- School of Basic Medical Sciences & Forensic Medicine, Hangzhou Medical College, Hangzhou, China
In recent years, the risk, such as hypertension, obesity and diabetes mellitus, of cardiovascular diseases has been increasing explosively with the development of living conditions and the expansion of social psychological pressure. The disturbance of glucose and lipid metabolism contributes to both collapse of myocardial structure and cardiac dysfunction, which ultimately leads to diabetic cardiomyopathy. The pathogenesis of diabetic cardiomyopathy is multifactorial, including inflammatory cascade activation, oxidative/nitrative stress, and the following impaired Ca2+ handling induced by insulin resistance/hyperinsulinemia, hyperglycemia, hyperlipidemia in diabetes. Some key alterations of cellular signaling network, such as translocation of CD36 to sarcolemma, activation of NLRP3 inflammasome, up-regulation of AGE/RAGE system, and disequilibrium of micro-RNA, mediate diabetic oxidative stress/inflammation related myocardial remodeling and ventricular dysfunction in the context of glucose and lipid metabolic disturbance. Here, we summarized the detailed oxidative stress/inflammation network by which the abnormality of glucose and lipid metabolism facilitates diabetic cardiomyopathy.
1 Introduction
There are three major nutrients, including carbohydrates, fats and proteins, for human body’s metabolism. The monosaccharide, especially glucose, hydrolyzed from carbohydrates provide indispensable energy for cellular function and survival. Diabetes mellitus is characterized by dysregulation of insulin pathway and insulin resistance leading to the disorder of carbohydrate metabolism and hyperglycemia. Microvascular and macrovascular abnormalities, resulting in diabetic retinopathy, nephropathy, neuropathy and cardiomyopathy, are primary causes of high disability and mortality in diabetes (1, 2). Diabetic cardiomyopathy (DCM) is confirmed to be inseparably related to the abnormal myocardial energy metabolism (3). With the development of diabetes, the inhibition of glucose utilization is accompanied with the increase of fatty acid β-oxidation to satisfy the cellular energy requirement (4). Lots of cellular signaling transduction pathways associating with inflammatory response and oxidative stress are abnormally regulated in the diabetic heart and cardiomyocyte because of the disturbance of glucose and lipid metabolism, which is involved in the development of cardiac hypertrophy, fibrosis and heart failure (5, 6). Here, we summarized the crosstalk between the abnormality of glucose and lipid metabolism and DCM focusing on oxidative stress and inflammation.
2 What is diabetic cardiomyopathy
It has been estimated in IDF Diabetes Atlas (the 10th edition) that the global number of diabetes in the age of 20-79 was 537 million in 2021 (an increase of 16% compared with that in 2019), which has been projected to reach 643 million by 2030, and more than 6.7 million adults have died (about 12.2% of global death) due to diabetes and diabetic complications in 2021 (http://diabetesatlas.org/atlas/tenth-edition/). Such a high prevalence of diabetes and a hug number of diabetes-related death confirm that diabetes is one of the fastest growing global health emergencies in the 21st century. Despite the different pathophysiology of type 1 diabetes mellitus (T1DM) and T2DM, chronic systemic hyperglycemia and the disorder of glucose and lipid metabolism ultimately result in severe complications, such as blindness, kidney failure, cardiomyopathy, stroke, and lower limb amputation (2). The cardiovascular system is one of the most vulnerable targets of diabetes and suffers from endothelial dysfunction, atherosclerosis, cardiomyopathy, and even heart failure, which accounts for the major cause of morbidity and mortality in diabetic patients (7). Diabetes confers about a two-fold excess risk for cardiovascular disease independently from other conventional risk factors (8) and three-fold higher cardiovascular mortality had been reported in diabetic subjects by the Framingham Heart Study (4, 9). DCM is defined as a chronic metabolic heart disease in the absence of congenital heart disease, coronary artery disease, cardiac valve disease, or hypertension and is one of the major causes of the mortality in diabetic patients (10, 11). The typical manifest of DCM includes cardiac remodeling (hypertrophy and fibrosis) and dysfunction (12). Left ventricular diastolic dysfunction is one of the earliest characteristics of the diabetic heart and systolic dysfunction, even heart failure, develop at a later stage of DCM independent of hypertension and ischemic coronary artery disease (4, 12, 13).
3 Metabolic disorder in the diabetic heart
Although the normal adult heart can use a variety of energy substrates, such as glucose, lactate, fatty acids, ketones, and amino acids for the continuous requirement of energy, but it primarily utilizes long-chain fatty acids (LCFA) to supply 50%-70% of energy (4, 14). Chronic alterations of myocardial substrate preference, which begins from the early stage of diabetes, can contribute to DCM. As shown in Figure 1, the decrease in utilization of glucose owing to insulin resistance promotes fatty acid β-oxidation to supply 90%-100% of energy associated with the translocation of cluster of differentiation 36 (CD36), a fatty acid transporter, into the sarcolemma and enhanced LCFA uptake in the cardiomyocyte, meanwhile leads to the lipotoxic cardiomyopathy in diabetes (14–16). Irreversible hyperglycemia and over-use of fatty acid β-oxidation, as forerunners in diabetes (13), break the cellular homeostasis of oxidation-reduction reaction and provoke cardiac inflammatory response, characterized by the over-production of reactive oxygen species (ROS) and pro-inflammatory factors, which contributes to the development of DCM, including ventricular remodeling, hypertrophy, myocardial stiffness, cardiac dysfunction, and heart failure (4, 13, 17). The impaired myocardial structure and function both contribute to further abnormalities of glucose and lipid metabolism in the diabetic heart, generating a positive feedback loop to exacerbate the cardiac injury in diabetes, which can not be ignored in the development of DCM (3, 18–20). Thus, rectifying metabolic disorder in the diabetic patient has been clinically accepted to attenuate cardiovascular complications.
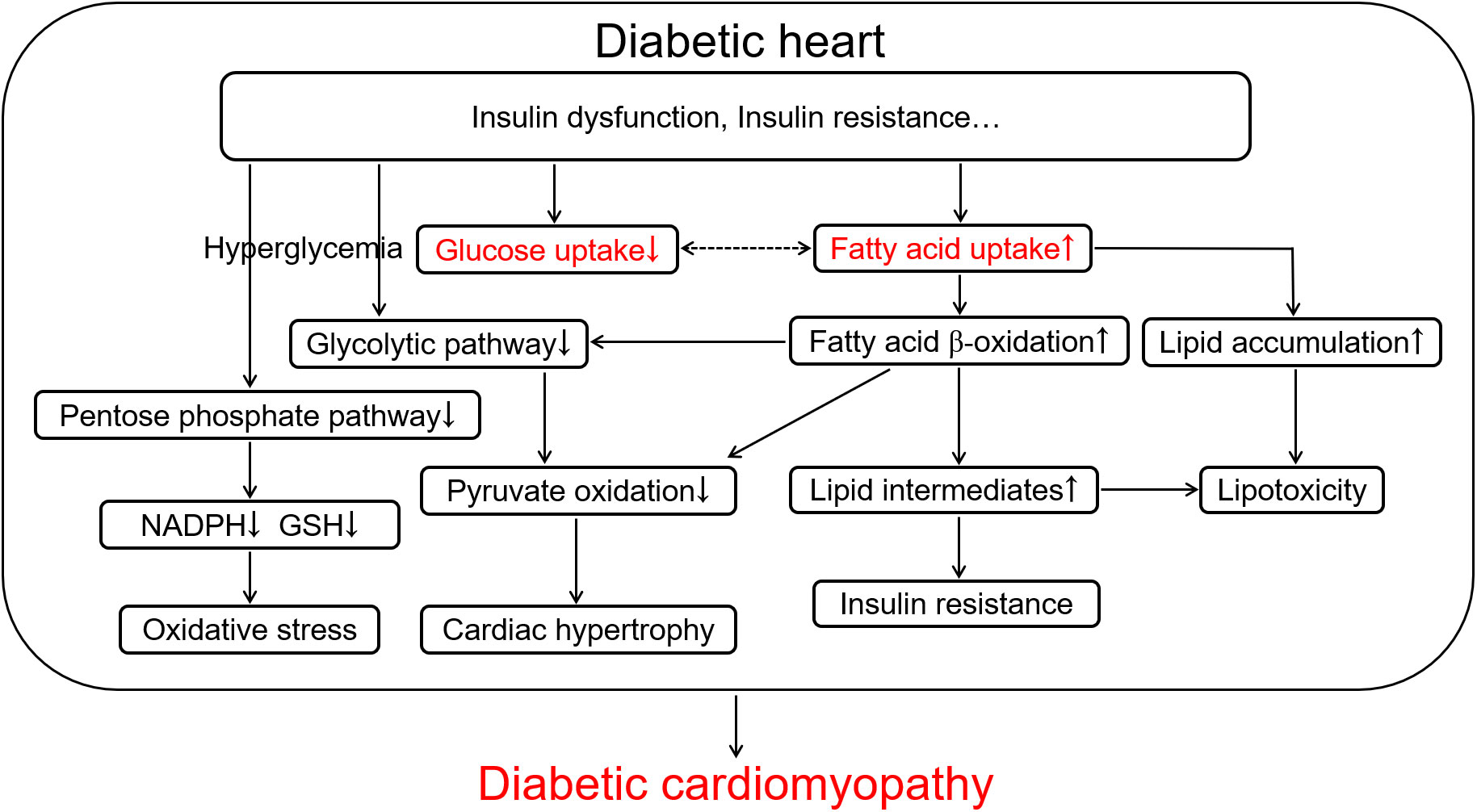
Figure 1 The scheme of glucose and lipid metabolism disorder in the diabetic heart contributing to diabetic cardiomyopathy. Diabetes-induced insulin dysfunction and insulin resistance cause the increase in fatty acid uptake characterized by up-regulation of fatty acid β-oxidation and lipid accumulation and the decrease in glucose uptake accompanying with hyperglycemia and down-regulation of pentose phosphate pathway and glycolytic pathway in the cardiomyocyte. Up-regulation of fatty acid β-oxidation further promotes the inhibition of glycolytic pathway and pyruvate oxidation, and increases lipid intermediates and lipotoxicity. These metabolic alterations in diabetes ultimately cause oxidative stress, further insulin resistance, and cardiac hypertrophy, which aggravates the development of diabetic cardiomyopathy. NADPH, reduced form of nicotinamide adenine dinucleotide phosphate; GSH, reduced glutathione.
3.1 Glycometabolism disorder and DCM
Hyperglycemia is an independent risk factor for DCM (5). The membrane glucose transporters (GLUTs) are responsible for the uptake of glucose into the cardiomyocyte. The shortage of insulin signaling or insulin resistance results in the internalization of GLUT4 and a marked down-regulation of membrane GLUT4 (Figure 2), which finally leads to the decreased glucose uptake in cells and hyperglycemia (21, 22) and compensatory increased LCFA uptake to break the equilibrium of myocardial energy metabolism in diabetes (5). Chronic hyperglycemia induces complicated alterations, including activation of sorbitol and hexosamine pathways, the increase of advanced glycation end-products (AGEs) and receptors for AGEs (RAGEs), and the explosion of ROS and proinflammatory factors, to result in cardiac remodeling and dysfunction in diabetes (Figure 2) (5, 13, 23).
