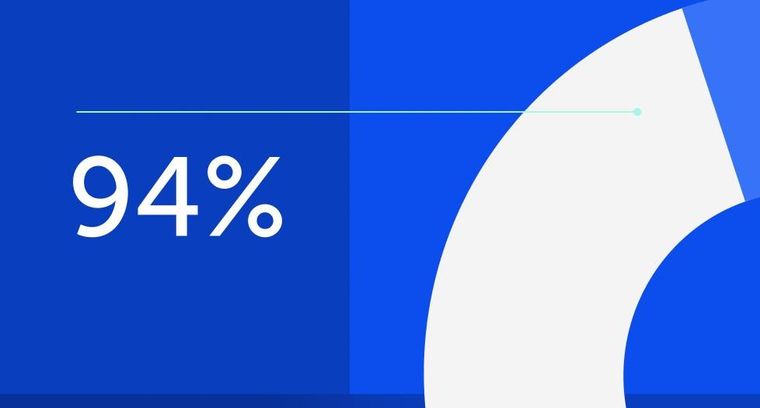
94% of researchers rate our articles as excellent or good
Learn more about the work of our research integrity team to safeguard the quality of each article we publish.
Find out more
REVIEW article
Front. Endocrinol., 04 October 2022
Sec. Experimental Endocrinology
Volume 13 - 2022 | https://doi.org/10.3389/fendo.2022.1023842
This article is part of the Research TopicWomen in Experimental Endocrinology 2022View all 8 articles
Feeding and growth are two closely related and important physiological processes in living organisms. Studies in mammals have provided us with a series of characterizations of neuropeptides and their receptors as well as their roles in appetite control and growth. The central nervous system, especially the hypothalamus, plays an important role in the regulation of appetite. Based on their role in the regulation of feeding, neuropeptides can be classified as orexigenic peptide and anorexigenic peptide. To date, the regulation mechanism of neuropeptide on feeding and growth has been explored mainly from mammalian models, however, as a lower and diverse vertebrate, little is known in fish regarding the knowledge of regulatory roles of neuropeptides and their receptors. In recent years, the development of omics and gene editing technology has accelerated the speed and depth of research on neuropeptides and their receptors. These powerful techniques and tools allow a more precise and comprehensive perspective to explore the functional mechanisms of neuropeptides. This paper reviews the recent advance of omics and gene editing technologies in neuropeptides and receptors and their progresses in the regulation of feeding and growth of fish. The purpose of this review is to contribute to a comparative understanding of the functional mechanisms of neuropeptides in non-mammalians, especially fish.
In recent ten years, the rapid development of omics technology has introduced us to the era of big data. The omics technology consists of four major groups, namely genomics, transcriptomics, proteomics and metabolomics (1). Genomics studies the genetic structure composition of biological systems (2); transcriptomics is about gene transcription in cells and the regulation of transcription at the overall level (3); proteomics investigates the proteins expressed by biological systems and their differences caused by external stimuli (4); and metabolomics examines the changes of metabolites produced by organisms under different conditions (5). Just as in the functional study of other target genes, the application of omics technology in the functional study of neuropeptides and their receptors also provides a large amount of data, opening up new directions for research in this field.
The establishment of gene editing technology is a landmark event in the life sciences. The success of specifically targeting DNA at any given location within the genome has enabled many interesting possibilities that have inspired scientists for decades. A variety of methods have been used in genetic engineering to modify DNA sequences in the genome. Gene targeting and gene trapping are two techniques for constructing knockout animals from embryonic stem cells (6). Gene targeting replaces the endogenous gene by homologous recombination to knock out the target gene, while gene trapping has two methods, promoter trapping and polyA trapping (7, 8). With the continuous development of life science, researchers have successively developed zinc finger nucleases (ZFNs) (9), transcription activator-like effector nucleases (TALENs) (10), and clustered regularly spaced short palindromes Repeat-CRISPR-associated 9 (CRISPR/Cas9) (11), which have provided a broad range of efficient tools for life and medical research.
Omics and gene editing approaches have been employed in the study of the mechanisms by which neuropeptides and their receptors regulate feeding and growth. For vertebrates, the ability to regulate food intake based on the energy storage status of the body is essential for growth and even survival. The physiological mechanisms controlling feeding and growth are relatively conserved in vertebrates, and many of the neuropeptides that centrally regulate feeding and growth in mammals have been identified successively in fish (12, 13). Fish are an extremely diverse group of species with great differences in their ecological niches, habitats, life histories and feeding behaviors at different life stages (14). In fish, many neuropeptides involved in feeding regulation have been isolated or their protein sequences have been deduced by cloning their cDNA sequences. Neuropeptide Y (NPY), isolated firstly from porcine brain, is a strong orexigenic neuropeptide in the hypothalamus (15), and other feeding-promoting neuropeptides such as orexin and ghrelin have also been identified in fish (16, 17). Somatostatin (SS) has been firstly known to inhibit the secretion of pituitary growth hormone (GH) and is later found to have feeding inhibitory effects as well (18). Other feeding-inhibiting neuropeptides, proopiomelanocortin (POMC) (19), corticotropin-releasing factor (CRF) (20), cholecystokinin (CCK) (21), and etc., have also been identified in fish. These neuropeptides act as feeding regulators by binding to the corresponding receptors and activating downstream signaling pathways, and the interactions between these neuropeptides have also been shown to regulate feeding in fish (22, 23).
Our objectives are firstly, to review the application and development of omics and gene editing technologies in the field of functional studies of neuropeptides and their receptors; secondly, to summarize the research progress of neuropeptides involved in fish feeding and growth regulation, focusing on their different types, distribution patterns and functional effects mediated by different receptors.
Neuropeptide research has been greatly promoted in recent years, due to the rapid emergence of omics technologies. Neuropeptides are mainly dependent on the corresponding receptors for executing their function. Therefore, the next section will explore the recent advances in the omics of neuropeptide and its receptors (Table 1 for detail).
Since the 1990s, transcriptomics technology has entered a period of vigorous development and has undergone Generation 1, 2 and 3 sequencing technologies, which have been widely used in biological, medical and clinical research as well as drug development (83). This technology has also been widely used in the study of neuropeptides, especially playing an important role in exploring the functions and mechanisms. Neuropeptides have been shown to play an important role in the regulation of physiological activities (84). The introduction of transcriptomics has helped the mechanistic exploration of neuropeptides at the RNA level and the network of interactions. NPY, SS, and CCK have been found to influence feeding behavior, and in recent years new evidence has been found in mammalian (24), avian (85), and fish species (27, 29, 53, 65) using transcriptomics. Among them, NPY is involved in promoting feeding, and analysis of the hypothalamus transcriptome of mice subjected to a daily 2-h heat treatment (36°C) for 14 d suggested that up-regulated neuropeptide Y acted to attenuate reduced food intake (24). It has been reported that in broiler chickens, dietary stevioside (STE) supplementation promoted feed intake, and the analysis of RNA-Seq in the hypothalamus of the STE-supplemented group compared with the control group showed that several appetite-related genes, such as NPY and NPY5R, were differentially expressed, and it was suggested that dietary STE supplementation promoted feed intake through the regulation of the hypothalamic neuroactive ligand-receptor interaction pathway and the alteration of intestinal microbiota composition (85). On the contrary, SS is involved in suppressing feeding, especially important in studying the mechanism of feed additives (28) and environmental influences on feeding (30). In yellow catfish (Tachysurus fulvidraco), it has been reported that sertraline (SER) increased shoaling, decreased food consumption, higher cannibalism rate and RNA-seq results showed that transcript levels of SS reduced in the brain following SER exposure, indicating that SER disturbs neuropeptides which may be unrelated to its antidepressant effects in vertebrates (30). Reproductive activity is endocrinologically regulated by many peptide hormones, and a more detailed transcriptome of the process of reproduction has been made in mammals (79, 80). Orexin is involved in the regulation of the reproductive system through the hypothalamic-pituitary-ovarian axis (79), and the transcriptome profile of the endometrium during early pregnancy in pigs has revealed that orexin affected the expression of a large number of genes differentially (79) while transcriptome of myometrial explants during the early implantation period suggested that orexin influenced the process involved in quiescence, proliferartion, remodeling and angiogenesis in myometrial explants during the peri-implantation period in the pig (80). NPY has also been found to be involved in gonadal development in crustaceans (31) and mammals (32). CCK receptor (CCKAR and CCKBR) deletion leads to abnormal cortical development, and using comparative transcriptomic analysis, transcripts of CXCL12, BMP7 are downregulated and may be target genes for abnormal cortical development (86). Neuropeptides are also involved in mood management, stress regulation. Transcriptome analysis of somatostatin interneurons in nucleus accumbens after cocaine exposure identified JUND transcription factor as a key regulator of cocaine (57) and CRF2 is also involved in cocaine-induced neuroexcitation (71). Somatostatin interneurons are involved in emotion regulation, and transcriptome analysis of somatostatin interneurons in male and female mice under chronic stress conditions separately revealed that chronic stress leads to gene dysregulation in several key pathways, including sex-specific differences in somatostatin interneuron profiles (87). These changes occurred mainly in the reduced expression of elongation initiation factor 2 (EIF2) signaling, suggesting that dysfunction of the translational machinery of somatostatin interneurons may be critical for the development of depressive behavior in males (87). In addition, somatostatin receptor (SSTR2) is also involved in neuro-emotional regulation (60). And, SSTR4 has a modulatory role in Alzheimer’s disease (88). CCK is involved in nociceptive perception, as the CCK2 receptor (CCKbR) was found to be a pain target in burn-induced nociception and is involved in reducing the effectiveness of opioids (49).
A wealth of functional genomic technologies has emerged as the focus of research has shifted to the gene specific function and understanding the regulation of each identified gene product. The goal of these efforts is to better understand how the information stored in the genome encodes all of the complexity required to sustain complex multicellular organisms (89). Despite the impressive advances made by these technologies, the interpretation of their results is limited without corresponding data on proteins (90). Neuropeptides have also been studied using proteomics and showing that they play an important role in the nervous system. In Parkinson’s disease, a disease of aging associated with vesicular transport dysfunction and neurotransmitter secretion, proteomic sequencing of secretory granules revealed reduced levels of NPY (33). In studies related to depression, NPY and NPY2R protein expression was downregulated in hippocampal proteins of mice in a model of social failure versus control mice (34). SST has been reported to be associated with Alzheimer’s disease, and identified by LC-MS/MS analysis, somatostatin-related amyloidosis is a novel restricted human amyloid type associated with somatostatin -producing enteropancreatic neuroendocrine tumors (36). LC-MS analysis using SST peptide (SST-scFv8D3) injected into the hippocampus of mice revealed the elevated degradation of NPY (35). Using proteomic analysis in renal failure studies, NPY-NPY2R system was found to predict nephrotoxicity and pathogenic effects in the glomerulus (91). Based on the brain proteomic analysis in silver carp (Hypophthalmichthys molitrix), it was revealed that hypoxia affected significantly the biochemical indices and neurotransmitter contents in the brain, and SST1A was upregulated as well (67).
Metabolites represent both downstream outputs from the genome and upstream ones from the environment. Thus, the study of metabolomics allows scientists to explore the relationships of gene-environment interactions. In comparison with genetic and genetic risk scores that can be used to indicate what is likely to happen, metabolic analysis and metabolic phenotyping indicate what is currently happening and what has already happened. In this regard, metabolomics is able to take a look at not only endogenous metabolites (gene-derived metabolites) and exogenous metabolites (environmentally derived metabolites) in the form of disease biomarkers, but also provides unique insights into the underlying causes of disease (92, 93). Retinal ischemia mouse cells treated with neuropeptide PACAP and the somatostatin analog octreotide and tested for anti-ischemic ability, metabolome results show reduced intravascular epidermal growth factor overexpression and glutamate release (69). Using metabolomics analysis, when NPY receptor Y2R activated by NPY, glycolysis is observed to increase while intracellular nutrients are depleted, which may be due to high rates of conversion of glucose to lactate, glycine and alanine. When the Y2R activation is blocked by BIIE0246, the metabolic responses reversed (37). To date, relatively few studies have been conducted on neuropeptides in metabolomics, and more studies are yet to be explored.
Genetic engineering has led to a better understanding of the genetic functions of neuropeptides and related receptors. Gene knockout technology is an irreplaceable part of transgenic technology, and conditional gene knockout technology, as a new generation gene knockout technology, has obvious advantages and wider application compared with the first-generation gene knockout technology (94). Next, advances in the study of neuropeptides and their receptors function using gene editing will be presented (Table 2 for detail).
Gene targeting technology is usually used to knock out the target gene, and its essence is to replace the endogenous gene through homologous recombination (172). Using the powerful technology, researchers constructed gene knockout models to explore the function of neuropeptides or their corresponding receptor. It has been reported that transgenic mice deleting NPY were used to investigate albuminuric kidney disease (91). And Y1R knockout mice have been reported to explore the role of Y1R in the regulation of bone and energy homeostasis (97). Ablating orexin in mice have been reported in the fields of sleep regulation, social fear, cataplexy and lipid metabolism (112, 113, 115, 118). Orexin receptor-deficient mice have been used to support the unique role of orexin receptors 1 and 2 in long-term energy metabolism (173). It has been reported that mice lacking SST were used to study the effect of SST in depression (87). In addition, mice deficient in the SST receptor, SSTR2, or SSTR4, have been reported to be models for studying behavioral changes induced by chronic stress (154–156). CCK knockout mice were reported to study the role of CCK in the lipid transport, gallbladder contractile and small intestinal motility (168, 169). Mouse with CCKAR knockout or CCKBR knockout or double knockout were used to study the role of CCK receptors in taste, fear, feeding and bone homeostasis (170, 171). Also, corticotropin-releasing hormone knockout (CRH-KO) mice have been reported to be used in the study of inflammatory bowel disease and stress (174, 175). It has been reported that CRF receptor 1 knockout mice were used in the study of cognitive and anxiety-like states and social behavior (160, 166), while CRF receptor 2 knockout mice were used in the study of inflammation, recognition memory and glucose metabolism (163, 164, 176).
CRISPR/Cas9 is a powerful gene editing tool with the advantages of being efficient, easy, fast and cheap (173). The CRISPR/Cas9 system consists of two components, Cas9, a signature nucleic acid endonuclease, and a guide RNA (gRNA) molecule. Cas9 cleaves the target sequence under the guidance of gRNA, resulting in DNA double-strand breaks. The broken double strand is repaired by homologous recombination, non-homologous end joining and other mechanisms, and abnormal bases are inserted or made absent at the break, resulting in code-shifting mutations, and finally the target gene is knocked out (173). It has been reported that the CRISPR/Cas9 system has been used for the construction of knockout models of neuropeptides and their receptors. In mammals, orexin 2 receptor knockout mice generated by CRISPR/Cas9 system were applied to investigate its role in the regulation of sleep/wake states (117). In zebrafish, CRISPR/Cas9 system was used to knock out NPY to study its effect on emotional behaviors (95, 96). Also, SS receptor 1 and 4 were reported to be knocked out using CRISPR/Cas9 to study their roles in growth and reproduction, respectively (152, 153).
Conditional gene knockout, an upgraded version of gene knockout, is a very powerful technique for studying the function of neuropeptides and their receptors in the nervous system. Particularly, condition-specific knockout mice based on the Cre-loxP system have been favored by many studies because of their good experimental results. Cre (Cre recombinase) is a protein with a relative molecular mass of 38000 Dalton composed of 343 amino acids encoded and expressed by the cre gene in bacteriophage P1 (177). The loxP (locus of x-over, P1) is a 34 bp sequence found from bacteriophage P1, consisting of two 13 bp inverted repeat sequences and an 8 bp spacer sequence in the middle (178). Cre recombinase can specifically recognize loxP sequences on intracellular genes or DNA, and mediate different specific recombination reactions according to the position of loxP sequences and the relationship between loxP sequences (179). Conditional knockout mice are usually generated by breeding the loxp mice in which loxp flanked alleles of interest gene with Cre mice expressing Cre recombinase under the control of a selected promoter that specifically targets the tissue or cell of interest. Tissue- or cell-specific expression promoters determine the location of Cre expression resulting in conditional gene knockout (180). The Cre-loxp system was first used to specifically knock out the DNA polymerase gene in T lymphocytes (181). Some studies have reported that neuropeptides such as NPY, orexin and their receptors have been conditional knockout in mice based on the Cre-lox system. The effect of NPY on feeding was investigated in mice with specific knockout of NPY on AGRP neurons (103). Male mice targeted disruption of the Npy1r gene in limbic areas were used to study the effect of NPY-Y1R system in energy balance and emotional behavior as well as diet-induced obesity (105). Another study applied mice with β-cell specific ablation of the Y1 receptor to investigate the effect of Y1 receptor on the glucose metabolism (110). Also, mice selectively disrupted the orexin receptor in noradrenaline neurons were generated to study the effect of orexin-to-noradrenaline signaling on the sleep behavior (129). Owing to the Cre-loxp system, researchers could perform specific knockout in the specific neurons of their interest. It has been reported that growth hormone receptor (GHR) or IGF-1 receptor (IGF1R) were ablated in SST neurons specifically to investigate whether GHR or IGF1R is required for the homeostatic control of GH secretion (58). And transgenic mice in which the vesicular glutamate transporter 2 gene was disrupted selectively in orexin-producing neurons were used to study the roles of orexin neurons in mediating methamphetamine-induced changes in body temperature and heart rate (119). It is well known that in the neuropeptide and receptor family, there are usually several receptors corresponding to one ligand, neuropeptides, such as NPY and somatostatin (182, 183). Therefore, the Cre-loxP conditional knockout system is very useful and efficient for identifying specific receptor types that mediate ligand function in specific tissues.
