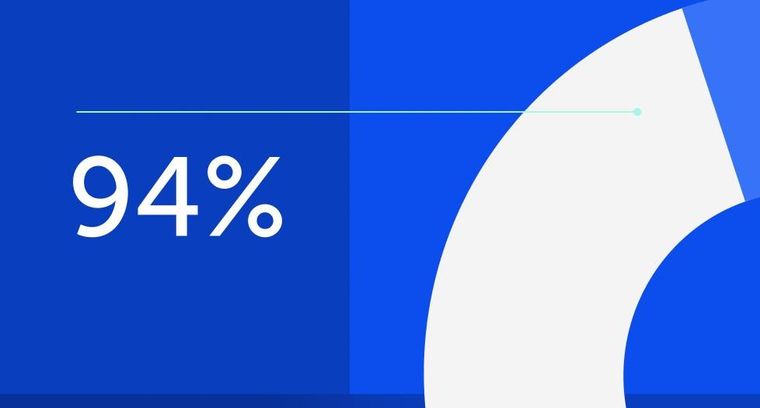
94% of researchers rate our articles as excellent or good
Learn more about the work of our research integrity team to safeguard the quality of each article we publish.
Find out more
REVIEW article
Front. Endocrinol., 17 January 2023
Sec. Reproduction
Volume 13 - 2022 | https://doi.org/10.3389/fendo.2022.970439
This article is part of the Research TopicA Year in Review: Discussions in ReproductionView all 7 articles
It is estimated that more and more couples suffer from fertility and pregnancy maintenance disorders. It is associated with impaired androgen secretion, which is influenced by many factors, ranging from genetic to environmental. It is also important to remember that fertility disorders can also result from abnormal anatomy of the reproductive male and female organ (congenital uterine anomalies – septate, unicornuate, bicornuate uterus; acquired defects of the uterus structure – fibroids, polyps, hypertrophy), disturbed hormonal cycle and obstruction of the fallopian tubes resulting from the presence of adhesions due to inflammation, endometriosis, and surgery, abnormal rhythm of menstrual bleeding, the abnormal concentration of hormones. There are many relationships between the endocrine organs, leading to a chain reaction when one of them fails to function properly. Conditions in which the immune system is involved, including infections and autoimmune diseases, also affect fertility. The form of treatment depends on infertility duration and the patient’s age. It includes ovulation stimulation with clomiphene citrate or gonadotropins, metformin use, and weight loss interventions. Since so many different factors affect fertility, it is important to correctly diagnose what is causing the problem and to modify the treatment regimen if necessary. This review describes disturbances in the hormone secretion of individual endocrine organs in the context of fertility and the maintenance of pregnancy.
Infertility is the inability to conceive within 12 months of regular intercourse (2-4 times a week) without using any contraceptive methods. Involuntary childlessness is a significant social problem faced by 20% of couples worldwide, and only in Poland the problem of infertility affects approximately 1.5 million couples each year (1).
Factors that influence fertility in both sexes include hyperprolactinemia, hypogonadotropic hypogonadism, infections, systemic diseases, and even lifestyle (2). More and more attention is also paid to the problem of obesity. Adipocytes act as an endocrine organ, and their excess promotes disorders of the hypothalamic-pituitary-ovarian axis. Secreted adipokines include leptin, adiponectin, resistin, interleukin 6, interleukin 1β, and tumor necrosis factor α (TNFα), involved in inflammatory processes and the regulation of metabolism (3).
According to the recommendations of the Fertility and Infertility Section at the Polish Society of Gynecologists and Obstetricians and the Polish Society of Reproductive Medicine and Embryology, there are several causes of male infertility (4): 1) pre-testicular, i.e. related to the malfunctioning of the endocrine system in terms of impaired secretion of luteinizing hormone, sex steroids – testosterone, inhibin, folliculostimulin or resulting from mutations which cause impaired sperm movement or chromosomal aberrations observed, e.g. in Klinefelter’s syndrome, 2) testicular, e.g. cryptorchidism, varicocele, infectious diseases; 3) extra-testicular, which include congenital absence of vas deferens, underdevelopment of seminal vesicles, epididymis defects, polycystic kidney disease, cystic fibrosis, diabetes; 4) sexual disorders – lack of erection or ejaculation, penis structure abnormalities (5). During the diagnosis of infertility, the assessment of male fertility should be obligatory, and the examination should be performed after maintaining a 2-7 day period of sexual abstinence (6).
On the other hand, infertility in women may be mainly due to: disturbances in the occurrence of ovulatory cycles, caused, inter alia, by woman’s age, hormonal disorders in the course of polycystic ovary syndrome (PCOS), hyperprolactinemia, abnormal anatomy of the reproductive organ (congenital uterine anomalies – septate, unicornuate, bicornuate uterus; acquired defects of the uterus structure – fibroids, polyps, hypertrophy), disturbed hormonal cycle and obstruction of the fallopian tubes resulting from the presence of adhesions due to inflammation, endometriosis, and surgery (7).
Diagnostics of female infertility should include: medical history regarding the regularity of menstruation, gynecological examination, determination of the concentration of selected sex hormones, including a single measurement of progesterone level 7 days before the planned menstruation to assess ovulation, ultrasound (USG) at the end of the follicular phase of the cycle (8, 9). However, in the case of ovulation disorders, manifested by an abnormal rhythm of menstrual bleeding, progesterone level lower than 2 ng/mL in the middle of the luteal phase, the diagnosis should be supplemented with the determination of the concentration of gonadotropins, androgens, thyroid-stimulating hormone (TSH), prolactin (PRL), anti-müllerian hormone (AMH) and assessment of the reproductive potential of the ovaries (10). The form of infertility treatment depends on its duration and the patient’s age. It includes ovulation stimulation with clomiphene citrate or gonadotropins, intrauterine insemination, in vitro fertilization (11).
The aim of this review was to present disturbances in the hormone secretion of individual endocrine organs in the context of fertility of both sexes and the maintenance of pregnancy (Figure 1).
Sex differentiation is a complex process that depends on the activity of many genes. The key to sex dimorphism is the SRY (sex-determining region on Y) gene, whose protein product initiates the cascade of expression of genes determining the formation of testicles. The lack of this protein allows development towards a female individual (12, 13).
The sex of the embryo is determined already during fertilization, but until the 7th week of development, the gonads do not show gender-related differences. Initially, the gonads are in the form of longitudinal folds, which are formed due to the proliferation of the body cavity epithelium and the thickening of the stromal mesenchyme. Primordial germ cells appear no earlier than the 6th week of development and it is important for the gonads development that they populate the genital crests. Genital crest epithelial cells proliferate and penetrate into the stromal mesenchyme, where they form irregularly shaped primary sex cords. In both female and male embryos, these cords maintain a connection to the surface epithelium. This makes it impossible to distinguish between male and female gonads, which is why these structures are referred to as undifferentiated gonads (14).
Female and male embryos have two pairs of ducts: mesonephric (Wolffian) and paramesonephric (Müllerian). The caudal part of the connected ducts is incorporated into the posterior wall of the urogenital sinus, forming a sinus tubercle.
At 3 weeks of development, the mesenchyme cells migrate around the cloacal membrane, forming a pair of slightly elevated cloacal folds. They join cranially to form the genital tubercle, and caudally to the urethral and the anal folds. At the same time, another pair of genital eminences is visible on either side of the urethral folds. Later, they form the scrotal eminences in men and the labia majora in women (15).
In female development, the primary sex cords break down into irregular clusters of cells. During further development, they disappear and are replaced by vascularized stroma tissue, forming the ovarian core. The surface epithelial cells of the female gonad (as opposed to the epithelial cells of the male gonad) continue to divide, resulting in the formation of cortical cords around the 7th week of development. In the third month, they disintegrate into separate cell foci forming follicular cells, creating, together with the oogonium, the primary (resting) follicle (16).
In the presence of estrogen, the main female genital ducts are formed from the paramesonephric duct. With the descent of the ovary, part of the ducts transform into the fallopian tube and uterine canal. The connected paramesonephric ducts become the corpus and cervix and the upper part of the vagina. In female embryos, the mesonephric ducts regress due to a lack of testosterone (16).
The development of female external genitalia is stimulated by estrogens. The genital tubercle lengthens to form the clitoris, and the folds of the urethra give rise to the labia minora. In turn, the vaginal prominences enlarge and produce the labia majora. The urethral groove remains open and forms the vestibule (17).
The genetically male embryo, under the influence of the SRY gene product, develops sex cords that penetrate deeper towards the core part of the gonad, forming medullary cords (13). These, in turn, continue to disintegrate, becoming the tubules of the testicle network. In further development, the testicular cords are separated from the surface epithelium by the tunica albuginea. In the fourth month, the testicular cords join the nucleus network. It is worth noting that the testicular cords retain the form of solid structures until puberty, and then transform into convoluted seminiferous tubules (14, 18).
They develop under the influence of testosterone and originate from part of the mesonephric organ. With the exception of the appendix of the epididymis, the mesonephric duct does not regress and forms the main genital ducts. Below the orifice of the efferent ducts, the epididymal duct is elongated and formed. The mesonephric duct from the tail of the epididymis to the protrusion of the seminal vesicle is called the vas deferens.
The development of male external genitalia is stimulated by androgens and is manifested by the rapid lengthening of the genital tubercle, the penis. In the process of elongation, the penis pulls on the folds of the urethra, which form the urethral grooves. The furthest part is the penis glans. The epithelial lining of the groove forms the urethral plate. At the end of the 3rd month, the folds of the urethra close above the plate, forming the spongy part of the urethra, then the urethral lumen is formed. In turn, the genital (scrotal) eminences move in the caudal direction during development and form the scrotum (17).
The hypothalamus and pituitary gland control and regulate the proper functioning of the endocrine glands. The gonadotropic pathway is especially important in the case of fertility and the maintenance of pregnancy (Tables 1, 2). The hypothalamus produces gonadotropin-releasing hormone (GnRH), the appropriate concentration of which is necessary for the secretion of gonadotropins, i.e. luteinizing hormone (LH) and follicle-stimulating hormone (FSH) by the anterior (glandular) lobe of the pituitary gland (22). Gonadotropins, in turn, have a direct influence on the production of sex hormones, sperm and ova, as well as the course of pregnancy. Thus, the malfunction of the hypothalamus or pituitary gland will be associated with low production of GnRH and gonadotropins, which in turn will lead to the gonadal failure, known as hypogonadotropic hypogonadism (23).
Other hormones produced by the anterior pituitary gland include adrenocorticotropic hormone (ACTH) and growth hormone (GH). ACTH is produced from the precursor hormone proopiomelanocortin (POMC), which stimulates the production of cortisol in the adrenal glands via melanocortin receptors. Corticotropin-releasing hormone (CRH), along with vasopressin (ADH), are the main hormones that control ACTH secretion. GH and ACTH secretion is pulsatile and subject to circadian rhythms. Factors that influence the increase in growth hormone secretion are sleep and physical activity, as well as fasting, hypoglycemia, hypovolemia, and surgery. Hyperglycemia initially reduces and then increases GH levels (rebound effect). GH secretion shows gender differences, in males it is pulsatile and in females secretion is continuous. GH levels decline with age and somatopause occurs in the elderly (24).
Growth hormone, by activating insulin-like growth factor 1 (IGF-1) synthesis, directly improves the quality of oocytes, and also increases FSH-induced ovarian steroidogenesis. Administration of GH during ovulation stimulation enhances the effectiveness of the in-vitro procedure by increasing the percentage of mature oocytes and embryos viable to the day of transfer. Daily administration of a low dose of growth hormone (0.5 IU) started on the day of GnRH agonist administration increases the pregnancy rate (34.4% vs. 0%), the percentage of good-quality embryos and cryopreserved embryos. In addition, the use of a low dose of GH (4IU/d) in patients with reduced response to GnRH antagonists preparing for the IVF/ICSI procedure decreases the effective dose of gonadotropins and the duration of stimulation, increasing the total number of oocytes and oocytes in metaphase II of meiosis (48).
In obese women, reduced fertility results, among others, from decreased LH levels. They experience accelerated sexual maturation, menstrual disorders, in particular prolongation of the follicular phase, indicating ovulation disorders, and a higher frequency of obstetric complications, including spontaneous miscarriages (49).
Interestingly, the serine protease inhibitor (SERPINA12, also known as Vaspin) plays a significant role in the pathogenesis of type 2 diabetes, inflammation and infertility. Its concentration increases with weight gain and the worsening of insulin resistance, and decreases with weight loss. Metformin reduces the serum concentration of Vaspin in patients with PCOS (50).
Too low body weight, intense exercise, chronic diseases, malabsorption disorders such as celiac disease, hyperthyroidism, and thus energy deficiencies lead to impaired pulsatile GnRH secretion, and as a result, the concentration of LH and FSH necessary for effective stimulation of the gonads is too low (51). Studies have also shown that women with a BMI below 18.5 have a 72% higher risk of miscarriage in the first trimester of pregnancy than women with a normal body weight (52).
Disturbance of the circadian rhythm including sleep quality, wakefulness and the above-related pulsatile hormone secretion plays a significant role in infertility. It is known that insomnia in female shift workers results in decreased melatonin production. Low melatonin concentration in Graaf vesicles is associated with higher generation of free oxygen radicals (ROS), and thus poorer quality of oocytes. Melatonin supplementation in patients undergoing the in vitro procedure increases its effectiveness by improving the quality of oocytes, increasing the percentage of fertilization and the quality of the emerging embryos (24).
Prolactin is a peptide hormone synthesized by lactotrophic cells of the anterior pituitary gland. Its secretion is mainly influenced by dopamine, which negatively influences its secretion through negative feedback. The main function of prolactin is to influence the development of the mammary glands during pregnancy and to support milk production after delivery. Plasma prolactin levels increase rapidly during pregnancy with an increase in the size and number of the anterior pituitary lactotrophic cells. During breastfeeding, the sucking of the nipple by the baby causes a rapid secretion of prolactin through a mechanism of rapid neuroendocrine reflex. In pathological situations, the symptoms of hyperprolactinemia include hypogonadotropic hypogonadism (menstrual disorders and infertility) or visual field disturbances due to the mass effect caused by prolactinoma, the most common pituitary tumor. Hyperprolactinemia is diagnosed by measuring plasma prolactin. A false-positive result may be due to the presence of macroprolactin in the plasma. Its verification is possible with the use of the polyethylene glycol (PEG) precipitation method, which allows to avoid unnecessary treatment (53).
Hypothyroidism leads to a compensatory increase in the production of thyrotropin-releasing hormone (TRH) by the hypothalamus, which in turn results in suppression of dopamine production, resulting in excessive prolactin secretion, i.e. hyperprolactinemia (54). Central nervous system (CNS) tumors that produce prolactin, called prolactinomas, also cause hyperprolactinemia. Hyperprolactinemia is of key importance in infertility as it inhibits the production of GnRH and, consequently, also of gonadotropins (LH, FSH) by the pituitary gland. The therapy most often uses bromocriptine, while the basic diagnostic imaging test is magnetic resonance imaging (MR) in the T1 sequence (19, 20, 55). Prolactinomas are the most common hormonally active tumors in pregnant women. A case of a 30-year-old woman in the 36th week of pregnancy with headaches and left-sided vision loss was described. MRI of the pituitary gland confirmed a 10x11mm suprasellar lesion. After delivery, the lesion was removed by endoscopic transnasal resection. Histopathological examination revealed a tissue of prolactinoma. Therefore, a number of potential causes, including prolactinoma, should be considered in the differential diagnosis of visual loss during pregnancy (56).
Studies on the neuropeptide kisspeptin encoded by the Kiss1 gene prove that it is a potent inducer of GnRH production. In animal models, mice with hyperprolactinemia were administered kisspeptin once a day for 20 days, which led to the restoration of estrus cycles and an increase in FSH and LH levels. Thus, kisspeptin may be used in the future to treat infertility associated with hyperprolactinemia (54). In studies with mice and sheep, paracrine substances secreted by the uterine glands have been shown to play a key role in implantation of the embryo and maintenance of pregnancy in the early stages. Therefore, problems with getting pregnant and with its maintenance may result from impaired functioning of the uterine glands. A thorough understanding and study of this issue may in the future contribute to improving the outcomes of infertility treatment as well as the maintenance of health by the mother and the fetus (57).
Multi-hormonal pituitary insufficiency is most often idiopathic and is associated with a mutation in one or several genes, among which the most important are transcription factors such as GLI2, LHX3, LHX4, HESX1, PROP1, POU1F1, SOX2, PITX2, OTX2, SOX3. Clinically, it is possible to encounter an isolated deficiency of one of the pituitary gland hormones or with a disturbance in the production of some or even all pituitary hormones, including gonadotrophins. It is therefore another potential cause of infertility (58). Postpartum pituitary hemorrhage, known as Sheehan’s syndrome, is a common cause of multi-hormonal pituitary insufficiency in tropical countries. Hypopituitarism also occurs in 10% of survivors of a poisonous snake. In turn, post-traumatic pituitary insufficiency accounts for approximately 7% of all cases (59). Empty sella syndrome can be another cause of hypopituitarism. It may be idiopathic or associated with postpartum hemorrhage, head trauma, CNS stroke, hormonally active pituitary microadenoma, and after radiation therapy or surgery. It manifests itself clinically with headaches, visual disturbances and hormonal insufficiency of the pituitary gland (60).
Autoimmune diseases, especially systemic lupus erythematosus (SLE) and rheumatoid arthritis (RA), as well as the toxicity of disease-modifying drugs, in particular non-steroidal anti-inflammatory drugs (NSAIDs) and glucocorticoids (GCs), also affect infertility. Although recently there has been an improvement, especially thanks to the use of biological drugs that reduce disease activity to a greater extent (61).
Infections in the hypothalamic-pituitary region account for less than 1% of the causes of its damage. The etiological factors can be bacteria, viruses, fungi and parasites. In imaging studies, infection may appear as a tumor in the area of the sella turcica, which raises the suspicion of a proliferative process. Risk factors for infection include meningitis, sinusitis, neurosurgery, and immune system disorders. Infection may develop in glands unchanged or damaged by previous diseases (adenomas, Rathke’s cyst, craniopharyngioma). Diagnostics is difficult due to the lack of specific symptoms. Patients may experience blurred vision or headache, fever and leukocytosis. During the acute phase of the disease, but also several months or years after successful antibiotic therapy, a significant proportion of patients develop symptoms of hypothalamic-pituitary insufficiency (62). Hypopituitarism can be caused by inflammation of the pituitary gland and can be primary or secondary. The most common form of primary inflammation is lymphocytic pituitary inflammation, which is most frequent in pregnant or postpartum women. In turn, immunotherapy, used in the treatment of neoplastic diseases, is important in secondary pituitary inflammation. Autoimmune complications, which can also affect the pituitary gland, are a side effect of such treatment. The most common clinical symptoms are headache, excessive thirst and visual disturbances, while laboratory deficiencies include ACTH, TSH, FSH, LH, GH and hyperprolactinemia. Although biopsy remains the gold standard in the diagnosis of pituitary inflammation, the most important in clinical practice is MRI, which also helps to differentiate pituitary inflammation from pituitary adenomas (63).
It is known that stress negatively influences the homeostasis of the circadian rhythm, which results in disturbance of the hypothalamic-pituitary-gonadal axis. In animal models, knock-out of clock genes such as period circadian regulator 1 and 2 (Per1, Per2), brain and muscle Arnt-like protein-1 (Bmal1) led to infertility in mice (64).
The female reproductive system is also affected by toxic substances such as pesticides, heavy metals, diethylstilbestrol, phenols, bisphenols, parabens. They can interfere with receptor binding, steroidogenesis and hormone metabolism. They also have a proven effect on the increased incidence of preterm labor, fetal growth disorders, miscarriages and difficulties in getting pregnant. Animal studies have shown that exposure to pesticides caused damage to oocytes, decreased production of steroid hormones by the ovaries, and lower fertility. Exposure to diethylstilbestrol suppressed gonadotropin production and caused damage to the pituitary gland and uterine tissue (65).
