- 1Reproduction Group, Department of Physiology and Biochemistry, School of Medicine, Universidad de Antioquia, Medellín, Colombia
- 2Department of Obstetrics and Gynecology, School of Medicine, Universidad de Antioquia, Medellín, Colombia
- 3Red Iberoamericana de Alteraciones Vasculares en Trastornos del Embarazo (RIVATREM), Chillan, Chile
- 4One Health and Veterinary Innovative Research & Development (OHVRI) Research Group, Escuela de Medicina Veterinaria, Universidad de Antioquia, Medellín, Colombia
- 5Grupo Infettare, Facultad de Medicina, Universidad Cooperativa de Colombia, Medellín, Colombia
Preeclampsia is a pregnancy-related multisystem disorder characterized by altered trophoblast invasion, oxidative stress, exacerbation of systemic inflammatory response, and endothelial damage. The pathogenesis includes hypertension and mild-to-severe microangiopathy in the kidney, liver, placenta, and brain. The main mechanisms involved in its pathogenesis have been proposed to limit trophoblast invasion and increase the release of extracellular vesicles from the syncytiotrophoblast into the maternal circulation, exacerbating the systemic inflammatory response. The placenta expresses glycans as part of its development and maternal immune tolerance during gestation. The expression profile of glycans at the maternal–fetal interface may play a fundamental role in physiological pregnancy changes and disorders such as preeclampsia. It is unclear whether glycans and their lectin-like receptors are involved in the mechanisms of maternal–fetal recognition by immune cells during pregnancy homeostasis. The expression profile of glycans appears to be altered in hypertensive disorders of pregnancy, which could lead to alterations in the placental microenvironment and vascular endothelium in pregnancy conditions such as preeclampsia. Glycans with immunomodulatory properties at the maternal–fetal interface are altered in early-onset severe preeclampsia, implying that innate immune system components, such as NK cells, exacerbate the systemic inflammatory response observed in preeclampsia. In this article, we discuss the evidence for the role of glycans in gestational physiology and the perspective of glycobiology on the pathophysiology of hypertensive disorders in gestation.
1 Introduction
Preeclampsia is the most common hypertensive disorder in pregnancy, characterized by mild-to-severe microangiopathy of the placenta, kidney, liver, and brain (1–3). Before clinical signs of the disease appear, altered placental development exacerbates the local and systemic inflammatory status targeting the vascular endothelium (4–6). The systemic inflammatory response and clinical signs of preeclampsia end shortly after the fetus and placenta are removed during cesarean section, though hypertensive episodes may persist in some patients beyond the postpartum period. This situation increases the risk of renal disease or new episodes of preeclampsia in the subsequent gestation (7–9).
Preeclampsia is defined as a new onset of maternal hypertension after 20 weeks of gestation and systemic endothelial dysfunction manifested by new onset proteinuria (though not in all patients), hepatic dysfunction, and thrombocytopenia, among other symptoms (10, 11). Furthermore, preeclampsia exhibits diverse clinical manifestations, such as mild or severe, early or late onset (>34 weeks), or the presence or absence of intrauterine growth restriction (12–14). Pathological features of preeclampsia include shallow trophoblast invasion and poor spiral artery remodeling, resulting in placental hypoperfusion and intrauterine growth restriction (15, 16). These events occur during the first trimester of pregnancy and initially compromise the maternal–fetal interface, resulting in increased anti-angiogenic (17) and inflammatory factor production (18–22). Two pathophysiological stages have been proposed (23) to understand better the underlying mechanisms of preeclampsia: The first stage includes poor placentation, placental hypoperfusion, hypoxia, and trophoblast oxidative damage, followed by endothelial dysfunction and hypertensive clinical signs in the second stage (21, 24–26). Endothelial activation is intrinsic to the exacerbated systemic inflammatory response in severe preeclampsia (27), which involves NK cell activation rather than monocyte or lymphocyte activation (28, 29), resulting in coagulation dysfunction, insulin resistance, and hyperlipidemia (30).
In this context, the etiopathogenesis of preeclampsia is unknown, and the factors triggering its onset and progression to a multisystemic syndrome are unpredictable. Preeclampsia pathophysiology has traditionally focused on abnormal trophoblastic invasion, vascular inflammation, and the systemic inflammatory response (Croci et al., 2014). A more comprehensive approach is required to understand the occurrence of the multisystem syndrome (31). In this review, we focused on glycan recognition by peripheral NK cells and modulation of activation toward the cytotoxic phenotype to uncover new insights into molecular communication mechanisms between the trophoblast and the peripheral innate immune system. In the last two decades, a glycobiological perspective on pregnancy has emerged (32–35), and these new approaches have the potential to provide critical insights into the pathogenesis of preeclampsia and the development of severe clinical forms of the disease (36–43).
1.1 The role of glycans in cell-to-cell communication
The glycocalyx is the cell’s outermost layer of glycoconjugates, mainly glycoproteins and proteoglycans. The glycans bound to these glycoconjugates participate in ligand–receptor interactions in biological processes involving cell-to-cell interaction and function as a barrier and filter in endothelia (44, 45). In turn, the set of glycoprotein modifications complements the glycocalyx, and its more complex structure is encoded by nearly 10% of the transcribed genes that comprise the so-called glycome (46). The glycocalyx conformation may vary depending on the cell type, its activation and differentiation state, the cellular microenvironment, and the physiological or pathological conditions of the cells (47). Therefore, two of the systems in which the physiological role of glycocalyx has been studied are the immune system (reviewed in 48) and the vascular endothelium (reviewed in 49), particularly in pathological conditions (reviewed in 47, 50).
1.2 Structure of glycans
The glycocalyx is made up of monosaccharides or carbohydrates. In this review, glycan is preferred over carbohydrates because, unlike carbohydrates, not all glycans are monomers. Additionally, carbohydrate is often used interchangeably with components of intermediary metabolism. Glycans are composed of one carbonyl group, and the rest of the carbons contain hydroxyl groups. Carbonyl position and hydroxyl group orientation of atoms in asymmetric carbon determine monosaccharide structure. The anomeric carbon is derived from the carbonyl group; depending on the orientation of the anomeric carbon’s hydroxyl group, the monosaccharide exists in either a or b anomer (48). The identity, variety, and functions of glycans are determined by chemical modifications of the hydroxyl groups, such as oxidation, N-acetylation, and sulfation (51, 52). Finally, when glycans combine to form linear and branched glycan structures, the variety of glycans exponentially increases. The diversity of glycans is estimated to be 1.05 × 1012 of linear and branched glycan structures (53). The most common monosaccharides found in mammalian cells include glucose (Glc), galactose (Gal), mannose (Man), N-acetylneuraminic acid (NeuAc), N-acetylglucosamine (GlcNAc), N-acetylgalactosamine (GalNAc), glucuronic acid (GlaA), xylose (Xyl), and fucose (Fuc) (48).
The DNA code does not determine protein modifications by enzymatic glycosylation because cellular enzymatic expression varies between species, cellular microenvironments, and cell types (54). Besides, glycosylation is a remarkably well-conserved enzymatic process (55). Specific changes in each glycan sequence will depend on the expression of glycosidases and glycosyltransferases, the availability of nucleotide sugar donor sources, and external influences mediated by cytokines and hormones in the cell microenvironment (56, 57). Glycosidases are enzymes that catalyze the hydrolysis of a bond linking a sugar of a glycoside to alcohol or another radical in the glycan molecule, whereas glycosyl transferases are enzymes that catalyze the transfer of glycosyl groups in biochemical reactions (58).
The structural diversity of the mammalian glycome is supported by the synthesis of glycans and their enzymatic regulation, which occurs in the endoplasmic reticulum and Golgi apparatus when added to proteins. If the glycan binds to the nitrogen of asparagine in the protein, it is called N-glycosylation or N-glycan; if it binds to the oxygen of a serine or threonine, it is called O-glycosylation or O-glycan (Figure 1A). In the case of proteoglycans, an unbranched polysaccharide chain composed of disaccharide units of a sulfated amino sugar (N-acetylglucosamine or N-acetylgalactosamine) and uronic acid (iduronic or glucuronic) is attached to a large protein core in a serine residue (59–61).
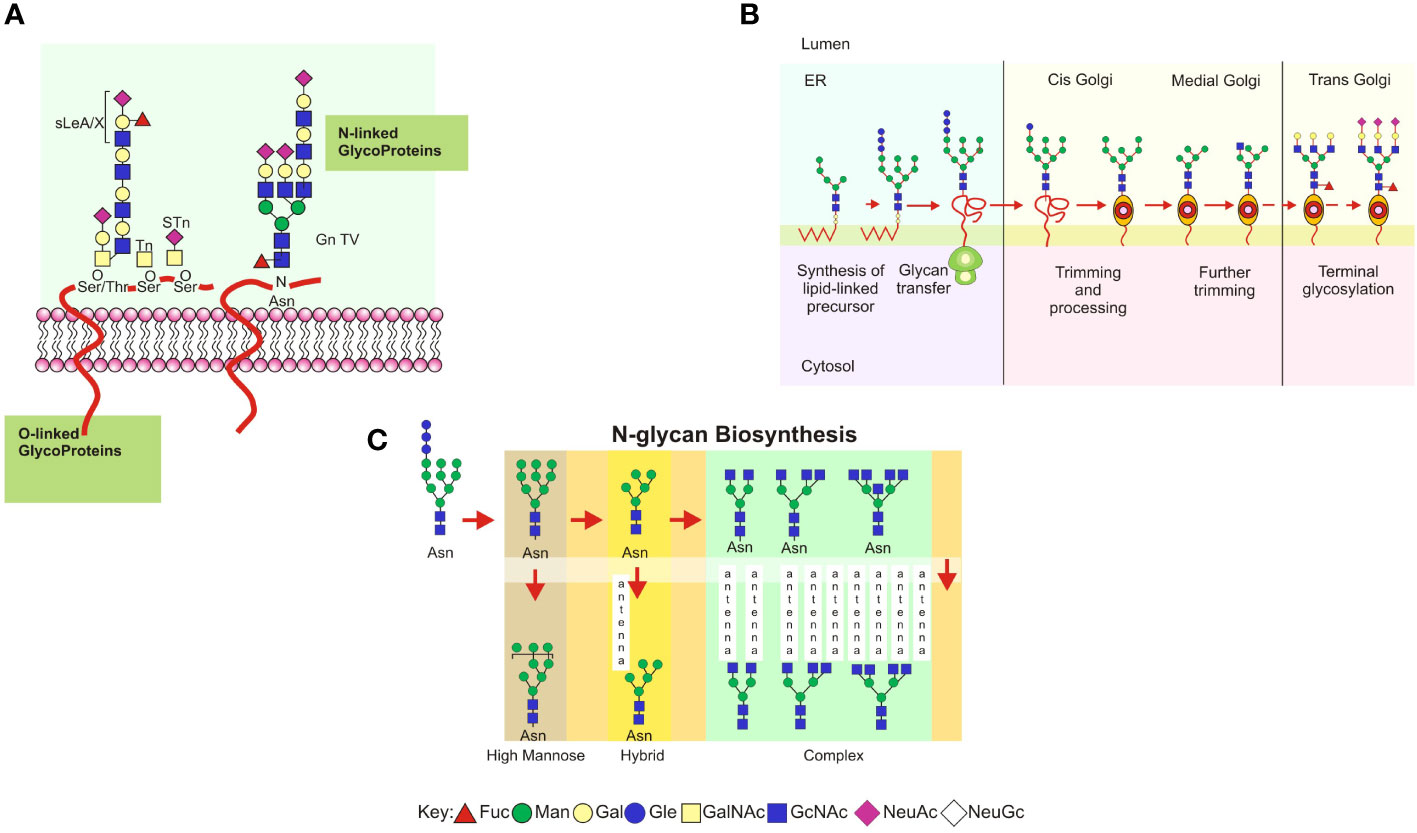
Figure 1 (A) Schematic representation of the main types of glycoproteins expressed in mammalian glycocalyx. Types of cell-surface glycans, including N-linked and O-linked glycans from glycoproteins. Each glycan has a common core whose terminal or internal sequences can vary, leading to a complex diversity among cells or defined microenvironments. (B) A consensus sequence for N-glycosylation (Asp-X-Ser/Thr, designated sequon) is used as an acceptor in polypeptide chains of a preformed oligosaccharide attached to a dolichol phosphate in the endoplasmic reticulum. The oligosaccharide glucose3–mannose9–N–acetylglucosamine2 is transferred to the protein chain by an oligosaccharyltransferase acting as a chaperone and modulating its folding to transport toward the Golgi complex. Trimming and processing of the attached glycan are performed in Golgi compartments by specific glycosyltransferases and glycosidases for each nucleotide sugar donor. It is in the Golgi apparatus that glycans become oligomeric and branched. (C) Eukaryotic N-glycan biosynthesis in the endoplasmic reticulum and Golgi complex. Processing intermediates of N-glycans preserve a unique sequence core Manα1-6 (Manα1-3) Manβ1-4GlcNAcβ1-4GlcNAcβ1-Asn-X-Ser/Thr. Three types of N-glycans are found: oligomannose, complex, and hybrid.
1.3 N-linked glycosylation
In N-glycosylation, a series of glycosyltransferases use nucleotide-activated sugar donors as substrates to form the mannose oligosaccharide 5-N-acetylglucosamine (Figure 1A). Oligosaccharide portions formed in the endoplasmic reticulum, specifically terminal glucose, and mannose, combined with molecular chaperones and thiol-disulfide oxidoreductases, help to fold newly synthesized proteins (62). The N-glycosylated polypeptide is delivered into the Golgi apparatus following interaction with cargo receptors in the vesicular transport system (63). If the protein is misfolded, it is translocated to the cytosol, deglycosylated by the enzyme peptide N-glycanase, or degraded by the ubiquitin/proteasome. In this pathway, glycosidases and mannosidases in the endoplasmic reticulum trim the N-glycans bound to the nascent protein, resulting in intermediate processing forms (Figure 1B; 64). The mannose residues are trimmed in the endoplasmic reticulum and subsequently in the Golgi complex, where the processed glycans undergo the addition of N-acetylglucosamine (N-acetylglucosaminylation), galactose (galactosylation), sialic acid (sialylation), or sulfate (sulfation). The intermediate forms of N-glycans share a common glycan core consisting of Manα1-6 (Manα1-3) Manβ1-4GlcNAcβ1-4GlcNAcβ1-Asn-X-Ser/Thr and have been classified into three types: (a) oligomannose, in which the mannose residues are attached to the core; (b) complex, in which the mannose residues are trimmed and replaced by N-acetylglucosamine, resulting in new elongated branches attached to the N-acetylglucosamine residues called “antennae”; and (c) a hybrid, in which only the mannose residues attached to Manα1-6 are conserved, while the mannose attached to Manα1-3 is trimmed and replaced in an antennae sequence by a branched N-acetylglucosamine (Figure 1C; reviewed in 64, 65).
1.4 O-linked glycosylation
O-linked glycosylation involves the formation of O-glycosidic bonds between oligosaccharide chains via N-acetylgalactosamine and GalNAc (1β-3), which is found in mucin glycoproteins (Figure 1A) (66). These glycans are mucins with GalNAc O-linked to Ser or Thr. O-glycosylation occurs in mucin domains containing many serine, threonine, and proline sequences. O-glycan processing occurs in the Golgi apparatus and has little impact on the early stages of protein folding. In mammalian mucins, the first attached GalNAc can be replaced by GlcNAc, GalNAc (linkage 1α-3), or Gal, forming up to eight different core structures: core 1: Galβ1-3GalNAc; core 2: GlcNAcβ1-6(Galb1-3)GalNAc; core 3: GlcNAcβ1-3GalNAc; core 4: GlcNAcβ1-6(GlcNAcβ1-3)GalNAca; core 5: GalNAcα1-3GalNAc; core 6: GlcNAcβ1-6GalNAc; core 7: GalNAcα1-6GalNAc; and core 8: Galα1-3GalNAc (67). The most common core sequences are 1 and 2; cores 3 and 4 are only found in mucins. The core structures can be substituted or elongated with sialic acid residues. O-glycan biosynthesis is initiated by the enzyme O-GalNAc transferase, which transfers the GalNAc residue of UDP-GalNAc to serine or threonine residues in a protein with the α-configuration (68). O-glycan processing does not require dolichol derivatives or specific glycosidases, and the mRNA levels of glycosyltransferases are critical factors for their assembly (69). Additionally, glycosyltransferases may form complexes with other proteins, influencing their activities, or metal ion concentrations in the Golgi apparatus may regulate them. The presence of an unsubstituted GalNAc on O-glycoproteins forms the Tn antigen in tumor metastasis (70), and GalNAC can be directly substituted with sialic acid to form the sialyl-Tn (STn) antigen. These glycans are truncated and not used in subsequent elongation reactions (68, 70–72).