The ligands of the NPY system, also known as NPY family peptides, are highly conserved in vertebrates in terms of their amino acid sequences and protein structures. PP, PYY and NPY are all composed of 36 amino acids with amidated C-terminus, classified into NPY family (184). In lower vertebrates, the discovery of NPY family polypeptides was mainly achieved through gene cloning. Only NPY and PYY have been cloned or isolated from both jawless and jawed cartilaginous fish, however, PP has not been found yet (185, 186). Among tetrapods, the presence of PP is found exclusively in Latimeria chalumnae (187). In summary, there are four NPY family peptides in teleost, namely NPYa, NPYb, PYYa and PYYb (Table 3). NPY of teleost is mainly expressed in the central nervous system (217, 229). In addition to the CNS, NPY mRNA levels has been detected in some peripheral tissues such as kidney, intestine, eye and pituitary (206, 210, 215). Studies have shown that PYYa of zebrafish (Danio rerio) (229), goldfish (197), grass carp (Ctenopharyngodon idellus) (193) and tilapia (220) is mainly expressed in the brain and spinal cord, while PYYb of grass carp (194), Japanese Flounder (Paralichthys olivaceus) (204), yellowtail (Seriola quinqueradiata) (230) and red-bellied piranha (Pygocentrus nattereri) (200) is mainly expressed in the foregut and midgut, and also expressed to a certain extent in the brain.
NPY family peptides exert their physiological functions by binding to their receptors, which are referred to as NPY family receptors. NPY family receptors are G protein-coupled receptors that consist of multiple members. In teleost, there are seven types of NPY receptors, which belong to Y1 family, Y1, Y4, Y8a and Y8b, and Y2 family, Y2, Y2-2, Y7, respectively (187). The y1 gene is only found in the zebrafish genome (189), speculating that most teleost lost Y1. Studies have revealed that Y4, Y8a, Y8b, Y2 and Y7 are all highly expressed in the brains (190, 218), eyes, heart, kidney, liver, intestine and gonads (182, 220). Research on the distribution of teleost NPY family receptors in brain regions, especially in various sub-regions other than the hypothalamus, remains to be studied.
The binding activity of NPY family receptors to ligands has been studied extensively in mammals, and a variety of receptor-specific agonists and antagonists have been screened, providing a basis for studying receptor-mediated physiological functions (231). However, knowledge about the ligand-binding activity of NPY family receptors is rare in teleost fish, and mainly focused on zebrafish and rainbow trout (Oncorhynchus mykiss) (190, 207). In zebrafish, Y4, Y8a, Y8b, and Y2-2 all have similar binding activities with NPY, PYYa, and PYYb (190, 191). While the affinity of Y2 with PYYb is about 50 times higher than that of PYYa and the affinity of Y7 with PYYa is about three times lower than that of NPY and PYYb (190, 191). In addition, unlike mammalian Y2, the two Y2 family receptors in rainbow trout, Y2 and Y7, are both sensitive to NPY with N-terminal deletion and have minimal binding capacity to NPY (13–24, 27–29, 31, 53, 65, 79, 80, 83–85) and NPY (18–24, 27–29, 31, 53, 65, 79, 80, 83–85), and neither binds to the mammalian Y2-specific antagonist BIIE0246 (207).
Research on the role of NPY in the regulation of bony fish feeding is mainly focused on NPYa. In the available literature, the orexigenic effects of NPY can be triggered by injection, feeding and immersion. Intracerebroventricular (icv) injection of NPYa in grass carp (195) and zebrafish (192), as well as intraperitoneal (ip) injection of NPYa in red tilapia (Oreochromis sp.) (232) and orange-spotted grouper (Epinephelus coioides) (223) stimulate food intake. Oral administration of synthetic NPYa significantly increased the growth rate, body weight gain and feed conversion ratio of orange-spotted grouper after feeding for 50 days (223), as well as in tilapia (233). In addition, immersing catfish (Clarias gariepinus) fry with recombinant NPYa can also increase their growth rate (234). There are relatively few reports on the regulation of feeding by PYY in teleost fish. In goldfish, PYYa ip (10 ng/g BW) or PYYa icv (5 ng/g BW) significantly reduced the food intake (197). Also, in Siberian sturgeon (Acipenser baerii), PYYa ip (10, 100 and 200 ng/g BW) significantly decreased food intake, while low dose of PYYa (1 ng/g BW) has no significant effect on feeding (227). Moreover, in tilapia, PYYa (50 ng/g BW) has no effect on food intake but PYYb icv (50 ng/g BW) significantly decreased feeding (220).
The role of NPY receptors in mediating NPY regulation in bony fish feeding is mainly verified based on the agonists and antagonists of mammalian NPY receptors. In goldfish and zebrafish, Y1 antagonist BIBP3226 icv inhibits the orexigenic effects of NPY (16, 192). ICV injection of Y1 agonist (Leu31, Pro34)-NPY and Y5 agonist (D-32Trp)-NPY promoted the food intake in goldfish, but neither the Y2 agonist NPY (2–24, 27–29, 31, 53, 65, 79, 80, 83–85) nor the (Pro13, Tyr36)-NPY (13–24, 27–29, 31, 53, 65, 79, 80, 83–85) has significant effect on feeding behaviors, suggesting that NPY receptors similar to mammalian Y1 and Y5 may be involved in feeding regulation in teleost (235, 236). On the other hand, in rainbow trout, ICV injection of both the Y1 agonist (Leu31, Pro34)-NPY and the Y2 agonist NPY (3–24, 27–29, 31, 53, 65, 79, 80, 83–85) stimulated food intake (208). Recently, it is reported that ICV injection of Y1 agonist (Leu31, Pro34)-NPY and Y2 agonist NPY (13–24, 27–29, 31, 53, 65, 79, 80, 83–85) both increased the appetite of Siberian sturgeon within 30 min (228). Unexpectedly, (Leu31, Pro34)-NPY does not specifically recognize NPY receptors in teleost (237), while BIBP3226 has an extremely low binding capacity with Y4, Y8a and Y8b in zebrafish (237). In addition, NPY (3–24, 27–29, 31, 53, 65, 79, 80, 83–85) and (13–24, 27–29, 31, 53, 65, 79, 80, 83–85) cannot specifically recognize Y2 of teleost and have poor binding capacity to Y2 in both rainbow trout and zebrafish (207) but have good binding capacity to Y4 of zebrafish (237). Therefore, the above studies can only infer the potential role of NPY receptors in teleost feeding by the performance of these agonists. The specific types of NPY family receptors involved in the regulation of teleost feeding remain to be studied.
In recent years, researchers have begun to use powerful gene editing techniques to study the function of NPY and its receptors. It has been reported that specific knockout of NPY in AGRP neurons reduced locomotion and energy expenditure and accelerated feeding and respiratory quotient in mice, and the study suggested that NPY originating from AGRP neurons is critical for initiating and sustaining feeding (103). Targeted disruption of the Npy1r gene in limbic areas revealed that limbic NPY-Y1R system was involved in energy balance and emotional behavior, and selective inactivation of limbic Npy1r gene increased susceptibility to diet-induced obesity in male mice (230). Another study showed that mice with β-cell specific ablation of the Y1 exhibit significantly upregulated serum insulin levels associated with increased body weight and adiposity (110). Zebrafish with global knockout of NPY have been found to exhibit some anxiety-like behaviors, indicating an important role of NPY in the regulation of emotional behaviors (95, 96).
Somatostatin (SS or SST), also known as somatotropin release-inhibiting factor (SRIF), is a cyclic polypeptide consisting of 14 amino acids. It was originally isolated from the hypothalamus of sheep and was named for its ability to inhibit the secretion of pituitary GH (18). SS is conservative and widespread in vertebrates, from fish to mammals, and it is also a multifunctional tetradecapeptide involved in a variety of physiological processes such as growth, development, metabolism, reproduction and immunity mediated by specific G protein-coupled receptors (238). Like other polypeptide hormones, SS is a mature peptide produced by tissue-specific enzymatic processing of its precursor protein (preprosomatostatin, PSS). In teleost, SS peptides were firstly identified from anglerfish (Lophius litulon) and spotted catfish (Anarrhichas minor), and subsequently their cDNAs were cloned (239, 240). Since then, multiple forms of SS peptides and their PSS cDNAs have been identified in different fish species (Table 4 for detail). Up to date, PSS is a recognized polygene family consisting of six homologous genes, namely pss1, pss2, pss3, pss4, pss5, and pss6 (270, 271). A comparative genomic approach reveals that pss1, pss2, and pss5 emerged from the second round of genome duplication early in vertebrate evolution; pss4 was a homolog of pss1 from the third round of genome doubling (3R) in most teleost; pss3 and pss6 arised from tandem duplication of pss1 and pss2, respectively (270, 271).
The function of SS is mediated by its seven transmembrane G protein-coupled receptors. Two members of the SSTR family, SSTR1 and SSTR2, were characterized from mice and human (272). SSTR3 was first reported in electric fish (Apteronotus albifrons) (273). It has been well reported that there are some receptor subtypes of SSTR in teleost. (For detail, Table 4). For example, the full-length cDNA sequences of four SS receptors (SSTR1, SSTR2, SSTR3 and SSTR5) were cloned from orange-spotted grouper (183). There were five SS receptors, SSTR2a (MW848786), SSTR2b (MW848787), SSTR3a (MW848788), SSTR3b (MW848789) and SSTR5 (MW848790), in Nile tilapia, which were highly homologous to the corresponding receptors of other known vertebrates.
Although SS is involved in a variety of physiological processes, its vital function is to inhibit the basal and induced GH secretion in the pituitary. Related research on SS function in teleost fish has mainly focused on the regulation of pituitary GH secretion. In vitro and in vivo studies in various teleost, including goldfish (244), tilapia (262), catfish (252) and rainbow trout (241), SS has been demonstrated to inhibit GH secretion. In goldfish, three precursor genes encoding SS (pss-Ⅰ, pss-Ⅱ and pss-III) showed different distribution patterns in regions of the hypothalamus associated with feeding and olfaction, hinting that SS may control food intake in fish (274). Treatment with SS14 has been observed to reduce food conversion, plasma IGF-I and INS levels, and further to causes growth retardation in rainbow trout (275, 276). In addition, SS may also indirectly influence feeding behavior. Reported as a major inhibitor of GH secretion, SS was ultimately found to increase food consumption due to its feeding-prolonging effect (277), which resulted from the direct function of SS to inhibit GH secretion (278). RNA-seq analysis of feeding and non-feeding anadromous fish (Coilia nasus) suggests that SS may be involved in the regulation of food intake and metabolic state (65).
Regarding the mechanism of SS on inhibiting growth, it has been revealed that SS inhibited GH secretion with the involvement of cAMP, protein kinase C (PKC), and Ca2+ in goldfish (279). Similarly, blockade using the PLC/IP/PKC pathway reversed SRIF-inhibited of GH release without affecting GHRH-stimulated of GH release in grouper (252). These studies suggest that SS inhibited GH secretion through the PKC pathway (280). However, in halibut (Psetta maxima), the PKC agonist TPA had no effect on GH secretion, and TPA promoted GH secretion only in the presence of SS (281). SS was found to have no effect on GH secretion induced by PKC agonists in chickens (282), and SS did not inhibit TPA-induced GH secretion in bovine pituitary cells (283). These results show a distinct species specific role for the PKC pathway, which is or is not involved in the process by which SS inhibits GH secretion. Generally, SS is considered as an inhibitor of GH secretion, however, studies in pigs and baboons have found that low concentrations of SS can also promote GH secretion (284, 285). In addition, studies in pigs found that the phospholipase C (PLC) inhibitor U73122 did not block SS-induced GH secretion (286). Similarly, in baboons, both U73122 and the PKC inhibitor Go6983 failed to block SS-induced GH secretion (284).
Studies in mammals have confirmed that activation of SSTRs inhibits cAMP levels and that the AC/cAMP/PKA pathway is involved in the physiological function of SS (287, 288). In goldfish, SS blocked GH secretion induced by the AC agonist forskolin and the cAMP analogue 8-Br-cAMP, suggesting that SS may inhibit GH secretion through the cAMP pathway (279). However, in halibut forskolin had no effect on GH secretion, and forskolin promoted GH secretion only in the presence of SS (281). In contrast, in pigs, SS increased cAMP levels and the AC/cAMP pathway mediated SS-induced GH secretion (286). Identically, SS promoted GH secretion via the AC/cAMP/PKA pathway in baboons (284). Taken together, the role of SS in regulating GH secretion differs among species, the AC/cAMP/PKA pathway (238) or the PLC/IP3/PKC pathway (240) is involved in SS-regulated GH secretion.
Nitric oxide (NO) is now recognized as an important signaling molecule involved in the regulation of SS function. NO is produced by NO synthase (NOS) in a reaction that catalyzes the conversion of arginine to citrulline (289). Typically, NO activates downstream ornithine cyclase (OC), which catalyzes the production of cGMP from GTP (290). NO promotes basal GH secretion in rat (291), pig (292), dog (293), and human (294). Conversely, NO was found to inhibit basal GH secretion in the mouse (295) and in the murine pituitary tumor cell line GH3 (296). In addition, it has also been reported that NO is not involved in basal GH secretion (297, 298). Interestingly, in human pituitary tumor cells, depending on its concentration, NO either promotes or inhibits basal GH secretion (299). There is abundant evidence confirming the involvement of NO in basal GH secretion, but its precise role and its mechanism remain highly controversial.
The regulation of feeding is a very complex process involving the modulation of various neuropeptides in the central nervous system. These neuropeptides are inextricably linked to each other. NPY, SS, orexin, etc. have been shown to be involved in feeding regulation, and whether there is a regulatory relationship between these neuropeptides has been a matter of interest to researchers. It is interesting to note that some functionally similar neuropeptides co-localize in brain regions. It has been reported that SS co-localizes with NPY in the mammalian amygdala (300). A double label in situ hybridization histochemistry has shown that NPY and SSTR1 are co-expressed in the arcuate nucleus (301). It has been shown that treatment of NPY and SS in the prepyramidal cortex alters the intake of essential amino acid deficient diets in rats, and the cytoarchitecture suggests that NPY and SS-containing neurons in the prepyramidal cortex may be synaptically or polysynaptically associated with local circuit. It is suggested a possible association between these two neuropeptides during electrical activity, olfactory information regulation and neural perception of essential amino acids (302, 303). Pharmacological evidence suggested a role for NPY in the feeding regulation of SS. An agonist of SS, ODT8-SST icv injection increased food intake in non-fasted rats during both the light and dark phases, whereas both the SSTR2 antagonist and the Y1 antagonist BIBP-3226 blocked the orexigenic effect of ODT8-SST (304), suggesting that ODT8-SST enhances feeding behavior through SSTR2 involving the activation of Y1. Similar to NPY, orexin is also involved in the feeding regulation of SS. It was found that the antagonist of the orexin receptor, SB-334867, completely blocked the orexigenic phenomenon induced by ODT8-SST (305). In contrast, pretreatment with the SSTR2 antagonist S-406-028 did not affect the orexigenic effect of orexin, indicating that the orexin system is part of the neural circuitry in the brain regions of the orexigenic response induced by icv injection of ODT8-SST, and that the orexigenic effect of orexin is independent of SSTR2 (305). In neonatal chicken, SS icv injection was shown to significantly increase food intake, and the Y1 antagonist B5063 and Y5 antagonist SML0891 alone showed a dose-dependent decrease in feeding, while the Y2 antagonist SF22 treatment showed a dose-dependent increase (306). The researchers found that co-injection of SS and Y1 antagonist B5063 significantly reduced food intake, however, Y2 antagonist SF22 and Y5 antagonist SML0891 had no significant effect on the feeding behavior induced by SS, these results suggest that the NPY-Y1 system is involved in the feeding regulation of SS (306).
There is no direct evidence in fish to suggest that there is a correlation between NPY and SS in feeding regulation. However, some indirect evidence in fish indicate that NPY and SS are interrelated. In green molly (Poecilia latipinna), SS-immunopositive neurons were also found to express NPY (307). In goldfish, NPY and SS were found to be highly co-localized in neurons in the ventrolateral telencephalon (VI), the entopenduncular nucleus (NE) and, to a lesser extent, the dorsocentral nucleus (Dc) of the telencephalon, and in the brainstem (308). Incubation of Spotted Sea Bass (Lateolabrax maculatus) brain cells with neuropeptide FF (NPFF) significantly upregulated orexin and npy mRNA levels and significantly downregulated ss mRNA levels (309). Antidepressant Sertraline (SER) stress in juvenile yellow catfish (Tachysurus fulvidraco) was found to affect feeding and growth by regulating transcript levels of npy, ss and gh (30).
Feeding and growth is regulated not only by the influence from complicated external environment, but also the integrate regulations at the intrinsic molecular level. In addition to the above-mentioned NPY family and SS family, there are other neuropeptides involved in fish feeding and growth regulation.
Cholecystokinin (CCK) is a brain-gut peptide secreted by both small intestinal mucosal I cell and central nervous cells. There are some forms of CCK precursor mRNA found in fish, which can be divided into two clusters by performing evolutionary tree analysis, so called CCK-1 and CCK-2 (310). CCK is an important peripheral inhibitor of feeding, and it may also be one of the factors that integrally regulate feeding and growth hormone release (311–313). CCK inhibited gastric emptying and promoted gallbladder contraction in rainbow trout and other salmonids (311, 312). The mRNA expression of cck was reduced during starvation and significantly increased after feeding in Atlantic salmon (Salmo salar) (311), Japanese flounder (314), winter skate (Raja ocellata) (315) and yellowtail (230). The mRNA expression of CCK in the pyloric caecum of goldfish and yellowtail was also elevated after feeding, and further research revealed that intraventricular and IP injection of CCK into goldfish could inhibit food intake (313). Oral ingestion of CCK reduced dietary intake in European sea bass (Dicentrarchus labrax), whereas CCK antagonists administered enhanced dietary intake in sea bass and rainbow trout (316). IP injections of CCK8 with 100 and 200 ng/g BW in Siberian sturgeon (Acipenser baerii Brandt) showed that CCK8 inhibited ingestion from 0-1 h and cumulative ingestion at 3 h. Chronic injections of 100 and 200 ng/g BW CCK8 at 7 d resulted in a significant reduction in daily and cumulative ingestion (317). These results suggest that CCK also acts as a satiety signal in fish and is one of the factors that integrally regulate fish food intake.
