- 1Department of Endocrinology and Metabolism, The Affiliated Hospital of Qingdao University, Qingdao, China
- 2Department of Nephrology, The Affiliated Hospital of Qingdao University, Qingdao, China
- 3Department of Geriatric Medicine, Yantai Yuhuangding Hospital Affiliated Hospital of Qingdao University, Yantai, China
Type 2 diabetes mellitus (T2DM) is a risk factor for osteoporosis. The effects of T2DM and anti-diabetic agents on bone and mineral metabolism have been observed. Sodium–glucose co-transporter 2 inhibitors (SGLT-2is) promote urinary glucose excretion, reduce blood glucose level, and improve the cardiovascular and diabetic nephropathy outcomes. In this review, we focused on the extraglycemic effect and physiological regulation of SGLT-2is on bone and mineral metabolism. SGLT-2is affect the bone turnover, microarchitecture, and bone strength indirectly. Clinical evidence of a meta-analysis showed that SGLT-2is might not increase the risk of bone fracture. The effect of SGLT-2is on bone fracture is controversial, and further investigation from a real-world study is needed. Based on its significant benefit on cardiovascular and chronic kidney disease (CKD) outcomes, SGLT-2is are an outstanding choice. Bone mineral density (BMD) and fracture risk evaluation should be considered for patients with a high risk of bone fracture.
Background
Type 2 diabetes mellitus (T2DM) is a common metabolic disease that affects individuals worldwide. T2DM is commonly associated with obesity, hypertension, heart failure, hyperuricemia, renal failure, proteinuria, and osteoporosis (1). Patients with T2DM show increased risks of cardiovascular or renal complications, resulting in the main causes of morbidity and mortality (2). Thus, the therapeutic strategy for T2DM concerns with the benefits on cardiovascular–renal complications. Among the glucose-lowering agents, sodium–glucose co-transporter inhibitors (SGLT-2is) are highly recommended for patients with T2DM, especially those with a high cardiovascular or renal risk, due to their remarkable cardiorenal protective effects (3, 4).
T2DM is one of the common risk factors associated with osteoporosis and osteoporotic fracture. As oral anti-diabetic agents, SGLT-2is inhibit the reabsorption of sodium and glucose, reduce the blood glucose level, and cause negative energy balance and body weight loss. However, the effects of SGLT-2is on bone and mineral metabolism and bone fracture risk remain controversial. In this review, we screened publications (Supplementary Figure S1) and focused on the extraglycemic effects of SGLT-2is on bone and mineral metabolism and osteoporotic fracture.
Glucose-Lowering Mechanism
The sodium–glucose linked transporter (SGLT) family has at least six isoforms. At present, SGLT-1 (encoded by the SGLT1 gene, also known as SLC5A1) and SGLT-2 (encoded by the SGLT2 gene, also known as SLC5A2) are known for their critical role in regulating the reabsorption and transportation of sodium and glucose (5). SGLT-1 accounts for most of the dietary glucose uptake by the intestinal tract and the transmembrane transport of glucose and galactose through the brush margin of the small intestine, while SGLT-2 is responsible for the majority of glucose reuptake in the tubular system of the kidney (6). There are two main types of glucose transporters: SGLTs, which co-transport sodium/glucose by an electrochemical gradient across the membrane, and glucose transporters (GLUTs), through which glucose is transported by facilitated diffusion. In proximal tubules of the kidney, SGLT-2 acts synergistically with GLUT-2. SGLT-2 reabsorbs sodium and glucose from the luminal side of proximal tubules. Glucose is transported into the blood via GLUT-2 located in the lateral basement membrane. SGLT-2is inhibit the reabsorption of sodium and glucose and increase the excretion of urine. On the other hand, the reduced reabsorption of sodium leads to an increased delivery of sodium to dense plaques. Due to the stimulation of the tubulo-glomerular feedback, as well as the contraction of the afferent glomerular arteriole, glomerular hyperperfusion and hyperfiltration are weakened (Figure 1) (7).
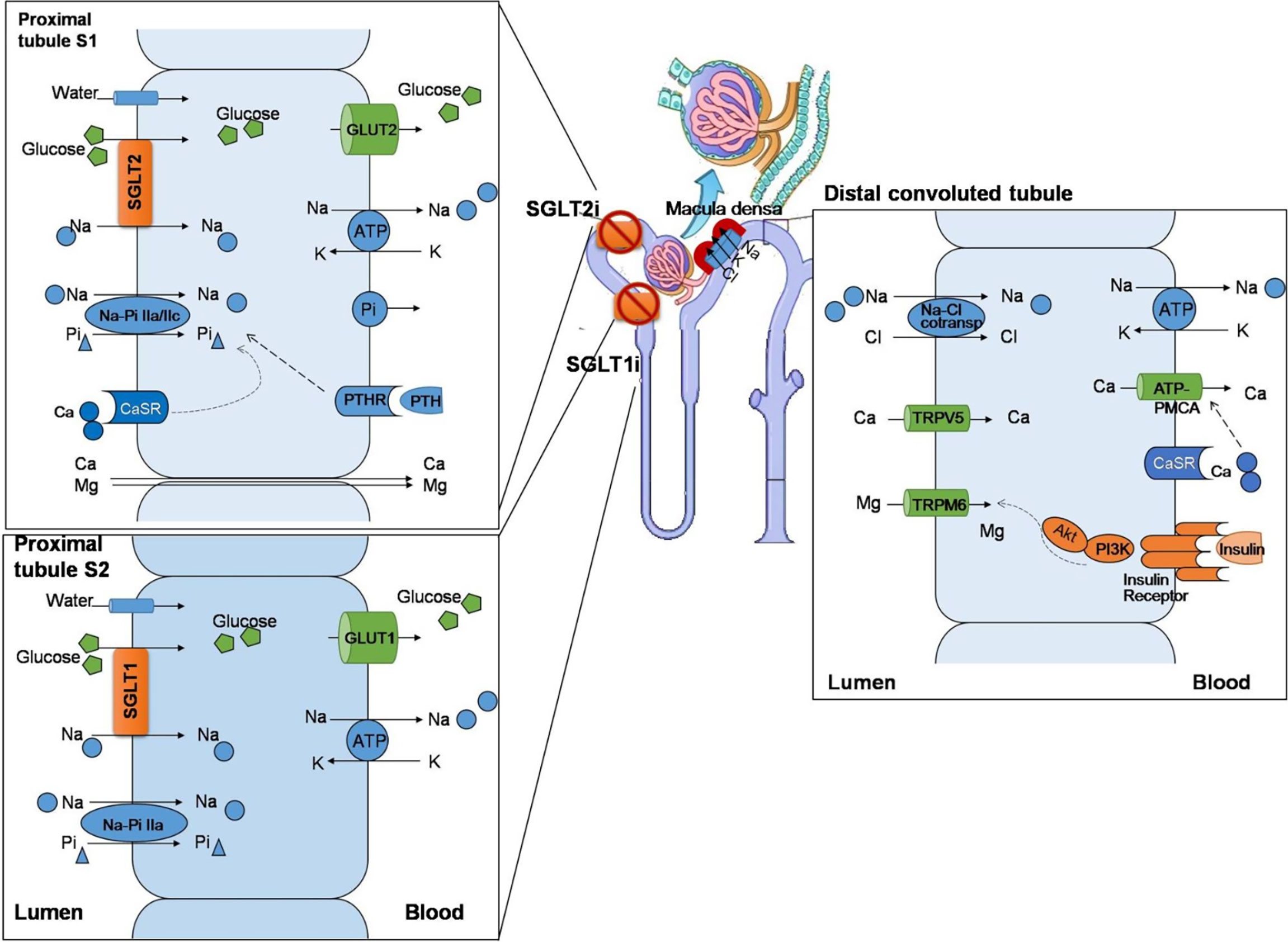
Figure 1 The effect of sodium–glucose co-transporters (SGLTs) on the regulation of mineral ions in renal tubules. The SGLT-1/SGLT-2 co-transporters are mainly expressed in proximal renal tubules, regulating the reabsorption of sodium and glucose. SGLT-2 reabsorbs sodium and glucose from the lumen side of proximal tubules and acts synergistically with glucose transporters (GLUTs) to transport glucose into the blood. In addition, the Na–Pi IIa/c co-transporters are expressed in the lumen side, which synergistically regulate the transport of sodium and phosphorus. Calcium and magnesium pass through intercellular channels. In proximal tubules, the calcium-sensing receptor (CaSR) is located on the lumen side, which senses the concentration of extracellular calcium and synergy with the parathyroid hormone receptor (PTHR), which is stimulated by the parathyroid hormone (PTH), to regulate the transporter function and maintain the hemostasis of calcium/phosphate. Sodium–glucose co-transporter 2 inhibitors (SGLT-2is) increase the excretion of urinary sodium and glucose. The increased sodium delivery in distal convoluted tubules stimulates dense plaques, triggers tubulo-glomerular feedback, and shrinks the afferent glomerular arteriole. In distal convoluted tubules, the insulin receptor is expressed in the blood side. Magnesium reabsorption passes via transient receptor potential melastatin type 6 (TRPM6), and the channel activity is suppressed by insulin binding to the insulin receptor via the PI3K/Akt signaling pathway. In this way, SGLT-1 and SGLT-2 play an important role in regulating mineral metabolism.