1.5 Glycan receptors and ligand-receptor interactions
Glycan receptors are proteins with carbohydrate recognition domains (CRDs) called lectins. Lectins bind to clustered CRDs with high affinity via (a) binding to multiple epitopes on a single oligosaccharide or polysaccharide, (b) multiple glycans attached to a single protein scaffold, and (c) binding to adjacent glycoproteins or glycolipids in the cell membrane. Plants produce the well-known lectins, followed by those isolated from animals and pathogenic microorganisms (73). The various types of CRDs are classified into four major lectin groups: (a) Siglecs (short for sialic acid binding immunoglobulin-type lectins) or I-type lectins, in which the CRDs are formed by an immunoglobulin domain fold (sialic acid is the major glycocalyx component related to immune system regulation); (b) galectins (predominantly binding to β-galactosidase), which contain CRDs formed from a beta-like fold of the protein; (c) C-type lectins, in which the sugars bind directly to a calcium ion attached to the CRD; and (d) lectins containing R-type CRDs, whose structure is related to the plant toxin ricin (reviewed in 74). The most important biological functions of ligand–receptor interactions between glycan ligands and lectin-like receptors include cell adhesion, intracellular trafficking, glycoprotein elimination and turnover, cell signaling, and pathogen recognition (48, 65, 74).
1.6 Trophoblast differentiation in human placental development, hypoxia, and glycan expression
The human placenta is an autonomous and transient anatomical structure that allows the mother and fetus to exchange nutritional, gas, and waste products. The trophoblast layer mediates fetal growth and maternal pregnancy adaptation generating a new vascular bed in the placental interface. These trophoblast cells are the first to separate from the developing blastocyst, giving rise to cytotrophoblast stem cells (75, 76). Placental villous tissue develops from primary to secondary and tertiary villous tissue with the progressive development of villous tree circulation complexity. Primary villi containing cytotrophoblast and syncytiotrophoblast cells predominate during the first four weeks of gestation. Secondary villi develop in the fifth week of gestation, with the extraembryonic mesoderm forming villi and covering the surface of the chorionic sac to provide a framework for intra-villi blood circulation. In the third stage of chorionic villi development, which occurs during the sixth week of gestation, mesenchyme differentiates into blood vessels and cells, resulting in the arteriocapillary framework that fuses with placental vessels into the connecting stalk (reviewed in 77). Besides, they develop with greater complexity, resulting in the formation of (a) stem villi or anchoring villi, from which cytotrophoblast cells migrate into the decidua and become extravillous cytotrophoblast (Figure 2A); (b) branched villi, which grow from the stem villi and represent the most critical portion for exchange with maternal blood from the placental bed through the intervillous spaces (Figure 2B); (c) terminal villi, which consist of protrusions caused by trophoblast proliferation due to the coiling of the fetal capillaries within the mature intermediate villi beginning in the third trimester of gestation; and (d) chorionic plate, which is the region at the base of the villi through which the placental arteries and vein pass (Figure 2A; 78–80). The villous cytotrophoblast stem cell population supports the differentiation of trophoblast into two major cell lineages, syncytiotrophoblast and the invasive trophoblast (named extravillous trophoblast [EVT]; 76, 81–83). The most invasive trophoblast wave occurs when EVT cells infiltrate the endothelium of maternal spiral arteries to come into direct contact with systemic circulation, forming placental villi and a maternal–fetal interface (78). The cell layer responsible for maternal–fetal nutrient and gas exchange is the well-differentiated syncytiotrophoblast cells, which cover the anchoring, branched, and terminal villi (84–86).
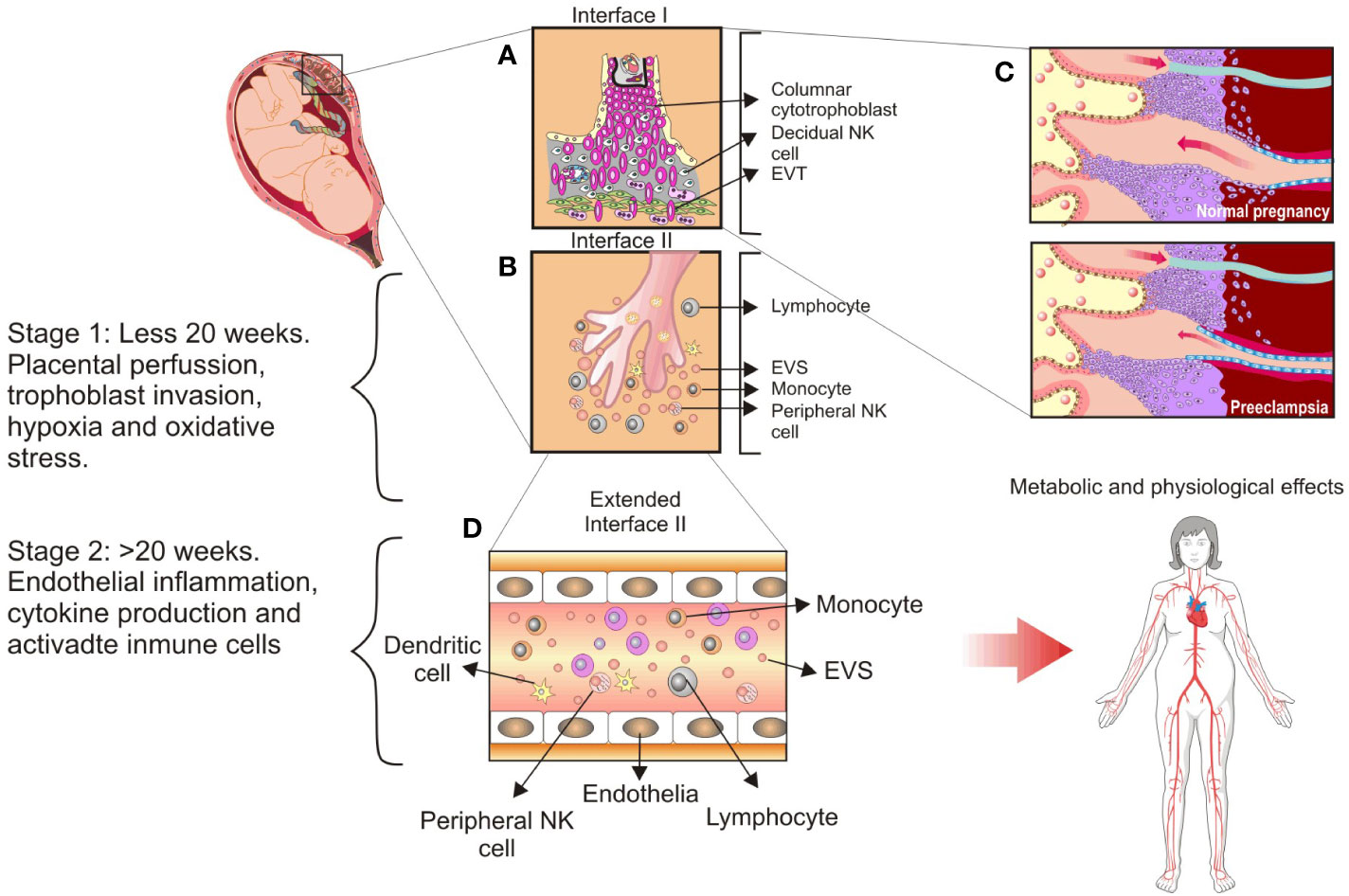
Figure 2 Main cellular components found at the maternal–fetal interface and the proposed stages of preeclampsia. (A), Interface I: Villous tree represents a tertiary villous and its predominant cellular components at interface: Columnar cytotrophoblast, anchoring trophoblast, and extravillous trophoblast subsets. (B), Interface II: Floating villi are in contact with maternal blood flow and release syncytiotrophoblast extracellular vesicles into circulation. Peripheral Natural Killer (NK) cells are highlighted as a significant maternal innate immune system component. (C), Spiral arteries remodeling in the placental bed occurs extensively in a normal pregnancy but not in preeclampsia. (D), Extended interface II: Syncytiotrophoblast extracellular vesicles are delivered into maternal circulation and promote the systemic inflammatory response observed during normal pregnancy. This inflammatory response is exacerbated in the case of women with preeclampsia, and it is linked to abnormal placental invasion, low placental perfusion, and increased oxidative stress accompanied by chronic hypoxia. CC, columnar cytotrophoblast; DC, dendritic cells; EVs, syncytiotrophoblast extracellular vesicles; EVT, extravillous trophoblast. This figure is adapted from Sargent et al. (232).
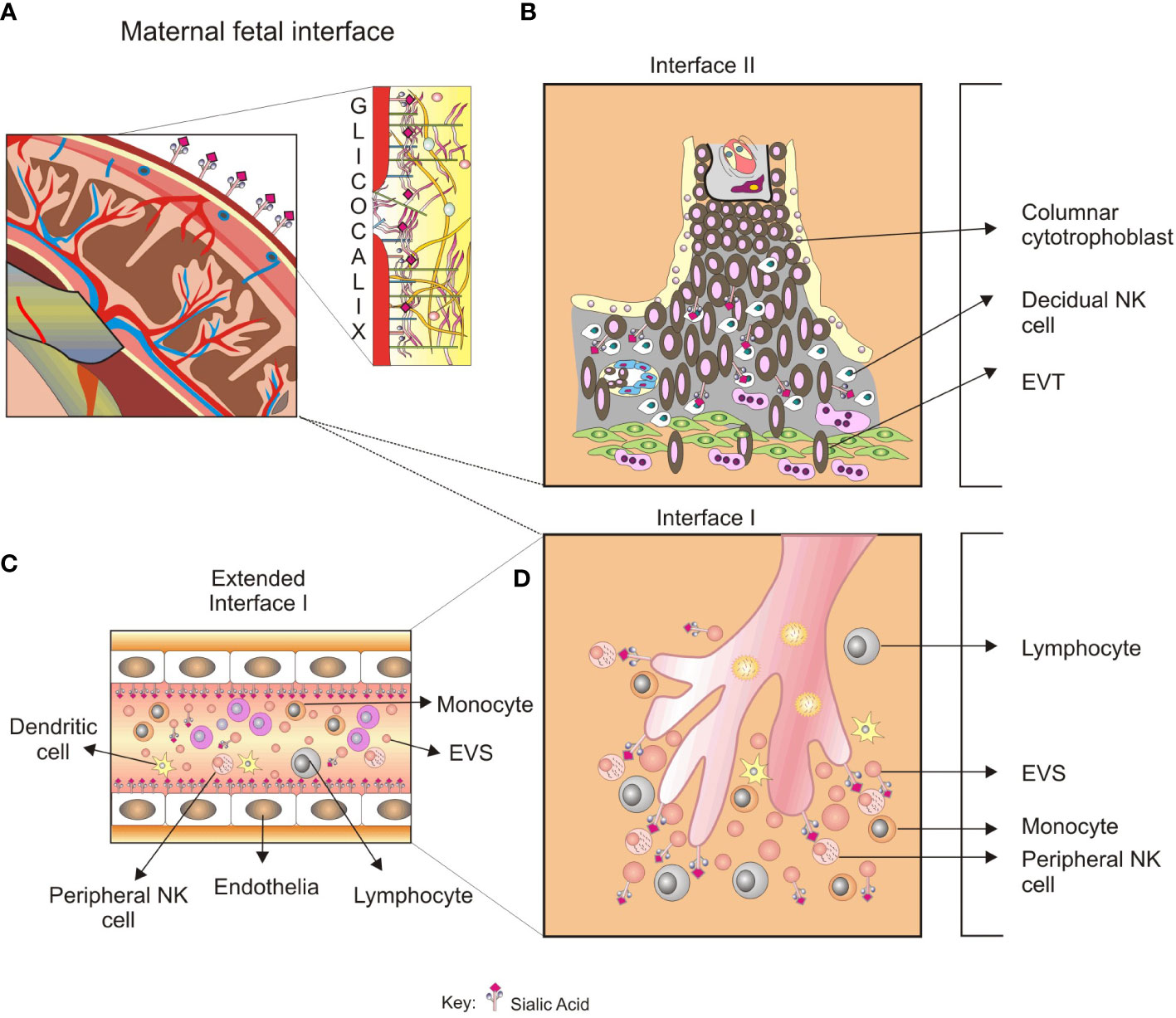
Figure 3 A glycobiological view of the systemic inflammatory response in preeclampsia. (A), Sialylated glycoproteins and glycoproteins with LacNAc glycotopes, among others glycoconjugates in the trophoblast glycocalyx, are expressed in maternal–fetal interface II. (B), The recognition by LacNAc by Gal-1 promotes angiogenesis in the placental bed, and impaired balance in the Gal-1 function results in a PE-like syndrome. Furthermore, defective trophoblast invasion has been associated with specific blocking of Gal-1. (C), Glycoconjugates could be recognized by peripheral NK cells in the maternal–fetal interface I, modulating the NK cell activity. The released syncytiotrophoblast EVs found in preeclampsia could transport glycoproteins with immunomodulatory activity and increase the systemic inflammatory response. (D), EVs released from maternal–fetal interface II spread into the systemic circulation and promote endothelial activation. The type and quantity of VES found in preeclampsia could initiate endothelial damage and exacerbate inflammation. The role of glycan recognition in syncytiotrophoblast VES-mediated endothelial damage is not elucidated yet.
One of the most intriguing aspects of placental development during the first trimester is that the partial occlusion of the maternal spiral artery lumen by the extravillous invading endothelial trophoblast (see 87) results in a temporary condition of placental hypoxia, with approximately 20-mm Hg O2 concentration during the first trimester compared with 60 and 40-mm Hg during the second and third trimesters, respectively (Figure 2C) (88). During the first trimester of gestation, hypoxia at the maternal–fetal interface shapes placental development, which involves cellular and molecular adaptations to compensate for the low oxygen tension. As a result, trophoblast cells are a highly dynamic cellular type responsible for proper placental development. However, these processes are disrupted during pathological gestation conditions, resulting in a loss of placental architecture and abnormalities in fetal growth and development (Figure 2C) (89).
Extravillous trophoblast cells exhibit an invasive phenotype that shares molecular mechanisms with tumor cells, such as binding to the extracellular matrix, extracellular matrix degradation by metalloproteases, and migration through the extracellular matrix mediated by N-linked complex-type glycans (90–92). Truncated forms of O-glycans, such as core 1 (the Thomsen–Friedenreich [T or TF] antigen) and core 1 with a sialic acid substitution at the α2-3 bond to Gal and at the α2-3 bond to GalNAc to form the sialyl-T antigens, are associated with metastasis in some tumors (93, 94). Furthermore, the environment of tumor cells and EVTs are hypoxic, which confers invasive characteristics. About 60% of solid tumors have an oxygen partial pressure of less than 10 mm Hg compared with 50- to 60-mm Hg observed in adjacent non-tumor tissues (95). These values are comparable with the hypoxic state of the maternal–fetal interface during the first trimester of gestation (96–99). Hung and Burton (100) and Robins et al. (99) identified fluctuations in placental oxygen tension as a determinant of trophoblast invasion and differentiation associated with maternal spiral artery endothelial remodeling and placental angiogenesis.
There is low intrauterine oxygen tension at early implantation, and these oxygen levels are maintained during the first trimester of pregnancy. However, when the placental vascular bed is established, the degree of differentiation of trophoblasts to EVTs and interstitial and endovascular EVT subsets between 8 and 24 weeks of gestation promote increased oxygen tension (97, 99, 101, 102). Besides, the microenvironment into which the EVT invades the decidual stroma influences the release of soluble factors (e.g., immunomodulatory, pro-inflammatory, and anti-inflammatory cytokines, growth, and angiogenic factors), and oxygen concentration influences changes in the invasion process. As previously mentioned, physiologically hypoxic conditions (2%–3% compared with the usual 20% oxygen) in the first trimester promote differentiation toward an invasive pathway and establish a new placental vascular bed (96, 103).
