- 1Diabetes Research Group, Division of Infection and Immunity, School of Medicine, Cardiff University, Cardiff, United Kingdom
- 2Systems Immunity Research Institute, School of Medicine, Cardiff University, Cardiff, United Kingdom
Type 2 diabetes mellitus, obesity and metabolic syndrome are becoming more prevalent worldwide and will present an increasingly challenging burden on healthcare systems. These interlinked metabolic abnormalities predispose affected individuals to a plethora of complications and comorbidities. Furthermore, diabetes is estimated by the World Health Organization to have caused 1.5 million deaths in 2019, with this figure projected to rise in coming years. This highlights the need for further research into the management of metabolic diseases and their complications. Studies on circadian rhythms, referring to physiological and behavioral changes which repeat approximately every 24 hours, may provide important insight into managing metabolic disease. Epidemiological studies show that populations who are at risk of circadian disruption such as night shift workers and regular long-haul flyers are also at an elevated risk of metabolic abnormalities such as insulin resistance and obesity. Aberrant expression of circadian genes appears to contribute to the dysregulation of metabolic functions such as insulin secretion, glucose homeostasis and energy expenditure. The potential clinical implications of these findings have been highlighted in animal studies and pilot studies in humans giving rise to the development of circadian interventions strategies including chronotherapy (time-specific therapy), time-restricted feeding, and circadian molecule stabilizers/analogues. Research into these areas will provide insights into the future of circadian medicine in metabolic diseases. In this review, we discuss the physiology of metabolism and the role of circadian timing in regulating these metabolic functions. Also, we review the clinical aspects of circadian physiology and the impact that ongoing and future research may have on the management of metabolic disease.
Introduction
Diabetes mellitus is estimated to affect 415 million adults worldwide, approximately 90% of whom have type 2 diabetes (1). Diabetes can lead to microvascular (e.g. retinopathy, nephropathy and neuropathy) and macrovascular complications which substantially increase the risk of developing cardiovascular disease and can substantially decrease the quality of life of these individuals (2). Managing diabetes and the associated complications will introduce more strain on healthcare systems worldwide as this disease becomes more prevalent (3). This highlights the need for both determining the importance of risk factors, which may modulate susceptibility to disease, and management of the metabolic syndrome (referring to metabolic abnormalities listed in Figure 1, which together increase the risk of cardiovascular diseases and type 2 diabetes), obesity and type 2 diabetes (T2DM).
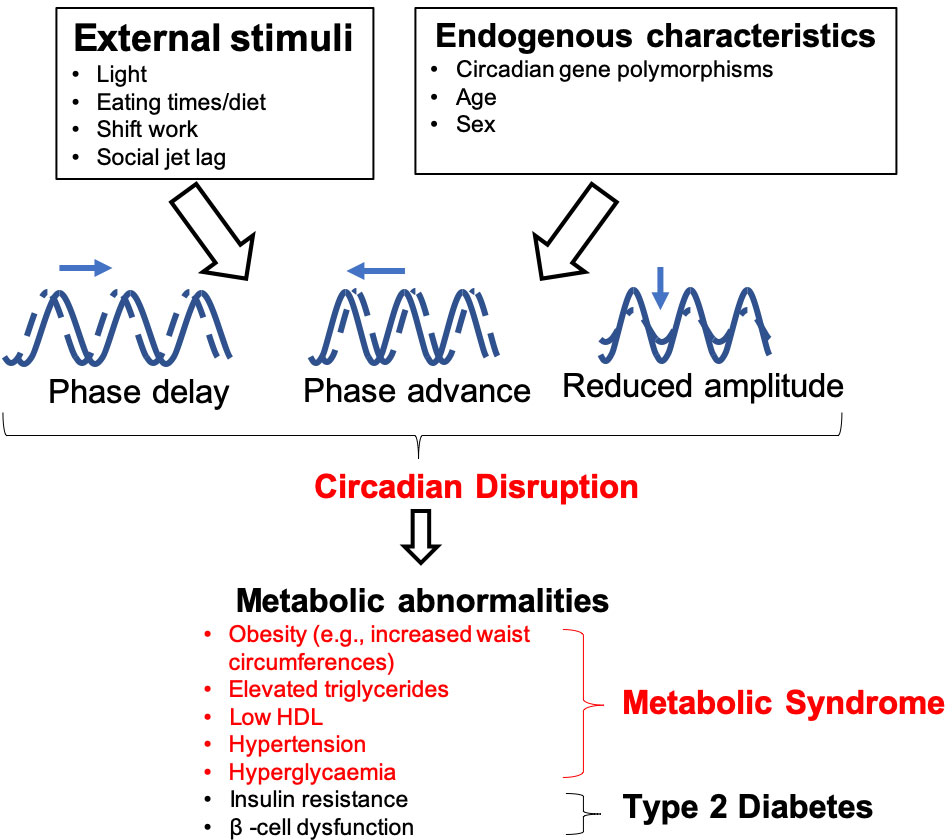
Figure 1 Influences on, and consequences of, circadian rhythm disruption. Both endogenous and external factors can predispose individuals to circadian disruption. This can cause dysfunction of peripheral oscillators, which are involved in the regulation of metabolic functions such as body weight homeostasis, glucose metabolism and β-cell function. Individuals who experience circadian disruption, which may be through phase delays/advances or by changes in amplitude (difference between peak and trough of the rhythms) are at an elevated risk of developing metabolic abnormalities, which can lead to metabolic syndrome and T2DM.
Many physiological processes, including energy expenditure and glucose homeostasis are regulated by circadian rhythms (4, 5), which are 24-hour daily cycles of physiological and behavioral patterns (6). The circadian rhythm apparatus consists of a central master clock located in the suprachiasmatic nuclei (SCN) within the hypothalamus, which synchronizes peripheral oscillators located in various tissues such as the liver, pancreas, adipose tissue and skeletal muscle. The SCN is entrained by light (7, 8), which allows synchronization with the external environment i.e. the 24-hour light-dark cycle governed by the Earth’s rotation. This directs the central and peripheral clocks to adapt to changes in light, optimizing physiological processes to these daily cycles. Through a number of regulatory mechanisms (e.g. endocrine, neurological, thermal), the SCN coordinates responses with the peripheral clocks, which have their own phases, to ensure synchronized daily rhythms are maintained (9). In addition, peripheral rhythms can also be modulated, for example, by nutrient sensing (i.e. from food intake), hormonal cues and temperature. Although the SCN acts as the master pacemaker in the human body, it is evident that circadian oscillations are observable in almost every cell of the body and these rhythms may persist in isolation from the SCN (10).
Disruption of circadian rhythms exacerbates metabolic diseases that include T2DM, obesity and metabolic syndrome in both animal models and humans (11–15) (Figure 1). Experimental and epidemiological studies show that night shift-workers are more likely to develop metabolic abnormalities, predisposing these individuals to developing T2DM compared to daytime workers (16–22). This elevated risk of developing T2DM is also seen in populations with social jetlag, a condition characterized by disruption to an individual’s sleeping pattern, and thus circadian rhythms, due to social engagements, leading to individuals feeling “jet lagged” or tired (20, 21, 23).
Many central and peripheral hormones that influence metabolism exhibit circadian rhythmicity. This review will focus on the current understanding of how circadian rhythms can influence pancreatic physiology and the consequent effects on metabolism and how this knowledge may be used to enhance clinical management of T2DM, obesity and metabolic syndrome. While we will focus on the pancreas in this review, other metabolic tissues e.g. liver, skeletal muscle and adipose tissue are also altered by circadian rhythms (Figure 2). While providing background information on blood glucose homeostasis and insulin resistance to those less familiar with these concepts Section 2: (Pancreatic physiology), we will also highlight key ways circadian rhythms are disrupted and how they interact to increase the risk of obesity and T2DM (Figure 3) discussed in Section 3 (Circadian rhythms in the pancreas), 4 (Molecular circadian rhythms in the pancreas) and 5 (Modulation of circadian rhythm).
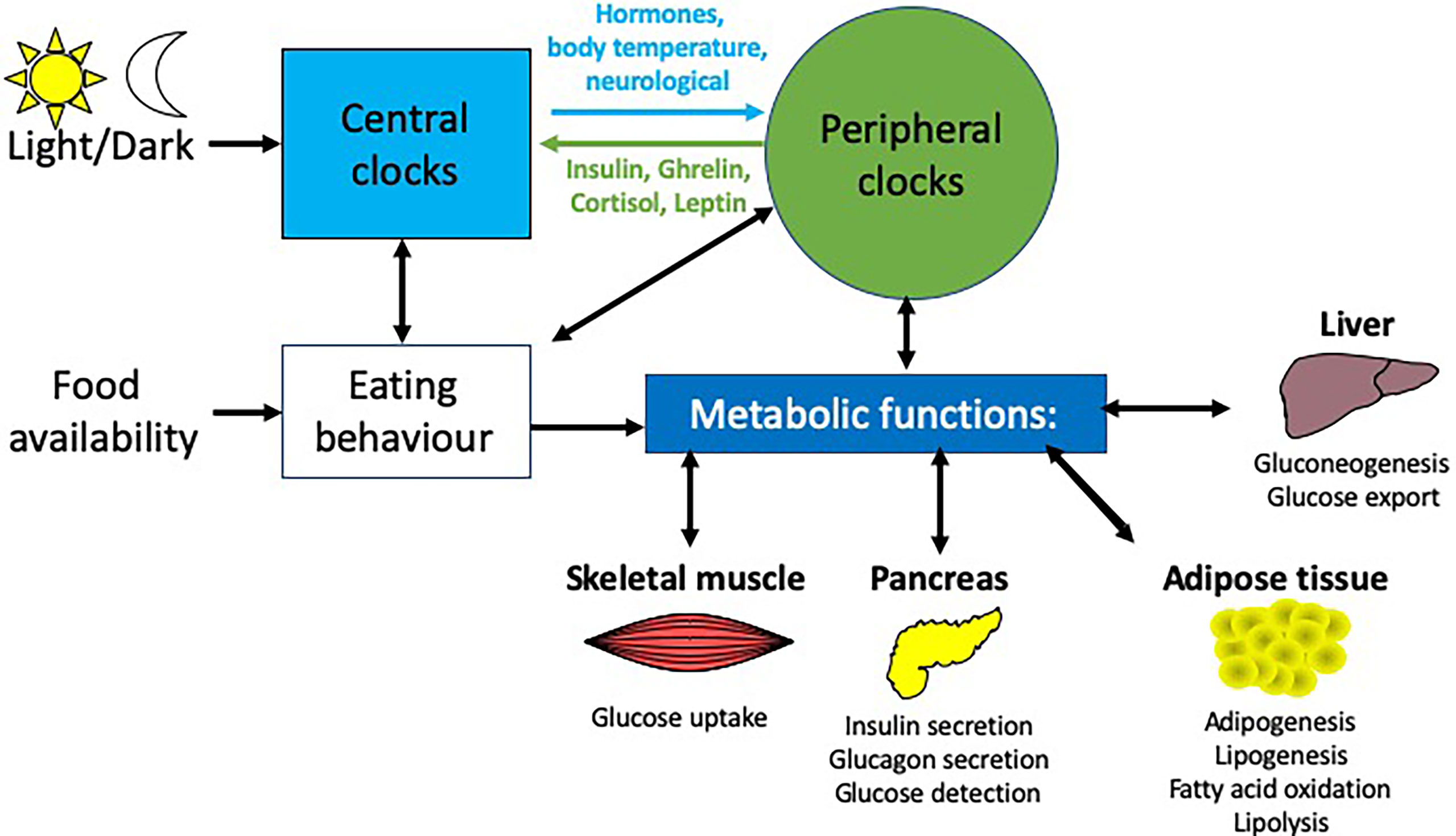
Figure 2 Circadian influences on different metabolic tissues. Light is the main entrainment factor for the SCN, the master pacemaker of the circadian system, which, through a number of signals e,g. hormones and neurotransmitters, synchronizes the circadian rhythms of peripheral tissues to light exposure. Crosstalk between these peripheral tissues and the brain enable feedback to modulate these rhythms e.g.the hormones insulin, ghrelin, leptin and cortisol provide feedback to the arcuate nucleus in the brain. There are many peripheral tissues, which regulate metabolic functions, including the liver, pancreas, skeletal muscle and adipose tissue, each of which exhibit their own rhythmicity. Together, these peripheral rhythms regulate many metabolic functions, including glucose homeostasis, insulin secretion and fatty acid metabolism.
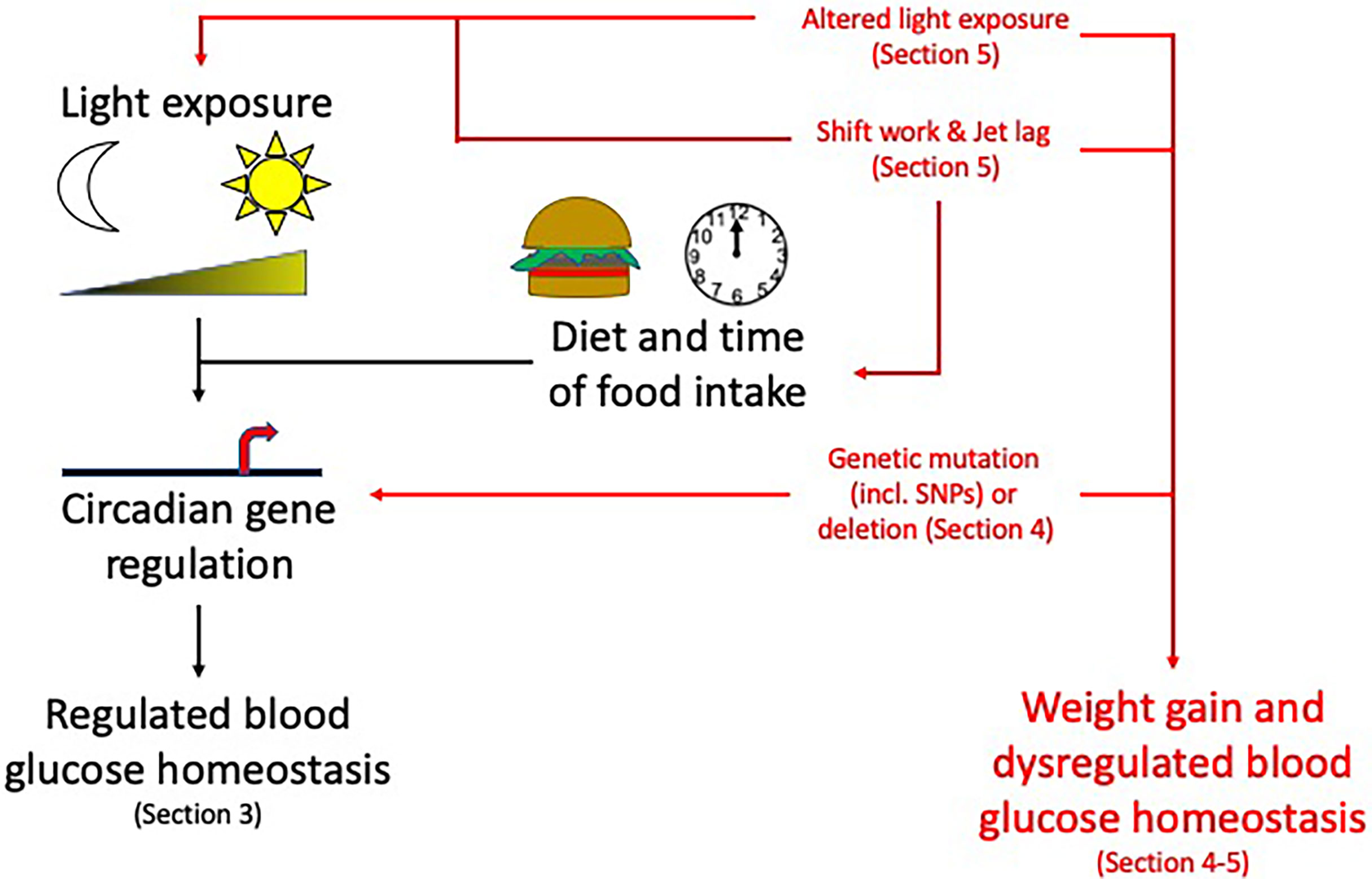
Figure 3 Circadian rhythms and their disruption: Topics to be discussed in this review. Circadian rhythms, influenced by light/dark cycles and diet and time of food intake, control many pancreas functions. Disruption to these circadian influences (altered light exposure, shift work/jet lag, genetic mutations) can lead to weight gain and dysregulated blood glucose homeostasis increasing the risk of developing obesity and T2DM. For information related to these factors altering the circadian rhythms please see the specific sections mentioned above within the review. Section 3: Circadian rhythms in the pancreas, Section 4: Molecular circadian rhythms in the pancreas and Section 5: Modulation of circadian rhythm.
