- 1State Key Laboratory of Biotherapy and Cancer Center, West China Hospital, and West China School of Basic Medical Sciences & Forensic Medicine, Sichuan University, and Collaborative Innovation Center for Biotherapy, Chengdu, China
- 2Department of Anesthesiology, The Affiliated Hospital of Medical School, Ningbo University, Ningbo, China
Ovarian cancer (OC) is one of the most lethal gynecologic malignancies globally. In spite of positive responses to initial therapy, the overall survival rates of OC patients remain poor due to the development of drug resistance and consequent cancer recurrence. Indeed, intensive studies have been conducted to unravel the molecular mechanisms underlying OC therapeutic resistance. Besides, emerging evidence suggests a crucial role for epigenetic modifications, namely, DNA methylation, histone modifications, and non-coding RNA regulation, in the drug resistance of OC. These epigenetic modifications contribute to chemoresistance through various mechanisms, namely, upregulating the expression of multidrug resistance proteins (MRPs), remodeling of the tumor microenvironment, and deregulated immune response. Therefore, an in-depth understanding of the role of epigenetic mechanisms in clinical therapeutic resistance may improve the outcome of OC patients. In this review, we will discuss the epigenetic regulation of OC drug resistance and propose the potential clinical implications of epigenetic therapies to prevent or reverse OC drug resistance, which may inspire novel treatment options by targeting resistance mechanisms for drug-resistant OC patients.
1 Introduction
With around 239,000 new cases and 152,000 deaths each year, ovarian cancer (OC) is the seventh most prevalent cancer and the second leading cause of death from gynecologic cancer (1). Despite advances in surgical procedures, platinum-based chemotherapy, targeted medicines, and immunotherapy in recent decades, patients with OC still have a poor prognosis due to advanced and extensive disseminated tumors (2–4). The high death rate of OC is partly attributable to its extremely invasive growth pattern, which is hard to detect in early stages and frequently resistant to drugs (5, 6).
Drug resistance is a significant barrier to treating OC and leads to a poor prognosis. While 80% of individuals initially diagnosed with OC respond to conventional first-line therapy, such as platinum-based chemotherapy and surgical cytoreduction, roughly 75% of patients with advanced OC relapse within three years, which is usually fatal (2, 5). With notable therapeutic advantages, poly (ADP-ribose) polymerase (PARP) inhibitors have emerged as the first targeted medicines for patients with platinum-sensitive recurrent OC (7, 8). However, the effectiveness of PARP inhibitors is severely limited in OC due to the narrow spectrum of administration and various resistance mechanisms (9, 10). To improve the prognosis of patients with OC, it is vital to understand the underlying mechanisms of treatment resistance in OC and develop techniques to postpone or overcome drug resistance (Figure 1).
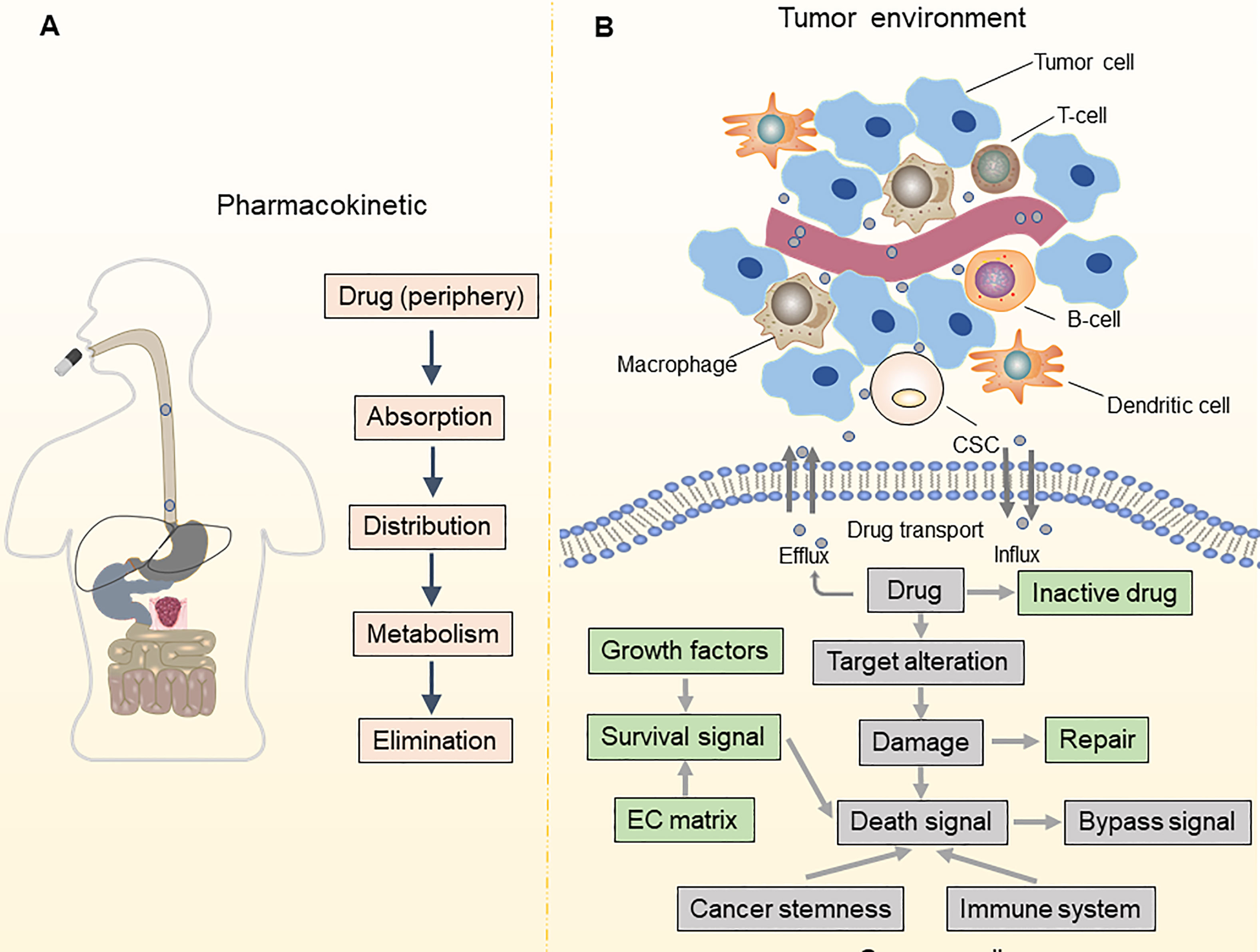
Figure 1 Pharmacokinetic and TME-associated factors contribute to drug resistance. (A) Mechanisms of drug resistance can be due to pharmacokinetics, including drug absorption, distribution, metabolism, and elimination; (B) Alterations in drug influx and efflux system impact the intracellular accumulation of anti-cancer drugs in tumor cells. The leading determinants of drug resistance are altered in drug targets, bypass signaling pathways, DNA damage and repair, and cell death signaling. Besides, maintenance of cancer stemness and a tumor-promoting immune microenvironment also contribute to drug resistance.
It is now generally accepted that drug resistance may arise from the diminished intracellular accumulation of drugs, alterations of drug targets, deregulation of immune response, and issues with the cell death executioner machinery, as well as the generation and maintenance of drug-resistant cells (11–15). Furthermore, a recent study suggested that epigenetic regulation (namely, DNA methylation, histone modifications, and non-coding RNA regulation) is one of the key mechanisms in OC that drives both intrinsic and acquired treatment resistance (16–19) (Figure 2). For instance, as one of the most effective broad-spectrum anti-cancer drugs, cisplatin kills tumor cells via DNA damage. However, epigenetic alterations are frequently observed in this process and are associated with cisplatin resistance (20, 21). For example, DNA methylation plays an indispensable role in OC drug resistance. Early studies suggested that hypermethylation of the BRCA1 gene in OC cells confers susceptibility to platinum-based chemotherapy (22, 23). At least 20 post-translational modifications occur in histone to govern the structures and activities of DNA. Accumulating evidence demonstrates that histone modifications, namely, acetylation, methylation, and phosphorylation, are linked to OC development and treatment resistance (24–26). Moreover, non-coding RNAs (ncRNAs), such as long non-coding RNAs (lncRNAs) and microRNAs (miRNAs), are currently recognized to be involved in various biological processes, including drug resistance (27, 28). As discussed above, we have summarized the epigenetic regulation in OC drug resistance (Table 1). Importantly, epigenetic alterations are reversible, and emerging epigenome-targeted therapy strategies can overcome OC drug resistance by reversing histone modifications and DNA methylation or by targeting ncRNAs. In this review, we will discuss the detailed mechanisms of epigenetic regulation that contribute to drug resistance in OC and highlight the advantages and challenges of epigenome-targeted therapy strategies for treating OC.
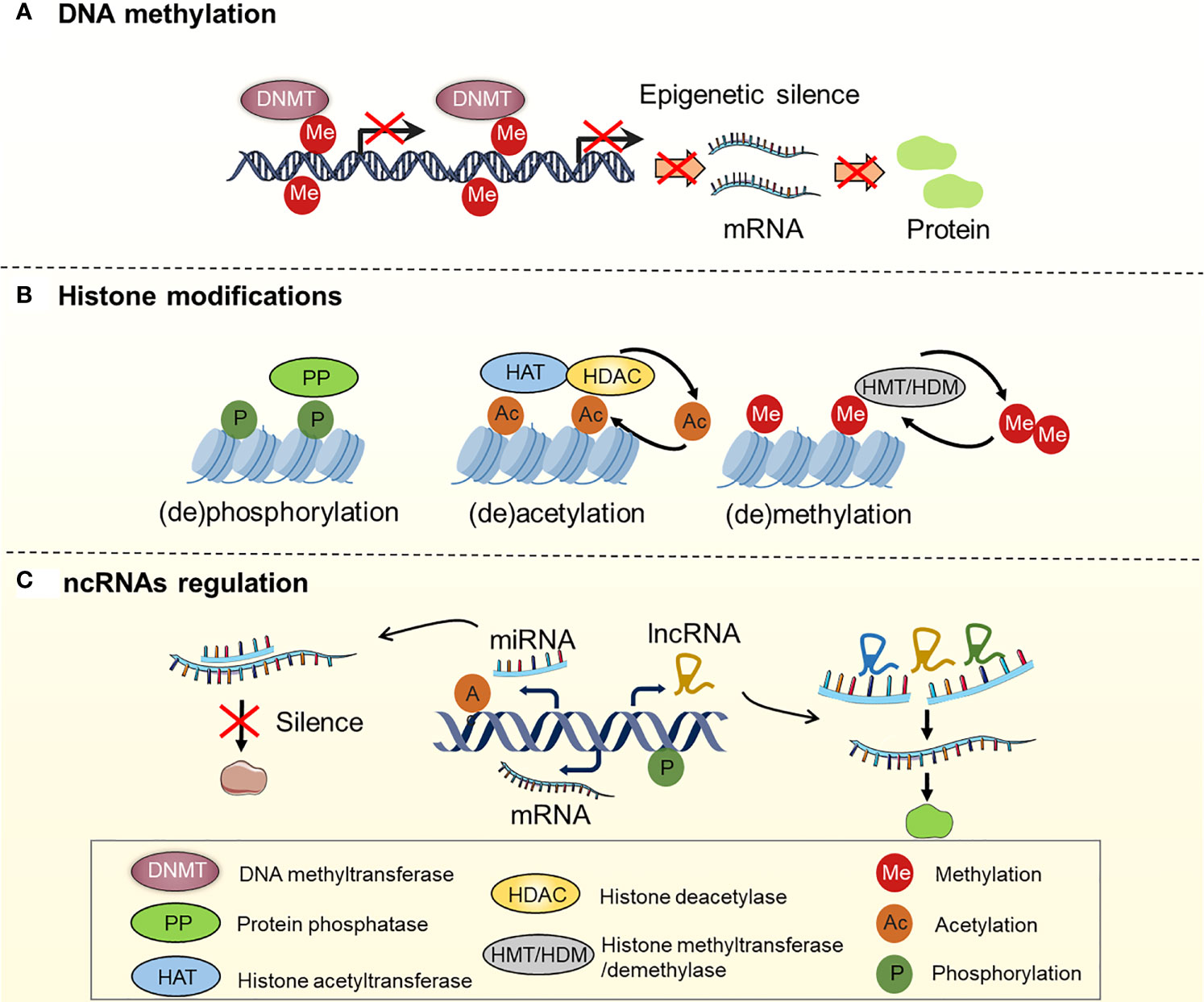
Figure 2 Regulation of DNA methylation, histone modifications and ncRNAs in OC. (A) Genes are silenced by hypermethylation, which is catalyzed by DNA methyltransferases (DNMTs); (B) Histone modifications, including histone (de)phosphorylation, (de)acetylation, (de)methylation, etc. Histone (de)phosphorylation is catalyzed by protein phosphatase (PP). Histone (de)acetylation is catalyzed by histone acetyltransferase (HAT) and histone deacetylase (HDAC). Histone (de)methylation is catalyzed by histone methyltransferase (HMT) and histone demethylase (HDM); (C) ncRNAs regulation: miRNAs and lncRNAs regulate gene expression by interacting with mRNA.
2 Epigenetic Modifications in OC Drug Resistance
Since the discovery of methylation of DNA repair genes by O6-methylguanine-DNA methyltransferase (MGMT), the role of epigenetic modifications in the context of inherent or acquired drug resistance has been extensively explored (46). Multiple mechanisms, namely, altered drug targets and bypass signaling pathways, enhanced drug efflux and metabolism, downstream adaptive responses, and maintenance of cancer stemness, are the primary causes of decreased anti-cancer drug effectiveness (47–50). Furthermore, emerging evidence suggests that the tumor microenvironment (TME) is crucial to multidrug resistance (MDR) in cancer cells (51–53). The molecular mechanisms of epigenetic regulation-mediated OC drug resistance, namely, enhanced drug efflux and metabolism, alteration of drug targets and bypass signaling pathways, downstream adaptive responses, maintenance of cancer stemness, and TME, are discussed here.
2.1 Epigenetic Modifications Involved in Drug Transport and Metabolism
Effective cytotoxic drug treatment requires a sufficient intracellular drug concentration in tumor cells. Drug concentration is coordinated by transporters mediating the influx and efflux of drugs and enzymes mediating drug metabolism. The effectiveness of chemotherapeutic and targeted drug delivery into cancer cells is determined by drug inflow and efflux transporters. The most known such transporters are the ATP-binding cassette (ABC) transporter family members, namely, ABCB1 (MDR1), ABCC2 (MRP-2), and ABCG2 (BCRP/MXR1), which have been widely shown to be associated with drug resistance (54, 55). The epigenetic control of ABC transporter-induced OC resistance has recently progressed significantly (Figure 3). ABCB1 is the first ABC transporter identified and plays a crucial role in determining drug sensitivity. The epigenetic regulation of ABCB1 is related to drug transportation in OC cells. For instance, paclitaxel therapy increased histone H3 acetylation and targeted the ABCB1 promoter in conjunction with the androgen receptor (AR), resulting in ABCB1 gene expression and the establishment of the paclitaxel resistance phenotype (56). Wu et al. found that overexpression of miR-873 improved the susceptibility of OC cells to paclitaxel and cisplatin by targeting ABCB1 (57). Besides, Tian et al. demonstrated that miR-490-3p enhances the sensitivity of OC cells to cisplatin by downregulating ABCC2 expression (58). Nevertheless, hnRNPA2B1 was shown to bind to the 5’UTR of ABCC2 mRNA and promote its translation, leading to cisplatin resistance in OC (59). Furthermore, ABCG2 is strongly expressed in cisplatin- or paclitaxel-resistant OC and OC stem cells, indicating the key role of ABCG2 in drug resistance and stemness acquisition in OC (60). Calcagno and colleagues discovered that elevated acetylation of histone H3 in the ABCG2 promoter is a cellular response to the treatment of doxorubicin, which underlies its doxorubicin resistance (61). Some lncRNAs and miRNAs found in extracellular vesicles (EVs) generated by drug-resistant cells control the expression of ABCG2, hence impacting tumor drug resistance (62–65). These investigations indicate that epigenetic regulation plays an essential role in OC drug resistance by modulating the ABC transporter family.
