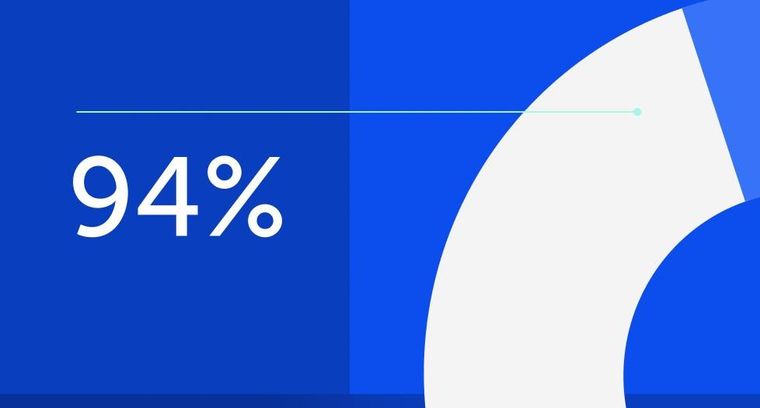
94% of researchers rate our articles as excellent or good
Learn more about the work of our research integrity team to safeguard the quality of each article we publish.
Find out more
REVIEW article
Front. Endocrinol., 09 March 2022
Sec. Reproduction
Volume 12 - 2021 | https://doi.org/10.3389/fendo.2021.799043
This article is part of the Research TopicDisruptors on Male Reproduction - Emerging Risk FactorsView all 19 articles
Per-fluoroalkyl and polyfluoroalkyl substances (PFAS) are a diverse group of synthetic fluorinated chemicals used widely in industry and consumer products. Due to their extensive use and chemical stability, PFAS are ubiquitous environmental contaminants and as such, form an emerging risk factor for male reproductive health. The long half-lives of PFAS is of particular concern as the propensity to accumulate in biological systems prolong the time taken for excretion, taking years in many cases. Accordingly, there is mounting evidence supporting a negative association between PFAS exposure and an array of human health conditions. However, inconsistencies among epidemiological and experimental findings have hindered the ability to definitively link negative reproductive outcomes to specific PFAS exposure. This situation highlights the requirement for further investigation and the identification of reliable biological models that can inform health risks, allowing sensitive assessment of the spectrum of effects of PFAS exposure on humans. Here, we review the literature on the biological effects of PFAS exposure, with a specific focus on male reproduction, owing to its utility as a sentinel marker of general health. Indeed, male infertility has increasingly been shown to serve as an early indicator of a range of co-morbidities such as coronary, inflammatory, and metabolic diseases. It follows that adverse associations have been established between PFAS exposure and the incidence of testicular dysfunction, including pathologies such as testicular cancer and a reduction in semen quality. We also give consideration to the mechanisms that render the male reproductive tract vulnerable to PFAS mediated damage, and discuss novel remediation strategies to mitigate the negative impact of PFAS contamination and/or to ameliorate the PFAS load of exposed individuals.
Perfluoroalkyl and polyfluoroalkyl substances (PFAS) are a diverse group of more than 4,700 synthetic, highly fluorinated, aliphatic chemicals with distinctive chemical properties [see review by Kirk et al. (1)], which render members of this chemical group incredibly stable and environmentally persistent (2, 3). Consequently, PFAS have been employed for a range of purposes including in the formulation of fire-fighting foams as well as in a variety of consumer products (4, 5), such as food packaging, cookware and water repellent clothing (6–9). Since the 1950s, the extensive manufacture, distribution, use and disposal of PFAS has resulted in the widespread environmental contamination and subsequent exposure of humans and animals. Despite endeavors to phase out the toxic eight chain PFAS initiated in 2000, the inherent stability of these compounds has resulted in omnipresence in the global environment (5, 9–11). Thus, many industrialized nations are seeking to implement measures to limit, detect and eradicate PFAS contamination (5, 9). Long-chain PFAS generally have longer environmental half-lives and a high propensity to accumulate in biological systems from which they may take many years to be fully excreted. For example, PFAS such as perfluorooctanoic acid (PFOA) and perfluorooctanesulfonic acid (PFOS) are the most extensively reported long-chain perfluoroalkyl acids described in scientific literature (5) and have a half-life in human serum of 3.8 and 5.4 years, respectively (Table 1) (13). Longer chain (≥ 6 carbon atoms) PFAS bioaccumulate to a greater extent than shorter chain analogues (14–17), and also possess longer half-lives (18, 19). Upon entering the body, PFAS bind to albumin in the blood stream and accumulate within the body’s protein-rich tissues (6, 20). Consequently, PFAS are readily detectable throughout the human body as well as accumulating to detectable levels in most bodily fluids, including urine, breast milk, blood, and seminal plasma (21, 22). Notably, in support of the notion that albumin binding is one of the key reasons that PFAS are slowly excreted in urine, Jain and Ducatman have shown that serum PFAS levels decrease under conditions of albuminuria (23). This pathology, during which albumin is able to escape into the urine as a consequence of renal dysfunction, is presumed to result in increased excretion of bound PFAS.
Table 1 Summary of a selection of common PFAS chemicals, detailing abbreviations, chemical formula, and half-life in humans.
PFOS and PFOA are the two most abundant PFAS found in human serum worldwide (7, 10, 24), with levels of each varying between countries, suggesting differences in the degree of exposure in each country (24, 25). Further, PFOS and PFOA have a propensity of accumulate in our food chains (10) and it is thought that dietary intake is a key pathway of exposure for the general population; either from food packaging or environmental contamination of food products (26–29). Other suggested routes of contamination include household dust (28, 30) or from the consumption of contaminated drinking water (29, 31, 32); although all paths of human exposure remain to be fully identified. Exposure levels vary between locations and individuals and range from background levels in the general population of up to around 14 ng/mL of PFOS and PFOA in the blood (33), through to considerably higher levels in individuals who have been occupationally exposed, or those who reside in contaminated areas (34). The highest concentrations have been detected in individuals employed in PFAS manufacturing facilities with a mean blood concentration of 1,000 to 2,000 ng/mL PFOS and 5,000 ng/mL PFOA (25, 35). Such findings are of particular concern in view of the potential of PFAS to elicit a range of adverse health outcomes.
Here, we review literature pertaining to the emerging threat posed by PFAS exposure, with a specific focus on the male reproductive tract and general male fertility, owing to its utility as a biomarker of general health. Indeed, in what has become a well-established paradigm, male infertility has been shown to serve as an early indicator of a range of co-morbidities such as coronary, inflammatory, and metabolic diseases; conditions that all have associated transgenerational effects (36–42). It follows that adverse associations have been established between PFAS and the incidence of testicular dysfunction, including pathologies such as testicular cancer (43–48) and a reduction in semen quality (35, 49, 50). Accordingly, we give consideration to the mechanisms that render the male reproductive tract vulnerable to PFAS mediated damage, as well as novel remediation strategies to mitigate the negative impact of PFAS contamination and/or ameliorate the concentration of PFAS that has accumulated in exposed individuals.
The term ‘fluorinated substances’ encompasses an extensive array of organic and inorganic chemicals that contain a minimum of one fluorine (F) atom, with each substance possessing different chemical, biological and physical properties (3). The properties of each compound are influenced by both the number of F atoms and their position in the molecule, with chemicals classed as partially fluorinated (polyfluoroalkyl), or fully fluorinated (perfluorinated) (4). The most common PFAS are the perfluorinated alkyl acids (PFAAs), which are amphiphilic and exhibit attraction to both aqueous and lipid media, mimicking phospholipid properties. Their structure contains a water-insoluble hydrophobic segment (the fluorinated carbon chain), and a water-soluble hydrophilic functional group such carboxylic acid or sulfonic acid (4). The structural formula of the resulting moiety is CnF2n+1-R, where R represents the functional group (Figure 1) (5). The PFAS moiety contains strong carbon-fluorine bonds conferring unique chemical properties that render these chemicals heat-resistant, water repellent, and exceptionally stable, to the point where they are almost indestructible under normal environmental conditions (5). The fluorination of the hydrocarbon chain drastically changes the chemical properties of the molecule, as the hydrophobic fluorinated segment repels water, while in parallel, the oleophobic properties also repel fat and oil (4). Thus, perfluorinated compounds can effectively lower surface tension and act as efficient surfactants for coatings on non-stick cookware and in food packaging and firefighting foam (1). Individual PFAS are distinguished from each other by 1) the properties of the functional group and 2) the length of the carbon backbone (Figure 1). However, PFAS molecules are also further categorized based on their usage, and the history of their manufacture. In this context, group members are described as either legacy PFAS, specifically those molecules with a long history of usage and/or environmental persistence, or as replacement PFAS, which include a new generation of compounds with different chemistries that were designed to replace the original and ‘more’ harmful legacy PFAS (51, 52).
Figure 1 Basic structure of perfluoroalkyl substances (PFAS), using perfluorooctanesulfonic acid (PFOS) as an example. Outlined in blue is the perfluoroalkyl tail (carbon/fluoride chain) and the functional group is outlined in red. All PFAS share these general features, with variation in the carbon chain length and functional group. Figure adapted from Blake and Fenton 2020 (51).
PFAS exposure can arise through several routes (Figure 2), with environmental contamination occurring at varying stages of production, usage, and waste disposal. In particular, PFAS have found application as a major component of aqueous film forming foams (AFFF) (53), which are widely used for firefighting, military training activities and at airports and thus has resulted in extensive contamination of nearby soil and waterways (31, 34, 54, 55). Elevated PFOS/PFOA levels have been detected in the serum of individuals living in areas with high levels of these chemicals in their drinking water (11, 31), including those communities located in close proximity to military bases, airports and PFAS manufacturing factories (31, 56). Not surprisingly, greater plasma contamination levels have also been detected in occupationally exposed individuals such as firefighters and factory workers manufacturing or using PFAS (31, 34, 55, 57, 58). Industry waste and AFFF usage has resulted in widespread contamination of groundwater, often used as drinking water, with dietary exposure suggested to be the main route of exposure for adults (29, 34, 56, 59). In addition, background levels of contamination are seen in the general population who are exposed to PFAS through drinking water (29, 60), house dust (61) and food consumption (27, 62, 63), with the latter arising from the extensive use of PFAS in consumer packaging. Compounding this situation, prenatal exposure can occur through the placenta (64, 65) and young babies can be exposed through breast milk (66, 67).
Figure 2 Schematic diagram illustrating the routes of human PFAS exposure. Following production, PFAS are used in consumer products such as food packaging, cookware, water repellent clothing and non-stick fry pans. PFAS are also a main component in firefighting foam, which can leach into the environment, or are otherwise disposed of as industrial waste. Human exposure may occur through use of consumer products or from contaminated water supplies. Accordingly, environmental exposure can occur as a result of waste products contaminating waterways and soil through leaching of firefighting foam and waste from industry and consumers.
PFAS enter the body through ingestion (31), inhalation (68) or dermal exposure (69). Once they have entered the bloodstream through gas-exchange or digestion, PFAS bind to serum proteins such as the major transport protein, human serum albumin (HSA) (70, 71). It appears PFOS has a greater binding affinity for HSA than PFOA, which correlates with the known longer half-life of PFOS (Table 1) (12, 72). Due to their biochemical stability, PFAS chemicals tend to accumulate within the body (1) and move from plasma into tissues, with the highest levels being found in human tissues with a larger blood supply such as the liver, lungs and kidneys (6, 20, 66). This is also reported in studies of mice where the highest accumulation of PFOS has been documented in the liver, lungs, kidney and bone marrow (73), as well as in primates, with the kidneys and blood also showing higher levels in comparison to other tissues (66). Notably, the tissue distribution of PFAS is influenced by multiple factors including species and gender, chemical characteristics such as chain length and functional group, as well as exposure dose (66, 74, 75). Most environmentally relevant PFAS have chain lengths between 4 and 13 fluorinated carbons (15, 76) giving rise to a variety of different structures such as branched forms (4, 76), although the most commonly detected PFAS in humans and wildlife are linear forms (Figure 1) (77). Longer chain PFAS have a greater potential to accumulate in living organisms than do shorter chain PFAS (<6 carbons) (14) due to the ability of longer chain PFAS such as PFOA, PFOS and perfluorohexanesulphonic acid (PFHxS) to bind to a wider range of serum proteins, including transferrin, plasma gamma-globulin and albumin (70). This is supported by evidence that PFAS accumulation occurs in protein-rich tissues such as the liver (66). Such evidence has led to the replacement of legacy and long-chain PFAS with structurally similar shorter chain variants, thought to be less toxic; for example, perfluorobutane sulfonic acid (PFBS) has been used to replace PFOS (51) and GenX (hexafluoropropylene oxide dimer acid) has replaced PFOA (16). Plasma concentrations support this rationale with longer chain PFAS such as PFOA and PFOS showing higher levels [3.9 and 20.7 µg/L, respectfully (21)] in comparison to the shorter chain replacements PFBS [typically below the detection limit of 4.2 µg/L (9)] and perfluorobutanoic acid (PFBA) [3.3 µg/L (78)]. However, little is currently known about the toxicology of these replacement chemicals (79). Additionally, bioaccumulation of PFAS appears to be influenced by the functional group(s) attached to the hydrocarbon backbone of each PFAS molecule. For example, compounds that harbor an attached carboxylic acid functional group have been shown to accumulate less in fish, than those with a sulfonate functional group of the same carbon chain length (14, 18). Although the exact mechanisms behind this disparity are currently unknown, it may be due to the stronger affinity for proteins seen with longer chain length and in sulfonic acids (71).
Increasing awareness of the dangers of PFAS and their propensity to bioaccumulate has led to a surge in scientific research and public interest, with PFAS being labelled as a potential risk for humans and the environment by the Scientific Committee on Health in 2018 (80). Studies have been conducted in both human and animal models to investigate possible health consequences arising from PFAS exposure (1, 43). The most commonly investigated PFAS with regards to human health are PFOS and PFOA (81), with a range of additional PFAS having been studied including PFHxS and PFBS (1) (Table 1). Mounting evidence from these studies supports an association between PFAS and an array of human diseases and disorders (1). However, it is somewhat difficult to definitively link causality to PFAS due to the variation in chemistries and potential biological activities between the different classes of PFAS, the duration and degree of exposure, potential synergistic or antagonistic effects of PFAS combinations in the body as well as the often-overlooked precursors of PFAS, which degrade to the terminal perfluoroalkyl acids (PFAAS) (82, 83). This situation is further compounded by the mechanisms of PFAS exposure, which vary both between and within communities, resulting in distinct PFAS profiles among individual subjects. Furthermore, disparities also exist in an individual's genetic and phenotypic constitution within affected populations, which could ultimately influence their PFAS clearance rates and susceptibility to the biological effects of these chemicals. Notwithstanding these limitations, the balance of evidence supports the potential for PFAS exposure to elicit adverse health sequelae at differing developmental stages and ages (66, 84–88). The C8 Health Project also bears out this conclusion; a comprehensive investigation of an entire community of 69,000 people exposed to PFAS via consumption of contaminated drinking water (43). This study revealed probable links between PFOA exposure and six diseases: kidney and testicular cancer, thyroid disease, high cholesterol, ulcerative colitis, and pregnancy-induced hypertension (43), findings that are supported by the International Agency for Research on Cancer evidence (48, 85). Although, it should be noted this evidence relates to PFOA exposure only, and therefore, further investigations of this nature are required for the remaining range of PFAS.
