- Reproductive Medicine Center, Tongji Hospital, Tongji Medical College, Huazhong University of Science and Technology, Wuhan, China
Reproductive disorders, including intrauterine adhesion (IUA), premature ovarian insufficiency (POI), and polycystic ovary syndrome (PCOS), are great threats to female reproduction. Recently, mesenchymal stem cells derived–extracellular vesicles (MSC-EVs) have presented their potentials to cure these diseases, not only for the propensity ability they stemmed from the parent cells, but also for the higher biology stability and lower immunogenicity, compared to MSCs. EVs are lipid bilayer complexes, functional as mediators by transferring multiple molecules to recipient cells, such as proteins, microRNAs, lipids, and cytokines. EVs appeared to have a therapeutic effect on the female reproductive disorder, such as repairing injured endometrium, suppressing fibrosis of endometrium, regulating immunity and anti-inflammatory, and repressing apoptosis of granulosa cells (GCs) in ovaries. Although the underlying mechanisms of MSC-EVs have reached a consensus, several theories have been proposed, including promoting angiogenesis, regulating immunity, and reducing oxidate stress levels. In the current study, we summarized the current knowledge of functions of MSC-EVs on IUA, POI, and PCOS. Given the great potentials of MSC-EVs on reproductive health, the critical issues discussed will guide new insights in this rapidly expanding field.
Introduction
Mesenchymal stem cells (MSCs), a type of adult stem cells, could be harvested from various tissues, including bone marrow, umbilical cord, menstrual blood, endometrial tissue, adipose tissue, etc. (1). Given the capacity of self-renewal and differentiation potentials, emerging researches have regarded MSCs as exciting candidates for cell therapy in regenerative medicine (2, 3). There are lots of pre-clinical and clinical trials confirming the efficacy of MSCs in a variety of diseases, such as cardiovascular disorders, diabetes, neurological diseases, renal fibrosis, and female reproductive disorders (4–8). However, stem cell therapy may raise some negative issues such as transplant rejection, inconvenience of transportation or storage, difficulties of commercialization, and still exhibit safety problems without proper monitoring tests (9, 10). EVs refer to lipid bilayer particles that release from cells into the microenvironment, serving as messengers by trafficking plenty of cargo, such as proteins, microRNAs, lipid and cytokines, and so on (11, 12). In contrast with MSCs, MSC derived extracellular vesicles (MSC-EVs) not only have similar functions with the parent cells, but also exhibit higher biology stability and lower immunogenicity (13, 14).
Female reproductive disorders are great threats to women’s reproductive health and contribute to infertility (15, 16). Although assisted reproductive techniques (ART) have made a great contribution to improving the pregnancy outcomes of infertile couples, women with intrauterine adhesion (IUA) or premature ovarian insufficiency (POI) are still difficult to conceive even with the help of ART (17, 18).
Considering the abovementioned advantages and great potentials in regenerative medicine, MSCs-EVs hold great promise as an alternative therapy for IUA and POI (19, 20). Moreover, recent evidence also indicated that MSC-EVs were functional in improving the ovarian health of women with polycystic ovarian syndrome (PCOS) (21). Accordingly, we draft this review to summarize the therapeutic effects and mechanisms of MSC-EVs on the abovementioned disorders. In this review, the latest studies on the therapeutic effect of MSC-EVs on these diseases are provided and the current limitations and future perspectives of MSC-EVs are discussed as well.
Methods
For this review, an extensive literature search was performed in PubMed, Embase, and Cochrane libraries. Literature published in English and available up to January 2021 was included.
The following keywords were used for the search, alone or in combination: mesenchymal stem cells, extracellular vesicles, exosomes, female reproductive diseases, intrauterine adhesion, thin endometrium, injured endometrium, endometrial fibrosis, premature ovarian insufficiency, premature ovarian failure, diminished ovarian reserve, polycystic ovary syndrome, follicles, in vitro fertilization treatment, angiogenesis, immune regulation, immunosuppressive, collagen remodel, anti-apoptosis, oxidative stress, embryo transfer. Then, the resulting articles were selected by screening titles and reviewing full-text of papers, only articles correlating to the interest topics and its relatives were selected for this review. In addition, we hand-searched references of relevant reviews, and included ongoing studies to locate other potentially eligible materials.
Extracellular Vesicles From Mesenchymal Stem Cell
Almost all cell types can generate EVs, and MSCs are no exception (22). There are three subtypes of EVs, including exosomes (50–150 nm), microvesicles (MVs) (100–1,000 nm), and apoptotic bodies (500–5,000 nm) (23). The biogenesis mechanism of these subtypes is different to some extent. Generally, the endocytosis and exocytosis account for the biogenesis of most exosomes: initially early endosome can be formed in endocytosis of plasma membrane; with subsequent inward budding of endosomal membrane, late endosome can arise and develop into multivesicular bodies (MVBs) filled with intraluminal vesicles (ILVs); then MVBs release ILVs upon fusion with cell membrane, which mediated by endosomal sorting complexes required for transport (ESCRT)-dependent pathway or ESCRT-independent pathway; finally the vesicles are secreted extracellularly as exosomes (24, 25) (Figure 1). As for MVs, the biogenesis is relatively simple, including directly budding from the plasma membrane (26) (Figure 1). In fact, evidence showed that exosomes could also originate in cell membrane protrusions (24, 25). Hence, the mechanism of EVs biogenesis needs to be investigated further. As aforementioned, the biogenesis of exosomes and MVs is not alike, their molecular contents or functions are thus dissimilar as well (27). For example, Phinney et al. pointed that the mitochondria could be transferred by MVs, not exosomes, to enhance mitochondrial bioenergetics in macrophages (27). Thus, the functional contents may be different between exosomes and MVs regarding the treatment of female reproductive disorders, which are worthy of exploration.
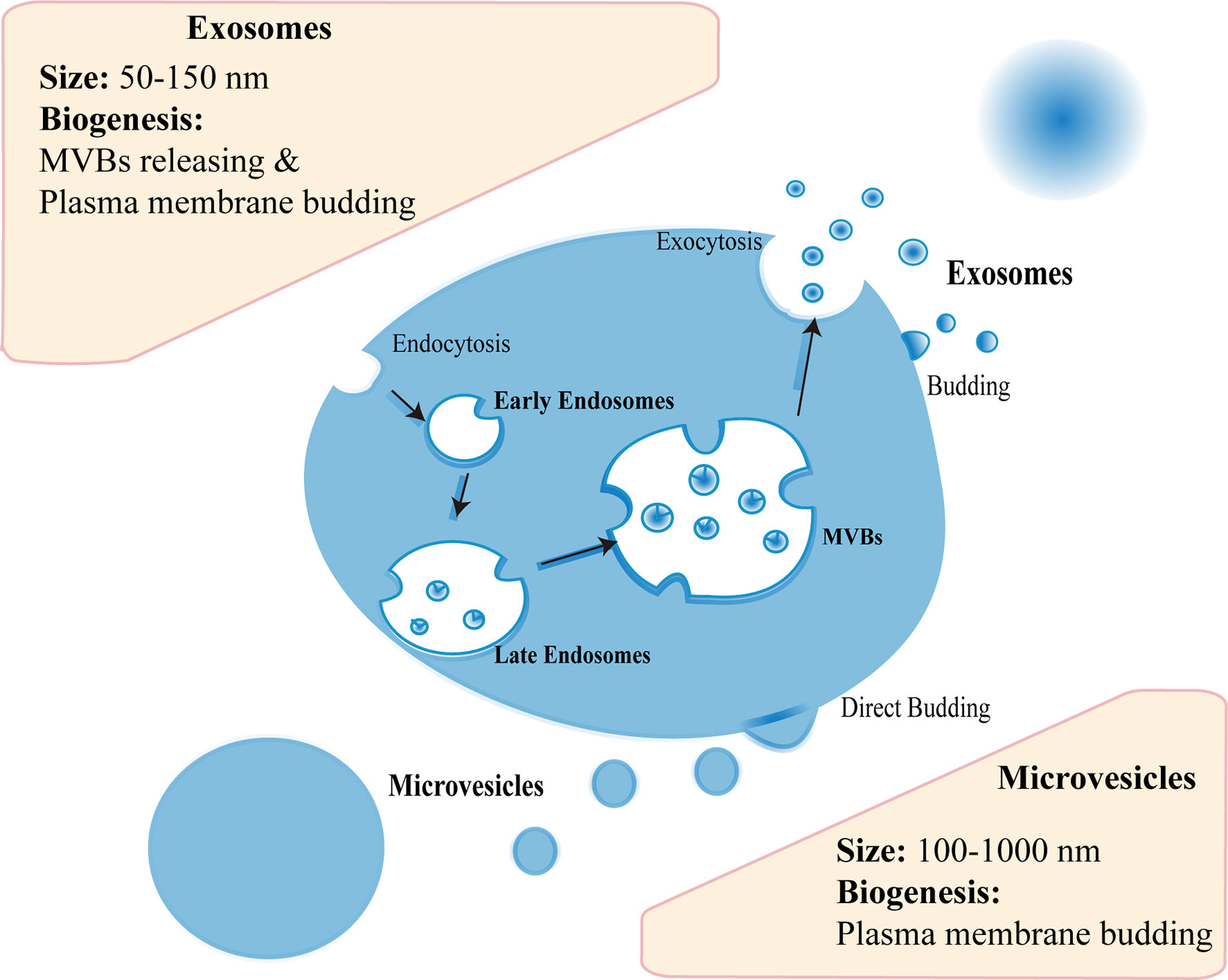
Figure 1 The biogenesis of extracellular vesicles. Exosomes are released upon fusion of MVBs with cell membrane or originate in cell membrane protrusions. Microvesicles bud from the plasma membrane.
According to the statement of the International Society for Extracellular Vesicles, ultracentrifugation has been the most commonly used technique to isolate exosomes or MVs, but the resultant EVs are a mixed population owing to the overlaps in the density or size of different EV types (23). As a consequence, it is of importance to characterize individual EV. Conventional means for identifying the characteristics of EVs usually include nanoparticle tracking analysis (NTA) and transmission electron microscope (TEM) observation for morphological information, or western blotting for membrane protein markers (19). Recently, atomic force microscope-infrared (AFM-IR) spectroscopy has been reported to be applied in characterizing individual EV structure and composition, which may provide us a vital tool for deeply understanding subtypes or individual EVs (20).
Generally, the function of EVs is up to their originating cells (13). The therapeutic use of MSCs was reported in female reproductive diseases, so did MSC-EVs (28–30). Table 1 summarized the major findings of EVs secreted from different categories of MSCs in terms of ameliorating female reproductive disorders. MSC-EVs with different origins exhibited diverse functions, such as repairing injured endometrium, suppressing endometrial fibrosis, regulating immunity, and anti-inflammatory, repressing apoptosis of damaged granulosa cells (GCs), and reducing reactive oxygen species (ROS) level of the ovary (Table 1). Although adipose-derived MSC (ADSC)/umbilical cord-derived MSC (UCMSC)/bone marrow MSC (BMSC)–EVs have shown their therapeutic potentials on intrauterine adhesion (IUA) or premature ovarian insufficiency (POI) in small animals (Table 1), further studies are required to determine the efficacy and safety of MSC-EVs in primates or even patients.
