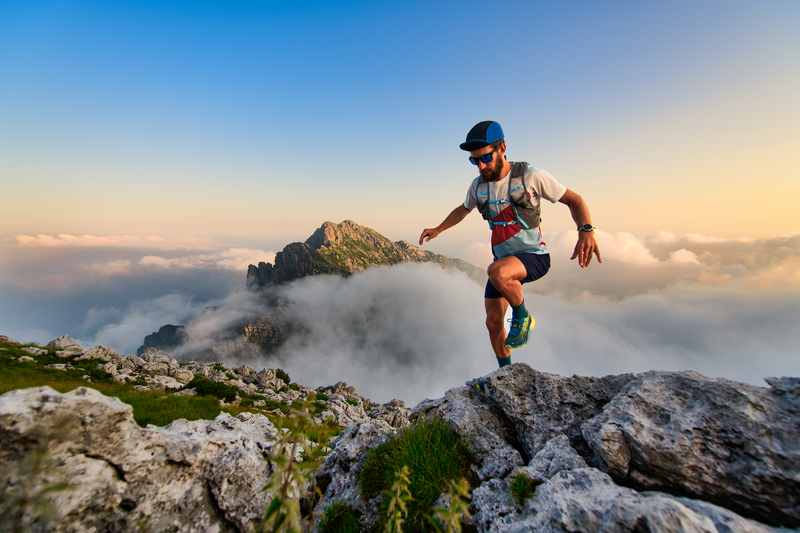
95% of researchers rate our articles as excellent or good
Learn more about the work of our research integrity team to safeguard the quality of each article we publish.
Find out more
REVIEW article
Front. Endocrinol. , 28 July 2022
Sec. Diabetes: Molecular Mechanisms
Volume 13 - 2022 | https://doi.org/10.3389/fendo.2022.927324
This article is part of the Research Topic Cellular Dysfunction and Cell Death in Diabetes (etio)Pathology: Novel Insights into Molecular Mechanisms and Therapeutic Targeting View all 6 articles
Diabetes is a complex metabolic disorder of carbohydrate metabolism, characterized by high blood glucose levels either due to an absolute deficiency of insulin secretion or an ineffective response of cells to insulin, a hormone synthetized by β-cells in the pancreas. Despite the current substantial progress of new drugs and strategies to prevent and treat diabetes, we do not understand precisely the exact cause of the failure and impairment of β-cells. Therefore, there is an urgent need to find new methods to restore β-cells. In recent years, pluripotent stem cells (PSCs) such as embryonic stem cells (ESCs) and induced pluripotent stem cells (iPSC) can serve as an ideal alternative source for the pancreatic β-cells. In this review, we systematically summarize the current progress and protocols of generating pancreatic β-cells from human PSCs. Meanwhile, we also discuss some challenges and future perspectives of human PSCs treatments for diabetes.
Diabetes has become more prevalent in recent years. Globally, more than 463 million adults are living with diabetes. It is estimated that over 693 million adults will be affected by 2045 (1). Diabetes is mainly classified into type 1 diabetes mellitus (T1DM), which is caused by absolute lack of insulin secretion, or type 2 diabetes mellitus (T2DM), which is mostly caused by insulin resistance in peripheral organs such as the liver muscle, and fat (2). In addition to T1DM and T2DM, diabetes also includes monogenic diabetes, gestational diabetes, and latent autoimmune diabetes. The burden of diabetes is increasing all over the world, which has a severe impact on the cost of medical care and human suffering.
New drugs for the treatment of diabetes are being developed. However, current drug treatments cannot entirely prevent or reverse the progression of diabetes. Furthermore, previous studies have shown that intensive insulin therapy has the potential to improve glycemic control and alleviate diabetes but also leads to some side effects, such as hypoglycemia and weight gain. Moreover, the whole-organ pancreas and islet transplantations represent a novel method of replacing beta cells. Once islet cells are transplanted successfully, the islet cells resume releasing insulin to maintain the patient’s blood glucose levels, which will significantly improve the quality of life of diabetic patients (3, 4). However, the success of pancreas or islet transplantation as a treatment for diabetes remains limited due to the scarcity of human suitable cadaveric islet donors, variability in donor pancreas quality, and immune rejection after islet transplantation. Therefore, the urgent need is to develop an alternative source of human islet cells for diabetes patients.
More recently, research has focused on developing regenerative approaches to treat diabetes patients, and pluripotent stem cell-derived cells therapy has become a viable therapy option. PSCs, which can be classified into hESCs and iPSCs, are likely to provide unlimited supplies of pancreatic β-cells that can respond to glucose and secret sufficient insulin. Initially obtained from mammalian blastocysts over two decades ago, hESCs have the potential to differentiate into any mature cell type of the three germ layers and the capacity for extensive self-renewal (5, 6). Notwithstanding, the utilization of hESCs is primarily restricted due to ethical concerns. In contrast, iPSCs, a breakthrough in the stem cell research field, are regarded to be more promising since they can be derived from varieties of human somatic cells in patients by using several transcription factors (7–9), thereby creating patient-specific cells that perfectly circumvent immune rejection after implantation, while resembling ESCs morphologically and functionally. Totally, the use of PSCs has generated significant outcomes in cell replacement therapy, drug screening, and disease modeling.
Tremendous progress has been made in generating glucose-responsive human stem cell-derived β-cells, which is an emerging promising therapeutic approach for diabetic patients. In this review, we summarize advances in the generation of pancreatic β-cells from human pluripotent stem cells, the differentiation of hESC/iPSC-derived cells into pancreatic β cells and islet-like organoids, and discuss the limitations and challenges for their successful generation and therapeutic application in diabetes.
The protocols for differentiation of PSCs into pancreatic β-cells are designed to mimic normal pancreatic development by sequential treatment of PSCs with various combinations of growth and signaling molecules added to the culture medium (10–13). Recent progress has crystallized in differentiation protocols that make possible the efficient differentiation of PSCs into pancreatic β-cells. See Table 1 and Figure 1 for an overview of the protocols for the generation of stem cell-derived beta cells.
Figure 1 Differentiation protocols of pancreatic β-cells from human iPSCs. Through mimicking the normal human pancreas development, directed differentiation protocols via adding different growth factors and small molecules can induce human iPSCs into pancreatic β-cells in vitro. This figure summarizes the 7-stage differentiation protocol and key markers of the differentiation pancreatic cells for evaluation of the consecutive stages of differentiation [(mainly based on references (20, 21)].
The initial discovery of spontaneous differentiation of hESCs into insulin-secreting cells (IPCs) that express vital genes, GLUT2 and islet-specific GK, in vitro has opened the possibility for directly differentiating PSCs into pancreatic β-cells in response to glucose for cell-based therapy in diabetes mellitus (29). Following the differentiation of embryoid bodies (EB) from hESCs, when growing the cells in suspension culture to promote the formation and maintenance of EB, the spontaneous process occurs much more frequently. The size of cell clusters and the addition of mesoderm-derived embryonic cells also play an essential role in the differentiation process (30). However, the transcriptional factors underlying this phenomenon are unknown, and the efficiency of the spontaneous process is low, which prompts the establishment of a standardized and productive protocol for transplantation therapy.
PSCs must undergo some key phases to mature into β-cells which include the formation of definite endoderm (DE), primitive gut tube, posterior foregut, pancreatic progenitors (PPs), and finally, hormone-expressing endocrine cell (ECs). The first step towards DE was accomplished in early studies with the use of activin A and decreased serum. Cell cultures containing ~80% SOX17 and FOXA2 dual positive definitive endoderm cells were successfully produced (31). A five-stage protocol was established by the same group the following year that recapitulates the pancreatic development in vivo (32). It is a milestone in the progress toward generating mature β-cells. Although an average of 7% of insulin-expressing cells are detected in the final product, more than one hormone is expressed in one single cell. In other words, most of them are polyhormonal instead of monohormonal, analogous to immature pancreatic ECs (33). Another evidence of the immaturity of the product is poor responsiveness to glucose, while glucose-stimulated insulin secretion (GSIS) is considered an important feature in mature β-cells. Besides, the expression of two key transcriptional factors, NKX6-1 and PDX1, cannot be observed in the final product (32). Later, improvements were made to this protocol, and ECs that exhibit GSIS were obtained after implanting stage-4 cells, namely pancreatic endoderm, into mice. The concentration of insulin stimulated by glucose resembles the control group in which nearly 3,000 human islets of Langerhans were transplanted. More strikingly, these cells are shown to ameliorate hyperglycemia and reverse diabetes in streptozotocin (STZ)-induced mice with considerable co-expression of NKX6-1 and PDX1 (34).
