- 1Department of Surgery, School of Veterinary Medicine and Animal Science, University of São Paulo, São Paulo, Brazil
- 2Centre for Natural and Human Sciences, Federal University of ABC, São Paulo, Brazil
Traditional therapeutic interventions aim to restore male fertile potential or preserve sperm viability in severe cases, such as semen cryopreservation, testicular tissue, germ cell transplantation and testicular graft. However, these techniques demonstrate several methodological, clinical, and biological limitations, that impact in their results. In this scenario, reproductive medicine has sought biotechnological alternatives applied for infertility treatment, or to improve gamete preservation and thus increase reproductive rates in vitro and in vivo. One of the main approaches employed is the biomimetic testicular tissue reconstruction, which uses tissue-engineering principles and methodologies. This strategy pursues to mimic the testicular microenvironment, simulating physiological conditions. Such approach allows male gametes maintenance in culture or produce viable grafts that can be transplanted and restore reproductive functions. In this context, the application of several biomaterials have been proposed to be used in artificial biological systems. From synthetic polymers to decellularized matrixes, each biomaterial has advantages and disadvantages regarding its application in cell culture and tissue reconstruction. Therefore, the present review aims to list the progress that has been made and the continued challenges facing testicular regenerative medicine and the preservation of male reproductive capacity, based on the development of tissue bioengineering approaches for testicular tissue microenvironment reconstruction.
1 Introduction
Recently, a worldwide decline of male fertility parameters has been observed, in both humans and animals. In humans, almost 50% of infertile couples have the male component as the major cause (1–3). Regarding other species, fertility preservation of endangered species has been the main goal, and the understanding of their reproductive biology assists on their conservation and management (4, 5).
Several factors may influence male fertility; however, there is no specific etiology for almost 40% of infertile men (6–8). Male infertility may occur due to several conditions, which include hormonal deficits (9), anatomical or genetic abnormalities (10), systemic illnesses, infections, traumas, intoxications, autoimmune diseases, environmental exposure or even lifestyle (11–13). Malignant testicular neoplasms are one of the greatest causes of testicular tissue degeneration, being the highest incident type of cancer in men, also having a high incidence in domestic species as dogs (14, 15). These neoplasms may occur due to hereditary mutations, but the greater percentage comes from environmental factors (16–20).
Considering that genome reprogramming steps take place during gametogenesis and early development, abnormal genome epigenetic reprogramming is highlighted as a contributing factor for male infertility (21, 22). Epigenetic processes are defined as hereditary alteration that affect gene expression, not modifying the DNA sequence (23). Among these alterations, there are DNA methylations, histones alterations and non-coding RNAs synthesis, which can be transmitted to the offspring (24, 25).
Facing this scenario, advances in male fertility preservation may guarantee the reestablishment of reproductive functions or germ cells safeguarding through the development of reproductive biotechnologies (26). One of the greatest achievements was the application of assisted reproduction technologies (ART) for recent generations of humans and the increase of livestock production (27, 28). More recently, due to environmental sustainability policies, such technologies have been devoted to endangered species conservation, seeking not only to maintain the current population, but to also preserve the genetic heritage (5, 29, 30).
Some of the most prominent approaches to preserve male fertility include sperm and testicular tissue cryopreservation; germ cells transplantation, and testicular grafts (31–34). An efficient germ cell maintenance is essential for in vitro fertilization as for intracytoplasmic sperm injection (ICSI), which is one of the most well-succeeded techniques in assisted reproduction (35, 36). When the spermatozoid production is not possible, the use of spermatogonial stem cells (SSCs) is considered a viable option (35), but due to several experimental difficulties as stem cells isolation, identification, purification and in vitro maintenance, this approach remains limited (36). Although its broad potential in the reproduction field, its effectiveness was reported in rodents, remaining a challenge in larger species (37, 38).
Essential processes of male reproductive physiology as spermatogenesis and maintenance of spermatic viability are highly dependent of the molecular microenvironment (39). Among the other elements that compound the tissue microenvironment as growth factors, hormones and other biofactors, the extracellular matrix (ECM) is highlighted due to its role in testicular tissue homeostasis (40, 41). ECM also provides three-dimensionality, which enhances the interaction between the cell and the extracellular environment, increasing cell susceptibility to molecular signaling from the ECM and other exogenous factors (42).
A promising approach both for degenerated testicular tissue replacement and for in vitro germ cells maintenance is the development of biomimetic testicular tissues that contain similar morphophysiological characteristics to those found in vivo (40). In this scenario, some bioengineering strategies that associate biomaterials, cells and bioactive factors have been proposed to provide greater complexity to the artificial tissues (41).
A biomaterial considered eligible to be applied in a biomimetic system, must have several physico-chemical properties as, suitable morphology, mechanic resistance, and porous structure (43–45). It must also be biocompatible and have an acceptable biodegradability that allows the interaction with cells (43–45). Synthetic biomaterials as polyesters and polyprolactone are viable alternatives to produce three-dimensional scaffolds that may be chemically altered to adapt for diverse contexts (39). However, due to their weak interaction with cell membrane adhesion proteins, such polymers are not able to fully mimic the ECM biological properties (39). Natural polymers, otherwise, due to their biological origin, present better cytocompatibility, which allows the development of a more reliable microenvironment, however, such components do not provide the entire ECM complexity (46). A more complex alternative to all these biomaterials is the application of decellularized matrixes, which, if well preserved, contain the main fibrillary and non-fibrillary components (4, 5, 47).
Therefore, this review aimed to describe the advances and challenges of tissue engineering for testicular tissue reconstruction and the development of artificial in vitro systems that can preserve and develop male germ cells, highlighting their advantages over the main methods of male fertility preservation. Furthermore, this article aims to discuss how bioengineering can be an important and innovative approach to andrological regenerative medicine, highlighting the role of the testicular microenvironment as a protagonist in the reproductive potential maintenance of both humans and other species.
2 Testicular morphophysiology
2.1 Testicular architecture, structure and ultrastructure
Anatomically, male reproductive tract is constituted by testis, epididymis, vas deferens, urethral adnexal glands (ampullae, vesicular glands, prostate, and bulbourethral gland) and the penis (48). The testis are paired organs located outside the abdominal cavity inside the scrotum. The testicular surface is covered by the tunica albuginea, with a thickness of 1 to 2 mm, composed of collagen fibers and containing the blood vessels (testicular artery and testicular vein) that are visible on the testicular surface, in which each species present a characteristic pattern (49, 50). Regarding muscle constitution, the testis is covered by the smooth muscle dartos tunic and suspended by the cremaster muscle, which contribute to testicular thermoregulation, moving the gonads away or closer to the inguinal-abdominal area (51). This mechanism is related to testicular temperature maintenance, which is essential for spermatogenesis to occur normally. In species that the testis are inside the scrotum, the temperature of the gonads must be between 4° and 7° C below body temperature (51, 52).
More externally, the testis are composed of a fibrous capsule called the tunica albuginea; more internally, there are the septa and the mediastinum, which make up the connective tissue (53). The tunica albuginea gives off the septa that spreads into the testicle. As the septa enters the testis, the testicular parenchyma divides into pyramidal lobes (54). These septa tend towards the central region forming the mediastinum of the testis, and their location can vary from axial to displaced towards the epididymis (55) (Figure 1).
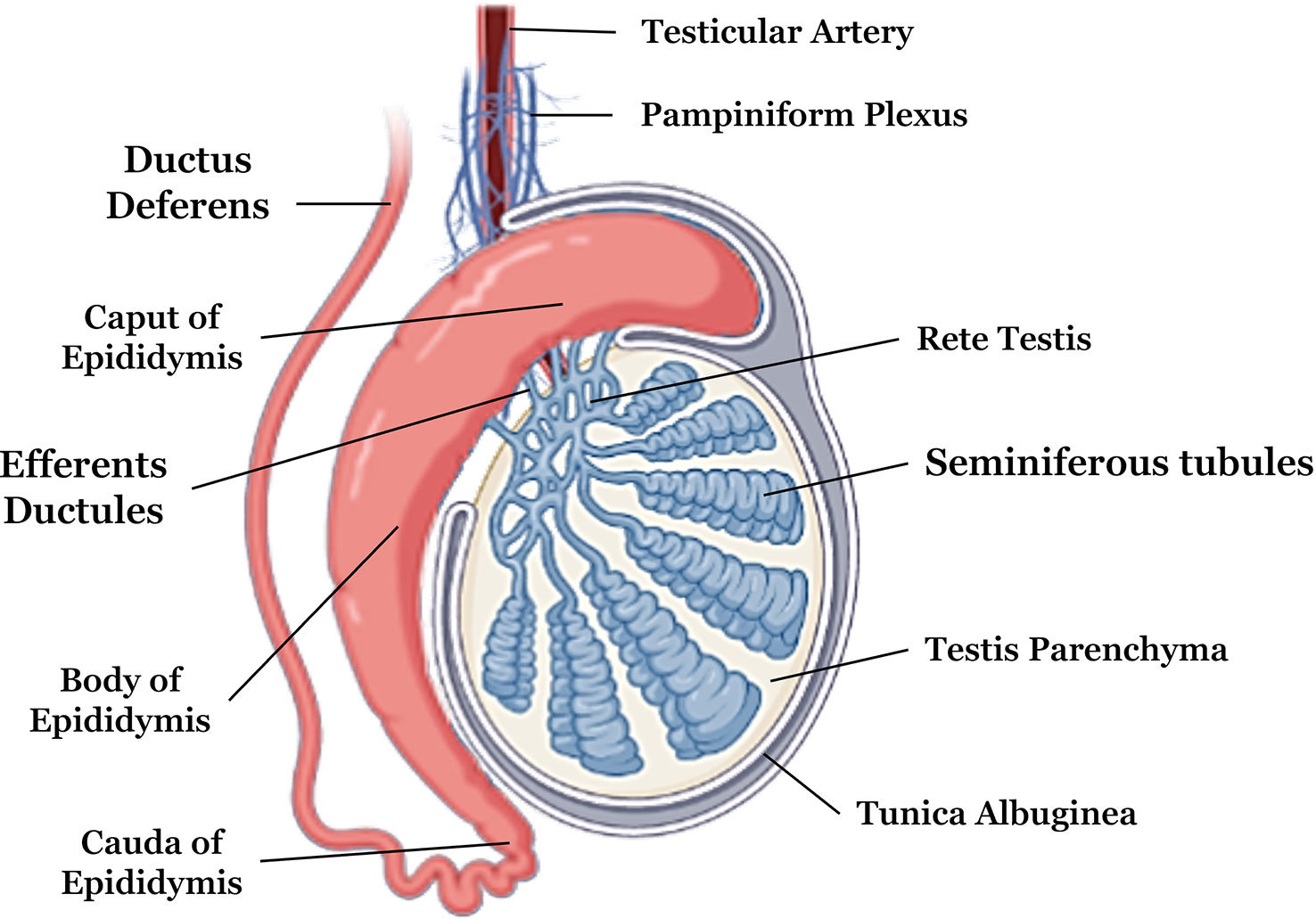
Figure 1 Anatomical description of testicular and epididymal structures. Adapted from Biorender .
Histologically, the testicular tissue has several cell types, which can be divided in germ cells lineage, from gonocytes to mature spermatozoids, support, and immune cells (56). The extracellular matrix is an association of structural and functional components that provide not only a structural network for cells but also a biochemical and biomechanical signaling that is able to impact on cell physiology directly (57, 58). The testicular ECM is formed by several components as collagen fibers, elastic fibers, glycosaminoglycans and proteoglycans (56) (Table 1).
Functionally, testicular ECM is organized into a specialized structure called the blood-testis barrier (BTB), formed mainly by type IV collagen, laminin, heparan sulfate proteoglycans, entactin and fibronectin (64). This barrier, along with the tight junctions of Sertoli cell membranes, restricts the flow of molecules from the bloodstream, selecting which components will come into the testicular parenchyma (65, 66).
In mammals, the testis consist of two compartments: the interstitial and seminiferous tubules compartments (67). The interstitial one contains nerves, blood, and lymphatic vessels (67). Its main cell type is the Leydig cells, that are responsible for testosterone synthesis. Other important cell types presented in this region are peritubular, endothelial, smooth muscle, perivascular cells, and testicular macrophages (68). Regarding the seminiferous tubules, several cell layers constitute them. In the periphery, there are Sertoli cells and spermatogonium. Inside the tubules, there are spermatic cells lineage, which includes spermatocyte I, spermatids and more centrally, spermatozoids (69).
The testicular microenvironment is essential for the spermatogenesis process to occur normally, providing favorable conditions for anchorage, cell growth, nutrient diffusion, and mechanical support necessary for tissue homeostasis (56). The complex structure of the testis directly acts on sperm maturation and the male hormones production (70). Sertoli cells are the main cell type responsible for the production and secretion of metal ion binding proteins, lipids, proteases, protease inhibitors, hormones, and growth factors. This secretion products act on germ cells, tissue remodeling, spermatid release, basement membrane formation and intercellular junctions (65). Another cell type essential for spermatogenesis is the Leydig cell, which acts directly on Sertoli cells and sperm development (65). Hormones such as testosterone and follicle-stimulating hormone (FSH) act on Sertoli cells gene expression, regulating their activity according to the spermatogenesis cycles (71).
Another important structure that is connected to the testicles is the epididymis, which is divided into caput, body, and cauda, situated longitudinally in the caudal portion of the testis (72). The caput of the epididymis is in the upper portion, while the cauda is located in the lower portion of the testis. The epididymis consists of a long tube that is coiled. In the caput the sperm maturation phase occurs, and in the body and cauda, sperm motility occurs (73, 74).
2.2 Spermatogenesis
The development of male gametes is a complex differentiation process that takes place in the testis and produces sperm (75, 76). Spermatogenesis begins with the proliferation and differentiation of diploid spermatogonial stem cells, followed by meiosis of spermatocytes that form round spermatids (77). In mammals, the spermatogenesis process is composed of three distinct phases: the mitotic or spermatogonial phase, in which the gonocytes or stem spermatogonia undergo mitotic divisions until the formation of primary spermatocytes; meiotic or spermatocyte phase, stage in which spermatocytes undergo reduction divisions that result in the formation of spermatids; and the spermiogenesis or differentiation phase, the period in which the morphological and functional changes of spermatids occur until the moment of their release into the lumen of the seminiferous tubule, where they become spermatozoa (38, 78, 79). The stages of differentiation of primordial germ cells into mature sperm are schematized in Figure 2.
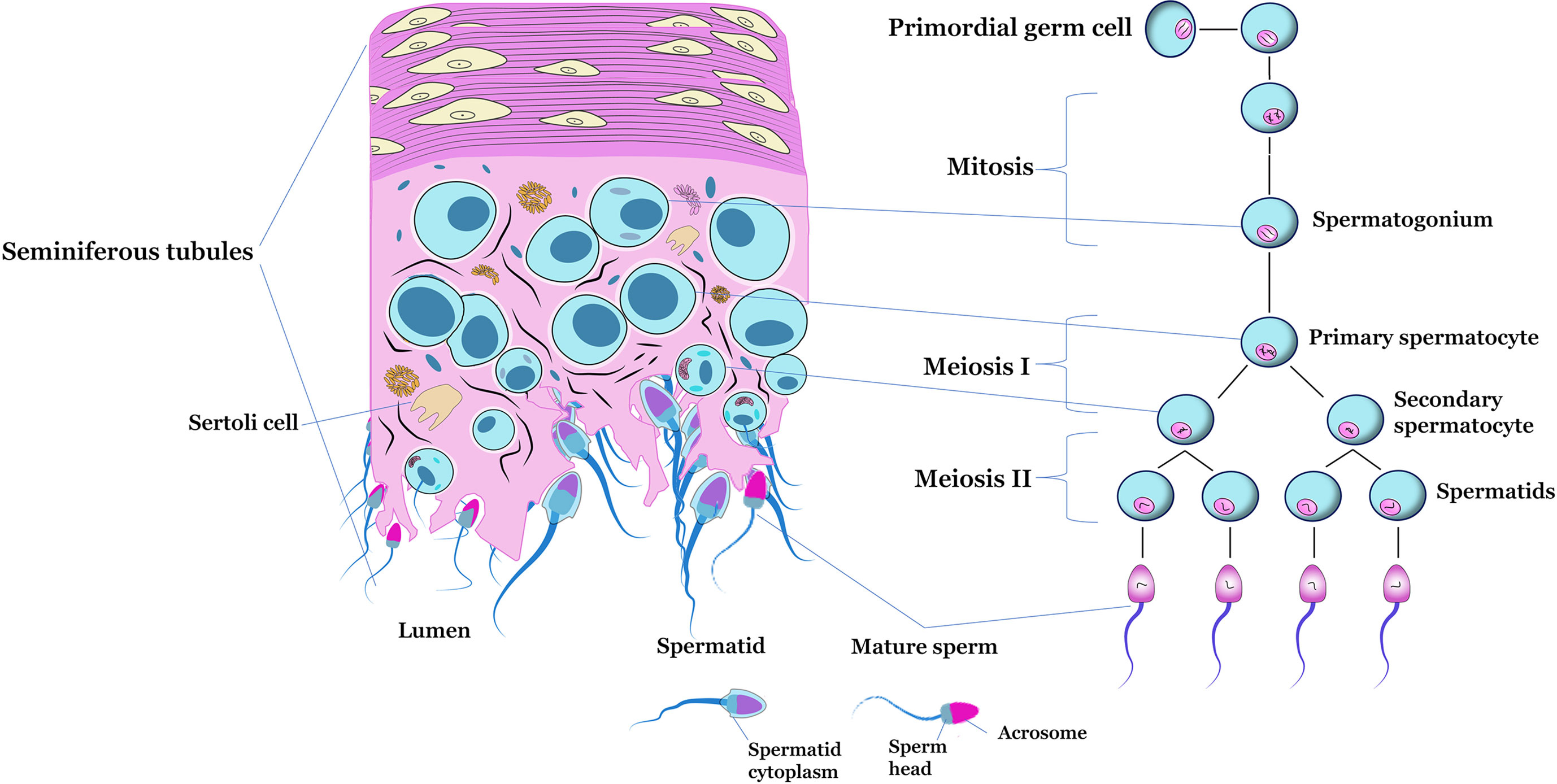
Figure 2 Schematic figure of a transverse section of the seminiferous tubule, highlighting the main cell types located inside (left). Representation of the of spermatogenesis (right).