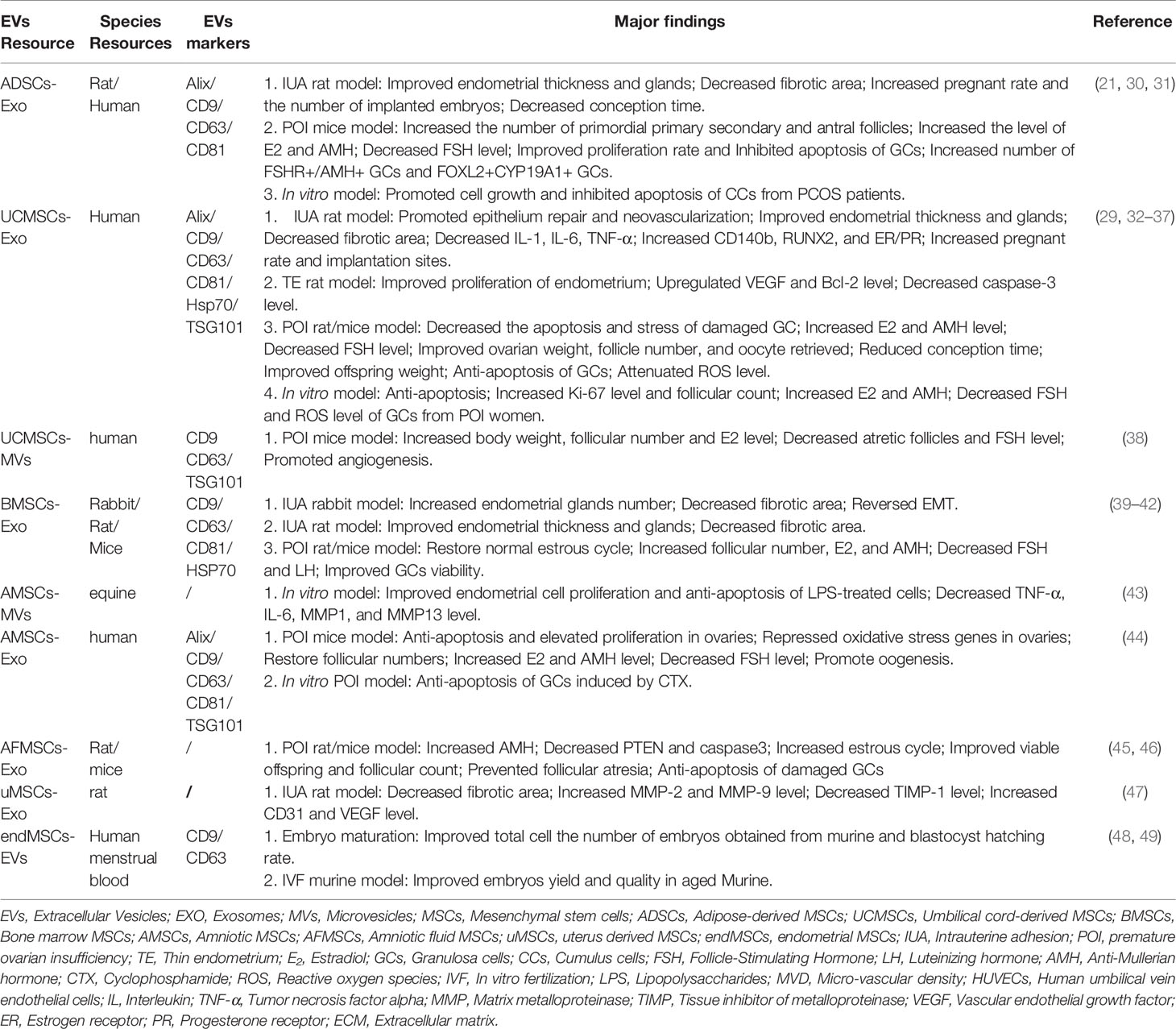
Table 1 Markers and therapeutic effects of reported MSC-derived EVs which contribute to ameliorating female reproductive disorders.
Compared with small animals, primates or human needs a higher dose of EVs. Currently, small-scale production of EVs is a restriction for the clinical application, thus, it is important to find new methods to scale up EV production (50). Previous evidence indicated that MSCs culture parameters were significant for EV production and functions. For example, Patel et al. found that enhanced production of EVs per cell of BMSCs resulted from lower cell seeding density in the culture flasks, and high vascularization bioactivity of EVs was within passage 4 of BMSCs (14). For 3D culture, Watson et al. utilized a hollow-fiber bioreactor to produce bioactive EVs (51). This bioreactor culture model yielded more than 40-fold EVs without serum protein contaminants in comparison with conventional cell culture. Besides, Haraszti et al. also developed a microcarrier-based 3D culture system in combination with tangential flow filtration as a scalable production method for MSC-EVs (52). Moreover, EV yield varies among different MSC cell types (52). With regard to the application method, we are presently witnessing the utilization of MSC-EVs with biomaterials developed rapidly, including collagen scaffold, extracellular matrix mimicking nanofibrous scaffolds, and 3D engineered scaffolds (32, 53, 54). All of them may be very useful for the future use of MSC-EVs in female reproductive diseases. In brief, in terms of clinical application of MSC-EVs, we should take several aspects into consideration, including MSC sources, MSC-EVs production, and usage approaches, which can help us to build a platform of MSC-EVs for clinical application and shorten the time from bench to bedside.
Therapeutic Effects of MSC-EVs on Female Reproductive Disorders
IUA
IUA, also known as Asherman’s syndrome (AS), is characterized by the damage of the endometrial basalis layer and consequent obliteration of endometrium by fibrous tissues (17). Patients with IUA presented with decreased volume of menstrual flow, recurrent pregnancy loss, aberrant placental implantation, and infertility (55). Besides, the endometrium of patients with recurrent IUA is usually very thin, that is, thin endometrium (TE) occurs (56). Multiple factors that interfere with the homeostasis of the uterine environment might be related to this condition, such as artificial abortion, curettage, chronic endometritis, and retained placenta (57). It is acknowledged that endometrial fibrosis is involved in the formation and progression of IUA (58). Initially, the impaired endometrium cannot be normally repaired, which may trigger immune activation and lead to inflammation response along with the over-deposition of extracellular matrix (ECM) protein (collagen and fibronectin) (58–60). As a result, the persistent inflammatory irritation promotes the formation of aberrant avascular fibrotic areas, which may cause tissue hypoxia and sequentially impede endometrial repair (60).
The pathogenesis of IUA is shown in Figure 2. Many studies indicated that the activation of transforming growth factor-β1 (TGF-β1)/Smad3 signaling pathway participated in the occurrence of IUA (61, 62). Moreover, inflammatory factor NF-κB was also identified as a risk factor for IUA (63). Xue et al. observed the expression of TGF-β and connective tissue growth factor-2 (CTGF/CCN2) were positively related to NF-κB pathway activity in the endometrium of IUA patients, and the expression of TGF-β was decreased after inhibiting NF-κB signaling pathway (64). Moreover, it has been reported that the aberrantly activated Wnt/β-catenin pathway was confirmed to stimulate TGF-β-mediated fibrosis and mediate fibrogenesis in the endometrium (65, 66). FOXF2 protein, which activated Wnt/β-catenin pathway and upregulated Collagen Type V Alpha 2 (COL5A2) transcription in the endometrium, was reported to promote fibrogenesis in the IUA as well (67, 68). Therefore, the interaction between many proteins or signaling pathways is of significance to the pathogenesis of IUA.
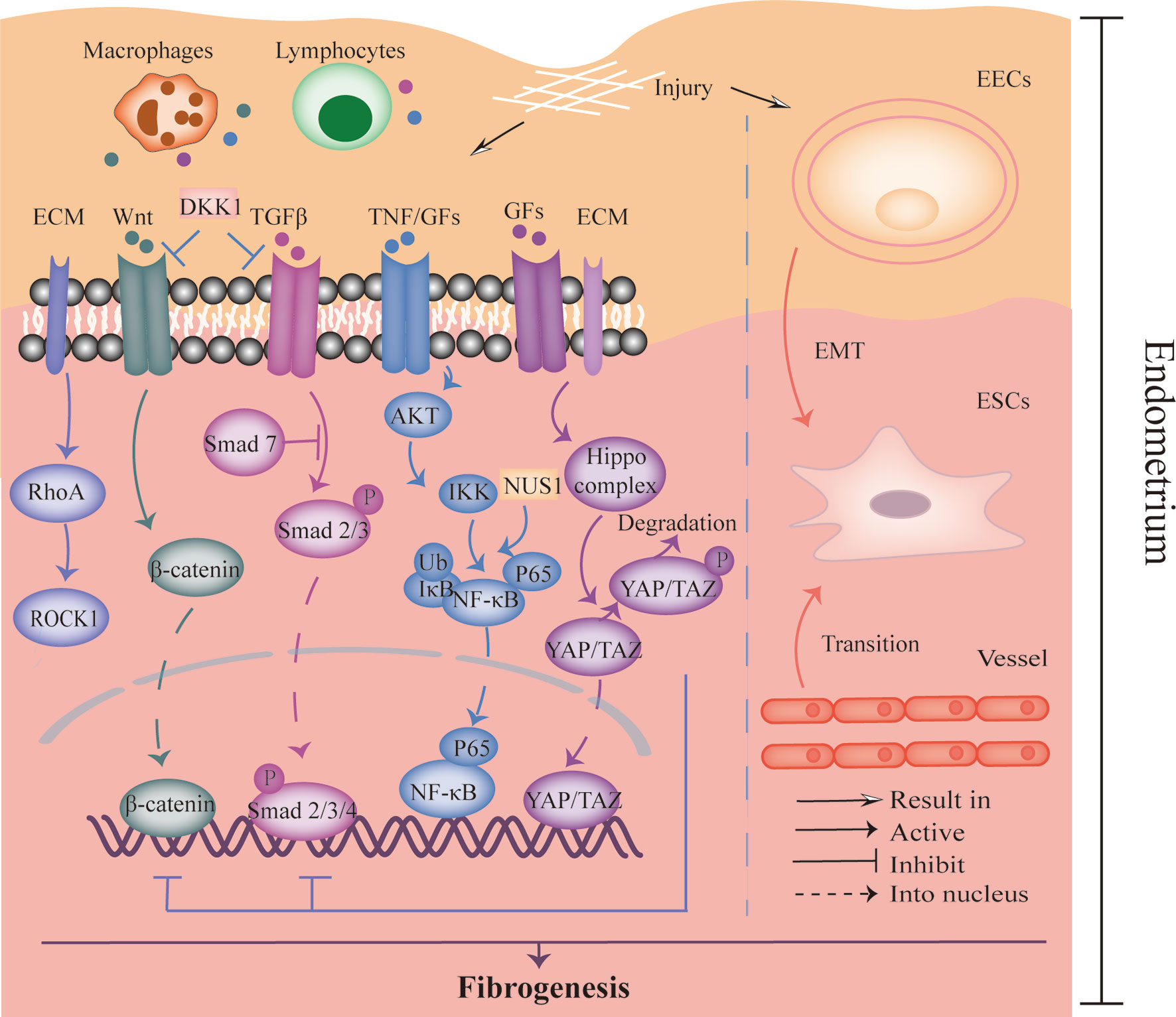
Figure 2 The pathogenesis of IUA. The left part showed the crosstalk of TGF-β, Wnt, NF-κB, Hippo, and RhoA/ROCK signaling pathways, which was relevant to endometrial fibrosis. The right part revealed the process of EMT and endothelial to mesenchymal transition that was involved in fibrogenesis. DKK-1, Dickkopf-1; ECM, Extracellular matrix; GFs, Growth factors; TNF, Tumor necrosis factor; EECs, Endometrial epithelial cells; ESCs, Endometrial stromal cells; EMT, Epithelial-mesenchymal transition.
Bioinformatic analysis revealed an evident cell heterogeneity in the uterus: endothelial cells, stromal cells, fibroblasts, M1 macrophages, mast cells, T cells, and smooth muscle cells (69). Understanding the intercellular interactions and cellular trans-differentiation may enable the pathogenesis of IUA to be elucidated and provide novel treatment targets. For instance, in this study, a heatmap showed the interaction between endothelial cells, especially endothelial cells in endothelial to mesenchymal transition stage, and fibroblasts or stromal cells was high, suggesting the communication might be involved in the progression of IUA. Moreover, the epithelial-to-mesenchymal transition (EMT) also plays an important role in IUA progression (65). A study with the use of IUA rat models revealed the EMT process was promoted by NUS1 protein overexpression via regulating AKT/NF-κB pathway, which could be attenuated by microRNA (miR)-466 (70). In contrast, another study showed that miR-1291 promoted endometrial fibrosis through acting ArhGAP29 negatively to upregulate RhoA/ROCK1 pathway that is also relevant to EMT (71). In addition, the Hippo/TAZ signaling pathway was also implicated in regulating EMT process negatively (65). Herein, activation of Hippo pathway would result in the phosphorylated TAZ, consequently inhibiting EMT process (65). Zhu et al. further found that Hippo pathway, which was stimulated by menstrual-blood derived stem cells (MenSCs), could inhibit TGFβ-mediated activation of myofibroblast phenotypes of endometrial stromal cells (ESCs) (72). Taken together, it is essential to treat AS via regulating the alternation of cell phenotypes in endometrium that is involved in IUA pathological process.
