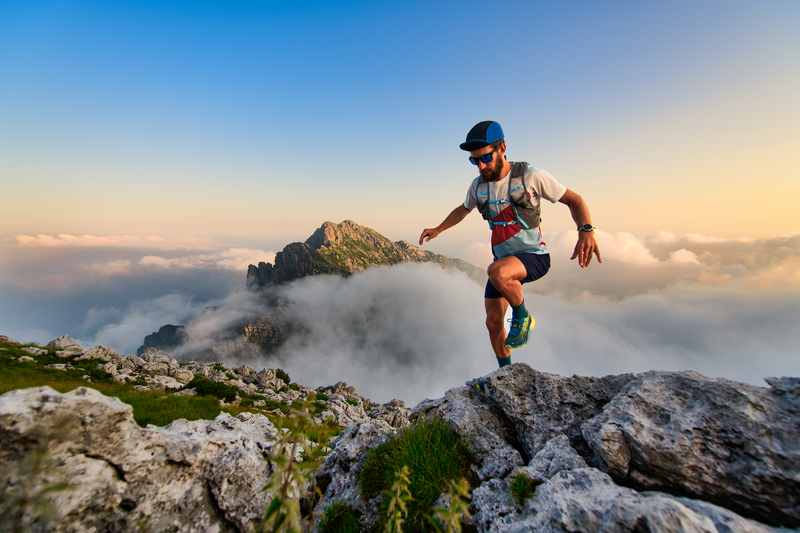
95% of researchers rate our articles as excellent or good
Learn more about the work of our research integrity team to safeguard the quality of each article we publish.
Find out more
REVIEW article
Front. Endocrinol. , 18 March 2022
Sec. Gut Endocrinology
Volume 13 - 2022 | https://doi.org/10.3389/fendo.2022.841703
This article is part of the Research Topic The Role of Bile Acid (BA) and Related Metabolites and Hormone Abnormalities in Metabolic Diseases View all 12 articles
The increasing prevalence of metabolic syndrome has become a serious public health problem. Certain bacteria-derived metabolites play a key role in maintaining human health by regulating the host metabolism. Recent evidence shows that indole-3-propionic acid content can be used to predict the occurrence and development of metabolic diseases. Supplementing indole-3-propionic acid can effectively improve metabolic disorders and is considered a promising metabolite. Therefore, this article systematically reviews the latest research on indole-3-propionic acid and elaborates its source of metabolism and its association with metabolic diseases. Indole-3-propionic acid can improve blood glucose and increase insulin sensitivity, inhibit liver lipid synthesis and inflammatory factors, correct intestinal microbial disorders, maintain the intestinal barrier, and suppress the intestinal immune response. The study of the mechanism of the metabolic benefits of indole-3-propionic acid is expected to be a potential compound for treating metabolic syndrome.
Metabolic syndrome is defined as a group of interrelated comprehensive diseases characterized by visceral obesity, hypertension, hyperlipidemia, atherosclerosis, and insulin resistance. Metabolic syndrome, including overweight/obesity, type 2 diabetes (T2D) (1, 2), non-alcoholic fatty liver disease (NAFLD) (3), and cardiovascular disease (CVD) (4) has become a severe public health problem (5). Accumulating evidence has linked intestinal microbe imbalance or compositional changes with the pathogenesis of metabolic diseases (6). Intestinal microbes produce functional metabolites that regulate intestinal endocrine function and neural signals, regulate energy metabolism, and affect host immune mechanisms and homeostasis (7). Functional metabolites serve as potential markers of disease and transfer to distant organs through the intestinal barrier–peripheral circulation, affecting the metabolic phenotype of the host (8–10). Therefore, the link between functional metabolites and metabolic diseases has received increasing attention.
Indole-3-propanoic acid (IPA) is a tryptophan (Trp) metabolite produced by intestinal bacteria that is closely associated with diet. IPA has received increasing attention in recent years because of its close correlation with metabolic diseases. Recent studies have found that IPA content can predict the occurrence of obesity (11), T2D (12), NAFLD (13), and CVD (14).
In recent years, supplementation with IPA has been shown to improve blood glucose, increase insulin sensitivity (15), inhibit liver lipid synthesis and inflammatory factors (16), correct intestinal microbial disorders (17), maintain the intestinal barrier, and suppress the intestinal immune response (18). Here, we systematically reviewed the latest research on IPA, its association with metabolic diseases, and its role in metabolic disorders, and discuss its future research directions.
IPA is a metabolite produced by the microflora of dietary Trp that accumulates in the host serum and exhibits high individual differences (19). Under physiological conditions, serum IPA concentrations range from 1 to 10 µM in humans (20, 21)
Trp is an essential amino acid from the host diet for use in protein synthesis (22). Trp is primarily metabolized through the 5-HT (23), canine uric acid (24), and intestinal microbial pathways. Indole-3-pyruvic acid (IPyA) is converted from Trp in the presence of an aromatic amino acid aminotransferase. IPyA is a precursor of indolelactic acid (ILA), and phenyllactate dehydrogenase is involved in this reduction reaction. Bacterial species containing phenyllactate dehydratase (fldBC) and its activator acyl-CoA ligase convert ILA to indoleacrylic acid (IA) through dehydration. IA can be further converted into IPA by acyl coenzyme A dehydrogenase, which is the final product of the reductive metabolism of Trp (Figure 1) (24–26). The most abundant metabolite of Trp in the intestine is indole, followed by indole-3-acetic acid and IPA (27, 28).
The metabolism of IPA in the body is affected by enzyme activity and intestinal microbes. Liquid chromatography–mass spectrometry (LC-MS) analysis was used to compare the plasma samples of sterile and conventional mice. The production of IPA was found to be entirely dependent on intestinal microbes. Colonization with Clostridium sporogenes and Clostridium botulinum can promote the concentration of IPA in the plasma (29, 30). Recently, the study found that, among 36 bacterial isolates cultured in Trp-containing medium, 4 (Peptostreptococcus anaerobius CC14N and 3 Clostridium cadaveris strains) were capable of producing IPA. Simultaneously, the presence of FLDC, a homologous cluster of the fldBC gene cluster, was found to be a reliable marker for IPA-producing bacteria (25). Other bacteria, such as Lechevalieria aerocolonigenes, can synthesize IPA through Trp deamination catalyzed by amino acid oxidase (31). Therefore, IPA is an important indicator of microbial metabolism.
In a study on the metabolic benefits of Trp, the Trp diet led to a decrease in mouse body weight (32); however, the mechanism was not elucidated. In another study, it was found that a diet supplemented with neomycin and Trp led to an increase in rat body weight, which was related to the significant change in the concentration of Trp-derived bacterial metabolites in the feces and blood. Further studies showed that the change in body weight increase was most relevant to the change in the concentration of the Trp metabolite IPA. The body weight gain in rats treated with IPA alone was two times lower than that in rats treated with the vehicle, suggesting that IPA might be an effector metabolite between a Trp-rich diet and lower body weight gain (33). Therefore, for the Trp-IPA metabolic pathway, the development of related probiotics, and the promotion of the production of IPA, we need to pay attention to the study of probiotics in the treatment of metabolic syndrome in the future.
Diet significantly impacts NAFLD, T2D, obesity, CVD, and metabolic disorders (34, 35). Therefore, we explored the relationship between IPA and diet. IPA was the metabolite most significantly and consistently related to both total carbohydrate and fiber intake (r = 0.28, p = 9.1 × 10−5 and r = 0.23, s = 0.001, respectively), including whole grain wheat, rye, and whole grain rye intake (12). In another study, 117 overweight adults were randomly divided into two groups. Based on the same diet, they were supplied with fried meat or not. The study found that the participants who consumed fried meat had higher lipopolysaccharide (LPS), tumor necrosis factor-α (TNF-α), interleukin-1β (IL-1β), and IL-10 levels (p < 0.05). Fried meat intake lowered microbial community richness and decreased Lachnospiraceae and Flavonifractor abundances while increasing Dialister, Dorea, and Veillonella abundances [p false discovery rate (FDR) < 0.05], which caused a significant decrease in the fecal metabolite IPA content (36).