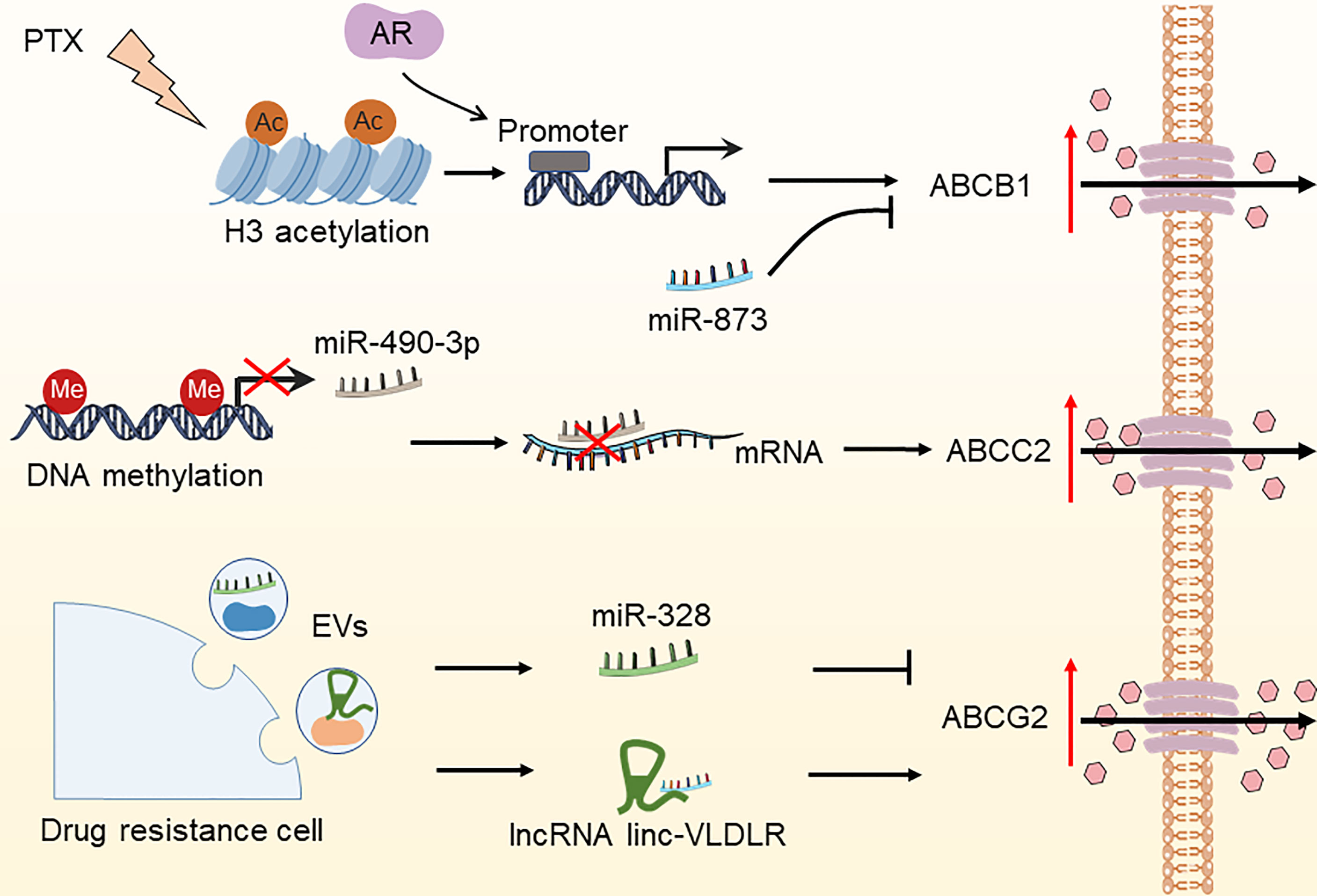
Figure 3 Epigenetic modifications regulate the expression of ABC transporters in OC. The DNA methylation of miR-490-3p, increased histone H3 acetylation due to paclitaxel treatment, miR-328, and lncRNA linc-VLDLR in EVs have been reported to increase drug efflux by regulating the expression of ABCC2, ABCB1, and ABCG2, thereby leading to OC drug resistance. PTX, paclitaxel; AR, androgen receptor; EVs, extracellular vesicles.
Drug metabolism regulates bioactivation, catabolism, conjugation, and excretion, determining drug clinical efficacy and toxicity (66). Drug metabolism enzymes can be affected by alterations in the expression level of affect drug metabolism. For instance, epigenetic alterations regulate the expression of the cytochrome P450 (CYP) enzyme family, which is the most well-known drug-metabolizing enzyme, thus affecting the metabolism of several anti-cancer medications. A recent study showed that histone modification enzyme G9a regulates the expression of CYP450 by affecting histone 3 lysine 4 and histone 3 lysine 27 methylation, suggesting G9a alters drug sensitivity (67). Luo and colleagues emphasized an unconventional epigenetic regulation mechanism of CYP gene expression; that is, miR-148a stimulates CYP2B6 expression by binding to the 3’UTR region to improve mRNA stability (68). Furthermore, miR-543 overexpression increases the production of CYP3A4, which is involved in the metabolism of ruxolitinib (69). Other drug-metabolizing enzymes are controlled by epigenetic alterations, which must be investigated further.
2.2 Epigenetic Modifications Regulate Drug Targets and Bypass Signaling Pathways
Over the past decade, targeted agents have been steadily introduced into clinical trials for treating recurrent OC, namely, the anti-VEGFR agent bevacizumab, PARP inhibitors (olaparib, niraparib, and rucaparib), the anti-MEK inhibitor trametinib, and anti-HER2 pertuzumab. However, chemotherapy resistance resulting from epigenetic alterations limits the effectiveness of these targeted treatments. Resistance to targeted therapy arises primarily through reduced expression of drug targets and activated bypass signaling pathways during treatment with targeted drugs (70–72). Here, we summarize the epigenetic modification-mediated drug resistance in OC, caused by the deregulated expression of drug targets, mutation of drug targets, and the persistent activation of bypass signaling pathways.
Angiogenesis is critical in the etiology of OC, which is associated with the generation of vascular endothelial growth factor (VEGF), linked to the progression of malignant ascites and OC (73, 74). In clinical trials, bevacizumab, an anti-VEGF monoclonal antibody, has been proven to improve outcomes in OC patients in clinical trials (75, 76). However, epigenetic modifications may diminish VEGF expression. Vesna and colleagues found twenty significant CpG sites in promoter regions, suggesting that the concentration of VEGF-A can be regulated by DNA methylation (77). Based on The Cancer Genome Atlas (TCGA) databases, Zhou and colleagues discovered that overexpression of insulin-like growth factor 2 mRNA-binding protein 3 (IGF2BP3) was related to cancer progression and poor survival of patients. Mechanistically, IGF2BP3 can bind with the mRNA of VEGF, where IGF2BP3 serves as a reader to recognize the m6A modification on VEGF mRNA. This effect controls both the production and stability of VEGF mRNA, which may affect drug sensitivity (78). Besides, VEGFA is the direct target of miR-652-5p, and miR-652-5p is down-regulated by hypermethylation at the upstream CpG site. Increasing VEGF synthesis is linked to tumor progression and metastasis (79). Moreover, it has been shown that cells with methylated BRCA1 have defective homologous recombination (HR) activity, thus being highly responsive to PARP inhibitors (olaparib, niraparib, and rucaparib), implying that BRCA1 inactivated by epigenetic mechanisms contributes to drug sensitivity (80–82).
In addition to alterations in drug targets, epigenetic modifications are implicated in the activation of bypass signaling pathways to regulate OC drug resistance. Although the Notch pathway is closely related to the growth of OC tumors, its clinical significance and molecular mechanisms remain unclear. Hu et al. found that alterations in the Notch pathway are prevalent and closely related to poor clinical outcomes in patients with OC (83, 84). For instance, epigenetic regulation of multiple Notch target genes (such as PPARG, CCND1, and RUNX1) can regulate the activation of the Notch pathway, which is associated with the prognosis of OC patients (85). Liu and colleagues found that Fas deficiency inhibits the release of miR-29b, thereby increasing intracellular miR-29b levels and subsequently downregulating DNA methyltransferase 1 (DNMT1) expression, which results in hypomethylation of the Notch1 promoter region and activation of Notch signaling (86). Hirsch et al. discovered that inhibition of histone deacetylase might alleviate abnormalities in the Notch and Eph axis in prion protein PrP deficient and prion-infected cells (87). Besides, studies have demonstrated that lncRNA HOTAIR induces OC drug resistance to cisplatin through activating the Wnt/β-catenin pathway (88). Further studies of epigenetic modifications that regulate drug targets and bypass signaling pathways may increase our understanding of the development of potential strategies to reverse drug resistance.
2.3 Epigenetic Modifications Modulate DNA Damage and Repair
Most chemotherapeutic drugs induce cell death through apoptosis due to DNA damage (89, 90). After treatment with cytotoxic agents, eukaryotic cells usually undergo damage repair to avoid apoptosis, leading to drug resistance (91). Therefore, components of the DNA repair system, such as O6-methylguanine-DNA methyltransferase (MGMT), can promote chemotherapeutic resistance. MGMT mediates the direct removal of O6-methylguanine (O6-MEG), a mark of DNA damage induced by temozolomide (TMZ), thereby facilitating DNA repair in melanoma cells. Other TMZ-induced lesions are mostly repaired by base excision repair (BER) or direct removal mechanisms catalyzed by DNA demethylase ALKBH2/3 (91, 92). Poly-ADP-ribose polymerase inhibitors (PARPis) are the most effective therapies approved for treating OC, and poly-ADP-ribose polymerase (PAPR) inhibitors (olaparib, niraparib, and rucaparib) are already being investigated in OC clinical trials. Indeed, all PARPis exhibit radio- or chemo-potentiation effects in vitro and in vivo, which is consistent with their ability to inhibit DNA damage repair (93, 94). According to a recent study by Nephew and colleagues, platinum-induced DNA damage contributes to the activation of the NF-κB pathway by the lncRNA HOTAIR and cellular senescence. Furthermore, DNA damage response activated NF-κB, which in turn triggered HOTAIR and created a positive-feedback loop, resulting in sustained NF-κB activation and persistent DNA damage signaling (40). Mutations in p53 are observed in 42% of human tumors (95). The regulation of RNA m6A on TP53 has been proven to overcome drug resistance by controlling downstream pathways and DNA damage repair, suggesting that it is a viable therapeutic approach (96). Meng et al. discovered that AZD1775, not only carboplatin, can increase sensitivity to gemcitabine and olaparib in TP53-mutated gynecologic cancer cells (97). WEE1 is a tyrosine kinase that blocks the CDK1/cyclin B complex by inducing CDK1 phosphorylation at tyrosine 15 (Y15), inducing cell cycle arrest and allowing DNA repair (98, 99). AZD1775 is a first-in-class, powerful, and selective WEE1 inhibitor that has shown a considerable anti-tumor effect in OC patients with TP53 mutations when combined with carboplatin (100). In conclusion, uncovering the mechanisms that influence epigenetic modification function in DNA damage repair may develop new strategies for the sensitization of OC cells to DNA damage inducers.
2.4 Epigenetic Modifications Activate Downstream Adaptive Responses
The interaction of anti-cancer drugs with corresponding cellular targets can induce cell death. However, a variety of adaptive cellular responses governed by epigenetic modifications are engaged to support the survival of tumor cells, which is the major target of cancer therapy. These include inhibition of apoptosis, initiation of autophagy, ferroptosis, and activation of pro-survival signals (101–103).
2.4.1 Apoptosis
After medication therapy, cancer cells with enhanced DNA repair capacities can survive drug-induced DNA damage. The anti-apoptotic proteins (Bcl-2), inhibitor of apoptosis proteins (IAPs), and FLICE inhibitor proteins (FLIP) are upregulated in tumor cells, which contributes to their medication resistance (90). As an anti-apoptotic member of the Bcl-2 family, MCL1 plays an important role in the advanced chemotherapy resistance of OC. Wu et al. found that the deubiquitinating enzyme 3 (DUB3) in the cytoplasm of OC cells interacts with and deubiquitinates MCL1, thereby protecting MCL1 from degradation. Furthermore, they discovered that histone deacetylase inhibitors (HDACis) could increase the expression level of MGMT/DUB3, and HDACis combined with PaTrin-2 treatment has a significant effect on OC (29). Zhu et al. found that ALKBH5 is a potential target for OC therapy, which activates the EGFR-PIK3CA-AKT-mTOR signaling pathway, enhancing the stability of BCL-2 mRNA and promoting the interaction between Bcl-2 and Beclin1 (104). Abedini et al. discovered that p53 could promote the ubiquitination and subsequent proteasomal degradation of FLIP in response to cisplatin treatment, leading to the apoptosis of OC cells, potentially improving the therapeutic effects of cisplatin on OC.
2.4.2 Autophagy
Autophagy partly enables tumor cells to cope with external stress, leading to the survival of cancer cells treated with anti-cancer drugs (105–107). Bi et al. have demonstrated that blocking autophagy can overcome resistance to HDAC inhibitors in gynecologic cancers (108). Autophagy can be activated by the upregulation of autophagy-related gene 14 (ATG14), and the abnormal expression of autophagy-related proteins contributes to drug resistance in OC. MiR-29c-3p inhibits autophagy and cisplatin resistance in part by downregulating the FOXP1/ATG14 pathway, suggesting that miR-29c-3p is a novel target for overcoming cisplatin resistance in OC (109). Bi et al. found that inhibition of methyltransferase-like 3 (METTL3) inhibits miR-126-5p’s upregulation of PTEN by regulating m6A modification, thereby preventing the activation of the PI3K/Akt/mTOR pathway and inhibiting the occurrence of OC. This finding highlights the role of m6A modification as a potential target for future OC treatment (110). O-Glcnacylation is a post-translational modification in which the O-GlCNAc transferase (OGT) transfers glucosamine (GlcNAc) to serine or threonine residues. Zhou et al. found that the levels of O-GlCNAC and OGT in OC chemically sensitive tissues were significantly higher than those in chemoresistant OC tissues, which reduced apoptotic cell death, resulting in increased resistance of OC cells to cisplatin (107). Together, the epigenetic modifications play an orchestrated role in regulating autophagy, and the effects of the epigenetic modifications on autophagy are cancer context-dependent. Additional studies are needed to elucidate other determinants of autophagy regulation related to epigenetic modifications.