Based on these mechanisms, SGLT-2is have been developed as oral anti-diabetic agents. They lower the renal threshold for glucose (RTG) and promote urinary glucose and sodium excretion and osmotic diuresis. SGLT-2is reduce the levels of blood glucose and glycosylated hemoglobin; they also lower the risk of major adverse cardiovascular events, including cardiovascular death, non-fatal myocardial infarction, non-fatal stroke, and risk of hospitalization for heart failure in T2DM patients with established cardiovascular diseases or risk factors (4). In addition, SGLT-2is reduce the progression to albuminuria, decrease the composite outcome of end-stage renal disease, and lower the need for renal replacement therapy or death from renal causes (8). They improve the outcomes of cardiovascular events and the prognosis of heart failure and diabetic kidney disease (DKD). Among the currently commercially available SGLT-2is, empagliflozin, dapagliflozin, ipragliflozin, ertugliflozin, luseogliflozin, and tofogliflozin can selectively inhibit the activity of SGLT-2, while canagliflozin and sotagliflozin also inhibit SGLT-1 (5). In this review, we aimed to provide an update on the regulatory mechanisms and clinical evidence on the bone and mineral effects of SGLT-2is.
Characteristics of the Bone Metabolism Abnormalities on Diabetic Bone Disease
In patients with T2DM, the bone mineral density (BMD) tends to be increased, but with an increased fracture risk. This phenomenon of a higher BMD may be related to the anabolic effect of hyperinsulinemia. However, an abnormal bone metabolism is characterized by a low bone turnover (9) and increased bone brittleness, leading to the high risk of fracture. The fracture risk could be caused by a decreased bone quality and strength, cortical bone porosity, and impaired bone microarchitecture. The decrease of bone quality in patients with diabetic bone disease (DBD) is accompanied by bone remodeling disorders (10). The pathophysiological mechanism underlying bone fragility in T2DM is complex. Possible mechanisms beyond bone include hyperglycemia, oxidative stress, and tissue-specific accumulation of advanced glycation end products (AGEs) that compromise collagen properties and increase bone marrow adiposity, releasing inflammatory factors and adipokines from visceral fat, chronic inflammatory microenvironment, and potentially altering the function of osteoblasts and osteocytes (11). In diabetic rats, the abnormal accumulation of AGEs in collagen promotes oxidative stress and local inflammatory response and induces the apoptosis of osteoblasts (12). T2DM complications such as retinopathy and cataracts, diabetic neuropathy, and postural hypotension may also contribute to the increased frequency of falls and the consequential bone fractures (13). In aging T2DM patients with fatigue or hypoglycemia, the increased propensity for falls increases the risk of fracture. Furthermore, some anti-diabetic agents may directly or indirectly affect BMD and bone turnover (14). For example, thiazolidinedione, the ligand of proliferator-activated receptor gamma (PPARγ), can directly inhibit the activity of osteoblasts, reduce bone formation and BMD, and increase the risk of fracture (15). Observational studies have shown that exposure to exogenous insulin increases the risk of hip and vertebral fracture, despite the established anabolic effects of insulin on bone matrix synthesis (13).
Effect of SGLT-2is on Mineral Metabolism
Based on the renal mechanism of SGLT-2is, they may have potential effects on electrolyte homeostasis and bone mineral metabolism. There are multiple interweaving networks of SGLT-2i on mineral and bone metabolism, including affecting the metabolism of electrolytes and calcium/phosphorus (Figure 1) and increasing the parathyroid hormone (PTH) and reducing 1,25-(OH)2-vitamin D levels (16) (Figure 2).
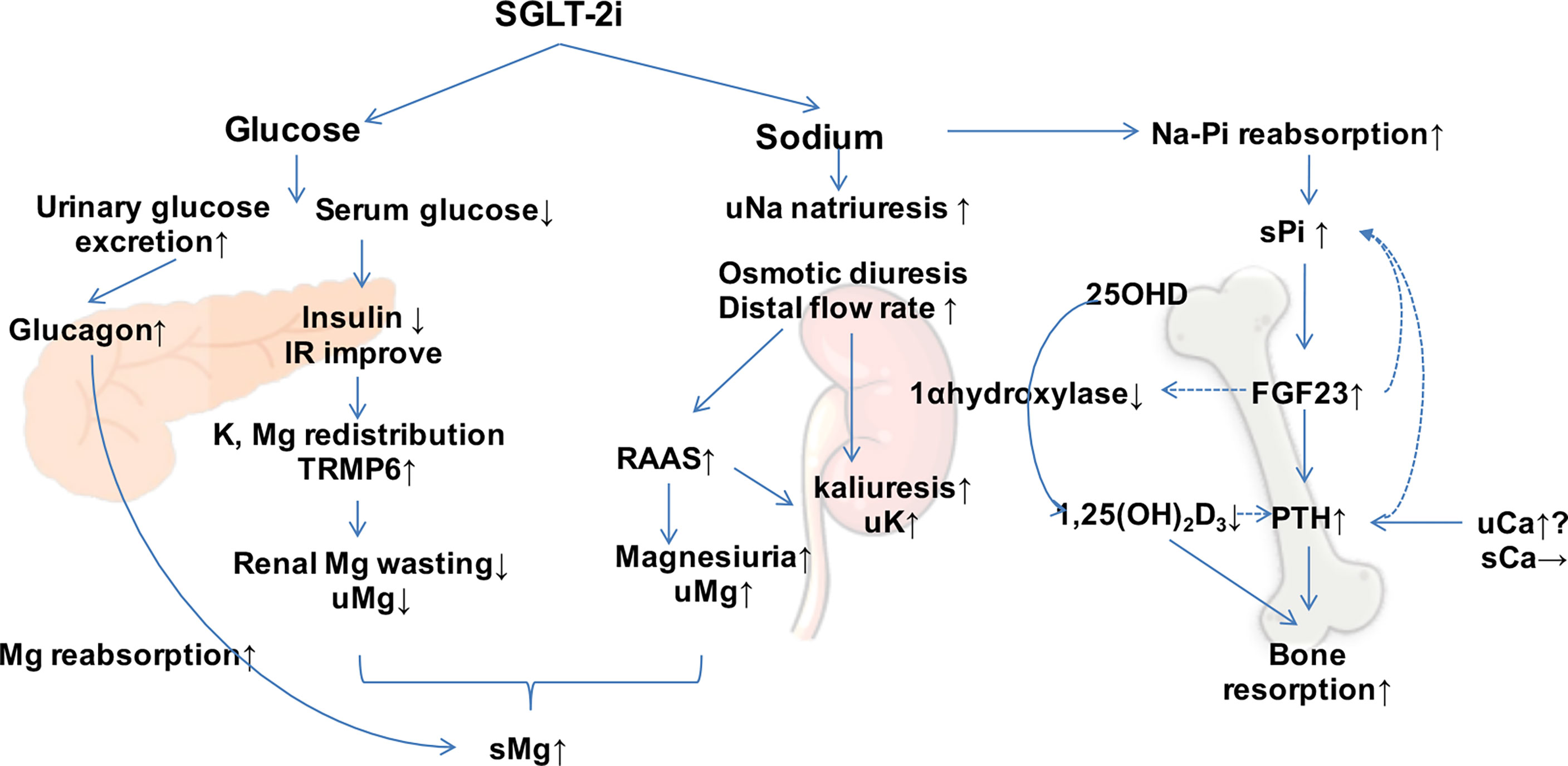
Figure 2 Effect of sodium–glucose co-transporter 2 inhibitors (SGLT-2is) on mineral metabolism. SGLT-2is increase the excretion of urinary glucose and sodium and regulate minerals directly and indirectly. Dotted arrows show suppressive effects. s, serum; u, urinary; Mg, magnesium; Ca, calcium; Na, sodium; Pi, phosphorus; PTH, parathyroid hormone; FGF23, fibroblast growth factor 23; IR, insulin resistance; RAAS, renin–angiotensin–aldosterone system.