In a diet study, 10 healthy participants were randomly fed a Western or Mediterranean diet for 4 days, and feces were collected for 16s RNA and metabolomics after 4 days. Different diets altered the intestinal flora structure. Simultaneously, IPA content in feces was significantly increased with the Mediterranean diet but decreased in the Western diet (37). This suggests that diet can affect the composition of intestinal microorganisms within a short time (38); however, the long-term effect and stability of the microbial structure are not apparent. Promoting the increase in IPA content may be an effective way to improve the metabolic benefits of the Mediterranean diet.
In the correlation experiment between 11 types of Trp metabolism levels and T2D events in the circulation of 9,180 participants from five cohorts, it was found that intake of fiber-rich foods, rather than protein/Trp-rich foods, and peripheral IPA content were positively correlated. Further research found that higher milk and fiber intake can improve the metabolism of Trp in the circulation of patients with T2D, but only in individuals with non-persistent genetic lactase (39). This suggests that diet can interfere with host–microbe interactions and affect the metabolism of Trp-IPA in the host. The effect of the metabolic benefits of a healthy diet is partly due to the promotion of IPA production in circulation.
We mainly discuss the relationship between IPA and various metabolic diseases, including obesity, T2D, NAFLD, and CVD, and focus on the potential connection between IPA and illness.
Obesity is a complex pathophysiological disease and one of the causes of metabolic syndrome, which is characterized by chronic low-grade inflammation. In 85 obese adults (average BMI = 40.48) and 42 non-obese control individuals (average BMI = 24.03), the serum IPA content was significantly lower in obese patients and was compared with BMI, serum high-sensitivity C-reactive protein (hsCRP), and high-sensitive interleukin 6 (hsIL-6), and the hsCRP and hsIL-6 levels were negatively correlated (40). This suggests that the indole metabolic pathway of Trp is affected in obese patients, which may be related to obesity-related systemic inflammation. However, in obese patients who underwent Roux-en-Y gastric bypass surgery (RYGB) operation, the level of IPA in the blood increased substantially 3 months post-surgery compared with 1 week post-surgery (40). This suggests that IPA content can be used as a marker for obesity. In future research, it will be necessary to perform correlation analyses between IPA and obesity-related complications to provide new diagnostic methods for invasive diagnosis of diseases and to predict obesity-related complications.
Current studies have found that IPA content is closely related to T2D and can predict the risk of T2D, distinguish different stages of T2D, and decrease with the improvement of T2D. IPA can be used as a biomarker of disease progression. When studying the brain–gut–microbiota characteristics of women with obesity and food addiction, a negative correlation was found between IPA in serum and food addiction (41). Bariatric surgery, such as RYGB, can improve T2D, obesity, NAFLD, and other metabolic diseases (42). In clinical studies, IPA content in the peripheral blood of obese patients with T2D was significantly lower than that in healthy participants. The IPA content in blood samples of these patients 3 months post-RYGB surgery was significantly higher than that in blood samples 1 week post-surgery (11).”XenoScan,” a metabolomics platform established by the University of California, Davis, used LC-MS to characterize a series of intestinal microflora metabolites and found that several metabolites, including IPA, could distinguish early T2D rats from rats 3 months after the onset of diabetes (43).
In a clinical trial, researchers used a non-targeted metabolomics approach to investigate whether serum metabolite profiles can predict the incidence of T2D in patients with impaired glucose tolerance. During the 15-year follow-up, patients with glucose tolerance who developed (n = 96) or did not develop (n = 104) T2D had lower and higher serum IPA levels, respectively. This suggests that higher serum IPA levels lead to a low risk of T2D (12). In a clinical study with a 7-year follow-up, it was verified that higher serum IPA levels were negatively correlated with the occurrence of T2D (OR [CI]: 0.86 [0.73–0.99], p = 0.04), directly correlated with insulin secretion (β = 0.10, p = 0.06), and negatively correlated with hsCRP when blood samples were collected (r = −0.22, p = 0.0001), and during follow-up visits (β = −0.19, p = 0.001). This suggests that IPA might be mediated by low-grade inflammation or enhance insulin sensitivity by protecting β-cell function to reduce the risk of T2D (21)
NAFLD manifests as liver fat accumulation, and the disease progresses to non-alcoholic steatohepatitis (NASH) or even hepatocellular carcinoma (HCC). Globally, the prevalence of NAFLD-related HCC may increase with an obesity epidemic (44).
IPA in the intestinal tract is absorbed by the intestinal epithelial cells and diffuses into the blood, which enters multiple target organs such as the liver after passing through the peripheral and portal circulation (30). This suggests that the liver may be a target organ for IPA biology. In 233 patients who underwent bariatric surgery and detailed liver histological examinations, the circulating IPA in patients with liver fibrosis was lower than that in those without fibrosis. Circulating IPA levels are also associated with the liver richness in genes that regulate hepatic stellate cell activation and liver fibrosis signaling. In vitro experiments have verified that IPA reduces the mRNA expression of fibrosis signaling markers such as COL1A2, aSMA, and ITGA3 in LX-2 cells (13).
Cholesterol is considered the primary lipotoxic molecule among liver lipids in NASH development (45–47). Lipotoxicity promotes the progression of NAFLD to NASH, liver cirrhosis, and even liver cancer (48, 49). Depletion of IPA was noted in both hypercholesterolemia-fed HCC mice and in sterile mouse serum transplanted with hypercholesterolemia-fed HCC mouse feces. In vitro experiments showed that IPA could inhibit the accumulation of triglycerides (TG) in the cholesterol-induced human normal hepatocyte line LO2 and inhibit the proliferation of NASH-HCC cell lines (HKCI-2 and HKCI10). Therefore, the partial reason for cholesterol-induced lipotoxicity is the damage to tryptophan metabolism in microorganisms and the reduced serum IPA content, thereby promoting the development of NASH-HCC (50).
CVD is a serious cause of death due to metabolic diseases (51, 52). In a cohort study from an advanced atherosclerosis (n = 100) and gender- and age-matched control group (n = 20), the level of IPA in plasma metabolites of the advanced atherosclerosis group was significantly reduced (0.41 [0.27–0.90] μM vs. 0.22 [0.16–0.34] μM; p < 0.01). In a study of risk factors for atherosclerosis, IPA (OR, 0.27; 95% CI, 0.019–0.91; p = 0.02) was negatively correlated with advanced atherosclerosis (14). In mice experiments, oral administration of IPA significantly reduced high-fat diet (HFD)-induced body weight gain and reduced serum total cholesterol (TC), low-density lipoprotein cholesterol (LDL-c), and TG levels, showing sufficient anti-hyperlipidemic effects (16).
In a 1-year follow-up study of patients with chronic kidney disease (CKD), the estimated glomerular filtration rate (eGFR) rapidly decreased by >20% (n = 10) and the control group (n = 10), and the eGFR decreased by <5%. It was found that IPA was the only metabolite that dropped significantly in eGFR rapidly decreased group of plasma. In cross-sectional clinical studies, it can also be found that the serum IPA content of the normal group was significantly higher than that of the CKD group (49.8 ± 15.9 vs. 34.7 ± 10.8 ng/ml; p < 0.01) (53). Intervention with IPA can also inhibit the gene expression of fibrosis and inflammation in proximal renal tubular cells induced by indophenol sulfate (54). In previous studies, oxidative stress was found to be associated with increased kidney damage (55), and IPA as a potent antioxidant may be an important bioprotective agent for CKD.