The corticotropin-releasing factor (CRF) system consists of four homologous lineages, two major receptors (CRF-R1 and CRF-R2) and a binding protein CRF-BP. The homologous gene lineages are corticotropin-releasing factor (CRF), urotensin I (UI)/sauvagine (SVG)/urocortin 1 (UCN1), urocortin 2 (UCN2) and urocortin 3 (UCN3), with UI, SVG and UCN1 as homologs (20, 318). It has been found that the CRF system is associated with fish feeding. The mRNA expression of crf was significantly down-regulated by fasting goldfish for 7 days and up-regulated by overfeeding (319). Pre- and post-prandial, fasting and refeeding researches on Dabry’s sturgeon (Acipenser dabryanus Dumeril) showed that crf mRNA elevated significantly at 1 h and 3 h after feeding, crf and crf -Rs transcripts significantly reduced at 10 d of fasting but elevated at day 10 of refeeding (320). ICV of CRF or UI treatment were found to reduce the feeding quantity in rainbow trout (321). On the other hand, the mRNA expression of crf decreased significantly when Schizothorax prenanti fasting for 7 days but increased significantly after re-feeding (322). IP injection of different doses of UCN3 into Siberian sturgeon showed a reduction in feeding from 0 to 6 hours, and with 7 days of injection, the cumulative amount of feeding reduced significantly compared to the control group (323). The above findings suggest that the CRF system may act as a feeding inhibitor in fish.
Two orexins (also known as hypothalamus), orexin-A (OXA) and -B (OXB), were first characterized from rat hypothalamus almost simultaneously by two teams (324, 325). Subsequently, orexin cDNA sequences have also been cloned successively from, zebrafish (326), Atlantic Cod (Gadus morhua) (327) and Winter Flounder (Pleuronectes americanus) (328). Fish orexin neurons concentrate in the hypothalamus and extend to other brain regions such as the ventral medial hypothalamus and dorsal mesencephalon (329). In zebrafish and goldfish, prolonged starvation significantly upregulated brain orexin mRNA expression (330). Goldfish ICV injections of either OXA or OXB can dose-dependently promote feeding, and the effect of OXA is stronger than that of OXB (17). IP injection of OXA in Thalassoma pavo also significantly stimulated feeding and locomotion (331). In Atlantic cod, orexin mRNA expression levels in the hypothalamus were significantly higher during ingestion than before and after ingestion (327). Orexin also interacts with other appetite-regulating peptides to regulate feeding. Blocking the orexin receptor pathway would decrease the NPY-induced pro-appetitive effect (22), in the same way, blocking the NPY receptor signaling pathway reduced the orexin-triggered pro-feeding effect (332). It suggests that orexin synergizes with NPY to promote feeding in fish.
POMC is a common precursor of adrenocorticotropic hormone (ATCH), melanocyte-stimulating hormones (including γ-MSHs, α-MSHs, β-MSHs, and δ-MSHs), β-endorphin (β-END) (19). POMC is mainly expressed in the pituitary gland and, together with its derived peptides, i.e., ATCH, MSHs and MSHs receptors such as MC4R, forms the melanocortin system, which is involved in food intake and energy balance (333). When POMC neurons receive stimulation, they release MSHs, which act with melanocortin receptors in the hypothalamus and other brain regions to reduce food intake and increase basal metabolic rate (334). In grass carp feeding experiment, feeding and weight gain rates increased significantly with enhancing levels of the diets, whereas restricted diets significantly reduced the transcript levels of hypothalamic POMC (335). Overfeeding of zebrafish larvae reduced POMC levels and declined activation of MC4R (336). Short-term fasting of snakeskin gourami (Trichopodus pectoralis) resulted in the lowest levels of POMC mRNAs in the telencephalon and mesencephalon as well as the pituitary gland at 12 h of fasting, while POMC transcripts reached their lowest point at 6 h of fasting (337). In addition, POMC as feeding-inhibiting neuropeptide in fish, displays a potential antagonistic relationship with other neuropeptides such as AgRP (Agouti Related protein) and NPY. Results from snakeskin gourami indicated that a decrease in MC4R expression was observed 1 h before the last meal of the day, while no such decrease was shown 1 h before the first meal of the day. Postprandial NPY expression decreases, with peak NPY expression occurring 1 hour before the first meal of the day (226). It has been reported that a unique mouse model (Pomc tm1Kgm) which was unable to generate desacetyl-α-MSH and α-MSH from ACTH 1-39 developed the characteristic melanocortin obesity phenotype, and desacetyl-α-MSH and α-MSH were found to regulate body weight and energy balance in mouse (213). Risperidone treatment was found to cause hyperphagia and induce weight gain, and transcriptomic analyses in the hypothalamus of risperidone-fed mice revealed that risperidone treatment reduced the expression of mc4r, and MC4R-specific agonist experiments indicated that the atypical antipsychotic risperidone targets hypothalamic MC4R to cause weight gain (338).
Food intake and growth are two physiological processes that are directly related and affect each other. NPY is an important orexigenic factor in the hypothalamus and has been shown to increase appetite, promote GH secretion and increase growth rate in fish (219, 223). SS is widely known for inhibiting the growth of animals earlier, but later studies show that SS also takes part in the regulation of feeding in fish (65, 274). Similar to SS, neuropeptides initially identified for other functions are later shown to be involved in feeding regulation in fish, such as CRF, which are originally known to regulate reproduction and promote corticotropin secretion, respectively (339).
Currently, studies on the regulation of feeding mechanisms in fish are still at infancy, and most of them are limited to the analysis of neuropeptides and receptors at the mRNA level, including the molecular cloning and tissue distribution characterization of neuropeptides and their receptors, but lacking the exploration of its feeding regulation mechanism. Moreover, the analysis of the tissue distribution of neuropeptides and receptors is relatively shallow. In the brain area, there are many feeding-related neuropeptides and receptors. However, most studies do not accurately delineate brain regions, but use the entire brain or the entire hypothalamus for tissue distribution analysis.
Although the basic mechanisms of food intake regulation in mammals and fish are relatively conserved, there are still huge physiological differences. In addition, teleost have also undergone the 3R (340). Compared with other groups, fish have more types of neuropeptides and receptors related to feeding. What’s more complicated is that multiple protein subtypes may have different physiological functions, which makes the study of their feeding regulation mechanism more challenging. There are still many gaps to be filled in understanding the regulation mechanism of fish ingestion.
Advances in omics and gene editing technologies have allowed us to study the function of neuropeptides and their receptors in greater depth. At present, the most used omics technology in the study of neuropeptide and its receptor function is transcriptomic technology, which mainly focuses on mammals. It is expected that researchers can focus more on non-mammals and use proteome or metabolome to explore the field of neuropeptides from multiple dimensions. Currently, both systemic knockout and conditional knockout transgenic animals are available for the study of neuropeptides and their receptors. Conditional knockout can be more targeted to study the function of neuropeptides and their receptors in a certain tissue or type of cells, but unfortunately, conditional knockout has only been reported in mice applying cre/loxp system. In the future, researchers may try to apply CRISP/Cas9 to perform tissue/cell-specific knockout of neuropeptides or their receptors in non-mammalian animals.
XY and HY contributed to the literature review and writing of the manuscript. WL contributed to review and editing of the manuscript. All authors contributed to the article and approved the submitted version.
This work was supported by the National Key R&D Program of China 2018YFD0900101, China Agriculture Research System (CARS-46), and National Science Foundation of China (32072968) to WL.
The authors declare that the research was conducted in the absence of any commercial or financial relationships that could be construed as a potential conflict of interest.
All claims expressed in this article are solely those of the authors and do not necessarily represent those of their affiliated organizations, or those of the publisher, the editors and the reviewers. Any product that may be evaluated in this article, or claim that may be made by its manufacturer, is not guaranteed or endorsed by the publisher.
3R, third round of genome doubling; CCK, cholecystokinin; CRF, corticotropin-releasing factor; GH, growth hormone; GHR1, growth hormone receptor 1; GHRH, growth hormone-releasing hormone; ICV, intracerebroventricular; IP, intraperitoneal; NPY, neuropeptide Y; POMC, proopiomelanocortin; PP, pancreatic polypeptide; PSS, preprosomatostatin; PYY, peptide YY; SS, somatostatin; SSTRs, somatostatin receptors; SVG, sauvagine; UCN1, urocortin 1; UCN2, urocortin 2; UCN3, urocortin 3; UI, urotensin I.
1. Kaur P, Singh A, Chana I. Computational techniques and tools for omics data analysis: State-of-the-Art, challenges, and future directions. Arch Comput Method E (2021) 28:4595–631. doi: 10.1007/s11831-021-09547-0
2. Del Giacco L, Cattaneo C. Introduction to genomics. Methods Mol Biol (2012) 823:79–88. doi: 10.1007/978-1-60327-216-2_6
3. Chambers DC, Carew AM, Lukowski SW, Powell JE. Transcriptomics and single-cell rna-sequencing. Respirology (2019) 24:29–36. doi: 10.1111/resp.13412
4. Aslam B, Basit M, Nisar MA, Khurshid M, Rasool MH. Proteomics: Technologies and their applications. J Chromatogr Sci (2017) 55:182–96. doi: 10.1093/chromsci/bmw167
5. Schrimpe-Rutledge AC, Codreanu SG, Sherrod SD, McLean JA. Untargeted metabolomics strategies-challenges and emerging directions. J Am Soc Mass Spectrom (2016) 27:1897–905. doi: 10.1007/s13361-016-1469-y
6. Gossler A, Joyner AL, Rossant J, Skarnes WC. Mouse embryonic stem cells and reporter constructs to detect developmentally regulated genes. Science (1989) 244:463–5. doi: 10.1126/science.2497519
7. Mansour SL, Thomas KR, Capecchi MR. Disruption of the proto-oncogene int-2 in mouse embryo-derived stem cells: A general strategy for targeting mutations to non-selectable genes. Nature (1988) 336:348–52. doi: 10.1038/336348a0
8. Stanford WL, Cohn JB, Cordes SP. Gene-trap mutagenesis: Past, present and beyond. Nat Rev Genet (2001) 2:756–68. doi: 10.1038/35093548
9. Porteus MH, Carroll D. Gene targeting using zinc finger nucleases. Nat Biotechnol (2005) 23:967–73. doi: 10.1038/nbt1125
10. Li T, Huang S, Jiang WZ, Wright D, Spalding MH, Weeks DP, et al. Tal nucleases (Talns): Hybrid proteins composed of tal effectors and foki DNA-cleavage domain. Nucleic Acids Res (2011) 39:359–72. doi: 10.1093/nar/gkq704
11. Wang H, Yang H, Shivalila CS, Dawlaty MM, Cheng AW, Zhang F, et al. One-step generation of mice carrying mutations in multiple genes by Crispr/Cas-mediated genome engineering. Cell (2013) 153:910–8. doi: 10.1016/j.cell.2013.04.025
12. MacDonald E, Volkoff H. Cloning, distribution and effects of season and nutritional status on the expression of neuropeptide y (Npy), cocaine and amphetamine regulated transcript (Cart) and cholecystokinin (Cck) in winter flounder (Pseudopleuronectes americanus). Horm Behav (2009) 56:58–65. doi: 10.1016/j.yhbeh.2009.03.002
13. Murashita K, Kurokawa T, Nilsen TO, Ronnestad I. Ghrelin, cholecystokinin, and peptide yy in Atlantic salmon (Salmo salar): Molecular cloning and tissue expression. Gen Comp Endocr (2009) 160:223–35. doi: 10.1016/j.ygcen.2008.11.024
14. Ronnestad I, Gomes AS, Murashita K, Angotzi R, Jonsson E, Volkoff H. Appetite-controlling endocrine systems in teleosts. Front Endocrinol (Lausanne) (2017) 8:73. doi: 10.3389/fendo.2017.00073
15. Tatemoto K, Carlquist M, Mutt V. Neuropeptide y–a novel brain peptide with structural similarities to peptide yy and pancreatic polypeptide. Nature (1982) 296:659–60. doi: 10.1038/296659a0
16. Narnaware YK, Peyon PP, Lin X, Peter RE. Regulation of food intake by neuropeptide y in goldfish. Am J Physiol Regul Integr Comp Physiol (2000) 279:1025–34. doi: 10.1152/ajpregu.2000.279.3.R1025
17. Volkoff H, Bjorklund JM, Peter RE. Stimulation of feeding behavior and food consumption in the goldfish, Carassius auratus, by orexin-a and orexin-b. Brain Res (1999) 846:204–9. doi: 10.1016/S0006-8993(99)02052-1
18. Brazeau P, Vale W, Burgus R, Ling N, Butcher M, Rivier J, et al. Hypothalamic polypeptide that inhibits the secretion of immunoreactive pituitary growth hormone. Science (1973) 179:77–9. doi: 10.1126/science.179.4068.77
19. Eipper BA, Mains RE. Structure and biosynthesis of pro-Adrenocorticotropin/Endorphin and related peptides. Endocr Rev (1980) 1:1–27. doi: 10.1210/edrv-1-1-1
20. Behan DP, Linton EA, Lowry PJ. Isolation of the human plasma corticotrophin-releasing factor-binding protein. J Endocrinol (1989) 122:23–31. doi: 10.1677/joe.0.1220023
21. Giannoulis EL, Barry RE. Bioassay of cholecystokinin. Gut (1982) 23:146–9. doi: 10.1136/gut.23.2.146
22. Volkoff H, Peter RE. Interactions between orexin a, npy and galanin in the control of food intake of the goldfish. Carassius Auratus Regul Pept (2001) 101:59–72. doi: 10.1016/S0167-0115(01)00261-0
23. Hoskins LJ, Xu M, Volkoff H. Interactions between gonadotropin-releasing hormone (Gnrh) and orexin in the regulation of feeding and reproduction in goldfish (Carassius auratus). Horm Behav (2008) 54:379–85. doi: 10.1016/j.yhbeh.2008.04.011
24. Han JL, Shao JJ, Chen Q, Sun HZ, Guan LL, Li YX, et al. Transcriptional changes in the hypothalamus, pituitary, and mammary gland underlying decreased lactation performance in mice under heat stress. FASEB J (2019) 33:12588–601. doi: 10.1096/fj.201901045R
25. Pan W, Adams JM, Allison MB, Patterson C, Flak JN, Jones J, et al. Essential role for hypothalamic calcitonin receptor-expressing neurons in the control of food intake by leptin. Endocrinology (2018) 159:1860–72. doi: 10.1210/en.2017-03259
26. Wray JR, Davies A, Sefton C, Allen TJ, Adamson A, Chapman P, et al. Global transcriptomic analysis of the arcuate nucleus following chronic glucocorticoid treatment. Mol Metab (2019) 26:5–17. doi: 10.1016/j.molmet.2019.05.008
27. Anderson KC, Knuckey R, Canepa M, Elizur A. A transcriptomic investigation of appetite-regulation and digestive processes in giant grouper Epinephelus lanceolatus during early larval development. J Fish Biol (2018) 93:694–710. doi: 10.1111/jfb.13798
28. Li ZJ, Liu XL, Zhang PP, Han RL, Sun GR, Jiang RR, et al. Comparative transcriptome analysis of hypothalamus-regulated feed intake induced by exogenous visfatin in chicks. BMC Genomics (2018) 19:1–17. doi: 10.1186/s12864-018-4644-7
