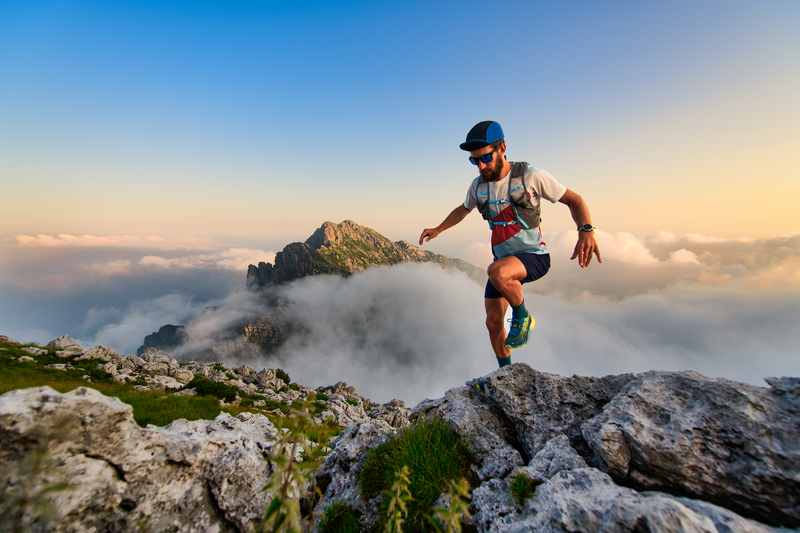
95% of researchers rate our articles as excellent or good
Learn more about the work of our research integrity team to safeguard the quality of each article we publish.
Find out more
ORIGINAL RESEARCH article
Front. Endocrinol. , 17 January 2023
Sec. Gut Endocrinology
Volume 13 - 2022 | https://doi.org/10.3389/fendo.2022.1105073
This article is part of the Research Topic Can Traditional Chinese Medicines affect Endocrine Diseases via Effects on the Intestinal Flora? View all 21 articles
Background: Grifola frondosa (G. frondosa) is a fungus with good economic exploitation prospects of food and medicine homologation. This study aims to investigate the effects of G. frondosa powder suspension (GFPS) on the intestinal contents microbiota and the indexes related to oxidative stress and energy metabolism in mice, to provide new ideas for developing G. frondosa weight loss products.
Methods: Twenty Kunming mice were randomly divided into control (CC), low-dose GFPS (CL), medium-dose GFPS (CM), and high-dose GFPS (CH) groups. The mice in CL, CM, and CH groups were intragastrically administered with 1.425 g/(kg·d), 2.85 g/(kg·d), and 5.735 g/(kg·d) GFPS, respectively. The mice in CC group were given the same dose of sterile water. After 8 weeks, liver and muscle related oxidative stress and energy metabolism indicators were detected, and the intestinal content microbiota of the mice was detected by 16S rRNA high-throughput sequencing.
Results: After eight weeks of GFPS intervention, all mice lost weight. Compared with the CC group, lactate dehydrogenase (LDH) and malondialdehyde (MDA) contents in CL, CM, and CH groups were increased, while Succinate dehydrogenase (SDH) and Superoxide Dismutase (SOD) contents in the liver were decreased. The change trends of LDH and SDH in muscle were consistent with those in the liver. Among the above indexes, the change in CH is the most significant. The Chao1, ACE, Shannon, and Simpson index in CL, CM, and CH groups were increased. In the taxonomic composition, after the intervention with GFPS, the short-chain fatty acid (SCFA)-producing bacteria such as unclassified Muribaculaceae, Alloprevotella, and unclassified Lachnospiraceae increased. In linear discriminant analysis effect size (LEfSe) analysis, the characteristic bacteria in CC, CL, CM, and CH groups showed significant differences. In addition, some characteristic bacteria significantly correlated with related energy metabolism indicators.
Conclusion: The preventive effect of G. frondosa on obesity is related to changing the structure of intestinal content microbiota and promoting the growth of SCFAs. While excessive intake of G. frondosa may not be conducive to the antioxidant capacity and energy metabolism.
G. frondosa [Grifola frondosa (Dicks.) Gray], also known as maitake, belongs to Basidiomycota, Hymenomycetes, Polyporales, Meruliaceae, and Ramalina. G. frondosa is a rare edible and medicinal fungus with effects of anti-obesity, anti-tumor, and regulating immune function, at the same time, it is rich in various bioactive components including polysaccharides, steroids and polyphenols (1). It has a long history of medicinal use in Oriental medicine in China, Japan, and India (2). In addition, G. frondosa has a delicious taste and is a good source of dietary fiber, protein, and carbohydrates (3).
The intestinal microbiota is a dynamic flora composed of 100 trillion microorganisms that inhabit the host’s intestinal tract (4). They play an irreplaceable role in various physiological activities, such as maintaining immune function, resisting colonization by pathogenic microorganisms, and assisting in nutrient absorption (5, 6). By secreting rich differential enzymes, some pharmaceutical components that do not have pharmacological activity can also be converted by the intestinal microbiota to form new active metabolites, which in turn have different biological effects on the body (7–9). More and more reports also indicated that intestinal microbiota might play a good intermediary role in the beneficial mechanism of G. frondosa (10). For example, Li et al. (11) reported that G. frondosa heteropolysaccharide could prevent non-alcoholic fatty liver disease by increasing the number of beneficial bacteria Allobaculum, Bacteroides, and Bifidobacterium. Chen et al. (12) reported that a new polysaccharide (GFP-N) extracted from G. frondosa could improve the intestinal microbiota of diabetic mice by increasing the abundance of Akkermansia, Lactobacillus, and Turicibacter. In addition, plant dietary fiber can be utilized and decomposed by the intestinal microbiota, partially absorbed by the microbiota itself, and partially converted into beneficial substances such as SCFAs (13). It has been reported that SCFAs can alleviate obesity, regulate intestinal pH, promote intestinal mucus production, and provide energy for epithelial cells (14–16). Among them, acetate, propionate, and butyrate are the intestines’ major SCFAs (14). Pan et al. (17) reported that the ethanol extract of G. frondosa can reduce the weight of rats fed with high-fat diet and increase the number of beneficial bacteria Intestinimonas and Butyricimonas, which are important producers of butyrate.