In 2014, a seven-stage protocol was built which successfully enabled the generation of β-cells that are monohormonal and possess the property of MAFA expression induced by the combination of R428, ALK5 inhibitor, and T3 in the last stage of the differentiation process in vitro, although delayed insulin release in response to glucose was observed due to a slower Ca2+ response (14). In the same year, another group proposed a protocol that also reached the goal of producing monohormonal β-cells that display GSIS in a 3D culture system, despite the differences in gene expression patterns from mature pancreatic islet cells (15). Both resulting cells are able to ameliorate hyperglycemia and reverse diabetes in STZ-induced mice within a short period, paving the way for follow-up studies and cell replacement therapy for patients suffering from diabetes. The protocol was further simplified by discarding the use of BMP inhibitors, thereby avoiding precocious endocrine induction with subsequent exposure to retinoic acid and EGF/KGF (35). Insulin-producing cells can also be obtained from human fibroblasts (36) and even patients with T1DM (37), resembling those from non-diabetic patients.
Since then, significant efforts have been made to optimize the protocol to enhance the efficiency of the generation of β-cells with a closer resemblance to bona fide pancreatic β-cells.
By modulating TGF-β signaling pathways, the differentiation into β-cells exhibiting GSIS is achieved successfully, indicating the critical role TGF-β plays in the differentiation process. It is shown that its blocking during stage 5 can improve the efficiency but hamper GSIS during stage 6. Notably, resizing the clusters in stage 6, which can decrease the average diameter by more than 50%, and an enriched serum-free media culture in this strategy also promote differentiation significantly (16). Another improvement to the differentiation protocol is to include a clustering/reaggregation step of immature β-cells in vitro to form enriched β-clusters which exhibit improved functional characteristics and key cell markers. The clustering/reaggregation process is reported to lead to enrichment in oxidative metabolic pathways and ERRγ, thereby maturing mitochondrial metabolism. Although a small proportion of dual-hormone-positive cells are also detected in addition to β-cells, tumors are not found 8 months after transplantation, indicating the increased safety of this approach (17). By utilizing such a reaggregation step, Liu et al. devised a protocol that efficiently produces β-cell islets from several pluripotent stem cell lines with a higher concentration of desired cell types. Remarkably, their guiding principles, including proper prolongation of specific differentiation steps to ensure the full acquisition of each cell state, and the employment of the late-stage gene markers referred to as the late-stage readout strategy, have successfully led to more promising outcomes (22).
In addition to the focus on constructing the mature structure of β-cells from iPSCs, scientists also attempt to illuminate their mature metabolic functionality. The metabolic immaturity of stem cell-derived islets includes 1) failure to respond to glucose in mitochondria; 2) reduced level of glucose-6-phosphate, 3-phosphoglycerate, phosphoenolpyruvate, and lactate, namely a bottleneck in glycolysis; 3) discrepant production of TCA-originated metabolites and redox shuttle components (25, 38). The induction of ERRγ can promote the activation of oxidative phosphorylation in mitochondria, thereby enhancing the production of ATP, which is indispensable for pancreatic β-cells to maintain high energy levels and secret insulin stimulated by glucose (39). It is reported that the overexpression of non-canonical WNT4 signaling can upregulate mitochondria-related genes through the ERRγ gene networks. In this study, iPSCs-derived pancreatic islets treated with WNT4 in the final maturation step have similar transcriptional patterns with human primary pancreatic islets, including key islet cell markers and, notably, ESRRG encoding ERRγ. The resulting islets show robust insulin secretion, highlighting the necessity of mature mitochondria in the regulation of GSIS in β-cells (19). Remarkably, based on an optimized protocol, Balboa et al. uncovered the functional mechanisms underlying the continuous maturation process by a series of analyses and assays, reporting the heterogenous maturation trajectory and the immaturity of the products in vitro. Compared to native pancreatic islets, SC-islets showed a reduced mitochondria TCA metabolite accumulation, lowered ATP/ADP ratio, and the disappearance of respiration spikes. Furthermore, the upregulation of MAFA and RBP4, markers for islet maturation, occurs 3 ~ 6 months post-transplantation (25).
The islet of Langerhans includes α-, β-, δ-, ϵ- and pancreatic polypeptide cells, which regulates the glucose level as a whole. For example, glucagon secreted by α-cells can increase the glucose concentration and regulate insulin secretion (13). The microenvironment surrounding the β-cells is vital to insulin secretion and maturation, including the cell-to-cell interaction and cell-to-matrix interaction (40). Thus, it is of paramount importance to understand and mimic the microenvironment to obtain fully mature β-cells that exhibit GSIS.
Extracellular matrix (ECM) proteins such as laminin and collagen can downregulate integrin α5 and thus induce endocrinogenesis. The contact of ECM with integrin α5 directs the PPs to ductal cells through the F-actin–YAP1–Notch mechanosignaling axis, while its inhibition can also promote the differentiation towards endocrine cells. It is shown that when exposed to a siRNA against ITGA5 or antibodies against integrin α5, NGN3, INS, and GCG are upregulated, indicating the functional maturation of hormone-expressing cells (41). The interaction with basement membrane proteins among all the ECM proteins is critical in activating integrin, forming focal adhesions, and thus maintaining the 3D structure of β-cells (42–44). Basement membrane proteins are found in the differentiated spheroids in a diffuse web-like network as a “by-product” with co-expression of fibroblast genes. In primary human islets, basement membrane proteins are secreted by vascular endothelial cells and originated from mesodermal lineage. However, the expression of CD31 and CD34, two endothelial markers, cannot be detected by a bioinformatic analysis and immunostaining in differentiated spheroids. It still remains elusive where basement membrane proteins in the spheroids come from, and further research needs to be done (18, 20). Despite the expression of polarity determinants and presynaptic proteins in β-cells within spheroids, they are disorganized, leading to unclear polarization of β-cells, while an organized polarity plays a vital role in the functionality of β-cells (45). Basement membrane proteins interact with the basal region, one of the three domains facing the capillaries, enriching presynaptic scaffold proteins which regulate GSIS (46, 47). Notably, if cultured on basement membrane proteins, the stimulation index is increased from 2.8 to 3.9-fold in spheroids containing PSCs-derived β-cells. Moreover, clear polarization and apparent segregation between the apical and basal poles can be detected in β-cells exposed to basement membrane proteins, suggesting the maturity of these β-cells derived from hESCs or iPSCs. By establishing ECM analogous to the native islet of Langerhans and the use of basement membrane proteins, the goal of generating mature β-cells for clinical use in regenerative medicine is coming closer (20).
The microenvironment in the pancreas offers key growth factors and signals to endocrine cells during their differentiation and maturing process (48), which are not fully elucidated. According to different phases of the process, the microenvironment presents different characteristics dynamically, mesenchyme and endothelium cells included (49, 50). Mesenchyme and endothelium cells (M-E cells) obtained from various endodermal organs and stages and divided into several groups (termed from M-E9 to M-E20) were co-cultured with hESC-derived PPs. It was revealed that M-E17 and M-E20 cells secreted an increased amount of C-peptide by 8.6~9.3 and 2.2~2.4-fold, respectively, compared to the control groups. Further investigation into the molecular mechanisms identified WNT5A as an initial activator through the non-canonical (JNK/c-JUN) WNT signaling pathway (26). Moreover, Ghezelayagh et al. also co-cultured human fetal pancreatic-derived mesenchymal cells (hFP-MCs) with hESC-derived PPs and discovered the upregulation of insulin secretion in co-cultured differentiated β-cell spheroids (27).
Integrins are transmembrane proteins that mediate the communication between ECM and actin cytoskeleton, which allow the movement and the possibility of changing the shape of cells. Via the role of integrins, ECM can regulate the recruitment of adhesion proteins and cytoskeletal polymerization and modulate the downstream signaling pathways, thereby influencing the differentiation direction of PSCs (21, 51–54). Recently, it was reported that NEUROG3 expression levels in PPs can be lowered by a polymerized cytoskeleton, inhibiting specification towards mature β-cells, which can be blocked by 24-hour treatment with latrunculin A, in conjunction with adequately timed cytoskeletal depolymerization. Previous studies favor the use of 3D culture to reconstruct the microenvironment surrounding β-cells to promote cell-to-cell and cell-to-matrix interaction. However, in this study, a planar culture protocol was applied to four cell lines, and the result was surprising. The resulting β-cells display dynamic GSIS and ameliorate diabetes in mice faster than those obtained in suspension cultures. It is even more surprising that cytoskeletal states can determine cell fate commitment in a wider range (21). Noteworthily, based on the theory mentioned above, this group proposed a protocol entailing the use of cytoskeletal depolymerizing compound latrunculin A completely on a planar culture (tissue culture polystyrene), which simplified the pancreatic differentiation process and made possible the scalable manufacture of stem cell-derived β-cells (23).
