- 1Instituto de Investigaciones Biomédicas “Alberto Sols”, Consejo Superior de Investigaciones Científicas (CSIC) y Universidad Autónoma de Madrid (UAM), Madrid, Spain
- 2Laboratorio de Investigación Aplicada en Enfermedades Neuromusculares, Unidad de Patología Neuromuscular, Servicio de Neuropediatría, Institut de Recerca Sant Joan de Déu, Esplugues de Llobregat, Spain
- 3Centro de Investigación Biomédica en Red (CIBERONC), Instituto de Salud Carlos III, Madrid, Spain
Thyroid differentiation of progenitor cells occurs during embryonic development and in the adult thyroid gland, and the molecular bases of these complex and finely regulated processes are becoming ever more clear. In this Review, we describe the most recent advances in the study of transcription factors, signaling molecules and regulatory pathways controlling thyroid differentiation and development in the mammalian embryo. We also discuss the maintenance of the adult differentiated phenotype to ensure the biosynthesis of thyroid hormones. We will focus on endoderm-derived thyroid epithelial cells, which are responsible for the formation of the thyroid follicle, the functional unit of the thyroid gland. The use of animal models and pluripotent stem cells has greatly aided in providing clues to the complicated puzzle of thyroid development and function in adults. The so-called thyroid transcription factors – Nkx2-1, Foxe1, Pax8 and Hhex – were the first pieces of the puzzle identified in mice. Other transcription factors, either acting upstream of or directly with the thyroid transcription factors, were subsequently identified to, almost, complete the puzzle. Among them, the transcription factors Glis3, Sox9 and the cofactor of the Hippo pathway Taz, have emerged as important players in thyroid differentiation and development. The involvement of signaling molecules increases the complexity of the puzzle. In this context, the importance of Bmps, Fgfs and Shh signaling at the onset of development, and of TSH, IGF1 and TGFβ both at the end of terminal differentiation in embryos and in the adult thyroid, are well recognized. All of these aspects are covered herein. Thus, readers will be able to visualize the puzzle of thyroid differentiation with most – if not all – of the pieces in place.
Introduction
Consistent with its important hormonal role in embryonic development, the thyroid gland is one of the first endocrine organs to differentiate. In mammals, the thyroid forms as an evagination of endodermal epithelial cells on the median surface of the developing pharyngeal floor. Two main cell types characterize the thyroid gland: epithelial or follicular cells and the parafollicular cells. The former, also known as thyrocytes, represent the majority of cells and are responsible for the synthesis and secretion of the thyroid hormones. The latter, also termed C-cells, are responsible for calcitonin synthesis and secretion. For many years it was believed that these two thyroid cell types derived from endoderm and neural crest, respectively (1). However, it is currently accepted that both are of endodermal origin (2), with the C-cell precursors arising from the ultimobranchial bodies that derive from the ventral recess of the fourth pharyngeal pouch (3).
In this review, we will focus on the follicular cells in mammals, with some illustrative examples from zebrafish and chicken, as these animal models have contributed substantially to our understanding of thyroid development. Polarized thyroid follicular cells, with a basal and an apical membrane, are responsible for the formation of the thyroid follicles. After its specification and budding in the pharynx floor, the thyroid primordium undergoes a series of events that involve complex morphological and differentiation processes (4). First, the thyroid follicular progenitor cells expand ventrally, as a diverticulum, and begin to migrate caudally downward from the bud to their tracheal position. Once the thyroid reaches its final position on both sides of the trachea, the thyroid lobes enlarge to form the distinctive bilobed (butterfly) shape. Subsequently, folliculogenesis takes place, which is a complex and essential mechanism for the terminal differentiation program, and occurs only when migration is complete and the follicles are formed.
At this time, thyroid cells start to express the thyroglobulin (Tg), thyroperoxidase (Tpo), TSH receptor (Tshr) and sodium (Na) iodide (I) symporter NIS (Slc5a5) genes, which are considered as the thyroid differentiation genes in this review. Together with other genes including dual oxidase 2 (Duox2), the membrane transporters pendrin (Slc26a4), monocarboxylate transporter 8 Mct8 (Slc16a2) and dehalogenase Dehal1 (IYD), these differentiation genes are required for the biosynthesis of the thyroid hormones triiodothyronine (T3) and tetraiodothyronine/thyroxine (T4). All stages of thyroid development are depicted in Figure 1, with the indication of the observed times at which they are expressed in mouse and human; the genes expressed in a differentiated thyroid follicular cell are depicted in Figure 2.
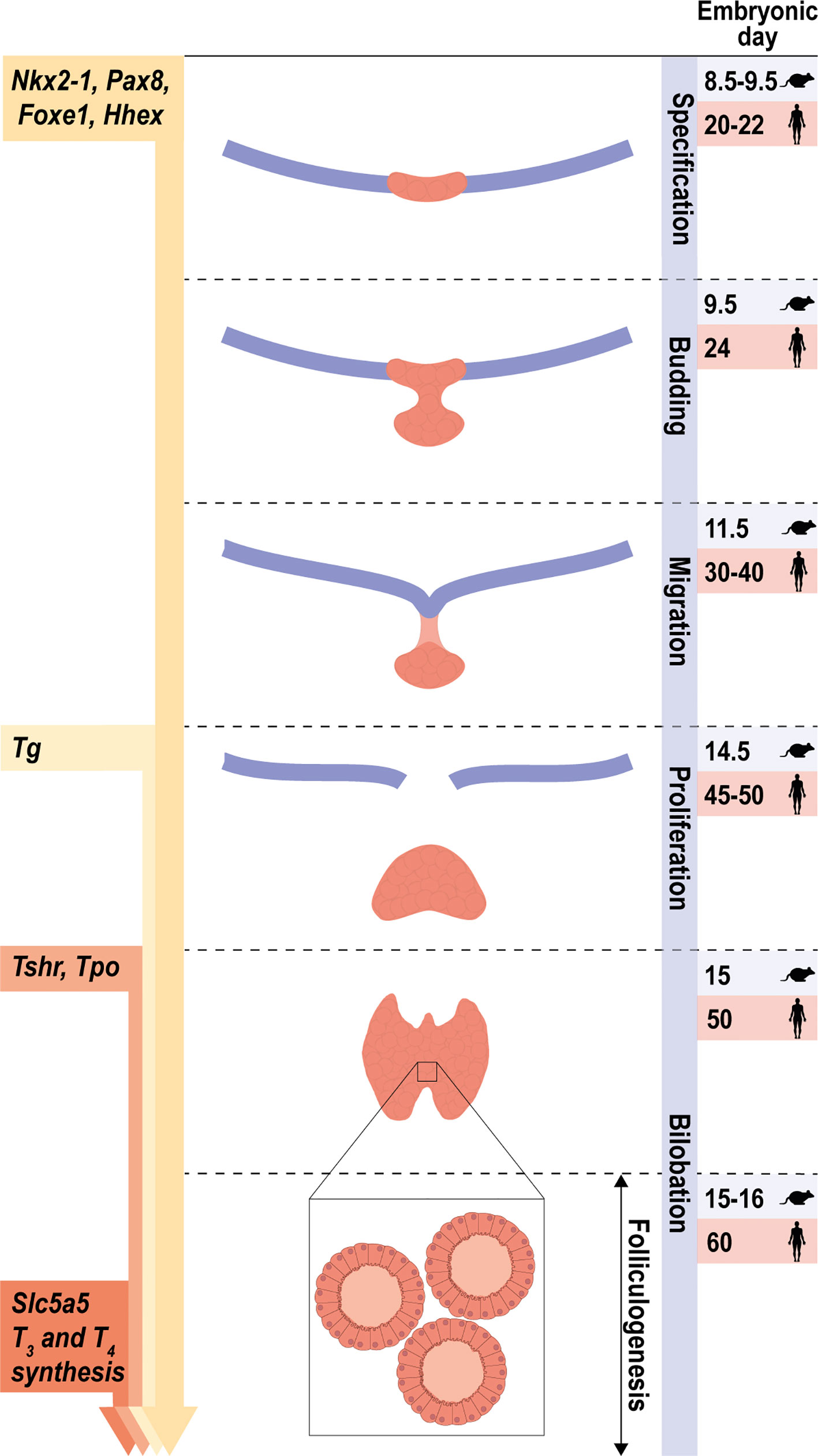
Figure 1 Stages of early thyroid development. Schematic representation of the main steps of thyroid morphogenesis indicating the corresponding embryonic or post-fertilization day in mice and humans, respectively. From an undifferentiated endoderm, the co-expression of Nkx2-1, Foxe1, Pax8 and Hhex defines the specification of thyroid progenitor cells. Arrows on the left represent the initial stage at which the expression of the thyroid transcription factors and their main target genes are first detected. The onset of thyroid hormone synthesis and Slc5a5 expression occurs at the final stage, once folliculogenesis has occurred and the thyroid gland is completely functional.
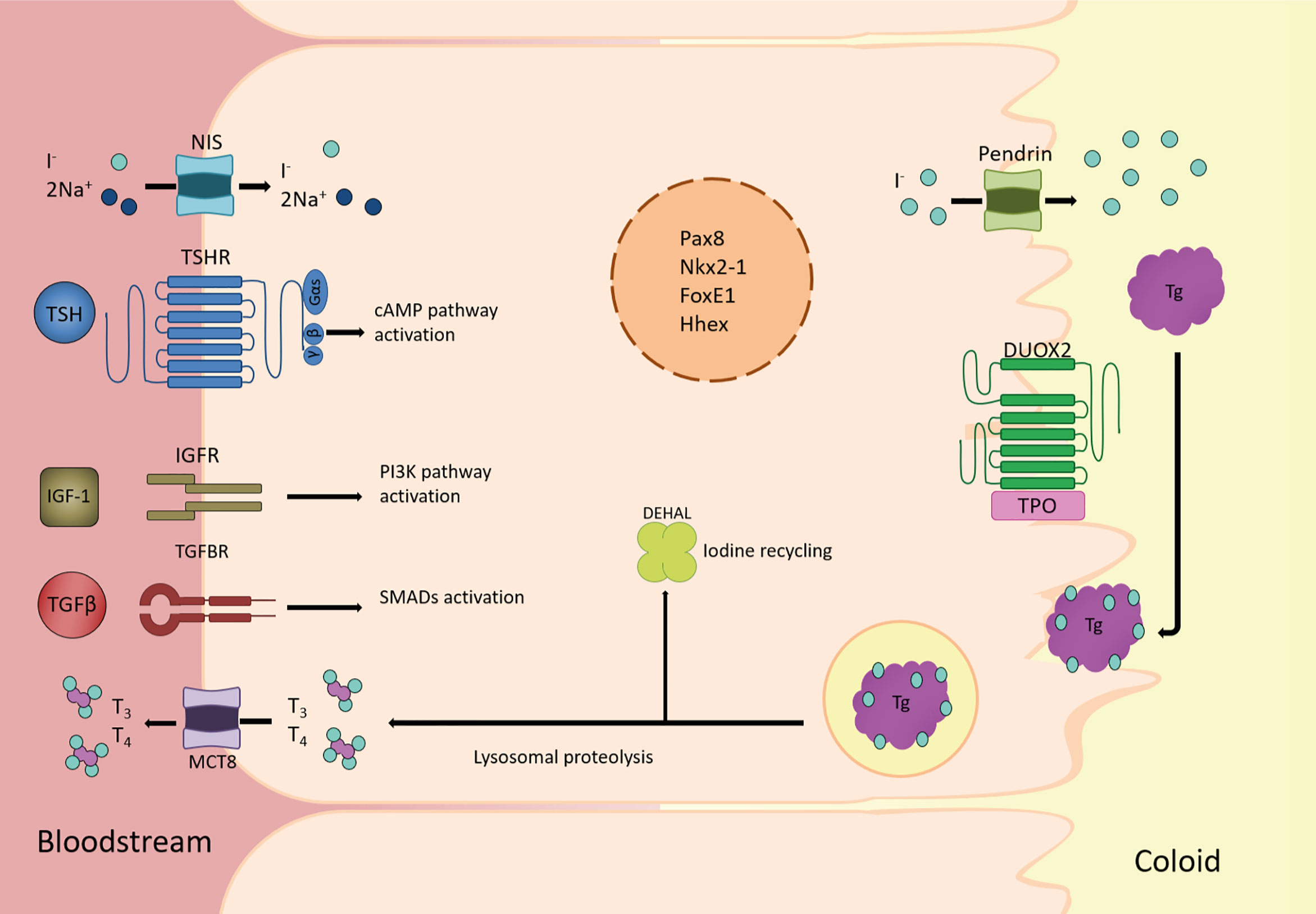
Figure 2 Overview of the main players in thyroid follicular cell function. Schematic diagram of a thyroid follicular cell and the main proteins involved in the synthesis, storage, and release into bloodstream of the thyroid hormones triiodothyronine (T3) and thyroxine (T4). At the basolateral membrane, circulating iodide (I-) is actively taken-up by the Nis symporter. It then passively diffuses to the apical membrane where it is actively transported to the lumen by Pendrin and other transporters. Once I- is oxidized by thyroid peroxidase (Tpo), using H2O2 generated by the oxidase Duox2, tyrosine residues in thyroglobulin (Tg) are iodinated to form mono-iodotyrosine (MIT) and/or di-iodotyrosine (DIT), whose coupling yields thyroid hormones. Colloid droplets rich in Tg are endocytosed into the thyroid follicular cell, where Tg lysosomal proteolysis releases MIT, DIT, T4, and T3. Thyroid hormones are transported to circulation by the monocarboxylate transporter 8 (Mct8), whereas I- from MIT and DIT is recycled by the iodotyrosine deiodinase (Dehal1). All of these processes rely on the co-existence of the thyroid transcription factors Nkx2-1, Foxe1, Pax8 and Hhex, in the nucleus of the thyroid follicular cell, from where they regulate the expression of genes such as Slc5a5, Tpo, Tg and Tshr. Also, in the basolateral membrane, TSH, IGF1 and TGFβ trigger the main pathways involved in adult maintenance of thyroid differentiation by cAMP, PI3K and SMAD activation, respectively.
Differentiated thyroid epithelial cells form follicular structures surrounding a lumen, with the resulting follicle considered as the functional unit of the thyroid gland. Recent studies, however, have revealed that the elements that comprise the functional unit of the thyroid are more complex than originally thought. In this regard, the concept of an angiofollicular unit has been proposed, which also includes endothelial cells of the blood vessels that sheath the thyroid follicles, and which is formed during embryonic development. The intimate relationship between the blood microvasculature and the thyroid follicle is essential for thyroid homeostasis and function (5). Differentiation is sustained throughout life, and must be finely regulated to maintain a controlled production of thyroid hormones. The mechanisms that control thyroid differentiation in the embryo occur simultaneously, with cross-talk between all the actors playing a role in this finely orchestrated process. To provide a more systematic and didactic review, we will organize the work by first defining the role of the thyroid transcription factors (TTFs) and then the signals that regulate the different steps in thyroid development. We will discuss the most current knowledge of new players in all the processes involved in achieving a differentiated thyroid functional unit in the embryo and the adult.