During embryonic development, primordial germ cells migrate from the yolk sac region to the undifferentiated gonads (80). After reaching the forming gonad, primordial cells begin the process of division before forming gonocytes. In males, these gonocytes undergo differentiation before puberty to form A0 spermatogonia, from which other germ cells originate (81). During the first phase of spermatogenesis, the proliferation and differentiation of spermatogonia occurs (75). The types of undifferentiated and differentiated spermatogonia vary according to the species (82).
In mice and rats, spermatogonia are divided into three types: A, Intermediate and B. Type A spermatogonia are subdivided, according to morphological criteria, into A isolated (Ai), A paired (Ap), A aligned (Aal), A1, A2, A3 and A4 (83). This classification varies according to the degree of differentiation, with Ai spermatogonia being the least differentiated and A4 the most differentiated within the type A subdivision. (84). Ap and Aal spermatogonia are called proliferative spermatogonia, A1 to A4 spermatogonia as in differentiation, Intermediate and B spermatogonia as differentiated (85). In humans, spermatogonia divide into dark type (Adark), pale type (Apale) and type B. Type Adark spermatogonia reproduce through mitosis that generate both Adark and Apale spermatogonia (81). During adult life, undifferentiated Apale spermatogonia divide, giving rise to B spermatogonia. Adark spermatogonia are quiescent reserve cells, as they show low proliferative capacity throughout spermatogenic activity, while Apale spermatogonia are in continuous proliferation (86). Although both types of spermatogonia (Adark and Apale) are in the basement membrane, they differ morphologically, with Adark being small, round, or slightly ovoid, while Apale are larger, oval or nearly round (87).
The period of spermatogenesis varies according to the species. In cattle, for example, the entire spermatogenesis process lasts an average of 61 days, divided into three phases (33). The initial phase, known as spermatocytogenesis, is the process by which germ cells undergo mitotic divisions, and after the first division there are germ stem cells (type A spermatogonia) and primary spermatocytes (from type B spermatogonia). To give rise to primary spermatocytes, the mitotic division of A1 spermatogonia into differentiated cells called A2, A3, intermediate, B1 and B2 spermatogonia must occur (88). During the second phase, there is a reduction in the number of chromosomes, originating a haploid cell, carrying out the recombination and segregation of the genetic material. In this way, the primary spermatocytes resulting from the first phase begin DNA replication, later entering the first meiotic division to produce spermatocytes. Secondary, such spermatocytes rapidly enter the second meiotic division resulting in rounded haploid spermatids (89, 90).
During the third phase, called spermiogenesis, no more cell division phases occur, so during this phase, spermatids suffer morphological changes, differentiating into spermatozoa (91). These changes include the formation and development of the acrosome and flagellum, chromatin condensation, remodeling, and elongation of the nucleus. Besides that, there is a cytoplasm removal, which occurs before the spermatid release during the spermiation, a phase characterized for the spermatozoa releasing into the seminiferous tubules lumen (92, 93). However, these spermatozoa do not have the ability to fertilize the oocyte, and it is necessary for these spermatozoa to pass into the straight tubules, reach the rete testis and reach the epididymis, where they start the maturation process (94, 95). Thus, in bulls the mitotic division phase lasts 21 days, the meiotic division phase 23 days and spermiogenesis 17 days, resulting in 61 days of spermatogenesis (88), and in humans and rats, the spermatogenesis process lasts 74 and 35 days, respectively (96, 97).
Spermatogenesis is also regulated by endocrine factors depending on the activity of the hypothalamic-pituitary-testicular axis, whereby Gonadotropin Releasing Hormone (GnRH) stimulates the anterior pituitary to release Luteinizing Hormone (LH) and Follicle Stimulating Hormone (FSH), that will produce stimuli to produce gonadal steroids and for the development of germ cells (98, 99). LH hormone receptors (LHR) stimulate the process of steroidogenesis and act during the development and maintenance of spermatogenesis (100), their expression takes place in Leydig cells and are essential for fertility in mammals (68).
During spermatogenesis, three hormones are essential for the process to occur without modification, namely testosterone, FSH and LH. The lack of any of these hormones cause germ cell apoptosis, and when administered, these hormones suppress apoptosis (101). Therefore, germ cells require the presence of these hormones for their survival. When there is a lack of testosterone, round spermatids do not complete the transition phase to elongated spermatids, as there is a loss of spermatids binding to Sertoli cells (102). FSH acts indirectly through Sertoli cells, being associated with early stages of spermatogenesis, especially during spermatocytogenesis and meiosis (103). On the other hand, testosterone is present in the later stages of spermatogenesis, such as in the spermatid differentiation stage and potentiating the effect of FSH (104).
In cattle, Leydig cells acquire the ability to respond to the LH stimulus at puberty (105), which begin to produce increasing amounts of testosterone, exerting control over Sertoli cell differentiation and, consequently, cell growth (101). Therefore, any interruption or alteration that occurs during the spermatogenesis process can generate changes in the pattern of cell development, affecting the reproductive capacity of animals (106). Testicular hormone regulation is summarized in Table 2.
3 Current strategies to reestablish testicular functionality
3.1 Testicular tissue cryopreservation
Testicular tissue cryopreservation is one of the alternatives to preserve human and animal fertility. Protection of male fertility can be performed by several techniques such as progenitor cells cryopreservation, testicular stem cells cryopreservation (spermatogonic stem cells or SSCs) or cryopreservation of testicular tissue fragments (107). The freezing of semen is the standard and most used technique for the preservation of male fertility in men and animals, being routinely performed in clinics and farms (108, 109). However, this technique is unfeasible when dealing with juvenile and prepubertal individuals, whose gonads have not yet started to produce sperm, or in adults in which mature sperm is not produced due to the occurrence of pathological and genetic disorders (110).
In such cases, testicular tissue cryopreservation is one of the viable alternatives (111, 112). The testis has a high number of germ cells, especially spermatogenic cells, which can offer an unlimited number of male gametes, when properly cultivated and preserved (33). This technique is used as treatment for several types of cancers, which mainly affect the spermatogenesis niche, and induce the death of spermatogonial stem cells (SSCs), reducing the sperm count in men (82). In addition to that, aiming to seek endangered species conservation, several studies have been carried out using this technique to try to preserve male reproductive tissue samples to further uses (32).
Studies have demonstrated that methods of collection and preservation of testis from sexually immature individuals, and from adult animals, alive or postmortem (113, 114). After immediate tissue recovery or cryopreservation, the fragments can be cultured in vivo or in vitro to obtain viable sperm (115). That is, the cryopreservation of testicular tissue fragments is used to preserve the fertility of prematurely dead animals, as well as those undergoing treatments that cause infertility, such as cancer treatment. The spermatogenic cells, present in the testicular fragments, can resume their functions in vitro after thawing, the genetic resources of high-value animals and the preservation of endangered species (116, 117).
Studies using both animal and human tissues generally advocate a DMSO-based cryopreservation medium for immature tissue cryopreservation and a glycerol-based medium for mature testicular tissues (35, 112, 118). Testicular tissue cryopreservation can be performed using techniques such as slow freezing, fast freezing, and vitrification; however, protocols for using these techniques are still being tested in different species (112, 119, 120).
Slow freezing is performed using a machine that gradually reduces the temperature until the frozen state of the testicular fragments is reached. The fragments are initially exposed to an equilibrium solution containing cryoprotective agent (CPAs) at 4° for 10 to 15 minutes, after which they are transferred to cryogenic flasks and stored in liquid nitrogen (121). This method allows the tissue to be less exposed to the deleterious effects of CPAs, however there is a high possibility of crystal formation during the process, which may invalidate the use of tissue after thawing (122). It is mostly used in immature human tissues and is associated with the survival of spermatogonia (35).
Vitrification is a technique widely used in the cryopreservation of female gonadal tissue, and in the research of this technique for the cryopreservation of testicular tissue, the technique used was solid surface vitrification. This technique consists of exposing the fragments to a vitrification solution, after which the fragments are placed in a metal cube above the liquid nitrogen, which allows the tissue to be cooled in an ultra-rapid way and, after freezing, they are stored in cryotubes and maintained in liquid nitrogen (123). Among the cryopreservation techniques, the most common methods are slow freezing and vitrification. Vitrification has the lowest operating cost and is easy to perform, in addition to avoiding crystallization in a more effective way than the other techniques due to the ultra-fast cooling (124).
During the freezing and thawing processes of the material, the loss of spermatogonia is inevitable, and to improve cell survival, cryoinjury caused by the formation of intracellular ice crystals must be avoided through the addition of cryoprotective agent and the control of freezing and thawing rates. Testicular cryopreservation is an economical and efficient method to preserve genetic material; however, its techniques are still being tested and improved, so there are several approaches that involve numerous positive and negative points to be improved. The procedure involves invasive surgery, therefore, it is extremely important to select patients, so that those who undergo testicular tissue cryopreservation are more likely to benefit from future applications, in addition, patients who need gonadotoxic therapy are at an additional risk of bleeding and infection, particularly those with hematologic disorders such as leukemia or aplastic anemia (118, 125–127).
3.2 Sperm cryopreservation
Sperm cryopreservation is an effective method used in the management and preservation of fertility of animals and humans through assisted reproduction techniques (ART) (128, 129). This technique is based on the freezing of sperm to maintain its viability and functionality, and when performed correctly, it allows long-term freezing, as it results in the arrest of cellular metabolism that prevents cellular aging, maintaining viability and fertilization potential, an essential part for ART (130). The preservation of spermatozoa by freezing has the first record in 1776, but it was only in 1949 that the cryopreservation technique had its scientific progress with the discovery of the cryoprotective properties of glycerol (131), this advance being a point of departure within the field of fertility preservation (132). From this advance, there were significant improvements in the cryopreservation of semen of several species (133) with the creation of sperm cryobanks that took place during the 1960s for bovine species and in the 1970s for humans, the constitution of genetic resource banks began (134).
There are several conventional methods available for cryopreservation of human and animal semen: slow, fast and ultra-rapid freezing (known as kinetic vitrification) (135). The slow freezing method consists of progressive cooling divided into two or three stages over a period of 2 to 4 hours (136). In the first stage, sperm collected by ejaculation or other techniques is kept at room temperature for 10 minutes (137). In the second step, there is a gradual cooling of the samples from a temperature of 20°C to 5°C with a cooling rate of 0.5-1°C/min. After reaching a temperature of 5°C the samples are cooled again from 5°C to -80°C at a rate of 1-10°C/min, and finally, in the third step, the samples are frozen in liquid nitrogen (138). However, slow freezing leads to the formation of ice crystals resulting in high concentrations of electrolytes inside the cell, causing physicochemical damage to spermatozoa (139).
The rapid freezing method is based on the direct contact of samples with liquid nitrogen vapor for at least 10 minutes, in this method the sperm are mixed with cryoprotective agent and placed in cryotubes that will be exposed to nitrogen vapors. After the vapor exposure phase; the samples are immersed in liquid nitrogen (140, 141). The addition of CPAs to the samples seeks to minimize osmotic damage and prevent intracellular and extracellular ice crystals from forming, however, CPAs have cytotoxic characteristics (142).
Vitrification is the process of solidifying a liquid substance at extremely high freezing rates, transforming the liquid sample into an amorphous solid state (143). This freezing process prevents the formation of ice crystals (144). In addition, unlike the slow and fast methods, during vitrification, CPAs use is eliminated, as this method is cryoprotectant free (145). When used as a cryopreservation method, vitrification is commonly used for oocytes and embryos (146), since sperm vitrification is still a challenge due to the greater osmotic fragility of sperm when compared to other reproductive tissues (147).
Sperm cryopreservation is a tool for fertility preservation, sought by men who wish to start ARTs, in some cases being the only opportunity for couples to have children in the future (148). In addition, it is considered before starting any medical procedure that may affect male fertility, as in the case of non-malignant and malignant diseases, where it is necessary to submit the patient to chemotherapy, local radiology or radical testicular surgery (138). This technique is also recommended for men who have had a vasectomy. More recently, transgender patients, who chose to save their gametes for later use (149), have used cryopreservation. When used in animal species, cryopreservation is used for artificial insemination by making use of frozen and thawed sperm to improve rates of genetic improvement (150). It is considered a valuable tool, as it allows the preservation of genetic material from endangered species, through the storage of these gametes in cryobanks (5, 151).
Despite the high success rates in fertilization using cryopreserved semen, the technique has limitations such as intracellular and extracellular ice formation, osmotic and oxidative stress and toxicity from the use of cryoprotectants. These factors are responsible for cellular damage in the cryopreservation and thawing process (93, 152). Damage occurs to a greater degree during thawing, causing an imbalance in reactive oxygen species that directly affects cell metabolism and signaling, as well as DNA integrity and plasma membrane function and integrity (153). Recent studies point out that non-coding RNA (ncRNA), chromatin remodeling, DNA methylation and post-translational histone modifications are among the epigenetic factors involved in gene expression that are affected by cryopreservation and the thawing process (21). Sperm motility is the morphological parameter most affected by cryopreservation and thawing, caused by mitochondrial damage, sperm tail deformities and sperm membrane alterations (140).
3.3 Spermatogonial stem cell transplantation
Germ cell transplantation is an innovative technique that began to be used in 1994. The technique consists of isolating spermatogonial stem cells (SSCs) from a donor animal of interest and transplanting these cells into the testis of the recipient animal. After transplantation, the transplanted germ cells will continue their development and form mature and fertile sperm bearing the genetic characteristics of the donor animal (154). In recent years, this technique has been applied to mammalian species, in order to understand the processes of spermatogenesis and the biological characteristics of stem cells (155). In addition to these applications, the technique has a high potential for use in research related to biotechnology, genetically modified animals, and preservation of genetic material from endangered species or animals of high economic interest (38).
The use of this technique demonstrated for the first time that germ cells could be transferred between species and between animals of the same species (65). When microinjection of donor germ cells is performed in the seminiferous tubules of infertile recipients, a part of the donor germ cells moves to the periphery of the seminiferous tubules, moving through the Sertoli cell junctions. Due to the absence of spermatogenesis in recipient males, donated germ cells can penetrate the epithelial layer of Sertoli cells and reach the basal lamina (84). Considering the importance of understanding the steps involved during the in vitro spermatogenesis process and seeking to establish a favorable environment for the development and maturation of SSCs, further studies are still needed to prove the efficiency of SSCT in animals of the same species and between animals of different species.
3.4 Testicular graft
Testicular tissue grafting is a technique that has been studied to restore fertility. When compared to other restoration methods, grafting has several advantages such as the SSCs remaining within their microenvironment (avoiding the need for isolation and cell expansion in vitro) and providing an in vivo environment for the complete proliferation, differentiation, and maturation of germ cells (156). The complete process of spermatogenesis by the testicular tissue graft technique, that is, by allografts and xenografts, showed promising results in non-human species such as mice (157), hamster (158, 159); rabbit (160); bovine (161); rhesus monkey (162); horse (163); cat (164); dog (119) and buffalo (165).
In 2019, the first female offspring were born using sperm from grafts from prepubertal rhesus macaques (32). Despite the great advance in non-human species, in humans, xenotransplantation of immature testicular tissue (ITT) with spermatogonial cells was not able to carry out complete spermatogenesis, not producing sperm (166). Autologous grafts in humans have not yet been reported, therefore, data on testicular tissue autotransplantation in animal species provide important knowledge for the future application of this technique in human fertility (167).
As with all reproductive technologies, testicular grafting also has disadvantages, for patients diagnosed with neoplasms, autograft presents the risk of reintroduction of residual malignant cells present in cryopreserved ITT fragments (168). Therefore, continuous research to overcome the limitations of testicular grafts and provide information for application in humans is essential so that in the future this technique provides chances of success for patients to have biological children (169).
4 Discussion
4.1 Bioengineering principles applied to reproduction
Conventional treatments for of male infertility and subfertility are still not effective related to problems associated to spermatogenesis disorders (170). Several testicular cell culture systems have been studied to mimetize the testicular microenvironment and restore fertility. An emerging field is reproductive tissue engineering (RTE), which is based on the same principles applied for vital organs as the heart, lungs, kidneys, liver, and skin (171–173). However, RTE must, in addition to reestablishing tissue integrity, generate a suitable microenvironment for the development of germ cells (174). Male reproductive tissues are under daily endocrine stimulation, with the rise and fall of testosterone, present a complex microarchitecture that changes due to intense hormonal flow (175). Aiming to mimetize these elements and testicular ECM, several biotechnological tools have been used to reconstruct testicular tissue and carry out the process of spermatogenesis in vitro (173) (Figure 3).