In another study, oral IPA supplementation reduced the systemic inflammation level in radiation-exposed mice, restored hematopoietic organs, relieved bone marrow suppression, and improved gastrointestinal function and epithelial integrity after irradiation, thereby exerting therapeutic effects on radiation toxicity (56). Supplementation with mouse probiotic Clostridia resulted in an increase in IPA production in the intestinal lumen and increased mitochondrial transcription factor A (Tfam) expression in osteoblasts by promoting Kdm6b/Jmjd3 histone demethylase, thereby inhibiting the epigenetic methylation of H3K27me3 at the Tfam promoter from preventing pathological bone loss in obese mice induced by a HFD (57). IPA is neuroprotective as a potent hydroxyl radical scavenger (58). IPA inhibits β-amyloid fibril formation, a potent neuroprotective agent, and is a potential drug for the treatment of Alzheimer’s disease (59). IPA also exhibits protective effects against streptozotocin-induced diabetic peripheral neuropathy in rats and high-glucose-induced neurotoxicity in neural 2a cells (60).
As mentioned above, IPA contributes to various metabolic diseases, and its mechanism is complex. It may be involved in the physiological and pathological processes of the disease through different pathways. Therefore, we comprehensively analyzed the mechanism of IPA in terms of glucose metabolism, insulin resistance, lipid synthesis, inflammatory reactions, and the intestinal microenvironment.
Impaired glucose tolerance and insulin tolerance are also pathogenic factors in metabolic syndrome. Rats fed a diet rich in IPA had improved glucose metabolism and significantly reduced HOMA index of fasting blood glucose, insulin, and insulin resistance (15).
Cognitive decline is a complication of T2D, and intermittent fasting (IF) is a dietary intervention used to alleviate the symptoms of T2D. In research of its mechanism, IF was found to improve cognition through the microorganism–metabolite–brain axis. Among the metabolites affected by IF, the complementary metabolite IPA showed similar results with IF. In db/db mice, IPA was found to improve cognitive function and insulin sensitivity, enhance mitochondrial biogenesis, and protect the ultrastructure of synapses (61). This may be related to IPA as an antioxidant, preventing neuronal death induced by amylin and β-amyloids and restoring mitochondrial function (62, 63).
First, the protective effect of serum IPA in T2D may be achieved through its efficacy in regulating the secretion of incretin, particularly glucagon-like peptide (GLP)-1 release by intestinal endocrine L cells (64). GLP-1 inhibits the occurrence of T2D by reducing B-cell apoptosis and increasing cell proliferation and regeneration (65)
Second, as a strong oxidant (62), IPA can protect β cells from damage related to metabolism and oxidative stress, and possibly from the accumulation of amyloid (66). These results suggest that IPA may be a promising candidate for the treatment of insulin-resistant metabolic disorders, including T2D.
IPA intervention can improve NASH model mice induced by a HFD through intestinal microenvironment homeostasis (17). In an in vitro experiment, supplementation with oleic acid (OA; 100 μM) resulted in significant accumulation of TG in a human hepatocarcinoma cell line of HepG2 cells, and IPA treatment significantly reduced OA-induced TG accumulation in a dose-dependent manner (10, 25, and 50 µM). Further research showed that IPA dose-dependently reduced the transcription of essential genes involved in fatty acid (Srebp1-c and Fas) and cholesterol biosynthesis (Srebp2 and Hmgr) in HepG2 cells (16).
In addition to inhibiting lipid synthesis in the liver, IPA intervention could inhibit the expression levels of pro-inflammatory cytokines such as TNF-α, IL-1β, and IL-6 in the liver of NASH rats induced by a HFD. In an in vitro LPS-induced mouse macrophage model, IPA also inhibited nuclear factor kappa B) NF-κB signaling, p65 phosphorylation, and the expression of NF-κB downstream target genes in a dose-dependent manner (17).
Excess free Fe(3+) and was also found to cause oxidative damage, which deteriorates NAFLD to NASH. In an in vitro experiment, FeCl(3+) (0.2 mM) was used to induce the isolated rat liver microsomes to simulate the oxidative damage model and then incubated with IPA. It was found that co-incubated IPA (concentrations of 10, 3, 2, and 1 mM) can prevent the decrease in cell membrane fluidity caused by Fe(3+). The increase in lipid peroxidation caused by Fe(3+) was only inhibited after incubation with the highest concentration (10 mM) of IPA (67). Moreover, IPA at a concentration of 5 mM was able to inhibit lipid peroxidation damage in hamster testes caused by iron ions (68). This suggests that IPA can act as an effective free radical scavenger to prevent iron-induced oxidative damage to cell membranes. The antioxidant effect of IPA is concentration dependent, which also explains the protective effect of high concentrations of IPA on the periphery of the body.
IPA maintains the stability of the intestinal microenvironment. Its primary mechanism is to correct the disordered intestinal microflora, repair the intestinal barrier, and inhibit the intestinal immune response.
The intestinal microbial structure can affect the host’s absorption of dietary monosaccharides and lipids, promoting the accumulation of TG in the adipose tissue and liver and causing metabolic disease (69, 70). An imbalance of intestinal microbes affects the TLR9- and TLR4-related inflammatory pathways in the liver (71). In multi-ethnic cohort studies, intestinal microbial α diversity was generally low in patients with metabolic diseases (72). In a clinical cohort study of 1,018 middle-aged women from TwinsUK, the relationship between serum IPA levels and gut microbial genes was evaluated, and a positive correlation between microbiota alpha diversity and serum IPA content was found (Shannon diversity: β [Shannon diversity: beta (95% CI] = 0.19 [0.13; 0.25], p = 6.41 × 10−10) (73).
In an 8-week NAFLD rat model induced by a HFD, IPA (20 mg/kg) was administered to rats for the 8-week experiment. The 16s rRNA method was used to detect rat feces, and principal coordinate and non-metric multidimensional scale analyses showed that the intestinal microbes of rats in the IPA administration group were significantly different from those in the model group. This suggests that IPA administration can improve the overall structure of the intestinal microbes in NAFLD rats. An increase and decrease in the abundance of Firmicutes and Bacteroidetes, respectively, were biomarkers of obesity (74, 75). Based on this feature, the authors analyzed the intestinal microbial composition, and an HFD was found to increase the ratio of Firmicutes to Bacteroidetes, which could be reversed by IPA treatment. The abundance of the two potential pathogenic bacteria Bacteroides and Streptococcus (76) was increased by an HFD and decreased by IPA treatment. It has also been reported that the abundance of the genus Parasutterella (77) associated with chronic intestinal inflammation was reduced by IPA treatment. In addition, the abundance of the two genera Oscillibacter and Odoribacter, which are important for maintaining intestinal homeostasis, were reduced in the HFD-fed group (78) and restored in the IPA group (17). In addition, IPA supplementation can inhibit the growth of Mycobacterium tuberculosis by blocking the synthesis of Trp in M. tuberculosis through the catalytic step of TrpE, thereby exerting an anti-tubercular effect (79).
Increased intestinal permeability and abnormal intestinal tight junctions caused by ecological imbalance are frequently observed in patients with metabolic diseases (80). Intestinal ecological imbalance leads to an increase in LPS and bile acid production, which is related to whole-body low-grade inflammation (81).
Treatment of HFD-fed mice with IPA reduced intestinal permeability (decreased circulating FITC-dextran) and reduced circulating LPS levels. In vitro, researchers used monolayers of T84 cells incubated with the pro-inflammatory cytokines interferon-γ (IFN-γ) and TNF-α. IPA was found to reduce the permeability of monolayers through an FITC-dextran permeability experiment (82).
The ratio of villi to crypts in the ileum of HFD-fed rats was reduced (83), and the villi height was restored by IPA treatment, which also promoted the protein expressions of zonula occludens-1 (ZO-1), occludin, and tight junction proteins in the rat ileum (17). The end of the afferent neurons of the vagus nerve is located in the intestinal mucosa, and the increase in LPS changes the afferent signals of the vagus nerve and reduces the satiety induced by cholecystokinin, thus promoting appetite and leading to obesity (84). Thus, IPA can inhibit appetite by inhibiting LPS levels in the plasma.