Besides the classical strategies for inducing mature β-cells, which commonly involve the modulation of key signaling pathways that determine β-cell specification, such as PI3K, FGF, BMP, WNT4, SIX2, and TGF-β (16, 19, 32, 55), small molecules are also of significant importance not only in the reprogramming of iPSCs but in the conversion of PSCs to mature β-cells. IDE1 and IDE2 were first identified to induce 70-80% of mouse ESCs into DE after screening 4,000 compounds (56). Follow-up studies discovered the capacity of (-)-indolactam V (ILV) to direct the induction of PDX1-expressing PPs from DE together with FGF10, probably due to the activation of protein kinase C (PKC) signaling (57). Forskolin, dexamethasone, and a TGF-β inhibitor are used as inducers to convert PPs to IPCs (58). Recently, a group found a compound, sodium cromoglicate (SCG), which can improve the differentiation efficiency by approximately 10% based on a refined version of a previous protocol (59). More recently, Liu et al. found that PPs treated with a combination consisting of ten chemical compounds, namely “PP-10C” after testing 21 combinations, can maintain the expression level of NKX6.1, a crucial marker for the production of monohormonal β-cells. Further differentiation into β-cells with increased efficiency requires the use of a combination of eight chemicals/factors (termed as “EP-8C”) at stage 6, a combination of nine chemicals/factors (termed as iβ-9C) at stage 7, and a combination of seven chemicals/factors (termed as Fβ-7C) at stage 8. These four cocktails of chemicals and/or factors support the generation of β-cells with higher yield. See Table 2 for a detailed description of the chemicals and factors in this protocol.
Mainstream studies commonly involve the use of Activin A to promote DE formation (15, 60). However Jiang et al. abandoned Activin A during DE stage and instead replaced it with dorsomorphin, an inhibitor of the BMP ALK2 receptor. Dorsomorphin, combined with CH, enhanced the number of the resulting DE that co-expressed SOX17 and FOXA2. Remarkably, DE obtained from this xeno-free strategy (termed as GiBi protocol) could be further induced into PPs and, finally pancreatic β-cells that displayed dynamic GSIS (24). Since this small molecule-based GiBi protocol omits high-cost Activin A, the costs decrease dramatically by 99% compared to published Activin A-involved protocols while tackling the problem of massive cell death (61), thereby paving the way for the clinical applications of cell-based therapy for diabetes. Meanwhile, by adding I-BET151 to inhibit bromodomain and extraterminal domain (BET), PPs with PDX1 and NKX6.1 dual positive can be maintained and expanded, providing novel supplies for regenerative research and transplantation therapy which is restricted by limited cadaveric donors (28).
Single-cell RNA sequencing (scRNA-seq) can analyze the transcriptional profiles of individual cells using a microfluidic droplet platform, thereby identifying the specific cell types of the PSCs-derived β-cells (62). This technology can unveil the heterogeneity of the cell clusters derived from the differentiation of PSCs and has been applied to various fields to gain a comprehensive view of β-cell differentiation (18, 55, 63–66).
Four types of cells were identified in the products of β-cell induction from iPSCs, including stem-cell-derived β (SC-β) cells co-expressing INS, NKX6.1, ISL1, and other β-cell markers, and three other non-β-cells (18). Polyhormonal stem-cell-derived α (SC-α) cells expressing GCG, ARX, IRX2, and also INS were characterized as α precursors in human fetal pancreatic development. A population of endocrine cells (SC-EC) similar to enterochromaffin cells, which serve as chemosensors in the intestine (67), co-express several marker genes related to enterochromaffin and the synthesis of serotonin such as TPH1, LMX1A, and SLC18A1. Surprisingly, a cluster of non-endocrine cells was observed with the absence of key endocrine markers and differentiated into the pancreatic exocrine cells (18). More importantly, the traits of GSIS and global transcriptional profiles were preserved in later studies, indicating that these traits do not require exogenous factors or serum (18). Of note, another team compared the gene expression profiles of PPs generated from PSCs based on three previously published protocols (14, 35, 66, 68). PPs generated based on two protocols (14, 68) resembled their counterparts obtained from human fetal pancreas. Therefore, scRNA-seq is promising in elucidating the molecular mechanisms underlying the differentiation and maturation of β-cells.
From the very beginning of generating pancreatic β-cells, much attention has been paid to converting iPSCs into functionally mature β-cells that display GSIS. A recent study showed that the morphology of the cell clusters had a significant impact on their differentiation into highly mature β-cells (69). Pancreatic islet organoids derived from PSCs and adult stem cells consist of all types of islet cells with similar 3D structures and functionality to native pancreatic islets. The generation of β-cells, which has been reviewed above, is at the core of islet organoids (see Figure 2). However, of significant importance is the generation of other islet cells, hormone-expressing, and non-hormone-expressing cells included, which altogether create an appropriate microenvironment for the survival and function of IPCs. Transplanting organoids provides protection to support the functionality of pancreatic β-cells, while mere β-cells can hardly survive or display efficient functions since they are normally transplanted into other sites instead of pancreatic islets. Such organoids provide an ideal disease model for the investigation into diabetes therapy and drug screening.
Figure 2 The generation of hPSC-derived islet spheroids and organoids on 2D or/and 3D culture. PSCs were grown, differentiated, and eventually turned into pancreatic precursor cells (PSCs-derived PP cells) in adherent culture. PSCs-derived PP cells were seeded to amikagel to form mature spheroids, or together with HUVEC cells to form islet-like organoids. Other methods, such as PSCs-derived PP cells placing in the gas-liquid surface or spinner culture flasks, form islet spheroids (mainly based on references 20, 21, 74 and 75). Abbreviation. Human endothelial cell, HUVEC.
In 2016, it was among the first to report that dissociated hormone-expressing cells differentiated from ESCs have the capacity to cluster spontaneously to form endocrine cell clusters (ECCs) in one day, with their size varying from 50~150 μm, analogous to native human islets whose diameter are approximately 100 μm. ECCs, containing several types of endocrine cells with α-cells as an exception which is an imperfection of the ECCs in this study, perform robust secretion of insulin and C-peptide, however, in response to stimuli including high-level glucose and the inhibition of potassium channel. On stimulation by glucose, an instant Ca2+ influx is detected, and insulin granules are observed in the cytoplasm of ECCs, indicating the rapid response of ECCs via Ca2+ oscillation. Moreover, hyperglycemia in STZ-induced mice suffering from diabetes is ameliorated within 3 days after transplantation with ECCs, faster than those with PSCs-derived endocrine cells, despite the low survival rate after day 12 (70). A revolutionary strategy involving an organ-on-a-chip system based on 3D suspension cultures was devised to produce islet organoids in situ. A perfusable multi-layer microfluidic chip composed of four parts that can accurately control the components of ECM, cell-to-matrix interaction, and circulatory flow was applied to the differentiation process of iPSCs (71). Dissociated iPSCs were injected into the top layer of the chip, followed by the addition of several growth factors known to induce the differentiation process efficiently. It was shown that the formed organoids tended to maintain their spherical shape and have smooth and closely connected surfaces with the upregulation of E-cadherin, a cell junction protein that regulates the formation of 3D structures in native islets, PDX1, and NKX6.1. Besides, the organoids cultured in the chips were found to react to glucose more sensitively and secrete a higher insulin level, due to a more immediate Ca2+ influx than static models.
Moreover, collagen-based scaffolds, which offer ECM compositions and allow the formation of 3D structures of iPSCs, with the combination of Matrigel consisting of several basement membrane proteins, provide a better alternative for the generation of islet organoids (C-M scaffolds) (72). An increase in the levels of the expression of pancreatic marker genes, including Sox17, Pdx1, Ngn3, Insulin, Glut2, and MAFA, was observed in the generated islet organoids in C-M scaffolds compared to 2D conditions. Notably, most insulin-producing cells were monohormonal which means somatostatin, glucagon, or PP were not expressed, and a 12-fold increase of insulin secretory granules was detected. These are indicators of the maturity of pancreatic β-cells. A hydrogel platform named Amikagel enabling the robust formation of islet organoids of precise size and cellular heterogeneity (73), and later a hydrogel system used for clustering islet cells originated from hESCs with endothelial cells (74), and recently a continuous microfluidic system that involved an array of droplets in generating hybrid hydrogel capsules containing islet organoids (75), all produced islet organoids with highly similar morphology and functionality to primary pancreatic islets and high level of the expression of β-cell markers. Cells cultured in microporous scaffolds also exhibit better GSIS by accurately modulating the size of the cell clusters at 250~425 µm (76). The perfusable organ-on-a-chip platform, which has been discussed above, also contains a through-hole PDMS layer and a polycarbonate porous membrane in the middle (71). Recently, Tao et al. proposed an innovative multi-organoid-on-chip system co-culturing liver and pancreatic islets, the two crucial organs in modulating circulation glucose levels and maintaining normoglycemia, for up to 30 days from iPSCs. The co-cultured organoids can improve hepatic glucose uptake and display more sensitive GSIS, which is not observed in mono-organoid culture. This new system provides a source for the research and therapy of T2DM (77). Consequently, transplanting islet organoids instead of pure β-cells into recipients is regarded as a more reasonable and appropriate strategy for treating patients suffering from diabetes.