We will mainly refer to data obtained from mice as the most recent advances come from studies in this model system. However, we will translate this information to humans when possible. We will follow the standard nomenclature; thus, proteins will be referred to in capital letters for humans and a capital followed by lowercase for mice. Italics will be used when referring to genes.
Thyroid Transcription Factors
Differentiated follicular cells express four genes encoding the so-called TTFs: these are Nkx2-1 (formerly known as thyroid transcription factor 1, Ttf1 or T/ebp), Foxe1 (formerly known as thyroid transcription factor 2, Ttf2), Pax8 and Hhex (hematopoietically-expressed homeobox protein) (4, 6, 7). These genes are members of the forkhead-, paired- and homeo-box family of transcription factors, and were initially identified through their promoter binding sites on thyroid differentiation genes such as Tg, Tpo, Tshr or Slc5a5 (7–9) (Figure 3). Most of the knowledge on the TTFs derives from the use of mouse models, embryonic stem (ESCs), induced pluripotent stem (iPSCs) and differentiated cell cultures, and advances in the understanding of their regulation and function have contributed to establishing the molecular mechanisms involved in thyroid differentiation. Although there are many reviews on TTFs, below we will provide a brief summary of each one, focusing mainly on its role in the complex differentiation processes that occur from embryos to adulthood for the correct function of the thyroid gland.
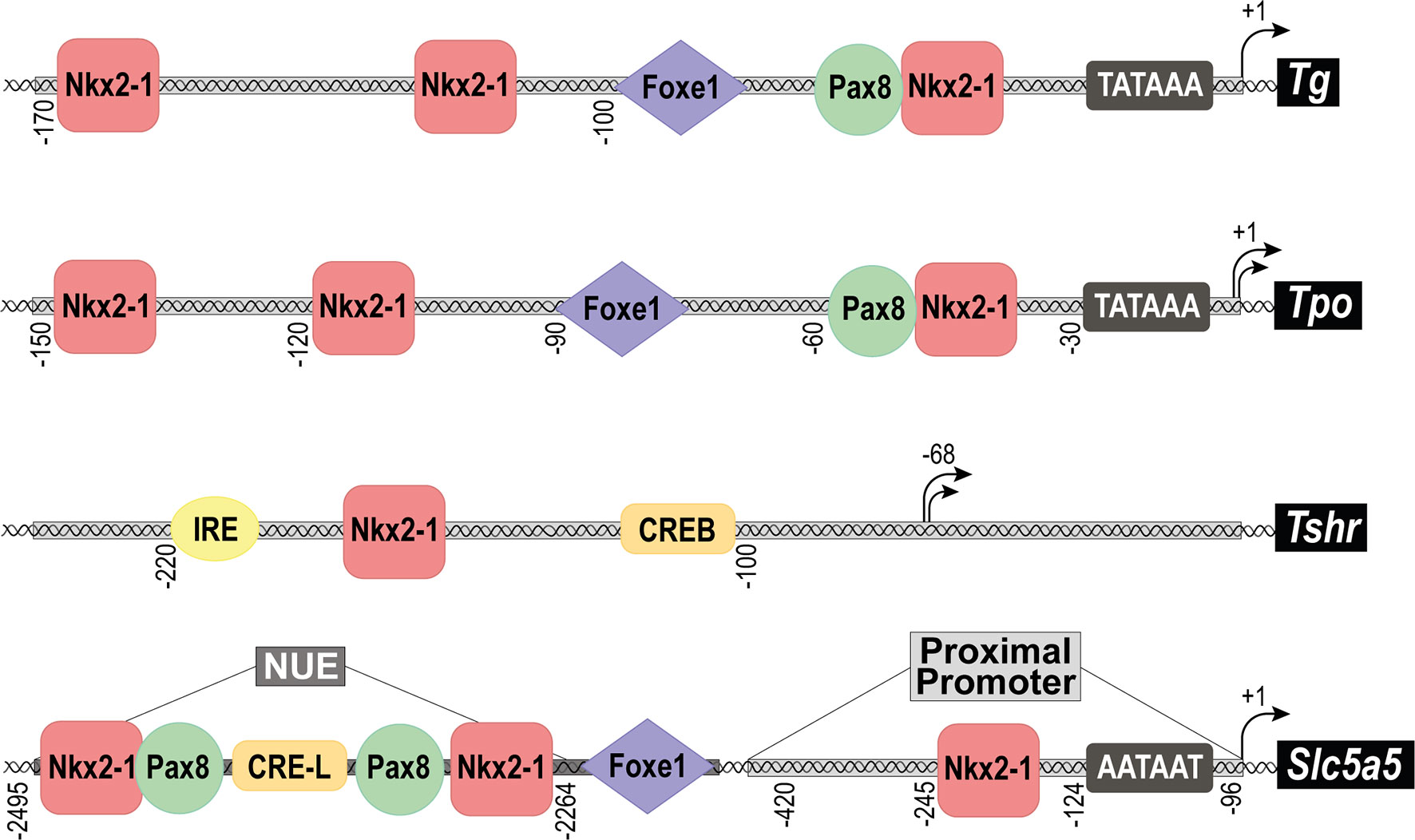
Figure 3 Structure of the promoter regions of the main thyroid differentiation genes. Schematic representation of the main transcription factor binding sites found in the promoter regions of the genes: Thyroglobulin (Tg), Thyroid peroxidase (Tpo), TSH receptor (Tshr) and Sodium/iodide symporter (Nis, Slc5a5). In the case of Slc5a5, the proximal promoter, and the Nis Upstream Enhancer (NUE) are separately indicated. Black arrows represent the transcriptional initiation site of each promoter and numbers define its position. The TATA box is indicated as well as the binding sites for transcription factors: Nkx2-1, Foxe1, Pax8, CREB, CRE-L (CRE-Like) and IRE (Insulin Respose Element).
Nk2 Homeobox 1, Nkx2-1
The homeodomain transcription factor Nkx2-1 was initially cloned and identified in rat thyroid cells and in mouse thyroid (10, 11), and was later observed to be widely distributed in other organs. It is expressed in both follicular and parafollicular cells, and also in other tissues such as lung, forebrain, and hypothalamus (12). Its expression in the mouse endoderm at embryonic day (E) 8.5 coincides with the recruitment of the cells from the adjacent endoderm that ultimately form the thyroid bud, prior to migration, and it remains expressed along embryonic development and in adult life. Nkx2-1-null mice die at birth from impairment of lung organogenesis and lack thyroid and pituitary glands, and exhibit severe abnormalities in the ventral forebrain (13). Although the thyroid is absent in Nkx2-1-null mice the bud still forms, but disappears later by apoptosis, pointing to a role for Nkx2-1 in the survival of thyroid precursor cells but not in their specification (14). In mice in which Nkx2-1 expression is inhibited at later stages of embryonic development, a structure can be observed with abnormal thyroid follicles, indicating that Nkx2-1 plays an important role in maintaining tissue architecture and cell function in the adult thyroid (15), as we will discuss later in the follicle formation section.
Forkhead Box E1, Foxe1
Foxe1 was initially cloned and identified in rat thyroid cells, and encodes a transcription factor containing a forkhead domain (16). Like Nkx2-1, it has a broad expression pattern in other embryonic tissues such as tongue, epiglottis, palate, hair follicles and pituitary, among others. It has also been detected in many adult organs, including testis, brain, pancreas kidney and intestine (7). Foxe1 is expressed at E8.5 in the endoderm lining the foregut and also in the cells that form the thickening primordium in the midline of the pharyngeal floor in the embryonic mouth cavity. Accordingly, its expression in the embryo is more evident in the posterior pharynx than in the thyroid precursor cells where it is maintained throughout development to adult life. Foxe1-null mice die shortly after birth due to a severe cleft palate phenotype. While the thyroid bud forms normally in these mice, the precursor cells are unable to migrate to the tracheal position at E9.5 and remain in the pharyngeal endoderm (6). Foxe1-null mice exhibit one of two thyroid phenotypes: athyreosis or ectopic thyroid (usually with a sublingual position). In contrast to mice, no thyroid ectopy has been observed in the absence of foxe1 in zebrafish (17). Furthermore, in humans no case of thyroid ectopy due to mutations in FOXE1 has been described to date, with the exception of polymorphism in its polyA tail (18). Based on this, it has been proposed that the differences between the two phenotypes might be due to stochastic events or to variations in the genetic backgrounds or sex-related factors of the mice (19). There is evidence, however, to support the potential role of Foxe1 in the migration of thyroid cell precursors from the anlage to their final position in the trachea. For instance, the reintroduction of Foxe1 exclusively in precursor cells recovers the proper gland position in Foxe1-null mice, indicating a role for this factor as the main regulator of thyroid migration (19). The active migration of the thyroid cell precursors is still a matter of controversy, as it has been described that these cells do not undergo the classical epithelial to mesenchymal transition during morphogenesis (20). But, there is other evidence to suggest that Foxe1 directly regulates the expression of Msx1 and Tgfb3, which are involved in the epithelial to mesenchymal transition and, consequently, in cell migration (21). It is plausible that both active migration by a novel mechanism and other morphogenetic events are involved in the final positioning of the thyroid gland. Thus, while further studies are needed there are strong indications to suggest that Foxe1 controls the migration of thyroid precursors to their definitive position in the anterior neck region on both sides of the trachea (6, 22). Another question that remains to be answered is whether migration is a necessary process for the terminal differentiation of thyroid gland as Foxe1-null mice, with a sublingual thyroid, express Tg (6).
Paired Box 8, Pax8
Pax8 was initially described in mouse kidney and later in thyroid, which is the only endoderm-derived organ where it is expressed. At E8.5, and coincident with the time of specification, Pax8 is expressed concomitantly with Nkx2-1 in the thickening primordium in the midline of the pharyngeal floor. Its expression is maintained in the thyroid, as well as in the kidney, ureter and genital tract, from all stages of embryonic development to adulthood. It is also expressed transiently in the brain during development but not at later, adult stages. Pax8-null mice are born alive, but show growth retardation likely due to low levels of thyroid hormones (23). The thyroid gland is devoid of follicular cells, and is therefore not functional, suggesting athyreosis. Pax8-null mice are also infertile and die at early stages unless administered T4, although this treatment does not recover the infertility due to a primary impairment in the reproductive system (24). The rudiment of the thyroid gland in Pax8-null mice contains only parafollicular cells expressing Nkx2-1 but none of the thyroid-specific genes, and the gland fails to expand laterally and the final lobular structure is lost. This phenotype demonstrates that, in addition to being necessary for the survival of thyroid precursors, Pax8 is essential for follicle formation and functional differentiation of thyroid cells at later stages of thyroid development. Accordingly, this gene is critical for the maintenance of the differentiated thyroid phenotype (25).
Hematopoietically-Expressed Homeobox, Hhex
Hhex is a transcription factor first described in hematopoietic cells (26) and is also expressed in endoderm during early mouse embryogenesis. At E8.5, Hhex delimits the primordium of several organs derived from the foregut such as thyroid, liver, thymus, pancreas and lung (27). Its expression is maintained from embryos to adults. Hhex-null mice die in middle gestation and show a broad phenotypic spectrum. In the more severe cases, embryos fail to express Foxe1 and Nkx2-1 and have thyroid agenesis, suggesting that Hhex plays an important role in thyroid specification. However, the defect is not specific to this gland and instead is due to a general defect in the anterior endoderm (28). Moderate phenotypes have some thyroid specification with a hypoplastic and ectopic gland, and with variable Foxe1 and Pax8 expression along embryonic development (29). Thus, while Hhex is not strictly necessary for thyroid specification, it is nevertheless essential for maintaining the expression of Pax8 and Foxe1 and is required for correct thyroid development and for cell mobility and migration during thyroid organogenesis (29, 30). The relation among these factors is even more complex as Hhex is not expressed in Nkx2-1- and Pax8-null mice. Thus, Hhex is critical for thyroid morphogenesis and for maintaining the expression of other factors.
The mechanisms and molecular signals involved in the control of the four TTFs during development and adulthood will be addressed below. These signals have been experimentally assessed during development mainly using in vitro murine ESC and iPSC models, which have provided invaluable information on Nkx2-1 and Pax8. Less information is available on Foxe1, and a paucity of studies has addressed the regulation of Hhex. In the adult context, the bulk of information has been provided using models of thyroid cells in culture.
New Transcription Factors Identified in Thyroid Differentiation
While the aforementioned TTFs define the fate of precursors cells toward a differentiated thyroid phenotype, new regulatory factors continue to be identified acting upstream or parallel to these, to control thyroid differentiation. Although their role in thyroid differentiation has yet to be defined in detail, these new factors have been identified through analysis of the thyroid phenotype in mice deficient for the genes, or through their conserved function in the development of endoderm-derived organs. Below, we will give a brief overview on the role of these new factors in thyroid development and what is currently known about their involvement in adult thyroid differentiation.
Among the factors that contribute to thyroid differentiation, genes of the Hox family of transcription factors play an important role in the foregut during embryonic development (31). Hoxa genes are expressed in the embryonic structures from which the thyroid gland is derived. Specifically, Hoxa3, and its paralogs Hoxb3 and Hoxd3 are not expressed in the thyroid epithelial cells, but instead play a role in the migration of the ultimobranchial bodies and, indirectly, in the development of the thyroid gland (32). Accordingly, mice deficient for Hoxa3, Hoxb3 and Hoxd3 show evident thyroid malformations with a displaced isthmus, and with only one lobe containing a low number of follicles (33). Also, Hoxb3 has been demonstrated to regulate the expression of Nkx2-1 (34). Finally, Hoxa5 is expressed in the developing thyroid at E12.5 and is maintained at E15.5, but disappears by E18.5 (35). This transcription factor plays an important role in thyroid development as adult Hoxa5-null mice present with hypothyroidism. Furthermore, although thyroid development is normal in the embryo, small and disorganized thyroid follicles are observed in later phases of gestation with reduced expression of thyroid transcription factors and Tg and Tpo (35, 36).
Concluding, the Hox genes provide positional information that contributes to the initiation of gene expression in the thyroid gland.
