- 1Circadian and Glial Biology Lab, Physiology Department, Molecular Medicine and Chronic Diseases Research Centre (CiMUS), University of Santiago de Compostela, Santiago de Compostela, Spain
- 2NeurObesity Lab, Physiology Department, Molecular Medicine and Chronic Diseases Research Centre (CiMUS), University of Santiago de Compostela, Santiago de Compostela, Spain
The endogenous timekeeping system evolved to anticipate the time of the day through the 24 hours cycle of the Earth’s rotation. In mammals, the circadian clock governs rhythmic physiological and behavioral processes, including the daily oscillation in glucose metabolism, food intake, energy expenditure, and whole-body insulin sensitivity. The results from a series of studies have demonstrated that environmental or genetic alterations of the circadian cycle in humans and rodents are strongly associated with metabolic diseases such as obesity and type 2 diabetes. Emerging evidence suggests that astrocyte clocks have a crucial role in regulating molecular, physiological, and behavioral circadian rhythms such as glucose metabolism and insulin sensitivity. Given the concurrent high prevalence of type 2 diabetes and circadian disruption, understanding the mechanisms underlying glucose homeostasis regulation by the circadian clock and its dysregulation may improve glycemic control. In this review, we summarize the current knowledge on the tight interconnection between the timekeeping system, glucose homeostasis, and insulin sensitivity. We focus specifically on the involvement of astrocyte clocks, at the organism, cellular, and molecular levels, in the regulation of glucose metabolism.
Functional Hierarchy Of the Timekeeping System
The circadian (in Latin “circa”, around; “diem”, day) clock is an endogenous and self‐sustaining oscillator that operates with a periodicity of 24 hours (h) to maintain proper rhythms of the vast majority of physiological and behavioral processes, including food intake, energy balance, sleep-wake cycles and many others (1). In mammals, the timekeeping system comprises a pacemaker located in the hypothalamic suprachiasmatic nucleus (SCN) (2, 3), as well as non-SCN brain and peripheral clocks and cell-autonomous oscillators within virtually every cell type of the body (4, 5). In the absence of any time cue from the environment, these clocks free run with a period close to 24 h. To compensate discrepancies between this intrinsic period and the environmental cycle, circadian clocks entrain to external Zeitgebers (ZT, in German “time giver”). The light entrains the SCN to local time which in turn, conveys the temporal information to other clocks in the brain and peripheral tissues via neuronal, hormonal, or behavioral activity rhythms, such as the feeding-fasting and sleep-wake cycles, which serve as entrainment signals for extra-SCN clocks (6, 7) (Figure 1).
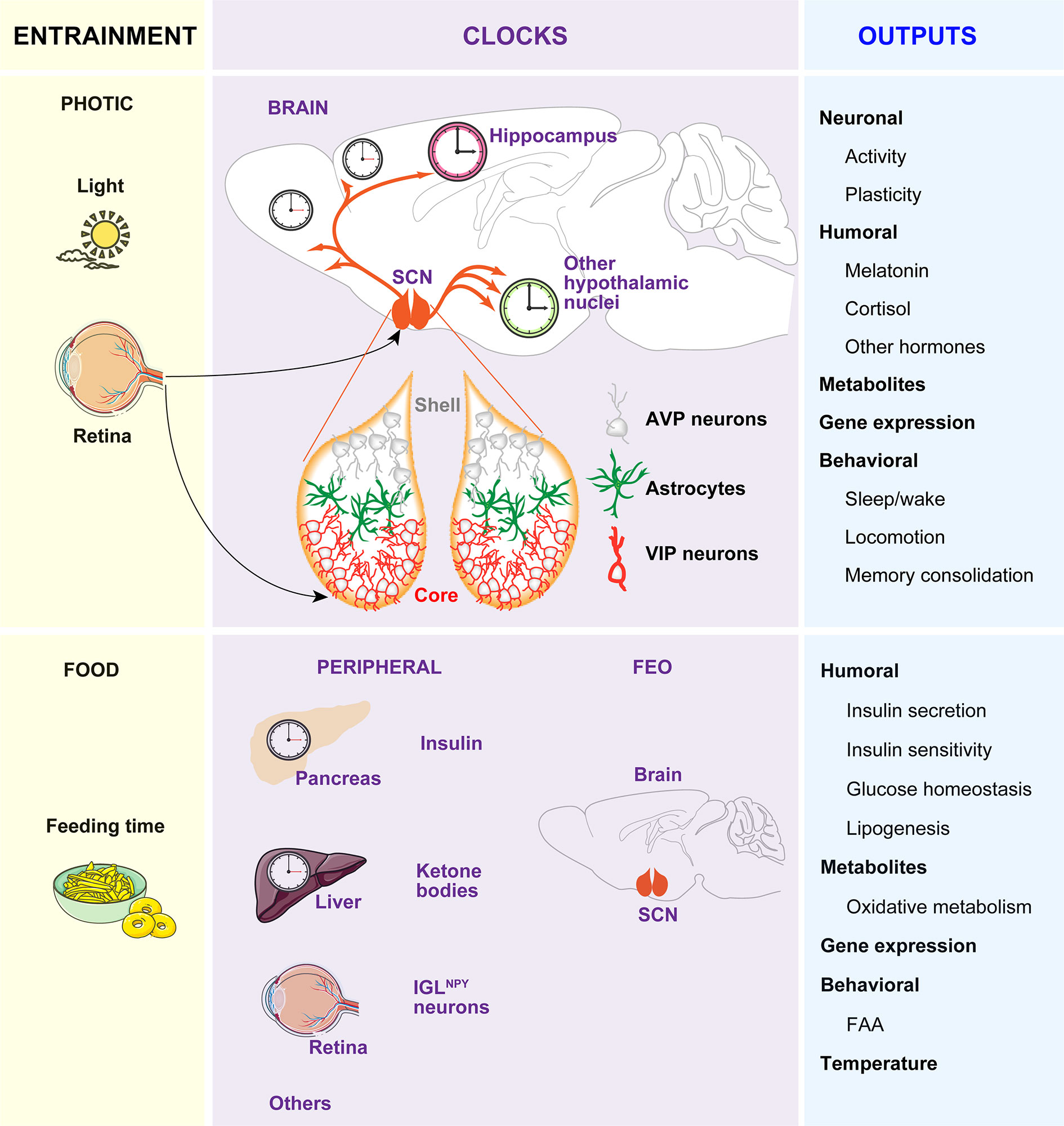
Figure 1 The circadian timing system. The timekeeping system is composed of two pacemakers (the SCN and the FEO), and peripheral clocks in other brain areas and peripheral tissues. Light inputs reaching the SCN via the retina and the retinohypothalamic tract, are the most important Zeitgeber for the SCN, which in turn synchronizes peripheral clocks through neural, endocrine, temperature, and behavioral signals. Feeding-related signals (INS and ketones bodies) generated by peripheral tissues and IGLNPY neurons entrain the FEO, which regulate the outputs such as FAA.
While a substantial amount of information is known about the neuroanatomy of the SCN, the mechanisms of light-affected entrainment and the transmission of time cues to other brain areas or peripheral clocks are not yet fully understood. Briefly, the SCN is a heterogeneous and complex bilateral nucleus (8, 9) comprising approximately 10,000 self-oscillating neurons on each side, in mice (10–12). Each part is divided into two functional subgroups, one in the ventrolateral region and the other in the dorsal SCN. The ventrolateral region or core receives direct photic inputs from the intrinsically photosensitive retinal ganglion cells (ipRGCs) (13–15). Activation of the retino hypothalamic tract by light increases the firing of vasoactive intestinal polypeptide (VIP)-expressing SCN cells and VIP release. VIP neurons set and phase-shift the circadian time by VIPergic and γ-aminobutyric acid (GABA)ergic signaling to arginine vasopressin (AVP)-expressing neurons within the second subgroup of cells located in the dorsal SCN or shell (Figure 1). Ultimately, this results in the induction of the so-called clock genes Period1 (Per1) and Period2 (Per2) (16) and subsequent time-of-day dependent phase responses of the SCN, thereby enabling entrainment to the light-dark (LD) cycle.
If food availability is restricted to a particular time of day or night (often referred to as time-restricted feeding, TRF), animals exhibit increased activity in anticipation of feeding (termed food anticipatory activity, FAA). Moreover, in this paradigm, the peripheral clocks shift their phase to preserve alignment with mealtime. The effects of TRF on peripheral clocks and/or behavior persist even when the feeding is out of phase with the LD cycle, and, indeed, can be uncoupled from the SCN, which remains synchronized to the light (17–19). Remarkably, in the absence of a functional SCN, FAA is preserved and food intake becomes an effective ZT capable of coordinating circadian rhythms of behavior, peripheral clock gene expression, and clock outputs, such as hormone secretion (20, 21). Thereby, the entrainment to TRF has been proposed to be independent of the SCN and driven by a food-entrainable oscillator (FEO) of still unknown location. A recent study reported that innervation of ipRGC to neuropeptide Y (NPY)-expressing intergeniculate leaflet (IGL) neurons in early postnatal stages, allow the entrainment to TRF in adults. In this model, TRF inhibitory signals from IGLNPY neurons modulate the SCN activity, allowing the FEO signals to influence FAA (22).
As hypothalamic lesions and gene knockouts (KOs) targeting specific cell types in the hypothalamus, brainstem, or forebrain areas also impair FAA (23–29), it is reasonable to hypothesize that the FEO might be functionally distributed among brain areas that are competent to drive changes in behavior instead to be restricted to a particular brain region. In line with this idea, it was recently reported that the action of insulin (INS) and insulin-like growth factor-1 (IGF-1) triggered after feeding, on different brain regions, are necessary and sufficient for both the FAA and the phase-shift of body clocks (7). Remarkably, an elegant study showed that the signal that generates FAA might be synthesized in the liver. Specifically, it was shown that liver PER2 is required for hepatic-derived ketone bodies production which in turn signals the brain to induce FAA (30). In sum, the FEO may not be in a single tissue but it might be of systemic nature. In this context, the feeding-related signals, such as INS and ketones generated in peripheral tissues, entrain different brain regions competent to drive changes in behavior. Among these regions is included the SCN, which is tuned by innervation of ipRGCs to IGL during development to allow non-photic entrainment to food (22) (Figure 1).
Astrocyte Circadian Clocks
How the activity of a small number of SCN neurons is translated into rhythmic behaviors or physiology at the organism level? The human brain contains more than 100 billion cells, the majority being glial cells, coordinated by this endogenous clock to determine alertness waxes and wanes in a highly predictable manner over the course of a 24 h day (31). However, how this clock signaling is orchestrated within so many brain cells that lead to the cycle-to-cycle precision of circadian rhythmicity is unknown. Consequently, we face a lack of knowledge on the mechanisms by which circadian dysfunction affects a wide range of physiological processes such as metabolic imbalance, premature aging, and reduced longevity (32–38).
Astrocytes have long lived in the shadow of the neurons as they were thought to have mainly a structural role in the central nervous system. The recent findings showing the critical role of the astrocyte clock in the control of SCN function and circadian behavior (39–43) is a game-changing discovery that offers radically new research directions for therapy of brain diseases originated by environmental miss functioning of circadian rhythms or genetic factors affecting clock genes or outputs. This glial cell type is highly diverse in its morphological appearance, functional properties, and distribution among and within different brain regions (44, 45). However, they share three anatomical features that are crucial to understand its functional contributions to the timekeeping system.
Firstly, the longstanding concept that astrocytic processes interdigitate to create a scaffold for the neuronal organization, has been challenged by several studies showing that, in vivo, astrocytes are organized in nonoverlapping domains, i.e, with little interaction between adjacent cells (46–48). Thus, one astrocyte can coordinate the activity of multiple sets of contiguous synapses, via regulation of neurotransmitters levels in the synaptic cleft, via control of the extracellular space, or by releasing chemical signals that actively modulate synaptic transmission, often referred to as gliotransmitters. Specifically, astrocytes play an essential role in the coupling of SCN neurons by controlling both glutamate and GABA levels (40, 42, 43, 49–51). Moreover, SCN astrocytes undergo rhythmic structural rearrangements (52), along with rhythms in GFAP expression (53), which allows differential day/night coverage of VIP neurons to facilitate entrainment to light (52, 54). Similarly, in response to metabolic cues, astrocytes undergo structural and morphological changes to influence the synaptic inputs within the hypothalamic melanocortin system, which might ultimately affect the feeding behavior (55–58). Astrocytes also regulate the extracellular space (59–62), enabling the exchange of solutes between the cerebrospinal fluid and the interstitial space, a system referred to as glymphatic clearance. As the diffusion of SCN output signals is sufficient for rhythmic behavior (63, 64), daily changes in the glymphatic system may underly the synchronization among different brain regions across the circadian cycle. On the other hand, in Drosophila, a glial-released factor was shown to be critical for normal rhythmicity by regulating a neurotransmitter, pigment dispersing factor, acting on a receptor similar to that for VIP in mammals (65–67). Similarly, in rodents, astrocytes release gliotransmitters, such as ATP, in a circadian manner (68), and arrhythmic astrocytes alter VIP expression in vivo (40). However, whether ATP release and/or astrocytic rhythmic metabolism impact the activity patterns of VIP neurons is still unknown.
Secondly, astrocytes form a syncytium, via gap junctions, that allow the propagation of small signaling molecules through the glial network (69). Pharmacological inhibition of gap junctions in SCN slides (70, 71) and mouse models with deletion of the neuronal connexin-36, impairs the circadian pattern of neuronal activity without affecting the long-term synchronization of clock gene expression (72) and with mild effect on behavioral rhythms (73). Similarly, studies in mouse models with deletion of astrocytic specific connexins indicate that the astrocytic coupling in the SCN is dispensable for circadian rhythm generation and light-entrainment (74). However, recently, a long-range function of astrocytes for the transmission of timing cues to distant neural populations was investigated in vitro with microfluidic devices that allowed compartmentalizing distinct neuronal populations connected through a network of astrocytes. In this paradigm, astrocytes were able to synchronize the clock of segregated cortical neuronal populations if intercellular communication between the glial network and/or calcium signaling were intact (75). Whether astrocytes are involved in the spatial transmission of timing cues in other extra-SCN brain regions in vivo is still unknown.
Thirdly, the exchange of metabolites and hormones through the blood-brain barrier (BBB) relies on astrocytes and is dependent both on the sleep/wake state and in the circadian clock (59, 76–80). As hypothalamic astrocytes have a crucial role in sensing nutrients such as glucose and fatty acids (56, 81–83) and express receptors for leptin (84, 85), IGF-1 (86), thyroid hormone (87), INS (57), and glucocorticoids (GCs) (88, 89) among others, they could link or coordinate peripheral and central oscillators. For example, astrocytes, as well‐known targets of GCs, might be sensitive to the negative feedback loop of the hypothalamus‐pituitary‐adrenal axis. It is widely accepted that GC signaling can reset peripheral clocks but not the central pacemaker because SCN neurons do not express the GC receptor (90). However, astrocytic feedback loops, via GC signaling, could explain the so far puzzling results showing that the Per1‐Luc phases of SCN were affected significantly when adrenalectomized animals were treated with hydrocortisone (6).