Building on this evidence, the greatest and most consistently reported metabolic consequence of PFAS exposure is dyslipidemia, with several notable studies finding links between serum PFAS and dysregulated lipid profiles (89), including increased low-density lipoprotein (90, 91), triglycerides (92) and total cholesterol (90, 91, 93, 94) in addition to diminished high-density lipoprotein (89). However, the extent of cholesterol dysregulation is variable across PFAS exposure levels as is the response to different forms of PFAS; with PFOA and PFOS demonstrating the most consistent effects between studies (62).
Epidemiological evidence has also linked PFAS exposure to the prevalence of testicular cancer, with the International Agency for Research on Cancer concluding PFOA is possibly carcinogenic to humans (48) and the United States Environment Protection Agency declaring it a likely carcinogen (95). In this context, studies by Barry et al. reported a strong association between testicular cancer and PFOA exposure (hazard ratio of 1.34) in adults exposed through drinking water assessed as part of the C8 Health Project cohort (43, 44). Similarly, Vieira et al. identified a positive correlation in individuals exposed to very high PFOS levels, with an adjusted odds ratio of 2.8; although a potential limitation of this study was the relatively small sample number (45). Confounding this situation, three additional studies focusing on PFOA exposure and mortality from testicular cancer all failed to identify an association in occupationally exposed workers (87, 96, 97). Thus, whilst not universally demonstrated, the potential significance of these positive associations is highlighted by parallel correlations between the widespread increase in worldwide PFAS usage and the rising prevalence of testicular cancer; a pathology that has significantly increased in recent times to become the most common malignancy in young men aged 20-40 years (98–101). Although the characterization of testicular cancer remains incomplete, there is speculation that environmental factors, as opposed to genetic factors, are a key contributor to the etiology of this form of cancer (98, 102).
Many challenges exist that have hindered attempts to fully assess PFAS effects on health, including those directly related to tracing the mode and levels of PFAS exposure in the general population, consequences of PFAS precursors, compound effects of PFAS mixtures, as well as nuances specific to studies of animal models (103). The use of the latter has proven invaluable for studying the toxicology of PFAS exposure, albeit with variable outcomes (66, 104, 105). Laboratory rodents are the chief animal model employed, with zebrafish also being utilized in recent times, particularly in the context of assessing the impacts of PFOA and PFOS exposure (12). Amongst animal models, considerable interspecies variation has been noted, but thus far, the mechanistic basis of such inconsistency has not been entirely resolved. Known variables include biological mechanisms of PFAS action within target tissues, rates of PFAS metabolism and elimination, as well as assessment of different disease endpoints (12, 29, 51, 103). For instance, the half-life of PFOA in mice, at 6 days, is much shorter than in rats at 16 to 22 days and is generally significantly longer in humans (~2.1 to 3.8 years) (12). These differences are further confounded by differential responses between genders, with PFOA being eliminated much quicker from female rats (2-4 hours) compared to their male counterparts (4-6 days) (12, 106, 107). Li et al. (108) have reported similar results in humans with a significantly lower PFOS half-life being seen in women (3.1 years) compared to males (4.6 years). Similarly, Zhang et al. (109) reported that PFOS half-life was shorter in women under 50 years of age (6.2 years) in comparison to women over 50 and for males of all assessed age groups (27 years), although, the same trend was not seen with PFOA. However, it should be noted that this estimated half-life of 27 years for PFOS is higher than that calculated in similar studies, and as such, should be considered an upper limit estimation.
The manufacture and pervasive use of PFAS began in the 1950s (4), meaning that virtually all humans born after this time have potentially been exposed to some degree of PFAS contamination. As a consequence, there is a genuine difficulty in identifying a naïve unexposed control cohort, a situation that hinders the reliability of epidemiological models and cohort studies used to compare exposure groups and evaluate the risk of disease associated with varying PFAS exposure levels (51). Further, direct evaluation of the health outcomes of an individual resulting from PFAS exposure provides challenges due to the wide array of PFAS profiles detected in individuals within the same community, and between geographically distinct communities (51). Indeed, an individual’s PFAS profile depends on several variables, such as the source of exposure, which can range from contamination associated with standard food packaging, through to the waste products encountered within the vicinity of a facility which manufactures PFAS (51). Exposure sources are also likely to differ over time due to fluctuations in PFAS usage as has been seen with the progressive phasing out of PFOS and PFOA chemicals in favor of alternative short chain PFAS derivatives (51). Additionally, possible synergistic or antagonist effects between different PFAS molecules may result in variable health outcomes between individuals (51), yet little is currently known of the repercussions of such chemical interplay (66). Variations in exposure also occur throughout the lifetime of an individual and can range from consistent chronic exposure to intermittent shorter periods of exposure. This exposure range has apparent consequences for an individual’s PFAS profile making attempts to relate PFAS exposure to incident health outcomes difficult. Indeed, PFAS-related health outcomes emerging in adults may be attributed to exposure at one or more key stages of development such as the in utero, childhood or puberty stages (110), or alternatively, to chronic life-long exposure. Additionally, it cannot be determined whether an individual’s PFAS contamination level is a result of a current exposure(s) or accumulation over a period of several years.
Another limitation of PFAS investigation is knowledge of the full assortment of contaminating PFAS chemicals. Initial identification of these chemicals in human serum was reported in 1980 when PFOA was discovered in a group of industrial plant workers exposed to fluorochemicals (111). A subsequent reduction in the manufacture, and phasing-out the use of PFAS classed as being damaging agents began in several countries in 2000 (5). Consequently, the original PFAS were replaced with other chemical analogues thought to be less toxic and which did not accumulate as readily in biological systems (79). Regrettably, the next generation of PFAS has subsequently been found to be detrimental to human health, with a prominent example being GenX, a branched short-chain PFAS that has subsequently been found to be more toxic than the PFOA it replaced (16). As a result, new PFAS are constantly being added to the list of hazardous and toxic chemicals (112, 113), which emphasizes the necessity for increased investment into PFAS-related research.
Currently, determination of the PFAS profile of an individual is limited by available testing methods. Serum testing is performed most often using mass spectrometry paired with liquid chromatography (78). Mass spectrometry identifies organic compounds based on their molecular mass and can detect and quantify compounds with a high level of sensitivity. Many methods measure a limited subset of 20-30 PFAS, which potentially leads to inaccurate PFAS profiles, and should be considered when comparing PFAS studies (114). The limit of detection varies between PFAS (e.g., 1.10 ng/L for perfluoroheptanoic acid (PFHpA) to 25.1 ng/L for PFBA), with the limit of quantitation being even greater (e.g. 3.3 ng/mL for PFHpA, to 75.3 ng/L for PFBA) (78). If a particular PFAS is present below the method reporting limit in a sample it would be assumed not to be present in the assessed sample, thus introducing inaccuracies in the assignment of biological effects. These limitations in measurement techniques highlight the need to identify and understand new and emerging fluorinated compounds to allow an accurate determination of exposed communities and the efficacy of remediation strategies to reduce exposure.
The confounders documented above highlight the requirement for reliable markers of general health with which to determine the risk posed by PFAS exposure. Here, we explore the utility of employing male reproductive health as one such indicator to understand the molecular pathways by which PFAS drive pathophysiological responses, a strategy that builds on evidence that the male germline is vulnerable to a variety of environmental toxicants (1, 115).
Infertility is a reproductive system disease that impacts 16 to 25% of couples, with almost half of all cases attributed to male reproductive issues (116). Such problems are often connected to semen abnormalities, key contributors to which include body mass, lifestyle, age, and environmental exposures (116). Over the past few decades, decreasing trends in semen quality have been reported but there remains no clear explanation for the underlying causes of this decline (116). In recent years there has also been increased understanding that the general health of a male is closely related to his reproductive health (116), with strong associations established between male infertility and future health; especially the development of testicular cancer (117–120), and chronic non-malignant diseases such as ischemic heart disease and diabetes (36, 37, 40, 42, 116, 121). It has been proposed that shared genetic pathways, lifestyle factors and the environment, possibly acting in utero, could play a key role (122). Mounting evidence implies that semen quality can serve as a biological marker for future male health, as multiple epidemiological studies of notable size (>50,000 men) describe consistent associations between reduced semen quality and mortality. For instance, diminished semen parameters, such as sperm count, concentration, motility, and morphology, are related to a 2.3-fold greater risk of death in the following eight years: factors comparable to the risk of death due to diabetes or smoking (36, 42, 117). These studies reveal that men with atypical semen characteristics commonly die due to a higher prevalence of testicular cancer and/or altered androgen signaling, which culminate in the onset of metabolic, cardiovascular, or inflammatory diseases (123, 124); a disease set not too dissimilar to those suggested to onset due to PFAS exposure (1, 43).
Hence, current epidemiological evidence aligns with the association between male infertility and PFAS exposure, as seen with the link between male infertility and risk of chronic disease and mortality. Nevertheless, the scarcity of prospective studies and insufficient adjustment of confounders hinder the ability to ascertain the causality of these associations, and the pathogenic pathways linking these conditions are still ambiguous (1). Despite this, male fertility, particularly the clinical assessment of basic sperm parameters, allows for readily accessible biomarkers and presents as a potentially important resource to identify diseases promptly and predict the long-term health of an individual (36, 39–42). The theory that male reproductive pathologies are triggered by environmental exposure is not a new concept. Indeed, several studies have investigated a range of environmental contaminants (115) such as pesticides and herbicides (125), acrylamide (126), and radiation (127) and their implications for male fertility. In addition, these studies provide an important precedent for equivalent research to be performed on PFAS.
Despite the publication of several studies exploring the relationship between PFAS exposure and male fertility, the evidence presented is often conflicting (81, 128), and further such studies are hindered by the nature of the chemicals assessed and the pre-existing history of worldwide PFAS exposure. Notwithstanding the limitations imposed by these confounders, male ailments such as testicular cancer are perceived as a prominent endpoint of PFAS exposure (1, 43–47) (Table 2). Further evidence of testicular dysfunction is supported by large cohort studies assessing semen quality (49, 140). In this context, a dose-response relationship may exist between chronic PFOA and PFOS exposure and sperm production. A 35% decline in normal sperm production was observed in the upper tertile of PFOS concentration (> 27.3 µg/L), compared to that of the first tertile (< 11.9 µg/L) (35). Similarly, a 40% decrease in normal sperm production was recorded in high PFOS and PFOA exposed individuals, compared to men classed as having low exposure levels (129). Moreover, in utero exposure to PFOA was shown to lower total sperm count (130). Further to this, recent studies have described a significant association between PFAS exposure and several indicators of human sperm quality (49, 134). For instance, Toft and colleagues (35) found no consistent associations between exposure to multiple PFAS and sperm concentration and count, or semen volume but did record a decrease in sperm cells with normal morphology in association with higher PFOS levels (35). Similarly, Joensen et al. reported equivalent results in their 2009 study with PFOA and PFOS (129), but these outcomes could not be reproduced in their later study published in 2013 (131), possibly due to lower levels of PFAS recorded in this later cohort (0.5% for PFOS), in which no participants were classified in the ‘high exposure’ category as per the criteria in the first study. Further studies also failed to find an association between PFAS and sperm quality (132, 133, 135–137); thus precluding the establishment of causative links (133, 136). Sperm motility has also been found to correlate both positively and negatively with PFOA exposure. One study investigating the implications of PFAS exposure on sperm parameters found greater sperm motility the study cohort with the highest serum PFOA levels (35). However, this observation was not consistent across all countries, nor the individual PFAS examined, and due to the many statistical tests performed in this study, the authors concluded such results might be due to chance events alone (35). In contrast, an independent study reported a significant negative correlation between several PFAS in semen, including PFOA, and sperm motility (78). This latter study also found associations between the PFAS concentration in semen and sperm motility, which indicates that seminal concentrations of PFAS may be more indicative of semen quality than that of serum PFAS levels. Such a finding has implications for the accuracy of extrapolating data from different sample sources across studies. Thus far, less than 15 studies have been conducted to investigate associations between PFAS levels and sperm parameters (1, 141) (Table 2). Half of these studies show no association while the other half report some associations. Yet, no studies consistently found the same set of altered sperm parameters due to PFAS exposure. Differing results may, in part, be attributed to the variation in the studied cohorts between countries; for example European and Arctic (35), versus Chinese populations (78), wherein participants are likely to have been exposed to different PFAS profiles depending on the source and route of contamination, not to mention other environmental factors to which they may be exposed, that could act synergistically or antagonistically. Although the variation in outcomes reported in these studies highlights the difficulties in directly comparing PFAS studies, the existence of positive correlations between PFAS exposure and abnormal sperm characteristics uphold the view that internalized PFAS do localize to the testis, along with other organs of the body, thereby forming a useful model to study PFAS-induced damage. However, it should be noted that using sperm parameters as a measure is quite a blunt tool and small changes are unlikely to be informative due to the wide variation seen between males and the low threshold for WHO defined parameters (142). Therefore, researchers should exercise caution when interpreting data on sperm parameter changes.
Table 2 Summary of outcomes from studies investigating the impact of PFAS on human male reproductive function.
The impact of PFAS on a variety of additional reproductive characteristics has also been investigated, including dysregulation of reproductive hormone profiles (141, 143, 144). In one such study, significantly lower serum testosterone levels were detected in male mice following 21 days of high (10 mg/kg) PFOS administration via oral gavage, compared to untreated controls (145). This finding supports previous evidence from studies of adult male rats exposed to PFOA by gavage at a concentration of 25 mg/kg/day for 14 days (146) and of mice treated for 28 days with PFOA (147). In the latter study, a dose-responsive reduction in testosterone and progesterone levels in the testis was revealed. These animal data are commensurate with some human investigations, which have also attributed reduced testosterone levels in men to high PFOS (131) and PFOA (139) levels. Furthermore, Luteinizing hormone (LH) and sex hormone binding globulin (SHBG) levels have been reported to correlate with increasing plasma PFOA concentrations in adult males (132, 133). Additionally, increased LH and follicle-stimulating hormone (FSH) were detected in men who experienced prenatal exposure to PFOA (130), indicating that this developmental phase may be particularly sensitive to maternal PFAS exposure. However, several conflicting studies fail to show any associations between PFAS exposure and plasma reproductive hormone levels in males (testosterone, LH, FSH, SHBG and estradiol) (129, 148, 149). Further, Nian et al. revealed a negative trend with FSH and PFBS in cord blood from newborns exposed to PFAS during pregnancy (138). As an additional caveat, animal study evidence should be interpreted cautiously as information on PFAS effects and male fertility is often not at environmentally relevant concentrations due to the much shorter half-life and faster elimination rates seen in animals, which result in lower internal levels at doses equivalent to human exposures.