Pancreatic physiology
The pancreas is a multifunctional organ which regulates metabolism and digestion through several endocrine and exocrine mechanisms (24). These tightly regulated, interrelated mechanisms are necessary for blood glucose homeostasis, lipolysis, and food intake (4, 5). Histologically, the pancreas contains clusters of exocrine cells known as acini which surround a network of interconnected ducts (25). The acini secrete inactive forms of pancreatic enzymes known as zymogens which subsequently enter the gut and become active digestive enzymes, including lipase, amylase and proteases. The endocrine cells of the pancreas are arranged in clusters known as the islets of Langerhans which contain α, β, γ and δ cells (26). Each of these cell populations within the islets secrete different hormones, as discussed later. Together, these pancreatic hormones regulate blood glucose homeostasis, food intake and insulin responses and are therefore integral to understanding T2DM and metabolic syndrome.
Introduction to blood glucose homeostasis
Blood glucose homeostasis is a highly regulated process, strongly influenced by both local (i.e. pancreas) and distal (i.e. liver, intestine, brain) signals (Figure 4). In this section, we discuss the role different hormones have on regulating blood glucose.
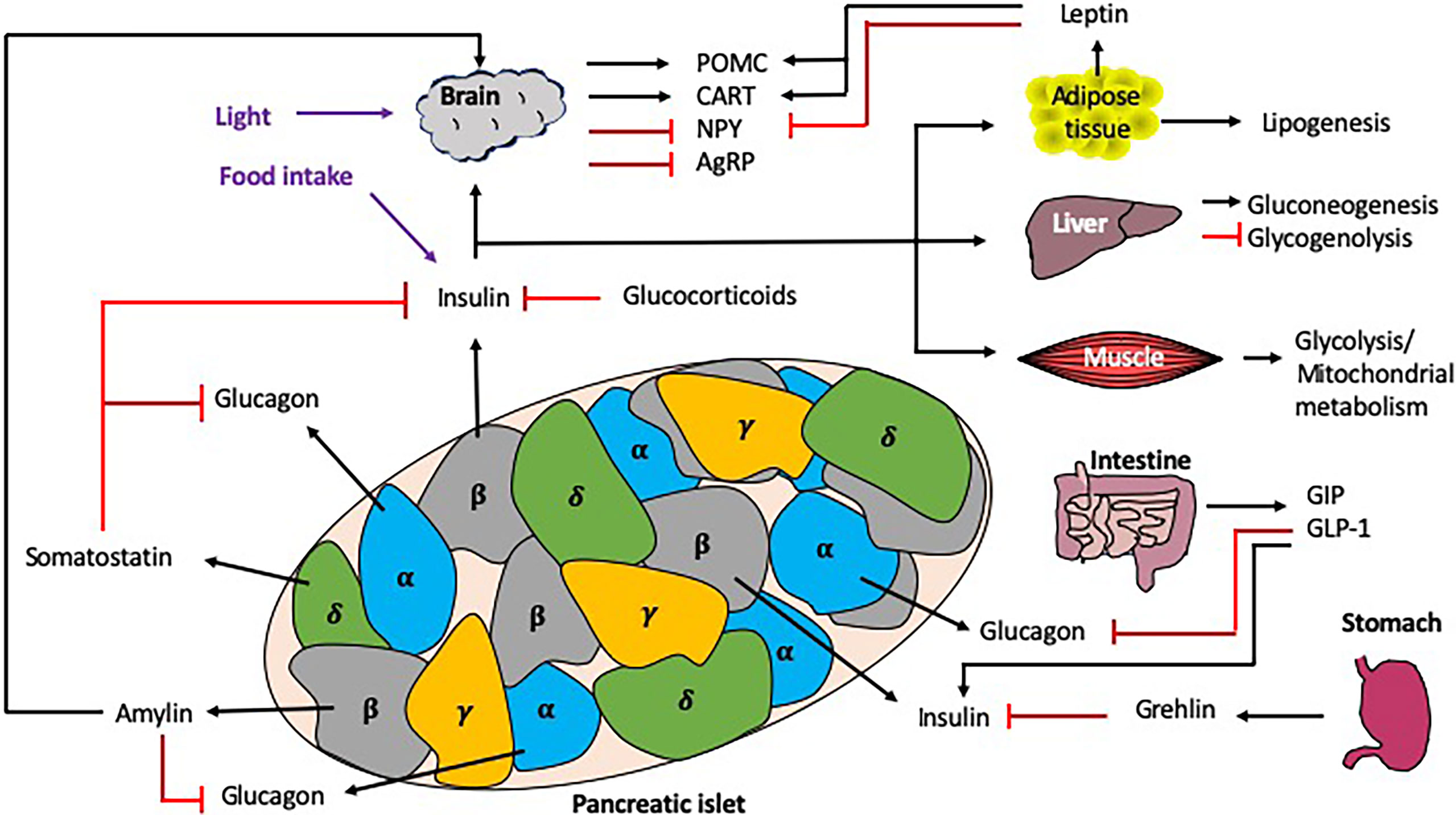
Figure 4 Interactions regulating blood glucose homeostasis. Blood glucose homeostasis is controlled by many signals both locally within the pancreas, and more distally, from the brain, liver, muscle, intestine, stomach and adipose tissue. Within the pancreatic islets, the α cells secrete glucagon, the β cells secrete insulin (and amylin), γcells secrete pancreatic polypeptide and the δ cells secrete somatostatin. In response to high glucose e.g. from dietary intake, the islet ββcells secrete insulin, which is detected by multiple tissues in the periphery, leading to the synthesis or induction of many molecules/pathways e.g. lipogenesis and gluconeogenesis, as well as the inhibition of others e.g. glycogenolysis. Importantly, there are many mechanisms of regulation to control the secretion of insulin both locally (e.g. glucagon and somatostatin) and more distally, e.g. intestine/stomach via hormones (e.g. grehlin and glucocorticoids) and incretins (GIP/GLP-1). Exogenous circadian modulation factors such as light and food intake (shown in purple) can also regulate blood glucose homeostasis, as can endogenous factors such as glucocorticoids, which also show rhythmicity. Black arrows indicate induction, red lines indicate inhibition. .
In normal pancreatic physiology, the β cells of the islets of Langerhans express glucose transporter type-2 (GLUT-2) molecules which detect changes in blood glucose levels, having high capacity and low affinity for glucose (27). Glucose enters the β cells through these high-capacity transporters and subsequently enters the glycolytic pathway and mitochondrial metabolism, which increases the cytoplasmic concentration of ATP leading to the gating of ATP-sensitive potassium channels (KATP). The subsequent plasma membrane depolarization opens voltage-dependent calcium channels (VDCC) and allows an influx of calcium into the cell. The increased intracellular concentration of calcium causes the fusion of insulin granules with the cell membrane and the secretion of insulin.
Insulin exerts its effect on cells by binding to insulin receptors on the cell surface. These insulin receptors are homodimers, consisting of two α and two β subunits. Insulin binds to the extracellular α subunits, leading to autophosphorylation of the β subunits that are tyrosine receptor kinases (RTKs). These RTKs phosphorylate insulin receptor substrate (IRS) that activates downstream pathways mediating the cellular effects (28). Insulin acts on several body tissues including the liver, adipose tissue and skeletal muscle to allow the entry of glucose into these cells to undergo glycolysis and mitochondrial metabolism or anabolic processes such as glycogenesis or lipogenesis (29). Importantly, insulin inhibits hepatic gluconeogenesis and glucose secretion, regulating blood glucose levels. The liver is also sensitive to decreased blood concentrations of insulin, and this stimulates glucose synthesis and secretion. Insulin receptors are expressed throughout different regions of the brain (30). In the arcuate nucleus of the hypothalamus (ARC), insulin modulates anorexigenic and orexigenic neuronal activity. Anorexigenic neurons (pro-opiomelanocortin (POMC) and cocaine–amphetamine-regulated-transcript (CART)) are stimulated by insulin whilst orexigenic neurons [neuropeptide Y (NPY) and agouti-related peptide (AgRP)] are inhibited (31). These actions simultaneously decrease food intake and increase energy expenditure. Leptin, a hormone released by adipose cells and also controlled in a circadian manner (32), exerts a similar effect by stimulating POMC and CART, whilst inhibiting NPY neurons (33). Together leptin and insulin act as signals of adiposity which allow the body to regulate adipose tissue mass (34).
Glucagon, another pancreatic hormone, is secreted by α cells in the pancreatic islets in response to decreased blood glucose levels (35). It opposes the action of insulin in glucose control by stimulating glucose synthesis and secretion. Glucagon also stimulates ketogenesis and lipolysis in the liver. Glucagon levels in the hepatic portal vein are detected by the liver and this signal is relayed centrally via the vagal afferents to the ARC to reduce meal sizes by stimulating postprandial satiety (36). Additionally, glucagon is able to cross the blood-brain barrier (BBB) and has been shown to activate GPCR pathways in the ARC in animal models (37). This implies that glucagon may have a direct effect on the CNS to regulate food intake.
The hormone amylin is co-secreted with insulin by the pancreatic β cells, reducing food intake by inhibiting orexigenic neurons in the ARC (38). Amylin also activates the area postrema (AP) in the medulla oblongata of the brainstem to slow gastric emptying, inhibit gastrointestinal secretions and inhibit the postprandial secretion of glucagon (39).
Somatostatin, a hormone secreted by pancreatic δ cells, regulates digestion, food intake and glucose metabolism through endocrine, exocrine and neurological mechanisms. This hormone inhibits the secretion of the insulin and glucagon as well as prolactin, thyroid stimulating hormone, gastrin and secretin. In the gut, somatostatin inhibits digestive secretions including pancreatic enzymes, gastric acid and bile.
Corticosteroids, produced in the adrenals, regulate a variety of physiological processes including stress responses, immune responses and inflammation, blood glucose homeostasis and electrolyte balance (40). The secretion of these hormones is also regulated by the circadian clock and follows a 24-hour cycle (41, 42). The two main classes of corticosteroids are mineralocorticoids and glucocorticoids (43). Whereas mineralocorticoids, such as aldosterone, regulate fluid and electrolyte balances by modulating the activity of the renal tubules (44), glucocorticoids have anti-inflammatory effects and also regulate carbohydrate, protein and lipid metabolism (45). Cortisol is the main endogenous glucocorticoid in human physiology and is also released as part of the stress response, which in the presence of hypoglycemia, increases blood glucose levels, in both stress and hypoglycaemia, by stimulating gluconeogenesis (46).
Incretins are peptide hormones that are secreted by gut cells postprandially to regulate blood glucose levels and nutrient absorption (47). The two main incretins are glucagon-like peptide-1 (GLP-1) and gastric inhibitory peptide (GIP) (48, 49), and they decrease blood glucose levels by facilitating the secretion of insulin from pancreatic β cells (50). GLP-1 also inhibits the secretion of glucagon by pancreatic α cells. In addition, incretins also slow the rate of gastric emptying to regulate the rate of nutrient absorption (51). Both GLP-1 and GIP are inactivated by dipeptidyl peptidase-4 (DPP-4) (47). Several GLP-1 analogues and DPP-4 inhibitors are used clinically in the management of T2DM (52). Preliminary reports indicate that GIP analogues may also be effective in the management of T2DM, although further investigation is needed to elucidate the clinical efficacy of these drugs (53–56).
Ghrelin initiates appetite by stimulating orexigenic NPY neurons and inhibiting POMC neurons in the ARC, and is also secreted by gastrointestinal cells, located predominantly in the stomach. Ghrelin also raises blood glucose levels by inhibiting glucose-stimulated insulin secretion (GSIS) and impairing glucose tolerance (57, 58).
An introduction to insulin resistance
Insulin resistance, a core component in the pathophysiology of T2DM, is associated with the metabolic syndrome (MS) and obesity (59), and influenced by many factors (Figure 5). Insulin resistance occurs in the presence of chronic energy excess, which leads to accumulation of ectopic lipids in hepatic and skeletal muscle tissue, impairing insulin signaling in these tissues, resulting in hyperglycemia. Although insulin resistance and obesity are strong risk factors for T2DM, these factors alone are not sufficient to produce hyperglycemia (60). β cell dysfunction in the islets of Langerhans is also required to produce T2DM, although the degree of β cell function and insulin resistance varies between individuals. β cell dysfunction results from an inability to detect elevated glucose levels to stimulate an appropriate secretion of insulin (59), which exacerbates hyperglycemia.
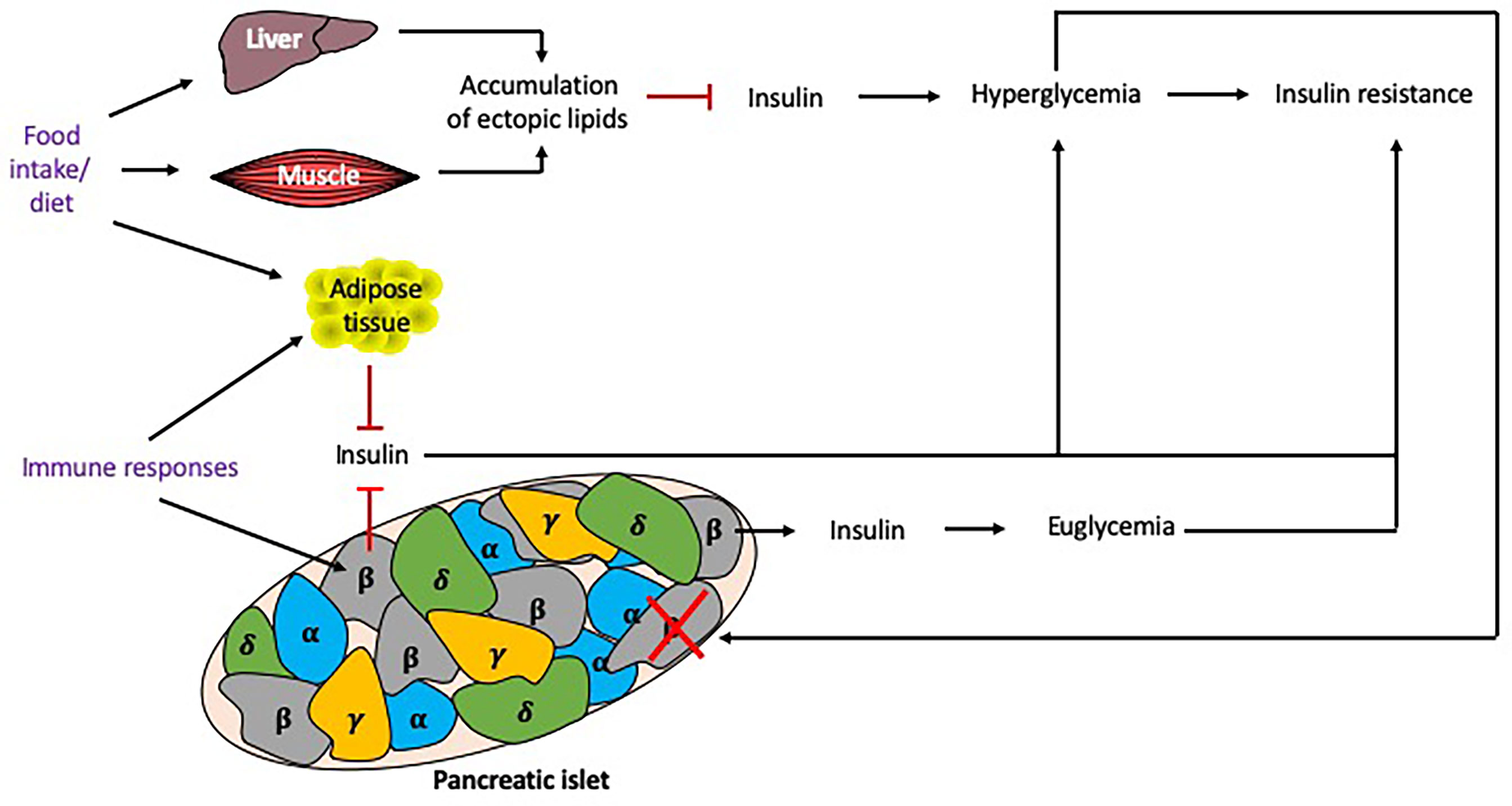
Figure 5 Factors influencing insulin resistance. Multiple factors may contribute to the development of insulin resistance. Excess food intake and the type of diet eaten can promote the accumulation of lipids in tissues, reducing insulin signaling and causing hyperglycemia and insulin resistance. Chronic hyperglycemia induces β cell stress, leading to β cells that fail to secrete sufficient insulin to maintain euglycemia, or exhausted/destroyed β cells, which do not secrete insulin, further promoting hyperglycemia. Genetics play an important role as some individuals may develop insulin resistance but remain euglycemic. Immune responses, particularly cytokines secreted from macrophages, can also promote adiposity and insulin resistance. Responses to food intake and immune responses (shown in purple) can be altered by the time of day and thus circadian rhythms may alter these responses. Black arrows indicate induction, red lines indicate inhibition.