Remarkably, Ramzy et al. and Shapiro et al. published their preliminary results from an ongoing phase I/II clinical trial using a device (VC-02) that allows the entry of capillaries launched by ViaCyte (NCT03163511), which is a pioneer in the field of transforming the success of utilizing PSCs-derived pancreatic islet cells to ameliorate hyperglycemia on diabetic animal models to T1DM patients (78, 79). The clinical trial was initiated in 2017 and was estimated to complete by November 2023 in which pancreatic endoderm cells (PEC-01) encapsulated within a device that permits vascular growth and promotes cell survival are transplanted subcutaneously, while C-peptide level is considered as a main indicator of the outcomes of the trial (80, 81). Thus, the use of immunosuppression becomes a necessity to avoid the attack of immune cells on transplanted sites since this encapsulation device cannot isolate PEC-01 from the hosts’ immune system.
In Shapiro’s multicenter study, 17 patients were enrolled, including 9 males and 8 females who had all suffered from T1DM for at least 8 years. The outcomes showed that 6 participants displayed increased C-peptide levels within 6 months at the earliest, and by immunohistochemical staining of the explants removed from the patients at certain points during the trial, the number of insulin-positive endocrine cells in the explants from responders (Circulation C-peptide > 0.1 ng/mL) is significantly higher than non-responders. Meanwhile, the safety and tolerability of VC-02 are also well tested in the trial, which reported participants with only mild adverse effects mostly correlated with surgical procedures and immunosuppressive drugs (79). Ramzy et al. further confirmed that the rise of C-peptide levels could be attributed to the implants due to the loss of meal-stimulated C-peptide in two patients after the removal of the implants and the existence of mature MAFA positive β-cells. In their trial, among the initial 15 participants (7 males and 8 females) from a single center, almost all exhibited increased C-peptide level 26 weeks post-implantation, while 4 patients reached 30 pM. In addition to side effects unassociated with implanted PEC-01, teratoma is also not found, providing solid evidence for the safety of VC-02 (78). Both studies showed the ability of PEC-01 to mature into insulin-secreting β-cells that enhance the concentration of C-peptide. However, participants did not achieve significant clinical benefits during the trial (79) or the connection between the improved insulin independence and the implanted grafts is unclear (78). It is suggested that by transplanting a larger number of PECs, enriching for the desired cell types, or improving cell survival, patients with T1DM can possibly have better clinical outcomes. It is also worth noting that ViaCyte launched its first clinical trial in 2014 involving VC-01, which includes an encapsulation device that inhibits exposure from immunocytes (82)(NCT02239354). Though the final results have not been released, some preliminary data has been published that reported the safety and efficacy of VC-01.
The two trials have provided substantial proof for translating the theory of PSCs-derived pancreatic islets into clinical application. Vertex, on the other hand, embarked on a new clinical trial from a different perspective, adopting the approach of injecting organoids generated from allogenic stem cells (termed as VX-880) into the portal vein (NCT04786262). VX-880, distinguished from VC-02, is fully differentiated islet-like organoids that possess the potential to restore normoglycemia which has been tested in animal models. Immunosuppression is also compulsory to ensure cell survival within VX-880 (83). These clinical trials bring hopes to millions of patients with T1DM. However, more is needed to employ stem cell-based therapy to exempt them from suffering.
Currently, tremendous endeavors have been made to recapitulate the development of the pancreas and generate β-cell clusters or pancreatic islet organoids that show significant similarities to native pancreatic islets in terms of morphology, functionality, and global gene expression profiles. Nonetheless, various issues remain, limiting the clinical applications of stem cell-derived pancreatic β-cells. See Table 3 for a summary of the limitations and their possible strategies.
Early studies mainly focused on producing pure β-cells, which ideally have the capacity to secrete sufficient insulin stimulated by glucose and ameliorate hyperglycemia. Microenvironment and ECM were then found to play a critical role in generating fully mature β-cells, necessitating the generation of islet organoids that contain all types of cells and exhibit better GSIS (72). Disappointingly, despite the closer resemblance to native β-cells discovered by scRNA-seq, some immature cells, and cells that cannot be found in human pancreatic islets, such as SC-EC cells (18), exist in the final product. Thus, further maturation in vivo is indispensable, entailing transplanting cell clusters at the final stage of the differentiation protocols into animal models. ScRNA-seq has proved that the grafted islet organoids display better GSIS after transplanting into mice (64), but the underlying cues from the local microenvironment and sophisticated mechanisms that promote β-cell maturation are not fully elucidated. The available alternatives of the sites of transplantation include portal infusion, subcutaneous, intramuscular, and omental/intraperitoneal sites. The limited amount of tissue that can be infused and the impracticability of retrieving islets that have been delivered, considering the possibility of forming teratoma, have restricted the clinical applications of portal fusion (84, 85). A more ideal site of transplantation is in need. Notably, the long duration of maturation cannot be neglected either. In a recent study, it takes 6 months for the grafts to mature.
Of note, subtypes of β-cells in human pancreatic islets of Langerhans were identified according to their differential expression levels of ST8SIA1 and CD9 (86), or Fltp, a marker gene (87). These subtypes co-exist in pancreatic islets and vary in the expression profiles and their functional characteristics, such as GSIS. However, the inappropriate heterogeneity, either in β-cell clusters or islet organoids, does not match the scenario in native islets, thereby hindering the development and maturation of β-cells.
Therefore, it is imperative to purify the generated cell clusters. In addition to improving and simplifying the protocols to generate sufficient supplies of β-cells, sorting skills that can filtrate undesirable cell types and immature cells and enrich the β-cell population are one of the strategies utilized to promote efficiency. CD49a, a positive cell surface marker, and CD9, a negative marker, were identified by scRNA-seq in two studies, which showed that CD49a+ CD9- cells displayed better GSIS (18, 65). Encoding suicide genes into the genome of PSCs is another direction of eliminating pluripotent cells or improperly differentiated cells in the final product (88, 89).
Disappointingly, although the generation of β-cell products has achieved tremendous success in recent years, it remains a primary hurdle to transplant the final product into patients while ensuring high survival rates simultaneously, which has hindered the clinical use of stem cell-based therapy. As a highly vascularized organ, β-cells rely strongly on capillaries to gain nutrients to survive and modulate blood glucose concentration (90). Thus, their sensitivity to hypoxia necessitates timely vascularization after transplantation. Methods aimed at overcoming this issue include co-transplantation with the parathyroid gland, incorporation of amniotic epithelial cells or human umbilical cord perivascular mesenchymal stromal cells (HUCPVCs) into islet organoids, and the use of growth factors, such as VEGF-A (91–94). Furthermore, when prevascularized with a catheter and transplanted with ∼500 pancreatic islets, the diabetic mice were found to retain euglycemia for >100 days (95).
Upon transplantation, the protection of β-cells from immune cells must be assured to achieve satisfying results. Immunosuppressive drugs are needed to prevent allo-rejection for life, but the severe side effects and low efficiency limit their clinical use. Currently, the fast development of immunotherapy has opened a new possibility for tackling autoimmune attacks. Regulatory T-cell therapy is considered a promising strategy that enhances graft survival rates (96, 97). The emergence of CRISPR-Cas9 also revolutionizes the field by ablating HLA-A/-B/-C and HLA class II in PSCs to generate immune tolerant β-cells (98). Notably, upregulation of the immune checkpoint protein PD-L1 also provides protection for the grafts from immune attack (19).
Moreover, encapsulation devices, which provide maximum immune protection with minimal impact on cell viability and function, are also a possible way to solve this issue. Encapsulation devices are used in the study of many diseases, such as cancer, kidney failure, and cartilage defects (99). The ideal cell dispensing device is like a reassembled Langerhans islet that is safe, does not interfere with normal cell physiology, has an optimal nutrient exchange and disposal of cellular waste and facilitates implantation into the recipient and recycling, and, more importantly, protects the cells therein from inflammation and immune system attack (100). The two most essential aspects in the research and fabrication of dispensing devices are physical and chemical properties. Physical properties include the size and shape of the biomaterial used in the dispensing device, material porosity, and surface mechanical properties. Chemical properties include the chemical composition of the biomaterial, the purity of the materials and the interactions between the materials, and the transport and binding capacity of different ions (101). According to their scale, there are mainly two types of encapsulation devices, including microencapsulation and macroencapsulation. Although microcapsules can maximize nutrition and oxygen usage to enhance cell survival since each islet is contained in one capsule, the difficulty of controlling membrane thickness and pore size, as well as the enormous number of microcapsules required for transplantation at one time, limits the application of microencapsulation. On the contrary, by sacrificing the efficiency of transporting soluble factors and metabolites, macroencapsulation can be better harnessed to facilitate the best clinical results (102). Macroencapsulation devices have been employed in clinical trials (NCT02239354, NCT03163511), in which PECs were wrapped by devices that prevent immune attacks and abandon the use of immunosuppressants and devices that allow direct vascular growth, respectively (79, 103, 104). However, fibrosis is observed around the implantation sites due to allogenic responses, which inhibits material exchange and cell survival. Hence, nanofibrous devices are devised to prevent the overgrowth of fibrous tissue that are safe, retrievable, and scalable, holding great promises for the cure of T1DM (105, 106).