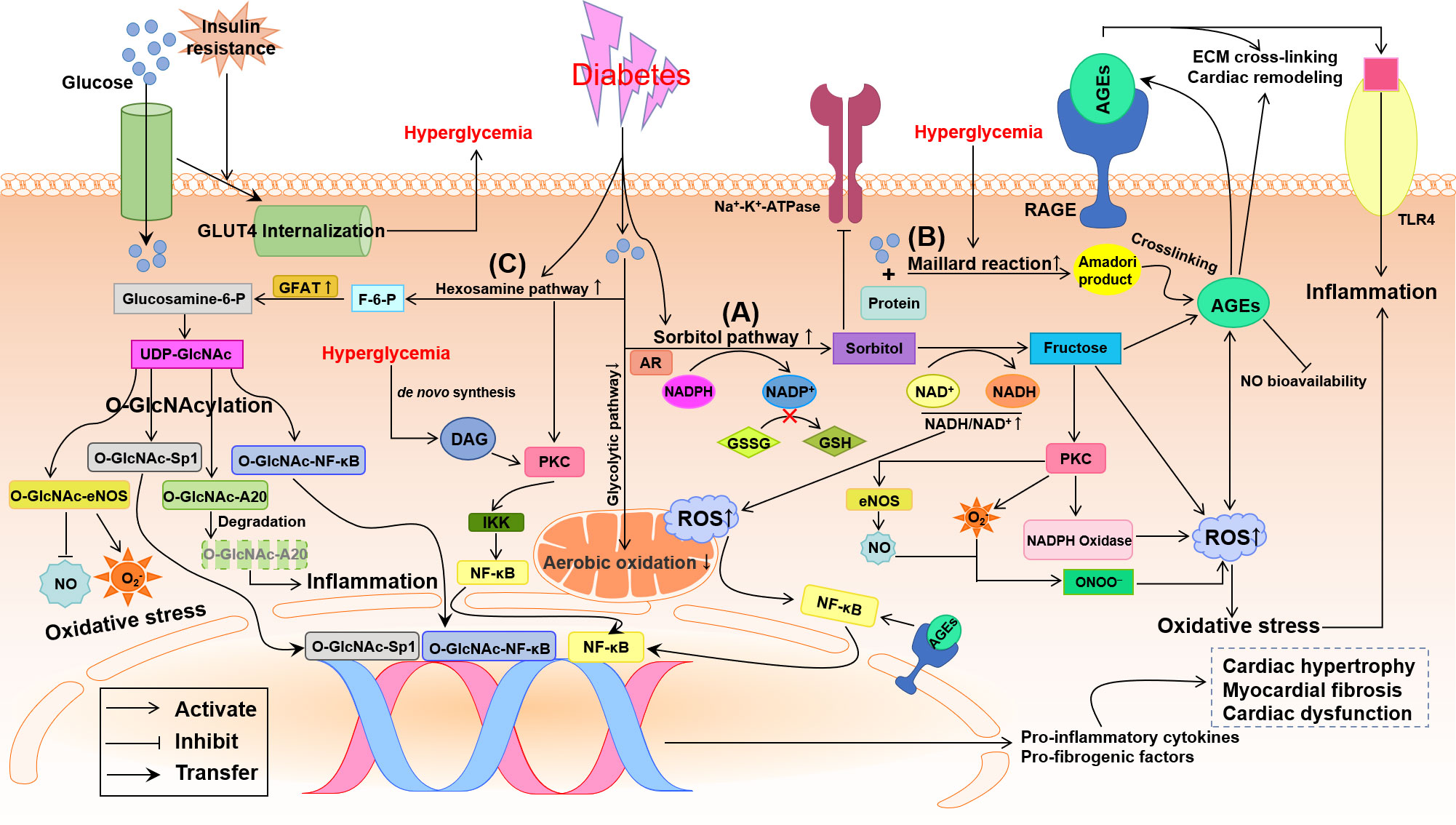
Figure 2 Potential pathways by which glycometabolism disorder damages the diabetic heart. Insulin resistance promotes the internalization of glucose transporter 4 (GLUT4) that means the decrease of transmembrane GLUT4 to inhibit glucose uptake in the diabetic cardiomyocyte. Oxidative stress and inflammation induced by the disturbance of glycometabolism involve the activation of three major pathways: sorbitol pathway, advanced glycation end-products (AGEs)/receptor of AGE (RAGE) and hexosamine pathway. (A) Up-regulation of sorbitol pathway augments oxidative stress through decreasing the reduced glutathione (GSH) and increasing reactive oxygen species (ROS), which causes nuclear factor κ-light-chain-enhancer of activated B cells (NF-κB)-induced inflammation. (B) Hyperglycemia boosts the formation AGEs and activates the AGE/RAGE system, which results in extracellular matrix (ECM) cross-linking, cardiac remodeling, nitric oxide (NO) inactivation and oxidative stress. (C) Up-regulation of hexosamine biosynthesis in diabetes leads to O-linked N-acetylglucosamine glycosylation (O-GlcNAcylation) modification of proteins, producing O-GlcNAc-A20, endothelial NO synthase (eNOS), Sp1 and NF-κB. O-GlcNAcylation of A20 accelerates its ubiquitination and proteasomal degradation resulting in inflammation. O-GlcNAcylation of Sp1 and NF-κB activate the transcriptional function to up-regulate pro-inflammatory and pro-fibrogenic factors. O-GlcNAc-eNOS decreases NO formation while increases superoxide anion (O2-) production. In addition, hyperglycemia may also activate protein kinase C (PKC), directly through de novo synthesis of diacylglycerol (DAG) and indirectly through increased flux of sorbitol pathway and hexosamine pathway, to promote inflammation and oxidative stress. All these glycometabolism disorders result in cardiac remodeling and dysfunction in diabetes. AR, aldose reductase; F-6-P, fructose 6-phosphate; GFAT, glutamine-fructose-6-phosphate amidotransferase; Glucosamine-6-P, glucosamine-6-phosphate; GSSH, oxidized glutathione; IKK, IκB kinase; NADH/NAD+, reduced/oxidized form of nicotinamide adenine dinucleotide; NADP+, nicotinamide adenine dinucleotide phosphate; NADPH, reduced form of NADP+; ONOO-, peroxynitrite; TLR4, toll-like receptor 4; UDP-GlcNAc, UDP-N-acetylglucosamine.
3.1.1 Up-regulation of sorbitol pathway
With the development of diabetes, aldose reductase (AR), a rate-limiting enzyme in sorbitol pathway, is up-regulated to convert glucose into sorbitol at the expense of reduced form of nicotinamide adenine dinucleotide phosphate (NADPH). Sorbitol is, in turn, converted into fructose by sorbitol dehydrogenase at the expense of oxidized form of nicotinamide adenine dinucleotide (NAD+) (Figure 2A) (24–26). Fructose can be further metabolized into fructose 3-phosphate and 3-deoxyglucosone, two potent nonenzymatic glycation agents, to augment the formation of AGEs, resulting in ROS production (25). Because NADPH is required for the shift of oxidized glutathione (GSSH) to reduced glutathione (GSH), an endogenous antioxidant, the consumption of NADPH by sorbitol pathway up-regulated by chronic hyperglycemia breaks the cellular antioxidant capacity (Figure 2A) (27). Thus the increase in intracellular sorbitol produced by sorbitol pathway has been identified as a biomarker of oxidative stress. Besides, the up-regulation of sorbitol pathway aberrant increases the activation of protein kinase C (PKC) (2). Accumulation of intracellular sorbitol causes hyperosmotic stress and the decreased activity of Na+-K+-ATPase (2, 23). An increase in cytosolic NADH/NAD+ triggers mitochondrial dependent ROS formation (27). All these alterations induced by the up-regulation of sorbitol pathway in diabetes augments oxidative stress and leads to diabetic cardiovascular complications (Figure 2A).
3.1.2 Accumulation of AGEs
The AGEs formation is closely related to hyperglycemia and involves the development of diabetic cardiovascular complications (28, 29). Under long-standing hyperglycemia, reducing sugars such as glucose and fructose non-enzymatically binds with amino-acid residues in proteins, lipids and nucleic acids to form Schiff bases and Amadori products, the so-called Maillard reaction (Figure 2B), resulting in the accumulation of AGEs (30). The connective tissue matrix and membrane are prime targets of advanced glycation, by which high concentrations of AGEs are accumulated in cardiac tissues characterized by extracellular matrix (ECM) cross-linking (4) and myocardial stiffness (31). Formation of AGEs also produces excess ROS, which positively promotes the surges of AGEs, and reduces the bioavailability of NO leading to oxidative stress. AGEs activate RAGE to amply the inflammatory response by modulating nuclear factor κ-light-chain-enhancer of activated B cells (NF-κB) signaling and toll-like receptor 4 (TLR4) pathway (Figure 2B) (5, 31). These changes jointly promote myocardial fibrosis and diastolic dysfunction in diabetes. The interaction of AGE with a pattern recognition receptor termed RAGE affects numerous cell signal pathways related to inflammation and oxidative stress (31). Blocking RAGE signaling or knockdown RAGE gene has been demonstrated to alleviate cardiac hypertrophy and fibrosis and prevent the diabetic heart from systolic and diastolic dysfunction (32, 33).
3.1.3 Up-regulation of hexosamine biosynthesis
Under physiological conditions, only 2%-5% of the total glucose is metabolized through the hexosamine pathway (2, 27). When exposed to persistent hyperglycemia, the hexosamine pathway and the rate-limiting enzyme, glutamine-fructose-6-phosphate amidotransferase (GFAT), are both over activated to convert glucose into excessive fructose 6-phosphate (F-6-P), glucosamine-6-phosphate and finally into UDP-N-acetylglucosamine (UDP-GlcNAc) (Figure 2C) (34). UDP-GlcNAc can donate N-acetylglucosamine to serine or threonine residues of target proteins within the nucleus and cytosol forming O-linked glycoproteins of proteins, which is called post-translational modification of proteins by single O-linked N-acetylglucosamine glycosylation (O-GlcNAcylation) (2, 34). The increased O-GlcNAcylation modification of proteins was found to alter gene expression in the diabetic heart and to be associated with DCM (2, 23, 35, 36). Abnormally elevated O-GlcNAc modification of endothelial nitric oxide synthase (eNOS) at Ser1177 deactivates eNOS and inhibits NO production (37), which impairs vasodilation, ECM remodeling and angiogenesis (Figure 2C). Importantly, several transcription factors, such as NF-κB (37, 38) and Sp1 (Figure 2C) (39), can be directly/indirectly activated by hyperglycemia-induced O-GlcNAcylation leading to the up-regulation of pro-inflammatory factors, including TGF-β, TNF-α and PAI-1, and down-regulation of SERCA2a leading to abnormal intracellular Ca2+ transient (38–40). Hyperglycemia clears anti-inflammatory and atheroprotective protein A20 via O-GlcNAcylation-dependent ubiquitination and proteasomal degradation (Figure 2C), which may be key to the cardiovascular system (37, 41).