The mechanisms of β cell dysfunction are not fully understood. A number of factors are believed to contribute to this phenomenon, including proinflammatory cytokines which are associated with obesity and induce mitochondrial stress in β cells (61). Macrophage infiltration into adipose tissue is considered to be the main source of cytokines in obese individuals, with both macrophage infiltration into the adipose tissue and cytokine secretion, shown to be modulated by circadian rhythms (62). Thus, their immune rhythms may also play an important role in β cell dysfunction. Chronic exposure to hyperglycemia causes oxidative stress which damages the organelles such as the mitochondria and endoplasmic reticula, leading to the apoptosis of these cells. Inflammation and oxidative stress contribute to the accumulation of reactive oxygen species in β cells, which hinders the mitochondrial electron transport chain and further damages organelles, thus exacerbating β cell dysfunction.
Although obesity and insulin resistance are risk factors for developing T2DM, not everyone who has these risk factors will progress to developing this condition (63). Indeed, some insulin resistant, obese individuals remain euglycemic because their β cells compensate by secreting more insulin. Genetic factors are determinants of whether or not β cell dysfunction develops in these individuals and there are several genetic variants which may protect or predispose to T2DM (64, 65).
Dietary factors are key risk factors for developing insulin resistance and β cell dysfunction (66). For example diets containing high amounts of saturated fats cause increased levels of circulating fatty acids, which is a risk factor for developing insulin resistance (67). Fatty acids compete with glucose for uptake and metabolism by tissues. Therefore, hyperglycemia will further increase free fatty acid concentrations in the blood, leading to a glucolipotoxic state which is toxic to β cells (59).
Circadian rhythms in the pancreas
Blood glucose homeostasis and insulin resistance are strongly influenced by both local (i.e. pancreas) and distal (i.e. liver, intestine, brain) circadian rhythms, impacting multiple cell types and the secretion of many hormones (Figures 4, 5).
It is clear that the secretion of insulin and glucagon, insulin sensitivity and glucose tolerance all display circadian rhythmicity (68–73), which can be disrupted in individuals with T2DM and their first degree relatives (74). Glucagon secretion is also controlled in a rhythmic manner; however, the circadian rhythms in both β and α cells are in different phases allowing them to respond accordingly to the local changes in glucose and insulin concentrations respectively (75). Bilateral thermic SCN ablation in rats has demonstrated the role of the central clock in glucose metabolism as in these rats, the diurnal patterns of glucose levels and insulin and glucagon secretion became arrythmic (70, 76). Furthermore, this SCN ablation caused desynchrony between peripheral clocks, indicating that the SCN master pacemaker maintains synchronization under normal physiological conditions (77, 78).
In addition to this, cortisol is secreted in a rhythmic, diurnal manner, with peak levels occurring shortly after waking in the morning (79, 80). Circadian disruption and misalignment are associated with aberrant cortisol secretion patterns (81), while a flattened diurnal cortisol curve and a diminished cortisol awakening response have both been associated with T2DM (82–84).
Both GIP and GLP-1 display circadian rhythmicity in humans and disruptions of these secretory patterns have been associated with obesity and T2DM (85–87). Furthermore, it has been postulated that GLP-1 is a key component of peripheral metabolic clocks, which entrains pancreatic, hepatic and gut clocks to daily patterns of nutrient intake (88, 89).
Ghrelin secretion oscillates in a circadian pattern which is reciprocally correlated to insulin secretion patterns (90). Immunolabelling studies show that ghrelin-responsive neurons in brain centers, including the ARC, receive direct synaptic input from the SCN, indicating that the downstream effects of ghrelin are regulated by the circadian timing system (91).
Thus, the regulation of blood glucose homeostasis is strongly influenced by circadian rhythms, both directly in the pancreas and through influences in other peripheral tissues i.e. the intestine, brain and liver. The successful coordination of these rhythms between the different tissues is paramount for maintaining good health. Preclinical animal model as well as human studies have been performed to investigate the role of the circadian molecular clock in regulating glucose homeostasis, insulin sensitivity and energy expenditure as discussed in more detail next.
Molecular circadian rhythms in the pancreas
Circadian rhythms are coordinated by tightly regulated central and peripheral clocks which respond to environmental and behavioral cues such as light, food intake and sleep-wake cycles (92). At the molecular level (Figure 6), Brain and Muscle Aryl hydrocarbon receptor nuclear translocator (Bmal) 1 and 2, Circadian Locomotor Output Cycles protein Kaput (Clock), Cryptochrome (Cry) 1 and 2, Period (Per) 1-3 genes regulate circadian rhythms via transcriptional-translational feedback loops (92). CLOCK and BMAL1 form heterodimers which bind to E-box sequences (CANNTG, where N is any nucleotide) to promote the transcription of Per and Cry genes. After translation, PER and CRY proteins form heterodimers in the cytoplasm and subsequently translocate into the nucleus to inhibit CLOCK : BMAL1 complexes from promoting further transcription. This cyclical regulation of transcription is achieved through modulating clock-specific and ubiquitous histone modifying factors. For example, CLOCK contains a histone-acetyltransferase (HAT) domain and also recruits histone 3 (H3) methyltransferase MLL1 and JARID1a, which inhibits histone deacetylase 1 (HDAC1) promoting CLOCK : BMAL1 activation (93–95), while PER1, recruits the SIN3A/HDAC1 complex which prevents CLOCK : BMAL1 complexes from binding to promoter regions (96).
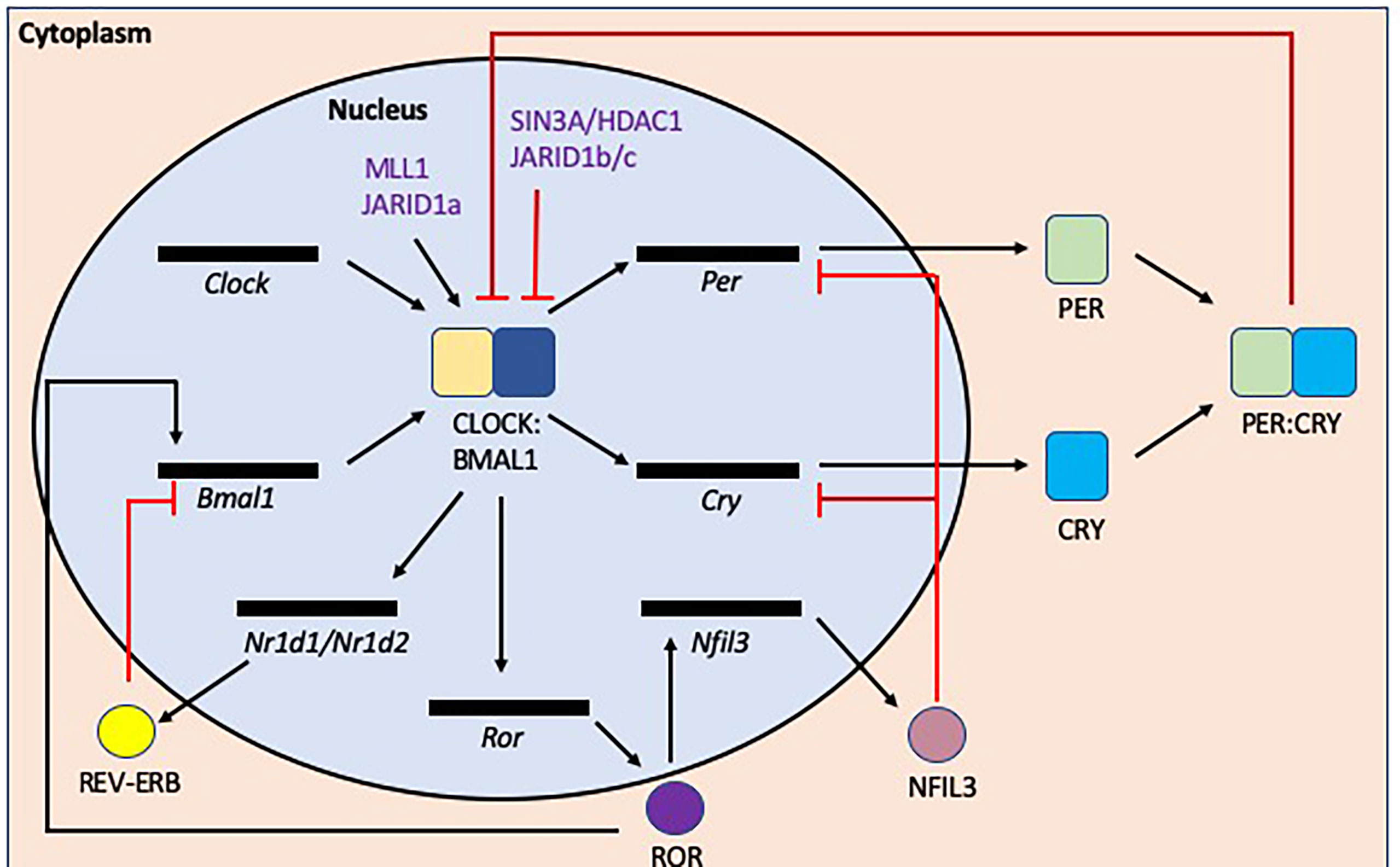
Figure 6 Transcription/translation feedback loops that modulate circadian rhythms at the molecular level. The induction of circadian rhythms relies on oscillations in gene expression and repression. In the initiation of the circadian rhythms, Bmal1 and Clock are transcribed/translated and then form a heterodimer. This CLOCK : BMAL1 heterodimer initiates the transcription of a number of genes including Per, Cry and Nr1d1/Nr1d2 (rev-erbα/β) genes, all which negatively repress the circadian initiators BMAL1 and/or CLOCK. In addition, CLOCK : BMAL1also activates Ror transcription, promoting transcription of Bmal1, while also inducing Nfil3 transcription/translation, which inhibits Per and Cry gene transcription. There are also epigenetic modulation factors e.g. (de)acetylation or (de)methylation that also regulate the circadian rhythm as shown in purple.
CLOCK : BMAL1 complexes also promote the transcription of the nuclear receptors REV-ERBα/β and retinoic acid receptor-related orphan receptors (RORs) α/β. ROR proteins encourage the transcription of BMAL1 whereas REV-ERB proteins inhibit transcription (97, 98). These opposing factors compete for the ROR Response Element (RORE) binding sites (AGGTCA preceded by a 5 base pair A/T rich sequence). ROR also promotes the transcription of Nuclear factor, interleukin 3 regulated (Nfil3), which suppresses the transcription of Per and Cry genes (99) and more recently has also been shown to influence intestinal lipid uptake and obesity (100). Thus, the circadian clock genes modulate susceptibility to metabolic disease, as shown in both mouse and human genetic studies.
Mouse circadian genetic studies
Studies of gene-deficient mice have identified that deletion, or mutation, of any core circadian gene can lead to altered glucose homeostasis or weight gain. In this section, we discuss the impact these genes individually have on metabolic circadian functions.
Studies on homozygous Clock mutant mice, characterized by lengthened circadian periods due to a deletion of exon 19 and amino acid 51 in the C-terminal activation domain of clock, show that circadian feedback loops have a considerable role in regulating glucose metabolism (101, 102). These mice demonstrate hyperphagia, dyslipidemia, hyperglycemia and hyperinsulinemia, all of which are associated with type 2 diabetes, obesity and metabolic syndrome. Interestingly, the clock mutation in these mice also reduces islet size and β cell proliferation (13), indicating an important role for circadian rhythms in both islet and β cell development. This suggests circadian rhythm modulation may promote β cell development and expansion, thus limiting metabolic dysfunction.
Mice deficient in bmal1 displayed impaired adipogenesis and hepatic carbohydrate metabolism (11, 12). Global bmal1 deficiency resulted in a blunted response to hypoglycemia, due to reduced hepatic gluconeogenesis, whereas liver-specific bmal1-deficiency resulted in impaired glucose tolerance (11, 103). Pancreas-specific bmal1 gene knock out (KO) models develop hyperglycaemia and hypoinsulinemia, both of which are characteristic of diabetes development (13). Together, these suggest fundamental roles for intrinsic circadian responses in individual cell types. Understanding the roles of individual cell types in modulating these rhythms, and how that impacts on crosstalk with other cell types, will be fundamental in developing targeted therapeutic strategies to be explored.
Per and cry are downstream target genes of Clock/Bmal1. Homozygous per2 knockdown mice had lower total triacylglycerol and non-esterified fatty acids compared with their wild-type (WT; i.e. per2-sufficient) counterparts; however there was no difference in the expression of other clock genes in white adipose tissue (WAT) (104). In this study, Grimaldi and colleagues found that Per2 is likely to regulate lipid metabolism through a PPARγ2-dependent mechanism (104). Per3 is also involved in lipid metabolism and Per3-deficient mice are more prone to weight gain, when exposed to a high-fat diet (HFD), compared to their WT counterparts (105).
Murine cry1 and cry2 gene deletions are associated with disruptions in the circadian rhythmicity of insulin and glucagon secretion, which in turn are associated with insulin resistance, hypertension and impaired glucose tolerance and dyslipidemia (106, 107). Similarly, agonists of Cry1 and Cry2 inhibit hepatic gluconeogenesis in vitro (108). In contrast, liver-specific expression of adenoviral encoded cry1, at a time when Cry1 is endogenously low, appeared to protect the mice from these metabolic risk factors and increased insulin sensitivity, whilst decreasing blood glucose (109). These differences may relate to cell-specific roles, differences in mice studied (food-restricted or not), timing and methodology of alteration (i.e. lifelong gene deficiency or induced expression following adenovirus delivery) or alterations in other circadian gene regulation or expression.