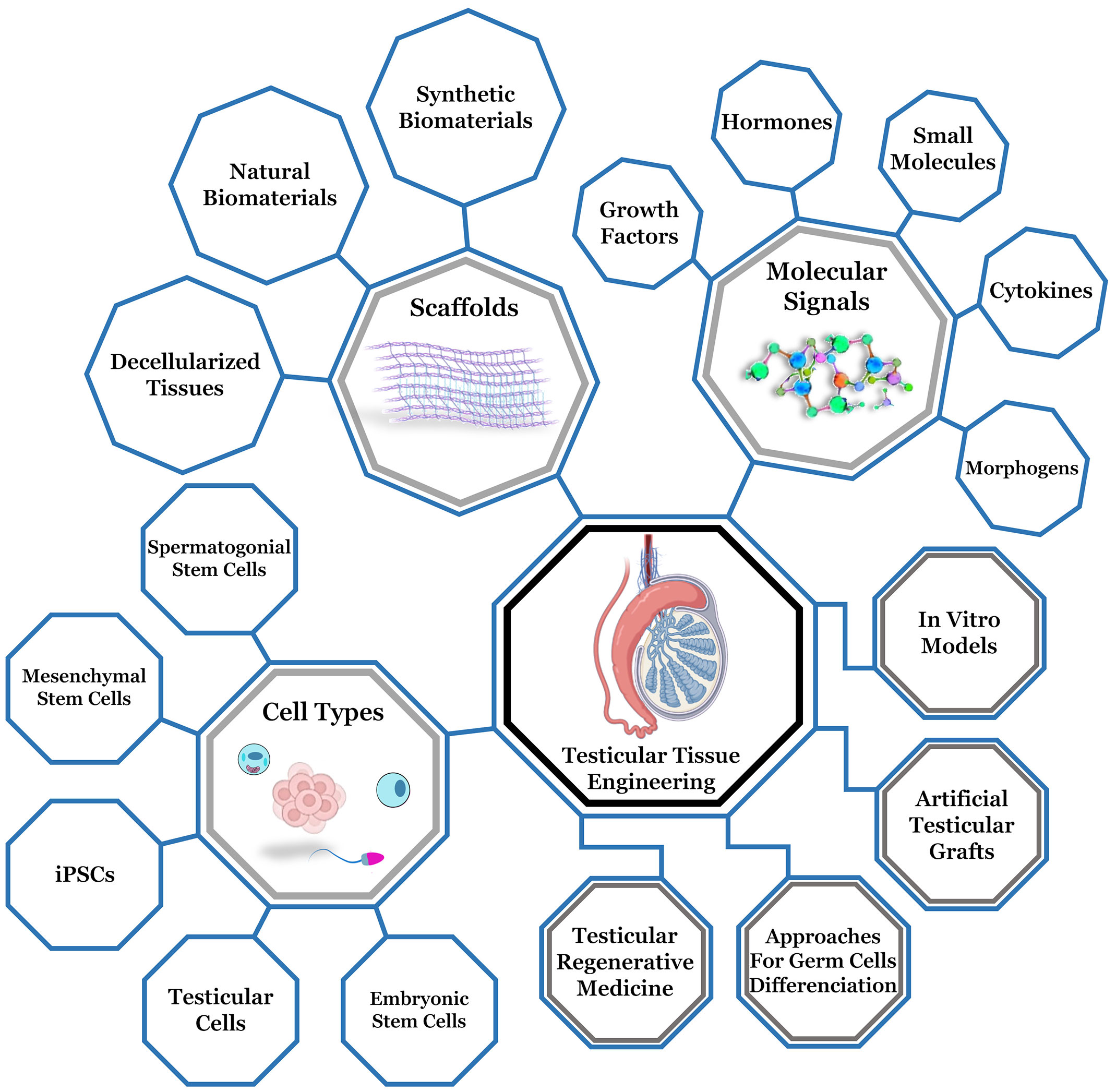
Figure 3 Representative diagram of testicular tissue engineering, highlighting the main components for testicular microenvironment reconstruction (biomaterials, cell types and molecular stimulation) and the main branches (testicular regenerative medicine, approaches for germ cells differentiation, development of artifical testicular grafts and production of in vitro models). Adapted from Biorender .
The understanding of the mechanisms involved during reproductive system development and how reproductive diseases occur has been of great value to develop in vitro models of reproductive tissues (41, 176). The advance of bioengineering has allowed the study of the male reproductive system in an innovative way by introducing new biomaterials that simulate organs and tissues that make up this system (177), by making bioprinted models (178–180). The microfluids (181) and biogels (58) production also may assist on cell development. The insertion of three-dimensional cell culture methods (182), maintaining physical and biochemical contact between cells and the tissue architecture brought the in vitro reality even closer to in vivo.
Studies report that cell-cell interaction in 3D culture systems influences the regulation and maturation of germ cells (183–186). 3D cultures favor the bidirectional communication between spermatozoa and the somatic cells that surround them, which are required for a proper testicular functioning and development (1, 187).
One of the limitations of in vitro spermatogenesis biotechnologies is to mimitize the testicular tissue microenvironment in vitro, as the interaction of gametes with the extracellular environment provides the necessary conditions to remain viable (188, 189). In search of alternatives to reproduce physiological conditions in vivo in the laboratory environment, tissue engineering approaches have been used to produce biomaterials that can reconstruct the structure of testicular tissue or assist in the process of spermatogenesis in vitro (190). Natural polymers such as collagen, fibrin and alginate have been explored because they are biomaterials with known biocompatibility and biodegradability (191). Synthetic polymers have also been studied to recompose the structural substrate for the maintenance of spermatogenesis, such as polyethylene glycol (PEG) and poly (epsilon-caprolactone) (PCL) (180, 192). Such materials can be chemically altered to adapt to certain conditions, however, as they are of synthetic origin, there are limitations in more accurately mimicking the extracellular environment (188).
Therefore, several synthetic and natural materials have been developed as extracellular matrix scaffolds, biogels, biodegradable polymers of gelatin, fibrin, collagen, hyaluronic acid, and poly (lactic-co-glycolic acid) that are widely used in tissue engineering (174, 193, 194).
4.1.1 Classification of biomaterials in male reproductive tissue engineering
Biomaterials applied for reproductive tissue engineering can be divided in synthetic polymers, natural polymers (scaffolds and biogels) and decellularized matrixes. The main experimental approaches in synthetic and natural polymers are summarized in Table 3. Some studies aimed to obtain efficient decellularized tissues to be used as three- dimensional platforms to reconstruct an in vitro testicular microenvironment, which may allow several applications in reproduction medicine (Table 4).
4.1.1.1 Synthetic polymers in testicular bioengineering
The use of synthetic polymers in tissue bioengineering is indicated because such polymers are biocompatible, have suitable physical-mechanical properties and elasticity to produce scaffolds. Among the synthetic materials used for the composition of scaffolds, there are the poly (L-lactic acid) (PLLA) (195, 213, 214), polypropylene, poly D, polycaprolactone (PCL) (215) and L-lactic-co-glycolic acid (208), which have already been used in the production of bone (227), cartilaginous (228), vascular (229) and dermal (230) scaffolds. Synthetic polymers can be combined with natural polymers, which improves their mechanical, physical and biocompatibility qualities.
However, even with favorable characteristics, few studies use synthetic polymers in male reproductive bioengineering. Among the studies found, Lee et al. (2011) (208) evaluated the ability of immature murine testicular cells to perform spermatogenesis in vitro when cultivated in biodegradable microporous scaffolds based on poly (D,L-lactic-co-glycolic acid) (PLGA). The results demonstrated that PLGA scaffold appears to provide a favorable microenvironment for spermatogenic germ cells to proliferate and differentiate into mature spermatids.
In the study of in vitro spermatogenesis, Ghorbani et al. (2019) (212) and Tseng et al. (2022) (214) used PLLA to produce scaffolds that were later cultured with spermatogonial cells. The results found showed that the use of PLLA in the manufacture of scaffolds could create a microenvironment like the native testis that promotes the growth and differentiation of spermatogonial stem cells.
The synthetic polymer polycaprolactone (PCL) was also used to study the proliferation and differentiation of spermatogonial stem cells, and the scaffolds produced from PCL were able to promote the expansion and differentiation of SSCs into spermatids (179). Thus, further studies are required to evaluate the advantages of synthetic polymers for the development of spermatogenesis in vitro models, since the results already obtained demonstrate that hydrogels based on synthetic polymers provide a favorable environment for germ cells to proliferate and differentiate into mature spermatids.
4.1.1.2 Natural biomaterials for testicular bioengineering
Natural polymers are often used in tissue engineering because they have functional properties like those of natural ECM, presenting characteristics that assist on cell behavior, cell adhesion, migration, and differentiation (231). Unlike synthetic polymers, natural polymers are more biodegradable and biocompatible and are widely used in male reproductive tissue engineering (232).
The main natural polymers used are alginate (211, 216), fibrin (201, 204), collagen (196, 233), Matrigel (203, 209, 210, 234), agarose (200, 205–207, 235) that are used as scaffolds to build a testicular microenvironment in vitro. The use of hydrogels has been the main method to reconstruct a three-dimensional testicular microenvironment, as it increases the contact surface between the material and the cells (236).
Several studies have used alginate for encapsulation of spermatogonial stem cells due to its cell compatibility, gelling property, biodegradability, and mechanical strength (237). When investigating the biocompatibility of alginate in the encapsulation of mouse spermatogonial stem cells, Jalayeri et al. (2017) (238) found that alginate hydrogel is a non-toxic compound that does not affect the viability and morphology of stem cells and can be used in the encapsulation of spermatogonial stem cells. Veisi et al. (2022) (239) used co-cultured spermatogonial stem cells encapsulated in alginate hydrogel with Sertoli cells and found that culturing SSCs in alginate hydrogel with Sertoli cells in a 3D culture can lead to efficient proliferation and maintenance of SSC and increase the efficiency of SSC transplantation. Poels et al. (2016) (201) evaluated two different compositions of hydrogels, one made of 1% alginate and the other made of fibrin (30 mg/mL fibrinogen/30 IU/mL thrombin), the results demonstrated an improvement in the survival of the spermatogonial subpopulation with the use of alginate matrix compared to fibrin gel.
Some studies have used fibrin as a scaffold for testicular reconstruction since this polymer assisted on wound healing and tissue regeneration by having bioactive factors such as fibronectin that act as a substrate for cell migration and anchorage (240). Ramzgouyan et al. (2015) (241) were able to differentiate germ cell-like cells on fibrin hydrogel, demonstrating the biocompatibility of the differentiated cells to the hydrogel. Although little studied, fibrin scaffolds have high porosity and biocompatibility, characteristics that can be used for the development of seminiferous tubule niches in testicular tissue (170).
Hydrogels based on collagen fibers have been used to develop methodologies for the cultivation and differentiation of male germ cells (198, 199, 242, 243). Studies using 3D structures based on collagen hydrogels report promising results regarding differentiation (242), maturation (198), maintenance of testicular cell viability (244) and provided support for in vitro spermatogenesis (199). The differentiation of spermatogonial cells in culture medium supplemented with collagen-based hydrogel is also pointed out (243).
Used as a support for the development of germ cells in vitro, Matrigel is a natural polymer derived from ECM, having several essential components such as laminin, collagen, and fibronectin. Its use in the cultivation of cells and tissues in vitro is due to its promoting effects on cell growth and differentiation (174). Matrigel has been used to differentiate functional haploid spermatids in previous studies. Sun et al. (2018) (234) reported that a three-dimensional induced system with Matrigel differentiated human SSCs into functional haploid spermatids. To evaluate differentiation, cell content and meiotic chromatin scattering assays were performed, which revealed that spermatocytes and haploid cells were effectively generated from human SSCs by the three-dimensional induced system with Matrigel. Another study reported the creation of a three-layer gradient system being one layer of Matrigel with testicular cells in the middle of two layers of Matrigel. The results demonstrated that testicular cells migrated within the Matrigel, forming testicular organoids with growing germ cells and presented a functional blood-testis barrier (245).
4.1.1.3 Decellularized ECM for testicular microenvironment reconstruction
Biological scaffolds from the decellularization process must present some characteristics to be considered ideal, such as: absence of toxicity, non-immunogenicity, non-pathogenicity and be biodegradable to allow cell adhesion and provide appropriate conditions for the creation of a biological scaffold microenvironment that can carry out cell growth, proliferation, and migration (77, 222). After the decellularization process, the bioactivity of growth factors present in acellular scaffolds such as VEGF, TGF-β and bFGF remains unchanged (246).
Several decellularization protocols reported range from chemical to enzymatic methods, depending on the type of tissue and its biological properties (220, 221). Obtaining decellularized scaffolds is commonly done by the agitation technique, however, recent studies have been using the technique of perfusion of cellular detergents via arterial route to break cellular bonds with the ECM and dissolve cellular materials and their debris from within the tissue or organ (225, 247). Among the most used detergents and solutions are sodium dodecyl sulfate (SDS), ethylenediaminetetraacetic acid (EDTA), sodium hypochlorite and Triton (X-100) (58).
It is known that ECM acts in the spermatogenesis process, through laminin and collagens that allow the differentiation of germ cells, promoting their change from the basal lamina to the lumen of the seminiferous tubules, through adjustments of the structural junction (66). Thus, the creation of a testicular scaffold from ECM that can support testicular cells may provide new information about the fundamental cell-matrix interactions that occur during spermatogenesis (64).
The interaction of male gametes with the microenvironment is essential for sperm development in testicular tissue (188, 218). The extracellular matrix is a major player in this process, as the communication and molecular signaling of the components of the ECM with the spermatozoon not only provides structural support to the gamete, but also plays an active role in maintaining its viability, as well as in the transport and distribution of essential substances to the gamete (248).
Decellularized extracellular matrix scaffolds can be biotransformed into biogels through solubilization followed by neutralization and gelation (249). Although the production of biogels from decellularized ECM scaffolds alters the three-dimensional conformation of the matrix, biogels can retain bioactive factors present in the ECM of native tissues, preserving the ability to guide and favor specific cellular behaviors through orientation by contact, and by fibrillation of proteins that interact with integrin receptors on the cell membrane (250, 251). In relation to scaffolds, biogels have some advantages, as they require the use of less invasive procedures for their deposition at the target site and facilitate the repair of irregular tissue surfaces due to their viscoelasticity, provided by collagen and other biomolecules that compose them (252). Thus, in addition to the new possibilities of cell culture to improve in vitro spermatogenesis rates, bioengineering proposes innovative methods of treatment for diseases that affect the reproductive system and the resumption of fertility, such as the application of decellularized matrix scaffolds or biogels (217, 253).
The reciprocal interactions performed by Sertoli cells, Leydig cells, germ cells, testicular endothelial cells, peritubular myoid cells and macrophages in the microenvironment in which they are found are of fundamental importance for the proper process of spermatogenesis (254). Various components of this microenvironment (e.g., growth factors, cytokines, hormones, and adjacent cells) and the way they orchestrate cellular development have been the subject of a lot of research over the last few years (255). On the other hand, the extracellular matrix that composes these tissues remained little explored, being recognized only as a passive component of cellular anchorage (256). With the discovery of the physicochemical properties of the extracellular matrix (ECM) of various organs, the hypothesis that this component plays the role of a simple cellular framework was abandoned, going from a mere protagonist to an active modulator of cellular functions that govern morphogenesis and tissue repair (257).
4.2 Techniques designed to preserve male fertility
4.2.1 Application of hydrogels in restoring male fertility
Providing a transitory tissue-mimicking environment for cell proliferation and differentiation to occur, hydrogels have high structural similarity to ECM, being used to encapsulate cells and tissues within reproductive bioengineering. Its composition is mostly water (90%), allowing the diffusion of nutrients, providing physical support for the cells (173). Recently, several studied applied hydrogels in testicular tissue and in cell culture focusing on the differentiation of spermatogonial stem cells into haploid spermatozoa (258). Hydrogels can be produced from natural and synthetic polymers; however, hydrogels from natural polymers are more used for their non-toxic properties and biocompatibility (259). As they have biodegradable and bioresorbable properties, these materials will provide functional 3D matrices for cells and tissues without inducing inflammation (260). Considering that the ECM is mainly composed of proteins and polysaccharides (257), two groups of polymers have been used for the spatial arrangement of testicular cells: proteins as collagen and polysaccharides such as chitosan and alginate (197).
As the most abundant structural protein, collagen is the most investigated polymer in the production of hydrogels to restore male fertility (199). Hydrogels produced from collagen provide a favorable environment for the differentiation and maturation of germ cells, offering a niche for the reassembly of testicular cells isolated from animals and humans (261). The use of collagen hydrogels alone or in combination with Matrigel allows germ cells to be in contact and actively interact with somatic cells and the ECM (262, 263). In a study by Lee et al. (2006) (242), rat testicular cells were cultured in collagen gel and collagen gel + Matrigel. The results showed that the matrices used showed the potential for reassembly of dissociated cells, provided meiotic support, post-meiotic progression and differentiated male germ cells.
Hydrogels are also being used to encapsulate spermatogonial stem cells during the cryopreservation process. Pirnia et al. (2017) (202) used alginate-based hydrogel for encapsulation of mouse spermatogonial stem cells during the cryopreservation process. The group performed a comparison of the colonization potential and degree of viability of SSCs before and after the freeze-thaw cycles. The results demonstrated that there were no differences in the freeze-thaw cycles, and after thawing there was a successful restoration of spermatogenesis.
4.2.2 Acellular ECM scaffolds
Biological scaffolds are generated by the process of tissue decellularization, which can occur both in the entire organ and in part of it (226, 264). These scaffolds act as the architecture for tissue formation and are normally seeded with cells and, occasionally, growth factors, or subjected to biophysical stimuli provided by a bioreactor, which consists of a device or system that applies different types of mechanical and chemical stimuli on the cell culture (265).
Therefore, the material that was previously decellularized is considered and used as a biological scaffold. After being inserted in vivo, the scaffolds generated by tissue decellularization, have properties similar to those of physiological tissues and provide the structural basis for aggregation to adjacent tissues (225, 266).