Sodium
SGLT-2 regulates sodium–glucose reabsorption. In a healthy population, more than 90% of glucose is absorbed through SGLT-2, with less than 10% through SGLT-1. However, the expressions of SGLT-2 and SGLT-1 are upregulated and activated in patients with T2DM, leading to the increased sodium reabsorption in proximal tubules. The excess sodium and glucose accumulate downstream and are reabsorbed supplementarily via other sodium transporters such as SGLT-1 and Na+/H+ exchange protein 3 (NHE3). Thus, sodium uptake is reduced in the dense plaque in order to stimulate the tubulo-glomerular balance feedback regulating system. On the other hand, the decreased synthesis of vasoconstrictor factors, which act on afferent glomerular arterioles, causes an increased renal blood flow and glomerular capillary hydrostatic pressure (17, 18). All of these mechanisms lead to an increased glomerular filtration, which is the pathophysiological basis of increased intraglomerular pressure and DKD. SGLT-2is can reverse these phenomena, regulate the co-transport of sodium–glucose, and modulate the excretion of sodium. In this manner, SGLT-2is play a vital role in reducing the volume load and lowering blood pressure.
One third of sodium is stored in the bone matrix, of which 40% is exchangeable with circulating sodium (19). It has been hypothesized that sodium is released from bones in an attempt to maintain sodium homeostasis, similar to the release of calcium during hypocalcemia (20). Bone resorption increases in the condition called relative hyponatremia, leading to the reduction of BMD and the increase of fracture risk. According to data from the U.S. National Health and Nutrition Examination Survey database, chronic hyponatremia increases the risk of hip fracture (21). In a hyponatremia animal model, a significant decrease in vertebral and cortical BMD was observed. The increased sensitivity of osteoclasts to extracellular sodium stimulates osteoclast differentiation and bone resorption, without a change in the osteoblast number or the bone mineralization rate (21). An in vitro study using murine pre-osteoclastic RAW 264.7 cells indicated that a decrease of the medium sodium level could increase the maturation and activity of osteoclasts. A low sodium concentration triggered osteoclast-mediated bone resorption mobilization (22). In addition, SGLT-2is induced hypovolemia, and relative “hyponatremia” may be accompanied by fatigue, weakness, or other psychosomatic and neurological symptoms, prone to coexist with cardiovascular, respiratory, and metabolic chronic diseases. All these factors increase the risk of falls and fractures.
Magnesium
SGLT-2is can elevate serum magnesium levels. A meta-analysis of 18 randomized controlled trials (RCTs) that involved 15,309 patients with T2DM administered four SGLT-2is (canagliflozin, empagliflozin, dapagliflozin, and ipragliflozin) showed that SGLT-2is can increase serum magnesium levels. Canagliflozin increased serum magnesium in a linear dose-dependent manner (23). Patients with T2DM often exhibit an increased urinary magnesium excretion, which leads to hypomagnesemia (24). Magnesium deficiency might affect BMD. Hypomagnesemia could reduce bone stiffness, increase osteoclast activity, and decrease osteoblast-induced bone formation. It also interferes with PTH and 1,25-(OH)2-vitamin D, reduces vitamin D synthesis and activation, and promotes inflammation/oxidative stress, which subsequently causes bone loss (25). Hypomagnesemia promotes inflammation, linking the relationship between chronic inflammation and bone loss (26). In magnesium-deficient rodents, the levels of TNF-α, IL-1, and IL-6 were increased both in serum and the bone marrow microenvironment (27). Furthermore, substance P is highly released during magnesium deficiency. To enhance the secretion of pro-inflammatory cytokines, substance P released on the nerve endings in bones stimulated the activity of osteoclasts (28). On the other hand, increased urinary magnesium may be associated with insulin resistance due to the decreased activity of the transient receptor potential melastatin type 6 (TRPM6) cation channel in distal renal collecting tubules (29). Type 2 obese diabetic rats showed downregulation of TRPM6 channel expression, hypermagneuria, and hypomagnesemia (30).
Clinical studies have suggested that SGLT-2is can increase the serum magnesium levels in patients with T2DM (31, 32). Osmotic diuresis results in a decrease in the extracellular volume load, which leads to a mild increase in blood magnesium levels (33). In addition, SGLT-2is induce elevated glucagon concentration and increase the magnesium reabsorption in distal tubules, also affecting magnesium homeostasis (34). SGLT-2is reduce the circulating insulin levels and improve insulin resistance, possibly leading to an increased TRPM6 activity and a reduced urinary magnesium excretion. Moreover, insulin promotes the transfer of magnesium into cells and interferes with the distribution of intercellular and extracellular magnesium (35). SGLT-2is also increase the concentration of aldosterone by natriuretic function and reduce the volume load, which has a direct effect on the transport of magnesium, causing its increased excretion (36). The complex mechanism of SGLT-2is on magnesium hemostasis has not been fully clarified. The regulatory mechanisms of magnesium ultimately result in a mild increase in serum magnesium levels.
Two-thirds of magnesium is stored in bone tissue. Magnesium directly regulates bone metabolism by modulating bone growth and bone strength. Magnesium deficiency results in a decreased osteoblast function and an increased osteoclast activity (28). An animal model of magnesium deficiency showed reduced trabecular number and thickness. The histological analysis revealed that the bone formation was reduced and the bone resorption was enhanced. On the contrary, the trabecular bone volume, maximum load, and elastic modulus were reduced (37). This may explain the mechanism of SGLT-2is improving bone metabolism by elevating the serum magnesium levels. On the other hand, magnesium indirectly influences bone metabolism by regulating the levels of PTH and 1,25-(OH)2-vitamin D. Basic research has suggested that magnesium is involved in improving the regulation of local inflammatory microenvironment and oxidative stress, which may indirectly affect bone metabolic processes (38).
Calcium
SGLT-2is cause a mild increase in urinary calcium excretion without obvious changes in the serum calcium levels (39). SGLT-2is inhibit the reabsorption of glucose in renal tubules, leading to osmotic diuresis, which increases tubular blood flow and reduces the calcium reabsorption in proximal tubules and the difference of the calcium gradient between tubules and the renal interstitium (40). A high urinary calcium is often observed in congenital transporter dysfunction, such as inherited SGLT-2 deficiency and familial renal glycosuria (41), similar to the pharmacological changes of SGLT-2is. An elevated urinary calcium excretion leads to a feedback increase in the PTH level, which stimulates bone turnover. PTH can increase serum calcium and decrease phosphorus by mobilizing bone osteolysis and enhancing urine phosphorus excretion. Patients with T2DM treated with canagliflozin showed reduced BMD in the hip. At pharmacological doses, canagliflozin did not significantly elevate urinary calcium or magnesium excretion. This might be due to the incomplete blockage of the transporter and compensation by SGLT-1, which increased the reabsorption of calcium from the distal nephron (42). SGLT-2is stimulate an increased urinary calcium excretion and increased renal phosphate reabsorption, leading to a decrease in the levels of active vitamin D, further reducing calcium absorption through the gastrointestinal tract. This possibly affects bone mineralization. However, more large-scale clinical trials are needed to confirm the link between calcium hemostasis affected by SGLT-2is.