Rev-erbα plays an important role in regulating insulin and glucagon secretion and pancreatic β cell proliferation (110). Downregulation of rev-erbα using siRNA in mouse pancreatic islet cells reduces glucose-stimulated insulin secretion (GSIS) in mouse models (111). The authors of this study found that exogenous leptin treatment enhanced rev-erbα expression in vitro and in vivo, whilst a HFD further downregulated rev-erbα expression. Mouse models lacking rorα and rev-erbα have lower high-density lipoprotein and decreased adiposity compared to their WT counterparts (97, 112). Solt and colleagues showed that Rev-erb agonists can decrease fat mass and total serum cholesterol in diet-induced obese mice (113). Similarly, Rorα inverse agonists are effective at preventing hyperglycaemia in mouse models of type 2 diabetes (114).
Thus, studies of mice have greatly helped to identify key mechanisms and cell-specific contributions that aid in the modulation of circadian rhythms, leading to altered susceptibility to obesity and diabetes development. It is clear that this is a growing field and more understanding is required of how cell intrinsic clocks impact on other cell types and how other environmental factors may alter peripheral oscillations, leading to altered susceptibility to obesity and diabetes development. Further knowledge of how we can modulate the circadian rhythms in humans will be vital, but animal models may be very helpful for developing preclinical therapies for translation into humans.
Human genetic studies
There are single nucleotide polymorphisms (SNPs) in humans that have been associated with the risk of developing metabolic dysbiosis, obesity and T2DM (Table 1). Clock SNPs can predispose individuals to developing obesity, metabolic syndrome and T2DM by altering the metabolism of fatty acids, as well as the monosaturated fatty acid content of red blood cells (115–118). Similarly, a SNP in NPAS2, a paralog of CLOCK, which can also bind to BMAL1 (126), has also been linked to risk factors (e.g. hypertension) for developing metabolic syndrome (119). SNPs in Bmal1 have also been associated with hypertension, hyperglycemia, T2DM and gestational diabetes (120, 121). In addition, SNPs in CLOCK : BMAL1-repressing genes, such as Cry and Per genes, have also been implicated in metabolic disease. Both Cry2 and Per2 SNPs have been associated with impaired glucose tolerance (119, 122), while Per2 SNPs have also been associated with binge eating and stress related to dieting, leading to increased weight gain (123). These core circadian rhythm-inducing genes, modulate the rhythmic expression of many other genes in the body. One example is the rhythmic secretion of melatonin, which in humans increases in the evening and decreases in the daytime, aiding in regulating our sleep/wake cycles (127). Interestingly, two SNPs in one of the melatonin receptors, the melatonin receptor 1B gene (Mtnr1b), have been associated with higher fasting glucose concentrations, reduced β cell function (as measured by homeostasis model assessment (HOMA)) and an increased risk of developing T2DM (124, 125). This SNP appears to influence the dynamics of melatonin secretion, which may modulate the susceptibility to developing T2DM (128). This suggests important roles for both the SNPs involved in the molecular circadian clock, but also their downstream genes in modulating susceptibility to metabolic syndrome, obesity and T2DM.
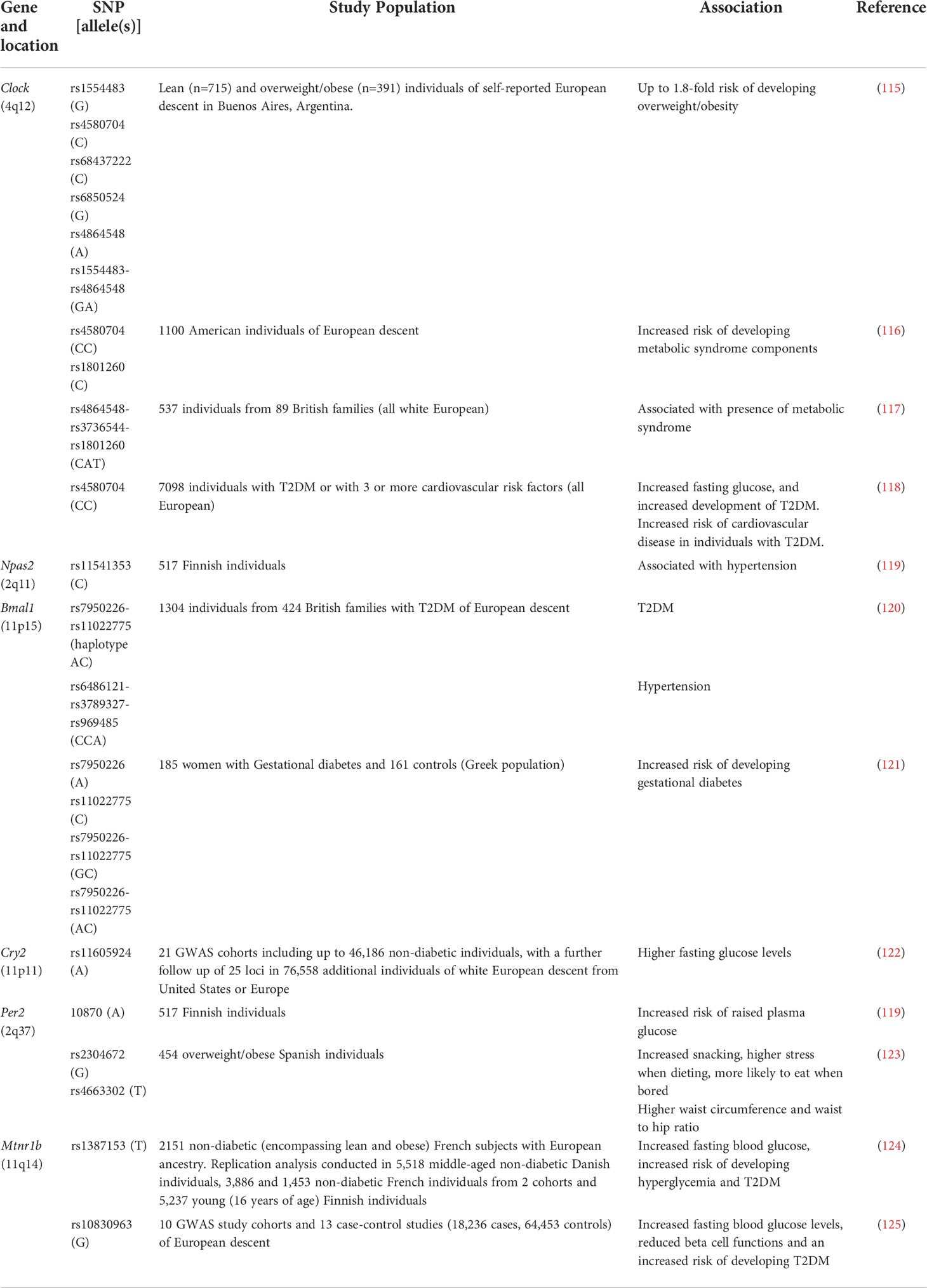
Table 1 SNPs in circadian rhythm-related genes associated with metabolic dysfunction, obesity and T2DM in humans.
Information on the expression of clock genes in human pancreatic islets is limited, but circadian genes are expressed in human islets (129). In individuals with T2DM, Cry2, Per2 and Per3 expression was reduced in the islets compared to islet donors without T2DM (130). Additionally, in vitro, islets cultured in glucolipotoxic conditions (16.7mmol/L glucose per 1mmol/L palmitate) for 48 hours downregulated the expression of Per3 in the pancreatic islets of individuals without T2DM (130). The aforementioned studies highlight the importance of circadian clock genes in regulating metabolic functions such as glucose tolerance and β cell function; however, many of these studies did not investigate the expression of these genes at the protein level. Although Stamenkovic and colleagues correlated mRNA expression to corresponding protein concentrations in human islets, post-transcriptional factors such as miRNA and post-translational modifications were not examined in this study (130). Future studies that address these interactions and mechanisms of regulation may provide additional insights into the relationship between the circadian clock and metabolic physiology. Additional studies, particularly in non-white European populations, with increased numbers of participants are also greatly needed.
Modulation of circadian rhythm
Misalignment between peripheral and central clocks is associated with insulin resistance, metabolic abnormalities and cardiovascular disease (17, 131, 132). This desynchrony can be achieved experimentally through a forced desynchronization (FD) protocol which involves altering behavioral patterns, such as feeding and sleep-wake cycles, so that they are substantially longer or shorter than 24 hours, whilst ensuring that the subjects are only exposed to dim light during their wake times (133). The aim of this is to desynchronize endogenous circadian rhythms from external influences e.g. food intake, light exposure as outlined in Figure 7. Buxton and colleagues demonstrated that a FD protocol (sleep restriction and circadian disruption) increased plasma glucose levels in human studies (134). Although the mechanism for this is unclear, a study which utilized human islet amyloid polypeptide (HIP) transgenic rats showed that circadian disruption accelerated the β cell loss and dysfunction in this model of T2DM (135). Furthermore, sleep deprivation studies demonstrated disrupted rhythmicity of insulin and glucagon levels, as well as insulin sensitivity and glucose tolerance (136–138). In these studies, circadian rhythm cycles have clearly influenced susceptibility to metabolic disease. In this section, we break down the different environmental cues that can significantly alter circadian rhythms in animal models and humans.
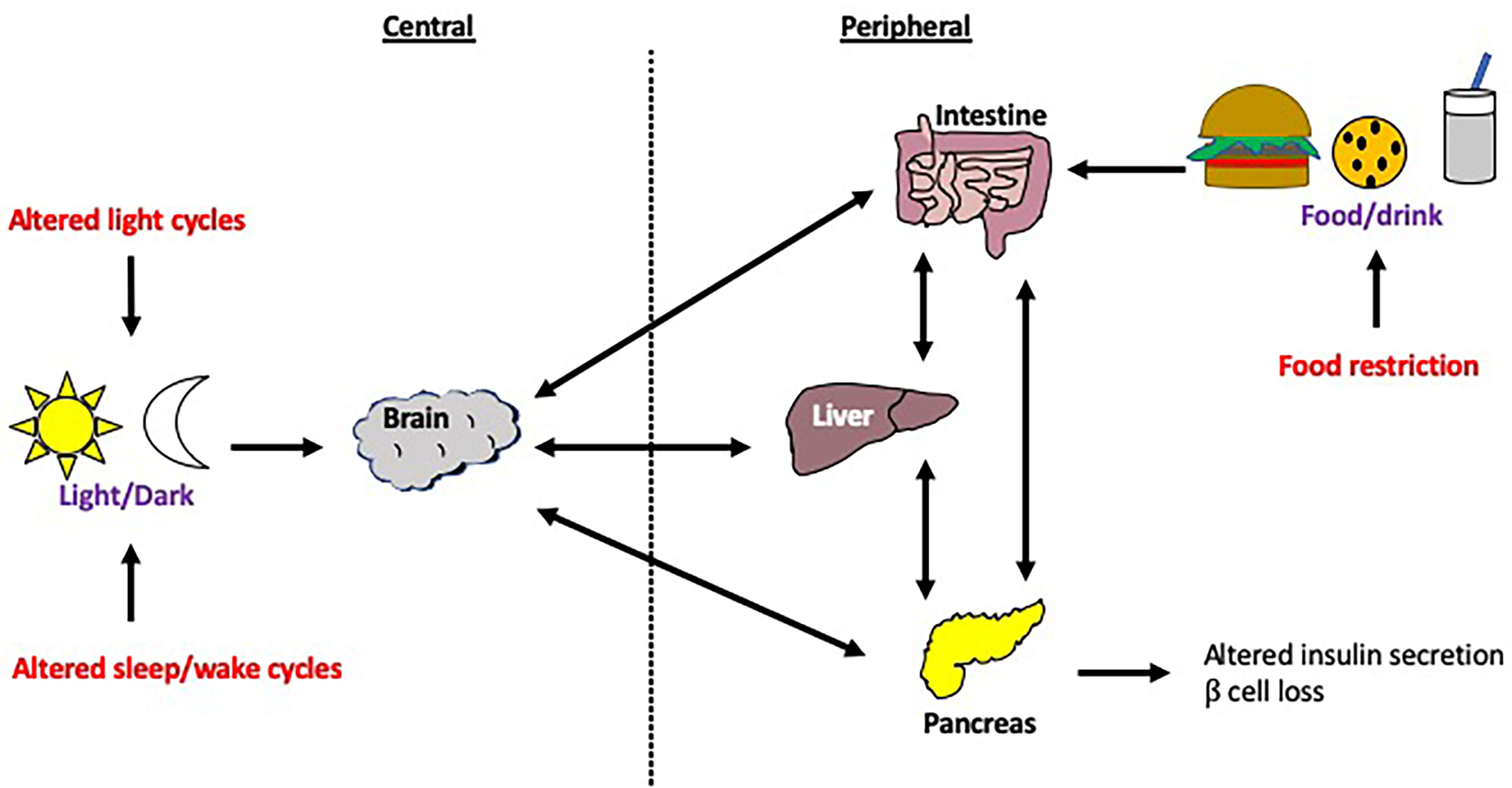
Figure 7 Modulation of host circadian rhythms. Circadian rhythms can be modulated through a number of interventions, including changes to the central clock via changes in the light/dark cycle or sleep/wake cycle, as well as changes to peripheral clocks e.g. food restriction. Disruption to the central clock can lead to disconnection from the peripheral clocks and vice versa. These changes to the circadian rhythm are often experienced by those with jet lag or in overnight shift workers. .
Altered light cycles
In mice, electrophysiological monitoring has shown that exposure to constant light reduced the amplitude (the difference between peak and trough) of SCN rhythmicity (139). This resulted in increased food intake, whilst energy expenditure was decreased. These mice also showed a complete loss of rhythmicity in insulin sensitivity, energy expenditure and food intake. In humans, light intensity has been shown to regulate postprandial glucose levels and triglycerides (140). In this study, healthy lean men and obese men with T2DM were exposed in the morning to 5 hours of either bright light (4000 lux) or dim light (10 lux), with a 600kcal meal given 1 hour after the start of the light exposure. While no changes were seen in the fasting or postprandial glucose levels of healthy lean men between dim or bright light exposure, obese men with T2DM had improved fasting and postprandial glucose levels, when exposed to bright light. In addition, healthy lean men exposed to bright light had higher fasting and postprandial plasma triglycerides, while in obese men with T2DM bright light only increased postprandial plasma triglycerides and did not change fasting triglyceride concentrations. Similar results were also seen in a study of individuals with insulin-resistance exposed to either bright day – dim evening light or dim day – bright evening light conditions (141). Thus, the light exposure can significantly impact our glucose homeostasis.
Altered diets and time of food intake
A high-fat diet also disrupts central and peripheral clocks in mouse models, including hypothalamic, adipose and hepatic clocks (142). The timing of food intake also affects circadian clocks. When kept in a 12-hour light/12-hour dark cycle (12:12h LD), mice consume the majority of their food during the dark phase when food is available ad libitum (143). In contrast, during timed food restriction, mice fed a HFD only during the light phase gained more weight than those fed during the dark phase (144). Similarly, mice fed a HFD restricted to the dark phase are less likely to develop metabolic abnormalities such as obesity and glucose intolerance than mice fed the same HFD ab libitum (145). Time-restricted feeding, where food is provided for a specific duration only, is also effective at preventing obesity and metabolic syndrome in circadian gene-deficient mice (whole body cry1/2-deficient mice and liver-specific bmal1 and rev-erbα/β-deficient mice) (146). Feeding restricted to the light phase also caused a desynchrony of peripheral clocks in the pancreas, liver, heart and kidneys by up to 12 hours, which did not affect the SCN (147). Mice exposed to calorific restriction in 12:12h LD cycles will become partly diurnal, as opposed to purely nocturnal and this is attributed to changes in peripheral clocks and the SCN (148, 149).
As in the animal studies, the timing of eating also influences risk of diabetes in humans. For example, a randomized crossover study showed that a later dinner was associated with impaired glucose tolerance in a subset of MTNR1B (melatonin receptor 1B) risk allele carriers (150). The postulated role of melatonin in this process agreed with a previous study showing that exogenous melatonin could also cause impaired glucose tolerance (151). Conversely, feeding restricted to 9 hours improved glycemic control in men with type 2 diabetes (152). Similarly, time-restricted eating (TRE) also improved metabolic parameters such as weight, visceral fat, atherogenic lipids and blood pressure in individuals with metabolic syndrome (153, 154); however, these benefits of TRE were observed in small sample sizes (n=15-20) and are currently under investigation larger cohorts (155, 156). Maintaining TRE after weight is potentially a challenge and further research would also be necessary.