Molecular Dynamics of the Clock
The Nobel Prize in Physiology or Medicine in 2017 was awarded to three Chronobiologists who first cloned the Droshopila Period gene in 1984 (91, 92). This finding allowed us to understand how the timekeeping system anticipates the environmental changes related to the Earth’s rotation in most, if not all, living organisms.
The molecular clock involves rhythmic and self‐sustained transcriptional–translational feedback loops (TTFLs) of clock genes/proteins (Figure 2). The E‐box specific transcription factors BMAL1 (Brain and muscle Arnt‐like protein‐1) and CLOCK (Circadian locomotor output cycles kaput) are the positive limb of the TTFL, which heterodimerize to activate transcription of the repressors Per1/2/3 and Cryptochrome genes (Cry1/2) (93, 94). The negative loop comprises PER/CRY heterocomplex that, upon accumulation, lead to the degradation of BMAL1/CLOCK dimers, thus inhibiting their own transcription (95). Hence, a new cycle of PER and CRY protein accumulation begins, generating rhythmic changes in the levels of the core clock transcripts and proteins that persist for approximately 24 h (96) (Figure 2). In a secondary feedback loop, the CLOCK- BMAL1 complex controls the rhythmic expression of the genes encoding the REV-ERB nuclear hormone receptors and ROR (97). In turn, REV-ERB and ROR compete for the same RORE elements within the Clock and Bmal1 promoter, repressing or activating, respectively, Clock and Bmal1 transcription (Figure 2).
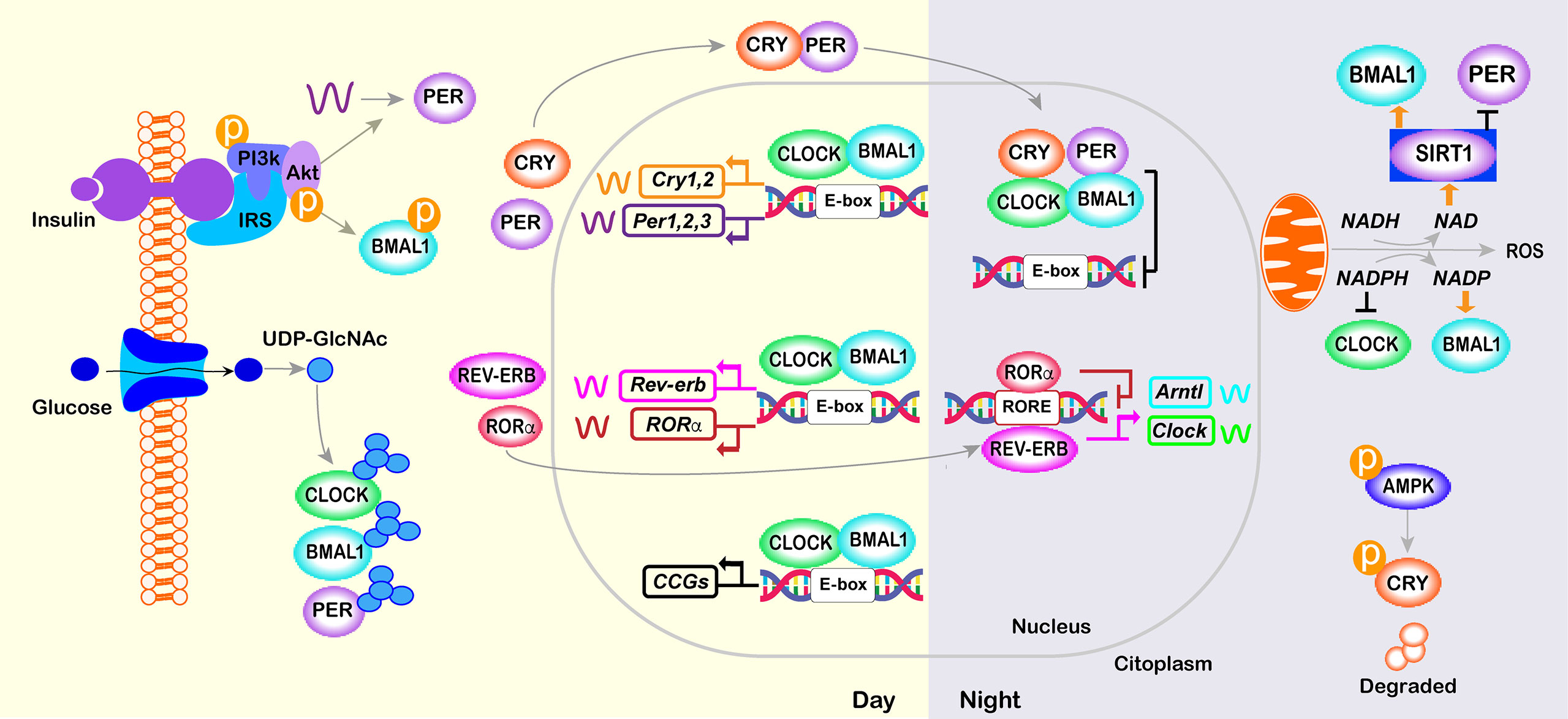
Figure 2 Core molecular clock network. The mammalian molecular clock consists of a transcriptional-translational feedback loop involving the clock proteins CLOCK, ARNTL, PER, and CRY and the nuclear receptors REV-ERB and ROR. The positive limb (CLOCK and BMAL1) heterodimerizes and activates the transcription of downstream genes, including Per, Cry, Rorα and Rev-erb. The negative limb proteins (PERs and CRYs) multimerize and inhibit CLOCK/BMAL1. In a secondary loop, Bmal1 and Clock are regulated by the repressor REV-ERB and its opposing nuclear receptor RORα, which bind competitively to the shared element RORE, thus repressing or activating the transcription of the Bmal1 or Clock gene, respectively. Post-translational modifications of core-clock factors can also regulate transcription (e.g., deacetylation of BMAL1 or PER2 by SIRT1, BMAL1 phosphorylation or PER2 translation by INS signaling; O- GlcNAcylation of CLOCK, BMAL1 and PER2; and CRY1 phosphorylation by AMPK).
Direct targets of CLOCK/BMAL1, referred to as clock-controlled genes (CCGs), include genes that are critically involved in rhythmic processes such as feeding behavior, sleep-wake cycle, and glucose homeostasis (34, 98) (Figure 2). In turn, metabolic state sensing pathways also alter the molecular clock in anticipation of the LD cycle. Specifically, during feeding, anabolic processes are triggered by the activation of the INS-AKT-mTOR pathway, whereas during fasting, AMP-activated protein kinase (AMPK) activation triggers catabolic processes and inhibits mTOR activity (99). BMAL1 phosphorylation and PER2 translation are regulated by the INS-AKT-mTOR pathway that is activated in the postprandial state (7, 100–102). Similarly, in peripheral tissues, AMPK1, which senses cellular ATP levels, modulate CRY1 phosphorylation and thus its rhythmic degradation (103). Additionally, high levels of glucose control the period length via O- GlcNAcylation of CLOCK, BMAL1, and PER2 (104–106). The molecular clocks are also sensitive to the ratio of reduced to oxidized nicotinamide adenine dinucleotide (NAD) and flavin adenine dinucleotide (FAD), which are indirect sensors of cellular energy status. Oxidation of NAD is under control of the clock and, in turn, prevents the deacetylation and this the transcriptional activity of CLOCK-BMAL1 complex by Sirtuin 1 (SIRT1) and poly-ADP-ribosylation mediated by poly(ADP-ribose) polymerase 1 (107–110) (Figure 2).
In summary, the circadian system ensures a temporal partitioning of catabolic and anabolic reactions synchronizing organism metabolism to the feeding-fasting cycle. However, as the connection of metabolism and the circadian clock works in both directions (111) is not surprising that animal models of genetic clock defects display metabolic alterations and that clock alterations can be found in metabolically challenged conditions (112).
Coordination of Glucose Homeostasis by Central and Peripheral Clocks
Glucose homeostasis is optimal when fasting-feeding and rest-activity cycles, hormonal rhythms, and central and peripheral clocks oscillate in synchrony with each other to ensure that the timing cues and tissue responsiveness are achieved at the right time. During the active phase, metabolic tissues such as the liver, muscle, and fat are very sensitive to INS to guarantee that glucose uptake is properly achieved after food intake. Conversely, these tissues are more resistant to the hormone during the fasting phase, to facilitate the endogenous glucose production and free fatty acid (FFA) secretion (113–115). In this section, we discuss the clocks in the tissues and organs involved in the control of glucose homeostasis and describe their role in the regulation of INS sensitivity and secretion.
The hypothalamus integrates glucose-sensing mechanisms with multiple effector pathways to precisely coordinate hepatic glucose production, muscle and fat glucose uptake, and endocrine pancreas function (Figure 3). Briefly, in the arcuate nucleus of the hypothalamus (ARC), orexigenic agouti-related peptide (AgRP)-producing neurons, and anorexigenic neurons releasing the pro-opiomelanocortin (POMC)-derived peptide, α-melanocyte-stimulating hormone, together with the neurons expressing the melanocortin 4 receptor (MC4R), are essential for glucose sensing (117). AgRP neurons are glucose-inhibited cells whereas POMC neurons are glucose-excited (118). In general terms, competitive binding of α-MSH and AgRP to MC4Rs defines the activation magnitude of downstream pathways and effectors. Furthermore, POMC and AgRP neurons project to numerous extrahypothalamic and hypothalamic regions, including the ventromedial nucleus (VMH) (119), which is crucial to initiate the glucose counter-regulatory response to hypoglycemia (120) (Figure 3). On the other hand, hypothalamic astrocytes respond to hyperglycemia by retraction of the coverage around POMC neurons to modify meal patterns (56). Consistently, astrocytes sense INS and leptin to co‐regulate behavioral responses and metabolic processes via the control of brain glucose uptake and the glial ensheathment of POMC neurons, respectively (57, 58). Moreover, deletion of leptin receptors in astrocytes reduces the physiological anorexigenic response to this hormone and enhances fasting or ghrelin-induced hyperphagia (58). Additionally, stimulation of astrocytes with ghrelin modify glutamate and glucose metabolism as well as glycogen storage by decreasing GLUT2, glutamine synthetase, and lactate dehydrogenase, and increasing glutamate uptake, glycogen phosphorylase, and lactate transporters, which might modulate the signals/nutrients reaching neighboring neurons (121). Finally, activated astrocytes release adenosine to inhibit AgRP neurons, thus suppressing the ghrelin-mediated increase of food intake (122, 123) (Figure 3).
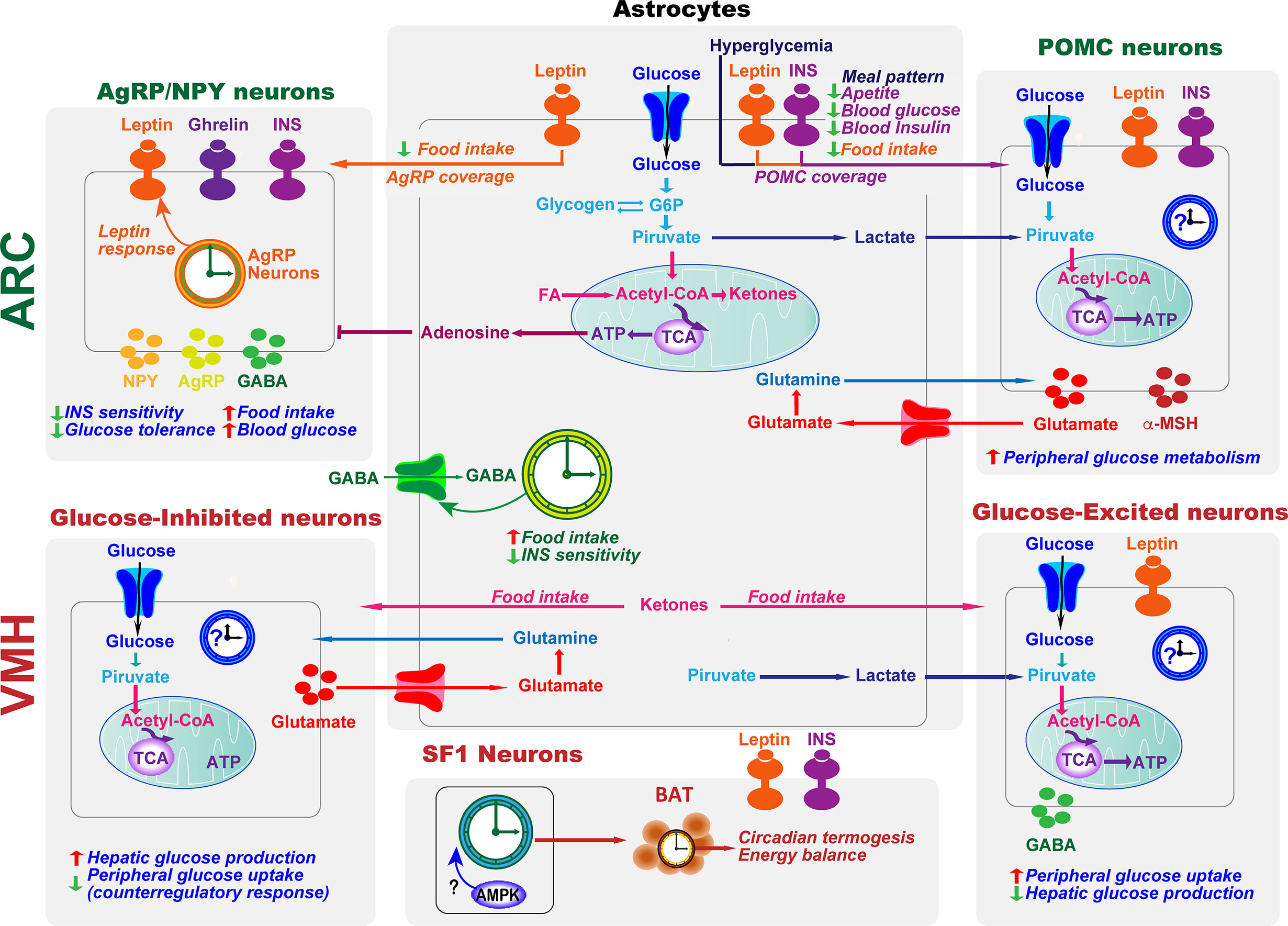
Figure 3 Astrocytes and clocks modulate hypothalamic glucose-sensing mechanisms. The ability of POMC and NPY/AgRP neuronal populations in ARC to alter energy metabolism is due to their sensitivity to several circulating signals, including hormones, such as leptin and insulin (INS), and nutrients. Hypothalamic astrocytes provide neurons with structural support and nutrients. Moreover, hyperglycemia, INS, and leptin signaling in this glial cell type lead to changes in the astrocytic coverage of POMC and/or AgRP neurons to regulate glucose sensing. Glucose transported into astrocytes can be metabolized to lactate, which is released and taken up by neurons and metabolized into pyruvate to serve as a glycolytic substrate. Astrocytes can also modulate synaptic transmission by uptake of neurotransmitters from the synaptic cleft (glutamate and GABA) and by releasing gliotransmitters such as adenosine, which inhibits AgRP neurons. In the ARC, the astrocyte circadian clock might control food intake and glucose homeostasis by regulating the uptake of GABA. On the other hand, the clock in AgRP neurons is required for coordinating leptin response and glucose metabolism. VMH neurons include glucose-sensing cells, referred to as glucose-excited and glucose-inhibited neurons. The activation of glucose-excited neurons leads to decreased hepatic glucose production and increased peripheral glucose uptake. VMH glucose-inhibited neurons are activated in response to hypoglycemia. In recurrent hypoglycemia, high accumulation of lactate enhances the glucose-excited neuronal activity and consequent GABA release, inhibiting the counterregulatory response. A high-fat diet increases astrocyte ketone bodies production, which are exported to VMH neurons to ultimately control food intake. Subsets of VMH neurons also express SF1. These SF1 neurons contain a clock that modulates energy expenditure by regulating cyclic thermogenesis in brown adipose tissue (BAT). As hypothalamic AMPK modulates BAT thermogenesis (116), and has a crucial role in the molecular clock, it would be interesting to investigate its involvement in the control of rhythmic BAT thermogenesis by the SF1 neuronal clock. TCA, tricarboxylic acid cycle.