The Caco-2/HT29 co-culture model was used to evaluate the effect of IPA on the intestinal barrier and explore its potential mechanism. Studies have shown that IPA increases transepithelial resistance and decreases paracellular permeability. Simultaneously, IPA enhances the mucus barrier by increasing the expression of mucins MUC2 and MUC4 and the goblet cell secretion products TFF3 and RELMβ. In addition, IPA reduces the expression of inflammatory factors in LPS-induced Caco-2/HT29 cells. These findings provide a new perspective for the intestinal microbial metabolite of Trp to improve the intestinal barrier (85). SLC2A5 (GLUT5-encoded) is the leading fructose absorption transporter in the kidneys, small intestine, and proximal tubules, and its overexpression causes metabolic syndrome by increasing fructose intake (86). Expression of the fructose transporter SLC2A5 mRNA was increased in IFN-γ-induced intestinal epithelial T84 cells, and IPA intervention reversed SLC2A5 mRNA expression (11).
Impaired intestinal barrier function and increased leakage of intestinal-derived antigens may lead to visceral lipid deposition and metabolic dysfunction (87). Serum IPA was reported to be decreased by approximately 60% in patients with active inflammatory bowel disease compared with that in healthy controls. During the recovery period of inflammatory bowel disease, the level of IPA in the serum gradually recovered (27, 88).
Administration of IPA showed significant induction of IL-10 receptor protein 1 expression in cultured intestinal epithelial cells T84 (27), based on a close correlation between epithelial IL-10 receptors and the maintenance and recovery of epithelial barrier function (89), which further supported the role of IPA in the maintenance of intestinal immunity.
Recent studies have suggested that IPA is an endogenous ligand for intestinal PXR. IPA induces the transcription of PXR target genes Mdr1, Cyp3a11, and Ugt1a1 mRNA in vivo (82). However, IPA alone is a weak human PXR ligand (82). Inoculated Clostridium sporogenes in germ-free mice accompanied with L-Trp-supplemented diets promoted the production of IPA to protect mice from dextran sulfate sodium-induced colitis through the PXR pathway (82). Studies have also confirmed that IPA improves intestinal permeability (FITC-dextran permeability) in a colitis (indomethacin-induced) mouse model with intestinal barrier defects. Intestinal TNF-α mRNA expression and p38-MAPK protein phosphorylation were inhibited, while in PXR-deficient (Nr1i2−/−) mice, the benefits of IPA were inhibited, suggesting that IPA improved the intestinal barrier via the PXR pathway (82). Furthermore, IPA can modulate vascular function by modulating PXR activity, and IPA exposure reduces the vasodilatory responses of nitric oxide-mediated muscarinic and protease-activated receptor 2-stimulated mouse aortic tissue (90).
In another study, IPA was shown to be an agonist of the aromatic meridian receptor (Ahr) of a commensal bacterial product (91), and Ahr activation was beneficial to the maintenance of intestinal homeostasis and the regulation of immunity (92, 93). Therefore, these studies indicated that promoting IPA formation by bacteria or the direct administration of IPA is beneficial for inflammatory bowel disease.
The intestinal microflora is a diverse microbial community that encodes functional genes several orders of magnitude higher than the human genome and can regulate human health (94). With the development of metabolomics, intestinal microbial metabolites play an increasingly important role in regulating host health and disease; however, the disturbance of metabolites is related to multiple chronic diseases (95). Therefore, research on bacteria-derived metabolites offers the possibility of personalized medicine for chronic diseases with complex pathogenesis. The study found that IPA, a metabolite produced by Trp under the action of intestinal microbes, was correlated with the occurrence and development of metabolic diseases (Figure 2). Metabolic diseases such as obesity, T2D, NAFLD, and CVD have been reported. The IPA content in the peripheral region was significantly consumed. After 3 months of bariatric surgery, it recovered, suggesting that IPA might be a potential biomarker for metabolic diseases. However, further studies have shown that IPA could be a potential biomarker of metabolic illnesses (Table 1). The intervention with IPA reduced the body weight and peripheral fat content; improved insulin resistance, liver lipid deposition, and peripheral blood lipid content; and maintained intestinal homeostasis, thereby improving metabolic syndrome (Table 2). The metabolic benefit mechanism of IPA may be predominantly related to its strong oxidant effect, which has an excellent antagonistic effect on chronic inflammation caused by metabolic diseases. Moreover, as a bacterial-derived metabolite (Table 3), IPA exerts its beneficial effects in regulating intestinal immune responses through Ahr and PXR ligand. Intestinal bacteria play an important role in the pathogenesis of metabolic diseases. In future research, we need to pay attention to the secondary metabolites produced by the interaction between IPA and the bacterial flora and its remote target organs to further study the mechanism of IPA.
However, the beneficial effects of IPA are all based on the HFD-induced NAFLD model mice, and the opposite result has been found in other models. In CCL4-induced liver fibrosis model mice, IPA aggravated CCL4-induced liver fibrosis injury through transforming growth factor-β1 (TGF-β1) and the Smad signaling pathway (98). Therefore, multiple models are required to verify the potential beneficial effects of IPA on metabolic diseases. At present, the therapeutic effects of IPA are primarily concentrated in basic animal experiments, and no clinical experiments have been performed. Therefore, an in-depth study on the toxicity and safe use of IPA is necessary to provide a sufficient theoretical basis for the development and utilization of IPA.
Furthermore, to fully exploit the potential of the intestinal microbiota in disease prevention, we need to understand in greater depth how dietary components and host genetics affect IPA production. Finally, these findings are converted into clinical practice and developed into clinical methods that can be widely used to predict the prognosis and outcome of diseases and even have diagnostic effects on some metabolic disorders. While leveraging metabolomics poses significant challenges in promoting human health, past studies have demonstrated that certain metabolites have considerable potential for the treatment of human diseases.
BZ and MJ: writing—original draft. These authors have contributed equally to this work. YS and JZ: writing—review and editing. WD and JS: funding acquisition. All authors contributed to the article and approved the submitted version.
This work was supported by the National Natural Science Foundation of China (No. 81570524); Key Medical Disciplines of Hangzhou; Zhejiang Province Basic Public Welfare Research Program Project "Relationship between cognitive impairment and gut microbial dysbiosis in patients with non-alcoholic fatty liver disease" (GF20H030035).
The authors declare that the research was conducted in the absence of any commercial or financial relationships that could be construed as a potential conflict of interest.
All claims expressed in this article are solely those of the authors and do not necessarily represent those of their affiliated organizations, or those of the publisher, the editors and the reviewers. Any product that may be evaluated in this article, or claim that may be made by its manufacturer, is not guaranteed or endorsed by the publisher.