In daily life, people usually eat G. frondosa after simple decocting, or grind the G. frondosa into powder and use it as a flavoring agent (3). At the same time, the components of glycoprotein, ergosterol and pyrrolefronine in G. frondosa also have pharmacological effects of anti-obesity, anti-tumor or anti-diabetes (18–20). However, at present, most studies are on the polysaccharide components and their functions in G. frondosa, and there is little research on the effect of direct intervention of G. frondosa on intestinal microbiota. SOD and MDA are usually one of the important indicators to measure the body’s ability to remove oxygen free radicals and the level of oxidative damage (21). Some reports have shown that G. frondosa polysaccharides and polyphenols have the effect of anti-oxidative stress (22–24). LDH is a regulatory enzyme produced by glycolysis of sugars in the body in the absence of oxygen, which converts pyruvic acid into lactic acid, with reversibility (25, 26). SDH is a marker enzyme reflecting mitochondrial function, which can provide electrons for cell mitochondria and the aerobic and productive respiratory chain (27). In his master’s degree thesis, Li BG (28) reported the good potential role of G. frondosa fermentation broth in anti-fatigue and promoting energy cycle. Therefore, this study intervened the mice with different doses of GFPS to explore its effects on intestinal content microbiota, body weight, energy metabolism or oxidative stress-related indicators of liver and muscle in mice. Aiming to provide new ideas for the development of G. frondosa weight loss products and suggestions for people’s daily consumption.
In order to eliminate the gender influence (29), this study selected 20 SPF-grade male Kunming mice (20 ± 2 g), purchased from Hunan Slaccas Jingda Laboratory Animal Company (Hunan, China). The animals were raised at a temperature 23-25°C and humidity of 47-53% in the Experimental Animal Center of the Hunan University of Chinese Medicine.
G. frondosa is produced in Qingyun County, Lishui City, Zhejiang Province, and is the first fruiting mushroom product of G. frondosa stick cultivation (30). Take a certain amount of G. frondosa, dry it in an oven at 105-110°C to constant weight, grind it into powder, and pass it through a 60-mesh sieve to obtain G. frondosa powder. A proper amount of G. frondosa powder was heated with distilled water, and boiled for 5 min. Then, concentrated into low, medium and high dose GFPS of 0.053 g/ml, 0.106 g/ml and 0.215 g/ml, respectively. The solutions were cooled and stored in a refrigerator at 4 °C for standby.
After 3 days of adaptive feeding, the mices were randomly divided into control group (CC), low-dose GFPS group (CL), medium-dose GFPS group (CM) and high-dose GFPS group (CH). The LD, MD and HD groups were given 0.4 mL low, medium and high dose GFPS by gavage, and CC Group was given the same frequency of sterile water by gavage, twice a day for 8 weeks. During this period, each mouse was weighed and recorded every week. The experimental procedures are shown in Figure 1. All animal experimental procedures were in the animal experimental protocol approved by the Institutional Animal Care and Use Committee of the Hunan University of Chinese Medicine.
At the end of the 8 weeks intervention, the mice were sacrificed on a sterile operating platform using cervical dislocation, and then the liver and muscles were taken out. According to the instructions of ELISA kits, the levels of SOD, MDA, LDH and SDH in the liver, and the levels of LDH and SDH in the muscle were detected using Rayto RT-6100 enzyme labeling analyzer. The kits were provided by Quanzhou kenuodi Biotechnology Co., LTD.
Under sterile conditions, the intestinal tissues from jejunum to ileum were longitudinally cut, and the intestinal contents were collected with forceps and stored at -80 °C for subsequent use (31).
The total microbial genomic DNA of the samples was extracted using a DNA extraction kit (MN NucleoSpin 96 So) through the steps of sample lysis, impurity removal by precipitation, inhibitor removal by filtration, DNA binding, membrane washing, drying, elution, etc. Using the extracted DNA as a template, the V3+V4 variable region of bacterial 16S rDNA was amplified with primers 338F (5’- ACTCCTACGGGAGGCAGCA-3’) and 806R (5’- GGACTACHVGGGTWTCTAAT-3’). The amplification reaction system consisted of 50 ng genomic DNA, 0.3 μL Vn F, 0.3 μL Vn R, 5 μL KOD FX Neo Buffer, 2 μL dNTP (2 mM each), and 0.2 μL KOD FX Neo, which were finally supplemented to 10 μl with ddH2O. Amplification conditions: denaturation at 95 °C for 5 min, rapid cooling to 50 °C, heating to 72 °C for 30 s, reacting for 40 s, then reacting at 72 °C for 7 min, and storing at 4 °C for 25 cycles. The amplified PCR products were purified, quantified, and homogenized. After the samples were mixed, they were subjected to column purification using OMEGA DNA purification column, and detected by 1.8% agarose gel electrophoresis. Use Monarch DNA glue recovery kit to cut glue and recover PCR products. The PCR products were sequenced by the Illumina Novaseq 6000 sequencing platform. All samples were processed by Beijing Biomarker Technologies Co, LTD.