Kang et al. (21) proposed that a failure of trophoblast invasion causes preeclampsia, and Goldman, Wohl, and Yagel (24) proposed that preeclampsia is caused by complex pathological events related to shallow invasion of endovascular and interstitial trophoblast, which reduces the transformation of spiral arteries into low-capacitance vessels (Figure 2C). Despite all evidence, it is inconclusive that the diseases have a distinct placental etiology. Thus, Staff (26) revised the concepts of the two-stage placental model of preeclampsia, which proposed a stage 1 caused by placental dysfunction and a stage 2 related to maternal clinical syndrome (25). In stage 1, maternal factors include a lack of tolerance to allogeneic trophoblasts, impaired placentation, altered spiral artery remodeling, and an oxidative stress state of the placenta. In stage 2, the impaired placenta and trophoblast stress send stress signals to the maternal endothelium, resulting in widespread vascular inflammation and the clinical signs of early-onset and late-onset preeclampsia (26). These concepts have recently been validated by evidence of atherosclerosis and spiral artery remodeling failure as determinants of preeclampsia and other pathological gestation conditions. Furthermore, Staff et al. (104) reaffirmed their proposal for a multistage placental model of preeclampsia.
1.7 HIF and hypoxia-related signaling
Low oxygen tension at the maternal–fetal interface promotes the accumulation of hypoxia-inducible factor, HIF-1, a regulator of oxygen homeostasis (105). HIF-1 is a heterodimeric protein composed of a constitutively expressed β subunit and an α subunit regulated by the partial oxygen pressure (pO2; 106). Low oxygen concentrations promote cell invasion and progressions by activating more than 60 putative genes after HIF-1 receptor activation (HIF-R) (107–109). Human cytotrophoblast cells express HIF-1α (20, 110), which regulates the expression of target genes in cells subjected to hypoxia, including transferrin receptor (TfR1), vascular endothelial growth factor (VEGF), erythropoietin, endothelin-1, leptin, and glucose transporter 1 (106). HIF-1α regulates the iron and 2-oxoglutarate-dependent dioxygenase family enzymes known as prolyl hydroxylases (PHD1, PHD2, and PHD3) and asparaginyl hydroxylases (95). The PHD2 enzyme is responsible for hydroxylating one of the two proline residues present in HIF-1α (pro402 and pro564), promoting its interaction with the von Hippel-Lindau protein (pVHL) and subsequent polyubiquitination and degradation in the proteasome (103). When a cell is deprived of its oxygen supply, hydroxylation, and subsequent degradation of HIF-1α are significantly reduced, resulting in accumulation (111). HIF-1α accumulates in the nucleus, where it forms a complex with HIF-1β (HIF-1α/HIF-1β heterodimer) that is selectively expressed in the placenta (112, 113). The HIF-1α/HIF-1β heterodimer binds to hypoxia-responsive elements, acting as transcription factors for genes involved in cellular stress response (114). Thus, hypoxia stimulates tumor cell and trophoblast migration and invasion, and the invasive phenotype is activated via multiple mechanisms, including direct and indirect regulation of the epithelial to mesenchymal transition and upregulation of proteolytic enzymes, primarily matrix metalloproteinases (115, 116).
During the first trimester of gestation, hypoxic conditions stimulate the production of soluble factors associated with placental angiogenesis and immune tolerance to trophoblast invasion at the maternal–fetal interface (117). However, hypoxia in the second or third trimester may promote an increase in placental apoptosis and a significant decrease in trophoblast invasion, exacerbating the pro-inflammatory state at the maternal–fetal interfaces (99, 101, 118). The significant reduction in maternal spiral artery remodeling during the trophoblastic invasion and the consequent decrease in placental perfusion could correlate with overexpression of HIF-1α, VEGF, soluble FMS-like tyrosine kinase (sFlt1), and soluble VEGF receptor (sVEGFR-1), all elicited by HIF (119, 120). Failure to detect low oxygen tension has been proposed as a cause of early-onset preeclampsia (121), supporting the concept of abnormal placental development as one of the triggering processes (122).
1.8 Glycans as mediators between the placenta and peripheral circulation
The glycosylation profile of trophoblast and glycans do change during endometrium trophoblast invasion. Because of their molecular versatility, they form new affinities and cell–to-cell crossovers and develop complex intercellular structures supporting trophoblast differentiation (123). Changes in glycan profiles could be new biomarkers for diseases such as preeclampsia (124, 125). These changes involve the underexpression and overexpression of naturally occurring glycans in response to the environment- or stress-induced signaling (126). Thus, glycosylation of glycoproteins may contain previously unknown patterns that control stem cell differentiation, phenotype changes, invasion, and mobilization (60, 127), vasculogenesis (128, 129), and extravillous trophoblast invasion (130). The maternal immune system regulates trophoblast differentiation and invasion at multiple levels: locally in the maternal–fetal interface I by uterine NK-to-dendritic cell interaction and systemically in the maternal–fetal interface II by trophoblast-derived extracellular vesicles (EVs) (Figure 2D) or in the vascular compartment by VEGF production (Figures 2A–D; 131, 132). In this regard, Clark et al. (133) proposed that the human embryo–fetus’ primary defense mechanism for protecting itself from maternal immune system recognition and rejection is the induction of specific immunosuppressive oligosaccharides in glycoconjugates of placental tissues (133). The syncytiotrophoblast in the full-term placenta expresses up to 95% N-linked oligosaccharides with complex sugars, with the remaining 5% being high mannose type (134). These glycan structures were immunosuppressive because the oligosaccharide membrane fraction isolated from syncytiotrophoblast decreased 3H thymidine uptake in a mixed leucocyte reaction (135).
Glycosylation changes can manifest in various ways, including impairing naturally occurring glycans through the influence of environmental or stress-induced signaling pathways. These changes can lead to either under-expression or overexpression of glycans. Additionally, the expression of glycans that are typically restricted to embryonic tissues can occur during the development of a tumor phenotype. (94). Changes in glycosyltransferase levels can lead to modifications in the core structure of N-linked and O-linked glycans, e. g. the oligosaccharide size and branching of N-linked glycans. The increased activity of N-acetylglucosaminyltransferase V (GlcNAc-TV, or MGAT5; the enzyme that leads to β1,6GlcNAc branching) promotes β1-6- linked branching and the invasive phenotype in transfected non-metastatic clones of murine mammary carcinoma (136). The placentation process resembles cancer metastasis in the invasion waves during the first trimester. Therefore, the expression of β1-6- linked branching increases in the trophoblast layer when it is detected by phytohemagglutinin lectin blotting (137). The increased branching creates additional sites for terminal sialic acid residues, which, in conjunction with the corresponding upregulation of sialyltransferases, ultimately leads to an increase in global sialylation. N-glycan structures obtained from syncytiotrophoblast proteins are highly sialylated during pregnancy, as was confirmed by mass spectrometry (43), which could explain syncytiotrophoblast resistance to NK cell—and other cytolytic leukocytes—mediated cytolysis (138). These patterns of glycosylation were confirmed later by Chen et al. (43), who proposed that biantennary bisecting type N-glycans(a particular type of glycan modification consisting of four-linked β1 GlcNAc structure attached to the core β-mannose residue expressed in syncytiotrophoblast), could protect class I MHC non-expressing trophoblast cells from NK cell-mediated cytotoxicity. However, further experimental evidence is needed to confirm this. A knockdown of the MGAT5 enzyme increases the migration capacities of first-trimester human placental villi, which are correlated with the upregulation of metalloproteases (MMP-9) and the downregulation of the invasion inhibitor TIMP1/2 (139). Furthermore, the ischemia-reperfusion insult of the placenta may promote the shallow trophoblastic invasion observed in preeclamptic pregnancies, whereas specific changes in N-glycans could be involved in migration and differentiation processes. Thus, N-acetylglucosaminyltransferase III (GnT-III) catalyzes the bisecting GlcNAcβ1 modification, which can suppress the processing and branching of the glycan catalyzed by GnT-V (140).
Reduction of biantennary de-sialylated non-fucosylated N-glycans was reported in first-trimester decidual tissue as a normal finding (141), but no reports on the expression by placental villi tissue were found. Furthermore, Whyte and Loke (142) reported increased sialylation of trophoblast glycoproteins compared with fetal and tumor cells. According to Jeschke et al. (143), one possible mechanism of immunosuppression in human gestation is the expression of TF antigen at sites of trophoblast invasion.
Placental proteins have different glycosylation patterns depending on gestational age or extracellular cell microenvironmental changes. For instance, Dell et al. (56) reported that glycodelin-A —a potent immunosuppressive protein in the human placenta— expresses biantennary N-glycans with the bisecting GlcNAc sequence and sialylated complex N-glycans (143–145). The syncytiotrophoblast layer of chorionic villi produces human chorionic gonadotrophin (hCG) throughout human pregnancy (146). hCG, an N-glycan glycoprotein, is also secreted by EVT during trophoblast invasion in a hyperglycosylated form, exerting potent immunomodulatory effects (147, reviewed in 146).
Changes in glycosylation profiles of placental glycoproteins associated with preeclampsia have been proposed to be related to oxidative stress-promoting hypoxia in the placental bed and the consequent accumulation of the HIF heterodimer, as mentioned above. For example, LewisY, a well-recognized glycan associated with angiogenesis, was found in cytotrophoblast cells and villous trophoblastic cells in cases of severe preeclampsia (148) and unexplained miscarriage (149). These results could be explained by a compensatory mechanism triggered by an increase in angiogenic factors, common in pregnancy-related hypertensive disorders, such as preeclampsia (28, 150). Besides, changes in glycan expression at the maternal–fetal interface caused by chronic hypoxia could be associated with increased lectin expression in preeclampsia. This is the case with galectins (Gal), soluble non-glycosylated proteins with a unique sequence motif in their CRDs that show an increased affinity for N-acetylated disaccharides such as N-acetyl-galactosamine (LacNAc; Galβ1,4GlcNAc) and similar structures. In murine models, Gal-1 exhibits proangiogenic functions during the early stages of pregnancy, promoting decidual vascular expansion via VEGF receptor 2 (VEGR2) signaling. A specific blockage of Gal-1 promotes defective trophoblast invasion and impaired maternal spiral artery remodeling, resulting in a PE-like syndrome (151). Gal-1 expression was significantly upregulated in the decidua of preeclamptic placentas and the villous trophoblast of placentas in women with hemolysis, elevated liver enzymes, and low platelet (HELLP), which is consistent with previous findings (144).
1.9 Changes in glycosylation are mediated by hypoxia and inflammation
As mentioned above, hypoxia affects metabolism cells and glycosyltransferase expression in placenta and tumors (139, 140, 152) such as colon cancer cell lines (153, 154) and the immortalized prostate cell line RWPE1 (155). It also promotes the transcription of genes encoding fucosyltransferases and sialyltransferases. Although the role of HIF-1α in placental glycosylation remains unknown, indirect evidence in tumor tissues indicates that HIF-1α upregulation increased fucosyltransferases (FUT)-1-2 mRNA levels and overexpression of fucosylated proteins on the surface of pancreatic adenocarcinoma cells (156). Hypoxia appears to alter endothelial cell glycosylation, inducing the expression of N-glycoproteins containing glycans with less α2,6-linked sialic acid, elongated poly-LacNAc (Gal-GlcNAc) residues, and β1,6-linked N-glycan structures branching (157, 158). Furthermore, pathophysiological changes of the endothelial surface layer occur following a variety of insults, among these, hypoxia associated with ischemia/reperfusion injury (159). The oxidative and inflammatory stress accompanying hypoxic insult promotes glycocalyx damage via reactive oxygen species (Ros), increasing vascular permeability and perivascular inflammation. These ROs-mediated changes to the glycocalyx are dependent on Ca2+ signaling and possibly matrix metalloproteinase activation (160). However, another signaling pathway could be implied in the hypoxic insult associated with glycosyltransferase expression.
Additionally, HIF-1 activates tumor and placental inflammatory signaling, particularly nuclear factor-kappa B (NF-κB) transcription. Inhibitory IκB dissociates from NF-κB during inflammation, allowing for nuclear translocation and multiple gene transcriptions, mainly IL-6, cycloxygenase-2, and matrix metalloproteinase 9 (161, 162). These findings imply that HIF-1 and NF-κB interaction activates the transcription factors of these genes. In preeclampsia, NFκB expression may stimulate pro-inflammatory and anti-angiogenic protein production, promoting oxidative stress, inflammation, and vascular dysfunction. On the contrary, in normal pregnancies, NFκB promotes placental cellular migration, invasion, and angiogenic protein production (163). In preeclamptic women, local placental inflammation and oxidative stress are associated with placental hyperactivation of NfκB and its release into the maternal circulation, with levels nearly 10-fold higher than in normal pregnancies (163, 164).
Abnormal placental blood perfusion increases hypoxia-induced placental-derived circulating agents and sFlt1 production (120). HIF-1α, the primary regulator of oxygen hemostasis in the placenta, induces TGF-β production (165, 166). The β3 isoform of TGF-β suppresses trophoblast trans-differentiation, reducing its differentiation toward EVT and invasive capacity (165). Therefore, reduced growth of maternal–fetal interfaces 1 and 2 could restrict blood perfusion and promote EV release into the general circulation, contributing to the exacerbated pro-inflammatory status observed after 20 weeks of gestation (167). Placental hypoxia occurs in diseases such as gestational iron deficiency anemia, associated with increased accumulation of HIF-1α, similar to preeclampsia. Additionally, alterations in iron status have been associated with hypertensive disorders of pregnancy (168–171). Glycosylation profiles induced by hypoxia and placental inflammation have been proposed to alter the exchange of macronutrients and micronutrients across the syncytiotrophoblast (172, 173). Iron is transferred from the mother to the fetus via the placenta after being taken up by TfR1 at the apical membrane of the syncytiotrophoblast (174–177). TfR1 contains three N-glycan and O-glycan chains in its extracellular domain (178–180). TfR1 expression may be altered in hypoxic conditions, such as in women with diabetes, due to post-translational modifications, including glycosylation (181). Thus, women with preeclampsia whose placenta is exposed to prolonged hypoxia may have elevated levels of TfR1, one of the HIF-1 target genes. In preeclamptic women, the galactose-N-acetylglucosamine and terminal mannose patterns detected by DSA and GNA lectins, respectively, were overexpressed in TfR1. In addition, TfR1 expressed α2-3 sialic acid rather than α2-6 sialic acid, and preeclamptic placentas overexpressed α2-3 sialic acid (182). Depleting α2-6 sialic acid may promote increased binding of gal-3 to terminal galactose in TfR1 and formation of the transferrin–iron–TfR1 complex during iron uptake (183, 184). Furthermore, overexpression of α2-3 sialic acid in TfR1 of preeclamptic placentas may be associated with resistance to cell membrane scission, which is consistent with the findings of Rutledce and Enns (185), where removal of α2-3 sialic acid released TfR1 protein into the culture medium. We wonder if differences in TfR1 glycosylation patterns in preeclamptic women have implications for TfR1 exportation to the cell membrane or ligand affinity modifications, events that could affect iron uptake by the placenta resulting in impaired fetal nutritional status and, in some cases, intrauterine growth restriction.
1.10 Role of glycan recognition by cells of innate immunity in the pathogenesis of the exacerbated systemic inflammatory response in preeclampsia
Because preeclampsia is a preclinical condition characterized by a prolonged hypoxic state, it may be associated with an increased release of EVs and soluble factors capable of initiating a systemic inflammatory response and impairing endothelial function (131). Murrieta-Coxca et al. (186) recently proposed that EVs could play a critical regulator of feto-maternal tolerance by transferring allogeneic material from fetal to maternal immune cells to remodel their function (in the context of fetal allograft tolerance) and fetal–maternal microchimerism. Darmochwal-Kolarz et al. (187) reported that in preeclamptic patients, there was an imbalance of pro-inflammatory cytokines (IL-2 and IFN-γ) compared with the anti-inflammatory profile (IL-4 and IL-10) characteristic of mitogen-activated CD4+/CD8+ T lymphocytes and NK cells. However, studies on cytokine production by NK cells in preeclampsia used chemical stimulation with Phorbol Myristate Acetate (PMA) and Ca2+ ionophores, which could alter the cytokine profile observed in these assays (188).