29. Anderson K, Kuo CY, Lu MW, Bar I, Elizur A. A transcriptomic investigation of digestive processes in orange-spotted grouper, Epinephelus coioides, before, during, and after metamorphic development. Gene (2018) 661:95–108. doi: 10.1016/j.gene.2018.03.073
30. Chen H, Liang X, Gu X, Zeng Q, Mao Z, Martyniuk CJ. Environmentally relevant concentrations of sertraline disrupts behavior and the brain and liver transcriptome of juvenile yellow catfish (Tachysurus fulvidraco): Implications for the feeding and growth axis. J Hazard Mater (2021) 409:124974. doi: 10.1016/j.jhazmat.2020.124974
31. Tsutsui N, Kobayashi Y, Izumikawa K, Sakamoto T. Transcriptomic analysis of the kuruma Prawnmarsupenaeus japonicus reveals possible peripheral regulation of the ovary. Front Endocrinol (2020) 11:541. doi: 10.3389/fendo.2020.00541
32. Nguyen LT, Reverter A, Canovas A, Venus B, Islas-Trejo A, Porto-Neto LR, et al. Global differential gene expression in the pituitary gland and the ovaries of pre- and postpubertal Brahman heifers. J Anim Sci (2017) 95:599–615. doi: 10.2527/jas.2016.0921
33. Wen GH, Pang H, Wu X, Jiang EZ, Zhang XQ, Zhan XN. Proteomic characterization of secretory granules in dnpaminergic neurons indicates Chromogranin/Secretogranin-meaiated protein processing impairment in parkinson's disease. Aging-Us (2021) 13:20335–58. doi: 10.18632/aging.203415
34. He Y, Li W, Tian Y, Chen X, Cheng K, Xu K, et al. Itraq-based proteomics suggests Lrp6, npy and Npy2r perturbation in the hippocampus involved in csds may induce resilience and susceptibility. Life Sci (2018) 211:102–17. doi: 10.1016/j.lfs.2018.09.016
35. Rofo F, Sandbaumhuter FA, Chourlia A, Metzendorf NG, Morrison JI, Syvanen S, et al. Wide-ranging effects on the brain proteome in a transgenic mouse model of alzheimer's disease following treatment with a brain-targeting somatostatin peptide. ACS Chem Neurosci (2021) 12:2529–41. doi: 10.1021/acschemneuro.1c00303
36. Muk T, Stensballe A, Pankratova S, Nguyen DN, Brunse A, Sangild PT, et al. Rapid proteome changes in plasma and cerebrospinal fluid following bacterial infection in preterm newborn pigs. Front Immunol (2019) 10:2651. doi: 10.3389/fimmu.2019.02651
37. Wang B, Sheriff S, Balasubramaniam A, Kennedy MA. Nmr based metabolomics study of Y2 receptor activation by neuropeptide y in the sk-N-Be2 human neuroblastoma cell line. Metabolomics (2015) 11:1243–52. doi: 10.1007/s11306-015-0782-y
38. Pottorf TS, Fagan MP, Burkey BF, Cho DJ, Vath JE, Tran PV. Metap2 inhibition reduces food intake and body weight in a ciliopathy mouse model of obesity. JCI Insight (2020) 5:e134278. doi: 10.1172/jci.insight.134278
39. Bao RY, Onishi KG, Tolla E, Ebling FJP, Lewis JE, Anderson RL, et al. Genome sequencing and transcriptome analyses of the Siberian hamster hypothalamus identify mechanisms for seasonal energy balance. P Natl Acad Sci USA (2019) 116:13116–21. doi: 10.1073/pnas.1902896116
40. Kumari R, Fazekas EA, Morvai B, Udvari EB, Dora F, Zachar G, et al. Transcriptomics of parental care in the hypothalamic-septal region of female zebra finch brain. Int J Mol Sci (2022) 23:2518. doi: 10.3390/ijms23052518
41. Hu ML, Zhu HM, Zhang QL, Liu JJ, Ding Y, Zhong JM, et al. Exploring the mechanisms of electroacupuncture-induced analgesia through rna sequencing of the periaqueductal Gray. Int J Mol Sci (2018) 19:2. doi: 10.3390/ijms19010002
42. Sharma A, Das S, Sur S, Tiwari J, Chaturvedi K, Agarwal N, et al. Photoperiodically driven transcriptome-wide changes in the hypothalamus reveal transcriptional differences between physiologically contrasting seasonal life-history states in migratory songbirds. Sci Rep-Uk (2021) 11:1–13. doi: 10.1038/s41598-021-91951-4
43. Michel L, Reygagne P, Benech P, Jean-Louis F, Scalvino S, So SLK, et al. Study of gene expression alteration in Male androgenetic alopecia: Evidence of predominant molecular signalling pathways. Brit J Dermatol (2017) 177:1322–36. doi: 10.1111/bjd.15577
44. Sharma NK, Comeau ME, Montoya D, Pellegrini M, Howard TD, Langefeld CD, et al. Integrative analysis of glucometabolic traits, adipose tissue DNA methylation, and gene expression identifies epigenetic regulatory mechanisms of insulin resistance and obesity in African americans. Diabetes (2020) 69:2779–93. doi: 10.2337/db20-0117
45. Jiang JL, Mao MG, Lu HQ, Wen SH, Sun ML, Liu RT, et al. Digital gene expression analysis of Takifugu rubripes brain after acute hypoxia exposure using next-generation sequencing. Comp Biochem Phys D (2017) 24:12–8. doi: 10.1016/j.cbd.2017.05.003
46. Espinosa R, Gutierrez K, Rios J, Ormeno F, Yanten L, Galaz-Davison P, et al. Palmitic and stearic acids inhibit chaperone-mediated autophagy (Cma) in pomc-like neurons in vitro. Cells-Basel (2022) 11:920. doi: 10.3390/cells11060920
47. Lam BYH, Cimino I, Polex-Wolf J, Kohnke SN, Rimmington D, Iyemere V, et al. Heterogeneity of hypothalamic pro-Opiomelanocortin-Expressing neurons revealed by single-cell rna sequencing. Mol Metab (2017) 6:383–92. doi: 10.1016/j.molmet.2017.02.007
48. Yu H, Rubinstein M, Low MJ. Developmental single-cell transcriptomics of hypothalamic pomc neurons reveal the genetic trajectories of multiple neuropeptidergic phenotypes. Elife (2022) 11:e72883. doi: 10.7554/eLife.72883
49. Yin K, Deuis JR, Lewis RJ, Vetter I. Transcriptomic and behavioural characterisation of a mouse model of burn pain identify the cholecystokinin 2 receptor as an analgesic target. Mol Pain (2016) 12:1744806916665366. doi: 10.1177/1744806916665366
50. Cao WX, Liu F, Li RW, Yang RL, Wang YM, Xue CH, et al. Triacylglycerol rich in docosahexaenoic acid regulated appetite Via the mediation of leptin and intestinal epithelial functions in high-fat, high-sugar diet-fed mice. J Nutr Biochem (2022) 99:108856. doi: 10.1016/j.jnutbio.2021.108856
51. Agrawal P, Kao D, Chung P, Looger LL. The neuropeptide drosulfakinin regulates social isolation-induced aggression in. Drosophila J Exp Biol (2020) 223:jeb207407. doi: 10.1242/jeb.207407
52. Hollander-Cohen L, Meir I, Shulman M, Levavi-Sivan B. Identifying the interaction of the brain and the pituitary in social- and reproductive- state of tilapia by transcriptome analyses. Neuroendocrinology (2022). doi: 10.1159/000524437
53. Qin CJ, Sun JX, Wen ZY, Han YW, Liu YF, Yuan DY, et al. Comparative transcriptome sequencing of the intestine reveals differentially expressed genes in. Pelteobagrus Vachellii Aquac Res (2018) 49:2560–71. doi: 10.1111/are.13718
54. Wang Y, Roth JD, Taylor SW. Simultaneous quantification of the glucagon-like peptide-1 (Glp-1) and cholecystokinin (Cck) receptor agonists in rodent plasma by on-line solid phase extraction and lc-Ms/Ms. J Chromatogr B (2014) 957:24–9. doi: 10.1016/j.jchromb.2014.02.040
55. Caron J, Cudennec B, Domenger D, Belguesmia Y, Flahaut C, Kouach M, et al. Simulated gi digestion of dietary protein: Release of new bioactive peptides involved in gut hormone secretion. Food Res Int (2016) 89:382–90. doi: 10.1016/j.foodres.2016.08.033
56. van der Stelt I, Hoevenaars F, Siroka J, de Ronde L, Friedecky D, Keijer J, et al. Metabolic response of visceral white adipose tissue of obese mice exposed for 5 days to human room temperature compared to mouse thermoneutrality. Front Physiol (2017) 8:179. doi: 10.3389/fphys.2017.00179
57. Ribeiro EA, Salery M, Scarpa JR, Calipari ES, Hamilton PJ, Ku SM, et al. Transcriptional and physiological adaptations in nucleus accumbens somatostatin interneurons that regulate behavioral responses to cocaine. Nat Commun (2018) 9:1–10. doi: 10.1038/s41467-018-05657-9
58. Chaves FM, Wasinski F, Tavares MR, Mansano NS, Frazao R, Gusmao DO, et al. Effects of the isolated and combined ablation of growth hormone and igf-1 receptors in somatostatin neurons. Endocrinology (2022) 163:bqac045. doi: 10.1210/endocr/bqac045
59. Adriaenssens A, Lam BYH, Billing L, Skeffington K, Sewing S, Reimann F, et al. A transcriptome-led exploration of molecular mechanisms regulating somatostatin-producing d-cells in the gastric epithelium. Endocrinology (2015) 156:3924–36. doi: 10.1210/en.2015-1301
60. Cruceanu C, Tan PPC, Rogic S, Lopez JP, Torres-Platas SG, Gigek CO, et al. Transcriptome sequencing of the anterior cingulate in bipolar disorder: Dysregulation of G protein-coupled receptors. Am J Psychiat (2015) 172:1131–40. doi: 10.1176/appi.ajp.2015.14101279
61. Salomon MP, Wang XW, Marzese DM, Hsu SC, Nelson N, Zhang X, et al. The epigenomic landscape of pituitary adenomas reveals specific alterations and differentiates among acromegaly, cushing's disease and endocrine-inactive subtypes. Clin Cancer Res (2018) 24:4126–36. doi: 10.1158/1078-0432.Ccr-17-2206
62. Tian CX, Zhang JP, Feng PZ, Lin XH, Ru XY, Zhu CH, et al. Comparative analysis of transcriptome responses to injected somatostatin 3 peptide in spotted scat (Scatophagus argus). Aquacult Rep (2022) 23:101022. doi: 10.1016/j.aqrep.2022.101022
63. Zhang DD, Thongda W, Li C, Zhao HG, Beck BH, Mohammed H, et al. More than just antibodies: Protective mechanisms of a mucosal vaccine against fish pathogen flavobacterium columnare. Fish Shellfish Immun (2017) 71:160–70. doi: 10.1016/j.fsi.2017.10.001
64. Powell D, Thoa NP, Nguyen NH, Knibb W, Elizur A. Transcriptomic responses of saline-adapted Nile tilapia (Oreochromis niloticus) to rearing in both saline and freshwater. Mar Genom (2021) 60:100879. doi: 10.1016/j.margen.2021.100879
65. Ma FJ, Yin DH, Fang DA, Yang YP, Jiang M, You L, et al. Insights into response to food intake in anadromous Coilia nasus through stomach transcriptome analysis. Aquac Res (2020) 51:2799–812. doi: 10.1111/are.14619
66. Pardo CAC, Massoz L, Dupont MA, Bergemann D, Bourdouxhe J, Lavergne A, et al. A delta-cell subpopulation with a pro-Beta-Cell identity contributes to efficient age-independent recovery in a zebrafish model of diabetes. Elife (2022) 11:e67576. doi: 10.7554/eLife.67576
67. Li X, Feng C, Sha H, Zhou T, Zou G, Liang H. Tandem mass tagging-based quantitative proteomics analysis reveals damage to the liver and brain of hypophthalmichthys molitrix exposed to acute hypoxia and reoxygenation. Antioxidants (Basel) (2022) 11:589. doi: 10.3390/antiox11030589
68. Xie Q, Liu TL, Ding J, Zhou NN, Meng XX, Zhu H, et al. Synthesis, preclinical evaluation, and a pilot clinical imaging study of [F-18]Alf-Nota-Jr11 for neuroendocrine neoplasms compared with [Ga-68]Ga-Dota-Tate. Eur J Nucl Med Mol I (2021) 48:3129–40. doi: 10.1007/s00259-021-05249-8
69. D'Alessandro A, Cervia D, Catalani E, Gevi F, Zolla L, Casini G. Protective effects of the neuropeptides pacap, substance p and the somatostatin analogue octreotide in retinal ischemia: A metabolomic analysis. Mol Biosyst (2014) 10:1290–304. doi: 10.1039/c3mb70362b
70. Zhang BX, Qi XJ, Cai Q. Metabolomic study of raw and bran-fried atractylodis rhizoma on rats with spleen deficiency. J Pharm BioMed Anal (2020) 182:112927. doi: 10.1016/j.jpba.2019.112927
71. Sharpe AL, Trzeciak M, Eliason NL, Blankenship HE, Byrd BAM, Douglas PD, et al. Repeated cocaine or methamphetamine treatment alters astrocytic Crf2 and glast expression in the ventral midbrain. Addict Biol (2022) 27:e13120. doi: 10.1111/adb.13120
72. McCullough KM, Chatzinakos C, Hartmann J, Missig G, Neve RL, Fenster RJ, et al. Genome-wide translational profiling of amygdala crh-expressing neurons reveals role for creb in fear extinction learning. Nat Commun (2020) 11:1–11. doi: 10.1038/s41467-020-18985-6
73. Wang BB, Wang PQ, Parobchak N, Treff N, Tao X, Wang JW, et al. Integrated rna-seq and chip-seq analysis reveals a feed-forward loop regulating H3k9ac and key labor drivers in human placenta. Placenta (2019) 76:40–50. doi: 10.1016/j.placenta.2019.01.010
74. Robinson NA, Johnsen H, Moghadam H, Andersen O, Tveiten H. Early developmental stress affects subsequent gene expression response to an acute stress in Atlantic salmon: An approach for creating robust fish for aquaculture? G3-Genes Genom Genet (2019) 9:1597–611. doi: 10.1534/g3.119.400152
75. Li YT, Pan L, Zeng XY, Zhang RX, Li X, Li JH, et al. Ammonia exposure causes the imbalance of the gut-brain axis by altering gene networks associated with oxidative metabolism, inflammation and apoptosis. Ecotox Environ Safe (2021) 224:112668. doi: 10.1016/j.ecoenv.2021.112668
76. Zong RR, Zhu FF, Han W, Wang YX, Wang GL, Wang YZ, et al. Tear dynamics testing and quantitative proteomics analysis in patients with chronic renal failure. J Proteomics (2021) 248:104351. doi: 10.1016/j.jprot.2021.104351
77. Li H, Cai J, Chen R, Zhao Z, Ying Z, Wang L, et al. Particulate matter exposure and stress hormone levels: A randomized, double-blind, crossover trial of air purification. Circulation (2017) 136:618–27. doi: 10.1161/CIRCULATIONAHA.116.026796
78. Wang Z, Zhang J, Wu P, Luo S, Li J, Wang Q, et al. Effects of oral monosodium glutamate administration on serum metabolomics of suckling piglets. J Anim Physiol Anim Nutr (Berl) (2020) 104:269–79. doi: 10.1111/jpn.13212
79. Dobrzyn K, Szeszko K, Kiezun M, Kisielewska K, Rytelewska E, Gudelska M, et al. In vitro effect of orexin a on the transcriptomic profile of the endometrium during early pregnancy in pigs. Anim Reprod Sci (2019) 200:31–42. doi: 10.1016/j.anireprosci.2018.11.008
80. Zaobidna E, Kiezun M, Dobrzyn K, Szeszko K, Rytelewska E, Kisielewska K, et al. The influence of orexin b on the transcriptome profile of porcine myometrial explants during early implantation. Theriogenology (2020) 156:205–13. doi: 10.1016/j.theriogenology.2020.07.002
81. Xie MY, Han D, Xu XJ. Orexin a promotes progesterone secretion in luteinized granulose cells of Mongolian ovis Aries ovary by Prrt2 and Abcg1 genes. Zygote (2021) 29:286–92. doi: 10.1017/S096719942000088x
82. Frederick AR, Heras J, Friedman CS, German DP. Withering syndrome induced gene expression changes and a de-Novo transcriptome for the Pinto abalone. Haliotis Kamtschatkana Comp Biochem Phys D (2022) 41:100930. doi: 10.1016/j.cbd.2021.100930
83. Hrdlickova R, Toloue M, Tian B. Rna-seq methods for transcriptome analysis. Wiley Interdiscip Rev RNA (2017) 8:e1364. doi: 10.1002/wrna.1364
84. Nassel DR, Zandawala M. Recent advances in neuropeptide signaling in Drosophila, from genes to physiology and behavior. Prog Neurobiol (2019) 179:101607. doi: 10.1016/j.pneurobio.2019.02.003
85. Jiang JL, Qi LN, Lv ZP, Wei QW, Shi FX. Dietary stevioside supplementation increases feed intake by altering the hypothalamic transcriptome profile and gut microbiota in broiler chickens. J Sci Food Agr (2021) 101:2156–67. doi: 10.1002/jsfa.10838
86. Nishimura S, Bilguvar K, Ishigame K, Sestan N, Gunel M, Louvi A. Functional synergy between cholecystokinin receptors cckar and cckbr in mammalian brain development. PloS One (2015) 10:e0124295. doi: 10.1371/journal.pone.0124295
87. Lin LC, Sibille E. Somatostatin, neuronal vulnerability and behavioral emotionality. Mol Psychiatr (2015) 20:377–87. doi: 10.1038/mp.2014.184
88. Weng GH, Zhou B, Liu T, Huang ZX, Huang SX. Tetramethylpyrazine improves cognitive function of alzheimer's disease mice by regulating Sstr4 ubiquitination. Drug Des Dev Ther (2021) 15:2385–99. doi: 10.2147/Dddt.S290030