As shown in Table 1, the application of MSC-EVs on AS has been investigated in animal and in vitro experiments (30, 32, 43). For example, Yao et al. found that BMSC-EVs promoted endometrial glands, decreased the fibrotic area, and even reversed EMT process in the rat IUA models (39). In this study, injection of BMSCs-EVs significantly declined vimentin (VIM) level and increased the cytokeratin (CK) 19 level. Besides, the expression of TGF-β1, TGF-β1R, and Smad2 was also lower in the treatment group, suggesting BMSC-EVs might repair endometrium by inhibiting TGF-β1/Smad2 signaling pathway (39). Additionally, in Saribas’ work, the injection of uterus-derived MSC_(uMSC)–EVs into the uterine cavity promoted angiogenesis in the IUA rats by increasing the expression of vascular marker CD31 and vascular endothelial growth factor receptor 1 (VEGFR1) (47). Recently, a conference paper reported that UCMSC-EVs promoted rat TE repair by upregulating VEGF, Bcl-2 level and decreasing fibrosis area, suggesting that the regeneration of endometrium could be improved by MSC-EVs (33). Moreover, MSC-EVs may impede IUA progress by inhibiting inflammation. UCMSC-EVs could repress the inflammatory factor interleukin (IL)-1β, IL-6, and tumor necrosis factor (TNF)-α, and increase anti-inflammatory factor IL-10 expression (32). In terms of fertility reservation, the implantation and pregnancy rates were analyzed in this study. Results revealed that UCMSC-EVs could improve these two rates respectively in IUA rats, indicating that UCMSC-EVs might be beneficial to restore infertility of IUA patients (32). Hence, MSC-EVs may serve as a new strategy for treating AS by ameliorating endometrial condition, impeding fibrosis process, promoting angiogenesis, and exerting immunomodulation effect.
However, the application of MSC-EVs in IUA patients is still in its infancy. Several challenges exist in terms of utilizing MSC-EVs in human-beings. First, the mechanisms of MSC-EVs on IUA have not been fully understood yet. Additionally, though the injured endometrium could be repaired by MSC-EVs in vivo, such therapeutic effects verified in animal models were in a short period. Whether MSC-EVs were functional in a long-lasting time, has not been explored yet. Furthermore, we could not mimic chronic IUA in animal models, the effect of MSCs on chronic IUA has not been determined. Actually, many patients with IUA have suffered for quite a long time, whether MSC-EVs are efficient in treating those patients demand more investigation. Besides, we only know few information of safety about MSC-EVs used in patients, so the safety of MSCs-EVs is required to study as well (73). Notably, Wu et al. reported clinic-grade human embryonic stem cells (hESCs)–derived immunity- and matrix-regulatory cells (IMRCs) that were verified to cure lung fibrosis, had an efficacy and safety profile in mice and primates (74). Moreover, IMRCs was demonstrated to be superior to UCMSCs as their higher expression of proliferative, immunomodulatory, and anti-fibrotic genes. Hence, it emphasized the safety of IMRCs, however, the therapeutic effects required to be determined in IUA models.
POI
POI is defined by senescence of ovarian function in women less than 40 years old and characterized by amenorrhea or oligomenorrhea for at least 4 months, an elevated FSH level (≥25 IU/L), and fluctuant reduction of estradiol (E2) (75–77). It affects approximately 1% of women under 40 years, which will result in poor infertility outcomes eventually and burden young couples (75, 78). POI might be caused by genetic abnormality, aberrant immunity, chemotherapy or radiotherapy, and environmental pollutants (79, 80). It is believed that primordial follicles cannot be generated and ovarian reservoir is determined at birth, so the ovarian function is easily compromised by accelerated activation of primordial follicles, depletion of ovarian follicles in the resting pool, and abnormal follicular atresia as shown in Figure 3 (81).
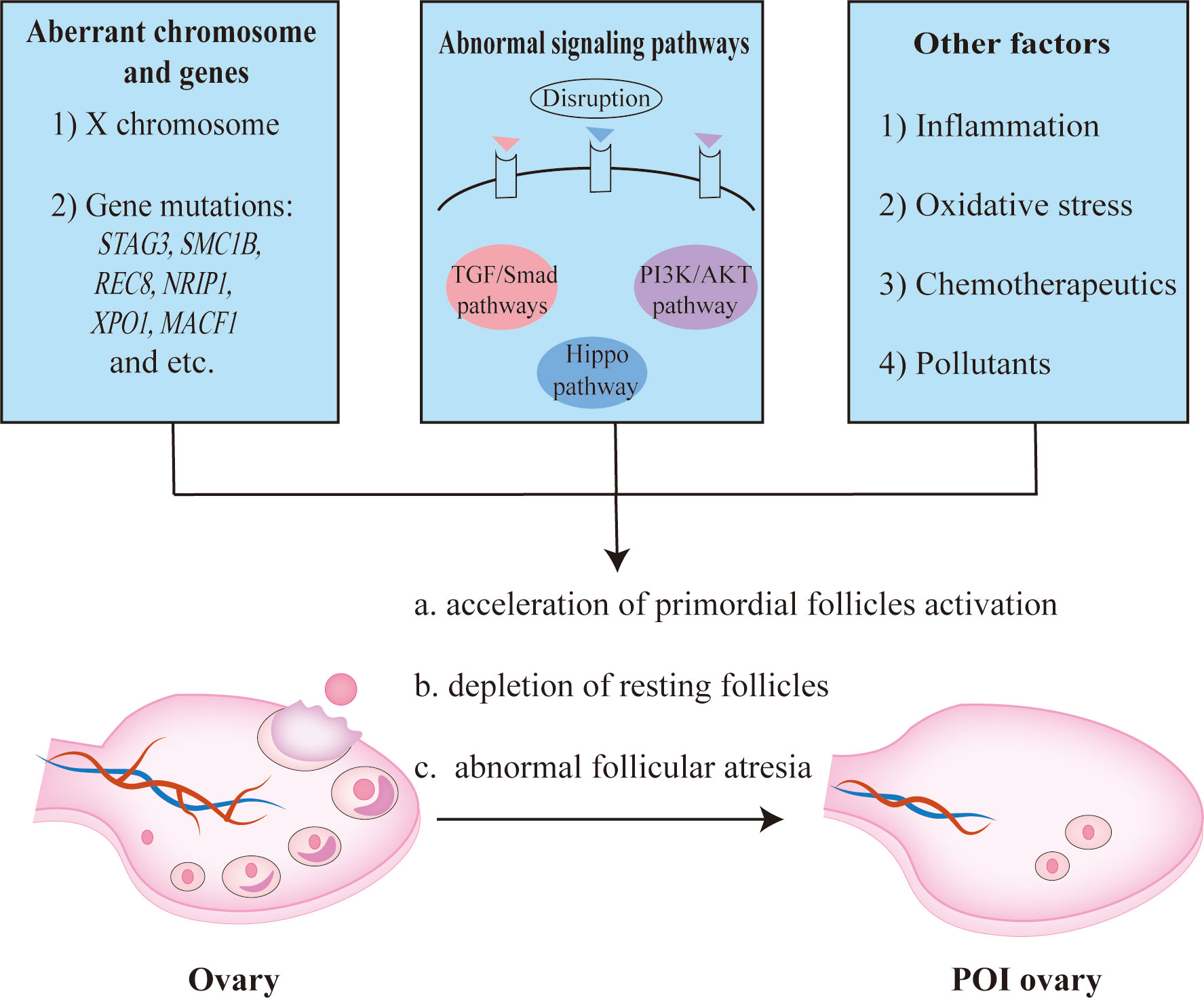
Figure 3 The pathogenesis of POI. Aberrant X chromosome and genes, abnormal signaling pathways, inflammation response, oxidative stress, etc. contributed to the occurrence of POI.
X chromosome and multiple genes are essential for follicle growth and development (82, 83). It has been reported that the inactivation of STAG3, SMC1B, or REC8 gene related to meiosis, would result in oocyte arrested with concomitant POI (84, 85). Using whole exome sequencing (WES) analysis, Jaillard et al. proposed new candidate POI genes, including NRIP1, XPO1, and MACF1 (86). In fact, it is hard to define a specific causative factor as different genes interplay intricately in the folliculogenesis (81). Apart from gene mutation, abnormal epigenetic modification might also be relevant to an increase of atresia follicles (87–89). Hence, aberrant gene expression or regulation in women can perturb folliculogenesis and bring about follicular atresia, which might lead to the occurrence of POI.
Signaling pathways play critical roles in the follicular development as well, such as PI3K/AKT/mTOR pathway, TGF-β pathway, and Hippo pathway (90, 91). Recently, Grosbois et al. found a synergistical effect of PI3K/AKT and Hippo signaling pathways, wherein primordial follicle recruitment was accelerated and consequently caused rapid depletion of follicles stock (91). Abnormal activation of PI3K pathway could induce the upregulation of AKT/mTOR, while the disruption of Hippo pathway would lead to the de-phosphorylation of YAP/TAZ, both of which resulted in a massive and precocious growth of primordial follicles (91). Whereas repressing PI3K/AKT pathway via depleting protein kinase Ck2 contributed to massive follicles atresia in mouse ovaries (92), indicating the significance of PI3K/AKT pathway equilibrium.
Besides, exaggerated autoimmune reaction or inflammatory response also accelerated follicular atresia (75, 93). For example, follicular atresia occurs when GCs continuously exposed to pro-inflammatory cytokines, such as IFN-γ (94). Apart from that, other factors, including unlimited ROS level, some chemotherapeutics, and environmental pollutants, might lead to follicular atresia or depletion as well (80, 81, 95). Notwithstanding, the exact pathogenesis of declined ovarian function remains an enigma, and the relatively complicated pathological mechanism of POI makes it difficult to cure this disease.
Ongoing researches demonstrated that MSC-EVs were able to rescue viability of GCs, suppress ROS level, and restore follicular number in POI animal models (Table 1) (31, 34, 38, 96). Recently, AFMSC-EVs were discovered to protect ovarian follicles against gonadotoxic effects of chemotherapy. Herein, miR-10a, a highly enriched miRNA in AFMSC-EVs, promoted resistance to GCs apoptosis or follicular atresia in chemotherapeutics-treated mice (45). After that, Sun et al. also found the UCMSC-EVs reduced cisplatin induced GCs apoptosis in vitro (35). And then a study in mice revealed higher level of AKT, P-AKT, VEGF, and IGF in UCMSC-MVs-treated groups compared to non-treated POI group, implying that UCMSC-MVs might induce angiogenesis and activate the PI3K/AKT signaling pathway in ovaries (38). Notably, PTEN, which regulated PI3K/AKT pathway negatively, was downregulated after injected BMSC-EVs or AFMSC-EVs in POI rat models (40, 46), suggesting that MSC-EVs might ameliorate GCs apoptosis in POI via PTEN-PI3K pathway. Moreover, MSC-EVs could suppress SIRT families (including SIRT4 or SIRT7) and P53 to reduce cisplatin or cyclophosphamide (CTX)-induced GCs apoptosis (36, 41, 44). Meanwhile, using mRNA and protein assay, Huang et al. found that ADSC-Exo regulated SMAD signaling pathway and rescue GCs from apoptosis (31).
Moreover, MSC-EVs improved offspring outcomes in POI models as well (37). In this study, Liu et al. found that POI mice in UCMSC-EVs transplantation group had higher fertility with less time-to-pregnancy and an increased number of offspring compared to the POI group. Besides, their offspring had nearly similar cognitive behaviors through assessing Y-maze test and novel object recognition task. It was demonstrated that MSC-EVs could improve the fertility of POI mice without adverse effects on the cognitive behavior of their offspring (37).