Other members of the Nkx and Fox family of transcription factors have been described in the endodermal layer of the developing pharynx. Nkx2-6¸ Nkx2-5 and Nkx2-3 are expressed in the endoderm layer of the primitive pharynx including the thyroid anlage. While the phenotype of Nkx2-6 and Nkx2-3 null mice does not suggest a thyroid defect, Nkx2-5-null mice present with a small thyroid bud expressing Nkx2-1, Foxe1 and Pax8 at E9.5, pointing to a role for Nkx2-5 in thyroid organogenesis. However, these mice die from other causes during embryonic development (19).
Similarly, Foxa2 (formerly called Hepatocyte nuclear factor-3β) is expressed in the primitive endoderm and is decisive for liver organogenesis (37). It is also expressed in the invaginating foregut endoderm and in endoderm-derived structures including the developing thyroid. It has been reported that changes in Foxa2 expression levels during the establishment of the anterior foregut endoderm determine thyroid fate. Specifically, high endodermal levels of Foxa2 are progressively downregulated to give rise to thyroid precursors (38). Accordingly, Foxa2 expression was found to be downregulated in the thyroid lineage at the bud stage (39), although its expression is maintained in adulthood and regulates the thyroid hormone synthesis-related gene Tpo (40). Beyond thyroid follicular cells, Foxa2 also plays an important role in the origin and differentiation of C-cells. In contrast to follicular cells, C-cells arise from precursors expressing Foxa2. Furthermore, the expression of this transcription factor is maintained in differentiated C-cells, and changes to its levels are involved in the progression of medullary thyroid cancer (2). For this reason, it can be acknowledged that Foxa2 plays an important role in the establishment of thyroid fate, both of the follicular cells and the C-cells of the endodermal precursors from which they derive.
In recent years, the transcription factor Glis3 (Gli-similar protein 3) has emerged as important in thyroid development, as mutations in this gene have been related to congenital endocrine defects, including thyroid alterations. Specifically GLIS3 mutations are associated with congenital hypothyroidism (41, 42).
Glis3 belongs to the Krüppel-like subfamily of zinc finger transcription factors (42) with variable-length transcripts generated by alternative splicing in a tissue-specific manner. The larger transcripts are highly expressed in kidney, thyroid and endocrine pancreas, whereas the smaller transcripts are expressed in kidney, heart and liver (43, 44). Beyond changes in the expression pattern, there is no evidence of functional differences between the splice variants. Similar to other transcription factors, most of the information regarding Glis3 comes from murine models. Glis3-null mice die soon after birth due to neonatal diabetes, as this factor is responsible for pancreatic β-cell development (45). In addition, Glis3-null mice have polycystic kidney disease, which suggests a role in renal function (46).
In the thyroid gland, Glis3 is expressed exclusively in follicular cells, and not in the parafollicular compartment, and it is required for TSH induction of thyroid hormone biosynthesis and thyroid cell proliferation in postnatal thyroid development. Although Glis3-null mice develop hypothyroidism, no other major complications have been found in gland development (47).
Glis3 encodes a protein of 90 kb, which acts as an activator of gene transcription by binding to specific sites present in the regulatory regions of target genes (48). One of these target genes is Slc5a5 (encoding the Nis symporter) and Glis3 binds to its promoter and functionally cooperates with Pax8 in a manner that does not involve the interaction between the two. Furthermore, the expression levels of other genes involved in thyroid hormone biosynthesis, such as Slc26a4, Slc16a4, Tpo, Tg, Tshr and Duoxa1 and 2, show some variability in null Glis3-null mice (47), as reviewed in (49).
Knockdown of glis3 in zebrafish embryos also results in thyroid specification defects related to the reduced expression of thyroid markers such as Tg and Nis. Indeed, this model recapitulates thyroid dysgenesis and demonstrates that Glis3 might determine endodermal cell fate by signaling downstream of the Sonic hedgehog (Shh) pathway (50). Several studies support the role of Glis3 in the Shh pathway. Glis3 degradation is prevented by its binding to suppressor of fused (Sufu), a negative regulator of the Shh pathway, and this interaction moderately inhibits its transcriptional activity (51). Both Shh and Glis3 are described to be localized to the primary cilia (46), which is suggested to play a role in thyroid follicular cell function due to its presence during development and permanence in adult life (52).
While thyroid cancer is not the focus of this review, it is interesting that the GLIS3-PAX8 fusion is associated with a rare thyroid neoplasm termed hyalinizing trabecular tumor, which is characterized by the development of a trabecular pattern and intra-trabecular hyalinization in the thyroid (53, 54). This inter-chromosomal rearrangement leads to the overexpression of GLIS portions with an intact DNA-binding domain, yielding higher GLIS3 transcriptional activity during the evolution of the pathology. Indeed, SLC5A5 and other genes related to thyroid hormone synthesis were found upregulated in hyalinizing trabecular tumors. Re-enforcing the link between GLIS3 and SHH, a recent study revealed that the oncogenic effects of the GLIS3-PAX8 fusion were mediated through the SHH pathway, and inhibition of this pathway partly rescued the malignant features of trabecular tumors (55). These data open the door to the investigation of Glis3 in thyroid development and function.
Sox9 is another transcription factor that has recently gained relevance in thyroid development, and is known to play a fundamental role in the development of numerous endoderm-derived organs. Sox9 belongs to the sex-determining region Y (SRY)-related high-mobility-group (HMG) box containing (SOX) family of transcription factors, and more specifically to the subgroup E (56).
The HMG box contains two nuclear localization signals and one nuclear export signal, which ensures a diverse subcellular distribution (57, 58). Sox9 plays a critical role in the development of the pancreas and lungs (59), and is important for maintaining a pool of undifferentiated progenitor cells in some adult tissues (60, 61).
Regarding thyroid development, the group of Nilsson has recently shed light on the function of Sox9 by showing that it is expressed at E9.5 in Nkx2-1-positive thyroid precursor cells. At E10.5, Sox9 expression is observed only in those cells that are part of the thyroid bud, specifically in the distal population, but not in those Nkx2-1 positive cells that have been retained in the thyroid placode and have not invaginated from the anterior endoderm. Thus, Sox9 is a transcription factor with a dynamic expression during thyroid budding, which confirms early ideas that essential transcription factors are differentially regulated in thyroid progenitors transiting from the placode to the bud (29, 62). In addition, it has been described that at this stage the mesenchyme surrounding the thyroid primordium expresses high levels of Sox9, which has led to propose that it could also be regulating thyroid development in a non-cell autonomous manner (62).
Branching is a fundamental mechanism in the embryonic development of various organs, typically in exocrine and ductal organs. Through this mechanism, they acquire the appropriate size and organization. Sox9 plays a role in the regulation of the developmental branching processes of several organs such as the lungs (63) and salivary glands (64). Developmental branching of the thyroid occurs after the migration of the gland to its final position, specifically after bilobation and fusion with ultimobranchial bodies, but prior to follicular cells differentiation and Tg production (62). In this process, the cells with the highest level of Sox9 expression are located in the distal zone of the expanding lobe, as occurs in other branching organs (63, 64). This disposition suggests that Sox9 has a role controlling the branching program of the developing thyroid gland during the stage of pre-follicular growth. This process has been recognized as reminiscent of the exocrine origin of the thyroid gland, as in the case of the endostyle of protochordates. This hypothesis is supported by the fact that thyroid follicular cells are most likely the only endocrine cells that present a clear apical-basal polarity and secrete their product to an interior space, in this case the lumen of the thyroid follicles (4). The branching process and Sox9 itself are finely regulated by growth factors. Among them, Fgf10, generated in the surrounding mesenchyme, and its receptor Fgfr2b, have been proposed to be involved in branching and organogenesis in several organs (65, 66) including the thyroid. Unlike what occurs in other endodermal organs, such as the pancreas, Fgf10 does not control the expression of Sox9 in the thyroid progenitors. However, the dual role of Fgf10 inducing the proliferation of progenitor cells and stimulating the branching of the developing thyroid has been shown to be essential (62). An animal model with a totally inhibited expression of Sox9 specifically in the thyroid is needed to study in-depth the role of Sox9 in the embryonic development of this gland. Currently available models, such as the generated with Cre under the control of the Nkx2-1 promoter (Nkx2-1Cre;Sox9fl/fl), have failed to inhibit Sox9 expression sufficiently to assess its function (62). In conclusion, a new and interesting role of Sox9 has been proposed in thyroid embryogenesis, which should fuel further study of the role this transcription factor not only in thyroid development but also in differentiation
In addition to the above transcription factors, other cofactors such as coactivators or corepressors also play important roles in thyroid differentiation, both in the embryo and in the adult. One of those cofactors is Eya1 (Eyes absent 1), which has a role in the morphogenesis of organs and tissues derived from the embryonic pharynx, including the thymus, parathyroid and the thyroid. Eya1 is expressed in the third and fourth pharyngeal region and in the ultimobranchial bodies. Eya1-null mice have persistent ultimobranchial bodies, hypoplasia of the thyroid lobes, no isthmus, and show a substantial reduction in the number of follicular cells and C-cells (67). Eya1 is not expressed in the thyroid diverticulum, which suggests that defects in follicular cells are due to the lack of fusion of the ultimobranchial bodies to the thyroid lobes. This idea is supported by the finding that Eya1-null mice do not show alterations in early thyroid development, with normal expression of Nkx2-1 and Pax8.
Interestingly the expression of Six1, the main transcription factor co-activated by Eya1, is severely reduced in the pharyngeal endoderm of Eya1-null embryos (67); however, its role in thyroid development remain to be elucidated.
Finally, another cofactor with an important role in thyroid function, both in development and adulthood, is Taz (transcriptional coactivator with PDZ-binding motif, encoded by the Wwtr1 gene), which is mainly recognized for driving the transcriptional output of the Hippo signaling pathway. Hippo cascade activation has a key role in controlling tissue homeostasis and organ growth by regulating many cell processes such as proliferation, apoptosis, and differentiation. Nucleus-cytoplasmic shuttling of TAZ ultimately determines its transcriptional activity and is dependent on its phosphorylation state. In its non-phosphorylated form, TAZ is located in the nucleus where, as a cofactor, it cannot directly bind DNA. Instead, TAZ interacts with different transcription factors to enhance or repress their transcriptional activity. Specifically, its main interactor partners are members of the Tead family of transcription factors (68). WWTR1 is expressed in all tissues, with the exception of the thymus and peripheral blood leukocytes (69). In rat thyroid follicular cells, Taz is found mainly in the nucleus where it has been described to interact with Pax8 and Nkx2-1 (70). During mouse thyroid development, Wwtr1 expression is detectable from E14.5 through adult life, concomitantly with Tg. Taz is described to co-activate both Pax8 and Nkx2-1 in the regulation of Tg, although the studies leading to these conclusions were performed exclusively in a heterologous system (70). TAZ nuclear enhancement has been used to promote thyroid differentiation in activin A-treated human ESCs, as TAZ activation by the DNA-intercalating agent ethacridine triggers upregulation of PAX8 and NKX2-1 expression (71).
Wwtr1-null mice show a polycystic kidney phenotype and alveolarization defects, and although no thyroid defects have been characterized in these animals, their small size suggests a hypothyroid phenotype (68).
Similar to what is seen in mice, taz expression can be observed in the thyroid primordium in zebrafish larvae. Morpholino-mediated knockdown of Wwtr1 in zebrafish results in a lower number of (smaller) thyroid follicles at later stages of development, suggesting that the co-factor is necessary for thyroid growth in this animal model. Nevertheless, its effects seemed to be unrelated to pax8 and nkx2-1 regulation, as its absence was found to trigger higher levels of thyroid-specific genes such as tg, slc5a5 or tpo (72). While the mechanism by which Taz transcriptionally enhances Pax8 and Nkx2-1 is not fully understood, analysis using an assay for transposase accessible chromatin sequencing (ATAC-seq) showed that chromatin accessibility of TAZ, PAX8 and NKX2-1 was enriched after TAZ activation by ethacridine exposure during human ESC differentiation (73). Interestingly, TAZ was described to interact with GLIS3 in kidney cells, and their co-expression was found to promote TAZ nuclear translocation and mediate co-activation of GLIS3 transcriptional activity (46). Considering the importance of both factors for the onset of thyroid differentiation, a possible interplay between these two proteins should be investigated. Despite the promising results indicating a role for Taz as an inductor of thyroid differentiation, there has been no characterization of its effects. Nevertheless, the available data suggest that Taz could be a modulator of thyroid morphogenesis, and may control the size of the gland, as has been described for the Hippo pathway in other organs (74–76). Further research is needed to confer a specific role for this co-factor and its paralog Yap in thyroid development.
Cross-Talk Between Transcription Factors Involved in Thyroid Development
Cross-communication between the four TTFs has been suggested. While it is clear that all four TTFs are expressed in thyroid precursors, it is not conclusively accepted whether all four are necessary for the commitment of the differentiation process from a precursor cell to a differentiated thyroid cell. The expression of Nkx2-1 and Pax8 is sufficient to drive differentiation to a thyroid lineage in murine ESC, and Nkx2-1 can induce Foxe1 expression (38, 77). This, together with the fact that Pax8 regulates Foxe1 in mice, suggests that this latter factor is downstream in the regulatory network (29). Because Foxe1 is considered as a pioneer factor with the intrinsic property to remodel chromatin (78), it likely instructs the thyroid differentiation program responsible for initiating the expression of thyroid differentiation genes. Furthermore, as we have observed an auto-regulatory mechanism for Nkx2-1 and Pax8 expression in thyroid cells in culture (unpublished data), a feed-forward transcriptional loop might be operating in thyroid development. Together, these data suggest the presence of a hierarchical network among the TTFs and a relationship between them (Figure 4). How other factors and cofactors such as Hox, Glis3, Sox9, Eya1 and Taz, and possibly others, participate in this network has not yet been studied in detail. In addition, genes acting upstream of TTFs in the regulatory network are not well known. Identifying these genes will be necessary to fully understand the early events that control thyroid differentiation in development.