3.2 Lipid metabolism disorder and DCM
Early in diabetes, abnormal glycometabolism grants the increase in fatty acid β-oxidation to compensate for a shortage of energy in the diabetic heart, which reduces mitochondrial oxidative capacity, and cardiac efficiency characterized by low ratio of myocardial ATP production/oxygen consumption and high mitochondria-derived superoxide anion (O2-) (42). As shown in Figure 3, the high fatty acid β-oxidation is accompanied with violent intracellular accumulation of toxic lipid metabolites, which precipitates DCM through multiple mechanisms including excessive generation of ROS, endoplasmic reticulum (ER) stress, and mitochondrial remodeling (43). Numerous studies using transgenic animal models have shown that up-regulation of myocardial fatty acid transporters such as CD36 and fatty acid transport protein 1 contribute to high fatty acid intake and lipotoxicity in the cardiomyocyte, which finally exacerbates the development of DCM (13, 44, 45).
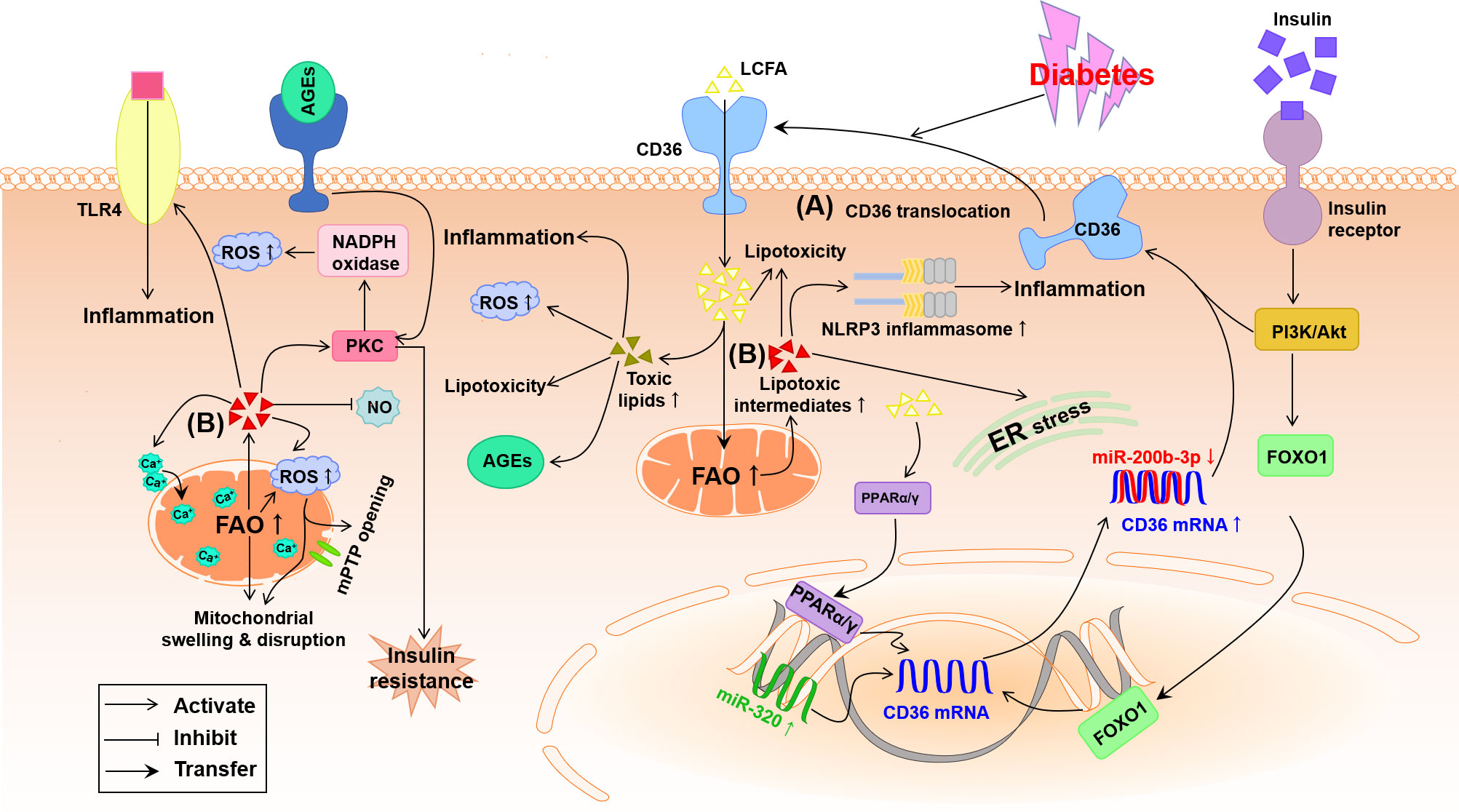
Figure 3 Potential pathways by which lipid metabolism disorder damages the diabetic heart. (A) Under diabetes conditions, the expression of cardiac PPARα/γ and FOXO1 is increased meanwhile miR-200b-3p is decreased and miR-320 is increased, which helps to up-regulation of CD36 causing the increase in LCFA uptake. (B) Abnormal FAO and accumulation of lipotoxic intermediates in the diabetic cardiomyocyte trigger oxidative stress, inflammation and ER stress. Furthermore, accumulation of lipotoxic intermediates in the diabetic cardiomyocyte increases mitochondrial uncoupling and impairment of mitochondria Ca2+ handing, leading to mitochondrial swelling and disruption through the opening of mitochondrial permeability transition pore (mPTP). AGEs, advanced glycation end-products; Akt, protein kinase B; CD36, cluster of differentiation 36; ER, endoplasmic reticulum; FAO, fatty acid oxidation; FOXO1, forkhead box protein O1; LCFA, long-chain fatty acid; NLRP3, nucleotide-binding domain, leucine-rich-containing family, pyrin domain containing 3; NO, nitric oxide; PI3K, phosphoinositide 3-kinase; PKC, protein kinase C; PPARα/γ, peroxisome proliferator-activated receptor α/γ; ROS, reactive oxygen species; TLR4, toll-like receptor 4.
3.2.1 Up-regulation of CD36
The LCFA transporter CD36, also named as scavenger receptor B2, has been evidenced to take oxidized low-density lipoproteins (oxLDLs) and phospholipids into myocytes and lipocytes of rodents and humans, and up to 70% of total fatty acids into cardiomyocytes (46, 47). The up-regulation of CD36 has been evidenced on the sarcolemma of cardiomyocytes in diabetic mice and patients with DCM, which is owing to both high membrane translocation and high expression of CD36 triggered by hyperinsulinemia, hyperglycemia and hyperlipidemia during the development of diabetes (5, 48). As suggested in Figure 3, high insulin at the beginning of diabetes up-regulates CD36 mRNA expression by activating the transcription factor forkhead box protein O1 (FOXO1) and strongly transports CD36 to sarcolemma by activating the PI3K-Akt pathway while chronic high glucose and high triglyceride (TG) in the advanced stage of diabetes further facilitate CD36 expression and sarcolemma distribution (48, 49). The recent study indicates that up-regulated nuclear micro-RNA (miR)-320 and down-regulated miR-200b-3p induced by diabetes directly promote CD36 transcription and translation, respectively, thus further promoting the sarcolemma distribution of CD36 (Figure 3) (48). At the same time, excessive intracellular fatty acid accumulation and β-oxidation and high oxLDL uptake due to the increased sarcolemma distribution of CD36 produce a great deal of ROS, which can advance inflammation and insulin resistance to worsen DCM (4, 49–51). The cardiac impairment induced by the high level of CD36 has been further confirmed by the fact that absence of CD36 inhibited the accumulation of cardiac lipotoxicity whereas improved the cellular utilization of glucose ultimately rescuing DCM (52, 53). Therefore, the increased CD36 during DCM in turn aggravates the heart injury. In addition, the transcription factor peroxisome proliferator-activated receptor (PPAR), a class of ligand activated nuclear receptor including PPARα, PPARβ/δ and PPARγ, is activated by CD36-induced high intracellular LCFA, which up-regulates the transcription and sarcolemma distribution of CD36 to irritate fatty acids uptake and lipotoxicity in the diabetic myocardium through a positive feed-back (Figure 3A) (13, 54).
3.2.2 Lipid accumulation-mediated myocardial injury
In the diabetic heart, insulin-dependent glucose intake is impaired while cardiac fatty acid influx and accumulation of lipids and lipotoxic intermediates are increased, resulting in cardiac lipotoxicity to play a causal role in the development of DCM (10, 13). Aberrant accumulation of lipids in diabetes usually leads to cardiovascular complications including DCM and heart failure (47). Excessive accumulation of lipids can facilitate numerous pathological processes linked to the development of DCM including mitochondrial dysfunction, ER stress, inflammation, and apoptosis (47, 55). The increase in myocardial fats especially TG (the other forms of LCFA) may exert deleterious effect on the left ventricular mass and diastolic function (56), which has been demonstrated in both ob/ob and db/db mice (57, 58). Furthermore, a constant myocardial influx of LCFA far exceeds cellular metabolic utilization, and the normal process of fatty acid β-oxidation is collapsed to generate plenty of mitochondrial derived ROS, which destroys cellular structure and function and promotes the process of DCM.
3.2.3 Lipotoxic intermediates-mediated myocardial injury
Intramyocardial accumulation of lipotoxic intermediates, such as ceramide, diacylglycerol (DAG) and fatty acyl-CoAs, caused by lipid metabolism disorder creates a lipotoxic microenvironment in the heart, that likely renders much of the cardiac injuries in diabetes (47). As indicated in Figure 3B, these lipotoxic intermediates have been identified to activate some serine/threonine kinases including PKC and TLR4-mediated innate immunity, causing plenty of proinflammatory cytokines, oxidative stress, apoptosis and hypertrophy in the diabetic heart (47, 59, 60). Briefly, ceramide, the precursor of sphingolipids, stimulates the assembly of the nucleotide-binding domain, leucine-rich-containing family, pyrin domain containing 3 (NLRP3) inflammasome, ER stress, insulin resistance, and myocardial death (Figure 3B) (60, 61). DAG can activate PKC to trigger insulin resistance, the release of ROS and dilated lipotoxic cardiomyopathy. In addition, both DAG and ceramides probably inhibit the production of NO, which is responsible for cardiovascular endothelial dysfunction in diabetes (Figure 3B) (49). The increase of LCFA uptake exceeds the limited oxidation capacity in the diabetic heart, which causes the overproduction of fatty acyl-CoAs that associated with cardiac lipotoxicity (62). The high fatty acid oxidation (FAO) and excess fatty acyl-CoAs may activate PKCθ leading to insulin resistance and impaired glucose metabolism ultimately metabolic disorder leading to DCM (Figure 3B) (63, 64).