Testicular dysgenesis syndrome (TDS) is a term that encompasses a range of male reproductive disorders originating from fetal development (150) and which are thought to be a consequence of environmental influences (150–152). Such issues share similarities with those attributed to PFAS exposure, such as undescended testes (cryptorchidism), fertility issues in adults, and testicular cancer (150, 151). Fetal development of the male reproductive system is sensitive to disturbance by environmental factors such as diethylstilbestrol, a synthetic estrogen prescribed to pregnant women in the mid-1900s (153) and cyclooxygenase inhibitors such as ibuprofen and paracetamol (154). An increased incidence of cryptorchidism has been identified in men exposed to these environmental toxicants in utero, with responses being particularly pronounced in those exposed during the critical programming windows of the first and second trimester (155, 156). Studies in rats have proposed the existence of male programming windows corresponding to weeks 8-14 of pregnancy in humans during which androgen-induced masculinization occurs, including programming of testes descent. Thus, environmental exposures encountered during this period have the potential to affect normal male reproductive development and reproductive hormone balance (156), suggesting a mechanism by which PFAS exposure during gestation may impact subsequent developmental events within the male reproductive system.
Endocrine disruptors are chemicals (both naturally occurring and synthetic) that interrupt the normal hormonal system of the body, either through direct hindrance of hormonal pathways or through mimicking the hormones within the endocrine system (157, 158). This can result in variable consequences such as dysregulation of immune, reproductive and developmental pathways (159–162). It follows that this diverse group of chemicals have been widely implicated in the development of reproductive abnormalities including TDS (150, 163–166). Indeed, the TDS hypothesis suggests that in utero exposure to endocrine disruptors damages testis development resulting in decreased function in adulthood, with symptoms ranging from moderately reduced semen quality through to the promulgation of testicular cancer (6, 167). PFAS display properties consistent with that expected of endocrine disruptors (1, 6, 168), and are widely reported as having endocrine disrupting actions (1, 6, 168–171). Accordingly, PFAS exposure often results in altered androgen and insulin-like factor 3 (INSL3) dependent processes (172–175) (Figure 3). There are two suggested mechanisms by which PFAS produce harmful endocrine effects: either by disturbing steroidogenesis (6) or by interfering with steroid hormone receptors (176, 177).
Figure 3 Proposed mechanisms of PFAS action pertaining to the male reproductive system. PFAS have the potential to enter the body through multiple routes. Following entry, PFAS are capable of binding to fatty acid binding proteins and transport proteins in the blood such as human serum albumin (HSA) and thereafter are thought to be transported throughout the body eliciting harmful endocrine effects via two possible mechanisms: disturbing steroidogenesis (e.g. via allosteric inhibition of vital enzymes) or directly interfering with steroid hormone receptors. This results in altered levels of reproductive hormones such as luteinizing hormone (LH), follicle stimulating hormone (FSH), sex hormone binding globulin (SHBG), testosterone (T) and insulin-like peptide 3 (INSL3), which has subsequent effects on male reproductive processes. PFAS also accumulate in protein rich tissues, including the testes, which is facilitated by the high expression of fatty acid binding proteins. Here, PFAS impact testicular cell function, namely Leydig and Sertoli cells. Altered Leydig cell function leads to reduced testosterone production resulting in altered sexual development, increased incidence of hyperplasia and adenomas and increased risk of cryptorchidism in the fetus. This reduction in testosterone leads to attendant impacts on Sertoli cell function by reducing Sertoli cell differentiation and precipitating compromise of spermatogenesis, reduced sperm count and altered sexual development. Gap junctions between Sertoli cells and developing germ cells are also affected by PFAS, which reduces communication between the cells, negatively affecting spermatogenesis and resulting in a range of defects in the mature spermatozoa.
Specifically, at least some of the pathologies attributed to in utero PFAS exposure are hypothesized to arise due to abnormal Leydig cell development and/or function (6, 176). Leydig cells are a vital component of the male reproductive system responsible for synthesizing the steroid hormone testosterone, which is essential for sexual development and testis decedent in the fetal period (178), and the support of normal sperm production in the adult (179). Biegel et al. reported altered Leydig cell function in vitro with cells isolated from untreated rats, in which a dose-dependent decrease in testosterone was seen following a 5-hour treatment with PFOA (IC50 approximately 200 μM) (146). Additionally, ex vivo investigations with Leydig cells isolated from rats gavaged for 14 days with 25 mg/kg/day of PFOA showed these alterations are reversible following cessation of PFOA treatment (146). Such effects may be attributed to PFAS interfering with one, or more, of the enzymes involved in steroidogenesis. By way of example, PFAS may directly inhibit the catalytic activity of the 3β-hydroxysteroid dehydrogenase (HSD3B) enzyme by competing against its native pregnenolone substrate, thereby limiting the production of testosterone in rat Leydig cells (180). In humans, PFOS displays non-competitive inhibition of HSD17B3, another enzyme required for testosterone synthesis (176). Interference with steroid hormone receptors has also been documented, with the binding of PFAS leading to antagonism of androgen receptors (50), thereby blocking their activation by androgens, such as testosterone, in a dose-dependent manner (181). Other studies have reported a reduction in fetal Leydig cell number in male offspring exposed in utero, with mothers gavaged with 5 or 20 mg/kg of PFOS daily from gestational day 11 to 19 (182), which provides a tenable explanation for the reduction in testosterone levels seen in independent studies of rat models (146). Alternatively, elevated exposure to PFAS has been positively correlated with increased serum cholesterol in humans (1), which may lead to an increase in the production of steroid hormones. Of concern, the consequences of alterations resulting from prenatal PFAS exposure have the potential to be passed on to offspring through epigenetic transgenerational inheritance modalities (183) and may thus increase the susceptibility of future generations to disease, as demonstrated with other environmental factors (121, 184).
Further to this, at least two studies have shown that high PFOS exposure in adult men results in a higher proportion of morphologically abnormal sperm cells (35, 129). However, Vested et al. failed to identify any such association between PFOA exposure in utero and the proportion of morphologically normal spermatozoa (130). The authors did, however, report associations between PFOA exposure and total sperm count and concentration (130), which would suggest that the timing of exposure plays a part in the mechanism by which PFAS affects the fidelity of sperm production, that is; whether PFAS exposure gives rise to defects in sperm morphology or sperm count. This reasoning is plausible since sperm morphology and motility are primarily determined during sperm production and maturation in adulthood. In contrast, the capacity for sperm production is determined during the fetal period of sexual organ development (130). Furthermore, the relationship between sperm count/concentration and PFOA exposure suggests an effect on Sertoli cell development during the fetal period, as failure of Sertoli cell maturation and consequential inability to support spermatogenesis invariably results in lower rates of sperm cell production (185). This is supported by evidence demonstrating that in vitro PFAS exposure disturbs Sertoli cell function by altering the gap junction network with implications for the intracellular communication and cell-cell interactions (186–188) that are necessary for the support of spermatogenesis (189–192). Abnormal Sertoli cell development during the initial in utero stages of male reproductive tract development will also, in turn, impact Leydig cell function and subsequent masculinization events (193).
An in vitro study using a human stem cell model of spermatogenesis discovered a reduction in both spermatogonia and primary spermatocyte markers when cultures were treated with a mixture of PFOA, PFOS, and PFNA at levels consistent with general population exposure and occupationally exposed individuals, suggesting a potential long-term effect on fertility through exhausting the spermatogonial stem cell pool, rather than directly affecting cell viability (194). In agreement with this notion, an in vivo study in mice revealed a reduction in sperm count and testicular weight upon treatment with PFOS over five weeks (195), while in a zebrafish study the gonadal structure of juvenile males was altered by a 5-month treatment period with PFOS, ultimately resulting in fewer spermatogonia (196). Such results have been attributed to a combination of increased apoptosis and reduced proliferation of germ cells (195). However, the effects of PFAS exposure on the reproductive system differ depending on the specific PFAS and the toxicokinetics of the species studied (197). For example, PFOA mainly accumulates in the plasma and liver of rats, with the half-life in female rats at 1 day being much shorter than in males at 15 days (106). By comparison, high PFOS accumulation has been detected in the liver and lungs of humans (20, 198), with no differences in elimination between genders (12). Another mechanism by which PFAS mediated testicular issues may arise is through the binding of fatty acid binding-proteins (FABP), a family of proteins that bind fatty acids to enhance their solubility, and to aid in both the intracellular and extracellular transport of fatty acids (199). The most common FABP is albumin, which binds and transports fatty acids within the plasma and interstitial fluid (200, 201). There are distinct types of FABP, with each type exhibiting certain tissue distribution patterns and named accordingly (202). For example, mammalian testicular cells express high levels of the Fabp9 gene (also known as testes FABP) (202), and Fabp12 gene expression has been detected in adult rat testis (203). Due to the distinct distribution and expression patterns of FABP encoding loci, it is suggested they may play a role in cell proliferation and differentiation (199, 204), specifically spermatogenesis in the testes (203). Therefore, the testes are a vulnerable organ for PFAS-mediated damage, owing to their abundant expression of FABPs that have a propensity to bind the perfluoroalkyl chains and lead to sequestration of PFAS.
Extensive worldwide use of PFAS has led to pervasive contamination of land and water, which demand remediation if we are to have any prospect of combating the adverse health outcomes attributed to these chemicals, both in humans and wildlife. Environmental matrices that require targeting for remediation include groundwater, drinking and surface water, as well as soil and sediments (66, 205). However, this diversity of substrates provides unique challenges considering that different PFAS do not all interact with different matrices in the same manner (206), nor do they behave like other environmental contaminants (207). It is thus imperative that chemical features specific to PFAS are taken into consideration when designing remediation strategies to ensure thorough and long-lasting removal is achieved. Similarly, the logistics of the treatment, accessibility, and safety measures need to be taken into consideration. Regrettably, options for PFAS remediation remain limited, with most current technologies having originally been developed for the removal of other contaminants (208). Thus, there is considerable scope for the development of novel technologies to facilitate PFAS remediation, perhaps even employing a combination of treatment processes tailored to the site and/or PFAS profile to achieve the most cost-effective and efficient treatment process for each site (209). Illustrated in Figure 4 are three remediation strategies that can be employed for effective treatment of PFAS water contamination (210–216). A novel technique has been proposed that utilizes plant proteins for effective removal of PFAS water contamination, through the pump-and-treat method (217). Such proteins contain both charged and uncharged residues on their surface, which allows them to form bonds with ligands through electrostatic interactions and/or hydrophobic/hydrophilic interactions (218), providing a method by which they could potentially remove PFAS contamination from water. Previous studies have shown PFOS forms a strong salt bridge with HSA, resulting in a high adsorption ratio of 45:1 PFOS to HSA (219). Building on these observations, Turner et al. (217) investigated the sequestration efficacy of six plant protein isolates and found that hemp protein has the highest removal rate for total PFAS at 92.5%, along with soy and pea proteins (around 82%), which is comparable to the granular activated carbon technique (95%). This technique provides a means by which plant proteins could be employed to reduce PFAS contamination within the body and thus warrants further investigation.
Figure 4 Schematic diagram of three possible treatment mechanisms for PFAS contaminated water. (A) Carbon-rich sorbents such as granular activated carbon (GAC) have a long history of being utilised to remove a variety of organic contaminants from water and as such are by far the best studied and most widely used sorption technology for treating PFAS contaminated water sources (208). Granular activated carbon has been shown to reliably remove PFOS with over 90% efficiency (59, 210) and is thus now the reference point for comparison of all new PFAS water sorption technologies. This technology can be employed to treat water before it reaches consumers, either as a single strategy, or as part of an integrated treatment programme. This treatment often involves the pump and treat method in which groundwater is extracted and filtered (208, 211), with the sorbent then being disposed of in landfill sites, provided certain risk criteria are met and the chemicals remain sequestered. International conventions state that waste materials containing > 50 mg/kg of PFAS must be treated in such a way as to destroy these chemicals, which is often accomplished by incinerating at high temperatures (over 1100°C) (208, 212). (B) Ion exchange uses anion exchange to target a wider range of PFAS, allowing for more efficient removal. Removal occurs via electrostatic interactions between the charged functional groups of PFAS chemicals and ions supported on an immobilized synthetic structure (213). In comparison to activated carbon treatment, this method has been shown to be more effective in removing PFAS, particularly the short chain variants (205). However, the efficiency of ion exchange technologies is dependent on several factors, such as the chemical composition of PFAS functional group, PFAS chain length and the ion exchange functional groups (the more hydrophobic, the better the sorption capacity of all chain lengths) (213). The success of almost all remediation strategies employed has been shown to depend on the perfluoroalkyl chain length, with increased efficacy seen with smaller chain length (214). (C) However, simply removing PFAS from water does not destroy the chemical, hence why further processing or incineration is required, making removal techniques lengthy and potentially hazardous. Sonochemical degradation has been shown to destroy aqueous PFOA and PFOA in laboratory conditions (213), demonstrating sonic irradiation can be effectively employed to reduce PFAS contamination at environmentally relevant levels (215). The degradation patterns of PFAS chemicals are influenced by their functional groups and chain lengths as well as physical variables such as temperature and pH (216). Utilizing the method of groundwater pumping is seen as a potentially endless endeavor due to continual contamination from untreated water sources. This, in turn, raises questions as to whether the pump and treat method is sustainable in the long-term treatment of PFAS contamination due to extensive resources and energy required (208).
Unlike other environmental toxicants, such as parabens (220), the body cannot metabolize or facilitate the rapid removal of PFAS. Thus far, there has been limited investigation into the possibility of sequestering PFAS chemicals to reduce bioaccumulation within contaminated individuals in order to mitigate negative health outcomes. However, the adaption of existing techniques developed for other environmental contaminants may provide opportunities by which to reduce PFAS accumulation. Unfortunately, most of these techniques are only newly recognized and as such are still at an early stage of research with inadequate evidence of efficacy (221).
One process investigated for detoxification in humans is the exploitation of the body’s natural excretion through perspiration, with an assortment of toxicants shown to be excreted in this manner, such as metals (222), phthalates (223) and bisphenol A (224). Studies have reported that induced perspiration treatment in individuals with toxicant accumulation, specifically polychlorinated biphenyls (PCBs) compounds, results in a statistically significant reduction in body burden (225, 226). Although promising, based on the limited available evidence, it seems that PFAS may not be readily excreted through sweat (227). Conversely, shorter half-lives are observed in women, which are proposed to result from increased PFAS elimination through menstruation, pregnancy or lactation (1, 109, 228). Accordingly, studies have shown regular phlebotomy or blood donation can also increase the elimination of PFAS (229, 230). Lorber et al. showed males undergoing regular blood withdrawals had 40% lower PFHxS, PFOA and PFOS levels in comparison to males in the general population (231), and suggested a 9% reduction in circulating blood volume was required to achieve significant reductions in PFAS levels. Additionally, evidence shows that bile acid sequestrants, such as cholestyramine, bind to PFAS toxicants within the gastrointestinal tract, thus preventing enterohepatic recirculation and increasing excretion of cholesterol and PFAS (227, 232). One case study with a single individual with high PFAS contamination showed increased levels of PFAS in stool samples following treatment with cholestyramine, with serum levels subsequently reducing with continued treatment (227). Ducatman and colleagues recently substantiated this evidence by analyzing the C8 Health Project Data (43) in which they found a reduction in serum PFAS levels (especially PFOS from 19 to 1 ng/mL) in individuals reporting to take regular cholestyramine medication, although data on duration and medication dosage administered was not reported (233). Of course, additional investigations are required to further corroborate these findings, but thus far evidence from these studies implies that utilizing cholestyramine treatment may allow for enhanced toxicant elimination.