Phosphorus
SGLT-2is inhibit sodium–glucose co-transport and reabsorption. Sodium-dependent sodium–phosphate transporters IIa (Na–Pi IIa, encoded by SLC24A1) and IIc (Na–Pi IIc, encoded by SLC34A3) are activated, and phosphate reabsorption is increased in proximal tubules to maintain the sodium concentration gradient in the tubule (43). In a single-blind, randomized crossover study, 25 healthy volunteers were observed to have a slight but significant increase in serum phosphorus levels after the administration of canagliflozin. On the other hand, the levels of serum fibroblast growth factor 23 (FGF23) and PTH were elevated, while that of 1,25-(OH)2-vitamin D showed a continuous reduction (44). A sustained increase in PTH enhances bone resorption and increases the risk of bone fracture. Similarly, increased levels of FGF23 are associated with bone metabolic disorders. Reduced 1,25-(OH)2-vitamin D levels diminished the absorption of calcium from the intestine and impaired bone calcification (43). FGF23 is an important hormone regulating the excretion of urinary phosphorus. However, it has adverse extrarenal outcomes such as heart failure and cardiovascular events. FGF23 is secreted by mature osteocytes to regulate intestinal phosphorus uptake and urinary excretion simultaneously and to maintain phosphorus hemostasis. Hyperphosphatemia stimulates the synthesis of FGF23 by osteocytes. FGF23 inhibits the reabsorption of phosphorus in proximal tubules, promotes the excretion of urinary phosphorus by downregulating the expression of the Na–Pi IIa transporter, and reduces the release of phosphate from the bone by inhibiting the secretion of PTH. Furthermore, FGF23 reduces the conversion of 25-hydroxyvitamin D (25-OHD) to active 1,25-(OH)2-vitamin D by inhibiting the activity of 1-α-hydroxylase, which results in a reduced phosphate absorption through the intestine (45). In a diabetic mouse model, canagliflozin induced a significant decrease in the messenger RNA (mRNA) expression of 25-OHD–1alpha hydroxylase (encoded by CYP27B1) (46). In turn, a low vitamin D level feedback stimulates the release of PTH and decreases the absorption of calcium through the intestine. It has been reported that the application of canagliflozin is associated with elevated blood phosphorus levels in diabetic patients (47). Treatment with dapagliflozin results in elevated serum phosphorus levels, accompanied by significant increases in the levels of PTH and serum FGF23 (48). SGLT-2is stimulate the reabsorption of renal phosphorus and the excretion of urinary calcium, leading to a feedback increase in the levels of FGF23 and PTH and a decrease in active vitamin D. However, the increased levels of PTH and FGF23 further promote the excretion of phosphorus. In T2DM patients with chronic renal insufficiency and renal bone disease, hyperphosphatemia causes dysfunction of the FGF23–1,25-(OH)2-vitamin D–PTH axis and exacerbates the risk of fracture (49) (Figure 2).
Effect of SGLT-2is on Bone Turnover
Bone turnover determines the dynamic balance between osteoclast-induced bone resorption and osteoblast-mediated bone formation, which is the key factor determining bone quality. Patients with DBD show bone metabolic abnormalities with features of low bone turnover (50). SGLT-1 and SGLT-2 are not expressed in mouse cranial osteoblasts, bone marrow macrophages, or mature osteoclasts (51). Therefore, the effects of SGLT-2is on bone metabolism and bone turnover may be indirect. The effect on bone remodeling differs from that of SGLT-2is. Canagliflozin increases the level of type I collagen carboxyl-terminal peptide β special sequence (β-CTX), a marker of bone resorption. At the same time, the bone formation marker osteocalcin was enhanced significantly (52). However, dapagliflozin and empagliflozin showed no significant effect on type I procollagen amino-terminal propeptide (PINP) or β-CTX (53). A subgroup analysis of a randomized controlled study was carried out to evaluate the effects of ipragliflozin on bone in Japanese patients with T2DM (baseline body mass index, ≥22 kg/m2; hemoglobin A1c, 7%–10%). The results showed that the SGLT-2i ipragliflozin had a negative effect on both bone and muscle content. The levels of tartrate-resistant acid phosphatase 5b (TRACP-5b), a bone resorption marker, increased from baseline between 12 and 24 weeks in the ipragliflozin group. The levels of bone-specific alkali phosphatase (BAP), a bone formation marker, were not significantly changed, suggesting that ipragliflozin promoted bone resorption, but not bone formation. However, there were no significant changes in vertebral BMD over 24 weeks. A long-term study is required for further understanding of the effects of ipragliflozin on BMD and bone remodeling (54). In addition, weight loss induced by SGLT-2is may also affect BMD and bone turnover. An RCT study showed that elderly obese patients exhibited reduced hip BMD after weight loss. On the other hand, the bone formation marker osteocalcin and the bone resorption marker β-CTX were simultaneously elevated, suggesting that body weight loss may enhance bone turnover and lead to negative bone regulation (55). Adiponectin, an anti-inflammatory cytokine, is upregulated after fat loss, which also modulates the osteoclast response activity by inhibiting the ratio of the receptor activator of NFκB ligand to osteoprotegerin (RANKL/OPG) (56). The activation of adiponectin receptors in osteoblasts inhibits sclerostin, the Wnt pathway inhibitor, and promotes osteoblast differentiation and maturation (57). The mechanism of the effect of SGLT-2is on bone turnover is complex and inconclusive. Firm evidence from larger-scale clinical trials is still needed.
Effect of SGLT-2is on Bone Microarchitecture and Bone Strength
Microstructure abnormalities in vertebral and cortical bone can be observed in postmenopausal patients with T2DM. The classic feature is characterized as an increased porosity in cortical bone (58), which disturbs bone strength and increases the risk of fracture. In a clinical study involving 716 patients with T2DM who received a 52-week administration of canagliflozin, there were no significant differences in vertebral and femoral neck BMD and bone strength compared to the placebo group (52). Japanese researchers recruited patients with T2DM and investigated whether the SGLT-2i luseogliflozin, with a higher SGLT-2 selectivity, could affect the bone microarchitecture. The effect of luseogliflozin on bone metabolism was evaluated using high-resolution peripheral quantitative computed tomography (HR-pqCT), which was expected to provide direct in vivo morphometric information about the bone microarchitecture (59). Due to the limitations of the specimen and the detection method, further clinical investigations of the effects of SGLT-2is on bone matrix mineralization, collagen synthesis, and biomechanics of the bone microstructure are expected in the future. Subsequently, the effects of SGLT-2is on bone microstructure and bone strength will be elucidated from molecular mechanism and animal studies to clinical outcomes in depth.
Effect of SGLT-2is on Bone in Animal Models
In animal models of diabetes, deficits in the trabecular bone microarchitecture can be observed, including decreased trabecular bone volume (BV/TV), trabecular number (Tb.N), and trabecular thickness (Tb.Th), with increased trabecular spacing (Tb.Sp) and changes in the trabecular shape (SMI). In addition, diabetic mice exhibited deficits in cortical bone content combined with increased cortical porosity and decreased material properties, bending strength, and toughness. The structural properties including structure strength and stiffness were reduced in mice with diabetes mellitus (DM). All these factors contribute to bone fracture (39). Slc5a2 mutant mice with SGLT2 deletion showed a reduction in cortical BMD, mild defects in cortical bone mineralization, and normal bone remodeling (60). In animal studies (Table 1), canagliflozin showed negative bone effects, including reduced femoral BV/TV and Tb.N and increased Tb.Sp. However, its administration combined with insulin prevented DM-related deterioration in the bone microarchitecture and bone strength (39, 61). In T2DM mouse models, canagliflozin partially improved the cortical and tracecular bone deficits (62). Dapagliflozin ameliorated bone tissue material properties, bone matrix strength, and matrix biomechanics (maximum load, indentation modulus, and hardness) (63).
Effect of SGLT-2is on the Risk of Fracture
The effects of SGLT-2is on the risk of fracture still remain controversial. They may vary across different agents (Tables 2 and 3). In the Canagliflozin Cardiovascular Assessment (CANVAS) Study, bone fractures and a decreased total hip BMD in the canagliflozin group were significantly higher than those in the placebo group. However, no increased risk was observed in the CANVAS—Renal (CANVAS-R) Study (hazard ratio, HR = 0.86) (65). In the Dapagliflozin Effect on Cardiovascular Events (DECLARE) Study including 17,160 patients with T2DM, the risk of fracture in the dapagliflozin group was comparable to that in the placebo group (66). Similarly, the EMPA-REG-OUTCOME [BI 10773 (Empagliflozin) Cardiovascular Outcome Event Trial in Type 2 Diabetes Mellitus Patients] Study showed that the risk of fracture was 1.4%–1.7% in the empagliflozin group at different doses (10 or 25 mg/day), both of which were comparable to those in the placebo group (67). In the VERTIS CV (Evaluation of Ertugliflozin Efficacy and Safety Cardiovascular Outcomes Trial) Study, the administration of ertugliflozin did not significantly increase the risk of fracture (68). Among the presently available SGLT-2is, canagliflozin has been reported to be associated with an increased risk of fracture (69).