In the process of obtaining iPSCs, retroviruses are the most frequently used vectors to deliver transgenes into the host, which, however, bring about an issue while providing high efficiency. The risk of insertional mutagenesis resulting from the use of retroviruses may disrupt transcriptional profiles of differentiating cells, leading to the production of unwanted cell types or a halt in the differentiation process. Moreover, the pluripotent state also increases the risk of forming teratoma in patients (107). From excision systems aimed at removing transgenes and non-integrative viruses such as Sendai viruses to the use of non-viral methods such as protein, RNA, and chemicals-based reprogramming, scientists have devised a number of strategies to avoid the use of viral vectors to decrease the possibility of teratoma formation and improve safety (108–113). Enrichment for the desired β-cells is also considered to be a promising approach to reducing teratoma.
The last decades have witnessed the considerable progress made in the field of pluripotent stem cell-derived β-cell therapy toward the cure for diabetes. Even though β-cells with close resemblance to bona fide human β-cells in native islets can be generated with stepwise protocols, barriers that limit their clinical applications still exist. More research is needed to conquer these barriers before cell-based therapy can be fully employed in the treatment of diabetes. To sum up, significant advancements with regard to understanding β-cell differentiation and producing pluripotent stem cell-derived pancreatic β-cells have been revealed, and preliminary data from ongoing PSCs-based clinical trials have confirmed the infinite possibility of this therapy. Although many challenges remain unanswered, we believe that the generation of pluripotent stem cell-derived pancreatic β-cells will represent a promising future for the treatment of diabetes.
XW and MG collected the literature and wrote the manuscript. YW and YZ wrote, conceived and reviewed the manuscript critically. All authors have read and agreed to the published version of the manuscript.
This research was funded by National Natural Science Foundation of China (81702896); Health Research Talent Project of Jilin Province (2020SCZ50); Science and Technology Plan Projects of Jilin Province (20200201539JC); Department of Education of Jilin Province (JJKH20180198KJ); China Scholarship Council (201706175044 and 201606175202); Jilin University Project (2019YX396).
The authors declare that the research was conducted in the absence of any commercial or financial relationships that could be construed as a potential conflict of interest.
All claims expressed in this article are solely those of the authors and do not necessarily represent those of their affiliated organizations, or those of the publisher, the editors and the reviewers. Any product that may be evaluated in this article, or claim that may be made by its manufacturer, is not guaranteed or endorsed by the publisher.
1. Cole JB, Florez JC. Genetics of diabetes mellitus and diabetes complications. Nat Rev Nephrol (2020) 16(7):377–90. doi: 10.1038/s41581-020-0278-5
2. Solis-Herrera C, Triplitt C, Reasner C, DeFronzo RA, Cersosimo E, Feingold KR, et al. Classification of diabetes mellitus. In: South Dartmouth. MA: MDText (2000).
3. Triolo TM, Bellin MD. Lessons from human islet transplantation inform stem cell-based approaches in the treatment of diabetes. Front Endocrinol (Lausanne) (2021) 12:636824. doi: 10.3389/fendo.2021.636824
4. Baeyens L, Lemper M, Staels W, De Groef S, De Leu N, Heremans Y, et al. (Re)Generating human beta cells: Status, pitfalls, and perspectives. Physiol Rev (2018) 98(3):1143–67. doi: 10.1152/physrev.00034.2016
5. Thomson JA, Itskovitz-Eldor J, Shapiro SS, Waknitz MA, Swiergiel JJ, Marshall VS, et al. Embryonic stem cell lines derived from human blastocysts. Science (1998) 282(5391):1145–7. doi: 10.1126/science.282.5391.1145
6. Cowan CA, Klimanskaya I, McMahon J, Atienza J, Witmyer J, Zucker JP, et al. Derivation of embryonic stem-cell lines from human blastocysts. N Engl J Med (2004) 350(13):1353–6. doi: 10.1056/NEJMsr040330
7. Takahashi K, Tanabe K, Ohnuki M, Narita M, Ichisaka T, Tomoda K, et al. Induction of pluripotent stem cells from adult human fibroblasts by defined factors. Cell (2007) 131(5):861–72. doi: 10.1016/j.cell.2007.11.019
8. Takahashi K, Yamanaka S. Induction of pluripotent stem cells from mouse embryonic and adult fibroblast cultures by defined factors. Cell (2006) 126(4):663–76. doi: 10.1016/j.cell.2006.07.024
9. Yu J, Vodyanik MA, Smuga-Otto K, Antosiewicz-Bourget J, Frane JL, Tian S, et al. Induced pluripotent stem cell lines derived from human somatic cells. Science (2007) 318(5858):1917–20. doi: 10.1126/science.1151526
10. Balboa D, Iworima DG, Kieffer TJ. Human pluripotent stem cells to model islet defects in diabetes. Front Endocrinol (Lausanne) (2021) 12:642152. doi: 10.3389/fendo.2021.642152
11. Gheibi S, Singh T, da Cunha J, Fex M, Mulder H. Insulin/Glucose-responsive cells derived from induced pluripotent stem cells: Disease modeling and treatment of diabetes. Cells (2020) 9(11):2465. doi: 10.3390/cells9112465
12. Shahjalal HM, Abdal Dayem A, Lim KM, Jeon TI, Cho SG. Generation of pancreatic beta cells for treatment of diabetes: Advances and challenges. Stem Cell Res Ther (2018) 9(1):355. doi: 10.1186/s13287-018-1099-3
13. Bourgeois S, Sawatani T, Van Mulders A, De Leu N, Heremans Y, Heimberg H, et al. Towards a functional cure for diabetes using stem cell-derived beta cells: Are we there yet? Cells (2021) 10(1):191. doi: 10.3390/cells10010191
14. Rezania A, Bruin JE, Arora P, Rubin A, Batushansky I, Asadi A, et al. Reversal of diabetes with insulin-producing cells derived in vitro from human pluripotent stem cells. Nat Biotechnol (2014) 32(11):1121–33. doi: 10.1038/nbt.3033
15. Pagliuca FW, Millman JR, Gurtler M, Segel M, Van Dervort A, Ryu JH, et al. Generation of functional human pancreatic beta cells in vitro. Cell (2014) 159(2):428–39. doi: 10.1016/j.cell.2014.09.040
16. Velazco-Cruz L, Song J, Maxwell KG, Goedegebuure MM, Augsornworawat P, Hogrebe NJ, et al. Acquisition of dynamic function in human stem cell-derived beta cells. Stem Cell Rep (2019) 12(2):351–65. doi: 10.1016/j.stemcr.2018.12.012
17. Nair GG, Liu JS, Russ HA, Tran S, Saxton MS, Chen R, et al. Recapitulating endocrine cell clustering in culture promotes maturation of human stem-Cell-Derived beta cells. Nat Cell Biol (2019) 21(2):263–74. doi: 10.1038/s41556-018-0271-4
18. Veres A, Faust AL, Bushnell HL, Engquist EN, Kenty JH, Harb G, et al. Charting cellular identity during human in vitro beta-cell differentiation. Nature (2019) 569(7756):368–73. doi: 10.1038/s41586-019-1168-5
19. Yoshihara E, O'Connor C, Gasser E, Wei Z, Oh TG, Tseng TW, et al. Immune-evasive human islet-like organoids ameliorate diabetes. Nature (2020) 586(7830):606–11. doi: 10.1038/s41586-020-2631-z
20. Singh R, Cottle L, Loudovaris T, Xiao D, Yang P, Thomas HE, et al. Enhanced structure and function of human pluripotent stem cell-derived beta-cells cultured on extracellular matrix. Stem Cells Transl Med (2021) 10(3):492–505. doi: 10.1002/sctm.20-0224
21. Hogrebe NJ, Augsornworawat P, Maxwell KG, Velazco-Cruz L, Millman JR. Targeting the cytoskeleton to direct pancreatic differentiation of human pluripotent stem cells. Nat Biotechnol (2020) 38(4):460–70. doi: 10.1038/s41587-020-0430-6
22. Liu H, Li R, Liao HK, Min Z, Wang C, Yu Y, et al. Chemical combinations potentiate human pluripotent stem cell-derived 3d pancreatic progenitor clusters toward functional beta cells. Nat Commun (2021) 12(1):3330. doi: 10.1038/s41467-021-23525-x