Thus, the development of biological and biocompatible scaffolds can present benefits for in vitro germ cell culture systems, recently, the use of these scaffolds for the in vitro spermatogenesis process has been considered promising (62, 224). The extracellular matrix is a tool to study disorders that affect spermatogenesis. Several biomaterials and scaffold manufacturing methods have been investigated for application in testicular tissue engineering (267). The development of scaffolds from biomaterials reduces problems associated with post-transplant complications, such as tissue deficiency and the use of immunosuppressive drugs.
The scaffold also provides a basis for performing tissue recellularization by culturing isolated cells in vitro or in the host in vivo by cell migration (223). Several types of stem cells can be used in the differentiation of germ cells, especially in cases of disturbances in spermatogonia (60).
Based on these characteristics, research has focused on optimizing protocols for decellularization of male reproductive tissues to obtain a scaffold that presents a three-dimensional structure that conforms to the components of the matrix preserved, thus enabling good development, adhesion, and cell proliferation in modalities of 3D cultivation (219, 262). Among the protocols that have been developed, the use of SDS and Triton X-100 at a concentration of 0.01% in the decellularization of immature swine testis fragments supported the cultivation of Sertoli cells. The data obtained showed that the scaffolds produced by the combination of two cellular detergents in low concentration preserved the functionality of vital testicular cells such as Leydig, peritubular, myoid cells and SSCs (221). Another study performed the cultivation of human Sertoli cells in decellularized ECM from porcine testis, pointing out that the use of decellularized porcine testis or testicular ECM from other animal species is viable in testicular bioengineering (60).
Recently, Vermeulen et al. (2019) (43) organized testicular organoids were generated in decellularized ECM-based hydrogels to restore male fertility. The system had an appropriate storage module (capacity to store energy in the elastic deformation of the material) for the porcine testicular organoid culture. The scaffold produced was able to form structures of the seminiferous tubule and showed that there was a preservation of growth factors within the organoids in addition to presenting regenerative capacity.
4.2.3 Approaches without using scaffolds
Traditionally tissue engineering involves a scaffold, bioactive factors, and cells. Materials used as scaffolds affect cell behavior, influence in their growth, proliferation, and differentiation (268). Despite this great stimulation, these approaches require complex and expensive techniques. As an alternative, scaffold-free methods have emerged. Through cell self-assembly technique, scaffold-free methods generate 3D multicellular aggregates that secrete their own matrices (269).
In vitro models of human testicular organoids that perform characteristic functions of testis have already been reported (270). However, morphologically there is no similarity of these organoids with testicular tissue. The models that most efficiently mimetize the testicular architecture is the suspension culture models that allow the expansion of germ cells and the incorporation of somatic cells. Pendergraft et al. (2017) (270), in non-human primates, did not achieve the progression of spermatogenesis, only the expansion of spermatogonia, raising the hypothesis that the addition of factors such as physiological microenvironments is necessary.
Soluble human testis ECM have been employed as an additive medium for the cultivation of human testicular organoids to mimetize the testicular microenvironment without providing a structural scaffold (271).
4.2.4 Microfluidic systems
In experiments using human materials, there are ethical and experimental limitations such as low availability of resources and difficulty in long-term in vivo maintenance of artificially produced tissues and organs. These limitations make in vitro human fertility research challenging. As an alternative to overcome such limitations, micro/nanofabrication techniques are being used, one of these techniques are microfluidics, which has the potential to promote a significant increase in techniques used in male reproduction clinics (272). The microfluidics technique is defined as the technology that designs, manufactures, and models devices for handling and analyzing small amounts of fluids (273).
With the use of microfluidic systems, male fertility restoration researchers can remove barriers that limit the results obtained, such as testicular cell death, limitation of primary testicular cells and the absence of new tools that can mimic the complexity of testicular tissue (99). Komeya et al. (2019) (274) cultured testicular tissue fragments from neonatal mice in a simple microfluidic device. The system was manufactured in a simple way and managed to maintain endocrine functions and spermatogenesis for 6 months. The microfluidic device used separated the testicular tissues and the fluid medium through a thin porous membrane while the culture medium flowed in channels that mimicked the capillaries.
In another experiment Kojima et al. (2018) (275), seeded neonatal mouse testis in agarose gel molded on a microfluidic chip. The polydimethylsiloxane (PDMS) present on the surface of the hydrogel showed high oxygen permeability and was able to support the transport of oxygen to the tissue layers, thus preventing central tissue necrosis and increasing cell growth during the 7 days of the experiment. In 2019, the group of Komeya et al. (2019) (274) carried out a follow-up work on the study cited above, where they placed the testicular tissues of immature mice in agarose gel blocks and forced the spread in monolayer through a microfluidic ceiling system. As a result, they observed that, when in the presence of the PMDS microfluidic device chip, the spermatogenesis process was initiated and maintained, followed by the increase in meiotic germ cells, the findings also demonstrated that the spermatogenesis process was able to differentiate the cells in the microfluidic system until the stage of rounded/elongated spermatids.
4.2.5 Bioprinting and 3D printed scaffolds
Through 3D printing, the fabrication of materials used in reproductive tissue bioengineering occurs with precision of the spatial geometry and internal microarchitecture of the pores, enabling the creation of a personalized biomimetic environment (175). In this way, this technology allows the integration of several biomaterials and multiple cell varieties, generating a 3D biomimetic structure. In male reproductive biotechnology, 3D-printed alginate scaffolds have been studied for the generation of organoids, however, a biomimetic morphology like the native testis was not observed (216).
3D bioprinting consists of the deposition of biocompatible materials, cell types and support components by computer generating complex 3D tissues. For the manufacture of 3D scaffolds, several layers of biological materials, biochemical compounds and cells are printed in sequence, making it possible to spatially control the positioning of the components used in bioprinting (276). Research with the application of 3D bioprinted materials is in early stages, however clinical applications with the generation and transplantation of bones and skin have already been reported (277).
It is essential to obtain a 3D structure that can be applied in bioengineering that the mechanical, structural, and functional components present characteristics like tissues in vivo (278). Therefore, the chosen biomaterials should always be chosen considering biocompatibility, easy handling, easy printing and maintenance of cell viability and function (279). However, some challenges are faced during 3D bioprinting such as the correct choice of design, as well as the choice of materials, cell types and growth and differentiation factors (269).
Baert et al. (2019) (216) performed the 3D bioprinting of alginate-based scaffolds for the study of spermatogenesis in vitro. After the bioprinting of the scaffolds manufactured, the authors carried out the cultivation in the scaffolds with testicular cells and observed that at the end of the days of cultivation there was a differentiation to the level of round spermatids and elongated spermatids, suggesting that the model created may be useful for studies (duck) of physiological and drug screening applications. Another study that used 3D bioprinting for in vitro production of spermatozoa was by Bashiri et al., 2022 (179). During the experiment, decellularized extracellular matrix of ram testis was used in 3D bioprinting, and these scaffolds were later manufactured and cultivated with spermatogonial stem cells. The results showed that 3D-printed scaffolds derived from decellularized extracellular matrix increased the viability and proliferation of spermatogonial stem cells, through the release of growth factors.
5 Future perspectives
When performed in vivo, spermatogenesis is a complex process being controlled by the endocrine system and totally dependent on the testicular microenvironment. Looking for solutions for the restoration of male fertility, reproductive bioengineering has been developing and introducing into research various types of biomaterials, whether synthetic, natural or from extracellular matrix. The projection of biomaterials for studies of infertility and recovery of male fertility is important since the number of cases of male infertility in humans and animals is increasing. Due to the structural and functional complexity of the testis that require specific hormones to carry out spermatogenesis, biomaterials that can promote structural regeneration and functional recovery of male gonads have not yet been developed. Currently, these biomaterials present a great potential as a tool for male reproductive medicine in applications such as screening assays in drug development and toxicology due to their adaptable and scalable resources. These new approaches may become more viable and efficient to be used as alternatives for male fertility preservation and restoration in comparison to other systems as in vitro spermatogenesis, culture of organotypic fragments. Seeking to overcome the challenges of spermatogenesis in vitro and in vivo, several advanced technologies are being used in the biomanufacturing of biomaterials for use in reproductive bioengineering. Among the techniques being designed to preserve male fertility are hydrogels, decellularized extracellular matrices, techniques without the use of scaffolds, microfluidic systems and 3D scaffold bioprinting. 3D bioprinting with the printing of cells on the scaffolds produced is a technique that has been growing within the field of bioengineering, with the advantage of controlling the spatial deposition of biomaterials and cell types. In addition, the application of microfluidic devices, chip platforms and other technologies have been employed in the construction of reproductive organoids. In future studies, such technologies can be combined with biomaterials that are being manufactured to mimic the testicular microenvironment.
Understanding the influence that the extracellular matrix performs on the testicular microenvironment makes it possible to choose the best biomaterial to be used for testicular regeneration and in the study of spermatogenesis in vitro. Thus, the use of biomaterials discussed in testicular bioengineering can still replace the requirement for experimental animals in in vitro spermatogenesis research, providing biomaterials that mimetize the testicular microenvironment.
Author contributions
Conceptualization, BiOH-P, LS, GA, AC and MM. Writing-original draft preparation, BiOH-P, LS, GA, Ba´OHPP, JF, MP and PD. Writing-review and editing, BiOH-P, Ba´OHPP, LS, GA, AC MM. Supervision, AC and MM. Funding acquisition, MM. All authors contributed to the article and approved the submitted version.
Funding
The São Paulo Research Foundation (FAPESP, grant number 2014/50844-3) and CAPES (Coordination for the Improvement of Higher Education Personnel).
Conflict of interest
The authors declare that the research was conducted in the absence of any commercial or financial relationships that could be construed as a potential conflict of interest.
Publisher’s note
All claims expressed in this article are solely those of the authors and do not necessarily represent those of their affiliated organizations, or those of the publisher, the editors and the reviewers. Any product that may be evaluated in this article, or claim that may be made by its manufacturer, is not guaranteed or endorsed by the publisher.
References
1. Ganjibakhsh M, Mehraein F, Koruji M, Aflatoonian R, Farzaneh P. Three-dimensional decellularized amnion membrane scaffold promotes the efficiency of male germ cells generation from human induced pluripotent stem cells. Exp Cell Res (2019) 384:111544. doi: 10.1016/J.YEXCR.2019.111544
2. Sun H, Gong TT, Jiang YT, Zhang S, Zhao YH, Wu QJ. Global, regional, and national prevalence and disability-adjusted life-years for infertility in 195 countries and territories, 1990–2017: Results from a global burden of disease study, 2017. Aging (Albany NY) (2019) 11:10952. doi: 10.18632/AGING.102497
3. Öztekin Ü, Caniklioğlu M, Sarı S, Selmi V, Gürel A, Işıkay L. Evaluation of Male infertility prevalence with clinical outcomes in middle Anatolian region. Cureus (2019) 11. doi: 10.7759/CUREUS.5122
4. Paulson RJ, Comizzoli P. Addressing challenges in developing and implementing successful in vitro fertilization in endangered species: An opportunity for humanity to “give back”. Fertil Steril (2018) 109:418–9. doi: 10.1016/j.fertnstert.2018.01.031
5. Comizzoli P, Holt W v. Recent progress in spermatology contributing to the knowledge and conservation of rare and endangered species. Annu Rev Anim Biosci (2022) 10:469–90. doi: 10.1146/ANNUREV-ANIMAL-020420-040600
6. Turner RM. Declining testicular function in the aging stallion: Management options and future therapies. Anim Reprod Sci (2019) 207:171–9. doi: 10.1016/J.ANIREPROSCI.2019.06.009
7. Sengupta P, Dutta S, Alahmar AT, D’souza UJA. Reproductive tract infection, inflammation and male infertility. Chem Biol (2020) 7:75–84.
8. Krausz C, Rosta V, Swerdloff RS, Wang C. Genetics of Male infertility. Emery Rimoin’s Principles Pract Med Genet Genomics (2022), 121–47. doi: 10.1016/B978-0-12-815236-2.00010-2
9. Nachtigall LB, Boepple PA, Pralong FP, Crowley WF Jr. Adult-onset idiopathic hypogonadotropic hypogonadism — a treatable form of Male infertility. New England Journal of Medicine (1997) 336:410–5. doi: 10.1056/NEJM199702063360604
10. Güney AI, Javadova D, Kirac D, Ulucan K, Koc G, Ergec D, et al. Detection of y chromosome microdeletions and mitochondrial DNA mutations in male infertility patients. Genet AND Mol Res (2012) 11:1039–48. doi: 10.4238/2012.APRIL.27.2
11. Orman D, Vardi N, Ates B, Taslidere E, Elbe H. Aminoguanidine mitigates apoptosis, testicular seminiferous tubules damage, and oxidative stress in streptozotocin-induced diabetic rats. Tissue Cell (2015) 47:284–90. doi: 10.1016/J.TICE.2015.03.006
12. Anway MD, Cupp AS, Uzumcu N, Skinner MK. Toxicology: Epigenetic transgenerational actions of endocrine disruptors and male fertility. Sci (1979) (2005) 308:1466–9. doi: 10.1126/SCIENCE.1108190/SUPPL_FILE/ANWAY.SOM.PDF
13. Thamer IK, Hussein FA, Khorsheed HH. Study the event cycles of spermatogenesis and spermogenesis in the testes of creeper, in: Euphrates Journal of agriculture science (2016). Available at: https://www.iasj.net/iasj/article/111735 (Accessed December 12, 2022).