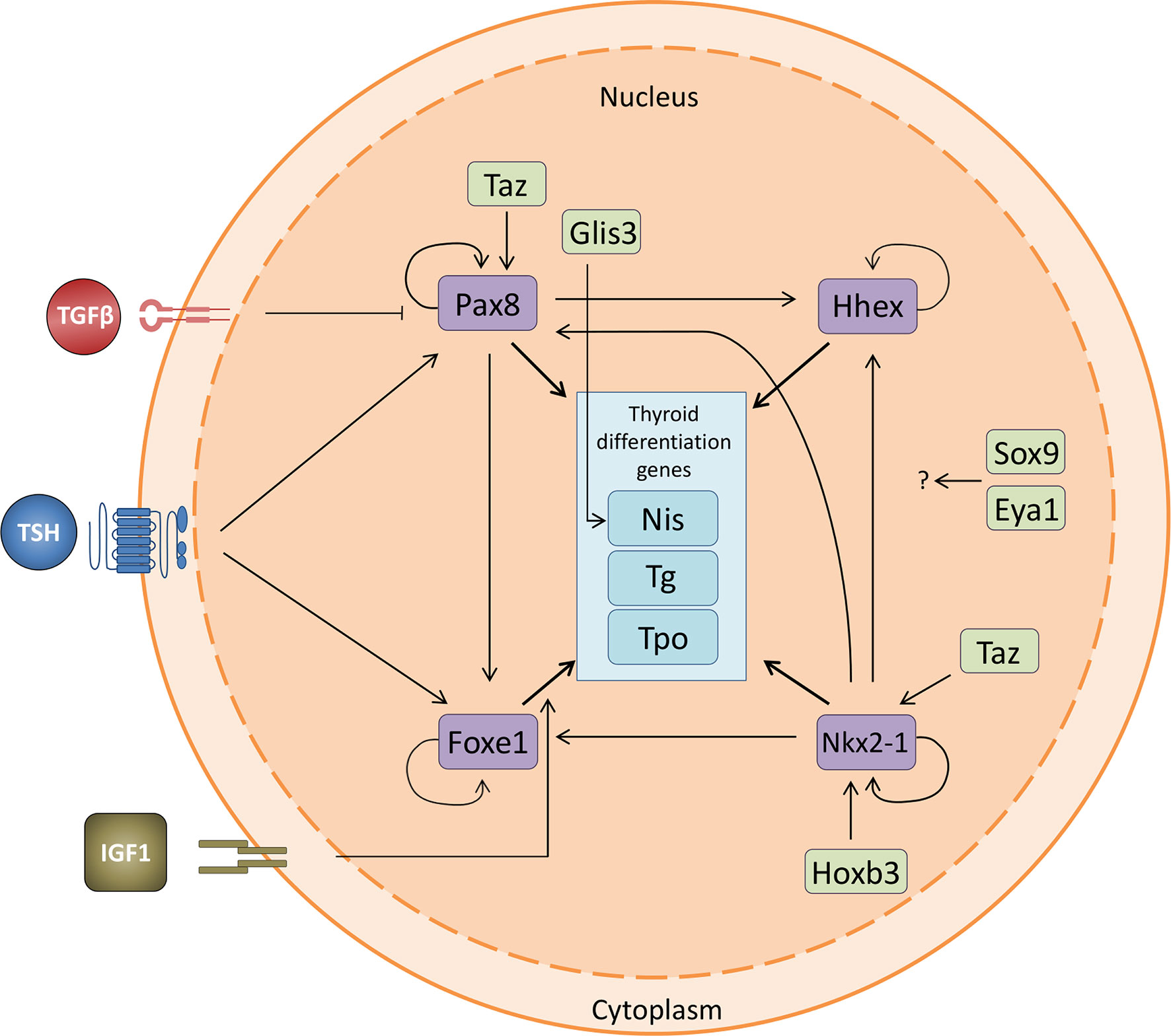
Figure 4 Thyroid transcription factor crosstalk. Relationship between the four thyroid transcription factors and the thyroid differentiation genes, including the interaction with the novel transcription factors involved in thyroid differentiation. Nkx2-1 is regulated by Taz and Hoxb3 and promotes the expression of the other three TTFs. Pax8 regulates the expression of Hhex and Foxe1 and is positively regulated both by TSH and Taz. It is also negatively regulated by TGFβ. Foxe1 is positively regulated by TSH and IGF1. All four TTFs promote the expression of the thyroid differentiation genes Nis (Slc5a5), Tg and Tpo. The effect of Sox9 and Eya1 remains to be fully elucidated. Pointed arrows denote activation while flat arrows indicate inhibition.
Molecules and Signaling Pathways Involved in Early Thyroid Differentiation During Embryonic Development
While the role of transcription factors in thyroid development is becoming ever more clear, the signals that regulate their expression and function in thyroid specification during embryonic development are less understood. TSH signaling, the major thyroid differentiation and proliferation stimulus for thyroid cells (79), appears not to be involved at this stage, as Tshr is not expressed until E15.5 in mice (80). There have been many advances in the elucidation of signals involved in early thyroid specification, budding, proliferation and folliculogenesis, but we are still far from knowing all the answers.
Because of the close proximity of the pharyngeal endoderm to the heart primordium, thyroid bud formation might be regulated by signals emerging from cardinogenic mesoderm, as has been described for other endoderm-derived organs (81). In this context, several factors have recently been identified in studies carried out in murine ESCs and iPSCs. Among them, fibroblast growth factors (Fgfs) and bone morphogenic proteins (Bmps) are inductive signals in early thyroid development. Treatment of mouse foregut explants with Fgf2 induces thyroid differentiation and, in zebrafish, Fgf1, Fgf2 and Fgf8 treatment rescues thyroid development in mutants lacking a thyroid (82). Furthermore, the use of an induction medium containing Fgf2 and Bmp4 stimulates functional thyroid differentiation in murine ESCs and iPSCs (83), whereas blocking both signals inhibits thyroid development. These results are in agreement with the finding that mice null for Fgf2-receptor and Fgf10 have athyreosis or thyroid rudiment, respectively (84, 85). In addition, the Notch pathway is involved in early thyroid development, as its disruption limits the number of progenitor cells. Notch also plays a central role during zebrafish thyroid development (86). Other morphogenic signals such as Wnt/βcatenin seem not to be involved, as thyroid development is not impaired in mice null for different components of this pathway (87). The combination of BMP4, Fgf2 and Wnt3a added to a medium developed for the directed differention of ESCs resulted in maximum efficiency of Nkx2-1-positive cell specification (83). Interestingly, Nkx2-1-positive cells cultured in the presence of Fgf2 and BMP4 express thyroid differentiation markers, whereas the same cells cultured with Wnt3a and BMP4 express lung differentiation markers (88). These data suggest a role for the Wnt pathway in lung differentiation.
Shh is not involved in thyroid specification since Shh-null mice present with a phenotype of thyroid hypoplasia and hemiagenesis (89). Shh is expressed in the ventral endoderm except in the areas forming the thyroid bud (29, 89). These data suggest that this signaling pathway regulates thyroid development indirectly by controlling the shape of the pharynx and its vasculature. Interestingly, in addition to thyroid hypoplasia the thyroid fails to fuse with the ultimobranchial bodies in Shh-null mice (4).
Likewise, the transcription factor Tbx1, regulated by Fgf8 in the developing mesoderm, stimulates the dissemination of thyroid precursors after they exit the endoderm (90, 91). In this regard, Fgf8-null mice show hypoplastic thyroid primordium, whereas fgf8-deficient zebrafish have a smaller thyroid with a lower number of follicles (82). Interestingly, the hypoplastic thyroid phenotype of Shh-null mice is similar to that of Tbx1-null embryos, indicating that the Shh gradient induced in the pharyngeal endoderm elicits distal effects that later promote proliferation of thyroid progenitors (4). It is important to note that Shh is expressed in the endoderm that surrounds the thyroid primordium during development, but not in the thyroid precursors themselves, and also that Shh-null mice – which show hypoplasia and hemiagenesis – express Tg (89). These data confirm that Shh signaling is involved in thyroid morphogenesis and localization, but not in the final differentiation program.
Once thyroid precursor cells have reached their final destination at the front of the trachea, the complex process of folliculogenesis begins. Despite the importance of follicle formation for thyroid differentiation, little is known about the signals involved in this process. It is currently accepted, however, that intrinsic transcription factors and extrinsic signals play an important role. Regarding transcription factors, the over-expression of Nkx2-1 and Pax8 in murine ESCs induces functional follicle formation in a 3D culture system (77). These data support earlier work showing that mice expressing Nkx2-1 mutated in phosphorylation sites present with a thyroid phenotype with impaired follicular organization and a reduction in the expression levels of Cadherin 16 (Cdh16) and other proteins essential for follicular formation (92). Pax8 also seems to play a role in folliculogenesis as Pax8-null mice lack follicles (23), and the absence of Pax8 in a polarized Fischer rat thyroid cell line hinders the acquisition of apical-basal polarity and results in impaired lumen formation (93). The control of thyroid polarity by Pax8 is mediated by Cdh16 and laminins, with the latter also involved in follicle development in the mouse embryo induced by Bmp through Smad1/5 (94). In addition to Bmp, signals originating from the surrounding thyroid bud are essential for folliculogenesis (19). Among others, vascular endothelial growth factor A (Vegfa) has an important role in this process. During embryonic development, reciprocal communication through signaling molecules between endothelial cells and thyroid precursors is essential for the maturation and formation of thyroid follicles (95). Thyroid progenitors have been reported to secrete VEGFA, inducing endothelial cell recruitment and the formation of the vascular network that surrounds the thyroid follicle. Endothelial cells establish the correct follicle-shaped structure of thyrocytes due to the transmission of molecular signals through extracellular vesicles. It has been shown that, in particular, laminin-α1 contained in the extracellular vesicles that are taken up by thyrocyte progenitors plays an important role in folliculogenesis and in the formation of the angiofollicular unit (96). Nevertheless, the mechanisms involved in follicle formation need to be studied in more depth.
The role of TSH in follicle formation remains controversial. While it seems to play no role in de novo follicle formation, as a correctly formed thyroid gland with follicles is observed in both Tsh- and Tshr-null mice (80), it is needed for follicle formation in murine ESCs cultured in 3D overexpressing Nkx2-1 and Pax8 (77, 83).
Control of Thyroid Differentiation at the Last Stages of Embryo Development and in Adult Follicular Cells
Once thyroid precursor cells have completed their migration to the trachea, and after bilobation and formation of follicles, the final process of thyroid differentiation ensues, which is maintained along adult life. Because thyroid function is under spatial and temporal control, thyroid differentiation genes are expressed following a strict developmental program: Tg is the first expressed at E14.5, Tpo and Tshr at E15 (12) and Slc5a5 and Duox2 at E15.5 (97, 98); T4 is detected at E16.5 (80). These embryonic periods in mice and the equivalent times in humans are depicted in Figure 1.
As explained earlier, the functional unit of the thyroid gland is the follicle, a structure formed by thyroid epithelial cells surrounding a lumen and tasked with the synthesis and secretion of T3 and T4 (Figure 2). As both T3 and T4 are iodinated hormones, iodide is the limiting factor for thyroid hormone synthesis. Iodide enters the thyroid cell from the bloodstream through a specific symporter located on the basolateral membrane of the follicular cell, Nis (97). From there, it is transported to the apical membrane, where it passes to the lumen by the action of Pendrin and other transporters that are still not well defined (99). In the apical membrane, iodide is oxidized by Tpo in an enzymatic reaction that requires H2O2 generated by Duox2. In the lumen, Tg synthesized by the follicular cells is iodinated and stored in the colloid. Iodinated Tg is the protein matrix for the formation of mono- and diiodotyrosine (MIT and DIT) and their coupling to generate T3 and T4. Depending on the need for thyroid hormones, iodinated Tg is endocytosed in the form of colloid drops, fused with lysosomes and proteolyzed to release MIT, DIT, T3 and T4. Iodide is recycled from MIT and DIT by Dehal1, encoded by Iyd1, and T3 and T4 are transported to the bloodstream by Mct8 located on the basal membrane.
Regulation of Thyroid Differentiation Genes
All processes involved in the synthesis and secretion of thyroid hormones require the exquisite regulation of the differentiation genes. The major regulator of thyroid function is the pituitary hormone TSH, and its role is co-ordinately controlled by other factors, among which are IGF1 and TGFβ.
Thyroid Stimulating Hormone (TSH)
TSH, a glycoprotein hormone synthesized and secreted by the anterior pituitary, is the major stimulus that controls proliferation and differentiation at late developmental stages and in the adult thyroid gland (79, 100). TSH controls thyroid hormone production and serum concentration through a negative feedback loop by binding to its receptor (TSHR) to signal through a number of pathways, targeting transduction and transcription effectors. TSHR belongs to the G protein-coupled receptor (GPCR) family and is anchored to the basal membrane of follicular thyroid cells. It is composed of two subunits, α and β, which are non-covalently linked (101, 102). Stimulation of TSHR induces dissociation of heterotrimeric G proteins leading to Gα and Gβγ activation. There are many subtypes of α and βγ subunits and their combinations activate different downstream pathways. In thyroid cells, Gα-induced subunits are mainly Gαs, whose activation “stimulates” adenylate cyclase to elevate cAMP production (103, 104). As a second messenger, cAMP has cell type-specific effects and acts principally through cAMP-dependent protein kinase A (PKA), which is necessary but not sufficient to reproduce the full mitogenic effect of cAMP in some thyroid cells (105). The catalytic subunit of PKA phosphorylates its target proteins including the transcription factor CREB, which is its classic nuclear target (106, 107). Phosphorylation of CREB recruits coactivators such as CBP (CREB-binding protein) and p300, enhancing the activity of cAMP response elements (CRE)-containing gene promoters. The transcriptional activity of CREB and, consequently, the activation of the TSH signaling pathway, are downregulated by its dephosphorylation, mediated by the action of PP1 and PP2A phosphatases (108). The cAMP-mediated TSH signaling pathway and the resulting activation of CREB is essential for the maintenance of the differentiated state of the follicular cell and therefore for the correct function of the thyroid (109). Interestingly, it has been described that mice with specific suppression of CREB lack thyroid follicular structures and show no expression of thyroid-specific proteins (110). Although the phosphorylation of CREB by cAMP/PKA is the primary event elicited by TSH, other transcription factors such as CREM can mediate the TSH/cAMP response in the regulation of Slc5a5 expression (111). As the TTFs are phosphoproteins (112–114), many studies have been performed to dissect the role of post-translational modification in the regulation of thyroid-specific genes. Thus, it has been reported that TSH, through cAMP, can induce the phosphorylation of TFFs and therefore their transcriptional activity. Nkx2.1 phosphorylation is important for its binding and activity to regulate TSHR transcription (115). However, other work reported that Nkx2.1 phosphorylation is not affected by cAMP treatment in dog thyrocytes (116). In addition, Nkx2.1 phosphorylation at serine 337, in response to an increase in cAMP levels, is not necessary for its activation of the Tg promoter (117). From the last two reports, it can be concluded that other transcription factor(s) or co-factor(s) are involved in the control elicited by cAMP/PKA on the expression of thyroid-specific genes. Pax8 is a nuclear phosphoprotein and it has been reported that its mRNA and protein levels are cAMP dependent; however, neither its phosphorylation nor its translocation to the nucleus are regulated by cAMP in dog thyrocytes (118). Furthermore, it has been shown that cAMP stimulation increases Pax8-specific transcription in rat thyroid cells, a mechanism that could be due to a direct target of PKA activating Pax8 (113). The phosphorylation of Foxe1 and Hhex in response to TSH/cAMP has been studied to a lesser extent and no information is yet available.