23. Hogrebe NJ, Maxwell KG, Augsornworawat P, Millman JR. Generation of insulin-producing pancreatic beta cells from multiple human stem cell lines. Nat Protoc (2021) 16(9):4109–43. doi: 10.1038/s41596-021-00560-y
24. Jiang Y, Chen C, Randolph LN, Ye S, Zhang X, Bao X, et al. Generation of pancreatic progenitors from human pluripotent stem cells by small molecules. Stem Cell Rep (2021) 16(9):2395–409. doi: 10.1016/j.stemcr.2021.07.021
25. Balboa D, Barsby T, Lithovius V, Saarimaki-Vire J, Omar-Hmeadi M, Dyachok O, et al. Functional, metabolic and transcriptional maturation of human pancreatic islets derived from stem cells. Nat Biotechnol (2022). doi: 10.1038/s41587-022-01219-z
26. Chmielowiec J, Szlachcic WJ, Yang D, Scavuzzo MA, Wamble K, Sarrion-Perdigones A, et al. Human pancreatic microenvironment promotes beta-cell differentiation Via non-canonical Wnt5a/Jnk and bmp signaling. Nat Commun (2022) 13(1):1952. doi: 10.1038/s41467-022-29646-1
27. Ghezelayagh Z, Zabihi M, Zarkesh I, Goncalves CAC, Larsen M, Hagh-Parast N, et al. Improved differentiation of hesc-derived pancreatic progenitors by using human fetal pancreatic mesenchymal cells in a micro-scalable three-dimensional Co-culture system. Stem Cell Rev Rep (2022) 18(1):360–77. doi: 10.1007/s12015-021-10266-z
28. Ma X, Lu Y, Zhou Z, Li Q, Chen X, Wang W, et al. Human expandable pancreatic progenitor-derived beta cells ameliorate diabetes. Sci Adv (2022) 8(8):eabk1826. doi: 10.1126/sciadv.abk1826
29. Assady S, Maor G, Amit M, Itskovitz-Eldor J, Skorecki KL, Tzukerman M. Insulin production by human embryonic stem cells. Diabetes (2001) 50(8):1691–7. doi: 10.2337/diabetes.50.8.1691
30. Xu X, Kahan B, Forgianni A, Jing P, Jacobson L, Browning V, et al. Endoderm and pancreatic islet lineage differentiation from human embryonic stem cells. Cloning Stem Cells (2006) 8(2):96–107. doi: 10.1089/clo.2006.8.96
31. D'Amour KA, Agulnick AD, Eliazer S, Kelly OG, Kroon E, Baetge EE. Efficient differentiation of human embryonic stem cells to definitive endoderm. Nat Biotechnol (2005) 23(12):1534–41. doi: 10.1038/nbt1163
32. D'Amour KA, Bang AG, Eliazer S, Kelly OG, Agulnick AD, Smart NG, et al. Production of pancreatic hormone-expressing endocrine cells from human embryonic stem cells. Nat Biotechnol (2006) 24(11):1392–401. doi: 10.1038/nbt1259
33. Riedel MJ, Asadi A, Wang R, Ao Z, Warnock GL, Kieffer TJ. Immunohistochemical characterisation of cells Co-producing insulin and glucagon in the developing human pancreas. Diabetologia (2012) 55(2):372–81. doi: 10.1007/s00125-011-2344-9
34. Kroon E, Martinson LA, Kadoya K, Bang AG, Kelly OG, Eliazer S, et al. Pancreatic endoderm derived from human embryonic stem cells generates glucose-responsive insulin-secreting cells in vivo. Nat Biotechnol (2008) 26(4):443–52. doi: 10.1038/nbt1393
35. Russ HA, Parent AV, Ringler JJ, Hennings TG, Nair GG, Shveygert M, et al. Controlled induction of human pancreatic progenitors produces functional beta-like cells in vitro. EMBO J (2015) 34(13):1759–72. doi: 10.15252/embj.201591058
36. Zhu S, Russ HA, Wang X, Zhang M, Ma T, Xu T, et al. Human pancreatic beta-like cells converted from fibroblasts. Nat Commun (2016) 7:10080. doi: 10.1038/ncomms10080
37. Millman JR, Xie C, Van Dervort A, Gurtler M, Pagliuca FW, Melton DA. Generation of stem cell-derived beta-cells from patients with type 1 diabetes. Nat Commun (2016) 7:11463. doi: 10.1038/ncomms11463
38. Davis JC, Alves TC, Helman A, Chen JC, Kenty JH, Cardone RL, et al. Glucose response by stem cell-derived beta cells in vitro is inhibited by a bottleneck in glycolysis. Cell Rep (2020) 31(6):107623. doi: 10.1016/j.celrep.2020.107623
39. Yoshihara E, Wei Z, Lin CS, Fang S, Ahmadian M, Kida Y, et al. Errgamma is required for the metabolic maturation of therapeutically functional glucose-responsive beta cells. Cell Metab (2016) 23(4):622–34. doi: 10.1016/j.cmet.2016.03.005
40. Bavamian S, Klee P, Britan A, Populaire C, Caille D, Cancela J, et al. Islet-Cell-To-Cell communication as basis for normal insulin secretion. Diabetes Obes Metab (2007) 9 Suppl 2:118–32. doi: 10.1111/j.1463-1326.2007.00780.x
41. Mamidi A, Prawiro C, Seymour PA, de Lichtenberg KH, Jackson A, Serup P, et al. Mechanosignalling Via integrins directs fate decisions of pancreatic progenitors. Nature (2018) 564(7734):114–8. doi: 10.1038/s41586-018-0762-2
42. Diaferia GR, Jimenez-Caliani AJ, Ranjitkar P, Yang W, Hardiman G, Rhodes CJ, et al. Beta1 integrin is a crucial regulator of pancreatic beta-cell expansion. Development (2013) 140(16):3360–72. doi: 10.1242/dev.098533
43. Rondas D, Tomas A, Halban PA. Focal adhesion remodeling is crucial for glucose-stimulated insulin secretion and involves activation of focal adhesion kinase and paxillin. Diabetes (2011) 60(4):1146–57. doi: 10.2337/db10-0946
44. Virtanen I, Banerjee M, Palgi J, Korsgren O, Lukinius A, Thornell LE, et al. Blood vessels of human islets of langerhans are surrounded by a double basement membrane. Diabetologia (2008) 51(7):1181–91. doi: 10.1007/s00125-008-0997-9
45. Gan WJ, Zavortink M, Ludick C, Templin R, Webb R, Webb R, et al. Cell polarity defines three distinct domains in pancreatic beta-cells. J Cell Sci (2017) 130(1):143–51. doi: 10.1242/jcs.185116
46. Ohara-Imaizumi M, Ohtsuka T, Matsushima S, Akimoto Y, Nishiwaki C, Nakamichi Y, et al. Elks, a protein structurally related to the active zone-associated protein cast, is expressed in pancreatic beta cells and functions in insulin exocytosis: Interaction of elks with exocytotic machinery analyzed by total internal reflection fluorescence microscopy. Mol Biol Cell (2005) 16(7):3289–300. doi: 10.1091/mbc.e04-09-0816
47. Ohara-Imaizumi M, Aoyagi K, Yamauchi H, Yoshida M, Mori MX, Hida Y, et al. Elks/Voltage-dependent Ca(2+) channel-beta subunit module regulates polarized Ca(2+) influx in pancreatic beta cells. Cell Rep (2019) 26(5):1213–26 e7. doi: 10.1016/j.celrep.2018.12.106
48. Cozzitorto C, Spagnoli FM. Pancreas organogenesis: The interplay between surrounding Microenvironment(S) and epithelium-intrinsic factors. Curr Top Dev Biol (2019) 132:221–56. doi: 10.1016/bs.ctdb.2018.12.005
49. Byrnes LE, Wong DM, Subramaniam M, Meyer NP, Gilchrist CL, Knox SM, et al. Lineage dynamics of murine pancreatic development at single-cell resolution. Nat Commun (2018) 9(1):3922. doi: 10.1038/s41467-018-06176-3
50. Scavuzzo MA, Hill MC, Chmielowiec J, Yang D, Teaw J, Sheng K, et al. Endocrine lineage biases arise in temporally distinct endocrine progenitors during pancreatic morphogenesis. Nat Commun (2018) 9(1):3356. doi: 10.1038/s41467-018-05740-1
51. Olson EN, Nordheim A. Linking actin dynamics and gene transcription to drive cellular motile functions. Nat Rev Mol Cell Biol (2010) 11(5):353–65. doi: 10.1038/nrm2890
52. Dupont S, Morsut L, Aragona M, Enzo E, Giulitti S, Cordenonsi M, et al. Role of Yap/Taz in mechanotransduction. Nature (2011) 474(7350):179–83. doi: 10.1038/nature10137
53. Swift J, Ivanovska IL, Buxboim A, Harada T, Dingal PC, Pinter J, et al. Nuclear lamin-a scales with tissue stiffness and enhances matrix-directed differentiation. Science (2013) 341(6149):1240104. doi: 10.1126/science.1240104
54. Janmey PA, Miller RT. Mechanisms of mechanical signaling in development and disease. J Cell Sci (2011) 124(Pt 1):9–18. doi: 10.1242/jcs.071001
55. Velazco-Cruz L, Goedegebuure MM, Maxwell KG, Augsornworawat P, Hogrebe NJ, Millman JR. Six2 regulates human beta cell differentiation from stem cells and functional maturation in vitro. Cell Rep (2020) 31(8):107687. doi: 10.1016/j.celrep.2020.107687