The obtained data were filtered by Trimmom (V0.33) (32), spliced by Usearch (v10.0) (33), and chimerism was removed by dada2 method (34) in QIIME2 (v2020.6) (35). Then effective sequences with similarity above 97% are clustered into an operational taxonomic unit (OTU), and the representative sequences of OTU are defined by classification. This study assessed the Alpha diversity of sample communities using ACE, Chao1, Simpson, and Shannon indices. The Beta diversity of sample communities was assessed using non-metric multidimensional scaling (NMDS) based on the unweighted unifrac distance. At the same time, the marked difference species in each group were screened by LEfSe, and the above visualization was completed with R v3.6.3.
The correlation between the two variables can be expressed by the correlation coefficient. The closer the correlation coefficient is to 1, the greater the correlation between two elements, and the closer the correlation coefficient is to 0, indicating that the two elements are more independent. R version 3.6.3 is used to calculate Spearman rank correlation coefficient and draw heat map, network map, and scatter map.
Experimental data were expressed as mean ± standard deviation. All the data were statistically analyzed by SPSS 21.0 statistical software. For comparison among multiple groups, the one-way analysis of variance was used to analyze the data that conformed to the normal distribution and homogeneity of variance, otherwise, the Kruskal-Wallis rank sum test was used. Pairwise comparison among multiple groups was performed using the LSD test. p < 0.05 indicated that the difference had statistical significance.
As shown in Figure 2, the body weight gain of mice after GFPS intervention was lower than that of CC group, and the CH and CM groups were significantly lower than CC group (pCH < 0.01, pCM < 0.05). Meanwhile, the trend of body weight gain in the CH group was the lowest among all GFPS intervention groups and significantly lower than that in the CL group (p < 0.05). This indicated that low, medium, and high doses of GFPS intervention had the effects of weight loss and lipid reduction, and the effect of high-dose of GFPS was the most significant.
Figure 2 Weight changes of mice. CC: control group, CL: low-dose GFPS group, CM: medium-dose GFPS group, CH: high-dose GFPS group.
LDH represented the anaerobic metabolism to some extent, while the up-regulation of SDH activity represented the acceleration of the tricarboxylic acid cycle and increase of Adenosine Triphosphate (25, 26). As shown in Figure 3A, LDH content in liver of different doses of GFPS intervention was significantly higher than that in CC group (p < 0.01). Meanwhile, LDH content in liver of CH group was significantly higher compared with the CL and CM groups. In terms of liver SDH content (Figure 3B), the SDH content after different doses of GFPS intervention was lower than that in the CC group, and the SDH content in the liver of the CM and CH groups was significantly lower than that in the CC group (p < 0.05). This indicated that the intervention of GFPS had an inhibitory effect on the energy metabolism level of liver cells in mice, and the intervention of high-dose of GFPS was the most effective. MDA is the final metabolite of lipid peroxidation, reflecting the body’s ability to be damaged by oxidation, while SOD indirectly reflects the body’s ability to remove oxygen free radicals (21). By comparing the levels of MDA and SOD activities, we could assess the effect of GFPS intervention on the antioxidant capacity of the liver in mice. As shown in Figures 3C, D, the MDA content in the CH group was significantly higher than that in other groups (pCC < 0.01, pCL < 0.01, pCM < 0.01), and SOD content was significantly lower than that in the CC group (p < 0.01). It indicated that a high-dose of GFPS intervention might not have a beneficial effect on the antioxidant capacity of the liver in mice.
Figure 3 LDH, SDH, SOD, and MDA content in liver. (A) LDH content. (B) SDH content. (C) MDA content. (D) SOD content. CC: control group, CL: low-dose GFPS group, CM: medium-dose GFPS group, CH: high-dose GFPS group. (*p < 0.05, **p < 0.01).
Figure 4 shows that SDH content in the muscle of mice decreases with the increase of GFPS dose. At the same time, the LDH content in different mouse groups ranked CC < CL < CM < CH. Among them, the CC group had significant differences with CM and CH groups (pCL < 0.05, pCH < 0.01), and CH group had significant differences with CL and CM groups (pCL < 0.01, pCM < 0.05). This is consistent with the changing trend of LDH and SDH in mouse liver after different doses of GFPS. It indicated that the intervention of GFPS inhibited the energy metabolism of muscle cells in mice, and the inhibition was enhanced with the increase of dose.
Figure 4 muscle SDH and LDH content. (A) SDH content. (B) LDH content. CC: control group, CL: low-dose GFPS group, CM: medium-dose GFPS group, CH: high-dose GFPS group. (*p < 0.05, **p < 0.01).
As shown in Figure 5A, the numbers of OTUs obtained in CC, CL, CM, and CH groups were 821, 913, 862, and 898, respectively. The unique OTU numbers of CC, CL, CM and CH groups are 58, 61, 54 and 57 respectively. The dilution curve was used to assess whether sequencing was sufficient to cover all taxa and indirectly reflect the abundance of species in the sample. When the curve flattens out, it can be considered that the sequencing depth has covered almost all the species in the sample (36). As can be seen from Figure 5B, the dilution curve sequences of the four groups of samples tended to be gentle when the number was 10000. It shows that the amount of sequencing data is enough for the next analysis.
Figure 5 OTUs number and dilution curve of intestinal microbiota of mice. (A) OTUs number. (B) dilution curve. CC: control group, CL: low-dose GFPS group, CM: medium-dose GFPS group, CH: high-dose GFPS group.
In Alpha diversity analysis, Chao 1, ACE, Simpson, and Shannon are often used to evaluate richness and diversity (37). It can be seen from Table 1 that the ACE, Chao 1, Simpson, and Shannon indexes of GFPS treated mice are slightly higher than CC group mice. The ACE and Chao 1 indexes of the CL group are higher than the CC, CM and CH groups, but there is no statistical significance. This result was consistent with the quantitative result of OTU, suggesting that the intervention of GFPS played a role in promoting the diversity and richness of intestinal content microbiota.