In vitro studies have shown that cannabinoids influence the functioning of the hypothalamus and pituitary gland through CB-1 receptors. Blocking these receptors results in an increase in the concentration of ACTH, GH, LH, and TSH, which proves that cannabinoids have an inhibitory effect on the activity of the hypothalamic-pituitary axis (66).
Gonadotropin-releasing hormone produced by the hypothalamus regulates the release and secretion of gonadotrophins that control the testes functioning, i.e. luteinizing hormone and follicle-stimulating hormone from the anterior pituitary. FSH receptors are located on Sertoli cells and LH receptors on Leydig cells. The effect of gonadotropins on testicular cells determines the proper course of testosterone synthesis, spermatogenesis, and the quality of sperm. The functioning of the male reproductive system is also influenced by hormones such as estradiol (E2) and prolactin. Estradiol, produced both by the testes and by the peripheral conversion of testosterone, is a potent inhibitor of LH and FSH. On the other hand, prolactin, by inhibiting the production of GnRH, reduces the concentration of LH and testosterone, which results in hypogonadism. In response to the generation of free oxygen radicals (ROS) by the male reproductive system cells, the hypothalamic-pituitary-adrenal axis is activated and cortisol is produced in response to stress. Cortisol, in turn, inhibits the production of gonadotropins, which causes a decrease in the testosterone production by Leydig cells (due to the lowered LH levels). Moreover, low FSH levels reduce the release of androgen-binding protein (ABP) by Sertoli cells, which further decreases circulating testosterone levels. Interestingly, obesity affects not only the hypothalamic-pituitary-adrenal and hypothalamic-pituitary-thyroid axes, but by generating ROS, it affects the increased production of leptin by adipocytes, which, together with insulin, reduces the concentration of triiodothyronine (T3), and thus adversely affects the testes functioning. Leptin is also an inhibitor of GnRH production by the hypothalamus (67). The pre-pubertal rise in leptin levels is responsible for the proper testes development. In turn, an increase in androgen concentration in adolescent boys causes a decrease in leptin concentration (68).
Obesity is not only associated with a higher risk of cardiovascular disease, stroke, type 2 diabetes, but also with disorders in the reproductive system in both women and men. It also leads to hyperinsulinemia, hyperlipidemia, hyperleptinemia and chronic inflammation. In obese men, decreased levels of testosterone, luteinizing hormone and sex hormone-binding globulin (SHBG) are observed. Increased amount of adipocytes negatively affects the quality and quantity of sperm, and as BMI increases, total sperm count decreases. A meta-analysis of 21 studies involving 13,000 men found a higher incidence of oligozoospermia and azoospermia in obese men. Moreover, the effectiveness of the IVF procedure decreases when the partner is obese (21).
In addition, studies in rats have shown that sleep disorders reduce testosterone levels, decrease sperm motility and apoptosis of Leydig cells in the testes. Moreover, it had a negative effect on the initiation of sexual behavior in males and on the percentage of ejaculation (69).
Infection with the severe acute respiratory syndrome coronavirus 2 (SARS-CoV2) is the cause of a cytokine storm that damages many organs. In addition to the well-known effects of SARS-CoV2 infection on the lungs, heart, and CNS, testicular damage is also under discussion. Evidence for the presence of SARS-CoV2 in sperm samples and in testicular tissue is limited, while an impaired immune system response, hyperthermia and oxidative stress caused by infection, damage testicular tissue. Testicular steroidogenesis defects related to SARS-CoV2 infection are the cause of decreased testosterone concentration, which leads to impaired spermatogenesis, impotence and, consequently, infertility in a significant percentage of convalescents. Increased levels of FSH and LH are biomarkers of testicular damage. Hypothalamic-pituitary-gonadal dysregulation due to SARS-CoV2 infection is also associated with the risk of acute renal failure and cirrhosis (70). It should also be noted that HIV infection is associated with an increased risk of pituitary gland stroke as well as pituitary lymphoma and may therefore lead to hypopituitarism, one of the effects of which is infertility (71).
The pineal gland is a relatively small gland located within the cranial cavity behind the third ventricle that connects to the cerebrum by a peduncle. It is a highly vascularized part of the brain not covered by the blood-brain barrier. 90-95% of the cells of this gland are pinacocytes, whose main function is the production of melatonin (N-acetyl-5-methoxytryptamine) (72). Its synthesis is regulated by the superior cervical ganglion, which receives information about the intensity of light through the suprachiasmatic nuclei of the hypothalamus, considered to be the anatomical center of the biological clock (73). The pineal gland, through melatonin, is responsible for controlling the regulation of the circadian rhythm, mainly sleep and wakefulness. Its synthesis and secretion significantly increases in the dark (74), and exposure to a light source causes a significant reduction in its synthesis (75). Melatonin acts through the MT1 and MT2 receptors located in the suprachiasmatic nucleus (SCN), reducing the activity of its neurons, which ultimately leads to the feeling of drowsiness and falling asleep (76). Another important function of melatonin is the inhibition of sexual maturation. In humans, the decline in the average daily melatonin production progresses with age and is associated with the progression on the Tanner scale (77, 78). This is due to the inhibition of kisspeptin expression by melatonin, which stimulates the hypothalamus cells to synthesize GnRH (79, 80). It has been proven that the administration of exogenous melatonin causes delayed puberty in children of both sexes (81). Melatonin is also produced in other organs, including the reproductive system (82–87), the retina and lens (88), the gastrointestinal tract (89, 90) and blood cells (91), acting as an autocrine or paracrine hormone (90). However, it most likely does not significantly affect the plasma concentration of melatonin as it was found to be undetectable in rats after pituitary removal (92). Melatonin is also considered to be a very effective antioxidant (73). By activating its MT1 and MT2 receptors, it stimulates the expression of superoxide dismutase (SOD), catalase (CAT), glutathione peroxidase (GPx), and glutathione reductase (GRd) (93). Another mechanism of antioxidant activity is the stimulation of the Nrf2 expression, which is a transcription factor for many genes encoding antioxidant enzymes (94). There are also many reports on the direct antioxidant activity of its metabolites: N1-acetyl-N2-formyl-5-methoxykynuramine (AFMK), N1-acetyl-5-methoxykynuramine (AMK) and cyclic 3-hydroxymelatonin (3-OHM) (95).
Melatonin appears to be an important hormone related to female fertility. Its higher plasma levels have been found in women with infertility caused by hypothalamus dysfunction (96, 97). At the same time, it has been shown that melatonin supplementation can positively affect fertility and improve the effectiveness of assisted reproductive protocols (25), which may be related to its influence on the increase of melatonin concentration in the follicular fluid (25, 98). Due to its antioxidant effect, melatonin has a positive effect on the quality of oocytes. Negative effects on fertility may be related to the inhibition of GnRH expression, which promotes ovulation by stimulating LH expression. Melatonin supplementation has also been shown to be beneficial in women suffering from polycystic ovary syndrome (26). This is due to a decrease in plasma testosterone concentration and elevated probability of ovulation (99). Studies carried out in a group of transgender men showed that testosterone inhibited the expression of gonadotropic hormones, which contributed to a strong suppression of ovulation (100). The reasons for the positive effect on the fertility of melatonin supplementation in PCOS patients are likely to be found in the antioxidant potential decrease (101).
Melatonin is associated not only with fertility, but also with the course of pregnancy. An increase in melatonin concentration during pregnancy and its sudden decrease after delivery have been shown (102). Furthermore, an increased risk of cholestasis has been demonstrated in women with a lower melatonin concentration (27) and the effectiveness of melatonin administration in lowering blood pressure and extending the duration of pregnancy in the course of pre-eclampsia has been confirmed (28). Studies are still needed to establish the relationship between melatonin levels and the course of pregnancy in more detail.
In animals, a strong relationship between melatonin and fertility has also been demonstrated. Melatonin deficiency is correlated with decreased fertility (29, 30), lower levels of sex steroid hormones (30), and decreased survival of embryos after fertilization (31). At the same time, a positive correlation was observed between serum melatonin concentration and fertility (32–36). Administration of exogenous melatonin is associated with a higher percentage of maintained pregnancies (37). Melatonin also participates in the activity changes of the female immune system during pregnancy in sheep, influencing the immune activity of the thymus, lymph nodes and spleen, which are involved in the process of fetal immune tolerance. The effect of melatonin on the immune system is complex and pleiotropic. At the beginning of the inflammatory reaction, it is immunostimulating, later it changes to immunosuppressive. The changes induced by melatonin in pregnancy are mainly related to the stimulation of the helper lymphocyte population by directly affecting the MT1 and MT2 receptors (38).
The relationship between melatonin receptors polymorphism and female fertility has been proven (32, 39–41). There are also numerous reports suggesting a protective effect of melatonin administration on fertility, maintenance of pregnancy and prevention of birth defects in females exposed to cytostatics, insecticides, herbicides and alcohol (42–46), which is directly related to its antioxidant nature.
In vitro studies focus mainly on the antioxidant activity of melatonin and its relationship with the production of gonads. In females, a positive correlation was demonstrated between the melatonin concentration in the follicular fluid and the markers of the ovarian reserve and the quality of oocytes (103–105).
Similarly in animal studies, the melatonin level in the follicular fluid correlated with the quality of oocytes and ovarian reserve (106, 107). In addition, melatonin seems to be a good component of cryopreserves used in the storage of the oocytes (108–110). The induction of estradiol production by granular cells was also confirmed (106).
In men, melatonin is also very important in terms of fertility. Similarly to women, melatonin causes a decrease in LH secretion, leading to lower testosterone secretion by Leydig cells in the testes, which has a negative impact on the intensity of spermatogenesis (107). A positive correlation was demonstrated between the concentration of melatonin and male fertility, both in the serum and in semen. Interestingly, an increased concentration of melatonin in the plasma and its decreased level in semen was demonstrated in infertile men (111). Studies have shown that the concentration of melatonin in semen inversely correlates with the frequency of sperm DNA damage, which is probably due to its antioxidant activity (112). It has also been reported that melatonin supplementation improves sperm quality (113, 114) and increases the chance of preserving fertility in patients with varicocele (115).
There are also studies linking melatonin to male fertility in animals (46). Protective effect on gonads has been demonstrated in the course of varicocele, radiotherapy and metabolic syndrome, exposure to cytostatics and heavy metals (47, 116–119). In addition, the synthesis of melatonin within the testes has been confirmed (120) and the positive effect of its concentration on semen quality was demonstrated (121).
The importance of the antioxidant activity of melatonin during spermatogenesis has also been shown in animal studies (122). Furthermore, melatonin seems to be a good component of cryopreserves used in the storage of sperm samples (123).
Inhibition of the secretion of thyroid hormones is associated with its hypothyroidism. In milder hypothyroidism, infertility is usually not achieved, but the risk of spontaneous miscarriage, premature births and stillbirths increases (124–126) (Table 3). Severe hypothyroidism may lead to infertility as a consequence of a direct inhibitory effect on the ovarian ovulatory activity as well as through an effect on the pituitary-ovarian axis. Decreased activity of sex hormone-binding globulin causes an increase in serum free testosterone and estradiol, moreover, metabolic clearance of androstenedione and estrone is decreased. The elevated level of thyrotropin-releasing hormone in primary hypothyroidism is responsible for the increase in serum prolactin levels and the delayed luteinizing hormone response to the stimulating effect of gonadotropin-releasing hormone, which in turn leads to luteal phase failure in women (124–126, 131, 132).
Due to the small number of in vitro studies and their contradiction regarding the influence of the role of thyroid hormones on reproductive function and semen parameters in humans, it is necessary to exercise caution when interpreting them. Kakita-Kobayashi et al. determined the effect of levothyroxine on decidualization in human endometrial stromal cells (hESCs) and the selection of key genes for this process in vitro. After exposure of cells to the drug, a significant increase in the temporal response was observed. Moreover, treatment with LT4 also influenced the regulation of many transcription factors important for decidualization. There was also an increase in type 3 deiodinase in the presence of thyroid hormones, which is an important element in the tissues of the fetus and placenta. In addition, it has been noted that the progesterone receptor and the ovarian steroid hormone receptor are involved in thyroid hormone-induced decidualization. Based on the conducted study, Kakita-Kobayashi et al. claim that impaired decidualization is a possible cause of infertility in patients with subliminal hypothyroidism (127).
It was shown that the trophoblast contains triiodothyronine receptors. In vitro studies indicate that thyroid hormones have a direct impact on the early development of the placenta by stimulating angiogenesis and by promoting the invasion and differentiation of embryonic cells (133). Thyroid receptors are found in the endometrium, and their highest levels are observed in the receptive endometrium (134). Barber et al., using immunohistochemical staining, localized specific isoforms of thyroid hormone receptors in extracellular trophoblasts in placenta biopsies in the first and second trimesters of pregnancy, which indicates a potential sensitivity of cells to T3. Epidermal growth factor (EGF) and T3 exhibited antiproliferative effects on the extravillous trophoblast cell line (SGHPL-4) while promoting proliferation in JEG-3 chorionic cancer cells. The study suggests that T3 and EGF may act synergistically, regulating both the proliferation and the differential function of human trophoblast (128).
In 2008, quercetin was shown to inhibit the dose-and time-dependent spread of Fischer rat thyroid cell line (FRTL-5) by inhibiting the insulin-regulated action of Akt kinase. Quercetin interferes with TSH-dependent NIS gene expression and transport in FRTL-5 cells. These observations may help to understand the molecular mechanism of quercetin’s anti-thyroid effects on cell growth and function. Even when taken from an in vitro thyroid cell line that does not have the characteristics of a transformed cell, these results led to the evaluation of quercetin as an anti-thyroid drug in hyperthyroidism (135, 136). In recent studies, quercetin appears to reduce the expression of the thyroid-stimulating hormone receptor, thyroid peroxidase (TPO) and thyroglobulin (Tg) genes. The anti-thyroid effect of quercetin was further assessed in vivo. It was administered (50 mg/kg) to a Sprague-Dawley rat, and after 14 days of treatment, the radioiodine uptake decreased significantly, indicating that quercetin may act as a thyroid disruptor (129, 135). Soybean extracts inhibit iodine uptake and increase the protein content of a known autoimmune Tg fragment in rat Fischer thyroid cells. These effects may be responsible for the link between more frequent soy consumption and thyroid disorders such as hypothyroidism, goiter, and autoimmune thyroid disease (135, 137). Among the flavonoids, epigallocatechin-3 gallate (EGCG), a catechin rich in green tea, when administered to male rats at doses of 25, 50 and 100 mg/kg of body weight, showed an antithyroid activity, which was manifested by reduced activity of thyroid peroxidase and 5′-deiodinase I and increased activity of the thyroid sodium-potassium pump. In addition, serum T3 and T4 levels were lowered while serum TSH was elevated in rats, demonstrating the possibility of goiter in vivo (135, 138).
Condorelli et al. assessed the effects of levothyroxine (LT4) on conventional and biofunctional sperm parameters and their impact on fertility. The authors obtained sperm from men with confirmed infertility and exposed them to LT4 in vitro. The results confirmed the effect of LT4 on an increase in the number of sperm with a high level of mitochondrial membrane potential (MMP), a reduction in the percentage of sperm with a low MMP and an increase in sperm motility. L4 was found to induce sperm proliferation and lipid peroxidation, thereby improving the chromatin tightness in the cell nucleus. According to the researchers, the results of the above-mentioned in vitro study may have clinical application in patients with idiopathic infertility due to the explanation of the influence of thyroid function on fertility in men (130).
So far, a key role of vitamin D in the male reproductive system has been suggested as its receptors and metabolizing enzymes have been shown to be expressed in the testes and sperm. Vitamin D metabolism is mainly regulated by parathyroid hormone (PTH), produced by the parathyroid glands, and fibroblast growth factor 23 (FGF23), synthesized by osteoblasts and osteoclasts. Decreased levels of circulating calcium and 25-hydroxyvitamin D3 increase PTH secretion, which stimulates 1-α-hydroxylase and inhibits the expression of 24-hydroxylase in the kidney, leading to higher levels of vitamin D and calcium (139, 140). In addition, elevated levels of phosphorus and 25-hydroxyvitamin D3 inhibit 1-α-hydroxylase and stimulate 24-hydroxylase, resulting in a reduction in vitamin D. To close the feedback loop when vitamin D and phosphorus levels decline, FGF23 is inhibited, leading to an increase in vitamin D levels (139, 141). The vitamin D receptor (VDR) and the enzymes that metabolize vitamin D are simultaneously expressed in Sertoli cells, germ cells, Leydig cells, sperm, and cells in the epithelial lining of the male reproductive system. The presence of vitamin D metabolizing enzymes suggests that the reproductive organs may modulate the local response to vitamin D in animals and humans. Nuclear somatic or embryonic cells appear to be able to synthesize and degrade vitamin D locally, independent of systemic vitamin D metabolism. Moreover, expression of VDR in the testes suggests that vitamin D may exert autocrine and paracrine effects, possibly playing a role in regulating testicular function, thus contributing to male infertility. The expression of VDR and vitamin D metabolizing enzymes in the male reproductive system has been extensively analyzed in animal and human studies. The VDR protein has been found in the prostate, seminal vesicles, epididymis, and also in germ cells, especially spermatogonia, spermatocytes, and Sertoli cells (139, 142). VDR protein expression has been found in animal sperm but has been suppressed in the tail of the epididymis (139, 143). In the same context, testosterone synthesis enzymes in the testes appeared to be reduced in mice fed the vitamin D deficiency (VDD) diet (144).
Changes in the immune system activity are necessary in the course of a healthy pregnancy as they help to avoid an immune response against an allogeneic fetus (145) (Table 4). One of the organs whose activity change is required for the development of immune tolerance during pregnancy is the thymus, in which the maturation and differentiation of helper T cells take place.
Maternal Th, Tc and NK cells express activation markers on their surface after blastocyst implantation in the uterine endometrium, which suggests rapid recognition of trophoblast by these cell populations (146). Many studies have shown changes in the population of T lymphocytes and their activity in normal pregnancy (147, 155–160). In addition, human placenta produces IL-35 with an immunosuppressive effect, stimulating the proliferation of regulatory T cells in the thymus (161, 162).
The activity of the thymus is also important in terms of the etiology of primary ovarian insufficiency (POI), where one of the causes may be an autoimmune reaction. An increased risk of POI in patients with autoimmune diseases and an increased risk of autoimmune diseases in patients with POI have been observed (163).
In animal studies, changes in the population of T lymphocytes mainly include an increase in the Th cell population during early pregnancy (149), a greater percentage of type 2 helper cells in relation to type 1 helper cells (148, 164), changes in the toll-like receptor (TLR) expression within thymic epithelial cells, and the synthesis of prostaglandins and proteins stimulated by interferon (150, 151). These changes are directly responsible for the phenomenon of fetal immunotolerance, inhibiting the development and activation of a subpopulation of T lymphocytes that can stimulate inflammatory reactions within the uterine endometrium, while maintaining the endometrial and trophoblast defense against pathogens (165). This is possible due to the activity of the placenta, which produces Th2-specific cytokines such as IL-4, IL-5 and IL-10 throughout the duration of pregnancy (164).
During pregnancy, there is both local (within the uterus) and general increase in the population of regulatory T cells specific for paternal antigens (166, 167), which is responsible for the inhibition of the immune response to them.
The activity of thymus is related not only to pregnancy, but also to the maturation of the ovaries and their activity. Studies in mice have shown that early removal of the thymus causes disorders in the ovarian development, failure of maturation and sterility. This effect was reversible by administering thymulin, although a decrease in fertility was observed due to the presence of autoreactive T cells (168, 169). It is related to the influence of thymulin on the expression of LH and FSH by the pituitary gland. It has been demonstrated that GnRH and thymulin act synergistically with the release of LH and additively with the FSH secretion (170). The variable effect of thymulin on LH and FSH expression depending on the time of the menstrual cycle has also been reported (171).