Overall, we can conclude that there is no clear demonstration that TSH/cAMP/PKA directly phosphorylates TTFs. Instead, the most plausible idea is that this signaling pathway could phosphorylate other factors co-operating with the TTFs.
Thyroid Differentiation Genes Regulated by TSH
As the main regulator of thyroid differentiation, TSH controls, at the transcriptional level, all of the genes that define the thyroid-differentiated phenotype: Tg, Tpo, Slc5a5, Tshr and Slc26a4 (7, 8, 119). As the bibliography on the transcriptional regulation of these genes is extensive, here we will discuss only the role of CREB and the TTFs in the TSH-mediated regulation of Tg, Tpo and Slc5a5, which has been studied more extensively in rat thyroid cells. Because TSH/cAMP regulates all three genes, they should contain CRE sites in their upstream regulatory region. Yet, no consensus CRE motif has been identified, except in Tg and in the Nis promoter/enhancer where a CRE-like motif has been described (9, 120–122). This would suggest that specific transcription factors modulate the TSH-regulated transcription of these genes.
In this regard, TSH induces the expression of the TTFs Pax8 (123) and Foxe1 (122, 124). Less clear is the role of TSH on Nkx2-1, as no effect (116) or a downregulation (125) has been reported. TSH not only regulates TTF gene expression but also the binding to their consensus sites within target promoters (9, 16, 80, 126–130). The mechanism of this regulation should be dependent on TTF phosphorylation; however as explained earlier it is currently not clear whether the phosphorylation state has a role to play. By contrast, what is accepted is the dependency of the redox state of these factors (131). For instance, the ability of Pax8 to bind to its consensus site in the Slc5a5 promoter depends on the reduction of two highly conserved cysteine residues that are found in all the proteins of the Pax family (132). Ape1 endonuclease (also known as Ref-1), whose expression is TSH-dependent, together with thioredoxin (Txn) and thioredoxin reductases (TxnRd1, 2 and 3), are the principal enzymes responsible for the reduction of Pax8 (133) and, consequently, the activation of the binding capacity of Pax8 to DNA through a selenium-dependent mechanism (134).
It is important to note that there are differences in the control of TSH action in the different mammalian species studied (135). With the exception of dog thyrocytes in culture, TSH also activates the phospholipase C signaling pathway, which leads to the organification of iodide. Furthermore, in some thyroid cell systems, TSH also activates MAPK and PI3K pathways; specifically, TSH has been shown to activate ERK (136), mTOR1 (137) and PI3K by signaling the RAS GPTase (138, 139). Furthermore, TSH induces the release of Gβγ dimers upon binding to its receptor, resulting in the activation of the PI3K/Akt pathway. Activation of this pathway homeostatically counteracts the effect induced by increased cAMP levels, as in the case of Nis (140).
This combined evidence indicates that the effects of TSH on the proliferation and differentiation of the thyroid follicle cell occurs via a complex network of cross-communication between different signaling pathways and their associated effectors, which is different among species. This is important to consider when evaluating the pathogenic effect that alterations of these signaling pathways has on each species, and is also important when extrapolating results between species.
Insulin-Like Growth Factor 1 (IGF1)
IGF1 is a growth factor similar in its molecular structure to insulin, and plays an important role in body growth by acting on almost every cell type. Many mechanisms are regulated by IGF1, including DNA synthesis, proliferation and differentiation (141–143). IGF1 binds to its transmembrane receptor tyrosine kinase (IGF1R) on the cell surface, which promotes its dimerization and autophosphorylation resulting in insulin receptor substrate-1 (IRS-1) and IRS-2 phosphorylation and PI3K recruitment to the cell membrane, in turn activating the PI3K signaling pathway (144–147). Active PI3K then catalyzes the formation of phosphatidyl-inositol triphosphate (PIP3), which leads to the recruitment of AKT and its activator PDK1 to the cell membrane. Two downstream components of this pathway – p27KIP1 and FOXO1– whose nuclear exclusion is a hallmark of the mechanism of action of IGF1, are involved in the function of IGF1 in thyroid cell proliferation and differentiation (148, 149).
Binding of IGF1 to its receptor also results in the phosphorylation of Src homology collagen (Shc), which leads to the activation of RAS and the subsequent activation not only of PI3K, but also of MAPK through the sequential activation of MEK and ERK. In turn, ERK activates several targets along the different compartments of the cell especially in the nucleus where it phosphorylates effectors related mainly to proliferation such as c-Fos, c-Jun, c-Myc or ELK1 (150).
Thyroid Differentiation Genes Regulated by IGF1
While little is known about the role of IGF1 in thyroid development, it is a recognized modulator of the TSH response in adult thyroid cell differentiation. IGF1 increases TG and TPO expression levels and has an additive effect with TSH (151, 152). This effect occurs at the transcriptional level, as IGF1 stimulates Tg and Tpo promoter activity via increased binding of Foxe1 to both promoters (126, 127). Accordingly, Foxe1 is at the center of the transcriptional control of both thyroid differentiation genes since, as explained above, it also mediates TSH regulation (122, 124).
However, IGF1 does not always play a positive role in the regulation of thyroid differentiation, as is the case for Slc5a5 in which IGF1 has been described to repress the TSH-induced expression involving the PI3K pathway (153). All of the aforementioned mechanisms of action of IGF1 make this growth factor an important component necessary for regulating follicular cell differentiation and modulating TSH action.
Transforming Growth Factor β (TGFβ)
TGFβ1 is a homodimeric protein of the TGFβ subfamily of cytokines, secreted by many cells types, that regulates cell proliferation, survival, adhesion, migration, differentiation and specification of developmental fate. TGFβ1 binds and brings together two different types of serine/threonine kinase receptors: TGFβR I and II. This allows TGFβRII to activate TGFβRI (also known as ALK5) by phosphorylation of specific cytoplasmic domains (154). In canonical TGFβ signaling, active TGFβRI phosphorylates the receptor-regulated SMADs (R-SMADs) 2 and 3 at two C-terminal serine residues. SMAD-4, the unique co-SMAD, binds to SMAD2/3 upon their phosphorylation, forming a heterotrimeric transcriptional complex that translocates to the nucleus (155). In the nucleus, SMADs bind to different DNA-regulatory regions by interacting with other transcription factors, transcriptional co-repressors, and co-activators, to inhibit or activate gene transcription. Furthermore, this signaling network is subject to negative feedback provided by the inhibitory SMADs (I-SMADs) such as SMAD-7, which suppresses R-SMAD and TGFβR activity by (156).
Thyroid Differentiation Genes Regulated by TGFβ
TGFβ1 is synthesized and secreted by thyroid follicular cells, where it blocks the effects of TSH/cAMP on cell proliferation (157–159), cell shape (160, 161) and differentiation (162), thus contributing to thyroid gland homeostasis. TGFβ diminishes the mRNA levels of the differentiation genes Tg, Tpo and Slc5a5 by a mechanism transcriptionally regulated by Pax8 downregulation (163–165). Smad2/Smad4 are the main players of this inhibition in the case of Tg, whereas Smad3-Pax8 interaction drives Slc5a5 downregulation. Additionally, TGFβ1 also affects Na+/K+ ATPase activity (166) and Tshr levels (167), both crucial for the iodide uptake needed for thyroid hormone synthesis. Overall, these data establish TGFβ1 as a negative regulator of differentiation maintenance in the adult thyroid.
TGFβ1 also participates in the de-differentiation processes that occur in papillary thyroid carcinomas. The BRAF-V600E oncogenic driver triggers TGFβ1 secretion, which represses SLC5A5 transcription by a mechanism that involves NOX4-derived reactive oxygen species generation by Smad signaling (168, 169). This contributes to impairment in iodide uptake, which leads to radioactive iodide refractory disease and a worse therapy outcome.
Activin A, another member of the TGFβ subfamily, is also expressed in thyroid follicular cells. By engagement with activin type IB and type II receptors, activin A is reported to induce SMAD2-3/4 complex translocation to the nucleus, thus interfering with the TSH-mediated cAMP response. The effects of activin A have been described to be milder than those of TGFβ1 (167).
Conclusions Remarks and Future Perspectives
The mechanisms involved in the development of the thyroid gland are dynamic and complex, and are increasingly better understood, particularly in terms of specific TTFs and other factors upstream or interacting with them to ultimately modulate their function. Knowledge of signaling factors has contributed to a better understanding of the development of this gland and, consequently, the formation of the thyroid hormones, and both animal models and stem cells have been critical for this advance. In this review, we have attempted to summarize the most current knowledge. Although much progress has been made, in our opinion there has been a paucity of studies in recent years. It is possible that this is because many research groups focus more on studies of thyroid pathologies. However, without basic studies, it is challenging to obtain information on these pathologies. Knowledge of thyroid development in animal models has contributed to a richer understanding of physiologic and pathologic processes, including congenital hypothyroidism and cancer. Accordingly, the future should be aimed at developing new animal and ESC models, and even 3D bioprinting of the thyroid gland. Indeed, this has already been developed (170, 171) and has the potential to greatly aid our understanding of all the associated processes, including the vascularization of the gland. Furthermore next generation sequencing will be instrumental in identifying new genes, and epigenetic analysis will help to create new concepts.
Author Contributions
AL-M, CC-L, and CF-M, wrote the different sections of the manuscript. PS prepared and edited the final manuscript. All authors contributed to the article and approved the submitted version.
Funding
This work was supported by grants PID2019-105303RB-I00 from Ministerio de Ciencia e Innovación (MICIN), Spain, S2017/BMD-3724 from Comunidad de Madrid, and GCB14142311CRES from Fundación Española Contra el Cáncer (AECC).
Conflict of Interest
The authors declare that the research was conducted in the absence of any commercial or financial relationships that could be constructed as a potential conflict of interest.
Acknowledgment
We thank Dr. Kenneth McCreath for his constructive comments on the manuscript.
References
1. Grapin-Botton A, Melton DA. Endoderm development: from patterning to organogenesis. Trends Genet (2000) 16(3):124–30. doi: 10.1016/s0168-9525(99)01957-5
2. Johansson E, Andersson L, Örnros J, Carlsson T, Ingeson-Carlsson C, Liang S, et al. Revising the embryonic origin of thyroid C cells in mice and humans. Development (2015) 142(20):3519–28. doi: 10.1242/dev.126581
3. Kameda Y. Morphological and molecular evolution of the ultimobranchial gland of nonmammalian vertebrates, with special reference to the chicken C cells. Dev Dyn (2017) 246(10):719–39. doi: 10.1002/dvdy.24534
4. Nilsson M, Fagman H. Development of the thyroid gland. Development (2017) 144(12):2123–40. doi: 10.1242/dev.145615
5. Colin IM, Denef JF, Lengelé B, Many MC, Gérard AC. Recent insights into the cell biology of thyroid angiofollicular units. Endocr Rev (2013) 34(2):209–38. doi: 10.1210/er.2012-1015
6. De Felice M, Ovitt C, Biffali E, Rodriguez-Mallon A, Arra C, Anastassiadis K, et al. A mouse model for hereditary thyroid dysgenesis and cleft palate. Nat Genet (1998) 19(4):395–8. doi: 10.1038/1289
7. Fernández LP, López-Márquez A, Santisteban P. Thyroid transcription factors in development, differentiation and disease. Nat Rev Endocrinol (2015) 11(1):29–42. doi: 10.1038/nrendo.2014.186
8. Damante G, Di Lauro R. Thyroid-specific gene expression. Biochim Biophys Acta (1994) 1218(3):255–66. doi: 10.1016/0167-4781(94)90176-7
9. Ohno M, Zannini M, Levy O, Carrasco N, di Lauro R. The paired-domain transcription factor Pax8 binds to the upstream enhancer of the rat sodium/iodide symporter gene and participates in both thyroid-specific and cyclic-AMP-dependent transcription. Mol Cell Biol (1999) 19(3):2051–60. doi: 10.1128/mcb.19.3.2051
10. Guazzi S, Price M, De Felice M, Damante G, Mattei MG, Di Lauro R. Thyroid nuclear factor 1 (TTF-1) contains a homeodomain and displays a novel DNA binding specificity. EMBO J (1990) 9(11):3631–9. doi: 10.1002/j.1460-2075.1990.tb07574.x
11. Mizuno K, Gonzalez FJ, Kimura S. Thyroid-specific enhancer-binding protein (T/EBP): cDNA cloning, functional characterization, and structural identity with thyroid transcription factor TTF-1. Mol Cell Biol (1991) 11(10):4927–33. doi: 10.1128/mcb.11.10.4927
12. Lazzaro D, Price M, de Felice M, Di Lauro R. The transcription factor TTF-1 is expressed at the onset of thyroid and lung morphogenesis and in restricted regions of the foetal brain. Development (1991) 113(4):1093–104.