89. Lander ES. Initial impact of the sequencing of the human genome. Nature (2011) 470:187–97. doi: 10.1038/nature09792
90. Pino LK, Searle BC, Bollinger JG, Nunn B, MacLean B, MacCoss MJ. The skyline ecosystem: Informatics for quantitative mass spectrometry proteomics. Mass Spectrom Rev (2020) 39:229–44. doi: 10.1002/mas.21540
91. Lay AC, Barrington AF, Hurcombe JA, Ramnath RD, Graham M, Lewis PA, et al. A role for npy-Npy2r signaling in albuminuric kidney disease. Proc Natl Acad Sci U.S.A. (2020) 117:15862–73. doi: 10.1073/pnas.2004651117
92. Wild CP, Scalbert A, Herceg Z. Measuring the exposome: A powerful basis for evaluating environmental exposures and cancer risk. Environ Mol Mutagen (2013) 54:480–99. doi: 10.1002/em.21777
93. Wishart DS. Emerging applications of metabolomics in drug discovery and precision medicine. Nat Rev Drug Discovery (2016) 15:473–84. doi: 10.1038/nrd.2016.32
94. Schmidt-Supprian M, Rajewsky K. Vagaries of conditional gene targeting. Nat Immunol (2007) 8:665–8. doi: 10.1038/ni0707-665
95. Shiozaki K, Kawabe M, Karasuyama K, Kurachi T, Hayashi A, Ataka K, et al. Neuropeptide y deficiency induces anxiety-like behaviours in zebrafish (Danio rerio). Sci Rep (2020) 10:5913. doi: 10.1038/s41598-020-62699-0
96. Kawabe M, Hayashi A, Komatsu M, Inui A, Shiozaki K. Ninjinyoeito improves anxiety behavior in neuropeptide y deficient zebrafish. Neuropeptides (2021) 87:102136. doi: 10.1016/j.npep.2021.102136
97. Baldock PA, Allison SJ, Lundberg P, Lee NJ, Slack K, Lin EJ, et al. Novel role of Y1 receptors in the coordinated regulation of bone and energy homeostasis. J Biol Chem (2007) 282:19092–102. doi: 10.1074/jbc.M700644200
98. Bertocchi I, Oberto A, Longo A, Palanza P, Eva C. Conditional inactivation of Npy1r gene in mice induces sex-related differences of metabolic and behavioral functions. Horm Behav (2020) 125:104824. doi: 10.1016/j.yhbeh.2020.104824
99. Malone IG, Hunter BK, Rossow HL, Herzog H, Zolotukhin S, Munger SD, et al. Y1 receptors modulate taste-related behavioral responsiveness in Male mice to prototypical gustatory stimuli. Horm Behav (2021) 136:105056. doi: 10.1016/j.yhbeh.2021.105056
100. Wee NKY, Sinder BP, Novak S, Wang X, Stoddard C, Matthews BG, et al. Skeletal phenotype of the neuropeptide y knockout mouse. Neuropeptides (2019) 73:78–88. doi: 10.1016/j.npep.2018.11.009
101. Goodman EK, Mitchell CS, Teo JD, Gladding JM, Abbott KN, Rafiei N, et al. The effect of insulin receptor deletion in neuropeptide y neurons on hippocampal dependent cognitive function in aging mice. J Integr Neurosci (2022) 21:6. doi: 10.31083/j.jin2101006
102. Mitchell CS, Goodman EK, Tedesco CR, Nguyen K, Zhang L, Herzog H, et al. The effect of dietary fat and sucrose on cognitive functioning in mice lacking insulin signaling in neuropeptide y neurons. Front Physiol (2022) 13:841935. doi: 10.3389/fphys.2022.841935
103. Qi Y, Lee NJ, Ip CK, Enriquez R, Tasan R, Zhang L, et al. Npy derived from agrp neurons controls feeding Via Y1 and energy expenditure and food foraging behaviour Via Y2 signalling. Mol Metab (2022) 59:101455. doi: 10.1016/j.molmet.2022.101455
104. Engstrom Ruud L, Pereira MMA, de Solis AJ, Fenselau H, Bruning JC. Npy mediates the rapid feeding and glucose metabolism regulatory functions of agrp neurons. Nat Commun (2020) 11:442. doi: 10.1038/s41467-020-14291-3
105. Paterlini S, Panelli R, Gioiosa L, Parmigiani S, Franceschini P, Bertocchi I, et al. Conditional inactivation of limbic neuropeptide y-1 receptors increases vulnerability to diet-induced obesity in Male mice. Int J Mol Sci (2021) 22:8745. doi: 10.3390/ijms22168745
106. Wee NKY, Vrhovac Madunic I, Ivanisevic T, Sinder BP, Kalajzic I. Divergent effects of peripheral and global neuropeptide y deletion. J Musculoskelet Neuronal Interact (2020) 20:579–90. doi: 10.1042/cs19980226
107. Lee NJ, Clarke IM, Zengin A, Enriquez RF, Nagy V, Penninger JM, et al. Rank deletion in neuropeptide y neurones attenuates oestrogen deficiency-related bone loss. J Neuroendocrinol (2019) 31:e12687. doi: 10.1111/jne.12687
108. Lee NJ, Clarke IM, Enriquez RF, Nagy V, Penninger J, Baldock PA, et al. Central rank signalling in npy neurons alters bone mass in Male mice. Neuropeptides (2018) 68:75–83. doi: 10.1016/j.npep.2018.02.004
109. Zhang L, Reed F, Herzog H. Leptin signalling on arcuate npy neurones controls adiposity independent of energy balance or diet composition. J Neuroendocrinol (2020) 32:e12898. doi: 10.1111/jne.12898
110. Loh K, Shi YC, Bensellam M, Lee K, Laybutt DR, Herzog H. Y1 receptor deficiency in beta-cells leads to increased adiposity and impaired glucose metabolism. Sci Rep (2018) 8:11835. doi: 10.1038/s41598-018-30140-2
111. Huang W, Zhang Q, Qi H, Shi P, Song C, Liu Y, et al. Deletion of neuropeptide y attenuates cardiac dysfunction and apoptosis during acute myocardial infarction. Front Pharmacol (2019) 10:1268. doi: 10.3389/fphar.2019.01268
112. Kaushik MK, Aritake K, Imanishi A, Kanbayashi T, Ichikawa T, Shimizu T, et al. Continuous intrathecal orexin delivery inhibits cataplexy in a murine model of narcolepsy. Proc Natl Acad Sci U.S.A. (2018) 115:6046–51. doi: 10.1073/pnas.1722686115
113. Zhou S, Yamashita A, Su J, Zhang Y, Wang W, Hao L, et al. Activity of putative orexin neurons during cataplexy. Mol Brain (2022) 15:21. doi: 10.1186/s13041-022-00907-w
114. Kakizaki M, Tsuneoka Y, Takase K, Kim SJ, Choi J, Ikkyu A, et al. Differential roles of each orexin receptor signaling in obesity. Iscience (2019) 20:1–13. doi: 10.1016/j.isci.2019.09.003
115. Faesel N, Kolodziejczyk MH, Koch M, Fendt M. Orexin deficiency affects sociability and the acquisition, expression, and extinction of conditioned social fear. Brain Res (2021) 1751:147199. doi: 10.1016/j.brainres.2020.147199
116. Mori T, Uzawa N, Masukawa D, Hirayama S, Iwase Y, Hokazono M, et al. Enhancement of the rewarding effects of 3,4-methylenedioxymethamphetamine in orexin knockout mice. Behav Brain Res (2021) 396:112802. doi: 10.1016/j.bbr.2020.112802
117. Yukitake H, Fujimoto T, Ishikawa T, Suzuki A, Shimizu Y, Rikimaru K, et al. Tak-925, an orexin 2 receptor-selective agonist, shows robust wake-promoting effects in mice. Pharmacol Biochem Behav (2019) 187:172794. doi: 10.1016/j.pbb.2019.172794
118. Mochizuki A, Nakayama K, Nakamura S, Dantsuji M, Kamijo R, Shioda S, et al. Involvement of orexin in lipid accumulation in the liver. J Oral Biosci (2018) 60:76–82. doi: 10.1016/j.job.2018.07.001
119. Miyata K, Ikoma Y, Murata K, Kusumoto-Yoshida I, Kobayashi K, Kuwaki T, et al. Multifaceted roles of orexin neurons in mediating methamphetamine-induced changes in body temperature and heart rate. IBRO Neurosci Rep (2022) 12:108–20. doi: 10.1016/j.ibneur.2022.01.002
120. Berteotti C, Lo Martire V, Alvente S, Bastianini S, Bombardi C, Matteoli G, et al. Orexin/Hypocretin and histamine cross-talk on hypothalamic neuron counts in mice. Front Neurosci (2021) 15:660518. doi: 10.3389/fnins.2021.660518
121. Berteotti C, Lo Martire V, Alvente S, Bastianini S, Matteoli G, Silvani A, et al. Effect of ambient temperature on sleep breathing phenotype in mice: The role of orexins. J Exp Biol (2020) 223:jeb219485. doi: 10.1242/jeb.219485
122. Khalil R, Fendt M. Increased anxiety but normal fear and safety learning in orexin-deficient mice. Behav Brain Res (2017) 320:210–8. doi: 10.1016/j.bbr.2016.12.007
123. Tabuchi S, Tsunematsu T, Black SW, Tominaga M, Maruyama M, Takagi K, et al. Conditional ablation of Orexin/Hypocretin neurons: A new mouse model for the study of narcolepsy and orexin system function. J Neurosci (2014) 34:6495–509. doi: 10.1523/JNEUROSCI.0073-14.2014
124. Steiner N, Rossetti C, Sakurai T, Yanagisawa M, de Lecea L, Magistretti PJ, et al. Hypocretin/Orexin deficiency decreases cocaine abuse liability. Neuropharmacology (2018) 133:395–403. doi: 10.1016/j.neuropharm.2018.02.010
125. Seifinejad A, Li S, Possovre ML, Vassalli A, Tafti M. Hypocretinergic interactions with the serotonergic system regulate rem sleep and cataplexy. Nat Commun (2020) 11:6034. doi: 10.1038/s41467-020-19862-y
126. Futatsuki T, Yamashita A, Ikbar KN, Yamanaka A, Arita K, Kakihana Y, et al. Involvement of orexin neurons in fasting- and central adenosine-induced hypothermia. Sci Rep (2018) 8:2717. doi: 10.1038/s41598-018-21252-w
127. Naganuma F, Bandaru SS, Absi G, Mahoney CE, Scammell TE, Vetrivelan R. Melanin-concentrating hormone neurons contribute to dysregulation of rapid eye movement sleep in narcolepsy. Neurobiol Dis (2018) 120:12–20. doi: 10.1016/j.nbd.2018.08.012
128. Roman A, Meftah S, Arthaud S, Luppi PH, Peyron C. The inappropriate occurrence of rapid eye movement sleep in narcolepsy is not due to a defect in homeostatic regulation of rapid eye movement sleep. Sleep (2018) 41:zsy046. doi: 10.1093/sleep/zsy046
129. Li S, Franken P, Vassalli A. Bidirectional and context-dependent changes in theta and gamma oscillatory brain activity in noradrenergic cell-specific Hypocretin/Orexin receptor 1-ko mice. Sci Rep (2018) 8:15474. doi: 10.1038/s41598-018-33069-8
130. Quaresma PGF, Teixeira PDS, Furigo IC, Wasinski F, Couto GC, Frazao R, et al. Growth Hormone/Stat5 signaling in proopiomelanocortin neurons regulates glucoprivic hyperphagia. Mol Cell Endocrinol (2019) 498:110574. doi: 10.1016/j.mce.2019.110574
131. Reilly AM, Zhou S, Panigrahi SK, Yan S, Conley JM, Sheets PL, et al. Gpr17 deficiency in pomc neurons ameliorates the metabolic derangements caused by long-term high-fat diet feeding. Nutr Diabetes (2019) 9:29. doi: 10.1038/s41387-019-0096-7
132. He Z, Lieu L, Dong Y, Afrin S, Chau D, Kabahizi A, et al. Perk in pomc neurons connects celastrol with metabolism. JCI Insight (2021) 6:e145306. doi: 10.1172/jci.insight.145306
133. Zhou Y, Rubinstein M, Low MJ, Kreek MJ. V1b receptor antagonist Ssr149415 and naltrexone synergistically decrease excessive alcohol drinking in Male and female mice. Alcohol Clin Exp Res (2018) 42:195–205. doi: 10.1111/acer.13544
134. Zhou Y, Leri F, Low MJ, Kreek MJ. Sex differences in the effect of bupropion and naltrexone combination on alcohol drinking in mice. Pharmacol Biochem Behav (2019) 181:28–36. doi: 10.1016/j.pbb.2019.04.004
135. Zhou Y, Liang YP, Low MJ, Kreek MJ. Nuclear transcriptional changes in hypothalamus of pomc enhancer knockout mice after excessive alcohol drinking. Genes Brain Behav (2019) 18:e12600. doi: 10.1111/gbb.12600
136. Yu H, Thompson Z, Kiran S, Jones GL, Mundada L, Surbhi, et al. Expression of a hypomorphic pomc allele alters leptin dynamics during late pregnancy. J Endocrinol (2020) 245:115–27. doi: 10.1530/JOE-19-0576
137. Deshpande D, Agarwal N, Fleming T, Gaveriaux-Ruff C, Klose CSN, Tappe-Theodor A, et al. Loss of pomc-mediated antinociception contributes to painful diabetic neuropathy. Nat Commun (2021) 12:426. doi: 10.1038/s41467-020-20677-0
138. Gao Y, Li J, Zhang Z, Zhang R, Pollock A, Sun T. Microrna mir-7 and mir-17-92 in the arcuate nucleus of mouse hypothalamus regulate sex-specific diet-induced obesity. Mol Neurobiol (2019) 56:7508–21. doi: 10.1007/s12035-019-1618-y
139. Wang ZG, Khor S, Cai DS. Age-dependent decline of hypothalamic Hif2 alpha in response to insulin and its contribution to advanced age-associated metabolic disorders in mice. J Biol Chem (2019) 294:4946–55. doi: 10.1074/jbc.RA118.005429
140. Brix LM, Hausl AS, Toksoz I, Bordes J, van Doeselaar L, Engelhardt C, et al. The Co-chaperone Fkbp51 modulates hpa axis activity and age-related maladaptation of the stress system in pituitary proopiomelanocortin cells. Psychoneuroendocrinology (2022) 138:105670. doi: 10.1016/j.psyneuen.2022.105670
141. Haissaguerre M, Ferriere A, Simon V, Saucisse N, Dupuy N, Andre C, et al. Mtorc1-dependent increase in oxidative metabolism in pomc neurons regulates food intake and action of leptin. Mol Metab (2018) 12:98–106. doi: 10.1016/j.molmet.2018.04.002
142. Jeong JH, Lee DK, Liu SM, Chua SC Jr., Schwartz GJ, Jo YH. Activation of temperature-sensitive Trpv1-like receptors in arc pomc neurons reduces food intake. PloS Biol (2018) 16:e2004399. doi: 10.1371/journal.pbio.2004399
143. Guo DF, Reho JJ, Morgan DA, Rahmouni K. Cardiovascular regulation by the neuronal bbsome. Hypertension (2020) 75:1082–90. doi: 10.1161/HYPERTENSIONAHA.119.14373
144. Liu ZY, Cheng YL, Luan Y, Zhong WL, Lai HJ, Wang H, et al. Short-term tamoxifen treatment has long-term effects on metabolism in high-fat diet-fed mice with involvement of Nmnat2 in pomc neurons. FEBS Lett (2018) 592:3305–16. doi: 10.1002/1873-3468.13240
145. Guo DF, Lin ZH, Wu YM, Searby C, Thedens DR, Richerson GB, et al. The bbsome in pomc and agrp neurons is necessary for body weight regulation and sorting of metabolic receptors. Diabetes (2019) 68:1591–603. doi: 10.2337/db18-1088
146. Surbhi, Wittmann G, Low MJ, Lechan RM. Adult-born proopiomelanocortin neurons derived from rax-expressing precursors mitigate the metabolic effects of congenital hypothalamic proopiomelanocortin deficiency. Mol Metab (2021) 53:101312. doi: 10.1016/j.molmet.2021.101312
147. Wang Z, do Carmo JM, da Silva AA, Bailey KC, Aberdein N, Moak SP, et al. Role of Socs3 in pomc neurons in metabolic and cardiovascular regulation. Am J Physiol-Reg I (2019) 316:338–51. doi: 10.1152/ajpregu.00163.2018
148. Timper K, Paeger L, Sanchez-Lasheras C, Varela L, Jais A, Nolte H, et al. Mild impairment of mitochondrial oxphos promotes fatty acid utilization in pomc neurons and improves glucose homeostasis in obesity. Cell Rep (2018) 25:383–97. doi: 10.1016/j.celrep.2018.09.034
149. Aberdein N, Dambrino RJ, do Carmo JM, Wang Z, Mitchell LE, Drummond HA, et al. Role of Ptp1b in pomc neurons during chronic high-fat diet: Sex differences in regulation of liver lipids and glucose tolerance. Am J Physiol-Reg I (2018) 314:478–88. doi: 10.1152/ajpregu.00287.2017
150. Yang YJ, He YL, Liu HL, Zhou WJ, Wang CM, Xu PW, et al. Hypothalamic steroid receptor coactivator-2 regulates adaptations to fasting and overnutrition. Cell Rep (2021) 37:110075. doi: 10.1016/j.celrep.2021.110075
151. Park S, Aintablian A, Coupe B, Bouret SG. The endoplasmic reticulum stress-autophagy pathway controls hypothalamic development and energy balance regulation in leptin-deficient neonates. Nat Commun (2020) 11:1––11. doi: 10.1038/s41467-020-15624-y
152. Zhao W, Chen J, Louro B, Cao L, Ma J, Martins RST, et al. Transcriptome sequencing analysis of somatostatin 1 gene mutation in zebrafish larvae. J Ocean Univ China (2021) 30:777–88. doi: 10.12024/jsou.20200403009
153. Sui C, Chen J, Ma J, Zhao W, Canario AVM, Martins RST. Somatostatin 4 regulates growth and modulates gametogenesis in zebrafish. Aquacult Fisheries (2019) 4:239–46. doi: 10.1016/j.aaf.2019.05.002
154. Scheich B, Cseko K, Borbely E, Abraham I, Csernus V, Gaszner B, et al. Higher susceptibility of somatostatin 4 receptor gene-deleted mice to chronic stress-induced behavioral and neuroendocrine alterations. Neuroscience (2017) 346:320–36. doi: 10.1016/j.neuroscience.2017.01.039
155. Prevot TD, Gastambide F, Viollet C, Henkous N, Martel G, Epelbaum J, et al. Roles of hippocampal somatostatin receptor subtypes in stress response and emotionality. Neuropsychopharmacol (2017) 42:1647–56. doi: 10.1038/npp.2016.281