Similarly, the clinical application of MSC-EVs in POI women is still rudimentary. On one hand, the mechanism of MSC-EVs therapeutic effect on POI is opaque, further researches are needed. On the other hand, the therapeutic effects of MSC-EVs verified on abdominal-injection-constructed animal models, could not totally extrapolate to human patients. Recently, a clinical trial reported a retrograde injection method was used to transplant MSCs based on collagen scaffold (CS) into ovaries of POI patients, suggested CS/MSC-EVs could also be transferred by intra-ovarian injection (97). However, the safety and efficacy of using this method remains to be studied. Additionally, Blazquez et al. noticed the higher blastomere count and hatching rate when murine embryos were exposed to endometrial MSCs (endMSC)-EVs (48, 98). Furthermore, endMSC-EVs were also verified to improve in vitro fertilization (IVF) outcome in aged murine model (49). IVF-embryo transfer (IVF-ET) was widely applied in POI patients for assisted conception (99). Perhaps IVF-ET combined with MSC-EVs might be a new method for helping POI patients to conceive, although the safety to offspring needs to be explored.
PCOS
PCOS is a common reproductive endocrine disorder characterized by hyperandrogenism, ovulatory dysfunction, polycystic ovarian morphology, obesity, and insulin resistance, which affects about 5–20% of women of reproductive age (98, 100, 101). Hyperandrogenism has been demonstrated as the essence of PCOS (102, 103). Herein, androgen excess was reported to initiate small antral follicle growth and trigger premature luteinization, which inhibited dominate follicle selection and consequently impaired ovulation (102, 103). Anovulatory infertility is a major challenge for women with PCOS, and assisted reproductive techniques are recognized as a last resort to conceive (104). Recently, it was found that in vitro maturation (IVM) protocol based on heterologous follicular fluid and GCs supernatant (HFF/GC-IVM protocol) could improve the maturation rate of immature denuded oocytes, fertilization rate, and hatched blastocysts rate for women with PCOS (105). In the meanwhile, ADSC-EVs were noticed to inhibit apoptosis and promote proliferation of cumulus cells (CCs) from PCOS patients, wherein elevated expression of miR-323-3p in exosomes works (21). However, the case of MSC-EVs used in treating PCOS is still few so far.
Possible Mechanism of Treatment
As described above, though the precise mechanisms of MSC-EVs on female reproductive diseases have not been elucidated yet, several hypotheses have been proposed, including promoting angiogenesis, regulating immunity, reducing oxidate stress level, etc. The functional contents related to above mechanisms in MSC-EVs were summarized in Table 2.
IUA
It has been well established that MSC-EVs stimulate neovascularization (111, 120, 137, 138). MSC-EVs promoted the formation of tube-like structure formation and spheroid-based sprouting of human umbilical vein endothelial cells (HUVECs) (102, 116, 118). Besides, hemoglobin or CD31+ cells were increased after injecting the mixture of Matrigel and MSC-EVs in mice subcutaneously, indicating the MSC-EVs promoted the formation of functional capillaries (102, 118). Similarly, MSC-EVs increased the expression of VEGF and CD31 in IUA model (29, 47, 139). The contents enclosed in the MSC-EVs might be responsible for such effects. MSC-EVs not only contained multiple pro-angiogenic proteins, such as VEGF and HGF (106). Moreover, several non-coding RNAs (nc-RNAs) enriched in the MSC-EVs, including miR-30b and miR-125a (102, 118). In addition, the following signaling pathways are greatly affected: a) Wnt4/β-catenin pathway (137), b) NF-κB signaling pathway (111), c) VEGF/VEGFR (106, 138), d) PI3K/AKT pathway (120, 140), e) ERK/AKT signaling pathway (114), f) DLL4/Notch signaling pathway (102, 118, 141). Interestingly, these signaling pathways not only were functional in promoting angiogenesis, but also exerted therapeutic effects via other mechanisms. For instance, Wnt/β-catenin pathway promoted angiogenesis, and was involved in TGF-β1-mediated fibrosis (67, 142). Therefore, further researches need to characterize the role of pathways in ameliorating IUA by MSC-EVs.
Some researchers proposed that MSC-EVs regulated cell phenotypes, like conferring plasticity of fibroblasts, or inducing mesenchymal-epithelial transition (MET) (39, 143). ADSC-EVs could induce the osteogenic and adipogenic differentiation of human dermal fibroblasts, via enhancing the expression of OCT4 and NANOG (143). It presents a new horizon in investigating the mechanism of MSC-EVs in terms of ameliorating IUA.
Besides, emerging studies supported that MSC-EVs had immunomodulatory properties (121, 144, 145). MSC-EVs could guide phenotypic switch of M1 to M2 macrophages in vivo and in vitro (146). Del Fattore et al. revealed MSC-EVs could promote the proliferation of regulatory T (Treg) cells, which repress immune response through Galectin-1 and PD-L1 (147). In addition, endMSC-EVs and Wharton’s Jelly-derived MSC (WJMSC)-EVs could suppress CD+4 T cell proliferation and activation (122, 123). Moreover, MSC-EVs could modulate immunology via regulating cytokines. MSC-EVs were able to downregulate IL-1β, IL-6, and TNF-α levels in LPS-treated endometrial cells (43). Herein, inflammatory pathway, such as NF-κB signaling pathway and JNK/P38 MAPK pathway, could be regulated negatively by MSC-EVs. Contents derived from MSC-EVs, like IL-10, KGF, and TSG-6, could alleviate inflammation as well (131, 133, 148). The role of such factors should be investigated in MSC-EV therapy for IUA (42, 74, 75, 107, 108, 112, 115, 117, 119, 124–126, 129, 131, 131, 149).
POI
Apart from promoting angiogenesis (150), MSC-EVs could ameliorate POI via reducing oxidative stress (44). Oxidative stress is a phenomenon resulted from accumulation of ROS, which impairs the function and structures of cells and tissues (136, 149). BMSC-EVs could protect cells from toxic effects of peroxide via reducing malondialdehyde (MDA) and increasing superoxide dismutase 1 (SOD1) and catalase expression (151). Moreover, MSC-EVs were likely to have a mitochondrial (MIT)-protective effect. The compromised mitochondrial membrane potential (MMP) or ATP level was rescued, and ROS level was reduced significantly after MSC-EVs treatment (151, 152). For this reason, studies about the mechanism of MSC-EVs in ameliorating POI, should not only focus on the effect of anti-apoptosis of GCs under lower levels of ROS, but also further explore the influence of MSC-EVs on MIT dysfunction of GCs or oocytes.
In addition, inflammation response in chemotherapeutic drugs-injured GCs was also inhibited by MSC-EVs, with the decreasing level of IL-6 and IL-1β. The survival rate of GCs was higher in MSC-EV-treated group compared to the model group (153). Collectively, MSC-EVs improve ovarian function mainly inducing angiogenesis, reducing oxidative stress, protecting MIT protective, and regulating inflammation.
PCOS
Currently, researches about the therapeutic effect of MSC-EVs on PCOS are still limited, thereby the mechanism of MSC-EVs om improving PCOS has not been elucidated yet. As above mentioned, the dysfunction of follicles is involved in the pathogenesis of PCOS. It has been demonstrated that the communication between oocytes and CCs plays a significant role in the development of follicles (154). Therefore, CCs dysfunction may be related to the decreased oocyte quality and poor pregnancy outcomes of women with PCOS. Zhao et al. found that miR-323-3p transferred by MSC-EVs could ameliorate PCOS via promoting growth and inhibiting apoptosis of CCs (21). Previous literature indicated that administration of functional CCs into IVM medium facilitated the oocyte meiosis and embryo development in women with PCOS. Therefore, MSC-EVs, which potentially improve CCs viability, might be a promising treatment for PCOS patients.
As stated above, the functions that MSC-EVs exert depend on the cell types, as the resources of MSC-EVs are heterogeneous. Hence, further studies regarding the therapeutic mechanisms and massive production of MSC-EVs, which may provide reliable evidence supporting clinical applications, are encouraged.
Conclusions and Future Perspective
MSC-EVs hold great prospects in treating female reproductive diseases, such as IUA, POI, and PCOS. The therapeutic mechanisms included pro-angiogenesis, immunomodulation, anti-fibrosis, and anti-oxidative stress. Although numerous studies confirm the efficacy of MSC-EVs on improving female fertility in in vitro and in vivo models, such effects may not fully extrapolate to humans. Besides, several questions need to be fully clarified before the application of MSC-EVs in clinic: a) standardized purification and identification protocols for MSC-EVs, b) convenient storage and transportation methods for MSC-EVs, c) determined cargo of large-scale generation of MSC-EVs, d) determined the exact mechanism of MSC-EVs treatment, e) safety issues of MSC-EVs (73). Limited yield is one of the most important problems that restrain the widespread application of MSC-EVs. The production of MSC-EVs might gain benefits from bioreactor culture models (11, 51). For example, hollow-fiber bioreactors or a microcarrier-based 3D culture system are reported to reach industrialized mass production of EVs (51, 52). Herein, a quality-control system should be established to monitor the process of production. Moreover, engineered MSC-EVs may also enhance the efficiency of delivering specific proteins to targeted cells (132, 155). It should be assessed from the effects and safety via long-term monitoring.
Author Contributions
ZL and CS performed data collection and outline design. ZL and CL drafted the manuscript, which was revised by HZ and LW. CL, CS, and HZ contributed equally to this manuscript. All authors contributed to the article and approved the submitted version.
Funding
This work was supported by National Natural Science Foundation of China (NSFC81901561 and NSFC81771582).
Conflict of Interest
The authors declare that the research was conducted in the absence of any commercial or financial relationships that could be construed as a potential conflict of interest.