A summary of the clinical evidence of the meta-analysis results of SGLT-2is on bone fracture is shown in Table 4 A systematic review and network meta-analysis compared the effects of anti-diabetic agents on fracture risk. The results indicated that empagliflozin and ertugliflozin may increase the risk of fracture, while dapagliflozin may show benefits (70). A study that included four SGLT-2is (dapagliflozin, empagliflozin, canagliflozin, and ertugliflozin) aimed to evaluate their safety in three chronic diseases: T2DM, chronic heart failure (CHF), and CKD. SGLT-2is showed increased trends of risk of fracture [risk ratio (RR) = 1.07, 95%CI = 0.99–1.16, p = 0.081] (71). The results of a meta-analysis of 38 RCTs showed fracture odds ratios (ORs) of canagliflozin, dapaglizin, and empagliflozin of 1.15, 0.68, and 0.93, respectively, compared to placebo (72). There was no increase in the risk of bone fracture observed among patients with T2DM treated with SGLT-2is compared with placebo. Another meta-analysis of 20 clinical studies also found similar results (73). A nationwide Medicare cohort of older patients with T2DM initiated on SGLT-2is did not show associations with an increased risk of fracture compared with the initiation of DPP-4i or GLP-1RA, with consistent results across categories of frailty, age, and insulin use (81). A meta-analysis of safety profiles comparing canagliflozin, empagliflozin, and tofogliflozin in Japanese patients with T2DM indicated that SGLT-2is were related to a similar risk of fracture to placebo (RR = 0.85, 95%CI = 0.20–3.61) (82). This is consistent with data on East Asian patients and 80% of Caucasian patients (72). However, a sub-analysis of the network meta-analysis showed that Asian populations exhibit a higher risk of fracture (82). Another study used a random effects model to estimate the RRs and summarized that the use of SGLT-2is (RR = 1.02, 95%CI = 0.91–1.16, n = 4) is not associated with the risk of fracture (74).
Based on the mechanism of SGLT-2is on bone mineral metabolism, they may cause secondary PTH increase, promote bone resorption, and cause bone microstructure damage, which are established fracture risks. Currently, among the main large-scale clinical trials that included dapagliflozin, empagliflozin, and canagliflozin, only canagliflozin in the CANVAS Study increased the risk of bone fracture and reduced BMD compared to placebo. Most of the participants in the CANVAS Study were with established cardiovascular disease, and these patients have a higher risk of fracture, which may lead to biased results. In the CANVAS-R study, which involved T2DM patients with albuminuric CKD or a reduced estimated glomerular filtration rate (eGFR), no significant differences in the rates of amputation or fracture were observed. In the same study, the participants may have had potential CKD–mineral and bone disorders (MBDs), which makes the bone and mineral metabolism more complex. In addition, the effect of canagliflozin, an SGLT-1 and SGLT-2 co-inhibitor, on BMD and bone fracture may be associated with the oversuppression of both SGLT-1 and SGLT-2, but other SGLT-2is only act on SGLT-2. Thus, the effect of another dual SGLT-1/SGLT-2 inhibitor, sotagliflozin, on bone fracture was expected.
According to meta-analysis evidence (70–74, 81, 82) (Table 4), most of the meta-analysis results indicated that the SGLT-2is did not increase the risk of bone fracture across categories of age, race, type of SGLT-2is, and underlying comorbidities. The fracture risk of SGLT-2is is controversial. Most clinical trials on SGLT-2is have focused on the primary endpoint of blood glucose control or cardiovascular renal outcomes and had short intervention and follow-up periods. Moreover, the negligible number of fractures may have caused statistical bias. To obtain firm evidence of the risk of fracture, BMD, and incidence of bone fracture, a longer follow-up time is needed. Furthermore, suitable participants, such as those with T2DM with established osteoporosis or osteopenia, and the inclusion and exclusion criteria should be designed to focus on parameters of bone fracture risk. Therefore, more large-scale clinical trials or real-world studies are needed to provide convincing evidence. In addition, SGLT-2is treatment may cause hypoglycemia, relative insufficiency of blood pressure and blood volume, and postural hypotension and may induce accidental falls and fractures (83). Based on its significant glucose-lowering effect and benefit on the cardiovascular renal outcomes and prognosis, SGLT-2is are an outstanding choice for T2DM. Full consideration of the advantages and disadvantages is necessary for T2DM patients with a high risk of falls and bone fracture, such as those who are aging, with weakness, osteoporosis, or osteopenia, those with previous fracture history, and those using other concomitant medications affecting fracture, among others. It is helpful to access BMD and fracture risk assessments when administering SGLT-2is to patients with a high risk of fracture.
Conclusion
In conclusion, SGLT-2is affect mineral homeostasis and bone/mineral-regulating hormone by promoting the excretion of sodium and glucose. The effect of SGLT-2is on bone fracture is controversial. In general, the use of SGLT-2is did not show an association with increased risk of bone fracture. SGLT-2is reduce blood glucose and effective circulating volume, which may lead to the risk of falls and fractures. Assessment of BMD and fracture risk should be considered, especially for patients with a high risk of fracture.
Author Contributions
BD and RL drafted the manuscript. BD designed and prepared the figures. JW, LC, ZW, and ZH provided helpful suggestions. YW conceived the study. LX designed the study and take responsibility for this study. All authors contributed to the article and approved the submitted version.
Funding
This work was supported by a grant from the National Natural Science Foundation of China (no. 81600691) and a China Postdoctoral Science Foundation-funded project (no. 2018M640615). The content of the article has not been influenced by the sponsors.
Conflict of Interest
The authors declare that the research was conducted in the absence of any commercial or financial relationships that could be construed as a potential conflict of interest.
Publisher’s Note
All claims expressed in this article are solely those of the authors and do not necessarily represent those of their affiliated organizations, or those of the publisher, the editors and the reviewers. Any product that may be evaluated in this article, or claim that may be made by its manufacturer, is not guaranteed or endorsed by the publisher.
Supplementary Material
The Supplementary Material for this article can be found online at: https://www.frontiersin.org/articles/10.3389/fendo.2022.918350/full#supplementary-material
Supplementary Figure 1 | Flowchart of the identification of eligible literatures. To estimate the effect of SGLT2is on BMD and bone fracture risk in patients with T2DM, we comprehensively searched Pubmed, Medline, Embase, the Cochrane Database of Systematic Reviews and ClinicalTrials.gov to identify all eligible trials. We searched various articles including the mechanism of SGLT-2is affecting bone metabolism, relevant clinical trials, animal studies, in vitro experiments and so on. Reference lists of relevant articles were also performed to retrieve additional studies. Two researchers searched and screened the literatures independently. The terms used for the research included “sodium–glucose co-transporter 2 inhibitors”, “SGLT-2 inhibitor”, “dapagliflozin”, “canagliflozin”, “empagliflozin”, “ipragliflozin”, “tofogliflozin”, “luseogliflozin”, “ertugliflozin”, “sotagliflozin”, “janagliflozin”, “bexagliflozin”, “licogliflozin”, “henagliflozin”, “remogliflozin”, “tianaglilflozin”, “bone and mineral metabolism”, “bone turnover”, “bone fracture”, “type 2 diabetes mellitus”, “T2DM” with no language restrictions. We screened and focused on clinical trials and studies, or animal studies, meta-analysis and systemic reviews and so on. We excluded duplicate publications, case reports, ongoing trials without results, not available full-texts literatures and those not associated with mineral bone metabolism or fracture.