156. Prevot TD, Viollet C, Epelbaum J, Dominguez G, Beracochea D, Guillou JL. Sst(2)-receptor gene deletion exacerbates chronic stress-induced deficits: Consequences for emotional and cognitive ageing. Prog Neuro-Psychoph (2018) 86:390–400. doi: 10.1016/j.pnpbp.2018.01.022
157. Li XJ. Effect of Sstr2 inhibition on growth hormone secretion in mice and its mechanism. Acta Med Mediterr (2021) 37:2765–70. doi: 10.19193/0393-6384_2021_5_426
158. Ezra-Nevo G, Prestori F, Locatelli F, Soda T, Ten Brinke MM, Engel M, et al. Cerebellar learning properties are modulated by the crf receptor. J Neurosci (2018) 38:6751–65. doi: 10.1523/JNEUROSCI.3106-15.2018
159. D'Costa S, Ayyadurai S, Gibson AJ, Mackey E, Rajput M, Sommerville LJ, et al. Mast cell corticotropin-releasing factor subtype 2 suppresses mast cell degranulation and limits the severity of anaphylaxis and stress-induced intestinal permeability. J Allergy Clin Immunol (2019) 143:1865–1877.e1864. doi: 10.1016/j.jaci.2018.08.053
160. Morisot N, Millan MJ, Contarino A. Crf1 receptor-deficiency induces anxiety-like vulnerability to cocaine. Psychopharmacol (Berl) (2014) 231:3965–72. doi: 10.1007/s00213-014-3534-1
161. Morisot N, Contarino A. The Crf1 and the Crf2 receptor mediate recognition memory deficits and vulnerability induced by opiate withdrawal. Neuropharmacology (2016) 105:500–7. doi: 10.1016/j.neuropharm.2016.02.021
162. Shemesh Y, Forkosh O, Mahn M, Anpilov S, Sztainberg Y, Manashirov S, et al. Ucn3 and crf-R2 in the medial amygdala regulate complex social dynamics. Nat Neurosci (2016) 19:1489–96. doi: 10.1038/nn.4346
163. Paruthiyil S, Hagiwara SI, Kundassery K, Bhargava A. Sexually dimorphic metabolic responses mediated by Crf2 receptor during nutritional stress in mice. Biol Sex Differ (2018) 9:49. doi: 10.1186/s13293-018-0208-4
164. Morisot N, Le Moine C, Millan MJ, Contarino A. Crf(2) receptor-deficiency reduces recognition memory deficits and vulnerability to stress induced by cocaine withdrawal. Int J Neuropsychopharmacol (2014) 17:1969–79. doi: 10.1017/S1461145714000625
165. Romero A, Garcia-Carmona JA, Laorden ML, Puig MM. Role of Crf1 receptor in post-incisional plasma extravasation and nociceptive responses in mice. Toxicol Appl Pharm (2017) 332:121–8. doi: 10.1016/j.taap.2017.04.021
166. Morisot N, Monier R, Le Moine C, Millan MJ, Contarino A. Corticotropin-releasing factor receptor 2-deficiency eliminates social behaviour deficits and vulnerability induced by cocaine. Br J Pharmacol (2018) 175:1504–18. doi: 10.1111/bph.14159
167. Miyamoto S, Shikata K, Miyasaka K, Okada S, Sasaki M, Kodera R, et al. Cholecystokinin plays a novel protective role in diabetic kidney through anti-inflammatory actions on macrophage: Anti-inflammatory effect of cholecystokinin. Diabetes (2012) 61:897–907. doi: 10.2337/db11-0402
168. King A, Yang Q, Huesman S, Rider T, Lo CC. Lipid transport in cholecystokinin knockout mice. Physiol Behav (2015) 151:198–206. doi: 10.1016/j.physbeh.2015.07.009
169. Wang HH, Liu M, Portincasa P, Tso P, Wang DQH. Lack of endogenous cholecystokinin promotes cholelithogenesis in mice. Neurogastroent Motil (2016) 28:364–75. doi: 10.1111/nmo.12734
170. Yoshida R, Shin M, Yasumatsu K, Takai S, Inoue M, Shigemura N, et al. The role of cholecystokinin in peripheral taste signaling in mice. Front Physiol (2017) 8:866. doi: 10.3389/fphys.2017.00866
171. Mohammad S, Ozaki T, Takeuchi K, Unno K, Yamoto K, Morioka E, et al. Functional compensation between cholecystokinin-1 and -2 receptors in murine paraventricular nucleus neurons. J Biol Chem (2012) 287:39391–401. doi: 10.1074/jbc.M112.416214
172. Thomas KR, Capecchi MR. Site-directed mutagenesis by gene targeting in mouse embryo-derived stem cells. Cell (1987) 51:503–12. doi: 10.1016/0092-8674(87)90646-5
173. Gupta D, Bhattacharjee O, Mandal D, Sen MK, Dey D, Dasgupta A, et al. Crispr-Cas9 system: A new-fangled dawn in gene editing. Life Sci (2019) 232:116636. doi: 10.1016/j.lfs.2019.116636
174. Tillinger A, Nostramo R, Kvetnansky R, Serova L, Sabban EL. Stress-induced changes in gene expression of urocortin 2 and other crh peptides in rat adrenal medulla: Involvement of glucocorticoids. J Neurochem (2013) 125:185–92. doi: 10.1111/jnc.12152
175. Giannogonas P, Apostolou A, Manousopoulou A, Theocharis S, Macari SA, Psarras S, et al. Identification of a novel interaction between corticotropin releasing hormone (Crh) and macroautophagy. Sci Rep (2016) 6:23342. doi: 10.1038/srep23342
176. Hasdemir B, Mhaske P, Paruthiyil S, Garnett EA, Heyman MB, Matloubian M, et al. Sex- and corticotropin-releasing factor receptor 2- dependent actions of urocortin 1 during inflammation. Am J Physiol Regul Integr Comp Physiol (2016) 310:1244–57. doi: 10.1152/ajpregu.00445.2015
177. Yang F, Liu C, Chen D, Tu M, Xie H, Sun H, et al. Crispr/Cas9-Loxp-Mediated gene editing as a novel site-specific genetic manipulation tool. Mol Ther Nucleic Acids (2017) 7:378–86. doi: 10.1016/j.omtn.2017.04.018
178. Kos CH. Cre/Loxp system for generating tissue-specific knockout mouse models. Nutr Rev (2004) 62:243–6. doi: 10.1301/nr2004.jun243-246
179. Sauer B, Henderson N. Site-specific DNA recombination in mammalian cells by the cre recombinase of bacteriophage P1. Proc Natl Acad Sci U.S.A. (1988) 85:5166–70. doi: 10.1073/pnas.85.14.5166
180. Gaveriaux-Ruff C, Kieffer BL. Conditional gene targeting in the mouse nervous system: Insights into brain function and diseases. Pharmacol Ther (2007) 113:619–34. doi: 10.1016/j.pharmthera.2006.12.003
181. Gu H, Marth JD, Orban PC, Mossmann H, Rajewsky K. Deletion of a DNA polymerase beta gene segment in T cells using cell type-specific gene targeting. Science (1994) 265:103–6. doi: 10.1126/science.8016642
182. Wang F, Chen WM, Lin HR, Li WS. Cloning, expression, and ligand-binding characterization of two neuropeptide y receptor subtypes in orange-spotted grouper. Epinephelus Coioides Fish Physiol Biochem (2014) 40:1693–707. doi: 10.1007/s10695-014-9960-5
183. Haiyan D, Wensheng L, Haoran L. Comparative analyses of sequence structure, evolution, and expression of four somatostatin receptors in orange-spotted grouper (Epinephelus coioides). Mol Cell Endocrinol (2010) 323:125–36. doi: 10.1016/j.mce.2010.03.016
184. Cerda-Reverter JM, Larhammar D. Neuropeptide y family of peptides: Structure, anatomical expression, function, and molecular evolution. Biochem Cell Biol (2000) 78:371–92. doi: 10.1139/bcb-78-3-371
185. Larsson TA, Tay BH, Sundstrom G, Fredriksson R, Brenner S, Larhammar D, et al. Neuropeptide y-family peptides and receptors in the elephant shark, Callorhinchus milii confirm gene duplications before the gnathostome radiation. Genomics (2009) 93:254–60. doi: 10.1016/j.ygeno.2008.10.001
186. Soderberg C, Pieribone VA, Dahlstrand J, Brodin L, Larhammar D. Neuropeptide role of both peptide yy and neuropeptide y in vertebrates suggested by abundant expression of their mrnas in a cyclostome brain. J Neurosci Res (1994) 37:633–40. doi: 10.1002/jnr.490370510
187. Larhammar D, Bergqvist CA. Ancient grandeur of the vertebrate neuropeptide y system shown by the coelacanth. Latimeria Chalumnae Front Neurosci (2013) 7:27. doi: 10.3389/fnins.2013.00027
188. Yang SY, Xu GC, Du FK, Xu P. Molecular cloning, tissue and embryonic development expression, and appetite regulatory effect of pancreatic peptide y in. Coilia Nasus Fisheries Sci (2016) 82:347–55. doi: 10.1007/s12562-015-0960-x
189. Salaneck E, Larsson TA, Larson ET, Larhammar D. Birth and death of neuropeptide y receptor genes in relation to the teleost fish tetraploidization. Gene (2008) 409:61–71. doi: 10.1016/j.gene.2007.11.011
190. Sundstrom G, Larsson TA, Xu B, Heldin J, Larhammar D. Interactions of zebrafish peptide yyb with the neuropeptide y-family receptors Y4, Y7, Y8a, and Y8b. Front Neurosci-Switz (2013) 7:29. doi: 10.3389/fnins.2013.00029
191. Fredriksson R, Sjodin P, Larson ET, Conlon JM, Larhammar D. Cloning and characterization of a zebrafish Y2 receptor. Regul Pept (2006) 133:32–40. doi: 10.1016/j.regpep.2005.09.013
192. Yokobori E, Azuma M, Nishiguchi R, Kang KS, Kamijo M, Uchiyama M, et al. Neuropeptide y stimulates food intake in the zebrafish. Danio Rerio J Neuroendocrinol (2012) 24:766–73. doi: 10.1111/j.1365-2826.2012.02281.x
193. Chen Y, Shen YB, Pandit NP, Fu JJ, Li D, Li JL. Molecular cloning, expression analysis, and potential food intake attenuation effect of peptide yy in grass carp (Ctenopharyngodon idellus). Gen Comp Endocr (2013) 187:66–73. doi: 10.1016/j.ygcen.2013.03.029
194. Chen Y, Pandit NP, Fu JJ, Li D, Li JL. Identification, characterization and feeding response of peptide yyb (Pyyb) gene in grass carp (Ctenopharyngodon idellus). Fish Physiol Biochem (2014) 40:45–55. doi: 10.1007/s10695-013-9822-6
195. Zhou Y, Liang XF, Yuan XC, Li J, He Y, Fang L, et al. Neuropeptide y stimulates food intake and regulates metabolism in grass carp. Ctenopharyngodon Idellus Aquacult (2013) 380:52–61. doi: 10.1016/j.aquaculture.2012.11.033
196. Kumar S, Singh U, Saha S, Singru PS. Tyrosine hydroxylase in the olfactory system, forebrain and pituitary of the Indian major carp, Cirrhinus cirrhosus: Organisation and interaction with neuropeptide y in the preoptic area. J Neuroendocrinol (2014) 26:400–11. doi: 10.1111/jne.12160
197. Gonzalez R, Unniappan S. Molecular characterization, appetite regulatory effects and feeding related changes of peptide yy in goldfish. Gen Comp Endocr (2010) 166:273–9. doi: 10.1016/j.ygcen.2009.09.008
198. Lopez-Patino MA, Guijarro AI, Isorna E, Delgado MJ, Alonso-Bedate M, de Pedro N. Neuropeptide y has a stimulatory action on feeding behavior in goldfish (Carassius auratus). Eur J Pharmacol (1999) 377:147–53. doi: 10.1016/S0014-2999(99)00408-2
199. Wei R, Zhou C, Yuan D, Wang T, Lin F, Chen H, et al. Characterization, tissue distribution and regulation of neuropeptidey in. Schizothorax Prenanti J Fish Biol (2014) 85:278–91. doi: 10.1111/jfb.12413
200. Volkoff H. Appetite regulating peptides in red-bellied piranha, pygocentrus nattereri: Cloning, tissue distribution and effect of fasting on mrna expression levels. Peptides (2014) 56:116–24. doi: 10.1016/j.peptides.2014.03.022
201. Wall A, Volkoff H. Effects of fasting and feeding on the brain mrna expressions of orexin, tyrosine hydroxylase (Th), pyy and cck in the Mexican blind cavefish (Astyanax fasciatus mexicanus). Gen Comp Endocrinol (2013) 183:44–52. doi: 10.1016/j.ygcen.2012.12.011
202. London S, Volkoff H. Cloning and effects of fasting on the brain expression levels of appetite-regulators and reproductive hormones in glass catfish (Kryptopterus vitreolus). Comp Biochem Phys A (2019) 228:94–102. doi: 10.1016/j.cbpa.2018.11.009
203. Cao L, Liang HW, Zhong LI, Wang XY, Ding WQ, XXXZ. GW. Cloning and expression analysis of npy gene in yellow catfish, Pelteobagrus fulvidraco. J Northwest A&F Univ (2013) 41:1–142. doi: 10.4172/2168-9652.1000202
204. Kurokawa T, Suzuki T. Development of neuropeptide y-related peptides in the digestive organs during the larval stage of Japanese flounder. Paralichthys Olivaceus Gen Comp Endocr (2002) 126:30–8. doi: 10.1006/gcen.2001.7774
205. Hosomi N, Furutani T, Takahashi N, Masumoto T, Fukada H. Yellowtail neuropeptide y: Molecular cloning, tissue distribution, and response to fasting. Fisheries Sci (2014) 80:483–92. doi: 10.1007/s12562-014-0711-4
206. Murashita K, Kurokawa T, Ebbesson LO, Stefansson SO, Rønnestad I. Characterization, tissue distribution, and regulation of agouti-related protein (Agrp), cocaine-and amphetamine-regulated transcript (Cart) and neuropeptide y (Npy) in Atlantic salmon (Salmo salar). Gen Comp Endocr (2009) 162:160–71. doi: 10.1016/j.ygcen.2009.03.015
207. Larsson TA, Larson ET, Fredriksson R, Conlon JM, Larhammar D. Characterization of npy receptor subtypes Y2 and Y7 in rainbow trout. Oncorhynchus Mykiss Peptides (2006) 27:1320–7. doi: 10.1016/j.peptides.2005.10.008
208. Aldegunde M, Mancebo M. Effects of neuropeptide y on food intake and brain biogenic amines in the rainbow trout (Oncorhynchus mykiss). Peptides (2006) 27:719–27. doi: 10.1016/j.peptides.2005.09.014
209. Velasco C, Blanco AM, Unniappan S, Soengas JL. The anorectic effect of central Pyy1-36 treatment in rainbow trout (Oncorhynchus mykiss) is associated with changes in mrnas encoding neuropeptides and parameters related to fatty acid sensing and metabolism. Gen Comp Endocr (2018) 267:137–45. doi: 10.1016/j.ygcen.2018.06.015
210. Kehoe AS, Volkoff H. Cloning and characterization of neuropeptide y (Npy) and cocaine and amphetamine regulated transcript (Cart) in Atlantic cod (Gadus morhua). Comp Biochem Phys A (2007) 146:451–61. doi: 10.1016/j.cbpa.2006.12.026
211. Arvidsson AK, Wraith A, Jonsson-Rylander AC, Larhammar D. Cloning of a neuropeptide Y/Peptide yy receptor from the Atlantic cod: The yb receptor. Regul Pept (1998) 75-76:39–43. doi: 10.1016/s0167-0115(98)00051-2
212. Tuziak SM, Rise ML, Volkoff H. An investigation of appetite-related peptide transcript expression in Atlantic cod (Gadus morhua) brain following a camelina sativa meal-supplemented feeding trial. Gene (2014) 550:253–63. doi: 10.1016/j.gene.2014.08.039
213. Mountjoy KG, Caron A, Hubbard K, Shome A, Grey AC, Sun B, et al. Desacetyl-Alpha-Melanocyte stimulating hormone and alpha-melanocyte stimulating hormone are required to regulate energy balance. Mol Metab (2018) 9:207–16. doi: 10.1016/j.molmet.2017.11.008
214. Wang Q, Tan XG, Du SJ, Sun W, You F, Zhang PJ. Characterization, tissue distribution, and expression of neuropeptide y in olive flounder. Paralichthys Olivaceus Chin J Oceanol Limn (2015) 33:553–8. doi: 10.1007/s00343-015-4090-1
215. Sundstrom G, Larsson TA, Brenner S, Venkatesh B, Larhammar D. Evolution of the neuropeptide y family: New genes by chromosome duplications in early vertebrates and in teleost fishes. Gen Comp Endocrinol (2008) 155:705–16. doi: 10.1016/j.ygcen.2007.08.016
216. Wang T, Liang J, Xiang X, Chen X, Zhang B, Zhou N, et al. Pharmacological characterization, cellular localization and expression profile of npy receptor subtypes Y2 and Y7 in Large yellow croaker, Larimichthys crocea. Comp Biochem Physiol B Biochem Mol Biol (2019) 238:110347. doi: 10.1016/j.cbpb.2019.110347
217. Kamijo M, Kojima K, Maruyama K, Konno N, Motohashi E, Ikegami T, et al. Neuropeptide y in tiger puffer (Takifugu rubripes): Distribution, cloning, characterization, and mrna expression responses to prandial condition. Zoolog Sci (2011) 28:882–90. doi: 10.2108/zsj.28.882
218. Larsson TA, Olsson F, Sundstrom G, Lundin LG, Brenner S, Venkatesh B, et al. Early vertebrate chromosome duplications and the evolution of the neuropeptide y receptor gene regions. BMC Evol Biol (2008) 8:184. doi: 10.1186/1471-2148-8-184
219. Li MJ, Tan XG, Sui YL, Jiao S, Wu ZH, Wang LJ, et al. The stimulatory effect of neuropeptide y on growth hormone expression, food intake, and growth in olive flounder (Paralichthys olivaceus). Fish Physiol Biochem (2017) 43:11–8. doi: 10.1007/s10695-016-0263-x
220. Yan P, Jia J, Yang G, Wang D, Sun C, Li W. Duplication of neuropeptide y and peptide yy in Nile tilapia Oreochromis niloticus and their roles in food intake regulation. Peptides (2017) 88:97–105. doi: 10.1016/j.peptides.2016.12.010