Increasing awareness of the potential health implications of PFAS and realization of the extent of environmental contamination has led to a rising demand for research into definitive health risks and effective remediation strategies. Animal models have been widely employed to investigate in vitro and in vivo consequences of PFAS exposure, as well as the toxicology of these chemicals. Such studies complement a growing body of evidence from human epidemiological studies. However, the literature abounds with conflicting evidence, and as such, it remains challenging to draw accurate conclusions regarding the causality of PFAS related health issues. This situation is exacerbated by the repeated demonstration that outcomes differ depending on factors such as the specific PFAS chemical(s) (of which there are over 4,700), stage of development (i.e., during fetal development or in later life) and duration of exposure, level and mix of contamination, route of exposure, and interaction with other environmental contaminants and toxicants, all of which are influenced by geographical location. These factors present significant difficulties for researchers in planning, executing, and interpreting studies, and thus hinder our ability to directly compare PFAS exposure studies. While standardization therefore remains an essential priority for future research, the identification of appropriate cellular model(s) with which to directly investigate and unlock the interaction of PFAS with the male reproductive system would also be advantageous. In addition, agreement is needed regarding endpoint measures, in which subtle changes, such as decreases in fertility or metabolic sequelae, may be used as early markers of PFAS-mediated health effects, rather than more extreme factors such as tumors. In this regard, the male reproductive system offers notable advantages as a sensitive marker of human disease and may ultimately provide a unique opportunity for assessing the emerging threat to human health posed by PFAS exposure. Indeed, this model draws on a growing body of evidence of a strong association between a male’s general health and reproductive potential, with infertility being strongly correlated with future health concerns such as testicular cancer, ischemic heart disease and diabetes.
We contend that the identification of a reliable indicator of PFAS exposure would allow for the identification of reproductive health conditions resulting from PFAS bioaccumulation and aid in identifying, with certainty, the mechanisms by which PFAS impacts male reproductive health. Exploiting male reproductive function and sperm biology as a non-invasive means by which to investigate health outcomes is justified due to the responsiveness and sensitivity of the male reproductive system to environmental toxicants. Indeed, previous studies have employed this system as a marker to define the health effects of environmental factors such as acrylamide (234, 235), mobile phone radiation (236), and heat (237, 238). Additionally, the male reproductive system is known to be an early indicator for the onset of chronic diseases such as coronary and inflammatory diseases, making it a suitable indicator of general health. Strong associations have been seen between PFAS exposure and testicular dysfunction, indicating the male reproductive system is vulnerable to PFAS-mediated damage. In this context, perhaps the most suitable human cohorts to study are those that have received occupational PFAS exposure, such as firefighters, to determine the common effects of exposure and gain insight into possible mechanisms of action. It would then be pertinent to study individuals with idiopathic infertility to identify if any clear associations can be drawn between their diagnosis and PFAS levels, through assessment of detailed life history and daily routine/exposure information. These factors could then be used to screen for affected individuals within the general population. In addition to elucidating the toxicological effects of PFAS chemicals in humans, there is a need for data on a wider range of PFAS in order to regulate, legislate and ban those that are harmful, thus preventing further contamination from replacement PFAS, which may be just as harmful as the legacy variants.
LC conceived of the idea and wrote the first draft of the manuscript. MG, GI, MD, BT, BC, AE, SR and BN conceived of the idea, sourced funding, and edited the manuscript. All authors approved the final version of the manuscript.
This work was supported by funding from a National Health & Medical Research Council of Australia (NHMRC) Targeted Call for Research into Per- and Poly-Fluoroalkylated Substances (APP1189415) awarded to BN, MG, GI, MD, BT, BC, AE, and SR. BN is the recipitent of an NHMRC Senior Research Fellowship (APP1154837). MD is the recipient of an NHMRC Investigator Grant (APP1173892) and a Defeat DIPG ChadTough New Investigator Fellowship.
The authors declare that the research was conducted in the absence of any commercial or financial relationships that could be construed as a potential conflict of interest.
All claims expressed in this article are solely those of the authors and do not necessarily represent those of their affiliated organizations, or those of the publisher, the editors and the reviewers. Any product that may be evaluated in this article, or claim that may be made by its manufacturer, is not guaranteed or endorsed by the publisher.
1. Kirk M, Smurthwaite K, Bräunig J, Trevenar S, D’Este C, Lucas R, et al. The PFAS Health Study: Systematic Literature Review. Canberra: The Australian National University. (2018). p. 1–256.
2. OECD, Organisation for Economic Co-operation and Development. Environment Directorate, Joint Meeting of the Chemicals Committee and the Working Party on Chemicals, Pesiticides and Biotechnology. Paris: Organisation for Economic Co-operation and Development (2018).
3. Banks RE, Smart BE, Tatlow J. Organofluorine Chemistry: Principles and Commercial Applications. Birmingham: Springer Science & Business Media (1994).
5. Buck RC, Franklin J, Berger U, Conder JM, Cousins IT, De Voogt P, et al. Perfluoroalkyl and Polyfluoroalkyl Substances in the Environment: Terminology, Classification, and Origins. Integrated Environ Assess Manage (2011) 7:513–41. doi: 10.1002/ieam.258
6. Jensen AA, Leffers H. Emerging Endocrine Disrupters: Perfluoroalkylated Substances. Int J Androl (2008) 31:161–9. doi: 10.1111/j.1365-2605.2008.00870.x
7. Lindstrom AB, Strynar MJ, Libelo EL. Polyfluorinated Compounds: Past, Present, and Future. Environ Sci Technol (2011) 45:7954–61. doi: 10.1021/es2011622
8. Renner R. Growing Concern Over Perfluorinated Chemicals. Environ Sci Technol (2001) 35:154a–60a. doi: 10.1021/es012317k
9. Olsen GW, Mair DC, Lange CC, Harrington LM, Church TR, Goldberg CL, et al. Per- and Polyfluoroalkyl Substances (PFAS) in American Red Cross Adult Blood Donors, 2000-2015. Environ Res (2017) 157:87–95. doi: 10.1016/j.envres.2017.05.013
10. Giesy JP, Kannan K. Global Distribution of Perfluorooctane Sulfonate in Wildlife. Environ Sci Technol (2001) 35:1339–42. doi: 10.1021/es001834k
11. Hölzer J, Midasch O, Rauchfuss K, Kraft M, Reupert R, Angerer J, et al. Biomonitoring of Perfluorinated Compounds in Children and Adults Exposed to Perfluorooctanoate-Contaminated Drinking Water. Environ Health Perspect (2008) 116:651–7. doi: 10.1289/ehp.11064
12. Fenton SE, Ducatman A, Boobis A, DeWitt JC, Lau C, Ng C, et al. Per- and Polyfluoroalkyl Substance Toxicity and Human Health Review: Current State of Knowledge and Strategies for Informing Future Research. Environ Toxicol Chem (2021) 40:606–30. doi: 10.1002/etc.4890
13. Olsen GW, Burris JM, Ehresman DJ, Froehlich JW, Seacat AM, Butenhoff JL, et al. Half-Life of Serum Elimination of Perfluorooctanesulfonate, Perfluorohexanesulfonate, and Perfluorooctanoate in Retired Fluorochemical Production Workers. Environ Health Perspect (2007) 115:1298–305. doi: 10.1289/ehp.10009
14. Martin JW, Mabury SA, Solomon KR, Muir DC. Dietary Accumulation of Perfluorinated Acids in Juvenile Rainbow Trout (Oncorhynchus Mykiss). Environ Toxicol Chem (2003) 22:189–95. doi: 10.1002/etc.5620220125
15. Conder JM, Hoke RA, De Wolf W, Russell MH, Buck RC. Are PFCAs Bioaccumulative? A Critical Review and Comparison With Regulatory Criteria and Persistent Lipophilic Compounds. Environ Sci Technol (2008) 42:995–1003. doi: 10.1021/es070895g
16. Gomis MI, Vestergren R, Borg D, Cousins IT. Comparing the Toxic Potency In Vivo of Long-Chain Perfluoroalkyl Acids and Fluorinated Alternatives. Environ Int (2018) 113:1–9. doi: 10.1016/j.envint.2018.01.011
17. Chambers WS, Hopkins JG, Richards SM. A Review of Per- and Polyfluorinated Alkyl Substance Impairment of Reproduction. Front Toxicol (2021) 3. doi: 10.3389/ftox.2021.732436
18. Martin JW, Mabury SA, Solomon KR, Muir DC. Bioconcentration and Tissue Distribution of Perfluorinated Acids in Rainbow Trout (Oncorhynchus Mykiss). Environ Toxicol Chem (2003) 22:196–204. doi: 10.1002/etc.5620220126
20. Pérez F, Nadal M, Navarro-Ortega A, Fàbrega F, Domingo JL, Barceló D, et al. Accumulation of Perfluoroalkyl Substances in Human Tissues. Environ Int (2013) 59:354–62. doi: 10.1016/j.envint.2013.06.004
21. Calafat AM, Wong LY, Kuklenyik Z, Reidy JA, Needham LL. Polyfluoroalkyl Chemicals in the U.S. Population: Data From the National Health and Nutrition Examination Survey (NHANES) 2003-2004 and Comparisons With NHANES 1999-2000. Environ Health Perspect (2007) 115:1596–602. doi: 10.1289/ehp.10598
22. Jian J-M, Chen D, Han F-J, Guo Y, Zeng L, Lu X, et al. A Short Review on Human Exposure to and Tissue Distribution of Per- and Polyfluoroalkyl Substances (PFASs). Sci Total Environ (2018) 636:1058–69. doi: 10.1016/j.scitotenv.2018.04.380
23. Jain RB, Ducatman A. Perfluoroalkyl Acids Serum Concentrations and Their Relationship to Biomarkers of Renal Failure: Serum and Urine Albumin, Creatinine, and Albumin Creatinine Ratios Across the Spectrum of Glomerular Function Among US Adults. Environ Res (2019) 174:143–51. doi: 10.1016/j.envres.2019.04.034
24. Kannan K, Corsolini S, Falandysz J, Fillmann G, Kumar KS, Loganathan BG, et al. Perfluorooctanesulfonate and Related Fluorochemicals in Human Blood From Several Countries. Environ Sci Technol (2004) 38:4489–95. doi: 10.1021/es0493446
25. Lau C, Butenhoff JL, Rogers JM. The Developmental Toxicity of Perfluoroalkyl Acids and Their Derivatives. Toxicol Appl Pharmacol (2004) 198:231–41. doi: 10.1016/j.taap.2003.11.031
26. Tittlemier SA, Pepper K, Edwards L. Concentrations of Perfluorooctanesulfonamides in Canadian Total Diet Study Composite Food Samples Collected Between 1992 and 2004. J Agric Food Chem (2006) 54:8385–9. doi: 10.1021/jf061713p
27. Tittlemier SA, Pepper K, Seymour C, Moisey J, Bronson R, Cao XL, et al. Dietary Exposure of Canadians to Perfluorinated Carboxylates and Perfluorooctane Sulfonate via Consumption of Meat, Fish, Fast Foods, and Food Items Prepared in Their Packaging. J Agric Food Chem (2007) 55:3203–10. doi: 10.1021/jf0634045
28. Haug LS, Huber S, Becher G, Thomsen C. Characterisation of Human Exposure Pathways to Perfluorinated Compounds–Comparing Exposure Estimates With Biomarkers of Exposure. Environ Int (2011) 37:687–93. doi: 10.1016/j.envint.2011.01.011
29. Vestergren R, Cousins IT. Tracking the Pathways of Human Exposure to Perfluorocarboxylates. Environ Sci Technol (2009) 43:5565–75. doi: 10.1021/es900228k
30. Shoeib M, Harner T, Webster MG, Lee SC. Indoor Sources of Poly- and Perfluorinated Compounds (PFCS) in Vancouver, Canada: Implications for Human Exposure. Environ Sci Technol (2011) 45:7999–8005. doi: 10.1021/es103562v
31. Emmett EA, Shofer FS, Zhang H, Freeman D, Desai C, Shaw LM. Community Exposure to Perfluorooctanoate: Relationships Between Serum Concentrations and Exposure Sources. J Occup Environ Med (2006) 48:759–70. doi: 10.1097/01.jom.0000232486.07658.74
32. Sinclair GM, Long SM, Jones OAH. What are the Effects of PFAS Exposure at Environmentally Relevant Concentrations? Chemosphere (2020) 258:127340. doi: 10.1016/j.chemosphere.2020.127340
33. Göckener B, Weber T, Rüdel H, Bücking M, Kolossa-Gehring M. Human Biomonitoring of Per- and Polyfluoroalkyl Substances in German Blood Plasma Samples From 1982 to 2019. Environ Int (2020) 145:106123. doi: 10.1016/j.envint.2020.106123
34. Fromme H, Tittlemier SA, Völkel W, Wilhelm M, Twardella D. Perfluorinated Compounds–Exposure Assessment for the General Population in Western Countries. Int J Hyg Environ Health (2009) 212:239–70. doi: 10.1016/j.ijheh.2008.04.007
35. Toft G, Jönsson BAG, Lindh CH, Giwercman A, Spano M, Heederik D, et al. Exposure to Perfluorinated Compounds and Human Semen Quality in Arctic and European Populations. Hum Reprod (2012) 27:2532–40. doi: 10.1093/humrep/des185
36. Jensen TK, Jacobsen R, Christensen K, Nielsen NC, Bostofte E. Good Semen Quality and Life Expectancy: A Cohort Study of 43,277 Men. Am J Epidemiol (2009) 170:559–65. doi: 10.1093/aje/kwp168
37. Del Giudice F, Kasman AM, Ferro M, Sciarra A, De Berardinis E, Belladelli F, et al. Clinical Correlation Among Male Infertility and Overall Male Health: A Systematic Review of the Literature. Investig Clin Urol (2020) 61:355–71. doi: 10.4111/icu.2020.61.4.355
38. Kasman AM, Del Giudice F, Eisenberg ML. New Insights to Guide Patient Care: The Bidirectional Relationship Between Male Infertility and Male Health. Fertil Steril (2020) 113:469–77. doi: 10.1016/j.fertnstert.2020.01.002
39. Glazer CH, Eisenberg ML, Tøttenborg SS, Giwercman A, Flachs EM, Bräuner EV, et al. Male Factor Infertility and Risk of Death: A Nationwide Record-Linkage Study. Hum Reprod (2019) 34:2266–73. doi: 10.1093/humrep/dez189
40. Eisenberg ML, Li S, Cullen MR, Baker LC. Increased Risk of Incident Chronic Medical Conditions in Infertile Men: Analysis of United States Claims Data. Fertil Steril (2016) 105:629–36. doi: 10.1016/j.fertnstert.2015.11.011
41. Latif T, Lindahl-Jacobsen R, Mehlsen J, Eisenberg ML, Holmboe SA, Pors K, et al. Semen Quality Associated With Subsequent Hospitalizations – Can the Effect be Explained by Socio-Economic Status and Lifestyle Factors? Andrology (2018) 6:428–35. doi: 10.1111/andr.12477
42. Eisenberg ML, Li S, Behr B, Cullen MR, Galusha D, Lamb DJ, et al. Semen Quality, Infertility and Mortality in the USA. Hum Reprod (2014) 29:1567–74. doi: 10.1093/humrep/deu106
43. Frisbee SJ, Brooks AP Jr, Maher A, Flensborg P, Arnold S, Fletcher T, et al. The C8 Health Project: Design, Methods, and Participants. Environ Health Perspect (2009) 117:1873–82. doi: 10.1289/ehp.0800379
44. Barry V, Winquist A, Steenland K. Perfluorooctanoic Acid (PFOA) Exposures and Incident Cancers Among Adults Living Near a Chemical Plant. Environ Health Perspect (2013) 121:1313–8. doi: 10.1289/ehp.1306615
45. Vieira VM, Hoffman K, Shin H-M, Weinberg JM, Webster TF, Fletcher T. Perfluorooctanoic Acid Exposure and Cancer Outcomes in a Contaminated Community: A Geographic Analysis. Environ Health Perspect (2013) 121:318–23. doi: 10.1289/ehp.1205829
46. Lin H-W, Feng H-X, Chen L, Yuan X-J, Tan Z. Maternal Exposure to Environmental Endocrine Disruptors During Pregnancy is Associated With Pediatric Germ Cell Tumors. Nagoya J Med Sci (2020) 82:323–33. doi: 10.18999/nagjms.82.2.323
47. Bartell SM, Vieira VM. Critical Review on PFOA, Kidney Cancer, and Testicular Cancer. J Air Waste Manage Assoc (2021) 71:663–79. doi: 10.1080/10962247.2021.1909668
48. IARC. International Agency for Research on Cancer, IARC, Monograph, Perfluorooctanoic Acid. Lyon: International Agency for Research on Cancer (2018).