The pro-inflammatory profile of NK cells could be induced by lectin-mediated glycan recognition at maternal–fetal interface 2, where peripheral immune cells come into contact with the syncytiotrophoblast layer in circulating microvilli. Under hypoxic conditions, for example, the glycan profile of invasive tumor cells and tumors may change, revealing truncated glycans such as Tn antigen or polysialylated N-glycan complexes (93, 189, 190). Recognition of these glycans by NK cells is mediated mainly by lectin-like and immunoglobulin-like receptors (called Siglecs; 191). On the other hand, the functional profile of peripheral NK cells is known to depend on their CD56 phenotype. Therefore, the CD56bright NK cell subset can promote the PMA and the Ca2+ ionophore-induced IFN-γ and TNF-α intracellular production (192). In non-pregnant women, the major NK cell subset is IFN-γ or TNF-α producing NK cells (NK1 profile), and IL-4, IL-5, and IL-13 producing NK cells (NK2 profile) or IL-10/TGF-β non-producing PMA-stimulated CD56bright and CD56dim NK cells (193–195). In pregnant women, the cytokine profile observed in NK cells changes to IL-10-producing NK cells; IFN-γ, TNF-α, IL-4, IL-5, or TGF β-producing CD56dim and CD56bright subsets corresponding to NK regulatory and NK3 profiles, respectively, with no difference between these subsets (194). This way, we evaluated the activation of peripheral NK cells before the more advanced stages of preeclampsia in women with early-onset severe preeclampsia without HELLP syndrome. Monocytes, T lymphocytes, NKT cells, and NK cell proportions were not significantly different compared with healthy pregnant women. Moreover, CD56dim and CD56bright NK cell subsets from the early-onset of severely preeclamptic women significantly produced more intracellular cytokines than NK cells from healthy pregnant women, with no cytokine imbalance. However, peripheral NK cells demonstrated increased cytotoxic activity in vitro (28), consistent with reports of increased NK cell cytotoxic activity in NK cells from mild preeclampsia cases (196). Besides, an increased percentage of NK cells bearing the CD96-NKGA/C receptors and CD56bright–NKG2C and NKG2A positive in NKdim and NKbright subsets (28). CD96-NKG2 heterodimers are lectin-like receptors that recognize protein-bound terminal glycans. They are members of the C-type lectin-like receptor superfamily, which includes six NKG2 molecular species (A, B, C, D, E, and H).
The heterodimer CD94/NKG2A is an inhibitory receptor with intracellular motifs that negatively regulates activation signaling for cytotoxic activity and anti-inflammatory cytokine production (197). Both CD94/NKG2 heterodimers can bind to non-classical HLA molecule HLA-E; however, their affinity varies: CD94/NKG2A binds to HLA-E with low affinity and very rapid association and dissociation rates, whereas CD94/NKG2C binds to HLA-E with nearly 10-fold lower affinity than CD94/NKG2A (198). This affinity is determined by the peptide sequence bound to HLA-E. However, evidence suggests a direct recognition of NKG2A/C receptors mediated by α2-3 sialic acid, a terminal residue of sialic acid monosaccharide-containing glycoproteins (199). NKG2A and NKG2C receptor expression on peripheral blood NK cells was reduced in women with preeclampsia (200). EA.hy296 endothelial cell line expresses HLA-E, and serum from severely preeclamptic women increased its expression (201). In severe preeclampsia, NK cell adhesion to the endothelium is significantly higher than in normal pregnancy, implying an interaction with an overexpressed HLA-E in the endothelial cell membrane (202). Endothelial HLA-E recognition by peripheral NK cells most likely modifies NK cell activity toward a low cytotoxic profile and promotes the production of pro-inflammatory cytokines such as TNF-α and IFN-γ (203, 204). A possible role of terminal α2-3 sialic acid in the HLA-E α1-domain could be relevant to explain this activation profile in peripheral NK cells mediated by NKG2 receptor activation in severe stages of preeclampsia. We wonder if changes in the endothelium and innate response cell (mainly NK cells) activation and cytokine influence the systemic inflammatory response observed during pregnancy. Although the putative role of cytokines produced by peripheral NK cells in the systemic inflammatory response of hypertensive disorders in pregnancy remains unknown, we propose that peripheral NK cells modulate their cytokine profile in preeclampsia via HLA-E signaling or lectin-like receptors such as members of NKG2 family, as previously reported (29).
There is little knowledge about glycan recognition in the context of NK cell activation. Previous studies identified glycophorin A, glycodelin-A, and sialic acid-related structures (sialyl Lewis X, among others) as negative modulators of NK cell-mediated cytolysis (205–207). Indirect evidence suggests that glycan recognition may activate NK cells during systemic inflammatory response in preeclampsia, including differential expression of lectin-like receptors or sialic acid-recognition receptors from the Siglec family (208, 209). Ibeto et al. (210) evaluated the glycosylation profile of hCG isolated in urine from women at 7 and 20 weeks of gestation (first and second trimesters, respectively) and urine samples from women suffering from gestational trophoblastic disease. Ibeto et al. (210) found mono-antennary, bi-antennary, tri-antennary, and tetra-antennary N-glycans in serum hCG from early and late gestation and significantly lower hCG containing bisected N-glycans in patients with the gestational trophoblastic disease. Ibeto et al. (210) suggested that hCG-related bisected type N-glycans may directly suppress NK cell cytotoxicity, consistent with the report of Chen et al. (43).
Campuzano et al. (211) investigated the effects of placental glycans on NK cell activity using a peripheral NK cell with a full-term human villous tissue co-culture system to assess whether changes in glycosylation profile could account for differences in cytokine production and cytotoxicity. Peripheral mononuclear leukocytes-containing NK cells co-cultured with fresh villous tissue or BeW0 cells showed that CD56Bright NK cells did significantly produce higher concentrations of IFN-γ and TGF-β than CD56Dim NK cells, consistent with a previous report in which circulating CD56dim and CD56bright NK cells exhibited predominantly cytotoxic and cytokine-producing phenotypes, respectively (211). Besides, blocking glycosylation in a BeWo cell syncytialization model using N-glycosylation inhibitors (e.g., castanomycin and tunicamycin) revealed differences in intracellular IFN-γ production by CD56Bright NK cells in co-culture (211). It demonstrated that co-culture of primary placental villous tissue with the choriocarcinoma cell line BeWo modulates NK cell function through specific glycan expression. Tunicamycin specifically inhibits N-linked glycosylation by preventing core oligosaccharide addition to the nascent protein, and thereby, it may interfere with specific ligand-receptor interaction by a decrease of the trophoblast protein synthesis(Surani, 1979). Additionally, castanospermine is a potent inhibitor of alpha- and beta-glucosidases and alters the distribution of insulin-like growth factor (IGF) receptors in villous tissue explants, which reduces the effect of IGF (212).
Based on this, it has been proposed that end-glycans of glycoproteins participate in the syncytiotrophoblast–NK cell interaction during pregnancy inflammatory response regulation (Figures 3A, B). Thus, increased expression of sialic acid binding immunoglobulin-like lectin-6, a unique sialic acid receptor in the placenta, was found during severe preeclampsia (213), and its concentrations were related to the severity of the pathology (214, 215). A case report of a woman presenting gestational diabetes mellitus, overweight, and severe PE, lower staining of Gal-GlcNAc (detected by the DSA lectin), mannose (detected by the GNA lectin), and Sia α2-3 (detected by the MAA lectin) was found in TfR. Accordingly, we propose that increased α2-3 sialyltransferase activity in this woman could explain the increased sialylation in TfR from placental villi (182).
The improved protein stability conferred by sialic acid may be advantageous because there is an increase in inflammatory status ROs production in preeclampsia (reviewed in 216–218). Protein stability is influenced by ROs (219). In placental malaria, increased chorionic villi α2,6 sialylation may influence intervillous placental parasite density as a compensatory response to Plasmodium falciparum infection (220). Preeclampsia and placental malaria are diseases with a placental and systemic inflammatory response in which NK cell activity increases progressively throughout gestation and may affect the communication between syncytiotrophoblast and immune cells (123, 221–223).
1.11 An emerging role of EVs in the physiology of the maternal-fetal interface and the exacerbated systemic inflammatory responses?
During the differentiation of cytotrophoblast and fusion to form the syncytiotrophoblast, glycosylation patterns change in a carbohydrate-driven pattern to provide the immunosuppressive microenvironment required for successful placentation, which appears impaired in pathological conditions of gestation. The released EVs from syncytiotrophoblast also carry various conjugated molecules in the maternofetal interface II context. Their size ranges from 30 to 150 nm, increasing in patients with an exacerbated systemic inflammatory response (224, 225). Studies have shown that levels of EVs increase in patients with systemic inflammatory response (224, 225), and this increase is even more pronounced in preeclampsia, where EVs have been found to increase three-fold compared to normal gestation (226, 227). To better understand the composition of EVs in placenta from normal and preeclamptic women, researchers have used perfusion systems to identify their main components (227). These EVs appear to play a role in the transfer of intercellular biomolecules such as flt1, the VEGF membrane receptor, as suggested by recent studies (228, 229). Thus, the exacerbated systemic inflammatory response in EP is associated with the number and variety of EVs found in healthy pregnancies (226, 227). The preeclampsia-associated chronic placental hypoxia and inflammatory status promote EVs shedding from syncytiotrophoblast to the maternal–fetal interface 2 and increase the systemic inflammatory response (230–233). Research suggests that glycans play a crucial role in EVs biogenesis, cellular recognition, and cell receptor interaction. In particular, mannosylated and sialylated N-glycans, with their antennae structures, are believed to mediate important biological functions such as inflammatory cascade activation and other paracrine events (234, 235) Thus, some glycan biomarkers, such as α-2-6 sialic acid and Gal or terminal GlcNAc in complex N-glycans, have been identified in syncytiotrophoblast-derived EVs (236). However, despite advancements in understanding the roles of syncytiotrophoblast-derived EVs glycans, their functional capabilities and interactions with target endothelial cells in both the glomerular filtration barrier and cerebral microcirculation remain largely unknown. Research conducted by our group has shown that serum from women with preeclampsia can affect the function of the glomerular filtration barrier in an in vitro model (237–239). This effect appears to be caused by a reduction in the anionic charge of the glomerular endothelium, allowing selective filtration of blood components into the urine. This raises the question of whether the glycans present in EVs from the placenta of women with preeclampsia could modify the permeability of the glomerular endothelium and cerebral microvasculature to albumin, and if they could affect endothelial integrity (43, 145, 240).
These EVs could explain the connection between the maternal–fetal interface and target organs such as the blood–brain barrier and the glomerular filtration barrier, where glycans are the most promising exchange molecules for research purposes. Further research is needed to fully decipher the complex mechanisms at play in these important physiological processes.
1.12 Perspectives on the role of glycans in gestation and preeclampsia: Connecting the network
Gérard Chaouat proposed nearly a decade ago that the specific immune system evolved to be adapted to vertebrate placentation (in the sense of subordination) as a critical feature of successful vertebrate reproduction (241). Decidual and circulating immune cells must undergo a series of molecular adaptations to avoid conceptus rejection and allow successful embryo and fetus development. The profile of glycan expression at the maternal–fetal interface, the phenotype and functional profile of innate immune cells present in the decidua, the presence of regulatory T cells (242, 243), and the production of soluble factors related to apoptosis, among others, may be responsible for such adaptation process (243–245).
The presence of immunomodulatory glycans in trophoblast is one fundamental mechanism supporting successful placental reproduction, along with the selective expression of “friendly” natural killer cells and low expression and activity of effector T cells capable of exerting cytotoxic activity. Most cellular and molecular mechanisms of the effector adaptive immune response are absent or regulated at the maternal–fetal interface. On the contrary, decidual NK cells are the primary immune cell population in up to 85% of decidua and are responsible for decidualization in early human pregnancy. Interestingly, one of the most abundant proteins produced by syncytiotrophoblast is β-hCG, a placental hormone sharing N-glycans coupled to asparagine residues that function as a potent immunomodulatory and angiogenic glycoprotein inducing decidual NK proliferation via mannose receptor activation (127, 246, 247). In healthy dynamics that support placental angiogenesis, decidualization, and establishing the maternal–fetal interface, uterine dendritic cells recruit decidual NK cells. Thus, the placenta of pregnant mice depleted of decidual NK cells shows a reduced expression of α2,3-sialylation and O-glycans, whereas the expression of branched N-glycans and Gal-1 increased. Ablation of decidual NK cells in the pre-implantation period could influence VEGF-mediated angiogenesis changes and depend on GAL-1 signaling. Experimental murine models of abnormal pregnancy show that changes in trophoblast glycosylation patterns precede poor pregnancy outcomes such as intrauterine growth restriction (248–250).
In conclusion, the fact that fetal and maternal cells exchange, at least in specific windows and compartments during the gestation period, adds to our understanding of the complex interplay between the maternal endocrine and immune systems as part of the maternal, placental, and fetal physiological environments and the maintenance of a balanced, functional immune response. Furthermore, evidence supports the theory of the involvement of the innate immune response in mediating critical processes required for early placental development and maintenance, such as decidual growth-supported placental development. In that context—and evolutionary highly conserved—glycans emerged as crucial innate immune cell regulators that support the maintenance of gestation. Because glycan expression profiles are essential modulators of adaptive and effector immune functions, critical advances in understanding successful human and mammalian gestation may provide a comprehensive understanding of normal and pathological pregnancy conditions (Figures 3C, D). We contributed to the discussion on the role of glycans in the pathophysiology of hypertensive disorders of gestation, as proposed by Gérard Chaouat in criticizing the pitfalls of the old immunotropic hypothesis. The main findings are summarized in the Table 1.
This manuscript was edited and proofread before being sent to Gerard Chaouat for final review and approval. However, we did have the misfortune of his death. Therefore, this is our tribute to “Gégé” (Gérard C. Chaouat, 1944–2021).
Author contributions
JB-S conceived and wrote the manuscript and designed the cited studies. AG-G performed cited studies and discussed their results. JM-E. participated in the critical review and reduction of the manuscript. JQ-C. participated in the critical review and reduction of the manuscript and study design. All authors contributed to the article and approved the submitted version.
Funding
Universidad de Antioquia, Vicerrectoría de Investigación, through the calls for support for consolidated research groups (Grupo Reproducción) and groups in consolidation 2020–2021 (Grupo OHVRI). This study was funded by Universidad Cooperativa de Colombia (INV2719-Dirección Nacional de Investigación), Sistema General de Regalías-Minciencias, Cod BPIN 2020000100488.
Acknowledgments
Authors thank the Office for Research and Development at the University of Antioquia (CODI and Vicerrectoría de Investigación, Estrategia de Consolidación de grupos 2020-2021), Corporación Universitaria Remington (CUR), and Universidad Cooperativa de Colombia (Dirección Nacional de Investigación) for funding research groups and projects supporting the cited papers. Special thanks to Minciencias, Colombia, for the financial support.
In Memoriam
In Memoriam: Gérard C. Chaouat (1944–2021).
Conflict of interest
The authors declare that the research was conducted in the absence of any commercial or financial relationships that could be construed as a potential conflict of interest.
Publisher’s note
All claims expressed in this article are solely those of the authors and do not necessarily represent those of their affiliated organizations, or those of the publisher, the editors and the reviewers. Any product that may be evaluated in this article, or claim that may be made by its manufacturer, is not guaranteed or endorsed by the publisher.
References
1. Gilstrap L, Ramin S. ACOG practice bulletin. diagnosis and management of preeclampsia and eclampsia. Obs. Gynecol (2002) 99:159–67.
2. Kornacki J, Boroń D, Gutaj P, Mantaj U, Wirstlein P, Wender-Ozegowska E. Diagnosis of preeclampsia in women with diabetic kidney disease. Hypertens Pregnancy (2021), 1–8. doi: 10.1080/10641955.2021.1987454
3. Magee LA, Nicolaides KH, von Dadelszen P. Preeclampsia. N Engl J Med (2022) 386:1817–32. doi: 10.1056/NEJMRA2109523
4. Brosens I, Puttemans P, Benagiano G. Placental bed research: I. the placental bed: from spiral arteries remodeling to the great obstetrical syndromes. Am J Obstet. Gynecol (2019) 221:437–56. doi: 10.1016/j.ajog.2019.05.044
5. Harris LK, Benagiano M, D'Elios MM, Brosens I, Benagiano G. Placental bed research: II. functional and immunological investigations of the placental bed. Am J Obstet Gynecol (2019) 221:457–69. doi: 10.1016/j.ajog.2019.07.010
6. Romero Infante XC, Uriel M, Rincón Franco. S, Ibáñez Pinilla EA, Rojas NA. First trimester placental growth factor in maternal blood and placenta related disorders. J Matern. Fetal. Neonatal Med (2021) 1:8. doi: 10.1080/14767058.2021.1960966
7. Yücesoy G, Ozkan S, Bodur H, Tan T, Calişkan E, Vural B, et al. Maternal and perinatal outcome in pregnancies complicated with hypertensive disorder of pregnancy: a seven year experience of a tertiary care center. Arch Gynecol. Obstet (2005) 273:43–9. doi: 10.1007/s00404-005-0741-3
8. Giannubilo SR, Landi B, Ciavattini A. Preeclampsia: what could happen in a subsequent pregnancy? Obstet. Gynecol. Surv (2014) 69:747–62. doi: 10.1097/OGX.0000000000000126
9. Coban U, Takmaz T, Unyeli OD, Ozdemir S. Adverse outcomes of preeclampsia in previous and subsequent pregnancies and the risk of recurrence. Sisli. Etfal. Hastan. Tip. Bul (2021) 55:426–31. doi: 10.14744/SEMB.2020.56650
10. ACOG (American College of Obstetris and Gynecology). Gestational hypertension and preeclampsia: ACOG Practice Bulletin, number 222. Obstet Gynecol (2020) 135:1492–5. doi: 10.1097/AOG.0000000000003892
11. Roberts JM, et al. Hypertension in pregnancy - ACOG (2013). Available at: https://www.acog.org/Clinical-Guidance-and-Publications/Task-Force-and-Work-Group-Reports/Hypertension-in-Pregnancy.