A positive correlation was demonstrated between the concentration of thymulin and LH, as well as a reduction in testosterone production with a decrease in thymulin concentration (152, 153). The presence of regulatory T cells within the testes has also been demonstrated (154, 172), which, through the secretion of IL-10, affect the ratio of the Th1 to Th2 subpopulation. Their presence is essential for the immunotolerance of spermatocyte antigens. In the case of disorders leading to a reduction in their population size, autoimmune orchitis may occur (154).
Disorders of lipid and glucose metabolism, recently associated with the increasingly frequent obesity, are a current medical problem. They not only pose a health risk, but also have an adverse effect on reproductive function (Table 5). Insulin resistance, i.e. reduced cell sensitivity to insulin and compensatory hyperinsulinemia, induce an early response to luteinizing hormones and cause premature differentiation of small follicles, resulting in anovulation. On the other hand, they adversely affects the functions and environment of the endometrium and are responsible for disturbances in embryo implantation (180). In addition, hyperinsulinemia disturbs the intra-follicular microenvironment during folliculogenesis, decreases the fertilization rate and the potential for embryonic development during natural ovarian stimulation cycles (173). Insulin primarily acts on its own receptors located on the theca cells surrounding the stromal and granulosa cells, thereby stimulating ovarian steroidogenesis (174). Research shows that inflammation (elevated levels of IL-6 and IL-17) accompanying insulin resistance and obesity affect ovulation and fertilization and increase the risk of early miscarriage. Moreover, obese people show increased levels of estrogens due to the overexpression of aromatase in adipose tissue, which in turn disturbs ovulation (173, 175).
Glucagon is a central mediator of glycemic control, released by pancreatic alpha cells in response to hypoglycemia. It is involved in insulin signaling and action, but also predisposes to adverse pregnancy outcomes. Interestingly, more and more reports confirm the modulating activity of glucagon-like peptide-1 (GLP-1) in reproduction. GLP-1 receptor antagonists play an important role in the treatment of type 2 diabetes and obesity by lowering glucose levels, reducing body weight and improving reproductive health. Obesity inhibits the hypothalamic-pituitary-gonadal axis, disrupting ovarian function, ovulation index and endometrial receptivity, and affects the molecular mechanisms that regulate the biological activity of the reproductive system (3, 181, 182).
Pancreatitis accompanying pregnancy is not common, but it carries a burden in terms of maternal mortality or perinatal mortality (183). Commonly used in hormone contraception, hormone replacement therapy (after menopause) or hormone therapy in in vitro fertilization (development of the endometrium in preparation for embryo transfer), estrogens may be one of the causes of acute drug-induced pancreatitis (184). These cases are rare and the clinical course is usually mild to moderate (185). The mechanism of acute estrogen-induced pancreatitis is not fully understood, but it is presumed to be due to hypertriglyceridemia. Mechanisms related to this phenomenon include an increase in the synthesis of TG in the liver, secretion of VLDL into the circulation, an increase in newly synthesized TG in the liver, excessive secretion of TG and apolipoprotein, and the effect of estrogens on the inhibition of lipoprotein lipase (LpL) promoter activity (176). Transported by chylomicrons and very low-density lipoproteins, triglycerides are hydrolyzed by high lipase concentrations in the pancreatic capillaries, where they form a large amount of toxic free fatty acids that cause lipotoxicity in acute pancreatitis (186). Hence, in the case of patients qualified for IVF, especially those from the high-risk group (such as diabetes, polycystic ovary syndrome, obesity, dyslipidemia), it is reasonable to screen for lipid abnormalities before starting the in vitro procedure and constantly monitor key biochemical parameters (176).
Studies in a mouse model (glucagon receptor knockout mice) showed that the lack of glucagon signaling did not alter the hypothalamic-pituitary-ovarian axis. Pregnant knockout female mice exhibited hypoglycemia and hyperglucagonemia accompanied by decreased fetal weight, increased late-stage fetal mortality, and placental abnormalities. Moreover, the lack of glucagon signaling significantly reduced the level of expression of genes controlling growth, adrenergic signaling, vascularization, oxidative stress and the activity of G-protein-coupled receptors (187). Studies on an animal model allow the conclusion that, similar to insulin, glucagon contributes to the proper reproductive function of women. It has also been shown that the lack of glucagon signaling creates a poor quality uterine environment for fetal growth. Animal studies have demonstrated that the lack of glucagon signaling during pregnancy was associated with reduced litter size, limited intrauterine growth, and increased neonatal mortality (177).
In a study of male patients with idiopathic infertility, the relationship of metabolic syndrome, obesity and diabetes mellitus was shown as factors contributing to the pathogenesis of male infertility. A strong association of insulin resistance has also been shown in patients with unexplained fertility problems, presuming that elevated blood insulin levels may impair spermatogenesis, and in patients with hyperinsulinemia and type 2 diabetes, also generate nuclear and mitochondrial DNA damage in sperm (178, 188). In the aspect of metabolic diseases, the relationship between sperm count and BMI was also investigated and it was shown that obese men had a twofold increase in the risk of oligozoospermia and increased scrotal temperature, leading to sperm dysfunction, reduced sperm count and mobility, and DNA damage (188).
Moreover, it has been confirmed that glucagon can directly stimulate the reproductive axis, which has not been observed in studies on men where there was no effect of glucagon administration on reproductive hormone levels (179). On the other hand, administration of glucagon receptor antagonists can cause metabolic effects (weight loss, i.e. alleviation of hypogonadism of the hypothalamus in obese men) without direct negative effects on the reproductive system (179).
Changes in the hypothalamic-pituitary-adrenal axis and the subsequent alterations in the concentration of circulating hormones constitute the body’s response to stressful challenges (Table 6). Mobilization of resources during the stress response suppresses the reproductive axis, which gives the survival of an individual higher priority than the preservation of the species (195). Stressors also affect the adrenal medulla, which secretes catecholamines, i.e. adrenaline and noradrenaline, and the adrenal cortex that secretes aldosterone, which is, next to cortisol, a key regulator of blood pressure (196).
Past studies have shown that patients with lower levels of adrenaline had a higher rate of implantation (the group of patients undergoing IVF), which suggests that the less stressful life they lead, the greater their reproductive success (189, 190). It has been found that stressors activate the hypothalamic-pituitary-adrenal axis, causing changes that have a significant impact on female fertility (197).
The IVF procedure itself may be a stressful condition. In a study by Smeenk et al. there was no difference in the stress response between women who became pregnant after treatment and those who did not. Little is known about the effects of catecholamines and cortisol on the physiological processes related to reproduction. It is concluded that catecholamines may affect fertility by altering uterine blood flow, while cortisol with immunosuppressive properties may additionally affect the immune states necessary for implantation. Studies have shown that a higher ratio of serum cortisol to follicular cortisol was associated with pregnancy, and that infertile women had higher levels of stress in terms of circulating prolactin and cortisol compared to fertile women in the control group (190).
Aldosterone, as a mineralocorticoid hormone secreted by the zona glomerulosa of the adrenal cortex, is responsible for the regulation of water and electrolyte balance and blood pressure. During physiological pregnancy, aldosterone levels increase, inducing elevated plasma volume, which is essential for the maintenance of circulating blood volume, blood pressure, and uteroplacental perfusion. Aldosterone levels remain high throughout pregnancy, suggesting a possible role in the regulation of placental and fetal development (198, 199). Recent studies have shown the possible involvement of aldosterone in some gynecological conditions and diseases, including endometriosis combined with infertility. Aldosterone may exacerbate systemic and local inflammatory states underlying endometriosis by activating mineralocorticoid receptors present in inflammatory cells (198).
High levels of the norepinephrine transporter (NET) have been documented in mammalian placenta tissue. Placental changes in NET were involved in the modification of reproductive function and fertility of the offspring caused by pregnancy stress (191). Studies in rats showed that exposure of pregnant rats to sympathetic stress affected the placental transport of norepinephrine, leading to a reduction in the ability of the placenta to remove norepinephrine from the fetus into the maternal circulation. It also resulted in impaired fertility of the offspring in adulthood (191). In addition, it has been observed that increased plasma levels of norepinephrine can result in the narrowing of the uterine arteries leading to failure of trophoblast invasion.
Pregnancy is particularly sensitive to stress factors that may lead to permanent modifications in the postpartum development of newborns, predisposing them to diseases that appear in adulthood (200, 201). In a guinea pig study, maternal access to nutrients was drastically restricted during the period of maximum fetal brain growth (70% of pregnancy), resulting in adult male offspring with elevated basal cortisol levels but normal adrenal stress response. However, the same stress applied to 90% of pregnancy results in male offspring with normal basal cortisol levels but increased responsiveness of the hypothalamic-pituitary-adrenal axis to challenge (192). Interspecies differences in the effects of prenatal stress on adult behavior may also be due to the unique development profiles of fetal bodies and brains. In humans, the rapid phase of fetal brain development occurs from about 27 to 30 weeks of gestation and extends to the postpartum period, while in rats or mice, maximum brain growth does not begin until postnatal life. Hence, the period of maternal stress in a rodent would probably correspond to a completely different phase in the human fetal brain.
Apart from oxidative stress, which has a negative impact on the quality of sperm, long-term stress manifested by an increased level of cortisol is also mentioned. This is related to male infertility due to the decreased conversion of androstenedione to testosterone, thereby reducing the volume and concentration of sperm in semen (202). Studies have shown that cortisol and adrenaline levels were significantly higher in the group of infertile men compared to the control group of men without fertility problems (194). Stress in this sense is both a physical and an emotional factor causing the activation of neurons that secrete the corticotropin-releasing hormone, leading to higher plasma cortisol levels. Excess cortisol reduces testosterone production, reducing sperm parameters. Cortisol may also directly reduce testosterone production by blocking the transcription of genes encoding enzymes necessary for testosterone synthesis (195).
High levels of adrenaline and associated low testosterone have also been observed in infertile golden hamsters (193). This phenomenon, also accompanying short-term stresses, results from the inhibitory effect of adrenaline and dopamine on testosterone production both by the HPA hypothalamic-pituitary axis and the peripheral blockade of testosterone release (203).
Estrogens belong to the family of steroid hormones produced mainly by the gonads and the placenta. In addition to the reproductive system, they play an important role in the immune, skeletal and neuroendocrine systems, therefore disturbances in their concentration or functioning are observed in pathological conditions and diseases such as infertility, cancer, obesity or osteoporosis (204). Estrogens have been shown to have negative and positive feedback on the hypothalamic-pituitary axis, and their action is possible by binding to the estrogen receptor alpha (ERα) and beta (ERβ). These receptors have different tissue expression patterns in both humans and rodents (205). Estrogen mediation in biological responses is possible through a genomic mechanism, usually occurring within hours in most tissues, and a non-genomic mechanism, occurring very rapidly within minutes of exposure to hormones (206).
Estrogen binds to the ER, which is mainly found in the nucleus of the target cell. The resulting complex can regulate gene activity by binding directly to DNA regulatory elements called estrogen response elements (EREs), leading to the recruitment of additional factors involved in the regulation of transcription (207). An alternative approach, also called indirect, involves interaction with transcription factors, including TF, AP-1, SP1, and NF-κB, which recruits chromatin-modifying coregulator proteins and enables activation or repression of ER target genes to direct cell proliferation (208). The non-genomic mechanism involves the interaction of the ER located in the plasma membrane or its vicinity with adapter proteins, including Shc and Src, as well as signaling through GPR30, resulting in activation of the MAPK cascades, PI3K, and adenylate cyclase (209).
Progesterone is an endogenous steroid hormone commonly produced by the cortex of the adrenal glands and the gonads. The ovarian follicles are the main source of peripheral progesterone from the late follicular to luteal phase (210). It is also secreted by the corpus luteum of the ovary, generally during the first ten weeks of pregnancy, and then through the placenta (211). The action of progesterone is based on binding to a receptor located in the cell cytoplasm. Then it dimerizes and translocates to the nucleus where it can bind to DNA, which enables the regulation of gene expression. There are three isoforms of progesterone receptors: PR-A, PR-B and PR-C (212).
Primary ovarian insufficiency (POI) is defined as the cessation or irregular menstrual cycles under the age of 40 in the presence of elevated serum FSH levels. This disorder is rare and affects approximately 1% of women. The causes of POIs can be spontaneous, genetic, environmental, infectious, autoimmune, surgical, chemotherapy or radiation related (213). The European Society of Human Reproduction and Embryology (ESHRE) recommends both of the following diagnostic criteria for POIs: hypomenorrhea/amenorrhea for at least four months, elevated follicle-stimulating hormone levels (> 25 mIU/mL) confirmed twice at an interval > 4 weeks (214). Serum FSH determination is the gold standard in the POI diagnosis. The anti-müllerian hormone can only be interpreted in conjunction with the FSH and estrogen levels. In the case of secondary amenorrhea, it is necessary to exclude pregnancy by testing the serum level of beta subunit of human chorionic gonadotropin (beta-HCG) and the concentration of thyroid stimulating hormone and prolactin, as endocrine diseases can lead to menstrual disorders (215). Chromosome analysis and fragile X premutation tests are recommended for all women with POI (216). As some cases of POI are autoimmune in nature, it is important to rule out other autoimmune diseases. According to ESHRE, it is necessary to assess the level of adrenal and thyroid antibodies. If the results are positive, it is imperative to monitor the function of these glands (214).
About 76% of POI patients maintain regular periods during adolescence and adulthood, followed by cycle disruptions (217). Ovarian function can be intermittent and unpredictable, with spontaneous ovulation in up to 20% and conception in approximately 5-10% of women (163). The symptoms these women experience are identical to those during menopause and can include hot flashes, night sweats, dyspareunia, vaginal dryness, sleep disturbances, mood changes, altered urination frequency, low libido, and a lack of energy. They are caused by a decrease in the production of estradiol in the ovaries. Symptoms may be transient or intermittent and may vary in severity due to fluctuations in ovarian activity during the spontaneous occurrence of POI (218). POI significantly reduces patients’ quality of life due to increased cardiovascular risk, decreased bone mineral density leading to osteoporosis and atrophic changes in the genitourinary system. The disease has a negative impact on the mental well-being of patients, is associated with pregnancy failures and lower sexual satisfaction (219).
Polycystic ovary syndrome (PCOS) is the most common endocrine disorder in women of childbearing age, affecting approximately 6–21% of women (220). PCOS can be diagnosed using the Rotterdam criteria, which require at least two of the three listed criteria: anovulation, clinical signs of hyperandrogenism and/or serological elevation of androgens, polycystic ovaries demonstrated by ultrasound. The National Institutes of Health criteria also require clinical or biochemical hyperandrogenism and oligo- or anovulation. The American Excess PCOS Society requires hyperandrogenism with one of the other two criteria. As this is an exclusion diagnosis, disorders that exhibit PCOS-like features must be ruled out. These include hyperprolactinemia, thyroid disease, non-classical congenital adrenal hyperplasia (221, 222).
In addition to infertility, many diseases are associated with PCOS, including endometrial cancer, type 2 diabetes, impaired glucose tolerance, metabolic syndrome, cardiovascular risk, non-alcoholic fatty liver disease/non-alcoholic steatohepatitis (NAFLD/NASH) (223). It has been estimated that about 50% of women with PCOS are overweight or obese and have reduced insulin sensitivity (224). Almost all causes of PCOS arise from functional ovarian hyperandrogenism (FOH). Two-thirds of cases have typical FOH, characterized by androgen secretion disruption with an excessive response of 17-hydroxyprogesterone to gonadotrophin stimulation. In other cases of PCOS, an increase in testosterone levels is observed, which can be detected after suppressing the production of androgens in the adrenal glands (225). The causes of dysregulation include excess insulin, which sensitizes the ovaries to luteinizing hormone, as well as an imbalance between the intra-ovarian regulatory systems.
The excess of androgens enhances the growth of primary follicles. At the same time, it initiates premature luteinization, making the selection of the dominant follicle difficult (226). It is estimated that about half of FOH patients have insulin-resistant hyperinsulinism, which prematurely luteinizes granulosa cells, increases steroidogenesis, and stimulates fat accumulation. Hyperandrogenemia causes LH excess, which acts on luteinized granulosa and theca cells (227). Hormonal dysregulation alters the pulsatile release of GnRH, resulting in increased biosynthesis and secretion of LH compared to FSH (228). It has been shown that LH stimulates the production of androgens in the ovaries, while a decrease in FSH levels leads to the inhibition of aromatase activity in granulosa cells, reducing the conversion of androgens to estradiol (229). Moreover, serum androgens are converted peripherally to estrogens. Since it occurs mainly in adipose tissue, estrogen production will be increased in obese PCOS patients. Furthermore, unbalanced estrogen stimulation can lead to endometrial hyperplasia and endometrial cancer (230).
Estrogens, in particular estradiol-17β, are essential fertility regulating hormones. This is related to their participation in the development of ovulatory follicles, induction of pre-ovulatory gonadotropin release in the middle of the cycle, or preparation of the uterine mucosa for implantation. Changes in the production and/or function of estrogen can therefore disrupt these processes, leading to infertility (207) (Table 7). Studies carried out so far in models using rats have shown that disruption of Esr1 causes infertility in both males and females (231). Rumi et al. also concluded that the proper functioning of Esr2 is the primary regulator of female fertility, but is not critical to male fertility (232).
Physiological estrogen replacement alleviates menopausal symptoms and may improve sexual dysfunction associated with vaginal dryness, dyspareunia, and decreased libido (251). Unfortunately, Hipp et al. showed that more than half of young women with POI either never use hormone replacement therapy (HRT) or start it many years after diagnosis and/or stop using it before the age of 45 (233). The current evidence supports transdermal or vaginal therapy with estradiol as the first line of HRT. They mimic the daily rate of ovarian estradiol production and achieve mean serum level of 100 pg/mL, which is the average level in women with normal ovarian function throughout the menstrual cycle (252). Oral estradiol may be an alternative, but this is associated with complications related to the hepatic first-pass effect, including increasing the risk of venous thromboembolism (234). The multicenter study of Estrogen and Thromboembolism Risk (ESTHER) confirmed that the odds ratio for this disease was 4.2 in oral estrogen users compared to 0.9 in transdermal estrogen users (235). Interestingly, Renoux et al. also noted that the use of oral HRT was associated with a higher rate of stroke in postmenopausal women (253). In addition, Langrish et al. conducted a study in which they compared the treatment effects of transdermal estradiol and cyclic progestins with treatment with combined oral contraceptives on the circulatory system of young women with POI. They noticed that 12-month transdermal HRT resulted in significantly lower blood pressure, better kidney function and reduced activation of the renin-angiotensin-aldosterone system, suggesting that it was more effective (236).
More and more studies reveal that reproductive disorders can have a negative impact on pregnancy, from implantation to delivery. Many patients with these conditions require assisted reproductive technology (ART), which may influence pregnancy outcomes. As a result, it is difficult to distinguish the contribution of certain factors to poor pregnancy outcomes (254). It has been shown that often multiple risk factors contribute to negative obstetric outcomes in women with reproductive disorders. In addition, many women of childbearing age have more than one reproductive disorder (255).
In the case of POI, the chances of a spontaneous pregnancy are very small. According to the ESHRE guidelines, there are no interventions that increase the chances of natural conception, so oocyte donation or reception is considered a reliable chance to become pregnant (214). Interestingly, most people with POI still have detectable follicles. The mechanism of follicle dysfunction was defined in the NIH study as its inappropriate luteinization due to tonically increased serum LH levels. HRT that suppress high LH levels, can increase the chances of ovulation and improve follicle function. Piedade et al. presented a case report where a patient chose intra-uterine insemination (IUI) in combination with follicle monitoring and HRT and successfully became pregnant (237). In turn, Pinelli et al. conducted a study in which women with reduced ovarian reserve were administered valerate estradiol (2 mg daily) adding dihydrogesterone (10 mg daily) in the luteal phase for 3 months prior to the standard short protocol with a GnRH antagonist for in vitro fertilization. They concluded that pretreatment with estrogen appeared to improve IVF outcomes (238).