In a FD protocol study involving 5 male and 5 female adults, increased blood glucose levels were coupled with a paradoxical rise in insulin secretion during the misalignment phase (where eating/sleeping is 12 hours out of synchrony with the normal schedule) (131). Another study which combined a FD protocol, with restricted sleeping hours (6.5 hours in a 28-hour day), showed a similar rise in glucose levels in the individuals, coupled with increased insulin secretion (134). A possible explanation for this may be reduced insulin sensitivity secondary to circadian misalignment. This is supported by a 12-hour rapid shift work protocol, which utilized the hyperinsulinemic-euglycemic clamp and showed that circadian misalignment is associated with decreased insulin sensitivity (157).
The timing of nutrient intake also alters the circadian rhythmicity of the gut bacterial composition in mice (158, 159). For example, bacterial species belonging to the phylum Firmicutes thrive postprandially in response to dietary glycan intake, whilst the phyla Bacteroidetes and Verrucomicrobia usually peak in numbers during fasting periods (158–160). As mentioned, the gut microbiota can oscillate, altering important metabolic functions in mice. In line with this, a recent study in humans identified a gut bacterial signature, encompassing 13 taxa with disrupted rhythmicity, which, in conjunction with BMI, could be used to predict individuals who would later develop T2DM (161). Thus, host-microbial rhythms may act as a biomarker for disease development. Interestingly, common gastric bypass procedures such as Roux-en-Y gastric bypass, which enable individuals to lose weight, are associated with altered microbial composition (162). It would be interesting to assess whether these procedures also alter host and microbial rhythmicity.
Shift work
As mentioned previously, circadian misalignment, and by extension shift-work, is a risk factor for developing metabolic syndrome, obesity and type 2 diabetes (18). Circadian misalignment as a risk factor for metabolic abnormalities has been corroborated in a real-life study that compared day-shift and night-shift workers (16). In this study, night-shift workers were found to have increased postprandial glucose and insulin levels as well as elevated triacylglyerol levels, compared to day-shift workers. A meta-analysis of 12 observational studies revealed that shift-work is associated with a 9% increase in the chance of developing T2DM compared to people who have not been exposed to shift work (19). Importantly, people who have rotating shift work are more at risk than those employed in constant shift work (22). This is likely due to exposure to both light and food intake at times-of-day different to when the body naturally anticipates these changes. Furthermore, a rat study using a simulated shift-work protocol (using rotating running wheels) was consistent with the human data and caused the animals to develop abdominal obesity and blunted rhythmicity of glucose levels (163). In rats, disruption of 12:12h LD cycles, with continuous light exposure, accelerated β cell dysfunction and loss, whilst impairing GSIS (135, 164). Studies on β cell-specific bmal1 knockout mice showed that Bmal1 plays an important role in adapting to circadian disruptions and preventing oxidative stress (14). Thus, a loss of function of this gene predisposes mice to β cell dysfunction and further to diabetes.
Jet lag
Jet lag is also associated with circadian misalignment and is characterized by a series of psychological and physiological symptoms such as low mood, impaired cognitive performance, loss of appetite, gastrointestinal disturbances and general malaise (165). Chronic jet lag may elevate the risk of developing cancer, cardiomyopathy and T2DM (166–168) and can be simulated in animal experiments by altering the duration of light or dark cycles (169). Studies utilizing this approach have found that there is extensive desynchrony between different body tissues and also variation in the time taken by different tissues to adjust to new light/dark cycles (170). Adrenal glucocorticoids (GCs) appear to play a key role in the re-entrainment process of circadian rhythms in jet lagged mice (171–173). Injection of metyrapone (MET; an inhibitor of corticosterone synthesis) prior to performing the jet lag protocol, was found to prolong re-entrainment when administered in the inactive phase and accelerate re-entrainment when given during the active phase (170). Since the SCN does not express GC receptors, GCs are unlikely to directly feedback to the SCN to regulate this process (173, 174). It has been hypothesized that dysregulation of the adrenal clock may cause aberrant secretion of other adrenal hormones such as aldosterone alongside GCs, which may feedback to the master pacemaker to regulate re-entrainment (175).
In jet-lagged mice, both the composition of the bacteria and metabolic functions were altered compared to control mice (158). These mice experienced impaired glucose tolerance and obesity caused by the bacteria as (a) these metabolic abnormalities were diminished following the eradication of the microbiota using antibiotics and (b) the transplantation of microbiota from these jet-lagged mice to germ-free mice, which lack all bacteria, transferred these metabolic abnormalities to the recipient mice. Together, these studies suggest that there is a functional relationship between host and bacterial rhythms which are important in modulating metabolic dysbiosis. Thus, alterations of the bacterial composition or oscillations could potentially be harnessed to prevent obesity, metabolic abnormalities or T2DM.
Social jet lag, defined as a temporal discrepancy between a person’s sleep pattern on working days and non-working days (23), has also been associated with diabetes susceptibility. Individuals with greater than 1 hour of social jet lag have a 75% greater chance of developing diabetes or prediabetes, compared to people with less than 1 hour of social jet lag (21).
Together, rodent and human studies have identified that the timing of food intake, nutrient content and light exposure are important stimuli in regulating the metabolic clock. These findings indicate that novel interventions such as time-specific therapy (chronotherapy) or interventions which target the circadian system such as synthetic circadian protein analogues may be beneficial in the future management of metabolic syndrome and T2DM (176).
Chronotherapy
Chronotherapy is the concept of administering drugs or other treatments at optimal times of the day in order to produce the most benefit (177). In clinical practice, statins are typically taken in the evening as their mechanism of action is to inhibit 3-hydroxy-3-methylglutaryl coenzyme A (HMG CoA) reductase, an enzyme which peaks in concentration in the night (178). There are many other examples of chronotherapy already in existence and its importance in relation to diabetes management as discussed below.
Bromocriptine is a dopamine agonist which is used as an adjunct in the treatment of T2DM (179). Dopaminergic activity in the hypothalamus follows a circadian rhythm and drives hepatic gluconeogenesis and adipocyte lipolysis. Bromocriptine is thought to reduce this drive when given within 2 hours of waking to prevent hyperglycaemia and dyslipidaemia. Although bromocriptine demonstrated efficacy in glycemic control and has been approved by the Food and Drug administration (FDA) in T2DM, this drug is infrequently used in clinical practice (52, 180, 181).
Despite preclinical studies showing that metformin interacts with molecular components of the circadian system and has time-dependent effects on blood glucose (182–184), there have not been any clinical studies to investigate the timing of metformin administration. This may be especially important given oscillations in both host and bacterial rhythms, as metformin alters the bacterial composition and function to mediate therapeutic effects of the drug (185). As metformin is a first line therapeutic for T2DM, it would seem particularly important to maximise efficacy. Similarly, the short-acting sulfonylurea drug tolbutamide appears to have time-dependent effects on insulin secretion but its chronotherapeutic potential is yet to be investigated (186).
Targeting circadian rhythm proteins may also be important for preventing/reversing metabolic dysbiosis. REV-ERBα agonist SR9011 and REV-ERBβ agonist SR9009 reduced obesity and hyperglycaemia in mouse models (113). Both of these synthetic REV-ERB agonists induced increased energy expenditure in white adipose tissue. Furthermore, the naturally-derived compound nobiletin activates circadian molecules RORα and RORγ and prevents metabolic syndrome from developing in diet-induced obese mice (187). Synthetic CRY stabilizers have also been reported to have a protective effect against diabetes by improving glucose tolerance in mice (188). In these rodent studies, these drugs have a short half-life and are typically administered by injection at intervals shorter than 3 hours to maintain suitable bioavailability (189). It has been hypothesized that humans would eliminate the active metabolites of these drugs even more quickly, therefore requiring more frequent injections (189). In a clinical context, this may not be practical. Therefore, this pharmacological obstacle must first be addressed before studies in humans can be carried out effectively. Novel therapies for T2DM, obesity and metabolic syndrome may be identified if these and similar drugs can demonstrate similar effects in human studies.
Future directions
In today’s industrialized world, only a minority of people have an internal sleep-wake cycle which is consistent with their social commitments (190). Therefore, it is unsurprising that social jetlag is common in the population (23). An individual’s personal circumstances has a substantial influence on many of the exogenous factors which influence circadian rhythms including sleep-wake cycles, exposure to light, eating times and activity level (191). These environmental factors, together with endogenous characteristics such as age, genetics and chronotype (i.e. the time of day people are most alert/sleep), influence the degree of circadian desynchrony which an individual experiences (9).
In clinical practice, there is currently neither a standardized scoring system which precisely encompasses the exogenous determinants of circadian activity, nor is there a genetic screening program which identifies carriers of genetic variations predisposing people to circadian disruption. The future development of these risk stratification tools may influence clinical practice by allowing disease management to be tailored to an individual’s circadian rhythmicity. For example, the timing of drug administration could be regulated in order to maximize efficacy, which may enable reduced drug concentrations to be used, thus limiting any toxicity or potential side effects. In order for this to be achieved, further epidemiological studies are necessary to quantify the relative risk of circadian disruption associated with different behavioral and genetic risk factors.
There are many exogenous factors which can alter host/microbial rhythmicity, which can then modulate susceptibility to metabolic dysfunction and T2DM. Recent evidence has shown arrhythmic bacterial signatures could be used as a biomarker to predict individuals who would later develop T2DM; thus, further investigation into host/microbial rhythms, or lack thereof, as biomarkers for predicting metabolic dysfunction or the onset of diabetes should be conducted. Non-pharmacological interventions can also be used to target the circadian system in metabolic disease. Currently, trials investigating the effects of time-restricted feeding are taking place on large cohorts with type 2 diabetes and metabolic syndrome (155, 156). The results from these studies will provide insight into the potential for simple lifestyle changes that can modulate circadian rhythms as a therapy. Understanding the mechanism behind these changes will be vital.
Summary
Human epidemiological and genetic studies have highlighted the importance of circadian rhythms in metabolic diseases such as T2DM and metabolic syndrome. This has led to extensive research in animal models and humans, which have concluded that circadian dysregulation and misalignment is associated with the development of metabolic abnormalities. Clinical applications of this knowledge may include the optimization of existing antidiabetic therapies such as metformin. Circadian molecules such as nobiletin, REV-ERB agonists and CRY stabilizers have demonstrated efficacy in preclinical studies and may lead to the development of novel treatments for diseases linked to circadian dysregulation. However, limitations in current knowledge mean that further research is required before these interventions can be used clinically.
Author contributions
KC and JP wrote the manuscript. FW and JP edited the manuscript. All authors contributed to the article and approved the submitted version.
Funding
This work was funded by a Medical Research Council Career Development Award (MR/T010525/1) and a JDRF UK small grant award (1-SGA-2021-0002) to JP and a Medical Research Council research grant (MR/K021141/1) to FW.
Conflict of interest
The authors declare that the research was conducted in the absence of any commercial or financial relationships that could be construed as a potential conflict of interest.
Publisher’s note
All claims expressed in this article are solely those of the authors and do not necessarily represent those of their affiliated organizations, or those of the publisher, the editors and the reviewers. Any product that may be evaluated in this article, or claim that may be made by its manufacturer, is not guaranteed or endorsed by the publisher.
References
1. Saeedi P, Petersohn I, Salpea P, Malanda B, Karuranga S, Unwin N, et al. Global and regional diabetes prevalence estimates for 2019 and projections for 2030 and 2045: Results from the international diabetes federation diabetes atlas, 9(th) edition. Diabetes Res Clin Pract (2019) 157:107843. doi: 10.1016/j.diabres.2019.107843
2. Papatheodorou K, Banach M, Bekiari E, Rizzo M, Edmonds M. Complications of diabetes 2017. J Diabetes Res (2018) 2018:3086167–. doi: 10.1155/2018/3086167
3. Khan MAB, Hashim MJ, King JK, Govender RD, Mustafa H, Al Kaabi J. Epidemiology of type 2 diabetes - global burden of disease and forecasted trends. J Epidemiol Glob Health (2020) 10(1):107–11. doi: 10.2991/jegh.k.191028.001
4. Mirzaei K, Xu M, Qi Q, de Jonge L, Bray GA, Sacks F, et al. Variants in glucose- and circadian rhythm-related genes affect the response of energy expenditure to weight-loss diets: the POUNDS LOST trial. Am J Clin Nutr (2014) 99(2):392–9. doi: 10.3945/ajcn.113.072066
5. Oishi K, Shirai H, Ishida N. CLOCK is involved in the circadian transactivation of peroxisome-proliferator-activated receptor alpha (PPARalpha) in mice. Biochem J (2005) 386(Pt 3):575–81. doi: 10.1042/bj20041150
6. Huang W, Ramsey KM, Marcheva B, Bass J. Circadian rhythms, sleep, and metabolism. J Clin Invest (2011) 121(6):2133–41. doi: 10.1172/jci46043
7. Meijer JH, Groos GA, Rusak B. Luminance coding in a circadian pacemaker: the suprachiasmatic nucleus of the rat and the hamster. Brain Res (1986) 382(1):109–18. doi: 10.1016/0006-8993(86)90117-4
8. Meijer JH, Rusak B, Gänshirt G. The relation between light-induced discharge in the suprachiasmatic nucleus and phase shifts of hamster circadian rhythms. Brain Res (1992) 598(1-2):257–63. doi: 10.1016/0006-8993(92)90191-B
9. Barclay JL, Tsang AH, Oster H. Interaction of central and peripheral clocks in physiological regulation. Prog Brain Res (2012) 199:163–81. doi: 10.1016/b978-0-444-59427-3.00030-7
10. Abraham U, Schlichting JK, Kramer A, Herzel H. Quantitative analysis of circadian single cell oscillations in response to temperature. PloS One (2018) 13(1):e0190004–e. doi: 10.1371/journal.pone.0190004
11. Rudic RD, McNamara P, Curtis A-M, Boston RC, Panda S, Hogenesch JB, et al. BMAL1 and CLOCK, two essential components of the circadian clock, are involved in glucose homeostasis. PloS Biol (2004) 2(11):e377–e. doi: 10.1371/journal.pbio.0020377
12. Shimba S, Ishii N, Ohta Y, Ohno T, Watabe Y, Hayashi M, et al. Brain and muscle arnt-like protein-1 (BMAL1), a component of the molecular clock, regulates adipogenesis. Proc Natl Acad Sci U S A (2005) 102(34):12071–6. doi: 10.1073/pnas.0502383102
13. Marcheva B, Ramsey KM, Buhr ED, Kobayashi Y, Su H, Ko CH, et al. Disruption of the clock components CLOCK and BMAL1 leads to hypoinsulinaemia and diabetes. Nature (2010) 466(7306):627–31. doi: 10.1038/nature09253
14. Lee J, Moulik M, Fang Z, Saha P, Zou F, Xu Y, et al. Bmal1 and β-cell clock are required for adaptation to circadian disruption, and their loss of function leads to oxidative stress-induced β-cell failure in mice. Mol Cell Biol (2013) 33(11):2327–38. doi: 10.1128/mcb.01421-12
15. Poggiogalle E, Jamshed H, Peterson CM. Circadian regulation of glucose, lipid, and energy metabolism in humans. Metabolism (2018) 84:11–27. doi: 10.1016/j.metabol.2017.11.017
16. Lund J, Arendt J, Hampton SM, English J, Morgan LM. Postprandial hormone and metabolic responses amongst shift workers in Antarctica. J Endocrinol (2001) 171(3):557–64. doi: 10.1677/joe.0.1710557
17. Gangwisch JE. Epidemiological evidence for the links between sleep, circadian rhythms and metabolism. Obes Rev (2009) 10 Suppl 2(0 2):37–45. doi: 10.1111/j.1467-789X.2009.00663.x
18. Monk TH, Buysse DJ. Exposure to shift work as a risk factor for diabetes. J Biol Rhythms (2013) 28(5):356–9. doi: 10.1177/0748730413506557
19. Gan Y, Yang C, Tong X, Sun H, Cong Y, Yin X, et al. Shift work and diabetes mellitus: a meta-analysis of observational studies. Occup Environ Med (2015) 72(1):72–8. doi: 10.1136/oemed-2014-102150
20. Lunn RM, Blask DE, Coogan AN, Figueiro MG, Gorman MR, Hall JE, et al. Health consequences of electric lighting practices in the modern world: A report on the national toxicology program's workshop on shift work at night, artificial light at night, and circadian disruption. Sci Total Environ (2017) 607-608:1073–84. doi: 10.1016/j.scitotenv.2017.07.056
21. Koopman ADM, Rauh SP, van 't Riet E, Groeneveld L, van der Heijden AA, Elders PJ, et al. The association between social jetlag, the metabolic syndrome, and type 2 diabetes mellitus in the general population: The new hoorn study. J Biol Rhythms (2017) 32(4):359–68. doi: 10.1177/0748730417713572
22. Vetter C, Dashti HS, Lane JM, Anderson SG, Schernhammer ES, Rutter MK, et al. Night shift work, genetic risk, and type 2 diabetes in the UK biobank. Diabetes Care (2018) 41(4):762–9. doi: 10.2337/dc17-1933
23. Wittmann M, Dinich J, Merrow M, Roenneberg T. Social jetlag: misalignment of biological and social time. Chronobiol Int (2006) 23(1-2):497–509. doi: 10.1080/07420520500545979
24. Yuan Q, Pan A, Fu Y, Dai Y. Chapter 1 - anatomy and physiology of the pancreas. In: Li M, Lu L, Xiao Y, Fu D, Zhang H, editors. Integrative pancreatic intervention therapy. (Amsterdam, Netherlands: Elsevier) (2021). p. 3–21.