In Beta diversity analysis, the nonlinear NMDS model can better reflect the nonlinear structure of ecological data (38). As shown in Figure 6, the distribution of samples in the CC and CH groups was relatively centralized, while that in CL and CM groups was relatively discrete. At the same time, the samples of the CC group are relatively separated from those of other groups, which indicated that the intervention of GFPS changed the community structure of the bacteria in the intestinal contents of mice.
Figure 6 The beta diversity of mice intestinal mucosal bacteria. Each point represents a sample, and samples of different groups are represented by different colors. The closer the distance between two points is, the higher the similarity is between two samples, and the smaller the difference is. CC: control group, CL: low-dose GFPS group, CM: medium-dose GFPS group, CH: high-dose GFPS group.
At the phylum level (Figure 7A), the intestinal microbiota in the CC group consisted mainly of Bacteroidota (53.94%), Firmicutes (44.11%), Actinobacteriota (0.48%), Desulfobacterota (0.55%), and other low abundance proportion taxa. The abundance of Firmicutes (44.11% vs 51.99%, 60.68%, 50.17%) and Actinobacteriota (0.48% vs 2.23%, 1.41%, 3.86%) was higher in the CL, CM, and CH groups compared with the CC group. The abundance of Firmicutes (44.11% vs 51.99%, 60.68%, 50.17%) and Actinobacteriota (0.48% vs 2.23%, 1.41%, 3.86%) was higher in the CL, CM, and CH groups compared with the CC group. Meanwhile, the abundance of Bacteroidota (53.94% vs 42.73%, 35.60%, 44.45%) is lower.
Figure 7 Relative abundance of bacteria in intestinal contents of mice after intervention with GFPS. (A) Level of phylum. (B) Level of genus. CC: control group, CL: low-dose GFPS group, CM: medium-dose GFPS group, CH: high-dose GFPS group.
At the genus level (Figure 7B), the dominant bacteria in CC, CL, CM, and CH groups were unclassified Muribaculaceae, accounting for 20.51%, 14.70%, 22.17%, and 22.27%, respectively. Alloprevotella was the second most common type, accounting for 10.84%, 11.00%, 10.92% and 6.68% respectively, while unclassified Lachnospiraceae accounted for 6.18%, 12.33%, 7.84% and 8.39% respectively.
In order to further identify the characteristic microbiota of GFPS intervention, LEfSe analysis was performed on the community composition at each taxonomic level in different treatment groups. Figure 8A shows the characteristic bacteria when the logarithmic LDA threshold is 2, and the characteristic bacteria in CC group include Frisingicoccus, Dorea, unclassified Butyricicoccaceae, Bacillus, Fusicatenibacter, Sellimonas. The characteristic bacterium in the CL group was Lachnospira. The characteristic bacteria in the CM group were unclassified UCG 010 and Caldicoprobacter. The characteristic bacterium in the CH group was Faecalibaculum. The above results could explain that different doses of GFPS could change the intestinal content microbiota. In different classification systems (Figure 8B), the characteristic bacteria among the four groups showed significant differences.
Figure 8 Characteristic bacteria of mice with GFPS intervention. (A) LDA score plots. (B) LEfSe analysis.
This study selected characteristic bacteria with logarithmic LDA threshold of 3 for correlation analysis between indicators. Figure 9A shows the correlation heat map between characteristic bacteria and liver indexes. LDH had a significant positive correlation with Faecalibaculum (p < 0.05) and a significant negative correlation with unclassified Butyricicoccaceae (p < 0.05). SDH was significantly positively correlated with Frisingicoccus, unclassified Butyricicoccaceae, Fusicatenibacter, Sellimonas, Dorea (p < 0.05). MDA was significantly negatively correlated with Sellimonas (p < 0.05). SOD had a significant positive correlation with unclassified Butyricicoccaceae (p < 0.05), and a significant negative correlation with Faecalibaculum and unclassified UCG 010 (p < 0.05). It could be seen from Figures 9B–F that LDH in muscle had a significant positive correlation with Faecalibaculum and Caldicoprobacter (p < 0.05). Muscle SDH significantly negatively correlated with Caldicoprobacter and Faecalibaculum (p < 0.05).
Figure 9 Correlation between characteristic bacteria of intestinal contents and indexes in mice. (A) Correlation heat map between characteristic bacteria and liver indexes. (B) Association network diagram of pathogenic bacteria and muscle indexes. (C) Scatter diagram of muscle LDH and Caldicoprobacter. (D) Scatter diagram of muscle LDH and Faecalibaculum. (E) Scatter diagram of muscle SDH and Caldicoprobacter. (F) Scatter diagram of muscle SHD and Faecalibaculum. CC: control group, CL: low-dose GFPS group, CM: medium-dose GFPS group, CH: high-dose GFPS group.
With the improvement of living standards and the strengthening of the concept of a healthy diet, people’s demand for healthy and functional foods is growing. Considering the side effects of some synthetic chemicals, multi-target and multi-channel natural plant products have broad application prospects in the development of functional foods and auxiliary drugs (39). Here, this study discussed the effects of GFPS intervention on oxidative stress, energy metabolism indicators, and intestinal microbiota in mice.