For overweight and obese women with PCOS, exercise and calorie restriction diets are the best first-line interventions for weight loss and impaired glucose tolerance (IGT) reduction. It is possible to alleviate the clinical symptoms of PCOS, including abnormal ovulation and hormone disturbances. Zhang et al. in their meta-analysis assessed the effectiveness of a low-carbohydrate diet in the treatment of PCOS and showed its beneficial effects (239). Treatments for menstrual disorders, hirsutism and acne include oral contraceptives, transdermal patch or vaginal ring. The progestogen component lowers LH levels, indirectly reducing the production of androgens in the ovaries and increasing the level of sex hormone binding globulin. Metformin is recommended in case of contraindications to the use of hormonal contraceptives. It has been shown to reduce the progression from impaired glucose tolerance to type 2 diabetes and also to improve menstrual cycles and vascular markers in non-obese women with PCOS (240).
Clomiphene citrate is considered the first-line treatment for infertility in PCOS patients. It is a selective estrogen receptor modulator (SERM) that is a competitive inhibitor of estrogen receptors. Clomiphene improves fertility and ovulation, especially by acting on the hypothalamus, where it binds to estrogen receptors. This causes a pulsatile release of GnRH, promoting the secretion of gonadotrophins from the anterior pituitary. Interestingly, up to 25% of women do not respond to the administration of clomiphene, which is associated with the implementation of gonadotropin therapy. However, Takasaki et al. proposed to modify the clomiphene treatment regimen. They used intermittent dosing, which was effective in approximately 80% of clomiphene-resistant patients (241). On the other hand, Homburg et al. showed that a small dose of FSH could be used instead of clomiphene (242). Another first-line drug for ovulation induction is letrozole. It is an aromatase inhibitor that blocks estrogen synthesis, reducing negative estrogen feedback in the pituitary gland. Waanbah et al. showed that treatment with letrozole, compared to clomiphene, was associated with higher rates of live births and ovulation among infertile women with polycystic ovary syndrome (243). Spironolactone, which is an antagonist of the mineralocorticoid receptor, is also used, as well as its combinations with metformin, which helped to improve the menstrual cycle, glucose and testosterone levels (244).
In PCOS patients, insulin sensitization is also introduced. It is associated with the administration of glucagon-like peptide 1 (GLP-1) agonists which, upon binding to the receptor, stimulate glucose-dependent insulin release from the pancreatic islets. This treatment reduced BMI and testosterone, and improved the ovulation rate in obese women with PCOS (256). Glintborg et al. showed that after treatment with oral contraceptives along with metformin, the level of GLP-1 in women with PCOS was comparable to that in healthy people (245). In addition, Devin et al. conducted studies using DPP4 inhibitors that have beneficial effects on weight loss and lowering blood glucose levels in obese women with PCOS. They reported that the effect of DPP4 inhibitors on the body weight of women with PCOS is based on the increase in the growth hormone levels, which are lowered in PCOS patients. This, in turn, reduces the mass of visceral adipose tissue (246). On the other hand, Javed et al. noted the promising effect of SGLT2 inhibitors in overweight and obese women with PCOS. After 12 weeks of treatment with empagliflozin, there was an improvement in body composition and anthropometric parameters compared to metformin treatment, however no changes in hormonal and metabolic parameters were observed (247). Interestingly, Benito et al. conducted a study in women with POCS who had undergone bariatric surgery. They observed a significant improvement in pregnancy and healthy birth rates compared to the control group (248). In turn, Toulis et al. showed that up to 40% of women with PCOS and glucose intolerance can develop gestational diabetes (GDM) (249). There was also an increased risk of pregnancy and neonatal complications, including premature pregnancy loss, gestational hypertension, preterm delivery, low birth weight and the need for cesarean delivery, regardless of obesity (250).
The testes are paired, oval glands located in the scrotum. Their main functions include the production of male gametes (sperm) and male sex hormones. Sperm formation is carried out by the germinal epithelium located in the convoluted tubules of the testes, while the production of testosterone, the main male sex hormone, is the responsibility of Leydig cells. These cells also produce a small amount of androstenedione (A4) (257). The functioning of the testes depends mainly on LH and FSH, as LH stimulates the production of testosterone, and FSH promotes the production of male gametes (258).
The testes are not only the site of testosterone synthesis, but also the main organ regulated by its activity. Testosterone stimulates spermatogenesis by affecting not only spermatogonial stem cells, but also Sertoli cells, which support spermatogenesis and create a blood-testis barrier within the epithelium (259). In addition, testosterone is the main hormone involved in male sexual maturation (260). Other functions of testosterone are the stimulation of bone and muscle mass growth, and the regulation of sexual drive (261). Unlike LH, FSH acts directly on epithelial cells. It regulates the function of Sertoli cells, stimulating them to paracrine activity, promoting the maturation of spermatogonia into spermatocytes (262). The large increase in testosterone production by the testes in adolescence is one of the main factors in stimulating puberty. During sexual maturation, its synthesis increases 20-30 times (263). Due to this, not only physical but also mental changes are induced, such as increased sex drive, tendencies towards non-physical aggressive behavior and domination (264).
The testes, although being the main androgen-producing organ, are not the only ones. Another source of androgens is the adrenal glands. They produce dehydroepiandrosterone (DHEA) and androstenedione (265). DHEA is an anabolic hormone and strongly influences cognitive functions such as mood and sex drive (266). A4 is a testosterone precursor and has an effect similar to testosterone, but weaker. Both hormones are involved in adrenarche, and the increase in their concentration at the age of 6-8 years is probably of key importance in the development of social and cultural skills (267). Impaired testosterone secretion, regardless of whether it results from primary organ failure (Klinefelter syndrome, Leydig cell aplasia, genetic or drug-induced defects) or a defect associated with the secretion of pituitary hormones (Kallmann’s syndrome, hypopituitarism, genetic or drug-induced defects in LH secretion) is a well-known cause of male infertility (268) (Table 8).
The percentage of infertile men ranges from 2.5-12% depending on the studied population (289). Infertile males account for 40-50% of couple infertility, and the trend is increasing (290). The main cause of male infertility is poor semen quality, i.e. changes in physical parameters and volume, a decrease in the number of sperm per unit volume, reduced sperm motility and viability, abnormal sperm morphology. There are many reasons for a decline in sperm quality, from primary and secondary testicular failure to numerous genetic, environmental and lifestyle factors (291).
The genetic factors include not only numerical and structural chromosomal aberrations, but also microdeletions of the Y chromosome (292) and mutations of autosomal genes (293). The genes whose mutations have been associated with the reduction of male fertility include methylenetetrahydrofolate reductase (MTHFR), which is important in DNA methylation in stem cells (294), steroid 5-alpha reductase responsible for the conversion of testosterone into dihydrotestosterone (DHT), i.e. a more active metabolite (295), protamines replacing histone proteins during the condensation of the nuclear chromatin of sperm (296), heat shock protein A2 (HSPA2) responsible for the protection of cells from exposure to stress factors (269).
Environmental and lifestyle factors are mainly related to the induction of oxidative stress (297). Reactive oxygen species disrupt sperm functions and their viability, damaging cell membranes, organelles and genetic material (298). It has been proven that oral administration of antioxidants may be effective in the treatment of some patients with infertility (299).
A positive correlation has also been demonstrated between high plasma testosterone concentration and fertility (300). An important finding is that the administration of exogenous testosterone, which reduces the production of endogenous hormone by suppressing the pituitary gland, correlates with a decrease in male fertility (301). However, it has been proven that early testosterone therapy after spinal cord injury increases the chances of preserving fertility in men (270).
Animal studies focus mainly on establishing the relationship of gene expression within the testes with fertility. It has been demonstrated with such genes as ADAD1 and ADAD2 (271), Kindlin-2 (272), Wip 1 (273), and Crybb2 (274). At the same time, the lack of influence on fertility of a dozen or so genes, which are expressed within the testes, has been confirmed (275–279).
Numerous studies have also focused on establishing the effects of various toxins on fertility. It has been shown that exposure to microcystin-leucine-arginine and T-2 toxin suppresses the release of FSH in the pituitary gland, which, by reducing testosterone levels, decreases fertility (280, 281). On the other hand, exposure to mequindox or Mn3O4 microparticles reduces fertility due to the induction of oxidative stress within the testes (282). Sleep deprivation has a similar effect, with a reversible decline in fertility due to increased oxidative stress (283).
There are also studies related to the treatment of male infertility. The use of metformin in obese males has been shown to increase fertility (284). The effectiveness of supplementation with apple star fruit in order to improve fertility has also been shown (285). It is probably related to the antioxidant activity of both substances (302).
Currently, in vitro research is focused on determining the relationship between gene expression in testicular cells and fertility. The expression of APP, a protein related to the etiology of Alzheimer’s disease, which influences numerous genes directly related to fertility, has been shown to be of great importance (286). Due to bioinformatic analysis, it was possible to determine the potential relationship of 30 genes with fertility and to exclude 54 genes from this group (287, 288).
Recently, increasing attention has been paid to the relationship between female fertility and blood levels of androgens (Table 9). Their sources are the ovaries, adrenal glands and the conversion of estrogen into androgens in other tissues (310). The concentration of this hormone is not subject to lifestyle fluctuations, except for smoking, which increases its level (311). The causes of the increase in androgen levels in women may include, apart from exogenous supplementation, polycystic ovary syndrome, adrenal hyperplasia, Cushing’s disease and neoplasms (312).
It has been shown that the increased concentration of androgens increases the frequency of anovulatory cycles (303). The effect of increased testosterone concentration on pregnancy is difficult to clearly establish, as both no effect on the course of pregnancy (304) and an increased risk of preeclampsia (305) have been demonstrated. An increased generation of pro-inflammatory factors within the uterine arteries was also observed, which may have a potentially negative impact on the course of pregnancy (306).
At the same time, it has been shown that the reduced testosterone concentration in women undergoing assisted reproductive protocols may be the cause of a poor response to ovarian stimulation (307). Optimal testosterone concentrations have been demonstrated in terms of the effectiveness of assisted reproductive protocols (308) and oral testosterone supplementation in order to increase the chances of success of such treatments (309).
More and more couples are affected by fertility and pregnancy maintenance disorders. It is estimated that male infertility accounts for 40-50% of couples’ infertility. It is associated with impaired testosterone secretion, which may result from primary organ failure or is associated with the disruption of the secretion of pituitary hormones. Normal ovarian function and placental secretion are essential for female fertility and the maintenance of pregnancy. The most common endocrine disorder in women of reproductive age is polycystic ovary syndrome, where androgen secretion is impaired, and an increase in testosterone levels is also possible. Such dysregulation results in a disruption of the pulsatile secretion of gonadotropin-releasing hormone by the hypothalamus, which in turn leads to abnormal gonadotropin secretion. There is also a rare disorder characterized by inhibition or irregular menstrual cycles known as primary ovarian insufficiency. Fertility is also influenced by autoimmune diseases, the toxicity of disease-modifying drugs, in particular non-steroidal anti-inflammatory drugs and glucocorticoids. Interestingly, HIV infection is associated with an increased risk of pituitary gland stroke and pituitary lymphoma, possibly leading to hypopituitarism, one of the effects of which is infertility. The pituitary-ovarian axis can also be influenced by thyroid hormones. Hypothyroidism leads to its inhibition, which increases the risk of miscarriage, premature births, stillbirths, and even infertility.
The form of infertility treatment depends on its duration and the patient’s age. It includes ovulation stimulation with clomiphene citrate or gonadotropins, intrauterine insemination, in vitro fertilization. Therapeutic regimens are modified and various methods of drug administration are tried to increase their effectiveness and minimize side effects. However, it is important to correctly diagnose the disorder and take the required medications. Obesity is also getting more and more attention, as it is an important factor contributing to the reduction of fertility and predisposing to adverse pregnancy outcomes. Weight loss interventions that include exercise and calorie restriction diets seem to be a good idea. In obese people, metformin is also often used, which improves menstrual cycles, reduces progression from impaired glucose tolerance to type 2 diabetes, and is also recommended in the case of contraindications to the use of hormonal contraceptives. Therefore, infertility as well as disorders of the maintenance of pregnancy and childbirth are a multifactorial issue related to numerous relationships between endocrine organs. Understanding these relationships as well as the importance of the influence of external factors is necessary to develop new and better diagnostic and therapeutic schemes.
All authors listed have made a substantial, direct, and intellectual contribution to the work and approved it for publication.
The authors declare that the research was conducted in the absence of any commercial or financial relationships that could be construed as a potential conflict of interest.
All claims expressed in this article are solely those of the authors and do not necessarily represent those of their affiliated organizations, or those of the publisher, the editors and the reviewers. Any product that may be evaluated in this article, or claim that may be made by its manufacturer, is not guaranteed or endorsed by the publisher.
1. Janicka A, Spaczynski RZ, Koziol K, Radwan M, Kurzawa R. Assisted reproductive medicine in Poland, 2013-2016: Polish society of reproductive medicine and embryology (PTMRiE) and fertility and sterility special interest group of the polish society of gynaecologists and obstetricians (SPiN PTGiP) report. Ginekol Pol (2021) 92:7–15. doi: 10.5603/GP.a2020.0142
2. Vander Borght M, Wyns C. Fertility and infertility: Definition and epidemiology. Clin Biochem (2018) 62:2–10. doi: 10.1016/j.clinbiochem.2018.03.012
3. Silvestris E, de Pergola G, Rosania R, Loverro G. Obesity as disruptor of the female fertility. Reprod Biol Endocrinol (2018) 16:22. doi: 10.1186/s12958-018-0336-z
4. Łukaszuk K, Kozioł K, Jakiel G, Jakimiuk A, Jędrzejczak P, Kuczyński W, et al. Diagnostyka i leczenie niepłodności — rekomendacje polskiego towarzystwa medycyny rozrodu i embriologii (PTMRiE) oraz polskiego towarzystwa ginekologów i położników (PTGP). Ginekol Perinatol Prakt (2018) 3:112–40.
5. Wdowiak A, Bazylewicz A, Dolzhenko MN, Nosenko NN, Hdyrya O. Technologie medyczne w diagnostyce męskiej niepłodności. Eur J Med Technol (2015) 1:7–17.
6. Khatun A, Rahman MS, Pang M-G. Clinical assessment of the male fertility. Obstet Gynecol Sci (2018) 61:179–91. doi: 10.5468/ogs.2018.61.2.179
7. Janicka A, Spaczyński RZ, Kurzawa R, Sp P, Clinics F. Assisted reproductive medicine in Poland – fertility and sterility special interest group of the polish gynaecological society (SPiN PTG) 2012 report. Ginekol Pol (2015) 86:932–39. doi: 10.17772/gp/60549
8. Practice committee of the American society for reproductive medicine. Diagnostic evaluation of the infertile female: a committee opinion. Fertil Steril (2015) 103:e44–50. doi: 10.1016/j.fertnstert.2015.03.019
9. van Rijswijk J, Pham CT, Dreyer K, Verhoeve HR, Hoek A, de Bruin J-P, et al. Oil-based or water-based contrast for hysterosalpingography in infertile women: a cost-effectiveness analysis of a randomized controlled trial. Fertil Steril (2018) 110:754–60. doi: 10.1016/j.fertnstert.2018.05.001
10. Tomassetti C, D’Hooghe T. Endometriosis and infertility: Insights into the causal link and management strategies. Best Pract Res Clin Obstet Gynaecol (2018) 51:25–33. doi: 10.1016/j.bpobgyn.2018.06.002
11. Practice committee of the American society for reproductive medicine. Electronic address:QVNSTUBhc3JtLm9yZyw= practice committee of the American society for reproductive medicine. The role of immunotherapy in in vitro fertilization: A guideline. Fertil Steril (2018) 110:387–400. doi: 10.1016/j.fertnstert.2018.05.009
12. Parma P, Radi O. Molecular mechanisms of sexual development. Sex Dev Genet Mol Biol Evol Endocrinol Embryol Pathol Sex Determ Differ (2012) 6:7–17. doi: 10.1159/000332209
13. Stévant I, Nef S. Genetic control of gonadal sex determination and development. Trends Genet TIG (2019) 35:346–58. doi: 10.1016/j.tig.2019.02.004
14. Biason-Lauber A. Control of sex development. Best Pract Res Clin Endocrinol Metab (2010) 24:163–86. doi: 10.1016/j.beem.2009.12.002
15. Makiyan Z. Studies of gonadal sex differentiation. Organogenesis (2016) 12:42–51. doi: 10.1080/15476278.2016.1145318
16. Lamothe S, Bernard V, Christin-Maitre S. Gonad differentiation toward ovary. Ann Endocrinol (2020) 81:83–8. doi: 10.1016/j.ando.2020.04.004
17. Cunha GR, Baskin LS. Development of the external genitalia. Differ Res Biol Divers (2020) 112:7–9. doi: 10.1016/j.diff.2019.10.008
18. Gustafson ML, Donahoe PK. Male Sex determination: current concepts of male sexual differentiation. Annu Rev Med (1994) 45:505–24. doi: 10.1146/annurev.med.45.1.505
19. Abbara A, Clarke SA, Nesbitt A, Ali S, Comninos AN, Hatfield E, et al. Interpretation of serum gonadotropin levels in hyperprolactinaemia. Neuroendocrinology (2018) 107:105–13. doi: 10.1159/000489264
20. Feng H, Zhong Q, Zhou H, Jiang X, Ding Y. The effects of combined bromocriptine and bu-shen-zhu-yun decoction on serum hormones, anxiety, and pregnancy in hyperprolactinemic infertility patients. Ann Palliat Med (2021) 10:12230–43. doi: 10.21037/apm-21-3111
21. Sermondade N, Faure C, Fezeu L, Shayeb AG, Bonde JP, Jensen TK, et al. BMI in relation to sperm count: an updated systematic review and collaborative meta-analysis. Hum Reprod Update (2013) 19:221–31. doi: 10.1093/humupd/dms050
22. Stamatiades GA, Kaiser UB. Gonadotropin regulation by pulsatile GnRH: Signaling and gene expression. Mol Cell Endocrinol (2018) 463:131–41. doi: 10.1016/j.mce.2017.10.015
23. Stamatiades GA, Carroll RS, Kaiser UB. GnRH-a key regulator of FSH. Endocrinology (2019) 160:57–67. doi: 10.1210/en.2018-00889
24. Lim CT, Khoo B. Normal physiology of ACTH and GH release in the hypothalamus and anterior pituitary in man. In: Feingold KR, Anawalt B, Boyce A, Chrousos G, de Herder WW, Dhatariya K, et al, editors. Endotext. South Dartmouth (MA: MDText.com, Inc (2000). Available at: http://www.ncbi.nlm.nih.gov/books/NBK279116/.
