s41574-020-0355-7
174. Khan S, Jena GB. Protective role of sodium butyrate, a HDAC inhibitor on beta-cell proliferation, function and glucose homeostasis through modulation of p38/ERK MAPK and apoptotic pathways: study in juvenile diabetic rat. Chem Biol Interact (2014) 213:1–12. doi: 10.1016/j.cbi.2014.02.001
175. Elgamal DA, Abou-Elghait AT, Ali AY, Ali M, Bakr MH. Ultrastructure characterization of pancreatic beta-cells is accompanied by modulatory effects of the HDAC inhibitor sodium butyrate on the PI3/AKT insulin signaling pathway in juvenile diabetic rats. Mol Cell Endocrinol (2020) 503:110700. doi: 10.1016/j.mce.2019.110700
176. Prause M, Pedersen SS, Tsonkova V, Qiao M, Billestrup N. Butyrate protects pancreatic beta cells from cytokine-induced dysfunction. Int J Mol Sci (2021) 22(19):10427. doi: 10.3390/ijms221910427
177. Hu S, Kuwabara R, de Haan BJ, Smink AM, de Vos P. Acetate and butyrate improve beta-cell metabolism and mitochondrial respiration under oxidative stress. Int J Mol Sci (2020) 21(4):1542. doi: 10.3390/ijms21041542
178. Yaribeygi H, Sathyapalan T, Atkin SL, Sahebkar A. Molecular mechanisms linking oxidative stress and diabetes mellitus. Oxid Med Cell Longev (2020) 2020:8609213. doi: 10.1155/2020/8609213
179. Powell EE, Wong VW-S, Rinella M. Non-alcoholic fatty liver disease. Lancet (2021) 39710290:2212–24. doi: 10.1016/s0140-6736(20)32511-3
180. Polyzos SA, Kountouras J, Mantzoros CS. Obesity and nonalcoholic fatty liver disease: From pathophysiology to therapeutics. Metabolism (2019) 92:82–97. doi: 10.1016/j.metabol.2018.11.014
181. Li H, Gao Z, Zhang J, Ye X, Xu A, Ye J, et al. Sodium butyrate stimulates expression of fibroblast growth factor 21 in liver by inhibition of histone deacetylase 3. Diabetes (2012) 614:797–806. doi: 10.2337/db11-0846
182. Zhang N, Qu Y, Qin B. Sodium butyrate ameliorates non-alcoholic fatty liver disease by upregulating miR-150 to suppress CXCR4 expression. Clin Exp Pharmacol Physiol (2021) 488:1125–36. doi: 10.1111/1440-1681.13497
183. Martins LB, Monteze NM, Calarge C, Ferreira AVM, Teixeira AL. Pathways linking obesity to neuropsychiatric disorders. Nutrition (2019) 66:16–21. doi: 10.1016/j.nut.2019.03.017
184. Tanaka H, Gourley DD, Dekhtyar M, Haley AP. Cognition, brain structure, and brain function in individuals with obesity and related disorders. Curr Obes Rep (2020) 94:544–9. doi: 10.1007/s13679-020-00412-y
185. O'Brien PD, Hinder LM, Callaghan BC, Feldman EL. Neurological consequences of obesity. Lancet Neurol (2017) 166:465–77. doi: 10.1016/s1474-4422(17)30084-4
Keywords: butyrate, SCFAs, gut microbiota, obesity, metabolic disease
Citation: Peng K, Dong W, Luo T, Tang H, Zhu W, Huang Y and Yang X (2023) Butyrate and obesity: Current research status and future prospect. Front. Endocrinol. 14:1098881. doi: 10.3389/fendo.2023.1098881
Received: 17 November 2022; Accepted: 07 February 2023;
Published: 24 February 2023.
Edited by:
Rodrigo Troncoso, University of Chile, ChileReviewed by:
Li Li, University of Texas Southwestern Medical Center, United StatesChengjun Hu, Chinese Academy of Tropical Agricultural Sciences, China
Copyright © 2023 Peng, Dong, Luo, Tang, Zhu, Huang and Yang. This is an open-access article distributed under the terms of the Creative Commons Attribution License (CC BY). The use, distribution or reproduction in other forums is permitted, provided the original author(s) and the copyright owner(s) are credited and that the original publication in this journal is cited, in accordance with accepted academic practice. No use, distribution or reproduction is permitted which does not comply with these terms.
*Correspondence: Yilan Huang, bHp5eHl5eHAxMjNAMTYzLmNvbQ==; Xuping Yang, eWFuZ3h1cGluZzY1NTJAMTYzLmNvbQ==