In the results of the liver oxidative stress index, the SOD contents decreased and MDA contents increased after the intervention with GFPS. Among them, the intervention of high-dose GFPS showed the most obvious change. This indicates that GFPS can inhibit the antioxidation of mouse liver. Notably, this differs from previous studies on the antioxidant effect of G. frondosa. For example, Men et al. (22) intervened the mice with acute liver injury with the polysaccharide extracted from the fruiting body of G. frondosa. They found that these G. frondosa polysaccharides not only had a protective effect on liver injury, but also decreased MDA content and increased SOD content in the liver. Another study (23) also found that G. frondosa polysaccharide intervention could significantly increase the liver antioxidant level of rats with hepatic fibrosis. We speculate that multiple complex components in G. frondosa may be the reason for this difference. It has been reported that Faecalibaculum has a negative correlation with SOD content, and can be used as a marker bacterium for intestinal oxidative stress (40). Oxidative stress improves cell permeability and causes LDH efflux from cells, thus leading to increased LDH activity (41). In experimental results, this was confirmed by increased LDH content in muscle and liver, which is also consistent with the significant positive correlation between Faecalibaculum and LDH in correlation analysis. and the contents of these enzymes in liver and muscle tissues have the LDH and SDH are representative enzymes of anaerobic respiration and aerobic respiration, respectively (42), same trend. This means that GFPS intervention reduced the energy metabolism of muscle and liver in mice to varying degrees, promoted anaerobic respiration, and had a certain dose dependence.
The diversity of intestinal microbiota is generally positively correlated with the quality of diet (43). The Alpha index and OTU results in this experiment showed that GFPS intervention promoted the diversity and richness of the intestinal content microbiota in mice. Meanwhile, the Beta analysis also showed that GFPS intervention changed the structure of intestinal microbiota. By further comparing the changes in the bacterial abundance of the intestinal contents among the four experimental groups, we could understand how GFPS affects the intestinal microbial environment. As the two largest taxonomic phylum in the intestinal microbiota, the increase in the ratio of Firmicutes to Bacteroidetes (F/B) is generally considered to be related to obesity (44). Two recent reports have shown that either G. frondosa polysaccharide or direct feeding G. frondosa can regulate lipid metabolism, reduce body weight, and is related to the TLR4/NF-κB signaling pathway (45, 46). In this research, the weight growth trend of mice after low, middle, and high doses of GFPS intervention decreased, which also proved this point. But interestingly, compared with the CC group, the F/B value of mouse microbiota in CL, CM, and CH groups increased.
From the perspective of taxonomic composition, the weight loss and intestinal health improvement effects of G. frondosa may be related to the promotion of the growth of SCFAs-producing bacteria, which can convert dietary fibers not absorbed by the body into metabolites SCFAs (47). SCFAs have been proved to stimulate energy consumption by promoting lipid oxidation, and the increase in SCFAs production can stimulate a large number of hormones and neural signals in different organs and tissue sites, thereby cumulatively inhibiting short-term appetite and energy intake (48, 49). In addition, SCFAs can also prevent diet-induced obesity by inhibiting the activity of Histone Deacetylase 3 in intestinal epithelial cells (15). Studies have shown that unclassified Muribacullaceae (50), unclassified lachnospiraceae (51), and Alloprevotella (52) are all intestinal SCFAs-producing bacteria. At the same time, compared to the CC group, the abundance of unclassified Muribaculaceae was increased in the CM and CH groups, and that of Alloprevotella was increased in the CL and CM groups. The abundance of unclassified Lachnospiraceae was increased after GFPS intervention. On the contrary, after high-dose intervention with GFPS, the abundance of Alloprevotella was decreased, but the characteristic bacterium Faecalibaculum in the CH group was also the producer of SCFAs (53). Studies have shown that Faecalibaculum has the effect of inhibiting the development of metabolic diseases (54). Combined with the results of oxidative stress, the MDA content of the CH group was significantly increased (p < 0.01), while the increased MDA content might damage the intestinal barrier (55), reduce the absorption of nutrients, and thus reduce body weight. This was also demonstrated by the lowest body weight in the CH group among the four groups (Figure 2). Furthermore, Lachnospira was a characteristic bacterium in the CL group, while Lachnospira could produce lactic acid and acetate, and lactic acid could become a metabolic substrate for bacteria to produce butyrate or propionate (56). The characteristic bacteria in LM group are Caldicoprobacter and unclassified UCG 010. Caldicoprobacter is a kind of bacteria that can degrade complex carbohydrates and ferment hemicellulose into lactic acid, ethanol, and hydrogen. Meanwhile, these products can be transformed into butyrate for colon cells to supply energy (57, 58).
In addition, there were some limitations in this study, such as the small sample size and the emphasis on the integrity and naturalness of G. frondosa in the experiment, and no specific discussion on the role of a component in G. frondosa. The intestinal microbiota is closely related to our health. In the future, metagenomic functional gene analysis will be used to further explore the relationship between G. frondosa and intestinal microbiota.
In summary, G. frondosa can promote health and prevent obesity by changing the structure of intestinal content microbiota, promoting microbiota diversity and richness, and increasing the beneficial bacteria producing SCFAs. However, excessive intake of G. frondosa may promote oxidative stress response in mice and inhibit energy metabolism in muscle and liver tissue. Therefore, low-dose (1.425 g/kg·d) of G. frondosa may be a good choice for further experiment or product development.
The datasets presented in this study can be found in online repositories. The names of the repository/repositories and accession number(s) can be found below: https://www.ncbi.nlm.nih.gov/, PRJNA903652.
The animal study was reviewed and approved by the Institutional Animal Care and Use Committee of the Hunan University of Chinese Medicine.
Experimental design, animal operation, data analysis, and paper writing were all performed by RH. The author confirms being the sole contributor of this work and has approved it for publication.
This research was financially supported by the Natural Science Foundation of Hunan Province (No. 2019JJ40166).
I thank the Natural Science Foundation of Hunan Province (No. 2019JJ40166) for the financial support of this study.
The author declares that the research was conducted in the absence of any commercial or financial relationships that could be construed as a potential conflict of interest.