56. Borowiak M, Maehr R, Chen S, Chen AE, Tang W, Fox JL, et al. Small molecules efficiently direct endodermal differentiation of mouse and human embryonic stem cells. Cell Stem Cell (2009) 4(4):348–58. doi: 10.1016/j.stem.2009.01.014
57. Chen S, Borowiak M, Fox JL, Maehr R, Osafune K, Davidow L, et al. A small molecule that directs differentiation of human escs into the pancreatic lineage. Nat Chem Biol (2009) 5(4):258–65. doi: 10.1038/nchembio.154
58. Kunisada Y, Tsubooka-Yamazoe N, Shoji M, Hosoya M. Small molecules induce efficient differentiation into insulin-producing cells from human induced pluripotent stem cells. Stem Cell Res (2012) 8(2):274–84. doi: 10.1016/j.scr.2011.10.002
59. Kondo Y, Toyoda T, Ito R, Funato M, Hosokawa Y, Matsui S, et al. Identification of a small molecule that facilitates the differentiation of human Ipscs/Escs and mouse embryonic pancreatic explants into pancreatic endocrine cells. Diabetologia (2017) 60(8):1454–66. doi: 10.1007/s00125-017-4302-7
60. Li QV, Dixon G, Verma N, Rosen BP, Gordillo M, Luo R, et al. Genome-scale screens identify jnk-jun signaling as a barrier for pluripotency exit and endoderm differentiation. Nat Genet (2019) 51(6):999–1010. doi: 10.1038/s41588-019-0408-9
61. Wang H, Luo X, Yao L, Lehman DM, Wang P. Improvement of cell survival during human pluripotent stem cell definitive endoderm differentiation. Stem Cells Dev (2015) 24(21):2536–46. doi: 10.1089/scd.2015.0018
62. Klein AM, Mazutis L, Akartuna I, Tallapragada N, Veres A, Li V, et al. Droplet barcoding for single-cell transcriptomics applied to embryonic stem cells. Cell (2015) 161(5):1187–201. doi: 10.1016/j.cell.2015.04.044
63. Russell R, Carnese PP, Hennings TG, Walker EM, Russ HA, Liu JS, et al. Loss of the transcription factor mafb limits beta-cell derivation from human pscs. Nat Commun (2020) 11(1):2742. doi: 10.1038/s41467-020-16550-9
64. Augsornworawat P, Maxwell KG, Velazco-Cruz L, Millman JR. Single-cell transcriptome profiling reveals beta cell maturation in stem cell-derived islets after transplantation. Cell Rep (2020) 32(8):108067. doi: 10.1016/j.celrep.2020.108067
65. Li X, Yang KY, Chan VW, Leung KT, Zhang XB, Wong AS, et al. Single-cell rna-seq reveals that Cd9 is a negative marker of glucose-responsive pancreatic beta-like cells derived from human pluripotent stem cells. Stem Cell Rep (2020) 15(5):1111–26. doi: 10.1016/j.stemcr.2020.09.009
66. Wesolowska-Andersen A, Jensen RR, Alcantara MP, Beer NL, Duff C, Nylander V, et al. Analysis of differentiation protocols defines a common pancreatic progenitor molecular signature and guides refinement of endocrine differentiation. Stem Cell Rep (2020) 14(1):138–53. doi: 10.1016/j.stemcr.2019.11.010
67. Bellono NW, Bayrer JR, Leitch DB, Castro J, Zhang C, O'Donnell TA, et al. Enterochromaffin cells are gut chemosensors that couple to sensory neural pathways. Cell (2017) 170(1):185–98 e16. doi: 10.1016/j.cell.2017.05.034
68. Nostro MC, Sarangi F, Yang C, Holland A, Elefanty AG, Stanley EG, et al. Efficient generation of Nkx6-1+ pancreatic progenitors from multiple human pluripotent stem cell lines. Stem Cell Rep (2015) 4(4):591–604. doi: 10.1016/j.stemcr.2015.02.017
69. Sharon N, Chawla R, Mueller J, Vanderhooft J, Whitehorn LJ, Rosenthal B, et al. A peninsular structure coordinates asynchronous differentiation with morphogenesis to generate pancreatic islets. Cell (2019) 176(4):790–804 e13. doi: 10.1016/j.cell.2018.12.003
70. Kim Y, Kim H, Ko UH, Oh Y, Lim A, Sohn JW, et al. Islet-like organoids derived from human pluripotent stem cells efficiently function in the glucose responsiveness in vitro and in vivo. Sci Rep (2016) 6:35145. doi: 10.1038/srep35145
71. Tao T, Wang Y, Chen W, Li Z, Su W, Guo Y, et al. Engineering human islet organoids from ipscs using an organ-On-Chip platform. Lab Chip (2019) 19(6):948–58. doi: 10.1039/c8lc01298a
72. Wang W, Jin S, Ye K. Development of islet organoids from H9 human embryonic stem cells in biomimetic 3d scaffolds. Stem Cells Dev (2017) 26(6):394–404. doi: 10.1089/scd.2016.0115
73. Candiello J, Grandhi TSP, Goh SK, Vaidya V, Lemmon-Kishi M, Eliato KR, et al. 3d heterogeneous islet organoid generation from human embryonic stem cells using a novel engineered hydrogel platform. Biomaterials (2018) 177:27–39. doi: 10.1016/j.biomaterials.2018.05.031
74. Augsornworawat P, Velazco-Cruz L, Song J, Millman JR. A hydrogel platform for in vitro three dimensional assembly of human stem cell-derived islet cells and endothelial cells. Acta Biomater (2019) 97:272–80. doi: 10.1016/j.actbio.2019.08.031
75. Liu H, Wang Y, Wang H, Zhao M, Tao T, Zhang X, et al. A droplet microfluidic system to fabricate hybrid capsules enabling stem cell organoid engineering. Adv Sci (Weinh) (2020) 7(11):1903739. doi: 10.1002/advs.201903739
76. Youngblood RL, Sampson JP, Lebioda KR, Shea LD. Microporous scaffolds support assembly and differentiation of pancreatic progenitors into beta-cell clusters. Acta Biomater (2019) 96:111–22. doi: 10.1016/j.actbio.2019.06.032
77. Tao T, Deng P, Wang Y, Zhang X, Guo Y, Chen W, et al. Microengineered multi-organoid system from hipscs to recapitulate human liver-islet axis in normal and type 2 diabetes. Adv Sci (Weinh) (2022) 9(5):e2103495. doi: 10.1002/advs.202103495
78. Ramzy A, Thompson DM, Ward-Hartstonge KA, Ivison S, Cook L, Garcia RV, et al. Implanted pluripotent stem-Cell-Derived pancreatic endoderm cells secrete glucose-responsive c-peptide in patients with type 1 diabetes. Cell Stem Cell (2021) 28(12):2047–61 e5. doi: 10.1016/j.stem.2021.10.003
79. Shapiro AMJ, Thompson D, Donner TW, Bellin MD, Hsueh W, Pettus J, et al. Insulin expression and c-peptide in type 1 diabetes subjects implanted with stem cell-derived pancreatic endoderm cells in an encapsulation device. Cell Rep Med (2021) 2(12):100466. doi: 10.1016/j.xcrm.2021.100466
80. Schulz TC. Concise review: Manufacturing of pancreatic endoderm cells for clinical trials in type 1 diabetes. Stem Cells Trans Med (2015) 4(8):927–31. doi: 10.5966/sctm.2015-0058
81. Schulz TC, Young HY, Agulnick AD, Babin MJ, Baetge EE, Bang AG, et al. A scalable system for production of functional pancreatic progenitors from human embryonic stem cells. PloS One (2012) 7(5):e37004. doi: 10.1371/journal.pone.0037004
82. Henry RR, Pettus J, Wilensky J, Shapiro AMJ, Senior PA, Roep B, et al. Initial clinical evaluation of vc-01tm combination product–a stem cell–derived islet replacement for type 1 diabetes (T1d). Diabetes (2018) 67(Supplement_1):138–OR. doi: 10.2337/db18-138-OR
83. de Klerk E, Hebrok M. Stem cell-based clinical trials for diabetes mellitus. Front Endocrinol (Lausanne) (2021) 12:631463. doi: 10.3389/fendo.2021.631463
84. Bertuzzi F, Colussi G, Lauterio A, De Carlis L. Intramuscular islet allotransplantation in type 1 diabetes mellitus. Eur Rev Med Pharmacol Sci (2018) 22(6):1731–6. doi: 10.26355/eurrev_201803_14588
85. Bluestone JA, Tang Q. Solving the puzzle of immune tolerance for beta-cell replacement therapy for type 1 diabetes. Cell Stem Cell (2020) 27(4):505–7. doi: 10.1016/j.stem.2020.09.008
86. Dorrell C, Schug J, Canaday PS, Russ HA, Tarlow BD, Grompe MT, et al. Human islets contain four distinct subtypes of beta cells. Nat Commun (2016) 7:11756. doi: 10.1038/ncomms11756
87. Bader E, Migliorini A, Gegg M, Moruzzi N, Gerdes J, Roscioni SS, et al. Identification of proliferative and mature beta-cells in the islets of langerhans. Nature (2016) 535(7612):430–4. doi: 10.1038/nature18624