3.3 Common downstream pathway and miRNA interference
3.3.1 PKC
PKC, a family of serine-threonine kinases composed of at least 12 isoforms, can phosphorylate target proteins and is essential for cell proliferation and differentiation in a tissue- and isoform-dependent manner (18, 65). Long-standing hyperglycemia-induced activation of PKC, through predominately a de novo synthesis of DAG, has been confirmed to thicken basement membrane, reduce blood flow and deposit ECM in the heart from both diabetic rodents and patients, which renders the development of DCM from cardiac inflammation, hypertrophy, fibrosis and diastolic dysfunction to heart failure (62, 66–69). Besides DAG, hyperglycemia may also activate PKC indirectly through increased flux of sorbitol pathway, increased flux of hexosamine pathway (Figure 2), and activated renin-angiotensin-aldosterone system, all of which result in the accumulation of pro-inflammatory cytokines and ROS in DCM (6, 34). PKC-induced overproduction of ROS mainly depends on the activation of NADPH oxidase (Figures 2, 3), which has been verified by the fact that treatment with the inhibitor of PKC reduced NADPH oxidase-produced ROS and rescued the heart from DCM (70–72). In addition, PKC can up-regulate eNOS to produce peroxynitrite (ONOO-), a potent ROS, under the reaction of NO with O2-, which results in a decreased bioavailability of NO and an increased oxidative stress in the diabetic vasculature (Figure 2) (73). Furthermore, PKC can activate NF-κB via IκB kinase (IKK)-dependent inactivation of inhibitor of NF-κB to up-regulate proinflammatory factors (Figure 2) (74, 75). The role of PKC in the development of DCM has been verified by several studies that high oxidative stress and inflammation triggered by activation of PKCθ and PKCβ2 is essential to the diabetic cardiac hypertrophy and fibrosis (76–78).
3.3.2 AMPK
AMP-activated protein kinase (AMPK) is an evolutionarily conserved serine-threonine kinase which regulates cellular energy homeostasis and coordinates multiple pathological processes, such as diabetes, cancer, cardiac hypertrophy and other chronic diseases (79, 80). Substantial evidence suggests that activating AMPK may be key to the function and survival of the diabetic cardiovascular system by balancing the utilization of intracellular energy substrates (such as glycolysis, TG synthesis and FAO) (80, 81), suppressing NLPR3 inflammasome-related inflammation and NADPH oxidase-related oxidative stress, and modulating autophagy and ER stress (82–88). In view of this, AMPK, for example, pharmacologically activated by metformin (80, 81, 86), appears to be a potential target for treating DCM.
3.3.3 miRNAs
MiRNAs are a group of short (22~25 nucleotides), single‐stranded and highly conserved RNAs and commonly act as post-transcriptional inhibitors of gene expression (89). A variety of miRNAs, including miR-1, miR-152, miR-187, miR-208a, miR-802, miR-126 and so on, have identified to modulate insulin production, energy metabolism (89, 90), and oxidative stress (89) resulting in DCM characterized by hypertrophy (89, 91), fibrosis (92) and heart failure (93–97). Recent studies have shown that miR-21 is abundantly expressed in the diabetic hearts (98–100), which accounts for the high oxidative stress and inflammation-related DCM probably through the insufficient activation of nuclear factor-E2 related factor 2 signaling pathway (100) and sprouty homolog 1/extracellular signal-regulated kinase/mammalian target of rapamycin (SPRY1/ERK/mTOR) pathway (99). The expression of miRNAs, such as miR-146a and miR-221, is disturbed to induce an enhancement of oxidative stress and inflammation response, which is acknowledged to promote the development of DCM. Several studies have showed that the significantly down-regulated miR-146a induced by longstanding hyperglycemia not only causes the overexpression of pro-inflammatory factors (IL-6, TNF-α and IL-1β) and NF-κB (101–103) but also generates excess ROS via NADPH oxidase 4 pathway, which impairs the diabetic cardiovascular system (101).
4 Inflammatory response and oxidative stress in DCM
Metabolic disturbance in the context of diabetes is associated with inflammation characterized by the excessive release of pro-inflammatory cytokines and activation of inflammatory cascade response (104). Pro-inflammatory cytokines, especially TNF-α, can lead to cardiac remodeling and dysfunction in the progression of diabetes. TNF-α inhibits tyrosine phosphorylation of insulin receptor substrate-1 aggravating insulin resistance and increases ventricular hypertrophy and vascular permeability leading to heart failure in diabetes (105). Inflammation and oxidative stress are closely interlinked (Figure 4). Both inflammatory response and overproduction of ROS/reactive nitrogen species (RNS) induced by metabolic disturbance in diabetes trigger NF-κB transcription and translocation to further arise pro-inflammatory cytokines and ROS/RNS, forming a vicious cycle of diabetic complications (106). Hyperglycemia and the increase of FAO produce excessive ROS/RNS to modify and blunt endogenous antioxidant defense systems, leading to deadly cell abnormalities such as mitochondrial membrane potential disruption (107), DNA double-strand break (27), and ER stress in diabetes (108). Eliminating pro-inflammatory factors and ROS/RNS is involved in the protection of correcting metabolic disturbance against DCM, which has been demonstrated by the curative effect of statins and active compounds in medicinal plants in diabetic heart diseases (109, 110).
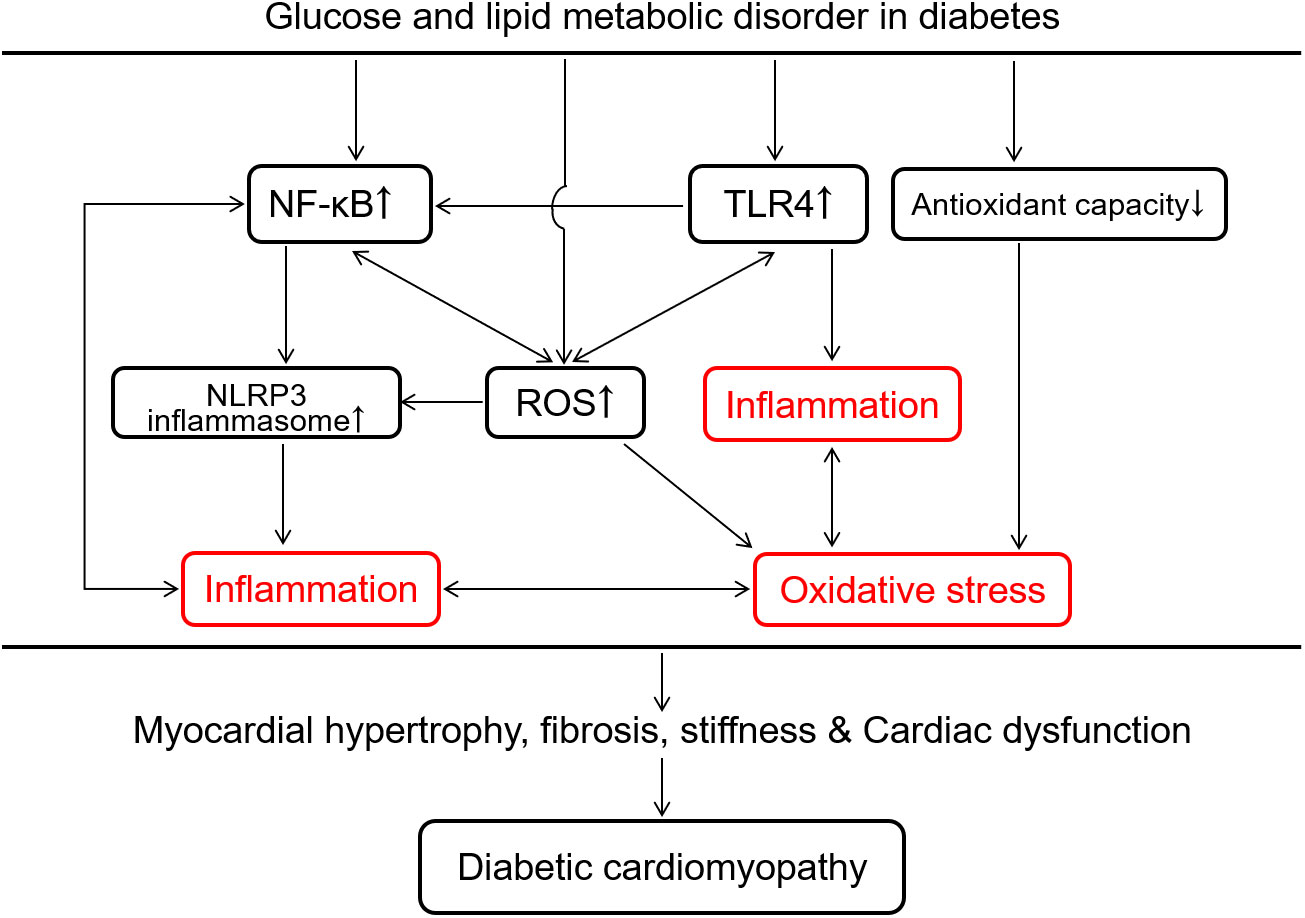
Figure 4 Overview of intracellular inflammation and oxidative stress interaction in response to glucose and lipid metabolic disorder in the development of diabetic cardiomyopathy. Glucose and lipid metabolic disorder in diabetes promotes cardiac inflammation via TLR4-mediated innate immunity, the surge of reactive oxygen species (ROS) and NF-κB-induced activation of NLRP3 inflammasome. The decrease in the antioxidant capacity and the increase in ROS production induced by the metabolic disorder both accelerate oxidative stress in the diabetic heart. Inflammation and oxidative stress promote each other to cause myocardial hypertrophy, fibrosis, stiffness and cardiac dysfunction, which is a potentially vicious cycle in the development of diabetic cardiomyopathy. NF-κB, nuclear factor κ-light-chain-enhancer of activated B cells; NLRP3, nucleotide-binding domain, leucine-rich-containing family, pyrin domain containing 3; TLR4, toll-like receptor 4.