As the SCN imposes the sleep-wake cycle and food intake occurs in the active period, the involvement of the pacemaker in controlling glucose homeostasis and systemic INS sensitivity is indirect (124). Indeed, BMAL1 deletion in SCN does not affect the body weight despite complete loss of rhythmic behavior (20). Moreover, the circadian locomotor activity but not the metabolic disturbances of Bmal1−/− mice were rescued by restoring BMAL1 expression in the SCN (125). Similarly, mice with astrocyte‐specific deletion of BMAL1 show altered energy balance and glucose homeostasis despite their circadian locomotor activity is not lost (39–43). Thus, peripheral and/or extra-SCN hypothalamic clocks, but not the SCN, might have a crucial role in developing glucose intolerance and INS resistance. In line with this idea, BMAL1 ablation within SF1 neurons in the VMH is sufficient to alter energy expenditure (126). Additionally, AgRP-specific ablation of BMAL1 increases hepatic gluconeogenesis (127). However, currently, little is known about the specific physiological functions of extra SCN brain clocks. This knowledge could be highly valuable for biomedical understanding and future therapeutic advancement in the metabolic imbalance associated with circadian disruption.
Metabolic tissues involved in glucose homeostasis also have autonomous clocks that govern and adjust their daily metabolic function or outputs. For example, in the liver, with an essential role as a buffer for glucose variations arising from rhythmic food consumption, ablation of the local clock leads to hypoglycemia restricted to the fasting phase and exaggerated glucose clearance (128). Moreover, it was reported that while hepatic glycogenesis is controlled by CLOCK (129), gluconeogenesis in the fasted state, is under the regulation of the repressor CRY1 (130–133). On the other hand, efficient glucose uptake by the hepatocytes at the beginning of the active phase depends on the rhythmic expression of glucose transporters and glucagon receptor (134, 135). Another tissue with a key role in the control of glucose homeostasis is the skeletal muscle. This tissue is responsible for 70-80% of INS-stimulated glucose uptake in the postprandial state (136). Interestingly, INS sensibility in muscle is controlled both by light and the local clock (137–140). Specifically, it was shown that photic inputs entrain diurnal changes in clock gene expression and INS sensitivity in muscle via SIRT1 in SF1 neurons (140). On the other hand, deletion of the autonomous clock in muscle is sufficient to cause local INS resistance (137). Glucose uptake is also dependent on rhythmic INS action in the white adipose tissue (WAT) (141). However, contrary to liver or skeletal muscle, ablation of the local clock in WAT do not impact glucose homeostasis and INS sensitivity (142), suggesting that lipid mobilization is mainly regulated by the hypothalamic actions of INS and leptin. Finally, brown adipose tissue (BAT), which relays in FFA and glucose supply to regulate thermogenesis, is highly flexible in terms of glucose uptake potential and can significantly contribute to whole-body glucose metabolism under some conditions. In this tissue, INS-stimulated glucose uptake is regulated by the VMH and AgRP neurons (143–145), as well as by the local clock (146, 147). Interestingly, mouse and human BAT express a red-light-sensitive protein, OPN3 (148), which increases glucose uptake upon red light stimulation. Recently, an elegant study demonstrated that animals reared without violet light show increased responses to β-agonists, which in humans activate BAT, lower blood glucose levels, and increase and INS sensitivity. This effect was mediated by a violet light-sensing photoreceptor Opsin 5 (OPN5) in glutamatergic warm-sensing hypothalamic preoptic area neurons (149). Altogether, these studies open the possibility of modulating glucose homeostasis by manipulating environmental light.
Altogether this suggests that perturbed rhythms of the central and/or tissue clocks might lead to a mismatch between hepatic glucose production, muscle glucose uptake, and carbohydrate intake which could contribute to elevated levels of glucose and an imbalance between lipid storage in WAT and lipid oxidation in the brown adipose tissue. Furthermore, hyperglycemia in diabetes is traditionally attributed to reduced INS sensitivity in skeletal muscle and liver but also coupled to decreased INS secretion by the pancreas. Not only glucose homeostasis and INS sensitivity is under control of the local clocks in most of the above-mentioned metabolic tissues, but the pancreatic clock also controls rhythmic INS secretion (150, 151). Indeed, ablation of the pancreatic clock, in mice, is sufficient to cause hypoinsulinemia and hyperglycemia (150, 152–154).
Interestingly, feeding-related hormones involved in the control of glucose homeostasis are timing cues for circadian behaviors. For example, leptin is involved in the regulation of sleep-wake cycles (155, 156); ghrelin, stimulate FAA in mice (157) and INS action, triggered after feeding, is a critical entrainment signal for the FEO (7). Thus, an intriguing unsolved question concerns how the neurocircuits involved in glucose homeostasis and the central or peripheral clocks crosstalk and coordinate appropriate metabolic and/or circadian responses.
Integration of Glucose Homeostasis by Astrocyte Clocks and Cellular Metabolism
As brain metabolic pathways are compartmentalized between astrocytes and neurons, the coordination of both cell types is needed to meet the high energy requirements of synaptic transmission and correct brain function (158). Thereby, it is not surprising that hypothalamic glucose sensing requires an intact metabolic coupling between astrocytes and neurons (159). Interestingly, a big percentage of components of cellular metabolic pathways are direct targets of the molecular clock (96, 111, 160). Together, this suggests that the close association between altered glucose homeostasis and circadian disruption may arise from a shared defect in the astrocyte-neuron metabolic coupling. In turn, this might impact the neurocircuitry governing energy and glucose homeostasis or alter metabolic adaptations to hypoglycemia in the diabetic brain. In this section, we discuss the current evidence that supports this hypothesis.
The major energy source for the brain is glucose, which is taken up by astrocytes and neurons via glucose transporters (GLUTs) (161–163). In the hypothalamus, the neuron-astrocyte glucose coupling expands beyond the accomplishment of energy requirements. For instance, astrocytes actively cooperate with hypothalamic neurons in detecting circulating glucose levels and in the generation of proper systemic metabolic responses (164). Not only astrocytic GLUT2 activity is involved in the regulation of systemic glucose homeostasis in rodents (165–167), but restoring astrocytic GLUT2 reestablish the counterregulatory response to low-glucose in GLUT2 deficient mice (165). Remarkably, rhythmic GLUTs expression (137, 168, 169) is impaired in the brain of experimental streptozotocin-induced diabetes rats (169). Moreover, 24 h oscillation in glucose levels may modulate the expression of clock genes and transcriptional outputs within hypothalamic neurons involved in glucose homeostasis (170). Whether astrocyte-neuron metabolism in hypothalamic glucose sensing and associated-systemic response in the normal or diabetic brain relies on the astrocyte molecular clock remains to be investigated. However, this idea is reinforced by the recent finding that deletion of Bmal1 in astrocytes impairs INS sensitivity and glucose homeostasis (39).
Further, glucose can be stored as glycogen or metabolized in the glycolytic pathway to produce pyruvate, which is either transferred into mitochondria or converted to lactate. According to the “Astrocyte-to-Neuron Lactate Shuttle” (ANLS) hypothesis (171), lactate is primarily produced by astrocytes and transferred to neurons, where it is converted to pyruvate for aerobic energy production in mitochondria (Figure 3). Thereby, the production and the release of lactate by astrocytes is directly linked to neuronal activity, as showed in orexin neurons (172, 173). Consistent with the ANLS hypothesis, brain lactate levels increase during the awake state when neuronal firing rates are higher and vice versa, leading to a 24 h rhythm of lactate concentration (174, 175). In turn, astrocytic lactate release regulates the sleep-wake cycle (74) and entrain forebrain oscillators between states of alertness and tiredness by controlling the DNA binding of CLOCK/BMAL1 (176). Remarkably, during hypoglycemia in diabetes patients, brain lactate levels drop while its infusion increased brain lactate levels compared to healthy subjects (177–179). These findings suggest increased lactate use as a metabolic substrate, impaired astrocyte lactate release, or perturbed compensatory metabolic mechanisms in the diabetic brain. Whether these effects underlie a potential astrocytic dysrhythmia is currently unknown.
More than half of the energy used by neurons during fasting derives from ketones bodies (180) synthesized by astrocytes. The astrocytic switch from glucose to FFA utilization (181, 182) to produce ketones is particularly enhanced in the hypothalamus (181), where stimulate neuropeptides critically involved in glucose sensing and energy homeostasis (83, 182, 183) (Figure 3). On the other hand, recurrent exposure to low glucose, mimicking variations often seen in patients with diabetes, results in increased astrocytic ketogenesis (184–186), likely to preserve brain ATP production (187). In turn, increased astrocytic ketogenesis alters INS signaling and consequently glucose homeostasis (183). Despite it was shown that Per2 controls the hepatic production of ketone bodies (30), whether hypothalamic ketogenesis is under the control of the astrocyte clock remains to be investigated. Similarly, whether arrhythmic astrocytes impair glucose homeostasis by contributing to the increased ketogenesis is unknown and could be crucial for therapeutic interventions involving the potentiation of the astrocyte clock.
On the other hand, glucose, as well as glutamine, can be metabolized to uridine diphosphate N-acetylglucosamine (UDP-GlcNAc) through the hexosamine biosynthetic pathway. The reversible enzymatic post-translational modification of proteins (on serine and threonine residues) with UDP-GlcNAc as glucose donor is termed O-GlcNAcylation. This process is conserved across species as occurs both in mouse brains and Drosophila neurons. While in conditions of glucose hypometabolism, brain levels of O-GlcNAc-modified proteins are reduced (188), hyperglycemia increases GlcNAcylation of proteins related to the INS pathway, thus contributing to INS resistance (189). Hyperinsulinemia is also associated with increased GlcNAcylation (189) of proteins involved in the pathology of diabetes, such as glycogen synthase, a major gatekeeper of glucose metabolism (190, 191). On the other hand, O-GlcNAcylation serves as a metabolic sensor to control the circadian period length via modification, and thus changes in the transcriptional activity of CLOCK and PER2 (104). As neurons depend on astroglial glucose and glutamine, this suggests that O-GlcNAcylation of the neuronal clocks might be coupled to astrocyte metabolism. However, further studies are needed to verify this hypothesis.
The metabolic endpoint of glycolysis and the mitochondrial metabolism is ATP generation, which apart from being used to fuel biological reactions is released to the extracellular space (eATP) (eATP) (192). Remarkably, INS stimulates ATP release from astrocytes (193). In turn, eATP leads to rapid upregulation of glycolysis (194) and promotes glucose uptake into both neurons and astrocytes (195). eATP is also a signaling molecule that acts on purinergic, ionotropic P2X, and G-protein coupled P2Y receptors to regulate neuronal activity (196). In the hypothalamus, NPY and AgRP neurons express P2X2R (197), whereas SF-1 neurons are excited by ATP via the P2X4 receptor (198). eATP released by astrocytes can also be metabolized to adenosine. While activation of the A1 receptor by adenosine inhibits appetite-stimulating AgRP neurons (122) (Figure 3), in astrocytes modulate sleep homeostasis (46). Thus, the circadian release of astrocytic ATP (199) and the circadian activity of enzymes involved in adenosine synthesis (200) suggest a central role of the astrocyte clock in modulating both processes. On the other hand, decreased ATP production activates the AMPK pathway to impact the circadian clock via degradation of CRY1 (103). Further investigation will clarify whether astrocytic rhythmic ATP release entrains the neuronal clocks via circadian activation of AMPK to control energy homeostasis and circadian sleep-wake changes in the brain.
Glutamate, the major excitatory neurotransmitter in the adult CNS, is released from neurons and recycled by astrocytes to form glutamine (Figure 3), which is returned to neurons and used as a precursor for synthesizing glutamate and GABA (201). The uptake of glutamate by astrocytes, critical for neuronal activity (202), is metabolically expensive and requires an increase in glycolysis and lactate production (171). Remarkably, control of glutamate and GABA levels, coupled to astrocyte rhythms (40–43, 203), is necessary for the generation of molecular and behavioral rhythms and, is also critically involved in the modulation of hypothalamic neural circuits controlling glucose homeostasis (39, 204, 205). However, excessive demands on astrocytes, in response to a decrease in glucose levels, impair glutamate uptake (206), altering the glutamatergic signaling to delay the onset of the normal counterregulatory response to hypoglycemia (206). Conversely, intake of an obesogenic diet rapidly increases hypothalamic glutamatergic signaling (207) and the expression of astrocytic glutamate transporters (208). It is reasonable to hypothesize that chronic elevated glutamatergic signaling, associated with diet-induced obesity, increases the metabolic demands on astrocytes to prevent glutamate-induced excitotoxicity. This in turn negatively impacts their ability to support neuronal activity thus, contributing to hypothalamic synaptic dysfunction and the death of POMC neurons (55, 209, 210). Altogether, this suggests that the regulation of glutamate and GABA levels might be a key astrocyte circadian function in normal physiology and likely involved in the alterations of the diabetic brain.
The enteric nervous system is gaining more attention in the last few years. While most of the research focused on the enteric neurons, less attention was directed towards the enteric glial cells (EGCs). Glucose enters the body via the gastrointestinal (GI) tract, and conversely, diabetes-induced GI dysfunction is related to increased apoptosis of EGC in the myenteric plexus (211). On the other hand, the gut clock synchronized by food intake (27, 212) regulates the expression of brush border disaccharidases and glucose absorption to the habitual feeding period (168). Moreover, Glucagon-like peptide-1secretion by enteroendocrine L-cells, with an important role in regulating glucose homeostasis (213, 214), is under control of the clock (215). In the gut, also anatomical and metabolome patterns of the microbiota undergo rhythmic fluctuations, resulting in system-wide effects on host circadian transcriptional, epigenetic, and metabolite cycles (216). Interestingly, repeated jet lag in mice disturbs the intestinal microbiome leading to reduced glucose tolerance (216). Similarly, fecal transfer from jet-lagged humans into germ-free mice impaired glucose tolerance (216). These findings suggest that the microbiome clock has an important role in the development of INS resistance due to repeated phase shifts. Altogether, this indicates that the contribution of the gut clock, specifically in the enteric glia, to the control of glucose homeostasis warrants further work.