Abbreviations
SGLT-2i, sodium-glucose co-transporter 2 inhibitor; PTH, parathyroid hormone; CKD, chronic kidney disease; T2DM, type 2 diabetes mellitus; GLUT, glucose transporters; Na, sodium; Ca, calcium; Mg, magnesium; Pi, phosphorus; BMD, bone mineral density; FGF23, fibroblast growth factor 23; IR, insulin resistance; RAAS, renin–angiotensin–aldosterone system
References
1. Wen Y, Li H, Zhang X, Liu P, Ma J, Zhang L, et al. Correlation of Osteoporosis in Patients With Newly Diagnosed Type 2 Diabetes: A Retrospective Study in Chinese Population. Front Endocrinol (Lausanne) (2021) 12:531904. doi: 10.3389/fendo.2021.531904
2. Lawson CA, Seidu S, Zaccardi F, McCann G, Kadam UT, Davies MJ, et al. Outcome Trends in People With Heart Failure, Type 2 Diabetes Mellitus and Chronic Kidney Disease in the UK Over Twenty Years. EClinicalMedicine (2021) 32:100739. doi: 10.1016/j.eclinm.2021.100739
3. Wilding JPH, Evans M, Fernando K, Gorriz JL, Cebrian A, Diggle J, et al. The Place and Value of Sodium-Glucose Cotransporter 2 Inhibitors in the Evolving Treatment Paradigm for Type 2 Diabetes Mellitus: A Narrative Review. Diabetes Ther (2022) 13(5):847–872. doi: 10.1007/s13300-022-01228-w
4. Cannon CP, Pratley R, Dagogo-Jack S, Mancuso J, Huyck S, Masiukiewicz U, et al. Cardiovascular Outcomes With Ertugliflozin in Type 2 Diabetes. N Engl J Med (2020) 383(15):1425–35. doi: 10.1056/NEJMoa2004967
5. Cefalo CMA, Cinti F, Moffa S, Impronta F, Sorice GP, Mezza T, et al. Sotagliflozin, the First Dual SGLT Inhibitor: Current Outlook and Perspectives. Cardiovasc Diabetol (2019) 18(1):20. doi: 10.1186/s12933-019-0828-y
6. Rieg T, Vallon V. Development of SGLT1 and SGLT2 Inhibitors. Diabetologia (2018) 61(10):2079–86. doi: 10.1007/s00125-018-4654-7
7. Cianciolo G, De Pascalis A, Capelli I, Gasperoni L, Di Lullo L, Bellasi A, et al. Mineral and Electrolyte Disorders With SGLT2i Therapy. JBMR Plus (2019) 3(11):e10242. doi: 10.1002/jbm4.10242
8. Perkovic V, Jardine MJ, Neal B, Bompoint S, Heerspink HJL, Charytan DM, et al. Canagliflozin and Renal Outcomes in Type 2 Diabetes and Nephropathy. N Engl J Med (2019) 380(24):2295–306. doi: 10.1056/NEJMoa1811744
9. Lin DPL, Dass CR. Weak Bones in Diabetes Mellitus: An Update on Pharma-Ceutical Treatment Options. J Pharm Pharmacol (2018) 70:1–17. doi: 10.1111/jphp.12808
10. Li CI, Liu CS, Lin WY, Meng NH, Chen CC, Yang SY, et al. Glycated Hemoglobin Level and Risk of Hip Fracture in Older People With Type 2 Diabetes: A Competing Risk Analysis of Taiwan Diabetes Cohort Study. J Bone Miner Res (2015) 30:1338–46. doi: 10.1002/jbmr.2462
11. Lecka-Czernik B. Diabetes, Bone and Glucose-Lowering Agents: Basic Biology. Diabetologia (2017) 60(7):1163–9. doi: 10.1007/s00125-017-4269-4
12. Ishibashi Y, Matsui T, Yamagishi S. Tofogliflozin, a Selective Inhibitor of Sodium-Glucose Transport 2, Suppresses Renal Damage in KKAy/Ta Mice, Obese and Type 2 Diabetic Animals. Diab Vasc Dis Res (2016) 13:438–41. doi: 10.1177/1479164116657304
13. Corrao G, Monzio Compagnoni M, Ronco R, Merlino L, Ciardullo S, Perseghin G, et al. Is Switching From Oral Antidiabetic Therapy to Insulin Associated With an Increased Fracture Risk? Clin Orthop Relat Res (2020) 478(5):992–1003. doi: 10.1097/CORR.0000000000001089
14. Meier C, Schwartz AV, Egger A, Lecka-Czernik B. Effects of Diabetes Drugs on the Skeleton. Bone (2016) 82:93–100. doi: 10.1016/j.bone.2015.04.026
15. Riche DM, King ST. Bone Loss and Fracture Risk Associated With Thiazolidinedione Therapy. Pharmacotherapy (2010) 30(7):716–27. doi: 10.1592/phco.30.7.716
16. Ye Y, Zhao C, Liang J, Yang Y, Yu M, Qu X. Effect of Sodium-Glucose Co-Transporter 2 Inhibitors on Bone Metabolism and Fracture Risk. Front Pharmacol (2019) 9:1517. doi: 10.3389/fphar.2018.01517
17. van Bommel EJ, Muskiet MH, Tonneijck L, Kramer MH, Nieuwdorp M, van Raalte DH. SGLT2 Inhibition in the Diabetic Kidney-From Mechanisms to Clinical Outcome. Clin J Am Soc Nephrol (2017) 12(4):700–10. doi: 10.2215/CJN.06080616
18. Alba M, Xie J, Fung A, Desai M. The Effects of Canagliflozin, a Sodium-Glucose Co-Transporter 2 Inhibitor, on Mineral Metabolism and Bone in Patients With Type 2 Diabetes Mellitus. Curr Med Res Opin (2016) 32:1375–85. doi: 10.1080/03007995.2016.1174841
19. Hannon MJ, Verbalis JG. Sodium Homeostasis and Bone. Curr Opin Nephrol Hypertens (2014) 23(4):370–6. doi: 10.1097/01.mnh.0000447022.51722.f4
20. Bergstrom WH, Wallace WM. Bone as a Sodium and Potassium Reservoir. J Clin Invest (1954) 33(6):867–73. doi: 10.1172/JCI102959
21. Verbalis JG, Barsony J, Sugimura Y, Tian Y, Adams DJ, Carter EA, et al. Hyponatremia-Induced Osteoporosis. J Bone Miner Res (2010) 25(3):554–63. doi: 10.1359/jbmr.090827
22. Barsony J, Sugimura Y, Verbalis JG. Osteoclast Response to Low Extracellular Sodium and the Mechanism of Hyponatremia-Induced Bone Loss. J Biol Chem (2011) 286(12):10864–75. doi: 10.1074/jbc.M110.155002
23. Filippatos TD, Tsimihodimos V, Liamis G, Elisaf MS. SGLT2 Inhibitors-Induced Electrolyte Abnormalities: An Analysis of the Associated Mechanisms. Diabetes Metab Syndr (2018) 12(1):59–63. doi: 10.1016/j.dsx.2017.08.003
24. Gommers LM. Hypomagnesemia in Type 2 Diabetes: A Vicious Circle? Diabetes (2016) 65(1):3–13. doi: 10.2337/db15-1028
25. Rondanelli M, Faliva MA, Tartara A, Gasparri C, Perna S, Infantino V, et al. An Update on Magnesium and Bone Health. Biometals (2021) 34(4):715–36. doi: 10.1007/s10534-021-00305-0
26. Baker-LePain JC, Nakamura MC, Lane NE. Effects of Inflammation on Bone: An Update. Curr Opin Rheumatol (2011) 23(4):389–95. doi: 10.1097/BOR.0b013e3283474dbe
27. Mazur A, Maier JA, Rock E, Gueux E, Nowacki W, Rayssiguier Y. Magnesium and the Inflammatory Response: Potential Physiopathological Implications. Arch Biochem Biophys (2007) 458(1):48–56. doi: 10.1016/j.abb.2006.03.031
28. Rude RK, Gruber HE, Wei LY, Frausto A, Mills BG. Magnesium Deficiency: Effect on Bone and Mineral Metabolism in the Mouse. Calcif Tissue Int (2003) 72(1):32–41. doi: 10.1007/s00223-001-1091-1
29. Günther T. The Biochemical Function of Mg2+ in Insulin Secretion, Insulin Signal Transduction and Insulin Resistance. Magnes Res (2010) 23(1):5–18. doi: 10.1684/mrh.2009.0195
30. Takayanagi K. Down-Regulation of Transient Receptor Potential M6 Channels as a Cause of Hypermagnesiuric Hypomagnesemia in Obese Type 2 Diabetic Rats. Am J Physiol Renal Physiol (2015) 308(12):F1386–1397. doi: 10.1152/ajprenal.00593.2013
31. Gilbert RE, Mende C, Vijapurkar U, Sha S, Davies MJ, Desai M. Effects of Canagliflozin on Serum Magnesium in Patients With Type 2 Diabetes Mellitus: A Post Hoc Analysis of Randomized Controlled Trials. Diabetes Ther (2017) 8:451–8. doi: 10.1007/s13300-017-0232-0