1. Bril F, Cusi K. Nonalcoholic Fatty Liver Disease: The New Complication of Type 2 Diabetes Mellitus. Endocrinol Metab Clinics North Am (2016) 45(4):765–81. doi: 10.1016/j.ecl.2016.06.005
2. Vilar-Gomez E, Calzadilla-Bertot L, Wai-Sun Wong V, Castellanos M, Aller-de la Fuente R, Metwally M, et al. Fibrosis Severity as a Determinant of Cause-Specific Mortality in Patients With Advanced Nonalcoholic Fatty Liver Disease: A Multi-National Cohort Study. Gastroenterology (2018) 155(2):443–457.e17. doi: 10.1053/j.gastro.2018.04.034
3. Lim S, Kim J, Targher G. Links Between Metabolic Syndrome and Metabolic Dysfunction-Associated Fatty Liver Disease. Trends Endocrinol Metabolism: TEM (2021) 32(7):500–14. doi: 10.1016/j.tem.2021.04.008
4. Yoneda M, Yamamoto T, Honda Y, Imajo K, Ogawa Y, Kessoku T, et al. Risk of Cardiovascular Disease in Patients With Fatty Liver Disease as Defined From the Metabolic Dysfunction Associated Fatty Liver Disease or Nonalcoholic Fatty Liver Disease Point of View: A Retrospective Nationwide Claims Database Study in Japan. J Gastroenterol (2021) 56(11):1022–32. doi: 10.1007/s00535-021-01828-6
5. Aguilar M, Bhuket T, Torres S, Liu B, Wong RJ. Prevalence of the Metabolic Syndrome in the United States, 2003-2012. JAMA (2015) 313(19):1973–4. doi: 10.1001/jama.2015.4260
6. Sharpton SR, Schnabl B, Knight R, Loomba R. Current Concepts, Opportunities, and Challenges of Gut Microbiome-Based Personalized Medicine in Nonalcoholic Fatty Liver Disease. Cell Metab (2021) 33(1):21–32. doi: 10.1016/j.cmet.2020.11.010
7. Fan Y, Pedersen O. Gut Microbiota in Human Metabolic Health and Disease. Nat Rev Microbiol (2021) 19(1):55–71. doi: 10.1038/s41579-020-0433-9
8. Bishai JD, Palm NW. Small Molecule Metabolites at the Host-Microbiota Interface. J Immunol (Baltimore Md 1950) (2021) 207(7):1725–33. doi: 10.4049/jimmunol.2100528
9. Koh A, Bäckhed F. From Association to Causality: The Role of the Gut Microbiota and Its Functional Products on Host Metabolism. Mol Cell (2020) 78(4):584–96. doi: 10.1016/j.molcel.2020.03.005
10. Nicholson JK, Holmes E, Kinross J, Burcelin R, Gibson G, Jia W, et al. Host-Gut Microbiota Metabolic Interactions. Science (2012) 336(6086):1262–7. doi: 10.1126/science.1223813
11. Jennis M, Cavanaugh CR, Leo GC, Mabus JR, Lenhard J, Hornby PJ. Microbiota-Derived Tryptophan Indoles Increase After Gastric Bypass Surgery and Reduce Intestinal Permeability In Vitro and In Vivo. Neurogastroenterol Motil (2018) 30(2):e13178. doi: 10.1111/nmo.13178
12. de Mello VD, Paananen J, Lindstrom J, Lankinen MA, Shi L, Kuusisto J, et al. Indolepropionic Acid and Novel Lipid Metabolites are Associated With a Lower Risk of Type 2 Diabetes in the Finnish Diabetes Prevention Study. Sci Rep (2017) 7:46337. doi: 10.1038/srep46337
13. Sehgal R, Ilha M, Vaittinen M, Kaminska D, Männistö V, Kärjä V, et al. Indole-3-Propionic Acid, a Gut-Derived Tryptophan Metabolite, Associates With Hepatic Fibrosis. Nutrients (2021) 13(10):3509. doi: 10.3390/nu13103509
14. Cason CA, Dolan KT, Sharma G, Tao M, Kulkarni R, Helenowski IB, et al. Plasma Microbiome-Modulated Indole- and Phenyl-Derived Metabolites Associate With Advanced Atherosclerosis and Postoperative Outcomes. J Vasc Surg (2018) 68(5):1552–1562.e7. doi: 10.1016/j.jvs.2017.09.029
15. Abildgaard A, Elfving B, Hokland M, Wegener G, Lund S. The Microbial Metabolite Indole-3-Propionic Acid Improves Glucose Metabolism in Rats, But Does Not Affect Behaviour. Arch Physiol Biochem (2018) 124(4):306–12. doi: 10.1080/13813455.2017.1398262
16. Li Y, Xu W, Zhang F, Zhong S, Sun Y, Huo J, et al. The Gut Microbiota-Produced Indole-3-Propionic Acid Confers the Antihyperlipidemic Effect of Mulberry-Derived 1-Deoxynojirimycin. mSystems (2020) 5(5):e00313–20. doi: 10.1128/mSystems.00313-20
17. Zhao ZH, Xin FZ, Xue Y, Hu Z, Han Y, Ma F, et al. Indole-3-Propionic Acid Inhibits Gut Dysbiosis and Endotoxin Leakage to Attenuate Steatohepatitis in Rats. Exp Mol Med (2019) 51(9):103. doi: 10.1038/s12276-019-0304-5
18. Hendrikx T, Schnabl B. Indoles: Metabolites Produced by Intestinal Bacteria Capable of Controlling Liver Disease Manifestation. J Internal Med (2019) 286(1):32–40. doi: 10.1111/joim.12892
19. Danaceau JP, Anderson GM, McMahon WM, Crouch DJ. A Liquid Chromatographic-Tandem Mass Spectrometric Method for the Analysis of Serotonin and Related Indoles in Human Whole Blood. J Analytical Toxicol (2003) 27(7):440–4. doi: 10.1093/jat/27.7.440
20. Negatu DA, Gengenbacher M, Dartois V, Dick T. Indole Propionic Acid, an Unusual Antibiotic Produced by the Gut Microbiota, With Anti-Inflammatory and Antioxidant Properties. Front Microbiol (2020) 11:575586. doi: 10.3389/fmicb.2020.575586
21. Tuomainen M, Lindström J, Lehtonen M, Auriola S, Pihlajamäki J, Peltonen M, et al. Associations of Serum Indolepropionic Acid, a Gut Microbiota Metabolite, With Type 2 Diabetes and Low-Grade Inflammation in High-Risk Individuals. Nutr Diabetes (2018) 8(1):35. doi: 10.1038/s41387-018-0046-9
22. Grifka-Walk HM, Jenkins BR, Kominsky DJ. Amino Acid Trp: The Far Out Impacts of Host and Commensal Tryptophan Metabolism. Front Immunol (2021) 12:653208. doi: 10.3389/fimmu.2021.653208
23. Bender DA. Biochemistry of Tryptophan in Health and Disease. Mol aspects Med (1983) 6(2):101–97. doi: 10.1016/0098-2997(83)90005-5
24. Keszthelyi D, Troost FJ, Masclee AA. Understanding the Role of Tryptophan and Serotonin Metabolism in Gastrointestinal Function. Neurogastroenterol Motil Off J Eur Gastrointestinal Motil Soc (2009) 21(12):1239–49. doi: 10.1111/j.1365-2982.2009.01370.x
25. Dodd D, Spitzer MH, Van Treuren W, Merrill BD, Hryckowian AJ, Higginbottom SK, et al. A Gut Bacterial Pathway Metabolizes Aromatic Amino Acids Into Nine Circulating Metabolites. Nature (2017) 551(7682):648–52. doi: 10.1038/nature24661
26. Kim J, Park W. Indole Inhibits Bacterial Quorum Sensing Signal Transmission by Interfering With Quorum Sensing Regulator Folding. Microbiol (Reading England) (2013) 159(Pt 12):2616–25. doi: 10.1099/mic.0.070615-0
27. Alexeev E, Lanis J, Kao D, Campbell E, Kelly C, Battista K, et al. Microbiota-Derived Indole Metabolites Promote Human and Murine Intestinal Homeostasis Through Regulation of Interleukin-10 Receptor. Am J Pathol (2018) 188(5):1183–94. doi: 10.1016/j.ajpath.2018.01.011