13. Kimura S, Hara Y, Pineau T, Fernandez-Salguero P, Fox CH, Ward JM, et al. The T/ebp null mouse: thyroid-specific enhancer-binding protein is essential for the organogenesis of the thyroid, lung, ventral forebrain, and pituitary. Genes Dev (1996) 10(1):60–9. doi: 10.1101/gad.10.1.60
14. Kimura S, Ward JM, Minoo P. Thyroid-specific enhancer-binding protein/thyroid transcription factor 1 is not required for the initial specification of the thyroid and lung primordia. Biochimie (1999) 81(4):321–7. doi: 10.1016/s0300-9084(99)80077-7
15. Kusakabe T, Kawaguchi A, Hoshi N, Kawaguchi R, Hoshi S, Kimura S. Thyroid-specific enhancer-binding protein/NKX2.1 is required for the maintenance of ordered architecture and function of the differentiated thyroid. Mol Endocrinol (2006) 20(8):1796–809. doi: 10.1210/me.2005-0327
16. Zannini M, Avantaggiato V, Biffali E, Arnone MI, Sato K, Pischetola M, et al. TTF-2, a new forkhead protein, shows a temporal expression in the developing thyroid which is consistent with a role in controlling the onset of differentiation. EMBO J (1997) 16(11):3185–97. doi: 10.1093/emboj/16.11.3185
17. Nakada C, Iida A, Tabata Y, Watanabe S. Forkhead transcription factor foxe1 regulates chondrogenesis in zebrafish. J Exp Zool B Mol Dev Evol (2009) 312(8):827–40. doi: 10.1002/jez.b.21298
18. Carré A, Castanet M, Sura-Trueba S, Szinnai G, Van Vliet G, Trochet D, et al. Polymorphic length of FOXE1 alanine stretch: evidence for genetic susceptibility to thyroid dysgenesis. Hum Genet (2007) 122(5):467–76. doi: 10.1007/s00439-007-0420-5
19. De Felice M, Di Lauro R. Thyroid development and its disorders: genetics and molecular mechanisms. Endocr Rev (2004) 25(5):722–46. doi: 10.1210/er.2003-0028
20. Fagman H, Grände M, Edsbagge J, Semb H, Nilsson M. Expression of classical cadherins in thyroid development: maintenance of an epithelial phenotype throughout organogenesis. Endocrinology (2003) 144(8):3618–24. doi: 10.1210/en.2003-0393
21. Venza I, Visalli M, Parrillo L, De Felice M, Teti D, Venza M. MSX1 and TGF-beta3 are novel target genes functionally regulated by FOXE1. Hum Mol Genet (2011) 20(5):1016–25. doi: 10.1093/hmg/ddq547
22. Fagman H, Andersson L, Nilsson M. The developing mouse thyroid: embryonic vessel contacts and parenchymal growth pattern during specification, budding, migration, and lobulation. Dev Dyn (2006) 235(2):444–55. doi: 10.1002/dvdy.20653
23. Mansouri A, Chowdhury K, Gruss P. Follicular cells of the thyroid gland require Pax8 gene function. Nat Genet (1998) 19(1):87–90. doi: 10.1038/ng0598-87
24. Chaves-Moreira D, Morin PJ, Drapkin R. Unraveling the mysteries of PAX8 in reproductive tract cancers. Cancer Res (2021) 81(4):806–10. doi: 10.1158/0008-5472.CAN-20-3173
25. Pasca di Magliano M, Di Lauro R, Zannini M. Pax8 has a key role in thyroid cell differentiation. Proc Natl Acad Sci USA (2000) 97(24):13144–9. doi: 10.1073/pnas.240336397
26. Crompton MR, Bartlett TJ, MacGregor AD, Manfioletti G, Buratti E, Giancotti V, et al. Identification of a novel vertebrate homeobox gene expressed in haematopoietic cells. Nucleic Acids Res (1992) 20(21):5661–7. doi: 10.1093/nar/20.21.5661
27. Bogue CW, Ganea GR, Sturm E, Ianucci R, Jacobs HC. Hex expression suggests a role in the development and function of organs derived from foregut endoderm. Dev Dyn (2000) 219(1):84–9. doi: 10.1002/1097-0177(2000)9999:9999<::AID-DVDY1028>3.0.CO;2-5
28. Martinez Barbera JP, Clements M, Thomas P, Rodriguez T, Meloy D, Kioussis D, et al. The homeobox gene Hex is required in definitive endodermal tissues for normal forebrain, liver and thyroid formation. Development (2000) 127(11):2433–45.
29. Parlato R, Rosica A, Rodriguez-Mallon A, Affuso A, Postiglione MP, Arra C, et al. An integrated regulatory network controlling survival and migration in thyroid organogenesis. Dev Biol (2004) 276(2):464–75. doi: 10.1016/j.ydbio.2004.08.048
30. Bort R, Signore M, Tremblay K, Martinez Barbera JP, Zaret KS. Hex homeobox gene controls the transition of the endoderm to a pseudostratified, cell emergent epithelium for liver bud development. Dev Biol (2006) 290(1):44–56. doi: 10.1016/j.ydbio.2005.11.006
31. Gordon J. Hox genes in the pharyngeal region: how Hoxa3 controls early embryonic development of the pharyngeal organs. Int J Dev Biol (2018) 62(11-12):775–83. doi: 10.1387/ijdb.180284jg
32. Chojnowski JL, Trau HA, Masuda K, Manley NR. Temporal and spatial requirements for Hoxa3 in mouse embryonic development. Dev Biol (2016) 415(1):33–45. doi: 10.1016/j.ydbio.2016.05.010
33. Manley NR, Capecchi MR. Hox group 3 paralogs regulate the development and migration of the thymus, thyroid, and parathyroid glands. Dev Biol (1998) 195(1):1–15. doi: 10.1006/dbio.1997.8827
34. Guazzi S, Lonigro R, Pintonello L, Boncinelli E, Di Lauro R, Mavilio F. The thyroid transcription factor-1 gene is a candidate target for regulation by Hox proteins. EMBO J (1994) 13(14):3339–47. doi: 10.1002/j.1460-2075.1994.tb06636.x
35. Meunier D, Aubin J, Jeannotte L. Perturbed thyroid morphology and transient hypothyroidism symptoms in Hoxa5 mutant mice. Dev Dyn (2003) 227(3):367–78. doi: 10.1002/dvdy.10325
36. Jeannotte L, Gotti F, Landry-Truchon K. Hoxa5: A Key Player in Development and Disease. J Dev Biol (2016) 4(13):1–18. doi: 10.3390/jdb4020013
37. Zaret KS. Regulatory phases of early liver development: paradigms of organogenesis. Nat Rev Genet (2002) 3(7):499–512. doi: 10.1038/nrg837
38. Dame K, Cincotta S, Lang AH, Sanghrajka RM, Zhang L, Choi J, et al. Thyroid Progenitors Are Robustly Derived from Embryonic Stem Cells through Transient, Developmental Stage-Specific Overexpression of Nkx2-1. Stem Cell Rep (2017) 8(2):216–25. doi: 10.1016/j.stemcr.2016.12.024
39. Fagman H, Amendola E, Parrillo L, Zoppoli P, Marotta P, Scarfò M, et al. Gene expression profiling at early organogenesis reveals both common and diverse mechanisms in foregut patterning. Dev Biol (2011) 359(2):163–75. doi: 10.1016/j.ydbio.2011.08.015
40. Sato K, Di Lauro R. Hepatocyte nuclear factor 3beta participates in the transcriptional regulation of the thyroperoxidase promoter. Biochem Biophys Res Commun (1996) 220(1):86–93. doi: 10.1006/bbrc.1996.0361
41. Dimitri P, Warner JT, Minton JA, Patch AM, Ellard S, Hattersley AT, et al. Novel GLIS3 mutations demonstrate an extended multisystem phenotype. Eur J Endocrinol (2011) 164(3):437–43. doi: 10.1530/EJE-10-0893
42. Lichti-Kaiser K, ZeRuth G, Jetten AM. Transcription Factor Gli-similar 3 (Glis3): Implications For The Development of Congenital Hypothyroidism. J Endocrinol Diabetes Obes (2014) 2(2):1024.
43. Kim YS, Nakanishi G, Lewandoski M, Jetten AM. GLIS3, a novel member of the GLIS subfamily of Krüppel-like zinc finger proteins with repressor and activation functions. Nucleic Acids Res (2003) 31(19):5513–25. doi: 10.1093/nar/gkg776
44. Senée V, Chelala C, Duchatelet S, Feng D, Blanc H, Cossec JC, et al. Mutations in GLIS3 are responsible for a rare syndrome with neonatal diabetes mellitus and congenital hypothyroidism. Nat Genet (2006) 38(6):682–7. doi: 10.1038/ng1802
45. Kang HS, Kim YS, ZeRuth G, Beak JY, Gerrish K, Kilic G, et al. Transcription factor Glis3, a novel critical player in the regulation of pancreatic beta-cell development and insulin gene expression. Mol Cell Biol (2009) 29(24):6366–79. doi: 10.1128/MCB.01259-09
46. Kang HS, Beak JY, Kim YS, Herbert R, Jetten AM. Glis3 is associated with primary cilia and Wwtr1/TAZ and implicated in polycystic kidney disease. Mol Cell Biol (2009) 29(10):2556–69. doi: 10.1128/MCB.01620-08
47. Kang HS, Kumar D, Liao G, Lichti-Kaiser K, Gerrish K, Liao XH, et al. GLIS3 is indispensable for TSH/TSHR-dependent thyroid hormone biosynthesis and follicular cell proliferation. J Clin Invest (2017) 127(12):4326–37. doi: 10.1172/JCI94417
48. Jetten AM. GLIS1-3 transcription factors: critical roles in the regulation of multiple physiological processes and diseases. Cell Mol Life Sci (2018) 75(19):3473–94. doi: 10.1007/s00018-018-2841-9
49. Rurale G, Di Cicco E, Dentice M, Salvatore D, Persani L, Marelli F, et al. Thyroid Hormone Hyposensitivity: From Genotype to Phenotype and Back. Front Endocrinol (Lausanne) (2019) 10:912. doi: 10.3389/fendo.2019.00912
50. Rurale G, Marelli F, Duminuco P, Persani L. as a Critical Regulator of Thyroid Primordium Specification. Thyroid (2020) 30(2):277–89. doi: 10.1089/thy.2019.0196
51. ZeRuth GT, Yang XP, Jetten AM. Modulation of the transactivation function and stability of Krüppel-like zinc finger protein Gli-similar 3 (Glis3) by Suppressor of Fused. J Biol Chem (2011) 286(25):22077–89. doi: 10.1074/jbc.M111.224964
52. Utrilla JC, Gordillo-Martínez F, Gómez-Pascual A, Fernández-Santos JM, Garnacho C, Vázquez-Román V, et al. Comparative study of the primary cilia in thyrocytes of adult mammals. J Anat (2015) 227(4):550–60. doi: 10.1111/joa.12360
53. Marchiò C, Da Cruz Paula A, Gularte-Merida R, Basili T, Brandes A, da Silva EM, et al. PAX8-GLIS3 gene fusion is a pathognomonic genetic alteration of hyalinizing trabecular tumors of the thyroid. Mod Pathol (2019) 32(12):1734–43. doi: 10.1038/s41379-019-0313-x
54. Nikiforova MN, Nikitski AV, Panebianco F, Kaya C, Yip L, Williams M, et al. GLIS Rearrangement is a Genomic Hallmark of Hyalinizing Trabecular Tumor of the Thyroid Gland. Thyroid (2019) 29(2):161–73. doi: 10.1089/thy.2018.0791
55. Basili T, Dopeso H, Kim SH, Ferrando L, Pareja F, Da Cruz Paula A, et al. Oncogenic properties and signaling basis of the PAX8-GLIS3 fusion gene. Int J Cancer (2020) 147(8):2253–64. doi: 10.1002/ijc.33040
56. Wegner M. From head to toes: the multiple facets of Sox proteins. Nucleic Acids Res (1999) 27(6):1409–20. doi: 10.1093/nar/27.6.1409
57. Südbeck P, Scherer G. Two independent nuclear localization signals are present in the DNA-binding high-mobility group domains of SRY and SOX9. J Biol Chem (1997) 272(44):27848–52. doi: 10.1074/jbc.272.44.27848
58. Gasca S, Canizares J, De Santa Barbara P, Mejean C, Poulat F, Berta P, et al. A nuclear export signal within the high mobility group domain regulates the nucleocytoplasmic translocation of SOX9 during sexual determination. Proc Natl Acad Sci USA (2002) 99(17):11199–204. doi: 10.1073/pnas.172383099
59. Jo A, Denduluri S, Zhang B, Wang Z, Yin L, Yan Z, et al. The versatile functions of Sox9 in development, stem cells, and human diseases. Genes Dis (2014) 1(2):149–61. doi: 10.1016/j.gendis.2014.09.004
60. Seymour PA. Sox9: a master regulator of the pancreatic program. Rev Diabetes Stud (2014) 11(1):51–83. doi: 10.1900/RDS.2014.11.51
61. Roche KC, Gracz AD, Liu XF, Newton V, Akiyama H, Magness ST. SOX9 maintains reserve stem cells and preserves radioresistance in mouse small intestine. Gastroenterology (2015) 149(6):1553–63.e10. doi: 10.1053/j.gastro.2015.07.004
62. Liang S, Johansson E, Barila G, Altschuler DL, Fagman H, Nilsson M. A branching morphogenesis program governs embryonic growth of the thyroid gland. Development (2018) 145(2):dev146829. doi: 10.1242/dev.146829
63. Chang DR, Martinez Alanis D, Miller RK, Ji H, Akiyama H, McCrea PD, et al. Lung epithelial branching program antagonizes alveolar differentiation. Proc Natl Acad Sci USA (2013) 110(45):18042–51. doi: 10.1073/pnas.1311760110
64. Chatzeli L, Gaete M, Tucker AS. Fgf10 and Sox9 are essential for the establishment of distal progenitor cells during mouse salivary gland development. Development (2017) 144(12):2294–305. doi: 10.1242/dev.146019
65. Abler LL, Mansour SL, Sun X. Conditional gene inactivation reveals roles for Fgf10 and Fgfr2 in establishing a normal pattern of epithelial branching in the mouse lung. Dev Dyn (2009) 238(8):1999–2013. doi: 10.1002/dvdy.22032
66. Kobberup S, Schmerr M, Dang ML, Nyeng P, Jensen JN, MacDonald RJ, et al. Conditional control of the differentiation competence of pancreatic endocrine and ductal cells by Fgf10. Mech Dev (2010) 127(3-4):220–34. doi: 10.1016/j.mod.2009.11.005
67. Xu PX, Zheng W, Laclef C, Maire P, Maas RL, Peters H, et al. Eya1 is required for the morphogenesis of mammalian thymus, parathyroid and thyroid. Development (2002) 129(13):3033–44.