25. Hedayat KM, Lapraz J-C, Schuff B. Chapter 14 - exocrine pancreas. In: Hedayat KM, Lapraz J-C, Schuff B, editors. The theory of endobiogeny. (Cambridge, Massachusetts, USA: Academic Press) (2020). p. 117–20.
26. Feher J. 9.4 - the endocrine pancreas and control of blood glucose. In: Feher J, editor. Quantitative human physiology. Boston: Academic Press (2012). p. 799–809.
27. Thorens B. GLUT2, glucose sensing and glucose homeostasis. Diabetologia (2015) 58(2):221–32. doi: 10.1007/s00125-014-3451-1
28. Boucher J, Kleinridders A, Kahn CR. Insulin receptor signaling in normal and insulin-resistant states. Cold Spring Harb Perspect Biol (2014) 6(1):a009191. doi: 10.1101/cshperspect.a009191
29. Petersen MC, Shulman GI. Mechanisms of insulin action and insulin resistance. Physiol Rev (2018) 98(4):2133–223. doi: 10.1152/physrev.00063.2017
30. Schulingkamp RJ, Pagano TC, Hung D, Raffa RB. Insulin receptors and insulin action in the brain: review and clinical implications. Neurosci Biobehav Rev (2000) 24(8):855–72. doi: 10.1016/S0149-7634(00)00040-3
31. Roh E, Song DK, Kim M-S. Emerging role of the brain in the homeostatic regulation of energy and glucose metabolism. Exp Mol Med (2016) 48(3):e216–e. doi: 10.1038/emm.2016.4
32. Kettner NM, Mayo SA, Hua J, Lee C, Moore DD, Fu L. Circadian dysfunction induces leptin resistance in mice. Cell Metab (2015) 22(3):448–59. doi: 10.1016/j.cmet.2015.06.005
33. Klok MD, Jakobsdottir S, Drent ML. The role of leptin and ghrelin in the regulation of food intake and body weight in humans: a review. Obes Rev (2007) 8(1):21–34. doi: 10.1111/j.1467-789X.2006.00270.x
34. Baskin DG, Figlewicz Lattemann D, Seeley RJ, Woods SC, Porte D, Schwartz MW. Insulin and leptin: dual adiposity signals to the brain for the regulation of food intake and body weight. Brain Res (1999) 848(1):114–23. doi: 10.1016/S0006-8993(99)01974-5
35. Briant L, Salehi A, Vergari E, Zhang Q, Rorsman P. Glucagon secretion from pancreatic α-cells. Ups J Med Sci (2016) 121(2):113–9. doi: 10.3109/03009734.2016.1156789
36. Al-Massadi O, Fernø J, Diéguez C, Nogueiras R, Quiñones M. Glucagon control on food intake and energy balance. Int J Mol Sci (2019) 20(16):3905. doi: 10.3390/ijms20163905
37. Quiñones M, Al-Massadi O, Gallego R, Fernø J, Diéguez C, López M, et al. Hypothalamic CaMKKβ mediates glucagon anorectic effect and its diet-induced resistance. Mol Metab (2015) 4(12):961–70. doi: 10.1016/j.molmet.2015.09.014
38. Lutz TA. Control of food intake and energy expenditure by amylin–therapeutic implications. Int J Obes (2009) 33(1):S24–S7. doi: 10.1038/ijo.2009.13
39. Wielinga PY, Löwenstein C, Muff S, Munz M, Woods SC, Lutz TA. Central amylin acts as an adiposity signal to control body weight and energy expenditure. Physiol Behav (2010) 101(1):45–52. doi: 10.1016/j.physbeh.2010.04.012
40. Waller DG, Sampson AP. 44 - corticosteroids (glucocorticoids and mineralocorticoids). In: Waller DG, Sampson AP, editors. Medical pharmacology and therapeutics (Fifth edition). (Amsterdam, Netherlands: Elsevier) (2018). p. 503–11.
41. Oster H, Damerow S, Kiessling S, Jakubcakova V, Abraham D, Tian J, et al. The circadian rhythm of glucocorticoids is regulated by a gating mechanism residing in the adrenal cortical clock. Cell Metab (2006) 4(2):163–73. doi: 10.1016/j.cmet.2006.07.002
42. Richards J, Cheng KY, All S, Skopis G, Jeffers L, Lynch IJ, et al. A role for the circadian clock protein Per1 in the regulation of aldosterone levels and renal na+ retention. Am J Physiol Renal Physiol (2013) 305(12):F1697–704. doi: 10.1152/ajprenal.00472.2013
43. Holt EH, Lupsa B, Lee GS, Bassyouni H, Peery HE. Chapter 4 - the adrenal glands. In: Holt EH, Lupsa B, Lee GS, Bassyouni H, Peery HE, editors. Goodman's basic medical endocrinology (Fifth edition). (Amsterdam, Netherlands: Elsevier) (2022). p. 101–43.
44. Zennaro MC, Caprio M, Fève B. Mineralocorticoid receptors in the metabolic syndrome. Trends Endocrinol Metab (2009) 20(9):444–51. doi: 10.1016/j.tem.2009.05.006
45. Nicolaides NC, Charmandari E, Chrousos GP. Overview of glucocorticoids. In: Huhtaniemi I, Martini L, editors. Encyclopedia of endocrine diseases (Second edition). Oxford: Academic Press (2018). p. 64–71.
46. Kuo T, McQueen A, Chen TC, Wang JC. Regulation of glucose homeostasis by glucocorticoids. Adv Exp Med Biol (2015) 872:99–126. doi: 10.1007/978-1-4939-2895-8_5
47. Deacon CF, Ahrén B. Physiology of incretins in health and disease. Rev Diabetes Stud (2011) 8(3):293–306. doi: 10.1900/RDS.2011.8.293
48. Anini Y, Brubaker PL. Glucagon-like peptides: GLP-1 and GLP-2. In: Henry HL, Norman AW, editors. Encyclopedia of hormones. New York: Academic Press (2003). p. 55–62.
49. Pederson RA, McIntosh CHS. GIP (Gastric inhibitory polypeptide). In: Martini L, editor. Encyclopedia of endocrine diseases. New York: Elsevier (2004). p. 202–7.
50. Kim W, Egan JM. The role of incretins in glucose homeostasis and diabetes treatment. Pharmacol Rev (2008) 60(4):470–512. doi: 10.1124/pr.108.000604
51. Wu T, Rayner CK, Jones K, Horowitz M. Chapter three - dietary effects on incretin hormone secretion. In: Litwack G, editor. Vitamins & hormones, vol. 84. (Cambridge, Massachusetts, USA: Academic Press) (2010). p. 81–110.
52. National Institute for H, Care E. National institute for health and care excellence: Clinical guidelines. type 2 diabetes in adults: management Vol. 2015. . London: National Institute for Health and Care Excellence (UK) Copyright National Institute for Health and Care Excellence (2015).
53. Frias JP, Nauck MA, Van J, Kutner ME, Cui X, Benson C, et al. Efficacy and safety of LY3298176, a novel dual GIP and GLP-1 receptor agonist, in patients with type 2 diabetes: a randomised, placebo-controlled and active comparator-controlled phase 2 trial. Lancet (2018) 392(10160):2180–93. doi: 10.1016/S0140-6736(18)32260-8
54. Bailey CJ. GIP analogues and the treatment of obesity-diabetes. Peptides (2020) 125:170202. doi: 10.1016/j.peptides.2019.170202
55. Coskun T, Sloop KW, Loghin C, Alsina-Fernandez J, Urva S, Bokvist KB, et al. LY3298176, a novel dual GIP and GLP-1 receptor agonist for the treatment of type 2 diabetes mellitus: From discovery to clinical proof of concept. Mol Metab (2018) 18:3–14. doi: 10.1016/j.molmet.2018.09.009
56. Frias JP, Bastyr EJ, Vignati L, Tschöp MH, Schmitt C, Owen K, et al. The sustained effects of a dual GIP/GLP-1 receptor agonist, NNC0090-2746, in patients with type 2 diabetes. Cell Metab (2017) 26(2):343–52.e2. doi: 10.1016/j.cmet.2017.07.011
57. Tong J, Prigeon RL, Davis HW, Bidlingmaier M, Kahn SE, Cummings DE, et al. Ghrelin suppresses glucose-stimulated insulin secretion and deteriorates glucose tolerance in healthy humans. Diabetes (2010) 59(9):2145–51. doi: 10.2337/db10-0504
58. Broglio F, Arvat E, Benso A, Gottero C, Muccioli G, Papotti M, et al. Ghrelin, a natural GH secretagogue produced by the stomach, induces hyperglycemia and reduces insulin secretion in humans. J Clin Endocrinol Metab (2001) 86(10):5083–6. doi: 10.1210/jcem.86.10.8098
59. Cerf ME. Beta cell dysfunction and insulin resistance. Front Endocrinol (Lausanne) (2013) 4:37. doi: 10.3389/fendo.2013.00037
60. Saisho Y. β-cell dysfunction: Its critical role in prevention and management of type 2 diabetes. World J Diabetes (2015) 6(1):109–24. doi: 10.4239/wjd.v6.i1.109
61. Gurgul-Convey E, Mehmeti I, Lortz S, Lenzen S. Cytokine toxicity in insulin-producing cells is mediated by nitro-oxidative stress-induced hydroxyl radical formation in mitochondria. J Mol Med (Berl) (2011) 89(8):785–98. doi: 10.1007/s00109-011-0747-1
62. Kim SM, Neuendorff N, Alaniz RC, Sun Y, Chapkin RS, Earnest DJ. Shift work cycle-induced alterations of circadian rhythms potentiate the effects of high-fat diet on inflammation and metabolism. FASEB J (2018) 32(6):3085–95. doi: 10.1096/fj.201700784R
63. Tam CS, Xie W, Johnson WD, Cefalu WT, Redman LM, Ravussin E. Defining insulin resistance from hyperinsulinemic-euglycemic clamps. Diabetes Care (2012) 35(7):1605–10. doi: 10.2337/dc11-2339
64. Gilbert ER, Liu D. Epigenetics: the missing link to understanding β-cell dysfunction in the pathogenesis of type 2 diabetes. Epigenetics (2012) 7(8):841–52. doi: 10.4161/epi.21238
65. Kettunen JLT, Tuomi T. Human physiology of genetic defects causing beta-cell dysfunction. J Mol Biol (2020) 432(5):1579–98. doi: 10.1016/j.jmb.2019.12.038
66. Ley SH, Hamdy O, Mohan V, Hu FB. Prevention and management of type 2 diabetes: dietary components and nutritional strategies. Lancet (2014) 383(9933):1999–2007. doi: 10.1016/S0140-6736(14)60613-9
67. Boden G. Free fatty acids, insulin resistance, and type 2 diabetes mellitus. Proc Assoc Am Physicians (1999) 111(3):241–8. doi: 10.1046/j.1525-1381.1999.99220.x
68. Freinkel N, Mager M, Vinnick L. Cyclicity in the interrelationships between plasma insulin and glucose during starvation in normal young men. J Lab Clin Med (1968) 71(1):171–8.