2.4.3 Ferroptosis
Using anti-cancer drugs to trigger apoptotic cell death is one of the principal ways to kill cancer cells. However, due to the acquired or intrinsic resistance of cancer cells to apoptosis, the effect of apoptosis inducers is limited (111, 112). Recently, a growing number of compounds and anti-cancer drugs kill tumor cells by ferroptosis (113–116). For instance, PARP inhibitors like olaparib kill BRCA mutant OC cells through ferroptosis (117). Ferroptosis is a newly discovered form of oxidative cell death caused by iron-dependent peroxidation of lipids. Thus, the redox balance controlled by various redox-active enzymes, which detoxify free radicals and lipid oxidation products, is critical for cells to avoid ferroptosis (118). Several regulatory molecules, such as GPX4, Nrf2, and members of the solute carrier (SLC) family of molecules, play essential roles in the aberrant iron metabolism and maladjustment of the antioxidant system. Studies have shown that epigenetic modification of these potent genes allows cancer cells to escape drug-induced ferroptosis, leading to drug resistance. Among them, ROS scavenger GPX4 is a key regulatory molecule of ferroptosis, which can convert lipid hydrogen peroxide into non-toxic lipid alcohols (119, 120). Due to upstream DNA hypomethylation and high levels of H3K27ac and H3K4me3, the expression level of GPX4 in OC tissues is higher than that in normal tissues, and it is negatively correlated with the prognosis of patients, indicating that aberrant GPX4 expression in cancer may result from epigenetic regulation (121). Additionally, they found that the GPX4 inhibitor RSL3 improves the anti-cancer effects of cisplatin by enhancing ferroptosis in vitro and in vivo (121, 122). Studies have shown that the activity of GPX4 depends on glutathione produced by system Xc (also known as SLC7A11 or xCT) (123). Indeed, p53 or the histone deubiquitinating enzyme BAP1 inhibiting SLC7A11 expression promotes ferroptosis (124, 125).
In addition to the above, the epigenetic alterations of transcription factor nuclear factor erythroid 2-related factor 2 (NRF2), a vital regulator of cellular antioxidant response and ferroptosis by upregulating SLC7A11, can affect the treatment resistance of OC (126, 127). For instance, hypermethylation of the gene promoter in the KEAP1/NRF2 axis has been described in various tumor tissues and is closely related to tumor recurrence and drug resistance (128–130). Van Jaarsveld et al. demonstrated that miR-141-mediated regulation of the KEAP1/NRF2 axis plays a crucial role in the OC response to cisplatin (131). Taken together, these findings provide evidence that epigenetic modifications play a significant role in ferroptosis and drug resistance of OC cells.
2.4.4 Pro-Survival Signaling
Cell survival and death are determined by the epidermal growth factor receptor (EGFR) and AKT (protein kinase B) signaling pathways. Cell survival and death (132, 133) are regulated by HR and non-homologous terminal junctions (NHEJ). The activation of EGFR and AKT can be induced by epigenetic alterations, which may lead to chemotherapy resistance in OC treatment. Overexpression or gene amplification of EGFR and HER2 is frequent in multiple cancers, including OC (134). Cao et al. found that EGFR, phosphorylated EGFR (P-EGFR), and phosphorylated AKT (P-Akt) were up-regulated in miR-125A-5p- and TAZ-transfected OC cells through m (6) A modification (135). Lin et al. discovered that the RNA methyltransferase METTL3, which is involved in mRNA biosynthesis, degradation, and translation regulation, enhances the translation of EGFR and the TAZ in human cancer cells (136). Furthermore, Luo et al. have identified that the RNA polymerase II transcriptional mediator subunit 12 (MED12) down-regulates EGFR expression by binding to the EGFR promoter and mediates chemotherapy resistance in OC (137). Therefore, targeting epigenetic modifications might be an effective strategy to sensitize tumor cells to DNA-damaging agents.
2.5 Epigenetic Modifications Involved in the Maintenance of Cancer Stem Cells
Cancer stem cells (CSCs) are a sub-population of tumor cells that are responsible for driving tumor growth, metastasis, and therapy resistance (52, 138, 139). Emerging results indicate that CSCs contribute to chemoresistance and poor clinical outcomes in various malignancies, including OC (140). Accumulating evidence has shown that CSCs display an innate predisposition to be chemotherapy-resistant and result in tumor relapse (141–143). Furthermore, ovarian cancer stem cells (OCSCs) contribute to resistance to chemotherapy (144). Therefore, solving this thorny problem might lead to the development of new strategies to tackle drug-resistant OC. Because epigenetic regulation plays an integral role in the control of normal stem cell differentiation, strategies to target cancer stem cells can be developed through epigenetics. Epigenetic modifications enable cancer cells to self-renew and generate CSCs, leading to drug resistance (140).
Wang and colleagues hypothesized that hypomethylation drugs might target resistant OCSCs to cure tumors along with chemotherapy drugs. In an orthotopic mouse model, they identified and analyzed ALDH (+) OCSC from OC cell lines and clinical samples, finding that ALDH (+) cells were more chemoresistant than ALDH (−) cells. Treatment with SGI-110, a second-generation DNA methyltransferase inhibitor (DNMTi), re-sensitizes OCSCs to platinum (140). Xu et al. demonstrated that STON2 could negatively regulate the stemness in OC cells by DNMT1-MUC1. STON2 plays a role in OCSC biology and could be used as a therapeutic target for OC treatment (145). Studies have shown that MYPT1 encoding myosin phosphatase target subunit 1 is down-regulated in OC, leading to resistance to platinum-based therapy (146). Similarly, miR-30b could target MYPT1 to lead to enhanced CSC-like properties in OC cells and activate the Hippo pathway. Moreover, inhibition of YAP sensitizes cells to platinum-based therapy (50). Taken together, these studies suggest that epigenetic modifications of these signaling pathways play an essential role in the development of drug resistance in OC.
2.6 Epigenetic Modifications and the Tumor Microenvironment
A tumor microenvironment (TME) refers to the ecological niche in which tumor cells interact with the host stroma, including various immune cells, endothelial cells, fibroblasts, tumor cells, and metabolites. It is now generally accepted that TME significantly influences the efficacy of anti-cancer drugs (51). TME reprogramming caused by epigenetic dysregulations has recently been recognized as an important factor in the progression and drug resistance of OC (2, 147). Here, we systematically generalize the epigenetic modifications of non-malignant OC-related microenvironment cells (namely, cancer-associated fibroblasts, mesenchymal stem cells, and tumor-associated macrophages), which is conducive to evaluating the therapeutic potential of epigenetic regulation of TME-associated cells (Figure 4).
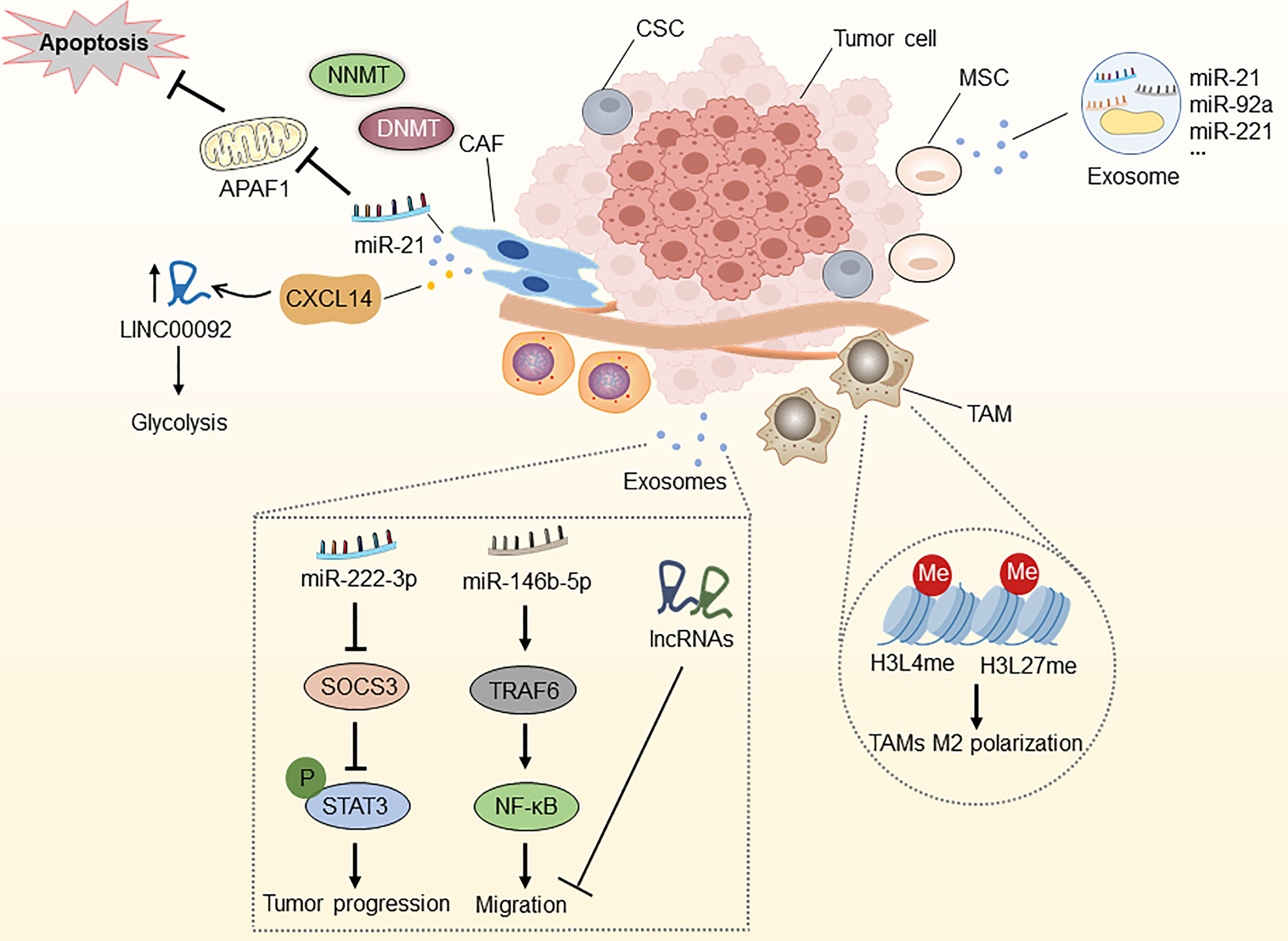
Figure 4 Epigenetic modifications in the tumor microenvironment. NNMT can diminish histone methylation in CAFs, and CAFs secrete miR-21 and CXCL14, which enhance OC development, metastasis, and therapy resistance. MiR-21, miR-92a, and miR-221, which are abundant in MSC-derived exosomes, are associated with OC development. Epigenetic alterations in histone H3 lysine4 and histone H3 lysine27 methylation in TAMs can induce macrophage M2 polarization. Epithelial ovarian cancer (EOC)-released exosomal miRNAs and lncRNAs via the SOCS3/STAT3 pathway and miR-146b-5P/TRAF6/NF-κB/MMP2 pathway regulate the progression, migration and drug resistance of OC. CAF, cancer-associated fibroblast; MSC, mesenchymal stem cell; TAM, tumor-associated macrophage; CSC, cancer stem cell; NNMT, nicotinamide N-methyltransferase; DNMT, DNA methyltransferase; APAF1, apoptosis protease activator-1; APAF6, TNF receptor associated factor 6.
2.6.1 Cancer-Associated Fibroblasts (CAFs)
Fibroblasts located in the TME, also termed CAFs, are the main components of host stromal cells and the main source of collagenous cells in solid tumors (148). They play an essential role in supporting tumors by reconstructing the extracellular matrix. Multiple studies have identified the important role of CAFs in tumor-stroma communication through the excretion of various growth factors and chemokines, contributing to tumor growth, immunosuppression, angiogenesis, cell stemness, and drug resistance (149, 150). Therefore, epigenetic modifications associated with CAFs may affect the resistance of OC.
Studies have reported that the DNA methylation state of genes in mesenchymal fibroblasts from various cancer tissues conforms to the methylation features identified in nearby malignant cells (151, 152). Furthermore, the expression of stromal nicotinamide N-methyltransferase (NNMT) is essential for the functional aspects of the CAF phenotype and supports the growth and metastasis of OC. Stromal NNMT expression in CAFs depletes S-adenosyl methionine and histone methylation, which is related to the global alternations of gene expression in the tumor stroma (153). Additionally, CAFs secrete miR-21, which targets apoptosis protease activator-1 (APAF1), leading to paclitaxel resistance in metastatic or recurrent OC (154). New evidence suggests that OC cells reprogram fibroblasts into CAFs through the action of miRNAs in the TME, including miR-31, miR-214, and miR-155. Targeting these miRNAs in stromal cells might be of therapeutic value, suggesting these miRNAs as novel therapeutic targets for halting OC progression (155). Curtis and colleagues discovered that CAF-secreted CXCL14 promoted OC development and invasion by interacting with the major restriction enzyme, 6-Phosphofructo-2-Kinase/Fructo-2,6-Biphosphatase 2 (PFKFB2) and boosting LINC0009, a long non-coding RNA, to accelerate glycolysis (156, 157). These observations suggest that molecular insights into the abnormally expressed lncRNAs in CAFs are essential for the further diagnostic and therapeutic strategies of OC.
2.6.2 Mesenchymal Stem Cells (MSCs)
MSCs contain various pluripotent cell subpopulations, and MSCs have been reported in most organs and tissues, including the ovaries (158). Because of their potential to develop into other active cancer-promoting stromal components (such as CAFs and cancer-associated adipocytes) and sustain cancer stem cell (CSC) populations, MSCs are strongly linked to cancer progression. In particular, OC-associated MSCs exhibit pluripotency and promote stem cell growth, increase resistance to platinum-based chemotherapy, and provide tumor matrix support and neovascularization (159–161). Although the great bulk of research has concentrated on understanding the impact of cellular signaling pathways, the epigenetic interactions between MSCs and OC remain largely unexplored.
Considering the function of epigenetic modifications in CSC reprogramming, MSCs can be efficiently transformed into CSCs by custom chromatin remodeling. Besides, MSCs can also differentiate into distinct stromal cells by epigenetic regulation (162–164). Through exosomal RNA sequencing, Reza et al. demonstrated numerous miRNAs that exhibit anti-cancer activity by targeting different molecules associated with OC survival (165). New evidence suggests that miR-21, miR-92a, and miR-221, abundant in MSC-derived extracellular vesicles, are associated with OC development (166). Taken together, the above findings revealed a direct or indirect epigenetic relationship between MSCs and OC cells, which should be investigated further and might lead to the identification of new therapeutic targets for OC.
2.6.3 Tumor-Associated Macrophages (TAMs)
TAMs are the most abundant myeloid cell type in the TME and are involved in cancer-related inflammation, matrix remodeling, angiogenesis, metastasis, cancer cell stemness, tumor immune escape, and drug resistance (167–170). The unique TME determines macrophage diversity and the ability to switch between M1 (pro-inflammatory with anti-tumor activity) and M2 (anti-inflammatory with pro-tumor activity) phenotypes (171, 172). Epigenetic regulation plays a significant role in TAM differentiation and activation, which is significantly associated with tumor drug resistance. For instance, studies have found that epithelial ovarian cancer (EOC)-released exosomal miR-222-3p activated M2 polarization and tumor-promoting capacities in ovarian TAMs by the SOCS3/STAT3 pathway. Similarly, exosomal miR-940 released from hypoxic epithelial ovarian tumors induces a shift in the macrophage M2 phenotype (173, 174). Furthermore, TAM-secreted exosomes prevented endothelial migration by targeting the miR-146b-5P/TRAF6/NF-κB/MMP2 pathway, and the lncRNAs in OC-secreted exosomes effectively inhibit migration (175). Ishii et al. described M2 macrophage polarization through STAT6-mediated reciprocal epigenetic alterations in histone H3 lysine4 and histone H3 lysine27 methylation, which resulted in transcriptional activation of particular M2 manufacturing genes (176). Together, these findings highlight the importance of TAM-regulated epigenetic alterations in OC, as they may reveal novel diagnostic and therapeutic approaches.
3 Therapeutic Targeting of the OC Epigenome
OC treatment prioritizes surgery and cytoreduction, followed by cytotoxic platinum and taxane chemotherapy. Although most OC patients respond effectively in the early phases of therapy, new therapeutic options are urgently needed to enhance outcomes for the most advanced patients (1). In the last decade, immunotherapy and targeted therapy have shown remarkable benefits for treating OC (177–179). Furthermore, due to the reversibility of epigenetic alterations, the prevention of treatment resistance through epigenetic drugs has become an attractive therapeutic concept, and the combination of epigenetic therapies with chemotherapy, immunotherapy, and molecularly targeted treatments holds a lot of promise for OC treatment. Based on this, epigenetic regulation, namely, DNA methylation, histone modifications, and ncRNA regulation, has emerged as a potential therapeutic target for OC (Figure 5).