88. Qadir MMF, Alvarez-Cubela S, Belle K, Sapir T, Messaggio F, Johnson KB, et al. A double fail-safe approach to prevent tumorigenesis and select pancreatic beta cells from human embryonic stem cells. Stem Cell Rep (2019) 12(3):611–23. doi: 10.1016/j.stemcr.2019.01.012
89. Liang Q, Monetti C, Shutova MV, Neely EJ, Hacibekiroglu S, Yang H, et al. Linking a cell-division gene and a suicide gene to define and improve cell therapy safety. Nature (2018) 563(7733):701–4. doi: 10.1038/s41586-018-0733-7
90. Cottle L, Gan WJ, Gilroy I, Samra JS, Gill AJ, Loudovaris T, et al. Structural and functional polarisation of human pancreatic beta cells in islets from organ donors with and without type 2 diabetes. Diabetologia (2021) 64(3):618–29. doi: 10.1007/s00125-020-05345-8
91. Staels W, Verdonck Y, Heremans Y, Leuckx G, De Groef S, Heirman C, et al. Vegf-a mrna transfection as a novel approach to improve mouse and human islet graft revascularisation. Diabetologia (2018) 61(8):1804–10. doi: 10.1007/s00125-018-4646-7
92. Lebreton F, Lavallard V, Bellofatto K, Bonnet R, Wassmer CH, Perez L, et al. Insulin-producing organoids engineered from islet and amniotic epithelial cells to treat diabetes. Nat Commun (2019) 10(1):4491. doi: 10.1038/s41467-019-12472-3
93. Forbes S, Bond AR, Thirlwell KL, Burgoyne P, Samuel K, Noble J, et al. Human umbilical cord perivascular cells improve human pancreatic islet transplant function by increasing vascularization. Sci Transl Med (2020) 12(526):eaan5907. doi: 10.1126/scitranslmed.aan5907
94. Kelly Y, Ward C, Duh Q-Y, Chang W, Peter S, Qizhi T. Sat-182 parathyroid Cd34+ cells induce angiogenesis of both donor and host as a mechanism for chimeric vessel formation. J Endocr Soc (2019) 3(Supplement_1):SAT–182. doi: 10.1210/js.2019-SAT-182
95. Pepper AR, Gala-Lopez B, Pawlick R, Merani S, Kin T, Shapiro AM. A prevascularized subcutaneous device-less site for islet and cellular transplantation. Nat Biotechnol (2015) 33(5):518–23. doi: 10.1038/nbt.3211
96. Pathak S, Meyer EH. Tregs and mixed chimerism as approaches for tolerance induction in islet transplantation. Front Immunol (2020) 11:612737. doi: 10.3389/fimmu.2020.612737
97. Choi B, Kim S-H. Regulatory T cells promote pancreatic islet function and viability Via tgf-β1 in vitro and in vivo. Korean J Clin Lab Sci (2018) 50:304–12. doi: 10.15324/kjcls.2018.50.3.304
98. Han X, Wang M, Duan S, Franco PJ, Kenty JH, Hedrick P, et al. Generation of hypoimmunogenic human pluripotent stem cells. Proc Natl Acad Sci U.S.A. (2019) 116(21):10441–6. doi: 10.1073/pnas.1902566116
99. Orive G, Santos E, Pedraz JL, Hernandez RM. Application of cell encapsulation for controlled delivery of biological therapeutics. Adv Drug Delivery Rev (2014) 67-68:3–14. doi: 10.1016/j.addr.2013.07.009
100. Marfil-Garza BA, Polishevska K, Pepper AR, Korbutt GS. Current state and evidence of cellular encapsulation strategies in type 1 diabetes. Compr Physiol (2020) 10(3):839–78. doi: 10.1002/cphy.c190033
101. Rokstad AM, Lacik I, de Vos P, Strand BL. Advances in biocompatibility and physico-chemical characterization of microspheres for cell encapsulation. Adv Drug Delivery Rev (2014) 67-68:111–30. doi: 10.1016/j.addr.2013.07.010
102. Desai T, Shea LD. Advances in islet encapsulation technologies. Nat Rev Drug Discovery (2017) 16(5):367. doi: 10.1038/nrd.2017.67
103. Agulnick AD, Ambruzs DM, Moorman MA, Bhoumik A, Cesario RM, Payne JK, et al. Insulin-producing endocrine cells differentiated in vitro from human embryonic stem cells function in macroencapsulation devices in vivo. Stem Cells Transl Med (2015) 4(10):1214–22. doi: 10.5966/sctm.2015-0079
104. Vegas AJ, Veiseh O, Gurtler M, Millman JR, Pagliuca FW, Bader AR, et al. Long-term glycemic control using polymer-encapsulated human stem cell-derived beta cells in immune-competent mice. Nat Med (2016) 22(3):306–11. doi: 10.1038/nm.4030
105. Liu W, Flanders JA, Wang LH, Liu Q, Bowers DT, Wang K, et al. A safe, fibrosis-mitigating, and scalable encapsulation device supports long-term function of insulin-producing cells. Small (2022) 18(8):e2104899. doi: 10.1002/smll.202104899
106. Wang X, Maxwell KG, Wang K, Bowers DT, Flanders JA, Liu W, et al. A nanofibrous encapsulation device for safe delivery of insulin-producing cells to treat type 1 diabetes. Sci Transl Med (2021) 13(596):eabb4601. doi: 10.1126/scitranslmed.abb4601
107. Miura K, Okada Y, Aoi T, Okada A, Takahashi K, Okita K, et al. Variation in the safety of induced pluripotent stem cell lines. Nat Biotechnol (2009) 27(8):743–5. doi: 10.1038/nbt.1554
108. Loh YH, Yang JC, De Los Angeles A, Guo C, Cherry A, Rossi DJ, et al. Excision of a viral reprogramming cassette by delivery of synthetic cre mrna. Curr Protoc Stem Cell Biol (2012) 21:4A.5.1–4A.5.16. doi: 10.1002/9780470151808.sc04a05s21
109. Fusaki N, Ban H, Nishiyama A, Saeki K, Hasegawa M. Efficient induction of transgene-free human pluripotent stem cells using a vector based on Sendai virus, an rna virus that does not integrate into the host genome. P Jpn Acad B-Phys (2009) 85(8):348–62. doi: 10.2183/pjab.85.348
110. Lee J, Sayed N, Hunter A, Au KF, Wong WH, Mocarski ES, et al. Activation of innate immunity is required for efficient nuclear reprogramming. Cell (2012) 151(3):547–58. doi: 10.1016/j.cell.2012.09.034
111. Liu C, Ameen M, Himmati S, Thomas D, Sayed N. Generation of human ipscs by protein reprogramming and stimulation of Tlr3 signaling. Methods Mol Biol (2021) 2239:153–62. doi: 10.1007/978-1-0716-1084-8_10
112. Li Y, Zhang Q, Yin X, Yang W, Du Y, Hou P, et al. Generation of ipscs from mouse fibroblasts with a single gene, Oct4, and small molecules. Cell Res (2011) 21(1):196–204. doi: 10.1038/cr.2010.142
113. Alvarez-Palomo AB, Requena-Osete J, Delgado-Morales R, Moreno-Manzano V, Grau-Bove C, Tejera AM, et al. A synthetic mrna cell reprogramming method using cyclin D1 promotes DNA repair generating improved genetically stable human induced pluripotent stem cells. Stem Cells (2021) 39(7):866–881. doi: 10.1002/stem.3358
Keywords: diabetes mellitus, stem cells, generation, human pluripotent stem cells, embryonic stem cells
Citation: Wang X, Gao M, Wang Y and Zhang Y (2022) The progress of pluripotent stem cell-derived pancreatic β-cells regeneration for diabetic therapy. Front. Endocrinol. 13:927324. doi: 10.3389/fendo.2022.927324
Received: 24 April 2022; Accepted: 27 June 2022;
Published: 28 July 2022.
Edited by:
Milica B. Markelic, Faculty of Biology, University of Belgrade, SerbiaReviewed by:
Nobuaki Shiraki, Tokyo Institute of Technology, JapanCopyright © 2022 Wang, Gao, Wang and Zhang. This is an open-access article distributed under the terms of the Creative Commons Attribution License (CC BY). The use, distribution or reproduction in other forums is permitted, provided the original author(s) and the copyright owner(s) are credited and that the original publication in this journal is cited, in accordance with accepted academic practice. No use, distribution or reproduction is permitted which does not comply with these terms.
*Correspondence: Yucheng Zhang, eXVjaGVuZ0BqbHUuZWR1LmNu; Yali Wang, d2FuZ3poYW5nNDA4M0BqbHUuZWR1LmNu
†These authors have contributed equally to this work
Disclaimer: All claims expressed in this article are solely those of the authors and do not necessarily represent those of their affiliated organizations, or those of the publisher, the editors and the reviewers. Any product that may be evaluated in this article or claim that may be made by its manufacturer is not guaranteed or endorsed by the publisher.
Research integrity at Frontiers
Learn more about the work of our research integrity team to safeguard the quality of each article we publish.