All claims expressed in this article are solely those of the authors and do not necessarily represent those of their affiliated organizations, or those of the publisher, the editors and the reviewers. Any product that may be evaluated in this article, or claim that may be made by its manufacturer, is not guaranteed or endorsed by the publisher.
1. Xiong WY, He JQ, Dai WZ, Wu YY, Liu B. Research progress on the functional components of grifola frondosa and their biological activities. Food Machinery (2022) 38:234–40. doi: 10.13652/j.spjx.1003.5788.2022.90086
2. Alonso EN, Ferronato MJ, Fermento ME, Gandini NA, Romero AL, Guevara JA, et al. Antitumoral and antimetastatic activity of maitake d-fraction in triple-negative breast cancer cells. Oncotarget (2018) 9:23396–412. doi: 10.18632/oncotarget.25174
3. He YL, Li XL, Hao C, Zeng PJ, Zhang M, Liu Y, et al. Grifola frondosa polysaccharide: A review of antitumor and other biological activity studies in China. Discovery Med (2018) 25:159–76.
4. Lepage P, Leclerc MC, Joossens M, Mondot S, Blottière HM, Raes J, et al. A metagenomic insight into our gut's microbiome. Gut (2013) 62:146–58. doi: 10.1136/gutjnl-01805
5. Vicentini FA, Keenan CM, Wallace LE, Woods C, Cavin JB, Flockton AR, et al. Intestinal microbiota shapes gut physiology and regulates enteric neurons and glia. Microbiome (2021) 9:210. doi: 10.1186/s40168-021-01165-z
6. Li XY, Peng XX, Guo KX, Tan ZJ. Bacterial diversity in intestinal mucosa of mice fed with dendrobium officinale and high-fat diet. 3 Biotech (2021) 11:22. doi: 10.1007/s13205-020-02558-x
7. Park EK, Shin J, Bae EA, Lee YC, Kim DH. Intestinal bacteria activate estrogenic effect of main constituents puerarin and daidzin of pueraria thunbergiana. Biol Pharm Bull (2006) 29:2432–5. doi: 10.1248/bpb.29.2432
8. Xie Y, Hu FD, Xiang DW, Lu H, Li WB, Zhao AP, et al. The metabolic effect of gut microbiota on drugs Drug Metab Rev. (2020) 52:139–56. doi: 10.1080/03602532.2020.1718691
9. Zhou K, Deng N, Yi X, Cai Y, Peng MJ, Xiao NQ. Baohe pill decoction for diarrhea induced by high-fat and high-protein diet is associated with the structure of lactase-producing bacterial community. Front Cell Infect Microbiol (2022) 12:1004845. doi: 10.3389/fcimb.2022.1004845
10. Wu JY, Siu KC, Geng P. Bioactive ingredients and medicinal values of grifola frondosa (Maitake). Foods (2021) 10:95. doi: 10.3390/foods10010095
11. Li X, Zeng F, Huang YF, Liu B. The positive effects of grifola frondosa heteropolysaccharide on NAFLD and regulation of the gut microbiota. Int J Mol Sci (2019) 20:5302. doi: 10.3390/ijms20215302
12. Chen YQ, Liu D, Wang DY, Lai SS, Zhong RT, Liu YY, et al. Hypoglycemic activity and gut microbiota regulation of a novel polysaccharide from grifola frondosa in type 2 diabetic mice. Food Chem Toxicol (2019) 126:295–302. doi: 10.1016/j.fct.2019.02.034
13. Holscher HD. Dietary fiber and prebiotics and the gastrointestinal microbiota. Gut bes (2017) 8:172–84. doi: 10.1080/19490976.2017.1290756
14. Martin-Gallausiaux C, Marinelli L, Blottière HM, Larraufie P, Lapaque N. SCFA: mechanisms and functional importance in the gut. Proc Nutr Soc (2021) 80:37–49. doi: 10.1017/S0029665120006916
15. You HM, Tan Y, Yu DW, Qiu ST, Bai Y, He JC, et al. The therapeutic effect of SCFA-mediated regulation of the intestinal environment on obesity. Front Nutr (2022) 9:886902. doi: 10.3389/fnut.2022.886902
16. Barrea L, Muscogiuri G, Annunziata G, Laudisio D, Pugliese G, Salzano C, et al. From gut obiota dysfunction to obesity: could short-chain fatty acids stop this dangerous course? Hormones (Athens) (2019) 18:245–50. doi: 10.1007/s42000-019-00100-0
17. Pan YY, Zeng F, Guo WL, Li TT, Jia RB, Huang ZR, et al. Effect of grifola frondosa 95% ethanol extract on lipid metabolism and gut microbiota composition in high-fat diet-fed rats. Food Funct (2018) 9:6268–78. doi: 10.1039/c8fo01116h
18. Cui F, Zan X, Li YF, Liu B. Purification and partial characterization of a novel anti-tumor glycoprotein from cultured mycelia of grifola frondosa. Int J Biol Macromol (2013) 62:684–90. doi: 10.1016/j.ijbiomac.2013.10.025
19. Chen SD, Yong TQ, Xiao C, Su JY, Zhang YF, Jiao CW, et al. Pyrrole alkaloids and ergosterols from grifola frondosa exert anti-α-glucosidase and anti-proliferative activities. J Funct Foods (2018) 43:196–205. doi: 10.1016/j.jff.2018.02.007
20. Zhuang C, Kawagishi H, Preuss HG. Glycoprotein with antidiabetic, antihypertensive, antiobesity and antihyperlipidemic effects from grifola frondosa, and a method for preparing same. United States patent (2005).