221. Grone BP, Carpenter RE, Lee M, Maruska KP, Fernald RD. Food deprivation explains effects of mouthbrooding on ovaries and steroid hormones, but not brain neuropeptide and receptor mrnas, in an African cichlid fish. Horm Behav (2012) 62:18–26. doi: 10.1016/j.yhbeh.2012.04.012
222. Babichuk NA, Volkoff H. Changes in expression of appetite-regulating hormones in the cunner (Tautogolabrus adspersus) during short-term fasting and winter torpor. Physiol Behav (2013) 120:54–63. doi: 10.1016/j.physbeh.2013.06.022
223. Wu S, Li B, Lin H, Li W. Stimulatory effects of neuropeptide y on the growth of orange-spotted grouper (Epinephelus coioides). Gen Comp Endocrinol (2012) 179:159–66. doi: 10.1016/j.ygcen.2012.08.010
224. Chen R, Li W, Lin H. Cdna cloning and mrna expression of neuropeptide y in orange spotted grouper, Epinephelus coioides. Comp Biochem Physiol B Biochem Mol Biol (2005) 142:79–89. doi: 10.1016/j.cbpc.2005.06.003
225. Nguyen MV, Jordal AE, Espe M, Buttle L, Lai HV, Ronnestad I. Feed intake and brain neuropeptide y (Npy) and cholecystokinin (Cck) gene expression in juvenile cobia fed plant-based protein diets with different lysine to arginine ratios. Comp Biochem Physiol A Mol Integr Physiol (2013) 165:328–37. doi: 10.1016/j.cbpa.2013.04.004
226. Boonanuntanasarn S, Jangprai A, Yoshizaki G. Characterization of neuropeptide y in snakeskin gourami and the change in its expression due to feeding status and melanocortin 4 receptor expression. Gen Comp Endocrinol (2012) 179:184–95. doi: 10.1016/j.ygcen.2012.07.024
227. Chen H, Zhang X, Hao J, Chen D, Liu J, Gao Y, et al. Molecular cloning, expression analysis, and appetite regulatory effect of peptide yy in Siberian sturgeon (Acipenser baerii). Gene (2015) 563:172–9. doi: 10.1016/j.gene.2015.03.028
228. Yuan DY, Gao YD, Zhang X, Wang B, Chen H, Wu YB, et al. Npy and npy receptors in the central control of feeding and interactions with cart and Mc4r in Siberian sturgeon. Gen Comp Endocr (2019) 284:113239. doi: 10.1016/j.ygcen.2019.113239
229. Soderberg C, Wraith A, Ringvall M, Yan YL, Postlethwait JH, Brodin L, et al. Zebrafish genes for neuropeptide y and peptide yy reveal origin by chromosome duplication from an ancestral gene linked to the homeobox cluster. J Neurochem (2000) 75:908–18. doi: 10.1046/j.1471-4159.2000.0750908.x
230. Murashita K, Fukada H, Hosokawa H, Masumoto T. Cholecystokinin and peptide y in yellowtail (Seriola quinqueradiata): Molecular cloning, real-time quantitative rt-pcr, and response to feeding and fasting. Gen Comp Endocr (2006) 145:287–97. doi: 10.1016/j.ygcen.2005.09.008
231. Lindner D, Stichel J, Beck-Sickinger AG. Molecular recognition of the npy hormone family by their receptors. Nutrition (2008) 24:907–17. doi: 10.1016/j.nut.2008.06.025
232. Carpio Y, Acosta J, Morales A, Herrera F, Gonzalez LJ, Estrada MP. Cloning, expression and growth promoting action of red tilapia (Oreochromis sp.) neuropeptide y. Peptides (2006) 27:710–8. doi: 10.1016/j.peptides.2005.08.013
233. Yu X, Xin Y, Cui L, Jia J, Yuan X, Fu S, et al. Effects of neuropeptide y as a feed additive on stimulating the growth of tilapia (Oreochromis niloticus) fed low fish meal diets. Peptides (2021) 138:170505. doi: 10.1016/j.peptides.2021.170505
234. Carpio Y, Leon K, Acosta J, Morales R, Estrada MP. Recombinant tilapia neuropeptide y promotes growth and antioxidant defenses in African catfish (Clarias gariepinus) fry. Aquaculture (2007) 272:649–55. doi: 10.1016/j.aquaculture.2007.08.024
235. de Pedro N, Lopez-Patino MA, Guijarro AI, Pinillos ML, Delgado MJ, Alonso-Bedate M. Npy receptors and opioidergic system are involved in npy-induced feeding in goldfish. Peptides (2000) 21:1495–502. doi: 10.1016/S0196-9781(00)00303-X
236. Narnaware YK, Peter RE. Neuropeptide y stimulates food consumption through multiple receptors in goldfish. Physiol Behav (2001) 74:185–90. doi: 10.1016/S0031-9384(01)00556-X
237. Berglund MM, Lundell I, Cabrele C, Serradeil-Le Gal C, Beck-Sickinger AG, Larhammar D. Binding properties of three neuropeptide y receptor subtypes from zebrafish: Comparison with mammalian Y1 receptors. Biochem Pharmacol (2000) 60:1815–22. doi: 10.1016/S0006-2952(00)00502-5
238. Li WS, Wang B. Mechanisms of actions of somatostatin on the regulation of pituitary growth hormone release in fish. J Fisheries China (2013) 37:1863–72. doi: 10.3724/SP.J.1231.2013.38776
239. Hobart P, Crawford R, Shen L, Pictet R, Rutter WJ. Cloning and sequence analysis of cdnas encoding two distinct somatostatin precursors found in the endocrine pancreas of anglerfish. Nature (1980) 288:137–41. doi: 10.1038/288137a0
240. Lin XW, Otto CJ, Peter RE. Evolution of neuroendocrine peptide systems: Gonadotropin-releasing hormone and somatostatin. Comp Biochem Physiol C Pharmacol Toxicol Endocrinol (1998) 119:375–88. doi: 10.1016/s0742-8413(98)00025-5
241. Eilertson CD, Kittilson JD, Sheridan MA. Effects of insulin, glucagon, and somatostatin on the release of somatostatin-25 and somatostatin-14 from rainbow trout, Oncorhynchus mykiss, pancreatic islets in vitro. Gen Comp Endocrinol (1995) 99:211–20. doi: 10.1006/gcen.1995.1104
242. Wang B, Qin CB, Zhang C, Jia JR, Sun CY, Li WS. Differential involvement of signaling pathways in the regulation of growth hormone release by somatostatin and growth hormone-releasing hormone in orange-spotted grouper (Epinephelus coioides). Mol Cell Endocrinol (2014) 382:851–9. doi: 10.1016/j.mce.2013.10.025
243. Wang B, Jia JR, Yang GK, Qin JK, Zhang C, Zhang QP, et al. In vitro effects of somatostatin on the growth hormone-Insulin-Like growth factor axis in orange-spotted grouper (Epinephelus coioides). Gen Comp Endocr (2016) 237:1–9. doi: 10.1016/j.ygcen.2015.10.014
244. Marchant TA, Fraser RA, Andrews PC, Peter RE. The influence of mammalian and teleost somatostatins on the secretion of growth hormone from goldfish (Carassius auratus l.) pituitary fragments in vitro. Regul Pept (1987) 17:41–52. doi: 10.1016/0167-0115(87)90031-0
245. Lin X, Otto CJ, Peter RE. Expression of three distinct somatostatin messenger ribonucleic acids (Mrnas) in goldfish brain: Characterization of the complementary deoxyribonucleic acids, distribution and seasonal variation of the mrnas, and action of a somatostatin-14 variant. Endocrinology (1999) 140:2089–99. doi: 10.1210/endo.140.5.6706
246. Lin XW, Peter RE. Somatostatin-like receptors in goldfish: Cloning of four new receptors. Peptides (2003) 24:53–63. doi: 10.1016/S0196-9781(02)00276-0
247. Morel A, Gluschankof P, Gomez S, Fafeur V, Cohen P. Characterization of a somatostatin-28 containing the (Tyr-7, gly-10) derivative of somatostatin-14: A terminal active product of prosomatostatin ii processing in anglerfish pancreatic islets. Proc Natl Acad Sci U.S.A. (1984) 81:7003–6. doi: 10.1073/pnas.81.22.7003
248. Ehrman MM, Melroe GT, Kittilson JD, Sheridan MA. Glucose-stimulated somatostatin gene expression in the brockmann bodies of rainbow trout (Oncorhynchus mykiss) results from increased mrna transcription and not from altered mrna stability. Zool Sci (2004) 21:87–91. doi: 10.2108/zsj.21.87
249. Gong JY, Kittilson JD, Slagter BJ, Sheridan MA. The two subtype 1 somatostatin receptors of rainbow trout, Tsst(1a) and Tsst(1b), possess both distinct and overlapping ligand binding and agonist-induced regulation features. Comp Biochem Phys B (2004) 138:295–303. doi: 10.1016/j.cbpc.2004.04.005
250. Devos N, Deflorian G, Biemar F, Bortolussi M, Martial JA, Peers B, et al. Differential expression of two somatostatin genes during zebrafish embryonic development. Mech Dev (2002) 115:133–7. doi: 10.1016/S0925-4773(02)00082-5
251. Feng X, Yu XM, Pang MX, Liu HY, Tong JO. Molecular characterization and expression of three preprosomatostatin genes and their association with growth in common carp (Cyprinus carpio). Comp Biochem Phys B (2015) 182:37–46. doi: 10.1016/j.cbpb.2014.12.001
252. Oyama H, Bradshaw RA, Bates OJ, Permutt A. Amino acid sequence of catfish pancreatic somatostatin I. J Biol Chem (1980) 255:2251–4. doi: 10.1016/S0021-9258(19)85880-5
253. Andrews PC, Dixon JE. Isolation and structure of a peptide hormone predicted from a mRNA sequence. a second somatostatin from the catfish pancreas. J Biol Chem (1981) 256:8267–70. doi: 10.1016/S0021-9258(19)68835-6
254. Hofmann HA, Fernald RD. Social status controls somatostatin neuron size and growth. J Neurosci (2000) 20:4740–4. doi: 10.1523/Jneurosci.20-12-04740.2000
255. Trainor BC, Hofmann HA. Somatostatin regulates aggressive behavior in an African cichlid fish. Endocrinology (2006) 147:5119–25. doi: 10.1210/en.2006-0511
256. Trainor BC, Hofmann HA. Somatostatin and somatostatin receptor gene expression in dominant and subordinate males of an African cichlid fish. Behav Brain Res (2007) 179:314–20. doi: 10.1016/j.bbr.2007.02.014
257. Dong HY, Chen WB, Sun C, Sun JW, Wang YL, Xie C, et al. Identification, characterization of selenoprotein W and its mrna expression patterns in response to somatostatin 14, cysteamine hydrochloride, 17 beta-estradiol and a binary mixture of 17 beta-estradiol and cysteamine hydrochloride in topmouth culter (Erythroculter ilishaeformis). Fish Physiol Biochem (2017) 43:115–26. doi: 10.1007/s10695-016-0272-9
258. Dong HY, Wei YH, Xie C, Zhu XX, Sun C, Fu QW, et al. Structural and functional analysis of two novel somatostatin receptors identified from topmouth culter (Erythroculter ilishaeformis). Comp Biochem Phys C (2018) 210:18–29. doi: 10.1016/j.cbpc.2018.04.004
259. Dai W, Zhao J, Liu J, Xue Y. Cloning,Tissue expression of three somatostatin genes of Siniperca chuatsi and their localizations in brain. J Fisheries China (2012) 36:1337–48. doi: 10.3724/SP.J.1231.2012.27887
260. Madeo M, Giusi G, Alo R, Facciolo RM, Carelli A, Canonaco M. Different somatostatin receptor subtypes are operating in the brain of the teleost fish, coris julis. J Exp Zool Part A (2005) 303a:406–13. doi: 10.1002/jez.a.184
261. Alo' R, Facciolo RM, Madeo M, Giusi G, Carelli A, Canonaco M. Effects of the xenoestrogen bisphenol a in diencephalic regions of the teleost fish Coris julis occur preferentially Via distinct somatostatin receptor subtypes. Brain Res Bull (2005) 65:267–73. doi: 10.1016/j.brainresbull.2005.01.006
262. Fryer JN, Nishioka RS, Bern HA. Somatostatin inhibition of teleost growth hormone secretion. Gen Comp Endocrinol (1979) 39:244–6. doi: 10.1016/0016-6480(79)90229-6
263. Nguyen TM, Wright JR Jr., Nielsen PF, Conlon JM. Characterization of the pancreatic hormones from the brockmann body of the tilapia: Implications for islet xenograft studies. Comp Biochem Physiol C Pharmacol Toxicol Endocrinol (1995) 111:33–44. doi: 10.1016/0742-8413(95)00023-z
264. Cruz EMV, Brown CL. The influence of social status on the rate of growth, eye color pattern and insulin-like growth factor-I gene expression in Nile tilapia. Oreochromis Niloticus Horm Behav (2007) 51:611–9. doi: 10.1016/j.yhbeh.2007.02.010
265. Conlon JM, Davis MS, Falkmer S, Thim L. Structural characterization of peptides derived from prosomatostatins I and ii isolated from the pancreatic islets of two species of teleostean fish: The daddy sculpin and the flounder. Eur J Biochem (1987) 168:647–52. doi: 10.1111/j.1432-1033.1987.tb13465.x
266. Conlon JM, Deacon CF, Hazon N, Henderson IW, Thim L. Somatostatin-related and glucagon-related peptides with unusual structural features from the European eel (Anguilla anguilla). Gen Comp Endocrinol (1988) 72:181–9. doi: 10.1016/0016-6480(88)90201-8
267. Rousseau K, Huang YS, Le Belle N, Vidal B, Marchelidon J, Epelbaum J, et al. Long-term inhibitory effects of somatostatin and insulin-like growth factor 1 on growth hormone release by serum-free primary culture of pituitary cells from European eel (Anguilla anguilla). Neuroendocrinology (1998) 67:301–9. doi: 10.1159/000054327
268. Sower SA, Chiang YC, Conlon JM. Polygenic expression of somatostatin in lamprey. Peptides (1994) 15:151–4. doi: 10.1016/0196-9781(94)90184-8
269. Tostivint H, Dettai A, Quan FB, Ravi V, Tay BH, Rodicio MC, et al. Identification of three somatostatin genes in lampreys. Gen Comp Endocr (2016) 237:89–97. doi: 10.1016/j.ygcen.2016.08.006
270. Liu Y, Lu D, Zhang Y, Li S, Liu X, Lin H. The evolution of somatostatin in vertebrates. Gene (2010) 463:21–8. doi: 10.1016/j.gene.2010.04.016
271. Tostivint H, Quan FB, Bougerol M, Kenigfest NB, Lihrmann I. Impact of Gene/Genome duplications on the evolution of the urotensin ii and somatostatin families. Gen Comp Endocrinol (2013) 188:110–7. doi: 10.1016/j.ygcen.2012.12.015
272. Yamada Y, Post SR, Wang K, Tager HS, Bell GI, Seino S. Cloning and functional characterization of a family of human and mouse somatostatin receptors expressed in brain, gastrointestinal tract, and kidney. Proc Natl Acad Sci U.S.A. (1992) 89:251–5. doi: 10.1073/pnas.89.1.251
273. Zupanc GK, Siehler S, Jones EM, Seuwen K, Furuta H, Hoyer D, et al. Molecular cloning and pharmacological characterization of a somatostatin receptor subtype in the gymnotiform fish. Apteronotus Albifrons Gen Comp Endocrinol (1999) 115:333–45. doi: 10.1006/gcen.1999.7316
274. Canosa LF, Cerda-Reverter JM, Peter RE. Brain mapping of three somatostatin encoding genes in the goldfish. J Comp Neurol (2004) 474:43–57. doi: 10.1002/cne.20097
275. Nelson LE, Sheridan MA. Regulation of somatostatins and their receptors in fish. Gen Comp Endocr (2005) 142:117–33. doi: 10.1016/j.ygcen.2004.12.002
276. Very NM, Sheridan MA. The role of somatostatins in the regulation of growth in fish. Fish Physiol Biochem (2002) 27:217–26. doi: 10.1023/B:FISH.0000032727.75493.e8
277. Abrahams MV, Sutterlin A. The foraging and antipredator behaviour of growth-enhanced transgenic Atlantic salmon. Anim Behav (1999) 58:933–42. doi: 10.1006/anbe.1999.1229
278. Silverstein JT, Wolters WR, Shimizu M, Dickhoff WW. Bovine growth hormone treatment of channel catfish: Strain and temperature effects on growth, plasma igf-I levels, feed intake and efficiency and body composition. Aquaculture (2000) 190:77–88. doi: 10.1016/S0044-8486(00)00387-2
279. Kwong P, Chang JP. Somatostatin inhibition of growth hormone release in goldfish: Possible targets of intracellular mechanisms of action. Gen Comp Endocrinol (1997) 108:446–56. doi: 10.1006/gcen.1997.6995
280. Yunker WK, Chang JP. Somatostatin actions on a protein kinase c-dependent growth hormone secretagogue cascade. Mol Cell Endocrinol (2001) 175:193–204. doi: 10.1016/s0303-7207(01)00386-0
281. Rousseau K, Le Belle N, Pichavant K, Marchelidon J, Chow BK, Boeuf G, et al. Pituitary growth hormone secretion in the turbot, a phylogenetically recent teleost, is regulated by a species-specific pattern of neuropeptides. Neuroendocrinology (2001) 74:375–85. doi: 10.1159/000054704
282. Donoghue DJ, Scanes CG. Possible involvement of adenylyl cyclase-Camp-Protein kinase a pathway in somatostatin inhibition of growth hormone release from chicken pituitary cells. Gen Comp Endocrinol (1991) 81:113–9. doi: 10.1016/0016-6480(91)90131-o