68. Varelas X. The Hippo pathway effectors TAZ and YAP in development, homeostasis and disease. Development (2014) 141(8):1614–26. doi: 10.1242/dev.102376
69. Kanai F, Marignani PA, Sarbassova D, Yagi R, Hall RA, Donowitz M, et al. TAZ: a novel transcriptional co-activator regulated by interactions with 14-3-3 and PDZ domain proteins. EMBO J (2000) 19(24):6778–91. doi: 10.1093/emboj/19.24.6778
70. Di Palma T, D’Andrea B, Liguori GL, Liguoro A, de Cristofaro T, Del Prete D, et al. TAZ is a coactivator for Pax8 and TTF-1, two transcription factors involved in thyroid differentiation. Exp Cell Res (2009) 315(2):162–75. doi: 10.1016/j.yexcr.2008.10.016
71. Ma R, Morshed SA, Latif R, Davies TF. TAZ Induction Directs Differentiation of Thyroid Follicular Cells from Human Embryonic Stem Cells. Thyroid (2017) 27(2):292–9. doi: 10.1089/thy.2016.0264
72. Pappalardo A, Porreca I, Caputi L, De Felice E, Schulte-Merker S, Zannini M, et al. Thyroid development in zebrafish lacking Taz. Mech Dev (2015) 138 Pt 3:268–78. doi: 10.1016/j.mod.2015.10.002
73. Ma R, Morshed S, Latif R, Davies TF. Epigenetic Changes During Human Thyroid Cell Differentiation. Thyroid (2020) 30(11):1666–75. doi: 10.1089/thy.2019.0772
74. Patel SH, Camargo FD, Yimlamai D. Hippo Signaling in the Liver Regulates Organ Size, Cell Fate, and Carcinogenesis. Gastroenterology (2017) 152(3):533–45. doi: 10.1053/j.gastro.2016.10.047
75. Koo JH, Plouffe SW, Meng Z, Lee DH, Yang D, Lim DS, et al. Induction of AP-1 by YAP/TAZ contributes to cell proliferation and organ growth. Genes Dev (2020) 34(1-2):72–86. doi: 10.1101/gad.331546.119
76. Yu FX, Zhao B, Guan KL. Hippo Pathway in Organ Size Control, Tissue Homeostasis, and Cancer. Cell (2015) 163(4):811–28. doi: 10.1016/j.cell.2015.10.044
77. Antonica F, Kasprzyk DF, Opitz R, Iacovino M, Liao XH, Dumitrescu AM, et al. Generation of functional thyroid from embryonic stem cells. Nature (2012) 491(7422):66–71. doi: 10.1038/nature11525
78. Cuesta I, Zaret KS, Santisteban P. The forkhead factor FoxE1 binds to the thyroperoxidase promoter during thyroid cell differentiation and modifies compacted chromatin structure. Mol Cell Biol (2007) 27(20):7302–14. doi: 10.1128/MCB.00758-07
79. Vassart G, Dumont JE. The thyrotropin receptor and the regulation of thyrocyte function and growth. Endocr Rev (1992) 13(3):596–611. doi: 10.1210/edrv-13-3-596
80. Postiglione MP, Parlato R, Rodriguez-Mallon A, Rosica A, Mithbaokar P, Maresca M, et al. Role of the thyroid-stimulating hormone receptor signaling in development and differentiation of the thyroid gland. Proc Natl Acad Sci USA (2002) 99(24):15462–7. doi: 10.1073/pnas.242328999
81. Wandzioch E, Zaret KS. Dynamic signaling network for the specification of embryonic pancreas and liver progenitors. Science (2009) 324(5935):1707–10. doi: 10.1126/science.1174497
82. Wendl T, Adzic D, Schoenebeck JJ, Scholpp S, Brand M, Yelon D, et al. Early developmental specification of the thyroid gland depends on han-expressing surrounding tissue and on FGF signals. Development (2007) 134(15):2871–9. doi: 10.1242/dev.02872
83. Kurmann AA, Serra M, Hawkins F, Rankin SA, Mori M, Astapova I, et al. Regeneration of Thyroid Function by Transplantation of Differentiated Pluripotent Stem Cells. Cell Stem Cell (2015) 17(5):527–42. doi: 10.1016/j.stem.2015.09.004
84. Revest JM, Spencer-Dene B, Kerr K, De Moerlooze L, Rosewell I, Dickson C. Fibroblast growth factor receptor 2-IIIb acts upstream of Shh and Fgf4 and is required for limb bud maintenance but not for the induction of Fgf8, Fgf10, Msx1, or Bmp4. Dev Biol (2001) 231(1):47–62. doi: 10.1006/dbio.2000.0144
85. Ohuchi H, Hori Y, Yamasaki M, Harada H, Sekine K, Kato S, et al. FGF10 acts as a major ligand for FGF receptor 2 IIIb in mouse multi-organ development. Biochem Biophys Res Commun (2000) 277(3):643–9. doi: 10.1006/bbrc.2000.3721
86. Marelli F, Persani L. Role of Jagged1-Notch pathway in thyroid development. J Endocrinol Invest (2018) 41(1):75–81. doi: 10.1007/s40618-017-0715-x
87. Goss AM, Tian Y, Tsukiyama T, Cohen ED, Zhou D, Lu MM, et al. Wnt2/2b and beta-catenin signaling are necessary and sufficient to specify lung progenitors in the foregut. Dev Cell (2009) 17(2):290–8. doi: 10.1016/j.devcel.2009.06.005
88. Serra M, Alysandratos KD, Hawkins F, McCauley KB, Jacob A, Choi J, et al. Pluripotent stem cell differentiation reveals distinct developmental pathways regulating lung- versus thyroid-lineage specification. Development (2017) 144(21):3879–93. doi: 10.1242/dev.150193
89. Fagman H, Grände M, Gritli-Linde A, Nilsson M. Genetic deletion of sonic hedgehog causes hemiagenesis and ectopic development of the thyroid in mouse. Am J Pathol (2004) 164(5):1865–72. doi: 10.1016/S0002-9440(10)63745-5
90. Lania G, Zhang Z, Huynh T, Caprio C, Moon AM, Vitelli F, et al. Early thyroid development requires a Tbx1-Fgf8 pathway. Dev Biol (2009) 328(1):109–17. doi: 10.1016/j.ydbio.2009.01.014
91. Fagman H, Liao J, Westerlund J, Andersson L, Morrow BE, Nilsson M. The 22q11 deletion syndrome candidate gene Tbx1 determines thyroid size and positioning. Hum Mol Genet (2007) 16(3):276–85. doi: 10.1093/hmg/ddl455
92. Silberschmidt D, Rodriguez-Mallon A, Mithboakar P, Calì G, Amendola E, Sanges R, et al. In vivo role of different domains and of phosphorylation in the transcription factor Nkx2-1. BMC Dev Biol (2011) 11:9. doi: 10.1186/1471-213X-11-9
93. Koumarianou P, Goméz-López G, Santisteban P. Pax8 controls thyroid follicular polarity through cadherin-16. J Cell Sci (2017) 130(1):219–31. doi: 10.1242/jcs.184291
94. Villacorte M, Delmarcelle AS, Lernoux M, Bouquet M, Lemoine P, Bolsée J, et al. Thyroid follicle development requires Smad1/5- and endothelial cell-dependent basement membrane assembly. Development (2016) 143(11):1958–70. doi: 10.1242/dev.134171
95. Hick AC, Delmarcelle AS, Bouquet M, Klotz S, Copetti T, Forez C, et al. Reciprocal epithelial:endothelial paracrine interactions during thyroid development govern follicular organization and C-cells differentiation. Dev Biol (2013) 381(1):227–40. doi: 10.1016/j.ydbio.2013.04.022
96. Degosserie J, Heymans C, Spourquet C, Halbout M, D’Auria L, Van Der Smissen P, et al. Extracellular vesicles from endothelial progenitor cells promote thyroid follicle formation. J Extracell Vesicles (2018) 7(1):1487250. doi: 10.1080/20013078.2018.1487250
97. Dohán O, De la Vieja A, Paroder V, Riedel C, Artani M, Reed M, et al. The sodium/iodide Symporter (NIS): characterization, regulation, and medical significance. Endocr Rev (2003) 24(1):48–77. doi: 10.1210/er.2001-0029
98. Milenkovic M, De Deken X, Jin L, De Felice M, Di Lauro R, Dumont JE, et al. Duox expression and related H2O2 measurement in mouse thyroid: onset in embryonic development and regulation by TSH in adult. J Endocrinol (2007) 192(3):615–26. doi: 10.1677/JOE-06-0003
99. De la Vieja A, Santisteban P. Role of iodide metabolism in physiology and cancer. Endocr Relat Cancer (2018) 25(4):R225–45. doi: 10.1530/ERC-17-0515
100. Brown RS. Minireview: developmental regulation of thyrotropin receptor gene expression in the fetal and newborn thyroid. Endocrinology (2004) 145(9):4058–61. doi: 10.1210/en.2004-0458
101. Loosfelt H, Pichon C, Jolivet A, Misrahi M, Caillou B, Jamous M, et al. Two-subunit structure of the human thyrotropin receptor. Proc Natl Acad Sci USA (1992) 89(9):3765–9. doi: 10.1073/pnas.89.9.3765
102. Urizar E, Montanelli L, Loy T, Bonomi M, Swillens S, Gales C, et al. Glycoprotein hormone receptors: link between receptor homodimerization and negative cooperativity. EMBO J (2005) 24(11):1954–64. doi: 10.1038/sj.emboj.7600686
103. Dumont JE. The action of thyrotropin on thyroid metabolism. Vitam Horm (1971) 29:287–412. doi: 10.1016/s0083-6729(08)60051-5
104. Calebiro D, Nikolaev VO, Gagliani MC, de Filippis T, Dees C, Tacchetti C, et al. Persistent cAMP-signals triggered by internalized G-protein-coupled receptors. PloS Biol (2009) 7(8):e1000172. doi: 10.1371/journal.pbio.1000172
105. Kimura T, Van Keymeulen A, Golstein J, Fusco A, Dumont JE, Roger PP. Regulation of thyroid cell proliferation by TSH and other factors: a critical evaluation of in vitro models. Endocr Rev (2001) 22(5):631–56. doi: 10.1210/edrv.22.5.0444
106. Gonzalez GA, Montminy: MR. Cyclic AMP stimulates somatostatin gene transcription by phosphorylation of CREB at serine 133. Cell (1989) 59(4):675–80. doi: 10.1016/0092-8674(89)90013-5
107. Cass LA, Summers SA, Prendergast GV, Backer JM, Birnbaum MJ, Meinkoth JL. Protein kinase A-dependent and -independent signaling pathways contribute to cyclic AMP-stimulated proliferation. Mol Cell Biol (1999) 19(9):5882–91. doi: 10.1128/mcb.19.9.5882
108. Lazzereschi D, Coppa A, Minicione G, Lavitrano M, Fragomele F, Colletta G. The phosphatase inhibitor okadaic acid stimulates the TSH-induced G1-S phase transition in thyroid cells. Exp Cell Res (1997) 234(2):425–33. doi: 10.1006/excr.1997.3627
109. Woloshin PI, Walton KM, Rehfuss RP, Goodman RH, Cone RD. 3’,5’-cyclic adenosine monophosphate-regulated enhancer binding (CREB) activity is required for normal growth and differentiated phenotype in the FRTL5 thyroid follicular cell line. Mol Endocrinol (1992) 6(10):1725–33. doi: 10.1210/mend.6.10.1333055
110. Nguyen LQ, Kopp P, Martinson F, Stanfield K, Roth SI, Jameson JL. A dominant negative CREB (cAMP response element-binding protein) isoform inhibits thyrocyte growth, thyroid-specific gene expression, differentiation, and function. Mol Endocrinol (2000) 14(9):1448–61. doi: 10.1210/mend.14.9.0516
111. Fenton MS, Marion KM, Hershman JM. Identification of cyclic adenosine 3’,5’-monophosphate response element modulator as an activator of the human sodium/iodide symporter upstream enhancer. Endocrinology (2008) 149(5):2592–606. doi: 10.1210/en.2007-1390
112. Zannini M, Acebron A, De Felice M, Arnone MI, Martin-Pérez J, Santisteban P, et al. Mapping and functional role of phosphorylation sites in the thyroid transcription factor-1 (TTF-1). J Biol Chem (1996) 271(4):2249–54. doi: 10.1074/jbc.271.4.2249
113. Poleev A, Okladnova O, Musti AM, Schneider S, Royer-Pokora B, Plachov D. Determination of functional domains of the human transcription factor PAX8 responsible for its nuclear localization and transactivating potential. Eur J Biochem (1997) 247(3):860–9. doi: 10.1111/j.1432-1033.1997.00860.x
114. Dathan N, Parlato R, Rosica A, De Felice M, Di Lauro R. Distribution of the titf2/foxe1 gene product is consistent with an important role in the development of foregut endoderm, palate, and hair. Dev Dyn (2002) 224(4):450–6. doi: 10.1002/dvdy.10118
115. Shimura H, Okajima F, Ikuyama S, Shimura Y, Kimura S, Saji M, et al. Thyroid-specific expression and cyclic adenosine 3’,5’-monophosphate autoregulation of the thyrotropin receptor gene involves thyroid transcription factor-1. Mol Endocrinol (1994) 8(8):1049–69. doi: 10.1210/mend.8.8.7997232
116. Van Renterghem P, Dremier S, Vassart G, Christophe D. Study of TTF-1 gene expression in dog thyrocytes in primary culture. Mol Cell Endocrinol (1995) 112(1):83–93. doi: 10.1016/0303-7207(95)03589-y
117. Feliciello A, Allevato G, Musti AM, De Brasi D, Gallo A, Avvedimento VE, et al. Thyroid transcription factor 1 phosphorylation is not required for protein kinase A-dependent transcription of the thyroglobulin promoter. Cell Growth Differ (2000) 11(12):649–54.