28. Pavlova T, Vidova V, Bienertova-Vasku J, Janku P, Almasi M, Klanova J, et al. Urinary Intermediates of Tryptophan as Indicators of the Gut Microbial Metabolism. Analytica Chimica Acta (2017) 987:72–80. doi: 10.1016/j.aca.2017.08.022
29. Elsden SR, Hilton MG, Waller JM. The End Products of the Metabolism of Aromatic Amino Acids by Clostridia. Arch Microbiol (1976) 107(3):283–8. doi: 10.1007/bf00425340
30. Wikoff WR, Anfora AT, Liu J, Schultz PG, Lesley SA, Peters EC, et al. Metabolomics Analysis Reveals Large Effects of Gut Microflora on Mammalian Blood Metabolites. Proc Natl Acad Sci USA (2009) 106(10):3698–703. doi: 10.1073/pnas.0812874106
31. Nishizawa T, Aldrich CC, Sherman DH. Molecular Analysis of the Rebeccamycin L-Amino Acid Oxidase From Lechevalieria Aerocolonigenes ATCC 39243. J bacteriology (2005) 187(6):2084–92. doi: 10.1128/jb.187.6.2084-2092.2005
32. Gartner SN, Aidney F, Klockars A, Prosser C, Carpenter EA, Isgrove K, et al. Intragastric Preloads of L-Tryptophan Reduce Ingestive Behavior via Oxytocinergic Neural Mechanisms in Male Mice. Appetite (2018) 125:278–86. doi: 10.1016/j.appet.2018.02.015
33. Konopelski P, Konop M, Gawrys-Kopczynska M, Podsadni P, Szczepanska A, Ufnal MJN. Indole-3-Propionic Acid, a Tryptophan-Derived Bacterial Metabolite, Reduces Weight Gain in Rats. Nutrients (2019) 11(3):591. doi: 10.3390/nu11030591
34. Panagiotakos DB, Pitsavos C, Arvaniti F, Stefanadis C. Adherence to the Mediterranean Food Pattern Predicts the Prevalence of Hypertension, Hypercholesterolemia, Diabetes and Obesity, Among Healthy Adults; the Accuracy of the MedDietScore. Prev Med (2007) 44(4):335–40. doi: 10.1016/j.ypmed.2006.12.009
35. Schulze MB, Hoffmann K, Manson JE, Willett WC, Meigs JB, Weikert C, et al. Dietary Pattern, Inflammation, and Incidence of Type 2 Diabetes in Women. Am J Clin Nutr (2005) 82(3):675–84; quiz 714-5. doi: 10.1093/ajcn.82.3.675
36. Gao J, Guo X, Wei W, Li R, Hu K, Liu X, et al. The Association of Fried Meat Consumption With the Gut Microbiota and Fecal Metabolites and Its Impact on Glucose Homoeostasis, Intestinal Endotoxin Levels, and Systemic Inflammation: A Randomized Controlled-Feeding Trial. Diabetes Care (2021) 44(9):1970–9. doi: 10.2337/dc21-0099
37. Zhu C, Sawrey-Kubicek L, Beals E, Rhodes CH, Houts HE, Sacchi R, et al. Human Gut Microbiome Composition and Tryptophan Metabolites Were Changed Differently by Fast Food and Mediterranean Diet in 4 Days: A Pilot Study. Nutr Res (2020) 77:62–72. doi: 10.1016/j.nutres.2020.03.005
38. Leeming ER, Johnson AJ, Spector TD, Le Roy CI. Effect of Diet on the Gut Microbiota: Rethinking Intervention Duration. Nutrients (2019) 11(12):2862. doi: 10.3390/nu11122862
39. Qi Q, Li J, Yu B, Moon JY, Chai JC, Merino J, et al. Host and Gut Microbial Tryptophan Metabolism and Type 2 Diabetes: An Integrative Analysis of Host Genetics, Diet, Gut Microbiome and Circulating Metabolites in Cohort Studies. Gut (2021) gutjnl-2021–324053. doi: 10.1136/gutjnl-2021-324053
40. Cussotto S, Delgado I, Anesi A, Dexpert S, Aubert A, Beau C, et al. Tryptophan Metabolic Pathways Are Altered in Obesity and Are Associated With Systemic Inflammation. Front Immunol (2020) 11:557. doi: 10.3389/fimmu.2020.00557
41. Dong TS, Mayer EA, Osadchiy V, Chang C, Katzka W, Lagishetty V, et al. A Distinct Brain-Gut-Microbiome Profile Exists for Females With Obesity and Food Addiction. Obes (Silver Spring Md.) (2020) 28(8):1477–86. doi: 10.1002/oby.22870
42. Schauer PR, Kashyap SR, Wolski K, Brethauer SA, Kirwan JP, Pothier CE, et al. Bariatric Surgery Versus Intensive Medical Therapy in Obese Patients With Diabetes. N Engl J Med (2012) 366(17):1567–76. doi: 10.1056/NEJMoa1200225
43. Mercer KE, Yeruva L, Pack L, Graham JL, Stanhope KL, Chintapalli SV, et al. Xenometabolite Signatures in the UC Davis Type 2 Diabetes Mellitus Rat Model Revealed Using a Metabolomics Platform Enriched With Microbe-Derived Metabolites. American Journal of Physiology. Gastrointestinal Liver Physiol (2020) 319(2):G157–g169. doi: 10.1152/ajpgi.00105.2020
44. Huang DQ, El-Serag HB, Loomba R. Global Epidemiology of NAFLD-Related HCC: Trends, Predictions, Risk Factors and Prevention. Nat Rev Gastroenterol Hepatol (2021) 18(4):223–38. doi: 10.1038/s41575-020-00381-6
45. Ioannou GN. The Role of Cholesterol in the Pathogenesis of NASH. Trends Endocrinol Metab (2016) 27(2):84–95. doi: 10.1016/j.tem.2015.11.008
46. McGettigan B, McMahan R, Orlicky D, Burchill M, Danhorn T, Francis P, et al. Dietary Lipids Differentially Shape Nonalcoholic Steatohepatitis Progression and the Transcriptome of Kupffer Cells and Infiltrating Macrophages. Hepatology (2019) 70(1):67–83. doi: 10.1002/hep.30401
47. Van Rooyen DM, Larter CZ, Haigh WG, Yeh MM, Ioannou G, Kuver R, et al. Hepatic Free Cholesterol Accumulates in Obese, Diabetic Mice and Causes Nonalcoholic Steatohepatitis. Gastroenterology (2011) 141(4):1393–403, 1403.e1-5. doi: 10.1053/j.gastro.2011.06.040
48. Jaeschke. Reactive oxygen H. And Mechanisms of Inflammatory Liver Injury: Present Concepts. J Gastroenterol Hepatol (2011) 26(Suppl 1):173–9. doi: 10.1111/j.1440-1746.2010.06592.x
49. Wong RJ, Cheung R, Ahmed A. Nonalcoholic Steatohepatitis is the Most Rapidly Growing Indication for Liver Transplantation in Patients With Hepatocellular Carcinoma in the U. S Hepatol (2014) 59(6):2188–95. doi: 10.1002/hep.26986
50. Zhang X, Coker OO, Chu ES, Fu K, Lau HCH, Wang YX, et al. Dietary Cholesterol Drives Fatty Liver-Associated Liver Cancer by Modulating Gut Microbiota and Metabolites. Gut (2021) 70(4):761–74. doi: 10.1136/gutjnl-2019-319664
51. Angulo P, Kleiner DE, Dam-Larsen S, Adams LA, Bjornsson ES, Charatcharoenwitthaya P, et al. Liver Fibrosis, But No Other Histologic Features, Is Associated With Long-Term Outcomes of Patients With Nonalcoholic Fatty Liver Disease. Gastroenterology (2015) 149(2):389–97.e10. doi: 10.1053/j.gastro.2015.04.043
52. Hagström H, Nasr P, Ekstedt M, Hammar U, Stål P, Hultcrantz R, et al. Fibrosis Stage But Not NASH Predicts Mortality and Time to Development of Severe Liver Disease in Biopsy-Proven NAFLD. J Hepatol (2017) 67(6):1265–73. doi: 10.1016/j.jhep.2017.07.027
53. Sun CY, Lin CJ, Pan HC, Lee CC, Lu SC, Hsieh YT, et al. Clinical Association Between the Metabolite of Healthy Gut Microbiota, 3-Indolepropionic Acid and Chronic Kidney Disease. Clin Nutr (Edinburgh Scotland) (2019) 38(6):2945–8. doi: 10.1016/j.clnu.2018.11.029