References
1. Vizoso FJ, Eiro N, Cid S, Schneider J, Perez-Fernandez R. Mesenchymal Stem Cell Secretome: Toward Cell-Free Therapeutic Strategies in Regenerative Medicine. Int J Mol Sci (2017) 18:1852. doi: 10.3390/ijms18091852
2. Ding D-C, Shyu W-C, Lin S-Z. Mesenchymal Stem Cells. Cell Transplant (2011) 20:5–14. doi: 10.3727/096368910X
3. Ullah I, Subbarao RB, Rho GJ. Human Mesenchymal Stem Cells - Current Trends and Future Prospective. Biosci Rep (2015) 35:e00191. doi: 10.1042/BSR20150025
4. Sneddon JB, Tang Q, Stock P, Bluestone JA, Roy S, Desai T, et al. Stem Cell Therapies for Treating Diabetes: Progress and Remaining Challenges. Cell Stem Cell (2018) 22:810–23. doi: 10.1016/j.stem.2018.05.016
5. Liu B, Ding F, Hu D, Zhou Y, Long C, Shen L, et al. Human Umbilical Cord Mesenchymal Stem Cell Conditioned Medium Attenuates Renal Fibrosis by Reducing Inflammation and Epithelial-to-Mesenchymal Transition via the TLR4/NF-κB Signaling Pathway In Vivo and In Vitro. Stem Cell Res Ther (2018) 9:7. doi: 10.1186/s13287-017-0760-6
6. Liang N, Trujillo CA, Negraes PD, Muotri AR, Lameu C, Ulrich H. Stem Cell Contributions to Neurological Disease Modeling and Personalized Medicine. Prog Neuropsychopharmacol Biol Psychiatry (2018) 80:54–62. doi: 10.1016/j.pnpbp.2017.05.025
7. Goradel NH, Hour FG, Negahdari B, Malekshahi ZV, Hashemzehi M, Masoudifar A, et al. Stem Cell Therapy: A New Therapeutic Option for Cardiovascular Diseases. J Cell Biochem (2018) 119:95–104. doi: 10.1002/jcb.26169
8. Du H, Taylor HS. Stem Cells and Female Reproduction. Reprod Sci (2009) 16:126–39. doi: 10.1177/1933719108329956
9. Liu F, Hu S, Yang H, Li Z, Huang K, Su T, et al. Hyaluronic Acid Hydrogel Integrated With Mesenchymal Stem Cell-Secretome to Treat Endometrial Injury in a Rat Model of Asherman’s Syndrome. Advanced Healthc Mater (2019) 8:e1900411. doi: 10.1002/adhm.201900411
10. Mendt M, Rezvani K, Shpall E. Mesenchymal Stem Cell-Derived Exosomes for Clinical Use. Bone Marrow Transplant (2019) 54:789–92. doi: 10.1038/s41409-019-0616-z
11. Ullah M, Qiao Y, Concepcion W, Thakor AS. Stem Cell-Derived Extracellular Vesicles: Role in Oncogenic Processes, Bioengineering Potential, and Technical Challenges. Stem Cell Res Ther (2019) 10:347. doi: 10.1186/s13287-019-1468-6
12. Théry C, Witwer KW, Aikawa E, Alcaraz MJ, Anderson JD, Andriantsitohaina R, et al. Minimal Information for Studies of Extracellular Vesicles 2018 (MISEV2018): A Position Statement of the International Society for Extracellular Vesicles and Update of the MISEV2014 Guidelines. J Extracell Vesicles (2018) 7:1535750. doi: 10.1080/20013078.2018.1535750
13. Zhao AG, Shah K, Cromer B, Sumer H. Mesenchymal Stem Cell-Derived Extracellular Vesicles and Their Therapeutic Potential. Stem Cells Int (2020) 2020:8825771. doi: 10.1155/2020/8825771
14. Patel DB, Gray KM, Santharam Y, Lamichhane TN, Stroka KM, Jay SM. Impact of Cell Culture Parameters on Production and Vascularization Bioactivity of Mesenchymal Stem Cell-Derived Extracellular Vesicles. Bioeng Transl Med (2017) 2:170–9. doi: 10.1002/btm2.10065
15. Vander Borght M, Wyns C. Fertility and Infertility: Definition and Epidemiology. Clin Biochem (2018) 62:2–10. doi: 10.1016/j.clinbiochem.2018.03.012
16. Zhao Y-X, Chen S-R, Su P-P, Huang F-H, Shi Y-C, Shi Q-Y, et al. Using Mesenchymal Stem Cells to Treat Female Infertility: An Update on Female Reproductive Diseases. Stem Cells Int (2019) 2019:9071720. doi: 10.1155/2019/9071720
17. Salazar CA, Isaacson K, Morris S. A Comprehensive Review of Asherman’s Syndrome: Causes, Symptoms and Treatment Options. Curr Opin Obstet Gynecol (2017) 29:249–56. doi: 10.1097/gco.0000000000000378
18. Sheikhansari G, Aghebati-Maleki L, Nouri M, Jadidi-Niaragh F, Yousefi M. Current Approaches for the Treatment of Premature Ovarian Failure With Stem Cell Therapy. BioMed Pharmacother (2018) 102:254–62. doi: 10.1016/j.biopha.2018.03.056
19. Lötvall J, Hill AF, Hochberg F, Buzás EI, Di Vizio D, Gardiner C, et al. Minimal Experimental Requirements for Definition of Extracellular Vesicles and Their Functions: A Position Statement From the International Society for Extracellular Vesicles. J Extracell Vesicles (2014) 3:26913. doi: 10.3402/jev.v3.26913
20. Kim SY, Khanal D, Kalionis B, Chrzanowski W. High-Fidelity Probing of the Structure and Heterogeneity of Extracellular Vesicles by Resonance-Enhanced Atomic Force Microscopy Infrared Spectroscopy. Nat Protoc (2019) 14:576–93. doi: 10.1038/s41596-018-0109-3
21. Zhao Y, Tao M, Wei M, Du S, Wang H, Wang X. Mesenchymal Stem Cells Derived Exosomal miR-323-3p Promotes Proliferation and Inhibits Apoptosis of Cumulus Cells in Polycystic Ovary Syndrome (PCOS). Artif Cells Nanomedicine Biotechnol (2019) 47:3804–13. doi: 10.1080/21691401.2019.1669619
22. Kourembanas S. Exosomes: Vehicles of Intercellular Signaling, Biomarkers, and Vectors of Cell Therapy. Annu Rev Physiol (2015) 77:13–27. doi: 10.1146/annurev-physiol-021014-071641
23. Qiu G, Zheng G, Ge M, Wang J, Huang R, Shu Q, et al. Functional Proteins of Mesenchymal Stem Cell-Derived Extracellular Vesicles. Stem Cell Res Ther (2019) 10:359. doi: 10.1186/s13287-019-1484-6
24. Pegtel DM, Gould SJ. Exosomes. Annu Rev Biochem (2019) 88:487–514. doi: 10.1146/annurev-biochem-013118-111902
25. Joo HS, Suh JH, Lee HJ, Bang ES, Lee JM. Current Knowledge and Future Perspectives on Mesenchymal Stem Cell-Derived Exosomes as a New Therapeutic Agent. Int J Mol Sci (2020) 21:22. doi: 10.3390/ijms21030727
26. Abbaszadeh H, Ghorbani F, Derakhshani M, Movassaghpour A, Yousefi M. Human Umbilical Cord Mesenchymal Stem Cell-Derived Extracellular Vesicles: A Novel Therapeutic Paradigm. J Cell Physiol (2020) 235:706–17. doi: 10.1002/jcp.29004
27. Phinney DG, Di Giuseppe M, Njah J, Sala E, Shiva S, St Croix CM, et al. Mesenchymal Stem Cells Use Extracellular Vesicles to Outsource Mitophagy and Shuttle microRNAs. Nat Commun (2015) 6:8472. doi: 10.1038/ncomms9472
28. Esfandyari S, Chugh RM, Park H-S, Hobeika E, Ulin M, Al-Hendy A. Mesenchymal Stem Cells as a Bio Organ for Treatment of Female Infertility. Cells (2020) 9:2253. doi: 10.3390/cells9102253
29. Ebrahim N, Mostafa O, El Dosoky RE, Ahmed IA, Saad AS, Mostafa A, et al. Human Mesenchymal Stem Cell-Derived Extracellular Vesicles/Estrogen Combined Therapy Safely Ameliorates Experimentally Induced Intrauterine Adhesions in a Female Rat Model. Stem Cell Res Ther (2018) 9:175. doi: 10.1186/s13287-018-0924-z
30. Zhao S, Qi W, Zheng J, Tian Y, Qi X, Kong D, et al. Exosomes Derived From Adipose Mesenchymal Stem Cells Restore Functional Endometrium in a Rat Model of Intrauterine Adhesions. Reprod Sci (2020) 27:1266–75. doi: 10.1007/s43032-019-00112-6
31. Huang B, Lu J, Ding C, Zou Q, Wang W, Li H. Exosomes Derived From Human Adipose Mesenchymal Stem Cells Improve Ovary Function of Premature Ovarian Insufficiency by Targeting SMAD. Stem Cell Res Ther (2018) 9:216. doi: 10.1186/s13287-018-0953-7
32. Xin L, Lin X, Zhou F, Li C, Wang X, Yu H, et al. A Scaffold Laden With Mesenchymal Stem Cell-Derived Exosomes for Promoting Endometrium Regeneration and Fertility Restoration Through Macrophage Immunomodulation. Acta Biomaterialia (2020) 113:252–66. doi: 10.1016/j.actbio.2020.06.029
33. Chang Y, Liu Y, Li X. Exosomes Derived From Human Umbilical Cord Mesenchymal Stem Cells Promote Proliferation of Endometrial Stromal Cell. Fertil Steril (2020) 114:e525. doi: 10.1016/j.fertnstert.2020.09.035
34. Zhang J, Yin H, Jiang H, Du X, Yang Z. The Protective Effects of Human Umbilical Cord Mesenchymal Stem Cell-Derived Extracellular Vesicles on Cisplatin-Damaged Granulosa Cells. Taiwan J Obstet Gynecol (2020) 59:527–33. doi: 10.1016/j.tjog.2020.05.010
35. Sun L, Li D, Song K, Wei J, Yao S, Li Z, et al. Exosomes Derived From Human Umbilical Cord Mesenchymal Stem Cells Protect Against Cisplatin-Induced Ovarian Granulosa Cell Stress and Apoptosis In Vitro. Sci Rep (2017) 7:2552. doi: 10.1038/s41598-017-02786-x
36. Ding C, Zhu L, Shen H, Lu J, Zou Q, Huang C, et al. Exosomal miRNA-17-5p Derived From Human Umbilical Cord Mesenchymal Stem Cells Improves Ovarian Function in Premature Ovarian Insufficiency by Regulating SIRT7. Stem Cells (2020) 38:1137–48. doi: 10.1002/stem.3204
37. Liu C, Yin H, Jiang H, Du X, Wang C, Liu Y, et al. Extracellular Vesicles Derived From Mesenchymal Stem Cells Recover Fertility of Premature Ovarian Insufficiency Mice and the Effects on Their Offspring. Cell Transplant (2020) 29:963689720923575. doi: 10.1177/0963689720923575
38. Yang Z, Du X, Wang C, Zhang J, Liu C, Li Y, et al. Therapeutic Effects of Human Umbilical Cord Mesenchymal Stem Cell-Derived Microvesicles on Premature Ovarian Insufficiency in Mice. Stem Cell Res Ther (2019) 10:250. doi: 10.1186/s13287-019-1327-5
39. Yao Y, Chen R, Wang G, Zhang Y, Liu F. Exosomes Derived From Mesenchymal Stem Cells Reverse EMT via TGF-β1/Smad Pathway and Promote Repair of Damaged Endometrium. Stem Cell Res Ther (2019) 10:225. doi: 10.1186/s13287-019-1332-8
40. Yang M, Lin L, Sha C, Li T, Zhao D, Wei H, et al. Bone Marrow Mesenchymal Stem Cell-Derived Exosomal miR-144-5p Improves Rat Ovarian Function After Chemotherapy-Induced Ovarian Failure by Targeting PTEN. Lab Invest (2020) 100:342–52. doi: 10.1038/s41374-019-0321-y
41. Sun B, Ma Y, Wang F, Hu L, Sun Y. miR-644-5p Carried by Bone Mesenchymal Stem Cell-Derived Exosomes Targets Regulation of p53 to Inhibit Ovarian Granulosa Cell Apoptosis. Stem Cell Res Ther (2019) 10:360. doi: 10.1186/s13287-019-1442-3