Altogether, this data indicates that further investigations about the role of the astrocyte clock in maintaining the cycle-to-cycle precision of cellular metabolism and neural rhythmic behavior could be crucial to counteract the systemic metabolic abnormalities associated with circadian disruption.
Circadian Disruption and Diabetes
In this section, we review the current evidence about the contribution of genetic or environmental factors (such as exposure to artificial light-dark cycles, disturbed sleep, shift work, and jet lag) that impact the timekeeping system to the development of insulin resistance and type 2 diabetes.
In humans, mutations in several clock genes are strongly associated with obesity, INS resistance, and type 2 diabetes. Specifically, it was reported associations between single nucleotide polymorphisms in ARNT and T2DM (217), specific haplotypes of CLOCK and obesity (218, 219), and between polymorphisms in CRY2 and elevated fasting glucose (220, 221). In line with the human clock gene mutation studies, rodent models with genetic deletions of core-clock genes (in either a whole-body or a tissue-specific manner) showed INS resistance, obesity, and type 2 diabetes (32, 125, 142, 222, 223). Remarkably, deletion of Bmal1 in astrocytes in mice is sufficient to phenocopy the obesity, INS resistance, and glucose intolerance of Bmal1-/- constitutive KO mice (39), suggesting that robust astrocyte circadian rhythms could preserve whole-body homeostasis and metabolic health.
The central pacemaker anticipates and synchronizes the daily function of peripheral tissues according to the entrainment by natural changes in light. With the advent of affordable artificial lighting and the 24/7 lifestyle of our society, humans began to experience increased exposure to artificial lights and irregular light schedules. These environmental changes lead to a desynchronization between the internal clock and the external ZT, a phenomenon referred to as circadian misalignment. Remarkably, human and animal studies have linked obesity and type 2 diabetes with increased light exposure during naturally dark hours (224–230). In turn, exposure to bright morning light increases fasting and postprandial glucose levels in patients with type 2 diabetes (230). Despite its relevance for health, the molecular and cellular mechanisms of normal and pathological phototransduction in the SCN are unclear. For instance, VIP rhythm, with a key role in synchronizing SCN neurons to each other and with the LD cycle (231, 232), is driven by the LD cycle and not by the circadian clock (233). Thereby, the mechanism by which deletion of Bmal1 in astrocytes constantly elevates VIP levels (40) remains unknown. Indeed, constant illumination increases VIP levels lengthening the circadian period and resulting in two or more peaks in daily activity (234), a circadian locomotor pattern that resembles that of mice with arrhythmic astrocytes (40). Consistently, studies in Drosophila showed that glial-specific genetic manipulations lead to circadian arrhythmicity due to alterations on a clock neuron peptide transmitter (pigment dispersing factor) that acts on a receptor similar to that for VIP in mammals (65, 66). These studies suggest that the astrocyte clock might facilitate the entrainment to light and therefore, to the light-induced phase shifts in physiology and behavior. Further investigations on the mechanism underlying circadian entrainment to light are critical for understanding why aberrant light exposure, disrupts circadian physiology leading to diabetes and INS resistance.
Evidence from epidemiological and experimental studies indicate that sleep restriction or disturbance, increases the risk of obesity and type 2 diabetes (235–239) likely due to increased food intake (237, 238), altered sympathovagal balance (240, 241), and increased circulating levels of catecholamines (242) or cortisol (241, 242). Interestingly, astrocytes modulate mammalian sleep homeostasis by controlling adenosine A1 receptors (243). The circadian release of the astrocytic transmitter ATP (199) as well as the circadian activity of enzymes involved in the synthesis of adenosine in areas of the brain related to sleep (200) suggests a central role of astrocytes in modulating circadian sleep-wake changes in the brain. Further studies will be needed to understand the importance of astrocyte clocks in the relationship between circadian sleep disorders and diabetes.
Whereas light is the dominant timing cue for the SCN, the time of meals represents the main ZT for peripheral clocks. Therefore is not surprising that extended/erratic eating patterns, such as in shift workers or subjects under experimental circadian misalignment, showed decreased glucose tolerance and insulin sensitivity (244–250). Indeed, a short-term circadian misalignment protocol of 8 days in humans is sufficient to cause higher blood glucose and insulin levels (249). It is reasonable to hypothesize that disturbance of nutrient fluxes or the misalignment of central and peripheral clock rhythms might contribute to the pathophysiology of insulin resistance at the tissue level. For instance, a mismatch between hepatic glucose production, muscle glucose uptake, and carbohydrate intake could contribute to elevated glucose levels, while an imbalance between lipid storage in WAT, lipid oxidation in BAT, and hepatic lipid production might contribute to ectopic lipid accumulation. However, to improve or prevent the metabolic alterations caused by circadian misalignment we need to further understand the mechanisms involved in the entrainment of both central and peripheral circadian clocks. Remarkably, as astrocytes are at the interface between vessels and neurons, they are in a privileged position to act as metabolic sensors of systemic cues that entrain the peripheral clocks, such as GCs, INS, or IGF1 (7, 40, 203). Further studies will clarify whether those metabolic cues might play a crucial role in communicating time-of-feeding to the astrocyte molecular clock linking the periphery and the CNS clocks.
Conclusion
A large body of evidence from human or animal studies demonstrated the circadian regulation of glucose homeostasis and INS sensitivity. However, the exact mechanisms involved in the metabolic derangements resulting from circadian disruption are not fully understood. Emerging groundbreaking findings, showing that astrocytes are pivotal for the circadian regulation of behavior and whole-body energy and glucose homeostasis, could provide a new cellular target to tune physiological responses operating on different timescales according to metabolic status. A key question that remains to be investigated is how the astrocyte clock is entrained to lead to the cycle-to-cycle precision of circadian rhythmicity in the SCN and/or in extra SCN clocks. Therefore, we face a lack of knowledge on the mechanisms by which astrocyte circadian dysfunction affects such a wide range of physiological processes. Understanding these mechanisms will be a challenge for years to come but a crucial aspect in designing better therapies, such as clock agonists, for diabetes. With this knowledge, the use of chronotherapies or temporally directed therapeutics to improve human metabolic health will be a matter of time.
Author Contribution
All authors contributed to the article and approved the submitted version. OB-M conceptualized and wrote the manuscript and made the figures. OB-M and ML discussed and edited the manuscript and the figures.
Conflict of Interest
The authors declare that the research was conducted in the absence of any commercial or financial relationships that could be construed as a potential conflict of interest.
Acknowledgements
OB-M is supported with a Ramón y Cajal award (RYC2018‐026293‐I.) from the Ministerio de Ciencia, Innovación y Universidades of Spain; by Spanish Agencia Estatal de Investigación (PID2019-109556RB-I00) and the Xunta de Galicia-Consellería de Cultura, Educación e Ordenación Universitaria (ED431F 2020/009).
References
1. Hastings MH, Maywood ES, Reddy AB. Two decades of circadian time. J Neuroendocrinol (2008) 20:812–9. doi: 10.1111/j.1365-2826.2008.01715.x
2. Moore RY, Eichler VB. Loss of a circadian adrenal corticosterone rhythm following suprachiasmatic lesions in the rat. Brain Res (1972) 42:201–6. doi: 10.1016/0006-8993(72)90054-6
3. Stephan FK, Zucker I. Circadian rhythms in drinking behavior and locomotor activity of rats are eliminated by hypothalamic lesions. Proc Natl Acad Sci U S A (1972) 69:1583–6. doi: 10.1073/pnas.69.6.1583
4. Nagoshi E, Saini C, Bauer C, Laroche T, Naef F, Schibler U. Circadian Gene Expression in Individual FibroblastsCell-Autonomous and Self-Sustained Oscillators Pass Time to Daughter Cells. Cell (2004) 119:693–705. doi: 10.1016/s0092-8674(04)01054-2
5. Welsh DK, Yoo SH, Liu AC, Takahashi JS, Kay SA. Bioluminescence imaging of individual fibroblasts reveals persistent, independently phased circadian rhythms of clock gene expression. Curr Biol (2004) 14:2289–95. doi: 10.1016/j.cub.2004.11.057
6. Pezük P, Mohawk JA, Wang LA, Menaker M. Glucocorticoids as entraining signals for peripheral circadian oscillators. Endocrinology (2012) 153:4775–83. doi: 10.1210/en.2012-1486
7. Crosby P, Hamnett R, Putker M, Hoyle NP, Reed M, Karam CJ, et al. Insulin/IGF-1 Drives PERIOD Synthesis to Entrain Circadian Rhythms with Feeding Time. Cell (2019) 177:896–909.e20. doi: 10.1016/j.cell.2019.02.017
8. Yan L, Karatsoreos I, LeSauter J, Welsh DK, Kay S, Foley D, et al. Exploring spatiotemporal organization of SCN circuits. Cold Spring Harbor Symp Quantitative Biol (2007) 72:527–41. doi: 10.1101/sqb.2007.72.037
9. Wen S, Ma D, Zhao M, Xie L, Wu Q, Gou L, et al. Spatiotemporal single-cell analysis of gene expression in the mouse suprachiasmatic nucleus. Nat Neurosci (2020) 23:456–67. doi: 10.1038/s41593-020-0586-x
10. Silver R, Schwartz WJ. The suprachiasmatic nucleus is a functionally heterogeneous timekeeping organ. Methods Enzymol (2005) 393:451–65. doi: 10.1016/S0076-6879(05)93022-X
11. Welsh DK, Logothetis DE, Meister M, Reppert SM. Individual neurons dissociated from rat suprachiasmatic nucleus express independently phased circadian firing rhythms. Neuron (1995) 14:697–706. doi: 10.1016/0896-6273(95)90214-7
12. Honma S, Nakamura W, Shirakawa T, Honma KI. Diversity in the circadian periods of single neurons of the rat suprachiasmatic nucleus depends on nuclear structure and intrinsic period. Neurosci Lett (2004) 358:173–6. doi: 10.1016/j.neulet.2004.01.022
13. Gooley JJ, Lu J, Chou TC, Scammell TE, Saper CB. Melanopsin in cells of origin of the retinohypothalamic tract. Nat Neurosci (2001) 4:1165. doi: 10.1038/nn768
14. Hattar S, Kumar M, Park A, Tong P, Tung J, Yau KW, et al. Central projections of melanopsin-expressing retinal ganglion cells in the mouse. J Comp Neurol (2006) 497:326–49. doi: 10.1002/cne.20970
15. Güler AD, Ecker JL, Lall GS, Haq S, Altimus CM, Liao HW, et al. Melanopsin cells are the principal conduits for rod-cone input to non-image-forming vision. Nature (2008) 453:102–5. doi: 10.1038/nature06829
16. Bae K, Jin X, Maywood ES, Hastings MH, Reppert SM, Weaver DR. Differential Functions of mPer1, mPer2, and mPer3 in the SCN Circadian Clock al The negative feedback loop involves the dynamic reg. Neuron (2001) 30:525–36. doi: 10.1016/S0896-6273(01)00302-6
17. Damiola F, Le Minli N, Preitner N, Kornmann B, Fleury-Olela F, Schibler U. Restricted feeding uncouples circadian oscillators in peripheral tissues from the central pacemaker in the suprachiasmatic nucleus. Genes Dev (2000) 14:2950–61. doi: 10.1101/gad.183500
18. Stokkan KA, Yamazaki S, Tei H, Sakaki Y, Menaker M. Entrainment of the circadian clock in the liver by feeding. Sci (80- ) (2001) 291:490–3. doi: 10.1126/science.291.5503.490
19. Hara R, Wan K, Wakamatsu H, Aida R, Moriya T, Akiyama M, et al. Restricted feeding entrains liver clock without participation of the suprachiasmatic nucleus. Genes to Cells (2001) 6:269–78. doi: 10.1046/j.1365-2443.2001.00419.x
20. Izumo M, Pejchal M, Schook AC, Lange RP, Walisser JA, Sato TR, et al. Differential effects of light and feeding on circadian organization of peripheral clocks in a forebrain Bmal1 mutant. Elife (2014) 3:e04617. doi: 10.7554/eLife.04617
21. Sheward WJ, Maywood ES, French KL, Horn JM, Hastings MH, Seck JR, et al. Entrainment to feeding but not to light: Circadian phenotype of VPAC 2 receptor-null mice. J Neurosci (2007) 27:4351–8. doi: 10.1523/JNEUROSCI.4843-06.2007
22. Fernandez DC, Komal R, Langel J, Ma J, Duy PQ, Penzo MA, et al. Retinal innervation tunes circuits that drive nonphotic entrainment to food. Nature (2020) 581:194–8. doi: 10.1038/s41586-020-2204-1
23. Davidson AJ. Lesion studies targeting food-anticipatory activity. Eur J Neurosci (2009) 30:1658–64. doi: 10.1111/j.1460-9568.2009.06961.x
24. Mistlberger RE. Neurobiology of food anticipatory circadian rhythms. Physiol Behav (2011) 104:535–45. doi: 10.1016/j.physbeh.2011.04.015
25. Challet E, Mendoza J, Dardente H, Pévet P. Neurogenetics of food anticipation. Eur J Neurosci (2009) 30:1676–87. doi: 10.1111/j.1460-9568.2009.06962.x
26. Butler AA, Girardet C, Mavrikaki M, Trevaskis JL, Macarthur H, Marks DL, et al. A life without hunger: The Ups (and downs) to modulating melanocortin-3 receptor signaling. Front Neurosci (2017) 11:128. doi: 10.3389/fnins.2017.00128
27. Davidson AJ, Cappendijk SLT, Stephan FK. Feeding-entrained circadian rhythms are attenuated by lesions of the parabrachial region in rats. Am J Physiol - Regul Integr Comp Physiol (2000) 278:R1296–304. doi: 10.1152/ajpregu.2000.278.5.r1296
28. Mendoza J, Pévet P, Felder-Schmittbuhl MP, Bailly Y, Challet E. The cerebellum harbors a circadian oscillator involved in food anticipation. J Neurosci (2010) 30:1894–904. doi: 10.1523/JNEUROSCI.5855-09.2010
29. Gallardo CM, Hsu CT, Gunapala KM, Parfyonov M, Chang CH, Mistlberger RE, et al. Behavioral and neural correlates of acute and scheduled hunger in C57BL/6 mice. PloS One (2014) 9:e95990. doi: 10.1371/journal.pone.0095990
30. Chavan R, Feillet C, Costa SSF, Delorme JE, Okabe T, Ripperger JA, et al. Liver-derived ketone bodies are necessary for food anticipation. Nat Commun (2016) 7:10580. doi: 10.1038/ncomms10580
31. Dunlap JC. Molecular bases for circadian clocks. Cell (1999) 96:271–90. doi: 10.1016/S0092-8674(00)80566-8
32. Shi SQ, Ansari TS, McGuinness OP, Wasserman DH, Johnson CH. Circadian disruption leads to insulin resistance and obesity. Curr Biol (2013) 23:372–81. doi: 10.1016/j.cub.2013.01.048
33. Stenvers DJ, Scheer FAJL, Schrauwen P, la Fleur SE, Kalsbeek A. Circadian clocks and insulin resistance. Nat Rev Endocrinol (2019) 15:75–89. doi: 10.1038/s41574-018-0122-1
34. Marcheva B, Ramsey KM, Affinati A, Bass J. Clock genes and metabolic disease. J Appl Physiol (2009) 107:1638–46. doi: 10.1152/japplphysiol.00698.2009
35. Bedrosian TA, Fonken LK, Nelson RJ. Endocrine Effects of Circadian Disruption. Annu Rev Physiol (2016) 78:109–31. doi: 10.1146/annurev-physiol-021115-105102
36. Terzibasi-Tozzini E, Martinez-Nicolas A, Lucas-Sánchez A. The clock is ticking. Ageing of the circadian system: From physiology to cell cycle. Semin Cell Dev Biol (2017) 70:164–76. doi: 10.1016/j.semcdb.2017.06.011
37. Uddin MS, Tewari D, Mamun AA, Kabir MT, Niaz K, Wahed MII, et al. Circadian and sleep dysfunction in Alzheimer’s disease. Ageing Res Rev (2020) 60:101046. doi: 10.1016/j.arr.2020.101046
38. Goh VHH, Tong TYY, Lee LKH. Sleep/wake cycle and circadian disturbances in shift work: Strategies for their management - A review. Ann Acad Med Singapore (2000) 29:90–6.