49. Louis GM, Chen Z, Schisterman EF, Kim S, Sweeney AM, Sundaram R, et al. Perfluorochemicals and Human Semen Quality: The LIFE Study. Environ Health Perspect (2015) 123:57–63. doi: 10.1289/ehp.1307621
50. Di Nisio A, Sabovic I, Valente U, Tescari S, Rocca MS, Guidolin D, et al. Endocrine Disruption of Androgenic Activity by Perfluoroalkyl Substances: Clinical and Experimental Evidence. J Clin Endocrinol Metab (2019) 104:1259–71. doi: 10.1210/jc.2018-01855
51. Blake BE, Fenton SE. Early Life Exposure to Per- and Polyfluoroalkyl Substances (PFAS) and Latent Health Outcomes: A Review Including the Placenta as a Target Tissue and Possible Driver of Peri- and Postnatal Effects. Toxicology (2020) 443:152565. doi: 10.1016/j.tox.2020.152565
52. Brendel S, Fetter É, Staude C, Vierke L, Biegel-Engler A. Short-Chain Perfluoroalkyl Acids: Environmental Concerns and a Regulatory Strategy Under REACH. Environ Sci Eur (2018) 30:9. doi: 10.1186/s12302-018-0134-4
53. Vecitis CD, Wang Y, Cheng J, Park H, Mader BT, Hoffmann MR. Sonochemical Degradation of Perfluorooctanesulfonate in Aqueous Film-Forming Foams. Environ Sci Technol (2010) 44:432–8. doi: 10.1021/es902444r
54. Wang Z, DeWitt JC, Higgins CP, Cousins IT. A Never-Ending Story of Per- and Polyfluoroalkyl Substances (PFASs)? Environ Sci Technol (2017) 51:2508–18. doi: 10.1021/acs.est.6b04806
55. Olsen GW, Burris JM, Burlew MM, Mandel JH. Epidemiologic Assessment of Worker Serum Perfluorooctanesulfonate (PFOS) and Perfluorooctanoate (PFOA) Concentrations and Medical Surveillance Examinations. J Occup Environ Med (2003) 45:260–70. doi: 10.1097/01.jom.0000052958.59271.10
56. Hu XC, Andrews DQ, Lindstrom AB, Bruton TA, Schaider LA, Grandjean P, et al. Detection of Poly- and Perfluoroalkyl Substances (PFASs) in U.S. Drinking Water Linked to Industrial Sites, Military Fire Training Areas, and Wastewater Treatment Plants. Environ Sci Technol Lett (2016) 3:344–50. doi: 10.1021/acs.estlett.6b00260
57. Tanner EM, Bloom MS, Wu Q, Kannan K, Yucel RM, Shrestha S, et al. Occupational Exposure to Perfluoroalkyl Substances and Serum Levels of Perfluorooctanesulfonic Acid (PFOS) and Perfluorooctanoic Acid (PFOA) in an Aging Population From Upstate New York: A Retrospective Cohort Study. Int Arch Occup Environ Health (2018) 91:145–54. doi: 10.1007/s00420-017-1267-2
58. Rotander A, Kärrman A, Toms LM, Kay M, Mueller JF, Gómez Ramos MJ. Novel Fluorinated Surfactants Tentatively Identified in Firefighters Using Liquid Chromatography Quadrupole Time-of-Flight Tandem Mass Spectrometry and a Case-Control Approach. Environ Sci Technol (2015) 49:2434–42. doi: 10.1021/es503653n
59. Eschauzier C, Beerendonk E, Scholte-Veenendaal P, De Voogt P. Impact of Treatment Processes on the Removal of Perfluoroalkyl Acids From the Drinking Water Production Chain. Environ Sci Technol (2012) 46:1708–15. doi: 10.1021/es201662b
60. Ericson I, Nadal M, van Bavel B, Lindström G, Domingo JL. Levels of Perfluorochemicals in Water Samples From Catalonia, Spain: Is Drinking Water a Significant Contribution to Human Exposure? Environ Sci Pollut Res Int (2008) 15:614–9. doi: 10.1007/s11356-008-0040-1
61. Björklund JA, Thuresson K, De Wit CA. Perfluoroalkyl Compounds (PFCs) in Indoor Dust: Concentrations, Human Exposure Estimates, and Sources. Environ Sci Technol (2009) 43:2276–81. doi: 10.1021/es803201a
62. Sunderland EM, Hu XC, Dassuncao C, Tokranov AK, Wagner CC, Allen JG. A Review of the Pathways of Human Exposure to Poly- and Perfluoroalkyl Substances (PFASs) and Present Understanding of Health Effects. J Expo Sci Environ Epidemiol (2019) 29:131–47. doi: 10.1038/s41370-018-0094-1
63. Begley TH, Hsu W, Noonan G, Diachenko G. Migration of Fluorochemical Paper Additives From Food-Contact Paper Into Foods and Food Simulants. Food Addit Contam Part A Chem Anal Control Expo Risk Assess (2008) 25:384–90. doi: 10.1080/02652030701513784
64. Johnson PI, Sutton P, Atchley DS, Koustas E, Lam J, Sen S, et al. The Navigation Guide - Evidence-Based Medicine Meets Environmental Health: Systematic Review of Human Evidence for PFOA Effects on Fetal Growth. Environ Health Perspect (2014) 122:1028–39. doi: 10.1289/ehp.1307893
65. Apelberg BJ, Witter FR, Herbstman JB, Calafat AM, Halden RU, Needham LL, et al. Cord Serum Concentrations of Perfluorooctane Sulfonate (PFOS) and Perfluorooctanoate (PFOA) in Relation to Weight and Size at Birth. Environ Health Perspect (2007) 115:1670–6. doi: 10.1289/ehp.10334
66. ATSDR. Toxicological Profile for Perfluoroalkyls: Draft for Public Comment, Agency for Toxic Substances and Disease Registry. Atlanta: Agency for Toxic Substances and Disease Registry (2018).
67. Tao L, Ma J, Kunisue T, Libelo EL, Tanabe S, Kannan K. Perfluorinated Compounds in Human Breast Milk From Several Asian Countries, and in Infant Formula and Dairy Milk From the United States. Environ Sci Technol (2008) 42:8597–602. doi: 10.1021/es801875v
68. Hinderliter PM, DeLorme MP, Kennedy GL. Perfluorooctanoic Acid: Relationship Between Repeated Inhalation Exposures and Plasma PFOA Concentration in the Rat. Toxicology (2006) 222:80–5. doi: 10.1016/j.tox.2006.01.029
69. Franko J, Meade BJ, Frasch HF, Barbero AM, Anderson SE. Dermal Penetration Potential of Perfluorooctanoic Acid (PFOA) in Human and Mouse Skin. J Toxicol Environ Health A (2012) 75:50–62. doi: 10.1080/15287394.2011.615108
70. Han X, Snow TA, Kemper RA, Jepson GW. Binding of Perfluorooctanoic Acid to Rat and Human Plasma Proteins. Chem Res Toxicol (2003) 16:775–81. doi: 10.1021/tx034005w
71. Jones PD, Hu W, De Coen W, Newsted JL, Giesy JP. Binding of Perfluorinated Fatty Acids to Serum Proteins. Environ Toxicol Chem (2003) 22:2639–49. doi: 10.1897/02-553
72. Beesoon S, Martin JW. Isomer-Specific Binding Affinity of Perfluorooctanesulfonate (PFOS) and Perfluorooctanoate (PFOA) to Serum Proteins. Environ Sci Technol (2015) 49:5722–31. doi: 10.1021/es505399w
73. Bogdanska J, Borg D, Sundström M, Bergström U, Halldin K, Abedi-Valugerdi M, et al. Tissue Distribution of 35S-Labelled Perfluorooctane Sulfonate in Adult Mice After Oral Exposure to a Low Environmentally Relevant Dose or a High Experimental Dose. Toxicology (2011) 284:54–62. doi: 10.1016/j.tox.2011.03.014
74. Kudo N, Sakai A, Mitsumoto A, Hibino Y, Tsuda T, Kawashima Y. Tissue Distribution and Hepatic Subcellular Distribution of Perfluorooctanoic Acid at Low Dose are Different From Those at High Dose in Rats. Biol Pharm Bull (2007) 30:1535–40. doi: 10.1248/bpb.30.1535
75. Cui L, Liao CY, Zhou QF, Xia TM, Yun ZJ, Jiang GB. Excretion of PFOA and PFOS in Male Rats During a Subchronic Exposure. Arch Environ Contam Toxicol (2010) 58:205–13. doi: 10.1007/s00244-009-9336-5
76. Prevedouros K, Cousins IT, Buck RC, Korzeniowski SH. Sources, Fate and Transport of Perfluorocarboxylates. Environ Sci Technol (2006) 40:32–44. doi: 10.1021/es0512475
77. De Silva AO, Mabury SA. Isolating Isomers of Perfluorocarboxylates in Polar Bears (Ursus Maritimus) From Two Geographical Locations. Environ Sci Technol (2004) 38:6538–45. doi: 10.1021/es049296p
78. Song X, Tang S, Zhu H, Chen Z, Zang Z, Zhang Y, et al. Biomonitoring PFAAs in Blood and Semen Samples: Investigation of a Potential Link Between PFAAs Exposure and Semen Mobility in China. Environ Int (2018) 113:50–4. doi: 10.1016/j.envint.2018.01.010
79. Wang Z, Cousins IT, Scheringer M, Hungerbühler K. Fluorinated Alternatives to Long-Chain Perfluoroalkyl Carboxylic Acids (PFCAs), Perfluoroalkane Sulfonic Acids (PFSAs) and Their Potential Precursors. Environ Int (2013) 60:242–8. doi: 10.1016/j.envint.2013.08.021
80. SCHEER, Scientific Committee on Health. Environmental and Emerging Risks, Statement on Emerging Health and Environmental Issues, Vol. 2018. Luxembourg City: Scientific Committee on Health, Environmental and Emerging Risks (2018).
81. Tarapore P, Ouyang B. Perfluoroalkyl Chemicals and Male Reproductive Health: Do PFOA and PFOS Increase Risk for Male Infertility? Int J Environ Res Public Health (2021) 18:3794. doi: 10.3390/ijerph18073794
82. Rand AA, Mabury SA. Is There a Human Health Risk Associated With Indirect Exposure to Perfluoroalkyl Carboxylates (PFCAs)? Toxicology (2017) 375:28–36. doi: 10.1016/j.tox.2016.11.011
83. Dagnino S, Strynar MJ, McMahen RL, Lau CS, Ball C, Garantziotis S, et al. Identification of Biomarkers of Exposure to FTOHs and PAPs in Humans Using a Targeted and Nontargeted Analysis Approach. Environ Sci Technol (2016) 50:10216–25. doi: 10.1021/acs.est.6b01170
84. N.T.P. (NTP), National Toxicology Program Monograph on Immunotoxicity Associated With Exposures to Pfoa and Pfos. Durham, North Carolina: National Toxicology Program (2016).