4.1 Inflammation in DCM
A chronic inflammatory response clearly persists in diabetes through deadly activating the innate immune system to damage the heart (20, 111). Some early-responding pro-inflammatory immune cells, like M1-polarized macrophages, lymphocytes and neutrophils, secrete IL-6, IL-18, TNF-α, IL-1β to attack vascular smooth muscle cells, fibroblasts and cardiomyocytes impairing cellular energy metabolism and cause cardiovascular dysfunction (112, 113). TLR4 can be activated by the excess free fatty acid and pathogen-associated molecular patterns (PAMPs) to induce the synthesis of pro-inflammatory cytokines, meanwhile the activation of NF-κB by TLR4 also amplify the inflammatory response and pathological alterations (Figure 4) (114). This up-regulation of TLR4-mediated inflammation has been found in the diabetic heart (115) and repression of TLR4 benefits to lower lipid accumulation and to ameliorate cardiac function in diabetes (13, 116). Additionally, NLRP3 inflammasome, assembled by NLRP3, caspase-1, and apoptosis-associated speck-like protein containing a caspase recruitment domain (ASC), has been considered as another inflammatory participant in the ventricular remodeling and dysfunction of cardiac disease such as acute myocardial infarction, DCM and heart failure (117–119). The deleterious effect of activating NLRP3 inflammasome in DCM includes inflammation, fibrosis, pyroptosis and apoptosis (119–122). In addition to DAMPs/PAMPs (119, 123, 124), the increased fatty acids (122, 125), lipid intermediates (122), glucose (119), ROS (37, 119) are also the positive regulator to boost NLRP3 inflammasome in DCM. Once exposed to diabetic or other nociceptive stimuli, the auto-oligomerization of NLRP3 inflammasome is rapidly initiated by connecting NLRP3 proteins with ASC through both PYD domains (119). The NLRP3-ASC complex recruits pro-caspase-1 to form NLRP3 inflammasome via CARD domain interaction (122). Subsequently, pro-caspase-1 is cleaved into activated caspase-1, which promotes the secretion of IL-1β and IL-18 leading to a novel cell death named pyroptosis (123, 126). NLRP3 inflammasome plays a vital role in the progression of inevitable cardiac fibrosis and collagen deposition via suppressing MAPK signaling pathway and the production of cAMP and in myocardial fibroblasts (127–129). Given such destructive effect, NLRP3 inflammasome is probably a new target for the treatment of DCM.
4.2 Oxidative stress in DCM
ROS are primarily produced by mitochondrial respiratory chain as an electron leakage due to the impairing electron transport in hyperglycemia (130). NADPH oxidase is another prominent source of ROS in diabetes (27, 131). The decline of endogenous antioxidant capacity by inactivating antioxidants (such as GSH and vitamin E) and/or down-regulating antioxidant enzymes (such as superoxide dismutase, peroxidase and catalase) is also involved in the oxidative stress to develop DCM (Figure 4) (2, 27, 71, 130). Excess ROS can break DNA double-strand and oxidize proteins, by which glyceraldehyde 3-phosphate dehydrogenase, a key glycolytic enzyme in the glycolysis process, is down-regulated leading to the inhibition of glycolysis and accumulation of glycolytic intermediates (37). High glucose powerfully hampers the pentose phosphate pathway via inhibiting its rate-limiting enzyme glucose-6-phosphate dehydrogenase, to decrease the production of NADPH and GSH resulting in a lower antioxidant defense with a higher ROS production in endothelial cells and cardiomyocytes (Figure 1) (132, 133). The excessive ROS in diabetes can modify the structure of lipids named lipid peroxidation such as formation of malondialdehyde, 4-hydroxynonenam and oxLDL. These oxidized lipids commonly enhance pro-inflammatory response and participate diabetic cardiovascular complications including atherosclerosis and DCM (50, 51). Hence, oxidative stress represents a major force in the development of diabetic inflammation and probably an important target in the treatment of DCM accompanied by disproportionate inflammation (Figure 4).
5 Conclusion
A variety of studies have confirmed that glucose and lipid metabolic disturbance plays a central role in the development of DCM through vicious oxidative stress and inflammatory response pathways. The polyol pathway, hexosamine biosynthesis, PCK activation, sarcolemmal translocation of CD36, up-regulation of AGE/RAGE system, and disequilibrium of micro-RNA are involved in the metabolic disturbance, all of which contribute to the enhancement of diabetic oxidative stress and inflammation finally resulting in DCM. Although several dependable first-line drugs targeting glucose/lipid metabolism such as metformin, thiazolidinediones and sodium-glucose transporter-2 inhibitors alleviate hyperglycemia, the exact effect of these agents on DCM still needs to be further explored in the diabetic animal model and patient.
Author contributions
M-YC wrote the manuscript. M-YC, X-FM, Y-PH, J-LY, CX, and L-BQ designed the figures and edited the manuscript. CX and L-BQ supervised the writing. All authors contributed to the article and approved the submitted version.
Funding
This work was supported by National Natural Science Foundation of China (81772035), Natural Science Foundation of Zhejiang Province (LQ20H150001), and Program of Cultivating Zhejiang Provincial High-level Personnel in Health (Innovative Talent in 2021).
Conflict of interest
The authors declare that the research was conducted in the absence of any commercial or financial relationships that could be construed as a potential conflict of interest.
Publisher’s note
All claims expressed in this article are solely those of the authors and do not necessarily represent those of their affiliated organizations, or those of the publisher, the editors and the reviewers. Any product that may be evaluated in this article, or claim that may be made by its manufacturer, is not guaranteed or endorsed by the publisher.
Abbreviations
AR, Aldose reductase; AGEs, Advanced glycation end-products; AMPK, AMP-activated protein kinase; ASC, Apoptosis-associated speck-like protein containing a caspase recruitment domain; CD36, Cluster of differentiation 36; DAG, Diacylglycerol; DCM, Diabetic cardiomyopathy; ECM, Extracellular matrix; eNOS, Endothelial nitric oxide synthase; ER, Endoplasmic reticulum; FAO, Fatty acid oxidation; F-6-P, Fructose 6-phosphate; FOXO1, Forkhead box protein O1; Glucosamine-6-P, Glucosamine-6-phosphate; GFAT, Glutamine-fructose-6-phosphate amidotransferase; GLUTs, Glucose transporters; GSH, Reduced glutathione; GSSH, Oxidized glutathione; IKK, IκB kinase; LCFA, Long-chain fatty acids; mPTP, Mitochondrial permeability transition pore; NAD+, Oxidized form of nicotinamide adenine dinucleotide; NADPH, Reduced form of nicotinamide adenine dinucleotide phosphate; NF-κB, Nuclear factor κ-light-chain-enhancer of activated B cells; NLRP3, Nucleotide-binding domain, leucine-rich-containing family, pyrin domain containing 3; O2-, Superoxide anion; O-GlcNAcylation, O-linked N-acetylglucosamine glycosylation; oxLDL, Oxidized low-density lipoprotein; PAMPs, Pathogen-associated molecular patterns; PKC, Protein kinase C; PPAR, Peroxisome proliferator-activated receptor; RAGE, Receptor for AGE; ROS, Reactive oxygen species; T1DM, Type 1 diabetes mellitus; TLR4, Toll-like receptor 4; UDP-GlcNAc, UDP-N-acetylglucosamine.
References
1. Marshall SM, Flyvbjerg A. Prevention and early detection of vascular complications of diabetes. BMJ (Clin Res ed) (2006) 333:475–80. doi: 10.1136/bmj.38922.650521.80
2. Forbes JM, Cooper ME. Mechanisms of diabetic complications. Physiol Rev (2013) 93:137–88. doi: 10.1152/physrev.00045.2011
3. Stanley WC, Lopaschuk GD, McCormack JG. Regulation of energy substrate metabolism in the diabetic heart. Cardiovasc Res (1997) 34:25–33. doi: 10.1016/s0008-6363(97)00047-3
4. Evangelista I, Nuti R, Picchioni T, Dotta F, Palazzuoli A. Molecular dysfunction and phenotypic derangement in diabetic cardiomyopathy. Int J Mol Sci (2019) 20:3264. doi: 10.3390/ijms20133264
5. Jia G, Adam WC, Sowers JR. Diabetic cardiomyopathy: A hyperglycaemia- and insulin-resistance-induced heart disease. Diabetologia (2018) 61:21–8. doi: 10.1007/s00125-017-4390-4
6. Jia G, Hill MA, Sowers JR. Diabetic cardiomyopathy: An update of mechanisms contributing to this clinical entity. Circ Res (2018) 122:624–38. doi: 10.1161/CIRCRESAHA.117.311586
7. Zheng Y, Ley SH, Hu FB. Global aetiology and epidemiology of type 2 diabetes mellitus and its complications. Nat Rev Endocrinol (2018) 14:88–98. doi: 10.1038/nrendo.2017.151
8. Sarwar N, Gao P, Seshasai SRK, Gobin R, Kaptoge S, Di Angelantonio E, et al. Diabetes mellitus, fasting blood glucose concentration, and risk of vascular disease: A collaborative meta-analysis of 102 prospective studies. Lancet (Lond Engl) (2010) 375:2215–22. doi: 10.1016/S0140-6736(10)60484-9
9. Mahmood SS, Levy D, Vasan RS, Wang TJ. The Framingham Heart Study and the epidemiology of cardiovascular disease: A historical perspective. Lancet (Lond Engl) (2014) 383:999–1008. doi: 10.1016/S0140-6736(13)61752-3