21. Jiang X, Chu QB, Li LZ, Qin LY, Hao J, Kou L, et al. The anti-fatigue activities of tuber melanosporum in a mouse model. Exp Ther Med (2018) 15:3066–73. doi: 10.3892/etm.2018.5793
22. Meng M, Zhang R, Han R, Kong Y, Wang RH, Hou LH. The polysaccharides from the grifola frondosa fruiting body prevent lipopolysaccharide/D-galactosamine-induced acute liver injury via the miR-122-Nrf2/ARE pathways. Food Funct (2021) 12:1973–82. doi: 10.1039/d0fo03327h
23. Li C, Meng M, Guo MZ, Wang MY, Ju AN, Wang CL. The polysaccharides from grifola frondosa attenuate CCl4-induced hepatic fibrosis in rats via the TGF-β/Smad signaling pathway. RSC Adv (2019) 9:33684–92. doi: 10.1039/c9ra04679h
24. Yeh JY, Hsieh LH, Wu KT, Tsai CF. Antioxidant properties and antioxidant compounds of various extracts from the edible basidiomycete grifola frondosa (Maitake). Molecules (2011) 16:3197–211. doi: 10.3390/molecules16043197
25. Valvona CJ, Fillmore HL, Nunn PB, Pilkington GJ. The regulation and function of lactate dehydrogenase a: Therapeutic potential in brain tumor. Brain Pathol (2016) 26:3–17. doi: 10.1111/bpa.12299
26. Wang J, Xue Z, Hua C, Lin J, Shen Z, Song Y, et al. Metabolomic analysis of the ameliorative effect of enhanced proline metabolism on hypoxia-induced injury in cardiomyocytes. Oxid Med Cell Longev (2020) 2020:8866946. doi: 10.1155/2020/8866946
27. Xu X, Dou D. The ginseng's fireness is associated with the lowering activity of liver na(+)-K(+)-ATPase. J Ethnopharmacol (2016) 190:241–50. doi: 10.1016/j.jep.2016.06.024
28. Li BG. Study on liquid fermentation culture and anti-fatigue activity of grifola frondosa. [Tianjin]: Tianjin University of Science & Technology (2018).
29. Wu Y, Peng XX, Li XY, Li DD, Tan ZJ, Yu R. Sex hormones influence the intestinal microbiota composition in mice. Front Microbiol (2022) 13:964847. doi: 10.3389/fmicb.2022.964847
30. Zhou ZQ, Ye CW, Mao KR, Lian CX. Stick cultivation of grifola frondosa and secondary fruiting technology with soil cover. Edible Fungi (2007) 3:43–7. doi: 10.3969/j.issn.1000-8357.2007.03.028
31. Shao HQ, Zhang CY, Xiao NQ, Tan ZJ. Gut microbiota characteristics in mice with antibiotic-associated diarrhea. BMC Microbiol (2020) 20:313. doi: 10.1186/s12866-020-01999-x
32. Bolger AM, Lohse M, Usadel B. Trimmomatic: a flexible trimmer for illumina sequence data. Bioinformatics (2014) 30:2114–20. doi: 10.1093/bioinformatics/btu170
33. Edgar RC. UPARSE: Highly accurate OTU sequences from microbial amplicon reads. Nat Methods (2013) 10:996–8. doi: 10.1038/nmeth.2604
34. Callahan BJ, McMurdie PJ, Rosen MJ, Han AW, Johnson AJ, Holmes SP. DADA2: High-resolution sample inference from illumina amplicon data. Nat Methods (2016) 13:581–3. doi: 10.1038/nmeth.3869
35. Bolyen E, Rideout JR, Dillon MR, Bokulich NA, Abnet CC, Al-Ghalith GA, et al. Reproducible, interactive, scalable and extensible microbiome data science using QIIME 2. Nat Biotechnol (2019) 37:852–7. doi: 10.1038/s41587-019-0209-9
36. Li XY, Deng N, Zheng T, Qiao B, Peng MJ, Xiao NQ, et al. Importance of dendrobium officinale in improving the adverse effects of high-fat diet on mice associated with intestinal contents microbiota. Front Nutr (2022) 9:957334. doi: 10.3389/fnut.2022.957334
37. Zhang CY, Shao HQ, Peng XX, Liu TH, Tan ZJ. Microbiotal characteristics colonized in intestinal mucosa of mice with diarrhoea and repeated stress. 3 Biotech (2020) 10:372. doi: 10.1007/s13205-020-02368-1
38. Yuan XL, Cao M, Liu XM, Du YM, Shen GM, Zhang ZF, et al. Composition and genetic diversity of the nicotiana tabacum microbiome in different topographic areas and growth periods. Int J Mol Sci (2018) 19:3421. doi: 10.3390/ijms19113421
39. Guo KX, Xu SS, Zhang QL, Peng MJ, Yang ZY, Li WG, et al. Bacterial diversity in the intestinal mucosa of mice fed with asparagus extract under high-fat diet condition. 3 Biotech (2020) 10:228. doi: 10.1007/s13205-020-02225-1
40. Ma H, Zhang B, Hu Y, Wang J, Liu J, Qin R, et al. Correlation analysis of intestinal redox state with the gut microbiota reveals the positive intervention of tea polyphenols on hyperlipidemia in high fat diet fed mice. J Agric Food Chem (2019) 67:7325–35. doi: 10.1021/acs.jafc.9b02211
41. Jovanovic P, Zoric L, Stefanovic I, Dzunic B, Djordjevic-Jocic J, Radenkovic M, et al. Lactate dehydrogenase and oxidative stress activity in primary open-angle glaucoma aqueous humour. Bosn J Basic Med Sci (2010) 10:83–8. doi: 10.17305/bjbms.2010.2743
42. Bao J, Li X, Xing Y, Feng C, Jiang H. Respiratory metabolism and antioxidant response in Chinese mitten crab eriocheir sinensis during air exposure and subsequent reimmersion. Front Physiol (2019) 10:907. doi: 10.3389/fphys.2019.00907