39. Barca-Mayo O, Boender AJ, Armirotti A, De Pietri Tonelli D. Deletion of astrocytic BMAL1 results in metabolic imbalance and shorter lifespan in mice. Glia (2020) 68:1131–47. doi: 10.1002/glia.23764
40. Barca-Mayo O, Pons-Espinal M, Follert P, Armirotti A, Berdondini L, De Pietri Tonelli D. Astrocyte deletion of Bmal1 alters daily locomotor activity and cognitive functions via GABA signalling. Nat Commun (2017) 8:14336. doi: 10.1038/ncomms14336
41. Tso CF, Simon T, Greenlaw AC, Puri T, Mieda M, Herzog ED. Astrocytes Regulate Daily Rhythms in the Suprachiasmatic Nucleus and Behavior. Curr Biol (2017) 27:1055–61. doi: 10.1016/j.cub.2017.02.037
42. Brancaccio M, Patton AP, Chesham JE, Maywood ES, Hastings MH. Astrocytes Control Circadian Timekeeping in the Suprachiasmatic Nucleus via Glutamatergic Signaling. Neuron (2017) 93:1420–1435.e5. doi: 10.1016/j.neuron.2017.02.030
43. Brancaccio M, Edwards MD, Patton AP, Smyllie NJ, Chesham JE, Maywood ES, et al. Cell-autonomous clock of astrocytes drives circadian behavior in mammals. Sci (80- ) (2019) 363:187–92. doi: 10.1126/science.aat4104
44. Ben Haim L, Rowitch DH. Functional diversity of astrocytes in neural circuit regulation. Nat Rev Neurosci (2016) 18:31–41. doi: 10.1038/nrn.2016.159
45. Zhang Y, Barres BA. Astrocyte heterogeneity: An underappreciated topic in neurobiology. Curr Opin Neurobiol (2010) 20:588–94. doi: 10.1016/j.conb.2010.06.005
46. Halassa MM, Fellin T, Haydon PG. The tripartite synapse: roles for gliotransmission in health and disease. Trends Mol Med (2007) 3:54–63. doi: 10.1016/j.molmed.2006.12.005
47. Haber M, Zhou L, Murai KK. Cooperative astrocyte and dendritic spine dynamics at hippocampal excitatory synapses. J Neurosci (2006) 26:8881–91. doi: 10.1523/JNEUROSCI.1302-06.2006
48. Bushong EA, Martone ME, Jones YZ, Ellisman MH. Protoplasmic astrocytes in CA1 stratum radiatum occupy separate anatomical domains. J Neurosci (2002) 22:183–92. doi: 10.1523/jneurosci.22-01-00183.2002
49. Spanagel R, Pendyala G, Abarca C, Zghoul T, Sanchis-Segura C, Magnone MC, et al. The clock gene Per2 influences the glutamatergic system and modulates alcohol consumption. Nat Med (2005) 11:35–42. doi: 10.1038/nm1163
50. Beaulé C, Swanstrom A, Leone MJ, Herzog ED. Circadian modulation of gene expression, but not glutamate uptake, in mouse and rat cortical astrocytes. PloS One (2009) 4:e7476. doi: 10.1371/journal.pone.0007476
51. Blanco ME, Mayo OB, Bandiera T, Tonelli DDP, Armirotti A. LC–MS/MS analysis of twelve neurotransmitters and amino acids in mouse cerebrospinal fluid. J Neurosci Methods (2020) 341:108760. doi: 10.1016/j.jneumeth.2020.108760
52. Becquet D, Girardet C, Guillaumond F, François-Bellan AM, Bosler O. Ultrastructural plasticity in the rat suprachiasmatic nucleus. Possible involvement in clock entrainment. Glia (2008) 56:294–305. doi: 10.1002/glia.20613
53. Monique L, Servière J. Circadian fluctuations in GFAP distribution in the syrian hamster suprachiasmatic nucleus. Neuroreport (1993) 4:1243–6. doi: 10.1097/00001756-199309000-00008
54. Girardet C, Blanchard MP, Ferracci G, Lévêque C, Moreno M, François-Bellan AM, et al. Daily changes in synaptic innervation of VIP neurons in the rat suprachiasmatic nucleus: Contribution of glutamatergic afferents. Eur J Neurosci (2010) 31:359–70. doi: 10.1111/j.1460-9568.2009.07071.x
55. Horvath TL, Sarman B, García-Cáceres C, Enriori PJ, Sotonyi P, Shanabrough M, et al. Synaptic input organization of the melanocortin system predicts diet-induced hypothalamic reactive gliosis and obesity. Proc Natl Acad Sci USA (2010) 107:14875–80. doi: 10.1073/pnas.1004282107
56. Nuzzaci D, Cansell C, Liénard F, Nédélec E, Ben Fradj S, Castel J, et al. Postprandial Hyperglycemia Stimulates Neuroglial Plasticity in Hypothalamic POMC Neurons after a Balanced Meal. Cell Rep (2020) 30:3067–3078.e5. doi: 10.1016/j.celrep.2020.02.029
57. García-Cáceres C, Quarta C, Varela L, Gao Y, Gruber T, Legutko B, et al. Astrocytic Insulin Signaling Couples Brain Glucose Uptake with Nutrient Availability. Cell (2016) 166:867–80. doi: 10.1016/j.cell.2016.07.028
58. Kim JG, Suyama S, Koch M, Jin S, Argente-Arizon P, Argente J, et al. Leptin signaling in astrocytes regulates hypothalamic neuronal circuits and feeding. Nat Neurosci (2014) 17:908–10. doi: 10.1038/nn.3725
59. Mendelsohn AR, Larrick JW. Sleep Facilitates Clearance of Metabolites from the Brain: Glymphatic Function in Aging and Neurodegenerative Diseases. Rejuvenation Res (2013) 16:518–23. doi: 10.1089/rej.2013.1530
60. Taoka T, Jost G, Frenzel T, Naganawa S, Pietsch H. Impact of the Glymphatic System on the Kinetic and Distribution of Gadodiamide in the Rat Brain: Observations by Dynamic MRI and Effect of Circadian Rhythm on Tissue Gadolinium Concentrations. Invest Radiol (2018) 53:529–34. doi: 10.1097/RLI.0000000000000473
61. Iliff JJ, Wang M, Liao Y, Plogg BA, Peng W, Gundersen GA, et al. A paravascular pathway facilitates CSF flow through the brain parenchyma and the clearance of interstitial solutes, including amyloid β. Sci Transl Med (2012) 4:147ra111. doi: 10.1126/scitranslmed.3003748
62. Murlidharan G, Crowther A, Reardon RA, Song J, Asokan A. Glymphatic fluid transport controls paravascular clearance of AAV vectors from the brain. JCI Insight (2016) 1:e88034. doi: 10.1172/jci.insight.88034
63. LeSauter J, Silver R. Output signals of the SCN. Chronobiol Int (1998) 15:535–50. doi: 10.3109/07420529808998706
64. Maywood ES, Chesham JE, O’Brien JA, Hastings MH. A diversity of paracrine signals sustains molecular circadian cycling in suprachiasmatic nucleus circuits. Proc Natl Acad Sci USA. (2011) 108:14306–11. doi: 10.1073/pnas.1101767108
65. Ng FS, Tangredi MM, Jackson FR. Glial cells physiologically modulate clock neurons and circadian behavior in a calcium-dependent manner. Curr Biol (2011) 21:625–34. doi: 10.1016/j.cub.2011.03.027
66. Suh J, Jackson FR. Drosophila Ebony Activity Is Required in Glia for the Circadian Regulation of Locomotor Activity. Neuron (2007) 55:435–47. doi: 10.1016/j.neuron.2007.06.038
67. Jackson FR. Glial cell modulation of circadian rhythms. Glia (2011) 59:1341–50. doi: 10.1002/glia.21097
68. Womac AD, Burkeen JF, Neuendorff N, Earnest DJ, Zoran MJ. Circadian rhythms of extracellular ATP accumulation in suprachiasmatic nucleus cells and cultured astrocytes. Eur J Neurosci (2009) 30:869–76. doi: 10.1111/j.1460-9568.2009.06874.x
69. Cornell-Bell AH, Finkbeiner SM, Cooper MS, Smith SJ. Glutamate induces calcium waves in cultured astrocytes: Long-range glial signaling. Sci (80- ) (1990) 247:470–3. doi: 10.1126/science.1967852
70. Shinohara K, Funabashi T, Mitushima D, Kimura F. Effects of gap junction blocker on vasopressin and vasoactive intestinal polypeptide rhythms in the rat suprachiasmatic nucleus in vitro. Neurosci Res (2000) 38:43–7. doi: 10.1016/S0168-0102(00)00141-3
71. Wang MH, Chen N, Wang JH. The coupling features of electrical synapses modulate neuronal synchrony in hypothalamic superachiasmatic nucleus. Brain Res (2014) 1550:9–17. doi: 10.1016/j.brainres.2014.01.007
72. Diemer T, Landgraf D, Noguchi T, Pan H, Moreno JL, Welsh DK. Cellular circadian oscillators in the suprachiasmatic nucleus remain coupled in the absence of connexin-36. Neuroscience (2017) 357:1–11. doi: 10.1016/j.neuroscience.2017.05.037
73. Long MA, Jutras MJ, Connors BW, Burwell RD. Electrical synapses coordinate activity in the suprachiasmatic nucleus. Nat Neurosci (2005) 8:61–6. doi: 10.1038/nn1361
74. Clasadonte J, Scemes E, Wang Z, Boison D, Haydon PG. Connexin 43-Mediated Astroglial Metabolic Networks Contribute to the Regulation of the Sleep-Wake Cycle. Neuron (2017) 95:1365–1380.e5. doi: 10.1016/j.neuron.2017.08.022
75. Giantomasi M, Malerba M, Barca-Mayo O, Miele E, De Pietri Tonelli D, Berdondini L. A microfluidic device to study molecular clocks synchronization among neuronal populations, in: 41st Annual International Conference of the IEEE Engineering in Medicine. (2019) IEEE. doi: 10.1109/EMBC.2019.8856999
76. Cirrito JR, Deane R, Fagan AM, Spinner ML, Parsadanian M, Finn MB, et al. P-glycoprotein deficiency at the blood-brain barrier increases amyloid-β deposition in an Alzheimer disease mouse model. J Clin Invest (2005) 115:3285–90. doi: 10.1172/JCI25247
77. Abbott NJ, Rönnbäck L, Hansson E. Astrocyte-endothelial interactions at the blood-brain barrier. Nat Rev Neurosci (2006) 7:41–53. doi: 10.1038/nrn1824
78. Xie L, Kang H, Xu Q, Chen MJ, Liao Y, Thiyagarajan M, et al. Sleep drives metabolite clearance from the adult brain. Sci (80- ) (2013) 342:373–7. doi: 10.1126/science.1241224
79. Nakazato R, Kawabe K, Yamada D, Ikeno S, Mieda M, Shimba S, et al. Disruption of Bmal1 impairs blood-brain barrier integrity via pericyte dysfunction. J Neurosci (2017) 37:10052–62. doi: 10.1523/JNEUROSCI.3639-16.2017
80. Zhang SL, Yue Z, Arnold DM, Artiushin G, Sehgal A. A Circadian Clock in the Blood-Brain Barrier Regulates Xenobiotic Efflux. Cell (2018) 173:130–139.e10. doi: 10.1016/j.cell.2018.02.017
81. Guillod-Maximin E, Lorsignol A, Alquier T, Pénicaud L. Acute intracarotid glucose injection towards the brain induces specific c-fos activation in hypothalamic nuclei: Involvement of astrocytes in cerebral glucose-sensing in rats. J Neuroendocrinol (2004) 16:464–71. doi: 10.1111/j.1365-2826.2004.01185.x
82. Gao Y, Layritz C, Legutko B, Eichmann TO, Laperrousaz E, Moullé VS, et al. Disruption of lipid uptake in astroglia exacerbates diet-induced obesity. Diabetes (2017) 66:2555–63. doi: 10.2337/db16-1278
83. Le Foll C, Levin BE. Fatty acid-induced astrocyte ketone production and the control of food intake. Am J Physiol - Regul Integr Comp Physiol (2016) 310:R1186–92. doi: 10.1152/ajpregu.00113.2016
84. Diano S, Kalra SP, Horvath TL. Leptin receptor immunoreactivity is associated with the Golgi apparatus of hypothalamic neurones and glial cells. J Neuroendocrinol (1998) 10:647–50. doi: 10.1046/j.1365-2826.1998.00261.x
85. Cheunsuang O, Morris R. Astrocytes in the arcuate nucleus and median eminence that take up a fluorescent dye from the circulation express leptin receptors and neuropeptide Y Y1 receptors. Glia (2005) 52:228–33. doi: 10.1002/glia.20239
86. Cardona-Gómez GP, Doncarlos L, Garcia-Segura LM. Insulin-like growth factor I receptors and estrogen receptors colocalize in female rat brain. Neuroscience (2000) 99:751–60. doi: 10.1016/S0306-4522(00)00228-1
87. Dezonne RS, Lima FRS, Trentin AG, Gomes FC. Thyroid Hormone and Astroglia: Endocrine Control of the Neural Environment. J Neuroendocrinol (2015) 27:435–45. doi: 10.1111/jne.12283
88. O’Banion MK, Young DA, Bohn MC. Corticosterone-responsive mRNAs in primary rat astrocytes. Mol Brain Res (1994) 22:57–68. doi: 10.1016/0169-328X(94)90032-9
89. Carter BS, Meng F, Thompson RC. Glucocorticoid treatment of astrocytes results in temporally dynamic transcriptome regulation and astrocyte-enriched mRNA changes in vitro. Physiol Genomics (2012) 44:1188–200. doi: 10.1152/physiolgenomics.00097.2012
90. Rosenfeld P, Van Eekelen JAM, Levine S, De Kloet ER. Ontogeny of the Type 2 glucocorticoid receptor in discrete rat brain regions: an immunocytochemical study. Dev Brain Res (1988) 470:119–27. doi: 10.1016/0165-3806(88)90207-6
91. Zehring WA, Wheeler DA, Reddy P, Konopka RJ, Kyriacou CP, Rosbash M, et al. P-element transformation with period locus DNA restores rhythmicity to mutant, arrhythmic drosophila melanogaster. Cell (1984) 39:369–76. doi: 10.1016/0092-8674(84)90015-1
92. Bargiello TA, Jackson FR, Young MW. Restoration of circadian behavioural rhythms by gene transfer in Drosophila. Nature (1984) 312:752–4. doi: 10.1038/312752a0