10. Bugger H, Abel ED. Molecular mechanisms of diabetic cardiomyopathy. Diabetologia (2014) 57:660–71. doi: 10.1007/s00125-014-3171-6
11. Dillmann WH. Diabetic cardiomyopathy. Circ Res (2019) 124:1160–2. doi: 10.1161/CIRCRESAHA.118.314665
12. Tate M, Grieve DJ, Ritchie RH. Are targeted therapies for diabetic cardiomyopathy on the horizon? Clin Sci (London Engl 1979) (2017) 131:897–915. doi: 10.1042/CS20160491
13. Tan Y, Zhang Z, Zheng C, Wintergerst KA, Keller BB, Cai L. Mechanisms of diabetic cardiomyopathy and potential therapeutic strategies: Preclinical and clinical evidence. Nat Rev Cardiol (2020) 17:585–607. doi: 10.1038/s41569-020-0339-2
14. Yan J, Young ME, Cui L, Lopaschuk GD, Liao R, Tian R. Increased glucose uptake and oxidation in mouse hearts prevent high fatty acid oxidation but cause cardiac dysfunction in diet-induced obesity. Circulation (2009) 119:2818–28. doi: 10.1161/CIRCULATIONAHA.108.832915
15. Lopaschuk GD, Ussher JR, Folmes CDL, Jaswal JS, Stanley WC. Myocardial fatty acid metabolism in health and disease. Physiol Rev (2010) 90:207–58. doi: 10.1152/physrev.00015.2009
16. Cai L, Kang YJ. Oxidative stress and diabetic cardiomyopathy: A brief review. Cardiovasc Toxicol (2001) 1:181–93. doi: 10.1385/ct:1:3:181
17. Aneja A, Tang WHW, Bansilal S, Garcia MJ, Farkouh ME. Diabetic cardiomyopathy: Insights into pathogenesis, diagnostic challenges, and therapeutic options. Am J Med (2008) 121:748–57. doi: 10.1016/j.amjmed.2008.03.046
18. Faria A, Persaud SJ. Cardiac oxidative stress in diabetes: Mechanisms and therapeutic potential. Pharmacol Ther (2017) 172:50–62. doi: 10.1016/j.pharmthera.2016.11.013
19. Birse RT, Bodmer R. Lipotoxicity and cardiac dysfunction in mammals and drosophila. Crit Rev Biochem Mol Biol (2011) 46:376–85. doi: 10.3109/10409238.2011.599830
20. Donath MY, Dinarello CA, Mandrup-Poulsen T. Targeting innate immune mediators in type 1 and type 2 diabetes. Nat Rev Immunol (2019) 19:734–46. doi: 10.1038/s41577-019-0213-9
21. Saw EL, Pearson JT, Schwenke DO, Munasinghe PE, Tsuchimochi H, Rawal S, et al. Activation of the cardiac non-neuronal cholinergic system prevents the development of diabetes-associated cardiovascular complications. Cardiovasc Diabetol (2021) 20:50. doi: 10.1186/s12933-021-01231-8
22. Begum N, Draznin B. Effect of streptozotocin-induced diabetes on GLUT-4 phosphorylation in rat adipocytes. J Clin Invest (1992) 90:1254–62. doi: 10.1172/JCI115988
23. Brownlee M. Biochemistry and molecular cell biology of diabetic complications. Nature (2001) 414:813–20. doi: 10.1038/414813a
24. Gabbay KH. The sorbitol pathway and the complications of diabetes. New Engl J Med (1973) 288:831–6. doi: 10.1056/NEJM197304192881609
25. Tang WH, Martin KA, Hwa J. Aldose reductase, oxidative stress, and diabetic mellitus. Front Pharmacol (2012) 3:87. doi: 10.3389/fphar.2012.00087
26. Obrosova IG. Increased sorbitol pathway activity generates oxidative stress in tissue sites for diabetic complications. Antioxid Redox Signaling (2005) 7:1543–52. doi: 10.1089/ars.2005.7.1543
27. Papachristoforou E, Lambadiari V, Maratou E, Makrilakis K. Association of glycemic indices (hyperglycemia, glucose variability, and hypoglycemia) with oxidative stress and diabetic complications. J Diabetes Res (2020) 2020:7489795. doi: 10.1155/2020/7489795
28. Goldin A, Beckman JA, Schmidt AM, Creager MA. Advanced glycation end products: Sparking the development of diabetic vascular injury. Circulation (2006) 114:597–605. doi: 10.1161/CIRCULATIONAHA.106.621854
29. Giacco F, Brownlee M. Oxidative stress and diabetic complications. Circ Res (2010) 107:1058–70. doi: 10.1161/CIRCRESAHA.110.223545
30. Singh R, Barden A, Mori T, Beilin L. Advanced glycation end-products: A review. Diabetologia (2001) 44:129–46. doi: 10.1007/s001250051591
31. Frati G, Schirone L, Chimenti I, Yee D, Biondi-Zoccai G, Volpe M, et al. An overview of the inflammatory signalling mechanisms in the myocardium underlying the development of diabetic cardiomyopathy. Cardiovasc Res (2017) 113:378–88. doi: 10.1093/cvr/cvx011
32. Ma H, Li SY, Xu P, Babcock SA, Dolence EK, Brownlee M, et al. Advanced glycation endproduct (AGE) accumulation and AGE receptor (RAGE) up-regulation contribute to the onset of diabetic cardiomyopathy. J Cell Mol Med (2009) 13:1751–64. doi: 10.1111/j.1582-4934.2008.00547.x
33. Nielsen JM, Kristiansen SB, Nørregaard R, Andersen CL, Denner L, Nielsen TT, et al. Blockage of receptor for advanced glycation end products prevents development of cardiac dysfunction in db/db type 2 diabetic mice. Eur J Heart Fail (2009) 11:638–47. doi: 10.1093/eurjhf/hfp070
34. Chiu CJ, Taylor A. Dietary hyperglycemia, glycemic index and metabolic retinal diseases. Prog retin eye Res (2011) 30:18–53. doi: 10.1016/j.preteyeres.2010.09.001
35. Kenny HC, Abel ED. Heart failure in type 2 diabetes mellitus. Circ Res (2019) 124:121–41. doi: 10.1161/CIRCRESAHA.118.311371
36. Hu Y, Belke D, Suarez J, Swanson E, Clark R, Hoshijima M, et al. Adenovirus-mediated overexpression of O-GlcNAcase improves contractile function in the diabetic heart. Circ Res (2005) 96:1006–13. doi: 10.1161/01.RES.0000165478.06813.58
37. Shah MS, Brownlee M. Molecular and cellular mechanisms of cardiovascular disorders in diabetes. Circ Res (2016) 118:1808–29. doi: 10.1161/CIRCRESAHA.116.306923
38. Yang WH, Park SY, Nam HW, Kim DH, Kang JG, Kang ES, et al. NFkappaB activation is associated with its O-GlcNAcylation state under hyperglycemic conditions. Proc Natl Acad Sci USA (2008) 105:17345–50. doi: 10.1073/pnas.0806198105
39. Du XL, Edelstein D, Rossetti L, Fantus IG, Goldberg H, Ziyadeh F, et al. Hyperglycemia-induced mitochondrial superoxide overproduction activates the hexosamine pathway and induces plasminogen activator inhibitor-1 expression by increasing Sp1 glycosylation. Proc Natl Acad Sci USA (2000) 97:12222–6. doi: 10.1073/pnas.97.22.12222
40. Fricovsky ES, Suarez J, Ihm SH, Scott BT, Suarez Ramirez JA, Banerjee I, et al. Excess protein O-GlcNAcylation and the progression of diabetic cardiomyopathy. Am J Physiol Regul Integr Comp Physiol (2012) 303:R689–R99. doi: 10.1152/ajpregu.00548.2011
41. Shrikhande GV, Scali ST, da Silva CG, Damrauer SM, Csizmadia E, Putheti P, et al. O-glycosylation regulates ubiquitination and degradation of the anti-inflammatory protein A20 to accelerate atherosclerosis in diabetic ApoE-null mice. PLoS One (2010) 5:e14240. doi: 10.1371/journal.pone.0014240
42. Kim JA, Wei Y, Sowers JR. Role of mitochondrial dysfunction in insulin resistance. Circ Res (2008) 102:401–14. doi: 10.1161/CIRCRESAHA.107.165472
43. Riehle C, Bauersachs J. Of mice and men: Models and mechanisms of diabetic cardiomyopathy. Basic Res Cardiol (2018) 114:2. doi: 10.1007/s00395-018-0711-0
44. Chiu HC, Kovacs A, Blanton RM, Han X, Courtois M, Weinheimer CJ, et al. Transgenic expression of fatty acid transport protein 1 in the heart causes lipotoxic cardiomyopathy. Circ Res (2005) 96:225–33. doi: 10.1161/01.RES.0000154079.20681.B9
45. Park TS, Hu Y, Noh HL, Drosatos K, Okajima K, Buchanan J, et al. Ceramide is a cardiotoxin in lipotoxic cardiomyopathy. J Lipid Res (2008) 49:2101–12. doi: 10.1194/jlr.M800147-JLR200
46. Pepino MY, Kuda O, Samovski D, Abumrad NA. Structure-function of CD36 and importance of fatty acid signal transduction in fat metabolism. Annu Rev Nutr (2014) 34:281–303. doi: 10.1146/annurev-nutr-071812-161220
47. Ritchie RH, Zerenturk EJ, Prakoso D, Calkin AC. Lipid metabolism and its implications for type 1 diabetes-associated cardiomyopathy. J Mol Endocrinol (2017) 58:R225–R40. doi: 10.1530/JME-16-0249
48. Shu H, Peng Y, Hang W, Nie J, Zhou N, Wang DW. The role of CD36 in cardiovascular disease. Cardiovasc Res (2022) 118:115–29. doi: 10.1093/cvr/cvaa319
49. Zhang X, Fan J, Li H, Chen C, Wang Y. CD36 signaling in diabetic cardiomyopathy. Aging Dis (2021) 12:826–40. doi: 10.14336/AD.2020.1217
50. Nègre Salvayre A, Augé N, Camaré C, Bacchetti T, Ferretti G, Salvayre R. Dual signaling evoked by oxidized LDLs in vascular cells. Free Radical Biol Med (2017) 106:118–33. doi: 10.1016/j.freeradbiomed.2017.02.006
51. Farhangkhoee H, Khan ZA, Barbin Y, Chakrabarti S. Glucose-induced up-regulation of CD36 mediates oxidative stress and microvascular endothelial cell dysfunction. Diabetologia (2005) 48:1401–10. doi: 10.1007/s00125-005-1801-8
52. Yang J, Sambandam N, Han X, Gross RW, Courtois M, Kovacs A, et al. CD36 deficiency rescues lipotoxic cardiomyopathy. Circ Res (2007) 100:1208–17. doi: 10.1161/01.RES.0000264104.25265.b6
53. Nagendran J, Pulinilkunnil T, Kienesberger PC, Sung MM, Fung D, Febbraio M, et al. Cardiomyocyte-specific ablation of CD36 improves post-ischemic functional recovery. J Mol Cell Cardiol (2013) 63:180–8. doi: 10.1016/j.yjmcc.2013.07.020
54. Febbraio M, Hajjar DP, Silverstein RL. CD36: A class B scavenger receptor involved in angiogenesis, atherosclerosis, inflammation, and lipid metabolism. J Clin Invest (2001) 108:785–91. doi: 10.1172/JCI14006