12. Sibai B, Dekker G, Kupferminc M. Pre-eclampsia. Lancet (2005) 365:785–99. doi: 10.1016/S0140-6736(05)17987-2
13. Robillard P-Y, Chaouat G, Le Bouteiller P, Fournier T, Barau G, Roman H, et al. [Current debates on immunology of preeclampsia. report of the sixth international workshop of reunion island (Indian ocean, December 2008)]. Gynécologie Obs. Fertil (2009) 37:570–8. doi: 10.1016/j.gyobfe.2009.03.028
14. Moussa HN, Sibai BM. Management of hypertensive disorders in pregnancy. Womens Health (Lond) (2014) 10:385–404. doi: 10.2217/whe.14.32
15. Roberts DJ, Post MD. The placenta in preeclampsia and intrauterine growth restriction. J Clin Pathol (2008) 61:1254–60. doi: 10.1136/JCP.2008.055236
16. Huppertz B. Maternal–fetal interactions, predictive markers for preeclampsia, and programming. J Reprod Immunol (2015) 108:26–32. doi: 10.1016/J.JRI.2014.11.003
17. Levine RJ, Lam C, Qian C, Yu KF, Maynard SE, Sachs BP, et al. Soluble endoglin and other circulating antiangiogenic factors in preeclampsia. N Engl J Med (2006) 355:992–1005. doi: 10.1056/NEJMoa055352
18. Lash GE, Schiessl B, Kirkley M, Innes BA, Cooper A, Searle RF, et al. Expression of angiogenic growth factors by uterine natural killer cells during early pregnancy. J Leukoc. Biol (2006) 80:572–80. doi: 10.1189/jlb.0406250
19. Schiessl B. Inflammatory response in preeclampsia. Mol Aspects Med (2007) 28:210–9. doi: 10.1016/j.mam.2007.04.004
20. Huppertz B. Placental origins of preeclampsia: challenging the current hypothesis. Hypertension (2008) 51:970–5. doi: 10.1161/HYPERTENSIONAHA.107.107607
21. Kang JH, Song H, Yoon JA, Park DY, Kim SH, Lee KJ, et al. Preeclampsia leads to dysregulation of various signaling pathways in placenta. J Hypertens (2011) 29:928–36. doi: 10.1097/HJH.0b013e328344a82c
22. Weiner E, Feldstein O, Tamayev L, Grinstein E, Barber E, Bar J, et al. Placental histopathological lesions in correlation with neonatal outcome in preeclampsia with and without severe features. Pregnancy Hypertens (2018) 12:6–10. doi: 10.1016/j.preghy.2018.02.001
23. Roberts JM, Hubel CA. The two stage model of preeclampsia: Variations on the theme. Placenta (2009) 30:S32. doi: 10.1016/J.PLACENTA.2008.11.009
24. Goldman-Wohl D, Yagel S. Preeclampsia–a placenta developmental biology perspective. J Reprod Immunol (2009) 82:96–9. doi: 10.1016/j.jri.2009.05.002
25. Staff AC, Johnsen GM, Dechend R, Redman CWG. Preeclampsia and uteroplacental acute atherosis: immune and inflammatory factors. J Reprod Immunol (2014) 101e2:120–6. doi: 10.1016/j.jri.2013.09.001
26. Staff AC. The two-stage placental model of preeclampsia: An update. J Reprod Immunol (2019) 134–135:1–10. doi: 10.1016/j.jri.2019.07.004
27. Redman CWG, Staff AC. Preeclampsia, biomarkers, syncytiotrophoblast stress, and placental capacity. Am J Obstet. Gynecol (2015) 213:S9.e1–4. doi: 10.1016/j.ajog.2015.08.003
28. Bueno-Sánchez JC, Agudelo-Jaramillo B, Escobar-Aguilerae LF, Lopera A, Cadavid-Jaramillo AP, Chaouat G, et al. Cytokine production by non-stimulated peripheral blood NK cells and lymphocytes in early-onset severe preeclampsia without HELLP. J Reprod Immunol (2013) 97:223–31. doi: 10.1016/j.jri.2012.11.007
29. Vinnars MT, Björk E, Nagaev I, Ottander U, Bremme K, Holmlund U, et al. Enhanced Th1 and inflammatory mRNA responses upregulate NK cell cytotoxicity and NKG2D ligand expression in human pre-eclamptic placenta and target it for NK cell attack. Am J Reprod Immunol (2018) 80:e12969. doi: 10.1111/aji.12969
30. Murthi P, Pinar AA, Dimitriadis E, Samuel CS. Inflammasomes-a molecular link for altered immunoregulation and inflammation mediated vascular dysfunction in preeclampsia. Int J Mol Sci (2020) 21:1406. doi: 10.3390/ijms21041406
31. Giachini FR, Galaviz-Hernandez C, Damiano AE, Viana M, Cadavid A, Asturizaga P, et al. Vascular dysfunction in mother and offspring during preeclampsia: Contributions from Latin-American countries. Curr Hypertension Rep (2017) 19(10):83. doi: 10.1007/s11906-017-0781-7
32. Clark GF. The role of glycans in immune evasion: The human fetoembryonic defence system hypothesis revisited. Mol Hum Reprod (2014) 20:185–99. doi: 10.1093/molehr/gat064
33. Ziganshina MM, Yarotskaya EL, Bovin NV, Pavlovich SV, Sukhikh GT. Can endothelial glycocalyx be a major morphological substrate in pre-eclampsia? Int J Mol Sci (2020) 21:3048. doi: 10.3390/IJMS21093048.b
34. Kulikova GV, Ziganshina MM, Shchegolev AI, Sukhikh GT. Comparative characteristics of the expression of fucosylated glycans and morphometric parameters of terminal placental villi depending on the severity of preeclampsia. Bull Exp Biol Med (2021) 172:90–5. doi: 10.1007/s10517-021-05338-6
35. Ziganshina MM, Dolgushina NV, Kulikova GV, Fayzullina NM, Yarotskaya EL, Khasbiullina NR, et al. Epithelial apical glycosylation changes associated with thin endometrium in women with infertility - a pilot observational study. Reprod Biol Endocrinol (2021) 19:73. doi: 10.1186/s12958-021-00750-z
36. Patankar MS, Ozgur K, Oehninger S, Dell A, Morris H, Seppala M, et al. Expression of glycans linked to natural killer cell inhibition on the human zona pellucida. Mol Hum Reprod (1997) 3:501–5. doi: 10.1093/molehr/3.6.501
37. Easton RL, Patankar MS, Clark GF, Morris HR, Dell A. Pregnancy-associated changes in the glycosylation of tamm-horsfall glycoprotein. expression of sialyl lewis(x) sequences on core 2 type O-glycans derived from uromodulin. J Biol Chem (2000) 275:21928–38. doi: 10.1074/jbc.M001534200
38. Jang-Lee J, North SJ, Sutton-Smith M, Goldberg D, Panico M, Morris H, et al. Glycomic profiling of cells and tissues by mass spectrometry: fingerprinting and sequencing methodologies. Methods Enzymol (2006) 415:59–86. doi: 10.1016/S0076-6879(06)15005-3
39. Pang P-C, Tissot B, Drobnis EZ, Sutovsky P, Morris HR, Clark GF, et al. Expression of bisecting type and Lewisx/Lewisy terminated N-glycans on human sperm. J Biol Chem (2007) 282:36593–602. doi: 10.1074/jbc.M705134200
40. Lee C-L, Pang P-C, Yeung WSB, Tissot B, Panico M, Lao TTH, et al. Effects of differential glycosylation of glycodelins on lymphocyte survival. J Biol Chem (2009) 284:15084–96. doi: 10.1074/jbc.M807960200
41. Clark GF, Schust DJ. Manifestations of immune tolerance in the human female reproductive tract. Front Immunol (2013) 4:26. doi: 10.3389/fimmu.2013.00026
42. Passaponti S, Pavone V, Cresti L, Ietta F. The expression and role of glycans at the feto-maternal interface in humans. Tissue Cell (2021) 73:101630. doi: 10.1016/j.tice.2021.101630
43. Chen Q, Pang P-C, Cohen ME, Longtine MS, Schust DJ, Haslam SM, et al. Evidence for differential glycosylation of trophoblast cell types. Mol Cell Proteomics (2016) 15:1857–66. doi: 10.1074/mcp.M115.055798
44. Jones CJP, Aplin JD. Reproductive glycogenetics-a critical factor in pregnancy success and species hybridisation. Placenta (2009) 30:216–9. doi: 10.1016/j.placenta.2008.12.005
45. Ziganshina MM, Kulikova GV, Fayzullina NM, Yarotskaya EL, Shchegolev AI, Le Pendu J, et al. Expression of fucosylated glycans in endothelial glycocalyces of placental villi at early and late fetal growth restriction. Placenta (2020) 90:98–102. doi: 10.1016/j.placenta.2019.12.005.a
46. Haslam SM, Julien S, Burchell JM, Monk CR, Ceroni A, Garden OA, et al. Characterizing the glycome of the mammalian immune system. Immunol Cell Biol (2008) 86:564–73. doi: 10.1038/icb.2008.54
47. Wautier JL, Wautier MP. Vascular permeability in diseases. Int J Mol Sci (2022) 23:3645. doi: 10.3390/ijms23073645
48. Möckl L. The emerging role of the mammalian glycocalyx in functional membrane organization and immune system regulation. Front Cell Dev Biol (2020) 8:253. doi: 10.3389/fcell.2020.00253
49. Jedlicka J, Becker BF, Chappell D. Endothelial glycocalyx. Crit Care Clin (2020) 36:217–32. doi: 10.1016/j.ccc.2019.12.007
50. Puchwein-Schwepcke A, Genzel-Boroviczény O, Nussbaum C. The endothelial glycocalyx: Physiology and pathology in neonates, infants and children. Front Cell Dev Biol (2021) 9:733557. doi: 10.3389/fcell.2021.733557
51. Pomin VH. Sulfated glycans in inflammation. Eur J Med Chem (2015) 92C:353–69. doi: 10.1016/j.ejmech.2015.01.002
52. Pomin VH. Phylogeny, structure, function, biosynthesis and evolution of sulfated galactose-containing glycans. Int J Biol Macromol (2016) 84:372–9. doi: 10.1016/J.IJBIOMAC.2015.12.035
53. Laine RA. A calculation of all possible oligosaccharide isomers both branched and linear yields 1.05 x 10(12) structures for a reducing hexasaccharide: the isomer barrier to development of single-method saccharide sequencing or synthesis systems. Glycobiology (1994) 4:759–67. doi: 10.1093/glycob/4.6.759
54. Määttänen P, Gehring K, Bergeron JJM, Thomas DY. Protein quality control in the ER: The recognition of misfolded proteins. semin. Cell Dev Biol (2010) 21:500–11. doi: 10.1016/j.semcdb.2010.03.006
55. Hirata T, Kizuka Y. N-Glycosylation. Adv Exp Med Biol (2021) 1325:3–24. doi: 10.1007/978-3-030-70115-4_1
56. Dell A, Morris HR, Easton RL, Panico M, Patankar M, Oehniger S, et al. Structural analysis of the oligosaccharides derived from glycodelin, a human glycoprotein with potent immunosuppressive and contraceptive activities. J Biol Chem (1995) 270:24116–26. doi: 10.1074/jbc.270.41.24116
57. Scott DW, Vallejo MO, Patel RP. Heterogenic endothelial responses to inflammation: Role for differential n-glycosylation and vascular bed of origin. J Am Hear. Assoc Cardiovasc Cerebrovasc. Dis (2013) 2:e000263. doi: 10.1161/JAHA.113.000263
58. Xu Y, Uddin N, Wagner GK. Covalent probes for carbohydrate-active enzymes: From glycosidases to glycosyltransferases. Methods Enzymol (2018) 598:237–65. doi: 10.1016/BS.MIE.2017.06.016
60. Dennis JW, Lau KS, Demetriou M, Nabi IR. Adaptive regulation at the cell surface by N-glycosylation. Traffic (2009) 10:1569–78. doi: 10.1111/j.1600-0854.2009.00981.x
61. Cummings RD, Pierce JM. The challenge and promise of glycomics. Chem Biol (2014) 21:1–15. doi: 10.1016/j.chembiol.2013.12.010
62. Mariño K, Bones J, Kattla JJ, Rudd PM. A systematic approach to protein glycosylation analysis: a path through the maze. Nat Chem Biol (2010) 6:713–23. doi: 10.1038/nchembio.437
63. Orberger G, Fuchs H, Geyer R, Gessner R, Köttgen E, Tauber R. Structural and functional stability of the mature transferrin receptor from human placenta. Arch Biochem Biophys (2001) 386:79–88. doi: 10.1006/abbi.2000.2177
64. Lattová; EL, Bryant J, Skř J, Zdráhal Z, et al. Efficient procedure for N-Glycan analyses and detection of endo h-like activity in human tumor specimens. Proteome Res (2016) 15:2777–86. doi: 10.1021/acs.jproteome.6b00346
65. Cummings RD. Stuck on sugars - how carbohydrates regulate cell adhesion, recognition, and signaling. Glycoconj J (2019) 36:241–57. doi: 10.1007/s10719-019-09876-0
66. Inoue M, Takahashi S, Yamashina I, Kaibori M, Okumura T, Kamiyama Y, et al. High density O-glycosylation of the MUC2 tandem repeat unit by N-acetylgalactosaminyltransferase-3 in colonic adenocarcinoma extracts. Cancer Res (2001) 61:950–6.
67. Wada Y, Dell A, Haslam SM, Tissot B, Canis K, Azadi P, et al. Comparison of methods for profiling O-glycosylation: Human proteome organisation human disease Glycomics/Proteome initiative multi-institutional study of IgA1. Mol Cel. Proteomics (2010) 9:719–27. doi: 10.1074/mcp.M900450-MCP200
68. Brockhausen I. Pathways of O-glycan biosynthesis in cancer cells. Biochim Biophys Acta (1999) 1473:67–95. doi: 10.1016/S0304-4165(99)00170-1
69. García-Vallejo JJ, Van Dijk W, Van Het Hof B, Van Die I, Engelse MA, Van Hinsbergh VWM, et al. Activation of human endothelial cells by tumor necrosis factor-alpha results in profound changes in the expression of glycosylation-related genes. J Cell Physiol (2006) 206:203–10. doi: 10.1002/jcp.20458
70. Brooks SA, Hall DM, Buley I. GalNAc glycoprotein expression by breast cell lines, primary breast cancer and normal breast epithelial membrane. Br J Cancer (2001) 85:1014–22. doi: 10.1038/sj.bjc.6692028
71. Brown NS, Bicknell R. Hypoxia and oxidative stress in breast cancer. oxidative stress: its effects on the growth, metastatic potential and response to therapy of breast cancer. Breast Cancer Res (2001) 3:323–7. doi: 10.1186/bcr315
72. Yue T, Goldstein IJ, Hollingsworth M, Kaul K, Brand RE, Haab BB. The prevalence and nature of glycan alterations on specific proteins in pancreatic cancer patients revealed using antibody-lectin sandwich arrays. Mol Cell Proteomics (2009) 8:1697–707. doi: 10.1074/mcp.M900135-MCP200
73. Taylor ME, Drickamer K, Schnaar RL, Etzler ME, Varki A. Discovery and classification of glycan-binding proteins. In: Varki A, Cummings RD, Esko JD, Stanley P, Hart GW, Aebi M, Darvill AG, Kinoshita T, Packer NH, Prestegard JH, Schnaar RL, Seeberger PH, editors. Essentials of glycobiology, 3rd ed. Cold Spring Harbor (NY: Cold Spring Harbor Laboratory Press (2017). p. 2015–7.