69. Boden G, Ruiz J, Urbain JL, Chen X. Evidence for a circadian rhythm of insulin secretion. Am J Physiol (1996) 271(2 Pt 1):E246–52. doi: 10.1152/ajpendo.1996.271.2.E246
70. La Fleur SE, Kalsbeek A, Wortel J, Buijs RM. A suprachiasmatic nucleus generated rhythm in basal glucose concentrations. J Neuroendocrinol (1999) 11(8):643–52. doi: 10.1046/j.1365-2826.1999.00373.x
71. Crosby P, Hamnett R, Putker M, Hoyle NP, Reed M, Karam CJ, et al. Insulin/IGF-1 drives PERIOD synthesis to entrain circadian rhythms with feeding time. Cell (2019) 177(4):896–909.e20. doi: 10.1016/j.cell.2019.02.017
72. Van Cauter E, Polonsky KS, Scheen AJ. Roles of circadian rhythmicity and sleep in human glucose regulation. Endocr Rev (1997) 18(5):716–38. doi: 10.1210/edrv.18.5.0317
73. Kalsbeek A, Yi CX, La Fleur SE, Fliers E. The hypothalamic clock and its control of glucose homeostasis. Trends Endocrinol Metab (2010) 21(7):402–10. doi: 10.1016/j.tem.2010.02.005
74. Boden G, Chen X, Polansky M. Disruption of circadian insulin secretion is associated with reduced glucose uptake in first-degree relatives of patients with type 2 diabetes. Diabetes (1999) 48(11):2182–8. doi: 10.2337/diabetes.48.11.2182
75. Petrenko V, Saini C, Giovannoni L, Gobet C, Sage D, Unser M, et al. Pancreatic α- and β-cellular clocks have distinct molecular properties and impact on islet hormone secretion and gene expression. Genes Dev (2017) 31(4):383–98. doi: 10.1101/gad.290379.116
76. Ruiter M, La Fleur SE, van Heijningen C, van der Vliet J, Kalsbeek A, Buijs RM. The daily rhythm in plasma glucagon concentrations in the rat is modulated by the biological clock and by feeding behavior. Diabetes (2003) 52(7):1709–15. doi: 10.2337/diabetes.52.7.1709
77. Sakamoto K, Nagase T, Fukui H, Horikawa K, Okada T, Tanaka H, et al. Multitissue circadian expression of rat period homolog (rPer2) mRNA is governed by the mammalian circadian clock, the suprachiasmatic nucleus in the brain. J Biol Chem (1998) 273(42):27039–42. doi: 10.1074/jbc.273.42.27039
78. Balsalobre A, Damiola F, Schibler U. A serum shock induces circadian gene expression in mammalian tissue culture cells. Cell (1998) 93(6):929–37. doi: 10.1016/s0092-8674(00)81199-x
79. Weitzman ED, Fukushima D, Nogeire C, Roffwarg H, Gallagher TF, Hellman L. Twenty-four hour pattern of the episodic secretion of cortisol in normal subjects. J Clin Endocrinol Metab (1971) 33(1):14–22. doi: 10.1210/jcem-33-1-14
80. Selmaoui B, Touitou Y. Reproducibility of the circadian rhythms of serum cortisol and melatonin in healthy subjects: a study of three different 24-h cycles over six weeks. Life Sci (2003) 73(26):3339–49. doi: 10.1016/j.lfs.2003.05.007
81. Wright KP Jr., Drake AL, Frey DJ, Fleshner M, Desouza CA, Gronfier C, et al. Influence of sleep deprivation and circadian misalignment on cortisol, inflammatory markers, and cytokine balance. Brain Behav Immun (2015) 47:24–34. doi: 10.1016/j.bbi.2015.01.004
82. Lederbogen F, Hummel J, Fademrecht C, Krumm B, Kühner C, Deuschle M, et al. Flattened circadian cortisol rhythm in type 2 diabetes. Exp Clin Endocrinol Diabetes (2011) 119(9):573–5. doi: 10.1055/s-0031-1275288
83. Bruehl H, Wolf OT, Convit A. A blunted cortisol awakening response and hippocampal atrophy in type 2 diabetes mellitus. Psychoneuroendocrinology (2009) 34(6):815–21. doi: 10.1016/j.psyneuen.2008.12.010
84. Hackett RA, Steptoe A, Kumari M. Association of diurnal patterns in salivary cortisol with type 2 diabetes in the Whitehall II study. J Clin Endocrinol Metab (2014) 99(12):4625–31. doi: 10.1210/jc.2014-2459
85. Mingrone G, Nolfe G, Gissey GC, Iaconelli A, Leccesi L, Guidone C, et al. Circadian rhythms of GIP and GLP1 in glucose-tolerant and in type 2 diabetic patients after biliopancreatic diversion. Diabetologia (2009) 52(5):873–81. doi: 10.1007/s00125-009-1288-9
86. Galindo Muñoz JS, Jiménez Rodríguez D, Hernández Morante JJ. Diurnal rhythms of plasma GLP-1 levels in normal and overweight/obese subjects: lack of effect of weight loss. J Physiol Biochem (2015) 71(1):17–28. doi: 10.1007/s13105-014-0375-7
87. Elliott RM, Morgan LM, Tredger JA, Deacon S, Wright J, Marks V. Glucagon-like peptide-1 (7-36)amide and glucose-dependent insulinotropic polypeptide secretion in response to nutrient ingestion in man: acute post-prandial and 24-h secretion patterns. J Endocrinol (1993) 138(1):159–66. doi: 10.1677/joe.0.1380159
88. Brubaker PL, Gil-Lozano M. Glucagon-like peptide-1: The missing link in the metabolic clock? J Diabetes Investig (2016) 7 Suppl 1(Suppl 1):70–5. doi: 10.1111/jdi.12477
89. Ando H, Ushijima K, Fujimura A. Indirect effects of glucagon-like peptide-1 receptor agonist exendin-4 on the peripheral circadian clocks in mice. PloS One (2013) 8(11):e81119. doi: 10.1371/journal.pone.0081119
90. Flanagan DE, Evans ML, Monsod TP, Rife F, Heptulla RA, Tamborlane WV, et al. The influence of insulin on circulating ghrelin. Am J Physiol Endocrinol Metab (2003) 284(2):E313–6. doi: 10.1152/ajpendo.00569.2001
91. Horvath TL, Abizaid A, Dietrich MO, Li Y, Takahashi JS, Bass J. Ghrelin-immunopositive hypothalamic neurons tie the circadian clock and visual system to the lateral hypothalamic arousal center. Mol Metab (2012) 1(1-2):79–85. doi: 10.1016/j.molmet.2012.08.003
92. Takahashi JS. Transcriptional architecture of the mammalian circadian clock. Nat Rev Genet (2017) 18(3):164–79. Epub 2016/12/19. doi: 10.1038/nrg.2016.150
93. Doi M, Hirayama J, Sassone-Corsi P. Circadian regulator CLOCK is a histone acetyltransferase. Cell (2006) 125(3):497–508. doi: 10.1016/j.cell.2006.03.033
94. Katada S, Sassone-Corsi P. The histone methyltransferase MLL1 permits the oscillation of circadian gene expression. Nat Struct Mol Biol (2010) 17(12):1414–21. doi: 10.1038/nsmb.1961
95. DiTacchio L, Le HD, Vollmers C, Hatori M, Witcher M, Secombe J, et al. Histone lysine demethylase JARID1a activates CLOCK-BMAL1 and influences the circadian clock. Science (2011) 333(6051):1881–5. doi: 10.1126/science.1206022
96. Duong HA, Robles MS, Knutti D, Weitz CJ. A molecular mechanism for circadian clock negative feedback. Science (2011) 332(6036):1436–9. doi: 10.1126/science.1196766
97. Preitner N, Damiola F, Lopez-Molina L, Zakany J, Duboule D, Albrecht U, et al. The orphan nuclear receptor REV-ERBalpha controls circadian transcription within the positive limb of the mammalian circadian oscillator. Cell (2002) 110(2):251–60. doi: 10.1016/s0092-8674(02)00825-5
98. Guillaumond F, Dardente H, Giguère V, Cermakian N. Differential control of Bmal1 circadian transcription by REV-ERB and ROR nuclear receptors. J Biol Rhythms (2005) 20(5):391–403. doi: 10.1177/0748730405277232
99. Mitsui S, Yamaguchi S, Matsuo T, Ishida Y, Okamura H. Antagonistic role of E4BP4 and PAR proteins in the circadian oscillatory mechanism. Genes Dev (2001) 15(8):995–1006. doi: 10.1101/gad.873501
100. Wang Y, Kuang Z, Yu X, Ruhn KA, Kubo M, Hooper LV. The intestinal microbiota regulates body composition through NFIL. Science (2017) 357(6354):912–6. doi: 10.1126/science.aan0677
101. Turek FW, Joshu C, Kohsaka A, Lin E, Ivanova G, McDearmon E, et al. Obesity and metabolic syndrome in circadian clock mutant mice. Science (2005) 308(5724):1043–5. doi: 10.1126/science.1108750
102. Vitaterna MH, Ko CH, Chang A-M, Buhr ED, Fruechte EM, Schook A, et al. The mouse <em<Clock</em< mutation reduces circadian pacemaker amplitude and enhances efficacy of resetting stimuli and phase–response curve amplitude. Proc Natl Acad Sci (2006) 103(24):9327. doi: 10.1073/pnas.0603601103
103. Lamia KA, Storch KF, Weitz CJ. Physiological significance of a peripheral tissue circadian clock. Proc Natl Acad Sci U S A (2008) 105(39):15172–7. doi: 10.1073/pnas.0806717105
104. Grimaldi B, Bellet MM, Katada S, Astarita G, Hirayama J, Amin RH, et al. PER2 controls lipid metabolism by direct regulation of PPARγ. Cell Metab (2010) 12(5):509–20. doi: 10.1016/j.cmet.2010.10.005
105. Dallmann R, Weaver DR. Altered body mass regulation in male mPeriod mutant mice on high-fat diet. Chronobiol Int (2010) 27(6):1317–28. doi: 10.3109/07420528.2010.489166
106. Okamura H, Doi M, Yamaguchi Y, Fustin JM. Hypertension due to loss of clock: novel insight from the molecular analysis of Cry1/Cry2-deleted mice. Curr Hypertens Rep (2011) 13(2):103–8. doi: 10.1007/s11906-011-0181-3
107. Barclay JL, Shostak A, Leliavski A, Tsang AH, Jöhren O, Müller-Fielitz H, et al. High-fat diet-induced hyperinsulinemia and tissue-specific insulin resistance in cry-deficient mice. Am J Physiol Endocrinol Metab (2013) 304(10):E1053–63. doi: 10.1152/ajpendo.00512.2012
108. Hirota T, Lee JW, St John PC, Sawa M, Iwaisako K, Noguchi T, et al. Identification of small molecule activators of cryptochrome. Science (2012) 337(6098):1094–7. doi: 10.1126/science.1223710
109. Zhang EE, Liu Y, Dentin R, Pongsawakul PY, Liu AC, Hirota T, et al. Cryptochrome mediates circadian regulation of cAMP signaling and hepatic gluconeogenesis. Nat Med (2010) 16(10):1152–6. doi: 10.1038/nm.2214
110. Vieira E, Merino B, Quesada I. Role of the clock gene rev-erbα in metabolism and in the endocrine pancreas. Diabetes Obes Metab (2015) 17 Suppl 1:106–14. doi: 10.1111/dom.12522
111. Vieira E, Marroquí L, Batista TM, Caballero-Garrido E, Carneiro EM, Boschero AC, et al. The clock gene rev-erbα regulates pancreatic β-cell function: modulation by leptin and high-fat diet. Endocrinology (2012) 153(2):592–601. doi: 10.1210/en.2011-1595
112. Lau P, Fitzsimmons RL, Raichur S, Wang SC, Lechtken A, Muscat GE. The orphan nuclear receptor, RORalpha, regulates gene expression that controls lipid metabolism: staggerer (SG/SG) mice are resistant to diet-induced obesity. J Biol Chem (2008) 283(26):18411–21. doi: 10.1074/jbc.M710526200
113. Solt LA, Wang Y, Banerjee S, Hughes T, Kojetin DJ, Lundasen T, et al. Regulation of circadian behaviour and metabolism by synthetic REV-ERB agonists. Nature (2012) 485(7396):62–8. doi: 10.1038/nature11030
114. Solt LA, Banerjee S, Campbell S, Kamenecka TM, Burris TP. ROR inverse agonist suppresses insulitis and prevents hyperglycemia in a mouse model of type 1 diabetes. Endocrinology (2015) 156(3):869–81. doi: 10.1210/en.2014-1677
115. Sookoian S, Gemma C, Gianotti TF, Burgueño A, Castaño G, Pirola CJ. Genetic variants of clock transcription factor are associated with individual susceptibility to obesity. Am J Clin Nutr (2008) 87(6):1606–15. doi: 10.1093/ajcn/87.6.1606
116. Garaulet M, Lee YC, Shen J, Parnell LD, Arnett DK, Tsai MY, et al. CLOCK genetic variation and metabolic syndrome risk: modulation by monounsaturated fatty acids. Am J Clin Nutr (2009) 90(6):1466–75. doi: 10.3945/ajcn.2009.27536
117. Scott EM, Carter AM, Grant PJ. Association between polymorphisms in the clock gene, obesity and the metabolic syndrome in man. Int J Obes (Lond) (2008) 32(4):658–62. doi: 10.1038/sj.ijo.0803778
118. Corella D, Asensio EM, Coltell O, Sorlí JV, Estruch R, Martínez-González M, et al. CLOCK gene variation is associated with incidence of type-2 diabetes and cardiovascular diseases in type-2 diabetic subjects: dietary modulation in the PREDIMED randomized trial. Cardiovasc Diabetol (2016) 15:4. doi: 10.1186/s12933-015-0327-8
119. Englund A, Kovanen L, Saarikoski ST, Haukka J, Reunanen A, Aromaa A, et al. NPAS2 and PER2 are linked to risk factors of the metabolic syndrome. J Circadian Rhythms (2009) 7:5. doi: 10.1186/1740-3391-7-5
120. Woon PY, Kaisaki PJ, Bragança J, Bihoreau MT, Levy JC, Farrall M, et al. Aryl hydrocarbon receptor nuclear translocator-like (BMAL1) is associated with susceptibility to hypertension and type 2 diabetes. Proc Natl Acad Sci U S A (2007) 104(36):14412–7. doi: 10.1073/pnas.0703247104
121. Pappa KI, Gazouli M, Anastasiou E, Iliodromiti Z, Antsaklis A, Anagnou NP. The major circadian pacemaker ARNT-like protein-1 (BMAL1) is associated with susceptibility to gestational diabetes mellitus. Diabetes Res Clin Pract (2013) 99(2):151–7. doi: 10.1016/j.diabres.2012.10.015
122. Dupuis J, Langenberg C, Prokopenko I, Saxena R, Soranzo N, Jackson AU, et al. New genetic loci implicated in fasting glucose homeostasis and their impact on type 2 diabetes risk. Nat Genet (2010) 42(2):105–16. doi: 10.1038/ng.520
123. Garaulet M, Corbalán-Tutau MD, Madrid JA, Baraza JC, Parnell LD, Lee YC, et al. PERIOD2 variants are associated with abdominal obesity, psycho-behavioral factors, and attrition in the dietary treatment of obesity. J Am Diet Assoc (2010) 110(6):917–21. doi: 10.1016/j.jada.2010.03.017
124. Bouatia-Naji N, Bonnefond A, Cavalcanti-Proença C, Sparsø T, Holmkvist J, Marchand M, et al. A variant near MTNR1B is associated with increased fasting plasma glucose levels and type 2 diabetes risk. Nat Genet (2009) 41(1):89–94. doi: 10.1038/ng.277
125. Prokopenko I, Langenberg C, Florez JC, Saxena R, Soranzo N, Thorleifsson G, et al. Variants in MTNR1B influence fasting glucose levels. Nat Genet (2009) 41(1):77–81. doi: 10.1038/ng.290
126. DeBruyne JP, Weaver DR, Reppert SM. CLOCK and NPAS2 have overlapping roles in the suprachiasmatic circadian clock. Nat Neurosci (2007) 10(5):543–5. doi: 10.1038/nn1884
127. Zisapel N. New perspectives on the role of melatonin in human sleep, circadian rhythms and their regulation. Br J Pharmacol (2018) 175(16):3190–9. doi: 10.1111/bph.14116
128. Lane JM, Chang AM, Bjonnes AC, Aeschbach D, Anderson C, Cade BE, et al. Impact of common diabetes risk variant in MTNR1B on sleep, circadian, and melatonin physiology. Diabetes (2016) 65(6):1741–51. doi: 10.2337/db15-0999
129. Pulimeno P, Mannic T, Sage D, Giovannoni L, Salmon P, Lemeille S, et al. Autonomous and self-sustained circadian oscillators displayed in human islet cells. Diabetologia (2013) 56(3):497–507. doi: 10.1007/s00125-012-2779-7
130. Stamenkovic JA, Olsson AH, Nagorny CL, Malmgren S, Dekker-Nitert M, Ling C, et al. Regulation of core clock genes in human islets. Metabolism (2012) 61(7):978–85. doi: 10.1016/j.metabol.2011.11.013
131. Scheer FA, Hilton MF, Mantzoros CS, Shea SA. Adverse metabolic and cardiovascular consequences of circadian misalignment. Proc Natl Acad Sci U S A (2009) 106(11):4453–8. doi: 10.1073/pnas.0808180106
132. Leproult R, Holmbäck U, Van Cauter E. Circadian misalignment augments markers of insulin resistance and inflammation, independently of sleep loss. Diabetes (2014) 63(6):1860–9. doi: 10.2337/db13-1546