32. Tang H, Zhang X, Zhang J, Li Y, Del Gobbo LC, Zhai S, et al. Elevated Serum Magnesium Associated With SGLT2 Inhibitor Use in Type 2 Diabetes Patients: A Meta- Analysis of Randomised Controlled Trials. Diabetologia (2016) 59(12):2546–51. doi: 10.1007/s00125-016-4101-6
33. Premaratne E, Verma S, Ekinci EI, Theverkalam G, Jerums G, MacIsaac RJ. The Impact of Hyperfiltration on the Diabetic Kidney. Diabetes Metab (2015) 41(1):5–17. doi: 10.1016/j.diabet.2014.10.003
34. Bankir L, Bouby N. Glucagon Actions on the Kidney Revisited: Possible Role in Potassium Homeostasis. Am J Physiol Renal Physiol (2016) 311(2):469–86. doi: 10.1152/ajprenal.00560.2015
35. Xu LH, Maalouf NM. Effect of Acute Hyperinsulinemia on Magnesium Homeostasis in Humans. Diabetes Metab Res Rev (2017) 33(2):e2486. doi: 10.1002/dmrr.2844
36. Ellison DH. Divalent Cation Transport by the Distal Nephron: Insights From Bartter's and Gitelman's Syndromes. Am J Physiol Renal Physiol (2000) 279(4):616–25. doi: 10.1152/ajprenal.2000.279.4.F616
38. Castiglioni S, Cazzaniga A, Albisetti W, Maier J. Magnesium and Osteoporosis: Current State of Knowledge and Future Research Directions. Nutrients (2013) 5(8):3022–33. doi: 10.3390/nu5083022
39. Thrailkill KM, Clay Bunn R, Nyman JS, Rettiganti MR, Cockrell GE, Wahl EC, et al. SGLT2 Inhibitor Therapy Improves Blood Glucose But Does Not Prevent Diabetic Bone Disease in Diabetic DBA/2J Male Mice. Bone (2016) 82:101–10. doi: 10.1016/j.bone.2015.07.025
40. Edwards A, Bonny O. A Model of Calcium Transport and Regulation in the Proximal Tubule. Am J Physiol Ren Physiol (2018) 315:F942–53. doi: 10.1152/ajprenal.00129.2018
41. Santer R, Calado J. Familial Renal Glucosuria and SGLT2: From a Mendelian Trait to a Therapeutic Target. Clin J Am Soc Nephrol (2010) 5:133–41. doi: 10.2215/CJN.04010609
42. Alexander RT, Dimke H. Effect of Diuretics on Renal Tubular Transport of Calcium and Magnesium. Am J Physiol Renal Physiol (2017) 312(6):F998–F1015. doi: 10.1152/ajprenal.00032.2017
43. Taylor SI, Blau JE, Rother KI. Possible Adverse Effects of SGLT2 Inhibitors on Bone. Lancet Diabetes Endocrinol (2015) 3:8–10. doi: 10.1016/S2213-8587(14)70227-X
44. Blau JE, Bauman V, Conway EM, Piaggi P, Walter MF, Wright EC, et al. Canagliflozin Triggers the FGF23/1,25- Dihydroxyvitamin D/PTH Axis in Healthy Volunteers in a Randomized Crossover Study. JCI Insight (2018) 3:19. doi: 10.1172/jci.insight.99123
45. Burnett SM, Gunawardene SC, Bringhurst FR, Juppner H, Lee H, Finkelstein JS. Regulation of C-Terminal and Intact FGF-23 by Dietary Phosphate in Men and Women. J Bone Miner Res (2016) 21:1187–96. doi: 10.1359/jbmr.060507
46. Masuyama R. Role of Local Vitamin D Signaling and Cellular Calcium Transport System in Bone Homeostasis. J Bone Miner Metab (2014) 32:1–9. doi: 10.1007/s00774-013-0508-z
47. Blevins TC, Farooki A. Bone Effects of Canagliflozin, a Sodium Glucose Co- Transporter 2 Inhibitor, in Patients With Type 2 Diabetes Mellitus. Postgr Med (2017) 129:159–68. doi: 10.1080/00325481.2017.1256747
48. Jong MA, Petrykiv SI, Laverman GD, van Herwaarden AE, de Zeeuw D, Bakker SJL, et al. Effects of Dapagliflozin on Circulating Markers of Phosphate Homeostasis. Clin J Am Soc Nephrol (2019) 14:66–73. doi: 10.2215/CJN.04530418
49. Quarles LD. Skeletal Secretion of FGF-23 Regulates Phosphate and Vitamin D Metabolism. Nat Rev Endocrinol (2012) 8:276–86. doi: 10.1038/nrendo.2011.218
50. Egger A, Kraenzlin ME, Meier C. Effects of Incretin-Based Therapies and SGLT2 Inhibitors on Skeletal Health. Curr Osteoporos Rep (2016) 14:345–50. doi: 10.1007/s11914-016-0337-9
51. Hygum K, Starup-Linde J, Harslof T, Vestergaard P, Langdahl BL. Mechanisms in Endocrinology: Diabetes Mellitus, a State of Low Bone Turnover - a Systematic Review and Meta-Analysis. Eur J Endocrinol (2017) 176:R137–57. doi: 10.1530/EJE-16-0652
52. Bilezikian JP, Watts NB, Usiskin K, Polidori D, Fung A, Sullivan D, et al. Evaluation of Bone Mineral Density and Bone Biomarkers in Patients With Type 2 Diabetes Treated With Canagliflozin. J Clin Endocrinol Metab (2016) 101:44–51. doi: 10.1210/jc.2015-1860
53. Ljunggren O, Bolinder J, Johansson L, Wilding J, Langkilde AM, Sjöström CD, et al. Dapagliflozin has No Effect on Markers of Bone Formation and Resorption or Bone Mineral Density in Patients With Inadequately Controlled Type 2 Diabetes Mellitus on Metformin. Diabetes Obes Metab (2012) 14:990–9. doi: 10.1111/j.1463-1326.2012.01630.x
54. Koshizaka M, Ishikawa K, Ishibashi R, Maezawa Y, Sakamoto K, Uchida D, et al. Effects of Ipragliflozin Versus Metformin in Combination With Sitagliptin on Bone and Muscle in Japanese Patients With Type 2 Diabetes Mellitus: Subanalysis of a Prospective, Randomized, Controlled Study (PRIME-V Study). J Diabetes Investig (2021) 12(2):200–6. doi: 10.1111/jdi.13340
55. Shah K, Armamento-Villareal R, Parimi N, Chode S, Sinacore DR, Hilton TN, et al. Exercise Training in Older Adults With Obesity Prevents Increase in Bone Turnover and Attenuates Decrease in Hip BMD Induced by Weight Loss Despite Decline in Bone-Active Hormones. J Bone Miner Res (2011) 26(12):2851–9. doi: 10.1002/jbmr.475
56. Campos RMDS, Masquio DCL, Corgosinho FC, Carvalho-Ferreira JP, Molin Netto BD, Clemente APG, et al. Relationship Between Adiponectin and Leptin on Osteocalcin in Obese Adolescents During Weight Loss Therapy. Arch Endocrinol Metab (2018) 62(3):275–84. doi: 10.20945/2359-3997000000039
57. Pal China S, Sanyal S, Chattopadhyay N. Adiponectin Signaling and its Role in Bone Metabolism. Cytokine (2018) 112:116–31. doi: 10.1016/j.cyto.2018.06.012
58. Burghardt AJ, Issever AS, Schwartz AV, Davis KA, Masharani U, Majumdar S, et al. High-Resolution Peripheral Quantitative Computed Tomographic Imaging of Cortical and Trabecular Bone Microarchitecture in Patients With Type 2 Diabetes Mellitus. J Clin Endocrinol Metab (2010) .95:5045–55. doi: 10.1210/jc.2010-0226
59. Haraguchi A, Shigeno R, Horie I, Morimoto S, Ito A, Chiba K, et al. The Effect of Luseogliflozin on Bone Microarchitecture in Older Patients With Type 2 Diabetes: Study Protocol for a Randomized Controlled Pilot Trial Using Second-Generation, High-Resolution, Peripheral Quantitative Computed Tomography (HR-pQCT). Trials (2020) 21(1):379. doi: 10.1186/s13063-020-04276-4
60. Gerber C, Wang X, David V, Quaggin SE, Isakova T, Martin A. Long-Term Effects of Sglt2 Deletion on Bone and Mineral Metabolism in Mice. JBMR Plus (2021) 5(8):e10526. doi: 10.1002/jbm4.10526
61. Thrailkill KM, Nyman JS, Bunn RC, Uppuganti S, Thompson KL, Lumpkin CJ, et al. The Impact of SGLT2 Inhibitors, Compared With Insulin, on Diabetic Bone Disease in a Mouse Model of Type 1 Diabetes. Bone (2017) 94:141–51. doi: 10.1016/j.bone.2016.10.026