85. Benbrahim-Tallaa L, Lauby-Secretan B, Loomis D, Guyton KZ, Grosse Y, El Ghissassi F, et al. Carcinogenicity of Perfluorooctanoic Acid, Tetrafluoroethylene, Dichloromethane, 1,2-Dichloropropane, and 1,3-Propane Sultone. Lancet Oncol (2014) 15:924–5. doi: 10.1016/S1470-2045(14)70316-X
86. Olsen GW, Ley CA. Prostate Cancer and PFOA. J Occup Environ Med (2015) 57:e60. doi: 10.1097/JOM.0000000000000446
87. Gilliland FD, Mandel JS. Mortality Among Employees of a Perfluorooctanoic Acid Production Plant. J Occup Med (1993) 35:950–4. doi: 10.1097/00043764-199309000-00020
88. Mastrantonio M, Bai E, Uccelli R, Cordiano V, Screpanti A, Crosignani P. Drinking Water Contamination From Perfluoroalkyl Substances (PFAS): An Ecological Mortality Study in the Veneto Region, Italy. Eur J Public Health (2017) 28:180–5. doi: 10.1093/eurpub/ckx066
89. Frisbee SJ, Shankar A, Knox SS, Steenland K, Savitz DA, Fletcher T, et al. Perfluorooctanoic Acid, Perfluorooctanesulfonate, and Serum Lipids in Children and Adolescents: Results From the C8 Health Project. Arch Pediatr Adolesc Med (2010) 164:860–9. doi: 10.1001/archpediatrics.2010.163
90. Sakr CJ, Kreckmann KH, Green JW, Gillies PJ, Reynolds JL, Leonard RC. Cross-Sectional Study of Lipids and Liver Enzymes Related to a Serum Biomarker of Exposure (Ammonium Perfluorooctanoate or APFO) as Part of a General Health Survey in a Cohort of Occupationally Exposed Workers. J Occup Environ Med (2007) 49:1086–96. doi: 10.1097/JOM.0b013e318156eca3
91. Steenland K, Tinker S, Frisbee S, Ducatman A, Vaccarino V. Association of Perfluorooctanoic Acid and Perfluorooctane Sulfonate With Serum Lipids Among Adults Living Near a Chemical Plant. Am J Epidemiol (2009) 170:1268–78. doi: 10.1093/aje/kwp279
92. Olsen GW, Zobel LR. Assessment of Lipid, Hepatic, and Thyroid Parameters With Serum Perfluorooctanoate (PFOA) Concentrations in Fluorochemical Production Workers. Int Arch Occup Environ Health (2007) 81:231–46. doi: 10.1007/s00420-007-0213-0
93. Sakr CJ, Leonard RC, Kreckmann KH, Slade MD, Cullen MR. Longitudinal Study of Serum Lipids and Liver Enzymes in Workers With Occupational Exposure to Ammonium Perfluorooctanoate. J Occup Environ Med (2007) 49:872–9. doi: 10.1097/JOM.0b013e318124a93f
94. Costa G, Sartori S, Consonni D. Thirty Years of Medical Surveillance in Perfluooctanoic Acid Production Workers. J Occup Environ Med (2009) 51:364–72. doi: 10.1097/JOM.0b013e3181965d80
95. EPA. EPA Advances Science to Protect the Public From PFOA and PFOS in Drinking Water, EPA. Washington, DC: Environmental Protection Agency (2021).
96. Steenland K, Woskie S. Cohort Mortality Study of Workers Exposed to Perfluorooctanoic Acid. Am J Epidemiol (2012) 176:909–17. doi: 10.1093/aje/kws171
97. Leonard RC, Kreckmann KH, Sakr CJ, Symons JM. Retrospective Cohort Mortality Study of Workers in a Polymer Production Plant Including a Reference Population of Regional Workers. Ann Epidemiol (2008) 18:15–22. doi: 10.1016/j.annepidem.2007.06.011
98. McIver SC, Roman SD, Nixon B, Loveland KL, McLaughlin EA. The Rise of Testicular Germ Cell Tumours: The Search for Causes, Risk Factors and Novel Therapeutic Targets. F1000Research (2013) 2:55. doi: 10.12688/f1000research.2-55.v1
99. Baade P, Carrière P, Fritschi L. Trends in Testicular Germ Cell Cancer Incidence in Australia. Cancer Causes Cont (2008) 19:1043–9. doi: 10.1007/s10552-008-9168-z
100. Skakkebaek NE, Rajpert-De Meyts E, Jørgensen N, Main KM, Leffers H, Andersson AM, et al. Testicular Cancer Trends as ‘Whistle Blowers’ of Testicular Developmental Problems in Populations. Int J Androl (2007) 30:198–204; discussion 204-5. doi: 10.1111/j.1365-2605.2007.00776.x
101. Walschaerts M, Huyghe E, Muller A, Bachaud JM, Bujan L, Thonneau P. Doubling of Testicular Cancer Incidence Rate Over the Last 20 Years in Southern France. Cancer Causes Cont (2008) 19:155–61. doi: 10.1007/s10552-007-9081-x
102. Richiardi L, Pettersson A, Akre O. Genetic and Environmental Risk Factors for Testicular Cancer. Int J Androl (2007) 30:230–40; discussion 240-1. doi: 10.1111/j.1365-2605.2007.00760.x
103. Post GB, Cohn PD, Cooper KR. Perfluorooctanoic Acid (PFOA), an Emerging Drinking Water Contaminant: A Critical Review of Recent Literature. Environ Res (2012) 116:93–117. doi: 10.1016/j.envres.2012.03.007
104. Lau C, Anitole K, Hodes C, Lai D, Pfahles-Hutchens A, Seed J. Perfluoroalkyl Acids: A Review of Monitoring and Toxicological Findings. Toxicol Sci (2007) 99:366–94. doi: 10.1093/toxsci/kfm128
105. OECD, Organisation for Economic Co-operation and Development. Co-Operation on Existing Chemicals. Hazard Assessment of Perfluorooctane Sulfonate (PFOS) and its Salts. Paris: Organisation for Economic Co-operation and Development (2002).
106. Vanden Heuvel JP, Kuslikis BI, Van Rafelghem MJ, Peterson RE, distribution T. Metabolism, and Elimination of Perfluorooctanoic Acid in Male and Female Rats. J Biochem Toxicol (1991) 6:83–92. doi: 10.1002/jbt.2570060202
107. Hanhijärvi H, Ophaug RH, Singer L. The Sex-Related Difference in Perfluorooctanoate Excretion in the Rat. Proc Soc Exp Biol Med (1982) 171:50–5. doi: 10.3181/00379727-171-41476
108. Li Y, Fletcher T, Mucs D, Scott K, Lindh CH, Tallving P, et al. Half-Lives of PFOS, PFHxS and PFOA After End of Exposure to Contaminated Drinking Water. Occup Environ Med (2018) 75:46–51. doi: 10.1136/oemed-2017-104651
109. Zhang Y, Beesoon S, Zhu L, Martin JW. Biomonitoring of Perfluoroalkyl Acids in Human Urine and Estimates of Biological Half-Life. Environ Sci Technol (2013) 47:10619–27. doi: 10.1021/es401905e
110. Barker DJ. The Developmental Origins of Chronic Adult Disease. Acta Paediatr Suppl (2004) 93:26–33. doi: 10.1111/j.1651-2227.2004.tb00236.x
111. Ubel FA, Sorenson SD, Roach DE. Health Status of Plant Workers Exposed to Fluorochemicals–a Preliminary Report. Am Ind Hyg Assoc J (1980) 41:584–9. doi: 10.1080/15298668091425310
112. Scheringer M, Trier X, Cousins IT, de Voogt P, Fletcher T, Wang Z, et al. Helsingør Statement on Poly- and Perfluorinated Alkyl Substances (PFASs). Chemosphere (2014) 114:337–9. doi: 10.1016/j.chemosphere.2014.05.044
113. Blum A, Balan SA, Scheringer M, Trier X, Goldenman G, Cousins IT, et al. The Madrid Statement on Poly- and Perfluoroalkyl Substances (PFASs). Environ Health Perspect (2015) 123:A107–11. doi: 10.1289/ehp.1509934
114. Coggan TL, Anumol T, Pyke J, Shimeta J, Clarke BO. A Single Analytical Method for the Determination of 53 Legacy and Emerging Per- and Polyfluoroalkyl Substances (PFAS) in Aqueous Matrices. Anal Bioanal Chem (2019) 411:3507–20. doi: 10.1007/s00216-019-01829-8
115. Krzastek SC, Farhi J, Gray M, Smith RP. Impact of Environmental Toxin Exposure on Male Fertility Potential. Transl Androl Urol (2020) 9:2797–813. doi: 10.21037/tau-20-685
116. Glazer CH, Bonde JP, Eisenberg ML, Giwercman A, Hærvig KK, Rimborg S, et al. Male Infertility and Risk of Nonmalignant Chronic Diseases: A Systematic Review of the Epidemiological Evidence. Semin Reprod Med (2017) 35:282–90. doi: 10.1055/s-0037-1603568
117. Eisenberg ML, Li S, Behr B, Pera RR, Cullen MR. Relationship Between Semen Production and Medical Comorbidity. Fertil Steril (2015) 103:66–71. doi: 10.1016/j.fertnstert.2014.10.017
118. Hanson HA, Anderson RE, Aston KI, Carrell DT, Smith KR, Hotaling JM. Subfertility Increases Risk of Testicular Cancer: Evidence From Population-Based Semen Samples. Fertil Steril (2016) 105:322–8.e1. doi: 10.1016/j.fertnstert.2015.10.027
119. Walsh TJ, Croughan MS, Schembri M, Chan JM, Turek PJ. Increased Risk of Testicular Germ Cell Cancer Among Infertile Men. Arch Intern Med (2009) 169:351–6. doi: 10.1001/archinternmed.2008.562
120. Møller H, Skakkebaek NE. Risk of Testicular Cancer in Subfertile Men: Case-Control Study. BMJ (1999) 318:559–62. doi: 10.1136/bmj.318.7183.559
121. Xavier MJ, Roman SD, Aitken RJ, Nixon B. Transgenerational Inheritance: How Impacts to the Epigenetic and Genetic Information of Parents Affect Offspring Health. Hum Reprod Update (2019) 25:519–41. doi: 10.1093/humupd/dmz017
122. Bonde JP, Flachs EM, Rimborg S, Glazer CH, Giwercman A, Ramlau-Hansen CH, et al. The Epidemiologic Evidence Linking Prenatal and Postnatal Exposure to Endocrine Disrupting Chemicals With Male Reproductive Disorders: A Systematic Review and Meta-Analysis. Hum Reprod Update (2016) 23:104–25. doi: 10.1093/humupd/dmw036
123. Araujo AB, Dixon JM, Suarez EA, Murad MH, Guey LT, Wittert GA. Clinical Review: Endogenous Testosterone and Mortality in Men: A Systematic Review and Meta-Analysis. J Clin Endocrinol Metab (2011) 96:3007–19. doi: 10.1210/jc.2011-1137
124. Yeap BB, Alfonso H, Chubb SA, Handelsman DJ, Hankey GJ, Almeida OP, et al. In Older Men an Optimal Plasma Testosterone is Associated With Reduced All-Cause Mortality and Higher Dihydrotestosterone With Reduced Ischemic Heart Disease Mortality, While Estradiol Levels do Not Predict Mortality. J Clin Endocrinol Metab (2014) 99:E9–18. doi: 10.1210/jc.2013-3272
125. Mehrpour O, Karrari P, Zamani N, Tsatsakis AM, Abdollahi M. Occupational Exposure to Pesticides and Consequences on Male Semen and Fertility: A Review. Toxicol Lett (2014) 230:146–56. doi: 10.1016/j.toxlet.2014.01.029
126. Nixon BJ, Stanger SJ, Nixon B, Roman SD. Chronic Exposure to Acrylamide Induces DNA Damage in Male Germ Cells of Mice. Toxicol Sci (2012) 129:135–45. doi: 10.1093/toxsci/kfs178
127. De Felice F, Marchetti C, Marampon F, Cascialli G, Muzii L, Tombolini V. Radiation Effects on Male Fertility. Andrology (2019) 7:2–7. doi: 10.1111/andr.12562
128. Bach CC, Vested A, Jørgensen KT, Bonde JP, Henriksen TB, Toft G. Perfluoroalkyl and Polyfluoroalkyl Substances and Measures of Human Fertility: A Systematic Review. Crit Rev Toxicol (2016) 46:735–55. doi: 10.1080/10408444.2016.1182117
129. Joensen UN, Bossi R, Leffers H, Jensen AA, Skakkebæk NE, Jørgensen N. Do Perfluoroalkyl Compounds Impair Human Semen Quality? Environ Health Perspect (2009) 117:923–7. doi: 10.1289/ehp.0800517
130. Vested A, Ramlau-Hansen CH, Olsen SF, Bonde JP, Kristensen SL, Halldorsson TI, et al. Associations of In Utero Exposure to Perfluorinated Alkyl Acids With Human Semen Quality and Reproductive Hormones in Adult Men. Environ Health Perspect (2013) 121:453–8. doi: 10.1289/ehp.1205118
131. Joensen UN, Veyrand B, Antignac JP, Blomberg Jensen M, Petersen JH, Marchand P, et al. PFOS (Perfluorooctanesulfonate) in Serum is Negatively Associated With Testosterone Levels, But Not With Semen Quality, in Healthy Men. Hum Reprod (2013) 28:599–608. doi: 10.1093/humrep/des425
132. Petersen MS, Halling J, Jørgensen N, Nielsen F, Grandjean P, Jensen TK, et al. Reproductive Function in a Population of Young Faroese Men With Elevated Exposure to Polychlorinated Biphenyls (PCBs) and Perfluorinated Alkylate Substances (PFAS). Int J Environ Res Public Health (2018) 15:1880. doi: 10.3390/ijerph15091880
133. Raymer JH, Michael LC, Studabaker WB, Olsen GW, Sloan CS, Wilcosky T, et al. Concentrations of Perfluorooctane Sulfonate (PFOS) and Perfluorooctanoate (PFOA) and Their Associations With Human Semen Quality Measurements. Reprod Toxicol (2012) 33:419–27. doi: 10.1016/j.reprotox.2011.05.024
134. Governini L, Guerranti C, De Leo V, Boschi L, Luddi A, Gori M, et al. Chromosomal Aneuploidies and DNA Fragmentation of Human Spermatozoa From Patients Exposed to Perfluorinated Compounds. Andrologia (2015) 47:1012–9. doi: 10.1111/and.12371
135. Specht IO, Hougaard KS, Spanò M, Bizzaro D, Manicardi GC, Lindh CH, et al. Sperm DNA Integrity in Relation to Exposure to Environmental Perfluoroalkyl Substances - A Study of Spouses of Pregnant Women in Three Geographical Regions. Reprod Toxicol (2012) 33:577–83. doi: 10.1016/j.reprotox.2012.02.008
136. Emerce E, Çetin Ö. Genotoxicity Assessment of Perfluoroalkyl Substances on Human Sperm. Toxicol Ind Health (2018) 34:884–90. doi: 10.1177/0748233718799191
137. Leter G, Consales C, Eleuteri P, Uccelli R, Specht IO, Toft G, et al. Exposure to Perfluoroalkyl Substances and Sperm DNA Global Methylation in Arctic and European Populations. Environ Mol Mutagen (2014) 55:591–600. doi: 10.1002/em.21874