25. Espino J, Macedo M, Lozano G, Ortiz Á, Rodríguez C, Rodríguez AB, et al. Impact of melatonin supplementation in women with unexplained infertility undergoing fertility treatment. Antioxidants (2019) 8:338. doi: 10.3390/antiox8090338
26. Mokhtari F, Akbari Asbagh F, Azmoodeh O, Bakhtiyari M, Almasi-Hashiani A. Effects of melatonin administration on chemical pregnancy rates of polycystic ovary syndrome patients undergoing intrauterine insemination: A randomized clinical trial. Int J Fertil Steril (2019) 13:225–9. doi: 10.22074/ijfs.2019.5717
27. Çelik S, Guve H, Çalışkan C, Çelik S. The role of melatonin, IL-8 and IL-10 in intrahepatic cholestasis of pregnancy. Z Geburtshilfe Neonatol (2021) 225:238–43. doi: 10.1055/a-1233-9084
28. Hobson SR, Gurusinghe S, Lim R, Alers NO, Miller SL, Kingdom JC, et al. Melatonin improves endothelial function in vitro and prolongs pregnancy in women with early-onset preeclampsia. J Pineal Res (2018) 65:e12508. doi: 10.1111/jpi.12508
29. Zhang C, Clough SJ, Adamah-Biassi EB, Sveinsson MH, Hutchinson AJ, Miura I, et al. Impact of endogenous melatonin on rhythmic behaviors, reproduction, and survival revealed in melatonin-proficient C57BL/6J congenic mice. J Pineal Res (2021) 71:e12748. doi: 10.1111/jpi.12748
30. Lv D, Tan T, Zhu T, Wang J, Zhang S, Zhang L, et al. Leptin mediates the effects of melatonin on female reproduction in mammals. J Pineal Res (2019) 66:e12559. doi: 10.1111/jpi.12559
31. Lombardo F, Giorgini E, Gioacchini G, Maradonna F, Ferraris P, Carnevali O. Melatonin effects on fundulus heteroclitus reproduction. Reprod Fertil Dev (2012) 24:794–803. doi: 10.1071/RD11267
32. Zhang L, Zhang Z, Wang J, Lv D, Zhu T, Wang F, et al. Melatonin regulates the activities of ovary and delays the fertility decline in female animals via MT1/AMPK pathway. J Pineal Res (2019) 66:e12550. doi: 10.1111/jpi.12550
33. Cosso G, Luridiana S, Pulinas L, Curone G, Pich G, Carcangiu V, et al. Melatonin treatment in rams and their replacement with novel treated rams advance first lambing and increase fertility in sarda ewe lambs. Anim Open Access J MDPI (2021) 11:1227. doi: 10.3390/ani11051227
34. Song C, Peng W, Yin S, Zhao J, Fu B, Zhang J, et al. Melatonin improves age-induced fertility decline and attenuates ovarian mitochondrial oxidative stress in mice. Sci Rep (2016) 6:35165. doi: 10.1038/srep35165
35. Dholpuria S, Vyas S, Purohit GN, Pathak KML. Sonographic monitoring of early follicle growth induced by melatonin implants in camels and the subsequent fertility. J Ultrasound (2012) 15:135–41. doi: 10.1016/j.jus.2012.02.008
36. Peng X, Cai X, Li J, Huang Y, Liu H, He J, et al. Effects of melatonin supplementation during pregnancy on reproductive performance, maternal-Placental-Fetal redox status, and placental mitochondrial function in a sow model. Antioxid Basel Switz (2021) 10:1867. doi: 10.3390/antiox10121867
37. Arend LS, Knox RV. Fertility responses of melatonin-treated gilts before and during the follicular and early luteal phases when there are different temperatures and lighting conditions in the housing area. Anim Reprod Sci (2021) 230:106769. doi: 10.1016/j.anireprosci.2021.106769
38. Bai J, Zhang L, Zhao Z, Li N, Wang B, Yang L. Expression of melatonin receptors and CD4 in the ovine thymus, lymph node, spleen and liver during early pregnancy. Immunology (2020) 160:52–63. doi: 10.1111/imm.13180
39. Gunwant P, Pandey AK, Kumar A, Singh I, Kumar S, Phogat JB, et al. Polymorphism of melatonin receptor (MTNR1A) gene and its association with seasonal reproduction in water buffalo (Bubalus bubalis). Anim Reprod Sci (2018) 199:51–9. doi: 10.1016/j.anireprosci.2018.10.006
40. Fathy HA, Gouda EM, Gafer JA, Galal MK, Nowier AM. Genetic polymorphism in melatonin receptor 1A and arylalkylamine n-acetyltransferase and its impact on seasonal reproduction in Egyptian sheep breeds. Arch Anim Breed (2018) 61:505–16. doi: 10.5194/aab-61-505-2018
41. Mura MC, Luridiana S, Bodano S, Daga C, Cosso G, Diaz ML, et al. Influence of melatonin receptor 1A gene polymorphisms on seasonal reproduction in sarda ewes with different body condition scores and ages. Anim Reprod Sci (2014) 149:173–7. doi: 10.1016/j.anireprosci.2014.07.022
42. do Nascimento Marinho KS, Lapa Neto CJC, de Sousa Coelho IDD, da Silva MA, Gomes Melo ME, Dos Santos KRP, et al. Genotoxic and mutagenic evaluation of the protective effect of exogenous melatonin in adult rats and their offspring exposed to the insecticides methomyl and cypermethrin during pregnancy. Mutat Res Genet Toxicol Environ Mutagen (2019) 848:503107. doi: 10.1016/j.mrgentox.2019.503107
43. Huang J, Shan W, Li N, Zhou B, Guo E, Xia M, et al. Melatonin provides protection against cisplatin-induced ovarian damage and loss of fertility in mice. Reprod BioMed Online (2021) 42:505–19. doi: 10.1016/j.rbmo.2020.10.001
44. de Almeida LL, Teixeira ÁAC, Soares AF, da Cunha FM, da Silva VA, Vieira Filho LD, et al. Effects of melatonin in rats in the initial third stage of pregnancy exposed to sub-lethal doses of herbicides. Acta Histochem (2017) 119:220–7. doi: 10.1016/j.acthis.2017.01.003
45. de Sousa Coelho IDD, Lapa Neto CJC, Souza TGDS, da Silva MA, Chagas CA, Santos KRPD, et al. Protective effect of exogenous melatonin in rats and their offspring on the genotoxic response induced by the chronic consumption of alcohol during pregnancy. Mutat Res Genet Toxicol Environ Mutagen (2018) 832–833:52–60. doi: 10.1016/j.mrgentox.2018.06.018
46. Wang Z, Teng Z, Wang Z, Song Z, Zhu P, Li N, et al. Melatonin ameliorates paclitaxel-induced mice spermatogenesis and fertility defects. J Cell Mol Med (2022) 26:1219–28. doi: 10.1111/jcmm.17177
47. Abo El Gheit RE, Soliman NA, Nagla SA, El-Sayed RM, Badawi GA, Emam MN, et al. Melatonin epigenetic potential on testicular functions and fertility profile in varicocele rat model is mediated by silent information regulator 1. Br J Pharmacol (2022) 179:3363–81. doi: 10.1111/bph.15804
48. Xu Y-M, Hao G-M, Gao B-L. Application of growth hormone in in vitro fertilization. Front Endocrinol (2019) 10:502. doi: 10.3389/fendo.2019.00502
49. Lainez NM, Coss D. Obesity, neuroinflammation, and reproductive function. Endocrinology (2019) 160:2719–36. doi: 10.1210/en.2019-00487
50. Kurowska P, Mlyczyńska E, Dawid M, Jurek M, Klimczyk D, Dupont J, et al. Review: Vaspin (SERPINA12) expression and function in endocrine cells. Cells (2021) 10:1710. doi: 10.3390/cells10071710
51. Roberts RE, Farahani L, Webber L, Jayasena C. Current understanding of hypothalamic amenorrhoea. Ther Adv Endocrinol Metab (2020) 11:2042018820945854. doi: 10.1177/2042018820945854
52. Shufelt CL, Torbati T, Dutra E. Hypothalamic amenorrhea and the long-term health consequences. Semin Reprod Med (2017) 35:256–62. doi: 10.1055/s-0037-1603581
53. Saleem M, Martin H, Coates P. Prolactin biology and laboratory measurement: An update on physiology and current analytical issues. Clin Biochem Rev (2018) 39:3–16.
54. Koyyada A, Orsu P. Role of hypothyroidism and associated pathways in pregnancy and infertility: Clinical insights. Tzu-Chi Med J (2020) 32:312–7. doi: 10.4103/tcmj.tcmj_255_19
55. Evanson J. Radiology of the pituitary. In: Feingold KR, Anawalt B, Boyce A, Chrousos G, de Herder WW, Dhatariya K, et al, editors. Endotext. South Dartmouth (MA: MDText.com, Inc (2000). Available at: http://www.ncbi.nlm.nih.gov/books/NBK279161/.
56. Bachmeier CAE, Snell C, Morton A. Visual loss in pregnancy. BMJ Case Rep (2019) 12:e228323. doi: 10.1136/bcr-2018-228323
57. Kelleher AM, DeMayo FJ, Spencer TE. Uterine glands: Developmental biology and functional roles in pregnancy. Endocr Rev (2019) 40:1424–45. doi: 10.1210/er.2018-00281
58. Ergun-Longmire B, Wajnrajch MP. Growth and growth disorders. In: Feingold KR, Anawalt B, Boyce A, Chrousos G, de Herder WW, Dhatariya K, et al, editors. Endotext. South Dartmouth (MA: MDText.com, Inc (2000). Available at: http://www.ncbi.nlm.nih.gov/books/NBK279142/.
59. Kumar S, Kumar KH. Pituitary diseases in the tropics. In: Feingold KR, Anawalt B, Boyce A, Chrousos G, de Herder WW, Dhatariya K, et al, editors. Endotext. South Dartmouth (MA: MDText.com, Inc (2000). Available at: http://www.ncbi.nlm.nih.gov/books/NBK568567/.
60. Miljic D, Pekic S, Popovic V. Empty sella. In: Feingold KR, Anawalt B, Boyce A, Chrousos G, de Herder WW, Dhatariya K, et al, editors. Endotext. South Dartmouth (MA: MDText.com, Inc (2000). Available at: http://www.ncbi.nlm.nih.gov/books/NBK532084/.
61. Somers EC, Marder W. Infertility – prevention and management. Rheum Dis Clin North Am (2017) 43:275–85. doi: 10.1016/j.rdc.2016.12.007
62. Pekic S, Miljic D, Popovic V. Infections of the hypothalamic-pituitary region. In: Feingold KR, Anawalt B, Boyce A, Chrousos G, de Herder WW, Dhatariya K, et al, editors. Endotext. South Dartmouth (MA: MDText.com, Inc (2000). Available at: http://www.ncbi.nlm.nih.gov/books/NBK532083/.
63. Prete A, Salvatori R. Hypophysitis, in: Endotext (2000). South Dartmouth (MA: MDText.com, Inc. Available at: http://www.ncbi.nlm.nih.gov/books/NBK519842/ (Accessed June 4, 2022).
64. Sciarra F, Franceschini E, Campolo F, Gianfrilli D, Pallotti F, Paoli D, et al. Disruption of circadian rhythms: A crucial factor in the etiology of infertility. Int J Mol Sci (2020) 21:3943. doi: 10.3390/ijms21113943
65. Rattan S, Zhou C, Chiang C, Mahalingam S, Brehm E, Flaws JA. Exposure to endocrine disruptors during adulthood: consequences for female fertility. J Endocrinol (2017) 233:R109–29. doi: 10.1530/JOE-17-0023
66. Borowska M, Czarnywojtek A, Sawicka-Gutaj N, Woliński K, Płazińska MT, Mikołajczak P, et al. The effects of cannabinoids on the endocrine system. Endokrynol Pol (2018) 69:705–19. doi: 10.5603/EP.a2018.0072
67. Darbandi M, Darbandi S, Agarwal A, Sengupta P, Durairajanayagam D, Henkel R, et al. Reactive oxygen species and male reproductive hormones. Reprod Biol Endocrinol RBE (2018) 16:87. doi: 10.1186/s12958-018-0406-2
68. Childs GV, Odle AK, MacNicol MC, MacNicol AM. The importance of leptin to reproduction. Endocrinology (2021) 162:bqaa204. doi: 10.1210/endocr/bqaa204
69. Lateef OM, Akintubosun MO. Sleep and reproductive health. J Circadian Rhythms (2020) 18:1. doi: 10.5334/jcr.190
70. Selvaraj K, Ravichandran S, Krishnan S, Radhakrishnan RK, Manickam N, Kandasamy M. Testicular atrophy and hypothalamic pathology in COVID-19: Possibility of the incidence of Male infertility and HPG axis abnormalities. Reprod Sci Thousand Oaks Calif (2021) 28:2735–42. doi: 10.1007/s43032-020-00441-x
71. Youssef J, Sadera R, Mital D, Ahmed MH. HIV And the pituitary gland: Clinical and biochemical presentations. J Lab Physicians (2021) 13:84–90. doi: 10.1055/s-0041-1723055
72. Møller M, Baeres FM. The anatomy and innervation of the mammalian pineal gland. Cell Tissue Res (2002) 309:139–50. doi: 10.1007/s00441-002-0580-5
73. Amaral FGdo, Cipolla-Neto J. A brief review about melatonin, a pineal hormone. Arch Endocrinol Metab (2018) 62:472–9. doi: 10.20945/2359-3997000000066
74. Arendt J, Aulinas A. Physiology of the pineal gland and melatonin. In: Feingold KR, Anawalt B, Boyce A, Chrousos G, de Herder WW, Dhatariya K, et al, editors. Endotext. South Dartmouth (MA): MDText.com, Inc (2000). Available at: http://www.ncbi.nlm.nih.gov/books/NBK550972/.
75. Reiter RJ, Tan D-X, Tamura H, Cruz MHC, Fuentes-Broto L. Clinical relevance of melatonin in ovarian and placental physiology: a review. Gynecol Endocrinol Off J Int Soc Gynecol Endocrinol (2014) 30:83–9. doi: 10.3109/09513590.2013.849238
76. Srinivasan V, Pandi-Perumal SR, Trahkt I, Spence DW, Poeggeler B, Hardeland R, et al. Melatonin and melatonergic drugs on sleep: possible mechanisms of action. Int J Neurosci (2009) 119:821–46. doi: 10.1080/00207450802328607
77. Crowley SJ, Acebo C, Carskadon MA. Human puberty: Salivary melatonin profiles in constant conditions. Dev Psychobiol (2012) 54:468–73. doi: 10.1002/dev.20605
78. Waldhauser F, Weiszenbacher G, Frisch H, Zeitlhuber U, Waldhauser M, Wurtman RJ. Fall in nocturnal serum melatonin during prepuberty and pubescence. Lancet Lond Engl (1984) 1:362–5. doi: 10.1016/s0140-6736(84)90412-4
79. Roy D, Belsham DD. Melatonin receptor activation regulates GnRH gene expression and secretion in GT1-7 GnRH neurons. Signal transduction mechanisms. J Biol Chem (2002) 277:251–8. doi: 10.1074/jbc.M108890200
80. Gingerich S, Wang X, Lee PKP, Dhillon SS, Chalmers JA, Koletar MM, et al. The generation of an array of clonal, immortalized cell models from the rat hypothalamus: analysis of melatonin effects on kisspeptin and gonadotropin-inhibitory hormone neurons. Neuroscience (2009) 162:1134–40. doi: 10.1016/j.neuroscience.2009.05.026
81. van Geijlswijk IM, Mol RH, Egberts TCG, Smits MG. Evaluation of sleep, puberty and mental health in children with long-term melatonin treatment for chronic idiopathic childhood sleep onset insomnia. Psychopharmacol (Berl) (2011) 216:111–20. doi: 10.1007/s00213-011-2202-y
82. Madalinski MH. Does a melatonin supplement alter the course of gastro-esophageal reflux disease? World J Gastrointest Pharmacol Ther (2011) 2:50–1. doi: 10.4292/wjgpt.v2.i6.50
83. Biran V, Phan Duy A, Decobert F, Bednarek N, Alberti C, Baud O. Is melatonin ready to be used in preterm infants as a neuroprotectant? Dev Med Child Neurol (2014) 56:717–23. doi: 10.1111/dmcn.12415
84. Bai J, Dong L, Song Z, Ge H, Cai X, Wang G, et al. The role of melatonin as an antioxidant in human lens epithelial cells. Free Radic Res (2013) 47:635–42. doi: 10.3109/10715762.2013.808743
85. Nawrot-Porąbka K, Jaworek J, Leja-Szpak A, Szklarczyk J, Konturek SJ, Reiter RJ. Luminal melatonin stimulates pancreatic enzyme secretion via activation of serotonin-dependent nerves. Pharmacol Rep PR (2013) 65:494–504. doi: 10.1016/s1734-1140(13)71025-9
86. Markus RP, Fernandes PA, Kinker GS, Cruz-Machado S da S, Marçola M. Immune-pineal axis – acute inflammatory responses coordinate melatonin synthesis by pinealocytes and phagocytes. Br J Pharmacol (2018) 175:3239. doi: 10.1111/bph.14083
87. Reiter RJ, Rosales-Corral SA, Manchester LC, Tan D-X. Peripheral reproductive organ health and melatonin: ready for prime time. Int J Mol Sci (2013) 14:7231–72. doi: 10.3390/ijms14047231
88. Reiter RJ, Tan DX, Osuna C, Gitto E. Actions of melatonin in the reduction of oxidative stress. A review. J BioMed Sci (2000) 7:444–58. doi: 10.1007/BF02253360
89. Chen C-Q, Fichna J, Bashashati M, Li Y-Y, Storr M. Distribution, function and physiological role of melatonin in the lower gut. World J Gastroenterol WJG (2011) 17:3888–98. doi: 10.3748/wjg.v17.i34.3888
90. Bubenik GA. Localization, physiological significance and possible clinical implication of gastrointestinal melatonin. Biol Signals Recept (2001) 10:350–66. doi: 10.1159/000046903
91. Carrillo-Vico A, Lardone PJ, Fernández-Santos JM, Martín-Lacave I, Calvo JR, Karasek M, et al. Human lymphocyte-synthesized melatonin is involved in the regulation of the interleukin-2/interleukin-2 receptor system. J Clin Endocrinol Metab (2005) 90:992–1000. doi: 10.1210/jc.2004-1429
92. Lewy AJ, Tetsuo M, Markey SP, Goodwin FK, Kopin IJ. Pinealectomy abolishes plasma melatonin in the rat. J Clin Endocrinol Metab (1980) 50:204–5. doi: 10.1210/jcem-50-1-204
93. Korkmaz A, Rosales-Corral S, Reiter RJ. Gene regulation by melatonin linked to epigenetic phenomena. Gene (2012) 503:1–11. doi: 10.1016/j.gene.2012.04.040
94. Li W, Kong A-N. Molecular mechanisms of Nrf2-mediated antioxidant response. Mol Carcinog (2009) 48:91–104. doi: 10.1002/mc.20465
95. Galano A, Tan DX, Reiter RJ. Cyclic 3-hydroxymelatonin, a key metabolite enhancing the peroxyl radical scavenging activity of melatonin. RSC Adv (2014) 4:5220–7. doi: 10.1039/C3RA44604B
96. Berga SL, Mortola JF, Yen SSC. Amplification of nocturnal melatonin secretion in women with functional hypothalamic amenorrhea. J Clin Endocrinol Metab (1988) 66:242–4. doi: 10.1210/jcem-66-1-242
97. Tang PL, Chan TY, Tang GWK, Pang SF. Plasma melatonin profile and hormonal interactions in the menstrual cycles of anovulatory infertile women treated with gonadotropins. Gynecol Obstet Invest (1998) 45:247–52. doi: 10.1159/000009977
98. Tamura H, Takasaki A, Taketani T, Tanabe M, Lee L, Tamura I, et al. Melatonin and female reproduction. J Obstet Gynaecol Res (2014) 40:1–11. doi: 10.1111/jog.12177
99. Jamilian M, Foroozanfard F, Mirhosseini N, Kavossian E, Aghadavod E, Bahmani F, et al. Effects of melatonin supplementation on hormonal, inflammatory, genetic, and oxidative stress parameters in women with polycystic ovary syndrome. Front Endocrinol (2019) 10:273. doi: 10.3389/fendo.2019.00273
100. Taub RL, Ellis SA, Neal-Perry G, Magaret AS, Prager SW, Micks EA. The effect of testosterone on ovulatory function in transmasculine individuals. Am J Obstet Gynecol (2020) 223:229.e1–8. doi: 10.1016/j.ajog.2020.01.059
101. Palacio JR, Iborra A, Ulcova-Gallova Z, Badia R, Martínez P. The presence of antibodies to oxidative modified proteins in serum from polycystic ovary syndrome patients. Clin Exp Immunol (2006) 144:217–22. doi: 10.1111/j.1365-2249.2006.03061.x
102. Ejaz H, Figaro JK, Woolner AMF, Thottakam BMV, Galley HF. Maternal serum melatonin increases during pregnancy and falls immediately after delivery implicating the placenta as a major source of melatonin. Front Endocrinol (2020) 11:623038. doi: 10.3389/fendo.2020.623038
103. Zheng M, Tong J, Li W-P, Chen Z-J, Zhang C. Melatonin concentration in follicular fluid is correlated with antral follicle count (AFC) and in vitro fertilization (IVF) outcomes in women undergoing assisted reproductive technology (ART) procedures. Gynecol Endocrinol Off J Int Soc Gynecol Endocrinol (2018) 34:446–50. doi: 10.1080/09513590.2017.1409713
104. Yang L, Wang Q, Cui M, Li Q, Mu S, Zhao Z. Effect of melatonin on the In vitro maturation of porcine oocytes, development of parthenogenetically activated embryos, and expression of genes related to the oocyte developmental capability. Anim Open Access J MDPI (2020) 10:E209. doi: 10.3390/ani10020209
105. Fathi M, Salama A, El-Shahat KH, El-Sherbiny HR, Abdelnaby EA. Effect of melatonin supplementation during IVM of dromedary camel oocytes (Camelus dromedarius) on their maturation, fertilization, and developmental rates. vitro. Theriogenology (2021) 172:187–92. doi: 10.1016/j.theriogenology.2021.05.021
106. Shu-Qin Z, Yong Z, Yuan G, Xiao-Pu Y, Zhen Y, Zhi-Jie Y. The in vitro effects of melatonin and cry gene on the secretion of estradiol from camel ovarian granulosa cells. Domest Anim Endocrinol (2021) 74:106497. doi: 10.1016/j.domaniend.2020.106497
107. Anderson RA, Lincoln GA, Wu FC. Melatonin potentiates testosterone-induced suppression of luteinizing hormone secretion in normal men. Hum Reprod Oxf Engl (1993) 8:1819–22. doi: 10.1093/oxfordjournals.humrep.a137940
108. Aghaz F, Vaisi-Raygani A, Khazaei M, Arkan E. Enhanced cryoprotective effect of melatonin and resveratrol by coencapsulation: Improved In vitro development of vitrified-warmed mouse germinal vesicle oocytes. Biopreservation Biobanking (2021) 19:184–93. doi: 10.1089/bio.2020.0102
109. Zhang Y, Li W, Ma Y, Wang D, Zhao X, Zeng C, et al. Improved development by melatonin treatment after vitrification of mouse metaphase II oocytes. Cryobiology (2016) 73:335–42. doi: 10.1016/j.cryobiol.2016.09.171
110. Tang Y, Zhang Y, Liu L, Yang Y, Wang Y, Xu B. Glycine and melatonin improve preimplantation development of porcine oocytes vitrified at the germinal vesicle stage. Front Cell Dev Biol (2022) 10:856486. doi: 10.3389/fcell.2022.856486
111. Awad H, Halawa F, Mostafa T, Atta H. Melatonin hormone profile in infertile males. Int J Androl (2006) 29:409–13. doi: 10.1111/j.1365-2605.2005.00624.x
112. Sharbatoghli M, Rezazadeh Valojerdi M, Bahadori MH, Salman Yazdi R, Ghaleno LR. The relationship between seminal melatonin with sperm parameters, DNA fragmentation and nuclear maturity in intra-cytoplasmic sperm injection candidates. Cell J (2015) 17:547–53. doi: 10.22074/cellj.2015.15
113. Ortiz A, Espino J, Bejarano I, Lozano GM, Monllor F, García JF, et al. High endogenous melatonin concentrations enhance sperm quality and short-term in vitro exposure to melatonin improves aspects of sperm motility. J Pineal Res (2011) 50:132–9. doi: 10.1111/j.1600-079X.2010.00822.x
114. Luboshitzky R, Shen-Orr Z, Nave R, Lavi S, Lavie P. Melatonin administration alters semen quality in healthy men. J Androl (2002) 23:572–8.