55. Wang J, Song Y, Wang Q, Kralik PM, Epstein PN. Causes and characteristics of diabetic cardiomyopathy. Rev Diabetes Stud (2006) 3:108–17. doi: 10.1900/RDS.2006.3.108
56. Rijzewijk LJ, van der Meer RW, Smit JWA, Diamant M, Bax JJ, Hammer S, et al. Myocardial steatosis is an independent predictor of diastolic dysfunction in type 2 diabetes mellitus. J Am Coll Cardiol (2008) 52:1793–9. doi: 10.1016/j.jacc.2008.07.062
57. Christoffersen C, Bollano E, Lindegaard MLS, Bartels ED, Goetze JP, Andersen CB, et al. Cardiac lipid accumulation associated with diastolic dysfunction in obese mice. Endocrinology (2003) 144:3483–90. doi: 10.1210/en.2003-0242
58. Demarco VG, Ford DA, Henriksen EJ, Aroor AR, Johnson MS, Habibi J, et al. Obesity-related alterations in cardiac lipid profile and nondipping blood pressure pattern during transition to diastolic dysfunction in male db/db mice. Endocrinology (2013) 154:159–71. doi: 10.1210/en.2012-1835
59. Shi H, Kokoeva MV, Inouye K, Tzameli I, Yin H, Flier JS. TLR4 links innate immunity and fatty acid-induced insulin resistance. J Clin Invest (2006) 116:3015–25. doi: 10.1172/JCI28898
60. Chaurasia B, Summers SA. Ceramides-lipotoxic inducers of metabolic disorders. Trends Endocrinol Metab (2015) 26:538–50. doi: 10.1016/j.tem.2015.07.006
61. Kolwicz SC, Purohit S, Tian R. Cardiac metabolism and its interactions with contraction, growth, and survival of cardiomyocytes. Circ Res (2013) 113:603–16. doi: 10.1161/CIRCRESAHA.113.302095
62. D'Souza K, Nzirorera C, Kienesberger PC. Lipid metabolism and signaling in cardiac lipotoxicity. Biochim Biophys Acta (2016) 1861:1513–24. doi: 10.1016/j.bbalip.2016.02.016
63. Park S-Y, Kim H-J, Wang S, Higashimori T, Dong J, Kim Y-J, et al. Hormone-sensitive lipase knockout mice have increased hepatic insulin sensitivity and are protected from short-term diet-induced insulin resistance in skeletal muscle and heart. Am J Physiol Endocrinol Metab (2005) 289:E30–E9. doi: 10.1152/ajpendo.00251.2004
64. Shulman GI. Cellular mechanisms of insulin resistance. J Clin Invest (2000) 106:171–6. doi: 10.1172/JCI10583
65. Drosatos K, Schulze PC. Cardiac lipotoxicity: Molecular pathways and therapeutic implications. Curr Heart fail Rep (2013) 10:109–21. doi: 10.1007/s11897-013-0133-0
66. Wakasaki H, Koya D, Schoen FJ, Jirousek MR, Ways DK, Hoit BD, et al. Targeted overexpression of protein kinase C beta2 isoform in myocardium causes cardiomyopathy. Proc Natl Acad Sci USA (1997) 94:9320–5. doi: 10.1073/pnas.94.17.9320
67. Farhangkhoee H, Khan ZA, Kaur H, Xin X, Chen S, Chakrabarti S. Vascular endothelial dysfunction in diabetic cardiomyopathy: Pathogenesis and potential treatment targets. Pharmacol Ther (2006) 111:384–99. doi: 10.1016/j.pharmthera.2005.10.008
68. Steinberg SF. Structural basis of protein kinase C isoform function. Physiol Rev (2008) 88:1341–78. doi: 10.1152/physrev.00034.2007
69. Das Evcimen N, King GL. The role of protein kinase C activation and the vascular complications of diabetes. Pharmacol Res (2007) 55:498–510. doi: 10.1016/j.phrs.2007.04.016
70. Guzik TJ, Mussa S, Gastaldi D, Sadowski J, Ratnatunga C, Pillai R, et al. Mechanisms of increased vascular superoxide production in human diabetes mellitus: Role of NAD(P)H oxidase and endothelial nitric oxide synthase. Circulation (2002) 105:1656–62. doi: 10.1161/01.cir.0000012748.58444.08
71. Inoguchi T, Sonta T, Tsubouchi H, Etoh T, Kakimoto M, Sonoda N, et al. Protein kinase C-dependent increase in reactive oxygen species (ROS) production in vascular tissues of diabetes: Role of vascular NAD(P)H oxidase. J Am Soc Nephrol JASN (2003) 14:S227–S32. doi: 10.1097/01.asn.0000077407.90309.65
72. Liu Y, Lei S, Gao X, Mao X, Wang T, Wong GT, et al. PKCβ inhibition with ruboxistaurin reduces oxidative stress and attenuates left ventricular hypertrophy and dysfunction in rats with streptozotocin-induced diabetes. Clin Sci (London Engl 1979) (2012) 122:161–73. doi: 10.1042/CS20110176
73. Cosentino F, Eto M, De Paolis P, van der Loo B, Bachschmid M, Ullrich V, et al. High glucose causes upregulation of cyclooxygenase-2 and alters prostanoid profile in human endothelial cells: Role of protein kinase C and reactive oxygen species. Circulation (2003) 107:1017–23. doi: 10.1161/01.cir.0000051367.92927.07
74. Min W, Bin ZW, Quan ZB, Hui ZJ, Sheng FG. The signal transduction pathway of PKC/NF-kappa B/c-fos may be involved in the influence of high glucose on the cardiomyocytes of neonatal rats. Cardiovasc Diabetol (2009) 8:8. doi: 10.1186/1475-2840-8-8
75. Wang M, Zhang W-B, Zhu J-H, Fu G-S, Zhou B-Q. Breviscapine ameliorates hypertrophy of cardiomyocytes induced by high glucose in diabetic rats via the PKC signaling pathway. Acta Pharmacol Sin (2009) 30:1081–91. doi: 10.1038/aps.2009.95
76. Lim JY, Park SJ, Hwang HY, Park EJ, Nam JH, Kim J, et al. TGF-beta1 induces cardiac hypertrophic responses via PKC-dependent ATF-2 activation. J Mol Cell Cardiol (2005) 39:627–36. doi: 10.1016/j.yjmcc.2005.06.016
77. Li Z, Abdullah CS, Jin ZQ. Inhibition of PKC-Θ preserves cardiac function and reduces fibrosis in streptozotocin-induced diabetic cardiomyopathy. Br J Pharmacol (2014) 171:2913–24. doi: 10.1111/bph.12621
78. Xia Z, Kuo KH, Nagareddy PR, Wang F, Guo Z, Guo T, et al. N-acetylcysteine attenuates PKCbeta2 overexpression and myocardial hypertrophy in streptozotocin-induced diabetic rats. Cardiovasc Res (2007) 73:770–82. doi: 10.1016/j.cardiores.2006.11.033
79. Qi D, Young LH. AMPK: Energy sensor and survival mechanism in the ischemic heart. Trends Endocrinol Metab (2015) 26:422–9. doi: 10.1016/j.tem.2015.05.010
80. Dolinsky VW, Dyck JRB. Role of AMP-activated protein kinase in healthy and diseased hearts. Am J Physiol Heart Circ Physiol (2006) 291:H2557–H69. doi: 10.1152/ajpheart.00329.2006
81. Ko HJ, Zhang Z, Jung DY, Jun JY, Ma Z, Jones KE, et al. Nutrient stress activates inflammation and reduces glucose metabolism by suppressing AMP-activated protein kinase in the heart. Diabetes (2009) 58:2536–46. doi: 10.2337/db08-1361
82. Xie Z, He C, Zou M-H. AMP-activated protein kinase modulates cardiac autophagy in diabetic cardiomyopathy. Autophagy (2011) 7:1254–5. doi: 10.4161/auto.7.10.16740
83. Zou M-H, Xie Z. Regulation of interplay between autophagy and apoptosis in the diabetic heart: New role of AMPK. Autophagy (2013) 9:624–5. doi: 10.4161/auto.23577
84. Li L, Chen X, Su C, Wang Q, Li R, Jiao W, et al. Si-Miao-Yong-An decoction preserves cardiac function and regulates GLC/AMPK/NF-κB and GLC/PPARα/PGC-1α pathways in diabetic mice. Biomed Pharmacother (2020) 132:110817. doi: 10.1016/j.biopha.2020.110817
85. Ma Z-G, Yuan Y-P, Xu S-C, Wei W-Y, Xu C-R, Zhang X, et al. CTRP3 attenuates cardiac dysfunction, inflammation, oxidative stress and cell death in diabetic cardiomyopathy in rats. Diabetologia (2017) 60:1126–37. doi: 10.1007/s00125-017-4232-4
86. Jeon S-M. Regulation and function of AMPK in physiology and diseases. Exp Mol Med (2016) 48:e245. doi: 10.1038/emm.2016.81
87. Song J, Li J, Hou F, Wang X, Liu B. Mangiferin inhibits endoplasmic reticulum stress-associated thioredoxin-interacting protein/NLRP3 inflammasome activation with regulation of AMPK in endothelial cells. Metabolism (2015) 64:428–37. doi: 10.1016/j.metabol.2014.11.008
88. Wang S, Zhang M, Liang B, Xu J, Xie Z, Liu C, et al. AMPKalpha2 deletion causes aberrant expression and activation of NAD(P)H oxidase and consequent endothelial dysfunction in vivo: Role of 26S proteasomes. Circ Res (2010) 106:1117–28. doi: 10.1161/CIRCRESAHA.109.212530
89. Fan B, Luk AOY, Chan JCN, Ma RCW. MicroRNA and diabetic complications: A clinical perspective. Antioxid Redox Signal (2018) 29:1041–63. doi: 10.1089/ars.2017.7318
90. Agbu P, Carthew RW. MicroRNA-mediated regulation of glucose and lipid metabolism. Nat Rev Mol Cell Biol (2021) 22:425–38. doi: 10.1038/s41580-021-00354-w
91. Rawal S, Manning P, Katare R. Cardiovascular MicroRNAs: As modulators and diagnostic biomarkers of diabetic heart disease. Cardiovasc Diabetol (2014) 13:44. doi: 10.1186/1475-2840-13-44
92. He X, Kuang G, Wu Y, Ou C. Emerging roles of exosomal miRNAs in diabetes mellitus. Clin Trans Med (2021) 11:e468. doi: 10.1002/ctm2.468
93. Zhang F, Ma D, Zhao W, Wang D, Liu T, Liu Y, et al. Obesity-induced overexpression of miR-802 impairs insulin transcription and secretion. Nat Commun (2020) 11:1822. doi: 10.1038/s41467-020-15529-w
94. Locke JM, da Silva Xavier G, Dawe HR, Rutter GA, Harries LW. Increased expression of miR-187 in human islets from individuals with type 2 diabetes is associated with reduced glucose-stimulated insulin secretion. Diabetologia (2014) 57:122–8. doi: 10.1007/s00125-013-3089-4
95. Ofori JK, Salunkhe VA, Bagge A, Vishnu N, Nagao M, Mulder H, et al. Elevated miR-130a/miR-130b/miR-152 expression reduces intracellular ATP levels in the pancreatic beta cell. Sci Rep (2017) 7:44986. doi: 10.1038/srep44986
96. Li Q, Song XW, Zou J, Wang GK, Kremneva E, Li XQ, et al. Attenuation of microRNA-1 derepresses the cytoskeleton regulatory protein twinfilin-1 to provoke cardiac hypertrophy. J Cell Sci (2010) 123:2444–52. doi: 10.1242/jcs.067165