93. Van Der Horst GTJ, Muijtjens M, Kobayashi K, Takano R, Kanno SI, Takao M, et al. Mammalian Cry1 and Cry2 are essential for maintenance of circadian rhythms. Nature (1999) 398:627–30. doi: 10.1038/19323
94. Zheng B, Albrecht U, Kaasik K, Sage M, Lu W, Vaishnav S, et al. Nonredundant roles of the mPer1 and mPer2 genes in the mammalian circadian clock. Cell (2001) 105:683–94. doi: 10.1016/S0092-8674(01)00380-4
95. Kume K, Zylka MJ, Sriram S, Shearman LP, Weaver DR, Jin X, et al. mCRY1 and mCRY2 are essential components of the negative limb of the circadian clock feedback loop. Cell (1999) 98:193–205. doi: 10.1016/S0092-8674(00)81014-4
96. Cox KH, Takahashi JS. Circadian clock genes and the transcriptional architecture of the clock mechanism. J Mol Endocrinol (2019) 63:R93–R102. doi: 10.1530/JME-19-0153
97. Preitner N, Damiola F, Lopez-Molina L-, Zakany J, Duboule D, Albrecht U, et al. The orphan nuclear receptor REV-ERBα controls circadian transcription within the positive limb of the mammalian circadian oscillator. Cell (2002) 110:251–60. doi: 10.1016/S0092-8674(02)00825-5
98. Richards J, Gumz ML. Advances in understanding the peripheral circadian clocks. FASEB J (2012) 26:3602–13. doi: 10.1096/fj.12-203554
99. Inoki K, Kim J, Guan KL. AMPK and mTOR in cellular energy homeostasis and drug targets. Annu Rev Pharmacol Toxicol (2012) 52:381–400. doi: 10.1146/annurev-pharmtox-010611-134537
100. Robles MS, Humphrey SJ, Mann M. Phosphorylation Is a Central Mechanism for Circadian Control of Metabolism and Physiology. Cell Metab (2017) 25:118–27. doi: 10.1016/j.cmet.2016.10.004
101. Dang F, Sun X, Ma X, Wu R, Zhang D, Chen Y, et al. Insulin post-transcriptionally modulates Bmal1 protein to affect the hepatic circadian clock. Nat Commun (2016) 7:12696. doi: 10.1038/ncomms12696
102. Luciano AK, Zhou W, Santana JM, Kyriakides C, Velazquez H, Sessa WC. CLOCK phosphorylation by AKT regulates its nuclear accumulation and circadian gene expression in peripheral tissues. J Biol Chem (2018) 293:9126–36. doi: 10.1074/jbc.RA117.000773
103. Lamia KA, Sachdeva UM, Di Tacchio L, Williams EC, Alvarez JG, Egan DF, et al. AMPK regulates the circadian clock by cryptochrome phosphorylation and degradation. Sci (80- ) (2009) 326:437–40. doi: 10.1126/science.1172156
104. Kaasik K, Kivimäe S, Allen JJ, Chalkley RJ, Huang Y, Baer K, et al. Glucose sensor O-GlcNAcylation coordinates with phosphorylation to regulate circadian clock. Cell Metab (2013) 17:291–302. doi: 10.1016/j.cmet.2012.12.017
105. Li MD, Ruan HB, Hughes ME, Lee JS, Singh JP, Jones SP, et al. O-GlcNAc signaling entrains the circadian clock by inhibiting BMAL1/CLOCK ubiquitination. Cell Metab (2013) 17:303–10. doi: 10.1016/j.cmet.2012.12.015
106. Ma YT, Luo H, Guan WJ, Zhang H, Chen C, Wang Z, et al. O-GlcNAcylation of BMAL1 regulates circadian rhythms in NIH3T3 fibroblasts. Biochem Biophys Res Commun (2013) 431:382–7. doi: 10.1016/j.bbrc.2013.01.043
107. Nakahata Y, Kaluzova M, Grimaldi B, Sahar S, Hirayama J, Chen D, et al. The NAD+-Dependent Deacetylase SIRT1 Modulates CLOCK-Mediated Chromatin Remodeling and Circadian Control. Cell (2008) 134:329–40. doi: 10.1016/j.cell.2008.07.002
108. Asher G, Gatfield D, Stratmann M, Reinke H, Dibner C, Kreppel F, et al. SIRT1 Regulates Circadian Clock Gene Expression through PER2 Deacetylation. Cell (2008) 134:317–28. doi: 10.1016/j.cell.2008.06.050
109. Foteinou PT, Venkataraman A, Francey LJ, Anafi RC, Hogenesch JB, Doyle FJ. Computational and experimental insights into the circadian effects of SIRT1. Proc Natl Acad Sci USA (2018) 115:11643–8. doi: 10.1073/pnas.1803410115
110. Asher G, Reinke H, Altmeyer M, Gutierrez-Arcelus M, Hottiger MO, Schibler U. Poly(ADP-Ribose) Polymerase 1 Participates in the Phase Entrainment of Circadian Clocks to Feeding. Cell (2010) 142:943–53. doi: 10.1016/j.cell.2010.08.016
111. Takahashi JS. Transcriptional architecture of the mammalian circadian clock. Nat Rev Genet (2017) 18:164–79. doi: 10.1038/nrg.2016.150
112. Eckel-Mahan K, Sassone-Corsi P. Metabolism and the circadian clock converge. Physiol Rev (2013) 93:107–35. doi: 10.1152/physrev.00016.2012
113. Sutton EF, Beyl R, Early KS, Cefalu WT, Ravussin E, Peterson CM. Early Time-Restricted Feeding Improves Insulin Sensitivity, Blood Pressure, and Oxidative Stress Even without Weight Loss in Men with Prediabetes. Cell Metab (2018) 27:1212–1221.e3. doi: 10.1016/j.cmet.2018.04.010
114. Carroll KF, Nestel PJ. Diurnal variation in glucose tolerance and in insulin secretion in man. Diabetes (1973) 22:333–48. doi: 10.2337/diab.22.5.333
115. Gibson T, Jarrett RJ. Diurnal Variation in Insulin Sensitivity. Lancet (1972) 2:947–8. doi: 10.1016/S0140-6736(72)92472-5
116. López M, Tena-Sempere M. Estradiol effects on hypothalamic AMPK and BAT thermogenesis: A gateway for obesity treatment? Pharmacol Ther (2017) 178:109–22. doi: 10.1016/j.pharmthera.2017.03.014
117. Timper K, Brüning JC. Hypothalamic circuits regulating appetite and energy homeostasis: Pathways to obesity. DMM Dis Model Mech (2017) 10:679–89. doi: 10.1242/dmm.026609
118. Routh VH, Hao L, Santiago AM, Sheng Z, Zhou C. Hypothalamic glucose sensing: Making ends meet. Front Syst Neurosci (2014) 8:236. doi: 10.3389/fnsys.2014.00236
119. Wang D, He X, Zhao Z, Feng Q, Lin R, Sun Y, et al. Whole-brain mapping of the direct inputs and axonal projections of POMC and AgRP neurons. Front Neuroanat (2015) 9:40. doi: 10.3389/fnana.2015.00040
120. Shimazu T, Fukuda A, Ban T. Reciprocal influences of the ventromedial and lateral hypothalamic nuclei on blood glucose level and liver glycogen content [38]. Nature (1966) 210:1178–9. doi: 10.1038/2101178a0
121. Fuente-Martín E, Garciá-Cáceres C, Argente-Arizón P, Diáz F, Granado M, Freire-Regatillo A, et al. Ghrelin Regulates Glucose and Glutamate Transporters in Hypothalamic Astrocytes. Sci Rep (2016) 6:23673. doi: 10.1038/srep23673
122. Yang L, Qi Y, Yang Y. Astrocytes Control Food Intake by Inhibiting AGRP Neuron Activity via Adenosine A1 Receptors. Cell Rep (2015) 11:798–807. doi: 10.1016/j.celrep.2015.04.002
123. Sweeney P, Qi Y, Xu Z, Yang Y. Activation of hypothalamic astrocytes suppresses feeding without altering emotional states. Glia (2016) 64:2263–73. doi: 10.1002/glia.23073
124. Bass J, Takahashi JS. Circadian integration of metabolism and energetics. Sci (80- ) (2010) 330:1349–54. doi: 10.1126/science.1195027
125. McDearmon EL, Patel KN, Ko CH, Walisser JA, Schook AC, Chong JL, et al. Dissecting the functions of the mammalian clock protein BMAL1 by tissue-specific rescue in mice. Sci (80- ) (2006) 314:1304–8. doi: 10.1126/science.1132430
126. Orozco-Solis R, Aguilar-Arnal L, Murakami M, Peruquetti R, Ramadori G, Coppari R, et al. The circadian clock in the ventromedial hypothalamus controls cyclic energy expenditure. Cell Metab (2016) 23:467–78. doi: 10.1016/j.cmet.2016.02.003
127. Cedernaes J, Huang W, Ramsey KM, Waldeck N, Cheng L, Marcheva B, et al. Transcriptional Basis for Rhythmic Control of Hunger and Metabolism within the AgRP Neuron. Cell Metab (2019) 29:1078–1091.e5. doi: 10.1016/j.cmet.2019.01.023
128. Lamia KA, Storch KF, Weitz CJ. Physiological significance of a peripheral tissue circadian clock. Proc Natl Acad Sci USA (2008) 105:15172–7. doi: 10.1073/pnas.0806717105
129. Doi R, Oishi K, Ishida N. CLOCK regulates circadian rhythms of hepatic glycogen synthesis through transcriptional activation of Gys2. J Biol Chem (2010) 285:22114–21. doi: 10.1074/jbc.M110.110361
130. Zhang EE, Liu Y, Dentin R, Pongsawakul PY, Liu AC, Hirota T, et al. Cryptochrome mediates circadian regulation of cAMP signaling and hepatic gluconeogenesis. Nat Med (2010) 16:1152–6. doi: 10.1038/nm.2214
131. Lamia KA, Papp SJ, Yu RT, Barish GD, Uhlenhaut NH, Jonker JW, et al. Cryptochromes mediate rhythmic repression of the glucocorticoid receptor. Nature (2011) 480:552–6. doi: 10.1038/nature10700
132. Toledo M, Batista-Gonzalez A, Merheb E, Aoun ML, Tarabra E, Feng D, et al. Autophagy Regulates the Liver Clock and Glucose Metabolism by Degrading CRY1. Cell Metab (2018) 28:268–81.e4. doi: 10.1016/j.cmet.2018.05.023
133. Vollmers C, Gill S, DiTacchio L, Pulivarthy SR, Le HD, Panda S. Time of feeding and the intrinsic circadian clock drive rhythms in hepatic gene expression. Proc Natl Acad Sci USA (2009) 106:21453–8. doi: 10.1073/pnas.0909591106
134. Storch KF, Lipan O, Leykin I, Viswanathan N, Davis FC, Wong WH, et al. Extensive and divergent circadian gene expression in liver and heart. Nature (2002) 417:78–83. doi: 10.1038/nature744
135. Panda S, Antoch MP, Miller BH, Su AI, Schook AB, Straume M, et al. Coordinated transcription of key pathways in the mouse by the circadian clock. Cell (2002) 109:307–20. doi: 10.1016/S0092-8674(02)00722-5
136. DeFronzo RA, Tripathy D. Skeletal muscle insulin resistance is the primary defect in type 2 diabetes. Diabetes Care (2009) Suppl 2:S157–63. doi: 10.2337/dc09-s302
137. Dyar KA, Ciciliot S, Wright LE, Biensø RS, Tagliazucchi GM, Patel VR, et al. Muscle insulin sensitivity and glucose metabolism are controlled by the intrinsic muscle clock. Mol Metab (2014) 3:29–41. doi: 10.1016/j.molmet.2013.10.005
138. Perrin L, Loizides-Mangold U, Chanon S, Gobet C, Hulo N, Isenegger L, et al. Transcriptomic analyses reveal rhythmic and CLOCK-driven pathways in human skeletal muscle. Elife (2018) 7:e34114. doi: 10.7554/eLife.34114
139. Hong S, Zhou W, Fang B, Lu W, Loro E, Damle M, et al. Dissociation of muscle insulin sensitivity from exercise endurance in mice by HDAC3 depletion. Nat Med (2017) 23:223–34. doi: 10.1038/nm.4245
140. Aras E, Ramadori G, Kinouchi K, Liu Y, Ioris RM, Brenachot X, et al. Light Entrains Diurnal Changes in Insulin Sensitivity of Skeletal Muscle via Ventromedial Hypothalamic Neurons. Cell Rep (2019) 27:2385–98.e3. doi: 10.1016/j.celrep.2019.04.093
141. Carrasco-Benso MP, Rivero-Gutierrez B, Lopez-Minguez J, Anzola A, Diez-Noguera A, Madrid JA, et al. Human adipose tissue expresses intrinsic circadian rhythm in insulin sensitivity. FASEB J (2016) 30:3117–23. doi: 10.1096/fj.201600269RR
142. Paschos GK, Ibrahim S, Song WL, Kunieda T, Grant G, Reyes TM, et al. Obesity in mice with adipocyte-specific deletion of clock component Arntl. Nat Med (2012) 18:1768–77. doi: 10.1038/nm.2979
143. Minokoshi Y, Haque MS, Shimazu T. Microinjection of leptin into the ventromedial hypothalamus increases glucose uptake in peripheral tissues in rats. Diabetes (1999) 18:1768–77. doi: 10.2337/diabetes.48.2.287
144. Morgan DA, McDaniel LN, Yin T, Khan M, Jiang J, Acevedo MR, et al. Regulation of glucose tolerance and sympathetic activity by MC4R signaling in the lateral hypothalamus. Diabetes (2015) 64:1976–87. doi: 10.2337/db14-1257
145. Coutinho EA, Okamoto S, Ishikawa AW, Yokota S, Wada N, Hirabayashi T, et al. Activation of SF1 neurons in the ventromedial hypothalamus by DREADD technology increases insulin sensitivity in peripheral tissues. Diabetes (2017) 66:2372–86. doi: 10.2337/db16-1344
146. Van Der Veen DR, Shao J, Chapman S, Leevy WM, Duffield GE. A diurnal rhythm in glucose uptake in brown adipose tissue revealed by in vivo PET-FDG imaging. Obesity (2012) 20:1527–9. doi: 10.1038/oby.2012.78
147. van den Berg R, Kooijman S, Noordam R, Ramkisoensing A, Abreu-Vieira G, Tambyrajah LL, et al. A Diurnal Rhythm in Brown Adipose Tissue Causes Rapid Clearance and Combustion of Plasma Lipids at Wakening. Cell Rep (2018) 22:3521–33. doi: 10.1016/j.celrep.2018.03.004
148. Nayak G, Zhang KX, Vemaraju S, Odaka Y, Buhr ED, Holt-Jones A, et al. Adaptive Thermogenesis in Mice Is Enhanced by Opsin 3-Dependent Adipocyte Light Sensing. Cell Rep (2020) 30:672–86.e8. doi: 10.1016/j.celrep.2019.12.043