138. Nian M, Luo K, Luo F, Aimuzi R, Huo X, Chen Q, et al. Association Between Prenatal Exposure to PFAS and Fetal Sex Hormones: Are the Short-Chain PFAS Safer? Environ Sci Technol (2020) 54:8291–9. doi: 10.1021/acs.est.0c02444
139. Cui Q, Pan Y, Wang J, Liu H, Yao B, Dai J. Exposure to Per- and Polyfluoroalkyl Substances (PFASs) in Serum Versus Semen and Their Association With Male Reproductive Hormones. Environ Pollut (2020) 266:115330. doi: 10.1016/j.envpol.2020.115330
140. Pan Y, Cui Q, Wang J, Sheng N, Jing J, Yao B, et al. Profiles of Emerging and Legacy Per-/Polyfluoroalkyl Substances in Matched Serum and Semen Samples: New Implications for Human Semen Quality. Environ Health Perspect (2019) 127:127005. doi: 10.1289/EHP4431
141. Green MP, Harvey AJ, Finger BJ, Tarulli GA. Endocrine Disrupting Chemicals: Impacts on Human Fertility and Fecundity During the Peri-Conception Period. Environ Res (2021) 194:110694. doi: 10.1016/j.envres.2020.110694
142. Cooper TG, Noonan E, von Eckardstein S, Auger J, Baker HW, Behre HM, et al. World Health Organization Reference Values for Human Semen Characteristics. Hum Reprod Update (2010) 16:231–45. doi: 10.1093/humupd/dmp048
143. Lopez-Espinosa M-J, Mondal D, Armstrong BG, Eskenazi B, Fletcher T. Perfluoroalkyl Substances, Sex Hormones, and Insulin-Like Growth Factor-1 at 6-9 Years of Age: A Cross-Sectional Analysis Within the C8 Health Project. Environ Health Perspect (2016) 124:1269–75. doi: 10.1289/ehp.1509869
144. Wang Y, Aimuzi R, Nian M, Zhang Y, Luo K, Zhang J. Perfluoroalkyl Substances and Sex Hormones in Postmenopausal Women: NHANES 2013-2016. Environ Int (2021) 149:106408. doi: 10.1016/j.envint.2021.106408
145. Wan HT, Zhao YG, Wong MH, Lee KF, Yeung WS, Giesy JP, et al. Testicular Signaling is the Potential Target of Perfluorooctanesulfonate-Mediated Subfertility in Male Mice. Biol Reprod (2011) 84:1016–23. doi: 10.1095/biolreprod.110.089219
146. Biegel LB, Liu RC, Hurtt ME, Cook JC. Effects of Ammonium Perfluorooctanoate on Leydig Cell Function: In Vitro, In Vivo, and Ex Vivo Studies. Toxicol Appl Pharmacol (1995) 134:18–25. doi: 10.1006/taap.1995.1164
147. Zhang H, Lu Y, Luo B, Yan S, Guo X, Dai J. Proteomic Analysis of Mouse Testis Reveals Perfluorooctanoic Acid-Induced Reproductive Dysfunction via Direct Disturbance of Testicular Steroidogenic Machinery. J Proteome Res (2014) 13:3370–85. doi: 10.1021/pr500228d
148. Olsen GW, Gilliland FD, Burlew MM, Burris JM, Mandel JS, Mandel JH. An Epidemiologic Investigation of Reproductive Hormones in Men With Occupational Exposure to Perfluorooctanoic Acid. J Occup Environ Med (1998) 40:614–22. doi: 10.1097/00043764-199807000-00006
149. Tsai M-S, Lin C-Y, Lin C-C, Chen M-H, Hsu SHJ, Chien K-L, et al. Association Between Perfluoroalkyl Substances and Reproductive Hormones in Adolescents and Young Adults. Int J Hyg Environ Health (2015) 218:437–43. doi: 10.1016/j.ijheh.2015.03.008
150. Skakkebaek NE, Rajpert-De Meyts E, Main KM. Testicular Dysgenesis Syndrome: An Increasingly Common Developmental Disorder With Environmental Aspects. Hum Reprod (2001) 16:972–8. doi: 10.1093/humrep/16.5.972
151. Rajpert-De Meyts E. Developmental Model for the Pathogenesis of Testicular Carcinoma in Situ: Genetic and Environmental Aspects. Hum Reprod Update (2006) 12:303–23. doi: 10.1093/humupd/dmk006
152. Toppari J, Larsen JC, Christiansen P, Giwercman A, Grandjean P, Guillette LJ Jr, et al. Male Reproductive Health and Environmental Xenoestrogens. Environ Health Perspect (1996) 104(Suppl 4):741–803. doi: 10.1289/ehp.96104s4741
153. Palmer JR, Herbst AL, Noller KL, Boggs DA, Troisi R, Titus-Ernstoff L, et al. Urogenital Abnormalities in Men Exposed to Diethylstilbestrol In Utero: A Cohort Study. Environ Health (2009) 8:37. doi: 10.1186/1476-069X-8-37
154. Jensen MS, Rebordosa C, Thulstrup AM, Toft G, Sørensen HT, Bonde JP, et al. Maternal Use of Acetaminophen, Ibuprofen, and Acetylsalicylic Acid During Pregnancy and Risk of Cryptorchidism. Epidemiology (2010) 21:779–85. doi: 10.1097/EDE.0b013e3181f20bed
155. Fudvoye J, Bourguignon JP, Parent AS. Endocrine-Disrupting Chemicals and Human Growth and Maturation: A Focus on Early Critical Windows of Exposure. Vitam Horm (2014) 94:1–25. doi: 10.1016/B978-0-12-800095-3.00001-8
156. Welsh M, Saunders PT, Fisken M, Scott HM, Hutchison GR, Smith LB, et al. Identification in Rats of a Programming Window for Reproductive Tract Masculinization, Disruption of Which Leads to Hypospadias and Cryptorchidism. J Clin Invest (2008) 118:1479–90. doi: 10.1172/JCI34241
157. Gore AC, Chappell VA, Fenton SE, Flaws JA, Nadal A, Prins GS, et al. EDC-2: The Endocrine Society’s Second Scientific Statement on Endocrine-Disrupting Chemicals. Endocr Rev (2015) 36:E1–e150. doi: 10.1210/er.2015-1010
158. Giulivo M, Lopez de Alda M, Capri E, Barceló D. Human Exposure to Endocrine Disrupting Compounds: Their Role in Reproductive Systems, Metabolic Syndrome and Breast Cancer. A Review Environ Res (2016) 151:251–64. doi: 10.1016/j.envres.2016.07.011
159. Damstra TB, Bergman S, Kavlock A, van der Kraak R, Van Der Kraak G. Global Assessment of the State-of-the-Science of Endocrine Disruptors. Geneva: World Health Organization (2002).
160. Alonso-Magdalena P, Vieira E, Soriano S, Menes L, Burks D, Quesada I, et al. Bisphenol A Exposure During Pregnancy Disrupts Glucose Homeostasis in Mothers and Adult Male Offspring. Environ Health Perspect (2010) 118:1243–50. doi: 10.1289/ehp.1001993
161. Ghassabian A, Trasande L. Disruption in Thyroid Signaling Pathway: A Mechanism for the Effect of Endocrine-Disrupting Chemicals on Child Neurodevelopment. Front Endocrinol (Lausanne) (2018) 9:204. doi: 10.3389/fendo.2018.00204
162. Kopp R, Martínez IO, Legradi J, Legler J. Exposure to Endocrine Disrupting Chemicals Perturbs Lipid Metabolism and Circadian Rhythms. J Environ Sci (2017) 62:133–7. doi: 10.1016/j.jes.2017.10.013
163. Sifakis S, Androutsopoulos VP, Tsatsakis AM, Spandidos DA. Human Exposure to Endocrine Disrupting Chemicals: Effects on the Male and Female Reproductive Systems. Environ Toxicol Pharmacol (2017) 51:56–70. doi: 10.1016/j.etap.2017.02.024
164. Abaci A, Demir K, Bober E, Buyukgebiz A. Endocrine Disrupters - With Special Emphasis on Sexual Development. Pediatr Endocrinol Rev (2009) 6:464–75.
165. Axelstad M, Hass U, Scholze M, Christiansen S, Kortenkamp A, Boberg J, et al. Reduced Sperm Counts in Rats Exposed to Human Relevant Mixtures of Endocrine Disrupters. Endocr Connect (2018) 7:139–48. doi: 10.1530/EC-17-0307
166. Skakkebaek NE. A Brief Review of the Link Between Environment and Male Reproductive Health: Lessons From Studies of Testicular Germ Cell Cancer. Horm Res Paediatr (2016) 86:240–6. doi: 10.1159/000443400
167. Thorup J, McLachlan R, Cortes D, Nation TR, Balic A, Southwell BR, et al. What is New in Cryptorchidism and Hypospadias–a Critical Review on the Testicular Dysgenesis Hypothesis. J Pediatr Surg (2010) 45:2074–86. doi: 10.1016/j.jpedsurg.2010.07.030
168. Coperchini F, Awwad O, Rotondi M, Santini F, Imbriani M, Chiovato L. Thyroid Disruption by Perfluorooctane Sulfonate (PFOS) and Perfluorooctanoate (PFOA). J Endocrinol Invest (2017) 40:105–21. doi: 10.1007/s40618-016-0572-z
169. Rickard BP, Rizvi I, Fenton SE. Per- and Poly-Fluoroalkyl Substances (PFAS) and Female Reproductive Outcomes: PFAS Elimination, Endocrine-Mediated Effects, and Disease. Toxicology (2021) 465:153031. doi: 10.1016/j.tox.2021.153031
170. Coperchini F, Croce L, Ricci G, Magri F, Rotondi M, Imbriani M, et al. Thyroid Disrupting Effects of Old and New Generation PFAS. Front Endocrinol (Lausanne) (2020) 11:612320. doi: 10.3389/fendo.2020.612320
171. Rosenmai AK, Taxvig C, Svingen T, Trier X, van Vugt-Lussenburg BM, Pedersen M, et al. Fluorinated Alkyl Substances and Technical Mixtures Used in Food Paper-Packaging Exhibit Endocrine-Related Activity In Vitro. Andrology (2016) 4:662–72. doi: 10.1111/andr.12190
172. Bay K, Main KM, Toppari J, Skakkebæk NE. Testicular Descent: INSL3, Testosterone, Genes and the Intrauterine Milieu. Nat Rev Urol (2011) 8:187–96. doi: 10.1038/nrurol.2011.23
173. Toft G, Jönsson BA, Bonde JP, Nørgaard-Pedersen B, Hougaard DM, Cohen A, et al. Perfluorooctane Sulfonate Concentrations in Amniotic Fluid, Biomarkers of Fetal Leydig Cell Function, and Cryptorchidism and Hypospadias in Danish Boys (1980-1996). Environ Health Perspect (2016) 124:151–6. doi: 10.1289/ehp.1409288
174. Zimmermann S, Steding G, Emmen JM, Brinkmann AO, Nayernia K, Holstein AF, et al. Targeted Disruption of the Insl3 Gene Causes Bilateral Cryptorchidism. Mol Endocrinol (1999) 13:681–91. doi: 10.1210/mend.13.5.0272
175. Nef S, Parada LF. Cryptorchidism in Mice Mutant for Insl3. Nat Genet (1999) 22:295–9. doi: 10.1038/10364
176. Ye L, Su ZJ, Ge RS. Inhibitors of Testosterone Biosynthetic and Metabolic Activation Enzymes. Molecules (2011) 16:9983–10001. doi: 10.3390/molecules16129983
177. Azhagiya Singam ER, Tachachartvanich P, Fourches D, Soshilov A, Hsieh JCY, La Merrill MA, et al. Structure-Based Virtual Screening of Perfluoroalkyl and Polyfluoroalkyl Substances (PFASs) as Endocrine Disruptors of Androgen Receptor Activity Using Molecular Docking and Machine Learning. Environ Res (2020) 190:109920. doi: 10.1016/j.envres.2020.109920
178. Huhtaniemi I, Pelliniemi LJ. Fetal Leydig Cells: Cellular Origin, Morphology, Life Span, and Special Functional Features. Proc Soc Exp Biol Med (1992) 201:125–40. doi: 10.3181/00379727-201-43493
179. Awoniyi CA, Santulli R, Sprando RL, Ewing LL, Zirkin BR. Restoration of Advanced Spermatogenic Cells in the Experimentally Regressed Rat Testis: Quantitative Relationship to Testosterone Concentration Within the Testis. Endocrinology (1989) 124:1217–23. doi: 10.1210/endo-124-3-1217
180. Zhao B, Hu GX, Chu Y, Jin X, Gong S, Akingbemi BT, et al. Inhibition of Human and Rat 3beta-Hydroxysteroid Dehydrogenase and 17beta-Hydroxysteroid Dehydrogenase 3 Activities by Perfluoroalkylated Substances. Chem Biol Interact (2010) 188:38–43. doi: 10.1016/j.cbi.2010.07.001
181. Kjeldsen LS, Bonefeld-Jørgensen EC. Perfluorinated Compounds Affect the Function of Sex Hormone Receptors. Environ Sci Pollut Res Int (2013) 20:8031–44. doi: 10.1007/s11356-013-1753-3
182. Zhao B, Li L, Liu J, Li H, Zhang C, Han P, et al. Exposure to Perfluorooctane Sulfonate In Utero Reduces Testosterone Production in Rat Fetal Leydig Cells. PloS One (2014) 9:e78888. doi: 10.1371/journal.pone.0078888
183. Kim S, Thapar I, Brooks BW. Epigenetic Changes by Per- and Polyfluoroalkyl Substances (PFAS). Environ Pollut (2021) 279:116929. doi: 10.1016/j.envpol.2021.116929
184. Nilsson EE, Sadler-Riggleman I, Skinner MK. Environmentally Induced Epigenetic Transgenerational Inheritance of Disease. Environ Epigenet (2018) 4:dvy016. doi: 10.1093/eep/dvy016
185. Sharpe RM, McKinnell C, Kivlin C, Fisher JS. Proliferation and Functional Maturation of Sertoli Cells, and Their Relevance to Disorders of Testis Function in Adulthood. Reproduction (2003) 125:769–84. doi: 10.1530/rep.0.1250769
186. Upham BL, Deocampo ND, Wurl B, Trosko JE. Inhibition of Gap Junctional Intercellular Communication by Perfluorinated Fatty Acids is Dependent on the Chain Length of the Fluorinated Tail. Int J Cancer (1998) 78:491–5. doi: 10.1002/(SICI)1097-0215(19981109)78:4<491::AID-IJC16>3.0.CO;2-9
187. Wan HT, Mruk DD, Wong CK, Cheng CY. Perfluorooctanesulfonate (PFOS) Perturbs Male Rat Sertoli Cell Blood-Testis Barrier Function by Affecting F-Actin Organization via P-FAK-Tyr(407): An In Vitro Study. Endocrinology (2014) 155:249–62. doi: 10.1210/en.2013-1657
188. Gao Y, Chen H, Xiao X, Lui W-Y, Lee WM, Mruk DD, et al. Perfluorooctanesulfonate (PFOS)-Induced Sertoli Cell Injury Through a Disruption of F-Actin and Microtubule Organization is Mediated by Akt1/2. Sci Rep (2017) 7:1110–0. doi: 10.1038/s41598-017-01016-8
189. Mruk DD, Cheng CY. Sertoli-Sertoli and Sertoli-Germ Cell Interactions and Their Significance in Germ Cell Movement in the Seminiferous Epithelium During Spermatogenesis. Endocrine Rev (2004) 25:747–806. doi: 10.1210/er.2003-0022
190. Cheng CY, Mruk DD. Cell Junction Dynamics in the Testis: Sertoli-Germ Cell Interactions and Male Contraceptive Development. Physiol Rev (2002) 82:825–74. doi: 10.1152/physrev.00009.2002