14. Tsili AC, Sofikitis N, Stiliara E, Argyropoulou MI. MRI Of testicular malignancies. Abdominal Radiol (2019) 44:1070–82. doi: 10.1007/S00261-018-1816-5/FIGURES/7
15. Smith ZL, Werntz RP, Eggener SE. Testicular cancer: Epidemiology, diagnosis, and management. Med Clinics North America (2018) 102:251–64. doi: 10.1016/j.mcna.2017.10.003
16. Litchfield K, Levy M, Orlando G, Loveday C, Law PJ, Migliorini G, et al. Identification of 19 new risk loci and potential regulatory mechanisms influencing susceptibility to testicular germ cell tumor. Nat Genet (2017) 49(7):1133–40. doi: 10.1038/ng.3896
17. de Padova S, Urbini M, Schepisi G, Virga A, Meggiolaro E, Rossi L, et al. Immunosenescence in testicular cancer survivors: Potential implications of cancer therapies and psychological distress. Front Oncol (2021) 10:564346/BIBTEX. doi: 10.3389/FONC.2020.564346/BIBTEX
18. de Angelis C, Galdiero M, Pivonello C, Salzano C, Gianfrilli D, Piscitelli P, et al. The environment and male reproduction: The effect of cadmium exposure on reproductive function and its implication in fertility. Reprod Toxicol (2017) 73:105–27. doi: 10.1016/J.REPROTOX.2017.07.021
19. Garolla A, Šabović I, Tescari S, de Toni L, Menegazzo M, Cosci I, et al. Impaired sperm function in infertile men relies on the membrane sterol pattern. Andrology (2018) 6:325–34. doi: 10.1111/ANDR.12468
20. Fuxjager MJ, Schuppe ER. Androgenic signaling systems and their role in behavioral evolution. J Steroid Biochem Mol Biol (2018) 184:47–56. doi: 10.1016/J.JSBMB.2018.06.004
21. McSwiggin HM, O’Doherty AM. Epigenetic reprogramming during spermatogenesis and male factor infertility. Reproduction (2018) 156:R9–R21. doi: 10.1530/REP-18-0009
22. Ge S, Zhao P, Liu X, Zhao Z, Liu M. Necessity to evaluate epigenetic quality of the sperm for assisted reproductive technology. Reprod Sci (2019) 26:315–22. doi: 10.1177/1933719118808907/ASSET/IMAGES/LARGE/10.1177_1933719118808907-FIG1.JPEG
23. Huntriss J, Balen AH, Sinclair KD, Brison DR, Picton HM. Epigenetics and reproductive medicine: Scientific impact paper no. 57. BJOG (2018) 125:e43–54. doi: 10.1111/1471-0528.15240
24. Skinner MK, ben Maamar M, Sadler-Riggleman I, Beck D, Nilsson E, McBirney M, et al. Alterations in sperm DNA methylation, non-coding RNA and histone retention associate with DDT-induced epigenetic transgenerational inheritance of disease. Epigenet Chromatin (2018) 11:1–24. doi: 10.1186/S13072-018-0178-0/FIGURES/10
25. Jenkins TG, Aston KI, Carrell DT. Sperm epigenetics and aging. Transl Androl Urol (2018) 7:S328. doi: 10.21037/TAU.2018.06.10
26. Borgström B, Fridström M, Gustafsson B, Ljungman P, Rodriguez-Wallberg KA. A prospective study on the long-term outcome of prepubertal and pubertal boys undergoing testicular biopsy for fertility preservation prior to hematologic stem cell transplantation. Pediatr Blood Cancer (2020) 67:e28507. doi: 10.1002/PBC.28507
27. Das M, Holzer HE. ART and Male infertility. Clin Manage Infertility (2021) 2:179–96. doi: 10.1007/978-3-030-71838-1_12
28. Pinto S, Carrageta DF, Alves MG, Rocha A, Agarwal A, Barros A, et al. Sperm selection strategies and their impact on assisted reproductive technology outcomes. Andrologia (2021) 53:e13725. doi: 10.1111/AND.13725
29. Herrick JR. Assisted reproductive technologies for endangered species conservation: Developing sophisticated protocols with limited access to animals with unique reproductive mechanisms. Biol Reprod (2019) 100:1158–70. doi: 10.1093/BIOLRE/IOZ025
30. Kochan J, Niżański W, Moreira N, Cubas ZS, Nowak A, Prochowska S, et al. ARTs in wild felid conservation programmes in Poland and in the world. J Veterinary Res (Poland) (2019) 63:457–64. doi: 10.2478/JVETRES-2019-0043
31. Kilcoyne KR, Mitchell RT. FERTILITY PRESERVATION: Testicular transplantation for fertility preservation: Clinical potential and current challenges. Reproduction (2019) 158:F1–F14. doi: 10.1530/REP-18-0533
32. Fayomi AP, Peters K, Sukhwani M, Valli-Pulaski H, Shetty G, Meistrich ML, et al. Autologous grafting of cryopreserved prepubertal rhesus testis produces sperm and offspring. Sci (1979) (2019) 363:1314–9. doi: 10.1126/SCIENCE.AAV2914/SUPPL_FILE/AAV2914_TABLES2.XLSX
33. Silva AMda, Pereira AF, Comizzoli P, Silva AR. Cryopreservation and culture of testicular tissues: An essential tool for biodiversity preservation. Biopreservation and Biobanking (2020) 18(3):235–43. doi: 10.1089/BIO.2020.0010
34. Pelzman DL, Orwig KE, Hwang K. Progress in translational reproductive science: testicular tissue transplantation and in vitro spermatogenesis. Fertil Steril (2020) 113:500–9. doi: 10.1016/J.FERTNSTERT.2020.01.038
35. Zarandi NP, Galdon G, Kogan S, Atala A, Sadri-Ardekani H. Cryostorage of immature and mature human testis tissue to preserve spermatogonial stem cells (SSCs): A systematic review of current experiences toward clinical applications. Stem Cells Cloning (2018) 11:23. doi: 10.2147/SCCAA.S137873
36. Binsila B, Selvaraju S, Ranjithkumaran R, Archana SS, Krishnappa B, Ghosh SK, et al. Current scenario and challenges ahead in application of spermatogonial stem cell technology in livestock. J Assist Reprod Genet (2021) 38:3155–73. doi: 10.1007/S10815-021-02334-7/TABLES/1
37. Ibtisham F, Zhao Y, Wu J, Nawab A, Mei X, Li GH, et al. The optimized condition for the isolation and in vitro propagation of mouse spermatogonial stem cells. Biol Futur (2019) 70:79–87. doi: 10.1556/019.70.2019.10
38. Ibtisham F, Honaramooz A. Spermatogonial stem cells for In vitro spermatogenesis and In vivo restoration of fertility. Cells (2020) 9:745. doi: 10.3390/CELLS9030745
39. Bhaskar R, Mishra B, Gupta MK. Engineering biomaterials for testicular tissue engineering and In vitro spermatogenesis. Eng Materials Stem Cell Regeneration (2021), 237–49. doi: 10.1007/978-981-16-4420-7_10
40. Porzionato A, Stocco E, Barbon S, Grandi F, Macchi V, de Caro R. Tissue-engineered grafts from human decellularized extracellular matrices: A systematic review and future perspectives. Int J Mol Sci (2018) 19:4117. doi: 10.3390/IJMS19124117
41. Ashammakhi N, Ghavaminejad A, Tutar R, Fricker A, Roy I, Chatzistavrou X, et al. Highlights on advancing frontiers in tissue engineering. Tissue Engineering Part B: Reviews (2022) 28:633–64. doi: 10.1089/TEN.TEB.2021.0012
42. Cortez J, Leiva B, Torres CG, Parraguez VH, de los Reyes M, Carrasco A, et al. Generation and characterization of bovine testicular organoids derived from primary somatic cell populations. Animals (2022) 12:2283. doi: 10.3390/ANI12172283
43. Vermeulen M, del Vento F, Kanbar M, Ruys SPD, Vertommen D, Poels J, et al. Generation of organized porcine testicular organoids in solubilized hydrogels from decellularized extracellular matrix. Int J Mol Sci (2019) 20:5476. doi: 10.3390/IJMS20215476
44. Meyer M. Processing of collagen based biomaterials and the resulting materials properties. Biomed Eng Online (2019) 18(1):74. doi: 10.1186/S12938-019-0647-0
45. Reddy MSB, Ponnamma D, Choudhary R, Sadasivuni KK. A comparative review of natural and synthetic biopolymer composite scaffolds. Polymers (2021) 13:1105. doi: 10.3390/POLYM13071105
46. Oliver E, Stukenborg JB. Rebuilding the human testis in vitro. Andrology (2020) 8:825–34. doi: 10.1111/ANDR.12710
47. Sainio A, Järveläinen H. Extracellular matrix-cell interactions: Focus on therapeutic applications. Cell Signal (2020) 66:109487. doi: 10.1016/J.CELLSIG.2019.109487
48. Obukohwo OM, Kingsley NE, Rume RA, Victor E. The concept of Male reproductive anatomy, in: Male Reproductive anatomy (2021). Available at: https://books.google.com.br/books?hl=pt-BR&lr=&id=N4JbEAAAQBAJ&oi=fnd&pg=PA3&dq=Obukohwo,+O.+M.,+Kingsley,+N.+E.,+Rume,+R.+A.,+%26+Victor,+E.+(2021).+The+Concept+of+Male+Reproductive+Anatomy.+In+Male+Reproductive+Anatomy.+IntechOpen.&ots=nGdnWTJnVq&sig=0EwPpgIiIE-UzzGfUC_-X095YZU#v=onepage&q&f=false (Accessed October 22, 2022).
49. Pathak D, Kapoor K, Gupta MK. Testicular stem cell niche. Stem Cells Veterinary Sci (2021), 161–82. doi: 10.1007/978-981-16-3464-2_10
50. Fahmy MAB. Anatomy of the scrotum. Normal Abnormal Scrotum (2022), 65–90. doi: 10.1007/978-3-030-83305-3_8
51. Ramírez-González JA, Sansone A. Male Reproductive system. Fertility Pregnancy Wellness (2022), 23–36. doi: 10.1016/B978-0-12-818309-0.00006-X
52. Raad G, Massaad V, Serdarogullari M, Bakos HW, Issa R, Khachan MJ, et al. Functional histology of human scrotal wall layers and their overlooked relation with infertility: a narrative review. Int J Impotence Res (2022), 1–11. doi: 10.1038/s41443-022-00573-5
53. Sofi SA, Zeebaree BK, Mohammed ZA. Histological and biometrical study of the adult local bull testis in duhok province, in: Journal homepage (2022). Available at: www.basjvet.org (Accessed October 22, 2022).
54. de Siqueira-Silva DH, da Silva Rodrigues M, Nóbrega RH. Testis structure, spermatogonial niche and sertoli cell efficiency in Neotropical fish. Gen Comp Endocrinol (2019) 273:218–26. doi: 10.1016/J.YGCEN.2018.09.004
55. Rebik K, Wagner JM, Middleton W. Scrotal ultrasound. Radiol Clin North Am (2019) 57:635–48. doi: 10.1016/j.rcl.2019.01.007
56. di Renzo L, de Lorenzo A, Fontanari M, Gualtieri P, Monsignore D, Schifano G, et al. Immunonutrients involved in the regulation of the inflammatory and oxidative processes: implication for gamete competence. J Assist Reprod Genet (2022) 39:817–46. doi: 10.1007/S10815-022-02472-6/FIGURES/2
57. Valiente-Alandi I, Schafer AE, Blaxall BC. Extracellular matrix-mediated cellular communication in the heart. J Mol Cell Cardiol (2016) 91:228–37. doi: 10.1016/J.YJMCC.2016.01.011
58. Yang Y, Lin Q, Zhou C, Li Q, Li Z, Cao Z, et al. A testis-derived hydrogel as an efficient feeder-free culture platform to promote mouse spermatogonial stem cell proliferation and differentiation. Front Cell Dev Biol (2020) 8:250/BIBTEX. doi: 10.3389/FCELL.2020.00250/BIBTEX
59. Mossadegh-Keller N, Sieweke MH. Testicular macrophages: Guardians of fertility. Cell Immunol (2018) 330:120–5. doi: 10.1016/J.CELLIMM.2018.03.009
60. Kargar-Abarghouei E, Vojdani Z, Hassanpour A, Alaee S, Talaei-Khozani T. Characterization, recellularization, and transplantation of rat decellularized testis scaffold with bone marrow-derived mesenchymal stem cells. Stem Cell Res Ther (2018) 9:1–16. doi: 10.1186/S13287-018-1062-3/FIGURES/14
61. Kauerhof AC, Nicolas N, Bhushan S, Wahle E, Loveland KA, Fietz D, et al. Investigation of activin a in inflammatory responses of the testis and its role in the development of testicular fibrosis. Hum Reprod (2019) 34:1536–50. doi: 10.1093/HUMREP/DEZ109
62. Gharenaz NM, Movahedin M, Mazaheri Z. Three-dimensional culture of mouse spermatogonial stem cells using a decellularised testicular scaffold. Cell J (Yakhteh) (2020) 21:410. doi: 10.22074/CELLJ.2020.6304
63. Li L, Li H, Wang L, Bu T, Liu S, Mao B, et al. A local regulatory network in the testis mediated by laminin and collagen fragments that supports spermatogenesis. Critical Reviews in Biochemistry and Molecular Biology (2021) 56:236–54. doi: 10.1080/10409238.2021.1901255
64. Siu MKY, Cheng CY. Extracellular matrix: Recent advances on its role in junction dynamics in the seminiferous epithelium during spermatogenesis. Biol Reprod (2004) 71:375–91. doi: 10.1095/BIOLREPROD.104.028225
65. Griswold MD. 50 years of spermatogenesis: Sertoli cells and their interactions with germ cells. Biol Reprod (2018) 99:87–100. doi: 10.1093/BIOLRE/IOY027
66. Cheng CY, Wong EWP, Yan HHN, Mruk DD. Regulation of spermatogenesis in the microenvironment of the seminiferous epithelium: New insights and advances. Mol Cell Endocrinol (2010) 315:49–56. doi: 10.1016/J.MCE.2009.08.004
67. Nakata H, Iseki S, Mizokami A. Three-dimensional reconstruction of testis cords/seminiferous tubules. Reprod Med Biol (2021) 20:402–9. doi: 10.1002/RMB2.12413
68. Heinrich A, DeFalco T. Essential roles of interstitial cells in testicular development and function. Andrology (2020) 8:903–14. doi: 10.1111/ANDR.12703
69. Yokonishi T, McKey J, Ide S, Capel B. Sertoli cell ablation and replacement of the spermatogonial niche in mouse. Nat Commun (2020) 11:1–11. doi: 10.1038/s41467-019-13879-8
70. Cham TC, Chen X, Honaramooz A. Current progress, challenges, and future prospects of testis organoids†. Biol Reprod (2021) 104:942–61. doi: 10.1093/BIOLRE/IOAB014
71. Wang JM, Li ZF, Yang WX, Tan FQ. Follicle-stimulating hormone signaling in sertoli cells: a licence to the early stages of spermatogenesis. Reprod Biol Endocrinol (2022) 20:1–18. doi: 10.1186/S12958-022-00971-W
72. Thakkar H, Bhushan P, Syed JS, Patwardhan S. Use of ultrasound in urology. Ultrasound Fundamentals (2021), 285–305. doi: 10.1007/978-3-030-46839-2_28
73. Lagarrigue M, Lavigne R, Guével B, Palmer A, Rondel K, Guillot L, et al. Spatial segmentation and metabolite annotation involved in sperm maturation in the rat epididymis by MALDI imaging mass spectrometry. J Mass Spectrometry (2020) 55:e4633. doi: 10.1002/JMS.4633
74. Choi HH, Taliaferro AS, Strachowski LM, Jha P. How common are traumatic injuries to the epididymis? A study of prevalence, imaging appearance, and management implications. Emerg Radiol (2021) 28:31–6. doi: 10.1007/S10140-020-01814-0/TABLES/4
75. Houda A, Nyaz S, Sobhy BM, Bosilah AH, Romeo M, Michael JP, et al. Seminiferous tubules and spermatogenesis, in: Male Reproductive anatomy (2021). Available at: https://books.google.com.br/books?hl=pt-BR&lr=&id=N4JbEAAAQBAJ&oi=fnd&pg=PA45&dq=Houda,+A.,+Nyaz,+S.,+Sobhy,+B.+M.,+Bosilah,+A.+H.,+Romeo,+M.,+Michael,+J.+P.,+%26+Eid,+H.+M.+(2021).+Seminiferous+Tubules+and+Spermatogenesis.+In+Male+Reproductive+Anatomy.+IntechOpen.&ots=nGdnWTHpXr&sig=6QQ7eSRGAI54Zn_gpkwYmRAwumE#v=onepage&q&f=false (Accessed October 22, 2022).
76. Boguenet M, Bouet PE, Spiers A, Reynier P, May-Panloup P. Mitochondria: their role in spermatozoa and in male infertility. Hum Reprod Update (2021) 27:697–719. doi: 10.1093/HUMUPD/DMAB001
77. Li H, Liu S, Wu S, Li L, Ge R, Yan Cheng C. Bioactive fragments of laminin and collagen chains: Lesson from the testis. Reproduction (2020) 159:R111–23. doi: 10.1530/REP-19-0288
78. Fok KL, Chan HC. Spermatogenesis: Biology and clinical implications (2018). Available at: https://books.google.com.br/books?hl=pt-BR&lr=&id=il0PEAAAQBAJ&oi=fnd&pg=PA94&dq=FOK+et+al.,+2018+spermatogenesis&ots=Hj2TcGuZtM&sig=yRQUbLvkmhzfpslbXNNiikNSEtk#v=onepage&q=FOK%20et%20al.%2C%202018%20spermatogenesis&f=false (Accessed October 24, 2022).
79. Khanehzad M, Abbaszadeh R, Holakuyee M, Modarressi MH, Nourashrafeddin SM. FSH regulates RA signaling to commit spermatogonia into differentiation pathway and meiosis. Reprod Biol Endocrinol (2021) 19:1–19. doi: 10.1186/S12958-020-00686-W
80. Silber S. Testis development, embryology, and anatomy. Fundamentals Male Infertility (2018), 3–12. doi: 10.1007/978-3-319-76523-5_1
81. Sharma S, Wistuba J, Pock T, Schlatt S, Neuhaus N. Spermatogonial stem cells: updates from specification to clinical relevance. Hum Reprod Update (2019) 25:275–97. doi: 10.1093/HUMUPD/DMZ006
82. Delessard M, Saulnier J, Rives A, Dumont L, Rondanino C, Rives N. Exposure to chemotherapy during childhood or adulthood and consequences on spermatogenesis and Male fertility. Int J Mol Sci (2020) 21:1454. doi: 10.3390/IJMS21041454
83. Oliveira PF, Alves MG. Sertoli cell metabolism and spermatogenesis. Sertoli Cell Metab Spermatogenesis (2015) 41–56. doi: 10.1007/978-3-319-19791-3
84. Kubota H, Brinster RL. Spermatogonial stem cells. Biol Reprod (2018) 99:52–74. doi: 10.1093/BIOLRE/IOY077
85. Fayomi AP, Orwig KE. Spermatogonial stem cells and spermatogenesis in mice, monkeys and men. Stem Cell Res (2018) 29:207–14. doi: 10.1016/J.SCR.2018.04.009
86. Caldeira-Brant AL, Martinelli LM, Marques MM, Reis AB, Martello R, Almeida FRCL, et al. A subpopulation of human adark spermatogonia behaves as the reserve stem cell. Reproduction (2020) 159:437–51. doi: 10.1530/REP-19-0254
87. Lall KR, Jones KR, Garcia GW. Reproductive technologies used in Male neo-tropical hystricomorphic rodents. Animals (2021) 12:34. doi: 10.3390/ANI12010034
88. Staub C, Johnson L. Review: Spermatogenesis in the bull. Animal (2018) 12:s27–35. doi: 10.1017/S1751731118000435
89. García-Rodríguez A, Gosálvez J, Agarwal A, Roy R, Johnston S. DNA Damage and repair in human reproductive cells. Int J Mol Sci (2018) 20:31. doi: 10.3390/IJMS20010031
90. Lyu R, Tsui V, McCarthy DJ, Crismani W. Personalized genome structure via single gamete sequencing. Genome Biol (2021) 22:1–19. doi: 10.1186/S13059-021-02327-W/FIGURES/4
91. Griswold MD. Spermatogenesis: The commitment to meiosis. Physiol Rev (2016) 96:1–17. doi: 10.1152/PHYSREV.00013.2015/ASSET/IMAGES/LARGE/Z9J0011627500008.JPEG
92. Teves ME, Roldan ERS, Krapf D, Strauss IJF, Bhagat V, Sapao P. Sperm differentiation: the role of trafficking of proteins. International journal of molecular sciences (2020) 21(10):3702 doi: 10.3390/ijms21103702
93. Ribeiro JC, Alves MG, Amado F, Ferreira R, Oliveira P. Insights and clinical potential of proteomics in understanding spermatogenesis. Expert Review of Proteomics (2021) 18:13–25. doi: 10.1080/14789450.2021.1889373
94. Elbashir S, Magdi Y, Rashed A, Henkel R, Agarwal A. Epididymal contribution to male infertility: An overlooked problem. Andrologia (2021) 53:e13721. doi: 10.1111/AND.13721
95. Kanazawa Y, Omotehara T, Nakata H, Hirashima T, Itoh M. Three-dimensional analysis and in vivo imaging for sperm release and transport in the murine seminiferous tubule. Reproduction (2022) 164:9–18. doi: 10.1530/REP-21-0400
96. Neto FTL, Bach PV, Najari BB, Li PS, Goldstein M. Spermatogenesis in humans and its affecting factors. Semin Cell Dev Biol (2016) 59:10–26. doi: 10.1016/J.SEMCDB.2016.04.009
97. Clouthier DE, Avarbock MR, Maika SD, Hammer RE, Brinster RL. Rat spermatogenesis in mouse testis. Nature (1996) 381:418–21. doi: 10.1038/381418a0
98. Huhtaniemi I. Role of gonadotropins in adult-onset functional hypogonadism. Controversies Testosterone Deficiency (2021), 23–34. doi: 10.1007/978-3-030-77111-9_3
99. Sharma S, Venzac B, Burgers T, le Gac S, Schlatt S. Microfluidics in male reproduction: is ex vivo culture of primate testis tissue a future strategy for ART or toxicology research? Mol Hum Reprod (2020) 26:179–92. doi: 10.1093/MOLEHR/GAAA006
100. Walker WH. Androgen actions in the testis and the regulation of spermatogenesis. Adv Exp Med Biol (2021) 1288:175–203. doi: 10.1007/978-3-030-77779-1_9/COVER
101. Sengupta P, Arafa M, Elbardisi H. Hormonal regulation of spermatogenesis. Mol Signaling Spermatogenesis Male Infertility (2019) 1:41–9. doi: 10.1201/9780429244216-5
102. Stukenborg JB, Jahnukainen K, Hutka M, Mitchell RT. Cancer treatment in childhood and testicular function: The importance of the somatic environment. Endocr Connect (2018) 7:R69–87. doi: 10.1530/EC-17-0382