42. Xiao B, Zhu Y, Huang J, Wang T, Wang F, Sun S. Exosomal Transfer of Bone Marrow Mesenchymal Stem Cell-Derived miR-340 Attenuates Endometrial Fibrosis. Biol Open (2019) 8:bio039958. doi: 10.1242/bio.039958
43. Perrini C, Strillacci MG, Bagnato A, Esposti P, Marini MG, Corradetti B, et al. Microvesicles Secreted From Equine Amniotic-Derived Cells and Their Potential Role in Reducing Inflammation in Endometrial Cells in an in-Vitro Model. Stem Cell Res Ther (2016) 7:169. doi: 10.1186/s13287-016-0429-6
44. Ding C, Qian C, Hou S, Lu J, Zou Q, Li H, et al. Exosomal miRNA-320a Is Released From hAMSCs and Regulates SIRT4 to Prevent Reactive Oxygen Species Generation in POI. Mol Ther Nucleic Acids (2020) 21:37–50. doi: 10.1016/j.omtn.2020.05.013
45. Xiao GY, Cheng CC, Chiang YS, Cheng WT, Liu IH, Wu SC. Exosomal miR-10a Derived From Amniotic Fluid Stem Cells Preserves Ovarian Follicles After Chemotherapy. Sci Rep (2016) 6:23120. doi: 10.1038/srep23120
46. Thabet E, Yusuf A, Abdelmonsif DA, Nabil I, Mourad G, Mehanna RA. Extracellular Vesicles miRNA-21: A Potential Therapeutic Tool in Premature Ovarian Dysfunction. Mol Hum Reprod (2020) 26:906–19. doi: 10.1093/molehr/gaaa068
47. Saribas GS, Ozogul C, Tiryaki M, Alpaslan Pinarli F, Hamdemir Kilic S. Effects of Uterus Derived Mesenchymal Stem Cells and Their Exosomes on Asherman’s Syndrome. Acta Histochem (2020) 122:151465. doi: 10.1016/j.acthis.2019.151465
48. Blázquez R, Sánchez-Margallo FM, Álvarez V, Matilla E, Hernández N, Marinaro F, et al. Murine Embryos Exposed to Human Endometrial MSCs-derived Extracellular Vesicles Exhibit Higher VEGF/PDGF AA Release, Increased Blastomere Count and Hatching Rates. PloS One (2018) 13:e0196080. doi: 10.1371/journal.pone.0196080
49. Marinaro F, Macías-García B, Sánchez-Margallo FM, Blázquez R, Álvarez V, Matilla E, et al. Extracellular Vesicles Derived From Endometrial Human Mesenchymal Stem Cells Enhance Embryo Yield and Quality in an Aged Murine Model†. Biol Reprod (2019) 100:1180–92. doi: 10.1093/biolre/ioy263
50. Maumus M, Rozier P, Boulestreau J, Jorgensen C, Noël D. Mesenchymal Stem Cell-Derived Extracellular Vesicles: Opportunities and Challenges for Clinical Translation. Front Bioengineering Biotechnol (2020) 8:997. doi: 10.3389/fbioe.2020.00997
51. Watson DC, Bayik D, Srivatsan A, Bergamaschi C, Valentin A, Niu G, et al. Efficient Production and Enhanced Tumor Delivery of Engineered Extracellular Vesicles. Biomaterials (2016) 105:195–205. doi: 10.1016/j.biomaterials.2016.07.003
52. Haraszti RA, Miller R, Stoppato M, Sere YY, Coles A, Didiot MC, et al. Exosomes Produced From 3D Cultures of MSCs by Tangential Flow Filtration Show Higher Yield and Improved Activity. Mol Ther (2018) 26:2838–47. doi: 10.1016/j.ymthe.2018.09.015
53. Hao D, Swindell HS, Ramasubramanian L, Liu R, Lam KS, Farmer DL, et al. Extracellular Matrix Mimicking Nanofibrous Scaffolds Modified With Mesenchymal Stem Cell-Derived Extracellular Vesicles for Improved Vascularization. Front Bioengineering Biotechnol (2020) 8:633. doi: 10.3389/fbioe.2020.00633
54. Diomede F, Gugliandolo A, Cardelli P, Merciaro I, Ettorre V, Traini T, et al. Three-Dimensional Printed PLA Scaffold and Human Gingival Stem Cell-Derived Extracellular Vesicles: A New Tool for Bone Defect Repair. Stem Cell Res Ther (2018) 9:104. doi: 10.1186/s13287-018-0850-0
56. Lo ST, Ramsay P, Pierson R, Manconi F, Munro MG, Fraser IS. Endometrial Thickness Measured by Ultrasound Scan in Women With Uterine Outlet Obstruction Due to Intrauterine or Upper Cervical Adhesions. Hum Reprod (2008) 23:306–9. doi: 10.1093/humrep/dem393
57. Xiao S, Wan Y, Xue M, Zeng X, Xiao F, Xu D, et al. Etiology, Treatment, and Reproductive Prognosis of Women With Moderate-to-Severe Intrauterine Adhesions. Int J Gynaecol Obstet (2014) 125:121–4. doi: 10.1016/j.ijgo.2013.10.026
58. Bai X, Liu J, Cao S, Wang L. Mechanisms of Endometrial Fibrosis and the Potential Application of Stem Cell Therapy. Discovery Med (2019) 27:267–79.
59. Wynn TA, Ramalingam TR. Mechanisms of Fibrosis: Therapeutic Translation for Fibrotic Disease. Nat Med (2012) 18:1028–40. doi: 10.1038/nm.2807
60. Santamaria X, Isaacson K, Simón C. Asherman’s Syndrome: It may Not be All Our Fault. Hum Reprod (2018) 33:1374–80. doi: 10.1093/humrep/dey232
61. Salma U, Xue M, Ali Sheikh MS, Guan X, Xu B, Zhang A, et al. Role of Transforming Growth Factor-β1 and Smads Signaling Pathway in Intrauterine Adhesion. Mediators Inflammation (2016) 2016:4158287. doi: 10.1155/2016/4158287
62. Abudukeyoumu A, Li M-Q, Xie F. Transforming Growth Factor-Beta 1 in Intrauterine Adhesion. Am J Reprod Immunol (2020) 84:e13262. doi: 10.1111/aji.13262
63. Wang X, Ma N, Sun Q, Huang C, Liu Y, Luo X. Elevated NF-κB Signaling in Asherman Syndrome Patients and Animal Models. Oncotarget (2017) 8:15399–406. doi: 10.18632/oncotarget.14853
64. Xue X, Chen Q, Zhao G, Zhao JY, Duan Z, Zheng PS. The Overexpression of TGF-β and CCN2 in Intrauterine Adhesions Involves the NF-κB Signaling Pathway. PloS One (2015) 10:e0146159. doi: 10.1371/journal.pone.0146159
65. Zhu H-Y, Ge T-X, Pan Y-B, Zhang S-Y. Advanced Role of Hippo Signaling in Endometrial Fibrosis: Implications for Intrauterine Adhesion. Chin Med J (2017) 130:2732–7. doi: 10.4103/0366-6999.218013
66. Akhmetshina A, Palumbo K, Dees C, Bergmann C, Venalis P, Zerr P, et al. Activation of Canonical Wnt Signalling is Required for TGF-β-Mediated Fibrosis. Nat Commun (2012) 3:735. doi: 10.1038/ncomms1734
67. Liu L, Chen G, Chen T, Shi W, Hu H, Song K, et al. si-SNHG5-FOXF2 Inhibits TGF-β1-Induced Fibrosis in Human Primary Endometrial Stromal Cells by the Wnt/β-Catenin Signalling Pathway. Stem Cell Res Ther (2020) 11:479. doi: 10.1186/s13287-020-01990-3
68. Chen G, Liu L, Sun J, Zeng L, Cai H, He Y. Foxf2 and Smad6 Co-Regulation of Collagen 5A2 Transcription is Involved in the Pathogenesis of Intrauterine Adhesion. J Cell Mol Med (2020) 24:2802–18. doi: 10.1111/jcmm.14708
69. Leung RK, Lin Y, Liu Y. Recent Advances in Understandings Towards Pathogenesis and Treatment for Intrauterine Adhesion and Disruptive Insights From Single-Cell Analysis. Reprod Sci (2020). doi: 10.1007/s43032-020-00343-y
70. Liu M, Zhao D, Wu X, Guo S, Yan L, Zhao S, et al. miR-466 and NUS1 Regulate the AKT/Nuclear Factor kappa B (NFκB) Signaling Pathway in Intrauterine Adhesions in a Rat Model. Med Sci Monit (2019) 25:4094–103. doi: 10.12659/msm.914202
71. Xu Q, Duan H, Gan L, Liu X, Chen F, Shen X, et al. MicroRNA-1291 Promotes Endometrial Fibrosis by Regulating the ArhGAP29-RhoA/ROCK1 Signaling Pathway in a Murine Model. Mol Med Rep (2017) 16:4501–10. doi: 10.3892/mmr.2017.7210
72. Zhu H, Pan Y, Jiang Y, Li J, Zhang Y, Zhang S. Activation of the Hippo/TAZ Pathway is Required for Menstrual Stem Cells to Suppress Myofibroblast and Inhibit Transforming Growth Factor β Signaling in Human Endometrial Stromal Cells. Hum Reprod (Oxford England) (2019) 34:635–45. doi: 10.1093/humrep/dez001
73. Gowen A, Shahjin F, Chand S, Odegaard KE, Yelamanchili SV. Mesenchymal Stem Cell-Derived Extracellular Vesicles: Challenges in Clinical Applications. Front Cell Dev Biol (2020) 8:149. doi: 10.3389/fcell.2020.00149
74. Wu J, Song D, Li Z, Guo B, Xiao Y, Liu W, et al. Immunity-and-Matrix-Regulatory Cells Derived From Human Embryonic Stem Cells Safely and Effectively Treat Mouse Lung Injury and Fibrosis. Cell Res (2020) 30:794–809. doi: 10.1038/s41422-020-0354-1
75. Domniz N, Meirow D. Premature Ovarian Insufficiency and Autoimmune Diseases. Best Pract Res Clin Obstet Gynaecol (2019) 60:42–55. doi: 10.1016/j.bpobgyn.2019.07.008
76. Tucker EJ, Grover SR, Bachelot A, Touraine P, Sinclair AH. Premature Ovarian Insufficiency: New Perspectives on Genetic Cause and Phenotypic Spectrum. Endocrine Rev (2016) 37:609–35. doi: 10.1210/er.2016-1047
77. Webber L, Davies M, Anderson R, Bartlett J, Braat D, Cartwright B, et al. ESHRE Guideline: Management of Women With Premature Ovarian Insufficiency. Hum Reprod (Oxford England) (2016) 31:926–37. doi: 10.1093/humrep/dew027
78. De Vos M, Devroey P, Fauser BC. Primary Ovarian Insufficiency. Lancet (2010) 376:911–21. doi: 10.1016/s0140-6736(10)60355-8
79. Huang B, Qian C, Ding C, Meng Q, Zou Q, Li H. Fetal Liver Mesenchymal Stem Cells Restore Ovarian Function in Premature Ovarian Insufficiency by Targeting MT1. Stem Cell Res Ther (2019) 10:362. doi: 10.1186/s13287-019-1490-8
80. Vabre P, Gatimel N, Moreau J, Gayrard V, Picard-Hagen N, Parinaud J, et al. Environmental Pollutants, a Possible Etiology for Premature Ovarian Insufficiency: A Narrative Review of Animal and Human Data. Environ Health (2017) 16:37. doi: 10.1186/s12940-017-0242-4
81. Ford EA, Beckett EL, Roman SD, McLaughlin EA, Sutherland JM. Advances in Human Primordial Follicle Activation and Premature Ovarian Insufficiency. Reprod (Cambridge England) (2020) 159:R15–29. doi: 10.1530/rep-19-0201
82. Huhtaniemi I, Hovatta O, La Marca A, Livera G, Monniaux D, Persani L, et al. Advances in the Molecular Pathophysiology, Genetics, and Treatment of Primary Ovarian Insufficiency. Trends Endocrinol Metab (2018) 29:400–19. doi: 10.1016/j.tem.2018.03.010
83. Wesevich V, Kellen AN, Pal L. Recent Advances in Understanding Primary Ovarian Insufficiency. F1000Res (2020) 9:F1000 Faculty Rev-1101. doi: 10.12688/f1000research.26423.1
84. Caburet S, Arboleda VA, Llano E, Overbeek PA, Barbero JL, Oka K, et al. Mutant Cohesin in Premature Ovarian Failure. N Engl J Med (2014) 370:943–9. doi: 10.1056/NEJMoa1309635