191. Skinner MK, Norton JN, Mullaney BP, Rosselli M, Whaley PD, Anthony CT. Cell-Cell Interactions and the Regulation of Testis Function. Ann N Y Acad Sci (1991) 637:354–63. doi: 10.1111/j.1749-6632.1991.tb27322.x
192. Jégou B. The Sertoli-Germ Cell Communication Network in Mammals. Int Rev Cytol (1993) 147:25–96. doi: 10.1016/S0074-7696(08)60766-4
193. Mackay S. Gonadal Development in Mammals at the Cellular and Molecular Levels. Int Rev Cytol (2000) 200:47–99. doi: 10.1016/S0074-7696(00)00002-4
194. Steves AN, Turry A, Gill B, Clarkson-Townsend D, Bradner JM, Bachli I, et al. Per- and Polyfluoroalkyl Substances Impact Human Spermatogenesis in a Stem-Cell-Derived Model. Syst Biol Reprod Med (2018) 64:225–39. doi: 10.1080/19396368.2018.1481465
195. Qu JH, Lu CC, Xu C, Chen G, Qiu LL, Jiang JK, et al. Perfluorooctane Sulfonate-Induced Testicular Toxicity and Differential Testicular Expression of Estrogen Receptor in Male Mice. Environ Toxicol Pharmacol (2016) 45:150–7. doi: 10.1016/j.etap.2016.05.025
196. Chen J, Wang X, Ge X, Wang D, Wang T, Zhang L, et al. Chronic Perfluorooctanesulphonic Acid (PFOS) Exposure Produces Estrogenic Effects in Zebrafish. Environ Pollut (2016) 218:702–8. doi: 10.1016/j.envpol.2016.07.064
197. Li K, Gao P, Xiang P, Zhang X, Cui X, Ma LQ. Molecular Mechanisms of PFOA-Induced Toxicity in Animals and Humans: Implications for Health Risks. Environ Int (2017) 99:43–54. doi: 10.1016/j.envint.2016.11.014
198. Maestri L, Negri S, Ferrari M, Ghittori S, Fabris F, Danesino P, et al. Determination of Perfluorooctanoic Acid and Perfluorooctanesulfonate in Human Tissues by Liquid Chromatography/Single Quadrupole Mass Spectrometry. Rapid Commun Mass Spectrom (2006) 20:2728–34. doi: 10.1002/rcm.2661
199. Glatz JFC, van der Vusse GJ. Cellular Fatty Acid-Binding Proteins: Their Function and Physiological Significance. Prog Lipid Res (1996) 35:243–82. doi: 10.1016/S0163-7827(96)00006-9
200. Hamilton JA. Binding of Fatty Acids to Albumin: A Case Study of Lipid-Protein Interactions. Physiology (1992) 7:264–70. doi: 10.1152/physiologyonline.1992.7.6.264
201. Spector AA. [17] Structure and Lipid Binding Properties of Serum Albumin. Methods Enzymol (1986) 128:320–39. doi: 10.1016/0076-6879(86)28077-5
202. Yamamoto T, Yamamoto A, Watanabe M, Matsuo T, Yamazaki N, Kataoka M, et al. Classification of FABP Isoforms and Tissues Based on Quantitative Evaluation of Transcript Levels of These Isoforms in Various Rat Tissues. Biotechnol Lett (2009) 31:1695–701. doi: 10.1007/s10529-009-0065-7
203. Liu R-Z, Li X, Godbout R. A Novel Fatty Acid-Binding Protein (FABP) Gene Resulting From Tandem Gene Duplication in Mammals: Transcription in Rat Retina and Testis. Genomics (2008) 92:436–45. doi: 10.1016/j.ygeno.2008.08.003
204. Storch J, Thumser AEA. The Fatty Acid Transport Function of Fatty Acid-Binding Proteins. Biochim Biophys Acta (BBA) - Mol Cell Biol Lipids (2000) 1486:28–44. doi: 10.1016/S1388-1981(00)00046-9
205. Gagliano E, Sgroi M, Falciglia PP, Vagliasindi FGA, Roccaro P. Removal of Poly- and Perfluoroalkyl Substances (PFAS) From Water by Adsorption: Role of PFAS Chain Length, Effect of Organic Matter and Challenges in Adsorbent Regeneration. Water Res (2020) 171:115381. doi: 10.1016/j.watres.2019.115381
206. Kah M, Sigmund G, Xiao F, Hofmann T. Sorption of Ionizable and Ionic Organic Compounds to Biochar, Activated Carbon and Other Carbonaceous Materials. Water Res (2017) 124:673–92. doi: 10.1016/j.watres.2017.07.070
207. Mueller B, Yingling G, Schlosser KE, Goodrow S. ITRC, PFAS Fact Sheets PFAS-1, Interstate Technology and Regulatory Council, Interstate Technology & Regulatory Council, PFAS Team. Washington, DC: Interstate Technology and Regulatory Counci (2018).
208. Ross I, McDonough J, Miles J, Storch P, Thelakkat Kochunarayanan P, Kalve E, et al. A Review of Emerging Technologies for Remediation of PFASs. Remediation J (2018) 28:101–26. doi: 10.1002/rem.21553
209. Lu D, Sha S, Luo J, Huang Z, Zhang Jackie X. Treatment Train Approaches for the Remediation of Per- and Polyfluoroalkyl Substances (PFAS): A Critical Review. J Hazard Mater (2020) 386:121963. doi: 10.1016/j.jhazmat.2019.121963
210. Ochoa-Herrera V, Sierra-Alvarez R. Removal of Perfluorinated Surfactants by Sorption Onto Granular Activated Carbon, Zeolite and Sludge. Chemosphere (2008) 72:1588–93. doi: 10.1016/j.chemosphere.2008.04.029
211. Nancy Merino YQ, Deeb RA, Hawley EL, Hoffmann MR, Mahendra S. Degradation and Removal Methods for Perfluoroalkyl and Polyfluoroalkyl Substances in Water. Environ Eng Sci (2016) 33:615–49. doi: 10.1089/ees.2016.0233
212. NEMP, PFAS National Environmental Management Plan Version 2.0 Heads of EPA Australia and New Zealand. Canberra, ACT: Department of Agriculture, Water and the Environment (2020).
213. Zaggia A, Conte L, Falletti L, Fant M, Chiorboli A. Use of Strong Anion Exchange Resins for the Removal of Perfluoroalkylated Substances From Contaminated Drinking Water in Batch and Continuous Pilot Plants. Water Res (2016) 91:137–46. doi: 10.1016/j.watres.2015.12.039
214. Dickenson E, Higgins C. Treatment Mitigation Strategies for Poly-and Perfluoroalkyl Substances. Water Res Foundation Web Rep (2016) 4322:241–54.
215. Vecitis CD, Park H, Cheng J, Mader BT, Hoffmann MR. Enhancement of Perfluorooctanoate and Perfluorooctanesulfonate Activity at Acoustic Cavitation Bubble Interfaces. J Phys Chem C (2008) 112:16850–7. doi: 10.1021/jp804050p
216. Cui J, Gao P, Deng Y. Destruction of Per- and Polyfluoroalkyl Substances (PFAS) With Advanced Reduction Processes (ARPs): A Critical Review. Environ Sci Technol (2020) 54:3752–66. doi: 10.1021/acs.est.9b05565
217. Turner BD, Sloan SW, Currell GR. Novel Remediation of Per-and Polyfluoroalkyl Substances (PFASs) From Contaminated Groundwater Using Cannabis Sativa L. (hemp) Protein Powder. Chemosphere (2019) 229:22–31. doi: 10.1016/j.chemosphere.2019.04.139
218. Parsons DF, Duignan TT, Salis A. Cation Effects on Haemoglobin Aggregation: Balance of Chemisorption Against Physisorption of Ions. Interface Focus (2017) 7:20160137. doi: 10.1098/rsfs.2016.0137
219. Zhang X, Chen L, Fei X-C, Ma Y-S, Gao H-W. Binding of PFOS to Serum Albumin and DNA: Insight Into the Molecular Toxicity of Perfluorochemicals. BMC Mol Biol (2009) 10:1–12. doi: 10.1186/1471-2199-10-16
220. Shin M-Y, Shin C, Choi JW, Lee J, Lee S, Kim S. Pharmacokinetic Profile of Propyl Paraben in Humans After Oral Administration. Environ Int (2019) 130:104917. doi: 10.1016/j.envint.2019.104917
221. Sears ME, Genuis SJ. Environmental Determinants of Chronic Disease and Medical Approaches: Recognition, Avoidance, Supportive Therapy, and Detoxification. J Environ Public Health (2012) 2012:356798. doi: 10.1155/2012/356798
222. Genuis SJ, Birkholz D, Rodushkin I, Beesoon S. Blood, Urine, and Sweat (BUS) Study: Monitoring and Elimination of Bioaccumulated Toxic Elements. Arch Environ Contam Toxicol (2011) 61:344–57. doi: 10.1007/s00244-010-9611-5
223. Genuis SJ, Beesoon S, Lobo RA, Birkholz D. Human Elimination of Phthalate Compounds: Blood, Urine, and Sweat (BUS) Study. ScientificWorldJournal (2012) 2012:615068. doi: 10.1100/2012/615068
224. Genuis SJ, Beesoon S, Birkholz D, Lobo RA. Human Excretion of Bisphenol A: Blood, Urine, and Sweat (BUS) Study. J Environ Public Health (2012) 2012:185731. doi: 10.1155/2012/185731
225. Dahlgren J, Cecchini M, Takhar H, Paepke O. Persistent Organic Pollutants in 9/11 World Trade Center Rescue Workers: Reduction Following Detoxification. Chemosphere (2007) 69:1320–5. doi: 10.1016/j.chemosphere.2006.05.127
226. David WS, Ben M, Megan GS. Body Burden Reductions of PCBs, PBBs and Chlorinated Pesticides in Human Subjects. Ambio (1984) 13:378–80.
227. Genuis SJ, Birkholz D, Ralitsch M, Thibault N. Human Detoxification of Perfluorinated Compounds. Public Health (2010) 124:367–75. doi: 10.1016/j.puhe.2010.03.002
228. Wong F, MacLeod M, Mueller JF, Cousins IT. Enhanced Elimination of Perfluorooctane Sulfonic Acid by Menstruating Women: Evidence From Population-Based Pharmacokinetic Modeling. Environ Sci Technol (2014) 48:8807–14. doi: 10.1021/es500796y
229. Genuis SJ, Liu Y, Genuis QI, Martin JW. Phlebotomy Treatment for Elimination of Perfluoroalkyl Acids in a Highly Exposed Family: A Retrospective Case-Series. PloS One (2014) 9:e114295. doi: 10.1371/journal.pone.0114295
230. Rotander A, Toms LM, Aylward L, Kay M, Mueller JF. Elevated Levels of PFOS and PFHxS in Firefighters Exposed to Aqueous Film Forming Foam (AFFF). Environ Int (2015) 82:28–34. doi: 10.1016/j.envint.2015.05.005
231. Lorber M, Eaglesham GE, Hobson P, Toms LM, Mueller JF, Thompson JS. The Effect of Ongoing Blood Loss on Human Serum Concentrations of Perfluorinated Acids. Chemosphere (2015) 118:170–7. doi: 10.1016/j.chemosphere.2014.07.093
232. Genuis SJ, Curtis L, Birkholz D. Gastrointestinal Elimination of Perfluorinated Compounds Using Cholestyramine and Chlorella Pyrenoidosa. ISRN Toxicol (2013) 2013:657849. doi: 10.1155/2013/657849
233. Ducatman A, Luster M, Fletcher T. Perfluoroalkyl Substance Excretion: Effects of Organic Anion-Inhibiting and Resin-Binding Drugs in a Community Setting. Environ Toxicol Pharmacol (2021) 85:103650. doi: 10.1016/j.etap.2021.103650
234. Katen AL, Stanger SJ, Anderson AL, Nixon B, Roman SD. Chronic Acrylamide Exposure in Male Mice Induces DNA Damage to Spermatozoa; Potential for Amelioration by Resveratrol. Reprod Toxicol (2016) 63:1–12. doi: 10.1016/j.reprotox.2016.05.004
235. Trigg NA, Skerrett-Byrne DA, Xavier MJ, Zhou W, Anderson AL, Stanger SJ, et al. Acrylamide Modulates the Mouse Epididymal Proteome to Drive Alterations in the Sperm Small Non-Coding RNA Profile and Dysregulate Embryo Development. Cell Rep (2021) 37:109787. doi: 10.1016/j.celrep.2021.109787
236. Houston BJ, Nixon B, McEwan KE, Martin JH, King BV, Aitken RJ, et al. Whole-Body Exposures to Radiofrequency-Electromagnetic Energy can Cause DNA Damage in Mouse Spermatozoa via an Oxidative Mechanism. Sci Rep (2019) 9:17478. doi: 10.1038/s41598-019-53983-9
237. Hamilton TRDS, Mendes CM, de Castro LS, de Assis PM, Siqueira AFP, Delgado J, et al. Evaluation of Lasting Effects of Heat Stress on Sperm Profile and Oxidative Status of Ram Semen and Epididymal Sperm. Oxid Med Cell Longev (2016) 2016:1687657–1687657. doi: 10.1155/2016/1687657
Keywords: male fertility, male infertility, male reproduction, perfluoroalkyl and polyfluoroalkyl substances, PFAS, sperm, toxicants
Citation: Calvert L, Green MP, De Iuliis GN, Dun MD, Turner BD, Clarke BO, Eamens AL, Roman SD and Nixon B (2022) Assessment of the Emerging Threat Posed by Perfluoroalkyl and Polyfluoroalkyl Substances to Male Reproduction in Humans. Front. Endocrinol. 12:799043. doi: 10.3389/fendo.2021.799043
Received: 21 October 2021; Accepted: 30 December 2021;
Published: 09 March 2022.
Edited by:
Claus Yding Andersen, University of Copenhagen, DenmarkReviewed by:
Jens Peter Bonde, University of Copenhagen, DenmarkCopyright © 2022 Calvert, Green, De Iuliis, Dun, Turner, Clarke, Eamens, Roman and Nixon. This is an open-access article distributed under the terms of the Creative Commons Attribution License (CC BY). The use, distribution or reproduction in other forums is permitted, provided the original author(s) and the copyright owner(s) are credited and that the original publication in this journal is cited, in accordance with accepted academic practice. No use, distribution or reproduction is permitted which does not comply with these terms.
*Correspondence: Brett Nixon, YnJldHQubml4b25AbmV3Y2FzdGxlLmVkdS5hdQ==
Disclaimer: All claims expressed in this article are solely those of the authors and do not necessarily represent those of their affiliated organizations, or those of the publisher, the editors and the reviewers. Any product that may be evaluated in this article or claim that may be made by its manufacturer is not guaranteed or endorsed by the publisher.
Research integrity at Frontiers
Learn more about the work of our research integrity team to safeguard the quality of each article we publish.