133. Czeisler CA, Duffy JF, Shanahan TL, Brown EN, Mitchell JF, Rimmer DW, et al. Stability, precision, and near-24-hour period of the human circadian pacemaker. Science (1999) 284(5423):2177–81. doi: 10.1126/science.284.5423.2177
134. Buxton OM, Cain SW, O'Connor SP, Porter JH, Duffy JF, Wang W, et al. Adverse metabolic consequences in humans of prolonged sleep restriction combined with circadian disruption. Sci Transl Med (2012) 4(129):129ra43. doi: 10.1126/scitranslmed.3003200
135. Gale JE, Cox HI, Qian J, Block GD, Colwell CS, Matveyenko AV. Disruption of circadian rhythms accelerates development of diabetes through pancreatic beta-cell loss and dysfunction. J Biol Rhythms (2011) 26(5):423–33. doi: 10.1177/0748730411416341
136. Boden G, Chen X, Urbain JL. Evidence for a circadian rhythm of insulin sensitivity in patients with NIDDM caused by cyclic changes in hepatic glucose production. Diabetes (1996) 45(8):1044–50. doi: 10.2337/diab.45.8.1044
137. Donga E, van Dijk M, van Dijk JG, Biermasz NR, Lammers GJ, van Kralingen K, et al. Partial sleep restriction decreases insulin sensitivity in type 1 diabetes. Diabetes Care (2010) 33(7):1573–7. doi: 10.2337/dc09-2317
138. Donga E, van Dijk M, van Dijk JG, Biermasz NR, Lammers GJ, van Kralingen KW, et al. A single night of partial sleep deprivation induces insulin resistance in multiple metabolic pathways in healthy subjects. J Clin Endocrinol Metab (2010) 95(6):2963–8. doi: 10.1210/jc.2009-2430
139. Coomans CP, van den Berg SA, Houben T, van Klinken JB, van den Berg R, Pronk AC, et al. Detrimental effects of constant light exposure and high-fat diet on circadian energy metabolism and insulin sensitivity. FASEB J (2013) 27(4):1721–32. doi: 10.1096/fj.12-210898
140. Versteeg RI, Stenvers DJ, Visintainer D, Linnenbank A, Tanck MW, Zwanenburg G, et al. Acute effects of morning light on plasma glucose and triglycerides in healthy men and men with type 2 diabetes. J Biol Rhythms (2017) 32(2):130–42. doi: 10.1177/0748730417693480
141. Harmsen JF, Wefers J, Doligkeit D, Schlangen L, Dautzenberg B, Rense P, et al. The influence of bright and dim light on substrate metabolism, energy expenditure and thermoregulation in insulin-resistant individuals depends on time of day. Diabetologia (2022) 65(4):721–32. doi: 10.1007/s00125-021-05643-9
142. Kohsaka A, Laposky AD, Ramsey KM, Estrada C, Joshu C, Kobayashi Y, et al. High-fat diet disrupts behavioral and molecular circadian rhythms in mice. Cell Metab (2007) 6(5):414–21. doi: 10.1016/j.cmet.2007.09.006
143. Ellacott KLJ, Morton GJ, Woods SC, Tso P, Schwartz MW. Assessment of feeding behavior in laboratory mice. Cell Metab (2010) 12(1):10–7. doi: 10.1016/j.cmet.2010.06.001
144. Arble DM, Bass J, Laposky AD, Vitaterna MH, Turek FW. Circadian timing of food intake contributes to weight gain. Obes (Silver Spring) (2009) 17(11):2100–2. doi: 10.1038/oby.2009.264
145. Hatori M, Vollmers C, Zarrinpar A, DiTacchio L, Bushong Eric A, Gill S, et al. Time-restricted feeding without reducing caloric intake prevents metabolic diseases in mice fed a high-fat diet. Cell Metab (2012) 15(6):848–60. doi: 10.1016/j.cmet.2012.04.019
146. Chaix A, Lin T, Le HD, Chang MW, Panda S. Time-restricted feeding prevents obesity and metabolic syndrome in mice lacking a circadian clock. Cell Metab (2019) 29(2):303–19.e4. doi: 10.1016/j.cmet.2018.08.004
147. Damiola F, Le Minh N, Preitner N, Kornmann B, Fleury-Olela F, Schibler U. Restricted feeding uncouples circadian oscillators in peripheral tissues from the central pacemaker in the suprachiasmatic nucleus. Genes Dev (2000) 14(23):2950–61. doi: 10.1101/gad.183500
148. Challet E, Solberg LC, Turek FW. Entrainment in calorie-restricted mice: conflicting zeitgebers and free-running conditions. Am J Physiol (1998) 274(6):R1751–61. doi: 10.1152/ajpregu.1998.274.6.R1751
149. Challet E. Interactions between light, mealtime and calorie restriction to control daily timing in mammals. J Comp Physiol B (2010) 180(5):631–44. doi: 10.1007/s00360-010-0451-4
150. Lopez-Minguez J, Saxena R, Bandín C, Scheer FA, Garaulet M. Late dinner impairs glucose tolerance in MTNR1B risk allele carriers: A randomized, cross-over study. Clin Nutr (2018) 37(4):1133–40. doi: 10.1016/j.clnu.2017.04.003
151. Rubio-Sastre P, Scheer FA, Gómez-Abellán P, Madrid JA, Garaulet M. Acute melatonin administration in humans impairs glucose tolerance in both the morning and evening. Sleep (2014) 37(10):1715–9. doi: 10.5665/sleep.4088
152. Hutchison AT, Regmi P, Manoogian ENC, Fleischer JG, Wittert GA, Panda S, et al. Time-restricted feeding improves glucose tolerance in men at risk for type 2 diabetes: A randomized crossover trial. Obes (Silver Spring) (2019) 27(5):724–32. doi: 10.1002/oby.22449
153. Wilkinson MJ, Manoogian ENC, Zadourian A, Lo H, Fakhouri S, Shoghi A, et al. Ten-hour time-restricted eating reduces weight, blood pressure, and atherogenic lipids in patients with metabolic syndrome. Cell Metab (2020) 31(1):92–104.e5. doi: 10.1016/j.cmet.2019.11.004
154. Chow LS, Manoogian ENC, Alvear A, Fleischer JG, Thor H, Dietsche K, et al. Time-restricted eating effects on body composition and metabolic measures in humans who are overweight: A feasibility study. Obes (Silver Spring) (2020) 28(5):860–9. doi: 10.1002/oby.22756
155. Quist JS, Jensen MM, Clemmensen KKB, Pedersen H, Bjerre N, Størling J, et al. Protocol for a single-centre, parallel-group, randomised, controlled, superiority trial on the effects of time-restricted eating on body weight, behaviour and metabolism in individuals at high risk of type 2 diabetes: the REStricted eating time (RESET) study. BMJ Open (2020) 10(8):e037166. doi: 10.1136/bmjopen-2020-037166
156. Świątkiewicz I, Mila-Kierzenkowska C, Woźniak A, Szewczyk-Golec K, Nuszkiewicz J, Wróblewska J, et al. Pilot clinical trial of time-restricted eating in patients with metabolic syndrome. Nutrients (2021) 13(2):346. doi: 10.3390/nu13020346
157. Wefers J, van Moorsel D, Hansen J, Connell NJ, Havekes B, Hoeks J, et al. Circadian misalignment induces fatty acid metabolism gene profiles and compromises insulin sensitivity in human skeletal muscle. Proc Natl Acad Sci U S A (2018) 115(30):7789–94. doi: 10.1073/pnas.1722295115
158. Thaiss CA, Zeevi D, Levy M, Zilberman-Schapira G, Suez J, Tengeler AC, et al. Transkingdom control of microbiota diurnal oscillations promotes metabolic homeostasis. Cell (2014) 159(3):514–29. doi: 10.1016/j.cell.2014.09.048
159. Zarrinpar A, Chaix A, Yooseph S, Panda S. Diet and feeding pattern affect the diurnal dynamics of the gut microbiome. Cell Metab (2014) 20(6):1006–17. doi: 10.1016/j.cmet.2014.11.008
160. Liang X, Bushman FD, FitzGerald GA. Rhythmicity of the intestinal microbiota is regulated by gender and the host circadian clock. Proc Natl Acad Sci United States America (2015) 112(33):10479–84. doi: 10.1073/pnas.1501305112
161. Reitmeier S, Kiessling S, Clavel T, List M, Almeida EL, Ghosh TS, et al. Arrhythmic gut microbiome signatures predict risk of type 2 diabetes. Cell Host Microbe (2020) 28(2):258–272. doi: 10.1016/j.chom.2020.06.004
162. Tremaroli V, Karlsson F, Werling M, Ståhlman M, Kovatcheva-Datchary P, Olbers T, et al. Roux-en-Y gastric bypass and vertical banded gastroplasty induce long-term changes on the human gut microbiome contributing to fat mass regulation. Cell Metab (2015) 22(2):228–38. doi: 10.1016/j.cmet.2015.07.009
163. Salgado-Delgado R, Angeles-Castellanos M, Saderi N, Buijs RM, Escobar C. Food intake during the normal activity phase prevents obesity and circadian desynchrony in a rat model of night work. Endocrinology (2010) 151(3):1019–29. doi: 10.1210/en.2009-0864
164. Qian J, Block GD, Colwell CS, Matveyenko AV. Consequences of exposure to light at night on the pancreatic islet circadian clock and function in rats. Diabetes (2013) 62(10):3469–78. doi: 10.2337/db12-1543
166. Mota MC, Silva CM, Balieiro LCT, Gonçalves BF, Fahmy WM, Crispim CA. Association between social jetlag food consumption and meal times in patients with obesity-related chronic diseases. PloS One (2019) 14(2):e0212126. doi: 10.1371/journal.pone.0212126
167. Mota MC, Silva CM, Balieiro LCT, Fahmy WM, Crispim CA. Social jetlag and metabolic control in non-communicable chronic diseases: a study addressing different obesity statuses. Sci Rep (2017) 7(1):6358. doi: 10.1038/s41598-017-06723-w
168. Penev PD, Kolker DE, Zee PC, Turek FW. Chronic circadian desynchronization decreases the survival of animals with cardiomyopathic heart disease. Am J Physiol (1998) 275(6):H2334–7. doi: 10.1152/ajpheart.1998.275.6.H2334
169. Casiraghi LP, Oda GA, Chiesa JJ, Friesen WO, Golombek DA. Forced desynchronization of activity rhythms in a model of chronic jet lag in mice. J Biol Rhythms (2012) 27(1):59–69. doi: 10.1177/0748730411429447
170. Kiessling S, Eichele G, Oster H. Adrenal glucocorticoids have a key role in circadian resynchronization in a mouse model of jet lag. J Clin Invest (2010) 120(7):2600–9. doi: 10.1172/jci41192
171. Schibler U, Ripperger J, Brown SA. Peripheral circadian oscillators in mammals: time and food. J Biol Rhythms (2003) 18(3):250–60. doi: 10.1177/0748730403018003007
172. Le Minh N, Damiola F, Tronche F, Schütz G, Schibler U. Glucocorticoid hormones inhibit food-induced phase-shifting of peripheral circadian oscillators. EMBO J (2001) 20(24):7128–36. doi: 10.1093/emboj/20.24.7128
173. Balsalobre A, Brown SA, Marcacci L, Tronche F, Kellendonk C, Reichardt HM, et al. Resetting of circadian time in peripheral tissues by glucocorticoid signaling. Science (2000) 289(5488):2344–7. doi: 10.1126/science.289.5488.2344
174. Rosenfeld P, Van Eekelen JA, Levine S, De Kloet ER. Ontogeny of the type 2 glucocorticoid receptor in discrete rat brain regions: an immunocytochemical study. Brain Res (1988) 470(1):119–27. doi: 10.1016/0165-3806(88)90207-6
175. Doi M, Takahashi Y, Komatsu R, Yamazaki F, Yamada H, Haraguchi S, et al. Salt-sensitive hypertension in circadian clock-deficient cry-null mice involves dysregulated adrenal Hsd3b6. Nat Med (2010) 16(1):67–74. doi: 10.1038/nm.2061
176. Panda S. The arrival of circadian medicine. Nat Rev Endocrinol (2019) 15(2):67–9. doi: 10.1038/s41574-018-0142-x
177. Adam D. Core concept: Emerging science of chronotherapy offers big opportunities to optimize drug delivery. Proc Natl Acad Sci United States America (2019) 116(44):21957–9. doi: 10.1073/pnas.1916118116
178. Mayer D. The circadian rhythm of synthesis and catabolism of cholesterol. Arch Toxicol (1976) 36(3-4):267–76. doi: 10.1007/bf00340534
179. Shivaprasad C, Kalra S. Bromocriptine in type 2 diabetes mellitus. Indian J Endocrinol Metab (2011) 15(Suppl 1):S17–24. doi: 10.4103/2230-8210.83058
180. Mahajan R. Bromocriptine mesylate: FDA-approved novel treatment for type-2 diabetes. Indian J Pharmacol (2009) 41(4):197–8. doi: 10.4103/0253-7613.56070
181. Vinik AI, Cincotta AH, Scranton RE, Bohannon N, Ezrokhi M, Gaziano JM. Effect of bromocriptine-QR on glycemic control in subjects with uncontrolled hyperglycemia on one or two oral anti-diabetes agents. Endocr Pract (2012) 18(6):931–43. doi: 10.4158/ep12187.Or
182. Henriksson E, Huber AL, Soto EK, Kriebs A, Vaughan ME, Duglan D, et al. The liver circadian clock modulates biochemical and physiological responses to metformin. J Biol Rhythms (2017) 32(4):345–58. doi: 10.1177/0748730417710348
183. Um JH, Yang S, Yamazaki S, Kang H, Viollet B, Foretz M, et al. Activation of 5'-AMP-activated kinase with diabetes drug metformin induces casein kinase iepsilon (CKIepsilon)-dependent degradation of clock protein mPer2. J Biol Chem (2007) 282(29):20794–8. doi: 10.1074/jbc.C700070200
184. Barnea M, Haviv L, Gutman R, Chapnik N, Madar Z, Froy O. Metformin affects the circadian clock and metabolic rhythms in a tissue-specific manner. Biochim Biophys Acta (2012) 1822(11):1796–806. doi: 10.1016/j.bbadis.2012.08.005
185. Wu H, Esteve E, Tremaroli V, Khan MT, Caesar R, Mannerås-Holm L, et al. Metformin alters the gut microbiome of individuals with treatment-naive type 2 diabetes, contributing to the therapeutic effects of the drug. Nat Med (2017) 23(7):850–8. doi: 10.1038/nm.4345
186. Baker IA, Jarrett RJ. Diurnal variation in the blood-sugar and plasma-insulin response to tolbutamide. Lancet (1972) 2(7784):945–7. doi: 10.1016/s0140-6736(72)92471-3
187. He B, Nohara K, Park N, Park YS, Guillory B, Zhao Z, et al. The small molecule nobiletin targets the molecular oscillator to enhance circadian rhythms and protect against metabolic syndrome. Cell Metab (2016) 23(4):610–21. doi: 10.1016/j.cmet.2016.03.007
188. Humphries PS, Bersot R, Kincaid J, Mabery E, McCluskie K, Park T, et al. Carbazole-containing sulfonamides and sulfamides: Discovery of cryptochrome modulators as antidiabetic agents. Bioorg Med Chem Lett (2016) 26(3):757–60. doi: 10.1016/j.bmcl.2015.12.102
189. Wang S, Li F, Lin Y, Wu B. Targeting REV-ERBα for therapeutic purposes: promises and challenges. Theranostics (2020) 10(9):4168–82. doi: 10.7150/thno.43834
190. Roenneberg T, Pilz LK, Zerbini G, Winnebeck EC. Chronotype and social jetlag: A (Self-) critical review. Biol (Basel) (2019) 8(3):54. doi: 10.3390/biology8030054
Keywords: circadian rhythm, pancreas, insulin, metabolism, obesity
Citation: Chan K, Wong FS and Pearson JA (2022) Circadian rhythms and pancreas physiology: A review. Front. Endocrinol. 13:920261. doi: 10.3389/fendo.2022.920261
Received: 14 April 2022; Accepted: 21 July 2022;
Published: 10 August 2022.
Edited by:
Undurti Narasimha Das, UND Life Sciences LLC, United StatesReviewed by:
Scott Deibel, Memorial University of Newfoundland, CanadaAgung Julius, Rensselaer Polytechnic Institute, United States
Copyright © 2022 Chan, Wong and Pearson. This is an open-access article distributed under the terms of the Creative Commons Attribution License (CC BY). The use, distribution or reproduction in other forums is permitted, provided the original author(s) and the copyright owner(s) are credited and that the original publication in this journal is cited, in accordance with accepted academic practice. No use, distribution or reproduction is permitted which does not comply with these terms.
*Correspondence: James Alexander Pearson, cGVhcnNvbmoxQGNhcmRpZmYuYWMudWs=