103. Walker WH. Androgen regulation of spermatogenesis. Spermatogenesis: Biol Clin Implications (2018) 1:40–51.
104. ben Maamar M, Sadler-Riggleman I, Beck D, Skinner MK. Epigenetic transgenerational inheritance of altered sperm histone retention sites. Sci Rep (2018) 8:1–10. doi: 10.1038/s41598-018-23612-y
105. Hannan MA, Fukami Y, Kawate N, Sakase M, Fukushima M, Pathirana IN, et al. Plasma insulin-like peptide 3 concentrations are acutely regulated by luteinizing hormone in pubertal Japanese black beef bulls. Theriogenology (2015) 84:1530–5. doi: 10.1016/J.THERIOGENOLOGY.2015.07.039
106. Gokhale G, Sharma GD. Adverse impact of heat stress on bovine development: Causes and strategies for mitigation (2021). Available at: https://books.google.com.br/books?hl=pt-BR&lr=&id=Ll5iEAAAQBAJ&oi=fnd&pg=PA125&dq=Gokhale,+G.,+%26+Sharma,+G.+D.+(2021).+Adverse+Impact+of+Heat+Stress+on+Bovine+Development:+Causes+and+Strategies+for+Mitigation.&ots=LafPRt6l64&sig=Bmc8IA5j3gGUuYcBMzYSbUU54FY#v=onepage&q&f=false (Accessed October 22, 2022).
107. Eugeni E, Arato I, del Sordo R, Sidoni A, Garolla A, Ferlin A, et al. Fertility preservation and restoration options for pre-pubertal Male cancer patients: Current approaches. Front Endocrinol (Lausanne) (2022) 13:877537/PDF. doi: 10.3389/FENDO.2022.877537/PDF
108. Anderson RA, Mitchell RT, Kelsey TW, Spears N, Telfer EE, Wallace WHB. Cancer treatment and gonadal function: experimental and established strategies for fertility preservation in children and young adults. Lancet Diabetes Endocrinol (2015) 3:556–67. doi: 10.1016/S2213-8587(15)00039-X
109. Khan IM, Cao Z, Liu H, Khan A, Rahman SU, Khan MZ, et al. Impact of cryopreservation on spermatozoa freeze-thawed traits and relevance OMICS to assess sperm cryo-tolerance in farm animals. Front Vet Sci (2021) 8:609180/BIBTEX. doi: 10.3389/FVETS.2021.609180/BIBTEX
110. Valli-Pulaski H, Peters KA, Gassei K, Steimer SR, Sukhwani M, Hermann BP, et al. Testicular tissue cryopreservation: 8 years of experience from a coordinated network of academic centers. Hum Reprod (2019) 34:966–77. doi: 10.1093/HUMREP/DEZ043
111. Bashawat M, Braun BC, Müller K. Cell survival after cryopreservation of dissociated testicular cells from feline species. Cryobiology (2020) 97:191–7. doi: 10.1016/J.CRYOBIOL.2020.03.001
112. Onofre J, Baert Y, Faes K, Goossens E. Cryopreservation of testicular tissue or testicular cell suspensions: A pivotal step in fertility preservation. Hum Reprod Update (2016) 22:744–61. doi: 10.1093/HUMUPD/DMW029
113. Lima DBC, da Silva TFP, Aquino-Cortez A, Leiva-Revilla J, da Silva LDM. Vitrification of testicular tissue from prepubertal cats in cryotubes using different cryoprotectant associations. Theriogenology (2018) 110:110–5. doi: 10.1016/J.THERIOGENOLOGY.2017.12.037
114. Lima DBC, da Silva LDM, Comizzoli P. Influence of warming and reanimation conditions on seminiferous tubule morphology, mitochondrial activity, and cell composition of vitrified testicular tissues in the domestic cat model. PloS One (2018) 13:e0207317. doi: 10.1371/JOURNAL.PONE.0207317
115. Patra T, Pathak D, Gupta MK. Strategies for cryopreservation of testicular cells and tissues in cancer and genetic diseases. Cell Tissue Res (2021) 385:1–19. doi: 10.1007/S00441-021-03437-4
116. Pukazhenthi BS, Nagashima J, Travis AJ, Costa GM, Escobar EN, França LR, et al. Slow freezing, but not vitrification supports complete spermatogenesis in cryopreserved, neonatal sheep testicular xenografts. PloS One (2015) 10:e0123957. doi: 10.1371/JOURNAL.PONE.0123957
117. Thuwanut P, Srisuwatanasagul S, Wongbandue G, Tanpradit N, Thongpakdee A, Tongthainan D, et al. Sperm quality and the morphology of cryopreserved testicular tissues recovered post-mortem from diverse wild species. Cryobiology (2013) 67:244–7. doi: 10.1016/J.CRYOBIOL.2013.07.002
118. Picton HM, Wyns C, Anderson RA, Goossens E, Jahnukainen K, et al. A European perspective on testicular tissue cryopreservation for fertility preservation in prepubertal and adolescent boys. Hum Reprod (2015) 30:2463–75. doi: 10.1093/HUMREP/DEV190
119. Abrishami M, Anzar M, Yang Y, Honaramooz A. Cryopreservation of immature porcine testis tissue to maintain its developmental potential after xenografting into recipient mice. Theriogenology (2010) 73:86–96. doi: 10.1016/J.THERIOGENOLOGY.2009.08.004
120. Buarpung S, Tharasanit T, Comizzoli P, Techakumphu M. Feline spermatozoa from fresh and cryopreserved testicular tissues have comparable ability to fertilize matured oocytes and sustain the embryo development after intracytoplasmic sperm injection. Theriogenology (2013) 79:149–58. doi: 10.1016/J.THERIOGENOLOGY.2012.09.022
121. Gurina TM, Pakhomov A v., Kyryliuk AL, Bozhok GA. Development of a cryopreservation protocol for testicular interstitial cells with the account of temperature intervals for controlled cooling below −60 °С. Cryobiology (2011) 62:107–14. doi: 10.1016/J.CRYOBIOL.2011.01.011
122. Wyns C. Fertility preservation: Current prospects and future challenges. Gynecological Endocrinology (2013) 29:403–7. doi: 10.3109/09513590.2012.754872
123. Lima DBC, Silva TFP, Morais GB, Aquino-Cortez A, Evangelista JSAM, Xavier JúniorFAF, et al. Different associations of cryoprotectants for testicular tissue of prepubertal cats submitted to vitrification. Reprod Domest Anim (2017) 52:235–41. doi: 10.1111/RDA.12833
124. Baert Y, Goossens E, van Saen D, Ning L, In’T Veld P, Tournaye H. Orthotopic grafting of cryopreserved prepubertal testicular tissue: In search of a simple yet effective cryopreservation protocol. Fertil Steril (2012) 97:1152–1157.e2. doi: 10.1016/J.FERTNSTERT.2012.02.010
125. Ginsberg JP, Li Y, Carlson CA, Gracia CR, Hobbie WL, Miller VA, et al. Testicular tissue cryopreservation in prepubertal male children: An analysis of parental decision-making. Pediatr Blood Cancer (2014) 61:1673–8. doi: 10.1002/PBC.25078
126. Uijldert M, Meißner A, de Melker AA, van Pelt AMM, van de Wetering MD, van Rijn RR, et al. Development of the testis in pre-pubertal boys with cancer after biopsy for fertility preservation. Hum Reprod (2017) 32:2366–72. doi: 10.1093/HUMREP/DEX306
127. Ming JM, Chua ME, Lopes RI, Maloney AM, Gupta AA, Lorenzo AJ. Cryopreservation of testicular tissue in pre-pubertal and adolescent boys at risk for infertility: A low risk procedure. J Pediatr Urol (2018) 14:274.e1–5. doi: 10.1016/J.JPUROL.2018.02.016
128. Pereira RMLN, Marques CC, Pimenta J, Barbas JP, Baptista MC, Diniz P, et al. Assisted reproductive technologies (ART) directed to germplasm preservation. Adv Anim Health Med Production (2020), 199–215. doi: 10.1007/978-3-030-61981-7_10
129. Hu H, Ji G, Shi X, Zhang J, Li M. Current status of Male fertility preservation in humans. Russian J Dev Biol (2022) 53:134–40. doi: 10.1134/S1062360422020060
130. Le MT, Nguyen TTT, Nguyen TT, van Nguyen T, Nguyen TAT, Nguyen QHV, et al. Does conventional freezing affect sperm DNA fragmentation? Clin Exp Reprod Med (2019) 46:67. doi: 10.5653/CERM.2019.46.2.67
131. Sztein JM, Takeo T, Nakagata N. History of cryobiology, with special emphasis in evolution of mouse sperm cryopreservation. Cryobiology (2018) 82:57–63. doi: 10.1016/J.CRYOBIOL.2018.04.008
132. Brannigan RE, Fantus RJ, Halpern JA. Fertility preservation in men: a contemporary overview and a look toward emerging technologies. Fertil Steril (2021) 115:1126–39. doi: 10.1016/J.FERTNSTERT.2021.03.026
133. Ugur MR, Saber Abdelrahman A, Evans HC, Gilmore AA, Hitit M, Arifiantini RI, et al. Advances in cryopreservation of bull sperm. Front Vet Sci (2019) 6:268/BIBTEX. doi: 10.3389/FVETS.2019.00268/BIBTEX
134. Hezavehei M, Sharafi M, Kouchesfahani HM, Henkel R, Agarwal A, Esmaeili V, et al. Sperm cryopreservation: A review on current molecular cryobiology and advanced approaches. Reprod BioMed Online (2018) 37:327–39. doi: 10.1016/J.RBMO.2018.05.012
135. Bóveda P, Toledano-Díaz A, Castaño C, Esteso MC, López-Sebastián A, Rizos D, et al. Ultra-rapid cooling of ibex sperm by spheres method does not induce a vitreous extracellular state and increases the membrane damages. PloS One (2020) 15:e0227946. doi: 10.1371/JOURNAL.PONE.0227946
136. Srinivasan G, Raja B. Heat and mass transfer analysis on multiport mini channel shelf heat exchanger for freeze-drying application. Sadhana - Acad Proc Eng Sci (2020) 45:1–14. doi: 10.1007/S12046-020-01496-X/FIGURES/12
137. Riva NS, Ruhlmann C, Iaizzo RS, López CAM, Martínez AG. Comparative analysis between slow freezing and ultra-rapid freezing for human sperm cryopreservation. JBRA Assist Reprod (2018) 22:331. doi: 10.5935/1518-0557.20180060
138. Martins AD, Agarwal A, Henkel R. Sperm cryopreservation. In Vitro Fertilization (2019), 625–42. doi: 10.1007/978-3-319-43011-9_51
139. Grötter LG, Cattaneo L, Marini PE, Kjelland ME, Ferré LB. Recent advances in bovine sperm cryopreservation techniques with a focus on sperm post-thaw quality optimization. Reprod Domest Anim (2019) 54:655–65. doi: 10.1111/RDA.13409
140. Le MT, Nguyen TTT, Nguyen TT, Nguyen VT, Nguyen TTA, Nguyen VQH, et al. Cryopreservation of human spermatozoa by vitrification versus conventional rapid freezing: Effects on motility, viability, morphology and cellular defects. Eur J Obstetrics Gynecology Reprod Biol (2019) 234:14–20. doi: 10.1016/J.EJOGRB.2019.01.001
141. Todorovic BP, Verheyen G, Vloeberghs V, Tournaye H. Sperm cryopreservation. Female Male Fertility Preservation (2022), 453–70. doi: 10.1007/978-3-030-47767-7_36
142. Matsumura K, Hayashi F, Nagashima T, Rajan R, Hyon SH. Molecular mechanisms of cell cryopreservation with polyampholytes studied by solid-state NMR. Commun Materials (2021) 2:1–12. doi: 10.1038/s43246-021-00118-1
143. Vanderzwalmen P, Ectors F, Panagiotidis Y, Schuff M, Murtinger M, Wirleitner B. The evolution of the cryopreservation techniques in reproductive medicine–exploring the character of the vitrified state intra- and extracellularly to better understand cell survival after cryopreservation. Reprod Med (2020) 1:142–57. doi: 10.3390/REPRODMED1020011
144. Chang CC, Shapiro DB, Nagy ZP. The effects of vitrification on oocyte quality. Biol Reprod (2022) 106:316–27. doi: 10.1093/BIOLRE/IOAB239
145. Li X, Long Xy, Xie Yj, Zeng X, Chen X, Mo Zc. The roles of retinoic acid in the differentiation of spermatogonia and spermatogenic disorders. Clinica Chimica Acta (2019) 497:54–60. doi: 10.1016/J.CCA.2019.07.013
146. Cai H, Niringiyumukiza JD, Li Y, Lai Q, Jia Y, Su P, et al. Open versus closed vitrification system of human oocytes and embryos: A systematic review and meta-analysis of embryologic and clinical outcomes. Reprod Biol Endocrinol (2018) 16:1–11. doi: 10.1186/S12958-018-0440-0/TABLES/3
147. Peris-Frau P, Soler AJ, Iniesta-Cuerda M, Martín-Maestro A, Sánchez-Ajofrín I, Medina-Chávez DA, et al. Sperm cryodamage in ruminants: Understanding the molecular changes induced by the cryopreservation process to optimize sperm quality. Int J Mol Sci (2020) 21:2781. doi: 10.3390/IJMS21082781
148. Bayefsky M, Vieira D, Caplan A, Quinn G. Navigating parent–child disagreement about fertility preservation in minors: Scoping review and ethical considerations. Hum Reprod Update (2022) 28:747–62. doi: 10.1093/HUMUPD/DMAC019
149. Tasker F, Gato J. Gender identity and future thinking about parenthood: A qualitative analysis of focus group data with transgender and non-binary people in the united kingdom. Front Psychol (2020) 11:865/BIBTEX. doi: 10.3389/FPSYG.2020.00865/BIBTEX
150. Yánez-Ortiz I, Catalán J, Rodríguez-Gil JE, Miró J, Yeste M. Advances in sperm cryopreservation in farm animals: Cattle, horse, pig and sheep. Anim Reprod Sci (2021) 246:106904. doi: 10.1016/J.ANIREPROSCI.2021.106904
151. Comizzoli P, Holt W v. Breakthroughs and new horizons in reproductive biology of rare and endangered animal species. Biol Reprod (2019) 101:514–25. doi: 10.1093/BIOLRE/IOZ031
152. Kumar A, Prasad JK, Srivastava N, Ghosh SK. Strategies to minimize various stress-related freeze-thaw damages during conventional cryopreservation of mammalian spermatozoa. Biopreserv Biobank (2019) 17:603–12. doi: 10.1089/BIO.2019.0037/ASSET/IMAGES/LARGE/BIO.2019.0037_FIGURE1.JPEG
153. Ezzati M, Shanehbandi D, Hamdi K, Rahbar S, Pashaiasl M. Influence of cryopreservation on structure and function of mammalian spermatozoa: An overview. Cell Tissue Bank (2020) 21:1–15. doi: 10.1007/S10561-019-09797-0/FIGURES/3
154. Huleihel M, Lunenfeld E. Approaches and technologies in Male fertility preservation. Int J Mol Sci (2020) 21:5471. doi: 10.3390/IJMS21155471
155. Sahare MG, Suyatno, Imai H. Recent advances of in vitro culture systems for spermatogonial stem cells in mammals. Reprod Med Biol (2018) 17:134–42. doi: 10.1002/RMB2.12087
156. Kanbar M, Delwiche G, Wyns C. Fertility preservation for prepubertal boys: are we ready for autologous grafting of cryopreserved immature testicular tissue? Ann Endocrinol (Paris) (2022) 83:210–7. doi: 10.1016/J.ANDO.2022.04.006
157. Schlatt S, Kim S. Spermatogenesis and steroidogenesis in mouse, hamster and monkey testicular tissue after cryopreservation and heterotopic grafting to castrated hosts, in: REPRODUCTION-CAMBRIDGE (2002). Available at: https://scholar.archive.org/work/umoclb675beslb4nauibbo5e7q/access/wayback/https://rep.bioscientifica.com/downloadpdf/journals/rep/124/3/339.pdf (Accessed October 24, 2022).