85. Bouilly J, Beau I, Barraud S, Bernard V, Azibi K, Fagart J, et al. Identification of Multiple Gene Mutations Accounts for a New Genetic Architecture of Primary Ovarian Insufficiency. J Clin Endocrinol Metab (2016) 101:4541–50. doi: 10.1210/jc.2016-2152
86. Jaillard S, Bell K, Akloul L, Walton K, McElreavy K, Stocker WA, et al. New Insights Into the Genetic Basis of Premature Ovarian Insufficiency: Novel Causative Variants and Candidate Genes Revealed by Genomic Sequencing. Maturitas (2020) 141:9–19. doi: 10.1016/j.maturitas.2020.06.004
87. Liu T, Liu Y, Huang Y, Chen J, Yu Z, Chen C, et al. miR-15b Induces Premature Ovarian Failure in Mice via Inhibition of α-Klotho Expression in Ovarian Granulosa Cells. Free Radic Biol Med (2019) 141:383–92. doi: 10.1016/j.freeradbiomed.2019.07.010
88. Zhang X, Dang Y, Liu R, Zhao S, Ma J, Qin Y. MicroRNA-127-5p Impairs Function of Granulosa Cells via HMGB2 Gene in Premature Ovarian Insufficiency. J Cell Physiol (2020) 235:8826–38. doi: 10.1002/jcp.29725
89. Wang X, Zhang X, Dang Y, Li D, Lu G, Chan WY, et al. Long Noncoding RNA HCP5 Participates in Premature Ovarian Insufficiency by Transcriptionally Regulating MSH5 and DNA Damage Repair Via YB1. Nucleic Acids Res (2020) 48:4480–91. doi: 10.1093/nar/gkaa127
90. Persani L, Rossetti R, Cacciatore C, Fabre S. Genetic Defects of Ovarian TGF-β-Like Factors and Premature Ovarian Failure. J Endocrinol Invest (2011) 34:244–51. doi: 10.1007/bf03347073
91. Grosbois J, Demeestere I. Dynamics of PI3K and Hippo Signaling Pathways During In Vitro Human Follicle Activation. Hum Reprod (Oxford England) (2018) 33:1705–14. doi: 10.1093/humrep/dey250
92. Liang QX, Wang ZB, Lin F, Zhang CH, Sun HM, Zhou L, et al. Ablation of Beta Subunit of Protein Kinase CK2 in Mouse Oocytes Causes Follicle Atresia and Premature Ovarian Failure. Cell Death Dis (2018) 9:508. doi: 10.1038/s41419-018-0505-1
93. Dragojević-Dikić S, Marisavljević D, Mitrović A, Dikić S, Jovanović T, Janković-Raznatović S. An Immunological Insight Into Premature Ovarian Failure (POF). Autoimmun Rev (2010) 9:771–4. doi: 10.1016/j.autrev.2010.06.008
94. Coulam CB, Stern JJ. Immunology of Ovarian Failure. Am J Reprod Immunol (New York NY 1989) (1991) 25:169–74. doi: 10.1111/j.1600-0897.1991.tb01089.x
95. Shen M, Jiang Y, Guan Z, Cao Y, Li L, Liu H, et al. Protective Mechanism of FSH Against Oxidative Damage in Mouse Ovarian Granulosa Cells by Repressing Autophagy. Autophagy (2017) 13:1364–85. doi: 10.1080/15548627.2017.1327941
96. Zhang S, Huang B, Su P, Chang Q, Li P, Song A, et al. Concentrated Exosomes From Menstrual Blood-Derived Stromal Cells Improves Ovarian Activity in a Rat Model of Premature Ovarian Insufficiency. Stem Cell Res Ther (2021) 12:178. doi: 10.1186/s13287-021-02255-3
97. Ding L, Yan G, Wang B, Xu L, Gu Y, Ru T, et al. Transplantation of UC-MSCs on Collagen Scaffold Activates Follicles in Dormant Ovaries of POF Patients With Long History of Infertility. Sci China Life Sci (2018) 61:1554–65. doi: 10.1007/s11427-017-9272-2
98. Azziz R, Carmina E, Chen Z, Dunaif A, Laven JSE, Legro RS, et al. Polycystic Ovary Syndrome. Nat Rev Dis Primers (2016) 2:16057. doi: 10.1038/nrdp.2016.57
99. Huang Y, Li J, Zhang F, Liu Y, Xu G, Guo J, et al. Factors Affecting the Live-Birth Rate in Women With Diminished Ovarian Reserve Undergoing IVF-ET. Arch Gynecol Obstet (2018) 298:1017–27. doi: 10.1007/s00404-018-4884-4
100. The Lancet Diabetes E. Empowering Women With PCOS. Lancet Diabetes Endocrinol (2019) 7:737. doi: 10.1016/S2213-8587(19)30289-X
101. Escobar-Morreale HF. Polycystic Ovary Syndrome: Definition, Aetiology, Diagnosis and Treatment. Nat Rev Endocrinol (2018) 14:270–84. doi: 10.1038/nrendo.2018.24
102. Liang X, Zhang L, Wang S, Han Q, Zhao RC. Exosomes Secreted by Mesenchymal Stem Cells Promote Endothelial Cell Angiogenesis by Transferring Mir-125a. J Cell Sci (2016) 129:2182–9. doi: 10.1242/jcs.170373
103. Rosenfield RL, Ehrmann DA. The Pathogenesis of Polycystic Ovary Syndrome (PCOS): The Hypothesis of PCOS as Functional Ovarian Hyperandrogenism Revisited. Endocrine Rev (2016) 37:467–520. doi: 10.1210/er.2015-1104
104. Balen AH, Morley LC, Misso M, Franks S, Legro RS, Wijeyaratne CN, et al. The Management of Anovulatory Infertility in Women With Polycystic Ovary Syndrome: An Analysis of the Evidence to Support the Development of Global WHO Guidance. Hum Reprod Update (2016) 22:687–708. doi: 10.1093/humupd/dmw025
105. Madkour A, Bouamoud N, Kaarouch I, Louanjli N, Saadani B, Assou S, et al. Follicular Fluid and Supernatant From Cultured Cumulus-Granulosa Cells Improve In Vitro Maturation in Patients With Polycystic Ovarian Syndrome. Fertil Steril (2018) 110:710–9. doi: 10.1016/j.fertnstert.2018.04.038
106. Han Y, Ren J, Bai Y, Pei X, Han Y. Exosomes From Hypoxia-Treated Human Adipose-Derived Mesenchymal Stem Cells Enhance Angiogenesis Through VEGF/VEGF-R. Int J Biochem Cell Biol (2019) 109:59–68. doi: 10.1016/j.biocel.2019.01.017
107. Gangadaran P, Rajendran RL, Lee HW, Kalimuthu S, Hong CM, Jeong SY, et al. Extracellular Vesicles From Mesenchymal Stem Cells Activates VEGF Receptors and Accelerates Recovery of Hindlimb Ischemia. J Control Release (2017) 264:112–26. doi: 10.1016/j.jconrel.2017.08.022
108. Wang H, Zheng R, Chen Q, Shao J, Yu J, Hu S. Mesenchymal Stem Cells Microvesicles Stabilize Endothelial Barrier Function Partly Mediated by Hepatocyte Growth Factor (HGF). Stem Cell Res Ther (2017) 8:211. doi: 10.1186/s13287-017-0662-7
109. Gonzalez-King H, García NA, Ontoria-Oviedo I, Ciria M, Montero JA, Sepúlveda P. Hypoxia Inducible Factor-1α Potentiates Jagged 1-Mediated Angiogenesis by Mesenchymal Stem Cell-Derived Exosomes. Stem Cells (Dayton, Ohio) (2017) 35:1747–59. doi: 10.1002/stem.2618
110. Lopatina T, Bruno S, Tetta C, Kalinina N, Porta M, Camussi G. Platelet-Derived Growth Factor Regulates the Secretion of Extracellular Vesicles by Adipose Mesenchymal Stem Cells and Enhances Their Angiogenic Potential. Cell Commun Signal (2014) 12:26. doi: 10.1186/1478-811X-12-26
111. Anderson JD, Johansson HJ, Graham CS, Vesterlund M, Pham MT, Bramlett CS, et al. Comprehensive Proteomic Analysis of Mesenchymal Stem Cell Exosomes Reveals Modulation of Angiogenesis via Nuclear Factor-KappaB Signaling. Stem Cells (2016) 34:601–13. doi: 10.1002/stem.2298
112. Zhang B, Wu X, Zhang X, Sun Y, Yan Y, Shi H, et al. Human Umbilical Cord Mesenchymal Stem Cell Exosomes Enhance Angiogenesis Through the Wnt4/β-Catenin Pathway. Stem Cells Transl Med (2015) 4:513–22. doi: 10.5966/sctm.2014-0267
113. Eirin A, Zhu X-Y, Puranik AS, Woollard JR, Tang H, Dasari S. Comparative Proteomic Analysis of Extracellular Vesicles Isolated From Porcine Adipose Tissue-Derived Mesenchymal Stem/Stromal Cells. Scientific Reports (2016) 6:36120. doi: 10.1038/srep36120
114. Vrijsen KR, Maring JA, Chamuleau SA, Verhage V, Mol EA, Deddens JC, et al. Exosomes From Cardiomyocyte Progenitor Cells and Mesenchymal Stem Cells Stimulate Angiogenesis Via Emmprin. Adv Healthc Mater (2016) 5:2555–65. doi: 10.1002/adhm.201600308
115. Merino-González C, Zuñiga FA, Escudero C, Ormazabal V, Reyes C, Nova-Lamperti E, et al. Mesenchymal Stem Cell-Derived Extracellular Vesicles Promote Angiogenesis: Potencial Clinical Application. Front Physiol (2016) 7:24. doi: 10.3389/fphys.2016.00024
116. McBride JD, Rodriguez-Menocal L, Guzman W, Candanedo A, Garcia-Contreras M, Badiavas EV. Bone Marrow Mesenchymal Stem Cell-Derived CD63(+) Exosomes Transport Wnt3a Exteriorly and Enhance Dermal Fibroblast Proliferation, Migration, and Angiogenesis In Vitro. Stem Cells Dev (2017) 26:1384–98. doi: 10.1089/scd.2017.0087
117. Shabbir A, Cox A, Rodriguez-Menocal L, Salgado M, Van Badiavas E. Mesenchymal Stem Cell Exosomes Induce Proliferation and Migration of Normal and Chronic Wound Fibroblasts, and Enhance Angiogenesis In Vitro. Stem Cells Dev (2015) 24:1635–47. doi: 10.1089/scd.2014.0316
118. Gong M, Yu B, Wang J, Wang Y, Liu M, Paul C, et al. Mesenchymal Stem Cells Release Exosomes That Transfer miRNAs to Endothelial Cells and Promote Angiogenesis. Oncotarget (2017) 8:45200–12. doi: 10.18632/oncotarget.16778
119. Wang N, Chen C, Yang D, Liao Q, Luo H, Wang X, et al. Mesenchymal Stem Cells-Derived Extracellular Vesicles, via miR-210, Improve Infarcted Cardiac Function by Promotion of Angiogenesis. Biochim Biophys Acta Mol Basis Dis (2017) 1863:2085–92. doi: 10.1016/j.bbadis.2017.02.023
120. Liu L, Liu Y, Feng C, Chang J, Fu R, Wu T, et al. Lithium-Containing Biomaterials Stimulate Bone Marrow Stromal Cell-Derived Exosomal miR-130a Secretion to Promote Angiogenesis. Biomaterials (2019) 192:523–36. doi: 10.1016/j.biomaterials.2018.11.007
121. Tsuji K, Kitamura S, Wada J. Immunomodulatory and Regenerative Effects of Mesenchymal Stem Cell-Derived Extracellular Vesicles in Renal Diseases. Int J Mol Sci (2020) 21:756. doi: 10.3390/ijms21030756
122. Crain SK, Robinson SR, Thane KE, Davis AM, Meola DM, Barton BA, et al. Extracellular Vesicles From Wharton’s Jelly Mesenchymal Stem Cells Suppress CD4 Expressing T Cells Through Transforming Growth Factor Beta and Adenosine Signaling in a Canine Model. Stem Cells Dev (2019) 28:212–26. doi: 10.1089/scd.2018.0097
123. Álvarez V, Sánchez-Margallo FM, Macías-García B, Gómez-Serrano M, Jorge I, Vázquez J, et al. The Immunomodulatory Activity of Extracellular Vesicles Derived From Endometrial Mesenchymal Stem Cells on CD4+ T Cells is Partially Mediated by TGFbeta. J Tissue Eng Regener Med (2018) 12:2088–98. doi: 10.1002/term.2743