115. Lu X-L, Liu J-J, Li J-T, Yang Q-A, Zhang J-M. Melatonin therapy adds extra benefit to varicecelectomy in terms of sperm parameters, hormonal profile and total antioxidant capacity: A placebo-controlled, double-blind trial. Andrologia (2018) 50:e13033. doi: 10.1111/and.13033
116. Cebi Sen C, Yumusak N, Atilgan HI, Sadic M, Koca G, Korkmaz M. The protective effect of melatonin on sperm quality in rat after radioiodine treatment. Andrologia (2018). doi: 10.1111/and.12962
117. Khalil SS, Aziz JA, Ismail KA, El-Malkey NF. Comparative protective effects of n-acetylcysteine and melatonin against obesity-induced testicular dysfunction in rats. Can J Physiol Pharmacol (2021) 99:708–19. doi: 10.1139/cjpp-2020-0499
118. Moradi M, Goodarzi N, Faramarzi A, Cheraghi H, Hashemian AH, Jalili C. Melatonin protects rats testes against bleomycin, etoposide, and cisplatin-induced toxicity via mitigating nitro-oxidative stress and apoptosis. BioMed Pharmacother Biomedecine Pharmacother (2021) 138:111481. doi: 10.1016/j.biopha.2021.111481
119. Venditti M, Ben Rhouma M, Romano MZ, Messaoudi I, Reiter RJ, Minucci S. Evidence of melatonin ameliorative effects on the blood-testis barrier and sperm quality alterations induced by cadmium in the rat testis. Ecotoxicol Environ Saf (2021) 226:112878. doi: 10.1016/j.ecoenv.2021.112878
120. Tijmes M, Pedraza R, Valladares L. Melatonin in the rat testis: evidence for local synthesis. Steroids (1996) 61:65–8. doi: 10.1016/0039-128x(95)00197-x
121. Nasiraei-Moghadam SN-M, Parivar K, Ahmadiani A, Movahhedin M, Vaez Mahdavi MR. Protective effect of melatonin against inequality-induced da mages on testicular tissue and sper m para meters. Int J Fertil Steril (2014) 7:313–22.
122. Deng S-L, Chen S-R, Wang Z-P, Zhang Y, Tang J-X, Li J, et al. Melatonin promotes development of haploid germ cells from early developing spermatogenic cells of Suffolk sheep under in vitro condition. J Pineal Res (2016) 60:435–47. doi: 10.1111/jpi.12327
123. Karimfar MH, Niazvand F, Haghani K, Ghafourian S, Shirazi R, Bakhtiyari S. The protective effects of melatonin against cryopreservation-induced oxidative stress in human sperm. Int J Immunopathol Pharmacol (2015) 28:69–76. doi: 10.1177/0394632015572080
124. Syrenicz A, Syrenicz M, Sworczak K, Garanty-Bogacka B, Zimnicka A, Walczak M. [Hashimoto disease and hypothyroidism in child-bearing period–essential problem for woman and her child]. Endokrynol Pol (2005) 56:1008–15.
125. Poppe K, Glinoer D. Thyroid autoimmunity and hypothyroidism before and during pregnancy. Hum Reprod Update (2003) 9:149–61. doi: 10.1093/humupd/dmg012
126. Vaquero E, Lazzarin N, De Carolis C, Valensise H, Moretti C, Ramanini C. Mild thyroid abnormalities and recurrent spontaneous abortion: diagnostic and therapeutical approach. Am J Reprod Immunol N Y N 1989 (2000) 43:204–8. doi: 10.1111/j.8755-8920.2000.430404.x
127. Kakita-Kobayashi M, Murata H, Nishigaki A, Hashimoto Y, Komiya S, Tsubokura H, et al. Thyroid hormone facilitates in vitro decidualization of human endometrial stromal cells via thyroid hormone receptors. Endocrinology (2020) 161:bqaa049. doi: 10.1210/endocr/bqaa049
128. Barber KJ, Franklyn JA, McCabe CJ, Khanim FL, Bulmer JN, Whitley GSJ, et al. The in vitro effects of triiodothyronine on epidermal growth factor-induced trophoblast function. J Clin Endocrinol Metab (2005) 90:1655–61. doi: 10.1210/jc.2004-0785
129. Giuliani C, Bucci I, Di Santo S, Rossi C, Grassadonia A, Piantelli M, et al. The flavonoid quercetin inhibits thyroid-restricted genes expression and thyroid function. Food Chem Toxicol Int J Publ Br Ind Biol Res Assoc (2014) 66:23–9. doi: 10.1016/j.fct.2014.01.016
130. Condorelli RA, La Vignera S, Mongioì LM, Alamo A, Giacone F, Cannarella R, et al. Thyroid hormones and spermatozoa: In VitroEffects on sperm mitochondria, viability and DNA integrity. J Clin Med (2019) 8:E756. doi: 10.3390/jcm8050756
131. Dallas JS. Autoimmune thyroid disease and pregnancy: relevance for the child. Autoimmunity (2003) 36:339–50. doi: 10.1080/08916930310001602993
132. Krassas GE. Thyroid disease and female reproduction. Fertil Steril (2000) 74:1063–70. doi: 10.1016/s0015-0282(00)01589-2
133. Colicchia M, Campagnolo L, Baldini E, Ulisse S, Valensise H, Moretti C. Molecular basis of thyrotropin and thyroid hormone action during implantation and early development. Hum Reprod Update (2014) 20:884–904. doi: 10.1093/humupd/dmu028
134. Stavreus Evers A. Paracrine interactions of thyroid hormones and thyroid stimulation hormone in the female reproductive tract have an impact on female fertility. Front Endocrinol (2012) 3:50. doi: 10.3389/fendo.2012.00050
135. Benvenga S, Ferrari SM, Elia G, Ragusa F, Patrizio A, Paparo SR, et al. Nutraceuticals in thyroidology: A review of in vitro, and in vivo animal studies. Nutrients (2020) 12:E1337. doi: 10.3390/nu12051337
136. Giuliani C, Noguchi Y, Harii N, Napolitano G, Tatone D, Bucci I, et al. The flavonoid quercetin regulates growth and gene expression in rat FRTL-5 thyroid cells. Endocrinology (2008) 149:84–92. doi: 10.1210/en.2007-0618
137. Tran L, Hammuda M, Wood C, Xiao CW. Soy extracts suppressed iodine uptake and stimulated the production of autoimmunogen in rat thyrocytes. Exp Biol Med Maywood NJ (2013) 238:623–30. doi: 10.1177/1535370213489488
138. Chandra AK, De N. Goitrogenic/antithyroidal potential of green tea extract in relation to catechin in rats. Food Chem Toxicol Int J Publ Br Ind Biol Res Assoc (2010) 48:2304–11. doi: 10.1016/j.fct.2010.05.064
139. Cito G, Cocci A, Micelli E, Gabutti A, Russo GI, Coccia ME, et al. Vitamin d and Male fertility: An updated review. World J Mens Health (2020) 38:164–77. doi: 10.5534/wjmh.190057
140. Brenza HL, DeLuca HF. Regulation of 25-hydroxyvitamin D3 1alpha-hydroxylase gene expression by parathyroid hormone and 1,25-dihydroxyvitamin D3. Arch Biochem Biophys (2000) 381:143–52. doi: 10.1006/abbi.2000.1970
141. Fukumoto S. Phosphate metabolism and vitamin d. BoneKEy Rep (2014) 3:497. doi: 10.1038/bonekey.2013.231
142. Zanatta L, Zamoner A, Gonçalves R, Zanatta AP, Bouraïma-Lelong H, Bois C, et al. Effect of 1α,25-dihydroxyvitamin D3 in plasma membrane targets in immature rat testis: ionic channels and gamma-glutamyl transpeptidase activity. Arch Biochem Biophys (2011) 515:46–53. doi: 10.1016/j.abb.2011.09.001
143. Mahmoudi AR, Zarnani AH, Jeddi-Tehrani M, Katouzian L, Tavakoli M, Soltanghoraei H, et al. Distribution of vitamin d receptor and 1α-hydroxylase in male mouse reproductive tract. Reprod Sci Thousand Oaks Calif (2013) 20:426–36. doi: 10.1177/1933719112459235
144. Fu L, Chen Y-H, Xu S, Ji Y-L, Zhang C, Wang H, et al. Vitamin d deficiency impairs testicular development and spermatogenesis in mice. Reprod Toxicol Elmsford N (2017) 73:241–9. doi: 10.1016/j.reprotox.2017.06.047
145. Kourtis AP, Read JS, Jamieson DJ. Pregnancy and infection. N Engl J Med (2014) 370:2211–8. doi: 10.1056/NEJMra1213566
146. Saito S, Nishikawa K, Morii T, Narita N, Enomoto M, Ichijo M. Expression of activation antigens CD69, HLA-DR, interleukin-2 receptor-alpha (IL-2R alpha) and IL-2R beta on T cells of human decidua at an early stage of pregnancy. Immunology (1992) 75:710–2.
147. Watanabe M, Iwatani Y, Kaneda T, Hidaka Y, Mitsuda N, Morimoto Y, et al. Changes in T, b, and NK lymphocyte subsets during and after normal pregnancy. Am J Reprod Immunol N Y N 1989 (1997) 37:368–77. doi: 10.1111/j.1600-0897.1997.tb00246.x
148. Li N, Wang L, Cao N, Zhang L, Han X, Yang L. Early pregnancy affects the expression of toll-like receptor pathway in ovine thymus. Reprod Biol (2020) 20:547–54. doi: 10.1016/j.repbio.2020.10.003
149. Zhang L, Zhao Z, Mi H, Liu B, Wang B, Yang L. Modulation of helper T cytokines in thymus during early pregnancy in ewes. Anim Open Access J MDPI (2019) 9:E245. doi: 10.3390/ani9050245
150. Yang L, Lv W, Liu Y, Chen K, Xue J, Wang Q, et al. Effect of early pregnancy on the expression of prostaglandin synthases in the ovine thymus. Theriogenology (2019) 136:166–71. doi: 10.1016/j.theriogenology.2019.06.040
151. Zhang L, Zhao Z, Wang Y, Li N, Cao N, Yang L. Changes in expression of interferon-stimulated genes and ubiquitin activating enzyme E1-like in ovine thymus during early pregnancy. Anim Reprod (2020) 17:e20190134. doi: 10.1590/1984-3143-AR2019-0134
152. Wise T. In vitro and in vivo effects of thymulin on rat testicular steroid synthesis. J Steroid Biochem Mol Biol (1998) 66:129–35. doi: 10.1016/s0960-0760(98)00045-4
153. Wise T, Ford JJ. Effects of the thymic peptide thymulin on in vitro and in vivo testicular steroid concentrations in white composite and meishan boars. J Anim Sci (1999) 77:2240–51. doi: 10.2527/1999.7782240x
154. Jacobo P, Guazzone VA, Jarazo-Dietrich S, Theas MS, Lustig L. Differential changes in CD4+ and CD8+ effector and regulatory T lymphocyte subsets in the testis of rats undergoing autoimmune orchitis. J Reprod Immunol (2009) 81:44–54. doi: 10.1016/j.jri.2009.04.005
155. Wegmann TG, Lin H, Guilbert L, Mosmann TR. Bidirectional cytokine interactions in the maternal-fetal relationship: is successful pregnancy a TH2 phenomenon? Immunol Today (1993) 14:353–6. doi: 10.1016/0167-5699(93)90235-D
156. Wegmann TG. Foetal protection against abortion: is it immunosuppression or immunostimulation? Ann Immunol (1984) 135D:309–12. doi: 10.1016/s0769-2625(84)81196-4
157. Szekeres-Bartho J, Wegmann TG. A progesterone-dependent immunomodulatory protein alters the Th1/Th2 balance. J Reprod Immunol (1996) 31:81–95. doi: 10.1016/0165-0378(96)00964-3
158. Piccinni MP, Beloni L, Livi C, Maggi E, Scarselli G, Romagnani S. Defective production of both leukemia inhibitory factor and type 2 T-helper cytokines by decidual T cells in unexplained recurrent abortions. Nat Med (1998) 4:1020–4. doi: 10.1038/2006
159. Saito S. Cytokine network at the feto-maternal interface. J Reprod Immunol (2000) 47:87–103. doi: 10.1016/s0165-0378(00)00060-7
160. Saito S, Sakai M, Sasaki Y, Tanebe K, Tsuda H, Michimata T. Quantitative analysis of peripheral blood Th0, Th1, Th2 and the Th1:Th2 cell ratio during normal human pregnancy and preeclampsia. Clin Exp Immunol (1999) 117:550–5. doi: 10.1046/j.1365-2249.1999.00997.x
161. Mao H, Gao W, Ma C, Sun J, Liu J, Shao Q, et al. Human placental trophoblasts express the immunosuppressive cytokine IL-35. Hum Immunol (2013) 74:872–7. doi: 10.1016/j.humimm.2013.04.010
162. Niedbala W, Wei X-Q, Cai B, Hueber AJ, Leung BP, McInnes IB, et al. IL-35 is a novel cytokine with therapeutic effects against collagen-induced arthritis through the expansion of regulatory T cells and suppression of Th17 cells. Eur J Immunol (2007) 37:3021–9. doi: 10.1002/eji.200737810
163. Komorowska B. Autoimmune premature ovarian failure. Przeglad Menopauzalny Menopause Rev (2016) 15:210–4. doi: 10.5114/pm.2016.65666
164. Lin H, Mosmann TR, Guilbert L, Tuntipopipat S, Wegmann TG. Synthesis of T helper 2-type cytokines at the maternal-fetal interface. J Immunol Baltim Md 1950 (1993) 151:4562–73.