158. Schlatt S. Spermatogonial stem cell preservation and transplantation. Mol Cell Endocrinol (2002) 187:107–11. doi: 10.1016/S0303-7207(01)00706-7
159. Ma P, Ge Y, Wang S, Ma J, Xue S, Han D. Spermatogenesis following syngeneic testicular transplantation in balb/c mice. Reproduction (2004) 128:163–70. doi: 10.1530/REP.1.00165
160. Shinohara T, Inoue K, Ogonuki N, Kanatsu-Shinohara M, Miki H, Nakata K, et al. Birth of offspring following transplantation of cryopreserved immature testicular pieces and in-vitro microinsemination. Hum Reprod (2002) 17:3039–45. doi: 10.1093/HUMREP/17.12.3039
161. Oatley JM, de Avila DM, Reeves JJ, McLean DJ. Spermatogenesis and germ cell transgene expression in xenografted bovine testicular tissue. Biol Reprod (2004) 71:494–501. doi: 10.1095/BIOLREPROD.104.027953
162. Honaramooz A, Li MW, Penedo MCT, Meyers S, Dobrinski I. Accelerated maturation of primate testis by xenografting into mice. Biol Reprod (2004) 70:1500–3. doi: 10.1095/BIOLREPROD.103.025536
163. Rathi R, Honaramooz A, Zeng W, Turner R, Dobrinski I. Germ cell development in equine testis tissue xenografted into mice. Reproduction (2006) 131:1091–8. doi: 10.1530/REP.1.01101
164. Kim Y, Selvaraj V, Pukazhenthi B, Travis AJ, Kim Y, Selvaraj V, et al. Effect of donor age on success of spermatogenesis in feline testis xenografts. Reprod Fertil Dev (2007) 19:869–76. doi: 10.1071/RD07056
165. Reddy N, Mahla RS, Thathi R, Suman SK, Jose J, Goel S. Gonadal status of male recipient mice influences germ cell development in immature buffalo testis tissue xenograft. Reproduction (2012) 143:59–69. doi: 10.1530/REP-11-0286
166. Ntemou E, Kadam P, van Saen D, Wistuba J, Mitchell RT, Schlatt S, et al. Complete spermatogenesis in intratesticular testis tissue xenotransplants from immature non-human primate. Hum Reprod (2019) 34:403–13. doi: 10.1093/HUMREP/DEY373
167. Wyns C, Kanbar M, Giudice MG, Poels J. Fertility preservation for prepubertal boys: lessons learned from the past and update on remaining challenges towards clinical translation. Hum Reprod Update (2021) 27:433–59. doi: 10.1093/HUMUPD/DMAA050
168. Barak S. Fertility preservation in male patients with cancer. Best Pract Res Clin Obstet Gynaecol (2019) 55:59–66. doi: 10.1016/J.BPOBGYN.2018.12.004
169. Bîcă O, Sârbu I, Ciongradi CI. Pediatric and adolescent oncofertility in Male patients–from alpha to omega. Genes (2021) 12:701. doi: 10.3390/GENES12050701
170. Bhaskar R, Gupta MK, Han SS. Tissue engineering approaches for the in vitro production of spermatids to treat male infertility: A review. Eur Polym J (2022) 174:111318. doi: 10.1016/J.EURPOLYMJ.2022.111318
171. Terada S, Sato M, Sevy A, Vacanti JP. Tissue engineering in the twenty-first century. Yonsei Med J (2009) 41:685–91. doi: 10.3349/YMJ.2000.41.6.685
172. Rajab TK, O’Malley TJ, Tchantchaleishvili V. Decellularized scaffolds for tissue engineering: Current status and future perspective. Artif Organs (2020) 44:1031–43. doi: 10.1111/AOR.13701
173. Eyni H, Ghorbani S, N H. Advanced bioengineering of male germ stem cells to preserve fertility. Journal of Tissue Engineering (2021) 12:1–25. doi: 10.1177/20417314211060590
174. Wang X, Wu D, Li W, Yang L. Emerging biomaterials for reproductive medicine. Engineered Regeneration (2021) 2:230–45. doi: 10.1016/J.ENGREG.2021.11.006
175. Gargus ES, Rogers HB, McKinnon KE, Edmonds ME, Woodruff TK. Engineered reproductive tissues. Nat Biomed Eng (2020) 4:381–93. doi: 10.1038/s41551-020-0525-x
176. Stejskalová A, Vankelecom H, Sourouni M, Ho MY, Götte M, Almquist BD. In vitro modelling of the physiological and diseased female reproductive system. Acta Biomater (2021) 132:288–312. doi: 10.1016/J.ACTBIO.2021.04.032
177. Dzobo K, Thomford NE, Senthebane DA, Shipanga H, Rowe A, Dandara C, et al. Advances in regenerative medicine and tissue engineering: Innovation and transformation of medicine. Stem Cells Int (2018) 2018. doi: 10.1155/2018/2495848
178. Bashiri Z, Amiri I, Gholipourmalekabadi M, Falak R, Asgari H, Maki CB, et al. Artificial testis: A testicular tissue extracellular matrix as a potential bio-ink for 3D printing. Biomater Sci (2021) 9:3465–84. doi: 10.1039/D0BM02209H
179. Bashiri Z, Gholipourmalekabadi M, Falak R, Amiri I, Asgari H, Chauhan NPS, et al. In vitro production of mouse morphological sperm in artificial testis bioengineered by 3D printing of extracellular matrix. Int J Biol Macromol (2022) 217:824–41. doi: 10.1016/J.IJBIOMAC.2022.07.127
180. Bashiri Z, Zahiri M, Allahyari H, Esmaeilzade B. Proliferation of human spermatogonial stem cells on optimized PCL/Gelatin nanofibrous scaffolds. Andrologia (2022) 54:e14380. doi: 10.1111/AND.14380
181. Naeemi S, Sabetkish S, Kiani MJ, Dehghan A, Kajbafzadeh AM. Ex-vivo and In-vivo expansion of spermatogonial stem cells using cell-seeded microfluidic testis scaffolds and animal model. Cell Tissue Bank (2022), 1–14. doi: 10.1007/S10561-022-10024-6
182. de Miguel‐Gómez L, López‐martínez S, Francés‐herrero E, Rodríguez‐eguren A, Pellicer A, Cervelló I. Stem cells and the endometrium: From the discovery of adult stem cells to pre-clinical models. Cells (2021) 10:595. doi: 10.3390/CELLS10030595
183. Gholami K, Pourmand G, Koruji M, Sadighigilani M, Navid S, Izadyar F, et al. Efficiency of colony formation and differentiation of human spermatogenic cells in two different culture systems. Reprod Biol (2018) 18:397–403. doi: 10.1016/J.REPBIO.2018.09.006
184. Sakib S, Voigt A, Goldsmith T, Dobrinski I. Three-dimensional testicular organoids as novel in vitro models of testicular biology and toxicology. Environ Epigenet (2019) 5:1–8. doi: 10.1093/EEP/DVZ011
185. Sakib S, Goldsmith T, Voigt A, Dobrinski I. Testicular organoids to study cell–cell interactions in the mammalian testis. Andrology (2020) 8:835–41. doi: 10.1111/ANDR.12680
186. Abe K, Kon S, Kameyama H, Zhang JD, Morohashi Ki, Shimamura K, et al. VCAM1-α4β1 integrin interaction mediates interstitial tissue reconstruction in 3-d re-aggregate culture of dissociated prepubertal mouse testicular cells. Sci Rep (2021) 11:1–13. doi: 10.1038/s41598-021-97729-y
187. Chen H, Alves MBR, Belleannée C. Contribution of epididymal epithelial cell functions to sperm epigenetic changes and the health of progeny. Hum Reprod Update (2021) 28:51–66. doi: 10.1093/HUMUPD/DMAB029
188. del Collado M, Andrade GM, Meirelles FV, da Silveira JC, Perecin F. Contributions from the ovarian follicular environment to oocyte function. Anim Reprod (2018) 15:261. doi: 10.21451/1984-3143-AR2018-0082
189. del Vento F, Vermeulen M, de Michele F, Giudice MG, Poels J, des Rieux A, et al. Tissue engineering to improve immature testicular tissue and cell transplantation outcomes: One step closer to fertility restoration for prepubertal boys exposed to gonadotoxic treatments. Int J Mol Sci (2018) 19:286. doi: 10.3390/IJMS19010286
190. Dadashzadeh A, Moghassemi S, Shavandi A, Amorim CA. A review on biomaterials for ovarian tissue engineering. Acta Biomater (2021) 135:48–63. doi: 10.1016/J.ACTBIO.2021.08.026
191. Dolmans MM, Amorim CA. FERTILITY PRESERVATION: Construction and use of artificial ovaries. Reproduction (2019) 158:F15–25. doi: 10.1530/REP-18-0536
192. Santi D, Crépieux P, Reiter E, Spaggiari G, Brigante G, Casarini L, et al. Follicle-stimulating hormone (FSH) action on spermatogenesis: A focus on physiological and therapeutic roles. J Clin Med (2020) 9:1014. doi: 10.3390/JCM9041014
193. Kashani MZ, Bagher Z, Asgari HR, Najafi M, Koruji M, Mehraein F. Differentiation of neonate mouse spermatogonial stem cells on three-dimensional agar/polyvinyl alcohol nanofiber scaffold. Syst Biol Reprod Med (2020) 66:202–15. doi: 10.1080/19396368.2020.1725927/SUPPL_FILE/IAAN_A_1725927_SM5260.DOCX
194. Perrard MH, Sereni N, Schluth-Bolard C, Blondet A, Giscard d’Estaing S, Plotton I, et al. Complete human and rat ex vivo spermatogenesis from fresh or frozen testicular tissue. Biol Reprod (2016) 95:1–10. doi: 10.1095/BIOLREPROD.116.142802/2883545
195. Eslahi N, Hadjighassem MR, Joghataei MT, Mirzapour T, Bakhtiyari M, Shakeri M, et al. The effects of poly l-lactic acid nanofiber scaffold on mouse spermatogonial stem cell culture. Int J Nanomedicine (2013) 8:4563–76. doi: 10.2147/IJN.S45535
196. Ito R, Abé SI. FSH-initiated differentiation of newt spermatogonia to primary spermatocytes in germ-somatic cell reaggregates cultured within a collagen matrix. Int J Dev Biol (2002) 43:111–6. doi: 10.1387/IJDB.10235386
197. Brown BN, Badylak SF. Extracellular matrix as an inductive scaffold for functional tissue reconstruction. Trans Res (2014) 163:268–85. doi: 10.1016/J.TRSL.2013.11.003
198. Lee JH, Gye MC, Choi KW, Hong JY, Lee YB, Park DW, et al. In vitro differentiation of germ cells from nonobstructive azoospermic patients using three-dimensional culture in a collagen gel matrix. Fertil Steril (2007) 87:824–33. doi: 10.1016/J.FERTNSTERT.2006.09.015
199. Khajavi N, Akbari M, Abolhassani F, Dehpour AR, Koruji M, Habibi Roudkenar M. Role of somatic testicular cells during mouse spermatogenesis in three-dimensional collagen gel culture system. Cell J (Yakhteh) (2014) 16:79.
200. Reda A, Hou M, Landreh L, Kjartansdóttir KR, Svechnikov K, Söder O, et al. In vitro spermatogenesis - optimal culture conditions for testicular cell survival, germ cell differentiation, and steroidogenesis in rats. Front Endocrinol (Lausanne) (2014) 5:21/BIBTEX. doi: 10.3389/FENDO.2014.00021/BIBTEX
201. Poels J, Abou-Ghannam G, Decamps A, Leyman M, Rieux Ad, Wyns C. Transplantation of testicular tissue in alginate hydrogel loaded with VEGF nanoparticles improves spermatogonial recovery. J Controlled Release (2016) 234:79–89. doi: 10.1016/J.JCONREL.2016.05.037
202. Pirnia A, Parivar K, Hemadi M, Yaghmaei P, Gholami M. Stemness of spermatogonial stem cells encapsulated in alginate hydrogel during cryopreservation. Andrologia (2017) 49:e12650. doi: 10.1111/AND.12650
203. Legendre A, Froment P, Desmots S, Lecomte A, Habert R, Lemazurier E. An engineered 3D blood-testis barrier model for the assessment of reproductive toxicity potential. Biomaterials (2010) 31:4492–505. doi: 10.1016/J.BIOMATERIALS.2010.02.029
204. Yadegar M, Hekmatimoghaddam SH, Saridar SN, Jebali A. The viability of mouse spermatogonial germ cells on a novel scaffold, containing human serum albumin and calcium phosphate nanoparticles. Iran J Reprod Med (2015) 13:141.
205. Yuan Y, Li L, Cheng Q, Diao F, Zeng Q, Yang X, et al. In vitro testicular organogenesis from human fetal gonads produces fertilization-competent spermatids. Cell Res (2020) 30:244–55. doi: 10.1038/s41422-020-0283-z
206. Mohaqiq M, Movahedin M, Mazaheri Z, Amirjannati N. In vitro transplantation of spermatogonial stem cells isolated from human frozen-thawed testis tissue can induce spermatogenesis under 3-dimensional tissue culture conditions. Biol Res (2019) 52:16. doi: 10.1186/S40659-019-0223-X/FIGURES/5
207. Gholami K, Pourmand G, Koruji M, Ashouri S, Abbasi M. Organ culture of seminiferous tubules using a modified soft agar culture system. Stem Cell Res Ther (2018) 9:1–8. doi: 10.1186/S13287-018-0997-8/FIGURES/4
208. Lee JH, Oh JH, Lee JH, Kim MR, Min CK. Evaluation of in vitro spermatogenesis using poly(D,L-lactic-co-glycolic acid) (PLGA)-based macroporous biodegradable scaffolds. J Tissue Eng Regener Med (2011) 5:130–7. doi: 10.1002/TERM.297
209. Alves-Lopes JP, Stukenborg JB. Testicular organoids: A new model to study the testicular microenvironment in vitro? Hum Reprod Update (2018) 24:176–91. doi: 10.1093/HUMUPD/DMX036
210. Gao H, Liu C, Wu B, Cui H, Zhao Y, Duan Y, et al. Effects of different biomaterials and cellular status on testicular cell self-organization. Adv Biosyst (2020) 4:1900292. doi: 10.1002/ADBI.201900292
211. Lee DR, Kaproth MT, Parks JE. In vitro production of haploid germ cells from fresh or frozen-thawed testicular cells of neonatal bulls. Biol Reprod (2001) 65:873–8. doi: 10.1095/BIOLREPROD65.3.873
212. Ghorbani S, Eyni H, Khosrowpour Z, Salari Asl L, Shabani R, Nazari H, et al. Spermatogenesis induction of spermatogonial stem cells using nanofibrous poly(l-lactic acid)/multi-walled carbon nanotube scaffolds and naringenin. Polym Adv Technol (2019) 30:3011–25. doi: 10.1002/PAT.4733
213. Slahi N, Hadjighassem MR, Joghataei MT, Bakhtiyari M, Ayyoubiyan M, Asadi MH, et al. The effects of plla nanofiber scaffold on proliferation of frozen-thawed neonate mouse spermatogonial stem cells. Anatomical Sci J (2012) 9:280–94.
214. Tseng H, Liu Y-L, Lu B-J, Kupiec-Weglinski W, Roselló-Catafau J, Adam R, et al. Immature testicular tissue engineered from weaned mice to adults for prepubertal fertility Preservation—An In vivo translational study. Int J Mol Sci (2022) 23:2042. doi: 10.3390/IJMS23042042
215. Talebi A, Ali Sadighi Gilani M, Koruji M, Ai J, Navid S, Jafar Rezaie M, et al. Proliferation and differentiation of mouse spermatogonial stem cells on a three-dimensional surface composed of PCL/Gel nanofibers proliferación y diferenciación de células madre espermatogónicas de ratón en una superficie tridimensional compuesta de nanofibras PCL / gel. Int J Morphol (2019) 37:1132–41.
216. Baert Y, Dvorakova-Hortova K, Margaryan H, Goossens E. Mouse in vitro spermatogenesis on alginate-based 3D bioprinted scaffolds. Biofabrication (2019) 11:035011. doi: 10.1088/1758-5090/AB1452
217. Kiani M, Movahedin M, Halvaei I, Soleimani M. Formation of organoid-like structures in the decellularized rat testis. Iran J Basic Med Sci (2021) 24:1523. doi: 10.22038/IJBMS.2021.58294.12948
218. Naeemi S, Eidi A, Khanbabaee R, Sadri-Ardekani H, Kajbafzadeh AM. Differentiation and proliferation of spermatogonial stem cells using a three-dimensional decellularized testicular scaffold: A new method to study the testicular microenvironment in vitro. Int Urol Nephrol (2021) 53:1543–50. doi: 10.1007/S11255-021-02877-9/FIGURES/8
219. Ashouri Movassagh S, Ashouri Movassagh S, Banitalebi Dehkordi M, Pourmand G, Gholami K, Talebi A, et al. Isolation, identification and differentiation of human spermatogonial cells on three-dimensional decellularized sheep testis. Acta Histochem (2020) 122:151623. doi: 10.1016/J.ACTHIS.2020.151623
220. Baert Y, Stukenborg JB, Landreh M, de Kock J, Jörnvall H, Söder O, et al. Derivation and characterization of a cytocompatible scaffold from human testis. Hum Reprod (2015) 30:256–67. doi: 10.1093/HUMREP/DEU330
221. Vermeulen M, del Vento F, de Michele F, Poels J, Wyns C. Development of a cytocompatible scaffold from pig immature testicular tissue allowing human sertoli cell attachment, proliferation and functionality. Int J Mol Sci (2018) 19:227. doi: 10.3390/IJMS19010227
222. Gharenaz NM, Movahedin M, Mazaheri Z. Comparison of two methods for prolong storage of decellularized mouse whole testis for tissue engineering application: An experimental study. Int J Reprod BioMed (2021) 19:321. doi: 10.18502/IJRM.V19I4.9058
223. Batista VF, de Sá Schiavo Matias G, Carreira ACO, Smith LC, Rodrigues R, Araujo MS, et al. Recellularized rat testis scaffolds with embryoid bodies cells: A promising approach for tissue engineering Taylor & FrancisEngland & Wales No. 30990675 Howick Place | London | SW1P 1WG, Vol. 68. (2022). pp. 44–54. doi: 10.1080/19396368.2021.2007554.