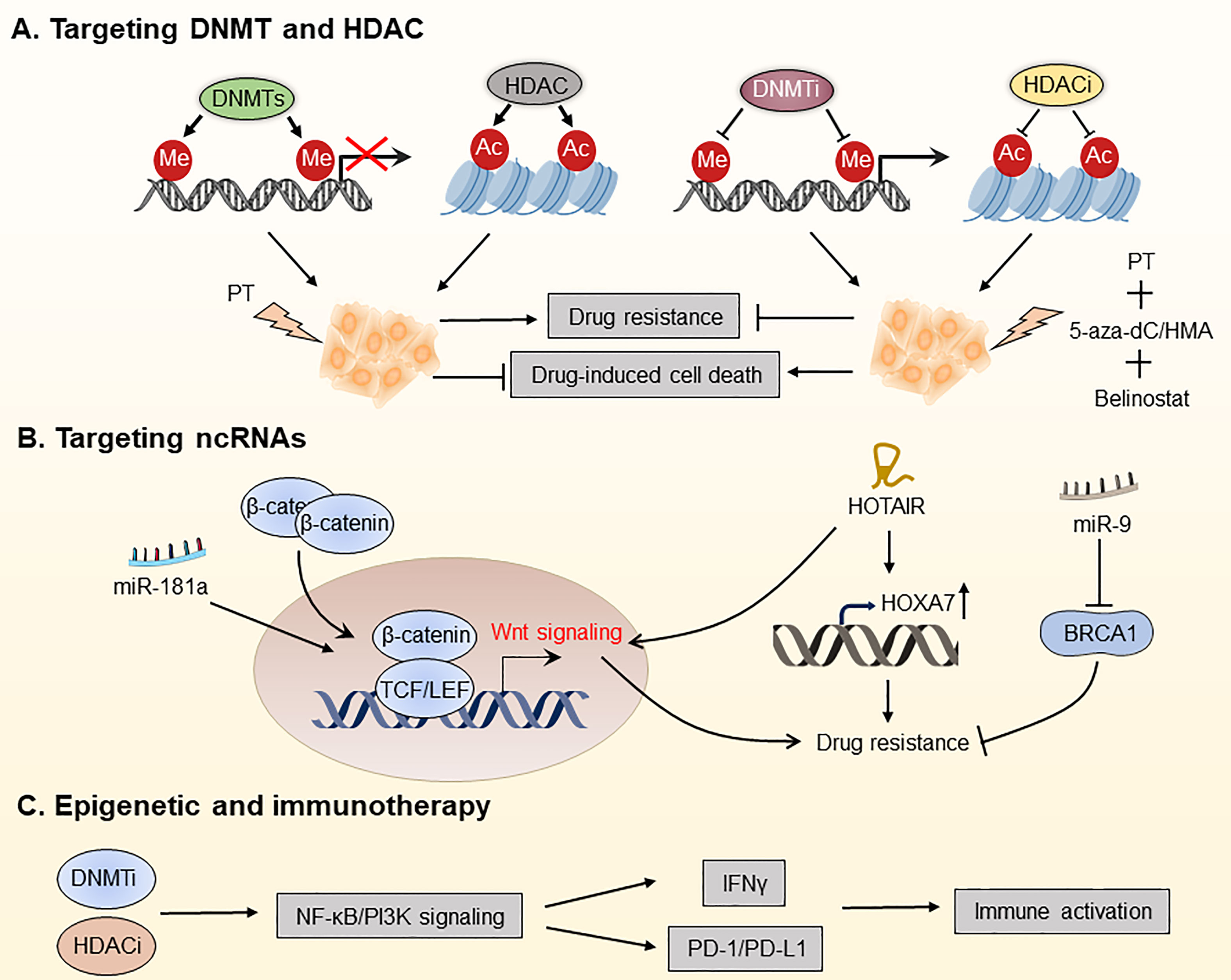
Figure 5 Targeting the OC epigenome and overcoming drug resistance in OC. (A) Platinum therapy combined with DNA methyltransferase inhibitors (DNMTis) and histone deacetylation inhibitors (HDACis) has been used for chemotherapeutic sensitization. (B) Overcoming OC drug resistance by targeting certain ncRNAs. (C) DNMTi and HDACi increased the responsiveness of OC to immune checkpoint therapy by reducing the immunosuppressive milieu via the type I IFN signaling pathway. PT, platinum; 5-aza-dC, 5-aza-2-deoxy-cytidine; HMA, hypomethylating agent; PD-1, programmed death protein 1; PD-L1, programmed death ligand 1.
3.1 Targeting DNMT
As one of the most well-known epigenetic modifications, DNA methylation plays an essential role in DNA repair, apoptosis, angiogenesis, gene expression regulation, and drug resistance. To date, aberrant DNA hypermethylation has been found in drug-resistant cancer cells, and drug-induced DNA hypermethylation has been proposed as a potential mechanism and biomarker of drug resistance. DNA methylation is mediated by three DNA methyltransferases (DNMTs), primarily by DNMT1, which mediates maintenance (one strand) methylation, and by DNMT3A and DNMT3B, which catalyze de novo methylation (180). The activity of DNMTs can be blocked by DNMT inhibitors (DNMTis), which are analogs of deoxycytosine and effectively block methyl transfer (181). 5-aza-2-deoxy-cytidine (decitabine) and 5-azacytidine were first successfully studied in hematological malignancies and myelodysplastic syndrome (MDS) and were approved by the FDA in 2006 for treating MDS, where they exhibit anti-tumor activity through the inhibition of DNA methylation (182).
To date, DNMTis has been successfully used for treating chemotherapy-resistant OC, restoring platinum sensitivity in refractory to standard chemotherapy of patients. DNA hypomethylation caused by DNMTi decitabine renders OC patients more susceptible to platinum treatment and is associated with a better prognosis. Matei et al. found that platinum resistance is mostly induced by epigenetic abnormalities, including abnormal DNA methylation. The regimen consists of a modest dosage of decitabine 5 days before the administration of carboplatin, which decreases toxicity and boosts the demethylation effect of decitabine, leading to the recovery of carboplatin sensitivity in patients with advanced OC (183). Decitabine has been shown in studies to be more effective than 5-azocytidine for treating platinum-resistant OC. It is capable of regulating the methylation status of the tumor antigen NY-ESO-1 to improve immunotherapy efficacy (184). Furthermore, decitabine improves responses of OC patients to platinum therapy by influencing signaling pathways that promote tumor progression, such as the TGF-β signaling pathway (185). Taken together, DNMTis plays a promising role in reversing or preventing chemotherapeutic and molecular-targeted drug resistance in OC patients.
3.2 Targeting HDAC
Histone deacetylase (HDAC) enzymes, which remove acetyl groups from histone and non-histone proteins, downregulate the transcription of genes (186). In cancer cells, HDAC inhibitors (HDACis) can restore transcriptional inhibition of tumor suppressor genes and generate an anti-cancer environment. Meng et al. showed that a combination of proteasome and HDAC inhibitors can inhibit gynecologic cancer growth (187). Inhibitors of enzyme-catalyzed histone modifications, among which HDACis are the most rapidly developed, have been investigated in solid tumors (188).
Fukumoto et al. found that ARID1A mutations confer sensitivity to pan-HDAC inhibitors such as SAHA in OC, which is associated with more significant effects of growth inhibition owing to suppression of HDAC2 activity (189). By suppressing HDAC6 with the small molecule ACY1215, Bitler et al. revealed that mice with ARID1A mutant tumors had dramatically increased survival. Mechanistically, HDAC6 deacetylates Lys120 on p53, thereby inactivating the pro-apoptotic function of p53 (190). However, not all HDAC subtypes are abnormally expressed in all malignancies, so pan-inhibition of HDAC is not an effective way to treat cancer (191). Besides, because of its poor activity as a single agent, HDACis have been investigated along with radiotherapy, chemotherapy, and other epigenetic drugs. For instance, belinostat re-sensitized drug-resistant OC cells to platinum, and the combination of decitabine and belinostat was more effective in re-sensitizing platinum than belinostat alone (192, 193). Furthermore, there is mounting evidence showing that HDACs-mediated deacetylation of non-histones is involved in many key cellular processes associated with drug resistance, such as apoptosis, suggesting that enhanced acetylation of non-histones using HDACis is promising for overcoming the drug resistance of OC. Taken together, targeting HDACs is an appealing strategy for OC treatment.
3.3 Targeting ncRNAs
Multiple ncRNAs, including miRNAs and lncRNAs, are increasingly implicated in the treatment resistance in OC (194, 195). Therefore, targeting tumor-specific miRNAs and lncRNAs is expected to overcome drug resistance of OC. Recently, miRNAs have received increasing attention as biomarkers and therapeutic targets for OC. For instance, Belur Nagaraj and colleagues found that miR-181a is an activator of Wnt/β-catenin signaling, driving stemness and chemotherapy resistance in high-grade serous ovarian cancer (HGSOC), thus being a potential target for treating recurrent OC (194). Using next-generation sequencing (NGS) techniques, Au Yeung et al. identified miR-21 in exosomes and tissue lysates isolated from cancer-associated adipocytes and fibroblasts, which is significantly higher than OC cells. They discovered that miR21 is transferred from CAAs or CAFs to OC cells, suppresses apoptosis and confers chemotherapeutic resistance in OC cells by binding with APAF1 (154). Additionally, Vescarelli et al. have demonstrated that miR-200c significantly enhanced the anti-cancer effect of the PARP inhibitor olaparib in drug-resistant OC cells (196). This finding indicates that combining olaparib with miRNA-based therapy is a potential therapeutic option for drug-resistant OC. Sun et al. found that miR-9 increases the sensitivity of OC cells to DNA damage by down-regulating BRCA1, thus improving the efficacy of chemotherapy (197).
In addition to miRNAs, LncRNAs are considered promising therapeutic targets for OC. For instance, the non-coding RNA HOTAIR has been shown to be a potential target for overcoming carboplatin resistance in OC (195). Furthermore, it has been discovered that HOTAIR is overexpressed in cisplatin-resistant OC cells, and knocking down HOTAIR enhances apoptosis in cisplatin-resistant OC cells by down-regulating HOXA7, thereby restoring cisplatin sensitivity (198, 199). Additionally, HOTAIR-mediated platinum resistance in OC can also be attributed to the upregulation of HOXA7 and activation of the Wnt/β-catenin pathway (88). These findings point to it as a possible target for re-sensitizing OC cells to platinum therapy. Besides, Wu et al. demonstrated that the lncRNA WDFY3-AS2 modulates the hsa-miR-139-5p/SDC4 axis and may play an essential role in the platinum resistance of OC (200). Taken together, the above studies show that it is promising to prevent or overcome OC resistance by targeting ncRNAs.
3.4 Epigenetic Therapy in Combination With Immunotherapy in OC
Antibodies against inhibitory immune receptors, namely, cytotoxic T-lymphocyte-associated protein 4 (CTLA-4/CD152), programmed death protein 1 (PD-1/CD279), and programmed death ligand 1 (PD-L1/B7H1/CD274), have become effective standard immunotherapy for many advanced malignancies (177, 201). However, its effectiveness has yet to be successfully applied to the treatment of OC (202). Although a small fraction of OC patients respond well to immunotherapy, most patients fail to respond or develop secondary resistance to immunotherapy. Therefore, a combinatorial strategy is needed to enhance the efficacy of immunotherapy for OC, which can be achieved by drug repurposing or de novo drug development that stimulates the immune response.
Initial findings showed that epigenetic repressive processes were linked to a “cold” immunological environment in OC. Based on them, epigenetic modifiers were used in several of preclinical and clinical trials to explore immune targeting techniques (188). For instance, Stone and colleagues have demonstrated that DNMTi 5-azacytidine (AZA) activates the type I interferon signaling pathway, boosts IFNγ+ T cells and natural killer (NK) cells, and reduces the percentage of macrophages in the TME. They also discovered that clinically relevant dosages of DNMTi and HDACi increased the responsiveness of OC to immune checkpoint therapy by reducing the immunosuppressive milieu via the type I IFN signaling pathway (203). The triple combination of DNMTi/HDACi with the immune checkpoint inhibitor α-PD-1 showed significantly better anti-tumor efficacy than DNMTi 5-azacytidine (AZA) alone or AZA along with HDACi, which may be a therapeutic option for treating OC. In a follow-up study, Travers et al. employed a hypomethylating agent (HMA) in conjunction with 2-difluoromethylornithine (DFMO) to rewire TME in OC, leading to a longer-lasting anti-tumor response. They found that AZA and DFMO, either alone or in combination, significantly recruited CD4+/CD8+ T and NK cells, decreased tumor burden, and improved the survival of OC patients (204). Another trial used decitabine in conjunction with paclitaxel and carboplatin to treat 55 patients with recurrent OC and found that the triple combination treatment group had a 58% overall response rate, which is superior to other groups (205). Therefore, combinational therapy with epigenetic modifiers may be able to avoid the loss of tumor antigens and expand the T cell pool by recognizing the initially targeted antigens and epitopes, showing a new and intriguing promise for vaccine and cell transfer platforms.
4 Conclusions and Perspectives
Although notable progress has been made in treating OC recently, most patients with advanced OC still relapse and eventually die from chemotherapy resistance, notably platinum. In fact, tumorigenesis, progression, and treatment resistance of OC are largely mediated by epigenetic regulation. These epigenetic alterations, such as DNA methylation and histone modifications, may occur before or during drug treatment and develop drug resistance by controlling several essential signaling pathways. Recently, with the improvement of our understanding of cancer-specific epigenetic alterations, targeting the epigenome to prevent or overcome OC drug resistance may be a viable therapeutic option.
In this article, we reviewed how epigenetic modifications play a key role in drug resistance in OC cells by affecting pivotal processes, namely, drug transport and metabolism, downstream signaling pathways, cancer stemness, and the immune microenvironment. Meanwhile, we also highlighted the biological functions of several key epigenetic modifiers, such as DNMT, HDAC, and ncRNAs. As the machinery embroidering epigenetic regulation continues to be deciphered, we conclude that the epigenome may emerge as a novel therapeutic target for OC. In fact, some small-molecule inhibitors targeting epigenetic alterations have entered the stage of clinical trials, which are expected to prevent or overcome OC resistance by changing the drug-responsive epigenome of OC. However, for complex reasons, the response rates of OC to monotherapy are typically modest. Although ongoing clinical trials have shown that epigenetic agents can significantly improve the drug sensitivity of OC when combined with targeted agents (e.g., PARP inhibitors) or immune drugs (e.g., anti-PD-1), leveraging the unique properties of epigenetics to obtain the optimal therapeutic advantage still requires considerable effort in the following research.
Author Contributions
Conceptualization, CH and LL. Investigation, YW. Writing-original draft preparation, YW and ZH. Writing-review and editing, YW, ZH, and BL. Visualization, YW and BL Supervision, CH and LL. All authors listed have made a substantial, direct, and intellectual contribution to the work and approved it for publication.
Funding
This work was supported by the National Natural Science Foundation of China (82003113, 82102738, 82103168).
Conflict of Interest
The authors declare that the research was conducted in the absence of any commercial or financial relationships that could be construed as a potential conflict of interest.
Publisher’s Note
All claims expressed in this article are solely those of the authors and do not necessarily represent those of their affiliated organizations, or those of the publisher, the editors and the reviewers. Any product that may be evaluated in this article, or claim that may be made by its manufacturer, is not guaranteed or endorsed by the publisher.