74. Taylor ME, Drickamer K. Mammalian sugar-binding receptors: known functions and unexplored roles. FEBS J (2019) 286(10):1800–14. doi: 10.1111/febs.14759
75. Bischof P, Irminger-Finger I. The human cytotrophoblastic cell, a mononuclear chameleon. int. j. biochem. Cell Biol (2005) 37:1–16. doi: 10.1016/j.biocel.2004.05.014
76. Lunghi L, Ferretti ME, Medici S, Biondi C, Vesce F. Control of human trophoblast function. Reprod Biol Endocrinol (2007) 5:6. doi: 10.1186/1477-7827-5-6
77. Kojima J, Ono M, Kuji N, Nishi H. Human chorionic villous differentiation and placental development. Int J Mol Sci (2022) 23:8003. doi: 10.3390/ijms23148003
78. Castellucci M, Kosanke G, Verdenelli F, Huppertz B, Kaufmann P. Villous sprouting: fundamental mechanisms of human placental development. Hum Reprod (2000) 6:485–94. doi: 10.1093/humupd/6.5.485
79. Caniggia I, Winter JL. Adriana and luisa castellucci award lecture 2001. hypoxia inducible factor-1: oxygen regulation of trophoblast differentiation in normal and preeclamptic pregnancies–a review. Placenta (2002) 23 Suppl A:S47–57. doi: 10.1053/plac.2002.0815
80. Gupta AK, Rusterholz C, Huppertz B, Malek A, Schneider H, Holzgreve W, et al. A comparative study of the effect of three different syncytiotrophoblast micro-particles preparations on endothelial cells. Placenta (2005) 26:59–66. doi: 10.1016/j.placenta.2004.04.004
81. Kliman HJ, Nestler JE, Sermasi E, Sanger JM, Strauss JF 3rd. Purification, characterization, and in vitro differentiation of cytotrophoblasts from human term placentae. Endocrinology (1986) 118:1567–82. doi: 10.1210/endo-118-4-1567
82. Tarrade A, Lai Kuen R, Malassiné A, Tricottet V, Blain P, Vidaud M, et al. Characterization of human villous and extravillous trophoblasts isolated from first trimester placenta. Lab Investig (2001) 81:1199–211. doi: 10.1038/labinvest.3780334
83. Gude NM, Roberts CT, Kalionis B, King RG. Growth and function of the normal human placenta. Thromb Res (2004) 114:397–407. doi: 10.1016/j.thromres.2004.06.038
84. Vargas A, Moreau J, Landry S, LeBellego F, Toufaily C, Rassart E, et al. Syncytin-2 plays an important role in the fusion of human trophoblast cells. J Mol Biol (2009) 392:301–18. doi: 10.1016/j.jmb.2009.07.025
85. Fischer I, Redel S, Hofmann S, Kuhn C, Friese K, Walzel H, et al. Stimulation of syncytium formation in vitro in human trophoblast cells by galectin-1. Placenta (2010) 31:825–32. doi: 10.1016/j.placenta.2010.06.016
86. Guo L, Gu F, Xu Y, Zhou C. Increased copy number of syncytin-1 in the trophectoderm is associated with implantation of the blastocyst. PeerJ (2020) 8:e10368. doi: 10.7717/PEERJ.10368
87. James JL, Boss AL, Sun C, Allerkamp HH, Clark AR. From stem cells to spiral arteries: A journey through early placental development. Placenta (2022) 125:68–77. doi: 10.1016/j.placenta.2021.11.004
88. Burton GJ, Cindrova-Davies T, Yung HW, Jauniaux E. Hypoxia and reproductive health: Oxygen and development of the human placenta. Reproduction (2021) 161:F53–65. doi: 10.1530/REP-20-0153
89. Soares MJ, Iqbal K, Kozai K. Hypoxia and placental development. Birth Defects Res (2017) 109:1309–29. doi: 10.1002/bdr2.1135
90. Graham CH, Hawley TS, Hawley RG, MacDougall JR, Kerbel RS, Khoo N, et al. Establishment and characterization of first trimester human trophoblast cells with extended lifespan. Exp Cell Res (1993) 206:204–11. doi: 10.1006/excr.1993.1139
91. Hamilton GS, Bond SL, McCrae KR, Shepherd T, Khoo NKS, Lala PK, et al. SV40 tag transformation of the normal invasive trophoblast results in a premalignant phenotype. i. mechanisms responsible for hyperinvasivess and resistance to anti-invasive action of TGFβ. Int J Cancer (2002) 77:429–39. doi: 10.1002/(sici)1097-0215(19980729)77:3<429::aid-ijc20>3.3.co;2-q
92. Lala PK, Lee BP, Xu G, Chakraborty C. Human placental trophoblast as an in vitro model for tumor progression 1 , 2. Society (2002) 149:142–9. doi: 10.1139/Y02-006
93. Rye PD, Fodstad O, Emilsen E, Bryne M. Invasion potential and N-acetylgalactosamine expression in a human melanoma model. Int J Cancer (1998) 75:609–14. doi: 10.1002/(sici)1097-0215(19980209)75:4<609::aid-ijc19>3.0.co;2-3
94. Dube DH, Bertozzi CR. Glycans in cancer and inflammation-potential for therapeutics and diagnostics. Nat Rev Drug Discovery (2005) 4:477–88. doi: 10.1038/nrd1751
95. Zhao HX, Li XY, Yao H, Wu Y, Yang R, Zhao RR, et al. The up-regulation of hypoxia-inducible factor-1alpha by hypoxic postconditioning reduces hypoxia/reoxygenation-induced injury in heart-derived H9c2 cells. Sheng Li Xue Bao (2013) 65:293–300.
96. Genbacev O, Joslin R, Damsky CH, Polliotti BM, Fisher SJ. Hypoxia alters early gestation human cytotrophoblast differentiation/invasion in vitro and models the placental defects that occur in preeclampsia. J Clin Invest (1996) 97:540–50. doi: 10.1172/JCI118447
97. Gratton RJ, Gandley RE, Genbacev O, McCarthy JF, Fisher SJ, McLaughlin MK. Conditioned medium from hypoxic cytotrophoblasts alters arterial function. Am J Obstet. Gynecol (2001) 184:984–90. doi: 10.1067/mob.2001.110499
98. Maltepe E, Krampitz GW, Okazaki KM, Red-Horse K, Mak W, Simon MC, et al. Hypoxia-inducible factor-dependent histone deacetylase activity determines stem cell fate in the placenta. Development (2005) 132:3393–403. doi: 10.1242/dev.01923
99. Robins JC, Heizer A, Hardiman A, Hubert M, Handwerger S. Oxygen tension directs the differentiation pathway of human cytotrophoblast cells. Placenta (2007) 28:1141–6. doi: 10.1016/j.placenta.2007.05.006
100. Hung TH, Burton GJ. Hypoxia and reoxygenation: a possible mechanism for placental oxidative stress in preeclampsia. Taiwan J Obs. Gynecol (2006) 45:189–200. doi: 10.1016/S1028-4559(09)60224-2
101. Benyo DF, Miles TM, Conrad KP. Hypoxia stimulates cytokine production by villous explants from the human placenta. J Clin Endocrinol Metab (1997) 82:1582–8. doi: 10.1210/jc.82.5.1582
102. Genbacev O, Zhou Y, Ludlow JW, Fisher SJ. Regulation of human placental development by oxygen tension. Science (1997) 277:1669–72. doi: 10.1126/science.277.5332.1669
103. Pringle KG, Kind KL, Sferruzzi-Perri AN, Thompson JG, Roberts CT. Beyond oxygen: complex regulation and activity of hypoxia inducible factors in pregnancy. Hum Reprod (2009) 16:415–31. doi: 10.1093/humupd/dmp046
104. Staff AC, Fjeldstad HE, Fosheim IK, Moe K, Turowski G, Johnsen GM, et al. Failure of physiological transformation and spiral artery atherosis: their roles in preeclampsia. Am J Obstet. Gynecol (2022) 226:S895–906. doi: 10.1016/j.ajog.2020.09.026
105. Ikeda R, Tabata S, Tajitsu Y, Nishizawa Y, Minami K, Furukawa T, et al. Molecular basis for the regulation of hypoxia-inducible factor-1alpha levels by 2-deoxy-D-ribose. Oncol Rep (2013) 30:1444–8. doi: 10.3892/or.2013.2572
106. Cowden Dahl KD, Fryer BH, Mack FA, Compernolle V, Maltepe E, Adelman DM, et al. Hypoxia-inducible factors 1alpha and 2alpha regulate trophoblast differentiation. Mol Cell Biol (2005) 25:10479–91. doi: 10.1128/MCB.25.23.10479-10491.2005
107. Wang GL, Semenza GL. Characterization of hypoxia-inducible factor 1 and regulation of DNA binding activity by hypoxia. J Biol Chem (1993) 268:21513–8. doi: 10.1016/S0021-9258(20)80571-7
108. Krishnamachary B, Berg-Dixon S, Kelly B, Agani F, Feldser D, Ferreira G, et al. Regulation of colon carcinoma cell invasion by hypoxia-inducible factor 1. Cancer Res (2003) 63:1138–43.
109. Gilkes DM, Semenza GL. Role of hypoxia-inducible factors in breast cancer metastasis. Futur Oncol (2013) 9:1623–36. doi: 10.2217/fon.13.92
110. Huppertz B, Gauster M, Orendi K, König J, Moser G. Oxygen as modulator of trophoblast invasion. J Anat (2009) 215:14–20. doi: 10.1111/j.1469-7580.2008.01036.x
111. Resnik ER, Herron JM, Lyu S-C, Cornfield DN. Developmental regulation of hypoxia-inducible factor 1 and prolyl-hydroxylases in pulmonary vascular smooth muscle cells. Proc Natl Acad Sci U. S. A (2007) 104:18789–94. doi: 10.1073/pnas.0706019104
112. Chung JW, Shin JE, Han KW, Ahn JH, Kim YJ, Park JW, et al. Up-regulation of hypoxia-inducible factor-1 alpha by cobalt chloride prevents hearing loss in noise-exposed mice. Env. Toxicol Pharmacol (2011) 31:153–9. doi: 10.1016/j.etap.2010.10.002
113. Shay JE, Imtiyaz HZ, Sivanand S, Durham AC, Skuli N, Hsu S, et al. Inhibition of hypoxia-inducible factors limits tumor progression in a mouse model of colorectal cancer. Carcinogenesis (2014) 35:1067–77. doi: 10.1093/carcin/bgu004
114. Min J-H, Yang H, Ivan M, Gertler F, Kaelin WGJ, Pavletich NP. Structure of an HIF-1alpha -pVHL complex: hydroxyproline recognition in signaling. Science (2002) 296:1886–9. doi: 10.1126/science.1073440
115. Airley R, Loncaster J, Davidson S, Bromley M, Roberts S, Patterson A, et al. Glucose transporter glut-1 expression correlates with tumor hypoxia and predicts metastasis-free survival in advanced carcinoma of the cervix. Clin Cancer Res (2001) 7:928–34.
116. Xing F, Okuda H, Watabe M, Kobayashi A, Pai SK, Liu W, et al. Hypoxia-induced Jagged2 promotes breast cancer metastasis and self-renewal of cancer stem-like cells. Oncogene (2011) 30:4075–86. doi: 10.1038/onc.2011.122
117. Skoura A, Sanchez T, Claffey K, Mandala SM, Proia RL, Hla T. Essential role of sphingosine 1-phosphate receptor 2 in pathological angiogenesis of the mouse retina. J Clin Invest (2007) 117:2506–16. doi: 10.1172/JCI31123
118. Schoots MH, Gordijn SJ, Scherjon SA, van Goor H, Hillebrands J-L. Oxidative stress in placental pathology. Placenta (2018) 69:153–61. doi: 10.1016/j.placenta.2018.03.003
119. Munaut C, Lorquet S, Pequeux C, Blacher S, Berndt S, Frankenne F, et al. Hypoxia is responsible for soluble vascular endothelial growth factor receptor-1 (VEGFR-1) but not for soluble endoglin induction in villous trophoblast. Hum Reprod (2008) 23:1407–15. doi: 10.1093/humrep/den114
120. Foidart JM, Schaaps JP, Chantraine F, Munaut C, Lorquet S. Dysregulation of antiangiogenic agents (sFlt-1, PLGF, and sEndoglin) in preeclampsia–a step forward but not the definitive answer. J Reprod Immunol (2009) 82:106–11. doi: 10.1016/j.jri.2009.09.001
121. Rolfo A, Many A, Racano A, Tal R, Tagliaferro A, Ietta F, et al. Abnormalities in oxygen sensing define early and late onset preeclampsia as distinct pathologies. PloS One (2010) 5:e13288. doi: 10.1371/journal.pone.0013288
122. Rajakumar A, Brandon HM, Daftary A, Ness R, Conrad KP. Evidence for the functional activity of hypoxia-inducible transcription factors overexpressed in preeclamptic placentae. Placenta (2004) 25:763–9. doi: 10.1016/J.PLACENTA.2004.02.011
123. Lee CL, Chiu PC, Pang PC, Chu IK, Lee KF, Koistinen R, et al. Glycosylation failure extends to glycoproteins in gestational diabetes mellitus: evidence from reduced alpha2-6 sialylation and impaired immunomodulatory activities of pregnancy-related glycodelin-A. Diabetes (2011) 60:909–17. doi: 10.2337/db10-1186
124. Godfrey-Smith P. Information in biology. In The Cambridge Guide to the Philosophy of Biology, ed. Hull DL, Ruse M. (2007) 103–19. Cambridge: Cambridge University Press.
125. Mian IS, Rose C. Communication theory and multicellular biology. Integr Biol (2011) 3:350–67. doi: 10.1039/c0ib00117a
126. Veillon L, Zhou S, Mechref Y. Quantitative glycomics: A combined analytical and bioinformatics approach. Methods Enzymol (2017) 585:431–77. doi: 10.1016/bs.mie.2016.11.006
127. Elliott MM, Kardana A, Lustbader JW, Cole LA. Carbohydrate and peptide structure of the alpha- and beta-subunits of human chorionic gonadotropin from normal and aberrant pregnancy and choriocarcinoma. Endocrine (1997) 7:15–32. doi: 10.1007/BF02778058
128. Harfouche R, Hentschel DM, Piecewicz S, Basu S, Print C, Eavarone D, et al. Glycome and transcriptome regulation of vasculogenesis. Circulation (2009) 120:1883–92. doi: 10.1161/CIRCULATIONAHA.108.837724
129. Piecewicz S, Sengupta S. The dynamic glycome microenvironment and stem cell differentiation into vasculature. Stem Cells Dev (2011) 20:749–58. doi: 10.1089/scd.2010.0454
130. Yamamoto E, Ino K, Miyoshi E, Inamori K, Abe A, Sumigama S, et al. N-acetylglucosaminyltransferase V regulates extravillous trophoblast invasion through glycosylation of alpha5beta1 integrin. Endocrinology (2009) 150:990–9. doi: 10.1210/en.2008-1005
131. Borzychowski AM, Sargent IL, Redman CW. Inflammation and pre-eclampsia. Semin Fetal Neonatal Med (2006) 11:309–16. doi: 10.1016/j.siny.2006.04.001
132. Huang S, Ernberg I, Kauffman S. Cancer attractors: A systems view of tumors from a gene network dynamics and developmental perspective. Semin Cell Dev Biol (2009) 20:869–76. doi: 10.1016/j.semcdb.2009.07.003
133. Clark GF, Oehninger S, Patankar MS, Koistinen R, Dell A, Morris HR, et al. A role for glycoconjugates in human development: the human feto-embryonic defence system hypothesis. Hum Reprod (1996) 11:467–73. doi: 10.1093/HUMREP/11.3.467
134. Arkwright PD, Redman CW, Williams PJ, Dwek RA, Rademacher TW. Syncytiotrophoblast membrane protein glycosylation patterns in normal human pregnancy and changes with gestational age and parturition. Placenta (1991) 12:637–51. doi: 10.1016/0143-4004(91)90498-5
135. Arkwright P, Rademacher T, Marshall J, Dwek R, Redman C. Glycoprotein glycosylation and the immunosuppressive effects of human pregnancy serum. J Reprod Immunol (1992) 21:97–102. doi: 10.1016/0165-0378(92)90043-4
136. Dennis JW, Laferté S. Recognition of asparagine-linked oligosaccharides on murine tumor cells by natural killer cells. Cancer Res (1985) 45:6034–40.