165. Wang W, Sung N, Gilman-Sachs A, Kwak-Kim J. T Helper (Th) cell profiles in pregnancy and recurrent pregnancy losses: Th1/Th2/Th9/Th17/Th22/Tfh cells. Front Immunol (2020) 11:2025. doi: 10.3389/fimmu.2020.02025
166. Kahn DA, Baltimore D. Pregnancy induces a fetal antigen-specific maternal T regulatory cell response that contributes to tolerance. Proc Natl Acad Sci U.S.A. (2010) 107:9299–304. doi: 10.1073/pnas.1003909107
167. Shima T, Inada K, Nakashima A, Ushijima A, Ito M, Yoshino O, et al. Paternal antigen-specific proliferating regulatory T cells are increased in uterine-draining lymph nodes just before implantation and in pregnant uterus just after implantation by seminal plasma-priming in allogeneic mouse pregnancy. J Reprod Immunol (2015) 108:72–82. doi: 10.1016/j.jri.2015.02.005
168. Garcia L, Hinojosa L, Dominguez R, Chavira R, Rosas P. Effects of infantile thymectomy on ovarian functions and gonadotrophin-induced ovulation in prepubertal mice: role of thymulin. J Endocrinol (2000) 166:381–7. doi: 10.1677/joe.0.1660381
169. Hinojosa L, Chavira R, Domínguez R, Rosas P. Effects of thymulin on spontaneous puberty and gonadotrophin-induced ovulation in prepubertal normal and hypothymic mice. J Endocrinol (1999) 163:255–60. doi: 10.1677/joe.0.1630255
170. Brown OA, Sosa YE, Dardenne M, Pléau JM, Goya RG. Studies on the gonadotropin-releasing activity of thymulin: changes with age. J Gerontol A Biol Sci Med Sci (2000) 55:B170–176. doi: 10.1093/gerona/55.4.b170
171. Hinojosa L, García L, Domínguez R, Romano MC, Damián-Matsumura PG, Castillo L, et al. Effects of thymulin and GnRH on the release of gonadotropins by in vitro pituitary cells obtained from rats in each day of estrous cycle. Life Sci (2004) 76:795–804. doi: 10.1016/j.lfs.2004.07.017
172. Duan Y-G, Yu C-F, Novak N, Bieber T, Zhu C-H, Schuppe H-C, et al. Immunodeviation towards a Th17 immune response associated with testicular damage in azoospermic men. Int J Androl (2011) 34:e536–545. doi: 10.1111/j.1365-2605.2010.01137.x
173. Wang H, Zhang Y, Fang X, Kwak-Kim J, Wu L. Insulin resistance adversely affect IVF outcomes in lean women without PCOS. Front Endocrinol (2021) 12:734638. doi: 10.3389/fendo.2021.734638
174. Mekaru K, Oishi S, Akamine K, Kinjo T, Heshiki C, Masamoto H, et al. The effect of insulin resistance on in-vitro fertilization-embryo transfer in women without polycystic ovary syndrome. Open J Obstet Gynecol (2016) 6:157–66. doi: 10.4236/ojog.2016.63020
175. Li Y, Lin H, Pan P, Yang D, Zhang Q. Impact of central obesity on women with polycystic ovary syndrome undergoing In vitro fertilization. Biores Open Access (2018) 7:116–22. doi: 10.1089/biores.2017.0040
176. Issa CM, Abu Khuzam RH. In vitro fertilization-induced hypertriglyceridemia with secondary acute pancreatitis and diabetic ketoacidosis. SAGE Open Med Case Rep (2017) 5:2050313X16689209. doi: 10.1177/2050313X16689209
177. Vuguin PM, Kedees MH, Cui L, Guz Y, Gelling RW, Nejathaim M, et al. Ablation of the glucagon receptor gene increases fetal lethality and produces alterations in islet development and maturation. Endocrinology (2006) 147:3995–4006. doi: 10.1210/en.2005-1410
178. Saleh AAEW, Amin EM, Elfallah AA, Hamed AM. Insulin resistance and idiopathic infertility: A potential possible link. Andrologia (2020) 52:e13773. doi: 10.1111/and.13773
179. Izzi-Engbeaya C, Jones S, Crustna Y, Machenahalli PC, Papadopoulou D, Modi M, et al. Acute effects of glucagon on reproductive hormone secretion in healthy men. J Clin Endocrinol Metab (2020) 105:dgaa164. doi: 10.1210/clinem/dgaa164
180. Sakumoto T, Tokunaga Y, Tanaka H, Nohara M, Motegi E, Shinkawa T, et al. Insulin resistance/hyperinsulinemia and reproductive disorders in infertile women. Reprod Med Biol (2010) 9:185–90. doi: 10.1007/s12522-010-0062-5
181. Gill L, Mackey S. Obstetrician-gynecologists’ strategies for patient initiation and maintenance of antiobesity treatment with glucagon-like peptide-1 receptor agonists. J Womens Health 2002 (2021) 30:1016–27. doi: 10.1089/jwh.2020.8683
182. Jensterle M, Janez A, Fliers E, DeVries JH, Vrtacnik-Bokal E, Siegelaar SE. The role of glucagon-like peptide-1 in reproduction: from physiology to therapeutic perspective. Hum Reprod Update (2019) 25:504–17. doi: 10.1093/humupd/dmz019
183. Eddy JJ, Gideonsen MD, Song JY, Grobman WA, O’Halloran P. Pancreatitis in pregnancy. Obstet Gynecol (2008) 112:1075–81. doi: 10.1097/AOG.0b013e318185a032
184. Seo D, Suh H, Lee JK, Jang DK, Kwon HY, Lee CH, et al. Estrogen-induced acute pancreatitis: A case report and literature review. Obstet Gynecol Sci (2017) 60:485–9. doi: 10.5468/ogs.2017.60.5.485
185. Badalov N, Baradarian R, Iswara K, Li J, Steinberg W, Tenner S. Drug-induced acute pancreatitis: an evidence-based review. Clin Gastroenterol Hepatol Off Clin Pract J Am Gastroenterol Assoc (2007) 5:648–61. doi: 10.1016/j.cgh.2006.11.023. quiz 644.
186. Gan S-I, Edwards A-L, Symonds C-J, Beck P-L. Hypertriglyceridemia-induced pancreatitis: A case-based review. World J Gastroenterol (2006) 12:7197–202. doi: 10.3748/wjg.v12.i44.7197
187. Ouhilal S, Vuguin P, Cui L, Du X-Q, Gelling RW, Reznik SE, et al. Hypoglycemia, hyperglucagonemia, and fetoplacental defects in glucagon receptor knockout mice: a role for glucagon action in pregnancy maintenance. Am J Physiol Endocrinol Metab (2012) 302:E522–531. doi: 10.1152/ajpendo.00420.2011
188. Kumar S, Agrawal D, Sharma K, Swain TR. Association of Male infertility to metabolic syndrome and other related disorders. J Integr Nephrol Androl (2015) 2:107. doi: 10.4103/2225-1243.168524
189. Csemiczky G, Landgren BM, Collins A. The influence of stress and state anxiety on the outcome of IVF-treatment: psychological and endocrinological assessment of Swedish women entering IVF-treatment. Acta Obstet Gynecol Scand (2000) 79:113–8. doi: 10.1034/j.1600-0412.2000.079002113.x
190. Smeenk JMJ, Verhaak CM, Vingerhoets AJJM, Sweep CGJ, Merkus JMWM, Willemsen SJ, et al. Stress and outcome success in IVF: the role of self-reports and endocrine variables. Hum Reprod Oxf Engl (2005) 20:991–6. doi: 10.1093/humrep/deh739
191. Piquer B, Fonseca JL, Lara HE. Gestational stress, placental norepinephrine transporter and offspring fertility. Reprod Camb Engl (2017) 153:147–55. doi: 10.1530/REP-16-0312
192. Kapoor A, Matthews SG. Short periods of prenatal stress affect growth, behaviour and hypothalamo-pituitary-adrenal axis activity in male guinea pig offspring. J Physiol (2005) 566:967–77. doi: 10.1113/jphysiol.2005.090191
193. Mayerhofer A, Bartke A, Steger RW. Catecholamine effects on testicular testosterone production in the gonadally active and the gonadally regressed adult golden hamster. Biol Reprod (1989) 40:752–61. doi: 10.1095/biolreprod40.4.752
194. Rehman R, Amjad S, Tariq H, Zahid N, Akhter M, Ashraf M. Oxidative stress and male infertility: a cross sectional study. JPMA J Pak Med Assoc (2020) 70:461–6. doi: 10.5455/JPMA.12992
195. Whirledge S, Cidlowski JA. Glucocorticoids, stress, and fertility. Minerva Endocrinol (2010) 35:109–25.
196. Ziaja J, Cholewa K, Mazurek U, Cierpka L. [Molecular basics of aldosterone and cortisol synthesis in normal adrenals and adrenocortical adenomas]. Endokrynol Pol (2008) 59:330–9.
197. Palomba S, Daolio J, Romeo S, Battaglia FA, Marci R, La Sala GB. Lifestyle and fertility: the influence of stress and quality of life on female fertility. Reprod Biol Endocrinol RBE (2018) 16:113. doi: 10.1186/s12958-018-0434-y
198. Pepin É, Dehboneh SS, Raguema N, Esfandarani MT, Lavoie JL. Role of the renin-angiotensin system in healthy and pathological pregnancies. IntechOpen (2017). doi: 10.5772/66748
199. Sabbadin C, Andrisani A, Ambrosini G, Bordin L, Donà G, Manso J, et al. Aldosterone in gynecology and its involvement on the risk of hypertension in pregnancy. Front Endocrinol (2019) 10:575. doi: 10.3389/fendo.2019.00575
200. Aranceta J, Serra-Majem L, Ribas L, Pérez-Rodrigo C. Breakfast consumption in Spanish children and young people. Public Health Nutr (2001) 4:1439–44. doi: 10.1079/phn2001235
201. Spergel DJ. Modulation of gonadotropin-releasing hormone neuron activity and secretion in mice by non-peptide neurotransmitters, gasotransmitters, and gliotransmitters. Front Endocrinol (2019) 10:329. doi: 10.3389/fendo.2019.00329
202. Ferlin A, Arredi B, Foresta C. Genetic causes of male infertility. Reprod Toxicol Elmsford N (2006) 22:133–41. doi: 10.1016/j.reprotox.2006.04.016
203. Whirledge S, Cidlowski JA. Glucocorticoids and reproduction: Traffic control on the road to reproduction. Trends Endocrinol Metab TEM (2017) 28:399–415. doi: 10.1016/j.tem.2017.02.005
204. Hamilton KJ, Hewitt SC, Arao Y, Korach KS. Estrogen hormone biology. Curr Top Dev Biol (2017) 125:109–46. doi: 10.1016/bs.ctdb.2016.12.005
205. Mueller SO, Korach KS. Estrogen receptors and endocrine diseases: lessons from estrogen receptor knockout mice. Curr Opin Pharmacol (2001) 1:613–9. doi: 10.1016/s1471-4892(01)00105-9
206. Lu Q, Schnitzler GR, Ueda K, Iyer LK, Diomede OI, Andrade T, et al. ER alpha rapid signaling is required for estrogen induced proliferation and migration of vascular endothelial cells. PloS One (2016) 11:e0152807. doi: 10.1371/journal.pone.0152807
207. Findlay JK, Liew SH, Simpson ER, Korach KS. Estrogen signaling in the regulation of female reproductive functions. Handb Exp Pharmacol (2010) 198:29–35. doi: 10.1007/978-3-642-02062-9_2
208. Palaniappan M, Nguyen L, Grimm SL, Xi Y, Xia Z, Li W, et al. The genomic landscape of estrogen receptor α binding sites in mouse mammary gland. PloS One (2019) 14:e0220311. doi: 10.1371/journal.pone.0220311
209. Stefkovich ML, Arao Y, Hamilton KJ, Korach KS. Experimental models for evaluating non-genomic estrogen signaling. Steroids (2018) 133:34–7. doi: 10.1016/j.steroids.2017.11.001
210. Wu S-P, Li R, DeMayo FJ. Progesterone receptor regulation of uterine adaptation for pregnancy. Trends Endocrinol Metab TEM (2018) 29:481–91. doi: 10.1016/j.tem.2018.04.001
211. Kumar P, Magon N. Hormones in pregnancy. Niger Med J J Niger Med Assoc (2012) 53:179–83. doi: 10.4103/0300-1652.107549
212. Taraborrelli S. Physiology, production and action of progesterone. Acta Obstet Gynecol Scand (2015) 94 Suppl 161:8–16. doi: 10.1111/aogs.12771
213. De Vos M, Devroey P, Fauser BCJM. Primary ovarian insufficiency. Lancet Lond Engl (2010) 376:911–21. doi: 10.1016/S0140-6736(10)60355-8
214. European Society for Human Reproduction and Embryology (ESHRE) Guideline Group on POI, Webber L, Davies M, Anderson R, Bartlett J, Braat D, et al. ESHRE guideline: management of women with premature ovarian insufficiency. Hum Reprod Oxf Engl (2016) 31:926–37. doi: 10.1093/humrep/dew027
215. Rebar RW, Connolly HV. Clinical features of young women with hypergonadotropic amenorrhea. Fertil Steril (1990) 53:804–10.
216. Chitayat D, Wyatt PR, SOCIETY OF OBSTETRICIANS AND GYNAECOLOGISTS OF CANADA GENETICS COMMITTEE, CANADIAN COLLEGE OF MEDICAL GENETICISTS PRENATAL DIAGNOSIS COMMITTEE. Fragile X testing in obstetrics and gynaecology in Canada. J Obstet Gynaecol Can JOGC J Obstet Gynecol Can JOGC (2008) 30:837–41. doi: 10.1016/S1701-2163(16)32949-8
217. Bachelot A, Rouxel A, Massin N, Dulon J, Courtillot C, Matuchansky C, et al. Phenotyping and genetic studies of 357 consecutive patients presenting with premature ovarian failure. Eur J Endocrinol (2009) 161:179–87. doi: 10.1530/EJE-09-0231
218. Lakhal B, Braham R, Berguigua R, Bouali N, Zaouali M, Chaieb M, et al. Cytogenetic analyses of premature ovarian failure using karyotyping and interphase fluorescence in situ hybridization (FISH) in a group of 1000 patients. Clin Genet (2010) 78:181–5. doi: 10.1111/j.1399-0004.2009.01359.x
219. Rudnicka E, Kruszewska J, Klicka K, Kowalczyk J, Grymowicz M, Skórska J, et al. Premature ovarian insufficiency - aetiopathology, epidemiology, and diagnostic evaluation. Przeglad Menopauzalny Menopause Rev (2018) 17:105–8. doi: 10.5114/pm.2018.78550
220. Varanasi LC, Subasinghe A, Jayasinghe YL, Callegari ET, Garland SM, Gorelik A, et al. Polycystic ovarian syndrome: Prevalence and impact on the wellbeing of Australian women aged 16-29 years. Aust N Z J Obstet Gynaecol (2018) 58:222–33. doi: 10.1111/ajo.12730
221. Rotterdam ESHRE/ASRM-Sponsored PCOS consensus workshop group. Revised 2003 consensus on diagnostic criteria and long-term health risks related to polycystic ovary syndrome (PCOS). Hum Reprod Oxf Engl (2004) 19:41–7. doi: 10.1093/humrep/deh098
222. Papadakis G, Kandaraki EA, Tseniklidi E, Papalou O, Diamanti-Kandarakis E. Polycystic ovary syndrome and NC-CAH: Distinct characteristics and common findings. A Systematic Review. Front Endocrinol (2019) 10:388. doi: 10.3389/fendo.2019.00388
223. Norman RJ, Teede HJ. A new evidence-based guideline for assessment and management of polycystic ovary syndrome. Med J Aust (2018) 209:299–300. doi: 10.5694/mja18.00635
224. Norman RJ, Noakes M, Wu R, Davies MJ, Moran L, Wang JX. Improving reproductive performance in overweight/obese women with effective weight management. Hum Reprod Update (2004) 10:267–80. doi: 10.1093/humupd/dmh018
225. Carvalho LML, Dos Reis FM, Candido AL, Nunes FFC, Ferreira CN, Gomes KB. Polycystic ovary syndrome as a systemic disease with multiple molecular pathways: a narrative review. Endocr Regul (2018) 52:208–21. doi: 10.2478/enr-2018-0026
226. Richards JS, Ren YA, Candelaria N, Adams JE, Rajkovic A. Ovarian follicular theca cell recruitment, differentiation, and impact on fertility: 2017 update. Endocr Rev (2018) 39:1–20. doi: 10.1210/er.2017-00164
227. Rosenfield RL. Current concepts of polycystic ovary syndrome pathogenesis. Curr Opin Pediatr (2020) 32:698–706. doi: 10.1097/MOP.0000000000000945
228. Chaudhari N, Dawalbhakta M, Nampoothiri L. GnRH dysregulation in polycystic ovarian syndrome (PCOS) is a manifestation of an altered neurotransmitter profile. Reprod Biol Endocrinol RBE (2018) 16:37. doi: 10.1186/s12958-018-0354-x
229. Ashraf S, Nabi M, Rasool S ul A, Rashid F, Amin S. Hyperandrogenism in polycystic ovarian syndrome and role of CYP gene variants: a review. Egypt J Med Hum Genet (2019) 20:25. doi: 10.1186/s43042-019-0031-4
230. Teng F, Ma X, Yu X, Yan Y, Zhao J, Gao J, et al. High serum androgen and insulin concentrations increase the tendency of endometrial carcinoma. J Cancer (2020) 11:5656–64. doi: 10.7150/jca.46391
231. Rumi MAK, Dhakal P, Kubota K, Chakraborty D, Lei T, Larson MA, et al. Generation of Esr1-knockout rats using zinc finger nuclease-mediated genome editing. Endocrinology (2014) 155:1991–9. doi: 10.1210/en.2013-2150
232. Rumi MAK, Singh P, Roby KF, Zhao X, Iqbal K, Ratri A, et al. Defining the role of estrogen receptor β in the regulation of female fertility. Endocrinology (2017) 158:2330–43. doi: 10.1210/en.2016-1916
233. Hipp HS, Charen KH, Spencer JB, Allen EG, Sherman SL. Reproductive and gynecologic care of women with fragile X primary ovarian insufficiency (FXPOI). Menopause N Y N (2016) 23:993–9. doi: 10.1097/GME.0000000000000658
234. Mohammed K, Abu Dabrh AM, Benkhadra K, Al Nofal A, Carranza Leon BG, Prokop LJ, et al. Oral vs transdermal estrogen therapy and vascular events: A systematic review and meta-analysis. J Clin Endocrinol Metab (2015) 100:4012–20. doi: 10.1210/jc.2015-2237
235. Canonico M, Oger E, Plu-Bureau G, Conard J, Meyer G, Lévesque H, et al. Hormone therapy and venous thromboembolism among postmenopausal women: impact of the route of estrogen administration and progestogens: the ESTHER study. Circulation (2007) 115:840–5. doi: 10.1161/CIRCULATIONAHA.106.642280
236. Langrish JP, Mills NL, Bath LE, Warner P, Webb DJ, Kelnar CJ, et al. Cardiovascular effects of physiological and standard sex steroid replacement regimens in premature ovarian failure. Hypertens Dallas Tex 1979 (2009) 53:805–11. doi: 10.1161/HYPERTENSIONAHA.108.126516
237. Piedade KC, Spencer H, Persani L, Nelson LM. Optimizing fertility in primary ovarian insufficiency: Case report and literature review. Front Genet (2021) 12:676262. doi: 10.3389/fgene.2021.676262
238. Pinelli S, Artini PG, Basile S, Obino MER, Sergiampietri C, Giannarelli D, et al. Estrogen treatment in infertile women with premature ovarian insufficiency in transitional phase: a retrospective analysis. J Assist Reprod Genet (2018) 35:475–82. doi: 10.1007/s10815-017-1096-y
239. Zhang X, Zheng Y, Guo Y, Lai Z. The effect of low carbohydrate diet on polycystic ovary syndrome: A meta-analysis of randomized controlled trials. Int J Endocrinol (2019) 2019:4386401. doi: 10.1155/2019/4386401
240. Glintborg D, Altinok ML, Mumm H, Hermann AP, Ravn P, Andersen M. Body composition is improved during 12 months’ treatment with metformin alone or combined with oral contraceptives compared with treatment with oral contraceptives in polycystic ovary syndrome. J Clin Endocrinol Metab (2014) 99:2584–91. doi: 10.1210/jc.2014-1135
241. Takasaki A, Tamura I, Okada-Hayashi M, Orita T, Tanabe M, Maruyama S, et al. Usefulness of intermittent clomiphene citrate treatment for women with polycystic ovarian syndrome that is resistant to standard clomiphene citrate treatment. Reprod Med Biol (2018) 17:454–8. doi: 10.1002/rmb2.12219
242. Homburg R, Hendriks ML, König TE, Anderson RA, Balen AH, Brincat M, et al. Clomifene citrate or low-dose FSH for the first-line treatment of infertile women with anovulation associated with polycystic ovary syndrome: a prospective randomized multinational study. Hum Reprod Oxf Engl (2012) 27:468–73. doi: 10.1093/humrep/der401
243. Waanbah BD, Joseph T, Rebekah G, Kunjummen AT, Kamath MS. Letrozole as first-line drug for ovulation induction in treatment-naïve infertile polycystic ovarian syndrome women. J Obstet Gynaecol Res (2021) 47:3583–9. doi: 10.1111/jog.14926
244. Ganie MA, Khurana ML, Nisar S, Shah PA, Shah ZA, Kulshrestha B, et al. Improved efficacy of low-dose spironolactone and metformin combination than either drug alone in the management of women with polycystic ovary syndrome (PCOS): a six-month, open-label randomized study. J Clin Endocrinol Metab (2013) 98:3599–607. doi: 10.1210/jc.2013-1040
245. Glintborg D, Mumm H, Holst JJ, Andersen M. Effect of oral contraceptives and/or metformin on GLP-1 secretion and reactive hypoglycaemia in polycystic ovary syndrome. Endocr Connect (2017) 6:267–77. doi: 10.1530/EC-17-0034
246. Devin JK, Nian H, Celedonio JE, Wright P, Brown NJ. Sitagliptin decreases visceral fat and blood glucose in women with polycystic ovarian syndrome. J Clin Endocrinol Metab (2020) 105:dgz028. doi: 10.1210/clinem/dgz028
247. Javed Z, Papageorgiou M, Deshmukh H, Rigby AS, Qamar U, Abbas J, et al. Effects of empagliflozin on metabolic parameters in polycystic ovary syndrome: A randomized controlled study. Clin Endocrinol (Oxf) (2019) 90:805–13. doi: 10.1111/cen.13968
248. Benito E, Gómez-Martin JM, Vega-Piñero B, Priego P, Galindo J, Escobar-Morreale HF, et al. Fertility and pregnancy outcomes in women with polycystic ovary syndrome following bariatric surgery. J Clin Endocrinol Metab (2020) 105:dgaa439. doi: 10.1210/clinem/dgaa439
249. Toulis KA, Goulis DG, Kolibianakis EM, Venetis CA, Tarlatzis BC, Papadimas I. Risk of gestational diabetes mellitus in women with polycystic ovary syndrome: a systematic review and a meta-analysis. Fertil Steril (2009) 92:667–77. doi: 10.1016/j.fertnstert.2008.06.045
250. De Frène V, Vansteelandt S, T’Sjoen G, Gerris J, Somers S, Vercruysse L, et al. A retrospective study of the pregnancy, delivery and neonatal outcome in overweight versus normal weight women with polycystic ovary syndrome. Hum Reprod Oxf Engl (2014) 29:2333–8. doi: 10.1093/humrep/deu154
251. Wierman ME, Basson R, Davis SR, Khosla S, Miller KK, Rosner W, et al. Androgen therapy in women: an endocrine society clinical practice guideline. J Clin Endocrinol Metab (2006) 91:3697–710. doi: 10.1210/jc.2006-1121
252. Sullivan SD, Sarrel PM, Nelson LM. Hormone replacement therapy in young women with primary ovarian insufficiency and early menopause. Fertil Steril (2016) 106:1588–99. doi: 10.1016/j.fertnstert.2016.09.046
253. Renoux C, Dell’aniello S, Garbe E, Suissa S. Transdermal and oral hormone replacement therapy and the risk of stroke: a nested case-control study. BMJ (2010) 340:c2519. doi: 10.1136/bmj.c2519
254. Talaulikar VS, Arulkumaran S. Reproductive outcomes after assisted conception. Obstet Gynecol Surv (2012) 67:566–83. doi: 10.1097/OGX.0b013e31826a5d4a
255. Holoch KJ, Savaris RF, Forstein DA, Miller PB, Higdon HL, Likes CE, et al. Coexistence of polycystic ovary syndrome and endometriosis in women with infertility. J Endometr Pelvic Pain Disord (2014) 6:79–83. doi: 10.5301/je.5000181
256. Niafar M, Pourafkari L, Porhomayon J, Nader N. A systematic review of GLP-1 agonists on the metabolic syndrome in women with polycystic ovaries. Arch Gynecol Obstet (2016) 293:509–15. doi: 10.1007/s00404-015-3976-7
257. Titi-Lartey OA, Khan YS. Embryology, testicle. Treasure Island (FL: StatPearls Publishing (2022). Available at: http://www.ncbi.nlm.nih.gov/books/NBK557763/.