Glossary
References
1. Lheureux S, Braunstein M, Oza AM. Epithelial Ovarian Cancer: Evolution of Management in the Era of Precision Medicine. CA: Cancer J Clin (2019) 69(4):280–304. doi: 10.3322/caac.21559
2. Jiang Y, Wang C, Zhou S. Targeting Tumor Microenvironment in Ovarian Cancer: Premise and Promise. Biochim Biophys Acta Rev Cancer (2020) 1873(2):188361. doi: 10.1016/j.bbcan.2020.188361
3. Cummings M, Freer C, Orsi NM. Targeting the Tumour Microenvironment in Platinum-Resistant Ovarian Cancer. Semin Cancer Biol (2021) 77:3–28. doi: 10.1016/j.semcancer.2021.02.007
4. Oza AM, Cook AD, Pfisterer J, Embleton A, Ledermann JA, Pujade-Lauraine E, et al. Standard Chemotherapy With or Without Bevacizumab for Women With Newly Diagnosed Ovarian Cancer (ICON7): Overall Survival Results of a Phase 3 Randomised Trial. Lancet Oncol (2015) 16(8):928–36. doi: 10.1016/S1470-2045(15)00086-8
5. Christie EL, Bowtell DDL. Acquired Chemotherapy Resistance in Ovarian Cancer. Ann Oncol (2017) 28(suppl_8):viii13–5. doi: 10.1093/annonc/mdx446
6. Moschetta M, George A, Kaye SB, Banerjee S. BRCA Somatic Mutations and Epigenetic BRCA Modifications in Serous Ovarian Cancer. Ann Oncol (2016) 27(8):1449–55. doi: 10.1093/annonc/mdw142
7. Mirza MR, Monk BJ, Herrstedt J, Oza AM, Mahner S, Redondo A, et al. Niraparib Maintenance Therapy in Platinum-Sensitive, Recurrent Ovarian Cancer. N Engl J Med (2016) 375(22):2154–64. doi: 10.1056/NEJMoa1611310
8. Mirza MR, Coleman RL, González-Martín A, Moore KN, Colombo N, Ray-Coquard I, et al. The Forefront of Ovarian Cancer Therapy: Update on PARP Inhibitors. Ann Oncol Off J Eur Soc Med Oncol (2020) 31(9):1148–59. doi: 10.1016/j.annonc.2020.06.004
9. Kwon JS, Daniels MS, Sun CC, Lu KH. Preventing Future Cancers by Testing Women With Ovarian Cancer for BRCA Mutations. J Clin Oncol Off J Am Soc Clin Oncol (2010) 28(4):675–82. doi: 10.1200/JCO.2008.21.4684
10. Slade D. PARP and PARG Inhibitors in Cancer Treatment. Genes Dev (2020) 34(5-6):360–94. doi: 10.1101/gad.334516.119
11. Vasconcelos MH, Caires HR, Ābols A, Xavier CPR, Linē A. Extracellular Vesicles as a Novel Source of Biomarkers in Liquid Biopsies for Monitoring Cancer Progression and Drug Resistance. Drug Resist Update (2019) 47:100647. doi: 10.1016/j.drup.2019.100647
12. Bar-Zeev M, Livney YD, Assaraf YG. Targeted Nanomedicine for Cancer Therapeutics: Towards Precision Medicine Overcoming Drug Resistance. Drug Resist Update (2017) 31:15–30. doi: 10.1016/j.drup.2017.05.002
13. Assaraf YG, Brozovic A, Gonçalves AC, Jurkovicova D, Linē A, Machuqueiro M, et al. The Multi-Factorial Nature of Clinical Multidrug Resistance in Cancer. Drug Resist Update (2019) 46:100645. doi: 10.1016/j.drup.2019.100645
14. Zhang Z, Qin S, Chen Y, Zhou L, Yang M, Tang Y, et al. Inhibition of NPC1L1 Disrupts Adaptive Responses of Drug-Tolerant Persister Cells to Chemotherapy. EMBO Mol Med (2022) 14(2):e14903. doi: 10.15252/emmm.202114903
15. Galluzzi L, Senovilla L, Vitale I, Michels J, Martins I, Kepp O, et al. Molecular Mechanisms of Cisplatin Resistance. Oncogene (2012) 31(15):1869–83. doi: 10.1038/onc.2011.384
16. Morel D, Jeffery D, Aspeslagh S, Almouzni G, Postel-Vinay S. Combining Epigenetic Drugs With Other Therapies for Solid Tumours - Past Lessons and Future Promise. Nat Rev Clin Oncol (2020) 17(2):91–107. doi: 10.1038/s41571-019-0267-4
17. Wilting RH, Dannenberg JH. Epigenetic Mechanisms in Tumorigenesis, Tumor Cell Heterogeneity and Drug Resistance. Drug Resist Update (2012) 15(1-2):21–38. doi: 10.1016/j.drup.2012.01.008
18. Li B, Jiang J, Assaraf YG, Xiao H, Chen ZS, Huang C, et al. Surmounting Cancer Drug Resistance: New Insights From the Perspective of N(6)-Methyladenosine RNA Modification. Drug Resist Update (2020) 53:100720. doi: 10.1016/j.drup.2020.100720
19. Konstantinopoulos PA, Ceccaldi R, Shapiro GI, D'Andrea AD. Homologous Recombination Deficiency: Exploiting the Fundamental Vulnerability of Ovarian Cancer. Cancer Discov (2015) 5(11):1137–54. doi: 10.1158/2159-8290.CD-15-0714
20. Shen DW, Pouliot LM, Hall MD, Gottesman MM. Cisplatin Resistance: A Cellular Self-Defense Mechanism Resulting From Multiple Epigenetic and Genetic Changes. Pharmacol Rev (2012) 64(3):706–21. doi: 10.1124/pr.111.005637
21. Li H, Yu G, Shi R, Lang B, Chen X, Xia D, et al. Cisplatin-Induced Epigenetic Activation of miR-34a Sensitizes Bladder Cancer Cells to Chemotherapy. Mol Cancer (2014) 13:8. doi: 10.1186/1476-4598-13-8
22. Bolton KL, Chenevix-Trench G, Goh C, Sadetzki S, Ramus SJ, Karlan BY, et al. Association Between BRCA1 and BRCA2 Mutations and Survival in Women With Invasive Epithelial Ovarian Cancer. JAMA (2012) 307(4):382–90. doi: 10.1001/jama.2012.20
23. Yang D, Khan S, Sun Y, Hess K, Shmulevich I, Sood AK, et al. Association of BRCA1 and BRCA2 Mutations With Survival, Chemotherapy Sensitivity, and Gene Mutator Phenotype in Patients With Ovarian Cancer. JAMA (2011) 306(14):1557–65. doi: 10.1001/jama.2011.1456
24. Yang Q, Yang Y, Zhou N, Tang K, Lau WB, Lau B, et al. Epigenetics in Ovarian Cancer: Premise, Properties, and Perspectives. Mol Cancer (2018) 17(1):109. doi: 10.1186/s12943-018-0855-4
25. Watson ZL, Yamamoto TM, McMellen A, Kim H, Hughes CJ, Wheeler LJ, et al. Histone Methyltransferases EHMT1 and EHMT2 (GLP/G9A) Maintain PARP Inhibitor Resistance in High-Grade Serous Ovarian Carcinoma. Clin Epigenet (2019) 11(1):165. doi: 10.1186/s13148-019-0758-2
26. Abbosh PH, Montgomery JS, Starkey JA, Novotny M, Zuhowski EG, Egorin MJ, et al. Dominant-Negative Histone H3 Lysine 27 Mutant Derepresses Silenced Tumor Suppressor Genes and Reverses the Drug-Resistant Phenotype in Cancer Cells. Cancer Res (2006) 66(11):5582–91. doi: 10.1158/0008-5472.CAN-05-3575
27. Zhang X, Xie K, Zhou H, Wu Y, Li C, Liu Y, et al. Role of Non-Coding RNAs and RNA Modifiers in Cancer Therapy Resistance. Mol Cancer (2020) 19(1):47. doi: 10.1186/s12943-020-01171-z
28. Wei L, Sun J, Zhang N, Zheng Y, Wang X, Lv L, et al. Noncoding RNAs in Gastric Cancer: Implications for Drug Resistance. Mol Cancer (2020) 19(1):62. doi: 10.1186/s12943-020-01185-7
29. Wu X, Luo Q, Zhao P, Chang W, Wang Y, Shu T, et al. MGMT-Activated DUB3 Stabilizes MCL1 and Drives Chemoresistance in Ovarian Cancer. Proc Natl Acad Sci USA (2019) 116(8):2961–6. doi: 10.1073/pnas.1814742116
30. Zhang X, et al. The Inhibition of UBC13 Expression and Blockage of the DNMT1-CHFR-Aurora A Pathway Contribute to Paclitaxel Resistance in Ovarian Cancer. Cell Death Dis (2018) 9(2):93. doi: 10.1038/s41419-017-0137-x
31. Han X, Liu D, Zhou Y, Wang L, Hou H, Chen H, et al. The Negative Feedback Between miR-143 and DNMT3A Regulates Cisplatin Resistance in Ovarian Cancer. Cell Biol Int (2021) 45(1):227–37. doi: 10.1002/cbin.11486
32. Li H, Lei Y, Li S, Li F, Lei J. MicroRNA-20a-5p Inhibits the Autophagy and Cisplatin Resistance in Ovarian Cancer via Regulating DNMT3B-Mediated DNA Methylation of RBP1. Reprod Toxicol (Elmsford N.Y.) (2022) 109:93–100. doi: 10.1016/j.reprotox.2021.12.011
33. Sharma SV, Lee DY, Li B, Quinlan MP, Takahashi F, Maheswaran S, et al. A Chromatin-Mediated Reversible Drug-Tolerant State in Cancer Cell Subpopulations. Cell (2010) 141(1):69–80. doi: 10.1016/j.cell.2010.02.027
34. Shang S, Yang J, Jazaeri AA, Duval AJ, Tufan T, Lopes Fischer N, et al. Chemotherapy-Induced Distal Enhancers Drive Transcriptional Programs to Maintain the Chemoresistant State in Ovarian Cancer. Cancer Res (2019) 79(18):4599–611. doi: 10.1158/0008-5472.CAN-19-0215
35. Jeong JY, Kang H, Kim TH, Kim G, Heo JH, Kwon AY, et al. MicroRNA-136 Inhibits Cancer Stem Cell Activity and Enhances the Anti-Tumor Effect of Paclitaxel Against Chemoresistant Ovarian Cancer Cells by Targeting Notch3. Cancer Lett (2017) 386:168–78. doi: 10.1016/j.canlet.2016.11.017
36. Wang Y, Bao W, Liu Y, Wang S, Xu S, Li X, et al. miR-98-5p Contributes to Cisplatin Resistance in Epithelial Ovarian Cancer by Suppressing miR-152 Biogenesis via Targeting Dicer1. Cell Death Dis (2018) 9(5):447. doi: 10.1038/s41419-018-0390-7
37. Li X, Chen W, Jin Y, Xue R, Su J, Mu Z, et al. miR-142-5p Enhances Cisplatin-Induced Apoptosis in Ovarian Cancer Cells by Targeting Multiple Anti-Apoptotic Genes. Biochem Pharmacol (2019) 161:98–112. doi: 10.1016/j.bcp.2019.01.009
38. Niu L, Ni H, Hou Y, Du Q, Li H. miR-509-3p Enhances Platinum Drug Sensitivity in Ovarian Cancer. Gene (2019) 686:63–7. doi: 10.1016/j.gene.2018.11.011
39. Zuo Y, Zheng W, Liu J, Tang Q, Wang SS, Yang XS, et al. MiR-34a-5p/PD-L1 Axis Regulates Cisplatin Chemoresistance of Ovarian Cancer Cells. Neoplasma (2020) 67(1):93–101. doi: 10.4149/neo_2019_190202N106
40. Özeş AR, Miller DF, Özeş ON, Fang F, Liu Y, Matei D, et al. NF-κb-HOTAIR Axis Links DNA Damage Response, Chemoresistance and Cellular Senescence in Ovarian Cancer. Oncogene (2016) 35(41):5350–61. doi: 10.1038/onc.2016.75
41. Yang C, Li H, Zhang T, Chu Y, Chen D, Zuo J, et al. miR-200c Overexpression Inhibits the Invasion and Tumorigenicity of Epithelial Ovarian Cancer Cells by Suppressing lncRNA HOTAIR in Mice. J Cell Biochem (2020) 121(2):1514–23. doi: 10.1002/jcb.29387
42. Li Z, Niu H, Qin Q, Yang S, Wang Q, Yu C, et al. lncRNA UCA1 Mediates Resistance to Cisplatin by Regulating the miR-143/FOSL2-Signaling Pathway in Ovarian Cancer. Mol Ther Nucleic Acids (2019) 17:92–101. doi: 10.1016/j.omtn.2019.05.007
43. Wu DI, Wang T, Ren C, Liu L, Kong D, Jin X, et al. Downregulation of BC200 in Ovarian Cancer Contributes to Cancer Cell Proliferation and Chemoresistance to Carboplatin. Oncol Lett (2016) 11(2):1189–94. doi: 10.3892/ol.2015.3983
44. Li J, Huang H, Li Y, Li L, Hou W, You Z, et al. Decreased Expression of Long Non-Coding RNA GAS5 Promotes Cell Proliferation, Migration and Invasion, and Indicates a Poor Prognosis in Ovarian Cancer. Oncol Rep (2016) 36(6):3241–50. doi: 10.3892/or.2016.5200
45. Long X, Li L, Zhou Q, Wang H, Zou D, Wang D, et al. Long Non-Coding RNA LSINCT5 Promotes Ovarian Cancer Cell Proliferation, Migration and Invasion by Disrupting the CXCL12/CXCR4 Signalling Axis. Oncol Lett (2018) 15(5):7200–6. doi: 10.3892/ol.2018.8241
46. Berdasco M, Esteller M. Clinical Epigenetics: Seizing Opportunities for Translation. Nat Rev Genet (2019) 20(2):109–27. doi: 10.1038/s41576-018-0074-2
47. Bivona TG, Doebele RC. A Framework for Understanding and Targeting Residual Disease in Oncogene-Driven Solid Cancers. Nat Med (2016) 22(5):472–8. doi: 10.1038/nm.4091
48. Li B, Jiang J, Assaraf YG, Xiao H, Chen ZS, Huang C, et al. Surmounting Cancer Drug Resistance: New Insights From the Perspective of N-Methyladenosine RNA Modification. Drug Resist Updates Rev Commentaries Antimicrob Anticancer Chemother (2020) 53:100720. doi: 10.1016/j.drup.2020.100720
49. Chen Z, Shi T, Zhang L, Zhu P, Deng M, Huang C, et al. Mammalian Drug Efflux Transporters of the ATP Binding Cassette (ABC) Family in Multidrug Resistance: A Review of the Past Decade. Cancer Lett (2016) 370(1):153–64. doi: 10.1016/j.canlet.2015.10.010
50. Muñoz‐Galván S, Felipe-Abrio B, Verdugo-Sivianes EM, Perez M, Jiménez-García MP, Suarez‐Martinez E, et al. Downregulation of MYPT1 Increases Tumor Resistance in Ovarian Cancer by Targeting the Hippo Pathway and Increasing the Stemness. Mol Cancer (2020) 19(1):7. doi: 10.1186/s12943-020-1130-z
51. Erin N, Grahovac J, Brozovic A, Efferth T. Tumor Microenvironment and Epithelial Mesenchymal Transition as Targets to Overcome Tumor Multidrug Resistance. Drug Resist Updates Rev Commentaries Antimicrob Anticancer Chemother (2020) 53:100715. doi: 10.1016/j.drup.2020.100715
52. Ahmed N, Escalona R, Leung D, Chan E, Kannourakis G. Tumour Microenvironment and Metabolic Plasticity in Cancer and Cancer Stem Cells: Perspectives on Metabolic and Immune Regulatory Signatures in Chemoresistant Ovarian Cancer Stem Cells. Semin Cancer Biol (2018) 53:265–81. doi: 10.1016/j.semcancer.2018.10.002
53. Qu Y, Dou B, Tan H, Feng Y, Wang N, Wang D, et al. Tumor Microenvironment-Driven Non-Cell-Autonomous Resistance to Antineoplastic Treatment. Mol Cancer (2019) 18(1):69. doi: 10.1186/s12943-019-0992-4
54. Li W, Zhang H, Assaraf YG, Zhao K, Xu X, Xie J, et al. Overcoming ABC Transporter-Mediated Multidrug Resistance: Molecular Mechanisms and Novel Therapeutic Drug Strategies. Drug Resist Update (2016) 27:14–29. doi: 10.1016/j.drup.2016.05.001
55. Fletcher JI, Williams RT, Henderson MJ, Norris MD, Haber M. ABC Transporters as Mediators of Drug Resistance and Contributors to Cancer Cell Biology. Drug Resist Update (2016) 26:1–9. doi: 10.1016/j.drup.2016.03.001
56. Sun NK, Kohli A, Huang SL, Chang TC, Chao CC. Androgen Receptor Transcriptional Activity and Chromatin Modifications on the ABCB1/MDR Gene Are Critical for Taxol Resistance in Ovarian Cancer Cells. J Cell Physiol (2019) 234(6):8760–75. doi: 10.1002/jcp.27535
57. Wu DD, Li XS, Meng XN, Yan J, Zong ZH. MicroRNA-873 Mediates Multidrug Resistance in Ovarian Cancer Cells by Targeting ABCB1. Tumour Biol (2016) 37(8):10499–506. doi: 10.1007/s13277-016-4944-y
58. Tian J, Xu YY, Li L, Hao Q. MiR-490-3p Sensitizes Ovarian Cancer Cells to Cisplatin by Directly Targeting ABCC2. Am J Transl Res (2017) 9(3):1127–38.