137. Tomiie M, Isaka S, Miyoshi E, Taniguchi N, Kimura T, Ogita K, et al. Elevated expression of N-acetylglucosaminyltransferase V in first trimester human placenta. Biochem Biophys Res Commun (2005) 330:999–1004. doi: 10.1016/j.bbrc.2005.02.186
138. Arkwright PD, Rademacher TW, Boutignon F, Dwek RA, Redman CW. Suppression of allogeneic reactivity in vitro by the syncytiotrophoblast membrane glycocalyx of the human term placenta is carbohydrate dependent. Glycobiology (1994) 4(1):39–47. doi: 10.1093/glycob/4.1.39
139. Deng Q, Chen Y, Yin N, Shan N, Luo X, Tong C, et al. N-acetylglucosaminyltransferase V inhibits the invasion of trophoblast cells by attenuating MMP2/9 activity in early human pregnancy. Placenta (2015) 36:1291–9. doi: 10.1016/j.placenta.2015.08.014
140. Deng Q, Liu X, Yang Z, Xie L. Expression of N-Acetylglucosaminyltransferase III Promotes Trophoblast Invasion and Migration in Early Human Placenta. Reprod Sci. (2019) 26:1373–81. doi: 10.1177/1933719118765967
141. Jones CJ, Aplin JD, Burton GJ. First trimester histiotrophe shows altered sialylation compared with secretory phase glycoconjugates in human endometrium. Placenta (2010) 31:576–80. doi: 10.1016/j.placenta.2010.04.011
142. Whyte A, Loke YW. Increased sialylation of surface glycopeptides of human trophoblast compared with fetal cells from the same conceptus. J Exp Med (1978) 148:1087–92. doi: 10.1084/jem.148.4.1087
143. Jeschke U, Richter DU, Hammer A, Briese V, Friese K, Karsten U. Expression of the thomsen-friedenreich antigen and of its putative carrier protein mucin 1 in the human placenta and in trophoblast cells in vitro. Histochem Cell Biol (2002) 117:219–26. doi: 10.1007/s00418-002-0383-5
144. Jeschke U, Mayr D, Schiessl B, Mylonas I, Schulze S, Kuhn C, et al. Expression of galectin-1, -3 (gal-1, gal-3) and the thomsen-friedenreich (TF) antigen in normal, IUGR, preeclamptic and HELLP placentas. Placenta (2007) 28:1165–73. doi: 10.1016/j.placenta.2007.06.006
145. Clark GF, Dell A, Morris HR, Patankar M, Oehninger S, Seppälä M. Viewing AIDS from a glycobiological perspective: potential linkages to the human fetoembryonic defence system hypothesis. Mol Hum Reprod (1997) 3:5–13. doi: 10.1093/molehr/3.1.5
146. Gridelet V, Perrier d'Hauterive S, Polese B, Foidart JM, Nisolle M, Geenen V. Human chorionic gonadotrophin: New pleiotropic functions for an "Old" hormone during pregnancy. Front Immunol (2020) 11:343. doi: 10.3389/fimmu.2020.00343
147. Norris W, Nevers T, Sharma S, Kalkunte S. Review: hCG, preeclampsia and regulatory T cells. Placenta (2011) 32 Suppl 2:S182–185. doi: 10.1016/j.placenta.2011.01.009
148. Minas V, Mylonas I, Schiessl B, Mayr D, Schulze S, Friese K, et al. Expression of the blood-group-related antigens sialyl Lewis a, sialyl Lewis x and Lewis y in term placentas of normal, preeclampsia, IUGR- and HELLP-complicated pregnancies. Histochem Cell Biol (2007) 128:55–63. doi: 10.1007/s00418-007-0293-7
149. Ma Z, Yang H, Peng L, Kuhn C, Chelariu-Raicu A, Mahner S, et al. Expression of the carbohydrate Lewis antigen, sialyl Lewis a, sialyl Lewis X, Lewis X, and Lewis y in the placental villi of patients with unexplained miscarriages. Front Immunol (2021) 12:679424. doi: 10.3389/fimmu.2021.679424
150. Jeschke U, Toth B, Scholz C, Friese K, Makrigiannakis A. Glycoprotein and carbohydrate binding protein expression in the placenta in early pregnancy loss. J Reprod Immunol (2010) 85:99–105. doi: 10.1016/j.jri.2009.10.012
151. Freitag N, Tirado-Gonzaĺez I, Barrientos G, Herse F, Thijssen VLJL, Weedon-Fekjær SM, et al. Interfering with gal-1-mediated angiogenesis contributes to the pathogenesis of preeclampsia. Proc Natl Acad Sci U. S. A (2013) 110:11451–6. doi: 10.1073/PNAS.1303707110
152. Ferrer CM, Lynch TP, Sodi VL, Falcone JN, Schwab LP, Peacock DL, et al. O-GlcNAcylation regulates cancer metabolism and survival stress signaling via regulation of the HIF-1 pathway. Mol Cell (2014) 54:820–31. doi: 10.1016/j.molcel.2014.04.026
153. Koike T, Kimura N, Miyazaki K, Yabuta T, Kumamoto K, Takenoshita S, et al. Hypoxia induces adhesion molecules on cancer cells: A missing link between warburg effect and induction of selectin-ligand carbohydrates. Proc Natl Acad Sci U. S. A (2004) 101:8132–7. doi: 10.1073/PNAS.0402088101
154. Miyata S, Noda A, Ozaki N, Hara Y, Minoshima M, Iwamoto K, et al. Insufficient sleep impairs driving performance and cognitive function. Neurosci Lett (2010) 469:229–33. doi: 10.1016/j.neulet.2009.12.001
155. Nonaka M, Fukuda MN, Gao C, Li Z, Zhang H, Greene MI, et al. Determination of carbohydrate structure recognized by prostate-specific F77 monoclonal antibody through expression analysis of glycosyltransferase genes. J Biol Chem (2014) 289:16478–86. doi: 10.1074/JBC.M114.559047
156. Belo AI, Van Vliet SJ, Maus A, Laan LC, Nauta TD, Koolwijk P, et al. Hypoxia inducible factor 1a down regulates cell surface expression of a1,2-fucosylated glycans in human pancreatic adenocarcinoma cells. (2015) 589:2359–66. doi: 10.1016/j.febslet.2015.07.035
157. Croci DO, Cerliani JP, Dalotto-Moreno T, Méndez-Huergo SP, Mascanfroni ID, Dergan-Dylon S, et al. Glycosylation-dependent lectin-receptor interactions preserve angiogenesis in anti-VEGF refractory tumors. Cell (2014) 156:744–58. doi: 10.1016/J.CELL.2014.01.043
158. Croci DO, Cerliani JP, Pinto NA, GMorosi L, Rabinovich GA. Regulatory role of glycans in the control of hypoxia-driven angiogenesis and sensitivity to antiangiogenic treatment. Glycobiology (2014) 24:1283–90. doi: 10.1093/glycob/cwu083
159. Alphonsus CS, Rodseth RN. The endothelial glycocalyx: a review of the vascular barrier. Anaesthesia (2014) 69:777–84. doi: 10.1111/anae.12661
160. Jackson-Weaver O, Friedman JK, Rodriguez LA, Hoof MA, Drury RH, Packer JT, et al. Hypoxia/reoxygenation decreases endothelial glycocalyx via reactive oxygen species and calcium signaling in a cellular model for shock. J Trauma Acute Care Surg (2019) 87:1070–6. doi: 10.1097/TA.0000000000002427
161. Haddad JJ, Harb HL. Cytokines and the regulation of hypoxia-inducible factor (HIF)-1alpha. Int Immunopharmacol (2005) 5:461–83. doi: 10.1016/j.intimp.2004.11.009
162. Bruning U, Fitzpatrick SF, Frank T, Birtwistle M, Taylor CT, Cheong A. NFκB and HIF display synergistic behaviour during hypoxic inflammation. Cell Mol Life Sci (2012) 69:1319–29. doi: 10.1007/s00018-011-0876-2
163. Vaughan JE, Walsh SW. Activation of NF-κB in placentas of women with preeclampsia. Hypertens Pregnancy (2012) 31:243–51. doi: 10.3109/10641955.2011.642436
164. Litang Z, Hong W, Weimin Z, Xiaohui T, Qian S. Serum NF-κBp65, TLR4 as biomarker for diagnosis of preeclampsia. Open Med (2017) 12:399–402. doi: 10.1515/med-2017-0057
165. Schäffer L, Scheid A, Spielmann P, Breymann C, Zimmermann R, Meuli M, et al. Oxygen-regulated expression of TGF-beta 3, a growth factor involved in trophoblast differentiation. Placenta (2003) 24:941–50. doi: 10.1016/s0143-4004(03)00166-8
166. Nishi H, Nakada T, Hokamura M, Osakabe Y, Itokazu O, Huang LE, et al. Hypoxia-inducible factor-1 transactivates transforming growth factor-beta3 in trophoblast. Endocrinology (2004) 145:4113–8. doi: 10.1210/en.2003-1639
167. Alijotas-Reig J, Palacio-Garcia C, Farran-Codina I, Ruiz-Romance M, Llurba E, Vilardell-Tarres M. Circulating cell-derived microparticles in severe preeclampsia and in fetal growth restriction. Am J Reprod Immunol (2012) 67:140–51. doi: 10.1111/j.1600-0897.2011.01072.x
168. Escudero C, Calle A. Hierro, oxígeno y desarrollo placentario en la génesis de la preeclampsia. Efectos de la altura en Ecuador [Iron, oxygen and placental development in the etiology of preeclampsia. Effects of high altitude in Ecuador]. Rev Med Chil (2006) 134:491–8. Spanish. doi: 10.4067/s0034-98872006000400014
169. Serdar Z, Gür E, Develioğlu O. Serum iron and copper status and oxidative stress in severe and mild preeclampsia. Cell Biochem Funct (2006) 24:209–15. doi: 10.1002/CBF.1235
170. Toldi G, Stenczer B, Molvarec A, Takáts Z, Beko G, Rigó J Jr., et al. Hepcidin concentrations and iron homeostasis in preeclampsia. Clin Chem Lab Med (2010) 48:1423–6. doi: 10.1515/CCLM.2010.290
171. Siddiqui IA, Jaleel A, Kadri HM, Saeed WA, Tamimi W. Iron status parameters in preeclamptic women. Arch Gynecol. Obstet (2011) 284:587–91. doi: 10.1007/S00404-010-1728-2
172. Gómez-Gutiérrez AM, Parra-Sosa BE, Bueno-Sánchez JC. Glicanos de la vellosidad trofoblástica en la anemia ferropénica y la preeclampsia grave. Rev Chil. Nutr (2015) 42:121–30. doi: 10.4067/S0717-75182015000200002
173. Gómez-Gutiérrez AM, Parra-Sosa BE, César Bueno-Sánchez J. Function of receptor 1 in uptaking transferrin and its relation to iron deficiency and iron gestational preeclampsia. Rev Cuba. Obstet. Ginecol (2013) 39:33–42.
174. Gambling L, Danzeisen R, Fosset C, Andersen HS, Dunford S, Srai SKS, et al. Iron and copper interactions in development and the effect on pregnancy outcome. J Nutr (2003) 133:1554S–6S. doi: 10.1093/jn/133.5.1554S
175. Bastin J, Drakesmith H, Rees M, Sargent I, Townsend A. Localisation of proteins of iron metabolism in the human placenta and liver. Br J Haematol (2006) 134:532–43. doi: 10.1111/J.1365-2141.2006.06216.X
176. McArdle HJ, Andersen HS, Jones H, Gambling L. Copper and iron transport across the placenta: regulation and interactions. J Neuroendocrinol (2008) 20:427–31. doi: 10.1111/j.1365-2826.2008.01658.x
177. Corrales-Agudelo V, Bueno-Sanchez J, Arboleda B--, Cardona-Ospina A, Lopez-Rojas E, Escobar L, et al. Transferrin receptor 1 and ferroportin expression in the full-term human placenta and its association with maternal and neonatal iron status: A pilot study. J Clin Nutr Metab (2018) 3:1–7. doi: 10.4172/JCNM.1000123
178. Ralton JE, Jackson HJ, Zanoni M, Gleeson PA. Effect of glycosylation inhibitors on the structure and function of the murine transferrin receptor. Eur J Biochem (1989) 186:637–47. doi: 10.1111/j.1432-1033.1989.tb15254.x
179. Do SI, Enns C, Cummings RD. Human transferrin receptor contains O-linked oligosaccharides. J Biol Chem (1990) 265:114–25. doi: 10.1016/S0021-9258(19)40203-2
180. Do S, Cummings RD. Presence of O-linked oligosaccharide on a threonine residue in the human transferrin receptor. Glycobiology (1992) 2:345–53. doi: 10.1093/GLYCOB/2.4.345
181. Georgieff MK, Petry CD, Mills MM, McKay H, Wobken JD. Increased N-glycosylation and reduced transferrin-binding capacity of transferrin receptor isolated from placentae of diabetic women. Placenta (1997) 18:563–8. doi: 10.1016/0143-4004(77)90011-x
182. Gómez-Gutiérrez AM, Parra-Sosa BE, Bueno-Sánchez JC. Glycosylation profile of the transferrin receptor in gestational iron deficiency and early-onset severe preeclampsia. J Pregnancy (2019) 2019:9514546. doi: 10.1155/2019/9514546
183. Zhuo Y, Bellis SL. Emerging role of α2,6-sialic acid as a negative regulator of galectin binding and function. J Biol Chem (2011) 286:5935. doi: 10.1074/JBC.R110.191429
184. Carlsson MC, Bengtson P, Cucak H, Leffler H. Galectin-3 guides intracellular trafficking of some human serotransferrin glycoforms. J Biol Chem (2013) 288:28398. doi: 10.1074/JBC.M113.487793
185. Rutledce EA, Enns CA. Cleavage of the transferrin receptor is influenced by the composition of the 0-linked carbohydrate at position 104. J Cell Physiol (1996) 168:284–293. doi: 10.1002/(SICI)1097-4652(199608)168:2
186. Murrieta-Coxca JM, Fuentes-Zacarias P, Ospina-Prieto S, Markert UR, Morales-Prieto DM. Synergies of extracellular vesicles and microchimerism in promoting immunotolerance during pregnancy. Front Immunol (2022) 13:837281. doi: 10.3389/fimmu.2022.837281
187. Darmochwal-Kolarz D, Rolinski J, Leszczynska-Goarzelak B, Oleszczuk J. The expressions of intracellular cytokines in the lymphocytes of preeclamptic patients. A. J Reprod Immunol (2002) 48:381–6. doi: 10.1034/j.1600-0897.2002.01089.x
188. Van Nieuwenhoven ALV, Moes H, Heineman MJ, Santema J, Faas MM. Cytokine production by monocytes, NK cells, and lymphocytes is different in preeclamptic patients as compared with normal pregnant women. Hypertension Pregnancy (2008) 27:207–24. doi: 10.1080/10641950701885006
189. Liao WC, Chen CH, Liu CH, Huang MJ, Chen CW, Hung JS, et al. Expression of GALNT2 in human extravillous trophoblasts and its suppressive role in trophoblast invasion. Placenta (2012) 33:1005–11. doi: 10.1016/j.placenta.2012.08.007
190. Hromatka BS, Drake PM, Kapidzic M, Stolp H, Goldfien GA, Shih I, et al. Polysialic acid enhances the migration and invasion of human cytotrophoblasts. Glycobiology (2013) 23:593–602. doi: 10.1093/glycob/cws162
191. Masilamani M, Nguyen C, Kabat J, Borrego F, Coligan JE. CD94/NKG2A inhibits NK cell activation by disrupting the actin network at the immunological synapse. J Immunol (2006) 177:3590–6. doi: 10.4049/jimmunol.177.6.3590
192. Jacobs R, Hintzen G, Kemper A, Beul K, Kempf S, Behrens G, et al. CD56 bright cells differ in their KIR repertoire and cytotoxic features from CD56 dim NK cells. Eur J Immunol (2001) 31:3121–7. doi: 10.1002/1521-4141(2001010)31:10<3121::aid-immu3121>3.0.co;2-4
193. Saito S, Sakai M. Th1/Th2 balance in preeclampsia. J Reprod Immunol (2003) 59:161–73. doi: 10.1016/S0165-0378(03)00045-7
194. Higuma-Myojo S, Sasaki Y, Miyazaki S, Sakai M, Siozaki A, Miwa N, et al. Cytokine profile of natural killer cells in early human pregnancy. Am J Reprod Immunol (2005) 54:21–9. doi: 10.1111/j.1600-0897.2005.00279.x
195. Saito S, Shigeru S, Nakashima A, Akitoshi N, Myojo-Higuma S, Subaru M-H, et al. The balance between cytotoxic NK cells and regulatory NK cells in human pregnancy. J Reprod Immunol (2008) 77:14–22. doi: 10.1016/j.jri.2007.04.007
196. Zhang Z, Gong F, Jia L, Chang C, Hou L, Yang R, et al. Studies on activity of NK cells in preeclampsia patients. J Huazhong Univ Sci Technolog. Med Sci (2004) 24:473–5.