124. Ti D, Hao H, Tong C, Liu J, Dong L, Zheng J, et al. LPS-Preconditioned Mesenchymal Stromal Cells Modify Macrophage Polarization for Resolution of Chronic Inflammation via Exosome-Shuttled Let-7b. J Transl Med (2015) 13:308. doi: 10.1186/s12967-015-0642-6
125. Harrell CR, Jovicic N, Djonov V, Arsenijevic N, Volarevic V. Mesenchymal Stem Cell-Derived Exosomes and Other Extracellular Vesicles as New Remedies in the Therapy of Inflammatory Diseases. Cells (2019) 8:1605. doi: 10.3390/cells8121605
126. Mardpour S, Hamidieh AA, Taleahmad S, Sharifzad F, Taghikhani A, Baharvand H. Interaction Between Mesenchymal Stromal Cell-Derived Extracellular Vesicles and Immune Cells by Distinct Protein Content. J Cell Physiol (2019) 234:8249–58. doi: 10.1002/jcp.27669
127. Spinosa M, Lu G, Su G, Bontha SV, Gehrau R, Salmon MD. Human Mesenchymal Stromal Cell-Derived Extracellular Vesicles Attenuate Aortic Aneurysm Formation and Macrophage Activation Via microRNA-147. FASEB J (2018) fj201701138RR. doi: 10.1096/fj.201701138RR
128. Zhao J, Li X, Hu J, Chen F, Qiao S, Sun X. Mesenchymal Stromal Cell-Derived Exosomes Attenuate Myocardial Ischaemia-Reperfusion Injury Through miR-182-Regulated Macrophage Polarization. Cardiovas Res (2016) 115:1205–16. doi: 10.1093/cvr/cvz040
129. Lo Sicco C, Reverberi D, Balbi C, Ulivi V, Principi E, Pascucci L, et al. Mesenchymal Stem Cell-Derived Extracellular Vesicles as Mediators of Anti-Inflammatory Effects: Endorsement of Macrophage Polarization. Stem Cells Transl Med (2017) 6:1018–28. doi: 10.1002/sctm.16-0363
130. Liu W, Rong Y, Wang J, Zhou Z, Ge X, Ji C. Exosome-Shuttled miR-216a-5p From Hypoxic Preconditioned Mesenchymal Stem Cells Repair Traumatic Spinal Cord Injury by Shifting Microglial M1/M2 Polarization. J Neuroinflamm (2020) 17:47. doi: 10.1186/s12974-020-1726-7
131. Chaubey S, Thueson S, Ponnalagu D, Alam MA, Gheorghe CP, Aghai Z, et al. Early Gestational Mesenchymal Stem Cell Secretome Attenuates Experimental Bronchopulmonary Dysplasia in Part via Exosome-Associated Factor TSG-6. Stem Cell Res Ther (2018) 9:173. doi: 10.1186/s13287-018-0903-4
132. Baek G, Choi H, Kim Y, Lee HC, Choi C. Mesenchymal Stem Cell-Derived Extracellular Vesicles as Therapeutics and as a Drug Delivery Platform. Stem Cells Transl Med (2019) 8:880–6. doi: 10.1002/sctm.18-0226
133. Park KS, Svennerholm K, Shelke GV, Bandeira E, Lässer C, Jang SC, et al. Mesenchymal Stromal Cell-Derived Nanovesicles Ameliorate Bacterial Outer Membrane Vesicle-Induced Sepsis via IL-10. Stem Cell Res Ther (2019) 10:231. doi: 10.1186/s13287-019-1352-4
134. Yang C, Lim W, Park J, Park S, You S, Song G. Anti-Inflammatory Effects of Mesenchymal Stem Cell-Derived Exosomal microRNA-146a-5p and microRNA-548e-5p on Human Trophoblast Cells. Mol Hum Reprod (2019) 25:755–71. doi: 10.1093/molehr/gaz054
135. Yang W, Zhang J, Xu B, He Y, Liu W, Li J, et al. Hucmsc-Derived Exosomes Mitigate the Age-Related Retardation of Fertility in Female Mice. Mol Ther (2020) 28:1200–13. doi: 10.1016/j.ymthe.2020.02.003
136. Bodart-Santos V, de Carvalho LRP, de Godoy MA, Batista AF, Saraiva LM, Lima LG, et al. Extracellular Vesicles Derived From Human Wharton’s Jelly Mesenchymal Stem Cells Protect Hippocampal Neurons From Oxidative Stress and Synapse Damage Induced by Amyloid-β Oligomers. Stem Cell Res Ther (2019) 10:332. doi: 10.1186/s13287-019-1432-5
137. Zhang B, Wang M, Gong A, Zhang X, Wu X, Zhu Y, et al. Hucmsc-Exosome Mediated-Wnt4 Signaling Is Required for Cutaneous Wound Healing. Stem Cells (2015) 33:2158–68. doi: 10.1002/stem.1771
138. Pizzicannella J, Gugliandolo A, Orsini T, Fontana A, Ventrella A, Mazzon E, et al. Engineered Extracellular Vesicles From Human Periodontal-Ligament Stem Cells Increase VEGF/VEGFR2 Expression During Bone Regeneration. Front Physiol (2019) 10:512. doi: 10.3389/fphys.2019.00512
139. Xiong Z-H, Wei J, Lu M-Q, Jin M-Y, Geng H-L. Protective Effect of Human Umbilical Cord Mesenchymal Stem Cell Exosomes on Preserving the Morphology and Angiogenesis of Placenta in Rats With Preeclampsia. Biomed Pharmacother = Biomed Pharmacother (2018) 105:1240–7. doi: 10.1016/j.biopha.2018.06.032
140. Liu X, Li Q, Niu X, Hu B, Chen S, Song W, et al. Exosomes Secreted From Human-Induced Pluripotent Stem Cell-Derived Mesenchymal Stem Cells Prevent Osteonecrosis of the Femoral Head by Promoting Angiogenesis. Int J Biol Sci (2017) 13:232–44. doi: 10.7150/ijbs.16951
141. Hellström M, Phng L-K, Hofmann JJ, Wallgard E, Coultas L, Lindblom P, et al. Dll4 Signalling Through Notch1 Regulates Formation of Tip Cells During Angiogenesis. Nature (2007) 445:776–80. doi: 10.1038/nature05571
142. Zhang Q, Xu M, Yao X, Li T, Wang Q, Lai D. Human Amniotic Epithelial Cells Inhibit Granulosa Cell Apoptosis Induced by Chemotherapy and Restore the Fertility. Stem Cell Res Ther (2015) 6:152. doi: 10.1186/s13287-015-0148-4
143. Tooi M, Komaki M, Morioka C, Honda I, Iwasaki K, Yokoyama N, et al. Placenta Mesenchymal Stem Cell Derived Exosomes Confer Plasticity on Fibroblasts. J Cell Biochem (2016) 117:1658–70. doi: 10.1002/jcb.25459
144. Ma D, Xu K, Zhang G, Liu Y, Gao J, Tian M, et al. Immunomodulatory Effect of Human Umbilical Cord Mesenchymal Stem Cells on T Lymphocytes in Rheumatoid Arthritis. Int Immunopharmacol (2019) 74:105687. doi: 10.1016/j.intimp.2019.105687
145. Nojehdehi S, Soudi S, Hesampour A, Rasouli S, Soleimani M, Hashemi SM. Immunomodulatory Effects of Mesenchymal Stem Cell-Derived Exosomes on Experimental Type-1 Autoimmune Diabetes. J Cell Biochem (2018) 119:9433–43. doi: 10.1002/jcb.27260
146. Wang J, Xia J, Huang R, Hu Y, Fan J, Shu Q, et al. Mesenchymal Stem Cell-Derived Extracellular Vesicles Alter Disease Outcomes via Endorsement of Macrophage Polarization. Stem Cell Res Ther (2020) 11:424. doi: 10.1186/s13287-020-01937-8
147. Del Fattore A, Luciano R, Pascucci L, Goffredo BM, Giorda E, Scapaticci M, et al. Immunoregulatory Effects of Mesenchymal Stem Cell-Derived Extracellular Vesicles on T Lymphocytes. Cell Transplant (2015) 24:2615–27. doi: 10.3727/096368915x687543
148. Monsel A, Zhu Y-G, Gennai S, Hao Q, Hu S, Rouby J-J, et al. Therapeutic Effects of Human Mesenchymal Stem Cell-Derived Microvesicles in Severe Pneumonia in Mice. Am J Respir Crit Care Med (2015) 192:324–36. doi: 10.1164/rccm.201410-1765OC
149. Pizzino G, Irrera N, Cucinotta M, Pallio G, Mannino F, Arcoraci V, et al. Oxidative Stress: Harms and Benefits for Human Health. Oxid Med Cell Longevity (2017) 2017:8416763. doi: 10.1155/2017/8416763
150. Yang Y, Lei L, Wang S, Sheng X, Yan G, Xu L, et al. Transplantation of Umbilical Cord-Derived Mesenchymal Stem Cells on a Collagen Scaffold Improves Ovarian Function in a Premature Ovarian Failure Model of Mice. In Vitro Cell Dev Biol Anim (2019) 55:302–11. doi: 10.1007/s11626-019-00337-4
151. Shi Z, Wang Q, Zhang Y, Jiang D. Extracellular Vesicles Produced by Bone Marrow Mesenchymal Stem Cells Attenuate Renal Fibrosis, in Part by Inhibiting the RhoA/ROCK Pathway, in a UUO Rat Model. Stem Cell Res Ther (2020) 11:253. doi: 10.1186/s13287-020-01767-8
152. Xia C, Zeng Z, Fang B, Tao M, Gu C, Zheng L, et al. Mesenchymal Stem Cell-Derived Exosomes Ameliorate Intervertebral Disc Degeneration Via Anti-Oxidant and Anti-Inflammatory Effects. Free Radic Biol Med (2019) 143:1–15. doi: 10.1016/j.freeradbiomed.2019.07.026
153. Deng T, He J, Yao Q, Wu L, Xue L, Wu M, et al. Human Umbilical Cord Mesenchymal Stem Cells Improve Ovarian Function in Chemotherapy-Induced Premature Ovarian Failure Mice Through Inhibiting Apoptosis and Inflammation Via a Paracrine Mechanism. Reprod Sci (2021) 28:1718–32. doi: 10.1007/s43032-021-00499-1
154. Das M, Djahanbakhch O, Hacihanefioglu B, Saridogan E, Ikram M, Ghali L, et al. Granulosa Cell Survival and Proliferation Are Altered in P olycystic Ovary Syndrome. J Clin Endocrinol Metab (2008) 93:881–7. doi: 10.1210/jc.2007-1650
155. Le Saux S, Aarrass H, Lai-Kee-Him J, Bron P, Armengaud J, Miotello G, et al. Post-Production Modifications of Murine Mesenchymal Stem Cell (mMSC) Derived Extracellular Vesicles (EVs) and Impact on Their Cellular Interaction. Biomaterials (2020) 231:119675. doi: 10.1016/j.biomaterials.2019.119675
Keywords: mesenchymal stem cells, extracellular vesicle, exosome, reproduction, infertility
Citation: Liao Z, Liu C, Wang L, Sui C and Zhang H (2021) Therapeutic Role of Mesenchymal Stem Cell-Derived Extracellular Vesicles in Female Reproductive Diseases. Front. Endocrinol. 12:665645. doi: 10.3389/fendo.2021.665645
Received: 08 February 2021; Accepted: 27 May 2021;
Published: 23 June 2021.
Edited by:
Richard Ivell, University of Nottingham, United KingdomReviewed by:
Kajal Khodamoradi, University of Miami, United StatesHuan Shen, Peking University, China
Copyright © 2021 Liao, Liu, Wang, Sui and Zhang. This is an open-access article distributed under the terms of the Creative Commons Attribution License (CC BY). The use, distribution or reproduction in other forums is permitted, provided the original author(s) and the copyright owner(s) are credited and that the original publication in this journal is cited, in accordance with accepted academic practice. No use, distribution or reproduction is permitted which does not comply with these terms.
*Correspondence: Chang Liu, bGljaGk2MDhAMTYzLmNvbQ==; Hanwang Zhang, aHd6aGFuZzYwNUAxMjYuY29t; Cong Sui, Y3N1aTA5MDRAMTYzLmNvbQ==