59. Wang JM, Liu BQ, Zhang Q, Hao L, Li C, Yan J, et al. ISG15 Suppresses Translation of ABCC2 via ISGylation of Hnrnpa2b1 and Enhances Drug Sensitivity in Cisplatin Resistant Ovarian Cancer Cells. Biochim Biophys Acta Mol Cell Res (2020) 1867(4):118647. doi: 10.1016/j.bbamcr.2020.118647
60. Zhang S, Balch C, Chan MW, Lai HC, Matei D, Schilder JM, et al. Identification and Characterization of Ovarian Cancer-Initiating Cells From Primary Human Tumors. Cancer Res (2008) 68(11):4311–20. doi: 10.1158/0008-5472.CAN-08-0364
61. Calcagno AM, Fostel JM, To KK, Salcido CD, Martin SE, Chewning KJ, et al. Single-Step Doxorubicin-Selected Cancer Cells Overexpress the ABCG2 Drug Transporter Through Epigenetic Changes. Br J Cancer (2008) 98(9):1515–24. doi: 10.1038/sj.bjc.6604334
62. Chen Y. Effects of(Linc-VLDLR) Existing in Extracellular Vesicles on the Occurrence and Multidrug Resistance of Esophageal Cancer Cells. Pathol Res Pract (2019) 215(3):470–7. doi: 10.1016/j.prp.2018.12.033
63. Dong Y, Lin Y, Gao X, Zhao Y, Wan Z, Wang H, et al. Targeted Blocking of Mir328 Lysosomal Degradation With Alkalized Exosomes Sensitizes the Chronic Leukemia Cells to Imatinib. Appl Microbiol Biotechnol (2019) 103(23-24):9569–82. doi: 10.1007/s00253-019-10127-3
64. Liang G, Zhu Y, Ali DJ, Tian T, Xu H, Si K, et al. Engineered Exosomes for Targeted Co-Delivery of miR-21 Inhibitor and Chemotherapeutics to Reverse Drug Resistance in Colon Cancer. J Nanobiotechnol (2020) 18(1):10. doi: 10.1186/s12951-019-0563-2
65. Liu T, Zhang X, Du L, Wang Y, Liu X, Tian H, et al. Exosome-Transmitted miR-128-3p Increase Chemosensitivity of Oxaliplatin-Resistant Colorectal Cancer. Mol Cancer (2019) 18(1):43. doi: 10.1186/s12943-019-0981-7
66. Thakur C, Chen F. Connections Between Metabolism and Epigenetics in Cancers. Semin Cancer Biol (2019) 57:52–8. doi: 10.1016/j.semcancer.2019.06.006
67. Pande P, Zhong X-B, Ku WW. Histone Methyltransferase G9a Regulates Expression of Nuclear Receptors and Cytochrome P450 Enzymes in HepaRG Cells at Basal Level and in Fatty Acid Induced Steatosis. Drug Metab Dispos: Biol Fate Chemicals (2020) 48(12):1321–9. doi: 10.1124/dmd.120.000195
68. Luo J, Xie M, Hou Y, Ma W, Jin Y, Chen J, et al. A Novel Epigenetic Mechanism Unravels hsa-miR-148a-3p-Mediated CYP2B6 Downregulation in Alcoholic Hepatitis Disease. Biochem Pharmacol (2021) 188:114582. doi: 10.1016/j.bcp.2021.114582
69. Fuentes-Mattei E, Bayraktar R, Manshouri T, Silva AM, Ivan C, Gulei D, et al. miR-543 Regulates the Epigenetic Landscape of Myelofibrosis by Targeting TET1 and TET2. JCI Insight (2020) 5(1):e121781. doi: 10.1172/jci.insight.121781
70. Luo M, Yang X, Chen HN, Nice EC, Huang C. Drug Resistance in Colorectal Cancer: An Epigenetic Overview. Biochim Biophys Acta Rev Cancer (2021) 1876(2):188623. doi: 10.1016/j.bbcan.2021.188623
71. Minari R, Bordi P, La Monica S, Squadrilli A, Leonetti A, Bottarelli L, et al. Concurrent Acquired BRAF V600E Mutation and MET Amplification as Resistance Mechanism of First-Line Osimertinib Treatment in a Patient With EGFR-Mutated NSCLC. J Thorac Oncol Off Publ Int Assoc Study Lung Cancer (2018) 13(6):e89–91. doi: 10.1016/j.jtho.2018.03.013
72. Nayar U, Cohen O, Kapstad C, Cuoco MS, Waks AG, Wander SA, et al. Acquired HER2 Mutations in ER Metastatic Breast Cancer Confer Resistance to Estrogen Receptor-Directed Therapies. Nat Genet (2019) 51(2):207–16. doi: 10.1038/s41588-018-0287-5
73. Monk BJ, Pujade-Lauraine E, Burger RA. Integrating Bevacizumab Into the Management of Epithelial Ovarian Cancer: The Controversy of Front-Line Versus Recurrent Disease. Ann Oncol Off J Eur Soc Med Oncol (2013) 24 Suppl 10:x53–8. doi: 10.1093/annonc/mdt472
74. Monk BJ, Dalton H, Farley JH, Chase DM, Benjamin I. Antiangiogenic Agents as a Maintenance Strategy for Advanced Epithelial Ovarian Cancer. Crit Rev Oncol Hematol (2013) 86(2):161–75. doi: 10.1016/j.critrevonc.2012.09.012
75. Ray-Coquard I, Pautier P, Pignata S, Pérol D, González-Martín A, Berger R, et al. Olaparib Plus Bevacizumab as First-Line Maintenance in Ovarian Cancer. N Engl J Med (2019) 381(25):2416–28. doi: 10.1056/NEJMoa1911361
76. Burger RA, Brady MF, Bookman MA, Fleming GF, Monk BJ, Huang H, et al. Incorporation of Bevacizumab in the Primary Treatment of Ovarian Cancer. N Engl J Med (2011) 365(26):2473–83. doi: 10.1056/NEJMoa1104390
77. Gorenjak V, Vance DR, Dade S, Stathopoulou MG, Doherty L, Xie T, et al. Epigenome-Wide Association Study in Healthy Individuals Identifies Significant Associations With DNA Methylation and PBMC Extract VEGF-A Concentration. Clin Epigenet (2020) 12(1):79. doi: 10.1186/s13148-020-00874-w
78. Yang Z, Wang T, Wu D, Min Z, Tan J, Yu B, et al. RNA N6-Methyladenosine Reader IGF2BP3 Regulates Cell Cycle and Angiogenesis in Colon Cancer. J Exp Clin Cancer Res CR (2020) 39(1):203. doi: 10.1186/s13046-020-01714-8
79. Gao P, Wang D, Liu M, Chen S, Yang Z, Zhang J, et al. DNA Methylation-Mediated Repression of Exosomal miR-652-5p Expression Promotes Oesophageal Squamous Cell Carcinoma Aggressiveness by Targeting PARG and VEGF Pathways. PloS Genet (2020) 16(4):e1008592. doi: 10.1371/journal.pgen.1008592
80. Integrated Genomic Analyses of Ovarian Carcinoma. Nature (2011) 474(7353):609–15. doi: 10.1038/nature10166
81. Fong PC, Yap TA, Boss DS, Carden CP, Mergui-Roelvink M, Gourley C, et al. Poly(ADP)-Ribose Polymerase Inhibition: Frequent Durable Responses in BRCA Carrier Ovarian Cancer Correlating With Platinum-Free Interval. J Clin Oncol (2010) 28(15):2512–9. doi: 10.1200/JCO.2009.26.9589
82. Audeh MW, Carmichael J, Penson RT, Friedlander M, Powell B, Bell-McGuinn KM, et al. Oral Poly(ADP-Ribose) Polymerase Inhibitor Olaparib in Patients With BRCA1 or BRCA2 Mutations and Recurrent Ovarian Cancer: A Proof-of-Concept Trial. Lancet (2010) 376(9737):245–51. doi: 10.1016/S0140-6736(10)60893-8
83. Hu W, Liu T, Ivan C, Sun Y, Huang J, Mangala LS, et al. Notch3 Pathway Alterations in Ovarian Cancer. Cancer Res (2014) 74(12):3282–93. doi: 10.1158/0008-5472.CAN-13-2066
84. McAuliffe SM, Morgan SL, Wyant GA, Tran LT, Muto KW, Chen YS, et al. Targeting Notch, a Key Pathway for Ovarian Cancer Stem Cells, Sensitizes Tumors to Platinum Therapy. Proc Natl Acad Sci USA (2012) 109(43):E2939–48. doi: 10.1073/pnas.1206400109
85. Ivan C, Hu W, Bottsford-Miller J, Zand B, Dalton HJ, Liu T, et al. Epigenetic Analysis of the Notch Superfamily in High-Grade Serous Ovarian Cancer. Gynecologic Oncol (2013) 128(3):506–11. doi: 10.1016/j.ygyno.2012.11.029
86. Liu S, Liu D, Chen C, Hamamura K, Moshaverinia A, Yang R, et al. MSC Transplantation Improves Osteopenia via Epigenetic Regulation of Notch Signaling in Lupus. Cell Metab (2015) 22(4):606–18. doi: 10.1016/j.cmet.2015.08.018
87. Hirsch TZ, Martin-Lannerée S, Reine F, Hernandez-Rapp J, Herzog L, Dron M, et al. Epigenetic Control of the Notch and Eph Signaling Pathways by the Prion Protein: Implications for Prion Diseases. Mol Neurobiol (2019) 56(3):2159–73. doi: 10.1007/s12035-018-1193-7
88. Li J, Yang S, Su N, Wang Y, Yu J, Qiu H, et al. Overexpression of Long non-Coding RNA HOTAIR Leads to Chemoresistance by Activating the Wnt/β-Catenin Pathway in Human Ovarian Cancer. Tumour Biol J Int Soc Oncodevelopmental Biol Med (2016) 37(2):2057–65. doi: 10.1007/s13277-015-3998-6
89. Brinkman JA, Liu Y, Kron SJ. Small-Molecule Drug Repurposing to Target DNA Damage Repair and Response Pathways. Semin Cancer Biol (2021) 68:230–41. doi: 10.1016/j.semcancer.2020.02.013
90. Carneiro BA, El-Deiry WS. Targeting Apoptosis in Cancer Therapy. Nat Rev Clin Oncol (2020) 17(7):395–417. doi: 10.1038/s41571-020-0341-y
91. Gobin M, Nazarov PV, Warta R, Timmer M, Reifenberger G, Felsberg J, et al. A DNA Repair and Cell-Cycle Gene Expression Signature in Primary and Recurrent Glioblastoma: Prognostic Value and Clinical Implications. Cancer Res (2019) 79(6):1226–38. doi: 10.1158/0008-5472.CAN-18-2076
92. Wu S, Li X, Gao F, de Groot JF, Koul D, Yung WKA, et al. PARP-Mediated PARylation of MGMT Is Critical to Promote Repair of Temozolomide-Induced O6-Methylguanine DNA Damage in Glioblastoma. Neuro Oncol (2021) 23(6):920–31. doi: 10.1093/neuonc/noab003
93. Calabrese CR, Almassy R, Barton S, Batey MA, Calvert AH, Canan-Koch S, et al. Anticancer Chemosensitization and Radiosensitization by the Novel Poly(ADP-Ribose) Polymerase-1 Inhibitor AG14361. J Natl Cancer Inst (2004) 96(1):56–67. doi: 10.1093/jnci/djh005
94. Mateo J, Lord CJ, Serra V, Tutt A, Balmaña J, Castroviejo-Bermejo M, et al. A Decade of Clinical Development of PARP Inhibitors in Perspective. Ann Oncol (2019) 30(9):1437–47. doi: 10.1093/annonc/mdz192
95. Cao X, Hou J, An Q, Assaraf YG, Wang X. Towards the Overcoming of Anticancer Drug Resistance Mediated by P53 Mutations. Drug Resist Updates Rev Commentaries Antimicrob Anticancer Chemother (2020) 49:100671. doi: 10.1016/j.drup.2019.100671
96. Uddin MB, Roy KR, Hosain SB, Khiste SK, Hill RA, Jois SD, et al. An N-Methyladenosine at the Transited Codon 273 of P53 pre-mRNA Promotes the Expression of R273H Mutant Protein and Drug Resistance of Cancer Cells. Biochem Pharmacol (2019) 160:134–45. doi: 10.1016/j.bcp.2018.12.014
97. Meng X, Bi J, Li Y, Yang S, Zhang Y, Li M, et al. AZD1775 Increases Sensitivity to Olaparib and Gemcitabine in Cancer Cells With P53 Mutations. Cancers (2018) 10(5):149. doi: 10.3390/cancers10050149
98. Squire CJ, Dickson JM, Ivanovic I, Baker EN. Structure and Inhibition of the Human Cell Cycle Checkpoint Kinase, Wee1A Kinase: An Atypical Tyrosine Kinase With a Key Role in CDK1 Regulation. Struct (London Engl 1993) (2005) 13(4):541–50. doi: 10.1016/j.str.2004.12.017
99. McGowan CH, Russell P. Human Wee1 Kinase Inhibits Cell Division by Phosphorylating P34cdc2 Exclusively on Tyr15. EMBO J (1993) 12(1):75–85. doi: 10.1002/j.1460-2075.1993.tb05633.x
100. Leijen S, van Geel RM, Sonke GS, de Jong D, Rosenberg EH, Marchetti S, et al. Phase II Study of WEE1 Inhibitor AZD1775 Plus Carboplatin in Patients With TP53-Mutated Ovarian Cancer Refractory or Resistant to First-Line Therapy Within 3 Months. J Clin Oncol Off J Am Soc Clin Oncol (2016) 34(36):4354–61. doi: 10.1200/JCO.2016.67.5942
101. Roos WP, Thomas AD, Kaina B. DNA Damage and the Balance Between Survival and Death in Cancer Biology. Nat Rev Cancer (2016) 16(1):20–33. doi: 10.1038/nrc.2015.2
102. Sabnis AJ, Bivona TG. Principles of Resistance to Targeted Cancer Therapy: Lessons From Basic and Translational Cancer Biology. Trends Mol Med (2019) 25(3):185–97. doi: 10.1016/j.molmed.2018.12.009
103. Vera-Ramirez L. Cell-Intrinsic Survival Signals. The Role of Autophagy in Metastatic Dissemination and Tumor Cell Dormancy. Semin Cancer Biol (2020) 60:28–40. doi: 10.1016/j.semcancer.2019.07.027
104. Zhu H, Gan X, Jiang X, Diao S, Wu H, Hu J. ALKBH5 Inhibited Autophagy of Epithelial Ovarian Cancer Through miR-7 and BCL-2. J Exp Clin Cancer Res CR (2019) 38(1):163. doi: 10.1186/s13046-019-1159-2
105. Piya S, Andreeff M, Borthakur G. Targeting Autophagy to Overcome Chemoresistance in Acute Myleogenous Leukemia. Autophagy (2017) 13(1):214–5. doi: 10.1080/15548627.2016.1245263
106. Zhitomirsky B, Assaraf YG. Lysosomes as Mediators of Drug Resistance in Cancer. Drug Resist Updates Rev Commentaries Antimicrob Anticancer Chemother (2016) 24:23–33. doi: 10.1016/j.drup.2015.11.004