197. Petrie EJ, Clements CS, Lin J, Sullivan LC, Johnson D, Huyton T, et al. CD94-NKG2A recognition of human leukocyte antigen (HLA) -e bound to an HLA class I leader sequence. J Exp Med (2008) 205:725–35. doi: 10.1084/jem.20072525
198. Valés-Gómez M, Reyburn HT, Erskine RA, López-Botet M, Strominger JL. Kinetics and peptide dependency of the binding of the inhibitory NK receptor CD94/NKG2-a and the activating receptor CD94/NKG2-c to HLA-e. EMBO J (1999) 18:4250–60. doi: 10.1093/emboj/18.15.4250
199. Xin X, Higai K, Imaizumi Y, Suzuki C, Ito K, Itoh A, et al. Natural killer group 2A (NKG2A) and natural killer group 2C (NKG2C) bind to sulfated glycans and a 2,3-NeuAc-containing glycoproteins. Biol Pharm Bull (2011) 34:480–5. doi: 10.1248/bpb.34.480
200. Hamad RR, Berg L, Bachmayer N, Sohlberg E, Sundstro Y, Sverremark-ekstro E, et al. Women with preeclampsia have an altered NKG2A and NKG2C receptor expression on peripheral blood natural killer cells. Am J Reprod Immunol (2009) 62:147–57. doi: 10.1111/j.1600-0897.2009.00724.x
201. Bueno-Sánchez JC, Peña-Alzate S, Peña RB, Agudelo-Jaramillo B, Cadavid-Jaramillo AP, Chaouat G, et al. Sera from early-onset, severely preeclamptic women directly modulate HLA-e expression in the EA.hy296 endothelial cell line. J Reprod Immunol (2014) 104-105:68–79. doi: 10.1016/j.jri.2014.03.004
202. Wei J, Satomi M. Effect of sera on the adhesion of natural killer cells to the endothelium in severe preeclampsia. J Obstet. Gynaecol. Res (2006) 32:443–8. doi: 10.1111/j.1447-0756.2006.00444.x
203. Damle NK, Doyle LV. IL-2-activated human killer lymphocytes but not their secreted products mediate increase in albumin flux across cultured endothelial monolayers. implications for vascular leak syndrome. J Immunol (1989) 142:2660–9. doi: 10.4049/jimmunol.142.8.2660
204. Coupel S, Moreau A, Hamidou M, Horejsi V, Soulillou J-P, Charreau B. Expression and release of soluble HLA-e is an immunoregulatory feature of endothelial cell activation. Blood (2007) 109:2806–14. doi: 10.1182/blood-2006-06-030213
205. Ohyama C, Tsuboi S, Fukuda M. Dual roles of sialyl Lewis X oligosaccharides in tumor metastasis and rejection by natural killer cells. EMBO J (1999) 18:1516–25. doi: 10.1093/emboj/18.6.1516
206. Sol M, Vacaresse N, Lule J, Davrinche C, Gabriel B, Teissie J, et al. N-linked oligosaccharides can protect target cells from the lysis mediated by NK cells but not by cytotoxic T lymphocytes: role of NKG2-a. Tissue Antigens (1999) 54:113–21. doi: 10.1034/j.1399-0039.1999.540201.x
207. Pang P, Drobnis EZ, Morris HR, Dell A, Clark GF. Analysis of the human seminal plasma glycome reveals the presence of immunomodulatory carbohydrate functional groups research articles. J Proteome Res (2009) 8:4906–15. doi: 10.1021/pr9001756
208. Moebius JM, Widera D, Schmitz J, Kaltschmidt C, Piechaczek C. Impact of polysialylated CD56 on natural killer cell cytotoxicity. BMC Immunol (2007) 8:13. doi: 10.1186/1471-2172-8-13
209. Pearce OMT, Läubli H. Sialic acids in cancer biology and immunity. Glycobiology (2016) 26:111–28. doi: 10.1093/glycob/cwv097
210. Ibeto L, Antonopoulos A, Grassi P, Pang PC, Panico M, Bobdiwala S, et al. Insights into the hyperglycosylation of human chorionic gonadotropin revealed by glycomics analysis. PloS One (2020) 15:e0228507. doi: 10.1371/JOURNAL.PONE.0228507
211. Campuzano M, Bueno-Sánchez J, Agudelo-Jaramillo B, Quintana-Castillo JC, Chaouat GC, Maldonado-Estrada JG. Glycan expression in chorionic villi from histocultures of women with early-onset preeclampsia: Immunomodulatory effects on peripheral natural killer cells. J Reprod Immunol (2020) 142:103212. doi: 10.1016/J.JRI.2020.103212
212. Saul R, Molyneux RJ, Elbein AD. Studies on the mechanism of castanospermine inhibition of alpha- and beta-glucosidases. Arch Biochem Biophys (1984) 230:668–75. doi: 10.1016/0003-9861(84)90448-x
213. Winn VD, Gormley M, Paquet AC, Kjaer-Sorensen K, Kramer A, Rumer KK, et al. Severe preeclampsia-related changes in gene expression at the maternal-fetal interface include sialic acid-binding immunoglobulin-like lectin-6 and pappalysin-2. Endocrinology (2009) 150:452–62. doi: 10.1210/en.2008-0990
214. Friese K, Schiessl B, Schulze S, Kuhn C, Jeschke U, Lomba I, et al. Expression of glycodelin a in decidual tissue of preeclamptic, HELLP and intrauterine growth-restricted pregnancies. Virchows Arch (2005) 446:360–8. doi: 10.1007/s00428-004-1201-3
215. Dundar B, Dincgez Cakmak B, Aydin Boyama B, Karadag B, Ozgen G. Maternal serum glycodelin levels in preeclampsia and its relationship with the severity of the disease. J Matern. Fetal. Neonatal Med (2018) 31:2884–92. doi: 10.1080/14767058.2017.1359530
216. Hussain T, Murtaza G, Metwally E, Kalhoro DH, Kalhoro MS, Rahu BA, et al. The role of oxidative stress and antioxidant balance in pregnancy. Mediators Inflammation (2021) 2021:9962860. doi: 10.1155/2021/9962860
217. Mukherjee I, Dhar R, Singh S, Sharma JB, Nag TC, Mridha AR, et al. Oxidative stress-induced impairment of trophoblast function causes preeclampsia through the unfolded protein response pathway. Sci Rep (2021) 11:18415. doi: 10.1038/s41598-021-97799-y
218. Vaka R, Deer E, Cunningham M, McMaster KM, Wallace K, Cornelius DC, et al. Characterization of mitochondrial bioenergetics in preeclampsia. J Clin Med (2021) 10:5063. doi: 10.3390/jcm10215063
219. Chandra PK, Cikic S, Rutkai I, Guidry JJ, Katakam PVG, Mostany R, et al. Effects of aging on protein expression in mice brain microvessels: ROS scavengers, mRNA/protein stability, glycolytic enzymes, mitochondrial complexes, and basement membrane components. Geroscience (2021) 44:371–88. doi: 10.1007/s11357-021-00468-1
220. Jones CJP, Owens S, Senga E, van Rheenen P, Faragher B, Denton J, et al. Placental expression of α2,6-linked sialic acid is upregulated in malaria. Placenta (2008) 29:300–4. doi: 10.1016/j.placenta.2007.12.007
221. Agudelo O, Bueno J, Villa A, Maestre A. High IFN-gamma and TNF production by peripheral NK cells of Colombian patients with different clinical presentation of plasmodium falciparum. Malar. J (2012) 11:38. doi: 10.1186/1475-2875-11-38
222. Agudelo OM, Aristizabal BH, Yanow SK, Arango E, Carmona-Fonseca J, Maestre A. Submicroscopic infection of placenta by plasmodium produces Th1/Th2 cytokine imbalance, inflammation and hypoxia in women from north-west Colombia. Malar J (2014) 13:122. doi: 10.1186/1475-2875-13-122
223. Arango EM, Samuel R, Agudelo OM, Carmona-Fonseca J, Maestre A, Yanow SK. Molecular detection of malaria at delivery reveals a high frequency of submicroscopic infections and associated placental damage in pregnant women from northwest Colombia. Am J Trop Med Hyg (2013) 89:178–83. doi: 10.4269/ajtmh.12-0669
224. Jin J, Menon R. Placental exosomes: A proxy to understand pregnancy complications. Am J Reprod Immunol (2018) 79:e12788. doi: 10.1111/aji.12788
225. Zhang Y, Zhao C, Wei Y, Yang S, Cui C, Yang J, et al. Increased circulating microparticles in women with preeclampsia. Int J Lab Hematol (2018) 40(3):352–8. doi: 10.1111/IJLH.12796
226. Sargent IL. Microvesicles and pre-eclampsia. pregnancy hypertension. Int J Women’s Cardiovasc Health (2013) 3(2):58. doi: 10.1016/J.PREGHY.2013.04.004
227. Dragovic RA, Collett GP, Hole P, Ferguson DJP, Redman CW, Sargent IL, et al. Isolation of syncytiotrophoblast microvesicles and exosomes and their characterisation by multicolour flow cytometry and fluorescence nanoparticle tracking analysis. Methods (2015) 87:64–74. doi: 10.1016/j.ymeth.2015.03.028
228. Condrat CE, Varlas VN, Duică F, Antoniadis P, Danila CA, Cretoiu D, et al. Pregnancy-related extracellular vesicles revisited. Int J Mol Sci (2021) 22:3904. doi: 10.3390/IJMS22083904
229. Laresgoiti-Servitje E, Gomez-Lopez N. The pathophysiology of preeclampsia involves altered levels of angiogenic factors promoted by hypoxia and autoantibody-mediated mechanisms. Biol Reprod (2012) 87:36. doi: 10.1095/biolreprod.112.099861
230. Goswami D, Tannetta DS, Magee LA, Fuchisawa A, Redman CWG, Sargent IL, et al. Excess syncytiotrophoblast microparticle shedding is a feature of early-onset pre-eclampsia, but not normotensive intrauterine growth restriction. Placenta (2006) 27(1):56–61. doi: 10.1016/j.placenta.2004.11.007
231. Redman CWG, Sargent IL. Immunology of pre-eclampsia. Am J Reprod Immunol (2010) 63:534–43. doi: 10.1111/j.1600-0897.2010.00831.x
232. Sargent IL, Borzychowski AM, Redman CWG. NK cells and human pregnancy–an inflammatory view. Trends Immunol (2006) 27:399–404. doi: 10.1016/j.it.2006.06.009
233. Sargent IL, Borzychowski AM, Redman CWG. Immunoregulation in normal pregnancy and pre-eclampsia: an overview. Reprod Biomed Online (2006) 13:680–6. doi: 10.1016/s1472-6483(10)60659-1
234. Williams C, Royo F, Aizpurua-Olaizola O, Pazos R, Boons GJ, Reichardt NC, et al. Glycosylation of extracellular vesicles: current knowledge, tools and clinical perspectives. J Extracell. Vesicles (2018) 7:1442985. doi: 10.1080/20013078.2018.1442985
235. Harada Y, Ohkawa Y, Maeda K, Kizuka Y, Taniguchi N. Extracellular vesicles and glycosylation. Adv Exp Med Biol (2021) 1325:37–149. doi: 10.1007/978-3-030-70115-4_6
236. Tannetta D, Masliukaite I, Vatish M, Redman C, Sargent I. Update of syncytiotrophoblast derived extracellular vesicles in normal pregnancy and preeclampsia. J Reprod Immunol (2017) 119:98–106. doi: 10.1016/J.JRI.2016.08.008
237. Henao DE, Mathieson PW, Saleem MA, Bueno JC, Cadavid A. A novel renal perspective of preeclampsia: a look from the podocyte. Nephrol Dial Transplant (2007) 22:1477. doi: 10.1093/ndt/gfl804
238. Henao DE, Saleem MA, Cadavid AP. Glomerular disturbances in preeclampsia: disruption between glomerular endothelium and podocyte symbiosis. Hypertens Pregnancy (2010) 29:10–20. doi: 10.3109/10641950802631036
239. Henao DE, Saleem MA. Proteinuria in preeclampsia from a podocyte injury perspective. Curr Hypertens Rep (2013) 15:600–5. doi: 10.1007/s11906-013-0400-1
240. Singh A, Satchell SC, Neal CR, McKenzie E, Tooke JE, Mathieson PW. Glomerular endothelial glycocalyx constitutes a barrier to protein permeability. J Am Soc Nephrol (2007) 18:2885–93. doi: 10.1681/ASN.2007010119
241. Chaouat G. Inflammation, NK cells and implantation: friend and foe (the good, the bad and the ugly?): replacing placental viviparity in an evolutionary perspective. J Reprod Immunol (2013) 97:2–13. doi: 10.1016/j.jri.2012.10.009
242. Chaouat G, Ledée-Bataille N, Dubanchet S. Immunological similarities between implantation and preeclampsia. Am J Reprod Immunol (2005) 53:222–9. doi: 10.1111/J.1600-0897.2005.00269.X
243. Clark DA, Chaouat G, Wong K, Gorczynski RM, Kinsky R. Tolerance mechanisms in pregnancy: a reappraisal of the role of class I paternal MHC antigens. Am J Reprod Immunol (2010) 63:93–103. doi: 10.1111/j.1600-0897.2009.00774.x
244. Chaouat G, Petitbarat M, Bulla R, Dubanchet S, Valdivia K, Ledée N, et al. Early regulators in abortion and implications for a preeclampsia model. J Reprod Immunol (2009) 82:131–40. doi: 10.1016/j.jri.2009.08.004
245. Robillard P, Dekker G, Chaouat G, Elliot MG, Scioscia M, Néonatologie S, et al. High incidence of early onset preeclampsia is probably the rule and not the exception worldwide . 20th anniversary of the reunion workshop . a summary. J Reprod Immunol (2019) 133:30–6. doi: 10.1016/j.jri.2019.05.003
246. Kane N, Kelly R, Saunders PTK, Critchley HOD. Proliferation of uterine natural killer cells is induced by human chorionic gonadotropin and mediated via the mannose receptor. Endocrinology (2009) 150:2882–8. doi: 10.1210/en.2008-1309
247. Berndt S, Blacher S, Munaut C, Detilleux J, Perrier d’Hauterive S, Huhtaniemi I, et al. Hyperglycosylated human chorionic gonadotropin stimulates angiogenesis through TGF-β receptor activation. FASEB J (2013) 27:1309–21. doi: 10.1096/fj.12-213686
248. Blois SM, Ilarregui JM, Tometten M, Garcia M, Orsal AS, Cordo-Russo R, et al. A pivotal role for galectin-1 in fetomaternal tolerance. Nat Med (2007) 131450–7. doi: 10.1038/nm1680
249. Molvarec A, Blois SM, Stenczer B, Toldi G, Tirado-Gonzalez I, Ito M, et al. Peripheral blood galectin-1-expressing T and natural killer cells in normal pregnancy and preeclampsia. Clin Immunol (2011) 139:48–56. doi: 10.1016/j.clim.2010.12.018
250. Borowski S, Tirado-Gonzalez I, Freitag N, Garcia MG, Barrientos G, Blois SM. Altered glycosylation contributes to placental dysfunction upon early disruption of the NK cell-DC dynamics. Front Immunol (2020) 11:1316. doi: 10.3389/fimmu.2020.01316
Keywords: glycosylation, preeclampsia, syncitiotrophoblast, NK cells activity, sialic acid (N-Acetyl neuraminic acid)
Citation: Bueno-Sánchez JC, Gómez-Gutiérrez AM, Maldonado-Estrada JG and Quintana-Castillo JC (2023) Expression of placental glycans and its role in regulating peripheral blood NK cells during preeclampsia: a perspective. Front. Endocrinol. 14:1087845. doi: 10.3389/fendo.2023.1087845
Received: 02 November 2022; Accepted: 03 March 2023;
Published: 03 May 2023.
Edited by:
Reinaldo Marín, Instituto Venezolano de Investigaciones Científicas (IVIC), VenezuelaReviewed by:
Frank Hills, Middlesex University, United KingdomLeonardo Ermini, University of Siena, Italy
Copyright © 2023 Bueno-Sánchez, Gómez-Gutiérrez, Maldonado-Estrada and Quintana-Castillo. This is an open-access article distributed under the terms of the Creative Commons Attribution License (CC BY). The use, distribution or reproduction in other forums is permitted, provided the original author(s) and the copyright owner(s) are credited and that the original publication in this journal is cited, in accordance with accepted academic practice. No use, distribution or reproduction is permitted which does not comply with these terms.
*Correspondence: Julio C. Bueno-Sánchez, anVsaW8uYnVlbm9AdWRlYS5lZHUuY28=