149. Zhang KX, D’Souza S, Upton BA, Kernodle S, Vemaraju S, Nayak G, et al. Violet-light suppression of thermogenesis by opsin 5 hypothalamic neurons. Nature (2020) 585:420–5. doi: 10.1038/s41586-020-2683-0
150. Perelis M, Marcheva B, Ramsey KM, Schipma MJ, Hutchison AL, Taguchi A, et al. Pancreatic b cell enhancers regulate rhythmic transcription of genes controlling insulin secretion. Sci (80- ) (2015) 350:aac4250. doi: 10.1126/science.aac4250
151. Peschke E, Peschke D. Evidence for a circadian rhythm of insulin release from perifused rat pancreatic islets. Diabetologia (1998) 41:1085–92. doi: 10.1007/s001250051034
152. Sadacca LA, Lamia KA, DeLemos AS, Blum B, Weitz CJ. An intrinsic circadian clock of the pancreas is required for normal insulin release and glucose homeostasis in mice. Diabetologia (2011) 54:120–4. doi: 10.1007/s00125-010-1920-8
153. Saini C, Petrenko V, Pulimeno P, Giovannoni L, Berney T, Hebrok M, et al. A functional circadian clock is required for proper insulin secretion by human pancreatic islet cells. Diabetes Obes Metab (2016) 18:355–65. doi: 10.1111/dom.12616
154. Lee J, Kim MS, Li R, Liu VY, Fu L, Moore DD, et al. Loss of Bmal1 leads to uncoupling and impaired glucose-stimulated insulin secretion in β-cells. Islets (2011) 3:381–8. doi: 10.4161/isl.3.6.18157
155. Laposky AD, Shelton J, Bass J, Dugovic C, Perrino N, Turek FW. Altered sleep regulation in leptin-deficient mice. Am J Physiol - Regul Integr Comp Physiol (2006) 290:R894–903. doi: 10.1152/ajpregu.00304.2005
156. Laposky AD, Bradley MA, Williams DL, Bass J, Turek FW. Sleep-wake regulation is altered in leptin-resistant (db/db) genetically obese and diabetic mice. Am J Physiol - Regul Integr Comp Physiol (2008) 295:R2059–66. doi: 10.1152/ajpregu.00026.2008
157. Lesauter J, Hoque N, Weintraub M, Pfaff DW, Silver R. Stomach ghrelin-secreting cells as food-entrainable circadian clocks. Proc Natl Acad Sci USA (2009) 106:13582–7. doi: 10.1073/pnas.0906426106
158. Bélanger M, Allaman I, Magistretti PJ. Brain energy metabolism: Focus on Astrocyte-neuron metabolic cooperation. Cell Metab (2011) 14:724–38. doi: 10.1016/j.cmet.2011.08.016
159. Leloup C, Allard C, Carneiro L, Fioramonti X, Collins S, Pénicaud L. Glucose and hypothalamic astrocytes: More than a fueling role? Neuroscience (2016) 323:110–20. doi: 10.1016/j.neuroscience.2015.06.007
160. Rey G, Cesbron F, Rougemont J, Reinke H, Brunner M, Naef F. Genome-wide and phase-specific DNA-binding rhythms of BMAL1 control circadian output functions in mouse liver. PloS Biol (2011) 9:e1000595. doi: 10.1371/journal.pbio.1000595
161. Morgello S, Uson RR, Schwartz EJ, Haber RS. The human blood-brain barrier glucose transporter (GLUT1) is a glucose transporter of gray matter astrocytes. Glia (1995) 14:43–54. doi: 10.1002/glia.440140107
162. De los Angeles García M, Millán C, Balmaceda-Aguilera C, Castro T, Pastor P, Montecinos H, et al. Hypothalamic ependymal-glial cells express the glucose transporter GLUT2, a protein involved in glucose sensing. J Neurochem (2003) 86:709–24. doi: 10.1046/j.1471-4159.2003.01892.x
163. Young JK, McKenzie JC. GLUT2 immunoreactivity in Gomori-positive astrocytes of the hypothalamus. J Histochem Cytochem (2004) 52:1519–24. doi: 10.1369/jhc.4A6375.2004
164. Oomura Y, Kimura K, Ooyama H, Maeno T, Matasaburo I, Kuniyoshi M. Reciprocal activities of the ventromedial and lateral hypothalamic areas of cats. Sci (80- ) (1964) 143:484–5. doi: 10.1126/science.143.3605.484
165. Marty N, Dallaporta M, Foretz M, Emery M, Tarussio D, Bady I, et al. Regulation of glucagon secretion by glucose transporter type 2 (glut2) and astrocytedependent glucose sensors. J Clin Invest (2005) 115:3545–53. doi: 10.1172/JCI26309
166. Bady I, Marty N, Dallaporta M, Emery M, Gyger J, Tarussio D, et al. Evidence from glut2-null mice that glucose is a critical physiological regulator of feeding. Diabetes (2006) 55:988–95. doi: 10.2337/diabetes.55.04.06.db05-1386
167. Stolarczyk E, Guissard C, Michau A, Even PC, Grosfeld A, Serradas P, et al. Detection of extracellular glucose by GLUT2 contributes to hypothalamic control of food intake. Am J Physiol - Endocrinol Metab (2010) 298:E1078–87. doi: 10.1152/ajpendo.00737.2009
168. Iwashina I, Mochizuki K, Inamochi Y, Goda T. Clock genes regulate the feeding schedule-dependent diurnal rhythm changes in hexose transporter gene expressions through the binding of BMAL1 to the promoter/enhancer and transcribed regions. J Nutr Biochem (2011) 22:334–43. doi: 10.1016/j.jnutbio.2010.02.012
169. Šoltésová D, Veselá A, Mravec B, Herichová I. Daily profile of glut1 and glut4 expression in tissues inside and outside the blood-brain barrier in control and streptozotocin-treated rats. Physiol Res (2013) 62:S115–24. doi: 10.33549/physiolres.932596
170. Lam TKT, Gutierrez-Juarez R, Pocai A, Rossetti L. Medicine: Regulation of blood glucose by hypothalamic pyruvate metabolism. Sci (80- ) (2005) 309:943–7. doi: 10.1126/science.1112085
171. Magistretti PJ, Pellerin L. Cellular Bases of Brain Energy Metabolism and Their Relevance to Functional Brain Imaging: Evidence for a Prominent Role of Astrocytes. Cereb Cortex (1996) 6:50–61. doi: 10.1093/cercor/6.1.50
172. Parsons MP, Hirasawa M. ATP-sensitive potassium channel-mediated lactate effect on orexin neurons: Implications for brain energetics during arousal. J Neurosci (2010) 30:8061–70. doi: 10.1523/JNEUROSCI.5741-09.2010
173. Burt J, Alberto CO, Parsons MP, Hirasawa M. Local network regulation of orexin neurons in the lateral hypothalamus. Am J Physiol - Regul Integr Comp Physiol (2011) 301:R572–80. doi: 10.1152/ajpregu.00674.2010
174. Lundgaard I, Lu ML, Yang E, Peng W, Mestre H, Hitomi E, et al. Glymphatic clearance controls state-dependent changes in brain lactate concentration. J Cereb Blood Flow Metab (2017) 37:2112–24. doi: 10.1177/0271678X16661202
175. Cespuglio R, Netchiporouk L, Shram N. Glucose and lactate monitoring across the rat sleep-wake cycle. in. Neuromethods (2013) 80:241–56. doi: 10.1007/978-1-62703-370-1-11
176. Rutter J, Reick M, Wu LC, McKnight SL. Regulation of crock and NPAS2 DNA binding by the redox state of NAD cofactors. Sci (80- ) (2001) 293:510–4. doi: 10.1126/science.1060698
177. Wiegers EC, Rooijackers HM, Tack CJ, Heerschap A, De Galan BE, Van Der Graaf M. Brain lactate concentration falls in response to hypoglycemia in patients with type 1 diabetes and impaired awareness of hypoglycemia. Diabetes (2016) 65:1601–5. doi: 10.2337/db16-0068
178. De Feyter HM, Mason GF, Shulman GI, Rothman DL, Petersen KF. Increased brain lactate concentrations without increased lactate oxidation during hypoglycemia in type 1 diabetic individuals. Diabetes (2013) 62:3075–80. doi: 10.2337/db13-0313
179. Geissler A, Fründ R, Schölmerich J, Feuerbach S, Zietz B. Alterations of Cerebral Metabolism in Patients with Diabetes Mellitus Studied by Proton Magnetic Resonance Spectroscopy. Exp Clin Endocrinol Diabetes (2003) 111:421–7. doi: 10.1055/s-2003-44289
180. George FC. Fuel metabolism in starvation. Annu Rev Nutr (2006) 26:1–22. doi: 10.1146/annurev.nutr.26.061505.111258
181. Le Foll C, Dunn-Meynell AA, Miziorko HM, Levin BE. Regulation of hypothalamic neuronal sensing and food intake by ketone bodies and fatty acids. Diabetes (2014) 63:1259–69. doi: 10.2337/db13-1090
182. Le Foll C, Dunn-Meynell AA, Miziorko HM, Levin BE. Role of VMH ketone bodies in adjusting caloric intake to increased dietary fat content in DIO and DR rats. Am J Physiol - Regul Integr Comp Physiol (2015) 308:R872–8. doi: 10.1152/ajpregu.00015.2015
183. Carneiro L, Geller S, Hébert A, Repond C, Fioramonti X, Leloup C, et al. Hypothalamic sensing of ketone bodies after prolonged cerebral exposure leads to metabolic control dysregulation. Sci Rep (2016) 6:34909. doi: 10.1038/srep34909
184. Hwang JJ, Jiang L, Hamza M, Sanchez Rangel E, Dai F, Belfort-DeAguiar R, et al. Blunted rise in brain glucose levels during hyperglycemia in adults with obesity and T2DM. JCI Insight (2017) 2:e95913. doi: 10.1172/jci.insight.95913
185. Kullmann S, Heni M, Hallschmid M, Fritsche A, Preissl H, Häring HU. Brain insulin resistance at the crossroads of metabolic and cognitive disorders in humans. Physiol Rev (2016) 96:1169–209. doi: 10.1152/physrev.00032.2015
186. Baker LD, Cross DJ, Minoshima S, Belongia D, Stennis Watson G, Craft S. Insulin resistance and alzheimer-like reductions in regional cerebral glucose metabolism for cognitively normal adults with prediabetes or early type 2 diabetes. Arch Neurol (2011) 68:51–7. doi: 10.1001/archneurol.2010.225
187. Weightman Potter PG, Vlachaki Walker JM, Robb JL, Chilton JK, Williamson R, Randall AD, et al. Basal fatty acid oxidation increases after recurrent low glucose in human primary astrocytes. Diabetologia (2019) 62:187–98. doi: 10.1007/s00125-018-4744-6
188. Dos Santos JPA, Vizuete A, Hansen F, Biasibetti R, Gonçalves CA. Early and Persistent O-GlcNAc Protein Modification in the Streptozotocin Model of Alzheimer’s Disease. J Alzheimer’s Dis (2018) 61:237–49. doi: 10.3233/JAD-170211
189. Dias WB, Hart GW. O-GlcNAc modification in diabetes and Alzheimer’s disease. Mol Biosyst (2007) 3:766–72. doi: 10.1039/b704905f
190. Parker GJ, Lund KC, Taylor RP, McClain DA. Insulin resistance of glycogen synthase mediated by O-linked N-acetylglucosamine. J Biol Chem (2003) 278:10022–7. doi: 10.1074/jbc.M207787200
191. Parker G, Taylor R, Jones D, McClain D. Hyperglycemia and inhibition of glycogen synthase in streptozotocin-treated mice: Role of O-linked N-acetylglucosamine. J Biol Chem (2004) 279:20636–42. doi: 10.1074/jbc.M312139200
192. Dosch M, Gerber J, Jebbawi F, Beldi G. Mechanisms of ATP release by inflammatory cells. Int J Mol Sci (2018) 19:1222. doi: 10.3390/ijms19041222
193. Cai W, Xue C, Sakaguchi M, Konishi M, Shirazian A, Ferris HA, et al. Insulin regulates astrocyte gliotransmission and modulates behavior. J Clin Invest (2018) 128:2914–26. doi: 10.1172/JCI99366
194. Juaristi I, Llorente-Folch I, Satrústegui J, del Arco A. Extracellular ATP and glutamate drive pyruvate production and energy demand to regulate mitochondrial respiration in astrocytes. Glia (2019) 67:759–74. doi: 10.1002/glia.23574
195. Lemos C, Pinheiro BS, Beleza RO, Marques JM, Rodrigues RJ, Cunha RA, et al. Adenosine A2B receptor activation stimulates glucose uptake in the mouse forebrain. Purinergic Signal (2015) 11:561–9. doi: 10.1007/s11302-015-9474-3
196. Jiang LH, Mousawi F, Yang X, Roger S. ATP-induced Ca2+-signalling mechanisms in the regulation of mesenchymal stem cell migration. Cell Mol Life Sci (2017) 74:3697–710. doi: 10.1007/s00018-017-2545-6
197. Colldén G, Mangano C, Meister B. P2X2 purinoreceptor protein in hypothalamic neurons associated with the regulation of food intake. Neuroscience (2010) 171:62–78. doi: 10.1016/j.neuroscience.2010.08.036
198. Jo YH, Donier E, Martinez A, Garret M, Toulmé E, Boué-Grabot E. Cross-talk between P2X4 and γ-aminobutyric acid, type A receptors determines synaptic efficacy at a central synapse. J Biol Chem (2011) 286:19993–20004. doi: 10.1074/jbc.M111.231324
199. Marpegan L, Swanstrom AE, Chung K, Simon T, Haydon PG, Khan SK, et al. Circadian regulation of ATP release in astrocytes. J Neurosci (2011) 31:8342–50. doi: 10.1523/JNEUROSCI.6537-10.2011
200. Mackiewicz M, Nikonova EV, Zimmerman JE, Galante RJ, Zhang L, Cater JR, et al. Enzymes of adenosine metabolism in the brain: Diumal rhythm and the effect of sleep deprivation. J Neurochem (2003) 85:348–57. doi: 10.1046/j.1471-4159.2003.01687.x
201. Verkhratsky A, Nedergaard M. Physiology of astroglia. Physiol Rev (2018) 98:239–389. doi: 10.1152/physrev.00042.2016