62. Thrailkill KM, Bunn RC, Uppuganti S, Ray P, Popescu I, Kalaitzoglou E, et al. Canagliflozin, an SGLT2 Inhibitor, Corrects Glycemic Dysregulation in TallyHO Model of T2D But Only Partially Prevents Bone Deficits. Bone (2020) 141:115625. doi: 10.1016/j.bone.2020.115625
63. Mieczkowska A, Millar P, Chappard D, Gault VA, Mabilleau G. Dapagliflozin and Liraglutide Therapies Rapidly Enhanced Bone Material Properties and Matrix Biomechanics at Bone Formation Site in a Type 2 Diabetic Mouse Model. Calcif Tissue Int (2020) 107(3):281–93. doi: 10.1007/s00223-020-00720-4
64. Suzuki M, Takeda M, Kito A, Fukazawa M, Yata T, Yamamoto M, et al. Tofogliflozin, a Sodium/Glucose Cotransporter 2 Inhibitor, Attenuates Body Weight Gain and Fat Accumulation in Diabetic and Obese Animal Models. Nutr Diabetes (2014) 4(7):e125. doi: 10.1038/nutd.2014.20
65. Neal B, Perkovic V, Mahaffey KW, de Zeeuw D, Fulcher G, Erondu N, et al. Canagliflozin and Cardiovascular and Renal Events in Type 2 Diabetes. N Engl J Med (2017) 377(7):644–57. doi: 10.1056/NEJMoa1611925
66. Wivioot SD, Raz I, Bonaca MP, Mosenzon O, Kato ET, Cahn A, et al. Dapagliflozin and Cardiovascular Outcomes in Type 2 Diabetes. N Engl J Med (2019) 380(4):347–57. doi: 10.1056/NEJMoa1812389
67. Zinman B, Lachin JM, Inzucchi SE. Empagliflozin, Cardiovascular Outcomes, and Mortality in Type 2 Diabetes. N Engl J Med (2015) 373:2117–28:21. doi: 10.1056/NEJMoa1504720
68. Cosentino F, Cannon CP, Cherney DZI, Masiukiewicz U, Pratley R, Dagogo-Jack S, et al. Efficacy of Ertugliflozin on Heart Failure-Related Events in Patients With Type 2 Diabetes Mellitus and Established Atherosclerotic Cardiovascular Disease: Results of the VERTIS CV Trial. Circulation (2020) 142(23):2205–15. doi: 10.1161/CIRCULATIONAHA.120.050255
69. Wolverton D, Blair MM. Fracture Risk Associated With Common Medications Used in Treating Type 2 Diabetes Mellitus. Am J Health Syst Pharm (2017) 74(15):1143–51. doi: 10.2146/ajhp160319
70. Zhang YS, Zheng YD, Yuan Y, Chen SC, Xie BC. Effects of Anti-Diabetic Drugs on Fracture Risk: A Systematic Review and Network Meta-Analysis. Front Endocrinol (Lausanne) (2021) 12:735824. doi: 10.3389/fendo.2021.735824
71. Qiu M, Ding LL, Zhang M, Zhou HR. Safety of Four SGLT2 Inhibitors in Three Chronic Diseases: A Meta-Analysis of Large Randomized Trials of SGLT2 Inhibitors. Diabetes Vasc Dis Res (2021) 18(2):14791641211011016. doi: 10.1177/14791641211011016
72. Tang HL, Li DD, Zhang JJ, Hsu YH, Wang TS, Zhai SD, et al. Lack of Evidence for a Harmful Effect of Sodium-Glucose Co-Transporter 2 (SGLT2) Inhibitors on Fracture Risk Among Type 2 Diabetes Patients: A Network and Cumulative Meta-Analysis of Randomized Controlled Trials. Diabetes Obes Metab (2016) 18(12):1199–206. doi: 10.1111/dom.12742
73. Ruanpeng D, Ungprasert P, Sangtian J, Harindhanavudhi T. Sodium-Glucose Contransporter 2 (SGLT2) Inhibitors and Fracture Risk in Patients With Type 2 Diabetes Mellitus: A Meta-Analysis. Diabetes Metab Res Rev 33:e2903. doi: 10.1002/dmrr.2903
74. Hidayat K, Du X, Shi BM. Risk of Fracture With Dipeptidyl Peptidase-4 Inhibitors, Glucagon-Like Peptide-1 Receptor Agonists, or Sodium-Glucose Cotransporter-2 Inhibitors in Real-World Use: Systematic Review and Meta-Analysis of Observational Studies. Osteoporos Int (2019) 30(10):1923–40. doi: 10.1007/s00198-019-04968-x
75. Kanie T, Mizuno A, Takaoka Y, Suzuki T, Yoneoka D, Nishikawa Y, et al. Dipeptidyl Peptidase-4 Inhibitors, Glucagon-Like Peptide 1 Receptor Agonists and Sodium-Glucose Co-Transporter-2 Inhibitors for People With Cardiovascular Disease: A Network Meta-Analysis. Cochrane Database Syst Rev (2021) 10(10):CD013650. doi: 10.1002/14651858.CD013650.pub2
76. Azharuddin M, Adil M, Ghosh P, Sharma M. Sodium-Glucose Cotransporter 2 Inhibitors and Fracture Risk in Patients With Type 2 Diabetes Mellitus: A Systematic Literature Review and Bayesian Network Meta-Analysis of Randomized Controlled Trials. Diabetes Res Clin Pract (2018) 146:180–90. doi: 10.1016/j.diabres.2018.10.019
77. Donnan JR, Grandy CA, Chibrikov E, Marra CA, Aubrey-Bassler K, Johnston K, et al. Comparative Safety of the Sodium Glucose Co-Transporter 2 (SGLT2) Inhibitors: A Systematic Review and Meta-Analysis. BMJ Open (2019) 9(1):e022577. doi: 10.1136/bmjopen-2018-022577
78. Shrestha DB, Budhathoki P, Sedhai YR, Karki P, Gurung S, Raut S, et al. Sodium-Glucose Cotransporter-2 Inhibitors in Heart Failure: An Updated Systematic Review and Meta-Analysis of 13 Randomized Clinical Trials Including 14,618 Patients With Heart Failure. J Cardiovasc Pharmacol (2021) 78(4):501–14. doi: 10.1097/FJC.0000000000001099
79. Cheng L, Li YY, Hu W, Bai F, Hao HR, Yu WN, et al. Risk of Bone Fracture Associated With Sodium-Glucose Cotransporter-2 Inhibitor Treatment: A Meta-Analysis of Randomized Controlled Trials. Diabetes Metab (2019) 45(5):436–45. doi: 10.1016/j.diabet.2019.01.010
80. Li X, Li T, Cheng Y, Lu Y, Xue M, Xu L, et al. Effects of SGLT2 Inhibitors on Fractures and Bone Mineral Density in Type 2 Diabetes: An Updated Meta-Analysis. Diabetes Metab Res Rev (2019) 35(7):e3170. doi: 10.1002/dmrr.3170
81. Zhuo M, Hawley CE, Paik JM, Bessette LG, Wexler DJ, Kim DH, et al. Association of Sodium-Glucose Cotransporter-2 Inhibitors With Fracture Risk in Older Adults With Type 2 Diabetes. JAMA Netw Open (2021) 4(10):e2130762. doi: 10.1001/jamanetworkopen.2021.30762
82. Mukai J, Kanno S, Kubota R. A Literature Review and Meta-Analysis of Safety Profiles of SGLT2 Inhibitors in Japanese Patients With Diabetes Mellitus. Sci Rep (2021) 11(1):13472. doi: 10.1038/s41598-021-92925-2
Keywords: sodium–glucose cotransporter-2 inhibitor, bone and mineral metabolism, bone turnover, bone fracture risk, type 2 diabetes mellitus
Citation: Dong B, Lv R, Wang J, Che L, Wang Z, Huai Z, Wang Y and Xu L (2022) The Extraglycemic Effect of SGLT-2is on Mineral and Bone Metabolism and Bone Fracture. Front. Endocrinol. 13:918350. doi: 10.3389/fendo.2022.918350
Received: 12 April 2022; Accepted: 30 May 2022;
Published: 07 July 2022.
Edited by:
Daniela Merlotti, University of Siena, ItalyReviewed by:
Stefano Ciardullo, University of Milano Bicocca, ItalyKalliopi Pafili, German Diabetes Center (DDZ), Germany
Copyright © 2022 Dong, Lv, Wang, Che, Wang, Huai, Wang and Xu. This is an open-access article distributed under the terms of the Creative Commons Attribution License (CC BY). The use, distribution or reproduction in other forums is permitted, provided the original author(s) and the copyright owner(s) are credited and that the original publication in this journal is cited, in accordance with accepted academic practice. No use, distribution or reproduction is permitted which does not comply with these terms.
*Correspondence: Lili Xu, qdfyxll@qdu.edu.cn; Yangang Wang, wangyg1966@126.com
†These authors have contributed equally to this work