283. Tanner JW, Davis SK, McArthur NH, French JT, Welsh TH Jr. Modulation of growth hormone (Gh) secretion and gh mrna levels by gh-releasing factor, somatostatin and secretagogues in cultured bovine adenohypophysial cells. J Endocrinol (1990) 125:109–15. doi: 10.1677/joe.0.1250109
284. Cordoba-Chacon J, Gahete MD, Culler MD, Castano JP, Kineman RD, Luque RM. Somatostatin dramatically stimulates growth hormone release from primate somatotrophs acting at low doses Via somatostatin receptor 5 and cyclic amp. J Neuroendocrinol (2012) 24:453–63. doi: 10.1111/j.1365-2826.2011.02261.x
285. Ramirez JL, Torronteras R, Castano JP, Sanchez-Hormigo A, Garrido JC, Garcia-Navarro S, et al. Somatostatin plays a dual, Stimulatory/Inhibitory role in the control of growth hormone secretion by two somatotrope subpopulations from porcine pituitary. J Neuroendocrinol (1997) 9:841–8. doi: 10.1046/j.1365-2826.1997.00650.x
286. Ramirez JL, Gracia-Navarro F, Garcia-Navarro S, Torronteras R, Malagon MM, Castano JP. Somatostatin stimulates gh secretion in two porcine somatotrope subpopulations through a camp-dependent pathway. Endocrinology (2002) 143:889–97. doi: 10.1210/en.143.3.889
287. Ben-Shlomo A, Melmed S. Pituitary somatostatin receptor signaling. Trends Endocrinol Metab (2010) 21:123–33. doi: 10.1016/j.tem.2009.12.003
288. Chang JP, Habibi HR, Yu Y, Moussavi M, Grey CL, Pemberton JG. Calcium and other signalling pathways in neuroendocrine regulation of somatotroph functions. Cell Calcium (2012) 51:240–52. doi: 10.1016/j.ceca.2011.11.001
289. Brunetti L. Nitric oxide: A gas as a modulator of neuroendocrine secretions. Clin Ter (1994) 144:147–53. doi: 10.1016/b978-012370420-7/50028-9
290. Koesling D, Russwurm M, Mergia E, Mullershausen F, Friebe A. Nitric oxide-sensitive guanylyl cyclase: Structure and regulation. Neurochem Int (2004) 45:813–9. doi: 10.1016/j.neuint.2004.03.011
291. Pinilla L, Tena-Sempere M, Aguilar E. Nitric oxide stimulates growth hormone secretion in vitro through a calcium- and cyclic guanosine monophosphate-independent mechanism. Horm Res (1999) 51:242–7. doi: 10.1159/000023378
292. Luque RM, Rodriguez-Pacheco F, Tena-Sempere M, Gracia-Navarro F, Malagon MM, Castano JP. Differential contribution of nitric oxide and cgmp to the stimulatory effects of growth hormone-releasing hormone and low-concentration somatostatin on growth hormone release from somatotrophs. J Neuroendocrinol (2005) 17:577–82. doi: 10.1111/j.1365-2826.2005.01345.x
293. Valverde I, Penalva A, Ghigo E, Casanueva FF, Dieguez C. Involvement of nitric oxide in the regulation of growth hormone secretion in dogs. Neuroendocrinology (2001) 74:213–9. doi: 10.1159/000054688
294. Rubinek T, Rubinfeld H, Hadani M, Barkai G, Shimon I. Nitric oxide stimulates growth hormone secretion from human fetal pituitaries and cultured pituitary adenomas. Endocrine (2005) 28:209–16. doi: 10.1385/ENDO:28:2:209
295. Kato M. Involvement of nitric oxide in growth hormone (Gh)-releasing hormone-induced gh secretion in rat pituitary cells. Endocrinology (1992) 131:2133–8. doi: 10.1210/endo.131.5.1330492
296. Tsumori M, Murakami Y, Koshimura K, Kato Y. Endogenous nitric oxide inhibits growth hormone secretion through cyclic guanosine monophosphate-dependent mechanisms in Gh(3) cells. Endocr J (1999) 46:779–85. doi: 10.1507/endocrj.46.779
297. Fisker S, Nielsen S, Ebdrup L, Bech JN, Christiansen JS, Pedersen EB, et al. The role of nitric oxide in l-Arginine-Stimulated growth hormone release. J Endocrinol Invest (1999) 22:89–93. doi: 10.1016/s1096-6374(99)80007-0
298. Korbonits M, Trainer PJ, Fanciulli G, Oliva O, Pala A, Dettori A, et al. L-arginine is unlikely to exert neuroendocrine effects in humans Via the generation of nitric oxide. Eur J Endocrinol (1996) 135:543–7. doi: 10.1530/eje.0.1350543
299. Bocca L, Valenti S, Cuttica CM, Spaziante R, Giordano G, Giusti M. Nitric oxide biphasically modulates gh secretion in cultured cells of gh-secreting human pituitary adenomas. Minerva Endocrinol (2000) 25:55–9. doi: 10.1210/endo.131.5.1330492
300. McDonald AJ, Zaric V. Extrinsic origins of the somatostatin and neuropeptide y innervation of the rat basolateral amygdala. Neuroscience (2015) 294:82–100. doi: 10.1016/j.neuroscience.2015.03.004
301. Fodor M, van Elk EJ, Huizinga CT, Prins T, Delemarre-van de Waal HA. Npy neurons express somatostatin receptor subtype 1 in the arcuate nucleus. Neuroreport (2005) 16:29–32. doi: 10.1097/00001756-200501190-00008
302. Cummings SL. Neuropeptide y, somatostatin, and cholecystokinin of the anterior piriform cortex. Cell Tissue Res (1997) 289:39–51. doi: 10.1007/s004410050850
303. Cummings SL, Truong BG, Gietzen DW. Neuropeptide y and somatostatin in the anterior piriform cortex alter intake of amino acid-deficient diets. Peptides (1998) 19:527–35. doi: 10.1016/s0196-9781(97)00468-3
304. Stengel A, Coskun T, Goebel M, Wang L, Craft L, Alsina-Fernandez J, et al. Central injection of the stable somatostatin analog Odt8-sst induces a Somatostatin2 receptor-mediated orexigenic effect: Role of neuropeptide y and opioid signaling pathways in rats. Endocrinology (2010) 151:4224–35. doi: 10.1210/en.2010-0195
305. Karasawa H, Yakabi S, Wang L, Tache Y. Orexin-1 receptor mediates the increased food and water intake induced by intracerebroventricular injection of the stable somatostatin pan-agonist, Odt8-sst in rats. Neurosci Lett (2014) 576:88–92. doi: 10.1016/j.neulet.2014.05.063
306. Yousefvand S, Hamidi F, Zendehdel M, Parham A. Interaction of neuropeptide y receptors (Npy1, Npy2 and Npy5) with somatostatin on somatostatin-induced feeding behaviour in neonatal chicken. Br Poult Sci (2019) 60:71–8. doi: 10.1080/00071668.2018.1547359
307. Batten TF, Cambre ML, Moons L, Vandesande F. Comparative distribution of neuropeptide-immunoreactive systems in the brain of the green molly. Poecilia Latipinna J Comp Neurol (1990) 302:893–919. doi: 10.1002/cne.903020416
308. Pickavance LC, Staines WA, Fryer JN. Distributions and colocalization of neuropeptide y and somatostatin in the goldfish brain. J Chem Neuroanat (1992) 5:221–33. doi: 10.1016/0891-0618(92)90047-t
309. Li Q, Wen H, Li Y, Zhang Z, Zhou Y, Qi X. Evidence for the direct effect of the npff peptide on the expression of feeding-related factors in spotted Sea bass (Lateolabrax maculatus). Front Endocrinol (Lausanne) (2019) 10:545. doi: 10.3389/fendo.2019.00545
310. Huong TTM, Murashita K, Senzui A, Matsumoto T, Fukada H. Cholecystokinin 1 and 2 in red seabreampagrus major: Molecular cloning, response to feeding, and a potential indicator of dietary protein source quality. Fisheries Sci (2020) 86:835–49. doi: 10.1007/s12562-020-01443-z
311. Einarsson S, Davies PS, Talbot C. Effect of exogenous cholecystokinin on the discharge of the gallbladder and the secretion of trypsin and chymotrypsin from the pancreas of the Atlantic salmon, Salmo salar l. Comp Biochem Physiol C Pharmacol Toxicol Endocrinol (1997) 117:63–7. doi: 10.1016/s0742-8413(96)00226-5
312. Olsson C, Aldman G, Larsson A, Holmgren S. Cholecystokinin affects gastric emptying and stomach motility in the rainbow trout. Oncorhynchus Mykiss J Exp Biol (1999) 202:161–70. doi: 10.1242/jeb.202.2.161
313. Himick BA, Peter RE. Cck/Gastrin-like immunoreactivity in brain and gut, and cck suppression of feeding in goldfish. Am J Physiol (1994) 267:841–51. doi: 10.1152/ajpregu.1994.267.3.R841
314. Suzuki T, Kurokawa T, McVey DC. Sequence and expression analyses of cholecystokinin (Cck) precursor cdna in the Japanese flounder (Paralichthys olivaceus). Fish Physiol Biochem (1999) 21:73–80. doi: 10.1023/A:1007703422249
315. MacDonald E, Volkoff H. Neuropeptide y (Npy), cocaine- and amphetamine-regulated transcript (Cart) and cholecystokinin (Cck) in winter skate (Raja ocellata): Cdna cloning, tissue distribution and mrna expression responses to fasting. Gen Comp Endocr (2009) 161:252–61. doi: 10.1016/j.ygcen.2009.01.021
316. Rubio VC, Sanchez-Vazquez FJ, Madrid JA. Role of cholecystokinin and its antagonist proglumide on macronutrient selection in European Sea bass Dicentrarchus labrax, l. Physiol Behav (2008) 93:862–9. doi: 10.1016/j.physbeh.2007.12.001
317. Zhang X, Tang N, Qi J, Wang S, Hao J, Wu Y, et al. Cck reduces the food intake mainly through Cck1r in Siberian sturgeon (Acipenser baerii Brandt). Sci Rep (2017) 7:12413. doi: 10.1038/s41598-017-12646-3
318. Lewis K, Li C, Perrin MH, Blount A, Kunitake K, Donaldson C, et al. Identification of urocortin iii, an additional member of the corticotropin-releasing factor (Crf) family with high affinity for the Crf2 receptor. P Natl Acad Sci USA (2001) 98:7570–5. doi: 10.1073/pnas.121165198
319. Maruyama K, Miura T, Uchiyama M, Shioda S, Matsuda K. Relationship between anorexigenic action of pituitary adenylate cyclase-activating polypeptide (Pacap) and that of corticotropin-releasing hormone (Crh) in the goldfish. Carassius Auratus Peptides (2006) 27:1820–6. doi: 10.1016/j.peptides.2006.01.013
320. Qi JW, Tang N, Wu YB, Chen H, Wang SY, Wang B, et al. The transcripts of crf and crf receptors under fasting stress in dabry's sturgeon (Acipenser dabryanus dumeril). Gen Comp Endocr (2019) 280:200–8. doi: 10.1016/j.ygcen.2019.05.005
321. Ortega VA, Lovejoy DA, Bernier NJ. Appetite-suppressing effects and interactions of centrally administered corticotropin-releasing factor, urotensin I and serotonin in rainbow trout (Oncorhynchus mykiss). Front Neurosci-Switz (2013) 7:196. doi: 10.3389/fnins.2013.00196
322. Wang T, Zhou CW, Yuan DY, Lin FJ, Chen H, Wu HW, et al. Schizothorax prenanti corticotropin-releasing hormone (Crh): Molecular cloning, tissue expression, and the function of feeding regulation. Fish Physiol Biochem (2014) 40:1407–15. doi: 10.1007/s10695-014-9935-6
323. Zhang X, Wu YB, Hao J, Zhu JEY, Tang N, Qi JW, et al. Intraperitoneal injection urocortin-3 reduces the food intake of Siberian sturgeon (Acipenser baerii). Peptides (2016) 85:80–8. doi: 10.1016/j.peptides.2016.09.007
324. Sakurai T, Amemiya A, Ishii M, Matsuzaki I, Chemelli RM, Tanaka H, et al. Orexins and orexin receptors: A family of hypothalamic neuropeptides and G protein-coupled receptors that regulate feeding behavior. Cell (1998) 92:573–85. doi: 10.1016/s0092-8674(00)80949-6
325. de Lecea L, Kilduff TS, Peyron C, Gao X, Foye PE, Danielson PE, et al. The hypocretins: Hypothalamus-specific peptides with neuroexcitatory activity. Proc Natl Acad Sci U.S.A. (1998) 95:322–7. doi: 10.1073/pnas.95.1.322
326. Faraco JH, Appelbaum L, Marin W, Gaus SE, Mourrain P, Mignot E. Regulation of hypocretin (Orexin) expression in embryonic zebrafish. J Biol Chem (2006) 281:29753–61. doi: 10.1074/jbc.M605811200
327. Xu MY, Volkoff H. Molecular characterization of prepro-orexin in Atlantic cod (Gadus morhua): Cloning, localization, developmental profile and role in food intake regulation. Mol Cell Endocrinol (2007) 271:28–37. doi: 10.1016/j.mce.2007.03.003
328. Buckley C, MacDonald EE, Tuziak SM, Volkoff H. Molecular cloning and characterization of two putative appetite regulators in winter flounder (Pleuronectes americanus): Preprothyrotropin-releasing hormone (Trh) and preproorexin (Ox). Peptides (2010) 31:1737–47. doi: 10.1016/j.peptides.2010.05.017
329. Huesa G. A.N. van den pol and T.E. finger. differential distribution of hypocretin (Orexin) and melanin-concentrating hormone in the goldfish brain. J Comp Neurol (2005) 492:380–1. doi: 10.1002/cne.20787
330. Novak CM, Jiang XL, Wang CF, Teske JA, Kotz CM, Levine JA. Caloric restriction and physical activity in zebrafish (Danio rerio). Neurosci Lett (2005) 383:99–104. doi: 10.1016/j.neulet.2005.03.048
331. Facciolo RM, Crudo M, Giusi G, Alo R, Canonaco M. Light- and dark-dependent orexinergic neuronal signals promote neurodegenerative phenomena accounting for distinct behavioral responses in the teleost. Thalassoma Pavo J Neurosci Res (2009) 87:748–57. doi: 10.1002/jnr.21886
332. Kojima K, Kamijo M, Kageyama H, Uchiyama M, Shioda S, Matsuda K. Neuronal relationship between orexin-a- and neuropeptide y-induced orexigenic actions in goldfish. Neuropeptides (2009) 43:63–71. doi: 10.1016/j.npep.2009.01.004
333. Bertile F, Raclot T. The melanocortin system during fasting. Peptides (2006) 27:291–300. doi: 10.1016/j.peptides.2005.03.063
334. Tao YX. The melanocortin-4 receptor: Physiology, pharmacology, and pathophysiology. Endocr Rev (2010) 31:506–43. doi: 10.1210/er.2009-0037
335. Gong YL, Chen WJ, Han D, Zhu XM, Yang YX, Jin JY, et al. Effects of food restriction on growth, body composition and gene expression related in regulation of lipid metabolism and food intake in grass carp. Aquaculture (2017) 469:28–35. doi: 10.1016/j.aquaculture.2016.12.003
336. Lohr H, Hess S, Pereira MMA, Reinoss P, Leibold S, Schenkel C, et al. Diet-induced growth is regulated Via acquired leptin resistance and engages a pomc-Somatostatin-Growth hormone circuit. Cell Rep (2018) 23:1728–41. doi: 10.1016/j.celrep.2018.04.018
337. Boonanuntanasarn S, Jangprai A, Yoshizaki G. Characterization of proopiomelanocortin in the snakeskin gourami (Trichopodus pectoralis) and its expression in relation to food intake. Domest Anim Endocrinol (2015) 50:1–13. doi: 10.1016/j.domaniend.2014.06.004
338. Li L, Yoo ES, Li X, Wyler SC, Chen X, Wan R, et al. The atypical antipsychotic risperidone targets hypothalamic melanocortin 4 receptors to cause weight gain. J Exp Med (2021) 218:e20202484. doi: 10.1084/jem.20202484
339. Conde-Sieira M, Libran-Perez M, Patino MAL, Miguez JM, Soengas JL. Crf treatment induces a readjustment in glucosensing capacity in the hypothalamus and hindbrain of rainbow trout. J Exp Biol (2011) 214:3887–94. doi: 10.1242/jeb.061564
Keywords: neuropeptidomics, gene editing, neuropeptide Y, somatostatin, feeding, growth
Citation: Yu X, Yan H and Li W (2022) Recent advances in neuropeptide-related omics and gene editing: Spotlight on NPY and somatostatin and their roles in growth and food intake of fish. Front. Endocrinol. 13:1023842. doi: 10.3389/fendo.2022.1023842
Received: 20 August 2022; Accepted: 20 September 2022;
Published: 04 October 2022.
Edited by:
Linyan Zhou, Southwest University, ChinaReviewed by:
Dong-Neng Jiang, Guangdong Ocean University, ChinaCopyright © 2022 Yu, Yan and Li. This is an open-access article distributed under the terms of the Creative Commons Attribution License (CC BY). The use, distribution or reproduction in other forums is permitted, provided the original author(s) and the copyright owner(s) are credited and that the original publication in this journal is cited, in accordance with accepted academic practice. No use, distribution or reproduction is permitted which does not comply with these terms.
*Correspondence: Wensheng Li, bHNzbHdzQG1haWwuc3lzdS5lZHUuY24=
†These authors have contributed equally to this work
Disclaimer: All claims expressed in this article are solely those of the authors and do not necessarily represent those of their affiliated organizations, or those of the publisher, the editors and the reviewers. Any product that may be evaluated in this article or claim that may be made by its manufacturer is not guaranteed or endorsed by the publisher.
Research integrity at Frontiers
Learn more about the work of our research integrity team to safeguard the quality of each article we publish.