43. Laitinen K, Mokkala K. Overall dietary quality relates to gut microbiota diversity and abundance. Int J Mol Sci (2019) 20:1835. doi: 10.3390/ijms20081835
44. Stojanov S, Berlec A, Štrukelj B. The influence of probiotics on the Firmicutes/Bacteroidetes ratio in the treatment of obesity and inflammatory bowel disease. Microorganisms (2020) 8:1715. doi: 10.3390/microorganisms8111715
45. Jiang X, Hao J, Zhu Y, Liu Z, Li L, Zhou Y, et al. The anti-obesity effects of a water-soluble glucan from grifola frondosa via the modulation of chronic inflammation. Front Immunol (2022) 13:962341. doi: 10.3389/fimmu.2022.962341
46. Jiang X, Hao J, Liu Z, Ma X, Feng Y, Teng L, et al. Anti-obesity effects of grifola frondosa through the modulation of lipid metabolism via ceramide in mice fed a high-fat diet. Food Funct (2021) 12:6725–39. doi: 10.1039/d1fo00666e
47. Chambers ES, Preston T, Frost G, Morrison DJ. Role of gut microbiota-generated short-chain fatty acids in metabolic and cardiovascular health. Curr Nutr Rep (2018) 7:198–206. doi: 10.1007/s13668-018-0248-8
48. Blaak EE, Canfora EE, Theis S, Frost G, Groen AK, Mithieux G, et al. Short chain fatty acids in human gut and metabolic health. Benef Microbes (2020) 11:411–55. doi: 10.3920/BM2020.0057
49. Chambers ES, Morrison DJ, Frost G. Control of appetite and energy intake by SCFA: what are the potential underlying mechanisms? Proc Nutr Soc (2015) 74:328–36. doi: 10.1017/S0029665114001657
50. Al-Bulish MSM, Cao WX, Yang RL, Wang YM, Xue CH, Tang QJ. Docosahexaenoic acid-rich fish oil alleviates hepatic steatosis in association with regulation of gut microbiome in ob/ob mice. Food Res Int (2022) 157:111373. doi: 10.1016/j.foodres.2022.111373
51. Yu GH, Ji XG, Huang JH, Liao AM, Pan L, Hou YC, et al. Immunity improvement and gut microbiota remodeling of mice by wheat germ globulin. World J Microbiol Biotechnol (2021) 37:64. doi: 10.1007/s11274-021-03034-1
52. Chen H, Zhang F, Zhang J, Zhang X, Guo Y, Yao Q. A holistic view of berberine inhibiting intestinal carcinogenesis in conventional mice based on microbiome-metabolomics analysis. Front Immunol (2020) 11:588079. doi: 10.3389/fimmu.2020.588079
53. Ye X, Liu Y, Hu J, Gao Y, Ma Y, Wen D. Chlorogenic acid-induced gut microbiota improves metabolic endotoxemia. Front Endocrinol (Lausanne) (2021) 12:762691. doi: 10.3389/fendo.2021.762691
54. Wang BT, Kong QM, Li X, Zhao JX, Zhang H, Chen W, et al. A high-fat diet increases gut microbiota biodiversity and energy expenditure due to nutrient difference. Nutrients (2020) 12:3197. doi: 10.3390/nu12103197
55. Zhang XY, Li S, Zhou YF, Su W, Ruan XZ, Wang B, et al. Ablation of cytochrome P450 omega-hydroxylase 4A14 gene attenuates hepatic steatosis and fibrosis. Proc Natl Acad Sci U.S.A. (2017) 114:3181–5. doi: 10.1073/pnas.1700172114
56. Jalanka J, Major G, Murray K, Singh G, Nowak A, Kurtz C, et al. The effect of psyllium husk on intestinal microbiota in constipated patients and healthy controls. Int J Mol Sci (2019) 20:433. doi: 10.3390/ijms20020433
57. Che J, Bai Y, Li X, Ye J, Liao H, Cui P, et al. Linking microbial community structure with molecular composition of dissolved organic matter during an industrial-scale composting. J Hazard Mater (2021) 405:124281. doi: 10.1016/j.jhazmat.2020.124281
Keywords: Grifola frondosa, intestinal contents, microbial diversity, short-chain fatty acid, anti-obesity
Citation: Hu R (2023) Grifola frondosa may play an anti-obesity role by affecting intestinal microbiota to increase the production of short-chain fatty acids. Front. Endocrinol. 13:1105073. doi: 10.3389/fendo.2022.1105073
Received: 22 November 2022; Accepted: 29 December 2022;
Published: 17 January 2023.
Edited by:
Xinhua Shu, Glasgow Caledonian University, United KingdomReviewed by:
Yueying Wu, Yunnan University, ChinaCopyright © 2023 Hu. This is an open-access article distributed under the terms of the Creative Commons Attribution License (CC BY). The use, distribution or reproduction in other forums is permitted, provided the original author(s) and the copyright owner(s) are credited and that the original publication in this journal is cited, in accordance with accepted academic practice. No use, distribution or reproduction is permitted which does not comply with these terms.
*Correspondence: Ruxiao Hu, MTczNDYzNzc4QHFxLmNvbQ==
Disclaimer: All claims expressed in this article are solely those of the authors and do not necessarily represent those of their affiliated organizations, or those of the publisher, the editors and the reviewers. Any product that may be evaluated in this article or claim that may be made by its manufacturer is not guaranteed or endorsed by the publisher.
Research integrity at Frontiers
Learn more about the work of our research integrity team to safeguard the quality of each article we publish.