97. Rawal S, Nagesh PT, Coffey S, Van Hout I, Galvin IF, Bunton RW, et al. Early dysregulation of cardiac-specific microRNA-208a is linked to maladaptive cardiac remodelling in diabetic myocardium. Cardiovasc Diabetol (2019) 18:13. doi: 10.1186/s12933-019-0814-4
98. Surina S, Fontanella RA, Scisciola L, Marfella R, Paolisso G, Barbieri M. MiR-21 in human cardiomyopathies. Front Cardiovasc Med (2021) 8:767064. doi: 10.3389/fcvm.2021.767064
99. Li X, Meng C, Han F, Yang J, Wang J, Zhu Y, et al. Vildagliptin attenuates myocardial dysfunction and restores autophagy via miR-21/SPRY1/ERK in diabetic mice heart. Front Pharmacol (2021) 12:634365. doi: 10.3389/fphar.2021.634365
100. Gao L, Liu Y, Guo S, Xiao L, Wu L, Wang Z, et al. LAZ3 protects cardiac remodeling in diabetic cardiomyopathy via regulating miR-21/PPARa signaling. Biochim Biophys Acta Mol basis Dis (2018) 1864:3322–38. doi: 10.1016/j.bbadis.2018.07.019
101. Wang HJ, Huang YL, Shih YY, Wu HY, Peng CT, Lo WY. microRNA-146a decreases high glucose/thrombin-induced endothelial inflammation by inhibiting NAPDH oxidase 4 expression. Mediators Inflammation (2014) 2014:379537. doi: 10.1155/2014/379537
102. Balasubramanyam M, Aravind S, Gokulakrishnan K, Prabu P, Sathishkumar C, Ranjani H, et al. Impaired miR-146a expression links subclinical inflammation and insulin resistance in type 2 diabetes. Mol Cell Biochem (2011) 351:197–205. doi: 10.1007/s11010-011-0727-3
103. Feng B, Chen S, Gordon AD, Chakrabarti S. MiR-146a mediates inflammatory changes and fibrosis in the heart in diabetes. J Mol Cell Cardiol (2017) 105:70–6. doi: 10.1016/j.yjmcc.2017.03.002
104. Wellen KE, Hotamisligil GS. Inflammation, stress, and diabetes. J Clin Invest (2005) 115:1111–9. doi: 10.1172/JCI25102
105. Calle MC, Fernandez ML. Inflammation and type 2 diabetes. Diabetes Metab (2012) 38:183–91. doi: 10.1016/j.diabet.2011.11.006
106. Zhang J, Wang X, Vikash V, Ye Q, Wu D, Liu Y, et al. ROS and ROS-mediated cellular signaling. Oxid Med Cell Longevity (2016) 2016:4350965. doi: 10.1155/2016/4350965
107. Kc S, Cárcamo JM, Golde DW. Vitamin C enters mitochondria via facilitative glucose transporter 1 (Glut1) and confers mitochondrial protection against oxidative injury. FASEB J (2005) 19:1657–67. doi: 10.1096/fj.05-4107com
108. Borradaile NM, Han X, Harp JD, Gale SE, Ory DS, Schaffer JE. Disruption of endoplasmic reticulum structure and integrity in lipotoxic cell death. J Lipid Res (2006) 47:2726–37. doi: 10.1194/jlr.M600299-JLR200
109. Al Rasheed NM, Al Rasheed NM, Hasan IH, Al Amin MA, Al Ajmi HN, Mohamad RA, et al. Simvastatin ameliorates diabetic cardiomyopathy by attenuating oxidative stress and inflammation in rats. Oxid Med Cell Longevity (2017) 2017:1092015. doi: 10.1155/2017/1092015
110. Xiao C, Xia ML, Wang J, Zhou XR, Lou YY, Tang LH, et al. Luteolin attenuates cardiac ischemia/reperfusion injury in diabetic rats by modulating Nrf2 antioxidative function. Oxid Med Cell Longevity (2019) 2019:2719252. doi: 10.1155/2019/2719252
111. Xu M, Liu PP, Li H. Innate immune signaling and its role in metabolic and cardiovascular diseases. Physiol Rev (2019) 99:893–948. doi: 10.1152/physrev.00065.2017
112. Saltiel AR, Olefsky JM. Inflammatory mechanisms linking obesity and metabolic disease. J Clin Invest (2017) 127:1–4. doi: 10.1172/JCI92035
113. Monnerat G, Alarcón ML, Vasconcellos LR, Hochman Mendez C, Brasil G, Bassani RA, et al. Author correction: Macrophage-dependent IL-1β production induces cardiac arrhythmias in diabetic mice. Nat Commun (2021) 12:7261. doi: 10.1038/s41467-021-27508-w
114. Rogero MM, Calder PC. Obesity, inflammation, toll-like receptor 4 and fatty acids. Nutrients (2018) 10:432. doi: 10.3390/nu10040432
115. Chiang CJ, Tsai BCK, Lu TL, Chao YP, Day CH, Ho TJ, et al. Diabetes-induced cardiomyopathy is ameliorated by heat-killed Lactobacillus reuteri GMNL-263 in diabetic rats via the repression of the toll-like receptor 4 pathway. Eur J Nutr (2021) 60:3211–23. doi: 10.1007/s00394-020-02474-z
116. Dong B, Qi D, Yang L, Huang Y, Xiao X, Tai N, et al. TLR4 regulates cardiac lipid accumulation and diabetic heart disease in the nonobese diabetic mouse model of type 1 diabetes. Am J Physiol Heart Circ Physiol (2012) 303:H732–H42. doi: 10.1152/ajpheart.00948.2011
117. Abbate A, Toldo S, Marchetti C, Kron J, Van Tassell BW, Dinarello CA. Interleukin-1 and the inflammasome as therapeutic targets in cardiovascular disease. Circ Res (2020) 126:1260–80. doi: 10.1161/CIRCRESAHA.120.315937
118. Toldo S, Abbate A. The NLRP3 inflammasome in acute myocardial infarction. Nat Rev Cardiol (2018) 15:203–14. doi: 10.1038/nrcardio.2017.161
119. Luo B, Huang F, Liu Y, Liang Y, Wei Z, Ke H, et al. NLRP3 inflammasome as a molecular marker in diabetic cardiomyopathy. Front Physiol (2017) 8:519. doi: 10.3389/fphys.2017.00519
120. Yang F, Qin Y, Wang Y, Meng S, Xian H, Che H, et al. Metformin inhibits the NLRP3 inflammasome via AMPK/mTOR-dependent effects in diabetic cardiomyopathy. Int J Biol Sci (2019) 15:1010–9. doi: 10.7150/ijbs.29680
121. Fender AC, Kleeschulte S, Stolte S, Leineweber K, Kamler M, Bode J, et al. Thrombin receptor PAR4 drives canonical NLRP3 inflammasome signaling in the heart. Basic Res Cardiol (2020) 115:10. doi: 10.1007/s00395-019-0771-9
122. Sharma A, Tate M, Mathew G, Vince JE, Ritchie RH, de Haan JB. Oxidative stress and NLRP3-inflammasome activity as significant drivers of diabetic cardiovascular complications: Therapeutic implications. Front Physiol (2018) 9:114. doi: 10.3389/fphys.2018.00114
123. Sharma BR, Kanneganti TD. NLRP3 inflammasome in cancer and metabolic diseases. Nat Immunol (2021) 22:550–9. doi: 10.1038/s41590-021-00886-5
124. Strowig T, Henao Mejia J, Elinav E, Flavell R. Inflammasomes in health and disease. Nature (2012) 481:278–86. doi: 10.1038/nature10759
125. Vandanmagsar B, Youm YH, Ravussin A, Galgani JE, Stadler K, Mynatt RL, et al. The NLRP3 inflammasome instigates obesity-induced inflammation and insulin resistance. Nat Med (2011) 17:179–88. doi: 10.1038/nm.2279
126. Wen H, Ting JPY, O'Neill LAJ. A role for the NLRP3 inflammasome in metabolic diseases–did Warburg miss inflammation? Nat Immunol (2012) 13:352–7. doi: 10.1038/ni.2228
127. Pinar AA, Scott TE, Huuskes BM, Tapia Cáceres FE, Kemp-Harper BK, Samuel CS. Targeting the NLRP3 inflammasome to treat cardiovascular fibrosis. Pharmacol Ther (2020) 209:107511. doi: 10.1016/j.pharmthera.2020.107511
128. Zhang X, Fu Y, Li H, Shen L, Chang Q, Pan L, et al. H3 relaxin inhibits the collagen synthesis via ROS- and P2X7R-mediated NLRP3 inflammasome activation in cardiac fibroblasts under high glucose. J Cell Mol Med (2018) 22:1816–25. doi: 10.1111/jcmm.13464
129. Shao BZ, Xu ZQ, Han BZ, Su DF, Liu C. NLRP3 inflammasome and its inhibitors: A review. Front Pharmacol (2015) 6:262. doi: 10.3389/fphar.2015.00262
130. Karam BS, Chavez Moreno A, Koh W, Akar JG, Akar FG. Oxidative stress and inflammation as central mediators of atrial fibrillation in obesity and diabetes. Cardiovasc Diabetol (2017) 16:120. doi: 10.1186/s12933-017-0604-9
131. Zhang Y, Murugesan P, Huang K, Cai H. NADPH oxidases and oxidase crosstalk in cardiovascular diseases: Novel therapeutic targets. Nat Rev Cardiol (2020) 17:170–94. doi: 10.1038/s41569-019-0260-8
132. Leverve XM, Guigas B, Detaille D, Batandier C, Koceir EA, Chauvin C, et al. Mitochondrial metabolism and type-2 diabetes: A specific target of metformin. Diabetes Metab (2003) 29:6S88–94. doi: 10.1016/s1262-3636(03)72792-x
133. González Domínguez Á, Visiedo F, Domínguez Riscart J, Ruiz Mateos B, Saez Benito A, Lechuga Sancho AM, et al. Blunted reducing power generation in erythrocytes contributes to oxidative stress in prepubertal obese children with insulin resistance. Antioxid (Basel Switzerland) (2021) 10:244. doi: 10.3390/antiox10020244
Keywords: disturbance of glucose metabolism, inflammation, oxidative stress, disturbance of lipid metabolism, diabetic cardiomyopathy
Citation: Chen M-Y, Meng X-F, Han Y-P, Yan J-L, Xiao C and Qian L-B (2022) Profile of crosstalk between glucose and lipid metabolic disturbance and diabetic cardiomyopathy: Inflammation and oxidative stress. Front. Endocrinol. 13:983713. doi: 10.3389/fendo.2022.983713
Received: 01 July 2022; Accepted: 16 August 2022;
Published: 15 September 2022.
Edited by:
Ying Xin, Jilin University, ChinaReviewed by:
Yi Tan, University of Louisville, United StatesXiaozhen Dai, Chengdu Medical College, China
Copyright © 2022 Chen, Meng, Han, Yan, Xiao and Qian. This is an open-access article distributed under the terms of the Creative Commons Attribution License (CC BY). The use, distribution or reproduction in other forums is permitted, provided the original author(s) and the copyright owner(s) are credited and that the original publication in this journal is cited, in accordance with accepted academic practice. No use, distribution or reproduction is permitted which does not comply with these terms.
*Correspondence: Ling-Bo Qian, YmlvcWlhbkAxNjMuY29t; Chi Xiao, eGNpZXJAMTYzLmNvbQ==