224. Movassagh SA, Dehkordi MB, Koruji M, Pourmand G, Farzaneh P, Movassagh SA, et al. In vitro spermatogenesis by three-dimensional culture of spermatogonial stem cells on decellularized testicular matrix. Galen Med J (2019) 8:e1565. doi: 10.31661/GMJ.V8I0.1565
225. Akbarzadeh A, Kianmanesh M, Fendereski K, Ebadi M, Daryabari SS, Masoomi A, et al. Decellularised whole ovine testis as a potential bio-scaffold for tissue engineering. Reprod Fertil Dev (2019) 31:1665–73. doi: 10.1071/RD19070
226. Majidi Gharenaz N, Movahedin M, Mazaheri Z. Production of biocompatible testis scaffold for use in tissue engineering. Razi J Med Sci (2020) 27:37–48.
227. Donnaloja F, Jacchetti E, Soncini M, Raimondi MT. Natural and synthetic polymers for bone scaffolds optimization. Polymers (2020) 12:905. doi: 10.3390/POLYM12040905
228. Wasyłeczko M, Sikorska W, Chwojnowski A. Review of synthetic and hybrid scaffolds in cartilage tissue engineering. Membranes (2020) 10:348. doi: 10.3390/MEMBRANES10110348
229. Joshi A, Xu Z, Ikegami Y, Yamane S, Tsurashima M, Ijima H. Co-Culture of mesenchymal stem cells and human umbilical vein endothelial cells on heparinized polycaprolactone/gelatin co-spun nanofibers for improved endothelium remodeling. Int J Biol Macromol (2020) 151:186–92. doi: 10.1016/J.IJBIOMAC.2020.02.163
230. Bacakova L, Zikmundova M, Pajorova J, Broz A, Filova E, Blanquer A, et al. Nanofibrous scaffolds for skin tissue engineering and wound healing based on synthetic polymers, in: Applications of nanobiotechnology (2019). Available at: https://books.google.com.br/books?hl=pt-BR&lr=&id=Kkv9DwAAQBAJ&oi=fnd&pg=PA33&dq=Bacakova,+L.,+Zikmundova,+M.,+Pajorova,+J.,+Broz,+A.,+Filova,+E.,+Blanquer,+A.,+.+%26+Sinica,+A.+(2019).+Nanofibrous+scaffolds+for+skin+tissue+engineering+and+wound+healing+based+on+synthetic+polymers.+Applications+of+nanobiotechnology,+1.&ots=xjZpisA0dP&sig=3XGSjH61NSKZyevclpvhQ-hx1i0#v=onepage&q&f=false (Accessed October 22, 2022).
231. Asadi N, del Bakhshayesh AR, Davaran S, Akbarzadeh A. Common biocompatible polymeric materials for tissue engineering and regenerative medicine. Mater Chem Phys (2020) 242:122528. doi: 10.1016/J.MATCHEMPHYS.2019.122528
232. Xin L, Lin X, Pan Y, Zheng X, Shi L, Zhang Y, et al. A collagen scaffold loaded with human umbilical cord-derived mesenchymal stem cells facilitates endometrial regeneration and restores fertility. Acta Biomater (2019) 92:160–71. doi: 10.1016/J.ACTBIO.2019.05.012
233. Richer G, Baert Y, Goossens E. In-vitro spermatogenesis through testis modelling: Toward the generation of testicular organoids. Andrology (2020) 8:879–91. doi: 10.1111/ANDR.12741
234. Sun M, Yuan Q, Niu M, Wang H, Wen L, Yao C, et al. Efficient generation of functional haploid spermatids from human germline stem cells by three-dimensional-induced system. Cell Death Differentiation (2018) 25:749–66. doi: 10.1038/s41418-017-0015-1
235. Silva AF, Escada-Rebelo S, Amaral S, Tavares RS, Schlatt S, Ramalho-Santos J, et al. Can we induce spermatogenesis in the domestic cat using an in vitro tissue culture approach? PloS One (2018) 13:e0191912. doi: 10.1371/JOURNAL.PONE.0191912
236. Wu X, Su J, Wei J, Jiang N, Ge X. Recent advances in three-dimensional stem cell culture systems and applications. Stem Cells Int (2021) 2021. doi: 10.1155/2021/9477332
237. Hemadi M, Assadollahi V, Saki G, Pirnia A, Alasvand M, Zendehdel A, et al. Use of alginate hydrogel to improve long-term 3D culture of spermatogonial stem cells: stemness gene expression and structural features. Zygote (2022) 30:312–8. doi: 10.1017/S0967199421000551
238. Jalayeri M, Pirnia A, Najafabad EP, Varzi AM, Gholami M. Evaluation of alginate hydrogel cytotoxicity on three-dimensional culture of type a spermatogonial stem cells. Int J Biol Macromol (2017) 95:888–94. doi: 10.1016/J.IJBIOMAC.2016.10.074
239. Veisi M, Mansouri K, Assadollahi V, Jalili C, Pirnia A, Salahshoor MR, et al. Evaluation of co-cultured spermatogonial stem cells encapsulated in alginate hydrogel with sertoli cells and their transplantation into azoospermic mice. Zygote (2022) 30:344–51. doi: 10.1017/S0967199421000733
240. Chiti MC, Dolmans MM, Donnez J, Amorim CA. Fibrin in reproductive tissue engineering: A review on its application as a biomaterial for fertility preservation. Ann BioMed Eng (2017) 45:1650–63. doi: 10.1007/S10439-017-1817-5/FIGURES/7
241. Roya Ramzgouyan M, Mohammad Tavangar S, Hadjati J, Amidi F. Human endometrial stem cells (hEnSCs) differentiation into germ cell-like cells by encapsulating in fibrin scaffold. Available at: https://www.researchgate.net/profile/Maryam-Roya-Ramzgouyan-3/publication/274781021_Human_endometrial_stem_cells_hEnSCs_differentiation_into_germ_cell-like_cells_by_encapsulating_in_fibrin_scaffold/links/554dc7a608ae12808b350d6b/Human-endometrial-stem-cells-hEnSCs-differentiation-into-germ-cell-like-cells-by-encapsulating-in-fibrin-scaffold.pdf (Accessed October 24, 2022).
242. Lee JH, Kim HJ, Kim H, Lee SJ, Gye MC. In vitro spermatogenesis by three-dimensional culture of rat testicular cells in collagen gel matrix. Biomaterials (2006) 27:2845–53. doi: 10.1016/J.BIOMATERIALS.2005.12.028
243. Zhang J, Hatakeyama J, Eto K, Abe SI. Reconstruction of a seminiferous tubule-like structure in a 3 dimensional culture system of re-aggregated mouse neonatal testicular cells within a collagen matrix. Gen Comp Endocrinol (2014) 205:121–32. doi: 10.1016/J.YGCEN.2014.03.030
244. Lee H, Lee J, Chansakul T, Yu C. Biomaterials JE-, 2006 undefined. collagen mimetic peptide-conjugated photopolymerizable PEG hydrogel . Elsevier. Available at: https://www.sciencedirect.com/science/article/pii/S0142961206005084 (Accessed October 24, 2022).
245. Alves-Lopes JP, Söder O, Stukenborg JB. Testicular organoid generation by a novel in vitro three-layer gradient system. Biomaterials (2017) 130:76–89. doi: 10.1016/J.BIOMATERIALS.2017.03.025
246. Ribeiro-Filho LA, Sievert KD. Acellular matrix in urethral reconstruction. Adv Drug Delivery Rev (2015) 82–83:38–46. doi: 10.1016/J.ADDR.2014.11.019
247. Rezaei Topraggaleh T, Rezazadeh Valojerdi M, Montazeri L, Baharvand H. A testis-derived macroporous 3D scaffold as a platform for the generation of mouse testicular organoids. Biomater Sci (2019) 7:1422–36. doi: 10.1039/C8BM01001C
248. Tosti E, Ménézo Y. Gamete activation: Basic knowledge and clinical applications. Hum Reprod Update (2016) 22:420–39. doi: 10.1093/HUMUPD/DMW014
249. Kim BS, Das S, Jang J, Cho DW. Decellularized extracellular matrix-based bioinks for engineering tissue- and organ-specific microenvironments. Chem Rev (2020) 120:10608–61. doi: 10.1021/ACS.CHEMREV.9B00808/ASSET/IMAGES/LARGE/CR9B00808_0018.JPEG
250. Neves SC, Moroni L, Barrias CC, Granja PL. Leveling up hydrogels: Hybrid systems in tissue engineering. Trends Biotechnol (2020) 38:292–315. doi: 10.1016/J.TIBTECH.2019.09.004
251. Almeida GHD, Iglesia RP, Araujo MS, Carreira ACO, Santos EX, Calomeno CVAQ, et al Uterine Tissue Engineering: Where We Stand and the Challenges Ahead. (2022) 28:861–890. Available at: https://home.liebertpub.com/teb.
252. Bertsch P, Diba M, Mooney DJ, Leeuwenburgh SCG. Self-healing injectable hydrogels for tissue regeneration. Chem Rev (2022) 2:834–73.
253. Sadri-Ardekani H, Atala A. Regenerative medicine and cell therapy. Stem Cell Nanoengineering (2015), 47–65. doi: 10.1002/9781118540640.CH4
254. Mäkelä JA, Hobbs RM. Molecular regulation of spermatogonial stem cell renewal and differentiation. Reproduction (2019) 158:R169–87. doi: 10.1530/REP-18-0476
255. Gattazzo F, Urciuolo A, Bonaldo P. Extracellular matrix: A dynamic microenvironment for stem cell niche. Biochim Biophys Acta (BBA) - Gen Subj (2014) 1840:2506–19. doi: 10.1016/J.BBAGEN.2014.01.010
256. Bachmann M, Kukkurainen S, Hytönen VP, Wehrle-Haller B. Cell adhesion by integrins. Physiol Rev (2019) 99:1655–99. doi: 10.1152/PHYSREV.00036.2018/ASSET/IMAGES/LARGE/Z9J0041929120009.JPEG
257. Nicolas J, Magli S, Rabbachin L, Sampaolesi S, Nicotra F, Russo L. 3D extracellular matrix mimics: Fundamental concepts and role of materials chemistry to influence stem cell fate. Biomacromolecules (2020) 21:1968–94. doi: 10.1021/ACS.BIOMAC.0C00045/ASSET/IMAGES/LARGE/BM0C00045_0012.JPEG
258. Vermeulen M, Poels J, de Michele F, des Rieux A, Wyns C. Restoring fertility with cryopreserved prepubertal testicular tissue: Perspectives with hydrogel encapsulation, nanotechnology, and bioengineered scaffolds. Ann BioMed Eng (2017) 45:1770–81. doi: 10.1007/S10439-017-1789-5/TABLES/3
259. Jafari M, Paknejad Z, Rad MR, Motamedian SR, Eghbal MJ, Nadjmi N, et al. Polymeric scaffolds in tissue engineering: A literature review. J BioMed Mater Res B Appl Biomater (2017) 105:431–59. doi: 10.1002/JBM.B.33547
260. Silva R, Fabry B, Boccaccini AR. Fibrous protein-based hydrogels for cell encapsulation. Biomaterials (2014) 35:6727–38. doi: 10.1016/J.BIOMATERIALS.2014.04.078
261. de Michele F, Vermeulen M, Wyns C. Fertility restoration with spermatogonial stem cells. Curr Opin Endocrinol Diabetes Obes (2017) 24:424–31. doi: 10.1097/MED.0000000000000370
262. Liu X, Wu K, Gao L, Wang L, Shi X. Biomaterial strategies for the application of reproductive tissue engineering. Bioact Mater (2022) 14:86–96. doi: 10.1016/J.BIOACTMAT.2021.11.023
263. Almeida MGHDR, Iglesia DRP, Rinaldi PJDC, Murai MMK, Calomeno MCVAQ, Junior MLNDS, et al Current Trends onBioengineering Approaches for Ovarian Microenvironment Reconstruction. (2022). Available at: https://home.liebertpub.com/teb.
264. Gilbert TW, Sellaro TL, Badylak SF. Decellularization of tissues and organs. Biomaterials (2006) 27:3675–83. doi: 10.1016/J.BIOMATERIALS.2006.02.014
265. Martin I, Wendt D, Heberer M. The role of bioreactors in tissue engineering. Trends Biotechnol (2004) 22:80–6. doi: 10.1016/J.TIBTECH.2003.12.001
266. Böer U, Lohrenz A, Klingenberg M, Pich A, Haverich A, Wilhelmi M. The effect of detergent-based decellularization procedures on cellular proteins and immunogenicity in equine carotid artery grafts. Biomaterials (2011) 32:9730–7. doi: 10.1016/J.BIOMATERIALS.2011.09.015
267. Jahanbani Y, Davaran S, Ghahremani-Nasab M, Aghebati-Maleki L, Yousefi M. Scaffold-based tissue engineering approaches in treating infertility. Life Sci (2020) 240:117066. doi: 10.1016/J.LFS.2019.117066
268. Ovsianikov A, Khademhosseini A, Mironov V. The synergy of scaffold-based and scaffold-free tissue engineering strategies. Trends Biotechnol (2018) 36:348–57. doi: 10.1016/J.TIBTECH.2018.01.005
269. Cui H, Nowicki M, Fisher JP, Zhang LG. 3D bioprinting for organ regeneration. Adv Healthc Mater (2017) 6:1601118. doi: 10.1002/ADHM.201601118
270. Pendergraft SS, Sadri-Ardekani H, Atala A, Bishop CE. Three-dimensional testicular organoid: A novel tool for the study of human spermatogenesis and gonadotoxicity in vitro. Biol Reprod (2017) 96:720–32. doi: 10.1095/BIOLREPROD.116.143446
271. Azizi H, Skutella T, Shahverdi A. Generation of mouse spermatogonial stem-Cell-Colonies in a non-adherent culture. Cell J (Yakhteh) (2017) 19:238. doi: 10.22074/CELLJ.2016.4184
272. Kashaninejad N, Shiddiky MJA, Nguyen NT. Advances in microfluidics-based assisted reproductive technology: From sperm sorter to reproductive system-on-a-Chip. Adv Biosyst (2018) 2:1700197. doi: 10.1002/ADBI.201700197
273. Daniele MA, Boyd DA, Adams AA, Ligler FS. Microfluidic strategies for design and assembly of microfibers and nanofibers with tissue engineering and regenerative medicine applications. Adv Healthc Mater (2015) 4:11–28. doi: 10.1002/ADHM.201400144
274. Komeya M, Yamanaka H, Sanjo H, Yao M, Nakamura H, Kimura H, et al. In vitro spermatogenesis in two-dimensionally spread mouse testis tissues. Reprod Med Biol (2019) 18:362–9. doi: 10.1002/RMB2.12291
275. Kojima K, Nakamura H, Komeya M, Yamanaka H, Makino Y, Okada Y, et al. Neonatal testis growth recreated in vitro by two-dimensional organ spreading. Biotechnol Bioeng (2018) 115:3030–41. doi: 10.1002/BIT.26822
276. Garreta E, Oria R, Tarantino C, Pla-Roca M, Prado P, Fernández-Avilés F, et al. Tissue engineering by decellularization and 3D bioprinting. Materials Today (2017) 20:166–78. doi: 10.1016/J.MATTOD.2016.12.005
277. Beheshtizadeh N, Lotfibakhshaiesh N, Pazhouhnia Z, Hoseinpour M, Nafari M. A review of 3D bio-printing for bone and skin tissue engineering: A commercial approach. J Materials Sci (2019) 55:3729–49. doi: 10.1007/S10853-019-04259-0
278. Gopinathan J, Noh I. Recent trends in bioinks for 3D printing. Biomaterials Res (2018) 22:1–15. doi: 10.1186/S40824-018-0122-1
Keywords: testis, biomaterials, spermatogenesis, bioengeneering, reproduction
Citation: Horvath-Pereira BdO, Almeida GHDR, Silva Júnior LNd, do Nascimento PG, Horvath Pereira BdO, Fireman JVBT, Pereira MLdRF, Carreira ACO and Miglino MA (2023) Biomaterials for Testicular Bioengineering: How far have we come and where do we have to go? Front. Endocrinol. 14:1085872. doi: 10.3389/fendo.2023.1085872
Received: 31 October 2022; Accepted: 24 February 2023;
Published: 16 March 2023.
Edited by:
Ludovic Dumont, Université de Rouen, FranceReviewed by:
Xiangguo Wang, Beijing University of Agriculture, ChinaLouise Saldutti, Merck, United States
Copyright © 2023 Horvath-Pereira, Almeida, Silva Júnior, do Nascimento, Horvath Pereira, Fireman, Pereira, Carreira and Miglino. This is an open-access article distributed under the terms of the Creative Commons Attribution License (CC BY). The use, distribution or reproduction in other forums is permitted, provided the original author(s) and the copyright owner(s) are credited and that the original publication in this journal is cited, in accordance with accepted academic practice. No use, distribution or reproduction is permitted which does not comply with these terms.
*Correspondence: Maria Angelica Miglino, bWlnbGlub0B1c3AuYnI=