54. Yisireyili M, Takeshita K, Saito S, Murohara T, Niwa T. Indole-3-Propionic Acid Suppresses Indoxyl Sulfate-Induced Expression of Fibrotic and Inflammatory Genes in Proximal Tubular Cells. Nagoya J Med Sci (2017) 79(4):477–86. doi: 10.18999/nagjms.79.4.477
55. Hosohata K, Jin D, Takai S. In Vivo and In Vitro Evaluation of Urinary Biomarkers in Ischemia/Reperfusion-Induced Kidney Injury. Int J Mol Sci (2021) 22(21):11448. doi: 10.3390/ijms222111448
56. Xiao HW, Cui M, Li Y, Dong JL, Zhang SQ, Zhu CC, et al. Gut Microbiota-Derived Indole 3-Propionic Acid Protects Against Radiation Toxicity via Retaining Acyl-CoA-Binding Protein. Microbiome (2020) 8(1):69. doi: 10.1186/s40168-020-00845-6
57. Behera J, Ison J, Voor MJ, Tyagi N. Probiotics Stimulate Bone Formation in Obese Mice via Histone Methylations. Theranostics (2021) 11(17):8605–23. doi: 10.7150/thno.63749
58. Poeggeler B, Pappolla MA, Hardeland R, Rassoulpour A, Hodgkins PS, Guidetti P, et al. Indole-3-Propionate: A Potent Hydroxyl Radical Scavenger in Rat Brain. Brain Res (1999) 815(2):382–8. doi: 10.1016/s0006-8993(98)01027-0
59. Bendheim PE, Poeggeler B, Neria E, Ziv V, Pappolla MA, Chain DG. Development of Indole-3-Propionic Acid (OXIGON) for Alzheimer’s Disease. J Mol Neurosci MN (2002) 19(1-2):213–7. doi: 10.1007/s12031-002-0036-0
60. Gundu C, Arruri VK, Sherkhane B, Khatri DK, Singh SB. Indole-3-Propionic Acid Attenuates High Glucose Induced ER Stress Response and Augments Mitochondrial Function by Modulating PERK-IRE1-ATF4-CHOP Signalling in Experimental Diabetic Neuropathy. Arch Physiol Biochem (2022), 1–14. doi: 10.1080/13813455.2021.2024577
61. Liu Z, Dai X, Zhang H, Shi R, Hui Y, Jin X, et al. Gut Microbiota Mediates Intermittent-Fasting Alleviation of Diabetes-Induced Cognitive Impairment. Nat Commun (2020) 11(1):855. doi: 10.1038/s41467-020-14676-4
62. Chyan YJ, Poeggeler B, Omar RA, Chain DG, Frangione B, Ghiso J, et al. Potent Neuroprotective Properties Against the Alzheimer Beta-Amyloid by an Endogenous Melatonin-Related Indole Structure, Indole-3-Propionic Acid. J Biol Chem (1999) 274(31):21937–42. doi: 10.1074/jbc.274.31.21937
63. Dragicevic N, Copes N, O’Neal-Moffitt G, Jin J, Buzzeo R, Mamcarz M, et al. Melatonin Treatment Restores Mitochondrial Function in Alzheimer’s Mice: A Mitochondrial Protective Role of Melatonin Membrane Receptor Signaling. J pineal Res (2011) 51(1):75–86. doi: 10.1111/j.1600-079X.2011.00864.x
64. Chimerel C, Emery E, Summers DK, Keyser U, Gribble FM, Reimann F. Bacterial Metabolite Indole Modulates Incretin Secretion From Intestinal Enteroendocrine L Cells. Cell Rep (2014) 9(4):1202–8. doi: 10.1016/j.celrep.2014.10.032
65. Garber AJ. Incretin Effects on β-Cell Function, Replication, and Mass: The Human Perspective. Diabetes Care (2011) 34 Suppl 2(Suppl 2):S258–63. doi: 10.2337/dc11-s230
66. Lutz TA, Meyer U. Amylin at the Interface Between Metabolic and Neurodegenerative Disorders. Front Neurosci (2015) 9:216. doi: 10.3389/fnins.2015.00216
67. Karbownik M, Reiter RJ, Garcia JJ, Cabrera J, Burkhardt S, Osuna C, et al. Indole-3-Propionic Acid, a Melatonin-Related Molecule, Protects Hepatic Microsomal Membranes From Iron-Induced Oxidative Damage: Relevance to Cancer Reduction. J Cell Biochem (2001) 81(3):507–13. doi: 10.1002/1097-4644(20010601)81:3<507::AID-JCB1064>3.0.CO;2-M
68. Karbownik M, Gitto E, Lewiñski A, Reiter RJ. Relative Efficacies of Indole Antioxidants in Reducing Autoxidation and Iron-Induced Lipid Peroxidation in Hamster Testes. J Cell Biochem (2001) 81(4):693–9. doi: 10.1002/jcb.1100
69. Bäckhed F, Ding H, Wang T, Hooper LV, Koh GY, Nagy A, et al. The Gut Microbiota as an Environmental Factor That Regulates Fat Storage. Proc Natl Acad Sci U.S.A. (2004) 101(44):15718–23. doi: 10.1073/pnas.0407076101
70. Bäckhed F, Ley RE, Sonnenburg JL, Peterson DA, Gordon JI. Host-Bacterial Mutualism in the Human Intestine. Science (2005) 307(5717):1915–20. doi: 10.1126/science.1104816
71. Henao-Mejia J, Elinav E, Jin C, Hao L, Mehal WZ, Strowig T, et al. Inflammasome-Mediated Dysbiosis Regulates Progression of NAFLD and Obesity. Nature (2012) 482(7384):179–85. doi: 10.1038/nature10809
72. Hullar MAJ, Jenkins IC, Randolph TW, Curtis KR, Monroe KR, Ernst T, et al. Associations of the Gut Microbiome With Hepatic Adiposity in the Multiethnic Cohort Adiposity Phenotype Study. Gut Microbes (2021) 13(1):1965463. doi: 10.1080/19490976.2021.1965463
73. Menni C, Hernandez MM, Vital M, Mohney RP, Spector TD, Valdes AM. Circulating Levels of the Anti-Oxidant Indoleproprionic Acid are Associated With Higher Gut Microbiome Diversity. Gut Microbes (2019) 10(6):688–95. doi: 10.1080/19490976.2019.1586038
74. Ley RE, Bäckhed F, Turnbaugh P, Lozupone CA, Knight RD, Gordon JI. Obesity Alters Gut Microbial Ecology. Proc Natl Acad Sci USA (2005) 102(31):11070–5. doi: 10.1073/pnas.0504978102
75. Turnbaugh PJ, Ley RE, Mahowald MA, Magrini V, Mardis ER, Gordon JI. An Obesity-Associated Gut Microbiome With Increased Capacity for Energy Harvest. Nature (2006) 444(7122):1027–31. doi: 10.1038/nature05414
76. Krzyściak W, Pluskwa KK, Jurczak A, Kościelniak D. The Pathogenicity of the Streptococcus Genus. Eur J Clin Microbiol Infect Dis Off Publ Eur Soc Clin Microbiol (2013) 32(11):1361–76. doi: 10.1007/s10096-013-1914-9
77. Hod K, Dekel R, Aviv Cohen N, Sperber A, Ron Y, Boaz M, et al. The Effect of a Multispecies Probiotic on Microbiota Composition in a Clinical Trial of Patients With Diarrhea-Predominant Irritable Bowel Syndrome. Neurogastroenterol Motil Off J Eur Gastrointestinal Motil Soc (2018) 30(12):e13456. doi: 10.1111/nmo.13456
78. Li J, Sung CY, Lee N, Ni Y, Pihlajamäki J, Panagiotou G, et al. Probiotics Modulated Gut Microbiota Suppresses Hepatocellular Carcinoma Growth in Mice. Proc Natl Acad Sci USA (2016) 113(9):E1306–15. doi: 10.1073/pnas.1518189113
79. Negatu DA, Yamada Y, Xi Y, Go ML, Zimmerman M, Ganapathy U, et al. Gut Microbiota Metabolite Indole Propionic Acid Targets Tryptophan Biosynthesis in Mycobacterium Tuberculosis. mBio (2019) 10(2):e02781–18. doi: 10.1128/mBio.02781-18
80. Mao JW, Tang HY, Zhao T, Tan XY, Bi J, Wang BY, et al. Intestinal Mucosal Barrier Dysfunction Participates in the Progress of Nonalcoholic Fatty Liver Disease. Int J Clin Exp Pathol (2015) 8(4):3648–58.