107. Zhou F, Yang X, Zhao H, Liu Y, Feng Y, An R, et al. Down-Regulation of OGT Promotes Cisplatin Resistance by Inducing Autophagy in Ovarian Cancer. Theranostics (2018) 8(19):5200–12. doi: 10.7150/thno.27806
108. Bi J, Zhang Y, Malmrose PK, Losh HA, Newtson AM, Devor EJ, et al. Blocking Autophagy Overcomes Resistance to Dual Histone Deacetylase and Proteasome Inhibition in Gynecologic Cancer. Cell Death Dis (2022) 13(1):59. doi: 10.1038/s41419-022-04508-2
109. Hu Z, Cai M, Zhang Y, Tao L, Guo R. miR-29c-3p Inhibits Autophagy and Cisplatin Resistance in Ovarian Cancer by Regulating FOXP1/ATG14 Pathway. Cell Cycle (Georgetown Tex.) (2020) 19(2):193–206. doi: 10.1080/15384101.2019.1704537
110. Bi X, Lv X, Liu D, Guo H, Yao G, Wang L, et al. METTL3-Mediated Maturation of miR-126-5p Promotes Ovarian Cancer Progression via PTEN-Mediated PI3K/Akt/mTOR Pathway. Cancer Gene Ther (2021) 28(3-4):335–49. doi: 10.1038/s41417-020-00222-3
111. Wong RS. Apoptosis in Cancer: From Pathogenesis to Treatment. J Exp Clin Cancer Res (2011) 30(1):87. doi: 10.1186/1756-9966-30-87
112. Hassannia B, Vandenabeele P, Vanden Berghe T. Targeting Ferroptosis to Iron Out Cancer. Cancer Cell (2019) 35(6):830–49. doi: 10.1016/j.ccell.2019.04.002
113. Chen P, Li X, Zhang R, Liu S, Xiang Y, Zhang M, et al. Combinative Treatment of β-Elemene and Cetuximab Is Sensitive to KRAS Mutant Colorectal Cancer Cells by Inducing Ferroptosis and Inhibiting Epithelial-Mesenchymal Transformation. Theranostics (2020) 10(11):5107–19. doi: 10.7150/thno.44705
114. Gao W, Huang Z, Duan J, Nice EC, Lin J, Huang C, et al. Elesclomol Induces Copper-Dependent Ferroptosis in Colorectal Cancer Cells via Degradation of ATP7A. Mol Oncol (2021) 15(12):3527–44. doi: 10.1002/1878-0261.13079
115. Conrad M, Angeli JP, Vandenabeele P, Stockwell BR. Regulated Necrosis: Disease Relevance and Therapeutic Opportunities. Nat Rev Drug Discov (2016) 15(5):348–66. doi: 10.1038/nrd.2015.6
116. Badgley MA, Kremer DM, Maurer HC, DelGiorno KE, Lee HJ, Purohit V, et al. Cysteine Depletion Induces Pancreatic Tumor Ferroptosis in Mice. Science (2020) 368(6486):85–9. doi: 10.1126/science.aaw9872
117. Hong T, Lei G, Chen X, Li H, Zhang X, Wu N, et al. PARP Inhibition Promotes Ferroptosis via Repressing SLC7A11 and Synergizes With Ferroptosis Inducers in BRCA-Proficient Ovarian Cancer. Redox Biol (2021) 42:101928. doi: 10.1016/j.redox.2021.101928
118. Tang D, Chen X, Kang R, Kroemer G. Ferroptosis: Molecular Mechanisms and Health Implications. Cell Res (2021) 31(2):107–25. doi: 10.1038/s41422-020-00441-1
119. Yang WS, Stockwell BR. Ferroptosis: Death by Lipid Peroxidation. Trends Cell Biol (2016) 26(3):165–76. doi: 10.1016/j.tcb.2015.10.014
120. Ursini F, Maiorino M. Lipid Peroxidation and Ferroptosis: The Role of GSH and Gpx4. Free Radic Biol Med (2020) 152:175–85. doi: 10.1016/j.freeradbiomed.2020.02.027
121. Zhang X, Sui S, Wang L, Li H, Zhang L, Xu S, et al. Inhibition of Tumor Propellant Glutathione Peroxidase 4 Induces Ferroptosis in Cancer Cells and Enhances Anticancer Effect of Cisplatin. J Cell Physiol (2020) 235(4):3425–37. doi: 10.1002/jcp.29232
122. Sui X, Zhang R, Liu S, Duan T, Zhai L, Zhang M, et al. RSL3 Drives Ferroptosis Through GPX4 Inactivation and ROS Production in Colorectal Cancer. Front Pharmacol (2018) 9:1371. doi: 10.3389/fphar.2018.01371
123. Chen X, Li J, Kang R, Klionsky DJ, Tang D. Ferroptosis: Machinery and Regulation. Autophagy (2021) 17(9):2054–81. doi: 10.1080/15548627.2020.1810918
124. Jiang L, Kon N, Li T, Wang SJ, Su T, Hibshoosh H, et al. Ferroptosis as a P53-Mediated Activity During Tumour Suppression. Nature (2015) 520(7545):57–62. doi: 10.1038/nature14344
125. Zhang Y, Shi J, Liu X, Feng L, Gong Z, Koppula P, et al. BAP1 Links Metabolic Regulation of Ferroptosis to Tumour Suppression. Nat Cell Biol (2018) 20(10):1181–92. doi: 10.1038/s41556-018-0178-0
126. Dodson M, Castro-Portuguez R, Zhang DD. NRF2 Plays a Critical Role in Mitigating Lipid Peroxidation and Ferroptosis. Redox Biol (2019) 23:101107. doi: 10.1016/j.redox.2019.101107
127. Liu N, Lin X, Huang C. Activation of the Reverse Transsulfuration Pathway Through NRF2/CBS Confers Erastin-Induced Ferroptosis Resistance. Br J Cancer (2020) 122(2):279–92. doi: 10.1038/s41416-019-0660-x
128. Muscarella LA, Barbano R, D'Angelo V, Copetti M, Coco M, Balsamo T, et al. Regulation of KEAP1 Expression by Promoter Methylation in Malignant Gliomas and Association With Patient’s Outcome. Epigenetics (2011) 6(3):317–25. doi: 10.4161/epi.6.3.14408
129. Yamamoto M, Kensler TW, Motohashi H. The KEAP1-NRF2 System: A Thiol-Based Sensor-Effector Apparatus for Maintaining Redox Homeostasis. Physiol Rev (2018) 98(3):1169–203. doi: 10.1152/physrev.00023.2017
130. Kansanen E, Kuosmanen SM, Leinonen H, Levonen AL. The Keap1-Nrf2 Pathway: Mechanisms of Activation and Dysregulation in Cancer. Redox Biol (2013) 1(1):45–9. doi: 10.1016/j.redox.2012.10.001
131. Fabrizio FP, Sparaneo A, Trombetta D, Muscarella LA. Epigenetic Versus Genetic Deregulation of the KEAP1/NRF2 Axis in Solid Tumors: Focus on Methylation and Noncoding RNAs. Oxid Med Cell Longevity (2018) 2018:2492063. doi: 10.1155/2018/2492063
132. Castejón-Griñán M, Herraiz C, Olivares C, Jiménez-Cervantes C, García-Borrón JC. cAMP-Independent Non-Pigmentary Actions of Variant Melanocortin 1 Receptor: AKT-Mediated Activation of Protective Responses to Oxidative DNA Damage. Oncogene (2018) 37(27):3631–46. doi: 10.1038/s41388-018-0216-1
133. Zhou Z, Lu H, Zhu S, Gomaa A, Chen Z, Yan J, et al. Activation of EGFR-DNA-PKcs Pathway by IGFBP2 Protects Esophageal Adenocarcinoma Cells From Acidic Bile Salts-Induced DNA Damage. J Exp Clin Cancer Res CR (2019) 38(1):13. doi: 10.1186/s13046-018-1021-y
134. Zanini E, Louis LS, Antony J, Karali E, Okon IS, McKie AB, et al. The Tumor-Suppressor Protein OPCML Potentiates Anti-EGFR- and Anti-HER2-Targeted Therapy in HER2-Positive Ovarian and Breast Cancer. Mol Cancer Ther (2017) 16(10):2246–56. doi: 10.1158/1535-7163.MCT-17-0081
135. Cao Y, Shen T, Zhang C, Zhang QH, Zhang ZQ. MiR-125a-5p Inhibits EMT of Ovarian Cancer Cells by Regulating TAZ/EGFR Signaling Pathway. Eur Rev Med Pharmacol Sci (2019) 23(19):8249–56. doi: 10.26355/eurrev_201910_19134
136. Lin S, Choe J, Du P, Triboulet R, Gregory RI. The M(6)A Methyltransferase METTL3 Promotes Translation in Human Cancer Cells. Mol Cell (2016) 62(3):335–45. doi: 10.1016/j.molcel.2016.03.021
137. Luo XL, Deng CC, Su XD, Wang F, Chen Z, Wu XP, et al. Loss of MED12 Induces Tumor Dormancy in Human Epithelial Ovarian Cancer via Downregulation of EGFR. Cancer Res (2018) 78(13):3532–43. doi: 10.1158/1538-7445.AM2018-5503
138. Ottevanger PB. Ovarian Cancer Stem Cells More Questions Than Answers. Semin Cancer Biol (2017) 44:67–71. doi: 10.1016/j.semcancer.2017.04.009
139. Li J, Condello S, Thomes-Pepin J, Ma X, Xia Y, Hurley TD, et al. Lipid Desaturation Is a Metabolic Marker and Therapeutic Target of Ovarian Cancer Stem Cells. Cell Stem Cell (2017) 20(3):303–14.e5. doi: 10.1016/j.stem.2016.11.004
140. Wang Y, Cardenas H, Fang F, Condello S, Taverna P, Segar M, et al. Epigenetic Targeting of Ovarian Cancer Stem Cells. Cancer Res (2014) 74(17):4922–36. doi: 10.1158/0008-5472.CAN-14-1022
141. Steinbichler TB, Dudás J, Skvortsov S, Ganswindt U, Riechelmann H, Skvortsova II, et al. Therapy Resistance Mediated by Cancer Stem Cells. Semin Cancer Biol (2018) 53:156–67. doi: 10.1016/j.semcancer.2018.11.006
142. Pieterse Z, Amaya-Padilla MA, Singomat T, Binju M, Madjid BD, Yu Y, et al. Ovarian Cancer Stem Cells and Their Role in Drug Resistance. Int J Biochem Cell Biol (2019) 106:117–26. doi: 10.1016/j.biocel.2018.11.012
143. Keyvani V, Farshchian M, Esmaeili SA, Yari H, Moghbeli M, Nezhad SK, et al. Ovarian Cancer Stem Cells and Targeted Therapy. J Ovarian Res (2019) 12(1):120. doi: 10.1186/s13048-019-0588-z
144. Wen Y, Hou Y, Yi X, Sun S, Guo J, He X, et al. EZH2 Activates CHK1 Signaling to Promote Ovarian Cancer Chemoresistance by Maintaining the Properties of Cancer Stem Cells. Theranostics (2021) 11(4):1795–813. doi: 10.7150/thno.48101
145. Xu S, Yue Y, Zhang S, Zhou C, Cheng X, Xie X, et al. STON2 Negatively Modulates Stem-Like Properties in Ovarian Cancer Cells via DNMT1/MUC1 Pathway. J Exp Clin Cancer Res CR (2018) 37(1):305. doi: 10.1186/s13046-018-0977-y
146. Pinheiro AS, Marsh JA, Forman-Kay JD, Peti W. Structural Signature of the MYPT1-PP1 Interaction. J Am Chem Soc (2011) 133(1):73–80. doi: 10.1021/ja107810r
147. Hansen JM, Coleman RL, Sood AK. Targeting the Tumour Microenvironment in Ovarian Cancer. Eur J Cancer (2016) 56:131–43. doi: 10.1016/j.ejca.2015.12.016
148. Bhowmick NA, Neilson EG, Moses HL. Stromal Fibroblasts in Cancer Initiation and Progression. Nature (2004) 432(7015):332–7. doi: 10.1038/nature03096
149. Kalluri R. The Biology and Function of Fibroblasts in Cancer. Nat Rev Cancer (2016) 16(9):582–98. doi: 10.1038/nrc.2016.73
150. Lin H-J, Lin J. Seed-In-Soil: Pancreatic Cancer Influenced by Tumor Microenvironment. Cancers (2017) 9(7):93. doi: 10.3390/cancers9070093
151. Hanson JA, Gillespie JW, Grover A, Tangrea MA, Chuaqui RF, Emmert-Buck MR, et al. Gene Promoter Methylation in Prostate Tumor-Associated Stromal Cells. J Natl Cancer Inst (2006) 98(4):255–61. doi: 10.1093/jnci/djj051
152. Hu M, Yao J, Cai L, Bachman KE, van denBrûle F, Velculescu V, et al. Distinct Epigenetic Changes in the Stromal Cells of Breast Cancers. Nat Genet (2005) 37(8):899–905. doi: 10.1038/ng1596
153. Eckert MA, Coscia F, Chryplewicz A, Chang JW, Hernandez KM, Pan S, et al. Proteomics Reveals NNMT as a Master Metabolic Regulator of Cancer-Associated Fibroblasts. Nature (2019) 569(7758):723–8. doi: 10.1038/s41586-019-1173-8
154. Au Yeung CL, Co NN, Tsuruga T, Yeung TL, Kwan SY, Leung CS, et al. Exosomal Transfer of Stroma-Derived Mir21 Confers Paclitaxel Resistance in Ovarian Cancer Cells Through Targeting APAF1. Nat Commun (2016) 7:11150. doi: 10.1038/ncomms11150
155. Mitra AK, Zillhardt M, Hua Y, Tiwari P, Murmann AE, Peter ME, et al. MicroRNAs Reprogram Normal Fibroblasts Into Cancer-Associated Fibroblasts in Ovarian Cancer. Cancer Discov (2012) 2(12):1100–8. doi: 10.1158/2159-8290.CD-12-0206
156. Curtis M, Kenny HA, Ashcroft B, Mukherjee A, Johnson A, Zhang Y, et al. Fibroblasts Mobilize Tumor Cell Glycogen to Promote Proliferation and Metastasis. Cell Metab (2019) 29(1):141–55.e9. doi: 10.1016/j.cmet.2018.08.007
157. Zhao L, Ji G, Le X, Wang C, Xu L, Feng M, et al. Long Noncoding RNA LINC00092 Acts in Cancer-Associated Fibroblasts to Drive Glycolysis and Progression of Ovarian Cancer. Cancer Res (2017) 77(6):1369–82. doi: 10.1158/0008-5472.CAN-16-1615
158. da Silva Meirelles L, Chagastelles PC, Nardi NB. Mesenchymal Stem Cells Reside in Virtually All Post-Natal Organs and Tissues. J Cell Sci (2006) 119(Pt 11):2204–13. doi: 10.1242/jcs.02932
159. McLean K, Gong Y, Choi Y, Deng N, Yang K, Bai S, et al. Human Ovarian Carcinoma–Associated Mesenchymal Stem Cells Regulate Cancer Stem Cells and Tumorigenesis via Altered BMP Production. J Clin Invest (2011) 121(8):3206–19. doi: 10.1172/JCI45273