258. Amory JK, Bremner WJ. Regulation of testicular function in men: implications for male hormonal contraceptive development. J Steroid Biochem Mol Biol (2003) 85:357–61. doi: 10.1016/s0960-0760(03)00205-x
259. Tanaka T, Kanatsu-Shinohara M, Lei Z, Rao CV, Shinohara T. The luteinizing hormone-testosterone pathway regulates mouse spermatogonial stem cell self-renewal by suppressing WNT5A expression in sertoli cells. Stem Cell Rep (2016) 7:279–91. doi: 10.1016/j.stemcr.2016.07.005
260. Tyagi V, Scordo M, Yoon RS, Liporace FA, Greene LW. Revisiting the role of testosterone: Are we missing something? Rev Urol (2017) 19:16–24. doi: 10.3909/riu0716
261. Walker WH. Testosterone signaling and the regulation of spermatogenesis. Spermatogenesis (2011) 1:116–20. doi: 10.4161/spmg.1.2.16956
262. O’Shaughnessy PJ, Monteiro A, Verhoeven G, De Gendt K, Abel MH. Effect of FSH on testicular morphology and spermatogenesis in gonadotrophin-deficient hypogonadal mice lacking androgen receptors. Reprod Camb Engl (2010) 139:177–84. doi: 10.1530/REP-09-0377
263. Nottelmann ED, Susman EJ, Inoff-Germain G, Cutler GB, Loriaux DL, Chrousos GP. Developmental processes in early adolescence: relationships between adolescent adjustment problems and chronologic age, pubertal stage, and puberty-related serum hormone levels. J Pediatr (1987) 110:473–80. doi: 10.1016/s0022-3476(87)80521-8
264. Rowe R, Maughan B, Worthman CM, Costello EJ, Angold A. Testosterone, antisocial behavior, and social dominance in boys: pubertal development and biosocial interaction. Biol Psychiatry (2004) 55:546–52. doi: 10.1016/j.biopsych.2003.10.010
265. Turcu A, Smith JM, Auchus R, Rainey WE. Adrenal androgens and androgen precursors-definition, synthesis, regulation and physiologic actions. Compr Physiol (2014) 4:1369–81. doi: 10.1002/cphy.c140006
266. de Menezes KJ, Peixoto C, Nardi AE, Carta MG, Machado S, Veras AB. Dehydroepiandrosterone, its sulfate and cognitive functions. Clin Pract Epidemiol Ment Health CP EMH (2016) 12:24–37. doi: 10.2174/1745017901612010024
267. Gray PB, McHale TS, Carré JM. A review of human male field studies of hormones and behavioral reproductive effort. Horm Behav (2017) 91:52–67. doi: 10.1016/j.yhbeh.2016.07.004
268. Verhoeven G, Willems A, Denolet E, Swinnen JV, De Gendt K. Androgens and spermatogenesis: lessons from transgenic mouse models. Philos Trans R Soc Lond B Biol Sci (2010) 365:1537–56. doi: 10.1098/rstb.2009.0117
269. Mohanty G, Jena SR, Kar S, Samanta L. Paternal factors in recurrent pregnancy loss: an insight through analysis of non-synonymous single-nucleotide polymorphism in human testis-specific chaperone HSPA2 gene. Environ Sci pollut Res Int (2021) 29:62219–34. doi: 10.1007/s11356-021-17799-3
270. Choobineh H, Kazemi M, Sadighi Gilani MA, Heydari T, Shokri S, Bazrafkan M, et al. Testosterone reduces spinal cord injury-induced effects on Male reproduction by preventing CADM1 defect. Cell J (2018) 20:138–49. doi: 10.22074/cellj.2018.5003
271. Snyder E, Chukrallah L, Seltzer K, Goodwin L, Braun RE. ADAD1 and ADAD2, testis-specific adenosine deaminase domain-containing proteins, are required for male fertility. Sci Rep (2020) 10:11536. doi: 10.1038/s41598-020-67834-5
272. Chi X, Luo W, Song J, Li B, Su T, Yu M, et al. Kindlin-2 in sertoli cells is essential for testis development and male fertility in mice. Cell Death Dis (2021) 12:604. doi: 10.1038/s41419-021-03885-4
273. Wei Y, Gao Q, Niu P, Xu K, Qiu Y, Hu Y, et al. Integrative proteomic and phosphoproteomic profiling of testis from Wip1 phosphatase-knockout mice: Insights into mechanisms of reduced fertility. Mol Cell Proteomics MCP (2019) 18:216–30. doi: 10.1074/mcp.RA117.000479
274. Xiang F, Cui B, Gao Q, Zhang J, Zhang J, Li W. Decreased levels of Ca2+-calmodulin-dependent protein kinase IV in the testis as a contributing factor to reduced fertility in male Crybb2–/– mice. Int J Mol Med (2012) 30:1145–51. doi: 10.3892/ijmm.2012.1116
275. Sun J, Lu Y, Nozawa K, Xu Z, Morohoshi A, Castaneda JM, et al. CRISPR/Cas9-based genome editing in mice uncovers 13 testis- or epididymis-enriched genes individually dispensable for male reproduction†. Biol Reprod (2020) 103:183–94. doi: 10.1093/biolre/ioaa083
276. Xia M, Xia J, Niu C, Zhong Y, Ge T, Ding Y, et al. Testis-expressed protein 33 is not essential for spermiogenesis and fertility in mice. Mol Med Rep (2021) 23:317. doi: 10.3892/mmr.2021.11956
277. Shah B, Khan R, Shah W, Aftab A, Khan M, Dil S, et al. Inactivation of testis-specific gene C4orf46 is dispensable for spermatogenesis and fertility in mouse. Mamm Genome Off J Int Mamm Genome Soc (2021) 32:364–70. doi: 10.1007/s00335-021-09879-z
278. Holcomb RJ, Oura S, Nozawa K, Kent K, Yu Z, Robertson MJ, et al. The testis-specific serine proteases PRSS44, PRSS46, and PRSS54 are dispensable for male mouse fertility†. Biol Reprod (2020) 102:84–91. doi: 10.1093/biolre/ioz158
279. He X, Xie W, Li H, Cui Y, Wang Y, Guo X, et al. The testis-specifically expressed gene Trim69 is not essential for fertility in mice. J BioMed Res (2020) 35:47–60. doi: 10.7555/JBR.34.20200069
280. Jia X, Liu Z, Lu X, Tang J, Wu Y, Du Q, et al. Effects of MCLR exposure on sex hormone synthesis and reproduction-related genes expression of testis in male rana nigromaculata. Environ pollut Barking Essex 1987 (2018) 236:12–20. doi: 10.1016/j.envpol.2018.01.057
281. Yang X, Zhang X, Yao Q, Song M, Han Y, Shao B, et al. T-2 toxin impairs male fertility by disrupting hypothalamic-pituitary-testis axis and declining testicular function in mice. Chemosphere (2019) 234:909–16. doi: 10.1016/j.chemosphere.2019.06.145
282. Zhang X, Yue Z, Zhang H, Liu L, Zhou X. Repeated administrations of Mn3O4 nanoparticles cause testis damage and fertility decrease through PPAR-signaling pathway. Nanotoxicology (2020) 14:326–40. doi: 10.1080/17435390.2019.1695976
283. Domínguez-Salazar E, Hurtado-Alvarado G, Medina-Flores F, Dorantes J, González-Flores O, Contis-Montes de Oca A, et al. Chronic sleep loss disrupts blood-testis and blood-epididymis barriers, and reduces male fertility. J Sleep Res (2020) 29:e12907. doi: 10.1111/jsr.12907
284. Ye J, Luo D, Xu X, Sun M, Su X, Tian Z, et al. Metformin improves fertility in obese males by alleviating oxidative stress-induced blood-testis barrier damage. Oxid Med Cell Longev (2019) 2019:9151067. doi: 10.1155/2019/9151067
285. Akomolafe SF, Odeniyi IA, Oyetayo FL, Ajayi OB. African Star apple fruit pulp-supplemented diet modulates fertility-related biomolecules in the testis and epididymis of high-fat diet/streptozotocin-induced diabetic rats. J Food Biochem (2019) 43:e12969. doi: 10.1111/jfbc.12969
286. Silva JV, Yoon S, Domingues S, Guimarães S, Goltsev AV, da Cruz E Silva EF, et al. Amyloid precursor protein interaction network in human testis: sentinel proteins for male reproduction. BMC Bioinf (2015) 16:12. doi: 10.1186/s12859-014-0432-9
287. Miyata H, Castaneda JM, Fujihara Y, Yu Z, Archambeault DR, Isotani A, et al. Genome engineering uncovers 54 evolutionarily conserved and testis-enriched genes that are not required for male fertility in mice. Proc Natl Acad Sci U.S.A. (2016) 113:7704–10. doi: 10.1073/pnas.1608458113
288. Lu Y, Oura S, Matsumura T, Oji A, Sakurai N, Fujihara Y, et al. CRISPR/Cas9-mediated genome editing reveals 30 testis-enriched genes dispensable for male fertility in mice†. Biol Reprod (2019) 101:501–11. doi: 10.1093/biolre/ioz103
289. Agarwal A, Mulgund A, Hamada A, Chyatte MR. A unique view on male infertility around the globe. Reprod Biol Endocrinol RBE (2015) 13:37. doi: 10.1186/s12958-015-0032-1
290. Kurkowska W, Bogacz A, Janiszewska M, Gabryś E, Tiszler M, Bellanti F, et al. Oxidative stress is associated with reduced sperm motility in normal semen. Am J Mens Health (2020) 14:1557988320939731. doi: 10.1177/1557988320939731
291. Kumar N, Singh AK. Trends of male factor infertility, an important cause of infertility: A review of literature. J Hum Reprod Sci (2015) 8:191–6. doi: 10.4103/0974-1208.170370
292. Tiepolo L, Zuffardi O. Localization of factors controlling spermatogenesis in the nonfluorescent portion of the human y chromosome long arm. Hum Genet (1976) 34:119–24. doi: 10.1007/BF00278879
293. Sharma N, Singh M, Acharya N, Singh SK, Thapa BR, Kaur G, et al. Implication of the cystic fibrosis transmembrane conductance regulator gene in infertile family members of Indian CF patients. Biochem Genet (2008) 46:847–56. doi: 10.1007/s10528-008-9199-x
294. Liu K, Zhao R, Shen M, Ye J, Li X, Huang Y, et al. Role of genetic mutations in folate-related enzyme genes on Male infertility. Sci Rep (2015) 5:15548. doi: 10.1038/srep15548
295. Xiao Q, Wang L, Supekar S, Shen T, Liu H, Ye F, et al. Structure of human steroid 5α-reductase 2 with anti-androgen drug finasteride. Res Sq (2020), rs.3.rs–40159. doi: 10.21203/rs.3.rs-40159/v1
296. Wang T, Gao H, Li W, Liu C. Essential role of histone replacement and modifications in Male fertility. Front Genet (2019) 10:962. doi: 10.3389/fgene.2019.00962
297. Choy JT, Eisenberg ML. Male Infertility as a window to health. Fertil Steril (2018) 110:810–4. doi: 10.1016/j.fertnstert.2018.08.015
298. Aitken RJ, Roman SD. Antioxidant systems and oxidative stress in the testes. Oxid Med Cell Longev (2008) 1:15–24. doi: 10.4161/oxim.1.1.6843
299. Torres-Arce E, Vizmanos B, Babio N, Márquez-Sandoval F, Salas-Huetos A. Dietary antioxidants in the treatment of Male infertility: Counteracting oxidative stress. Biology (2021) 10:241. doi: 10.3390/biology10030241
300. Di Guardo F, Vloeberghs V, Bardhi E, Blockeel C, Verheyen G, Tournaye H, et al. Low testosterone and semen parameters in Male partners of infertile couples undergoing IVF with a total sperm count greater than 5 million. J Clin Med (2020) 9:E3824. doi: 10.3390/jcm9123824
301. Crosnoe LE, Grober E, Ohl D, Kim ED. Exogenous testosterone: a preventable cause of male infertility. Transl Androl Urol (2013) 2:106–13. doi: 10.3978/j.issn.2223-4683.2013.06.01
302. Shiming Z, Mak K-K, Balijepalli MK, Chakravarthi S, Pichika MR. Swietenine potentiates the antihyperglycemic and antioxidant activity of metformin in streptozotocin induced diabetic rats. BioMed Pharmacother Biomedecine Pharmacother (2021) 139:111576. doi: 10.1016/j.biopha.2021.111576
303. Sjaarda LA, Mumford SL, Kuhr DL, Holland TL, Silver RM, Plowden TC, et al. Association of testosterone and antimüllerian hormone with time to pregnancy and pregnancy loss in fecund women attempting pregnancy. Fertil Steril (2018) 109:540–548.e1. doi: 10.1016/j.fertnstert.2017.11.014
304. Lathi RB, Dahan MH, Reynolds-May MF, Milki AA, Behr B, Westphal LM. The role of serum testosterone in early pregnancy outcome: a comparison in women with and without polycystic ovary syndrome. J Obstet Gynaecol Can JOGC J Obstet Gynecol Can JOGC (2014) 36:811–6. doi: 10.1016/S1701-2163(15)30483-7
305. Valdimarsdottir R, Wikström A-K, Kallak TK, Elenis E, Axelsson O, Preissl H, et al. Pregnancy outcome in women with polycystic ovary syndrome in relation to second-trimester testosterone levels. Reprod BioMed Online (2021) 42:217–25. doi: 10.1016/j.rbmo.2020.09.019
306. Chinnathambi V, Blesson CS, Vincent KL, Saade GR, Hankins GD, Yallampalli C, et al. Elevated testosterone levels during rat pregnancy cause hypersensitivity to angiotensin II and attenuation of endothelium-dependent vasodilation in uterine arteries. Hypertens Dallas Tex 1979 (2014) 64:405–14. doi: 10.1161/HYPERTENSIONAHA.114.03283
307. Sun B, Wang F, Sun J, Yu W, Sun Y. Basal serum testosterone levels correlate with ovarian response but do not predict pregnancy outcome in non-PCOS women undergoing IVF. J Assist Reprod Genet (2014) 31:829–35. doi: 10.1007/s10815-014-0246-8
308. Chen Z, Zhang D, Sun Z, Yu Q. A proper increasing in the testosterone level may be associated with better pregnancy outcomes for patients with tubal or Male infertility during in vitro Fertilization/Intracytoplasmic sperm injection. Front Physiol (2021) 12:696854. doi: 10.3389/fphys.2021.696854
309. Saharkhiz N, Zademodares S, Salehpour S, Hosseini S, Nazari L, Tehrani HG. The effect of testosterone gel on fertility outcomes in women with a poor response in in vitro fertilization cycles: A pilot randomized clinical trial. J Res Med Sci Off J Isfahan Univ Med Sci (2018) 23:3. doi: 10.4103/jrms.JRMS_864_17
310. Burger HG. Androgen production in women. Fertil Steril (2002) 77 Suppl 4:S3–5. doi: 10.1016/s0015-0282(02)02985-0
311. Sowers MF, Beebe JL, McConnell D, Randolph J, Jannausch M. Testosterone concentrations in women aged 25-50 years: associations with lifestyle, body composition, and ovarian status. Am J Epidemiol (2001) 153:256–64. doi: 10.1093/aje/153.3.256
Keywords: endocrine disorders, fertility, infertility, pregnancy, reproductive health, hormones
Citation: Bendarska-Czerwińska A, Zmarzły N, Morawiec E, Panfil A, Bryś K, Czarniecka J, Ostenda A, Dziobek K, Sagan D, Boroń D, Michalski P, Pallazo-Michalska V and Grabarek BO (2023) Endocrine disorders and fertility and pregnancy: An update. Front. Endocrinol. 13:970439. doi: 10.3389/fendo.2022.970439
Received: 15 June 2022; Accepted: 28 December 2022;
Published: 17 January 2023.
Edited by:
Surasak Saokaew, University of Phayao, ThailandReviewed by:
Olarik Musigavong, Ministry of Public Health, ThailandCopyright © 2023 Bendarska-Czerwińska, Zmarzły, Morawiec, Panfil, Bryś, Czarniecka, Ostenda, Dziobek, Sagan, Boroń, Michalski, Pallazo-Michalska and Grabarek. This is an open-access article distributed under the terms of the Creative Commons Attribution License (CC BY). The use, distribution or reproduction in other forums is permitted, provided the original author(s) and the copyright owner(s) are credited and that the original publication in this journal is cited, in accordance with accepted academic practice. No use, distribution or reproduction is permitted which does not comply with these terms.
*Correspondence: Anna Bendarska-Czerwińska, Y3plcndpbnNrYWEwMDJAZ21haWwuY29t; Nikola Zmarzły, bmlrb2xhLnptYXJ6bHlAZ21haWwuY29t; Beniamin Oskar Grabarek, QmdyYWJhcmVrN0BnbWFpbC5jb20=
Disclaimer: All claims expressed in this article are solely those of the authors and do not necessarily represent those of their affiliated organizations, or those of the publisher, the editors and the reviewers. Any product that may be evaluated in this article or claim that may be made by its manufacturer is not guaranteed or endorsed by the publisher.
Research integrity at Frontiers
Learn more about the work of our research integrity team to safeguard the quality of each article we publish.