81. Plaza-Díaz J, Solis-Urra P, Aragón-Vela J, Rodríguez-Rodríguez F, Olivares-Arancibia J, Álvarez-Mercado AI. Insights Into the Impact of Microbiota in the Treatment of NAFLD/NASH and Its Potential as a Biomarker for Prognosis and Diagnosis. Biomedicines (2021) 9(2). doi: 10.3390/biomedicines9020145
82. Venkatesh M, Mukherjee S, Wang H, Li H, Sun K, Benechet AP, et al. Symbiotic Bacterial Metabolites Regulate Gastrointestinal Barrier Function via the Xenobiotic Sensor PXR and Toll-Like Receptor 4. Immunity (2014) 41(2):296–310. doi: 10.1016/j.immuni.2014.06.014
83. Rahman K, Desai C, Iyer SS, Thorn NE, Kumar P, Liu Y, et al. Loss of Junctional Adhesion Molecule A Promotes Severe Steatohepatitis in Mice on a Diet High in Saturated Fat, Fructose, and Cholesterol. Gastroenterology (2016) 151(4):733–746.e12. doi: 10.1053/j.gastro.2016.06.022
84. de La Serre CB, de Lartigue G, Raybould HE. Chronic Exposure to Low Dose Bacterial Lipopolysaccharide Inhibits Leptin Signaling in Vagal Afferent Neurons. Physiol Behav (2015) 139:188–94. doi: 10.1016/j.physbeh.2014.10.032
85. Li J, Zhang L, Wu T, Li Y, Zhou X, Ruan Z. Indole-3-Propionic Acid Improved the Intestinal Barrier by Enhancing Epithelial Barrier and Mucus Barrier. J Agric Food Chem (2021) 69(5):1487–95. doi: 10.1021/acs.jafc.0c05205
86. Soleimani M. Dietary Fructose, Salt Absorption and Hypertension in Metabolic Syndrome: Towards a New Paradigm. Acta physiologica (Oxford England) (2011) 201(1):55–62. doi: 10.1111/j.1748-1716.2010.02167.x
87. Gummesson A, Carlsson LM, Storlien LH, Bäckhed F, Lundin P, Löfgren L, et al. Intestinal Permeability is Associated With Visceral Adiposity in Healthy Women. Obes (Silver Spring Md.) (2011) 19(11):2280–2. doi: 10.1038/oby.2011.251
88. Wlodarska M, Luo C, Kolde R, d’Hennezel E, Annand JW, Heim CE, et al. Indoleacrylic Acid Produced by Commensal Peptostreptococcus Species Suppresses Inflammation. Cell Host Microbe (2017) 22(1):25–37.e6. doi: 10.1016/j.chom.2017.06.007
89. Kominsky DJ, Campbell EL, Ehrentraut SF, Wilson KE, Kelly CJ, Glover LE, et al. IFN-γ-Mediated Induction of an Apical IL-10 Receptor on Polarized Intestinal Epithelia. J Immunol (Baltimore Md 1950) (2014) 192(3):1267–76. doi: 10.4049/jimmunol.1301757
90. Pulakazhi Venu VK, Saifeddine M, Mihara K, Tsai YC, Nieves K, Alston L, et al. The Pregnane X Receptor and its Microbiota-Derived Ligand Indole 3-Propionic Acid Regulate Endothelium-Dependent Vasodilation. Am J Physiol Endocrinol Metab (2019) 317(2):E350–e361. doi: 10.1152/ajpendo.00572.2018
91. Rothhammer V, Mascanfroni ID, Bunse L, Takenaka MC, Kenison JE, Mayo L, et al. Type I Interferons and Microbial Metabolites of Tryptophan Modulate Astrocyte Activity and Central Nervous System Inflammation via the Aryl Hydrocarbon Receptor. Nat Med (2016) 22(6):586–97. doi: 10.1038/nm.4106
92. Hubbard TD, Murray IA, Perdew GH. Indole and Tryptophan Metabolism: Endogenous and Dietary Routes to Ah Receptor Activation. Drug Metab disposition: Biol fate chemicals (2015) 43(10):1522–35. doi: 10.1124/dmd.115.064246
93. Lanis JM, Alexeev EE, Curtis VF, Kitzenberg DA, Kao DJ, Battista KD, et al. Tryptophan Metabolite Activation of the Aryl Hydrocarbon Receptor Regulates IL-10 Receptor Expression on Intestinal Epithelia. Mucosal Immunol (2017) 10(5):1133–44. doi: 10.1038/mi.2016.133
94. Turnbaugh PJ, Ley RE, Hamady M, Fraser-Liggett CM, Knight R, Gordon JI. The Human Microbiome Project. Nature (2007) 449(7164):804–10. doi: 10.1038/nature06244
95. Lynch SV, Pedersen O. The Human Intestinal Microbiome in Health and Disease. New Engl J Med (2016) 375(24):2369–79. doi: 10.1056/NEJMra1600266
96. Bansal T, Alaniz RC, Wood TK, Jayaraman A. The Bacterial Signal Indole Increases Epithelial-Cell Tight-Junction Resistance and Attenuates Indicators of Inflammation. Proc Natl Acad Sci USA (2010) 107(1):228–33. doi: 10.1073/pnas.0906112107
97. Chappell CL, Darkoh C, Shimmin L, Farhana N, Kim DK, Okhuysen PC, et al. Fecal Indole as a Biomarker of Susceptibility to Cryptosporidium Infection. Infect Immun (2016) 84(8):2299–306. doi: 10.1128/iai.00336-16
Keywords: metabolic syndrome, indole-3-propanoic acid, obesity, type 2 diabetes, non-alcoholic fatty liver disease, cardiovascular diseases
Citation: Zhang B, Jiang M, Zhao J, Song Y, Du W and Shi J (2022) The Mechanism Underlying the Influence of Indole-3-Propionic Acid: A Relevance to Metabolic Disorders. Front. Endocrinol. 13:841703. doi: 10.3389/fendo.2022.841703
Received: 22 December 2021; Accepted: 21 February 2022;
Published: 18 March 2022.
Edited by:
Fang Zhongze, Tianjin Medical University, ChinaReviewed by:
Elena Rampanelli, Amsterdam University Medical Center, NetherlandsCopyright © 2022 Zhang, Jiang, Zhao, Song, Du and Shi. This is an open-access article distributed under the terms of the Creative Commons Attribution License (CC BY). The use, distribution or reproduction in other forums is permitted, provided the original author(s) and the copyright owner(s) are credited and that the original publication in this journal is cited, in accordance with accepted academic practice. No use, distribution or reproduction is permitted which does not comply with these terms.
*Correspondence: Weidong Du, aHphZHVAMTYzLmNvbQ==; Junping Shi, MjAxMzEwMDRAaHpudS5lZHUuY24=
†These authors have contributed equally to this work
Disclaimer: All claims expressed in this article are solely those of the authors and do not necessarily represent those of their affiliated organizations, or those of the publisher, the editors and the reviewers. Any product that may be evaluated in this article or claim that may be made by its manufacturer is not guaranteed or endorsed by the publisher.
Research integrity at Frontiers
Learn more about the work of our research integrity team to safeguard the quality of each article we publish.