160. Lis R, Touboul C, Mirshahi P, Ali F, Mathew S, Nolan DJ, et al. Tumor Associated Mesenchymal Stem Cells Protects Ovarian Cancer Cells From Hyperthermia Through CXCL12. Int J Cancer (2011) 128(3):715–25. doi: 10.1002/ijc.25619
161. Cho JA, Park H, Lim EH, Kim KH, Choi JS, Lee JH, et al. Exosomes From Ovarian Cancer Cells Induce Adipose Tissue-Derived Mesenchymal Stem Cells to Acquire the Physical and Functional Characteristics of Tumor-Supporting Myofibroblasts. Gynecologic Oncol (2011) 123(2):379–86. doi: 10.1016/j.ygyno.2011.08.005
162. Muñoz P, Iliou MS, Esteller M. Epigenetic Alterations Involved in Cancer Stem Cell Reprogramming. Mol Oncol (2012) 6(6):620–36. doi: 10.1016/j.molonc.2012.10.006
163. Teven CM, Liu X, Hu N, Tang N, Kim SH, Huang E, et al. Epigenetic Regulation of Mesenchymal Stem Cells: A Focus on Osteogenic and Adipogenic Differentiation. Stem Cells Int (2011) 2011:201371. doi: 10.4061/2011/201371
164. Meyer MB, Benkusky NA, Sen B, Rubin J, Pike JW. Epigenetic Plasticity Drives Adipogenic and Osteogenic Differentiation of Marrow-Derived Mesenchymal Stem Cells. J Biol Chem (2016) 291(34):17829–47. doi: 10.1074/jbc.M116.736538
165. Reza AMMT, Choi YJ, Yasuda H, Kim JH. Human Adipose Mesenchymal Stem Cell-Derived Exosomal-miRNAs Are Critical Factors for Inducing Anti-Proliferation Signalling to A2780 and SKOV-3 Ovarian Cancer Cells. Sci Rep (2016) 6:38498. doi: 10.1038/srep38498
166. Lopatina T, Gai C, Deregibus MC, Kholia S, Camussi G. Cross Talk Between Cancer and Mesenchymal Stem Cells Through Extracellular Vesicles Carrying Nucleic Acids. Front Oncol (2016) 6:125. doi: 10.3389/fonc.2016.00125
167. Shu Y, Cheng P. Targeting Tumor-Associated Macrophages for Cancer Immunotherapy. Biochim Biophys Acta Rev Cancer (2020) 1874(2):188434. doi: 10.1016/j.bbcan.2020.188434
168. Pan Y, Yu Y, Wang X, Zhang T. Tumor-Associated Macrophages in Tumor Immunity. Front Immunol (2020) 11:583084. doi: 10.3389/fimmu.2020.583084
169. Cortese N, Carriero R, Laghi L, Mantovani A, Marchesi F. Prognostic Significance of Tumor-Associated Macrophages: Past, Present and Future. Semin Immunol (2020) 48:101408. doi: 10.1016/j.smim.2020.101408
170. Li C, Xu X, Wei S, Jiang P, Xue L, Wang J, et al. Tumor-Associated Macrophages: Potential Therapeutic Strategies and Future Prospects in Cancer. J Immunother Cancer (2021) 9(1):e001341. doi: 10.1136/jitc-2020-001341
171. Lawrence T, Natoli G. Transcriptional Regulation of Macrophage Polarization: Enabling Diversity With Identity. Nat Rev Immunol (2011) 11(11):750–61. doi: 10.1038/nri3088
172. Xue J, Schmidt SV, Sander J, Draffehn A, Krebs W, Quester I, et al. Transcriptome-Based Network Analysis Reveals a Spectrum Model of Human Macrophage Activation. Immunity (2014) 40(2):274–88. doi: 10.1016/j.immuni.2014.01.006
173. Ying X, Wu Q, Wu X, Zhu Q, Wang X, Jiang L, et al. Epithelial Ovarian Cancer-Secreted Exosomal miR-222-3p Induces Polarization of Tumor-Associated Macrophages. Oncotarget (2016) 7(28):43076–87. doi: 10.18632/oncotarget.9246
174. Chen X, Ying X, Wang X, Wu X, Zhu Q, Wang X, et al. Exosomes Derived From Hypoxic Epithelial Ovarian Cancer Deliver microRNA-940 to Induce Macrophage M2 Polarization. Oncol Rep (2017) 38(1):522–8. doi: 10.3892/or.2017.5697
175. Wu Q, Wu X, Ying X, Zhu Q, Wang X, Jiang L, et al. Suppression of Endothelial Cell Migration by Tumor Associated Macrophage-Derived Exosomes Is Reversed by Epithelial Ovarian Cancer Exosomal lncRNA. Cancer Cell Int (2017) 17:62. doi: 10.1186/s12935-017-0430-x
176. Ishii M, Wen H, Corsa CA, Liu T, Coelho AL, Allen RM, et al. Epigenetic Regulation of the Alternatively Activated Macrophage Phenotype. Blood (2009) 114(15):3244–54. doi: 10.1182/blood-2009-04-217620
177. Odunsi K. Immunotherapy in Ovarian Cancer. Ann Oncol Off J Eur Soc Med Oncol (2017) 28(suppl_8):viii1–7. doi: 10.1093/annonc/mdx444
178. Pujade-Lauraine E. New Treatments in Ovarian Cancer. Ann Oncol Off J Eur Soc Med Oncol (2017) 28(suppl_8):viii57–60. doi: 10.1093/annonc/mdx442
179. Kuroki L, Guntupalli SR. Treatment of Epithelial Ovarian Cancer. BMJ (Clin Res ed.) (2020) 371:m3773. doi: 10.1136/bmj.m3773
180. Cai Y, Tsai HC, Yen RC, Zhang YW, Kong X, Wang W, et al. Critical Threshold Levels of DNA Methyltransferase 1 Are Required to Maintain DNA Methylation Across the Genome in Human Cancer Cells. Genome Res (2017) 27(4):533–44. doi: 10.1101/gr.208108.116
181. Lyko F, Brown R. DNA Methyltransferase Inhibitors and the Development of Epigenetic Cancer Therapies. J Natl Cancer Inst (2005) 97(20):1498–506. doi: 10.1093/jnci/dji311
182. Mahfouz RZ, Jankowska A, Ebrahem Q, Gu X, Visconte V, Tabarroki A, et al. Increased CDA Expression/Activity in Males Contributes to Decreased Cytidine Analog Half-Life and Likely Contributes to Worse Outcomes With 5-Azacytidine or Decitabine Therapy. Clin Cancer Res an Off J Am Assoc Cancer Res (2013) 19(4):938–48. doi: 10.1158/1078-0432.CCR-12-1722
183. Matei D, Fang F, Shen C, Schilder J, Arnold A, Zeng Y, et al. Epigenetic Resensitization to Platinum in Ovarian Cancer. Cancer Res (2012) 72(9):2197–205. doi: 10.1158/0008-5472.CAN-11-3909
184. Griffiths EA, Srivastava P, Matsuzaki J, Brumberger Z, Wang ES, Kocent J, et al. NY-ESO-1 Vaccination in Combination With Decitabine Induces Antigen-Specific T-Lymphocyte Responses in Patients With Myelodysplastic Syndrome. Clin Cancer Res an Off J Am Assoc Cancer Res (2018) 24(5):1019–29. doi: 10.1158/1078-0432.CCR-17-1792
185. Cicek MS, Koestler DC, Fridley BL, Kalli KR, Armasu SM, Larson MC, et al. Epigenome-Wide Ovarian Cancer Analysis Identifies a Methylation Profile Differentiating Clear-Cell Histology With Epigenetic Silencing of the HERG K+ Channel. Hum Mol Genet (2013) 22(15):3038–47. doi: 10.1093/hmg/ddt160
186. Kim H-J, Bae S-C. Histone Deacetylase Inhibitors: Molecular Mechanisms of Action and Clinical Trials as Anti-Cancer Drugs. Am J Trans Res (2011) 3(2):166–79. doi: 10.1007/978-1-4419-6612-4_66
187. Meng X, Yang S, Li Y, Li Y, Devor EJ, Bi J, et al. Combination of Proteasome and Histone Deacetylase Inhibitors Overcomes the Impact of Gain-Of-Function P53 Mutations. Dis Markers (2018) 2018:3810108. doi: 10.1155/2018/3810108
188. Matei D, Nephew KP. Epigenetic Attire in Ovarian Cancer: The Emperor’s New Clothes. Cancer Res (2020) 80(18):3775–85. doi: 10.1158/0008-5472.CAN-19-3837
189. Fukumoto T, Park PH, Wu S, Fatkhutdinov N, Karakashev S, Nacarelli T, et al. Repurposing Pan-HDAC Inhibitors for ARID1A-Mutated Ovarian Cancer. Cell Rep (2018) 22(13):3393–400. doi: 10.1016/j.celrep.2018.03.019
190. Bitler BG, Wu S, Park PH, Hai Y, Aird KM, Wang Y, et al. ARID1A-Mutated Ovarian Cancers Depend on HDAC6 Activity. Nat Cell Biol (2017) 19(8):962–73. doi: 10.1038/ncb3582
191. Shetty MG, Pai P, Deaver RE, Satyamoorthy K, Babitha KS. Histone Deacetylase 2 Selective Inhibitors: A Versatile Therapeutic Strategy as Next Generation Drug Target in Cancer Therapy. Pharmacol Res (2021) 170:105695. doi: 10.1016/j.phrs.2021.105695
192. Qian X, LaRochelle WJ, Ara G, Wu F, Petersen KD, Thougaard A, et al. Activity of PXD101, a Histone Deacetylase Inhibitor, in Preclinical Ovarian Cancer Studies. Mol Cancer Ther (2006) 5(8):2086–95. doi: 10.1158/1535-7163.MCT-06-0111
193. Steele N, Finn P, Brown R, Plumb JA. Combined Inhibition of DNA Methylation and Histone Acetylation Enhances Gene Re-Expression and Drug Sensitivity In Vivo. Br J Cancer (2009) 100(5):758–63. doi: 10.1038/sj.bjc.6604932
194. Belur Nagaraj A, Knarr M, Sekhar S, Connor RS, Joseph P, Kovalenko O, et al. The miR-181a-SFRP4 Axis Regulates Wnt Activation to Drive Stemness and Platinum Resistance in Ovarian Cancer. Cancer Res (2021) 81(8):2044–55. doi: 10.1158/0008-5472.CAN-20-2041
195. Teschendorff AE, Lee SH, Jones A, Fiegl H, Kalwa M, Wagner W, et al. HOTAIR and Its Surrogate DNA Methylation Signature Indicate Carboplatin Resistance in Ovarian Cancer. Genome Med (2015) 7:108. doi: 10.1186/s13073-015-0233-4
196. Vescarelli E, Gerini G, Megiorni F, Anastasiadou E, Pontecorvi P, Solito L, et al. MiR-200c Sensitizes Olaparib-Resistant Ovarian Cancer Cells by Targeting Neuropilin 1. J Exp Clin Cancer Res CR (2020) 39(1):3. doi: 10.1186/s13046-019-1490-7
197. Sun C, Li N, Yang Z, Zhou B, He Y, Weng D, et al. miR-9 Regulation of BRCA1 and Ovarian Cancer Sensitivity to Cisplatin and PARP Inhibition. J Natl Cancer Inst (2013) 105(22):1750–8. doi: 10.1093/jnci/djt302
198. Liu S, Lei H, Luo F, Li Y, Xie L. The Effect of lncRNA HOTAIR on Chemoresistance of Ovarian Cancer Through Regulation of HOXA7. Biol Chem (2018) 399(5):485–97. doi: 10.1515/hsz-2017-0274
199. Wang Y, Wang H, Song T, Zou Y, Jiang J, Fang L, et al. HOTAIR is a Potential Target for the Treatment of Cisplatin−Resistant Ovarian Cancer. Mol Med Rep (2015) 12(2):2211–6. doi: 10.3892/mmr.2015.3562
200. Wu Y, Wang T, Xia L, Zhang M. LncRNA WDFY3-AS2 Promotes Cisplatin Resistance and the Cancer Stem Cell in Ovarian Cancer by Regulating hsa-miR-139-5p/SDC4 Axis. Cancer Cell Int (2021) 21(1):284. doi: 10.1186/s12935-021-01993-x
201. Olino K, Park T, Ahuja N. Exposing Hidden Targets: Combining Epigenetic and Immunotherapy to Overcome Cancer Resistance. Semin Cancer Biol (2020) 65:114–22. doi: 10.1016/j.semcancer.2020.01.001
202. McCaw TR, Randall TD, Arend RC. Overcoming Immune Suppression With Epigenetic Modification in Ovarian Cancer. Trans Res J Lab Clin Med (2019) 204:31–8. doi: 10.1016/j.trsl.2018.06.003
203. Stone ML, Chiappinelli KB, Li H, Murphy LM, Travers ME, Topper MJ, et al. Epigenetic Therapy Activates Type I Interferon Signaling in Murine Ovarian Cancer to Reduce Immunosuppression and Tumor Burden. Proc Natl Acad Sci USA (2017) 114(51):E10981–90. doi: 10.1073/pnas.1712514114
204. Travers M, Brown SM, Dunworth M, Holbert CE, Wiehagen KR, Bachman KE, et al. DFMO and 5-Azacytidine Increase M1 Macrophages in the Tumor Microenvironment of Murine Ovarian Cancer. Cancer Res (2019) 79(13):3445–54. doi: 10.1158/0008-5472.CAN-18-4018
Keywords: ovarian cancer, drug resistance, cancer epigenetics, DNA methylation, histone modifications, non-coding RNA, epigenetic therapy
Citation: Wang Y, Huang Z, Li B, Liu L and Huang C (2022) The Emerging Roles and Therapeutic Implications of Epigenetic Modifications in Ovarian Cancer. Front. Endocrinol. 13:863541. doi: 10.3389/fendo.2022.863541
Received: 06 February 2022; Accepted: 30 March 2022;
Published: 10 May 2022.
Edited by:
Xianquan Zhan, Shandong First Medical University, ChinaReviewed by:
Na Li, Shandong Cancer Hospital, ChinaJianling Bi, The University of Iowa, United States
Copyright © 2022 Wang, Huang, Li, Liu and Huang. This is an open-access article distributed under the terms of the Creative Commons Attribution License (CC BY). The use, distribution or reproduction in other forums is permitted, provided the original author(s) and the copyright owner(s) are credited and that the original publication in this journal is cited, in accordance with accepted academic practice. No use, distribution or reproduction is permitted which does not comply with these terms.
*Correspondence: Lin Liu, MTA0Nzk3ODA5QHFxLmNvbQ==; Canhua Huang, aGNhbmh1YUBzY3UuZWR1LmNu
†These authors have contributed equally to this work