202. Young JK, Baker JH, Montes MI. The brain response to 2-deoxy glucose is blocked by a glial drug. Pharmacol Biochem Behav (2000) 67:233–9. doi: 10.1016/S0091-3057(00)00315-4
203. Barca Mayo O, Berdondini L, De Pietri Tonelli D. “Astrocytes and circadian rhythms: An emerging astrocyte–neuron synergy in the timekeeping system,” in. Methods Mol Biol (2019) 1938:131–54. doi: 10.1007/978-1-4939-9068-9_10
204. Zhang Y, Reichel JM, Han C, Zuniga-Hertz JP, Cai D. Astrocytic Process Plasticity and IKKβ/NF-κB in Central Control of Blood Glucose, Blood Pressure, and Body Weight. Cell Metab (2017) 25:1091–102.e4. doi: 10.1016/j.cmet.2017.04.002
205. Chen N, Sugihara H, Kim J, Fu Z, Barak B, Sur M, et al. Direct modulation of GFAP-expressing glia in the arcuate nucleus bi-directionally regulates feeding. Elife (2016) 5:e18716. doi: 10.7554/eLife.18716
206. Chowdhury GMI, Wang P, Ciardi A, Mamillapalli R, Johnson J, Zhu W, et al. Impaired glutamatergic neurotransmission in the ventromedial hypothalamus may contribute to defective counterregulation in recurrently hypoglycemic rats. in. Diabetes (2017) 66:1979–89. doi: 10.2337/db16-1589
207. Guyenet SJ, Matsen ME, Morton GJ, Kaiyala KJ, Schwartz MW. Rapid glutamate release in the mediobasal hypothalamus accompanies feeding and is exaggerated by an obesogenic food. Mol Metab (2013) 2:116–22. doi: 10.1016/j.molmet.2013.02.001
208. Fuente-Martiń E, García-Cáceres C, Granado M, De Ceballos ML, Sánchez-Garrido MÁ, Sarman B, et al. Leptin regulates glutamate and glucose transporters in hypothalamic astrocytes. J Clin Invest (2012) 122:3900–13. doi: 10.1172/JCI64102
209. Thaler JP, Yi CX, Schur EA, Guyenet SJ, Hwang BH, Dietrich MO, et al. Obesity is associated with hypothalamic injury in rodents and humans. J Clin Invest (2012) 122:153–62. doi: 10.1172/JCI59660
210. Reis WL, Yi CX, Gao Y, Tschöp MH, Stern JE. Brain innate immunity regulates hypothalamic arcuate neuronal activity and feeding behavior. Endocrinology (2015) 156:1303–15. doi: 10.1210/en.2014-1849
211. Chen Y, Liu G, He F, Zhang L, Yang K, Yu H, et al. MicroRNA 375 modulates hyperglycemia-induced enteric glial cell apoptosis and Diabetes-induced gastrointestinal dysfunction by targeting Pdk1 and repressing PI3K/Akt pathway. Sci Rep (2018) 8:12681. doi: 10.1038/s41598-018-30714-0
212. Konturek PC, Brzozowski T, Konturek SJ. Gut clock: Implication of circadian rhythms in the gastointestinal tract. J Physiol Pharmacol (2011) 62:139–50. doi: 10.2337/db13-1501
213. Gil-Lozano M, Mingomataj EL, Wu WK, Ridout SA, Brubaker PL. Circadian secretion of the intestinal hormone GLP-1 by the rodent L cell. Diabetes (2014) 63:3674–85. doi: 10.2337/db13-1501
214. Brubaker PL, Gil-Lozano M. Glucagon-like peptide-1: The missing link in the metabolic clock? J Diabetes Investig (2016) 7:70–5. doi: 10.1111/jdi.12477
215. Martchenko A, Oh RH, Wheeler SE, Gurges P, Chalmers JA, Brubaker PL. Suppression of circadian secretion of glucagon-like peptide-1 by the saturated fatty acid, palmitate. Acta Physiol (2018) 222:e13007. doi: 10.1111/apha.13007
216. Thaiss CA, Levy M, Korem T, Dohnalová L, Shapiro H, Jaitin DA, et al. Microbiota Diurnal Rhythmicity Programs Host Transcriptome Oscillations. Cell (2016) 167:1495–510.e12. doi: 10.1016/j.cell.2016.11.003
217. Woon PY, Kaisaki PJ, Bragança J, Bihoreau MT, Levy JC, Farrall M, et al. Aryl hydrocarbon receptor nuclear translocator-like (BMAL1) is associated with susceptibility to hypertension and type 2 diabetes. Proc Natl Acad Sci USA (2007) 104:14412–7. doi: 10.1073/pnas.0703247104
218. Scott EM, Carter AM, Grant PJ. Association between polymorphisms in the Clock gene, obesity and the metabolic syndrome in man. Int J Obes (2008) 32:658–62. doi: 10.1038/sj.ijo.0803778
219. Sookoian S, Gemma C, Gianotti TF, Burgueño A, Castaño G, Pirola CJ. Genetic variants of Clock transcription factor are associated with individual susceptibility to obesity. Am J Clin Nutr (2008) 87:1606–15. doi: 10.1093/ajcn/87.6.1606
220. Dupuis J, Langenberg C, Prokopenko I, Saxena R, Soranzo N, Jackson AU, et al. New genetic loci implicated in fasting glucose homeostasis and their impact on type 2 diabetes risk. Nat Genet (2010) 42:105–16. doi: 10.1038/ng.520
221. Barker A, Sharp SJ, Timpson NJ, Bouatia-Naji N, Warrington NM, Kanoni S, et al. Association of genetic loci with glucose levels in childhood and adolescence: A meta-analysis of over 6,000 children. Diabetes (2011) 60:1805–12. doi: 10.2337/db10-1575
222. Marcheva B, Ramsey KM, Buhr ED, Kobayashi Y, Su H, Ko CH, et al. Disruption of the clock components CLOCK and BMAL1 leads to hypoinsulinaemia and diabetes. Nature (2010) 466:627–31. doi: 10.1038/nature09253
223. Hogenboom R, Kalsbeek MJ, Korpel NL, de Goede P, Koenen M, Buijs RM, et al. Loss of arginine vasopressin- and vasoactive intestinal polypeptide-containing neurons and glial cells in the suprachiasmatic nucleus of individuals with type 2 diabetes. Diabetologia (2019) 62:2088–93. doi: 10.1007/s00125-019-4953-7
224. Fonken LK, Nelson RJ. The effects of light at night on circadian clocks and metabolism. Endocr Rev (2014) 35:648–70. doi: 10.1210/er.2013-1051
225. Fonken LK, Workman JL, Walton JC, Weil ZM, Morris JS, Haim A, et al. Light at night increases body mass by shifting the time of food intake. Proc Natl Acad Sci USA (2010) 107:18664–9. doi: 10.1073/pnas.1008734107
226. Obayashi K, Saeki K, Iwamoto J, Ikada Y, Kurumatani N. Independent associations of exposure to evening light and nocturnal urinary melatonin excretion with diabetes in the elderly. Chronobiol Int (2014) 31:394–400. doi: 10.3109/07420528.2013.864299
227. Obayashi K, Saeki K, Iwamoto J, Okamoto N, Tomioka K, Nezu S, et al. Exposure to light at night, nocturnal urinary melatonin excretion, and obesity/dyslipidemia in the elderly: A cross-sectional analysis of the HEIJO-KYO study. J Clin Endocrinol Metab (2013) 98:337–44. doi: 10.1210/jc.2012-2874
228. McFadden E, Jones ME, Schoemaker MJ, Ashworth A, Swerdlow AJ. The relationship between obesity and exposure to light at night: Cross-sectional analyses of over 100,000 women in the breakthrough generations study. Am J Epidemiol (2014) 180:245–50. doi: 10.1093/aje/kwu117
229. Cheung IN, Zee PC, Shalman D, Malkani RG, Kang J, Reid KJ. Morning and Evening Blue-Enriched Light Exposure Alters Metabolic Function in Normal Weight Adults. PloS One (2016) 11:e0155601. doi: 10.1371/journal.pone.0155601
230. Versteeg RI, Stenvers DJ, Visintainer D, Linnenbank A, Tanck MW, Zwanenburg G, et al. Acute Effects of Morning Light on Plasma Glucose and Triglycerides in Healthy Men and Men with Type 2 Diabetes. J Biol Rhythms (2017) 32:130–42. doi: 10.1177/0748730417693480
231. Aton SJ, Colwell CS, Harmar AJ, Waschek J, Herzog ED. Vasoactive intestinal polypeptide mediates circadian rhythmicity and synchrony in mammalian clock neurons. Nat Neurosci (2005) 8:476–83. doi: 10.1038/nn1419
232. Colwell CS, Michel S, Itri J, Rodriguez W, Tam J, Lelievre V, et al. Disrupted circadian rhythms in VIP- and PHI-deficient mice. Am J Physiol - Regul Integr Comp Physiol (2003) 285:R939–49. doi: 10.1152/ajpregu.00200.2003
233. Takahashi Y, Okamura H, Yanaihara N, Hamada S, Fujita S, Ibata Y. Vasoactive intestinal peptide immunoreactive neurons in the rat suprachiasmatic nucleus demonstrate diurnal variation. Brain Res (1989) 497:374–7. doi: 10.1016/0006-8993(89)90283-7
234. Ohta H, Yamazaki S, McMahon DG. Constant light desynchronizes mammalian clock neurons. Nat Neurosci (2005) 8:267–9. doi: 10.1038/nn1395
235. Shan Z, Ma H, Xie M, Yan P, Guo Y, Bao W, et al. Sleep duration and risk of type 2 diabetes: A meta-analysis of prospective studies. Diabetes Care (2015) 38:529–37. doi: 10.2337/dc14-2073
236. Cappuccio FP, D’Elia L, Strazzullo P, Miller MA. Quantity and quality of sleep and incidence of type 2 diabetes: A systematic review and meta-analysis. Diabetes Care (2010) 33:414–20. doi: 10.2337/dc09-1124
237. Bosy-Westphal A, Hinrichs S, Jauch-Chara K, Hitze B, Later W, Wilms B, et al. Influence of partial sleep deprivation on energy balance and insulin sensitivity in healthy women. Obes Facts (2008) 1:266–73. doi: 10.1159/000158874
238. Nedeltcheva AV, Kilkus JM, Imperial J, Kasza K, Schoeller DA, Penev PD. Sleep curtailment is accompanied by increased intake of calories from snacks. Am J Clin Nutr (2009) 89:126–33. doi: 10.3945/ajcn.2008.26574
239. Reutrakul S, Mokhlesi B. Obstructive Sleep Apnea and Diabetes: A State of the Art Review. Chest (2017) 152:1070–86. doi: 10.1016/j.chest.2017.05.009
240. Spiegel K, Leproult R, Van Cauter E. Impact of sleep debt on metabolic and endocrine function. Lancet (1999) 354:1435–9. doi: 10.1016/S0140-6736(99)01376-8
241. Stamatakis KA, Punjabi NM. Effects of sleep fragmentation on glucose metabolism in normal subjects. Chest (2010) 137:95–101. doi: 10.1378/chest.09-0791
242. Rao MN, Neylan TC, Grunfeld C, Mulligan K, Schambelan M, Schwarz JM. Subchronic sleep restriction causes tissue-specific insulin resistance. J Clin Endocrinol Metab (2015) 100:1664–71. doi: 10.1210/jc.2014-3911
243. Halassa MM, Fellin T, Haydon PG. Tripartite synapses: Roles for astrocytic purines in the control of synaptic physiology and behavior. Neuropharmacology (2009) 57:343–6. doi: 10.1016/j.neuropharm.2009.06.031
244. Vetter C, Dashti HS, Lane JM, Anderson SG, Schernhammer ES, Rutter MK, et al. Night shift work, genetic risk, and type 2 diabetes in the UK biobank. Diabetes Care (2018) 41:762–9. doi: 10.2337/dc17-1933
245. Gan Y, Yang C, Tong X, Sun H, Cong Y, Yin X, et al. Shift work and diabetes mellitus: A meta-analysis of observational studies. Occup Environ Med (2015) 72:72–8. doi: 10.1136/oemed-2014-102150
246. Leproult R, Holmbäck U, Van Cauter E. Circadian misalignment augments markers of insulin resistance and inflammation, independently of sleep loss. Diabetes (2014) 63:1860–9. doi: 10.2337/db13-1546
247. Qian J, Dalla Man C, Morris CJ, Cobelli C, Scheer FAJL. Differential effects of the circadian system and circadian misalignment on insulin sensitivity and insulin secretion in humans. Diabetes Obes Metab (2018) 20:2481–5. doi: 10.1111/dom.13391
248. Wefers J, Van Moorsel D, Hansen J, Connell NJ, Havekes B, Hoeks J, et al. Circadian misalignment induces fatty acid metabolism gene profiles and compromises insulin sensitivity in human skeletal muscle. Proc Natl Acad Sci USA (2018) 115:7789–94. doi: 10.1073/pnas.1722295115
249. Scheer FAJL, Hilton MF, Mantzoros CS, Shea SA. Adverse metabolic and cardiovascular consequences of circadian misalignment. Proc Natl Acad Sci USA (2009) 106:4453–8. doi: 10.1073/pnas.0808180106
Keywords: astrocytes, circadian clock, metabolism, diabetes, glucose homeostasis
Citation: Barca-Mayo O and López M (2021) Astrocyte Clocks and Glucose Homeostasis. Front. Endocrinol. 12:662017. doi: 10.3389/fendo.2021.662017
Received: 31 January 2021; Accepted: 01 March 2021;
Published: 18 March 2021.
Edited by:
Cristina García Cáceres, Ludwig Maximilian University of Munich, GermanyReviewed by:
Alexandre Benani, Centre National de la Recherche Scientifique (CNRS), FrancePaulo Kofuji, University of Minnesota Twin Cities, United States
Copyright © 2021 Barca-Mayo and López. This is an open-access article distributed under the terms of the Creative Commons Attribution License (CC BY). The use, distribution or reproduction in other forums is permitted, provided the original author(s) and the copyright owner(s) are credited and that the original publication in this journal is cited, in accordance with accepted academic practice. No use, distribution or reproduction is permitted which does not comply with these terms.
*Correspondence: Olga Barca-Mayo, b2xnYS5iYXJjYS5tYXlvQHVzYy5lcw==