118. Van Renterghem P, Vassart G, Christophe D. Pax 8 expression in primary cultured dog thyrocyte is increased by cyclic AMP. Biochim Biophys Acta (1996) 1307(1):97–103. doi: 10.1016/0167-4781(96)00018-8
119. Jang D, Marcus-Samuels B, Morgan SJ, Klubo-Gwiezdzinska J, Neumann S, Gershengorn MC. Thyrotropin regulation of differentiated gene transcription in adult human thyrocytes in primary culture. Mol Cell Endocrinol (2020) 518:111032. doi: 10.1016/j.mce.2020.111032
120. Christophe D, Gérard C, Juvenal G, Bacolla A, Teugels E, Ledent C, et al. Identification of a cAMP-responsive region in thyroglobulin gene promoter. Mol Cell Endocrinol (1989) 64(1):5–18. doi: 10.1016/0303-7207(89)90060-9
121. Berg V, Vassart G, Christophe D. Identification of a thyroid-specific and cAMP-responsive enhancer in the upstream sequences of the human thyroglobulin promoter. Biochim Biophys Acta (1996) 1307(1):35–8. doi: 10.1016/0167-4781(96)00044-9
122. López-Márquez A, Fernández-Méndez C, Recacha P, Santisteban P. Regulation of Foxe1 by Thyrotropin and Transforming Growth Factor Beta Depends on the Interplay Between Thyroid-Specific, CREB and SMAD Transcription Factors. Thyroid (2019) 29(5):714–25. doi: 10.1089/thy.2018.0136
123. Medina DL, Santisteban P. Thyrotropin-dependent proliferation of in vitro rat thyroid cell systems. Eur J Endocrinol (2000) 143(2):161–78. doi: 10.1530/eje.0.1430161
124. Ortiz L, Zannini M, Di Lauro R, Santisteban P. Transcriptional control of the forkhead thyroid transcription factor TTF-2 by thyrotropin, insulin, and insulin-like growth factor I. J Biol Chem (1997) 272(37):23334–9. doi: 10.1074/jbc.272.37.23334
125. Saito T, Endo T, Nakazato M, Kogai T, Onaya T. Thyroid-stimulating hormone-induced down-regulation of thyroid transcription factor 1 in rat thyroid FRTL-5 cells. Endocrinology (1997) 138(2):602–6. doi: 10.1210/endo.138.2.4918
126. Santisteban P, Acebrón A, Polycarpou-Schwarz M, Di Lauro R. Insulin and insulin-like growth factor I regulate a thyroid-specific nuclear protein that binds to the thyroglobulin promoter. Mol Endocrinol (1992) 6(8):1310–7. doi: 10.1210/mend.6.8.1406708
127. Aza-Blanc P, Di Lauro R, Santisteban P. Identification of a cis-regulatory element and a thyroid-specific nuclear factor mediating the hormonal regulation of rat thyroid peroxidase promoter activity. Mol Endocrinol (1993) 7(10):1297–306. doi: 10.1210/mend.7.10.8264661
128. Espinoza CR, Schmitt TL, Loos U. Thyroid transcription factor 1 and Pax8 synergistically activate the promoter of the human thyroglobulin gene. J Mol Endocrinol (2001) 27(1):59–67. doi: 10.1677/jme.0.0270059
129. Marians RC, Ng L, Blair HC, Unger P, Graves PN, Davies TF. Defining thyrotropin-dependent and -independent steps of thyroid hormone synthesis by using thyrotropin receptor-null mice. Proc Natl Acad Sci USA (2002) 99(24):15776–81. doi: 10.1073/pnas.242322099
130. Fernández LP, López-Márquez A, Martínez AM, Gómez-López G, Santisteban P. New insights into FoxE1 functions: identification of direct FoxE1 targets in thyroid cells. PloS One (2013) 8(5):e62849. doi: 10.1371/journal.pone.0062849
131. Kambe F, Nomura Y, Okamoto T, Seo H. Redox regulation of thyroid-transcription factors, Pax-8 and TTF-1, is involved in their increased DNA-binding activities by thyrotropin in rat thyroid FRTL-5 cells. Mol Endocrinol (1996) 10(7):801–12. doi: 10.1210/mend.10.7.8813721
132. Cao X, Kambe F, Ohmori S, Seo H. Oxidoreductive modification of two cysteine residues in paired domain by Ref-1 regulates DNA-binding activity of Pax-8. Biochem Biophys Res Commun (2002) 297(2):288–93. doi: 10.1016/s0006-291x(02)02196-4
133. Tell G, Pellizzari L, Cimarosti D, Pucillo C, Damante G. Ref-1 controls pax-8 DNA-binding activity. Biochem Biophys Res Commun (1998) 252(1):178–83. doi: 10.1006/bbrc.1998.9548
134. Leoni SG, Sastre-Perona A, De la Vieja A, Santisteban P. Selenium Increases Thyroid-Stimulating Hormone-Induced Sodium/Iodide Symporter Expression Through Thioredoxin/Apurinic/Apyrimidinic Endonuclease 1-Dependent Regulation of Paired Box 8 Binding Activity. Antioxid Redox Signal (2009) 24(15):855–66. doi: 10.1089/ars.2014.6228
135. Song Y, Massart C, Chico-Galdo V, Jin L, De Maertelaer V, Decoster C, et al. Species specific thyroid signal transduction: conserved physiology, divergent mechanisms. Mol Cell Endocrinol (2010) 319(1-2):56–62. doi: 10.1016/j.mce.2010.01.024
136. Nellore A, Paziana K, Ma C, Tsygankova OM, Wang Y, Puttaswamy K, et al. Loss of Rap1GAP in papillary thyroid cancer. J Clin Endocrinol Metab (2009) 94(3):1026–32. doi: 10.1210/jc.2008-1042
137. Brewer C, Yeager N, Di Cristofano A. Thyroid-stimulating hormone initiated proliferative signals converge in vivo on the mTOR kinase without activating AKT. Cancer Res (2007) 67(17):8002–6. doi: 10.1158/0008-5472.CAN-07-2471
138. Cass LA, Meinkoth JL. Ras signaling through PI3K confers hormone-independent proliferation that is compatible with differentiation. Oncogene (2000) 19(7):924–32. doi: 10.1038/sj.onc.1203393
139. Pham N, Cheglakov I, Koch CA, de Hoog CL, Moran MF, Rotin D. The guanine nucleotide exchange factor CNrasGEF activates ras in response to cAMP and cGMP. Curr Biol (2000) 10(9):555–8. doi: 10.1016/s0960-9822(00)00473-5
140. Zaballos MA, Garcia B, Santisteban P. Gbetagamma dimers released in response to thyrotropin activate phosphoinositide 3-kinase and regulate gene expression in thyroid cells. Mol Endocrinol (2008) 22(5):1183–99. doi: 10.1210/me.2007-0093
141. Kineman RD, Del Rio-Moreno M, Sarmento-Cabral A. 40 YEARS of IGF1: Understanding the tissue-specific roles of IGF1/IGF1R in regulating metabolism using the Cre/loxP system. J Mol Endocrinol (2018) 61(1):T187–98. doi: 10.1530/JME-18-0076
142. LeRoith D, Yakar S. Mechanisms of disease: metabolic effects of growth hormone and insulin-like growth factor 1. Nat Clin Pract Endocrinol Metab (2007) 3(3):302–10. doi: 10.1038/ncpendmet0427
143. Butler AA, LeRoith D. Minireview: tissue-specific versus generalized gene targeting of the igf1 and igf1r genes and their roles in insulin-like growth factor physiology. Endocrinology (2001) 142(5):1685–8. doi: 10.1210/endo.142.5.8148
144. Rabiee A, Krüger M, Ardenkjær-Larsen J, Kahn CR, Emanuelli B. Distinct signalling properties of insulin receptor substrate (IRS)-1 and IRS-2 in mediating insulin/IGF-1 action. Cell Signal (2018) 47:1–15. doi: 10.1016/j.cellsig.2018.03.003
145. White MF. The IRS-1 signaling system. Curr Opin Genet Dev (1994) 4(1):47–54. doi: 10.1016/0959-437x(94)90090-6
146. Vottero A, Guzzetti C, Loche S. New aspects of the physiology of the GH-IGF-1 axis. Endocr Dev (2013) 24:96–105. doi: 10.1159/000342573
147. Weeks KL, Bernardo BC, Ooi JYY, Patterson NL, McMullen JR. The IGF1-PI3K-Akt Signaling Pathway in Mediating Exercise-Induced Cardiac Hypertrophy and Protection. Adv Exp Med Biol (2017) 1000:187–210. doi: 10.1007/978-981-10-4304-8_12
148. Medina DL, Velasco JA, Santisteban P. Somatostatin is expressed in FRTL-5 thyroid cells and prevents thyrotropin-mediated down-regulation of the cyclin-dependent kinase inhibitor p27kip1. Endocrinology 140(1):87–95. doi: 10.1210/endo.140.1.6426
149. Zaballos MA, Santisteban P. FOXO1 controls thyroid cell proliferation in response to TSH and IGF-I and is involved in thyroid tumorigenesis. Mol Endocrinol (2013) 27(1):50–62. doi: 10.1210/me.2012-1032
150. Zaballos MA, Santisteban P. Key signaling pathways in thyroid cancer. J Endocrinol (2017) 235(2):R43–61. doi: 10.1530/JOE-17-0266
151. Santisteban P, Kohn LD, Di Lauro R. Thyroglobulin gene expression is regulated by insulin and insulin-like growth factor I, as well as thyrotropin, in FRTL-5 thyroid cells. J Biol Chem (1987) 262(9):4048–52. doi: 10.1016/S0021-9258(18)61309-2
152. Zarrilli R, Formisano S, Di Jeso B. Hormonal regulation of thyroid peroxidase in normal and transformed rat thyroid cells. Mol Endocrinol (1990) 4(1):39–45. doi: 10.1210/mend-4-1-39
153. García B, Santisteban P. PI3K is involved in the IGF-I inhibition of TSH-induced sodium/iodide symporter gene expression. Mol Endocrinol (2002) 16(2):342–52. doi: 10.1210/mend.16.2.0774
154. Wrana JL, Attisano L, Wieser R, Ventura F, Massagué J. Mechanism of activation of the TGF-beta receptor. Nature (1994) 370(6488):341–7. doi: 10.1038/370341a0
155. Nakao A, Imamura T, Souchelnytskyi S, Kawabata M, Ishisaki A, Oeda E, et al. TGF-beta receptor-mediated signalling through Smad2, Smad3 and Smad4. EMBO J (1997) 16(17):5353–62. doi: 10.1093/emboj/16.17.5353
156. Massagué J, Seoane J, Wotton D. Smad transcription factors. Genes Dev (2005) 19(23):2783–810. doi: 10.1101/gad.1350705
157. Grubeck-Loebenstein B, Buchan G, Sadeghi R, Kissonerghis M, Londei M, Turner M, et al. Transforming growth factor beta regulates thyroid growth. Role in the pathogenesis of nontoxic goiter. J Clin Invest (1989) 83(3):764–70. doi: 10.1172/JCI113955
158. Pang XP, Park M, Hershman JM. Transforming growth factor-beta blocks protein kinase-A-mediated iodide transport and protein kinase-C-mediated DNA synthesis in FRTL-5 rat thyroid cells. Endocrinology (1992) 131(1):45–50. doi: 10.1210/endo.131.1.1612026
159. Carneiro C, Alvarez CV, Zalvide J, Vidal A, Domínguez F. TGF-beta1 actions on FRTL-5 cells provide a model for the physiological regulation of thyroid growth. Oncogene (1998) 16(11):1455–65. doi: 10.1038/sj.onc.1201662
160. Taton M, Lamy F, Roger PP, Dumont JE. General inhibition by transforming growth factor beta 1 of thyrotropin and cAMP responses in human thyroid cells in primary culture. Mol Cell Endocrinol (1993) 95(1-2):13–21. doi: 10.1016/0303-7207(93)90024-e
161. Toda S, Matsumura S, Fujitani N, Nishimura T, Yonemitsu N, Sugihara H. Transforming growth factor-beta1 induces a mesenchyme-like cell shape without epithelial polarization in thyrocytes and inhibits thyroid folliculogenesis in collagen gel culture. Endocrinology (1997) 138(12):5561–75. doi: 10.1210/endo.138.12.5613
162. Kawaguchi A, Ikeda M, Endo T, Kogai T, Miyazaki A, Onaya T. Transforming growth factor-beta1 suppresses thyrotropin-induced Na+/I- symporter messenger RNA and protein levels in FRTL-5 rat thyroid cells. Thyroid (1997) 7(5):789–94. doi: 10.1089/thy.1997.7.789
163. Nicolussi A, D’Inzeo S, Santulli M, Colletta G, Coppa A. TGF-beta control of rat thyroid follicular cells differentiation. Mol Cell Endocrinol (2003) 207(1-2):1–11. doi: 10.1016/s0303-7207(03)00238-7
164. Costamagna E, García B, Santisteban P. The functional interaction between the paired domain transcription factor Pax8 and Smad3 is involved in transforming growth factor-beta repression of the sodium/iodide symporter gene. J Biol Chem (2004) 279(5):3439–46. doi: 10.1074/jbc.M307138200
165. Kang HC, Ohmori M, Harii N, Endo T, Onaya T. Pax-8 is essential for regulation of the thyroglobulin gene by transforming growth factor-beta1. Endocrinology (2001) 142(1):267–75. doi: 10.1210/endo.142.1.7918
166. Pekary AE, Levin SR, Johnson DG, Berg L, Hershman JM. Tumor necrosis factor-alpha (TNF-alpha) and transforming growth factor-beta 1 (TGF-beta 1) inhibit the expression and activity of Na+/K(+)-ATPase in FRTL-5 rat thyroid cells. J Interferon Cytokine Res (1997) 17(4):185–95. doi: 10.1089/jir.1997.17.185
167. Franzén A, Piek E, Westermark B, ten Dijke P, Heldin NE. Expression of transforming growth factor-beta1, activin A, and their receptors in thyroid follicle cells: negative regulation of thyrocyte growth and function. Endocrinology (1999) 140(9):4300–10. doi: 10.1210/endo.140.9.6961
168. Riesco-Eizaguirre G, Rodríguez I, De la Vieja A, Costamagna E, Carrasco N, Nistal M, et al. The BRAFV600E oncogene induces transforming growth factor beta secretion leading to sodium iodide symporter repression and increased malignancy in thyroid cancer. Cancer Res (2009) 69(21):8317–25. doi: 10.1158/0008-5472.CAN-09-1248
169. Azouzi N, Cailloux J, Cazarin JM, Knauf JA, Cracchiolo J, Al Ghuzlan A, et al. NADPH Oxidase NOX4 Is a Critical Mediator of BRAF. Antioxid Redox Signal (2017) 26(15):864–77. doi: 10.1089/ars.2015.6616
170. Bulanova EA, Koudan EV, Degosserie J, Heymans C, Pereira FD, Parfenov VA, et al. Bioprinting of a functional vascularized mouse thyroid gland construct. Biofabrication (2017) 9(3):034105. doi: 10.1088/1758-5090/aa7fdd
Keywords: transcription factors, signaling pathways, development, differentiation, thyroid
Citation: López-Márquez A, Carrasco-López C, Fernández-Méndez C and Santisteban P (2021) Unraveling the Complex Interplay Between Transcription Factors and Signaling Molecules in Thyroid Differentiation and Function, From Embryos to Adults. Front. Endocrinol. 12:654569. doi: 10.3389/fendo.2021.654569
Received: 16 January 2021; Accepted: 29 March 2021;
Published: 20 April 2021.
Edited by:
Mikael Nilsson, University of Gothenburg, SwedenReviewed by:
Anton M. Jetten, National Institute of Environmental Health Sciences (NIEHS), United StatesRobert Opitz, Charity University Medicine Berlin, Germany
Copyright © 2021 López-Márquez, Carrasco-López, Fernández-Méndez and Santisteban. This is an open-access article distributed under the terms of the Creative Commons Attribution License (CC BY). The use, distribution or reproduction in other forums is permitted, provided the original author(s) and the copyright owner(s) are credited and that the original publication in this journal is cited, in accordance with accepted academic practice. No use, distribution or reproduction is permitted which does not comply with these terms.
*Correspondence: Pilar Santisteban, cHNhbnRpc3RlYmFuQGlpYi51YW0uZXM=